- 1Department of Biology, College of Science, United Arab Emirates University, Al-Ain, United Arab Emirates
- 2Khalifa Center for Genetic Engineering and Biotechnology, United Arab Emirates University, Al-Ain, United Arab Emirates
- 3Harry Butler Institute, Murdoch University , Murdoch, WA, Australia
Mangrove (Avicennia marina) is a “green lung” tree growing along the Arabian Gulf coastline in the United Arab Emirates. Here, we aimed to determine the impact of the application of a commercial seaweed extract (SWE) biostimulant and endophytic actinobacterial isolates on growth performance and endogenous hormonal levels of mangroves. Therefore, we isolated endophytic plant growth-promoting (PGP) actinobacteria (PGPA) from mangrove roots and evaluated their potential as biological inoculants on mangrove seedlings under greenhouse and open-field nursery conditions. Seven salt-tolerant isolates had the ability to produce different levels of in vitro plant growth regulators (PGRs) and 1-aminocyclopropane-1-carboxylic acid (ACC) deaminase (ACCD) and to solubilize phosphorus. Accordingly, only one isolate, Streptomyces tubercidicus UAE1 (St), was selected based on its relative superiority in displaying multiple modes of action and in successfully colonizing mangrove tissues for 15 weeks. In the greenhouse experiments, plants treated with St and SWE significantly (p < 0.05) improved dry biomass by 40.2% and 55.1% in roots and 42.2% and 55.4% in shoots, respectively, compared to seawater-irrigated non-treated mangrove plants (control). However, St+SWE caused a greater significant (p < 0.05) increase in dry weight of roots (67.6%) and shoots (65.7%) than did control plants. Following the combined treatment of St+SWE, in planta PGR levels were found to be greatly enhanced over the non-treated control plants grown in non-SWE supplemented sediments, or plants inoculated with only St without the supplementation with SWE, or with non-inoculated plants grown in sediments supplied with SWE only. This was evident from the significant (p < 0.05) increases in the photosynthetic pigments and production of PGRs, as well as the reduction in the endogenous ACC levels of plant tissues compared to those in other treatments. Tissue nutrient contents of seedlings also increased by at least two-fold in St+SWE treatment as compared to control. Similar effects were observed on all growth parameters under natural open-field nursery conditions. Combining St with SWE not only stimulates plant growth but also potentially has additive effects on mangrove ecosystem productivity in nutrient-impoverished soils in the Arabian coastal areas. This report is the first in the field of marine agriculture that uses SWE as a nutrient base for actinobacteria capable of producing PGRs and ACCD.
1 Introduction
The Arabian Gulf is a marginal and semi-enclosed sea situated in the subtropical region of the Middle East. The “Gulf” is naturally stressed due to the seasonal fluctuations in surface temperatures (15°C–36°C), the elevated levels of salinity (>43 practical salinity unit), and the low annual precipitation (Naser, 2014). In addition to these factors, the Arabian Gulf is characterized by relative shallowness and high evaporation rates (Barth and Khan, 2008). Despite these extremely harsh environmental conditions, the Arabian Gulf supports a broad range of marine and coastal ecosystems such as mangrove creeks and channels, mud and sand flats, seagrass beds, and coral reefs (Naser, 2011). These ecosystems maintain genetic biodiversity in the marine environment, help in the conservation of threatened species, provide valuable services for fisheries and tourism, and support economic functions for oil and gas production (Hamza and Munawar, 2009; Samara et al., 2020).
Mangroves are unique tree species that help reduce the impact of climate change, act as a nursery to fish stocks, improve coastal water, and protect coastlines (Hutchison et al., 2014; Spalding and Parrett, 2019). Despite these advantages, mangrove habitats are globally in decline (Polidoro et al., 2010). This can be attributed to the coastal development and land reclamation, aquaculture, oil spills, and coastal pollution as well as climate change effects (Ellison and Farnsworth, 1996). According to the Food and Agriculture Organization (FAO), mangrove areas in the United Arab Emirates (UAE) have, however, increased over the last 30 years (FAO, 2020). This is partly due to localized planting activities, alteration of shorelines, water-flow patterns, and increased public awareness and conservation efforts (Moore et al., 2015; Elmahdy et al., 2020). The highly salt-tolerant gray mangrove (Avicennia marina (Forsk.) Vierh.) is the most common mangrove species in the UAE (Dodd et al., 1999; Moore et al., 2015). Within the UAE, mangrove plantations appear to be widely abundant in the emirate of Abu Dhabi. Even though the mangrove ecosystem is very rich in microbial diversity, less than 5% of species have been described (Thatoi et al., 2013).
Biostimulants are natural or synthetic substances that can be applied to seeds, plants, and soils. In addition to their role in reducing the need for chemical fertilizers, biostimulants have been recognized for their efficiency in enhancing growth, improving stress tolerance, and increasing the productivity of plants (Shukla et al., 2019; Ashour et al., 2021; Hassan et al., 2021). Seaweeds, also known as marine macroalgae or kelp, are sessile multicellular photosynthetic eukaryotes that can be differentiated from plants by their lack of specialized tissues such as root systems and vascular structures (Graham and Wilcox, 2000). Seaweeds play a key role in marine ecosystems, mainly on rocky shores in coastal temperate marine environments. They provide food and space for marine microorganisms and higher organisms, act as nurseries and shelters for many invertebrate species, and maintain the overall biodiversity structure (Schiel and Lilley, 2007; Egan et al., 2013). Commercially, the growth of seaweed aquaculture has recently increased, particularly in food markets, feedstocks, and biofuel production (Borines et al., 2011; Jacob et al., 2016). In agriculture, seaweed extracts (SWEs) are currently used as biostimulants in organic farming (Chami and Galli, 2020; Mukherjee and Patel, 2020; Ali et al., 2021). Despite the extensive literature reports about using SWE in crop management, no study to date has deployed SWE in marine agriculture to promote the growth of mangroves or Salicornia under greenhouse and/or open-field conditions.
Many attempts have focused on the microbial diversity in mangrove ecosystems to explore their potential applications in agricultural, environmental, industrial, and medical fields (Bashan et al., 2000; Holguin et al., 2001; Bashan and Holguin, 2002; Hong et al., 2009; Allard et al., 2020). Yet little is known about the bacterial community living in mangroves, particularly actinobacteria, with the potential to stimulate plant growth. Microbial endophytes are found in almost all plant species, thus colonizing their internal tissues. Plant growth-promoting (PGP) rhizobacteria (PGPR), including PGP actinobacteria (PGPA), are also known to affect plant fitness and soil quality, thereby increasing the productivity of agriculture and the stability of soils (Rai et al., 2018; El-Tarabily et al., 2019; El-Tarabily et al., 2020; Mathew et al., 2020).
Endophytic PGPR stimulates plant growth directly by facilitating resource acquisition needed by plants, modulating the levels of plant growth regulators (PGRs; Santoyo et al., 2016), or indirectly by decreasing the inhibitory effects of pathogens on plants (Khare et al., 2018). PGPR possessing the 1-aminocyclopropane-1-carboxylic acid (ACC) deaminase (ACCD) enzyme can also facilitate growth and induce tolerance to environmental stresses in plants by hydrolyzing ACC into α-ketobutyrate and ammonia (NH3), thus decreasing ethylene (ET) levels in plant tissues (Glick, 2015; Olanrewaju et al., 2017). Previously, endophytic actinobacteria have been isolated from mangroves to study their antimicrobial activities (Gayathri and Muralikrishnan, 2013; Jiang et al., 2018; Chen et al., 2021b). Except for El-Tarabily et al. (2021a), there are no reports about endophytic actinobacterial strains isolated from mangrove tissues to study their potential in the plant growth activities of mangroves.
It has been reported that a combination of natural SWE and PGPR can improve plant growth, increase crop production and quality, and ameliorate stress effects. For example, the combined Bacillus licheniformis and Pseudomonas fluorescens with Kelpak® (a seaweed-derived extract) improved the production and mineral contents of leafy vegetables (Ngoroyemoto et al., 2020). In addition, supplying plants with SWE, PGPR (Bacillus amyloliquefaciens subsp. plantarum, Bacillus simplex, and Pseudomonas sp.) and micronutrients improved tolerance to cold stress during the early growth of maize (Bradáčová et al., 2016). To date, however, there are no data in the literature to determine the impact of combining rhizosphere or endophytic PGPA with SWE treatments on the growth of halophytic plants (e.g., mangrove) under greenhouse or field conditions.
Due to the increasing interest in maintaining healthy forests of mangroves in the UAE (Alsumaiti et al., 2017; Samara et al., 2020), we hypothesized that the combined effect of SWE application derived from kelp and PGPA inoculation with multiple PGP traits would boost the growth of mangroves compared to the single PGPA or SWE treatment. In this context, we used different treatments of the identified endophytic actinobacterial isolate and SWE, either individually or in combination. The objectives of the present investigation were to i) isolate endophytic actinobacteria from mangrove roots capable of producing multiple PGP compounds and ii) determine the response of mangrove plants to inoculation with the most promising PGPA isolate and SWE under greenhouse and natural open-field nursery conditions. In addition to plant growth, we assessed the endogenous levels of PGRs and ACC in tissues in response to PGPA, SWE, and PGPA+SWE. Overall, we determined—for the first time—the role of SWE in association with endophytic PGPA in improving the growth performance of mangroves under controlled greenhouse and non-controlled open-field nursery conditions.
2 Materials and methods
2.1 Sediment Collection, Plant Material, and Seaweed Extract
In the current study, dark grayish-black sediments were collected from the east coast of Abu Dhabi-UAE (24°26′48.5″N; 54°26′40.6″E). Viviparous propagules of gray mangrove (A. marina) were obtained from either mother trees or freshly fallen ones collected from the same abovementioned location. Similar sized propagules were surface-sterilized using 70% ethyl alcohol (Sigma-Aldrich Chemie GmbH, Taufkirchen, Germany) and 20% Clorox bleach. All surface-sterilized propagules were then washed ten times with 0.22-μm filter-sterilized (Millipore Corporation, Burlington, MA, USA) full-strength seawater (salinity of 40) and left to air-dry for 30 min.
In the current study, a commercial Acadian soluble SWE powder (Acadian Seaplants Limited, Dartmouth, NS, Canada) was used as a biostimulant. This organic seaweed concentrate is derived from the kelp, Ascophyllum nodosum. Physiochemical properties, macronutrients, and micronutrients of SWE can be found in Table S1. Mangrove sediment was amended with 400 ml of SWE pot−1 according to the manufacturer’s recommended rate (0.3 g SWE L−1 water) every 14 days for the entire period of the in vivo experiments.
2.2 Isolation of Endophytic Actinobacteria
Mangrove propagules (Section 2.1) sown in plastic pots (23 cm diameter × 17 cm depth) containing sediment from the area described above were watered daily with full-strength seawater under greenhouse conditions (temperature of 25°C ± 2°C; relative humidity of 60% ± 5%; average daily photosynthetic photon flux density of 700 ± 150 μmol m−2 s−1). Eight pots (each containing two propagules) were prepared.
After 5 weeks, 16 seedlings from eight pots were collected and transferred to the laboratory. Roots were cut and washed, and fresh weight (FW) was recorded. Roots were soaked in sterile phosphate-buffered saline (PBS) solution (pH 7.0) for 10 min (Rennie et al., 1982) and surface-disinfested. For the surface sterilization, roots were first exposed to propylene oxide (Sigma-Aldrich) vapor for 25 min (Sardi et al., 1992). Roots were soaked in 70% ethyl alcohol for 4 min and 1.05% NaOCl for 4 min, followed by rinsing ten times in PBS.
Sterility checks were carried out for each sample before root maceration (Hallmann et al., 1997). The slurry was filtered through a sterile cotton cloth, and the filtrate was serially diluted (10−2, 10−3, and 10−4). Aliquots (200 μl) were spread on plates containing inorganic salt starch agar (ISSA; Küster, 1959). For each root sample dilution, three replicated plates were dried for 15 min followed by 7-day incubation at 28°C ± 2°C in the dark (El-Tarabily et al., 2019). Population density (PD; log10 colony-forming units (cfu) g root FW−1) of endophytic actinobacteria was calculated (Hallmann et al., 1997). Colonies of streptomycete actinobacteria (SA) and non-streptomycete actinobacteria (NSA) were purified and identified on oatmeal agar plates supplemented with 0.1% yeast extract (OMYEA; ISP medium 3; Shirling and Gottlieb, 1966). Hyphae/spores of actinobacterial isolates were stored in 20% glycerol at −70°C (Wellington and Williams, 1977).Culture characteristics such as the color of aerial and substrate mycelia, and the production of diffusible pigments in addition to the presence or absence of aerial mycelia, the distribution of spores on both aerial and substrate mycelia, the formation of sporangia, and the stability/fragmentation of substrate mycelia were used to differentiate between SA and NSA (Cross, 1989). Filter-sterilized full-strength seawater was used in the preparation of all microbiological media in the current study.
2.3 In Vitro Screening for Plant Growth-Promoting Traits
All endophytic isolates were streaked on an ISSA medium supplemented with 80 g L−1 (8%) of NaCl (Williams et al., 1972). Plates were incubated at 28°C in the dark for 7 days. Strong growth and heavy sporulation of actinobacterial isolates indicated high salt tolerance.
The high salt-tolerant SA and NSA were preliminarily tested for the production of indole-3-acetic acid (IAA). Briefly, 2 ml of each isolate (108 cfu ml−1) was incubated on a 250-rpm orbital shaker incubator in flasks containing 50 ml of inorganic salt starch broth (ISSB; Küster, 1959) supplied with 5 ml of 5% l-tryptophan (Sigma-Aldrich) for 7 at 28°C in the dark (Khalid et al., 2004; El-Tarabily et al., 2019). Suspensions were centrifuged at 12,000 × g, and 4 ml of the Salkowski reagent was added to the collected supernatants (Gordon and Weber, 1951). IAA-equivalents (µg ml−1) were quantitatively determined using a spectrophotometer (UV-2101/3101 PC; Shimadzu Corporation, Analytical Instruments Division, Kyoto, Japan) at 530 nm.
All promising IAA-producing isolates were further grown for 10 days at 28°C in the dark in glucose peptone broth (di Menna, 1957) supplemented with 5 ml of 5% l-tryptophan to detect IAA and indole-3-pyruvic acid (IPYA) and on Strzelczyk and Pokojska-Burdziej (1984) medium to detect gibberellic acid (GA3) and cytokinins (CKs) including isopentenyl adenine (iPa), isopentenyl adenoside (iPA), and zeatin (Z), using reverse-phase high-performance liquid chromatography (HPLC; SpectraLab Scientific Inc., Ontario, Canada). Auxins, GAs, and CKs were separated by using two isocratic solvent systems, according to Tien et al. (1979). Waters Associates HPLC with a differential ultraviolet detector was used to analyze the chromatograms, which were created by injecting 10 µl of the methanol dissolved extract onto a 10-m reverse-phase column (Waters Associates Bondapak C18, 4 mm × 30 cm) (El-Tarabily et al., 2020). In order to calculate the concentrations of PGRs in the unknown sample, their respective peak areas were compared with those obtained with authentic samples (Sigma-Aldrich) of a known concentration.
For ACCD assay, all isolates were plated on Dworkin and Foster’s (DF) salts minimal agar medium (Dworkin and Foster, 1958) supplemented with either 0.3033 g l−1 of ACC (DF-ACC; Sigma-Aldrich) or 2 g l−1 of ammonium sulfate (DF-(NH4)2SO4; control) for 7 days at 28°C in the dark (El-Tarabily et al., 2019). Growth/sporulation on DF-ACC plates indicated that the isolate could produce ACCD. We also quantified the enzymatic activity of ACCD by measuring the amount of α-keto-butyrate (Honma and Shimomura, 1978). Protein concentrations were determined according to Bradford (1976).
To test the putrescine (Put) production, plates containing Moeller’s decarboxylase agar medium (MDAM) were supplemented with 2 g l−1 of l-arginine-monohydrochloride (Sigma-Aldrich) and phenol red (Sigma-Aldrich) (Arena and Manca de Nadra, 2001). MDAM plates were incubated for 2 days in the dark at 28°C (El-Tarabily et al., 2020). The dark red halo surrounding the colonies indicated a Put-producing isolate. We also quantitatively test these Put-producing actinobacterial isolates for their production of the polyamines Put, spermidine (Spd), and spermine (Spm) using reverse-phase HPLC (Marino et al., 2000). Positive Put producers were placed in Moeller’s decarboxylase broth medium (MDBM) amended with 2 g l−1 of l-arginine-monohydrochloride (Arena and Manca de Nadra, 2001). With the use of a 254-nm UV detector (Smith and Davies, 1985), an aliquot of 10 µl of the sample was injected onto a Bondapak C18 column (4 mm × 30 cm) in a liquid chromatograph (Waters Associates) as described by Marino et al. (2000).
For siderophore production, plates of chrome azurol S (CAS) agar (Schwyn and Neilands, 1987) were inoculated with isolates and incubated for 3 days at 28°C in dark. Actinobacterial isolates that were considered siderophore producers developed a yellow-orange halo zone around the colony.
Phosphate-solubilizing actinobacteria (PSA) were assayed using Pikovskaya (PVK) agar medium (Pikovskaya, 1948) supplemented with rock phosphate (Tianjin Crown Champion International Co. Limited, Tianjin, China) and amended with bromophenol blue. The appearance of a clear zone underneath the colony indicated a PSA isolate. The same isolates were also grown in 20 ml of sterilized National Botanical Research Institute Phosphate (NBRIP; Nautiyal, 1997) broth at 28°C for 2 days on a shaker at 150 rpm. For each isolate, aliquots (1 ml) were transferred to a flask containing 250 ml of NBRIP medium and incubated with continuous shaking at 28°C. Sterilized un-inoculated medium served as a control. After 3 days, a 10-ml sample of each culture or control was centrifuged at 12,000 × g for 15 min. The supernatant was used to determine the drop in pH and the amount of P released into the medium. The pH was recorded using a pH meter, whereas P availability was determined using the phospho-molybdate blue color method (Murphy and Riley, 1962).
Acetylene-reduction assays (Dye, 1962) and Nessler’s reagent (Holguin et al., 1992) were used to measure nitrogenase activity and NH3 production, respectively. In all in vitro assays, eight independent replicates were used for each strain.
2.4 Evaluation of Plant Growth-Promoting Parameters of Plant Growth-Promoting Actinobacteria Isolates Under Gnotobiotic Conditions
Of all PGP traits tested in vitro, the strongest PGPA isolates (#11, #12, and #20) were selected for the preliminary growth promotion experiment under gnotobiotic conditions. In a greenhouse, surface-sterilized mangrove propagules were sown in plastic pots containing sediment for 10 days and watered daily with full-strength seawater.
In order to prepare the inoculum for all the gnotobiotic experiments, 4 ml of aliquots of 20% glycerol suspension of the selected endophytes were individually inoculated into 250 ml of ISSB and shaken at 250 rpm on an orbital shaker incubator for 5 days. Cells were centrifuged at 12,000 × g for 15 min at 20°C, and the pellet was suspended in 10 ml of PBS and re-centrifuged (El-Tarabily et al., 2019). For each suspension, 0.1 ml of each of the dilutions of 10−3, 10−4, 10−5, and 10−6 was made in PBS and spread on ISSA. After 5 days of incubation, a final concentration of ~108 cfu ml−1 of each isolate was used as an inoculum.
Selected endophytic actinobacterial isolates were introduced inside the young seedlings using the pruned-root dip method (Musson et al., 1995). Briefly, root tips (3 mm) from germinated propagules were trimmed, and young seedlings were placed in sterile plastic cups containing the inoculum suspension (108 cfu ml−1) of each isolate for 3 h at 25°C. Seedlings of mangroves with or without the actinobacterial inoculum were aseptically planted into glass tubes (300 × 35 mm) filled with sediment and moistened with seawater. The control treatment was represented by seedlings that were placed in autoclaved ISSB.
All seedlings maintained in a growth chamber (day-time cycle, 16/8-h light/night; temperature, 25°C/20°C; fluorescent light, 180–200 µmol m−2 s−1) were irrigated daily with full-strength sterilized seawater. After 6 weeks of transplantation, plants were harvested, washed, and separated into roots and shoots. Dry weight (DW; g) and length (cm) of the shoot and root tissues were measured. Each treatment representing one seedling was independently replicated eight times.
In Planta Population Density of Selected Actinobacterial Isolates
Rifampicin resistant mutants of the promising PGPA (#11, #12, and #20) and non-PGPA positive control (#7) isolates were selected on ISSA medium supplemented with rifampicin (100 µg ml−1; Sigma-Aldrich) and compared to the corresponding wild-type strains (Misaghi and Donndelinger, 1990). Features, such as morphology growth and PGP, of these mutants were found to be similar to the parental strains.
The pruned-root dip method was used to inoculate 10-day-old seedlings of mangroves with the endophyte inoculum (Section 2.4) in order to evaluate the colonization of internal root and stem tissues by isolates. Free-draining pots (36 cm in diameter) were filled with 14 kg of sediments (Section 2.1) and watered daily with full-strength seawater to container capacity in the greenhouse (Section 2.2). Roots and stems were sampled, washed, and surface-sterilized (Section 2.1) every 3 weeks (for 15 weeks) after planting. Samples were homogenized to determine the PD of isolates on ISSA amended with rifampicin. Each replicate represents a single pot containing one seedling, and each treatment was replicated eight times.
For light microscopy (LM) and transmission electron microscopy (TEM), specimens (6-week-old mangrove seedlings inoculated with the selected PGPA isolate) were fixed in freshly prepared Karnovsky’s fixative (2% paraformaldehyde (Sigma-Aldrich) + 2.5% glutaraldehyde (Sigma-Aldrich) in 0.17 M of Sorensen’s phosphate buffer, pH 7.2) for 24 h at 4°C. After being washed three times with the buffer, tissues were post-fixed with 1% aqueous osmium tetroxide for 2 h at 25°C, dehydrated with ascending grades of ethanol (30%–100%), and dipped into the propylene oxide (Sigma-Aldrich). Finally, samples were infiltrated, embedded in epoxy resin (Epon 812, Agar Scientific, UK), and polymerized at 60°C in an embedding oven for 24 h (Millonig, 1976). Blocks were trimmed into semi-thin sections (1.5 µm) and ultra-thin sections (95 nm) with Leica EM7 ultra microtome (Vienna, Austria). Slides of selected heat-dried, semi-thin sections stained with 1% toluidine blue and 1% borax (Sigma-Aldrich) were examined using Olympus BH-2 (Olympus Optical Co. Ltd, Tokyo, Japan) LM equipped with a digital camera and software (Jenoptik ProgRes Camera, C12plus, Frankfurt, Germany). For the TEM study, ultra-thin sections were collected on 200 mesh copper grids, stained with 10% uranyl acetate and 3% lead citrate, and examined using Tecnai Spirit G2 Biotwin TEM (FEI Co., Eindhoven, Netherlands).
2.6 Identification of the Most Potent Plant Growth-Promoting Endophytic Isolate
The most potent isolate was identified based on the 16S rRNA gene sequencing, performed by Deutsche Sammlung von Mikroorganismen und Zellkulturen GmbH (DSMZ), Germany. Primers targeting 16S rRNA gene: 900R (5′-CCGTCAATT CATTTGAGTTT-3′), 800F (5′-ATTAGATACCCTGGTAG-3′), and 357F (5′-TACGGGAGGCAGCAG-3′) were used (Rainey et al., 1996; Saeed et al., 2017; Kamil et al., 2018). All 16S rRNA gene sequences of 16 representatives from the genus Streptomyces were retrieved from the National Center for Biotechnology Information (NCBI) database (http://www.ncbi.nlm.nih.gov/nucleotide/). Multiple sequence alignment of 16S rRNA genes was carried out using CLUSTAL-X (Thompson et al., 1997) implemented in the Molecular Evolutionary Genetics Analysis 7.0 (MEGA7) software with default parameters (Kumar et al., 2016). A phylogenetic tree of 16S rRNA genes was reconstructed using the maximum likelihood (ML) method (Felsenstein, 1981) implemented in the MEGA 7.0 software. Bootstrap values were calculated with 1,000 resamples.
The spore chain morphology and spore surface were examined using Philips XL-30 scanning electron microscopy (SEM; FEI Co., Eindhoven, Netherlands) of 15-day-old cultures grown on ISP-3 (Kumar et al., 2011).
2.7 Assessment of Growth Promotion, Photosynthetic Pigments, and Endogenous Plant Growth Regulators in Mangrove Under Greenhouse Conditions
In free-draining pots filled with sediments (Section 2.5), seedlings were inoculated with the most promising endophytic isolate #12 using the pruned-root dip method (Section 2.4). A total of four treatments were carried out: 1) control (seedlings inoculated with autoclaved ISSB medium only; neither SWE nor isolate was applied), 2) St (seedlings inoculated with endophytic isolate #12), 3) SWE (seedlings supplemented with only SWE), and 4) St+SWE (seedlings inoculated with endophytic isolate #12 in a sediment supplemented with SWE). SWE was added as per the manufacturer’s recommended rate (Section 2.1). Seedlings were watered daily with full-strength seawater to container capacity (Section 2.5). In the greenhouse, a randomized complete block design (RCBD) was used, where each replicate (total of eight replicates) was determined by a single pot containing one seedling. Trials were independently repeated twice with similar results.
The DW and length of roots and shoots, number of branches, and leaf surface area (cm2) were recorded at the end of 9 months post-planting (mpp) of propagules. Chlorophyll (Chl) fluorescence measurements were performed at 645 (for Chl a) and 663 nm (for Chl b) (Holden, 1965). Carotenoid pigments were measured at 470 nm according to Davies (1965).
The PAs (Put, Spd, and Spm) extracted from tissues of apical root and shoot tissues (Flores and Galston, 1982) were quantitatively determined using benzoyl chloride (Sigma-Aldrich) and normal internal standard of PAs (Redmond and Tseng, 1979). Reverse-phase HPLC chromatograms were produced onto a 10-µm reverse-phase column (Marino et al., 2000; Section 2.3).
To quantitatively measure the endogenous PGRs (auxins, CKs, GA3, and abscisic acid [ABA]), crude extracts from mangrove root and shoot tissues (Shindy and Smith, 1975; Guinn et al., 1986; Machàckovà et al., 1993) were detected with the UV at 254 nm using reversed-phase HPLC (Waters Associates). This method provides quantification of phytohormones in a single run from 50 mg of fresh plant tissue. ACC content was assayed by the method of Lizada and Yang (1979). Derivatization of ACC was carried out by the addition of phenylisothiocyanate (Sigma-Aldrich), and the reverse-phase HPLC chromatograms were produced as described by Lanneluc-Sanson et al. (1986).
All measurements were taken on seedlings at the end of 9 mpp of propagules. Sixteen replicate samples from two independently repeated experiments were analyzed for all the tested parameters for each treatment.
2.8 Analyses of Plant Nutrients
We selected the tissues of the terminal part of the root and shoot systems at the end of 9 mpp of propagules, washed them in deionized water, and cut them into small pieces, which were then dried overnight at 70°C. Mineral nutrients were then analyzed in the roots and shoots, as follows: nitrogen was measured using a LECO FP-428 nitrogen analyzer by combusting finely ground plant material at 950°C in oxygen. As the sample passed through a thermal conductivity cell, the amount of N released from it was measured (Sweeney and Rexroad, 1987). Plant samples were digested in a 9:1 solution of nitric acid and perchloric acid to measure phosphorus (P), K, sulfur (S), magnesium (Mg), calcium (Ca), sodium (Na), Fe, manganese (Mn), copper (Cu), and zinc (Zn) (McQuaker et al., 1979). For the measurement of boron (B), plant material was dry-ashed and extracted with dilute acid, and B was colorimetrically measured with azomethine H (Gaines and Mitchell, 1979). Analyses were performed for all the nutrients in 16 replicates from two independently repeated experiments.
2.9 Assessment of In vivo Growth Promotion Under Open-Field Nursery Conditions
In free-draining pots filled with sediments (Section 2.5), seedlings were inoculated with the promising endophytic isolate #12 using the pruned-root dip method (Section 2.4). A total of four treatments were carried out as described above (Section 2.7). For each treatment, eight separate pots each containing one seedling were arranged in an RCBD. The container open-field nursery experiments were carried out on the Arabian Gulf coast of Abu Dhabi in the same location described in Section 2.1, and the nursery trials were independently repeated twice.
Control and inoculated seedlings were maintained under natural conditions between February and October (relative humidity range = 22%–43%; daytime length range = 11.0–13.5 h day; average temperature = 35.0°C ± 9°C day/23.0°C ± 8.0°C night; average precipitation = 6.7 mm). Irrigation was carried out naturally from the sea during the high and low tide times of the day. The DW and length of roots and shoots, number of branches, and leaf surface area (cm2) were recorded from 16 samples from two independently repeated experiments at the end of 12 mpp of propagules.
2.10 Estimation of the Sediment Total Microbial Activity
To study the effect of SWE on the growth of PGPA, including Streptomyces tubercidicus UAE1, a new SWE agar (SWEA) medium was developed. This medium was prepared by dissolving 40 ml of SWEA in 1 l of filter-sterilized full-strength seawater (pH 7.5). All endophytic isolates were streaked on SWEA, and the plates were incubated at 28°C in the dark for 7 days. Strong growth and sporulation of actinobacterial isolates on SWEA indicated the ability of SWE to support the growth of the endophytic isolates.
In order to compare the effect of SWE individually or in combination with S. tubercidicus UAE1 on the mangrove sediment ecosystem, total microbial activity was assessed at the end of the greenhouse and open-field nursey experiments. The microbial activity was measured using the fluorescein diacetate (FDA) hydrolysis technique (Schnurer and Rosswall, 1982). Briefly, 5 g of each sediment was added to 20 ml of 60 mM potassium phosphate buffer (8.7 g of K2HPO4 and 1.3 g of KH2PO4 in 1 l of distilled water, pH 7.6) in 250-ml flasks. The FDA was dissolved in acetone and stored as a stock solution (2 mg ml−1) at −20°C. The reaction was started by adding 0.2 ml of FDA (400 µg) from the stock solution to a buffer–sediment mix. The reaction flasks were shaken (250 rpm) at 25°C for 20 min on an orbital shaker incubator. The reaction was then stopped by adding 20 ml of acetone to all samples. Sediment residues were centrifuged at 12,000 × g for 15 min and filtered using Whatman filter paper (Whatman, Maidstone, UK). The filtrate was collected in a test tube, covered with parafilm, and placed into an ice bath to reduce the volatilization of the acetone. The concentration of fluorescein was determined by reading the optical density at 490 nm using a spectrophotometer (Shimadzu Corporation). For each treatment, the background absorbance was corrected with the blank sample run under identical conditions but without the addition of FDA. Standard curves were prepared according to Chen et al. (1988). The results were converted to μg hydrolyzed FDA g dry sediment−1. All analyses were collected from sediments at the end of 9 and 12 mpp of propagules of the greenhouse and open-field nursery experiments, respectively. Sixteen replicate samples from two independently repeated experiments were analyzed for each treatment.
2.11 Statistical Analyses
In all experiments, RCBD was performed. Gnotobiotic greenhouse and nursery experiments were repeated with similar results. All data were combined and analyzed using the ANOVA procedure of SAS Software version 9 (SAS Institute Inc., NC, USA). Mean values of treatments were compared using Fisher’s protected least significant difference (LSD) test at p = 0.05 levels. The PD of the selected PGPA isolates was transformed into log10 cfu g root or stem FW−1.
3 Results
3.1 In Vitro Assessment of Plant Growth-Promoting Traits of Purified Endophytic Actinobacteria
The purified endophytic actinobacterial isolates were in vitro assessed for their salinity tolerance and different PGP traits. From the 26 endophytic actinobacteria, 20 SA and 6 NSA were isolated from the surface-sterilized root samples, and seven (6 SA and 1 NSA) isolates (#3, #11, #12, #17, #20, #23, and #25) were able to perform different PGP activities with an ability also to tolerate 8% NaCl (Table 1; Figure S1). Regardless of their PGP traits, the rest of the isolates were not included in further experiments because they did not grow or sporulate on ISSA containing 8% NaCl.
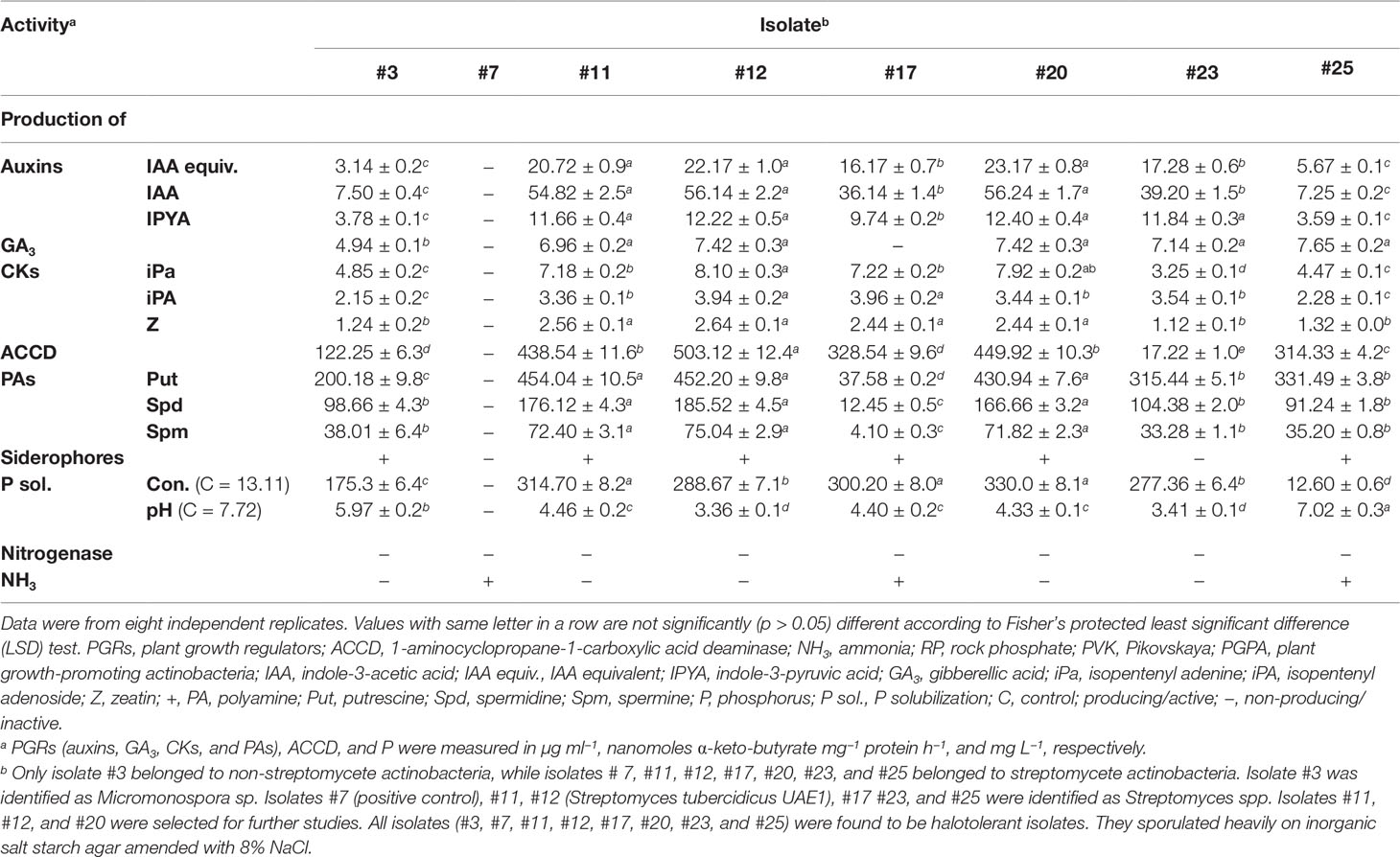
Table 1 In vitro production of PGRs, ACCD, siderophores, nitrogenase enzyme, and NH3 and effect of inoculation with RP-amended PVK broth with the selected halotolerant endophytic PGPA strains isolated from within surface-sterilized mangrove roots.
We first tested the highly salt-tolerant isolates for the production of PGRs, ACCD, siderophores, nitrogenase, and NH3 and for their ability to solubilize insoluble P. The identified isolates synthesized significantly (p < 0.05) different levels of auxins (IAA and IPYA), GA3, and CKs (iPa, iPA, and Z) (Table 1). Three isolates (#11, #12, and #20) produced higher amounts of auxins (Table 1; Figure S1), whereas five (#11, #12, #20, #23, and #25) produced higher levels of GA3 than any other tested isolates (Table 1). Although isolate #3 produced the lowest levels of auxins and GA3, isolate #17 did not produce GA3 in the culture extracts. By comparing the in vitro production of CKs among the tested actinobacteria, isolate #12 produced the highest amounts of the three types (Table 1). Thus, other isolates synthesized comparable amounts of iPa (#20) and iPA (#17) to isolate #12. Except for #3, #23, and #25, all other isolates produced significantly (p < 0.05) high levels of Z (Table 1).
The production of enzymes ACCD and nitrogenase known for their PGP activities (El-Tarabily et al., 2019) by the actinobacterial isolates were also assessed in vitro. Although isolates #11, #12, #17, #20, and #25 produced the highest level of ACDD (Table 1; Figure S1), none of these isolates produced nitrogenase (Table 1).
We also qualitatively and quantitatively determined the production of PAs on culture extracts of the seven isolates. The amounts of Put, Spd, and Spm synthesized by these isolates significantly (p < 0.05) varied (Table 1). By using the HPLC, isolates #11, #12, #20, #23, and #25 produced significantly (p < 0.05) higher levels of Put than the other tested isolates (Table 1; Figure S1). Isolates #11, #12, and #20 synthesized the highest amounts of Spd and Spm (Table 1). When we checked the production of siderophores and NH3in vitro, #17 and #25 were the only actinobacterial isolates that showed these PGP traits. The remaining produced either one or none of the two traits (Table 1).
Six isolates (#3, #11, #12, #17, #20, and #23) were able to solubilize P (Table 1; Figure S1). Since we tend to find the isolate(s) showing most, if not all, PGP activities, with the highest values in all the PGRs and ACCD tested, only isolates #11, #12, and #20 were further included for their endophytic existence and abundance in planta.
3.2 Growth Promotion and Tissue-Specific Colonization of Selected Endophytic Plant Growth-Promoting Actinobacteria
The strongest actinobacterial isolates showing multiple in vitro PGP traits were selected to determine the growth promotion of mangroves under gnotobiotic conditions. First, the chemical characteristics of the sediment collected earlier (Section 2.1) were analyzed and recorded as follows: pH (8.36), electrical conductivity (5.81 dS m−1), organic carbon (6.24%), total P (85 and 8.83 mg kg−1 sediment), available P (8.83 mg kg−1), N (4 and 6.4 mg kg−1 as nitrate and ammonium, respectively), bicarbonate extractable potassium (K; 241 mg kg−1), amorphous iron (Fe) oxides (331 mg kg−1), and sulfate (414 mg kg−1).
In the greenhouse, endophytic isolates #11, #12, and #20 showed variable effects on mangrove growth. The three isolates significantly (p < 0.05) increased lengths and DWs of root and shoot as compared to non-inoculated (negative control) or inoculated with isolate #7 (positive control) treatments (Table S2). The measured lengths of roots and stems and DWs of mangrove seedlings treated with the endophytic isolate #12 were significantly (p < 0.05) higher than those non-treated control or treated with isolates #11 or #20. Although plants inoculated with isolate #11 showed significantly (p < 0.05) increased lengths and DWs of shoots, we did notice the same effects on roots, compared to those inoculated with isolate #20.
To determine the longevity of the promising endophytic isolates in planta, we compared their PD in the internal tissues of mangrove roots and stems on a triweekly basis (up to 15 weeks). In our greenhouse experiments, isolates #11, #12, and #20 recovered in all tissues albeit at the sampling time. This suggests an endophytic nature of these PGPA isolates without causing harm to the host plant, i.e., mangrove. The total population of isolates, except for #11, increased significantly (p < 0.05) from the beginning until the end of the experiment in both tissues (Figure 1). Following the significant (p < 0.05) increase in the first 6 weeks of colonization, there was a drop in PD of isolate #11 inside root and stem tissues of mangroves by the end of the experiment. We also noticed that the mean of the total population of isolate #20 in mangrove stems in Weeks 6–15 was insignificant (p > 0.05; Figure 1). Together, our data suggested that isolate #11 did not sufficiently recover from the tissues of mangrove seedlings after Week 6 and that isolate #20 inefficiently colonized stem tissues. Thus, the two isolates were excluded from further experiments. Our results imply, however, that #12 can be a potential PGPA isolate to be considered as a potential endophytic PGPA isolate. This was evident from the PD of this isolate that strikingly (p < 0.05) increased for the period of colonization in root and stem tissues throughout the greenhouse trials (Figure 1). Compared to isolate #12, we noticed similar increase patterns in the PD of non-PGPA isolate #7 (control). Overall, the increased growth and high PD consistently found in seedlings by isolate #12 under controlled gnotobiotic and greenhouse conditions, respectively, made this isolate ideal for a large-scale experiment, i.e., a non-controlled open-field nursery.
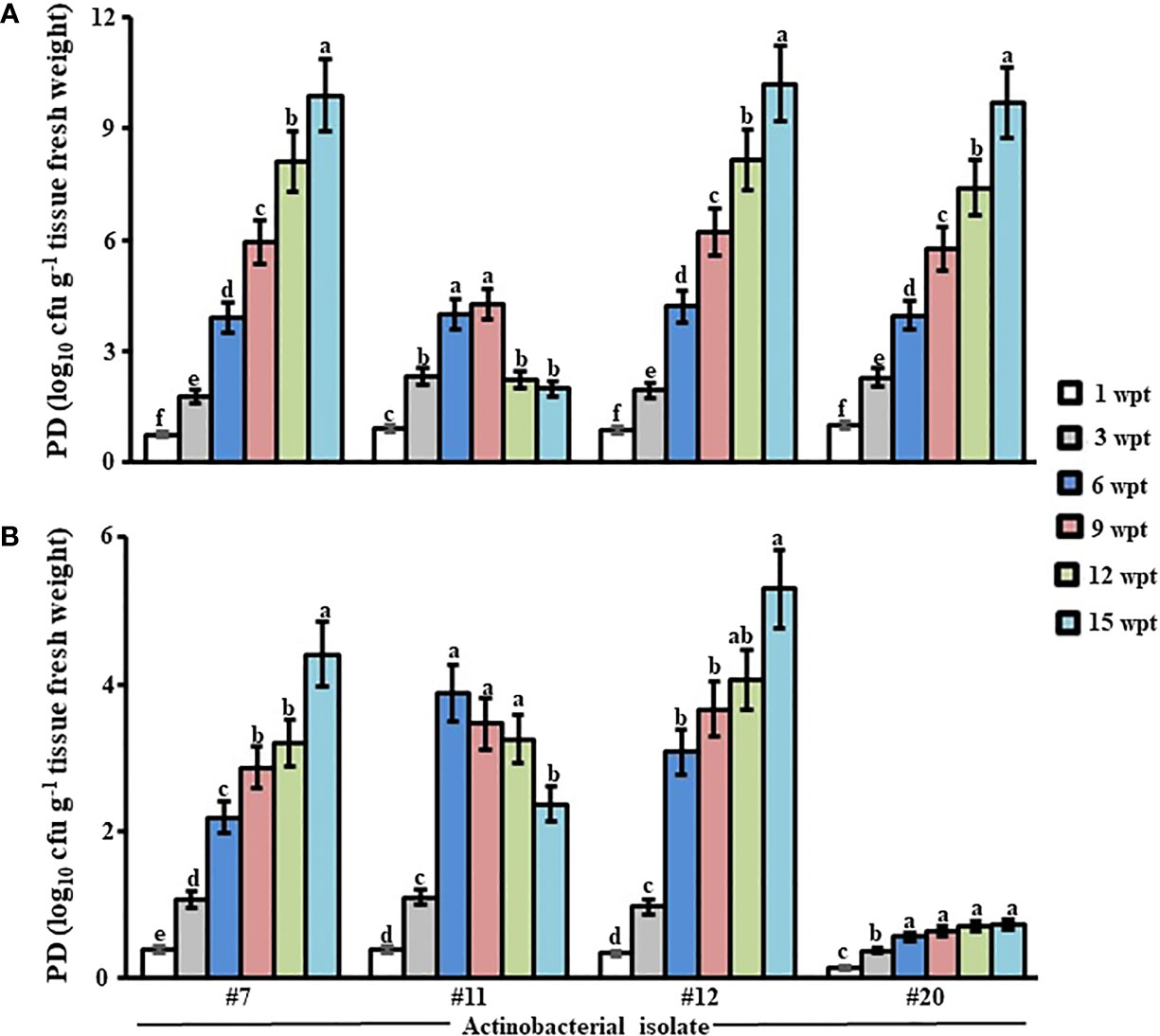
Figure 1 Population density of the promising endophytic actinobacteria within mangrove tissues. Total population of the endophytic isolates (#11, #12, and #20) showing multiple PGP traits in vitro residing in (A) root and (B) stem tissues of gray mangrove grown under greenhouse conditions. Tissues were sampled every 3 wpt. Values are means of eight replicates ± SE for each sampling per treatment. Mean values followed by different letters are significantly (p < 0.05) different from PD of each strain in that particular tissue according to Fisher’s protected LSD test. Isolate #7 represents the endophytic Streptomyces sp. that does not show any PGP trait in vitro, while isolate #12 represents Streptomyces tubercidicus UAE1. Isolates #11 and #20 belonged to Streptomyces spp. PGP, plant growth promoting; PD, population density; wpt, weeks post treatment; LSD, least significant difference.
To confirm the endophytic lifestyle of isolate #12, we used microscopic analyses to visually investigate the presence of spores and/or mycelia within the internal tissues of mangrove roots and stems at 6 weeks post-inoculation (wpi). With the use of LM, the spores of actinobacterial isolate #12 colonized the root tissues intercellularly within the parenchyma cells of the cortex and the xylem (Figure 2A). In addition, mycelial growth carrying the spiral spore chains of isolate #12 was detected within the cortical cells of roots (Figure 2B). Interestingly, the germinated spores and the formed germ tubes indicated the ability of isolate #12 to colonize the intracellular spaces of root cells (Figure S2). Similarly, the endophytic isolate could successfully reside within the stem cells (Figure 2C). This was confirmed by the growth and survival of isolate #12 as part of its life cycle in vascular structures with its host plant (Figure 2D). The obtained results suggested that isolate #12 can translocate between the root and shoot systems through conductive tissues of the xylem.
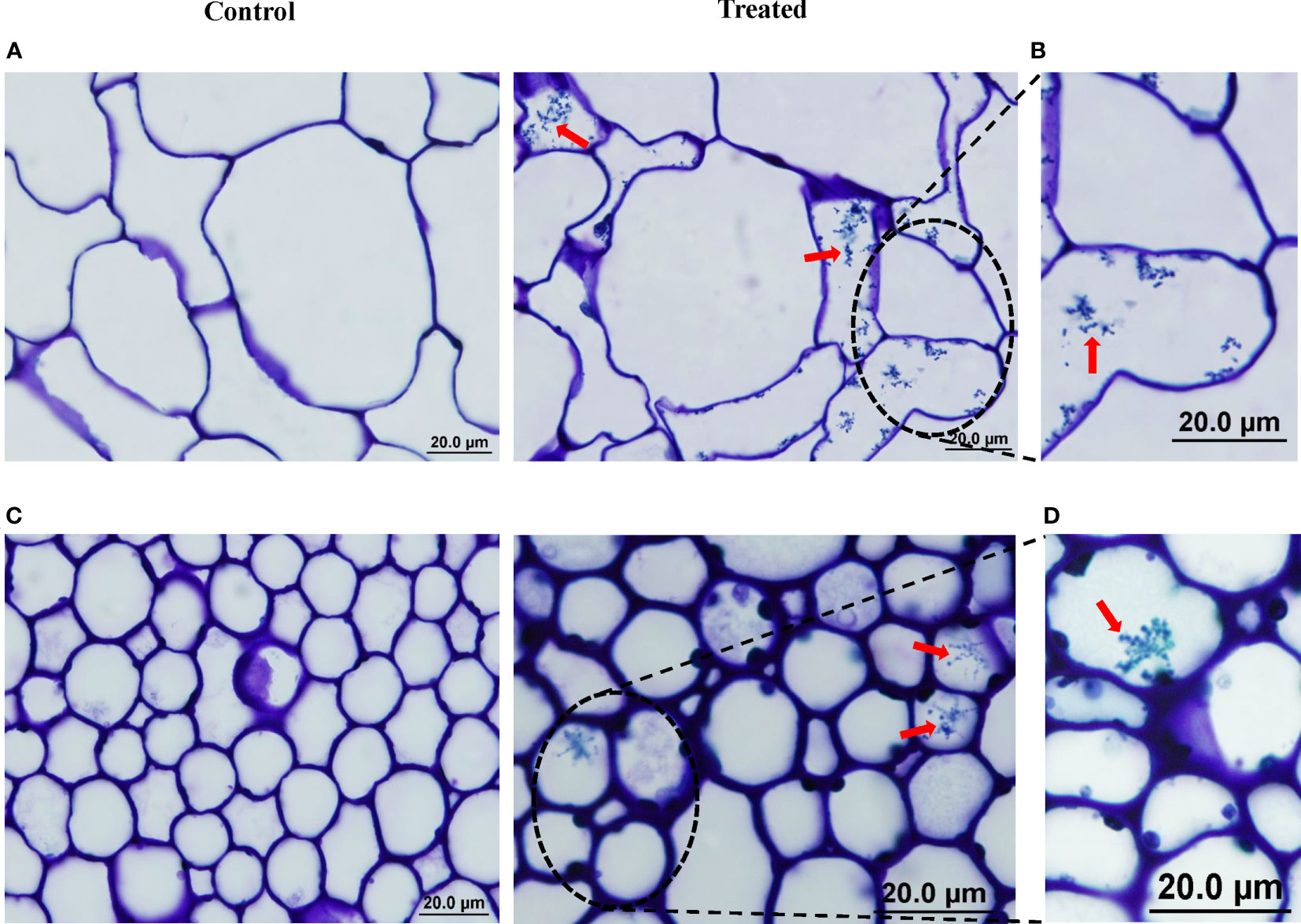
Figure 2 Colonization of mangrove tissues by actinobacterial isolate #12. Light micrograph of semi-thin sections of mangrove (A) root and (C) stem not inoculated (control; left) or inoculated with isolate #12 (treated; right) (×1,000). Close-up views of mangrove (B) root and (D) stem inoculated with isolate #12 (×1,000). (A–D) All sections were stained with 0.1% toluidine blue showing the distribution of spores (red arrows) within cells of root and shoot tissues of mangrove. Bars, 20 µm. Isolate #12 represents Streptomyces tubercidicus UAE1.
With the use of TEM, the presence of the spore chains and substrate mycelia of isolate #12 was determined within the cortex of roots (Figure 3A) and stems (Figure 3B). We also figured out individual spores colonizing the cortical cells (Figure 3C) and attaching the cell membrane of host plant roots (Figure 3C) and stems (Figure 3D). This indicates a beneficial association between isolate #12 and mangrove seedlings. It is worth mentioning that we did not observe plant cell defects in any of the imaged samples in these microscopic studies. Overall, our data suggested that isolate #12 is an actinobacterial endophyte that inhabits the living root and stem tissues of mangroves.
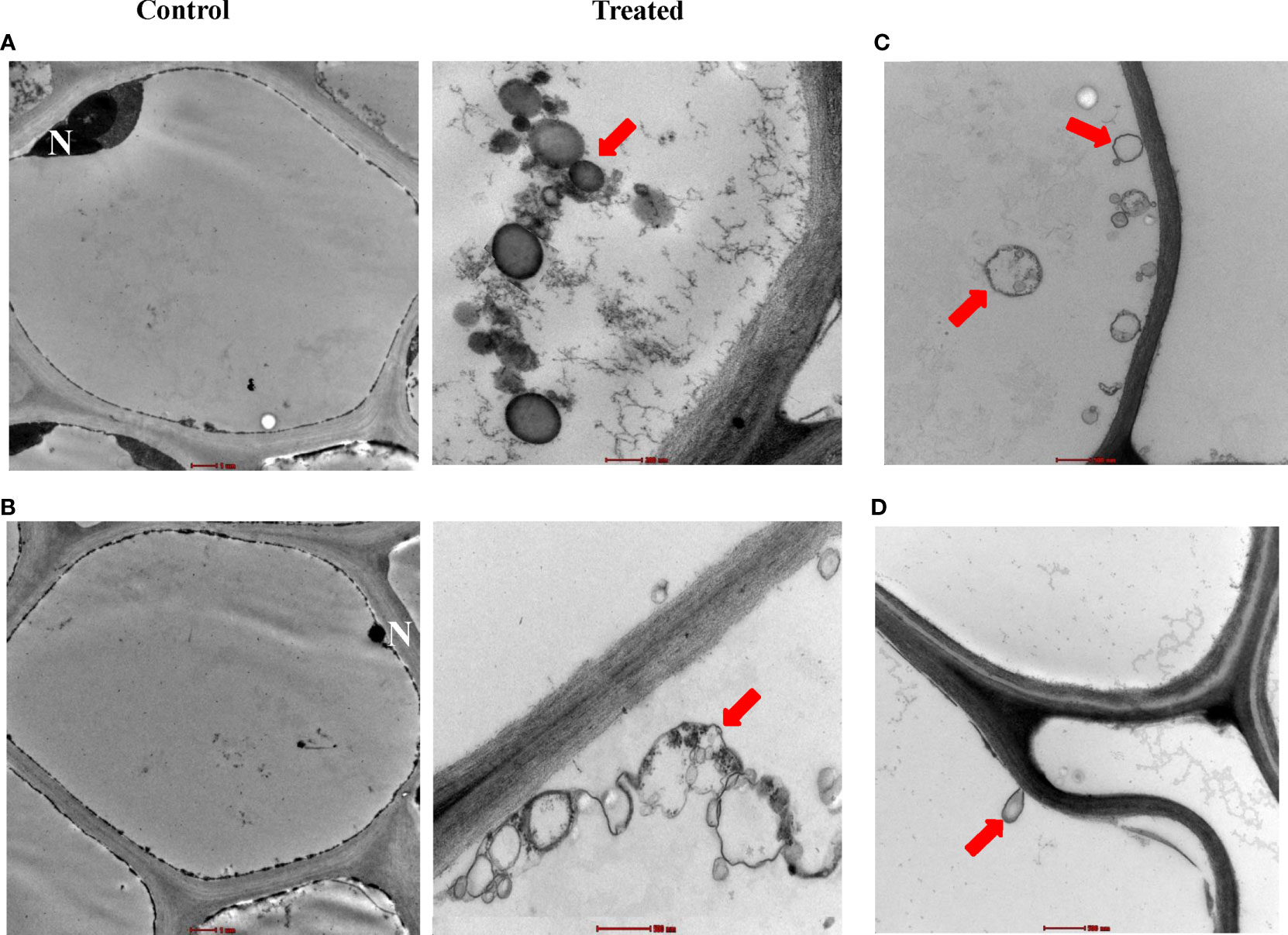
Figure 3 Intercellular colonization of mangrove root and stem tissues by actinobacterial isolate #12. Transmission electron micrograph of ultra-thin sections of mangrove (A) root and (B) stem not inoculated (×6,000; control; left) or inoculated (treated; right) with isolate #12 (top, ×43,000; bottom, ×26,500). (C) Distribution of individual spores in root cells (×16,500) and (D) attachment of spores on the cell membrane (×20,500). (A–D) All sections were stained with uranyl acetate and lead citrate showing the distribution of spore chains (red arrows). Bars: (A, left; B, left) 1 µm, (B, right, C, D) 500 nm, and (A, right) 200 nm. Isolate #12 represents Streptomyces tubercidicus UAE1. N, nucleus.
3.3 Taxonomic, Cultural, and Morphological Identification of Streptomyces tubercidicus UAE1
Genomic DNA was extracted from the promising endophytic actinobacterial isolate, and the 16S rRNA gene was amplified (1,523 bp), sequenced, and deposited in GenBank under accession number MT883495. Next, the amplified fragment was used to perform a comparative sequence analysis with sequences available in GenBank. Our results identified actinobacterial species belonging to the genus Streptomyces. The identity between the 16S rRNA gene sequences obtained from isolate #12 and those available in GenBank ranged from 97.9% to 100.0%, of which Streptomyces nigrescens NBRC12894, Streptomyces libani NBRC13452, and S. tubercidicus NBRC13090 showed the highest similarities (Figure 4A). To distinguish it from other Streptomyces species, we described the pure cultures of this particular strain and the morphological characteristics of its spore chains. On ISP medium 3, the actinobacterial isolate developed light brownish gray mass color and yellowish-brown substrate mycelium with the production of yellow pigment on the reverse side of cultures (Figure 4B). The spore chains belonged to section Spirales, consisting of 3–10 mature, smooth-surfaced spores per chain (Figure 4C). Together, the molecular phylogeny, culture characteristics, and morphology of spores classified isolate #12 as S. tubercidicus Nakamura, 1961 Strain UAE1.
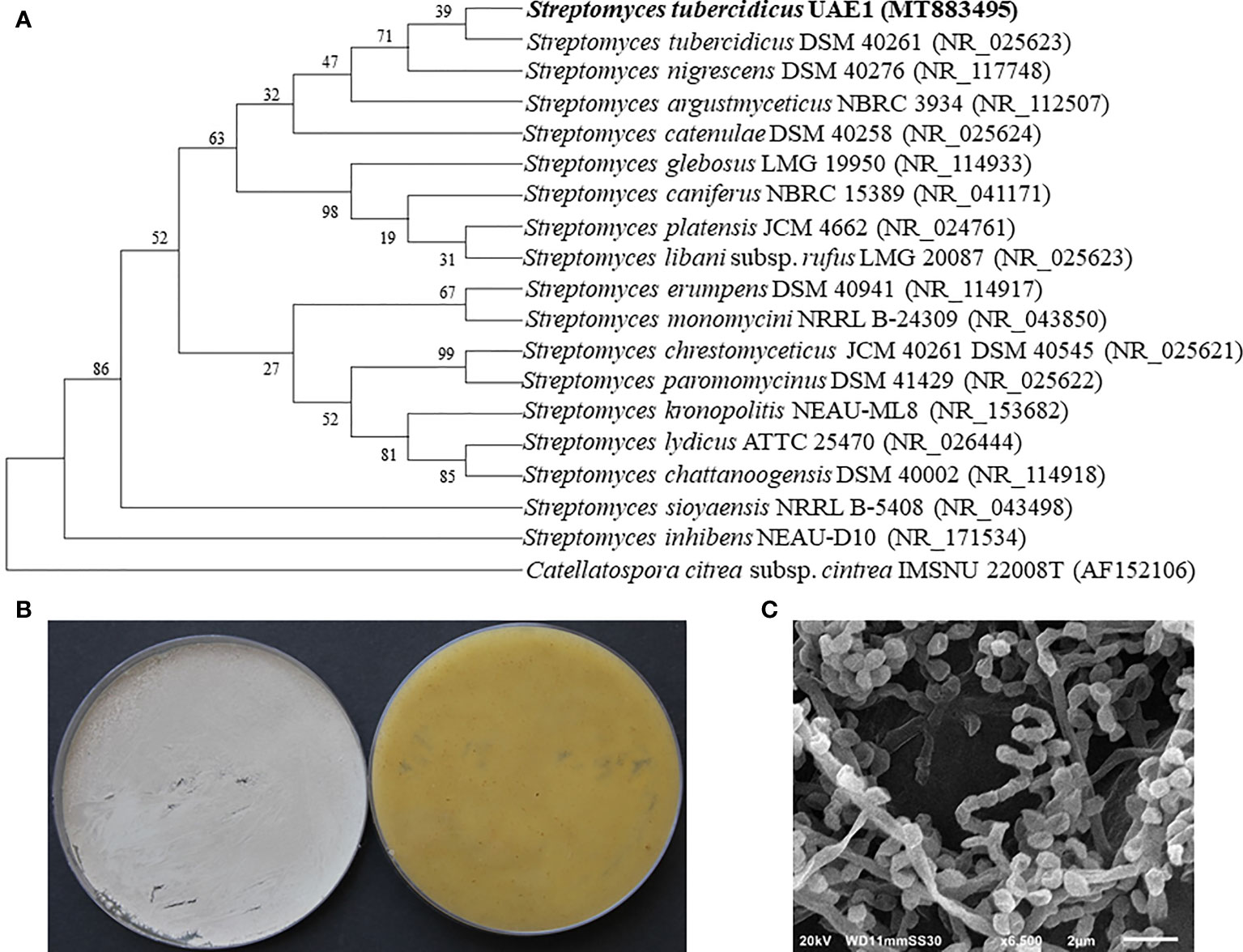
Figure 4 Taxonomic determination of Streptomyces tubercidicus UAE1, based on phylogenetic, cultural, and morphological characteristics. (A) The tree showing the phylogenetic relationships between S. tubercidicus UAE1 (MT883495; 1,523 bp) and other members of Streptomyces species on the basis of 16S rRNA sequences. (B) Aerial mycelia (left) and substrate mycelia (right) growing on ISP medium 3 supplemented with yeast extract, and (C) scanning electron micrograph (×6,500) of the spiral-shaped chains and smooth-surfaced spores of the strain of S. tubercidicus UAE1. (A) Numbers at nodes indicate percentage levels of bootstrap support based on a maximum likelihood analysis of 1,000 resampled datasets. Catellatospora citrea subsp. citrea IMSNU 22008 (AF52106) was used as an outgroup. GenBank accession numbers are given in parentheses.
3.4 Evaluation of Growth Promotion of Mangrove Seedlings Under Greenhouse Conditions
To determine their effect on growth promotion, mangrove seedlings were grown in sediment supplemented with SWE and/or inoculated with S. tubercidicus UAE1 (St) under greenhouse conditions. After SWE application or St inoculation, mangroves grew and developed into healthy plants (Figure 5A). The DW (Figure 5B) and length (Figure 5C) of roots and shoots apparently increased with either SWE or St compared to non-treated plants at the end of 9 mpp of the inoculated mangrove propagules, thus significantly (p < 0.05) varied between the two treatments. Seedlings of mangroves treated with the combination of SWE+St, however, showed the greatest DW and length of root and shoot systems. In line with that, the number of branches significantly (p < 0.05) increased by 40.6% and 55.3% in St-inoculated plants and SWE-treated, respectively (Figure 5D). We also noticed that the total leaf area was larger in plants treated with SWE by 29.0% or St by 18.3% than in non-inoculated plants (Figure 5E). The number of branches and total leaf area per plant were recorded at the highest values with SWE+St by 64.8% and 38.0%, respectively.
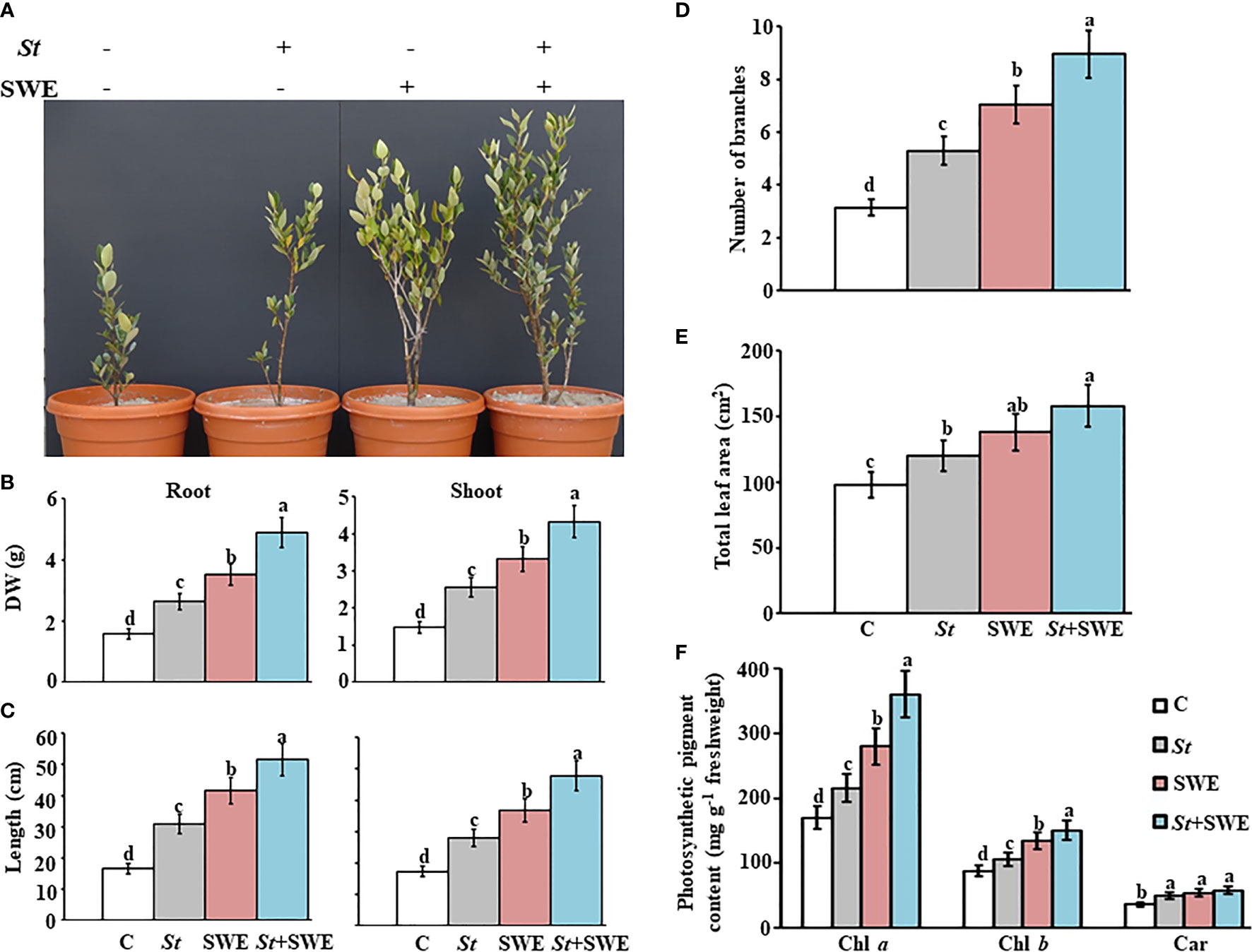
Figure 5 Effect of the application of seaweed extract and Streptomyces tubercidicus UAE1 on mangrove growth under greenhouse conditions. Effect of the inoculation of the endophytic isolate Streptomyces tubercidicus UAE1 (St) and application of SWE biostimulant, on the (A) formation of the vegetative growth; (B) DW and (C) length of root (left panel) and shoot (right panel); (D) number of branches; (E) total leaf area; and (F) photosynthetic pigment contents of Chl a, Chl b, and Car of mangrove. (A–F) Non-supplemented/non-inoculated seedlings with either St or SWE were used as the control treatment. (B–F) Measurements were taken at the end of 9 months after planting the inoculated mangrove propagules. Values are means of 16 replicates ± SE for each sampling from two independent experiments. Mean values followed by different letters are significantly (p < 0.05) different from each other according to Fisher’s protected least significant difference (LSD) test. Bars represent SE. C, control (non-inoculated inorganic salt starch broth); St, Streptomyces tubercidicus UAE1 (isolate #12); SWE, seaweed extract; St+SWE, combination of S. tubercidicus UAE1 and SWE; DW, dry weight; Chl a/b, chlorophyll a/b, Car, carotenoids.
In planta photosynthetic pigments largely determine the photosynthetic capacity and hence plant growth (Li et al., 2018). Our results showed that contents of Chl a and Chl b pigments were significantly (p < 0.05) higher in SWE-treated plants than in non-inoculated or St-inoculated plants at 9 mpp in the inoculated mangrove propagules (Figure 5F). Among all the treatments, the combined treatment of SWE and St had the highest chlorophyll contents. Although there was no significant difference in the amounts of carotenoids in plants treated with SWE, St, or SWE+St, we observed that any of these treatments significantly (p < 0.05) stimulated the production of this particular photosynthetic pigment as compared to the control. Our data suggested that the growth of mangroves can be enhanced as a result of the photosynthesis and biomass production, more in plants treated with the combined treatment than the individual treatments of SWE or St.
3.5 Effect of Seaweed Extract and St on Plant Growth Regulators and Nutrient Contents in Mangrove Tissues
In the greenhouse, we also studied the effect of SWE and/or St application on the endogenous contents of PGRs (phytohormones and ACCD) and mineral nutrients in roots and shoots at 9 mpp in the inoculated propagules associated with the growth promotion of mangroves. In general, all PGR levels investigated in this study were higher in shoot than in root tissues. The concentration of auxins (IAA and IPYA) in the tissues of plants grown in sediment supplemented with SWE, inoculated with St or both, were significantly (p < 0.05) different from the control treatment and each other (Figure 6A; Figure S3). Seedlings grown in sediment supplemented with SWE or inoculated with St were characterized by about 28.3%–30.6% or 16.7%–17.3% higher IAA concentration in root and shoot, respectively, than those in control treatments. IAA levels increased by 42% in root and 33% in shoot tissues in plants supplied with the combined two treatments. Compared to control, there were greater contents of IPYA in roots (22.3%–41.4%) and shoots (18.0%-28.0%) in seedlings supplied with any of the three treatments, of which SWE+St was the highest (Figure 6A; Figure S3). Similarly, the concentration of three types of CKs (iPA, iPa, and Z) varied significantly (p < 0.05) among all treatments in root tissues (Figure S3). Although iPA and iPa contents increased significantly (p < 0.05) by SWE, St, and SWE+St treatments, we did not find significant (p > 0.05) differences in Z concentrations between control and St in shoots (Figure 6B). Treatments of SWE or SWE+St on mangrove seedlings increased Z in shoot tissues to similar levels, which were significantly (p < 0.05) higher than those with or without St (Figure 6B). There was no significant (p > 0.05) difference in the endogenous levels of ABA in mangrove root and shoot tissues in the four treatments (Figure S4). Our data suggested that the growth promotion of mangroves can be increased by the application of SWE or inoculation of St by increasing the endogenous levels of auxins and CKs in tissues; thus, these can be greatly enhanced by SWE+St treatment.
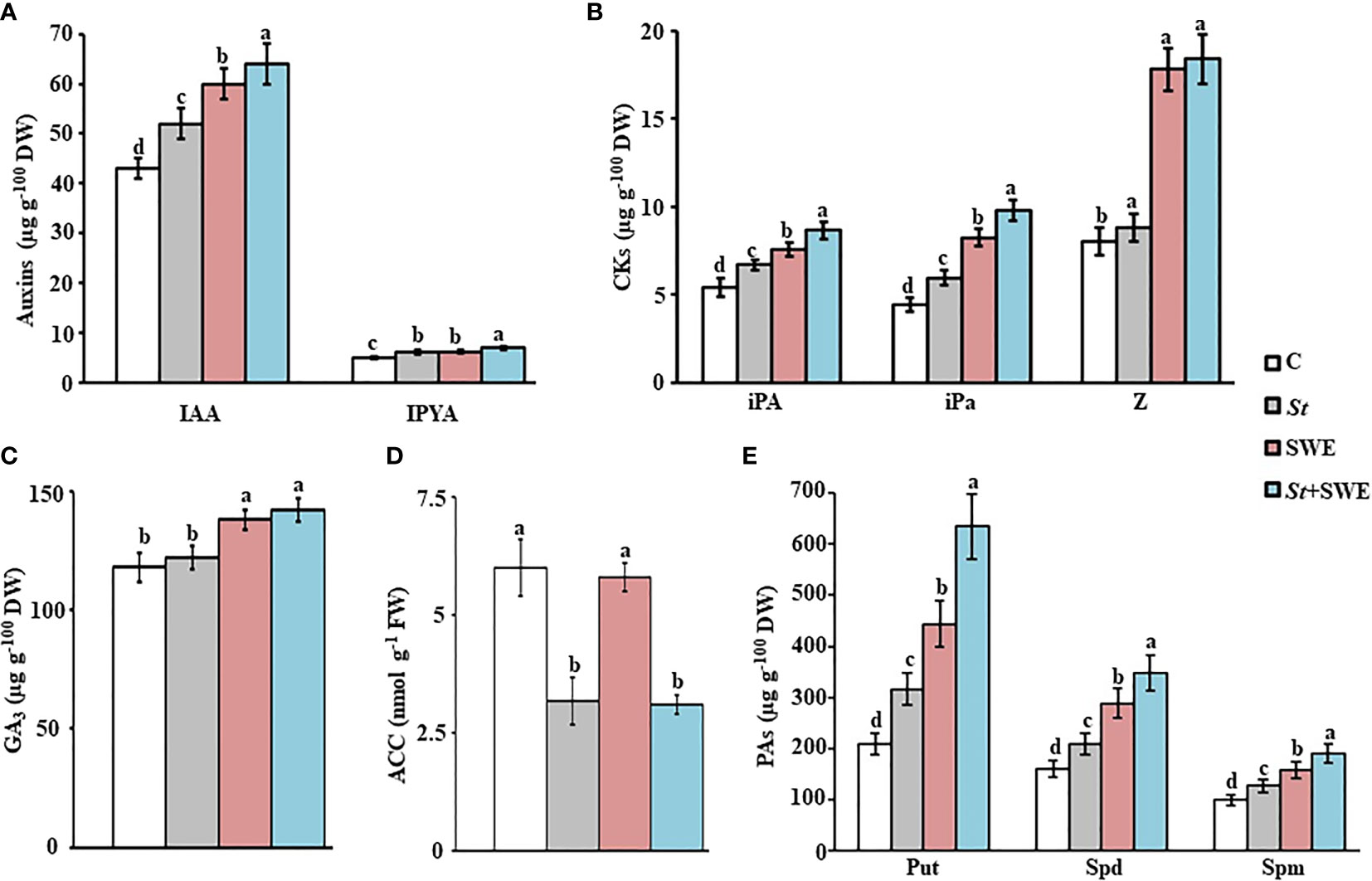
Figure 6 Effects of Streptomyces tubercidicus UAE1 and seaweed extract on PGRs in mangrove shoots. Endogenous contents of (A) auxins, (B) CKs, (C) GA3, (D) ACC, and (E) PAs in mangrove shoot tissues after treatment with SWE and/or St. Mangrove seedlings were grown in an evaporative-cooled greenhouse and maintained at 30°C ± 2°C. Values are means ± SE of 16 replicates for each treatment from two different independent experiments. Mean values followed by different letters are significantly (p < 0.05) different from each other according to Fisher’s protected LSD test. Bars represent SE. Endogenous contents of all PGRs were measured at the end of 9 months after planting the inoculated mangrove propagules. C, control (non-inoculated inorganic salt starch broth); St, Streptomyces tubercidicus UAE1 (isolate #12); SWE, seaweed extracts; St+SWE, combination of S. tubercidicus UAE1 and SWE; DW, dry weight; IAA, indole-3-acetic acid; IPYA, indole-3-pyruvic acid; iPA, isopentenyl adenine; iPa, isopentenyl adenoside; Z, zeatin; GA3, gibberellic acid, ACC, 1-aminocyclopropane-1-carboxylic; PA, polyamine, Put, putrescine; Spd, spermidine; Spm, spermine; LSD, least significant difference.
In addition, the contents of GA3 increased and ACC decreased significantly (p < 0.05) by St whether it was individually inoculated or combined with SWE in the examined tissues of mangroves (Figures 6C, D; S3). In general, there was a drop in ACC contents of 40%–50% in root and shoot tissues upon applying St. This suggests that the ACCD secreted by the local strain of S. tubercidicus UAE1 efficiently relieves plants from stress.
Under controlled conditions, there was a significant (p < 0.05) difference in the endogenous levels of Put, Spd, and Spm in tissues of all treatments (Figures 6E; S3). When St was inoculated or SWE was applied in pots containing seedlings of mangroves, roots had significantly (p < 0.05) higher levels of Put (34.2% or 53.2%, respectively), Spd (23.4% or 44.4%, respectively), and Spm (22% or 37.3%, respectively) than those grown without any biostimulant/bioinoculant. The treatment of SWE+St, however, increased Put by 62.7%, Spd by 61.1%, and Spm by 47.0% in the same tissue. Likewise, the pattern of increase in the three PAs was clearly demonstrated in shoot tissues as follows (from the highest to the lowest): SWE+St > SWE > St > control (Figures 6E; S3).
In addition, we examined if other growth-promoting substances (e.g., macro- and micronutrients) of St and SWE have a role in plant growth and development. In general, we found significant (p < 0.05) differences in the tissue contents of all examined macro- and micronutrients in plants treated with SWE (Table 2). We also noticed that there could be an additive effect of SWE when provided simultaneously with St. For instance, SWE or St significantly (p < 0.05) increased N in both tested tissues as compared to control plants (Table 2). Thus, these three nutrients increased to the highest levels when SWE and St were applied together compared to their corresponding individual treatments. Notably, there was a significant (p < 0.05) increase in the measured available P in sediments and contents of P and K in roots and shoots in plants treated with SWE and St+SWE compared to those non-treated (control) or inoculated with St only (Table 2). This suggests that SWE, but not St, can positively regulate nutrient (e.g., P) availability in sediments, thus increasing P and other nutrients in mangrove tissues for improved growth characteristics.
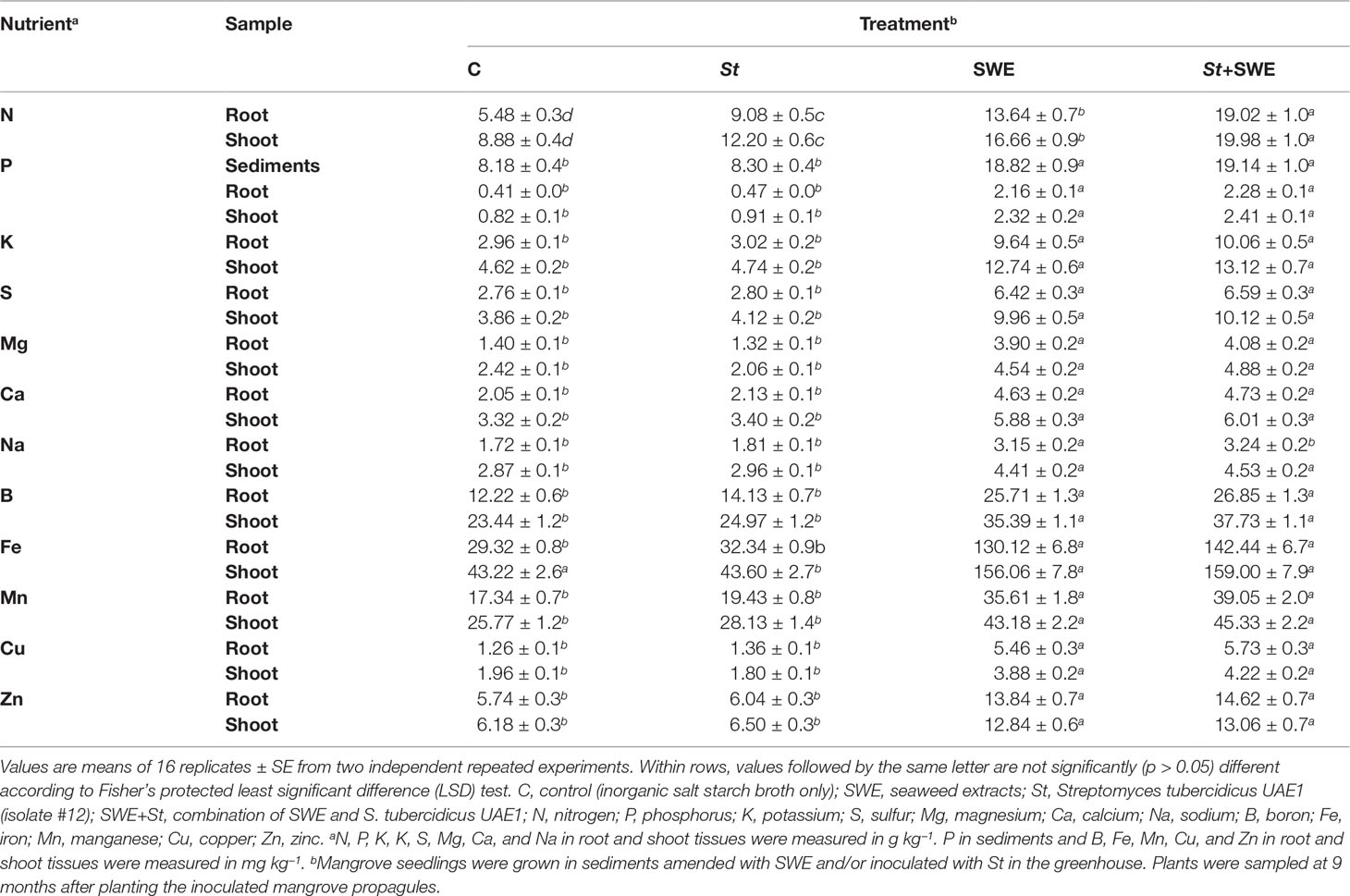
Table 2 Effect of seaweed extracts and/or Streptomyces tubercidicus UAE1 on available P concentration in sediments and tissue nutrient contents in mangrove grown in the greenhouse.
In addition, there were significant (p < 0.05) differences in the concentrations of S, Mg, Ca, Na, B, Fe, Mn, Cu, and Zn in the shoots and roots of SWE- or St+SWE-treated plants as compared to those non-inoculated or inoculated with St (Table 2), although all the measured macro- and micro-elements were insignificantly (p > 0.05) different between control and St treatments. This suggests that St does not have a direct effect on the uptake of these mineral nutrients. In general, St+SWE-treated plants showed a comparable effect in the concentrations of the abovementioned elements in tissues compared to those SWE treated. Our data indicated that the biostimulant SWE and the bioinoculant S. tubercidicus UAE1 can compensate for the lack/deficiency of mineral nutrients in sediments and regulate endogenous PGRs in planta, thus enhancing the growth of mangroves and improving the efficiency of photosynthesis.
3.6 Mangrove Growth Promotion Under Non-Controlled Open-Field Nursey Conditions
The DW and length of roots and shoots were clearly increased with either St or SWE compared to non-treated plants at 12 mpp of the inoculated mangrove propagules under non-controlled open-field nursery conditions; thus, these parameters significantly (p < 0.05) varied between the two treatments (Table 3). Mangrove plants treated with St+SWE demonstrated, however, the greatest effect on their DW and length. Along with that, the number of branches significantly (p < 0.05) increased by 1.7 and 2.2 times in St-inoculated and SWE-treated plants, respectively (Table 3). In addition, the total leaf area was larger in plants treated with St by 21.6% or SWE by 38.1% times than in non-inoculated plants. The number of branches and total leaf area per plant were recorded as the highest with St+SWE, reaching threefold and 63.1%, respectively.
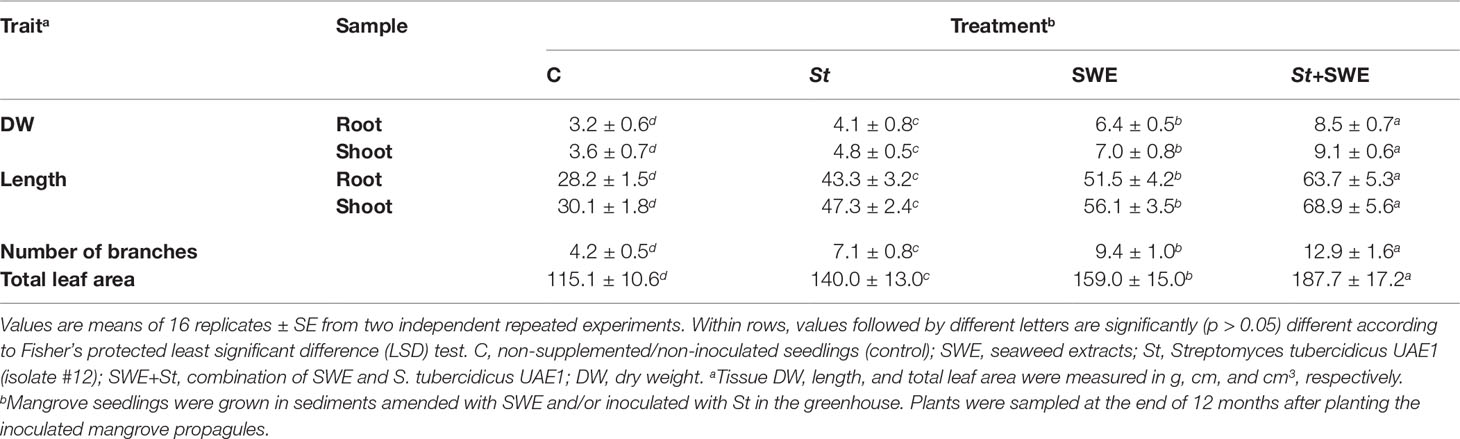
Table 3 Effect of the application of seaweed extracts and Streptomyces tubercidicus UAE1 on the growth of mangrove under non-controlled open-field nursery conditions.
3.7 Stimulation of Microbial Activity in Mangrove Sediments Upon Seaweed Extract Treatment
Endophytic PGPA isolates grew and sporulated on SWEA. This suggests that SWE can serve as a nutrient/food base for the growth of PGPA isolates without showing any adverse effect on the growth and multiplication of isolates. Microbial activity in sediment amended with SWE was found to be significantly (p < 0.05) higher than in the non-amended control sediment in both the greenhouse and open-field nursery experiments (Table S3). An increase of 66.4% after 9 mpp in the greenhouse and 75.3% at 12 mpp was observed in the open-field nursery of mangrove seedlings supplemented with SWE compared to the control sediment. Thus, there was no significant (p > 0.05) difference between SWE treatments, with or without inoculation with St. When comparing St-inoculated and non-inoculated (control) sediments under controlled (i.e., greenhouse) and non-controlled (i.e., open-field nursery) conditions, no significant (p > 0.05) difference was detected in the microbial activities (Table S3). This suggests that SWE can increase the number of soil microbiota, in addition to its growth benefits when applied to marine plants, such as mangroves.
4 Discussion
Biostimulants are widely studied for their role in improving plant growth and productivity. They are derived from a range of natural resources including manure compost, SWE, and beneficial PGPR (Yakhin et al., 2017) and are mostly applied solely to plants. However, the combined effects of different biostimulants are seldom investigated. In our efforts to preserve and increase mangrove forest coverage in the UAE and the Arabian Gulf, we determined—for the first time—the interactive effect of the endophytic actinobacterial isolate S. tubercidicus UAE1 and the commercial SWE-based biostimulant, applied alone and in combination on mangrove plants. Our aim was to evaluate the effect of St and SWE on the growth characteristics and phytochemical contents of mangrove plants.
Previously, in vitro investigations followed by preliminary in vivo studies have successfully identified rhizosphere-competent and endophytic PGPA of various mechanisms in Salicornia (El-Tarabily et al., 2019; Mathew et al., 2020), mangroves (El-Tarabily et al., 2021a; El-Tarabily et al., 2021b), and other plant species (Al Hamad et al., 2021; Al Raish et al., 2021; Alblooshi et al., 2022). An extensive in vitro screening was carried out in the current study to find the suitable salt-tolerant endophytic PGPA strains producing high levels of PGRs (auxins, CKs, GA3, and PAs) and possessing ACCD activity. The potential isolates were also assessed for their effects on growth as well as their endophytic nature in plant tissues under gnotobiotic and greenhouse conditions. Accordingly, S. tubercidicus UAE1 was identified based on the similarity of the 16S rRNA gene to others of Streptomyces species. Consistent with the positive effects of PGPA on the growth of mangroves as a halophytic plant in the current study, other reports have shown the involvement of PGPR in the growth promotion of non-halophytic plant species. For example, Ansari and Ahmad (2019) have demonstrated that inoculation with B. licheniformis and P. fluorescens can improve growth and photosynthetic efficiency in wheat. In alignment with that, P. fluorescens-inoculated plants enhanced yields and resistance against pathogens in rice (Nehal, 2015).
The rise of the global seaweed industry and its environmental consequences has, with no doubt, positive impacts on agriculture. SWE-based biostimulants have been commercially used for their plant growth promotion as fertilizers and soil conditioning agents (Rouphael and Colla, 2020). The application of A. nodosum-based biostimulants can stimulate plant growth, stress tolerance, and disease management (Shukla et al., 2019). However, the role of SWE on marine plant species has not been elucidated yet. In the current study, a commercial A. nodosum-based SWE was tested on mangrove seedlings, thus suggesting its contribution to plant growth promotion. This could be attributed to the presence of various nutrients, organic matter, and PGRs in SWE. Previously, SWE-treated okra (Abelmoschus esculentus) showed increased length and weight of shoots and numbers of leaves and roots under P and K deficiencies (Papenfus et al., 2013). Spraying of SWE on sugarcane seedlings enhanced growth and increased cane yield and sucrose content (Chen et al., 2021a). The SWE, originally derived from Sargassum horneri, also increased yield and fruit hardness and shortened ripening time, thus achieving high net returns for tomatoes (Yao et al., 2020). SWE can also promote chlorophyll biosynthesis or minimize its breakdown (Sharma et al., 2014). In the current study, contents of chlorophyll and carotenoids were highly abundant in SWE-treated seedlings of mangroves (Figure 5F), which could potentially increase photosynthetic rates in leaves (Bulgari et al., 2019). Similarly, Rengasamy et al. (2016) have detected an elevation in photosynthetic pigments in cabbage treated with eckol (a phenolic SWE compound).
Plant improvement can be associated with the application of the seaweed fertilizer by enhancing N uptake and synthesis of chlorophyll to increase photosynthetic rates of Arachis hypogaea (Prakash et al., 2014). In the present study, SWE-treated plants showed an increase in tissue N and S acquisition (Table 2). In addition, the roots and shoots were able to uptake more P, K, Mg, Ca, Na, B, Fe, Mn, Cu, and Zn after SWE application than in control or St-treated seedlings of mangroves. Similar observations have been reported in corn leaves that absorbed more nutrients in plants treated with SWE originated from A. nodosum or Laminaria spp. than in non-treated control plants (Ertani et al., 2018). Mustard (Brassica rapa L. ssp. sylvestris) and switchgrass (Panicum virgatum L.) supplied with SWE significantly increased K uptake in leaves (Di Stasio et al., 2017; Fei et al., 2017). Brown and Saa (2015) have proposed that SWE-based biostimulants are not nutrients per se; instead, they facilitate the uptake of nutrients or contribute to growth promotion or stress resistance. We argue that SWE supplemented with mangrove sediments can enhance soil nutrient contents and stimulate soil microbiota (Table S3). Hence, this improves plant health and aids plant response during periods of stress. It has also been reported that SWE can cause a reduction in electrolyte leakage and lipid peroxidation, decreased Na+/K+ ratio, and increased Ca2+ content, thus reducing ionic disparity (Ali et al., 2021). In line with that, we noticed a decrease in Na+/K+ ratio and an increase in Ca2+ concentration by at least twofold in seedlings treated with St+SWE compared to the control. In general, the increased biomass, lengths, branching, and total leaf area observed in mangrove seedlings upon the application of SWE (Figure 5) may lower the atmospheric CO2, thus contributing to global carbon sequestration and sustainable marine ecosystems (Troell et al., 2022). Future research will focus on measuring the atmospheric CO2 concentrations upon SWE treatment.
Compared to individual treatments, St+SWE had additive and synergistic effects to improve growth and productivity in mangroves. Similar observations have been reported in crop plants using a combination of PGPR bioinoculants and SWE biostimulants (Ngoroyemoto et al., 2020; Aremu et al., 2022). Despite the benefits of PGPR on the growth and yield of Salicornia and mangroves (Bashan et al., 2000; Komaresofla et al., 2019; Mathew et al., 2020; El-Tarabily et al., 2021a), the impact of the co-application of SWE and rhizosphere and/or endophytic PGPA in marine agriculture is still meager. Thus, the novelty of the present study was the use of SWE combined with S. tubercidicus UAE1 to provide more nutritional values and PGRs to govern all the factors of growth and development within mangroves for sustainable marine farming. The overlapping growth promotion and stress relief effects between these two stimulants may offer an opportunity for synergy if applied together. Actinoplanes deccanensis, Streptomyces euryhalinus, Streptomyces polychromogenes, and Streptomyces bacillaris are PGPA strains that promote growth and enhance biochemical properties in Salicornia and mangroves by stimulating the endogenous levels of PGRs (El-Tarabily et al., 2020; El-Tarabily et al., 2021a). Aligned with that, the endophytic S. tubercidicus UAE1 developed its abilities to produce relatively high levels of PGRs and increased the enzymatic activity of ACCD. Similar to other ACCD-producing PGPA isolates (El-Tarabily et al., 2019; Mathew et al., 2020; El-Tarabily et al., 2021b), St reduced ACC levels in both shoot and root tissues in mangrove plants. This suggests that ACCD is a major mechanism utilized by St to lower ET levels and reduce environmental stresses in planta; thus, this is in agreement with other reports (Glick et al., 2007; El-Tarabily et al., 2019).
The nutrient uptake was, however, most pronounced in tissues of plants treated with St+SWE (Table 2). This indicates that SWE can enhance plant growth (Figure 5) directly through the regulation of macro- and micronutrients (Table 2) and endogenous PGRs (Figure 6; Figure S3) or indirectly through the enhancement of other microorganisms in the rhizosphere to promote growth (Table S3). This was evident when P levels significantly (p < 0.05) increased in sediments and plant tissues upon the application of SWE with or without St (Table 2). Some studies have suggested that SWE treatments may cause significant changes in the microbiome of the soil and plant, thus contributing to plant growth (Renaut et al., 2019). For instance, SWE supplements enrich the diversity of rhizosphere bacteria, which, in turn, enhances soil nutrient levels and increases the yield and quality of rice (Chen et al., 2022). The current study not only demonstrated the potential of SWE to serve as a nutrient base for PGPA but also supported the overall increase of the microbial activity in mangrove sediments (Table S3). These results were in agreement with a previous study on the biostimulant fish emulsion, which was successfully used as a food base for PGPR/PGPA to promote the growth and productivity of radish (El-Tarabily et al., 2003). The combined treatment of St+SWE, used in the present study, resulted in a significant improvement in the growth of mangroves, indicating that the positive effect of SWE complemented that of the PGPA isolate.
It is worth mentioning that not all combinations of biostimulants are synergistic; they might have antagonistic effects instead. For example, the combination of either B. licheniformis or P. fluorescens and smoke-water (containing naturally occurring stimulant karrikinolide) were antagonistic, albeit there was slight rooting improvement of grapevine cuttings when applied individually (Papenfus et al., 2015). This might be attributed to the overlapping modes of action, thus disrupting hormone levels in the plant. In the current study, the presumed mode of action involved in the stimulation of growth performance and chlorophyll biosynthesis was linked to enhanced nutrient availability and PGRs driven by the synergistic action of St and SWE applied in combination. Thus, this could be due to the different types of signal transduction pathways activated by the two stimulants in order to mediate shared signaling components (e.g., hormones) and to regulate the expression level of a pathway-specific component by the other pathway (Mengiste et al., 2010; AbuQamar et al., 2021). Elucidating regulatory mechanisms employed by the endophytic St and biostimulant SWE in enhancing the growth of mangroves is on top of our priorities.
5 Conclusion
The current study demonstrated, for the first time, the remarkable effect of S. tubercidicus UAE1 (an endophytic PGPA isolate) and A. nodosum (a commercial SWE-based biostimulant) on the growth of mangroves. The individual St and SWE treatment had varying effects on growth, endogenous PGRs, and mineral element content in mangrove tissues. Compared to St treatment, SWE treatment enhanced growth parameters, photosynthetic pigments, PGRs levels (IAA, CKs, GA3, and PAs), and nutritional quality of mangroves. Even though St enhanced growth to a lesser extent than SWE, it did not inhibit the growth parameters relative to control. Overall, St had a positive impact on mangrove growth due to the increased auxins, iPA, iPa, PAs, and N, and decreased ACC in planta, thus mitigating the adverse effects of stress-generating ET. However, the combined treatments of St+SWE were relatively superior in enhancing the growth of mangroves over any of the single treatments. The novelty of this research lies in the fact that SWE can act as a conducive environment for PGPR/PGPA to grow. This research also proves that mangrove plants treated with St and SWE can increase nutrients and endogenous PGRs levels in plant tissues, for better growth of marine plants (e.g., gray mangrove) under greenhouse and open-field nursery conditions. Thus, this novel combination can be used on a commercial applied basis. Our long-run objective is to foster the propagation of mangroves in open areas along the Arabian Gulf coastline, thus helping the UAE implement a mangrove reforestation program.
Data Availability Statement
The datasets presented in this study can be found in online repositories. The names of the repository/repositories and accession number(s) can be found below: NCBI [accession: MT883495.1].
Author Contributions
KE-T and SA designed and conceived the research. KE-T and SA supervised the study. AA and AT performed greenhouse experiments. SA developed the phylogenetic analyses. GR and KE-T performed microscopic experiments. AA, KE-T, and SA analyzed the data. AA, GR, and AT assisted with experiments and/or data evaluation. KE-T and SA wrote the manuscript. All authors critically revised the manuscript and approved the final version.
Funding
This project was funded by Abu Dhabi Award for Research Excellence—Department of Education and Knowledge (Grant #21S105) to KE-T and Khalifa Center for Biotechnology and Genetic Engineering-UAEU (Grant #31R286) to SA.
Conflict of Interest
The authors declare that the research was conducted in the absence of any commercial or financial relationships that could be construed as a potential conflict of interest.
Publisher’s Note
All claims expressed in this article are solely those of the authors and do not necessarily represent those of their affiliated organizations, or those of the publisher, the editors and the reviewers. Any product that may be evaluated in this article, or claim that may be made by its manufacturer, is not guaranteed or endorsed by the publisher.
Supplementary Material
The Supplementary Material for this article can be found online at: https://www.frontiersin.org/articles/10.3389/fmars.2022.896461/full#supplementary-material
References
AbuQamar S. F., El-Tarabily K. A., Sham A. (2021). “Co-expression Networks in Predicting Transcriptional Gene Regulation,” in Modeling Transcriptional Regulation: Methods and Protocols, Methods in Molecular Biology, vol. 2328 . Ed. Mukhtar S. (New York, USA: Springer Science + Business Media L.C.C., Humana Press), 1–11.
Alblooshi A. A., Purayil G. P., Saeed E. E., Ramadan G. A., Tariq S., Altaee A. S., et al. (2022). Biocontrol potential of endophytic actinobacteria against Fusarium solani, the causal agent of sudden decline syndrome on date palm in the UAE. J. Fungi 8, 8.
Al Hamad B. M., Al Raish S. M., Ramadan G. A., Saeed E. E., Alameri S. S. A., Al Senaani S. S., et al. (2021). Effectiveness of augmentative biological control of Streptomyces griseorubens UAE2 depends on 1-aminocyclopropane-1-carboxylic acid deaminase activity against Neoscytalidium dimidiatum. J. Fungi 7, 885. doi: 10.3390/jof7110885
Ali O., Ramsubhag A., Jayaraman J. (2021). Biostimulant properties of seaweed extracts in plants: implications towards sustainable crop production. Plants 10, 531. doi: 10.3390/plants10030531
Allard S. M., Costa M. T., Bulseco A. N., Helfer V., Wilkins L. G. E., Hassenrück C., et al. (2020). Introducing the mangrove microbiome initiative: identifying microbial research priorities and approaches to better understand, protect, and rehabilitate mangrove ecosystems. mSystems 5, e00658-20. doi: 10.1128/mSystems.00658-20
Al Raish S. M., Saeed E. E., Alyafei D. M., El-Tarabily K. A., AbuQamar S. F. (2021). Evaluation of streptomycete actinobacterial isolates as biocontrol agents against royal poinciana stem canker disease caused by Neoscytalidium dimidiatum. Biol. Control. 164, 104783. doi:10.1016/j.biocontrol.2021.104783
Alsumaiti T. S., Hussein K., Al-Sumaiti A. S. (2017). Mangroves of Abu Dhabi Emirate, UAE, in a global context: A review. Int. J. Environ. Sci. 6, 110–121.
Ansari F. A., Ahmad I. (2019). Fluorescent Pseudomonas -FAP2 and Bacillus licheniformis interact positively in biofilm mode enhancing plant growth and photosynthetic attributes. Sci. Rep. 9, 4547. doi:10.1038/s41598-019-40864-4
Aremu A. O., Makhaye G., Tesfay S. Z., Gerrano A. S., Du Plooy C. P., Amoo S. O. (2022). Influence of commercial seaweed extract and microbial biostimulant on growth, yield, phytochemical content, and nutritional quality of five Abelmoschus esculentus genotypes. Agronomy 12, 428. doi: 10.3390/agronomy12020428
Arena M. E., Manca de Nadra M. C. (2001). Biogenic amine production by Lactobacillus.J. Appl. Microbiol. 90, 158–162. doi: 10.1046/j.1365-2672.2001.01223.x
Ashour M., Hassan S. M., Elshobary M. E., Ammar G. A. G., Gaber A., Alsanie W. F., et al. (2021). Impact of commercial seaweed liquid extract (TAM®Q4) biostimulant and its bioactive molecules on growth and antioxidant activities of hot pepper (Capsicum annuum).Plants 10, 1045.
Barth H., Khan N. (2008). “Biogeophysical setting of the Gulf,” in Protecting the Gulf’s Marine Ecosystems from Pollution, Eds. Abuzinada A., Barth H., Krupp F., Boer B., Abdessalam T. (Basel, Switzerland: Brikhauser Verlag), 1–21.
Bashan Y., Moreno M., Troyo E. (2000). Growth promotion of the seawater-irrigated oilseed halophyte Salicornia bigelovii inoculated with mangrove rhizosphere bacteria and halotolerant Azospirillum spp. Biol. Fertil. Soils 32, 265–272. doi: 10.1007/s003740000246
Bashan Y., Holguin G. (2002). Plant growth-promoting bacteria: a potential tool for arid mangrove reforestation. Trees 16, 159–166. doi: 10.1007/s00468-001-0152-4
Borines M. G., McHenry M. P., de Leon R. L. (2011). Integrated macroalgae production for sustainable bioethanol, aquaculture and agriculture in Pacific island nations. Biofuels Bioprod. Biorefin. 5, 599–608. doi: 10.1002/bbb.310
Bradáčová K., Weber N. F., Morad-Talab N., Asim M., Imran M., Weinmann M., et al. (2016). Micronutrients (Zn/Mn), seaweed extracts, and plant growth-promoting bacteria as cold-stress protectants in maize. Chem. Biol. Technol. Agric. 3, 19. doi: 10.1186/s40538-016-0069-1
Bradford M. (1976). A rapid and sensitive method for the quantitation of microgram quantities of protein utilizing the principle of protein-dye binding. Anal. Biochem. 72, 248–258. doi: 10.1016/0003-2697(76)90527-3
Brown P., Saa S. (2015). Biostimulants in agriculture. Front. Plant Sci. 6, 671. doi: 10.3389/fpls.2015.00671
Bulgari R., Franzoni G., Ferrante A. (2019). Biostimulants application in horticultural crops under abiotic stress conditions. Agronomy 9, 306. doi: 10.3390/agronomy9060306
Chami D. E., Galli F. (2020). An assessment of seaweed extracts: Innovation for sustainable agriculture. Agronomy 10, 1433. doi: 10.3390/agronomy10091433
Chen W., Hoitink H. A. J., Madden L. V. (1988). Microbial activity and biomass in container media for predicting suppressiveness to damping-off caused by Pythium ultimum. Phytopathology 78, 1447–1450. doi: 10.1094/Phyto-78-1447
Chen D., Zhou W., Yang J., Ao J., Huang Y., Shen D., et al. (2021a). Effects of seaweed extracts on the growth, physiological activity, cane yield and sucrose content of sugarcane in China. Front. Plant Sci. 12, 659130. doi: 10.3389/fpls.2021.659130
Chen M. S., Chen X. H., Huang Z. H., Yan X. R., Tuo L. (2021b). Phycicoccus avicenniae sp. nov., a novel endophytic actinomycete isolated from a branch of Avicennia mariana. Antonie Van Leeuwenhoek. 114, 1431–1442. doi: 10.1007/s10482-021-01614-7
Chen C.-L., Song W.-L., Sun L., Qin S., Ren C.-G., Yang J.-C., et al. (2022). Effect of seaweed extract supplement on rice rhizosphere bacterial community in tillering and heading stages. Agronomy 12, 342. doi: 10.3390/agronomy12020342
Davies B. H. (1965). “Analysis of carotenoid pigments,” in Chemistry and Biochemistry of Plant Pigments, vol. pp . Ed. Goodwin T. W. (London, UK: Academic Press), 489–532.
di Menna M. E. (1957). The isolation of yeasts from soil. J. Gen. Microbiol. 17, 678–688. doi: 10.1099/00221287-17-3-678
Di Stasio E., Rouphael Y., Colla G., Raimondi G., Giordano M., Pannico A., et al. (2017). The influence of Ecklonia maxima seaweed extract on growth, photosynthetic activity and mineral composition of Brassica rapa L. ssp. sylvestris under nutrient stress conditions. Eur. J. Hortic. Sci. 82, 286–293. doi: 10.17660/eJHS.2017/82.6.3
Dodd R. S., Blasco F., Rafi Z. A., Torquebiau E. (1999). Mangroves of the United Arab Emirates: Ecotypic diversity in cuticular waxes at the bioclimatic extreme. Aquat. Bot. 63, 291–304. doi: 10.1016/S0304-3770(98)00124-7
Dworkin M., Foster J. (1958). Experiments with some microorganisms which utilize ethane and hydrogen. J. Bacteriol. 75, 592–601. doi: 10.1128/jb.75.5.592-603.1958
Dye D. W. (1962). The inadequacy of the usual determinative tests for identification of Xanthomonas spp. NZT Sci. 5, 393–416.
Egan S., Harder T., Burke C., Steinberg P., Kjelleberg S., Thomas T. (2013). The seaweed holobiont: understanding seaweed–bacteria interactions. FEMS Microbiol. Rev. 37, 462–476. doi: 10.1111/1574-6976.12011
Ellison A. M., Farnsworth E. J. (1996). Anthropogenic disturbance of Caribbean mangrove ecosystems: Past impacts, present trends, and future predictions. Biotropica 28, 549–565. doi: 10.2307/2389096
Elmahdy S. I., Ali T. A., Mohamed M. M., Howari F. M., Abouleish M., Simonet D. (2020). Spatiotemporal mapping and monitoring of mangrove forests changes from 1990 to 2019 in the Northern Emirates, UAE using random forest, kernel logistic regression and naive bayes tree models. Front. Environ. Sci. 8, 102. doi: 10.3389/fenvs.2020.00102
El-Tarabily K. A., Nassar A. H., Hardy G.E.St., Sivasithamparam K. (2003). Fish emulsion as a food base for rhizobacteria promoting growth of radish (Raphanus sativus L. var. sativus) sandy soil. Plant Soil 252, 397–411. doi: 10.1023/A:1024729620154
El-Tarabily K. A., AlKhajeh A. S., Ayyash M. M., Alnuaimi L. H., Sham A., ElBaghdady K. Z., et al. (2019). Growth promotion of Salicornia bigeloviiby Micromonospora chalcea UAE1, an endophytic 1-aminocyclopropane-1-carboxylic acid deaminase-producing actinobacterial isolate. Front. Microbiol. 10, 1694. doi: 10.3389/fmicb.2019.01694
El-Tarabily K. A., ElBaghdady K. Z., AlKhajeh A. S., Ayyash M. M., Aljneibi R. S., El-Keblawy A., et al. (2020). Polyamine-producing actinobacteria enhance biomass production and seed yield in Salicornia bigelovii. Biol. Fertil. Soils 56, 499–519. doi: 10.1007/s00374-020-01450-3
El-Tarabily K. A., Ramadan G. A., Elbadawi A. A., Hassan A. H., Tariq S., Ghazal E. W., et al. (2021a). The marine endophytic polyamine-producing Streptomyces mutabilis UAE1 isolated from extreme niches in the Arabian Gulf promotes the performance of mangrove (Avicennia marina) seedlings under greenhouse conditions. Front. Mar. Sci. 8, 710200. doi: 10.3389/fmars.2021.710200
El-Tarabily K. A., Sham A., Elbadawi A. A., Hassan A. H., Alhosani B. K. K., El-Esawi M. A., et al. (2021b). A consortium of rhizosphere-competent actinobacteria exhibiting multiple plant growth-promoting traits improves the growth of Avicennia marina in the United Arab Emirates. Front. Mar. Sci. 8, 715123. doi:10.3389/fmars.2021.715123
Ertani A., Francioso O., Tinti A., Schiavon M., Pizzeghello D., Nardi S. (2018). Evaluation of seaweed extracts from Laminaria and Ascophyllumnodosum spp. as biostimulants in Zea mays L. using a combination of chemical, biochemical and morphological approaches. Front. Plant Sci. 9, 428.
FAO (2020). Global Forest Resources Assessment Main Report (Rome, Italy: Food and Agriculture Organization of the United Nations), 184. doi: 10.4060/ca9825en
Fei H., Crouse M., Papadopoulos Y., Vessey J. K. (2017). Enhancing the productivity of hybrid poplar (Populus × Hybrid) and switchgrass (Panicum virgatum L.) by the application of beneficial soil microbes and a seaweed extract. Biomass Bioenergy 107, 122–134. doi: 10.1016/j.biombioe.2017.09.022
Felsenstein J. (1981). Evolutionary trees from DNA sequences: a maximum likelihood approach. J. Mol. Evol. 17, 368–376. doi:10.1007/BF01734359
Flores H. E., Galston A. W. (1982). Analysis of polyamines in higher plants by high performance liquid chromatography. Plant Physiol. 69, 701–706. doi: 10.1104/pp.69.3.701
Gaines T. P., Mitchell G. A. (1979). Boron determination in plant tissue by the azomethine-H method. Commun. Soil Sci. Plan. 10, 1099–1108. doi: 10.1080/00103627909366965
Gayathri P., Muralikrishnan V. (2013). Isolation and characterization of endophytic actinomycetes from mangrove plant for antimicrobial activity. Int. J. Curr. Microbiol. App. Sci. 2, 78–89.
Glick B. R. (2015). “Introduction to plant growth promoting bacteria,” in Beneficial Plant-Bacterial Interactions, vol. pp . Ed. Glick B. R. (Switzerland: Springer International Publishing), 1–28.
Glick B. R., Todorovic B., Czarny J., Cheng Z., Duan J., McConkey B. (2007). Promotion of plant growth by bacterial ACC deaminase. Crit. Rev. Plant Sci. 26, 227–242. doi: 10.1080/07352680701572966
Gordon S. A., Weber R. P. (1951). Colorimetric estimation of indoleacetic acid. Plant Physiol. 26, 192–195. doi: 10.1104/pp.26.1.192
Guinn G., Brummett D. L., Beier R. C. (1986). Purification and measurement of abscisic acid and indole-acetic acid by high performance liquid chromatography. Plant Physiol. 81, 997–1002. doi: 10.1104/pp.81.4.997
Hallmann J., Quadt-Hallmann A., Mahaffee W. F., Kloepper J. W. (1997). Bacterial endophytes in agricultural crops. Can. J. Microbiol. 43, 895–914. doi: 10.1139/m97-131
Hamza W., Munawar M. (2009). Protecting and managing the Arabian Gulf: past, present and future. Aquat. Ecosyst. Health Manage. 12, 429–439. doi: 10.1080/14634980903361580
Hassan S. M., Ashour M., Sakai N., Zhang L., Hassanien H. A., Ammar G. A. G., et al (2021). Impact of seaweed liquid extract biostimulant on growth, yield, and chemical composition of cucumber (Cucumis sativus).Agriculture 11, 320. doi: 10.3390/agriculture11040320
Holden M. (1965). “Chlorophylls,” in Chemistry and Biochemistry of Plant Pigments, vol. pp . Ed. Goodwin T. W. (London, UK: Academic Press), 462–488.
Holguin G., Bashan Y., Vazquez P. (2001). The role of sediment microorganisms in the productivity, conservation, and rehabilitation of mangrove ecosystems: an overview. Biol. Fert. Soils 33, 265–278. doi: 10.1007/s003740000319
Holguin G., Guzman M. A., Bashan Y. (1992). Two new nitrogen-fixing bacteria from the rhizosphere of mangrove trees: their isolation, identification and in vitro interaction with rhizosphere Staphylococcus sp. FEMS Microbiol. Ecol. 101, 207–216. doi: 10.1111/j.1574-6968.1992.tb05777.x
Hong K., Gao A. H., Xie Q. Y., Gao H., Zhuang L., Lin H. P., et al. (2009). Actinobacteria for marine drug discovery isolated from mangrove soils and plants in China. Mar. Drugs 7, 24–44. doi: 10.3390/md7010024
Honma M., Shimomura T. (1978). Metabolism of 1-aminocyclopropane-1-carboxylic acid. Agric. Biol. Chem. 42, 1825–1831. doi: 10.1080/00021369.1978.10863261
Hutchison J., Spalding M., zu Ermgassen P. (2014). The Role of Mangroves in Fisheries Enhancement Vol. pp (Cambridge, UK: The Nature Conservancy and Wetlands International), 54.
Jacob A., Xia A., Gunning D., Burnell G., Murphy J. D. (2016). Seaweed biofuel derived from integrated multi-trophic aquaculture. Int. J. Environ. Sci. Dev. 7, 805–809. doi: 10.18178/ijesd.2016.7.11.885
Jiang Z.-k., Tuo L., Huang D. L., Osterman I. A., Tyurin A. P., Liu S. W., et al. (2018). Diversity, novelty, and antimicrobial activity of endophytic actinobacteria from mangrove plants in Beilun Estuary National Nature Reserve of Guangxi, China. Front. Microbiol. 9, 868. doi: 10.3389/fmicb.2018.00868
Kamil F. H., Saeed E. E., El-Tarabily K. A., AbuQamar S. F. (2018). Biological control of mango dieback disease caused by Lasiodiplodia theobromae using streptomycete and non-streptomycete actinobacteria in the United Arab Emirates. Front. Microbiol. 9, 829. doi: 10.3389/fmicb.2018.00829
Khalid A., Arshad M., Zahir Z. A. (2004). Screening plant growth-promoting rhizobacteria for improving growth and yield of wheat. J. Appl. Microbiol. 96, 473–480. doi: 10.1046/j.1365-2672.2003.02161.x
Khare E., Mishra J., Arora N. K. (2018). Multifaceted interactions between endophytes and plant: Developments and prospects. Front. Microbiol. 9, 2732. doi: 10.3389/fmicb.2018.02732
Komaresofla B. R., Alikhani H. A., Etesami H., Khoshkholgh-Sima N. A. (2019). Improved growth and salinity tolerance of the halophyte Salicornia sp. by co–inoculation with endophytic and rhizosphere bacteria. Appl. Soil Ecol. 138, 160–170. doi: 10.1016/j.apsoil.2019.02.022
Kumar V., Bharti A., Gusain O., Bisht G. S. (2011). Scanning electron microscopy of Streptomyces without use of any chemical fixatives. Scanning 33, 1–4. doi: 10.1002/sca.20261
Kumar S., Stecher G., Tamura K. (2016). MEGA7: Molecular evolutionary genetics analysis version 7.0 for bigger datasets. Mol. Biol. Evol. 33, 1870–1874. doi: 10.1093/molbev/msw054
Küster E. (1959). Outline of a comparative study of criteria used in characterization of the actinomycetes. Int. Bull. Bact. Nomen. Taxon. 9, 97–104.
Lanneluc-Sanson D., Phan C. T., Granger R. L. (1986). Analysis by reverse-phase high-pressure liquid chromatography of phenylisothiocyanate-deriderivatized 1-aminocyclopropane-1-carboxylic acid in apple extracts. Anal. Biochem. 155, 322–327. doi: 10.1016/0003-2697(86)90441-0
Li Y., He N., Hou J., Xu L., Liu C., Zhang J., et al. (2018). Factors influencing leaf chlorophyll content in natural forests at the biome scale. Front. Ecol. Evol. 6, 64. doi: 10.3389/fevo.2018.00064
Lizada M. C., Yang S. F. (1979). A simple and sensitive assay for 1-aminocyclopropane-1-carboxylic acid. Anal. Biochem. 100, 140–145. doi: 10.1016/0003-2697(79)90123-4
Machàckovà I., Krekule J., Eder J., Seidlovà F., Strnad M. (1993). Cytokinins in photoperiodic induction of flowering Chenopodium species. Physiol. Plantarum 87, 160–166. doi: 10.1111/j.1399-3054.1993.tb00138.x
Marino M., Maifreni M., Moret S., Rondinini G. (2000). The capacity of Enterobacteriaceae species to produce biogenic amines in cheese. Lett. Appl. Microbiol. 31, 169–173. doi: 10.1046/j.1365-2672.2000.00783.x
Mathew B. T., Torky Y., Amin A., Mourad A. I., Ayyash M. M., El-Keblawy A., et al. (2020). Halotolerant marine rhizosphere-competent actinobacteria promote Salicornia bigelovii growth and seed production using seawater irrigation. Front. Microbiol. 11, 552. doi: 10.3389/fmicb.2020.00552
McQuaker N. R., Brown D. F., Kluckner P. D. (1979). Digestion of environmental materials for analysis by inductively coupled plasma-atomic emission spectrometry. Anal. Chem. 51, 1082–1084. doi: 10.1021/ac50043a071
Mengiste T., Laluk K., AbuQamar S. (2010). “Mechanisms of induced resistance against B. cinerea,” in Post-Harvest Pathology, Plant Pathology in The 21st Century, vol. 2pp . Eds. Prusky D., Gullino M. L. (Dordrecht, The Netherlands: Springer Science Business Media B.V.), 13–30.
Millonig G. (1976). “Laboratory Manual of Biological Electron Microscopy’,. Ed. Saviolo M.(Vercelli, Italy), 1–67.
Misaghi I. J., Donndelinger I. J. (1990). Endophytic bacteria in symptom-free cotton plants. Phytopathol. 80, 808–811. doi: 10.1094/Phyto-80-808
Moore G. E., Grizzle R. E., Ward K. M., Alshihi R. M. (2015). Distribution, pore-water chemistry, and stand characteristics of the mangroves of the United Arab Emirates. J. Coast. Res. 31, 957–963. doi: 10.2112/JCOASTRES-D-14-00142.1
Mukherjee A., Patel J. S. (2020). Seaweed extract: biostimulator of plant defense and plant productivity. Int. J. Environ. Sci. Technol. 17, 553–558. doi: 10.1007/s13762-019-02442-z
Murphy J., Riley J. P. (1962). A modified single solution method for the determination of phosphate in natural waters. Anal. Chim. Acta 27, 31–36. doi: 10.1016/S0003-2670(00)88444-5
Musson G., McInroy J. A., Kloepper J. W. (1995). Development of delivery systems for introducing endophytic bacteria into cotton. Biocontrol Sci. Technol. 5, 407–416. doi: 10.1080/09583159550039602
Nakamura G. (1961). Studies on antibiotic actinomycetes. II. On Streptomyces producing a new antibiotic tubercidin. J. Antibiot. (Tokyo) Ser. A 14, 90–93. doi: 10.11554/antibioticsa.14.2_90
Naser H. A. (2011). “Human impacts on marine biodiversity: macrobenthos in Bahrain, Arabian Gulf,” in The Importance of Biological Interactions in The Study of Biodiversity, vol. pp . Ed. Lopez-Pujol J. (Rijeka, Croatia: InTech Publishing), 109–126.
Naser H. A. (2014). “Marine Ecosystem Diversity in the Arabian Gulf: Threats and Conservation,” in Biodiversity - The Dynamic Balance of the Planet, vol. pp . Ed. Grillo O. (Rijeka, Croatia: InTech Publishing), 297–327.
Nautiyal C. S. (1997). A method for selection and characterization of rhizosphere-competent bacteria of chickpea. Curr. Microbiol. 34, 12–17. doi: 10.1007/s002849900136
Nehal M. E. (2015). Efficiency of Pseudomonas fluorescens as plant growth promoting rhizobacteria (PGPR) for the enhancement of seedling vigor, nitrogen uptake, yield and its attributes of rice (Oryza sativa L). Int. J. Sci. Res. Agric. Sci. 2, 57–67.
Ngoroyemoto N., Kulkarni M. G., Stirk W. A., Gupta S., Finnie J. F., van Staden J. (2020). Interactions between microorganisms and a seaweed-derived biostimulant on the growth and biochemical composition of Amaranthus hybridus L. Nat. Prod. Commun. 15, 1–11. doi: 10.1177/1934578X20934228
Olanrewaju O. S., Glick B. R., Babalola O. O. (2017). Mechanisms of action of plant growth promoting bacteria. World J. Microbiol. Biotechnol. 33, 197. doi: 10.1007/s11274-017-2364-9
Papenfus H. B., Kulkarni M. G., Stirk W. A., Finnie J. F., Van Staden J. (2013). Effect of a commercial seaweed extract (Kelpak®) and polyamines on nutrient-deprived (N, P and K) okra seedlings. Sci. Hortic. 151, 142–146. doi: 10.1016/j.scienta.2012.12.022
Papenfus H. B., Kulkarni M. G., Stirk W. A., Rengasamy K. R. R., Salomon M. V., Piccoli P., et al. (2015). Interactions between a plant growth-promoting rhizobacterium and smoke-derived compounds and their effect on okra growth. J. Plant Nutr. Soil Sci. 178, 741–747. doi: 10.1002/jpln.201400556
Pikovskaya R. I. (1948). Mobilization of phosphorus in soil in connection with the vital activity of some microbial species. Microbiology 17, 362–370.
Polidoro B., Carpenter K., Collins L., Duke N., Ellison A., Ellison J., et al. (2010). The loss of species: Mangrove extinction risk and failure of critical ecosystem services. PloS One 5, e10095. doi: 10.1371/journal.pone.0010095
Prakash P., Medhi S., Saikia S., Narendrakumar G., Thirugnanasambandam T., Abraham L. S. (2014). Production, formulation and application of seaweed liquid fertilizer using humic acid on growth of Arachis hypogaea. Biosci. Biotechnol. Res. Asia 11, 1515–1519. doi: 10.13005/bbra/1546
Rainey F. A., Ward-Rainey N., Kroppenstedt R. M., Stackebrandt E. (1996). The genus Nocardiopsis represents a phylogenetically coherent taxon and a distinct actinomycete lineage: Proposal of Nocardiopsaceae fam. nov. Int. J. Syst. Bacteriol. 46, 1088–1092. doi: 10.1099/00207713-46-4-1088
Rai A., Rai P. K., Singh S. (2018). Characterization of phosphate solubilizing fluorescent pseudomonads from the rhizosphere of Aloe vera (L.). Arch. Agron. Soil Sci. 64, 1032–1040. doi: 10.1080/03650340.2017.1407869
Redmond J. W., Tseng A. (1979). High-pressure liquid chromatographic determination of putrescine, spermidine and spermine. J. Chromatogr. 170, 479–481. doi: 10.1016/S0021-9673(00)95481-5
Renaut S., Masse J., Norrie J. P., Blal B., Hijri M. A. (2019). Commercial seaweed extract structured microbial communities associated with tomato and pepper roots and significantly increased crop yield. Microb. Biotechnol. 12, 1346–1358. doi: 10.1111/1751-7915.13473
Rengasamy K. R. R., Kulkarni M. G., Pendota S. C., Van Staden J. (2016). Enhancing growth, phytochemical constituents and aphid resistance capacity in cabbage with foliar application of eckol–a biologically active phenolic molecule from brown seaweed. N. Biotechnol. 33, 273–279. doi: 10.1016/j.nbt.2015.11.002
Rennie R. J., De Freitas J. R., Ruschel A. P., Vose P. B. (1982). Isolation and identification of N2-fixing bacteria associated with sugar cane (Saccharum sp.). Can. J. Microbiol. 28, 462–467. doi: 10.1139/m82-070
Rouphael Y., Colla G. (2020). Editorial: Biostimulants in agriculture. Front. Plant Sci. 11, 40. doi: 10.3389/fpls.2020.00040
Saeed E. E., Sham A., Salmin Z., Abdelmowla Y., Iratni R., El-Tarabily K. A., et al. (2017). Streptomyces globosus UAE1, a potential effective biocontrol agent for black scorch disease in date palm plantations. Front. Microbiol. 8, 1455. doi: 10.3389/fmicb.2017.01455
Samara F., Solovieva N., Ghalayini T., Nasrallah Z. A., Saburova M. (2020). Assessment of the environmental status of the mangrove ecosystem in the United Arab Emirates. Water 12, 1623. doi: 10.3390/w12061623
Santoyo G., Moreno-Hagelsieb G., Orozco-Mosqueda Mdel C., Glick B. R. (2016). Plant growth-promoting bacterial endophytes. Microbiol. Res. 183, 92–99. doi: 10.1016/j.micres.2015.11.008
Sardi P., Saracchi M., Quaroni S., Petrolini B., Borgonovi G. E., Merli S. (1992). Isolation of endophytic Streptomyces strains from surface-sterilized roots. Appl. Environ. Microbiol. 58, 2691–2693. doi: 10.1128/aem.58.8.2691-2693.1992
Schiel D. R., Lilley S. (2007). Gradients of disturbance to an algal canopy and the modification of an intertidal community. Mar. Ecol. Prog. Ser. 339, 1–11. doi: 10.3354/meps339001
Schnurer J., Rosswall T. (1982). Fluorescein diacetate hydrolysis as a measure of total microbial activity in soil and litter. Appl. Environ. Microbiol. 6, 1256–1261. doi: 10.1128/aem.43.6.1256-1261.1982
Schwyn B., Neilands J. B. (1987). Universal chemical assay for the detection and determination of siderophores. Anal. Biochem. 160, 47–56. doi: 10.1016/0003-2697(87)90612-9
Sharma H. S. S., Fleming C., Selby C., Rao J. R., Martin T. (2014). Plant biostimulants: a review on the processing of macroalgae and use of extracts for crop management to reduce abiotic and biotic stresses. J. Appl. Phycol. 26, 465–490. doi: 10.1007/s10811-013-0101-9
Shindy W. W., Smith O. E. (1975). Identification of plant hormones from cotton ovules. Plant Physiol. 55, 550–554. doi: 10.1104/pp.55.3.550
Shirling E. B., Gottlieb D. (1966). Methods for characterization of Streptomycesspecies. Int. J. Syst. Bacteriol. 16, 313–340. doi: 10.1099/00207713-16-3-313
Shukla P. S., Mantin E. G., Adil M., Bajpai S., Critchley A. T., Prithiviraj B. (2019). Ascophyllum nodosum-based biostimulants: Sustainable applications in agriculture for the stimulation of plant growth, stress tolerance, and disease management. Front. Plant Sci. 10, 655. doi: 10.3389/fpls.2019.00655
Smith M. A., Davies P. J. (1985). Separation and quantitation of polyamines in plant tissue by high performance liquid chromatography of their dansyl derivatives. Plant Physiol. 78, 89–91. doi: 10.1104/pp.78.1.89
Spalding M., Parrett C. L. (2019). Global patterns in mangrove recreation and tourism. Mar. Policy 110, 103540. doi: 10.1016/j.marpol.2019.103540
Strzelczyk E., Pokojska-Burdziej A. (1984). Production of auxins and gibberellin-like substances by mycorrhizal fungi, bacteria and actinomycetes isolated from the soil and the mycorrhizosphere of pine (Pinus silvestris L.). Plant Soil 81, 185–194. doi: 10.1007/BF02197150
Sweeney R. A., Rexroad P. R. (1987). Comparison of LECO FP-228 ‘nitrogen determinator’ with AOAC copper catalyst Kjeldahl method for crude protein. J. Assoc. Off. Anal. Chem. 70, 1028–1030. doi: 10.1093/jaoac/70.6.1028
Thatoi H., Behera B. C., Mishra R. R., Dutta S. K. (2013). Biodiversity and biotechnological potential of microorganisms from mangrove ecosystems: a review. Ann. Microbiol. 63, 1–19. doi: 10.1007/s13213-012-0442-7
Thompson J. D., Gibson T. J., Plewniak F., Jeanmougin F., Higgins D. G. (1997). The CLUSTAL_X windows interface: flexible strategies for multiple sequence alignment aided by quality analysis tools. Nucleic Acids Res. 25, 4876–4882. doi: 10.1093/nar/25.24.4876
Tien T. M., Gaskings M. H., Hubbell D. H. (1979). Plant growth substances produced by Azospirillum brasilense and their effect on the growth of pearl millet (Pennisetum americanum L.). Appl. Environ. Microbiol. 37, 1016–1024. doi: 10.1128/aem.37.5.1016-1024.1979
Troell M., Henriksson P. J. G., Buschmann A. H., Chopin T., Quahe S. (2022). Farming the ocean – seaweeds as a quick fix for the climate? Rev. Fish. Sci. Aquac. doi: 10.1080/23308249.2022.2048792
Wellington E. M. H., Williams S. T. (1977). Preservation of actinomycete inoculum in frozen glycerol. Microbios Lett. 6, 151–157.
Williams S. T., Shameemullah M., Watson E. T., Mayfield C. I. (1972). Studies on the ecology of actinomycetes in soil. VI. The influence of moisture tension on growth and survival. Soil Biol. Biochem. 4, 215–225.k. doi: 10.1016/0038-0717(72)90014-4
Yakhin O. I., Lubyanov A. A., Yakhin I. A., Brown P. H. (2017). Biostimulants in plant science: A global perspective. Front. Plant Sci. 7, 2049. doi: 10.3389/fpls.2016.02049
Keywords: Arabian Gulf, marine agriculture, mangrove, nutrient base, plant growth promotion, seaweed extract, seawater irrigation
Citation: Alkaabi AK, Ramadan GA, Elddin AMT, El-Tarabily KA and AbuQamar SF (2022) The Multifarious Endophytic Actinobacterial Isolate, Streptomyces tubercidicus UAE1, Combined With the Seaweed Biostimulant Further Promotes Growth of Avicennia marina. Front. Mar. Sci. 9:896461. doi: 10.3389/fmars.2022.896461
Received: 15 March 2022; Accepted: 23 June 2022;
Published: 25 July 2022.
Edited by:
Shady A. Amin, New York University Abu Dhabi, United Arab EmiratesReviewed by:
Mahmoud Yaish, Sultan Qaboos University, OmanPradeep Kumar Rai, Indian Farmers Fertilizer Cooperative Limited, India
Copyright © 2022 Alkaabi, Ramadan, Elddin, El-Tarabily and AbuQamar. This is an open-access article distributed under the terms of the Creative Commons Attribution License (CC BY). The use, distribution or reproduction in other forums is permitted, provided the original author(s) and the copyright owner(s) are credited and that the original publication in this journal is cited, in accordance with accepted academic practice. No use, distribution or reproduction is permitted which does not comply with these terms.
*Correspondence: Khaled A. El-Tarabily, a3RhcmFiaWx5QHVhZXUuYWMuYWU=; Synan F. AbuQamar, c2FidXFhbWFyQHVhZXUuYWMuYWU=