- 1Pure Salmon Kaldnes AS, Sandefjord, Norway
- 2Department of Biotechnology and Food Science, NTNU - Norwegian University of Science and Technology, Trondheim, Norway
Several industries, including aquaculture, produce effluents with high or varying salt concentrations. The treatment of such effluents by the biological nitrification process can be challenging, as the microbes performing this process are sensitive to salinity. In certain cases, such as in recirculating aquaculture systems (RAS), it is essential to maintain high nitrification efficiency during salinity changes to prevent ammonia and nitrite toxicity. Therefore, suitable strategies are required to make nitrifying bioreactors tolerant to salinity variations. Although salinity changes can impact the nitrification performance, it has been shown that nitrifying bioreactors can acclimate to salinity variations over several days. This acclimation can be due to the physiological adaptation of the existing microorganisms or due to selection for microorganisms adapted to that salinity regime. Other factors, such as the biofilm matrix, can also play a role in salinity acclimation. Recent studies have shown that microbial management strategies can be applied to improve the salinity tolerance and reduce the recovery time of nitrifying bioreactors. Here, we discuss the existing knowledge on salinity acclimation in nitrifying systems, and recent advances in strategies to make nitrifying biofilms more tolerant to salinity variations. We also propose directions for future research to improve our understanding of the salinity acclimation mechanisms in nitrifying systems.
Introduction
Worldwide, more than 5% of effluents are saline or hypersaline (Lefebvre et al., 2007). This will increase in the future as seawater may be utilized to fulfil freshwater shortages. Saline effluents are produced by several industries, such as petroleum refineries, leather, food and aquaculture (Intrasungkha et al., 1999; Lefebvre and Moletta, 2006). Wastewater treatment plants in coastal cities may also receive saline water from seawater flushing (Vyrides, 2015). In many systems, large variations in salinity are common due to process fluctuations, rather than a gradual increase (Lefebvre and Moletta, 2006). Moreover, salinity may change temporally (Vyrides, 2015). High or variable salinity can impact biological water treatment processes such as nitrification, as the osmotic stress can inhibit the activity of the microorganisms (Sleator and Hill, 2002; Madigan et al., 2018).
Nitrification is a two-step microbiological process where ammonia (NH3) is first oxidized to nitrite (NO2-) and subsequently to nitrate (NO3-). The two steps are typically performed by two microbial guilds: 1) ammonia oxidizing microorganisms (AOM) that include ammonia oxidizing bacteria (AOB) and archaea (AOA), and 2) nitrite oxidizing bacteria (NOB), respectively (Madigan et al., 2018). Bacteria capable of complete oxidation of ammonia to nitrate (comammox) were recently discovered within the genus Nitrospira (Van Kessel et al., 2015). Despite the advantages of more recently discovered processes like anammox, nitrification is still commonly used to convert ammonia in water treatment plants. This is likely because the nitrifying microorganisms grow faster than anammox, and can function efficiently across a wider spectrum of environmental conditions. Nitrification is especially important in recirculating aquaculture systems (RAS). RAS are land-based fish farms with treatment systems to reuse the water. In RAS, nitrification is essential for keeping the concentration of ammonia and nitrite below toxic levels for the fish. Thus, it is vital to maintain high nitrification efficiency during salinity variations.
Although some literature reviews briefly discuss the impact of salinity on nitrification (Lefebvre and Moletta, 2006; Lay et al., 2010; Vyrides and Stuckey, 2017; Zhao et al., 2020), we are not aware of any reviews that describe strategies for salinity acclimation in nitrification processes,. Thus, here we present existing knowledge on the impact of salinity on nitrification and discuss recent advances in salinity acclimation strategies for nitrifying systems.
Salinity Adaption Mechanisms in Microorganisms
Salinity changes in the environment of a microbe can disrupt the osmotic balance. This osmotic pressure difference causes an instantaneous efflux or influx of water and/or a cell response to regulate the cellular osmolarity (Csonka, 1989; Sleator and Hill, 2002). The nature of these processes depends on whether the salinity shock is hyper- (salinity increase) or hypoosmotic (salinity decrease). Hypoosmotic shock is less severe as the rigid bacterial cell walls can withstand some increase in pressure caused by water influx (Csonka, 1989; Gonzalez-Silva et al., 2021). In contrast, hyperosmotic shock causes dehydration and plasmolysis (cell shrinkage), which can inhibit nutrient uptake and growth (Csonka, 1989; Madigan et al., 2018).
If the hyperosmotic shock is not severe, the cells can adapt to the higher salinity by increasing the internal osmolarity (Csonka, 1989). To accomplish this without losing water, the cells utilize either (i) the salt-in cytoplasm strategy, or (ii) the organic osmolyte (compatible solute) strategy (Csonka, 1989; Oren, 1999; Sleator and Hill, 2002). The salt-in strategy requires extensive structural adaptations, and is therefore only adopted by obligate halophiles (Sleator and Hill, 2002; Oren, 2011). The osmolyte strategy involves a bi-phasic response with an increase in K+, followed by an increase of osmolytes in the cytoplasm (Sleator and Hill, 2002). Despite being more energy-intensive than the salt-in strategy, the osmolyte strategy is adapted by most halotolerant microorganisms, as it offers a higher degree of flexibility to combat variations in the external osmolarity (Sleator and Hill, 2002; Oren, 2011). The ability of a microorganism to survive in an environment with high/variable salinity depends on the energy generated during dissimilatory metabolism and the mode of osmotic adaptation (Oren, 2011). Autotrophic nitrifiers generate relatively little energy, and growth at elevated salinities can be challenging (Oren, 2011).
Phylogeny and Salinity Tolerance of Nitrifying Microorganisms
Ammonia oxidizing bacteria belong to three genera within β- and γ-proteobacteria: Nitrosomonas, Nitrosospira and Nitrosococcus (Prosser et al., 2014). The salt tolerance of the species within Nitrosomonas and Nitrosospira varies greatly (Table 1). For example, whereas Nitrosomonas oligotropha has a maximum salt tolerance of ~100 mM (~6‰), other species such as N. marina, N. aestuarii, and N. cryotolerans are obligate halophiles (Koops et al., 2006). In contrast, Nitrosococcus has only been found in marine environments and is reported to be obligately halophilic (Koops et al., 2006).
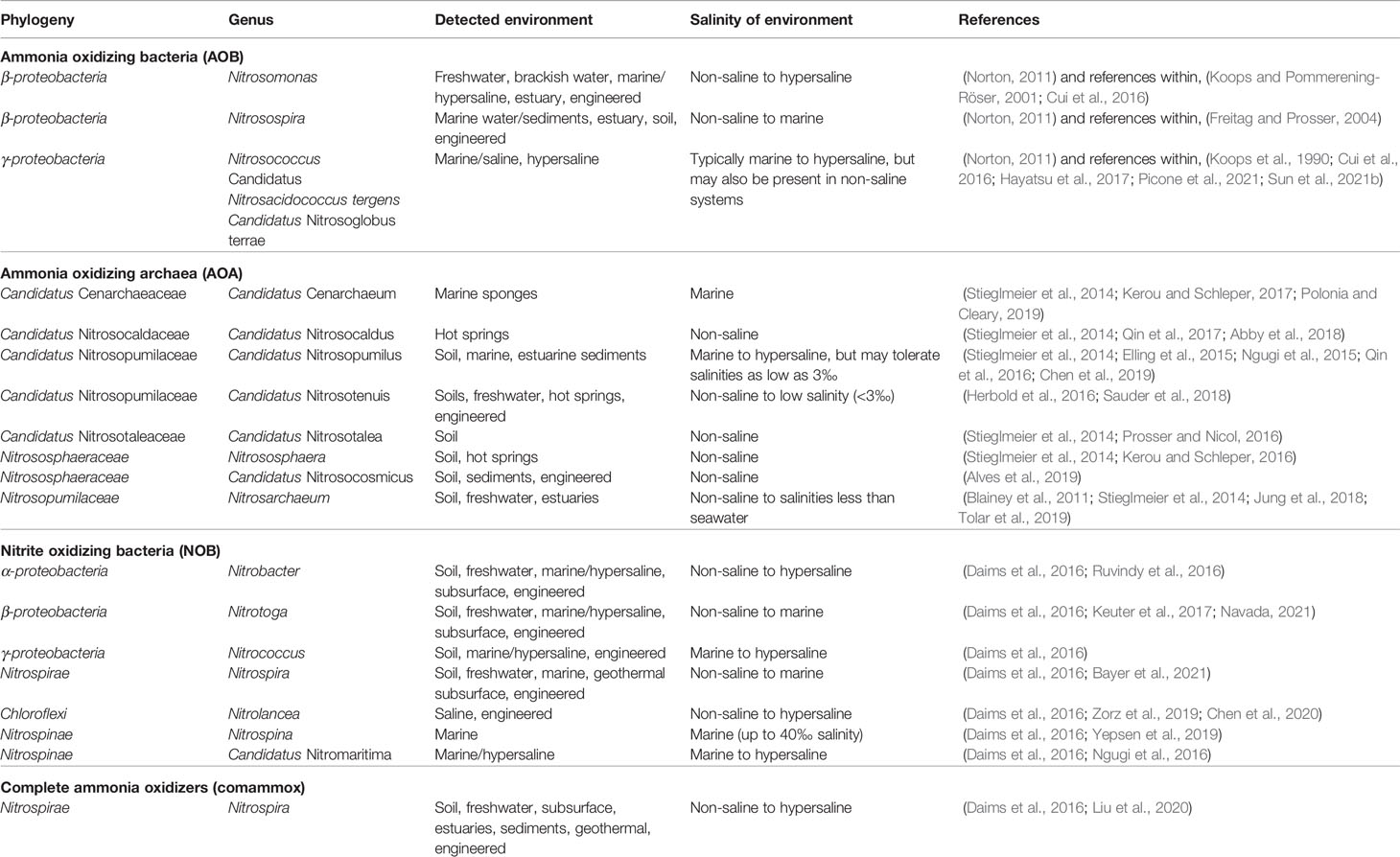
Table 1 Environmental distribution and salinity of environment for genera of ammonia oxidizing bacteria (AOB), ammonia oxidizing archaea (AOA), nitrite oxidizing bacteria (NOB) and comammox bacteria.
Ammonia oxidizing archaea are phylogenetically restricted to the phylum Thaumarchaeota. AOA are abundant across a wide range of salinities (Table 1), both in natural and man-made systems (Francis et al., 2005; Bernhard and Bollmann, 2010; Sauder et al., 2011; Bartelme et al., 2017; Bartelme et al., 2019; Kitzinger et al., 2020). Moreover, AOA have been detected in estuaries, indicating that they can adapt to variable salinity (Bernhard et al., 2010; Santos et al., 2020). AOA are more abundant than AOB in ecosystems with low ammonia concentration (Bernhard et al., 2007; Ward et al., 2007; Bernhard et al., 2010; Nicol et al., 2011; Stieglmeier et al., 2014). Thus, AOA may be an important player in RAS (Sauder et al., 2011), where the ammonium concentration is typically low (<2 mgN L-1). Despite the high relative abundance, the contribution of AOA to the overall nitrification activity is not well understood (Bernhard and Bollmann, 2010; Hatzenpichler, 2012).
The known nitrite oxidizers belong to seven genera within six bacterial phyla. All these genera have been detected in saline environments (Table 1), indicating that they contain species that are at least halotolerant, if not halophilic. Members of Nitrospinae have only been detected in saline systems, suggesting a halophilic lifestyle (Daims et al., 2016). Comammox Nitrospira are mainly found in non-saline to low salinity environments (Daims et al., 2016; Sun et al., 2021a), but were recently detected in estuarine sediments with salinities as high as 55‰ (Liu et al., 2020).
Impact of Salinity Change on Nitrification Activity
Several studies have investigated the impact of salinity on the nitrification process across a wide range of systems – activated sludge (Moussa et al., 2006a; Moussa et al., 2006b; Bassin et al., 2012; He et al., 2017), aerobic granular sludge (Bassin et al., 2011; Wang et al., 2017), fixed bed biofilters (Nijhof and Bovendeur, 1990; Sudarno, 2011; Cortes-Lorenzo et al., 2015), and moving bed biofilm reactors (MBBR) (Gonzalez-Silva, 2016). There is consensus that an increase in salinity generally inhibits nitrification. However, a salinity increase from 0 to ~10‰ appears to have a slight positive or no impact on the ammonia oxidation rate (Sudarno, 2011; Aslan and Simsek, 2012; Bassin et al., 2012; Cortes-Lorenzo et al., 2015; Navada et al., 2019), although not without exceptions (Sánchez et al., 2004; Kinyage et al., 2019). This is likely because the energy required for osmoregulation is lowest at salinities close to the isotonic point (~9‰), leaving more energy for growth and metabolism (Oren, 2011; He et al., 2017). A significant difference in microbial community composition and species inventory was observed between fresh- and brackish water (12‰ salinity) biofilms subjected to similar start-up conditions (Navada et al., 2020a). Furthermore, there was no drop in nitrification activity when salinity was increased in brackish water biofilms (12‰) (Navada et al., 2020b). This indicates that 9-12‰ is a critical salinity for adaptation in nitrifiers. This is corroborated by several studies where the nitrification activity dropped significantly at salinities >8-15‰ (Bassin et al., 2011; Bassin et al., 2012; Gonzalez-Silva et al., 2016; Kinyage et al., 2019; Navada et al., 2019; Navada et al., 2020b; Fossmark et al., 2021). Thus, in the present article, salinity adaptation implies salinities >10‰, unless specified otherwise.
Salinity change can impact AOM and NOB to different extents. Some studies found that AOM are more influenced than NOB by a salinity increase (Hunik et al., 1993; Moussa et al., 2006b; Sharrer et al., 2007), whereas others report the opposite (Nijhof and Bovendeur, 1990; Bassin et al., 2011; Sudarno, 2011; Aslan and Simsek, 2012). The discrepancy may be due to differences in experimental conditions, initial microbial community, salinity change method or biofilm history (Navada et al., 2020b). Several studies report nitrite accumulation after a salinity increase (Nijhof and Bovendeur, 1990; Hovanec et al., 1998; Dinçer and Kargi, 1999; Bassin et al., 2011; Cortes-Lorenzo et al., 2015; Gonzalez-Silva, 2016; Navada et al., 2020b). The lower energy yield and slower growth rate of NOB compared to AOM makes them more susceptible to stress, and nitrite oxidation may not generate sufficient energy for osmoregulation at elevated salinities (Oren, 2011). Some studies show that the proportion of NOB is lower than AOB in saline biofilms (Roalkvam et al., 2020; Navada, 2021), whereas others report the opposite (Gonzalez-Silva, 2016; Fossmark et al., 2021). The fragile mutualism between AOM and NOB is likely prone to chaotic instability, where minor perturbations can escalate the instability and lead to nitrite accumulation (Graham et al., 2007). Thus, nitrite concentration should be monitored closely during and after salinity variations.
Many studies report a shift in the microbial community composition after salinity increase (Sudarno, 2011; Bassin et al., 2012; Cortes-Lorenzo et al., 2015; Gonzalez-Silva, 2016; Luo et al., 2016). This community shift likely eliminates microorganisms that cannot survive at higher salinities, and selects for halotolerant or halophilic microorganisms. Shifts in the nitrifying community composition are also reported, with the appearance or disappearance of certain taxa at higher salinities (Moussa et al., 2006b; Bassin et al., 2011; Cortes-Lorenzo et al., 2015). For instance, increasing the salinity to ~33‰ resulted in a loss of Nitrosomonas oligotropha (Moussa et al., 2006b). In the same study, Nitrosomonas europaea was detected at salinities as high as 66‰. Similarly, the NOB Nitrospira disappeared at high salinities, and resulted in nitrite accumulation (Moussa et al., 2006b; Bassin et al., 2011; Rud et al., 2017). In our studies, Nitrotoga was the dominant NOB across salinities ranging from freshwater to seawater (Navada, 2021), suggesting that this NOB genus contains species that are highly adaptable to varying salinities.
Salinity changes have more complex effects on biofilms than in monocultures with free-living cells. Extracellular polymeric substances (EPS) in the biofilm matrix can retain water and protect the cells against desiccation (Flemming et al., 2016). Thus, a salinity increase can induce EPS formation as a defense mechanism (Wang et al., 2015; Corsino et al., 2017). Future studies should investigate the role of EPS and the possibility to manipulate it to improve salinity adaptation in biofilms. This requires a better understanding of the interactions between EPS-producing heterotrophs and nitrifying bacteria. Salt can also strengthen the biofilm structure due to better settling characteristics and ionic interactions (Goode and Allen, 2011). As nitrifiers are physiologically diverse, functional redundancy in biofilms may facilitate stable nitrification under osmotic stress. Biofilms can respond to prolonged salinity changes by physiological adaptation of the existing microbes, and through shifts in the microbial community composition by selection of microbes that are more suited to that salinity regime. The adaptation strategy will depend on the intensity and duration of the change (Shade et al., 2012). For example, small salinity increments led to a larger shift in community composition than large increments (Navada et al., 2019).
Acclimation Strategies to Salinity Change
Several factors can influence salinity acclimation, and these may be manipulated to develop salinity acclimation strategies (Table 2). Not only the salinity, but also the salinity change regime – shock (discrete steps) or gradual – may impact the nitrification performance (Moussa et al., 2006b). Although the nitrification activity can recover after a few days, a large shock change in salinity causes a drastic reduction in activity during the initial days (Nijhof and Bovendeur, 1990; Gonzalez-Silva et al., 2016; Gonzalez-Silva et al., 2021). The salinity can be increased in smaller steps and the system can be acclimated over several days/months with almost no reduction in nitrification activity (Sharrer et al., 2007; Bassin et al., 2011; Bassin et al., 2012). This is a common strategy to adapt the microbes, but the adaptation period can be very long (weeks to months) (Bassin et al., 2012; Vyrides, 2015; Gonzalez-Silva, 2016). Another method is to increase the salinity gradually by increasing the influent salinity. This strategy may be more conducive to the bacteria than shock/step changes in salinity, as it allows the bacteria to adapt to the gradually increasing salinity by K+ uptake or through the synthesis of osmolytes. Indeed, whereas shock transfers from freshwater to seawater caused >95% inhibition (Gonzalez-Silva, 2016; Kinyage et al., 2019), gradual increments in salinity over 2-3 days caused only a 55-75% inhibition (Navada et al., 2019; Navada et al., 2020b; Navada et al., 2021).
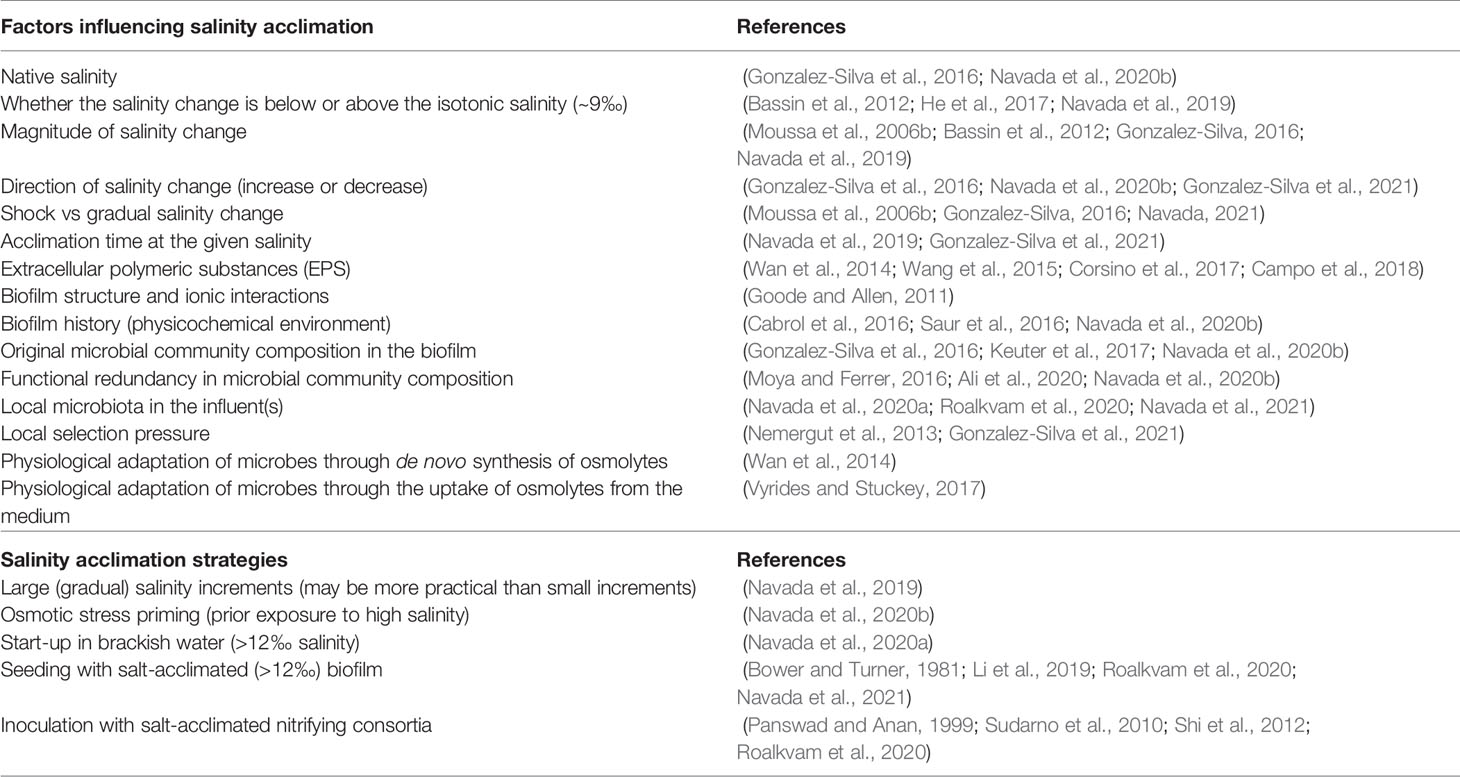
Table 2 Factors influencing salinity acclimation and feasible salinity acclimation strategies for nitrifying bioreactors.
One of our studies showed that irrespective of the rate of gradual salinity increment, the ammonia oxidation capacity decreased by 50-90% when the salinity increased from freshwater to seawater (Navada et al., 2019). The nitrification capacity was mainly dependent on the salinity (∼2.7% decrease per 1‰ increase) and the recovery time in seawater was independent of the salinity increase regime (∼2.1% increase in activity per day). Thus, large salinity increments can be more practical than small increments. Nitrifying bioreactors can adapt to a prolonged exposure to salinity (Sharrer et al., 2007; Bassin et al., 2012). Further, the ammonia oxidation capacity in bioreactors acclimated to seawater was comparable to or higher than those in freshwater (Navada, 2021). These findings contradict the traditional view that the nitrification rate is lower in seawater than in freshwater (Nijhof and Bovendeur, 1990; Chen et al., 2006; Rusten et al., 2006).
Early perturbations in young biofilms are influential in structuring the biofilm and affecting the microbial community composition (Cabrol et al., 2016; Saur et al., 2016). Thus, strategies adopted during the start-up of nitrifying bioreactors can be effective in molding the community composition and the consequent microbial functionality. This strategy was employed by Navada et al. (2020b) to show that osmotic stress priming by seawater exposure could increase the salinity tolerance of freshwater biofilms. We speculate that seawater exposure led either to physiological priming of the nitrifying community, or strengthened the biofilm structure through EPS formation. Both factors could make the biofilms more tolerant to future salinity changes. Future studies should investigate the long-term impacts of seawater priming to reveal whether seawater tolerance is sustained over periods longer than a few weeks. The success of the osmotic priming strategy suggests that newly-started bioreactors are the most susceptible to drastic drops in nitrification due to salinity increase. Thus, suitable start-up strategies should be employed to make bioreactors tolerant to salinity variations.
Brackish water (12-22‰) biofilms can be more tolerant to salinity increase than freshwater biofilms (Gonzalez-Silva et al., 2016; Navada et al., 2020b). Further, although biofilms started up in brackish water had lower nitrification capacity than in freshwater, complete nitrification was established in both bioreactors within 60 days (Navada et al., 2020a). This suggests that start-up in 12‰ brackish water could be a practical strategy. Seeding with brackish biofilm can improve salinity tolerance in bioreactors (Navada et al., 2021). Nitrifying bioreactors seeded with brackish biofilm had only a ∼20% reduction in ammonia oxidation capacity compared to 65-75% in bioreactors seeded with freshwater biofilm. Notably, a salinity decrease can also impact nitrification. In our study, a ∼50% drop in nitrification capacity was observed when the salinity was reduced from 12 or 32‰ to freshwater, and approximately two weeks were required for acclimation to the new salinity (Navada et al., 2020b).
Inoculation with salt-acclimated biomass or nitrifying consortia can improve salinity adaptation (Panswad and Anan, 1999; Sudarno et al., 2010; Shi et al., 2012; Cui et al., 2016; Vyrides and Stuckey, 2017). However, this strategy may not work during sudden increases in salinity and suitable inocula can be expensive or difficult to procure (Vyrides, 2015). Moreover, the original community composition may be modified through community assembly processes such as dispersal, drift and selection (Nemergut et al., 2013). Thus, the inoculum may be outcompeted by the local microbiota, and the final microbial community will be established primarily based on the local species pool and selection pressure. Although some studies suggest the opposite (Wittebolle et al., 2009; Keuter et al., 2017), this was the case for Navada et al. (2020a). Recent studies show that seeding with local biofilm carriers was more successful than commercial inocula (Roalkvam et al., 2020; Navada et al., 2021). This is likely because the species on biofilm carriers are already adapted to life in a biofilm and the local environment. Thus, local salinity acclimation strategies appear more beneficial than the addition of commercial inocula in nitrifying bioreactors, and should be further investigated.
Halotolerant microorganisms typically utilize the osmolyte strategy to adapt to an increase in salinity (Sleator and Hill, 2002). This strategy can be implemented either by de novo synthesis or uptake of osmolyte molecules from the medium (osmoprotectants) (Sleator and Hill, 2002; Oren, 2011). Osmoprotectant uptake is energetically cheaper than de novo synthesis (Sleator and Hill, 2002; Oren, 2011), and has been effective for a range of microorganisms (Vyrides and Stuckey, 2017). Thus, the exogenous addition of osmolytes was tested as a feasible strategy for salinity adaptation in nitrifying microorganisms. Contrary to our hypothesis, osmoprotectants did not improve salinity adaptation in nitrifying biofilms, likely due to their uptake by the heterotrophs instead of the nitrifiers (Navada, 2021).
In conclusion, physiological adaptation rather than an alteration in the nitrifying taxa appears to be the dominant mechanism for salinity acclimation in biofilms (Gonzalez-Silva et al., 2016; Navada, 2021). Moreover, several nitrifying taxa have been detected across different salinities, both in man-made and natural ecosystems (Ward et al., 2007; Bernhard and Bollmann, 2010; Gonzalez-Silva, 2016; Santos et al., 2018; Fossmark et al., 2021; Navada, 2021). This suggests that several nitrifiers are halotolerant and can adapt to varying salinities. Future studies should test individual osmolytes at different concentrations, accompanied by metaproteomics. This could further our understanding of transporter genes and facilitate the identification of osmolytes that are preferentially taken up by nitrifiers for salinity acclimation. The effect and contribution of archaea to the overall nitrification performance during salinity variations should be investigated. Estuarine systems should also be studied in combination with engineered systems to advance our understanding of salinity acclimation in nitrifiers.
Data Availability Statement
The original contributions presented in the study are included in the article/supplementary material. Further inquiries can be directed to the corresponding author.
Author Contributions
This paper is the result of discussions the authors had during the PhD study of SN. SN wrote the first version of the manuscript and the final version is the result of interactions between the authors. All authors contributed to the article and approved the submitted version.
Funding
The Industry PhD of SN was a part of CtrlAQUA SFI, Center for research-based innovation funded by the Research Council of Norway (#237856, #270888) and the Center partners. This study is partly supported by the ERA-Net Bluebio project “Microbial management in RAS for sustainable aquaculture production” (RASbiome), Research Council of Norway (#311886).
Conflict of Interest
SN is employed by Pure Salmon Kaldnes AS.
The remaining author declares that the research was conducted in the absence of any commercial or financial relationships that could be construed as a potential conflict of interest.
Publisher’s Note
All claims expressed in this article are solely those of the authors and do not necessarily represent those of their affiliated organizations, or those of the publisher, the editors and the reviewers. Any product that may be evaluated in this article, or claim that may be made by its manufacturer, is not guaranteed or endorsed by the publisher.
Acknowledgments
This work was largely adapted from the doctoral thesis of SN. In addition to OV, the PhD was supervised by Frederic Gaumet (Pure Salmon Kaldnes AS), Jelena Kolarevic (University of Tromsø) and Øyvind Mikkelsen (NTNU), whom we gratefully acknowledge.
References
Abby S. S., Melcher M., Kerou M., Krupovic M., Stieglmeier M., Rossel C., et al. (2018). Candidatus Nitrosocaldus Cavascurensis, an Ammonia Oxidizing, Extremely Thermophilic Archaeon With a Highly Mobile Genome. Front. Microbiol. 9. doi: 10.3389/fmicb.2018.00028
Ali M., Shaw D. R., Albertsen M., Saikaly P. E. (2020). Comparative Genome-Centric Analysis of Freshwater and Marine ANAMMOX Cultures Suggests Functional Redundancy in Nitrogen Removal Processes. Front. Microbiol. 11. doi: 10.3389/fmicb.2020.01637
Alves R. J. E., Kerou M., Zappe A., Bittner R., Abby S. S., Schmidt H. A., et al. (2019). Ammonia Oxidation by the Arctic Terrestrial Thaumarchaeote Candidatus Nitrosocosmicus Arcticus is Stimulated by Increasing Temperatures. Front. Microbiol. 10. doi: 10.3389/fmicb.2019.01571
Aslan S., Simsek E. (2012). Influence of Salinity on Partial Nitrification in a Submerged Biofilter. Bioresour. Technol. 118, 24–29. doi: 10.1016/j.biortech.2012.05.057
Bartelme R. P., McLellan S. L., Newton R. J. (2017). Freshwater Recirculating Aquaculture System Operations Drive Biofilter Bacterial Community Shifts Around a Stable Nitrifying Consortium of Ammonia-Oxidizing Archaea and Comammox Nitrospira. Front. Microbiol. 8. doi: 10.3389/fmicb.2017.00101
Bartelme R. P., Smith M. C., Sepulveda-Villet O. J., Newton R. J. (2019). Component Microenvironments and System Biogeography Structure Microorganism Distributions in Recirculating Aquaculture and Aquaponic Systems. mSphere 4, 1–15. doi: 10.1128/msphere.00143-19
Bassin J. P., Kleerebezem R., Muyzer G., Rosado A. S., Van Loosdrecht M. C. M., Dezotti M. (2012). Effect of Different Salt Adaptation Strategies on the Microbial Diversity, Activity, and Settling of Nitrifying Sludge in Sequencing Batch Reactors. Appl. Microbiol. Biotechnol. 93, 1281–1294. doi: 10.1007/s00253-011-3428-7
Bassin J. P., Pronk M., Muyzer G., Kleerebezem R., Dezotti M., van Loosdrecht M. C. M. (2011). Effect of Elevated Salt Concentrations on the Aerobic Granular Sludge Process: Linking Microbial Activity With Microbial Community Structure. Appl. Environ. Microbiol. 77, 7942–7953. doi: 10.1128/AEM.05016-11
Bayer B., Saito M. A., Mcilvin M. R., Lücker S., Moran D. M., Lankiewicz T. S., et al. (2021). Metabolic Versatility of the Nitrite-Oxidizing Bacterium Nitrospira Marina and its Proteomic Response to Oxygen-Limited Conditions. ISME J. 15, 1025–1039. doi: 10.1038/s41396-020-00828-3
Bernhard A. E., Bollmann A. (2010). Estuarine Nitrifiers: New Players, Patterns and Processes. Estuar. Coast. Shelf Sci. 88, 1–11. doi: 10.1016/j.ecss.2010.01.023
Bernhard A. E., Landry Z. C., Blevins A., de la Torre J. R., Giblin A. E., Stahl D. A. (2010). Abundance of Ammonia-Oxidizing Archaea and Bacteria Along an Estuarine Salinity Gradient in Relation to Potential Nitrification Rates. Appl. Environ. Microbiol. 76, 1285–1289. doi: 10.1128/AEM.02018-09
Bernhard A. E., Tucker J., Giblin A. E., Stahl D. A. (2007). Functionally Distinct Communities of Ammonia-Oxidizing Bacteria Along an Estuarine Salinity Gradient. Environ. Microbiol. 9, 1439–1447. doi: 10.1111/j.1462-2920.2007.01260.x
Blainey P. C., Mosier A. C., Potanina A., Francis C. A., Quake S. R. (2011). Genome of a Low-Salinity Ammonia-Oxidizing Archaeon Determined by Single-Cell and Metagenomic Analysis. PloS One. 6(2), e16626. doi: 10.1371/journal.pone.0016626
Bower C. E., Turner D. T. (1981). Accelerated Nitrification in New Seawater Culture Systems: Effectiveness of Commercial Additives and Seed Media From Established Systems. Aquaculture 24, 1–9.
Cabrol L., Poly F., Malhautier L., Pommier T., Lerondelle C., Verstraete W., et al. (2016). Management of Microbial Communities Through Transient Disturbances Enhances the Functional Resilience of Nitrifying Gas-Biofilters to Future Disturbances. Environ. Sci. Technol. 50, 338–348. doi: 10.1021/acs.est.5b02740
Campo R., Corsino S. F., Torregrossa M., Di Bella G. (2018). The Role of Extracellular Polymeric Substances on Aerobic Granulation With Stepwise Increase of Salinity. Sep. Purif. Technol. 195, 12–20. doi: 10.1016/j.seppur.2017.11.074
Chen Z., Chang Z., Zhang L., Wang J., Qiao L., Song X., et al. (2020). Effects of Carbon Source Addition on Microbial Community and Water Quality in Recirculating Aquaculture Systems for Litopenaeus Vannamei. Fish. Sci. 86, 507–517. doi: 10.1007/s12562-020-01423-3
Chen C., Liang H., Gao D. (2019). Community Diversity and Distribution of Ammonia-Oxidizing Archaea in Marsh Wetlands in the Black Soil Zone in North-East China. Front. Environ. Sci. Eng. 13. doi: 10.1007/s11783-019-1146-z
Chen S., Ling J., Blancheton J. P. (2006). Nitrification Kinetics of Biofilm as Affected by Water Quality Factors. Aquac. Eng. 34, 179–197. doi: 10.1016/j.aquaeng.2005.09.004
Corsino S. F., Capodici M., Torregrossa M., Viviani G. (2017). Physical Properties and Extracellular Polymeric Substances Pattern of Aerobic Granular Sludge Treating Hypersaline Wastewater. Bioresour. Technol. 229, 152–159. doi: 10.1016/j.biortech.2017.01.024
Cortes-Lorenzo C., Rodriguez-Diaz M., Sipkema D., Juarez-Jimenez B., Rodelas B., Smidt H., et al. (2015). Effect of Salinity on Nitrification Efficiency and Structure of Ammonia-Oxidizing Bacterial Communities in a Submerged Fixed Bed Bioreactor. Chem. Eng. J. 266, 233–240. doi: 10.1016/j.cej.2014.12.083
Csonka L. N. (1989). Physiological and Genetic Responses of Bacteria to Osmotic Stress. Microbiol. Rev. 53, 121–147.
Cui Y. W., Zhang H. Y., Ding J. R., Peng Y. Z. (2016). The Effects of Salinity on Nitrification Using Halophilic Nitrifiers in a Sequencing Batch Reactor Treating Hypersaline Wastewater. Sci. Rep. 6, 1–11. doi: 10.1038/srep24825
Daims H., Lücker S., Wagner M. (2016). A New Perspective on Microbes Formerly Known as Nitrite-Oxidizing Bacteria. Trends Microbiol. 24, 699–712. doi: 10.1016/j.tim.2016.05.004
Dinçer A. R., Kargi F. (1999). Salt Inhibition of Nitrification and Denitrification in Saline Wastewater. Environ. Technol. 20, 1147–1153. doi: 10.1080/09593332008616912
Elling F. J., Könneke M., Mußmann M., Greve A., Hinrichs K. U. (2015). Influence of Temperature, Ph, and Salinity on Membrane Lipid Composition and TEX86 of Marine Planktonic Thaumarchaeal Isolates. Geochim. Cosmochim. Acta 171, 238–255. doi: 10.1016/j.gca.2015.09.004
Flemming H. C., Wingender J., Szewzyk U., Steinberg P., Rice S. A., Kjelleberg S. (2016). Biofilms: An Emergent Form of Bacterial Life. Nat. Rev. Microbiol. 14, 563–575. doi: 10.1038/nrmicro.2016.94
Fossmark R. O., Attramadal K. J. K., Nordøy K., Østerhus S. W., Vadstein O. (2021). A Comparison of Two Seawater Adaptation Strategies for Atlantic Salmon Post-Smolt (Salmo Salar) Grown in Recirculating Aquaculture Systems (RAS): Nitrification, Water and Gut Microbiota, and Performance of Fish. Aquaculture 532, 735973. doi: 10.1016/j.aquaculture.2020.735973
Francis C. A., Roberts K. J., Beman J. M., Santoro A. E., Oakley B. B. (2005). Ubiquity and Diversity of Ammonia-Oxidizing Archaea in Water Columns and Sediments of the Ocean. Proc. Natl. Acad. Sci. 102 (41), 14683–8. doi: 10.1073/pnas.0506625102
Freitag T. E., Prosser J. I. (2004). Differences Between Betaproteobacterial Ammonia-Oxidizing Communities in Marine Sediments and Those in Overlying Water. Appl. Environ. Microbiol. 70 (6), 3789–3793. doi: 10.1128/AEM.70.6.3789-3793.2004
Gonzalez-Silva B. M. (2016). Salinity as a Driver for Microbial Community Structure in Reactors for Nitrification and Anammox (Doctoral Dissertation). Trondheim, Norway: Norwegian Institute of Science and Technology
Gonzalez-Silva B. M., Jonassen K. R., Bakke I., Østgaard K., Vadstein O. (2016). Nitrification at Different Salinities: Biofilm Community Composition and Physiological Plasticity. Water Res. 95, 48–58. doi: 10.1016/j.watres.2016.02.050
Gonzalez-Silva B. M., Jonassen K. R., Bakke I., Østgaard K., Vadstein O. (2021). Understanding Structure/Function Relationships in Nitrifying Microbial Communities After Cross-Transfer Between Freshwater and Seawater. Sci. Rep. 11, 2979. doi: 10.1038/s41598-021-82272-7
Goode C., Allen D. G. (2011). Effect of Calcium on Moving-Bed Biofilm Reactor Biofilms. Water Environ. Res. 83, 220–232. doi: 10.2175/106143010x12780288628255
Graham D. W., Knapp C. W., Van Vleck E. S., Bloor K., Lane T. B., Graham C. E. (2007). Experimental Demonstration of Chaotic Instability in Biological Nitrification. ISME J. 1, 385–393. doi: 10.1038/ismej.2007.45
Hatzenpichler R. (2012). Diversity, Physiology, and Niche Differentiation of Ammonia-Oxidizing Archaea. Appl. Environ. Microbiol. 78, 7501–7510. doi: 10.1128/AEM.01960-12
Hayatsu M., Tago K., Uchiyama I., Toyoda A., Wang Y., Shimomura Y., et al. (2017). An Acid-Tolerant Ammonia-Oxidizing γ -Proteobacterium From Soil. ISME J. 11, 1130–1141. doi: 10.1038/ismej.2016.191
He H., Chen Y., Li X., Cheng Y., Yang C., Zeng G. (2017). Influence of Salinity on Microorganisms in Activated Sludge Processes: A Review. Int. Biodeterior. Biodegrad. 119, 520–527. doi: 10.1016/j.ibiod.2016.10.007
Herbold C. W., Lebedeva E., Palatinszky M., Wagner M. (2016). “Candidatus Nitrosotenuis,” in Bergey’s Manual of Systematics of Archaea and Bacteria eds. Trujillo M. E., Dedysh S., DeVos P., Hedlund B., Kämpfer P., Rainey F. A., et al. (John Wiley & Sons, Inc). doi: 10.1002/9781118960608.gbm01291
Hovanec T. A., Taylor L. T., Blakis A., Delong E. F. (1998). Nitrospira-Like Bacteria Associated With Nitrite Oxidation in Freshwater Aquaria. Appl. Environ. Microbiol. 64 (1), 258–264. doi: 10.1128/AEM.64.1.258-264.1998
Hunik J. H., Meijer H. J. G., Tramper J. (1993). Kinetics of Nitrobacter Agilis at Extreme Substrate , Product and Salt Concentrations. Appl. Environ. Microbiol. 40, 442–448. doi: 10.1007/BF00170408
Intrasungkha N., Keller J., Blackall L. L. (1999). Biological Nutrient Removal Efficiency in Treatment of Saline Wastewater. Water Sci. Technol. 39, 183–190. doi: 10.1016/S0273-1223(99)00138-9
Jung M. Y., Islam M. A., Gwak J. H., Kim J. G., Rhee S. K. (2018). Nitrosarchaeum Koreense Gen. Nov., Sp. Nov., an Aerobic and Mesophilic, Ammonia-Oxidizing Archaeon Member of the Phylum Thaumarchaeota Isolated From Agricultural Soil. Int. J. Syst. Evol. Microbiol. 68, 3084–3095. doi: 10.1099/ijsem.0.002926
Kerou M., Schleper C. (2016). “Nitrososphaera” in Bergey’s Manual of Systematics of Archaea and Bacteria. eds. Trujillo M. E, Dedysh S., DeVos P., Hedlund B., Kämpfer P., Rainey F. A., et al. (John Wiley & Sons, Inc). doi: 10.1002/9781118960608.gbm01294
Kerou M., Schleper C. (2017). “Candidatus Cenarchaeum” in Bergey’s Manual of Systematics of Archaea and Bacteria. eds. Trujillo M. E, Dedysh S., DeVos P., Hedlund B., Kämpfer P., Rainey F. A., et al. (John Wiley & Sons, Inc). doi: 10.1002/9781118960608.gbm01288
Keuter S., Beth S., Quantz G., Schulz C., Eva S. (2017). Longterm Monitoring of Nitrification and Nitrifying Communities During Biofilter Activation of Two Marine Recirculation Aquaculture Systems (RAS). Int. J. Aquac. Fish. Sci. 3, 051–061. doi: 10.17352/2455-8400.000029
Kinyage J. P. H., Pedersen P. B., Pedersen L. F., Peter J., Kinyage H., Bovbjerg P., et al. (2019). Effects of Abrupt Salinity Increase on Nitrification Processes in a Freshwater Moving Bed Biofilter. Aquac. Eng. 84, 91–98. doi: 10.1016/j.aquaeng.2018.12.005
Kitzinger K., Marchant H. K., Bristow L. A., Herbold C. W., Padilla C. C., Kidane A. T., et al. (2020). Single Cell Analyses Reveal Contrasting Life Strategies of the Two Main Nitrifiers in the Ocean. Nat. Commun. 11, 767. doi: 10.1038/s41467-020-14542-3
Koops H. P., Bottcher B., Moller U. C., Pommerening-Roser A., Stehr G. (1990). Description of a New Species of Nitrosococcus. Arch. Microbiol. 154, 244–248. doi: 10.1007/BF00248962
Koops H. P., Pommerening-Röser A. (2001). Distribution and Ecophysiology of the Nitrifying Bacteria Emphasizing Cultured Species. FEMS Microbiol. Ecol. 37, 1–9. doi: 10.1016/S0168-6496(01)00137-4
Koops H.-P., Purkhold U., Pommerening-Röser A., Timmermann G., Wagner M. (2006). “The Lithoautotrophic Ammonia-Oxidizing Bacteria,” in The Prokaryotes New York, NY: Springer, vol. 778–811. doi: 10.1007/0-387-30745-1_36
Lay W. C. L., Liu Y., Fane A. G. (2010). Impacts of Salinity on the Performance of High Retention Membrane Bioreactors for Water Reclamation: A Review. Water Res. 44, 21–40. doi: 10.1016/j.watres.2009.09.026
Lefebvre O., Moletta R. (2006). Treatment of Organic Pollution in Industrial Saline Wastewater: A Literature Review. Water Res. 40, 3671–3682. doi: 10.1016/j.watres.2006.08.027
Lefebvre O., Quentin S., Torrijos M., Godon J. J., Delgenès J. P., Moletta R. (2007). Impact of Increasing NaCl Concentrations on the Performance and Community Composition of Two Anaerobic Reactors. Appl. Microbiol. Biotechnol. 75, 61–69. doi: 10.1007/s00253-006-0799-2
Li C., Liang J., Lin X., Xu H., Tadda M. A., Lan L., et al. (2019). Fast Start-Up Strategies of MBBR for Mariculture Wastewater Treatment. J. Environ. Manage. 248, 109267. doi: 10.1016/j.jenvman.2019.109267
Liu Z., Zhang C., Wei Q., Zhang S., Quan Z., Li M. (2020). Temperature and Salinity Drive Comammox Community Composition in Mangrove Ecosystems Across Southeastern China. Sci. Total Environ. 742, 140456. doi: 10.1016/j.scitotenv.2020.140456
Luo W., Phan H. V., Hai F. I., Price W. E., Guo W., Ngo H. H., et al. (2016). Effects of Salinity Build-Up on the Performance and Bacterial Community Structure of a Membrane Bioreactor. Bioresour. Technol. 200, 305–310. doi: 10.1016/j.biortech.2015.10.043
Madigan M. T., Bender K. S., Buckley D. H., Sattley W. M., Stahl D. A. (2018). Brock Biology of Microorganisms. 15th ed (Pearson Education Limited).
Moussa M. S., Garcia Fuentes O., Lubberding H. J., Hooijmans C. M., Van Loosdrecht M. C. M., Gijzen H. J. (2006a). Nitrification Activities in Full-Scale Treatment Plants With Varying Salt Loads. Environ. Technol. 27, 635–643. doi: 10.1080/09593332708618673
Moussa M. S., Sumanasekera D. U., Ibrahim S. H., Lubberding H. J., Hooijmans C. M., Gijzen H. J., et al. (2006b). Long Term Effects of Salt on Activity, Population Structure and Floc Characteristics in Enriched Bacterial Cultures of Nitrifiers. Water Res. 40, 1377–1388. doi: 10.1016/j.watres.2006.01.029
Moya A., Ferrer M. (2016). Functional Redundancy- Induced Stability of Gut Microbiota Subjected to Disturbance. Trends Microbiol. 24, 402–413. doi: 10.1016/j.tim.2016.02.002
Navada S. (2021). Salinity Acclimation Strategies for Nitrifying Bioreactors in Recirculating Aquaculture Systems (Doctoral dissertation). Trondheim, Norway: Norwegian Institute of Science and Technology. 540. doi: 10.1016/j.aquaculture.2021.736663
Navada S., Gaumet F., Tveten A.-K., Kolarevic J., Vadstein O. (2021). Seeding as a Start-Up Strategy for Improving the Acclimation of Freshwater Nitrifying Bioreactors to Salinity Stress. Aquacult..
Navada S., Sebastianpillai M., Kolarevic J., Fossmark R. O., Tveten A. K., Gaumet F., et al. (2020a). A Salty Start: Brackish Water Start-Up as a Microbial Management Strategy for Nitrifying Bioreactors With Variable Salinity. Sci. Total Environ. 739, 139934. doi: 10.1016/j.scitotenv.2020.139934
Navada S., Vadstein O., Gaumet F., Tveten A.-K. K., Spanu C., Mikkelsen Ø., et al. (2020b). Biofilms Remember: Osmotic Stress Priming as a Microbial Management Strategy for Improving Salinity Acclimation in Nitrifying Biofilms. Water Res. 176, 115732. doi: 10.1016/j.watres.2020.115732
Navada S., Vadstein O., Tveten A.-K., Verstege G. C., Terjesen B. F., Mota V. C., et al. (2019). Influence of Rate of Salinity Increase on Nitrifying Biofilms. J. Clean. Prod. 238, 117835. doi: 10.1016/j.jclepro.2019.117835
Nemergut D. R., O’Neill S. P., Bilinski T. M., Stanish L. F., Knelman J. E., Wickey P., et al. (2013). Patterns and Processes of Microbial Community Assembly. Microbiol. Mol. Biol. Rev. 77, 342–356. doi: 10.1128/MMBR.00051-12
Ngugi D. K., Blom J., Alam I., Rashid M., Ba-Alawi W., Zhang G., et al. (2015). Comparative Genomics Reveals Adaptations of a Halotolerant Thaumarchaeon in the Interfaces of Brine Pools in the Red Sea. ISME J. 9, 396–411. doi: 10.1038/ismej.2014.137
Ngugi D. K., Blom J., Stepanauskas R., Stingl U. (2016). Diversification and Niche Adaptations of Nitrospina - Like Bacteria in the Polyextreme Interfaces of Red Sea Brines. ISME J. 10, 1383–1399. doi: 10.1038/ismej.2015.214
Nicol G. W., Leininger S., Schleper C. (2011). “Distribution and Activity of Ammonia Oxidizing Archaea in Natural Environments” in Nitrification. Eds. Ward B. B., Arp D. J., Klotz M. G. (Washington D.C., U.S.A).
Nijhof M., Bovendeur J. (1990). Fixed Film Nitrification Characteristics in Sea-Water Recirculation Fish Culture Systems. Aquaculture 87, 133–143. doi: 10.1016/0044-8486(90)90270-W
Norton J. M. (2011). “Diversity and Environmental Distribution of Ammonia-Oxidizing Bacteria” in Nitrification. Eds. Ward B. B., Arp D. J., Klotz M. G.. doi: 10.1128/9781555817145.ch3
Oren A. (2011). Thermodynamic Limits to Microbial Life at High Salt Concentrations. Environ. Microbiol. 13, 1908–1923. doi: 10.1111/j.1462-2920.2010.02365.x
Panswad T., Anan C. (1999). Impact of High Chloride Wastewater on an Anaerobic/Anoxic/Aerobic Process With and Without Inoculation of Chloride Acclimated Seeds. Water Res. 33, 1165–1172. doi: 10.1016/S0043-1354(98)00314-5
Picone N., Pol A., Mesman R., van Kessel M. A. H. J., Cremers G., Gelder A. H., et al. (2021). Ammonia Oxidation at pH 2 . 5 by a New Gammaproteobacterial Ammonia-Oxidizing Bacterium. ISME J. 15, 1150–1164. doi: 10.1038/s41396-020-00840-7
Polonia A. R. M., Cleary D. F. R. (2019). Archaeal Communities in Sponge, Sediment and Water From Marine Lakes and Open Water Habitats. Mar. Biol. Res. 15, 259–274.
Prosser J., Head I., Stein L. (2014). “The Family Nitrosomononadaceae” in The Prokaryotes. eds. Rosenberg E., Delong E. F., Lory S., Stackebrandt E., Thompson F.. (Berlin, Heidelberg: Springer), 901–918.
Prosser J. I., Nicol G. W. (2016). “Candidatus Nitrosotalea” in Bergey’s Manual of Systematics of Archaea and Bacteria. eds. Trujillo M. E, Dedysh S., DeVos P., Hedlund B., Kämpfer P., Rainey F. A., et al. (John Wiley & Sons, Inc). doi: 10.1002/9781118960608.gbm01292
Qin W., Jewell T. N. M., Russell V. V., Hedlund B. P., de la Torre J. R., Stahl D. A. (2017). “Candidatus Nitrosocaldus” in Bergey’s Manual of Systematics of Archaea and Bacteria. eds. Trujillo M. E, Dedysh S., DeVos P., Hedlund B., Kämpfer P., Rainey F. A., et al. (John Wiley & Sons, Inc), 1–5. doi: 10.1002/9781118960608.gbm01287
Qin W., Martens-Habbena W., Kobelt J. N., Stahl D. A. (2016). “Candidatus Nitrosopumilus”, in Bergey’s Manual of Systematics of Archaea and Bacteria. eds. Trujillo M. E, Dedysh S., DeVos P., Hedlund B., Kämpfer P., Rainey F. A., et al. (John Wiley & Sons, Inc), 1–9. doi: 10.1002/9781118960608.gbm01290
Roalkvam I., Drønen K., Dahle H., Inger H., Wergeland H. I. (2020). Comparison of Active Biofilm Carriers and Commercially Available Inoculum for Activation of Biofilters in Marine Recirculating Aquaculture Systems (RAS). Aquaculture 514, 734480. doi: 10.1016/j.aquaculture.2019.734480
Rud I., Kolarevic J., Holan A. B., Berget I., Calabrese S., Terjesen B. F. (2017). Deep-Sequencing of the Bacterial Microbiota in Commercial-Scale Recirculating and Semi-Closed Aquaculture Systems for Atlantic Salmon Post-Smolt Production. Aquac. Eng. 78, 50–62. doi: 10.1016/j.aquaeng.2016.10.003
Rusten B., Eikebrokk B., Ulgenes Y., Lygren E. (2006). Design and Operations of the Kaldnes Moving Bed Biofilm Reactors. Aquac. Eng. 34, 322–331. doi: 10.1016/j.aquaeng.2005.04.002
Ruvindy R., Allen R., Iii W., Neilan B. A., Burns B. P. (2016). Unravelling Core Microbial Metabolisms in the Hypersaline Microbial Mats of Shark Bay Using High-Throughput Metagenomics. ISME J. 10, 183–196. doi: 10.1038/ismej.2015.87
Sánchez O., Aspé E., Martí M. C., Roeckel M. (2004). The Effect of Sodium Chloride on the Two-Step Kinetics of the Nitrifying Process. Water Environ. Res. 76, 73–80. doi: 10.2175/106143004X141609
Santos J. P., Mendes D., Monteiro M., Ribeiro H., Baptista M. S., Borges M. T., et al. (2018). Salinity Impact on Ammonia Oxidizers Activity and amoA Expression in Estuarine Sediments. Estuar. Coast. Shelf Sci. 211, 177–187. doi: 10.1016/j.ecss.2017.09.001
Santos J. P., Sousa A. G. G., Ribeiro H., Magalhães C. (2020). The Response of Estuarine Ammonia-Oxidizing Communities to Constant and Fluctuating Salinity Regimes. Front. Microbiol. 11. doi: 10.3389/fmicb.2020.574815
Sauder L. A., Engel K., Lo C., Chain P., Neufeld J. D. (2018). “Candidatus Nitrosotenuis Aquarius” an Ammonia-Oxidizing Archaeon From a Freshwater Aquarium Biofilter. Appl. Environ. Microbiol. 84(19), e01430–18. doi: 10.1128/AEM.01430-18
Sauder L. A., Engel K., Stearns J. C., Masella A. P., Pawliszyn R., Neufeld J. D. (2011). Aquarium Nitrification Revisited: Thaumarchaeota are the Dominant Ammonia Oxidizers in Freshwater Aquarium Biofilters. PloS One 6(8), e23281. doi: 10.1371/journal.pone.0023281
Saur T., Escudié R., Santa-Catalina G., Bernet N., Milferstedt K. (2016). Conservation of Acquired Morphology and Community Structure in Aged Biofilms After Facing Environmental Stress. Water Res. 88, 164–172. doi: 10.1016/j.watres.2015.10.012
Shade A., Peter H., Allison S. D., Baho D. L., Berga M., Bürgmann H., et al. (2012). Fundamentals of Microbial Community Resistance and Resilience. Front. Microbiol. 3. doi: 10.3389/fmicb.2012.00417
Sharrer M. J., Tal Y., Ferrier D., Hankins J. A., Summerfelt S. T. (2007). Membrane Biological Reactor Treatment of a Saline Backwash Flow From a Recirculating Aquaculture System. Aquac. Eng. 36, 159–176. doi: 10.1016/j.aquaeng.2006.10.003
Shi K., Zhou W., Zhao H., Zhang Y. (2012). Performance of Halophilic Marine Bacteria Inocula on Nutrient Removal From Hypersaline Wastewater in an Intermittently Aerated Biological Filter. Bioresour. Technol. 113, 280–287. doi: 10.1016/j.biortech.2012.01.117
Sleator R. D., Hill C. (2002). Bacterial Osmoadaptation: The Role of Osmolytes in Bacterial Stress and Virulence. FEMS Microbiol. Rev. 26, 49–71. doi: 10.1016/S0168-6445(01)00071-7
Stieglmeier M., Alves R., Schleper C. (2014). “The Phylum Thaumarchaeota”, in The Prokaryotes. Eds. Rosenberg E., Delong E. F., Lory S., Stackebrandt E., Thompson F. (Springer), 348–362.
Sudarno U. (2011). Nitrification in Fixed Bed Reactors Treating Saline Wastewater (Doctoral dissertation). Karlsruhe, Germany:Karlsruhe Institute of Technology.
Sudarno U., Bathe S., Winter J., Gallert C. (2010). Nitrification in Fixed-Bed Reactors Treating Saline Wastewater. Appl. Microbiol. Biotechnol. 85, 2301–2304. doi: 10.1007/s00253-009-2301-4
Sun D., Zhao M., Tang X., Liu M., Hou L., Zhao Q., et al. (2021a). Niche Adaptation Strategies of Different Clades of Comammox Nitrospira in the Yangtze Estuary. Int. Biodeterior. Biodegradation 164, 105286. doi: 10.1016/j.ibiod.2021.105286
Sun X., Zhao J., Zhou X., Bei Q., Xia W., Zhao B., et al. (2021b). Salt Tolerance-Based Niche Differentiation of Soil Ammonia Oxidizers. ISME J 16, 412–422. doi: 10.1038/s41396-021-01079-6
Tolar B. B., Mosier A. C., Lund M. B., Francis C. A. (2019). “Nitrosarchaeum”, in Bergey’s Manual of Systematics of Archaea and Bacteria. eds. Trujillo M. E, Dedysh S., DeVos P., Hedlund B., Kämpfer P., Rainey F. A., et al. (John Wiley & Sons, Inc). doi: 10.1002/9781118960608.gbm01289
Van Kessel M. A. H. J., Speth D. R., Albertsen M., Nielsen P. H., Op Den Camp H. J. M., Kartal B., et al. (2015). Complete Nitrification by a Single Microorganism. Nature 528, 555–559. doi: 10.1038/nature16459
Vyrides I. (2015). “Anaerobic Treatment of Organic Saline Waste/Wastewater: Overcome Salinity Inhibition by Addition of Compatible Solutes,”. In Sukla L., Pradhan N., Panda S., Mishra B.. (eds) Environmental Microbial Biotechnology (Springer) 45, 105–117. doi: 10.1007/978-3-319-19018-1_6
Vyrides I., Stuckey D. C. (2017). Compatible Solute Addition to Biological Systems Treating Waste/Wastewater to Counteract Osmotic and Other Environmental Stresses: A Review. Crit. Rev. Biotechnol. 37, 865–879. doi: 10.1080/07388551.2016.1266460
Wang Z., Gao M., She Z., Wang S., Jin C., Zhao Y., et al. (2015). Effects of Salinity on Performance, Extracellular Polymeric Substances and Microbial Community of an Aerobic Granular Sequencing Batch Reactor. Sep. Purif. Technol. 144, 223–231. doi: 10.1016/j.seppur.2015.02.042
Wang Z., van Loosdrecht M. C. M., Saikaly P. E. (2017). Gradual Adaptation to Salt and Dissolved Oxygen: Strategies to Minimize Adverse Effect of Salinity on Aerobic Granular Sludge. Water Res. 124, 702–712. doi: 10.1016/j.watres.2017.08.026
Wan C., Yang X., Lee D. J., Liu X., Sun S., Chen C. (2014). Partial Nitrification of Wastewaters With High NaCl Concentrations by Aerobic Granules in Continuous-Flow Reactor. Bioresour. Technol. 152, 1–6. doi: 10.1016/j.biortech.2013.10.112
Ward B. B., Eveillard D., Kirshtein J. D., Nelson J. D., Voytek M. A., Jackson G. A. (2007). Ammonia-Oxidizing Bacterial Community Composition in Estuarine and Oceanic Environments Assessed Using a Functional Gene Microarray. Environ. Microbiol. 9, 2522–2538. doi: 10.1111/j.1462-2920.2007.01371.x
Wittebolle L., Verstraete W., Boon N. (2009). The Inoculum Effect on the Ammonia-Oxidizing Bacterial Communities in Parallel Sequential Batch Reactors. Water Res. 43, 4149–4158. doi: 10.1016/j.watres.2009.06.034
Yepsen D. V., Levipan H. A., Molina V. (2019). Nitrospina Bacteria in a Rocky Intertidal Habitat ( Quintay Bay , Central Chile ). Microbiologyopen 8, 2045–8827. doi: 10.1002/mbo3.646
Zhao Y., Zhuang X., Ahmad S., Sung S., Ni S. Q. (2020). Biotreatment of High-Salinity Wastewater: Current Methods and Future Directions. World J. Microbiol. Biotechnol. 36, 37. doi: 10.1007/s11274-020-02815-4
Keywords: osmotic stress, osmoregulation, ammonia oxidizing archaea, seawater, halotolerance, compatible solute, osmolyte, wastewater
Citation: Navada S and Vadstein O (2022) Salinity Acclimation Strategies in Nitrifying Bioreactors. Front. Mar. Sci. 9:867592. doi: 10.3389/fmars.2022.867592
Received: 01 February 2022; Accepted: 14 March 2022;
Published: 11 April 2022.
Edited by:
Wei Huang, Ministry of Natural Resources, ChinaReviewed by:
Musa Abubakar Tadda, Bayero University Kano, NigeriaJohan Verreth, Wageningen University and Research, Netherlands
Copyright © 2022 Navada and Vadstein. This is an open-access article distributed under the terms of the Creative Commons Attribution License (CC BY). The use, distribution or reproduction in other forums is permitted, provided the original author(s) and the copyright owner(s) are credited and that the original publication in this journal is cited, in accordance with accepted academic practice. No use, distribution or reproduction is permitted which does not comply with these terms.
*Correspondence: Sharada Navada, c2hhcmFkYS5uYXZhZGFAbnRudS5ubw==