- Immunology and Genomics Group, Instituto de Investigaciones Marinas (IIM), Consejo Superior de Investigaciones Científicas (CSIC), Vigo, Spain
Aeromonas salmonicida subsp. salmonicida is the causative agent of furunculosis, a disease affecting numerous fish species worldwide. It is a highly pathogenic bacterium for turbot, whose farming production represents an important economic activity in several European countries and China. To better understand the response of this organism to A. salmonicida, we conducted RNA-Seq analysis of the head kidney from experimentally infected and uninfected turbot juveniles at 24 hours post-infection (hpi). As expected, among the differentially expressed genes (DEGs) between infected and uninfected fish, we observed the modulation of a multitude of immune-related genes but also a high representation of genes linked to metabolism. Interestingly, one of the most upregulated genes was that encoding the hormone leptin. Leptin is a multifunctional hormone/cytokine that has been shown to play roles in the immune system, stress response, food intake, metabolism and energy balance. We used recombinant human leptin to elucidate its role during infection with A. salmonicida in turbot (anorexigenic activity, ability to modulate metabolism and the immune response, and its effect on survival and bacterial load during infection). We found that the intraperitoneal administration of leptin was able to alter the response to the bacteria at the immune level, but especially at the metabolic level, which resulted in a higher survival rate without affecting the bacterial load. Based on this, we hypothesized that leptin could offer great potential as a therapeutic treatment during furunculosis outbreaks by reducing the impact of sepsis. Our results reveal the complex interplay between bacterial activity and the regulation of food intake, metabolism and inflammation.
Introduction
Bacterial pathogens are a major cause of infectious diseases and mortality in fish and cause important economic losses in aquaculture (Meyer, 1991). The Gram-negative bacterium Aeromonas salmonicida subsp. salmonicida is the aetiologic agent of classical furunculosis, a disease characterized, among other signs, by sepsis, muscular lesions and haemorrhages and death in fish populations (McCarthy and Roberts, 1980). This pathogen is a serious concern, especially for salmonid species such as Atlantic salmon (Salmo salar) and rainbow trout (Oncorhynchus mykiss) (Austin and Austin, 2007). However, several studies have shown that A. salmonicida can infect many other economically relevant fish species (Nougayrede et al., 1990; Willumsen, 1990; Real et al., 1994; El Morabit et al., 2004; Magariños et al., 2011; Fernández-Álvarez et al., 2016) and even cause serious furunculosis outbreaks in some of them, including turbot (Scophthalmus maximus) (Toranzo and Barja, 1992; Pedersen and Larsen, 1996).
Turbot aquaculture is an important economic activity in China and certain European countries, especially Spain (APROMAR, 2020). Epizootic outbreaks of acute furunculosis in turbot farms have been reported in Spain, Denmark, France, Norway and China (Nougayrede et al., 1990; Toranzo and Barja, 1992; Pedersen et al., 1994; Lillehaug et al., 2003; Fan et al., 2005). Because aquaculture is probably the fastest growing food-producing sector in the world, there is an urgent need to find effective treatments against the different pathogens affecting farmed fish. Bacterial outbreaks are usually controlled with antibiotics, which are often neither totally effective nor consumer/environmentally friendly, and there is a potential danger of antibiotic resistance development in aquatic bacteria (Defoirdt et al., 2011; Romero et al., 2012). Therefore, alternative treatments aimed at controlling bacterial diseases are required to guarantee the sustainable development of the aquaculture industry. During the last decade, considerable effort has been devoted to increasing genomic and transcriptomic resources to better understand the immune reactions of fish against bacterial fish pathogens, which is pivotal for developing new antibacterial treatments.
In recent years, a growing interest has emerged in studying the importance of the different metabolic pathways in the regulation of immune responses against pathogens in mammals (O'Neill et al., 2016). Immune cell function and metabolism are closely linked, and alterations in cellular metabolism influence immune cell function; conversely, immune cell function determines the cellular metabolic state (O'Neill et al., 2016). Indeed, currently, it is well known that upon pathogen recognition by immune cell types, cellular metabolic pathways undergo shifts that alter their functionality (O'Neill et al., 2016). On the other hand, viruses and intracellular bacterial pathogens are able to reprogram the metabolism of host cells to favour their own replication (Eisenreich et al., 2019).
However, the effects of metabolism on immune function are not limited to cellular metabolism, but also involve systemic metabolism (Matarese and La Cava, 2004; Wensveen et al., 2019). The neuroendocrine system, metabolism and immune function are intricately linked, with several cytokines, hormones, neuropeptides and transcription factors performing pivotal functions in food intake, metabolic processes and immune response (Matarese and La Cava, 2004). Among the metabolic hormones, leptin is a well-described adipocyte-derived hormone/cytokine with hormonal and immune actions that link all of those processes (Matarese and La Cava, 2004; Lam and Lu, 2007). The leptin receptor is expressed at high levels in the hypothalamus, but also in endothelial cells, pancreatic β-cells, ovaries and different immune cell types, such as monocytes/macrophages and T and B cells (Matarese and La Cava, 2004). Fat accumulation in adipocytes promotes the synthesis of leptin, which acts as an anorexigenic hormone by signalling satiety to the brain and resulting in the inhibition of food intake (Matarese and La Cava, 2004). At the metabolic level, leptin induces lipolysis, inhibits lipogenesis and systemically increases the metabolism of glucose (Reidy and Weber, 2000). As an immune regulator, leptin promotes the activation of monocytes/macrophages, chemotaxis and activation of neutrophils, development and activation of natural killer cells, T-helper 1 responses and activation and proliferation of B cells, among other immune functions (Matarese and La Cava, 2004; Lam and Lu, 2007).
In fish, duplicated leptin (lep) genes and a single leptin receptor (lepr) gene are usually present, with some exceptions (Blanco and Soengas, 2021). Nevertheless, as in mammals, alternative splicing of the lepr gene can generate a variety of receptor isoforms with different characteristics (Blanco and Soengas, 2021), which could exert a clear influence on leptin functionality. However, teleost lep orthologues are not primarily expressed in adipocytes, with the liver being the main leptin-secreting tissue (Blanco and Soengas, 2021). Numerous publications confirmed a similar role of leptin in teleosts compared to tetrapods: regulation of food intake, metabolism, stress, growth and reproduction (Copeland et al., 2011). However, the effect of this hormone against infection and its effect on metabolism-immune system interplay remain practically unexplored in fish.
By analysing the transcriptome response of head kidney samples of juvenile turbot to A. salmocida, we found that, in addition to a multitude of immune genes, one of the genes which was more overexpressed at 24 hpi was lep. Therefore, because recombinant human leptin has been previously demonstrated to reproduce some of its effects in fish (Peyon et al., 2001; Weil et al., 2003; de Pedro et al., 2006; Gorissen et al., 2012), we administered it to turbot to explore the biological significance of lep overexpression during an infection with bacteria. We found that human leptin was able to reduce food intake, modulate metabolism, and alter the expression of certain metabolism- and immune-related genes in turbot. The activity mediated by recombinant human leptin also significantly increased the survival of the A. salmonicida-infected turbot, which highlights the potential of this type of metabolic treatment to ameliorate the outcome of bacterial infections.
Materials and Methods
Fish, Bacteria and Recombinant Leptin
Juvenile turbot were obtained from a commercial fish farm (Insuiña S.L., Galicia, Spain). Fish were maintained in 500 L fibreglass tanks with a recirculating saline water system (total salinity approximately 35 g/L) and a 12 L:12 D photoperiod at 15°C. The fish mean weight was 2.5 g ± 0.3 g. Prior to the experiments, fish were acclimatized to laboratory conditions for 2 weeks. Animals were fed daily to satiety with a commercial dry diet (LARVIVA-BioMar). For fish injections, animals were anaesthetized with MS-222 (50 mg/L); before samplings and at the end of the survival experiments, fish were sacrificed via MS-222 overdose (500 mg/L). Fish care and challenge experiments were reviewed and approved by the CSIC National Committee on Bioethics under approval number ES3605702020012020/13/FUN.01/INM06/BNG.
Aeromonas salmonicida subsp. salmonicida (strain VT 45.1, kindly provided by Dr. M.L. Lemos; University of Santiago de Compostela, Spain) was cultured in tryptic soy agar (TSA) plates at 22°C for 24 h before use. A bacterial suspension was prepared in 1x phosphate buffered saline (PBS) immediately before inoculation. Serial dilutions were performed to calculate the number of colony forming units (CFU)/mL.
Recombinant human leptin was purchased from Sigma–Aldrich (L-4146) and dissolved in PBS to the desired concentration. Leptin dose (1 μg/g body weight) and administration procedure (daily intraperitoneal injection) were adapted from previous studies (de Pedro et al., 2006).
Fish Infection and Sampling for RNA−Seq
After two weeks of acclimation, fish were randomly assigned to 60 L experimental tanks. Sedated specimens were intraperitoneally (i.p.) injected with a volume of 50 µL of PBS (control) or with A. salmonicida (5.3 × 107 CFU/mL). The head kidneys from 9 fish per condition were sampled at 24 hpi under RNase-free conditions. The same quantity of tissue from 3 animals was pooled, performing 3 biological replicates (3 fish/replicate). Samples were stored at −80°C until RNA isolation.
RNA Isolation and High-Throughput Transcriptome Sequencing
RNA isolation was performed with the Maxwell 16 LEV simplyRNA tissue kit (Promega). The RNA concentration and purity were measured with a spectrophotometer (ND-1000; Nanodrop Technologies Inc., Wilmington, DE, USA), and RNA integrity was analysed in an Agilent 2100 Bioanalyzer (Agilent Technologies Inc., Santa Clara, CA, USA) according to the manufacturer’s instructions. All the samples showed an RNA integrity number (RIN) over 8.0 and were therefore used for Illumina library preparation. Double-stranded cDNA libraries were constructed using the TruSeq RNA Sample Preparation Kit v2 (Illumina, San Diego, CA, USA), and sequencing was performed using Illumina HiSeq 4000 technology at Macrogen Inc., Korea (Seoul, Republic of Korea). The read sequences were deposited in the Sequence Read Archive (SRA) under accession number PRJNA810312.
Bioinformatics Analyses
CLC Genomics Workbench v. 11.0.2 (CLC Bio, Aarhus, Denmark) was used to filter and perform the RNA-Seq and statistical analyses. Raw reads were trimmed to remove low-quality sequences (quality score limit 0.01 = PHRED 20), and the high-quality reads were used for further analysis. These reads were mapped against the turbot genome (Figueras et al., 2016) with the following parameters: length fraction = 0.8, similarity fraction = 0.8, mismatch cost = 2, insertion cost = 3 and deletion cost = 3. Expression levels were calculated as transcripts per million (TPM) values. To identify and quantify the directions of variability in the data, a principal component analysis (PCA) plot was constructed by using the TPM values for all the genes and with the web tool Clustvis (Metsalu and Vilo, 2015) (https://biit.cs.ut.ee/clustvis/). To determine statistically significant differences between infected and uninfected turbot, a differential expression analysis test was conducted. Genes showing a fold-change>|2| and an FDR<0.05 were selected as differentially expressed genes (DEGs). Gene Ontology (GO) and Kyoto Encyclopedia of Genes and Genomes (KEGG) enrichment analyses were performed using OmicsBox software (https://www.biobam.com/omicsbox), and an FDR corrected p value<0.01 was employed. Using the TPM values of the selected DEGs, heatmaps were constructed using the average linkage method with Euclidean distance, also using the web tool Clustvis.
Food Intake Assay
Changes in food intake after bacterial infection and/or leptin administration were evaluated in juvenile turbot. For this, four groups of 10 fish/tank were i.p. inoculated with 50 μL of PBS (controls), leptin (1 μg/g), an A. salmonicida suspension (4.3 × 108 CFU/mL per fish) or leptin + A. salmonicida. The experiment was conducted in triplicate. Food intake was registered for 3 days before treatment (to define basal line data) and during the subsequent three days after the administration of the treatments. Food was supplied daily in batches of approximately 5 g every 2 min until satiation. After feeding, the uneaten food remaining at the tank bottom and feed waste were withdrawn, dried and weighed. The amount of food consumed by fish in each tank was calculated as the difference between total food offered and uneaten food. Food intake values registered after treatment are referred to as those of basal values, and the results are shown as the mean ± SEM of the data obtained in three different tanks per treatment.
Survival Assay and Sampling for Metabolite Quantification and Gene Expression Analyses
A schematic representation of the experimental design is shown in Figure 1. Forty fish were randomly divided into 2 batches of 20 turbot each. One batch was i.p. injected with 50 μL of PBS, and the other batch was injected with 50 μL of leptin (1 μg/g) every day for one week. After this period, half of the fish from the PBS group (n=10) were i.p. injected with 50 µl of PBS (control group) or an A. salmonicida solution (4.3 × 108 CFU/mL) (A. salmonicida group). For the leptin-stimulated group, half of the turbot were i.p. injected with leptin (leptin group), and the remaining 10 fish were injected with leptin and A. salmonicida (leptin + A. salmonicida group). Then, fish were i.p. injected every day with PBS (control and A. salmonicida groups) or leptin (leptin and leptin + A. salmonicida groups), and mortality was recorded every day. The survival assay was repeated three times, with two replicates per group.
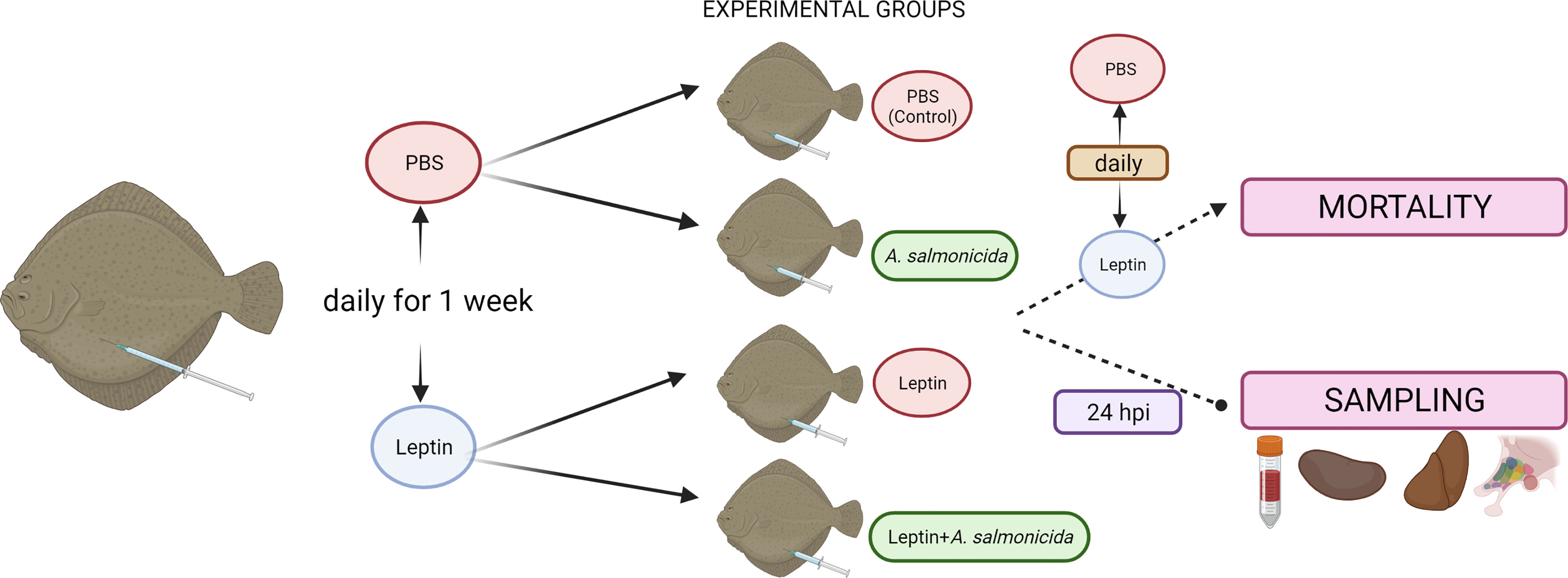
Figure 1 Experimental design of the treatment with human recombinant leptin. For the mortality assay, two replicates of 10 fish were used per condition (control, leptin, A. salmonicida, Leptin + A. salmonicida), and the assay was repeated three times. For tissue sampling, fourteen fish were used per experimental condition (8 fish for assessment of the metabolite levels and 6 fish for gene expression analysis). Figure created with BioRender.com.
For tissue sampling, a parallel experiment was conducted (Figure 1). Groups of 28 turbot were also inoculated daily for a week with leptin or the same volume of PBS. Then, each group was divided into two groups of 14 fish. The fish inoculated with PBS were inoculated again with PBS (control group) or with the bacteria (A. salmonicida group). Among the leptin-administered groups, one of them was i.p. injected with leptin (leptin group), and the other one was treated with leptin + A. salmonicida. At 24 hpi, fish were anaesthetized with MS-222, and blood was collected by caudal puncture with heparinized syringes. Plasma samples were obtained after blood centrifugation at 400 xg for 10 min, deproteinized immediately (using 0.6 M perchloric acid (PCA)) and neutralized (using 1 M potassium bicarbonate) before their freezing and storage at -80°C until further assay. Fish were sacrificed, and liver, head kidney and hypothalamus samples were taken, snap-frozen in dry ice, and stored at -80°C. Samples from a total of 8 fish were used to assess metabolite levels, whereas the remaining 6 fish were used for gene expression analysis through qPCR.
Assessment of Metabolite Levels
Lactate, glucose and triglyceride levels were determined enzymatically using commercial kits (Spinreact, Barcelona, Spain) adapted to a microplate format. Tissue samples used to assess metabolite levels were homogenized by mechanical disruption using a potter linked to a motor pestle in 7.5 vol of ice-cooled 6% PCA and neutralized (using 1 mol/L potassium bicarbonate). The homogenate was centrifuged at 16,000 xg for 4 min, and the supernatant was collected to determine metabolite levels.
RNA Isolation, cDNA Synthesis and qPCR Analysis
Total RNA was extracted from head kidney, liver and hypothalamus using the Maxwell 16 LEV simplyRNA tissue kit (Promega) with the automated Maxwell 16 instrument in accordance with instructions provided by the manufacturer. cDNA synthesis was performed with the NZY First-Strand cDNA Synthesis Kit (NZYtech) using 500 ng of RNA. The quantitative analysis of gene expression was determined using the 7300 Real Time PCR System (Applied Biosystems). Specific qPCR primers for turbot genes were designed based on sequences obtained from the turbot genome (Figueras et al., 2016) or transcriptome (Pereiro et al., 2012) by using the Primer3 program (Rozen and Skaletsky, 2000). Their amplification efficiency was calculated using seven serial five-fold dilutions of head kidney cDNA from unstimulated turbot with the threshold cycle (CT) slope method (Pfaffl, 2001). For the detection of A. salmonicida subsp. salmonicida, we used specific primers designed on the basis of the publication of Balcázar et al. (2007) but including some modifications. Analyses were performed on 1 µL of cDNA using SYBR GREEN PCR Master Mix (Applied Biosystems) in a total PCR volume of 25 µL containing 10 µM of each primer. Sequences of the forward and reverse primers used for each gene expression analysis are shown in Supplementary Table S1. Thermal cycling was initiated with a denaturation step (95°C, 10 min) followed by 40 cycles of a denaturation step (95°C, 15 s) and a hybridization-elongation step (60°C, 1 min). The mRNA levels of the target genes were normalized to the reference gene eukaryotic translation elongation factor 1 alpha (eef1a), which was stably expressed in this experiment. Expression levels were calculated following the Pfaffl method (Pfaffl, 2001).
Statistics
Comparisons among groups were carried out using Student’s t test (paired comparisons) or one-way ANOVA (multiple comparisons) followed by a Student-Newman–Keuls test, and differences were considered statistically significant at p<0.05. When necessary, data were log-transformed to fulfil the conditions of the analysis of variance.
In survival experiments, Kaplan–Meier cumulative survival curves were analysed for statistical significance with the log-rank (Mantel–Cox) test, and significant differences are displayed as *** (0.0001 < p < 0.001), ** (0.001 < p < 0.01) or * (0.01 < p < 0.05).
Results
Transcriptome Modulation in Turbot After A. salmonicida Infection
Using the TPM values obtained from RNA-Seq analyses, PCA was performed to determine the sample distribution and to identify the presence of outliers (Figure 2A). The PCA plot showed that the control samples were very similar to each other compared with the A. salmonicida samples. Figure 2B shows the distribution and fold-change magnitude of the differentially expressed genes (DEGs) in the head kidney from infected animals compared to the controls. A total of 2,056 genes were differentially regulated, with 1,142 upregulated and 914 downregulated at 24 hpi (Figure 2B; Supplementary File S1).
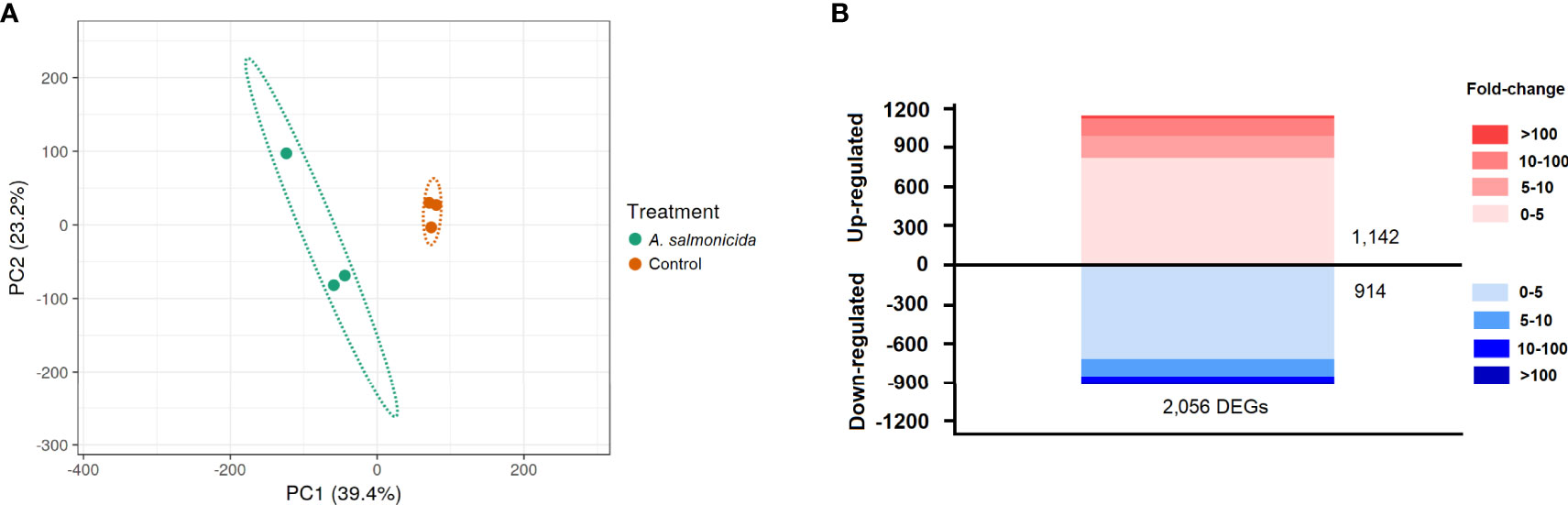
Figure 2 RNA-Seq analysis of the head kidney transcriptome response to A salmonicida subsp. salmonicida infection in turbot. (A) Principal component analysis (PCA) of the samples. (B) Stacked column chart showing the number and intensity of the up- and downregulated DEGs after A salmonicida infection.
To better elucidate the biological processes in which these DEGs were involved, GO enrichment analyses were carried out. A total of 221 GO terms were significantly enriched according to an FDR<0.01 (Supplementary File S2), although only the 40 most statistically significant terms are shown in the graph (Figure 3A). As expected, among the most significantly enriched biological processes, a multitude of immune-related terms were represented, including those related to pathogen recognition via toll-like receptors (TLRs), macrophage activation, cytokine activities, antigen presentation and inflammation (Figure 3A; Supplementary File S2). However, several metabolic processes involved in nucleotide, carbohydrate, lipid and amino acid metabolism were also significantly enriched (Supplementary File S2). This fact was also manifested in the KEGG pathway analysis, which highlighted the modulation of different metabolic routes after A. salmonicida challenge (Figure 3B). Among these, amino acid, lipid, nucleotide and carbohydrate metabolism were the most enriched KEGG pathways.
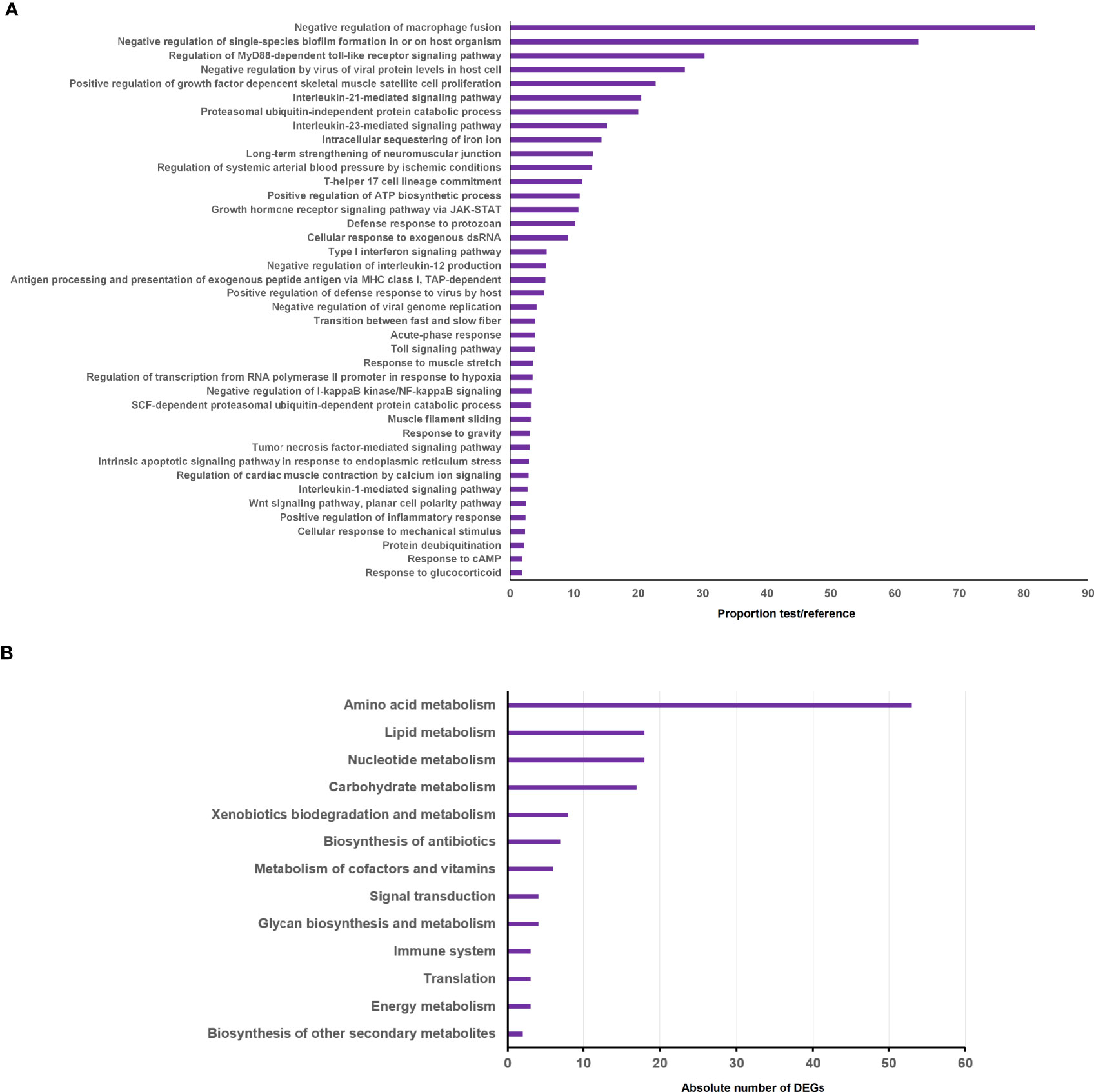
Figure 3 Enrichment analysis of the genes differentially expressed in head kidney after A salmonicida challenge compared to the uninfected controls. (A) GO enrichment analysis showing the 40 most significantly enriched biological processes. (B) KEGG pathways enriched for the significantly modulated genes.
Since glucose and lipid metabolism are intimately linked and have been described as the major metabolic processes impacted upon host responses to infection (Gleeson and Sheedy, 2016), heatmaps were constructed to show the alteration in the gene expression profiles of both metabolic processes after A. salmonicida infection. When those DEGs related to carbohydrate metabolism were represented, we observed that, in general terms, glycogenolysis and glycolysis seemed to be inhibited at 24 hpi (Figure 4A). Nevertheless, the existence of different isoforms for some glycolytic enzymes, such as hexokinases and 6-phosphofructo-2-kinase/fructose-2,6-biphosphatases, with alternative functions, reflects an opposite regulation in head kidney. On the other hand, when hormones and hormone receptors playing major roles in glucose and lipid metabolism were analysed, downregulation of both insulin and growth hormone receptors (insr and ghr) was observed after bacterial challenge, whereas the leptin (lep) gene was highly induced by A. salmonicida (Figure 4B). Finally, certain genes involved in lipid metabolic processes were also affected by challenge with the bacteria (Figure 4C).
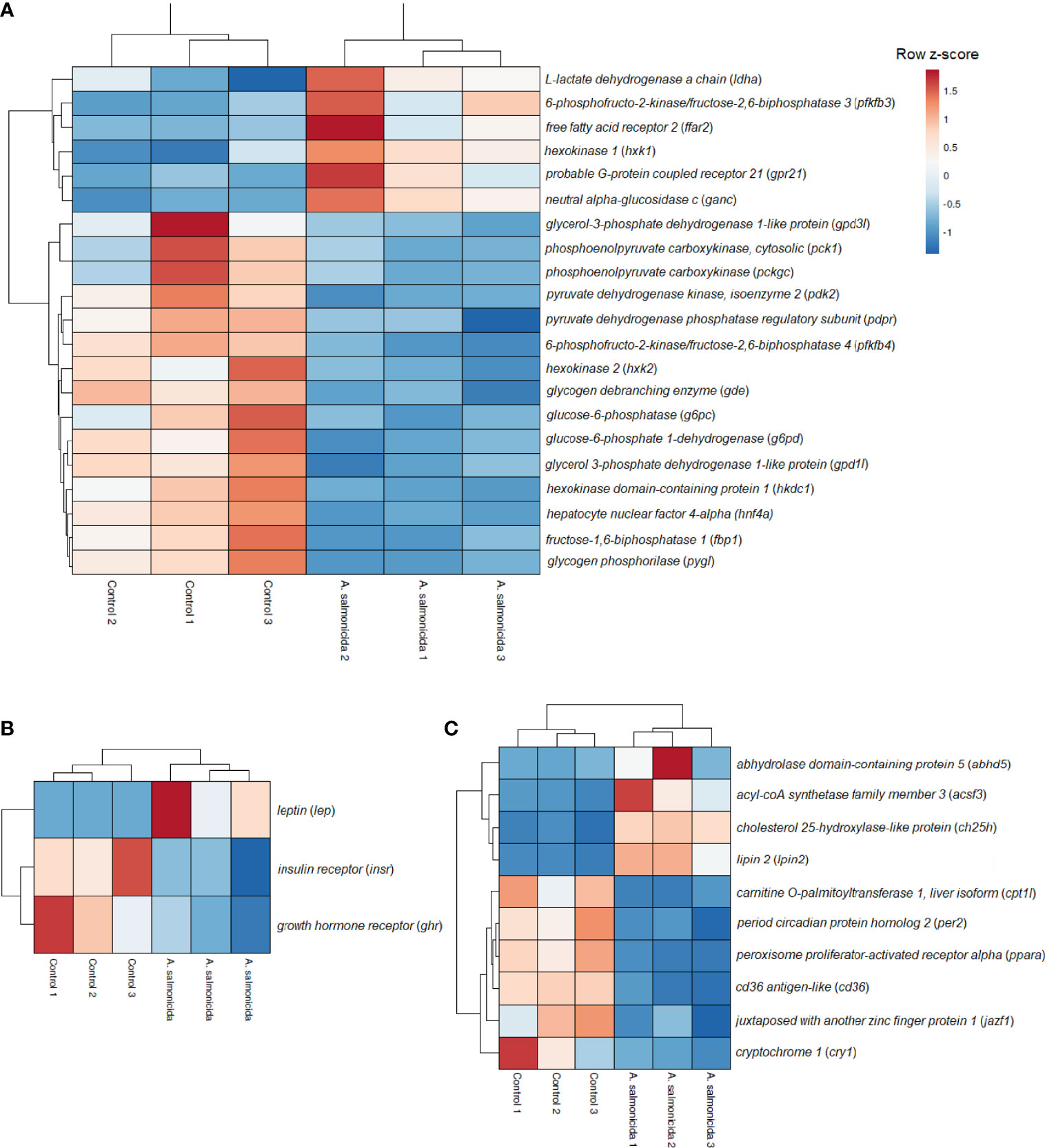
Figure 4 Analysis of the carbohydrate and lipid metabolism-related genes modulated in head kidney samples from A salmonicida-infected turbot. Heatmaps representing the TPM values of (A) carbohydrate metabolism-related genes, (B) hormones and hormone receptors and (C) lipid metabolism-related genes differentially expressed in turbot head kidney at 24 hpi with the bacteria A salmonicida compared to the uninfected controls. The colour scale represents gene expression, ranging from blue (less expressed) to red (more expressed).
Among the annotated DEGs more substantially modulated in head kidney after A. salmonicida infection (Table 1), a significant number of immune genes were represented among the upregulated DEGs. Interestingly, the fifth most upregulated gene was the lep gene, with an FC=270.89. Among the top 25 downregulated and annotated DEGs, an important representation of cytoskeleton organization proteins was observed, including different myosin and troponin members (Table 1).
Protective Effect of Leptin During Infection With A. salmonicida and Modulation of the Immune Response
To better elucidate the potential role of leptin during infection with A. salmonicida, we evaluated whether recombinant human leptin showed any protective or pernicious effect during a challenge with the bacteria. After infection, those fish inoculated with A. salmonicida alone showed a survival rate of 20.78%, whereas those turbot infected but treated with leptin achieved a survival rate of 54.17% (Figure 5A). No mortality events were registered for the uninfected groups. Interestingly, the quantification of the bacteria through qPCR in head kidney samples at 24 hpi revealed that there were no significant differences in the specific detection of A. salmonicida subsp. salmonicida between those fish receiving leptin and the bacteria and those inoculated with the bacteria alone (Figure 5B). Indeed, the results showed a tendency towards higher detection in those animals treated with leptin.
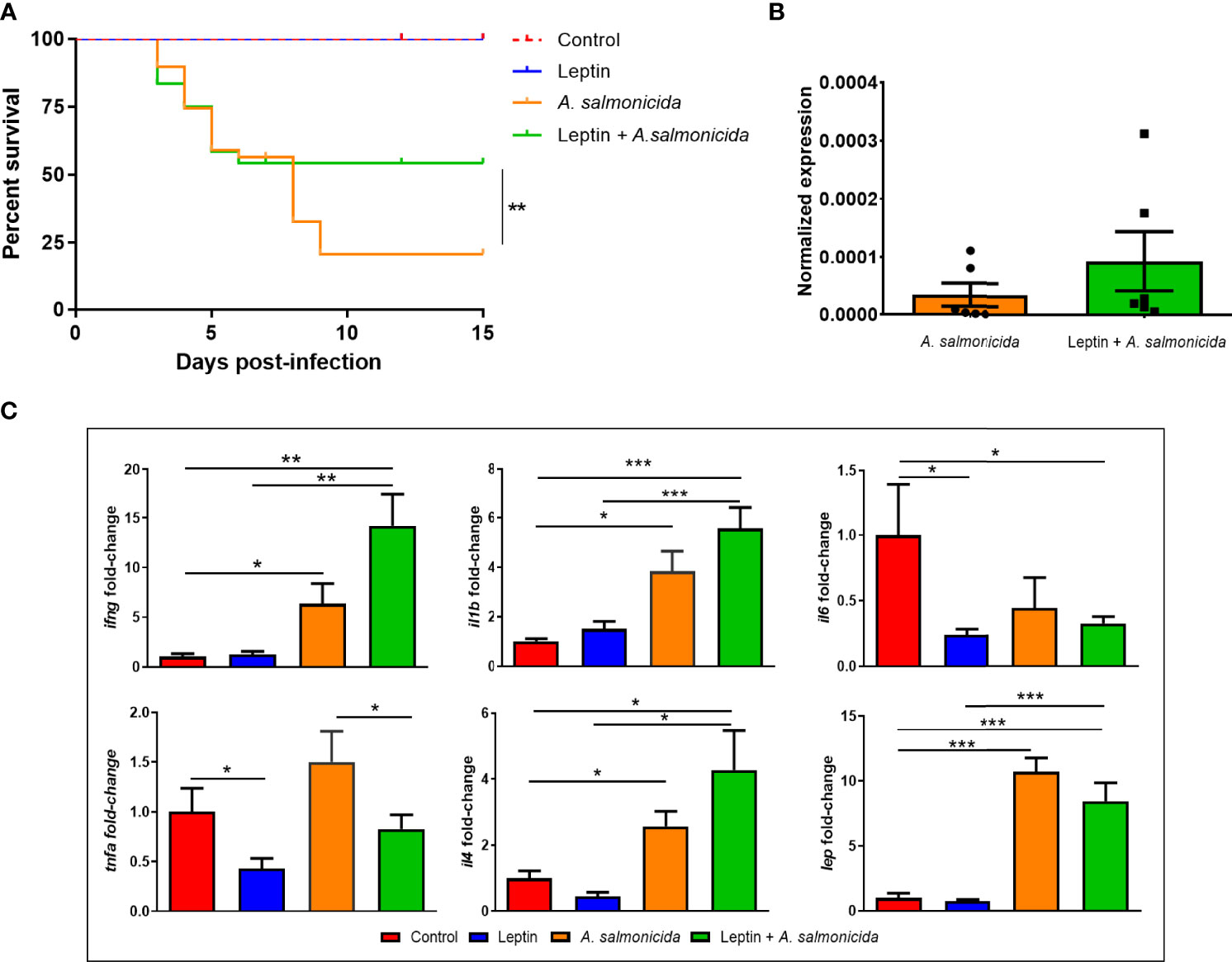
Figure 5 Effect of the chronic administration of human recombinant leptin on the survival, bacterial load and expression of inflammation-related genes in head kidney during an infection with A salmonicida in juvenile turbot. (A) Kaplan–Meier survival curves of fish infected with the bacteria and treated or not treated with leptin and the corresponding uninfected controls. The graph represents the results of one of the three independent experiments conducted (n = 20). Survival curves were analysed for statistical significance with the log-rank (Mantel–Cox) test. Statistically significant differences are displayed as ** (0.01≤ p ≥ 0.001). (B) qPCR detection of A salmonicida subsp. salmonicida in head kidney samples from infected fish at 24 hpi. The bacterial signal was normalized to the expression of eef1a. (C) Gene expression analysis of inflammation-related cytokines in head kidney at 24 hpi. The expression level of each gene was normalized to the expression of eef1a and expressed as the fold-change with respect to the levels detected in the control group. Data represent the mean + SEM of 6 individuals. Statistically significant differences are displayed as *(p ≤ 0.05), **(p ≤ 0.01) or ***(p ≤ 0.001).
To determine whether the continuous administration of human leptin had the ability to modulate the transcription of the turbot lep gene and the immune response in head kidney by itself or during an infection with A. salmonicida, we conducted qPCR analyses of selected inflammation-related genes (Figure 5C). The exogenous administration of human leptin did not affect the expression of the turbot lep gene, which was only induced as a consequence of bacterial infection. Whereas the challenge with A. salmonicida significantly induced the expression of ifng, il1b and il4, leptin treatment significantly reduced the expression of il6 and tnfa compared with the untreated controls (Figure 5C). Interestingly, recombinant leptin also significantly reduced the transcription level of tnfa in those animals receiving leptin + A. salmonicida treatment compared to those inoculated with the bacteria alone (Figure 5C).
Recombinant Human Leptin Shows Anorexigenic Effects in Turbot
We also sought to elucidate whether recombinant human leptin is able to affect food intake control in turbot. For this, food intake, gene expression of some anorexigenic and orexigenic neuropeptides and glucose and lactate levels in turbot hypothalamus were determined (Figure 6). Leptin significantly decreased food intake after 1, 3 and 5 days postinfection (dpi) compared with the untreated controls, whereas infection with A. salmonicida significantly reduced food intake after 3 and 5 dpi (Figure 6A). Furthermore, the decrease was greater in the group treated with leptin and infected with A. salmonicida at the three sampling points, reflecting a synergic effect (Figure 6A).
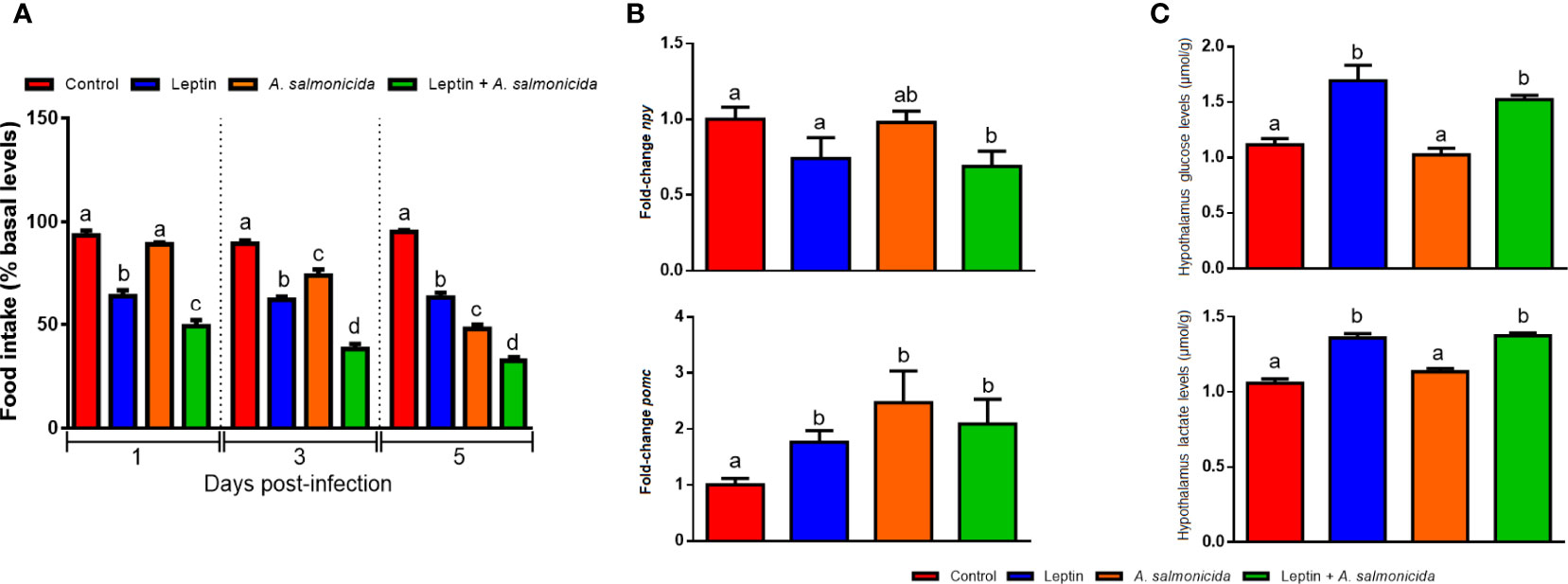
Figure 6 Anorexigenic effects of recombinant human leptin. (A) Effect of the chronic administration of leptin on food intake in the absence or presence of A salmonicida infection. Food intake is represented as the percentage of food ingested with respect to basal levels (calculated as the average of food intake during the three days prior to the experiment). The results are shown as the mean + SEM of the results obtained in three different experiments in which 10 fish were used per group in each experiment. Different letters indicate significant differences (p < 0.05) among treatments at the same post-treatment time. (B) Gene expression analysis of npy and pmoc and (C) glucose and lactate levels in the hypothalamus at 24 h after the i.p. administration of saline solution (control), leptin, A salmonicida or leptin + A salmonicida. For the gene expression data, the expression level of each gene was normalized to the expression of eef1a and expressed as the fold-change with respect to the levels detected in the control group. Each value represents the mean + SEM of n = 6 fish per treatment. Different letters indicate significant differences (p < 0.05) among treatments.
Since it is known that leptin inhibits the expression of orexigenic neuropeptides, such as the neuropeptide y gene (npy) but induces the expression of anorexigenic genes, such as the proopiomelanocortin gene (pomc) at hypothalamic level, we analyzed the modulation of these two genes after A. salmonicida infection and/or leptin administration. In the hypothalamus, treatment with leptin in infected fish significantly decreased the mRNA levels of the npy gene compared with those in controls and infected fish (Figure 6B). In contrast, leptin and bacteria alone or in combination were able to significantly increase the mRNA levels of the pomc gene (Figure 6B). The glucose levels in the hypothalamus increased after treatment with leptin when compared with those in the other groups, and lactate levels increased after treatment with leptin and leptin + A. salmonicida (Figure 6C).
Leptin and A. salmonicida Induce Changes in the Levels of Metabolites and in the Expression of Glucose Regulator Genes
Since a multitude of metabolism-related genes were found to be altered in the RNA-Seq analyses conducted to compare A. salmonicida-infected and uninfected turbot, and due to the involvement of leptin in metabolic regulation, we evaluated how both treatments or their combination affected different metabolic parameters (Figure 7). The measurement of the levels of metabolites (glucose, lactate and triglycerides) in plasma, head kidney and liver revealed interesting modulations of energy expenditure (Figure 7A). Those turbot treated with leptin showed that glucose levels significantly increased in plasma and head kidney, whereas the glucose level was reduced in liver; lactate was only affected in plasma, with a significant increase in those animals receiving leptin (Figure 7A). In contrast, triglyceride levels were reduced in plasma, head kidney and liver by leptin administration (Figure 7A). Interestingly, A. salmonicida challenge reduced the glucose levels in plasma and liver, but the decrease in the plasma glucose levels after infection was counteracted in the presence of leptin, which elevated the levels of plasma glucose even beyond the level of the absolute control (Figure 7A). On the other hand, lactate and triglyceride levels were not significantly affected by the bacterial challenge. In general terms, the presence of leptin during the infection tended to modulate the metabolite levels towards those observed in the presence of leptin alone (Figure 7A). Leptin treatment (in the presence or absence of infection) induced the upregulation of the glucose transporter 2 (glut2) gene in the liver, especially with the combination of both treatments (Figure 7B). On the other hand, the expression of the glycolysis initiating enzyme glucokinase (gck) gene in the liver was significantly reduced by the bacteria, but especially by the administration of leptin (Figure 7B). The fatty acid synthase (fasn) gene was inhibited both by leptin administration and bacteria, whereas carnitine palmitoyltransferase 1a (cpt1a) was inhibited by the bacteria alone but induced by leptin administration (in the presence or absence of infection) (Figure 7B).
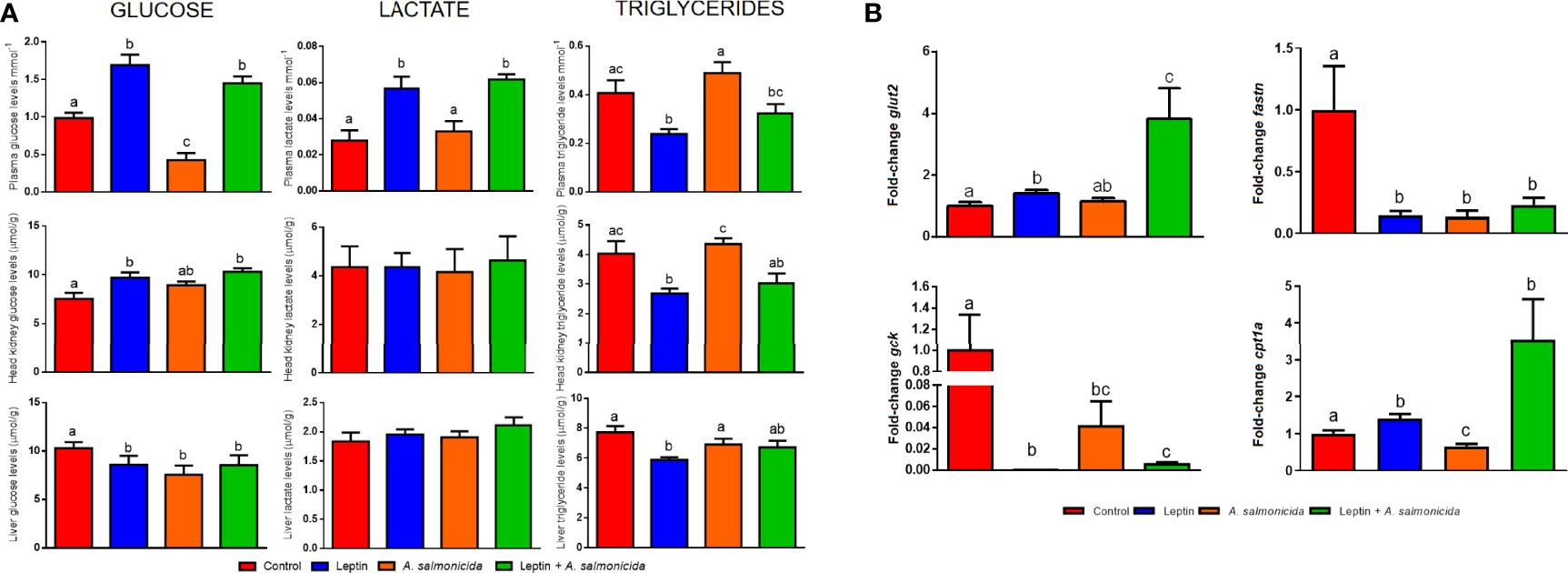
Figure 7 Measurement of metabolic regulation induced by leptin and A salmonicida. (A) Levels of glucose, lactate and triglycerides in plasma, head kidney and liver, and (B) gene expression analysis of glut2, gck, fasn and cpt1a in liver of turbot chronically treated with leptin in the absence or presence of infection. Fish were sampled at 24 hpi, where 8 individuals were used for assessment of the metabolite levels and 6 fish were used for gene expression analysis. For the gene expression data, the expression level of each gene was normalized to the expression of eef1a and expressed as the fold-change with respect to the levels detected in the control group. Graphs represent the mean + SEM of 8 or 6 fish per treatment, respectively. Different letters indicate significant differences (p < 0.05) among treatments.
Discussion
High-throughput molecular analyses (genomics, transcriptomics, proteomics or metabolomics) allow us to obtain a diversity of information that can be used to further develop potential preventive strategies or treatments against infectious diseases. For that reason, we wanted to study the overall transcriptome response of turbot to infection with the bacterium A. salmonicida, one of the most threatening pathogens in the culture of this important commercial fish species.
Some studies have used microarrays or high-throughput RNA sequencing (RNA-Seq) to understand the effects of A. salmonicida subsp. salmonicida infection in fish, including rainbow trout (Rebl et al., 2014; Long et al., 2015; Ji et al., 2020), Atlantic salmon (Ewart et al., 2005; Xia et al., 2022) and turbot (Millán et al., 2011). Millán et al. (2011), using an immune-enriched oligo-microarray, partially characterized the transcriptome response of different tissues (spleen, liver and head kidney) from turbot to infection with A. salmonicida. However, that microarray was biased towards certain immune-related genes, and the overall response remained unexplored. Therefore, the present study is the first to conduct in-depth transcriptome analysis in turbot after infection with A. salmonicida.
As expected, RNA-Seq analysis of head kidney samples from A. salmonicida-infected and uninfected turbot revealed a multitude of immune DEGs upregulated during the infection (interleukins, chemokines, toll-like receptors, antigen-presentation proteins, complement components, etc.), as well as numerous enriched biological processes related to different aspects of the immune response. These modulations were compatible with the activation of a wide range of antibacterial mechanisms (Magnadóttir, 2006). However, the most downregulated genes were mainly involved with the cytoskeleton (myosins, actins, troponins) and extracellular matrix organization. This fact is frequently observed during infection with pathogenic bacteria, which use bacterial virulence factors to hijack the host cell machinery and manipulate cytoskeleton and tight junction integrity to facilitate their attachment, entry into cells, movement within and between cells, and avoidance of phagocytosis, among other processes (Navarro-García et al., 2013).
Interestingly, a multitude of metabolism-related genes were also differentially modulated after infection, reflecting the tight link between metabolism and immunity. The metabolic gene showing the greatest modulation was the leptin (lep) gene, which was overexpressed 271-fold in A. salmonicida-infected turbot compared to the controls. Leptin is a pleiotropic hormone involved in the regulation of food intake and body fat through its effects on the central nervous system (CNS) via neuroendocrine signalling systems (Francisco et al., 2018). However, leptin also exerts powerful peripheral modulations on immune cells (Francisco et al., 2018; Maurya et al., 2018). Consequently, it is considered to participate in the interplay between the immune response and metabolism (Lam and Lu, 2007; Francisco et al., 2018). In humans, this hormone is secreted mainly by white adipose tissue, while the main tissue involved in leptin synthesis in teleost fish is the liver, and its levels are positively correlated with the amount of body fat (Considine et al., 1996; Blanco and Soengas, 2021). Mammalian immune cells express leptin receptors, and therefore, leptin is able to exert a variety of immune functions, such as activation of monocytes/macrophages and dendritic cells, enhancement of NK-cell cytotoxicity, cytokine induction, chemotaxis, shift of T cells towards the Th1 phenotype and proliferation of naïve T cells (Francisco et al., 2018). Most of these immune effects are mediated by the induction of certain proinflammatory cytokines, such as TNF-α, IL-1β, IL-6 or IFN-γ, and the inhibition of anti-inflammatory cytokines, such as IL-4 (Francisco et al., 2018; Maurya et al., 2018).
In murine models, leptin deficiency has been associated with increased susceptibility to bacterial infections and mortality, whereas leptin supplementation had the opposite effect (Ikejima et al., 2005; Wieland et al., 2005; Hsu et al., 2007; Tschöp et al., 2010). In this work, we sought to determine whether lep overexpression after A. salmonicida infection in turbot could be related to infection prognosis. Although teleost and mammalian leptins show very low sequence identity, their tertiary structures and key amino acids required for their biological activity are highly conserved (Blanco and Soengas, 2021). Because of this, we used recombinant human leptin to study its effect in turbot and its contribution to the bacterial infection outcome. We found that the chronic administration of recombinant human leptin for one week before bacterial challenge and during the course of bacterial infection significantly increased survival after A. salmonicida infection. Curiously, the detection of A. salmonicida in head kidney samples did not show significant differences between those animals receiving leptin and those inoculated with PBS, reflecting that the protective mechanisms induced by leptin could not be related to a boosted immune response. Those turbot infected with the bacteria showed a substantial increase in the expression of the proinflammatory cytokines ifng, il1b and tnfa. However, contrary to what was expected due to the proinflammatory effects described for leptin, this hormone downregulated the expression of il6 and tnfa in the absence or presence of infection, which could indicate an anti-inflammatory role of leptin. Moreover, leptin was able to counteract the overexpression of tnfa induced by the bacteria. Because of this, the protective effect mediated by leptin seems not to be linked, or is at least not restricted, to the activation of the immune response and could induce a protective effect against sepsis-induced mortality. This protective effect of leptin against septic shock has been previously documented in murine models (2000; Faggioni et al., 1999; Madiehe et al., 2003; Wang et al., 2004; Tschöp et al., 2010; Dong et al., 2013; Landgraf et al., 2014; Vallejos et al., 2018). Indeed, TNF-α administration induces anorexia (among other mechanisms through the activation of leptin synthesis), metabolic alteration, inflammation and even septic shock and death in mice (Tracey, 1992; Takahashi et al., 1999), which could be consistent with the transcriptome data observed in turbot after A. salmonicida infection. Overproduction of TNF-α during septic shock triggers tissue injury, whereas lower levels of this cytokine are related to effective tissue homeostasis and wound healing (Tracey, 1992). More interestingly, anti-TNF-α therapy for patients with sepsis was demonstrated to improve survival (Lv et al., 2014). Since it has been described that leptin mediates protective effects against the toxicity exerted by TNF-α (Takahashi et al., 1999), our results could reflect that the downregulation of the tnfa gene in those animals receiving exogenous leptin could be one of the protective effects induced by leptin during infection with A. salmonicida. However, we cannot discard a certain effect on the immune response as a consequence of the administration of a non-self protein. Nevertheless, we would expect an increase in the inflammatory response as a consequence of this.
Anorexia is a highly conserved central process of sickness behaviour during infection regulated by immune–endocrine interactions (Wensveen et al., 2019). Fasting has been proposed to be pivotal for host defence against bacterial pathogens, while nutritional supplementation is detrimental in bacterial sepsis (Wang et al., 2016; Wensveen et al., 2019). As expected, A. salmonicida infection reduced food intake in turbot, and this effect was potentiated by the administration of human leptin. However, in addition to metabolic alterations as a consequence of reduced food intake, leptin also regulates carbohydrate and lipid metabolism centrally and peripherally (Reidy and Weber, 2000). Evidence indicates that proinflammatory cytokines released upon infection, such as TNF-α, IL-1β or IL-6, contribute to the development of alterations in glucose and lipid metabolism homeostasis (Shi et al., 2019; Wensveen et al., 2019). Bacterial sepsis affects the ability of host tissues to use glucose via glycolysis and alternative fuel sources, such as ketone bodies and free fatty acids, via oxidative phosphorylation (Agwunobi et al., 2000). These metabolic alterations, frequently observed during sepsis, represent a prognostic marker for sepsis severity (Wasyluk and Zwolak, 2021). Leptin switches the fuel source from carbohydrate (glucose) to lipid (fatty acids), serving as a powerful inductor of lipolysis and fatty acid oxidation (Reidy and Weber, 2000), and this shift has been shown to be protective during bacterial sepsis (Wang et al., 2016).
We found that A. salmonicida infection impacted the expression of a multitude of metabolism-related genes and significantly diminished the liver and plasma levels of glucose. Leptin administration restored and even increased the plasma circulating levels of glucose with respect to the control turbot. Leptin also increased the glucose and lactate levels in the hypothalamus, and glucose in the head kidney, but lower levels of this metabolite were observed in the liver. Additionally, the expression of the gene gck, encoding the enzyme catalysing the first reaction of the glycolytic pathway, was inhibited by leptin, and the gene glut2, encoding a bidirectional glucose transporter across hepatocytes, was induced by leptin treatment. These expression results could be compatible with glycolysis inhibition by leptin and the release of glucose from the liver to the circulation. On the other hand, although plasma, head kidney and liver triglyceride levels were not affected by the bacteria, leptin decreased their levels, probably due to its role as an inducer of fatty acid oxidation, which is also consistent with the downregulation of fasn and the upregulation of cpt1a, which are involved in fatty acid synthesis and fatty acid oxidation, respectively.
The higher plasma glucose levels observed due to leptin administration could therefore be a consequence of the shift from glycolysis to fatty acid oxidation, reducing glucose utilization. Indeed, treatment with the glucose analogue 2-deoxy-D-glucose (2DG) improves the survival of mice suffering LPS-induced endotoxaemia or infected with the bacterium Listeria monocytogenes by inducing tissue tolerance to inflammatory damage and consequently reducing organ failure (Wang et al., 2016). On the other hand, a glucose surplus promoted tissue damage in endotoxaemia (Wang et al., 2016). However, higher plasma triglyceride levels were also associated with a protective effect in host tissues during sepsis (Luan et al., 2019). Most likely due to the higher fatty acid oxidation and anorexia induced by leptin, triglyceride levels were lower in plasma, head kidney and liver from fish treated with this hormone. Nevertheless, this reduction in triglyceride levels could result in a benefit for the host in the context of leptin treatment, since triglycerides induce leptin resistance by inhibiting leptin transport across the blood–brain barrier (Banks et al., 2004). Therefore, as was previously observed for the anorexigenic cytokine growth differentiation factor-15 (GDF15) both in mammals (Luan et al., 2019) and fish (Pereiro et al., 2020), leptin could also induce protective effects after bacterial infection without affecting the bacterial load by acting as a sepsis tolerance mediator that protects host tissues from exacerbated inflammation through the regulation of metabolic fluxes. The metabolic regulation mediated by leptin could also be involved in the inhibition of tnfa gene expression, since TNF-α is also a potent regulator of lipid metabolism (Chen et al., 2009). Interestingly, overexpression of gdf15 also reduced the expression of tnfa in zebrafish (Pereiro et al., 2020). Since TNF-α increases fatty acid production and, consequently, plasma triglycerides, the lower levels of triglycerides observed in turbot inoculated with recombinant leptin could also be a consequence of tnfa downregulation.
Conclusions
This is the first time that the overall transcriptomic response of turbot infected with A. salmonicida has been described. In addition to the expected immune response induced by the bacteria, numerous metabolic genes were found to be altered by the infection. Among these, the gene encoding the hormone leptin showed strong upregulation, which highlights the relevance of metabolic regulation during infection. Treatment with recombinant human leptin before and during the course of infection with A. salmonicida significantly increased the survival of the treated turbot, but without affecting the bacterial load. Recombinant leptin significantly reduced food intake and potentiated anorexia in the infected turbot. Moreover, exogenous leptin restored the plasma glucose level reduced by the bacteria and even elevated it over that observed in the control fish, which could indicate leptin mediation of glycolysis inhibition. On the other hand, leptin inhibited the level of circulating triglycerides, which could be linked to the inhibition of tnfa gene expression, among other modulatory effects. The main effects mediated by leptin in turbot and described in this work were summarized in Figure 8. Based on these results, it seems that leptin protected turbot from A. salmonicida infection through the regulation of the interplay among immunity, food intake and systemic metabolism, probably resulting in an increase in sepsis tolerance. More investigations are needed to better elucidate the complete repertoire of mechanisms mediated by leptin in the context of bacterial infection. However, leptin administration could be suggested as an interesting therapeutic treatment for bacterial disease outbreaks in aquaculture.
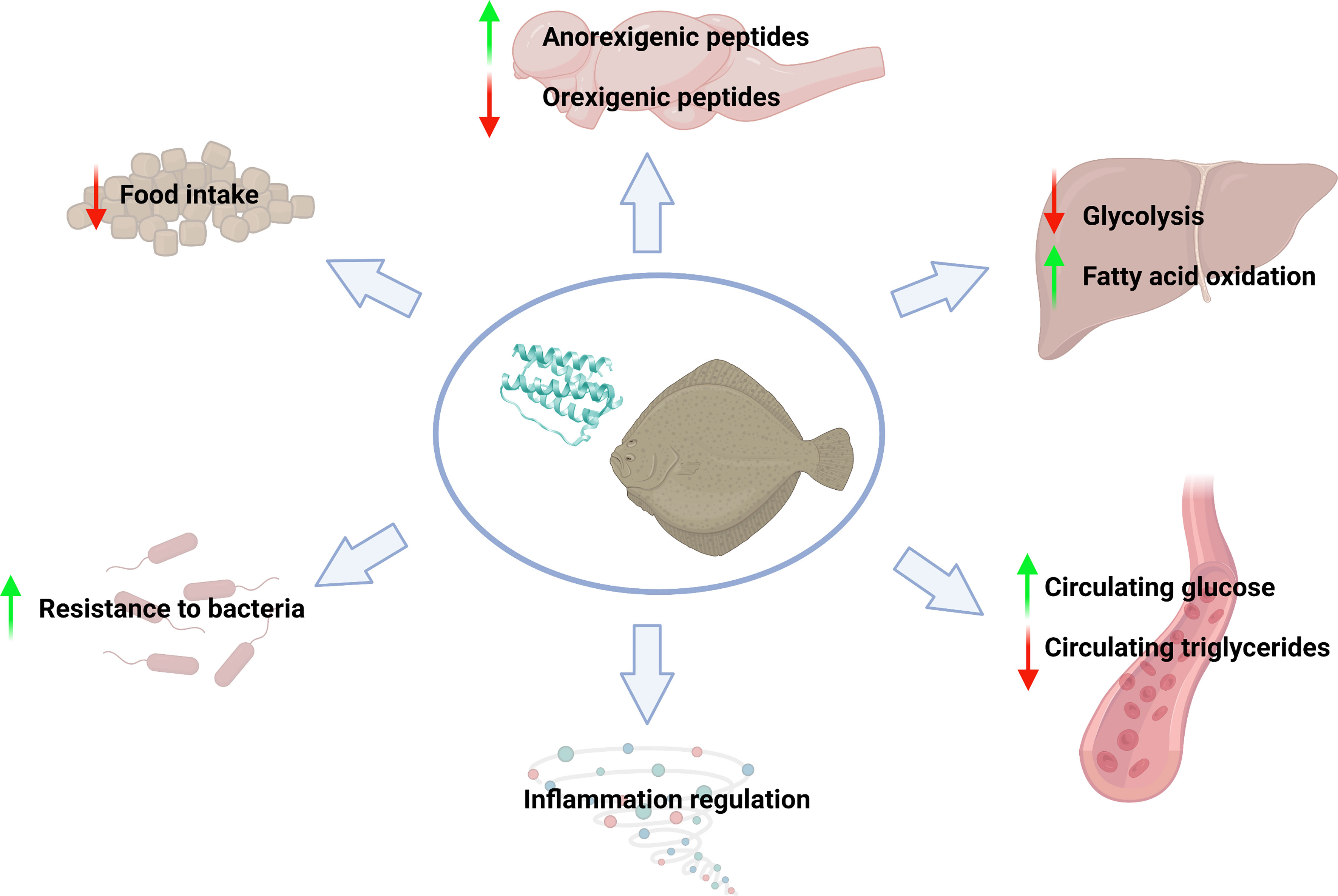
Figure 8 Summary of the main effects mediated by recombinant human leptin administration in juvenile turbot. Figure created with BioRender.com.
Data Availability Statement
The datasets presented in this study can be found in online repositories. The names of the repository/repositories and accession number(s) can be found below: NCBI [accession: PRJNA810312].
Ethics Statement
The animal study was reviewed and approved by CSIC National Committee on Bioethics under approval number ES3605702020012020/13/FUN.01/INM06/BNG.
Author Contributions
ML-P and PP conducted the experimental assays. AF and PP conducted the bioinformatic analyses. ML-P and PP analysed the data, prepared the figures and wrote the manuscript. AF and BN conceptualized and supervised the study and edited and reviewed the manuscript. All authors contributed to the article and approved the submitted version.
Funding
Our laboratory is funded by projects PID2020-119532RB-I00 from Ministerio de Ciencia e Innovación, MetDisFish from Ministerio de Agricultura, Pesca y Alimentación (MAPA) and European Maritime, Fisheries and Aquaculture Fund (EMFAF), 0474_BLUEBIOLAB from EU FEDER Programa Interreg España-Portugal and IN607B 2019/01 from Consellerıa de Economıa, Emprego e Industria (GAIN), Xunta de Galicia. ML-P and PP wish to thank the Ministerio de Ciencia e Innovación and Axencia Galega de Innovación (GAIN, Xunta de Galicia), respectively, for their postdoctoral contracts (IN606B-2018/010; IN606B-2018/010). We acknowledge support of the publication fee by the CSIC Open Access Publication Support Initiative through its Unit of Information Resources for Research (URICI).
Conflict of Interest
The authors declare that the research was conducted in the absence of any commercial or financial relationships that could be construed as a potential conflict of interest.
The handling editor BC declared a past co-authorship with the author PP.
Publisher’s Note
All claims expressed in this article are solely those of the authors and do not necessarily represent those of their affiliated organizations, or those of the publisher, the editors and the reviewers. Any product that may be evaluated in this article, or claim that may be made by its manufacturer, is not guaranteed or endorsed by the publisher.
Acknowledgments
We want to thank Judit Castro and the aquarium staff for their technical assistance.
Supplementary Material
The Supplementary Material for this article can be found online at: https://www.frontiersin.org/articles/10.3389/fmars.2022.888115/full#supplementary-material
Supplementary File S2 | Biological process GO terms enriched for the comparison A. salmonicida-infected vs. control turbot (FDR<0.01).
References
Agwunobi A. O., Reid C., Maycock P., Little R. A., Carlson G. L. (2000). Insulin Resistance and Substrate Utilization in Human Endotoxemia. J. Clin. Endocrinol. Metab. 85, 3770–3778. doi: 10.1210/jcem.85.10.6914
APROMAR. (2020). Aquaculture in Spain. Available at: https://apromar.es/sites/default/files/2020/APROMAR%20Report%20AQUACULTURE%20IN%20SPAIN%202020_0.pdf (Accessed February 24, 2022).
Austin B., Austin D. A. (2007). Bacterial Fish Pathogens: Diseases of Farmed and Wild Fish (Chichester: Springer).
Balcázar J. L., Vendrell D., de Blas I., Ruiz-Zarzuela I., Gironés O., Múzquiz J. L. (2007). Quantitative Detection of Aeromonas Salmonicida in Fish Tissue by Real-Time PCR Using Self-Quenched, Fluorogenic Primers. J. Med. Microbiol. 56, 323–328. doi: 10.1099/jmm.0.46647-0
Banks W. A., Coon A. B., Robinson S. M., Moinuddin A., Shultz J. M., Nakaoke R., et al. (2004). Triglycerides Induce Leptin Resistance at the Blood-Brain Barrier. Diabetes 53, 1253–1260. doi: 10.2337/diabetes.53.5.1253
Blanco A. M., Soengas J. L. (2021). Leptin Signalling in Teleost Fish With Emphasis in Food Intake Regulation. Mol. Cell. Endocrinol. 526, 111209. doi: 10.1016/j.mce.2021.111209
Chen X., Xun K., Chen L., Wang Y. (2009). TNF-Alpha, a Potent Lipid Metabolism Regulator. Cell Biochem. Funct. 27, 407–416. doi: 10.1002/cbf.1596
Considine R. V., Sinha M. K., Heiman M. L., Kriauciunas A., Stephens T. W., Nyce M. R., et al. (1996). Serum Immunoreactive-Leptin Concentrations in Normal-Weight and Obese Humans. N. Engl. J. Med. 334, 292–295. doi: 10.1056/NEJM199602013340503
Copeland D. L., Duff R. J., Liu Q., Prokop J., Londraville R. L. (2011). Leptin in Teleost Fishes: An Argument for Comparative Study. Front. Physiol. 2. doi: 10.3389/fphys.2011.00026
Defoirdt T., Sorgeloos P., Bossier P. (2011). Alternatives to Antibiotics for the Control of Bacterial Disease in Aquaculture. Curr. Opin. Microbiol. 14, 251–258. doi: 10.1016/j.mib.2011.03.004
de Pedro N., Martínez-Alvarez R., Delgado M. J. (2006). Acute and Chronic Leptin Reduces Food Intake and Body Weight in Goldfish (Carassius Auratus). J. Endocrinol. 188, 513–520. doi: 10.1677/joe.1.06349
Dong H. Y., Xu M., Ji Z. Y., Wang Y. X., Dong M. Q., Liu M. L., et al. (2013). Leptin Attenuates Lipopolysaccharide or Oleic Acid-Induced Acute Lung Injury in Mice. Am. J. Respir. Cell Mol. Biol. 49, 1057–1063. doi: 10.1165/rcmb.2012-0301OC
Eisenreich W., Rudel T., Heesemann J., Goebel W. (2019). How Viral and Intracellular Bacterial Pathogens Reprogram the Metabolism of Host Cells to Allow Their Intracellular Replication. Front. Cell. Infect. Microbiol. 9. doi: 10.3389/fcimb.2019.00042
El Morabit A., García-Márquez S., Santos Y. (2004). Is Sea Lamprey a Potential Source of Infection With Aeromonas Salmonicida for Wild and Farmed Fish? Bull. Eur. Assoc. Fish. Pathol. 24, 100–103.
Ewart K. V., Belanger J. C., Williams J., Karakach T., Penny S., Tsoi S. C., et al. (2005). Identification of Genes Differentially Expressed in Atlantic Salmon (Salmo Salar) in Response to Infection by Aeromonas Salmonicida Using cDNA Microarray Technology. Dev. Comp. Immunol. 29, 333–347. doi: 10.1016/j.dci.2004.08.004
Faggioni R., Fantuzzi G., Gabay C., Moser A., Dinarello C. A., Feingold K. R., et al. (1999). Leptin Deficiency Enhances Sensitivity to Endotoxin-Induced Lethality. Am. J. Physiol. 276, R136–R142. doi: 10.1152/ajpregu.1999.276.1.R136
Faggioni R., Moser A., Feingold K. R., Grunfeld C. (2000). Reduced Leptin Levels in Starvation Increase Susceptibility to Endotoxic Shock. Am. J. Pathol. 156, 1781–1787. doi: 10.1016/S0002-9440(10)65049-3
Fan W. H., Huang J., Wang X. H., Shi C. Y., Liu L. (2005). Identification and Phylogenetic Study of Pathogenic Bacteria Causing Ulcer Disease of Cultured Turbot (Scophthalmus Maximus). Wei Sheng Wu Xue Bao. 45, 665–670.
Fernández-Álvarez C., Gijón D., Álvarez M., Santos Y. (2016). First Isolation of Aeromonas Salmonicida Subspecies Salmonicida From Diseased Sea Bass, Dicentrarchus Labrax (L.), Cultured in Spain. Aquac. Rep. 4, 36–41. doi: 10.1016/j.aqrep.2016.05.006
Figueras A., Robledo D., Corvelo A., Hermida M., Pereiro P., Rubiolo J. A., et al. (2016). Whole Genome Sequencing of Turbot (Scophthalmus Maximus; Pleuronectiformes): A Fish Adapted to Demersal Life. DNA Res. 23, 181–192. doi: 10.1093/dnares/dsw007
Francisco V., Pino J., Campos-Cabaleiro V., Ruiz-Fernández C., Mera A., Gonzalez-Gay M. A., et al. (2018). Obesity, Fat Mass and Immune System: Role for Leptin. Front. Physiol. 9. doi: 10.3389/fphys.2018.00640
Gleeson L. E., Sheedy F. J. (2016). Metabolic Reprogramming & Inflammation: Fuelling the Host Response to Pathogens. Semin. Immunol. 28, 450–468. doi: 10.1016/j.smim.2016.10.007
Gorissen M., Bernier N. J., Manuel R., de Gelder S., Metz J. R., Huising M. O., et al. (2012). Recombinant Human Leptin Attenuates Stress Axis Activity in Common Carp (Cyprinus Carpio L.). Gen. Comp. Endocr. 178, 75–81. doi: 10.1016/j.ygcen.2012.04.004
Hsu A., Aronoff D. M., Phipps J., Goel D., Mancuso P. (2007). Leptin Improves Pulmonary Bacterial Clearance and Survival in Ob/Ob Mice During Pneumococcal Pneumonia. Clin. Exp. Immunol. 150, 332–339. doi: 10.1111/j.1365-2249.2007.03491.x
Ikejima S., Sasaki S., Sashinami H., Mori F., Ogawa Y., Nakamura T., et al. (2005). Impairment of Host Resistance to Listeria Monocytogenes Infection in Liver of Db/Db and Ob/Ob Mice. Diabetes 54, 182–189. doi: 10.2337/diabetes.54.1.182
Ji L., Sun G., Li X., Liu Y. (2020). Comparative Transcriptome Analysis Reveals the Mechanism of β-Glucan in Protecting Rainbow Trout (Oncorhynchus Mykiss) From Aeromonas Salmonicida Infection. Fish Shellfish Immunol. 98, 87–99. doi: 10.1016/j.fsi.2019.12.022
Landgraf M. A., Silva R. C., Corrêa-Costa M., Hiyane M. I., Carvalho M. H. C., Landgraf R. G., et al. (2014). Leptin Downregulates LPS-Induced Lung Injury: Role of Corticosterone and Insulin. Cell Physiol. Biochem. 33, 835–846. doi: 10.1159/000358656
Lillehaug A., Lunestad B. T., Grave K. (2003). Epidemiology of Bacterial Diseases in Norwegian Aquaculture—A Description Based on Antibiotic Prescription Data for the Ten-Year Period 1991 to 2000. Dis. Aquat. Org. 53, 115–125. doi: 10.3354/dao053115
Long M., Zhao J., Li T., Tafalla C., Zhang Q., Wang X., et al. (2015). Transcriptomic and Proteomic Analyses of Splenic Immune Mechanisms of Rainbow Trout (Oncorhynchus Mykiss) Infected by Aeromonas Salmonicida Subsp. Salmonicida. J. Proteomics. 122, 41–54. doi: 10.1016/j.jprot.2015.03.031
Luan H. H., Wang A., Hilliard B. K., Carvalho F., Rosen C. E., Ahasic A. M., et al. (2019). GDF15 is an Inflammation-Induced Central Mediator of Tissue Tolerance. Cell 178, 1231–1244.e11. doi: 10.1016/j.cell.2019.07.033
Lv S., Han M., Yi R., Kwon S., Dai C., Wang R. (2014). Anti-TNF-α Therapy for Patients With Sepsis: A Systematic Meta-Analysis. Int. J. Clin. Pract. 68, 520–528. doi: 10.1111/ijcp.12382
Madiehe A. M., Mitchell T. D., Harris R. B. S. (2003). Hyperleptinemia and Reduced TNF-α Secretion Cause Resistance of Db/Db Mice to Endotoxin. Am. J. Physiol. Regul. Integr. Comp. Physiol. 284, R763–R770. doi: 10.1152/ajpregu.00610.2002
Magariños B., Devesa S., González A., Castro N., Toranzo A. E. (2011). Furunculosis in Senegalese Sole (Solea Senegalensis) Cultured in a Recirculation System. Vet. Rec. 168, 431b. doi: 10.1136/vr.c6754
Magnadóttir B. (2006). Innate Immunity of Fish (Overview). Fish. Shellfish. Immunol. 20, 137–151. doi: 10.1016/j.fsi.2004.09.006
Matarese G., La Cava A. (2004). The Intricate Interface Between Immune System and Metabolism. Trends Immunol. 25, 193–200. doi: 10.1016/j.it.2004.02.009
Maurya R., Bhattacharya P., Dey R., And Nakhasi H. L. (2018). Leptin Functions in Infectious Diseases. Front. Immunol. 9, 2741. doi: 10.3389/fimmu.2018.02741
McCarthy D. H., Roberts R. J. (1980). “Furunculosis of Fish: The Present State of Our Knowledge,” in Advances in Aquatic Microbiology, vol. vol. 2 . Eds. Droop M. R., Jannasch H. W. (London, UK: Academic Press), 293–341.
Metsalu T., Vilo J. (2015). ClustVis: A Web Tool for Visualizing Clustering of Multivariate Data Using Principal Component Analysis and Heatmap. Nucleic Acids Res. 43, W566–W570. doi: 10.1093/nar/gkv468
Meyer F. P. (1991). Aquaculture Disease and Health Management. J. Anim. Sci. 69, 4201–4208. doi: 10.2527/1991.69104201x
Millán A., Gómez-Tato A., Pardo B. G., Fernández C., Bouza C., Vera M., et al. (2011). Gene Expression Profiles of the Spleen, Liver, and Head Kidney in Turbot (Scophthalmus Maximus) Along the Infection Process With Aeromonas Salmonicida Using an Immune-Enriched Oligo-Microarray. Mar. Biotechnol. 13, 1099–1114. doi: 10.1007/s10126-011-9374-7
Navarro-Garcia F., Serapio-Palacios A., Ugalde-Silva P., Tapia-Pastrana G., Chavez-Dueñas L. (2013). Actin Cytoskeleton Manipulation by Effector Proteins Secreted by Diarrheagenic Escherichia Coli Pathotypes. Biomed. Res. Int. 2013, 374395. doi: 10.1155/2013/374395
Nougayrede P., Sochon E., Vuiiaume A. (1990). Isolation of Aeromonas Subspecies Salmonicida in Farmed Turbot (Psetta Maxima) In France. Bull. Eur. Ass. Fish. Pathol. 10, 139–140.
O'Neill L. A., Kishton R. J., Rathmell J. (2016). A Guide to Immunometabolism for Immunologists. Nat. Rev. Immunol. 16, 553–565. doi: 10.1038/nri.2016.70
Pedersen K., Kofod H., Dalsgaard I., Larsen J. L. (1994). Isolation of Oxidase-Negative Aeromonas Salmonicida From Diseased Turbot Scophthalmus Maximus. Dis. Aquat. Org. 18, 149–154. doi: 10.3354/dao018149
Pedersen K., Larsen J. L. (1996). First Report on Outbreak of Furunculosis in Turbot, Scophthalmus Maximus Caused by Aeromonas Salmonjcida Subsp. Salmonjcida in Denmark. Bull. Eur. Ass. Fish. Pathol. 16, 129–133.
Pereiro P., Balseiro P., Romero A., Dios S., Forn-Cuní G., Fuste B., et al. (2012). High-Throughput Sequence Analysis of Turbot (Scophthalmus Maximus) Transcriptome Using 454-Pyrosequencing for the Discovery of Antiviral Immune Genes. PloS One 7, e35369. doi: 10.1371/journal.pone.0035369
Pereiro P., Librán-Pérez M., Figueras A., Novoa B. (2020). Conserved Function of Zebrafish (Danio Rerio) Gdf15 as a Sepsis Tolerance Mediator. Dev. Comp. Immunol. 109, 103698. doi: 10.1016/j.dci.2020.103698
Peyon P., Zanuy S., Carrillo M. (2001). Action of Leptin on In Vitro Luteinizing Hormone Release in the European Sea Bass (Dicentrarchus Labrax). Biol. Reprod. 65, 1573–1578. doi: 10.1095/biolreprod65.5.1573
Pfaffl M. W. (2001). A New Mathematical Model for Relative Quantification in Real-Time RT-PCR. Nucleic Acids Res. 29, e45. doi: 10.1093/nar/29.9.e45
Real F., Acosta B., Déniz S., Oros J., Rodriguez E. (1994). Aeromonas Salmonicida Infection in Sparus Aurata in the Canaries. Bull. Eur. Assoc. Fish. Pathol. 14, 153–155.
Rebl A., Korytář T., Köbis J. M., Verleih M., Krasnov A., Jaros J., et al. (2014). Transcriptome Profiling Reveals Insight Into Distinct Immune Responses to Aeromonas Salmonicida in Gill of Two Rainbow Trout Strains. Mar. Biotechnol. 16, 333–348. doi: 10.1007/s10126-013-9552-x
Reidy S. P., Weber J. (2000). Leptin: An Essential Regulator of Lipid Metabolism. Comp. Biochem. Physiol. A Mol. Integr. Physiol. 125, 285–198. doi: 10.1016/s1095-6433(00)00159-8
Romero J., Feijoó C. G., Navarrete P. (2012). “Antibiotics in Aquaculture - Use, Abuse and Alternatives,” in Health and Environment in Aquaculture. Eds. Carvalho E. D., David J. S., Silva R. J. (London, UK: IntechOpen), 159–198. doi: 10.5772/28157
Rozen S., Skaletsky H. (2000). Primer3 on the WWW for General Users and for Biologist Programmers. Methods Mol. Biol. 132, 365–386. doi: 10.1385/1-59259-192-2:365
Shi J., Fan J., Su Q., Yang Z. (2019). Cytokines and Abnormal Glucose and Lipid Metabolism. Front. Endocrinol. 10. doi: 10.3389/fendo.2019.00703
Takahashi N., Waelput W., Guisez Y. (1999). Leptin Is an Endogenous Protective Protein Against the Toxicity Exerted by Tumor Necrosis Factor. J. Exp. Med. 189, 207–212. doi: 10.1084/jem.189.1.207-a
Toranzo A. E., Barja J. L. (1992). First Report of Furunculosis in Turbot Reared in Floating Cages in Northwest Spain. Bull. Eur. Ass. Fish. Pathol. 12, 147–149.
Tracey K. J. (1992). “The Acute and Chronic Pathophysiologic Effects of TNF: Mediation of Septic Shock and Wasting (Cachexia),” in Tumor Necrosis Factors. The Molecules and Their Emerging Role in Medicine. Ed. Beutler B. (New York, NY: Raven Press), 255–273.
Tschöp J., Nogueiras R., Haas-Lockie S., Kasten K. R., Castañeda T. R., Huber N., et al. (2010). and Survival in Sepsis. J. Neurosci. 30, 6036–6047. doi: 10.1523/JNEUROSCI.4875-09.2010
Vallejos A., Olivares P., Varela D., Echeverria C., Cabello-Verrugio C., Pérez-Leighton C., et al. (2018). Preventive Leptin Administration Protects Against Sepsis Through Improving Hypotension, Tachycardia, Oxidative Stress Burst, Multiple Organ Dysfunction, and Increasing Survival. Front. Physiol. 9. doi: 10.3389/fphys.2018.01800
Wang A., Huen S. C., Luan H. H., Yu S., Zhang C., Gallezot J. D., et al. (2016). Opposing Effects of Fasting Metabolism on Tissue Tolerance in Bacterial and Viral Inflammation. Cell 166, 1512–1525.e12. doi: 10.1016/j.cell.2016.07.026
Wang W., Poole B., Mitra A., Falk S., Fantuzzi G., Lucia S., et al. (2004). Role of Leptin Deficiency in Early Acute Renal Failure During Endotoxemia in Ob/Ob Mice. J. Am. Soc Nephrol. 15, 645–649. doi: 10.1097/01.asn.0000113551.14276.0b
Wasyluk W., Zwolak A. (2021). Metabolic Alterations in Sepsis. J. Clin. Med. 10, 2412. doi: 10.3390/jcm10112412
Weil C., Le Bail P. Y., Sabin N., Le Gac F. (2003). In Vitro Action of Leptin on FSH and LH Production in Rainbow Trout (Onchorynchus Mykiss) at Different Stages of the Sexual Cycle. Gen. Comp. Endocr. 130, 2–12. doi: 10.1016/s0016-6480(02)00504-x
Wensveen F. M., Šestan M., Turk Wensveen T., Polić B. (2019). Beauty and the Beast' in Infection: How Immune-Endocrine Interactions Regulate Systemic Metabolism in the Context of Infection. Eur. J. Immunol. 49, 982–995. doi: 10.1002/eji.201847895
Wieland C. W., Florquin S., Chan E. D., Leemans J. C., Weijer S., Verbon A., et al. (2005). Pulmonary Mycobacterium Tuberculosis Infection in Leptin-Deficient Ob/Ob Mice. Int. Immunol. 17, 1399–1408. doi: 10.1093/intimm/dxh317
Willumsen B. (1990). Aeromonas Salmonicida Subsp. Salmonicida Isolated From Atlantic Cod and Coalfish. Bull. Eur. Assoc. Fish. Pathol. 10, 62–63.
Keywords: turbot, Aeromonas salmonicida, RNA-Seq, leptin, immunometabolism
Citation: Librán-Pérez M, Pereiro P, Figueras A and Novoa B (2022) Transcriptome Analysis of Turbot (Scophthalmus maximus) Infected With Aeromonas salmonicida Reveals a Direct Effect on Leptin Synthesis as a Neuroendocrine Mediator of Inflammation and Metabolism Regulation. Front. Mar. Sci. 9:888115. doi: 10.3389/fmars.2022.888115
Received: 02 March 2022; Accepted: 09 May 2022;
Published: 09 June 2022.
Edited by:
Benjamin Costas, University of Porto, PortugalReviewed by:
Monica Fengsrud Brinchmann, Nord University, NorwayTomáš Korytář, University of South Bohemia, Czechia
Copyright © 2022 Librán-Pérez, Pereiro, Figueras and Novoa. This is an open-access article distributed under the terms of the Creative Commons Attribution License (CC BY). The use, distribution or reproduction in other forums is permitted, provided the original author(s) and the copyright owner(s) are credited and that the original publication in this journal is cited, in accordance with accepted academic practice. No use, distribution or reproduction is permitted which does not comply with these terms.
*Correspondence: Beatriz Novoa, YmVhdHJpem5vdm9hbEBpaW0uY3NpYy5lcw==