- 1School of Life Sciences, University of Essex, Colchester, United Kingdom
- 2Archipelagos Institute of Marine Conservation, Pythagorio, Greece
- 3Institute of Marine Sciences, School of Biological Sciences, University of Portsmouth, Portsmouth, United Kingdom
Seagrass meadows’ ability to capture carbon through sequestering autochthonous carbon via photosynthesis means they could represent a potential nature-based solution to rising carbon emissions. In multispecies seagrass communities, and due to species introduction or predicted range shifts, it is important to know which species deliver different carbon sequestration gains to inform conservation actions. Large benthic chamber experiments (volume = 262L) assessed the seasonal and spatial variation in metabolism dynamics of the endemic and dominant Mediterranean seagrass, P. oceanica whilst small benthic chamber experiments (volume = 7L) compared the dynamics between, P. oceanica the native C. nodosa and non-native H. stipulacea. Within shallow P. oceanica edge habitat lower Net Apparent Productivity (NAP) occurs in autumn ( = 1.3, SD ± 2.95 O2 mmol m-2 d-1) compared to summer ( = 9.9, SD ± 2.75 O2 mmol m-2 d-1 corresponding with periods of light limiting and light saturating conditions, but it remains overall autotrophic annually (2.3 C mol m-2 yr-1). However, spatial heterogeneity exists, the center areas of P. oceanica were more productive (NAP =19.7, SD± 3.83 O2 mmol m-2 d-1) compared to edge habitat with spatial changes in productivity relating to plant surface area (96%), shoot density (81%), blade length (72%) and seagrass percentage cover (64%). Under comparative conditions in a sparse multispecies area of the meadow the species demonstrated different capacities for carbon fixation. H. stipulacea was carbon positive and P. oceanica fluctuated between positive and negative carbon balance suggesting both can maintain a balance between carbon fixation and carbon utilised for metabolic activity. In contrast the C. nodosa here would be expected to deteriorate as it was utilising carbon more than it was fixing (NAPN2 ( = -0.0012, SD ± 0.0007 O2 mmol cm-2 d-1). This study demonstrates that not all seagrass habitat is equal. If seagrass meadows are to play a part in mitigating CO2 emissions, variability in primary productivity within seagrass meadows needs to be accounted for to produce accurate total fixed carbon estimates, and subsequently autochthonous carbon sequestration estimates. This means seagrass meadow species composition and the condition of these meadows must be better understood.
1 Introduction
Seagrass meadows act as a major global carbon sink (Duarte et al., 2013), therefore restoration or expansion of seagrass beds represent a potential nature-based solution to rising carbon emissions. Seagrasses capture carbon through actively sequestering autochthonous carbon by photosynthesis and passively trapping allochthonous carbon within their architectural structure. Allochthonous carbon is typically considered more labile, therefore deposits of autochthonous carbon are those expected to lead to long-term stable carbon deposits (Mazarrasae et al., 2018). The metabolic rates of global seagrass communities favour net autotrophy, with temperate meadows typically favoured to have a higher net autotrophy than tropical meadows (Duarte et al., 2010), suggesting not all seagrass is equal in its ability to sequester carbon.
The temperate seagrass Posidonia oceanica forms vast monospecific meadows in the Mediterranean and is unique in its ability to form vertical mattes that can store sedimentary carbon for millennia (Mateo et al., 1997). It is perhaps why some herald these meadows to represent the global maximum in carbon sequestration among seagrasses (Lavery et al., 2013). Unlike terrestrial soils, Posidonia sediments have the potential to not become saturated with carbon over time because they can accrete vertically (Mateo et al., 1997; Samper-Villarreal et al., 2018). If the vertical accretion matches the rate of sea level rise, they potentially have a limitless capacity, which in part demonstrates their suitability for climate mitigation policy efforts (McKee et al., 2007; Howard et al., 2017). However, P. oceanica meadows have undergone severe regression in the last 50 years (34% loss), with only localised areas of persistence and growth (Telesca et al., 2015). The causes of decline for P. oceanica include water quality degradation, coastal modification, mechanical damage (i.e., bottom trawling, anchoring, mooring, culture farm occupation), extreme weather events and non-native macroalgae invasion (Santos et al., 2019).
At the Mediterranean scale, P. oceanica populations in the Western and Eastern basins maintain genetic differentiation due to present-day dispersal limits (Arnaud-Haond et al., 2007). There is a greater distribution of P. oceanica in the Eastern basin (713,992 ha) compared to the Western basin (510,715 ha) (Telesca et al., 2015). Despite the genetic variation between basins and larger coverage in the Eastern Mediterranean basin, the majority of P. oceanica metabolism estimates have come from the Western Mediterranean basin (Frankignoulle and Bouquegneau, 1987; Holmer et al., 2004; Gazeau et al., 2005; Barron and Duarte, 2009; Olive et al., 2015; Champenois and Borges, 2018). There is a single published study on P. oceanica metabolism in the Eastern Mediterranean basin, in the western region of the Aegean Sea (Apostolaki et al., 2010). Metabolism and carbon sequestration estimations for P. oceanica are considered one of the most well researched amongst seagrass species (Nordlund et al., 2018), yet there are distinct local knowledge gaps and spatial biases, particularly within the Eastern Mediterranean basin that need to be addressed. In the Eastern Mediterranean basin, the sea surface has warmed by 0.05 ± 0.009°C yr-1 compared to just 0.03 ± 0.008°C yr-1 in the Western Mediterranean basin from 1985 to 2006 (Nykjaer, 2009). Given the Eastern Mediterranean basin is warming faster than the Western Mediterranean basin understanding if the metabolism and drivers of productivity are different in Eastern vs Western Mediterranean seagrass is important.Seagrass metabolism is influenced by multiple variables including light (Champenois and Borges, 2018), nutrient availability (Holmer et al., 2008; Apostolaki et al., 2010), and temperature and ocean acidification (Berg et al., 2019); which leads to seasonal fluctuations in productivity. Seagrass depth distribution is determined by light availability, as under insufficient light conditions the plant does not meet the photosynthetic requirements needed to maintain positive metabolic and carbon balance (Ralph et al., 2007), moving away from a state of net autotrophy and net carbon storage. Although seagrass presence is a function of the accumulative light availability throughout the year as this will change seasonally from periods where light is limiting to periods where it is potentially light saturating. The optimal thermal conditions for P. oceanica are between 17 - 20°C (Champenois and Borges, 2018), therefore its metabolism both above and below these conditions, is not optimal for maximum oxygen production and is more likely to become heterotrophic. Annual patterns of P. oceanica metabolism therefore comprise periods that alternate from negative to positive carbon balance as temperature and light availability change with seasons (Alcoverro et al., 2001). Further to this P. oceanica meadows are known to undergo seasonal senescence, leading to the shedding of >85% of leaf material at the end of Summer (Ott, 1980; Cebrian and Duarte, 2001). If metabolism estimates were produced only during the summer months it would overestimate the meadow’s net carbon sequestration capacity (Champenois and Borges, 2012). Notably as stable carbon stocks rely on autochthonous carbon deposits, we will also assess key environmental parameters that influence photosynthesis in these different seasons: for example change in light and temperature.Seagrass meadows are not always a uniform habitat and whilst P. oceanica meadows can form continuous meadows, patchy coverage across meadows is common. Patchy P. oceanica creates complex seascapes, which are mosaics including habitats of sand, P. oceanica dead matte and live P. oceanica (Borg et al., 2006; Abadie et al., 2015). This complexity also acts within a P. oceanica meadow, because at the junction between the seagrass and adjacent habitats there are considerable edge effects, resulting in distinct areas of central and edge P. oceanica habitat (Abadie et al., 2018). Meadows that experience a wave exposure gradient from low to high energy develop patchier meadow formation (Folkard, 2005; Pace et al., 2016). Anthropogenic factors have also increased patchiness including anchoring, impact from historic military activity, fishing practices and fish farming (Montefalcone et al., 2009; Abadie et al., 2015). When a P. oceanica meadow is described as patchy it has lower overall cover, more complex patch shapes and reduced within-patch architectural complexity (Pace et al., 2016). This patchiness influences the available surface of photosynthetically active plant material. It is therefore important that in this study we consider the spatial variation in canopy architecture of P. oceanica when considering its area based carbon sequestration potential, and not just take estimates from pristine intact meadows.The Mediterranean temperate-tropical combination of seagrass species is considered a unique bioregion of seagrass diversity (Short et al., 2007). Alongside the dominant endemic P. oceanica, the next most prevalent species in the Aegean Sea is the native Cymodocea nodosa. The eastern region of the Aegean Sea also sits at a crossroad for alien species expansion (Pancucci-Papadopoulou et al., 2012), including the non-native seagrass Halophila stipulacea, introduced to the Mediterranean and first reported in 1894 off the Island of Rhodes in the south-eastern region of the Aegean Sea (Fritsch, 1895; also see Halophila decipiens, Gerakaris et al., 2019). H. stipulacea was listed amongst the ‘100 Worst Invasive Species’ in the Mediterranean (Streftaris and Zenetos, 2006). However, there are discrepancies in whether it should be considered ‘invasive’ or not as no ecological consequences of its introduction and spread in the Mediterranean have been reported (Williams, 2007). Scarce research has focused on its potential impact or contribution to ecosystem services within Mediterranean coastal ecosystems, yet H. stipulacea habitat was recently suggested to support carbon sequestration (Apostolaki et al., 2019; Wesselmann et al., 2021). Given H. stipulacea is one of the longest monitored non-native species in the Mediterranean, there has been a clear lag from reporting its presence and rate of expansion, to understanding its impact within the communities where it has successfully established.In the Mediterranean H. stipulacea can be found in single species meadows, multi-species meadows with the native Cymodocea nodosa, in the free spaces between patches of P. oceanica or in habitats previously devoid of seagrass (Boudouresque et al., 2009). Whilst there are reports of its ‘invasive’ behaviour on sandy substrata when it exists in high abundances, no displacement of native species has yet been reported (Tsiamis et al., 2010). Given that H. stipulacea has various contexts in which it can be found in the Mediterranean its contribution to community productivity may be context dependent. When H. stipulacea has colonised areas previously absent of seagrass its presence has increased the distribution of seagrass habitat in the Mediterranean, this is one reason why it can be considered a new potential blue carbon sink habitat (Apostolaki et al., 2019; Wesselmann et al., 2021). The potential of H. stipulacea to also influence the net productivity of mixed seagrass habitats, especially considering evidence it can colonise patchy areas of bare ground where P. oceanica may have been present in the past (Telesca et al., 2015), will also be assessed in this study.This study will firstly determine if seasonal differences in metabolism measurements occur for shallow P. oceanica meadows in the eastern region of the Aegean Sea and the relative influence of the concurrent seasonal changes in light, temperature, and seagrass canopy height, on net productivity. These seasonal measurements will provide a conservative estimate of annual total fixed carbon. Secondly in the summer we will assess if spatial variation in productivity exists between edge and central P. oceanica habitat and if various plant biometrics can be used to describe any variation in spatial plant productivity. Finally, we assess if variation in species-specific metabolism exists between both native seagrass species P. oceanica and C. nodosa in direct comparison to the non-native seagrass species H. stipulacea. Our results will contribute to suitable Mediterranean scale carbon budgets which take into account the variation in total fixed carbon produced by Mediterranean seagrass.
2 Methods
2.1 Study Site
This study took place at Vroulia Bay (37.317460° N, 26.724704° E), Lipsi Island, which is part of the Dodecanese Islands, in the eastern region of the Greek Aegean Sea. The bay consists of a multispecies seagrass meadow, largely dominated by monospecific areas of P. oceanica. However, the bay also houses small monospecific patches of C. nodosa and H. stipulacea, as well as areas where two or all three of the species form mixed meadows (Supplementary Figure 1).
2.2 Experimental Design
This metabolism study exists in two parts. Firstly, and primarily, large benthic chamber experiments focus on the seasonal and spatial metabolism dynamics of P. oceanica from within monospecific shallow P. oceanica (2m depth). Seasonal P. oceanica sampling occurred in autumn (3rd – 9th November 2018), spring (12th – 19th April 2019) and summer (2nd - 12th July 2019); whilst spatial P. oceanica sampling occurred only in summer (2nd July – 12th August 2019).
Secondly small benthic chamber experiments from a deeper area (7m depth) of the same meadow where all three seagrass species are found mixed focus on comparative metabolism dynamics of P. oceanica, C. nodosa and H. stipulacea, in summer (20th June – 17th July 2019) (Full sampling regime available in; Supplementary Table 1).
2.2.1 Seasonal and Spatial P. oceanica Metabolism -Large Benthic Chamber Setup
Large dome-shaped clear PVC benthic chambers (diameter = 1 m, height = 50 cm, benthic surface area = 0.79 m2, volume = 262 L) were deployed via free divers (Figure 1) in shallow monospecific P. oceanica (1.6 - 2 m depth). PME miniDOT® loggers were fitted to the apex of the chamber, to record dissolved oxygen and temperature in 10 minutes intervals. HOBO Pedant® loggers were fixed and weighted flat to the seafloor within the benthic chambers to record relative irradiance in 5 minutes intervals. The HOBO loggers were calibrated under controlled light and temperature facilities in Essex before being sent out to the field site. In our experience pre-calibration differences between newly purchased HOBO loggers are always minimal. A submersible pump was secured to the inside of the chamber 30 cm from the seafloor to mix water at a flow rate of 480 L hr-1 in 10 minute intervals (10 minutes on followed by 10 minutes off), as such the overall flow rate within the chamber was considered to be 240 L hr-1. The benthic chambers were held in place by large chains and sank 5 cm into the sediment to create a seal. Given the morphology and typically large aboveground biomass of P. oceanica, the large benthic chambers combined with internal mixing support sufficient volume of water inside the chamber relative to vegetative biomass to prevent oxygen saturation (Olive et al., 2015), and prevent oxygen saturation (Supplementary Figure 2). The benthic chambers were deployed within the same 2 hour timeframe each morning (10:45 am -12:45 pm) then left in situ for 24 hour (autumn and summer) or 23 hour (spring) incubations.
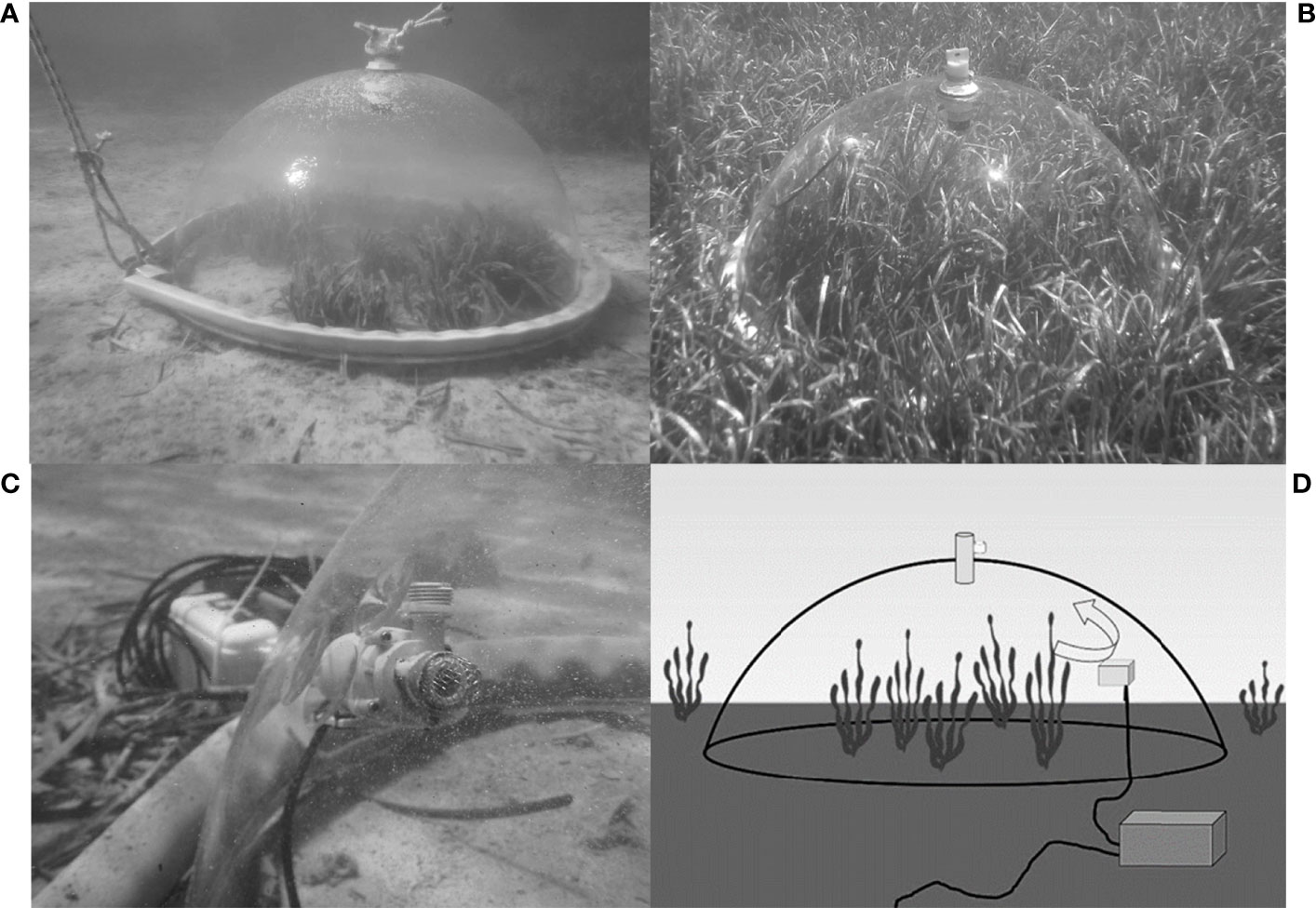
Figure 1 Large benthic chamber setup (A) Deployed in autumn over P. oceanica edge habitat (B) Deployed in summer over dense central P. oceanica meadow. (C) Setup of the internal water pump (foreground) within bare sand incubation. External submersible pump battery pack in background. (D) Illustration of large benthic chamber set-up. Photographs © Emma A Ward, Illustration © Tom Wade.
The large in situ benthic chambers were deployed over edge habitat in autumn, spring and summer. For this study we consider patchy edge habitat to be within the fringing 1m of P. oceanica before the transition point from continuous P. oceanica (> 2m) to sand or the entirety of small P. oceanica patches (< 2m), because patches of P. oceanica< 2m in size are assumed to consist entirely of edge habitat due to the proximity of the centre of the small patch to the edge. Edge habitat creates a good seal at the base of the large benthic chambers because of the high proportion of sand to low proportion of rhizome. Simultaneously a large benthic chamber was placed over the adjacent unvegetated habitat to collect bare sand incubations. We have used a sand-based control given we are studying patchy and shallow beds and therefore they are not sitting on as established matte, however a dead seagrass matte control achieved by cutting live material away from a chamber’s area would be an alternative, in our case using bare sand incubations also limits any impacts to our study meadow. If it was not possible to simultaneously deploy the bare sand incubations, they were deployed the day after the first incubation was completed, although this only occurred once (Supplementary Table 1).To achieve a comparison between fragmented edge seagrass habitat and the continuous central meadow, in summer benthic chambers were placed over P. oceanica within the meadow centre in addition to those deployed over edge habitat. Within dense areas of P. oceanica the rigid PVC benthic chamber likely cut the rhizomes and roots, however the stress caused is generally considered marginal (Champenois and Borges, 2012). The summer bare sand incubations collected simultaneously to the summer P. oceanica edge habitat (n = 4) were used as the control to summer central measurements (Full sampling regime available in; Supplementary Table 1).
2.2.2 Species Specific Productivity -Small Benthic Chamber Setup
An area within Vroulia bay was chosen where all three species were in proximity, therefore maintaining as much as possible similar in situ environmental conditions for each species (appx. 7m depth). An area of patchy mixed meadow transitioned in areas from P. oceanica dominant to C. nodosa dominant, with scarce intermittent presence of clumps of H. stipulacea, as such this was chosen for the metabolism measurements focusing on P. oceanica and C. nodosa (Supplementary Figure 1). The use of the small chambers allowed for complimentary experimental questions to occur in the summer 2019 field season, but also enabled sampling to take place in the deeper multispecies area of the meadow where we could not safely deploy the large chambers with the equipment we had.
Care was taken to avoid placing benthic chambers over mixed species compositions and generally the benthic chambers only encompassed the intended dominant species. Two of five C. nodosa chambers covered a small amount of either P. oceanica or H. stipulacea. Nevertheless, in those instances C. nodosa represented 93% and 96% of the total seagrass plant surface area within the chamber and therefore these two replicates were still considered representative of C. nodosa patches. One of six P. oceanica chambers covered a small amount of H. stipulacea but P. oceanica represented 98% of the total plant surface area within the chamber and therefore was also considered representative of P. oceanica. Directly adjacent to the P. oceanica and C. nodosa was a monospecific area of H. stipulacea, which was utilised for the metabolism measurements focused on this species.
Three smaller clear dome PVC benthic chambers (diameter = 30 cm, height = 15 cm, benthic surface area = 0.071 m2, volume = 7 L) were deployed by free divers, secured with a small chain and sank into the sediment to create a seal (Figure 2). The benthic incubations took place for each seagrass species consecutively; H. stipulacea 20th June – 5th July, C nodosa 10th – 14th July and P. oceanica 14th – 17th July. A bare sand incubation was simultaneously deployed every day. The same oxygen, light and temperature loggers and temporal sampling methods were used as per the large domes described above. The benthic chambers were deployed within the same half an hour timeframe each morning (11:00 - 11:30 am) and left in situ for ~ 23 hours. As H. stipulacea and C. nodosa are smaller seagrass species with lower above ground biomass, the small benthic chamber design should sufficiently provide a suitable volume of water to vegetative biomass to prevent oxygen saturation. The P. oceanica within this area of the bay is naturally patchy and short, which should also accommodate the smaller chamber size and volume (Figure 2B). There was no mixing present inside these small benthic chambers, given water motion reduces the thickness of the diffusion boundary-layer which in turn allows for a higher carbon availability on the plant surface (Koch, 1993), these smaller benthic chambers likely underestimate the carbon flux taking place in situ with water motion present.
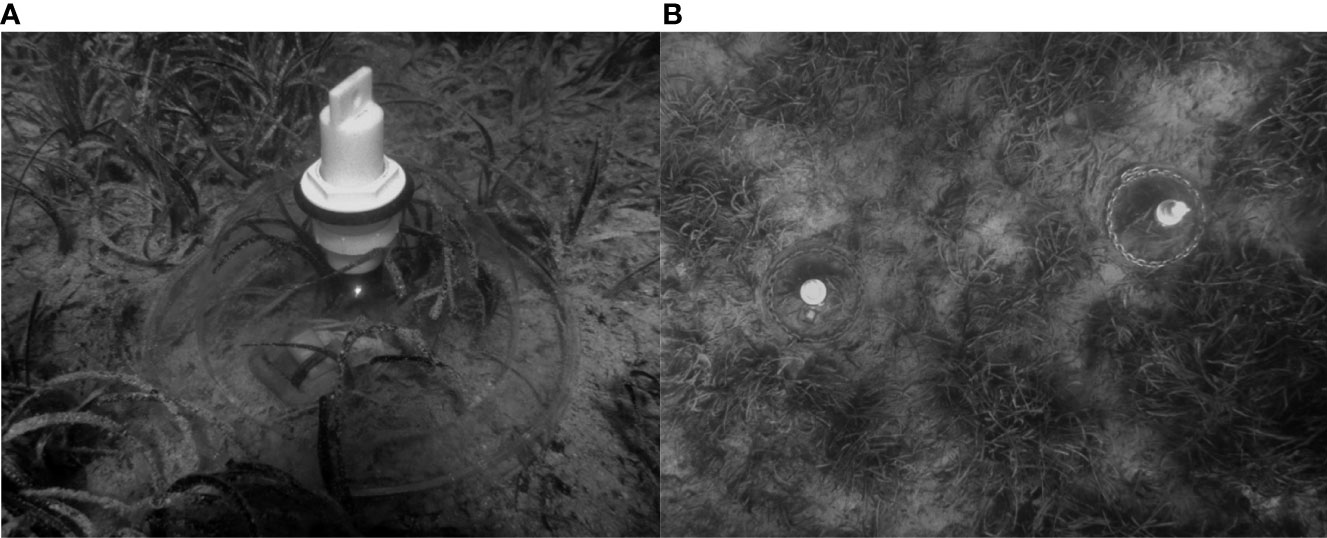
Figure 2 Small benthic chamber setup (A) Deployed over short patchy P. oceanica habitat (B) Aerial view above patchy P. oceanica chambers deployed. Photographs; A and B © Jente van Langerak.
2.2.3 Quantifying Seagrass Canopy Within the Benthic Chamber
The quantity of seagrass canopy enclosed within all benthic chambers was quantified; large benthic chambers a representative sample was selected, measured in situ and scaled up to reflect the full chamber; small chambers had all the leaf material collected and measured back at the lab.
For seasonal large benthic chambers, blade length (Blength) (cm) from 5 – 6 randomly selected blades were measured from the P. oceanica enclosed within the chambers to reflect canopy height and broadly represent the biomass enclosed within the chambers across seasons. In the summer several additional measurements were taken from the seagrass within the benthic chambers. P. oceanica coverage (Cover) (%) was estimated once the benthic chamber was deployed reflecting the percentage of seagrass cover within the entirety of the chamber. When the benthic incubations were complete three 20 x 20 cm quadrats were placed on the P. oceanica enclosed within the chamber to determine shoot density (ShootD) (m-2). To establish a proxy of the plant surface area for individual P. oceanica shoots enclosed (ShootSA) the following was also acquired; the number of blades (BNumber) (shoot-1) from 5 - 6 randomly selected shoots; blade length (Blength) and blade width (Bwidth) (cm) from 5 – 6 randomly selected blades:
Seagrass cover (%), ShootD and Blength representative of canopy height were all used as standalone plant biometrics. However, in combination these seagrass biometrics and ShootSA determine plant surface area (PlantSA) (cm2 m-2) within each chamber, calculated as:
For the small benthic chambers all leaf material from within the chambers was collected by cutting the leaves leaving the rhizome and roots, this along with the small sample size within these chambers’ limits damage to the overall sampling area. The aboveground leaf material was transported back to the lab where they were separated from any dead leaf litter and all individual leaves were photographed using a digital camera, on a white background. The planar surface area of all seagrass leaves within corresponding benthic chambers were determined, by Image J software, (modified from, Schneider et al., 2012). This was converted into plant surface area (PlantSA) (cm2 m-2) by doubling the planar surface area and dividing by the chamber benthic surface area (A) (m2).
2.3 Community Metabolism Calculations
The record from the PME oxygen logger was used to calculate net community productivity for every 10-minute interval over each 24-hour period (NCP24) (modified from Cole and Pace, 2000):
where DO is dissolved oxygen. This was applicable to the autumn and summer large benthic chamber P. oceanica samples when 24-hour incubations were completed.
The spring P. oceanica and mixed species incubations were completed across 23-hour incubations missing one hour of daylight incubation. HOBO Pendant® light intensity data was used to distinguish hours of daytime (Hd) from hours of night (Hn). Daytime NCP (NCPDaytime) (Champenois and Borges, 2012; Rodriguez et al., 2016) was calculated whereby Hi is the daylight incubation time:
The change that occurred during the night-time dark period (Hn) were summed over the whole night to calculate CRNight, which has a negative sign, as follows:
For 23-hour incubations the combination of the net community change during the daytime (NCPDaytime) and night-time dark period (CRNight) determine NCP across 24 hours (NCP24):
Since the benthic chamber incubations do not acquire a direct measurement of CRDaytime, we assume the hourly value of CRNight and CRDaytime are equal (Cole and Pace, 2000):
This allows daily community respiration (CR24) to be found:
GPP24 is then calculated as:
Measurements of metabolism (with units of O2 mg L-1 d-1) were converted to measurements of flux (with units of O2 mol m−2 d−1) (Olive et al., 2015), by multiplying by volume (V) and dividing by area (A) of the benthic chamber.
The photosynthetic and respiratory quotient of 1 mol of O2: 1 mol CO2 is applied to terrestrial plants that use starch and sugars as respiratory substrates, as observed in P. oceanica (Alcoverro et al., 2001). The photosynthetic and respiratory quotient of 1 mol of O2: 1 mol CO2 is conservatively applied to a wide number of seagrass species (Duarte et al., 2010) and therefore also used for H. stipulacea and C. nodosa. Therefore, the metabolism values are interchangeable as units of oxygen or carbon (O2 mol m−2 d−1 and C mol m−2 d−1).
2.4 Net Apparent Productivity Calculations
The net apparent productivity (NAP) of the seagrass and epiphytes (modified from Murray and Wetzel, 1987), was calculated as the difference between estimates for the NCP24 of the seagrass benthic chambers (NCPSG) and the average NCP24 of the bare sand incubations (NCPBS) (Murray and Wetzel, 1987) from the same corresponding season. Whilst there may be differences between the habitats beyond the presence and absence of vegetation the calculation of NAP is made on the assumption the prime difference between the vegetated and unvegetated benthic chambers is the presence of the seagrass and epiphytes. In fact, the expected contribution of epiphyte productivity is deemed minimal due to the overall high biomass of P. oceanica to that of the epiphytes (Cox et al., 2015), therefore the large benthic chamber NAP is assumed to primarily reflect the productivity of the P. oceanica.
To account for seasonal changes in seagrass canopy the seasonal NAP measurements were also standardised by the concurrent seasonal canopy height (Blength) within the large benthic chambers and presented separately as NAP canopy-height-normalised flux (NAPN) (O2 mmol m−2 cm−1 d−1).
Accounting for the metabolism of the community within a seagrass meadow is important to determine the net productivity at a community level, but as the autotrophy within the community is largely attributed to the primary productivity of the seagrasses (Hemminga and Duarte, 2000), distinguishing the NAP and NAPN enables a clearer determination of the influences driving this metabolic component of the community. Justifying the approach to distinguish between NCP (proxy of community metabolism) and NAP (proxy of plant productivity) within the seagrass meadow ecosystem, as seasonal community metabolism may partially mask the drivers in seagrass productivity. However, NCP estimates are widely accepted for benthic chamber metabolism studies within seagrass meadows (Duarte et al., 2010; Olive et al., 2015), because they clearly quantify the extent to which the overall community is a carbon sink or source. Hence, we also chose to present our results in NCP as it allows for closer comparisons to existing NCP estimates.To determine the NAP of the small benthic chambers the average NCP of the bare sand incubations deployed at the same time as the corresponding seagrass species were used. Given each seagrass species were deployed consecutively the corresponding bare sand incubations were grouped; thus, 20th June – 5th July (n = 6) corresponding to H. stipulacea, 10th – 14th July (n = 3) for C. nodosa and 14th – 17th July (n = 3) for P. oceanica. Given the differences between these species typical above ground biomass comparative NAP is most appropriately discussed when accounting for these differences in biomass. NAP is therein presented standardised by the respective plant surface area as NAP plant-surface-area-normalised flux NAPN2 (O2 mmol cm−2 d−1), calculated with the plant surface area from within the small benthic chambers (cm2 m−2).
2.5 Data Analysis
2.5.1 Seasonal P. oceanica NCP, NAP and NAPN
The three samples from winter and spring and four samples in summer, meant finding an appropriate data distribution suitable for the seasonal P. oceanica benthic chamber data is challenging, but Shapiro-Wilk tests confirmed that when grouped by Season both the NCP and NAP conform to normal distribution (autumn W = 0.9903741, P > 0.05; spring W = 0.8167365, P > 0.05; summer W = 0.9591216, P > 0.05) (Supplementary Figure 3). Whilst Bartlett’s test confirmed equal variances across both the NCP and NAP seasonal groups (K = 0.00952, P > 0.05). Therefore, ANOVAs were appropriate and were used to assess if there was a significant seasonal difference in P. oceanica NCP, NAP and NAPN. Tukey post hoc tests were used in each case to test for pairwise differences between the seasons.
2.5.2 Influence of Environmental Conditions on P. oceanica NAP
The mean PME miniDOT water temperature was calculated across each incubation period. HOBO logger irradiance (lux) were converted to PAR (photosynthetic active radiation), according to the conversion factor appropriate to the light source ‘daylight’ (Thimijan and Heins, 1983), this converts the light intensity into a more useful measure than the standard lux reported by the logger. The Instantaneous PAR with the unit μmol s-1 m-2 is used to calculate Daily Light integral (DLI) given the time interval in seconds (t) between each PAR reading over each 24-hour period:
The DLI is presented with the unit mol m-2 d-1.To examine the change in P. oceanica NAP relative to the changing light environment, the photosynthesis-irradiance relationship was fit with a hyperbolic tangent function (Jassby and Platt, 1976), modified to account for respiration (Rheuban et al., 2014), as used for the seagrass species in question (Koopmans et al., 2020). The model fitted was:
where Pmax, Ik and RI are fitting constants; Pmax represents the maximum photosynthetic rate, Ik is the saturation irradiance, RI is respiration, and I is available light. The parameters were estimated by non-linear least squares approach (nls function, R version 3.5.1), estimating approximate start values. The irradiance compensation point is the irradiance at which net oxygen production equals zero. If no light saturation is occurring, this function converges to a linear function, (Rheuban et al., 2014). To determine the most appropriate model for both the saturation curve and linear model an Akaike Information Criterion approach was used (Burnham and Anderson, 2002).
As there was no significant interaction between temperature and seasonal NAP (F1,4 = 0.189, P > 0.05), or significant effect when temperature is assessed as a covariate for change in seasonal NAP by ANCOVA (F1,6 = 2.1154, P > 0.05) (Supplementary Figure 4). The maximal model to assess for significant effect of temperature on NAP was by ANOVA.
2.5.3 Annual P. oceanica Productivity
Annual NAP and NCP was estimated by scaling up the three average seasonal daily NAP and NCP measurements; the November measurement was chosen to represent the period between October to January (123 days), April represented the period from February to May (120 days) and July represented June to September (122 days):
2.5.4 Influence of Spatial Dynamics on P. oceanica NAP
A Welch’s t-test, to account for unequal sample size, is applied to determine if the NAP differs between the central meadow area and the meadow edge. Then linear regressions are applied to the summer NAP measurements to determine if the difference in central meadow and meadow edge relate to changes in plant surface area, percentage cover, shoot density and blade length. Comparison of the R2 values provides an understanding of which seagrass biometric account for the variation in NAP and are the best spatial predictor for P. oceanica NAP.
2.5.5 Species Specific Productivity Comparison
The comparative species-specific NAPN2 data conforms to a normal distribution (W1,7 = 0.922, P > 0.05) and an ANOVA was used to assess if there was a significant difference in NAPN2 between seagrass species during summer. The NAP standardised for plant surface area also confirmed to normal distribution (W1,7 = 0.968, P > 0.05) therefore an ANOVA was used here also, and Tukey post hoc tests confirmed any pairwise differences between species.
3 Results
3.1 Seasonal P. oceanica NCP, NAP and NAPN
The P. oceanica meadow NCP is greater than the bare sand incubations in every season (Figure 3A). In autumn, the P. oceanica community is overall heterotrophic, as CR is greater than GPP (. 3 B and C). However, the oxygen deficit is still greater in the community without seagrass (NCP ( = -3.1, SD ± 7.62 O2 mmol m-2 d-1), than in the P. oceanica community (NCP = -1.8, SD ± 2.95 O2 mmol m-2 d-1) (Figure 3A). There is a significant seasonal influence on P. oceanica NCP (F2,7 = 10.924, P< 0.05). Pairwise post hoc comparisons show NCP is significantly higher in spring (NCP = 7.6, SD ± 2.84 O2 mmol m-2 d-1) and summer (NCP = 7.1, SD ± 2.75 O2 mmol m-2 d-1), compared to autumn when it is heterotrophic (Tukey: P< 0.05). There is no significant difference in NCP between the spring and summer.
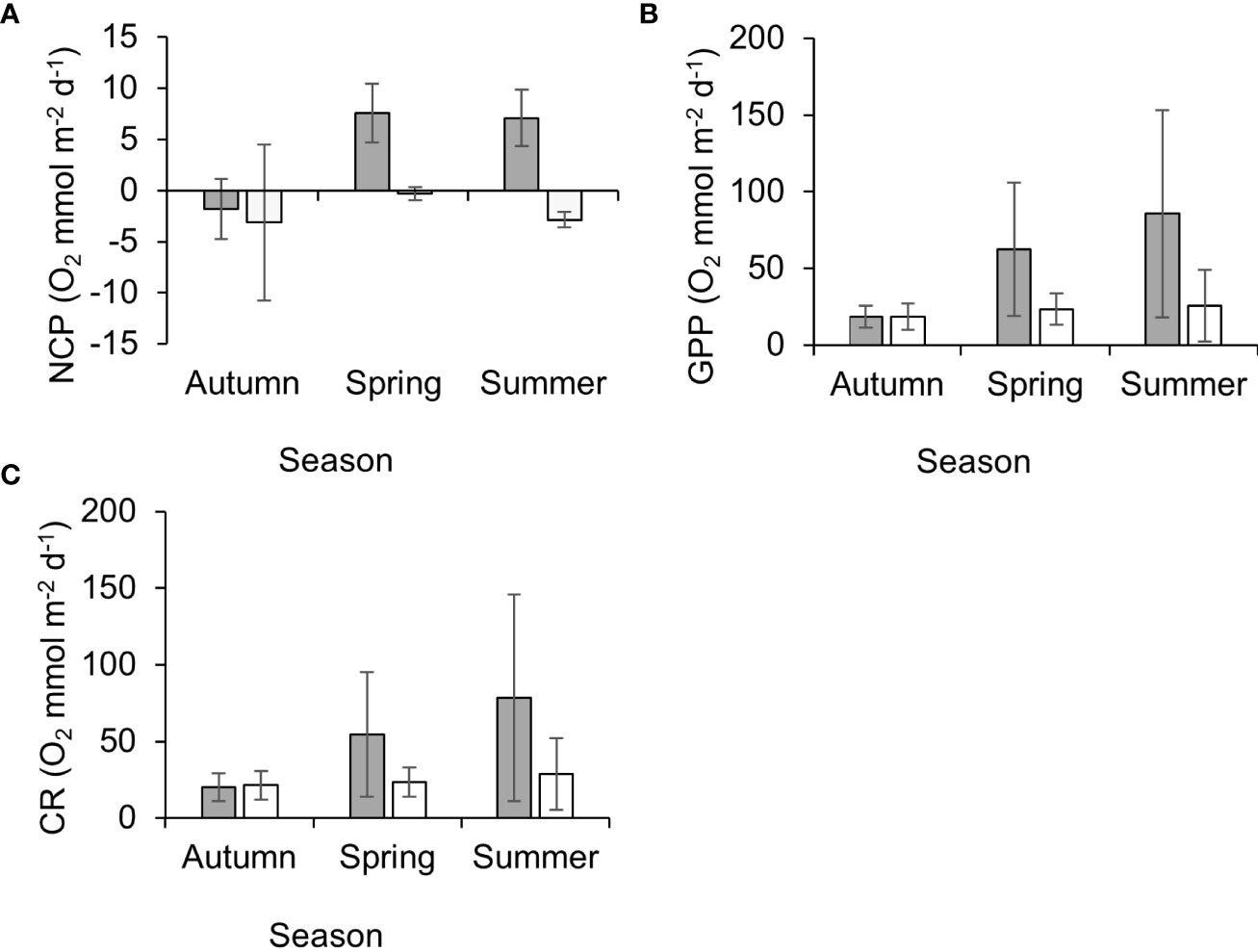
Figure 3 (A) Net community productivity (NCP), (B) Gross primary productivity (GPP) and (C) Community respiration (CR) of P. oceanica meadow edge (grey) and bare sand incubations (White) determined across seasons with large benthic chambers; autumn (November), spring (April) and summer (July). Error bars represent standard deviation (autumn and spring n = 3, summer n = 4).
During the transition from autumn to spring there is a large increase in GPP (GPP = 18.3, SD ± 7.11 O2 mmol m-2 d-1 to GPP = 62.3, SD ± 43.53 O2 mmol m-2 d-1, Figure 3B) and a simultaneous increase in CR (CR = 20.2, SD ± 9.21 O2 mmol m-2 d-1 to CR = 54.7, SD ± 40.75 O2 mmol m-2 d-1, Figure 3C). However, the increase in GPP is greater than the increase in CR transitioning the P. oceanica meadow from heterotrophic in autumn to autotrophic in spring (Figures 3B, C). The P. oceanica meadow stays in an autotrophic state in the Summer (Figures 3B, C) registering its highest GPP (GPP = 85.6, SD ± 67.52 O2 mmol m-2 d-1, Figure 3B), but also its highest CR (CR = 78.6, SD ± 67.51O2 mmol m-2 d-1, Figure 3C). The increase in GPP is not as great as the increase in CR, therefore the NCP is lower in summer compared to spring (Figure 3A). Further to this, bare sand incubations have a higher metabolic rate in the summer (NCP = -2.9, SD ± 0.74 O2 mmol m-2 d-1) compared to the spring (NCP = -0.3, SD ± 0.65 O2 mmol m-2 d-1, Figure 3A).Whilst the P. oceanica NCP is highest in spring, P. oceanica NAP is highest in summer (NAP = 9.9, SD ± 2.75 O2 mmol m-2 d-1) (Figure 4A). There remains a significant seasonal difference in P. oceanica NAP (F2,7 = 8.3885, P< 0.05). However, Tukey post hoc comparisons found NAP is only significantly lower in autumn compared to summer (Tukey: P< 0.05). Although there is an observational difference in NAP between the autumn (NAP = 1.3, SD ± 2.95 O2 mmol m-2 d-1) and spring (NAP = 7.9, SD ± 2.84 O2 mmol m-2 d-1) the difference is not significant. There remains no significant difference in NAP between the periods of highest productivity in spring and summer.
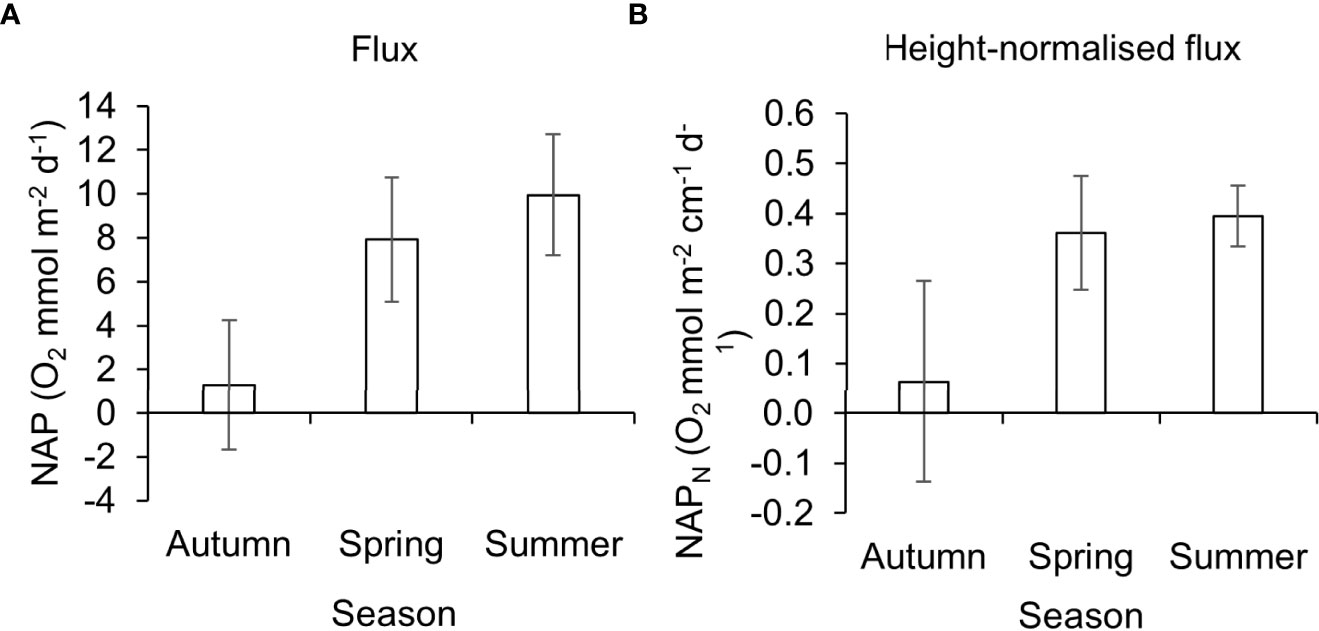
Figure 4 (A) Net Apparent Productivity (NAP) and (B) NAP canopy-height-normalised flux (NAPN) of P. oceanica meadow edge determined across seasons with large benthic chambers. Autumn and spring n = 3, summer n = 4. Error bars represent standard deviation.
The canopy height within the edge P. oceanica habitat is lowest during autumn ( = 15.4, SD ± 2.84 cm), this increases into spring ( = 22.3, SD ± 4.77 cm) and peaks during summer ( = 25.1, SD ± 5.90 cm). P. oceanica NAPN is lowest in autumn and most variable within autumn (NAPN = 0.01, SD ± 0.201 O2 mmol m-2 cm-1 d-1) (Figure 4B). In comparison P. oceanica NAPN in Summer (NAPN = 0.4, SD ± 0.06 O2 mmol m-2 cm-1 d-1), is less varied and similar to spring (NAPN = 0.4, SD ± 0.121 O2 mmol m-2 cm-1 d-1) (Figure 4B). Thus, the significant seasonal difference in NAPN remains, between autumn and summer (F2,7 = 6.3149 P< 0.05). There is no significant difference in NAPN between autumn and spring or spring and summer.
3.2 Influence of Environmental Conditions on P. oceanica NAP
The daily light integral ranged from 4.7 mol photons m–2 d–1 during autumn to 25.2 mol photons m-2 d-1 in the summer. Variation in environmental conditions within each season allowed for some overlap in the irradiance encountered between seasons and the P. oceanica NAP resembled a saturation curve when plotted as a function of irradiance (Figure 5A), as did the curve for NAPN (Figure 5B). When fit to the NAP dataset the AIC value of the hyperbolic tangent function versus linear model (AIC = 58.77 vs 61.72) confirmed the use of a light saturation model for demonstrating the presence of light saturation opposed to that of a linear function. The model fitting constants for NAP are Pmax= 64.853, Ik = 3.609 and RI = 55.646. The DLI conditions in autumn (minimum = 4.7 mol photons m–2 d–1) sit near the predicted irradiance compensation point (IC= 4.6 mol photons m–2 d–1) as such within the autumnal DLI range there are conditions where the plant may only just or may not maintain metabolic requirements due to low irradiance. In spring the seagrass transitions between light limiting and light saturating levels. The light saturating conditions occur throughout summer, when NAP (NAP = 9.9, SD ± 2.75 O2 mmol m-2 d-1Figure 4A) sits near the predicted maximum (9.21 O2 mmol m-2 d-1, Figure 5A). The changes in the DLI account for 71% of the variation in P. oceanica NAP (Figure 4A). The period of summer light saturation also corresponds to when NAPN is less varied (Figure 4B).
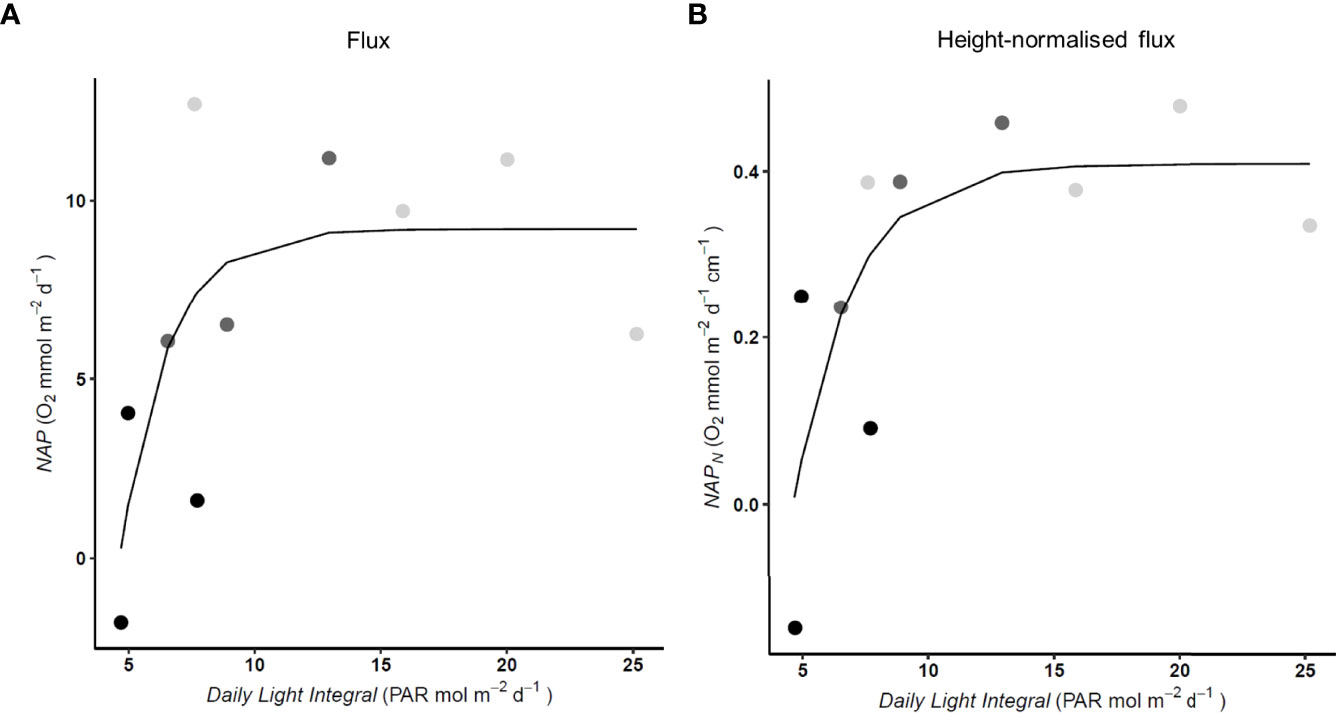
Figure 5 (A) Net Apparent Productivity (NAP) (R2 = 0.713) and (B) NAP canopy-height-normalised flux (NAPN) (R2 = 0.711) presented as a function of PAR given as the Daily Light Integral, for P. oceanica meadow edge determined across seasons with large benthic chambers. The irradiance compensation point (IC) was 4.6 mol photons m–2 d–1. Replicate season denoted for visual reference autumn (●), spring () and summer (
).
The water temperature was lowest in spring ( = 17.7, SD ± 0.13 °C), increasing to a summer high ( = 23.6, SD ± 0.40 °C). The autumn temperatures encountered sat at a relative mid-point ( = 20.7, SD ± 0.45 °C) between spring and summer, yet the NAP was lowest in autumn. Therefore, the change in NAP and was not relative to the change in water temperature and not a statistically significant factor in our model (Supplementary Figure 4), with season (Temperature F1,6 = 2.1154, P > 0.05) or without season (Temperature F1,8 = 0.7265, P > 0.05).
3.3 Annual Productivity of P. oceanica Edge Habitat
The annual NAP of the P. oceanica edge habitat in this meadow was estimated at 2.3 C mol m-2 yr-1. However, the organisms present within the P. oceanica meadow have their own metabolic requirements (NCP without seagrass present -0.8 C mol m-2 yr-1), as such the net carbon gain at the community level for this P. oceanica meadow was less than the NAP produced annually by the P. oceanica (NCP = 1.5 C mol m-2 yr-1).
3.4 Influence of Spatial Dynamics on P. oceanica NAP
The P. oceanica NAP in summer is significantly different between the central areas of the meadow and the meadow edge (t (1,4) = 3.7647, P< 0.05). (Figure 6). The P. oceanica NAP is highest in the central areas of the meadow (NAP =19.7, SD± 3.83 O2 mmol m-2 d-1) and approximately two-fold that of the edge habitat (NAP =9.9, SD± 2.75 O2 mmol m-2 d-1). The P. oceanica NAP increases relative to the plant surface area of P. oceanica (LM: F1,5 = 132.5, P< 0.01) (Figure 6A). The plant surface area was the best predictor of NAP and accounted for more than 96% of the variation in NAP. As standalone biometrics of seagrass meadow metabolism, shoot density (m2), blade length (cm) and seagrass cover (%), were all significant predictors of NAP (shoot density, LM: F1,5 = 21.13, P< 0.01; blade length, LM: F1,5 = 12.98, P< 0.05; percentage cover, LM: F1,5 = 8.78, P< 0.05) but they only accounted for 81%, 72% and 64% (Figures 6B−D) of the variation in NAP of the P. oceanica.
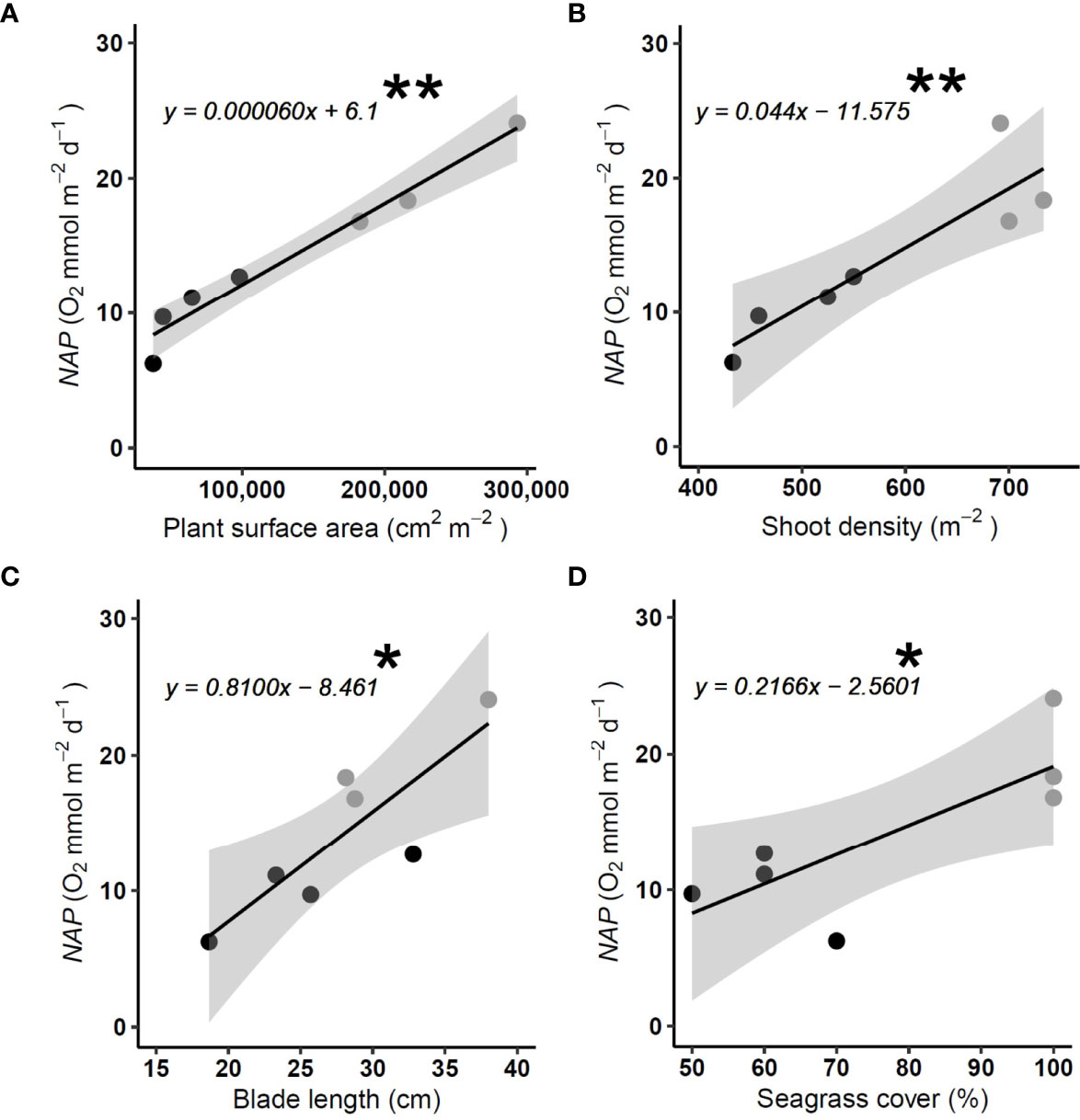
Figure 6 Net apparent productivity (NAP) of P. oceanica meadow edge (Black) and meadow center (grey) determined in Summer with large benthic chambers, in relation to (A) P. oceanica blade surface area (B) P. oceanica shoot density (m-2) (C) P. oceanica blade length (cm) and (D) P. oceanica cover (%). Central meadow n = 3, meadow edge n = 4. Significant linear regression from edge and central meadow combined * = P < 0.05, ** = P < 0.01. Grey bands reflect the 95% confidence level interval for predictions from a linear model.
3.5 Species Specific Productivity
The seagrass benthic communities at 7m depth are all heterotrophic at the community level, ranging from the least heterotrophic with H. stipulacea present (NCP = -4.8, SD ± 4.7 O2 mmol m-2 d-1), followed by the community with P. oceanica present (NCP = -7.5, SD ± 8.9 O2 mmol m-2 d-1) and finally the most heterotrophic the C. nodosa dominant community (NCP = -10.5, SD ± 6.0 O2 mmol m-2 d-1) (Figure 7A). The difference spatially in above ground vegetative material between species is demonstrated through the increasing total plant surface between species from H. stipulacea ( = 5.1, SD ± 1.4 x10-3 cm2 m-2), to C. nodosa ( = 10.6, SD ± 9.4 x10-3cm2 m-2) and culminating in P. oceanica ( = 17.6, SD ± 7.3 x10-3cm2 m-2) (Figure 7B). However, the plant surface area is also more uniform for H. stipulacea spatially across the habitat sampled, in comparison the plant surface area of both C. nodosa and P. oceanica show higher variation between samples (Figure 7B).
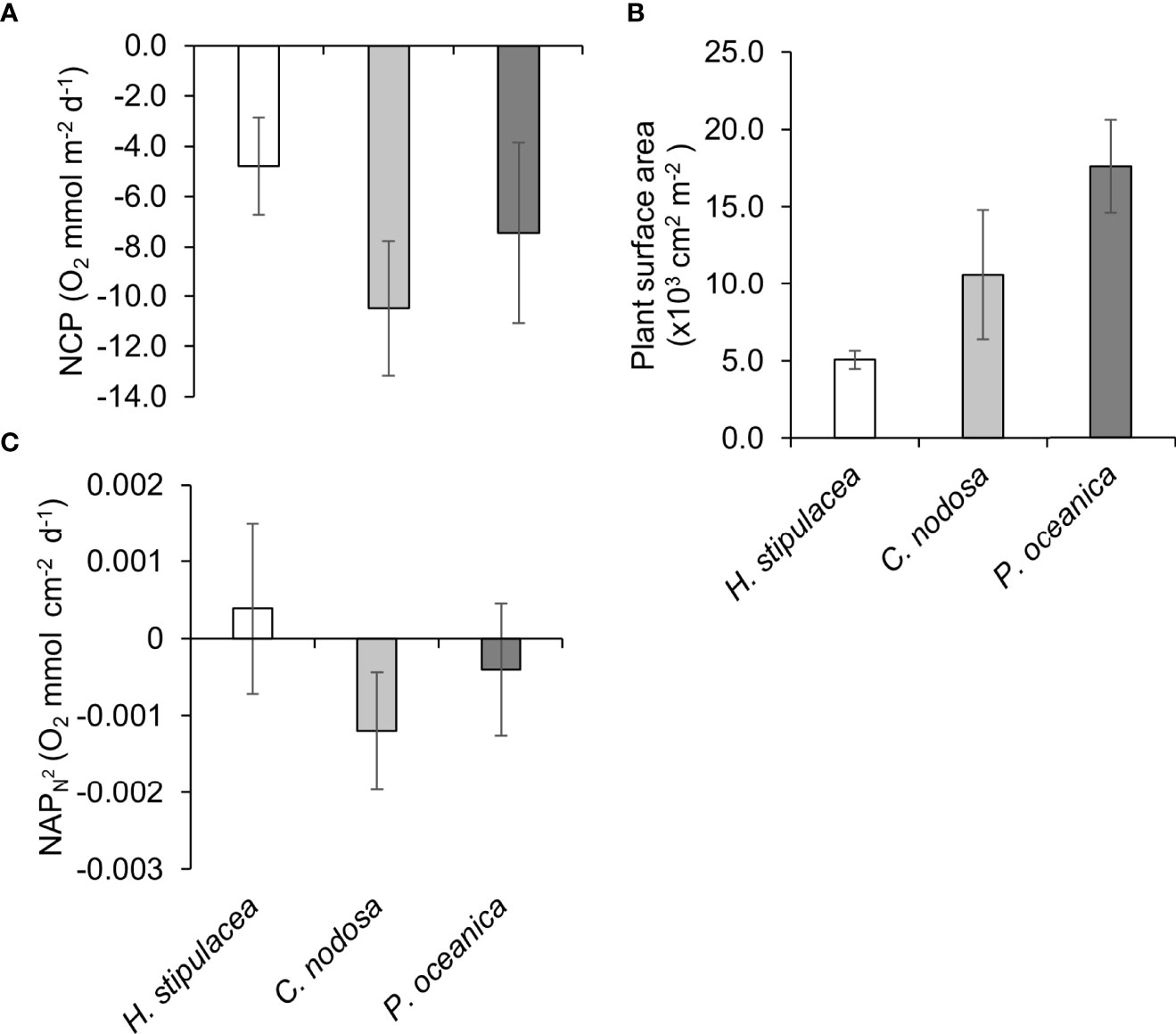
Figure 7 (A) Net community productivity (NCP), (B) Plant surface Area (C) NAP plant-surface area-normalised flux (NAPN2), for sparse H. stipulacea (n = 6), C. nodosa (n = 5) and P. oceanica (n = 6) determined in summer by small benthic chambers. Error bars represent standard deviation.
H. stipulacea NAPN2 appears to be the highest comparatively and the only seagrass species to on average maintain positive carbon balance (O2 > 0) (Figure 7C). However, variation in NAPN2 for H. stipulacea (NAPN2 ( = 0.0004, SD ± 0.0011 O2 mmol cm-2 d-1) and P. oceanica (NAPN2 ( = -0.0004, SD ± 0.0009 O2 mmol cm-2 d-1) show both species fluctuate between positive and negative carbon balance within this area of the bay in Summer (Figure 7C). Whilst C. nodosa NAPN2 is the lowest and consistently negative (NAPN2 ( = -0.0012, SD ± 0.0007 O2 mmol cm-2 d-1). A significant difference in NAPN2 does occur between species (F2,14 = 4.1922, P< 0.05). Tukey post hoc comparisons confirm C. nodosa NAPN2 to be significantly lower than H. stipulacea NAPN2 (Tukey: P< 0.05). But there was no significant difference between the native species C. nodosa and P. oceanica NAPN2 and also no significant difference in NAPN2 between H. stipulacea and P. oceanica.
4 Discussion
4.1 Seasonal P. oceanica NCP, NAP and NAPN
We found strong seasonality in P. oceanica productivity, at both the community (NCP Spring = 7.6, SD ± 2.84; Autumn = -1.8, SD ± 2.95 O2 mmol m-2 d-1) and plant level (NAP Summer = 9.9, SD ± 2.75; Autumn ( = 1.3, SD ± 2.95 O2 mmol m-2 d-1), which reinforces the need for seagrass carbon sequestration studies to consider year-round dynamics, thereby ensuring carbon storage estimates are not exaggerated by only reflecting summer peaks. Similar conclusions from studies of net ecosystem productivity in freshwater lakes (Brentrup et al., 2020), temperate saltmarshes (Vazquez-Lule & Vargas, 2021) and seagrass habitat (Champenois and Borges, 2012; Caffray et al., 2014), suggest that this should be the norm. Strong seasonality of P. oceanica NCP in the Western Mediterranean typically occurs after an October minimum coinciding with increases in aboveground biomass, followed by a decrease through summer and autumn when aboveground biomass is stable and then decreases (Champenois and Borges, 2012). Similarly in the Eastern Mediterranean but within the western region of the Aegean Sea strong seasonality in NCP is apparent with a marked decrease from June to August, before reaching its minimum in October. Therefore, our study adds to the evidence that single season measures of seagrass NCP are inappropriate, given autumnal lows are a typical artifact of P. oceanica studies across the Mediterranean.
Multi-season approaches enable the assessment of seasonal drivers such as irradiance (Gazeau et al., 2005; Champenois and Borges, 2012). During autumn, the overall irradiance reaching the plant is lower as the daylight period is shorter, the likelihood of cloud cover increases, and increased frequency of stormy conditions results in greater hydrodynamic activity reducing in-water visibility. Subsequently autumnal photosynthetic activity occurs when conditions are light limiting, promoting a greater variability in productivity. In contrast light saturating levels present throughout summer, lead to a lower P. oceanica photosynthetic efficiency and saturation is evident in this study given the low variation in summer productivity. Long-term exposure to saturating irradiance decreases plant productivity and can lead to photoinhibition (Ralph and Burchett, 1995), though a decline from photoinhibition is not considered in this study. The saturation response alone has important considerations for modelling total fixed carbon, re-emphasising the importance of seasonality which is dependent on the concurrent irradiance levels. Physical disturbance to seagrass meadows such as autumnal and winter storms will seasonally reduce the available photosynthetically active plant material, i.e., as storms tear seagrass blades. Although we have considered seasonal changes in seagrass productivity (NAP) and presented this standardised by any seasonal changes in seagrass canopy height (NAPN), we have not directly measured vegetative export from the meadow over winter, which can be vast enough to create seagrass exported onshore forms called banquettes (Gomez-Pujol et al., 2013); in short – large, heaped mounds of decaying seagrass on the shoreline. The seasonal storm export of biomass from these meadows needs to be determined, because the retention of plant material is intrinsically linked to its carbon sequestration potential. Senescence the shedding and loss of leaf material at the end of the summer season which can be at a scale of >85% of P. oceanica leaf production (Cebrian and Duarte, 2001), would also lead to reduced productivity in autumn as less photosynthetically active material would remain within the meadow. In fact the canopy height of the shallow sparse P. oceanica within this meadow is notably reduced in the autumn with the loss of more mature longer blades and likely a contributary factor to the observed low productivity.Given P. oceanica meadows within the eastern region of the Aegean Sea have been noted to be fragmented in response to elevated sea surface temperatures (Chefaoui et al., 2017), it is surprising we found no statistically significant influence of temperature on the productivity of shallow water P. oceanica. Other temperate seagrass species have been shown to transition to a negative carbon balance when exposed to high summer water temperatures (Marsh Jr. et al., 1986). Although our highest average daily water temperature was above optimal thermal conditions (23.9 vs 17 - 20 °C) for P. oceanica (Champenois and Borges, 2018), this was well below P. oceanica critical temperature limits (> 29 °C). The in-situ nature of this study does not allow for the independent control of water temperature or light intensity encountered at any time, and so they are often correlated making it challenging to differentiate between them. Shallow water P. oceanica has a greater tolerance to increased water temperatures than deeper P. oceanica (Marin-Guirao et al., 2016). This heat acclimation in shallow P. oceanica emerges by stabilising respiration, thereby establishing respiratory homeostasis (i.e., balanced photosynthetic carbon gains and respiratory carbon losses; Marin-Guirao et al., 2016). This suggests that shallow P. oceanica may maintain net productivity gains at higher water temperatures for longer periods. It is also possible that the P. oceanica within the Eastern Mediterranean may have a greater acclimation to increased water temperature, as the Eastern Mediterranean basin has been exposed to higher sea surface temperatures for longer than the Western basin (since 1985) (Nykjaer, 2009). Ultimately any temperature effect on P. oceanica metabolism in this study was confounded by the strong irradiance effect and thus could not be disentangled.
4.2 Annual P. oceanica NCP and NAP
The annual net community productivity for P. oceanica meadows in our study was lower than recorded for two P. oceanica meadows previously studied in the western region of the Aegean Sea, even one negatively impacted by eutrophication (4.83 C mol m-2 yr-1) (Apostolaki et al., 2010), however that study used smaller chambers, which are more likely to cause oxygen saturation which can overestimate productivity. The annual NCP in our study was conservative as it was obtained from patchy P. oceanica edge habitat, this underestimates the meadows total fixed carbon which consisted of both dense and patchy habitat. However, in shallow water meadows, patchy edge habitat is a more prominent feature (Montefalcone et al., 2009), therefore it is an appropriate conservative annual NCP estimate. The annual NCP of 1.5 C mol m-2 yr-2 calculated for shallow P. oceanica in the Eastern Mediterranean would benefit from sampling at a bimonthly or monthly regime to better ascertain the switch point when the meadow transitions from autotrophic to heterotrophic. However annual metabolism rates of P. oceanica in the Eastern Mediterranean are rare and therefore this estimation remains important.
Our measures of P. oceanica meadow centre productivity was two-fold that of the edge habitat, therefore shallow P. oceanica meadow can support annual total fixed carbon at between 2.3 - 4.6 C mol m-2 yr-2. The carbon sequestration potential of seagrass meadows ultimately relates to how much of the total fixed carbon produced by the seagrass, i.e., the productivity, accumulates as refractory material in the seagrass meadow. It has been estimated that P. oceanica has the capacity to sequester in the sedimentary compartment around 21% of the carbon fixed by primary production (Pergent-Martini et al., 2021). However, reduced current attenuation should promote significantly higher organic carbon concentration with distance from the edge (Oreska et al., 2017). The patchiness of shallow meadows may therefore render them less effective at attenuating currents and promoting settlement of organic particles leading to a lower capacity for the sequestration of photosynthetically fixed carbon. Despite a lower potential for patchy P. oceanica to fix and sequester the fixed carbon compared to other seagrass meadows they still actively contribute to carbon acquisition within the carbon cycle. If shallow patchy P. oceanica meadows transitioned to an unvegetated state this would result in the loss of this photosynthetic carbon fixation, transitioning from an autotrophic habitat to one that functions as a carbon source (i.e., bare sand habitat). Given a total of 7139.92 km2 of P. oceanica is estimated to occur in the Eastern Mediterranean Basin (Telesca et al., 2015), and seagrass coverage within Greek waters has been estimated at 2619 km2 (Topouzelis et al., 2018), P. oceanica within Greek waters represents around 36.7% of the total P. oceanica in the Eastern Mediterranean. The quantity of P. oceanica habitat within the Aegean Sea and Eastern Mediterranean means it represents a substantial blue carbon habitat irrespective of its fragmented nature (Chefaoui et al., 2018). The further loss of this habitat would risk current climate change targets therefore seagrass meadows need distinct conservation management strategies given their proximity to current threats including impacts from human infrastructure and coastal activities (Giakoumi et al., 2015).
4.3 Spatial Dynamics of P. oceanica NAP
Metabolism measurements are typically presented as an area-based unit (Apostolaki et al., 2010; Duarte et al., 2010; Olive et al., 2015). We have shown Net Apparent Productivity to be affected by spatial variation in plant aboveground biomass, this would suggest that reported estimates may benefit from additional information on canopy height and shoot density, particularly in the case of P. oceanica. Estimates should therefore potentially move away from denoting discrete areal measurements for meadows. Instead acquiring and mapping large scale data on canopy height (shoot size) and shoot density to enable comprehensive habitat wide total fixed carbon estimates. Acquiring seagrass biometric data to support productivity estimates requires greater effort than collating presence-absence or coverage data, but simple plant biometrics of shoot density and leaf area index have been demonstrated as standalone predictors of carbon sequestration and community composition (Samper-Villarreal et al., 2016; Collier et al., 2021). High shoot density in shallow areas with a high degree of patchiness creates very specific nest-like patterns, these have their highest shoot density in the centre and decrease in density radially towards the edge of the patches (Zupo et al., 2005). Therefore, this distinction in spatial productivity between edge and central areas of P. oceanica may be a specific characteristic to consider for shallow meadows because overall shoot densities are highest in the shallow and decrease with depth (Olesen et al., 2002; Zupo et al., 2005). More work would be needed to determine if the same distinctions in shoot density and subsequent primary productivity exist between the edge and central areas of P. oceanica meadows in deeper waters. The use of chambers with a larger benthic surface area has a greater representation of community-scale metabolism, as trialled for heterogenic coral reef habitats (Yates and Halley, 2003). The use of a larger benthic chamber for P. oceanica metabolism measurements in this study (1m diameter) comprises community metabolism measurements influenced by fine scale variation in shoot density, compared to previous studies using smaller chambers (0.18m diameter), which house only a few shoots at similar densities within the benthic chamber (Gazeau et al., 2005; Barron et al., 2006).
4.4 Species Specific NCP and NAPN2
Whilst we found shallow central and edge P. oceanica meadow (2m) to be autotrophic in the Summer, P. oceanica at 7m depth, where it is found mixed with C. nodosa and H. stipulacea, fluctuated between positive and negative carbon balance. Such heterogeneity within the same bay highlights that adjacent P. oceanica patches may simultaneously undergo growth and loss. Given the health of the P. oceanica at 7m depth was decreased indicated by the reduced plant surface area ( = 17.6, SD ± 7.3 x10-3 cm2 m-2) relative to the shallow area where the plant surface area was higher (Ranging from 37.6 – 293.0 x10-3 cm2 m-2) and given plant surface area acts as a predictor of productivity in the shallower areas, lower productivity and in this case the heterotrophic nature may be explained by these characteristics. It must also be considered that our results for this comparison are influenced by differences in chamber area, volume, and incubation time.
Based on our productivity assessments alone, the non-native H. stipulacea ability to fix carbon was comparable to P. oceanica (NAPN2 ( = 0.0004, SD ± 0.0011 vs ( = -0.0004, SD ± 0.0009 O2 mmol m-2 d-1). H. stipulacea’s NAP is positive and the P. oceanica fluctuates between positive and negative carbon balance, suggesting that both can maintain a balance between carbon fixation and carbon utilised for carbon metabolic activity. Which explains why H. stipulacea has the capability to opportunistically colonise space previously occupied by P. oceanica. With regression of P. oceanica meadows well documented (Telesca et al., 2015), alongside H. stipulacea range expansion (Georgiou et al., 2016), maintenance of Mediterranean seagrass meadow areal cover through replacement of current seagrass species cover is likely. Whilst H. stipulacea has persisted within the Mediterranean since 1894 and expanded its known range (Fritsch, 1895; van der Velde and den Hartog, 1992; Gambi et al., 2009; Tsiamis et al., 2010; Sghaier et al., 2011; Georgiou et al., 2016). H. stipulacea is known to disappear and recolonise as per a metapopulation (Gambi et al., 2009), making the persistence of its sedimentary carbon stocks questionable. While questions remain, H. stipulacea is considered to be making positive contributions to community blue carbon in the eastern Aegean (Apostolaki et al., 2019). In fact, carbon sequestration into the sedimentary compartment by H. stipulacea has been estimated to be greater than P. oceanica in the Eastern Mediterranean (Wesselmann et al., 2021). However, H. stipulacea carbon sequestration is not yet greater than P. oceanica carbon sequestration estimates in the Western Mediterranean (Mazarrasa et al., 2017). H. stipulacea productivity in the Mediterranean is lower than its native range, given irradiance was the driving factor in H. stipulacea productivity within its home range in the Gulf of Aquaba and its generally tropical origins (Cardini et al., 2018), under future climate change predictions of a warming Mediterranean H. stipulacea has potential to increase its productivity, whilst the same cannot be said for P. oceanica.The replacement of P. oceanica by C. nodosa is well documented (Montefalcone et al., 2006; Montefalcone et al., 2007). This replacement is typically considered a result of C. nodosa being more tolerant to varying environmental conditions. Compared against six dominant native Mediterranean macrophytes C. nodosa presented the highest thermal optima (Savva et al., 2018). But the substitution of P. oceanica for C. nodosa is not exclusive, replacement of P. oceanica dead matte to algae dominated Caulerpa spp. habitats also occurs (Montefalcone et al., 2007). C. nodosa may not always be the ‘winner’ following loss or fragmentation of P. oceanica meadows. Whilst the C. nodosa and H. stipulacea in the deeper part of this meadow had different plant surface areas (C. nodosa = 10.6, SD ± 9.4 cm2; H. stipulacea = = 5.1, SD ± 1.4 cm2), when their biomass is comparable C. nodosa would be expected to deteriorate as it is utilising carbon more than it is fixing, whilst H. stipulacea would maintain a positive carbon balance. In the Southern Mediterranean replacement of C. nodosa by H. stipulacea under warmer environmental conditions has already been demonstrated to occur (Sghaier et al., 2014). Very shallow water will be readily heated by the sun, in the North Mediterranean this may provide warmer conditions closer to the tropical native range of H.stipulacea. Within our study site, but at a shallower location (0.5m depth), H. stipulacea was found mixed within dense C. nodosa beds and growing multiple paired leaves off a vertical stem (Pers. obv., Supplementary Figure 5). This is important given lateral growth is more typical of this species (Posluszny and Tomlinson, 1991; Winters et al., 2020). Vertical growth would give H. stipulacea the potential to increase its biomass relative to C. nodosa. However, C. nodosa colonisation has been shown to shift the benthic sediment community from autotrophic (e.g., microphytobenthic) to strongly heterotrophic when associated with mature stands of C. nodosa (Barron et al., 2004). Therein the negative productivity documented here in association with C. nodosa could be representative of a strong benthic community shift towards respiration within the C. nodosa enclosed benthic chambers.
5 Conclusions
This study establishes that not all seagrass habitat is equal in its capacity to fix carbon, but the variability can in part be accounted for seasonally and spatially; using environmental predictors such as irradiance; plant biometrics such as plant surface area; and determining species specific assessments particularly in mixed species seagrass meadows. Here seagrass meadows in the eastern Mediterranean have been used as an archetype to demonstrate the high levels of spatio-temporal variability in these plants ability to fix carbon. Which highlights the importance of protecting Eastern Mediterranean seagrass meadows for their capacity to naturally fix carbon, even when shallow and patchy in nature. If the true value of natural ecosystems such as seagrass meadows are to be understood particularly for their capacity to sequester carbon, finetuning knowledge to account for the natural spatial and seasonal variation in these ecosystems needs to take place.
Data Availability Statement
The raw data supporting the conclusions of this article is available to access at the following link: http://researchdata.essex.ac.uk/153/.
Author Contributions
EW, TC, TW, CA, TT, and AM contributed to the study conception and design. EW and CA led the field-based studies, supported by TT and AM. TW led a preliminary pilot study integral to our final experimental design. EW and TC led on data analysis and the manuscript first draft. All authors were involved in the revision and final approval of this version of the manuscript.
Funding
The Erasmus+ Programme provided funding for TW and EW to complete student placements with Archipelagos Institute of Marine Conservation (AIMC). The University of Essex supported CA. AIMC, the University of Essex and the UKRI Natural Environment Research Council (NE/R016569) funded the collaboration, purchase of field equipment and time spent by TT, AM, and TCC on this project.
Conflict of Interest
The authors declare that the research was conducted in the absence of any commercial or financial relationships that could be perceived as a potential conflict of interest.
Publisher’s Note
All claims expressed in this article are solely those of the authors and do not necessarily represent those of their affiliated organizations, or those of the publisher, the editors and the reviewers. Any product that may be evaluated in this article, or claim that may be made by its manufacturer, is not guaranteed or endorsed by the publisher.
Acknowledgments
Thank you to Jack Wade for technical support related to the design, assembly, and maintenance of the submersible pump system. The following interns from AIMC assisted with fieldwork operations: Camilla Hansen, Jente van Langerak, Nikos Papagiannopoulos and Saulė Medelytė;. The works presented here formed part of the study towards a Masters by Dissertation (EW) and Masters of Science (CA and TW) at the University of Essex.
Supplementary Material
The Supplementary Material for this article can be found online at: https://www.frontiersin.org/articles/10.3389/fmars.2022.891467/full#supplementary-material
References
Abadie A., Gobert S., Bonacorsi M., Lejeune P., Pergent G., Pergent-Martini C. (2015). Marine Space Ecology and Seagrasses. Does Patch Types Matter in Posidonia Oceanica Seascapes? Ecol. Indic. 57, 435–446. doi: 10.1016/j.ecolind.2015.05.020
Abadie A., Pace M., Gobert S., Borg J. A. (2018). Seascape Ecology in Posidonia Oceanica Seagrass Meadows: Linking Structure and Ecological Processes for Management. Ecol. Indic. 87, 1–13. doi: 10.1016/j.ecolind.2017.12.029
Alcoverro T., Manzanera M., Romero J. (2001). Annual Metabolic Carbon Balance of the Seagrass Posidonia Oceanica: The Importance of Carbohydrate Reserves. Mar. Ecol. Prog. Ser. 211, 105–116. doi: 10.3354/meps211105
Apostolaki E. T., Holmer M., Marba N., Karakassis I. (2010). Metabolic Imbalance in Coastal Vegetated (Posidonia Oceanica) and Unvegetated Benthic Ecosystems. Ecosystems 13, 459–471. doi: 10.1007/s10021-010-9330-9
Apostolaki E. T., Vizzini S., Santinelli V., Kaberi H., Andolina C., Papathanassiou E. (2019). Exotic Halophila Stipulacea is an Introduced Carbon Sink for the Eastern Mediterranean Sea. Nat. Sci. Rep. 9, 9643. doi: 10.1038/s41598-019-45046
Arnaud-Haond S., Migliaccio M., Diaz-Almela E., Teixeira S., van de Vliet M. S., Alberto F., et al. (2007). Vicariance Patterns in the Mediterranean Sea: East-West Cleavage and Low Dispersal in the Endemic Seagrass Posidonia Oceanica. J. biogeography 34, 963–976. doi: 10.1111/j.1365-2699.2006.01671.x
Barron C., Duarte C. M. (2009). Dissolved Organic Matter (DOM) Release in a Posidonia Oceanica Meadow. Mar. Ecol. Ser. 374, 75–84. doi: 10.3354/meps07715
Barron C., Duarte C. M., Frankignoulle M., Borges A. V. (2006). Organic carbon metabolism and carbonate dynamics in a Mediterranean seagrass (Posidonia oceanica) meadow. Estuaries and Coasts, 29, 417–426.
Barron C., Marbe N., Terrados J., Kennedy H., Duarte C. M. (2004). Community Metabolism and Carbon Budget Along a Gradient of Seagrass (Cymodocea Nodosa) Colonization. Limnol. Oceanogr. 49, 1642–1651. doi: 10.4319/lo.2004.49.5.1642
Berg P., Delgard M. L., Polsenaere P., McGlathery K. J., Doney S. C., Berger A. C. (2019). Dynamics of Benthic Metabolism, O2 and Pco2 in a Temperate Seagrass Meadow. Assoc. Sci. Limnol. Oceanogr. 64, 2586–2604. doi: 10.1002/lno.11236
Borg J. A., Rowden A. A., Attrill M. J., Schembri P. J., Jones M. B. (2006). Wanted Dead or Alive: High Diversity of Macroinvertebrates Associated With Living and ‘Dead’ Posidonia Oceanica Matte. Mar. Biol. 149, 667–677. doi: 10.1007/s00227-006-0250-3
Boudouresque C. F., Bernaud G., Pergent G., Shili A., Verlaque M. (2009). Regression of Mediterranean Seagrasses Caused by Natural Processes and Anthropogenic Disturbances and Stress: A Critical Review. Botanica Marina 52, 395–418. doi: 10.1515/BOT.2009.057
Brentrup J. A., Richardson D. C., Carey C. C., Ward N. K., Bruesewitz D. A., Weathers K. C. (2020). Under-Ice Respiration Rates Shift the Annual Carbon Cycle in the Mixed Layer of an Oligotrophic Lake From Autotrophy to Heterotrophy. Inland waters 11, 114–123. doi: 10.1080/20442041.2020.1805261
Burnham K. P., Anderson D. R. (2002). Model Selection and Multimodel Inference: A Practical Information-Theoretic Approach. 2nd ed (New York: Springer).
Caffray J. M., Murrell M. C., Amacker K. S., Harper J. W., Phipps S., Woodrey M. S. (2014). Seasonal and Inter-Annual Patterns in Primary Production, Respiration, and Net Ecosystem Metabolism in Three Estuaries in the Northeast Gulf of Mexico. Estuaries Coasts 37, 222–241. doi: 10.1007/s12237-013-9701-5
Cebrian J., Duarte C. M. (2001). Detrital Stocks and Dynamics of the Seagrass Posidonia Oceanica (L.) Delile in the Spanish Mediterranean. Aquat. Bot. 70, 295–309. doi: 10.1016/S0304-3770(01)00154-1
Champenois W., Borges A. V. (2012). Seasonal and Inter-Annual Variations of Community Metabolism Rates of a Posidonia Oceanica Seagrass Meadow. Limnol. Oceanogr. 57, 347–361. doi: 10.4319/lo.2012.57.1.0347
Champenois W., Borges A. V. (2018). Inter-Annual Variations Over a Decade of Primary Production of the Seagrass Posidonia Oceanica. Limnol. Oceanogr. 64, 32–45. doi: 10.1002/lno.11017
Cardini U., van Hoytema N., Bednarz V. N., Al-Rshaidat M. M. D., Wild C. (2018). N2 fixation and primary productivity in a red sea Halophila stipulacea meadow exposed to seasonality. Limnology and Oceanography, 63, 786–798.
Chefaoui R. M., Duarte C. M., Serrão E. A. (2017). Paleoclimatic Conditions in the Mediterranean Explain Genetic Diversity of Posidonia Oceanica Seagrass Meadows. Nat. Sci. Rep. 7, 2732. doi: 10.1038/s41598-017-03006-2
Chefaoui R. M., Duarte C. M., Serrao E. A. (2018). Dramatic loss of seagrass habitat under projected climate change in the Mediterranean Sea. Global Change Biology, 24, 4919–4928.
Cole J. J., Pace M. L. (2000). Persistence of Net Heterotrophy in Lakes During Nutrient Addition and Food Web Manipulations. Limnol. Oceanogr. 45, 1718–1730. doi: 10.4319/lo.2000.45.8.1718
Collier C. J., Langlois L. M., McMahon K. M., Udy J., Rasheed M., Lawrence E., et al. (2021). What Lies Beneath: Predicting Seagrass Below-Ground Biomass From Above-Ground Biomass, Environmental Conditions and Seagrass Community Composition. Ecol. Indic. 121, 107156. doi: 10.1016/j.ecolind.2020.107156
Cox T. E., Schenone S., Delille J., Diaz-Castaneda V., Alliouane S., Gattuso J.-P., et al. (2015). Effects of Ocean Acidification on Posidonia Oceanica Epiphytic Community and Shoot Production. J. Ecol. 103, 1594–1609. doi: 10.1111/1365-2745.12477
Duarte C. M., Losada I. J., Hendriks I. E., Mazarrasa I., Marba N. (2013). The Role of Coastal Plant Communities for Climate Change Mitigation and Adaptation. Nat. Climate Change 3, 961–968. doi: 10.1038/nclimate1970
Duarte C. M., Marba N., Gacia E., Fourqurean J. W., Beggins J., Barron C., et al. (2010). Seagrass Community Metabolism: Assessing the Carbon Sink Capacity of Seagrass Meadows. Global Biogeochemical Cycles 24, GB4032. doi: 10.1029/2010GB003793
Folkard A. M. (2005). Hydrodynamics of Model P. Oceanica Patches in Shallow Water. Limnol. Oceanogr. 50, 1592–1600. doi: 10.4319/lo.2005.50.5.1592
Frankignoulle M., Bouquegneau J. M. (1987). Seasonal Variation of the Diel Carbon Budget of a Marine Macrophyte Ecosystem. Mar. Ecol. Prog. Ser. 38, 297–299. doi: 10.3354/meps038197
Fritsch C. (1895). Uber Die Auffindung Einer Marinen Hydrocharidae Im Mittelmeer. Verhandlungen der Zoologisch Botanischen gesamten Wien 45, 104–106.
Gambi M. C., Barbieri F., Bianchi C. N. (2009). New Record of the Alien Seagrass Halophila Stipulacea (Hydrocharitaceae) in the Western Mediterranean: A Further Clue to Changing Mediterranean Sea Biogeography. Mar. Biodiversity Records 2, e84. doi: 10.1017/S175526720900058X
Gazeau F., Duarte C. M., Gattuso J.-P., Barron C., Navarro N., Ruiz S., et al. (2005). Whole System Metabolism and CO2 Fluxes in a Mediterranean Bay Dominated by Seagrass Beds (Palma Bay, NW Mediterranean). Biogeosciences 2, 43–60. doi: 10.5194/bg-2-43-2005
Georgiou D., Alexandre A., Luis J., Santos R. (2016). Temperature is Not a Limiting Factor for the Expansion of Halophila Stipulacea Throughout the Mediterranean Sea. Mar. Ecol. Prog. Ser. 544, 159–167. doi: 10.3354/meps11582
Gerakaris V., Lardi P., Issaris Y. (2019). First Record of the Tropical Seagrass Species Halophila Decipiens Ostenfeld in the Mediterranean Sea. Aquat. Bot. 160, 103151. doi: 10.1016/j.aquabot.2019.103151
Giakoumi S., Halpern B. S., Michel L. N., Gobert S., Sini M., Boudouresque C.-F., et al. (2015). Towards a Framework for Assessment and Management of Cumulative Human Impacts on Marine Food Webs. Conserv. Biol. 29, 1228–1234. doi: 10.1111/cobi.12468
Gomez-Pujol L., Orfila A., Alvarez-Ellacuriaa A., Terrados J., Tintore J. (2013). Posidonia Oceanica Beach-Cast Litter in Mediterranean Beaches: A Coastal Video Monitoring Study. J. Coast. Res. 65, 1768–1773. doi: 10.2112/SI65-299.1
Holmer M., Argyrou M., Dalsgaard T., Danovaro R., Diaz-Almela E., Duarte C. M., et al. (2008). Effects of Fish Farm Waste on Posidonia Oceanica Meadows: Synthesis and Provision of Monitoring and Management Tools. Mar. pollut. 56, 1618–1629. doi: 10.1016/j.marpolbul.2008.05.020
Holmer M., Duarte C. M., Boschker H. T. S., Barron C. (2004). Carbon Cycling and Bacterial Carbon Sources in Pristine and Impacted Mediterranean Seagrass Sediments. Aquat. Microbial Ecol. 36, 227–237. doi: 10.3354/ame036227
Howard J., Sutton-Grier A., Herr D., Kleypas J., Landis E., McLeod E., et al. (2017). Clarifying the Role of Coastal and Marine Systems in Climate Mitigation. Front. Ecol. Environ. 15, 42–50. doi: 10.1002/fee.1451
Jassby A. D., Platt T. (1976). Mathematical Formulation of the Relationship Between Photosynthesis and Light for Phytoplankton. Limnol. Oceanogr. 21, 540–547. doi: 10.4319/lo.1976.21.4.0540
Koch E. W. (1993). Hydrodynamics, Diffusion-Boundary Layers and Photosynthesis of the Seagrasses Thalassia Testidinum and Cymodocea Nodosa. Mar. Biol. 118, 767–776. doi: 10.1007/BF00347527
Koopmans D., Holtappels M., Chennu A., Weber M., de Beer D. (2020). High Net Primary Proution of Mediterranean Seagrass (Posidonia Oceanica) Meadows Determined With Aquatic Eddy Covariance. Front. Mar. Sci. 7. doi: 10.3389/fmars.2020.00118
Lavery P. S., Mateo M.-A., Serrano O., Rozaimi M. (2013). Variability in the Carbon Storage of Seagrass Habitats and Its Implications for Global Estimates of Blue Carbon Ecosystem Service. PloS One 8, e73748. doi: 10.1371/journal.pone.0073748
Marin-Guirao L., Ruiz J. M., Dattolo E., Garcia-Munoz R., Procccini G. (2016). Physiological and Molecular Evidence of Differential Short-Term Heat Tolerance in Mediterranean Seagrasses. Nat. Sci. Rep. 6, 28615. doi: 10.1038/srep28615
Marsh J. A. Jr., Dennison W. C., Alberte R. S. (1986). Effects of Temperature on Photosynthesis and Respiration in Eelgrass (Zostera Marina L.). J. Exp. Mar. Biol. Ecol. 101, 257–267. doi: 10.1016/0022-0981(86)90267-4
Mateo M. A., Romero J., Pérez M., Littler M. M., Littler D. S. (1997). Dynamics of Millenary Organic Deposits Resulting From the Growth of the Mediterranean Seagrass Posidonia Oceanica. Estuar. Coast. Shelf Sci. 44, 103–110. doi: 10.1006/ecss.1996.0116
Mazarrasae I., Samper-Villarreal J., Serrano O., Lavery P. S., Lovelock C. E., Marba N., et al. (2018). Habitat Characteristics Provide Insights of Carbon Storage in Seagrass Meadows. Mar. pollut. Bull. 134, 106–117. doi: 10.1016/j.marpolbul.2018.01.059
Mazarrasa I., Marba N., Garcia-Orellana J., Masque P., Arias-Ortiz A., Duarte C. M. (2017). Effect of Environmental Factors (Wave Exposure and Depth) and Anthropogenic Pressure in the C Sink Capacity of Posidonia Oceanica Meadows. Limnol. Oceanogr. 62, 1436–1450. doi: 10.1002/lno.10510
McKee K. L., Cahoon D. R., Feller I. C. (2007). Caribbean Mangroves Adjust to Rising Sea Level Rise Through Biotic Controls on Change in Soil Elevation. Global Ecol. Biogeography 16, 545–556. doi: 10.1111/j.1466-8238.2007.00317.x
Montefalcone M., Albertelli G., Bianchi C. N., Mariani M., Morri C. (2006). A New Synthetic Index and a Protocol for Monitoring the Status of Posidonia Oceanica Meadows: A Case Study at Sanremo (Ligurian Sea, NW Mediterranean). Aquat. Conservation: Mar. Freshw. Syst. 16, 29–42. doi: 10.1002/aqc.688
Montefalcone M., Morri C., Peirano A., Albertelli G., Bianchi C. N. (2007). Substitution and Phase Shift Within the Posidonia Oceanica Seagrass Meadows of NW Mediterranean Sea. Estuarine Coast. Shelf Sci. 75, 63–71. doi: 10.1016/j.ecss.2007.03.034
Montefalcone M., Parravicini V., Vacchi M., Alvertelli G., Ferrari M., Morri C., et al. (2009). Human Influence on Seagrass Habitat Fragmentation in NW Mediterranean Sea. Estuarine Coast. shelf Sci. 86, 292–298. doi: 10.1016/j.ecss.2009.11.018
Murray L., Wetzel R. L. (1987). Oxygen Production and Consumption Associated With the Major Autotrophic Components in Two Temperate Seagrass Communities. Mar. Ecol. Prog. Ser. 38, 231–239. doi: 10.3354/meps038231
Nordlund L. M., Jackson E. L., Nakaoka M., Samper-Villarreal J., Beco-Carretero P., Creed J. C. (2018). Seagrass Ecosystem Services – What’s Next? Mar. Pollut. Bull. 134, 145–151. doi: 10.1016/j.marpolbul.2017.09.014
Nykjaer L. (2009). Mediterranean Sea Surface Warming 1985-2006. Climate Res. 39, 11–17. doi: 10.3354/cr00794
Olesen B., Enriquez s., Duarte C. M., Sand-Jensen K. (2002). Depth Acclimation of Photosynthesis, Morphology and Demography of Posidonia Oceanica and Cymodocea Nodosa in the Spanish Mediterranen Sea. Mar. Ecol. Prog. Ser. 236, 89–97. doi: 10.3354/meps236089
Olive I., Silva J., Costa M. M., Santos R. (2015). Estimating Seagrass Community Metabolism Using Benthic Chambers: The Effect of Incubation Time. Estuaries Coasts 39, 138–144. doi: 10.1007/s12237-015-9973-z
Oreska M. P. J., McGlathery K. J., Porter J. H. (2017). Seagrass Blue Carbon Spatial Patterns at the Meadow-Scale. PloS One 12, e0176630 doi: 10.1371/journal.pone.0176630
Ott J. A. (1980). Growth and Production in Posidonia Oceanica (L.) Delile. Mar. Ecol. 1, 47–64. doi: 10.1111/j.1439-0485.1980.tb00221.x
Pace M., Borg J. A., Galdies C., Malhotra A. (2016). Influence of Wave Climate on Architecture and Landscape Characteristics of Posiddonia Oceanica Meadows. Mar. Ecol. 38, e12387. doi: 10.1111/maec.12387
Pancucci-Papadopoulou M. A., Raitsos D. E., Corsini-Foka M. (2012). Biological Invasions and Climatic Warming: Implications for South-Eastern Aegean Ecosystem Functioning. J. Mar. Biol. Assoc. United Kingdom 92, 777–789. doi: 10.1017/S0025315411000981
Pergent-Martini C., Pergent G., Monnier B., Boudouresque C.-F., Mori C., Valetter-Sansevin A. (2021). Contribution of Posidonia Oceanica Meadows in the Context of Climate Change Mitigation in the Mediterranean Sea. Mar. Environ. Res. 165, 105236. doi: 10.1016/j.marenvres.2020.105236
Posluszny U., Tomlinson P. B. (1991). Shoot Organization in the Seagrass Halophila (Hydrocharitaceae). Can. J. Bot. 69, 1600–1615. doi: 10.1139/b91-204
Ralph P. J., Burchett M. D. (1995). Photosynthetic Responses of the Seagrass Halophila Ovalis (R. Br.) Hook. F. @ to High Irradiance Stress, Using Chlorophyll a Fluorescence. Aquat. Bot. 51, 55–66. doi: 10.1016/0304-3770(95)00456-A
Ralph P. J., Durako M. J., Enriquez S., Collier C. J., Doblin M. A. (2007). Impact of Light Limitation on Seagrasses. J. Exp. Mar. Biol. Ecol. 350, 176–193. doi: 10.1016/j.jembe.2007.06.017
Rheuban J. E., Berg P., McGlathery K. J. (2014). Ecosystem Metabolism Along a Colonization Gradient of Eelgrass (Zostera Marina) Measured by Eddy Correlation. Limnol. Oceanogr. 59, 1376–1387. doi: 10.4319/lo.2014.59.4.1376
Rodriguez P., Bystrom P., Geibrink E., Hedstrom P., Vasconcelos F. R., Karlsson J. (2016). Do Warming and Humic River Runoff Alter the Metabolic Balance of Lake Ecosystems. Aquat. Sci. 78, 717–725. doi: 10.1007/s00027-015-0463-y
Samper-Villarreal J., Lovelock C. E., Saunders M. I., Roelfsema C., Mumby P. J. (2016). Organic Carbon in Seagrass Sediments is Influenced by Seagrass Canopy Complexity, Turbidity, Wave Height, and Water Depth. Limnol. Oceanogr. 61, 938–952. doi: 10.1002/lno.10262
Schneider C. A., Rasband W. S.,, Eliceiri K. W. (2012) NIH Image to ImageJ: 25 years of Image Analysis. Nature Methods. 9, 671−675.
Samper-Villarreal J., Mumby P. J., Saunders M. I., Baryy L. A., Zawadzki A., Heijnis H., et al. (2018). Vertical Accretion and Carbon Rates in Subtropical Seagrass Meadows Increased Following Anthropogenic Pressure From European Colonisation. Estuarine Coast. Shelf Sci. 202, 40–53. doi: 10.1016/j.ecss.2017.12.006
Santos C. B., Krause-Jensen D., Alcoverro T., Marba N., Duarte C. M., Katwijk M. M., et al. (2019). Recent Trend Reversal for Declining European Seagrass Meadows. Nat. Commun. 10, 3356. doi: 10.1038/s41467-019-11340-4
Savva I., Bennett S., Roca G., Jorda G., Marba N. (2018). Thermal Tolerance of Mediterranean Marine Macrophytes: Vulnerability to Global Warming. Ecol. Evol. 8, 12032–12043. doi: 10.1002/ece3.4663
Sghaier Y. R., Zakhama-Sraieb R., Benamer I., Charfi-Cheikhrouha F. (2011). Occurrence of the Seagrass Halophila Stipulacea (Hydrocharitaceae) in the Southern Mediterranean Sea. Botanica Marina 54, 575–582. doi: 10.1515/BOT.2011.061
Sghaier Y. R., Zakhama-Sraieb R., Charfi-Cheikhrouha F. (2014). “Effects of the Invasive Seagrass Halophila Stipulacea on the Native Seagrass Cymodocea Nodosa,” in Proceedings of the Fifth Mediterranean Symposium on Marine Vegetation (Portorož, SL: UNEP), 167–171.
Short F., Carruthers T., Dennison W., Waycott M. (2007). Global Seagrass Distribution and Diversity: A Bioregional Model. J. Exp. Mar. Biol. Ecol. 350, 3–20. doi: 10.1016/j.jembe.2007.06.012
Streftaris N., Zenetos A. (2006). Alien Marine Species in the Mediterranean –the 100 ‘Worst Invasives’ and Their Impact. Mediterr. Mar. Sci. 7, 87–118. doi: 10.12681/mms.180
Telesca L., Belluscio A., Criscoli A., Ardizzone G., Apostolaki E. T., Fraschetti S., et al. (2015). Seagrass Meadows (Posidonia Oceanica) Distribution and Trajectories of Change. Nat. Sci. Rep. 5, 12505. doi: 10.1038/srep12505
Thimijan R. W., Heins R. D. (1983). Photometric, Radiometric, and Quantum Light Unit of Measure: A Review of Procedures for Interconversion. Am. Soc. Hortic. Sci. 18, 818–822.
Topouzelis K., Makri D., Stoupas N., Papakonstantinou A., Katsanevakis S. (2018). Seagrass mapping in Greek territorial waters using Landsat-8 satellite images. International Journal of applied earth observation and geoinformation, 67, 98–113.
Tsiamis K., Montesanto B., Panayotidis P., Katsaros C., Verlaque M. (2010). Updated Records and Range Expansion of Alien Marine Macrophytes in Greece, (2009). Mediterr. Mar. Sci. 11, 61–79. doi: 10.12681/mms.91
van der Velde G., den Hartog C. (1992). Continuing Range Extension of Halophila Stipulacea (Forssk.) Aschers. (Hydrocharitaceae) in the Mediterranean -Now Found at Kefallinia and Ithaki (Ionian Sea). Acta Botanica neerlandica 41, 345–348. doi: 10.1111/j.1438-8677.1992.tb01341.x
Vazquez-Lule A., Vargas R. (2021). Biophysical Drivers of Net Ecosystem and Methane Exchange Across Phenological Phases in a Tidal Salt Marsh. Agric. For. Meteorology 300, 108309. doi: 10.1016/j.agrformet.2020.108309
Wesselmann M., Geraldi N. R., Duarte C. M., Garcia-Orellana J., Diaz-Rua R., Arias-Ortiz A., et al. (2021). Seagrass (Halophila Stipulacea) Invasion Enhances Carbon Sequestration in the Mediterranean Sea. Global Change Biol. 27, 2592–2607. doi: 10.1111/gcb.15589
Williams S. L. (2007). Introduced Species in Seagrass Ecosystems: Status and Concerns. J. Exp. Mar. Biol. 350, 89–110. doi: 10.1016/j.jembe.2007.05.032
Winters G., Beer S., Willette D. A., Viana I. G., Chiquillo K. L., Beco-Carretero P., et al. (2020). The Tropical Seagrass Halophila Stipulacea Reviewing What We Know From its Native and Invasive Habitats, Alongside Identifying Knowledge Gaps. Front. Mar. Sci. 7. doi: 10.3389/fmars.2020.00300
Yates K. K., Halley R. B. (2003) Measuring Coral Reef Community Metabolism Using New Benthic Chamber Technology. Coral reefs 22, 247–255. doi: 10.1007/s00338-003-0314-5
Zupo V., Mazzella L., Buia M. C., Gambi M. C., Lorenti M., Scipione M. B., et al. (2005). A Small-Scale Analysis of the Spatial Structure of a Posidonia Oceanica Meadow Off the Island of Ischia (Gulf of Naples, Italy): Relationship With the Seafloor Morphology. Aquat. Bot. 84, 101–109. doi: 10.1016/j.aquabot.2005.08.006
Keywords: seagrass, productivity, carbon, nature based solutions, blue carbon, Mediterranean, restoration
Citation: Ward EA, Aldis C, Wade T, Miliou A, Tsimpidis T and Cameron TC (2022) Is All Seagrass Habitat Equal? Seasonal, Spatial, and Interspecific Variation in Productivity Dynamics Within Mediterranean Seagrass Habitat. Front. Mar. Sci. 9:891467. doi: 10.3389/fmars.2022.891467
Received: 07 March 2022; Accepted: 09 June 2022;
Published: 25 July 2022.
Edited by:
Hilary Anne Kennedy, Bangor University, United KingdomReviewed by:
Gregory N. Nishihara, Nagasaki University, JapanDirk Jacob Koopmans, University of Virginia, United States
Copyright © 2022 Ward, Aldis, Wade, Miliou, Tsimpidis and Cameron. This is an open-access article distributed under the terms of the Creative Commons Attribution License (CC BY). The use, distribution or reproduction in other forums is permitted, provided the original author(s) and the copyright owner(s) are credited and that the original publication in this journal is cited, in accordance with accepted academic practice. No use, distribution or reproduction is permitted which does not comply with these terms.
*Correspondence: Emma A. Ward, ZW1tYS53YXJkM0BteXBvcnQuYWMudWs=; Anastasia Miliou, YS5taWxpb3VAYXJjaGlwZWxhZ28uZ3I=; Tom C. Cameron, dGNhbWVyb25AZXNzZXguYWMudWs=