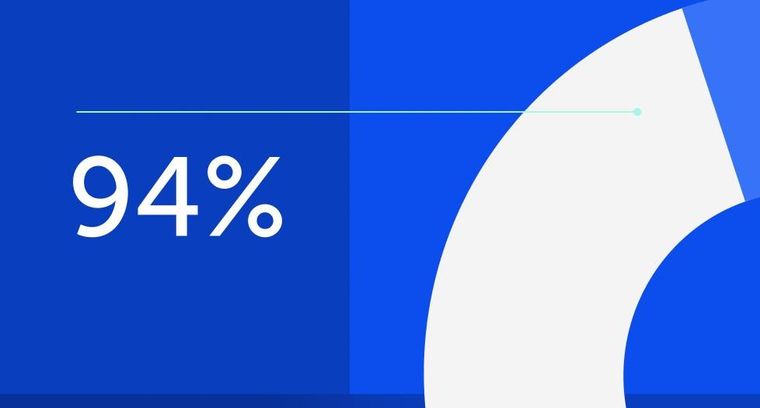
94% of researchers rate our articles as excellent or good
Learn more about the work of our research integrity team to safeguard the quality of each article we publish.
Find out more
REVIEW article
Front. Mar. Sci., 27 June 2022
Sec. Marine Molecular Biology and Ecology
Volume 9 - 2022 | https://doi.org/10.3389/fmars.2022.874534
This article is part of the Research TopicMarine Biological Materials: Functional Mechanisms and Environmental Impacts from the Molecular to the Macro-ScaleView all 11 articles
The field of biomineralization is an inspiration for human design across disciplines, e.g. biomimetic materials, environmental and biomedical treatments, etc. Having a coherent understanding of the basic science sets the pillars for these fields that will impact human welfare. Intensive studies lead to great progress in unraveling the molecular mechanism underlying molluscan shell formation, especially in the past three decades. However, some problems remain, and discrepancy exists in varied studies. In this review, we pay attention to some issues which have been overlooked and warranted more in-depth studies, and pointed out that considerations should be seriously taken when looking into the cellular and molecular events in shell formation. We first consider the evolution of shell mineralogy and organic matrix by emphasizing the great impact of sea water chemistry. Secondly, we discussed the recent progress on the shell matrix protein (SMP) characterization and pointed out environmental and physiological conditions should be taken into account when studying the SMP functions. Finally, we highlighted some ambiguous issues in the less studied mineralizing tissues and cells, and the underlying cellular control on shell formation. New researchers in this field should keep in mind that early geochemistry in vitro research has mostly failed to address the in vivo context of biomineralization in cells and tissues. Therefore, the more biologically relevant experiments are still needed for future research.
In nature, biominerals are synthesized by myriads of living organisms for body support, protection, feeding and perception (Addadi and Weiner, 2014; Clark, 2020). Molluscs are among the most exceptional for their ability to fabricate tremendous shells, leading molluscs to be the second largest phylum in invertebrates with more than 70, 000 living species (Rosenberg, 2014). The field of biomineralization is an inspiration for human design across disciplines, e.g. biomimetic materials, tissue engineering in medicine, energy production, environmental treatments, etc. Having a coherent understanding of the basic science sets the pillars for these fields that will impact human welfare.
The primary role of the shell is to protect the soft tissues, and in particular cases, the shell may serve as a buoyancy regulator in squids (Yang et al., 2020) or burrowing tool in shipworms (Distel et al., 2017). Some classes and genera completely lost their shells, such as slugs and sea hares in Gastropoda and octopus in Cephalopoda. The shells are composed of more than 95% inorganic minerals and a small amount of organic matrix (Marin et al., 2012; Checa, 2018). CaCO3 in shells is commonly found as calcite and aragonite in adult individuals and often amorphous calcium carbonate (ACC) in juvenile animals (Lowenstam and Weiner, 1989; Weiss et al., 2002; McDougall and Degnan, 2018). In contrast to the limited forms of inorganic constituents, the organic matrix exhibits vast diversity and contains polysaccharides, lipids and proteins. Shell proteins play a central role in regulating CaCO3 precipitation, including nucleation, polymorph selection, and morphology modification by self-assembly and bind to the polysaccharide framework (Marin et al., 2008; Marin et al., 2012). Since the pioneering work of identifying Lustrin A, MSI60 and MSI30 in the 1990s (Shen et al., 1997; Sudo et al., 1997), hundreds of shell matrix proteins (SMPs) have been characterized. Recombinant SMPs expressed and purified in Escherichia coli further shed light on their vital role and location in the shell layers (Pan et al., 2014; Bahn et al., 2017; Kong et al., 2018), although they may not fully reveal the exact role of the corresponding natural matrix proteins due to their lack of post-translational modifications and the absence of other SMPs.
Both inorganic constituents and organic matrix are secreted by the mineralizing cells lining the outer surface of the mantle which is a compartmentalized membrane-shape tissue. Typically, the mantle edge has three folds (Figure 1): inner fold for muscular movement, middle fold for sensing, and outer fold for shell secreting (Taylor and Kennedy, 1969). The groove between the middle and outer folds is responsible for synthesizing periostracum which is a tanned organic membrane covering the inner calcified shell layers (Checa, 2000; Yue et al., 2019). The mineralized shells usually have several layers with dramatically different morphologies and textures. The multilayers may be ascribed to the compartmentalization of the mantle tissue (Figure 1). For example, in the pearl oyster Pterioida, the mantle edge and submarginal zone are responsible for the prismatic layer secretion, while the pallial zone and central zone secrete the nacreous layer (Marie et al., 2012; Liu et al., 2015). In addition, hemocytes in bivalves have been found to participate in shell formation (Mount et al., 2004; Li et al., 2016).
Figure 1 Hematoxylin-eosin staining of the mantle tissue and decalcified shell in the pearl oyster Pinctada fucata. (A) holistic view of the mantle and the underlying shell layers. (B-E) magnifications of the black frames. (B) the mantle edge and shell edge. Note that the periostracum membrane (pe) secreted in the periostracum groove is visible. (C) submarginal zone of the mantle and prismatic layer. (D) transition zone of the prismatic layer and nacreous layer. (E) pallial zone of the mantle and nacreous layer. pe, periostracum; pg, periostracum groove; of, outer fold; mf, middle fold; if, inner fold; EPS, extrapallial space; PL, prismatic layer; NL, nacreous layer. The blue arrowhead indicates the growth front of the nacreous layer.
More and more evidence shows that the shell biomineralization is a complex process involving exquisite regulation on cellular and molecular levels. And there are some overlooked aspects in recent studies. In vitro CaCO3 crystallization experiment on SMPs could lead to misleading conclusions as these reactions were not properly set under physiologically relevant conditions. In this comment, we summarize some unsolved issues which warrant more in-depth research in the future, and make the community aware that there are some problems which should be carefully appreciated.
Calcite and aragonite are the two most used calcium carbonate polymorphs in mollusk shells. In natural environment and inorganic chemical reaction under abiotic conditions, the formation of calcite or aragonite is mainly controlled by Mg/Ca ratio of the solution and the reaction temperature (Balthasar and Cusack, 2015). As shown in Figure 2, relatively higher content of magnesium ions can strongly promote the formation of aragonite. The current seawater has a high Mg/Ca ratio (~ 5.2), so the naturally formed calcium carbonate at mild temperature would have been 100% aragonite. In addition, modern seawater contains a large amount of which can also promote aragonite precipitation (Vavouraki et al., 2008). Therefore, the modern ocean belongs to the aragonite sea. In this case, the deposition of aragonite such as nacreous shell layer in mollusks or exoskeleton in scleractinian corals is thermodynamically favored.
Figure 2 Influence of temperature and Mg/Ca ratio on aragonite deposition [Taken from Balthasar & Cusack (Balthasar and Cusack, 2015), with permission of GSA]. The Mg/Ca ratio and temperature in the reaction system affect the content of aragonite in CaCO3 crystals. The number in the white circle indicates the proportion of aragonite in the total CaCO3 sediment, and the black circle indicates that more than 99% of the formed CaCO3 is aragonite. Note that the Mg/Ca ratio of current sea water is around 5.2 (corresponding to the right of the graph).
However, most CaCO3 produced by living organisms is definitely under biologically controlled. It has been found that organic matrices extracted from aragonite shells promote aragonite formation, while those extracted from calcite shells promote the precipitation of calcite (Falini et al., 1996; Takeuchi et al., 2008; Ponce and Evans, 2011; Fang et al., 2012; Rivera-Perez and Hernandez-Saavedra, 2021). It should be noted that most of the studies were based on results from in vitro CaCO3 crystallization experiments, and these results do not necessarily reflect the exact physiological functions of SMPs in vivo. For example, PfN44 localized in both prismatic and nacreous layers, and could promote deposition of high Mg calcite at high Mg/Ca ratio (Pan et al., 2014). Clearly, this is unlikely the role PfN44 plays in the aragonitic nacre. Moreover, many SMPs were found to promote aragonite deposition in vitro (Suzuki et al., 2009; Ponce and Evans, 2011; Fang et al., 2012), but synthesizing proteins to facilitate aragonite deposition in modern aragonite sea is energetically costly and evolutionarily unfavorable. Therefore, the function of some SMPs should be carefully reconsidered. Moreover, relatively high level of Ca2+ solutions (mostly 10 mM) are used indiscriminatingly in most crystallization experiments, but that is quite different from the physiological context of freshwater molluscs, which may alter the experimental output.
Study of the mollusk exoskeleton evolution has shown that calcite and aragonite seas do have a strong influence on carbonate skeletal mineralogy at the time mineralized skeletons first evolve (Porter, 2010). In that case, the carbonate skeletons evolved in the aragonite seas were primarily aragonite, while those evolved in the calcite seas were primarily calcite (Figure 3). Interestingly, the mineralogy of most calcified shell layers appeared to remain unchanged once determined evolutionarily, even in the face of dramatic seawater chemistry changes (Porter, 2010). This may be due to the high energy cost of changing the shell mineralogy, so maintaining the original mineralogy was energetically more favorable.
Figure 3 Effect of seawater chemistry on calcium carbonate mineralogy in calcified skeleton evolution [Taken from S. Porter (2010), with permission of Wiley]. The first emergence time and predicted primary biomineral of the carbonate-mineralizing clades. Calcite seas and aragonite seas reconstructed from Mg/Ca ratios in non-skeletal carbonates and fluid inclusions. T, Tommotian Stage; A, Atdabanian Stage; Bot / Toy, Botomian and Toyonian stages.
We speculate that the aragonite-promoting or calcite-promoting SMPs evolved as the mineralized skeletons first appeared, and their primary functions remain unchanged across Phanerozoic times. For example, when a mollusk species evolved a calcified skeleton in a calcite sea, calcite rather than aragonite would stochastically precipitate due to thermodynamics. Simultaneously, those calcite-promoting extracellular organic matrices rapidly evolved under evolutionary pressure. Very likely, these calcite-promoting extracellular organic matrices could specifically bind and interact with calcite, and ultimately be integrated into the calcite crystals (Addadi et al., 1987). In this situation, the mineralogy may serve as the main effector that determined the evolution of organic matrices. In turn, the matrices, such as Asp-rich matrix proteins further promote calcite deposition (Politi et al., 2007; Takeuchi et al., 2008). When the seawater chemistry changed (into an aragonite sea), the cost might have been too high to evolve an aragonite formation secretome. Instead, the species retained the calcite formation secretome, although it cost much energy to overcome the inhibitory effect of high Mg/Ca ratio. Occasionally, some species have lost their original shell layers during the long history of evolution (Hautmann, 2001).
In addition to the regulation of shell matrices, molluscs may determine the CaCO3 mineralogy by regulating the ion concentrations in vivo (Robert and Lorens, 1980). This strategy has been found to be used by foraminifera to deposit calcite (de Nooijer et al., 2009). In addition to actively removing Mg from vacuolized seawater, some foraminifera are able to elevate the pH at the calcification site, thus overcoming inhibitory effect of ambient Mg concentrations (de Nooijer et al., 2009). However, the cellular and molecular mechanisms by which they operate is not fully understood, nor it is clear that the molluscs use a similar mechanism. New techniques such as cryo-SEM-EDS and in situ atomic force microscopy are helpful for us to further understand the regulation mechanism. These techniques can be used to elucidate the cellular processes involved in biomineralization and examine the sample in situ (Hendley et al., 2015; Sviben et al., 2016; Choudhary et al., 2020).
Prismatic layers are frequently found in Palaeoheterodonta and Pteriomorphia. They are the outer most mineral layers and composed of polygonal prisms. Although the morphologies are quite similar, prismatic layers in Palaeoheterodonta (freshwater bivalves) are all composed of aragonite, whereas those in Pteriomorphia (seawater bivalves) are exclusive calcite (Lowenstam and Weiner, 1989; Dauphin, 2003; Marie et al., 2007; Okumura et al., 2010). This scenario is puzzling, considering the high Mg/Ca ratio in the seawater and low Mg/Ca ratio in the freshwater (Table 1). Such contradictory results may be ascribed to the different ambient environments where freshwater bivalves and marine bivalves first evolved prismatic layers. It is likely that freshwater bivalve ancestors evolved the outer prismatic layer in the aragonite sea before they moved into freshwater systems. Indeed, in the ‘living fossil’ bivalve Neotrigonia, which belongs to marine palaeoheterodont, the shell contained prismatic layer and nacreous layer of aragonite (Checa et al., 2014), suggesting that the ancestor of Neotrigonia and Unionoida have already evolved the aragonite prismatic layer. And the evolution of prismatic layers in marine bivalves should have occurred in calcite sea. In that case, the prismatic layers were independently evolved in the ancestors of current freshwater and marine bivalves.
Table 1 Comparison of the shell layers in freshwater bivalve Hyriopsis Cumingii and marine bivalve Pinctada fucata and the corresponding ambient environment.
The exact time when bivalve prismatic layers evolved is not clear. Until now, the earliest known freshwater bivalve is Archanodon catskillensis, presumed to be Unionoida genus of Devonian age, according to the fossil record found in New York (Friedman and Chamberlain, 1995). However, there is no evidence showing the fine microstructure of the shell layer of this species, leading to the uncertainty of the existence of its prismatic layer. Because the prismatic layers of modern Unionidae are all aragonite, their ancestor might have evolved prismatic layer in aragonite sea before entering the freshwater habitats. Moreover, within the Unionidae, Hyriopsis cumingii F and Anodonta woodiana F split in early Permian (Figure 4), and both of them have aragonitic prisms (Liu and Li, 2015; Zhang et al., 2021). Therefore, the acquisition of prismatic layer in the Unionidae likely occurred during the early Mississippian to the early Permian, corresponding to the aragonite sea II period (Figure 3). As for the marine bivalves, typical columnar prismatic layers are present in the Ostreoida and Pteriida of Pteriomorphia (Checa et al., 2009; Okumura et al., 2010), but not in Pectinoidea and Mytilidae (Grefsrud et al., 2008; Gao et al., 2015). Therefore, the time when the Ostreoida and Pteriida evolved prismatic layer might be between the late Cambrian and early Devonian, right after the radiation of the Pteriomorphia (Figure 4). Alternatively, the prismatic microstructure might represent a convergent evolution during the Jurassic and Cretaceous, which is less likely. Comprehensive studies on the mantle transcriptome and shell protein proteome may provide insight into the evolution of the prismatic layers, both in the freshwater Unionidae and marine Pteriomorphia.
Figure 4 Bivalve phylogeny coupled with the geologic time scale showing the evolution of the selected bivalve genera (Modified from Plazzi and Passamonti (2010), with permission of Elsevier). Ca, Cambrian; Or, Ordovician; Si, Silurian; De, Devonian; Mi, Mississippian; Pn, Pennsylvanian; Pr, Permian; Tr, Triassic; Ju, Jurassic; Cr, Cretaceous; Ce, Cenozoic; Ma, million years.
Molluscan shells are composed of more than 95% CaCO3 and 1-5% organic matrices (Marin et al., 2008; Checa et al., 2016). The organic matrices contain polysaccharides, lipids and proteins and play a crucial role in regulating shell formation. The functions of polysaccharides and lipids are not understood at all. Most studies focused on the protein components which have been shown to control CaCO3 precipitation, including nucleation, morphological modification, mineralogy selection, crystal growth and inhibition (Weiner and Dove, 2003; Marin et al., 2012; Checa, 2018). Hundreds of shell matrix proteins have been identified in the past three decades, especially after the application of high through-put omics methods (Liu and Zhang, 2021). However, attention should be taken to the contamination of residual proteins from soft tissues, as suggested by Marie et al. (Marie et al., 2013). Moreover, the extrapallial fluids contain large amounts of cellular proteins and immune components (Hattan et al., 2001) which may be adsorbed to the inner shell surface and lead to false detection if the samples were not carefully prepared.
Although most cellular proteins in shell matrix proteomic studies are regarded as contamination of residual proteins from attached tissues, their presence in the shell layers has been shown by some robust evidence. Drake et al. (Drake et al., 2013) show that the cell-free skeleton of the hermatypic coral Stylophora pistillata has some cellular components such as Actin and Glyceraldehyde 3-phosphatase dehydrogenase. In our recent work, prismatic layers from pearl oyster P. fucata were completely digested with HClO (Liu et al., 2021), resulting in removal of cellular components and extraction of mineral-occluded organic matrix. Although cellular proteins commonly found in other studies such as Actin and Paramyosin were absent, certain cellular proteins were found in the intra-mineral matrix (such Isocitrate lyase and Toll-like receptor 6) (Liu et al., 2021). In this case, we speculate that some cellular proteins are indeed incorporated into the mineral phase of the shell, but this does not necessarily lead to their indispensable function in shell formation. Take it a step further, some proteins previously identified in the shell may not be true shell matrix proteins even though they have been characterized to some certain extent. We suggest that these proteins should be regarded as shell proteins which integrate into the shell minerals but actually do not participate in shell mineralization, such as the immune components and some commonly found cellular proteins.
Nevertheless, it should be noted that there are some biomineralization-related proteins which are not incorporated into the shell layers. Amorphous calcium carbonate binding protein (ACCBP) was identified by our group from the extrapallial fluids of P. fucata (Ma et al., 2007). ACCBP is secreted by mantle epithelial cells and can stabilize ACC in vitro, suggesting its important role in shell formation. Strikingly, ACCBP has never been found in any shell layers. The way by which it participates in biomineralization remains unclear. Similarly, EP28, the most abundant EPF protein (account for half of the total EPF proteins) in Mytilus edulis is absent in the mussel shell, although it has calcium binding affinity and is supposed to participate in shell mineralization (Hattan et al., 2001). Very likely, these components, together with other biomineralization-related proteins such as Calmodulin (Li et al., 2005), Calcineurin (Buddawong et al., 2021) and ferritin (Varney et al., 2021) are involved in the metabolism of calcium and/or other ions. Alternatively, EPF proteins ACCBP and EP28 may inhibit undesired mineralization in the hemolymph and other body fluids considering their broad tissue distributions (Hattan et al., 2001; Ma et al., 2007).
Although hundreds of SMPs have been identified in molluscan shells, only a small part of them are characterized with full-length amino acid sequence and known function. Because the genomes of most molluscs have not been sequenced, except several species including the pearl oyster P. fucata (Takeuchi et al., 2012), Pacific oyster Crassostrea gigas (Zhang et al., 2012), blue mussel Mytilus spp (Murgarella et al., 2016; Yang et al., 2021)., and scallops (Wang et al., 2017; Li et al., 2018). For those without available genome information, the full-length amino acid sequence of an SMP is deduced from its coding cDNA obtained by routine RACE (rapid-amplification of cDNA ends) (Fang et al., 2012; Jia et al., 2015). Their involvement in shell formation can be speculated from the tissue distribution of the gene expression and localization of the mature protein. In this case, if the coding gene of a protein is specifically expressed in the mantle epithelia and/or the mature protein is detected in the shell layer, for example by gold particle labeling (Suzuki et al., 2009), this protein would be considered to participate in shell formation. Moreover, the potential function of the protein can be speculated from its amino acid sequence via homologous blast search against public database (Finn et al., 2014). But a large number of SMPs are unique in molluscs and have no homologs in other animals. In some cases, the predicted SMPs are heterogeneously expressed in E. coli, and the recombinant SMPs are purified and studied via in vitro CaCO3 crystallization. Until now, dozens of recombinant SMPs have been published and found to fulfill important roles in shell formation (Rivera-Perez and Hernandez-Saavedra, 2021).
However, due to the lack of cell lines, traditional and advanced techniques in cell biology are seldom used in studying the exact physiological function of the SMPs. Moreover, in situ observation of shell mineralization is rather difficult, especially in adult animals. These situations greatly hinder the unraveling of cellular and molecular processes in shell mineralization and lead to some principle perspectives largely uncertain and under debate. Moreover, the functions of SMPs are usually not explored under physiological conditions. For example, some SMPs from marine molluscs are found to promote aragonite precipitation in the absence of magnesium (calcite-promoting condition) (Suzuki et al., 2009; Fang et al., 2012). Because the ion composition in marine molluscs is similar to the ambient sea water, aragonite deposition would be favored under physiological conditions (Crenshaw, 1972; Nair and Robinson, 1998; Balthasar and Cusack, 2015). In this scenario, aragonite-promoting would be redundant for marine molluscs. We surmise that the results of in vitro CaCO3 crystallization do not necessarily link to the exact physiological role of the SMPs.
Another problem of the in vitro CaCO3 crystallization experiment is the effects of impurity. Most of the characterized SMPs are found to inhibit CaCO3 growth in vitro (Miyamoto et al., 2005; Samata et al., 2008; Pan et al., 2014; Liang et al., 2015; Rivera-Perez and Hernandez-Saavedra, 2021), regardless of their chemical properties. Even the carbonic anhydrase domain-containing Nacrein was found to be a negative regulator in CaCO3 crystallization according to the in vitro experiments (Miyamoto et al., 2005). Considering the hypersaturation in CaCO3 crystallization system, the effect of impurity may greatly influence the results, and lead to the observed inhibitory effect of SMPs. Therefore, new criteria are urgently needed in assessing SMPs functions.
As aforementioned, seawater chemistry had a great impact on the evolved marine shells when they first emerged (Porter, 2010). But the evolution of SMPs and their relations to shell evolution remain elusive. We propose that the SMPs evolution coordinate with the shell evolution: the mineralogy of the evolved shell may serve as the main effector that determines the corresponding SMPs, rather than the specific SMPs determining the shell mineralogy (Figure 5). For example, when the calcified shell evolved in an aragonite sea, aragonite-promoting SMPs would rapidly evolve and further accelerate aragonite precipitation in the shell. These aragonite-promoting SMPs (such nacre proteins Pif, N16 and PfN23) have strong binding ability to aragonite and may have special domains or motifs (e.g. Gln-Gly-Gln tandem repeats) which can be inserted into the lattice of aragonite crystal (Ponce and Evans, 2011; Fang et al., 2012; Bahn et al., 2017), thus facilitating aragonite nucleation. When the sea water chemistry changes, the aragonite-promoting SMPs (together with other organic matrices) are conserved under unknown evolutionary pressure, and in turn determine the unchanged shell mineralogy (Figure 5, lower panel). It is the same case for calcite-promoting proteins (Figure 5, upper panel).
Figure 5 Schematic illustration of proposed shell matrix evolution in molluscs. The progenitor of the shell matrix evolved in accordance with the seawater chemistry when the calcified skeleton first emerged. When the seawater chemistry changed, for example from calcite sea to aragonite sea, the evolved calcite-associated shell matrices promote calcite deposition in the aragonite sea.
Extrapallial fluids (EPF) are the body fluids in the extrapallial space enclosed by the mantle tissue and the forming shell. The chemical composition of the EPF is similar to the hemolymph (Crenshaw, 1972), indicating the close relationship between them. In marine molluscs, the concentration of inorganic ions in EPF is consistent with those in the ambient environment, while in freshwater molluscs, calcium and carbonates of the EPF have higher content (>110 ppm) than the ambient environment (around 30 ppm) (Pietrzak et al., 1976), suggesting that an active uptake and retention of calcium in the EPF in these animals.
The primary role of the EPF is supposed to maintain the microenvironment for shell mineralization. Any disturbance in the EPF might lead to abnormal CaCO3 deposition. Xie et al. (Xie et al., 2016) found that when the protein content of the EPF was reduced, the nacreous layers were disordered, and calcite was randomly deposited. Moreover, some pathogenic microbes may invade the extrapallial space and cause serious infection. Indeed, the EPF was found to exert immune reaction to clear the pathogens, thus ensuring the biomineralization process (Allam et al., 2000; Huang et al., 2018). In addition, EPF is believed to contain the precursor of shell matrices. Liu et al. (Liu et al., 2018) used pearl nucleus (same as those in pearl aquaculture) as a template and found that EPF of P. fucata could promote the growth of nacre-like multilayer structures, suggesting that EPF might directly participate in shell formation. However, in-depth analysis of the EPF composition turned out to be quite confusing. Only a few SMPs were identified by LC/MS-MS, and when the EPF were incubated with aragonite crystals, the eluate did not contain aragonite-binding SMPs such as Pif and N16 (Xie, 2016). These puzzling results made us revisit the exact role of EPF.
More than a decade ago, Steve Werner and his colleagues (Addadi et al., 2006) questioned the role of EPF and proposed that epithelial cells deposit calcium carbonate directly on the shell surface and that EPF plays a very limited role in shell formation. Although ACCBP and another calcium-binding protein have been identified in mollusk EPF, these proteins were not SMPs (see discussion in Shell Matrix Proteins Versus Shell Proteins). Considering that the majority of EPF proteins were cellular components and immune factors, the primary function of EPF may be exerting immune reaction, together with the hemocytes in the extrapallial space (Huang et al., 2018).
Nevertheless, it is important to note that the role of EPF may be different for various molluscs. For example, in freshwater mussels, the extrapallial space is filled with a large amount of EPF (personal observation), so the mantle (central region) is unlikely to contact the nacreous layer for direct mineralization. Therefore, the nacre of freshwater mussels should be directed by EPF. Unfortunately, information about the EPF composition in the freshwater molluscs is scarce, which warrants more studies in the future.
Because the mineral assembly and growth appear to occur extracellularly, the shell matrices secreted by the mantle cells should undergo self-assembly and guide the CaCO3 precipitation independently. However, up to now, how these events happen remains largely unknown. Levi-Kalisman et al. (Levi-Kalisman et al., 2001) proposed that the shell matrix forms a hydrogel where the chitin fibers are arranged as the main framework which is bound by silk-like matrix proteins. More and more evidence supports the principle idea of this model. Indeed, many SMPs have modular arrangement in their amino acid sequences. For example, the nacre protein Pif of P. fucata, which plays a vital role in nacreous layer deposition, has a typical arrangement of a chitin binding domain and an acidic domain which can bind calcium ion (Suzuki et al., 2009). Other shell matrix proteins may have VWA domain mediating protein-protein interaction with each other (McDougall and Degnan, 2018; Liu and Zhang, 2021).
One of the most impressive features of SMPs is the presence of low complexity regions (LCR) in their sequence (Marin et al., 2008). LCR usually forms random coil and does not have secondary structure, which makes it difficult to predict their functions. Moreover, the LCRs in SMPs are unique in that they have hardly any homologies with known proteins and are clade-specific (McDougall et al., 2013). It should be noted that SMPs from similar shell microstructures (such as nacre tablets in the pearl oyster and Mytilus spp.) may vary dramatically in their LCRs (Liu et al., 2015; Gao et al., 2015). Stephen Weiner and Leroy Hood’s pioneering work revealed that the soluble proteins in the mollusk shell matrix were enriched in Asp, Gly and Ser (Weiner and Hood, 1975). However, the insoluble fractions of the shell matrix from many genera contained proteins enriched in Gly and basic amino acids such as Lys and Arg (Zhang et al., 2006), indicating that the shell matrices undergo self-assembly through intermolecular interactions. Indeed, our group recently found that electrostatic interaction and hydrophobicity play a key role in the shell matrix organization of the prismatic layers in pearl oyster P. fucata (Figure 6) (Huang et al., 2021). Furthermore, the lipids (very likely derived from secreted vesicles) fused with the hydrophobic fraction of the matrix and in return increase the hydrophobicity of the organic mixture.
Figure 6 Schematic model of the prismatic column formation controlled by shell matrix (Huang et al., 2021). I. Shell matrices secreted by the mantle cells contain both eosinophilic matrix and basophilic matrix. II. Calcium and carbonate are recruited by the shell matrix and concentrated until saturation state is reached. Eosinophilic matrix which contains more acidic amino acids and sulfonate serves as nucleation sites and crystallizes with calcium carbonate, while the basophilic matrix which contains numerous hydrophobic amino acids is excluded outside the mineral. III. As the crystals grow, the excluded basophilic matrix is squeezed and hardened, thus forming the polygonal framework.
Liquid-liquid phase separation (LLPS) of the shell matrix may add to another driving force of self-assembly. LLPS of proteins is crucial for the formation of membrane-less organelles, as well as some pathological progresses (Brangwynne et al., 2015; Boeynaems et al., 2017; Kanaan et al., 2020). The proteins undergo LLPS via intra-chain interaction (such as charge-charge and hydrogen bonding), hydrophobic effect (especially via aromatic groups), and multivalent interaction via folded domains (Alberti et al., 2019; Dzuricky et al., 2020). Interestingly, many SMPs share similar features in amino acid sequences. Indeed, Pif80 and Pif97 from nacreous layers of P. fucata formed liquid droplets under high concentration of salt (Bahn et al., 2015; Bahn et al., 2017). We proposed that many SMPs would undergo LLPS, and SMPs with LCRs comprising similar amino acid compositions or interactive domains may fuse via LLPS.
As seawater is rich in Ca2+, and CaCO3 is in a supersaturated state, calcium transportation may not be a limiting factor for shell deposition in marine molluscs (Sillanpaa et al., 1891). Moreover, seawater bivalves balance calcium ions between hemolymph and EPF through channels in the mantle cells (Bleher and Machado, 2004). Given that CaCO3 is continuously deposited on the shell surface, calcium from the hemolymph can reach the EPF by simple diffusion or passive transport (Figure 7). It should be noted that, in the cultured mantle cells, Xiang Liang et al. (Xiang et al., 2014) found that the mantle cells could de novo synthesize ACC in the cytosol. ACC has been proposed to be the precursor of shell minerals and has been found in the larval and adult shells (Weiss et al., 2002; Gal et al., 2014). Is the ACC precursor synthesized by the mantle cells and then secreted to the shell growth front? Moreover, hemocytes bearing CaCO3 crystals have been reported in several bivalves (Li et al., 2016), and particularly in the eastern oyster C. virginica, CaCO3 crystals were secreted onto the shell surface by the hemocytes (Silverman. et al., 1988; Mount et al., 2004). However, how crystalline calcium carbonate is transported across the cell membrane is unclear.
Figure 7 Schematic model showing the possible secretary pathways of the organic materials and inorganic constituents by the mantle cell. Calcium and may be secreted directly or via ACC formed in the cytosol. Organic materials are shown as blue dots and maybe secreted via diverse pathways, namely (1) ACC binding matrix, (2) exocytosis; (3) multivesicular body; (4) shedding microvesicle. The solid arrow indicates pathway which is supported by published work, and dotted arrow indicates potential pathway which warrant more evidence.
Despite the intensive studies on the characterization of the shell matrix, especially the SMPs, there is limited information on the way shell matrices are secreted (Figure 7). Most of the identified SMPs have signal peptides, suggesting their secretion via classical endoplasmic reticulum-Golgi complex pathway (Davis and Tai, 1980). However, as we mentioned in Section 3.3, SMPs were not the main components in the EPF and could hardly be detected in the EPF, making their secreting pathway a mystery. Moreover, some shell proteins (not classical matrix proteins) have no signal peptides, indicating that non-classical secretion pathways are involved (Huang et al., 2022). In fact, Xiaotong Wang et al. (Wang et al., 2013) proposed diverse origins of shell proteins from mantle cells, hemocytes and other organs.
The microstructures of the molluscan shells display dramatic diversity, including cross-lamella, polygonal prisms, sheets, granules, etc (Marin et al., 2012). Although the shell microstructures in various genera have been explored and characterized for decades, the mechanism by which these microstructures are determined is still under debate. Take the prismatic layer in bivalves as an example. Both cellular control and physical control were thought to serve as the determinant for the polygonal arrangement. Bayerlein et al. (Bayerlein et al., 2014) used synchrotron-radiation-based micro-CT to reveal the evolution process of polygon domains in Pinna nobilis, and found that the domain evolution can be well predicted by using grain growth theory, indicating a passive adaptation of the organic matrix to the mineral domains. On the other hand, Checa et al. (Checa et al., 2016) reported in some bivalves, the prism domain pattern could not be explained by the grain growth theory, but was rather controlled, as the authors argued, by the membranes (organic matrix). How to reconcile these contradictory mechanisms is tough and challenging.
SMPs are believed to play central roles in regulating CaCO3 precipitation, including crystal morphology. Indeed, dozens of extracted or recombinant SMPs could modify the appearance of calcite or aragonite in vitro, which may be due to the specific binding of the SMPs to the lattice plane or crystal axis (Rivera-Perez and Hernandez-Saavedra, 2021). Furthermore, inhibition of these SMPs by neutralizing antibodies or RNAi knock-down showed disturbance on the shell microstructures (Suzuki et al., 2009). These results pointed to the important role of SMPs in regulating microstructure. However, due to the lack of direct in vivo evidence, seldom did any study come to an unequivocal conclusion that a certain SMP plays a designated role in shell deposition. For example, until now, no SMP has been found to be responsible for the formation of hexagonal tablets in nacre. Moreover, helical arrangements of nacre tablets have been found in some genera and ascribed to helical dislocations in crystallography (Yao et al., 2006). It is worth paying more attention to applying classical theories of thermodynamics and crystallography in shell biomineralization.
Nevertheless, the mineralizing cells themselves have been proposed to control shell microstructure directly. M.B. Johnstone et al. (Johnstone et al., 2015) examined the metal alloy surfaces implanted under the outer mantle epithelial cells (OME) of Crassostrea virginica and found the extracellular matrix walls appeared to be originated from the OME surface, indicating direct orchestration of the shell microstructure by the OME. Furthermore, Checa et al. suggested that direct subcellular activity in the formation of the helical fibrous microstructure in cavolinioidean gastropods (Checa et al., 2016), where the mantle cells dynamically recognize the positions of the fiber tips and secrete shell constituents. However, in this scenario, the mantle cells have to displace the fiber tips along circular routes to achieve a spiral path. Direct observation of the matrix secretion across the outer cell membrane would underpin this innovative idea, although it may be technically difficult.
After the pioneering work published by Andrew Mount’ group showing that hemocytes (granulocytes in particular) might mediate a special mineralization pathway in the eastern oyster C. virginica (Figures 8A, B) (Mount et al., 2004), the exact role of types of hemocytes in shell formation is still poorly studied. In the previous study, granulocytes were found to deliver calcium, in CaCO3 crystalline form, to the shell growth front during shell repair (Mount et al., 2004). Later, two hemocyte-secreted matrix proteins (48kDa and 55kDa phosphoproteins) were identified and localized in the circulatory system using immuno-fluorescent probe (Johnstone et al., 2008). Moreover, hemocytes’ participation in shell formation (especially in calcium supply) has been reported in several bivalves (Figure 8) (Silverman. et al., 1988; Kádár, 2008; Trinkler et al., 2011; Li et al., 2016). However, there is no robust evidence showing that the hemocytes are indispensable for shell formation. Meanwhile, it is difficult to rule out the participation of hemocytes, because hemocytes are commonly present in the shell growth front, and we could not block the migration of hemocytes into the mantle tissue.
Figure 8 Hemocytes in bivalve molluscs may participate in shell calcification. (A, B) comparison of agranulocytes, granulocytes and REF granulocytes in the eastern oyster C. virginica [Taken from Mount et al. (Mount et al., 2004), with permission of Science]. Note that the REF granulocytes were highly refractive under DIC microscopy. (C, D) hemocytes colored with Von Kossa stain in the clam Venerupis philippinarum during shell repair [Taken from Trinkler et al. (Trinkler et al., 2011), ©Inter-Research 2011, with permission of Inter-Research]. Hemocytes in (C) were collected from hemolymph, and hemocytes in (D) were collected from central extrapallial fluid. The insoluble calcium carbonate salts are dark (brown) colored.
The presence of hemocytes in the EPF has been reported in many bivalves. Considering their large numbers, the exact way they get into the extrapallial space is not fully understood. In a previous study, we found that there were many pore structures on the outer surface of mantle tissue, and some hemocytes were observed nearby (Li et al., 2016). However, when using a milder fixation method, we could not observe these pore structures, which was further supported by histochemical staining and transmission electron microscopy of the mantle tissues. Moreover, P. S. Nair and W. E. Robinson (Nair and Robinson, 1998) examined the material interchange between the EPF and hemolymph in Mercenaria mercenaria. It was found that small molecules, such as tyrosine, can quickly reach equilibrium between the two body fluids, while macromolecules, such as proteins (bovine serum albumin) can never reach equilibrium, but are confined in EPF. Therefore, it is unlikely that the hemocytes migrate into the EPF via holes on the mantle epithelium, and the exact way remains to be further explored.
Nevertheless, the presence of calcium carbonate crystals inside the hemocytes is fascinating. Although most molluscs appeared to assemble and grow calcified shells extracellularly, many other non molluscan species have been shown to produce intracellular compartment for crystals nucleation to overcome the thermodynamic barrier of Ca supersaturation states required (Hayes and Goreau, 1977; Sorrosa et al., 2005). In bivalves, intracellular calcium carbonate is often supposed to serve as a temporary storage for calcium (Silverman et al., 1983), but its formation remains a great mystery. Our group found that in P. fucata, crystal-bearing hemocytes have numerous calcium-rich particles (Li et al., 2016; Huang et al., 2018), consistent with the granulocytes reported in the eastern oyster and clam (Mount et al., 2004; Trinkler et al., 2011). Moreover, many genes related to calcium metabolism and carbonic anhydrase gene were highly expressed in the hemocytes, indicating that some hemocytes may accumulate calcium in the form of calcium carbonate and ultimately produce CaCO3 crystals (Huang et al., 2018). If these hemocytes are responsible for calcium storage and transportation, there should be a reverse process for the release of calcium (or calcium carbonate), which is largely unknown.
Great progress has been made to elucidate cellular and molecular events which lead to the final delicate shells. In this review, we underscored some specific considerations that should be taken seriously when studying shell biomineralization. Due to the lack of proper research models for in situ observation, a large part of the knowledge was obtained from indirect evidence. In vitro CaCO3 crystallization experiments facilitate elucidation of SMP functions, which, however, would lead to misleading conclusions if the reaction conditions were not properly set. Moreover, studies resolving biomineralization mechanism are limited within only a few model species. More representative species should be included to fulfill the whole map of shell formation and evolution.
JH contributed to the design of the study, drafted the manuscript and provided financial support; RZ provided financial support and revised the manuscript. All authors gave final approval for publication.
This work was supported by the National Natural Science Foundation of China Grants 32072951 and 42106091, and the Fundamental Research Funds for the Central Universities, Sun Yat-sen University, 2021qntd13.
The authors declare that the research was conducted in the absence of any commercial or financial relationships that could be construed as a potential conflict of interest.
All claims expressed in this article are solely those of the authors and do not necessarily represent those of their affiliated organizations, or those of the publisher, the editors and the reviewers. Any product that may be evaluated in this article, or claim that may be made by its manufacturer, is not guaranteed or endorsed by the publisher.
Addadi L., Joester D., Nudelman F., Weiner S. (2006). Mollusk Shell Formation: A Source of New Concepts for Understanding Biomineralization Processes. Chem-Eur. J. 12 (4), 981–987. doi: 10.1002/chem.200500980
Addadi L., Moradian J., Shay E., Maroudas N. G., Weiner S. (1987). A Chemical Model for the Cooperation of Sulfates and Carboxylates in Calcite Crystal Nucleation: Relevance to Biomineralization. Proc. Natl. Acad. Sci. U. S. A. 84 (9), 2732–2736. doi: 10.1073/pnas.84.9.2732
Addadi L., Weiner S. (2014). Biomineralization: Mineral Formation by Organisms. Phys. Scripta. 89 (9). doi: 10.1088/0031-8949/89/9/098003
Alberti S., Gladfelter A., Mittag T. (2019). Considerations and Challenges in Studying Liquid-Liquid Phase Separation and Biomolecular Condensates. Cell 176 (3), 419–434. doi: 10.1016/j.cell.2018.12.035
Allam B., Paillard C., Auffret M. (2000). Alterations in Hemolymph and Extrapallial Fluid Parameters in the Manila Clam, Ruditapes Philippinarum, Challenged With the Pathogen Vibrio Tapetis. J. Invertebr. Pathol. 76 (1), 63–69. doi: 10.1006/jipa.2000.4940
Bahn S. Y., Jo B. H., Choi Y. S., Cha H. J. (2017). Control of Nacre Biomineralization by Pif80 in Pearl Oyster. Sci. Adv. 3 (8). doi: 10.1126/sciadv.1700765
Bahn S. Y., Jo B. H., Hwang B. H., Choi Y. S., Cha H. J. (2015). Role of Pif97 in Nacre Biomineralization: In Vitro Characterization of Recombinant Pif97 as a Framework Protein for the Association of Organic-Inorganic Layers in Nacre. Cryst. Growth Des. 15 (8), 3666–3673. doi: 10.1021/acs.cgd.5b00275
Balthasar U., Cusack M. (2015). Aragonite-Calcite Seas-Quantifying the Gray Area. Geology 43 (2), 99–102. doi: 10.1130/G36293.1
Bayerlein B., Zaslansky P., Dauphin Y., Rack A., Fratzl P., Zlotnikov I. (2014). Self-Similar Mesostructure Evolution of the Growing Mollusc Shell Reminiscent of Thermodynamically Driven Grain Growth. Nat. Mat. 13 (12), 1102–1107. doi: 10.1038/Nmat4110
Bleher R., Machado J. (2004). Paracellular Pathway in the Shell Epithelium of Anodonta Cygnea. J. Exp. Zool. Part A. 301a (5), 419–427. doi: 10.1002/jez.a.20065
Boeynaems S., Bogaert E., Kovacs D., Konijnenberg A., Timmerman E., Volkov A., et al. (2017). Phase Separation of C9orf72 Dipeptide Repeats Perturbs Stress Granule Dynamics. Mol. Cell. 65 (6), 1044–1055. doi: 10.1016/j.molcel.2017.02.013
Brangwynne C. P., Tompa P., Pappu R. V. (2015). Polymer Physics of Intracellular Phase Transitions. Nat. Phys. 11 (11), 899–904. doi: 10.1038/Nphys3532
Buddawong T., Asuvapongpatana S., Suwannasing C., Habuddha V., Sukonset C., Sombutkayasith C., et al. (2021). Calcineurin Subunit B is Involved in Shell Regeneration in Haliotis Diversicolor. Peerj 9. doi: 10.7717/peerj.10662
Checa A. (2000). A New Model for Periostracum and Shell Formation in Unionidae (Bivalvia, Mollusca). Tissue Cell. 32 (5), 405–416. doi: 10.1054/tice.2000.0129
Checa A. G. (2018). Physical and Biological Determinants of the Fabrication of Molluscan Shell Microstructures. Front. Mar. Sci. 5. doi: 10.3389/fmars.2018.00353
Checa A. G., Esteban-Delgado F. J., Ramirez-Rico J., Rodriguez-Navarro A. B. (2009). Crystallographic Reorganization of the Calcitic Prismatic Layer of Oysters. J. Struct. Biol. 167 (3), 261–270. doi: 10.1016/j.jsb.2009.06.009
Checa A. G., Macias-Sanchez E., Harper E. M., Cartwright J. H. (2016). Organic Membranes Determine the Pattern of the Columnar Prismatic Layer of Mollusc Shells. Proc. Biol. Sci. 283 (1830). doi: 10.1098/rspb.2016.0032
Checa A. G., Macias-Sanchez E., Ramirez-Rico J. (2016). Biological Strategy for the Fabrication of Highly Ordered Aragonite Helices: The Microstructure of the Cavolinioidean Gastropods. Sci. Rep. 6. doi: 10.1038/srep25989
Checa A. G., Salas C., Harper E. M., Bueno-Perez J. D. (2014). Early Stage Biomineralization in the Periostracum of the 'Living Fossil' Bivalve Neotrigonia. PloS One 9 (2). doi: 10.1371/journal.pone.0090033
Choudhary M. K., Jain R., Rimer J. D. (2020). In Situ Imaging of Two-Dimensional Surface Growth Reveals the Prevalence and Role of Defects in Zeolite Crystallization. P. Natl. Acad. Sci. U.S.A. 117 (46), 28632–28639. doi: 10.1073/pnas.2011806117
Clark M. S. (2020). Molecular Mechanisms of Biomineralization in Marine Invertebrates. J. Exp. Biol. 223 (Pt 11). doi: 10.1242/jeb.206961
Crenshaw M. A. (1972). The Inorganic Composition of Molluscan Extrapallial Fluid. Biol. Bull. 143 (3), 506–512. doi: 10.2307/1540180
Dauphin Y. (2003). Soluble Organic Matrices of the Calcitic Prismatic Shell Layers of Two Pteriomorphid Bivalves - Pinna Nobilis and Pinctada Margaritifera. J. Biol. Chem. 278 (17), 15168–15177. doi: 10.1074/jbc.M204375200
Davis B. D., Tai P. C. (1980). The Mechanism of Protein Secretion Across Membranes. Nature 283 (5746), 433–438. doi: 10.1038/283433a0
de Nooijer L. J., Toyofuku T., Kitazato H. (2009). Foraminifera Promote Calcification by Elevating Their Intracellular Ph. P. Natl. Acad. Sci. U.S.A. 106 (36), 15374–15378. doi: 10.1073/pnas.0904306106
Distel D. L., Altamia M. A., Lin Z. J., Shipway J. R., Han A., Forteza I., et al. (2017). Discovery of Chemoautotrophic Symbiosis in the Giant Shipworm Kuphus Polythalamia (Bivalvia: Teredinidae) Extends Wooden-Steps Theory. P. Natl. Acad. Sci. U.S.A. 114 (18), E3652–E36E8. doi: 10.1073/pnas.1620470114
Drake J. L., Mass T., Haramaty L., Zelzion E., Bhattacharya D., Falkowski P. G. (2013). Proteomic Analysis of Skeletal Organic Matrix From the Stony Coral Stylophora Pistillata. P. Natl. Acad. Sci. U.S.A. 110 (10), 3788–3793. doi: 10.1073/pnas.1301419110
Dzuricky M., Rogers B. A., Shahid A., Cremer P. S., Chilkoti A. (2020). De Novo Engineering of Intracellular Condensates Using Artificial Disordered Proteins. Nat. Chem. 12 (9), 814–825. doi: 10.1038/s41557-020-0511-7
Falini G., Albeck S., Weiner S., Addadi L. (1996). Control of Aragonite or Calcite Polymorphism by Mollusk Shell Macromolecules. Science 271 (5245), 67–69. doi: 10.1126/science.271.5245.67
Fang D., Pan C., Lin H., Lin Y., Zhang G., Wang H., et al. (2012). Novel Basic Protein, PfN23, Functions as Key Macromolecule During Nacre Formation. J. Biol. Chem. 287 (19), 15776–15785. doi: 10.1074/jbc.M112.341594
Finn R. D., Bateman A., Clements J., Coggill P., Eberhardt R. Y., Eddy S. R., et al. (2014). Pfam: The Protein Families Database. Nucleic Acids Res. 42 (D1), D222–DD30. doi: 10.1093/nar/gkt1223
Friedman G. M., Chamberlain J. A. Jr. (1995). Archanodon Catskillensis (Vanuxem): Fresh-Water Clams From One of the Oldest Back-Swamp Fluvial Facies (Upper Middle Devonian), Catskill Mountains, New York. Northeastern. Geol. Environ. Sci. 17 (4), 431–443.
Gal A., Kahil K., Vidavsky N., DeVol R. T., Gilbert P. U. P. A., Fratzl P., et al. (2014). Particle Accretion Mechanism Underlies Biological Crystal Growth From an Amorphous Precursor Phase. Adv. Funct. Mater. 24 (34), 5420–5426. doi: 10.1002/adfm.201400676
Gao P., Liao Z., Wang X. X., Bao L. F., Fan M. H., Li X. M., et al. (2015). Layer-By-Layer Proteomic Analysis of Mytilus Galloprovincialis Shell. PloS One 10 (7). doi: 10.1371/journal.pone.0133913
Grefsrud E. S., Dauphin Y., Cuif J. P., Denis A., Strand O. (2008). Modifications in Microstructure of Cultured and Wild Scallop Shells (Pecten Maximus). J. Shellfish. Res. 27 (4), 633–641. doi: 10.2983/0730-8000(2008)27[633:Mimoca]2.0.Co;2
Hattan S. J., Laue T. M., Chasteen N. D. (2001). Purification and Characterization of a Novel Calcium-Binding Protein From the Extrapallial Fluid of the Mollusc, Mytilus Edulis. J. Biol. Chem. 276 (6), 4461–4468. doi: 10.1074/jbc.M006803200
Hautmann M. (2001). Taxonomy and Phylogeny of Cementing Triassic Bivalves (Families Prospondylidae, Plicatulidae, Dimyidae and Ostreidae). Palaeontology 44, 339–373. doi: 10.1111/1475-4983.00183
Hayes R. L., Goreau N. I. (1977). Intracellular Crystal-Bearing Vesicles in the Epidermis of Scleractinian Corals, Astrangia Danae (Agassiz) and Porites Porites (Pallas). Biol. Bull. 152 (1), 26–40. doi: 10.2307/1540724
Hendley C. T., Tao J. H., Kunitake J. A. M. R., De Yoreo J. J., Estroff L. A. (2015). Microscopy Techniques for Investigating the Control of Organic Constituents on Biomineralization. Mrs. Bulletin. 40 (6), 480–489. doi: 10.1557/mrs.2015.98
Huang J., Li L., Jiang T., Xie L., Zhang R. (2022). Mantle Tissue in the Pearl Oyster Pinctada Fucata Secretes Immune Components via Vesicle Transportation. Fish. Shellfish. Immunol. 121, 116–123. doi: 10.1016/j.fsi.2022.01.001
Huang J., Li S., Liu Y., Liu C., Xie L., Zhang R. (2018). Hemocytes in the Extrapallial Space of Pinctada Fucata are Involved in Immunity and Biomineralization. Sci. Rep. 8 (1), 4657. doi: 10.1038/s41598-018-22961-y
Huang J., Liu Y., Liu C., Xie L., Zhang R. (2021). Heterogeneous Distribution of Shell Matrix Proteins in the Pearl Oyster Prismatic Layer. Int. J. Biol. Macromol. 189, 641–648. doi: 10.1016/j.ijbiomac.2021.08.075
Jia G., Liang J., Xie J., Wang J., Bao Z., Xie L., et al. (2015). cfMSP-1, an Extremely Acidic Matrix Protein Involved in Shell Formation of the Scallop Chlamys Farreri. Comp. Biochem. Physiol. B. Biochem. Mol. Biol. 185, 34–41. doi: 10.1016/j.cbpb.2015.03.004
Johnstone M. B., Ellis S., Mount A. S. (2008). Visualization of Shell Matrix Proteins in Hemocytes and Tissues of the Eastern Oyster, Crassostrea Virginica. J. Exp. Zool. Part B. 310b (3), 227–239. doi: 10.1002/jez.b.21206
Johnstone M. B., Gohad N. V., Falwell E. P., Hansen D. C., Hansen K. M., Mount A. S. (2015). Cellular Orchestrated Biomineralization of Crystalline Composites on Implant Surfaces by the Eastern Oyster, Crassostrea Virginica (Gmelin, 1791). J. Exp. Mar. Biol. Ecol. 463, 8–16. doi: 10.1016/j.jembe.2014.10.014
Kádár E. (2008). Haemocyte Response Associated With Induction of Shell Regeneration in the Deep-Sea Vent Mussel Bathymodiolus Azoricus (Bivalvia: Mytilidae). J. Exp. Mar. Biol. Ecol. 362 (2), 71–78. doi: 10.1016/j.jembe.2008.05.014
Kanaan N. M., Hamel C., Grabinski T., Combs B. (2020). Liquid-Liquid Phase Separation Induces Pathogenic Tau Conformations In Vitro. Nat. Commun. 11 (1). doi: 10.1038/s41467-020-16580-3
Kong J. J., Liu C., Yang D., Yan Y., Chen Y., Huang J. L., et al. (2018). Alv Protein Plays Opposite Roles in the Transition of Amorphous Calcium Carbonate to Calcite and Aragonite During Shell Formation. Cryst. Growth Des. 18 (7), 3794–3804. doi: 10.1021/acs.cgd.8b00025
Levi-Kalisman Y., Falini G., Addadi L., Weiner S. (2001). Structure of the Nacreous Organic Matrix of a Bivalve Mollusk Shell Examined in the Hydrated State Using Cryo-TEM. J. Struct. Biol. 135 (1), 8–17. doi: 10.1006/jsbi.2001.4372
Liang J., Xu G., Xie J., Lee I., Xiang L., Wang H., et al. (2015). Dual Roles of the Lysine-Rich Matrix Protein (KRMP)-3 in Shell Formation of Pearl Oyster, Pinctada Fucata. PloS One 10 (7), e0131868. doi: 10.1371/journal.pone.0131868
Li S., Liu Y., Liu C., Huang J., Zheng G., Xie L., et al. (2016). Hemocytes Participate in Calcium Carbonate Crystal Formation, Transportation and Shell Regeneration in the Pearl Oyster Pinctada Fucata. Fish. Shellfish. Immunol. 51, 263–270. doi: 10.1016/j.fsi.2016.02.027
Li C., Liu X., Liu B., Ma B., Liu F. Q., Liu G. L., et al. (2018). Draft Genome of the Peruvian Scallop Argopecten Purpuratus. Gigascience 7 (4). doi: 10.1093/gigascience/giy031
Liu C., Ji X., Huang J., Wang Z., Liu Y., Hincke M. T. (2021). Proteomics of Shell Matrix Proteins From the Cuttlefish Bone Reveals Unique Evolution for Cephalopod Biomineralization. ACS Biomat. Sci. Eng. doi: 10.1021/acsbiomaterials.1c00693
Liu X. J., Li J. L. (2015). Formation of the Prismatic Layer in the Freshwater Bivalve Hyriopsis Curninqii: The Feedback of Crystal Growth on Organic Matrix. Acta Zool-Stockholm. 96 (1), 30–36. doi: 10.1111/azo.12048
Liu C., Li S. G., Kong J. J., Liu Y. J., Wang T. P., Xie L. P., et al. (2015). In-Depth Proteomic Analysis of Shell Matrix Proteins of Pinctada Fucata. Sci. Rep. 5. doi: 10.1038/srep17269
Liu C., Xu G. R., Du J. Z., Sun J., Wan X., Liu X. J., et al. (2018). Mineralization of Nacre-Like Structures Mediated by Extrapallial Fluid on Pearl Nucleus. Cryst. Growth Des. 18 (1), 32–36. doi: 10.1021/acs.cgd.7b01316
Liu C., Zhang R. Q. (2021). Biomineral Proteomics: A Tool for Multiple Disciplinary Studies. J. Proteomics 238. doi: 10.1016/j.jprot.2021.104171
Li S., Xie L. P., Ma Z. J., Zhang R. Q. (2005). cDNA Cloning and Characterization of a Novel Calmodulin-Like Protein From Pearl Oyster Pinctada Fucata. FEBS J. 272 (19), 4899–4910. doi: 10.1111/j.1742-4658.2005.04899.x
Ma Z., Huang J., Sun J., Wang G., Li C., Xie L., et al. (2007). A Novel Extrapallial Fluid Protein Controls the Morphology of Nacre Lamellae in the Pearl Oyster, Pinctada Fucata. J. Biol. Chem. 282 (32), 23253–23263. doi: 10.1074/jbc.M700001200
Marie B., Joubert C., Tayale A., Zanella-Cleon I., Belliard C., Piquemal D., et al. (2012). Different Secretory Repertoires Control the Biomineralization Processes of Prism and Nacre Deposition of the Pearl Oyster Shell. Proc. Natl. Acad. Sci. U. S. A. 109 (51), 20986–20991. doi: 10.1073/pnas.1210552109
Marie B., Luquet G., De Barros J. P. P., Guichard N., Morel S., Alcaraz G., et al. (2007). The Shell Matrix of the Freshwater Mussel Unio Pictorum (Paleoheterodonta, Unionoida). FEBS J. 274 (11), 2933–2945. doi: 10.1111/j.1742-4658.2007.05825.x
Marie B., Ramos-Silva P., Marin F., Marie A. (2013). Proteomics of CaCO3 Biomineral-Associated Proteins: How to Properly Address Their Analysis. Proteomics 13 (21), 3109–3116. doi: 10.1002/pmic.201300162
Marin F., Luquet G., Marie B., Medakovic D. (2008). Molluscan Shell Proteins: Primary Structure, Origin, and Evolution. Curr. Top. Dev. Biol. 80, 209–276. doi: 10.1016/S0070-2153(07)80006-8
Marin F., Roy N. L., Marie B. (2012). The Formation and Mineralization of Mollusk Shell. Front. Biosci. S4, 1099–1125. doi: 10.2741/321
McDougall C., Aguilera F., Degnan B. M. (2013). Rapid Evolution of Pearl Oyster Shell Matrix Proteins With Repetitive, Low-Complexity Domains. J. R. Soc. Interface. 10 (82). doi: 10.1098/rsif.2013.0041
McDougall C., Degnan B. M. (2018). The Evolution of Mollusc Shells. Wire. Dev. Biol. 7 (3). doi: 10.1002/wdev.313
Miyamoto H., Miyoshi F., Kohno J. (2005). The Carbonic Anhydrase Domain Protein Nacrein is Expressed in the Epithelial Cells of the Mantle and Acts as a Negative Regulator in Calcification in the Mollusc Pinctada Fucata. Zool. Sci. 22 (3), 311–315. doi: 10.2108/zsj.22.311
Mount A. S., Wheeler A. P., Paradkar R. P., Snider D. (2004). Hemocyte-Mediated Shell Mineralization in the Eastern Oyster. Science 304 (5668), 297–300. doi: 10.1126/science.1090506
Murgarella M., Puiu D., Novoa B., Figueras A., Posada D., Canchaya C. (2016). A First Insight Into the Genome of the Filter-Feeder Mussel Mytilus Galloprovincialis. PloS One 11 (3). doi: 10.1371/journal.pone.0151561
Nair P. S., Robinson W. E. (1998). Calcium Speciation and Exchange Between Blood and Extrapallial Fluid of the Quahog Mercenaria Mercenaria (L.). Biol. Bull. 195 (1), 43–51. doi: 10.2307/1542774
Okumura T., Suzuki M., Nagasawa H., Kogure T. (2010). Characteristics of Biogenic Calcite in the Prismatic Layer of a Pearl Oyster, Pinctada Fucata. Micron 41 (7), 821–826. doi: 10.1016/j.micron.2010.05.004
Pan C., Fang D., Xu G., Liang J., Zhang G., Wang H., et al. (2014). A Novel Acidic Matrix Protein, PfN44, Stabilizes Magnesium Calcite to Inhibit the Crystallization of Aragonite. J. Biol. Chem. 289 (5), 2776–2787. doi: 10.1074/jbc.M113.504027
Pietrzak J. E., Bates J. M., Scott R. M. (1976). Constituents of Unionid Extrapallial Fluid. Ii Ph and Metal Ion Composition. Hydrobiologia 50, 89–93. doi: 10.1007/BF00016846
Plazzi F., Passamonti M. (2010). Towards a Molecular Phylogeny of Mollusks: Bivalves' Early Evolution as Revealed by Mitochondrial Genes. Mol. Phylogenet. Evol. 57 (2), 641–657. doi: 10.1016/j.ympev.2010.08.032
Politi Y., Mahamid J., Goldberg H., Weiner S., Addadi L. (2007). Asprich Mollusk Shell Protein: In Vitro Experiments Aimed at Elucidating Function in CaCO3 Crystallization. Crystengcomm 9 (12), 1171–1177. doi: 10.1039/b709749b
Ponce C. B., Evans J. S. (2011). Polymorph Crystal Selection by N16, an Intrinsically Disordered Nacre Framework Protein. Cryst. Growth Des. 11 (10), 4690–4696. doi: 10.1021/cg201015w
Porter S. M. (2010). Calcite and Aragonite Seas and the De Novo Acquisition of Carbonate Skeletons. Geobiology 8 (4), 256–277. doi: 10.1111/j.1472-4669.2010.00246.x
Rivera-Perez C., Hernandez-Saavedra N. Y. (2021). Review: Post-Translational Modifications of Marine Shell Matrix Proteins. Comp. Biochem. Phys. B. 256. doi: 10.1016/j.cbpb.2021.110641
Robert B., Lorens M. L. B. (1980). The Impact of Solution Chemistry on Mytilus Edulis Calcite and Aragonite. Geochim. Cosmochim. Ac. 44 (9), 1265–1278. doi: 10.1016/0016-7037(80)90087-3
Rosenberg G. (2014). A New Critical Estimate of Named Species-Level Diversity of the Recent Mollusca. Am. Malacol. Bull. 32 (2), 308–322. doi: 10.4003/006.032.0204
Samata T., Ikeda D., Kajikawa A., Sato H., Nogawa C., Yamada D., et al. (2008). A Novel Phosphorylated Glycoprotein in the Shell Matrix of the Oyster Crassostrea Nippona. FEBS J. 275 (11), 2977–2989. doi: 10.1111/j.1742-4658.2008.06453.x
Shen X., Belcher A. M., Hansma P. K., Stucky G. D., Morse D. E. (1997). Molecular Cloning and Characterization of Lustrin A, a Matrix Protein From Shell and Pearl Nacre of Haliotis Rufescens. J. Biol. Chem. 272 (51), 32472–32481. doi: 10.1074/jbc.272.51.32472
Sillanpaa J. K., Sundh H., Sundell K. S. (1891). Calcium Transfer Across the Outer Mantle Epithelium in the Pacific Oyster, Crassostrea Gigas. P. Roy. Soc. B-Biol. Sci. 2018, 285. doi: 10.1098/rspb.2018.1676
Silverman H., Richard P. E., Goddard R. H., Dietz T. H. (1988). Intracellular Formation of Calcium Concretions by Phagocytic Cells in Freshwater Mussels. Can. J. Zool. 67 (1), 198–207. doi: 10.1139/z89-027
Silverman H., Steffens W. L., Dietz T. H. (1983). Calcium Concretions in the Gills of a Freshwater Mussel Serve as a Calcium Reservoir During Periods of Hypoxia. J. Exp. Zool. 227 (2), 177–189. doi: 10.1002/jez.1402270203
Sorrosa J. M., Satoh M., Shiraiwa Y. (2005). Low Temperature Stimulates Cell Enlargement and Intracellular Calcification of Coccolithophorids. Mar. Biotechnol. 7 (2), 128–133. doi: 10.1007/s10126-004-0478-1
Sudo S., Fujikawa T., Nagakura T., Ohkubo T., Sakaguchi K., Tanaka M., et al. (1997). Structures of Mollusc Shell Framework Proteins. Nature 387 (6633), 563–564. doi: 10.1038/42391
Suzuki M., Saruwatari K., Kogure T., Yamamoto Y., Nishimura T., Kato T., et al. (2009). An Acidic Matrix Protein, Pif, is a Key Macromolecule for Nacre Formation. Science 325 (5946), 1388–1390. doi: 10.1126/science.1173793
Sviben S., Gal A., Hood M. A., Bertinetti L., Politi Y., Bennet M., et al. (2016). A Vacuole-Like Compartment Concentrates a Disordered Calcium Phase in a Key Coccolithophorid Alga. Nat. Commun. 7. doi: 10.1038/ncomms11228
Takeuchi T., Kawashima T., Koyanagi R., Gyoja F., Tanaka M., Ikuta T., et al. (2012). Draft Genome of the Pearl Oyster Pinctada Fucata: A Platform for Understanding Bivalve Biology. DNA Res. 19 (2), 117–130. doi: 10.1093/dnares/dss005
Takeuchi T., Sarashina I., Iijima M., Endo K. (2008). In Vitro Regulation of CaCO3 Crystal Polymorphism by the Highly Acidic Molluscan Shell Protein Aspein. FEBS Lett. 582 (5), 591–596. doi: 10.1016/j.febslet.2008.01.026
Taylor J. D., Kennedy W. J. (1969). The Influence of the Periostracum on the Shell Structure of Bivalve Molluscs. Calcif. Tissue Res. 3 (3), 274–283. doi: 10.1007/BF02058669
Trinkler N., Bardeau J. F., Marin F., Labonne M., Jolivet A., Crassous P., et al. (2011). Mineral Phase in Shell Repair of Manila Clam Venerupis Philippinarum Affected by Brown Ring Disease. Dis. Aquat. Organ. 93 (2), 149–162. doi: 10.3354/dao02288
Varney R. M., Speiser D. I., McDougall C., Degnan B. M., Kocot K. M. (2021). The Iron-Responsive Genome of the Chiton Acanthopleura Granulata. Genome Biol. Evol. 13 (1). doi: 10.1093/gbe/evaa263
Vavouraki A. I., Putnis C. V., Putnis A., Koutsoukos P. G. (2008). An Atomic Force Microscopy Study of the Growth of Calcite in the Presence of Sodium Sulfate. Chem. Geol. 253 (3-4), 243–251. doi: 10.1016/j.chemgeo.2008.05.013
Wang X. T., Li L., Zhu Y. B., Du Y. S., Song X. R., Chen Y. X., et al. (2013). Oyster Shell Proteins Originate From Multiple Organs and Their Probable Transport Pathway to the Shell Formation Front. PloS One 8 (6). doi: 10.1371/journal.pone.0066522
Wang S., Zhang J. B., Jiao W. Q., Li J., Xun X. G., Sun Y., et al. (2017). Scallop Genome Provides Insights Into Evolution of Bilaterian Karyotype and Development. Nat. Ecol. Evol. 1 (5). doi: 10.1038/s41559-017-0120
Weiner S., Dove P. M. (2003). An Overview of Biomineralization Processes and the Problem of the Vital Effect. Rev. Mineral. Geochem. 54, 1–29. doi: 10.2113/0540001
Weiner S., Hood L. (1975). Soluble Protein of the Organic Matrix of Mollusk Shells: A Potential Template for Shell Formation. Science 190 (4218), 987–989. doi: 10.1126/science.1188379
Weiss I. M., Tuross N., Addadi L., Weiner S. (2002). Mollusc Larval Shell Formation: Amorphous Calcium Carbonate is a Precursor Phase for Aragonite. J. Exp. Zool. 293 (5), 478–491. doi: 10.1002/jez.90004
Xiang L., Kong W., Su J. T., Liang J., Zhang G. Y., Xie L. P., et al. (2014). Amorphous Calcium Carbonate Precipitation by Cellular Biomineralization in Mantle Cell Cultures of Pinctada Fucata. PloS One 9 (11). doi: 10.1371/journal.pone.0113150
Xie J. (2016). The Mechanism Study of Extrapallial Fluid Protein in the Shell Formation of Pinctada Fucata (Beijing: Tsinghua University).
Xie J., Liang J., Sun J., Gao J., Zhang S. R., Liu Y. J., et al. (2016). Influence of the Extrapallial Fluid of Pinctada Fucata on the Crystallization of Calcium Carbonate and Shell Biomineralization. Cryst. Growth Des. 16 (2), 672–680. doi: 10.1021/acs.cgd.5b01203
Yang J. L., Feng D. D., Liu J., Xu J. K., Chen K., Li Y. F., et al. (2021). Chromosome-Level Genome Assembly of the Hard-Shelled Mussel Mytilus Coruscus, a Widely Distributed Species From the Temperate Areas of East Asia. Gigascience 10 (4). doi: 10.1093/gigascience/giab024
Yang T., Jia Z., Chen H. S., Deng Z. F., Liu W. K., Chen L. N., et al. (2020). Mechanical Design of the Highly Porous Cuttlebone: A Bioceramic Hard Buoyancy Tank for Cuttlefish. P. Natl. Acad. Sci. U.S.A. 117 (38), 23450–23459. doi: 10.1073/pnas.2009531117
Yao N., Epstein A., Akey A. (2006). Crystal Growth via Spiral Motion in Abalone Shell Nacre. J. Mat. Res. 21 (8), 1939–1946. doi: 10.1557/Jmr.2006.0252
Yue X., Zhang S. J., Yu J. J., Liu B. Z. (2019). Identification of a Laccase Gene Involved in Shell Periostracal Tanning of the Clam Meretrix Petechialis. Aquat. Biol. 28, 55–65. doi: 10.3354/ab00709
Zhang G., Fang X., Guo X., Li L., Luo R., Xu F., et al. (2012). The Oyster Genome Reveals Stress Adaptation and Complexity of Shell Formation. Nature 490 (7418), 49–54. doi: 10.1038/nature11413
Zhang X., Xia Z. H., Liu X. J., Li J. L. (2021). The Novel Matrix Protein Hic7 of Hyriopsis Cumingii Participates in the Formation of the Shell and Pearl. Comp. Biochem. Phys. B. 256. doi: 10.1016/j.cbpb.2021.110640
Keywords: mollusk, mineralogy, organic matrix, shell matrix protein (SMPs), evolution
Citation: Huang J and Zhang R (2022) The Mineralization of Molluscan Shells: Some Unsolved Problems and Special Considerations. Front. Mar. Sci. 9:874534. doi: 10.3389/fmars.2022.874534
Received: 12 February 2022; Accepted: 18 May 2022;
Published: 27 June 2022.
Edited by:
Gary H. Dickinson, The College of New Jersey, United StatesReviewed by:
Frederic Marin, Délégation Centre-Est (CNRS), FranceCopyright © 2022 Huang and Zhang. This is an open-access article distributed under the terms of the Creative Commons Attribution License (CC BY). The use, distribution or reproduction in other forums is permitted, provided the original author(s) and the copyright owner(s) are credited and that the original publication in this journal is cited, in accordance with accepted academic practice. No use, distribution or reproduction is permitted which does not comply with these terms.
*Correspondence: Jingliang Huang, aHVhbmdqbGlhbmczQG1haWwuc3lzdS5lZHUuY24=; Rongqing Zhang, cnF6aGFuZ0BtYWlsLnRzaW5naHVhLmVkdS5jbg==
Disclaimer: All claims expressed in this article are solely those of the authors and do not necessarily represent those of their affiliated organizations, or those of the publisher, the editors and the reviewers. Any product that may be evaluated in this article or claim that may be made by its manufacturer is not guaranteed or endorsed by the publisher.
Research integrity at Frontiers
Learn more about the work of our research integrity team to safeguard the quality of each article we publish.