- Simon F.S. Li Marine Science Laboratory, School of Life Sciences, The Chinese University of Hong Kong, Shatin, Hong Kong SAR, China
Tidal flats are widely distributed and provide a variety of ecosystem services. Nevertheless, the consequences of tidal flat loss and implications for services such as carbon (C) sequestration have not been assessed. In unvegetated tidal flat ecosystems, sediment is the most important carbon reservoir, similar to that of vegetated coastal wetlands (i.e., mangroves, salt marshes, and seagrass). We examined the C stocks and C accumulation rate (CAR) reported from 123 locations of tidal flat around the world and compared these results with data from mangroves, salt marshes, and seagrass meadows. The global average CAR of tidal flats is 129.8 g C m-2 yr-1, with the top-meter sediments containing on average 86.3 Mg C ha-1. Globally, tidal flat can bury 6.8 Tg C (24.9 Tg CO2) per year and can store 0.9 Pg C (3.3 Pg CO2) in the top meter sediment. Assuming the same rate of loss tidal flats as in the past three decades and that all disturbed sediment C is remineralized, 4.8 Tg C will be lost from tidal flat sediments every year, equivalent to an emission of 17.6 Tg CO2 to the water column and atmosphere.
Introduction
The emission of greenhouse gases (GHG), especially carbon dioxide (CO2), from human activities, is the main driver of climate change. Reducing GHG emissions as well as capturing and storing carbon (C) could mitigate the progression of climate change (Yang et al., 2020) and ensure warming does not exceed 2°C above pre-industrial levels. Nature-based climate solutions, involving both terrestrial and marine ecosystems, have great potential in C sequestration (Griscom et al., 2017; Macreadie et al., 2021). Coastal wetlands (e.g., mangroves, salt marshes and seagrasses), also known as “blue carbon” ecosystems can store large amounts of autochthonous C and trap allochthonous C produced by other ecosystems (Macreadie et al., 2021). With high C sequestration capacity, coastal wetlands represent long-term atmospheric C sinks (Zhao et al., 2020) and sediment C can be preserved for long periods because of their anoxic sediment environment (Mcleod et al., 2011; Donato et al., 2011; Fourqurean et al., 2012; Duarte et al., 2013). C sequestration by coastal wetlands (e.g., mangrove forests) is also more effective than by terrestrial forests (Mcleod et al., 2011; Alongi, 2012). For example, although mangroves only occupy 0.1% of the continental surface, they support a global C stock of 1.9 to 2.2 Pg C in the top-meter sediment (Ouyang and Lee, 2020) and 49-98% of the ecosystem C pool is preserved in sediments from 0.5 to 3 m (Donato et al., 2011). Moreover, blue carbon ecosystems significantly contribute to global oceanic C accumulation. Despite covering only < 0.5% of the ocean floor, vegetated coastal wetlands bury nearly 50% of the oceanic C every year (Duarte et al., 2005; Fourqurean et al., 2012; Duarte et al., 2013).
Unvegetated tidal flats are generally considered as adjacent habitats to vegetated coastal wetlands. They are areas of intertidal sand or mud accumulation on gently sloping coastlines with heavy sediment inflows, at the interface between land and ocean (Hua et al., 2017). Most tidal flat sediments originate from both riverine and marine sources (Roberts et al., 2015). They provide critical ecosystem services to coastal communities, such as food production, and storm and shoreline protection (Barbier et al., 2011; Lovelock and Reef, 2020). The producer assemblage of tidal flats is largely restricted to micro-autotrophs (e.g., microalgae) with high turnover and mineralization due to their high palatability, resulting in less labile allochthonous C (e.g., from adjacent mangroves and salt marshes) being the dominant source of sediment C (Mueller et al., 2017). Daily net primary productivity on tidal flats is only 10 to 20% of that of vegetated coastal wetlands (Lin et al., 2020). However, tidal flats also have high C sequestration capacity similar to that of vegetated coastal ecosystems, especially in estuaries where the hydrodynamic environment promotes C burial and riverine sediment supply provides large quantities of organic matter (Choi and Wang, 2004; Roberts et al., 2015; Chen et al., 2020).
On global coastlines from 60°CN to 60°CS, at least 127,921 km2 of tidal flats exist, with nearly 70% located in Asia, North America, and South America (Murray et al., 2019). Compared to other coastal ecosystems, tidal flats have an areal extent far larger than that of salt marshes (41,657 km2) (Ouyang and Lee, 2014), similar with mangroves (81,485 to 137,600 km2) (Hamilton and Casey, 2016; Bunting et al., 2018) but less than that of seagrasses (160,387 km2) (McKenzie et al., 2020). As tidal flats occur across wide latitudinal ranges, including locations where vegetated wetlands are absent, making sediment C a significant component of the coastal C pool. For example, as the most widely distributed coastal ecosystems in China, tidal flats store nearly 78 Tg C within the sediments, which is about four times higher than that of mangroves and salt marshes in the country (Chen et al., 2020). Nevertheless, global data on C sequestration in tidal flats are scarce compared to those on vegetated coastal wetlands (Phang et al., 2015; Lin et al., 2020). Few records are available on tidal flat sediment C stock and carbon accumulation rate (CAR), hampering an assessment of their contribution to global coastal sediment C sequestration relative to the vegetated habitats.
With the continuing development of coastal areas (Arkema et al., 2013), sea level rise and coastal erosion (Passeri et al., 2015), the extent of global tidal flats has reduced by 16.02% (>20,000 km2) since 1984 (Murray et al., 2019), and widespread degradation has reduced their capacity for services, e.g., to protect and stabilize shorelines (Blum and Roberts, 2009; Murray et al., 2019; Chen et al., 2020). In the fast-developing East Asia region, the loss of tidal flats is particularly significant in both qualitative and quantitative terms (Lee and Khim, 2017). Concurrently, the degradation of global vegetated coastal ecosystems has weakened sediment C storage and driven significant net GHG emissions (Duarte et al., 2013; Lovelock and Reef, 2020). Despite these threats, the role of tidal flats in global sediment C sequestration has rarely been investigated, and the change in C emission caused by habitat loss and vegetated degradation is largely unknown. Therefore, an assessment of sediment C sequestration by tidal flat ecosystems is important not only for assessing their capacity for climate change mitigation but also for estimating the potential C emission following their future loss. This information is essential to establishing the baseline for future management of coastal CO2 emissions.
Here we compiled global data on sediment C sequestration from peer-reviewed studies of tidal flats. We evaluated the C stock and CAR of tidal flats and compared these with those reported from mangroves, salt marshes, and seagrass meadows. We also estimated C emissions from future loss of tidal flats and their contribution to coastal C sequestration.
Methods
Data Collection
To collect the data on C sequestration of tidal flats, peer-reviewed research papers were searched on the Web of Science using the following search clauses [(“unvegetated flat” OR “unvegetated flats” OR “tidal wetland” OR “tidal wetlands” OR “sand flat” OR “sand flats” OR “mud flat” OR “mud flats” OR “sandflat” OR “sandflats” OR “mudflat” OR “mudflats” OR “tidal flat” OR “tidal flats” OR “intertidal flat” OR “intertidal flats”) AND (“carbon sequestration” OR “carbon storage” OR “carbon stock” OR “carbon accumulation rate” OR “blue carbon” OR “carbon burial”)]. The last search date was made on 23rd December 2021. A total of 204 potentially relevant papers were identified during the search. These papers were then examined for information about C stocks and CAR data on tidal flats in their titles, abstracts, and main texts. Only 44 papers were selected after further review. Figure S1 shows the PRISMA flow diagram for selection of papers. The global C stock and CAR data on mangroves, salt marshes, and seagrass meadows were also collected from peer-reviewed journal articles, reviews and books for comparison.
Raw data, such as information about location (site, country, latitude and longitude), length of sediment cores, sediment type (muddy or sandy); bulk density, sediment C content, C stock and CAR were extracted from each selected paper and recorded in the dataset. Before comparison, all data of coastal sediment C stocks and CAR were converted to Mg C ha-1 and g C m-2 yr-1, respectively. In this study, the C stock in the top-meter of tidal flat sediments was used for comparison, as this part of the sediment C is more susceptible to anthropogenic disturbance and is also the common depth range used for assessing coastal sediment C stocks. However, the sediment cores collected for the individual studies ranged in length from 2 to 300 cm. Accordingly, to standardize sediment C stock for comparison, raw data from samples that were not 100 cm long were standardized as follows:
Where CS1m is the predicted top-meter sediment C stock (Mg C ha-1), CS is the actual sediment C stock (Mg C ha-1), and L represents the core length in cm.
Some studies only recorded the mean sediment bulk density (BD) (g cm-3 or kg m-3), C content (SC) (%) and C density (CD) (g C cm-3 or kg C m-3). Therefore, the mean C stock (Mg C ha-1) value at the top meter was calculated as
Or
The sediment types of tidal flats were classified as sand, mixed, or mud. For sediment types that are not specified in the papers, they were classified according to their grain size. Sandy, mixed and muddy sediments were defined where silt and clay content was less than 25%, between 25% to 75%, and greater than 75%, respectively (Flemming, 2000). If there is no information about the sediment in the publication, sediment type was assigned as “unknown”.
Data Analysis
All data analyses were conducted using R (Version 4.1.0). One-way ANOVA was used to test the difference of C stocks and CAR among different sediment types and latitudinal positions of tidal flats. We first tested the assumptions on normality and homogeneity of variance by using the Shapiro-Wilk and Levene’s tests, respectively. Raw data were log-transformed if they did not meet the assumption of normality. The nonparametric Kruskal-Wallis test was applied if the transformed data still failed the tests above. If a significant difference was detected (p<0.05), the Mann-Whitney U test was applied to compare differences between groups. Linear regression was applied to understand the relationship between latitudinal range and C sequestration of tidal flats.
Results
The Global Distribution of Carbon Sequestration of Tidal Flats
We extracted data on 123 sites from 44 peer-reviewed studies, covering 76° of latitude between 25°S and 51°N. A total of 104 and 49 sites provided C stock and CAR data, respectively. Although tidal flats can be observed in most coastline, relative studies are still insufficient compared to mangroves, salt marshes and seagrasses. Our analysis suggested that C stock in the top meter reported by these studies showed significant variations among locations and sediment types (Figure 1A). Approximately 87% of C stocks were < 150 Mg C ha-1 at the top meter of the sediment (Figure 1B), while 67% of the CAR were < 100 g C m-2 yr-1 (Figure 1C). Studies on C sequestration of tidal flats were concentrated in Asia (~75%). Although nearly 46% and 20% of the C sequestration data on tidal flats originated from China and South Korea, respectively (Figure 1A), they only occupied 11% of the global area extent.
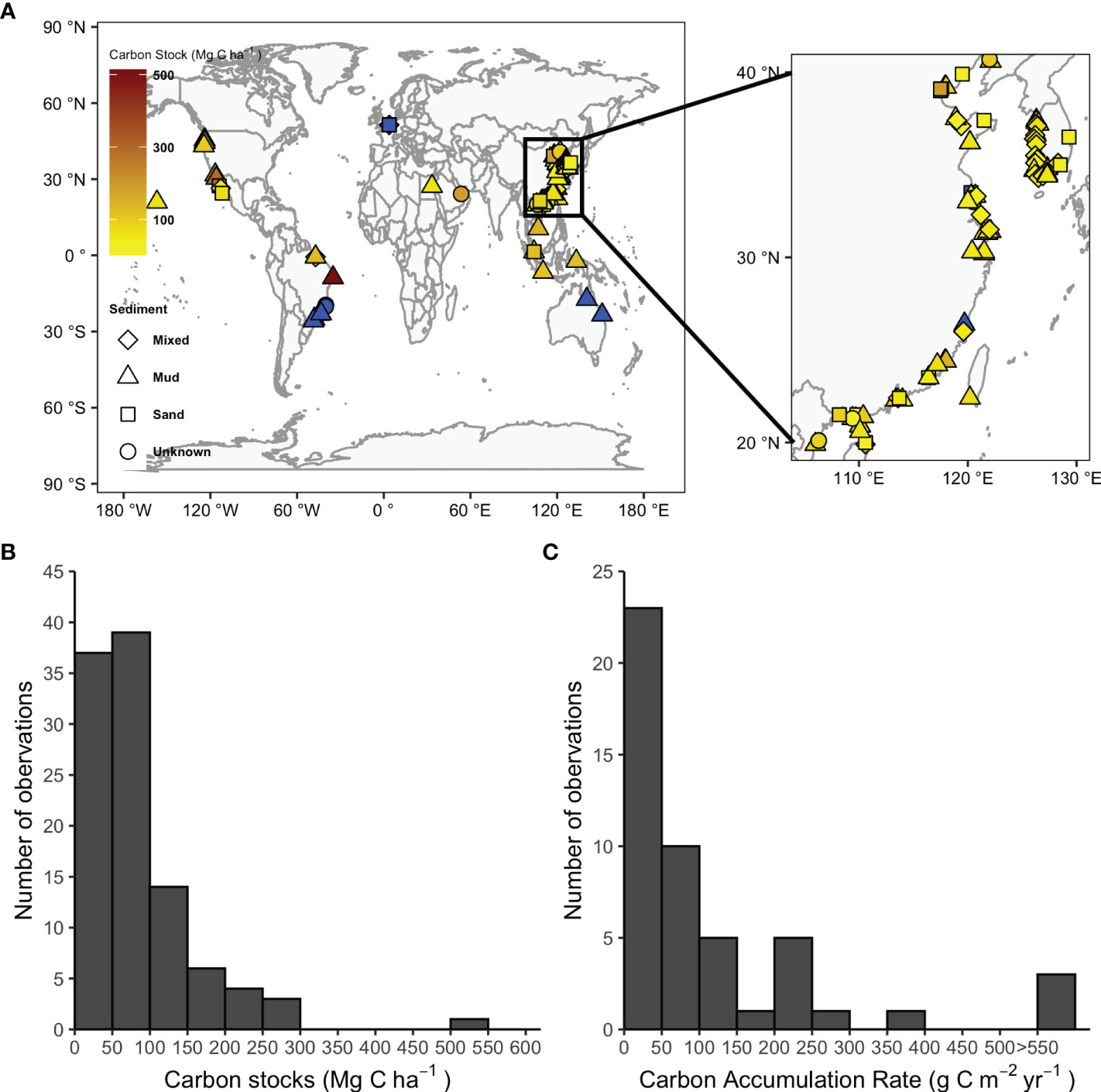
Figure 1 Locations of studies on tidal flats sediment C sequestration and frequency histogram of the values of sediment carbon stock and carbon accumulation rate in tidal flats. (A) Studies without C stock information are indicated in blue. (B) Sediment carbon stock from 104 sites; (C) Carbon accumulation rate from 49 sites.
Carbon Stocks and the Carbon Accumulation Rate of Tidal Flats
With a mean ( ± SE) value of 86.3 ± 7.6 Mg C ha-1 (Table 1), the global sediment C stock of tidal flats ranges from 1.4 to 514.2 Mg C ha-1 (Table S1). The mean C stock value of unvegetated tidal flats is between 2 to 4 times lower than that in vegetated ecosystems (293.9 Mg C ha-1 for mangroves, 317.2 Mg C ha-1 for salt marshes and 194.2 Mg C ha-1 for seagrass) (Fourqurean et al., 2012; Alongi, 2018; Ouyang and Lee, 2020) (Figure 2A and Table 1). As the data distribution did not conform to normality, the median value (67.9 Mg C ha-1) is used to avoid the effects of extreme outliners. Sedimentation rates estimated using radioisotope dating (e.g., 137Cs and 210Pb) (Howe et al., 2009) suggest that tidal flats exhibit significant but variable CAR (Table S1). The mean ( ± SE) and median values of CAR of tidal flats were 129.8 ± 29.9 and 53 g C m-2 yr-1, respectively (Table 1). Although the mean CAR value of tidal flats is similar to that of seagrass meadows (Figure 2B), the median value of CAR is still much lower than those of mangroves and salt marshes (Table 1), which are 230.9 and 244.7 g C m-2 yr-1, respectively (Breithaupt et al., 2012; Ouyang and Lee, 2014). However, tidal flat CAR also exhibited a high value, which was up to 1129 g C m-2 yr-1 in a mudflat adjacent to a Brazilian mangrove (Sanders et al., 2010).
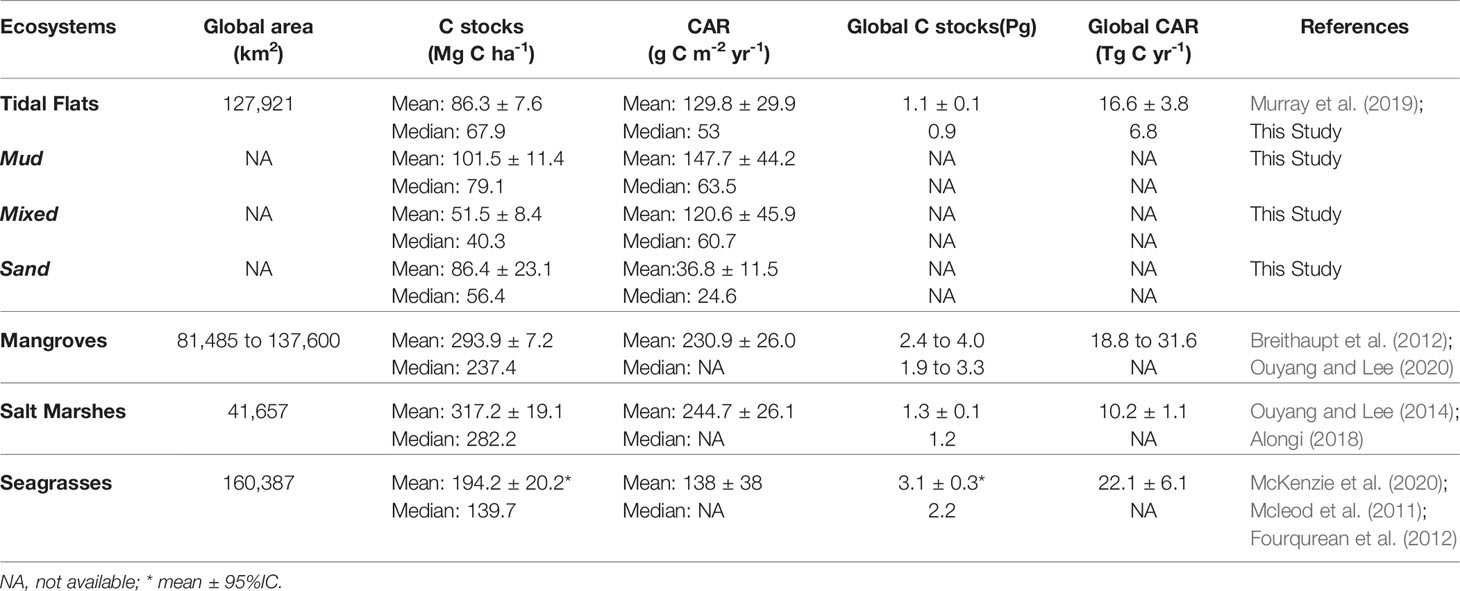
Table 1 Comparison of rate of tidal flat C sequestration (mean ± SE) estimated in this study with other published data on vegetated coastal wetlands.
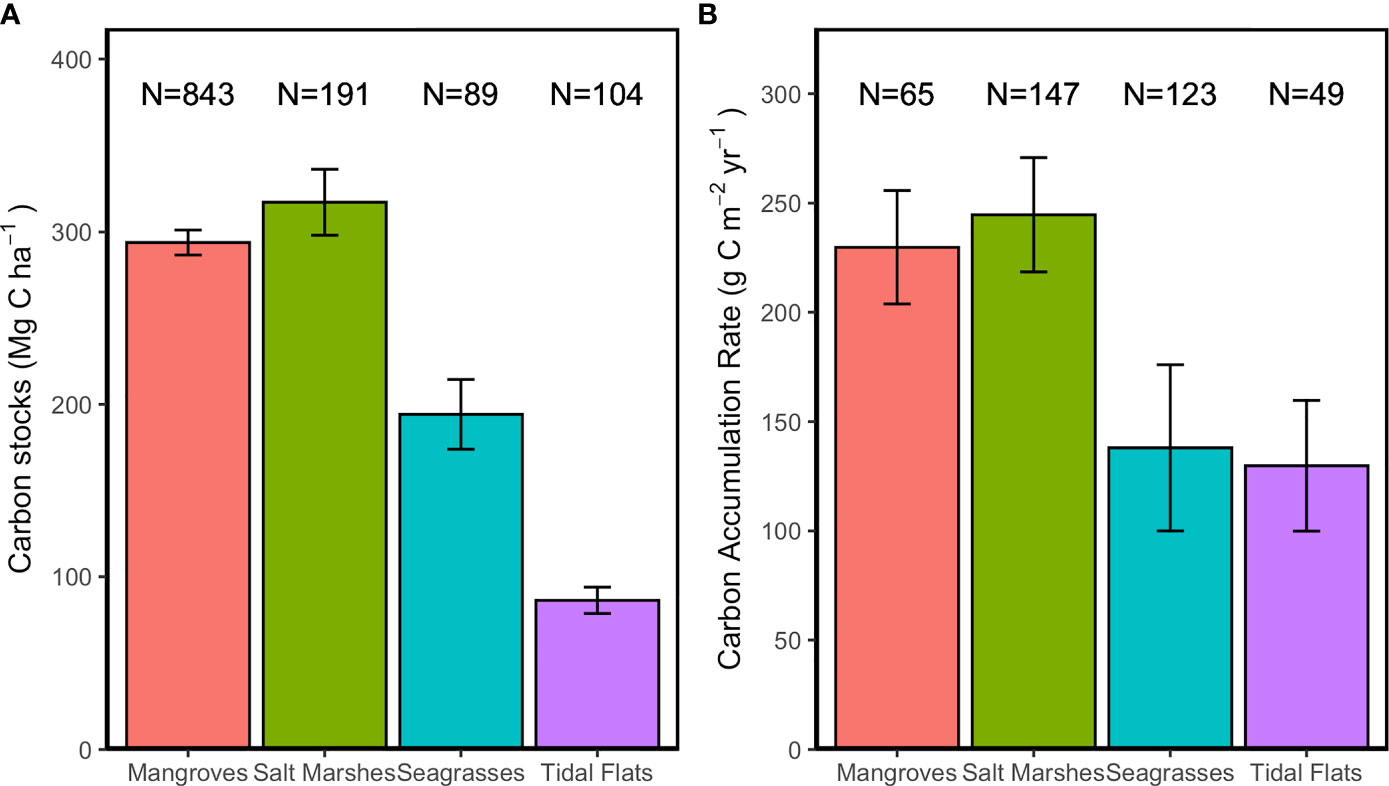
Figure 2 Comparison of sediment C sequestration in global mangroves, salt marshes and tidal flats. (A) Comparison of sediment C stocks (mean ± SE) in mangroves, salt marshes, seagrass meadows and tidal flats. C stocks data of seagrass is presented in C stock (mean ± 95% CI). (B) Comparison of CAR (mean ± SE) in mangroves (OC), salt marshes, seagrass meadows and tidal flats.
Substrate and Regional Differences in Carbon Sequestration of Tidal Flats
Our analysis indicated that the mean and median sediment C stock values of mudflats (101.5 and 79.1 Mg C ha-1, respectively) were higher than those of mixed flats (51.5 and 40.3 Mg C ha-1, respectively) or sandflats (86.4 and 23.1 Mg C ha-1, respectively). However, the sediment C stocks of tidal flats were only significantly different between mudflats and mixed flats (Kruskal-Wallis test, P<0.001) (Figure 3A and Table 1). There are no significant differences in CAR among the three sediment types (P>0.05), although the mean value of CAR was higher in mudflats (147.7 g C m-2 yr-1) than in mixed flats (120.6 g C m-2 yr-1) or sandflats (36.8 g C m-2 yr-1) (Figure 3B and Table 1).
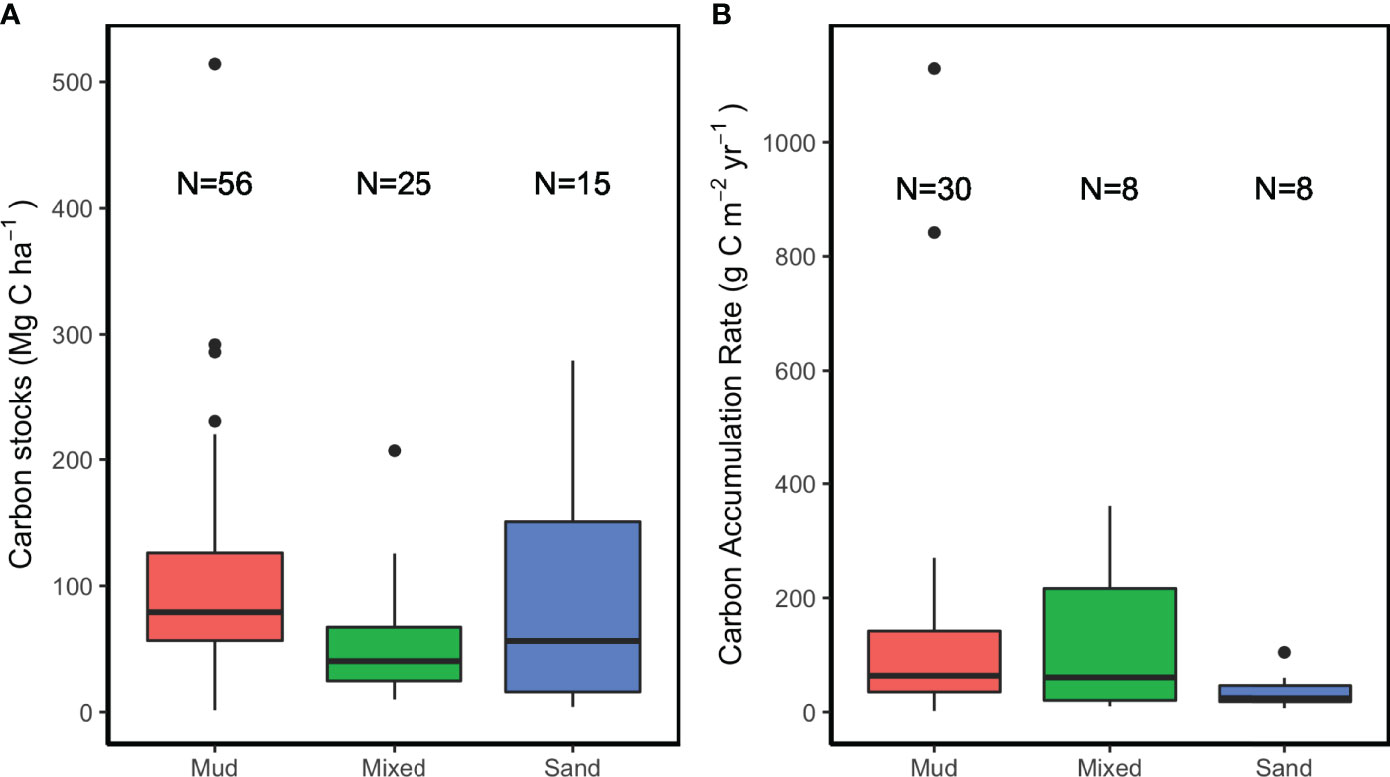
Figure 3 Comparison of sediment C sequestration in tidal flats of different sediment types. Comparison of sediment C stock and CAR in mudflats and sandflats are shown in (A, B), respectively. Studies without information on grain sizes are not included in the comparison.
In general, the top-meter sediment C stock and CAR of tidal flats showed a decline pattern along latitudinal range. Overall, tidal flats at low latitudes are more effective at sequestering C. The highest top-meter sediment C stock of tidal flats was in the 0 to 10° region, which was significantly higher than those at 20 to 30°and 30 to 40° latitudinal ranges (Mann-Whitney U tests, P<0.01). The lowest sediment C stock was found in 30 to 40° latitudinal ranges (Figure 4A). However, the mean sediment C stock increases from 30-40° to 40-50° (Figure 4C). As with C stocks, CAR of tidal flats showed similar decline patterns with latitudes (R2 = 0.12, P<0.01, Figure 4D) and there is a significant difference of CAR in each latitudinal range (P<0.05, Figure 4B).
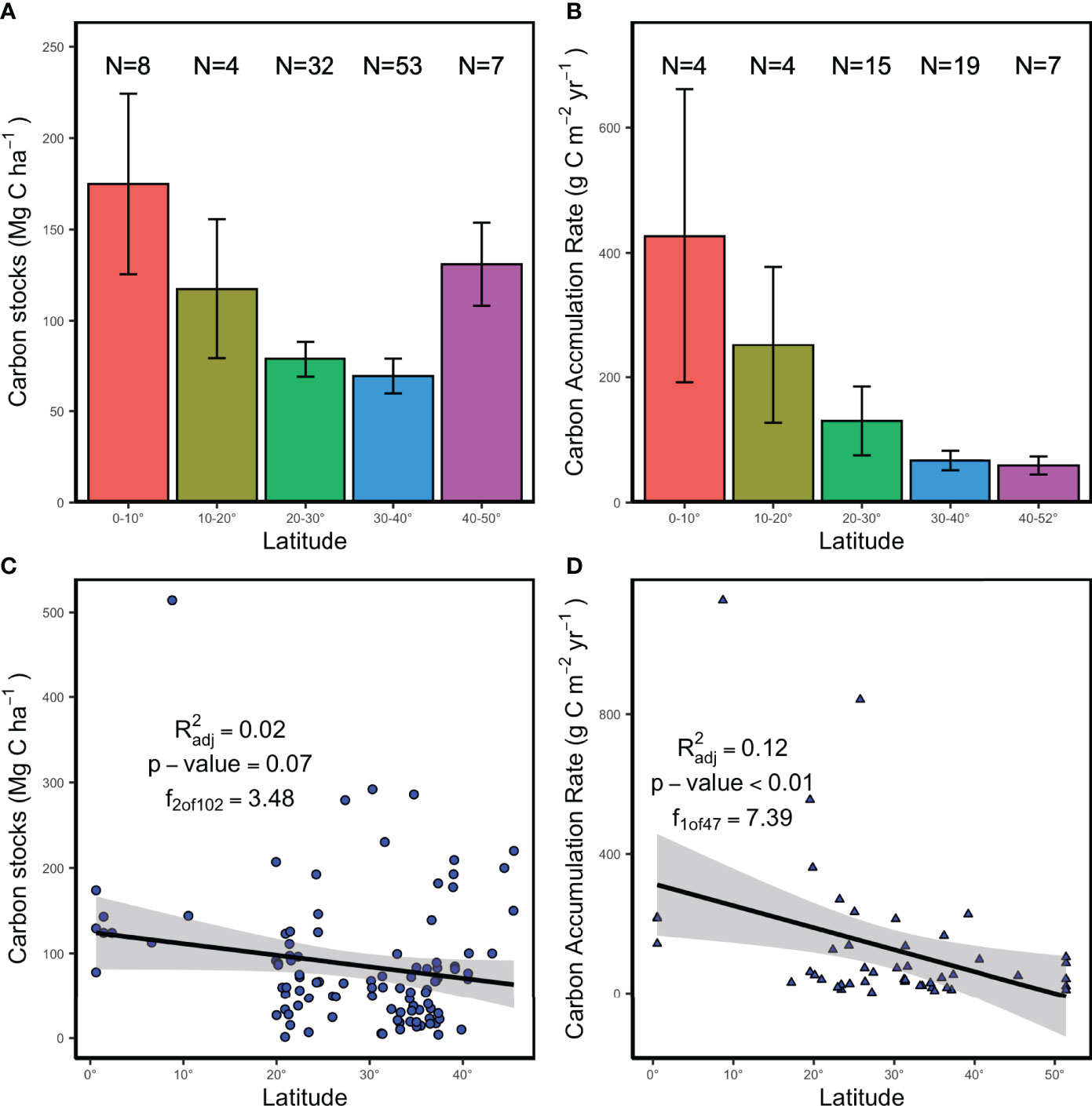
Figure 4 Latitudinal variation of sediment C sequestrations in tidal flats. Latitudinal comparison of sediment C stock (mean ± SE) and CAR (mean ± SE) are shown in (A, B), respectively. The solid line and the shadow area in (C, D) represent the best fitting line and 95% CI, respectively.
Discussion
Impact Factors of Carbon Sequestration in Tidal Flats
The low sediment C stock in tidal flats is related to lower availability of vascular plant detritus (Dinakaran et al., 2014; Zhang et al., 2017). With high primary productivity and slow remineralization, coastal vegetated wetlands have greater C sequestration rates than terrestrial ecosystems and unvegetated tidal flats (Mcleod et al., 2011; Davis et al., 2015). This is not unexpected, as vegetative structures such as aerial roots and shoots can weaken waves, reduce currents (Hendriks et al., 2008), promoting organic matter trapping (Kamal et al., 2017; Martin et al., 2019; Chen and Lee, 2021) and storage (Yang et al., 2014; Krauss et al., 2018). Furthermore, autochthonous C from the root and litter decomposition of coastal vascular plants or allochthonous C input from upstream forests (e.g., woody tissues and leaf litter) also contribute to the sediment C pool (Ouyang et al., 2017; Krauss et al., 2018; Lovelock and Reef, 2020). The microphytobenthos (MPB) are known to be the dominant primary producers in tidal flats and serve as a major nutrient source for the benthic food web (MacIntyre et al., 1996; Lee et al., 2021). The abundance of MPB will also affect the source of organic matter in the sediments of tidal flats. They can modify the quality and quantity of sediment organic matter (Hardison et al., 2013) and stabilize sediments through the production of extracellular polymeric substances, as well as affect the flow and cycling of C (Fernández et al., 2021).
However, when riverine sediment input is high, tidal flats can also be important C storages, for example, in Amazon River estuaries (514.2 Mg C ha-1) (Sanders et al., 2010). C from hydrologically connected mangroves, seagrass and macroalgae can also be buried in the sediment of tidal flats (Alongi, 2012) and increase their sediment C stocks, with vascular plant detritus being the main contributor (Endo and Otani, 2019). Sedimentation on tidal flats is strongly related to the fluvial process. Estuaries with high loads of suspended sediments and nutrient input are the major source of organic matter in tidal flats (Howarth et al., 1991). Rapid and high rates of sediment deposition in estuaries can promote high C storage and burial (Choi and Wang, 2004), especially in deltaic geomorphologic settings, where terrigenous riverine rather than oceanic sources may be the main contributor to the sediment C pool (Roberts et al., 2015).
Sediment type also influences C sequestration in tidal flats. During the sedimentation process, finer particles trap and bury more suspended organic matter than coarser sediments (Burdige, 2007) due to their higher relative sediment surface area (Lee et al., 2019). Finer sediments usually exhibit a more anoxic environment than a coarser sediment environment, which may promote preservation of C by reducing the remineralization rate (Burdige, 2007; Lee et al., 2019). Additionally, estuarine mudflats are more affected by sediment input from terrigenous sources. Therefore, a higher sedimentation rate in estuarine tidal flats may generally result in higher C burial than at oceanic sites. There is a regional difference in sediment C sequestration on tidal flats. It potentially attributable to increased allochthonous input from connected vegetated habitats (e.g., mangroves) in the tropics. Despite the absence of vegetation, their CAR is still much greater than that of terrestrial forests (0.7 to 13.1 g C m−2 yr−1) (Zehetner, 2010; Mcleod et al., 2011; Lovelock and Reef, 2020).
Estimates of Global Tidal Flat Carbon Stocks and Burial Rates
Although our analysis revealed that the C sequestration in tidal flat sediments is much lower than that reported for mangroves, salt marshes, and seagrass meadows, considering their wide distribution, tidal flats are still important C reservoir. Tidal flats should not be ignored when estimating regional and global coastal C sequestration, especially in areas with few vegetated ecosystems. The total C sequestration of tidal flats in our study is estimated by multiplying the median value of C stock (top-meter sediment) and CAR with their global area (Table 1), as the values of C stock and CAR of global tidal flats do not follow a normal distribution. This estimation approach means that outliers, such as the high C sequestration of the Brazilian tidal flat (Sanders et al., 2010), will not unduly affect the estimate. Despite covering only < 0.04% of the global marine sediment area extent (Duarte et al., 2005; Atwood et al., 2020), globally, tidal flats could store 0.9 Pg C (3.3 Pg CO2). Recent estimates of ecosystem sediment C stocks in global mangroves range from 1.9 Pg C (mangrove area = 81,485km2) to 3.3 Pg C (mangrove area = 137,600km2) (Ouyang and Lee, 2020). This amount is larger than that of our sediment C stock estimate for tidal flats. However, our estimate is limited to the top-meter of tidal flats and may therefore underestimate their global total C stock. For example, the Amazon River estuaries receive a large sediment supply and accretion during the wet season. Tidal flats usually have a rapid annual sedimentation rate and could result in accretion of up to more than one meter (Allison et al., 1995). The low porosity, water-logged and anoxic conditions in the deep tidal flat sediment beyond the resuspension and bioturbation zones also help protect sediment C from remineralization (Endo and Otani, 2019; Chen et al., 2020). In our estimate, the global C burial estimate for salt marshes is 10.2 Tg C yr-1, which is higher than the corresponding value for tidal flats (6.8 Tg C yr-1) (Table 1). This difference is attributed to the higher CAR of salt marshes, even though they have a smaller global coverage than tidal flats.
With high primary productivity and a slow remineralization rate, coastal blue carbon ecosystems can store 10 to 24 Pg C within their sediment and biomass (Duarte et al., 2013). Vegetated coastal habitats, such as mangroves, salt marshes and seagrass bury nearly half of the annual ocean sediment C (Duarte et al., 2005). The lack of a comprehensive assessment of tidal flats has led to a gap in global coastal sediment C estimates. According to the difference estimate of mangroves extent, global sediment C stock of coastal wetlands range from 6.2 to 7.6 Pg C and their global C burial rate can range from 57.9 to 70.9 Tg C yr−1 (Table 1). In our estimate, tidal flats contribute 11.8 to 14.5% and 9.6 to 11.7% of the global coastal sediment C stock and annual burial, respectively. However, large uncertainties (e.g., the definition and areal extent of tidal flats (Murray et al., 2019), insufficient studies of C sequestration) exist in estimates of tidal flat C sequestration compared to better-studied mangroves and other coastal vegetated wetlands.
Estimates of Potential Carbon Loss From Tidal Flats
Tidal flats are one of the most threatened coastal ecosystems, along with mangroves, salt marshes and seagrass meadows. It is predicted that, by the 2030s, globally 900 million people will live in the low-elevation coastal zones (Neumann et al., 2015), which include tidal flats. Therefore, anthropogenic impacts, such as reclamation are the main causes of extensive losses (Lee and Khim, 2017). Meanwhile, the direct loss due to sea-level rise, shoreline erosion, and reduction in estuarine sediment supply also contribute to the decline of tidal flats. The estimated annual instantaneous loss rate of tidal flats is around 0.55% from 1984 to 2016 (Murray et al., 2019). However, how tidal flat decline may impact the global tidal flat sediment C stock still needs to be assessed.
On most accreting coasts, colonization by mangrove or salt marsh plants promotes tidal flat loss. In contrast with losses caused by human activities, habitat transitions accompanying macrophyte colonization contribute to an increase in sediment C (Ye et al., 2014). Increased shoreline sediment erosion will occur if vertical sedimentation at the tidal flats cannot keep pace with the expected sea-level rise (Choi and Wang, 2004). Extreme weather events like heat (or cold) waves will also directly increase thermal stress in tidal flats, salt marshes and mangroves (Cabral et al., 2019) and the changing oceanic conditions will further reduce the stability of the sediment environment (Van de Broek et al., 2018). Urbanization and reclamation also contribute to coastal habitat loss. Coastal infrastructure and urbanization could reduce the accommodation space of coastal habitats, driving or limiting habitat transitions (Schuerch et al., 2018). These strong anthropogenic impacts modify the C cycle (Kuwae et al., 2016) and reduce the frequency of the sediment dry-rewetting process, which will increase the loss of sediment C (Li et al., 2020). However, the impact of global habitat loss on C emissions from tidal flats is still unquantified.
Assuming all the C in the top-meter sediment of those lost habitats were oxidized as CO2 and released back into the water column and atmosphere, based on our estimates of the median value of global sediment C stocks, 0.14 Pg C (0.51 Pg CO2) has already been lost with the decline in tidal flats (16.02%) in the past three decades. According to the current degradation rate (0.55%), global tidal flat C loss is estimated as 4.8 Tg C yr-1 (17.6 Tg CO2 yr-1). Coastal habitat C loss is not limited to tidal flats. From 2000 to 2012, around 2% of global mangrove carbon was lost and led to a potential emission of 0.32 Pg CO2 (Hamilton and Friess, 2018). A greater annual mangrove deforestation rate (1-2%) (Duke et al., 2007) may result in an emission rate of 20 to 120 Tg C yr-1 (73.3 to 440 Tg CO2 yr-1) (Donato et al., 2011), which is 4 to 24 times higher than that from tidal flats. It should be noted that tidal flat decline due to sea-level rise or reclamation will not release all stored C back into the environment. Furthermore, sediment C stocks may increase when the tidal flats are resorted and colonized by mangroves or salt marshes (Lunstrum and Chen, 2014; Xiang et al., 2015). The estimated maximum restorable areal extent of mangroves, salt marshes and seagrasses ranged from 175,000 to 416,000 km2 (Griscom et al., 2017; Worthington and Spalding, 2018). However, in most cases, native tidal flats are altered by exotic species. Although a case study indicates the sediment C content in mudflats increased 26% after colonized by exotic Spartina alterniflora (Ye et al., 2014), the consequence of occupying the niche of local species, changing and even diminishing biodiversity in tidal flats should also be serious discussed (Wan et al., 2009). Furthermore, the data of coastal wetlands colonization is still unknown, minimizing further decline of tidal flats is a priority. Our estimation highlights the significance and potential of tidal flats in global C storage and climate change migration, and the need for more data to inform future management.
The current data on sediment C burial and emission in coastal zones are insufficient to properly assess sediment C budgets and may result in significant errors when assessing the capacity of tidal flat sediments to sequester C on a global scale. Knowledge about GHG, especially CO2 emissions, from lost tidal flat sediments is particularly limited. Further chronological studies are needed to evaluate how C dynamics of tidal flats respond to long-term geologic (e.g., sedimentation rate) and environmental (e.g., catchment land use) changes. Apart from precise information about C sequestration, assessing the temporal trajectories of regional tidal flat distribution and the cause of their loss and degradation will also improve the estimation of C loss. A better understanding of these dynamics will contribute to improved future management and conservation of these important but under-studied coastal wetlands.
Conclusion
Vegetated coastal ecosystems (e.g., mangroves, seagrass meadows and salt marshes) are better studied and their role as important C reservoirs is well established, but similar assessments of tidal flats are lacking. Notwithstanding, the tidal flat is also the most vulnerable coastal ecosystem under the threats of climate change and anthropogenic degradation. Similar to mangroves, salt marshes and seagrass meadows, thick and anoxic tidal flat sediments also serve as large long-term C reservoirs and significantly contribute to the global sediment C stock in coastal wetlands. There is a knowledge gap for assessing their ecological functions, particularly in terms of C sequestration, storage, and emission. There is also an urgent need to renew our knowledge of the C dynamics of tidal flats (e.g., C emission dynamics) and establish the baseline contributions from different, particularly allochthonous and autochthonous, sources to their C pool.
Data Availability Statement
The original contributions presented in the study are included in the article/Supplementary Material. Further inquiries can be directed to the corresponding authors.
Author Contributions
ZC and SL contributed to the conceptualization of the study. ZC contributed to data analysis and drafted the manuscript. SL contributed to manuscript editing and supervision. All authors read and approved the final manuscript.
Funding
This study was supported by a postgraduate studentship of The Chinese University of Hong Kong awarded to ZC.
Conflict of Interest
The authors declare that the research was conducted in the absence of any commercial or financial relationships that could be construed as a potential conflict of interest.
Publisher’s Note
All claims expressed in this article are solely those of the authors and do not necessarily represent those of their affiliated organizations, or those of the publisher, the editors and the reviewers. Any product that may be evaluated in this article, or claim that may be made by its manufacturer, is not guaranteed or endorsed by the publisher.
Acknowledgments
We sincerely acknowledge Dr Xiaoguang Ouyang for help in reviewing the manuscript.
Supplementary Material
The Supplementary Material for this article can be found online at: https://www.frontiersin.org/articles/10.3389/fmars.2022.900896/full#supplementary-material
References
Allison M. A., Nittrouer C. A., Kineke G. C. (1995). Seasonal Sediment Storage on Mudflats Adjacent to the Amazon River. Mar. Geolog. 125, 303–328. doi: 10.1016/0025-3227(95)00017-S
Alongi D. M. (2012). Carbon Sequestration in Mangrove Forests. Carb. Manage. 3, 313–322. doi: 10.4155/cmt.12.20
Arkema K. K., Guannel G., Verutes G., Wood S. A., Guerry A., Ruckelshaus M., et al. (2013). Coastal Habitats Shield People and Property From Sea-Level Rise and Storms. Nat. Climate Change 3, 913–918. doi: 10.1038/nclimate1944
Atwood T. B., Witt A., Mayorga J., Hammill E., Sala E. (2020). Global Patterns in Marine Sediment Carbon Stocks. Front. Mar. Sci. 7, 165. doi: 10.3389/fmars.2020.00165
Barbier E. B., Hacker S. D., Kennedy C., Koch E. W., Stier A. C., Silliman B. R. (2011). The Value of Estuarine and Coastal Ecosystem Services. Ecol. Monogr. 81, 169–193. doi: 10.1890/10-1510.1
Blum M. D., Roberts H. H. (2009). Drowning of the Mississippi Delta Due to Insufficient Sediment Supply and Global Sea-Level Rise. Nat. Geosci. 2, 488–491. doi: 10.1038/ngeo553
Breithaupt J. L., Smoak J. M., Smith T. J. III, Sanders C. J., Hoare A. (2012). Organic Carbon Burial Rates in Mangrove Sediments: Strengthening the Global Budget. Global Biogeochem. Cycle. 26, GB3011. doi: 10.1029/2012GB004375
Bunting P., Rosenqvist A., Lucas R. M., Rebelo L., Hilarides L., Thomas N., et al. (2018). The Global Mangrove Watch—A New 2010 Global Baseline of Mangrove Extent. Remote Sens. 10, 1669. doi: 10.3390/rs10101669
Burdige D. J. (2007). Preservation of Organic Matter in Marine Sediments: Controls, Mechanisms, and an Imbalance in Sediment Organic Carbon Budgets? Chem. Rev. 107, 467–485. doi: 10.1021/cr050347q
Cabral H., Fonseca V., Sousa T., Costa Leal M. (2019). Synergistic Effects of Climate Change and Marine Pollution: An Overlooked Interaction in Coastal and Estuarine Areas. Int. J. Environ. Res. Public Health 16, 2737. doi: 10.3390/ijerph16152737
Chen Z., Lee S. Y. (2021). Contribution of Microplastics to Carbon Storage in Coastal Wetland Sediments. Environ. Sci. Technol. Lett. 8, 1045–1050. doi: 10.1021/acs.estlett.1c00784
Chen J., Wang D., Li Y., Yu Z., Chen S., Hou X., et al. (2020). The Carbon Stock and Sequestration Rate in Tidal Flats From Coastal China. Global Biogeochem. Cycle 34, e2020GB006772. doi: 10.1029/2020GB006772
Choi Y., Wang Y. (2004). Dynamics of Carbon Sequestration in a Coastal Wetland Using Radiocarbon Measurements. Global Biogeochem. Cycle. 18, GB4016. doi: 10.1029/2004GB002261
Davis J. L., Currin C. A., O’Brien C., Raffenburg C., Davis A. (2015). Living Shorelines: Coastal Resilience With a Blue Carbon Benefit. PLoS One 10 (11), e0142595. doi: 10.1371/journal.pone.0142595
Dinakaran J., Hanief M., Meena A., Rao K. S. (2014). The Chronological Advancement of Soil Organic Carbon Sequestration Research: A Review. Proc. Natl. Acad. Sci. Ind. Sec. B.: Biol. Sci. 84, 487–504. doi: 10.1007/s40011-014-0320-0
Donato D. C., Kauffman J. B., Murdiyarso D., Kurnianto S., Stidham M., Kanninen M. (2011). Mangroves Among the Most Carbon-Rich Forests in the Tropics. Nat. Geosci. 4, 293–297. doi: 10.1038/ngeo1123
Duarte C. M., Losada I. J., Hendriks I. E., Mazarrasa I., Marbà N. (2013). The Role of Coastal Plant Communities for Climate Change Mitigation and Adaptation. Nat. Climate Change 3, 961–968. doi: 10.1038/nclimate1970
Duarte C. M., Middelburg J. J., Caraco N. (2005). Major Role of Marine Vegetation on the Oceanic Carbon Cycle. Biogeosciences 2, 1–8. doi: 10.5194/bg-2-1-2005
Duke N. C., Meynecke J., Dittmann S., Ellison A. M., Anger K., Berger U., et al. (2007). A World Without Mangroves? Science 317, 41–42. doi: 10.1126/science.317.5834.41b
Endo T., Otani S. (2019). “Carbon Storage in Tidal Flats,” in Blue Carbon in Shallow Coastal Ecosystems (Singapore: Springer), 129–151.
Fernández C., Lara R. J., Parodi E. R. (2021). Influence of Microphytobenthos on the Sedimentary Organic Matter Composition in Two Contrasting Estuarine Microhabitats. Environ. Monitor. Assess. 193, 1–20. doi: 10.1007/s10661-021-08888-4
Flemming B. W. (2000). A Revised Textural Classification of Gravel-Free Muddy Sediments on the Basis of Ternary Diagrams. Continent. Shelf. Res. 20, 1125–1137. doi: 10.1016/S0278-4343(00)00015-7
Fourqurean J. W., Duarte C. M., Kennedy H., Marbà N., Holmer M., Mateo M. A., et al. (2012). Seagrass Ecosystems as a Globally Significant Carbon Stock. Nat. Geosci. 5, 505–509. doi: 10.1038/ngeo1477
Griscom B. W., Adams J., Ellis P. W., Houghton R. A., Lomax G., Miteva D. A., et al. (2017). Natural Climate Solutions. Proc. Natl. Acad. Sci. 114, 11645–11650. doi: 10.1073/pnas.1710465114
Hamilton S. E., Casey D. (2016). Creation of a High Spatio-Temporal Resolution Global Database of Continuous Mangrove Forest Cover for the 21st Century (CGMFC-21). Global Ecol. Biogeogr. 25, 729–738. doi: 10.1111/geb.12449
Hamilton S. E., Friess D. A. (2018). Global Carbon Stocks and Potential Emissions Due to Mangrove Deforestation From 2000 to 2012. Nat. Climate Change 8, 240–244. doi: 10.1038/s41558-018-0090-4
Hardison A. K., Canuel E. A., Anderson I. C., Tobias C. R., Veuger B., Waters M. N. (2013). Microphytobenthos and Benthic Macroalgae Determine Sediment Organic Matter Composition in Shallow Photic Sediments. Biogeosciences 10, 5571–5588. doi: 10.5194/bg-10-5571-2013
Hendriks I. E., Sintes T., Bouma T. J., Duarte C. M. (2008). Experimental Assessment and Modeling Evaluation of the Effects of the Seagrass Posidonia Oceanica on Flow and Particle Trapping. Mar. Ecol. Prog. Ser. 356, 163–173. doi: 10.3354/meps07316
Howarth R. W., Fruci J. R., Sherman D. (1991). Inputs of Sediment and Carbon to an Estuarine Ecosystem: Influence of Land Use. Ecol. Appl. 1, 27–39. doi: 10.2307/1941845
Howe A. J., Rodríguez J. F., Saco P. M. (2009). Surface Evolution and Carbon Sequestration in Disturbed and Undisturbed Wetland Soils of the Hunter Estuary, Southeast Australia. Estua. Coast. Shelf. Sci. 84, 75–83. doi: 10.1016/j.ecss.2009.06.006
Hua J., Feng Y., Jiang Q., Bao X., Yin Y. (2017). Shift of Bacterial Community Structure Along Different Coastal Reclamation Histories in Jiangsu, Eastern China. Sci. Rep. 7, 1–10. doi: 10.1038/s41598-017-10608-3
Kamal S., Warnken J., Bakhtiyari M., Lee S. Y. (2017). Sediment Distribution in Shallow Estuaries at Fine Scale: In Situ Evidence of 3D Structural Complexity Effects by Mangrove Pneumatophores. Hydrobiologia 803, 121–132. doi: 10.1007/s10750-017-3178-3
Krauss K. W., Noe G. B., Duberstein J. A., Conner W. H., Stagg C. L., Cormier N., et al. (2018). The Role of the Upper Tidal Estuary in Wetland Blue Carbon Storage and Flux. Global Biogeochem. Cycle. 32, 817–839. doi: 10.1029/2018GB005897
Kuwae T., Kanda J., Kubo A., Nakajima F., Ogawa H., Sohma A., et al. (2016). Blue Carbon in Human-Dominated Estuarine and Shallow Coastal Systems. Ambio 45, 290–301. doi: 10.1007/s13280-015-0725-x
Lee S. Y., Khim J. S. (2017). Hard Science is Essential to Restoring Soft-Sediment Intertidal Habitats in Burgeoning East Asia. Chemosphere 168, 765–776. doi: 10.1016/j.chemosphere.2016.10.136
Lee J., Kwon B., Kim B., Noh J., Hwang K., Ryu J., et al. (2019). Natural and Anthropogenic Signatures on Sedimentary Organic Matters Across Varying Intertidal Habitats in the Korean Waters. Environ. Int. 133, 105166. doi: 10.1016/j.envint.2019.105166
Lee I. O., Noh J., Lee J., Kim B., Hwang K., Kwon B., et al. (2021). Stable Isotope Signatures Reveal the Significant Contributions of Microphytobenthos and Saltmarsh-Driven Nutrition in the Intertidal Benthic Food Webs. Sci. Tot. Environ. 756, 144068. doi: 10.1016/j.scitotenv.2020.144068
Lin W., Wu J., Lin H. (2020). Contribution of Unvegetated Tidal Flats to Coastal Carbon Flux. Global Change Biol. 26, 3443–3454. doi: 10.1111/gcb.15107
Li J., Qu W., Han G., Lu F., Zhou Y., Song W., et al. (2020). Effects of Drying-Rewetting Frequency on Vertical and Lateral Loss of Soil Organic Carbon in a Tidal Salt Marsh. Wetlands 40, 1433–1443. doi: 10.1007/s13157-020-01286-5
Lovelock C. E., Reef R. (2020). Variable Impacts of Climate Change on Blue Carbon. One Earth 3, 195–211. doi: 10.1016/j.oneear.2020.07.010
Lunstrum A., Chen L. (2014). Soil Carbon Stocks and Accumulation in Young Mangrove Forests. Soil Biol. Biochem. 75, 223–232. doi: 10.1016/j.soilbio.2014.04.008
MacIntyre H. L., Geider R. J., Miller D. C. (1996). Microphytobenthos: The Ecological Role of the “Secret Garden” of Unvegetated, Shallow-Water Marine Habitats. I. Distribution, Abundance and Primary Production. Estuaries 19, 186–201. doi: 10.2307/1352224
Macreadie P. I., Costa M. D., Atwood T. B., Friess D. A., Kelleway J. J., Kennedy H., et al. (2021). Blue Carbon as a Natural Climate Solution. Nat. Rev. Earth Environ. 2, 826–839. doi: 10.1038/s43017-021-00224-1
Martin C., Almahasheer H., Duarte C. M. (2019). Mangrove Forests as Traps for Marine Litter. Environ. Poll. 247, 499–508. doi: 10.1016/j.envpol.2019.01.067
McKenzie L. J., Nordlund L. M., Jones B. L., Cullen-Unsworth L. C., Roelfsema C., Unsworth R. K. (2020). The Global Distribution of Seagrass Meadows. Environ. Res. Lett. 15, 074041. doi: 10.1088/1748-9326/ab7d06
Mcleod E., Chmura G. L., Bouillon S., Salm R., Björk M., Duarte C. M., et al. (2011). A Blueprint for Blue Carbon: Toward an Improved Understanding of the Role of Vegetated Coastal Habitats in Sequestering CO2. Front. Ecol. Environ. 9, 552–560. doi: 10.1890/110004
Mueller P., Granse D., Nolte S., Do H. T., Weingartner M., Hoth S., et al. (2017). Top-Down Control of Carbon Sequestration: Grazing Affects Microbial Structure and Function in Salt Marsh Soils. Ecol. Appl. 27, 1435–1450. doi: 10.1002/eap.1534
Murray N. J., Phinn S. R., DeWitt M., Ferrari R., Johnston R., Lyons M. B., et al. (2019). The Global Distribution and Trajectory of Tidal Flats. Nature 565, 222–225. doi: 10.1038/s41586-018-0805-8
Neumann B., Vafeidis A. T., Zimmermann J., Nicholls R. J. (2015). Future Coastal Population Growth and Exposure to Sea-Level Rise and Coastal Flooding-a Global Assessment. PLoS One 10 (3), e0118571. doi: 10.1371/journal.pone.0118571
Ouyang X., Lee S. Y. (2014). Updated Estimates of Carbon Accumulation Rates in Coastal Marsh Sediments. Biogeosciences 11, 5057–5071. doi: 10.5194/bg-11-5057-2014
Ouyang X., Lee S. Y. (2020). Improved Estimates on Global Carbon Stock and Carbon Pools in Tidal Wetlands. Nat. Commun. 11, 1–7. doi: 10.1038/s41467-019-14120-2
Ouyang X., Lee S. Y., Connolly R. M. (2017). The Role of Root Decomposition in Global Mangrove and Saltmarsh Carbon Budgets. Earth-Sci. Rev. 166, 53–63. doi: 10.1016/j.earscirev.2017.01.004
Passeri D. L., Hagen S. C., Medeiros S. C., Bilskie M. V., Alizad K., Wang D. (2015). The Dynamic Effects of Sea Level Rise on Low-Gradient Coastal Landscapes: A Review. Earth. Future 3, 159–181. doi: 10.1002/2015EF000298
Phang V. X., Chou L. M., Friess D. A. (2015). Ecosystem Carbon Stocks Across a Tropical Intertidal Habitat Mosaic of Mangrove Forest, Seagrass Meadow, Mudflat and Sandbar. Earth Surf. Proc. Landf. 40, 1387–1400. doi: 10.1002/esp.3745
Roberts H. H., DeLaune R. D., White J. R., Li C., Sasser C. E., Braud D., et al. (2015). Floods and Cold Front Passages: Impacts on Coastal Marshes in a River Diversion Setting (Wax Lake Delta Area, Louisiana). J. Coast. Res. 31, 1057–1068. doi: 10.2112/JCOASTRES-D-14-00173.1
Sanders C. J., Smoak J. M., Naidu A. S., Sanders L. M., Patchineelam S. R. (2010). Organic Carbon Burial in a Mangrove Forest, Margin and Intertidal Mud Flat. Estua. Coast. Shelf. Sci. 90, 168–172. doi: 10.1016/j.ecss.2010.08.013
Schuerch M., Spencer T., Temmerman S., Kirwan M. L., Wolff C., Lincke D., et al. (2018). Future Response of Global Coastal Wetlands to Sea-Level Rise. Nature 561, 231–234. doi: 10.1038/s41586-018-0476-5
Van de Broek M., Vandendriessche C., Poppelmonde D., Merckx R., Temmerman S., Govers G. (2018). Long-Term Organic Carbon Sequestration in Tidal Marsh Sediments is Dominated by Old-Aged Allochthonous Inputs in a Macrotidal Estuary. Global Change Biol. 24, 2498–2512. doi: 10.1111/gcb.14089
Wan S., Qin P., Liu J., Zhou H. (2009). The Positive and Negative Effects of Exotic Spartina Alterniflora in China. Ecol. Eng. 35, 444–452. doi: 10.1016/j.ecoleng.2008.05.020
Worthington T., Spalding M. (2018). Mangrove Restoration Potential: A Global Map Highlighting a Critical Opportunity. doi: 10.17863/CAM.39153
Xiang J., Liu D., Ding W., Yuan J., Lin Y. (2015). Invasion Chronosequence of Spartina Alterniflora on Methane Emission and Organic Carbon Sequestration in a Coastal Salt Marsh. Atmos. Environ. 112, 72–80. doi: 10.1016/j.atmosenv.2015.04.035
Yang J., Gao J., Liu B., Zhang W. (2014). Sediment Deposits and Organic Carbon Sequestration Along Mangrove Coasts of the Leizhou Peninsula, Southern China. Estua. Coast. Shelf. Sci. 136, 3–10. doi: 10.1016/j.ecss.2013.11.020
Yang H., Tang J., Zhang C., Dai Y., Zhou C., Xu P., et al. (2020). Enhanced Carbon Uptake and Reduced Methane Emissions in a Newly Restored Wetland. J. Geophys. Res: Biogeosciences 12, e2019JG005222. doi: 10.1029/2019JG005222
Ye X., Wang A., Chen J. (2014). Distribution and Deposition Characteristics of Carbon and Nitrogen in Sediments in a Semi-Closed Bay Area, Southeast China. Continent. Shelf. Res. 90, 133–141. doi: 10.1016/j.csr.2014.07.015
Zehetner F. (2010). Does Organic Carbon Sequestration in Volcanic Soils Offset Volcanic CO2 Emissions? Quaternar. Sci. Rev. 2, 1313–1316. doi: 10.1016/j.quascirev.2010.03.003
Zhang T., Chen H., Cao H., Ge Z., Zhang L. (2017). Combined Influence of Sedimentation and Vegetation on the Soil Carbon Stocks of a Coastal Wetland in the Changjiang Estuary. Chin. J. Oceanol. Limnol. 35, 833–843. doi: 10.1007/s00343-017-6054-0
Keywords: tidal flats, coastal wetlands, carbon stock, carbon accumulation rate, carbon loss, blue carbon
Citation: Chen ZL and Lee SY (2022) Tidal Flats as a Significant Carbon Reservoir in Global Coastal Ecosystems. Front. Mar. Sci. 9:900896. doi: 10.3389/fmars.2022.900896
Received: 21 March 2022; Accepted: 22 April 2022;
Published: 30 May 2022.
Edited by:
Xinping Hu, Texas A&M University Corpus Christi, United StatesReviewed by:
Marcus Sheaves, James Cook University, AustraliaJong Seong Khim, Seoul National University, South Korea
Copyright © 2022 Chen and Lee. This is an open-access article distributed under the terms of the Creative Commons Attribution License (CC BY). The use, distribution or reproduction in other forums is permitted, provided the original author(s) and the copyright owner(s) are credited and that the original publication in this journal is cited, in accordance with accepted academic practice. No use, distribution or reproduction is permitted which does not comply with these terms.
*Correspondence: Zhao Liang Chen, emhhb2xpYW5nQ0hFTkBsaW5rLmN1aGsuZWR1Lmhr; Shing Yip Lee, am9lc3lsZWVAY3Voay5lZHUuaGs=
†ORCID: Zhao Liang Chen, orcid.org/0000-0002-5755-574X
Shing Yip Lee, orcid.org/0000-0001-9336-2323