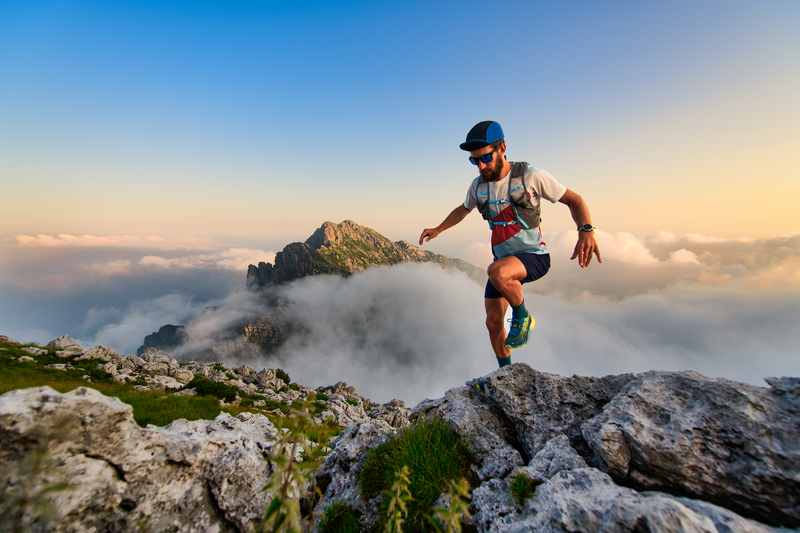
94% of researchers rate our articles as excellent or good
Learn more about the work of our research integrity team to safeguard the quality of each article we publish.
Find out more
ORIGINAL RESEARCH article
Front. Mar. Sci. , 22 July 2022
Sec. Deep-Sea Environments and Ecology
Volume 9 - 2022 | https://doi.org/10.3389/fmars.2022.867656
Cold-water coral (CWC) systems are hotspots of biodiversity that need protection from the increasing human impacts and global climate change. The restoration of degraded cold-water coral reefs may be conducted through transplantation of nubbins. To do so, we need to set up the optimal conditions for CWCs livelihood in an aquarium setting. Here we investigated the food selection of three cold-water coral species inhabiting the NE Atlantic Ocean and the Mediterranean Sea to identify the optimal feeding conditions to rear corals, by means of stable isotope analysis (δ15N and δ13C) and of prey-capture rates. Colonies of Desmophyllum pertusum, Madrepora oculata and Dendrophyllia cornigera were collected in the Mediterranean Sea and nourished in mesocosms with a) nauplii of Artemia salina, b) the green algae Tetraselmis subcordiformis, c) two rotifer species (Brachionus plicatilisand B. rotundiformis) and d) mysids of the species Mysis relicta. Prey-capture rates coupled with isotope analysis revealed that M. relictawas the preferred food source even if it was provided as a frozen item, followed by the live-items A. salina and Brachionus spp. Isotopic analyses allowed to determine that Particulate Organic Matter (POM) appears to contribe to a large portion of the isotopic composition of the coral tissue and also suggested that M. oculata has the most opportunistic behaviour among the three target coral species. This study confirms that it is possible to optimize CWCs livelihood in aquaria choosing the right food sources during their maintenance, also in preparation to their transplant in degraded habitats during future projects of active restoration.
Cold-water corals (CWCs) are important habitat-forming species including azooxanthellate stony corals (Scleractinia), gorgonians (Alcyonacea), black corals (Antipatharia) and hydrocorals (Stylasteridae) (Roberts et al., 2006). CWCs and their habitats are receiving great interest from the scientific community due to their high ecological value (Roberts et al., 2009). Cold-water reefs formed by stony corals are complex three-dimensional structures and are biodiversity hotspots (Henry and Roberts, 2007; Hovland, 2008; Bongiorni et al., 2010; Mastrototaro et al., 2010; Rueda et al., 2019) acting as nurseries, refugia or feeding grounds for several species of fishes and invertebrates (Baillon et al., 2012; D’Onghia, 2019).
CWCs are threatened by several anthropogenic activities (D’Onghia et al., 2016; Ragnarsson et al., 2016; Montseny et al., 2021). Among them, deep-sea fisheries directly affect CWC reefs (D’Onghia, 2019), up to an estimated 95-98% of the total coral cover (Gianni, 2004). Oil and gas exploitation is extending at deeper depths, increasing the risks of oil-spill accidents or dispersal near CWC reefs (Cordes et al., 2016). Also, deep-sea mining might affect CWC reefs that are often located near sites of exploitation (Roberts and Cairns, 2014; Ragnarsson et al., 2016). In addition, global climate change represents a serious concern for CWCs as the reduction of pH determines a decrease of the depth of aragonite saturation horizon (Ragnarsson et al., 2016; Sweetman et al., 2017) and increasing rates of skeletal dissolution (Gómez et al., 2018). Acidification and higher water temperatures are proved to affect cold-water corals also by increasing their vulnerability to other environmental stressors like chemical pollution (Weinnig et al., 2020). Projections indicate that 70% of the actual locations of CWC ecosystems could become under-saturated by 2099 (Guinotte et al., 2006).
Ecosystem restoration is a key action of several frameworks and conventions both at European and global level. Restoration of damaged CWCs ecosystems will be increasingly required in the future, even because sequestration of carbon dioxide from seawater by CWCs (Turley et al., 2007) constitutes an essential regulating service and, because of this, the deep sea has been proposed as storage environment for the surplus CO2 produced by humans with the use of fossil fuels (Davies et al., 2007).
Considering that the research field on ecological restoration of deep-sea ecosystems is still in its infancy (Da Ros et al., 2019), only few studies focus on CWCs active restoration actions, as reported in the literature (Montseny et al., 2021). The transplantation of nubbins taken from healthy colonies or reared in aquaria into degraded coral grounds is the preferred restoration action (Da Ros et al., 2019 and references therein). Pilot experiments on transplantation of nubbins of Desmophyllum pertusum were carried out in the Gulf of Mexico, in the North Sea and off Central California, with encouraging results of high survival rates for D. pertusum (Brooke and Young, 2009; Dahl, 2013; Boch et al., 2019). Recently, in the Mediterranean Sea and in the Atlantic (Azores Archipelago), several CWCs, including fan corals, were transplanted to evaluate their survival rate (Linares et al., 2020).
The success of maintenance of CWCs in aquaria can be affected by variations of physical parameters (mainly flow velocities and temperature) that can also influence the feeding behaviour of CWCs like widely demonstrated in literature (Purser et al., 2010; Gori et al., 2015; Orejas et al., 2016).
Artemia salina is often used for keeping CWCs in aquaria and for conducting feeding experiments because it has the same size of target copepods (Orejas et al., 2019 and references therein). Usually, rotifers in aquaria are provided as feeding supplementary to corals characterised by very small polyp size, like black corals (e.g., Bathypathes sp. and Leiopathes glaberrima) or gorgonians (es Dentomuricea meteor) (Orejas et al., 2019). In captivity, nauplii of A. salina (Linnaeus, 1758) and algae are routinely used to also feed D. pertusum (Orejas et al., 2019), while Mysis sp. (Latreille, 1802) and adults of A. salina are used to feed D. cornigera (Gori et al., 2015; Orejas et al., 2019).
In the Mediterranean Sea, the most common reef-building CWCs are the ‘white corals’ Desmophyllum pertusum (Linnaues 1758), formerly known as Lophelia pertusa (Addamo et al., 2016), and Madrepora oculata (Linnaeus, 1758). These two taxa often co-occur and engineer the CWC-grounds with maximum areal occupancy in the coral provinces identified in the central and western Mediterranean Sea, between ca. 200-800 m (Fanelli et al., 2017; Taviani et al., 2017; Chimienti et al., 2019; Taviani et al., 2019) at a temperature comprised between 11 and 13.9°C (Naumann et al., 2014). In-situ observations carried out through ROVs along Bari canyon and on the Apulian Plateau showed that M. oculata is the dominant species of CWCs in the Central Mediterranean Sea (Freiwald et al., 2009), probably due to the environmental conditions that in this basin are closed to the ecological limits of this species (Davies et al., 2008). The “yellow coral” Dendrophyllia cornigera (Lamarck, 1816) forms colonies from the mesophotic zone down to bathyal depths, where it often mingles with white corals (Castellan et al., 2019). In the Mediterranean Sea, this eurybathic species lives in a temperature range between 7 and 16°C (Castellan et al., 2019).
Here we investigated the feeding preferences of D. pertusum, M. oculata and D. cornigera to establish the best conditions to keep them in captivity and for maintaining them on-board during the oceanographic campaigns. Four food sources were chosen to feed the corals and establish their food preferences: 1) nauplii of A. salina, 2) the green algae Tetraselmis subcordiformis, (Wille) Butcher 1959, 3) two species of rotifers (Brachionus plicatilis, Müller, 1786, and B. rotundiformis, Tschugunoff, 1921) and 4) the crustacean mysid M. relicta (Lovén, 1862).
The main goal of this study is to determine the best conditions for successfully and effectively keeping CWCs alive in aquaria, in face of increasing request of such kind of knowledge for restoration of marine degraded habitats as promoted by the UN Decade on Ecosystem Restoration (UNGA, 2019) at global level and by the EU 2030 Biodiversity Strategy (EC, 2020) at European level. Specific aims of this study are: 1) to determine the isotopic signatures of the exposed specimens to discriminate between ingested vs. assimilated food items and their resource partitioning (i.e., which of the captured preys were preferentially assimilated by the different species) and 2) to increase our understanding on the feeding ecology of CWCs occurring in the Mediterranean Sea.
Three species of cold-water corals were sampled using a ROV (Remotely Operated Vehicle), during two oceanographic campaigns conducted in 2016 in the Mediterranean Sea on-board of RV Minerva Uno. In order to reflect the cold-water coral community structure of the Central Mediterranean Sea, one live colony of D. pertusum (made up of 280 polyps) and 4 colonies of M. oculata (with a total of 1501 polyps) were collected during the SIRIAD16 oceanographic campaign at depth of 244 and 400 m, respectively, in the south-western Adriatic Sea (Figure 1). One colony of D. cornigera (made up of 9 polyps) was collected at 139 m depth, during the RISD_16 campaign, in the northern Ionian Sea (Figure 1). Corals were kept alive inside 20 L PVC darkened aquaria filled with bottom seawater (previously filtered with a 20 µm-mesh) collected simultaneously to corals, without feeding them due to the logistical limitations on board, according to literature (Orejas et al., 2019).
Figure 1 The map shows the points in which corals were collected. The location of sites is discussed in this study. “Area 1” highlights the zone of Bari canyon in which Desmophyllum pertusum and Madrepora oculata were collected. “Area 2” highlights the position of the collecting site of Dendrophyllia cornigera. Bathymetry from EMODnet (European Marine Observation and Data Network) Bathymetry portal (http://www.emodnet-bathymetry.eu).
Once in the laboratory, the colonies were kept in different aquaria (one tank for each species, a 30 L tank for D. cornigera, two 50 L tanks for D. pertusum and M. oculata, with the 4 colonies of M. oculata kept together in the same aquaria to better simulate the community structure) filled with bottom seawater (previously filtered with a 20 µm-mesh). Before choosing how to divide the colonies and the volumes of the tanks to use, we checked that the estimated total dry weights of the collected species were similar, according to dry weights values reported in Maier et al., 2012 for D. pertusum and M. oculata and in Gori et al., 2014 for D. cornigera.
Corals were maintained in the dark and at a temperature of 13 ± 0.5°C. Although corals were collected in different sites, the in-situ temperature was similar, (Table S1), thus we set the temperature at ca. 13°C for all the three species as this is the mean temperature of the deep waters in the Western and Central Mediterranean Sea (Danovaro et al., 2010), with the maximum recorded temperature for the occurrence of D. pertusum at 13.8°C in the Ionian Sea (Freiwald et al., 2004). Temperature was maintained constant through a common water bath and a refrigerator (TECO SeaChill Chiller TR5). Seawater was sampled in the central Adriatic Sea and filtered with a 20 µm-mesh prior to gradually mix it with the bottom seawater of the aquaria (final salinity 37 ± 0.2 PSU). Subsequently, ~60% of the seawater in the tanks was exchanged every 10 days. Every day, the bottom of the tank was cleaned with an aquarium siphon to ensure build-up of detritus to be minimal.
Due to the difficulties in replicating the natural complexity of the environments from which the corals were collected in laboratory settlings, and differently from previous studies that focused on the effects of current velocities on their feeding capacity, here we maintained a constant water recirculation using submersible pumps with a flow rate of ~2000 L h−1 (Orejas et al., 2019). The flow velocity was maintained in a range of 5-10 cm s-1 to better simulate the natural conditions of collection areas (Turchetto et al., 2007; Langone et al., 2016) with peaks of ~20 cm s-1 in the tank of D. cornigera (Davies et al., 2009). Pumps water-outlets were coated with a 20 µm-NITEX nylon mesh in order to avoid harming of prey items.
An air stone oxygenated the seawater and was placed at the top of the aquaria preventing any influence on polyps’ activity. The oxygen concentration was maintained at in-situ conditions (5.2 ± 0.2 mL L-1) (Davies et al., 2008). We started the experiment when the colonies completely extended their polyps without showing stress signals (e.g., mucus production) (Murray et al., 2019). This acclimation period lasted 4 weeks for D. pertusum and M. oculata (Chapron et al., 2018) and 2 weeks for D. cornigera. Since the filtered seawater still contained sufficient organic particles, no extra food had to be provided during the acclimation period (Van Oevelen et al., 2018). Food was provided when at least the 90% of the polyps of the colonies were open.
Corals’ behaviour and their response to presence of food or light were observed and these qualitative data were collected to gather essential information at best maintenance in aquaria. Twice a day, at 9 a.m. and at 6 p.m., polyps’ activity and tissue conditions were monitored to exclude stress signs like tissue sloughing and loss, and extensive mucus production (Orejas et al., 2019). During the first twenty minutes from the provisioning of food, corals’ reaction to the presence of food items was observed. During all the monitoring, we used a weak lighting screened with red filters to reduce possible impacts by the necessary light exposure (Orejas et al., 2019).
Artemia’s dry cysts (1 g) were placed inside a conical Artemia hatchery filled with 2 L of pre-filtered 0.7 µm seawater (filtered using Whatman GF/F filters) with intensive light. After 24 hours, nauplii were hatched and used for the experiment. The green algae T. subcordiformis was cultured in 500 mL Erlenmeyer flasks filled with sterile F/2 medium (Guillard, 1975). The cultures were maintained at 21°C, lightened by a continuous light with a photon flux density of 100 µmol m-2 s-1 (400-700 nm). B. plicatilis and B. rotundiformis were cultured at 21°C in a tank with mechanical aeration filled with pre-filtered 0.7 µm seawater at a salinity of 33 (Yufera et al., 1997). Rotifers were fed with baker’s yeast (Orejas et al., 2019). These food sources were selected for the highly standardised rearing protocols and the variety of the composition, which can simulate a wide range of potential coral preys (Orejas et al., 2019). On the contrary, mysids are not easy to rear due to the common cannibalism of the adults towards juveniles (Mauchline, 1980). For this reason, M. relicta was supplied from frozen stocks and kept in suspension using an intense water recirculation (Orejas et al., 2019).
For each species, each food source was provided in the same total amount (total biomass expressed as µg of C). D. pertusum and M. oculata (for both species, around 75 µgC L-1 from each food source, a biomass determined considering the amount of nauplii of A. salina provided by Larsson et al., 2013) were fed twice a week, while food items were provided to D. cornigera three times a week (around 250 µgC L-1 for each food source, a biomass determined considering the amount of nauplii of A. salina provided by Gori et al., 2015). Food sources’ biomass was determined using literature data (T. subcordiformis) and bio-volumetric measurements (zooplankton). Body volumes of M. relicta, A. salina, Brachionus spp. were determined with a stereomicroscope (LEICA WILD L3B) with micrometric grids. The biovolume and the biomass of rotifers was calculated accordingly to Gradinger et al. (1999). For crustaceans, the biovolume was calculated from the body width (W) and length (L) (ten specimens for each food source) using the formula V=L×W2×C, where C is an a-dimensional factor (Gambi et al., 2019). We assumed an average density of 1.13 g cm-3 to calculate the wet biomass and then the dry weight (μg dry weight: μg wet weight = 0.25) (Wieser, 2007). The carbon content was considered as the 40% of the dry weight (Higgins and Thiel, 1988).
Stable-nitrogen (δ15N) and stable-carbon (δ13C) isotope ratios were determined in the three species. These values can provide useful information in dietary studies and are commonly applied to analyse marine food webs (DeNiro and Epstein, 1978; Post, 2002; Fry, 2006; Layman et al., 2012) providing an indication of the origin and transformations of organic matter (i.e., food assimilated) (Peterson and Fry, 1987; Layman et al., 2007; Newsome et al., 2007). The δ15N in tissues of consumers are typically greater by 2–3‰ relative to their prey so that δ15N data can be used to estimate the trophic levels of organisms (Owens, 1988). δ13C may act as a useful indicator of primary organic carbon sources of an animal’s diet, as tissues tend to be rather weakly enriched in 13C at progressively higher trophic levels (less than 1‰; DeNiro and Epstein, 1978). Stable Isotope Analysis (SIA) results allowed also to better understand the trophic ecology of the investigated species in the Central Mediterranean Sea.
Once arrived at the laboratory, one third of the sampled colonies was immediately frozen at –20°C (T0). SIA was conducted only on the soft bodies of the animals to avoid the interference of the C signal provided by the analysis of the entire calyx (Sherwood et al., 2008). Samples from the different cultures of the food sources were collected and frozen. At the end of the experiment, that lasted 30 days (Tf), samples of each of the coral species (at least n=5 samples with at least 3 polyps for each species) were collected and immediately frozen. Samples were then dried for 24 h at 60°C and ground to a fine powder with a mortar and a pestle (Fanelli et al., 2011). Except for algae and rotifers, subsamples were acidified adding drop by drop HCl 1M (Sigma-Aldrich, CAS Number 7647-01-0) to remove inorganic carbonates. Cessation of bubbling was used as signal of completion of the reaction. These subsamples were dried again at 60°C for 24 h (Jacob et al., 2005). Some samples were acidified (and dried) once again until complete removal of inorganic carbonates. All the samples were weighed (ca. 1 mg of dry weight) in tin capsules (Elemental Microanalysis Tin Capsules Pressed, Standard Weight 5 x 3.5 mm). Stable isotope measurements were carried out by an elemental analyser coupled to an isotope ratio mass spectrometer (ThermoFisher Flash EA 1112 elemental analyzer coupled to a Thermo Electron Delta Plus XP isotope ratio mass spectrometer, IRMS) according to standard protocols (Fanelli et al., 2009; Fanelli et al., 2011; Rumolo et al., 2016). Briefly, the samples were run against blank cups and known urea standards. Three capsules of urea were analysed at the beginning of each sequence and one every six samples as a quality control measure and to compensate for potential machine drift. Experimental precision (based on the standard deviation of replicates of the internal standard) was <0.1 ‰ for δ15N and <0.2 ‰ for δ13C. The δ15N and δ13C values were obtained in parts per thousand (‰) relative to Vienna Pee Dee Belemnite (VPDB) and atmospheric N2 standards, respectively, according to the following formula:
where R=13C/12C or 15N/14N. At least three replicates for samples were analysed.
The prey-capture rate was evaluated for each of the three species. For this experiment, we followed the protocol reported in Tsounis et al. (2010). Fresh living zooplankton and phytoplankton were mixed and added in the aquaria, twice a week (for 30 days) for D. pertusum and M. oculata, and three times a week for D. cornigera. Frozen individuals of M. relicta were added at the same time. Seawater in the aquaria was gently mixed by a continuous slight aeration (Piccinetti et al., 2016) and by the submersible recirculation pumps (~2000 L h-1). Seawater was vigorously mixed, and samples (100 mL for 3 replicates) were taken from each tank after a couple of seconds and after 5 hours (Orejas et al., 2016). Samples were preserved with 4% formaldehyde (Sigma-Aldrich, CAS Number 50-00-0) (Orejas et al., 2016) and, after 24 hours, individuals of A. salina and Brachionus spp. were counted using a Dolphus curve (Tsounis et al., 2010) and a stereomicroscope (LEICA WILD L3B). For counting T. subcordiformis cells, replicates of 60 mL of seawater were collected at the same time from each tank and preserved for 24 hours with 2% formaldehyde. Replicated subsamples of 1 mL of seawater were observed using a Sedgewick-rafter counting chamber and algae cells were counted under a microscope (Orejas et al., 2016). Averaged prey-capture rate was normalized to the number of polyps present in each mesocosm to determine the number of nauplii captured by each polyp at each hour. Each time after feeding, the uneaten food was removed (Orejas et al., 2019). Control experiments were run under the same conditions but without corals to determine the percentage loss of prey items in each different aquarium and to correct the values of the determined prey-capture rates.
Statistical analyses were carried out using R (4.0.5 version R Development Core Team, 2021). After testing the homogeneity of variances using Bartlett’s test, one-way analysis of variance (ANOVA) was performed with the R function “aov” to test for differences in δ13C and δ15N contents of each species between the two sampling periods. same approach was used to test differences among the assumption of C from the different food sources by the three coral species. When significant differences were encountered with ANOVA, a Tukey’s post-hoc comparison test was performed with the R function “TukeyHSD” to ascertain differences among the contributions of C provided by the different food sources. For all the analyses, p<0.05 was considered the significant threshold.
To provide an estimate of the relative contributions of the different sources to the isotopic content of the samples, the package Stable Isotope Mixing Models in R (simmr) was used (Parnell, 2021). Simmr is designed as an upgrade of the package SIAR (Stable Isotope Analysis in R) (Parnell and Jackson, 2013) and it is designed to solve mixing equations for stable isotopic data within a Bayesian framework. The standard deviation depends on the intraspecific variability among the individuals and on the uncertainty of fractionation corrections. In this study, we used for 13C the Trophic Enrichment Factor (TEF) of 1.0 ± 0.1 ‰ (Ferrier-Pagès et al., 2011) and for 15N the TEF of several consumers’ diets that is 2.5 ± 0.1 ‰ (Carlier et al., 2009).
Before running the model, the isotopic values of the sources and of the three species of corals were plotted together, applying the correct TEFs to determine the mixing polygon (Smith et al., 2013; Phillips et al., 2014) of the CWCs community. We excluded T. subcordiformis as food source due to its very high δ15N isotope value which remains far outside the mixing polygon (Jackson et al., 2011; Phillips et al., 2014). The corals isotopic values did not fall completely within the range of the food source isotopic values, so we decided to use data from the literature to better construct the mixing polygon and to define the sources which to run the model with (Phillips et al., 2014) (Figure S1). One of the potential food sources for CWCs is the particulate organic matter (POM) contained in the seawater (Mueller et al., 2014). In this study, we used two different POM values as inputs in simmr, based on the hypothesis that CWCs can be influenced by both the Bari Canyon POM during the collection, transportation, and the beginning of the experiment, and by the Northern Adriatic Sea POM for the other part of the experiment, as we used Adriatic seawater for maintaining corals in our laboratory. The values of the isotopic content of POM of the Bari Canyon (δ15N = –2.6 ‰ and δ13C = –21.7 ‰) and POM of the Adriatic Sea (summer period, δ15N = 7 ‰ and δ13C = –22 ‰) were taken from the literature (respectively from Carlier et al., 2009 and from Faganeli et al., 2009).
To examine the trophic targets of each species, SIBER package (Stable Isotope Bayesian Ellipses in R) was used (Jackson et al., 2011). SIBER allowed to determine the trophic preference of the three species. Layman metrics, which provide quantitative measures of the trophic structure of a community, were also calculated in SIBER, specifically: δ13C range (CR), δ15N range (NR), total area of the convex hull (TA), mean distance to centroid (CD) (Layman et al., 2007). CR provides information on the diversity of the resources at the base of the trophic web with higher values that indicate multiple basal carbon sources; NR gives information on the trophic length of the community and CD estimates trophic diversity within a food web and is a function of the degree of species spacing lower numbers indicate that distinct taxa are exhibiting similar ecological functions (Jackson et al., 2011). TA gives an indication of the variety of food items but is highly sensitive to sample size (Layman et al., 2007). Simmr and SIBER were used also to calculate the corrected Standard Ellipse Areas (SEAC) that is the sample-size corrected population isotopic targets (Jackson et al., 2011) allowing the comparison between the preferences of the three species (classified as groups of the same community). It contains approximately 40% of the data within a set of bivariate data and thus represents the core area for a population or community (Layman et al., 2007; Jackson et al., 2011). Overlap of isotopic data suggests, at least in part, an overlap of resource usage by the groups (Layman et al., 2007; Jackson et al., 2011). The percent overlap is given by the percent of the overlapping area over the total area covered by the two ellipses (Krumsick and Fisher, 2019). All the analyses were carried out using R.
Even if this experiment was not set to study corals polyp activity and behaviour, these observations were reported to provide additional information on corals’ feeding behaviour. Among the three species, D. cornigera was the most reactive one, with all the polyps opened after 2 weeks of acclimation. It reacted to each food provisioning by moving tentacles to capture preys. Also M. oculata expanded the polyps’ tentacles when it detected the presence of food items, while D. pertusum seemed to be the most light-sensible species, withdrawing polyps and producing mucus even when exposed to a weak light (used during sampling operations). At the end of the experiment, the corals did not display signals of stress.
The isotopic content of the food items provided to the three coral species, varied from 6.41 ‰ (in M. relicta) to 14.58 ‰ (in T. subcordiformis) for δ15N, and from –20.5 ‰ (in M. relicta) to –14.5 ‰ (in Brachionus spp.) for δ13C (Table 1).
At the end of the experiment (Tf, after 30 days from the beginning of the feeding experiment) 15N values were more enriched in all the species. D. pertusum showed a significant increase in the δ15N value of 2.9 ‰ (from 3.4 ‰ ± 0.4 to 6.3 ‰ ± 0.5, p<0.001). In M. oculata the increase was of 0.8 ‰ (from 3.3‰ ± 0.9 to 4.1‰ ± 1.8, p>0.05, not significant) and in D. cornigera the significant increment was of 1.2 ‰ (from 4.8‰ ± 0.6 to 6.0 ‰ ± 0.6, p<0.05) (Figure 2A and Table 2). The isotopic signals in D. pertusum and M. oculata were 13C-depleted (respectively from –19.9 ± 0.3 ‰ to –20.7 ± 0.8 ‰ and from –19.5 ± 1.9 ‰ to –20 ± 1.7 ‰, p>0.05, not significant) but for D. cornigera the values of δ13C showed an increase (from –21 ± 0.5 ‰ to –20.4 ± 1.7 ‰, p>0.05, not significant) (Figure 2B and Table 2).
Figure 2 Boxplots showing the values of δ15N (A) and δ13C (B) of the specimens of corals used in the experiment at T0 (green boxes) and Tf (violet boxes) with significant differences indicated by the stars. The horizontal line within the box represents the median, the boundaries of the boxes represent the first and second quartiles and the whiskers of the boxes represent the 95 % credibility interval. *p<0.05; ***p<0.001.
Table 2 Mean δ15N and δ13C of the corals at the beginning (T0) and at the end (Tf) of the experiment.
Simmr provided the proportional contribution of each food source to the diet of the three CWC species (Figure 3). At T0, the major contribution to the isotopic composition of the three species was given by the POM of Bari canyon (means were of 64.9% in D. pertusum, 65.7% in M. oculata, 48.3% in D. cornigera) (Figure 3). After 30 days, the proportion of the contribution of POM of Bari canyon to CWC’s diet decreased (30.6% in D. pertusum, 54.1% in M. oculata, 32.8% in D. cornigera), while that of North Adriatic Sea POM increased (64.9 ± 0.04% in D. pertusum, 12.6% in M. oculata, 48.3% in D. cornigera) together with the contribution of M. relicta (19% in D. pertusum, 12.6% in M. oculata, 18.6% in D. cornigera) (Figure 3), A. salina (12.1% in D. pertusum, 9% in M. oculata, 12.6% in D. cornigera) and Brachionus spp (9.3% in D. pertusum, 7.6% in M. oculata, 10.7% in D. cornigera). The simmr output is presented as the full distribution of the prior and posterior probability density function (Figure S2).
Figure 3 Posterior probabilities for the proportional contribution of each food source to the diet of (A) D. pertusum, (B) M. oculata and (C) D. cornigera obtained with stable isotope analysis mixing models. Each plot shows proportions for each food source at the beginning (figures on the left, T0) and at the end (figures on the right, Tf) of the experiment. The horizontal line within the box represents the median, the boundaries of the boxes represent the first and second quartiles and the whiskers of the boxes represent the 95% credibility interval. POM BariC = Particulate Organic Matter of Bari’s canyon; POM N_Adr = Particulate Organic Matter of the North Adriatic Sea.
Standard ellipses showed that M. oculata has the widest isotopic variability and D. cornigera the smallest one (Figure 4). Additionally, Layman metrics (Table 3) indicated that D. cornigera had the smallest total area (TA) (3.12 ‰2), followed by D. pertusum (4.12 ‰2), while M. oculata showed a TA of 16.2 ‰2. The corresponding values of the different SEAC are 2.75, 2.38 and 5.83 ‰2, for D. pertusum, D. cornigera and M. oculata respectively. While the SEAC of M. oculata and D. cornigera are expanded along the x-axis (pointing out to a wider δ13C range), the SEAC of D. pertusum is stretched along the y-axis (corresponding to a greater δ15N range) (Figure 4 and Table 3).
Figure 4 δ13C - δ15N scatterplot with standard ellipses corrected for small sample size population (SEAC) overlaid for the three CWC species (p interval=0.4).
Table 3 Total area (TA, ‰2), Standard ellipse area (SEAC,‰2) and Layman metrics calculated for each species.
M. oculata has the greatest δ13C and δ15N ranges and mean CD, which is a proxy of trophic diversity (Table 4). Overall, there is a partial overlap of the SEAc of the three species. The SEAC of D. pertusum and M. oculata overlapped for 16.1%, while those of D. pertusum and that of D. cornigera for 15.4%. There is no overlap of the SEAC of M. oculata and D. cornigera.
Table 4 Different prey-capture rates of the three species of corals and total quantity of carbon captured per polyp.
Each polyp of D. pertusum captured an average of 3.6 ± 1.8 (SE) nauplii of A. salina per hour, 3.7 ± 2 (SE) individuals of Brachionus spp. and about 3.2 ± 0.7 × 103 (SE) cells of T. subcordiformis. Each polyp of M. oculata captured an average 1 ± 0.3 (SE) nauplii of A. salina per hour, 2.1 ± 0.7 (SE) rotifers and about 9.8 ± 2.6 × 103 (SE) cells of the algae. Each polyp of D. cornigera preyed, on average, 2 × 103 ± 53 (SE) nauplii per hour, 1.6 × 103 ± 5.6 × 102 (SE) individuals of Brachionus spp. and about 4.8 ± 1.9 ×104 (SE) cells of algae (Table 4). Specimens of M. relicta were almost completely removed each time by all the three species of corals.
In terms of biomass, most of the organic carbon (µg C polyp-1 h-1) was obtained by all the species from the largest preys (M. relicta, p<0.01 in the Tukey’s post-hoc contrast tests; Table 4). Both D. pertusum and D. cornigera preyed a similar number of nauplii of A. salina and specimens of Brachionus spp., but the mass of carbon supplied by the crustaceans was higher (p>0.05, not significant in the Tukey’s post-hoc contrast tests) than that of the rotifers. All coral species fed also upon T. subcordiformis, which provided a food supply higher (p>0.05, not significant in the Tukey’s post-hoc contrast tests) than that of the rotifers for the species D. pertusum and M. oculata.
This study investigated the food preferences of the CWCs, maintained in aquaria at conditions like those encountered during their life in the deep Mediterranean Sea. D. cornigera was the most reactive species, especially after the food supply that stimulates its polyps’ reaction (Orejas et al., 2019). D. pertusum was the most sensitive species to the exposure, even to brief and weak-light expositions, such as those occurring during sampling operations. Being D. pertusum the species most closed to its ecological upper-temperature limit, probably it reacted stronger than the other species to this environmental stressor. Further studies are needed to further explore the role of light. Moreover, this species reacted slowly at the presence of food, confirming previous observations (Mortensen, 2001). Behaviour differences among species could possibly also reflect their reaction to variations in pressure from the site of collection to aquaria conditions, in the order of ca. 20 bars for D. pertusum and ca. 40 for M. oculata (Orejas et al., 2019). The eurybathic D. cornigera was instead collected at ca. 14 bars, thus facing lower pressure variations, in principle less stressing for the animal. M. oculata shows, in general, much higher tolerance to environmental fluctuations (Wienberg et al., 2009), while D. pertusum is a species more commonly found in cold waters with temperatures between 4-12°C (Freiwald et al., 2004). In the central Mediterranean Sea, temperatures measured in live coral habitats during oceanographic campaigns range between 13.4 and 13.9°C (Freiwald et al., 2009). These temperatures are close to the ecological limit of D. pertusum (Brooke et al., 2013; Matos et al., 2021) while the optimal temperatures for this species were estimated to be around 6.2-6.7°C (Davies et al., 2008). These behavioural observations support the hypothesis that the temperature used during the experiments (13°C) was likely more suitable for M. oculata and D. cornigera (Naumann et al., 2014; Gori et al., 2015) than for D. pertusum.
During the whole experiment, the three CWC species captured all types of preys. Available literature data on feeding rates (Table S2) show that prey-capture rates differ among the three CWC species, depending also on the type of prey and on the different life stage of the specimens offered as food (e.g., naupliar stages vs. adults) as well as the temperature and the flow velocity under with the experiments are run.
In our study, prey-capture rates of the three species of CWCs showed a preference for the mysid M. relicta, and subordinately for the branchiopod A. salina. Several studies reported that the diet of CWCs in the field is based on zooplankton, such as copepods (Henrich and Freiwald, 1997; Kiriakoulakis et al., 2005; Naumann et al., 2015). A. salina has the same size of these target copepods (Orejas et al., 2019 and references therein). Among the three different species, M. oculata is the one with smallest polyps (5-10 mm in diameter) (Orejas et al., 2019), so the greater capture rates for rotifers (around 350 µm in length) appears as the result of the selection of a prey with a suitable size compared to the size of the corals’ polyps.
Comparing measurements of prey-capture rates from different experiments is difficult because capture rates may overestimate the real ingestion rate if the prey is not efficiently transferred to the gut (Purser et al., 2010). Trapped food may be partly lost due to the sloppy feeding (Møller, 2004) or could not be assimilated, so prey-capture rates cannot directly be translated into ingestion rates or even in assimilation rates (Orejas et al., 2019). Because of this, we integrated capture-rates results with SIA outcomes to determine the assimilated food.
The values of δ13C measured at T0 in this study for the three CWCs species (from –21 to –19.5 ‰) fit well with that of North Atlantic CWCs (from –22.2 to –19.3 ‰) (Duineveld et al., 2004; Sherwood et al., 2008). These values are slightly more negative than that for CWCs from the Strait of Sicily, South of Malta (from –18.9 to –18.2 ‰, CNR cruise CORAL of the RV ‘Urania’, 450–600 m) (pers. comm. M. Taviani), but this can be justified by the different characteristics of the Adriatic basin and of the Sicily Strait (higher salinity and temperature of this latter, Simoncelli et al., 2014), which typically influence the isotopic composition of the POM (Fanelli et al., 2013; Conese et al., 2019). Our results are consistent also with data from the CWCs coral province of Santa Maria di Leuca for D. pertusum and M. oculata (δ13C from –19 to –21 ‰) (Carlier et al., 2009). In our experiment, a decrease in δ13C in D. pertusum and M. oculata was observed at Tf, although δ13C values remain like those reported for the Ionian Sea (Carlier et al., 2009). These results likely support the hypothesis of a high similarity, in terms of food sources, between the South Adriatic (Bari Canyon) and S. Maria di Leuca CWC provinces. On the contrary, an increase in δ13C for D. cornigera was observed probably due to the preferential ingestion of M. relicta. The polyps of this coral measure 20-40 mm in diameter (Orejas et al., 2019) and mysids are likely more suitable to the dimensions of this species (Gori et al., 2015; Orejas et al., 2019).
The values of δ15N at T0 in all the species were more negative (about halved) than those obtained for D. pertusum and M. oculata analysed in the Santa Maria di Leuca CWC province (values of δ15N from 6.9 to 10.1 ‰) (Carlier et al., 2009) or in the Atlantic (Duineveld et al., 2004; Kiriakoulakis et al., 2005). The 15N-depletion could be caused by both thermal shocks that occurred during the recovery from the seabed, and/or by light exposure and decompression (Orejas et al., 2019). In fact, corals started eating only after a period of acclimation. The results from the simmr mixing model show that at T0, all the three investigated species had an isotopic composition close to that of the POM of the region (i.e., the Bari Canyon; Carlier et al., 2009). POM is easier to consume and less energy-expensive to capture compared to whole animal preys (living or frozen), so our results confirm that CWCs are able to use also fine organic particles as food source (Mortensen, 2001; Mueller et al., 2014; Orejas et al., 2016). These results suggest that corals filtered POM from surrounding seawater. It is probable that CWCs can sustain their basal metabolism feeding only on POM when other food sources are not available. As an example, the values of δ15N of D. pertusum and M. oculata in the Rockall Bank are very close to that of obligate filter-feeding taxa like tunicates and bivalves that are known to feed only on organic particles (Duineveld et al., 2007). In our experiment, after 30 days, the isotopic values of corals reared in aquaria were closer to those of POM of the northern Adriatic Sea that is probably incorporated in their soft bodies faster than the other food sources. Among them, according to simmr results, the mysids M. relicta contributed to the corals’ diet for ~17%. This prey seemed to be the preferred one, also in terms of feeding rates. This crustacean is a member of the family Mysidae, the same family of Boreomysis arctica (Krøyer, 1861) and B. megalops (G.O. Sars, 1872) that are among the most abundant supra-benthic species on the upper and middle slope of the Ionian (Madurell and Cartes, 2003) and the Catalan Sea (Cartes et al., 2011), and are likely among the main natural preys of CWCs living in these areas. Considering together results of prey-capture rates and results of SIA allows to suggest that the maintenance in aquaria of CWCs could be optimised by a diet based on the supplying of frozen Mysis sp. instead of the most common used and live-prey A. salina. Supplying the corals with Mysis sp. can also solve the problem of the depletion of some components, like fatty acids, in the tissue of corals fed only with A. salina (Larsson et al., 2013). Discrepancies between prey-capture rates and the degree of assimilation of the food sources in the soft bodies of the corals should be correlated with the utilization of the ingested food for respiration, for maintenance and growth, tissue growth and storage, reproduction and the release of mucus as dissolved organic matter (Orejas et al., 2019) that can also be re-ingested ad assimilated as a strategy to withstand several months without food supply (Mueller et al., 2014).
M. oculata seems to be a more generalist species, with the greatest trophic diversity, as evidenced by the wide δ13C range and the greatest CD values, and the highest feeding plasticity compared to the other two CWC species. A generalist behaviour in deep-sea ecosystems represents an advantage, as the species can rely on available food source (POM, zooplankton, phytodetritus) in an environment where food availability may be heterogeneous in time and space (Gori et al., 2018). Opportunistic feeding behaviour was previously reported for D. pertusum (Mortensen, 2001; van Oevelen et al., 2009; Mueller et al., 2014; Orejas et al., 2016), M. oculata and D. cornigera (Gori et al., 2018). In the Mediterranean basin, M. oculata seems to be the most abundant CWC species: it is up to 50 times more abundant than D. pertusum in Cap de Creus and Lacaze-Duthiers canyons in the Gulf of Lion (Gori et al., 2013), exclusively present in the eastern Ligurian sea (Fanelli et al., 2017), and dominates the CWC communities in the Santa Maria di Leuca coral province (Vertino et al., 2010), in Bari Canyon (Freiwald et al., 2009) and in the Alboran Sea (Corbera et al., 2019). The higher abundance of this species in the basin may be related also to its greater range of food items and its wider ability to exploit different food sources.
There is growing evidence that conservation measures alone, such as the creation of offshore MPAs or Fishery Restricted Areas (FRAs), albeit necessary to avoid the negative effects of the bottom trawling (Huvenne et al., 2016), are not sufficient to protect these vulnerable habitats from the numerous synergistic impacts that threaten them (Ragnarsson et al., 2016). Moreover, it is known that CWC colonies are slow growing species, which require decades to reach a diameter of 1.5-2 m and possibly thousands of years to build a reef (10-30 m thick; Fosså et al., 2002). Due to their low growth rates, the expected natural recovery rates can be very slow.
Active restoration actions for CWC reefs will require rearing and/or maintenance in aquaria of nubbins taken from healthy donor colonies and transplantation of these fragments into degraded mounds (Van Dover et al., 2014). Promising results of pilot transplantation experiments have been reported in literature for D. pertusum (Da Ros et al., 2019 and references therein). The outcomes of our experiments confirm that it is possible to maintain CWCs in aquaria and allow us to identify the best feeding conditions to keep them in aquaria. Our study provides novel information on the trophic items preferred by D. pertusum, M. oculata and D. cornigera in the Mediterranean Sea. Maintaining corals in aquaria and improving their wellness in captivity may positively impinge on their growth or reproduction success, which may turn into the generation of nubbins successfully transplantable in degraded reefs. Additionally, transplanting healthy corals will increase the chances of obtaining a positive outcome of the effort made, thus contributing to the achievement of the goal of a successful restoration of degraded ecosystem as several frameworks and directives foresee for the next future.
The original contributions presented in the study are included in the article/Supplementary Material. Further inquiries can be directed to the corresponding authors.
RD, MT, AD’A conceived the study. RD, MT, EF and AD’A designed the sampling strategy. LA and MT performed the sampling. ZDR and EF analysed the samples. ZDR and EF performed the statistical analyses. ZDR wrote the original draft of the paper, with contributions from EF, RD, MT, AD’A and LA revised the original draft. All authors have read and agreed to the published version of the manuscript.
This research was carried out within the frame of the MIUR “Flagship project RITMARE” and the European Union Horizon 2020 projects, Marine Ecosystem Restoration in Changing European Seas (MERCES), grant agreement No. 689518.
The authors declare that the research was conducted in the absence of any commercial or financial relationships that could be construed as a potential conflict of interest.
All claims expressed in this article are solely those of the authors and do not necessarily represent those of their affiliated organizations, or those of the publisher, the editors and the reviewers. Any product that may be evaluated in this article, or claim that may be made by its manufacturer, is not guaranteed or endorsed by the publisher.
We thank Captain, crew and scientific staff for their assistance during the oceanographic missions the R/V Minerva Uno, and M. Lo Martire for his help in collecting and maintaining D. cornigera in the on-board aquarium. Special thanks to G. Barone for helping with the preparation of the map in Figure 1. ISMAR-CNR scientific contribution. 2017.
The Supplementary Material for this article can be found online at: https://www.frontiersin.org/articles/10.3389/fmars.2022.867656/full#supplementary-material
Addamo A. M., Vertino A., Stolarski J., García-Jiménez R., Taviani M., Machordom A. (2016). Merging Scleractinian Genera: The Overwhelming Genetic Similarity Between Solitary Desmophyllum and Colonial Lophelia. BMC Evolution. Biol. 16(2016). doi: 10.1186/s12862-016-0654-8
Baillon S., Hamel J.-F., Wareham V. E., Mercier A. (2012). Deep Cold-Water Corals as Nurseries for Fish Larvae. Front. Ecol. Environ. 10, 351–356. doi: 10.1890/120022
Boch C. A., Devogelaere A., Burton E., King C., Lord J., Lovera C., et al. (2019). Coral Translocation as a Method to Restore Impacted Deep-Sea Coral Communities. Front. Mar. Sci. 6(540). doi: 10.3389/fmars.2019.00540
Bongiorni L., Mea M., Gambi C., Pusceddu A., Taviani M., Danovaro R. (2010). Deep-Water Scleractinian Corals Promote Higher Biodiversity in Deep-Sea Meiofaunal Assemblages Along Continental Margins. Biol. Conserv. 143, 1687–1700. doi: 10.1016/j.biocon.2010.04.009
Brooke S., Ross S. W., Bane J. M., Seim H. E., Young C. M. (2013). Temperature Tolerance of the Deep-Sea Coral Lopheliapertusa From the Southeastern United States. Deep-Sea Res. Part II: Topical. Stud. Oceanogr. 92, 240–248. doi: 10.1016/j.dsr2.2012.12.001
Brooke S., Young C. M. (2009). In Situ Measurement of Survival and Growth of Lopheliapertusa in the Northern Gulf of Mexico. Mar. Ecol. Prog. Ser. 397, 153–161. doi: 10.3354/meps08344
Carlier A., Le Guilloux E., Olu K., Sarrazin J., Mastrototaro F., Taviani M., et al. (2009). Trophic Relationships in a Deep Mediterranean Cold-Water Coral Bank (Santa Maria Di Leuca, Ionian Sea). Mar. Ecol. Prog. Ser. 397, 125–137. doi: 10.3354/meps08361
Cartes J. E., Mamouridis V., Fanelli E. (2011). Deep-Sea Suprabenthos Assemblages (Crustacea) Off the Balearic Islands (Western Mediterranean): Mesoscale Variability in Diversity and Production. J. Sea. Res. 65, 340–354. doi: 10.1016/j.seares.2011.02.002
Castellan G., Angeletti L., Taviani M., Montagna P. (2019). The Yellow Coral Dendrophylliacornigera in a Warming Ocean. Front. Mar. Sci. 6. doi: 10.3389/fmars.2019.00692
Chapron L., Peru E., Engler A., Ghiglione J. F., Meistertzheim A. L., Pruski A. M., et al. (2018). Macro and Microplastics Affect Cold-Water Corals Growth, Feeding and Behaviour. Sci. Rep. 8, 15299. doi: 10.1038/s41598-018-33683-6
Chimienti G., Bo M., Taviani M., Mastrototaro F. (2019). “Occurrence and Biogeography of Mediterranean Cold-Water Corals,” in Mediterranean Cold-Water Corals: Past, Present and Future: Understanding the Deep-Sea Realms of Coral, vol. 19 . Eds. Orejas C., Jiménez C. (Cham, Switzerland: Springer International Publishing AG), Pages 213–243.
Conese I., Fanelli E., Miserocchi S., Langone L. (2019). Food Web Structure and Trophodynamics of Deep-Sea Plankton From the Bari Canyon and Adjacent Slope (Southern Adriatic, Central Mediterranean Sea). Prog. Oceanogr. 175, 92–104. doi: 10.1016/j.pocean.2019.03.011
Corbera G., Lo Iacono C., Gràcia E., Grinyó J., Pierdomenico M., Huvenne V. A. I., et al. (2019). Ecological Characterisation of a Mediterranean Cold-Water Coral Reef: Cabliers Coral Mound Province (Alboran Sea, Western Mediterranean). Prog. Oceanogr. 175, 245–262. doi: 10.1016/j.pocean.2019.04.010
Cordes E. E., Jones D. O. B., Schlacher T. A., Amon D. J., Bernardino A. F., Brooke S., et al. (2016). Environmental Impacts of the Deep-Water Oil and Gas Industry: A Review to Guide Management Strategies. Front. Environ. Sci. 4. doi: 10.3389/fenvs.2016.00058
Dahl M. (2013). Conservation Genetics of Lophelia pertusa. PhD thesis. Department of Biological and Environmental Sciences, University of Gothenburg, Gothenburg.
Danovaro R., Company J. B., Corinaldesi C., D’Onghia G., Galil B., Gambi C., et al. (2010). Deep-Sea Biodiversity in the Mediterranean Sea: The Known, the Unknown, and the Unknowable. PLoS One 5, e11832. doi: 10.1371/journal.pone.0011832
Da Ros Z., Dell’Anno A., Morato T., Sweetman A. K., Carreiro-Silva M., Smith C. J., et al. (2019). The Deep Sea: The New Frontier for Ecological Restoration. Mar. Policy 108. doi: 10.1016/j.marpol.2019.103642
Davies A. J., Duineveld G. C. A., Lavaleye M. S. S., Bergman M. J. N., van Haren H., Murray Roberts J. (2009). Downwelling and Deep-Water Bottom Currents as Food Supply Mechanisms to the Cold-Water Coral Lophelia pertusa (Scleractinia) at the Mingulay Reef Complex. Limnol. Oceanogr. 54, 620–629. doi: 10.4319/lo.2009.54.2.0620
Davies A. J., Roberts J. M., Hall-Spencer J. (2007). Preserving Deep-Sea Natural Heritage: Emerging Issues in Offshore Conservation and Management. Biol. Conserv. 138, 299–312. doi: 10.1016/j.biocon.2007.05.011
Davies A. J., Wisshak M., Orr J. C., Murray Roberts J. (2008). Predicting Suitable Habitat for the Cold-Water Coral Lopheliapertusa (Scleractinia). Deep-Sea Res. Part I: Oceanogr. Res. Papers 55, 1048–1062. doi: 10.1016/j.dsr.2008.04.010
DeNiro M. J., Epstein S. (1978). Influence of Diet on the Distribution of Carbon Isotopes in Animals. Geochimica. Cosmochimica. Acta 42 (5), 495–506. doi: 10.1016/0016-7037(78)90199-0
D’Onghia G. (2019). “Cold-Water Corals as Shelter, Feeding and Life-History Critical Habitats for Fish Species: Ecological Interactions and Fishing Impact,” in Mediterranean Cold-Water Corals: Past, Present and Future: Understanding the Deep-Sea Realms of Coral. Eds. Orejas C., Jiménez C. (Cham, Switzerland: Springer International Publishing AG), Pages 335–356.
D’Onghia G., Calculli C., Capezzuto F., Carlucci R., Carluccio A., Grehan A., et al. (2016). Anthropogenic Impact in the Santa Maria Di Leuca Cold-Water Coral Province (Mediterranean Sea): Observations and Conservation Straits. Deep-Sea Res. Part II: Topical. Stud. Oceanogr. 145, 87–101. doi: 10.1016/j.dsr2.2016.02.012
Duineveld G. C. A., Lavaleye M. S. S., Berghuis E. M. (2004). Particle Flux and Food Supply to a Seamount Cold-Water Coral Community (Galicia Bank, NW Spain). Mar. Ecol. Prog. Ser. 277, 13–23. doi: 10.3354/meps277013
Duineveld G. C. A., Lavaleye M. S. S., Bergman E. M., De Stigter H., Mienis F. (2007). Trophis Structure of a Cold-Water Coral Mound Community (Rockall Bank, NE Atlantic) in Relation to the Near-Bottom Particle Supply and Current Regime. Bull. Mar. Sci. 81, 449–467.
European Community (2020). COM(2020) 380 Final: EU Biodiversity Strategy for 2030. (European Community,Brussels), 20.05.2020.
Faganeli J., Ogrinc N., Kovac N., Kukovec K., Falnoga I., Mozetic P., et al. (2009). Carbon and Nitrogen Isotope Composition of Particulate Organic Matter in Relation to Mucilage Formation in the Northern Adriatic Sea. Mar. Chem. 114, 102–109. doi: 10.1016/j.marchem.2009.04.005
Fanelli E., Cartes J. E., Rumolo P., Sprovieri M. (2009). Food-Web Structure and Trophodynamics of Mesopelagic–Suprabenthic Bathyal Macrofauna of the Algerian Basin Based on Stable Isotopes of Carbon and Nitrogen. Deep-Sea Res. Part I: Oceanogr. Res. Papers 56, 1504–1520. doi: 10.1016/j.dsr.2009.04.004
Fanelli E., Delbono I., Ivaldi R., Pratellesi M., Cocito S., Peirano A. (2017). Cold-Water Coral Madrepora oculata in the Eastern Ligurian Sea (NW Mediterranean): Historical and Recent Findings. Aquat. Conserv.: Mar. Freshw. Ecosyst. 27, 965–975. doi: 10.1002/aqc.2751
Fanelli E., Papiol V., Cartes J. E., Rumolo P., Brunet C., Sprovieri M. (2011). Food Web Structure of the Epibenthic and Infaunal Invertebrates on the Catalan Slope (NW Mediterranean): Evidence From δ13C and δ15N Analysis. Deep-Sea Res. Part I: Oceanogr. Res. Papers 58, 98–109. doi: 10.1016/j.dsr.2010.12.005
Fanelli E., Papiol V., Cartes J. E., Rumolo P., López-Pérez C. (2013). Trophic Webs of Deep-Sea Megafauna on Mainland and Insular Slopes of the NW Mediterranean: A Comparison by Stable Isotope Analysis. Mar. Ecol. Prog. Ser. 490, 199–221. doi: 10.3354/meps10430
Ferrier-Pagès C., Peirano A., Abbate M., Cocito S., Negri A., Rottier C., et al. (2011). Summer Autotrophy and Winter Heterotrophy in the Temperate Symbiotic Coral Cladocora caespitosa. Limnol. Oceanogr. 56, 1429–1438. doi: 10.4319/lo.2011.56.4.1429
Fosså J. H., Mortensen P. B., Furevik D. M. (2002). The Deep-Water Coral Lophelia pertusa in Norwegian Waters: Distribution and Fishery Impacts. Hydrobiologia 471, 1–12. doi: 10.1023/A:1016504430684
Freiwald A., Beuck L., Ruggeberg A., Taviani M., Hebbeln D.R/V Meteor Cruise M70-1 Participants (2009). The White Coral Community in the Central Mediterranean Sea Revealed by ROV Surveys. Oceanography. 22
Freiwald A., Fosså J. H., Grehan A., Koslow T., Roberts J. M. (2004). Cold-Water Coral Reefs (UK: Cambridge).
Gambi C., Carugati L., Lo Martire M., Danovaro R. (2019). Biodiversity and Distribution of Meiofauna in the Gioia, Petrace and Dohrn Canyons (Tyrrhenian Sea). Prog. Oceanogr. 171, 162–174. doi: 10.1016/j.pocean.2018.12.016
Gianni M. (2004). High Seas Bottom Trawl Fisheries and Their Impacts on the Biodiversity of Vulnerable Deep-Sea Ecosystems (Gland, Switzerland: IUCN).
Gómez C. E., Wickes L., Deegan D., Etnoyer P. J., Cordes E. E. (2018). Growth and Feeding of Deep-Sea Coral Lophelia pertusa From the California Margin Under Simulated Ocean Acidification Conditions. PeerJ 9:e5671. doi: 10.7717/peerj.5671
Gori A., Grover R., Orejas C., Sikorski S., Ferrier-Pagès C. (2014). Uptake of Dissolved Free Amino Acids by Four Cold-Water Coral Species From the Mediterranean Sea. Deep-Sea Res. Part II: Topical. Stud. Oceanogr. 99, 42–50. doi: 10.1016/j.dsr2.2013.06.007
Gori A., Orejas C., Madurell T., Bramanti L., Martins M., Quintanilla E., et al. (2013). Bathymetrical Distribution and Size Structure of Cold-Water Coral Populations in the Cap De Creus and Lacaze-Duthiers Canyons (Northwestern Mediterranean). Biogeosciences 10, 2049–2060. doi: 10.5194/bg-10-2049-2013
Gori A., Reynaud S., Orejas C., Ferrier-Pagès C. (2015). The Influence of Flow Velocity and Temperature on Zooplankton Capture Rates by the Cold-Water Coral Dendrophyllia cornigera. J. Exp. Mar. Biol. Ecol. 466, 92–97. doi: 10.1016/j.jembe.2015.02.004
Gori A., Tolosa I., Orejas C., Rueda L., Viladrich N., Grinyó J., et al. (2018). Biochemical Composition of the Cold-Water Coral Dendrophyllia cornigeraUnder Contrasting Productivity Regimes: Insights From Lipid Biomarkers and Compound-Specific Isotopes. Deep-Sea Res. Part I: Oceanogr. Res. Papers 141, 106–117. doi: 10.1016/j.dsr.2018.08.010
Gradinger R., Friedrich C., Spindler M. (1999). Abundance, Biomass and Composition of the Sea Ice Biota of the Greenland Sea Pack Ice. Deep-Sea Res. Part II 46 (6-7), 1457–1472. doi: 10.1016/S0967-0645(99)00030-2
Guillard R. R. L. (1975). “Culture of Phytoplankton for Feeding Marine Invertebrates,” in Culture of Marine Invertebrate Animals. Eds. Smith W. L., Chanley M. H. (Boston, MA, USA: Springer), Pages 29–60.
Guinotte J. M., Orr J., Cairns S., Freiwald A., Morgan L., George R. (2006). Will Human-Induced Changes in Seawater Chemistry Alter the Distribution of Deep-Sea Scleractinian Corals? Front. Ecol. Environ. 4, 141–146. doi: 10.1890/1540-9295(2006)004[0141:WHCISC]2.0.CO;2
Henry L.-A., Roberts J. M. (2007). Biodiversity and Ecological Composition of Macrobenthos on Cold-Water Coral Mounds and Adjacent Off-Mound Habitat in the Bathyal Porcupine Seabight, NE Atlantic. Deep-Sea Res. Part I: Oceanogr. Res. Papers 54, 654–672. doi: 10.1016/j.dsr.2007.01.005
Higgins R., Thiel H. (1988). Introduction to the Study of Meiofauna (Washington, D.C: Smithsonian Institution Press).
Huvenne V. A. I., Bett B. J., Masson D. G., Le Bas T. P., Wheeler A. J. (2016). Effectiveness of a Deep-Sea Cold-Water Coral Marine Protected Area, Following Eight Years of Fisheries Closure. Biol. Conserv. 200, 60–69. doi: 10.1016/j.biocon.2016.05.030
Jackson A. L., Inger R., Parnell A. C., Bearhop S. (2011). Comparing Isotopic Niche Widths Among and Within Communities: SIBER - Stable Isotope Bayesian Ellipses in R. J. Anim. Ecol. 80, 595–602. doi: 10.1111/j.1365-2656.2011.01806.x
Jacob U., Mintenbeck K., Brey T., Knust R., Beyer K. (2005). Stable Isotope Food Web Studies: A Case for Standardized Sample Treatment. Mar. Ecol. Prog. Ser. 287, 251–253. doi: 10.3354/meps287251
Kiriakoulakis K., Fisher E., Wolff G. A., Freiwald A., Grehan A., Roberts J. M. (2005). “Lipids and Nitrogen Isotopes of Two Deep-Water Corals From the North-East Atlantic: Initial Results and Implications for Their Nutrition,” in Cold-Water Corals and Ecosystems. Eds. Freiwald A., Roberts J. M. (Heidelberg, Germany: Springer-Verlag), Pages 715–729.
Krumsick K. J., Fisher J. A. D. (2019). Spatial and Ontogenetic Variation in Isotopic Niche Among Recovering Fish Communities Revealed by Bayesian Modeling. PLoS One 14, e0215747. doi: 10.1371/journal.pone.0215747
Langone L., Conese I., Miserocchi S., Boldrin A., Bonaldo D., Carniel S., et al. (2016). Dynamics of Particles Along the Western Margin of the Southern Adriatic: Processes Involved in Transferring Particulate Matter to the Deep Basin. Mar. Geol. 375, 28–43. doi: 10.1016/j.margeo.2015.09.004
Larsson A. I., Lundälv T., Van Oevelen D. (2013). Skeletal Growth, Respiration Rate and Fatty Acid Composition in the Cold-Water Coral Lophelia pertusa Under Varying Food Conditions. Mar. Ecol. Prog. Ser. 483, 169–184. doi: 10.3354/meps10284
Layman C. A., Araujo M. S., Boucek R., Hammerschlag-Peyer C. M., Harrison E., Jud Z. R., et al. (2012). Applying Stable Isotopes to Examine Food-Web Structure: An Overview of Analytical Tools. Biol. Rev. 87, 545–562. doi: 10.1111/j.1469-185X.2011.00208.x
Layman C. A., Quattrochi J. P., Peyer C. M., Allgeier J. E. (2007). Niche Width Collapse in a Resilient Top Predator Following Ecosystem Fragmentation. Ecol. Lett. 10, 937–944. doi: 10.1111/j.1461-0248.2007.01087.x
Linares C., Gori A., Carreiro-Silva M., Montseny M., Bilan M., Afonso P., et al. (2020). Deliverable 4.3. Effectiveness of tools/techniques for restoration in the deep-sea. Marine Ecosystem Restoration in Changing European Seas. MERCES project. Grant agreement n. 689518.
Madurell T., Cartes J. E. (2003). The Suprabenthic Peracarid Fauna Collected at Bathyal Depths in the Ionian Sea (Eastern Mediterranean). Crustaceana 76, 61–624. doi: 10.1163/156854003322316245
Maier C., Watremez P., Taviani M., Weinbauer M. G., Gattuso J.-P. (2012). Seawater Carbonate Chemistry, Nutrients and Calcification During Experiments With Cold-Water Scleractinian Corals (Lophelia pertusa, Madrepora oculata and Desmophyllum dianthus). PANGAEA2011, 1716–1723.
Mastrototaro F., D’Onghia G., Corriero G., Matarrese A., Maiorano P., Panetta P., et al. (2010). Biodiversity of the White Coral Bank Off Cape Santa Maria Di Leuca (Mediterranean Sea): An Update. Deep-Sea Res. Part II: Topical. Stud. Oceanogr. 57, 412–430. doi: 10.1016/j.dsr2.2009.08.021
Matos F. L., Company J. B., Cunha M. R. (2021). Mediterranean Seascape Suitability for Lophelia pertusa: Living on the Edge. Deep-Sea Res. Part I: Oceanogr. Res. Papers 170, 103496. doi: 10.1016/j.dsr.2021.103496
Mauchline J. (1980). “The Biology of Mysids and Euphausiids,” in Advances in Marine Biology. Eds. Blaxter J. H. S., Russell F. S., Yonge M. ( Academic Press: London) 3–369.
Møller E. F. (2004). Sloppy Feeding in Marine Copepods: Prey-Size-Dependent Production of Dissolved Organic Carbon. J. Plankton Res. 27, 27–35. doi: 10.1093/plankt/fbh147
Montseny M., Linares C., Carreiro-Silva M., Henry L.-A., Billett D., Cordes E. E., et al. (2021). Active Ecological Restoration of Cold-Water Corals: Techniques, Challenges, Costs and Future Directions. Front. Mar. Sci. 8. doi: 10.3389/fmars.2021.621151
Mortensen P. B. (2001). Aquarium Observations on the Deep-Water Coral Lophelia pertusa (L. 1758) (Scleractinia) and Selected Associated Invertebrates. Ophelia 54, 83–104. doi: 10.1080/00785236.2001.10409457
Mueller C. E., Larsson A. I., Veuger B., Middelburg J. J., Van Oevelen D. (2014). Opportunistic Feeding on Various Organic Food Sources by the Cold-Water Coral Lophelia pertusa. Biogeosciences 11, 123–133. doi: 10.5194/bg-11-123-2014
Murray F., De Clippele L. H., Hiley A., Wicks L., Roberts J. M., Hennige S. (2019). Multiple Feeding Strategies Observed in the Cold-Water Coral Lophelia pertusa. J. Mar. Biol. Assoc. United Kingdom 99, 1281–1283. doi: 10.1017/S0025315419000298
Naumann M. S., Orejas C., Ferrier-Pagès C. (2014). Species-Specific Physiological Response by the Cold-Water Corals Lophelia pertusa and Madrepora oculata to Variations Within Their Natural Temperature Range. Deep-Sea Res. Part II: Topical. Stud. Oceanogr. 99, 36–41. doi: 10.1016/j.dsr2.2013.05.025
Naumann M. S., Tolosa I., Taviani M., Grover R., Ferrier-Pagès C. (2015). Trophic Ecology of Two Cold-Water Coral Species From the Mediterranean Sea Revealed by Lipid Biomarkers and Compound-Specific Isotope Analyses. Coral Reefs 34, 1165–1175. doi: 10.1007/s00338-015-1325-8
Newsome S. D., Martinez Del Rio C., Bearhop S., Phillips D. L. (2007). A Niche for Isotopic Ecology. Front. Ecol. Environ. 5 (8), 429–436. doi: 10.1890/060150.1
Orejas C., Gori A., Rad-Menéndez C., Last K. S., Davies A. J., Beveridge C. M., et al. (2016). The Effect of Flow Speed and Food Size on the Capture Efficiency and Feeding Behaviour of the Cold-Water Coral Lophelia pertusa. J. Exp. Mar. Biol. Ecol. 481, 34–40. doi: 10.1016/j.jembe.2016.04.002
Orejas C., Taviani M., Ambroso S., Andreou V., Bilan M., Bo M., et al. (2019). “Cold-Water Coral in Aquaria: Advances and Challenges. A Focus on the Mediterranean,” in Mediterranean Cold-Water Corals: Past, Present and Future. Eds. Orejas C., Jìmenez C. (Cham, Switzerland: Springer International Publishing AG), Pages: 435–471.
Owens N. J. P. (1988). “Natural Variations in 15N in the Marine Environment,” in Advances in Marine Biology. Eds. Blaxter J. H. S., Southward A. J. ( Academic Press:London), Pages 389–451.
Parnell A. (2021) Simmr: A Stable Isotope Mixing Model. R Package Version 0.4.5. Available at: https://CRAN.R-project.org/package=simmr.
Parnell A. C., Inger R., Bearhop S., Jackson A. L. (2010). Source Partitioning Using Stable Isotopes: Coping With Too Much Variation. PLoS One 5, e9672. doi: 10.1371/journal.pone.0009672
Parnell A., Jackson A., 2013. SIAR: Stable Isotope Analysis in R. R package version 4.2. https://CRAN.R-project.org/package=siar
Peterson B. J., Fry B. (1987). Stable Isotopes in Ecosystem Studies. Annu. Rev. Ecol. System. 18 (1), 293–320. doi: 10.1146/annurev.es.18.110187.001453
Phillips D. L., Inger R., Bearhop S., Jackson A. L., Moore J. W., Parnell A. C., et al. (2014). Best Practices for Use of Stable Isotope Mixing Models in Food-Web Studies. Can. J. Zool. 92, 823–835. doi: 10.1139/cjz-2014-0127
Piccinetti C. C., Ricci R., Pennesi C., Radaelli G., Totti C., Norici A., et al. (2016). Herbivory in the Soft Coral Sinularia flexibilis (Alcyoniidae). Sci. Rep. 6, 22679. doi: 10.1038/srep22679
Post D. (2002). Using Stable Isotopes to Estimate Trophic Position: Models, Methods and Assumptions. Ecology 83, 703–718. doi:10.1890/0012-9658(2002)083[0703:USITET]2.0.CO;2
Purser A., Larsson A. I., Thomsen L., Van Oevelen D. (2010). The Influence of Flow Velocity and Food Concentration on Lophelia pertusa (Scleractinia) Zooplankton Capture Rates. J. Exp. Mar. Biol. Ecol. 395, 55–62. doi: 10.1016/j.jembe.2010.08.013
Ragnarsson S., Burgos J., Kutti T., Van Den Beld I., Egilsdottir H., Arnaud-Haond S., et al. (2016). “The Impact of Anthropogenic Activity on Cold-Water Corals,” in Marine Animal Forests ( Springer International Publishing:Switzerland), Pages 1–35.
R Development Core Team (2021). R: A Language and Environment for Statistical Computing (Vienna: R Foundation for Statistical Computing). Available at: http://www.R-project.org.
Roberts J. M., Cairns S. D. (2014). Cold-Water Corals in a Changing Ocean. Curr. Opin. Environ. Sustain. 7, 118–126. doi: 10.1016/j.cosust.2014.01.004
Roberts J., Wheeler A., Freiwald A. (2006). Reefs of the Deep: The Biology and Geology of Cold-Water Coral Ecosystems. Science 312, 543–547. doi: 10.1126/science.1119861
Roberts J., Wheeler A., Freiwald A., Cairns S. (2009). Cold Water Corals: The Biology and Geology of Deep-Sea Coral Habitats. ( Cambridge University Press: Cambridge) Pages: 1–350.
Rueda J. L., Urra J., Aguilar R., Angeletti L., Bo M., García-Ruiz C., et al. (2019). “Cold-Water Coral Associated Fauna in the Mediterranean Sea and Adjacent Areas,” in Mediterranean Cold-Water Corals: Past, Present and Future: Understanding the Deep-Sea Realms of Coral. Eds. Orejas C., Jiménez C. (Cham, Switzerland: Springer International Publishing AG), Pages 295–333.
Rumolo P., Bonanno A., Barra M., Fanelli E., Calabro M., Genovese S., et al. (2016). Spatial Variations in Feeding Habits and Trophic Levels of Two Small Pelagic Fish Species in the Central Mediterranean Sea. Mar. Environ. Res. 115, 65–77. doi: 10.1016/j.marenvres.2016.02.004
Sherwood O. A., Jamieson R. E., Edinger E. N., Wareham V. E. (2008). Stable C and N Isotopic Composition of Cold-Water Corals From the Newfoundland and Labrador Continental Slope: Examination of Trophic, Depth and Spatial Effects. Deep-Sea Res. Part I: Oceanogr. Res. Papers 55, 1392–1402. doi: 10.1016/j.dsr.2008.05.013
Simoncelli S., Schaap D., Schlitzer R. (2014). Mediterranean Sea - Temperature and Salinity Observation Collection V1.1. doi: 10.12770/cd552057-b604-4004-b838-a4f73cc98fcf
Smith J. A., Mazumder D., Suthers I. M., Taylor M. D., Bowen G. (2013). To Fit or Not to Fit: Evaluating Stable Isotope Mixing Models Using Simulated Mixing Polygons. Methods Ecol. Evol. 4, 612–618. doi: 10.1111/2041-210X.12048
Sweetman A. K., Thurber A. R., Smith C. R., Levin L. A., Mora C., Wei C.-L., et al. (2017). Major Impacts of Climate Change on Deep-Sea Benthic Ecosystems. ELEMENTA Sci. Anthropocene 5,4.
Taviani M., Angeletti L., Canese S., Cannas R., Cardone F., Cau A., et al. (2017). The “Sardinian Cold-Water Coral Province” in the Context of the Mediterranean Coral Ecosystems. Deep-Sea Res. Part II: Topical. Stud. Oceanogr. 145, 61–78. doi: 10.1016/j.dsr2.2015.12.008
Taviani M., Angeletti L., Cardone F., Montagna P., Danovaro R. (2019). A Unique and Threatened Deep Water Coral-Bivalve Biotope New to the Mediterranean Sea Offshore the Naples Megalopolis. Sci. Rep. 9,3411. doi: 10.1038/s41598-019-39655-8
Tsounis G., Orejas C., Reynaud S., Gili J. M., Allemand D., Ferrier-Pagès C. (2010). Prey-Capture Rates in Four Mediterranean Cold Water Corals. Mar. Ecol. Prog. Ser. 398, 149–155. doi: 10.3354/meps08312
Turchetto M., Boldrin A., Langone L., Miserocchi S., Tesi T., Foglini F. (2007). Particle Transport in the Bari Canyon (Southern Adriatic Sea). Mar. Geol. 246, 231–247. doi: 10.1016/j.margeo.2007.02.007
Turley C. M., Roberts J. M., Guinotte J. M. (2007). Corals in Deep-Water: Will the Unseen Hand of Ocean Acidification Destroy Cold-Water Ecosystems? Coral Reefs 26, 445–448. doi: 10.1007/s00338-007-0247-5
United Nations General Assembly (2019). “Resolution A/RES/73/284,” in 69th plenary meeting, , 1 March 2019. ( United Nations General Assembly: New York).
Van Dover C. L., Aronson J., Pendleton L., Smith S., Arnaud-Haond S., Moreno-Mateos D., et al. (2014). Ecological Restoration in the Deep Sea: Desiderata. Mar. Policy 44, 98–106. doi: 10.1016/j.marpol.2013.07.006
van Oevelen D., Duineveld G., Lavaleye M., Mienis F., Soetaert K., Heip C. H. R. (2009). The Cold-Water Coral Community as Hotspot of Carbon Cycling on Continental Margins: A Food-Web Analysis From Rockall Bank (Northeast Atlantic). Limnol. Oceanogr. 54, 1829–1844. doi: 10.4319/lo.2009.54.6.1829
Van Oevelen D., Mueller C. E., Lundälv T., Van Duyl F. C., De Goeij J. M., Middelburg J. J. (2018). Niche Overlap Between a Cold-Water Coral and an Associated Sponge for Isotopically-Enriched Particulate Food Sources. PLoS One. doi: 10.1371/journal.pone.0194659
Vertino A., Savini A., Rosso A., Di Geronimo I., Mastrototaro F., Sanfilippo R., et al. (2010). Benthic Habitat Characterization and Distribution From Two Representative Sites of the Deep-Water SML Coral Province (Mediterranean). Deep-Sea Res. Part II: Topical. Stud. Oceanogr. 57, 380–396. doi: 10.1016/j.dsr2.2009.08.023
Weinnig A. M., Gomez C. E., Hallaj A., Cordes E. E. (2020). Cold-Water Coral (Lophelia pertusa) Response to Multiple Stressors: High Temperature Affects Recovery From Short-Term Pollution Exposure. Sci. Rep. 10, 1768. doi: 10.1038/s41598-020-58556-9
Wienberg C., Hebbeln D., Fink H. G., Mienis F., Dorschel B., Vertino A., et al. (2009). Scleractinian Cold-Water Corals in the Gulf of Cádiz—First Clues About Their Spatial and Temporal Distribution. Deep-Sea Res. Part I: Oceanogr. Res. Papers 56, 1873–1893. doi: 10.1016/j.dsr.2009.05.016
Wieser W. (2007). “Die Beziehung Zwischen Mundhöhlengestalt, Ernährungsweise Und Vorkommen Bei Freilebenden Marinen Nematoden : Eine Ökologisch-Morphologische Studie,” in Arkiv För Zoologi 2, vol. 4. ( Universitätsbibliothek Johann Christian Senckenberg:Frankfurt, Germany), 439–484.
Keywords: cold-water corals, Desmophyllum pertusum, Madrepora oculata, Dendrophyllia cornigera, food selection, stable isotopes, restoration, Mediterranean Sea
Citation: Da Ros Z, Dell’Anno A, Fanelli E, Angeletti L, Taviani M and Danovaro R (2022) Food Preferences of Mediterranean Cold-Water Corals in Captivity. Front. Mar. Sci. 9:867656. doi: 10.3389/fmars.2022.867656
Received: 01 February 2022; Accepted: 20 June 2022;
Published: 22 July 2022.
Edited by:
Clara F. Rodrigues, University of Aveiro, PortugalReviewed by:
Alexis M. Weinnig, United States Geological Survey (USGS), United StatesCopyright © 2022 Da Ros, Dell’Anno, Fanelli, Angeletti, Taviani and Danovaro. This is an open-access article distributed under the terms of the Creative Commons Attribution License (CC BY). The use, distribution or reproduction in other forums is permitted, provided the original author(s) and the copyright owner(s) are credited and that the original publication in this journal is cited, in accordance with accepted academic practice. No use, distribution or reproduction is permitted which does not comply with these terms.
*Correspondence: Antonio Dell’Anno, YS5kZWxsYW5ub0B1bml2cG0uaXQ=; Emanuela Fanelli, ZS5mYW5lbGxpQHVuaXZwbS5pdA==
Disclaimer: All claims expressed in this article are solely those of the authors and do not necessarily represent those of their affiliated organizations, or those of the publisher, the editors and the reviewers. Any product that may be evaluated in this article or claim that may be made by its manufacturer is not guaranteed or endorsed by the publisher.
Research integrity at Frontiers
Learn more about the work of our research integrity team to safeguard the quality of each article we publish.