- School of Earth Sciences, University of Bristol, Bristol, United Kingdom
Climate change is impacting organisms in every region of the world ocean by acting though on individuals in response to their local environments. Given projected future risks derived from these changes, it is becoming increasingly important to understand regional signals of how organisms respond to facilitate their governance and protection. Benthic organisms structure ecological compositions and ecosystem dynamics, therefore not only providing insights into their own response to climate change but also how ecosystems might respond to future conditions. European seas are transitional areas including boreal, warm-temperate, and subarctic waters with organisms frequently at limits of their distributions. Here, we use a meta-analytical approach to assess how calcification, growth, metabolism, photosynthesis, reproduction, and survival in European benthic organisms respond to ocean acidification and warming. Using meta-regression, we examine how study design factors influence effect-size outcomes. Longer experimental periods generally amplified the effects of climate change on taxonomic groupings and related physiological traits and against expectation do not result in acclimation. In agreement with global studies, we find that impacts vary considerably on different taxonomic groupings and their physiological traits. We found calcifying organisms are an at-risk taxon in European waters, with climate stressors decreasing growth rates, reproduction, and survival rates. Fleshy algal species demonstrate resilience to climate stressors, suggesting future European benthic ecosystems will undergo restructuring based on current climate emission pathways.
1 Introduction
Atmospheric carbon dioxide concentration is currently ~412.5ppm (Friedlingstein et al., 2020), the highest level in 3.6 million years (Martínez-Botí et al., 2015). Depending on emission scenarios, likely global atmospheric air warming is projected to be 2.5°C – 4°C by 2100 (IPCC, 2021). These emissions drive changes to ocean properties, chiefly ocean acidification from excess CO2 interacting with seawater (Doney et al., 2009) and increases to ocean temperature from absorbing excess atmospheric heat (IPCC, 2021). Such warming is amplified in higher latitudes (Wu et al., 2011; Singh et al., 2017) but lower in the ocean due oceanic to thermal inertia (Abdussamatov et al., 2012). High latitudes are absorbing most CO2 as cold water can naturally store more gasses (Cooley et al., 2022).
These changes in the ocean physico-chemical properties have consequences for marine organisms including changes to growth, calcification, reproduction, metabolic processes, and survival; these effects though are highly variable across taxonomic groups (Kroeker et al., 2013; Cattano et al., 2018; Bindoff et al., 2019; Sampaio et al., 2021). While the development of the climatic drivers over the next decades is broadly well understood (Cooley et al., 2022) and a vast range of responses of individuals and their developmental stages exemplified, upscaling to the response of larger groups, ecosystems and their services is a vast challenge, despite scientific literature on individual studies accumulating (Bass et al., 2021).
Meta-analyses help focus knowledge on how marine organisms may respond by condensing many independent studies into a standardised and comparable format allowing patterns to emerge, despite the variation between experiments, across taxonomic groups and latitudes. Agenda setting studies showed how climate change interacts with organisms and the physiological responses at a global scale (Hendriks et al., 2010; Harvey et al., 2013; Kroeker et al., 2013). These studies identified potential generalised trends like calcifying organisms’ growth rates decreasing under ocean acidification.
Organism responses to climate change though are not homogenous and interregional variability is expected (Krumhansl et al., 2016). For example, a meta-analysis showed coral calcification rates to ocean acidification increase in the tropics, decreases in the subtropics, and are unaffected in temperate ecosystems in response to climate scenarios for 2100 (Kornder et al., 2018). The natural variability of the climate and environment, as well as the synergies between drivers, might predispose organisms to a wider range of impacts. Temperate regions, for example, record greater annual variability in temperature than tropical regions which may lead to different responses to climate stressors (Sheldon et al., 2011; Vergés et al., 2014).
Two recent meta-analyses have taken a different regional approach focussing on ocean acidification in the Southern Ocean (Hancock et al., 2020; Figuerola et al., 2021) finding potential to alter ecosystem interactions. Bove et al. (2020) investigated Caribbean coral calcification indicating ocean warming reduces calcification while ocean acidification and ocean acidification with warming, does not. A study of Mediterranean benthic organism responses to ocean acidification showed a replacement of calcifying algae and corals by fleshy algae (Zunino et al., 2017). Regional studies find trends that are less evident using global analyses (Parker et al., 2013) such as resilience in calcification or growth responses (Goethel et al., 2017; Zunino et al., 2017; Kornder et al., 2018; Bove et al., 2020) which are not identifiable when pooling studies globally (Hendriks et al., 2010; Kroeker et al., 2013; Harvey et al., 2013).
Given projected future risks derived from these changes, it is becoming increasingly important to understand regional signals of how organisms response. Here we use the approach of global studies in the context of European waters to determine vulnerability or resilience of taxonomic groupings to multiple climate stressors. This understanding will be important to prioritise conservation efforts and identify governance needs in an international context. Across Europe multiple biogeographic regions are in close proximity with organisms at the edges of their distributions (Narayanaswamy et al., 2013; Kotta et al., 2017). Large volumes of experimental work analysing organism responses to climate change exist for Europe, augmented by long-time series data sets assessing natural variation of presence and absence e.g. MarClim in the UK (Mieszkowska et al., 2006), and the Helgoland Roads time series (Wiltshire et al., 2010). Despite the large volume of data, studies assessing interactions across multiple stressors and their potential cumulative impacts within the wider context of European waters are lacking. It is not clear which habitats are most vulnerable or which services are most under pressure which hinders the effectiveness to conserve European marine ecosystems through protected area networks (Mazaris et al., 2019), and the sustainable development of coastal resources (Qiu and Jones, 2013).
We focus on benthic organisms as these are habitat formers, keystone species, and provide regulating and provisioning services which are fundamental in structuring ecosystems. Molluscs are food sources for other organisms (Coen et al., 2007), form biogenic habitats (Koivisto and Westerbom, 2010), and key economic resources (Mangi et al., 2018). Crustaceans are key components of food webs often serving as a trophic link between benthic and pelagic species (Szaniawska, 2018). Algal species fix carbon via photosynthesis (Tsai et al., 2017), stabilise shorelines (Currin et al., 2010), contribute to nutrient cycles (Smale et al., 2013), and provide habitat for epiphytic communities (Bates and DeWreede, 2007; King et al., 2021). Echinoderms are dominant grazing species in ecosystems that structure habitats (Norderhaug and Christie, 2009) and drive trophic interactions (Hughes et al., 2012). Calcifying algae build habitats both via their physical structures (Hofmann et al., 2012) and shaping surrounding sediment profiles (Coniglio and James, 1985), and host epiphytic species (Nelson, 2009).
Additionally, meta-analyses have moved on technically since earlier seminal studies. While the importance of study design in experimental outcomes has been acknowledged and suggestions for standardisation have been made (Riebesell et al., 2011), its relevance has rarely been explored in meta-analysis. The substantial increase in understanding of impacts of climate change on marine organisms since publication of earlier agenda setting work offers the chance to incorporate a larger volume of data and develop meta-analysis studies to improve understanding of the underlying mechanisms that may drive observations. Duration of exposure and acclimation periods are thought to impact how organisms respond to climate stressors (Evans and Hofmann, 2012; Griffith and Gobler, 2017). Short term experiments, often without days in the laboratory conditions allowing equilibration, are perceived as shock to the physiology of the study organisms (Li et al., 2015) while long-term response allow for acclimation and therefore are considered to give a better idea of the responses to long term climate change (Leung et al., 2021). New longer duration datasets allow us to analyse the importance of these designs of experimental datasets expanding from previous meta-analyses.
Using a meta-analytical approach, we examine peer-reviewed literature focusing on how European benthic marine organisms respond to climate change drivers. We focus our analysis on organism calcification, growth, metabolism, reproduction, photosynthesis, and survival. Based on global analyses and understanding of the physiology of these taxa, we hypothesise that calcifying organism will be impacted more strongly, and that growth is negatively impacted while photosynthesis is enhanced. These traits capture a range of key organism physiological traits providing insights into organism fitness and allow of comparisons with previous meta-analyses. Using meta-regression, we examine how study design factors influence effect-size outcomes.
2 Methods
2.1 Study and Selection Criteria
This study gathered articles published between 1st January 2000 – 1st January 2020 to cover most ocean acidification (OA) research articles (Riebesell and Gattuso, 2015). Web of Science and Scopus were used to find articles covering climate change impacts on native European benthic marine organisms using the keywords: “ocean acidification”, “acidification”, “ocean warming”, “climate change”, “global warming”, and combinations/permutations of these. The results were snowballed following recommendations made by Orr et al. (2020); article lists from previous comprehensive reviews (Harvey et al., 2013; Kroeker et al., 2013) and their reference lists were used to locate additional studies. Article collection occurred between March 2019 – Jan 2020, article list available in Supplementary Data.
Data were limited to native European species (Figure 1), excluding invasive species. Minimum standards for reporting of meta data were: control condition, acidification and or warming manipulated individually or synergistically within possible for conditions for 2100 conditions in European seas. Plausible conditions were determined based on author knowledge of likely conditions at a study site or species might experience, allowing inclusion of local contexts not identifiable in large, averaged projections. We exclude unrealistic stress (i.e., conditions beyond high end emissions scenarios by 2100) experiments in the context of climate change designed to improve our understanding of physiology. Studies assessing interaction with other stressors (such as heavy metal pollution or nutrients) were included if they analysed the climate stressors separately. Studies examining multiple species, biological responses, seasons, or geographical locations were included if they could be disaggregated.
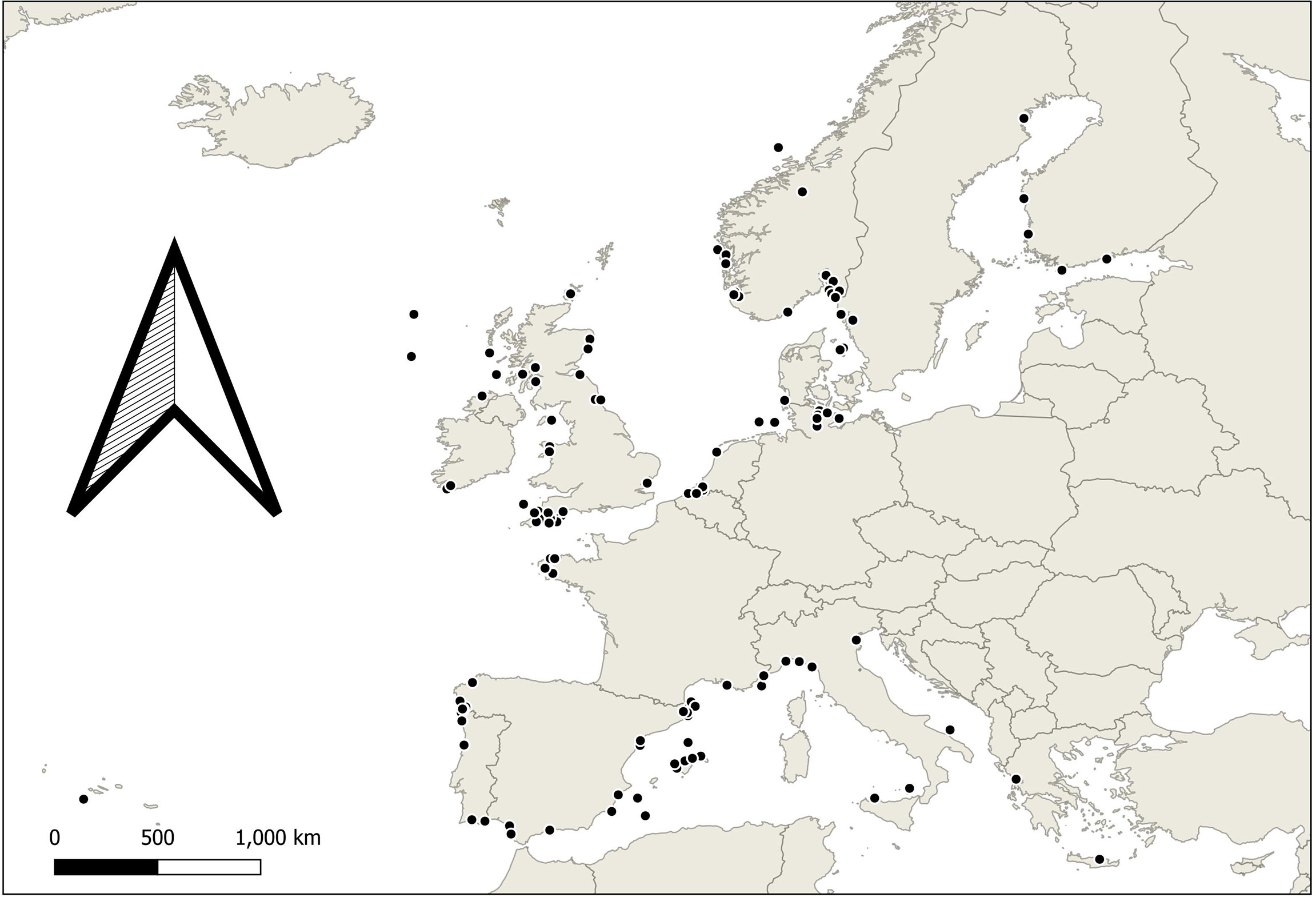
Figure 1 A map of Europe detailing approximate collection locations of study organisms in identified articles for meta-analysis.
Data on calcification, growth, metabolism, reproduction, photosynthesis, and survival were extracted (Supplementary Data). When the same biological trait was measured in multiple ways (e.g. growth as organism length and biomass), one was chosen at random following standard methodology (Harvey et al., 2013; Kroeker et al., 2013; Sampaio et al., 2021). To avoid higher weighting, one parameter was chosen when multiple biological responses (e.g. length and biomass to determine growth) were reported. Survival data were converted from mortality for standardisation. Many identified articles reported survival rates. However, these where often reported as percentages and thereby did not provide variance, preventing us from computing an effect size consistent with the rest of these meta-analyses, resulting in exclusion from our study. Time-series data were attributed to the endpoint of that experiment.
Datapoints, a form or variance (standard error, confidence intervals, or standard deviation), and sample size were extracted directly from either text or tables. Graphs were data mined using WebPlotDigitizer (Rohatgi,1 2019, See SI). Acclimation period (experimental period whereby organisms were transitioned to climate stressors e.g., increasing water temperature by 0.5°C daily to reach temperature increase value), study duration (length of organism exposure to climate stressor excluding acclimation period), organism developmental stage (categorised as Egg/larva, Juvenile, and Adult), pH change or temperature change, organisms’ size were extracted when possible. Size at the start of the experiment was converted to grams [g] or millimetres [mm]) based on the sample mean and log transformed. Insufficient data were extracted to allow for taxonomic-biological trait responses to organism size, so data were pooled combining biological traits to identify general trends on traits alone.
2.2 Statistical Analyses
All analyses were conducted using the R (version 4.0.0; R Development Core Team). The package “Metafor” (Viechtbauer, 2010) was used to calculate effect size using the “escalc” function and conduct the meta-analyses. Log Response Ratio (LnRR) was chosen to measure effect size (Koricheva et al., 2013).
Multivariate meta-analysis models (function “rma.mv”) were used to calculate the mean effect and sampling variance of a treatment on a biological response. Random intercepts of article and species for each treatment were incorporated to account for possible results correlation due to studies from the same article or organism and account for pseudo-replication. Data were subsetted into taxonomic groupings, calcifiers vs non-calcifiers, heterotrophic vs autotrophic, and developmental stage when possible. Climate stressors were used as model moderators (mods = stressor -1). A result is considered significant if the 95% confidence interval for the overall mean calculated by a model does not overlap zero. Tests for residual heterogeneity (QE) were used to ascertain if additional moderators not considered in the analysis might be influencing study results (Hedges and Olkin, 2014).
To examine the impact of study duration and acclimation period we modified the multivariate meta-analysis models to include either of these as moderators within the models. E.g: [model <- rma.mv (yi, vi, method=“REML”, test=“t”, random = ~1|Article/species, data=data, mods = ~duration: stressor -1)].
2.3 Publication Bias
Standardized diagnostic tests for multivariate meta-analytical methods are still evolving (Viechtbauer and Cheung, 2010; Habeck and Schultz, 2015) and currently only applicable for univariate models (e.g. models of class “rma”). To detect publication bias within our data we modified our multivariate models in accordance with Habeck and Schultz (2015) to calculate Egger’s regression. Egger’s regression detects funnel plot asymmetry, a common measure of publication bias, by looking if there are statistically absent studies based on the distribution of studies within meta-analysis datasets using linear regression (Egger et al., 1997). Multivariate meta-analysis models were extended to include the square root on effect size variance as a model moderator variable to perform a regression test (Habeck and Schultz, 2015). When this regression test intercept significantly deviates from zero, a data set is considered asymmetrical, thus biased (Sterne et al., 2005). Following previous recommendations (Egger et al., 1997; Habeck and Schultz, 2015) an analysis is considered biased if the intercept differs from zero at P = 0.10. Results from tests for residual heterogeneity (QE) and publication bias are reported in Supplementary Information.
3 Results
171 articles matched our inclusion criteria (Figure 2), totalling 791 experiments for meta-analysis (Supplementary Data). Most studies were molluscs (n = 173), acidification (n = 423) and the traits of calcification (n = 126), growth (n = 266), and metabolic response (n = 202). Average experimental duration was 86.3 ( ± 102.1) days, acclimation period 7.1 ( ± 20.4) days, pH change 0.26 ( ± 0.17) and temperature change = 2.69°C ( ± 2).
The responses of benthic organisms to climate stressors were highly variable across taxonomic groups (Figure 3). No stressor consistently produced positive or negative changes. Crustaceans were the most impacted with combined ocean acidification and warming decreasing calcification (LnRR = -0.1448, P = 0.0111, 95% confidence interval -0.2509 - -0.0387), reproduction (LnRR = -0.144, P = 0.0014, 95% confidence interval -0.2277 - -0.0604), and survival (LnRR = -0.4057, P = 0.0001, 95% confidence interval -0.4086 - -0.3604), but increasing metabolic rate (LnRR = 0.2211, P = 0.0188, 95% confidence interval 0.0425 – 0.3996). Ocean acidification alone lowered reproduction (LnRR = -0.111, P = 0.0109, 95% confidence interval -0.1945 - -0.0276). Warming increased metabolic rates (LnRR = 0.3993, P = 0.0001, 95% confidence interval 0.2344 – 0.5641) but decreased reproduction (LnRR = -0.1272, P = 0.0042, 95% confidence interval -0.2108 - -0.0436), and survival (LnRR = -0.269, P = 0.0053). Most calcifying organisms exhibit statistically significant responses when taxa were examined individually (Figure 3) and when data were aggregated to compare with non-calcifying organisms with respect to growth rates and metabolism (Figure 4).
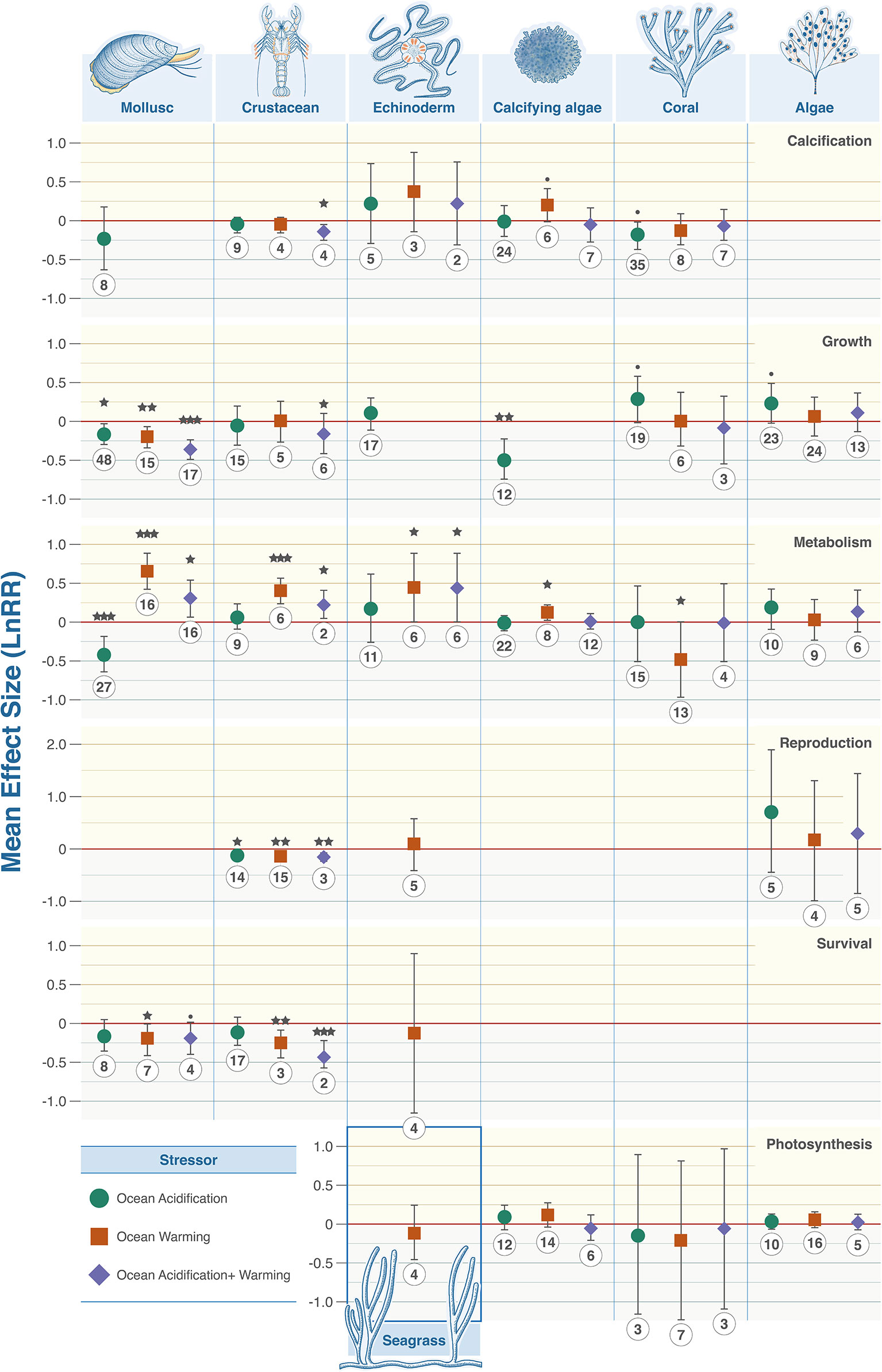
Figure 3 Taxonomic grouping and associated biological trait responses to marine climate change stressors. From top to bottom: Calcification, growth, metabolism, photosynthesis, reproduction, and survival. Colour denotes the driver with green representing OA, orange warming and purple their combination. Effect size calculated as Log Response Ratio (LnRR), points denote mean effect size and error bars ± 95% CL. Red dashed lines (R = 0) indicates no effect, (R = <0) indicates a negative effect, (R = >0) indicates a positive effect. P value indicators: <.0001 = ‘***’, 0.001 = ‘**’, 0.01 = ‘*’, 0.05 = ‘·’.
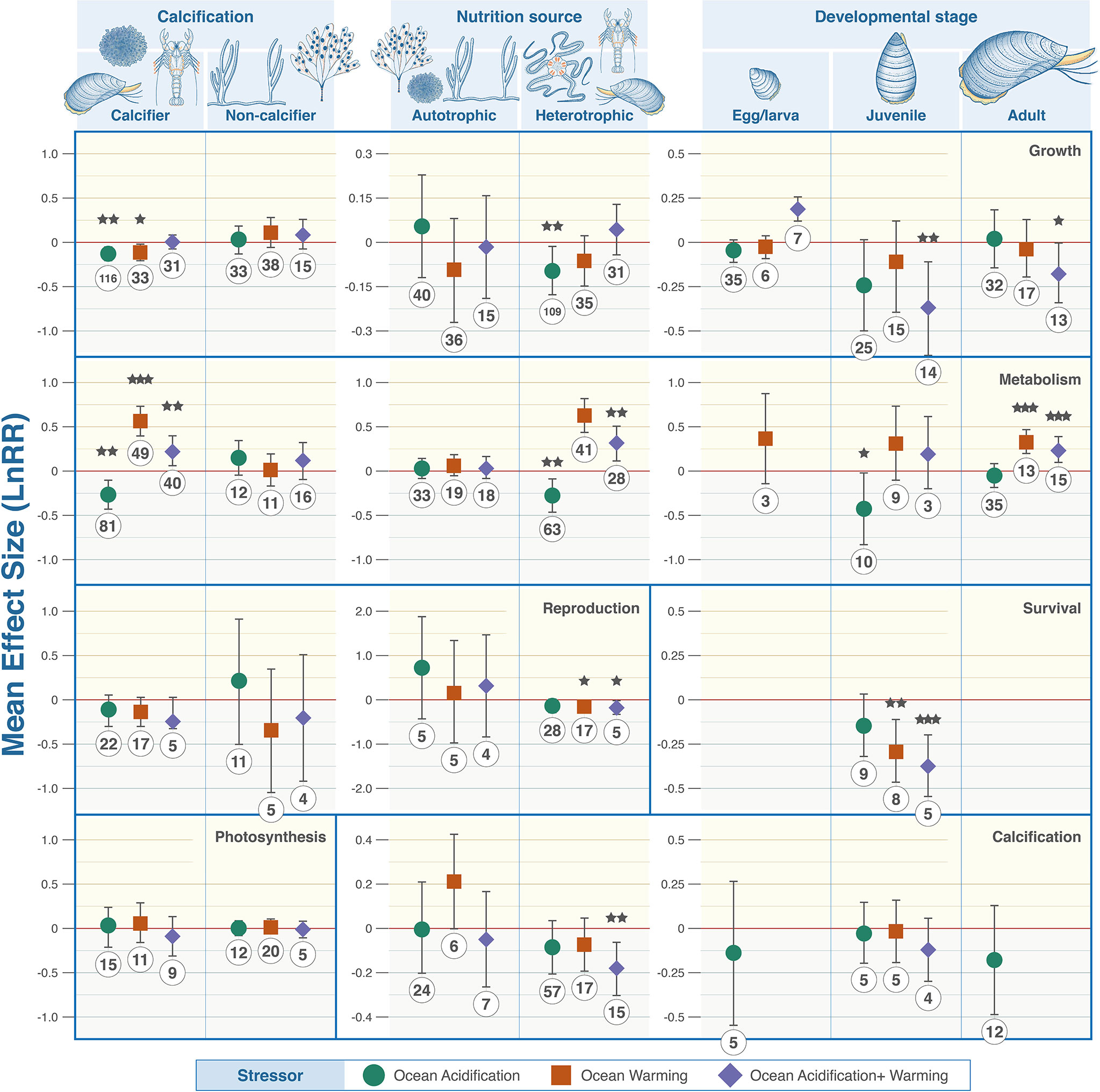
Figure 4 Wider organism grouping and associated biological trait responses to marine climate change stressors. From top to bottom: Calcification, growth, metabolism, photosynthesis, reproduction, and survival. Colour denotes the driver with green representing OA, orange warming and purple their combination. Effect size calculated as Log Response Ratio (LnRR), points denote mean effect size and error bars ± 95% CL. Red dashed lines (R = 0) indicates no effect, (R = <0) indicates a negative effect, (R = >0) indicates a positive effect. P value indicators: <.0001 = ‘***’, 0.001 = ‘**’, = 0.01 ‘*’.
Metabolism was both negatively and positively affected by at least one climate stressor in every taxon except algae (9 statistically significant observations in 5 taxa) (Figure 3). Fleshy algae are the most resilient with no statistically significant signals in growth, metabolism, photosynthesis, and reproduction (Figure 3). Ocean acidification caused decreases in metabolism in calcifying and heterotrophic organisms (Figure 4). Warming individually, and combined with ocean acidification, increase metabolic rates except corals where metabolic rate decreased and algae where no statistical signal was detected. Egg/larval growth rates across all taxa increased in response to combined acidification and warming (LnRR = 0.1935, P = 0.0001, 95% confidence interval 0.1268 – 0.2603), but decreased in juveniles and adults (LnRR = -0.3701, P = 0.0058, 95% confidence interval -0.6283 - -0.1118; LnRR = -0.1715, P = 0.0404, 95% confidence interval -0.3353 - -0.0078 respectively) (Figure 4).
Experimental design greatly influenced the observed impacts of organism responses to climate change stressors (Supplementary Material) as revealed by meta-regression. Study duration was important for responses of taxonomic groups while acclimation period mostly had no statistical significance suggesting that the current practise eliminates impacts on study design (Supplementary Table). Increased study duration period generally amplified responses, suggesting that European benthic organisms do not readily acclimate to acidification and/or warming within the same generation. Metabolic responses increased with study durations (Table 1). Survival was the only trait not significantly impacted by experimental duration or acclimation periods (Table 1).
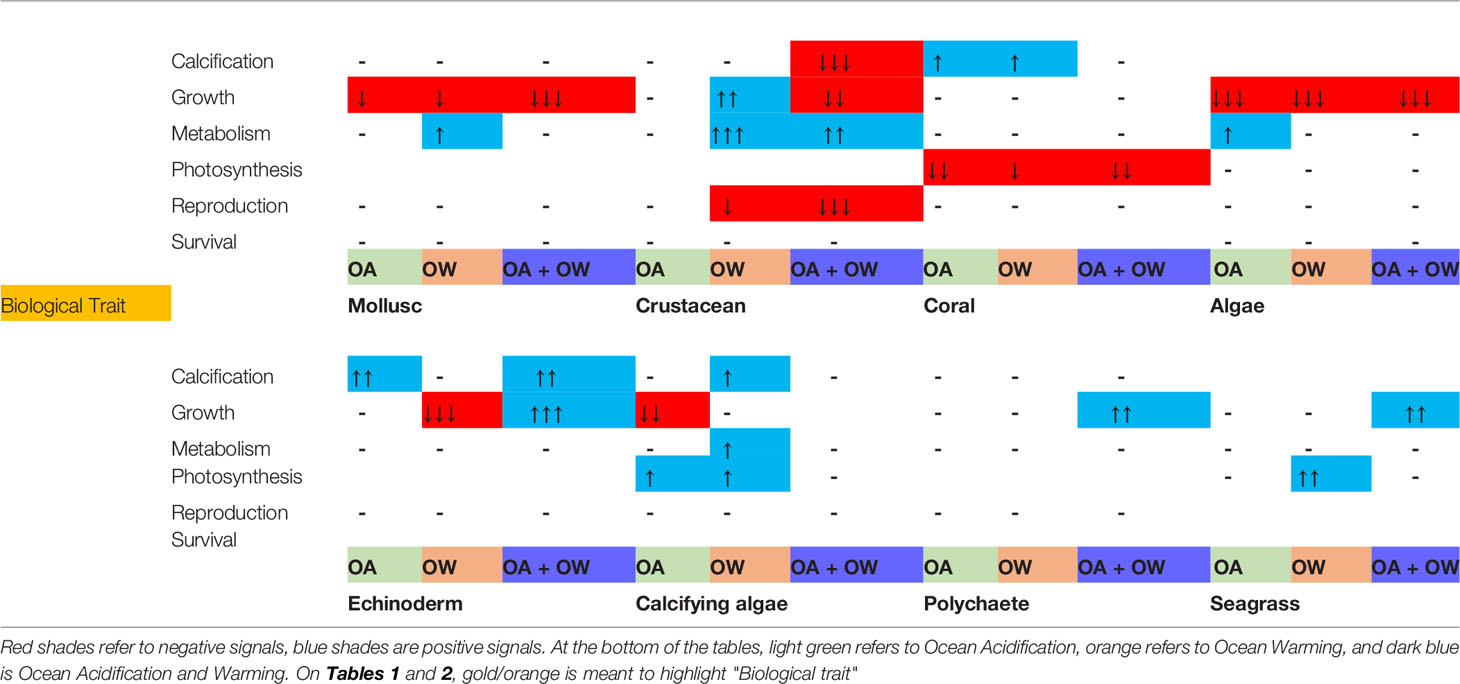
Table 1 Study duration period impact on effect size (LnRR) for biological traits and climate change stressor treatment.
The impact on taxa scales with the degree of environmental change with larger driver change resulting in higher impacts. There are expectations for example metabolic activity for molluscs and algae were metabolism decreased in response to OA (Table 2). Climate stress negatively impacted growth across all taxa except for polychaeta growth which showed an increase.
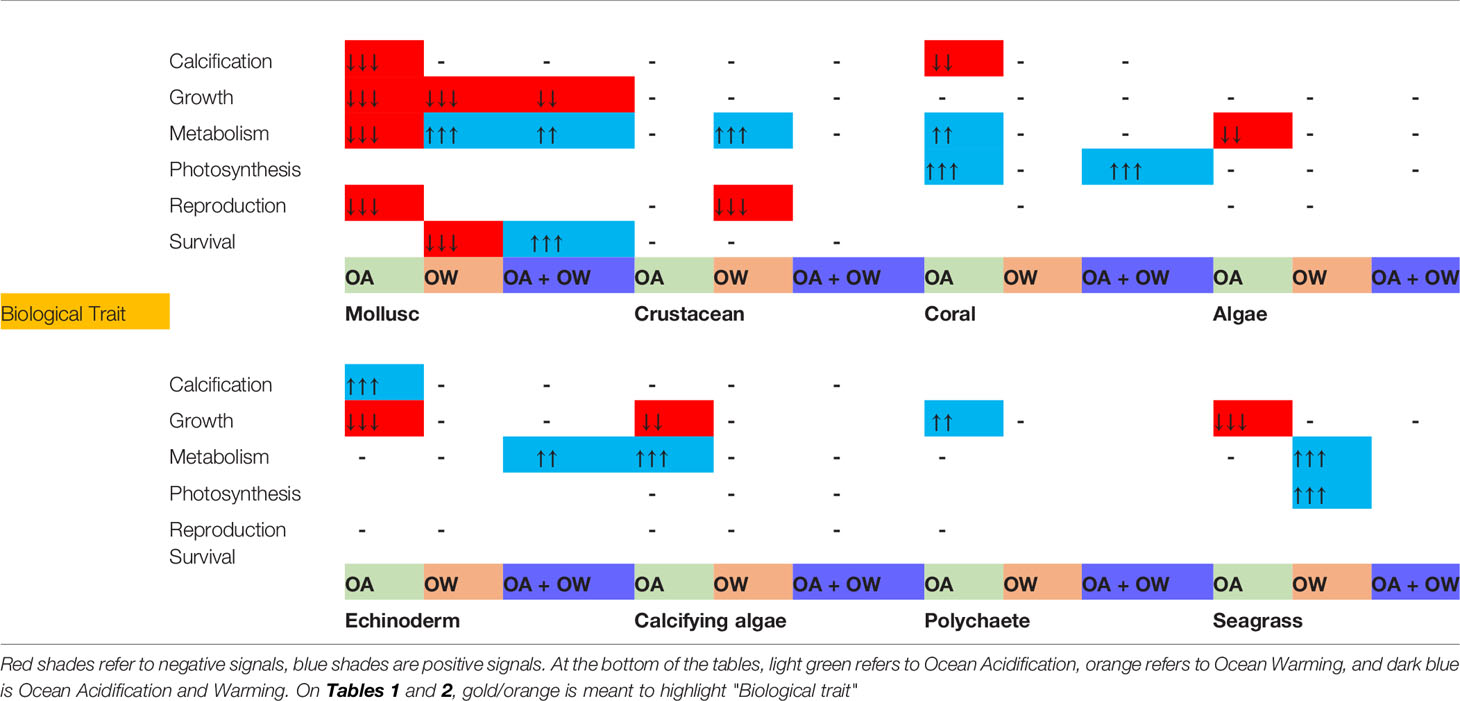
Table 2 Stressor severity impact on effect size (LnRR) for biological traits and climate change stressor treatment (OA = Ocean Acidification, OW = Ocean Warming, OA + OW = Ocean Acidification and Warming). Direction of response indicated by arrows, boxes with (-) indicate no statistically significant effect, blank boxes indicate insufficient/no data for meta-regression. P values denoted by number of arrows; <0.0001 = 3 arrows, < 0.001 = 2 arrows, <0.01 = 1 arrow.
Interestingly, results suggest that larger organisms do not increase metabolisms in response to climate stressors as larger organisms grew more, while smaller organism’s growth rate was lower (Table 3). Impacts on survival are not clear; for larger organisms determined by volume or weight (g) survival rates were negatively impacted by OA, but when organisms were measured by length larger organism had higher survival with increasing size.
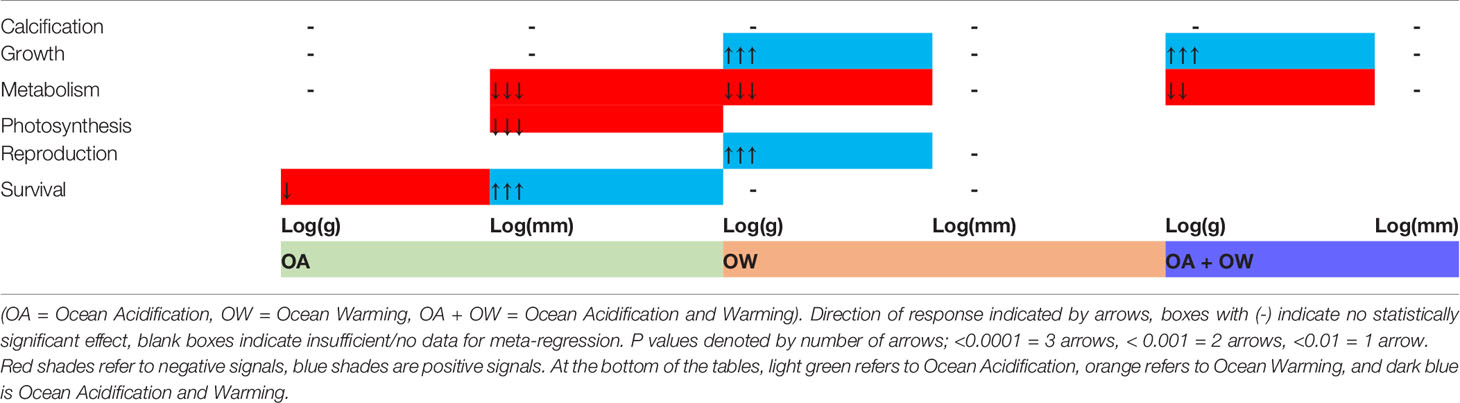
Table 3 The effect of organism size at the start of an experiment on effect size (LnRR) for biological traits and climate change stressor treatment.
4 Discussion
While there have been meta-analyses of climate change impacts on marine organisms in the past, climate change is acting on a local to regional scale. Projected future risks originating from these changes make it is increasingly important to identify regional signals of organismal responses to guide conservation strategy and governance. We chose European waters due to the geographic proximity of different biogeographic regions driven by environmental differences. Such analysis is fundamental to design effective conservation strategies by providing granularity that is not present in overarching datasets. In agreement with many experiments on individual taxa, we found highly variable impacts. Metabolic rates of molluscs, crustaceans, echinoderms, and calcifying algae increased under ocean warming and combined ocean acidification and warming, while mollusc and coral metabolic rates decreased under ocean acidification and ocean warming respectively (Figure 2). The physiology of calcifying organisms is significantly impacted with decreased growth rates of molluscs under all examined climate stressors and calcifying algae under ocean acidification, crustacean calcification decreased under combined ocean acidification and warming, while fleshy algal species appear resilient to the tested combinations of climate stressors. Using meta-regression, we found against expectation that longer experimental periods generally amplified the effects of climate change and did not result in acclimation.
4.1 Impacts on Habitat Formers
Growth and reproduction of calcifying organisms in particular were reduced in response to warming and lowering of pH. However not all calcifying taxa experienced calcification reduction. Molluscs show negative impacts on calcification, growth and increases in metabolic costs, adding to the body of studies showing molluscs are a highly at-risk taxon globally (Parker et al., 2013; Kroeker et al., 2013; Goethel et al., 2017; Figuerola et al., 2021). Our findings contrast recent work on the Mediterranean Sea and a global analysis which suggested mollusc growth is unaffected by climate stressors (Zunino et al., 2017; Sampaio et al., 2021). Our results likely differs from the signal observed by Zunino et al. (2017) due to a larger sample size encompassing a wider range of taxa and growth responses. We interpret this apparent disagreement with the global analysis as indication that European mollusc species are particularly sensitive to climate stressors; a suggestion which is corroborated by global studies which have a strong bias towards temperate molluscan species in North Atlantic waters resulting in a similar interpretation (Kroeker et al., 2013; Harvey et al., 2013). European species have long been reported to be altering distributions in response to climate change (Helmuth et al., 2006) highlighting species at the edge of distributions are sensitive to climate stressors.
Reductions in growth rates will not only affect size and fitness, but also reduce molluscs ability to form biogenic structures, limiting their ability to support biodiversity (Strain et al., 2021). Many habitats created by these taxa are protected due to the associated high biodiversity (Donnarumma et al., 2018). Evidence suggests high food availability can buffer against climate stressors (Thomsen et al., 2013), but primary productivity globally is predicted to decrease by ~8.6% under business-as-usual scenarios (Bopp et al., 2013) thereby potentially amplifying the impacts depending on local habitat and other inputs into coastal ecosystems.
Reductions in mollusc growth rates and survival paired with reductions in crustacean survival and reproduction would impact commercial fishing in Europe by lowering catch potential and impacting livelihoods of European fishers. France, the Netherlands, and Spain annually produce approximately half of consumed European molluscs (Narita and Rehdanz, 2017) suggesting these regions are particularly susceptible to the economic repercussions of climate impacts on mollusc species. Additionally, the resilience of mollusc and crustacean populations against climate change has been reduced by destructive fishing and pollution for decades (De Groot, 1984). There are attempts to restore habitats in line with historical distribution, e.g., recreation of oyster beds around the UK (Harding et al., 2016) or red gorgonian in the Mediterranean (Linares et al., 2008). Restoring historical habitats that are reliant on calcifying organisms as keystone species may be a risky approach and not provide longevity due to observed impacts on growth rates, reproduction, and survival. Assessing local environmental conditions, the vulnerability of specific taxa, and options for reducing secondary local stressors are all needed to improve the long-term viability of these habitats.
Other ecosystem builders are similarly impacted, increasing the risk for coastal biodiversity. The decrease growth rates of calcifying algae are supported by other studies, highlighting the vulnerability of this group (Brodie et al., 2014; Chan et al., 2020). As they lose suitable environmental conditions (Simon-Nutbrown et al., 2020), calcifying algae are predicted to experience increased breakage due to increasingly intense weather, reducing structural complexity of Mearl beds (Melbourne et al., 2018). The reduced growth rates of calcifying algae suggested in our analysis will compound these problems (Ragazzola et al., 2013; Marchini et al., 2019). Furthermore, future sea level rise and changes in riverine input into coastal setting due to changes in precipitation and land use all threaten light attenuation in some habitats, for example where habitats cannot migrate landwards due to artificial coastal structures or cliffs, which exacerbates the impacts.
Our data suggest European corals are mostly unaffected by climate stressors, likely because most studies investigated the cold-water coral Lophelia pertusa which have been found in experiments to be resilient to projected end of century ocean conditions (Form and Riebesell, 2012; Wall et al., 2015; Büscher et al., 2017; Varnerin et al., 2020; Wang et al., 2021). While symbiont bearing tropical coral ecosystems are projected to be lost with only small amounts of additional warming (Hoegh-Guldberg et al., 2017), temperate deeper-water taxa appear more resilient (Kornder et al., 2018, this study). However, the 3D structure generated by deep water corals will be impacted by dissolution after death and thereby reduce the habitat and associated biodiversity (Hennige et al., 2020).
Our analysis suggests that autotrophic species, in our dataset mostly fleshy algae, will benefit from climate change. We echo the findings that algal species can display resilience to climate change stressors, in both regional (Strain et al., 2015; Phelps et al., 2017) and global analyses (Krumhansl et al., 2016). This finding is supported by field observations of intertidal habitats in Scotland which have observed increases in canopy-forming algal species (Burrows et al., 2017). However, the focus on the physiology masks species interactions which suggest that temperate algal communities may undergo simplification with decreases in structural complexity and diversity as climate change amplifies (Brodie et al., 2014; Agostini et al., 2021; King et al., 2021). Algal species frequently grow in high energy environments which experience rapid daily variations in tides and temperature. Therefore, they have evolved rapid growth responses to compensate frequent breakage in these environments (Rai and Gaur, 2012) which may also benefit under global change conditions. Due to their apparent resilience, associated ecosystem services like helping shoreline stabilisation and nutrient cycling may continue despite warming and acidification. However, the complexity of these systems is overlooked by our analysis of individual taxa as associated epifaunal species might be negatively impacted and thereby reduce biofim production on the algae (Berner et al., 2015). It is important to note though that eutrophication, pollution and habitat destruction from shoreline protection, fishing or future sea level rise were not part of this assessment. This apparent resilience may support endeavours to grow kelp or seagrass for carbon sequestration (Unsworth et al., 2018; Bach and Boyd, 2021), or grow algae as part of the blue economy (Araújo et al., 2021). Our results suggest efforts to restore algal habitats, such as kelp beds around Norway (Hynes et al., 2021), might benefit from the sugested resilience to climate stressors.
Our findings identify gaps in the experiments on some European benthic organisms. For example, while there is a high abundance of experiments on mollusc growth rates comparatively less is known for crustaceans or calcifying algae. This bias is replicated on a global scale (Kroeker et al., 2013; Harvey et al., 2013; Sampaio et al., 2021). Limited work on polychaetes and bryozoans hindered inclusion in our analysis expect for the generalised categories (calcifiers vs non-calcifiers, heterotrophs vs autotrophs). To gain a more differentiated understanding of what European seascapes may look like in the future and which services they can provide, greater efforts need to be taken to determine impacts on less studied taxonomic groups. Despite a clear importance of seasonal variation of environmental conditions, for example marine heat waves (Oliver et al., 2018), most studies were performed under stable environmental conditions resulting in insufficient data to analyse potential seasonal impacts on organism physiology. Such extreme conditions and their projected increasing frequency will threaten communities as the reducing time periods between these extreme events reduce opportunities for communities to recover (Bednar-Friedl et al., 2022).
4.2 Physiological Responses
The observed increases in algal metabolism in our analysis show an increased demand for oxygen, indicating that climate change is increasing standard physiological rates (Pörtner, 2008). Whether these increases are beneficial or detrimental depends on the relative deviation from optimal environmental conditions for temperature and oxygen (Pörtner, 2002; Pörtner and Farrell, 2008). The detected impacts on the metabolic responses of calcifiers corroborate Lefevre (2016) who found calcifying organisms consumed significantly more oxygen when exposed to climate stressors. In contrast, previous meta-analysis suggested that ocean acidification does not impact metabolic responses (Kroeker et al., 2013; Sampaio et al., 2021) while warming increased them (Sampaio et al., 2021). One potential interpretation of this difference is the nature of the regional marine environment. European waters experience large temperature fluctuations and organisms in these environments have shown to have limited ability to acclimate to climate stressors (Seebacher et al., 2015). Increased physiological demands can be compensated, for example by higher food intake at warmer temperature or to facilitate calcification at lower pH. However, future oxygen losses in the ocean (Schmidtko et al., 2017) could prevent organisms increasing their metabolic rates sufficiently. Therefore, interpretations on metabolic responses must be viewed in a wider biological context as increases in metabolism may result in negative responses in other parameters like growth rates (Kordas et al., 2011) and depend on the relative change in environmental conditions relative to critical and optimum values at their respective life stages (Pörtner and Farrell, 2008).
Organism size has been shown to greatly influence ectotherm survival (Peralta-Maraver and Rezende, 2021). Dynamic energy budget research shows organism metabolic rates generally decrease concurrently with size (Ren and Ross, 2001; Agüera et al., 2015). Increasing organism size decreases surface area to volume ratio, and oxygen uptake generally decreases relative to size as organism size increases (Lefevre et al., 2017). In general, metabolism increases in response to climate stressors. Meta-regression revealed that increasing organism size leads to negative metabolism responses (Figure 3), but this is likely an artifact of established metabolic theory. This result highlights the complexity of impacts on organisms, and potentially explains discrepancies between results of different studies. We were unable to explore this in greater detail due to studies infrequently reporting organism size and encourage experimentalist to make this part of their data reporting.
4.3 Increased Stressor Exposure Duration Amplifies Risk
Study duration (the period an organism was continuously exposured a to climate stressor) clearly impacts organism physiological responses to climate change. Using meta-regression to examine how study duration period changed trait responses to climate stressors suggests that impacts amplify the longer organisms are exposed. We expect effect size signals to shift towards zero if acclimation would occur. Our data though for growth and metabolism generally increased their response the longer experments were conducted (Table 1). We suggest that European benthic organisms have limited capacity for acclimation over the time of the experiments. While we also detected many statistically insignificant signals, it is important to note that this does not indicate acclimation; it only suggests that study duration did not have a statistical relationship with effect size.
The mean experimental duration period across our data was only 86.3 days representing short-term exposure in contrast to climate change over decades. Long term experiments assessing acclimation are rare and typically conducted on organisms with fast reproduction time such as Pseudokirchneriella or Emiliania huxleyi (Schlüter et al., 2016; Limberger and Fussmann, 2021). Study length has not increased much since the meta-analysis Kroeker et al. (2013) clearly limiting our knowledge of the true long-term ecological impacts. Our selection of organisms may have a bias towards species which are adapted to deal with acute stress such as exposure to air, and highly variable environments. For example, photosynthetic performance of Fucus serratus in Spain when exposed to sudden thermal stress recovered within 24hrs, while populations from Norway, Denmark, and France did not recover (Jueterbock et al., 2014). Our results raise concerns that even organisms currently considered resilient and associated biological traits like algal growth rates can be overwhelmed by prolonged exposure to climate stressors. Chronic stress has been documented to lower the acclimation ability of marine organisms, reducing the upper thermal tolerances in mussels (Sorte et al., 2011) and coralline algae (Page et al., 2021).
Experiments used in this meta-analysis maintained stable conditions. Climate change will be associated with increasing frequency and duration of extreme events. Marine heat waves have led to mass mortality (Garrabou et al., 2009; Smale et al., 2019) and ecosystem restructuring (Wernberg et al., 2016; Ainsworth et al., 2020). Such extreme events have the potential to alter many currently statistically neutral responses) and are projected to increase in the future with climate change (Ranasinghe et al., 2021), thereby reducing time intervals which facilitate recovery (Cooley et al., 2022). Compounded by destructive fishing (Pedersen et al., 2017) and habitat fragmentation this increasing complexity of the currently observed risks (Simpson et al., 2021) highlights our still limited ability to project the true extent of the challenges marine ecosystems will face.
5 Conclusions
These results suggest European benthic organisms have highly variable responses to ocean acidification and warming. Future European benthic ecosystems will undergo restructuring based on current climate emission pathways. We highlight the vulnerability of calcifying organisms, and the potential resilience of fleshly algae. This study highlights the importance of experimental design parameters when investigating climate stressor interactions with marine organisms. Study duration in particular is highlighted as amplifiying observed organism physiological responses to climate change, but average study durations are still limited in the context of decadal changes to ocean chemistry. Our understanding of how European marine ecosystems may evolve under future conditions are still limited due to focuses on specific organisms like molluscs. Future experimental studies on marine organism responses to climate change need to expand to under represented groups like polychaetes.
Data Availability Statement
The original contributions presented in the study are included in the article/Supplementary Material. Further inquiries can be directed to the corresponding author.
Author Contributions
GH, and DNS conceptulized and designed the study. GH collected the data and preformed the statistical analysis. All authors contributed to manuscript revision, read, and approved the submitted version. All authors contributed to the article and approved the submitted version.
Conflict of Interest
The authors declare that the research was conducted in the absence of any commercial or financial relationships that could be construed as a potential conflict of interest.
Publisher’s Note
All claims expressed in this article are solely those of the authors and do not necessarily represent those of their affiliated organizations, or those of the publisher, the editors and the reviewers. Any product that may be evaluated in this article, or claim that may be made by its manufacturer, is not guaranteed or endorsed by the publisher.
Acknowledgments
GH is funded by NERC scholarship grant number NE/L002434/1, DS is supported by the Leverhulme Trust grant RF-2021-489\4. Figures 3, 4 were produced with help from Dr Nuria Melisa Morales García.
Supplementary Material
The Supplementary Material for this article can be found online at: https://www.frontiersin.org/articles/10.3389/fmars.2022.896157/full#supplementary-material
References
Abdussamatov H. I., Khankov S. I., Lapovok Y. V. (2012). The Thermal Inertia Characteristics of the System Ocean-Atmosphere. J. Geograph. Inf. System 4 (5), 479–482. doi: 10.4236/jgis.2012.45052
Agostini S., Harvey B. P., Milazzo M., Wada S., Kon K., Floc’h N., et al. (2021). Simplification, Not “Tropicalization”, of Temperate Marine Ecosystems Under Ocean Warming and Acidification. Global Change Biol. 27 (19), 4771–84. doi: 10.1111/gcb.15749.
Agüera A., Collard M., Jossart Q., Moreau C., Danis B. (2015). Parameter Estimations of Dynamic Energy Budget (DEB) Model Over the Life History of a Key Antarctic Species: The Antarctic Sea Star Odontaster Validus Koehler 1906. PloS One 10 (10), e0140078. doi: 10.1371/journal.pone.0140078
Ainsworth T. D., Hurd C. L., Gates R. D., Boyd P. W. (2020). How do We Overcome Abrupt Degradation of Marine Ecosystems and Meet the Challenge of Heat Waves and Climate Extremes? Global Change Biol. 26 (2), 343–354.
Araújo R., Vázquez Calderón F., Sánchez López J., Azevedo I. C., Bruhn A., Fluch S., et al. (2021). Current Status of the Algae Production Industry in Europe: An Emerging Sector of the Blue Bioeconomy. Front. Mar. Sci. 7, 1247. doi: 10.3389/fmars.2020.626389
Bach L. T., Boyd P. W. (2021). Seeking Natural Analogs to Fast-Forward the Assessment of Marine CO2 Removal. Proc. Natl. Acad. Sci. United States America 118 (40), 1–8. doi: 10.1073/pnas.2106147118.
Bass A., Wernberg T., Thomsen M. S., Smale D. (2021). Another Decade of Marine Climate Change Experiments: Trends, Progress and Knowledge Gaps. Front. Mar. Sci. 8, 714462. doi: 10.3389/fmars.2021.714462
Bates C. R., DeWreede R. E. (2007). Do Changes in Seaweed Biodiversity Influence Associated Invertebrate Epifauna? J. Exp. Mar. Biol. Ecol. 344 (2), 206–214. doi: 10.1016/j.jembe.2007.01.002
Bednar-Friedl B., Biesbroek R., Schmidt D. N. (2022). IPCC Sixth Assessment Report (AR6): Climate Change 2022-Impacts, Adaptation and Vulnerability: Regional Factsheet Europe. Cambridge: Cambridge University Press.
Berner F., Heimann K., Sheehan M. (2015). Microalgal Biofilms for Biomass Production. J. Appl. Phycology. 27 (5), 1793–1804. doi: 10.1007/s10811-014-0489-x
Bindoff N. L., Cheung W. W., Kairo J. G., Arstegui J., Guinder V. A., Hallberg R., et al. (2019). “Changing Ocean, Marine Ecosystems, and Dependent Communities,” in IPCC Special Report on the Ocean and Cryosphere in a Changing Climate.
Bopp L., Resplandy L., Orr J. C., Doney S. C., Dunne J. P., Gehlen M., et al. (2013). Multiple Stressors of Ocean Ecosystems in the 21st Century: Projections With CMIP5 Models. Biogeosciences 10 (10), 6225–6245. doi: 10.5194/bg-10-6225-2013
Bove C. B., Umbanhowar J., Castillo K. D. (2020). Meta-Analysis Reveals Reduced Coral Calcification Under Projected Ocean Warming But Not Under Acidification Across the Caribbean Sea. Front. Mar. Sci. 7. doi: 10.3389/fmars.2020.00127
Brodie J., Williamson C. J., Smale D. A., Kamenos N. A., Mieszkowska N., Santos R., et al. (2014). The Future of the Northeast Atlantic Benthic Flora in a High CO2 World. Ecol. Evol. 4 (13), 2787–2798. doi: 10.1002/ece3.1105
Burrows M. T., Twigg G., Mieszkowska N., Harvey R. (2017). Marine Biodiversity and Climate Change (MarClim), Scotland 2014/15. Scottish Natural Heritage.
Büscher J. V., Form A. U., Riebesell U. (2017). Interactive Effects of Ocean Acidification and Warming on Growth, Fitness and Survival of the Cold-Water Coral Lophelia Pertusa Under Different Food Availabilities. Front. Mar. Sci. 4, 101. doi: 10.3389/fmars.2017.00101
Cattano C., Claudet J., Domenici P., Milazzo M. (2018). Living in a High CO2 World: A Global Meta-Analysis Shows Multiple Trait-Mediated Fish Responses to Ocean Acidification. Ecol. Monogr. 88 (3), 320–335. doi: 10.1002/ecm.1297
Chan P. T. W., Halfar J., Adey W. H., Lebednik P. A., Steneck R., Norley C. J. D., et al. (2020). Recent Density Decline in Wild-Collected Subarctic Crustose Coralline Algae Reveals Climate Change Signature. Geology 48 (3), 226–230. doi: 10.1130/G46804.1
Coen L. D., Brumbaugh R. D., Bushek D., Grizzle R., Luckenbach M. W., Posey M. H., et al. (2007). Ecosystem Services Related to Oyster Restoration. Mar. Ecol. Prog. Ser. 341, 303–307. doi: 10.3354/meps341303
Coniglio M., James N. P. (1985). Calcified Algae as Sediment Contributors to Early Paleozoic Limestones; Evidence From Deep-Water Sediments of the Cow Head Group, Western Newfoundland. J. Sediment. Res. 55 (5), 746–754. doi: 10.1306/212F87D8-2B24-11D7-8648000102C1865D
Cooley S., Schoeman D., Bopp L., Boyd P., Donner S., IGhebrehiwet D. Y., et al. (2022). Ocean and Coastal Ecosystems and their Services. In: Pörtner H.-O., Roberts D.C., Tignor M., Poloczanska E.S., Mintenbeck K., Alegría A., et al Climate Change 2022: Impacts, Adaptation, and Vulnerability. Contribution of Working Group II to the Sixth Assessment Report of the Intergovernmental Panel on Climate Change. Cambridge University Press.
Currin C. A., Chappell W. S., Deaton A. (2009). Developing Alternative Shoreline Armoring Strategies: The Living Shoreline Approach in North Carolina. In:Shipman H, Dethier MN, Gelfenbaum G, Fresh KL, Dinicola RS Puget Sound Shorelines and the Impacts of Armoring, Proceedings of a State of the Science Workshop (US Geological Survey, Reston, VA), pp 91–102
De Groot S. J. (1984). The Impact of Bottom Trawling on Benthic Fauna of the North Sea. Ocean Manage. 9 (3-4), 177–190. doi: 10.1016/0302-184X(84)90002-7
Doney S. C., Fabry V. J., Feely R. A., Kleypas J. A. (2009). Ocean Acidification: The Other CO2 Problem. Annu. Rev. Mar. Sci. 1, 169–192. doi: 10.1146/annurev.marine.010908.163834
Donnarumma L., Sandulli R., Appolloni L., Russo G. F. (2018). Assessing Molluscs Functional Diversity Within Different Coastal Habitats of Mediterranean Marine Protected Areas. Ecol. Questions 29 (3), pp.35–pp.51. doi: 10.12775/EQ.2018.021
Egger M., Smith G. D., Schneider M., Minder C. (1997). Bias in Meta-Analysis Detected by a Simple, Graphical Test. Bmj 315 (7109), 629–634. doi: 10.1136/bmj.315.7109.629
Evans T. G., Hofmann G. E. (2012). Defining the Limits of Physiological Plasticity: How Gene Expression can Assess and Predict the Consequences of Ocean Change. Philos. Trans. R. Soc. B: Biol. Sci. 367 (1596), 1733–1745. doi: 10.1098/rstb.2012.0019
Figuerola B., Hancock A. M., Bax N., Cummings V. J., Downey R., Griffiths H. J., et al. (2021). A Review and Meta-Analysis of Potential Impacts of Ocean Acidification on Marine Calcifiers From the Southern Ocean. Front. Mar. Sci. 8, 24. doi: 10.3389/fmars.2021.584445
Form A. U., Riebesell U. (2012). Acclimation to Ocean Acidification During Long-Term CO2 Exposure in the Cold-Water Coral Lophelia Pertusa. Global Change Biol. 18 (3), 843–853. doi: 10.1111/j.1365-2486.2011.02583.x
Friedlingstein P., O'sullivan M., Jones M. W., Andrew R. M., Hauck J., Olsen A., et al. (2020). Global Carbon Budget 2020. Earth System Sci. Data 12 (4), 3269–3340. doi: 10.5194/essd-12-3269-2020
Garrabou J., Coma R., Bensoussan N., Bally M., Chevaldonné P., Cigliano M., et al. (2009). Mass Mortality in Northwestern Mediterranean Rocky Benthic Communities: Effects of the 2003 Heat Wave. Global Change Biol. 15 (5), 1090–1103. doi: 10.1111/j.1365-2486.2008.01823.x
Goethel C. L., Grebmeier J. M., Cooper L. W., Miller T. J. (2017). Implications of Ocean Acidification in the Pacific Arctic: Experimental Responses of Three Arctic Bivalves to Decreased pH and Food Availability. Deep Sea Res. Part II: Topical Stud. Oceanog. 144, 112–124. doi: 10.1016/j.dsr2.2017.08.013
Griffith A. W., Gobler C. J. (2017). Transgenerational Exposure of North Atlantic Bivalves to Ocean Acidification Renders Offspring More Vulnerable to Low pH and Additional Stressors. Sci. Rep. 7 (1), 1–11. doi: 10.1038/s41598-017-11442-3
Habeck C. W., Schultz A. K. (2015). Community-Level Impacts of White-Tailed Deer on Understorey Plants in North American Forests: A Meta-Analysis. AoB Plants 7, plv119. doi: 10.1093/aobpla/plv119
Hancock A. M., King C. K., Stark J. S., McMinn A., Davidson A. T. (2020). Effects of Ocean Acidification on Antarctic Marine Organisms: A Meta-Analysis. Ecol. Evol. 10 (10), 4495–4514. doi: 10.1002/ece3.6205
Harding S., Nelson L., Glover T. (2016). Solent Oyster Restoration Project Management Plan. Blue Mar. Foundation 47.
Harvey B. P., Gwynn-Jones D., Moore P. J. (2013). Meta-Analysis Reveals Complex Marine Biological Responses to the Interactive Effects of Ocean Acidification and Warming. Ecol. Evol. 3 (4), 1016–1030. doi: 10.1002/ece3.516
Hedges L. V., Olkin I. (2014). Statistical Methods for Meta-Analysis (Orlando, Florida: Academic press).
Helmuth B., Mieszkowska N., Moore P., Hawkins S. J. (2006). Living on the Edge of Two Changing Worlds: Forecasting the Responses of Rocky Intertidal Ecosystems to Climate Change. Annu. Rev. Ecol. Evol. Syst. 37, 373–404. doi: 10.1146/annurev.ecolsys.37.091305.110149
Hendriks I. E., Duarte C. M., Álvarez M. (2010). Vulnerability of Marine Biodiversity to Ocean Acidification: A Meta-Analysis. Estuarine Coast. Shelf Sci. 86 (2), 157–164. doi: 10.1016/j.ecss.2009.11.022
Hennige S. J., Wolfram U., Wickes L., Murray F., Roberts J. M., Kamenos N. A., et al. (2020). Crumbling Reefs and Cold-Water Coral Habitat Loss in a Future Ocean: Evidence of “Coralporosis” as an Indicator of Habitat Integrity. Front. Mar. Sci. 7 (668). doi: 10.3389/fmars.2020.00668
Hoegh-Guldberg O., Poloczanska E. S., Skirving W., Dove S. (2017). Coral Reef Ecosystems Under Climate Change and Ocean Acidification. Front. Mar. Sci. 4, 158. doi: 10.3389/fmars.2017.00158
Hofmann L. C., Yildiz G., Hanelt D., Bischof K. (2012). Physiological Responses of the Calcifying Rhodophyte, Corallina Officinalis (L.), to Future CO 2 Levels. Mar. Biol. 159 (4), 783–792. doi: 10.1007/s00227-011-1854-9
Hughes A. D., Brunner L., Cook E. J., Kelly M. S., Wilson B. (2012). Echinoderms Display Morphological and Behavioural Phenotypic Plasticity in Response to Their Trophic Environment. PLoS ONE. 7(1), e41243. doi: 10.1371/journal.pone.0041243
Hynes S., Chen W., Vondolia K., Armstrong C., O'Connor E. (2021). Valuing the Ecosystem Service Benefits From Kelp Forest Restoration: A Choice Experiment From Norway. Ecol. Economics 179, 106833. doi: 10.1016/j.ecolecon.2020.106833
IPCC (2021). Climate Change 2021: The Physical Science Basis. Contribution of Working Group I to the Sixth Assessment Report of the Intergovernmental Panel on Climate Change. Eds. Masson-Delmotte V., Zhai P., Pirani A., Connors S. L., Péan C., Berger S., et al (Cambridge: Cambridge University Press).
Jueterbock A., Kollias S., Smolina I., Fernandes J. M., Coyer J. A., Olsen J. L., et al. (2014). Thermal Stress Resistance of the Brown Alga Fucus Serratus Along the North-Atlantic Coast: Acclimatization Potential to Climate Change. Mar. Genomics 13, 27–36. doi: 10.1016/j.margen.2013.12.008
King N. G., Moore P. J., Wilding C., Jenkins H. L., Smale D. A. (2021). Multiscale Spatial Variability in Epibiont Assemblage Structure Associated With Stipes of Kelp Laminaria Hyperborea in the Northeast Atlantic. Mar. Ecol. Prog. Ser. 672, 33–44. doi: 10.3354/meps13794
Koivisto M. E., Westerbom M. (2010). Habitat Structure and Complexity as Determinants of Biodiversity in Blue Mussel Beds on Sublittoral Rocky Shores. Mar. Biol. 157 (7), 1463–1474. doi: 10.1007/s00227-010-1421-9
Kordas R. L., Harley C. D., O'Connor M. I. (2011). Community Ecology in a Warming World: The Influence of Temperature on Interspecific Interactions in Marine Systems. J. Exp. Mar. Biol. Ecol. 400 (1-2), 218–226. doi: 10.1016/j.jembe.2011.02.029
Koricheva J., Gurevitch J., Mengersen K. (Eds.) (2013). Handbook of Meta-Analysis in Ecology and Evolution (Princeton, New Jersey: Princeton University Press).
Kornder N. A., Riegl B. M., Figueiredo J. (2018). Thresholds and Drivers of Coral Calcification Responses to Climate Change. Global Change Biol. 24 (11), 5084–5095. doi: 10.1111/gcb.14431
Kotta J., Orav-Kotta H., Jänes H., Hummel H., Arvanitidis C., Van Avesaath P., et al. (2017). Essence of the Patterns of Cover and Richness of Intertidal Hard Bottom Communities: A Pan-European Study. J. Mar. Biol. Assoc. United Kingdom 97 (3), 525–538. doi: 10.1017/S0025315416001351
Kroeker K. J., Kordas R. L., Crim R., Hendriks I. E., Ramajo L., Singh G. S., et al. (2013). Impacts of Ocean Acidification on Marine Organisms: Quantifying Sensitivities and Interaction With Warming. Global Change Biol. 19 (6), 1884–1896. doi: 10.1111/gcb.12179
Krumhansl K. A., Okamoto D. K., Rassweiler A., Novak M., Bolton J. J., Cavanaugh K. C., et al. (2016). Global Patterns of Kelp Forest Change Over the Past Half-Century. Proc. Natl. Acad. Sci. 113 (48), 13785–13790. doi: 10.1073/pnas.1606102113
Lefevre S. (2016). Are Global Warming and Ocean Acidification Conspiring Against Marine Ectotherms? A Meta-Analysis of the Respiratory Effects of Elevated Temperature, High CO2 and Their Interaction. Conserv. Physiol. 4 (1), cow009. doi: 10.1093/conphys/cow009
Lefevre S., McKenzie D. J., Nilsson G. E. (2017). Models Projecting the Fate of Fish Populations Under Climate Change Need to be Based on Valid Physiological Mechanisms. Global Change Biol. 23 (9), 3449–3459. doi: 10.1111/gcb.13652
Leung J. Y., Russell B. D., Coleman M. A., Kelaher B. P., Connell S. D. (2021). Long-Term Thermal Acclimation Drives Adaptive Physiological Adjustments of a Marine Gastropod to Reduce Sensitivity to Climate Change. Sci. Total Environ. 771, 145208. doi: 10.1016/j.scitotenv.2021.145208
Li W., Han G., Dong Y., Ishimatsu A., Russell B. D., Gao K. (2015). Combined Effects of Short-Term Ocean Acidification and Heat Shock in a Benthic Copepod Tigriopus Japonicus Mori. Mar. Biol. 162 (9), 1901–1912. doi: 10.1007/s00227-015-2722-9
Limberger R., Fussmann G. F. (2021). Adaptation and Competition in Deteriorating Environments. Proc. R. Soc. B 288 (1946), 20202967. doi: 10.1098/rspb.2020.2967
Linares C., Coma R., Zabala M. (2008). Restoration of Threatened Red Gorgonian Populations: An Experimental and Modelling Approach. Biol. Conserv. 141 (2), 427–437. doi: 10.1016/j.biocon.2007.10.012
Mangi S. C., Lee J., Pinnegar J. K., Law R. J., Tyllianakis E., Birchenough S. N. (2018). The Economic Impacts of Ocean Acidification on Shellfish Fisheries and Aquaculture in the United Kingdom. Environ. Sci. Policy 86, 95–105. doi: 10.1016/j.envsci.2018.05.008
Marchini A., Ragazzola F., Vasapollo C., Castelli A., Cerrati G., Gazzola F., et al. (2019). Intertidal Mediterranean Coralline Algae Habitat is Expecting a Shift Toward a Reduced Growth and a Simplified Associated Fauna Under Climate Change. Front. Mar. Sci. 6, 106. doi: 10.3389/fmars.2019.00106
Martínez-Botí M. A., Foster G. L., Chalk T. B., Rohling E. J., Sexton P. F., Lunt D. J., et al. (2015). Plio-Pleistocene Climate Sensitivity Evaluated Using High-Resolution CO2 Records. Nature 518 (7537), 49–54. doi: 10.1038/nature14145
Mazaris A. D., Kallimanis A., Gissi E., Pipitone C., Danovaro R., Claudet J., et al. (2019). Threats to Marine Biodiversity in European Protected Areas. Sci. Total Environ. 677, 418–426. doi: 10.1016/j.scitotenv.2019.04.333
Melbourne L. A., Denny M. W., Harniman R. L., Rayfield E. J., Schmidt D. N. (2018). The Importance of Wave Exposure on the Structural Integrity of Rhodoliths. J. Exp. Mar. Biol. Ecol. 503, 109–119. doi: 10.1016/j.jembe.2017.11.007
Mieszkowska N., Leaper R., Moore P., Kendall M. A., Burrows M. T., Lear D., et al. (2006). Marine Biodiversity and Climate Change: Assessing and Predicting the Influence of Climatic Change Using Intertidal Rocky Shore Biota Vol. 20 (Plymouth: Occasional Publication of the Marine Biological Association).
Narayanaswamy B. E., Coll M., Danovaro R., Davidson K., Ojaveer H., Renaud P. E. (2013). Synthesis of Knowledge on Marine Biodiversity in European Seas: From Census to Sustainable Management. PloS One 8 (3), e58909. doi: 10.1371/journal.pone.0058909
Narita D., Rehdanz K. (2017). Economic Impact of Ocean Acidification on Shellfish Production in Europe. J. Environ. Plann. Manage. 60 (3), 500–518. doi: 10.1080/09640568.2016.1162705
Nelson W. A. (2009). Calcified Macroalgae–Critical to Coastal Ecosystems and Vulnerable to Change: A Review. Mar. Freshw. Res. 60 (8), 787–801. doi: 10.1071/MF08335
Norderhaug K. M., Christie H. C. (2009). Sea Urchin Grazing and Kelp Re-Vegetation in the NE Atlantic. Mar. Biol. Res. 5 (6), 515–528. doi: 10.1080/17451000902932985
Oliver E. C., Donat M. G., Burrows M. T., Moore P. J., Smale D. A., Alexander L. V., et al. (2018). Longer and More Frequent Marine Heatwaves Over the Past Century. Nat. Commun. 9 (1), 1–12. doi: 10.1038/s41467-018-03732-9
Orr J. A., Vinebrooke R. D., Jackson M. C., Kroeker K. J., Kordas R. L., Mantyka-Pringle C., et al. (2020). Towards a Unified Study of Multiple Stressors: Divisions and Common Goals Across Research Disciplines. Proc. R. Soc. B 287 (1926), 20200421. doi: 10.1098/rspb.2020.0421
Page T. M., Bergstrom E., Diaz-Pulido G. (2021). Acclimation History of Elevated Temperature Reduces the Tolerance of Coralline Algae to Additional Acute Thermal Stress. Front. Mar. Sci. 8, 511. doi: 10.3389/fmars.2021.660196
Parker L. M., Ross P. M., O'Connor W. A., Pörtner H. O., Scanes E., Wright J. M. (2013). Predicting the Response of Molluscs to the Impact of Ocean Acidification. Biology 2 (2), 651–692. doi: 10.3390/biology2020651
Pedersen E. J., Thompson P. L., Ball R. A., Fortin M. J., Gouhier T. C., Link H., et al. (2017). Signatures of the Collapse and Incipient Recovery of an Overexploited Marine Ecosystem. R. Soc. Open Sci. 4 (7), 170215. doi: 10.1098/rsos.170215
Peralta-Maraver I., Rezende E. L. (2021). Heat Tolerance in Ectotherms Scales Predictably With Body Size. Nat. Climate Change 11 (1), 58–63. doi: 10.1038/s41558-020-00938-y
Phelps C. M., Boyce M. C., Huggett M. J. (2017). Future Climate Change Scenarios Differentially Affect Three Abundant Algal Species in Southwestern Australia. Mar. Environ. Res. 126, 69–80. doi: 10.1016/j.marenvres.2017.02.008
Pörtner H. O. (2002). Climate Variations and the Physiological Basis of Temperature Dependent Biogeography: Systemic to Molecular Hierarchy of Thermal Tolerance in Animals. Comp. Biochem. Physiol. Part A: Mol. Integr. Physiol. 132 (4), 739–761. doi: 10.1016/S1095-6433(02)00045-4
Pörtner H. O. (2008). Ecosystem Effects of Ocean Acidification in Times of Ocean Warming: A Physiologist’s View. Mar. Ecol. Prog. Ser. 373, 203–217. doi: 10.3354/meps07768
Pörtner H. O., Farrell A. P. (2008). Physiology and Climate Change. Science 322 (5902), 690–692. doi: 10.1126/science.116315
Qiu W., Jones P. J. (2013). The Emerging Policy Landscape for Marine Spatial Planning in Europe. Mar. Policy 39, 182–190. doi: 10.1016/j.marpol.2012.10.010
Ragazzola F., Foster L. C., Form A. U., Büscher J., Hansteen T. H., Fietzke J. (2013). Phenotypic Plasticity of Coralline Algae in a High CO 2 World. Ecol. Evol. 3 (10), 3436–3446. doi: 10.1002/ece3.723
Rai L. C., Gaur J. P. (2012). Algal Adaptation to Environmental Stresses: Physiological, Biochemical and Molecular Mechanisms (Heidelberg: Springer Science & Business Media).
Ranasinghe R., Ruane A. C., Vautard R., Arnell N., Coppola E., Cruz F. A., et al. (2021). “Chapter 12. Climate Change Information for Regional Impact and for Risk Assessment,” in Climate Change. (Cambridge: Cambridge University Press)
Ren J. S., Ross A. H. (2001). A Dynamic Energy Budget Model of the Pacific Oyster Crassostrea Gigas. Ecol. Model. 142 (1-2), 105–120. doi: 10.1016/S0304-3800(01)00282-4
Riebesell U., Gattuso J.P. (2015). Lessons Learned From Ocean Acidification Research. Nat. Clim. Chang. 5 (1), 12–4.
Riebesell U., Fabry V. J., Hansson L., Gattuso J. P. (2011). Guide to Best Practices for Ocean Acidification Research and Data Reporting (Luxembourg: Office for Official Publications of the European Communities).
Rohatgi A. (2019). WebPlotDigitalizer: HTML5 Based Online Tool to Extract Numerical Data From Plot Images. Version 4.2. Available at: https://automeris.io/WebPlotDigitizer/index.html (Accessed November 2019).
Sampaio E., Santos C., Rosa I. C., Ferreira V., Pörtner H. O., Duarte C. M., et al. (2021). Impacts of Hypoxic Events Surpass Those of Future Ocean Warming and Acidification. Nat. Ecol. Evol. 5 (3), 311–321. doi: 10.1038/s41559-020-01370-3.
Schlüter L., Lohbeck K. T., Gröger J. P., Riebesell U., Reusch T. B. (2016). Long-Term Dynamics of Adaptive Evolution in a Globally Important Phytoplankton Species to Ocean Acidification. Sci. Adv. 2 (7), e1501660. doi: 10.1126/sciadv.1501660
Schmidtko S., Stramma L., Visbeck M. (2017). Decline in Global Oceanic Oxygen Content During the Past Five Decades. Nature 542 (7641), 335–339. doi: 10.1038/nature21399
Seebacher F., White C. R., Franklin C. E. (2015). Physiological Plasticity Increases Resilience of Ectothermic Animals to Climate Change. Nat. Climate Change 5 (1), 61–66. doi: 10.1038/nclimate2457
Sheldon K. S., Yang S., Tewksbury J. J. (2011). Climate Change and Community Disassembly: Impacts of Warming on Tropical and Temperate Montane Community Structure. Ecol. Lett. 14 (12), 1191–1200. doi: 10.1111/j.1461-0248.2011.01689.x
Simon-Nutbrown C., Hollingsworth P. M., Fernandes T. F., Kamphausen L., Baxter J. M., Burdett H. L. (2020). Species Distribution Modeling Predicts Significant Declines in Coralline Algae Populations Under Projected Climate Change With Implications for Conservation Policy. Front. Mar. Sci. 7, 758. doi: 10.3389/fmars.2020.575825
Simpson N. P., Mach K. J., Constable A., Hess J., Hogarth R., Howden M., et al. (2021). A Framework for Complex Climate Change Risk Assessment. One Earth 4 (4), 489–501. doi: 10.1016/j.oneear.2021.03.005
Singh H. A., Rasch P. J., Rose B. E. J. (2017). Increased Ocean Heat Convergence Into the High Latitudes With CO2 Doubling Enhances Polar-Amplified Warming. Geophysic. Res. Lett. 44 (20), 10–583. doi: 10.1002/2017GL074561
Smale D. A., Burrows M. T., Moore P., O'Connor N., Hawkins S. J. (2013). Threats and Knowledge Gaps for Ecosystem Services Provided by Kelp Forests: A Northeast Atlantic Perspective. Ecol. Evol. 3 (11), 4016–4038. doi: 10.1002/ece3.774
Smale D. A., Wernberg T., Oliver E. C. J., Thomsen M., Harvey B. P., Straub S. C., et al. (2019). Marine Heatwaves Threaten Global Biodiversity and the Provision of Ecosystem Services. Nat. Climate Change 9 (4), 306–312. doi: 10.1038/s41558-019-0412-1
Sorte C. J., Jones S. J., Miller L. P. (2011). Geographic Variation in Temperature Tolerance as an Indicator of Potential Population Responses to Climate Change. J. Exp. Mar. Biol. Ecol. 400 (1-2), 209–217. doi: 10.1016/j.jembe.2011.02.009
Sterne J. A., Becker B. J., Egger M. (2005). Publication Bias in Meta-Analysis: Prevention, Assessment, and Adjustments. The Funnel Plot 75–98.
Strain E. M., Steinberg P. D., Vozzo M., Johnston E. L., Abbiati M., Aguilera M. A., et al. (2021). A Global Analysis of Complexity–Biodiversity Relationships on Marine Artificial Structures. Global Ecol. Biogeography 30 (1), 140–153. doi: 10.1111/geb.13202
Strain E. M., van Belzen J., van Dalen J., Bouma T. J., Airoldi L. (2015). Management of Local Stressors can Improve the Resilience of Marine Canopy Algae to Global Stressors. PloS One 10 (3), e0120837. doi: 10.1371/journal.pone.0120837
Szaniawska A. (2018). “Function and Importance of Crustaceans,” in Baltic Crustaceans (Switzerland: Springer, Cham), 185–188.
Thomsen J., Casties I., Pansch C., Körtzinger A., Melzner F. (2013). Food Availability Outweighs Ocean Acidification Effects in Juvenile Mytilus Edulis: Laboratory and Field Experiments. Global Change Biol. 19 (4), 1017–1027. doi: 10.1111/gcb.12109
Tsai D. D. W., Chen P. H., Ramaraj R. (2017). The Potential of Carbon Dioxide Capture and Sequestration With Algae. Ecol. Eng. 98, 17–23. doi: 10.1016/j.ecoleng.2016.10.049
Unsworth R. K. F., McKenzie L. J., Nordlund L. M., Cullen-Unsworth L. C. (2018). A Changing Climate for Seagrass Conservation? Curr. Biol. 28 (21), R1229–R1232. doi: 10.1016/j.cub.2018.09.027
Varnerin B. V., Hopkinson B. M., Gleason D. F. (2020). Recruits of the Temperate Coral Oculina Arbuscula Mimic Adults in Their Resilience to Ocean Acidification. Mar. Ecol. Prog. Ser. 636, 63–75. doi: 10.3354/meps13228
Vergés A., Steinberg P. D., Hay M. E., Poore A. G., Campbell A. H., Ballesteros E., et al. (2014). The Tropicalization of Temperate Marine Ecosystems: Climate-Mediated Changes in Herbivory and Community Phase Shifts. Proc. R. Soc. B: Biol. Sci. 281 (1789), 20140846. doi: 10.1098/rspb.2014.0846
Viechtbauer W. (2010). Conducting Meta-Analyses in R With the Metafor Package. J. Stat. software 36 (3), 1–48. doi: 10.18637/jss.v036.i03
Viechtbauer W., Cheung M. W. L. (2010). Outlier and Influence Diagnostics for Meta-Analysis. Res. synthesis Methods 1 (2), 112–125. doi: 10.1002/jrsm.11
Wall M., Ragazzola F., Foster L. C., Form A., Schmidt D. N. (2015). pH Up-Regulation as a Potential Mechanism for the Cold-Water Coral Lophelia Pertusa to Sustain Growth in Aragonite Undersaturated Conditions. Biogeosciences 12 (23), 6869–6880. doi: 10.5194/bg-12-6869-2015
Wang C., Arneson E. M., Gleason D. F., Hopkinson B. M. (2021). Resilience of the Temperate Coral Oculina Arbuscula to Ocean Acidification Extends to the Physiological Level. Coral Reefs 40 (1), 201–214. doi: 10.1007/s00338-020-02029-y
Wernberg T., Bennett S., Babcock R. C., De Bettignies T., Cure K., Depczynski M., et al. (2016). Climate-Driven Regime Shift of a Temperate Marine Ecosystem. Science 353 (6295), 169–172. doi: 10.1126/science.aad8745
Wiltshire K. H., Kraberg A., Bartsch I., Boersma M., Franke H. D., Freund J., et al. (2010). Helgoland Roads, North Sea: 45 Years of Change. Estuaries Coasts 33 (2), 295–310. doi: 10.1007/s12237-009-9228-y
Wu P., Jackson L., Pardaens A., Schaller N. (2011). Extended Warming of the Northern High Latitudes Due to an Overshoot of the Atlantic Meridional Overturning Circulation. Geophysic. Res. Lett. 38 (24) , L24704. doi: 10.1029/2011GL049998
Keywords: climate change, benthic organisms, ocean acidification, ocean warming, meta-analysis, european ecosystems
Citation: Hoppit G and Schmidt DN (2022) A Regional View of the Response to Climate Change: A Meta-Analysis of European Benthic Organisms’ Responses. Front. Mar. Sci. 9:896157. doi: 10.3389/fmars.2022.896157
Received: 14 March 2022; Accepted: 23 May 2022;
Published: 27 June 2022.
Edited by:
Iris Eline Hendriks, Spanish National Research Council (CSIC), SpainReviewed by:
Simon Morley, British Antarctic Survey (BAS), United KingdomJacqueline Mary Grebmeier, University of Maryland, United States
Copyright © 2022 Hoppit and Schmidt. This is an open-access article distributed under the terms of the Creative Commons Attribution License (CC BY). The use, distribution or reproduction in other forums is permitted, provided the original author(s) and the copyright owner(s) are credited and that the original publication in this journal is cited, in accordance with accepted academic practice. No use, distribution or reproduction is permitted which does not comply with these terms.
*Correspondence: George Hoppit, Z2VvcmdlLmhvcHBpdEBicmlzdG9sLmFjLnVr