- 1Department of Marine Sciences, University of Gothenburg, Göteborg, Sweden
- 2Department of Marine Sciences, Tjärnö Marine Laboratory, University of Gothenburg, Strömstad, Sweden
- 3Centre for Ocean Life, DTU Aqua, Technical University of Denmark, Lyngby, Denmark
Phytoplankton induce defensive traits in response to chemical alarm signals from grazing zooplankton. However, these signals are potentially vulnerable to changes in pH and it is not yet known how predator recognition may be affected by ocean acidification. We exposed four species of diatoms and one toxic dinoflagellate to future pCO2 levels, projected by the turn of the century, in factorial combinations with predatory cues from copepods (copepodamides). We measured the change in growth, chain length, silica content, and toxin content. Effects of increased pCO2 were highly species specific. The induction of defensive traits was accompanied by a significant reduction in growth rate in three out of five species. The reduction averaged 39% and we interpret this as an allocation cost associated with defensive traits. Copepodamides induced significant chain length reduction in three of the four diatom species. Under elevated pCO2 Skeletonema marinoi reduced silica content by 30% and in Alexandrium minutum the toxin content was reduced by 30%. Using copepodamides to induce defensive traits in the absence of direct grazing provides a straightforward methodology to assess costs of defense in microplankton. We conclude that copepodamide signalling system is likely robust to ocean acidification. Moreover, the variable responses of different taxa to ocean acidification suggest that there will be winners and losers in a high pCO2 world, and that ocean acidification may have structuring effects on phytoplankton communities.
Introduction
Ocean acidification (OA) is caused by increased atmospheric CO2 concentrations dissolving into surface waters, thus driving the equilibrium of the carbonate system towards more acidic conditions (Sabine et al., 2004). The present-day atmospheric CO2 concentration is 400 µatm, but this is projected to increase to 1000 µatm by the year 2100 (RCP 8.5, IPCC, 2013). This has resulted in an increased concentration of surface water hydrogen ions of 30% since the industrial revolution, corresponding to a pH reduction of 0.1 units (Raven et al., 2005). This decrease is projected to continue and results in a decrease of 0.4 units by year 2050 and 0.7 units by year 2300 (Caldeira and Wickett, 2003; Orr et al., 2005). The majority of the pH drop takes place in the upper mixed layer but will eventually reach deeper water as well (Sabine et al., 2004).
The photic zone of the ocean is home to the vast majority of marine primary production, and more than half of global primary productivity (Field et al., 1998; Falkowski et al., 1998). Phytoplankton, marine photosynthesizers that form the basis of most marine food webs, typically reside in the upper surface layers and utilize CO2 for growth through photosynthesis. Changes in pCO2 have both positive and negative effects on photosynthetic carbon fixation rate in marine plankton (Riebesell, 2004). Calcifying organisms such as coccolithophorids appear to be carbon limited at present-day pH, whilst diatoms and Phaeocystis are at or close to CO2 saturation (Riebesell et al., 2000; Zondervan et al., 2001; Burkhardt et al., 2001). Phytoplankton have optimal growth rate at different CO2 ranges (Burkhardt et al., 1999; Riebesell et al., 2000; Gervais and Riebesell, 2001; Barker et al., 2003; Boyd and Doney, 2003; Nielsen et al., 2010). Deviations from optimal CO2 concentrations have the ability to alter organisms’ physiological responses which can result in large scale shifts in biogeochemical cycling (Riebesell, 2004). The shift in pCO2 may limit the activity of individuals and may ultimately favour those species which are more adaptable.
Copepods are a common group of microalgae grazers, which release copepodamides, a unique combination of polar lipids whose concentrations have been shown to correlate to copepod density (Selander et al., 2015; Selander et al., 2019). Copepodamides induce defensive responses in both dinoflagellates and diatoms (Selander et al., 2011; Selander et al., 2015; Lindström et al., 2017; Grønning and Kiørboe, 2020; Rigby and Selander, 2021). Two out of three diatom species shortened their chain length when exposed to copepodamides (Rigby and Selander, 2021) and six of seven species of diatoms showed a decrease in growth rate accompanied by an increase in silica content in response to copepodamides (Grønning and Kiørboe, 2020). It is not yet known how these interactions are affected by ocean acidification.
Changes in pH have the potential to disrupt chemical signalling systems and interfere with chemically mediated behavioural responses (Hanazato, 1999; Hanazato, 2001; Gutierrez et al., 2012). Altered pH can for example result in protonation or deprotonation of odorant ligands or change the tertial structure of the receptor proteins (Leduc et al., 2013; Roggatz et al., 2016). In female sticklebacks an increase in pH led to enhanced olfactory communication from males, resulting in gravid females being more attracted to males (Heuschele and Candolin, 2007). While others have reported infodisruption with impaired chemoreception in term of foraging in crayfish, suppression of feeding behaviour in newt larvae and impaired foraging abilities in gold mollies (Allison et al., 1992; Griffiths, 1993; Tembo, 2009). While infodisruption is more extensively studied in snails, fish, and crustaceans it is not yet known how it may affect chemical signalling in other taxa such as phytoplankton (Cothran et al., 2021). However, ocean acidification has been shown to change the perception of odours by copepods (Maibam et al., 2015).
Future increase in pCO2 has been hypothesized to lead to increased phytoplankton growth rate (Beardall and Raven, 2004; Beardall et al., 2009). However, past mesocosm experiments show that phytoplankton exhibit different responses to increased pCO2, including both negative and positive effects on growth rate (Kim et al., 2006; Bach et al., 2017; Riebesell et al., 2017; Dörner et al., 2020) and primary production (Schulz et al., 2013; Liu et al., 2017; Bach et al., 2019). Phytoplankton stoichiometry suggests that inorganic carbon will rarely be the limiting element in sea water. Normal concentration is around 2 mM, orders of magnitude higher than Redfield ratios with respect to nitrogen and phosphate (Redfield, 1958; Libes, 2011). While inorganic carbon is not limiting in seawater it is primarily present as hydrogen carbonate. This cannot be used for growth without first being brought into a cell using a carbon concentration mechanism (CCM, Giordano et al., 2005). The increased pCO2 associated with OA allows greater passive intake of carbon, which frees more energy for growth; energy that would otherwise be invested in CCM (Giordano et al., 2005). Furthermore, it has been suggested that some species of phytoplankton are able to up-regulate their inorganic carbon transport system as a response to changes in pCO2 (Tortell et al., 2008). Previous studies on diatoms have differentiated between OA-sensitive and OA-insensitive species (e.g., Kim et al., 2006). In a 14-day long experiment, growth rate in Skeletonema costatum increased with increasing levels of pCO2 whereas Nitzschia spp. did not show any difference in growth rate across treatments (Kim et al., 2006). This suggests that Nitzschia spp. are insensitive to changes in pCO2 and that the different responses between species to future elevated pCO2 can change the structure of the diatom population. These changes in community composition can alter the bottom-up processes and have the potential to influence food web dynamics (Hays et al., 2005).
The specific responses to elevated pCO2 are unknown for many phytoplankton species. Here we explored the interactive effects of ocean acidification and simulated predation risk with regards to toxin content, chain length, and silica content on the dinoflagellate Alexandrium minutum, and the diatoms Skeletonema marinoi, Thalassiosira rotula, Chaetoceros curvisetus, and Chaetoceros affinis. We expose cells to elevated (1100 µatm) and ambient (400 µatm) pCO2 levels for a 48-hour period with and without copepodamides. We then analyze the growth rate and toxin content in A. minutum, as well as the growth rate, silica content, and chain length in S. marinoi, T. rotula, C. curvisetus, and C. affinis. We hypothesize that all taxa will increase growth rate in response to the increased concentration of available inorganic carbon. We further hypothesize that copepodamides will initiate grazer induced responses also in future pCO2 levels and impaired copepodamide signalling would manifest in interactive effects between copepodamide addition and pCO2 level.
Materials and Methods
Phytoplankton Cultivation
Stock cultures of Alexandrium minutum strain GUMACC #83 (also known as AL1V, CCMP113), Thalassiosira rotula strain CCAP1085/20, Chaetoceros curvisetus strain RCC6895, Chaetoceros affinis strain CCAP1010/27, and Skeletonema marinoi strain GF 04-7D were grown at 26 PSU, 12:12 light:dark cycle 100 µmol photons m-2 s-1, and 16°C prior to the experiment. A. minutum was cultured with L1 metals (Guillard and Hargraves, 1993) and f/2 nutrients (Guillard & Ryther, 1962) and the diatom species were grown with f/2 media enriched with silica at a salinity of 26 PSU. All algal strains were obtained from GUMACC (Gothenburg University Marine Culture Collection, Sweden).
Experimental Design
Control cultures of phytoplankton were maintained in ambient pCO2 (400 µatm) while experimental cultures were gradually exposed to increased levels of pCO2 over five days until experimental conditions were reached (1100 µatm; corresponding to the projected value at the end of this century). Treatments were regulated using solenoid valves controlled by pH-computers (Aqua Medic, Germany, NBS-calibrated) which mixed CO2 (AGA, Sweden) with air that had CO2 removed to reach desired levels. The pCO2 was monitored with LI-850 CO2/H2O Gas Analyzer (LI-COR Biosciences, USA). Salinity, temperature, pCO2, and pH were measured at the beginning of the experiment, pH was also measured at the end of the experiment (Table S1). Total alkalinity was estimated from salinity using long-term salinity:alkalinity relationship data (Eriander et al., 2016; Falkenberg et al., 2019). All seawater was filtered (0.2 µm) and autoclaved, nutrients were added to the cultures before the experiment started. The cultures were split into four groups (n=6): “ambient pCO2”, “elevated pCO2”, “ambient pCO2 x 5nM copepodamides”, and “elevated pCO2 x 5nM copepodamides”. The average effective concentration is 1%– 2% of the nominal concentration corresponding to ~ 55 pM (Selander et al., 2019). At the end of the experiment copepodamide concentrations were measured in 3 replicates from each treatment and there was no significant difference between the treatment groups (p=0.8).
Alexandrium minutum cultures were diluted into twenty-four 310 mL bottles with either ambient pCO2 or elevated pCO2 autoclaved seawater with L1 metals and f/2 nutrients to a starting concentration 300 cells mL-1. Diatoms were diluted into 25 mL vials with f/4 nutrients + Si to 1000 cells mL-1, 6 replicates per treatment. All cell counts from start and end of all the experiments (48 hours) were obtained by placing 1 mL of well mixed culture with acidic lugol on a Sedgewick rafter chamber where at least 0.1 mL per replicate was counted. A. minutum bottles and diatom bottles were placed in a thermo-constant room at 16°C with a 12:12 light cycle with intensity at 22-32 µmol m-2s-1. The diatom vials were placed on a plankton wheel (0.5 rpm). Chain lengths were sampled at the start and end by gently pipetting a 1 mL sample into a 48 well plate. Then the first 50 observed chains from a random location in the well were counted from each replicate. Chain length is determined by the number of cells in an individual chain.
Toxin Analysis
Toxin content of A. minutum was determined by suction filtering 300 mL culture of a known cell concentration from each replicate onto 25 mm Whatman GF/F filters. The filters were transferred to 2 mL Eppendorf tubes, frozen (-20°C), and freeze dried for 45 minutes. The dry filters were soaked in 750 µL 0.05 M acetic acid (aq) and subjected to three freeze-thaw cycles to facilitate extraction of toxins. The samples were filtered (GF/F) into 1.5 mL glass HPLC vials and stored frozen until analysis.
The A. minutum strain used here produce Gonyautoxins 1-4 (Selander et al., 2006), which were analyzed using high performance liquid chromatography (Agilent 1200 series fitted with an Agilent InfinityLab Poroshell 120 HILIC-Z, 2.1 x 50mm 2.7µm) column coupled to an Agilent 6410 triple quadrupole (Turner and Tölgyesi, 2019). Eluent A consisted of 500 mL water + 75 µL formic acid + 300 µL ammonium hydroxide. Eluent B consisted of 850 mL acetonitrile + 150 mL water + 100 µL formic acid + 300 µL ammonium hydroxide. Concentrations were determined against authentic standards from certified reference materials program, Canadian National Research Council (Halifax, Canada).
Biogenic Silica
Biogenic silica in the four diatom species was determined following the method of Paashe (1980) as modified by Grønning and Kiørboe (2020). Subsamples of 20 mL were filtered onto 3 µm polycarbonate filters and washed twice with acidic milliQ, to minimize dissolution of silica. The filters were then dried for 90 minutes at 65°C and stored at -20°C until analysis. Biogenic silica on the filters were later dissolved in 15 mL 0.5% (w/v) sodium carbonate solution, heated at 85°C for 90 minutes and cooled. The pH was then adjusted to 5.0-6.0 by adding concentrated sulfuric acid, and the reactive silica analyzed following the method of Strickland and Parsons (1972) using a SmartChem 200 wet chemistry analyser (Unity Scientific, MA).
Statistical Analysis
Growth rates, silica and toxin content were tested for equality of variances with a Levene’s test and for normality with Shapiro–Wilk’s test. When these assumptions were not met, data were analyzed with Aligned Rank Transform for nonparametric factorial ANOVAs (ART). Chain lengths were count data and therefore analyzed with a generalized linear mixed model with a Poisson distribution. C. curvisetus growth rate and A. minutum toxin content passed the equality and normality tests and were analyzed using a 2-way ANOVA. In all models copepodamide and pCO2 were treated as fixed factors (i.e., presence or absence of copepodamides; current or future pCO2 concentrations). Silica data was analyzed with a generalized linear model. Two outliers, 8 and 14 times lower than treatment average, were identified as failed measurements and were removed from further analysis. Significant differences between means were further analyzed post-hoc with Tukey’s HSD (honestly significant differences) test. All analyses were performed with R and R studio version 4.1.0.
Results
Growth Rate
Growth rates of microalgae were generally lower in copepodamide exposed cultures suggesting an allocation cost associated with expression of defensive traits. T. rotula, C. affinis and A. minutum showed significantly lower growth in copepodamide exposed cultures and there was a trend towards reduced growth rate also in C. curvisetus against their respective controls (p=0.33 ambient pCO2 & p=0.06 elevated pCO2, Figure 1). There was a significant interaction between pCO2 level and copepodamides on growth rate in S. marinoi (p=0.04). However, the post-hoc test revealed only a significant effect of pCO2 with 42% higher growth rate in elevated pCO2 compared to ambient pCO2 treatments. In the other three diatom species there were negative main effects of elevated pCO2 as well as copepodamides on growth rate (p ≤ 0.006 for all, Figure 1), with the overall pattern of elevated pCO2 and copepodamides resulting in lower growth rate, which was the most suppressed under the combined treatment of elevated pCO2 and copepodamides. The dinoflagellate A. minutum grew 133% faster in the “elevated pCO2’’ treatment compared to the other three groups (p=0.01, Figure 1), however in the “elevated pCO2 x copepodamide” treatment growth was 43% lower when compared to “elevated pCO2” (p=0.002, Figure 1).
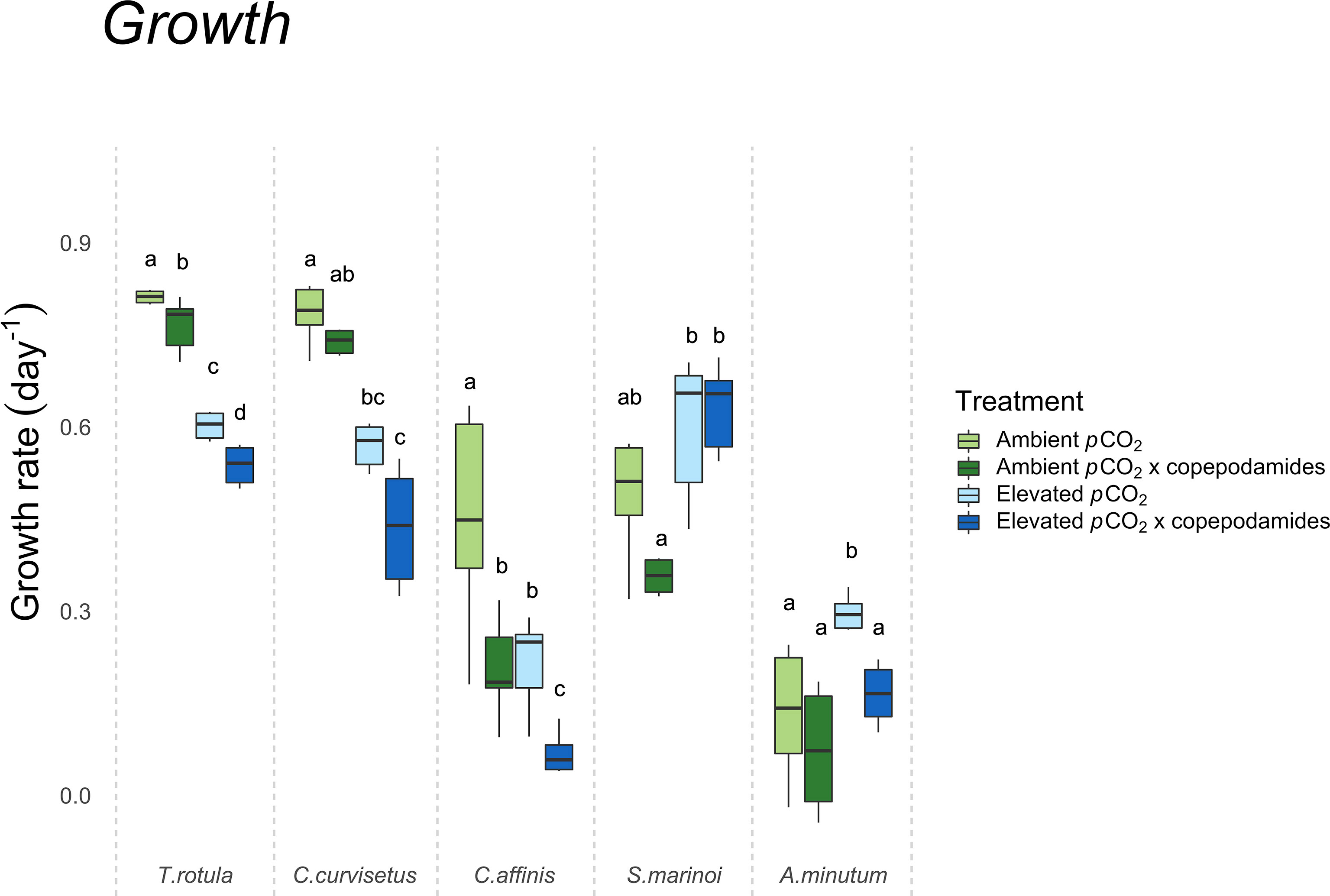
Figure 1 Box plot showing the specific growth rates (day−1) for T. rotula, C. curvisetus, C. affinis, S. marinoi and A. minutum after 48 hours of exposure to one of four treatments (“ambient pCO2”, “ambient pCO2 x copepodamide”, “elevated pCO2” or “elevated pCO2 x copepodamide”). Solid line inside the box signifies the median, and box signifies the lower and upper quartile ranges; letters denote significance (p< 0.05), n = 6.
Induced Defences
Copepodamides and pCO2 had an interactive effect on chain length in T. rotula (p=0.03, Figure 2). Chain length was slightly more reduced in ambient (70%) than in elevated pCO2 (64%) when exposed to copepodamides. The reason, however, is that the cultures without copepodamides were 26% shorter in the elevated pCO2 treatment. In C. affinis there was a weak interaction (p=0.05, Figure 2). However, this was not confirmed by post-hoc tests which only show a significant chain length shortening (31%) in response to elevated pCO2 regardless of copepodamide concentration. For both C. curvisetus and S. marinoi there was a significant main effect of copepodamides (p<0.001 for both; Figure 2) showing that chain length was 29% and 67% shorter in the presence of copepodamides regardless of pCO2.
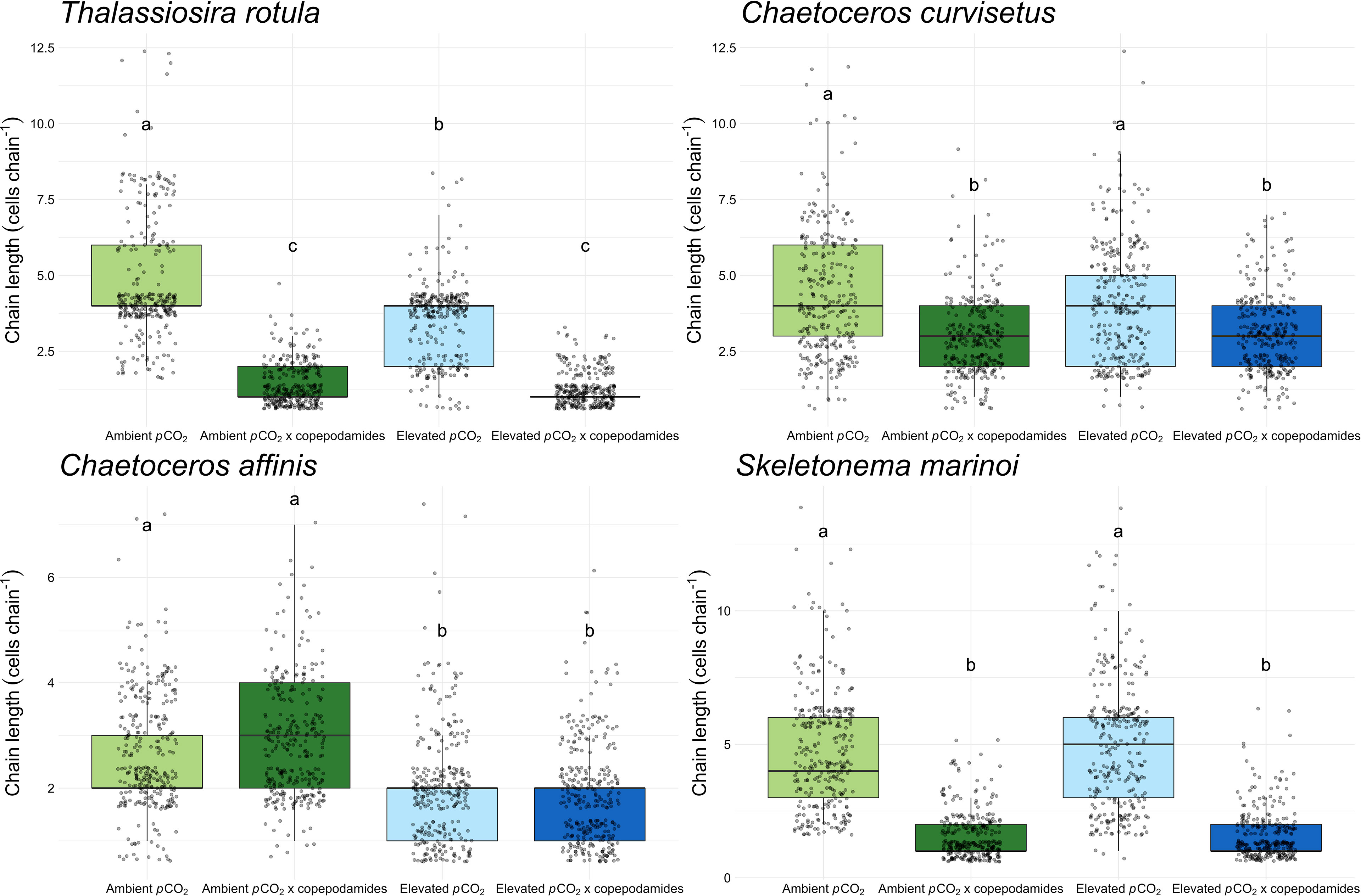
Figure 2 Boxplots showing chain length of four species of chain-forming diatoms (T. rotula, C. curvisetus, C. affinis, S. marinoi) after 48 hours of exposure to one of four treatments (“ambient pCO2”, “ambient pCO2 x copepodamide”, “elevated pCO2” or “elevated pCO2 x copepodamide”). In total, 300 observations were made per species and treatment (6 replicates of 50 chain length measurements). Solid line inside the box signifies the median, box contains the lower and upper quartile ranges, and dots represent individual observations; letters denote significance (p< 0.05), n = 6.
Silica content was only affected in S. marinoi which contained 30% less silica per cell when grown under elevated pCO2 (p<0.001, Figure 3) regardless of whether copepodamides were present or not. The analysis of toxin content in A. minutum showed reduced toxicity in elevated pCO2 (p=0.005, Figure 4) whereas copepodamide exposure led to increased toxin content (p=0.03, Figure 4). The highest toxin content was found in the “ambient pCO2 x copepodamide” treatment and the lowest toxin content was measured in the “elevated pCO2” treatment (46% lower).
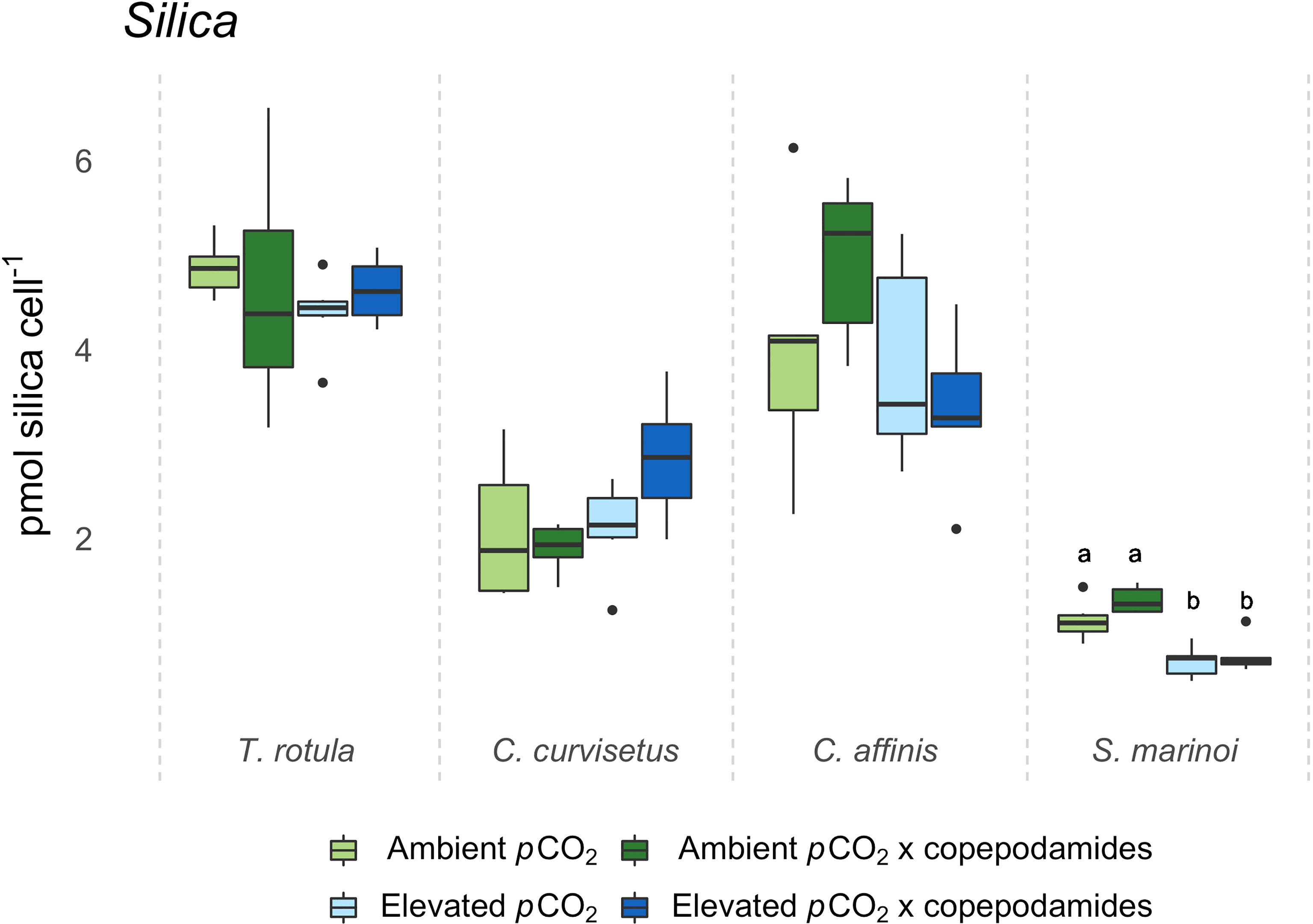
Figure 3 Box plot of silica content (pmol silica cell-1) for Thalassiosira rotula, Chaetoceros curvisetus, Chaetoceros affinis and Skeletonema marinoi after 48 hours exposure to one of four treatments (“ambient pCO2”, “ambient pCO2 x copepodamide”, “elevated pCO2” or “elevated pCO2 x copepodamide”). Solid line inside the box signifies the median, box contains the lower and upper quartile ranges; letters denote significance (p< 0.05), n = 6, for all groups but C. affinis “ambient pCO2” and S. marinoi “elevated pCO2” where n = 5.
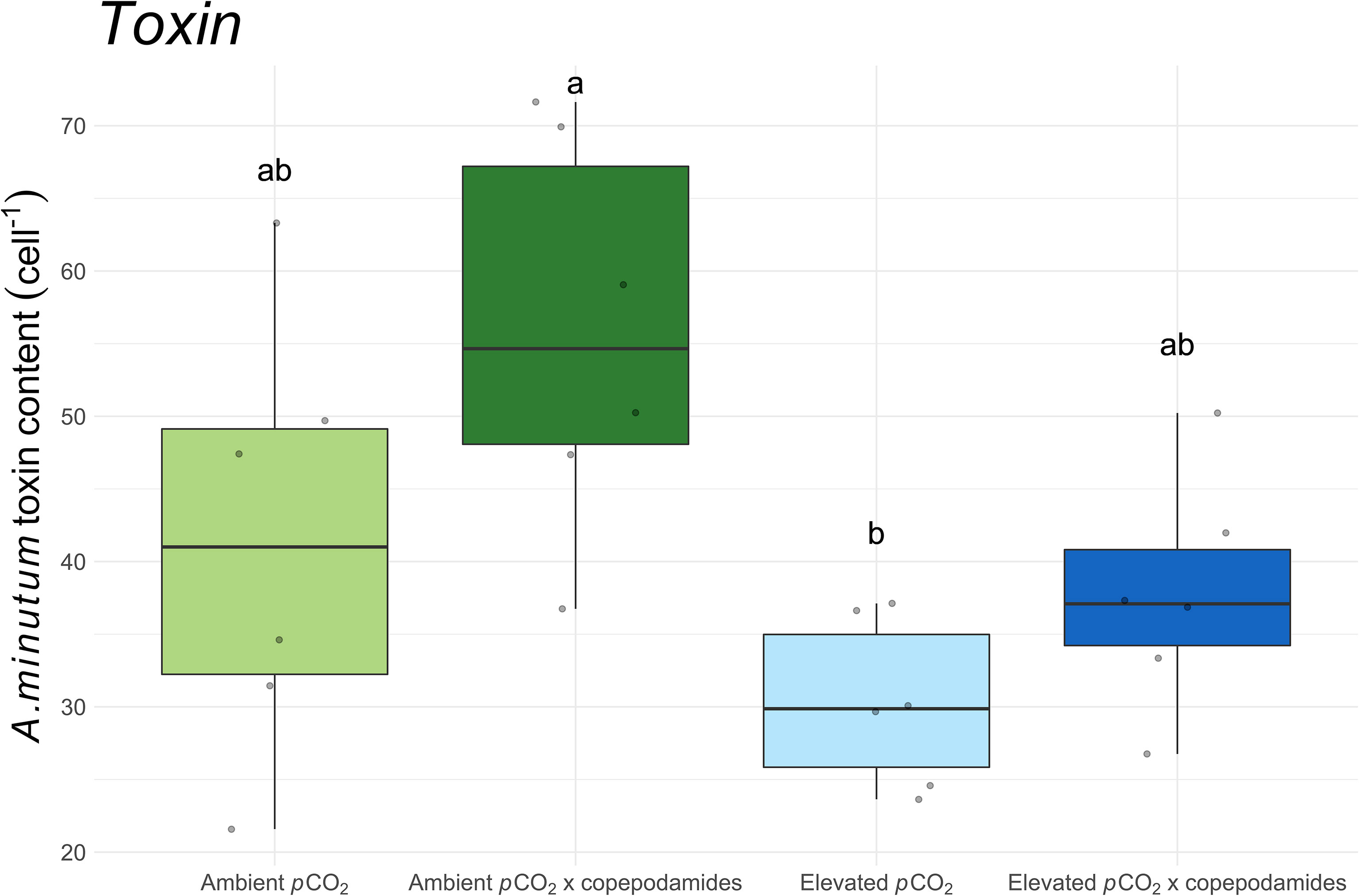
Figure 4 Box plot of Alexandrium minutum toxin content (cell-1) after 48 hours exposure to one of four treatments (“ambient pCO2”, “ambient pCO2 x copepodamide”, “elevated pCO2” or “elevated pCO2 x copepodamide”). Solid line inside the box signifies the median, box contains the lower and upper quartile ranges, and dots represent individual observations; letters denote significance (p< 0.05), n = 6.
Discussion
We show that growth was suppressed (between 27 and 63%) in three out of five species under elevated pCO2 but increased in the other two (Figure 1). This suggests that the response to increased pCO2 is species or even strain specific. Previous studies also show variable results and report higher growth rates in S. marinoi in elevated pCO2 (Scheinin et al., 2015). Although closely related species grew slower: Ihnken et al. (2011) found a 10% decrease in growth in Chaetoceros muelleri. Mejía et al. (2013) found that Thalassiosira weissflogii had a decreased growth rate and 90% decrease in silica when exposed to elevated pCO2.
Growth rate was lower in all treatments exposed to copepodamides when compared to their respective controls, although this difference was not always significant. Theoretically there should be a cost associated with inducible defensive traits (Tollrian and Harvell, 1999), while defensive traits without costs are more likely to become constitutive (i.e., always present). However, some studies have been unable to establish costs of grazer induced traits in terms of reduced growth rate (Blossom et al., 2019; Ryderheim et al., 2021; but see Grønning and Kiørboe, 2020; Park and Dam, 2021). Our findings of reduced growth rate consequently suggests the presence of a direct allocation cost associated with expression of defensive traits, particularly in diatoms. Inducible defenses offer effective protection in microplankton (Bergkvist et al., 2012; Prevett et al., 2019; Ryderheim et al., 2021) thus providing a clear benefit. However, the benefit must be larger than the cost to motivate the investment in defensive traits which should only be induced when grazers are abundant.
One of the species that grew faster in this study was the dinoflagellate A. minutum. At a first glance this suggests that future pCO2 would favour A. minutum harmful algal blooms, but the increased growth was accompanied by a 30% reduction in cell toxin content (Figure 4). Therefore, the amount of toxin per unit volume will be partially counterbalanced by the lower toxin content but will eventually lead to higher volume specific toxin content with time. Less toxic cells are, however, grazed at higher rates which will reduce the benefits of increased growth (Teegarden, 1999; Selander et al., 2006; Ryderheim et al., 2021). It is consequently hard to predict if the higher growth rate in future pCO2 levels will favour harmful algal bloom formation of A. minutum. Reduction in toxin content when exposed to elevated pCO2 has also been seen in Pseudo-nitzschia and Karlodinium veneficum (Lundholm et al., 2004; Fu et al., 2010). This reduced production of deterring compounds has also been seen for phlorotannin content in macroalgae (Kinnby et al., 2021a; Kinnby et al., 2021b) and phenolics in seagrass (Arnold et al, 2012).
In addition, the effect of high pCO2 seen on toxin production in this study was relatively small compared to the well-established effects of nitrogen or phosphate-availability and grazer cues (Griffin et al., 2019; Brandenburg et al., 2020). Phosphate limitation may for example trigger an order of magnitude higher toxin content in Alexandrium catenella compared to nutrient replete controls Tatters et al. (2013). Thus, pCO2 is likely not among the most important drivers controlling toxin production in paralytic shellfish toxin producing algae (Brandenburg et al., 2019). Effects from ocean acidification on toxin formation have further been found to weaken in high temperatures (Tatters et al., 2013).
We found that copepodamide-induced toxin production was similar in elevated and ambient pCO2 conditions (Figure 4). Copepodamides have previously been shown to increase silica content and induce chain length shortening in diatoms and increase toxin production in dinoflagellates (Selander et al., 2015; Selander et al., 2019; Grønning and Kiørboe, 2020; Rigby and Selander, 2021). The results from this study imply that under future pCO2 conditions, copepodamides will still be able to induce chain shortening and an increase in toxin production, even though alterations in growth rate may be accompanied by slight changes in the magnitude of the induction. Dinoflagellates follow a typical eukaryotic cell cycle and the production of PST toxins mainly occurs during the G1 (Taroncher-Oldenburg et al., 1999), and G2 phase of the cell cycle (Harlow et al., 2007). An alteration in the length of these phases could consequently affect toxin production. The dinoflagellate K. mikimotoi reduced the percentage of cells in the G2 phase by up to 26% when exposed to elevated pCO2 (Li et al., 2021). Van De Waal et al. (2014) report up to a 26% reduction in toxin content from two Alexandrium tamarense strains in response to elevated pCO2. Since A. minutum grew faster in high pCO2, it is likely that the reduced toxin content resulted from shorter time spent in the G1-2 phase.
Elevated pCO2 levels have previously been shown to have an indirect effect on diatoms (Spisla et al., 2021) as the cell volume positively correlates with growth rate and elevated pCO2, this then leads to a shift towards larger diatom species (Wu et al., 2014; Bach et al., 2019). Through bottom-up processes, even small-scale changes in community composition have the capacity to alter the food web structure. For instance, we found a reduction in silica content in S. marinoi (Figure 3), suggesting a reduction in its ballast potential which could result in lower sinking rates (Petrou et al., 2019). Understanding how individual species will react to an increase in pCO2 is necessary to disentangle how and why communities might change in a future environment. This study was carried out under laboratory conditions with single species cultures testing two different pCO2 levels. On the one hand an increase in carbon enhances growth; on the other hand, there will be induced stress of lowered pH on species and strains. In different conditions we may experience different responses, each increment of pH may have a distinctive effect on each species and strain.
Ocean acidification has varying effects even within strains of species: Kremp and colleagues (2012) found that the response to increased pCO2 is different among strains in Alexandrium ostenfeldii. Three out of eight strains showed no significant increase in growth from pCO2 alone and toxins were significantly lower in elevated pCO2 in one strain while there was an overall trend towards increasing cell toxin content with increasing pCO2 and temperature. Multiple strains of Alexandrium fundyense were shown to have an overall trend towards faster growth but only one from Northport Bay, New York became more toxic while another from the Bay of Fundy showed no change with increased pCO2 (Hattenrath-Lehmann et al., 2015). This shows that there is a wide variation of growth rate and toxin production responses in experiments that focus on OA. Thus, demonstrating the variability within species and the winner and loser concept may be equally valid on strains rather than just at the species level.
We see an overall effect from copepodamides creating shorter chains, trends of higher toxin content, and lower growth, thus we conclude that copepodamide signalling is likely to be robust to future pCO2 changes. The variable response of pCO2 suggests that some species, or strains of species, will be favoured in a high pCO2 world. However, in nature the conditions are much more diverse and any general patterns arising from single species laboratory experiments calls for validation in more natural and complex settings, such as large scale mesocosms.
Data Availability Statement
The original contributions presented in the study are included in the article/Supplementary Material. Further inquiries can be directed to the corresponding author.
Author Contributions
KR and AK designed the experiment, KR, AK, JG, FR, GC, and ES conducted the experiment and conducted lab analyses. KR, AK, and EB performed statistical analyses. KR, AK, and ES wrote the draft and all authors contributed to revisions. All authors contributed to the article and approved the submitted version.
Funding
This work was supported by Swedish Research Council VR 2019- 05238 (to ES) and the Centre for Ocean Life, a VKR Centre for Excellence funded by the Villum Foundation (to JG, FR).
Conflict of Interest
The authors declare that the research was conducted in the absence of any commercial or financial relationships that could be construed as a potential conflict of interest.
Publisher’s Note
All claims expressed in this article are solely those of the authors and do not necessarily represent those of their affiliated organizations, or those of the publisher, the editors and the reviewers. Any product that may be evaluated in this article, or claim that may be made by its manufacturer, is not guaranteed or endorsed by the publisher.
Acknowledgments
We thank Olga Kourtchenko for the algae cultures and Ellika Faust for help with scripting figures.
Supplementary Material
The Supplementary Material for this article can be found online at: https://www.frontiersin.org/articles/10.3389/fmars.2022.875858/full#supplementary-material
References
Allison V., Dunham D. W., Harvey H. H. (1992). Low Ph Alters Response to Food in the Crayfish Cambarus Bartoni. Can. J. Zool. 70 (12), 2416–2420. doi: 10.1139/z92-324
Arnold T., Mealey C., Leahey H., Miller A. W., Hall-Spencer J. M., Milazzo M., et al. (2012). Ocean Acidification and the Loss of Phenolic Substances in Marine Plants. PloS One 7 (4), e35107. doi: 10.1371/journal.pone.0035107
Bach L. T., Alvarez-Fernandez S., Hornick T., Stuhr A., Riebesell U. (2017). Simulated Ocean Acidification Reveals Winners and Losers in Coastal Phytoplankton. PloS One 12 (11), e0188198. doi: 10.1371/journal.pone.0188198
Bach L. T., Hernández-Hernández N., Taucher J., Spisla C., Sforna C., Riebesell U., et al. (2019). Effects of Elevated CO2 on a Natural Diatom Community in the Subtropical NE Atlantic. Front. Marine Sci. 6. doi: 10.3389/fmars.2019.00075
Barker S., Higgins J. A., Elderfield H. (2003). The Future of the Carbon Cycle: Review, Calcification Response, Ballast and Feedback on Atmospheric CO2. Phil Trans. R. Soc. A 361 (1810), 1977–1999. doi: 10.1098/rsta.2003.1238
Beardall J., Raven J. A. (2004). The Potential Effects of Global Climate Change on Microalgal Photosynthesis, Growth and Ecology. Phycologia 43 (1), 26–40. doi: 10.2216/i0031-8884-43-1-26.1
Beardall J., Stojkovic S., Larsen S. (2009). Living in a High CO2 World: Impacts of Global Climate Change on Marine Phytoplankton. Plant Ecol. Divers. 2 (2), 191–205. doi: 10.1080/17550870903271363
Bergkvist J., Thor P., Jakobsen H. H., Wängberg S.Å., Selander E. (2012). Grazer Induced Chain Length Plasticity Reduces Grazing Risk in a Marine Diatom. Limnol. Oceanogr. 57 (1), 318–324. doi: 10.4319/lo.2012.57.1.0318
Blossom H. E., Markussen B., Daugbjerg N., Krock B., Norlin A., Hansen P. J. (2019). The Cost of Toxicity in Microalgae: Direct Evidence From the Dinoflagellate Alexandrium. Front. Microbiol. 1065. doi: 10.3389/fmicb.2019.01065
Boyd P. W., Doney S. C. (2003). “The Impact of Climate Change and Feedback Processes on the Ocean Carbon Cycle,” in Ocean Biogeochemistry. Eds. Fasham M. J. R. (Berlin, Heidelberg: Springer-Verlag). p. 157–193.
Brandenburg K., Siebers L., Keuskamp J., Jephcott T. G., Van de Waal D. B. (2020). Effects of Nutrient Limitation on the Synthesis of N-Rich Phytoplankton Toxins: A Meta-Analysis. Toxins 12 (4), 221. doi: 10.3390/toxins12040221
Brandenburg K. M., Velthuis M., Van de Waal D. B. (2019). Meta-Analysis Reveals Enhanced Growth of Marine Harmful Algae From Temperate Regions With Warming and Elevated CO2 Levels. Global Change Biol. 25 (8), 2607–2618. doi: 10.1111/gcb.14678
Burkhardt S., Amoroso G., Riebesell U., Sültemeyer D. (2001). CO2 and HCO3 † Uptake in Marine Diatoms Acclimated to Different CO2 Concentrations. Limnol. Oceanogr. 46 (6), 1378–1391. doi: 10.4319/lo.2001.46.6.1378
Burkhardt S., Riebesell U., Zondervan I. (1999). Effects of Growth Rate, CO2 Concentration, and Cell Size on the Stable Carbon Isotope Fractionation in Marine Phytoplankton. Geochimica Cosmochimica Acta 63 (22), 3729–3741. doi: 10.1016/S0016-7037(99)00217-3
Caldeira K., Wickett M. E. (2003). Anthropogenic Carbon and Ocean Ph. Nature 425 (6956), 365–365. doi: 10.1038/425365a
Cothran R. D., Monahan P. J., Relyea R. A. (2021). Antipredator Behaviour Affected by Prey Condition, Food Availability and Ph-Mediated Info-Disruption. Anim. Behaviour 171, 111–118. doi: 10.1016/j.anbehav.2020.11.007. Elsevier Ltd.
Dörner I., Hauss H., Aberle N., Lohbeck K., Spisla C., Riebesell U., et al. (2020). Ocean Acidification Impacts on Biomass and Fatty Acid Composition of a Post-Bloom Marine Plankton Community. Marine Ecol. Prog. Ser. 647, 49–64. doi: 10.3354/meps13390
Eriander L., Wrange A.-L., Havenhand J. N. (2016). Simulated Diurnal Ph Fluctuations Radically Increase Variance in—But Not the Mean of—Growth in the Barnacle Balanus Improvisus. ICES J. Marine Sci. 73, 596–603. doi: 10.1093/icesjms/fsv214
Falkenberg L. J., Styan C. A., Havenhand J. N. (2019). Sperm Motility of Oysters From Distinct Populations Differs in Response to Ocean Acidification and Freshening. Sci. Rep. 9 (1), 1–7. doi: 10.1038/s41598-019-44321-0
Falkowski P. G., Barber R. T., Smetacek V. (1998). Biogeochemical Controls and Feedbacks on Ocean Primary Production. Science 281 (5374), 200–206. doi: 10.1126/science.281.5374.200
Field C. B., Behrenfeld M. J., Randerson J. T., Falkowski P. (1998). Primary Production of the Biosphere: Integrating Terrestrial and Oceanic Components. Science 281 (5374), 237–240. doi: 10.1126/science.281.5374.237
Fu F. X., Place A. R., Garcia N. S., Hutchins D. A. (2010) CO2 and Pphosphate Availability Control the Toxicity of the Harmful Bloom Dinoflagellate Karlodinium veneficum. Aquat. Microb. Ecol. 59, 55–65. doi: 10.3354/ame01396
Gervais F., Riebesell U. (2001). Effect of Phosphorus Limitation on Elemental Composition and Stable Carbon Isotope Fractionation in a Marine Diatom Growing Under Different CO2 Concentrations. Limnol. Oceanogr. 46, 497–504. doi: 10.4319/lo.2001.46.3.0497
Giordano M., Beardall J., Raven J. A. (2005). CO2 Concentrating Mechanisms in Algae: Mechanisms, Environmental Modulation, and Evolution. Annu. Rev. Plant Biol. 56, 99–131. doi: 10.1146/annurev.arplant.56.032604.144052
Grønning J., Kiørboe T. (2020). Diatom Defence: Grazer Induction and Cost of Shell-Thickening. Funct. Ecol. 34 (9), 1790–1801. doi: 10.1111/1365-2435.13635
Griffin J. E., Park G., Dam H. G. (2019). Relative Importance of Nitrogen Sources, Algal Alarm Cues and Grazer Exposure to Toxin Production of the Marine Dinoflagellate Alexandrium Catenella. Harmful Algae 84 (2018), 181–187. doi: 10.1016/j.hal.2019.04.006
Griffiths R. A. (1993). The Effect of Ph on Feeding Behaviour in Newt Larvae (Triturus: Amphibia). J. Zool. 231, 285–290. doi: 10.1111/j.1469-7998.1993.tb01918.x
Guillard R. R. L., Hargraves P. E. (1993). Stichochrysis Immobilis is a Diatom, Not a Chrysophyte. Phycologia 32:3, 234–236. doi: 10.2216/i0031-8884-32-3-234.1
Guillard R. R. L., Ryther J. H. (1962). Studies of Marine Planktonic Diatoms. I. Cyclotella Nana Hustedt and Detonula Confervacea Cleve. Can. J. Microbiol. 8, 229– 239. doi: 10.1139/m62-029
Gutierrez M. F., Paggi J. C., Gagneten A. M. (2012). Infodisruptions in Predator-Prey Interactions: Xenobiotics Alter Microcrustaceans Responses to Fish Infochemicals. Ecotoxicol. Environ. Saf 81, 11–16. doi: 10.1016/j.ecoenv.2012.04.001
Hanazato T. (1999). Anthropogenic Chemicals (Insecticides) Disturb Natural Organic Chemical Communication in the Plankton Community. Environ. Pollut. 105, 137–142. doi: 10.1016/S0269-7491(98)00203-6
Hanazato T. (2001). Pesticide Effects on Freshwater Zooplankton: An Ecological Perspective. Environ. Pollut. 112, 1–10. doi: 10.1016/S0269-7491(00)00110-X
Harlow L. D., Negri A., Hallegraeff G. M., Koutoulis A. (2007). Sam, Sahh and Map Gene Expression During Cell Division and Paralytic Shellfish Toxin Production of Alexandrium Catenella (Dinophyceae). Phycologia 46 (6), 666–674. doi: 10.2216/07-25.1
Hattenrath-Lehmann T. K., Smith J. L., Wallace R. B., Merlo L. R., Koch F., Mittelsdorf H., et al. (2015). The Effects of Elevated CO2 on the Growth and Toxicity of Field Populations and Cultures of the Saxitoxin-Producing Dinoflagellate, Alexandrium Fundyense.Limnol. Oceanogr. 60 (1), 198–214. doi: 10.1002/lno.10012
Hays G. C., Richardson A. J., Robinson C. (2005). Climate Change and Marine Plankton. Trends Ecol. Evol. 20 (6), 337–344. doi: 10.1016/j.tree.2005.03.004
Heuschele J., Candolin U. (2007). An Increase in Ph Boosts Olfactory Communication in Sticklebacks. Biol. Lett. 3, 411–413. doi: 10.1098/rsbl.2007.0141
Ihnken S., Roberts S., Beardall J. (2011). Differential Responses of Growth and Photosynthesis in the Marine Diatom Chaetoceros Muelleri to CO2 and Light Availability. Phycologia 50, 182–193. doi: 10.2216/10-11.1
IPCC. (2013). Summary for Policymakers Climate Change 2013: The Physical Science Basis. Contribution of Working Group I to the Fifth Assessment Report of the Intergovernmental Panel on Climate Change. Eds. Stocker T. F., Qin D., Plattner G.-K., Tignor M., Allen S. K., Boschung J., Nauels A., Xia Y., Bex V., Midgley P. M. (Cambridge UK: Cambridge University Press).
Kim J. M., Lee K., Shin K., Kang J. H., Lee H. W., Kim M., et al. (2006). The Effect of Seawater CO2 Concentration on Growth of a Natural Phytoplankton Assemblage in a Controlled Mesocosm Experiment. Limnol. Oceanogr. 51 (4), 1629–1636. doi: 10.4319/lo.2006.51.4.1629
Kinnby A., Toth G. B., Pavia H. (2021b). Climate Change Increases Susceptibility to Grazers in a Foundation Seaweed. Front. Marine Sci. 8. doi: 10.3389/fmars.2021.688406
Kinnby A., White J. C., Toth G. B., Pavia H. (2021a). Ocean Acidification Decreases Grazing Pressure But Alters Morphological Structure in a Dominant Coastal Seaweed. PloS One 16 (1), e0245017. doi: 10.1371/journal.pone.0245017
Kremp A., Godhe A., Egardt J., Dupont S., Suikkanen S., Casabianca S., et al. (2012). Intraspecific Variability in the Response of Bloom-Forming Marine Microalgae to Changed Climate Conditions. Ecol. Evol. 2 (6), 1195–1207. doi: 10.1002/ece3.245
Leduc A. O. H. C., Munday P. L., Brown G. E., Ferrari M. C. O. (2013). Effects of Acidification on Olfactory Mediated Behaviour in Freshwater and Marine Ecosystems: A Synthesis. Philos. Trans. R. Soc. B Biol. Sci. 368 (1627), 20120447. doi: 10.1098/rstb.2012.0447
Lindström J., Grebner W., Rigby K., Selander E. (2017). Effects of Predator Lipids on Dinoflagellate Defence Mechanisms-Increased Bioluminescence Capacity. Sci. Rep. 7 (1), 13104. doi: 10.1038/s41598-017-13293-4
Liu N., Tong S., Yi X., Li Y., Li Z., Miao H., et al. (2017). Carbon Assimilation and Losses During an Ocean Acidification Mesocosm Experiment, With Special Reference to Algal Blooms. Mar. Environ. Res. 129, 229–235. doi: 10.1016/j.marenvres.2017.05.003
Li Y., Zhou Z., Li Y., Wang Y., Xu M., Zhou B., et al. (2021). The Bloom-Forming Dinoflagellate Karenia Mikimotoi Adopts Different Growth Modes When Exposed to Short or Long Period of Seawater Acidification. Toxins 13 (9), 629. doi: 10.3390/toxins13090629
Lundholm N., Hansen P. J., Kotaki Y. (2004). Effect of Ph on Growth and Domoic Acid Production by Potentially Toxic Diatoms of the Genera Pseudo-Nitzschia and Nitzschia. Mar. Ecol. Prog. Ser. 273, 1–15. doi: 10.3354/meps273001
Maibam C., Fink P., Romano G., Buia M. C., Butera E., Zupo V. (2015). Centropages Typicus (Crustacea, Copepoda) Reacts to Volatile Compounds Produced by Planktonic Algae. Marine Ecol. 36 (3), 819–834. doi: 10.1111/maec.12254
Mejía L. M., Isensee K., Méndez-Vicente A., Pisonero J., Shimizu N., González C., et al. (2013). B Content and Si/C Ratios From Cultured Diatoms (Thalassiosira Pseudonana and Thalassiosira Weissflogii): Relationship to Seawater Ph and Diatom Carbon Acquisition. Geochimica Cosmochimica Acta 123, 322–337. doi: 10.1016/j.gca.2013.06.011
Nielsen L. T., Jakobsen H. H., Hansen P. J. (2010). High Resilience of Two Coastal Plankton Communities to Twenty-First Century Seawater Acidification: Evidence From Microcosm Studies. Marine Biol. Res. 6 (6), 542–555. doi: 10.1080/17451000903476941
Orr J. C., Fabry V. J., Aumont O., Bopp L., Doney S. C., Feely R. A., et al. (2005). Anthropogenic Ocean Acidification Over the Twenty-First Century and its Impact on Calcifying Organisms. Nature 437 (7059), 681–686. doi: 10.1038/nature04095
Paasche E. (1980). Silicon Content of Five Marine Plankton Diatom Species Measured With a Rapid Filter Method. Limnol. Oceanogr. 25, 474–480. doi: 10.4319/lo.1980.25.3.0474
Park G., Dam H. G. (2021). Cell-Growth Gene Expression Reveals a Direct Fitness Cost of Grazer-Induced Toxin Production in Red Tide Dinoflagellate Prey. Proc. R. Soc. B 288 (1944), 20202480. doi: 10.1098/rspb.2020.2480
Petrou K., Baker K. G., Nielsen D. A., Hancock A. M., Schulz K. G., Davidson A. T. (2019). Acidification Diminishes Diatom Silica Production in the Southern Ocean. Nat. Climate Change 9 (10), 781–786. doi: 10.1038/s41558-019-0557-y
Prevett A., Lindström J., Xu J., Karlson B., Selander E. (2019). Grazer-Induced Bioluminescence Gives Dinoflagellates a Competitive Edge. Curr. Biol. 29 (12), R564–R565. doi: 10.1016/j.cub.2019.05.019
Raven J., Caldeira K., Elderfield H., Hoegh-Guldberg O., Liss P., Riebesell U., et al. (2005). Acidification Due to Increasing Carbon Dioxide. Policy Document 12/05 (London: The Royal Society).
Redfield A. C. (1958). The Biological Control of Chemical Factors in the Environment. Am. Sci 46 (3), 230A–2221.
Riebesell U. (2004). Effects of CO2 Enrichment on Marine Phytoplankton. J. Oceanogr. 60, 719–729. doi: 10.1007/s10872-004-5764-z
Riebesell U., Bach L. T., Bellerby R. G., Monsalve J. R. B., Boxhammer T., Czerny J., et al. (2017). Competitive Fitness of a Predominant Pelagic Calcifier Impaired by Ocean Acidification. Nat. Geosci. 10 (1), 19–23. doi: 10.1038/ngeo2854
Riebesell U., Zondervan I., Rost B., Tortell P. D., Zeebe R. E., Morel F. M. (2000). Reduced Calcification of Marine Plankton in Response to Increased Atmospheric CO 2. Nature 407 (6802), 364–367. doi: 10.1038/35030078
Rigby K., Selander E. (2021). Predatory Cues Drive Colony Size Reduction in Marine Diatoms. Ecol. Evol. 11 (16), 11020–11027. doi: 10.1002/ece3.7890
Roggatz C. C., Lorch M., Hardege J. D., Benoit D. M. (2016). Ocean Acidification Affects Marine Chemical Communication by Changing Structure and Function of Peptide Signalling Molecules. Global Change Biol. 22 (12), 3914–3926. doi: 10.1111/gcb.13354
Ryderheim F., Selander E., Kiørboe T. (2021). Predator-Induced Defence in a Dinoflagellate Generates Benefits Without Direct Costs. ISME J. 15, 2107–2116. doi: 10.1038/s41396-021-00908-y
Sabine C. L., Feely R. A., Gruber N., Key R. M., Lee K., Bullister J. L., et al. (2004). The Oceanic Sink for Anthropogenic CO2. Science 305 (5682), 367–371. doi: 10.1126/science.1097403
Scheinin M., Riebesell U., Rynearson T. A., Lohbeck K. T., Collins S. (2015). Experimental Evolution Gone Wild. J. R. Soc. Interface 12 (106), 20150056. doi: 10.1098/rsif.2015.0056
Schulz K. G., Bellerby R. G. J., Brussaard C. P., Büdenbender J., Czerny J., Engel A., et al. (2013). Temporal Biomass Dynamics of an Arctic Plankton Bloom in Response to Increasing Levels of Atmospheric Carbon Dioxide. Biogeosciences 10 (1), 161–180. doi: 10.5194/bg-10-161-2013
Selander E., Berglund E. C., Engström P., Berggren F., Eklund J., Harðardóttir S., et al. (2019). Copepods Drive Large-Scale Trait-Mediated Effects in Marine Plankton. Sci. Adv. 5 (2), eaat5096. doi: 10.1126/sciadv.aat5096
Selander E., Jakobsen H. H., Lombard F., Kiørboe T. (2011). Grazer Cues Induce Stealth Behavior in Marine Dinoflagellates. Proc. Natl. Acad. Sci. 108 (10), 4030–4034. doi: 10.1073/pnas.1011870108
Selander E., Kubanek J., Hamberg M., Andersson M. X., Cervin G., Pavia H. (2015). Predator Lipids Induce Paralytic Shellfish Toxins in Bloom-Forming Algae. Proc. Natl. Acad. Sci. 112 (20), 6395–6400. doi: 10.1073/pnas.1420154112
Selander E., Thor P., Toth G. B., Pavia H. (2006). Copepods Induce Paralytic Shellfish Toxin Production in Marine Dinoflagellates. Proc. R. Soc. Lond. Ser. B-Biol. Sci. 273, 1673–1680. doi: 10.1098/rspb.2006.3502
Spisla C., Taucher J., Bach L. T., Haunost M., Boxhammer T., King A. L., et al. (2021). Extreme Levels of Ocean Acidification Restructure the Plankton Community and Biogeochemistry of a Temperate Coastal Ecosystem: A Mesocosm Study. Front. Marine Sci. 7. doi: 10.3389/fmars.2020.611157
Strickland J. D. H., Parsons T. R. (1972). A Practical Handbook of Sea- Water Analysis. 2nd ed (Ottawa, Canada: Fisheries Research Board of Canada).
Taroncher-Oldenburg G., Kulis D. M., Anderson D. M. (1999). Coupling of Saxitoxin Biosynthesis to the G1 Phase of the Cell Cycle in the Dinoflagellate Alexandrin Fundyense: Temperature and Nutrient Effects. Nat. Toxins 7 (5), 207–219. doi: 10.1002/1522-7189(200009/10)7:5<207::AID-NT61>3.0.CO;2-Q
Tatters A. O., Flewelling L. J., Fu F., Granholm A. A., Hutchins D. A. (2013). High CO2 Promotes the Production of Paralytic Shellfish Poisoning Toxins by Alexandrium Catenella From Southern California Waters. Harmful Algae 30, 37–43. doi: 10.1016/j.hal.2013.08.007
Teegarden G. J. (1999). Copepod Grazing Selection and Particle Discrimination on the Basis of PSP Toxin Content. Marine Ecology-Progress Ser. 181, 163–176. doi: 10.3354/meps181163
Tembo R. N. (2009). The Sublethal Effects of Low-Ph Exposure on the Chemoreception of Poecilia Sphenops. Arch. Environ. Contam. Toxicol. 57, 157. doi: 10.1007/s00244-008-9255-x
Tollrian R., Harvell C. D. (1999). The Ecology and Evolution of Inducible Defences (Princeton: Princeton University Press).
Tortell P. D., Payne C., Gueguen C., Strzepek R. F., Boyd P. W., Rost B. (2008). Inorganic Carbon Uptake by Southern Ocean Phytoplankton. Limnol. Oceanogr. 53 (4), 1266–1278. doi: 10.4319/lo.2008.53.4.1266
Turner A. D., Tölgyesi L. (2019). Determination of Paralytic Shellfish Toxins and Tetrodotoxin in Shellfish using HILIC. MS/MS (Application Note No. 5994-0967EN).
Van De Waal D. B., Eberlein T., John U., Wohlrab S., Rost B. (2014). Impact of Elevated Pco2 on Paralytic Shellfish Poisoning Toxin Content and Composition in Alexandrium Tamarense. Toxicon 78, 58–67. doi: 10.1016/j.toxicon.2013.11.011
Wu Y., Campbell D. A., Irwin A. J., Suggett D. J., Finkel Z. V. (2014). Ocean Acidification Enhances the Growth Rate of Larger Diatoms. Limnol. Oceanogr. 59 (3), 1027–1034. doi: 10.4319/lo.2014.59.3.1027
Keywords: chemical defenses, chemical ecology, ocean acidification, inducible defense, plankton ecology, predator-prey interactions, pCO2
Citation: Rigby K, Kinnby A, Grønning J, Ryderheim F, Cervin G, Berdan EL and Selander E (2022) Species Specific Responses to Grazer Cues and Acidification in Phytoplankton- Winners and Losers in a Changing World. Front. Mar. Sci. 9:875858. doi: 10.3389/fmars.2022.875858
Received: 14 February 2022; Accepted: 11 March 2022;
Published: 07 April 2022.
Edited by:
Jorg D. Hardege, University of Hull, United KingdomReviewed by:
Elizabeth Harvey, University of New Hampshire, United StatesVittoria Roncalli, Stazione Zoologica Anton Dohrn Napoli, Italy
Valerio Zupo, Stazione Zoologica Anton Dohrn Napoli, Italy
Copyright © 2022 Rigby, Kinnby, Grønning, Ryderheim, Cervin, Berdan and Selander. This is an open-access article distributed under the terms of the Creative Commons Attribution License (CC BY). The use, distribution or reproduction in other forums is permitted, provided the original author(s) and the copyright owner(s) are credited and that the original publication in this journal is cited, in accordance with accepted academic practice. No use, distribution or reproduction is permitted which does not comply with these terms.
*Correspondence: Kristie Rigby, a3Jpc3RpZS5yaWdieUBnbWFpbC5jb20=
†These authors have contributed equally to this work and share first authorship