- 1School of Interdisciplinary Arts and Sciences, University of Washington, Bothell, WA, United States
- 2School of Marine and Atmospheric Sciences, Stony Brook University, Stony Brook, NY, United States
- 3Department of Biology, Old Dominion University, Norfolk, VA, United States
- 4Wildlife Conservation Society, Gabon-Program, Libreville, Gabon
- 5British Trust for Ornithology, The Nunnery, Thetford, Norfolk, United Kingdom
- 6South Atlantic Environmental Research Institute, Stanley FIQQ1ZZ, Falkland Islands
- 7Department of Biological Sciences, Macquarie University, Sydney, NSW, Australia
- 8Marine Mammal and Turtle Division, Southwest Fisheries Science Center, National Marine Fisheries Service, National Oceanic and Atmospheric Administration, Moss Landing, CA, United States
- 9Moss Landing Marine Laboratories, San Jose State University, Moss Landing, CA, United States
- 10Hopkins Marine Station, Stanford University, Pacific Grove, CA, United States
- 11National Oceanic and Atmospheric Administration, Southwest Fisheries Science Center, Environmental Research Division, Monterey, CA, United States
- 12Chicago Zoological Society’s Sarasota Dolphin Research Program, c/o Mote Marine Laboratory, Sarasota, FL, United States
- 13Population Ecology Division, Bedford Institute of Oceanography, Department of Fisheries and Oceans, Dartmouth, NS, Canada
- 14Biology Department, Dalhousie University, Halifax, NS, Canada
- 15School of Biological Science (Zoology), University of Aberdeen, Aberdeen, United Kingdom
- 16Instituto de Investigación y Desarrollo Pesquero (INIDEP), Mar del Plata, Argentina
- 17Instituto de Investigaciones Marinas y Costeras (UNMdP-CONICET), Mar del Plata, Argentina
- 18Department of Biology, University of Washington, Bothell, WA, United States
- 19Department of Biology, Sonoma State University, Sonoma, CA, United States
- 20Department of Ecology and Evolutionary Biology, University of California Santa Cruz, Santa Cruz, CA, United States
- 21Department of Biology, University of Central Florida, Orlando, FL, United States
- 22Division of Avian Conservation, Yamashina Institute for Ornithology, Abiko, Chiba, Japan
- 23National Oceanic and Atmospheric Administration, Southwest Fisheries Science Center, Fisheries Resources Division, La Jolla, CA, United States
- 24Centre for Ecology & Conservation, College of Life & Environmental Sciences, University of Exeter, Penryn Campus, Cornwall, United Kingdom
- 25African Aquatic Conservation Fund, Chilmark, MA, United States
- 26MarAlliance, Ciudad del Saber, Panama City, Panama
- 27Division of Sea Turtle Science and Recovery, Padre Island National Seashore, Corpus Christi, TX, United States
- 28Wetland and Aquatic Research Center, U.S. Geological Survey, Davie, FL, United States
- 29University of Exeter, College of Life and Environmental Sciences, Hatherly Laboratories, Prince of Wales Road, Exeter, United Kingdom
- 30Scottish Natural Heritage, Inverness, Scotland
- 31Groundswell Coastal Ecology, Davenport CA, United States
- 32Marine Mammal Institute and Department of Fisheries, Wildlife and Conservation, Hatfield Marine Science Center, Oregon State University, Newport, OR, United States
- 33Department of Biology, Baylor University, Waco, TX, United States
- 34National Oceanic and Atmospheric Administration Alaska Fisheries Science Center, Marine Mammal Laboratory, Seattle, WA, United States
- 35Department of Biology, Dalhousie University, Halifax, NS, Canada
- 36Department of Fisheries and Wildlife, Oregon State University, Hatfield Marine Science Center, Newport, OR, United States
- 37Marine Conservation Institute, Seattle, WA, United States
- 38Wildlife Conservation Society, Ocean Giants Program, Bronx, NY, United States
- 39Department of Biological Sciences, San Jose State University, San Jose, CA, United States
- 40Marine Mammal Commission, Marine Mammal Commission, Bethesda, MD, United States
- 41Cherokee Nations Technology, Contracted to the U.S. Geological Survey, Davie, FL, United States
- 42Aventures Sans Frontières, Libreville, Gabon
- 43Agence Nationale des Parcs Nationaux, Libreville, Gabon
- 44Auke Bay Laboratories, Alaska Fisheries Science Center, NOAA Fisheries, Juneau, AK, United States
- 45National Institute of Water and Atmospheric Research Ltd., Hataitai, Wellington, New Zealand
- 46Joint Nature Conservation Committee, Peterborough, United Kingdom
- 47Department of Ecology and Marine Biology, University of California Santa Barbara, Santa Barbara, CA, United States
- 48British Antarctic Survey, Cambridge, United Kingdom
- 49Office of Naval Research, Arlington, VA, United States
- 50Department of Forestry and Environmental Conservation, South Carolina Cooperative Fish and Wildlife Research Unit, Clemson University, Clemson, SC, United States
Marine protected areas (MPAs), particularly large MPAs, are increasing in number and size around the globe in part to facilitate the conservation of marine megafauna under the assumption that large-scale MPAs better align with vagile life histories; however, this alignment is not well established. Using a global tracking dataset from 36 species across five taxa, chosen to reflect the span of home range size in highly mobile marine megafauna, we show most MPAs are too small to encompass complete home ranges of most species. Based on size alone, 40% of existing MPAs could encompass the home ranges of the smallest ranged species, while only < 1% of existing MPAs could encompass those of the largest ranged species. Further, where home ranges and MPAs overlapped in real geographic space, MPAs encompassed < 5% of core areas used by all species. Despite most home ranges of mobile marine megafauna being much larger than existing MPAs, we demonstrate how benefits from MPAs are still likely to accrue by targeting seasonal aggregations and critical life history stages and through other management techniques.
Introduction
One of the perceived benefits of marine protected areas (MPAs) has been for the conservation of megafauna (Hooker and Gerber, 2004). Many of these species are or have been targets of human exploitation or disturbance, have less resilient life histories than smaller species, and have been depleted across much of the planet (Dirzo et al., 2014; Ripple et al., 2014; Carman et al., 2015; McCauley et al., 2015). Marine megafauna are protected under a variety of international and domestic laws to conserve their important roles in maintaining the structure and function of their associated ecosystems (Estes et al., 2011; McCauley et al., 2015). Many proponents of large MPAs point to their size as a way to protect marine megafauna and their habitats (e.g., Game et al., 2009; Toonen et al., 2013; O’Leary et al., 2018; Gallagher et al., 2020). Moreover, many MPA guidelines (Lewis et al., 2017; Smyth and Hanich, 2019) as well as official MPA declarations explicitly cite megafauna protection as a key driver of establishment for large-scale MPAs, such as the Ross Sea Marine Protected Area (Commission for the Conservation of Antarctic Marine Living Resources, 2016), and expansion of Papahānaumokuākea (Executive Office of the US President, 2016) and the Pacific Remote Islands (Executive Office of the US President, 2014) Marine National Monuments in the US, though many smaller, coastal MPAs are created for other reasons. Although the protection of key habitat is a primary tool in the conservation and management of marine megafauna (Hooker et al., 2011), this perceived benefit may come with significant socioeconomic costs. This is especially true for large MPAs as they can restrict large areas of various human uses, such as fishing, mineral extraction, and vessel activity to a larger degree than smaller MPAs (Ban et al., 2017; O’Leary et al., 2018). These costs demand that the benefits of MPAs for wide-ranging megafauna be demonstrated.
Global targets for MPAs have been proposed and continue to be refined. The United Nations (UN) is actively negotiating the implementation of MPAs in regions beyond national jurisdictions (i.e., the ‘High Seas’) (United Nations, 2017), regions that are particularly important for marine megafauna (Harrison et al., 2018; Beal et al., 2021; Davies et al., 2021b). Moreover, in 2016 the International Union for the Conservation of Nature (IUCN) called to increase the UN Convention on Biological Diversity Aichi Biodiversity Target 11 from 10% to up to 40% of the ocean to be protected by 2030 (Hilborn, 2016; IUCN, 2016; Jefferson et al., 2021), and many countries and Tribal nations have come out in support of these goals (Allen et al., 2021; Sullivan-Stack et al., 2022). Large MPAs (> 100,000 km2) have increasingly emerged over the last decade, in part to achieve those targets (O’Leary et al., 2018), though many of the large, highly protected MPAs are placed in remote regions where threats and human conflicts are limited, while MPAs closer to human populations are often more limited in protections offered (Sullivan-Stack et al., 2022). In addition to achieving targets, the push to establish larger MPAs has been fueled by i) the successes of coastal MPAs, ii) a recognition that most of the global ocean is not coastal, and iii) the need to protect large scale processes that characterize oceanic systems (e.g., long-distance animal movements) (Longhurst, 2010; Block et al., 2011). The various purported benefits of large MPAs also include their ability to protect entire functional ecosystems, and to help buffer these systems from climate change, large spatial-scale disturbances, and edge effects of smaller MPAs (Toonen et al., 2013; Wilhelm et al., 2014).
Despite purported benefits of MPAs, and especially large MPAs, to highly mobile marine megafauna, there remains much uncertainty about their effectiveness, in part due to observed or theorized mismatches in scale between MPA sizes and megafauna ranges or critical habitats in specific regions and cases (e.g., Agardy et al., 2011; ICES, 2011). Thus, there is a need for a comprehensive, empirical effort to explicitly evaluate the adequacy of existing MPA sizes as they relate to highly mobile marine megafauna movements. This critical first step is necessary to determine if MPAs as they are currently designed are spatially equipped to offer the protection that is necessary for highly mobile marine megafauna, and to evaluate how MPAs might be designed to be most spatially compatible.
Quantifying and understanding MPA effectiveness for megafauna will require a multi-faceted approach, requiring studies both at global scales and at smaller, regional scales. Here, our objective is to understand the extent to which global MPA boundaries align in spatial scale with the movements of highly mobile marine megafauna. This evaluation is particularly timely as the push for large MPAs continues and as an agreement for protecting biodiversity on the high seas under the UN Law of the Sea Convention (UNCLOS) develops. Here, we compiled an extensive tracking dataset from 36 species of highly mobile marine megafauna from five higher taxa (cetaceans, elasmobranchs [sharks and rays], pinnipeds, chelonioids [sea turtles], and seabirds) to quantify the congruence of global MPA sizes with home ranges and to evaluate how this congruence may fluctuate across the annual cycle and species life history. Our results identify key considerations for MPA design and management necessary to amplify the effectiveness of MPAs for marine megafauna conservation.
Methods
Data analysis
We used the following packages in R 3.5.3 (R Core Team, 2019) for all data manipulation and analyses: ‘tidyverse’ (Wickham, 2017); spatial analysis: ‘adehabitatLT’ (Calenge, 2012), ‘adehabitatHR’ (Calenge, 2006), ‘geosphere’ (Hijmans et al., 2017), ‘maptools’ (Bivand and Lewin-Koh, 2017), ‘raster’ (Hijmans and van Etten, 2018), ‘rgeos’ (Bivand, 2018), ‘sp’ (Pebesma, 2018), ‘sf’ (Pebesma, 2018), and ‘spatstat’ (Baddeley, 2015); and visualization: ‘ggplot2’ (Wickham et al., 2018), ‘scales’ (Wickham and Seidel, 2015), ‘smoothr’ (Strimas-Mackey, 2018).
Animal tracking data
We collected a large tracking dataset of targeted species that represented the breadth of movement patterns in high mobile marine megafauna across taxa. To do this, we gathered 43 tracking datasets from 36 species across five major taxonomic groupings of marine megafauna (elasmobranchs, cetaceans, pinnipeds, seabirds, and sea turtles) between 2001-2018 and from four of the planet’s five major oceans and a few minor seas, gulfs, and channels (Figure 1; Table 1). Bony fishes were excluded due to the lack of datasets with spatial resolution that matched the rest of the dataset (e.g., bony fish locations are primarily derived from geolocation calculations vs. satellite-derived locations). Location data were obtained from species equipped with Argos satellite-linked (n=25 species), GPS (n=12 species), a combination of GPS+geolocation (GLS) (n=1 species), or GLS-only (n=1 species) tracking devices. Three species (Laysan [Phoebastria immutabilis], black-footed [P. nigripes], and short-tailed [P. albatrus] albatross) were tracked with both Argos and GPS devices, and one species (basking sharks [Cetorhinus maximus]) was tracked with Argos and GLS+GPS devices. Methodological details on specific deployments are available for most tracking datasets in previously published work (Supplemental Table S1); however, if not previously described, we provide additional deployment details in Supplemental Table S1.
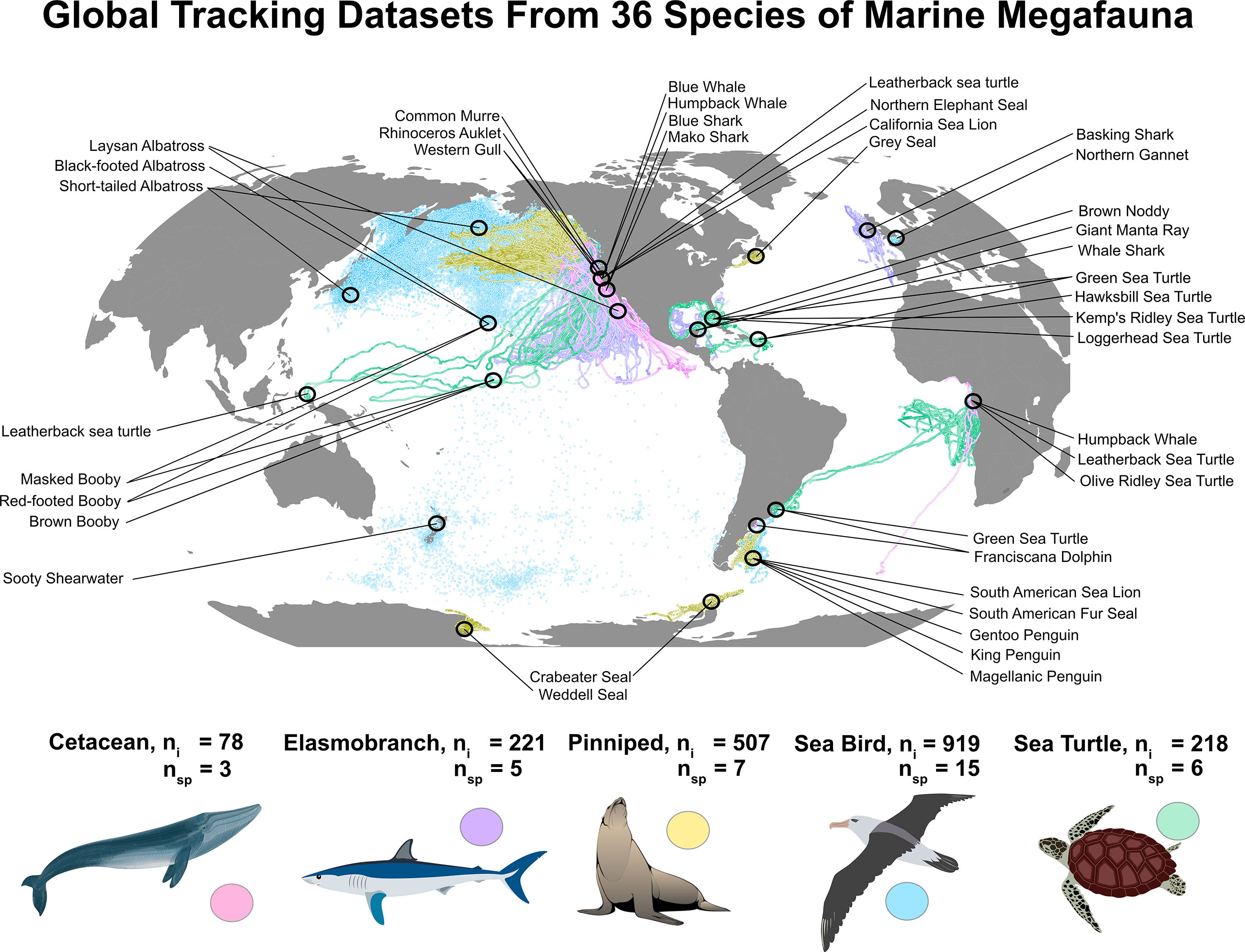
Figure 1 We gathered 43 tracking datasets from 1,943 individuals of 36 species representing five mobile marine taxa (elasmobranchs, cetaceans, pinnipeds, sea birds, and sea turtles). Location data were collected from marine vertebrates equipped with tracking devices (Argos satellite-linked (n=25), GPS (n=12), a combination of GPS+geolocation (GLS) (n=1, basking sharks), or GLS only (n=1, sooty shearwaters)) from four out of five of Earth’s five major oceans and from a few minor seas, gulfs, and channels between 2001-2018. Some species (n=4) were tracked with a mixture of Argos, GPS, and GLS devices.
Nearly all datasets were filtered and processed as described in published work (Supplemental Table S1). In brief, locations from Argos transmitters were processed through various state-space or non-state-based random walk models (Jonsen et al., 2005; Johnson et al., 2008; Tremblay et al., 2009); both types of methods provided robust estimates of animal tracks by linking mechanistic movement models with observation error. Location data estimated from geolocation (GLS) contain large spatial errors that, even after post-processing, can be orders of magnitude greater than those of Argos satellite and GPS data (mean error, in general: 10s to 100s of kilometres (GLS), < 50 kilometres (Argos), and 10s of meters (GPS) (Wilson et al., 2002; Phillips et al., 2004; Shaffer et al., 2005; Dujon et al., 2014)). Thus we limited our inclusion of GLS data to (1) a subset of basking shark tracks (n=13 out of 43 basking shark individuals) that were processed in a robust movement model that corrected GLS locations with a subset of available GPS locations (Doherty et al., 2017), and (2) a single case study of sooty shearwaters (Ardenna grisea), with which we evaluated relative, not absolute, change in home range size across an annual cycle.
Location data from colony-based, central-place foragers (e.g., terrestrial breeding seabirds and pinnipeds) can artificially inflate the influence of colony location due to repeated visits to and long times spent on-land at these locations. Our focus was limited to movements at sea, so all locations from central-place foragers that fell within a designated radius from the colony/tagging location were removed following standard practice (e.g., Maxwell et al., 2016). Radius distances were determined according to spatial error of different tag types and movement characteristics specific to each species and ranged from as low as 0.25 km for brown noddies (Anous stolidus) and up to 2 km for albatross species. Resulting tracks were linearly interpolated. Two interpolation schemes were used given the extremely disparate movement rates and trip durations among study taxa. Species that moved rapidly (mean speed > 40 km/hr) and had short duration trips (mean < 8 hours) were interpolated at 10-minute intervals while all other datasets were interpolated to 30-minute intervals (Supplemental Table S1). The smaller interval for high-speed, small-ranged species allowed for a sufficient sample size of locations to enable home range analyses. A handful of species (basking sharks, blue sharks, whale sharks [Rhincodon typus], and short-tailed albatross) had large gaps in data (weeks to months) in individual tracks. For gaps greater than a week, we did not interpolate between the contiguous portions of the track to avoid introducing erroneous boundaries in our home range estimates.
Home range utilization distributions
To determine patterns of marine space use, we conducted home range analyses using a gridded utilization distribution (UD) method which resulted in the probability of finding an animal in a defined area within its home range (Millspaugh and Marzluff, 2001; Maxwell et al., 2011; Maxwell et al., 2013). The N% UD is the smallest area in which the pixels of the UD sum to N%, and thus there is a cumulative N% probability of finding the animal within that cell at a random time. Home ranges were defined as the 90% UD to encapsulate the majority of areas visited while excluding spurious movements. Core areas were defined as areas with significant visitation and were quantified as the UD where a curve of total cumulative area used deviated the greatest from random, following Seaman & Powell (1990), indicating the UD contour where space use patterns changed distinctly. Core area UDs varied among datasets and ranged from the 75% – 90% UD depending on how uniform or clumped a population’s space use was across the seascape (Table 2 and Supplemental Figure S1). Geo-computation methods were used to compute all distances and areas from animal location data. We used Haversine great circle distances to compute geographical distances on a uniform sphere. UD polygons were transformed to planar coordinates with a Lambert Azimuthal Equal Area projection centred on the mean latitude and mean longitude from each respective dataset when calculating home range and core areas.
Due to the large differences in home range size across datasets, an initial analysis of home range used an adjustable grid size that scaled with the range size of each species’ dataset to avoid overly pixelated or smoothed UDs (Supplemental Figure S1). Adjustable grid sizes were equivalent to 5% of the population’s median maximum distance (km) from the initial tagging location. For the initial gridded utilization distribution analysis, dataset-specific adjusted grid sizes ranged from 1.2 km2 for the highly resident Franciscan dolphins to 163.3 km2 in the extremely vagile leatherback sea turtle. Tracks were normalized before gridded UD analyses to account for variable biases in tracking data (Block et al., 2011; Maxwell et al., 2011; Maxwell et al., 2013). For species with nomadic-type movement patterns and abbreviated tracking data (whales, elasmobranchs, and sea turtles), the unnatural abbreviation of trips due to tag failure or tag loss can result in a positive bias for areas near the tagging location. To correct for this potential bias, we weighted each location by the inverse number of individuals in that dataset who had locations for the same relative duration of their track (Block et al., 2011), which resulted in lower weights in locations close to the initial tagging location. This basic time-weighting scheme was modified using the 85th percentile of track lengths for a given dataset, which minimizes bias in spatial density patterns in datasets with smaller sample sizes (Block et al., 2011). Central-place foragers (pinnipeds and seabirds in our study) incur a different kind of bias since they are often tracked across multiple trips to sea from the breeding colony. This leads to a locational bias in species that exhibit at-sea site fidelity (common in seabird and pinniped species) for which we have a greater number of trips for some individuals. To correct for this potential bias in central place foragers, we weighted each location in an individual’s dataset by the inverse number of trips taken by that individual. For species with datasets sourced from different colonies (e.g., different geographic origins) (n=7, Table 1), we calculated UDs separately for each dataset with the exception of Pacific Ocean leatherback sea turtles. Pacific leatherbacks from multiple colonies (tagged in both Papua Barat, Indonesia and the West Coast USA) were pooled into a single dataset, because turtle movements from both tagging origins overlapped across the entire North Pacific basin. Despite these corrections, some bias will still exist based on the region of tagging, however, random sampling of individuals and study regions within tagging studies is often either cost-prohibitive or unfeasible. Thus, the home range estimates we present here largely reflect those of the tagged population, rather than the species as a whole.
Sampling adequacy and final home range classification
To evaluate sampling bias in our home range estimates, we measured how home range area changed within a population with increasing sample sizes (sampling adequacy), following Lascelles et al. (2016) and Soanes et al. (2013). In brief, we selected random individuals starting with a sample size of one, with sample size increasing incrementally by one in each subsequent iteration until reaching the true sample size of the dataset. From the ‘selected’ individuals, we calculated the area (km2) of the 90% UD using the gridded utilization distribution method described above. For each iteration of sample size, we repeated this calculation 100 times, with a different set of randomly selected individuals. For central place foragers with individuals tracked across multiple trips, we selected a single trip (that with the largest maximum range) per individual for this analysis. A nonlinear regression fitting home range area as a function of n/n+1 (n=sample size) was then used to estimate how representative the tracking dataset was to its population (Supplemental Figure S2). We approximated the nonlinear fit to a sample size of 100 individuals and calculated the ratio of the home range area estimated from the true sample size to the home range area of 100 individuals estimated by the ensuing function. The resulting percentage conveyed the degree of sampling bias in home range estimates and was used to evaluate both the robustness of each dataset also as a correction factor to adjust home range size estimates by multiplying the original home range area with the correction factor fraction (Table 2 and Supplemental Figure S2).
Nonlinear regression functions were used to visualize and define home range size classes. These functions were calculated separately for each species’ dataset, and then visualized together on a single axis in log-scale (Figure 2). We did not observe distinct groupings of home range size in the aggregated plot, so we defined four home range size classes by order of magnitude, as the following: ‘localized’ (0–10,000 km2), ‘intermediate’ (10,000–100,000 km2), ‘large’ (100,000–1M km2), and ‘vast’ (> 1M km2). Final home ranges, and final core areas, were calculated for all datasets using a grid size defined by the home range size class of each dataset. We used 5, 10, 25, and 50 km2 grids for ‘localized’, ‘intermediate’, ‘large’, and ‘vast’ home range size classes, respectively. One exception was made for Pacific leatherback sea turtles (Dermochelys coricea) which required a larger grid size (75 km2) than all other species because of their expansive, trans-ocean basin movements.
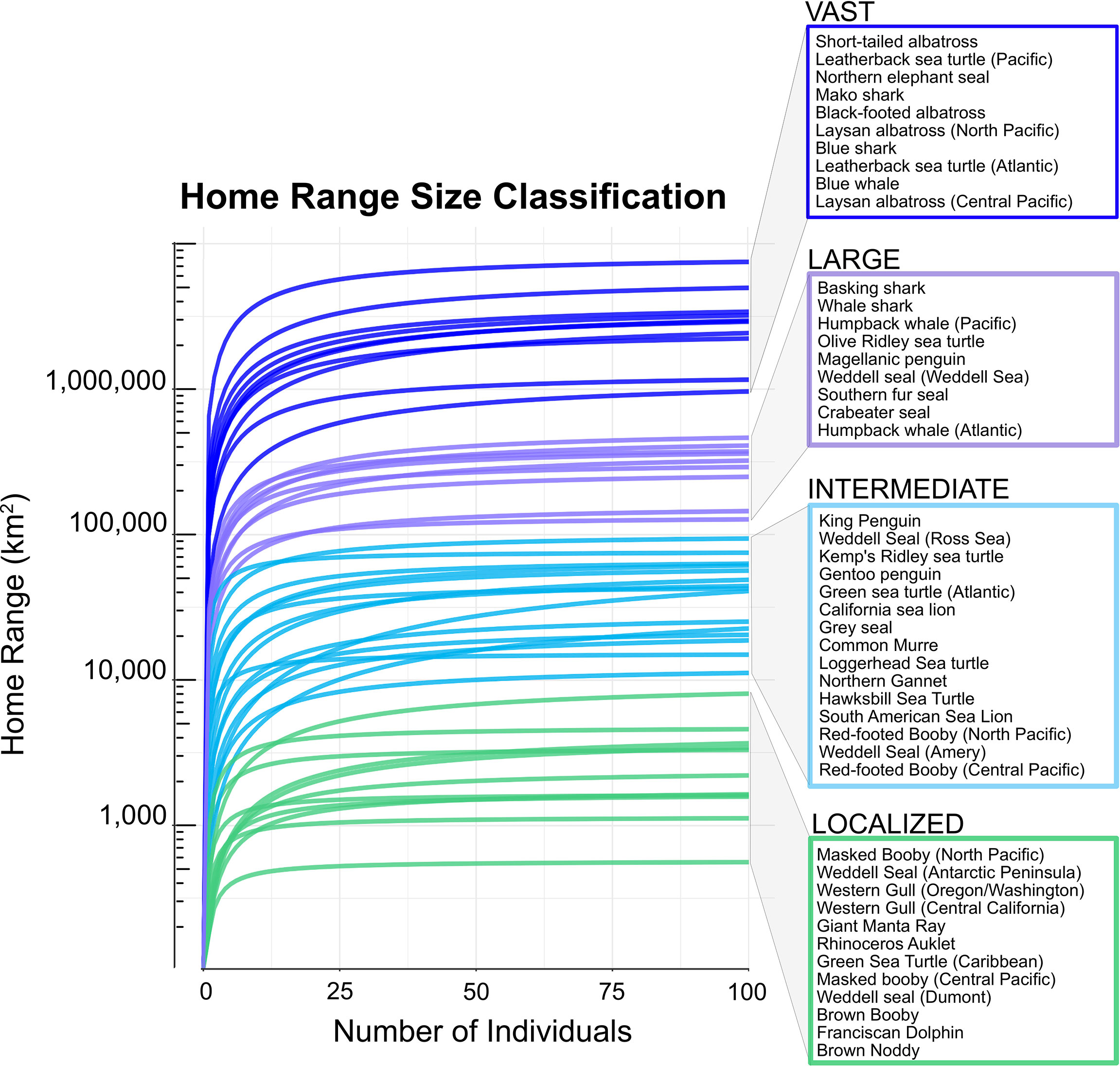
Figure 2 Species home ranges (HRs) were classified into one of four home range size-classes based on the asymptotic area of their sampling adequacy curve: ‘localized’ (100<HR<10,000), ‘intermediate’ (10,000<HR<100,000), ‘large’ (100,000<HR<1,000,000), or ‘vast’ (HR >1,000,000). Note log scale on the y-axis.
Spatial scales of global MPAs and highly mobile marine megafauna
MPA boundaries and metadata were sourced from the ‘Atlas of Marine Protection’ database (www.mpatlas.org) maintained by Marine Conservation Institute, accessed June 2018. Although this database is derived from the Protected Planet database at the United Nations Environment World Conservation Monitoring Centre, it is specific to marine protected areas and subjected to more rigorous quality control than the Protected Planet database (www.protectedplanet.net) in determining if areas meet IUCN guidance for an MPA (Morgan et al., 2018). We limited our dataset to those MPAs defined as ‘designated’ and ‘implemented’ by the ‘Atlas of Marine Protection’ (Morgan et al., 2018). Boundaries of current MPAs less than 100 km2 in size were excluded from analyses because 100 km2 was smaller than the smallest core area across all species (brown noddies: 191 km2) and would have negligible impacts on results, and because the vast majority of MPAs were < 100km2, a spatial scale minimally relevant to that of highly mobile marine megafauna core and home ranges. We acknowledge that MPAs even as small as < 100km2 may influence megafauna protection, particularly those that may target specific critical threats within populations that occur on smaller spatial scales; however, our broader-scale objectives required a focus on larger MPA boundaries. The boundaries of MPAs that were contained within another MPA, or had a boundary immediately adjacent to another MPA, were merged, so that one size was calculated for contiguous MPAs. This resulted in 456 discrete MPA polygons for analysis. To conceptualize the global congruence between MPA size and marine megafauna space use, binned distributions of global MPA sizes (km2) were used to quantify the percentage of MPAs large enough to encompass home ranges of highly mobile marine megafauna in each home range size class.
Overlap of megafauna community aggregate home ranges with MPA networks
To evaluate overlap between megafauna communities and MPAs in real space and time, we isolated three regions in our dataset where communities of tracked megafauna species co-occurred with networks of MPAs: (i) the U.S. West Coast National Marine Sanctuary complex (USNMS) where MPAs overlapped with 12 tagged species); (ii) the confluence of the Gulf of Mexico and the Caribbean Sea (GOM) where MPAs overlapped with 6 tagged species; and (iii) the Pacific Remote Islands (PRI) where MPAs overlapped with 6 tagged species. In these regions, a community-aggregate UD was calculated from merged tracking data following methods of Maxwell et al. (2013) and overlap between individual and community-aggregate UDs and MPA boundaries was calculated. To define core areas of species aggregates, we used the 50% UD contour since individual species display different distribution patterns and core area UD thresholds calculated within individual species varied. We summed the UDs within each cell across all species, and then normalized the summed layer by maximum UD values across all cells. UDs were weighted by multiplying the grid value by the number of species that occurred in each cell to emphasize regions of greater species overlap. We then calculated the percentage of home range and core areas that occurred within and outside the boundaries of any MPA that overlapped with the range of the species aggregate.
The influence of the annual cycle and Life history on spatial scales of protection
To evaluate the influence of seasonal cycles and discrete population subsectors (e.g., sex and age classes) on megafauna movement patterns, we targeted a subset of datasets (n=14 species) that represented multiple taxa and that contained sufficient tracking data from enough individuals across population subsectors or seasonal cycles (Supplemental Table S2). For central place foragers (albatrosses, gulls, auklets, penguins, sea lions) we identified individuals with tracking data from different parts of the breeding cycle (e.g. incubation, brood-guard, chick-rearing, post-breeding in albatrosses). A single central-place forager dataset (sooty shearwater) was composed of tracking data from individuals that spanned an entire annual cycle: breeding (Nov–Mar), the spring migration (Apr-May), moulting/post-breeding grounds (May–Sep), and the fall migration (Sep–Oct). For non-central-place foragers (sea turtles, elephant seals, and whales), the metrics ‘residence time’ and ‘day of year’ were used to determine nesting/interesting movements (sea turtles only) versus migrating movements (low residence time) versus foraging movements (high residence time). Residence time is a scale-dependent metric that imposes a virtual circle with a user-defined radius over each consecutive location and sums the time spent along all track segments within the circle, both forward and backward (Barraquand and Benhamou, 2008). Residence time was calculated using a 50 km radius and a maximum time outside the circle of 24 hours. Tracks were interpolated to 1 point a day and transformed to a Lambert’s Equal Area projection before residence time analysis. For elephant seals that forage continuously along their migrations to their core feeding grounds (Robinson et al., 2010; Robinson et al., 2012), ‘high’ residence time was considered to be the top 30th percentile of all residence time values; for whales and turtles that have a more discrete migration before exhibiting more localized movements, ‘high’ residence time was considered to be the top 50th percentile of all residence time values. Once datasets were divided into different life history segments, we ran the gridded utilization distribution analysis to determine home range and core area of species under different sex, age, and seasonal conditions. For all species, except for sooty shearwaters (due to a spatial resolution limitation), we then calculated the percentage of current MPAs that were theoretically large enough to encompass the core area of a population across different life history conditions. For all but sooty shearwaters, core areas were used to highlight the times and places where marine megafauna distributions aggregate and are thus, in theory, more feasible to protect spatially. Individual home ranges, rather than core areas, were used in the sooty shearwater case study to emphasize dramatic seasonal changes in the overall space use of individuals.
Results
Animal tracking data and home ranges
In total, our study included 1,709,042 raw locations from 1,943 individuals and 36 species in 43 population-level datasets, ranging from 78°S to 66°N latitude and over 220 degrees of longitude (Figure 1, Table 1). Seabirds were the most extensively represented taxa (15 species; 919 individuals; average track length: 40 d ± 79), followed by pinnipeds (7 species; 507 individuals; average track length: 108 d ± 72), sea turtles (6 species; 218 individuals; average track length: 279 d ± 156), elasmobranchs (5 species; 221 individuals; average track length: 166 d ± 126), and cetaceans (3 species; 78 individuals; average track length: 75 d ± 34). Estimated megafauna home ranges varied from <1,000 km2 for breeding brown noddies (Anous stolidus) to just over 12,000,000 km2 for Pacific leatherback sea turtles (Dermochelys coriacea) (Table 2).
Spatial scales of global MPAs and highly mobile marine megafauna
Less than 1% of all existing MPAs were large-scale MPAs (>100,000 km2). Indeed, the vast majority (n=8,788, 86.8%) were considered too small (< 100 km2) to be relevant to the scale of mobile marine megafauna ranges and were subsequently excluded. Our final MPA dataset of 456 MPA boundaries ranging in size from 100 km2 (Pensacola Bay, USA) to 1,513,723 km2 (Ross Sea Protected Area, Antarctica), with a median size (5th percentile, 95th percentile) of 727 km2 (130, 93626). After excluding MPAs < 100 km2, 43% of MPAs were large enough to encompass localized species ranges while only 12, 5, and 1% were large enough to encompass the home ranges of intermediate-, large-, and vast-ranging species, respectively (Figure 3). Home ranges of megafauna community aggregates overlapped minimally (< 5%) with their associated MPA networks. Home range overlap was <1, 4, and 1%, for the USNMS, GOM, and PRI, respectively (Figure 4). These small levels of overlap were primarily driven by the occurrence of large- and vast-ranging species in each region. While community-level MPA overlap was low, MPA overlap of many individual species was high (Figure 4 inlaid barplot, Table 2).
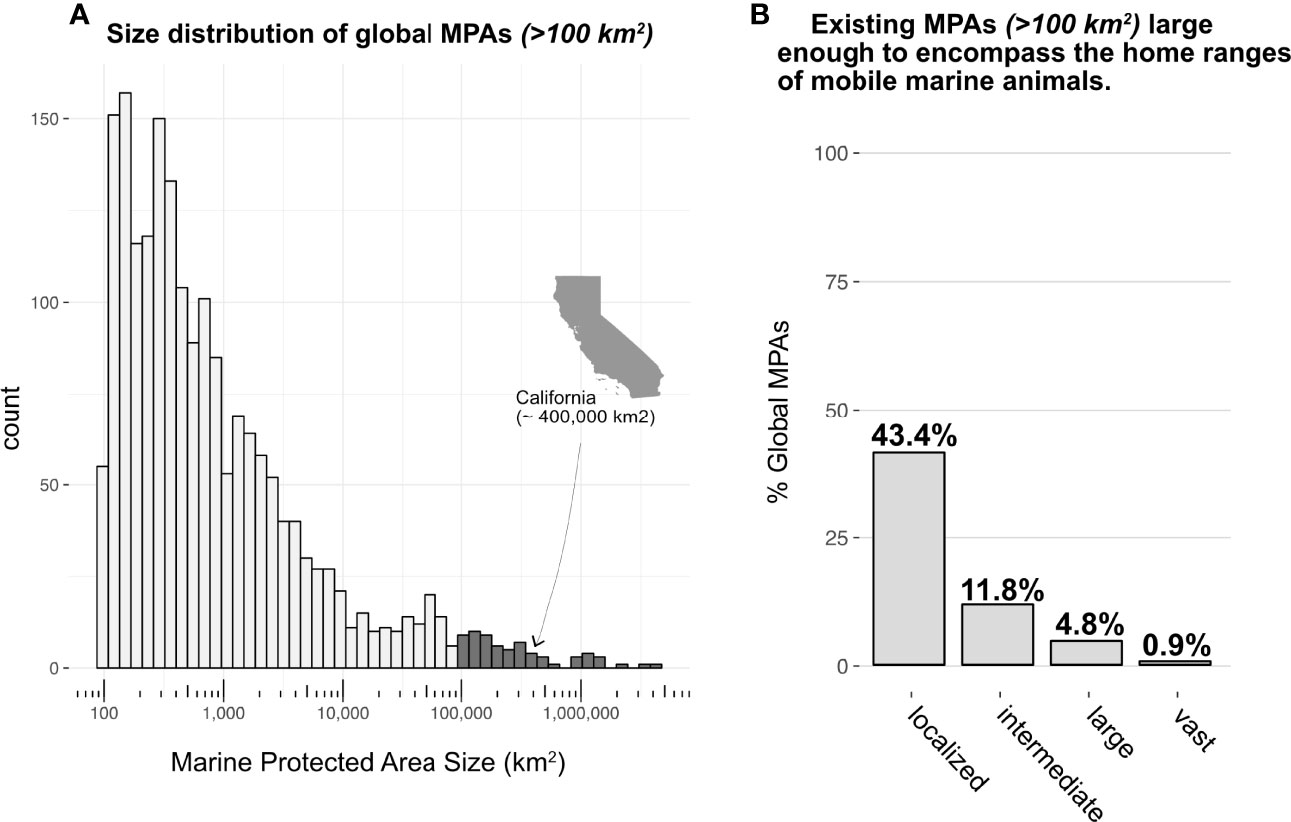
Figure 3 Size distributions of existing marine protected areas relative to home range sizes of mobile marine species. Less than 1% of all existing MPAs of all sizes (including those < 100 km2 – not shown in histogram) can be classified as large MPAs (greater than 100,000 km2, dark grey shading, (A); California is included as a point of reference for size for large MPAs. Excluding MPAs that are less than 100 km2, just over 43% of remaining MPAs were large enough to encompass the home ranges of ‘localized’ species, but MPA coverage dropped off rapidly for species with ‘intermediate’, ‘large’, and ‘vast’ home ranges (B).
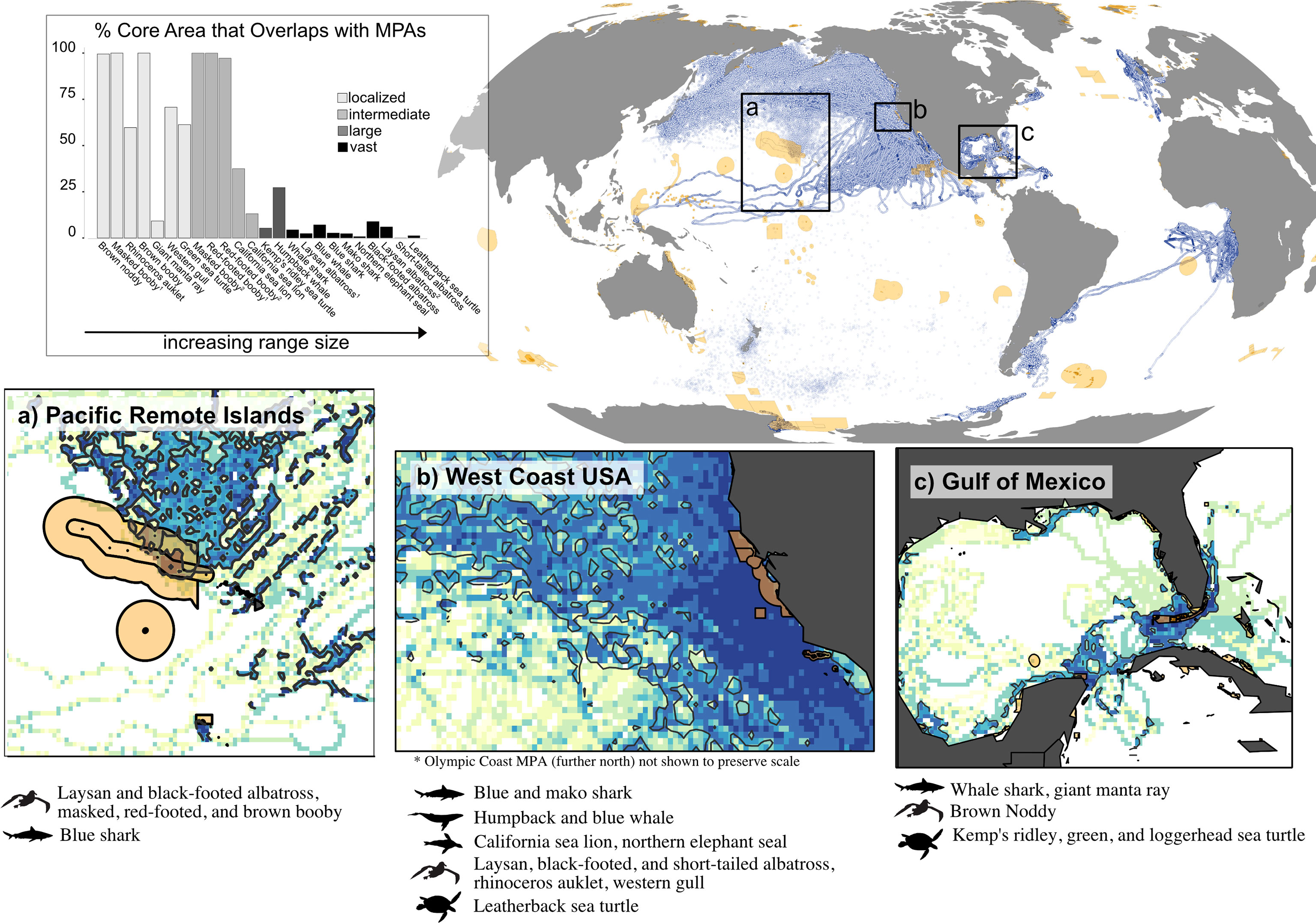
Figure 4 Global distribution of MPAs greater than 100 km2 (orange polygons) and animal tracking datasets (blue points). Insets (A-C) display regions that contained overlap of MPAs and the home ranges of at least five species. For these regions, gridded utilization distributions (UDs; shown in insets) were calculated on a normalized species aggregate; contours of the core UDs of the species aggregate are outlined in black. The inlaid barplot demonstrates a sharp decrease in coverage of mobile marine taxa with increasing home range size, particularly when transitioning from ‘intermediate’ to ‘large’ home range area. Species whose ranges did not overlap with existing MPAs in the case study regions are not included.
The influence of the annual cycle and life history on spatial scales of protection
Movement patterns often changed markedly with season, sex, and life stage in some cases resulting in marked expansion or contraction of home range size (Figure 5, Supplemental Table S2). The most pronounced change in the congruence of spatial scales of MPAs and home ranges was that for olive ridley sea turtles (Lepidochelys olivacea), where > 50% of existing MPAs were large enough to encompass turtle core areas during nesting, compared to only 5% of MPAs during migration and only 8% while turtles were on their feeding grounds. The influence of life stage, season, and sex on other datasets was highly variable, and in general, less pronounced than seen in olive ridley sea turtles. For some species (e.g., elephant seals, short-tailed albatross), the potential for spatial protection through MPAs remained low across sexes, seasons, and life stages (Figure 5).
Discussion
MPAs and marine megafauna conservation at scale
Despite the recent global increase in large MPAs, there remains a considerable mismatch between the size of marine areas used by megafauna species and the size of marine areas managed for biodiversity protection. For these highly mobile species, size does indeed matter; however, MPA size quickly hits a ceiling of what is practical and affordable to implement. For example, in the Papahānaumokuākea Marine National Monument (PMNM), one of the largest MPAs worldwide, the home ranges of three intermediate-ranged breeding seabird species were nearly fully enclosed in protected waters (Figure 4 inlaid barplot, Table 2).
Nonetheless, this expansive MPA with a footprint greater than 1.5 million km2, remained too small to provide much at-sea protection for the two vast-ranging albatross species that breed there, despite protection of critical seabird habitat being cited as part of the designation of this MPA (Executive Office of the US President, 2016).
While this would seem to bode poorly for the utility of MPAs in the conservation and management of megafauna, more nuanced considerations suggest that MPAs can have an important role. For example, while PMNM alone did not provide significant at-sea protection for black-footed albatross, this species was additionally found in the West Coast National Marine Sanctuary network, an important foraging destination, which includes some protections for seabirds as part of its regulations (NOAA, 2008). These MPAs are separated by half an ocean but combined they overlapped with 11.8% of the albatross’s total home range, and 15.0% of their core area, a sizable amount for a species with a total home range of over 4 million km2 (Table 2). Despite this, many black-footed albatross breeding populations additionally depend on habitats that fall under the sovereignty of Japan and Russia, as well as international waters, exemplifying that cooperation within and among nations may be necessary for successful conservation outcomes for some wide-ranging species (Harrison et al., 2018; Beal et al., 2021; Davies et al., 2021a).
Not all marine megafauna move at this basin-level scale, however, and over 40% of existing MPAs would be large enough to encompass the entire home ranges of localized species, for example masked boobies (Sula dactylatra) and Franciscana dolphins (Pontoporia blainvillei). This suggests that with proper placement and appropriate management measures, single, ‘average-sized’ MPAs could still play an important role in the conservation of some megafauna populations. Indeed, developing and enforcing protected areas and no-take zones are among the priority actions in the International Whaling Commission’s conservation management plan for Franciscana dolphins (IWC, 2016). Furthermore, the potential for comprehensive inclusion in protected MPA waters isn’t wholly limited to localized populations. Nearly 12% of existing MPAs are large enough to encompass the home ranges of intermediate-ranged species and there are some MPAs large enough encompass the home ranges of even large-and vast-ranged species (Figure 3). However, a clear incongruence in the sizes of megafauna home range and existing MPAs becomes plainly evident around spatial scales greater than 10,000 km2.
Placement and connectivity of MPAs for marine megafauna
Placement of MPAs is critical for their effectiveness; and, when done effectively, MPAs can support even large-ranged species. For example, U.S. National Marine Sanctuaries encompassed nearly 30% of the core area of large-ranged humpback whales (Megaptera novaeangliae) in stark contrast to large-ranged Kemp’s ridley sea turtles (L. kempii), whose core areas overlapped less than 5% with the MPA network in the Gulf of Mexico (Figure 4). Even with suitable placement, potential for overlap with MPAs may remain minimal for those species with vast ranges. For example, movements of Pacific blue whales (Balaenoptera musculus) paralleled the west coast of the US, as did nearby MPAs, but their core areas only overlapped with these MPAs by only 7% (Table 2). Thus, even for species with movement patterns amenable to protection (e.g., those that parallel coastlines and thus are within national waters), the spatial scale of their movements – even core areas – may be well beyond that of most available MPAs, though international collaboration may aid in protection.
This underscores the importance of identifying critical habitats throughout annual cycles and understanding migratory connectivity of populations for effective area-based conservation measures for marine megafauna (e.g., Dunn et al., 2019). Networks of MPAs have been shown to be effective for less mobile species, particularly fishes (Gaines et al., 2010), and may similarly prove effective for megafauna because many, if not most, large- and vast-ranged species aggregate seasonally and by life stage, sex, or season, and these aggregations that occur at smaller spatial scales have greater potential to benefit from MPAs (Hooker et al., 2011; Carneiro et al., 2020). For instance, the average individual home range of sooty shearwaters was 80% smaller during the vulnerable moulting period and 50% smaller during the breeding period than during peak trans-equatorial migrations (Figure 5D). Spatial protection can be particularly effective for central-place foragers that return repeatedly to the same location (Table 2) (Young et al., 2015; Maxwell et al., 2016; Handley et al., 2020; Gilmour et al., 2022). Across taxa, ranges typically contracted during breeding seasons (Figure 5A), increasing the capacity for spatial protection during this critical life stage. For example, the highly restricted ranges of nesting sea turtles are in strong contrast to their long post-breeding migratory journeys (Witt et al., 2011; Dawson et al., 2017). Indeed, over half the core area of nesting olive ridley turtles in this study overlapped with MPAs in Gabonese waters, likely a direct result of both their smaller ranges during this life stage and that their tracking data was explicitly incorporated into the MPA siting process with the Gabonese government (Maxwell et al., 2011; Dawson et al., 2017; Hays et al., 2019; Metcalfe et al., 2022). Conservation impacts from MPAs may compound when there is potential to protect multiple species during critical life history stages, such as in the Gulf of Mexico where loggerhead (Caretta caretta), green (Chelonia mydas), and hawksbill sea turtles (Eretmochelys imbricata) were found to select for areas within protected boundaries while transiting and foraging, despite having alternate habitat available to them (Roberts et al., 2021).
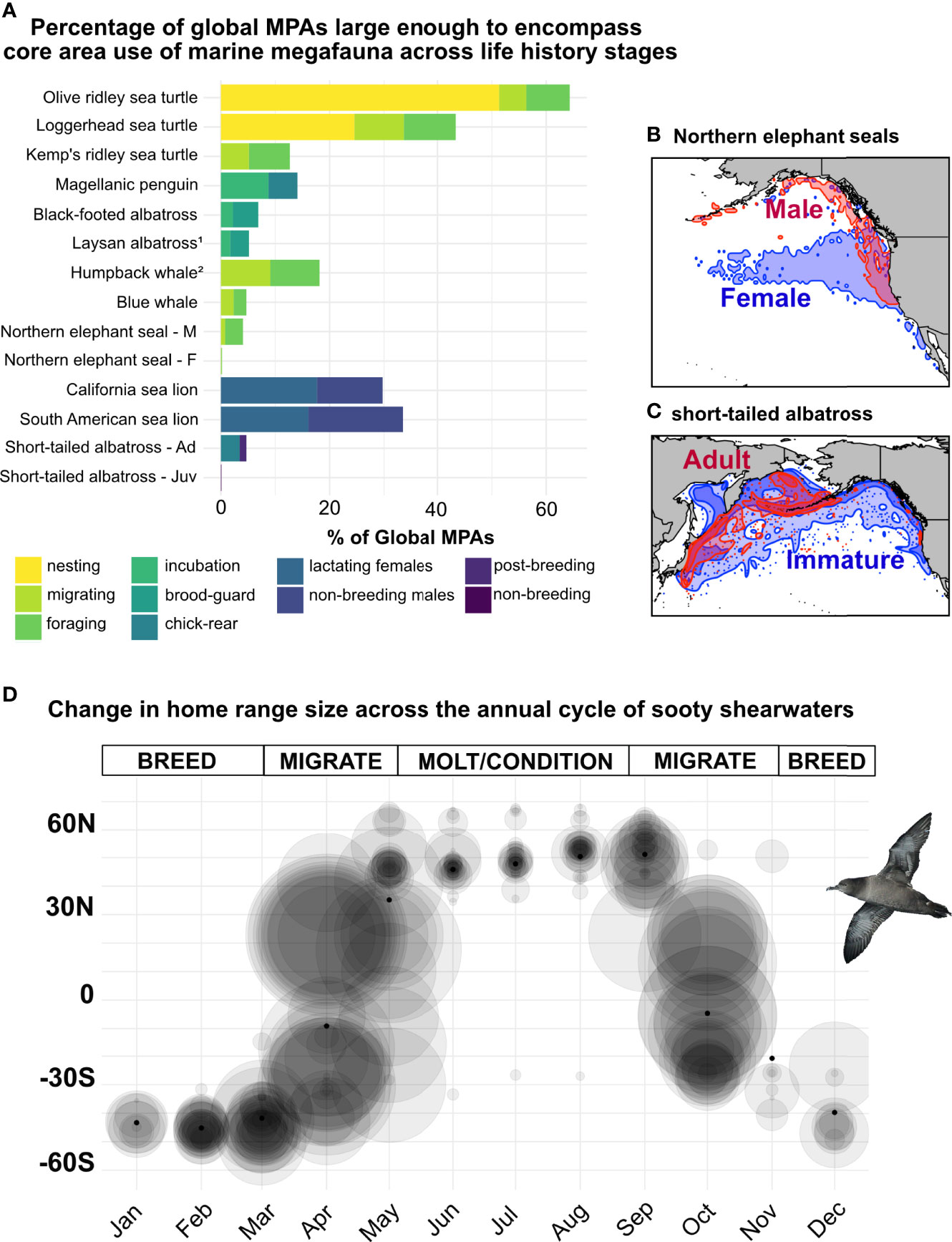
Figure 5 Tracking datasets from fifteen species demonstrate how the size distribution of current MPAs relates to the potential spatial protection of marine megafauna species under different sex, age, and seasonal contexts (panel A). Male northern elephant seals strictly forage along the northeast perimeter of the North Pacific Ocean, along the continental shelf, while females primarily target the pelagic North Pacific Transition Zone, far offshore (panel B). Immature short-tailed albatrosses are far more pelagic than their adult conspecifics, resulting in larger home range and core use areas (panel C). The annual cycle of sooty shearwaters includes vast trans-equatorial migrations in which birds travel from breeding grounds in the high latitudes of the south Pacific to their foraging and molting grounds in high latitudes of the North Pacific in the boreal summer (panel D) (Shaffer et al., 2006). Individual shearwater home ranges are plotted, by month, as a transparent grey circle whose radius was scaled to home range size; each individual home range circle was plotted monthly with their position on the y-axis displaying the average monthly latitude of that individual. Mean latitude of all shearwaters was overlaid on each month as a black dot. 1 Tagging Site, Tern Island; 2 Tagging Site, West Coast USA.
Similarly, age-classes and sexes within populations may also translate into varying capacity for area-based conservation. This may be particularly relevant to conservation planning when sub-sectors act as bottlenecks in population recovery or stability (Crouse et al., 1987). For example, male northern elephant seals (Mirounga angustirostris) primarily ranged along the continental shelf, close to coastal waters, and thus may be easier to protect through area-based conservation measures than their highly pelagic female counterparts (Figure 5B) (Robinson et al., 2012; Kienle et al., 2022). Similarly, adult short-tailed albatrosses (P. albatrus) formed dense aggregations around the continental shelf year-round, while juveniles ranged transiently across the entire North Pacific basin (Figure 5C) (Suryan et al., 2007; Orben et al., 2018). Despite relatively smaller ranges of male seals and adult albatross than their intra-specific counterparts, the spatial scale of these populations still far exceeded that of the majority of existing MPAs (Figure 5A). For many vast-ranged populations, sector-based conservation measures, such as bycatch mitigation policies or increasing prey stocks through sustainable fisheries management, may have a greater impact than area-based measures.
Conservation measures for marine megafauna: Beyond MPAs
A critical consideration is the level of protection afforded by MPAs, as well as what threats they limit, as MPAs vary widely in the protections they offer or enhanced. For example, in the US, 96% of the largest MPAs are highly protected meaning they limit extractive activities, however these occur almost exclusively in remote Pacific regions where extractive activities were limited to begin with (Sullivan-Stack et al., 2022). Additionally, in some cases, populations of the species studied herein are increasing (e.g., northern elephant seals), despite mismatches in scale of MPA protections, suggesting that other, non-MPA management interventions are positively impacting their recoveries. For those species for which area-based measures are a tractable option, MPAs may not confer benefits if they don’t (or can’t) effectively address key threats to the population. For example, ship strikes are a primary threat to many baleen whale populations, but even highly protected MPAs rarely exclude shipping transport (Wiley et al., 2011), though modifications to shipping lanes or ship speeds have occurred within MPAs (Freedman et al., 2017). Pollution (e.g., plastics, persistent organic pollutants, ghost nets, noise) is another key threat to many marine megafauna populations (Maxwell et al., 2013; Nelms et al., 2021), but the diffusive nature of pollutants often belies MPA boundaries. Despite limitations in the protective capacity of MPAs and the reality that their benefits will vary across species and regions, area-based conservation measures, including MPAs, have been repeatedly identified as a key strategy for the protection of all marine megafauna taxa presented here (e.g. Scott et al., 2012; Lascelles et al., 2014; Gallagher et al., 2020; Handley et al., 2021; Nelms et al., 2021). As larger MPAs become increasingly common and established, it will become critical to quantify explicit population-level impacts of these MPAs on marine megafauna, as has been done for less mobile species (e.g., Halpern, 2003). This currently remains challenging given the extreme longevities, low intrinsic rates of increase, and a host of issues in effective sampling and population estimation unique to highly mobile megafauna. However, if MPAs provide mechanisms to reduce human impacts and/or strengthen the prey base used by megafauna, a population-level impact is likely. The most effective strategy for most highly mobile marine megafauna species may be a combination of traditional area-based conservation measures (e.g., MPAs) with alternative strategies, such as those that are sector-based and target specific threats across space (e.g., bycatch mitigation techniques, rerouting shipping lanes). Complicating matters, effects of climate change on megafauna distributions and habitat may necessitate an adaptive approach to MPA boundaries through time (Bruno et al., 2018). Dynamic management (Maxwell et al., 2015) or mobile MPAs (Maxwell et al., 2020) may prove to be particularly effective in buffering effects of climate change for marine megafauna, particularly those that require large, dynamic habitats, such as the high seas.
Conclusion
Tracking data, such as those used here, have advanced megafauna conservation around the world, including in the creation of MPAs (Hays et al., 2019). Using tracking data to identify areas of significant importance to multi-species megafauna assemblages in the high seas [e.g., the North Atlantic Current and Evlanov Seamount proposed MPA (Davies et al., 2021b); Areas of Ecological Significance in the Southern Ocean Ecosystem (Hindell et al., 2020)] is becoming more commonplace as data across megafauna species accumulates over time and across regions (Sequeira et al., 2019). Given the wide-ranging nature of most marine megafauna species, a first step for managers and decision-makers in making management decisions to protect marine megafauna is to gather datasets that link spatial data on animal ranges with existing metadata on colony, sex, age, and life stage (Carneiro et al., 2020). Though gathering datasets with this level of detail can be a challenge, large, global databases of aggregated tracking data are multiplying [e.g., Movebank (ca. 2007), MegaMove (ca. 2021)] along with repeated calls for establishing a standardized framework for the collection and aggregation of tracking data to optimize conservation gains (Campbell et al., 2016; Sequeira et al., 2021). It is important to recognize that in this study, our tracking dataset was not exhaustive and datasets were selected based on availability and on those that, together, represented the range of movement patterns observed across marine megafauna taxa; thus, not all regions or life history stages are represented for all species and taxa. Furthermore, the species and MPAs in this study reflect a diversity of marine systems, human impacts, and conservation strategies; in some cases, MPAs were established with megafauna in mind using the tracking data herein to design MPAs (e.g., Metcalfe et al., 2022), while in other cases megafauna were not included in the planning or justification of MPAs. Our objective was not to consider specific conservation goals of individual MPAs and region/species-specific threats, but rather to: 1) evaluate the agreement or mismatch of spatial scales between global MPAs and those of highly mobile marine megafauna; and, 2) to highlight spatial considerations needed for area-based conservation measures to effectively support highly mobile marine megafauna. Despite some evidence that even large- and vast-ranged species could benefit from MPAs when properly sized, placed, and networked, these findings underscore that MPAs, as currently designed, may not be effective for many megafauna species by virtue of just existing, even if deliberately large. This point is underscored by Mason et al. (2018) who demonstrated that large MPAs in Australia did not necessarily protect critically-endangered albatrosses simply as a result of being large, and rather, performed more poorly at protecting critical habitat than those located randomly.
Data availability statement
All major scripts, functions, utilization distributions (core and home range) for all species and MPA shapefiles used in this study will be available online at https://github.com/melindaconners/megafauna_mpas. Most of the raw tracking datasets are archived in public data repositories and available by request; see Supplementary Table S1 for database location and dataset IDs. Tracking data will not be made public for some species to protect sensitive information (e.g., species that could be exploited by use of the fine-scale resolution of tracking data), however the utilization distributions for these species are still provided.
Ethics statement
The animal study was reviewed and approved by relevant ethics committee for individual studies of datasets included.
Author contributions
MC conducted analyses and led manuscript creation; MC and SM conceived and executed the study; NS uploaded datasets to various data repositories. All authors contributed data and contributed to the writing and editing of the manuscript.
Funding
This work was funded by a grant from Pew Charitable Trusts, with additional funding from a fellowship from the Alfred P Sloan Foundation awarded to SMM. Individual data sets were funded by the Busch Gardens Sea World Conservation Fund, Old Dominion University, Tagging of Pacific Predators program, Achievement Rewards for College Scientists, European Association of Zoos and Aquaria (EAZA) ShellShock Campaign, the Department for Environment Food and Rural Affairs (Defra) Darwin Initiative, Natural Environment Research Council (NERC), Disney Conservation Fund, Tullow Oil Inc, Natural Sciences and Engineering Research Council (NSERC) of Canada, Canadian Foundation for Innovation, Department of Fisheries and Oceans, Canada, Moore, Packard, and Sloan Foundations, Office of Naval Research, NOAA-National Marine Fisheries Service, National Park Service, U.S. Geological Survey, Point Blue’s contribution and support for rhinoceros auklet and western gull research, National Geographic Society, California State University Council on Ocean Affairs, Science, and Technology, Myers Oceanographic and Marine Biology Trust, Scottish Natural Heritage, Marine Conservation Society UK, Natural England, National Fish and Wildlife Foundation, US Fish and Wildlife Service, Japan Ministry of Environment, North Pacific Research Board, Japan Ministry of Education, Culture, Sports, Science and Technology, Suntory Fund for Bird Conservation, Asahi Newspaper Company, Mitsui & Co., Ltd. Environmental Fund, The National Science Foundation, the Wildlife Conservation Society, Inter-American Institute for Global Change Research (IAI), Bureau of Ocean and Energy Management, and donors to the Oregon State University Endowed Marine Mammal Institute and donors Wildlife Conservation Society. The scientific results and conclusions, as well as any views or opinions expressed herein, are those of the author(s) and the U.S. Geological Survey but do not necessarily reflect those of NOAA or the Department of Commerce. Any use of trade, firm, or product names is for descriptive purposes only and does not imply endorsement by the U.S. Government.
Conflict of interest
The authors declare that the research was conducted in the absence of any commercial or financial relationships that could be construed as a potential conflict of interest.
Publisher’s note
All claims expressed in this article are solely those of the authors and do not necessarily represent those of their affiliated organizations, or those of the publisher, the editors and the reviewers. Any product that may be evaluated in this article, or claim that may be made by its manufacturer, is not guaranteed or endorsed by the publisher.
Acknowledgments
The authors thank multiple reviewers for suggestions leading to the improvement of this manuscript. We also thank James Estes for reading and editing earlier drafts of this work. We are grateful for the multitudes of field assistants providing support for deploying and recovering tracking devices in the field, as well as the multiple state and federal agencies involved in permitting research activities leading to these datasets.
Supplementary material
The Supplementary Material for this article can be found online at: https://www.frontiersin.org/articles/10.3389/fmars.2022.897104/full#supplementary-material.
References
Agardy T., Di Sciara G. N., Christie P. (2011). Mind the gap: addressing the shortcomings of marine protected areas through large scale marine spatial planning. Mar. Policy 35, 226–232. doi: 10.1016/j.marpol.2010.10.006
Allen R., Cromwell B., Forsman L., Ramirez D., Andres-Maltais C., Franklin R., et al. (2021) Tribal leader statement on 30x30 proposed policy. Available at: https://nativephilanthropy.org/wp-content/uploads/2021/05/FINAL-Tribal-Leader30x30-Statement-5.6.21.pdf.
Ban N. C., Davies T. E., Aguilera S. E., Brooks C., Cox M., Epstein G., et al. (2017). Social and ecological effectiveness of large marine protected areas. Global Environ. Change 43, 82–91. doi: 10.1016/j.gloenvcha.2017.01.003
Barraquand F., Benhamou S. (2008). Animal movements in heterogeneous landscapes: identifying profitable places and homogeneous movement bouts. Ecology 89, 3336–3348. doi: 10.1890/08-0162.1
Beal M., Dias M. P., Phillips R. A., Oppel S., Hazin C., Pearmain E. J., et al. (2021). Global political responsibility for the conservation of albatrosses and large petrels. Sci. Adv. 7, eabd7225. doi: 10.1126/sciadv.abd7225
Bivand R. (2018). Rundel C. rgeos: Interface to Geometry Engine-Open Source (GEOS). 2016. R package version 0.3–4.
Bivand R. S., Lewin-Koh N. (2017). Maptools: Tools for reading and handling spatial objects. R package V. 0.8-41.
Block B. A., Jonsen I. D., Jorgensen S. J., Winship A. J., Shaffer S. A., Bograd S. J., et al. (2011). Tracking apex marine predator movements in a dynamic ocean. Nature 475, 86–90. doi: 10.1038/nature10082
Bruno J. F., Bates A. E., Cacciapaglia C., Pike E. P., Amstrup S. C., Van Hooidonk R., et al. (2018). Climate change threatens the world’s marine protected areas. Nat. Climate Change 8, 499–503. doi: 10.1038/s41558-018-0149-2
Calenge C. (2012) Package ‘“adehabitatLT”‘for the R software: Analysis of animal movements. Available at: https://www.faunalia.it/animove/trac.
Calenge C. (2006)The package adehabitat for the R software: a tool for the analysis of space and habitat use by animals. Ecological Modelling, 197, 516–519
Campbell H. A., Urbano F., Davidson S., Dettki H., Cagnacci F. (2016). A plea for standards in reporting data collected by animal-borne electronic devices. Anim. Biotelemetry 4, 1. doi: 10.1186/s40317-015-0096-x
Carman V. G., Machain N., Campagna C. (2015). Legal and institutional tools to mitigate plastic pollution affecting marine species: argentina as a case study. Mar. pollut. Bull. 92, 125–133. doi: 10.1016/j.marpolbul.2014.12.047.
Carneiro A. P. B., Pearmain E. J., Oppel S., Clay T. A., Phillips R. A., Bonnet-Lebrun A., et al. (2020). A framework for mapping the distribution of seabirds by integrating tracking, demography and phenology. J. Appl. Ecol. 57, 514–525. doi: 10.1111/1365-2664.13568
Commission for the Conservation of Antarctic Marine Living Resources (2016). Ross sea region marine protected area. Conservation measure.
Crouse D. T., Crowder L. B., Caswell H. (1987). A stage-based population model for loggerhead sea turtles and implications for conservation. Ecology 68, 1412–1423. doi: 10.2307/1939225
Davies T. E., Carneiro A. P. B., Campos B., Hazin C., Dunn D. C., Gjerde K. M., et al. (2021a). Tracking data and the conservation of the high seas: opportunities and challenges. J. Appl. Ecol. doi: 10.1111/1365-2664.14032
Davies T. E., Carneiro A. P. B., Tarzia M., Wakefield E., Hennicke J. C., Frederiksen M., et al. (2021b). Multispecies tracking reveals a major seabird hotspot in the North Atlantic. Conserv. Lett. 14. 58, 2703–2710. doi: 10.1111/conl.12824
Dawson T. M., Formia A., Agamboue P. D., Asseko G. M., Boussamba F., Cardiec F., et al. (2017). Informing marine protected area designation and management for nesting olive ridley sea turtles using satellite tracking. Front. Mar. Sci. 4, 312. doi: 10.3389/fmars.2017.00312
Dirzo R., Young H. S., Galetti M., Ceballos G., Isaac N. J. B., Collen B. (2014). Defaunation in the anthropocene. Science 401, 401–406. doi: 10.1126/science.1251817
Doherty P. D., Baxter J. M., Godley B. J., Graham R. T., Hall G., Hall J., et al. (2017). Testing the boundaries: Seasonal residency and inter-annual site fidelity of basking sharks in a proposed marine protected area. Biol. Conserv. 209, 68–75. doi: 10.1016/j.biocon.2017.01.018
Dujon A. M., Lindstrom R. T., Hays G. C. (2014). The accuracy of fastloc-GPS locations and implications for animal tracking. Methods Ecol. Evol. 5, 1162–1169. doi: 10.1111/2041-210X.12286
Dunn D. C., Harrison A.-L., Curtice C., DeLand S., Donnelly B., Fujioka E., et al. (2019). The importance of migratory connectivity for global ocean policy. Proc. R. Soc. B 286, 20191472. doi: 10.1098/rspb.2019.1472
Estes J. A., Terborgh J., Brashares J. S., Power M. E., Berger J., Bond W. J., et al. (2011). Trophic downgrading of planet earth. Science 333, 301–306. doi: 10.1126/science.1205106
Executive Office of the US President (2014). Proclamation No. 9173: Pacific remote islands marine national monument expansion Vol.79 (Washington DC, United States, Federal register), 58645–58653.
Executive Office of the US President (2016). Proclamation No. 9478: Papahānaumokuākea marine national monument expansion Vol. 81 (Washington DC, United States, Federal register), 0227–60234.
Freedman R., Herron S., Byrd M., Birney K., Morten J., Shafritz B., et al. (2017). The effectiveness of incentivized and non-incentivized vessel speed reduction programs: Case study in the santa barbara channel. Ocean Coast. Manage. 148, 31–39. doi: 10.1016/j.ocecoaman.2017.07.013
Gaines S. D., White C., Carr M. H., Palumbi S. R. (2010). Designing marine reserve networks for both conservation and fisheries management. Proc. Natl. Acad. Sci. U.S.A. 107, 18286–18293. doi: 10.1073/pnas.0906473107
Gallagher A. J., Amon D., Bervoets T., Shipley O. N., Hammerschlag N., Sims D. W. (2020). The caribbean needs big marine protected areas. Science, 367(6479), 749–749. doi: 10.1126/science.abb0650
Game E. T., Grantham H. S., Hobday A. J., Pressey R. L., Lombard A. T., Beckley L. E., et al. (2009). Pelagic protected areas: the missing dimension in ocean conservation. Trends Ecol. Evol. 24, 360–369. doi: 10.1016/j.tree.2009.01.011
Gilmour M. E., Adams J., Block B. A., Caselle J. E., Friedlander A. M., Game E. T., et al. (2022). Evaluation of MPA designs that protect highly mobile megafauna now and under climate change scenarios. Global Ecol. Conserv. 35, e02070. doi: 10.1016/j.gecco.2022.e02070
Halpern B. S. (2003). The impact of marine reserves: Do reserves work and does reserve size matter? Ecol. Appl. 13, 117–137. doi: 10.1890/1051-0761(2003)013[0117:TIOMRD]2.0.CO;2
Handley J. M., Pearmain E. J., Oppel S., Carneiro A. P. B., Hazin C., Phillips R. A., et al. (2020). Evaluating the effectiveness of a large multi-use MPA in protecting key biodiversity areas for marine predators1–15. Divers Distrib; 26: 715–729. doi: 10.1111/ddi.13041
Handley J., Rouyer M. M., Pearmain E. J., Warwick-Evans V., Teschke K., Hinke J. T., et al. (2021). Marine important bird and biodiversity areas for penguins in Antarctica, targets for conservation action. Front. Mar. Sci. 7. doi: 10.3389/fmars.2020.602972
Harrison A.-L., Costa D. P., Winship A. J., Benson S. R., Bograd S. J., Antolos M., et al. (2018). The political biogeography of migratory marine predators. Nat. Ecol. Evol. 2, 1571–1578. doi: 10.1038/s41559-018-0646-8
Hays G. C., Bailey H., Bograd S. J., Bowen W. D., Campagna C., Carmichael R. H., et al. (2019). Translating marine animal tracking data into conservation policy and management. Trends Ecol. Evol. 34, 459–473. doi: 10.1016/j.tree.2019.01.009
Hijmans R. J., van Etten J. (2018). Raster: Geographic analysis and modeling with raster data (Austria: R Foundation for Statistical Computing).
Hilborn R. (2016). Policy: Marine biodiversity needs more than protection. Nat. News 535, 224. doi: 10.1038/535224a
Hindell M. A., Reisinger R. R., Ropert-Coudert Y., Hückstädt L. A., Trathan P. N., Bornemann H., et al. (2020). Tracking of marine predators to protect southern ocean ecosystems. Nature 580, 87–92. doi: 10.1038/s41586-020-2126-y
Hooker S. K., Cañadas A., Hyrenbach K. D., Corrigan C., Polovina J. J., Reeves R. R. (2011). Making protected area networks effective for marine top predators. Endangered Species Res. 13, 203–218. doi: 10.3354/esr00322
Hooker S. K., Gerber L. R. (2004). Marine reserves as a tool for ecosystem-based management: The potential importance of megafauna. Bioscience 54, 27–39. doi: 10.1641/0006-3568(2004)054[0027:mraatf]2.0.co;2
ICES (2011). Report of the working group on marine mammal ecology (WGMME), 21-24 February (Berlin, Germany, International Council for the Exploration of the Sea), 204 pp. ICES CM 2011/ACOM:25.
IUCN (2016). WCC-2016-Res-050-EN-Increasing marine protected area coverage for effective marine biodiversity conservation.
IWC (2016). A conservation management plan for franciscana (Pontoporia Blainvillei). IWC/66/CC11. Agenda item 7.4.1. International whaling commission.
Jefferson T., Costello M. J., Zhao Q., Lundquist C. J. (2021). Conserving threatened marine species and biodiversity requires 40% ocean protection. Biol. Conserv. 264, 109368. doi: 10.1016/j.biocon.2021.109368
Johnson D. S., London J. M., Lea M. A., Durban J. W. (2008). Continuous-time correlated random walk model for animal telemetry data. Ecology 89, 1208–1215. doi: 10.1890/07-1032.1
Jonsen I. D., Flenming J. M., Myers R. A. (2005). Robust state-space modeling of animal movement data. Ecology 86, 2874–2880. doi: 10.1890/04-1852
Kienle S. S., Friedlaender A. S., Crocker D. E., Mehta R. S., Costa D. P. (2022). Trade-offs Between Foraging Reward and Mortality Risk Drive Sex-Specific Foraging Strategies in Sexually Dimorphic Northern Elephant Seals. Royal Society open science, 9(1), 210522
Lascelles B., Notarbartolo Di Sciara G., Agardy T., Cuttelod A., Eckert S., Glowka L., et al. (2014). Migratory marine species: Their status, threats and conservation management needs. Aquat. Conservation: Mar. Freshw. Ecosyst. 24, 111–127. doi: 10.1002/aqc.2512
Lascelles B. G., Taylor P. R., Miller M. G. R., Dias M. P., Oppel S., Torres L., et al. (2016). Applying global criteria to tracking data to define important areas for marine conservation. Diversity Distributions. 22: 422–431. doi: 10.1111/ddi.12411
Lewis N., Day J. C., Wilhelm A., Wagner D., Gaymer C., Parks J., et al. (2017). Large-scale marine protected areas: guidelines for design and management (Gland, Switzerland: IUCN).
Mason C., Alderman R., McGowan J., Possingham H. P., Hobday A. J., Sumner M., et al. (2018). Telemetry reveals existing marine protected areas are worse than random for protecting the foraging habitat of threatened shy albatross (Thalassarche cauta). Divers. Distrib. 24, 1744–1755. doi: 10.1111/ddi.12830
Maxwell S. M., Breed G. A., Nickel B. A., Makanga-Bahouna J., Pemo-Makaya E., Parnell R. J., et al. (2011). Using satellite tracking to optimize protection of long-lived marine species: Olive ridley sea turtle conservation in central Africa. PLoS One 6, e19905. doi: 10.1371/journal.pone.0019905
Maxwell S. M., Conners M. G., Sisson N. B., Dawson T. M. (2016). Potential benefits and shortcomings of marine protected areas for small seabirds revealed using miniature tags. Front. Mar. Sci. 3, 264. doi: 10.3389/fmars.2016.00264
Maxwell S. M., Gjerde K. M., Conners M. G., Crowder L. B. (2020). Mobile protected areas for biodiversity on the high seas. Science 367, 252–254. DOI: 10.1126/science.aaz9327
Maxwell S. M., Hazen E. L., Bograd S. J., Halpern B. S., Breed G. A., Nickel B., et al. (2013). Cumulative human impacts on marine predators. Nat. Commun. 4, 2688. doi: 10.1038/ncomms3688
Maxwell S. M., Hazen E. L., Lewison R. L., Dunn D. C., Bailey H., Bograd S. J., et al. (2015). Dynamic ocean management: Defining and conceptualizing real-time management of the ocean. Mar. Policy 58, 42–50. doi: 10.1016/j.marpol.2015.03.014
McCauley D. J., Pinsky M. L., Palumbi S. R., Estes J. A., Joyce F. H., Warner R. R. (2015). Marine defaunation: Animal loss in the global ocean. Science 347, 1255641. doi: 10.1126/science.1255641
Metcalfe K., White L., Lee M. E., Fay J. M., Abitsi G., Parnell R. J., et al. (2022). Fulfilling global marine commitments; lessons learned from gabon. Conserv. Lett. doi: 10.1111/conl.12872
Millspaugh J. J., Marzluff J. M. (2001). “Radio-tracking and animal populations”, in Radio tracking and animal populationspast trends and future needs. In Radio tracking and animal populations (pp. 383–393). Academic Press, 383–393. doi: 10.1016/B978-012497781-5/50016-5
Morgan L., Pike E., Moffitt R. (2018). How much of the ocean is protected? Biodiversity 19, 148–151.
Nelms S. E., Alfaro-Shigueto J., Arnould J. P. Y., Avila I. C., Nash S. B., Campbell E., et al. (2021). Marine mammal conservation: Over the horizon. Endangered Species Res. 44, 291–325. doi: 10.3354/ESR01115
NOAA (2008). Gulf of the farallones national marine sanctuary regulations; Moneterey bay national marine sanctuary regulations; and Cordell Bank National Marine Sanctuary Regulations. Federal Register 73, 70488–70540.
O’Leary B. C., Ban N. C., Fernandez M., Friedlander A. M., García-Borboroglu P., Golbuu Y., et al. (2018). Addressing Criticisms of large-scale marine protected areas. Bioscience 68, 359–370. doi: 10.1093/biosci/biy021
Orben R. A., O’Connor A. J., Suryan R. M., Ozaki K., Sato F., Deguchi T. (2018). Ontogenetic changes in at-sea distributions of immature short-tailed albatrosses phoebastria albatrus. Endangered Species Res. 35, 23–37. doi: 10.3354/esr00864
Pebesma E., 2018. Simple Features for R: Standardized Support for Spatial Vector Data. The R. Journal. 10(1), 439–446, doi: 10.32614/RJ-2018-009
Phillips R. A., Silk J. R. D., Croxall J. P., Afanasyev V., Briggs D. R. (2004). Accuracy of geolocation estimates for flying seabirds. Mar. Ecol. Prog. Ser. 266, 265–272. doi: 10.3354/meps266265
Ripple W. J., Estes J. A., Beschta R. L., Wilmers C. C., Ritchie E. G., Hebblewhite M., et al. (2014). Status and ecological effects of the world’s largest carnivores. Science 343, 1241484. DOI: 10.1126/science.1241484
Roberts K. E., Smith B. J., Burkholder D., Hart K. M. (2021). Evaluating the use of marine protected areas by endangered species: A habitat selection approach. Ecol. Solutions Evidence 2, e12035 doi: 10.1002/2688-8319.12035
Robinson P. W., Costa D. P., Crocker D. E., Gallo-Reynoso J. P., Champagne C. D., Fowler M. A., et al. (2012). Foraging behavior and success of a mesopelagic predator in the northeast pacific ocean: insights from a data-rich species, the northern elephant seal. PLoS One 7, e36728. doi: 10.1371/journal.pone.0036728
Robinson P. W., Simmons S. E., Crocker D. E., Costa D. P. (2010). Measurements of foraging success in a highly pelagic marine predator, the northern elephant seal. J. Anim. Ecol. 79, 1146–1156. doi: 10.1111/j.1365-2656.2010.01735.x
Scott R., Hodgson D. J., Witt M. J., Coyne M. S., Adnyana W., Blumenthal J. M., et al. (2012). Global analysis of satellite tracking data shows that adult green turtles are significantly aggregated in marine protected areas. Global Ecol. Biogeography 21, 1053–1061. doi: 10.1111/j.1466-8238.2011.00757.x
Seaman D. E., Powell R. A. (1990). Identifying patterns and intensity of home range use. Bears: Their Biol. Manage. 8, 243. doi: 10.2307/3872925
Sequeira A. M. M., Hays G. C., Sims D. W., Eguíluz V. M., Rodríguez J. P., Heupel M. R., et al. (2019). Overhauling ocean spatial planning to improve marine megafauna conservation. Front. Mar. Sci. 6. doi: 10.3389/fmars.2019.00639
Sequeira A. M. M., O’Toole M., Keates T. R., McDonnell L. H., Braun C. D., Hoenner X., et al. (2021). A standardisation framework for bio-logging data to advance ecological research and conservation. Methods Ecol. Evol. 12, 996–1007. doi: 10.1111/2041-210X.13593
Shaffer S. A., Tremblay Y., Awkerman J. A., Henry R. W., Teo S. L. H., Anderson D. J., et al. (2005). Comparison of light- and sst-based geolocation with satellite telemetry in free-ranging albatrosses. Mar. Biol. 147, 833–843. doi: 10.1007/s00227-005-1631-8
Shaffer S. A., Tremblay Y., Weimerskirch H., Scott D., Thompson D. R., Sagar P. M., et al. (2006). Migratory shearwaters integrate oceanic resources across the Pacific Ocean in an endless summer. Proceedings of the National Academy of Sciences of the United States of America.103, 12799–12802. doi: 10.1073/pnas.0603715103
Smyth C., Hanich Q. A. (2019). Large scale marine protected areas: Current status and consideration of socio-economic dimensions (Washington D.C,Pew Charitable Trusts).
Soanes L. M., Arnould J. P. Y., Dodd S. G., Sumner M. D., Green J. A. (2013). How many seabirds do we need to track to define home-range area? J. Appl. Ecol. 50, 671–679. doi: 10.1111/1365-2664.12069
Sullivan-Stack J., Aburto-Oropeza O., Brooks C. M., Cabral R. B., Caselle J. E., Chan F., et al. (2022). a scientific synthesis of marine protected areas in the united states: status and recommendations. Front. Mar. Sci. 9. doi: 10.3389/fmars.2022.849927
Suryan R. M., Dietrich K. S., Melvin E. F., Balogh G. R., Sato F., Ozaki K. (2007). Migratory routes of short-tailed albatrosses: use of exclusive economic zones of north pacific rim countries and spatial overlap with commercial fisheries in Alaska. Biol. Conserv. 137, 450–460. doi: 10.1016/j.biocon.2007.03.015
Toonen R. J., Wilhelm T., Maxwell S. M., Wagner D., Bowen B. W., Sheppard C. R. C., et al. (2013). One size does not fit all: the emerging frontier in large-scale marine conservation. Mar. pollut. Bull. 77, 7–10. doi: 10.1016/j.marpolbul.2013.10.039
Tremblay Y., Robinson P. W., Costa D. P. (2009). A parsimonious approach to modeling animal movement data. PLoS One 4, e4711. doi: 10.1371/journal.pone.0004711
United Nations (2017). International legally binding instrument under the United Nations convention on the law of the sea on the conservation and sustainable use of marine biological diversity of areas beyond national jurisdiction. United Nations Gen. Assembly Resolution 72, 4.
Wickham H., Seidel D. (2015). Scales: Scale Functions for Visualization. R package version 1.2.0.https://CRAN.R-project.org/package=scales>.
Wickham H., Chang W., Henry L. (2018). R package ‘Ggplot2’. Create elegant data visualisations using the grammar of graphics, version 3.0. 0.
Wiley D. N., Thompson M., Pace R. M. III, Levenson J. (2011). Modeling speed restrictions to mitigate lethal collisions between ships and whales in the stellwagen bank national marine sanctuary, USA. Biol. Conserv. 144, 2377–2381. doi: 10.1016/j.biocon.2011.05.007
Wilhelm T., Sheppard C. R. C., Sheppard A. L. S., Gaymer C. F., Parks J., Wagner D., et al. (2014). Large marine protected areas–advantages and challenges of going big. Aquat. Conservation: Mar. Freshw. Ecosyst. 24, 24–30. doi: 10.1002/aqc.2499
Wilson R. P., Gremillet D., Syder J., Kierspel M. A. M., Garthe S., Weimerskirch H., et al. (2002). Remote-sensing systems and seabirds: Their use, abuse and potential for measuring marine environmental variables. Mar. Ecol. Prog. Ser. 228, 241–261. doi: 10.3354/meps228241
Witt M. J., Augowet Bonguno E., Broderick A. C., Coyne M. S., Formia A., Gibudi A., et al. (2011). Tracking leatherback turtles from the world’s largest rookery: Assessing threats across the South Atlantic. Proc. R. Soc. B: Biol. Sci. 278, 2338–2347. doi: 10.1098/rspb.2010.2467
Keywords: dynamic ocean management, home range, life history, marine predators, marine protected areas, migratory connectivity, mobile marine protected areas, pelagic conservation
Citation: Conners MG, Sisson NB, Agamboue PD, Atkinson PW, Baylis AMM, Benson SR, Block BA, Bograd SJ, Bordino P, Bowen WD, Brickle P, Bruno IM, González Carman V, Champagne CD, Crocker DE, Costa DP, Dawson TM, Deguchi T, Dewar H, Doherty PD, Eguchi T, Formia A, Godley BJ, Graham RT, Gredzens C, Hart KM, Hawkes LA, Henderson S, Henry RW III, Hückstädt LA, Irvine LM, Kienle SS, Kuhn CE, Lidgard D, Loredo SA, Mate BR, Metcalfe K, Nzegoue J, Kouerey Oliwina CK, Orben RA, Ozaki K, Parnell R, Pike EP, Robinson PW, Rosenbaum HC, Sato F, Shaffer SA, Shaver DJ, Simmons SE, Smith BJ, Sounguet G-P, Suryan RM, Thompson DR, Tierney M, Tilley D, Young HS, Warwick-Evans V, Weise MJ, Wells RS, Wilkinson BP, Witt MJ and Maxwell SM (2022) Mismatches in scale between highly mobile marine megafauna and marine protected areas. Front. Mar. Sci. 9:897104. doi: 10.3389/fmars.2022.897104
Received: 15 March 2022; Accepted: 15 June 2022;
Published: 20 July 2022.
Edited by:
Ryan Rudolf Reisinger, University of Southampton, United KingdomReviewed by:
Bradley W Barr, University Centre of the Westfjords, IcelandEaston R. White, University of New Hampshire, United States
Copyright © 2022 Conners, Sisson, Agamboue, Atkinson, Baylis, Benson, Block, Bograd, Bordino, Bowen, Brickle, Bruno, González Carman, Champagne, Crocker, Costa, Dawson, Deguchi, Dewar, Doherty, Eguchi, Formia, Godley, Graham, Gredzens, Hart, Hawkes, Henderson, Henry, Hückstädt, Irvine, Kienle, Kuhn, Lidgard, Loredo, Mate, Metcalfe, Nzegoue, Kouerey Oliwina, Orben, Ozaki, Parnell, Pike, Robinson, Rosenbaum, Sato, Shaffer, Shaver, Simmons, Smith, Sounguet, Suryan, Thompson, Tierney, Tilley, Young, Warwick-Evans, Weise, Wells, Wilkinson, Witt and Maxwell. This is an open-access article distributed under the terms of the Creative Commons Attribution License (CC BY). The use, distribution or reproduction in other forums is permitted, provided the original author(s) and the copyright owner(s) are credited and that the original publication in this journal is cited, in accordance with accepted academic practice. No use, distribution or reproduction is permitted which does not comply with these terms.
*Correspondence: Melinda G. Conners, Y29ubmVyc21AZ21haWwuY29t
†Deceased