- 1Department of Marine Science and Convergence Technology, Hanyang University, Ansan, South Korea
- 2Division of Ocean Sciences, Korea Polar Research Institute, Incheon, South Korea
- 3Marine Environmental Research Center, Korea Institute of Ocean Science & Technology, Busan, South Korea
We investigated changes in heterotrophic bacterial metabolic activities and associated carbon cycles in response to a change in dominant phytoplankton communities during two contrasting environmental conditions in austral summer in the Amundsen Sea polynya (ASP), Antarctica: the closed polynya condition in 2014 (ANA04) and the open polynya condition in 2016 (ANA06). In ANA04, Phaeocystis antarctica predominated phytoplankton biomass, comprising 78% of total phytoplankton carbon biomass, whereas diatoms and Dictyocha speculum accounted for 45% and 48% of total phytoplankton carbon biomass, respectively, in ANA06. Bacterial production (BP) showed a significant positive correlation with only chlorophyll-a (Chl-a, rho = 0.66, p < 0.001) in P. antarctica-dominated ANA04, whereas there were significant positive relationships of BP with various organic carbon pools, such as chromophoric dissolved organic matter (CDOM, rho = 0.84, p < 0.001), Chl-a (rho = 0.59, p < 0.001), and dissolved organic carbon (DOC, rho = 0.51, p = 0.001), in ANA06 when diatoms and D. speculum co-dominated. These results indicate that BP depended more on DOC directly released from P. antarctica in ANA04, but was supported by DOC derived from various food web processes in the diatom-dominated system in ANA06. The BP to primary production (BP : PP) ratio was three-fold higher in P. antarctica-dominated ANA04 (BP: PP = 0.09), than in diatom- and D. speculum-co-dominated ANA06 (BP : PP = 0.03). These results suggested that the microbial loop is more significant in Phaeocystis-dominated conditions than in diatom-dominated conditions. In addition, the decreases in BP : PP ratio and bacterial respiration with increasing diatom proportion in the surface mixed layer indicated that the change from P. antarctica to diatom predominance enhanced biological carbon pump function by increasing particulate organic carbon export efficiency. Consequently, our results suggest that bacterial metabolic response to shifts in phytoplankton communities could ultimately affect larger-scale ecological and biogeochemical processes in the water column of the ASP.
Introduction
Heterotrophic prokaryotes, hereafter bacteria as the traditional ecological term, are a major ecological and biogeochemical component of microbial food web processes and biogeochemical carbon and nutrient cycles in the ocean (Kirchman, 2008; Herndl and Reinthaler, 2013; Cavan and Boyd, 2018). Heterotrophic bacteria are responsible for the solubilization of sinking and suspended particles (Arístegui et al., 2009; Giering et al., 2014), and exclusively consume dissolved organic carbon (DOC) from various sources (Nagata, 2008; Carlson and Hansell, 2015). The DOC assimilated by these bacteria is either transferred to a higher trophic level via the microbial loop (Azam et al., 1983; Sherr et al., 1988) or respired to CO2 during microbial metabolic processes in the water column (Ducklow et al., 1986; Williams and del Giorgio, 2005), which ultimately determines the significance of the microbial loop and the efficiency of carbon sequestration via the biological pump (Legendre and Le Fèvre, 1995; Karl, 1999). Therefore, it is essential to quantify heterotrophic bacterial biomass and metabolic activities and to identify controlling factors in order to construct a biogeochemical and ecological model for carbon cycles and microbial food web processes at the local or global scale (Ducklow, 2000; del Giorgio and Williams, 2005).
The Southern Ocean, which accounts for approximately 20% of atmospheric CO2 uptake of global ocean, plays a significant role as a major carbon sink (Arrigo et al., 2008; Takahashi et al., 2009). During austral summer, the Antarctic coastal ocean is characterized by the development of polynyas, a seasonally recurring area of open water surrounded by sea ice (Yager et al., 2012). Polynyas exhibit high biological production because of the enhanced light and iron availability resulting from ice melting (Arrigo and van Dijken, 2003). Since they are the first areas to be exposed to the atmosphere with the reduction of sea ice coverage (Arrigo and van Dijken, 2003), polynyas act as windows for understanding ecosystem changes and anticipating ocean’s carbon sink function associated with climate change in polar seas (Smith and Barber, 2007). Among the 37 polynyas formed along the Antarctic coast, the Amundsen Sea polynya (ASP) is considered the most biologically productive region in the Southern Ocean, representing high net community production (NCP, 85 ± 56 mmol C m-2 d-1, Hahm et al., 2014) and primary production (PP, 2.1 ± 0.7 g C m-2 d-1, Arrigo and van Dijken, 2003; 2.2 ± 1.4 g C m-2 d-1, Lee et al., 2012; 234 ± 51 mmol C m-2 d-1, Yager et al., 2016).
Phytoplankton blooms in the ASP are dominated by haptophyte Phaeocystis antarctica and various diatoms, and their relative proportions might vary by timing and location. For example, in Ross Sea polynya, P. antarctica blooms occur during the early austral summer from December to early January in the center of the polynya, while diatom blooms are observed after mid-January near the sea ice edge (Arrigo and van Dijken, 2003; Smith et al., 2010). In the Southern Ocean, where allochthonous input of DOC is negligible, heterotrophic bacterial metabolic activities rely primarily on DOC produced by phytoplankton (Ducklow et al., 2012; Hyun et al., 2016; William et al., 2016). The quantity and quality of phytoplankton-derived dissolved organic matter (DOM) varies with phytoplankton composition (Biersmith and Benner, 1998; Aluwihare and Repeta, 1999; Solomon et al., 2003; Alderkamp et al., 2007; Romera-Castillo et al., 2011; Kinsey et al., 2018; Mühlenbruch et al., 2018). For example, organic matter produced by picophytoplankton with a slow sinking rate is rapidly recycled in the surface water column, while large phytoplankton export more bio-decomposable organic matter to deeper layers (Herndl and Reinthaler, 2013; McDonnell et al., 2015). Therefore, shifts in the dominant phytoplankton community can have a significant impact on the microbially mediated biogeochemical carbon cycle and biological carbon pump function.
West Antarctica, including the Amundsen Sea and the Bellingshausen Sea, has undergone rapid climatic warming in recent decades (Montes-Hugo et al., 2009; Turner et al., 2014; Brown et al., 2019). The continuous intrusion of warm Circumpolar Deep Water (CDW) into the narrow Antarctic continental shelves has led to massive glacier melting (Thoma et al., 2008; Jacobs et al., 2012). The average mass loss rate of the Antarctic ice sheet was 109 ± 56 Gt yr-1 during the past 25 years (1992 – 2017), with mass loss of the West Antarctic ice sheet accounting for approximately 86% of the total mass loss (The IMBIE Team, 2018). Particularly in the Amundsen Sea, thinning of the ice sheet has accelerated, with losses of 70 – 80 m in thickness from 1995 – 2008 (Jenkins et al., 2010; Jenkins et al., 2018), and ice discharge from the Amundsen Sea Embayment has increased by 73% since 1973 (Mouginot et al., 2014). In this context, the ASP is considered a key coastal environment to understand the biogeochemical responses of the Southern Ocean to ocean warming (Yager et al., 2012; Meredith et al., 2016). Thus far, differences in NCP and carbon sequestration according to phytoplankton communities (DeJong et al., 2017) and close associations between bacterial parameters (i.e., biomass and production) and Phaeocystis blooms have been well established in the Southern Ocean (Cota et al., 1990; Lochte et al., 1997; Ducklow et al., 1999; Morán et al., 2001; Hyun et al., 2016; Williams et al., 2016). Despite these clear relationships between heterotrophic bacteria and phytoplankton, however, changes in bacterial metabolic activities in response to shifts in phytoplankton communities have not yet been investigated in the ASP.
During the research expedition in January 2014 and 2016, we encountered uniquely contrasting conditions in the ASP; the ASP was closed to the open sea by thick marginal sea ice in 2014, whereas it was opened to the open sea in 2016 (Figure 1). Interestingly, phytoplankton carbon biomass was dominated by P. antarctica in 2014, while it was co-dominated by diatoms and chrysophyte Dictyocha speculum in 2016 (Lee et al., 2016b; Lee et al., 2022). Given the rapid climate warming in West Antarctica (Turner et al., 2014; Brown et al., 2019), we speculated that this change in major phytoplankton communities might take place occasionally in the future as warming temperature-induces a continuous decrease in sea ice in the ASP (Petrou et al., 2016; Deppeler and Davidson, 2017; Tréquer et al., 2017). Considering this, we further hypothesized that the associated change in phytoplankton communities would ultimately regulate bacterial metabolic activities, thereby affecting ecological and biogeochemical processes in the water column of the ASP. Despite the importance of heterotrophic bacteria in the biogeochemical carbon cycle, few studies have investigated the effects of warming-induced changes on bacterial production or respiration in polar regions (Kirchman et al., 2009b; Li et al., 2009; Cavan and Boyd, 2018; Vaqué et al., 2019). The objectives of this study are: (1) to elucidate changes in heterotrophic bacterial parameters (i.e., abundance, production, and respiration) in response to change in major phytoplankton communities; and (2) to understand how heterotrophic metabolic response affects the importance of the microbial loop and efficiency of biological carbon pump in the ASP, the forefront where rapid climate change-induced variations in ocean-atmosphere interaction occur.
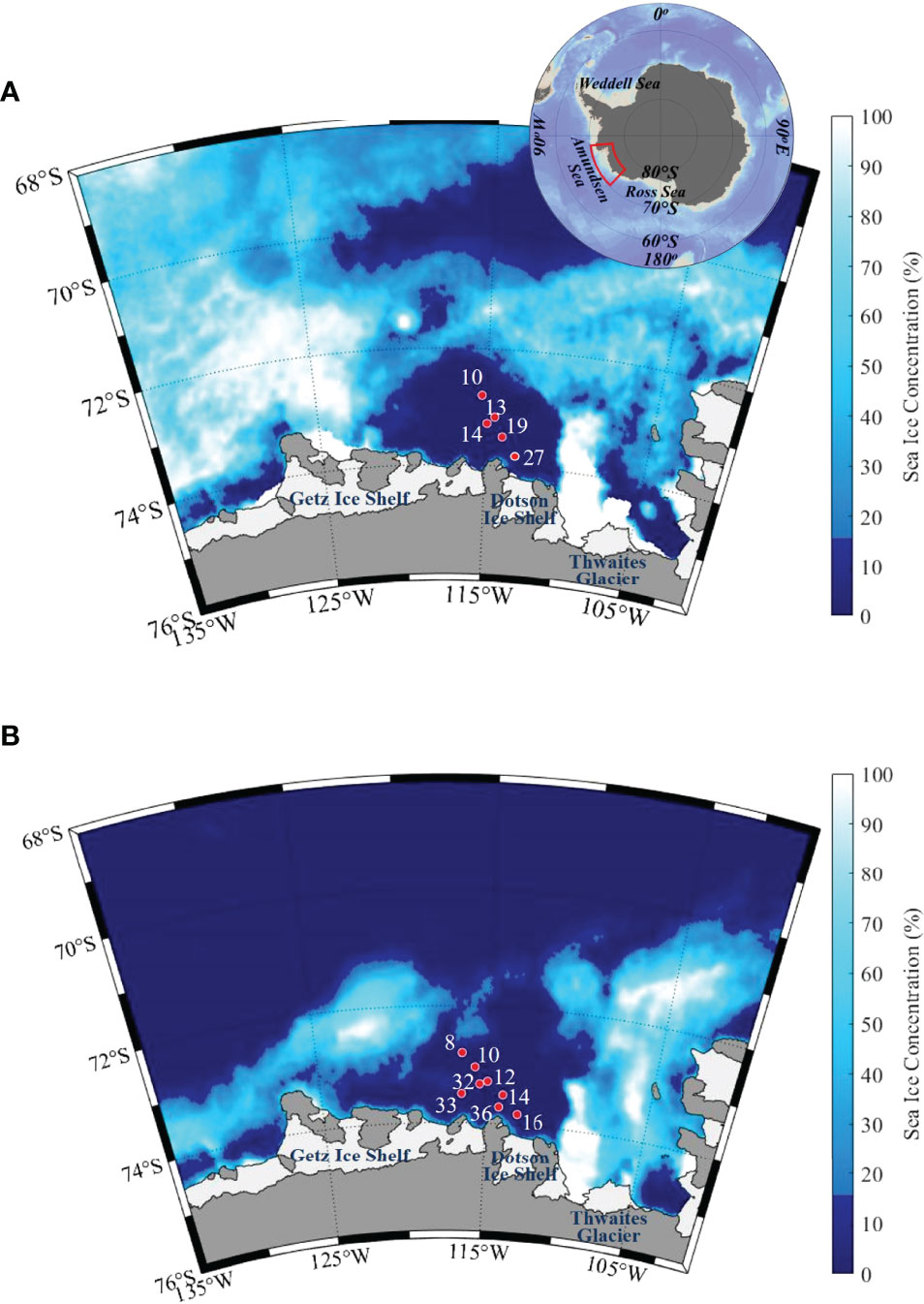
Figure 1 Maps showing sea ice coverage and sampling sites in the ASP during the (A) ANA04 and (B) ANA06 cruises.
Materials and Methods
Study Area and Sampling
The coastal polynya in the Amundsen Sea generally forms in November and closes again by March (Arrigo et al., 2012). The peak phytoplankton bloom in the ASP generally occurs in January and then declines from February to March (Arrigo and van Dijken, 2003; Yager et al., 2016). This study was conducted during the phytoplankton bloom period on board the IBRV Araon from January 5 to 10, 2014 (ANA04) and from January 17 to 24, 2016 (ANA06). During the study period, the ASP was closed to the open sea in ANA04 but not in ANA06, resulting in an expanded ice-free area in ANA06 (Figure 1). Water samples for physico-chemical and microbiological analysis were collected at five and eight stations during ANA04 and ANA06, respectively (Table 1 and Figure 1).
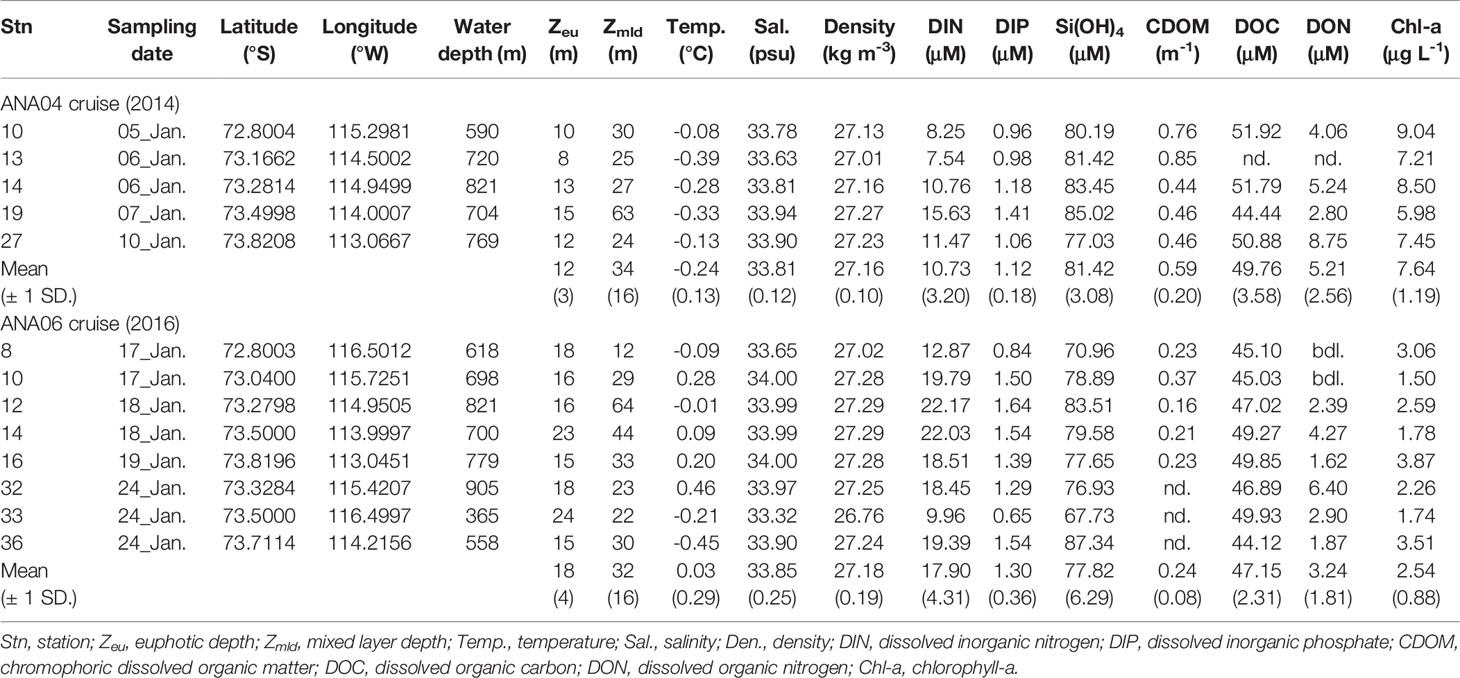
Table 1 Oceanographic parameters in surface water from the Amundsen Sea polynya during ANA04 and ANA06.
Physico-Chemical Parameters
Water temperature, salinity, density, and photosynthetically active radiation (PAR) were measured using a conductivity-temperature-depth (CTD) probe (SBE 911 Plus, Seabird Electronics, USA). Euphotic depth was defined as the depth of 1% penetration of the surface on PAR. Mixed layer depth (MLD) was defined as the depth at which the density was 0.05 kg m-3 higher than the 10-m value (Venables and Moore, 2010). Water samples were collected from 7 – 8 depths in the upper 100 m using Niskin bottles attached to a CTD rosette sampler. The sample bottles were first washed with 10% HCl and rinsed three times with Milli-Q water.
Concentrations of inorganic nutrients were measured onboard using a 4-channel continuous Auto-Analyzer (QuAAtro, Seal Analytical). The precisions for the NOX, , , and Si(OH)4 measurements were ± 0.14, ± 0.02, ± 0.18, and ± 0.29 μmol L-1, respectively. Samples of chromophoric dissolved organic matter (CDOM) were filtered through a syringe filter (Adventec, 0.2-μm pore size) that had been pre-washed ultra-pure Milli-Q water, and then stored in 150-mL amber bottles in the dark at 2 – 4°C in a refrigerator (Stedmon and Markager, 2001). The absorbance of the samples was measured on board using a double-beam Shimadzu UV-1800 spectrophotometer with a 10-cm quartz cell in the spectral range of 350 – 900 nm. A quartz cell filled with pre-filtered Milli-Q water was used as the reference for all samples. The absorbance at 350 nm relative to distilled water was measured, and then the absorption coefficient at 350 nm (CDOM m-1) was determined using the following equation (Kowalczuk et al., 2005):
where a(λ) is the absorption coefficient (m-1), A(λ) is the absorption coefficient at the reference wavelength λ (m-1), 2.303 is the conversion factor from log10 to log units, and l is the length of the optical path (m).
Samples for DOC and dissolved organic nitrogen (DON) analysis were filtered through pre-combusted (at 550°C for 6 hours) GF/F filters (0.7-μm pore size), followed by hermetic sealing in pre-combusted (at 550°C for 6 hours) 20-mL glass ampoules and were preserved at -24°C until analysis in the laboratory. The concentrations of DOC and total dissolved nitrogen were determined via high temperature combustion on a Shimadzu TOC-L analyzer (Shimadzu Co.) fitted with a chemiluminescence (CLS) detector that was incorporated into the total nitrogen microanalyzer. For the accuracy of the DOC concentration, Milli-Q water blanks and consensus reference material (CRM, 42–45 M, University of Miami) were measured every sixth analysis. The concentration of DON was obtained as the difference between total dissolved nitrogen and dissolved inorganic nitrogen (i.e., , , and ) concentrations.
Phytoplankton Parameters
Chl-a was extracted with 90% acetone for 24 h in the dark after filtration through a GF/F filter (0.7-μm pore size) (Parsons et al., 1984). The Chl-a concentration was determined using a fluorometer (Turner Designs Trilogy).
To determine phytoplankton abundance, water samples were preserved with glutaraldehyde (final concentration, 1%) and stored at 4°C before filtration and staining. The water samples (50 - 150 mL) were filtered through Nuclepore filters (0.8-μm pore size, black, 25-mm diameter) until 5 mL remained in the filtration tower. Then, concentrated DAPI (4’,6-diamidino-2-phenyl-indole) solution (50 μg mL-1 final concentration) was added, and briefly incubated (5 s) before filtration (Taylor et al., 2011). The filters were mounted on slide glass with immersion oil. Phytoplankton cells were counted using an epifluorescence microscope (Olympus BX 51). Carbon (C) biomass was estimated from the cell biovolume using modified Eppley equations (Smayda, 1978; Hillebrand et al., 1999) as follows: for diatoms, log10 C (pg) = 0.76 log10 [cell volume (μm3)] − 0.352; for other phytoplankton, log10 C (pg) = 0.94 log10 [cell volume (μm3)] − 0.60. The conversion factor used to transform cell numbers of solitary P. antarctica into carbon biomass was 3.33 pg C cell−1 (Mathot et al., 2000).
To elucidate the major phytoplankton groups (Dunbar et al., 2003), disappearance ratios of NOX (ΔN), (ΔP) and Si(OH)4 (ΔSi) were calculated as the difference between the concentration within the upper 100 m and the concentration at 500-m depth as a proxy for the pre-bloom concentration within the upper 100 m (Lee et al., 2016a). A ΔN:ΔP ratio of 19.2 was used as a proxy for the P. antarctica bloom, whereas ΔN:ΔP of 9.69 was used for the diatom bloom (Arrigo et al., 1999). Similarly, ΔSi:ΔN of < 0.9 was used for the P. antarctica bloom, whereas ΔSi:ΔN of > 2.15 was used for the diatom bloom (Dunbar et al., 2003).
Microbiological Parameters
Samples for bacterial abundance (BA) were preserved with glutaraldehyde at a final concentration of 1% and stored at -20 °C (Hyun and Yang, 2003). Samples were stained with DAPI solution (Porter and Feig, 1980), filtered through a Nuclepore filter (0.2-µm pore size, black), and mounted on slide glass with immersion oil (Cargille type A). Bacterial cells were counted using an epifluorescence microscope (Eclipse 80i, Nikon, Tokyo, Japan) equipped with a mercury lamp (HB-10101 AF), an ultraviolet (UV) excitation filter, and a BA 420 barrier filter.
Heterotrophic bacterial production (BP) was determined from bacterial incorporation of 3H-thymidine (3H-TdR) (Fuhrman and Azam, 1980; Fuhrman and Azam, 1982). Duplicate 20-mL water samples were incubated in disposable plastic tubes for 30 min at in situ water temperatures under dark conditions with 3H-TdR (MT-6034; final concentration, 10 nM, Moravek Biochemicals, Inc., Brea, CA, USA). Incubation was stopped by adding cold trichloroacetic acid (TCA, final concentration 5%), and cold TCA-insoluble materials were precipitated for 15 min. Samples were collected by vacuum filtration on 0.2-μm cellulose nitrate membrane filters and rinsed three times with 80% cold ethanol. The filters were placed in scintillation vials. In the lab, after scintillation cocktail (Lumagel Safe; Lumac-LSC, Groningen, The Netherlands) was added to the vials, the activity of the cold TCA-insoluble macromolecules was determined using a liquid scintillation counter (Tri-Carb 2910TR; PerkinElmer, Waltham, MA, USA). Samples treated with 5% TCA solution before the addition of 3H-TdR were used as killed controls. A conversion factor of 8.6×1017 cells mol-1 (Ducklow et al., 1999) was used to convert 3H-TdR measurements into bacterial cell production estimates. Bacterial carbon biomass was calculated using a conversion factor of 10 fg C cell-1 (Fukuda et al., 1998).
Total microbial community respiration rates were calculated from the consumption of dissolved oxygen (DO) over time in ca. 300-mL water samples maintained in BOD bottles (Pomeroy et al., 1994). DO concentration was measured using the Winkler titration method for the ANA04 cruise, while both Winkler titration and the O2-optode method were applied for samples collected on the ANA06 cruise. In the Winkler titration method, after water was subsampled into six BOD bottles at each depth, duplicate water samples were immediately fixed with Winkler reagent to estimate initial DO concentration. The remaining bottles were incubated at in situ water temperatures under dark conditions. After 12 and 24 h, duplicate water samples were fixed with Winkler reagent, and DO concentrations were determined at a wavelength of 466 nm on a spectrophotometer (Shimadzu UV-1800) (Labasque et al., 2004). In the O2-optode method (Wikner et al., 2013), DO concentrations were measured using a fiber-optical oxygen meter (FireStingO2, PyroScience GmbH, Germany). The BOD bottles for O2-optode measurements were incubated for 8 – 14 h in the dark at in situ temperature using a water bath. Prior to measurement, two-point calibration (0 and 100%) was performed. The 0% oxygen saturation was calibrated by adding sodium dithionite (Na2S2O4) to water and the 100% oxygen saturation was calibrated using air-saturated water. The respiration rates measured using the O2-optode method showed no significant difference from those measured by Winkler titration (p = 0.209, Supplementary Table 1). BR was estimated using a bacteria respiration proportion of 0.45 from total microbial community respiration (Robinson, 2008). Bacterial community growth rates were calculated by dividing bacterial production by bacterial carbon biomass (Kirchman et al., 2009a). Bacterial carbon demand (BCD) and bacterial growth efficiency (BGE) were calculated from the following equation: BCD = BP + BR; BGE = BP/BCD (del Giorgio and Cole, 1998; del Giorgio and Cole, 2000).
Statistics
Statistical analyses were conducted in R (R version 4.0.2, R core Team, using R Studio v.1.3.1073). Data normality was tested using Shapiro-Wilk test. Mann-Whitney U-test was used to compare mean values for chemical and biological parameters between the two periods (ANA04 and ANA06). Spearman’s rho correlation was conducted to examine the relationship between bacterial production and environmental parameters. p < 0.05 was considered significant.
Results
Physical Parameters
Surface water temperature, salinity, and density exhibited different ranges between the ANA04 and ANA06 periods (Table 1). In ANA04, water temperature, salinity, and density ranged from -0.39 to -0.08°C (average, -0.24 ± 0.13°C), from 33.63 to 33.94 psu (average, 33.81 ± 0.12 psu), and from 27.01 to 27.27 kg m-3 (average, 27.16 ± 0.10 kg m-3), respectively. Average water temperature (0.03 ± 0.29 °C) in ANA06 was slightly higher than that of ANA04, but average salinity (33.85 ± 0.25 psu) and density (27.18 ± 0.19 kg m-3) did not show significant difference between ANA04 and ANA06 (p > 0.05). Average mixed layer depth was not significantly different between ANA04 and ANA06 (p = 0.69, Table 1). Euphotic depth in ANA06 (18 m on average) was slightly deeper than that observed in ANA04 (12 m on average) (p = 0.005, Table 1), but the median value of surface PAR during the sampling period was six times lower in ANA06 (52 μmol photons m-2 s-1) than in ANA04 (290 μmol photons m-2 s-1, Supplementary Figure 1).
Chemical Parameters
Chemical parameters within the upper 100 m varied in ANA04 and ANA06 (Figures 2, 3). DIN and DIP concentrations increased with depth (Figures 2A, B) and were lower in range in ANA04 (average, 18.15 ± 8.15 μM and 1.48 ± 0.40 μM, respectively) than in ANA06 (average, 24.12 ± 4.95 μM and 1.72 ± 0.40 μM, respectively) (Figures 3A, B). Concentrations of CDOM, DOC, and DON were greater in ANA04 than in ANA06 (Figures 2C, D, E). CDOM concentrations measured within the upper 50 m were higher in ANA04 (average, 0.53 ± 0.19 m-1) than in ANA06 (average, 0.23 ± 0.12 m-1) (p < 0.001, Figure 3C). DOC concentrations were generally homogenously distributed and DOC measured during ANA04 (average, 50.24 ± 8.09 μM) was higher than that of ANA06 (average, 45.26 ± 3.00 μM) (p = 0.021, Figure 3D). DON concentrations varied, but were slightly higher in ANA04 (average, 5.65 ± 2.56 μM) than in ANA06 (average, 2.80 ± 1.90 μM) (p = 0.003, Figure 3E). The range of DOC and DON concentrations for both periods were slightly lower than those reported during the early-mid phytoplankton bloom in the Amundsen Sea (Yager et al., 2016).
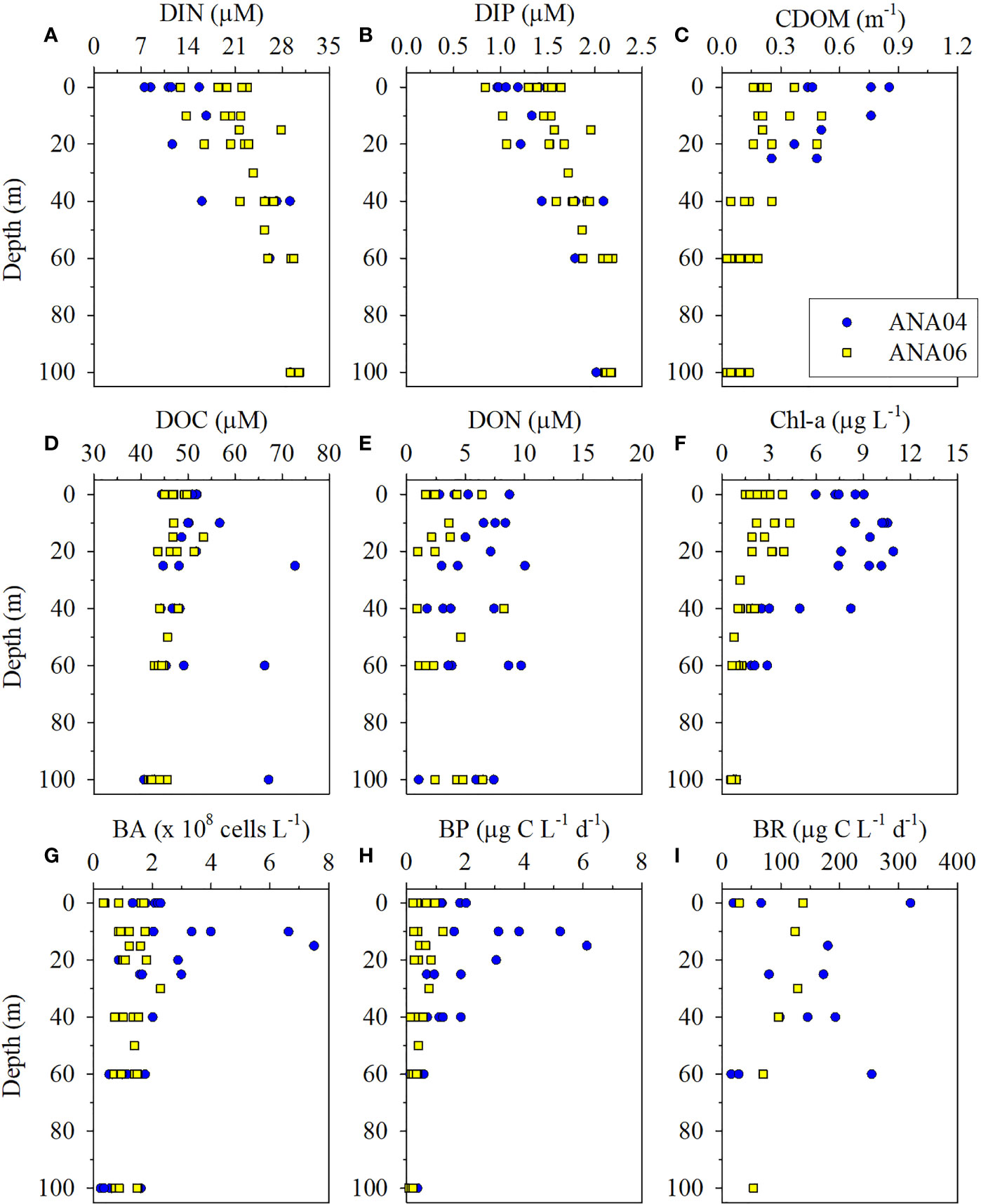
Figure 2 Depth profile of chemical and biological parameters during ANA04 and ANA06. Dissolved inorganic nitrogen (DIN) (A), dissolved inorganic phosphate (DIP) (B), chromophoric dissolved organic matter (CDOM) (C), dissolved organic carbon (DOC) (D), dissolved organic nitrogen (DON) (E), chlorophyll-a (Chl-a) (F), bacterial abundance (BA) (G), bacterial production (BP) (H), and bacterial respiration (BR) (I).
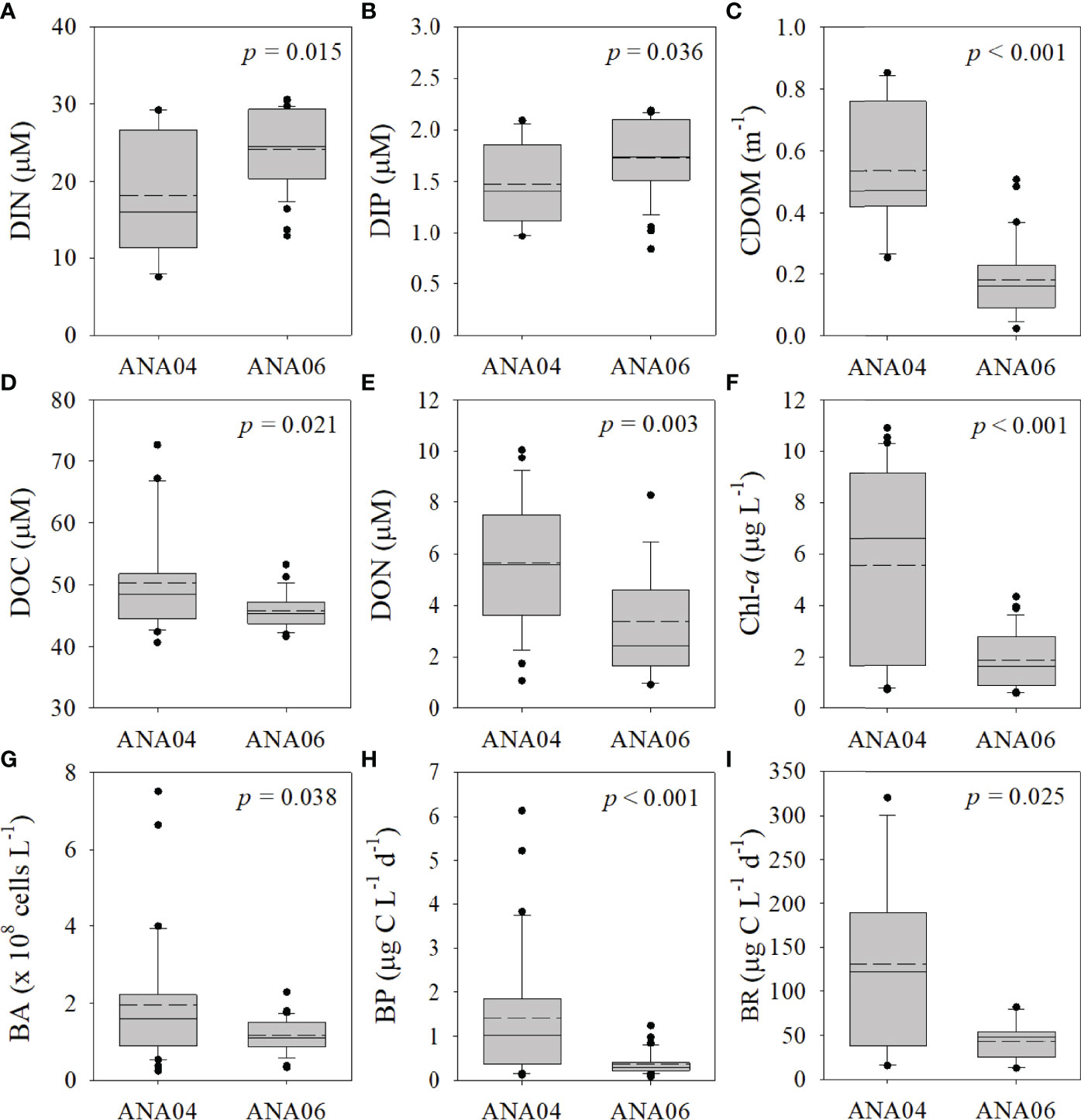
Figure 3 Box plot of chemical and biological parameters within the upper 100 m of the water column in ANA04 and ANA06. Dissolved inorganic nitrogen (DIN) (A), dissolved inorganic phosphate (DIP) (B), chromophoric dissolved organic matter (CDOM) (C), dissolved organic carbon (DOC) (D), dissolved organic nitrogen (DON) (E), chlorophyll a (Chl-a) (F), bacterial abundance (BA) (G), production (BP) (H) and respiration (BR) (I). The solid and dashed lines are the median and average, respectively. The top and bottom of the box are the 25th and 75th percentiles, and the ends of the whiskers represent the 5th and 95th percentiles.
Phytoplankton Community Structure
Concentrations of Chl-a were distributed differently in ANA04 and ANA06 (Figure 2F). Chl-a concentrations measured during ANA04 (average, 5.55 ± 3.78 μg L-1) with lower DIN and DIP concentrations (Figures 2A, B) were three times higher than that of ANA06 (average, 1.90 ± 1.24 μg L-1) (Figure 3F).
Major phytoplankton groups in the ASP consist of P. antarctica, diatoms, and D. speculum (Figure 4). P. antarctica dominated carbon biomass in ANA04, but diatoms and D. speculum co-dominated in ANA06 (Figure 4A). The relative contribution of P. antarctica was higher in ANA04 (78 ± 14%), but it decreased to 3 ± 2% in ANA06 (Figure 4B). In contrast, the relative contribution of diatoms was lower in ANA04 (11 ± 12%), but increased to 45 ± 25% in ANA06 (Figure 4B). In ANA06, the average carbon biomass of diatoms (143 ± 94 μg C L-1) was 12-fold higher than that of P. antarctica (12 ± 7 μg C L-1) (Figure 4A) because of their larger size and higher carbon content (Lee et al., 2016b). In ANA06, D. speculum also exhibited high relative carbon biomass, comprising 48 ± 25% of the total carbon biomass (Figure 4B).
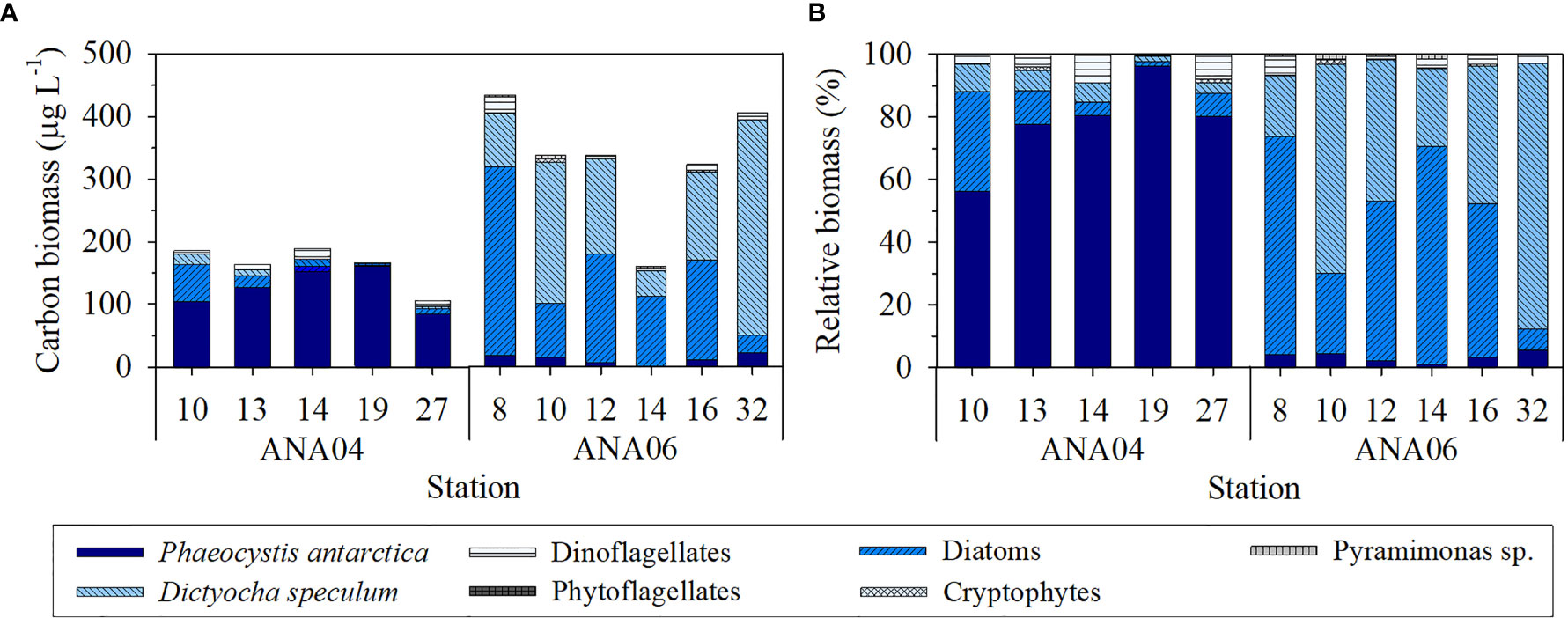
Figure 4 Carbon biomass (A) and relative biomass (B) of the major phytoplankton groups in ANA04 and ANA06 (recalculated from Lee et al., 2016b; Lee et al., 2022).
Bacterial Abundance, Production, and Respiration
BA ranged from 0.25 to 7.50 × 108 cells L-1 during ANA04 and ANA06 (Figure 2G). In ANA04, maximum BA was observed at 15-m depth and decreased rapidly with increasing depth, while BA in ANA06 was distributed relatively consistently with depth. In the upper 100 m, BA in ANA04 (1.95 ± 1.66 x 108 cells L-1) was slightly higher than that in ANA06 (1.49 ± 0.96 x 108 cells L-1) (p = 0.038; Figure 3G). BA was positively correlated with BP (rho = 0.64, p < 0.001), CDOM (rho = 0.51, p = 0.001), and Chl-a (rho = 0.40, p < 0.001), but negatively correlated with NOx (rho = - 0.30, p = 0.014) (Supplementary Figure 2). Depth-integrated bacterial carbon biomass (BCB) from the surface down to mixed layer depth in ANA04 (99 ± 46 mg C m-2) was two times higher than estimated in ANA06 (45 ± 21 mg C m-2) (Table 2).
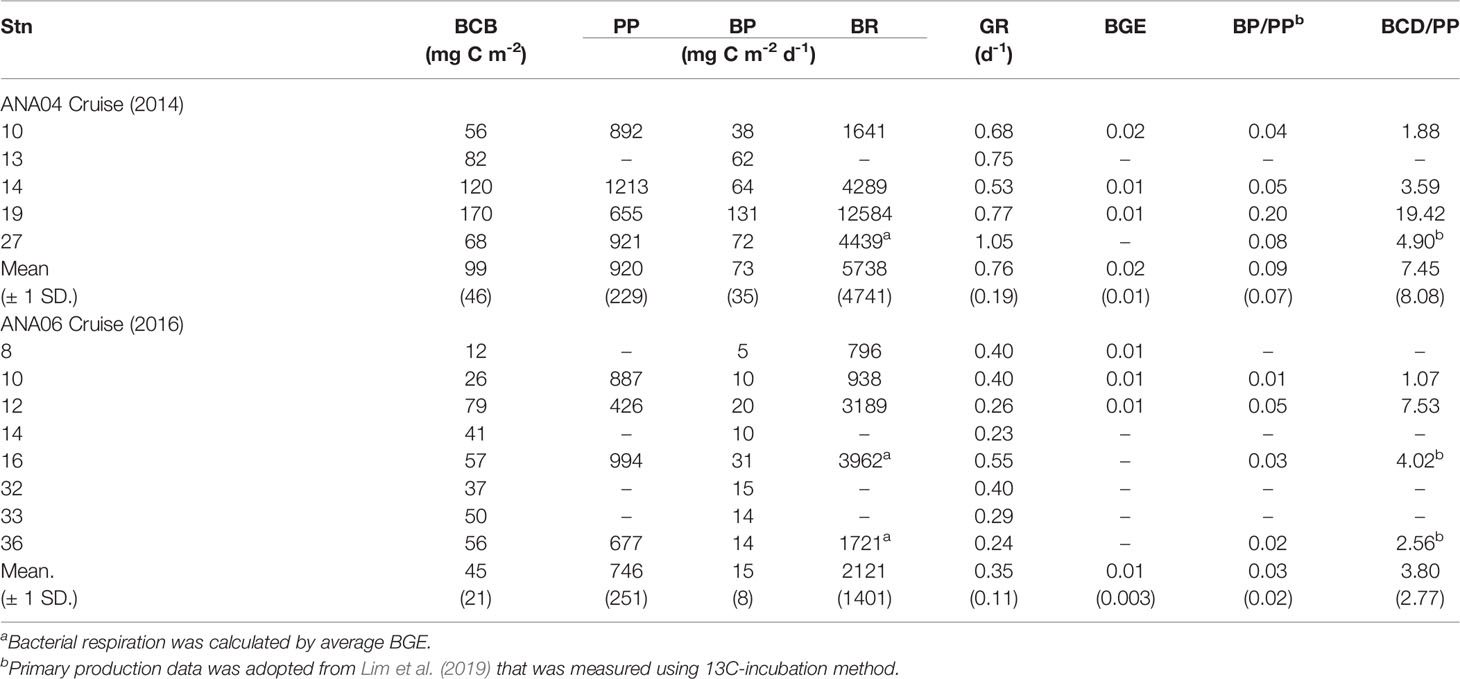
Table 2 Depth-integrated (down to mixed layer depth) bacterial carbon biomass (BCB), bacterial production (BP), primary production (PP), bacterial production (BP), bacterial respiration (BR), bacterial growth rates (GR), the ratio of bacterial production (BP) to primary production (PP) and the ratio of bacterial carbon demand (BCD) to PP.
BP ranged from 0.08 to 6.13 µg C L-1 d-1 during ANA04 and ANA06 (Figure 2H). Like BA, maximum BP in ANA04 was observed at 15-m depth and decreased rapidly with depth, while BP declined only slightly with depth in ANA06. In the upper 100 m of the ASP, BP was four times higher in ANA04 (1.41 ± 1.50 µg C L-1 d-1) than in ANA06 (0.39 ± 0.25 µg C L-1 d-1) (p <0.001; Figure 3H). BP was positively correlated with CDOM (rho = 0.90, p < 0.001), Chl-a (rho = 0.74, p < 0.001), DOC (rho = 0.50, p < 0.001), DON (rho = 0.32, p = 0.013), and temperature (rho = 0.38, p = 0.001), but negatively correlated with NOx (rho = -0.62, p < 0.001), (rho = -0.63, p < 0.001), salinity (rho = -0.67, p < 0.001), and Si(OH)4 (rho = -0.39, p = 0.001) (Table 3 and Supplementary Figure 2). When integrated from surface to mixed layer depth, BP was five times higher in ANA04 (73 ± 35 mg C m-2 d-1) than in ANA06 (15 ± 8 mg C m-2 d-1) (Table 2). The bacterial growth rate in ANA04 (0.76 ± 0.19 d-1) was two-fold higher than that in ANA06 (0.35 ± 0.11 d-1) (Table 2).
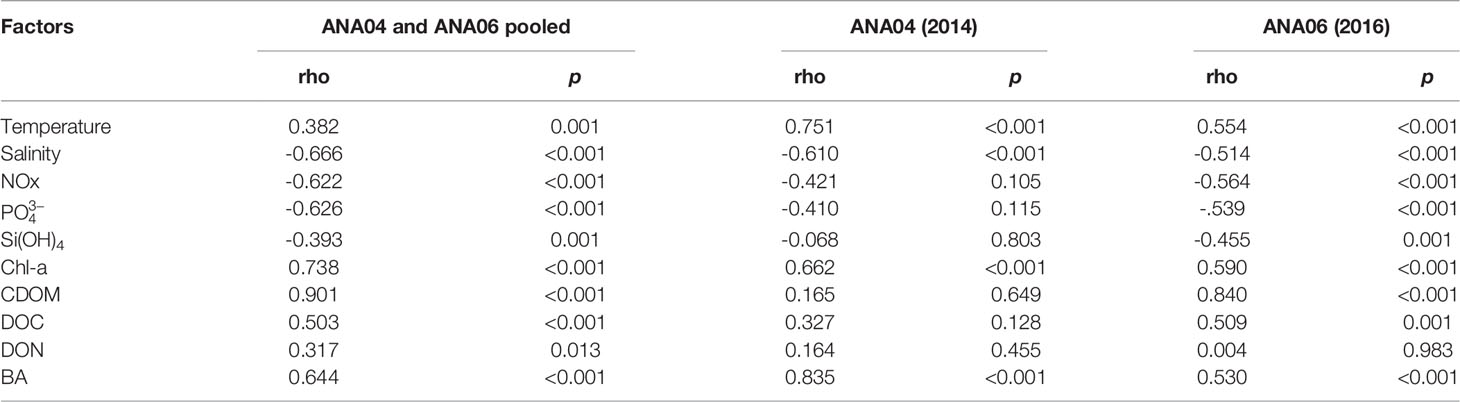
Table 3 Spearman’s correlation between bacterial production and environmental factors in ANA04 and ANA06 (upper 100 m).
Unlike BP, BR did not show notable vertical variation (Figure 2I). The average BR in ANA04 (131 ± 96 µg C L-1 d-1) was three-fold higher than that observed in ANA06 (43 ± 20 µg C L-1 d-1) (p = 0.025, Figure 3I). In this study, BR ranged from 12.85 to 320 µg C L-1 d-1 in the upper 100 m of the ASP, which was much higher than previously reported (10 – 53 µg C L-1 d-1; William et al., 2016).
Discussion
Change in Major Phytoplankton Group
The dominant phytoplankton group changed from P. antarctica in ANA04 to a mixed community of diatoms and D. speculum in ANA06 (Figure 4). In ANA06, large pennate diatoms (Proboscia alata, Plagiotropus gaussi, and Corethron pennatum) and centric diatoms (Chaetoceros spp. and Thalassiosira spp.) were the major diatom species, rather than small diatoms (Fragilariopsis spp. and Pseudonitzschia spp.) (Lee et al., 2022). The change in dominant phytoplankton biomass from P. antarctica in ANA04 to diatoms and D. speculum in ANA06 was also confirmed using the disappearance ratio of inorganic nutrients (ΔN:ΔP and ΔSi:ΔN) between the two periods. The ΔN:ΔP ratio was 19.5 in ANA04 (Figure 5A) similar with the ratio in previous research on P. antarctica-dominant communities (19.2; Arrigo et al., 1999). However, the ΔN:ΔP ratio in ANA06 decreased to 13.4 (Figure 5A), which was between the value for a P. antarctica-dominated system and diatom bloom conditions (9.69; Arrigo et al., 1999). In addition, the ΔSi:ΔN ratio changed from 0.25 in ANA04 to 0.96 in ANA06 (Figure 5B). The ΔSi:ΔN ratio of < 0.9 represents a P. antarctica-dominated system, whereas the ratio of 0.9 – 2.15 in the water column suggests a mixed phytoplankton community (Dunbar et al., 2003; Fragoso and Smith, 2012). Both microscopic quantification (Lee et al., 2016b; Lee et al., 2022) and chemical proxies using ΔN:ΔP and ΔSi:ΔN ratios clearly revealed a change in the dominant phytoplankton from P. antarctica in ANA04 to diatoms and D. speculum in ANA06.
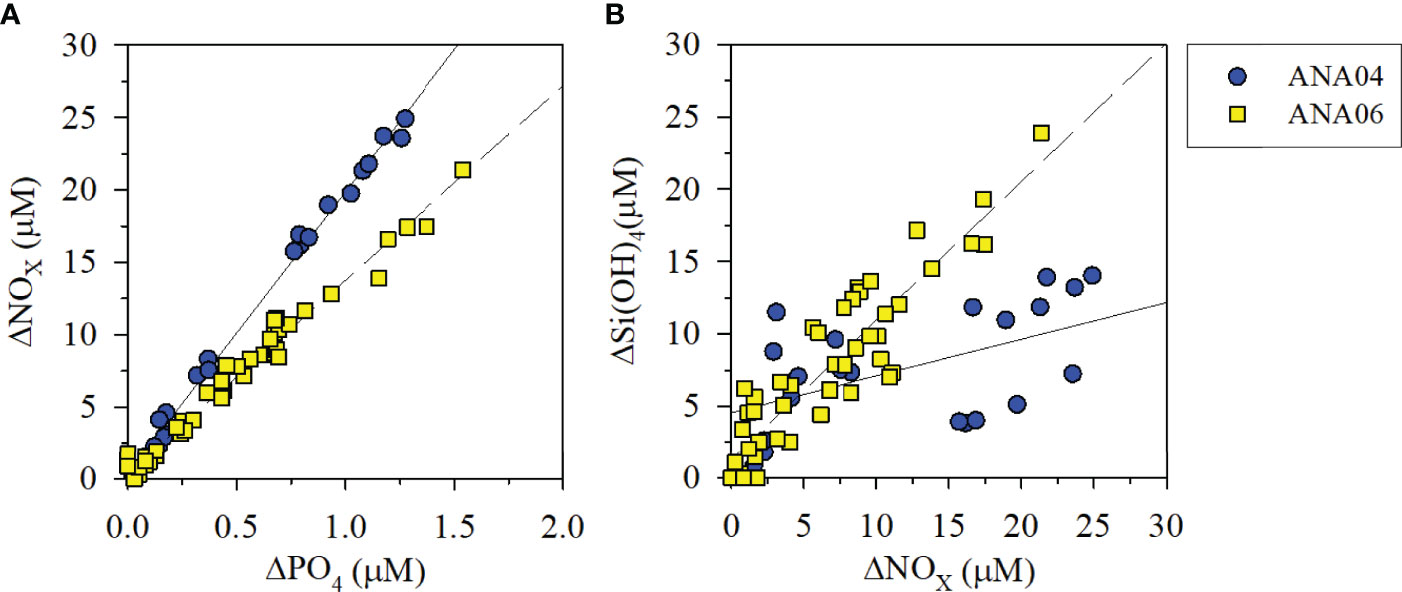
Figure 5 Disappearance ratio of nitrate + nitrite (NOX) versus phosphate (PO4) concentrations (A, ANA04: y = 19.53x + 0.42, r2 = 0.99, p < 0.001; ANA06: y = 13.38x + 0.44, r2 = 0.98, p < 0.001) and silicate [Si(OH)4] versus NOX concentrations (B, ANA04: y = 0.25x + 4.56, r2 = 0.27, p = 0.015; ANA06: y = 0.96x + 1.31, r2 = 0.82, p < 0.001) during the ANA04 and ANA06 periods.
The change in phytoplankton communities between the two periods was likely driven by changes in light intensity and iron availability, as previously reported in the Southern Ocean (Timmermans et al., 2004; Tagliabue and Arrigo, 2005; Kropuenske et al., 2009; Lee et al., 2022). Previous studies found that P. antarctica can thrive under dynamic light conditions (Kropuenske et al., 2009), while the growth rate of large diatoms and D. speculum were lower than that of P. antarctica at high light intensity (Biggs et al., 2019; Lee et al., 2022). In the present study, surface light intensity was approximately six times lower in ANA06 than in ANA04 (Supplementary Figure 1), and thus phytoplankton biomass was dominated by large pennate diatoms (31%), centric diatoms (14%), and D. speculum (48%) rather than P. antarctica (3%) in ANA06 (Lee et al., 2022).
In addition, several studies reported that P. antarctica has a lower iron requirement than diatoms (Arrigo et al., 2003; Tagliabue and Arrigo, 2005), while the iron requirement of diatoms declines with decreasing cell size (Timmermans et al., 2004). In the present study, the maximum quantum efficiency (Fv/Fm), which is used as an indicator of phytoplankton status to iron stress (Falkowski et al., 2004), was higher in diatom-dominated ANA06 (> 0.5) than in P. antarctica-dominated ANA04 (0.27–0.36) (Lee, 2017). In previous studies, Fv/Fm was approximately 0.55 in the iron-replete Southern Ocean (Coale et al., 2004), but was under 0.35 in the iron-depleted ASP (Alderkamp et al., 2015; Park et al., 2017). Thus, the growth of large diatoms with higher iron requirements is likely to be favored in ANA06 with higher Fv/Fm.
Bacterial Production According to Change in Dominant Phytoplankton
Bacterial metabolism in the ocean is primarily controlled by temperature and the availability of organic matter (Pomeroy and Wiebe, 2001). In cold polar seas, despite low temperatures, BP during phytoplankton blooms is as high as that reported in temperate waters (Kirchman et al., 2009b). Likewise, in the present study, although the temperature was lower in ANA04 than in ANA06, Chl-a was higher in ANA04 (Table 1), and BP was also four times higher in ANA04 compared to ANA06 (Figure 3H). Therefore, bacterial metabolic activities in the ASP are primarily controlled by the availability of organic matter produced by phytoplankton rather than temperature (Kirchman et al., 2009a; Kirchman et al., 2009b; Ducklow et al., 2012; Hyun et al., 2016).
Interestingly, correlation analysis revealed that the dependence of BP on organic matter source varied between ANA04 and ANA06. BP was significantly correlated with only Chl-a (rho = 0.66, p < 0.001) during ANA04, whereas BP in ANA06 showed a significant correlation with CDOM (rho = 0.84, p < 0.001), Chl-a (rho = 0.59, p < 0.001), and DOC (rho = 0.51, p = 0.001) (Table 3). The difference between the two periods was attributed to variation in DOM quality resulting from the change in the dominant phytoplankton community from P. antarctica in ANA04 to diatoms in ANA06 (Figure 4). A large fraction of the DOM exudated by phytoplankton is in the form of polysaccharides, but the main components of these polysaccharides vary by phytoplankton community (Mühlenbruch et al., 2018). Several previous studies found that Phaeocystis spp. excretes more bioavailable forms of polysaccharides than diatoms (Biersmith and Benner, 1998; Aluwihare and Repeta, 1999; Solomon et al., 2003; Alderkamp et al., 2007). Kinsey et al. (2018) also reported that labile DOM concentration and bacterial abundance were highest in Phaeocystis among phytoplankton culture experiments, including various diatoms, which implies that Phaeocystis supplies more bioavailable DOM for bacterial metabolism than diatoms. Our results also revealed that bacterial growth rates in Phaeocystis-dominated ANA04 (0.76 d-1 on average) were two-fold that measured in diatom and D. speculum co-dominated ANA06 (0.35 d-1 on average) (Table 2). In the same period Fang et al. (2020) analyzed 14C-DOC analysis in surface water and also found a greater amount of freshly produced DOC in ANA04 than in ANA06. Given that BP was significantly correlated with only Chl-a in ANA04 (Table 3), the DOC released directly from P. antarctica is likely a major source of organic matter supporting BP (Ducklow et al., 2012).
In contrast to ANA04, BP in ANA06 was positively correlated not only with Chl-a but also with CDOM and DOC (Table 3). CDOM can be produced via various pathways including direct release from phytoplankton, photodegradation of DOM, microbial degradation, sloppy feeding or excretion by zooplankton, and viral lysis (Moran et al., 2000; Carlson and Hansell, 2015; Lee et al., 2016a; Guallar and Flos, 2019). Although diatoms generate less bioavailable DOM compared with P. antarctica (Biersmith and Benner, 1998; Aluwihare and Repeta, 1999; Solomon et al., 2003; Alderkamp et al., 2007), more zooplankton grazing occurs on diatoms than on Phaeocystis spp. (Stelfox-Widdicombe et al., 2004; Nejstgaard et al., 2007), and the presence of grazing stimulates DOM release from phytoplankton (Strom et al., 1997). Therefore, CDOM might be generated from more diverse sources within food web processes under diatom-dominated conditions in ANA06, providing more support for bacterial metabolic activities.
Control of the Microbial Loop and Export Flux
The quantitative significance of the microbial loop is often evaluated using the BP : PP ratio, in which a higher BP : PP ratio implies that relatively more PP is channeled to nano- and microzooplankton via microbial food web processes and/or mineralized to CO2 within the microbial loop (Kirchman et al., 2009b). In the present study, the BP : PP ratio was three-fold higher in Phaeocystis-dominated ANA04 (0.09 on average) than in diatom- and D. speculum-co-dominated ANA06 (0.03 on average) (Table 2), which indicated that the microbial loop is relatively more significant under Phaeocystis-dominated conditions than diatom-dominated conditions. The results are consistent with a previous study on the ASP (Yager et al., 2016) that reported a relatively lower BP : PP ratio at diatom-dominated stations (0.03) than in P. antarctica-dominated stations (0.06). Considered with respiration, the BCD : PP ratio was 7.45 in ANA04 and 3.80 in ANA06, BCD exceeded PP in both periods, and BGE was very low (0.02 in ANA04, 0.01 in ANA06)(Table 2). The BCD : PP ratio and BGE in the present study were much higher and lower, respectively, than the previously reported values in ASP (BCD : PP = 0.43, BGE = 0.11; Williams et al., 2016). These results indicate that bacteria utilize a large amount of semilabile DOM for respiration. According to Chen et al. (2019), although massive labile CDOM production occurs in ASP, semilabile DOC accumulation is low due to the high bioavailability and rapid turnover of DOM. Williams et al. (2016) also suggested that the low DOM accumulation in ASP resulted from rapid DOM turnover by bacteria, and thus a high CDOM production and rapid DOM turnover could explain high BR and low BGE. Consequently, although semilabile DOM consumption by bacterial respiration is significantly large in ASP, the relatively higher BP : PP and BCD : PP ratios in ANA 04 than ANA06 indicate that carbon consumption through the microbial loop is more active during the P. antarctica-dominated condition. As the BP : PP ratio and export flux are inversely related (Cho et al., 2001; Kirchman, 2008), the BP : PP ratio also provides quantitative information about the efficiency of export flux. In the present study, we found a clear inverse relationship between BP : PP ratio and the diatom carbon biomass (Figure 6A). The high BP : PP ratio in the Phaeocystis-dominated system and low ratio in the diatom-dominated system strongly implies that the significance of the microbial loop and the efficiency of export flux (i.e., the biological pump) are directly controlled by changes in dominant phytoplankton group. DOM excreted from P. antarctica is rapidly utilized by bacteria compared to diatom-derived DOM (Biersmith and Benner, 1998; Aluwihare and Repeta, 1999; Kinsey et al., 2018). In addition, the zooplankton grazing rate on P. antarctica is lower (Tagliabue and Arrigo, 2003; Tang et al., 2008) and its sinking rate slower (Becquevort and Smith, 2001; Reigstad and Wassmann, 2007) than those of diatoms. Thus, under P. antarctica-dominated conditions, most DOC derived from P. antarctica is rapidly mineralized by bacteria within the water column before it reaches the seafloor (Kirchman et al., 2001). In contrast, more fresh DOC is likely to be transported to deeper ocean during a fast-sinking diatom bloom (Alldredge and Gotschalk, 1989). Fang et al. (2020) during the same periods also found that a greater amount of fresh DOC was transported to the deeper ocean in ANA06 (i.e., the diatom-dominated condition) than in ANA04 (the P. antarctica-dominated condition). These results suggest that as the carbon biomass of diatoms increases, less organic carbon is degraded by bacterial metabolism in the euphotic layer, which results in an increase of POC export flux.
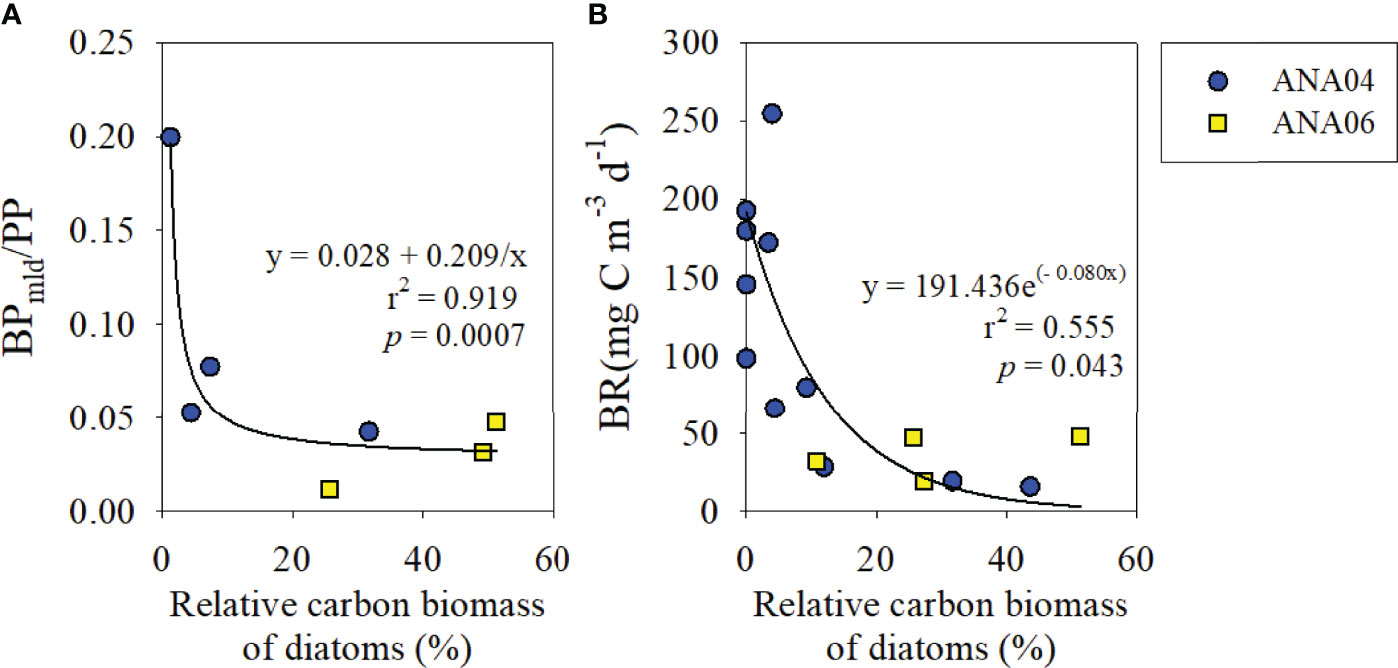
Figure 6 The relationship between the mixed-layer depth integrated BP to PP ratio and relative carbon biomass of diatoms (%) (A), and the relationship between BR and relative carbon biomass of diatoms (%) (B). PP data were adopted from Lim et al. (2019).
Previous studies in the ASP also revealed that the POC flux efficiency varied depending on the dominant phytoplankton species in the ASP (Ducklow et al., 2015; Kim et al., 2015). In the P. antarctica-dominant polynya, only 1.6% of net primary production (NPP) is delivered to the deep layer, and most of the exported organic matter is mineralized between depths of 60 and 150 m via microbial respiration (Ducklow et al., 2015). However, in the sea ice zone, where diatoms were a dominant phytoplankton group, approximately 18% of NPP is delivered to the deep layer (Kim et al., 2015). Similarly, in the Ross Sea, DeJong et al. (2017) reported that carbon export flux in diatom-dominated regions (7.3 ± 0.9 mol C m-2 d-1) was two times higher than that measured in Phaeocystis-dominated regions (3.4 ± 0.8 mol C m-2 d-1). In the present study, BR also showed a significant decreasing trend with increasing diatom proportion (y=191.44e(-0.08x), r2 = 0.55, p = 0.043; Figure 6B). These results imply that the change in major phytoplankton communities from P. antarctica to diatoms weakens the role of the microbial loop and enhances the efficiency of biological pump (i.e., POC export flux) (Passow et al., 2007).
Conclusion
In recent decades, West Antarctica has undergone rapid warming with decreasing sea ice (Turner et al., 2014; Brown et al., 2019). Increased light and iron availability resulting from this sea ice reduction are major factors increasing primary production (Gerringa et al., 2012; Duprat et al., 2016; Kaufman et al., 2017; Wu and Hou, 2017; Brown et al., 2019). Recent studies revealed that warming and iron availability could stimulate diatom blooms over Phaeocystis blooms (Boyd et al., 2016; Petrou et al., 2016; Tréquer et al., 2017; Nissen and Vogt, 2021). If climate change induced a shift in phytoplankton communities, this would ultimately affect not only POC export flux (Boyd and Newton, 1999; Henson et al., 2012), but also food web structure (Feng et al., 2010; Marañón, 2015). The nutritional quality of the food web depends on grazing efficiency, and is linked to trophic efficiency (Mangoni et al., 2017). For example, in a P. antarctica-dominated system, the majority of photosynthetically fixed organic carbon is remineralized by bacterial metabolic activity even before reaching the mesopelagic zone (Ducklow et al., 2015). When P. antarctica colonies are formed, the removal by zooplankton grazing is less efficient (Nejstgaard et al., 2007). However colony residence time is long in the euphotic layer due to the foam-like structures (Becquevort and Smith, 2001; Alderkamp et al., 2007), and they are mostly consumed through a microbial loop (e.g., microbial decomposition) (Ducklow et al., 2015). In contrast, when P. antarctica exists as a single cell, a significant amount is removed by microzooplankton and delivered to the microbial food web (Yang et al., 2016). Since the microbial food web is more complex than grazing food chain, the intensified microbial loop ultimately weakens food web efficiency (Legendre and Le Fèvre, 1995). Conversely, food web efficiency is relatively high during diatom blooms due to the high zooplankton grazing rate (Deppeler and Davidson, 2017). Consequently, considering the low BP : PP ratio with increasing diatom biomass (Figure 6A), as well as higher carbon export under diatom bloom conditions (DeJong et al., 2017), if climate change induced shift in phytoplankton communities from P. antarctica to diatoms, this would weaken the importance of the microbial loop and further enhance the function of biological pump as well as food web efficiency in the ASP.
Data Availability Statement
The original contributions presented in the study are included in the article/Supplementary Material. Further inquiries can be directed to the corresponding author.
Author Contributions
BK and J-HH as the first and corresponding author, designed the study and wrote the manuscript. S-HK, J-OM, and J-HH performed the field and microbial analysis. YL and EY carried out analysis of Chl-a and phytoplankton community. JJ provided chemical parameter data. T-WK provided physical parameter data. JL contributed to the design of a respiration analysis method using an optode. SL and JP were the leader of the Korean Antarctic Research Program and provided scientific advice. All authors contributed to the discussion of the results.
Funding
This study was supported by the Microbially mediated ecological and biogeochemical process study in the Arctic Ocean funded by the Korean Ministry of Oceans and Fisheries (20220554), Korea Polar Research Institute (KOPRI, PE21110), and a grant from the National Research Foundation of Korea (NRF) funded by the Korean Ministry of Science and Information Communication Technology (NRF-2018R1A2B2006340).
Conflict of Interest
The authors declare that the research was conducted in the absence of any commercial or financial relationships that could be construed as a potential conflict of interest.
Publisher’s Note
All claims expressed in this article are solely those of the authors and do not necessarily represent those of their affiliated organizations, or those of the publisher, the editors and the reviewers. Any product that may be evaluated in this article, or claim that may be made by its manufacturer, is not guaranteed or endorsed by the publisher.
Acknowledgments
The authors would like to thank the captain and crew of the IBRV Araon who supported all shipboard operations.
Supplementary Material
The Supplementary Material for this article can be found online at: https://www.frontiersin.org/articles/10.3389/fmars.2022.872052/full#supplementary-material
References
Alderkamp A.-C., Buma A. G. J., van Rijssel M. (2007). The Carbohydrates of Phaeocystis and Their Degradation in the Microbial Food Web. Biogeochemistry 83, 99–118. doi: 10.1007/s10533-007-9078-2
Alderkamp A.-C., van Dijken G. L., Lowry K. E., Connelly T. L., Larerström M., Sherrell R. M. (2015). Fe Availability Drives Phytoplankton Photosynthesis Rates During Spring Bloom in the Amundsen Sea Polynya, Antarctica. Elem. Sci. Anth. 3, 43. doi: 10.12952/journal.elementa.000043
Alldredge A. L., Gotschalk C. C. (1989). Direct Observations of the Mass Flocculation of Diatom Blooms: Characteristics, Settling Velocities and Formation of Diatom Aggregates. Deep-Sea Res. Part A 36, 159–171. doi: 10.1016/0198-0149(89)90131-3
Aluwihare L. I., Repeta D. J. (1999). A Comparison of the Chemical Characteristics of Oceanic DOM and Extracellular DOM Produced by Marine Algae. Mar. Ecol. Prog. Ser. 186, 105–117. doi: 10.3354/meps186105
Arístegui J., Gasol J. M., Duarte C. M., Herndl G. J. (2009). Microbial Oceanography of the Dark Ocean’s Pelagic Realm. Limnol. Oceanogr. 54 (5), 1501–1529. doi: 10.4319/lo.2009.54.5.1501
Arrigo K. R., Lowry K. E., van Dijken G. L. (2012). Annual Changes in Sea Ice and Phytoplankton in Polynyas of the Amundsen Sea, Antarctica. Deep-Sea Res. II 71–67, 5–15. doi: 10.1016/j.dsr2.2012.03.006
Arrigo K. R., Robinson D. H., Worthen D. L., Dunbar R. B., DiTullio G. R., VanWoert M., et al. (1999). Phytoplankton Community Structure and the Drawdown of Nutrients and CO2 in the Southern Ocean. Science 283, 365–367. doi: 10.1126/science.283.5400.365
Arrigo K. R., van Dijken G. L. (2003). Phytoplankton Dynamics Within 37 Antarctic Coastal Polynya Systems. J. Geophys. Res. 108: 3271. doi: 10.1029/2002JC001739
Arrigo K. R., van Dijken G. L., Long M. (2008). Coastal Southern Ocean: A Strong Anthropogenic CO2 Sink. Geophys. Res. Lett. 35, L21602. doi: 10.1029/2008GL035624
Arrigo K. R., Worthen D. L., Robinson D. H. (2003). A Coupled Ocean-Ecosystem Model of the Ross Sea: 2. Iron Regulation of Phytoplankton Taxonomic Variability and Primary Production. J. Geophys. Res. 108 (C7), 3231. doi: 10.1029/2001JC000856
Azam F., Fenchel T., Field J. G., Gray J. S., Meyer-Reil L. A., Thingsted F. (1983). The Ecological Role of Water-Column Microbes in the Sea. Mar. Ecol. Prog. Ser. 10, 257–263. doi: 10.3354/meps010257
Becquevort S., Smith W. O. Jr. (2001). Aggregation, Sedimentation and Biodegradability of Phytoplankton-Derived Material During Spring in the Ross Sea, Antarctica. Deep-Sea Res. II. 48, 4155–4178. doi: 10.1016/S0967-0645(01)00084-4
Biersmith A., Benner R. (1998). Carbohydrates in Phytoplankton and Freshly Produced Dissolved Organic Matter. Mar. Chem. 63, 131–144. doi: 10.1016/S0304-4203(98)00057-7
Biggs T. E. G., Alvarez-Fernandez S., Evans C., Mojica K. D. A., Rozema P. D., Venables H. J., et al. (2019). Antarctic Phytoplankton Community Composition and Size Structure Importance of Ice Type and Temperature as Regulatory Factors. Pol. Biol. 42, 1997–2015. doi: 10.1007/s00300-019-02576-3
Boyd P. W., Dillingham P. W., McGraw C. M., Armstrong E. A., Cornwall C. E., Feng Y.-y., et al. (2016). Physiological Responses of a Southern Ocean Diatom to Complex Future Ocean Conditions. Nat. Clim. Change. 6, 207–213. doi: 10.1038/NCLIMATE2811
Boyd P. W., Newton P. P. (1999). Dose Planktonic Community Structure Determine Downward Particulate Organic Carbon Flux in Different Oceanic Provinces? Deep-Sea. Res. Part I. 46, 63–91. doi: 10.1016/S0967-0637(98)00066-1
Brown M. S., Munro D. R., Feehan C. J., Sweeney C., Ducklow H. W., Schofield O. M. (2019). Enhanced Oceanic CO2 Uptake Along the Rapidly Changing West Antarctic Peninsula. Nat. Clim. Change 9, 678–683. doi: 10.1038/s41558-019-0552-3
Carlson C. A., Hansell D. A. (2015). “DOM Sources, Sinks, Reactivity, and Budgets”, in Biogeochemistry of Marine Dissolved Organic Matter, 2nd ed. Eds. Hansell D. A., Carlson C. A. (San Diego, CA: Academic Press), 66–127.
Cavan E. L., Boyd P. W. (2018). Effect of Anthropogenic Warming on Microbial Respiration and Particulate Organic Carbon Export Rates in the Sub-Antarctic Southern Ocean. Aquat. Microb. Ecol. 82, 111–127. doi: 10.3354/ame01889
Chen M., Jung J., Lee Y. K., Kim T.-W., Hur J. (2019). Production of Tyrosine-Like Fluorescence and Labile Chromophoric Dissolved Organic Matter (DOM) and Low Surface Accumulation of Low Molecular Weight-Dominant DOM in a Productive Antarctic Sea. Mar. Chem. 213, 40–48. doi: 10.1016/j.marchem.2019.04.009
Cho B. C., Pack M. G., Shim J. H., Choi D. H. (2001). Sea-Surface Temperature and F-Ratio Explain Large Variability in the Ratio of Bacterial Production to Primary Production in the Yellow Sea. Mar. Ecol. Prog. Ser. 216, 31–41. doi: 10.3354/meps216031
Coale K. H., Johnson K. S., Chavez F. P., Buesseler K. O., Barber R. T., Brzezinski M. A. (2004). Southern Ocean Iron Enrichment Experiments: Carbon Cycling in High- and Low-Si Waters. Science 304, 408–414. doi: 10.1126/science.1089778
Cota G. F., Kottmeier S. T., Robinson D. H., Smith W. O. Jr., Sullivan C. W. (1990). Bacterioplankton in the Marginal Ice Zone of the Weddell Sea: Biomass, Production and Metabolic Activities During Austral Autumn. Deep-Sea. Res. 37 (7), 1145–1167. doi: 10.1016/0198-0149(90)90056-2
DeJong H. G., Dunbar R. B., Koweek D. A., Mucciarone D. A., Bercovici S. K., Hansell D. A. (2017). Net Community Production and Carbon Export During the Late Summer in the Ross Sea, Antarctica. Global Biogeochem. Cy. 31, 473–491. doi: 10.1002/2016GB005417
del Giorgio P. A., Cole J. J. (1998). Bacterial Growth Efficiency in Natural Aquatic Systems. Annu. Rev. Ecol. Evol. Syst. 29 (1), 503–541. doi: 10.1146/annurev.ecolsys.29.1.503
del Giorgio P. A., Cole J. J. (2000). “Bacterial Energetics and Growth Efficiency,” in Microbial Ecology of the Oceans, 1st ed. Ed. Kirchman D. L., (New York:Willy-Liss) 289–325.
del Giorgio P. A., Williams P. (2005). “The Global Significance of Respiration in Aquatic Ecosystems: From Single Cell to the Biosphere”, in Respiration in Aquatic Ecosystems. Eds. del Giorgio P. A., Williams P. (New York: Oxford University Press), 267–303.
Deppeler S. L., Davidson A. T. (2017). Southern Ocean Phytoplankton in a Changing Climate. Front. Mar. Sci. 4. doi: 10.3389/fmars.2017.00040
Ducklow H. W. (2000). “Bacterial Production and Biomass in the Oceans”, in Microbial Ecology of the Oceans. Ed. Kirchman D. L. (New York: Wiley-Liss), 85–120.
Ducklow H. G., Carlson C., Smith W. O. Jr. (1999). Bacterial Growth in Experimental Plankton Assemblages and Seawater Cultures From the Phaeocystis Antarctica Bloom in the Ross Sea, Antarctica. Aquat. Microb. Ecol. 19, 215–227. doi: 10.3354/ame019215.
Ducklow H. W., Purdie D. A., Williams P., Davis J. M. (1986). Bacterioplankton: A Sink for Carbon in a Coastal Marine Plankton Community. Science 232 (4752), 865–867. doi: 10.1126/science.232.4752.865.
Ducklow H. W., Scofield O., Vernet M., Stammerjohn S., Erickson M. (2012). Multiscale Control of Bacterial Production by Phytoplankton Dynamics and Sea Ice Along the Western Antarctic Peninsula: A Regional and Decadal Investigation. J. Mar. Syst. 98–99, 26–39. doi: 10.1016/j.jmarsys.2012.03.003
Ducklow H. W., Wilxon S. E., Post A. F., Stammerjohn S. E., Erickson M., Lee S. H., et al. (2015). Particle Flux on the Continental Shelf in the Amundsen Sea Polynya and Western Antacrtic Penensula. Elem. Sci. Anth. 3, 46. doi: 10.12952/journal.elementa.000046
Dunbar R. B., Arrigo K. R., Lutz M., Ditullio G. R., Leventer A. R., Lizotte M. P., et al. (2003). Non-Redfield Production and Export of Marine Organic Matter: A Reccurent Part of the Annual Cycle in the Ross Sea, Antarctica. Biogeochem. Ross. Sea. 78, 179–196. doi: 10.1029/078ARS11
Duprat L. P. A. M., Bigg G. R., Wilton D. J. (2016). Enhanced Southern Ocean Marine Productivity Due to Fertilization by Giant Icebergs. Nat. Geosci. 9, 219–221. doi: 10.1038/NGEO2633
Falkowski P. G., Katz M. E., Knoll A. H., Quigg A., Raven J. A., Schofield O., et al. (2004). The Evolution of Modern Eukaryotic Phytoplankton. Science 305, 354–360. doi: 10.1126/science.1095964
Fang L., Lee S. H., Lee S.-A., Hahm D., Kim G., Druffel E. R. M., et al. (2020). Removal of Refractory Dissolved Organic Carbon in the Amundsen Sea, Antarctica. Sci. Rep. 10, 1213. doi: 10.1038/s41598-020-57870-6
Feng Y., Hare C. E., Rose J. M., Handy S. M., DiTullio G. R., Lee P. A., et al. (2010). Interactive Effects of Iron, Irradiance and CO2 on Ross Sea Phytoplankton. Deep-Sea. Res. I. 57, 368–383. doi: 10.1016/j.dsr.2009.10.013
Fragoso G. M., Smith W. O. Jr. (2012). Influence of Hydrography on Phytoplankton Distribution in the Amundsen and Ross Seas, Antarctica. J. Mar. Syst. 89, 19–29. doi: 10.1016/j.jmrsys.2011.07.008
Fuhrman J. A., Azam F. (1980). Bacterioplankton Secondary Production Estimates for Coastal Waters of British Columbia Antarctica, and California. Appl. Environ. Microbiol. 39 (6), 1085–1095. doi: 10.1128/aem.39.6.1085-1095.1980
Fuhrman J. A., Azam F. (1982). Thymidine Incorporation as a Measure of Heterotrophic Bacterioplankton Production in Marine Surface Waters: Evaluation and Field Results. Mar. Biol. 66 (2), 109–120. doi: 10.1007/BF00397184
Fukuda R., Ogawa H., Nagata T., Koike I. (1998). Direct Determination of Carbon and Nitrogen Contents of Natural Bacterial Assemblages in Marine Environments. Appl. Environ. Microbiol. 64, 3352–3358. doi: 10.1128/AEM.64.9.3352-3358.1998
Gerringa L. J. A., Alderkamp A.-C., Laan P., Thuroczy C.-E., De Baar H. J. W., Mills M. M., et al. (2012). Iron From Melting Glaciers Fuels the Phytoplankton Blooms in Amundsen Sea (Southern Ocean): Iron Biogeochemistry. Deep-Sea Res. II 71–76, 16–31. doi: 10.1016/j.dsr2.2012.03.007
Giering S. L., Sanders R., Lampitt R. S., Anderson T. R., Tamburini C., Boutrif M., et al. (2014). Reconciliation of the Carbon Budget in the Ocean’s Twilight Zone. Nature 507, 480–483. doi: 10.1038/nature13123
Guallar C., Flos J. (2019). Linking Phytoplankton Primary Production and Chromophoric Dissolved Organic Matter in the Sea. Prog. Oceanogr. 176, 102116. doi: 10.1016/j.pocean.2019.05.008
Hahm D., Rhee T. S., Kim H.-C., Park J., Kim Y.-N., Shin H. C., et al. (2014). Spatial and Temporal Variabion of Net Community Production and Its Regulating Factors in the Amundsen Sea, Antarcitica. J. Geophys. Res. Ocean. 119, 2815–2826. doi: 10.1002/2013JC009762
Henson S., Lampitt R., Johns D. (2012). Variability in Phytoplankton Community Structure in Response to the North Atlantic Oscillation and Implications for Organic Carbon Flux. Limnol. Oceanogr. 57 (6), 1591–1601. doi: 10.4319/lo.2012.57.6.1591
Herndl G. J., Reinthaler T. (2013). Microbial Control of the Dark End of the Biological Pump. Nat. Geosci. 6 (9), 718–724. doi: 10.1038/ngeo1921
Hillebrand H., Dürselen C. D., Kirschtel D., Pollingher U., Zohary T. (1999). Biovolume Calculation for Pelagic and Benthic Microalgae. J. Phycol. 35, 403–424. doi: 10.1046/j.1529-8817.1999.3520403.x
Hyun J.-H., Kim S.-H., Yang E. J., Choi A., Lee S. H. (2016). Biomass, Production, and Control of Heterotrophic Bacterioplankton During a Late Phytoplankton Bloom in the Amundsen Sea Polynya, Antarctica. Deep-Sea. Res. II. 123, 102–112. doi: 10.1016/j.dsr2.2015.10.001
Hyun J.-H., Yang E. J. (2003). Freezing Seawater for the Long-Term Storage of Bacterial Cells for Microscopic Enumeration. J. Microbiol. 41 (3), 262–265.
Jacobs S. S., Jenkins A., Hellmer H., Giulivi C., Nitsche F., Huber B., et al. (2012). The Amundsen Sea and the Antarctic Ice Sheet. Oceanography 25, 154–163. doi: 10.5670/oceanog.2012.90
Jenkins A., Dutrieux P., Jacobs S. S., McPhail S. D., Perrett J. R., Webb A. T., et al. (2010). Observations Beneath Pine Island Glacier in West Antarctica and Implications for Its Retreat. Nat. Geosci. 3, 468–472. doi: 10.1038/NGEO890
Jenkins A., Shoosmith D., Dutrieux P., Jacobs S., Kim T. W., Lee S. H., et al. (2018). West Antarctic Ice Sheet Reteat in the Amundsen Sea Driven by Decadal Oceanic Variability. Nat. Geosci. 11, 733–738. doi: 10.1038/s41561-018-0207-4
Karl D. M. (1999). A Sea of Change: Biogeochemical Variability in the North Pacific Subtropical Gyre. Ecosystems 2, 181–214. doi: 10.1007/s100219900068
Kaufman D. E., Friedrichs M. A. M., Smith W. O. Jr., Hofmann E. E., Dinniman M. S., Hemmings C. P. (2017). Climate Change Impacts on Southern Ross Sea Phytoplankton Composition, Productivity, and Export. Geophys. Res. Ocean. 122, 2339–2359. doi: 10.1002/1026JC012514
Kim M., Hwang J., Kim H. J., Kim D., Yany E. J., Ducklow H. W., et al. (2015). Sinking Particle Flux in the Sea Ice Zone of the Amundsen Shelf, Antarctica. Deep-Sea Res. I 101, 110–117. doi: 10.1016/j.dsr.2015.04.002
Kinsey J. D., Corradino G., Ziervogel K., Schnetzer A., Osburn C. L. (2018). Formation of Chromophoric Dissolved Organic Matter by Bacterial Degradation of Phytoplankton-Derived Aggregates. Front. Mar. Sci. 4. doi: 10.3389/fmars.2017.00430
Kirchman D. L. (2008). “Introduction and Overview”, in Microbial Ecology of the Oceans, 2nd ed. Ed. Kirchman D. L. (New Jersey: Wiley-Liss), 299–334.
Kirchman D. L., Hill V., Cottrell M. T., Gradinger R., Malmstrom R. R., Parker A. (2009a). Standing Stocks, Production, and Respiration of Phytoplankton and Heterotrophic Bacteria in the Western Arctic Ocean. Deep-Sea Res. II. 56, 1237–1248. doi: 10.1016/j.dsr2.2008.10.018
Kirchman D. L., Meon B., Ducklow H. W., Carlson C. A., Hansell D. A., Steward G. F. (2001). Glucose Fluxes and Concentrations of Dissolved Combined Neutral Sugars (Polysaccharides) in the Ross Sea and Polar Front Zone, Antarctica. Deep-Sea Res. II 48, 4179–4197. doi: 10.1016/S0967-0645(01)00085-6
Kirchman D. L., Morán X. A. G., Ducklow H. (2009b). Microbial Growth in the Polar Oceans – Role of Temperature and Potential Impact of Climate Change. Nat. Rev. Microbiol. 7, 451–459. doi: 10.1038/nrmicro2115
Kowalczuk P., Stoń-Egiert J., Copper W. J., Whitehead R. F., Durako M. J. (2005). Characterization of Chromophoric Dissolved Organic Matter (CDOM) in the Baltic Sea by Excitation Emission Matrix Fluorescence Spectroscopy. Mar. Chem. 96, 273–292. doi: 10.1016/j.marchem.2005.03.002
Kropuenske L. R., Mills M. M., van Dijken G. L., Bailey S., Robinson D. H., Welschmeyer N. A., et al. (2009). Photophysiology in Two Major Southern Ocean Phytoplankton Taza: Photoprotection in Phaeocystis Antarctica and Fragilatiopsis Cylindrus. Limnol. Oceanogr. 54 (40), 1176–1196. doi: 10.4319/lo.2009.54.4.1176
Labasque T., Chaumery C., Aminot A., Kergoat G. (2004). Spectrophotometric Winkler Determination of Dissolved Oxygen: Reexamination of Factors and Reliability. Mar. Chem. 88, 53–60. doi: 10.1016/j.marchem.2004.03.004
Lee Y., Jung J., Kim T. W., Yang E. J., Park J. (2022). Phytoplankton Growth Rates in the Amundsen Sea (Antarctica) During Summer: The Role of Light. Environ. Res. 207, 112165. doi: 10.1016/j.envres.2021.112165
Lee S. (2017). Physical and Bio-geochemical Processes in the Amundsen Sea : Their Roles & Responses in Global Climate Change.
Lee S. H., Kim B., Yun M. S., Joo H. T., Yang E. J., Kim Y. N., et al. (2012). Spatial Distribution of Phytoplankton Productivity in the Amundsen Sea, Antarctica. Pol. Biol. 35, 1721–1733. doi: 10.1007/s00300-012-1220-5
Lee Y. C., Park M. O., Jung J., Yang E. J., Lee S. H. (2016a). Taxonomic Variability of Phytoplankton and Relationship With Production of CDOM in the Polynya of the Amundsen Sea, Antarctica. Deep-Sea Res. II 123, 30–41. doi: 10.1016/j.dsr2.2015.09.002
Lee Y., Yang E. J., Park J., Jung J., Kim T. W., Lee S. H. (2016b). Physical-Biological Coupling in the Amundsen Sea, Antarctica: Influence of Physical Factors on Phytoplankton Community Structure and Biomass. Deep-Sea. Res. I. 117, 51–60. doi: 10.1016/j.dsr.2016.10.001
Legendre L., Le Fèvre J. (1995). Microbial Food Webs and the Export of Biogenic Carbon in Oceans. Aquat. Microb. Ecol. 9, 69–77. doi: 10.3354/ame009069
Li W. K. W., McLaughlin F. A., Lovejoy C., Carmack E. C. (2009). Smallest Algae Thrive as the Arctic Ocean Freshens. Science 326, 539. doi: 10.1126/science.1179798
Lim Y. J., Kim T.-W., Lee S. H., Lee D., Park J., Kim B. K., et al. (2019). Seasonal Variations in the Small Phytoplankton Contribution to the Total Primary Production in the Amundsen Sea, Antarctica. J. Geophys. Res. Ocean. 124. doi: 10.1029/2019JC015305
Lochte K., Bjornsen P. K., Giesenhageni H., Webers A. (1997). Bacterial Standing Stock and Production and Their Relation to Phytoplankton in the Southern Ocean. Deep-Sea. Res. II. 44, 321–340. doi: 10.1016/S0967-0645(96)00081-1
Mangoni O., Saggiomo V., Bolinesi F., Margiotta F., Budillon G., Cotroneo Y., et al. (2017). Phytoplankton Blooms During Austral Summer in the Ross Sea, Antarctica: Driving Factors and Trophic Implications. PloS One 12 (4), e0176033. doi: 10.1371/journal.pone.0176033
Marañón E. (2015). Cell Size as a Key Determinant of Phytoplankton Metabolism and Community Structure. Annu. Rev. Mar. Sci. 7, 241–264. doi: 10.1146/annurev-marine-010814-015955
Mathot S., Smith W. O., Carlson C. A., Garrison D. L., Gowing M. M., Vickers C. L. (2000). Carbon Partitioning Within Phaeocystis Antarctica (Prymnesiophyceae) Colonies in the Ross Sea, Antarctica. J. Phycol. 36, 1049–1056. doi: 10.1046/j.1529-8817.2000.99078.x
McDonnell A. M. P., Boyd P. W., Buesseler K. O. (2015). Effects of Sinking Velocities and Microbial Respiration Rates on the Attenuation of Particulate Carbon Fluxes Through the Mesopelagic Zone. Global Biogeochem. Cycle. 29, 175–193. doi: 10.1002/2014GB004935
Meredith M. P., Ducklow H. W., Schofield O., Wåhlin A., Newman L., Lee S. (2016). The Interdisciplinary Marine System of the Amundsen Sea, Southern Ocean: Recent Advances and the Need for Sustained Observations. Deep-Sea. Res. II. 123, 1–6. doi: 10.1016/j.dsr2.2015.12.002
Montes-Hugo M., Doney S. C., Ducklow H. W., Fraser W., Martinson D., Stammerjohn S. E., et al. (2009). Recent Changes in Phytoplankton Communities Associated With Rapid Regional Climate Change Along the Western Antarctic Peninsula. Science 323, 1470–1473. doi: 10.1126/science.1164533
Morán X. A. G., Gasol J. M., Pedrós-Alió C., Estrada M. (2001). Dissolved and Particulate Primary Production and Bacterial Production in Offshore Antarctic Waters During Austral Summer: Coupled or Uncoupled? Mar. Ecol. Prog. Ser. 222, 25–39. doi: 10.3354/meps222025
Moran M. A., Sheldon W. M., Zepp R. G. (2000). Carbon Loss and Optical Property Changes During Long-Term Photochemical and Biological Degradation of Estuarine Dissolved Organic Matter. Limnol. Oceanogr. 45 (6), 1254–1264. doi: 10.4319/lo.2000.45.6.1254
Mouginot J., Rignot E., Scheuchl B. (2014). Sustained Increase in Ice Discharge From the Amundsen Sea Embayment, West Antarctica, From 1973 to 2013. Geophys. Res. Lett. 41, 1576–1584. doi: 10.1002/2013GL059069
Mühlenbruch M., Grossart H.-P., Eigemann F., Voss M. (2018). Mini-Review: Phytoplankton-Derived Polysaccharides in the Marine Environment and Their Interactions With Heterotrophic Bacteria. Environ. Microbiol. 20 (8), 2671–2685. doi: 10.1111/1462-2920.14302
Nagata T. (2008). “Organic Matter-Bacteria Interactions in Seawater”, in Microbial Ecology of the Oceans, 2nd ed. Ed. Kirchman D. L. (New Jersey:Wiley-Liss), 207–241.
Nejstgaard J. C., Tang K. W., Steinke M., Dutz J., Koski M., Antajan E., et al. (2007). Zooplankton Grazing on Phaeocystis: A Quantitative Review and Future Challenges. Biogeochemistry 83, 147–172. doi: 10.1007/s10533-007-9098-y
Nissen C., Vogt M. (2021). Factors Controlling the Competition Between Phaeocystis and Diatoms in the Southern Ocean and Implications for Carbon Export Fluxes. Biogeosciences 18, 251–283. doi: 10.5194/bg-18-251-2021
Park J., Kuzminov F. I., Bailleul B., Yang E. J., Lee S., Falkowski P. G., et al. (2017). Light Availability Rather Than Fe Controls the Magnitude of Massive Phytoplankton Bloom in the Amundsen Sea Polynyas, Antarctica. Limnol. Oceanogr. 62, 2260–2276. doi: 10.1002/lno.10565
Parsons T. R., Maita Y., Lalli C. M. (1984). A Manual of Chemical and Biological Methods for Seawater Analysis (Oxford: Pergamon Press).
Passow U., de la Rocha C. L., Arnosti C., Grossart H.-P., Murray A. E., Engel A. (2007). Microbial Dynamics in Autotrophic and Heterotrophic Seawater Mesocosms. I. Effect of Phytoplankton on the Microbial Loop. Aquat. Microb. Ecol. 49, 109–121. doi: 10.3354/ame01138
Petrou K., Kranz S. A., Trimborn S., Hassler C. S., Ameijeiras S. B., Sackett O., et al. (2016). Southern Ocean Phytoplankton Physiology in a Changing Climate. J. Plant Physiol. 203, 135–150. doi: 10.1016/j.jplph.2016.05.004
Pomeroy L. R., Sheldon J. E., Sheldon W. M. Jr. (1994). Changes in Bacterial Numbers and Leucine Assimilation During Estimations of Microbial Respiratory Rates in Seawater by the Precision Winkler Method. Appl. Environ. Microbiol. 60 (1), 328–332. doi: 10.1128/aem.60.1.328-332.1994
Pomeroy L. R., Wiebe W. J. (2001). Temperature and Substrates as Interactive Limiting Factors for Marine Heterotrophic Bacteria. Aquat. Microb. Ecol. 23, 187–204. doi: 10.3354/ame023187
Porter K. G., Feig Y. S. (1980). The Use of DAPI for Identifying and Counting Aquatic Microflora. Limnol. Oceanogr. 25 (5), 943–948. doi: 10.4319/lo.1980.25.5.0943
Reigstad M., Wassmann P. (2007). Does Phaeocystis Spp. Contribute Significantly to Vertical Export of Organic Carbon? Biogeochemistry 83, 217–234. doi: 10.1007/s10533-007-9093-3
Robinson C. (2008). “Heterotrophic Bacterial Respiration”, in Microbial Ecology of the Oceans, 2nd ed. Ed. Kirchman D. L. (New Jersey: Wiley-Liss), 299–334.
Romera-Castillo C., Sarmento H., Álvarez-Salgado X. A., Gasol J. M., Marrasé C. (2011). Net Production and Composition of Fluorescent Colored Dissolved Organic Matter by Natural Bacterial Assemblages Growing on Marine Phytoplankton Exudates. Appl. Environ. Microbiol. 77 (21), 7490–7498. doi: 10.1128/AEM.00200-11
Sherr B. F., Sherr E. B., Hopkinson C. S. (1988). Trophic Interactions Within Pelagic Microbial Communities: Indications of Feedback Regulation of Carbon Flow. Hydrobiologia 159, 19–26. doi: 10.1007/BF00007364
Smayda T. J. (1978). “From Phytoplankters to Biomass”, in Phytoplankton Manual. Ed. Sournia A. (Paris: UNESCO), 273–279.
Smith J. W. O., Barber D. G. (Eds.) (2007). “Polynyas” in Windows to the World (Amsterdam: Elsevier).
Smith W. O. Jr., Dinniman M. S., Tozzi S., DiTullio G. R., Mangoni O., Modigh M., et al. (2010). Phytoplankton Photosynthetic Pigments in the Ross Sea: Patterns and Relationships Among Functional Groups. J. Mar. Syst. 82, 177–185. doi: 10.1016/j.jmarsys.2010.04.014
Solomon C. M., Lessard E. J., Keil R. G., Foy M. S. (2003). Characterization of Extracellular Polymers of Phaeocystis Globose and P. Antarctica. Mar. Ecol. Prog. Ser. 250, 81–89. doi: 10.3354/meps250081
Stedmon C. A., Markager S. (2001). The Optics of Chromophoric Dissolved Organic Matter (CDOM) in the Greenland Sea: An Algorithm for Differentiation Between Marine and Terrestrially Derived Organic Matter. Limnol. Oceanogr. 46, 2087–2093. doi: 10.4319/lo.2001.46.8.2087
Stelfox-Widdicombe C. E., Archer S. D., Burkill P. H., Stefels J. (2004). Microzooplankton Grazing in Phaeocystis and Diatom-Dominated Waters in the Southern North Sea in Spring. J. Sea. Res. 51, 37–51. doi: 10.1016/j.seares.2003.04.004
Strom S. L., Benner R., Ziegler S., Dagg M. J. (1997). Planktonic Grazers are a Potentially Important Source of Marine Dissolved Organic Carbon. Limnol. Oceanogr. 42, 1364–1374. doi: 10.4319/lo.1997.42.6.1364
Tagliabue A., Arrigo K. R. (2005). Iron in the Ross Sea: 1. Impact on CO2 Fluxes via Variation in Phytoplankton Functional Group and non-Redfield Stoichiometry. J. Geophys. Res. 110, C03009. doi: 10.1029/2004JC002531
Tagllabue A., Arrigo K. R. (2003). Anomalously Low Zooplankton Abundance in the Ross Sea: An Alternative Explanation. Limnol. Oceanogr. 48 (2), 686–699. doi: 10.4319/lo.2003.48.2.0686
Takahashi T., Sutherland S. C., Wanninkhof R., Sweeney C., Feely R. A., Chipman D. W., et al. (2009). Climatological Mean and Decadal Change in Surface Ocean pCO2, and Net Sea–Air CO2 Flux Over the Global Oceans. Deep-Sea Res. II. 56, 554–577. doi: 10.1016/j.dsr2.2008.12.009
Tang K. W., Smith W. O. Jr., Elliott D. T., Shields A. R. (2008). Colony Size of Phaeocytis Antarctica (Prymnesiophyceae) as Influenced by Zooplankton Grazers. J. Phycol. 44, 1372–7387. doi: 10.1111/j.1529-8817.2008.00595.x
Taylor A. G., Landry M. R., Selph K. E., Yang E. J. (2011). Biomass, Size Structure and Depth Distributions of the Microbial Community in the Eastern Equatorial Pacific. Deep-Sea Res. I. 58, 342–357. doi: 10.1016/j.dsr2.2010.08.017
The IMBIE Team (2018). Mass Balance of the Antarctic Ice Sheet From 1992 to 2017. Nature 558 (7709), 219–222. doi: 10.1038/s41586-018-0179-y
Thoma M., Jenkins A., Holland D., Jacobs S. (2008). Modelling Circumpolar Deep Water Intrusions on the Amundsen Sea Continental Shelf, Antarctica. Geophys. Res. Lett. 35, L18602. doi: 10.1029/2008GL034939
Timmermans K. R., van der Wagt B., de Baar H. J. W. (2004). Growth Rates, Half-Saturation Constants, and Silicate, Nitrate, and Phosphate Depletion in Related to Iron Availability for Four Large, Open-Ocean Diatoms From the Southern Ocean. Limnol. Oceanogr. 49 (6), 2141–2151. doi: 10.4319/lo.2004.49.6.2141
Tréguer P., Bowler C., Moriceau B., Dutkiewicz S., Gehlen M., Aumont O., et al. (2017). Influence of Diatom Diversity on the Ocean Biological Carbon Pump. Nat. Geosci. 11, 27–37. doi: 10.1038/s41561-017-0028-x
Turner J., Barrand N. E., Bracegirdle T. J., Convey P., Hodgson D. A., Jarvis M., et al. (2014). Antarctic Climate Change and the Environment: An Update. Pol. Rec. 50 (254), 237–259. doi: 10.1017/S003224741300296
Vaqué D., Lara E., Arrieta J. M., Holding J., Sà E. L., Hendriks I. E., et al. (2019). Warming and CO2 Enhance Arctic Heterotrophic Microbial Activity. Front. Microbiol. 10. doi: 10.3389/fmicb.2019.00494
Venables H., Moore C. M. (2010). Phytoplankton and Light Limitation in the Southern Oceans: Learning From High-Nutrient, High-Chlorophyll Areas. J. Geophys. Res. Ocean. 115 (C2). doi: 10.1029/2009JC005361
Wikner J., Panigrahi S., Nydahl A., Lundberg E., Bamstedt U., Tengberg A. (2013). Precise Continuous Measurements of Pelagic Respiration in Coastal Waters With Oxygen Optodes. Limnol. Oceanogr. Methods 11, 1–15. doi: 10.4319/lom.2013.11.1
Williams P., del Giorgio P. A. (2005). “Respiration in Aquatic Ecosystems: History and Background.”, in Respiration in Aquatic Ecosystems. Eds. del Giorgio P. A., Williams P. (New York: Oxford University Press), 1–17.
Williams C. M., Dupont A. M., Loevenich J., Post A. F., Dinasquet J., Yager P. L. (2016). Pelagic Microbial Heterotrophy in Response to a Highly Productive Bloom of Phaeocystis Antarctica in the Amundsen Sea Polynya, Antarctica. Elem. Sci. Anth. 4, 102. doi: 10.12952/journal.elementa.000102
Wu S.-Y., Hou S. (2017). Impact of Icebergs on Net Primary Productivity in the Southern Ocean. Cryosph 11, 707–722. doi: 10.5194/tc-11-707-2017
Yager P. L., Sherrell R. M., Stammerjohn S. E., Alderkamp A.-C., Schofield O., Abrahamsen E. P., et al. (2012). ASPIRE: The Amundsen Sea Polynya International Research Expedition. Oceanography 25 (3), 40–53. doi: 10.5670/oceanog.2012.73
Yager P. L., Sherrell R. M., Stammerjohn S. E., Ducklow H. W., Schofield O. M. E., Ingall E. D., et al. (2016). A Carbon Budget for the Amundsen Sea Polynya, Antarctica: Estimating Net Community Production and Export in a Highly Productive Polar Ecosystem. Elem. Sci. Anth. 4, 140. doi: 10.12952/journal.elementa.000140
Keywords: bacterial production, bacterial respiration, phytoplankton community composition, microbial loop, biological pump, Amundsen Sea polynya, climate change
Citation: Kim B, Kim S-H, Min J-O, Lee Y, Jung J, Kim T-W, Lee JS, Yang EJ, Park J, Lee S and Hyun J-H (2022) Bacterial Metabolic Response to Change in Phytoplankton Communities and Resultant Effects on Carbon Cycles in the Amundsen Sea Polynya, Antarctica. Front. Mar. Sci. 9:872052. doi: 10.3389/fmars.2022.872052
Received: 09 February 2022; Accepted: 28 April 2022;
Published: 02 June 2022.
Edited by:
Jin Zhou, Tsinghua University, ChinaReviewed by:
Ilka Peeken, Alfred Wegener Institute Helmholtz Centre for Polar and Marine Research (AWI), GermanyJulie Dinasquet, University of California, San Diego, United States
Copyright © 2022 Kim, Kim, Min, Lee, Jung, Kim, Lee, Yang, Park, Lee and Hyun. This is an open-access article distributed under the terms of the Creative Commons Attribution License (CC BY). The use, distribution or reproduction in other forums is permitted, provided the original author(s) and the copyright owner(s) are credited and that the original publication in this journal is cited, in accordance with accepted academic practice. No use, distribution or reproduction is permitted which does not comply with these terms.
*Correspondence: Jung-Ho Hyun, aHl1bmpoQGhhbnlhbmcuYWMua3I=