- 1State Key Laboratory for Conservation and Utilization of Subtropical Agro-Bioresources, Guangxi University, Nanning, China
- 2Guangxi Key Laboratory of Forest Ecology and Conservation, College of Forestry, Guangxi University, Nanning, China
- 3Guangxi Key Laboratory of Marine Environmental Science, Guangxi Beibu Gulf Marine Research Center, Guangxi Academy of Sciences, Nanning, China
Phaeocystis globosa has a haplo-diplontic alternative life cycle and is an important causative species of harmful algal blooms. Diploid solitary cells of Phaeocystis can form colonies and bloom in the surface water. However, haploid cells are abundant in deep water rather than surface water. We hypothesize that the haploid cells of Phaeocystis globosa could better adapt to deep dim water than its diploid cells. Haploid and diploid solitary cells of P. globosa were cultured with eutrophic medium (f/2) under moderately low (15 µmol photons m-2 s-1), extremely low (5 µmol photons m-2 s-1) and normal (60 µmol photons m-2 s-1) irradiance conditions. The results showed that irradiances used in this study did not induce reactive oxygen species (ROS) damage in either haploid or diploid cells. Both haploid and diploid solitary cells grew faster at higher irradiance during the initial exponential growth phase. However, the haploid abundances under moderately and extremely low irradiances were higher than that under normal irradiance after the exponential growth phase, but diploid cells formed more colonies at higher irradiances. An increase in the photosynthetic pigments (PSC) ratio combined with a reduction in photoprotective pigments (PPC) ratio were found in both ploidies with decreasing irradiance, but the ratios of PSC and PPC and xanthophyll cycle pigments were significantly higher in haploid cells than in diploid cells. For haploids, the highest potential photochemistry efficiency of photosystems П was found under extremely low irradiance, but for diploids, it was observed under moderately low irradiance. The results suggest that both haploid and diploid solitary cells of P. globosa in eutrophic water can survive under low-light conditions, but haploid cells have an advantage in extremely low irradiance.
Introduction
Phaeocystis is a cosmopolitan genus known for its capacity to form large scale blooms in the coastal water (Baumann et al., 1994; Peperzak, 2000). Phaeocystis has drawn extensive public concern since it appears mainly in the form of visible colonies in surface water during blooms, which have caused serious ecological hazards and economic losses (Schoemann et al., 2005; Blauw et al., 2010; Wang et al., 2022). This genus is also an important producer of dimethylsulfide (DMS), which connects sulfur cycling between the ocean and atmosphere (Rousseau et al., 1990; Arrigo et al., 1999; Hamm and Rousseau, 2003; Smith et al., 2003). To date, harmful algal blooms caused by this genus have only been reported in three species, i.e., Phaeocystis globosa, Phaeocystis pouchetii and Phaeocystis antarctica in which P. globosa is the most widespread species and produces blooms in both temperate and tropical coastal water (Rousseau et al., 1994; Peperzak and Gäbler-Schwarz, 2012).
Light is an important factor regulating the growth of phytoplankton and every phytoplankton species has a specific irradiance range for survival (Falkowski and Raven, 2007). The success of Phaeocystis partly because it was able to grow in a wide range of irradiances (Moisan and Mitchell, 1999; Moisan et al., 2006; Liu et al., 2017; Xu et al., 2017; Wang et al., 2022). Phytoplankton living in the wide range of irradiances has evolved a series of physiological mechanisms to adapt to this light variation. For example, Phaeocystis changed its chloroplast under low-light conditions by increasing the numbers of thylakoids (Moisan et al., 2006). In fact, most phytoplankton, including Phaeocystis, increase their light absorption capabilities under low-light conditions by increasing the content of light-harvesting pigments combined with a reduction in photoprotective pigments (PPC) (Dubinsky and Stambler, 2009; Liu et al., 2011; Pinchasov-Grinblat et al., 2011; Ramakrishnan, 2018). In particular, Phaeocystis has relatively efficient xanthophyll cycling, i.e., interconversion between diadinoxanthin (dd) and diatoxanthin (dt), by which it can undergo a rapid response to variable light quality or light intensity without extra energy costs for new pigment synthesis (Moisan et al., 1998; Meyer et al., 2000). In addition, the accumulation of reactive oxygen species (ROS) by phytoplankton is unavoidable during photosynthesis (Hajiboland, 2014), which may cause irreversible damage to a cell (Fridovich, 1998; Nishiyama et al., 2006; Falkowski and Raven, 2007). In response to light stress, algal cells increase the activities of antioxidant enzymes for the removal of excess ROS (Asada, 1999). Therefore, the specific irradiance range for phytoplankton survival is also dependent on its physiological capabilities.
Light in water decreases with depth by several orders of magnitude because of light attenuation by water molecules and other absorbing materials. Less than one percent of sunlight in the surface of euphotic zone can reach the disphotic zone (Dubinsky and Schofield, 2010; Ramakrishnan, 2018). For most of phytoplankton species, the photosynthesis rate in disphotic zone is less than the rate of respiration. However, some species can survive at this low irradiance conditions (Overmann and Garcia-Pichel, 2006; Raven and Cockell, 2006). In fact, the nutrient levels in deep dim water are often high, and the phytoplankton species of that acclimate to low-light conditions in the sea water often have a deeper vertical distribution and more chances of using nutrients in the low-light layer (Dubinsky and Schofield, 2010). Field investigations have confirmed that Phaeocystis could survive from surface water to disphotic zone (at a depth of 300 m), suggesting their extraordinary ability to cope well with both high- and low-irradiance environments (El-Sayed et al., 1983; Palmisano et al., 1986). Indeed, P. globosa, the most widespread marine Prymnesiophyceae, has been documented to be able to grow in a wide range of irradiances from approximately 20 to 500 µmol photons m-2 s-1. However, the knowledge about the response of P. globosa to low irradiances less than 20 µmol photons m-2 s-1, such as those in deep water, is still limited (Moisan and Mitchell, 1999; Moisan et al., 2006; Liu et al., 2011; Xu et al., 2017; Wang et al., 2022).
In fact, P. globosa has an alternating life cycle of diploid and haploid phases (Vaulot et al., 1994; Dutz and Koski, 2006; Peperzak and Gäbler-Schwarz, 2012). The survival strategies of these two cell types are different (Rousseau et al., 2007), and light requirements of Phaeocystis also depends on cell type (Cota et al., 1994). For example, colonies formation of diploid cells can increase buoyancy by which Phaeocystis blooming in illuminated surface water (Skreslet, 1988; Peperzak et al., 2003). In contrast, haploid cells exhibited negative phototaxis (Cheng et al., 2015) and are abundant in deep water rather than in surface water (Davies et al., 1992; Peperzak, 1993). Although the differences in extreme low-light adaptation between diploid and haploid P. globosa are still unknown, the studies on other phytoplankton species of Prymnesiophyceae demonstrated that haploid cells are able to adapt to unfavorable environments (Green et al., 1996; Houdan et al., 2005; D’Amario et al., 2017; de Vries et al., 2021). Therefore, we hypothesize that the haploid of P. globosa has an advantage in extremely low-light environments under eutrophic conditions over diploid cells. This paper evaluates the low-light adaptation ability of different ploidy levels of P. globosa and will shed light on how the haplo-diplontic life cycle contributes to P. globosa success.
Material and Methods
Strains and Culture Conditions
P. globosa employed in this study was isolated from coastal water of the Beibu Gulf in southern China and supplied by the Culture Collection of Marine Algae, Guangxi Academy of Science. This strain was maintained in tubes with f/2 medium (-Si) under 60 µmol photons m-2 s-1 irradiance with a 12 h:12 h light:dark cycle (Guillard, 1975). The salinity of the medium was 30 psu, and the culture temperature was 20°C. Diploid cells of P. globosa can form colonies, but haploid cells cannot. During the culture of P. globosa, the cells that could not form colonies were identified as haploid cells (Li et al., 2020). Previous studies have reported that some diploid strains sometimes turn into haploid solitary cells during culture instead of forming colonies (Janse et al., 1996; Mars Brisbin and Mitarai, 2019; Li et al., 2020). A pure culture of haploid cells was obtained by isolating 1 haploid cell from a noncolony-forming tube with a micropipette, and spreading cultivation of haploid cells (diameter of ~ 4.22 µm) was conducted in the following experiments. To obtain a pure culture of diploid cells, 1 colony from the colony-forming tube was pipetted out and recultured in fresh medium. The diploid solitary cells (diameter of ~ 6.40 µm) used in the following experiments were obtained by filtering the colonies through a 10 µm mesh via gravity. The abundance and ploidy of solitary cells were examined every day by flow cytometry (Beckman Coulter, Brea, California, USA) following the methods of Vaulot et al. (1994). The abundance of diploid cells in this study means that the abundance of diploid solitary cells and colonial cells was not included. The number of diploid solitary cells in the sample was counted after removal of colonies by gentle filtration via gravity using 10 µm meshes. No interconversion between diploid and haploid solitary cells was found in the following experiments.
Experimental Design
72 days culture experiments were performed to compare the low-light adaptation of diploid and haploid cells of P. globosa. First, diploid and haploid solitary cells were introduced into 20 l glass carboys (GX-Farred, Guangxi, China) with an initial concentration of 0.22 µg C ml-1, which was equal to a haploid cell abundance of 4.73 ×104 cells ml-1 or a diploid cell abundance of 1.99 ×104 cells ml-1. Culture conditions were the same as mentioned above for strain maintenance except for the light intensities, which were set as 5 µmol photons m-2 s-1, 15 µmol photons m-2 s-1 and 60 µmol photons m-2 s-1. Phaeocystis can distribute from surface to disphotic zone in natural water (El-Sayed et al., 1983; Palmisano et al., 1986). 5 µmol photons m-2 s-1 irradiance was set as a representative of the irradiance level at disphotic zone, and this irradiance was also approximately the lowest irradiance for the survival of most phytoplankton (Overmann and Garcia-Pichel, 2006). 15 µmol photons m-2 s-1 is the low irradiance used in most previous studies for the light adaptation of P. globosa and was set here as a representative of the irradiance level at the bottom of euphotic zone (Moisan and Mitchell, 1999; Moisan et al., 2006; Liu et al., 2011; Xu et al., 2017; Wang et al., 2022). Although 60 µmol photons m-2 s-1 is lower than the irradiance level of most surface water but it was known as the saturating irradiance for the growth of P. globosa in the coastal water of the southern China Sea (Xu et al., 2017). Light was provided by cool white fluorescent tubes. To ensure that the samples can be analyzed in time, we did not setup replicates in the long-term culture experiment, but instead conducted a dense sampling for improvement. Sampling was performed at approximately 10:00 am, samples for abundance analysis were taken every day, and samples for nutrients and the maximum quantum yield of photosystem II (Fv/Fm) were taken every two days. Cell abundance was counted as mentioned above. The specific growth rate of diploid and haploid solitary cells were determined at the first five days during the exponential growth phase before the colony formation by means of liner regression. Nutrient concentrations and Fv/Fm value during the culture experiment were measured as described below. Accompanied with this experiment, another triplicate cultivation (2.5 l) of diploid solitary cells using a conical flask was set for the colony count at the end of the experiment. Colonies and the number of colonial cells per colony were enumerated using an inverted microscope (Nikon, TE 300), and colony size was measured with the software. To obtain a reliable estimation, at least 100 colonies were measured per sample.
After the long-term cultivation, another three 20 l cultivations of haploid or diploid cells under the different light treatments same as mentioned above were conducted with three replicates to compare the differences in pigment compositions and antioxidative enzyme activities between haploid and diploid solitary cells of P. globosa. The initial abundance and culture conditions, including light intensities, were the same as those in the long-term culture experiments mentioned above. Samples for pigment analysis were taken at day 0, day 3 and day 20, and those for measurement of antioxidative enzyme activities was taken at 0 h, 4 h, 8 h, 24 h, 48 h, 96 h, 144 h and 192 h. The details for pigment analysis and measurement of antioxidative enzyme activities are described below.
Measurement of Fv/Fm
A water sample (5 ml) was taken every day for Fv/Fm measurement. It should be noted that colonies were gently removed before measuring the Fv/Fm value of diploid solitary cells. The Fv/Fm value was measured using a Phyto-PAM fluorometer (Heinz Walz GmbH, Effeltrich, Germany). The minimum (Fo) and maximum fluorescence (Fm) were recorded after 10 min of dark adaptation. The sample was introduced into the measuring chamber and allowed to stabilize at modulated (non-actinic) light (470 nm). Fo was determined by a weak modulated measuring light, and then a short saturating pulse (wavelength pear: 655nm; intensity: 4000 µmol photons m-2 s-1; duration: 200 ms) was applied to determine Fm. Fv/Fm, the maximum quantum yield of PSII, was calculated as (Fm- Fo)/Fm.
Nutrient Analyses
Samples (25 ml) for nutrient determination were filtered through Whatman GF/F filters. The filtrate was kept at -20°C until analysis. The concentrations of nitrate (NO3-) and phosphate (PO43-) were measured using a Skalar SANplus autoanalyzer, following the methods of Hansen and Koroleff (1999).
Measurement of Antioxidative Enzyme Activities
Algae samples (200 ml) were collected by centrifuging cultivation mixtures at 12,000 × g for 15 min at 4°C and then sonicating for 5 min after adding 1.5 ml of phosphate buffer solution (pH=7.8). After sonication, the liquid was centrifuged, and the supernatant was kept at -80°C until analysis. Superoxide dismutase (SOD) and catalase (CAT) activities were measured according to the method of Nounjan et al. (2012).
HPLC Pigment Analysis
Samples (400 ml) for pigment analysis were filtered through Whatman GF/F filters (0.7 µm), subsequently frozen in liquid nitrogen and then stored at -80°C until analysis. Pigments were analyzed by an Agilent series 1100 HPLC equipped with a G1314A detector and Waters Symmetry C8 column (150 mm×4.6 mm, 3.5 µm particle size, 100 Å pore size) according to the method of Zapata et al. (2000). Pigment peaks were identified based on absorbance spectra and retention times at 440 nm, and retention times were compared with those of authentic standards obtained from DIH Inc. (Horsholm, Denmark), which included chlorophyll a (Chl a), chlorophyll c1 (Chl c1), chlorophyll c2 (Chl c2), chlorophyll c3 (Chl c3), pheophorbide a (Pheide a), pheophythin a (Phe a), Mg-2,4-divinylpheoporphyrin (Mg DVP), peridinin (Peri), fucoxanthin (Fuco), 19-but-fucoxanthin (But-Fuco), 19´-hex-fucoxanthin (Hex-Fuco), prasinoxanthin (Pras), dd, dt and β-carotene (β-Car). Pheide a, Phe a, and Pras were not detected; Mg DVP was not included because of the inability to replicate its separation. PPC were the sum of dd, dt and β-Car; photosynthetic pigments (PSC) were the sum of chlorophyll c, Peri, Fuco and Hex-Fuco. Pigment data were normalized to Chl a.
Statistical Analysis
TWO-way ANOVA and least significant difference (LSD) tests for multiple comparisons were performed to evaluate the differences in antioxidative enzyme activities and pigment compositions among different light treatments. The difference in colonial density between ≤100 µm colonies and >100 µm colonies under the same irradiance treatments was assessed using a t-test. We did not conduct statistical analyses on culture experimental data due to the lack of replication. All of the analyses were performed using the statistical program SPSS 16.0, with a significance level of 5%.
Results
Abundance Variations in Haploid and Diploid Solitary Cells
The statistical significance among different treatments was not tested as lack of replicate. However, the slow growths of both haploid and diploid solitary cells with decreasing light intensity during the exponential growth phase were clearly found. For haploid cells, the specific growth rate in the exponential phase was 0.18 d-1, 0.29 d-1 and 0.32 d-1 at 5, 15 and 60 µmol photons m-2 s-1 treatments, respectively. Haploid abundance at 60 µmol photons m-2 s-1 remained relatively stable after day 9, with a mean value of 35.64×104 cells ml-1 ± 6.41 ×104 cells ml-1 (Figure 1A). The abundance of haploid cells under 5 and 15 µmol photons m-2 s-1 irradiance continued to increase after day 9 and reached a higher level than that of cells cultured under an irradiance of 60 µmol photons m-2 s-1. Although the abundance of haploids between the 5 and 15 µmol photons m-2 s-1 treatments was similar during the stable stage, the cell abundance at 15 µmol photons m-2 s-1 reached the stable stage earlier than that at 5 µmol photons m-2 s-1. For diploid solitary cells, the specific growth rates during the exponential phase were 0.07 d-1, 0.13 d-1 and 0.43 d-1 at 5, 15 and 60 µmol photons m-2 s-1, respectively. Unlike that of haploid cells, the abundance of diploid solitary cells fluctuated after early rapid growth, and no obvious differences were found among the different irradiance treatments after the rapid growth phase (Figure 1B).
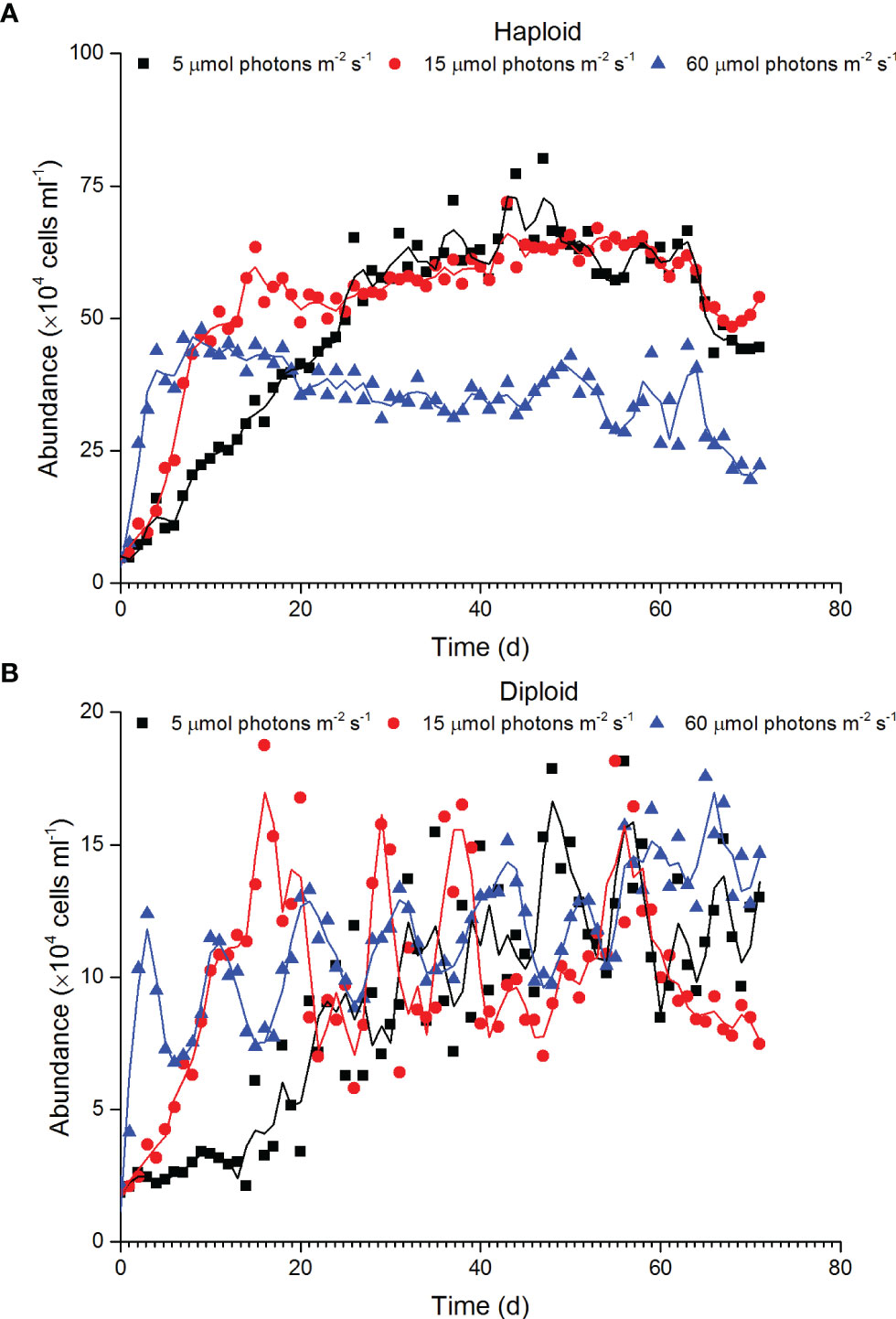
Figure 1 Variation in the abundance of haploid (A) and diploid solitary cells (B) of P. globosa under different low-light intensities.
At the end of diploid culture experiments, no significant differences in the density of small colonies were found (<100 µm) between the 5 and 15 µmol photons m-2 s-1 treatments, but the density of total colonies and > 100 µm colonies significantly increased with increasing irradiance (p<0.05, Figure 2). The colony size was significantly linearly correlated with the number of colonial cells under each irradiance condition. The regression slopes at 5 and 15 µmol photons m-2 s-1 were similar but the regression slope of the sample at 60 µmol photons m-2 s-1 was significantly greater than those at the other two irradiances (p<0.05), indicating denser colonial cells in mature colonies under the 60 µmol photons m-2 s-1 treatment (Figure 3).
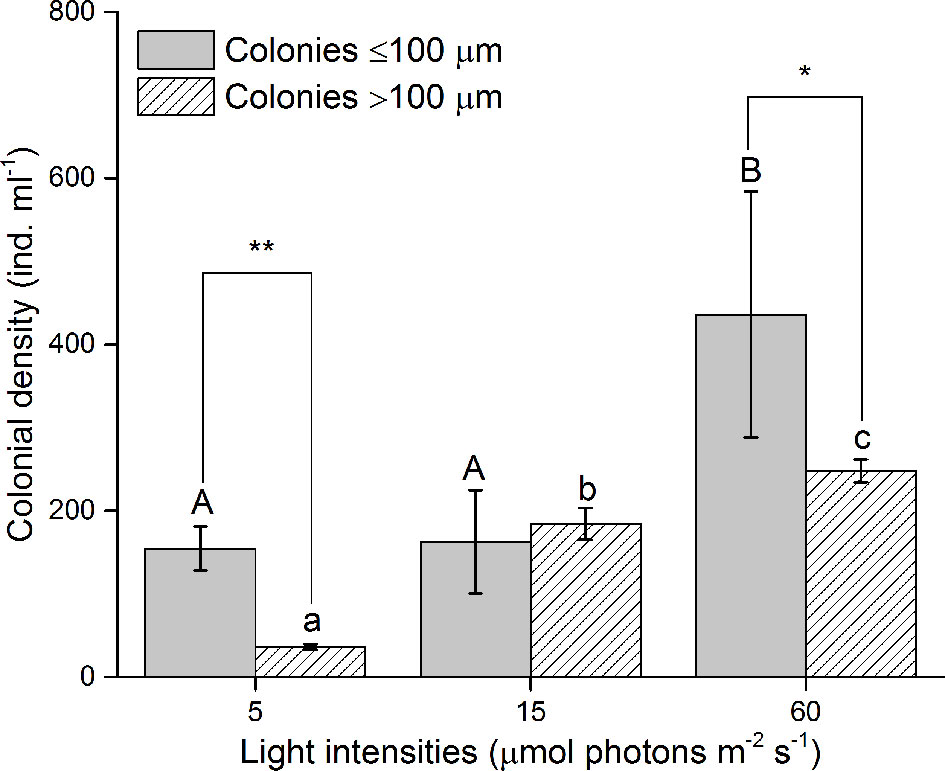
Figure 2 Colonial density under different light intensities at the end of diploid cell culture experiments. * and **, significant difference (p < 0.05 and p < 0.01) between the density of ≤ 100 µm and > 100 µm colonies in the same light intensities. Different uppercase and lowercase letters indicate significant differences among the three light intensities for ≤ 100 µm and > 100 µm colonies, respectively.
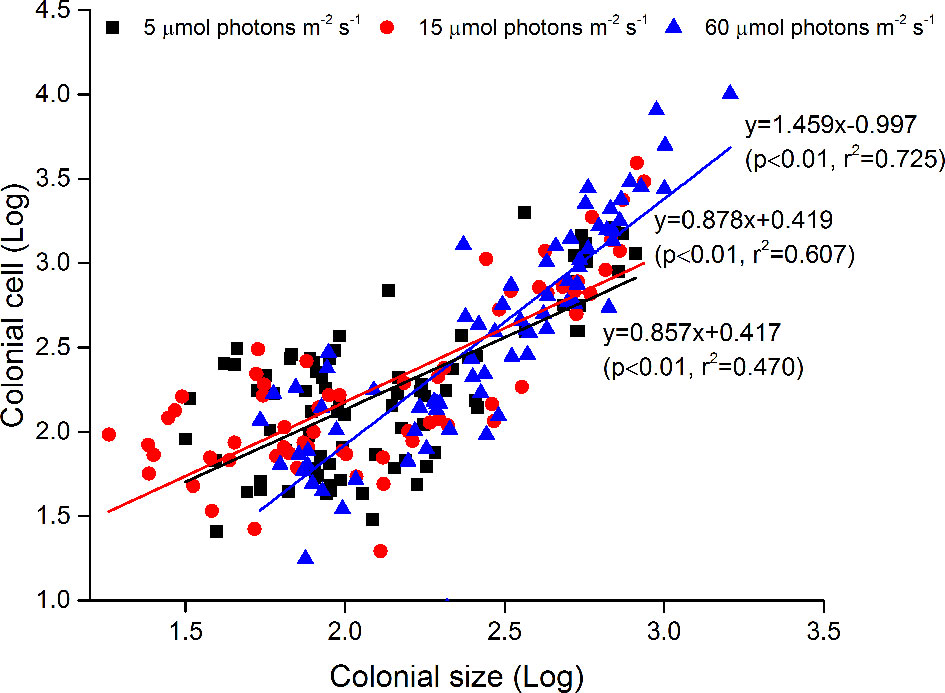
Figure 3 Relationships between colony size (log) and the number of colonial cells per colony (log). Data were obtained at the end of diploid culture experiments.
Nutrient Concentrations
The concentrations of phosphate and nitrate at the start of the experiment were 36.19 ± 0.85 µmol l-1 and 886.00 ± 0.25 µmol l-1, respectively. The concentrations of phosphate and nitrate obviously decreased during the culture experiments, but the nutrient consumption by haploid cells was greater than that by diploid cells. At the end of the experiments, the phosphate concentration in the haploid group decreased to 20.56, 12.14 and 12.86 µmol l-1 under 5, 15 and 60 µmol photons m-2 s-1 irradiance, respectively, and the nitrate concentration in the haploid groups decreased to 676.50, 527.24 and 586.70 µmol l-1 under 5, 15 and 60 µmol photons m-2 s-1 irradiance, respectively. However, the phosphate concentration in the diploid group decreased to 30.91, 25.35 and 20.31 µmol l-1 at the end of the experiment under irradiance treatments of 5, 15 and 60 µmol photons m-2 s-1, respectively, and the nitrate concentration in the diploid group decreased to 817.99, 722.98, and 655.09 µmol l-1 at the end of the experiment under irradiances of 5 s-1, 15 and 60 µmol photons m-2 s-1, respectively (Figure 4).
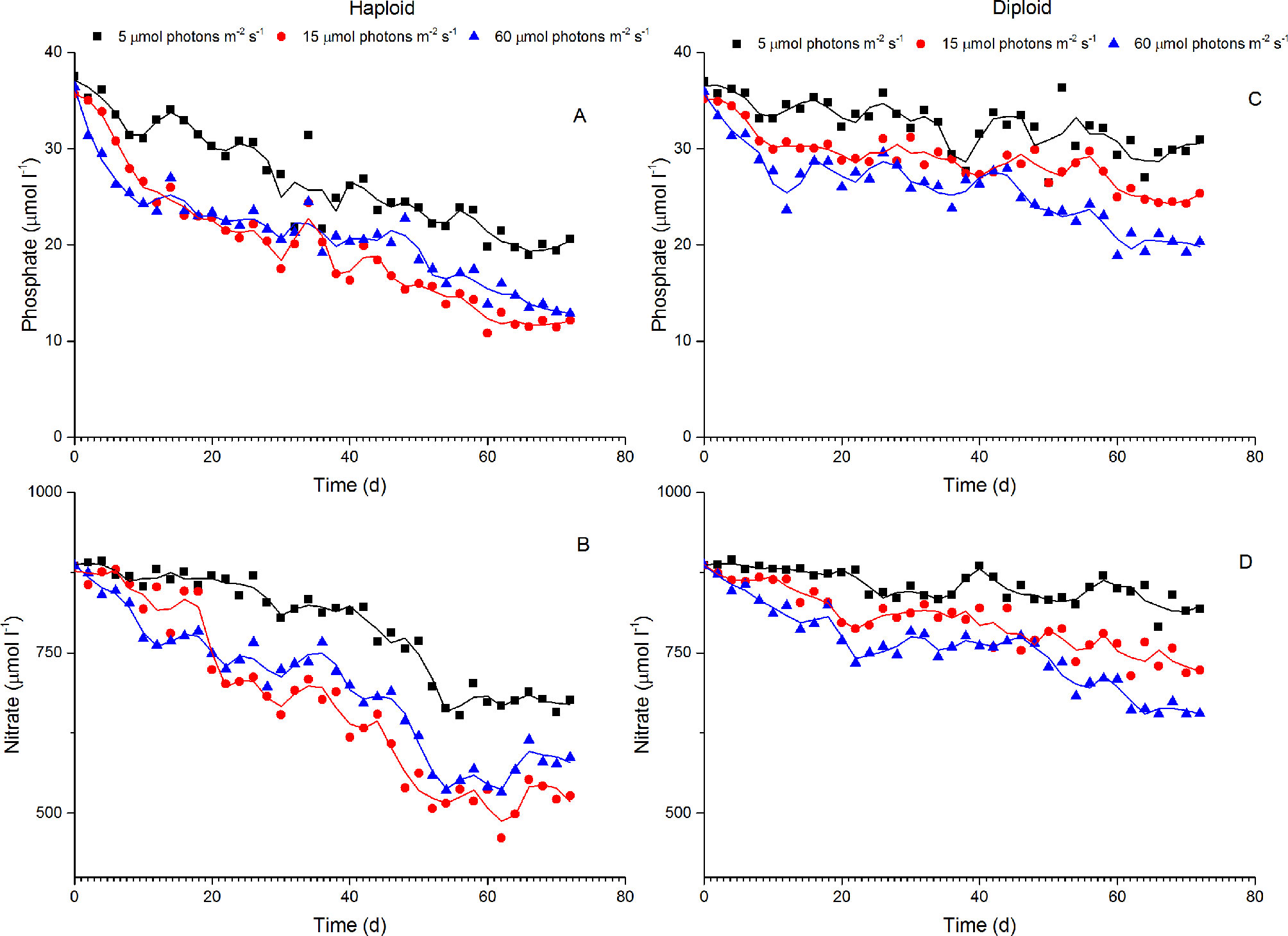
Figure 4 Variations in phosphate and nitrate concentrations in the culture of haploid and diploid cells of P. globosa under different light intensities. (A, B) phosphate and nitrate concentrations in haploid cultures; (C, D) phosphate and nitrate concentrations in diploid cultures.
Fv/Fm Value of Haploid and Diploid Solitary Cells
The Fv/Fm value of haploid cells ranged from 0.37 to 0.66 with a mean value of 0.54 ± 0.08, and that of diploid solitary cells ranged from 0.24 to 0.66 with a mean value of 0.53 ± 0.11. In haploid cells, the Fv/Fm value decreased with increasing irradiances, indicating that haploid cells preferred an irradiance of 5 µmol photons m-2 s-1 over irradiances of 15 and 60 µmol photons m-2 s-1. In contrast, the highest Fv/Fm value in diploid appeared at an irradiance of 15 µmol photons m-2 s-1. The Fv/Fm value of diploid solitary cells in the 5 µmol photons m-2 s-1 treatment was significantly decreased in comparison with those under the irradiances of 15 and 60 µmol photons m-2 s-1, indicating the stress of the deficit of light energy under 5 µmol photons m-2 s-1 for diploid solitary cells (Figure 5).
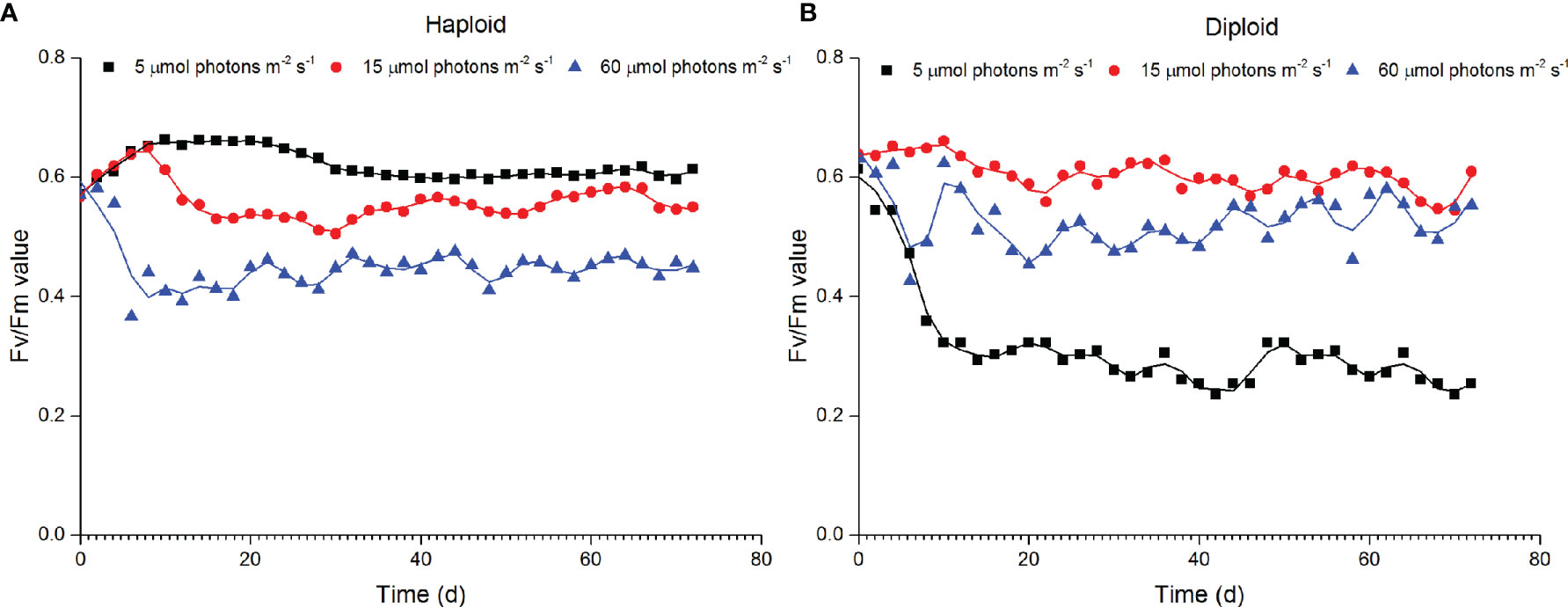
Figure 5 Variations in the maximum PSII quantum yield (Fv/Fm) of haploid (A) and diploid solitary cells (B) of P. globosa under different light intensities.
Antioxidant Enzyme Activities
The ratio of CAT and SOD in haploid cells to that in diploid solitary cells were 2.08 and 2.5 at the start of the experiment (Figure 6). The activities of CAT and SOD of haploid cells in all treatments decreased with the duration of the experiment; however, the activities of CAT and SOD in the treatment of 5 µmol photons m-2 s-1 were significantly higher (p < 0.05) than under the other two irradiances after 48 and 24 h. Unlike haploid cells, the activities of CAT and SOD of diploid solitary cells remained relatively stable except for those in the 60 µmol photons m-2 s-1 treatment after 8 h. The activities of CAT and SOD of diploid solitary cells in the 60 µmol photons m-2 s-1 treatment increased from 8 h to the peak value at 24 h and then gradually decreased to approximately normal values at the end of the experiments (192 h), and the activities of CAT and SOD of diploid solitary cells during these processes were significantly higher in the 60 µmol photons m-2 s-1 treatment than in the 5 and 15 µmol photons m-2 s-1 treatments (Figure 6).
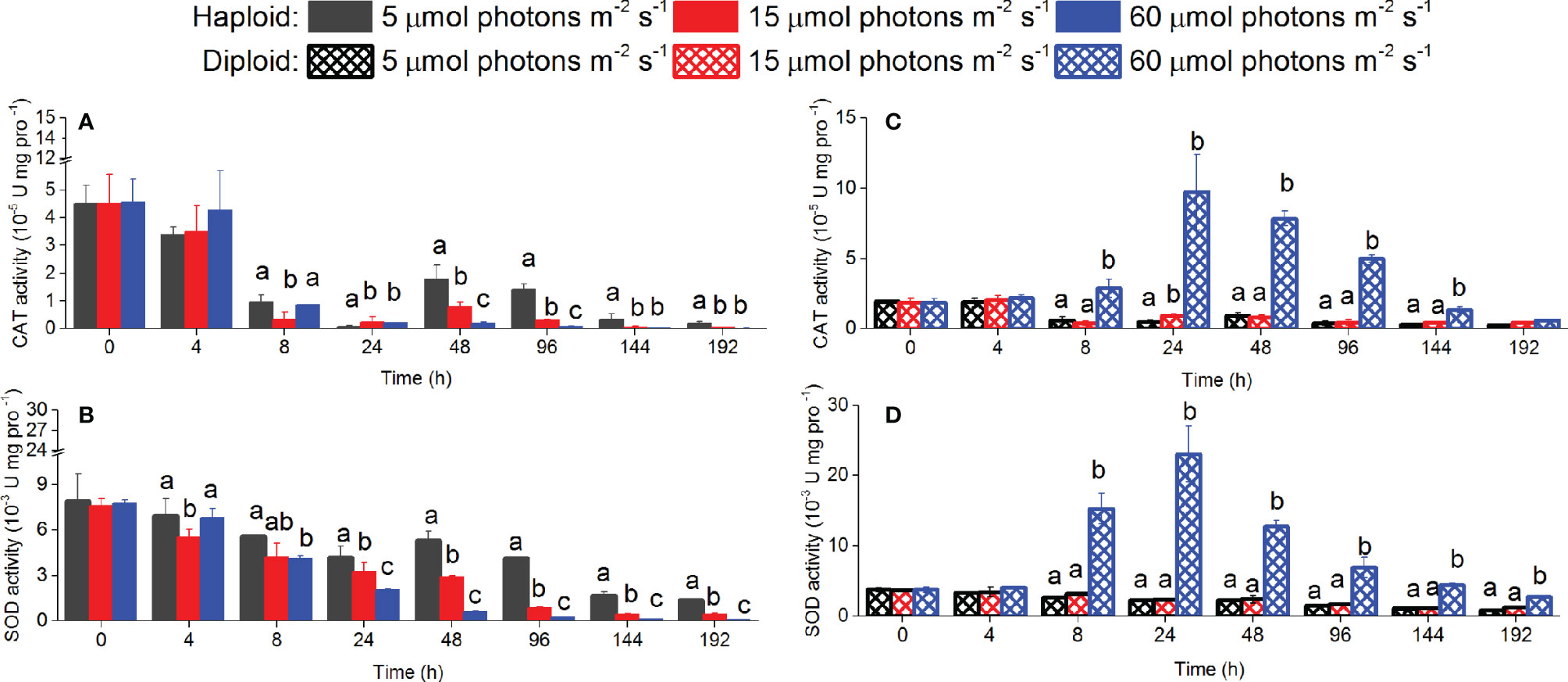
Figure 6 CAT and SOD activities in haploid and diploid solitary cells of P. globosa at 0 h, 4 h, 8 h, 24 h, 48 h, 96 h, 144 h and 192 h under different irradiance treatments. Different lowercase letters indicate significant differences among the three light intensities at the same time. (A, B) for haploid cells; (C, D) for diploid solitary cells.
Pigment Composition
The pigments contents of dd, dt and the sum of dd+dt were significantly higher in haploid cells than diploid solitary cells during the experiments (p<0.05). The xanthophyll cycling pool (dd+dt) ratio of haploid solitary cells to diploid solitary cells was 1.72~2.18. However, no significant differences were found among the different light treatments except for dt in haploid cells at day 20 and dd+dt in diploid solitary cells at day 3 (Figure 7). Both PSC and PPC contents were significantly higher in haploid cells than diploid solitary cells during the experiment (p<0.05). The PSC and PPC content ratio of haploid solitary cells to diploid solitary cells was 2.20~2.46 and 2.54~4.50 during the experiment. Moreover, the PSC content significantly increased with decreasing irradiance in both haploid and diploid solitary cells (p<0.05), and in contrast, the PPC content significantly decreased with decreasing irradiance in both haploid and diploid solitary cells during experiments (p<0.05, Figure 8).
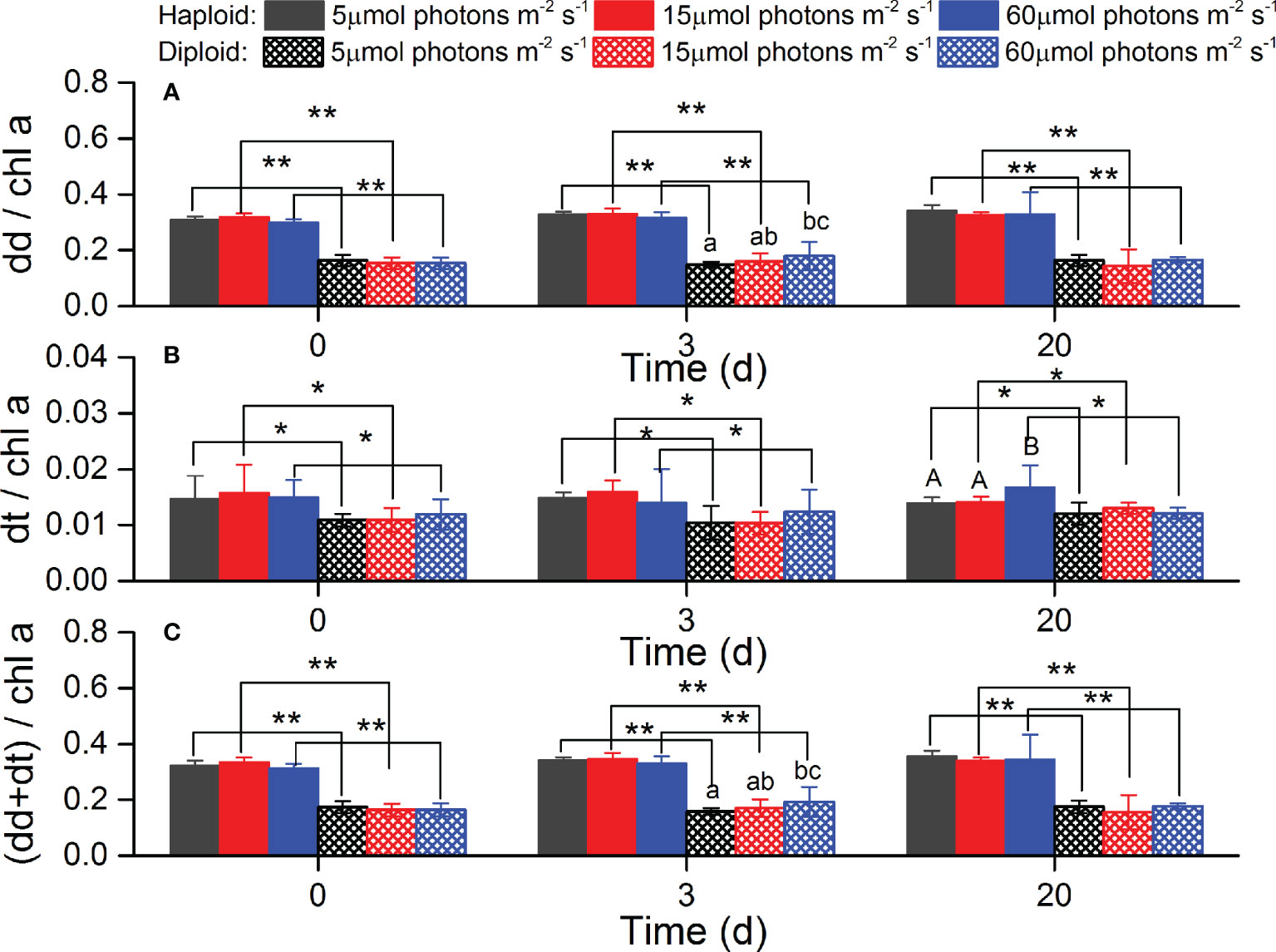
Figure 7 Average contents of diadinoxanthin (dd, (A)), diatoxanthin (dt, (B)) and sum of dd and dt (C) for haploid and diploid solitary cells of P. globosa under different light intensities at day 0, day 3 and day 20. Data are normalized to the Chl a content. * and ** indicate significant differences (p < 0.05 and p < 0.01) between haploid and diploid solitary cells on the same day. Different uppercase and lowercase letters indicate significant differences among the three light intensities for haploid and diploid solitary cells, respectively.
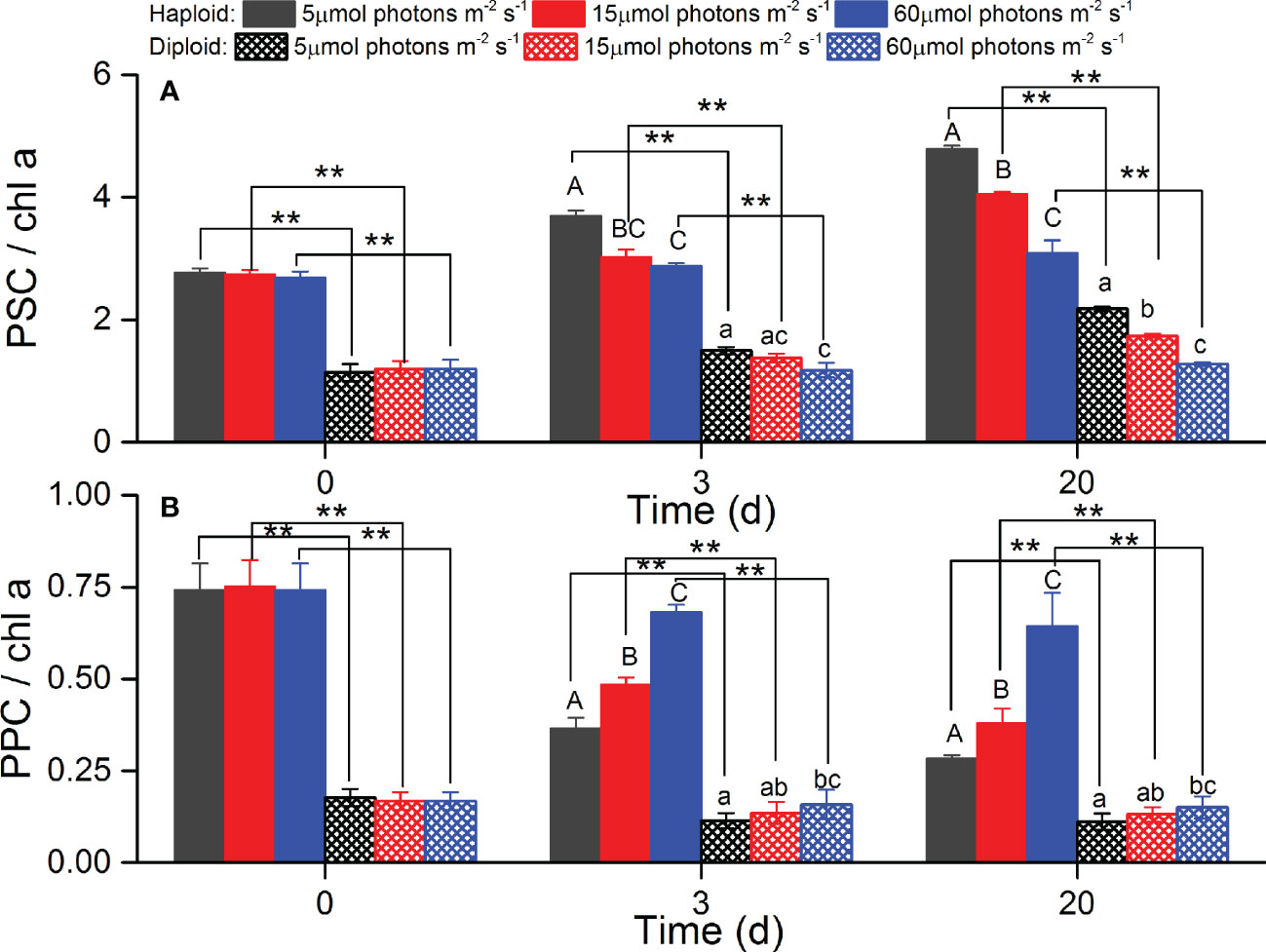
Figure 8 Averages of photosynthetic pigment (PSC, A) and photoprotective pigment (PPC, B) contents for haploid and diploid solitary cells of P. globosa under different light intensities at day 0, day 3 and day 20. Data are normalized to the Chl a content. ** indicates a significant difference (p < 0.01) between haploid and diploid solitary cells on the same day. Different uppercase and lowercase letters indicate significant differences among the three light intensities for haploid and diploid solitary cells, respectively.
Discussion
Growth and Colony Formation of P. Globosa under Low-Light Conditions
Previous studies have reported that irradiances govern the growth and colony formation of Phaeocystis. The saturating irradiance of the P. globosa strain isolated from coastal water of southern China was 60 µmol photons m-2 s-1 (Xu et al., 2017). Generally, the growth of Phaeocystis cultured at light lower than saturating irradiance increases with light intensity (Peperzak, 1993; Liu et al., 2011; Xu et al., 2017; Wang et al., 2022). Consistent with previous studies, the growth of both diploid and haploid solitary cells increased with increasing irradiance ranging from 5 to 60 µmol photons m-2 s-1 during the initial exponential phase (Figure 1). However, the variation in the abundance of both diploid and haploid solitary cells after the exponential phase exhibited different patterns, and the highest abundance did not appear under an irradiance of 60 µmol photons m-2 s-1 (Figure 1).
The abundance of haploid cells after the exponential phase remained higher at 5 and 15 µmol photons m-2 s-1 than at 60 µmol photons m-2 s-1 (Figure 1), indicating that haploid cells prefer relatively low light intensities in long-term cultivation. This is consistent with field investigation that a high density of haploid cell appeared at deep water rather than at the surface water (Davies et al., 1992; Peperzak, 1993). Although some species can survive at irradiances less than 2 µmol photons m-2 s-1 (Raven and Cockell, 2006), the minimum irradiance required by most phytoplankton is approximately 2 µmol photons m-2 s-1 (Overmann and Garcia-Pichel, 2006). The lowest irradiance used in this study was 5 µmol photons m-2 s-1, close to the minimum irradiance need of most phytoplankton. Algae cope with low-light conditions through physiological adjustments, such as increasing the content of PSC to capture light, and this process is sensitive to nutrient supplies, especially the availability of nitrogen, phosphorus and iron (Garcia et al., 2009; Dubourg et al., 2015; Bender et al., 2018).
The lowest concentrations of nitrogen and phosphorus were still higher than 500 µmol l-1 and 12 µmol l-1 at the end of the experiments (Figure 4). It was reported that the growth of P. globosa was not inhibited at nitrogen and phosphorus concentrations higher than 1.7 µmol l-1 and 1 µmol l-1, respectively (Riegman and Boekel, 1996; Xu et al., 2017). Although the iron concentration was not measured in this study, the iron concentration in our experiment was approximately 11.6 nmol l-1 at the start of the experiment according to the f/2 medium. A concentration of 0.2~0.4 nmol l-1 iron was shown to be sufficient to support Phaeocystis growth under low-light conditions (Sedwick et al., 2007; Garcia et al., 2009; Bender et al., 2018). Given that the f/2 medium is eutrophic for algae growth and that algae assimilate micronutrients in a certain ratio with macronutrients (nitrogen and phosphorus), not only iron but also other nutrients were sufficient to support the growth of P. globosa in our experiments.
The self-shading effect should also be considered in the research on the light effect, but haploids were more abundant under low-light conditions (2 and 5 µmol photons m-2 s-1), which indicated that abundance variation did not contribute to self-shading effects. Haploid cells of P. globosa cultured at a relatively high but lower than saturating irradiance grew faster during short-term cultivation, but for long-term cultivation, haploid cells preferred lower light intensities. This is probably a result of long evolutionary adaptation rather than nutrient limitation or self-shading effects. Moreover, the Fv/Fm value, a sensitive indicator of environmental stress (Sharma et al., 2015; Zha et al., 2017; Jamali Jaghdani et al., 2021), also confirmed the stress of 15 and 60 µmol photons m-2 s-1 on haploid cells (Figure 5).
Unlike that of haploid cells, the abundance of diploid solitary cells fluctuated after the exponential phase, and abundance differences among different irradiance treatments were hard to identify (Figure 1). This observation did not indicate a negligible effect of irradiances on diploid solitary cells because the Fv/Fm value indicated that diploid solitary cells were clearly inhibited at 5 and 60 µmol photons m-2 s-1 (Figure 5). In contrast, the fluctuations in abundance of diploid solitary cell may be attributed to new colony formation from diploid solitary cells and the release of diploid solitary cells into water from the splitting of aged colonies (Rousseau et al., 1994; Rousseau et al., 2007). This process certainly caused fluctuations in the abundance of diploid solitary cells in water and masked the irradiance impact on the abundance. However, the abundance of diploid cells showed more regular fluctuation in 15 and 60 µmol photons m-2 s-1 treatments than in 5 µmol photons m-2 s-1 treatment probably because the rapid growth of colonies in higher irradiances, which may increase the frequency of the colony formation and splitting process. In fact, the effects of light intensities on diploid cells were found in more abundant colonies and denser colonial cells in mature colonies under higher irradiance treatments of 15 and 60 µmol photons m-2 s-1 rather than under the extreme low-light conditions of 5 µmol photons m-2 s-1 (Figures 2, Figure 3), which, together with the Fv/Fm value, indicated that diploid solitary cells could also survive under extreme low-light conditions (5 µmol photons m-2 s-1), but photosynthesis and colony formation were inhibited under extremely low irradiances. The higher irradiance preference of diploid cells was also supported by field observation that diploid solitary cells can increase their buoyancy by forming colonies which ascend diploid cells to illuminated surface water and forming bloom (Skreslet, 1988; Peperzak et al., 2003).
In fact, irradiance is considered one of the most important triggers for the colony formation of P. globosa. It has been reported that P. globosa did not form colonies when the light intensity is less than ~38 µmol photons m-2 s-1 (Peperzak, 1993; Wang et al., 2022). Similar results were also reported by other researchers (Peperzak et al., 1998; Blauw et al., 2010), who found that P. globosa colonies occurred only when irradiance exceeded 25-35 µmol photons m-2 s-1. The P. globosa strain from Shantou coastal waters in southeastern China required higher irradiance for colony formation and failed to develop colonies when the irradiance was less than 50 µmol photons m-2 s-1 (reviewed by Wang et al., 2022). However, colony formation was also observed at lower intensities of 10-20 µmol photons m-2 s-1 (Riegman and Boekel, 1996). Diploid solitary cells in this study formed colonies in all irradiance treatments. It is likely that the irradiance threshold for colony formation is highly strain-dependent, and the strain used in this study coped well with relatively low irradiance levels. Nevertheless, the increase in colony density with light level has been widely reported in the studies mentioned above and was also confirmed by this study (Figure 2). Interestingly, no ploidy transformation was found in our study, indicating that low irradiance was not a trigger for ploidy transformation of P. globosa, at least for this strain in a nutrient-sufficient system under a 12 h:12 h light/dark cycle.
Different Physiological Responses Between Haploid and Diploid Solitary Cells Under Low-Light Conditions
Acclimation to light involves an array of cellular responses, one of which is the pigment ratio, which is affected at extreme light levels (Ramakrishnan, 2018). It has been repeatedly demonstrated in laboratory experiments and field observations that the main adjustment to low light by P. globosa and other phytoplankton is an increase in their light absorption capabilities by an increase in the content of light-harvesting pigments (PSC) and a reduction in PPC (Dubinsky and Stambler, 2009; Pinchasov-Grinblat et al., 2011; Ramakrishnan, 2018). Consistent with previous studies, the PSC content of both diploid and haploid solitary cells in the present study increased with declining irradiance, and PPC decreased with decreasing light intensity (Figure 8). However, the PSC content of haploid cells was significantly higher than that of diploid solitary cells (p<0.05), indicating the advantage of haploid cells in coping with low-light conditions over diploid cells. Moreover, the PPC content of haploid cells was also significantly higher than diploid solitary cells, implying a wider tolerance range for irradiances in haploid cells. Consistent with our study, although ploidy was not examined in their study, Xu et al. (2017) suggested a wide irradiance tolerance range of flagellate cells, which were probably haploid cells based on their descriptions.
Xanthophyll cycling through interconversion between dd and dt has been observed in Phaeocystis (Moisan et al., 1998; Meyer et al., 2000). The conversion from dd to dt during the transition from low-light to relatively high-light conditions via a de-epoxidation reaction involves excess light energy dissipation leading to nondestructive thermal de-excitation of pigments (Olaizola et al., 1992; Brunet et al., 1993; Schubert et al., 1994; Meyer et al., 2000). The advantages of this mechanism are that it rapidly occurs in response to abrupt light change, lessens the cost of other pigment synthesis and does not affect the light harvesting efficiency (Brunet et al., 1993; Schubert et al., 1994). Therefore, the pigment pool associated with xanthophyll pigment cycling (dd+dt) has been used as an indirect measure of photoacclimation. Previous studies suggested that xanthophyll cycling in Phaeocystis was important for its bloom (Moisan and Mitchell, 1999; Meyer et al., 2000). However, the differences in the xanthophyll pool between haploid and diploid solitary cells of P. globosa have not been reported. In this study, we demonstrated that the xanthophyll pool of haploid cells was significantly greater than that of diploid solitary cells (p<0.05, Figure 7), implying that haploid cells not only cope well with extremely low irradiance conditions but also have competitive advantages in coping well with higher-light stress and responding to abrupt irradiance changes in comparison with diploid solitary cells. However, the effect of light intensity on conversion between dd and dt in both haploid and diploid solitary cells was not clearly observed in this study (Figure 7) probably because the response of the xanthophyll cycle is rapid and often happens within several hours (Schubert et al., 1994; Moisan et al., 1998; Meyer et al., 2000), but the dd and dt contents in this study were examined only at day 0, day 3 and day 20 (Figure 7).
The degree of ROS damage to phytoplankton could be indirectly measured by measuring antioxidant enzymes or peroxidation products, such as SOD and CAT activity (Dat et al., 2000; Liu et al., 2017). For haploid cells, no significant increase in SOD or CAT activity was found during experiments in all light treatments (Figure 6), indirectly indicating that no ROS-induced damage was found in haploid cells even under the irradiance of 60 µmol photons m-2 s-1 (Figure 6). However, the activities of SOD and CAT were higher at 0 h than at other sampling times under all irradiance treatments (Figure 6), which is likely a normal physiological response when the cells were introduced to new culture conditions because the ROS contents of normal cells temporarily accumulate in response to abrupt environmental changes (Mittler, 2002). Unlike haploid cells, diploid solitary cells were obtained by breaking colonies before introduction into new culture conditions. These colonies were originally cultured at 60 µmol photons m-2 s-1, but the light actually acquired by colonial cells was lower than 60 µmol photons m-2 s-1 because of the shading effects of the colonial membrane. Diploid solitary cells in 60 µmol photons m-2 s-1 treatments suffered higher irradiance stress than others when they were introduced to new culture conditions. Therefore, the SOD and CAT activities for diploid solitary cells significantly increased within 24 h only when they were cultured at 60 µmol photons m-2 s-1 but not in the lower irradiance treatments of 5 and 15 µmol photons m-2 s-1. The SOD and CAT activity of diploid solitary cells at 60 µmol photons m-2 s-1 treatment gradually decreased to normal levels after 24 h (Figure 6), indicating an absence of ROS damage to both haploid and diploid solitary cells.
Ecological Significance of Different Low-Light Responses in Diploid and Haploid Solitary Cells
Both haploid and diploid solitary cells could survive in extreme low-light conditions in this study, but haploid cells exhibited more flexibility in response to light variations, which allowed for better performance of haploid cells in using light and nutrients in different layers of the water column. First, compared to diploid solitary cells, haploid cells preferred extreme low-light conditions in long-term cultivation. Nutrient levels are often high in the dim zone of the water column (Dubinsky and Schofield, 2010). Haploid cells exhibited negative phototaxis in a nutrient limitation condition (Cheng et al., 2015). Therefore, haploid cells may sink to the dim layer and survive well for a long time when nutrients are depleted in surface water. Field investigations indeed reported that a high density of haploid cells appeared at deep water rather than at the surface water (Davies et al., 1992; Peperzak, 1993). Second, haploid cells equipped with flagellates (Rousseau et al., 2007; Peperzak and Gäbler-Schwarz, 2012) may swim to a specific water layer and stay there by flagellate movement, which allows for better utilization of solar energy and nutrients. The more efficient xanthophyll cycle and higher PPC content of haploid cells than of diploid solitary cells are helpful for vertical migration because they have to deal with the stress of frequent light variation and sometimes even photoinhibition.
However, haploid cells are vulnerable to predators because they cannot form colonies, which is a useful strategy for defending against predators (Rousseau et al., 2007). Diploid cells require more solar energy because they need extra carbon for colony production (Schoemann et al., 2005). Therefore, diploid solitary cells may prefer higher irradiance conditions than haploid cells and are probably more suitable in the upper water layer. Overall, the diploid and haploid cells have different light utilization modes by which P. globosa might have a deeper vertical distribution and result in an increasing span of their ecological niches. However, the differences in the vertical distribution of haploid and diploid solitary cells in the water column should be clarified in future studies to make better projections.
Conclusion
Both haploid and diploid solitary cells of P. globosa in eutrophic water can survive under low-light conditions, but haploid cells have an advantage in extremely low irradiances. Haploid helps P. globosa distribute to deeper dim water and result in an increasing span of their ecological niches.
Data Availability Statement
The original contributions presented in the study are included in the article/supplementary material. Further inquiries can be directed to the corresponding author.
Author Contributions
K-FC, JLu and JLZ conceived the ideas, designed the experiments, and wrote the manuscript. JLZ carried out the experiments. All authors contributed the analysis of the data, read and approved the final manuscript.
Funding
This study was supported by the Guangxi Natural Science Foundation (No.2018GXNSFAA138194) and the Science and Technology Major Project of Guangxi (No.AA17202020).
Conflict of Interest
The authors declare that the research was conducted in the absence of any commercial or financial relationships that could be construed as a potential conflict of interest.
Publisher’s Note
All claims expressed in this article are solely those of the authors and do not necessarily represent those of their affiliated organizations, or those of the publisher, the editors and the reviewers. Any product that may be evaluated in this article, or claim that may be made by its manufacturer, is not guaranteed or endorsed by the publisher.
Acknowledgments
We would like to thank thank Zhiming Zhao and Tian Xi for their work on the cultivation of P. globosa.
References
Arrigo K. R., Robinson D. H., Worthen D. L., Dunbar R. B., DiTullio G. R., VanWoert M., et al. (1999). Phytoplankton Community Structure and the Drawdown of Nutrients and CO 2 in the Southern Ocean. Science 283, 365–367. doi: 10.1126/science.283.5400.365
Asada K. (1999). The Water-Water Cycle in Chloroplasts: Scavenging of Active Oxygens and Dissipation of Excess Photons. Annu. Rev. Plant Physiol. Plant Mol. Biol. 50, 601–639. doi: 10.1146/annurev.arplant.50.1.601
Baumann M. E. M., Lancelot C., Brandini F. P., Sakshaug E., John D. M. (1994). The Taxonomic Identity of the Cosmopolitan Prymnesiophyte Phaeocystis: A Morphological and Ecophysiological Approach. J. Marine Syst. 5, 5–22. doi: 10.1016/0924-7963(94)90013-2
Bender S. J., Moran D. M., McIlvin M. R., Zheng H., McCrow J. P., Badger J., et al. (2018). Colony Formation in Phaeocystis Antarctica : Connecting Molecular Mechanisms With Iron Biogeochemistry. Biogeosciences 15, 4923–4942. doi: 10.5194/bg-15-4923-2018
Blauw A. N., Los F. J., Huisman J., Peperzak L. (2010). Nuisance Foam Events and Phaeocystis Globosa Blooms in Dutch Coastal Waters Analyzed With Fuzzy Logic. J. Marine Syst. 83, 115–126. doi: 10.1016/j.jmarsys.2010.05.003
Brunet C., Brylinski J., Lemoine Y. (1993). In Situ Variations of the Xanthophylls Diatoxanthin and Diadinoxanthin: Photoadaptation and Relationships With a Hydrodynamical System in the Eastern English Channel. Mar. Ecol. Pro. Ser. 102, 69–77. doi: 10.3354/meps102069
Cheng J.-X., Li W.-B., Liao X.-S., Lin C.-X. (2015). Negative Phototaxis and Low-Nitrate Induced Flagellum Abscission in Phaeocystis Globosa. J. Hydroecol. 36, 58–62. doi: 10.15928/j.1674-3075.2015.05.009
Cota G. F., Smith W. O., Mitchell B. G. (1994). Photosynthesis of Phaeocystis in the Greenland Sea. Limnol. Oceanogr. 39, 948–953. doi: 10.4319/lo.1994.39.4.0948
D’Amario B., Ziveri P., Grelaud M., Oviedo A., Kralj M. (2017). Coccolithophore Haploid and Diploid Distribution Patterns in the Mediterranean Sea: Can a Haplo-Diploid Life Cycle be Advantageous Under Climate Change? J. Plankton. Res. 39, 781–794. doi: 10.1093/plankt/fbx044
Dat J., Vandenabeele S., Vranova E., Van Montagu M., Van Breusegem F. (2000). Dual Action of the Active Oxygen Species During Plant Stress Responses. Cell Mol. Life Sci. 57, 779–795. doi: 10.1007/s000180050041
Davies A. G., De Madariaga I., Bautista B., Fernandez E., Harbour D. S., Serret P., et al. (1992). The Ecology of a Coastal Phaeocystis Bloom in the North-Western English Channel in 1990. J. Mar. Biol. Assoc. U.K. 72, 691–708. doi: 10.1017/S0025315400059452
De Vries J., Monteiro F., Wheeler G., Poulton A., Godrijan J., Cerino F., et al. (2021). Haplo-Diplontic Life Cycle Expands Coccolithophore Niche. Biogeosciences 18, 1161–1184. doi: 10.5194/bg-18-1161-2021
Dubinsky Z., Schofield O. (2010). From the Light to the Darkness: Thriving at the Light Extremes in the Oceans. Hydrobiologia 639, 153–171. doi: 10.1007/s10750-009-0026-0
Dubinsky Z., Stambler N. (2009). Photoacclimation Processes in Phytoplankton: Mechanisms, Consequences, and Applications. Aquat. Microb. Ecol. 56, 163–176. doi: 10.3354/ame01345
Dubourg P., North R. L., Hunter K., Vandergucht D. M., Abirhire O., Silsbe G. M., et al. (2015). Light and Nutrient Co-Limitation of Phytoplankton Communities in a Large Reservoir: Lake Diefenbaker, Saskatchewan, Canada. J. Great Lakes Res. 41, 129–143. doi: 10.1016/j.jglr.2015.10.001
Dutz J., Koski M. (2006). Trophic Significance of Solitary Cells of the Prymnesiophyte Phaeocystis Globosa Depends on Cell Type. Limnol. Oceanogr. 51, 1230–1238. doi: 10.4319/lo.2006.51.3.1230
El-Sayed S. Z., Biggs D. C., Holm-Hansen O. (1983). Phytoplankton Standing Crop, Primary Productivity, and Near-Surface Nitrogenous Nutrient Fields in the Ross Sea, Antarctica. Deep-Sea Res. Pt I 30, 871–886. doi: 10.1016/0198-0149(83)90005-5
Falkowski P. G., Raven J. A. (2007). Aquatic Photosynthesis: Second Edition (New Jersey, USA: Princeton University Press). doi: 10.1515/9781400849727
Fridovich I. (1998). Oxygen Toxicity: A Radical Explanation. J. Exp. Biol. 201, 1203–1209. doi: 10.1242/jeb.201.8.1203
Garcia N., Sedwick P., DiTullio G. (2009). Influence of Irradiance and Iron on the Growth of Colonial Phaeocystis Antarctica: Implications for Seasonal Bloom Dynamics in the Ross Sea, Antarctica. Aquat. Microb. Ecol. 57, 203–220. doi: 10.3354/ame01334
Green J. C., Course P. A., Tarran G. A. (1996). The Life-Cycle of Emiliania Huxleyi: A Brief Review and a Study of Relative Ploidy Levels Analysed by Flow Cytometry. J. Marine Syst. 9, 33–44. doi: 10.1016/0924-7963(96)00014-0
Guillard R. R. L. (1975). “"Culture of Phytoplankton for Feeding Marine Invertebrates,",” in Culture of Marine Invertebrate Animals. Eds. Smith W. L., Chanley M. H. (New York: Plenum Press), 26–60.
Hajiboland R. (2014). “"Reactive Oxygen Species and Photosynthesis,",” in Oxidative Damage to Plants:antioxidant Networks and Signaling. Ed. Ahmad P. (London,Waltham: Academic Press), 1–63.
Hamm C. E., Rousseau V. (2003). Composition, Assimilation and Degradation of Phaeocystis Globosa-Derived Fatty Acids in the North Sea. J. Sea Res. 50, 271–283. doi: 10.1016/S1385-1101(03)00044-3
Hansen H. P., Koroleff F. (1999). “"Determination of Nutrients,",” in Methods of Seawater Analysis. Eds. Grasshoff K., Kremling K., Ehrhardt M. (Weinheim, Germany: Wiley-VCH), 159–228.
Houdan A., Probert I., Van Lenning K., Lefebvre S. (2005). Comparison of Photosynthetic Responses in Diploid and Haploid Life-Cycle Phases of Emiliania Huxleyi (Prymnesiophyceae). Mar. Ecol. Prog. Ser. 292, 139–146. doi: 10.3354/meps292139
Jamali Jaghdani S., Jahns P., Tränkner M. (2021). Mg Deficiency Induces Photo-Oxidative Stress Primarily by Limiting CO2 Assimilation and Not by Limiting Photosynthetic Light Utilization. Plant Sci. 302, 110751. doi: 10.1016/j.plantsci.2020.110751
Janse I., van Rijssel M., Gottschal J., Lancelot C., Gieskes W. (1996). Carbohydrates in the North Sea During Spring Blooms of Phaeocystis: A Specific Fingerprint. Aquat. Microb. Ecol. 10, 97–103. doi: 10.3354/ame010097
Li J., Lu J., Wang J., Wang Y., Lai J., Yu K. (2020). Do Diploid and Haploid Solitary Cells of Phaeocystis Globosa Support the Same Copepod Grazing, Survival, Egg Production, and Hatching Success? J. Coastal Res. 37, 113–121. doi: 10.2112/JCOASTRES-D-20-00052.1
Liu S., Yu Z., Song X., Cao X. (2017). Effects of Modified Clay on the Physiological and Photosynthetic Activities of Amphidinium Carterae Hulburt. Harmful Algae 70, 64–72. doi: 10.1016/j.hal.2017.10.007
Liu S., Yu Z., Yao P., Zheng Y., Li D. (2011). Effects of Irradiance on Pigment Signatures of Harmful Algae During Growth Process. Acta Oceanol. Sin. 30, 46–57. doi: 10.1007/s13131-011-0160-1
Mars Brisbin M., Mitarai S. (2019). Differential Gene Expression Supports a Resource-Intensive, Defensive Role for Colony Production in the Bloom-Forming Haptophyte, Phaeocystis Globosa. J. Eukaryot. Microbiol. 66, 788–801. doi: 10.1111/jeu.12727
Meyer A. A., Tackx M., Daro N. (2000). Xanthophyll Cycling in Phaeocystis Globosa and Thalassiosira Sp.: A Possible Mechanism for Species Succession. J. Sea Res. 43, 373–384. doi: 10.1016/S1385-1101(00)00031-9
Mittler R. (2002). Oxidative Stress, Antioxidants and Stress Tolerance. Trends Plant Sci. 7, 405–410. doi: 10.1016/S1360-1385(02)02312-9
Moisan T. A., Ellisman M. H., Buitenhuys C. W., Sosinsky G. E. (2006). Differences in Chloroplast Ultrastructure of Phaeocystis Antarctica in Low and High Light. Mar. Biol. 149, 1281–1290. doi: 10.1007/s00227-006-0321-5
Moisan T. A., Mitchell B. G. (1999). Photophysiological Acclimation of Phaeocystis Antarctica Karsten Under Light Limitation. Limnol. Oceanogr. 44, 247–258. doi: 10.4319/lo.1999.44.2.0247
Moisan T., Olaizola M., Mitchell B. (1998). Xanthophyll Cycling in Phaeocystis Antarctica:Changes in Cellular Fluorescence. Mar. Ecol. Prog. Ser. 169, 113–121. doi: 10.3354/meps169113
Nishiyama Y., Allakhverdiev S. I., Murata N. (2006). A New Paradigm for the Action of Reactive Oxygen Species in the Photoinhibition of Photosystem II. BBA-Bioenergetics 1757, 742–749. doi: 10.1016/j.bbabio.2006.05.013
Nounjan N., Nghia P. T., Theerakulpisut P. (2012). Exogenous Proline and Trehalose Promote Recovery of Rice Seedlings From Salt-Stress and Differentially Modulate Antioxidant Enzymes and Expression of Related Genes. J. Plant Physiol. 169, 596–604. doi: 10.1016/j.jplph.2012.01.004
Olaizola M., Bienfang P. K., Ziemann D. A. (1992). Pigment Analysis of Phytoplankton During a Subarctic Spring Bloom: Xanthophyll Cycling. J. Exp. Mar. Bio. Ecol. 158, 59–74. doi: 10.1016/0022-0981(92)90308-W
Overmann J., Garcia-Pichel F. (2006). “"The Phototrophic Way of Life,",” in The Prokaryotes:A Handbook on the Biology of Bacteria. Eds. Dworkin M., Falkow S., Rosenburg E., Schleifer K. H., Stackebrandt E. (Heidelberg,Germany: Springer), 32–85.
Palmisano A. C., SooHoo J. B., SooHoo S. L., Kottmeier S. T., Craft L. L., Sullivan C. W. (1986). Photoadaptation in Phaeocystis Pouchetii Advected Beneath Annual Sea Ice in McMurdo Sound, Antarctica. J. Plankton Res. 8, 891–906. doi: 10.1093/plankt/8.5.891
Peperzak L. (1993). Daily Irradiance Governs Growth Rate and Colony Formation of Phaeocystis (Prymnesiophyceae). J. Plankton Res. 15, 809–821. doi: 10.1093/plankt/15.7.809
Peperzak L. (2000). Observations of Flagellates in Colonies of Phaeocystis Globosa (Prymnesiophyceae); a Hypothesis for Their Position in the Life Cycle. J. Plankton Res. 22, 2181–2203. doi: 10.1093/plankt/22.12.2181
Peperzak L., Colijin F., Koeman R., Gieskes W. W. C., Joordens A. (2003). Phytoplankton Sinking Rates in the Rhine Region of Freshwater Influence. J. Plankton Res. 25, 365–383. doi: 10.1093/plankt/25.4.365
Peperzak L., Colijn F., Gieskes W. W. C., Peeters J. C. H. (1998). Development of the Diatom- Phaeocystis Spring Bloom in the Dutch Coastal Zone of the North Sea: The Silicon Depletion Versus the Daily Irradiance Threshold Hypothesis. J. Plankton Res. 20, 517–537. doi: 10.1093/plankt/20.3.517
Peperzak L., Gäbler-Schwarz S. (2012). Current Knowledge of the Life Cycles of Phaeocystis Globosa and Phaeocystis Antarctica (Prymnesiophyceae). J. Phycol. 48, 514–517. doi: 10.1111/j.1529-8817.2012.01136.x
Pinchasov-Grinblat Y., Hoffman R., Dubinsky Z. (2011). The Effect of Photoacclimation on Photosynthetic Energy Storage Efficiency, Determined by Photoacoustics. Open J. Marine Sci. 1, 43–49. doi: 10.4236/ojms.2011.12005
Ramakrishnan R. (2018). Low Light Phytoplankton Genera Observed in the Coastal and Estuarine Waters of Goa, India. Appl. Ecol. Env. Res. 16, 1783–1796. doi: 10.15666/aeer/1602_17831796
Raven J. A., Cockell C. S. (2006). Influence on Photosynthesis of Starlight, Moonlight, Planetlight, and Light Pollution (Reflections on Photosynthetically Active Radiation in the Universe). Astrobiology 6, 668–675. doi: 10.1089/ast.2006.6.668
Riegman R., Boekel W. V. (1996). The Ecophysiology of Phaeocystis Globosa: A Review. J. Sea Res. 35, 235–242. doi: 10.1016/S1385-1101(96)90750-9
Rousseau V., Chrétiennot-Dinet M.-J., Jacobsen A., Verity P., Whipple S. (2007). The Life Cycle of Phaeocystis: State of Knowledge and Presumptive Role in Ecology. Biogeochemistry 83, 29–47. doi: 10.1007/s10533-007-9085-3
Rousseau V., Mathot S., Lancelot C. (1990). Calculating Carbon Biomass of Phaeocystis Sp. From Microscopic Observations. Mar. Biol. 107, 305–314. doi: 10.1007/BF01319830
Rousseau V., Vaulot D., Casotti R., Cariou V., Lenz J., Gunkel J., et al. (1994). The Life Cycle of Phaeocystis (Prymnesiophyceae): Evidence and Hypotheses. J. Marine Syst. 5, 23–39. doi: 10.1016/0924-7963(94)90014-0
Schoemann V., Becquevort S., Stefels J., Rousseau V., Lancelot C. (2005). Phaeocystis Blooms in the Global Ocean and Their Controlling Mechanisms: A Review. J. Sea Res. 53, 43–66. doi: 10.1016/j.seares.2004.01.008
Schubert H., Kroon B. M., Matthijs H. C. (1994). In Vivo Manipulation of the Xanthophyll Cycle and the Role of Zeaxanthin in the Protection Against Photodamage in the Green Alga Chlorella Pyrenoidosa. J. Biol. Chem. 269, 7267–7272. doi: 10.1016/S0021-9258(17)37278-2
Sedwick P., Garcia N., Riseman S., Marsay C., DiTullio G. (2007). Evidencefor High Iron Requirements of Colonial Phaeocystis Antarctica at Low Irradiance. Biogeochemistry 83, 83–97. doi: 10.1007/s10533-007-9081-7
Sharma D. K., Andersen S. B., Ottosen C.-O., Rosenqvist E. (2015). Wheat Cultivars Selected for High Fv /Fm Under Heat Stress Maintain High Photosynthesis, Total Chlorophyll, Stomatal Conductance, Transpiration and Dry Matter. Physiol. Plantarum 153, 284–298. doi: 10.1111/ppl.12245
Skreslet S. (1988). Buoyancy in Phaeocystis Pouchetii (Hariot) Lagerheim. J. Exp. Mar. Ecol. 119, 157–166. doi: 10.1016/0022-0981(88)90230-4
Smith W. O., Dennett M. R., Mathot S., Caron D. A. (2003). The Temporal Dynamics of the Flagellated and Colonial Stages of Phaeocystis Antarctica in the Ross Sea. Deep-Sea Res. Pt II 50, 605–617. doi: 10.1016/S0967-0645(02)00586-6
Vaulot D., Birrien J.-L., Marie D., Casotti R., Veldhuis M. J., Kraay G. W., et al. (1994). Morphology, Ploidy, Pigment Composition, and Genome Size of Cultured Strains of Phaeocystis (Prymnesiophyceae). J. Phycol. 30, 1022–1035. doi: 10.1111/j.0022-3646.1994.01022.x
Wang J.-X., Kong F.-Z., Geng H.-X., Zhao Y., Guan W.-B., He C., et al. (2022). Pigment Characterization of the Giant Colony-Forming Haptophyte Phaeocystis Globosa in the Beibu Gulf Reveals Blooms of Different Origins. Appl. Environ. Microb 88, e01654-21. doi: 10.1128/AEM.01654-21. AEM.01654-21.
Xu N., Huang B., Hu Z., Tang Y., Duan S., Zhang C. (2017). Effects of Temperature, Salinity, and Irradiance on the Growth of Harmful Algal Bloom Species Phaeocystis Globosa Scherffel (Prymnesiophyceae) Isolated From the South China Sea. Chin. J. Ocean. Limnol. 35, 557–565. doi: 10.1007/s00343-017-5352-x
Zapata M., Rodríguez F., Garrido J. (2000). Separation of Chlorophylls and Carotenoids From Marine Phytoplankton:a New HPLC Method Using a Reversed Phase C8 Column and Pyridine-Containing Mobile Phases. Mar. Ecol. Prog. Ser. 195, 29–45. doi: 10.3354/meps195029
Zha T.-S., Wu Y. J., Jia X., Zhang M. Y., Bai Y. J., Liu P., et al. (2017). Diurnal Response of Effective Quantum Yield of PSII Photochemistry to Irradiance as an Indicator of Photosynthetic Acclimation to Stressed Environments Revealed in a Xerophytic Species. Ecol. Indic. 74, 191–197. doi: 10.1016/j.ecolind.2016.11.027
Keywords: phaeocystis globose, low irradiances, haploid cells, diploid cells, growth, physiological response
Citation: Zhuang J, Lu J, Cao K-F and Li J (2022) Haploid Helps Phaeocystis Globosa Distribute to Deeper Dim Water, as Evidenced by Growth and Photosynthetic Physiology. Front. Mar. Sci. 9:902330. doi: 10.3389/fmars.2022.902330
Received: 23 March 2022; Accepted: 16 May 2022;
Published: 06 June 2022.
Edited by:
Fernando P. Lima, Centro de Investigacao em Biodiversidade e Recursos Geneticos (CIBIO-InBIO), PortugalCopyright © 2022 Zhuang, Lu, Cao and Li. This is an open-access article distributed under the terms of the Creative Commons Attribution License (CC BY). The use, distribution or reproduction in other forums is permitted, provided the original author(s) and the copyright owner(s) are credited and that the original publication in this journal is cited, in accordance with accepted academic practice. No use, distribution or reproduction is permitted which does not comply with these terms.
*Correspondence: Jiachang Lu, amlhY2hhbmdsdWd4QDE2My5jb20=; Kun-Fang Cao, a3VuZmFuZ2Nhb0BneHUuZWR1LmNu