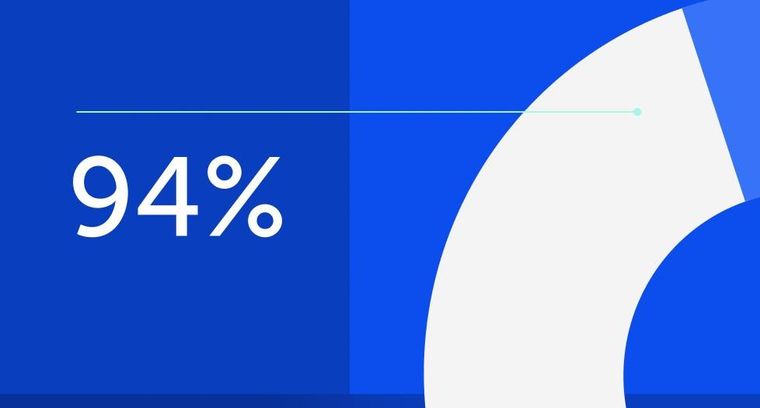
94% of researchers rate our articles as excellent or good
Learn more about the work of our research integrity team to safeguard the quality of each article we publish.
Find out more
ORIGINAL RESEARCH article
Front. Mar. Sci., 20 April 2022
Sec. Marine Biology
Volume 9 - 2022 | https://doi.org/10.3389/fmars.2022.869619
This article is part of the Research TopicBiological Invasions in the Mediterranean SeaView all 13 articles
Biological invasions of jellyfish may critically affect ecosystems and ecosystem services, yet their complex life cycle makes tracking their origins and dispersal vectors a challenging task. Here we combine citizen science observations, oceanographic modeling, and population genetics to track swarms of the invasive nomad jellyfish, Rhopilema nomadica, across the Eastern Mediterranean Sea. Jellyfish observations were recorded by citizens from two Israeli beaches in two consecutive years. A Lagrangian model coupled with a high-resolution 3D hydrodynamic model (SINMOD) was then used to simulate drift of ephyrae from probable polyp bed locations. Finally, mitochondrial DNA (mtDNA) sequence was constructed to examine swarm connectivity. Temporal (both seasonal and interannual) variation in observed swarms generally exceeded spatial differences between the two surveyed beaches. Early detection of swarms by citizens in offshore waters and the higher offshore particle distribution shown by the drift model, point to considerable offshore transport of the swarms. However, a higher probability was found for a nearshore location of the polyp beds, as nearshore origins were more closely correlated to hits on target beaches. R. nomadica released as ephyrae in early spring were likely to reach target beaches 200-300 km down current within two to three months as swarms of young adults in the early summer bathing season. R. nomadica populations exhibited little temporal or spatial genetic differentiation, a typical feature of a species that has recently undergone rapid population expansion. The offshore transport, the lack of genetic structure, and the interannual differences in both hydrodynamics and citizen scientist observations, all indicate decentralized swarm origins. This type of interdisciplinary approach can thus provide viable tools to track bloom formations. Understanding the complexity of jellyfish swarm dynamics supports future management strategies such as forecasting, preparedness and public education.
Jellyfish blooms are a global natural phenomenon that is sometimes considered to be exacerbated by human activities (Purcell et al., 2007). Jellyfish are typically perceived as a nuisance, negatively affecting human interests such as coastal leisure, tourism, fisheries, aquaculture, power, and desalination plants (Purcell et al., 2007; Edelist et al., 2021). They also play an important role in marine ecosystems and can be considered as a boon for fishers, a valuable source of food and other products when harvested sustainably (Edelist et al., 2021). For all these reasons, understanding jellyfish swarm dynamics and tracking their origins are important. Rhopilema nomadica is the largest, most venomous, and most prominent jellyfish species in the Eastern Mediterranean (Galil et al., 1990; Edelist et al., 2020). This species may have entered the Mediterranean Sea via the Suez Canal (Lessepsian migration), though it was rarely recorded outside the Mediterranean. R. nomadica was first recorded in the Mediterranean Sea in 1977 (Lotan et al., 1994), and its population has increased considerably since the early 1980s. Appearing in Israel first (Galil et al., 1990), and to date Israeli beaches are the only ones where polyps of R. nomadica were found in situ (albeit only on settlement plates, see Nakar, 2011; Dror, unpublished), large swarms were then recorded in Lebanon and Syria (Lotan et al., 1994), Turkey, Greece, Malta (Brotz et al., 2012) Egypt (Abu El-Regal and Temraz, 2016; Madkour et al., 2019) and most recently Tunisia, Sardinia and Sicily (Balistreri et al., 2017). R. nomadica swarms affect tourism by stinging bathers, thwart fishing operations by clogging nets and power and desalination plants by blocking seawater intake gear (Edelist et al., 2020). This species is therefore regarded as one of the worst invasive species in the Mediterranean Sea (Streftaris and Zenetos, 2006).
Like most coastal scyphozoan jellyfish species, R. nomadica has a metagenic life cycle, alternating between a free-swimming, sexually reproducing medusa stage, and an asexual benthic polyp stage. Jellyfish polyps may survive over many years (Purcell et al., 2007) and it is thus plausible that swarms will display some degree of spatial consistency (Fleming et al., 2013). R. nomadica polyps were shown to strobilate mostly in mild temperatures of 20-26°C when reared in laboratory conditions (Lotan et al., 1994), they may produce ephyrae almost year-round, and their activity in nature remains unknown. The life cycle of medusae from sessile polyps to sexually mature jellyfish and on to new polyps relies on circulation patterns that provide them with a suitable environment and disperses them over vast expanses of ocean (Johnson et al., 2001; Moon et al., 2010). However, our knowledge of polyp bed locations and the distances travelled by adult medusae are still very limited (Schnedler-Meyer et al., 2018).
Oceanographic models have played a key role in facilitating our understanding of oceanic processes, including jellyfish swarms (e.g. Kawahara et al., 2006; Moon et al., 2010). Shelf currents were among the main factors determining jellyfish distribution and swarm dynamics in the case of Nemopilema nomurai in the East China Sea and East/Japan Sea (Moon et al., 2010). In the eastern Mediterranean the Libyo-Egyptian Current is a major vector spreading jellyfish from the North African coast toward Turkey, via Israeli coastal waters (Edelist et al., 2020). Anticyclonic eddies may develop along this route, to the west of the Israeli coast (Rosentraub and Brenner, 2007), impacting the along and cross-slope transport routes. South of Cyprus, an anticyclonic eddy (Cyprus Eddy, Figure 1) is a fairly persistent feature that significantly impacts Levantine water transport (Mauri et al., 2019). In addition, the surface currents outside the Israeli coastal zone include cyclonic and anticyclonic eddies associated with the Shikmona Gyre system (Figure 1). South-East of Cyprus, there is an eddy system (Latakya Eddy) that can be either cyclonic or anticyclonic. Offshore transport by eddies may thus help explain the large seasonal and interannual fluctuations and complex dynamics in R. nomadica swarming (Edelist et al., 2020).
Figure 1 Study area: Bathymetric map of the Mediterranean Sea, with main circulation patterns (blue arrows) and release and target beaches explored in the study (red circles).
In the absence of frequent oceanographic surveys of gelatinous zooplankton in both coastal and offshore waters, our ability to follow the jellyfish swarm dynamics in situ is often highly limited. Here, reports by the public are essential to complement scientific knowledge. Citizen science initiatives are flourishing in recent years (Guerrini et al., 2018; Johansen et al., 2021). Today, smartphone apps and social networks facilitate collection of local ecological knowledge from the public in unprecedented quantity and quality (Newman et al., 2012). Such endeavors have been in use for observing species distribution and invasive species in general (Giovos et al., 2019) and jellyfish swarms particularly in the Mediterranean for more than a decade (Fleming et al., 2013; Edelist et al., 2020; Marambio et al., 2021).
To be able to understand jellyfish swarm dynamics, it is crucial to focus on the origin of the bloom and the connectivity of the populations in time and space; whether the individuals we observe in a swarm are from common local origins, have drifted from another region, or have accumulated in a specific region from multiple sources (Dawson & Hamner, 2008). R. nomadica adults were recently shown to have a considerable swimming ability (Malul et al., 2019). Adult jellyfish do not only passively drift (horizontally) but may travel up and down in the water column or, to a limited extent, with or even against the current (Fossette et al., 2015), while juvenile stages exhibit a more passive drift behavior. Therefore, oceanographic current models can be useful in studying swarms especially for ephyrae and juvenile stages, even though they predominately assume a passive drift. Similarly, when locations of the polyp populations are not known, as is the case with R. nomadica in the Mediterranean, the population genetic approach using adult specimens forming the swarm can provide valuable information on the scale and origin of the swarm. Population genetics, using either mitochondrial DNA cytochrome oxidase I (mtCOI) as a marker or a microsatellite approach, have been used to clarify the connectivity or distinctness of jellyfish aggregations for several jellyfish taxa (e.g., Stopar et al., 2010; Lee et al., 2013). For some taxa, the population genetic approach enabled clear population subdivisions defining locations where swarms occur among geographically isolated populations (Lee et al., 2013). Conversely, some taxa have shown low genetic diversity and lack of genetic structure (Stopar et al., 2010). For R. nomadica, several microsatellite loci have been identified by a whole genome sequencing approach (Douek et al., 2020). Long-term changes in population genetic features of R. nomadica were recently studied by looking at the mtCOI haplotype diversity in the Eastern Mediterranean (Giallongo et al., 2021). The Giallongo et al. (2021) dataset concluded that all specimens of R. nomadica collected in the area belong to a single population, lacking clear genetic structure and there was no significant difference in the four seasons along a 12-year sampling period.
In the present study, we applied a high-resolution numerical model of Eastern Mediterranean current vectors to estimate distribution time and track distance scales from probable polyp habitats to areas in which mature R. nomadica swarms were observed by a citizen science project. In addition, we used a complementary population genetic approach, to determine the homogeneity of these R. nomadica swarms observed in the coast of Israel.
In the following we describe methodology for: a) distribution of jellyfish using data from the Israeli citizen science project Meduzot Ba’am (“Jellyfish Inc.”), b) hydrodynamic tracking used to simulate dispersal from ephyra release to adult swarming along the Levantine coast and c) population genetics of medusae collected along the coast.
Jellyfish sightings have been recorded by volunteer reporters (citizens) along the entire Israeli coastline since 2011, highlighting massive swarms in the June-July bathing season (Edelist et al., 2020). A subset of R. nomadica observations was thus extracted to focus on the two popular urban bathing beaches of Dado (Haifa) and Gordon (Tel Aviv) for the bathing season (calendar weeks 18-40) of 2017 and 2018. Observations were recorded via a dedicated internet website (www.meduzot.co.il) and the subset was extracted from Pangaea - an Open Access data archiving and publication repository (https://doi.pangaea.de/10.1594/PANGAEA.897389). For the present study, R. nomadica was chosen since it is the most common, conspicuous, stinging, and recognizable species in the Levant. Across the entire eight-year database, out of 6,033 sightings of eight scyphozoan jellyfish species, R. nomadica was by far the most common with 4,031 sightings (66.8%). Moreover, other scyphomedusa species in the region, mainly Rhizostoma pulmo, Phyllorhiza punctata, Aurelia sp., Marivagia stellata and Cotylorhiza spp., typically co-occur and intermix with R. nomadica during summer swarms (Edelist et al., 2020). The locations were chosen to represent main urban bathing beaches with sufficient spatial and temporal reporting coverage. Detailed information facilitating species identification was provided on the website. The distance from shore, type of activity, jellyfish species encountered, their quantity and size, whether stranded jellyfish were observed on the beach and if volunteer reporters were stung by jellyfish were also recorded (See Edelist et al., 2020).
The relative abundances of jellyfish observed by volunteers was ranked according to categorical values of “0”, “2”, “10” and “50”, denoting “zero”, “few”, “medium” and “swarm” situations respectively. The average number of jellyfish per report per week [Eq. (1)] was used to create JSI - a Jellyfish Swarm Index (Edelist et al., 2020), defined as:
where Ai is the binned quantity (0, 2, 10 or 50) of R. nomadica per report i, and n is the number of reports per week. The main modification in the JSI calculated here (in comparison to the JSI defined in Edelist et al., 2020) was that the JSI was calculated per week rather than per month, in order to match the higher resolution of the oceanographic model. Differences between mean ranked abundances were further tested by Kruskal-Wallis test – a nonparametric one-way ANOVA on ranks.
The present study examines how currents along nearshore areas in the Eastern Mediterranean Sea can affect horizontal drift of particles with a 40 m depth threshold, targeting beaches located down current from the release sites (Figure 1).
Jellyfish may modulate their swimming behavior in relation to currents, thereby increasing swarm formation or reducing the probability of stranding (Fossette et al., 2015). Despite the fact that large scyphozoan jellyfish like R. nomadica show a certain degree of active mobility (Malul et al., 2019), a passive drift in relation to Levantine currents was assumed here, as summer swarms of R. nomadica are comprised of more juvenile stages than winter swarms (Edelist et al., 2020). Using passive drifting as a proxy to track jellyfish blooms is a likely assumption especially for ephyrae and young medusae, since drift is the principal dispersal mechanism for early medusae life stages (Moon et al., 2010).
In the present study, the SINMOD model framework has been used to track the movement of passive tracers to the Israeli shores. SINMOD is a coupled 3D model developed over the past 30 years to simulate physical (hydrodynamic) and biological processes (see Wassmann et al., 2010). The model’s hydrodynamic component is based on the primitive equations that are solved using a z*-coordinate regular grid with square cells.
A model domain covering the Mediterranean Sea with 4 km horizontal resolution and 43 vertical layers was run from 2010 to 2018. Tides from TPXO (www.txpo.net/global), freshwater supply from various sources and atmospheric forcing (ERA5 from European Centre for Medium-Range Weather Forecasts, ECMWF) were applied. A high-resolution model with 0.8 km resolution was nested in the 4 km model and run for 2017 and 2018. The high-resolution model is computationally expensive to run but gives a good representation of ocean dynamics including gyres, coastal currents, and eddies. The spatial scale of the dynamic mesoscale field can be calculated from the internal Rossby radius of deformation and was previously found to be 5-12 km in the Mediterranean Sea (Pinardi and Masetti, 2000), hence the high resolution model will significantly improve representation of mesoscale variability.
A Lagrangian particle tracking approach allows examination of multiple release points for simulated R. nomadica ephyrae. The particles (ephyrae) drift passively with currents horizontally but are allowed to migrate upwards if they were mixed or advected below 40 m depth. Particles were released at both 8 m and 15 m depths at 269 positions (in total 538 positions) along the Egyptian coast between Alexandria and Port Said. The position of the particles was updated every timestep (80 s) and saved every hour for later analysis. It has been shown that R. nomadica accelerates strobilation rates when Levantine waters begin to warm, generally around mid-April (Lotan et al., 1994). We therefore chose April 18th as the start date for release of the particles both in 2017 and 2018, and the simulation was allowed to run until August 31st. The simulations were run for 2017 and 2018, releasing 538 new particles every hour. The particles were not allowed to grow or die during the simulations and were tracked from release to the end of the simulations.
To date Israeli beaches are the only ones where polyps of R. nomadica were found in situ (Nakar, 2011; Dror, unpublished). We therefore ran a second release from Israel. Particles were released from 55 locations (at 8m and at 15m for each, in total 110 particles released per hour) near the coast off Dado beach in Israel starting on April 18th to the end of August. The aim was to test further spread of the particles to Eastern Turkey - an area where R. nomadica is long known to swarm (Avsar et al., 1996). In addition, we calculated the drift time from the Dado release sites, until the particles return to Dado by recirculation in an eddy offshore Dado. While Iskenderun Bay only receives a fraction of the longshore counterclockwise current, R. nomadica has long been known to swarm beaches in this region (Avsar et al., 1996; Duysak et al., 2013).
Rhopilema nomadica were sampled in the Eastern Mediterranean Sea in different seasons in 2020-2021 and from three locations along the Israeli coast. For the analysis we defined those areas as South and Centre (relating to the Tel-Aviv region), and North (relating to the Haifa region). We focus on two time periods: summer (June-July 2020) and winter (January-March 2021 (for details see Supplementary Material). Most medusae were hand-collected from a small boat, 0.5-1.5 nautical miles offshore where bottom depth ranged between 5 to 35m by means of a dip net and 20 L buckets. Tissue samples were also taken from several freshly stranded medusae that had washed to shore. Whenever possible, bell diameter, weight, sex and sea surface temperature were documented. Pieces of gonads or oral arms were removed and immediately fixed in 70% ethanol. DNA from specimens was extracted using a modified Chelex rapid-boiling procedure as explained in Granhag et al. (2012). The mtCOI gene was amplified using primers FFDL and FRDL (Armani et al., 2013). The polymerase chain reaction (PCR) was conducted in a total volume of 20 µl, containing 1.0 µl of DNA template, 0.4 μL Phire® Hot Start DNA polymerase, 4 μL of Phire ® reaction buffer, 1μL of each primer (final concentration 0.2 mmol), 0.4 μL of DNTP, 0.6 μL of 3% DMSO, and 1.6 μL nuclease-free water. PCR conditions consisted of an initial cycle at 95°C for 5 min, 40 cycles of 94°C for 30 sec, 50°C for 30 sec, 72°C for 90, and a final extension at 72°C for 10 min. PCR products that yielded a clear band on 1.5% agarose gel by electrophoresis were sent to Macrogen Europe for purification and sequencing. All samples were sequenced in the forward and reverse direction to assure the accuracy of description for each polymorphic site and nucleotide haplotype. The resulting nucleotide sequence electropherograms were checked by eye for poor base calls using Chromas Lite 2.1 (Technelysium Pty Ltd). During the process misreads were corrected, and poorly resolved terminal portions of sequences were discarded. High-quality sequences were assembled using BioEdit software (Hall, 1999), and identities of sequences were confirmed by BLAST search in GenBank (>98% identity). Individual sequences were aligned with the MAFFT online service (Katoh et al., 2019). Genetic diversity within populations was estimated by computing haplotype diversity (H) and nucleotide diversity (π) (Nei, 1987) using DnaSP v5 (Librado and Rozas, 2009). A median-joining network showing the relationships between the mtDNA haplotypes was constructed using the PopART (http://popart.otago.ac.nz/howtocite.shtml; Bandelt et al., 2000). Genetic differentiation was calculated by means of pairwise FST values using 10,000 permutations in ARLEQUIN 3.1 (Excoffier et al., 1992) within the analysis of molecular variance (AMOVA) framework (Excoffier et al., 1992). The neutrality statistics Tajima’s D and Fu’s FS were used to test constant population size versus population growth (Ramos-Onsins and Rozas, 2002) using DnaSP v5. Also, mismatch distributions (Rogers and Harpending, 1992) were generated in DnaSP. Although there is a mismatch between the years used for the simulation of jellyfish distribution (2017-2018) and the years when jellyfish were collected for genetic analyses (2020-2021), we assume the current regimes do not change on an annual basis and that the genetic makeup of R. nomadica in this region is stable and does not change rapidly, as also noted in Giallongo et al. (2021). The sequences reported in the study have been deposited in the European Nucleotide Archive repository with accession numbers: OW055719-OW055735.
Jellyfish reports in the two study beaches for the study period yielded 701 observations of either “R. nomadica” (n=198) or “zero jellyfish” (n=503). Most reports were submitted for the inshore zone, from the coastline to a distance of 200 m from shore, with Haifa beaches characterized by a higher quantity and proportion of offshore reports in both study years (Table 1).
Table 1 Number of inshore and offshore observations of R. nomadica in 2017 and 2018 in weeks 18-40 (May to September) in Haifa and Tel Aviv beaches.
Both Haifa and Tel Aviv beaches were impacted by jellyfish swarms in both study years. However, a longer and more intense early summer swarm was recorded in 2017; while 2018 was characterized by a shorter early summer swarm followed by a later autumn swarm in mid-September (Figure 2). Juveniles (0-10 cm) were only observed in the late summer 2018 swarm and jellyfish typically arrived as young adults (11-30cm) to full adult (30-60) sizes, with a few rare observations of larger specimens in 2017 (Figure 2, although large specimens >60 were viewed in the summer and other seasons as well – see Edelist et al., 2020).
Figure 2 Weekly Jellyfish Swarm Index (JSI) in Tel Aviv and Haifa beaches in the summers of 2017 and 2018 (A), and total number of R. nomadica reported per week, ranked by size (bell diameter) distribution (B).
Swarms were detected at the northern Dado Beach (Haifa) before they were observed in the southern (Tel Aviv) Gordon Beach (Figure 2A). In 2017, swarms arrived earlier and were larger and more diverse in size composition, including extra-large specimens >60cm, while the 2018 autumn swarm consisted of predominately smaller individuals (Figure 2B). On average, more R. nomadica individuals per report were sighted offshore (>200 m from the beach) than inshore at both beaches, in both study years (Figure 3), and distance from shore was found to be a statistically significant factor in determining swarm probability (Kruskal-Wallis H(2) = 70.46, P = 5.551e-16).
Figure 3 Mean number of jellyfish per observation (JSI) in inshore (0-200 m, n=612) mid-range (200 m – 1,000 m, n=71) and offshore (1,000 m – 10,000 m, n=17) observations in the two study years.
Over the two study periods, significant differences in current patterns were simulated. The dynamic structure of Levantine eddies/gyres and currents are known to vary seasonally and interannually (Özsoy et al., 1993). The weak longshore current recorded in May from Egypt towards Israel intensified considerably in June in 2018 and less so in 2017 (Figure 4).
Figure 4 Monthly average surface current velocity and direction used in the particle model for May (left) and June (right) in 2017 (top) and 2018 (bottom).
The Levantine longshore currents either entered the Shikmona eddy, meandering clockwise south of Cyprus, or flowed northwards closer to shore carrying particles (and jellyfish) towards Turkey (Figure 4). Looking into current maps for surface, 8m and 15m depth from May to August, we generally found that the current is a bit weaker at 15m than at shallower depths for the release area and eastwards. This is mainly valid for the shallow shelf and less so for deeper parts of the study area. When we compared the particle drift patterns for particles released at 8m and 15m, only few differences were discernible in the model results, and we used an averaging of results from both depths in further analysis.
A hindcasting approach was used here, based on citizen reports of adult medusae. These led to postulated ephyra release locations, from which the drift of particles was simulated to produce “hits” on the target beaches. Nearshore particle released from the continental shelf (<200m depth) in the Eastern region of Bardawil and Port-Said to Damietta (Egypt) were shown to have the highest correlation with hits on both target beaches in both study years (Figure 5).
Figure 5 Colour-coded release points off the Egyptian coast according to particle hit distribution on Israeli target beaches in 2017 and 2018, averaged across both release depths of 8 m and 15 m. The numbers refer to hits at Gordon (Tel Aviv) and Dado (Haifa) for every release point.
Interannual differences in the current regime may affect results somewhat (in 2018 stronger currents could have widened hindcasting range westwards), but overall offshore release points and release from points further west in the Baltim-Alexandria region west of the Nile delta showed a significantly lower hit correlation with the two target beaches than the Bardawil-Port Said-Damietta area, (Figure 5).
The final (August 18th) distribution of released particles is shown in Figure 6. The highest number of hits was found in the shelf area along the coasts of Egypt and Israel, while some particles meandered offshore and into the gyres. Fewer particles drifted with the longshore currents to the coasts of Lebanon, Syria and Turkey (Figure 6).
Figure 6 Total number of particle hits within each model gridpoint, released from April 18th to August 31st 2018. Release locations along the Egyptian coast are shown in Figure 5.
Overall, the inshore areas east of Alexandria, including the nutrient rich Nile delta were tracked as the probable origins of Israeli summer swarms (Figure 5). The early summer swarms probably emanate from enhanced strobilation and release as water begins to warm in April, reaching Tel Aviv and Haifa in 47.1 and 58.3 days respectively (Figure 7).
Figure 7 Particle hit distribution on target beaches and mean drift time in days from release locations along the Egyptian coast (Between Alexandria and Bardawil) to Gordon (Tel Aviv) and Dado (Haifa) beaches in 2017 and 2018.
Most particles released from the Israeli coast hit the beaches of Lebanon and Syria, and lower numbers reached Turkey. There was also considerable meandering of particles westwards towards the gyres and the Cypriot coast (Figure 8).
Figure 8 Total number of particle visits within each model gridpoint, released from Dado beach (Haifa) from April 18th to August 31st of 2018. Insets: colour-coded release points off the coast of Haifa according to particle hit distribution on target beaches in 2017 (upper, left) and 2018 (lower, left), averaged across both release depths of 8m and 15m. Inset numbers refer to hits at Iskenderum from the release points, so the red points are connected to the target beach with more than 31 particles (2017) and small black points less than two particles (2018).
In 2018, there was a significantly lower hit correlation to Iskenderun than in 2017, and a longer mean duration of 93.6 days of particle drift time from Haifa to Iskenderun (Figure 9 and Table 2).
Figure 9 Particle hit distribution on target beaches and mean drift time in days from release locations in Haifa region from April 18th to August 31st to Iskenderun, Turkey.
In the simulation from April 2017 and 2018, particles were released from 55 points in northern Israel (Figure 8). In 2017, there were high hit rates of particles reaching Iskenderun (Figure 8) and the mean transportation time was 69.1 days (Table 2 and Figure 9).
Some particles released near Haifa did not follow the coast northwards as Figure 8 indicates, but instead went into the Shikmona eddy or southwestwards before returning to Haifa. Counting returned particles older than average drifttime from offshore Egypt (57 days), gave mean/standard deviation of 69.4 d/14.4 d drift time for 2017 and 72.1 d/16.9 d for 2018, indicating the probability of cyclic swarming dynamics. In 2017 the number of paticles from Haifa to Iskenderun was higher than particles from Haifa to Haifa, but for 2018 the situation was reversed.
To assess the genetic diversity of R. nomadica, a sequence length of 433 bp of the mtCOI gene was analysed from a total of 42 R. nomadica individuals sampled from the three areas during the summer of 2020 and winter of 2021). Over the whole data set collected in this study, 22 polymorphic sites, of which 9 were parsimony informative, were identified leading to the definition of 18 haplotypes. Haplotype richness was high (h = 0.90 ± 0.03 on average), but differentiation among haplotypes was modest (π (%) = 0.49 ± 0.5). All geographical regions showed similar haplotype and nucleotide diversity (Table 3).
Table 3 Sample sizes and standard diversity indices for partial mtCOI sequences of Rhopilema nomadica sampled at three locations (North, Centre and South) and two seasons (summer 2020 and winter 2021).
A haplotype network based on mtCOI supports the non-differentiation of R. nomadica in the various regions along the Mediterranean coast of Israel. Analysis of molecular variance (AMOVA) showed that maximum variance was obtained within geographic regions (100%) while the variation among populations displayed negligible variance. This picture was concordant with pairwise FST values (Table 4).
Table 4 Pairwise FST values among samples of Rhopilema nomadica in three locations the coast of Israel (p>0.05).
Similarly, when comparing specimens collected from the coast of Israel in summer 2020 and the same region in winter 2021 (n=39), 22 polymorphic sites, of which 8 were parsimony informative, were identified leading to the definition of 18 haplotypes (Figure 10) and no significant inter-population genetic divergence was observed (FST=-0.01, p> 0.05). Moreover, all measures of genetic diversity and population structure calculated across populations pointed to low genetic structure regarding seasonal variations.
Figure 10 Median-joining network showing the relationships between 18 haplotypes detected by sequencing R. nomadica collected from the coast of Israel during summer 2020 and winter 2021 for the partial mitochondrial DNA cytochrome oxidase I (mtCOI) region. Circle sizes are approximately proportional to haplotype frequency: the smallest circle represents a single individual; the largest circle represents 10 individuals. Each connection represents a single mutation, and small open black dots represent missing intermediate haplotypes.
Despite the low sample number, the overall mismatch distributions of mtCOI haplotypes from the coast of Israel displayed unimodal distributions similar to the curve expected when a population has undergone rapid population growth in the past (Roman and Darling, 2007). Moreover, tests of neutrality also showed low and significant values of Fu’s FS (−11.93, p < 0.05) and Tajima’s D (−1.099, p < 0.05), further pointing to a recent demographic expansion. The low subdivision observed across space and time was also recorded across sizes, further strengthening the notion of a single unstructured population.
Currents are an important factor that can alter the formation of jellyfish swarms, in addition to productivity, storms, light, moon phase, substrate availability, salinity, and temperature (Gibbons et al., 2016). It was suggested that in the Levant, swarming synchronicity of R. nomadica is triggered by temperature (Lotan et al., 1994). However, the presence of large swarms both in winter (Edelist et al., 2020) and/or autumn, as shown in this study (Figure 2), suggests that the process is more complex. Here, our model suggests that timing of R. nomadica swarm arrival is congruent with ephyrae releases hundreds of km up current, and our observations suggest that the typical expected drifting period of early life-stages of R. nomadica is two to three months, before swarming is recorded at the target beaches. The growth rates of R. nomadica in the wild are unknown, and as ephyrae of metagenic jellyfish species may spend their early life period below the benthic boundary layer (Higgins et al., 2008), an earlier release and delayed transport may also occur. In the 2017 bathing season, a long, dense swarm of R. nomadica of mixed sized specimens was recorded in both Israeli beaches from mid-June to late July, while in 2018 a low-density swarm was recorded in early July followed by another low-density autumn swarm in September (Figure 2). Such autumn swarms are relatively rare and have only been recorded twice (2013 and 2018) over the past decade, while winter swarms comprised of large adult specimens are much more common (Edelist et al., 2020). In 2017, currents were strong in April and May and weakened in June while 2018 showed an opposite trend. In this study, we tackled the question - did the weaker longshore current in May 2018 lead to a smaller and later swarm? Temperatures in the Levant were relatively high throughout the winter of 2018 (Herut et al., 2019), so is there a logical explanation for the smaller, later swarm? Usually, a single factor is too simplistic to explain interannual variations in jellyfish phenology and swarming, and other factors like productivity, salinity, and storm conditions need to be taken into consideration to understand the full complexity of bloom dynamics (Boero et al., 2008). Here, we propose that such complexity may involve the entry of the jellyfish into offshore eddies (Figures 6, 8) or alternative factors affecting strobilation in the polyp beds. While no observations of R. nomadica in the offshore Levantine gyres are known from literature, their presence in offshore pelagic and mesopelagic realms should not be excluded a priori and further studies investigating the presence of R. nomadica in open offshore waters are needed.
The present study suggests that ephyrae of R. nomadica travel hundreds of kilometers over a period of 2-3 months to reach beaches down-current as young adults during the bathing season. The simulations of current-induced particle drift provide scenarios for the nearshore jellyfish observations in the Eastern Mediterranean Sea, as well as some offshore meandering, triggered by a complex system of eddies and gyres (Figures 4, 6 and 8). A recent study (Kuplik et al., 2021) has shown that R. nomadica is able to successfully utilize scarce resources found in hyper-oligotrophic environments, such as the Levantine offshore waters. This property of R. nomadica may allow survival during extended offshore migrations, e.g. in the Shikmona-Cyprus eddies, or the counterclockwise transport from the Levant longshore to Turkey and then back through the gyre system. The arrival of large specimens in winter (Edelist et al., 2020) and the genetic connectivity between summer and winter specimens may support this notion. However, for the early summer bathing season during which the large dense mixed-size swarms are recorded, nearshore releases were shown to have a higher hit probability on target beaches than offshore releases (Figure 5). Ephyrae released from the Bardawil-Damietta area will typically reach Israeli beaches located 200-300 km down current in 20-40 days, while release from the Alexandria-Baltim area located 300-600 km up current from target beaches will take up to 90 days. This may of course change with variation in current velocities, but an overall mean arrival time of 55-60 days (Figure 8 and Table 2) was found for the study duration in the spring. Similar results are attained when particles are released from Israeli beaches, reaching areas 200 km down current within a month and 400 km (Iskenderun) within three months (Figure 8 and Table 2).
Surprisingly, jellyfish were occasionally spotted in the north (Haifa) before they were reported 100km up current in Tel Aviv (Figure 2). When observing the Meduzot Citizen Science database we find that the reason for this may be that at Dado Beach in Haifa, many of the reporters are long-distance swimmers that spotted the jellyfish offshore (twice that of Tel Aviv – Table 1) while most Tel Aviv Beach observations were submitted by inshore bathers. This pattern of an offshore positioning of the swarm (>1km from shore) before actually hitting the beaches is also in line with the oceanographic data that predicted a larger number of hits in offshore locations (Figure 6).
Where jellyfish swarm, they are likely to leave polyps on the seabed if the right conditions and suitable habitat exist. The present study only models about a quarter of the Mediterranean distribution area of R. nomadica (see Balistreri et al., 2017; Edelist et al., 2020), and while polyp beds located in Egypt may lead to outbreaks on Israeli beaches, Tunisian polyp beds could cause swarms along the Libyan or Egyptian coasts. In 2017, R. nomadica swarms were reported from the Israeli coast from June to July while they occurred along the Egyptian coast in mid-June at Port Said (Madkour et al., 2019) and at Baltim and Alexandria from late June to early July (http://www.egyptindependent.com/sea-turtlesto-counter-jellyfish-on-shores/). A swarm covering the entire South-Eastern Mediterranean simultaneous basin thus remains a viable possibility. The availability of observations in the present study was limited to Israeli beaches, and the oceanographic model that tracked them up-current inevitably pointed at potential polyps located in Northern Sinai and the Nile delta region. Similarly, particles released from the Israeli coast showed how Israeli polyp beds may support swarm formation impacting beaches in East Turkey, or even returning (Figure 8).
One of the outstanding features of R. nomadica in the winter months is their exceptionally large size, up to 90 cm bell diameter as observed in Israeli coastal waters (Edelist et al., 2020). This size disparity may be a result of currents that carry the summer populations into the gyres and return them to the Levantine shores in winter. The population genetic data found in this study supports this possibility since the specimens sampled did not show any strong temporal or spatial genetic differentiation of the populations. Another possibility is that such large specimens (>60cm) could have been released as ephyrae in Western Egypt, Libya or even Tunisia (where autumn R. nomadica swarms have been reported in recent years by Balistreri et al., 2017). Peculiarly, R. nomadica swarms are seldom reported in Cyprus (www.ciesm.org/gis/JW/build/JellyBlooms.php) despite currents that may carry them both north and south of the Island (Figures 4, 8). A wider population genetic study with specimens collected from these regions is imperative for the study of both origins and spread of R. nomadica in the region.
Kawahara et al. (2006) and Moon et al. (2010) showed that Nemopilema nomurai swarms can travel thousands of km in the Tsushima current along Japanese coasts from July to November. R. nomadica may similarly traverse the ~2000km distance from Tunisia to Israel, or the ~3000km long Egypt-Israel-Lebanon-Syria-Turkey-Rhodes-Egypt circuit (Figure 6). Said et al. (2013) showed that drogue drifters released at 15 m depth near the Rhodes gyre may reach the Egyptian longshore current and drift eastwards at mean velocities of 20-25 cm/s (17.2-21.6 km/day). These values change geographically and throughout the year and the longshore current may at times be an order of magnitude slower. Mean monthly current velocities over the Israeli shelf as measured by Rosentraub and Brenner (2007) were only 0.86 km/day (July) to 10.36 km/day (February). If jellyfish do travel with the currents at these velocities, it should take a specimen only 18 days in February and 30 days in July to cover a distance of 200 km.
Initially, low winter temperatures were thought to be the main barrier to westward spread of R. nomadica beyond Greece in the northern Mediterranean, and an absence of R. nomadica from the coastal waters of Egypt was thought to result from lack of sufficient rocky substrates necessary for polyp settlement (Lotan et al., 1992). Since then, however, these supposed barriers proved no match for R. nomadica, which is now known to swarm beaches as far west as Sardinia and Tunisia (Balistreri et al., 2017) with numerous recent reports of swarms off Egyptian coasts (Abu El-Regal and Temraz, 2016; Madkour et al., 2019). Swarms may thus be much larger than the 100 km estimated by Galil and Zenetos (2002) as Alexandria and Baltim are located >500 km up current from Israel. Moreover, a synchronous bloom across the entire SE Mediterranean basin also seems reasonable, with numerous polyp beds contributing to these pan-basin blooms. Seascape genetics can potentially align hydrodynamic modelling with population genetic data and help identify population differentiation in swarms over local vs. broader spatial scales. Tools that are more advanced and better suited for populations genetic studies than mtCOI exist today and can be applied. For R. nomadica, future studies should also extend to pan-basin and cross-basin swarm dynamics, including reports from more target beaches over longer periods and sampling of specimens from countries across the Mediterranean for a full population genetics study.
A recent genetic study of R. nomadica populations indicates that the Israeli medusae are all part of a single Levantine population (Giallongo et al., 2021), with phylogenetic trees showing no geographical or temporal partitioning, but haplotype numbers indicating a small north-south gradient. Our findings agree with these results, and no significant spatial and temporal gradients were found in the medusae analysed. Some scyphozoan species, such as Pelagia noctiluca (Stopar et al., 2010), Nemopilema nomurai (Dong et al., 2016) and Periphylla periphylla (Majaneva pers. comm), have shown similar lack of genetic structure at comparable geographical scales over several hundred kilometres. However, other species have shown a clear geographically structured pattern; for example, Cyanea capillata and Aurelia aurita (Majaneva et al. In Prep)., particularly when observations cover different bodies of water (e.g. the Baltic and North Sea). The single unstructured Israeli population, and high number of haplotypes and singletons in R. nomadica were proposed by Giallongo et al. (2021) to indicate recurring independent introductions, or an open corridor augmenting gene flow. While this may be the case inside the Mediterranean, R. nomadica is unique in the sense that large swarms have never been recorded anywhere outside the Mediterranean, and the nearest ‘origin’ specimens ever recorded were >2,000 km away from the mouth of the supposed invasion vector, the Suez-Canal. The unimodal distribution found here for mtCOI haplotypes resembles that of a population that has experienced a recent demographic expansion (Roman and Darling, 2007; Giallongo et al., 2021). Therefore, while it is still unknown how (and whether) R. nomadica first entered the Mediterranean, ballast water or hull fouling scenarios should be favoured over direct drift\swimming through the canal. Recurring wide scale swarm observations and the oceanographic modelling of the currents driving them from various locations suggest a decentralized, inshore origin for R. nomadica swarms in the Mediterranean, rather than a single polyp bed.
The many unresolved questions regarding R. nomadica’s successful ongoing colonization of the Mediterranean merit a wide spatial future study, that includes examination of specimens from all Mediterranean areas alongside Indo-Pacific ‘origin’ populations, if such can be found. As shown here, a combination of citizen-science with oceanographic and molecular approaches can be instrumental in such applications.
The datasets presented in this study can be found in online repositories. The names of the repository/repositories and accession number(s) can be found below: European Nucleotide Archive OW055719-OW055735.
DE and DA drafted the manuscript and oversaw acquisition of jellyfish observation data, ØK and IE carried out oceanographic modelling and spatial analysis, NA, SM and HD carried out genetic sampling and analysis. All authors contributed to manuscript writing and editing.
This work was supported by the GoJelly Project, EU Horizon 2020 [grant number 774499].
The authors declare that the research was conducted in the absence of any commercial or financial relationships that could be construed as a potential conflict of interest.
All claims expressed in this article are solely those of the authors and do not necessarily represent those of their affiliated organizations, or those of the publisher, the editors and the reviewers. Any product that may be evaluated in this article, or claim that may be made by its manufacturer, is not guaranteed or endorsed by the publisher.
We would like to thank all the citizens who contributed jellyfish observations. We further wish to thank Dr. Zafrir Kuplik, who commented on the manuscript and helped improve it.
The Supplementary Material for this article can be found online at: https://www.frontiersin.org/articles/10.3389/fmars.2022.869619/full#supplementary-material
Abu El-Regal M., Temraz T.. (2016). Blooming of Nomad Jelly Fish Rhopilema Nomadica Along the Egyptian Mediterranean Coasts. Commun. Int. Explor. Sci. Mer Mediterr. Rapp 41, 489–490
Armani A., Tinacci L., Giusti A., Castigliego L., Gianfaldoni D., Guidi A. (2013). What is Inside the Jar? Forensically Informative Nucleotide Sequencing (FINS) of a Short Mitochondrial COI Gene Fragment Reveals a High Percentage of Mislabeling in Jellyfish Food Products. Food Res. Int. 54 (2), 1383–1393. doi: 10.1016/j.foodres.2013.10.003
Avsar D., Çevik C., Türeli C. (1996). İskenderun Körfezi Için Yeni Bir Tür Olan (Rhopilema Nomadica)’nın Biyometrisi Ve Yumurtalık Koyundaki Bulunurluğu. XIII. Ulusal Biyoloji Kongresi, 17–20.
Balistreri P., Spiga A., Deidun A., Gueroun S. K. M., Nejib M., Yahia D. (2017). Further Spread of the Venomous Jellyfish Rhopilema Nomadica Galil, Spannier and Ferguson 1990 (Rhizostomeae, Rhizostomatidae) in the Western Mediterranean. BioInvasions Rec 6. doi: 10.3391/bir.2017.6.1.04
Bandelt H. J., Macaulay V., Richards M. (2000). Median Networks: Speedy Construction and Greedy Reduction, One Simulation, and Two Case Studies From Human mtDNA. Mol. Phylogenet. Evol. 16 (1), 8–28. doi: 10.1006/mpev.2000.0792
Boero F., Bouillon J., Gravili C., Miglietta M. P., Parsons T., Piraino S. (2008). Gelatinous Plankton: Irregularities Rule the World (Sometimes). Mar. Ecol. Prog. Ser. 356, 299–310. doi: 10.3354/meps07368
Brotz L., Cheung W. W. L., Kleisner K., Pakhomov E., Pauly D. (2012). Increasing Jellyfish Populations: Trends in Large Marine Ecosystems. Hydrobiologia 690, 3–20. doi: 10.1007/s10750-012-1039-7
Dawson M., Hamner W. (2008). A Character-Based Analysis of the Evolution of Jellyfish Blooms: Adaptation and Exaptation. Hydrobiologia 616, 193–215. doi: 10.1007/s10750-008-9591-x
Dong Z., Liu Z., Liu D., Liu Q., Sun T. (2016). Low Genetic Diversity and Lack of Genetic Structure in the Giant Jellyfish Nemopilema Nomurai in Chinese Coastal Waters. Mar. Biol. Res. 12, 769–75. doi: 10.1080/17451000.2016.1196818
Douek J., Harbuzov Z., Galil B. S., Rinkevich B. (2020). Developing Novel Microsatellite Markers by NGS Technology for Rhopilema Nomadica, an Invasive Jellyfish. Mol. Biol. Rep. 47, 4821–4825. doi: 10.1007/s11033-020-05533-0
Duysak Ö., Yılmaz A., Mazlum Y. (2013). Metal Concentrations in Different Tissues of Jellyfish (Rhopilema Nomadica Gali) in Iskenderun Bay, Northeastern Mediterranean. Int. J. Anim. Vet. Adv. 12, 1109–1113. doi: 10.3923/javaa.2013.1109.1113
Edelist D., Angel D. L., Canning-Clode J., Gueroun S. K. M., Aberle N., Javidpour J., et al. (2021). Jellyfishing in Europe: Current Status, Knowledge Gaps, and Future Directions Towards a Sustainable Practice. Sustainability 13, 12445. doi: 10.3390/su132212445
Edelist D., Guy-Haim T., Kuplik Z., Zuckerman N., Nemoy P., Angel D. L. (2020). Phenological Shift in Swarming Patterns of Rhopilema Nomadica in the Eastern Mediterranean Sea. J. Plankt. Res. 42 (2), 211–219. doi: 10.1093/plankt/fbaa008
Excoffier L., Smouse P. E., Quattro J. M. (1992). Analysis of Molecular Variance Inferred From Metric Distances Among DNA Haplotypes: Application to Human Mitochondrial DNA Restriction Data. Genetics 131 (2), 479–491. doi: 10.1093/genetics/131.2.479
Fleming N. E. C., Harrod C., Houghton J. D. R. (2013). Identifying Potentially Harmful Jellyfish Blooms Using Shoreline Surveys. Aquacult. Environ. Interact. 4, 263–272. doi: 10.3354/aei00086
Fossette S., Gleiss A. C., Karpytchev M., Hays G. C. (2015). Current-Oriented Swimming by Jellyfish and Its Role in Bloom Maintenance. Curr Biol. 25, 342–347. doi: 10.1016/j.cub.2014.11.050
Galil B. S., Spanier E., Ferguson W. W. (1990). The Scyphomedusae of the Mediterranean Coast of Israel, Including 2 Lessepsian Migrants New to the Mediterranean. Zool. Med. 64, 95–105.
Galil B. S., Zenetos A. (2002). “A Sea Change – Exotics in the Eastern Mediterranean,” in In Invasive Aquatic Species of Europe: Distributions, Impacts and Management, vol. pp . Eds. Leppäkoski E., Olenin S., Golasch S. (Dordrecht, the Netherlands: Kluwer Academic Publishers), 325–336. doi: 10.1007/978-94-015-9956-6_33
Giallongo G., Douek J., Harbuzov Z., Galil B., Rinkevich B. (2021). Long-Term Changes in Population Genetic Features of a Rapidly Expanding Marine Invader: Implication for Invasion Success. Biol. Invas 23 (8), 2541–2552. doi: 10.1007/s10530-021-02521-8
Gibbons M. J., Boero F., Brotz L. (2016). We Should Not Assume That Fishing Jellyfish Will Solve Our Jellyfish Problem. ICES J. Mar. Sci. 73, 1012–1018. doi: 10.1093/icesjms/fsv255
Giovos I., Kleitou P., Poursanidis D., Batjakas I., Bernardi G., Crocetta F., et al. (2019). Citizen-Science for Monitoring Marine Invasions and Stimulating Public Engagement: A Case Project From the Eastern Mediterranean. Biol. Invas. 21, 3707–3721. doi: 10.1007/s10530-019-02083-w
Granhag L., Majaneva S., Moller L. F. (2012). First Recordings of the Ctenophore Euplokamis Sp (Ctenophora, Cydippida) in Swedish Coastal Waters and Molecular Identification of This Genus. Aquat. invas. 7 (4), 455–463. doi: 10.3391/ai.2012.7.4.002
Guerrini C. J., Majumder M. A., Lewellyn M. J., McGuire A. L. (2018). Citizen Science, Public Policy New Research Models may Benefit From Policy Modifications. Science 361 (6398), 134–136. doi: 10.1126/science.aar8379
Hall T. A. (1999). BioEdit: A User-Friendly Biological Sequence Alignment Editor and Analysis Program for Windows 95/98/NT. Nucleic Acids Symp. Ser. 41, 95–98.
Herut B., Rahav E., IOLR group (2019). The National Monitoring Program of Israel's Mediterranean Waters – Scientific Report on Climate Change and Hydrography for 2018-2019, Israel Oceanographic and Limnological Research. IOLR Rep, H61, 37.
Higgins J. E., Ford M. D., Costello J. H. (2008). Transitions in Morphology, Nematocyst Distribution, Fluid Motions, and Prey Capture During Development of the Scyphomedusa Cyanea Capillata. Biol. Bull. 214, 29–41. doi: 10.2307/25066657
Johansen E., Aberle N., Østensen M. A., Majaneva S. (2021). Assessing the Value of a Citizen Science Approach for Ctenophore Identification. Front. Mar. Sci. 8. doi: 10.3389/fmars.2021.772851
Johnson D. R., Perry H. M., Burke W. D. (2001). Developing Jellyfish Strategy Hypotheses Using Circulation Models. Hydrobiologia 451, 213–221. doi: 10.1023/A:1011880121265
Katoh K., Rozewicki J., Yamada K. D. (2019). MAFFT Online Service: Multiple Sequence Alignment, Interactive Sequence Choice and Visualization. Brief Bioinform. 20 (4), 1160–1166. doi: 10.1093/bib/bbx108
Kawahara M., Uye S., Ohtsu K., Izumi H. (2006). Unusual Population Explosion of the Giant Jellyfish Nemopilemia Nomurai in East Asian Waters. Mar. Ecol. Prog. Ser. 307, 161–173. doi: 10.3354/meps307161
Kuplik Z., Kerem D., Angel D. L. (2021). Respiration Rates, Metabolic Demands and Feeding of Ephyrae and Young Medusae of the Rhizostome Rhopilema Nomadica. Diversity 13, 320. doi: 10.3390/d13070320
Lee P. L. M., Dawson M. N., Neill S. P., Robins P. E., Houghton J. D. R., Doyle T. K., et al. (2013). Identification of Genetically and Oceanographically Distinct Blooms of Jellyfish. J. R. Soc. 10, 1–11. doi: 10.1098/rsif.2012.0920
Librado P., Rozas J. (2009). DnaSP V5: A Software for Comprehensive Analysis of DNA Polymorphism Data. Bioinformatics 25 (11), 1451–1452. doi: 10.1093/bioinformatics/btp187
Lotan A., Ben-Hillel R., Loya Y. (1992). Life Cycle of Rhopilema Nomadica: A New Immigrant Scyphomedusan in the Mediterranean. Mar. Biol. 112, 237–242. doi: 10.1007/BF00702467
Lotan A., Fine M., Ben-Hillel R. (1994). Synchronization of the Life Cycle and Dispersal Pattern of the Tropical Invader Scyphomedusan Rhopilema Nomadica is Temperature Dependent. Mar. Ecol. Prog. Ser. 109, 59–65. doi: 10.3354/meps109059
Madkour F. F., Hanafy M. H., Zaghloul W. S. (2019). Record of Aggregation of Alien Tropical Schyphozoan Rhopilema Nomadica Galiin the Mediterranean Coast of Egypt. Int. Mar. Sci. J. 2, 1–7. doi: 10.14302/issn.2643-0282.imsj-19-2672
Malul D., Lotan T., Makovsky Y., Holzman R., Shavit U. (2019). The Levantine Jellyfish Rhopilema Nomadica and Rhizostoma Pulmo Swim Faster Against the Flow Than With the Flow. Sci. Rep. 9 (1), 20337. doi: 10.1038/s41598-019-56311-3
Marambio M., Canepa A., Lòpez L., Gauci A. A., Gueroun S. K. M., Zampardi S., et al. (2021). Unfolding Jellyfish Bloom Dynamics Along the Mediterranean Basin by Transnational Citizen Science Initiatives. Diversity 13, 274. doi: 10.3390/d13060274
Mauri E., Sitz L., Gerin R., Poulain P. M., Hayes D., Gildor H. (2019). On the Variability of the Circulation and Water Mass Properties in the Eastern Levantine Sea Between September 2016–August 2017. Water 11, 1741. doi: 10.3390/w11091741
Moon J. H., Pang I. C., Yang J. Y., Yoon W. D. (2010). Behavior of the Giant Jellyfish Nemopilema Nomurai in the East China Sea and East/Japan Sea During the Summer of 2005: A Numerical Model Approach Using a Particle-Tracking Experiment. J. Mar. Syst. 80 (1–2), 101–114. doi: 10.1016/j.jmarsys.2009.10.015
Nakar N. (2011). Economic Evaluation of Jellyfish Effects on the Fishery Sector- Case Study From the Eastern Mediterranean (University of Haifa: M.A. Dissertation), 95 p.
Nei M. (1987). Molecular Evolutionary Genetics (New York: Colombia University Press), 512 p. doi: 10.1002/ajpa.1330750317
Newman G., Wiggins A., Crall A., Graham E., Newman S., Crowston K. (2012). The Future of Citizen Science: Emerging Technologies and Shifting Paradigms. Front. Ecol. Environ. 10 (6), 298–304. doi: 10.1890/110294
Özsoy E., Hecht A., Ünlüata Ü., Brenner S., Sur H. I., Bishop J., et al. (1993). A Synthesis of the Levantine Basin Circulation and Hydrograph–1990, Deep Sea Research Part Ii. Topical Stud. Oceanogr. 40 (6), 1075–1119. doi: 10.1016/0967-0645(93)90063-S
Pinardi N., Masetti E. (2000). Variability of the Large Scale General Circulation of the Mediterranean Sea From Observations and Modelling: A Review. Palaeogeogr. Palaeoclimatology Palaeoecol. 158 (3–4), 153–173. doi: 10.1016/S0031-0182(00)00048-1
Purcell J. E., Uye S., Lo W. T. (2007). Anthropogenic Causes of Jellyfish Blooms and Their Direct Consequences for Humans: A Review. Mar. Ecol. Prog. Ser. 350, 153–174. doi: 10.3354/meps07093
Ramos-Onsins S. E., Rozas J. (2002). Statistical Properties of New Neutrality Tests Against Population Growth. Mol. Biol. Evol. 19 (12), 2092–2100. doi: 10.1093/oxfordjournals.molbev.a004034
Rogers A. R., Harpending H. (1992). Population Growth Makes Waves in the Distribution of Pairwise Genetic Differences. Mol. Biol. Evol. 9 (3), 552–569. doi: 10.1093/oxfordjournals.molbev.a040727
Roman J., Darling J. A. (2007). Paradox Lost: Genetic Diversity and the Success of Aquatic Invasions. Trends Ecol. Evol. 22, 454–464. doi: 10.1016/j.tree.2007.07.002
Rosentraub Z., Brenner S. (2007). Circulation Over the SouthEastern Continental Shelf and Slope of the Mediterranean Sea: Direct Current Measurements, Winds, and Numerical Model Simulations. J. Geophys. Res. 112, 1–21. doi: 10.1029/2006JC003775
Said M. A., El-Gindy A., Radwan A. A. (2013). Surface Circulation of the Egyptian Mediterranean Waters Using Satellite-Tracked Lagrangian Drifters. J.K.A.U. Mar. Sci. 24, 69–81. doi: 10.4197/Mar.24-1.6
Schnedler-Meyer N. A., Kiørboe T., Mariani P. (2018). Boom and Bust: Life History, Environmental Noise, and the (Un)Predictability of Jellyfish Blooms. Front. Mar. Sci. 5. doi: 10.3389/fmars.2018.00257
Stopar K., Ramšak A., Trontelj P., Malej A. (2010). Lack of Genetic Structure in the Jellyfish Pelagia Noctiluca (Cnidaria: Scyphozoa: Semaeostomeae) Across European Seas. Mol. Phylogenet. Evol. 57, 417–428. doi: 10.1016/j.ympev.2010.07.004
Streftaris N., Zenetos A. (2006). Alien Marine Species in the Mediterranean - the 100 'Worst Invasives' and Their Impact. Med. Mar. Sci. 7 (1), 87–118. doi: 10.12681/mms.180
Keywords: biological invasion, jellyfish tracking, citizen science (CS), oceanographic modeling, Mediterranean Sea-Eastern, population genetics-empirical
Citation: Edelist D, Knutsen Ø, Ellingsen I, Majaneva S, Aberle N, Dror H and Angel DL (2022) Tracking Jellyfish Swarm Origins Using a Combined Oceanographic-Genetic-Citizen Science Approach. Front. Mar. Sci. 9:869619. doi: 10.3389/fmars.2022.869619
Received: 04 February 2022; Accepted: 17 March 2022;
Published: 20 April 2022.
Edited by:
Periklis Kleitou, Marine and Environmental Research Lab (MER), CyprusReviewed by:
Song Feng, Chinese Academy of Sciences (CAS), ChinaCopyright © 2022 Edelist, Knutsen, Ellingsen, Majaneva, Aberle, Dror and Angel. This is an open-access article distributed under the terms of the Creative Commons Attribution License (CC BY). The use, distribution or reproduction in other forums is permitted, provided the original author(s) and the copyright owner(s) are credited and that the original publication in this journal is cited, in accordance with accepted academic practice. No use, distribution or reproduction is permitted which does not comply with these terms.
*Correspondence: Dror L. Angel, ZGFuZ2VsQHVuaXYuaGFpZmEuYWMuaWw=; Ingrid Ellingsen, SW5ncmlkLkVsbGluZ3NlbkBzaW50ZWYubm8=
Disclaimer: All claims expressed in this article are solely those of the authors and do not necessarily represent those of their affiliated organizations, or those of the publisher, the editors and the reviewers. Any product that may be evaluated in this article or claim that may be made by its manufacturer is not guaranteed or endorsed by the publisher.
Research integrity at Frontiers
Learn more about the work of our research integrity team to safeguard the quality of each article we publish.