- Centre for Coastal Biogeochemistry, Faculty of Science and Engineering, Southern Cross University, Lismore, NSW, Australia
Research related to the potential for coral reef-derived dimethylsulfide (DMS) oxidation products to regulate the regional climate of the Great Barrier Reef (GBR) according to the CLAW hypothesis is summarized in this mini review. The GBR has been indicated as a region of high DMS production where atmospheric emissions may be increased when corals are subject to environmental stresses associated with low tide. During low wind speeds over aerially exposed coral reefs, plumes of atmospheric DMS and new sulfate-containing nano-particle production under photo-oxidative conditions have been detected on the GBR. Hygroscopic growth of these particles in combination with coagulation and condensation processes could potentially provide a coral-mediated mechanism of new aerosol for seeding low-level stratocumulus clouds. Fine mode aerosol optical depth over GBR coral reefs has been correlated with low wind speeds and a coral stress metric formulated as a function of irradiance, water clarity, and tide height. This correlation has been proposed as a possible mechanism by which the GBR might alter the optical properties of the overlying atmosphere to attenuate local insolation leading to regional climate regulation. However, recent regional-scale aerosol-climate modeling indicates that the potential for GBR regional climate regulation via DMS atmospheric oxidation products is weak under current anthropogenic conditions which have instigated mass coral bleaching events along the entire length of the GBR between 1998 and 2022. This increased bleaching indicates that DMS oxidation products are insufficient to regulate the regional climate of the GBR according to the CLAW hypothesis under current global warming conditions.
Introduction
It is now fifty years since Lovelock et al. (1972) proposed that dimethylsulfide (DMS) was the missing link in the natural biogeochemical sulfur cycle capable of annually cycling megatonnes of sulfur from the sea back onto the land. Later, in 1987, DMS was assigned as the driving force in a mechanism by which the ocean and the atmosphere were hypothesized to be dynamically coupled in a global system that had the potential to affect and possibly even regulate climate (Charlson et al., 1987). This hypothesis, which was conceived almost by accident in 1985 during a visit by James Lovelock to Robert Charlson at the University of Washington in Seattle (Liss and Lovelock, 2007), has become known as the CLAW hypothesis, an acronym of the four contributing authors’ surnames. CLAW hypothesized that the Earth’s radiation balance may be modulated by reflection or absorption of solar radiation by clouds seeded by the oxidation products of the marine volatile DMS, an algal metabolic by-product that is constantly exchanged from the oceans to the atmosphere. Consequential changes in sea surface temperate (SST) could affect algal growth and hence DMS emissions, thereby providing a potential climate feedback loop where surface ocean primary productivity is dynamically linked to marine cloud production and albedo. Regarding this CLAW mechanism, it has more recently been stated by Liss and Lovelock (2007) that any climate feedback effect could be stabilizing (i.e. negative) or destabilizing (i.e. positive) and the extent, or even its existence, to play any part in the present day climate is in question. What makes the CLAW hypothesis so scientifically compelling is that it continues to be possible to provide empirical evidence for various processes in the proposed mechanism (Figure 1). For example, in Southern Ocean clean marine air sampled at Cape Grim in north-west Tasmania it has been possible to show coherence in the seasonal cycles of atmospheric DMS (DMSa) and its major oxidation products methanesulfonic acid (MSA) and non-sea-salt sulfate (nss-SO4) (Ayers et al., 1991). Additionally, cloud condensation nuclei (CCN) number concentrations, modeled cloud droplet concentrations, and mean cloud droplet effective radii were also shown to have the same seasonal phase cycles as DMS (Boers et al., 1994). These early data strongly indicated a connection between DMS emissions, aerosol particle chemistry, and CCN and cloud droplet concentrations (Ayers and Gillett, 2000). Other data have shown a strong relationship between DMS and solar radiation dose over the global surface ocean, providing support for a negative climate feedback (Vallina and Simó, 2007).
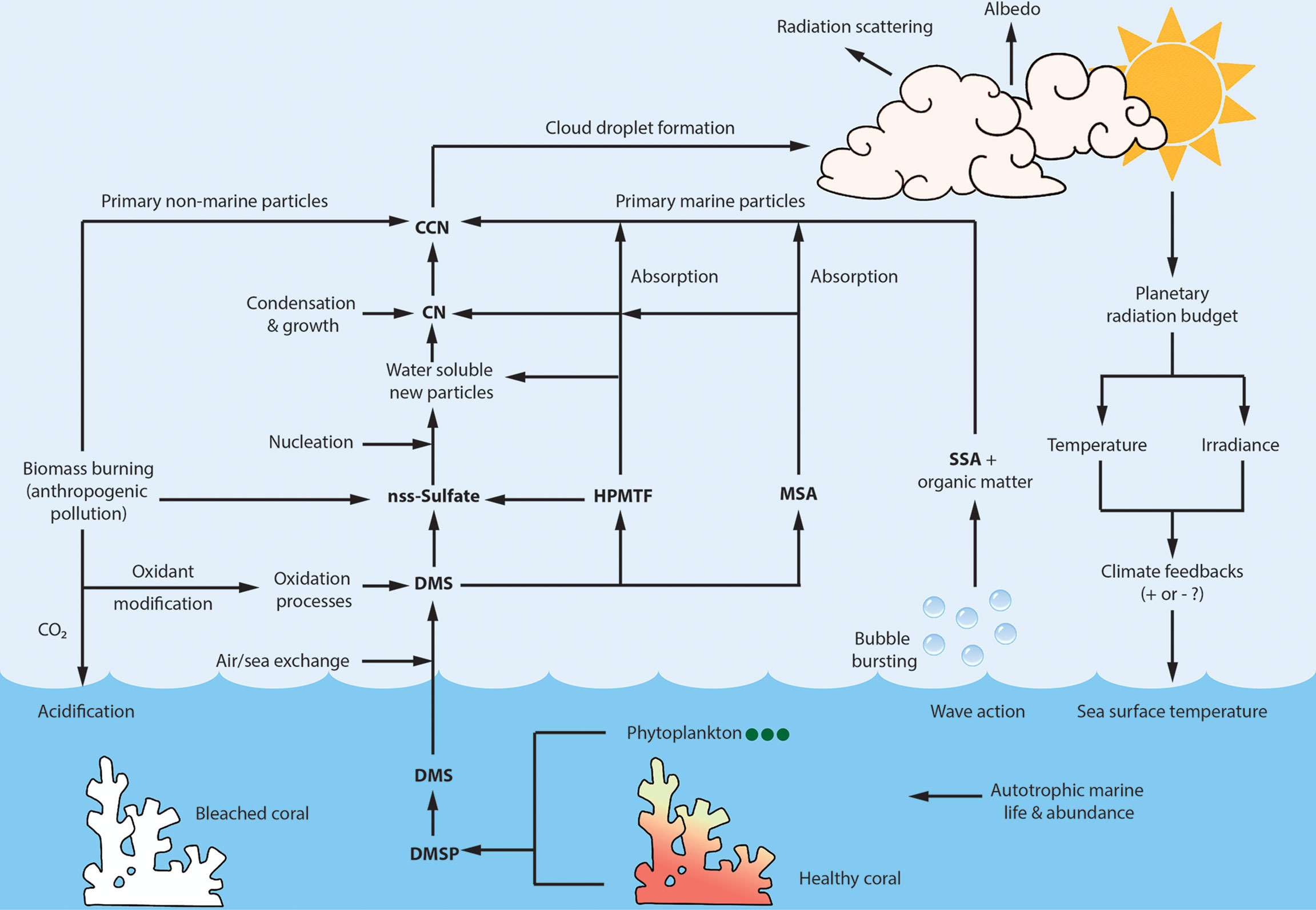
Figure 1 Schematic of the proposed climate feedback loop according to the CLAW hypothesis (Charlson et al., 1987) adapted to the GBR with some updated information. DMSP, produced by coral and phytoplankton, is the dominant source of DMS which on exchange to the atmosphere can be oxidized to products such as nss-SO4 and HPMTF which are involved in new particle production, while MSA primarily contributes to the growth of existing particles. CCN formed from these DMS oxidation products can generate marine clouds with increased albedo, which may alter the Earth’s radiation budget leading to the hypothesized climatic feedback. The proposed biogenic feedback loop may be perturbed by primary marine sources of CCN such as SSA and organic matter released from surface waters by bubble bursting. Anthropogenic forcing over the GBR may contribute to recurrent coral bleaching and loss of DMS production to dampen the feedback loop. Additionally, regional biomass burning may contribute nss-sulfate and primary particles in the CCN size range (Dp ~50-100 nm) (Zaveri et al., 2022) as well as terrestrially-derived oxidants that may alter the background marine atmospheric oxidation processing capacity (Mauldin III et al., 2012; Zhu et al., 2021). Condensation nuclei (CN), Cloud condensation nuclei (CCN), Dimethylsulfide (DMS), Dimethylsulfoniopropionate (DMSP), Particle diameter (Dp), Great Barrier Reef (GBR), Hydroperoxymethyl thioformate (HPMTF), Methanesulfonic acid (MSA), non sea salt sulfate (nss-Sulfate), Sea spray aerosol (SSA).
The CLAW hypothesis has been experimentally shown to be far more complex than first proposed, and despite much research it has not been possible to quantitatively demonstrate the relationship between the mass air-sea flux of DMS-derived sulfate and the number concentration of CCN (Ayers and Cainey, 2007). Failure to demonstrate this relationship led Quinn and Bates (2011) to suggest that it might be time to retire the CLAW hypothesis with over 20 years of collated evidence that DMS bio-regulation of climate is prevented by weak sensitivity to change in each step of the CLAW hypothesis feedback loop (Figure 1). DMS is, however, the major reduced sulfur-containing volatile emitted from the global oceans, currently estimated to be 27.1 Tg (as S) annually (Hulswar et al., 2021). This massive amount of biogenic sulfur is suspected to be the origin of the globally dominant nss-SO4 contribution to CCN over the global oceans between 70°S and 80°N (Quinn et al., 2017); thus, signaling that DMS oxidation products and CCN are connected with the potential to influence Earth’s energy budget, and ultimately climate. According to James Lovelock, demonstration of climate regulation via cloud albedo control linked to DMS emissions is now only probable, if at all, in the southern hemisphere because of excessive anthropogenic sulfate pollution in the northern hemisphere (Lovelock, 2009). Given that global realization of CLAW is now most likely impossible, a relatively unpolluted location in the southern hemisphere such as the GBR remains a last marine frontier where research has been directed to possibly demonstrate regional climate regulation according to the CLAW hypothesis.
Production of Dimethylsulfide and Dimethylsulfoniopropionate (DMS/P) by Scleractinian Corals
It has been known since the early 1990s that coral reef ecosystems can be significant sessile sources of dimethylsulfoniopropionate (DMSP), the major marine precursor of DMS (Jones et al., 1994; Hill et al., 1995). Initially, the algal zooxanthellae endosymbionts (Symbiodium sp.) were presumed and also indicated to be the coral’s source of DMS/P because cellular or particulate DMSP was typically correlated with Symbiodium number (Broadbent and Jones, 2004; Jones et al., 2007; Van Alstyne et al., 2008); however, it is now recognized that the coral polyp also has the ability to produce DMSP (Raina et al., 2013). Just as the symbiotic union of the zooxanthellae and the polyp provides a very efficient metabolic system enabling coral to flourish in oligotrophic tropical waters, this union may also provide the basis for the very high concentrations of DMS/P that have been measured in coral and its products. For example, mucus ropes exuded from the staghorn coral Acropora formosa have been reported to contain 18.7 µM DMS and 54.4 µM DMSP (Broadbent and Jones, 2004), which are some of the highest concentrations of DMS/P measured in any natural marine material. Zooxanthellae DMSP cellular concentrations of up to 686 mmol L-1 (cell volume) (Yost and Mitchelmore, 2009) and 7590 mmol L-1 (cell volume) (Broadbent et al., 2002) are large by comparison with DMSP cellular concentrations reported in other marine dinoflagellates (Caruana and Malin, 2014). High zooxanthellae densities typically of 1‐4 x 106 Symbiodinium cells cm-2 coral surface area in healthy Acropora sp. (Moothien-Pillay et al., 2005) make these abundant branching corals throughout the GBR highly concentrated sources of DMS/P. Consequently, the GBR has been described as a DMS hotspot (Jones et al., 2017; Jones et al., 2018); however, it is not identified as such among the 56 biogeochemical global ocean provinces defined by Hulswar et al. (2021), probably because the GBR is a relatively under-sampled location.
Conditions That Promote DMS Transfer From Coral Reefs to the Atmosphere
In chamber experiments with three Indo-Pacific coral species, it was shown that gas phase DMS increased by an order of magnitude when the corals were exposed to air, and this was followed by an additional rise in gas phase DMS on their re-submersion (Hopkins et al., 2016). In the environment, atmospheric DMS (DMSa) was observed to peak over coral reefs in the northern GBR at, or shortly after, low tide when the reefs were exposed to the atmosphere (Jones and Trevena, 2005). This was also observed at Heron Island, a coral cay in the southern GBR, where it was possible to demonstrate that median DMSa mixing ratios were a function of tide height, which controls the extent and duration of coral reef aerial exposure, Figure 2 (Swan, 2017). A combination of DMSa source signals are shown in Figure 2, where DMSa derived from the coral reef is the tidally-induced contribution above the oceanic background DMSa continuum. When a coral reef is aerially exposed, DMS can diffuse from the mucus covered coral surfaces directly to the atmosphere, a process that circumvents DMS air-sea exchange; hence, low-tide DMS emissions may result in rapidly released plumes. These were observed as intermittent DMSa time-line spikes in the Heron Island datasets, which were in most instances detected under low wind speeds (WS < 2 m s-1) when atmospheric mixing with oceanic air was least (Swan et al., 2017). Coral reefs emit DMS at low tide in response to environmental stresses when corals can be subject to elevated solar irradiance, heating and hypoxia in shallow pooled water, and dehydration due to air exposure (Deschaseaux et al., 2014).
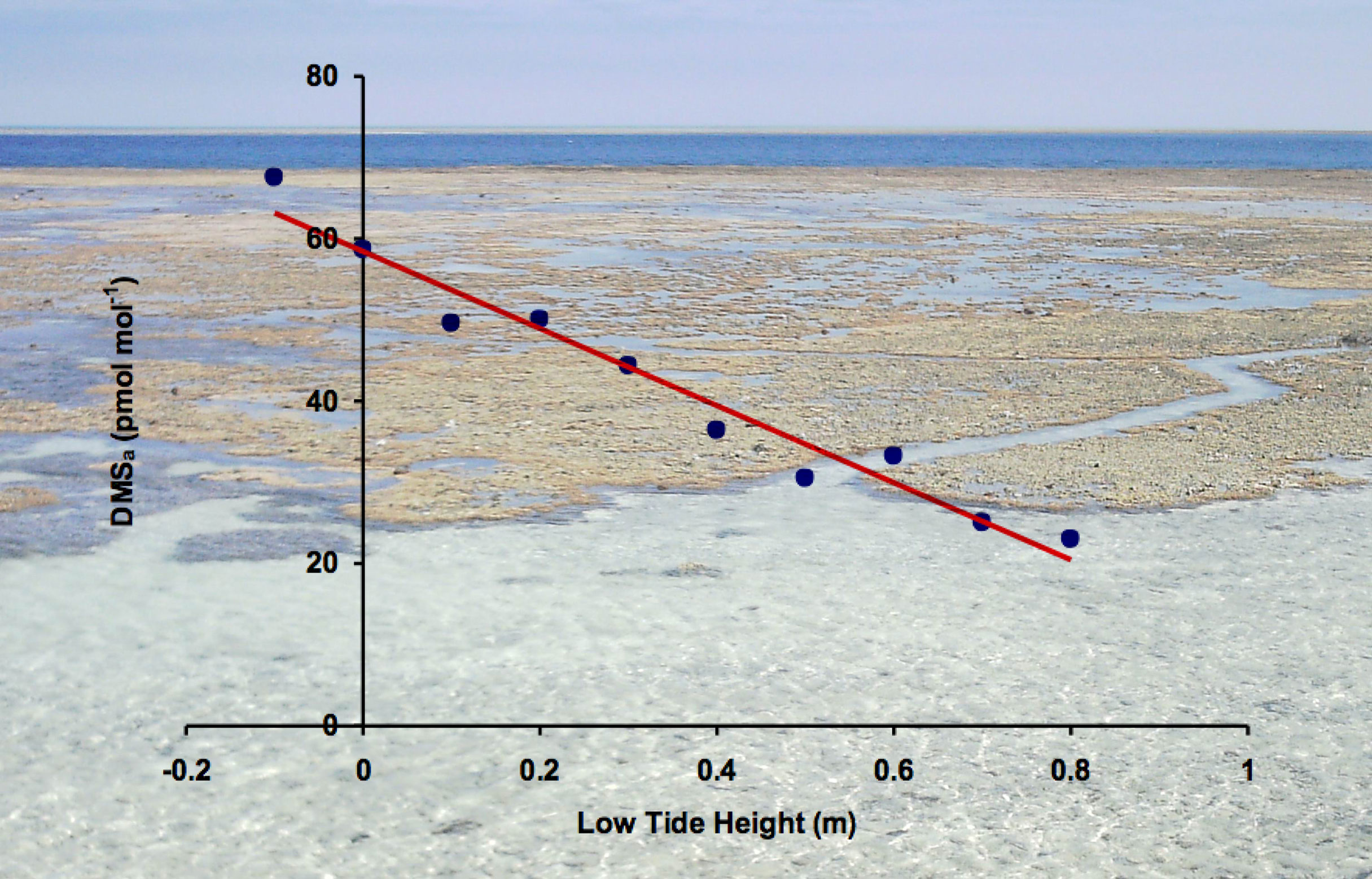
Figure 2 Median atmospheric DMS mixing ratios plotted against low tide seawater height at Heron Island (23.44°S, 151.91°E) on the southern GBR, 18 Jul - 5 Aug 2013 (austral winter), derived from 30 min resolution measurements of DMSa. The plotted median values are for measurements made in a time window approximately ± 1 h either side of the low tide height range that, to varying extents, aerially exposed the 27 km2 lagoonal platform coral reef surrounding Heron Island shown in the photo (H.B. Swan). According to the dominant south-easterly trade winds at this location, the Heron Reef and the adjacent Wistari Reef, 1 km to the south, are expected to be the sources of DMSa in excess of the approximately 20 pmol mol-1 DMSa background wintertime mixing ratio.
DMS Flux and Emission Estimates From the GBR
Seasonally averaged DMS flux estimates for five coral reefs across the GBR, determined from spatially and temporally combined data, have been reported by Jones et al. (2018). These averaged fluxes, estimated from dissolved DMS (DMSw) and WS using the gradient approach of Liss and Merlivat (1986), were 6.4 and 2.4 µmol m-2 d-1 for summer (Oct-Mar, n = 237) and winter (Apr-Sep, n = 156), respectively. Seasonal fluxes at Heron Island, estimated from DMSa using the mass balance-photochemical box model of Ayers et al. (1995), were 5.0 and 1.4 µmol m-2 d-1 for the 2012 summer wet season (n = 651) and the 2013 winter dry season (n = 923), respectively (Swan et al., 2017). For those Heron Island field campaigns, the coral reef surrounding the island was estimated to contribute 4% during the summer and 14% during the winter to the background oceanic DMS flux sourced from the dominant south-easterly trade winds. A proxy for DMSw across the GBR was derived by Jackson et al. (2021) using a multiple linear regression model, satellite-derived photosynthetically active radiation, SST data, and DMSw field data compiled by Jones et al. (2018). Using that modeled DMSw data, a climatology of DMS air-sea flux across the GBR was calculated using three gradient approach parameterizations. Average (± 1σ) seasonal DMS fluxes were 7.3 ± 1.6 and 3.1 ± 0.3 µmol m-2 d-1 in late summer and late winter, respectively, where inclusion of an estimate for DMS release from exposed corals at low tide increased the average flux by 1.5 µmol m-2 d-1. DMS emission across the 347,000 km2 area of the GBR is estimated by Jackson et al. (2021) to be 0.03–0.05 Tg yr-1 of DMS (1,500–2,100 mol km2 yr‐1), which represents 0.06-0.1% of the 52.5 Tg yr‐1 of DMS estimated on average to be transferred from the global oceans to the atmosphere (Hulswar et al., 2021). Reported DMS fluxes from the GBR are not extraordinary; they are within the 0-10 µmol S m-2 d-1 range emitted from 93% of the world’s oceans according to Hulswar et al. (2021).
Aerosol Optical Depth and Cloud Cover Over the GBR
The direct effect of marine aerosol to alter the extent that the atmosphere reflects and absorbs solar radiation can be evaluated by aerosol optical depth (AOD). A 16-year (2000-2015) satellite-derived record of fine mode AOD (0.1-0.25 μm radius) for an area of the GBR centred over Heron Island, was correlated with a coral reef stress metric formulated as a function of irradiance, water clarity, and tide height (Cropp et al., 2018). The correlation, which assumes that biogenic aerosol derived from the GBR was primarily linked to AOD, was strongest at low WS when biogenic aerosol is least advected away from its source point, and there is less sea spray aerosol generation. This correlation, which was consistent with field observations of DMSa plumes at Heron Island at low-tide under low WS, was proposed as a possible mechanism by which coral reefs might alter the optical properties of the overlying atmosphere to attenuate local insolation (Cropp et al., 2018). This analysis was extended by Jackson et al. (2018) who analyzed fine mode AOD (2000-2017) over the entire 2,300 km length of the GBR and found AOD to be positively correlated with both SST and the coral reef stress metric. However, a thermal tipping point in the coral stress metric was identified where it became uncoupled from AOD, this being interpreted as a threshold for coral bleaching based on the assumption that bleaching leads to a reduction in emission of biogenic aerosol. The thermal threshold that leads to coral bleaching, separation of Symbiodinium from its polyp host and cessation of coral DMS production, has been reported to be when SST exceeds 30°C for an extended period (Fischer and Jones, 2012; Jones et al., 2017; Jones et al., 2018). Such marine heatwaves are increasing in frequency and, from a culmination of events in 1998, 2002, 2016, 2017 and 2020, have now resulted in mass coral bleaching along the entire GBR (Hughes et al., 2021). Increased summer cloud cover may alleviate coral bleaching by surface cooling, an effect that has been quantified over the GBR using satellite imagery and in-situ temperature and light loggers (Leahy et al., 2013). In that study, cloud cover alone was responsible for up to 32% of the variation in SST, although there was a 3-day lag between a change in cloud cover and a change in SST. Local-scale cloud cover over shallow GBR waters was recently reported to be more highly correlated with SST cooling than the larger-scale regional modulation of cloud cover linked to the El Niño–Southern Oscillation (Zhao et al., 2021). This cloud cover-SST relationship has been proposed to operate as an ocean thermostat in the western Pacific warm pool to the north of Australia where coral bleaching events have been relatively few in number despite the region having the highest average SST of all ocean regions (Kleypas et al., 2008). It has been proposed that DMS atmospheric oxidation products might promote a similar cloud cooling ocean thermostat effect over the GBR (Fischer and Jones, 2012), although this phenomenon may be explained by physical processes alone (Johnson et al., 2001; Takahashi et al., 2010).
Modelling the CLAW Hypothesis Globally and Regionally on the GBR
Application of aerosol microphysics models to calculate the sensitivity of CCN, cloud fraction and surface incoming short-wave radiation to change due to varying DMS air-sea emissions over a wide range of climatologies have indicated the role of DMS in climate regulation to be globally weak (Woodhouse et al., 2010; Fiddes et al., 2018). These modeled outcomes are underpinned by observational evidence showing (i) gas-to-particle nucleation of DMS-derived H2SO4 does not commonly occur in the marine boundary layer (MBL) (Clarke et al., 1998; Gras et al., 2009; Kerminen et al., 2018); (ii) ultra-fine sea salt (Clarke et al., 2006; Smith, 2007; Xu et al., 2022) and other primary marine-derived materials (Leck and Bigg, 2007; Hawkins and Russell, 2010; Zelenyuk et al., 2010) can effectively act as CCN; and (iii) there are other marine-derived volatile organic compounds in addition to DMS that can form secondary organic aerosol (Meskhidze and Nenes, 2006; Exton et al., 2015; Swan et al., 2016). DMS may, however, have more importance regionally than globally to attenuate insolation according to large spatial and temporal differences in DMSw concentrations and air-sea fluxes among the 56 global oceans provinces of Hulswar et al. (2021). Modelling of CCN production per unit mass of DMS emitted sulfur has been found to vary regionally by a factor of 20, suggesting that DMS emissions in some marine locations might provide regional climate regulation (Woodhouse et al., 2013). A recent GBR modeling study that added a coral-derived DMS surface emission of 0.3 Tg yr-1 S (1.7% of the global DMS sulfur emission) concluded that this superambient input of atmospheric sulfur into the model provided no robust effect on the regional climate of the GBR from direct and indirect aerosol effects (Fiddes et al., 2021). Another study that examined the effect of sub-daily changes in coral reef-derived DMS in a regional-scale climate model that was constrained using data gained from a recent field study (Sep-Oct 2016) on the GBR similarly found no significant changes in sulfate aerosol mass or total aerosol number to promote regional climate regulation (Fiddes et al., 2022). In that study, it was suggested that the close proximity of anthropogenic aerosol sources, such as power stations and other biomass burning sources, prevents the GBR from having any significant influence on the regional sulfate aerosol burden. The field study data used to constrain the model was collected following the 2016 summer of mass coral bleaching across the northern third of the GBR (Hughes et al., 2017). Although the field study was conducted on a section of the GBR that was less impacted by bleaching, uncertainty exists about the health of the GBR study zone at that time. Continuing bleaching of the GBR is closing the window of opportunity to research this topic (Jackson et al., 2020).
Concluding Comments
Periodic new sulfate-containing particle production (Dp < 20 nm) in the MBL has been indicated from the GBR (Modini et al., 2009) and on the GBR (Vaattovaara et al., 2013). These nano-particles can grow to the climatically active CCN size range (Dp ~50-100 nm) attenuating irradiance and contributing to marine cloud formation. However, aerosol contributions to CCN and cloud formation over the GBR have not been clearly identified and quantified, so it remains uncertain if the proposed ocean thermostat effect from low-level clouds to cool GBR SST is actually a negative feedback driven by coral-derived DMS oxidation products. Additionally, while clouds can reflect incoming sunlight leading to localized 3‐day delayed temporal surface cooling, they also trap outgoing infrared radiation; hence, net radiative forcing by clouds over the GBR remains an uncertain complex component of the planetary greenhouse effect. While aerosol-climate models are important tools to assess the potential for DMS-facilitated climate regulation on the GBR, all are restricted by limited multiphase DMS chemistry, nucleation and nanoparticle growth mechanisms as well as other inherent short-comings and biases in meteorological, physical and chemical processes (Lee et al., 2019). DMS oxidation is far more complex than first imagined by Charlson et al. (1987) and continues to be elucidated (Von Glasow and Crutzen, 2004; Hoffmann et al., 2016). Notably, a previously unrecognized stable DMS oxidation product, hydroperoxymethyl thioformate (HPMTF, HOOCH2SCHO) is currently not widely incorporated into atmospheric models. More than 30% of oceanic DMS emissions may form HPMTF, a major reservoir of sulfur in the MBL that is involved in new particle formation and growth (Veres et al., 2020). Mindful of these limitations, recent modeling (Fiddes et al., 2022; Jackson et al., 2022) indicates that DMS emissions from the GBR are currently insufficient, relative to background anthropogenic sulfur emissions, to significantly enhance sulfate aerosol and CCN number concentrations according to CLAW. Perhaps in pre-industrial times coral reef-derived DMS emissions may have exerted sufficient influence to regulate GBR regional climate (Fung et al., 2022). However, the GBR is no longer a pristine environment; it is impacted by regional pollution (Chen et al., 2019) and is also under immediate threat from global warming (Smith, 2019; Osman et al., 2021). Under current anthropogenic driven radiative forcing, the option to geo-engineer marine stratocumulus cloud albedo using ultra-fine sea salt, known as marine cloud brightening (MCB), has been proposed as a solution to protect the GBR. Modeling has indicated that MCB has the potential to sufficiently cool SST to limit coral bleaching on the GBR under a scenario of doubled atmospheric CO2 levels (Latham et al., 2013). There are, however, logistical difficulties, uncertainties and risks of applying MCB (Stuart et al., 2013). Given that since 1998 only 2% of the GBR now remains untouched by bleaching (Hughes et al., 2021), which has again occurred in the 2021-22 summer during a La Niña year (GBRMPA et al., 2022), it is now apparent that it will not be DMS but an immediate coordinated global reduction of green house gas emissions that will save the GBR from continuing decline.
Author Contributions
HS declares that he is the sole author of this mini review and has done his best to correctly summarize all cited research. The author agrees to be accountable for the content of this work and has approved the submitted version.
Funding
The Faculty of Science and Engineering, Southern Cross University, has provided financial support to publish this mini review. Australian Research Council grant DP150101649 for a Discovery Project titled, “The Great Barrier Reef as a significant source of climatically relevant aerosol particles” has assisted recent research cited in this mini review.
Conflict of Interest
The author declares that the research was conducted in the absence of any commercial or financial relationships that could be construed as a potential conflict of interest.
Publisher’s Note
All claims expressed in this article are solely those of the authors and do not necessarily represent those of their affiliated organizations, or those of the publisher, the editors and the reviewers. Any product that may be evaluated in this article, or claim that may be made by its manufacturer, is not guaranteed or endorsed by the publisher.
Acknowledgments
I would like to thank the topic editors for facilitating the Frontiers Research Topic which led to this mini review. I would also like to thank Edith Olsen for assistance with preparation of Figure 1 and the Faculty of Science and Engineering, Southern Cross University for an adjunct position that provides on-going association for work such as this to be published.
References
Ayers G. P., Cainey J. M. (2007). The CLAW Hypothesis: A Review of the Major Developments. Environ. Chem. 4, 366–374. doi: 10.1071/EN07080
Ayers G. P., Gillett R. W. (2000). DMS and its Oxidation Products in the Remote Marine Atmosphere: Implications for Climate and Atmospheric Chemistry. J. Sea Res. 43, 275–286. doi: 10.1016/S1385-1101(00)00022-8
Ayers G. P., Gillett R. W., Ivey J. P., Schäfer B., Gabric A. (1995). Short-Term Variability in Marine Atmospheric Dimethylsulfide Concentration. Geophysical Res. Lett. 22, 2513–2516. doi: 10.1029/95GL02484
Ayers G. P., Ivey J. P., Gillett R. W. (1991). Coherence Between Seasonal Cycles of Dimethylsulphide, Methanesulphonate and Sulphate in Marine Air. Nature 329, 404–406. doi: 10.1038/349404a0
Boers R., Ayres G. P., Gras J. L. (1994). Coherence Between Seasonal Variation in Satellite-Derived Cloud Optical Depth and Boundary Layer CCN Concentrations at a Mid-Latitude Southern Hemisphere Station. Tellus 46B, 123–131. doi: 10.3402/tellusb.v46i2.15757
Broadbent A. D., Jones G. B. (2004). DMS and DMSP in Mucus Ropes, Coral Mucus, Surface Films and Sediment Pore Waters From Coral Reefs in the Great Barrier Reef. Mar. Freshw. Res. 55, 849–855. doi: 10.1071/MF04114
Broadbent A. D., Jones G. B., Jones R. J. (2002). DMSP in Corals and Benthic Algae From the Great Barrier Reef. Estuarine Coast. Shelf Sci. 55, 547–555. doi: 10.1006/ecss.2002.1021
Caruana A. M. N., Malin G. (2014). The Variability in DMSP Content and DMSP Lyase Activity in Marine Dinoflagellates. Prog. Oceanography 120, 410–424. doi: 10.1016/j.pocean.2013.10.014
Charlson R. J., Lovelock J. E., Andreae M. O., Warren S. G. (1987). Oceanic Phytoplankton, Atmospheric Sulphur, Cloud Albedo and Climate. Nature 326, 655–661. doi: 10.1038/326655a0
Chen Z., Schofield R., Rayner P., Zhang T., Liu C., Vincent C., et al. (2019). Characterization of Aerosols Over the Great Barrier Reef: The Influence of Transported Continental Sources. Sci. Total Environ. 690, 426–437. doi: 10.1016/j.scitotenv.2019.07.007
Clarke A. D., Owens S. R., Zhou J. (2006). An Ultrafine Sea-Salt Flux From Breaking Waves: Implications for Cloud Condensation Nuclei in the Remote Marine Atmosphere. J. Geophysical Res. 111, D06202. doi: 10.1029/2005JD006565
Clarke A. D., Varner J. L., Eisele F., Mauldin R. L., Tanner D., Litchy M. (1998). Particle Production in the Remote Marine Atmosphere: Cloud Outflow and Subsidence During Ace1. J. Geophysical Res. 103, 16397–16409. doi: 10.1029/97JD02987
Cropp R., Gabric A., Van Tran D., Jones G., Swan H., Butler H. (2018). Coral Reef Aerosol Emissions in Response to Irradiance Stress in the Great Barrier Reef, Australia. Ambio 47, 671–681. doi: 10.1007/s13280-018-1018-y
Deschaseaux E. S. M., Jones G. B., Deseo M. A., Shepherd K. M., Kiene R. P., Swan H. B., et al. (2014). Effects of Environmental Factors on Dimethylated Sulfur Compounds and Their Potential Role in the Antioxidant System of the Coral Holobiont. Limnology Oceanography 59, 758–768. doi: 10.4319/lo.2014.59.3.0758
Exton D. A., Mcgenity T. J., Steinke M., Smith D. J., Suggett D. J. (2015). Uncovering the Volatile Nature of Tropical Coastal Marine Ecosystems in a Changing World. Global Change Biol. 21, 1383–1394. doi: 10.1111/gcb.12764
Fiddes S. L., Woodhouse M. T., Lane T. P., Schofield R. (2021). Coral-Reef-Derived Dimethyl Sulfide and the Climatic Impact of the Loss of Coral Reefs. Atmospheric Chem. Phys. 21, 5883–5903. doi: 10.5194/acp-21-5883-2021
Fiddes S. L., Woodhouse M. T., Nicholls Z., Lane T. P., Schofield R. (2018). Cloud, Precipitation and Radiation Responses to Large Perturbations in Global Dimethyl Sulfide. Atmospheric Chem. Phys. 18, 10177–10198. doi: 10.5194/acp-18-10177-2018
Fiddes S. L., Woodhouse M. T., Utembe S., Schofield R., Alroe J., Chambers S. D., et al. (2022). The Contribution of Coral Reef-Derived Dimethyl Sulfide to Aerosol Burden Over the Great Barrier Reef: A Modelling Study. Atmospheric Chem. Phys. 22, 2419–2445. doi: 10.5194/acp-22-2419-2022
Fischer E., Jones G. (2012). Atmospheric Dimethylsulphide Production From Corals in the Great Barrier Reef and Links to Solar Radiation, Climate and Coral Bleaching. Biogeochemistry 110, 31–46. doi: 10.1007/s10533-012-9719-y
Fung K. M., Heald C. L., Kroll J. H., Wang S., Jo D. S., Gettelman A., et al. (2022). Exploring Dimethyl Sulfide (DMS) Oxidation and Implications for Global Aerosol Radiative Forcing. Atmospheric Chem. Phys. 22, 1549–1573. doi: 10.5194/acp-22-1549-2022
Gras J. L., Jimi S. I., Siems S. T., Krummel P. B. (2009). Postfrontal Nanoparticles at Cape Grim: Observations. Environ. Chem. 6, 508–514. doi: 10.1071/EN09075
Great Barrier Reef Marine Park Authority, Australian Institute of Marine Science, CSIRO. (2022). Reef Snapshot: Summer 2021-22. Available at: https://elibrary.gbrmpa.gov.au/jspui/bitstream/11017/3916/3/Reef-summer-snapshot-2021-22.pdf.
Hawkins L. N., Russell L. M. (2010). Polysaccharides, Proteins, and Phytoplankton Fragments: Four Chemically Distinct Types of Marine Primary Organic Aerosol Classified by Single Particle Spectromicroscopy. Adv. Meteorology 612132, 1–14. doi: 10.1155/2010/612132
Hill R. W., Dacey J. W. H., Krupp D. A. (1995). Dimethylsulfoniopropionate in Reef Corals. Bull. Mar. Sci. 57, 489–494.
Hoffmann E. H., Tilgner A., Schrödner R., Bräuer P., Wolke R., Herrmann H. (2016). An Advanced Modeling Study on the Impacts and Atmospheric Implications of Multiphase Dimethyl Sulfide Chemistry. Proc. Natl. Acad. Sci. 113, 11776–11781. doi: 10.1073/pnas.1606320113
Hopkins F. E., Bell T. G., Yang M., Suggett D. J., Steinke M. (2016). Air Exposure of Coral Is a Significant Source of Dimethylsulfide (DMS) to the Atmosphere. Sci. Rep. 6, 36031. doi: 10.1038/srep36031
Hughes T. P., Kerry J. T., Álvarez-Noriega M., Álvarez-Romero J. G., Anderson K. D., Baird A. H., et al. (2017). Global Warming and Recurrent Mass Bleaching of Corals. Nature 543, 373–377. doi: 10.1038/nature21707
Hughes T. P., Kerry J. T., Connolly S. R., Álvarez-Romero J. G., Eakin M., Heron S. F., et al. (2021). Emergent Properties in the Responses of Tropical Corals to Recurrent Climate Extremes. Curr. Biol. 31, p5393–5399. doi: 10.1016/j.cub.2021.10.046
Hulswar S., Simo R., Galí M., Bell T., Lana A., Inamdar S., et al. (2021). Third Revision of the Global Surface Seawater Dimethyl Sulfide Climatology (DMS-Rev3). Earth System Sci. Data Discussions. doi: 10.5194/essd-2021-236
Jackson R., Gabric A., Cropp R. (2018). Effects of Ocean Warming and Coral Bleaching on Aerosol Emissions in the Great Barrier Reef, Australia. Sci. Rep. 8, 14048. doi: 10.1038/s41598-018-32470-7
Jackson R. L., Gabric A. J., Cropp R., Woodhouse M. T. (2020). Dimethylsulfide (DMS), Marine Biogenic Aerosols and the Ecophysiology of Coral Reefs. Biogeosciences 17, 2181–2204. doi: 10.5194/bg-17-2181-2020
Jackson R. L., Gabric A. J., Matrai P. A., Woodhouse M. T., Cropp R., Jones G. B., et al. (2021). Parameterizing the Impact of Seawater Temperature and Irradiance on Dimethylsulfide (DMS) in the Great Barrier Reef and the Contribution of Coral Reefs to the Global Sulfur Cycle. J. Geophysical Research: Oceans 126, e2020JC016783. doi: 10.1029/2020JC016783
Jackson R. L., Woodhouse M. T., Gabric A. J., Cropp R. A., Swan H. B., Deschaseaux E. S. M., et al. (2022). Modeling The Influence of Coral Reef-Derived Dimethylsulfide on the Atmosphere of the Great Barrier Reef, Australia. Front. Mar. Sci.
Johnson R. H., Ciesielski P. E., Cotturone J. A. (2001). Multiscale Variability of the Atmospheric Mixed Layer Over the Western Pacific Warm Pool. J. Atmospheric Sci. 58, 2729–2750. doi: 10.1175/1520-0469(2001)058<2729:MVOTAM>2.0.CO;2
Jones G. B., Curran M. A. J., Broadbent A. D. (1994). “Dimethylsulphide in the South Pacific,” in Recent Advances in Marine Science and Technology 1994. Eds. Bellwood O., Choat H., Saxena N. (Queensland, Australia: Townsville: James Cook University Press), 183–190.
Jones G., Curran M., Broadbent A., King S., Fischer E., Jones R. (2007). Factors Affecting the Cycling of Dimethylsulfide and Dimethylsulfoniopropionate in Coral Reef Waters of the Great Barrier Reef. Environ. Chem. 4, 310–322. doi: 10.1071/EN06065
Jones G., Curran M., Deschaseaux E., Omori Y., Tanimoto H., Swan H., et al. (2018). The Flux and Emission of Dimethylsulfide From the Great Barrier Reef Region and Potential Influence on the Climate of NE Australia. J. Geophysical Research: Atmospheres 123, 13835–13856. doi: 10.1029/2018JD029210
Jones G., Curran M., Swan H., Deschaseaux E. (2017). Dimethylsulfide and Coral Bleaching: Links to Solar Radiation, Low Level Cloud and the Regulation of Seawater Temperatures and Climate in the Great Barrier Reef. Am. J. Climate Change 6, 328–359. doi: 10.4236/ajcc.2017.62017
Jones G. B., Trevena A. J. (2005). The Influence of Coral Reefs on Atmospheric Dimethylsulphide Over the Great Barrier Reef, Coral Sea, Gulf of Papua and Solomon and Bismarck Seas. Mar. Freshw. Res. 56, 85–93. doi: 10.1071/MF04097
Kerminen V.-M., Chen X., Vakkari V., Petäjä T., Kulmala M., Bianchi F. (2018). Atmospheric New Particle Formation and Growth: Review of Field Observations. Environ. Res. Lett. 13, 103003. doi: 10.1088/1748-9326/aadf3c
Kleypas J. A., Danabasoglu G., Lough J. M. (2008). Potential Role of the Ocean Thermostat in Determining Regional Differences in Coral Reef Bleaching Events. Geophysical Res. Lett. 35, L03613. doi: 10.1029/2007GL032257
Latham J., Kleypas J., Hauser R., Parkes B., Gadian A. (2013). Can Marine Cloud Brightening Reduce Coral Bleaching? Atmospheric Sci. Lett. 14, 214–219. doi: 10.1002/asl2.442
Leahy S. M., Kingsford M. J., Steinberg C. R. (2013). Do Clouds Save the Great Barrier Reef? Satellite Imagery Elucidates the Cloud-SST Relationship at the Local Scale. PloS One 8, e70400. doi: 10.1371/journal.pone.0070400
Leck C., Bigg E. K. (2007). A Modified Aerosol-Cloud-Climate Feedback Hypothesis. Environ. Chem. 4, 400–403. doi: 10.1071/EN07061
Lee S. H., Gordon H., Yu H., Lehtipalo K., Haley R., Li Y., et al. (2019). New Particle Formation in the Atmosphere: From Molecular Clusters to Global Climate. J. Geophysical Research: Atmospheres 124, 7098–7146. doi: 10.1029/2018JD029356
Liss P. S., Lovelock J. E. (2007). Climate Change: The Effect of DMS Emissions. Environ. Chem. 4, 377–378. doi: 10.1071/EN07072
Liss P. S., Merlivat L., Dordrecht, Netherlands: Reidel (1986). “Air-Sea Exchange Rates: Introduction and synthesis,” in The Role of Air-Sea Exchange in Geochemical Cycling. Ed. Buat-Menard P.. (Dordrecht, Netherlands: Reidel Publishing Co.) 113–127.
Lovelock J. E. (2009). The Vanishing Face of Gaia: A Final Warning (London, UK: Allen Lane, Penguin Group).
Lovelock J. E., Maggs R. J., Rasmussen R. A. (1972). Atmospheric Dimethylsulphide and the Natural Sulphur Cycle. Nature 237, 452–453. doi: 10.1038/237452a0
Mauldin R. L. III, Berndt T., Sipilä M., Paasonen P., Petäjä T., Kim S., et al. (2012). A New Atmospherically Relevant Oxidant of Sulfur Dioxide. Nature 488, 193–197. doi: 10.1038/nature11278
Meskhidze N., Nenes A. (2006). Phytoplankton and Cloudiness in the Southern Ocean. Science 314, 1419–1423. doi: 10.1126/SCIENCE.1131779
Modini R. L., Ristovski Z. D., Johnson G. R., He C., Surawski N., Morawska L., et al. (2009). New Particle Formation and Growth at a Remote, Subtropical Coastal Location. Atmospheric Chem. Phys. 9, 7607–7621. doi: 10.5194/acp-9-7607-2009
Moothien-Pillay R., Willis B., Terashima H. (2005). Trends in the Density of Zooxanthellae in Acropora Millepora (Ehrenberg 1834) at the Palm Island Group, Great Barrier Reef, Australia. Symbiosis 38, 209–226. Available at: https://researchonline.jcu.edu.au/4563/1/4563_Pillay_et_al...2005.pdf
Osman M. B., Tierney J. E., Zhu J., Tardif R., Hakim G. J., King J., et al. (2021). Globally Resolved Surface Temperatures Since the Last Glacial Maximum. Nature 599, 239–244. doi: 10.1038/s41586-021-03984-4
Quinn P. K., Bates T. S. (2011). The Case Against Climate Regulation via Oceanic Phytoplankton Sulphur Emissions. Nature 480, 51–56. doi: 10.1038/nature10580
Quinn P. K., Coffman D. J., Johnson J. E., Upchurch L. M., Bates T. S. (2017). Small Fraction of Marine Cloud Condensation Nuclei Made Up of Sea Spray Aerosol. Nat. Geosci. 10, 674–679. doi: 10.1038/ngeo3003
Raina J.-B., Tapiolas D. M., Foret S., Lutz A., Abrego D., Ceh J., et al. (2013). DMSP Biosynthesis by an Animal and its Role in Coral Thermal Stress Response. Nature 502, 677–680. doi: 10.1038/nature12677
Smith M. H. (2007). Sea-Salt Particles and the CLAW Hypothesis. Environ. Chem. 4, 391–395. doi: 10.1071/EN07071
Smith G. A. (2019). Seasonal Climate Summary for the Southern Hemisphere (Autumn 2017): The Great Barrier Reef Experiences Coral Bleaching During El Niño–Southern Oscillation Neutral Conditions. J. South. Hemisphere Earth Syst. Sci. 69, 310–330. doi: 10.1071/ES19006
Stuart G. S., Stevens R. G., Partanen A. I., Jenkins A. K. L., Korhonen H., Forster P. M., et al. (2013). Reduced Efficacy of Marne Cloud Brightening Geoengineering Due to in-Plume Aerosol Coagulation: Parameterization and Global Implications. Atmospheric Chem. Phys. 13, 10385–10396. doi: 10.5194/acp-13-10385-2013
Swan H. B. (2017). Investigation of Dimethylsulfide Biogeochemistry Relevant to the CLAW Hypothesis at Heron Island, Southern Great Barrier Reef (Southern Cross University: Doctor of Philosophy (PhD) Dissertation).
Swan H. B., Crough R. W., Vaattovaara P., Jones G. B., Deschaseaux E. S. M., Eyre B. D., et al. (2016). Dimethyl Sulfide and Other Biogenic Organic Compound Emissions From Branching Coral and Reef Seawater: Potential Sources of Secondary Aerosol Over the Great Barrier Reef. J. Atmospheric Chem. 73, 303–328. doi: 10.1007/s10874-016-9327-7
Swan H. B., Jones G. B., Deschaseaux E. S. M., Eyre B. D. (2017). Coral Reef Origins of Atmospheric Dimethylsulfide at Heron Island, Southern Great Barrier Reef, Australia. Biogeosciences 14, 229–239. doi: 10.5194/bg-14-229-2017
Takahashi Y., Okazaki Y., Sato M., Miyahara H., Sakanoi K., Hong P. K., et al. (2010). 27-Day Variation in Cloud Amount in the Western Pacific Warm Pool Region and Relationship to the Solar Cycle. Atmospheric Chem. Phys. 10, 1577–1584. doi: 10.5194/acp-10-1577-2010
Vaattovaara P., Swan H. B., Jones G. B., Deschaseaux E., Miljevic B., Laaksonen A., et al. (2013). The Contribution of Sulfate and Oxidized Organics in Climatically Important Ultrafine Particles at a Coral Reef Environment. Int. J. Mar. Environ. Sci. 7, 720–724. Available at: https://publications.waset.org/9998064/pdf
Vallina S. M., Simó R. (2007). Strong Relationship Between DMS and the Solar Radiation Dose Over the Global Ocean. Science 315, 506. doi: 10.1126/science.1133680
Van Alstyne K. L., Dominique V. J., Muller-Parker G. (2008). Is Dimethylsulfoniopropionate (DMSP) Produced by the Symbionts or the Host in an Anemone-Zooxanthella Symbiosis? Coral Reefs 28, 167–176. doi: 10.1007/s00338-008-0443-y
Veres P. R., Neuman J. A., Bertram T. H., Assaf E., Wolfe G. M., Williamson C. J., et al. (2020). Global Airborne Sampling Reveals a Previously Unobserved Dimethyl Sulfide Oxidation Mechanism in the Marine Atmosphere. Proc. Natl. Acad. Sci. 117, 4505–4510. doi: 10.1073/pnas.1919344117
Von Glasow R., Crutzen P. J. (2004). Model Study of Multiphase DMS Oxidation With a Focus on Halogens. Atmospheric Chem. Phys. 4, 589–608. doi: 10.5194/acp-4-589-2004
Woodhouse M. T., Carslaw K. S., Mann G. W., Vallina S. M., Vogt M., Halloran P. R., et al. (2010). Low Sensitivity of Cloud Condensation Nuclei to Changes in the Sea-Air Flux of Dimethyl-Sulphide. Atmospheric Chem. Phys. 10, 7545–7559. doi: 10.5194/acp-10-7545-2010
Woodhouse M. T., Mann G. W., Carslaw K. S., Boucher O. (2013). Sensitivity of Cloud Condensation Nuclei to Regional Changes in Dimethyl-Sulphide Emissions. Atmospheric Chem. Phys. 13, 2723–2733. doi: 10.5194/acp-13-2723-2013
Xu W., Ovadnevaite J., Fossum K. N., Lin C., Huang R.-J., Ceburnis D., et al. (2022). Sea Spray as an Obscured Source for Marine Cloud Nuclei. Nat. Geosci. 15, 282–286. doi: 10.1038/s41561-022-00917-2
Yost D. M., Mitchelmore C. L. (2009). Dimethylsulfoniopropionate (DMSP) Lyase Activity in Different Strains of the Symbiotic Alga Symbiodinium Microadriaticum. Mar. Ecol. Prog. Ser. 386, 61–70. doi: 10.3354/meps08031
Zaveri R. A., Wang J., Fan J., Zhang Y., Shilling J. E., Zelenyuk A., et al. (2022). Rapid Growth of Anthropogenic Organic Nanoparticles Greatly Alters Cloud Life Cycle in the Amazon Rainforest. Sci. Adv. 8, eabj0329. doi: 10.1126/sciadv.abj0329
Zelenyuk A., Imre D., Earle M., Easter R., Korolev A., Leaitch R., et al. (2010). In Situ Characterization of Cloud Condensation Nuclei, Interstitial, and Background Particles Using the Single Particle Mass Spectrometer, SPLAT II. Analytical Chem. 82, 7943–7951. doi: 10.1021/ac1013892
Zhao W., Huang Y., Siems S., Manton M. (2021). The Role of Clouds in Coral Bleaching Events Over the Great Barrier Reef. Geophysical Res. Lett. 48, e2021GL093936. doi: 10.1029/2021GL093936
Keywords: dimethylsulfide (DMS), Great Barrier Reef (GBR), climate, oxidation, sulfate
Citation: Swan HB (2022) The Potential for Great Barrier Reef Regional Climate Regulation via Dimethylsulfide Atmospheric Oxidation Products. Front. Mar. Sci. 9:869166. doi: 10.3389/fmars.2022.869166
Received: 03 February 2022; Accepted: 23 May 2022;
Published: 20 June 2022.
Edited by:
Gabrielle Nevitt, University of California, Davis, United StatesReviewed by:
Stephen D. Archer, Bigelow Laboratory For Ocean Sciences, United StatesMike Harvey, National Institute of Water and Atmospheric Research (NIWA), New Zealand
Copyright © 2022 Swan. This is an open-access article distributed under the terms of the Creative Commons Attribution License (CC BY). The use, distribution or reproduction in other forums is permitted, provided the original author(s) and the copyright owner(s) are credited and that the original publication in this journal is cited, in accordance with accepted academic practice. No use, distribution or reproduction is permitted which does not comply with these terms.
*Correspondence: Hilton B. Swan, aGlsdG9uLnN3YW5Ac2N1LmVkdS5hdQ==