- Key Laboratory of Pollution Ecology and Environmental Engineering, Institute of Applied Ecology, Chinese Academy of Sciences, Shenyang, China
Microbial reductive dechlorination has been considered an effective process for the clean-up of organohalide-contaminated sites. Heavy metal ions are commonly present as co-contaminants in various organohalide-contaminated sites. To understand the impacts of heavy metal ions on the environmental fate of organohalides, we investigated the effects of Zn2+, Cu2+ and Cd2+ on reductive dechlorination of tetrachloroethene (PCE) and 1,2-dichloroethane (1,2-DCA) in sediment microcosms and transferred enrichment cultures. PCE and 1,2-DCA-dechlorinating enrichment cultures could be consecutively transferred in the presence of up to 10 mg/L Cu2+ or 10 mg/L Zn2+; by comparison, up to 50 mg/L Cd2+ had minor impacts on the microbial reductive dechlorination of PCE and 1,2-DCA. The inhibitory effects of tested heavy metal ions on microbial reductive dechlorination ranked in descending order are Zn2+, Cu2+, and Cd2+. Community profiling and principal component analysis indicate that the concentration and type of contaminants (e.g., heavy metal ions, organohalides) shaped the microbial community structure, an observation similar to a prior report. The enrichment of certain organohalide-respring bacteria (e.g., Dehalococcoides, Dehalogenimonas) during continuous transfers exposed to heavy metal ions suggests that they are capable of tolerating high concentrations of heavy metal ions. Our findings provide insights into the impacts of heavy metal ions on microbial reductive dechlorination and may be helpful for in situ bioremediation at sites contaminated with organohalides and heavy metals.
Introduction
Organohalides have been produced on a massive scale for a variety of industrial and agricultural applications such as solvents, pesticides, degreasing agents, and active ingredients (Doherty, 2000; He et al., 2021). Improper handling and uncontrolled discharge have resulted in the accumulation of organohalides in various anoxic environments (e.g., groundwater, sediments). Due to severe toxicity, recalcitrance and tendency to accumulate in lipid-rich organs, many organohalides pose great threats to human health and ecosystem functions (Loganathan and Kannan, 1994; Atashgahi et al., 2018b; Potapowicz et al., 2020; Girones et al., 2021). To mitigate the hazardous impacts of toxic organohalides on natural environments, (bio)degradation and (bio)transformation of anthropogenic organohalides have been intensively studied in the past few decades (Stroo and Ward, 2010; Stroo et al., 2012; Atashgahi et al., 2016). One of the notable findings is the discovery of specialized anaerobic bacteria that can couple the reductive dechlorination of organohalides with energy conservation (i.e., organohalide respiration) (de Bruin et al., 1992; Mohn and Tiedje, 1992; Adrian and Löffler, 2016). Microbial reductive dechlorination has also been demonstrated as a cost-effective and environmentally friendly method for in situ remediations of organohalide contaminants (Ellis et al., 2000; Ferguson and Pietari, 2000; Devlin et al., 2004; Aulenta et al., 2006; He et al., 2021).
Various biogeochemical factors can influence the success of contaminated site clean-up. Organohalides are frequently found to co-exist with other volatile organic compounds (e.g., trihalomethane) (Squillace et al., 2002) and nitrate (Nelson et al., 2002), and these chemicals have been demonstrated of negative effects on microbial reductive dechlorination processes (Kuo and Genthner, 1996; Pardue et al., 1996; Zhang et al., 2012). Other overlooked factors that can affect microbial reductive dechlorination are heavy metals [e.g., lead (Pb), chromium (Cr), arsenic (As), zinc (Zn), cadmium (Cd), copper (Cu)] (Arjoon et al., 2012; Lu et al., 2020). Heavy metals are mainly released to soil and subsurface environments from fertilizers, pesticides, industrial wastewater, and metal mining processes, and often co-mingled with anthropogenic organohalides in groundwater plumes (Wuana and Okieimen, 2011; Zhang et al., 2012; Deng et al., 2018; Wu et al., 2019). Despite that heavy metals (e.g., Ni, Cu, Zn) can serve as enzyme cofactors for various biochemical reactions, many of them often exhibit toxic effects on microbial processes at elevated concentrations (Silver, 1996; Juliastuti et al., 2003; Li and Fang, 2007; Altas, 2009; Rahman and Singh, 2020). Several molecular mechanisms, such as (i) substitutive metalligand binding, (ii) reaction of metal ions with cellular thiols, and (iii) participation of transition metals in Fentontype reactions have been proposed to explain such inhibitory effects (Harrison et al., 2007; Roane et al., 2015; Prabhakaran et al., 2016). Heavy metals also can negatively affect key enzymes involved in microbial biotransformation, resulting in stalled biodegradation of contaminants and extended periods for site clean-up (Kuo and Genthner, 1996). For instance, biodegradation of 3-chlorobenzoate and phenol have been found susceptible to several heavy metal ions (e.g., Cd2+, Cr6+, Cu2+, Hg2+) (Kuo and Genthner, 1996). Inhibition of 2,3,4-trichloroaniline biodegradation at the presence of 10 ~ 200 μg/L Cd2+ has also been demonstrated (Pardue et al., 1996).
Organohalide-respiring bacteria (OHRB) are microbial specialists that can utilize organohalides as electron acceptors for energy conservation under anoxic conditions (Atashgahi et al., 2016). OHRB have been frequently detected in various contaminated and pristine environments, and may play indispensable but underestimated roles in the natural attenuation and recycling of organohalides (Atashgahi et al., 2016; Atashgahi et al., 2018a; Yang et al., 2020). For instance, members within OHRB genera Dehalococcoides, Dehalogenimonas and Desulfoluna have been frequently found to inhabit marine environments (e.g., marine intertidal sediments, estuary sediments) (Zanaroli et al., 2015; Peng et al., 2020; Xu et al., 2022). Coastal and marine environments are increasingly contaminated by heavy metals due to a variety of anthropogenic activities (e.g., industrial effluents, oil pollution) (Naser, 2013). How heavy metal ions affect certain marine OHRB is not well elucidated. Recently, Lu et al. investigated the inhibitory effects of heavy metal ions (e.g., Cu2+, Cd2+, Cr3+, Pb2+) on the activities of several OHRB, and found that OHRB in mixed cultures generally had a higher tolerance to the toxicity of heavy metal ions compared to the OHRB in pure cultures. For instance, Dehalococcoides-containing microcosms exhibited higher tolerance to heavy metal ions compared to the pure culture of Dehalococcoides strain CG1 (Lu et al., 2020). Furthermore, some OHRB (e.g., Dehalococcoides) have a relatively higher level of tolerance to metal ions than the non-dechlorinating populations (e.g., fermenters, acetogens, methanogens) in the dechlorinating microbial consortia (Lu et al., 2020), suggesting that the addition of a metal ion (e.g., Pb2+, Cu2+) may be used as a strategy to stimulate and enrich certain OHRB. Nonetheless, the enrichment processes and evolution of microbial communities at the presence of heavy metal ions require further investigation.In this study, we investigated the impacts of different concentrations of Cd2+, Cu2+ and Zn2+ on reductive dechlorination of tetrachloroethene (PCE) and 1,2-dichloroethane (1,2-DCA). Enrichment cultures were consecutively transferred to evaluate the effects of heavy metal ions on the evolution of microbial communities and to identify OHRB phylotypes that can tolerate high concentrations of heavy metal ions. Our findings demonstrate that heavy metal ions extended the dechlorination period but increased the relative abundances of several OHRB including Dehalococcoides and Dehalogenimonas. We also obtained 1,2-DCA- and PCE-dechlorinating consortia that can tolerate as high as 50 mg/L Cd2+. This study expands current understanding of the effects of heavy metal ions on microbial reductive dechlorination with an overarching goal for cost-effective remediation at sites where heavy metals are present as co-contaminants.
Materials and Methods
Chemicals
PCE, trichloroethylene (TCE), cis-1,2-dichloroethylene (cDCE), 1,2-DCA (all ≥99%) were purchased from Macklin Biochemical Co., Ltd (Shanghai, China). Vinyl chloride (VC) and ethene (both ≥99%) were purchased from Dalian Special Gases Co., Ltd (Dalian, China). CuCl2·2H2O, and ZnCl2 were purchased from Sigma-Aldrich (St. Louis, MO, USA). CdCl2 was purchased from Shanghai Aladdin Biochemical Technology Co., Ltd (Shanghai, China). All other chemicals used in this study were analytical grade or of higher purity.
Microcosm Setup and Enrichment Cultures
Medium preparation and anaerobic cultivation were performed following estabolished protocols (Löffler et al., 2005; Yang et al., 2017a; Yang et al., 2017b). Briefly, reduced, bicarbonate-buffered mineral salts medium was boiling under an atmosphere of N2 to remove dissolved oxygen, cooled down to room temperature and then dispensed into serum bottles flushed with N2/CO2 (80/20, v/v). Sediment samples were collected from the Xi River, Shenyang, Liaoning Province, China (41°44′41″N, 123°17′35″E) as described (Jiang et al., 2022). Microcosms were constructed inside an anoxic chamber (Coy Laboratory Inc., MI, USA) filled with N2/H2 (97/3, v/v). An aliquot of 2 mL sediment sludge was pipetted into 160 mL glass serum bottles prefilled with 100 mL of medium mentioned above as described (Yang et al., 2017b; Yang et al., 2020). Bottles were sealed with butyl rubber stoppers (Fushiyuan rubber and plastic products factory, Shenzhen, Guangdong, China) and crimped with aluminum caps (Hongpu Instrument Technology, Ningbo, Zhejiang, China). Lactate (10 mM) was provided as the carbon source and electron donor. A dose of 5 μL neat PCE (ca. 0.31 mM or 51.41 mg/L aqueous phase concentration) or 5 μL neat 1,2-DCA (ca. 0.62 mM or 61.36 mg/L aqueous phase concentration) was added as the electron acceptor. All bottles were amended with Wolin vitamin mix (Wolin et al., 1963). A heavy metal ion (e.g., Cu2+, Cd2+, Zn2+) was added into each bottle from a stock solution. For each heavy metal ion, three different concentrations (5, 10, and 50 mg/L) were examined. Following the complete dechlorination of PCE or 1,2-DCA to ethene, 1 mL culture suspensison was transferred into fresh medium following the same procedures described above. Additional dechlorinating microcosms and enrichment cultures that did not receive the amenedment of a heavy metal ion were established as controls. The bottles were incubated statically in the dark at 30°C. Microcosms and enrichment cultures were established in duplicate bottles.
16S rRNA Gene Amplicon Sequencing and Bioinformatics
Cells were harvested from 5 mL culture suspension via vacuum filtration onto 25 mm diameter 0.22 μm pore-size membrane filters (Merck Millipore Ltd, Darmstadt, Germany). Genomic DNA was extracted from the filters using the Soil DNA Kit (Tiangen Biotech, Beijing, China) following the manufacturer’s instructions. DNA concentrations were determined using a Qubit 3.0 fluorometer (Invitrogen, Carlsbad, CA, USA). Amplicon sequencing was performed by Suzhou Genewiz Biotechnology (Suzhou, Jiangsu, China). For 16S rRNA gene amplicon sequencing, the primer set Pro341F (5’-CCTACGGRRBGCASCAGKVRVGAAT-3’) and Pro806R (5’-GGACTACNVGGGTWTCTAATCC-3’) modified by Genewiz for relatively conserved regions within the V3 and V4 hypervariable regions were used to generate amplicons (Fu et al., 2016). The quality and quantity of DNA libraries were evaluated using an Agilent 2100 Bioanalyzer (Agilent Technologies, Palo Alto, CA, USA) and a Qubit 2.0 Fluorometer, respectively. DNA libraries were multiplexed and loaded on an Illumina MiSeq instrument (Illumina, San Diego, CA, USA) following the manufacturer’s instructions. Sequencing was performed using a 2x250 paired-end (PE) configuration and image analysis and base calling were conducted using the MiSeq Control Software (MCS) embedded in the MiSeq instrument. The raw data were processed using Cutadapt (v1.9.1), VSEARCH (v1.9.6), and QIIME (v1.9.1) with default parameters to obtain clean reads (Caporaso et al., 2010; Bhute et al., 2016). Following quality control, sequences were grouped into operational taxonomic units (OTUs) using VSEARCH and QIIME at a 97% sequence identity. All OTUs were assigned to the lowest possible taxonomic rank using RDP classifier 2.2 and Silva_132 16S rRNA database (Wang et al., 2007; Edgar, 2010). Number of the obtained sequences in each sample was between 58,306 and 182,628, and a total of 686 OTUs were clustered from all the samples. Amplicon sequencing reads were deposited into Figshare repository with the following access address: https://doi.org/10.6084/m9.figshare.19212618.v1.
Analytical Methods
Ethene and chlorinated compounds were analyzed using an Agilent 7890B gas chromatography equipped with an Agilent 7697A automatic headspace sampler, a flame ionization detector (FID) (method detection limit ~ 0.2μM) and an Agilent DB-624 capillary column (60 m length x 0.32 mm inner diameter x 1.8 μm film thickness) as described (Yang et al., 2017b). Oven temperature was initially held at 60°C for 2 min, increased to 200°C at 25°C/min, and held at 200°C for 1 min. Inlet and FID temperatures were set at 200°C and 300°C, respectively.
Data Analysis
Principal component analysis (PCA) is a widely used statistical technique for dimension reduction and can be used for resolving differences among samples with distinct treatments. PCA was performed using the PCA plug-in (version 1.50) in OriginPro 2021 (OriginLab Corp. Northampton, MA, USA). Heatmaps of microbial communities were plotted using the ggplot2 packages (Wickham, 2011) embedded in R language (version 4.1.1) (R Core Team, 2020).
Results
Microbial Dechlorination of PCE and 1,2-DCA and Microbial Community Profiles
PCE and 1,2-DCA dechlorinating cultures were enriched from urban river sediments with lactate as the electron donor and carbon source. The enrichment cultures maintained stepwise PCE-to-ethene dechlorination and 1,2-DCA-to-ethene dihaloelimination after two consecutive transfers (Figure 1A). In the PCE-dechlorinating enrichment cultures, the initial 46.40 ± 0.72 μmol PCE was dechlorinated to stoichiometric amounts of ethene within 30 days via hydrogenolysis with TCE, cDCE, and VC being sequentially produced. Geobacter and Dehalococcoides were the dominant OHRB genera in the second transferred enrichment cultures with relative abundances of 11.12% and 3.42%, respectively. Geobacter lovleyi strain SZ, isolated from Su-Zi Creek sediment of Korea in 2005, and Geobacter lovleyi strain LYY, isolated from black-odorous urban river sediment of China, are capable of PCE-to-cDCE dechlorination (Sung et al., 2006; Amos et al., 2007; Liang et al., 2021). This information suggested that the Geobacter population in the PCE-dechlorinating enrichment was responsible for the reductive dechlorination of PCE to cDCE, which was futher dechlorinated to ethene by Dehalococcoides.
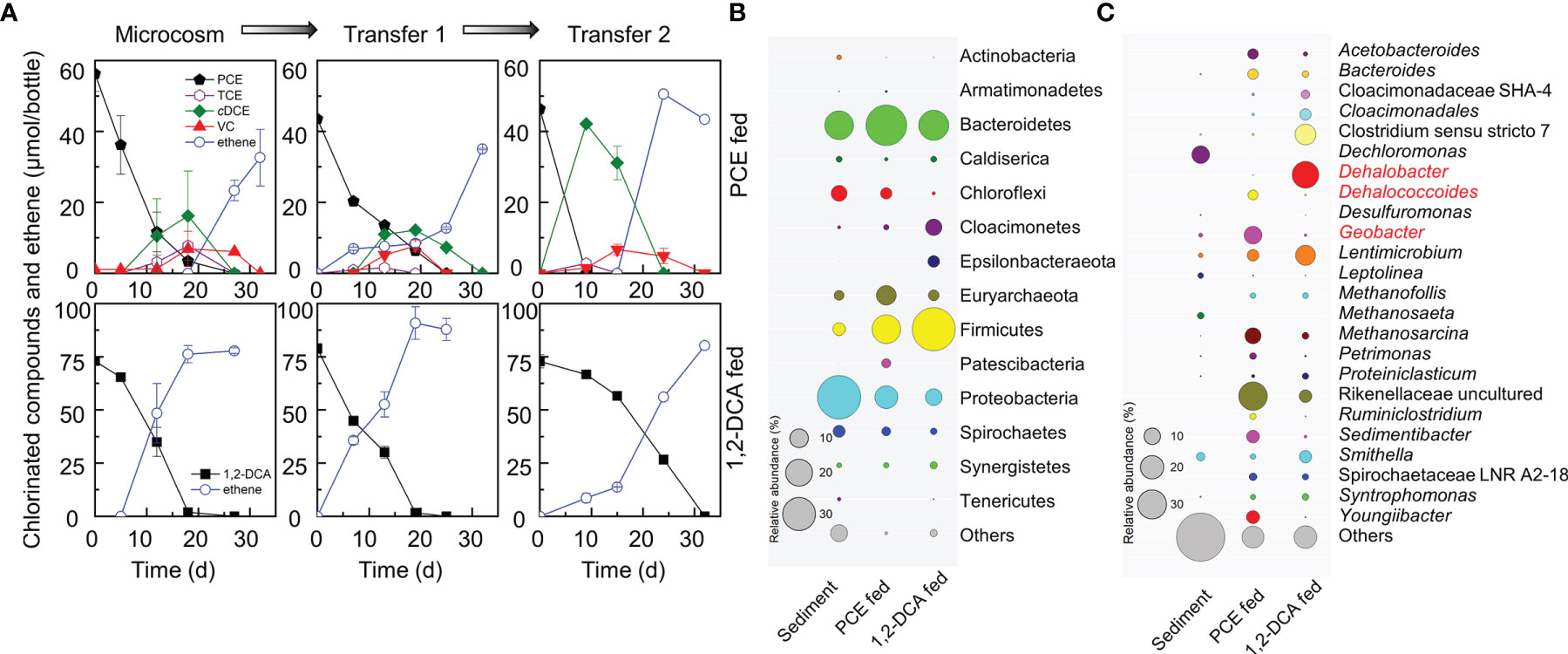
Figure 1 PCE and 1,2-DCA dechlorination by microcosms and transferred enrichment cultures (A), and the microbial structures of the second transferred PCE- and 1,2-DCA-fed enrichment cultures at the phylum level (B) and the genus level (C). PCE-fed and 1,2-DCA-fed enrichment cultures serving as the positive controls, maintained PCE-to-ethene stepwise hydrogenolysis and 1,2-DCA-to-ethene dihaloelimination, respectively. Representative microorganisms potentially performing reductive dechlorination are highlighted in red. The sizes of circles represent relative abundances.
In the 1,2-DCA-dechlorinating enrichment cultures, the intial 72.86 ± 3.09 μmol 1,2-DCA was dihaloeliminated to stoichiometric amounts of ethene within 30 days. Dehalobacter was the most abundant OHRB genus in the second transfer cultures with a relative abundance of 24.52% (Figure 1C), indicating that Dehalobacter may be responsible for dihaloelimination of 1,2-DCA. Dehalobacter sp. strain WL is able to dechlorinate 1,2-DCA to ethene using a novel reductive dehalogenase (RDase), which shares a 92% similarity to the dihaloeliminating DcaA of Desulfitobacterium dichloroeliminans strain DCA1 (Grostern and Edwards, 2006; van der Zaan et al., 2009). To date, the ability to perform dihaloelimination only has been identified in four organohalide-respiring genera including Dehalobacter, Desulfitobacterium, Geobacter and Dehalogenimonas (De Wildeman et al., 2003; Grostern and Edwards, 2009; Yan et al., 2009; Jiang et al., 2022).
Differential Effects of Heavy Metal Ions on PCE Dechlorination
To investigate the effects of heavy metal ions on dechlorination processes, sediment microcosms were set up with urban river sediments to dechlorinate PCE or 1,2-DCA at the presence of elevated concentration (i.e., 5, 10 or 50 mg/L) of a heavy metal ion (i.e., Zn2+, Cu2+ or Cd2+). In control cultures (i.e., without the addition of heavy metal ions), complete PCE-to-ethene dechlorination was achieved in microcosms and the transferred enrichment cultures within one month (Figure 1A). Complete dechlorination of PCE to ethene was also accomplished within one month in sediment microcosms amended with a heavy metal ion (i.e., Zn2+, Cu2+ or Cd2+) at a concentration up to 50 mg/L (Figure 2A), suggesting that the tolerance of OHRB to heavy metal ions can be greatly enhanced with protective solid matrix (e.g., minerals). Subsequent transfers of the PCE-dechlorinating enrichment cultures at the presence of relatively low concentrations (i.e., 5 or 10 mg/L) of a heavy metal ion were successful (Figure 2B), indicating that the enrichment cultures inherited the ability to tolerate the toxicity of Zn2+, Cu2+ and Cd2+ in low concentrations. By comparison, PCE-to-ethene dechlorination could not be sustained and stalled activity was observed in the first and second transfer cultures when exposed to 50 mg/L Zn2+ or 50 mg/L Cu2+ during a 80 days’ incubation (Figure 2A). PCE was completely dechlorinated to ethene in the transferred enrichment cultures exposed to 50 mg/L Zn2+ after incubated for about 200 days; nevertheless, complete PCE dechlorination did not occur in transferred enrichment cultures at the presence of 50 mg/L Cu2+ after incubated for one and half years (data not shown). In the first transfer, reduced PCE dechlorination rate (i.e., 40 days for complete dechlorination) was observed in enrichment cultures at the presence of 5 or 10 mg/L Zn2+. When the concentration of Zn2+ was increased to 50 mg/L, 7.04 ± 1.97 μmol TCE, negligible cDCE and VC were detected in the first transfer on day 68 (Figure 2A). The inhibitory effects of 50 mg/L Cu2+ on the dechlorination of PCE were similar to Zn2+ (Figure 2A). Unexpectedly, up to 50 mg/L Cd2+ did not affect PCE-to-ethene dechlorination activity, and complete PCE dechlorination to stoichiometric amounts of ethene was accomplished within 40 days (Figures 2A, B).
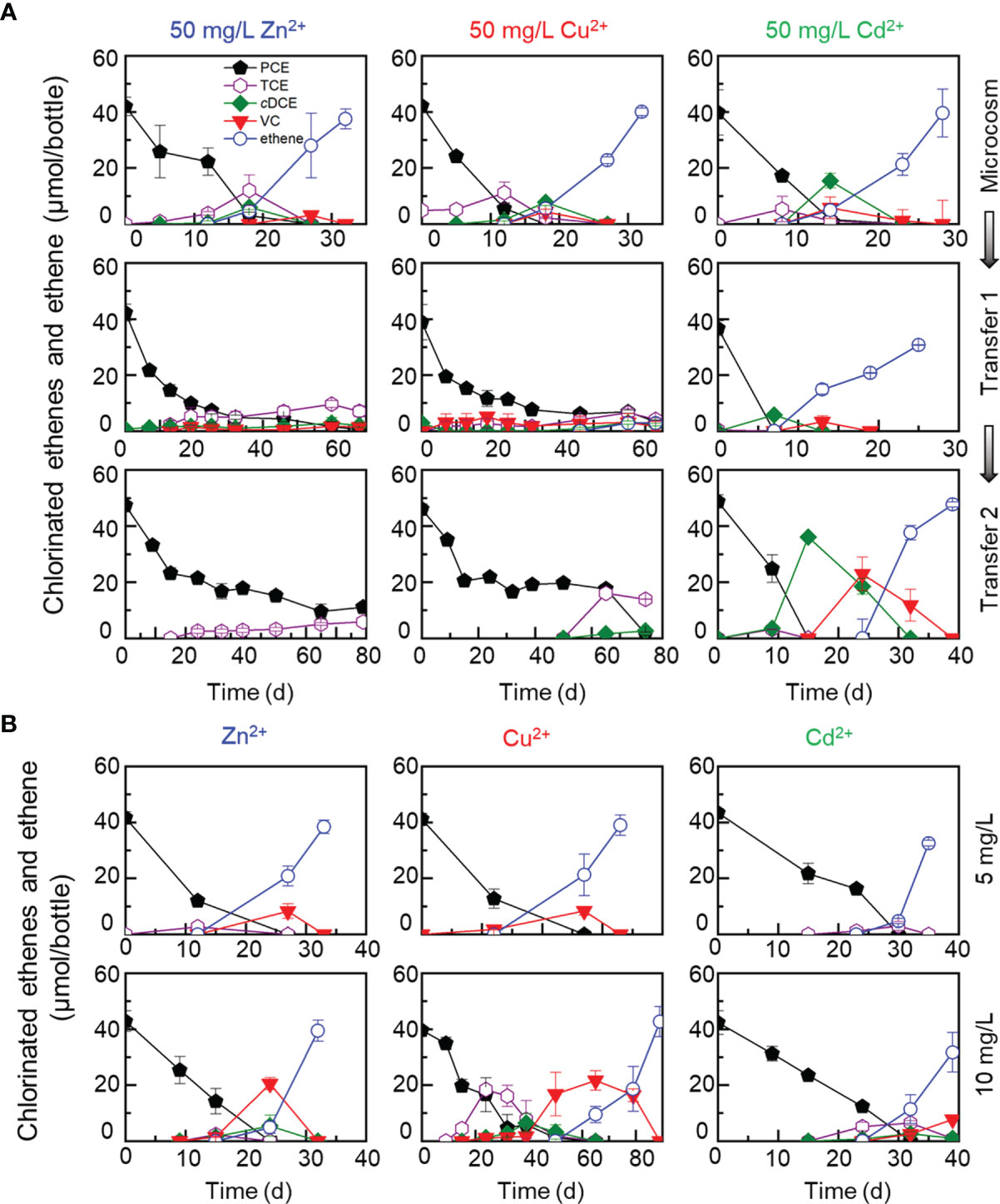
Figure 2 Effects of metal ions (50 mg/L Zn2+, Cu2+, Cd2+) on dechlorination of PCE during transferred enrichment processes (A), and effects of metal ions (5 and 10 mg/L Zn2+, Cu2+, Cd2+) on dechlorination of PCE in the second transferred enrichment cultures (B). High concentrations of Zn2+ and Cu2+ inhibited the PCE-ethene dechlorination, and no daughter products were detected or detected in small amounts in the transferred enrichment cultures. The decrease of PCE concentrations in transferred enrichment cultures exposed to 50 mg/L Cu2+ or Zn2+ was partly due to abiotic loss (e.g., adsorption to the butyl rubber stoppers).
Differential Effects of Heavy Metal Ions on 1,2-DCA Dihaloelimination
Without the addition of a heavy metal ion, sediment microcosms and transferred enrichment cultures completely dechlorinated the initial 72.86 ± 3.09 μmol 1,2-DCA to stoichiometric amounts of ethene within 25 days (Figure 1A). In sediment microcosms amended with up to 50 mg/L of a heavy metal ion (e.g., Cd2+, Zn2+ or Cu2+), 1,2-DCA could also be dihaloeliminated to ethene within 30 days (Figure 3A), indicating that these heavy metal ions at a concentration less than 50 mg/L had no apparent inhibitory effects on the reductive dechlorination of 1,2-DCA. Nevertheless, 1,2-DCA-to-ethene dechlorination could not be sustained and was completely lost in the first transfer cultures exposed to 50 mg/L Zn2+ and the second transfer cultures exposed to 50 mg/L Cu2+ (Figure 3A). Unlike the PCE-dechlorinating enrichment cultures, 1,2-DCA was not dechlorinated to ethene after prolonged incubation in the transfer cultures amended with 50 mg/L Zn2+ or Cu2+ (data not shown). By comparison, up to 10 mg/L Zn2+ or Cu2+ had little impacts on the dechlorination of 1,2-DCA in the second transfer cultures, and 72.86 ± 3.09 μmol 1,2-DCA was completely dechlorinated to ethene within 30 days (Figure 3B). Surprisingly, Cd2+ had little inhibitory effects on the dechlorination of 1,2-DCA as well, and 1,2-DCA was completely dechlorinated to ethene within 30 days in microcosms and transfer cultures at the presence of as high as 50 mg/L Cd2+ (Figures 3A, B).
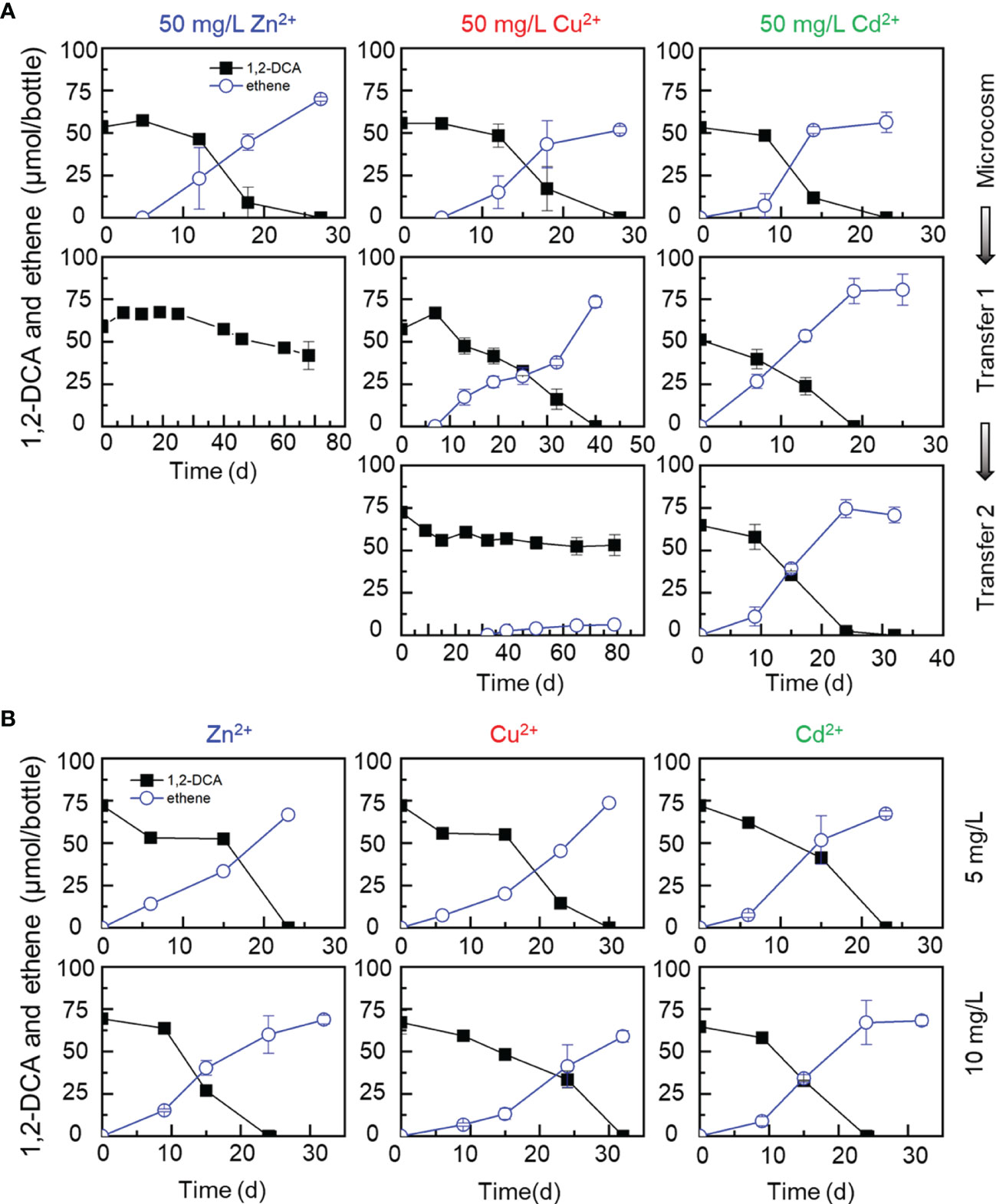
Figure 3 Effects of metal ions (50 mg/L Zn2+, Cu2+, Cd2+) on dihaloelimination of 1,2-DCA during transferred enrichment processes (A) and effects of metal ions (5 and 10 mg/L Zn2+, Cu2+, Cd2+) on dechlorination of 1,2-DCA in the second transferred enrichment cultures (B).
Impacts of Heavy Metal Ions on Microbial Community Structure
To evaluate effects of heavy metal ions on the dechlorinating communities, 16S rRNA gene amplicon sequencing was applied to samples collected from PCE- or 1,2-DCA-dechlorinating microcosms and transfer cultures exposed to different concentrations of heavy metal ions. Proteobacteria, Bacteroidetes, Firmicutes and Chloroflexi were the dominant phyla in sediment microcosms and enrichment cultures. The abundances of Bacteroidetes and Firmicutes increased when PCE or 1,2-DCA was provided (Figure 1B). Geobacter and Dehalococcoides were the main OHRB genera in the second transfer of PCE-dechlorinating cultures where a heavy metal ion was not added. Of note, the relative abundances of Geobacter and Dehalococcoides were 0.58% and undetectable, respectively, in the river sediment sample (Figure 1C). Dehalobacter was the most abundant OHRB genus in the second transfer of 1,2-DCA-dechlorinating enrichment cultures without the addition of a heavy metal ion;however, Dehalobacter was not detected (i.e., below the detection limit) in the river sediment sample (Figure 1C). Rikenellaceae, Lentimicrobium, Sedimentibacter, and Youngiibacter were likely the major fermenters in PCE-dechlorinating microcosms that produced acetate and H2 to supply Dehalococcoides and Geobacter. By comparison, Clostridium, Lentimicrobium, Rikenellaceae, Smithella and Acetobacteroides were the most abundant fermenters potentially producing acetate and H2 for Dehalobacter in the 1,2-DCA-dechlorinating enrichment cultures.
Principal component analysis (PCA) demonstrated that the concentrations and types of heavy metal ions and the types of organohalides were the predominant factors shaping the community structures (Figure 4). Geobacter, Dehalococcoides, Dehalogenimonas, and Desulfovibrio were clustered under the PCE-dechlorinating condition, while Dehalobacter was enriched under the 1,2-DCA-dechlorinating condition (Figure 4A). Moreover, community structures of the enrichment cultures received an addition of 50 mg/L Cu2+ or 50 mg/L Zn2+ differed greatly from the enrichment cultures that did not receive the addition of a heavy metal ion and other enrichment cultures, especially the enrichment cultures amended with Cd2+ (Figures 4B, C).
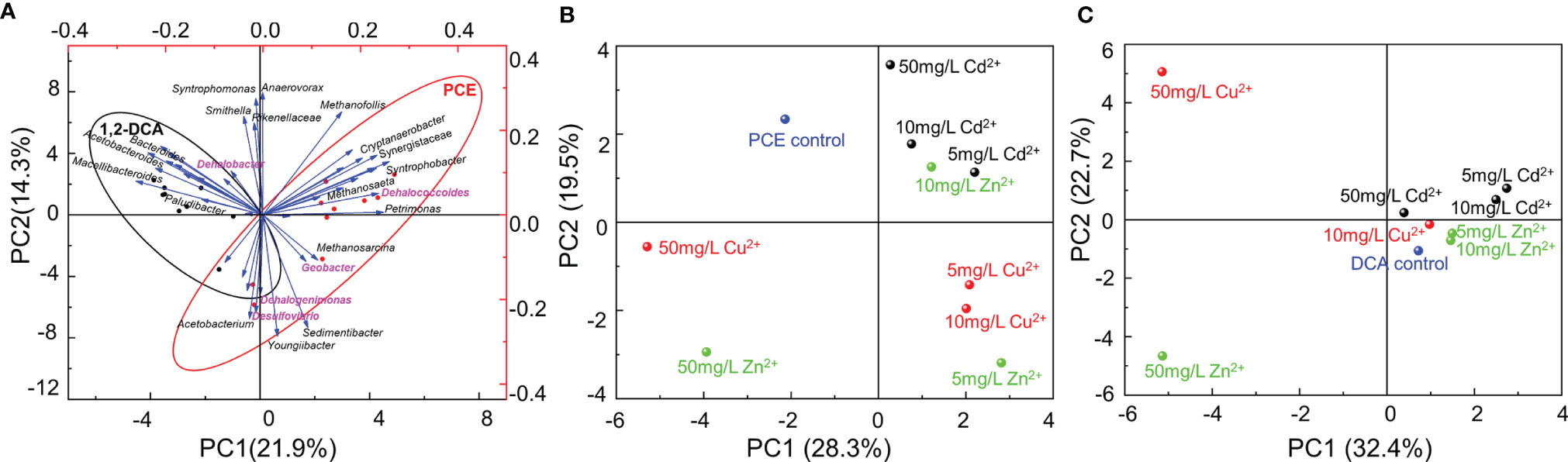
Figure 4 Principal component analysis (PCA) of the microbial communities in PCE- and 1,2-DCA-fed second transferred enrichment cultures amended with gradient concentrations of heavy metal ions: (A) PCE- and 1,2-DCA-fed second transferred enrichment cultures; (B) PCE-fed second transferred enrichment cultures with different types and concentrations of metal ions; (C) 1,2-DCA-fed second transferred enrichment cultures with different types and concentrations of metal ions. Representative microorganisms potentially performing reductive dechlorination are highlighted in purple (Panel A).
We also observed that the addition of a heavy metal ion (i.e., Zn2+, Cu2+, Cd2+) likely promoted the enrichment of OHRB, especially the obligate OHRBs (Figures 5, 6). In the second transfer of PCE-dechlorinating enrichment cultures amended with a heavy metal ion, the relative abundances of Dehalobacter increased from 0.02% in the control cultures to 4.5% in the enrichment cultures amended with 5 mg/L Zn2+, and 17.2% in the enrichment cultures amended with 10 mg/L Zn2+. Dehalococcoides, Dehalogenimonas, and Desulfovibrio were also enriched with the addition of a heavy metal ion. For instance, the relative abundances of Dehalogenimonas, of which certain member is capable of dechlorinating TCE to ethene (Yang et al., 2017b), increased to 48.1% in the enrichment cultures exposed to 50 mg/L Zn2+ from 0.1% in the control cultures. Likewise, the relative abundances of Dehalococcoides was 11.2% in the enrichment cultures exposed to 10 mg/L Cu2+, compared with 3.5% in control cultures and 5.7% in cultures amended with 5 mg/L Cu2+. In contrast, decreasing abundances of Geobacter was found in the enrichment cultures exposed to a heavy metal ion during consective transfers. The relative abundances of Geobacter decreased from 11.1% in the control cultures to 6.2% in the enrichment cultures exposed to 50 mg/L Cd2+. The fermenter Lentimicrobium was seemingly tolerant to heavy metal ions, and the relative abundances were 4.5%, 8.9% and 15.7% in control cultures, the second transfer cultures exposed to 10 mg/L Zn2+ and 10 mg/L Cd2+, respectively.
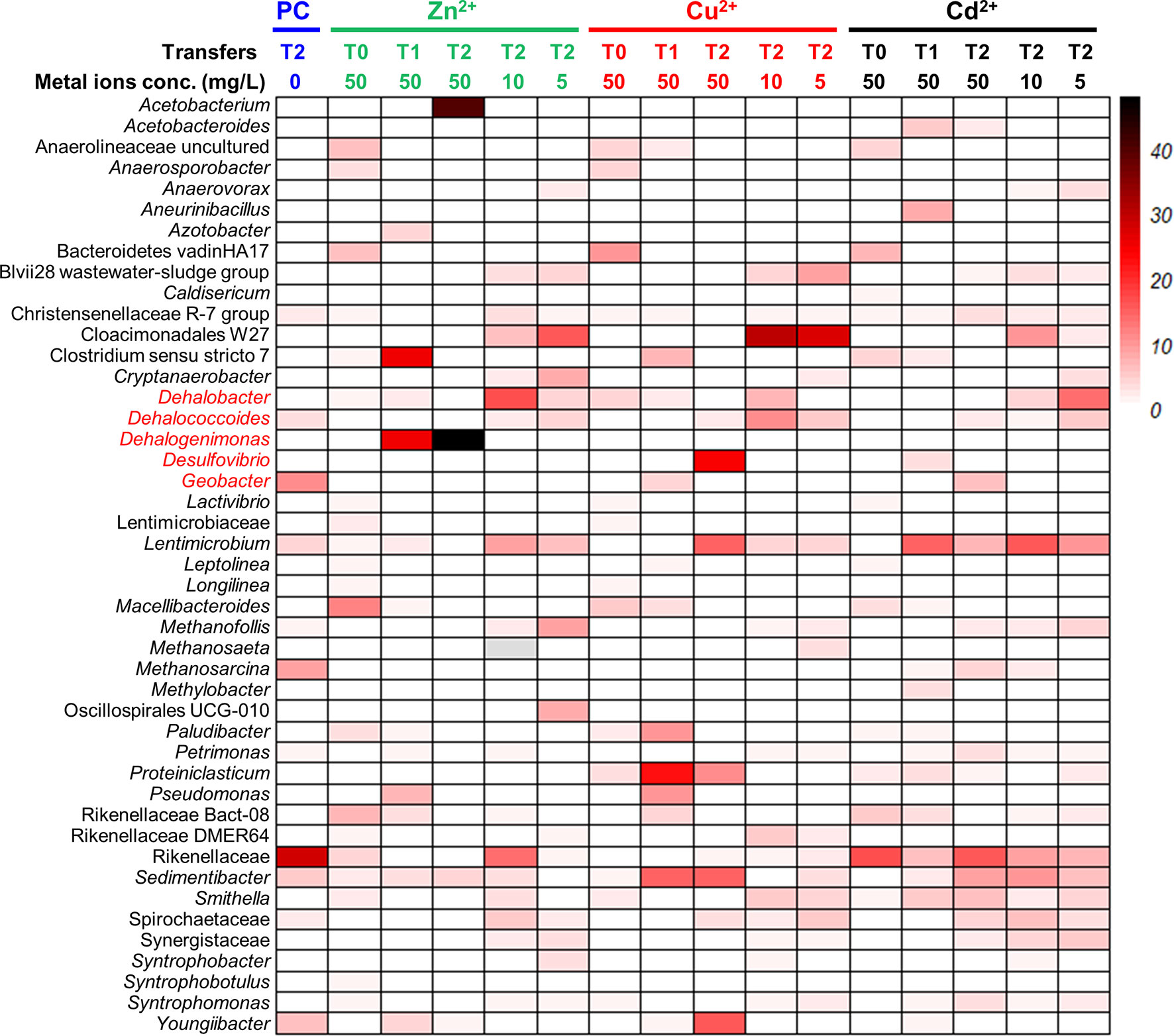
Figure 5 Microbial communities profiling of PCE-fed enrichment cultures amended with gradient concentrations of heavy metal ions. PC represents biotic controls without heavy metal amendments. T0: sediment microcosms; T1: the first transferred enrichment cultures; T2: the second transferred enrichment cultures. The numbers below the transfers represent the concentrations of heavy metal ions with the unit “mg/L”.
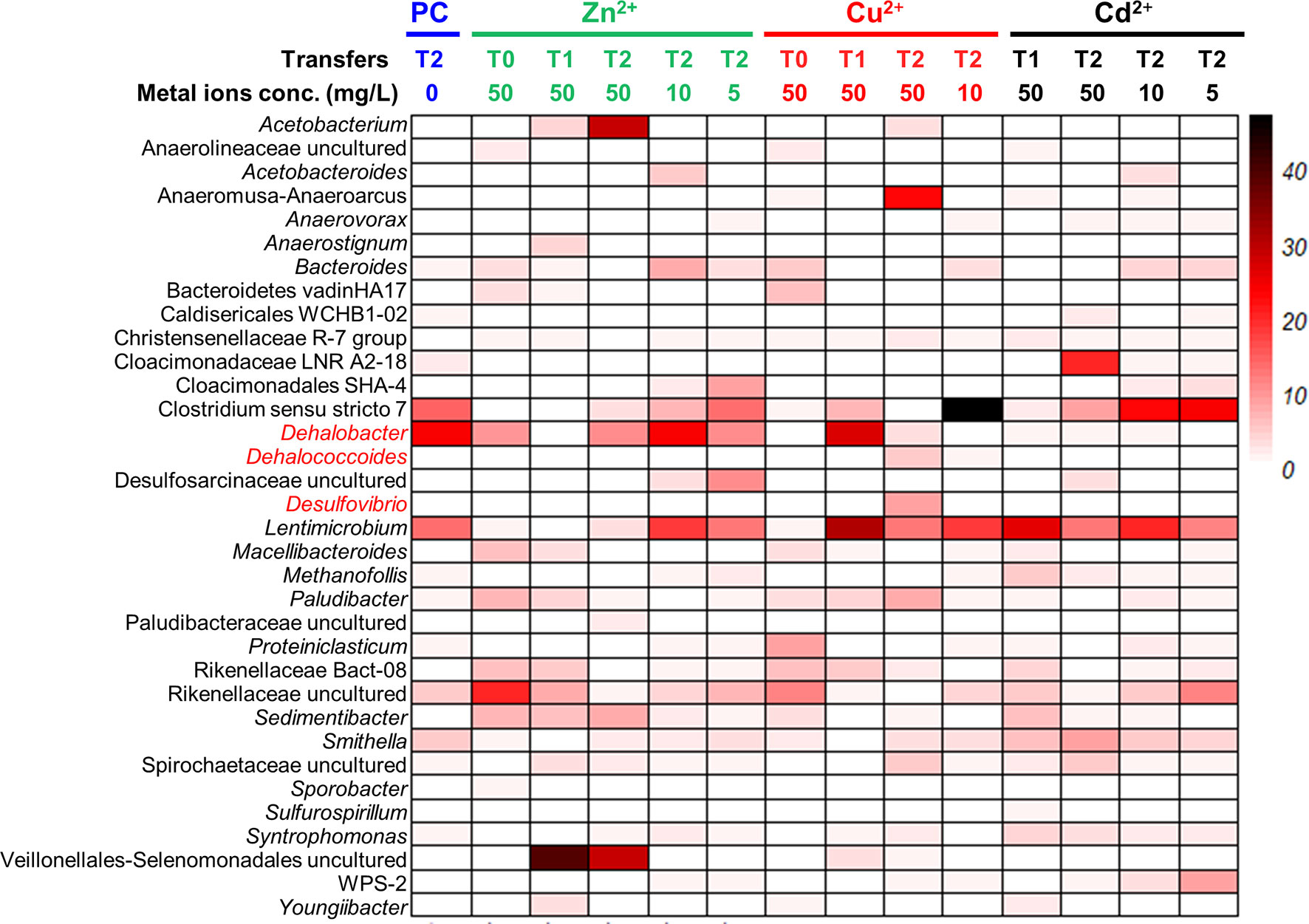
Figure 6 Microbial communities profiling of 1,2-DCA-fed enrichment cultures amended with gradient concentrations of heavy metal ions. PC represents biotic controls without heavy metal amendments. T0: sediment microcosms; T1: the first transferred enrichment cultures; T2: the second transferred enrichment cultures. The numbers below the transfers represent the concentrations of heavy metal ions with the unit “mg/L”.
In the 1,2-DCA-dechlorinating cultures, Dehalococcoides and Desulfovibrio were greatly enriched in Cu2+ amended enrichment cultures. The relative abundance of Dehalococcoides in the second transfer of 1,2-DCA-dechlorinating enrichment cultures amended with 50 mg/L Cu2+ was 5.7% compared with 0.3% in the control cultures. The relative abundance of Desulfovibrio in the second transfer of 1,2-DCA-dechlorinating enrichment cultures amended with 10 mg/L Cu2+ was 8.7%, compared with 0.2% in the control cultures. Similar to the PCE-dechlorinating enrichment cultures, Lentimicrobium was enriched in 1,2-DCA-dechlorinating enrichment cultures exposed to a heavy metal ion, and the relative abundances were 14.0%, 18.3%, 18.5% and 20.8% in control cultures, the second transfer of enrichment cultures exposed to 10 mg/L Zn2+, 10 mg/L Cu2+ and 10 mg/L Cd2+, respectively.
Discussion
In the PCE-dechlorinating enrichment cultures, Geobacter and Dehalococcoides may play key roles for the sequential dechlorination of PCE to ethene. Cross-feeding interactions between Geobacter and Dehalococcoides, not limited to electron acceptor dependency, have been frequently observed and investigated. For instance, Geobacter lovleyi strain SZ can fulfill the cobamide requirement of Dehalococcoides strains (Yan et al., 2012). Geobacter can also supply carbon source to Dehalococcoides strains by fermenting lactate to acetate (Liang et al., 2021). Also worth mentioning is that, without dynamic monitoring, the abundance and function of Geobacter may be underestimated since community structures were only analyzed at the completion of reductive dechlorination. To date, Geobacter isolates capable of respiring chlorinated ethenes only couple growth with PCE-to-cDCE dechlorination but inactive during the stage of cDCE-to-ethene dechlorination, probably due to the depletion of an utilizable electron acceptor (e.g., PCE, TCE) (Lu et al., 2021).
Concerns have been raised over the global contamination of heavy metals and organohalides. An improved understanding of how heavy metal ions can impact OHRB and the associated dechlorination processes is critical for the successful bioremediation at the contaminated sites. Our study revealed that the types (i.e., Zn2+, Cu2+, Cd2+) and concentrations of heavy metal ions posed differential effects on the microbial dechlorination of PCE and 1,2-DCA. A previous microcosm study showed minor inhibitory effects of heavy metal ions (i.e., Cd2+, Cr3+, Pb2+) at concentrations less than 20 mg/L on the PCE dechlorination in sediment microcosms (Lu et al., 2020), which was not completely in line with the observation in this study. Such inhibitory effects of heavy metal ions on the dechlorination processes have been reported (Said and Lewis, 1991; Pardue et al., 1996; Kong, 1998). The inconsistency between our study and previous studies may be caused by differential geochemical and microbial compositions in source materials (e.g., sediments) used for microcosm construction. Therefore, conclusions regarding the tolerance of dechlorinating microcosms or pure cultures should be evaluated with precaution and a number of factors (e.g., minerals, pH, salinity, ionic strength) should be considered.
Cd2+ is detrimental to various biological functions, such as competition with Zn2+ for the active sites of proteins and enzymes, interference with calcium metabolism, and covalently binding to the -SH group (Hall, 2002). The inhibitive concentration of Cd2+ on trichloroaniline degradation could be as low as 0.01 mg/L in a mineral-dominated soil (Pardue et al., 1996). Previous studies suggested that Cd2+ had inhibitory effects on 1,2-DCA degradation and the presence of 33.7 mg/L Cd2+ resulted in partial 1,2-DCA degradation (Arjoon et al., 2015). Recently, another study also demonstrated that Cd2+ was one of the most toxic heavy metal ions that inhibited the PCE-to-ethene dechlorination process in pure cultures, mixed cultures, and microcosms (Lu et al., 2020). Unexpectedly, we did not observe significant inhibition of up to 50 mg/L Cd2+ on the dechlorination of PCE or 1,2-DCA in the microcosms and enrichment cultures. Such discrepancy and, especially, the mechanisms behind the tolerance of organohalide-respiring Dehalococcoidia to Cd2+requires further investigation.
Certain OHRB can have a relatively higher tolerance to heavy metal ions, and the relative abundances of OHRB in the microbial community increases when exposed to a heavy metal ion. For instance, 50 mg/L Zn2+ may promote the growth of Dehalogenimonas (PCE-dechlorinating cultures), 50 mg/L Cu2+ may be conducive to the enrichment of Dehalococcoides (PCE- and 1,2-DCA-dechlorinating cultures) and Dehalobacter (1,2-DCA-dechlorinating cultures), and 50 mg/L Cd2+ may enhance the growth of Geobacter (PCE-dechlorinating cultures). In the meantime, non-dechlorinating populations such as Acetobacterium, Sedimentibacter, Rikenellaceae, Lentimicrobium, Youngiibacter in the PCE-dechlorinating cultures, and Acetobacterium and Lentimicrobium in the 1,2-DCA-dechlorinating cultures were also enriched when exposed to a heavy metal ion. How heavy metal ions affect the interaction of syntrophic metabolic networks involving OHRB and other beneficial populations (e.g., Desulfovibrio, Acetobacterium, Methanosarcina) for interspecies carbon, electron, and cobamide transfers (Men et al., 2014; Wang et al., 2019; Lu et al., 2020) is still largely unknown. Synthetic and systematic studies using omics tools may assist us in unraveling the syntrophy mechanisms.In summary, our study demonstrated that: (1) the inhibitions of heavy metal ions on PCE and 1,2-DCA reductive dechlorination depend on the concentrations and types of the heavy metal ions, an observation similar to a previous study (Lu et al., 2020); (2) certain OHRB had a relatively high tolerance to heavy metal ions, of which Zn2+ may be the most toxic heavy metal ion to reductive dechlorination; and (3) the 1,2-DCA- and PCE-dechlorinating enrichment cultures can tolerate as high as 50 mg/L Cd2+.
Data Availability Statement
The datasets presented in this study can be found in online repositories. The names of the repository/repositories and accession number(s) can be found below: https://figshare.com/, doi.org/10.6084/m9.figshare.19212618.v1.
Author Contributions
JW, XL, JY, and YY contributed to the conception and design of the study. JW and XL performed the experiments and data analysis. JW and YY wrote the first draft of the manuscript. . All authors contributed to manuscript revision, read, and approved the submitted version.
Funding
This work was supported by the National Key Research and Development Program of China (2019YFC1804400), National Natural Science Foundation of China (grant nos. 41907287, 41977295, 41907220, 42177220), and Key Research Program of Frontier Sciences, Chinese Academy of Sciences (CAS), Grant No. ZDBS-LY-DQC038.
Conflict of Interest
The authors declare that the research was conducted in the absence of any commercial or financial relationships that could be construed as a potential conflict of interest.
Publisher’s Note
All claims expressed in this article are solely those of the authors and do not necessarily represent those of their affiliated organizations, or those of the publisher, the editors and the reviewers. Any product that may be evaluated in this article, or claim that may be made by its manufacturer, is not guaranteed or endorsed by the publisher.
References
Altas L. (2009). Inhibitory Effect of Heavy Metals on Methane-Producing Anaerobic Granular Sludge. J. Hazard. Mater. 162 (2-3), 1551–1556. doi: 10.1016/j.jhazmat.2008.06.048
Amos B. K., Christ J. A., Abriola L. M., Pennell K. D., Löffler F. E. (2007). Experimental Evaluation and Mathematical Modeling of Microbially Enhanced Tetrachloroethene (PCE) Dissolution. Environ. Sci. Technol. 41 (3), 963–970. doi: 10.1021/es061438n
Arjoon A., Olaniran A. O., Pillay B. (2012). Co-Contamination of Water With Chlorinated Hydrocarbons and Heavy Metals: Challenges and Current Bioremediation Strategies. Int. J. Environ. Sci. Technol. 10 (2), 395–412. doi: 10.1007/s13762-012-0122-y
Arjoon A., Olaniran A. O., Pillay B. (2015). Kinetics of Heavy Metal Inhibition of 1,2-Dichloroethane Biodegradation in Co-Contaminated Water. J. Basic. Microbiol. 55 (3), 277–284. doi: 10.1002/jobm.201200776
Atashgahi S., Häggblom M. M., Smidt H. (2018a). Organohalide Respiration in Pristine Environments: Implications for the Natural Halogen Cycle. Environ. Microbiol. 20 (3), 934–948. doi: 10.1111/1462-2920.14016
Atashgahi S., Lu Y., Smidt H. (2016). “Overview of Known Organohalide-Respiring Bacteria—Phylogenetic Diversity and Environmental Distribution,” in Organohalide-Respiring Bacteria. Eds. Adrian L., Löffler F. E. (Berlin, Heidelberg: Springer Berlin Heidelberg), 63–105.
Atashgahi S., Shetty S. A., Smidt H., de Vos W. M. (2018b). Flux, Impact, and Fate of Halogenated Xenobiotic Compounds in the Gut. Front. Physiol. 9. doi: 10.3389/fphys.2018.00888
Aulenta F., Potalivo M., Majone M., Papini M. P., Tandoi V. (2006). Anaerobic Bioremediation of Groundwater Containing a Mixture of 1,1,2,2-Tetrachloroethane and Chloroethenes. Biodegradation 17 (3), 193–206. doi: 10.1007/s10532-005-4218-7
Bhute S., Pande P., Shetty S. A., Shelar R., Mane S., Kumbhare S. V., et al. (2016). Molecular Characterization and Meta-Analysis of Gut Microbial Communities Illustrate Enrichment of Prevotella and Megasphaera in Indian Subjects. Front. Microbiol. 7. doi: 10.3389/fmicb.2016.00660
Caporaso J. G., Kuczynski J., Stombaugh J., Bittinger K., Bushman F. D., Costello E. K., et al. (2010). QIIME Allows Analysis of High-Throughput Community Sequencing Data. Nat. Methods 7 (5), 335–336. doi: 10.1038/nmeth.f.303
de Bruin W. P., Kotterman M. J., Posthumus M. A., Schraa G., Zehnder A. J. (1992). Complete Biological Reductive Transformation of Tetrachloroethene to Ethane. Appl. Environ. Microbiol. 58 (6), 1996–2000. doi: 10.1128/aem.58.6.1996-2000.1992
Deng M., Kuo D. T. F., Wu Q., Zhang Y., Liu X., Liu S., et al. (2018). Organophosphorus Flame Retardants and Heavy Metals in Municipal Landfill Leachate Treatment System in Guangzhou, China. Environ. pollut. 236, 137–145. doi: 10.1016/j.envpol.2018.01.042
Devlin J. F., Katic D., Barker J. F. (2004). In Situ Sequenced Bioremediation of Mixed Contaminants in Groundwater. J. Contam. Hydrol. 69 (3-4), 233–261. doi: 10.1016/S0169-7722(03)00156-6
De Wildeman S., Diekert G., Van Langenhove H., Verstraete W. (2003). Stereoselective Microbial Dehalorespiration With Vicinal Dichlorinated Alkanes. Appl. Environ. Microbiol. 69 (9), 5643–5647. doi: 10.1128/aem.69.9.5643-5647.2003
Doherty R. E. (2000). A History of the Production and Use of Carbon Tetrachloride, Tetrachloroethylene, Trichloroethylene and 1,1,1-Trichloroethane in the United States: Part 1–Historical Background; Carbon Tetrachloride and Tetrachloroethylene. Environ. Forensics. 1 (2), 69–81. doi: 10.1006/enfo.2000.0010
Edgar R. C. (2010). Search and Clustering Orders of Magnitude Faster Than BLAST. Bioinformatics 26 (19), 2460–2461. doi: 10.1093/bioinformatics/btq461
Ellis D., Lutz E., Odom J. M., Buchanan R., Bartlett C. (2000). Bioaugmentation for Accelerated in Situ Anaerobic Bioremediation. Environ. Sci. Technol. 34, 2254–2260. doi: 10.1021/es990638e. 11.
Ferguson J. F., Pietari J. M. H. (2000). Anaerobic Transformations and Bioremediation of Chlorinated Solvents. Environ. pollut. 107 (2), 209–215. doi: 10.1016/S0269-7491(99)00139-6
Fu S., Wang F., Shi X., Guo R. (2016). Impacts of Microaeration on the Anaerobic Digestion of Corn Straw and the Microbial Community Structure. Chem. Eng. J. 287, 523–528. doi: 10.1016/j.cej.2015.11.070
Girones L., Oliva A. L., Negrin V. L., Marcovecchio J. E., Arias A. H. (2021). Persistent Organic Pollutants (POPs) in Coastal Wetlands: A Review of Their Occurrences, Toxic Effects, and Biogeochemical Cycling. Mar. pollut. Bull. 172, 112864. doi: 10.1016/j.marpolbul.2021.112864
Grostern A., Edwards E. A. (2006). Growth of Dehalobacter and Dehalococcoides Spp. During Degradation of Chlorinated Ethanes. Appl. Environ. Microbiol. 72 (1), 428–436. doi: 10.1128/AEM.72.1.428-436.2006
Grostern A., Edwards E. A. (2009). Characterization of a Dehalobacter Coculture That Dechlorinates 1,2-Dichloroethane to Ethene and Identification of the Putative Reductive Dehalogenase Gene. Appl. Environ. Microbiol. 75 (9), 2684–2693. doi: 10.1128/AEM.02037-08
Hall J. L. (2002). Cellular Mechanisms for Heavy Metal Detoxification and Tolerance. J. Exp. Bot. 53 (366), 1–11. doi: 10.1093/jexbot/53.366.1
Harrison J. J., Ceri H., Turner R. J. (2007). Multimetal Resistance and Tolerance in Microbial Biofilms. Nat. Rev. Microbiol. 5 (12), 928–938. doi: 10.1038/nrmicro1774
He H., Li Y., Shen R., Shim H., Zeng Y., Zhao S., et al. (2021). Environmental Occurrence and Remediation of Emerging Organohalides: A Review. Environ. pollut. 290, 118060. doi: 10.1016/j.envpol.2021.118060
Jiang L., Yang Y., Jin H., Wang H., Swift C. M., Xie Y., et al. (2022). Geobacter Sp. Strain IAE Dihaloeliminates 1,1,2-Trichloroethane and 1,2-Dichloroethane. Environ. Sci. Technol. 56 (6), 3430–3440. doi: 10.1021/acs.est.1c05952
Juliastuti S. R., Baeyens J., Creemers C., Bixio D., Lodewyckx E. (2003). The Inhibitory Effects of Heavy Metals and Organic Compounds on the Net Maximum Specific Growth Rate of the Autotrophic Biomass in Activated Sludge. J. Hazard. Mater. 100 (1-3), 271–283. doi: 10.1016/s0304-3894(03)00116-x
Kong I. C. (1998). Metal Toxicity on the Dechlorination of Monochlorophenols in Fresh and Acclimated Anaerobic Sediment Slurries. Water Sci. Technol. 38 (7), 143–150. doi: 10.1016/S0273-1223(98)00616-7
Kuo C., Genthner B. (1996). Effect of Added Heavy Metal Ions on Biotransformation and Biodegradation of 2-Chlorophenol and 3-Chlorobenzoate in Anaerobic Bacterial Consortia. Appl. Environ. Microbiol. 62 (7), 2317–2323. doi: 10.1128/aem.62.7.2317-2323.1996
Liang Y., Lu Q., Liang Z., Liu X., Fang W., Liang D., et al. (2021). Substrate-Dependent Competition and Cooperation Relationships Between Geobacter and Dehalococcoides for Their Organohalide Respiration. ISME. Commun. 1 (1), 23. doi: 10.1038/s43705-021-00025-z
Li C., Fang H. H. P. (2007). Inhibition of Heavy Metals on Fermentative Hydrogen Production by Granular Sludge. Chemosphere 67 (4), 668–673. doi: 10.1016/j.chemosphere.2006.11.005
Löffler F. E., Sanford R. A., Ritalahti K. M. (2005). Enrichment, Cultivation, and Detection of Reductively Dechlorinating Bacteria. Method. Enzymol. 397, 77–111. doi: 10.1016/S0076-6879(05)97005-5
Loganathan B. G., Kannan K. (1994). Global Organochlorine Contamination Trends: An Overview. Ambio 23 (3), 187–191.
Lu Q., Liu J., He H., Liang Z., Qiu R., Wang S. (2021). Waste Activated Sludge Stimulates in Situ Microbial Reductive Dehalogenation of Organohalide-Contaminated Soil. J. Hazard. Mater. 411, 125189. doi: 10.1016/j.jhazmat.2021.125189
Lu Q., Zou X., Liu J., Liang Z., Shim H., Qiu R., et al. (2020). Inhibitory Effects of Metal Ions on Reductive Dechlorination of Polychlorinated Biphenyls and Perchloroethene in Distinct Organohalide-Respiring Bacteria. Environ. Int. 135, 105373. doi: 10.1016/j.envint.2019.105373
Men Y., Seth E. C., Yi S., Allen R. H., Taga M. E., Alvarez-Cohen L. (2014). Sustainable Growth of Dehalococcoides Mccartyi 195 by Corrinoid Salvaging and Remodeling in Defined Lactate-Fermenting Consortia. Appl. Environ. Microbiol. 80 (7), 2133–2141. doi: 10.1128/AEM.03477-13
Mohn W. W., Tiedje J. M. (1992). Microbial Reductive Dehalogenation. Microbiol. Rev. 56 (3), 482–507. doi: 10.1128/mr.56.3.482-507.1992
Naser H. A. (2013). Assessment and Management of Heavy Metal Pollution in the Marine Environment of the Arabian Gulf: A Review. Mar. pollut. Bull. 72 (1), 6–13. doi: 10.1016/j.marpolbul.2013.04.030
Nelson D. K., Hozalski R. M., Clapp L. W., Semmens M. J., Novak P. J. (2002). Effect of Nitrate and Sulfate on Dechlorination by a Mixed Hydrogen-Fed Culture. Bioremediat. J. 6 (3), 225–236. doi: 10.1080/10889860290777585
Pardue J. H., Kongara S., Jones J. W. (1996). Effect of Cadmium on Reductive Dechlorination of Trichloroaniline. Environ. Toxicol. Chem. 15 (7), 1083–1088. doi: 10.1002/etc.5620150710
Peng P., Goris T., Lu Y., Nijsse B., Burrichter A., Schleheck D., et al. (2020). Organohalide-Respiring Desulfoluna Species Isolated From Marine Environments. ISME. J. 14 (3), 815–827. doi: 10.1038/s41396-019-0573-y
Potapowicz J., Lambropoulou D., Nannou C., Koziol K., Polkowska Z. (2020). Occurrences, Sources, and Transport of Organochlorine Pesticides in the Aquatic Environment of Antarctica. Sci. Total. Environ. 735, 139475. doi: 10.1016/j.scitotenv.2020.139475
Prabhakaran P., Ashraf M., Wan Mohd Noor W. S. A. (2016). Microbial Stress Response to Heavy Metal in the Environment. RSC. Adv. 6, 109862–109877. doi: 10.1039/C6RA10966G
Rahman Z., Singh V. P. (2020). Bioremediation of Toxic Heavy Metals (THMs) Contaminated Sites: Concepts, Applications and Challenges. Environ. Sci. pollut. Res. 27 (22), 27563–27581. doi: 10.1007/s11356-020-08903-0
R Core Team (2020). R: A Language and Environment for Statistical Computing (Vienna, Austria: R Foundation for Statistical Computing). Available at: https://www.r-project.org/.
Roane T. M., Pepper I. L., Gentry T. J., Pepper I. L., Gerba C. P., Gentry T. J. (2015). “Microorganisms and Metal Pollutants,” in Environmental Microbiology (Third Edition) (San Diego: Academic Press), 415–439.
Said W. A., Lewis D. L. (1991). Quantitative Assessment of the Effects of Metals on Microbial Degradation of Organic Chemicals. Appl. Environ. Microbiol. 57 (5), 1498–1503. doi: 10.1128/aem.57.5.1498-1503.1991
Silver S. (1996). Bacterial Resistances to Toxic Metal Ions - a Review. Gene 179 (1), 9–19. doi: 10.1016/S0378-1119(96)00323-X
Squillace P. J., Scott J. C., Moran M. J., Nolan B. T., Kolpin D. W. (2002). VOCs, Pesticides, Nitrate, and Their Mixtures in Groundwater Used for Drinking Water in the United States. Environ. Sci. Technol. 36, 1923–1930. doi: 10.1021/es015591n
Stroo H. F., Leeson A., Ward C. H. (2012). Bioaugmentation for Groundwater RemediationIn Situ Remediation of Chlorinated Solvent Plumes (New York: Springer).
Stroo H. F., Ward C. H. (2010). “In Situ Remediation of Chlorinated Solvent Plumes,” (New York: Springer).
Sung Y., Fletcher K. E., Ritalahti K. M., Apkarian R. P., Ramos-Hernandez N., Sanford R. A., et al. (2006). Geobacter Lovleyi Sp. Nov. Strain SZ, a Novel Metal-Reducing and Tetrachloroethene-Dechlorinating Bacterium. Appl. Environ. Microbiol. 72 (4), 2775–2782. doi: 10.1128/aem.72.4.2775-2782.2006
van der Zaan B., de Weert J., Rijnaarts H., de Vos W. M., Smidt H., Gerritse J. (2009). Degradation of 1,2-Dichloroethane by Microbial Communities From River Sediment at Various Redox Conditions. Water Res. 43 (13), 3207–3216. doi: 10.1016/j.watres.2009.04.042
Wang S., Chen C., Zhao S., He J. (2019). Microbial Synergistic Interactions for Reductive Dechlorination of Polychlorinated Biphenyls. Sci. Total. Environ. 666, 368–376. doi: 10.1016/j.scitotenv.2019.02.283
Wang Q., Garrity G. M., Tiedje J. M., Cole J. R. (2007). Naive Bayesian Classifier for Rapid Assignment of rRNA Sequences Into the New Bacterial Taxonomy. Appl. Environ. Microbiol. 73 (16), 5261–5267. doi: 10.1128/AEM.00062-07
Wolin E. A., Wolin M. J., Wolfe R. S. (1963). Formation of Methane by Bacterial Extracts. J. Biol. Chem. 238, 2882–2886. doi: 10.1016/S0021-9258(18)67912-8
Wuana R. A., Okieimen F. E. (2011). Heavy Metals in Contaminated Soils: A Review of Sources, Chemistry, Risks and Best Available Strategies for Remediation. ISRN. Ecol. 2011, 402647. doi: 10.5402/2011/402647
Wu Q., Du Y., Huang Z., Gu J., Leung J. Y. S., Mai B., et al. (2019). Vertical Profile of Soil/Sediment Pollution and Microbial Community Change by E-Waste Recycling Operation. Sci. Total. Environ. 669, 1001–1010. doi: 10.1016/j.scitotenv.2019.03.178
Xu G., Zhang N., Zhao X., Chen C., Zhang C., He J. (2022). Offshore Marine Sediment Microbiota Respire Structurally Distinct Organohalide Pollutants. Environ. Sci. Technol. 56 (5), 3065–3075. doi: 10.1021/acs.est.1c06680
Yang Y., Capiro N. L., Marcet T. F., Yan J., Pennell K. D., Löffler F. E. (2017a). Organohalide Respiration With Chlorinated Ethenes Under Low pH Conditions. Environ. Sci. Technol. 51 (15), 8579–8588. doi: 10.1021/acs.est.7b01510
Yang Y., Higgins S. A., Yan J., Simsir B., Chourey K., Iyer R., et al. (2017b). Grape Pomace Compost Harbors Organohalide-Respiring Dehalogenimonas Species With Novel Reductive Dehalogenase Genes. ISME. J. 11 (12), 2767–2780. doi: 10.1038/ismej.2017.127
Yang Y., Sanford R., Yan J., Chen G., Capiro N. L., Li X., et al. (2020). Roles of Organohalide-Respiring Dehalococcoidia in Carbon Cycling. mSystems 5 (3), e00757–e00719. doi: 10.1128/mSystems.00757-19
Yan J., Rash B. A., Rainey F. A., Moe W. M. (2009). Isolation of Novel Bacteria Within the Chloroflexi Capable of Reductive Dechlorination of 1,2,3-Trichloropropane. Environ. Microbiol. 11 (4), 833–843. doi: 10.1111/j.1462-2920.2008.01804.x
Yan J., Ritalahti K. M., Wagner D. D., Löffler F. E. (2012). Unexpected Specificity of Interspecies Cobamide Transfer From Geobacter Spp. To Organohalide-Respiring Dehalococcoides Mccartyi Strains. Appl. Environ. Microbiol. 78 (18), 6630–6636. doi: 10.1128/AEM.01535-12
Zanaroli G., Negroni A., Häggblom M. M., Fava F. (2015). Microbial Dehalogenation of Organohalides in Marine and Estuarine Environments. Curr. Opin. Biotechnol. 33, 287–295. doi: 10.1016/j.copbio.2015.03.013
Keywords: reductive dechlorination, tetrachlorethene, 1,2-dichloroethane, heavy metal ions, Dehalococcoidia
Citation: Wang J, Li X, Yan J and Yang Y (2022) Effects of Heavy Metal Ions on Microbial Reductive Dechlorination of 1, 2-Dichloroethane and Tetrachloroethene. Front. Mar. Sci. 9:881950. doi: 10.3389/fmars.2022.881950
Received: 23 February 2022; Accepted: 08 June 2022;
Published: 13 July 2022.
Edited by:
Zhisong Cui, Ministry of Natural Resources, ChinaReviewed by:
Shanquan Wang, Sun Yat-sen University, ChinaMengyan Li, New Jersey Institute of Technology, United States
Copyright © 2022 Wang, Li, Yan and Yang. This is an open-access article distributed under the terms of the Creative Commons Attribution License (CC BY). The use, distribution or reproduction in other forums is permitted, provided the original author(s) and the copyright owner(s) are credited and that the original publication in this journal is cited, in accordance with accepted academic practice. No use, distribution or reproduction is permitted which does not comply with these terms.
*Correspondence: Yi Yang, eWFuZ3lpQGlhZS5hYy5jbg==
†ORCID ID:Yi Yang, orcid.org/0000-0002-3519-5472
Jun Yan, orcid.org/0000-0001-6883-8529
Xiuying Li, orcid.org/0000-0003-3555-741