- 1Department of Biological Sciences, Sungkyunkwan University, Suwon, South Korea
- 2Department of Marine Sciences and Convergent Technology, Hanyang University, Ansan, South Korea
Kelp forests have declined gradually all over the world. Understanding the trophic structure of such a productive and diverse ecosystem is crucial for its restoration and effective management. Few studies, however, have focused on the trophic structure and functional recovery of kelp forests in the process of restoration. This study was conducted in the eastern coast of Korea where kelp bed restoration was in process with the removal of sea urchins. In addition to quantitative measurement for recovery using common community parameters, we investigated how fast the stability of a food web structure could be established in the newly restored kelp beds with an initially barren condition, using stable isotope analysis, in comparison with a nearby natural bed and barren site. At the restored bed, total algal biomass and diversity reached the levels of the natural bed within 1 year. While the δ13C and δ15N values of macroalgae and organic matter were separated isotopically, they were similar among sites, excluding organic matter at the natural bed in 2019. Most consumers showed similar isotopic values among sites, with overlapping range for δ13C of producers. However, some herbivores showed higher δ15N values as predators/omnivores, particularly at barrens, which could be explained by trophic plasticity depending on the macroalgal structure. In the restored bed, for the first year, higher trophic diversity (CR, CD) and lower trophic redundancy (MNND, SDNND) showed non-overlapping >50% CIs among sites. However, this distinctive stage moved toward the natural bed upon entering the second year as trophic diversity decreased and trophic redundancy increased while natural bed overlapped at<50% CIs. The recovery speed in this system was fast (1 year for quantitative perspectives and 2 years for functional completion). After removing the sea urchins, recruitment of macroalgae quickly increased concomitantly with consumer groups, boosting the diversity and trophic structure of the restored bed. The trophic structure of the first year of restoration was not an intermediate stage toward the completion, but a transient over-shooting state. This might have been triggered by the fast introduction of diverse macroalgae to the urchin-free bare rock space, providing an interesting finding needed to be tested in other temperate marine systems.
Introduction
Kelps are large, brown macroalgae belonging to the genera Laminaria, Ecklonia, and Macrocystis, among others. They constitute marine subtidal forests in temperate and subpolar latitudes around the world (Shannon and Weaver, 1949; Steneck et al., 2002; Smale and Wernberg, 2013; Krumhansl et al., 2016; Wernberg et al., 2016; Layton et al., 2019). Kelps are key components as foundational species and food resources (Steneck et al., 2002; Graham, 2004) for a myriad of biota, ranging from small invertebrates to large mammals and other macroalgae (Mann, 1973; Christie et al., 2003). Unfortunately, kelp forests have been threatened by coastline urbanization, sewage outfalls, global warming, and ocean acidification (Filbee-Dexter and Scheibling, 2014) along with extension of destructive grazing by urchins and herbivorous fish (Ling et al., 2009; Wernberg et al., 2016). Kelp forests have declined substantially over the past half-century, with approximately 38% loss of kelp forest areas globally (Krumhansl et al., 2016). Recent studies have revealed that kelp deforestation can trigger a cascade of impacts on energy flow (Moore and De Ruiter, 2012; Thompson et al., 2012; Eger et al., 2021; Gabara et al., 2021), biodiversity, and ecosystem services (Graham, 2004; Edwards et al., 2020; Spector and Edwards, 2020). Considering the highly valued roles of kelp forests, there have been many efforts to mitigate kelp deforestation and its notable impacts on kelp-associated ecosystems.
Diverse kelp restoration studies have been conducted to understand how well algal beds and ecological functions can re-develop in deforested grounds (Ling et al., 2015; Teagle et al., 2017; Layton et al., 2019). Generally, biomass and density in the recovered site have been simultaneously compared with those in the reference (or damaged) habitat to determine the recovery success (Graham, 2004; Rooney et al., 2008). However, there might be a limit with only a numeric approach (e.g., biomass and density) to prove trophic relationships between food source and its consumers (Baskett and Salomon, 2010; Salomon et al., 2010; Leleu et al., 2012). Furthermore, the recovery timing and procedure are not simply predictable due to temporal and regional variability caused by fluctuating environmental conditions and biotic factors (Krumhansl et al., 2016). This is a critical point for kelp restoration projects because re-developing kelps can exert a bottom-up regulation for consumer populations in complex ways, ranging from direct effects on diet availability for consumers to indirect effects on overall food web functioning (Moore and De Ruiter, 2012; Thompson et al., 2012; Clasen and Shurin, 2015; Miller et al., 2018). Examining the trophic relation and diets of consumers, particularly in kelp ecosystems, can provide crucial insights into the recovery mechanisms underlying the ecological functioning and recommendations for sustainable management actions at local and regional levels (Graham, 2004; Rooney et al., 2008). Nevertheless, limited studies have been conducted to evaluate food web structure and functional recovery in the process of kelp forest restoration (Neckles et al., 2002; Kang et al., 2008).
Stable isotope ratio analysis has become one of the most common tools to understand trophic connectivity, food web structure, and ecosystem functioning (Bearhop et al., 2004; Newsome et al., 2007; Abrantes and Sheaves, 2009; Rader et al., 2017; Nielsen et al., 2018; James et al., 2020; Gabara et al., 2021). This is because the stable isotope ratios of carbon (δ13C) and nitrogen (δ15N) in consumer’s tissues can reflect its food resources in a predictable manner (Deniro and Epstein, 1978; Fry and Sherr, 1989; Hobson and Welch, 1992), showing negligible values for δ13C or quite stable stepwise enrichment for δ15N with a trophic transfer (Minagawa and Wada, 1984; Post, 2002). Ultimately, the combination of δ13C and δ15N values is a useful tool to indicate the strength and weight of a trophic network more objectively and effectively (Wozniak et al., 2006; Nordström et al., 2015). Recently, stable isotope signatures with Bayesian ellipses have provided diverse information such as available resources, resource contribution, trophic niche overlapping, and trophic diversity (Bearhop et al., 2004; Newsome et al., 2007; Abrantes and Sheaves, 2009; Demopoulos et al., 2017; Rader et al., 2017; Nielsen et al., 2018; James et al., 2020). In this respect, isotope tools have been applied to understand food web changes in response to several restoration management actions (or plans) in diverse damaged habitats (e.g., salt marsh and estuary), particularly for macrofauna and fish. However, most subtidal restoration programs have focused less on functional recovery and its procedure for macrobenthic consumers in a kelp-associated community (but see Kang et al., 2008; Cresson et al., 2014), although how fast the newly restored kelp community can achieve functional recovery and food web stability remains unclear.As a part of kelp restoration projects conducted in the east coast of Korea with sea urchin’s removal on barren sites, we selected sites where successful restoration was achieved and investigated the food web structure of the first year and that of the second year of restored algal beds in two consecutive years. We also determined how the temporal change of the food web structure, including the macrobenthic consumer community, would occur during the restoration status of kelp beds. A comparative assessment using stable isotope analysis was made with the nearby existing natural kelp bed and the barren site as reference sites.
Materials and methods
Study Area and Experimental Conditions
This study was part of Korea’s nationwide kelp restoration project (Hong et al., 2021). This particular work was focused on the restoration of kelp forests by controlling sea urchin density and the recovery of food web structure in the restored kelp beds. The study site was located at the shallow coastal area in Jangho, Samcheok City, on the eastern coast of Korea (37°17′1.5″ N, 129°19′21.48″ E, Figure 1). The restoration experiment was conducted in the subtidal zone (depth range: 7–10 m), which contained multiple rock beds mostly separated by sand. These rocky substrates were completely barren with a high density (average: 7.5 individuals m-2) of sea urchins, Mesocentrotus nudus and Strongylocentrotus intermidius, before the initiation of this study. Our previous survey (Jeon et al., 2015) done at the same site indicated that the subtidal macroalgal community showed a distinctive assemblage with a gradient of sea urchin density. In the present survey before the initiation of sea urchin control, the low-urchin zone (0–2 individuals m-2) was dominated by brown macroalgae (such as Undaria pinnatifida and Sargassum horneri) and red alga (Pachymeniopsis spp.), showing a typical macroalgal forests in this area in contrast with the barren urchin zone (>8 individual m-2) which showed a little coverage of folious algae with the spontaneous appearance of Colpomenia claytoniae, Dictyota coriacea, and Ulva australis.
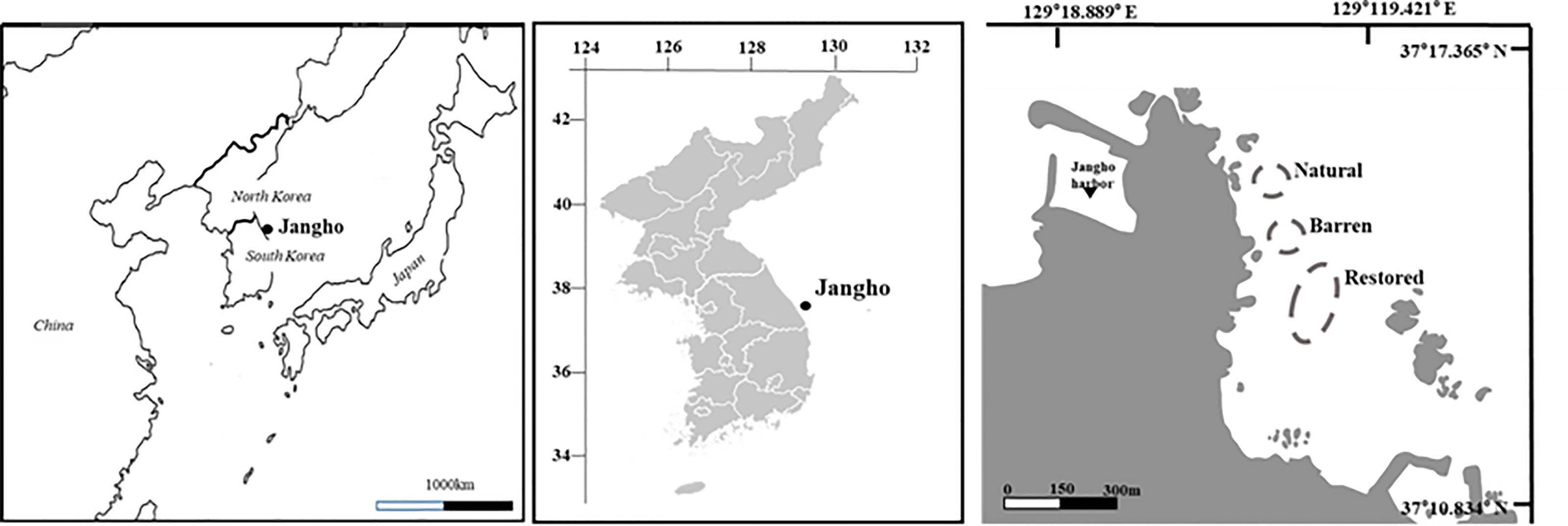
Figure 1 Map of the study area in Jangho-ri, Samcheock-si, South Korea (37°17′1.5″ N, 129°19′21.48″ E).
The experiment was started in August 2017 with the first sea urchin removal in the restored bed. It was ended in August 2019. In the barren zone, we divided the area into two sites, one for no-urchin zone (named “restored bed” hereafter) and the other for sea-urchin-present control zone (“barren site”). We removed the sea urchins by SCUBA diving continuously at an interval of 2 to 3 months during the study, leaving other herbivores (such as Aplysia kurodai, Chlorostoma lischkei, and Haliotis discus) uncontrolled because their densities were low. This manipulation was effective in keeping the sea urchin density at less than 2 individuals m-2 because the existing sandy substratum between bedrocks made it hard for the sea urchins to move across. The natural kelp beds (“natural bed”) located nearby—180 m away from the barren site (also 285 m away from the restored bed)—was chosen as the reference site. During the study, a total of 12 species of benthic consumers were present, including 7 species of herbivores (Acmaea pallida, Aplysia kurodai, Chlorostoma lischkei, Haliotis discus, Mesocentrotus nudus, Stichopus japonicus, and Strongylocentrotus intermedius) and 6 species of secondary consumers and omnivores (Asterias amurensis, Asterina pectinifera, Cellana grata, Ceratostoma fournieri, Kelletia lischkei, and Thais bronni). The flora and fauna in this site have also been reported previously (Choi et al., 2006; Kang, 2010).
Sample Collection and Processing
During our study period, macroalgae, organic matter (OM), and benthic invertebrates were sampled in June of 2018 and 2019 by SCUBA diving at the restored bed, the natural bed, and the barren site. The sampling month in June of 2018 and 2019 is the end of spring, which has been frequently observed as the most productive season for macroalgae in the area. All visible macroalgal species with five replicate thalli were collected at each of the three sites for stable isotope analysis. After the epiphytes were carefully removed, the macroalgae were then rinsed in the field. In addition, periphytonic OM was sampled by scraping with a brush (area of 100 cm2) the rock surface underwater. The sampled periphytonic OM attached to the brush was resuspended in distilled water. After a pre-filtration procedure through a 100-μm sieve to remove large particles, OM was collected on pre-combusted GF/F 0.7-μm glass fiber filters. For consumer samples, the replicate individuals of macroinvertebrates were hand-picked in all three sites to secure enough volume for analysis. The samples of macroalgae and macroinvertebrates were sorted and identified to the lowest taxonomic level in the lab.
Stable Isotope Analysis
To reduce any possible variations of algal tissue types to appear on stable isotopic analysis, mature and healthy-looking individuals (e.g., excluding the thallus with epiphytes or reproductive organs such as sporangia or shedding parts) were used. For consumers, the most abundant size classes were chosen to reduce the variability of stable isotope value in response to the size and the development stage of the consumer (Fredriksen, 2003; Sun et al., 2012) rather than targeting mixed sizes and classes. Then, consumer samples were dissected to obtain primarily muscle tissues such as the jaw muscles of Aristotle’s lanterns of sea urchins, podia of sea star, foot tissue of gastropods, and body walls of sea cucumbers. All samples from primary producer groups (macroalgae and OM) and consumer groups (benthic macroinvertebrates) were freeze-dried. Biota samples were then ground to a fine powder with mortar and pestle. The pulverized samples, approximately 5 mg for algal sample and 1 mg for consumers, were weighed into tin capsules for nitrogen isotope analysis (N = 3). Samples containing inorganic carbonates were pretreated with HCl. Approximately 1 mg of algal sample and 0.5 mg of consumer sample were then used for carbon isotope analysis (N = 3).
Both carbon and nitrogen isotope ratios were analyzed using an elemental analyzer (Euro EA3028, EuroVector, Milan, Italy) coupled to an isotope ratio mass spectrometer (Isoprime, GV Instruments, Manchester, UK). Stable isotope ratios were expressed as relative ratio to the standard in permillage (‰) with a standard δ notation:
where X was 13C or 15N and R was the corresponding ratio of 13C/12C or 15N/14N relative to the Vienna Pee Dee Belemnite standard and atmospheric N2, respectively. We performed triplicate measurements for each sample.
Recovery of Macroalgal Community in Trophic Base
The characteristics of the macroalgal assemblage were measured with several indices, including the Shannon–Wiener species diversity index (H′, Shannon and Weaver, 1949), based on algal taxa specific biomass and total biomass. Differences in macroalgal abundance (total biomass) among three sites (N = 8 for restored bed, N = 5 for natural bed, and N = 5 for barren site) and between two sampling years (2018 and 2019) were compared using a two-way analysis of variance (ANOVA) followed by Tukey HSD multiple comparison. The normality and homogeneity of variance of all data were evaluated using Levene’s test prior to ANOVA. Differences of macroalgal assemblages among the three sites from 2018 and 2019 (as two fixed factors) were tested with PERMANOVA and visually represented by non-metric multidimensional scaling based on similarity (PRIMER statistical package, Clarke and Warwick, 1994).
Effects of Basal Algal Source Availability on Herbivores
To explore the patterns of δ13C and δ15N signatures in macroalgae and consumers, we performed one-way ANOVA among sites, followed by Tukey HSD multiple comparison. If some species were observed in less than three cases, we used independent t-tests. All data were examined for normality and heteroscedasticity using Levene’s tests (Zar, 1999). To assess the effect of macroalgal assemblage differences across sites on consumers, we compared the trophic position of herbivores at these sites. To calculate the trophic position, the equation of TP = [(δ15Norg - δ15Nbase)/trophic discrimination factor (TDF)] + TPbase with the nitrogen value of each species was used (Post et al., 2000). TDF was assumed to be 3.40 ± 1.00‰ for δ15N (Post, 2002). When trophic base as a nitrogen range of primary producers varied, the value of herbivores could substitute that of a primary producer (Hussey et al., 2014; Kang et al., 2016; Lake et al., 2019). The use of primary consumers helps to establish the most accurate δ15N baseline value and their TP base fitted to a local environment for estimating the consumer TP of interest. Therefore, in this study, we used TPbase of 2 and δ15Nbase of the mean of nitrogen isotope value of herbivores in each community for estimating the trophic position of consumers.
Community Iso-space Metrics
Carbon and nitrogen isotopic signatures of macroinvertebrate consumers (grouped by taxa and feeding guild) were used to investigate the structure of trophic webs in each site using community-wide metrics (Layman et al., 2007a). Overall, 13 consumer species were sampled for community metrics analysis. Those species were grouped by trophic guilds according to their feeding habits into herbivores, omnivores, and predators (Kang et al., 2008). Three Layman’s community metrics suggested trophic diversity derived from δ13C–δ15N bi-plots in each site. δ13C range (CR) and δ15N range (NR) gave an estimate of diversity of basal resources and trophic length of the community, respectively. Mean distance to centroid (CD), measured as the average of Euclidean distance of each consumer’s group from δ13C–δ15N centroid, gave a measure of the average degree of trophic diversity. Two other Layman’s metrics, mean nearest neighbor distance (MNND) and standard deviation of the nearest neighbor distance (SDNND), represented trophic redundancy. Low values of MNND indicated a large proportion of members with similar trophic preferences. SDNND was a measure of evenness of community component packing, with low values representing a more uniform distribution of trophic niches (Layman et al., 2007a; Layman et al., 2007b). Layman’s community-wide metrics with Bayesian inference were analyzed using the SIBER package in R (Jackson et al., 2011). To clarify isotopic niches, we used standard ellipse area (SEA) to compare communities. SEA was calculated with the SIAR package in R (Jackson et al., 2011).
Results
Macroalgal Structure and Diversity
Twenty-nine macroalgal species (4 Chlorophyta, 9 Phaeophyta, 16 Rhodophyta, and one Magnoliophyta) were found at the three sites (restored bed, natural bed, and barren site) during the study period (Table 1). In 2018, as the first year of recovery, 19 algal species were present in the restored bed, which had higher richness and diversity (H′ = 0.75) than the natural bed (6 species, H′ = 0.36) and the barren site (5 species, H′ = 0.40). The high richness pattern that appeared in the restored bed in 2018 continued to 2019, the second year of recovery. On the other hand, the diversity index dropped to 0.40, similar to that of the natural bed (0.35). The recovery pattern of macroalgal abundance in the restored bed is shown in Figure 2 in comparison with the natural bed and the barren site. In 2018, the macroalgal biomass of the restored bed (1,568.3 ± 277.47 g wet weight m-2) reached a level comparable to that of the natural bed (1,446.7 ± 385.00 g wet weight m-2, p = 0.807), which was about 5.7 times higher than that of the barren site. This fast recovery of total biomass of macroalgae within 1 year at the restored bed was also found for the annual pattern in 2019, having a macroalgal biomass similar to that of the natural bed (Figure 2).
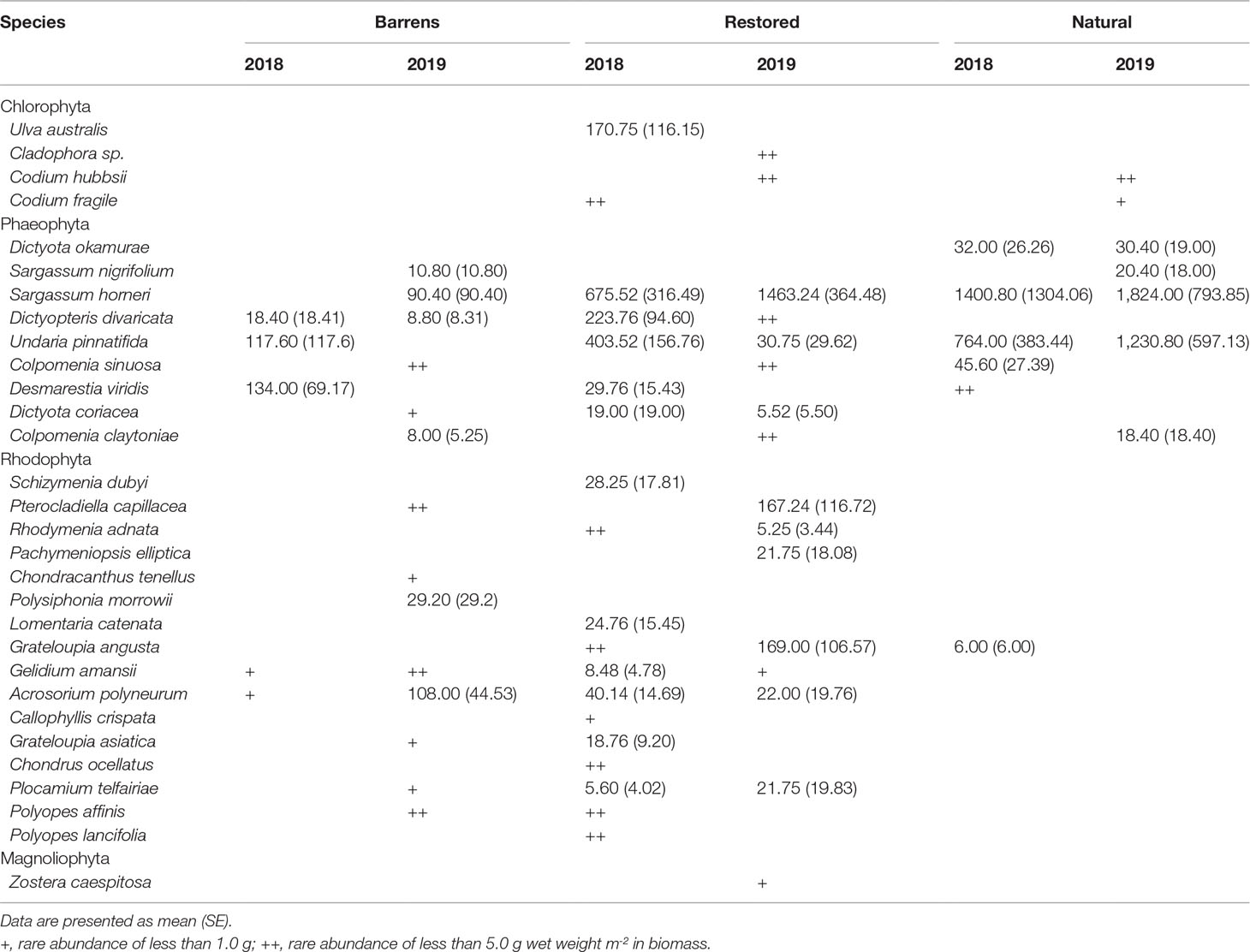
Table 1 Spatial and temporal changes of biomass (g wet weight m-2) of macroalgae in barrens, restored bed, and natural bed.
From MDS analysis (Figure 3), there were significant separations by sites, years, and their interactions (all p< 0.05), whereas the natural bed showed a tight clustering in 2018 and 2019 (p = 0.481), indicating no substantial change in its natural community assemblage. Distinctive separations were observed in the restored bed and the barren site between both years, respectively (all p< 0.05). Compared to the 1-year-old restored bed, a tighter clustering between the 2-year-old restored bed and the natural bed was observed (average similarity of 20.936 in 2018 vs. 38.577 in 2019), indicating that a significant recovery process was shown in the restored bed from 2018 to 2019 with respect to macroalgal biomass, diversity, and community structure (Figures 2, 3; Table 1). Both restored bed and natural bed shared the same top two dominant species (Sargassum horneri and Undaria pinnatifida, comprising around 40 and 30% of total algal biomass, respectively) in both years, indicating that the newly recovered algal assemblage had already exhibited a mature natural form (Table 1). Such brown algal dominance did not appear in the barren site. Moreover, several red algae (including Acrosorium polyneurum and Gelidium amansii) and green algae (such as Ulva australis) were found (1–5%) in the restored bed. However, those were rare (< 1%) in the natural bed for both years, whereas they were found in the barren site particularly in 2019 (2%).
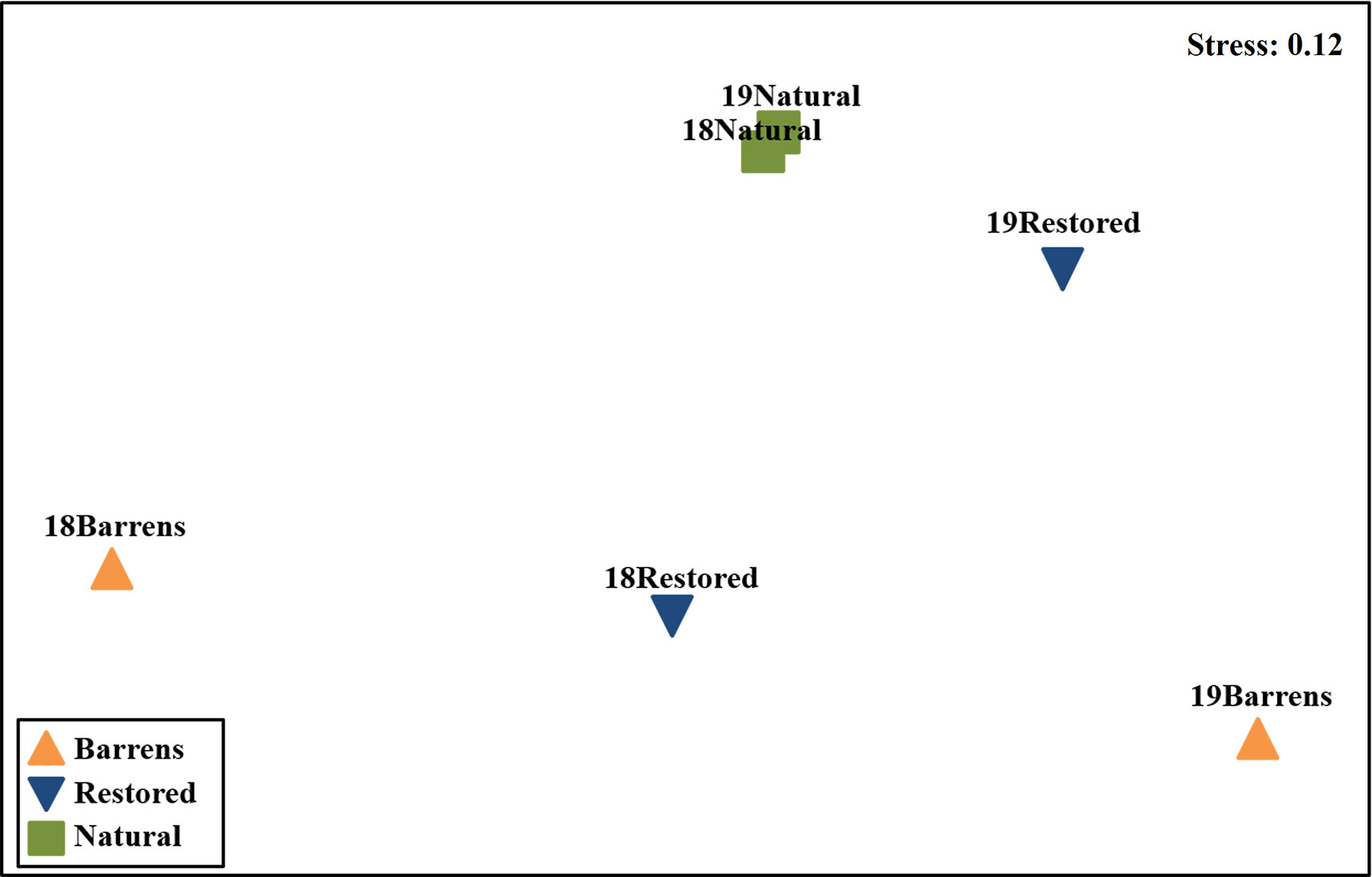
Figure 3 Multidimensional scaling analysis for comparing macroalgae assemblages based on Bray–Curtis similarities of square-root-transformed total biomass.
Contribution of Macroalgal Sources to Consumers
Isotope Variability of Producers among Habitats
We analyzed 39 different macroalgal species as well as periphytonic OM considered as primary producers for herbivores. The results of δ13C and δ15N compositions of primary producers among the three sites in 2018 and 2019 are shown in Supplementary Table S1and Figure 4. Rhodophyta (e.g., Acrosorium polynerum) showed the lowest δ13C value, while Chlorophyta (e.g., Ulva. australis in 2018 and Codium hubbsii in 2019) showed the highest value. The values of Phaeophyta (e.g., Sargassum horneri) and OM tended to overlap or appear between the range of Rhodophyta and Chlorophyta
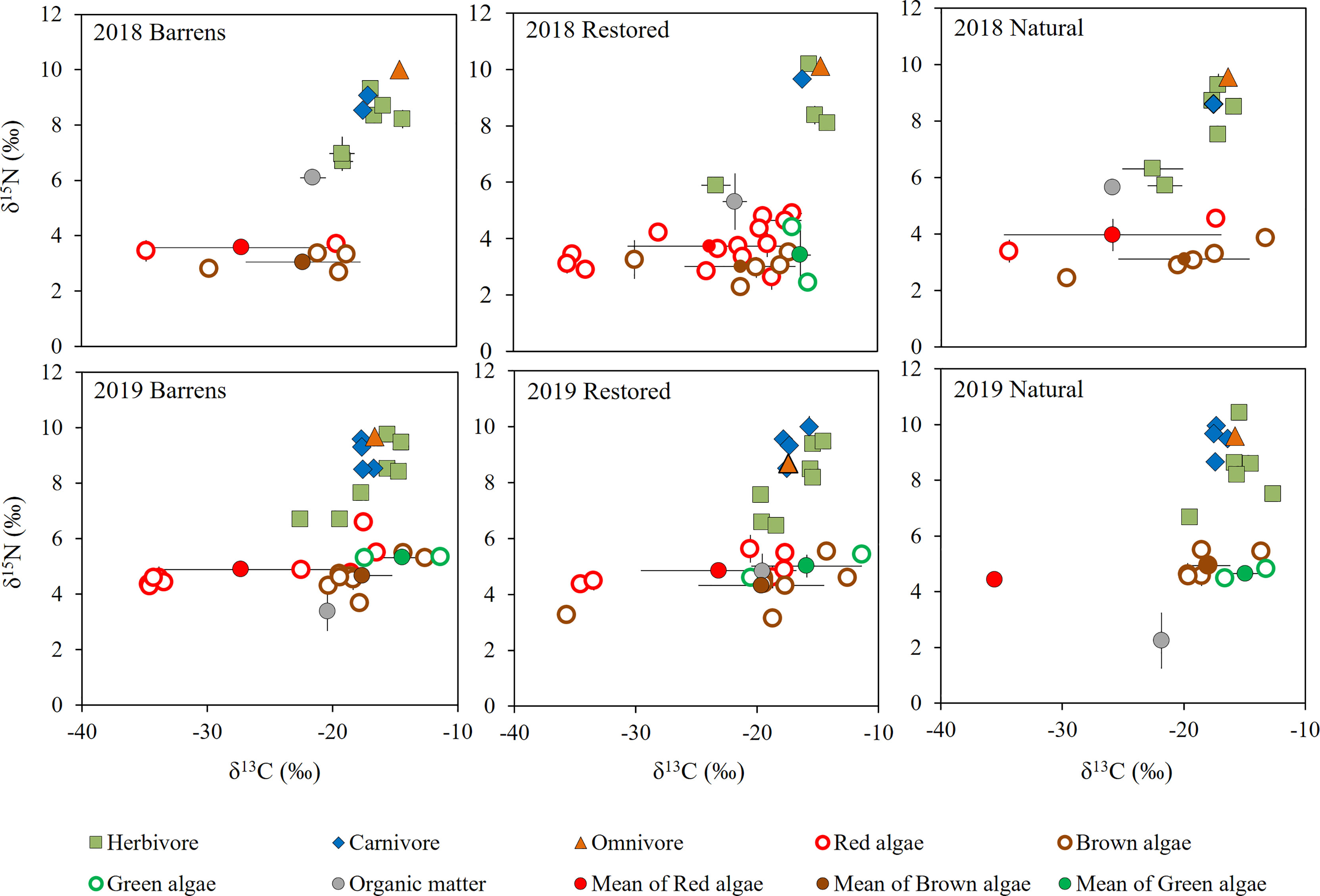
Figure 4 Bi-plots of mean δ13C and δ15N values of potential diet resources and consumers in each year and site in this study. Vertical and horizontal error bars represent the standard errors of each sample (n = 3). Filled circles indicate the average of overall chlorophytes, phaeophytes, and rhodophytes occurring in barrens, restored bed, and natural bed in 2018 and 2019, respectively.
Of diverse macroalgal species, Acrosorium polynerum, Undaria pinnatifida, and Sargassum horneri were dominant in 2018 (listed in Supplementary Table S1). Their δ13C and δ15N values from the natural bed were similar to those from the barren site and the restored bed, showing only<0.5‰ difference on average. In addition, the δ13C values of OM in 2018 were not significantly different among sites (p = 0.801, Supplementary Table S1). In 2019, the δ13C and δ15N values for most of the common macroalgal species, such as U. pinnatifida (p = 0.770 for carbon, p = 0.453 for nitrogen, Supplementary Table S1), were not significantly different among habitats. Contrarily, OM in 2019 showed significantly lower δ13C and δ15N values for those from the natural bed (2.2‰ for δ13C, p = 0.003, Supplementary Table S1 and 2.6‰ for δ15N, p = 0.024, Supplementary Table S1) relative to the restored bed isotope variability of consumers among habitats.
Isotope Variability of Vonsumers Among Habitats
The isotope compositions of herbivores and non-herbivorous consumers are shown in Supplementary Table S2 and Figure 4. Herbivores showed relatively lower δ15N values than predators/omnivores (i.e., at least 0.77‰ difference), whereas trophic step increases of approximately 3.40 ± 1.00‰ are commonly reported (Post, 2002). The consumers for δ13C values changed little through food webs, as generally accepted (Deniro and Epstein, 1978). In 2018, there was a low isotopic variation of overall consumers in the barren site (standard deviation 0.3 for δ13C and 1.1‰ for δ15N) compared to the restored bed and the natural bed (standard deviation over 0.4‰ for δ13C and over 1.4‰ for δ15N) (Figure 4). At the species level, Acmaea pallida showed a significant difference in δ13C value among the three sites, showing the following order: barren site = restored bed > natural bed (3.5‰ on average) (p< 0.001, Supplementary Table S2). Other herbivores (including Aplysia kurodai and Haliotis discus) at the barren site (or restored bed) were higher (from 2.5 to 3.5‰ on average) than those in the natural bed. However, their differences were not statistically significant. Furthermore, such isotope variability in δ13C value among habitats was not seen for δ15N. Strongylocentrotus intermedius and H. discus had similar δ13C values (< 1.0‰ difference) at the barren site and the natural bed. In 2019, the δ13C values of A. pallida and A. kurodai in the barren site were slightly but significantly higher (0.7 and 2.9‰ on average) than those in the restored bed (A. kurodai, p< 0.001; A. pallida, p = 0.008, Supplementary Table S2). However, such δ13C difference among habitats was not detected for other herbivore species. Moreover, slight but significant increases (i.e., > 0.7‰) of δ15N values were found for Mesocentrotus nudus and Chlorostoma lischkei at the barren site in 2019 (M. nudus, p = 0.027; C. lischkei, p = 0.032, Supplementary Table S2), but not for other herbivore species.
Trophic Position Shifts of Herbivores
To distinguish the effects of basal food source availability on the upper trophic levels, we compared the trophic positions (TPs) of direct consumer groups among sites and between years (Figure 5). When determining the TP changes of herbivores, the δ15N values of common herbivores were used as trophic base (TP = 2). The baseline δ15N values for estimating trophic length/position in 2018 and 2019 were 8.03 and 8.17‰ at the barren site, 8.13 and 8.02‰ at the restored bed, and 7.68 and 8.34‰ at the natural bed, respectively. In 2018, the TPs of Haliotis discus and Acmaea pallida were higher (0.2 and 0.3, respectively) at the barren site (or restored bed) than at the natural bed (H. discus, p< 0.001; A. pallida, p = 0.026) (natural bed > barren site > restored bed, Figure 5). However, such difference was not detected for other herbivores such as Mesocentrotus nudus or Strongylocentrotus intermedius. Consistently, in 2019, there was no significant TP variability for M. nudus or S. intermedius among habitats. In 2019, the TPs of A. pallida, Chlorostoma lischkei, and Stichopus japonicus at both the barren site and the restored bed were higher (over 0.3) than those at the natural bed on average (A. pallida, p = 0.009) (barren site > restored bed > natural bed; C. lischkei, p = 0.010, natural bed > barren site > restored bed; S. japonicus, p = 0.037, Figure 5).
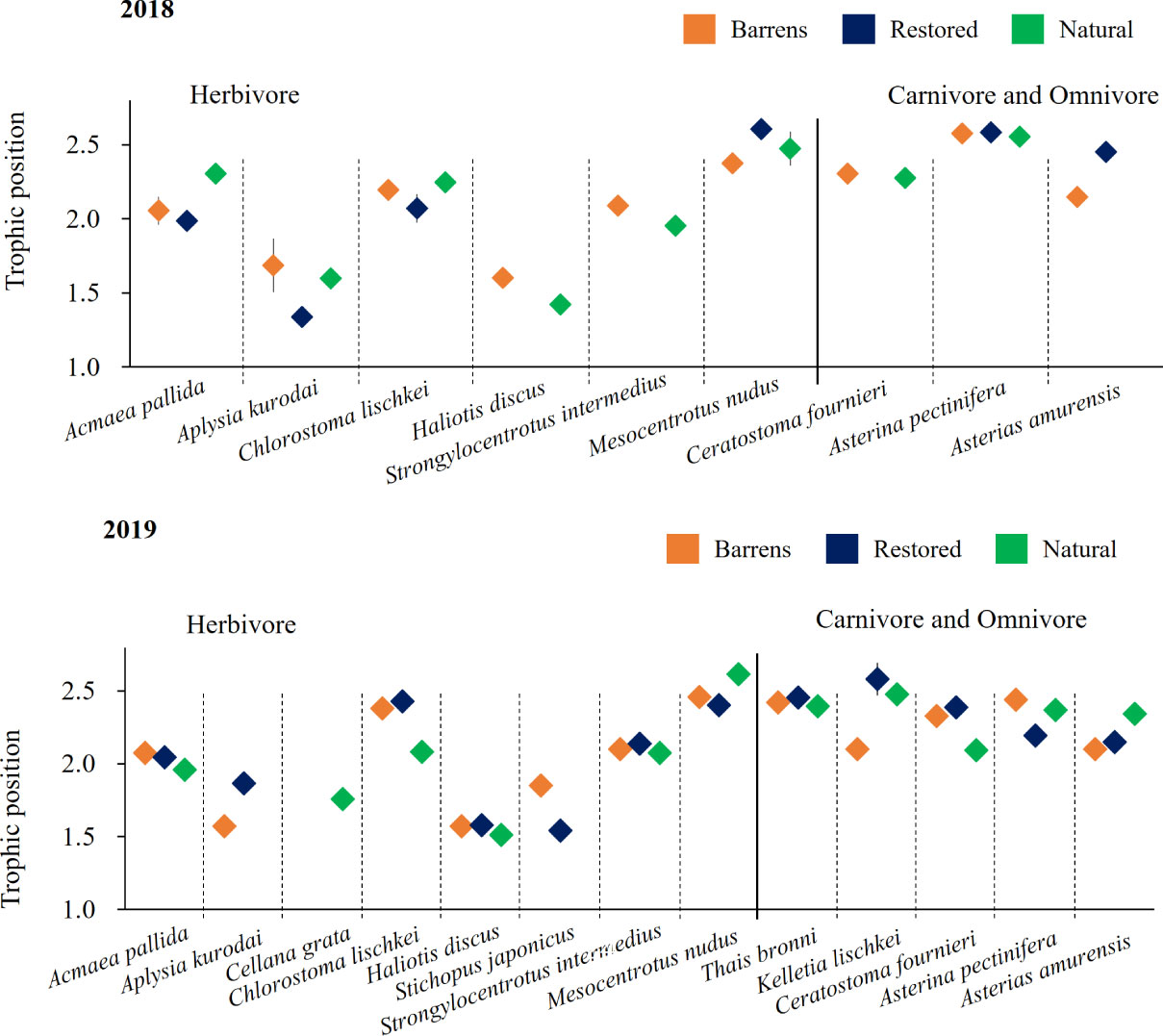
Figure 5 Trophic positions of consumers at each year and site (represented by colored squares). The symbol and the vertical error bar represent the average and the standard error of each sample, respectively (n = 3).
Trophic Structure and Isotopic Niche of Consumers
Spatial Differences in Trophic Structure
The changes of trophic structure among sites and years based on the community metrics and SEA are shown in Figures 6, 7. These results were represented by mode, 50, 75, and 95% confidence intervals (CI), and then graphically compared between sites and years based on the overlapping degree to indicate similarities/dissimilarities. In 2018, there were notable differences in community metrics except for δ15N range among sites. At the restored bed, all community metrics were especially higher than those at other sites without overlap at >50% CIs. In addition, the restored bed had the largest SEA and CD, suggesting a higher trophic diversity and a less compact food web than the other two sites. At the barren site, trophic diversity (particularly, lower CD) was smaller than that of the natural bed with overlap at >75% CIs. However, the community metrics of trophic redundancy (MNND and SDNND) showed a level similar to that of the natural bed with overlap at<50% CIs, which means that the barren site had a less diverse food web structure than the natural bed (Figures 6, 7). In 2019, all community metrics including trophic diversity (i.e., CR, CD, and SEA) and redundancy (i.e., MNND and SDNND) in the restored bed decreased to the levels of the natural bed with overlapping at<50% CIs, indicating that the community structure of benthic consumers became close to that of the natural bed in a period of 2 years, whereas the SEA and community metrics at the barren site in 2019 increased slightly compared to those in 2018. CR and CD for the restored bed especially showed an analogous tendency in 2018. The increased trophic niche at the barren site in 2019 might be due to an increase of the CR value.
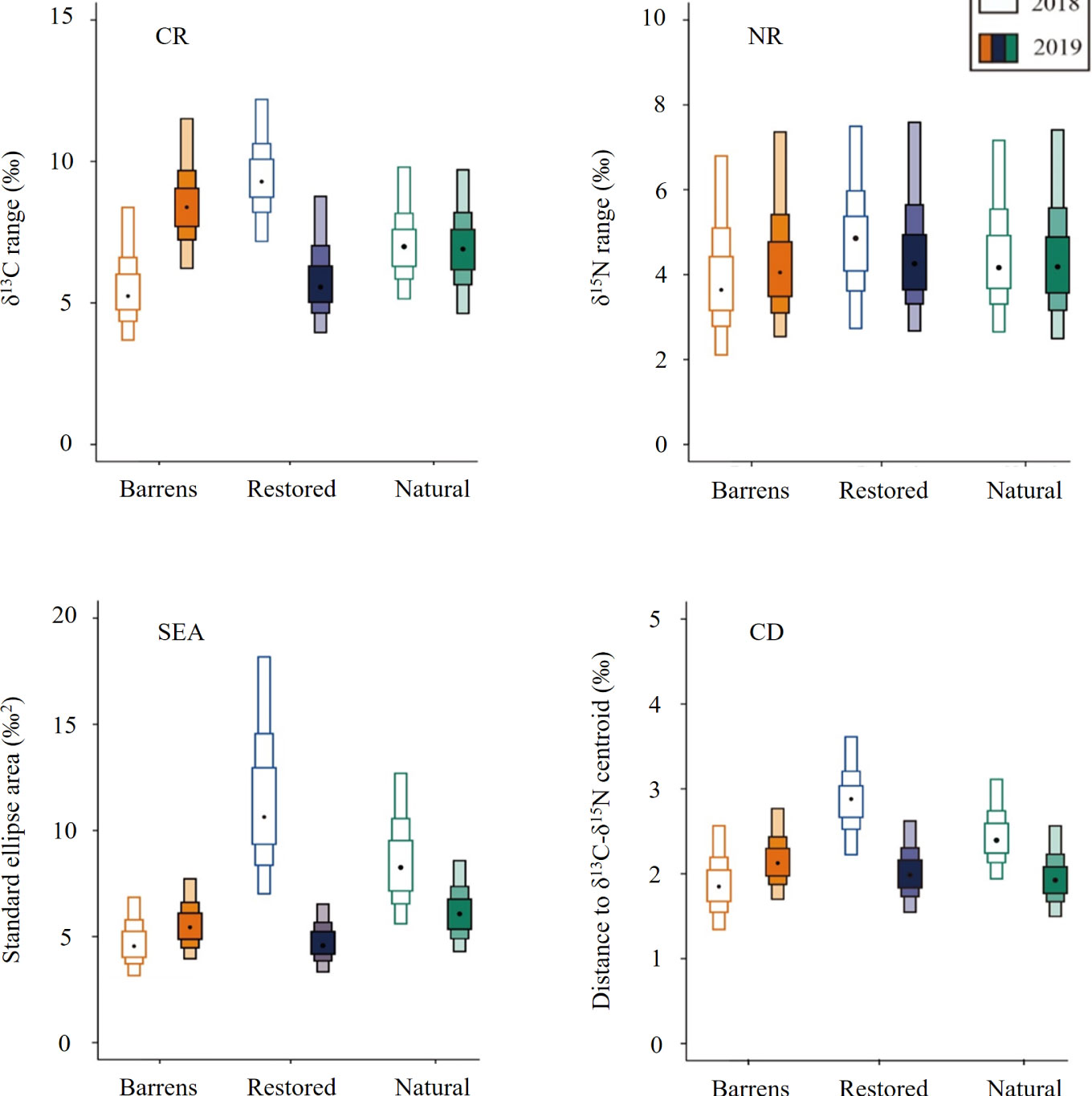
Figure 6 Three of the community metrics and SEA inferred by Bayesian, providing information on trophic diversity within a food web—CR (δ13C range = maximum δ13C − minimum δ13C, ‰), NR (δ15N range = maximum δ15N − minimum δ15N, ‰), SEA (standard ellipse areas, calculated from the variance and covariance of δ13C and δ15N, ‰2), and the mean CD (distance to the centroid, calculated from the mean Euclidian distance of each individual to the δ13C–δ15N centroid for target population or community, ‰). Black dots indicate the mode. Boxes indicate 50, 75, and 95% of credibility intervals for each site (shown in axis) and year. 2018 data and 2019 data are represented by empty squares and colored squares, respectively.
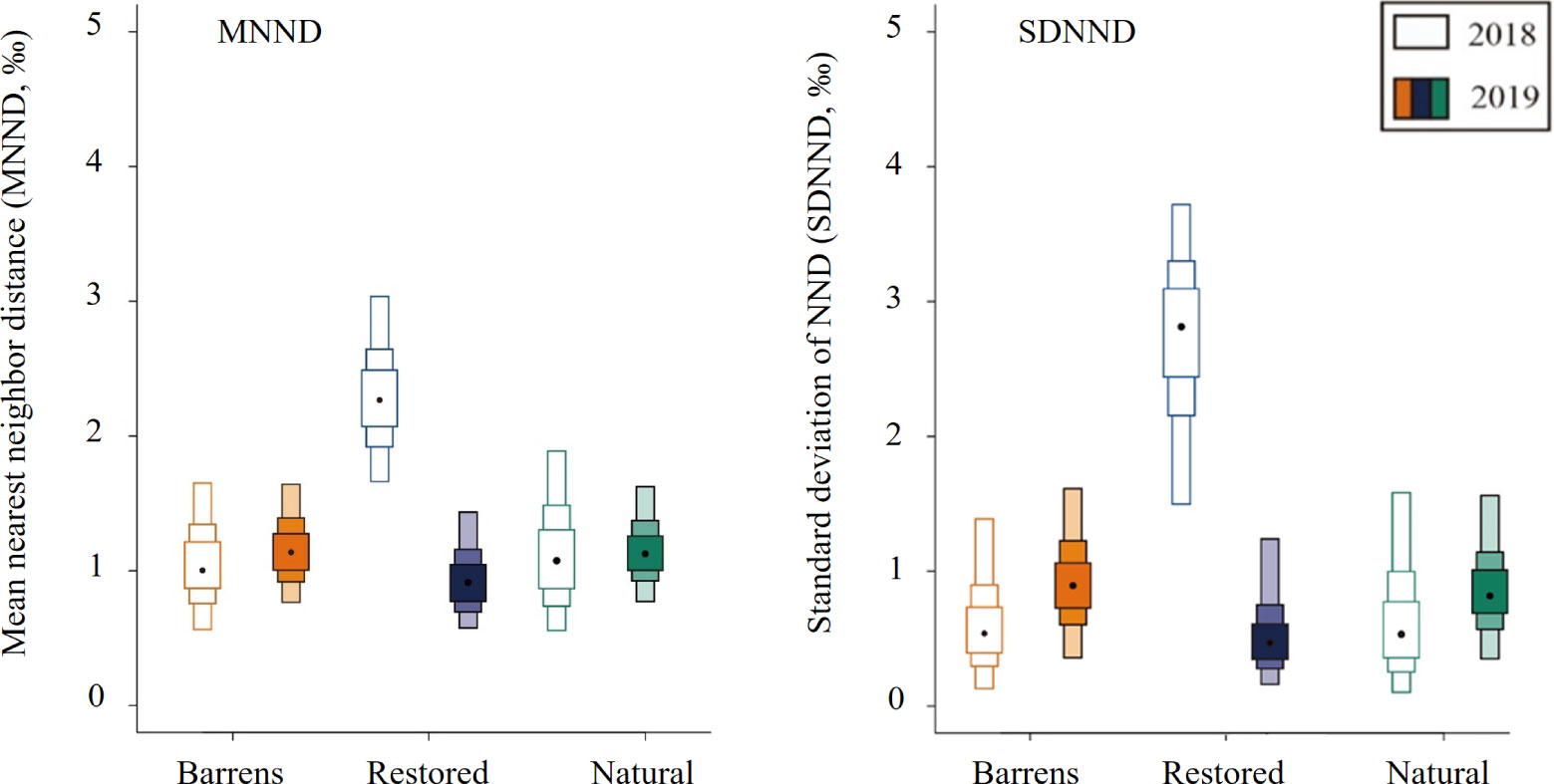
Figure 7 Two community metrics (mean nearest neighbor distance and standard deviation of the nearest neighbor distance) in a Bayesian model presenting information on trophic redundancy within a food web. 2018 data and 2019 data are respectively represented by empty squares and colored squares.
Annual Changes in Trophic Structure
At the restored bed, SEA in 2019 became low without overlapping at 95% CIs compared to SEA in 2018. The other four metrics (CR, CD, MNND, and SDNND) in 2019 also became low to non-overlapping at 75 and 95% of CIs, respectively (Figures 6, 7). The decreased SEA in 2019 might be due to the decreased CR, indicating a low number of species with depleted carbon value. Moreover, the decreased community metrics at the restored bed in 2019 were comparable to those of the natural bed overlapping at 50% CIs, indicating that the over-shooting value of the trophic structure at the restored bed in 2018, the first year of restoration, was at a transient state (Figures 6, 7). However, the tendency observed in the restored bed did not appear at the barren site. The SEA and community metrics at the barren site in 2019 were increased slightly compared to those in 2018, especially for CR and CD, which showed an analogous tendency to those of the restored bed in 2018. The increased trophic niche might be due to the increased CR value in 2019. Unlike the restored bed or the barren site, the natural bed showed no annual difference in trophic structure. A low MNND at the natural bed indicated relatively similar trophic ecologies with a high trophic redundancy (Figures 6, 7).
Discussion
Our results revealed that functional recovery based on food web structure during the restoration of kelp beds showed an unexpectedly fast (within 2 years) but interesting pattern rarely reported in previous works. The continuous removal of sea urchins dramatically changed the community structure of the barren site to the existing natural macroalgal bed level, which took 1 year for quantitative perspectives and 2 years for trophic structure. In quantitative terms, total algal biomass, regardless of its assemblage, achieved the level of the natural bed within 1 year, indicating the effectiveness of controlling herbivores, specifically sea urchins, in this system. The trophic structure in the restored bed became similar to its corresponding natural bed in the second year with herbivore control, indicating that the functional recovery of kelp beds took longer than 1 year, approximately 2 years. The restored forest especially showed, in the first year, a rather unexpected pop-up with higher trophic diversity and complexity than the forest of the natural bed instead of showing a transitional stage toward the completion of stabilization of the trophic structure.
Characteristics of Algal Assemblage During Recovery
Macroalgal biomass in the restored bed reached a level comparable to that of the natural bed in 2018, also sharing the same dominant group with the natural bed community. This indicated that restoration was completed in quantitative perspectives in 1 year after sea urchin control, consistent with the result of our pilot experiment conducted 21 km away from the present study site (JH Kim, personal communication). Several studies have reported a phase shift between barrens and kelp forests (Gagnon et al., 2004; Yoo et al., 2004; Kim et al., 2012; Piazzi and Ceccherelli, 2019). Many studies have focused on the effects of sea urchin removal and changes in the composition of the algal community. When the grazing pressure from a high density of sea urchins is released, the chance for additional producers, including fast-growing, opportunistic algae and diatoms, to emerge within days could be high. Kelp recruitment is also increased within several years (Airoldi, 2000; Filbee-Dexter and Scheibling, 2014). According to Gagnon et al. (2004) and Guarnieri et al. (2020), the increase of early macroalgal colonists is fast. Further introduction of habitat-forming kelp species is delayed until 3 years later. Our result could be one of the fastest cases of phase shift from barrens to kelp beds among published studies that mostly show a recovery after more than 2 years (Ling et al., 2015; Tracey et al., 2015; Guarnieri et al., 2020).
Our results also indicated that species diversity had the highest level in the 2018 restored community among all sites during the entire period. This pop-up increase of diversity in the first year of recovery might be due to the introduction of new colonists (such as Chondrus ocellatus, Gelidium amansii, Grateloupia asiatica, and Ulva australis) that were not major components in the existing natural bed. The high recruitment of these members mostly observed in the first year at the restored bed followed by a decrease when entering the second year to the natural bed level might need further explanations. First, these competitively recessive species could be recruited without the presence of existing competitive canopy-forming superiors (e.g., Sargassum and Undaria species) in the first year at the restored bed since this site has been barren before the initiation of the experiment. Second, these recessive species might have been provided for an equal opportunity of establishment with superiors, benefiting from enough bare space and simultaneous recruitment timing [see also Airoldi (2000)]. Canopy-forming species are usually slow-growing types, requiring a longer time to be recruited compared to opportunistic and ephemeral algae (Thibaut et al., 2014). However, once the canopy-forming species are colonized, opportunistic pioneer species will have reduced recruitment opportunity (Benedetti-Cecchi, 2000; Thibaut et al., 2014). Not all the recessive members in our study may not be opportunistic; however, this explanation can be supportive, in part, to our result.
Isotopic Signature of Potential Food Sources and Consumers
The macroalgae showed a wide range of δ13C values in each site, similar to the pattern shown by Kang et al. (2008). Most macroalgae that co-existed at the restored bed and the natural bed showed a similar level of isotopic signature of carbon and nitrogen within the range of -35 to -13‰ for δ13C and 2 to 6‰ for δ15N (Supplementary Table S1 and Figure 4). Specifically, red algae represented the most depleted δ13C (around -35‰). The most enriched δ13C was found for green algae at around -15‰. The distribution of δ13C values of red algae also showed a wide gap between brown and green algae. With depleted δ13C values, red algae contributed to a small amount of whole biomass. Hence, red algae are viewed as minor dietary sources for grazing herbivores (e.g., Choi et al., 2006; Kang et al., 2008). However, Fredriksen (2003) and Andrade et al. (2016) have reported that grazing gastropods use red algae as a main food source. In our study, a number of red algal species appeared in substantial amounts during the study period, contributing to consumers as potential food sources. Therefore, the low δ13C values of Aplysia kurodai could reflect its significant consumption on red algae. Further investigation is needed to clarify the relationship between the feeding habits of consumers and red algae within the food web.
OM was also considered in this study as a food source or part of the food web base. It included debris from macroalgae, suspended particulate organic matter, sediment, and other matters. The δ13C of OM in 2018 was comparable to that of brown algae specifically. It was also similar among sites, implying that the isotopic value of OM could be affected by the distributed macroalgae on each site. However, the δ15N value of OM was about 3‰ higher than that of brown algae, separating from other primary producers. The higher δ15N in OM relative to other sources (macroalgae) might be related to the production of waste matter from consumers and their microbial colonization, which would contribute as an additional pool of OM available in ecosystems (Deither et al., 2019). This difference seemed to influence the value of consumers as well. Specifically, sea urchins (i.e., Mesocentrotus nudus) showed differences among sites regarding isotopic signatures. Fredriksen (2003) has stated that sea urchins presumably feed on diverse food resources including macroalgae as well as epilithic microalgae, even encrusting animals on the barren ground. Furthermore, sea urchins help in creating OM by spreading macroalgal particulates, which become available for benthic consumers (Yorke et al., 2019). The OM that originated from macroalgae and other resources is an important food source to consumers, particularly on the barren ground (Bustamante et al., 1995; Bustamante and Branch, 1996; Yorke et al., 2019). Therefore, in the sea urchin-dominated barren site, OM should be a responsible component for sea urchins’ survival in the food web when not much preferred food choice is available. Based on the indispensable contribution of OM as a nutrient source, it is expected that OM can influence the overall consumers’ isotopic values.
Although the δ13C values of some consumers were not distributed within the range of macroalgae or OM at the barren site or the restored bed in 2018, most herbivores showed high δ13C isotopic signatures in 2019, which were within the δ13C range of potential food resources (Figure 4). Some herbivores were Acmaea pallida, Chlorostoma lischkei, and Mesocentrotus nudus in this study. These species showed a slight variation in the isotopic compositions of consumers among sites and between years. This unexpected phenomenon could be due to the non-sampled resources within the food web (e.g., epiphytes and different macroalgae species), as pointed by Catry et al. (2016). Potentially, Codium fragile with an unusually high δ13C value was notably sampled in 2019, but not in 2018. Thus, the use of this algal species by herbivores might have caused δ13C range shift and increased the trophic niche of herbivores in 2019. Such trophic shift of consumers (e.g., Acmaea pallida and Chlorostoma lischkei) could also be due to changes in macroalgal structure at the barren site and the restored bed in 2019 based on PERMANOVA result (p = 0.003). Particularly, Ulva australis was notably decreased in 2019 compared to that in 2018. Thus, the use of this species with high δ13C values by herbivores generated a broader δ13C range for herbivores in 2019. Chlorophyte C. fragile and U. australis might be food sources available for herbivores.The isotopic signatures of δ13C and δ15N and the trophic position of benthic herbivores in this study indicated that they might have a different trophic ecology although they share the same feeding strategy. As herbivores, gastropods Aplysia kurodai, Cellana grata, Haliotis discus and the holothruoidea Stichopus japonicus showed corresponding δ13C values for macroalgae (approximately -24 to -12‰) at all sites and years (Figure 4). Their δ15N values were about 3‰ higher than those of the macroalgal group (approximately 5 to 8.5‰), suggesting that these four species could graze directly on macroalgae. However, other gastropods (Acmaea pallida and Chlorostoma lischkei) and Echinoidea Mesocentrotus nudus, known as herbivores, showed a similar range of δ13C values to the macroalgal group but much higher δ15N values at the restored bed in 2019 and at the barren site in both 2018 and 2019. Such δ15N value difference relative to the average of the entire macroalgal resources as potential dietary resources (i.e., approximately 4‰) is beyond the trophic enrichment factor of 3.4‰ suggested by Post (2002). Across sites, the trophic position of M. nudus was recorded from 2.38 (2018 barren site) to 2.62 (2019 natural bed). These results suggest that this species might have a similar trophic position as omnivores or predators (Figure 5). According to Mottet (1976) and Himmelman and Steele (1971), sea urchins can feed on various resources, especially when the macroalgal abundance is low, implying that sea urchins behave like generalist feeders that cover a wide variability of prey, including algal species, debris originated from kelps, animal carcass, and even crustose coralline algae on rocks. Our results might support the case claimed by Mottet (1976) and Himmelman and Steele (1971).
Changes in the Food Web Structure During Recovery
The changes in the trophic structure at the restored bed showed an interesting pattern during the two years of restoration. It was surprising that the food web structure in the first year was not at transient level forwarding to the second year’s stabilizing state. It was comparable to that of the natural bed. However, it showed a pop-up increment represented by an increase of trophic diversity (supported by increases of CR, CD, and SEA) and a decrease in trophic redundancy (supported by increases of MNND and SDNND). This phenomenon coincides with the over-shooting level of macroalgal diversity in the first year at the restored bed, although its biomass did not exceed that of the natural bed, indicating that producer diversity, not abundance, was the key factor responsible for the unexpected increase in trophic diversity. When the sea urchin density dropped quickly, the grazing pressure was released. Then, filamentous algae and benthic diatoms could occur within days, laying the foundation for the development of algal canopies with increased kelp recruitment within 1–3 years (Gagnon et al., 2004; Yoo et al., 2004; Wakanuki et al., 2010; Filbee-Dexter and Scheibling, 2014). Under a barren condition without sea urchins, it is likely that diverse algal species are free to occupy plenty of bare spots without much competitive pressure. Turning to the second year, the space for new-arrival species could have been limited due to the occupancy of existing settlers. Thus, those competitive recessives might have been excluded by dominant components, causing a reduction in diversity. When the resource diversity fell to a low level, it might promote consumers to change their feeding strategies, leading to a high trophic redundancy. These changes could be due to the consumers’ adaptation to decreased resource diversity (Winemiller and Pianka, 1990; Costa-Pereira et al., 2017; Pool et al., 2017; Mcmeans et al., 2019).
In our results, the MNND for the barren site and the natural bed in 2018 and 2019 was low and similar to the restored bed (only in 2019). The similar variation of MNND might be related to differences in sources of nutrition supporting the consumers of trophic ecologies in the barren and the natural bed, respectively. At the same time, there was a slight increase of food web complexity (i.e., CR, SEA, and CD) in the second year at the barren site, with its biomass level being consistently low in the first year. The yearly variation of MNND and food web complexity in the barren site suggests the input of nutritional sources like a transient increase of diversity level of macroalgae that affects the food web structure for that particular timing. In fact, recruits of small-sized algae such as Acrosorium polyneurum, Colpomenia claytoniae, and Pterocladiella capillacea (Table 1) that did not appear in the previous year were found in the barren site, surely contributing more to the diversity index but less to biomass or percent covers. A transient change in sea urchin density for that particular place and time was not detected in our data with a 2-month interval. This phenomenon re-confirmed two findings in our study: first, food web indices based on stable isotope analysis were more sensitive to the diversity of the algal community rather than biomass to determine the criteria of recovery and, second, our result of the stable isotope analysis on the second year at the barren site showed a somewhat analogous pattern to the restored bed in the first year, a boosting start-up from barrens to recovery.
Ultimately, adaptive feeders with diverse diet choices may improve the long-term stability of complex food webs by compensating low resources in environments and by increasing trophic redundancy, but without losing consumer diet connectivity (Kondoh, 2003; Thompson et al., 2012; Matich et al., 2017; Wootton, 2017; Mcmeans et al., 2019)—that is, the trophic plasticity of some consumers could reflect flexible trophic shifts, which can loosen the strengths of trophic interaction and release low-density prey from predation pressure to promote food web stability, especially with the prevalence of omnivores depending on resource diversity (Mccann and Hastings, 1997; Kondoh, 2003; Mcmeans et al., 2019). Such consumer response to fluctuating diet abundance was observed in a few gastropod species, Mesocentrotus nudus, and omnivores in our study as well. Hence, the notable changes in algal species’ diversity are likely due to the control of sea urchin which might allow the introduction of a wide range of producers, thus supporting the introduction of more diverse consumers. Therefore, diverse and abundant food sources could lead to a higher trophic diversity and a lower trophic redundancy of the consumer community at the restored bed of the first year (e.g., Layman et al., 2007b; Abrantes and Sheaves, 2009; Wang et al., 2016; Park et al., 2020). Abrantes et al. (2014) have demonstrated that the low trophic diversity and SEA in estuarine fish food webs are due to a low producer availability caused by the high level of loaded suspended sediments, which can restrict primary productivity in the water. However, in our study, the abundance of primary producers was constant in 2018 and 2019. The only notable change observed was the diversity of macroalgae. Therefore, in the process of restoration, changes of food web structure are likely to be caused by changes of food source diversity rather than food abundance (Hogsden and Harding, 2014; Park et al., 2020; Gabara et al., 2021).
Quantitative Recovery vs. Functional Recovery
Most previous studies regarding the restoration of kelp forests have focused on quantitative recovery, such as abundance, diversity, and other community parameters (Wozniak et al., 2006; Marzinelli et al., 2016; Layton et al., 2019). The functional recovery of a community as a stable ecosystem could be measured by the trophic structure and food web stability. However, such approach has been empirically rare. This study reports the first case of fieldwork using recently developed community-wide isotopic metrics to describe the trophic structure as well as to demonstrate the functional recovery of kelp beds along with quantitative recovery. Since stable isotope analysis is considered as one of the most powerful tools to examine the food web structure according to Abrantes et al. (2014), our results of the isotope matrix provide a better understanding about how the trophic structure is changed from being barren to a forest, suggesting a promising tool for the functional diagnosis of a newly established habitat in the ecosystem. About 2 years after the initiation of sea urchin density control, the barren site reached the level of completion of recovery equivalent to the state of the natural kelp bed nearby for both macroalgal abundance and food web structure. However, the trophic structure and stability of the restored system were not similar to the natural forests, although the macroalgal biomass recovered to the level of the natural forest in the first year. As Graham (2004) and Leclerc et al. (2015) stated, understanding the structural complexity and functional relatedness such as energy flow through the food web is required for a better understanding of kelp forests.
In this study, the change of species diversity and food complexity in sea urchin-free space was unexpectedly fast, and this site became similar, in terms of ecological status, to our natural bed by removing sea urchin regularly from the barren site. Such effort to reduce the ecological impacts in the barren areas needs to be tested in a wider area via a long-term study to confirm that such actions are needed in the future. We can forecast that similar results would be expected if the main cause of the barren site is sea urchins. Otherwise, if the cause is pollution, water temperature, and others, it can be predicted that the restoration process would be gradual and without pop-up trends as witnessed in our study. This study also reports that the speed of phase shift from being barren to a forest can be much more rapid (within 1 year for quantitative recovery and within 2 years for qualitative recovery when sea urchins are controlled) than any other ecosystems, although further evidence is needed for generalization.
Data Availability Statement
The original contributions presented in the study are included in the article/Supplementary Material. Further inquiries can be directed to the corresponding authors.
Author Contributions
JHK: designed the research. MJK, HYY, KHS and JHK, and JK: conducted the research. MJK and HYY: analyzed the specimens and data. MK, HY, and JHK: wrote the manuscript. MK, HY, K-HS, and JHK: provided critical review. JHK had primary responsibility for the final content. All authors contributed to the article and approved the submitted version.
Funding
This study was supported by a NRF grant (2018R1D1A1 B07048996) to JHK and by the MOF (the Ministry of Oceans and Fisheries, Republic of Korea) grant (20220526) to KHS.
Conflict of Interest
The authors declare that the research was conducted in the absence of any commercial or financial relationships that could be construed as a potential conflict of interest.
Publisher’s Note
All claims expressed in this article are solely those of the authors and do not necessarily represent those of their affiliated organizations, or those of the publisher, the editors and the reviewers. Any product that may be evaluated in this article, or claim that may be made by its manufacturer, is not guaranteed or endorsed by the publisher.
Acknowledgments
We are grateful to Min Seob Kim (National Institute of Environmental Research) for his technical support. We also thank Dong Seok Lee, Daniela Macias, Kwon Mo Yang, and Seokwoo Hong, members of the Marine Ecology Lab of Sungkyunkwan University, for their help in the field.
Supplementary Material
The Supplementary Material for this article can be found online at: https://www.frontiersin.org/articles/10.3389/fmars.2022.885676/full#supplementary-material
References
Abrantes K. G., Barnett A., Bouillon S. (2014). Stable Isotope-Based Community Metrics as a Tool to Identify Patterns in Food Web Structure in East African Estuaries. Funct. Ecol. 28, 270–282. doi: 10.1111/1365-2435.12155
Abrantes K., Sheaves M. (2009). Food Web Structure in a Near-Pristine Mangrove Area of the Australian Wet Tropics. Estuar. Coast. Shelf Sci. 82, 597–607. doi: 10.1016/j.ecss.2009.02.021
Airoldi L. (2000). Responses of Algae With Different Life Histories to Temporal and Spatial Variability of Disturbance in Subtidal Reefs. Mar. Ecol. Prog. Ser. 195, 81–92. doi: 10.3354/meps195081
Andrade C., Ríos C., Gerdes D., Brey T. (2016). Trophic Structure of Shallow-Water Benthic Communities in the Sub-Antarctic Strait of Magellan. Polar Biol. 39, 2281–2297. doi: 10.1007/s00300-016-1895-0
Baskett M. L., Salomon A. K. (2010). Recruitment Facilitation can Drive Alternative States on Temperate Reefs. Ecology 91, 1763–1773. doi: 10.1890/09-0515.1
Bearhop S., Adams C. E., Waldron S., Fuller R. A., Macleod H. (2004). Determining Trophic Niche Width: A Novel Approach Using Stable Isotope Analysis. J. Anim. Ecol. 73, 1007–1012. doi: 10.1111/j.0021-8790.2004.00861.x
Benedetti-Cecchi L. (2000). Priority Effects, Taxonomic Resolution, and the Prediction of Variable Patterns of Colonisation of Algae in Littoral Rock Pools. Oecologia 123, 265–274. doi: 10.1007/s004420051013
Bustamante R. H., Branch G. M. (1996). The Dependence of Intertidal Consumers on Kelp-Derived Organic Matter on the West Coast of South Africa. J. Exp. Mar. Biol. Ecol. 196, 1–28. doi: 10.1016/0022-0981(95)00093-3
Bustamante R. H., Branch G. M., Eekhout S. (1995). Maintenance of an Exceptional Intertidal Grazer Biomass in South Africa: Subsidy by Subtidal Kelps. Ecology 76, 2314–2329. doi: 10.2307/1941704
Catry T., Lourenço P. M., Lopes R. J., Carneiro C., Alves J. A., Costa J., et al. (2016). Structure and Functioning of Intertidal Food Webs Along an Avian Flyway: A Comparative Approach Using Stable Isotopes. Funct. Ecol. 30, 468–478. doi: 10.1111/1365-2435.12506
Choi C. G., Kwak S. N., Sohn C. H. (2006). Community Structure of Subtitdal Marine Algae at Uljin on the East Coast of Korea. Algae 21, 463–470. doi: 10.4490/ALGAE.2006.21.4.463
Christie H., Jørgensen N. M., Norderhaug K. M., Waage-Nielsen E. (2003). Species Distribution and Habitat Exploitation of Fauna Associated With Kelp (Laminaria Hyperborea) Along the Norwegian Coast. J. Mar. Biol. Assoc. U.K. 83, 687–699. doi: 10.1017/S0025315403007653h
Clarke K.R., Warwick R.M. (1994) Changes in Marine Communities: An Approach to Statistical Analyses and Interpretation.Natural Environment Research Council, Plymouth
Clasen J. L., Shurin J. B. (2015). Kelp Forest Size Alters Microbial Community Structure and Function on Vancouver Island, Canada. Ecology 96, 862–872. doi: 10.1890/13-2147.1
Costa-Pereira R., Tavares L. E. R., De Camargo P. B., Araújo M. S. (2017). Seasonal Population and Individual Niche Dynamics in a Tetra Fish in the Pantanal Wetlands. Biotropica 49, 531–538. doi: 10.1111/btp.12434
Cresson P., Ruitton S., Harmelin M. (2014). Artificial Reefs do Increase Secondary Biomass Production: Mechanisms Evidenced by Stable Isotopes. Mar. Ecol. Prog. Ser. 509, 15–26. doi: 10.3354/meps10866
Deither M. N., Hoins G., Kobelt J., Lowe A. T., Galloway A. W. E., Schram J. B., et al. (2019). Feces as Food: The Nutritional Value of Urchin Feces and Implications for Benthic Food Webs. J. Exp. Mar. Biol. Ecology. 514 (515), 95–102. doi: 10.1016/j.jembe.2019.03.016
Demopoulos A., Mcclain-Counts J., Ross S., Brooke S., Mienis F. (2017). Food-Web Dynamics and Isotopic Niches in Deep-Sea Communities Residing in a Submarine Canyon and on the Adjacent Open Slopes. Mar. Ecol. Prog. Ser. 578, 19–33. doi: 10.3354/meps12231
Deniro M. J., Epstein S. (1978). Influence of Diet on the Distribution of Carbon Isotopes in Animals. Geochimica Cosmochimica Acta 42, 495–506. doi: 10.1016/0016-7037(78)90199-0
Edwards M. S., Konar B. K., Kim J. H., Gabara S., Sullaway G. (2020). Marine Deforestation Leads to Widespread Loss of Ecosystem Function. PLos One. doi: 10.1371/journal.pone.022617315(3): e0226173
Eger A. M., Marzinelli E. M., Baes R., Blain C., Blamey L., Carnell P. E., et al. (2021). The Economic Value of Fisheries, Blue Carbon, and Nutrient Cycling in Global Marine Forests. EcoEvoRxiv. doi: 10.32942/osf.io/n7kjs
Filbee-Dexter K., Scheibling R. (2014). Sea Urchin Barrens as Alternative Stable States of Collapsed Kelp Ecosystems. Mar. Ecol. Prog. Ser. 495, 1–25. doi: 10.3354/meps10573
Fredriksen S. (2003). Food Web Studies in a Norwegian Kelp Forest Based on Stable Isotope (Delta C-13 and Delta N-15) Analysis. Mar. Ecol. Prog. Ser. 260, 71–81. doi: 10.3354/meps260071
Fry B., Sherr E. B. (1989). δ13c Measurements as Indicators of Carbon Flow in Marine and Freshwater Ecosystems. doi: 10.1007/978-1-4612-3498-2_12
Gabara S. S., Konar B. H., Edwards M. S. (2021). Biodiversity Loss Leads to Reductions in Community-Wide Trophic Complexity. Ecoshpore 12, 1–15. doi: 10.1002/ecs2.3361
Gagnon P., Himmelman J. H., Johnson L. E. (2004). Temporal Variation in Community Interfaces: Kelp-Bed Boundary Dynamics Adjacent to Persistent Urchin Barrens. Mar. Biol. 144, 1191–1203. doi: 10.1007/s00227-003-1270-x
Graham M. H. (2004). Effects of Local Deforestation on the Diversity and Structure of Southern California Giant Kelp Forest Food Webs. Ecosystems 7, 341–357. doi: 10.1007/s10021-003-0245-6
Guarnieri G., Bevilacqua S., Figueras N., Tamburello L., Fraschetti S. (2020). Large-Scale Sea Urchin Culling Drives the Reduction of Subtidal Barren Grounds in the Mediterranean Sea. Front. Mar. Sci. 7. doi: 10.3389/fmars.2020.00519
Himmelman J. H., Steele D. H. (1971). Foods and Predators of the Green Sea Urchin Strongylocentrotus Droebachiensis in Newfoundland Waters. Mar. Biol. 9, 315–322. doi: 10.1007/BF00372825
Hobson K., Welch H. E. (1992). Determination of Trophic Relationships Within a High Marine Food Web Using Delta-C-13 and Delta-N-15N Analysis. Mar. Ecology-Progress Ser. 84, 9–18. doi: 10.3354/meps084009
Hogsden K. L., Harding J. S. (2014). Isotopic Metrics as a Tool for Assessing the Effects of Mine Pollution on Stream Food Webs. Ecol. Indic. 36, 339–347. doi: 10.1016/j.ecolind.2013.08.003
Hong S., Kim J., Ko Y. W., Yang K. M., Macias D., Kim J. H. (2021). Effects of Sea Urchin and Herbivorous Gastropod Removal, Coupled With Transplantation, on Seaweed Forest Restoration. Botanica Marina 64, 427–438. doi: 10.1515/bot-2021-0043
Hussey N. E., Macneil M. A., McMeans B. C., Olin J. A., Dudley S. F. J., Cliff G., et al. (2014). Rescaling the Trophic Structure of Marine Food Webs. Ecol. Lett. 17, 239–250. doi: 10.1111/ele.12226
Jackson A. L., Inger R., Parnell A. C., Bearhop S. (2011). Comparing Isotopic Niche Widths Among and Within Communities: SIBER – Stable Isotope Bayesian Ellipses in R. J. Anim. Ecol. 80, 595–602. doi: 10.1111/j.1365-2656.2011.01806.x
James W. R., Lesser J. S., Litvin S. Y., Nelson J. A. (2020). Assessment of Food Web Recovery Following Restoration Using Resource Niche Metrics. Sci. Total Environ. 711, 134801. doi: 10.1016/j.scitotenv.2019.134801
Jeon B. H., Yang K. M., Kim J. H. (2015). Changes in Macroalgal Assemblage With Sea Urchin Density on the East Coast of South Korea. Algae 30, 139–146. doi: 10.4490/algae.2015.30.2.139
Kang R. S. (2010). A Review of Destruction of Seaweed Habitats Along the Coast of the Korean Peninsula and its Consequences. Bull. Fisheries Res. Agency 32, 25–31.
Kang S., Choi B., Han Y., Shin K.-H. (2016). Ecological Importance of Benthic Microalgae in the Intertidal Mud Flat of Yeongheung Island; Application of Stable Isotope Analysis (SIA). Korean J. Ecol. Environ. 49, 80–88. doi: 10.11614/KSL.2016.49.2.080
Kang C. K., Choy E., Son Y., Lee J.-Y., Kim J. K., Kim Y., et al. (2008). Food Web Structure of a Restored Macroalgal Bed in the Eastern Korean Peninsula Determined by C and N Stable Isotope Analyses. Mar. Biol. 153, 1181–1198. doi: 10.1007/s00227-007-0890-y
Kim Y. D., Park M. S., Yoo H. I., Min B. H., Jin H.-J. (2012). Seasonal Variations of Seaweed Community Structure at the Subtidal Zone of Bihwa on the East Coast of Korea. Korean J. Fisheries Aquat. Sci. 45, 262–270. doi: 10.5657/KFAS.2012.0262
Kondoh M. (2003). Foraging Adaptation and the Relationship Between Food-Web Complexity and Stability. Science 299, 1388–1391. doi: 10.1126/science.1079154
Krumhansl K. A., Okamoto D. K., Rassweiler A., Novak M., Bolton J. J., Cavanaugh K. C., et al. (2016). Global Patterns of Kelp Forest Change Over the Past Half-Century. Proc. Natl. Acad. Sci. 113, 13785–13790. doi: 10.1073/pnas.1606102113
Lake J. L., Serbst J. R., Kuhn A., Smucker N. J., Edwards P., Libby A., et al. (2019). Use of Stable Isotopes in Benthic Organic Material as a Baseline for Estimating Fish Trophic Positions in Lakes. Can. J. Fisheries Aquat. Sci. 76, 1227–1237. doi: 10.1139/cjfas-2017-0381
Layman C. A., Arrington D. A., Montaña C. G., Post D. M. (2007a). Can Stable Isotope Ratios Provide for Community-Wide Measures of Trophic Structure? Ecology 88, 42–48. doi: 10.1111/j.1461-0248.2007.01087.x
Layman C. A., Quattrochi J. P., Peyer C. M., Allgeier J. E. (2007b). Niche Width Collapse in a Resilient Top Predator Following Ecosystem Fragmentation. Ecol. Lett. 10, 937–944. doi: 10.1111/j.1461-0248.2007.01087.x
Layton C., Shelamoff V., Cameron M. J., Tatsumi M., Wright J. T., Johnson C. R. (2019). Resilience and Stability of Kelp Forests: The Importance of Patch Dynamics and Environment-Engineer Feedbacks. PLos One 14, e0210220. doi: 10.1371/journal.pone.0210220
Leclerc J.-C., Riera P., Laurans M., Leroux C., Lévêque L., Davoult D. (2015). Community, Trophic Structure and Functioning in Two Contrasting Laminaria Hyperborea Forests. Estuarine Coast. Shelf Sci. 152, 11–22. doi: 10.1016/j.ecss.2014.11.005
Leleu K., Remy-Zephir B., Grace R., Costello M. J. (2012). Mapping Habitats in a Marine Reserve Showed How a 30-Year Trophic Cascade Altered Ecosystem Structure. Biol. Conserv. 155, 193–201. doi: 10.1016/j.biocon.2012.05.009
Ling S. D., Johnson C. R., Frusher S. D., Ridgway K. R. (2009). Overfishing Reduces Resilience of Kelp Beds to Climate-Driven Catastrophic Phase Shift. Proc. Natl. Acad. Sci. 106, 22341–22345. doi: 10.1073/pnas.0907529106
Ling S. D., Scheibling R. E., Rassweiler A., Johnson C. R., Shears N., Connell S. D., et al. (2015). Global Regime Shift Dynamics of Catastrophic Sea Urchin Overgrazing. Philos. Trans. R. Soc. B: Biol. Sci. 370, 20130269. doi: 10.1098/rstb.2013.0269
Mann K. H. (1973). Seaweeds: Their Productivity and Strategy for Growth. Science 182, 975–981. doi: 10.1126/science.182.4116.975
Marzinelli E. M., Leong M. R., Campbell A. H., Steinberg P. D., Vergés A. (2016). Does Restoration of a Habitat-Forming Seaweed Restore Associated Faunal Diversity? Restor. Ecol. 24, 81–90. doi: 10.1111/rec.12292
Matich P., Kiszka J. J., Gastrich K. R., Heithaus M. R. (2017). Trophic Redundancy Among Fishes in an East African Nearshore Seagrass Community Inferred From Stable-Isotope Analysis. J. Fish Biol. 91, 490–509. doi: 10.1111/jfb.13354
Mccann K., Hastings A. (1997). Re-Evaluating the Omnivory–Stability Relationship in Food Webs. Proc. R. Soc. B: Biol. Sci. 264, 1249–1254. doi: 10.1098/rspb.1997.0172
Mcmeans B. C., Kadoya T., Pool T. K., Holtgrieve G. W., Lek S., Kong H., et al. (2019). Consumer Trophic Positions Respond Variably to Seasonally Fluctuating Environments. Ecology 100, e02570. doi: 10.1002/ecy.2570
Miller R. J., Lafferty K. D., Lamy T., Kui L., Rassweiler A., Reed D. C. (2018). Giant Kelp, Macrocystis Pyrifera, Increases Faunal Diversity Through Physical Engineering. Proc. R. Soc B 285, 20172571. doi: 10.1098/rspb.2017.2571
Minagawa M., Wada E. (1984). Stepwise Enrichment of 15N Along Food Chains: Further Evidence and the Relation Between δ15n and Animal Age. Geochimica Cosmochimica Acta 48, 1135–1140. doi: 10.1016/0016-7037(84)90204-7
Moore J. C., De Ruiter P. C. (2012). Energetic Food Webs: An Analysis of Real and Model Ecosystems New York: (Oxford University Press).
Mottet M. G. (1976). The Fishery Biology of Sea Urchins in the Family Strongylocentrotidae (State of Washington, Dept. of Fisheries, Shellfish Division). No. 20
Neckles H. A., Dionne M., Burdick D. M., Roman C. T., Buchsbaum R., Hutchins E. (2002). A Monitoring Protocol to Assess Tidal Restoration of Salt Marshes on Local and Regional Scales. Restor. Ecol. 10, 556–563. doi: 10.1046/j.1526-100X.2002.02033.x
Newsome S. D., Martinez Del Rio C., Bearhop S., Phillips D. L. (2007). A Niche for Isotopic Ecology. Front. Ecol. Environ. 5, 429–436. doi: 10.1890/1540-9295(2007)5[429:ANFIE]2.0.CO;2
Nielsen J. M., Clare E. L., Hayden B., Brett M. T., Kratina P. (2018). Diet Tracing in Ecology: Method Comparison and Selection. Methods Ecol. Evol. 9, 278–291. doi: 10.1111/2041-210X.12869
Nordström M. C., Demopoulos A. W. J., Whitcraft C. R., Rismondo A., Mcmillan P., Gonzalez J. P., et al. (2015). Food Web Heterogeneity and Succession in Created Saltmarshes. J. Appl. Ecol. 52, 1343–1354. doi: 10.1111/1365-2664.12473
Park H. J., Kwak J. H., Lee Y. J., Kang H. Y., Choy E. J., Kang C. K. (2020). Trophic Structures of Two Contrasting Estuarine Ecosystems With and Without a Dike on the Temperate Coast of Korea as Determined by Stable Isotopes. Estuaries Coasts 43, 560–577. doi: 10.1007/s12237-019-00522-4
Piazzi L., Ceccherelli G. (2019). Effect of Sea Urchin Human Harvest in Promoting Canopy Forming Algae Restoration. Estuarine Coast. Shelf Sci. 219, 273–277. doi: 10.1016/j.ecss.2019.02.028
Pool T., Holtgrieve G., Elliott V., Mccann K., Mcmeans B., Rooney N., et al. (2017). Seasonal Increases in Fish Trophic Niche Plasticity Within a Flood-Pulse River Ecosystem (Tonle Sap Lake, Cambodia). Ecosphere 8, e01881. doi: 10.1002/ecs2.1881
Post D. M. (2002). Using Stable Isotopes to Estimate Trophic Position: Models, Methods, and Assumptions. Ecology 83, 703–718. doi: 10.1890/0012-9658(2002)083[0703:USITET]2.0.CO;2
Post D. M., Pace M. L., Hairston N. G. (2000). Ecosystem Size Determines Food-Chain Length in Lakes. Nature 405, 1047–1049. doi: 10.1038/35016565
Rader J. A., Newsome S. D., Sabat P., Chesser R. T., Dillon M. E., Martínez Del Rio C. (2017). Isotopic Niches Support the Resource Breadth Hypothesis. J. Anim. Ecol. 86, 405–413. doi: 10.1111/1365-2656.12629
Rooney N., Mccann K. S., Moore J. C. (2008). A Landscape Theory for Food Web Architecture. Ecol. Lett. 11, 867–881. doi: 10.1111/j.1461-0248.2008.01193.x
Salomon Y., Connolly S. R., Bode L. (2010). Effects of Asymmetric Dispersal on the Coexistence of Competing Species. Ecol. Lett. 13, 432–441. doi: 10.1111/j.1461-0248.2009.01436.x
Shannon C., Weaver W. (1949). A Mathematical Model of CommunicationUrbana and Chicago. ( University of Illinois Press).
Smale D. A., Wernberg T. (2013). Extreme Climatic Event Drives Range Contraction of a Habitat-Forming Species. Proc. R. Soc. B: Biol. Sci. 280, 20122829. doi: 10.1098/rspb.2012.2829
Spector M., Edwards M. S. (2020). Modelling the Impacts of Kelp Deforestation on Benthic Primary Production on Temperate Rocky Reefs. Algae 35, 237–252. doi: 10.4490/algae.2020.35.8.19
Steneck R. S., Graham M. H., Bourque B. J., Corbett D., Erlandson J. M., Estes J. A., et al. (2002). Kelp Forest Ecosystems: Biodiversity, Stability, Resilience and Future. Environ. Conserv. 29, 436–459. doi: 10.1017/S0376892902000322
Sun Z. L., Gao Q. F., Dong S. L., Shin P. K. S., Wang F. (2012). Estimates of Carbon Turnover Rates in the Sea Cucumber Apostichopus Japonicus (Selenka) Using Stable Isotope Analysis: The Role of Metabolism and Growth. Mar. Ecol. Prog. Ser. 457, 101–112. doi: 10.3354/meps09760
Teagle H., Hawkins S. J., Moore P. J., Smale D. A. (2017). The Role of Kelp Species as Biogenic Habitat Formers in Coastal Marine Ecosystems. J. Exp. Mar. Biol. Ecol. 492, 81–98. doi: 10.1016/j.jembe.2017.01.017
Thibaut T., Blanfune A., Boudoureque C.-F., Verlaque M. (2014). Decline and Local Extinction of Fucales in French Riviera: The Harbinger of Future Extinctions? Mediterr. Mar. Sci. 16, 206–224. doi: 10.12681/mms.1032
Thompson R. M., Brose U., Dunne J. A., Hall R. O., Hladyz S., Kitching R. L., et al. (2012). Food Webs: Reconciling the Structure and Function of Biodiversity. Trends Ecol. Evol. 27, 689–697. doi: 10.1016/j.tree.2012.08.005
Tracey S. R., Baulch T., Hartmann K., Ling S. D., Lucieer V., Marzloff M. P., et al. (2015). Systematic Culling Controls a Climate Driven, Habitat Modifying Invader. Biol. Invasions 17, 1885–1896. doi: 10.1007/s10530-015-0845-z
Wakanuki A., Aota T., Otsuka E., Kawai T., Iwahashi Y., Kuwahara H., et al. (2010). Restoration of Kelp Beds on an Urchin Barren: Removal of Sea Urchins by Citizen Divers in Southwestern Hokkaido. Bull. Fisheries Res. Agency 32, 83–87.
Wang J., Li L., Xu J., Gu B. (2016). Initial Response of Fish Trophic Niche to Hydrological Alteration in the Upstream of Three Gorges Dam. Ecol. Processes 5, 11. doi: 10.1186/s13717-016-0055-3
Wernberg T., Bennett S., Babcock R. C., De Bettignies T., Cure K., Depczynski M., et al. (2016). Climate-Driven Regime Shift of a Temperate Marine Ecosystem. Science 353, 169–172. doi: 10.1126/science.aad8745
Winemiller K. O., Pianka E. R. (1990). Organization in Natural Assemblages of Desert Lizards and Tropical Fishes. Ecol. Monogr. 60, 27–55. doi: 10.2307/1943025
Wootton K. L. (2017). Omnivory and Stability in Freshwater Habitats: Does Theory Match Reality? Freshw. Biol. 62, 821–832. doi: 10.1111/fwb.12908
Wozniak A. S., Roman C. T., Wainright S. C., Mckinney R. A., James-Pirri M.-J. (2006). Monitoring Food Web Changes in Tide-Restored Salt Marshes: A Carbon Stable Isotope Approach. Estuaries Coasts 29, 568–578. doi: 10.1007/BF02784283
Yoo J.-W., Son Y.-S., Lee C.-G., Kim J.-S., Han C.-H., Kim C.-S., et al. (2004). Distribution Pattern of the Sea Urchin Strongylocentrotus Nudus in Relation to Predation Pressure in Hosan, the East Coast of Korea. J. Korean Soc. Oceanography 9, 40–49.
Yorke C. E., Page H. M., Miller R. J. (2019). Sea Urchins Mediate the Availability of Kelp Detritus to Benthic Consumers. Proc. R. Soc B. 286, 20190846. doi: 10.1098/rspb.2019.0846
Keywords: community wide metrics, food web/trophic structure, kelp beds, stable isotope analysis, restoration
Citation: Kim MJ, Yun HY, Shin K-H and Kim JH (2022) Evaluation of Food Web Structure and Complexity in the Process of Kelp Bed Recovery Using Stable Isotope Analysis. Front. Mar. Sci. 9:885676. doi: 10.3389/fmars.2022.885676
Received: 28 February 2022; Accepted: 14 June 2022;
Published: 26 July 2022.
Edited by:
Matthew Edwards, San Diego State University, United StatesReviewed by:
Scott Gabara, University of Alaska System, United StatesRodrigo Beas-Luna, Universidad Autónoma de Baja California, Mexico
Copyright © 2022 Kim, Yun, Shin and Kim. This is an open-access article distributed under the terms of the Creative Commons Attribution License (CC BY). The use, distribution or reproduction in other forums is permitted, provided the original author(s) and the copyright owner(s) are credited and that the original publication in this journal is cited, in accordance with accepted academic practice. No use, distribution or reproduction is permitted which does not comply with these terms.
*Correspondence: Kyung-Hoon Shin, c2hpbmtoQGhhbnlhbmcuYWMua3I=; Jeong Ha Kim, amhrYmlvQHNra3UuZWR1
†These authors share first authorship