- Stony Brook University, School of Marine and Atmospheric Sciences, Southampton NY, United States
Coastal zones can be focal points of acidification where the influx of atmospheric CO2 can be compounded by additional sources of acidity that may collectively impair calcifying organisms. While the photosynthetic action of macrophytes may buffer against coastal ocean acidification, such activity has not been well-studied, particularly among aquacultured seaweeds. Here, we report on field and laboratory experiments performed with North Atlantic populations of juvenile hard clams (Mercenaria mercenaria), eastern oysters (Crassostrea virginica), and blue mussels (Mytilus edulis) grown with and without increased CO2 and with and without North Atlantic kelp (Saccharina latissima) over a range of aquaculture densities (0.3 – 2 g L-1). In all laboratory experiments, exposure to elevated pCO2 (>1,800 µatm) resulted in significantly reduced shell- and/or tissue-based growth rates of bivalves relative to control conditions. This impairment was fully mitigated when bivalves were exposed to the same acidification source but also co-cultured with kelp. Saturation states of aragonite were transformed from undersaturated to saturated in the acidification treatments with kelp present, while the acidification treatments remained undersaturated. In a field experiment, oysters grown near aquacultured kelp were exposed to higher pH waters and experienced significantly faster shell and tissue based growth rates compared to individuals grown at sites away from kelp. Collectively, these results suggest that photosynthesis by S. latissima grown at densities associated with aquaculture increased pH and decreased pCO2, fostering a carbonate chemistry regime that maximized the growth of juvenile bivalves. As S. latissima has been shown to benefit from increased CO2, growing bivalves and kelp together under current or future acidification scenarios may be a synergistically beneficial integrated, multi-trophic aquaculture approach.
Introduction
The continued delivery of CO2 into surface oceans is expected to alter pools of inorganic carbon (increased pCO2 and , decreased and saturation states (Ω) of calcite and aragonite), and coastal zones are particularly prone to enhanced acidification due to processes such as upwelling, riverine discharge, and eutrophication (Meehl et al., 2007; Feely et al., 2009; Cai et al., 2011; Melzner et al., 2013). Specifically, eutrophication-enhanced microbial respiration is a strong regional source of CO2 in coastal zones on diel and seasonal timescales that can result in pCO2 levels that far exceed end of the century projections for the open ocean (>1,000 µatm; Cai et al., 2011; Melzner et al., 2013; Wallace et al., 2014). Present and future acidification is expected to have varying effects on marine flora and fauna, with the reduced saturation states negatively affecting the growth and survival of calcifying organisms (Gazeau et al., 2007; Talmage and Gobler, 2010; Doney et al., 2020) and the higher CO2 levels benefitting some, but not all, photosynthetic organisms (Palacios and Zimmerman, 2007; Koch et al., 2013; Hattenrath-Lehmann et al., 2015b; Young and Gobler, 2016).
Ocean acidification is a significant environmental threat to early life stage bivalves. Acidification-induced reductions in Ωaragonite and Ωcalcite can lower the survivorship and growth of larval- and juvenile-stage bivalves (Green et al., 2009; Talmage and Gobler, 2011; Gobler et al., 2014; Waldbusser G. et al., 2015). The shells of early life-stage bivalves are composed partly or completely of aragonite (Stenzel, 1964; Carriker, 1996; Talmage and Gobler, 2009), making them vulnerable to reductions in specifically Ωaragonite (Gazeau et al., 2007; Gazeau et al., 2013). While Ω values that exceed 1.0 favor the mineral precipitation of calcium carbonate, successful larval bivalve growth and survival may require Ωaragonite ≥ 1.6, owing to the energetics of biocalcification (Barton et al., 2012; Waldbusser et al., 2013).
In contrast to bivalves, elevated pCO2 has been shown to enhance the growth of some species of macroalgae (Gao et al., 1993; Olischläger et al., 2013; Young et al., 2021) including species grown in aquaculture (Gao et al., 1993; Bartsch et al., 2010; Young and Gobler, 2016). Aquacultured macroalgae may provide a myriad of ecosystem services, such as carbon sequestration (Chung et al., 2011) and absorption of excessive nitrogen (Marinho-Soriano et al., 2009), with harvesting of the macroalgae representing removal of the excess carbon and nitrogen from the ecosystem. In addition, the aquaculture of seaweeds may provide a chemical refuge against acidified conditions (Anthony et al., 2013; Wahl et al., 2018; Young and Gobler, 2018), sometimes to the benefit of bivalves (Anthony et al., 2013; Wahl et al., 2018; Young and Gobler, 2018; Xiao et al., 2021).
Saccharina latissima (sugar kelp) is a bladed, cold-water brown macroalgae that can be found across the North Atlantic, Pacific, and Arctic Oceans (Brinkhuis et al., 1983; Sivertsen and Bjørge, 2014). Beyond the numerous ecosystems services (e.g., nursery habitat, predation refuge, coastal defense, carbon and nitrogen sequestration, food source) provided by kelp species such as S. latissima (Norderhaug et al., 2005; Chung et al., 2013; Smale et al., 2013), the commercial and aquacultural importance of kelp has expanded in the Americas and Europe (Grebe et al., 2019), In the US, there is growing interest in kelp aquaculture (Marinho et al., 2015), primarily in colder regions such as Alaska, Maine, and the Northeast U.S. (Kim et al., 2019). When grown in an aquaculture setting, particularly in eutrophic estuaries, S. latissima can grow robustly and extract CO2 and nutrients from the water (Kim et al., 2015; Jiang et al., 2020). While recent studies have demonstrated S. latissima benefit from elevated levels of pCO2 through enhanced growth and reduced herbivory (Young et al., 2021), the potential for S. latissima or any kelp to benefit co-cultured bivalves is generally unknown.
The prime objective for this study, therefore, was to assess how elevated pCO2 and the presence of Saccharina latissima influence the growth of juvenile bivalves indigenous to the North Atlantic, including eastern oysters (Crassostrea virginica), blue mussels (Mytilus edulis), and hard clams (Mercenaria mercenaria) using laboratory experiments. The second objective was to determine if the co-culture of bivalves with S. latissima in an ecosystem-based aquaculture setting influences bivalve growth and settlement. Shell- and tissue-based growth of bivalves were quantified along with carbonate chemistry within experimental vessels in the laboratory and in open waters at field sites where shellfish and S. latissimi were cultivated using standard aquaculture practices.
Methods
Collection and Preparation of Bivalves and Saccharina latissima
Hatchery produced and wild-collected bivalves were used for experiments. Mercenaria mercenaria were produced by the Stony Brook University shellfish hatchery in Southampton, NY (40.89° N, 72.44° W) using broodstock collected from Shinnecock Bay, NY, USA. Crassostrea virginica were provided by the Aeros Cultured Oyster hatchery, NY, USA (41.05° N, 72.40° W) using broodstock from the Peconic Estuary, NY, USA. Mytilus edulis were collected from wild populations in Old Fort Pond, NY, USA (40.89° N, 72.44° W) and Moriches Bay, NY, USA (40.78° N, 72.78° W; Supplementary Figure S1). S. latissima used for all experiments were grown on horizontal longlines at a commercial oyster farm (Great Gun Shellfish) in Moriches Bay, NY, USA (40.78° N, 72.78° W; Supplementary Figure S1), a lagoon contiguous with Shinnecock Bay to the east, using kelp seedstock derived from wild populations in the Long Island Sound, CT, USA. S. latissima was grown throughout winter and spring 2021, and was collected from early March to late May, during which laboratory and field experiments were performed. Densities of kelp on this shallow (~2 m) farm have previously estimated to range from 0.6 g L-1 during high tide in the early growing season to 4.4 g L-1 during low tide at the end of the growing season (Sylvers and Gobler, 2021). For experiments, large, well-pigmented blades of the macroalga were chosen from samples collected by hand at low tide. S. latissima blades were cut from their holdfasts as close to the longlines as possible and were immediately placed in seawater-filled containers and transported to the Stony Brook Marine Science Center within 30 minutes of collection. Upon arrival to the facility, S. latissima were placed in a large, round ~2000 L tank filled with flowing, 1 µm filtered seawater from Shinnecock Bay.
Laboratory Experiments With Bivalves and Saccharina latissima
Six laboratory experiments were performed to assess the effects of elevated pCO2 and the presence of S. latissima on the growth rates of M. mercenaria, C. virginica, and M. edulis. Prior to the laboratory experiments with bivalves and S. latissima, a preliminary experiment was performed to assess the ability of S. latissima to alter pH in experimental containers. All experiments were performed in 1 L polycarbonate containers, which were acid-washed (10% HCl) and liberally rinsed with deionized water prior to use. Experimental containers were placed in an environmental control chamber set to a consistent temperature (18.6 ± 0.3°C), light intensity (~200 µmol photons m-2 s-1) and photoperiod (14 h: 10 h light:dark cycle). The light intensity and photoperiod of the environmental control chamber mimicked conditions observed at the S. latissima collection site during the time of collection (see above) while temperatures were indicative of the latter half of the growing season (May). Containers were filled with filtered (0.2 µm polysulfone filter capsule, Pall©) seawater to maximum capacity and covered with plexiglass with pre-drilled holes to allow delivery of dissolved gases and minimize interaction with the environment. Dissolved gases were delivered into each experimental container by aeration via a 3.8 x 1.3 cm air diffuser (Pentair) connected to a 1 mL, polystyrene serological pipette inserted through the pre-drilled holes in the plexiglass covers to the bottom of each container and connected via Tygon tubing to an air source. The containers were then randomly assigned, in quadruplicate, to each treatment (see below). Containers subjected to elevated (~2,000 µatm) CO2 levels utilized a multitube gas proportionator system (Cole Parmer® Flowmeter system, multitube frame) that mixed ambient air with 5% CO2 gas (Talmage and Gobler, 2010). The pCO2 levels in the elevated treatments were higher than levels present at the S. latissima collection site but are consistent with levels present in eutrophic US East Coast estuaries (Wallace et al., 2014; Baumann et al., 2015; Wallace and Gobler, 2015) including those near the oyster farm (Wallace and Gobler, 2021; Wallace et al., 2021). Containers subjected to ambient (~400 µatm) CO2 levels utilized a single tube proportioner system to introduce only ambient air into containers. The gases were mixed through gang valves and were delivered at a flow rate of 1000 ± 5 mL min-1 to experimental containers through serological pipettes inserted through the plexiglass covers. The bubbling rates in the containers turned over the volume ~100 times d-1, and bubbling was initiated at least 2 – 3 d prior to the beginning of experiments to allow CO2 levels and carbonate chemistry to reach a state of equilibrium. Continuous and discrete measurements of pH were made using an Orion Star A321 Plus electrode ( ± 0.001 pH unit, NBS scale) calibrated prior to each use using National Institute of Standards and Technology (NIST) traceable standards.
Dissolved inorganic carbon (DIC) within experimental containers was measured directly from water samples collected at the beginning and end of experiments, which were preserved using a saturated mercuric chloride (HgCl2) solution and stored at ~4°C until they were analyzed on a VINDTA 3D (Versatile Instrument for the Determination of Total inorganic carbon) delivery system coupled within a UIC Inc. coulometer (model CM5017O) as per Young and Gobler (2018). Total DIC, total alkalinity, pCO2, , Ωaragonite, and Ωcalcite (Table 1) were calculated from measured DIC levels, pH, temperature, and salinity, as well as the first and second dissociation constants of carbonic acid in seawater (Millero, 2010) using the program CO2SYS (http://cdiac.ess-dive.lbl.gov/ftp/co2sys/). For quality assurance, DIC levels and pH within certified reference material (provided by Andrew Dickson of the University of California, San Diego, Scripps Institution of Oceanography; batches 180 = 2021.87 µmol kgSW-1) were measured during analyses of each sample set. Analyses of samples only continued when complete recovery (>99.9%) of certified reference material was attained.
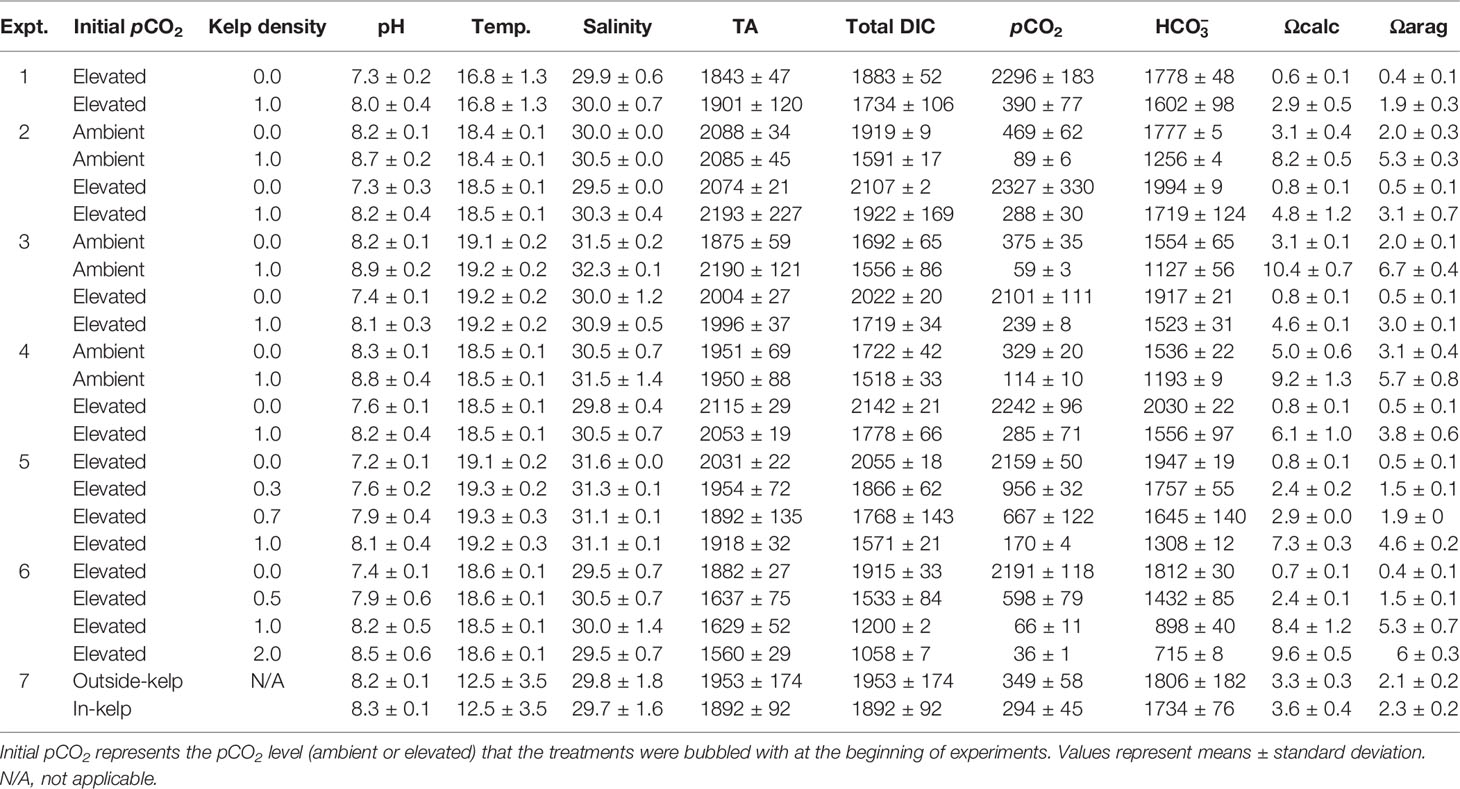
Table 1 Values of S. latissima density (g L-1), mean pH (NBS scale), temperature (°C), salinity (g kg-1), pCO2 (µatm), total alkalinity (TA; µmol kgSW-1), total DIC (µmol kgSW-1), (µmol kgSW-1), Ωaragonite, and Ωcalcite for all experiments in the study (n=4 for all treatments).
For all experiments, individual S. latissima blades were prepared by cutting the stipe 2.5 cm below the blade-stipe interface. Cuts were made above the blade-stipe interface in order to obtain the desired fresh weight, depending on the experiment being performed (see below). This was done to standardize initial S. latissima blade tissue type and size (Boderskov et al., 2016). All samples were extensively rinsed with filtered (0.2 µm) seawater, spun in a salad spinner to remove debris and epiphytes and excess seawater, rinsed and spun again, and weighed on a Scientech ZSA 120 digital microbalance ( ± 0.0001 g) to obtain initial fresh weight in grams.
An initial experiment was performed to assess the ability of S. latissima to alter pH in experimental conditions similar to the later laboratory experiments with bivalves and S. latissima. For this experiment, there were two treatments: a control with increased CO2 without S. latissima and a treatment with increased delivery of CO2 and S. latissima.
Four experiments (Experiments 1 – 4; Table 2) were performed to assess the effects of elevated pCO2 and the presence of S. latissima on the growth of C. virginica, M. edulis, and M. mercenaria. For the first experiment, C. virginica (~2.6 mm) were placed, in quadruplicate, in one of two treatments: a control with elevated pCO2 (~1700 – 1800 µatm) without S. latissima and a treatment with elevated pCO2 and S. latissima added at a level within the range of densities found on the kelp farm (1.0 g L-1; Sylvers and Gobler, 2021). The second, third, and fourth experiments placed C. virginica (~2.8 mm), M. edulis (~2.3 mm), and M. mercenaria (~1.1 m), respectively, in quadruplicate, in one of four treatments: a control with ambient pCO2 (~360 – 500 µatm) without S. latissima, a treatment with ambient pCO2 and S. latissima (1.0 g L-1) added, a treatment with elevated pCO2 (~2,000 µatm) without S. latissima, and a treatment with elevated pCO2 and S. latissima (1.0 g L-1) added. Two experiments (Experiments 5 and 6; Table 2) were performed to assess the minimum S. latissima biomass required to alter the growth of M. edulis and C. virginica when grown under elevated pCO2. For the fifth experiment (Experiment 5; Table 2), M. edulis (~5.7 mm) were placed, in quadruplicate, in one of four treatments: a control with elevated pCO2 (~2,700 µatm) without S. latissima, a treatment with elevated pCO2 and 0.3 g L-1 S. latissima, a treatment with elevated pCO2 and 0.7 g L-1 S. latissima, and a treatment with elevated pCO2 and 1.0 g L-1 S. latissima. For the sixth experiment (Experiment 6; Table 2), M. edulis (~6.5 mm) and C. virginica (~5.1 mm) were placed, in quadruplicate, in one of four treatments: a control with elevated pCO2 (~3,500 µatm) without S. latissima, a treatment with elevated pCO2 and 0.5 g L-1 S. latissima, a treatment with elevated pCO2 and 1.0 g L-1 S. latissima, and a treatment with elevated pCO2 and 2.0 g L-1 S. latissima. Across all experiments, bivalves were fed a mixture of Isochrysis galbana and Chaetoceros muelleri ad libitum (4 x 104 cells mL-1 d-1; Helm et al., 2004). In the preliminary experiment, the same microalgal mixtures were added to treatments with and without S. latissima and demonstrated that microalgae did not have a discernable impact on pH levels in the experimental containers. Microalgal cultures were maintained in exponential phase growth in f/2 media using standard culturing conditions (Helm et al. (2004).
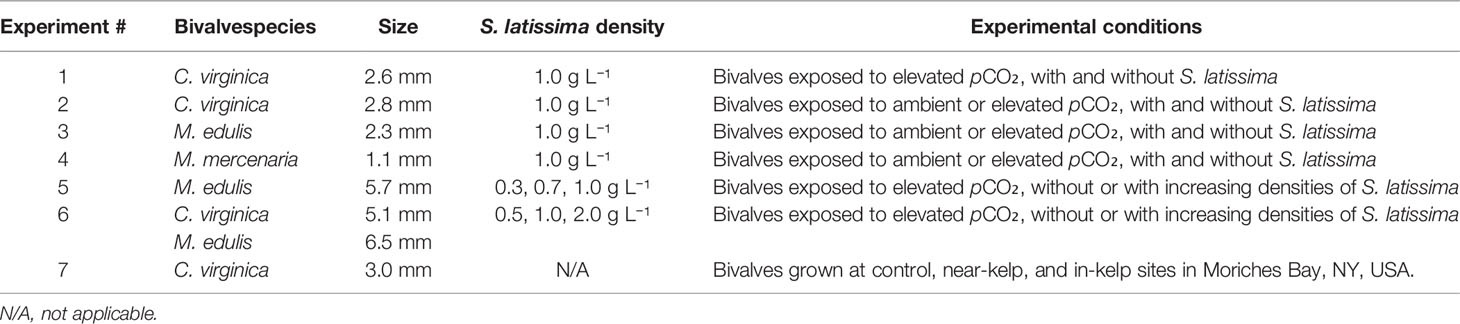
Table 2 List of experiments with their respective bivalve species and size (as shell height for C. virginica and M. edulis or shell length for M. mercenaria), S. latissima density, and conditions.
Experiments began with the introduction of bivalves and S. latissima into polycarbonate containers fitted with continuous pH electrodes prepared as described above. Initial DIC samples were collected and discrete measurements of pH and temperature were taken and were made daily for the duration of experiments. Each experiment persisted for ~two weeks, with the exception of the preliminary experiment and Experiment 1, which persisted for ~one week and ~three weeks, respectively. At the beginning of each experiment, 15 – 20 individuals from each bivalve cohort were placed in each experimental container, in quadruplicate, with a set of 15 – 20 individuals from the same cohort put aside to obtain initial measurements of shell height (for C. virginica and M. edulis) or length (for M. mercenaria) and tissue weight. Bivalve dimensions were determined by analysis of digital images using the software ImageJ (Young and Gobler, 2018). At the end of each week, a complete water change was performed for all containers using water bubbled in 20-L polycarbonate containers with the same gas mixtures for ambient and elevated pCO2 treatments as described above to ensure that bivalves were exposed to the target pCO2 levels for the duration of the experiment. At the end of experiments, final pH, temperature, and salinity measurements were made and final DIC samples were collected and analyzed and bivalves were collected on a 500 µm sieve, transferred to a graduated laminated grid sheet, and digitally imaged for shell measurements.
Field Experiment
Two field experiments (Experiment 7; Table 2) were conducted to assess the impacts of S. latissima on bivalves. For Experiment 7, the growth of C. virginica was monitored at the Great Gun Shellfish farm in Moriches Bay, NY, USA (40.78° N, 72.78° W), where S. latissima was collected for the laboratory experiments (Experiments 1 – 6). S. latissima was cultivated along four 30m horizontal longlines that were staked ~0.4 m above the bay bottom and spaced ~2 m apart (Supplementary Figure S2). The spacing of the lines in shallow water depths (~0.6 m MLW; ~2.0 m MHW) has been employed in other seaweed farms (Mongin et al., 2016).
Experimental oysters were placed at one of three locations that differed in proximity to the lines of S. latissima: in-kelp, near-kelp, and outside-kelp sites (Supplementary Figure S2). The in-kelp location was positioned in the center of the four-line kelp array, the near kelp location was positioned ~3 m away from the end of the kelp lines, and the outside kelp location was positioned ~50 m away from the kelp lines. At each location triplicate cages were each stocked with 100 experimental oysters (~3.0 mm). For each replicate, oysters were placed in a spat bag (1.0 mm mesh) housed in a floating oyster cage composed of a standard oyster ‘grow-out’ bag (36” x 18” x 3”) made of semi-rigid polyethylene mesh (4 mm mesh size), with plastic, air-filled, cylindrical floats (3” diameter) attached to each long side. The bags were secured directly to the kelp lines in the ‘in-kelp’ treatment with a bridle-clip system and were attached to lines without kelp in the ‘near-kelp’ and ‘outside-kelp’ treatments. Prior to deployment, a subset (n = 100) of oysters were digitally photographed for measurement of shell height and width using ImageJ (see above), and then frozen for later analysis of tissue weight (see below).
YSI EXO3 multi-parameter sondes were deployed to continuously monitor pH (NBS scale), which internally logged data every 10 min. Calibration of the pH sensors was performed by use of three NIST pH buffers with known pH levels of 4, 7, and 10. While not shown here, temperature (°C) and conductivity (used to measure salinity) were also continuously monitored by the EXO3. Temperature was measured with a highly stable and aged thermistor that requires no prior calibration, while conductivity was calibrated using a conductivity standard with a recommended standard of 1 mS cm-1 (1000 µS cm-1) for the highest stability. Sondes were deployed at the outside-kelp and in-kelp sites. At both sites, triplicate DIC samples were collected at the beginning, middle, and end of the field experiment. The collection, preservation, and analysis of DIC samples for the field experiment followed the same procedure as for the laboratory experiments, described above.
Post-Experimental Analyses
At the conclusion of experiments, the initial and final shell height and shell width of experimental bivalves was measured and averaged to obtain the mean shell height and width for each experimental container of the lab experiments (n = 4) or cage of the field experiment (n = 3). Shell-based growth (mm d-1) was determined from the changes in shell dimensions during the experiment. Tissue weights were obtained by weighing bivalves after drying at 60°C for 72 h, combusting them at 450°C for 4 h, weighing them again, and subtracting the combusted weight from the dry weight. All individuals from each experimental container or cage were combined for drying and combustion to obtain collective weights for each replicate. Tissue-based growth (mg bivalve-1 d-1) was determined by subtracting the initial tissue weight (from the initially-collected subset of bivalves) from the final tissue weight and dividing by the duration of the experiment in days, for each individual from each replicate container, whereby tissue weight was determined by subtracting the combusted weight from the dry weight. One-way ANOVAs were performed with SigmaPlot 11.0 to assess significant differences in growth rates (Experiments 1, and 5 – 7) and M. edulis abundances (Experiment 7) between treatments. Two-way ANOVAs were performed to assess significant differences in growth rates (Experiments 2 – 4) where the main treatment effects were pCO2 level (ambient or elevated) and S. latissima (with and without the macroalga). Normality and equal variance were tested via the use of Shapiro-Wilk and Levene tests within SigmaPlot 11.0; assumptions of equal variance and normality were met for all data. If significant differences were detected, a Tukey’s Honest Significant Difference (HSD) test using R v.3.4.0 within RStudio v.1.0.143 was performed.
Results
Experiments With and Without Saccharina latissima
An initial experiment was conducted to assess how Saccharina latissima would alter pH levels within experimental vessels that had been bubbled with CO2 and began with a pH of ~7.2 (Figure 1). In the treatment with S. latissima, pH levels increased during the light portion of the photoperiod by ~0.4 units for the first two days, ~0.3 units the next two days, and more slowly thereafter reaching a maximum of ~8.8 on day 7 (Figure 1). In contrast, pH levels in the treatment without S. latissima increased more slowly and steadily, peaking at only 7.5 on the seventh day (Figure 1).
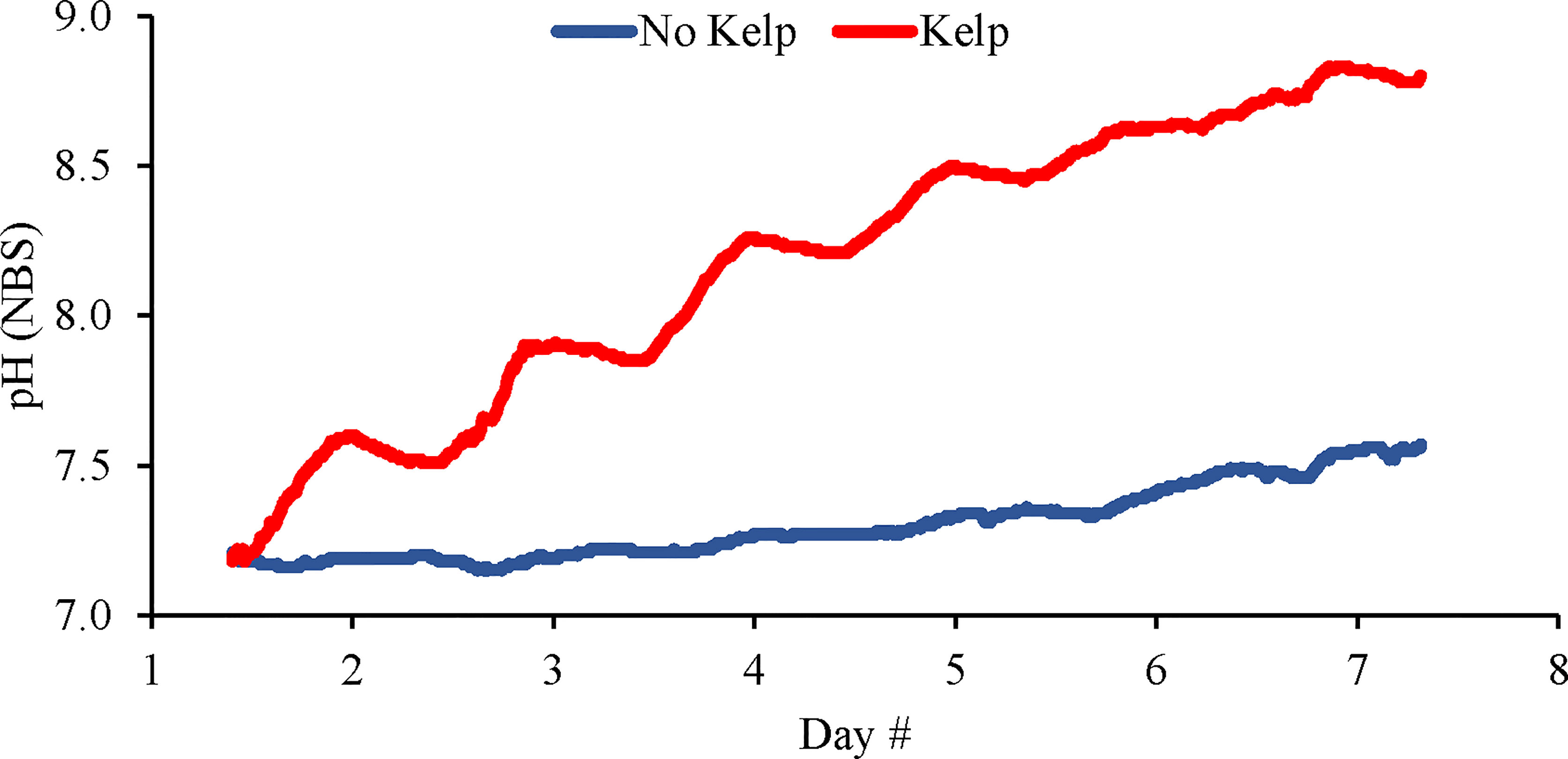
Figure 1 Continuous pH (NBS scale) measurements made in elevated pCO2 treatments with and without kelp (S. latissima; 1 g L-1).
During the first experiment with bivalves using C. virginica (Table 2 and Figure 2), pH values in the treatments with and without S. latissima ranged 7.44 – 8.48 and 7.02 – 7.69, respectively, and pH was, on average, 7.94 ± 0.17 and 7.27 ± 0.19, respectively (Table 1 and Figure 2A) and Ωaragonite was 1.9 ± 0.3 and 0.4 ± 0.1 (Table 1 and Figure 2B). Shell-based growth rates of C. virginica were significantly and 40% higher in the presence of S. latissima than the treatment without S. latissima (One-way ANOVA; p < 0.05; Figure 2C and Supplementary Table S1). Tissue-based C. virginica growth rates were significantly higher by 50% in the treatment with S. latissima compared to the treatment without S. latissima (One-way ANOVA; p < 0.05; Figure 2D and Supplementary Table S1).
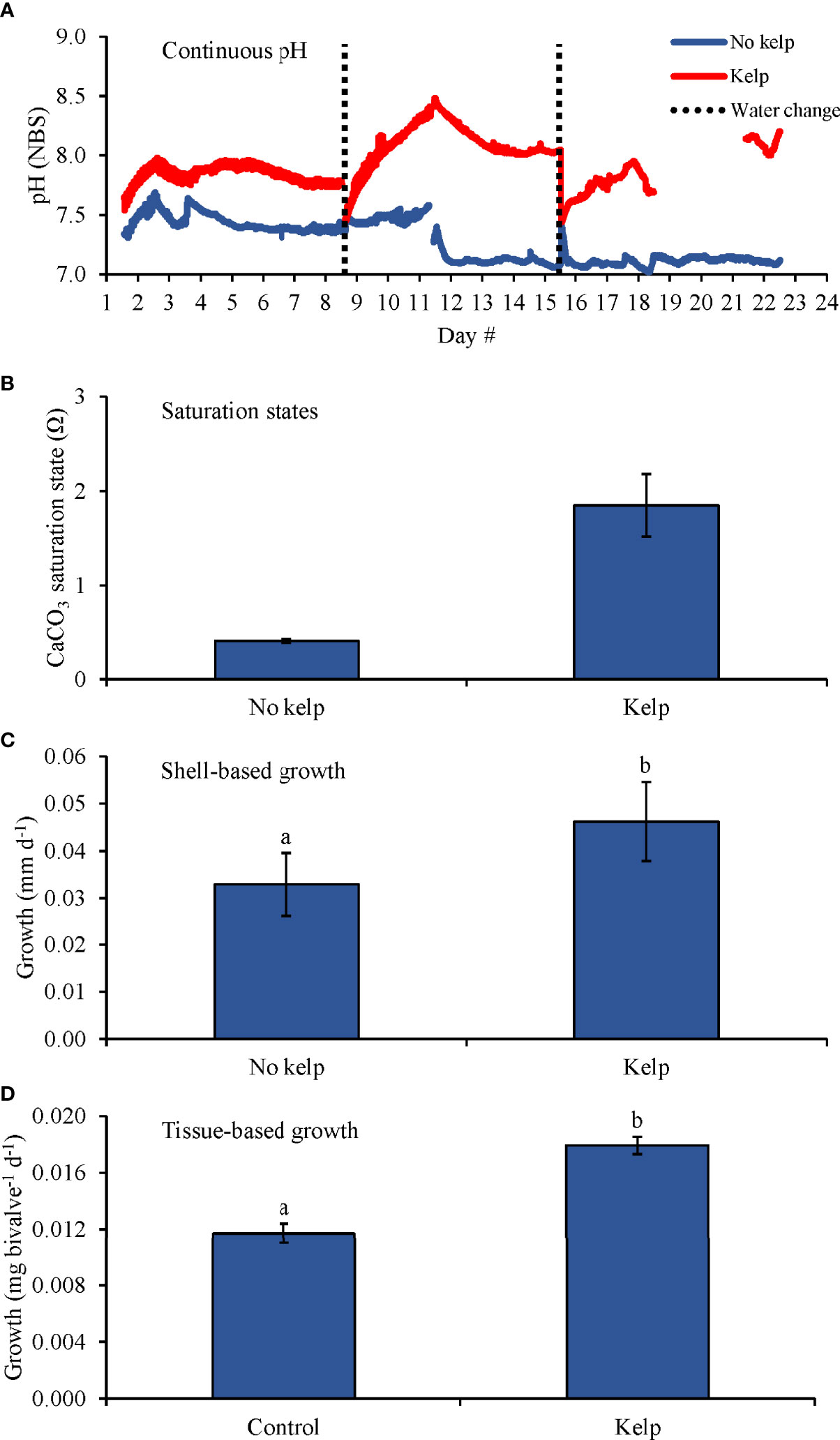
Figure 2 (A) Continuous pH measurements, (B) Saturation states, (C) Shell-based growth, and (D) Tissue-based growth rates of C. virginica grown in elevated pCO2, with and without kelp (S. latissima; 1 g L-1). Letters above columns represent Tukey HSD results.
In the second bivalve experiment with C. virginica (Table 2 and Figure 3), pH levels in the elevated pCO2 treatments were 8.25 ± 0.15 and 7.34 ± 0.32, respectively (Figure 3A) while Ωaragonite in the ambient pCO2 treatments with and without S. latissima were 5.2 ± 0.3 and 2.0 ± 0.3, respectively, and were 3.1 ± 0.7 and 0.5 ± 0.1 in the elevated pCO2 treatments with and without S. latissima, respectively (Table 1; Figure 3B). There was a significant interaction in this experiment as the effects of pCO2 on C. virginica (~2.8 mm) shell-based growth rates were dependent upon the presence of S. latissima (Two-way ANOVA; p < 0.05; Figure 3C). Comparing specific treatments, shell-based growth rates were significantly lower in the elevated pCO2 treatment without S. latissima compared to the elevated pCO2 treatment with S. latissima and the ambient pCO2 treatments with and without S. latissima by 64, 60, and 58%, respectively (Tukey HSD; p < 0.05 for all; Figure 3C and Supplementary Table S3). There were no significant differences in tissue-based growth rates in this experiment (Figure 3D and Supplementary Table S2).
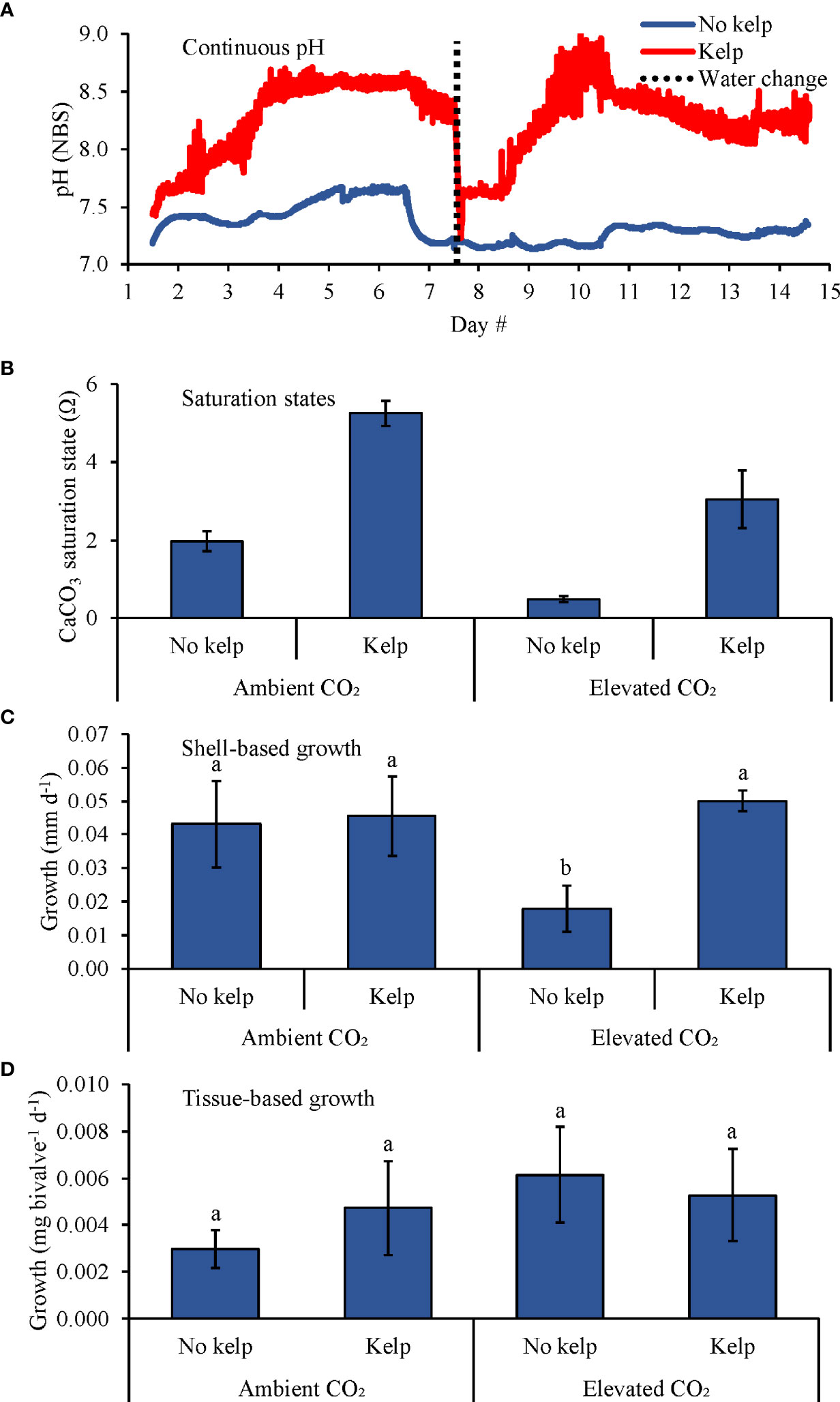
Figure 3 (A) Continuous pH measurements, (B) Saturation states, (C) Shell-based growth, and (D) Tissue-based growth rates of C. virginica grown in ambient and elevated pCO2, with and without kelp (S. latissima; 1 g L-1). Letters above columns represent Tukey HSD results.
During the experiment with Mytilus edulis (Table 2 and Figure 4), average pH levels in the ambient pCO2 treatments with and without S. latissima and the elevated pCO2 treatments with and without S. latissima were 8.88 ± 0.16, 8.31 ± 0.12, 8.14 ± 0.28, and 7.36 ± 0.10, respectively (Figure 4A). Average Ωaragonite in the ambient pCO2 treatments with and without S. latissima was 6.7 ± 0.4 and 2.0 ± 0.1, respectively, and was 3.0 ± 0.1 and 0.5 ± 0.1 in the elevated pCO2 treatments with and without S. latissima, respectively (Figure 4B). There was a significant interaction between S. latissima and pCO2 on shell growth for M. edulis (Two-way ANOVA; p < 0.05). Comparing specific treatments, shell-based growth rates were significantly lower in the elevated pCO2 treatment without S. latissima than the elevated pCO2 treatment with S. latissima and the ambient pCO2 treatments with and without S. latissima by 36, 29, and 31%, respectively (Tukey HSD; p < 0.05 for all; Figure 4C and Supplementary Table S5). Shell-based growth rates were not different between the elevated pCO2 treatment with S. latissima and ambient pCO2 treatments with and without kelp (Tukey HSD; p > 0.05 for all; Figure 4C and Supplementary Table S5). Tissue-based growth was significantly higher in the presence of S. latissima by ~47% (Two-way ANOVA; p < 0.05; Figure 4D and Supplementary Table S4), and there was no significant effect of pCO2 on tissue-based growth and no interaction detected (Tukey HSD; p > 0.05; Figure 4D and Supplementary Table S5).
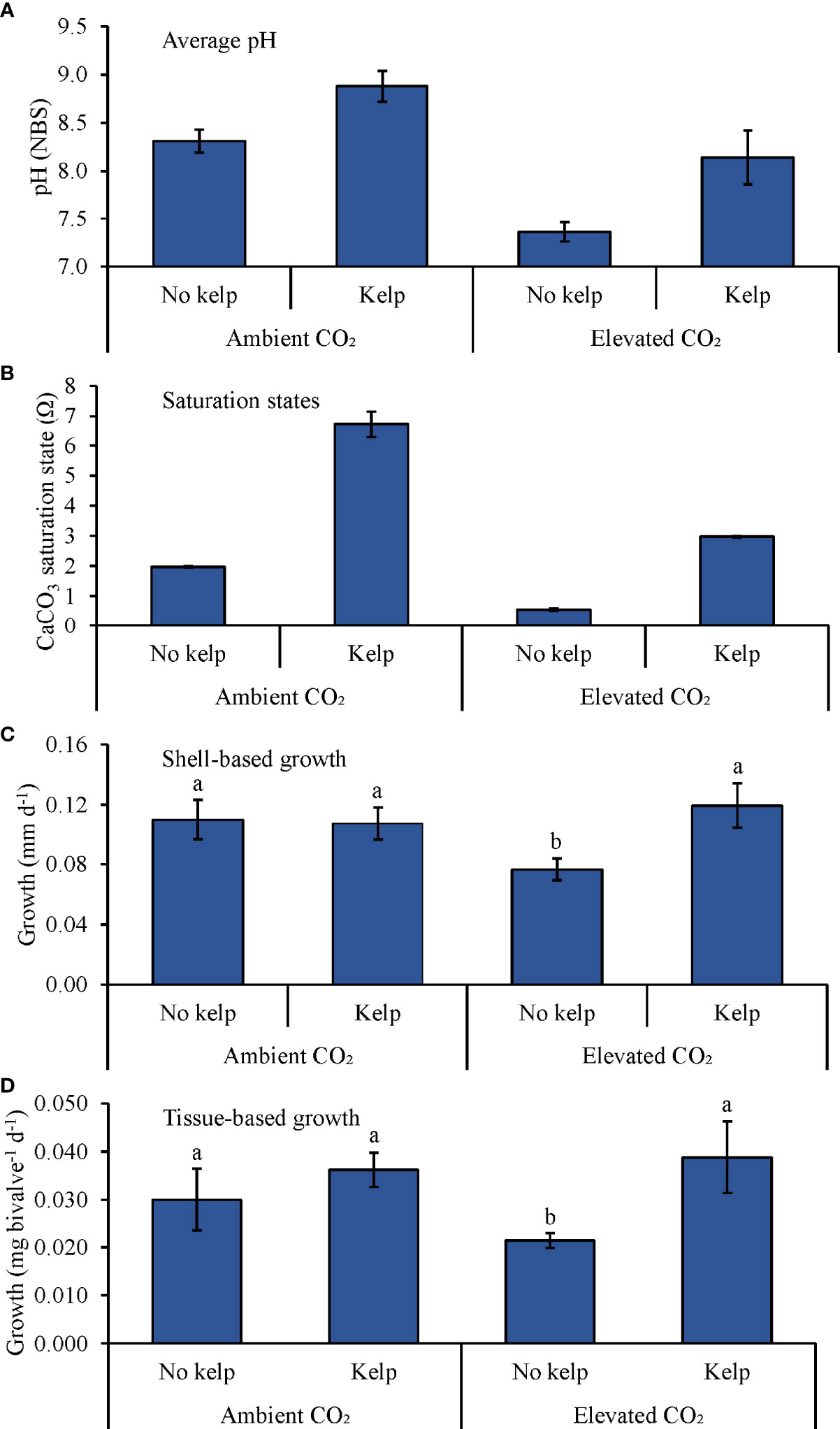
Figure 4 (A) Average pH measurements, (B) Saturation states, (C) Shell-based growth, and (D) Tissue-based growth rates of M. edulis grown in ambient and elevated pCO2, with and with kelp (S. latissima; 1 g L-1). Letters above columns represent Tukey HSD results.
During the experiment with Mercenaria mercenaria (Table 2 and Figure 5), average pH levels in the ambient pCO2 treatments without and with S. latissima and the elevated pCO2 treatments without and with S. latissima were 8.45 ± 0.19, 8.79 ± 0.35, 7.55 ± 0.07, and 8.18 ± 0.40, respectively (Figure 5A). Ωaragonite in the ambient pCO2 treatments with and without S. latissima was 5.7 ± 0.8 and 2.1 ± 0.2, respectively, and was 3.8 ± 0.6 and 0.5 ± 0.1 in the elevated pCO2 treatments with and without S. latissima, respectively (Table 1 and Figure 5B). There was a significant interaction between pCO2 and S. latissima for shell- and tissue-based growth rates of hard clams (Two-way ANOVA; p < 0.05). Shell-based growth rates of M. mercenaria (~1.1 mm) were significantly faster in the elevated pCO2 treatment with S. latissima, and the ambient pCO2 treatments with and without S. latissima than in the elevated pCO2 treatment without S. latissima by ~100, ~110, and ~90%, respectively (Two-way ANOVA and Tukey HSD; p < 0.05 for all; Figure 5C and Supplementary Tables S6, S7); no other significant differences were detected. between treatments for shell growth. Tissue-based growth rates were significantly (two-fold) higher in the elevated pCO2 treatment with S. latissima than the elevated pCO2 treatment without S. latissima (Two-way ANOVA and Tukey HSD; p < 0.05; Figure 5D and Supplementary Tables S6, S7). In the ambient pCO2 treatments with and without S. latissima, tissue-based growth was significantly higher by 100 and 150%, respectively, than in the elevated pCO2 treatment without S. latissima (Two-way ANOVA and Tukey HSD; p < 0.05 for both; Figure 5D and Supplementary Tables S6, S7). There were no significant differences in tissue-based growth between the ambient pCO2 treatments and the elevated pCO2 treatment with S. latissima (Two-way ANOVA and Tukey HSD; p > 0.05 for all; Figure 5D and Supplementary Tables S6, S7).
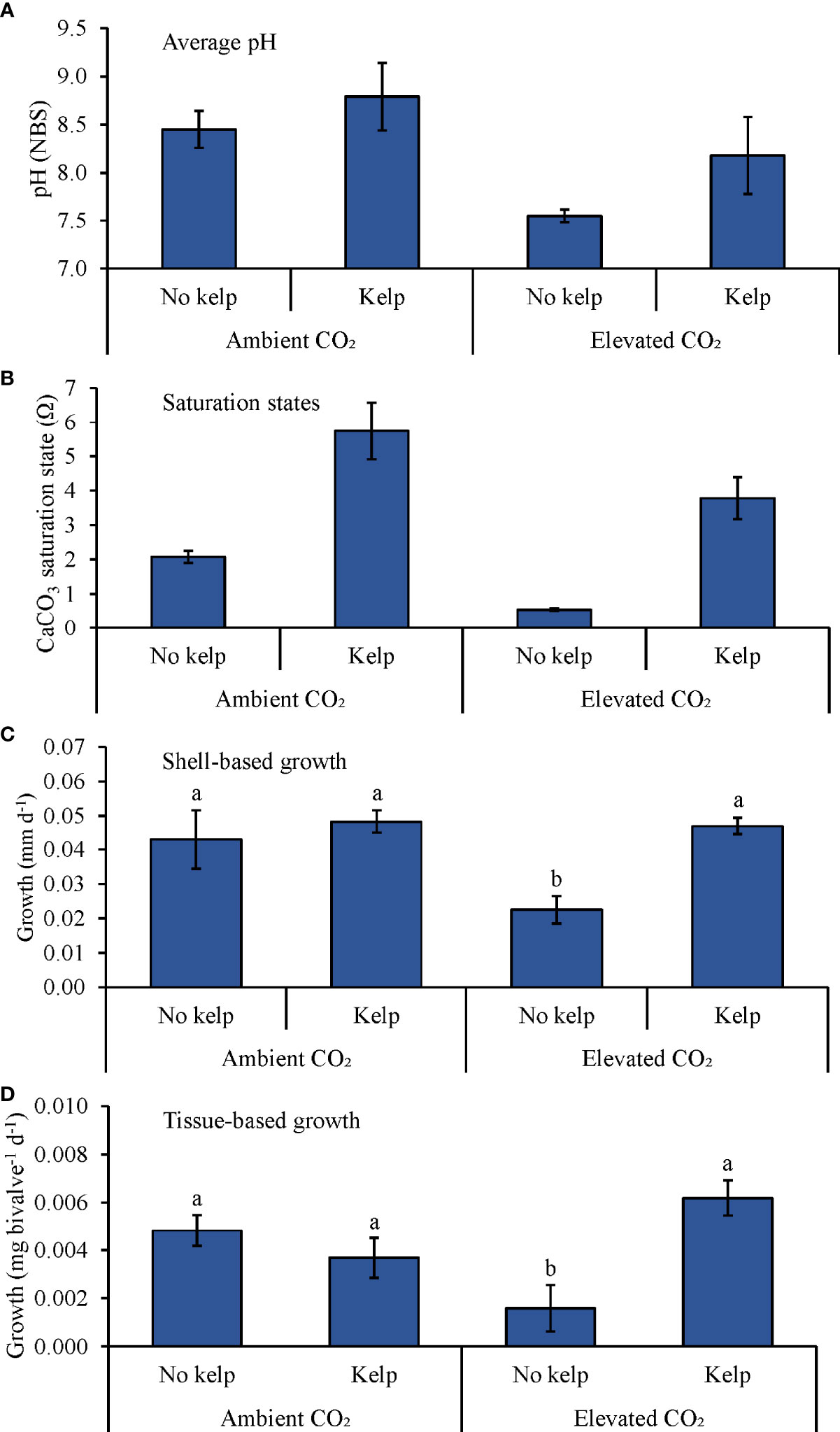
Figure 5 (A) Continuous pH measurements, (B) Saturation states, (C) Shell-based growth, and (D) Tissue-based growth rates of M. mercenaria grown in ambient and elevated pCO2, with and without kelp (S. latissima; 1 g L-1). Letters above columns represent Tukey HSD results.
Experiments With Multiple Levels of Saccharina latissima
During the fifth experiment growing M. edulis with four levels of kelp (Table 2 and Figure 6), pH levels in the 0.0 (control), 0.3, 0.7, and 1.0 g L-1 were, on average, 7.44 ± 0.10, 7.74 ± 0.15, 8.01 ± 0.24, and 8.42 ± 0.33, respectively (Figure 6A), while Ωaragonite values were 0.5 ± 0.1, 1.5 ± 0.1, 1.9 ± 0.1, and 4.6 ± 0.2, respectively (Table 1 and Figure 6B). Shell-based growth rates were significantly higher in the 0.3, 0.7, and 1.0 g L-1 treatments than in the control by ~50, ~55, and ~70%, respectively, but there were no differences in shell-based growth between the 0.3, 0.7, and 1.0 g L-1 treatments (One-way ANOVA and Tukey HSD; p < 0.05 for all significant differences; Figure 6C; Supplementary Tables and S8 and S9). Tissue-based growth rates were significantly higher in the 1.0 g L-1 treatment than in the control, 0.3, and 0.7 g L-1 treatments by ~45, ~40, and ~40%, respectively; there were no other significant differences among tissue-based growth rates (One-way ANOVA and Tukey HSD; p < 0.05 for all significant differences; Figure 6D; Supplementary Tables and S8 and S9).
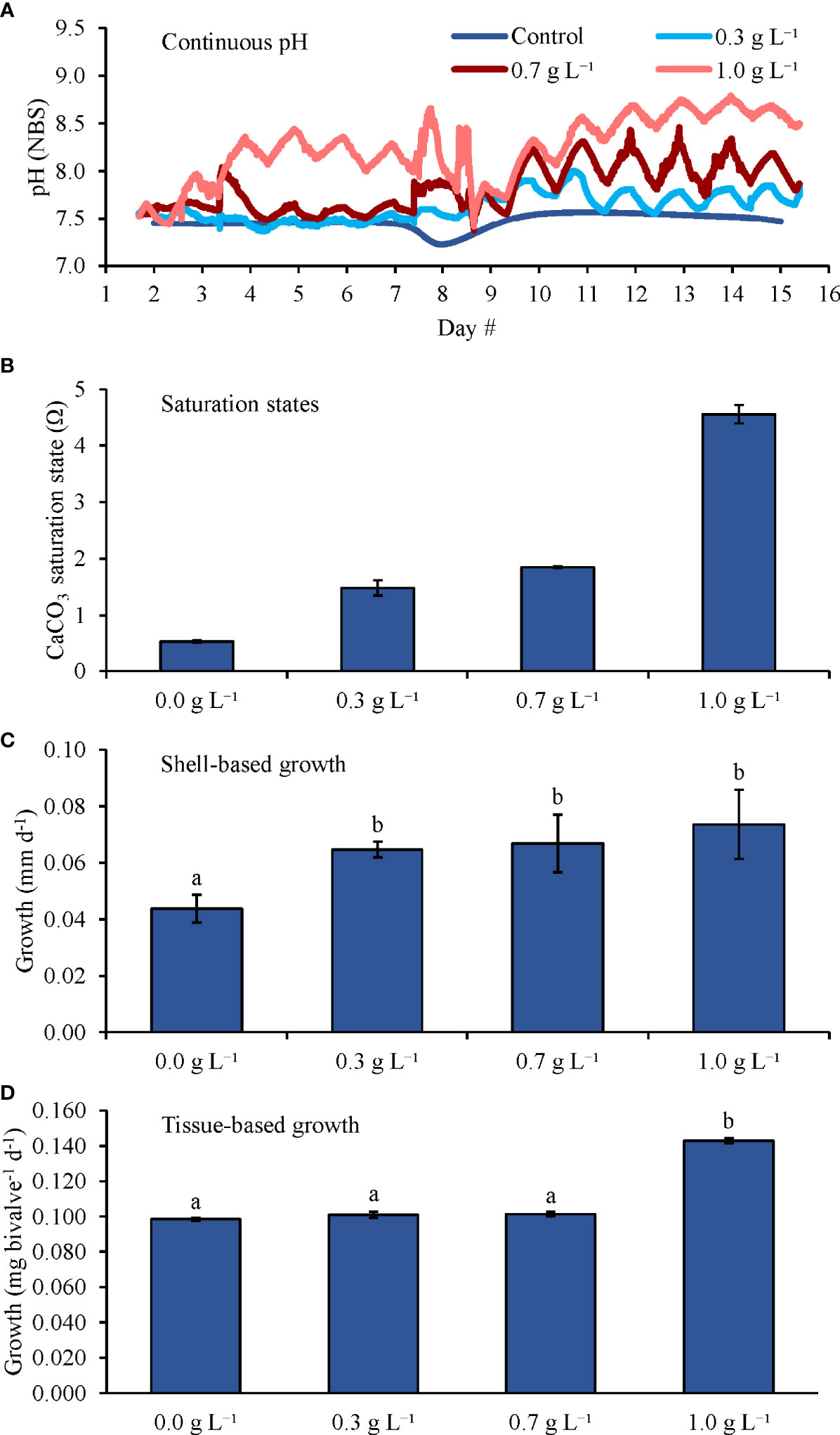
Figure 6 (A) Continuous pH measurements, (B) Saturation states, (C) Shell-based growth, and (D) Tissue-based growth rates of M. edulis grown in elevated pCO2, with increasing densities of kelp (S. latissima). Letters above columns represent Tukey HSD results.
During the sixth and final experiment that grew C. virginica and M. edulis with four levels of kelp (Table 2 and Figure 7), pH levels in the 0.0 (control), 0.5, 1.0, and 2.0 g L-1 treatments were, on average, 7.37 ± 0.10, 7.66 ± 0.40, 8.19 ± 0.51, and 8.53 ± 0.39, respectively (Figure 7A), while Ωaragonite values were 0.4 ± 0.1, 1.5 ± 0.1, 5.3 ± 0.7, and 6.0 ± 0.3, respectively (Table 1 and Figure 7B). Shell-based growth rates of C. virginica (~5.1 mm) were significantly higher in the 0.5, 1.0, and 2.0 g L-1 treatments compared to the 0.0 g L-1 treatment by ~140, ~280, and ~290%, respectively (One-way ANOVA and Tukey HSD; p < 0.05 for all; Figure 7C and Supplementary Tables S10, S11). Additionally, C. virginica shell-based growth rates were significantly higher in the 1.0 and 2.0 g L-1 treatments than in the 0.5 g L-1 treatment by ~60% but did not differ between the former two treatments (One-way ANOVA and Tukey HSD; p < 0.05 for all significant differences; Figure 7C and Supplementary Tables S10, S11). Tissue-based growth rates for C. virginica were significantly higher in the 1.0 and 2.0 g L-1 treatments than in the control by ~160 and ~150%, respectively, but there were no differences detected between other treatment combinations (One-way ANOVA and Tukey HSD; p < 0.05 for all significant differences; Figure 7D and Supplementary Tables S10, S11). For M. edulis (~6.5 mm), shell-based growth rates were significantly higher in the 0.5, 1.0, and 2.0 g L-1 treatments than in the control by ~90, 100, and ~130%, respectively (One-way ANOVA and Tukey HSD; p < 0.05 for all; Figure 7C and Supplementary Tables S12, S13). There were no differences in M. edulis shell-based growth between the 0.5, 1.0, and 2.0 g L-1 treatments (One-way ANOVA and Tukey HSD; p > 0.05 for all; Figure 7C and Supplementary Tables S12, S13). Tissue-based growth rates for M. edulis were significantly higher in the 0.5, 1.0, and 2.0 g L-1 treatments than in the control by ~135, ~110, and ~150%, respectively, but there were no significant differences in M. edulis tissue-based growth rates between the 0.5, 1.0, and 2.0 g L-1 treatments (One-way ANOVA and Tukey HSD; p < 0.05 for all significant differences; Figure 7D and Supplementary Tables S12, S13).
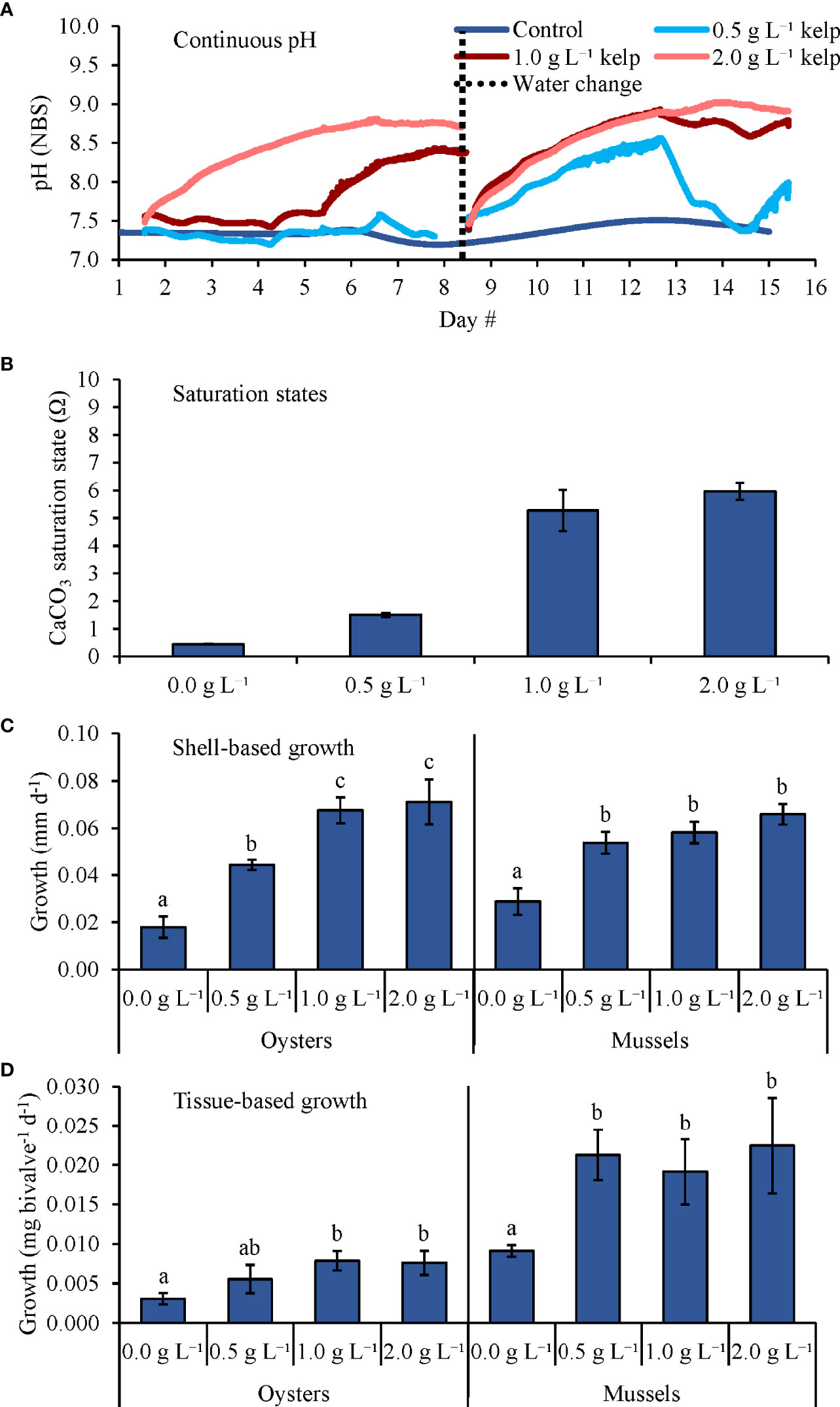
Figure 7 (A) Continuous pH measurements, (B) Saturation states, (C) Shell-based growth, and (D) Tissue-based growth rates of C. virginica and M. edulis grown in elevated pCO2, with increasing densities of kelp (S. latissima). Letters above columns represent Tukey HSD results. Tukey HSD tests were performed for C. virginica and M. edulis separately.
Field Experiment
For the one-month field experiment (Experiment 7; Table 2 and Figure 8), pH levels at the outside-kelp and in-kelp sites were, on average, 7.71 ± 0.16 and 7.86 ± 0.18, respectively (Figure 8A) while average Ωaragonite values were 2.1 ± 0.2 and 2.3 ± 0.2, respectively (Table 1 and Figure 8B). pH differences between within and outside of the kelp were pronounced for the first two weeks of the experiment, small for the third week, and near absent for the final week (Fig, 8A). C. virginica (~3.0 mm) shell-based growth rates were significantly faster in the in- and near-kelp sites than at the outside-kelp site by ~270 and ~90%, respectively (One-way ANOVA and Tukey HSD; p < 0.05 for both; Figure 8C and Supplementary Tables S14, S15). Shell-based growth rates were significantly faster at the in-kelp site than at the near-kelp site by ~90% (One-way ANOVA and Tukey HSD; p < 0.05; Figure 8C and Supplementary Tables S14, S15). Tissue-based growth rates were also significantly higher at the in-kelp site than at the outside-kelp and near-kelp sites by 160 and ~55%, respectively (One-way ANOVA and Tukey HSD; p < 0.05 for both; Figure 8D and Supplementary Tables S14, S15). Additionally, tissue-based growth was significantly higher at the near-kelp site than at the outside-kelp site by 70% (One-way ANOVA and Tukey HSD; p < 0.05; Figure 8D and Supplementary Tables S14, S15).
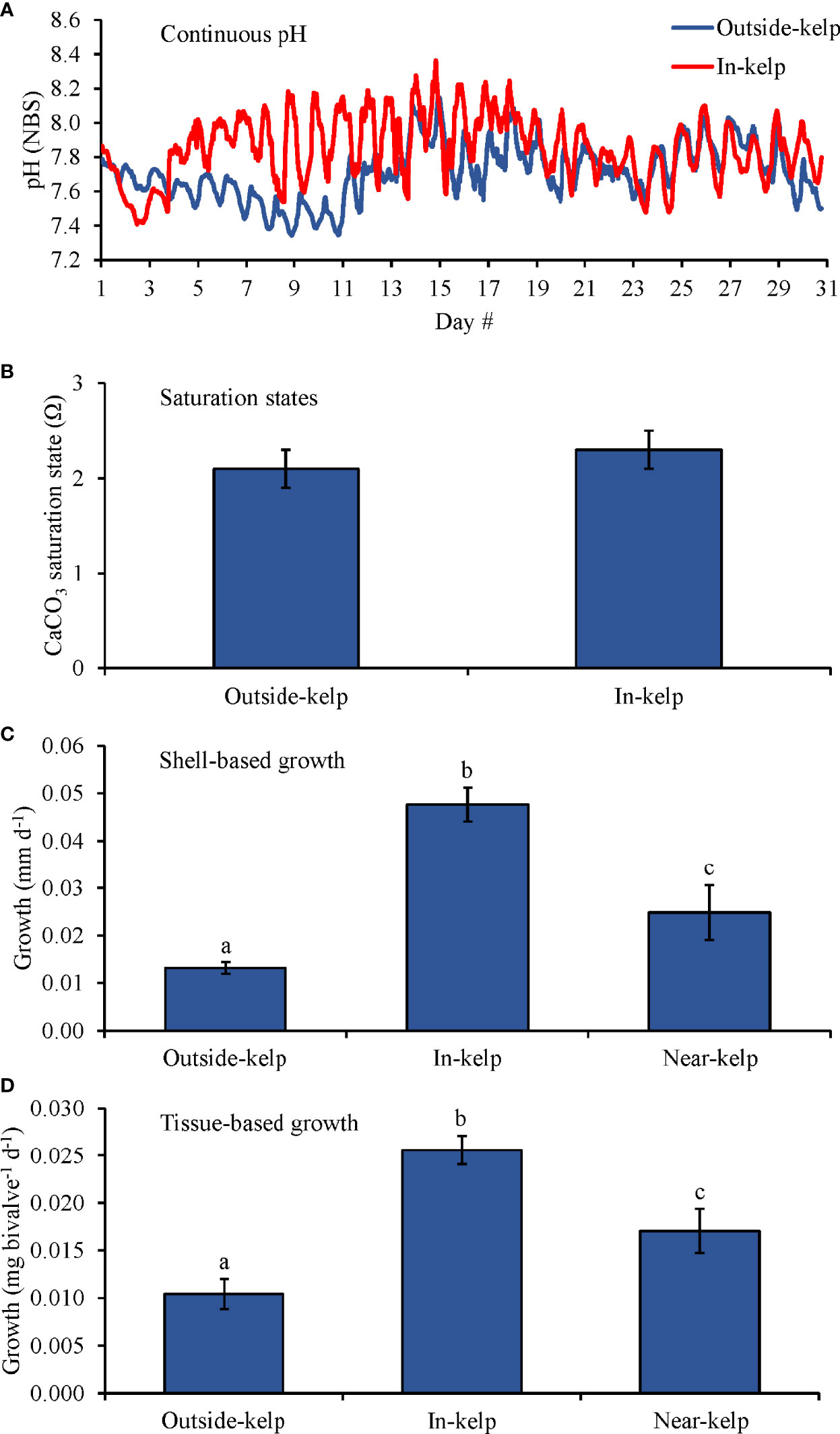
Figure 8 (A) Continuous pH measurements, (B) Saturation states, (C) Shell-based growth, and (D) Tissue-based growth rates of C. virginica grown at outside-kelp (control), on-kelp, and off-kelp (S. latissima) sites at the Great Gun oyster farm in Center Moriches, NY, USA during May 2021. Letters above columns represent Tukey HSD results.
Discussion
During this study, exposure of bivalves to elevated pCO2 (>1,800 µatm) resulted in significantly reduced shell- and tissue-based growth rates for all bivalve species (Crassostrea virginica, Mytilus edulis, and Mercenaria mercenaria) relative to control conditions in all experiments. The co-exposure of these bivalves to Saccharina latissima, however, fully ameliorated the negative effects of the same source of pCO2, resulting in growth rates of bivalves that were no different than control conditions. Improvements in growth rates were likely a consequence of changes in carbonate chemistry as Ωaragonite was transformed from undersaturated to saturated by the presence of kelp. When grown on an aquaculture farm, the growth rates of C. virginica increased with increasing proximity to aquacultured kelp. Collectively, these findings provide insight regarding the ability of kelps such as S. latissima to mitigate the deleterious effects of ocean acidification on bivalves with practical implications for aquaculture.
The negative effects of acidification on the growth and survival of bivalves have been well documented. Consistent with previous studies that have gauged the response of juvenile bivalves to increased levels of pCO2 (Gazeau et al., 2007; Green et al., 2009; Talmage and Gobler, 2011; Young & Gobler, 2018), all species of bivalves in the present study experienced significantly slower shell- and tissue-based growth rates under elevated pCO2 relative to their counterparts in ambient pCO2 treatments. Elevated pCO2 treatments without S. latissima contained average Ωcalcite and Ωaragonite values that < 1.0 in all experiments. Early-life-stage bivalves are particularly vulnerable to the undersaturation of aragonite due to their shells consisting partially or completely of aragonite (Stenzel, 1964; Carriker, 1996; Talmage and Gobler, 2009). While the formation of calcium carbonate is thermodynamically favored when Ω is greater than 1.0, biotic aragonite is less crystalline than non-biogenic aragonite (Weiss et al., 2002; Ramesh et al., 2018), and Ωaragonite values exceeding 1.6 may be required to yield successful growth and survival of bivalves (Barton et al., 2012) with enhanced growth under increasingly higher Ωaragonite values (Talmage and Gobler, 2010). Beyond reductions in shell growth rates, tissue-based growth rates were reduced in most experiments under elevated pCO2 levels without S. latissima compared to ambient pCO2 treatments, which is consistent with Beniash et al. (2010), who found significantly lower soft body mass of juvenile C. virginica under hypercapnia (pH = 7.5). The proposed mechanism for reduced bivalve growth and calcification under increased pCO2 is that decreased Ωaragonite increases the amount of energy used by bivalves for shell formation, which diverts energy away from maintaining homeostasis and other metabolic processes, including those that contribute toward growth (Beniash et al., 2010; Waldbusser G. G. et al., 2015). Bivalve responses to acidification can, however, be more nuanced and complex at later life stages and across generations. For example, transgenerational acclimation to ocean acidification varies among bivalve species (Griffith and Gobler, 2017; Zhao et al., 2019; Zhao et al., 2020). Further research is required to determine if the results of the present study would translate to later life stages or across generations of the bivalve species utilized.
The culture of kelp was consistently capable of fully ameliorating acidified conditions and significantly increasing bivalve growth rates during this study. In experiments 2-4 that exposed bivalves to ambient or elevated pCO2 with and without S. latissima (Table 2), the presence of S. latissima yielded higher pH, pCO2 values in-line with control values, and Ωaragonite values that always exceeded 2.0 with a mean value of ~4.6 (Table 1). In experiments where bivalves were exposed to increasing densities of S. latissima (Experiments 5 and 6; Table 2), even the lowest densities of the macroalga (0.3 – 0.5 g L-1) were capable of yielding Ωaragonite values of 1.5 and improving growth rates while the higher densities (1.0 – 2.0 g L-1) yielded values of ≥4.6 (Table 1). In both sets of experiments, Ωaragonite in the combined kelp-enhanced CO2 delivery treatments frequently exceeded the thresholds for maximal growth rates in early-life-stage bivalves (Talmage and Gobler, 2010; Barton et al., 2012). Additionally, in the field experiment of the present study, bivalve growth rates significantly increased with increasing proximity to aquacultured S. latissima, which may be the result of minor but sustained improvements to carbonate chemistry that can significantly affect growth of juvenile bivalves (Talmage and Gobler, 2011; Waldbusser G. et al., 2015).
Aquacultured macroalgae such as kelp may buffer carbonate chemistry to the benefit of nearby aquacultured bivalves. Previous laboratory studies have demonstrated that primary productivity by macroalgae can promote the growth and survival of calcifying organisms, even under acidified conditions, by increasing pH and Ω (Wahl et al., 2018; Young and Gobler, 2018). Continuous pH measurements from the field experiment reported here demonstrated that pH values were, on average, ~0.15 units higher within kelp lines compared to the control site, presumably due to increased primary productivity at the in-kelp site. While the control site pH (7.8) during this experiment was lowered than the open ocean global average (8.1), it is consistent with levels measure in many estuaries which experience acidification due eutrophication (Wallace et al., 2014; Cai et al., 2017; Wallace and Gobler, 2021; Wallace et al., 2021). In a manner similar to the observations here, Xiao et al. (2021) reported a 0.10 pH unit increase and 58.7 ± 15.9 µatm decrease within a Saccharina japonica aquaculture area compared to a control site. While the mean pCO2 levels were 20% lower at the ‘in kelp site’ and Ωaragonite was 10% higher evidencing the photosynthetic influence of the kelp, Ωaragonite was saturated (>3) suggesting this field site did not experiencing extreme acidification used in labortatory experiments. Still, unlike pH that was measured many times a day for one month, only three DIC samples were collected. Daytime primary productivity within kelp beds has been shown to significantly increase pH and reduce pCO2 compared to outside the bed across horizontal and vertical gradients on diel and even seasonal timescales (Delille et al., 2000; Delille et al., 2009; Hofmann et al., 2011). Conversely, respiration during the night by macrophyte assemblages release CO2 (Hofmann et al., 2011; Cornwall et al., 2013), thereby reducing pH and potentially lowering the potential for calcification during the night (Saderne et al., 2015), a patterna reflected in the continuous pH measurements, but not captured by carbonate chemistry measurements. Given this, it is likely that Ωaragonite was lower at night during this study and that the extent of acidification observed in the pH record would have been reflected in the carbonate chemistry record had nocturnal samples or if more samples had been collected. Regardless, during the present study, oysters had significantly higher growth rates at the in-kelp site than the outside-kelp site, a pattern mimicking trends in pH and, to a lesser extent, carbonate chemistry. Beyond S. latissima buffering carbonate chemistry, kelp detritus has been shown to be a potential food source for bivalves (Duggins et al., 1989; Duggins and Eckman, 1997; Levinton and Shumway, 2002). Hence, kelp may enhance the growth of bivalves by mitigation acidification and/or, potentially, by enhancing the nutritional status of bivalves.
Eutrophication can act as a driver of acidification and hypoxia in coastal zones (Wallace et al., 2014; Baumann et al., 2015; Wallace and Gobler, 2021), which can negatively affect the growth and survival of bivalves (Gobler et al., 2014; Stevens and Gobler, 2018). Some species of kelp, including S. latissima, experience enhanced growth under elevated nutrient conditions (Xu et al., 2011; Young et al., 2021) and grow robustly when aquacultured in eutrophic estuaries (Kim et al., 2015; Jiang et al., 2020). Given that harmful algal blooms (HABs) flourish in eutrophic zones, (Anderson et al., 2002; Anderson et al., 2008), the application of kelp in aquaculture to remove excess nutrients may reduce the intensity of HABs, which may indirectly benefit bivalves that are directly harmed by such events (Shumway, 1990). Kelp and other aquacultured macroalgae may also benefit nearby bivalves by directly reducing densities of HAB species. Various species of red, green, and brown macroalgae have been shown to directly reduce HAB-forming microalgae through allelopathy (Wang et al., 2007; Tang and Gobler, 2011; Tang et al., 2015; Sylvers and Gobler, 2021; Benitt et al., 2022). Sylvers and Gobler (2021) found that S. latissima reduced densities of the HAB-forming dinoflagellate Alexandrium catenella by 50 – 95% within an experimental setting and reduced the accumulation of saxitoxin in M. edulis below the US FDA closure limit for bivalves. Additionally, the mitigation of HABs (i.e., A. catenella) by kelp could also benefit shellfish industries that are damaged by toxin-producing dinoflagellates due to closures and contamination (Hoagland et al., 2002).
In present and future acidification scenarios, macroalgae such as kelp may directly benefit from increased pCO2. In a previous study (Young et al., 2021), S. latissima was shown to benefit directly from elevated pCO2 due to enhanced growth rates and indirectly due to reduced grazing pressure by gastropods. When exposed to elevated pCO2 levels, macroalgae may be relieved of carbon limitation (assuming its inorganic carbon uptake is not substrate-saturated) and/or may downregulate carbon-concentrating mechanisms used to convert to CO2, thus allowing for additional energy to be available for vegetative growth (Mercado et al., 1998; Koch et al., 2013). While global distributions of S. latissima are declining due to warming oceans (Filbee-Dexter et al., 2016), eutrophication (Moy and Christie, 2012), and overfishing of predators that prey on kelp grazers (Steneck et al., 2002), increased growth rates by exposure to elevated pCO2 may counteract these processes (Hepburn et al., 2011; Young et al., 2021) and, in turn, may provide localized benefits to calcifying organisms.
This study has additional broad implications for ecosystems where kelp and bivalves are aquacultured together. The harvest of aquacultured macroalgae, such as S. latissima, represents the direct removal of sequestered carbon and nitrogen (Marinho-Soriano et al., 2009; Chung et al., 2011), rather than the return of these elements back into the ecosystem through the eventual degradation of the macroalgae (Bricker et al., 2008). Beyond nutrient assimilation by kelp, aquacultured bivalves have the capacity to remove excess nutrients by harvesting of the bivalves as well as by denitrification of particulate organic nitrogen (PON) transferred to the sediment surface via biodeposition (Newell, 2004; Shpigel, 2005). Additionally, suspension-feeding activity by bivalves can reduce phytoplankton biomass in the water column and increase light penetration to the benthos, which benefits seagrass (Newell, 1988; Wall et al., 2008). Finally, cultivated bivalves, kelp, and associated aquaculture gear can provide forage and breeding habitats, as well as a predation refuge for marine life (Walls et al., 2016; O'Brien et al., 2018; Theuerkauf et al., 2021).
In the future, the benefits of kelp aquaculture to bivalves may become more habitat specific. While the net growth rates of kelp accelerate in reponse to high CO2 (Young et al., 2021), rising temperarures will continue to force kelp into higher latitude environments this century (Wernberg et al., 2010; Filbee-Dexter et al., 2016). Warming and acidification represent a dual threat to coastal ecosystems, in general, (Agostini et al., 2021), and to bivalves in particular, (Talmage and Gobler, 2011; Matoo et al., 2013). Hence, the ability of kelp to serves as a biogenic buffer again ocean acidification for bivalves may be most probable to continue in coastal zones where temperatures remain optimal for both kelp and bivalves for extended periods of the year.
In conclusion, primary productivity by S. latissima significantly lowered pH, increased Ωaragonite and enhanced shell- and tissue-based growth rates of bivalves by mitigating the deleterious effects of high CO2. Even the lowest densities of S. latissima (0.3 – 0.5 g L-1) were able to increase Ωaragonite to ~1.5 and significantly increase bivalve growth rates. In the field, primary productivity by aquacultured S. latissima increased pH by ~0.15 units relative to the control site and oyster growth rates significantly increased with increasing proximity to kelp. This study, therefore, demonstrates that the purposeful deployment of kelp, such as S. latissima, in an aquaculture setting is a beneficial strategy for protecting bivalves against acidification and may have additional ecosystem and aquaculture benefits including the sequestration and extraction of carbon and nitrogen and the mitigation of HABs. Collectively, the cultivation of kelp constitutes an environmentally-friendly means of protecting shellfisheries against present and future ocean acidification and other coastal stressors.
Data Availability Statement
The original contributions presented in the study are included in the article/Supplementary Material. Further inquiries can be directed to the corresponding author.
Author Contributions
CG, CY, and MD conceived and designed the experiments. CY and AL performed the experiments and post-experimental analyses. CY, LS, SJT, and CS collected data for the field experiment. CY, CG, MD, LS, and SJT analysed the data. CG contributed reagents, materials, and analysis tools. CY and CG wrote the paper. All authors contributed to the article and approved the submitted version.
Funding
This work was supported by New York Sea Grant (R/ATD-14), the Long Island Sound Study, the Chicago Community Trust, and the Moore Good Foundation.
Conflict of Interest
The authors declare that the research was conducted in the absence of any commercial or financial relationships that could be construed as a potential conflict of interest.
Publisher’s Note
All claims expressed in this article are solely those of the authors and do not necessarily represent those of their affiliated organizations, or those of the publisher, the editors and the reviewers. Any product that may be evaluated in this article, or claim that may be made by its manufacturer, is not guaranteed or endorsed by the publisher.
Acknowledgments
We thank Karen Rivara of Aeros Cultured Oyster hatchery in Southold, NY for suppling Crassostrea virginica, Brooke Morell and Marissa Velasquez for their efforts in growing and collecting Saccharina latissima in Moriches Bay, NY, and Paul McCormick at the Great Gun Shellfish Farm in Moriches Bay, NY for use of his farm and assistance in cultivating kelp used in these experiments. We greatly appreciate the logistic support provided by the Stony Brook Southampton Marine Science Center staff throughout this study.
Supplementary Material
The Supplementary Material for this article can be found online at: https://www.frontiersin.org/articles/10.3389/fmars.2022.881254/full#supplementary-material
References
Agostini S., Harvey B. P., Milazzo M., Wada S., Kon K., Floc'h N., et al. (2021). Simplification, Not ‘Tropicalization’, of Temperate Marine Ecosystems Under Ocean Warming and Acidification. Global Change Biol. 27 (19), 4771–4784. doi: 10.1111/gcb.15749
Anderson D. M., Burkholder J. M., Cochlan W. P., Glibert P. M., Gobler C. J., Heil C. A., et al. (2008). Harmful Algal Blooms and Eutrophication: Examining Linkages From Selected Coastal Regions of the United States. Harm. Algae. 8, 39–53. doi: 10.1016/j.hal.2008.08.017
Anderson D. M., Glibert P. M., Burkholder J. M. (2002). Harmful Algal Blooms and Eutrophication: Nutrient Sources, Composition, and Consequences. Estuaries 25, 704–726. doi: 10.1007/BF02804901
Anthony K. R. N., Diaz-Pulido G., Verlinden N., Tilbrook B., Andersson A. J. (2013). Benthic Buffers and Boosters of Ocean Acidification on Coral Reefs. Biogeosciences 10, 4897–4909. doi: 10.5194/bg-10-4897-2013
Barton A., Hales B., Waldbusser G. G., Langdon C., Feely R. A. (2012). The Pacific Oyster, Crassostrea Gigas, Shows Negative Correlation to Naturally Elevated Carbon Dioxide Levels: Implications for Near-Term Ocean Acidification Effects. Limnol. Oceanograph. 57 (3), 698–710. doi: 10.4319/lo.2012.57.3.0698
Bartsch I., Lars G., Olischläger M., Rahmann M. M., Roleda M. Y., Sarker Y., et al. (2010). “Ocean Acidification Effects on Growth, Photosynthesis and Trophic Status of Seaweeds”, in Joint EPOCA, BIOACID and UKOARP Meeting (Bremerhaven).
Baumann H., Wallace R. B., Tagliaferri T., Gobler C. J. (2015). Large Natural Ph, CO2 and O2 Fluctuations in a Temperate Tidal Salt Marsh on Diel, Seasonal, and Interannual Time Scales. Estuaries. Coast. 38 (1), 220–231. doi: 10.1007/s12237-014-9800-y
Beniash E., Ivanina A., Lieb N. S., Kurochkin I., Sokolova I. M. (2010). Elevated Level of Carbon Dioxide Affects Metabolism and Shell Formation in Oysters Crassostrea Virginica. Mar. Ecol. Prog. Ser. 419, 95–108. doi: 10.3354/meps08841
Benitt C., Young C. S., Sylvers P., Gobler C. J. (2022). Inhibition of Harmful Algal Blooms Caused by Aureococcus Anophagefferens (Pelagophyceae) Using Native (Gracilaria Tikvahiae) and Invasive (Dasysiphonia Japonica) Red Seaweeds From North America. J. Appl. Phycol. (2022) 34, 965–983 doi: 10.1007/s10811-021-02677-9
Boderskov T., Schmedes P. S., Bruhn A., Rasmussen M. B., Nielsen M. M., Pedersen M. F. (2016). The Effect of Light and Nutrient Availability on Growth, Nitrogen, and Pigment Contents of Saccharina Latissima (Phaeophyceae) Grown in Outdoor Tanks, Under Natural Variation of Sunlight and Temperature, During Autumn and Early Winter in Denmark. J. Appl. Phycol. 28 (2), 1153–1165. doi: 10.1007/s10811-015-0673-7
Bricker S. B., Longstaff B., Dennison W., Jones A., Boicourt K., Wicks C., et al. (2008). Effects of Nutrient Enrichment in the Nation's Estuaries: A Decade of Change. Harm. Algae. 8, 21–32. doi: 10.1016/j.hal.2008.08.028
Brinkhuis B. H., Breda V. A., Tobin S., Macler B. A. (1983). New York Marine Biomass Program-Culture of Laminaria Saccharina. J. World Mariculture. Soc. 14, 360–379. doi: 10.1111/j.1749-7345.1983.tb00090.x
Cai W.-J., Huang W.-J., Luther G. W., Pierrot D., Li M., Testa J., et al. (2017). Redox Reactions and Weak Buffering Capacity Lead to Acidification in the Chesapeake Bay. Nat. Commun. 8 (1), 369. doi: 10.1038/s41467-017-00417-7
Cai W.-J., Hu X., Huang W.-J., Murrell M. C., Lehrter J. C., Lohrenz S. E., et al. (2011). Acidification of Subsurface Coastal Waters Enhanced by Eutrophication. Nat. Geosci. 4, 766–770. doi: 10.1038/ngeo1297
Carriker M. R. (1996). “"The Shell and Ligment,",” in The Eastern Oyster: Crassostrea Virginica. Eds. Kennedy V. S., Newell R. I. E., Eble A. E. (Maryland Sea Grant College: University of Maryland System), 75–168.
Chung I. K., Beardall J., Mehta S., Sahoo D., Stojkovic S. (2011). Using Marine Macroalgae for Carbon Sequestration: A Critical Appraisal. J. Appl. Phycol. 23, 877–886. doi: 10.1007/s10811-010-9604-9
Chung I. K., Oak J. H., Lee J. A., Shin J. A., Kim J. G., Park K.-S. (2013). Installing Kelp Forests/Seaweed Beds for Mitigation and Adaptation Against Global Warming: Korean Project Overview. ICES. J. Mar. Sci. 70 (5), 1038–1044. doi: 10.1093/icesjms/fss206
Cornwall C. E., Hepburn C. D., McGraw C. M., Currie K. I., Pilditch C. A., Hunter K. A., et al. (2013). “Diurnal Fluctuations in Seawater pH Influence the Response of a Calcifying Macroalga to Ocean Acidification,” in Proceedings of the Royal Society B: Biological Sciences, Vol. 280. 20132201. doi: 10.1098/rspb.2013.2201
Delille B., Borges A. V., Delille D. (2009). Influence of Giant Kelp Beds (Macrocystis Pyrifera) on Diel Cycles of Pco2 and DIC in the Sub-Antarctic Coastal Area. Estuarine. Coast. Shelf. Sci. 81 (1), 114–122. doi: 10.1016/j.ecss.2008.10.004
Delille B., Delille D., Fiala M., Prevost C., Frankignoulle M. (2000). Seasonal Changes of Pco2 Over a Subantarctic Macrocystis Kelp Bed. Polar. Biol. 23, 706–716. doi: 10.1007/s003000000142
Doney S. C., Busch D. S., Cooley S. R., Kroeker K. J. (2020). The Impacts of Ocean Acidification on Marine Ecosystems and Reliant Human Communities. Annu. Rev. Environ. Resour. 45, 83–112. doi: 10.1146/annurev-environ-012320-083019
Duggins D. O., Eckman J. E. (1997). Is Kelp Detritus a Good Food for Suspension Feeders? Effects of Kelp Species, Age, and Secondary Metabolites. Mar. Biol. 128, 489–495. doi: 10.1007/s002270050115
Duggins D. O., Simenstad C. A., Estes J. A. (1989). Magnification of Secondary Production by Kelp Detritus in Coastal Marine Ecosystems. Science 245 (4914), 170–173. doi: 10.1126/science.245.4914.170
Feely R. A., Doney S. C., Cooley S. R. (2009). Ocean Acidification: Present Conditions and Future Changes in a High-CO2 World. Oceanography 22 (4), 36–47. doi: 10.5670/oceanog.2009.95
Filbee-Dexter K., Feehan C. J., Scheibling R. E. (2016). Large-Scale Degradation of a Kelp Ecosystem in an Ocean Warming Hotspot. Mar. Ecol. Prog. Ser. 543, 141–152. doi: 10.3354/meps11554
Gao K., Aruga Y., Asada K., Kiyoharda M. (1993). Influence of Enhanced CO2 on Growth and Photosynthesis of the Red AlgaeGracilaria Sp. And G. Chilensis. J. Appl. Phycol. 5 (6), 563–571. doi: 10.1007/BF02184635
Gazeau F., Parker L. M., Comeau S., Gattuso J.-P., O'Conner W. A., Martin S., et al. (2013). Impacts of Ocean Acidification on Marine Shelled Molluscs. Mar. Biol. 160, 2207–2245. doi: 10.1007/s00227-013-2219-3
Gazeau F., Quiblier C., Jansen J. M., Gattuso J.-P., Middelburg J. J., Heip C. H. R. (2007). Impact of Elevated CO2 on Shellfish Calcification. Geophy. Res. Lett. 34, L07603. doi: 10.1029/2006GL028554
Gobler C. J., DePasquale E. L., Griffith A. W., Baumann H. (2014). Hypoxia and Acidification Have Additive and Synergistic Negative Effects on the Growth, Survival, and Metamorphosis of Early Life Stage Bivalves. PloS One 9 (1), e83648. doi: 10.1371/journal.pone.0083648
Grebe G. S., Byron C. J., Gelais A. S., Kotowicz D. M., Olson T. K. (2019). An Ecosystem Approach to Kelp Aquaculture in the Americas and Europe. Aquaculture. Rep. 15, 100215. doi: 10.1016/j.aqrep.2019.100215
Green M. A., Waldbusser G. G., Reilly S. L., Emerson K., O'Donnell S. (2009). Death by Dissolution: Sediment Saturation State as a Mortality Factor for Juvenile Bivalves. Limnol. Oceanograph. 54, 1037–1047. doi: 10.4319/lo.2009.54.4.1037
Griffith A. W., Gobler C. J. (2017). Transgenerational Exposure to North Atlantic Bivalves to Ocean Acidification Renders Offspring More Vulnerable to Low pH and Additional Stressors. Sci. Rep. 7 (1), 11394. doi: 10.1038/s41598-017-11442-3
Hattenrath-Lehmann T. K., Smith J. L., Wallace R. B., Merlo L. R., Koch F., Mittelsdorf H., et al. (2015b). The Effects of Elevated CO2 on the Growth and Toxicity of Field Populations and Cultures of the Saxitoxin-Producing Dinoflagellate, Alexandrium Fundyense. Limnol. Oceanograph. 60, 198–214. doi: 10.1002/lno.10012
Helm M. M., Bourne N., Lovatelli A. (2004). Hatchery Culture of Bivalves: A Practical Manual (Rome: Food and Agriculture Organization of the United Nations).
Hepburn C. D., Pritchard D. W., Cornwall C. E., McLeod R. J., Beardall J., Raven J. A. (2011). Diversity of Carbon Use Strategies in a Kelp Forest Community: Implications for a High CO2 Ocean. Global Change Biol. 17 (7), 2488–2497. doi: 10.1111/j.1365-2486.2011.02411.x
Hoagland P., Anderson D. M., Kaoru Y., White A. W. (2002). The Economic Effects of Harmful Algal Blooms in the United States: Estimates, Assessment Issues, and Information Needs. Estuaries 25, 819–837. doi: 10.1007/BF02804908
Hofmann G. E., Smith J. E., Johnson K. S., Send U., Levin L. A., Micheli F., et al. (2011). High-Frequency Dynamics of Ocean Ph: A Multi-Ecosystem Comparison. PloS One 6 (12), e28983. doi: 10.1371/journal.pone.0028983
Jiang Z., Liu J., Li S., Chen Y., Du P., Zhu Y., et al. (2020). Kelp Cultivation Effectively Improves Water Quality and Regulates Phytoplankton Community in a Turbid, Highly Eutrophic Bay. Sci. Total. Environ. 707, 135561. doi: 10.1016/j.scitotenv.2019.135561
Kim J. K., Kraemer G. P., Yarish C. (2015). Use of Sugar Kelp Aquaculture in Long Island Sound and the Bronx River Estuary for Nutrient Extraction. Mar. Ecol. Prog. Ser. 531, 155–166. doi: 10.3354/meps11331
Kim J., Stekoll M., Yarish C. (2019). Opportunities, Challenges and Future Directions of Open-Water Seaweed Aquaculture in the United States. Phycologia 58 (5), 446–461. doi: 10.1080/00318884.2019.1625611
Koch M., Bowes G., Ross C., Zhang X.-H. (2013). Climate Change and Ocean Acidification Effects on Seagrasses and Marine Macroalgae. Global Change Biol. 19, 103–132. doi: 10.1111/j.1365-2486.2012.02791.x
Levinton J. S., Shumway S. E. (2002). Feeding Responses of the Bivalves Crassostrea Gigas and Mytilus Trossulus to Chemical Composition of Fresh and Aged Kelp Detritus. Mar. Biol. 141, 367–376. doi: 10.1007/s00227-002-0830-9
Marinho G., Holdt S. L., Birkeland M. J., Angelidaki I. (2015). Commercial Cultivation and Bioremediation Potential of Sugar KelpSaccharina Latissima, in Danish Waters. J. Appl. Phycol. 27, 1963–1973. doi: 10.1007/s10811-014-0519-8
Marinho-Soriano E., Nunes S. O., Carneiro M. A. A., Pereira D. C. (2009). Nutrients' Removal From Aquaculture Wastewater Using the Macroalgae Gracilaria Birdiae. Biomass Bioenergy 33 (2), 327–331. doi: 10.1016/j.biombioe.2008.07.002
Matoo O. B., Ivanina A. V., Ullstad C., Beniash E., Sokolova I. M. (2013). Interactive Effects of Elevated Temperature and CO2 Levels on Metabolism and Oxidative Stress in Two Common Marine Bivalves (Crassostrea Virginica and Mercenaria Mercenaria). Comp. Biochem. Physiol. Part A 164, 545–553. doi: 10.1016/j.cbpa.2012.12.025
Meehl G. A., Stocker T. F., Collins W. D., Friedlingstein P., Gaye A. T., Gregory J. M., et al. (2007). “"Global Climate Projections",” in Climate Change 2007: The Physical Science Basis. Contribution of Working Group I to the Fourth Assessment Report of the Intergovernmental Panel on Climate Change. Eds. Solomon S. D., Qin M. M., Chen Z., Marquis M., Averyt K. B., Tignor M., Miller H. L. (Cambridge: Cambridge University Press).
Melzner F., Jörn T., Koeve W., Oschlies A., Gutowska M. A., Bange H. W., et al. (2013). Future Ocean Acidification Will be Amplified by Hypoxia in Coastal Habitats. Mar. Biol. 160, 1875–1888. doi: 10.1007/s00227-012-1954-1
Mercado J. M., Gordillo F. J. L., Niella F. X., Figueroa F. L. (1998). External Carbonic Anhydrase and Affinity for Inorganic Carbon in Intertidal Macroalgae. J. Exp. Mar. Biol. Ecol. 221, 209–220. doi: 10.1016/S0022-0981(97)00127-5
Millero F. J. (2010). History of the Equation of State of Seawater. Oceanography 23 (3), 18–33. doi: 10.5670/oceanog.2010.21
Mongin M., Baird M. E., Hadley S., Lenton A. (2016). Optimising Reef-Scale CO2 Removal by Seaweed to Buffer Ocean Acidification. Environ. Res. Lett. 11, 34023. doi: 10.1088/1748-9326/11/3/034023
Moy F.E., Christie H. (2012). Large-Scale Shift From Sugar Kelp (Saccharina latissima) to Ephemeral Algae Along the South and West Coast of Norway. Mar. Biol. Res. 8 (4), 309–321. doi: 10.1080/17451000.2011.637561
Newell R. I. E. (1988). Ecological Changes in Chesapeake Bay: Are They the Result of Overharvesting the American Oyster, Crassostrea Virginica? Understand. Estuary.: Adv. Chesapeake. Bay. Res. 129, 536–546.
Newell R. I. (2004). Ecosystem Influences of Natural and Cultivated Populations of Suspension-Feeding Bivalve Molluscs: A Review. J. Shellfish. Res. 23 (1), 51–62.
Norderhaug K. M., Christie H., Fosså J. H., Fredriksen S. (2005). Fish-Macrofauna Interactions in a Kelp (Laminaria Hyperborea) Forest. J. Mar. Biol. Assoc. Unite. Kingdom. 85, 1279–1286. doi: 10.1017/S0025315405012439
O'Brien B. S., Mello K., Litterer A., Dijkstra J. A. (2018). Seaweed Structure Shapes Trophic Interactions: A Case Study Using a Mid-Trophic Level Fish Species. J. Exp. Mar. Biol. Ecol. 506, 1–8. doi: 10.1016/j.jembe.2018.05.003
Olischläger M., Bartsch I., Gutow L., Wiencke C. (2013). Effects of Ocean Acidification on Growth and Physiology of Ulva Lactuca (Chlorophyta) in a Rockpool-Scenario. Phycol. Res. 61 (3), 180–190. doi: 10.1111/pre.12006
Palacios S. L., Zimmerman R. C. (2007). Response of Eelgrass Zostera Marina to CO2 Enrichment: Possible Impacts of Climate Change and Potential for Remediation of Coastal Habitats. Mar. Ecol. Prog. Ser. 344, 1–13. doi: 10.3354/meps07084
Ramesh K., Melzner F., Griffith A. W., Gobler C. J., Rouger C., Tasdemir D., et al. (2018). In Vivo Characterization of Bivalve Larval Shells: A Confocal Raman Microscopy Study. J. R. Soc. Interface 15 (141), 20170723. doi: 10.1098/rsif.2017.0723
Saderne V., Fietzek P., Aßmann S., Körtzinger A., Hiebenthal C. (2015). Seagrass Beds as Ocean Acidification Refuges for Mussels? High Resolution Measurements of Pco2 and O2 in a Zostera Marina and Mytilus Edulis Mosaic Habitat. Biogeosci. Discuss. 12 (14), 11423–11461. doi: 10.5194/bgd-12-11423-2015
Shpigel M. (2005). “"Bivalves as Biofilters and Valuable Byproducts in Land-Based Aquaculture Systems,",” in The Comparative Roles of Suspension-Feeders in Ecosystems. NATO Science Series IV: Earth and Environmental Series. Eds. Dame R. F., Olenin S. (Dordrecht: Springer).
Shumway S. E. (1990). A Review of the Effects of Algal Blooms on Shellfish and Aquaculture. J. World Aquaculture. Soc. 21 (2), 65–104. doi: 10.1111/j.1749-7345.1990.tb00529.x
Sivertsen K., Bjørge A. (2014). On the Brink of the Arctic: Unusual Intertidal Sub-Arctic Kelp Associations in the Porsangerfjord, North Norway. Mar. Biol. Res. 11 (4), 405–413. doi: 10.1080/17451000.2014.940975
Smale D. A., Burrows M. T., Moore P., O'Connor N., Hawkins S. J. (2013). Threats and Knowledge Gaps for Ecosystem Services Provided by Kelp Forests: A Northeast Atlantic Perspective. Ecol. Evol. 3 (11), 4016–4038. doi: 10.1002/ece3.774
Steneck R. S., Graham M. H., Bourque B. J., Corbett D., Erlandson J. M., Estes J. A., et al. (2002). Kelp Forest Ecosystems: Biodiversity, Stability, Resilience and Future. Environ. Conserv. 29 (4), 436–459. doi: 10.1017/S0376892902000322
Stenzel H. B. (1964). Oysters: Composition of the Larval Shell. Science 304, 1005–1008. doi: 10.1126/science.145.3628.155
Stevens A. M., Gobler C. J. (2018). Interactive Effects of Acidification, Hypoxia, and Thermal Stress on Growth, Respiration, and Survival of Four North Atlantic Bivalves. Mar. Ecol. Prog. Ser. 604, 143–161. doi: 10.3354/meps12725
Sylvers P., Gobler C. J. (2021). Mitigation of Harmful Algal Blooms Caused by Alexandrium Catenella and Reduction in Saxitoxin Accumulation in Bivalves Using Cultivable Seaweeds. Harm. Algae. 105, 102056. doi: 10.1016/j.hal.2021.102056
Talmage S. C., Gobler C. J. (2009). The Effects of Elevated Carbon Dioxide Concentrations on the Metamorphosis, Size, and Survival of Larval Hard Clams (Mercenaria Mercenaria), Bay Scallops (Argopecten Irradians), and Eastern Oysters (Crassostrea Virginica). Limnol. Oceanograph. 54 (6), 2072–2080. doi: 10.4319/lo.2009.54.6.2072
Talmage S. C., Gobler C. J. (2010). “Effects of Past, Present, and Future Ocean Carbon Dioxide Concentrations on the Growth and Survival of Larval Shellfish,” in Proceedings of the National Academy of Sciences of the United States of America, Vol. 107. 17246–17251. doi: 10.1073/pnas.0913804107
Talmage S. C., Gobler C. J. (2011). Effects of Elevated Temperature and Carbon Dioxide on the Growth and Survival of Larvae and Juveniles of Three Species of Northwest Atlantic Bivalves. PloS One 6 (10), e26941. doi: 10.1371/journal.pone.0026941
Tang Y. Z., Gobler C. J. (2011). The Green MacroalgaUlva Lactuca, Inhibits the Growth of Seven Common Harmful Algal Bloom Species via Allelopathy. Harm. Algae. 10 (5), 480–488. doi: 10.1016/j.hal.2011.03.003
Tang Y. Z., Kang Y., Berry D., Gobler C. J. (2015). The Ability of the Red Macroalga, Porphyra Purpurea (Rhodophyceae) to Inhibit the Proliferation of Seven Common Harmful Microalgae. J. Appl. Phycol. 27 (1), 531–544. doi: 10.1007/s10811-014-0338-y
Theuerkauf S. J., Barrett L. T., Alleway H. K., Costa-Pierce B. A., St. Gelais A., Jones R. C. (2021). Habitat Value of Bivalve Shellfish and Seaweed Aquaculture for Fish and Invertebrates: Pathways, Synthesis and Next Steps. Rev. Aquaculture. 14 (1), 54–72. doi: 10.1111/raq.12584
Wahl M., Schneider Covachã S., Saderne V., Hiebenthal C., Müller J. D., Pansch C., et al. (2018). Macroalgae may Mitigate Ocean Acidification Effects on Mussel Calcification by Increasing pH and its Fluctuations. Limnol. Oceanograph. 63 (1), 3–21. doi: 10.1002/lno.10608
Waldbusser G. G., Brunner E. L., Haley B. A., Hales B., Langdon C. J., Prahl F. G. (2013). A Developmental and Energetic Basis Linking Larval Oyster Shell Formation to Acidification Sensitivity. Geophy. Res. Lett. 40 (10), 2171–2176. doi: 10.1002/grl.50449
Waldbusser G., Hales B., Langdon C. J., Haley B. A., Schrader P., Brunner E. L., et al. (2015). Saturation-State Sensitivity of Marine Bivalve Larvae to Ocean Acidification. Nat. Climate Change 5, 273–280. doi: 10.1002/grl.50449
Waldbusser G. G., Hales B., Langdon C., Haley B. A., Schrader P., Brunner E. L., et al. (2015). Ocean Acidification has Multiple Modes of Action on Bivalve Larvae. PloS One 10 (6), e0128376. doi: 10.1371/journal.pone.0128376
Wallace R. B., Baumann H., Grear J. S., Aller R. C., Gobler C. J. (2014). Coastal Ocean Acidification: The Other Eutrophication Problem. Estuarine. Coast. Shelf. Sci. 148, 1–13. doi: 10.1016/j.ecss.2014.05.027
Wallace R. B., Gobler C. J. (2015). Factors Controlling Blooms of Microalgae and Macroalgae (Ulva Rigida) in a Eutrophic, Urban Estuary: Jamaica Bay, NY, USA. Estuaries. Coast. 38 (2), 519–533. doi: 10.1007/s12237-014-9818-1
Wallace R. B., Gobler C. J. (2021). The Role of Algal Blooms and Community Respiration in Controlling the Temporal and Spatial Dynamics of Hypoxia and Acidification in Eutrophic Estuaries. Mar. Poll. Bull. 172, 112908. doi: 10.1016/j.marpolbul.2021.112908
Wallace R. B., Peterson B. J., Gobler C. J. (2021). Ecosystem Metabolism Modulates the Dynamics of Hypoxia and Acidification Across Temperate Coastal Habitat Types. Front. Mar. Sci (8), 611781. doi: 10.3389/fmars.2021.611781
Wall C. C., Peterson B. J., Gobler C. J. (2008). Facilitation of Seagrass Zostera Marina Productivity by Suspension-Feeding Bivalves. Mar. Ecol. Prog. Ser. 357, 165–174. doi: 10.3354/meps07289
Walls A. M., Kennedy R., Fitzgerald R. D., Blight A. J., Johnson M. P., Edwards M. D. (2016). Potential Novel Habitat Created by Holdfasts From Cultivated Laminaria Digitata: Assessing the Macroinvertebrate Assemblages. Aquaculture. Environ. Interact. 8, 157–169. doi: 10.3354/aei00170
Wang R., Xiao H., Wang Y., Zhou W., Tang X. (2007). Effects of Three Macroalgae, Ulva Linza (Chlorophyta), Corallina Pilulifera (Rhodophyta) and Sargassum Thunbergii (Phaeophyta) on the Growth of the Red Tide Microalga Prorocentrum Donghaiense Under Laboratory Conditions. J. Sea. Res. 58 (3), 189–197. doi: 10.1016/j.seares.2007.03.002
Weiss I. M., Tuross N., Addadi L., Weiner S. (2002). Mollusc Larval Shell Formation: Amorphous Calcium Carbonate is a Precursor Phase for Aragonite. J. Exp. Zoology. 293, 478–491. doi: 10.1002/jez.90004
Wernberg T., Thomsen M. S., Tuya F., Kendrick G. A., Staehr P. A., Toohey B. D. (2010). Decreasing Resilience of Kelp Beds Along a Latitudinal Temperature Gradient: Potential Implications for a Warmer Future. Ecol. Lett. 13 (6), 685–694. doi: 10.1111/j.1461-0248.2010.01466.x
Xiao X., Agustí S., Yu Y., Huang Y., Chen W., Hu J., et al. (2021). Seaweed Farms Provide Refugia From Ocean Acidification. Sci. Total. Environ. 776, 145192. doi: 10.1016/j.scitotenv.2021.145192
Xu D., Gao Z., Zhang X., Qi Z., Meng C., Zhuang Z., et al. (2011). Evaluation of the Potential Role of the Macroalga Laminaria Japonica for Alleviating Coastal Eutrophication. Bioresource. Technol. 102 (21), 9912–9918. doi: 10.1016/j.biortech.2011.08.035
Young C. S., Doall M. H., Gobler C. J. (2021). The Dual Benefit of Ocean Acidification for the Laminarialean Kelp, Saccharina Latissima: Enhanced Growth and Reduced Herbivory. Mar. Ecol. Prog. Ser. 664, 87–102. doi: 10.3354/meps13659
Young C. S., Gobler C. J. (2016). Ocean Acidification Accelerates the Growth of Two Bloom-Forming Macroalgae. PloS One 11 (5), e0155152. doi: 10.1371/journal.pone.0155152
Young C. S., Gobler C. J. (2018). The Ability of Macroalgae to Mitigate the Negative Effects of Ocean Acidification on Four Species of North Atlantic Bivalve. Biogeosciences 15 (20), 6167–6183. doi: 10.5194/bg-15-6167-2018
Zhao L., Liu L., Liu B., Liang J., Lu Y., Yang F. (2019). Antioxidant Responses to Seawater Acidification in an Invasive Fouling Mussel are Alleviated by Transgenerational Acclimation. Aquat. Toxicol. 217, 105331. doi: 10.1016/j.aquatox.2019.105331
Keywords: ocean acidification, macroalgae, bivalves, aquaculture, chemical refuge
Citation: Young CS, Sylvers LH, Tomasetti SJ, Lundstrom A, Schenone C, Doall MH and Gobler CJ (2022) Kelp (Saccharina latissima) Mitigates Coastal Ocean Acidification and Increases the Growth of North Atlantic Bivalves in Lab Experiments and on an Oyster Farm. Front. Mar. Sci. 9:881254. doi: 10.3389/fmars.2022.881254
Received: 22 February 2022; Accepted: 21 March 2022;
Published: 19 April 2022.
Edited by:
Menghong Hu, Shanghai Ocean University, ChinaReviewed by:
Liqiang Zhao, Guangdong Ocean University, ChinaJason Michael Hall-Spencer, University of Plymouth, United Kingdom
Copyright © 2022 Young, Sylvers, Tomasetti, Lundstrom, Schenone, Doall and Gobler. This is an open-access article distributed under the terms of the Creative Commons Attribution License (CC BY). The use, distribution or reproduction in other forums is permitted, provided the original author(s) and the copyright owner(s) are credited and that the original publication in this journal is cited, in accordance with accepted academic practice. No use, distribution or reproduction is permitted which does not comply with these terms.
*Correspondence: Christopher J. Gobler, Q2hyaXN0b3BoZXIuZ29ibGVyQHN0b255YnJvb2suZWR1