- Department of Chemical Engineering, Birla Institute of Science and Technology (BITS) Pilani, Goa, India
Stressors like microplastics (MPs) cause proliferating environmental pollution globally. Since plastics are continuously introduced into water bodies through numerous paths, novel solutions are required to segregate as well as decline their quantity in various environmental sectors. Numerous techniques have been used and proposed in the last 10 years to screen and enumerate MPs, define the particle’s properties, for instance form, color, or size, and recognize the polymer material. This critical review aims to provide an overview of advanced procedures in MP investigation, provides illustrations of probable routes forward and lingering challenges, and categorizes present approaches as per their underlying research question. Methods presently employed for MP sampling, extraction, identification, characterization, and quantification were evaluated. Studies proposing use of precursors for removal of MPs from water via the sol–gel process were reviewed. Research on microfluidics systems finds application in environmental and industrial fields and has gained momentum in concentrating, sorting, classifying, focusing, and desegregating MPs. This review briefly discusses active and passive label-free microfluidic methods that are efficient in executing the desired particle separation and are gaining momentum in the ecological analysis of MPs. Although some sets of preliminary data of MPs at selected regions across the globe have been studied and obtained, the degree of MP contamination in most important rivers, nearshore inland areas, and air is yet to be understood completely. Along the Charleston Harbor Estuary, the MP concentration in intertidal sediment was found to be 0 to 652 MPs/m2. In Asia, at the South Korean region, western Pacific Ocean, a high plastic concentration of 15–9,400 particles/m3 was reported. In India, the MP concentration was identified as 288 pieces/m3 in the Netravati River. In Turkey, ingestion of MPs was reported to be found in 458 out of 1,337 fish samples, indicating the polluted situation of the Mediterranean Sea. Despite the rapid development in MP analysis, no standardized technique for sampling along with separation has been approved. Therefore, for attaining a more inclusive picture of MPs’ fate and abundance, this study highlights the importance of a standardized procedure for MP research that can be used globally and adequately enables comparisons around the world.
1 Introduction
Across the world, microplastics (MPs) are currently a major pollutant, especially in marine ecosystems. Plastics are synthetic polymers obtained from fossil fuels such as crude oil and natural gas which are the utmost prevalent type of marine debris found in oceans. Two major characteristics of plastics are durability and light weight, making them widely used. There has been a rise in global plastic production—up to 360 million tons of plastic production in 2018 from 1.5 million tons in 1950—and a portion of these plastics ranging from as high as 12.7 million tons to as low as 4.8 million tons through different pathways makes its way into the ocean. Hence, by 2050, production of plastics is expected to intensify up to 2,000 million tons (Barra et al., 2018). According to the U.S. National Oceanic and Atmospheric Administration (NOAA) and the European Chemicals Agency (EChA), any type of broken-down plastic fragment less than 5 mm in length is classified as “MP” (Dey et al., 2021). MPs are minuscule pieces of plastic that contaminate the environment and are far more pervasive than plastics which can be attributed to their persistent nature, imposing on marine, freshwater, and terrestrial ecosystems across the globe. The history of MPs is depicted in the timeline, as shown in Figure 1. Owing to the non-biodegradability of MPs and their minimal size, they are easily ingested by low trophic fauna. Apart from having adverse consequences on the organism ingesting it directly, it has far reached impacts on other organisms and ecosystems as low trophic fauna are almost always a part of a much longer food chain or food web (Veerasingam et al., 2020).
Plastics, including MPs depending on the polymer’s physical and chemical characteristics and the environmental conditions, undergo biotic and abiotic processes of degradation (Sascha et al., 2018; Ali et al., 2020; Oliveira et al., 2020). Under favorable conditions, the general degradation processes of MPs include weathering triggered mechanical disintegration, photo- or thermal degradation linked to oxidation, and microbial and enzymatic degradation. Nevertheless, these become insignificant in the aquatic environment and additionally the complete breakdown or final state of reduced plastic particles is still not accurately understood; instead, they are assumed to be a constant (Ali et al., 2020).
MPs can further be classified into primary and secondary MPs (Duis and Coors, 2016; Smith et al., 2018). Primary MPs are MPs that are purposefully commercially manufactured. They are used in facial cleansers and other cosmetic products. Microfibers shed from clothes and other textiles such as fishing nets fall in the same category. Secondary MPs are obtained from larger plastic pieces or debris. Through fragmentation of such larger plastics by either physical or chemical or biological or photodegradation, these pieces lose their structural integrity and are reduced to sizes that are undetectable by the naked eye.
In modern times, plastics contribute an essential part of human society; their versatility, durability, and endurance are chief reasons for their widespread applications. The applications of plastics include making water bottles, tanks, containers, insulations, implants, and packaging material being, among others, composed of varied shapes (like pellets, fragments, films, foams), colors, sizes, polymer types, and specific densities (Hidalgo-Ruz et al., 2012). A significant constituent of electronic waste (e-waste) is plastic material. Recycling of e-waste frequently entails shredding and burning plastics, especially in developing nations, resulting in the release of MPs and additives locally (Hale et al., 2020). Chai et al. used FTIR spectroscopy to establish a new technique for assessing MPs in complex soil substrates and identified MPs in an e-waste dismantling location in Guangdong Province, China, suggesting that e-waste disposal sites have become MPs hotspots (Chai et al., 2020). E-waste generation is estimated to reach 52.2 million tons per year by the end of 2021 that will contribute as another source for MPs. Imminent technologies such as microfactories focus attention on a series of small devices and machines that use patented knowledge to accomplish restructuring of dilapidated commodity into novel and functioning products. This 3D printing technology is being used for recycling plastics into value-added products like industrial-grade ceramics, green steel, and plastic filaments (Sahajwalla and Gaikwad, 2018).
COVID-19 triggered a worldwide surge in single-use plastic and unrecyclable personal protection equipment, clogging sewage channels and ending up in seas and oceans via surface flooding and canals. According to WHO, almost 89 million procedural masks were required each month to prevent COVID-19, increasing the global manufacturing of face masks made from polymeric materials to an unprecedented level (Aragaw, 2020). The improper and unregulated disposal of face masks, composed of polypropylene (PP) and polyethylene (PE), as well as other polymeric materials including nylon and polystyrene, releases micro- and nanosized plastic fibers and silicon grains (Sullivan et al., 2021). Morgana et al. observed in their study that despite an overall modest level of fabric degradation (average 1.2 ± 1.3% of the initial weight), a consistently large number of micro/nanoplastics can be released from a single mask by replicating weathering circumstances under realistic intensity levels of mechanical deterioration (Morgana et al., 2021). Apart from face masks, personal protective equipment (PPEs), gloves, face shields, and many single-use plastics (SUPs) are not recycled or handled appropriately, resulting in mismanaged plastic waste (MMPW). Peng et al. also estimated that pandemic-associated MMPW would equal 11 million tons, resulting in a worldwide riverine discharge to the ocean of 34,000 tons (Peng et al., 2021).
Developing countries usually have a high percentage of mismanaged plastic waste and are the main contributors to plastic pollution in the marine atmosphere. Municipalities in these countries are often underfunded, lack institutional organization and interest, and have inadequate waste collection equipment; even when reuse and recycling activities exist, they sometimes lack a legal base and are thus carried out on an ad hoc basis. As a result, a large amount of solid trash winds up in landfills or is illegally dumped, and plastic garbage near freshwater systems has the potential to reach the aquatic environment, where further breakdown can create MPs (Wagner et al., 2018). For example, the current state of MP contamination in African countries’ water systems results from poor management and weak implementation of applicable rules and regulations. To combat this, a top-down sustainability method has been proposed, which includes developing/adopting established policies and frameworks, and both corporate and government/public entities participate in microplastic research (Alimi et al., 2021; Aragaw, 2021; Deme et al., 2022). The geographical location and size of the water bodies had little effect on the dispersion of microplastic pollution (Chen et al., 2022).
Further, many chemicals and additives are added to plastics for altering their mechanical properties with approximately 4,000 chemicals in plastics used for food packaging alone. As consumption of plastic increases, accordingly concentrations of the debris and waste have increased as well, after the plastics have been used and discarded. MPs have been found and reported as early as the 1970s, and today it has become a major contributor to the plastic debris generated. MPs are being ingested by numerous smaller organisms like planktons and higher organisms such as fishes and thus entering the MP into the food chain (Andrady, 2011; Smith et al., 2018). Dumping of plastic litter off marine vessels remains the chief source of plastic debris in the marine environment. Despite regulations, dumping of plastic litter contributed as much as 6.5 million tons of plastic to the oceans in the early 1990s (Cole et al., 2011). Other sources include discarded fishing gears, microbeads from cosmetic and healthcare products running off via domestic or industrial drainage, and aquaculture. A large proportion of the plastics are present in the form of secondary MPs generated by abrasion and fragmentation of more significant plastic fragments. Cole et al. assessed that there were additionally 5 trillion plastic pieces of debris afloat on the sea, 90% of which were secondary MPs derived from fragmentation (Cole et al., 2011).
Although sewage treatment facilities are not designed to remove MPs, an average removal value of 88% for wastewater treatment plants (WWTPs) using preliminary/primary plus secondary treatment and 94% for WWTPs using preliminary/primary plus secondary and tertiary treatment has been reported, providing a clearer understanding of how WWTPs manage MP load and to what degree MPs reach river systems through WWTPs. The majority of the elimination occurs during the preliminary and main therapy stages. Although the overall removal is substantial, the residual quantity in treated effluent (10% of the MPs in influent wastewater) constitutes a significant release of MPs to the aquatic environment, considering the massive quantities of effluent involved (Iyare et al., 2020). The settling stage was shown to be responsible for a significant reduction in MPs reaching later stages of wastewater treatment. Tagg et al. observed that MPs larger than 600 μm were more likely to be removed at this stage, while a total of 1.5 MPs/l was identified in the final effluent (Tagg et al., 2020). In a similar study, Conley et al. assessed the removal efficiencies of three WWTPs discharging into Charleston Harbor, USA, over the course of a year. The authors calculated a total of 500–1,000 million MPs per day present in the effluent load of the three WWTPs combined. This resulted in an estimated 0.34–0.68 g MP per capita per year in treated wastewater, accounting for <0.1% of plastic debris input to the metropolitan area water bodies (Conley et al., 2019).
Gela et al. in their investigation to evaluate MPs from the urban ditches in Bahir Dar, Ethiopia, recorded a total of 239 MP particles, demonstrating that a high quantity of MPs would wind up in the neighboring water bodies (Mhiret Gela and Aragaw, 2022). Sun et al. reviewed the removal of MPs in wastewater treatment plants. MPs have been found in both the influent and effluent of WWTPs, with reported influent quantities ranging from 1 to 10,044 particles/L and effluent concentrations ranging from 0 to 447 particles/l. Despite the comparatively low concentration of MPs in WWTP effluent, the overall discharge of MPs from WWTPs has been observed to have a median value of 2 × 106 particles/day, equating to an average annual efflux of 5 × 107 m3/year (Sun et al., 2019). Figure 2 indicates the steps and techniques used in WWTP for detection of MPs.
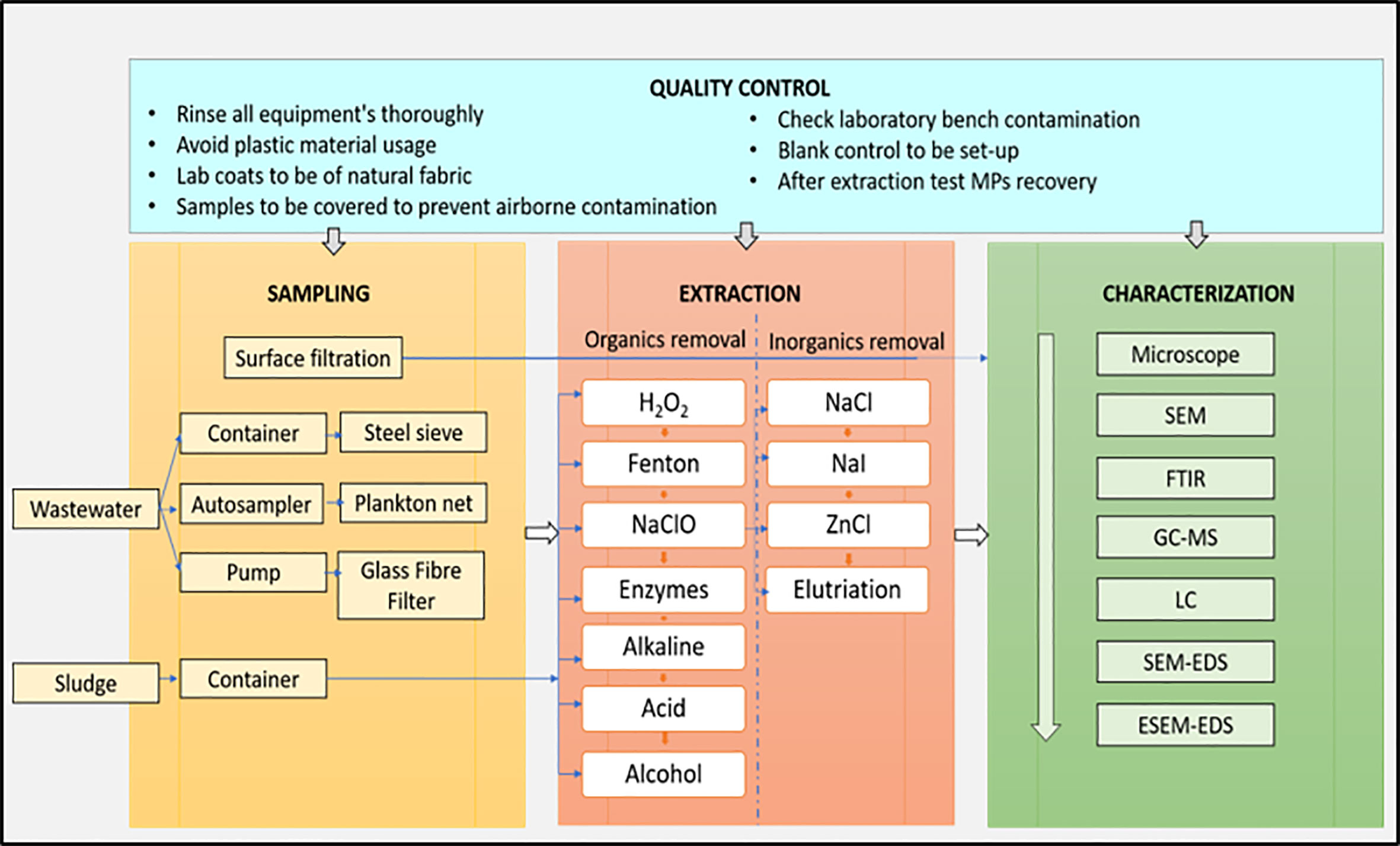
Figure 2 Diagram encapsuling the various steps and techniques used in WWTPs for microplastics detection (Modified from (Sun et al., 2018).
Although there are several techniques and methods present to analyze MPs, a lack of standardized and uniform protocol makes it difficult to monitor MP samples of various sizes and types. Sascha et al. reported size ranges of MPs according to the sampling methods assumed in field studies (Sascha et al., 2018; Thomas et al., 2020).
2 Survey on the occurrence of MP
A literature survey was conducted on the presence of MPs in sediments, water, and biota samples across the globe. Retrieved articles were screened by study area, and only studies pertaining to beaches, rivers, estuaries, bays, and lakes were selected. A total of 74 reports were considered for this study, as represented on the world map in Figure 3. The geographical distribution of MP abundance observed in sediments, water, and biota across the globe is summarized in Tables 1–3.
Out of the 74 reports, 33 studies investigated the occurrence and distribution of MPs in the water column across the globe. A total of 50 papers have looked into the abundance of MPs in sediments from beaches, coasts, islands, and lakes. Lastly, there are 24 studies from field and laboratory investigations around the world that addressed the topic of MPs in various aquatic biota (zooplankton, fish, shrimps, mussel, oyster, bivalves, and invertebrates). The abundance of MPs in the subtidal sediment of the Charleston Harbor Estuary (3–4,375 MPs/kg wet weight) in South Carolina, USA, was higher than that recorded in the Kelvin River, UK (161–432 items/kg of dry sediment). In Europe, the River Elbe in Germany had the highest MP abundance in sediments (3.35 × 106 ± 6.60 × 106 particles/m3). China had the highest MP abundance in sediments in Asia (Vila Bay and Mila Bay: 33–33,300 particles/kg and 450–15,167 particles/kg). Based on the sample methods used, quantitative values of MPs in water are reported in various quantities (items/L, items/km2, items/m3, and percent). As a result, comparing the data is challenging. MPs in surface water along Rapa Nui, Chile, USA (64,907.5 ± 18,296.5 particles/km2), are lower than those observed in the Mediterranean Sea, Turkey (16,339 to 5,20,213 items/km2). The reporting units for MP abundance in biota are “items/individual” and “items/g”; fishes were frequently utilized to study MP intake in most of the publications analyzed. The South African coastline from the mouth of the Orange River to Mossel Bay had a 68% MP ingestion rate, while the Kerala coast in India had a 21% MP ingestion rate. Fish species in the Brazos River Basin, Texas, ingested 44.9 percent of the MP (Central Texas).
From the reports, it was observed that fragments, fibers, pellets, films, and foams have been found in diverse environmental matrices across the globe. The most common morphologies observed in research were fibers and fragments. According to the physical characterization of MPs, the majority of MPs identified were secondary MPs (fragments, fibers, films, and foams) rather than primary MPs (pellets), which might be generated by fragmentation of larger plastic products. In general, the shape of MPs is utilized to determine where they came from. MP fibers come from the use of degraded fishing gear (ropes, lines, and nets) at sea and land-based washing of synthetic fabrics, whereas films come from plastic bags and agricultural films. Both land-based (packing containers) and sea-based (thermocol buoys) sources are used to make the foams. Primary MPs (pellets) were discovered to be more abundant in beach sediments than in water and biota around the world. The most common MP forms detected in water and sediment samples are fibers and pieces. Fibers are the most common MPs in biota.
3 Various methods for analysis of MP samples
3.1 Sampling methods
3.1.1 Water column
There are different methods of sampling to get the sample for processing in the laboratory from water streams. For collection of MPs, water is first sampled using nets with a standard mesh size of either 335 (Bakir et al., 2020), 333 (Xiong et al., 2018), 330 (Ory et al., 2017), or 200 µm from depths of 10-cm to 200-m range. This choice is mainly due to the lower size limit for MPs of 333-µm size proposed by NOAA, USA. To quantify the volume of water sampled, a flow meter was mounted to the mouth of manta trawl nets. However, several studies did not specify whether a flow meter was put on the net, and measuring just the distance during sampling could result in significant errors if the net is not fully immersed or hindered by an abundance of suspended and floating objects. If the net is completely submerged in water, it may miss the surface layer, which is likely to contain a substantial number of floating MPs. Furthermore, sampling from the windward side or the back of a boat has an impact on quantification. The net was towed at a pace of one to four knots for 10–20 min in the majority of the investigations. Trawl nets have been frequently employed for collecting MPs in aquatic habitats since they enable the relatively rapid sampling of broad surfaces or quantities of water to get a representative sample of the investigated site (Sutton et al., 2016). The accuracy of the trawl, however, was discovered to be mostly dependent on the capacity to keep it level in the water and visually assess the water height during sampling. The actual trawled volume will also be influenced by the current and wind conditions at the time of sampling. This volume uncertainty is most likely the most significant uncertainty in trawl sampling (Karlsson et al., 2019). The Manta trawl is presently the most extensively used tool for surface sampling of MPs in saltwater and freshwater settings (Pasquier et al., 2022). The Neuston net has been the most routinely utilized trawl net in the marine environment for sampling and monitoring MP contamination after the Manta net and is used to collect samples located in the first few centimeters of the water column (Neuston net - Aquatic BioTechnology, 2022). The primary contrast between the nets is the height of the sampled water layer: Manta samples typically the first 15–25 cm, whereas the Neuston net samples a greater water layer (generally slightly less than 50 cm) (Pasquier et al., 2022). Other methods involve the surface microlayer process, hand-net array, and sampling of bulk water (Eriksen et al., 2018). Units used to express the quantity of MPs in water samples were, for instance, items/m3 (Kazour et al., 2019), items/L (Su et al., 2016), and items/km2 (Xiong et al., 2018). Hence, for sampling and data collection, vessels of diverse speeds, dimensions, and types are used.
3.1.2 Sediment sampling
Currently, MPs are more frequently analyzed in sediments or beaches than in water columns.
MPs were collected in sediments from beaches and coastal areas by laying a metal or wooden frame on the sediment surface, pushing it down to a depth of 1–5 cm, scooping out the material, and removing the sample using a steel spoon or shovel. For sediment sampling, the frame sizes used are 25 × 25 cm, 30 × 30 cm, 50 × 50 cm, 100 × 100 cm, and 200 × 200 cm, with sampling depths ranging from 0 to 5 cm. MP pellets were handpicked or collected using stainless tweezers. Sampling location plays a vital role in determining the approaches for sampling, i.e., subtidal sediment sampling from a ship or sediment sampling directly on the beaches. Units used to express the occurrence of MPs in sediment samples were items/g (Lots et al., 2017; Dowarah and Devipriya, 2019), items/m2 (Jayasiri et al., 2013; Cheung et al., 2016; Sruthy and Ramasamy, 2017; Robin et al., 2020), and items/kg (Patterson et al., 2019; Sathish et al., 2019; Sarkar et al., 2019; S. K. and Varghese, 2019; Tiwari et al., 2019; He et al., 2020).
3.1.3 Biota sampling
The ingestion of MPs by different aquatic invertebrates and vertebrates has been documented under laboratory and on-site conditions. Fishing nets, trawl nets, cages, and hand collection were used to acquire biota samples. Moreover, market-purchased biota samples were utilized. The biota samples were frozen at –20°C until further analysis. This review offers a brief overview of likely specimen species, emphasizing on-site sampling for the ingestion of MPs, as sampling approaches are complex and greatly rely on the targeted organism (Cole et al., 2013). Miniscule plastic beads of familiar polymer source are sometimes used in research work on the marine biota’s consumption of MPs that can quickly be reconsidered and calculated in gut contents and excretions under the microscope or, in the organism itself, in the case of transparent planktonic species (Kumar et al., 2018; James et al., 2020). The recovery and enumeration of the MP particles are possible by the use of fluorescent particles (Dowarah et al., 2020). Study into the biota’s intake of MPs on-site requires considerably greater exertion, and thus, studies are rare in this field of research. Digestive tract material or excretion of an organism is the target for sampling. Primarily fishes are sampled for MPs in larger species typically sampled by traps or networks.
In order to minimize contamination during a field campaign, intertidal sediment or sea surface microlayer samples are collected and stored using stainless steel, glass, or wooden containers to reduce contamination at each collection site. To reduce potential air deposition, sampling is carried out during calm conditions or from the site’s downwind side. To prevent plastic contamination from clothing, nitrile gloves and white cotton laboratory jackets are worn at all times during sampling and in the lab. Blanks are used to measure potential plastic contamination in the lab.
3.2 Extraction techniques
After sampling, samples collected from water, sediment, and biota need to be segregated to extract the MPs present in the samples. MPs that are large in size can be extracted through visual sorting using tweezers, while small-sized MPs are extracted by size fractionation and density separation techniques. Popular consumer plastic polymers have densities ranging from 0.8 g cm-3 (silicone) to 1.4 g cm-3, for example, polyvinyl chloride (PVC) and polyethylene terephthalate (PET), whereas long-drawn-out plastic foams, for instance, <0.05 g cm-3 expanded polystyrene (EPS), only have a fraction of the original polymer density. Isolation of MP particles can thus be carried out by flotation by saturated salt solutions such as NaCl, CaCl2, NaI, and ZnCl2 of high density from higher-density matrices, such as sediments with a 2.65-g cm−3 density (Patterson et al., 2019; Sathish et al., 2019; Sundar et al., 2020; Tiwari et al., 2019; Robin et al., 2020). For a certain period of time, dried sediment sample is combined with the concentrated salt solution and gets agitated (e.g., by stirring, shaking, aeration). Plastic particles remain dispersed in suspension or float to the surface.
3.2.1 Size fractionation
The fractionation of samples (sediment, biota, water) into at the minimum two divisions of a scale, for example, <500 and >500 μm, is appropriate regardless of the technique used for later detection of MPs. Recently, EU suggested a break into fractions of 20 μm–1 mm and 1–5 mm for monitoring purposes. The water samples can easily be fractionated through the sieving method. In case of large quantities in biotic form, for instance, large plankton, tissue, and gut contents obstructing the sieve, a purification process can be advantageous before the sieving step (Cole et al., 2013; Kumar et al., 2018; Patterson et al., 2019; Dowarah et al., 2020; James et al., 2020; Sathish et al., 2020). After extraction, MPs are readily size-fractionated from sediment samples. The fractionation step fails to distinguish between organic matter and MPs (Elkhatib and Oyanedel-Craver, 2020). Fractionation should be followed by the density separation method for effective extraction of MPs.
3.2.2 Density separation
Considering the nature of polymer in addition to the production method, MPs vary from 0.01 to 2.30 g cm-3 density. While organic matter and phyllosilicate minerals, for example, micas and clay minerals, can be witnessed dispersed apart from the particles, these values are typically lower than other minerals from the sediment. In particular, the process of density separation is necessary for sediments to differentiate between the residual of sample and MPs. Salinity-based density segregation employing an aqueous salt suspension promotes isolation of higher-density particles and inorganic material solids and flotation on the supernatant of the solution by lower-density MP particles (Veerasingam et al., 2020). Generally, for density separation processes, glass-separating funnels are used. The ability to extract and isolate MPs depends on their density, cost, and toxicity differences. The limitation of digestion and density separation technique is that they are time-consuming and expensive compared to other extraction techniques (Elkhatib and Oyanedel-Craver, 2020).
3.2.3 Biological digestion
In brief, the soft flesh of fishes is rinsed with purified, distilled water to eliminate any MP that may have accumulated on the outside. After that, the tissue samples are kept in a glass bottle with 10% KOH added for digestion. The bottles are placed in an incubator and covered with aluminum foil till a clear solution is formed. After the digesting phase is completed, each bottle is filled with NaCl, CaCl2, NaI, and ZnCl2, which causes the MP particles to float due to density separation. The upper half of the solution is separated through filter paper after the samples have been allowed to remain at room temperature for 24 h, and the retrieved MPs are dried and stored for subsequent analysis. In 69 investigations, 30% H2O2, KOH, or NaOH was used to digest organic matter before or after density separation to dissolve it. After density separation and digestion, the supernatant was filtered through a variety of mesh sizes, including 0.22 µm [12 studies], 0.45 µm [18 studies], 0.7 µm [9 studies], 0.8 µm [7 studies], 1.2 mm [5 studies], and 38 µm [5 studies], and then dried naturally or in an oven for microscopic and spectroscopic investigation.
3.3 Characterization and quantification of MPs
Once MPs have been extracted, they need to be quantified and characterized. Occurrence and distribution of large MPs (1–5 mm) were recorded chiefly on beaches and, to a lesser degree, in surface waters before the word “MPs” became common. Due to this relatively vast size range accompanied by chemical characterization for confirmation of plastics, Figure 4 indicates various techniques available for MP detection and identification with respect to time, cost, and precision per sample analyzed. There are advantages and limitations of each technique and different combinations.
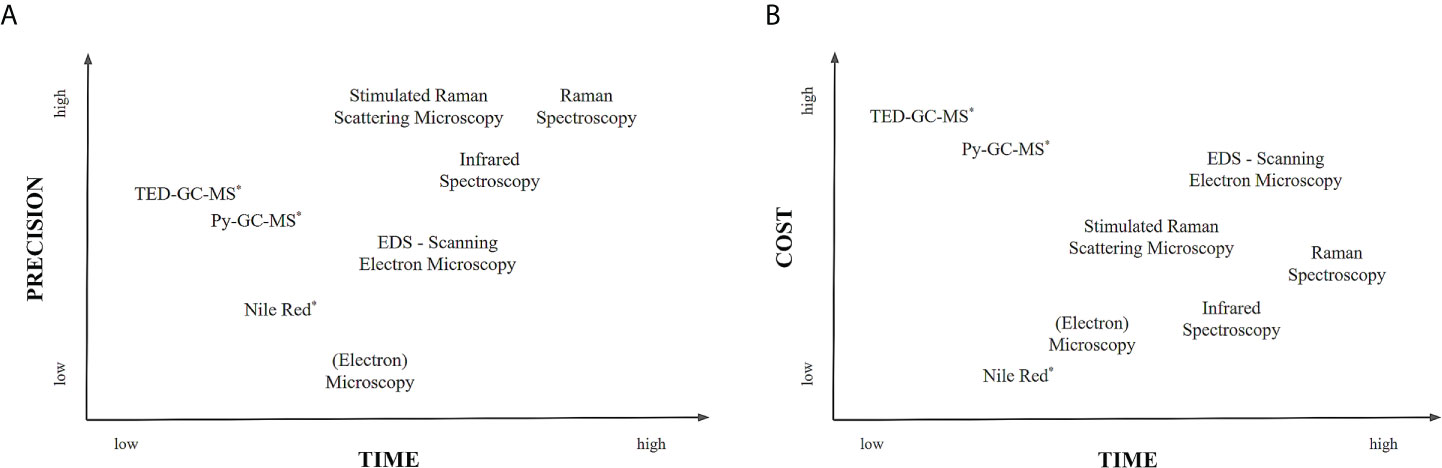
Figure 4 Identification and quantification techniques of microplastics based on precision/degree of detail, time and cost per sample to be analyzed. (A) A comparison between precision and time and (B) a comparison between cost and time. [The relative location of methods is based on literature data on required time for sample processing. Methods marked with an asterisk are destructive because they either pyrolyze the sample (Py-GC-MS, TDS-GC-MS) or stain the microplastic particles (Nile Red)]. [Modified from (Zarfl, 2019), (Primpke et al., 2020)].
3.3.1 Visual sorting
In order to perform the analysis, MPs must be categorized from the residuary plastics after the completion of the purification and separation step. Noticeably, minute-sized MPs necessitate additional monitoring with the help of an optical microscope, while large plastics can be sorted out directly. The MPs were identified visually by their uniform color, brightness, and lack of cellular features. Even though visual sorting is performed, it is not always a reliable technique. Few studies have coupled visual sorting with hot needle tests to confirm the presence of MP (Sathish et al., 2019; Jeyasanta et al., 2020). Visual inspection is used to characterize MPs based on their size, color, and shape, as well as inferring their origin. Although visual sorting saves time when counting MPs, it can lead to dramatic over- or underestimation of plastic content based on plastic size ranges, as well as the possibility of counting non-plastic particles as plastic. Hence, Fourier transform-infrared spectroscopy (FT-IR) and Raman spectroscopy are done for further identification.
3.3.2 FT-IR spectroscopy
The FT-IR approach was employed in 70% of the 74 articles reviewed to detect the polymer types of MPs in various environmental matrices. FT-IR spectroscopy and its optimized technologies, such as µ-FT-IR, attenuated total reflectance (ATR) FT-IR (Ory et al., 2017), and focal plane array detector-based µ-FT-IR imaging, are most widely used to identify and quantify MPs (Krishnakumar et al., 2018; Sathish et al., 2019; Tiwari et al., 2019; S. K. and Varghese, 2019; Jeyasanta et al., 2020; Maharana et al., 2020; Robin et al., 2020). In MP research, the mid-infrared band (400–4,000 cm-1) was the most commonly used FT-IR spectral region. MPs show absorption peaks during analysis due to rocking deformation and CO stretching symmetric and asymmetric CH (-CH2 or –CH3) stretching, deformation, and bending whose characteristic wave numbers vary from material to material (Veerasingam et al., 2016). The most common FT-IR spectroscopy modes are attenuated total reflectance (ATR) and transmission. The big-size MPs (>500 mm) in sediments and water were characterized using the ATR-FT-IR method. The polymer types of tiny size MPs (500 mm) in biota were identified using FT-IR and a confocal microscope (known as µ-FT-IR imaging or chemical imaging). In addition to MP identification and characterization, the carbonyl index values were used to analyze the weathering pattern or ageing of MPs using the FT-IR technique. The µ-FTIR approach has grown in popularity to characterize materials that are too minute to be chemically examined using traditional FT-IR techniques. It gathers infrared signals with excellent spatial resolution (beam size as tiny as 5 µm) and has significant promise for the characterization of compositionally complex substances. More crucially, µ-FT-IR allows for in situ, non-destructive analysis without the need for sample purification and concentration, which is required in traditional FTIR analysis and almost always results in specimen loss and chemical property changes (Chen et al., 2015). Nonetheless, applying FT-IR for assessing fine plastic particles, for example, <1-μm-sized particles in addition to categorizing types of polymers from ecological samples, remains a challenge. Prior to FT-IR analysis, manual sorting is additionally required. Despite FT-IR being a promising advancement, additional optimization is essential for precise MP analysis (Xu et al., 2019).
3.3.3 Raman spectroscopy
Raman spectroscopy is a light scattering technique which provides in-depth information of the material under analysis. Details such as structure, crystallinity, molecular interactions and phase are obtained from this chemical analysis. The Raman technique (spectroscopy and microscope) is frequently used for identifying polymer kinds of MPs, and it was utilized in seven studies around the world to detect MPs in sediment and biota, with spectra ranging from 200 to 3,500 cm-1. Raman spectroscopy is another imminent investigative method for MP recognition apart from the FT-IR technique (Sruthy and Ramasamy, 2017; Dowarah et al., 2020; Primpke et al., 2020; Sathish et al., 2020). Raman spectroscopy has a higher lateral resolution (1 vs. 20 mm) and a greater spectral coverage with a highly distinct fingerprint spectrum and less interference from water than FTIR spectroscopy. Raman spectroscopy can be used to analyze small particles up to 1 μm size. Another benefit is that in comparison to other analytical methods, Raman spectroscopy has improved responses to functional groups like non-polar plastic clusters (Xu et al., 2019). Micro-Raman is an optimized technology which couples Raman spectroscopy to an optical microscope, thereby enabling higher resolution of a sample (Di et al., 2019). The drawback, however, is that an FT-IR is more economically preferred than a Raman laser spectrometer.
3.3.4 Pyr-GC/MS and TDS-GC/MS
Organic additives as well as polymer type of MPs can be simultaneously analyzed by pyrolysis gas chromatography with mass spectrometry (Pyr-GC/MS) (Scherer et al., 2020). In order to determine the composition of each particle of the polymer by GC/MS, plastic particles are thermally decomposed, upon being removed from the environmental matrices. Further analysis of the MP composition in environmental samples is performed by the thermal desorption gas chromatography with mass spectrometry (TDS-GC/MS) method. The merits of TDS-GC/MS compared with Pyr-GC/MS are that it can measure more complex matrices and process larger sample mass. However, this technique has drawbacks such as it permits only a single unit at a period of time to drive through the pyrolysis since it is restricted by the tube’s aperture size and is a time-consuming process. For a comprehensive analysis of MPs, TDS-GC/MS or Pyr-GC/MS may be used as opposed to FT-IR spectroscopy or Raman spectroscopy (Fries et al., 2013; Xu et al., 2019).
3.3.5 SEM/EDS
A scanning electron microscope coupled with an energy-dispersive X-ray spectrometer (SEM/EDS) provides topographical, elemental information, origin, and aging of MPs. SEM generates images of samples by scanning the surface with a focused beam of electrons. SEM additionally offers high-resolution data on surface condition as well as qualitative information on chemical composition. SEM/EDS was used to characterize MPs drawn out from water samples (Su et al., 2016; Patterson et al., 2019), sediment samples (Su et al., 2016; Patterson et al., 2019; Sathish et al., 2019; Tiwari et al., 2019), and biota samples (Su et al., 2016; Patterson et al., 2019; Sathish et al., 2020). SEM/EDX is a time-consuming and costly technique. Furthermore, because the separation of the MP is dependent on the researcher’s expertise, chemical characterization may be prone to selection bias. Table 4 indicates the frequency of various plastic types identified using numerous characterization techniques across the globe. In most of the research examined, spectroscopic approaches were used to confirm the polymer types of MPs in various environmental matrices. Polyethylene (PE), polypropylene (PP), and polystyrene (PS) were the most frequent polymer types, as expected given that they accounted for 74% of worldwide plastic production (in 2015) and were commonly employed in short life-cycle products. Other polymers described in some research were polyethylene terephthalate (PET), polyvinyl chloride (PVC), nylon (NY), polyamide (PA), and polyurethane (PU). Since the major polymers (PE and PP) have a lower density than seawater (1.02 g cm-3), they are widely disseminated in the water and its accompanying biota. Thus, based on literature Figure 5 summarizes in the form of a schematic representation how various analytical methods are required for the analysis of MPs.
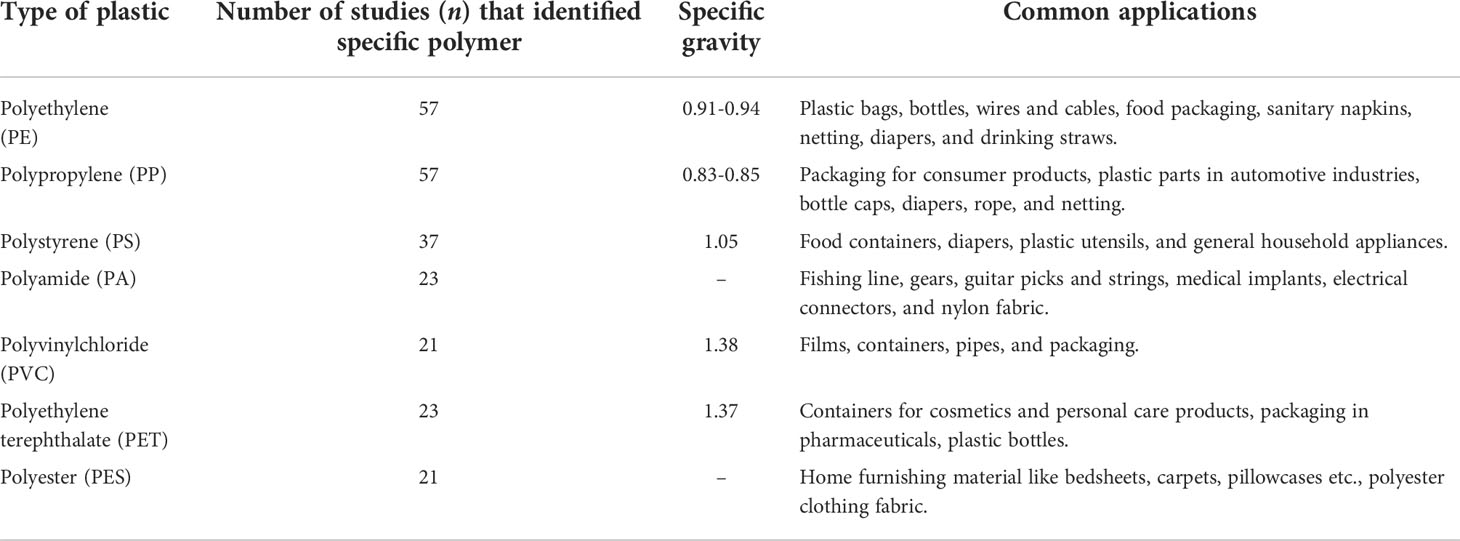
Table 4 Frequency of type of plastic as observed in the sediments, water, and biota samples across the globe and their common application (61 out of 74 studies reported the polymer type).
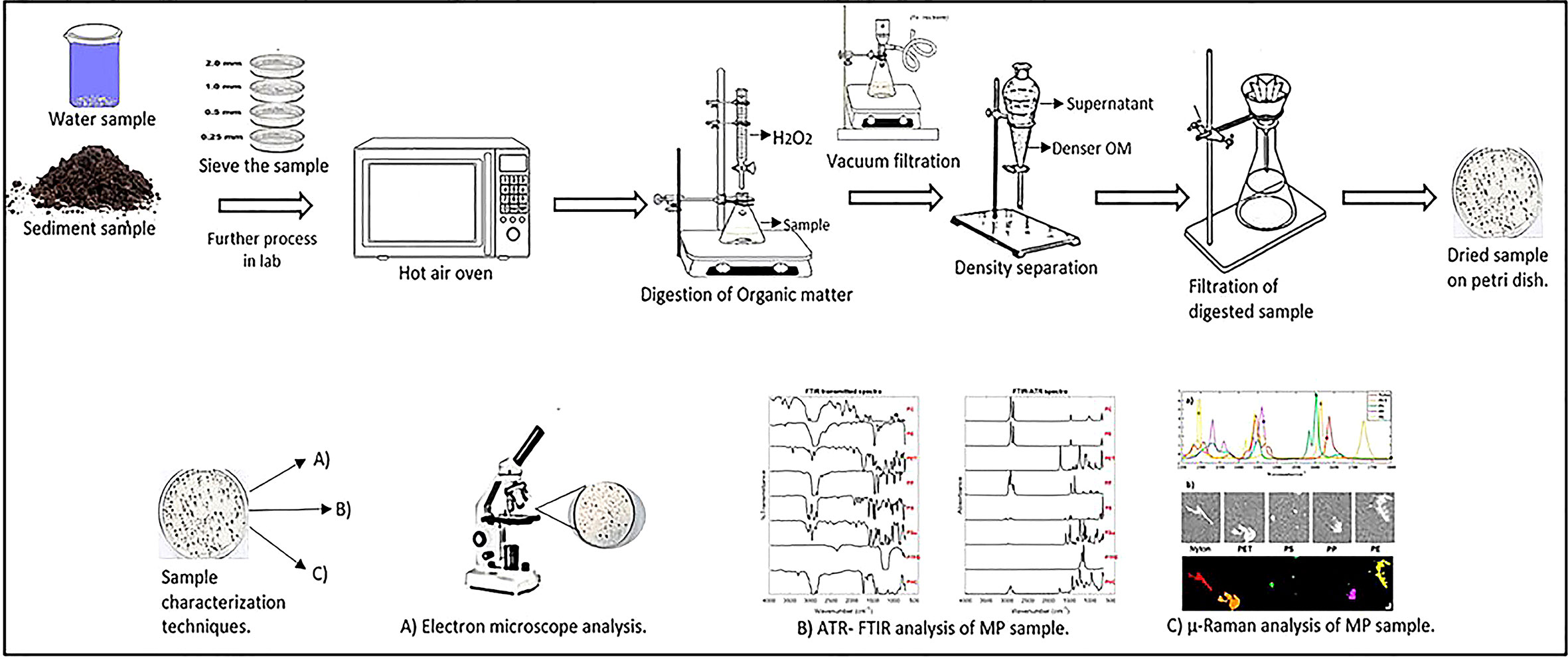
Figure 5 Schematic representation of various methods for analysis of MPs. Number of studies identifying the polymer type: 61 out of 74 studies reported polymer type.
4 Discussion
4.1 Effect of various parameters on MP collection
4.1.1 Temperature and weathering
Degree of weathering of MPs is governed by hydrodynamic conditions such as wind, waves, currents, photo-oxidation by the UV rays from the sun, and biofouling. Figure 6 exhibits changes in primary MPs subjected to different weathering conditions (Andrady, 2017). The SEM characterization helps in estimating the extent of MP weathering. FT-IR is used to determine the extent of weathering/aging of MP based on carbonyl index value. For instance, Veerasingam et al. assessed the value of carbonyl index (CI values) to quantify photo-oxidation brought about by light in the marine environment (Veerasingam et al., 2016). Sathish et al. evaluated values of CI, with respect to the methylene group and carbonyl group area ratio in MPs (Sathish et al., 2019). Most studies use visual identification techniques despite the availability of sophisticated techniques such as FT-IR or Raman spectroscopy which is much more efficient and reliable, especially for small-sized MPs (Veerasingam et al., 2020).
4.1.2 Acoustic behavior of MP
In most of the cases, MPs are primarily segregated using sieving, digestion, and filtration step, but theoretical and practical views of acoustics for collection of MPs show great potential (Akiyama et al., 2020). Akiyama et al., in an experiment, used a microfluidic device with a bulk acoustic wave (BAW), and Oleh et al. used a device with a standing acoustic wave (SAW) for separation of MPs such as Nylon 6, polystyrene, and PET based on the principle of acoustic focusing using a piezo element attached to the microfluidic device (Akiyama et al., 2020; Oleh et al., 2020). Based on their physical properties and in accordance with theoretical considerations, MPs can be concentrated using acoustic focusing, and therefore acoustics can be used for separation of MPs of approximately 5-μm size. It also has potential to filter smaller-sized particles (<5μm) in aqueous suspensions such as laundry effluents.
4.1.3 Electrostatic behavior of MP
MP extraction becomes difficult as MPs come in different sizes, shapes, and properties. The acoustic properties of MPs can be used to filter the MPs below 5 μm, but for MPs of size >5 μm, the BAW device cannot be used. In such cases, the electrostatic properties of MPs can be used to filter MPs from the sample (Felsing et al., 2018). This process uses Hamo’s electrostatic metals/plastic separator, which is known as the Korona–Walzen–Scheider (KWS) separator. The KWS separator uses a dry separation process where metal particles and other conductive materials introduced in the sample are separated from non-conductive materials like plastics of size range >5 μm in diameter. The absolute prerequisite is for samples to be dried well thoroughly before the separation. This process has high efficiency (has 99% yield), reduced time consumption, and manpower efficiency (Enders et al., 2020).
4.2 Additives and metal interaction with MPs
Plastic fragments can contain a wide range of chemicals which can be attributed to ingredients present in the plastic and chemicals that are absorbed. Chemicals or persistent organic pollutants (POPs) that are usually present in these plastic fragments are ultraviolet (UV) stabilizers, hydrocarbons, plasticizers, antioxidants, flame retardants, intermediates, lubricants, degradation products, and compounds of dyes and inks. These plastic-associated chemicals or additives can be analyzed by X-ray fluorescence analysis, chromatographic techniques, or mass spectroscopy–gas chromatography (MS/GC) (Hong et al., 2017). However, studies are limited to assessing adsorbed additives or POPs in MPs such as polychlorinated biphenyls (PCBs), organochlorine pesticides (OCPs) like dichlorodiphenyltrichloroethane (DDT), hexachlorocyclohexane (HCHs), and polycyclic aromatic hydrocarbons (PAHs) (Ogata et al., 2009; Jayasiri et al., 2015; Sharma et al., 2020). It was reported that the frequency of detection and the total PCB concentration decreased with the increase in the IUPAC number of PCBs except for PCB-153, as shown in Figure 7. When plastics are suspended in a marine environment for an extended period of time, although inherently neutral, they tend to acquire a charge. The presence of metal elements such as Bi, Br, Cl, Cr, Cu, Fe, Ni, Pb, and Zn were noted in most of the MPs extracted from beaches (Robin et al., 2020). In another study, gastrointestinal tracts of fish revealed the presence of organic elements like Fe, Ni, As, Na, Sr, Ti, Mg, Si, S, Cl, and Ca present on the surface of fiber and fragment MPs (Sathish et al., 2020). The traces of these metal elements in MPs can be attributed to the fact that they are used as stabilizers, colorants, antioxidants, flame retardants, pigments, and catalysts, which pose harm to the environment.
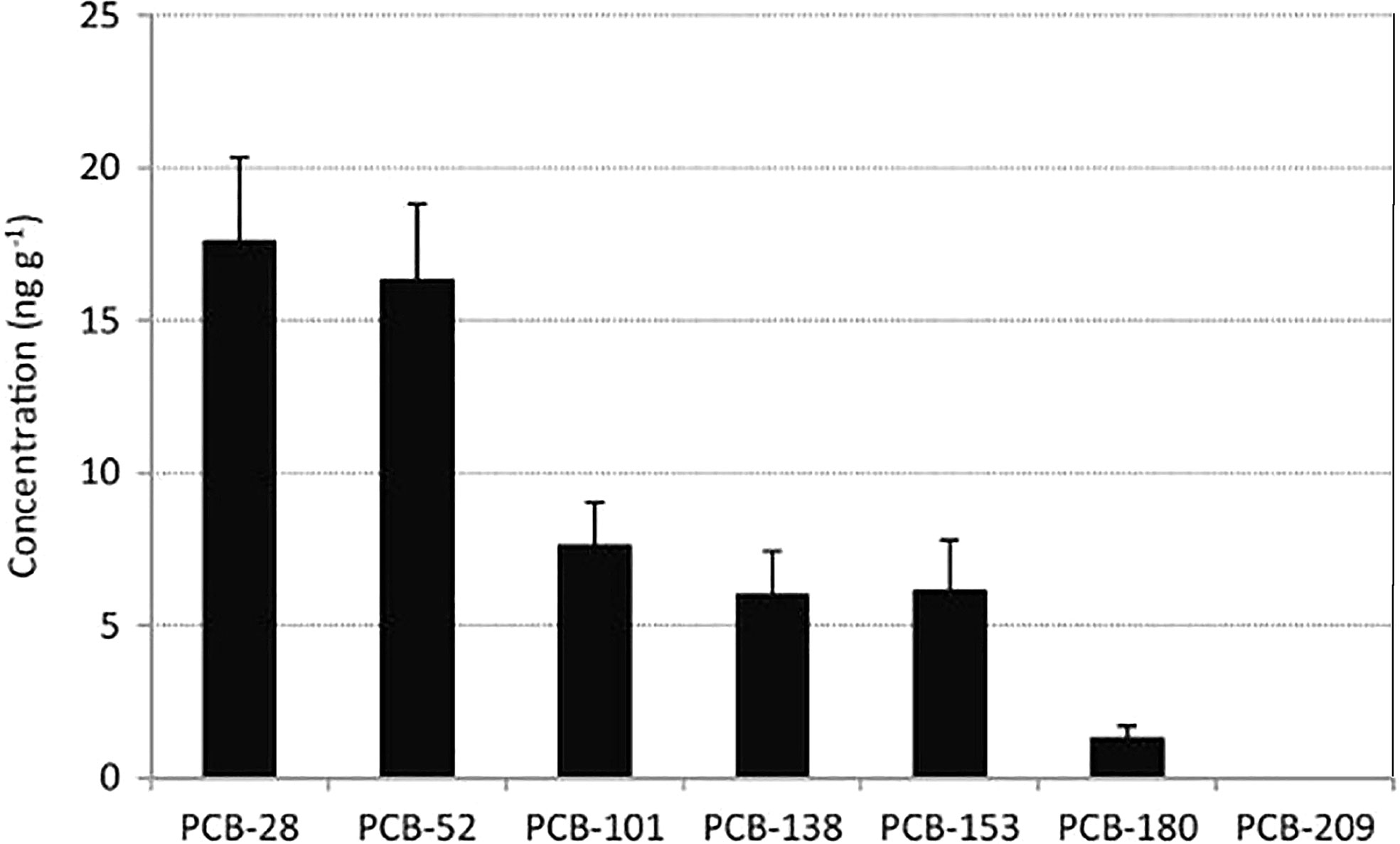
Figure 7 Figure represents the mean concentration of PCBs in plastic pellets (n=72) [Imprinted from (Jayasiri et al., 2015)].
4.3 Separation of MPs
4.3.1 Removal of MPs by agglomeration
Herbort and Schuhen presented a novel idea where silicon-based precursors were used for agglomeration of MPs in water. This concept proposed to affix organic–inorganic hybrid silica gels via the sol–gel method as a precursor to remove hydrophobic stressors (MPs) from water in a cost-effective and straightforward approach. The proposed mechanism is shown in Figure 8 (Herbort and Schuhen, 2016). Another study presented the use of different branched and linear alkyl groups (chain length 1–18 C-atom) of alkyltrichlorosilanes to agglomerate MPs, a mixture of polyethylene (PE) and polypropylene (PP). This study evaluated the influence of the alkyl group in the precursor on the agglomeration behavior and reaction rate of the sol–gel process. Results of the study suggested that alkyl groups with intermediate chain length (three to five C-atoms) were best suited for removal of a synthetically prepared MP sample solution with removal efficiency >95%. The challenge is to transfer this concept to real-time samples (Sturm et al., 2020).
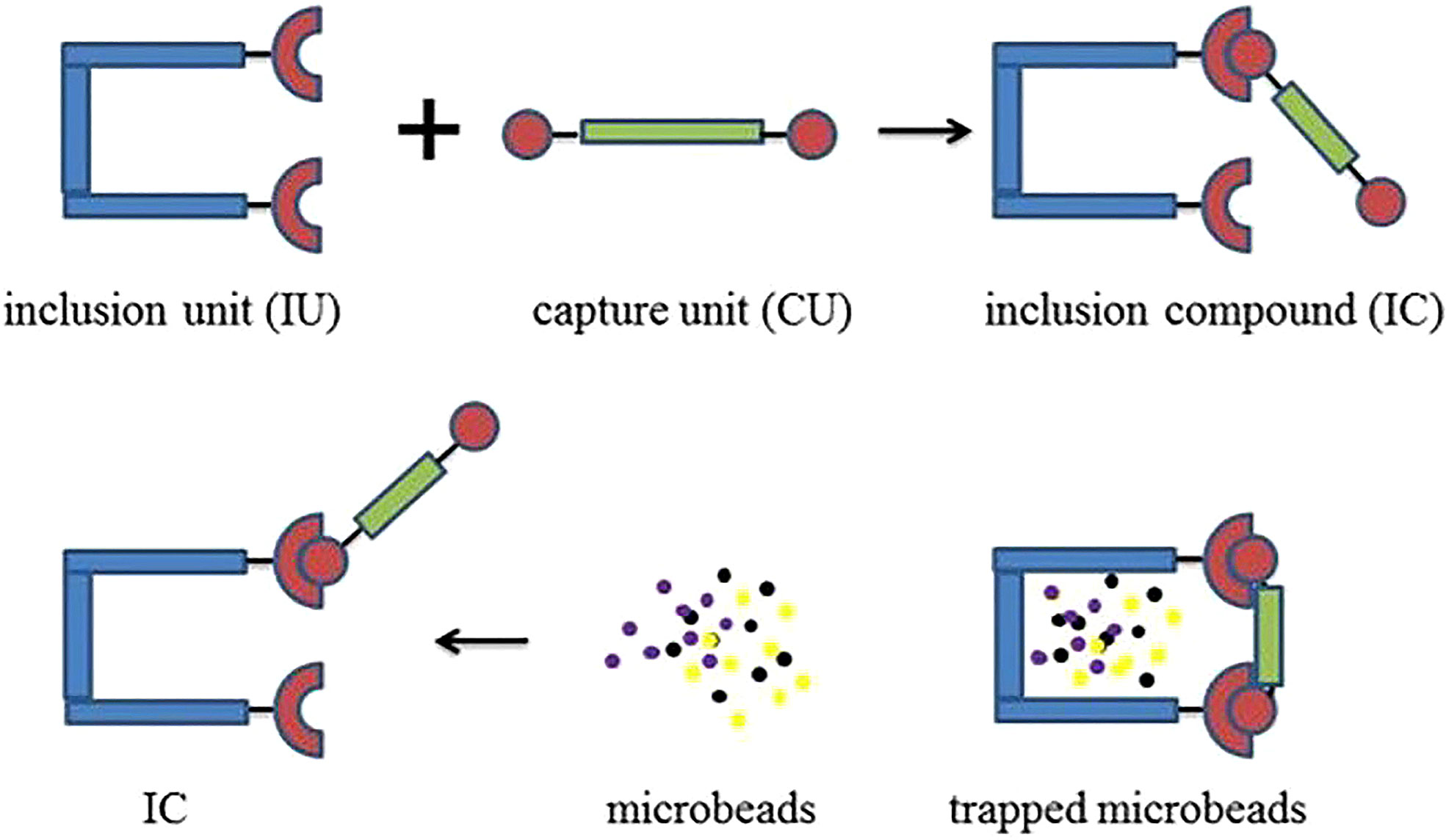
Figure 8 Schematic showing the capture mechanism of hydrophobic stressors (Imprinted from (Herbort and Schuhen, 2016).
4.3.2 Sorting methods for MPs
The need to sort MPs in environmental, biomedical, and industrial applications is paving a way for the development of new microfluidic devices. Active sorting methods such as acoustophoresis or electrophoresis require the use of external differentiating forces to control sorting of particles in a microfluidic device. Passive sorting methods, on the other hand, sort particles under the influence of hydrodynamic forces due to an intense particle interaction suspended in the channel or actual structure of the microfluidic channel. The passive sorting approach is cost-effective, label-free, and straightforward in comparison to active sorting approaches. Notable techniques in this group include hydrodynamic filtration (HDF) (Yamada and Seki, 2005), pinched flown fractionation (PFF) (Yamada et al., 2004), cross flow filtration (CFF) (Altmann and Ripperger, 1997), deterministic lateral displacement (DLD) (Huang, 2004), inertial microfluidic (iMF) separation (Zhou et al., 2013), and shear-induced diffusion (SID) (Zhou et al., 2018). These techniques use physical properties such as density, shape, deformability, roughness, and size for sorting of micron-sized particles. The versatile mechanisms used in label-free passive techniques for particle sorting comprises intense particle interactions with a microfluidic structure or channel walls as in the case of DLD, with flow as observed in iMF, with fluid as witnessed in viscoelastic flow, and with other particles in SID or their combinations as noted in CFF, PFF, and HDF. Most common physical markers utilized in these passive sorting techniques are the differences in size. Out of these, high-purity separation >90% has been accomplished in DLD, viscoelastic iMF devices. The drawbacks of these passive methods are the on-demand separation activation and adaptability of parameters of separation, for instance, cutoff size. There is difficulty in separation with respect to the passive method since there are no external forces that can be adjusted. Hence, when flow conditions stabilize, the separation occurs. The challenges in using passive label-free microfluidic devices are that they have extensively been used only in biomedical practices. In areas like therapeutics, cell biology, and diagnostics (Gossett et al., 2010), passive sorting is yet to be utilized in the separation of MPs (Zhou et al., 2019).
4.4 Future challenges
Since MPs have been found in a variety of aquatic components, such as surface waters, oceans, and sediments, and even in the ice core of the polar regions due to their persistent nature, they have been a cause of great concern due to potential risks and toxicity posed to the organisms living in these ecosystems (Tang et al., 2021). Regardless of their density, MPs can act as colloid particles and remain suspended in the water bodies and their distribution depends on their hydrophobicity, surface currents, and wind forces (Wang et al., 2020). Hence, the presence of MPs in the marine environment makes it available to a wide range of aquatic organisms inhabiting in these ecosystems. Due to their small size, MPs are generally ingested by various aquatic organisms, including zooplankton, invertebrates, fishes, and seabirds. Lower trophic organisms, due to their indiscriminate feeding and limited ability to differentiate food from other particles, are more likely to ingest MPs (Cole et al., 2011). Figure 9 shows the interaction between organisms and MPs. Exposure to fishes occurs for the reason that they consume such MPs mistaking them for prey or through consumption of organisms that have themselves consumed MPs. An investigation carried out on the insides of gastrointestinal tracts of fish provided a conclusive proof regarding intake of MPs by fish species (Wang et al., 2020).
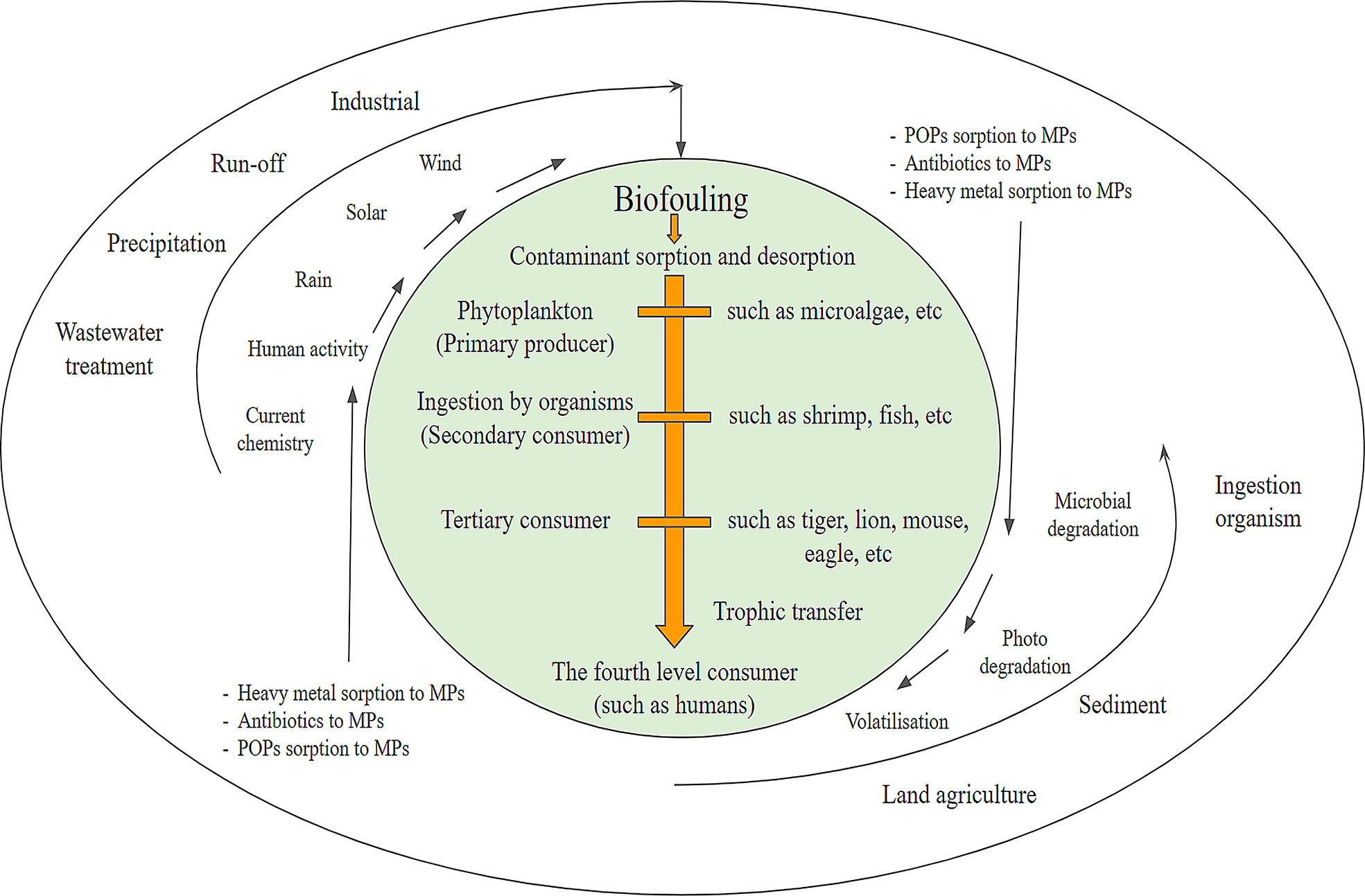
Figure 9 Diagram showing interaction between organisms and microplastics [Modified from (Tang et al., 2021)].
Many studies have been conducted under laboratory conditions to understand the toxicity effects of MPs in fishes and other organisms. Toxicity effects may include obstructions in the digestive system, inflammation, oxidative stress, and distress in the immune system. Studies have also shown that intake of MPs can have an impact on energy homeostasis and lead to reduced feeding activities in worms and crabs. Additives added to enhance plastic properties are introduced in the biological matrix of these organisms when ingested along with MPs and could lead to additional consequences. However, the extent of contribution to the transportation of lethal chemicals via MPs across diverse trophic levels are yet to reach a technical agreement (Wang et al., 2020). Furthermore, in the natural environment, an assortment of MPs is present, the overall toxicity of which has not been thoroughly studied under laboratory conditions. Therefore, this is an area for future work intending to obtain a more conclusive data on the toxicity of MPs in the environment. To address and overcome some of the problems highlighted thus far, the following section discusses the possible use of flow sensors and micro-liquid handling systems, which when implemented with the appropriate variables can detect and analyze MPs with high efficiency and precision.
4.4.1 Flow sensors
Flow sensors are devices used to regulate or measure flow of fluids through systems of pipes and tubes. They are connected to either digital devices or gauges to give the physical values of flow rate measured by electrical or mechanical means. Although there are many types of flow sensors, the working principle remains more or less the same. The flow sensors measure fluid flow rate in terms of either mass or volume (All About Flow Sensors, 2022). As fluid passes through the sensor, changes in velocity or area displayed help to determine and control the flow rate.
4.4.1.1 Types of flow sensors
Flow sensors can be broadly divided into two categories: contact and non-contact flow sensors. Processes where contact between the fluid being utilized and moving parts of the flow sensor can be tolerated are termed contact flow sensors. Non-contact flow sensors have no moving parts, hence used when risk of contamination of the liquid or gas would be high; otherwise, contact flow sensors are used. These sensors are used primarily in the food industry, where such risks cannot be permitted (What is a Flow Sensor?, 2022). Several parameters like the liquid or gas being measured, its temperature and pressure, corrosiveness, viscosity, and cost are taken into consideration for selecting a flow sensor in any particular process (Liquid Flowmeters, 2022).
4.4.1.2 Flow sensor and MPs
Recently, there has been thriving interest in the research and development of micro-liquid handling systems. The amount of fluid to be regulated in such systems is of utmost importance. Hence, flow sensors form an essential part of micro-liquid handling systems (SJ Lammerink et al., 1993). Extensive research has shown that the integration of microfluidics with sensor applications can be used to detect MPs effectively.
One such example that utilizes this very concept is microelectromechanical systems (MEMS) and nanoelectromechanical systems (NEMS). These devices can deal with fluids in small amounts, typically in micro- and nanoliters, and are used in separation, transport, purification, and sensing (Ashraf et al., 2011). MEMS devices have already been used successfully in various applications such as drug delivery systems, gas flow monitoring in industries, and marine hydrodynamic sensing technology. Small size and high sensitivity are what make them an attractive alternative to traditional sensing devices. In addition, various sensing principles can be used as thermal flow sensors, piezoresistive flow sensors, and piezoelectric flow sensors (Ejeian et al., 2019).
Since microfluidic devices deal with fluids at microscale, the behavior of fluid is quite different from its behavior in bulk systems. Several factors come into play that would otherwise have been negligible such as interaction of MPs with confining surfaces of the device, surface tension, and flow regime (Ashraf et al., 2011; Georgiev et al., 2020). Thus, detection and separation have become quite challenging in the field of MPs. Hence, a complete understanding of fluid behavior is necessary while designing a flow sensor for microfluidic applications. Moreover, precise flow control is essentially needed in automated meso–microfluidic applications. However, flow sensors are currently being used due to their large size and limited sensitivity that are inadequate for such applications. A trade-off is then made between the speed of a sensor and its specificity (Wu et al., 2001). Therefore, further research in this field is required to sense MP, and once sensed, additional research on whether the quality or quantity of MP can be determined in combination with sensing is an area that can be explored in the future.
Our understanding of MPs in the environment is fast evolving, as seen by information presented in this review. Nevertheless, there are significant knowledge gaps and numerous unanswered concerns. In summary, the following are the most pressing concerns:
1. How severe is MP pollution in diverse habitats right now, and how does it correlate with known contaminants in aquatic environments? Which polymers are the most abundant, and how does this differ depending on habitat and region?
2. To what degree do environmental variables and the properties of various plastic materials influence MP behavior and bioaccessibility in terrestrial and freshwater environments?
3. Do possible consequences result primarily from particle physical impacts, chemical toxicity, or a combined effect, and does this differ with polymer and species? Are there any parallels between what we know about the mechanisms of action of some nanoparticles and what we know about the mechanisms of action of others?
4. What are the potential ecological outcomes of plastics under practical exposure settings (i.e., MPs of the types and concentrations that organisms are likely to encounter)?
5 Conclusion
Over the last decade, a variety of techniques for sampling, extraction, identification, and quantification of MPs have been developed. Although a number of procedures and techniques are available for MP analysis, applied methods are subject to uncertainties, limitations being the structural resolution which dismisses miniscule MPs and restrictions in identifying natural particles from MP particles. This review offers a summary of the advanced procedures in MP analysis and the effect of various parameters on MP and gives examples of possible ways forward and challenges to be encountered ahead. Gaps in research in comprehending MPs’ origin, its outcome, different conveyance pathways, standard operating procedures for analysis of MPs, and toxic effects of MPs in addition to the MP-related metal and POP’s pollutants within the marine ecosystem still persist. The highly systematic separation of MPs is possible by meticulous manipulation of a particle in a microfluidic channel. MPs are heterogenous in composition and widely vary in size range. Therefore, MP particle separation necessitates tremendous effort in taking novel separation concepts into efficient, reliable sorting methods. In order to achieve separation of MPs, the integration of active and passive sorting methods is required to enable a more sophisticated and valuable platform. In an effort to obtain reliable data, it is recommended to use alternatives for plastic materials and to instead opt for glass- or metal-based materials for storage of samples, to use procedural blanks, and to use high-quality glass fiber filters. In the last decade, considerable progress has been made globally in understanding the toxicologic effects of MPs; the comprehension of MPs in water bodies across the globe is rather partial, and this offers room for research in the future. Therefore, intensive research for MP detection and separation is the need of the hour that paves a way for the development of a novel, rapid, and robust system for the same.
Author contributions
PP wrote the first draft of the manuscript. KP and PP wrote sections of the manuscript. JK contributed to early conception of the study. PP and KP organized the database. All authors contributed to manuscript revision. JK contributed for the funding source and approved the submitted version. All authors contributed to the article and approved the submitted version.
Funding
The corresponding author of this work have been funded by Birla Institute of Technology and Science Pilani, K K Birla Goa Campus, India
Conflict of interest
The authors declare that the research was conducted in the absence of any commercial or financial relationships that could be construed as a potential conflict of interest.
Publisher’s note
All claims expressed in this article are solely those of the authors and do not necessarily represent those of their affiliated organizations, or those of the publisher, the editors and the reviewers. Any product that may be evaluated in this article, or claim that may be made by its manufacturer, is not guaranteed or endorsed by the publisher.
References
Abidli S., Toumi H., Lahbib Y., Trigui El Menif N. (2017). The first evaluation of microplastics in sediments from the complex lagoon-channel of bizerte (Northern Tunisia). Water Air Soil pollut. 228, 1–10. doi: 10.1007/s11270-017-3439-9
Akiyama Y., Egawa T., Koyano K., Moriwaki H. (2020). Acoustic focusing of microplastics in microchannels: A promising continuous collection approach. Sensors Actuators B: Chem. 304, 127328. doi: 10.1016/j.snb.2019.127328
Alfonso M., Scordo F., Seitz C., Mavo Manstretta G., Ronda A., Arias A., et al. (2020). First evidence of microplastics in nine lakes across Patagonia (South America). Sci. Total Environ. 733, 139385. doi: 10.1016/j.scitotenv.2020.139385
Alimi O., Fadare O., Okoffo E. (2021). Microplastics in African ecosystems: Current knowledge, abundance, associated contaminants, techniques, and research needs. Sci. Total Environ. 755, 142422. doi: 10.1016/j.scitotenv.2020.142422
Ali C., Moon H., Zheng J., Qiu Y., Tabassum T., Jang J., et al. (2020). Degradation rates of plastics in the environment. ACS Sustain. Chem. Eng. 8, 3494–3511. doi: 10.1021/acssuschemeng.9b06635
Altmann J., Ripperger S. (1997). Particle deposition and layer formation at the crossflow microfiltration. J. Membrane Sci. 124, 119–128. doi: 10.1016/s0376-7388(96)00235-9
Amrutha K., Warrier A. (2020). The first report on the source-to-sink characterization of microplastic pollution from a riverine environment in tropical India. Sci. Total Environ. 739, 140377. doi: 10.1016/j.scitotenv.2020.140377
Andrady A. (2011). Microplastics in the marine environment. Mar. pollut. Bull. 62, 1596–1605. doi: 10.1016/j.marpolbul.2011.05.030
Andrady A. (2017). The plastic in microplastics: A review. Mar. pollut. Bull. 119, 12–22. doi: 10.1016/j.marpolbul.2017.01.082
Aragaw T. (2020). Surgical face masks as a potential source for microplastic pollution in the COVID-19 scenario. Mar. pollut. Bull. 159, 111517. doi: 10.1016/j.marpolbul.2020.111517
Aragaw T. (2021). Microplastic pollution in African countries’ water systems: a review on findings, applied methods, characteristics, impacts, and managements. SN Appl. Sci. 3, 1–30. doi: 10.1007/s42452-021-04619-z
Ashraf M., Tayyaba S., Afzulpurkar N. (2011). Micro electromechanical systems (MEMS) based microfluidic devices for biomedical applications. Int. J. Mol. Sci. 12, 3648–3704. doi: 10.3390/ijms12063648
Ashwini S. K., Varghese G. (2019). Environmental forensic analysis of the microplastic pollution at “Nattika” beach, kerala coast, India. Environ. Forensics 21, 21–36. doi: 10.1080/15275922.2019.1693442
(2022) All about flow sensors. Available at: https://www.thomasnet.com/articles/instruments-controls/all-about-flow-sensors/ (Accessed February 7, 2022). Thomasnet.com.
(2022) Liquid flowmeters. Available at: https://www.omega.com/en-us/resources/flow-meter-types (Accessed February 7, 2022). https://www.omega.com/en-us.
Bakir A., Desender M., Wilkinson T., Van Hoytema N., Amos R., Airahui S., et al. (2020). Occurrence and abundance of meso and microplastics in sediment, surface waters, and marine biota from the south pacific region. Mar. pollut. Bull. 160, 111572. doi: 10.1016/j.marpolbul.2020.111572
Bakir A., van der Lingen C., Preston-Whyte F., Bali A., Geja Y., Barry J., et al. (2020). Microplastics in commercially important small pelagic fish species from south Africa. Front. Mar. Sci. 7. doi: 10.3389/fmars.2020.574663
Barra R., Leonard S., Whaley C., Bierbaum R. (2018). “Plastics and the circular economy: A STAP document,” in Scientific and technical advisory panel (STAP) to the global environment facility (GEF), UN environment. Available at: https://www.thegef.org/sites/default/files/publications/PLASTICS%20for%20posting.pdf.
Barrett J., Chase Z., Zhang J., Holl M., Willis K., Williams A., et al. (2020). Microplastic pollution in deep-Sea sediments from the great Australian bight. Front. Mar. Sci. 7. doi: 10.3389/fmars.2020.576170
Blair R., Waldron S., Phoenix V., Gauchotte-Lindsay C. (2019). Microscopy and elemental analysis characterisation of microplastics in sediment of a freshwater urban river in Scotland, UK. Environ. Sci. pollut. Res. 26, 12491–12504. doi: 10.1007/s11356-019-04678-1
Bridson J., Patel M., Lewis A., Gaw S., Parker K. (2020). Microplastic contamination in Auckland (New Zealand) beach sediments. Mar. pollut. Bull. 151, 110867. doi: 10.1016/j.marpolbul.2019.110867
Cai M., He H., Liu M., Li S., Tang G., Wang W., et al. (2018). Lost but can’t be neglected: Huge quantities of small microplastics hide in the south China Sea. Sci. Total Environ. 633, 1206–1216. doi: 10.1016/j.scitotenv.2018.03.197
Chai B., Wei Q., She Y., Lu G., Dang Z., Yin H. (2020). Soil microplastic pollution in an e-waste dismantling zone of China. Waste Manage. 118, 291–301. doi: 10.1016/j.wasman.2020.08.048
Chen M., Jin M., Tao P., Wang Z., Xie W., Yu X., et al. (2018). Assessment of microplastics derived from mariculture in xiangshan bay, China. Environ. pollut. 242, 1146–1156. doi: 10.1016/j.envpol.2018.07.133
Chen H., Qin Y., Huang H., Xu W. (2022). A regional difference analysis of microplastic pollution in global freshwater bodies based on a regression model. Water 12, 1889. doi: 10.3390/w12071889
Chen Y., Zou C., Mastalerz M., Hu S., Gasaway C., Tao X. (2015). Applications of micro-Fourier transform infrared spectroscopy (FTIR) in the geological sciences–a review. Int. J. Mol. Sci. 16, 30223–30250. doi: 10.3390/ijms161226227
Cheung P., Cheung L., Fok L. (2016). Seasonal variation in the abundance of marine plastic debris in the estuary of a subtropical macro-scale drainage basin in south China. Sci. Total Environ. 562, 658–665. doi: 10.1016/j.scitotenv.2016.04.048
Cole M., Lindeque P., Fileman E., Halsband C., Goodhead R., Moger J., et al. (2013). Microplastic ingestion by zooplankton. Environ. Sci. Technol. 47, 6646–6655. doi: 10.1021/es400663f
Cole M., Lindeque P., Halsband C., Galloway T. (2011). Microplastics as contaminants in the marine environment: A review. Mar. pollut. Bull. 62, 2588–2597. doi: 10.1016/j.marpolbul.2011.09.025
Conley K., Clum A., Deepe J., Lane H., Beckingham B. (2019). Wastewater treatment plants as a source of microplastics to an urban estuary: Removal efficiencies and loading per capita over one year. Water Res. X 3, 100030. doi: 10.1016/j.wroa.2019.100030
Deme G., Ewusi-Mensah D., Olagbaju O., Okeke E., Okoye C., Odii E., et al. (2022). Macro problems from microplastics: Toward a sustainable policy framework for managing microplastic waste in Africa. Sci. Total Environ. 804, 150170. doi: 10.1016/j.scitotenv.2021.150170
Dey T., Uddin M., Jamal M. (2021). Detection and removal of microplastics in wastewater: evolution and impact. Environ. Sci. pollut. Res. 28, 16925–16947. doi: 10.1007/s11356-021-12943-5
Di M., Liu X., Wang W., Wang J. (2019). Manuscript prepared for submission to environmental toxicology and pharmacology pollution in drinking water source areas: Microplastics in the danjiangkou reservoir, China. Environ. Toxicol. Pharmacol. 65, 82–89. doi: 10.1016/j.etap.2018.12.009
Di M., Wang J. (2018). Microplastics in surface waters and sediments of the three gorges reservoir, China. Sci. Total Environ. 616-617, 1620–1627. doi: 10.1016/j.scitotenv.2017.10.150
Dodson G., Shotorban A., Hatcher P., Waggoner D., Ghosal S., Noffke N. (2020). Microplastic fragment and fiber contamination of beach sediments from selected sites in Virginia and north Carolina, USA. Mar. pollut. Bull. 151, 110869. doi: 10.1016/j.marpolbul.2019.110869
Dowarah K., Devipriya S. (2019). Microplastic prevalence in the beaches of puducherry, India and its correlation with fishing and tourism/recreational activities. Mar. pollut. Bull. 148, 123–133. doi: 10.1016/j.marpolbul.2019.07.066
Dowarah K., Patchaiyappan A., Thirunavukkarasu C., Jayakumar S., Devipriya S. (2020). Quantification of microplastics using Nile red in two bivalve species perna viridis and meretrix from three estuaries in pondicherry, India and microplastic uptake by local communities through bivalve diet. Mar. pollut. Bull. 153, 110982. doi: 10.1016/j.marpolbul.2020.110982
Duis K., Coors A. (2016). Microplastics in the aquatic and terrestrial environment: sources (with a specific focus on personal care products), fate and effects. Environ. Sci. Europe 28, 1–25. doi: 10.1186/s12302-015-0069-y
Ejeian F., Azadi S., Razmjou A., Orooji Y., Kottapalli A., Ebrahimi Warkiani M., et al. (2019). Design and applications of MEMS flow sensors: A review. Sensors Actuators A: Phys. 295, 483–502. doi: 10.1016/j.sna.2019.06.020
Elkhatib D., Oyanedel-Craver V. (2020). A critical review of extraction and identification methods of microplastics in wastewater and drinking water. Environ. Sci. Technol. 54, 7037–7049. doi: 10.1021/acs.est.9b06672
Enders K., Tagg A., Labrenz M. (2020). Evaluation of electrostatic separation of microplastics from mineral-rich environmental samples. Front. Environ. Sci. 8. doi: 10.3389/fenvs.2020.00112
Eo S., Hong S., Song Y., Han G., Seo S., Shim W. (2021). Prevalence of small high-density microplastics in the continental shelf and deep sea waters of East Asia. Water Res. 200, 117238. doi: 10.1016/j.watres.2021.117238
Eriksen M., Liboiron M., Kiessling T., Charron L., Alling A., Lebreton L., et al. (2018). Microplastic sampling with the AVANI trawl compared to two neuston trawls in the bay of Bengal and south pacific. Environ. Pollut. 232, 430–439. doi: 10.1016/j.envpol.2017.09.058
Felsing S., Kochleus C., Buchinger S., Brennholt N., Stock F., Reifferscheid G. (2018). A new approach in separating microplastics from environmental samples based on their electrostatic behavior. Environ. pollut. 234, 20–28. doi: 10.1016/j.envpol.2017.11.013
Frank Y., Vorobiev E., Vorobiev D., Trifonov A., Antsiferov D., Soliman Hunter T., et al. (2020). Preliminary screening for microplastic concentrations in the surface water of the ob and tom rivers in Siberia, Russia. Sustainability 13, 80. doi: 10.3390/su13010080
Fries E., Dekiff J., Willmeyer J., Nuelle M., Ebert M., Remy D. (2013). Identification of polymer types and additives in marine microplastic particles using pyrolysis-GC/MS and scanning electron microscopy. Environ. Sci.: Processes Impacts 15, 1949. doi: 10.1039/c3em00214d
Gallagher A., Rees A., Rowe R., Stevens J., Wright P. (2016). Microplastics in the Solent estuarine complex, UK: An initial assessment. Elsiever. doi: 10.1016/j.marpolbul.2015.04.002
Georgiev R., Toscano S., Uspal W., Bet B., Samin S., van Roij R., et al. (2020). Universal motion of mirror-symmetric microparticles in confined stokes’s flow. Proc. Natl. Acad. Sci. 117, 21865–21872. doi: 10.1073/pnas.2005068117
Gossett D., Weaver W., Mach A., Hur S., Tse H., Lee W., et al. (2010). Label-free cell separation and sorting in microfluidic systems. Analytical Bioanalytical Chem. 397, 3249–3267. doi: 10.1007/s00216-010-3721-9
Güven O., Gökdağ K., Jovanović B., Kıdeyş A. (2017). Microplastic litter composition of the Turkish territorial waters of the Mediterranean Sea, and its occurrence in the gastrointestinal tract of fish. Environ. pollut. 223, 286–294. doi: 10.1016/j.envpol.2017.01.025
Hale R., Seeley M., La Guardia M., Mai L., Zeng E. (2020). A global perspective on microplastics. J. Geophysical Res.: Oceans 125, 1–40. doi: 10.1029/2018jc014719
He B., Goonetilleke A., Ayoko G., Rintoul L. (2020). Abundance, distribution patterns, and identification of microplastics in Brisbane river sediments, Australia. Sci. Total Environ. 700, 134467. doi: 10.1016/j.scitotenv.2019.134467
Herbort A., Schuhen K. (2016). A concept for the removal of microplastics from the marine environment with innovative host-guest relationships. Environ. Sci. pollut. Res. 24, 11061–11065. doi: 10.1007/s11356-016-7216-x
Hidalgo-Ruz V., Gutow L., Thompson R., Thiel M. (2012). Microplastics in the marine environment: A review of the methods used for identification and quantification. Environ. Sci. Technol. 46, 3060–3075. doi: 10.1021/es2031505
Hong S., Shim W., Hong L. (2017). Methods of analysing chemicals associated with microplastics: A review. Analytical Methods 9, 1361–1368. doi: 10.1039/c6ay02971j
Horton A., Jürgens M., Lahive E., van Bodegom P., Vijver M. (2018). The influence of exposure and physiology on microplastic ingestion by the freshwater fish rutilus rutilus (roach) in the river Thames, UK. Environ. pollut. 236, 188–194. doi: 10.1016/j.envpol.2018.01.044
Huang L. (2004). Continuous particle separation through deterministic lateral displacement. Science 304, 987–990. doi: 10.1126/science.1094567
Iyare P., Ouki S., Bond T. (2020). Microplastics removal in wastewater treatment plants: a critical review. Environ. Sci.: Water Res. Technol. 6, 2664–2675. doi: 10.1039/d0ew00397b
Jahan S., Strezov V., Weldekidan H., Kumar R., Kan T., Sarkodie S., et al. (2019). Interrelationship of microplastic pollution in sediments and oysters in a seaport environment of the eastern coast of Australia. Sci. Total Environ. 695, 133924. doi: 10.1016/j.scitotenv.2019.133924
James K., Vasant K., Padua S., Gopinath V., K.S. A., R. J., et al. (2020). An assessment of microplastics in the ecosystem and selected commercially important fishes off kochi, south eastern Arabian Sea, India. Mar. pollut. Bull. 154, 111027. doi: 10.1016/j.marpolbul.2020.111027
Jayasiri H., Purushothaman C., Vennila A. (2013). Quantitative analysis of plastic debris on recreational beaches in Mumbai, India. Mar. pollut. Bull. 77, 107–112. doi: 10.1016/j.marpolbul.2013.10.024
Jayasiri H., Purushothaman C., Vennila A. (2015). Bimonthly variability of persistent organochlorines in plastic pellets from four beaches in Mumbai coast, India. Environ. Monit. Assess. 187, 1–13. doi: 10.1007/s10661-015-4531-5
Jeyasanta K., Sathish N., Patterson J., Edward J. (2020). Macro-, meso- and microplastic debris in the beaches of tuticorin district, southeast coast of India. Mar. pollut. Bull. 154, 111055. doi: 10.1016/j.marpolbul.2020.111055
Jiang C., Yin L., Wen X., Du C., Wu L., Long Y., et al. (2018). And Microplastics in sediment and surface water of West dongting lake and south dongting lake: Abundance, source and composition. Int. J. Environ. Res. Public Health 15, 2164. doi: 10.3390/ijerph15102164
Jones K., Hartl M., Bell M., Capper A. (2020). Microplastic accumulation in a zostera marina l. bed at deerness sound, Orkney, Scotland. Mar. pollut. Bull. 152, 110883. doi: 10.1016/j.marpolbul.2020.110883
Jualaong S., Pransilpa M., Pradit S., Towatana P. (2021). Type and distribution of microplastics in beach sediment along the coast of the Eastern gulf of Thailand. J. Mar. Sci. Eng. 9, 1405. doi: 10.3390/jmse9121405
Karlsson T., Kärrman A., Rotander A., Hassellöv M. (2019). Comparison between manta trawl and in situ pump filtration methods, and guidance for visual identification of microplastics in surface waters. Environ. Sci. pollut. Res. 27, 5559–5571. doi: 10.1007/s11356-019-07274-5
Kazour M., Jemaa S., Issa C., Khalaf G., Amara R. (2019). Microplastics pollution along the Lebanese coast (Eastern Mediterranean basin): Occurrence in surface water, sediments and biota samples. Sci. Total Environ. 696, 133933. doi: 10.1016/j.scitotenv.2019.133933
Kobayashi T., Yagi M., Kawaguchi T., Hata T., Shimizu K. (2021). Spatiotemporal variations of surface water microplastics near Kyushu, Japan: A quali-quantitative analysis. Mar. pollut. Bull. 169, 112563. doi: 10.1016/j.marpolbul.2021.112563
Krishnakumar S., Srinivasalu S., Saravanan P., Vidyasakar A., Magesh N. (2018). A preliminary study on coastal debris in nallathanni island, gulf of mannar biosphere reserve, southeast coast of India. Mar. pollut. Bull. 131, 547–551. doi: 10.1016/j.marpolbul.2018.04.026
Kumar V., Ravikumar G., Jeyasanta K. (2018). Occurrence of microplastics in fishes from two landing sites in tuticorin, south east coast of India. Mar. pollut. Bull. 135, 889–894. doi: 10.1016/j.marpolbul.2018.08.023
Lammerink S. J., Tas N. R., Elwenspoek M., HJ Fluitman J. (1993). Micro-liquid flow sensor. Sensors Actuators A: Phys. 37, 45–50. doi: 10.1016/0924-4247(93)80010-E
Leads R., Weinstein J. (2019). Occurrence of tire wear particles and other microplastics within the tributaries of the Charleston harbor estuary, south Carolina, USA. Mar. pollut. Bull. 145, 569–582. doi: 10.1016/j.marpolbul.2019.06.061
Leslie H., Brandsma S., van Velzen M., Vethaak A. (2017). Microplastics en route: Field measurements in the Dutch river delta and Amsterdam canals, wastewater treatment plants, north Sea sediments and biota. Environ. Int. 101, 133–142. doi: 10.1016/j.envint.2017.01.018
Li J., Green C., Reynolds A., Shi H., Rotchell J. (2018). Microplastics in mussels sampled from coastal waters and supermarkets in the united kingdom. Environ. pollut. 241, 35–44. doi: 10.1016/j.envpol.2018.05.038
Li Y., Lu Z., Zheng H., Wang J., Chen C. (2020). Microplastics in surface water and sediments of chongming island in the Yangtze estuary, China. Environ. Sci. Europe 32, 1–12. doi: 10.1186/s12302-020-0297-7
Li H., Ma L., Lin L., Ni Z., Xu X., Shi H., et al. (2018). Microplastics in oysters saccostrea cucullata along the pearl river estuary, China. Environ. pollut. 236, 619–625. doi: 10.1016/j.envpol.2018.01.083
Li Y., Wolanski E., Dai Z., Lambrechts J., Tang C., Zhang H. (2018). Trapping of plastics in semi-enclosed seas: Insights from the bohai Sea, China. Mar. pollut. Bull. 137, 509–517. doi: 10.1016/j.marpolbul.2018.10.038
Li J., Zhang H., Zhang K., Yang R., Li R., Li Y. (2018). Characterization, source, and retention of microplastic in sandy beaches and mangrove wetlands of the qinzhou bay, China. Mar. pollut. Bull. 136, 401–406. doi: 10.1016/j.marpolbul.2018.09.025
Lots F., Behrens P., Vijver M., Horton A., Bosker T. (2017). A large-scale investigation of microplastic contamination: Abundance and characteristics of microplastics in European beach sediment. Mar. pollut. Bull. 123, 219–226. doi: 10.1016/j.marpolbul.2017.08.057
Lusher A., Buenaventura N., Eidsvol D., Thrane J., Økelsrud A., Jartun M. (2018). Freshwater microplastics in Norway a first look at sediment, biota and historical plankton samples from lake mjøsa and lake femunden (Norway: Norwegian Institute for Water Research). Available at: https://niva.brage.unit.no/niva-xmlui/handle/11250/2588713.
Maes T., van der Meulen M., Devriese L., Leslie H., Huvet A., Frère L., et al. (2017). Microplastics baseline surveys at the water surface and in sediments of the north-East Atlantic. Front. Mar. Sci. 4. doi: 10.3389/fmars.2017.00135
Maharana D., Saha M., Dar J., Rathore C., Sreepada R., Xu X., et al. (2020). Assessment of micro and macroplastics along the west coast of India: Abundance, distribution, polymer type and toxicity. Chemosphere 246, 125708. doi: 10.1016/j.chemosphere.2019.125708
Martin J., Lusher A., Thompson R., Morley A. (2017). The deposition and accumulation of microplastics in marine sediments and bottom water from the Irish continental shelf. Sci. Rep. 7, 1–9. doi: 10.1038/s41598-017-11079-2
Mhiret Gela S., Aragaw T. (2022). Abundance and characterization of microplastics in main urban ditches across the bahir dar city, Ethiopia. Front. Environ. Sci. 10. doi: 10.3389/fenvs.2022.831417
Morgana S., Casentini B., Amalfitano S. (2021). Uncovering the release of micro/nanoplastics from disposable face masks at times of COVID-19. J. Hazardous Mater. 419, 126507. doi: 10.1016/j.jhazmat.2021.126507
Nel H., Froneman P. (2015). A quantitative analysis of microplastic pollution along the south-eastern coastline of south Africa. Marine Pollution Bulletin 101, 274–279. doi: 10.1016/j.marpolbul.2015.09.043
Ogata Y., Takada H., Mizukawa K., Hirai H., Iwasa S., Endo S., et al. (2009). International pellet watch: Global monitoring of persistent organic pollutants (POPs) in coastal waters. 1. initial phase data on PCBs, DDTs, and HCHs. Mar. Pollut. Bull. 58, 1437–1446. doi: 10.1016/j.marpolbul.2009.06.014
Oleh M., Klymkovych T., Bokla N., Lobur M., Melnyk M., Timofiejczuk A. (2020). “Simulation of acoustophoretic separation of microplastic particles in mkFluidic Lab-chip,” in 2020 IEEE XVIth international conference on the perspective technologies and methods in MEMS design (MEMSTECH) (Ukraine, IEEE), 123–126. doi: 10.1109/MEMSTECH49584.2020.9109452
Oliveira J., Belchior A., da Silva V., Rotter A., Petrovski Ž., Almeida P., et al. (2020). Marine environmental plastic pollution: Mitigation by microorganism degradation and recycling valorization. Front. Mar. Sci. 7. doi: 10.3389/fmars.2020.567126
Ory N., Sobral P., Ferreira J., Thiel M. (2017). Amberstripe scad decapterus muroadsi (Carangidae) fish ingest blue microplastics resembling their copepod prey along the coast of rapa nui (Easter island) in the south pacific subtropical gyre. Sci. Total Environ. 586, 430–437. doi: 10.1016/j.scitotenv.2017.01.175
Pasquier G., Doyen P., Kazour M., Dehaut A., Diop M., Duflos G., et al. (2022). Manta net: The golden method for sampling surface water microplastics in aquatic environments. Front. Environ. Sci. 10. doi: 10.3389/fenvs.2022.811112
Patterson J., Jeyasanta K., Sathish N., Booth A., Edward J. (2019). Profiling microplastics in the Indian edible oyster, magallana bilineata collected from the tuticorin coast, gulf of mannar, southeastern India. Sci. Total Environ. 691, 727–735. doi: 10.1016/j.scitotenv.2019.07.063
Peng Y., Wu P., Schartup A., Zhang Y. (2021). Plastic waste release caused by COVID-19 and its fate in the global ocean. Proc. Natl. Acad. Sci. 118. doi: 10.1073/pnas.2111530118
Peters C., Bratton S. (2016). Urbanization is a major influence on microplastic ingestion by sunfish in the brazos river basin, central Texas, USA. Environ. pollut. 210, 380–387. doi: 10.1016/j.envpol.2016.01.018
Primpke S., Christiansen S., Cowger W., De Frond H., Deshpande A., Fischer M., et al. (2020). Critical assessment of analytical methods for the harmonized and cost-efficient analysis of microplastics. Appl. Spectrosc. 74, 1012–1047. doi: 10.1177/0003702820921465
Ram B., Kumar M. (2020). Correlation appraisal of antibiotic resistance with fecal, metal and microplastic contamination in a tropical Indian river, lakes and sewage. NPJ Clean Water 3, 1–12. doi: 10.1038/s41545-020-0050-1
Robin R., Karthik R., Purvaja R., Ganguly D., Anandavelu I., Mugilarasan M., et al. (2020). Holistic assessment of microplastics in various coastal environmental matrices, southwest coast of India. Sci. Total Environ. 703, 134947. doi: 10.1016/j.scitotenv.2019.134947
Rodrigues M., Abrantes N., Gonçalves F., Nogueira H., Marques J., Gonçalves A. (2018). Spatial and temporal distribution of microplastics in water and sediments of a freshwater system (Antuã river, Portugal). Sci. Total Environ. 633, 1549–1559. doi: 10.1016/j.scitotenv.2018.03.233
Sahajwalla V., Gaikwad V. (2018). The present and future of e-waste plastics recycling. Curr. Opin. Green Sustain. Chem. 13, 102–107. doi: 10.1016/j.cogsc.2018.06.006
Samandra S., Johnston J., Jaeger J., Symons B., Xie S., Currell M., et al. (2022). Microplastic contamination of an unconfined groundwater aquifer in Victoria, Australia. Sci. Total Environ. 802, 149727. doi: 10.1016/j.scitotenv.2021.149727
Sarkar D., Das Sarkar S., Das B., Manna R., Behera B., Samanta S. (2019). Spatial distribution of meso and microplastics in the sediments of river ganga at eastern India. Sci. Total Environ. 694, 133712. doi: 10.1016/j.scitotenv.2019.133712
Sathish N., Jeyasanta K., Patterson J. (2019). Abundance, characteristics and surface degradation features of microplastics in beach sediments of five coastal areas in Tamil nadu, India. Mar. pollut. Bull. 142, 112–118. doi: 10.1016/j.marpolbul.2019.03.037
Sathish M., Jeyasanta I., Patterson J. (2020). Occurrence of microplastics in epipelagic and mesopelagic fishes from tuticorin, southeast coast of India. Sci. Total Environ. 720, 137614. doi: 10.1016/j.scitotenv.2020.137614
Scherer C., Weber A., Stock F., Vurusic S., Egerci H., Kochleus C., et al. (2020). Comparative assessment of microplastics in water and sediment of a large European river. Sci. Total Environ. 738, 139866. doi: 10.1016/j.scitotenv.2020.139866
Sharma M., Elanjickal A., Mankar J., Krupadam R. (2020). Assessment of cancer risk of microplastics enriched with polycyclic aromatic hydrocarbons. J. Hazardous Mater. 398, 122994. doi: 10.1016/j.jhazmat.2020.122994
Smith M., Love D., Rochman C., Neff R. (2018). Microplastics in seafood and the implications for human health. Curr. Environ. Health Rep. 5, 375–386. doi: 10.1007/s40572-018-0206-z
Sparks C., Awe A., Maneveld J. (2021). Abundance and characteristics of microplastics in retail mussels from cape town, south Africa. Mar. pollut. Bull. 166, 112186. doi: 10.1016/j.marpolbul.2021.112186
Sruthy S., Ramasamy E. (2017). Microplastic pollution in vembanad lake, kerala, India: The first report of microplastics in lake and estuarine sediments in India. Environ. pollut. 222, 315–322. doi: 10.1016/j.envpol.2016.12.038
Sturm M., Herbort A., Horn H., Schuhen K. (2020). Comparative study of the influence of linear and branched alkyltrichlorosilanes on the removal efficiency of polyethylene and polypropylene-based microplastic particles from water. Environ. Sci. pollut. Res. 27, 10888–10898. doi: 10.1007/s11356-020-07712-9
Su L., Cai H., Kolandhasamy P., Wu C., Rochman C., Shi H. (2018). Using the Asian clam as an indicator of microplastic pollution in freshwater ecosystems. Environ. pollut. 234, 347–355. doi: 10.1016/j.envpol.2017.11.075
Sullivan G., Delgado-Gallardo J., Watson T., Sarp S. (2021). An investigation into the leaching of micro and nano particles and chemical pollutants from disposable face masks - linked to the COVID-19 pandemic. Water Res. 196, 117033. doi: 10.1016/j.watres.2021.117033
Su L., Nan B., Hassell K., Craig N., Pettigrove V. (2019). Microplastics biomonitoring in Australian urban wetlands using a common noxious fish (Gambusia holbrooki). Chemosphere 228, 65–74. doi: 10.1016/j.chemosphere.2019.04.114
Sun J., Dai X., Wang Q., van Loosdrecht M., Ni B. (2019). Microplastics in wastewater treatment plants: Detection, occurrence and removal. Water Res. 152, 21–37. doi: 10.1016/j.watres.2018.12.050
Sundar S., Chokkalingam L., Roy P., Usha T. (2020). Estimation of microplastics in sediments at the southernmost coast of India (Kanyakumari). Environ. Sci. pollut. Res. 28, 18495–18500. doi: 10.1007/s11356-020-10333-x
Sun X., Liang J., Zhu M., Zhao Y., Zhang B. (2018). Microplastics in seawater and zooplankton from the yellow Sea. Environ. pollut. 242, 585–595. doi: 10.1016/j.envpol.2018.07.014
Sutton R., Mason S., Stanek S., Willis-Norton E., Wren I., Box C. (2016). Microplastic contamination in the San Francisco bay, California, USA. Mar. pollut. Bull. 109, 230–235. doi: 10.1016/j.marpolbul.2016.05.077
Su L., Xue Y., Li L., Yang D., Kolandhasamy P., Li D., et al. (2016). Microplastics in taihu lake, China. Environ. pollut. 216, 711–719. doi: 10.1016/j.envpol.2016.06.036
Tagg A., Sapp M., Harrison J., Sinclair C., Bradley E., Ju-Nam Y., et al. (2020). Microplastic monitoring at different stages in a wastewater treatment plant using reflectance micro-FTIR imaging. Front. Environ. Sci. 8. doi: 10.3389/fenvs.2020.00145
Tang Y., Liu Y., Chen Y., Zhang W., Zhao J., He S., et al. (2021). A review: Research progress on microplastic pollutants in aquatic environments. Sci. Total Environ. 766, 142572. doi: 10.1016/j.scitotenv.2020.142572
Thomas D., Schütze B., Heinze W., Steinmetz Z. (2020). Sample preparation techniques for the analysis of microplastics in soil–a review. Sustainability 12, 9074. doi: 10.3390/su12219074
Tiwari M., Rathod T., Ajmal P., Bhangare R., Sahu S. (2019). Distribution and characterization of microplastics in beach sand from three different Indian coastal environments. Mar. pollut. Bull. 140, 262–273. doi: 10.1016/j.marpolbul.2019.01.055
Vaughan R., Turner S., Rose N. (2022). Microplastics in the sediments of a UK urban lake. Environmental Pollution 229, 10–18. doi: 10.1016/j.envpol.2017.05.057
Veerasingam S., Mugilarasan M., Venkatachalapathy R., Vethamony P. (2016). Influence of 2015 flood on the distribution and occurrence of microplastic pellets along the chennai coast, India. Mar. pollut. Bull. 109, 196–204. doi: 10.1016/j.marpolbul.2016.05.082
Veerasingam S., Ranjani M., Venkatachalapathy R., Bagaev A., Mukhanov V., Litvinyuk D., et al. (2020). Microplastics in different environmental compartments in India: Analytical methods, distribution, associated contaminants and research needs. TrAC Trends Analytical Chem. 133, 116071. doi: 10.1016/j.trac.2020.116071
Veerasingam S., Saha M., Suneel V., Vethamony P., Rodrigues A., Bhattacharyya S., et al. (2016). Characteristics, seasonal distribution and surface degradation features of microplastic pellets along the goa coast, India. Chemosphere 159, 496–505. doi: 10.1016/j.chemosphere.2016.06.056
Vidyasakar A., Krishnakumar S., Kasilingam K., Neelavannan K., Bharathi V., Godson P., et al. (2020). Characterization and distribution of microplastics and plastic debris along silver beach, southern India. Mar. pollut. Bull. 158, 111421. doi: 10.1016/j.marpolbul.2020.111421
Viet Dung L., Huu Duc T., Thi Khanh Linh L., Thi Dieu Ly T., Anh Duong H., Thi My Hao N. (2021). Depth profiles of microplastics in sediment cores from two mangrove forests in northern Vietnam. J. Mar. Sci. Eng. 9, 1381. doi: 10.3390/jmse9121381
Wang W., Ge J., Yu X. (2020). Bioavailability and toxicity of microplastics to fish species: A review. Ecotoxicol. Environ. Saf. 189, 109913. doi: 10.1016/j.ecoenv.2019.109913
Wagner M., Lambert S. (2018). Freshwater microplastics: challenges for regulation and management. Freshwater Microplastics 58, 239–272. doi: 10.1007/978-3-319-61615-5.
Wu S., Lin Q., Yuen Y., Tai Y. (2001). MEMS flow sensors for nano-fluidic applications. Sensors Actuators A: Phys. 89, 152–158. doi: 10.1016/s0924-4247(00)00541-0
Xiong X., Zhang K., Chen X., Shi H., Luo Z., Wu C. (2018). Sources and distribution of microplastics in china’s largest inland lake – qinghai lake. Environ. pollut. 235, 899–906. doi: 10.1016/j.envpol.2017.12.081
Xu J., Thomas K., Luo Z., Gowen A. (2019). FTIR and raman imaging for microplastics analysis: State of the art, challenges and prospects. TrAC Trends Analytical Chem. 119, 115629. doi: 10.1016/j.trac.2019.115629
Yamada M., Nakashima M., Seki M. (2004). Pinched flow fractionation: Continuous size separation of particles utilizing a laminar flow profile in a pinched microchannel. Analytical Chem. 76, 5465–5471. doi: 10.1021/ac049863r
Yamada M., Seki M. (2005). Hydrodynamic filtration for on-chip particle concentration and classification utilizing microfluidics. Lab. Chip 5, 1233. doi: 10.1039/b509386d
Yonkos L., Friedel E., Perez-Reyes A., Ghosal S., Arthur C. (2014). Microplastics in four estuarine rivers in the Chesapeake bay, U.S.A. Environ. Sci. Technol. 48, 14195–14202. doi: 10.1021/es5036317
Zarfl C. (2019). Promising techniques and open challenges for microplastic identification and quantification in environmental matrices. Analytical Bioanalytical Chem. 411, 3743–3756. doi: 10.1007/s00216-019-01763-9
Zhou J., Giridhar P., Kasper S., Papautsky I. (2013). Modulation of aspect ratio for complete separation in an inertial microfluidic channel. Lab. Chip 13, 1919. doi: 10.1039/c3lc50101a
Zhou J., Mukherjee P., Gao H., Luan Q., Papautsky I. (2019). Label-free microfluidic sorting of microparticles. APL Bioeng. 3, 041504. doi: 10.1063/1.5120501
Zhou J., Tu C., Liang Y., Huang B., Fang Y., Liang X., et al. (2018). Isolation of cells from whole blood using shear-induced diffusion. Sci. Rep. 8, 1–13. doi: 10.1038/s41598-018-27779-2
Keywords: microplastics, global coastline, standard analytical methods, separation techniques, flow sensors
Citation: Prabhu PP, Pan K and Krishnan JN (2022) Microplastics: Global occurrence, impact, characteristics and sorting. Front. Mar. Sci. 9:893641. doi: 10.3389/fmars.2022.893641
Received: 10 March 2022; Accepted: 07 September 2022;
Published: 27 September 2022.
Edited by:
Bernardo Duarte, Center for Marine and Environmental Sciences (MARE), PortugalReviewed by:
Muhammad Reza Cordova, Indonesian Institute of Sciences, IndonesiaTadele Assefa Aragaw, Bahir Dar University, Ethiopia
Copyright © 2022 Prabhu, Pan and Krishnan. This is an open-access article distributed under the terms of the Creative Commons Attribution License (CC BY). The use, distribution or reproduction in other forums is permitted, provided the original author(s) and the copyright owner(s) are credited and that the original publication in this journal is cited, in accordance with accepted academic practice. No use, distribution or reproduction is permitted which does not comply with these terms.
*Correspondence: Jegatha Nambi Krishnan, amVnYXRoYWtAZ29hLmJpdHMtcGlsYW5pLmFjLmlu