- 1College of Fisheries, Guangdong Ocean University, Zhanjiang, China
- 2Key Laboratory of Aquatic, Livestock and Poultry Feed Science and Technology in South China, Ministry of Agriculture, Zhanjiang, China
- 3Aquatic Animals Precision Nutrition and High Efficiency Feed Engineering Research Center of Guangdong Province, Zhanjiang, China
The effects of dietary zymosan A on the growth performance and histological structure, digestive capacity, and microbiota were investigated in Litopenaeus vannamei, an important shrimp species used in aquaculture. L. vannamei (initial body weight = 0.41 ± 0.05 g) were fed diets supplemented with zymosan-A at doses of 0 mg/kg (Z0, control), 1 mg/kg (Z1), 5 mg/kg (Z5), 25 mg/kg (Z25), 125 mg/kg (Z125), or 625 mg/kg (Z625) for 8 weeks. The results showed that doses of 25 and 125 mg/kg significantly increased the final body weight, weight gain rate (WGR), and specific growth rate (SGR) and significantly decreased the feed conversion rate compared with the controls (p < 0.05). Analyses of the WGR and SGR revealed that the optimum dietary zymosan-A levels were 308.56 and 300.00 mg/kg, respectively. Compared with the controls, the intestinal villus height was significantly increased by the addition of zymosan-A at doses ≥25 mg/kg (p < 0.05). An obvious upregulation of the activities of trypsin and amylase was observed in all groups that received zymosan-A supplementation, while the activity of lipase was significantly increased in groups Z125 and Z625 (p < 0.05). Consistently, the gene expressions of trypsin and lipase were significantly higher in group Z125, while the gene expression of amylase was significantly increased in all zymosan-A-supplemented groups (p < 0.05). Analysis of the intestinal microbiota showed significant differences in the alpha diversity between group Z125 and controls. The supplemented groups showed altered intestinal bacterial community structures and compositions at the phylum, family, and genus levels, with statistical differences observed in the relative abundances of some dominant species. Tax4Fun predictions of the microbiota indicated that multiple intestinal functions were significantly altered in all zymosan-A-supplemented groups, except Z1. Among these groups, the functions related to transport and catabolism, substance dependence, cardiovascular disease, and signaling molecules and interactions were obviously increased by the addition of zymosan-A at different levels. In conclusion, dietary supplementation of the optimum amount of zymosan-A can improve growth and intestinal function in L. vannamei, which will be valuable in aquaculture.
Introduction
Litopenaeus vannamei is a decapod crustacean species of the Penaeidae family that is economically important in global aquaculture. Shrimp production has increased with the rapid development of shrimp farming; however, diseases such as white spot disease (WSD) and acute hepatopancreatic necrosis disease (AHPND) have caused huge economic losses and resource wastage in this industry (Thitamadee et al., 2016). Antibiotics are a common form of disease control in aquaculture, yet may enhance the resistance of numerous pathogenic microorganisms, reduce the quality and safety of shrimp products, and threaten human health (Holmstrom et al., 2003). Alternatively, immunostimulants such as probiotics, prebiotics, hormones, cytokines, and polysaccharides, which can improve growth and immune responses while controlling disease, may be a better choice for use in aquaculture (Carbone and Faggio, 2016; Dawood et al., 2018; Mohan et al., 2019).
β-glucan is a type of polysaccharide present in the cell walls of bacteria, fungi, algae, and plants. It has various structural types consisting of β-(1,3/1,4) or β-(1,3/1,6)-D-linked glucose units (Barsanti et al., 2011). β-glucan is a well-known immunomodulator that has been administered to invertebrates and vertebrates via bath treatment, injection, and diet (Kim et al., 2000; De Oliveira et al., 2019). In humans, β-glucan has been reported to have anticancer, antidiabetic, anti-inflammatory, and immunomodulating effects (Jayachandran et al., 2018). Recent findings have suggested that β-glucan has significant impact on changes in the gut microbiota and, in turn, on the health of humans, pigs, and mice (Xu et al., 2020; Golisch et al., 2021; Wu et al., 2021). Currently, β-glucan is used as an immunomodulatory food supplement that is beneficial to immunity in commercial aquaculture species (Rodrigues et al., 2020). The beneficial effects of dietary β-glucan on growth, health status, and immunity have been reported in Oreochromis niloticus (Fabiana et al., 2017), Paramisgurnus dabryanus (Zhu and Wu, 2018), L. vannamei (Li et al., 2019), and Cyprinus carpio (Harris et al., 2020). Intake of β-glucan can also optimize the intestinal microbiota of aquatic animals, including O. niloticus, C. carpio, Scophthalmus maximus, Apostichopus japonicus, and L. vannamei (Kühlwein et al., 2014; Yang et al., 2015; Miest et al., 2016; Li et al., 2019; Xu et al., 2020). However, the structures of β-glucan have not been classified in most of the previous studies and their sources are also diverse.
Zymosan-A is a purified β-1,3-glucan obtained from a naturally insoluble polysaccharide macromolecule extracted from the cell walls of Saccharomyces cerevisiae. As a type of β-glucan, zymosan-A has been reported to play an important role in regulating the immune response of several species (Stuyven et al., 2009; Lei et al., 2013; Yu et al., 2014). In mice, zymosan-A enhances the mucosal adjuvant activity of poly(I:C) in a nasal influenza vaccine and also affects the composition and biodiversity of the gut microbiota (Ainai et al., 2010; Jeong et al., 2021). However, studies using zymosan-A as a dietary supplement for aquatic animals are rare. In addition, knowledge of its effects on the intestinal microbiota of aquatic animals remains lacking.
In this study, the effects of dietary supplementation of zymosan-A on the growth performance, histological structure, digestive enzyme activities and gene expressions, and microbiotic structure of L. vannamei were analyzed to evaluate the growth and intestinal functional responses to zymosan-A. These results have implications for improvement of the nutritional regulation of shrimp farming.
Materials and Methods
Diets and Experimental Design
Six experimental diets were formulated; a proximate analysis is given in Table 1. Group Z0 was used as the control, while groups Z1, Z5, Z25, Z125, and Z625 were supplemented with graded levels of zymosan-A (1, 5, 25, 125, or 625 mg/kg, respectively; Z4250; supplied by Sigma-Aldrich, St. Louis, MO, USA). Dietary ingredients were ground through an 80-mesh screen, weighed, and thoroughly mixed to homogeneity (M-256, South China University of Technology, Guangzhou, China). Each diet was weighed according to the test formula and mixed step by step using a twin-screw extruder (F-26, South China University of Technology, Guangzhou, China) to make pellets with particle sizes of 1.0 and 1.5 mm. The pellets were heated in an electric oven at 60°C for 30 min, then dried at room temperature to a moisture content of about 10%, sealed in Ziploc bags, and stored at −20°C until required.
Shrimp Feeding Trial and Sample Collection
The entire experiment was carried out in an indoor breeding system at the Marine Biology Research Base of Guangdong Ocean University (Zhanjiang, China). L. vannamei, with average body weight 0.41 ± 0.05 g, were obtained from Guangdong Haixing Agriculture Group Co., Ltd. (Zhanjiang, China). Before starting the feeding experiment, the shrimp were allowed to adapt to the experimental conditions for 1 week before feeding with commercial feed. The acclimated shrimp were randomly distributed into 300-L fiberglass tanks at 40 shrimp per tank. Shrimp were fed the experimental diets to apparent satiation four times daily (0700, 1100, 1700, and 2100 hours) for 8 weeks. During the experimental period, the temperature range was 29.0–30.0°C, salinity was 27–30 g/L, the dissolved oxygen level was at least 6.0 mg/L, the pH value was 7.7–8.0, and the ammonia nitrogen level was <0.05 mg/L. During the trial, each tank was individually aerated and 60% of the water was exchanged daily.
At the end of the 8-week period, shrimp were fasted for 24 h before collection of samples. After being counted and weighed to determine survival and weight gain, six shrimp from each tank were randomly sampled for each of the following analyses: histological sectioning, digestive enzyme activity analysis, gene expression analysis, and intestinal microbiome analysis. The samples were rapidly removed and frozen in liquid nitrogen until analysis.
Histological Structure of the Intestine
At the end of the feeding trial, the midguts were sampled from four shrimp from each tank and fixed with 4% paraformaldehyde in 5-ml Eppendorf tubes to remove the intestinal content. Following fixation, the intestinal tissue was embedded in paraffin, sliced, and stained with hematoxylin–eosin (H&E) using standard histological techniques, then examined for intestinal morphology parameters under an optical microscope (Olympus BX51, serial no. 9K18395, Tokyo, Japan). The electronic images were further analyzed using ImageJ software to assess the dimensions of intestinal villus height (VH), villus width (VW), and muscle thickness (MT).
Digestive Enzyme Analysis
Intestine samples were homogenized in ice-cold phosphate buffer (1:10 dilution). The homogenate was then centrifuged for 20 min (4°C, 3,000 rpm), and aliquots of the supernatant were used to quantify the digestive enzymes. All indices, including amylase (AMS), lipase, and trypsin, were measured with commercial assay kits (Nanjing Jian Cheng Bioengineering Institute, Nanjing, China) in accordance with the manufacturer’s instructions.
Gene Expression Analysis
Total RNA was extracted from the intestines without feces of three shrimp from each tank using TransZol Up Plus RNA kits (TransGen, Beijing, China) following the manufacturer’s protocol. Spectrophotometric analysis (Nanodrop 2000) was used to assess the RNA quality and concentration. Complementary DNA (cDNA) was synthesized using a PrimeScriptTM RT reagent kit with gDNA Eraser (Takara, Shiga, Japan) according to the manufacturer’s instructions. Total RNA (1 μg) was reverse-transcribed to the first-strand cDNA using reverse transcriptase (Accurate Biology, Changsha, China) following the manufacturer’s instructions.
Real-time PCR for the target genes was performed using a SYBR® Green Premix Pro Taq HS qPCR Kit II (Accurate Biology, Changsha, China) and quantified on a LightCycler 480 (Roche Applied Science, Mannheim, Germany) using the following program: 0.5 μM of forward and reverse specific primers, 5 μl of 2× SYBR® Green Pro Taq HS Premix II, 10 ng of cDNA template, and nuclease-free water to make a final volume of 10 μl; denaturation at 95°C for 30 s, 40× 5 s amplification cycles, denaturation at 95°C, and 30 s annealing at 60°C, followed by melting curve analysis and cooling to 4°C. The elongation factor 1α (EF1α; GenBank accession no. GU136229) was used as the internal control. All samples were tested in triplicate. The primer sequences are listed in Table 2.
Intestinal Microbial Analysis
The total genomic DNA of microbes from the intestinal samples was extracted using HiPure Soil DNA Kits (or HiPure Stool DNA Kits; Magen, Guangzhou, China) according to the manufacturer’s protocols. Then, the V3+V4 region of the bacterial 16S ribosomal RNA (rDNA) gene was amplified using a pair of barcoded specific primers: 341F (5′-CCTACGGGNGGCWGCAG-3′) and 806R (5′-GGACTACHVGGGTATCTAAT-3′). The PCR amplification began with 2 min at 94°C, followed by 30 cycles at 98°C for 10 s, 62°C for 30 s, 68°C for 30 s, and a final extension at 68°C for 5 min. PCR reactions were performed in triplicate 50 μl mixtures containing 5 μl of 10× KOD buffer, 5 μl of 2 mM dNTPs, 3 μl of 25 mM MgSO4, 1.5 μl of each primer (10 mM), 1 μl of KOD polymerase, and 100 ng of template DNA. Related PCR reagents were obtained from Toyobo (Osaka, Japan). The amplified products were purified using the AxyPrep DNA Gel Extraction Kit (Axygen Biosciences, Union City, CA, USA) according to the manufacturer’s instructions. The purified and amplified products were mixed equivalently and ligated with the sequencing adapter, then sequenced with a Hiseq2500 PE250 machine (Illumina, San Diego, CA, USA). The raw data have been deposited in the NCBI GenBank (http://www.ncbi.nlm.nih.gov/genbank/).
The data were filtered for noisy sequences, checked for the presence of chimaeras, and clustered with a threshold of 97% sequence similarity. To determine the level of sequencing depth, rarefaction curves were produced by plotting the number of observed operational taxonomic units (OTUs) against the number of sequences. The tag sequence with the highest abundance was selected as a representative sequence within each cluster. Between-group Venn analysis was performed in R software (version 3.4.1) to identify unique and common OTUs. The representative sequences were classified into organisms by a naive Bayesian model using the RDP classifier, version 2.2 (Wang, 2007), based on the SILVA database (https://www.arb-silva.de/) (Quast et al., 2012), with confidence threshold values of 0.8–1. Alpha diversity indices [observed species (Sobs), Shannon, Simpson, Chao1, and the abundance-based coverage estimator (ACE)] were calculated using QIIME software. The Kyoto Encyclopedia of Genes and Genomes (KEGG) pathway analysis of the OTUs was inferred using Tax4Fun, version 1.0 (Aßhauer et al., 2015).
Statistical Analysis and Calculations
The experimental results are presented as the mean ± standard deviation (SD). Statistically significant differences were established using one-way analysis of variance (ANOVA) at a 5% level of probability, and differences between means were compared using Tukey’s tests. Statistical analysis was carried out in SPSS for Windows, version 22 (SPSS Inc., Chicago, IL, USA). The formulae employed were as follows:
Results
Growth Performance
After the feeding experiment, all L. vannamei had a high SR, which was unaffected by supplementation with zymosan-A (Table 3). The final body weight (FBW), WGR, and SGR were significantly higher and the FCR significantly lower in groups Z25 and Z125 than those in group Z0 (p < 0.05). According to the line chart analysis using WGR or SGR as the evaluation index, the optimal amounts of zymosan-A supplementation were 308.56 and 300.00 mg/kg, respectively (Figure 1).
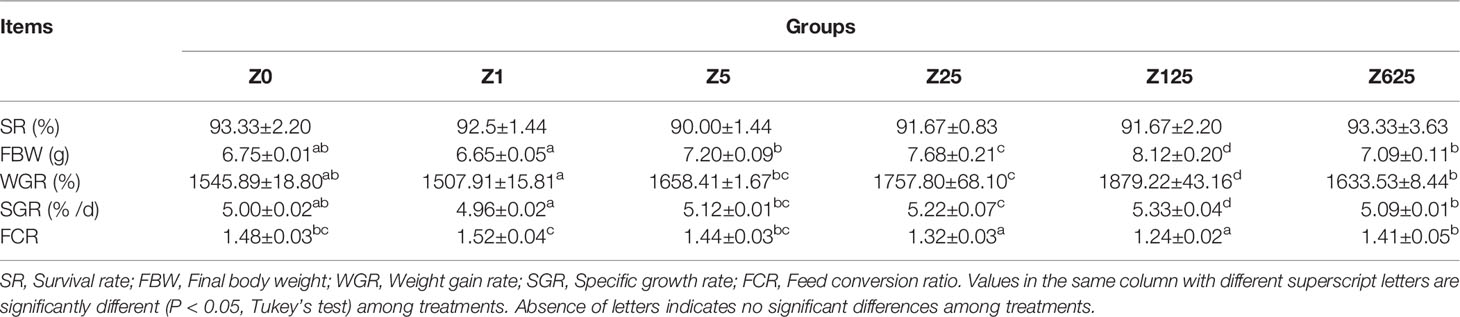
Table 3 Growth performance and feed utilization of L. vannamei fed the experimental diets for 8 weeks.
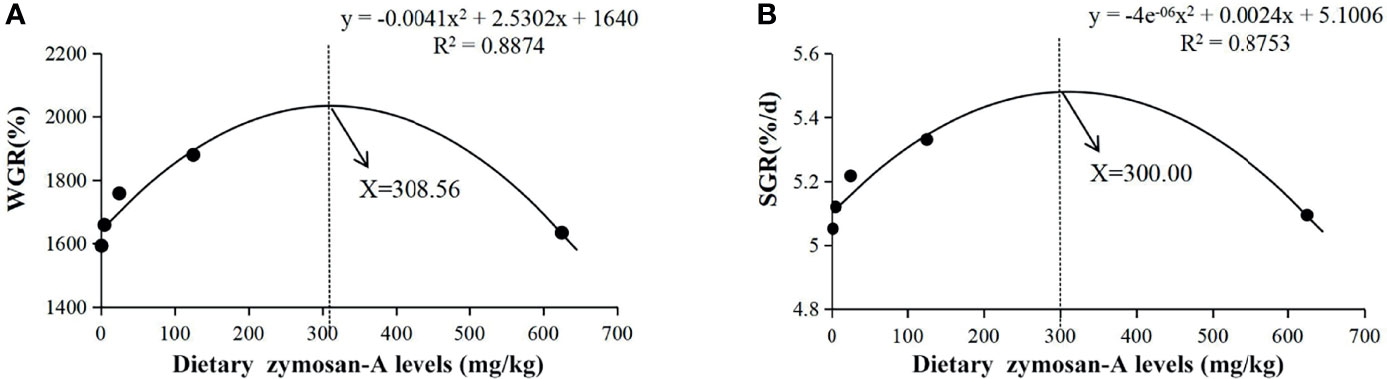
Figure 1 Relationships between the level of dietary zymosan-A and weight gain rate (WGR) (A) and specific growth rate (SGR) (B) in Litopenaeus vannamei fed experimental diets for 8 weeks (with fitted second-order polynomial models).
Histological Observation of the Intestine
The effects of dietary zymosan-A supplementation on the intestinal morphological parameters are shown in Figure 2. Photomicrographs of cross-sections of the intestinal tract are shown in Table 4. When the amount of zymosan-A reached 25 mg/kg, the intestinal VH in L. vannamei was significantly higher compared to that of group Z0 (p < 0.05). Zymosan-A supplementation did not obviously change the intestinal VW and MT. In addition, the intestinal structure was destroyed in group Z625.
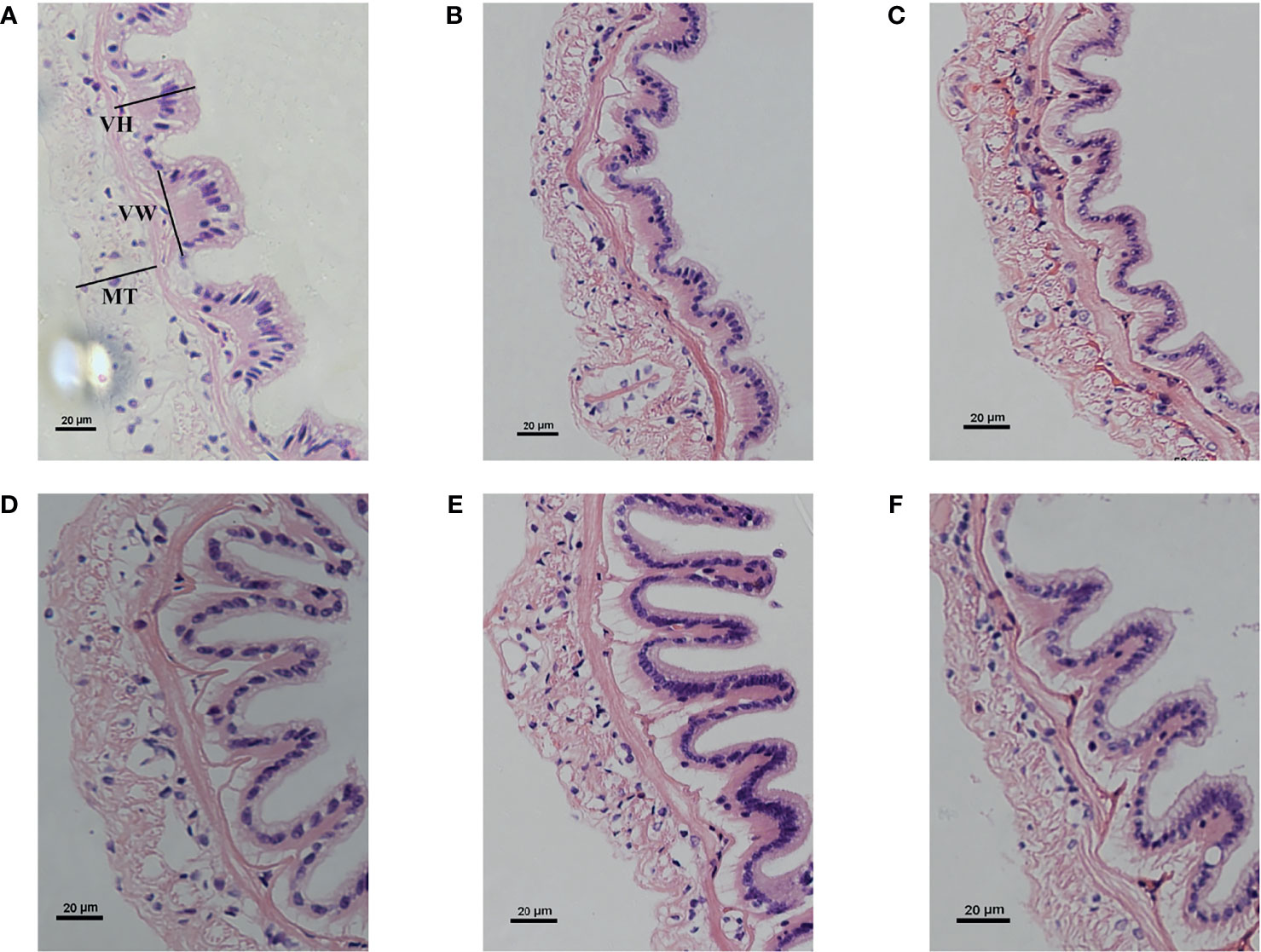
Figure 2 Intestinal histology of Litopenaeus vannamei fed different levels of zymosan-A. (A–F) Groups Z0 (control), Z1, Z5, Z25, Z125, and Z625, respectively. VH, villus height; VW, villus width; MT, muscle thickness.
Digestive Enzyme Activities in the Intestine
As shown in Figures 3A, C, the activities of both the intestinal trypsin and amylase were significantly increased in all zymosan-A-supplemented groups (p < 0.5), with the highest levels in group Z125, followed by group Z25. For lipase activity, compared with group Z0, obvious increases were only observed in groups Z125 and Z625, being highest in group Z125 (Figure 3B).
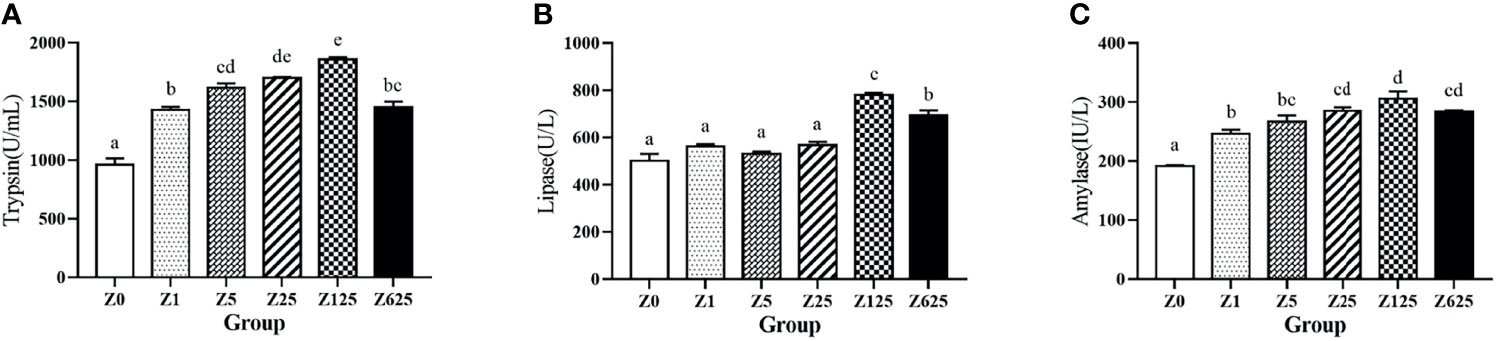
Figure 3 Effect of different levels of zymosan-A on the activities of trypsin (A), lipase (B), and amylase (C) in the intestine of Litopenaeus vannamei. Different letters indicate significant differences between treatments (p < 0.05).
Gene Expressions of Digestive Enzymes in the Intestine
Compared with the controls, the relative expression levels of the digestive-related genes, including trypsin, lipase, and amylase, were increased by supplementation with zymosan-A (Figure 4). The gene expressions of both trypsin and lipase were significantly induced in group Z125, while amylase expression was obviously higher in all zymosan-A-supplemented groups than those in controls (p < 0.5).
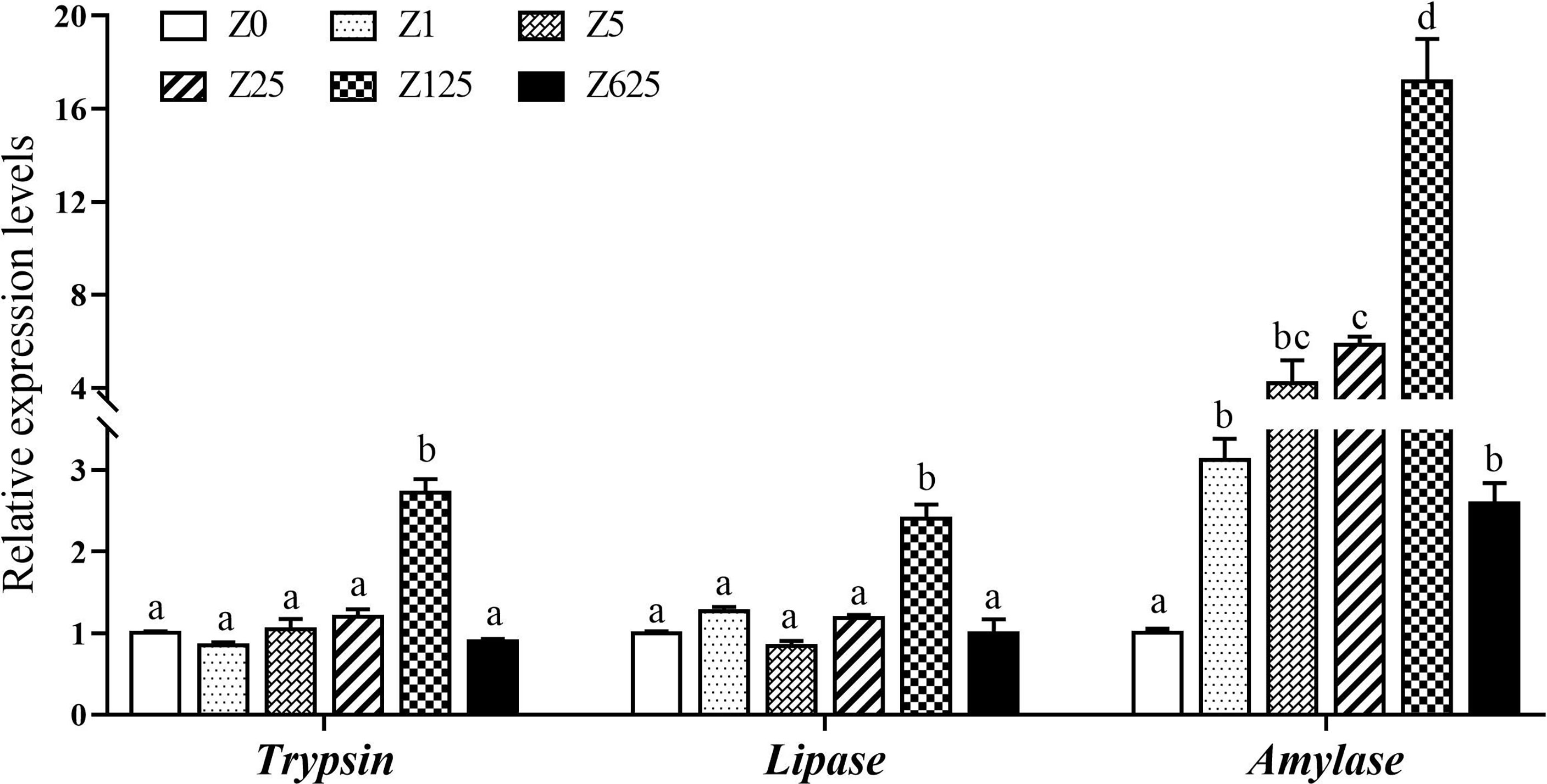
Figure 4 Effect of different levels of zymosan-A on the expressions of trypsin, lipase, and amylase genes in the intestine of Litopenaeus vannamei. Different letters indicate significant differences between treatments (p < 0.05).
Microbiota Community Characterization in the Intestine
The raw data of this study have been deposited in the NCBI’s Sequence Read Archive (SRA) database with the accession number PRJNA808041. After quality control and read assembly, the effective tags were >90%. All OTUs of the comparison group with an average abundance >1 were selected for Venn diagram analysis. A Venn diagram was constructed to identify the core and different OTUs existing in different groups of shrimp samples. In this regard, 432 OTUs were shared among all the intestine samples (Figure 5). In contrast, 341, 158, 261, 272, 321, and 281 OTUs were unique to groups Z0, Z1, Z5, Z25, Z125, and Z625, respectively. Group Z1 had the minimum number of unique OTUs, while group Z125 had the maximum.
As shown in Table 5, significant differences in the diversity and richness of the intestinal microbiota were observed among the dietary treatments, as evaluated using the alpha indices, including the observed species (Sobs), Chao1, Shannon, and Simpson indices, and ACE. Compared to those in group Z0, the Sobs and Chao1 were significantly higher in groups with zymosan-A addition of >5 mg/kg (p < 0.05). Similarly, only the Shannon and Simpson indices of group Z125 were significantly higher than those of group Z0 (p < 0.05). The ACE values were significantly higher in each zymosan-A-supplemented group than those in group Z0 (p < 0.05).
At the phylum level, the average intestinal microbiotic community of L. vannamei was dominated by Proteobacteria (48.29%), Bacteroidetes (25.32%), Planctomycetes (7.97%), and Verrucomicrobia (7.20%). Actinobacteria (3.99%) and Chlamydiae (2.83%) were subdominant (Figure 6A). Moreover, Acidobacteria, Cyanobacteria, Firmicutes, and Chloroflexi were also among the top 10 phyla in the intestine. Of the top 10 phyla, Verrucomicrobia, Acidobacteria, Cyanobacteria, and Chloroflexi were significantly different in the zymosan-A-supplemented groups compared with the controls (Figure 6B). The relative abundance of Verrucomicrobia was significantly greater in groups Z1 and Z625 than in controls. The relative abundance of Acidobacteria was obviously higher in all zymosan-A-supplemented groups, except group Z1, being highest in group Z125. The relative abundances of Cyanobacteria and Chloroflexi were significantly reduced and increased in all zymosan-A-supplemented groups, respectively.
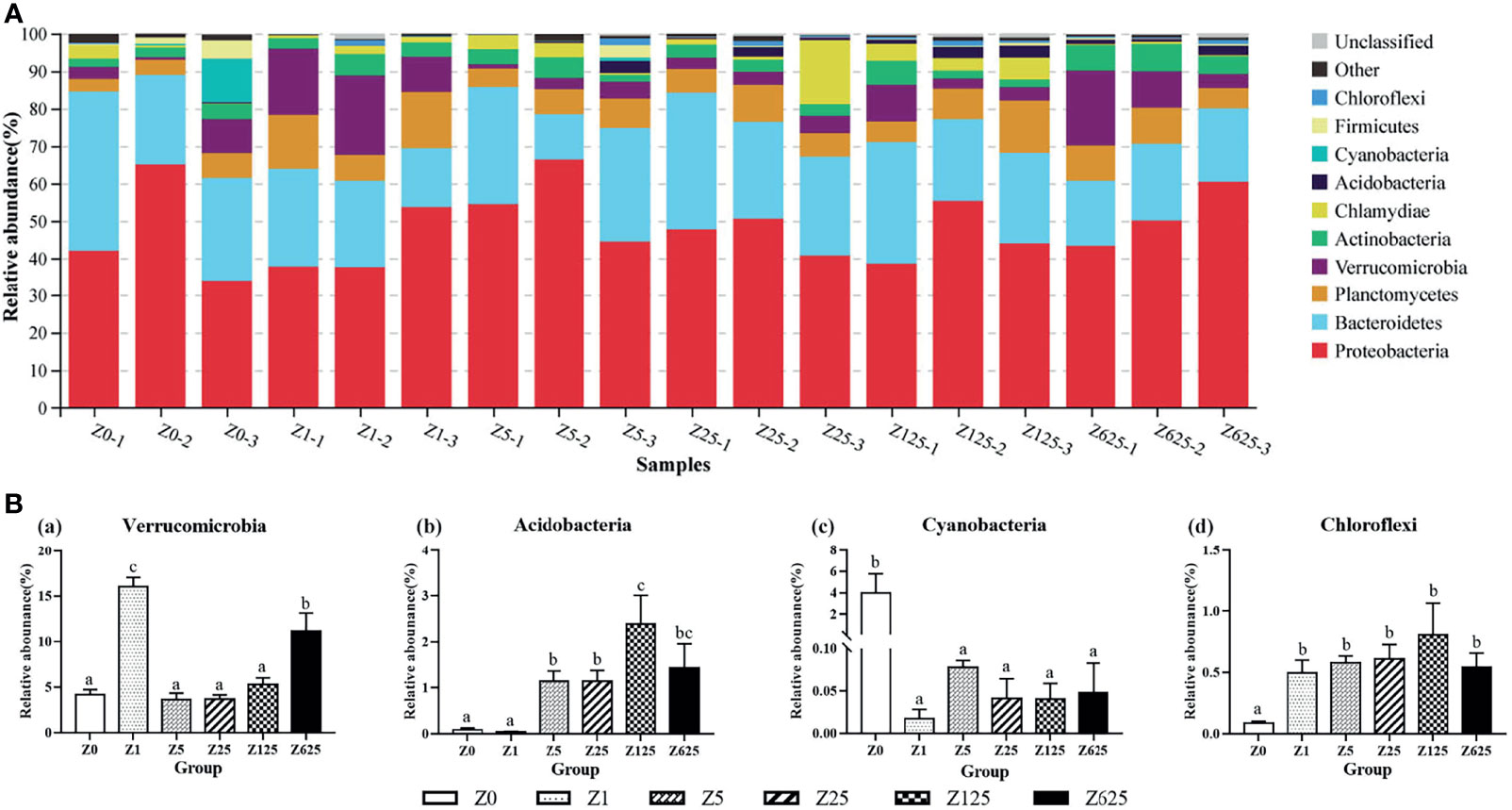
Figure 6 Structure and composition of the intestinal bacterial communities in Litopenaeus vannamei fed different levels of zymosan-A (phylum level). (A) Species distribution stacking of the treatment groups. (B) Relative abundances of the top 10 phyla. Different letters indicate significant differences (p < 0.05).
At the family level, the nine known families of the top 10 families, from high to low relative abundance, were Flavobacteriaceae (20.50%), Rhodobacteraceae (17.77%), Vibrionaceae (9.34%), Rubritaleaceae (6.54%), Pirellulaceae (4.51%), Halieaceae (4.38%), Rubinisphaeraceae (2.71%), Demequinaceae (2.44%), and Psychromonadaceae (2.33%) (Figure 7A). As Figure 7B shows, there were significant between-group differences in the relative abundance rates of Rhodobacteraceae, Vibrionaceae, Rubinisphaeraceae, and Psychromonadaceae (P < 0.05). Unlike the various degrees of significant increases in Rhodobacteraceae, Rubinisphaeraceae, and Psychromonadaceae in the zymosan-A-supplemented groups, the relative abundance of Vibrionaceae was significantly reduced in all supplemented groups compared with the controls.
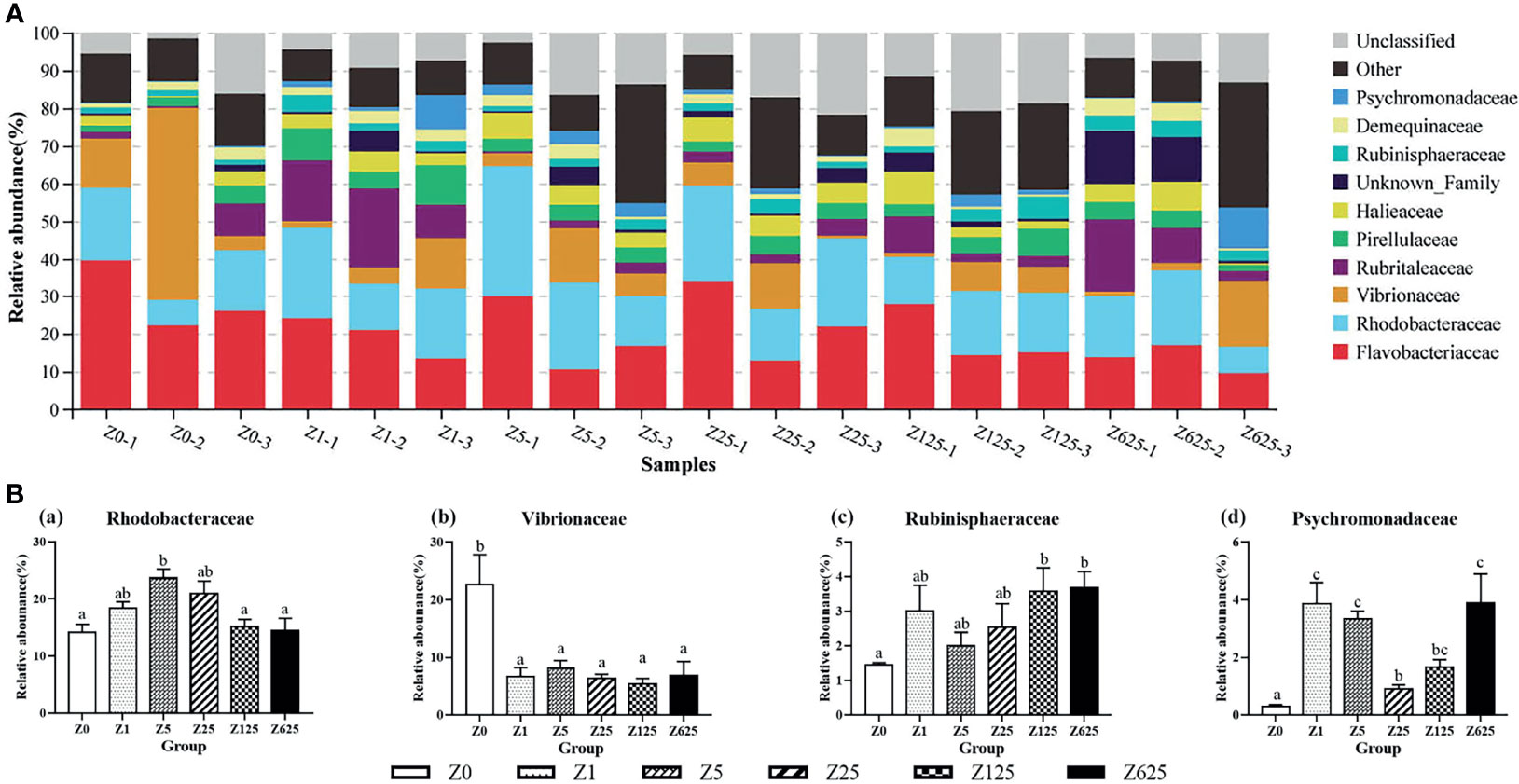
Figure 7 Structure and composition of the intestinal bacterial communities in Litopenaeus vannamei fed different levels of zymosan-A (family level). (A) Species distribution stacking of the treatment groups. (B) Relative abundances of the top 4 families. Different letters indicate significant differences (p < 0.05).
At the genus level, nearly half of the genera (49.27%) were unclassified. The top 10 genera were Vibrio, Haloferula, Ruegeria, Hoppeia, Pir4_lineage, Tenacibaculum, Demequina, Motilimonas, Actibacter, and Pseudoalteromonas, with average community richness values of 8.60%, 5.89%, 4.37%, 2.67%, 2.64%, 2.46%, 2.18%, 2.12%, 1.63%, and 1.63%, respectively (Figure 8A). Vibrio, Haloferula, Ruegeria, Motilimonas, and Pseudoalteromonas were the five genera with significant differences between the zymosan-A-supplemented and control groups (Figure 8B). Vibrio was the only genus that was significantly reduced in all zymosan-A-supplemented groups.
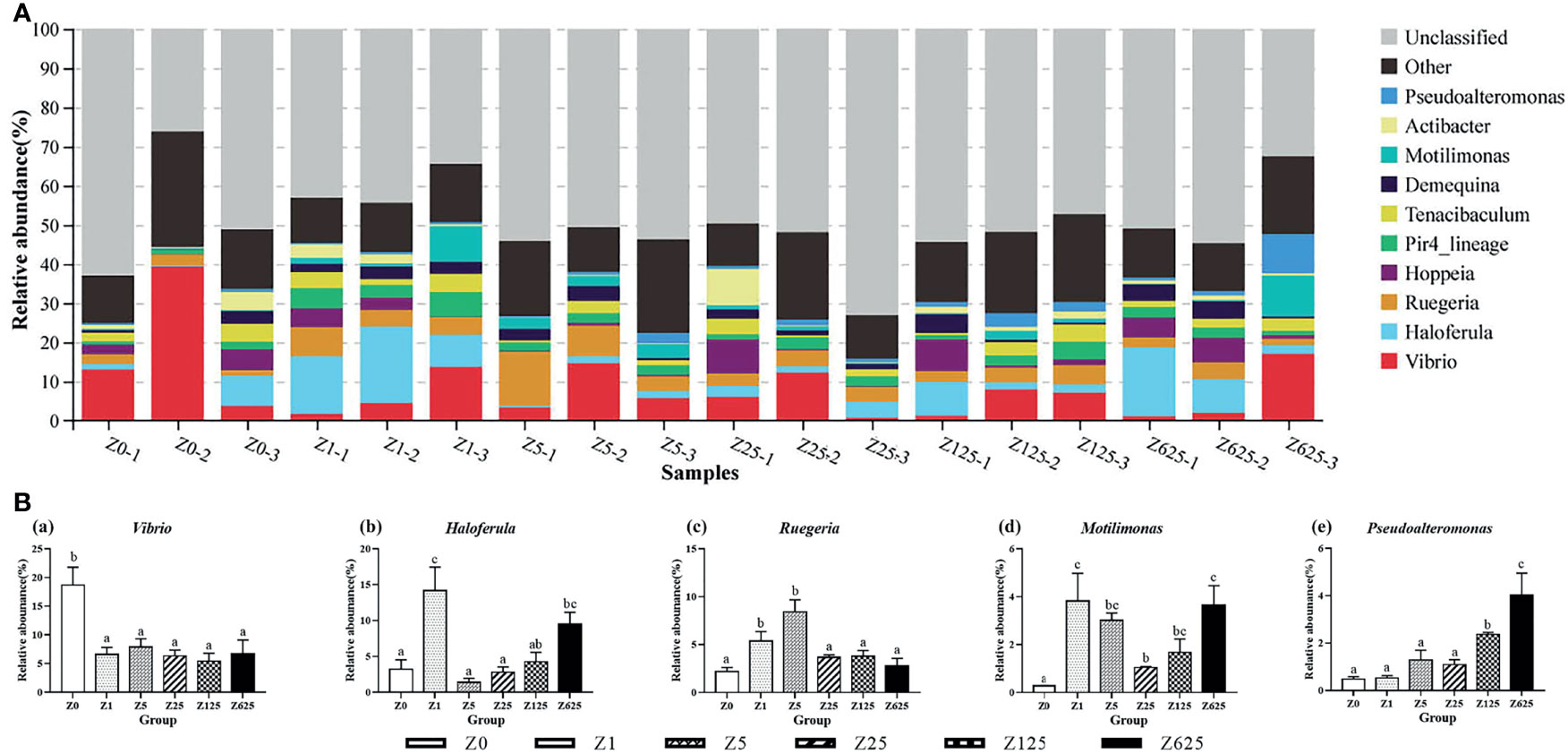
Figure 8 Structure and composition of the intestinal bacterial communities in Litopenaeus vannamei fed different levels of zymosan-A (genus level). (A) Species distribution stacking of the treatment groups. (B) Relative abundances of the top 5 genera. Different letters indicate significant differences (p < 0.05).
Functional Prediction of the Intestinal Microbiota
Changes in the presumptive functions of the intestinal microflora were examined using Tax4Fun software to predict the metagenomes. As shown in Figure 9A, the top 10 predicted functions with their relative abundance rates were as follows: membrane transport (13.2%–13.8%), carbohydrate metabolism (12.8%–13.1%), amino acid metabolism (12.2%–12.5%), signal transduction (7.3%–7.5%), metabolism of cofactors and vitamins (6.9%–7.1%), energy metabolism (6.8%–6.9%), nucleotide metabolism (5.2%–5.3%), translation (4.1%–4.2%), xenobiotic biodegradation and metabolism (3.8%–4.1%), and replication and repair (3.8%–3.9%). Welch’s t-tests showed that there were no significantly changed functions between groups Z0 and Z1. In the other four zymosan-A-supplemented groups, several predicted pathways were significantly enriched in the microbiota in KEGG level 2 (95% confidence intervals, p < 0.05). Compared with group Z0, transport and catabolism were significantly enriched in group Z5, while endocrine and metabolic diseases were the opposite (Figure 9B). In group Z25, transport and catabolism and substance dependence were the only two significantly enriched functions (Figure 9C). Comparison of groups Z0 and Z125 indicated that the functions of the nervous system, signaling molecules and interaction, cardiovascular diseases, and cell communication were significantly enriched in group Z125 (Figure 9D). As Figure 9E shows, folding, sorting and degradation, transport and catabolism, environmental adaptation, substance dependence, excretory system, cardiovascular diseases, and signaling molecule functions were significantly enriched in group Z625 compared with the controls.
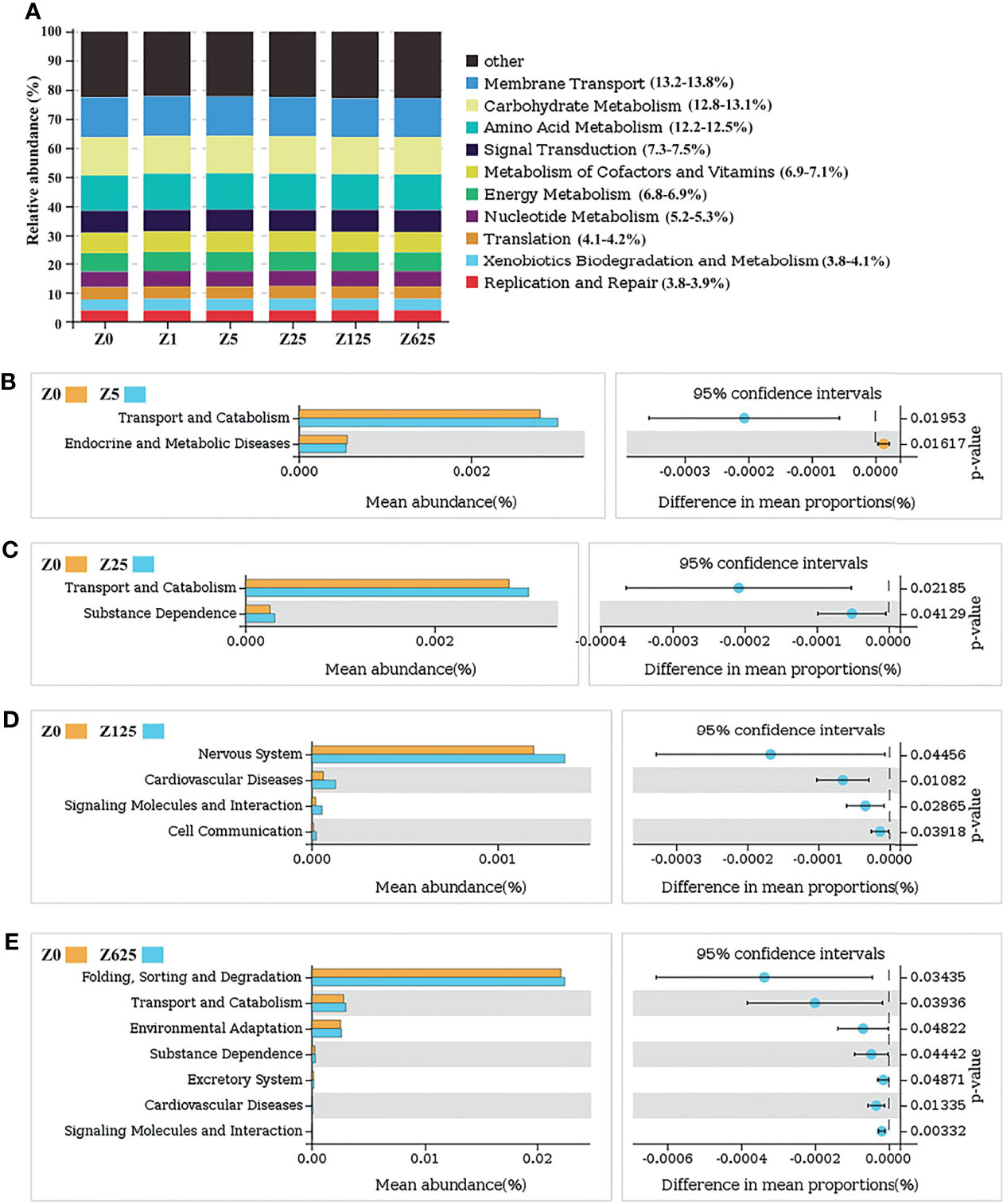
Figure 9 (A) Relative abundances of the top 10 predicted functions. (B–E) Welch’s t-tests of the significantly different functions at level 2.
Discussion
Several studies have established the beneficial effects of β-glucan supplementation in aquatic animals (Fabiana et al., 2017; Ji et al., 2017; Nieves-Rodríguez et al., 2018; Zhu and Wu, 2018; Harris et al., 2020). However, the complexity of the structure of β-glucan and the diversity of its sources are often ignored. Furthermore, studies using high-throughput sequencing to assess the impact of β-glucan on the intestinal flora are also uncommon. In this study, the effects of zymosan-A, which is a purified β-1,3-glucan, on L. vannamei were investigated. The results indicated that an optimum amount of dietary zymosan-A improves growth performance and intestinal function from the aspects of histological structure, digestive capacity, and microbiota.
Numerous studies have indicated a positive role of β-glucan in aquatic animal growth. (Ji et al., 2017) confirmed that Oncorhynchus mykiss fed 100–200 mg/kg β-glucan showed significantly improved growth parameters and feeding efficiency. Additionally, dietary supplementation with 200 mg/kg β-glucan promoted growth in O. niloticus, while supplementation with 50 and 100 mg/kg β-glucan significantly enhanced the growth and survival rates in Trachinotus ovatus (Linnaeus) (Whittington et al., 2005; Do Huu et al., 2016). Furthermore, L. vannamei fed 200–400 mg/kg β-glucan exhibited higher growth performance (Bai et al., 2010; Boonanuntanasarn et al., 2016; Li et al., 2019). Consistent with previous research, the present study found that diets supplemented with zymosan-A not only increased the growth performance but also decreased the FCR of L. vannamei. The optimal supplementation amount was approximately 300 mg/kg using either WGR or SGR as the evaluation index. The results indicated that zymosan-A, which is a purified β-1,3-glucan, possesses the same functionality as other types of β-glucan in improving growth. The optimal amount identified in the present study for L. vannamei is consistent with those of previous studies.
Several parameters are closely related to growth performance, including intestinal structure and digestive enzymes. An increased intestinal villus length increases the area of contact between the intestine and ingested nutrients, which, in turn, improves growth performance (Kühlwein et al., 2014; Sewaka et al., 2019). In the present study, dietary zymosan-A supplementation increased the intestinal villus height and body weight. This is consistent with observations in L. vannamei, O. niloticus, and Tarphops oligolepis (Bleeker, 1858) fed dietary β-glucan (Boonanuntanasarn et al., 2016; Dawood et al., 2019; Gu et al., 2021). Another factor closely related to growth parameters is digestive enzyme activity, which reflects the degree of feed utilization and digestion. There are three key enzymes known to be related to nutrient metabolism in shrimp (Wang, 2007; Anand et al., 2013): trypsin (which catalyzes the hydrolysis of proteins into smaller peptides), lipase (known to catalyze the hydrolysis of fats/lipids), and amylase (known to catalyze the hydrolysis of starch into sugars). In the present study, the activities of trypsin, amylase, and lipase in the zymosan-A-supplemented groups were positively correlated with the concentration of zymosan-A in the diet. These results are similar to those of (Li et al., 2019), in which dietary addition of 400 mg/kg β-glucan improved the activities of protease and amylase in L. vannamei. However, our study found that 125 and 625 mg/kg zymosan-A can also improve lipase activity. Our results also agree with studies on fish. Lutjanus peru fed 0.2% β-glucan had greater aminopeptidase, trypsin, and chymotrypsin activities than those fed 0.1% β-glucan diet (Guzmán-Villanueva et al., 2014). In Atractosteus tropicus, diets supplemented with 1.0% and 1.5% β-glucan increased the activity of chymotrypsin (Nieves-Rodríguez et al., 2018). The increased activities of digestive enzymes were further confirmed by the gene expressions of the intestinal digestive enzymes; hence, the gene expressions of trypsin, lipase, and amylase could be significantly induced by optimum addition of zymosan-A.
It is well known that the intestinal microbiota plays a vital role in the digestion and absorption processes of shrimp, as well as a supportive role in intestinal digestive activities, which, in turn, determine their growth traits (Dai et al., 2017; Xiong et al., 2017). In the present study, Illumina sequencing showed that the intestinal microbial diversity and richness were higher in zymosan-A-supplemented groups than those in controls. At the phylum level, Proteobacteria, Bacteroidetes, and Planctomycetes were dominant in the intestines, which is consistent with previous studies on fish and shrimp (Rungrassamee et al., 2014; Xiong et al., 2015; Dehler et al., 2017; Amoah et al., 2019). The distinguishing feature of the changes in the intestinal microbiota, both at the family and genus levels, is that pathogenic bacteria decreased significantly while beneficial bacteria increased significantly. The study of Yang et al. showed that the intestinal microbial community in β-glucan-fed A. japonicus was widely dominated by Flavobacteriaceae, Rhodobacteraceae, and Vibrionaceae, while dietary β-glucan supplementation reduced the relative abundance of Flavobacteriaceae and promoted Rhodobacteraceae (Yang et al., 2015). This is consistent with the present study, where Flavobacteriaceae, Rhodobacteraceae, and Vibrionaceae were the top 3 dominant families in L. vannamei and where the relative abundances of Flavobacteriaceae and Rhodobacteraceae were reduced and increased by the addition of zymosan-A, respectively. Furthermore, the dominant family Vibrionaceae was significantly decreased, while Rhodobacteraceae, Rubinisphaeraceae, and Psychromonadaceae were significantly increased. It is worth noting that Vibrionaceae and Rhodobacteraceae are considered pathogenic bacteria and are probiotics in aquatic animals (Zhou et al., 2007; Suphoronski et al., 2019). At the genus level, the microbial community was consistent with those at the family and phylum levels. The most obvious result was that the dominant genus Vibrio (family Vibrionaceae) was significantly reduced in all zymosan-A-supplemented groups. A common bacteria in aquatic animals, Vibrio has been characterized as the dominant member of the shrimp gastrointestinal tract, while Vibrio infection can cause serious diseases in shrimp (Rungrassamee et al., 2014; Tzuc et al., 2014; Huang et al., 2016; Zoqratt et al., 2018; Suphoronski et al., 2019). The significantly decreased abundance of Vibrio suggests that dietary zymosan-A could effectively reduce the risk of disease in L. vannamei. In addition, Haloferula (phylum Verrucomicrobia) was significantly increased by zymosan-A supplementation. In general, a low abundance of Haloferula in the intestine indicates an interrupted intestinal function that may lead to an energy imbalance (Zeng et al., 2021). In the present study, the increased abundance of Haloferula in supplemented L. vannamei indicates that zymosan-A might be helpful in maintaining normal intestinal function in this species. In addition, the predicted functions of the intestinal microflora showed that metabolic-related functions comprised most of the top 10 functions and are closely related to improved growth in L. vannamei. Overall, the changes in the intestinal microbiota showed that dietary zymosan-A can improve the intestinal microbial community and, in turn, promote growth by regulating metabolism in L. vannamei.
Considering all the observed effects of dietary zymosan-A on L. vannamei, this study provides further evidence that β-glucan plays a positive role in improving growth performance. This is probably due to the regulation of intestinal function in terms of morphology, digestive capacity, and the microbial community. Considering that zymosan-A is a purified form of β-1,3-glucan, it can be speculated that β-1,3-glucan is an important part of the function of β-glucan.
Conclusion
The present study demonstrated that dietary supplementation of zymosan-A improved not only the growth performance but also the intestinal morphology and the activities and gene expressions of digestion enzymes and the microbiome. Using the growth index as the evaluation factor, the optimal amount of zymosan-A addition to the diet of L. vannamei was approximately 300 mg/kg. These results provided detailed information for using β-glucan in shrimp and for achieving precise nutritional regulation in aquaculture.
Data Availability Statement
The datasets presented in this study can be found in online repositories. The names of the repository/repositories and accession number(s) can be found below: NCBI [accession: PRJNA808041].
Author Contributions
SZ and BT conceived and designed the experiments. YZ analyzed the data and wrote the paper. YZ, CH, ZY, JC, and HW collected samples and performed the experiments. All authors contributed to the article and approved the submitted version.
Funding
This work was supported by the National Key R&D Program of China (grant no. 2019YFD0900200), National Natural Science Foundation of China (grant no. 32072988), General Program of Natural Science Foundation of Guangdong Province, China (grant no. 2020A1515010319), and Project of Zhanjiang Key Laboratory Construction (grant no. 2020A05003).
Conflict of Interest
The authors declare that the research was conducted in the absence of any commercial or financial relationships that could be construed as a potential conflict of interest.
Publisher’s Note
All claims expressed in this article are solely those of the authors and do not necessarily represent those of their affiliated organizations, or those of the publisher, the editors and the reviewers. Any product that may be evaluated in this article, or claim that may be made by its manufacturer, is not guaranteed or endorsed by the publisher.
References
Aßhauer K. P., Wemheuer B., Daniel R., Meinicke P. (2015). Tax4Fun: Predicting Functional Profiles from Metagenomic 16S rRNA Data. Bioinformatics 31 (3), 2882–2884. doi: 10.1093/bioinformatics/btv287
Ainai A., Ichinohe T., Tamura S., Kurata T., Sata T., Tashiro M., et al. (2015). Zymosan Enhances the Mucosal Adjuvant Activity of Poly(I:C) in a Nasal Influenza Vaccine. J. Med. Virol. 82 (3), 476–484. doi: 10.1002/jmv.21694
Amoah K., Huang Q. C., Tan B. P., Zhang S., Chi S. Y., Yang Q. H., et al. (2019). Dietary Supplementation of Probiotic Bacillus Coagulans ATCC 7050, Improves the Growth Performance, Intestinal Morphology, Microflora, Immune Response, and Disease Confrontation of Pacific White Shrimp, Litopenaeus Vannamei. Fish. Shellfish. Immunol. 87, 796–808. doi: 10.1016/j.fsi.2019.02.029
Anand P. S., Kohli M., Roy S. D., Sundaray J., Kumar S., Sinha A., et al. (2013). Effect of Dietary Supplementation of Periphyton on Growth Performance and Digestive Enzyme Activities in Penaeus Monodon. Aquaculture 392, 59–68. doi: 10.1016/j.aquaculture.2013.09.051
Bai N., Zhang W., Mai K., Wang X., Xu W., Ma H. J. A. (2010). Effects of Discontinuous Administration of β-Glucan and Glycyrrhizin on the Growth and Immunity of White Shrimp Litopenaeus Vannamei. Aquaculture 306, 218–224. doi: 10.1016/j.aquaculture.2010.06.017
Barsanti L., Passarelli V., Evangelista V., Frassanito A. M., Gualtieri P. (2011). Chemistry, Physico-Chemistry and Applications Linked to Biological Activities of β-Glucans. Nat. Prod. Rep. 28, 457–466. doi: 10.1039/c0np00018c
Boonanuntanasarn S., Wongsasak U., Pitaksong T., Chaijamrus S. (2016). Effects of Dietary Supplementation With β-Glucan and Synbiotics on Growth, Haemolymph Chemistry, and Intestinal Microbiota and Morphology in the Pacific White Shrimp. Aquac. Nutr. 22, 837–845. doi: 10.1111/anu.12302
Carbone D., Faggio C. (2016). Importance of Prebiotics in Aquaculture as Immunostimulants: Effects on Immune System of Sparus Aurata and Dicentrarchus Labrax. Fish. Shellfish. Immunol. 54, 172–178. doi: 10.1016/j.fsi.2016.04.011
Dai W., Yu W., Zhang J., Zhu J., Tao Z., Xiong J. B. (2017). The Gut Eukaryotic Microbiota Influences the Growth Performance Among Cohabitating Shrimp. Appl. Microbiol. Biotechnol. 101, 6447–6457. doi: 10.1007/s00253-017-8388-0
Dawood M. A., Koshio S., Esteban M. A. (2018). Beneficial Roles of Feed Additives as Immunostimulants in Aquaculture: A Review. Rev. Aquacult. 10, 950–974. doi: 10.1111/raq.12209
Dawood M. A., Magouz F. I., Salem M. F., Elbialy Z. I., Abdel-Daim H., Proteins A. (2019). Synergetic Effects of Lactobacillus Plantarum and β-Glucan on Digestive Enzyme Activity, Intestinal Morphology, Growth, Fatty Acid, and Glucose-Related Gene Expression of Genetically Improved Farmed Tilapia. Probiotics. Antimicro. 4, 1–11. doi: 10.1007/s12602-019-09552-7
Dehler C. E., Secombes C. J., Martin S. A. M. (2017). Environmental and Physiological Factors Shape the Gut Microbiota of Atlantic Salmon Parr (Salmo Salar L.). Aquaculture. 467, 149–157. doi: 10.1016/j.aquaculture.2016.07.017
De Oliveira C. A. F., Vetvicka V., Zanuzzo F. S. (2019). β-Glucan Successfully Stimulated the Immune System in Different Jawed Vertebrate Species. Comp. Immunol. Microbiol. Infect. Dis. 62, 1–6. doi: 10.1016/j.cimid.2018.11.006
Do Huu H., Sang H. M., Thuy N. T., Immunology S. (2016). Dietary β-Glucan Improved Growth Performance, Vibrio Counts, Haematological Parameters and Stress Resistance of Pompano Fish, Trachinotus Ovatus Linnaeus 1758. Fish. Shellfish. Immunol. 54, 402–410. doi: 10.1016/j.fsi.2016.03.161
Fabiana P., Oliveira C., Souza F. P. (2017). Different β-Glucans Improve the Growth Performance and Bacterial Resistance in Nile Tilapia. Fish. Shellfish. Immunol. 70, 25–29. doi: 10.1016/j.fsi.2017.06.059
Golisch B., Lei Z., Tamura K., Brumer H. (2021). Configured for the Human Gut Microbiota: Molecular Mechanisms of Dietary β-Glucan Utilization. ACS. Chem. Biol. 16, 2087–2102. doi: 10.1021/acschembio.1c00563
Gu M., Pan S., Li Q., Qi Z., Deng W., Chen C., et al. (2021). Evaluation and Compare of Yeast β-Glucan and Carboxymethylglucan to Improve the Immunity and Gut Health of Turbot Fed Diet Containing 400 G Kg-1 of Soybean Meal. Aquacult. Rep. 21, 100882. doi: 10.1016/j.aqrep.2021.100882
Guzmán-Villanueva L. T., Ascencio-Valle F., Macías-Rodríguez M. E., Tovar-Ramírez D. (2014). Effects of Dietary β-1, 3/1, 6-Glucan on the Antioxidant and Digestive Enzyme Activities of Pacific Red Snapper (Lutjanus Peru) After Exposure to Lipopolysaccharides. Fish. Physiol. Biochem. 40, 827–837. doi: 10.1007/s10695-013-9889-0
Harris S. J., Bray D. P., Adamek M., Hulse D. R., Steinhagen D., Hoole D. (2020). Effect of β-1/3, 1/6-Glucan Upon Immune Responses and Bacteria in the Gut of Healthy Common Carp (Cyprinus Carpio). J. Fish. Biol. 96, 444–455. doi: 10.1111/jfb.14222
Holmstrom K., Graslund S., Wahlstrom A., Poungshompoo S., Bengtsson B. E., Kautsky N. (2003). Antibiotic Use in Shrimp Farming and Implications for Environmental Impacts and Human Health. Int. J. Food. Sci. Tech. 38, 255–266. doi: 10.1046/j.1365-2621.2003.00671.x
Huang Z., Li X., Wang L., Shao Z. (2016). Changes in the Intestinal Bacterial Community During the Growth of White Shrimp, Litopenaeus Vannamei. Aquacult. Res. 47, 1737–1746. doi: 10.1111/are.12628
Jayachandran M., Chen J., Chung S., Xu B. (2018). A Critical Review on the Impacts of β-Glucans on Gut Microbiota and Human Health. J. Nutri. Biochem. 61, 101–110. doi: 10.1016/j.jnutbio.2018.06.010
Jeong H., Kim I. Y., Bae E. K., Jeon C. H., Ahn K. S., Cha H. S. (2021). Selective Estrogen Receptor Modulator Lasofoxifene Suppresses Spondyloarthritis Manifestation and Affects Characteristics of Gut Microbiota in Zymosan-Induced SKG Mice. Sci. Rep. 11, 11923. doi: 10.1038/s41598-021-91320-1
Ji L., Sun G., Li J., Wang Y., Du Y., Li X., et al. (2017). Effect of Dietary β-Glucan on Growth, Survival and Regulation of Immune Processes in Rainbow Trout (Oncorhynchus Mykiss) Infected by Aeromonas Salmonicida. Fish. Shellfish. Immunol. 64, 56–67. doi: 10.1016/j.fsi.2017.03.015
Kim Y. S., Ryu J. H., Han S. J., Choi K. H., Nam K. B., Jang I. H., et al. (2000). Gram-Negative Bacteria-Binding Protein, a Pattern Recognition Receptor for Lipopolysaccharide and β-1, 3-Glucan That Mediates the Signaling for the Induction of Innate Immune Genes in Drosophila Melanogaster Cells. J. Biol. Chem. 275, 32721–32727. doi: 10.1074/jbc.M003934200
Kühlwein H., Merrifield D. L., Rawling M. D., Foey A. D., Davies S. J. (2014). Effects of Dietary β-(1, 3) (1, 6)-D-Glucan Supplementation on Growth Performance, Intestinal Morphology and Haemato-Immunological Profile of Mirror Carp (Cyprinus Carpio L.). J. Anim. Physiol. An. N. 98, 279–289. doi: 10.1111/jpn.12078
Lei C. L., Dong G. Z., Jin L., Zhang S., Zhou J. (2013). Effects of Dietary Supplementation of Montmorillonite and Yeast Cell Wall on Lipopolysaccharide Adsorption, Nutrient Digestibility and Growth Performance in Beef Cattle. Livest. Sci. 158 (1-3), 57–63. doi: 10.1016/j.livsci.2013.08.019
Li H., Xu C., Zhou L., Dong Y., Su Y., Wang X., et al. (2019). Beneficial Effects of Dietary β-Glucan on Growth and Health Status of Pacific White Shrimp Litopenaeus Vannamei at Low Salinity. Fish. Shellfish. Immunol. 91, 315–324. doi: 10.1016/j.fsi.2019.05.052
Miest J. J., Arndt C., Adamek M., Steinhagen D., Reusch T. B. (2016). Dietary β-Glucan (MacroGard®) Enhances Survival of First Feeding Turbot (Scophthalmus Maximus) Larvae by Altering Immunity, Metabolism and Microbiota. Fish. Shellfish. Immunol. 48, 94–104. doi: 10.1016/j.fsi.2015.11.013
Mohan K., Ravichandran S., Muralisankar T., Uthayakumar V., Chandirasekar R., Seedevi P., et al. (2019). Application of Marine-Derived Polysaccharides as Immunostimulants in Aquaculture: A Review of Current Knowledge and Further Perspectives. Fish. Shellfish. Immunol. 86, 1177–1193. doi: 10.1016/j.fsi.2018.12.072
Nieves-Rodríguez K. N., Álvarez-González C. A., Peña-Marín E. S., Vega-Villasante F., Martínez-García R., Camarillo-Coop S., et al. (2018). Effect of β-Glucans in Diets on Growth, Survival, Digestive Enzyme Activity, and Immune System and Intestinal Barrier Gene Expression for Tropical Gar (Atractosteus Tropicus) Juveniles. Fishes 3, 27. doi: 10.3390/fishes3030027
Quast C., Pruesse E., Yilmaz P., Gerken J., Schweer T., Yarza P., et al. (2012). The SILVA Ribosomal RNA Gene Database Project: Improved Data Processing and Web-Based Tools. Nucleic. Acids. Res. 41, D590–D596. doi: 10.1093/nar/gks1219
Rodrigues M. V., Zanuzzo F. S., Koch J. F. A., de Oliveira C. A. F., Sima P., Vetvicka V. (2020). Development of Fish Immunity and the Role of β-Glucan in Immune Responses. Molecules 25, 5378. doi: 10.3390/molecules25225378
Rungrassamee W., Klanchui A., Maibunkaew S., Chaiyapechara S., Jiravanichpaisal P., Karoonuthaisiri N. (2014). Characterization of Intestinal Bacteria in Wild and Domesticated Adult Black Tiger Shrimp (Penaeus Monodon). PloS One 9, e91853. doi: 10.1371/journal.pone.0091853
Sewaka M., Trullas C., Chotiko A., Rodkhum C., Chansue N., Boonanuntanasarn S., et al. (2019). Efficacy of Synbiotic Jerusalem Artichoke and Lactobacillus Rhamnosus GG-Supplemented Diets on Growth Performance, Serum Biochemical Parameters, Intestinal Morphology, Immune Parameters and Protection Against Aeromonas Veronii in Juvenile Red Tilapia (Oreochromis Spp.). Fish. Shellfish. Immunol. 86, 260–268. doi: 10.1016/j.fsi.2018.11.026
Stuyven E., Cox E., Vancaeneghem S., Arnouts S., Deprez P., Goddeeris B. M. (2009). Effect of Beta-Glucans on an ETEC Infection in Piglets. Vet. Immunol. Immunop. 128, 60–66. doi: 10.1016/j.vetimm.2008.10.311
Suphoronski S. A., Chideroli R. T., Facimoto C. T., Mainardi R., Souza F., Lopera-Barrero N., et al. (2019). Effects of a Phytogenic, Alone and Associated With Potassium Diformate, on Tilapia Growth, Immunity, Gut Microbiome and Resistance Against Francisellosis. Sci. Rep. 9, 6045. doi: 10.1038/s41598-019-42480-8
Thitamadee S., Prachumwat A., Srisala J., Jaroenlak P., Salachan P. V., Sritunyalucksana K., et al. (2016). Review of Current Disease Threats for Cultivated Penaeid Shrimp in Asia. Aquaculture 452, 69–87. doi: 10.1016/j.aquaculture.2015.10.028
Tzuc J. T., Escalante D. R., Rojas Herrera R., Gaxiola Cortés G., Ortiz M. L. A. (2014). Microbiota From Litopenaeus Vannamei: Digestive Tract Microbial Community of Pacific White Shrimp (Litopenaeus Vannamei). SpringerPlus. 3, 1–10. doi: 10.1186/2193-1801-3-280
Wang Y. B. (2007). Effect of Probiotics on Growth Performance and Digestive Enzyme Activity of the Shrimp Penaeus Vannamei. Aquaculture 269, 259–264. doi: 10.1016/j.aquaculture.2007.05.035
Whittington R., Lim C., Klesius P. H. (2005). Effect of Dietary β-Glucan Levels on the Growth Response and Efficacy of Streptococcus Iniae Vaccine in Nile Tilapia, Oreochromis Niloticus. Aquaculture. 248, 217–225. doi: 10.1016/j.aquaculture.2005.04.013
Wu Y., Li X., Liu H., Du Y., Zhou J., Zou L., et al. (2021). A Water-Soluble β-Glucan Improves Growth Performance by Altering Gut Microbiome and Health in Weaned Pigs. Animal. Nutri. 7 (4), 1345–1351. doi: 10.1016/j.aninu.2021.04.006
Xiong J., Dai W., Zhu J., Liu K., Dong C., Qiu Q. F. (2017). The Underlying Ecological Processes of Gut Microbiota Among Cohabitating Retarded, Overgrown and Normal Shrimp. Microb. Ecol. 73, 988–999. doi: 10.1007/s00248-016-0910-x
Xiong J., Wang K., Wu J., Qiuqian L., Yang K., Qian Y., et al. (2015). Changes in Intestinal Bacterial Communities Are Closely Associated With Shrimp Disease Severity. Appl. Microbiol. Biot. 99, 6911–6919. doi: 10.1007/s00253-015-6632-z
Xu M., Mo X., Huang H., Chen X., Liu H., Peng Z., et al. (2020). Yeast β-Glucan Alleviates Cognitive Deficit by Regulating Gut Microbiota and Metabolites in Aβ1-42-Induced AD-Like Mice. Int. J. Biol. Macromol. 161, 258–270. doi: 10.1016/j.ijbiomac.2020.05.180
Yang G., Xu Z., Tian X., Dong S., Peng M. (2015). Intestinal Microbiota and Immune Related Genes in Sea Cucumber (Apostichopus Japonicus) Response to Dietary β-Glucan Supplementation. Biochem. Bioph. Res. Co. 458, 98–103. doi: 10.1016/j.bbrc.2015.01.074
Yu H. H., Han F., Xue M., Wang J., Tacon P., Zheng Y. H., et al. (2014). Efficacy and Tolerance of Yeast Cell Wall as an Immunostimulant in the Diet of Japanese Seabass (Lateolabrax Japonicus). Aquaculture. 432, 217–224. doi: 10.1016/j.aquaculture.2014.04.043
Zeng F., Rabbi M. H., Hu Y., Li Z., Ren X., Han Y., et al. (2021). Synergistic Effects of Dietary Selenomethionine and Vitamin C on the Immunity, Antioxidant Status, and Intestinal Microbiota in Sea Cucumber (Apostichopus Japonicus). Biol. Trace. Elem. Res. 199, 3905–3917. doi: 10.1007/s12011-020-02483-3
Zhou X. X., Pan Y. J., Wang Y. B., Li W. F. (2007). In Vitro Assessment of Gastrointestinal Viability of Two Photosynthetic Bacteria, Rhodopseudomonas Palustris and Rhodobacter Sphaeroides. J. Zhejiang. Uni-Sci. B. 8 (9), 686–692. doi: 10.1631/jzus.2007.B0686
Zhu M., Wu S. (2018). The Growth Performance and Nonspecific Immunity of Loach Paramisgurnus Dabryanus as Affected by Dietary β-1, 3-Glucan. Fish. Shellfish. Immunol. 83, 368–372. doi: 10.1016/j.fsi.2018.09.049
Keywords: Litopenaeus vannamei, zymosan-A, growth performance, digestive enzyme, intestinal microbiota
Citation: Zheng Y, Hou C, Yan Z, Chen J, Wang H, Tan B and Zhang S (2022) Effects of Dietary Zymosan-A on the Growth Performance and Intestinal Morphology, Digestive Capacity, and Microbial Community in Litopenaeus vannamei. Front. Mar. Sci. 9:877865. doi: 10.3389/fmars.2022.877865
Received: 17 February 2022; Accepted: 09 March 2022;
Published: 19 April 2022.
Edited by:
Jin Niu, Sun Yat-sen University, ChinaReviewed by:
Yihong Chen, South China Normal University, ChinaMuting Yan, South China Agricultural University, China
Copyright © 2022 Zheng, Hou, Yan, Chen, Wang, Tan and Zhang. This is an open-access article distributed under the terms of the Creative Commons Attribution License (CC BY). The use, distribution or reproduction in other forums is permitted, provided the original author(s) and the copyright owner(s) are credited and that the original publication in this journal is cited, in accordance with accepted academic practice. No use, distribution or reproduction is permitted which does not comply with these terms.
*Correspondence: Shuang Zhang, enNodWFuZ0BnZG91LmVkdS5jbg==