- 1Centro de Investigação em Biodiversidade e Recursos Genéticos (CIBIO), Centro de Investigação em Biodiversidade e Recursos Genéticos, InBIO Laboratório Associado, Vairão, Portugal
- 2Program in Genomics, Biodiversity and Land Planning (BIOPOLIS), Biodiversity and Land Planning, Campus de Vairão, Vairão, Portugal
Global warming has been causing severe impacts on marine ecosystems, a notorious one being shifts in the geographical ranges of species. The north-western coast of the Iberian Peninsula is an especially interesting zone to study distributional shifts as it has a strong latitudinal thermal gradient, is influenced by the Canary upwelling system (which partially cancels coastal warming) and holds some of the most diverse macroalgae communities in Europe. Notably, it is within this region that many cold-water species, common in northern Europe, have their southernmost distribution refuge. Recent studies hypothesize that the environmental conditions may be nonetheless changing and already threatening this biodiversity hotspot. The main goal of this study was to carry out a fine-scale assessment of the distributional limits of several macroalgae in North-western Iberia, as well as identify possible population and range shifts using historical data (2001-2005) as reference. In addition, non-indigenous species were also surveyed. We also assessed if the regions of (i) Galicia, (ii) Northern Portugal, and (iii) Central Portugal displayed distinctive characters regarding macroalgae composition and abundance. We identified an increase in abundance of some non-indigenous macroalgae as well as a decrease in the abundance of some cold-water species. In the most severe cases, cold-water species were extirpated along hundreds of km. The compounded effect of the decrease in the abundance of cold-water species and the increase in the abundance of non-indigenous species is leading to the homogenization of macroalgae communities in north-western Iberia.
1 Introduction
Climate change is affecting the biodiversity, structure and function of coastal ecosystems, especially in rocky intertidal zones (Jurgens et al., 2015; Poloczanska et al., 2016; Ullah et al., 2018). One of the most pervasive effects of climate change in marine species has been the change in their distributional ranges, with species of temperate or boreal origin disappearing from sites where temperatures surpass their higher thermal tolerance limits, and with tropical or warm-temperate species reaching locations once unfavorably cold. While the first is concerning because we risk losing biodiversity and/or genetic diversity with cascading effects throughout the ecosystem, the second could also be damaging, especially in the case of invasive species, owing to their characteristic potential to alter entire communities (Stachowicz et al., 2002; Hawkins et al., 2009; Antão et al., 2020).
Near the distribution limit of a species, populations are expected to experience sub-optimal conditions that might render them more vulnerable than populations in the center of the distribution (Hampe and Petit, 2005). This leads to greater impacts and lower recovery capacity of these populations in the case of changes at large spatial scales, such as those caused by climate change (Pearson et al., 2009; Viana et al., 2014; Rubal et al., 2015). In this respect, macroalgae are relevant organisms to study since temperature is an important driver of their key life cycle events, regulating reproduction and growth (Harley et al., 2012). In addition, macroalgae play a pivotal role in coastal ecosystems, given that they are primary producers, provide habitat and nursery grounds for a wide variety of species (of which many are economically relevant), and their canopies offer a buffer from the harsh physical conditions of the intertidal (Smale et al., 2013; Seitz et al., 2014; Bulleri et al., 2018).
In parallel, marine non-indigenous species (NIS sensu Richardson et al., 2011) are spreading at an ever-faster pace due to the increase in vectors of introduction (mainly maritime traffic and marine aquaculture) and the facilitation from climate change (McKnight et al., 2021). Monitoring biological introductions is necessary as some invasive macroalgae are at the forefront of ecological change by outcompeting native taxa, modifying the habitat and threatening biodiversity (Mineur et al., 2015; McKnight et al., 2021).
Northern Portugal is a relevant area to study the effects of climate change in the biodiversity and biogeography of intertidal macroalgae because it is a transition zone – representing the rear edge for several cold-temperate species and the leading edge or biogeographical gap for warm-water species. In particular, for several cold-water macroalgae typical of higher latitudes, northern Portugal is the southern edge of their distribution in Europe resulting in a regional biodiversity hotspot (Van den Hoek and Donze, 1967). From a climatic and oceanographic standpoint, northern Portugal and Galicia (north-western Iberia) are remarkable due to their markedly colder sea surface summer temperatures in comparison with neighboring regions, a pattern driven by the Canary Upwelling System (Fraga, 1981; Fiuza, 1983). Nevertheless, an overall increase in air temperature (Lima et al., 2007), associated with more intense heat waves and less prominent cold spells have been observed in the last decades in the region (Espírito Santo et al., 2014). In the sea, average and extreme temperatures have been increasing as well (Lima and Wethey, 2012) with some evidence suggesting that the buffering effect of cold upwelling may be slowing down (Seabra et al., 2019). Heat stress, either when caused by desiccation and elevated air temperatures during low tide (Pearson et al, 2009), by warm high-tide water temperatures (Mota et al., 2018) or by a combination of both (Román et al., 2020), is known to negatively affect the physiology of intertidal macroalgae. Work published thus far already indicates changes in the distribution and abundance of intertidal taxa in Northern Portugal, and further changes are expected under current climate projections (Lima et al., 2006; Wethey et al., 2011; Rubal et al., 2013; Casado-Amezúa et al., 2019), though not always in the direction expected given recent local patterns of climatic changes (Lima et al., 2007; Lima et al., 2009; Seabra et al., 2015).
Here we analyze the distribution and abundance of a selected group of macroalgae in northern Portugal with a fine-scale ad-libitum timed search designed to capture the small-scale variability between sites and robustly identify abundances at or near the distribution limits of selected species. These data were then contrasted against historical surveys in the area (Lima et al., 2007; Pereira et al., 2021a) to assess possible range shifts of warm-water, cold-water and thermally neutral species. Additionally, we also explored if the regions of Galicia, northern Portugal, and central Portugal display distinctive characters regarding macroalgae composition and abundance, and how those characters might have been recently changing.
2 Material and methods
2.1 Selection of species and sites
To detail distributional limits of intertidal macroalgae species in northern Portugal and neighboring regions - Galicia and central Portugal - we selected a list of 34 species to be surveyed with high spatial resolution (52 locations over 750 km). We then applied a survey method (ad-libitum timed search with in-situ determination of abundance on a semi-logarithmic scale - SACFOR) appropriate to detect rare species, from our list of selected species (such as cold-water species disappearing from a region or warm-water species recently arriving), in highly variable environments (such as the intertidal), see description in 2.3.
Species were selected based on previous studies (Veiga et al., 2014; Pereira et al., 2021a) that identified macroalgae with range limits in the area (either rear- or poleward-edge) or that reached neighboring regions and thus could be in the process of migrating into the area facilitated by ongoing environmental changes and/or anthropogenic disturbance (e.g., NIS, Supplementary Table 1 and Pereira et al., 2022). Importantly, Lima and colleagues have previously described that while warm-water species in this region had been moving northwards, cold-water species had been moving on both directions, with no clear trend (Lima et al., 2007). Hence, to better understand the direction of change and to compare our results with those previous findings, we classified species as having “cold-water”, “warm-water” or “neutral” affinity. The classification was based on how their Species Temperature Index (STI) compared with temperatures in the region - if it was lower, higher, or in-between the range of average temperatures in surveyed sites, respectively. The STI is the median of temperatures recorded in the area where the species is known to occur (Burrows et al., 2020), here calculated for the North Atlantic only. Then, to compare between regions and between historical and present-day surveys, we computed the average STI of all surveyed species, per site. For details, see Pereira et al. (2022).
Sites surveyed included nearly all wave-exposed rocky shores (38) in our region of interest – northern Portugal. In addition, four sites in central Portugal and 11 in Galicia (north-west Spain) were used to determine the uniqueness of northern Portugal relative to its neighboring regions, in a total of 52 sites (Figure 1; Supplementary Table 2). Twenty-three of the locations had been surveyed in the past (2001-2005) by the same research group (Pereira et al., 2021a; Pereira et al., 2021b; Pereira et al., 2021c, unpublished data). Sampling occurred between October 2020 and August 2021. To account for seasonality in species presence and abundance, some sites (6) were visited twice at different seasons. Furthermore, we sampled 18 additional sites with artificial hard substrate to finely track the ongoing early-stage invasion of the kelp Undaria pinnatifida (already reported in 2 locations in Portugal at the beginning of our surveys, Veiga et al., 2014). This subset of sites where all located within large stretches of sandy coast, and some were in proximity of marinas and harbors, Undaria’s likely entry points (Pereira et al., 2022).
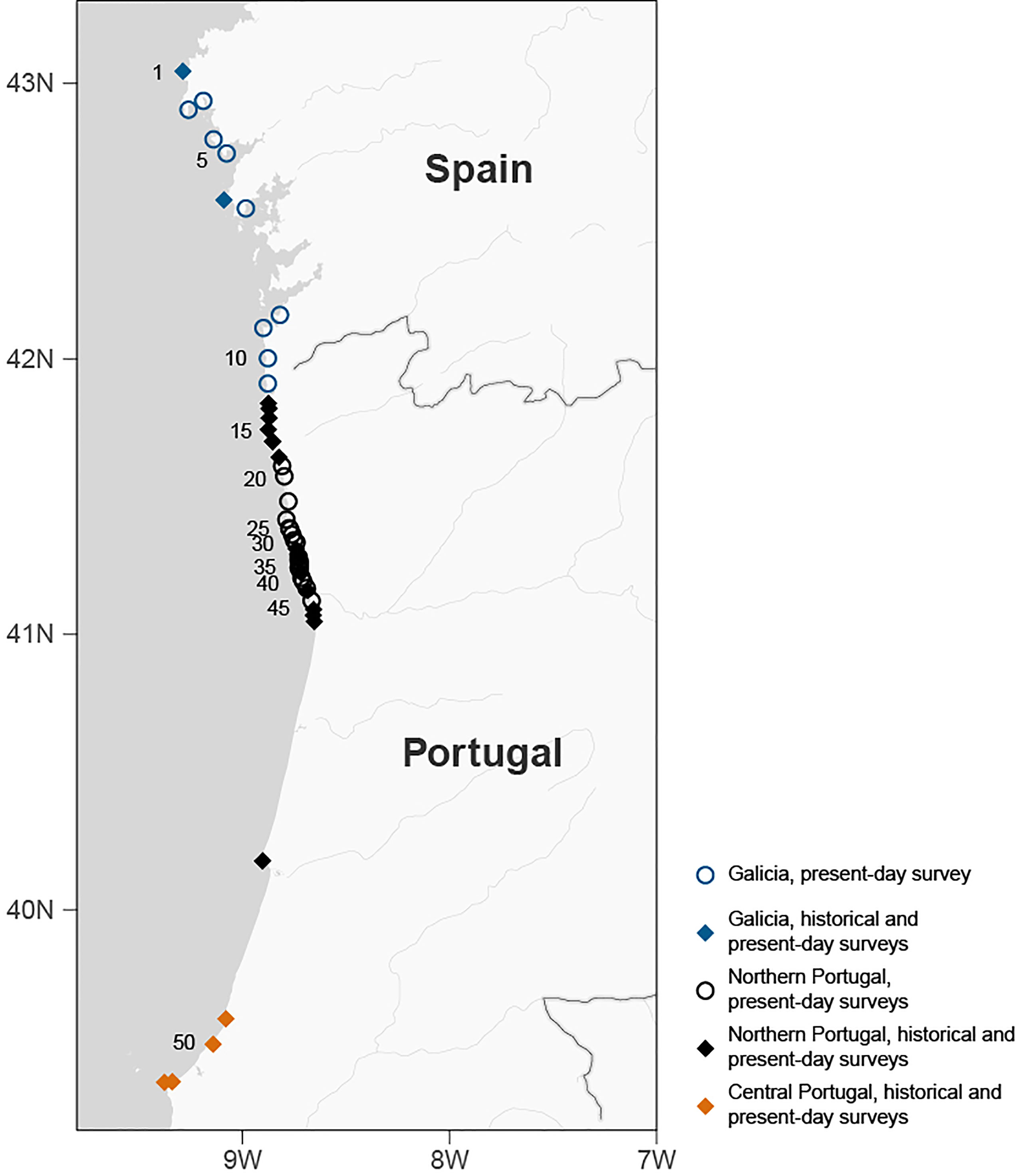
Figure 1 Map of the surveyed sites. Locations are numbered in multiples of 5, from the northernmost (1) to the southernmost site (52). For more details on the sites see Supplementary Table 2. Blue symbols correspond to sites in Galicia, black symbols to sites in northern Portugal and orange symbols to sites in central Portugal. Open circles indicate locations only visited in 2020-2021 while closed diamonds indicate locations visited both in 2001-2005 and 2020-2021.
2.2 Data collection
The quadrat sampling methodology is a staple method in ecology, which owing to its inherent objectivity and precision in the assessment of the percentage cover of species, is widely used to quantify the occurrence and abundance of sessile marine species (Boaventura et al., 2002; Bertocci et al., 2012; Franklin et al., 2013). In addition, it can be easily integrated with automated methodologies (e.g., quadrats can be photographed and pictures automatically processed, Bravo et al., 2021). It does not, however, efficiently detect rare species or species inside tide pools or in otherwise hard to reach surfaces (such as rock overhangs, slippery slopes, or areas exposed to the surf during the surveys). In turn, ad-libitum timed search for selected species, quantifying and recording their abundance in a simple, semi-logarithmic scale (i.e., the SACFOR scale, Southward et al., 1995; Burrows et al., 2008) is, at least in theory, a more appropriate method to pinpoint the distribution limits of species, where it is more likely that populations will occur with low densities or even cryptically in specific microhabitats.
All locations were surveyed during low tides by a two-person team. The region has a semi-diurnal, meso-tidal regime, the largest tidal range is 3.5–4 m during spring tides. Each site was surveyed for 60 minutes. Whenever possible due to geographical proximity, the team surveyed two locations during the same low tide. At each site, the team carefully searched for each of the selected species and recorded the abundance based on the SACFOR scale (Hiscock, 1981; Burrows et al., 2008). This scale grades abundances as Superabundant (more than 90% cover), Abundant (60-90% cover), Common (widespread on the shore, 30-59%), Frequent (patches apparent, up to 30% cover), Occasional (3 to 20 individuals scattered) and Rare (only 1 or 2 individuals). Absent species are recorded as Not Found. In the case of locations surveyed more than once during this study, the higher SACFOR abundance was kept in all analyses.
The identification of the selected species was based on Chapman and Goudey (1983); Campbell et al. (1994); Molenaar et al. (1996); Stuart et al. (1999); Faes & Viejo (2003); Aziza et al. (2008); Araújo et al. (2009; 2011); Bárbara (2009; 2013); Vieira et al. (2010); Edwards et al. (2012); Bunker et al. (2017); Poza et al. (2017); Benita et al. (2018). For further details on the species surveyed, locations visited, and specific methodology refer to Pereira et al. (2022).
2.3 Data Analysis
Data analysis was divided into (i) the analysis of present-day data (years 2020 and 2021) from all 52 locations, and (ii) a comparative analysis between present-day data and historical data (recorded between 2001 and 2005) from the 22 locations for which historical data was available (Supplementary Table 2). Data analyses were performed in R (R Core Team, 2020).
2.3.1 Present-day data (2020 and 2021)
A preliminary analysis was done by creating species distribution and abundance maps using the packages ggplot2, PBSmapping, and ggrepel (Schnute et al., 2004; Wickham et al., 2016; Slowikowski et al., 2018). Coastline information was based on the GSHHG (Global Self-consistent, Hierarchical, High-resolution Geography Database) downloaded from https://www.ngdc.noaa.gov/mgg/shorelines/. Similar maps were created for historical and present-day distribution data of all selected species (Supplementary Table 1). Then, using the packages viridis, ggplot2, reshape2 and hrbrthemes (Wickham, 2012; Wickham et al., 2016; Garnier et al., 2018; Rudis, 2020), a heatmap was created representing the abundance and distribution of all surveyed species in 2020/2021. To facilitate recognition and interpretation of patterns and differences among regions, non-metric multi-dimensional scaling (NMDS) plots were produced using the vegan “metaMDS” function based on Bray-Curtis dissimilarity values, calculated from untransformed data by the function “vegdist”, also from the package vegan (Oksanen et al., 2022). The site “Areia” (ID 27) was not included in this analysis as it was an extreme outlier. Finally, using the “pairwiseAdonis” function (Arbizu, 2020), a wrapper function for multilevel pairwise comparison for the “adonis” function from the package vegan (Oksanen et al., 2022), we performed a nonparametric MANOVA (Anderson, 2001) to test the significance of the distinction between regions identified in the NMDS ordination plot, using the Bray-Curtis dissimilarity index and 999 permutations, followed by pairwise comparisons between each group.
Indicator species for each region (Galicia, northern Portugal, central Portugal) were identified using a multilevel pattern analysis with the function “IndVal.g” from the R package “indicspecies” (De Cáceres et al., 2016). IndVal.g corrects the phi coefficient for the fact that some regions have more sites than others and returns the better matching species patterns, assessing the predictive values of species as indicators of the conditions prevailing within each group of locations. This function also outputs specificity (“A”) and fidelity (“B”) values, the specificity component being the estimate of the probability that the surveyed site belongs to the target site group given the fact that the species has been found, and the fidelity component being the estimate of the probability of finding a given species in sites belonging to the target site group.
To test for differences in the averaged STI between the regions of Galicia, northern Portugal, and central Portugal, a Krustal-Wallis test was performed using the “multcomp” package (Hothorn et al., 2016), followed by a pairwise comparison using the Wilcoxon rank sum exact test.
2.3.2 Temporal analysis (historical and present-day data)
NMDS plots were performed to facilitate recognition and interpretation of patterns between historical and present-day data, following the procedure described above. To test for a possible trend towards community homogenization manifested in the reduction of the average Bray-Curtis dissimilarity from historical to present-day data, a permutation test was employed (Petrov, 2021). Indicator species for the historical and present-day group were also identified using, as before, the function “IndVal.g” from the R package “indicspecies” (De Cáceres et al., 2016). A one-way ANOVA test was used to test for differences in the average STI of the selected species, also using R.
3 Results
3.1 Present-day data
3.1.1 Graphical representation and permanova analysis
The heatmap plot in Figure 2 shows an overview of the occurrence and abundance of the 34 species surveyed in the 52 locations in north-western Iberia in 2020/2021. Despite having met the criteria for inclusion in this survey, the species with warm-water affinity Valonia utricularis, Phyllophora crispa, Hypnea musciformis, Halopithys incurva, and the cold-water affinity Phycodrys rubens, Dumontia contorta and Delesseria sanguinea were not found in any of the locations surveyed.
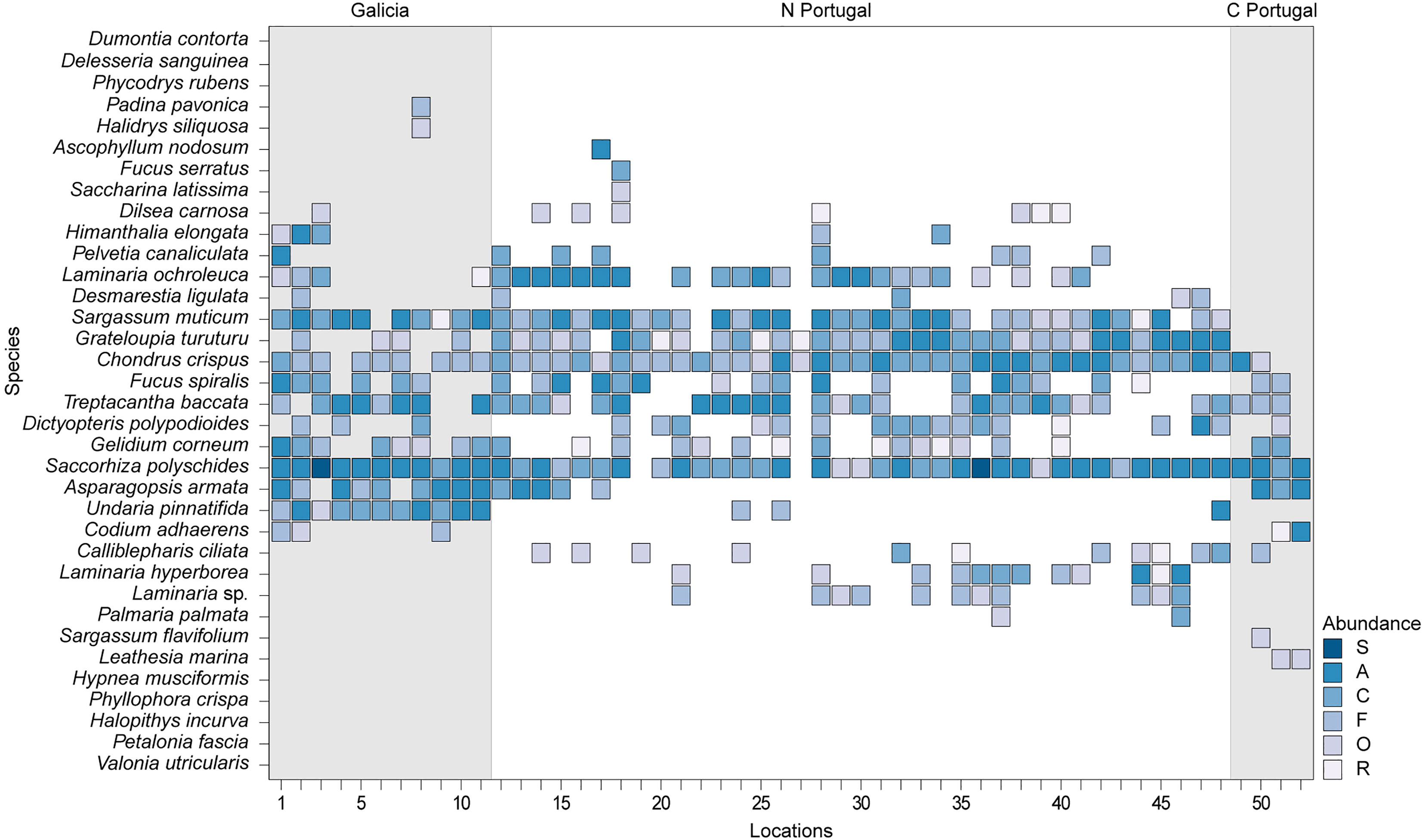
Figure 2 Abundance of selected species (y-axis) in North-western Iberia. In the x-axis, locations are identified by an ID number, from the northernmost (1) to the southernmost site (52). For more details see Supplementary Table 2. Color gradient indicates abundance based on the SACFOR abundance scale. S, Super Abundant; A, Abundant; C, common; F, frequent; O, occasional; R, rare.
The NMDS of the present-day data (Figure 3) revealed a distinct separation in the multidimensional space between sites from the regions of Galicia, northern Portugal, and central Portugal, which displayed significantly different macroalgae communities between every region (nonparametric MANOVA, p < 0.05, Supplementary Tables 3 and 4). Even though the nonparametric MANOVA is quite robust to unbalanced designs, we tested its sensitivity with 5000 bootstrapped MANOVAs based on five locations randomly selected with substitution from each region. 85.64% of the tests between Galicia and northern Portugal, 79.00% of the tests between Galicia and central Portugal, and 89.08% of the tests between northern and central Portugal yielded significant (p<0.05) results.
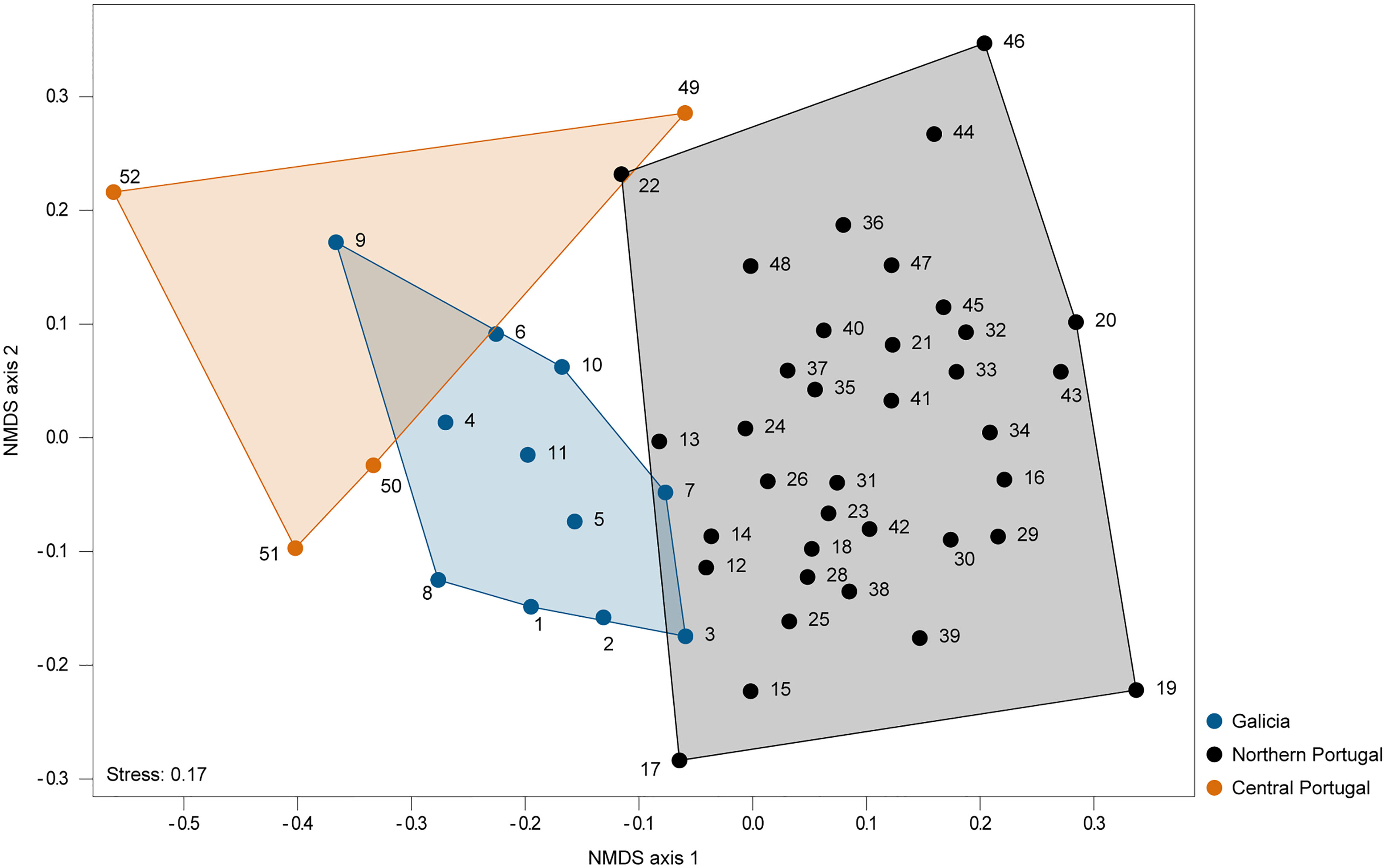
Figure 3 NMDS ordination plot of all sites based on the Bray-Curtis dissimilarity index. Blue symbols and blue polygon correspond to sites in Galicia, black symbols and black polygon correspond to sites in northern Portugal and orange symbols and orange polygon correspond to sites in central Portugal. Locations are depicted by an ID number, for more details see Supplementary Table 2.
3.1.2 Indicator species
Using a Multilevel Pattern Analysis, we extracted information on the indicator species characteristic of a region or group of regions (Supplementary Table 5). For each region, A and B vary from 0 to 1, where species with specificity value (A) equal to 1 occur only in this region, and species with a fidelity value (B) = 1 occur in all sites within a region. Undaria pinnatifida (Supplementary Figure 1B) was the only species significantly associated with Galicia. It showed a high specificity value (A = 0.93), as it occurred almost exclusively within that region, with a few exceptions in northern Portugal. U. pinnatifida also displayed a high-fidelity value (B = 1), as it was present in most locations in Galicia that were sampled in this study.
Regarding northern Portugal, the analysis could not identify significant representative species. However, five species, all with cold-water affinity - Laminaria hyperborea, Palmaria palmata, Ascophyllum nodosum, Fucus serratus, and Saccharina latissima - were flagged as associated with this region because they occurred almost exclusively in northern Portugal (thus receiving high values of specificity - A), although their occurrence was not consistent among all sites (hence, receiving relatively low values of fidelity - B).
The species that significantly separated central Portugal from the other two regions were the cold-water Leathesia marina (Supplementary Figure 2B) and the warm-water Codium adhaerens (Supplementary Figure 3B), which are specific to this region.
Considering region pairs, both Sargassum muticum (Supplementary Figure 4B) and Grateloupia turuturu (Supplementary Figure 5B) held high values of specificity (A = 0.91 and 0.81, respectively) in Galicia and northern Portugal, significantly representing both regions. The species significantly associated with Galicia and central Portugal were Asparagopsis armata (Supplementary Figure 6B) and Gelidium corneum (Supplementary Figure 7B), mostly due to their high values of specificity (A = 0.92 and 0.83 respectively), indicating that they were either rare or non-existent in northern Portugal. Both are warm-water species in the study area. There were no species significantly associated with both northern and central Portugal. This indicates that, regarding the surveyed species, these are two distinct regions, a pattern corroborated by the NMDS and multivariate analysis (Figure 3, Supplementary Tables 3 and 4).
3.1.3 Average species temperature index
The average Species Temperature Index (avg STI) was significantly different between regions (Chi square = 8.86, p = 0.011, df = 2, Supplementary Table 6). Galicia and central Portugal exhibited the same average STI (15°C), significantly higher than that found for northern Portugal (14°C) (Wilcoxon rank sum exact test, p-value = 0.009; Supplementary Table 7).
3.2 Temporal analysis – historical and present-day data
Table 1 summarizes the information on the species that increased, decreased, or maintained their average abundance between historical and present-day surveys. Among those species that increased their distribution, 4 were warm-water species, 6 were cold-water species and 3 were neutral species. In addition, 4 species were NIS. Among the species that decreased their distribution, 3 were warm-water, 2 were neutral species, and 10 were cold-water species. In other words, when compared to past data and considering only species that changed their distribution and abundance within the study area, more cold-water species decreased than increased (10 to 6 out of 18) while more warm-water species increased than decreased (4 to 3 out of 10).
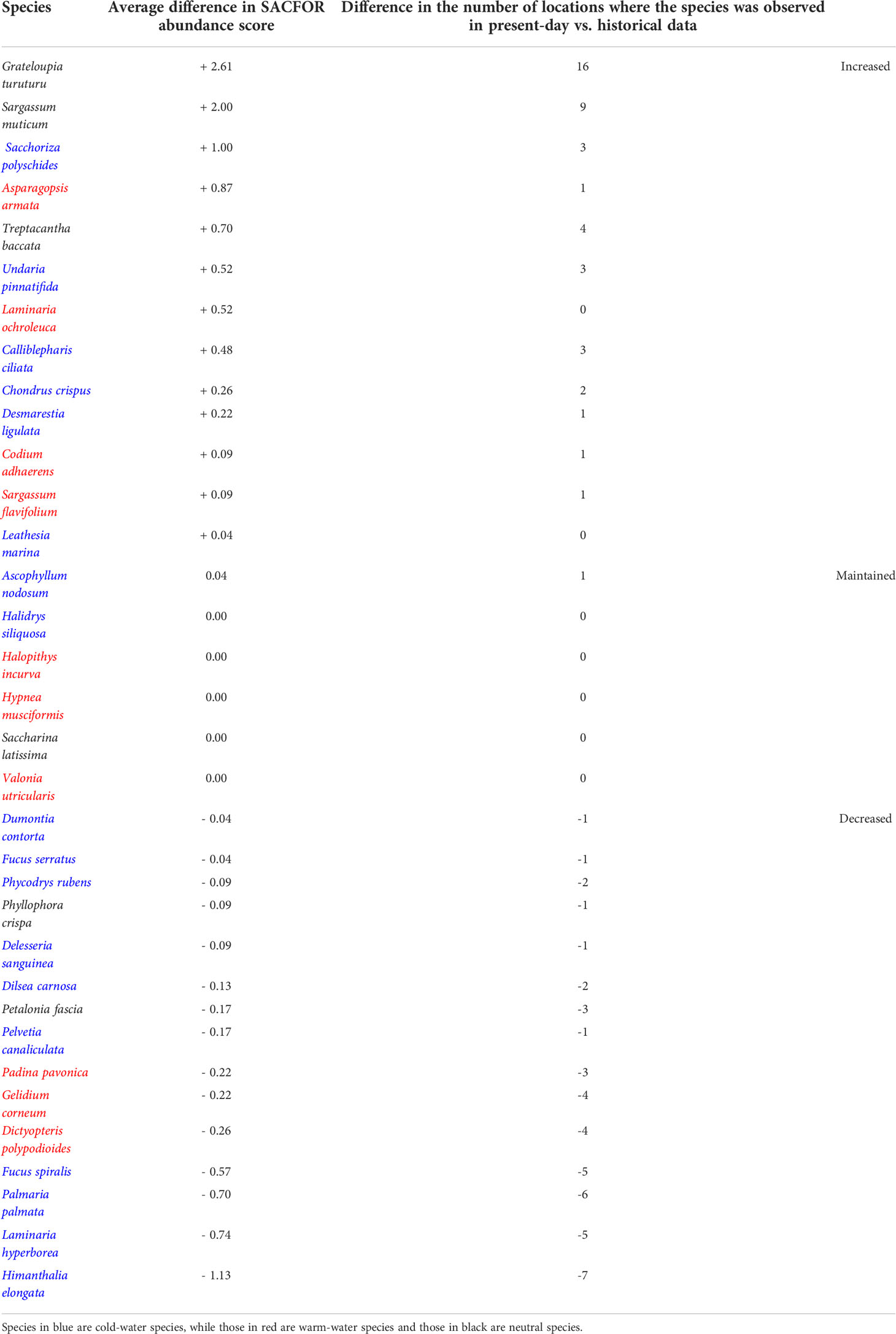
Table 1 Description of species that increased, decreased, or maintained their average abundance and geographical distribution from historical (2001-2005) to present-day data (2020-2021).
3.2.1 Graphical representation and permutation analysis
The presence and abundance of selected macroalgae species significantly differed between historical (2001-2005) and present-day (2020-2021) datasets (PERMANOVA, p = 0.001, Supplementary Table 8).
The NMDS presented in Figure 4 shows that the average dispersion of locations around their centroid, based on each site’s species composition, is smaller in present-day data than in historical data although, probably owing to the high variability within each group, the trend is not statistically significant (t (36.057) = 1.4812, p = 0.1472).
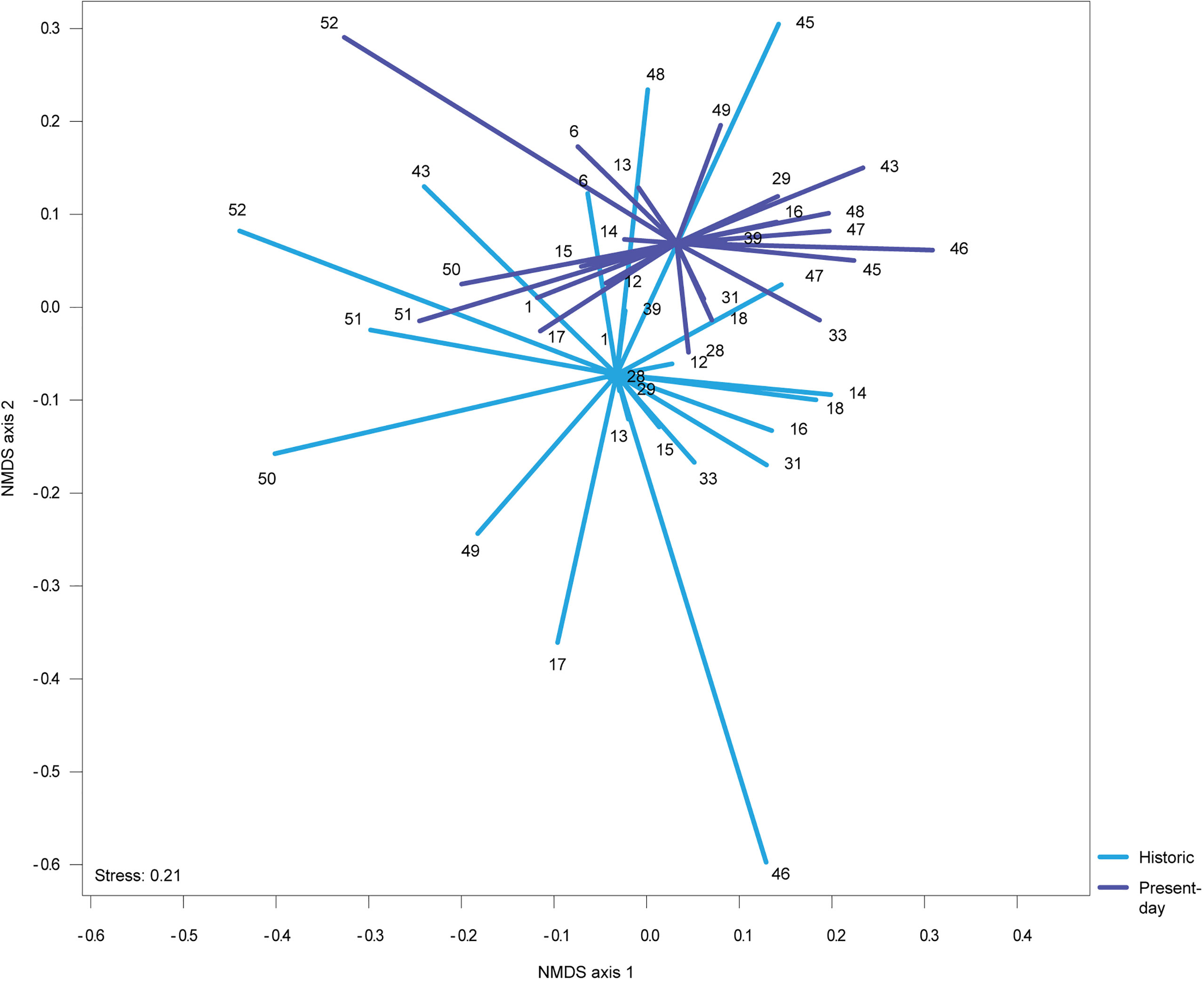
Figure 4 NMDS ordination plot based on the Bray-Curtis dissimilarity index for the list of common sites between present-day (2020-2021) and historical (2001-2005) sampling campaigns. Blue lines connect each historic location to its centroid and the purple lines connect each present-day location to its centroid. Locations are depicted by an ID number, for more details see Supplementary Table 2.
Average fidelity across all sites, computed through indicator species analysis, was higher in 2020-2021 (Average fidelity = 0.383; Standard deviation = 0.335) than in 2001-2005 (Average fidelity = 0.136; Standard deviation = 0.136), which also suggests a tendency towards homogenization of the macroalgae communities, albeit not significant.
3.2.2 Indicator species
Himanthalia elongata (Supplementary Figure 9) and Palmaria palmata (Supplementary Figure 10) were highlighted as significantly characteristic species in the historical surveys (Supplementary Table 9). Although found at relatively high abundance in numerous locations in Portugal ~15 years ago, these species have since gone extinct almost everywhere within the study area. Currently, H. elongata can only be found at three sites in Galicia and in two sites in Portugal (Praia Central – 34; Mindelo - 28), while P. palmata can only be found in a single location in Northern Portugal (Senhor da Pedra - 46).
Grateloupia turuturu (Supplementary Figure 5) and Sargassum muticum (Supplementary Figure 4) are NIS that have recently expanded their geographical distribution and increased in abundance in the Portuguese coast, resulting in their significant contribution to the 2020/2021 group (Supplementary Table 9).
3.2.3 Average species temperature index
The analysis revealed that most locations have increased their average STI from 2001-2005 to 2020-2021 (i.e., locations above the y = x line in Figure 5). Every location either maintained or increased their average STI except for the sites Senhor da Pedra (ID-46) and São Martinho do Porto (ID-50). Still, this trend was not significant overall (F (1,44) = 0.947, p = 0.34).
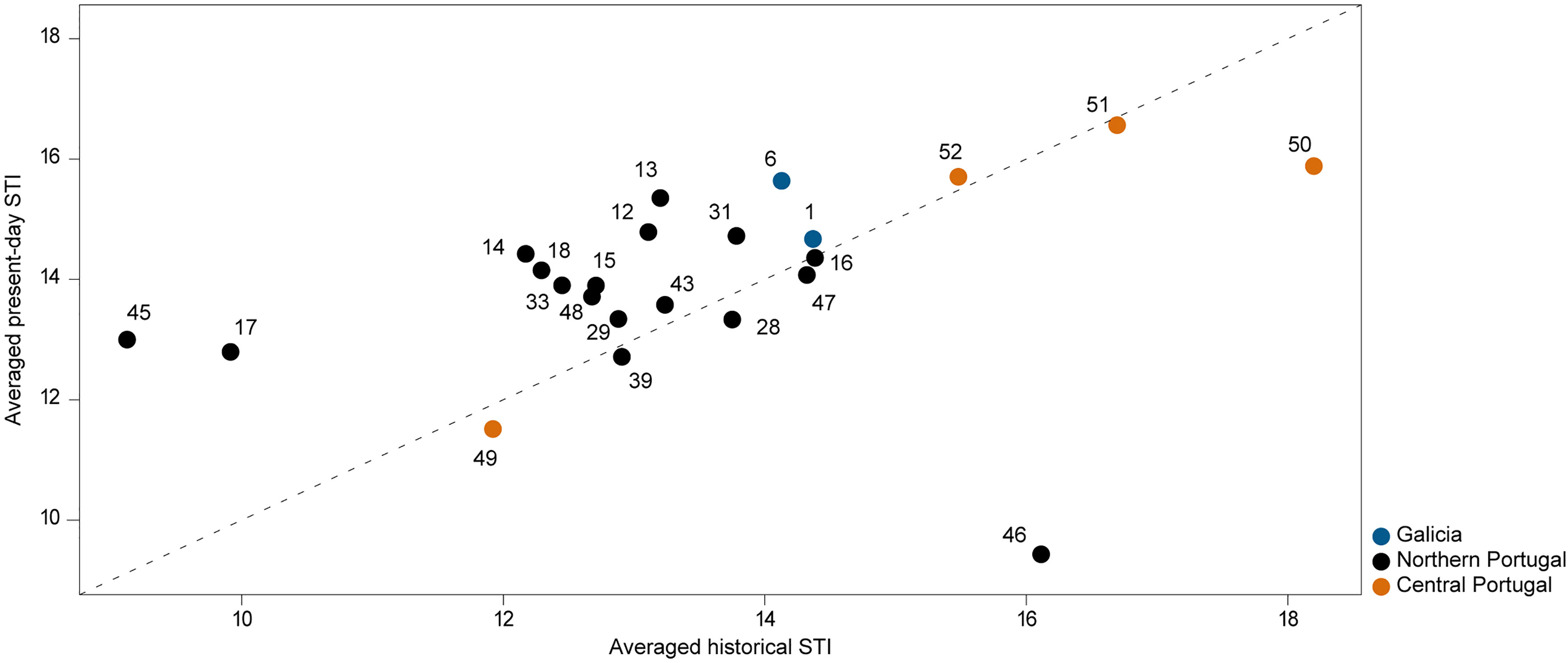
Figure 5 Comparison between averaged Species Temperature Index of sites in the present (averaged present-day STI) with averaged Species Temperature Index of sites in the past (averaged historical STI). Orange circles represent sites in central Portugal, black circles represent sites in northern Portugal and blue circles represent sites in Galicia. The line y = x shows the theoretical position of points if there were no changes in average STI. Locations are depicted by an ID number, for more details see Supplementary Table 2.
4 Discussion
4.1 Regional variation in the north-western Iberian Peninsula
Considering macroalgae composition and abundance, our results show that the regions of Galicia, northern Portugal and central Portugal are all significantly different from each other (Figure 3). The distinction between central Portugal and other regions was expected, since it is geographically isolated by a long stretch of sand and is less affected than the other regions by the seasonal upwelling of cold and nutrient-rich waters (Fraga, 1981; Fiuza, 1983; Lima et al., 2007; Seabra et al., 2015). Previous studies on the distribution of intertidal species along the Portuguese coast had also classified northern and central Portugal as distinct biogeographic regions (Van den Hoek and Donze, 1967; Boaventura et al., 2002; Lima et al., 2007).
Remarkably, this study also suggests that Galicia and northern Portugal are significantly different in their seaweed composition and abundance despite being geographically very close (they are part of the same continuum of rocky coast) and having (at least apparently, and based on remote sensing data) similar sea surface temperature profiles (Banzon et al., 2016). The underlying reason for these differences is not evident as the two regions have rarely been surveyed and compared in a standardized way until now. One hypothesis is that macroalgae communities in Galicia and northern Portugal are distinct due to small-scale temperature variability which is not easily detected from remotely sensed data due to its insufficient resolution (Meneghesso et al., 2020). In reality, it would be surprising if the contrasting topography and geomorphology contexts associated with each of the regions would not create dissimilar environmental conditions, not only in relation to water and atmospheric temperatures, but also regarding water salinity, wave exposure, tidal regime, current speed, nutrient availability, or magnitude of upwelling, all of which have the potential to influence local biodiversity. Specifically, the coast of Galicia is characterized by the occurrence of Rias (large coastal inlets formed by the partial submergence of unglaciated river valleys), while the coast of Northern Portugal is linear, with a north to south orientation, and absent of sheltered sites.
Another hypothesis is that the differences detected could be related with the different anthropogenic pressures exerted in these regions. The aquaculture production of seaweed and bivalves (Minchin and Nunn, 2014; James and Shears, 2016) and the heavy traffic of leisure and fishing vessels in the Rias might represent vectors for biological invasions (Blanco et al., 2021). Several non-indigenous species (NIS) have higher local abundances and are distributed more widespread in Galicia than in northern Portugal (e.g., Undaria pinnatifida – Supplementary Figure 1 and Asparagopsis armata – Supplementary Figure 6).
Interestingly, Galicia and central Portugal have a higher average species temperature index than northern Portugal. Additionally, all indicator species that significantly represent both Galicia and central Portugal are considered warm-water species in Northern Portugal (Asparagopsis armata and Gelidium corneum), thus supporting the hypothesis that temperature may play an important role in the patterns here described. Our results suggest that Northern Portugal is an important thermal refugia for several cold-water species (e.g., Laminaria hyperborea, Ascophyllum nodosum or Fucus serratus) which, considering the surveyed locations, were only found there. Nevertheless, for some species such as Himanthalia elongata or Halidrys siliquosa, the potential thermal refugia of this region seems to be eroding. It will soon be possible to test this hypothesis through the analysis of high-resolution intertidal temperature profiles currently being collected by autonomous temperature loggers (Lima and Wethey, 2012) at the same locations where the biodiversity surveys were carried out.
There is high variability in species composition and abundance in northern Portugal, as revealed by the lack of significant representative species (Supplementary Table 5) and large dispersion among sites within the region (Figure 3). Notwithstanding the fact that the sampling effort in this region was much higher, the within-region biodiversity variability suggests that even a seemingly linear and homogeneous coastline holds significant high-frequency spatial variability. One explanation for this pattern is that biodiversity variability is simply mirroring the micro-climate variability created by subtle, yet biologically important differences in solar radiation exposure, salinity, topography, wave exposure and hydrodynamics (Seabra et al., 2011; Lima et al., 2016). Combinations of these different environmental conditions create a complex mosaic of micro-habitats, even among neighboring locations, which might explain the high biodiversity variability (Helmuth et al., 2006; Potter et al., 2013). Thus, the northern Portuguese coast is a good case study to explore how the effects of small-scale environmental variability interact with large-scale patterns, namely global changes in sea temperature.
In conclusion, our analyses indicate that the regions of Galicia and central Portugal, although geographically separated, share a regionally warm-water species pool. In turn, Northern Portugal is, at least from the standpoint of the average Species Temperature Index and from the distribution of cold-water species, the coldest region, highlighting its potential importance as a climate change refugia that may be sustaining the equatorial range edge of several macroalgae. This calls for protection of the rocky intertidal in this region since climate change refugia are increasingly considered in conservation priorities (Morelli et al., 2020).
4.2 Temporal variation between present-day and historical data
Macroalgae occurrence and abundance have changed considerably in the 15 years that mediate our historical (2000-2005) and present-day surveys (2020-2021). Currently, macroalgae communities seem to be more homogeneous, although the effect is small and not significant (Figure 4). This homogenization, also evident in subtidal macroalgae communities along the coast of Portugal (de Azevedo, 2019), could lead to impacts in ecosystem functioning, productivity and ecosystem services (Clavel et al., 2011).
In addition, we recorded a decrease in presence in abundance and/or distributional range of 15 species, of which 10 were cold-water species (Table 1), and among these 2 were significantly associated with historical surveys (section 3.2.2). Species with warm-water affinity exhibited a mixed response, with some increasing (4), 3 maintaining their distribution and abundance and 3 showing declines in present-day data. Importantly, species whose abundance increased the most were non-indigenous macroalgae (Table 1), of which 2 neutral ones were significantly associated with present-day data. This contrasts with the results from Lima et al. (2007), that observed a clear trend of northward migration of warm-water species and mixed responses of cold-water species (some were retreating northwards while others were advancing southwards). Given that this work comprises two datapoints in time separated by 15 years, and the reduced geographical area surveyed when compared with Lima et al. (2007), we cannot confirm that a shift in the overall direction of change in communities is occurring. Still, our results are concerning especially given the accentuated decrease in some cold-water (foundation) species and the parallel increase of some warm-water (NIS) macroalgae, both with the potential to disproportionately impact the entire ecosystem.
Several macroalgae for which we report decreases in presence and abundance are canopy-forming brown algae that play an important role in coastal biodiversity as foundation species (sensu Dayton, 1972), providing food, shelter, and habitat to other species of flora and fauna, as well as protection from desiccation, temperature extremes, and wave action (Smale et al., 2013; Seitz et al., 2014; Bulleri et al., 2018). Similar declines have been reported in other temperate regions of the Atlantic, with impacts on the structure and functioning of coastal ecosystems (Smale, 2020, Yesson et al., 2015). This is the case for Himanthalia elongata (Creed, 1995) which, among all the species here surveyed, and in agreement with previous studies (Piñeiro-Corbeira et al., 2016; Casado-Amezúa et al., 2019; Barrientos et al., 2020), showed the greatest populational and geographic declines in Galicia and Portugal (it was present in less 7 sites and showed an average 1.13 reduction in SACFOR score). Currently, it can only be found in two locations south of the NW corner of the Iberian Peninsula – one healthy population in Praia Central (ID – 34) and a very small population in Mindelo (ID – 28) with just a few scattered individuals (Supplementary Figure 9). Similarly, a noticeable decrease of the foundation species Laminaria hyperborea was also evident (a it occurred in less 5 sites and an average 0.74 reduction in SACFOR score, Supplementary Figure 11). This kelp forms marine forests and sustains a diverse community along the north-east Atlantic coast (Christie et al., 2003; Norderhaug et al., 2021). In parallel, the warm-water counterpart L. ochroleuca increased slightly in abundance, though not enough to replace L. hyperborea. It is important to note that even if successful in replacing L. hyperborea, L. ochroleuca supports a significantly less diverse community and has lower biomass (Teagle and Smale, 2018).
In addition, the decrease of Laminaria hyperborea could have contributed to the decrease of Palmaria palmata (Supplementary Figure 10), as this red algae occurs often as an epiphyte of L. hyperborea towards the rear edge of its distribution (Whittick, 1983). If confirmed, this would be a prime example of how changes in the distribution of one species impact another, having cascading effects in the community. The decrease in these canopy-forming algae could lead to negative ecological and economic outcomes since they simultaneously are at the basis of the food-web and are cultivated and harvested with applications in several industries (Plaza et al., 2008). Moreover, populations at the rear-edge are often relict populations, holding unique genetic diversity that might be key for a species’ persistence under current global change trends (Provan and Maggs, 2012).
Contrastingly, some species highlighted in this study as indicator species have recently increased their distributional range and abundance in the region. The NIS warm-water macroalgae Grateloupia turuturu (Supplementary Figure 5) and the NIS, neutral species Sargassum muticum (Supplementary Figure 4) have both greatly increased their distribution in the last 15 years. S. muticum exhibits extremely high growth rates during spring and is a strong competitor – limiting the distribution and replacing native species, which once established can have a drastic impact in ecosystem flora and fauna composition (Critchley et al., 1986; Boudouresque et al., 1995; Stæhr et al., 2000; Sanchez and Fernandez, 2005). Additionally, the kelp Undaria pinnatifida, an non-indigenous cold-water species which was never recorded during the surveys of 2000-2005 and since then had only been recorded in Portugal in Buarcos (ID – 48) and within a marina in Viana do Castelo (Báez et al., 2010; Veiga et al., 2014), was now observed to be spreading rather quickly. It is currently present in four more sites, two with artificial substrate (a breakwater in Cabedelo and the northern breakwater at Barra), and two with natural rocky substrate (24 – Carvalhido and 26 – Forte São João). This species has a year-round presence in non-native areas, where it competes with native species with seasonal reproductive, growth and senescence stages, resulting in a successful establishment and spread in cold to temperate systems (Epstein and Smale, 2017). It is considered one of the top-100 worst invaders (Lowe et al., 2000), causing severe ecological damages in some regions, however with rather neutral and even benign effects in others (South et al., 2017), making it very difficult to predict ecosystem-wide effects of its current spreading in north-western Iberia.
The dramatic changes in the distribution of some non-indigenous species are cause for concern and call for a close and continuous monitoring of these macroalgae in north-western Iberia. Considering that their increase in abundance led to significant ecological disturbance in other regions (Stæhr et al., 2000; Casas et al., 2004; Sanchez and Fernandez, 2005; Williams and Smith, 2007; Silva et al., 2021), it is likely that they will too significantly and negatively impact ecosystem functioning in north-western Iberia (Mineur et al., 2015). Moreover, NIS such as G. turuturu in northern Portugal (Araújo et al., 2011) could also be taking the role of passenger of ecological change rather than driver, benefiting from the loss of algae canopies and relying on the disruption of native assemblages to spread (Mulas and Bertocci, 2016), in which case, these species can be important indicators of ecosystem health.
Temperature is a fundamental driver of change in marine systems, and metrics based on species thermal affinities have successfully been used to track community-wide changes over large geographical areas (see, for example, Burrows et al., 2019). Community Temperature Index (CTI) integrates in a single index four distinct processes – increase/decrease in the abundance of warm-affinity species (tropicalization and detropicalization, respectively), and decrease/increase of cold-affinity species (deborealization and borealization, respectively). This complexity is further increased by a variety of factors related to environmental conditions, human impacts and to the community structure itself (Mclean et al., 2021). Still, deborealization has been described as an important component frequently leading to increasing CTI (Mclean et al., 2021). In this study, however, and despite the decrease in abundance of several cold-water species over the last 15 years, the average Species Temperature Index (STI) did not increase significantly across the study area. This may be related to the fact that we only analyzed a restricted subset of species among all possible, and thus we may not have had enough resolution to find significant differences. Indeed, it has been shown for coastal communities of NW Atlantic that the utility of CTI analyses may be limited when a reduced number of species is used, especially if individual species have non-linear responses to temperature (Flanagan et al., 2019). In addition, it may happen that the macroalgae community as a whole is less responsive to changes in temperature, which would explain the marginal increase in the average STI in this work. In fact, a different study in the NE Atlantic including substantially more species showed that CTI is increasing as sea surface temperature increases, though the CTI of animals is responding more readily to changes in temperature than that of macroalgae (Burrows et al., 2020).
4.3 Fine-scale sampling
We used a fine-scale survey strategy that allowed us to obtain distribution and abundance data at an average resolution of 3.4 ± 5.9 km within the region of highest interest (northern Portugal), a value higher than what is typically provided in similar studies (Boaventura et al., 2002; Araújo et al., 2009). Such high-resolution distribution data proved instrumental, particularly in circumstances where certain species were found to have disappeared from historical sites only to be recorded in other locations just a few hundreds of meters away (e.g., Pelvetia canaliculata, which disappeared from Cabo do Mundo (ID-39) but was found 2.2 kms away at site Memória (ID-38)). This means that the most common long-term monitoring strategy, which is to target all the effort towards revisiting the same sites repeatedly (Mieszkowska et al., 2021) may yield oversimplified datasets, and be insufficient to accurately pinpoint the distribution limits of certain species or to detect and therefore track fast changes. Moreover, previous studies have already demonstrated that species distribution models based on fine-scale surveys result in different patterns from those fed with data obtained from coarser surveys (Trivedi et al., 2008; Gastón and García-Viñas, 2010), yielding qualitatively distinct predictions with important consequences for the conservation and management of biodiversity (Franklin et al., 2013).
By adopting the ad-libitum timed search for selected species and recording their abundance in a simple, semi-logarithmic scale (i. e., the SACFOR scale, Southward et al., 1995; Burrows et al., 2008), we successfully mitigate the drawbacks associated with the commonly-used quadrat sampling method. In particular, while the combined ad-libitum timed search with SACFOR recording can be regarded as a less objective or accurate method which often requires expert knowledge (and thus is harder to train for, Mieszkowska et al., 2006), our study confirms that it can efficiently detect rare species or species in hard-to-reach microhabitats, unlike the quadrat method. Furthermore, our data strongly suggests that the use of intensive, fine-scale surveys was essential to accurately assess the distribution of the studied species, which in turn allowed us to formally test hypotheses on effects of warming on species abundances at or near their range edges. The presence and abundance of some macroalgae species varies with the season. Although we visited some sites more than once and found similar results between the two sampling events, we do expect that for some species seasonal variation in abundance may be greater than long-term change, and thus suggest that future studies strive to survey consecutive seasons along the year. This clearly highlights the importance of taking into consideration the overall goals of any assessment of intertidal biodiversity when choosing the spatial coverage required and the sampling method to be used.
4.4 Conclusions
In conclusion, the contrasts between historical and present-day biodiversity and biogeography patterns suggest that a homogenization of the macroalgae communities may be underway across the north-western Iberia. We found that the average community dissimilarity seems to have been decreasing over the last two decades, both within and among regions. Some cold-water species are becoming rarer while warm-water counterparts are found over larger spans of coast. Another factor of homogenization is the increasing prevalence of some NIS, which already dominate some microhabitats and/or sites. Hence, a higher effort in conservation and restoration is vital to protect the health of these coastal ecosystems and the communities that depend on them (García Molinos et al., 2016). Although this work did not formally explore the role ongoing changes in temperature may be playing in these alterations, both the net decline of cold-water species and the net increase in warm-water species, as well as the higher prevalence of NIS is congruent with the effects expected from an increase in temperature driven by climate change. These effects could soon be exacerbated by any decrease in the magnitude of the upwelling in the region, which mitigates further warming (Seabra et al., 2019; Sousa et al., 2020). Further studies that explicitly analyze the joint change in biodiversity and temperature are urgently needed to test some of the hypotheses here discussed.
Data availability statement
The datasets presented in this study can be found in online repositories. The names of the repository/repositories and accession number(s) can be found below:
http://ipt.gbif.pt/ipt/resource?r=ibpc,
http://ipt.gbif.pt/ipt/resource?r=2021_iberianpeninsula,
http://ipt.gbif.pt/ipt/resource?r=herbarium.
Author contributions
CM: Fieldwork, data curation, data analyses, writing – original draft and review and editing, supervision. JP: Fieldwork, data curation, data analyses, writing – original draft and review and editing. RS: Data curation, writing – review and editing, funding acquisition. FL: Conceptualization, writing – review and editing, funding acquisition, supervision, project administration. All authors contributed to the article and approved the submitted version.
Funding
This work was supported by ERDF funds through COMPETE (grant numbers POCI-01-0145-FEDER-031088, POCI -01-0145-FEDER-031893), through POR Norte (grant numbers NORTE-01-0145-FEDER-031053), by Portuguese national funds through FCT (grant numbers PTDC/BIA-BMA/31088/2017, PTDC/BIA-BMA/31053/2017, PTDC/BIA-BMA/31893/2017), and by European Union’s Horizon 2020 Research and Innovation Programme FutureMARES (Grant Agreement no. 869300) and BIOPOLIS (Grant Agreement Number 857251). RS was supported through CEECIND/01424/2017 and FL through CEECIND/03185/2018.
Acknowledgments
We thank Francisco Arenas Parra for his support in taxonomical identification and for revising the manuscript. We thank José Carneiro for his support during fieldwork in Galiza.
Conflict of interest
The authors declare that the research was conducted in the absence of any commercial or financial relationships that could be construed as a potential conflict of interest.
Publisher’s note
All claims expressed in this article are solely those of the authors and do not necessarily represent those of their affiliated organizations, or those of the publisher, the editors and the reviewers. Any product that may be evaluated in this article, or claim that may be made by its manufacturer, is not guaranteed or endorsed by the publisher.
Supplementary material
The Supplementary Material for this article can be found online at: https://www.frontiersin.org/articles/10.3389/fmars.2022.880074/full#supplementary-material
References
Anderson M. J. (2001). A new method for non-parametric multivariate analysis of variance. Austral Ecol. 26, 32–46. doi: 10.1111/j.1442-9993.2001.01070.pp.x
Antão L. H., Bates A. E., Blowes S. A., Waldock C., Supp S. R., Magurran A. E., et al. (2020). Temperature-related biodiversity change across temperate marine and terrestrial systems. Nat. Ecol. Evol. 4, 927–933. doi: 10.1038/s41559-020-1185-7
Araújo R., Bárbara I., Tibaldo M., Berecibar E., Tapia P. D., Pereira R., et al. (2009). Checklist of benthic marine algae and cyanobacteria of northern Portugal. Bot. Mar. 52, 24–46. doi: 10.1515/BOT.2009.026
Araújo R., Violante J., Pereira R., Abreu H., Arenas F., Sousa-Pinto I. (2011). Distribution and population dynamics of the introduced seaweed Grateloupia turuturu (Halymeniaceae, rhodophyta) along the Portuguese coast. Phycologia 50, 392–402. doi: 10.2216/10-65.1
Arbizu P. M. (2020). pairwiseAdonis: Pairwise multilevel comparison using adonis. r package version 0.4.
Aziza M., Givernaud T., Chikhaoui-khay M., Bennasser L. (2008):509–514. Seasonal variation of the growth, chemical composition and carrageenan extracted from Hypnea musciformis (Wulfen) lamouroux harvested along the Atlantic coast of Morocco. Sci. Res. Essays 3. doi: 10.5897/SRE.9000823
Báez J. C., Olivero J., Peteiro C., Ferri-Yáñez F., Garcia-Soto C., Real R. (2010). Macro-environmental modelling of the current distribution of Undaria pinnatifida (Laminariales, ochrophyta) in northern Iberia. Biol. Invasions 12, 2131–2139. doi: 10.1007/s10530-009-9614-1
Banzon V., Smith T. M., Chin T. M., Liu C., Hankins W. (2016). A long-term record of blended satellite and in situ sea-surface temperature for climate monitoring, modeling and environmental studies. Earth Syst. Sci. Data 8, 165–176. doi: 10.5194/essd-8-165-2016
Bárbara I. (2009). Especies invasoras en Galicia e introducción a súa problemática: Medio mariño, flora (Spain, Universidad de A Coruña).
Bárbara I. (2013). Algas marinas y salores de Galicia y norte de españa: Parte 2 (Facultad de Ciencias, Universidad de A Coruña: Laboratorio de Algas Marinas).
Barrientos S., Barreiro R., Cremades J., Piñeiro-Corbeira C. (2020). Setting the basis for a long-term monitoring network of intertidal seaweed assemblages in northwest Spain. Mar. Environ. Res. 160, 105039. doi: 10.1016/j.marenvres.2020.105039
Benita M., Dubinsky Z., Iluz D. (2018). Padina pavonica: Morphology and calcification functions and mechanism. Am. J. Plant Sci. 09, 1156–1168. doi: 10.4236/ajps.2018.96087
Bertocci I., Dominguez R., Freitas C., Sousa-Pinto I. (2012). Patterns of variation of intertidal species of commercial interest in the parque litoral norte (north Portugal) MPA: Comparison with three reference shores. Mar. Environ. Res. 77, 60–70. doi: 10.1016/j.marenvres.2012.02.003
Blanco A., Larrinaga A. R., Neto J. M., Troncoso J., Méndez G., Domínguez-Lapido P., et al. (2021). Spotting intruders: Species distribution models for managing invasive intertidal macroalgae. J. Environ. Manage. 281, 111861. doi: 10.1016/j.jenvman.2020.111861
Boaventura D., Ré P., Cancela da Fonseca L., Hawkins S. J. (2002). Intertidal rocky shore communities of the continental Portuguese coast: Analysis of distribution patterns. Mar. Ecol. 23, 69–90. doi: 10.1046/j.1439-0485.2002.02758.x
Boudouresque C. F., Meinesz A., Ribera M. A., Ballesteros E. (1995). Spread of the green alga Caulerpa taxifolia (Caulerpales, chlorophyta) in the Mediterranean: possible consequences of a major ecological event. Sci. Mar. 21–29.
Bravo G., Moity N., Londoño-Cruz E., Muller-Karger F., Bigatti G., Klein E., et al. (2021). Robots versus humans: Automated annotation accurately quantifies essential ocean variables of rocky intertidal functional groups and habitat state. Front. Mar. Sci. 8, 1366. doi: 10.3389/fmars.2021.691313
Bulleri F., Eriksson B. K., Queirós A., Airoldi L., Arenas F., Arvanitidis C., et al. (2018). Harnessing positive species interactions as a tool against climate-driven loss of coastal biodiversity. PloS Biol. 16, e2006852. doi: 10.1371/journal.pbio.2006852
Bunker F. S. D., Maggs C. A., Brodie J. A., Bunker A. R. (2017). Seaweeds of Britain and Ireland (Plymouth, Uk: Wild Nature Press). S. Edition.
Burrows M. T., Bates A. E., Costello M. J., Edwards M., Edgar G. J., Fox C. J., et al. (2019). Ocean community warming responses explained by thermal affinities and temperature gradients. Nat. Climate Change. 9, 959–963. doi: 10.1038/s41558-019-0631-5
Burrows M., Harvey R., Robb L. (2008). Wave exposure indices from digital coastlines and the prediction of rocky shore community structure. Mar. Ecol. Prog. Ser. 353, 1–12. doi: 10.3354/meps07284
Burrows M. T., Hawkins S. J., Moore J. J., Adams L., Sugden H., Firth L., et al. (2020). Global-scale species distributions predict temperature-related changes in species composition of rocky shore communities in Britain. Glob. Change Biol. 26, 2093–2105. doi: 10.1111/gcb.14968
Campbell A., Selvagens F.-F., para a P d. A., Múrias A., Santos P. T., Soares M. (1994). Fauna e flora do litoral de Portugal e Europa (FAPAS). Portugal.
Casado-Amezúa P., Araújo R., Bárbara I., Bermejo R., Borja Á., Díez I., et al. (2019). Distributional shifts of canopy-forming seaweeds from the Atlantic coast of southern Europe. Biodivers. Conserv. doi: 10.1007/s10531-019-01716-9
Casas G., Scrosati R., Luz Piriz M. (2004). The invasive kelp Undaria pinnatifida (Phaeophyceae, laminariales) reduces native seaweed diversity in nuevo gulf (Patagonia, Argentina). Biol. Invasions 6, 411–416. doi: 10.1023/B:BINV.0000041555.29305.41
Chapman A. R. O., Goudey C. L. (1983). Demographic study of the macrothallus of Leathesia difformis (Phaeophyta) in Nova Scotia. Can. J. Bot. 61, 319–323. doi: 10.1139/b83-035
Christie H., Jorgensen N. M., Norderhaug K. M., Waage-Nielsen E. (2003). Species distribution and habitat exploitation of fauna associated with kelp (Laminaria hyperborea) along the Norwegian coast. J. Mar. Biol. Assoc. UK 83, 687–699. doi: 10.1017/S0025315403007653h
Clavel J., Julliard R., Devictor V. (2011). Worldwide decline of specialist species: toward a global functional homogenization? Front. Ecol. Environ. 9, 222–228. doi: 10.1890/080216
Creed J. C. (1995). Spatial dynamics of a Himanthalia elongata (Fucales, phaeophyta) population. J. Phycol. 31, 851–859. doi: 10.1111/j.0022-3646.1995.00851.x
Critchley A. T., Farnham W. F., Morrell S. L. (1986). An account of the attempted control of an introduced marine alga, Sargassum muticum, in southern England. Biol. Conserv. 35, 313–332. doi: 10.1016/0006-3207(86)90092-3
Dayton P. K. (1972). “Toward an understanding of community resilience and the potential effects of enrichments to the benthos at McMurdo sound, Antarctica,” in Proc. coll. on conser. prob. in Antarctica (Kansas, USA: Allen Press Lawrence), 81–96.
de Azevedo J. (2019). What has changed in the macroalgal communities of the Portuguese coast over a 6-year period? master the. Portugal, University of Porto
De Cáceres M., Jansen F., De Cáceres M. M. (2016). Package ‘indicspecies.’ indicators 8, 1. doi: 10.1111/j.1600-0706.2010.18334.x
Edwards M., Hanniffy D., Heesch S., Harnández-kantún J., Moniz M., Quéguineus B., et al. (2012). Macroalgae fact-sheets. Eds. Soler-Vila Moniz A., Ryan M., Institute. Ireland, Ryan Institute.
Epstein G., Smale D. A. (2017). Undaria pinnatifida: A case study to highlight challenges in marine invasion ecology and management. Ecol. Evol. 7, 8624–8642. doi: 10.1002/ece3.3430
Espírito Santo F., de Lima M. I. P., Ramos A. M., Trigo R. M. (2014). Trends in seasonal surface air temperature in mainland Portugal, since 1941. Int. J. Climatol. 34, 1814–1837. doi: 10.1002/joc.3803
Faes V. A., Viejo R. M. (2003). Structure and dynamics of a population of Palmaria palmata (Rhodophyta) in northern Spain. J. Phycol. 39, 1038–1049. doi: 10.1111/j.0022-3646.2003.02-142.x
Fiuza A. F. G. (1983). Upwelling patterns off Portugal. NATO Conf. Ser. 4 Mar. Sci. 10 A, 85–98. doi: 10.1007/978-1-4615-6651-9_5
Flanagan P. H., Jensen O. P., Morley J. W., Pinsky M. L. (2019). Response of marine communities to local temperature changes. Ecography 42, 214–224. doi: 10.1111/ecog.03961
Fraga F. (1981). “Upwelling off the galacian coast, northwest Spain,” in Coastal upwelling (USA, American Geophysical Union (AGU), 176–182. doi: 10.1029/co001p0176
Franklin J., Davis F. W., Ikegami M., Syphard A. D., Flint L. E., Flint A. L., et al. (2013). Modeling plant species distributions under future climates: how fine scale do climate projections need to be? Glob. Change Biol. 19, 473–483. doi: 10.1111/gcb.12051
García Molinos J., Halpern B. S., Schoeman D. S., Brown C. J., Kiessling W., Moore P. J., et al. (2016). Climate velocity and the future global redistribution of marine biodiversity. Nat. Clim. Change 6, 83–88. doi: 10.1038/nclimate2769
Garnier S., Ross N., Rudis B., Sciaini M., Scherer C. (2018). Viridis: Default color maps from ‘matplotlib.’. R Packag. version 0.5 1, 2018.
Gastón A., García-Viñas J. I. (2010). Updating coarse-scale species distribution models using small fine-scale samples. Ecol. Modell. 221, 2576–2581. doi: 10.1016/j.ecolmodel.2010.07.016
Hampe A., Petit R. J. (2005). Conserving biodiversity under climate change: the rear edge matters. Ecol. Lett. 8, 461–467. doi: 10.1111/j.1461-0248.2005.00739.x
Harley C. D. G., Anderson K. M., Demes K. W., Jorve J. P., Kordas R. L., Coyle T. A., et al. (2012). Effects of climate change on global seaweed communities. J. Phycol. 48, 1064–1078. doi: 10.1111/j.1529-8817.2012.01224.x
Hawkins S. J., Sugden H. E., Mieszkowska N., Moore P. J., Poloczanska E., Leaper R., et al. (2009). Consequences of climate-driven biodiversity changes for ecosystem functioning of north European rocky shores. Mar. Ecol. Prog. Ser. 396, 245–259. doi: 10.3354/meps08378
Helmuth B., Broitman B. R., Blanchette C. A., Gilman S., Halpin P., Harley C. D. G., et al. (2006). Mosaic patterns of thermal stress in the rocky intertidal zone: implications for climate change. Ecol. Monogr. 76, 461–479. doi: 10.1890/0012-9615(2006)076[0461:MPOTSI]2.0.CO;2
Hiscock K. (1981). The rocky shore ecology of sullom voe. Proc. R. Soc Edinburgh Sect. B Biol. Sci. 80, 219–240.
Hothorn T., Bretz F., Westfall P., Heiberger R. M., Schuetzenmeister A., Scheibe S., et al. (2016). Package ‘multcomp.’ simultaneous inference gen Vienna, Austria, Parametr. Model. Proj. Stat. Comput.
James K., Shears N. T. (2016). Proliferation of the invasive kelp Undaria pinnatifida at aquaculture sites promotes spread to coastal reefs. Mar. Biol. 163, 34. doi: 10.1007/s00227-015-2811-9
Jurgens L. J., Rogers-Bennett L., Raimondi P. T., Schiebelhut L. M., Dawson M. N., Grosberg R. K., et al. (2015). Patterns of mass mortality among rocky shore invertebrates across 100 km of northeastern pacific coastline. PloS One 10, e0126280. doi: 10.1371/journal.pone.0126280
Lima F. P., Gomes F., Seabra R., Wethey D. S., Seabra M. I., Cruz T., et al. (2016). Loss of thermal refugia near equatorial range limits. Glob. Change Biol. 22, 254–263. doi: 10.1111/gcb.13115
Lima F. P., Queiroz N., Ribeiro P. A., Hawkins S. J., Santos A. M. (2006). Recent changes in the distribution of a marine gastropod, Patella rustica Linnaeus 1758, and their relationship to unusual climatic events. J. Biogeogr. 33, 812–822. doi: 10.1111/j.1365-2699.2006.01457.x
Lima F. P., Queiroz N., Ribeiro P. A., Xavier R., Hawkins S. J., Santos A. M. (2009). First record of Halidrys siliquosa on the Portuguese coast: counter-intuitive range expansion? Mar. Biodivers. Rec. 2, e1. doi: 10.1017/S1755267208000018
Lima F. P., Ribeiro P. A., Queiroz N., Hawkins S. J., Santos A. M. (2007). Do distributional shifts of northern and southern species of algae match the warming pattern? Glob. Change Biol. 13, 2592–2604. doi: 10.1111/j.1365-2486.2007.01451.x
Lima F. P., Wethey D. S. (2012). Three decades of high-resolution coastal sea surface temperatures reveal more than warming. Nat. Commun. 3, 1–13. doi: 10.1038/ncomms1713
Lowe S., Browne M., Boudjelas S., De Poorter M. (2000). 100 of the world’s worst invasive alien species: a selection from the global invasive species database (New Zealand, Invasive Species Specialist Group Auckland).
McKnight E., Spake R., Bates A., Smale D. A., Rius M. (2021). Non-native species outperform natives in coastal marine ecosystems subjected to warming and freshening events. Glob. Ecol. Biogeogr. doi: 10.1111/geb.13318
Mclean M., Mouillot D., Maureaud A. A., Engelhard G., Pinsky M., Correspondence A. A. (2021). Disentangling tropicalization and deborealization in marine ecosystems under climate change. Curr. Biol. 31:1–7. doi: 10.1016/j.cub.2021.08.034
Meneghesso C., Seabra R., Broitman B. R., Wethey D. S., Burrows M. T., Chan B. K. K., et al. (2020). Remotely-sensed L4 SST underestimates the thermal fingerprint of coastal upwelling. Remote Sens. Environ. 237, 111588. doi: 10.1016/j.rse.2019.111588
Mieszkowska N., Burrows M. T., Hawkins S. J., Sugden H. (2021). Impacts of pervasive climate change and extreme events on rocky intertidal communities: Evidence from long-term data. Front. Mar. Sci. 8. doi: 10.3389/fmars.2021.642764
Mieszkowska N., Kendall M. A., Hawkins S. J., Leaper R., Williamson P., Hardman-Mountford N. J., et al. (2006). “Changes in the range of some common rocky shore species in Britain–a response to climate change?” in Marine biodiversity (Netherlands, Springer), 241–251.
Minchin D., Nunn J. (2014). The invasive brown alga Undaria pinnatifida (Harvey) suringar 1873 (Laminariales: Alariaceae), spreads northwards in Europe. BioInvasions Rec. 3, 57–63. doi: 10.3391/bir.2014.3.2.01
Mineur F., Arenas F., Assis J., Davies A. J., Engelen A. H., Fernandes F., et al. (2015). European Seaweeds under pressure: Consequences for communities and ecosystem functioning. J. Sea Res. 98, 91–108. doi: 10.1016/j.seares.2014.11.004
Molenaar F. J., Venekamp L. A. H., Breeman A. M. (1996). Life-history regulation in the subtidal red algae Calliblepharis ciliata. Eur. J. Phycol. 31, 241–247. doi: 10.1080/09670269600651441
Morelli T. L., Barrows C. W., Ramirez A. R., Cartwright J. M., Ackerly D. D., Eaves T. D., et al. (2020). Climate-change refugia: biodiversity in the slow lane. Front. Ecol. Environ. 18, 228–234. doi: 10.1002/fee.2189
Mota C. F., Engelen A. H., Serrao E. A., Coelho M. A. G., Marbà N., et al. (2018). Differentiation in fitness-related traits in response to elevated temperatures between leading and trailing edge populations of marine macrophytes. PloS One 13, e0203666. doi: 10.1371/journal.pone.0203666
Mulas M., Bertocci I. (2016). Devil’s tongue weed ( Grateloupia turuturu yamada) in northern Portugal: Passenger or driver of change in native biodiversity? Mar. Environ. Res. 118, 1–9. doi: 10.1016/j.marenvres.2016.04.007
Norderhaug K. M., Nedreaas K., Huserbråten M., Moland E. (2021). Depletion of coastal predatory fish sub-stocks coincided with the largest sea urchin grazing event observed in the NE Atlantic. Ambio 50, 163–173. doi: 10.1007/s13280-020-01362-4
Oksanen J., Simpson G. L., Blanchet F. G., Kindt R., Legendre P., Minchin P. R., et al. (2022). Package ‘vegan.’ community ecology package version 2.6-2. 1–295.
Pearson G. A., Lago-Leston A., Mota C. (2009). Frayed at the edges: selective pressure and adaptive response to abiotic stressors are mismatched in low diversity edge populations. J. Ecol. 97, 450–462. doi: 10.1111/j.1365-2745.2009.01481.x
Pereira J., Monteiro C., Santos A. M., Seabra R., Ribeiro P., Lima F. P. (2021a). Intertidal biodiversity along the Portuguese coast, (2001-2002). GBIF.org. doi: 10.15468/mbg5p3
Pereira J., Monteiro C., Seabra R., Lima F., Lima F. P. (2022). Fine-scale abundance of rocky shore macroalgae species with distribution limits in NW Iberia in 2020/2021. Biodivers. Data J. 2, e80942. doi: 10.3897/BDJ.10.e80798
Pereira J., Monteiro C., Seabra R., Santos Múrias A., Lima F. P. (2021b). Intertidal macroalgae species distribution along the northwestern Iberian coast in 2020/2021. GBIF.org. doi: 10.15468/247z4g
Pereira J., Ribeiro P. A., Santos A. M., Monteiro C., Seabra R., Lima F. P. (2021c). A comprehensive assessment of the intertidal biodiversity along the Portuguese coast in the early 2000s. Biodivers. Data J. 9:1–31. doi: 10.3897/BDJ.9.e72961
Petrov S. (2021). Permutation hypothesis test in r. exploring a powerful simulation | by serafim petrov | towards data science.
Piñeiro-Corbeira C., Barreiro R., Cremades J. (2016). Decadal changes in the distribution of common intertidal seaweeds in Galicia (NW Iberia). Mar. Environ. Res. 113, 106–115. doi: 10.1016/j.marenvres.2015.11.012
Plaza M., Cifuentes A., Ibáñez E. (2008). In the search of new functional food ingredients from algae. Trends Food Sci. Technol. 19, 31–39. doi: 10.1016/j.tifs.2007.07.012
Poloczanska E. S., Burrows M. T., Brown C. J., García Molinos J., Halpern B. S., Hoegh-Guldberg O., et al. (2016). Responses of marine organisms to climate change across oceans. Front. Mar. Sci. 3. doi: 10.3389/fmars.2016.00062
Potter K. A., Arthur Woods H., Pincebourde S. (2013). Microclimatic challenges in global change biology. Glob. Change Biol. 19, 2932–2939. doi: 10.1111/gcb.12257
Poza A. M., Gauna M. C., Escobar J. F., Parodi E. R. (2017). Heteromorphic phases of Leathesia marina (Ectocarpales, ochrophyta) over time from northern Patagonia, Argentina. Phycologia 56, 579–589. doi: 10.2216/16-117.1
Provan J., Maggs C. A. (2012). Unique genetic variation at a species’ rear edge is under threat from global climate change. Proc. R. Soc B Biol. Sci. 279, 39–47. doi: 10.1098/rspb.2011.0536
Richardson D. M., Pyšek P., Carlton J. T. (2011). A compendium of essential concepts and terminology in invasion ecology. Fifty years invasion Ecol. Leg. Charles Elt. 1, 409–420. doi: 10.1002/9781444329988.ch30
Román M., Román S., Vázquez E., Troncoso J., Olabarria C. (2020). Heatwaves during low tide are critical for the physiological performance of intertidal macroalgae under global warming scenarios. Sci. Rep. 10, 1–14. doi: 10.1038/s41598-020-78526-5
Rubal M., Veiga P., Cacabelos E., Moreira J., Sousa-Pinto I. (2013). Increasing sea surface temperature and range shifts of intertidal gastropods along the Iberian peninsula. J. Sea Res. 77, 1–10. doi: 10.1016/j.seares.2012.12.003
Rubal M., Veiga P., Maldonado C., Torres C., Moreira J. (2015). Population attributes and traits of Siphonaria pectinata (Mollusca: Siphonariidae) in range-edge and non range-edge populations at its Eastern Atlantic northern distribution boundary. J. Exp. Mar. Bio. Ecol. 471, 41–47. doi: 10.1016/j.jembe.2015.05.015
Rudis B. (2020). Additional themes, theme components and utilities for “ggplot2” [R package hrbrthemes version 0.8.0].
Sanchez I., Fernandez C. (2005). Impact of the invasive seaweed Sargassum muticum (Phaeophyta) on an intertidal macroalgal assemblage. J. Phycol. 41, 923–930. doi: 10.1111/j.1529-8817.2005.00120.x
Schnute J., Boers N., Haigh R. (2004). PBS mapping 2: User’s guide. Can. Tech. Rep. Fish. Aquat. Sci. 2549, viii + 126.
Seabra R., Varela R., Santos A. M., Gómez-Gesteira M., Meneghesso C., Wethey D. S., et al. (2019). Reduced nearshore warming associated with Eastern boundary upwelling systems. Front. Mar. Sci. 6. doi: 10.3389/fmars.2019.00104
Seabra R., Wethey D. S., Santos A. M., Lima F. P. (2011). Side matters: microhabitat influence on intertidal heat stress over a large geographical scale. J. Exp. Mar. Bio. Ecol. 400, 200–208. doi: 10.1016/j.jembe.2011.02.010
Seabra R., Wethey D. S., Santos A. M., Lima F. P. (2015). Understanding complex biogeographic responses to climate change. Sci. Rep. 5, 1–6. doi: 10.1038/srep12930
Seitz R. D., Wennhage H., Bergström U., Lipcius R. N., Ysebaert T. (2014). Ecological value of coastal habitats for commercially and ecologically important species. ICES J. Mar. Sci. 71, 648–665. doi: 10.1093/icesjms/fst152
Silva C. O., Lemos M. F. L., Gaspar R., Gonçalves C., Neto J. M. (2021). The effects of the invasive seaweed Asparagopsis armata on native rock pool communities: Evidences from experimental exclusion. Ecol. Indic. 125, 107463. doi: 10.1016/j.ecolind.2021.107463
Slowikowski K., Schep A., Hughes S., Lukauskas S., Irisson J.-O., Kamvar Z. N., et al. (2018). Package ggrepel. autom. position non-overlapping text labels with ‘ggplot2.
Smale D. A. (2020). Impacts of ocean warming on kelp forest ecosystems. New Phytol. 225, 1447–1454. doi: 10.1111/nph.16107
Smale D. A., Burrows M. T., Moore P., O’Connor N., Hawkins S. J. (2013). Threats and knowledge gaps for ecosystem services provided by kelp forests: a northeast Atlantic perspective. Ecol. Evol. 3, 4016–4038. doi: 10.1002/ece3.774
Sousa M. C., Ribeiro A., Des M., Gomez-Gesteira M., deCastro M., Dias J. M. (2020). NW Iberian Peninsula coastal upwelling future weakening: Competition between wind intensification and surface heating. Sci. Total Environ. 703, 134808. doi: 10.1016/j.scitotenv.2019.134808
South P. M., Floerl O., Forrest B. M., Thomsen M. S. (2017). A review of three decades of research on the invasive kelp Undaria pinnatifida in Australasia: An assessment of its success, impacts and status as one of the world’s worst invaders. Mar. Environ. Res. 131, 243–257. doi: 10.1016/j.marenvres.2017.09.015
Southward A. J., Hawkins S. J., Burrows M. T. (1995). Seventy years’ observations of changes in distribution and abundance of zooplankton and intertidal organisms in the western English channel in relation to rising sea temperature. J. Therm. Biol. 20, 127–155. doi: 10.1016/0306-4565(94)00043-I
Stachowicz J. J., Terwin J. R., Whitlatch R. B., Osman R. W. (2002). Linking climate change and biological invasions: Ocean warming facilitates nonindigenous species invasions. Proc. Natl. Acad. Sci. 99, 15497 LP–15500. doi: 10.1073/pnas.242437499
Stæhr P. A., Pedersen M. F., Thomsen M. S., Wernberg T., Krause-Jensen D. (2000). Invasion of Sargassum muticum in limfjorden (Denmark) and its possible impact on the indigenous macroalgal community. Mar. Ecol. Prog. Ser. 207, 79–88. doi: 10.3354/meps207079
Stuart M. D., Hurd C. L., Brown M. T. (1999). “Effects of seasonal growth rate on morphological variation of undaria pinnatifida (Alariaceae, phaeophyceae),” in Sixteenth international seaweed symposium. Eds. Kain J. M., Brown M. T., Lahaye M. (Dordrecht: Springer Netherlands), 191–199.
Teagle H., Smale D. A. (2018). Climate-driven substitution of habitat-forming species leads to reduced biodiversity within a temperate marine community. Divers. Distrib. 24, 1367–1380. doi: 10.1111/ddi.12775
Trivedi M., Berry P., Morecroft M., Dawson T. (2008). Spatial scale affects bioclimate model projections of climate change impacts on mountain plants. Glob. Change Biol. 14, 1089–1103. doi: 10.1111/j.1365-2486.2008.01553.x
Ullah H., Nagelkerken I., Goldenberg S. U., Fordham D. A. (2018). Climate change could drive marine food web collapse through altered trophic flows and cyanobacterial proliferation. PloS Biol. 16, e2003446. doi: 10.1371/journal.pbio.2003446
Van den Hoek C., Donze M. (1967). Algal phytogeography of the European Atlantic coasts. Blumea Biodiversity Evol. Biogeogr. Plants 15, 63–89.
Veiga P., Torres A. C., Rubal M., Troncoso J., Sousa-Pinto I. (2014). The invasive kelp Undaria pinnatifida (Laminariales, ochrophyta) along the north coast of Portugal: Distribution model versus field observations. Mar. pollut. Bull. 84, 363–365. doi: 10.1016/j.marpolbul.2014.05.038
Viana I. G., Bode A., Fernández C. (2014). Growth and production of new recruits and adult individuals of Ascophyllum nodosum in a non-harvested population at its southern limit (Galicia, NW Spain). Mar. Biol. 161, 2885–2895. doi: 10.1007/s00227-014-2553-0
Vieira R., Pereira R., Arenas F., Araujo R., Pinto I. S. (2010). Espécies intertidais características da costa norte de Portugal. Ed. SerSilito Portugal, CIIMAR.
Wethey D. S., Woodin S. A., Hilbish T. J., Jones S. J., Lima F. P., Brannock P. M. (2011). Response of intertidal populations to climate: effects of extreme events versus long term change. J. Exp. Mar. Bio. Ecol. 400, 132–144. doi: 10.1016/j.jembe.2011.02.008
Whittick A. (1983). Spatial and temporal distributions of dominant epiphytes on the stipes of Laminaria hyperborea (Gunn.) fosl. (Phaeophyta:Laminariales) in S.E. Scotland. J. Exp. Mar. Bio. Ecol. 73, 1–10. doi: 10.1016/0022-0981(83)90002-3
Wickham H. (2012). reshape2: Flexibly reshape data: a reboot of the reshape package. r packag. version 1.
Wickham H., Chang W., Henry L., Pedersen T. L., Takahashi K., Wilke C., et al. (2016). ggplot2: Create elegant data visualisations using the grammar of graphics. r packag. version 2.
Williams S. L., Smith J. E. (2007). A global review of the distribution, taxonomy, and impacts of introduced seaweeds. Annu. Rev. Ecol. Evol. Syst. 38, 327–359. doi: 10.1146/annurev.ecolsys.38.091206.095543
Keywords: range shifts, intertidal, seaweed, rocky shores, North-western Iberia, upwelling system, biodiversity
Citation: Monteiro C, Pereira J, Seabra R and Lima FP (2022) Fine-scale survey of intertidal macroalgae reveals recent changes in a cold-water biogeographic stronghold. Front. Mar. Sci. 9:880074. doi: 10.3389/fmars.2022.880074
Received: 21 February 2022; Accepted: 27 June 2022;
Published: 26 July 2022.
Edited by:
Katerina Vasileiadou, Hellenic Centre for Marine Research (HCMR), GreeceReviewed by:
Gerardo Zardi, Rhodes University, South AfricaBarbara Spiecker, University of California, Santa Barbara, United States
Copyright © 2022 Monteiro, Pereira, Seabra and Lima. This is an open-access article distributed under the terms of the Creative Commons Attribution License (CC BY). The use, distribution or reproduction in other forums is permitted, provided the original author(s) and the copyright owner(s) are credited and that the original publication in this journal is cited, in accordance with accepted academic practice. No use, distribution or reproduction is permitted which does not comply with these terms.
*Correspondence: Fernando P. Lima, ZnBsaW1hQGdtYWlsLmNvbQ==
†These authors have contributed equally to this work and share first authorship