- 1CAS Key Laboratory of Tropical Marine Bio-resources and Ecology, Guangdong Provincial Key Laboratory of Applied Marine Biology, South China Sea Institute of Oceanology, Chinese Academy of Sciences, Guangzhou, China
- 2Sanya National Marine Ecosystem Research Station, Tropical Marine Biological Research Station in Hainan, Chinese Academy of Sciences, Sanya, China
- 3Innovation Academy of South China Sea Ecology and Environmental Engineering, Chinese Academy of Sciences, Guangzhou, China
- 4School of Earth and Planetary, University of Chinese Academy of Sciences, Beijing, China
- 5Chinese Academy of Sciences-The Hong Kong University of Science and Technology (CAS-HKUST) Sanya Joint Laboratory of Marine Science Research, Key Laboratory of Tropical Marine Biotechnology of Hainan Province, Sanya Institute of Oceanology, South China Sea Institute of Oceanology (SCSIO), Sanya, China
Reef-building corals present various colony morphologies that may greatly influence their nutritional ecology. Fatty acids (FAs) and lipids are important components of corals and have been increasingly used to research the nutritional ecology of corals. In this study, we examined the symbiodiniaceae density, corallite area, total lipid content, and FAs composition of 14 species of corals with different colony morphologies. The results showed that the different colony morphology of coral was significantly correlated with the corallite area but not with the symbiodiniaceae density. Massive corals, with a large corallite area (7.16 ± 6.29 mm2), could ingest a high quantity of food, leading to high levels of total lipid content and unsaturated FAs [particularly n-6 polyunsaturated FAs (PUFAs) and monounsaturated FAs]. For branching corals, the total lipid content and saturated FAs (SFAs, 16:0 and 18:0) were significantly positively correlated with the Symbiodiniaceae density, indicating that branching corals are predominantly autotrophic. Moreover, compared with healthy corals, bleached corals consume larger amounts of stored energy (such as lipids and SFAs) to maintain their normal physiological functions. Although bleached corals may obtain PUFAs from heterotrophic assimilation or biosynthesize, the efficiency is too low to sufficiently replenish essential PUFAs in a short time. Overall, massive corals with more initial total lipid content and PUFAs exhibit an advantage under adverse environmental conditions.
1 Introduction
Reef ecosystems are among the world’s most productive and biodiverse marine ecosystems (Hughes et al., 2017; Conti-Jerpe et al., 2020). Reef-building corals, or scleractinians, are distinct organisms in reef ecosystems and play fundamental roles as ecosystem engineers (Jones and Ray, 2011; Martínez-Castillo et al., 2020). Scleractinian skeletons are mainly composed of calcium carbonate (aragonite), which maintains the shape of their polyps. They also exhibit various colony morphologies, such as massive, branching, columnar, laminar, corymbose, and digitate (Veron, 2000). The different colony morphologies of scleractinians often show diverse symbiodiniaceae density (Thornhill et al., 2011), corallite width (Loya et al., 2001), and tissue thickness (Edmunds et al., 2014). Importantly, these differences may strongly influence coral nutritional ecology parameters, such as energy storage (Pupier et al., 2021), trophic strategies (Radice et al., 2019; Conti-Jerpe et al., 2020), and even response mechanisms to environmental changes (Loya et al., 2001; Hughes et al., 2017), which can affect the evolution of coral communities.
Lipid reserves serve as a universal proxy for the health status of corals (Conlan et al., 2017; Bhojoo et al., 2018). Fatty acids (FAs), the primary components of lipids, play important roles in energy storage, cell membrane structure, and overall fitness (Berge and Barnathan, 2005; Farre et al., 2010). Therefore, lipid content and FAs composition have been investigated as tools for understanding the resistance and resilience of corals under environmental stress (Baumann et al., 2014; Grottoli et al., 2014; Schoepf et al., 2015; Chapron et al., 2022). For instance, numerous studies demonstrated that corals depend on initial lipid stores to survive during bleaching events (Rodrigues et al., 2008; Baumann et al., 2014; Tagliafico et al., 2017). Chapron et al. (2022) also found that corals with higher overall energy reserves and heterotrophic contributions to tissues can better buffer against environmental stress. Moreover, Teece et al. (2011) demonstrated that the supplement of n-3 polyunsaturated FAs (PUFAs) and saturated FAs (SFAs) is important for coral to sustain growth under turbid conditions. As such, analyses of lipid content and FAs are important aspects of understanding coral nutritional ecology.
Because of the influence of climate change and intensified anthropogenic factors, numerous coral bleaching events and the resultant mortality have been reported in various geographical areas (Loya et al., 2001; Grottoli et al., 2006; Hughes et al., 2017). Notably, changes in the community structure and species diversity of corals before and after a bleaching event are morphology specific. Kayanne et al. (2010) found that massive Porites had higher bleaching resilience or recovery capacity than branching Acropora and Porites in the 1998 bleaching event on Ishigaki Island. Moreover, Loya et al. (2001) suggested that colony morphology affected bleaching vulnerability and subsequent coral mortality; massive corals are the “winners,” and branching corals are the “losers” in coral bleaching. Although colony morphology of coral affects bleaching vulnerability and subsequent mortality, few studies have focused on these effects from the perspective of nutritional ecology (Baker et al., 2015; Conti-Jerpe et al., 2020).
Therefore, in the present study, we assessed Symbiodiniaceae density, corallite area, total lipid content, and FAs composition of corals with different colony morphology, including healthy and bleached samples. This study aimed to determine the differences in total lipid content and FAs composition between massive and branching corals, and between healthy and bleached samples, to understand the morphology specificity of coral nutritional ecology. These findings could provide a theoretical basis for evaluating and predicting the changes in the community structure and species diversity of corals.
2 Materials and Methods
2.1 Corals Sampling
On November 2–3, 2018, 14 specimens of reef-building corals (Table 1) belonging to nine genera (seven families) were collected by SCUBA in the Luhuitou fringing reef (3 m depth) at the Tropical Marine Biological Research Station in Hainan, Chinese Academy of Sciences (18°14′N, 109°28′E). Moreover, three bleached Acropora spp. (A. florida, A. nana, and A. intermedia) were also sampled after a tropical cyclone on December 28, 2018. Images were collected using a camera (TG-6, Tokyo, Olympus, Japan) before sampling. All coral specimens were identified by taxonomists at the South China Sea Institute of Oceanology, Chinese Academy of Sciences. From October 2018 to January 2019, the temperature (°C) and light intensity (lux) of the sampling area were monitored continuously at 2-h intervals using a HOBO logger (UA-002-64, Onset Computer, Bourne, MA, USA) (Figure 1). The HOBO logger was cleaned weekly to reduce the interference of algae, sediment, and other fouling organisms as much as possible.
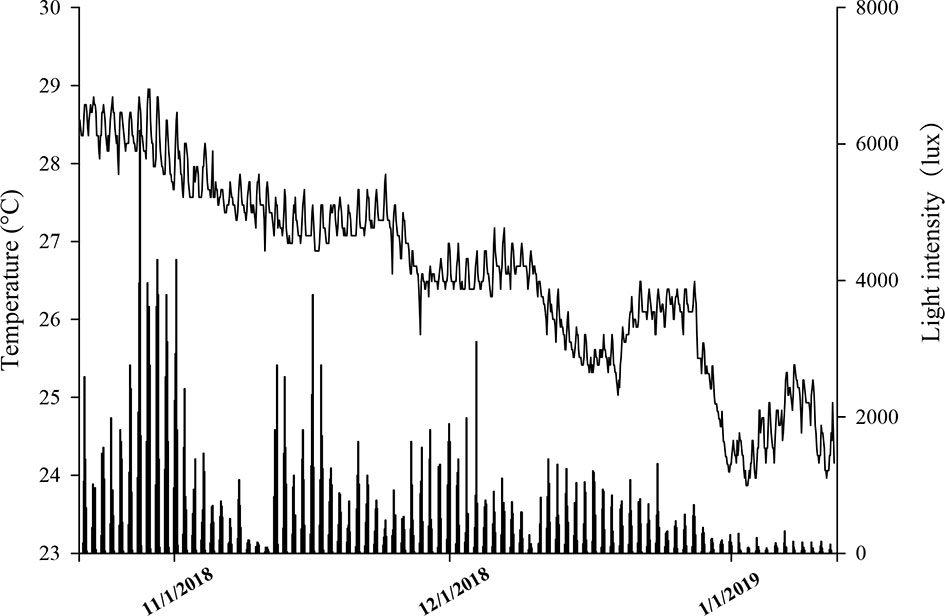
Figure 1 The temperature (°C) and light intensity (lux) of Luhuitou fringing reef (3m) from October 2018 to January 2019.
Healthy (n = 8) and bleached (n = 3) samples from different colonies (3–5 cm fragments) of each coral species were collected and immediately transported to the laboratory, where they were divided into two subsamples. One subsample (healthy, n = 5; bleached, n = 3) was freeze-dried in a vacuum freezing dryer (Biosafer-21A, Duhong Biological Technology Co., Ltd., Guangzhou, China), crushed into 0.1–0.3-mm pieces, and then frozen at −80°C until analyses of the total lipid content and FA composition. The other subsample (healthy, n = 3) was stored at −20°C until analyses of the Symbiodiniaceae density and corallite area.
2.2 Symbiodiniaceae Density
The Symbiodiniaceae density in the coral tissues was evaluated as described by Martínez-Castillo et al. (2020) with some modifications. Coral tissues were removed from the coral fragment using jets of pressurized air in filtered seawater (~1 ml) until only the white coral skeleton was visible. The tissue slurry was homogenized using a hand-held blender (MY-10, Jingxin Technology, Jiangsu, China) for 45 s and centrifuged (3,000×g, 5 min; Microfuge 20, Beckman Coulter, Brea, CA, USA); the supernatant was then removed. The process was repeated three times. The number of Symbiodiniaceae per slurry volume was counted using hemocytometer plates with four replicate counts. The total number of Symbiodiniaceae per area was calculated based on the volume of the homogenate and coral surface area (×106 cells cm−2). The coral surface area was measured using a structured light 3D scanner (JTscan-MS, Guangzhou Jeatech Electronics Technology Co., Ltd., Guangdong, China).
2.3 Corallite Area
Given that the shape of coral corallite is irregular, the area of the corallite (mm2) was measured as described by Conti-Jerpe et al. (2020). Images of the skeleton of each sample were captured under a microscope (CX31, Olympus, Japan) after determining the Symbiodiniaceae density, and ImageJ software (version 1.8.0; NIH, Bethesda, MD, USA) was used to calculate the area of the coral corallite (mm2) for 10 replicates.
2.4 Total Lipid Content
To ensure the accuracy of the measurement of the total lipid content and prevent the oxidation of FAs, total lipid content and FA composition were assessed separately. The crushed samples (approximately 0.5 g) were packed in a filter paper, and lipids were extracted using a Soxhlet extractor (AG-SXT-06, Shanghai Ouge Electronics Co., Ltd., Shanghai, China). Petroleum ether (boiling point, 60°C–90°C) was used as the solvent, and a thermostat-heating mantle was used to maintain 90°C for 5 h. The total lipid content was considered as the difference in sample weight between before and after the extraction.
Total lipid content was measured in crushed whole coral samples (skeleton, animal tissue, and Symbiodiniaceae), normalized to the dry weight, and expressed as a percentage (g lipid/g dry weight).
2.5 Fatty Acid Methyl Esters Obtention and Analysis
Approximately 1-g sample (dry weight) from each sample was analyzed. The samples were homogenized, and each lipid fraction was extracted using chloroform/methanol (2:1, v/v) containing 0.01% butylated hydroxytoluene as an antioxidant, as previously described by Liu et al. (2019). The chloroform layer was separated from the methanol layer and dried to a constant weight under a stream of nitrogen to obtain the total lipid content.
Fatty acid methyl esters (FAMEs) were obtained by esterification with 2 ml methyl esterification reagent (hydrochloric acid/methanol, 1:5, v/v) at 90°C for 3 h, as described by Liu et al. (2018). The upper phase was dried under nitrogen and resuspended in hexane.
FAMEs were quantified by injecting 1 μl of sample into a gas chromatograph instrument (GC-2010 Plus; Shimadzu, Kyoto, Japan) equipped with a flame-ionization detector (GC-2010; Shimadzu, Kyoto, Japan) and an RTX-WAX fused-silica capillary column (length, 30 m; internal diameter, 0.25 mm; thickness, 0.25 μm; Phenomenex, Torrance, CA, USA). The gradient temperature program was set as follows: (i) initial temperature of 60°C for 1.0 min; (ii) increase at a rate of 10°C min−1 to 190°C; (iii) increase at 2.0°C min−1 to 260°C; and (iv) hold at 260°C for 0.6 min. The FAMEs were identified and quantified by comparing the retention times (identification) and peak areas (quantification) with the 37-FAME Mix calibration solution (Supelco, Bellefonte, PA, USA).
2.6 Statistical Analyses
R software version 3.5.2 (The R Project for Statistical Computing, Vienna, Austria) was used for statistical analyses. The distribution of the data was evaluated for normality using the Kolmogorov–Smirnov test (p > 0.05). Levene’s test (p > 0.05) was used to assess the homogeneity of variance. Data of massive and branching samples were then evaluated by t-test analysis to identify significant differences (p < 0.05). The correlation of total lipid content between the Symbiodiniaceae density and corallite area was determined using Pearson’s product-moment correlation with the package “corrplot,” and the FA composition of corals between massive and branching corals was characterized using multivariate principal component analyses with the package “vegan” (Oksanen et al., 2010). Graphs were prepared using the ggplot2 package (Wickham, 2016).
To facilitate a comparison of FA compositions, we calculated the unsaturation index (UI) and the unsaturated-to-saturated fatty acid ratio (U/S) as reported by Snyder et al. (2012) and Wallaert and Babin (1994). The UI and U/S algorithms were as follows:
where monoenes, dienes, and trienes … are FAs containing 1, 2, 3…double bonds, respectively; % is the weight percentage; UFA indicates unsaturated FA; and SFA indicates saturated FA.
3 Results
3.1 Symbiodiniaceae Density and Corallite Area
The Symbiodiniaceae density and corallite area are shown in Tables 2, 3. The Symbiodiniaceae density ranged from 1.96 × 106 (A. nana) to 1.02 × 106 cells cm−2 (Platygyra acuta). The average Symbiodiniaceae density of the massive and branching corals were 1.50 ± 0.26×106 and 1.55 ± 0.36×106 cells cm−2, respectively. There was no significant difference (p > 0.05) in the Symbiodiniaceae density between the massive and branching corals.
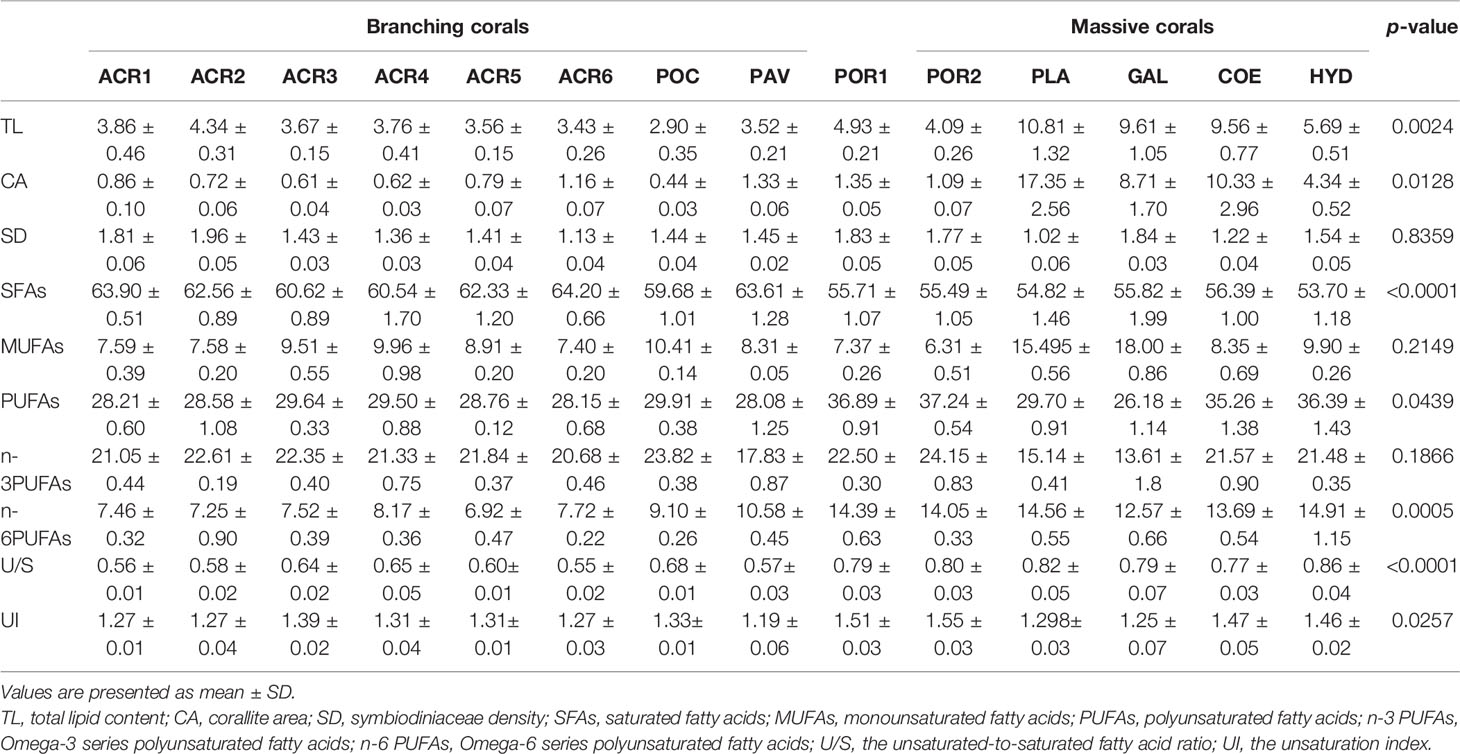
Table 2 Levels of TL (%), CA (mm2), SD (×106cells cm-2), SFAs (%), MUFAs (%), PUFAs (%), n-3 PUFAs (%), n-6 PUFAs (%), U/S, and UI of massive and branching corals.
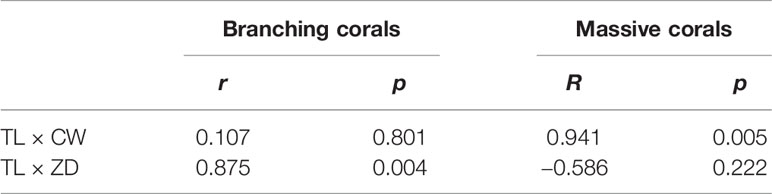
Table 3 Correlations of total lipid content (TL) of massive and branching corals with corallite area (CA) and Symbiodiniaceae density (SD).
The corallite area of massive corals (7.19 ± 6.24 mm2) was significantly larger (p < 0.05) than that of the branching corals (0.69 ± 0.25 mm2). Platygyra acuta showed the largest corallite area (17.35 ± 5.11 mm2), followed by Coelastrea aspera (10.33 ± 2.02 mm2), and Pocillopora damicornis showed the smallest area (0.44 ± 0.12 mm2).
3.2 Total Lipid Content
The total lipid content of the 14 species of scleractinians are shown in Table 2. Among all species, the highest total lipid content was observed in P. acuta (10.81 ± 0.96%), followed by Galaxea fascicularis (9.61 ± 1.16%), whereas P. damicornis showed the lowest total lipid content (2.90 ± 0.20%). The total lipid content varied with the colony morphology. Overall, the branching corals (3.60 ± 0.36%) showed lower lipid content than massive corals (7.45 ± 2.87%) (Table 2). Moreover, the total lipid content of branching corals was positively correlated with the Symbiodiniaceae density but unrelated to the corallite area. In contrast, the total lipid content of massive corals was positively correlated with the corallite area but not with the symbiodiniaceae density (Table 3).
Compared to healthy corals, a significant decrease in total lipid content was found in the bleached coral samples (Figure 2), which accounted for only approximately 25% of the total lipid content of the healthy group. The average total lipid content was 3.84 ± 0.49% for healthy corals and 1.01 ± 0.16% for bleached corals.
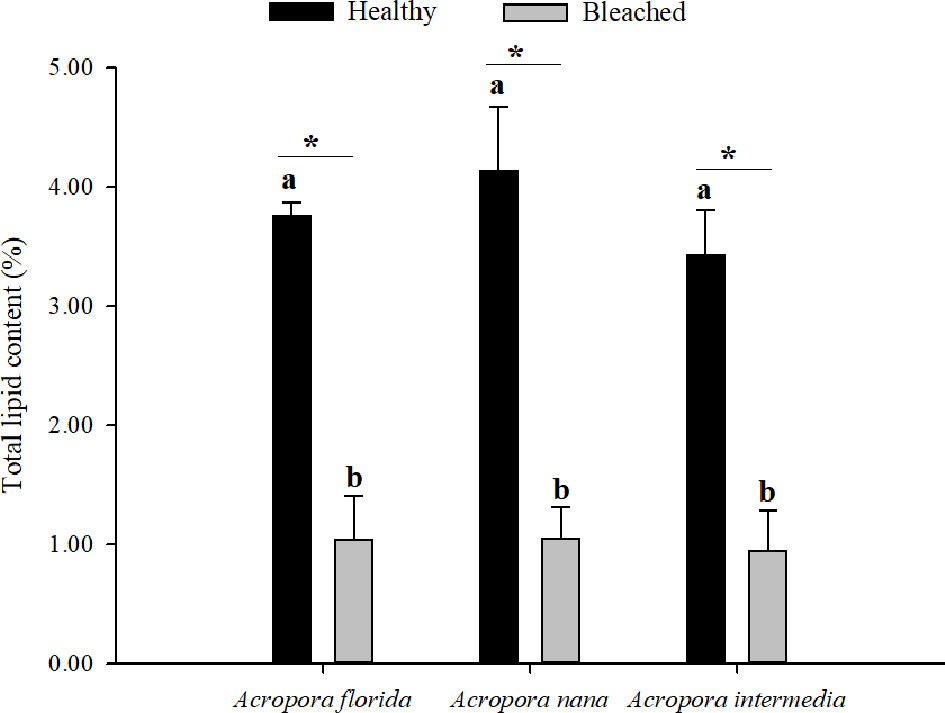
Figure 2 Total lipid content (%) of healthy (n = 5) and bleaching (n = 3) corals. Note: Values are mean ± SD. The different letters mean a significant difference between healthy and bleaching coral for all samples (p < 0.05); the “*” means a significant difference between healthy and bleaching samples for each species (p < 0.05).
3.3 FA Composition
3.3.1 FA Composition of Branching and Massive Corals
A total of 18–25 FAs were found in all corals, including 6–9 SFAs, 3–6 monounsaturated fatty acids (MUFAs), and 8–10 PUFAs. For all corals, the proportion of SFAs, MUFAs, and PUFAs were 55.49%–64.20%, 6.31%–17.84%, and 25.11%–38.21%, respectively. Moreover, 16:0 and 18:1n9 were predominant in SFAs and MUFAs, respectively. However, the largest proportion of PUFAs is significantly different among the varieties of coral species.
Most specimens studied belonged to two colony morphologies (branching and massive), making them suitable for examining the relationship between the coral FA composition and colony morphology. Therefore, all FA compositions were used for principal coordinate analysis (PCA), and the results are shown in Figure 3. The first two components explained 81.15% of the total data variance. Branching corals showed great clustering, mainly because of differences in the levels of SFAs (such as 16:0 and 14:0) and n-3 PUFAs (such as 20:5n3). However, massive corals were subdivided into two parts; G. fascicularis, C. aspera, and P. acuta have high proportions of MUFAs (such as 16:1n7 and 18:1n9), whereas Hydnophora exesa, Porites lutea, and Porites lobata showed high levels of PUFAs, (18:3n3, 18:2n6, and 22:6n3). Hence, both U/S and UI of massive corals (except for G. fascicularis) were higher than those of branching corals. Generally, the colony morphology of coral was significantly correlated with SFAs, n-6PUFAs, and PUFAs (Table 2). Compared with branching corals, massive corals showed higher levels of PUFAs (particularly n-6 PUFAs) and lower levels of SFAs.
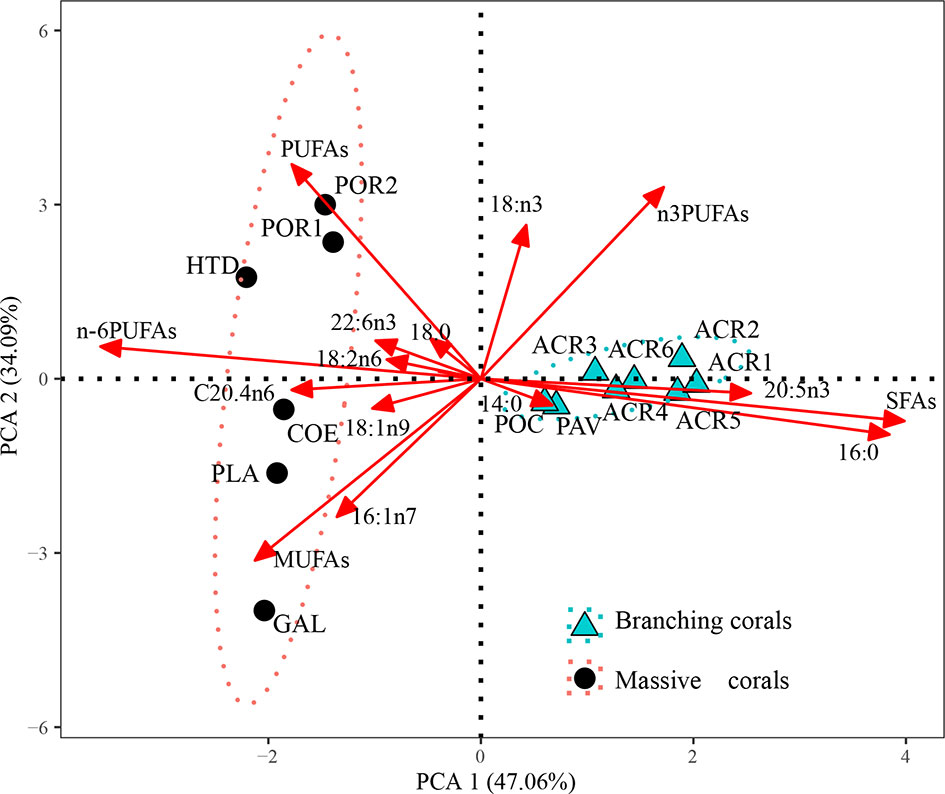
Figure 3 Principal component analysis (PCA) of fatty acids (% of total fatty acids) of massive (black round) and branching (green triangle) corals (only healthy corals).
3.3.2 FA Composition of Healthy and Bleached Corals
The differences in the FA composition between healthy and bleached corals are shown in Table 4 and Figure 4. The three species of bleached corals exhibited the same trends in the changes in FAs. The concentrations of both SFAs (such as 14:0, 16:0, and 18:0) and UFAs (such as 16:1n7, 18:1n9, 20:3n3, 20:5n3, and 22:6n3) of the bleached corals were significantly lower than those of healthy corals, especially SFAs, showing reductions of nearly 50% (average value: healthy, 1,743.23 µg g−1; bleached, 865.13 µg g−1). Changes in the PUFAs of bleached corals were even greater. In bleached corals, the levels of C20–22 PUFAs (such as 20:3n3, 20:5n3, and 22:6n3) were significantly lower (average value: healthy, 549.41 µg g−1; bleached, 223.50 µg g−1), whereas those of C18 PUFAs (such as 18:2n6, 18:3n3, and 18:3n6) (average value: healthy, 263.27 µg g−1; bleached, 273.53 µg g−1) were significantly higher than in healthy corals.
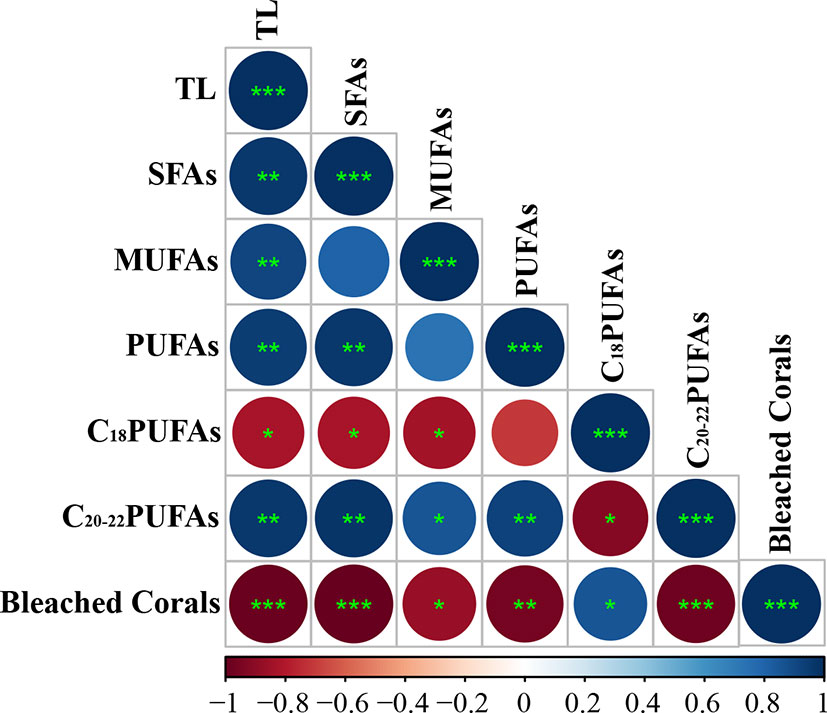
Figure 4 Heatmap of the correlation relationship between bleached corals and FAs composition (SFA, PUFAs, MUFAs, C18 PUFAs, and C20–22 PUFAs, %) and total lipid content (TL, %). Note: MUFA, monounsaturated fatty acid; PUFA, polyunsaturated fatty acid; SFA, saturated fatty acid; C18PUFA, 18-carbon chain PUFA; C20–22PUFA, 20–22-carbon chain PUFA. The symbols “*”,”**,” and “***” represent statistically significant results “p < 0.05,” “p < 0.01,” and “p < 0.001,” respectively. Heatmap color is presented as Pearson correlation coefficients whereby 1 (blue) is a total positive linear correlation, 0 (white) is no linear correlation, and −1 (red) is total negative linear correlation.
4 Discussion
4.1 Total Lipid Content and FAs Composition in Branching and Massive Corals
4.1.1 Total Lipid Content
The total lipid content of massive corals was higher than that of the branching corals. Corroborating the present research, Rotmann and Thomas (2012) and Yost et al. (2013) demonstrated that massive corals stored more energy-source molecules, such as lipids, than branching corals did. This may be because of the morphology specificity of the trophic pattern. Although autotrophy is the main lipid source of corals, there is also a non-negligible lipid input through heterotrophy (Rossi et al., 2020; Pupier et al., 2021). Corals can take up plankton, suspended particles, and dissolved organic material, particularly poor-swimming and mid-sized (200–400 μm) zooplankton organisms, which are important sources of lipid supplementation for corals (Palardy et al., 2005; Palardy et al., 2008; Houlbrèque and Ferrier-Pagès, 2009). Furthermore, the corallite area of massive corals was larger than that of branching corals (Table 2) and was positive with total lipid content (Table 3). Corallites are cup-like skeletal structures containing individual coral polyps, and thus, the corallite area is a good proxy for polyp size—a key functional trait linked to nutrient acquisition (Todd et al., 2004; Conti-Jerpe et al., 2020). Palardy et al. (2005) found that the feeding rates in the mounding (or massive) coral Pavona clavus were higher than in the branching coral P. damicornis. Therefore, massive corals, with large corallite area, present a high heterotrophic capacity, which may lead to higher total lipid content than that of branching corals.
4.1.2 FA Composition
Colony morphology was significantly correlated with SFAs and PUFAs (mainly in n-6 PUFAs). The composition of SFAs (such as 14:0, 16:0, and 18:0) were more than 50% for all samples, particularly in branching corals (>60%), which is consistent with previous studies (Iluz and Dubinsky, 2015; Conlan et al., 2017). Photosynthesis of Symbiodiniaceae is the most important SFA source (Yamashiro et al., 1999). Bishop and Kenrick (1980) demonstrated that approximately 20%–40% of SFAs (such as 10:0, 16:0, and 18:0) in corals are obtained from Symbiodiniaceae. Moreover, in our study, the total lipid content was positively correlated with the Symbiodiniaceae density in branching corals, indicating that branching corals are predominantly autotrophic.
Branching corals contained a high level of 20:5n3, whereas 18:3n3, 20:4n6, and 22:6n3 were abundant in massive corals (Figure 3). The PUFAs profile of coral was significantly affected by Symbiodiniaceae (Teece et al., 2011; Pupier et al., 2021). Zhukova and Titlyanov (2003) demonstrated that Symbiodiniaceae isolated from P. damicornis had higher 20:5n3 (11.2%) and 22:6n3 (10.7%), whereas those isolated from Millepora intricata had higher 18:4n3 (26.2%). Furthermore, biosynthesis of PUFAs by Symbiodiniaceae is a complex and variable process (Neuringer et al., 1988; Chen et al., 2015), and it can also be regulated by the coral hosts (Imbs et al., 2007). Overall, the specific UFAs in coral hosts and Symbiodiniaceae lead to the differences in UFAs in different corals.
FAs composition of corals has not only different biosynthetic origins but also different biological sources. Various foods consumed by corals have different characteristics FAs (Kattner and Hagen, 2009; Imbs et al., 2010). The diversity in quantity and quality of food and randomness of food uptake result in uncertainty in the FAs composition of coral. Therefore, massive corals have a large corallite area, which takes up a wide range of foods, leading to FA composition more discrete than that of branching corals. Furthermore, three large corallite massive corals (G. fascicularis, C. aspera, and P. acuta) showed higher proportions of MUFAs (16:1n7 and 18:1n9) compared to other corals. MUFAs have been confirmed as the trophic marker of herbivorous microzooplankton (Dalsgaard et al., 2003; Imbs et al., 2010). A similar result for this species was reported by Radice et al. (2019), who showed that G. fascicularis contains a high proportion of MUFAs derived from copepods.
4.2 Total Lipid Content and FAs Composition in Bleached and Healthy Corals
Most corals maintain an endosymbiotic association with algae of the family Symbiodiniaceae (Radice et al., 2019). However, the symbiotic relationship is fragile and may collapse because of adverse environmental changes, provoking coral bleaching (whitening of corals caused by the loss of symbiotic dinoflagellates) and even mortality (Hughes et al., 2017; Tagliafico et al., 2017).
Lipids are involved in most biochemical and physiological processes in corals; thus, these may reflect the health status of corals. We found that bleached corals lost more than 50% and 75% of their original SFAs and total lipid content, respectively, which is common following bleaching events (Yamashiro et al., 1999; Imbs and Yakovleva, 2012). Symbiodiniaceae inherently contain considerable amounts of lipids and SFAs (Chen et al., 2015; Conlan et al., 2017). Therefore, the loss of symbiodiniaceae directly leads to decreased levels of lipids and SFAs in corals. Under bleached conditions, corals may rely more heavily on stored lipid to sustain their normal physiological functions (Tolosa et al., 2011). Similar reductions in lipid levels of bleached corals in the Caribbean (Porter et al., 1989), Okinawa (Yamashiro et al., 2005), and Hawaii (Rodrigues et al., 2008) were attributed to decreases in translocated carbon from their symbionts. Moreover, marine organisms tend to preferentially metabolize SFAs to protect long-chain PUFAs (such as 20:4n6 and 22:6n3) from oxidation during starvation (Tocher, 2003; Liu et al., 2020). Thus, a large quantity of SFAs, as the preferred source of metabolic energy, was oxidized to provide energy for bleaching corals.
Interestingly, C20–22 PUFAs were significantly reduced in bleached corals, whereas C18 PUFAs were increased significantly. The reduction in PUFAs in corals was reported following bleaching events (Tolosa et al., 2011; Tagliafico et al., 2017). Bachok et al. (2006) showed that completely bleached coral lost more than 70% of their PUFAs. Symbiodiniaceae transfer sufficient PUFAs for the hosts’ requirements under ideal conditions (Chen et al., 2015), whereas in bleaching situations, the host just acquires PUFAs from heterotrophic feeding (Grottoli et al., 2006). Moreover, recent works showed that Cnidaria, including scleractinian corals, contain genes for de novo biosynthesis of PUFAs (Kabeya et al., 2018; Monroig and Naoki, 2018). C18 PUFAs, such as 18:2n6 and 18:3n3, are key precursors in the biosynthesis of C20–22 PUFAs in marine invertebrates. Therefore, C18 PUFAs were abundant in bleached corals, indicating that corals could obtain PUFAs from biosynthesize or heterotrophic assimilation, but with low efficiency. Furthermore, 18:3n-6 and 18:2n6 and their metabolites can affect the expression of genes associated with immunity and apoptosis (Rocker et al., 2019), such as the aryl hydrocarbon receptor (AhR). Overall, considering the large consumption of lipids and SFAs and the inability to rapidly replenish essential FAs (long-chain PUFAs), long-term bleaching of corals inevitably leads to coral death.
4.3 Response to Environmental Changes of Branching and Massive Corals From the Perspective of Nutritional Ecology
Indeed, the nutritional status of corals can affect their resistance and resilience to bleaching. Branched corals have low total lipid content but high surface areas to volume ratio; hence, branched corals have lower levels of stored lipid than do massive corals. Such levels significantly influence the timing of onset of bleaching and subsequent mortality (Anthony and Willis, 2002; Grottoli et al., 2004). Conlan et al. (2017) suggested that corals with “full” initial lipid stores survive twice as long as those with low lipid stores under high bleaching rates. Therefore, branching corals with low initial lipid stores are disadvantaged in the face of environmental stress. Moreover, the ability to obtain extra lipids from the environment is also extremely important for corals under adverse conditions (Houlbrèque and Ferrier-Pagès, 2009; Grottoli et al., 2014). Heterotrophy accounts for 0%–66% of the fixed carbon incorporated into coral skeletons and can meet 15%–35% of the daily metabolic requirements in healthy corals and up to 100% in bleached corals (Houlbrèque and Ferrier-Pagès, 2009; Conti-Jerpe et al., 2020). Yamashiro et al. (2005) found that massive corals were relatively rich in lipids even after a bleaching event, which was attributed to increased feeding rates. Massive corals, predominantly heterotrophic or mixotrophic, are less susceptible to bleaching and show higher resilience to stress than branching corals do.
FAs are the primary constituents of the cell and subcellular organelle membranes in organisms. Differences in FA compositions can affect membrane-associated physical features and biological functions such as fluidity, permeability, and related enzyme activity (Tchernov et al., 2004; Ernst et al., 2016; Liu et al., 2020). We found that massive corals have a higher composition of UFAs than that of branching corals. Compared to SFAs, homologous UFAs have a lower melting point and occupy a larger space within the membrane lipid bilayer to enhance their fluidity and stability (Hazel, 1979; Yeagle, 1989; Liu et al., 2018). Increased biomembrane fluidity may facilitate membrane-bound enzyme activity and material exchange (Conlan et al., 2020; Liu et al., 2020) and help minimize the influence of environmental stress. Tchernov et al. (2004) demonstrated that high levels of unsaturated in the membrane are a key determinant of thermal-stress sensitivity in symbiotic algae of cnidarians. Furthermore, PUFAs (such as 20:4n6, and 22:6n3) exert immune and reproductive functions and are used as precursors of some hormones (Berge and Barnathan, 2005). Therefore, massive corals with more PUFAs may have a survival advantage during environmental changes.
Data Availability Statement
The original contributions presented in the study are included in the article/supplementary material. Further inquiries can be directed to the corresponding author.
Author Contributions
CL, YZ, and HH designed experiments. CL, YZ, YS, LJ, YL, and XY carried out experiments. CL, YZ, SL, LH, and HH analyzed experimental results and wrote the manuscript. All authors contributed to the article and approved the submitted version.
Funding
This study was jointly funded by the National Natural Science Foundation of China (NSFC) (Nos. 41906097 and 41976120); Science and Technology Planning Project of Guangdong Province, China (No. 2020B1212060058); Innovation Academy of South China Sea Ecology and Environmental Engineering, Chinese Academy of Sciences (No. ISEE2018PY01); Key Research and Development Project of Hainan Province, China (No. ZDYF2020200); and Research Project of Sanya Yazhou-Bay SCI-TECH City Administration (No. SKJC-2020-01-010).
Conflict of Interest
The authors declare that the research was conducted in the absence of any commercial or financial relationships that could be construed as a potential conflict of interest.
Publisher’s Note
All claims expressed in this article are solely those of the authors and do not necessarily represent those of their affiliated organizations, or those of the publisher, the editors and the reviewers. Any product that may be evaluated in this article, or claim that may be made by its manufacturer, is not guaranteed or endorsed by the publisher.
Acknowledgments
The authors would like to express our gratitude to the staff of the Hainan Tropical Marine Biological Research Station for providing technical assistance and facilities for conducting the work, and to those who critically reviewed this manuscript.
References
Anthony K., Willis C. (2002). Comparative Analysis of Energy Allocation to Tissue and Skeletal Growth in Corals. Limnol. Oceanogr. 47, 1417–1429. doi: 10.4319/lo.2002.47.5.1417
Bachok Z., Mfilinge P., Tsuchiya M. (2006). Characterization of Fatty Acid Composition in Healthy and Bleached Corals From Okinawa, Japan. Coral. Reef. 25, 545–554. doi: 10.1007/s00338-006-0130-9
Baker D., Freeman C. J., Knowlton N., Thacker R., Fogel M., Kim K.. (2015). Productivity Links Morphology, Symbiont Specificity and Bleaching in the Evolution of Caribbean Octocoral Symbioses. ISME. J. Emultidiscip. J. Microbial. Ecol 9, 2620–2629. doi: 10.1038/ismej.2015.71
Baumann J., Grottoli A. G., Hughes A. D., Matsui Y. (2014). Photoautotrophic and Heterotrophic Carbon in Bleached and non-Bleached Coral Lipid Acquisition and Storage. J. Exp. Mar. Biol. Ecol. 461, 469–478. doi: 10.1016/j.jembe.2014.09.017
Berge J. P., Barnathan G. (2005). Fatty Acids From Lipids of Marine Organisms: Molecular Biodiversity, Roles as Biomarkers, Biologically Active Compounds, and Economical Aspects. Adv. Biochem. Eng. Biot. 96, 49–125. doi: 10.1007/b135782
Bhojoo U., Chen M., Zou S. (2018). Temperature Induced Lipid Membrane Restructuring and Changes in Nanomechanics. Biochim. Biophys. Acta (BBA) - Biomembr. 1860, 700–709. doi: 10.1016/j.bbamem.2017.12.008
Bishop D. G., Kenrick J. R. (1980). Fatty Acid Composition of Symbiotic Zooxanthellae in Relation to Their Hosts. Lipids 15, 799–804. doi: 10.1007/BF02534368
Chapron L., Schoepf V., Levas S. J., Aschaffenburg M. D., Warner M. E., Grottoli A. G. (2022). Natural Variability in Caribbean Coral Physiology and Implications for Coral Bleaching Resilience. Front. Mar. Sci. 8, 811055. doi: 10.3389/fmars.2021.811055
Chen H.-K., Song S.-N., Wang L.-H., Mayfield A. B., Chen Y. J., Chen W.-N. U., et al. (2015). A Compartmental Comparison of Major Lipid Species in a Coral-Symbiodinium Endosymbiosis: Evidence That the Coral Host Regulates Lipogenesis of its Cytosolic Lipid Bodies. PLoS One 10, e0132519. doi: 10.1371/journal.pone.0132519
Conlan J. A., Bay L. K., Jones A., Thompson A., Francis D. S. (2020). Seasonal Variation in the Lipid Profile of Acropora Millepora at Halfway Island, Great Barrier Reef. Coral. Reef. 39, 1753–1765. doi: 10.1007/s00338-020-02001-w
Conlan J. A., Rocker M. M., Francis D. S. (2017). A Comparison of Two Common Sample Preparation Techniques for Lipid and Fatty Acid Analysis in Three Different Coral Morphotypes Reveals Quantitative and Qualitative Differences. PeerJ 5, e3645. doi: 10.7717/peerj.3645
Conti-Jerpe I. E., Thompson P. D., Wong C. W. M., Oliveira N. L., Duprey N. N., Moynihan M. A., et al. (2020). Trophic Strategy and Bleaching Resistance in Reef-Building Corals. Sci. Adv. 6, eaaz5443. doi: 10.1126/sciadv.aaz5443
Dalsgaard J., St. John M., Kattner G., Müller-Navarra D., Hagen W. (2003). Fatty Acid Trophic Markers in the Pelagic Marine Environment. Adv. Mar. Biol. 46, 225–340. doi: 10.1016/S0065-2881(03)46005-7
Edmunds P. J., Burgess S. C., Putnam H. M., Baskett M. L., Bramanti L., Fabina N. S., et al. (2014). Evaluating the Causal Basis of Ecological Success Within the Scleractinia: An Integral Projection Model Approach. Mar. Biol. 161, 2719–2734. doi: 10.1007/s00227-014-2547-y
Ernst R., Ejsing C. S., Antonny B. (2016). Homeoviscous Adaptation and the Regulation of Membrane Lipids. J. Mol. Biol. 428, 4776–4791. doi: 10.1016/j.jmb.2016.08.013
Farre B., Cuif J. P., Dauphin Y. (2010). Occurence and Diversity of Lipids in Modern Coral Skeletons. Zoology 113, 250–257. doi: 10.1016/j.zool.2009.11.004
Grottoli A. G., Rodrigues L. J., Juarez C. (2004). Lipids and Stable Carbon Isotopes in Two Species of Hawaiian Corals, Porites Compressa and Montipora Verrucosa, Following a Bleaching Event. Mar. Biol. 145, 621–631. doi: 10.1007/s00227-004-1337-3
Grottoli A. G., Rodrigues L. J., Palardy J. E. (2006). Heterotrophic Plasticity and Resilience in Bleached Corals. Nature 440, 1186–1189. doi: 10.1038/nature04565
Grottoli A. G., Warner M. E., Levas S. J., Aschaffenburg M. D., Schoepf V., McGinley M., et al. (2014). The Cumulative Impact of Annual Coral Bleaching can Turn Some Coral Species Winners Into Losers. Global Change Biol. 20, 3823–3833. doi: 10.1111/gcb.12658
Hazel J. R. (1979). Influence of Thermal Acclimation on Membrane Lipid Composition of Rainbow Trout Liver. Proc. Natl. Acad. Sci. U. S. A. 236, R91. doi: 10.1152/ajpregu.1979.236.1.R91
Houlbrèque F., Ferrier-Pagès C. (2009). Heterotrophy in Tropical Scleractinian Corals. Biol. Rev. 84, 1–17. doi: 10.1111/j.1469-185X.2008.00058.x
Hughes T. P., Kerry J. T., Álvarez-Noriega M., Álvarez-Romero J. G., Anderson K. D., Baird A. H., et al. (2017). Global Warming and Recurrent Mass Bleaching of Corals. Nature 543, 373–377. doi: 10.1038/nature21707
Iluz D., Dubinsky Z. (2015). Coral Photobiology: New Light on Old Views. Zoology 118, 71–78. doi: 10.1016/j.zool.2014.08.003
Imbs A. B., Demidkova D. A., Latypov Y. Y., Pham L. Q. (2007). Application of Fatty Acids for Chemotaxonomy of Reef-Building Corals. Lipids 42, 1035–1046. doi: 10.1007/s11745-007-3109-6
Imbs A. B., Latyshev N. A., Dautova T. N., Latypov Y. Y. (2010). Distribution of Lipids and Fatty Acids in Corals by Their Taxonomic Position and Presence of Zooxanthellae. Mar. Ecol. Prog. Ser. 409, 65–75. doi: 10.3354/meps08622
Imbs A. B., Yakovleva I. (2012). Dynamics of Lipid and Fatty Acid Composition of Shallow-Water Corals Under Thermal Stress: An Experimental Approach. Coral. Reef. 31, 41–53. doi: 10.1007/s00338-011-0817-4
Jones A. M., Ray B. (2011). Tradeoffs to Thermal Acclimation: Energetics and Reproduction of a Reef Coral With Heat Tolerant Symbiodinium Type-D. J. Mar. Biol. 12, 1–12. doi: 10.1155/2011/185890
Kabeya N., Fonseca M. M., Ferrier D. E. K., Navarro J. C., Bay L. K., Francis D. S., et al. (2018). Genes for De Novo Biosynthesis of Omega-3 Polyunsaturated Fatty Acids are Widespread in Animals. Sci. Adv. 4, eaar6849. doi: 10.1126/sciadv.aar6849
Kattner G., Hagen W. (2009). Lipids in Marine Copepods: Latitudinal Characteristics and Perspective to Global Warming. J. Plankton Res. 31, 257–280. doi: 10.1007/978-0-387-89366-2_11
Kayanne H., Harii S., Yamano H., Tamura M., Ide Y., Akimoto F. (2010). Changes in Living Coral Coverage Before and After the 1998 Bleaching Event on Coral Reef Flats of Ishigaki Island, Ryukyu Islands. J. Jap. Coral. Reef. Soc. 1999, 73–82. doi: 10.3755/jcrs.1999.73
Liu C., Dong S., Zhou Y., Shi K., Pan Z., Sun D., et al. (2019). Temperature-Dependent Fatty Acid Composition Change of Phospholipid in Steelhead Trout (Oncorhynchus Mykiss) Tissues. J. Ocean. Univ. China 18, 519–527. doi: 10.1007/s11802-019-3775-z
Liu C., Ge J., Zhou Y., Thirumurugan R., Gao Q. (2020). Effects of Decreasing Temperature on Phospholipid Fatty Acid Composition of Different Tissues and Hematology in Atlantic Salmon (Salmo Salar). Aquaculture 515, 734587–734587. doi: 10.1016/j.aquaculture.2019.734587
Liu C., Zhou Y., Dong K., Sun D., Gao Q., Dong S. (2018). Differences in Fatty Acid Composition of Gill and Liver Phospholipids Between Steelhead Trout (Oncorhynchus Mykiss) and Atlantic Salmon (Salmo Salar) Under Declining Temperatures. Aquaculture 495, 815–822. doi: 10.1016/j.aquaculture.2018.06.045
Loya Y., Sakai K., Yamazato K., Nakano Y., Sambali H., van Woesik R. (2001). Coral Bleaching: The Winners and the Losers. Ecol. Lett. 4, 122–131. doi: 10.1046/j.1461-0248.2001.00203.x
Martínez-Castillo V., Rodríguez-Troncoso A. P., Santiago-Valentín J. D., Cupul-Magaña A. L. (2020). The Influence of Urban Pressures on Coral Physiology on Marginal Coral Reefs of the Mexican Pacific. Coral. Reef. 39, 625–637. doi: 10.1007/s00338-020-01957-z
Monroig Ó., Naoki K. (2018). Desaturases and Elongases Involved in Polyunsaturated Fatty Acid Biosynthesis in Aquatic Invertebrates: A Comprehensive Review. Fish. Sci. 84, 911—928. doi: 10.1007/s12562-018-1254-x
Neuringer M. G., Anderson G. J., Connor W. E. (1988). The Essentiality of N-3 Fatty Acids for the Development and Function of the Retina and Brain. Annu. Rev. Nutr. 8, 517—541. doi: 10.1146/annurev.nu.08.070188.002505
Oksanen J., Blanchet F. G., Kindt R., Legendre P., Wagner H. (2010).Vegan: Community Ecology Package. Available at: http://cran.r-project.org/package=vegan
Palardy J., Grottoli A. G., Matthews K. A. (2005). Effects of Upwelling, Depth, Morphology and Polyp Size on Feeding in Three Species of Panamanian Corals. Mar. Ecol. Prog. Ser. 300, 79—89. doi: 10.3354/meps300079
Palardy J., Rodrigues L., Grottoli A. (2008). The Importance of Zooplankton to theDailyMetabolic Carbon Requirements of Healthy and Bleached Corals at Two Depths. J. Exp. Mar. Biol. Ecol. 367, 180—188. doi: 10.1016/j.jembe.2008.09.015
Porter J. W., Fitt W. K., Spero H. J., White R. (1989). Bleaching in Reef Corals: Physiological and Stable Isotopic Responses. Proc. Natl. Acad. Sci. U. S. A. 86, 9342–9346. doi: 10.1073/pnas.86.23.9342
Pupier C. A., Mies M., Fine M., Francini‐Filho R. B., Brandini F. P., Zambotti‐Villela L., et al. (2021). Lipid Biomarkers Reveal the Trophic Plasticity of Octocorals Along a Depth Gradient. Limnol. Oceanogr. 66, 2078–2087.doi: 10.1002/lno.11746
Radice V. Z., Brett M. T., Fry B., Fox M. D., Dove S. G. (2019). Evaluating Coral Trophic Strategies Using Fatty Acid Composition and Indices. PLoS One 14, e0222327. doi: 10.1371/journal.pone.0222327
Rocker M. M., Kenkel C. D., Francis D. S., Willis B. L., Bay L. K. (2019). Plasticity in Gene Expression and Fatty Acid Profiles of Acropora Tenuis Reciprocally Transplanted Between Two Water Quality Regimes in the Central Great Barrier Reef, Australia. J. Exp. Mar. Biol. Ecol. 511, 40–53. doi: 10.1016/j.jembe.2018.11.004
Rodrigues L. J., Grottoli A. G., Pease T. K. (2008). Lipid Class Composition of Bleached and Recovering Porites Compressa Dana 1846 and Montipora Capitata Dana 1846 Corals From Hawaii. J. Exp. Mar. Biol. Ecol. 358, 136–143. doi: 10.1016/j.jembe.2008.02.004
Rossi S., Schubert N., Brown D., Gonzalez-Posada A., Soares M. O. (2020). Trophic Ecology of Caribbean Octocorals: Autotrophic and Heterotrophic Seasonal Trends. Coral. Reef. 39, 433–449. doi: 10.1007/s00338-020-01906-w
Rotmann S., Thomas S. (2012). Coral Tissue Thickness as a Bioindicator of Mine-Related Turbidity Stress on Coral Reefs at Lihir Island, Papua New Guinea. Oceanography 25, 52–63. doi: 10.2307/24805627
Schoepf V., Grottoli A. G., Levas S. J., Aschaffenburg M., Baumann J. H., Matsui Y., et al. (2015). Annual Coral Bleaching and the Long-Term Recovery Capacity of Coral. Proc. Biol. Sci. 282, 1887–1896. doi: 10.1098/rspb.2015.1887
Snyder R. J., Schregel W. D., Wei Y. (2012). Effects of Thermal Acclimation on Tissue Fatty Acid Composition of Freshwater Alewives (Alosa Pseudoharengus). Fish. Physiol. Biochem. 38, 363–373. doi: 10.1007/s10695-011-9513-0
Tagliafico A., Rudd D., Rangel M. S., Kelaher B. P., Benkendorff K. (2017). Lipid-Enriched Diets Reduce the Impacts of Thermal Stress in Corals. Mar. Ecol. Prog. Ser. 573, 129–141. doi: 10.3354/meps12177
Tchernov D., Gorbunov M. Y., Vargas C.d., Yadav S. N., Milligan A. J., Ha¨ggblom M, et al. (2004). Membrane Lipids of Symbiotic Algae are Diagnostic of Sensitivity to Thermal Bleaching in Corals. Proc. Natl. Acad. Sci. U. S. A. 101, 13531–13535. doi: 10.1073/pnas.0402907101
Teece M. A., Estes B., Gelsleichter E., Lirman D. (2011). Heterotrophic and Autotrophic Assimilation of Fatty Acids by Two Scleractinian Corals, Montastraea Faveolata and Porites Astreoides. Limnol. Oceanogr. 56, 1285–1296. doi: 10.4319/lo.2011.56.4.1285
Thornhill D. J., Rotjan R. D., Todd B. D., Chilcoat G. C., Iglesias-Prieto R., Kemp D. W., et al. (2011). A Connection Between Colony Biomass and Death in Caribbean Reef-Building Corals. PLoS One 537, 129–141. doi: 10.1371/journal.pone.0029535
Tocher D. (2003). Metabolism and Functions of Lipids and Fatty Acids in Teleost Fish. Rev. Fish. Sci. 11, 107–184. doi: 10.1080/713610925
Todd P. A., Ladle R. J., Lewin-Koh N., Chou L. M. (2004). Flesh or Bone? Quantifying Small-Scale Coral Morphology Using With-Tissue and Without-Tissue Techniques. Mar. Biol. 145, 323–328. doi: 10.1007/s00227-004-1324-8
Tolosa I., Treignier C., Grover R., Ferrier-Pagès C. (2011). Impact of Feeding and Short-Term Temperature Stress on the Content and Isotopic Signature of Fatty Acids, Sterols, and Alcohols in the Scleractinian Coral Turbinaria Reniformis. Coral. Reef. 30, 763–774. doi: 10.1007/s00338-011-0753-3
Veron J. (2000). Corals of the World (Australian Institute of Marine Science and CCR Qld Pty Ltd: Townsville).
Wallaert C., Babin P. J. (1994). Thermal Adaptation Affects the Fatty Acid Composition of Plasma Phospholipids in Trout. Lipids 29, 373–376. doi: 10.1007/BF02537193
Wickham H. (2016). Ggplot2: Elegant Graphics for Data Analysis (New York: Springer). Available at: http://ggplot2.org.
Yamashiro H., Oku H., Higa H., Chinen I., Sakai K. (1999). Composition of Lipids, Fatty Acids and Sterols in Okinawan Corals. Comp. Biochem. Physiol. B. 122, 379–407. doi: 10.1016/S0305-0491(99)00014-0
Yamashiro H., Oku H., Onaga K. (2005). Effect of Bleaching on Lipid Content and Composition of Okinawan Corals. Fish. Sci. 71, 448–453. doi: 10.1111/j.1444-2906.2005.00983.x
Yeagle P. L. (1989). Lipid Regulation of Cell Membrane Structure and Function. FASEB J. 3, 1833–1842. doi: 10.1096/fasebj.3.7.2469614
Yost D. M., Wang L. H., Fan T. Y., Chen C. S., Gates R. D. (2013). Diversity in Skeletal Architecture Influences Biological Heterogeneity and Symbiodinium Habitat in Corals. Zoology 116, 262–269. doi: 10.1016/j.zool.2013.06.001
Keywords: colony morphology, reef-building coral, fatty acids, nutritional ecology, total lipid content
Citation: Liu C, Zhang Y, Huang L, Yu X, Luo Y, Jiang L, Sun Y, Liu S and Huang H (2022) Differences in Fatty Acids and Lipids of Massive and Branching Reef-Building Corals and Response to Environmental Changes. Front. Mar. Sci. 9:882663. doi: 10.3389/fmars.2022.882663
Received: 24 February 2022; Accepted: 19 April 2022;
Published: 26 May 2022.
Edited by:
Charles Alan Jacoby, St. Johns River Water Management District, United StatesReviewed by:
Christine Ferrier-Pagès, Centre Scientifique de Monaco, MonacoJoshua Patterson, University of Florida, United States
Copyright © 2022 Liu, Zhang, Huang, Yu, Luo, Jiang, Sun, Liu and Huang. This is an open-access article distributed under the terms of the Creative Commons Attribution License (CC BY). The use, distribution or reproduction in other forums is permitted, provided the original author(s) and the copyright owner(s) are credited and that the original publication in this journal is cited, in accordance with accepted academic practice. No use, distribution or reproduction is permitted which does not comply with these terms.
*Correspondence: Hui Huang, aHVhbmdodWlAc2NzaW8uYWMuY24=
†These authors share first authorship