- 1Ifremer, Département REM, Plouzané, France
- 2Department of Earth Science and Centre for Deep Sea Research, University of Bergen, Bergen, Norway
- 3Ocean Networks Canada, University of Victoria, Victoria, BC, Canada
- 4Equipe de Géosciences Marines, Université de Paris, Institut de Physique du Globe de Paris, UMR CNRS, Paris, France
- 5School of Oceanography, University of Washington, Seattle, WA, United States
- 6Department of Marine Zoology, Senckenberg Research Institute and Natural History Museum, Frankfurt am Main, Germany
- 7Géosciences Environnement Toulouse CNRS UMR IRD/UPS/CNES, Université de Toulouse, Toulouse, France
- 8Instituto de Investigação em Ciências do Mar - Okeanos, Universidade dos Açores, Horta, Portugal
- 9Laboratoire de Géologie, CNRS, UMR, École Normale Supérieure, PSL University, Paris, France
- 10Universidad Nacional Autónoma de México, Instituto de Ciencias del Mar y Limnología, Ciudad Universitaria Mexico City, Mexico
- 11Geology and Geophysics Department, Woods Hole Oceanographic Institution, Woods Hole, MA, United States
- 12Institute of Geophysics, Center for Earth System Research and Sustainability, Hamburg University, Hamburg, Germany
- 13Marine Chemistry and Geochemistry, Woods Hole Oceanographic Institution, Woods Hole, MA, United States
- 14Center for Marine Biodiversity and Conservation, Scripps Institution of Oceanography, UC San Diego, La Jolla, CA, United States
- 15Department of Earth and Spatial Sciences, University of Idaho, Moscow, ID, United States
- 16L@BISEN, ISEN Yncréa Ouest, Brest, France
- 17Norwegian Research Center, NORCE, Bergen, Norway
- 18Ocean Observation Systems, National Institute of Ocean Technology, Chennai, India
- 19Univ. Brest, CNRS, IRD, Ifremer, Laboratoire d’Océanographie Physique et Spatiale, Plouzané, France
The unique ecosystems and biodiversity associated with mid-ocean ridge (MOR) hydrothermal vent systems contrast sharply with surrounding deep-sea habitats, however both may be increasingly threatened by anthropogenic activity (e.g., mining activities at massive sulphide deposits). Climate change can alter the deep-sea through increased bottom temperatures, loss of oxygen, and modifications to deep water circulation. Despite the potential of these profound impacts, the mechanisms enabling these systems and their ecosystems to persist, function and respond to oceanic, crustal, and anthropogenic forces remain poorly understood. This is due primarily to technological challenges and difficulties in accessing, observing and monitoring the deep-sea. In this context, the development of deep-sea observatories in the 2000s focused on understanding the coupling between sub-surface flow and oceanic and crustal conditions, and how they influence biological processes. Deep-sea observatories provide long-term, multidisciplinary time-series data comprising repeated observations and sampling at temporal resolutions from seconds to decades, through a combination of cabled, wireless, remotely controlled, and autonomous measurement systems. The three existing vent observatories are located on the Juan de Fuca and Mid-Atlantic Ridges (Ocean Observing Initiative, Ocean Networks Canada and the European Multidisciplinary Seafloor and water column Observatory). These observatories promote stewardship by defining effective environmental monitoring including characterizing biological and environmental baseline states, discriminating changes from natural variations versus those from anthropogenic activities, and assessing degradation, resilience and recovery after disturbance. This highlights the potential of observatories as valuable tools for environmental impact assessment (EIA) in the context of climate change and other anthropogenic activities, primarily ocean mining. This paper provides a synthesis on scientific advancements enabled by the three observatories this last decade, and recommendations to support future studies through international collaboration and coordination. The proposed recommendations include: i) establishing common global scientific questions and identification of Essential Ocean Variables (EOVs) specific to MORs, ii) guidance towards the effective use of observatories to support and inform policies that can impact society, iii) strategies for observatory infrastructure development that will help standardize sensors, data formats and capabilities, and iv) future technology needs and common sampling approaches to answer today’s most urgent and timely questions.
Introduction
Over the past ~70 years, since the revolution in Earth and Ocean sciences precipitated by the confirmation of seafloor spreading and plate tectonics, the technological challenges, limited access, and high costs associated with deep-sea studies led to significant gaps in understanding spatial and temporal changes associated with chemical, biological and physical phenomena in the deep ocean. This has been especially true of seasonal processes that occur over a wide range of spatial and temporal scales throughout the global oceans (e.g., Glover et al., 2010). To date, deep-sea time-series investigations have documented notable environmental and biological variations from seconds to decades in a wide range of ecosystems from bathyal and abyssal zones (e.g., Hartman et al., 2012; Barreyre et al., 2014b; Cuvelier et al., 2014b; Doya et al., 2014; Matabos et al., 2014; Sarrazin et al., 2014; Cuvelier et al., 2017; Lelièvre et al., 2017; Taylor et al., 2017; Chavagnac et al., 2018a; Taylor et al., 2018; Durden et al., 2020). Increasing societal interest in deep-ocean resources has been accompanied by a growing recognition of the urgent need for a comprehensive assessment of the status and health of deep-sea ecosystems (Rogers et al., 2015; Franke et al., 2020). Robust environmental and biological baselines as well as understanding of their natural dynamics are key to assessing and predicting the impacts and responses of interconnected ecosystems to large-scale disturbances caused by natural processes (e.g., earthquakes and volcanic eruptions), and anthropogenic activities (e.g., ocean acidification and warming and their climate and environmental impacts) (e.g., Ramirez-Llodra et al., 2010; Danovaro et al., 2017b; Danovaro et al., 2020). Multi-decadal and multidisciplinary time-series studies at variable spatial scales are essential for discriminating between human-induced impacts, and long-term natural periodic and episodic variability, and inform our assessment of the environmental drivers and their biological consequences.
The need for long-term, time-series measurements of key ocean variables with a specific focus on the deep-sea (Thiel et al., 1994), has been identified as a critical topic since the 1990s (National Research Council, 2000), and has led to the development of seafloor-water column observatories. The Committee on Seafloor Observatories, bringing together scientists from all fields of Ocean and Earth sciences, gathered in 2000 to provide a vision for a global Seafloor Observatory Network including the risks and benefits of ocean observation platforms (National Research Council, 2000). They defined “seafloor observatories” as an autonomous “system of instruments, sensors, and command modules connected either acoustically or via a seafloor junction box to a surface buoy or a fiber optic cable to land”. This comprehensive report highlighted scientific questions that observatories could specifically address. Observatories were foreseen as requiring a major investment of resources over many decades. In the following two decades, a number of observatories have developed around the world, with instruments distributed from seafloor to the water column (e.g., Kelley et al., 2014; Smith et al., 2018; Dañobeitia et al., 2020). More recently an observatory was defined as an “integrated observing, monitoring, and experimenting infrastructure which aims to collect high-resolution data in a restricted geographical region” (Crise et al., 2018). One of the first areas of interest for observatories has been highly-dynamic mid-ocean ridges and back-arc spreading centres, where seafloor volcanism and tectonic activity predominate.
Mid-Ocean Ridge (MOR) and back-arc spreading systems host unique ecosystems and biodiversity (Tunnicliffe, 1991; Ramirez-Llodra et al., 2010; Mullineaux et al., 2018; Chapman et al., 2019). Hydrothermal circulation within these environments is responsible for significant transfer of heat and chemical constituents between the oceanic lithosphere and the overlying hydrosphere, thus controlling the thermo-mechanical state of the crust, its formation and deformation, rock alteration, and influencing element balances in the ocean. (e.g., Boyd and Ellwood, 2010; Fitzsimmons et al., 2014). Hydrothermal circulation also supports high-biomass chemosynthesis-based ecosystems that sharply contrast with surrounding deep-sea habitats (e.g., Kelley et al., 2005; Lutz et al., 2008; Nees et al., 2008; Dick, 2019). Hydrothermal vents sustain dense populations of endemic faunal species that rely on microbial dark CO2 fixation (Tunnicliffe, 1991; Tunnicliffe et al., 2003) that is coupled to the oxidation of reducing elements (e.g., HS-, H2, CH4, etc.) in discharging hydrothermal fluids (Le Bris et al., 2019). Its footprint impacts neighbouring water and seafloor and marine ecosystems (Levin et al., 2016). Vent fauna communities are influenced by local population’s demography and connected by dispersing planktonic larvae, which impact the dynamics of the vent metacommunity at a regional scale (Mullineaux et al., 2018). Challenging physico-chemical conditions at seafloor vents constrain faunal diversity (Tunnicliffe, 1991), whereas highly diverse prokaryotes exploit a broad range of habitats (Dick, 2019). These ecosystems broaden our understanding of the potential origins of life on Earth (Martin et al., 2008; Barge et al., 2019) and of the possibilities for life on other worlds (e.g., Hand and German, 2018). Furthermore, adaptations of vent fauna and microorganisms to extreme conditions have been a source of inspiration for biotechnology, but also in Design and Art (reviewed in Van Dover, 2014). Growing interests in the mining of polymetallic sulphide deposits raise concerns about the potential impacts of mineral extraction on these unique ecosystems, stressing the need for research to inform environmental policy and management strategies (Boschen et al., 2013; Van Dover, 2019; Orcutt et al., 2020; Smith et al., 2020; Van Dover et al., 2020). Climate change is also expected to affect the deep sea through increased bottom temperatures, loss of oxygen in certain areas of the ocean, and modifications to deep water circulation (Levin and Le Bris, 2015; Sweetman et al., 2017; Galaasen et al., 2020; Levin et al., 2020). Understanding how these changes will affect ecosystem integrity and function requires detailed baseline knowledge of natural processes, with an understanding of the mechanisms involved.
Questions remain regarding the evolution of biological communities through time including non-directional change (i.e., change in species compositions, symbiosis, and abundances due to immigration, extinction, competition, predation), as well as on directional change (i.e., succession after natural and/or anthropogenic disturbance). Those processes can only be addressed with robust baseline knowledge provided by time series observations. Since the discovery of low-hydrothermal vents in 1977 (Ballard and Van Andel, 1977; Corliss et al., 1979), only a few areas have been repeatedly visited (Glover et al., 2010; Fornari et al., 2012). Long-term time-series acquired at these sites, including 9°N or 13°N along the East Pacific Rise, allowed significant advances in our understanding of community dynamics and successional processes (e.g., Sarrazin et al., 1997; Shank et al., 1998; Marcus et al., 2009; Cuvelier et al., 2011; Du Preez and Fisher, 2018; Mullineaux et al., 2020). However understanding episodic and periodic hydrothermal and sub-seafloor processes that operate over time scales of seconds to years (e.g., Tivey et al., 2002; Scheirer et al., 2006; Barreyre et al., 2014b; Barreyre et al., 2022), and the associated faunal and microbial responses require continuous or at least annual in situ monitoring and observations (e.g., Huber et al., 2003).
Cabled and autonomous deep-sea observatories can provide long-term time-series collection of multi-disciplinary data (e.g., geological, physical, chemical, biological) at high temporal resolution and over years to decades. Repeated submersible visits to study sites, combined with observatory maintenance, have documented submarine volcanic eruptions and associated changes to hydrothermal vent fields (e.g., Chadwick et al., 2012; Fornari et al., 2012; Chadwick et al., 2013; Nooner and Chadwick, 2016; Wilcock et al., 2016; Chadwick et al., 2022; Barreyre et al., 2022), changes in community structure and activity (Shank et al., 1998; Fortunato et al., 2018; Rommevaux et al., 2019) as well as interactions with other nearby chemosynthetic and peripheral surrounding ecosystems (Levin et al., 2016). Together, these data contribute to answering a number of questions across various disciplines from geophysics to microbiology:
● How are hydrothermal heat, mass and chemical fluxes to the ocean impacted by seismicity, volcanic activity and ground deformation at a diverging plate boundary?
● Does tectonic setting impact the long-term stability or temporal variability of these systems?
● How do steep axial valley topography and hydrothermal buoyancy flux affect the dynamics of water masses, and how do local dynamics impact the dispersion of hydrothermal effluents?
● How do species compositions differ between habitats, geological settings, and ridges?
● How do pioneering species colonise new sites and which processes are involved?
● What are the impacts of telluric, climatic, and anthropogenic changes on deep seafloor ecosystems and hydrothermal communities?
There are currently three observatory installations at hydrothermally active MOR systems. Plans for a fourth at the Mohn Ridge (EMSO-Mohn), north of Jan Mayen, are ongoing (https://emso.eu/observatories-node/nordic-seas/). In concert, these observatories provide Ocean and Earth scientists, engineers, educators, policy makers, and the public opportunities to deepen multidisciplinary understanding of myriad processes operating at MOR environments and utilization and advancement of deep-sea technology. The InterRidge Working Group on Integrating Multidisciplinary Observations in Vent Environments (IMOVE; https://www.interridge.org/working-group/wg_imove/) was created to advance and coordinate the integration of observatory research at deep-sea vent fields. InterRidge (IR - www.interridge.org) is an international non-profit organization created in 1992 that brings together ca. 1600 scientists from 20 nations involved in MOR research. IR aims to (i) facilitate interdisciplinary, international collaboration; (ii) coordinate scientific exchange - including information, technology and facilities; (iii) encourage the protection and environmental management of ridge environments; and (iv) promote communication between scientists and members of the society through coordinated outreach efforts. InterRidge Working Groups (WGs) are formed to support the implementation of these objectives in priority areas. The IMOVE WG was formed to advance multi-disciplinary research within and across the three MOR observatories.
A large volume of temporally- and spatially-variable multidisciplinary data has been collected at the three presently active MOR vent observatories (Figure 1), requiring considerable support from funding agencies. One of the goals of the IMOVE WG is to advance the integration of data from different disciplines into quantitative, cross-disciplinary models relevant to hydrothermal processes on the global MOR system. Coordinating the efforts of the scientific communities working at MOR observatories has the potential to produce transformative research outcomes and major knowledge advancements. The IMOVE WG held two international workshops (2019 and 2020) to set the stage for such a global effort. The results of these workshops, including recommendations for future MOR observatory science, are presented in this paper (IMOVE, working group, 2019).
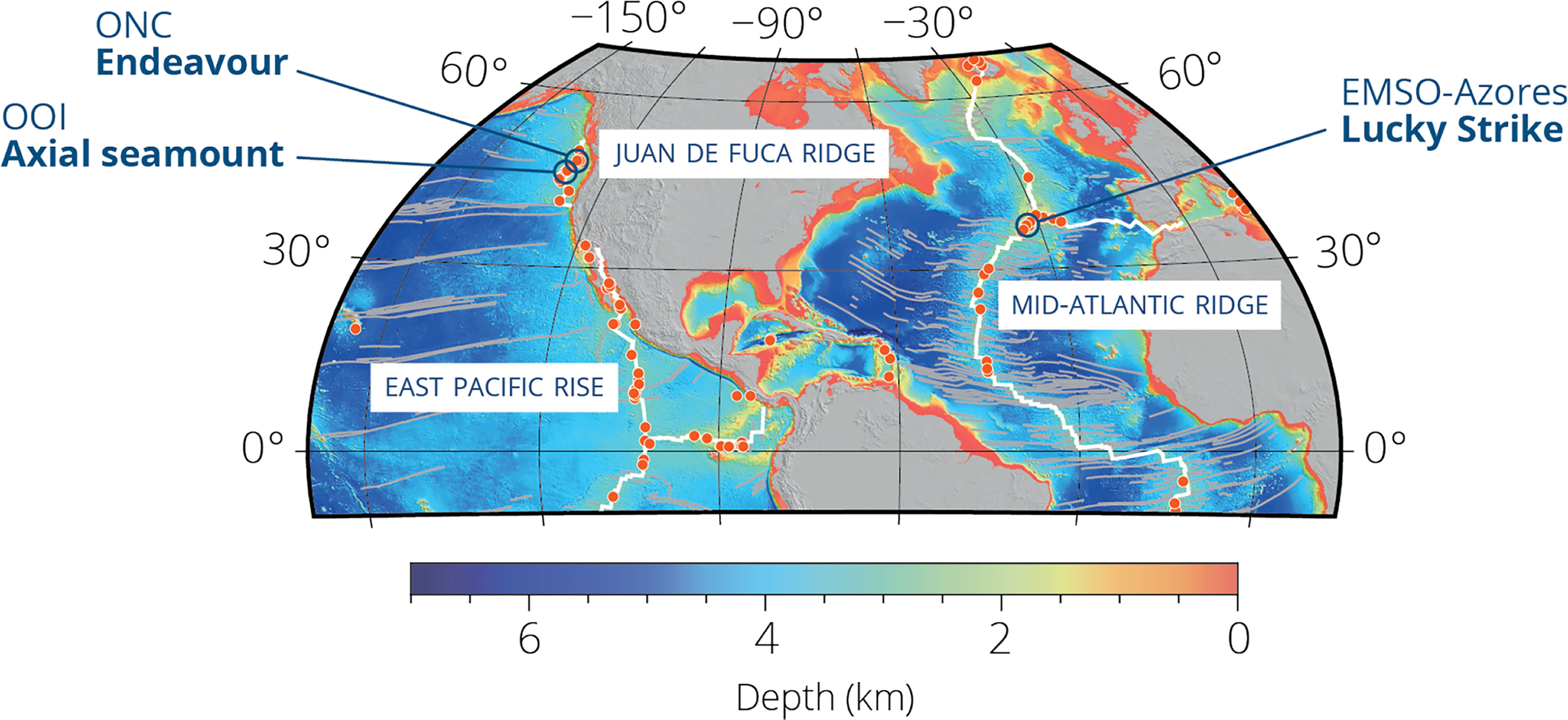
Figure 1 Location of the three currently operating seafloor and water column mid-ocean ridges ocean observatories in the Pacific and Atlantic oceans. Orange dots represent active known vent fields.
Current Observatories at Mid-Ocean Ridges
The three currently operating MOR ocean observatories are the Azores component of the European Multidisciplinary Seafloor and water column Observatory (EMSO), the Endeavour node of Ocean Networks Canada (ONC) and the Axial Seamount array of Ocean Observatory Initiative (OOI) (Figure 1, Box 1). EMSO-Azores, part of the EMSO European Research Infrastructure Consortium (ERIC), is located on the slow-spreading Mid-Atlantic Ridge (MAR), at the summit of a magmatically robust central volcano hosting the Lucky Strike hydrothermal field (Blandin et al., 2010; Colaço et al., 2011; Cannat et al., 2016) (Figure 2). The two other observatories include submarine high power and bandwidth cables spanning the intermediate-spreading Juan de Fuca Ridge (JdFR) in the NE Pacific. ONC-Endeavour, located on the intensely hydrothermally-active Endeavour Segment, is significantly influenced by seismicity, extensional tectonics and faulting and is operated by ONC (Kelley et al., 2012; Kelley et al., 2014) (Figure 3). OOI-Axial Seamount, located on the magmatically robust and volcanically active Axial volcano is operated by the University of Washington for the US OOI (Kelley et al., 2014; Delaney et al., 2016), as part of the OOI Regional Cable Array (RCA, Figure 4). All three observatories support research aimed at understanding second, to daily, to multidecadal geophysical and geochemical processes occurring at these submarine volcanoes and their vent systems, and, in turn, how these processes affect the water column and drive biological responses in time and space, below, at, and above the seafloor. The multidisciplinary observatories host sensors to characterize crustal dynamics, hydrothermal circulation, fluid dynamics and composition both below and above ground, and biological activity.
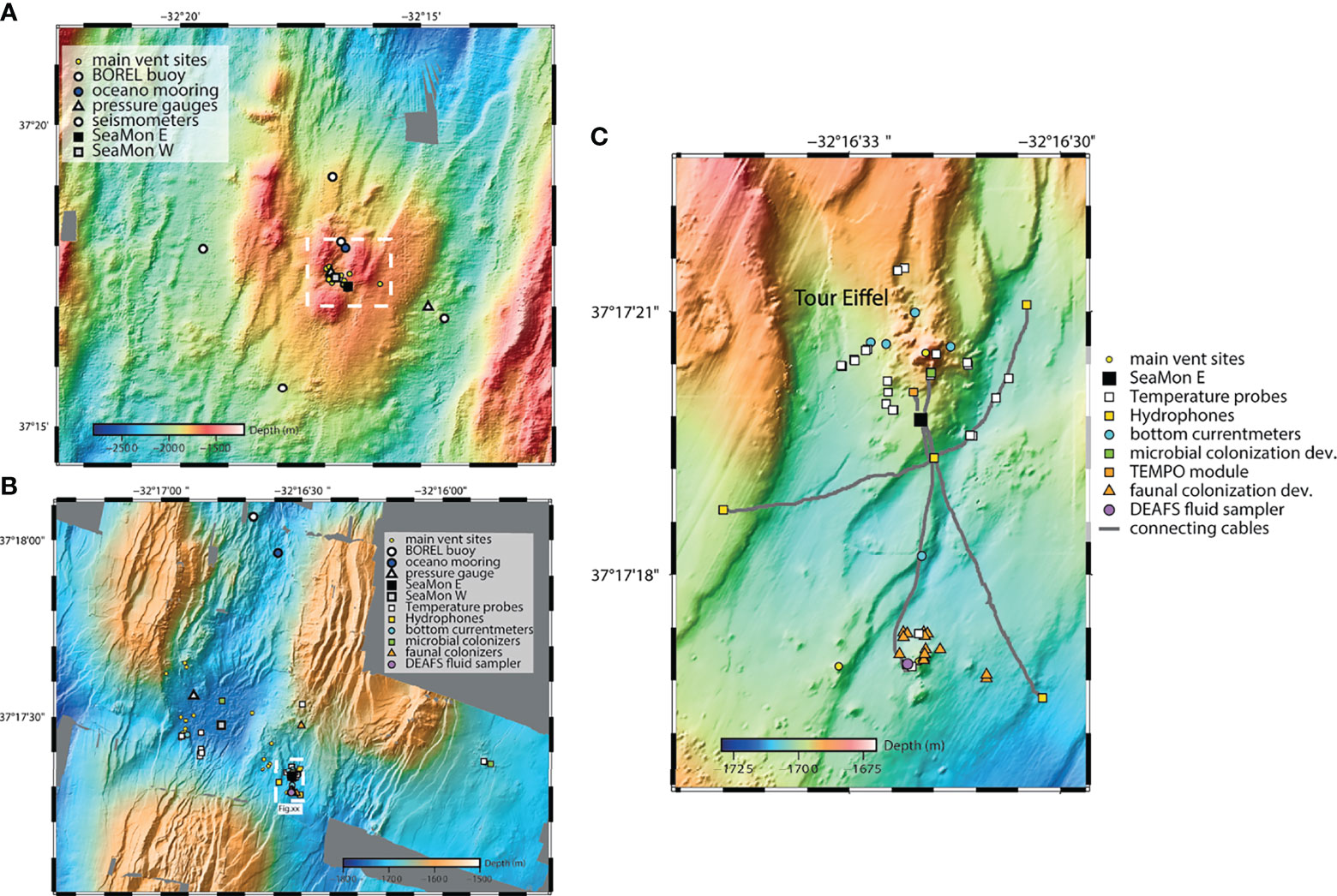
Figure 2 The EMSO-Azores observatory displaying instruments location. (A) Segment of the Mid-Atlantic Ridge showing the location of the Lucky Strike vent field. (B) The Lucky Strike vent field showing the location of the EMSO-Azores observatory (area represented by the white dashed box in A). (C) Detail of the instrumentation at Tour Eiffel (area represented by the white dashed box in B).
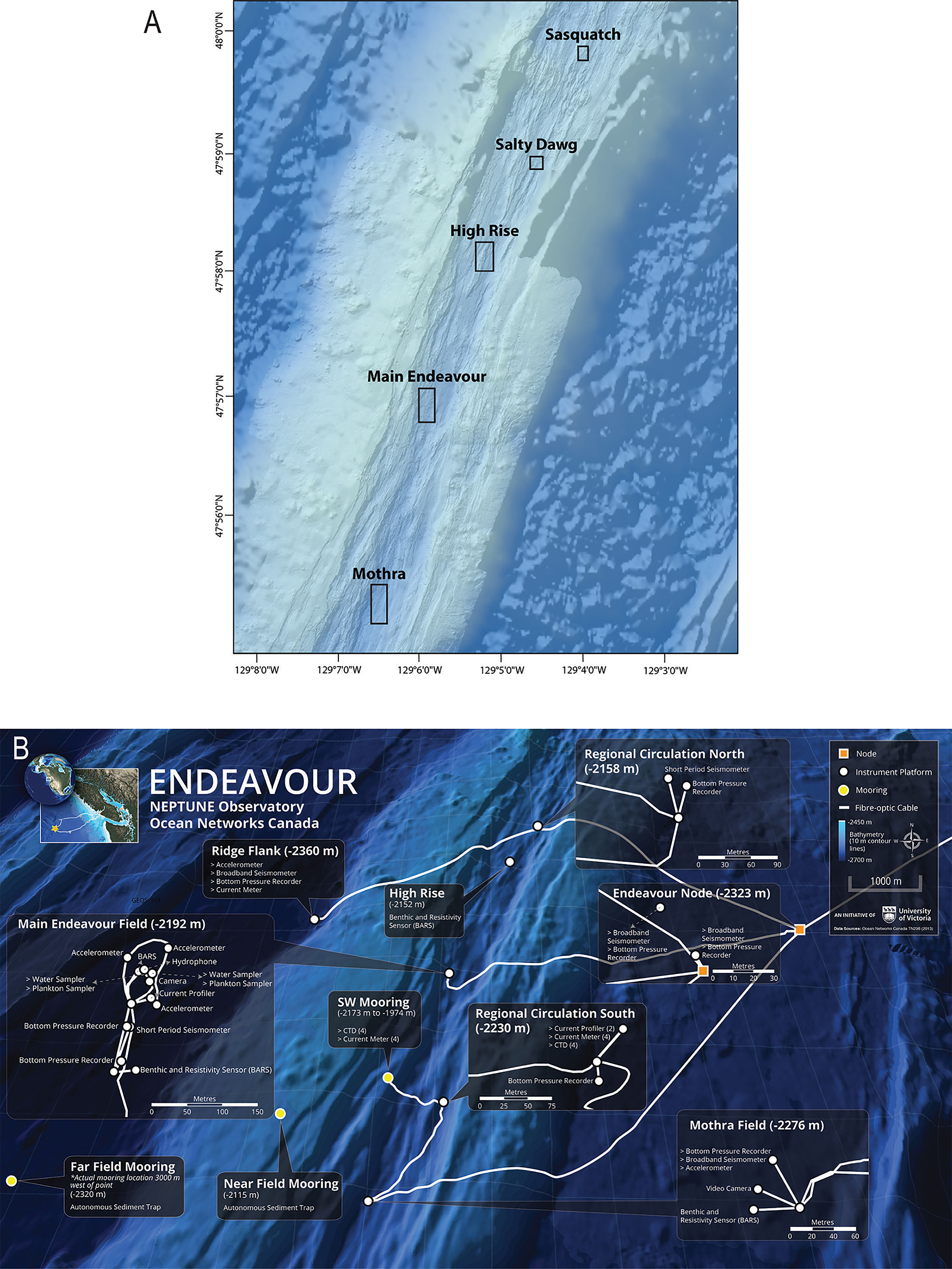
Figure 3 The Ocean Networks Canada on the Endeavour segment of the Juan de Fuca Ridge. (A) Regional bathymetry showing the main hydrothermal vent fields. (B) Details of the instruments location at the Endeavour node.
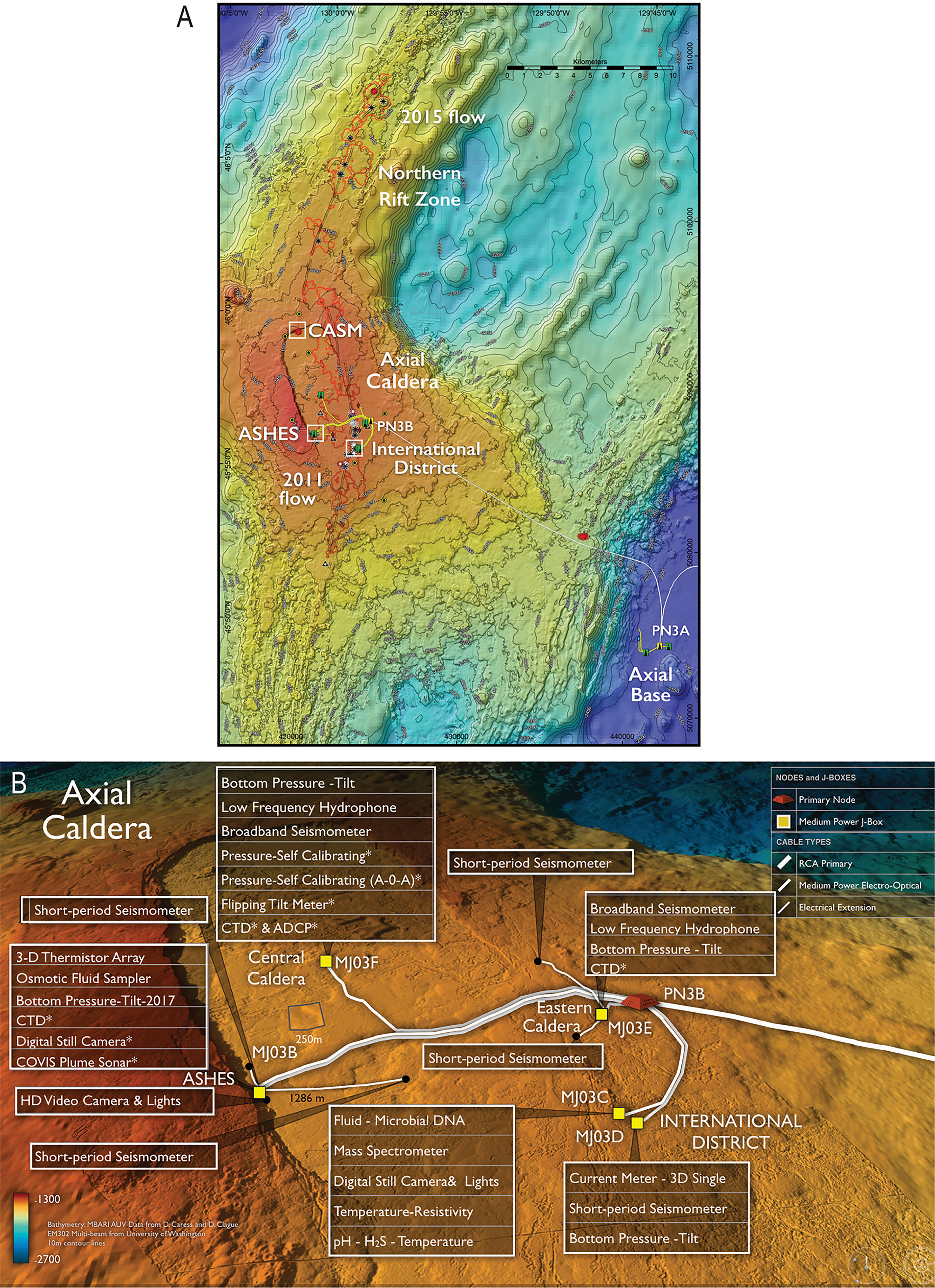
Figure 4 The Ocean Observatory Initiative Axial Seamount array on the Juan de Fuca Ridge. (A) Regional bathymetry showing the main hydrothermal vent fields. (B) Details of the instruments locations within the axial caldera. * indicate instruments funded outside of OOI.
BOX 1 – Observatories at Mid Ocean Ridges and Vents
Currently three observatories operate at vents on the Mid-Atlantic and Juan de Fuca Ridges including EMSO-Azores, part of the European Multidisciplinary Seafloor and water column Observatory Research Infrastructure (EMSO-ERIC), the Endeavour node of the Ocean Networks Canada NEPTUNE observatory (ONC) and the Axial Seamount component of the Ocean Observatories Initiative Regional Cabled Array (OOI-RCA). They provide real-time to nearly real-time open access data, tools for data visualization and download and resources for training and outreach.
EMSO-Azores
EMSO-ERIC website: http://emso.eu/
EMSO-Azores: https://www.emso-fr.org/EMSO-Azores
Data download: https://www.emso-fr.org/EMSO-Azores/Data-download
Data visualization tool: http://www.emso-fr.org/charts/azores/
Infrastructure design: https://www.emso-fr.org/EMSO-Azores/Infrastructure-2021-2022
Maintenance cruises: https://campagnes.flotteoceanographique.fr/series/130/
ONC-Endeavour
Ocean Networks Canada website https://www.oceannetworks.ca/
Data download: https://data.oceannetworks.ca/DataSearch
Data visualization: https://data.oceannetworks.ca/home?TREETYPE=1&LOCATION=11&TIMECONFIG=0
Infrastructure: https://www.oceannetworks.ca/observatories/pacific/endeavour
Regional Cabled Array – Axial Seamount
OOI website: https://oceanobservatories.org/
University of Washington Educational Site: https://interactiveoceans.washington.edu/
Data access and visualization: https://dataexplorer.oceanobservatories.org/
Axial seamount
Live video stream https://oceanobservatories.org/streaming-underwater-video/
The EMSO-Azores Observatory, Lucky Strike Vent Field, Mid-Atlantic Ridge
EMSO-Azores sits atop an active volcano close to the Azores Triple Junction on the northern part of the Mid-Atlantic Ridge (MAR). The volcano hosts Lucky Strike, one of the largest active ridge-related hydrothermal vent fields (~1 km2, Langmuir et al., 1997; Barreyre et al., 2012; Beaulieu and Szafranski, 2020). This basalt-hosted field, situated at an average water depth of 1700 m, contains over twenty active hydrothermal edifices distributed around a fossilized lava lake (Ondréas et al., 2009; Barreyre et al., 2012), and overlying a magma chamber at ~3 km depth below seafloor (Singh et al., 2006). Part of the venting area is included in a Marine Protected Area (MPA) in the Portuguese Exclusive Economic Zone (EEZ) (Menini and Van Dover, 2019). Planning for this observatory began in October 1998 at an Interridge workshop dedicated to the long-term Monitoring of the Mid-Atlantic Ridge (MoMAR). A series of follow-up MoMAR workshops (Santos et al., 2002; Barriga et al., 2005) established scientific objectives, technical goals, and experimental designs. These plans served as a basis for the implementation of the observatory, funded for the most part by the French ministry of research with support over the years of several EC research grants. The autonomous observing system EMSO-Azores, first deployed in 2010 (Cannat et al., 2011; Colaço et al., 2011; Legrand et al., 2019), comprises two Sea Monitoring Nodes (SeaMoN) that provide local electrical power (28V), sensor control, and data archiving, and limited satellite data transmission to shore via an acoustic connection to a surface buoy (Figure 2). It supports research focused on understanding hydrothermal circulation above the magmatic chamber to the seafloor, and its influence on deep-sea ecosystem functioning and water column processes in the context of a magmatically robust slow-spreading ridge environment. This multidisciplinary array has evolved over its, now, 11 years of continuous recording and the following description concerns its present-day configuration.
The first node, SeaMoN west, is deployed at a former lava lake that formed within a series of rifted volcanic cones at the summit of the central volcano (e.g., Ondréas et al., 2009; Escartı́n et al., 2014). This node measures seafloor seismic activity, vertical seafloor motion, and is presently also equipped with the multisensor platform EGIM (EMSO Generic Instrumentation Module) that comprises a turbidimeter, a CTD, an ADCP, one hydrophone, an Oxygen optode, and a pressure gauge (Lantéri et al., 2022). The SeaMoN East node is deployed at the base of the Tour Eiffel active hydrothermal edifice and is dedicated to physical, chemical and ecological studies. An High-Definition (HD) video camera module (Sarrazin et al., 2007), chemical sensor (Laës-Huon et al., 2020), and thermistor string, support studies of the dynamics of mussel assemblages and their environment. Biological and chemical properties of high-temperature fluids are monitored with the microbial colonization module CISICS (Connected In Situ Instrumented Colonizing System), and the in situ automated sampling of high-temperature hydrothermal fluid device DEAFS (DEep sea Autonomous Fluid Sampler). The two nodes are acoustically linked to a surface relay instrumented buoy, ensuring satellite communication to the land base station in Brest, France. Data are archived, published with a DOI, and available on the EMSO-Azores web page (Box 1).
In addition to these nodes, the observatory setup comprises several sets of autonomous instruments, with data recovered during yearly maintenance cruises. The autonomous instruments deployed at Lucky Strike include 4 short-period Ocean Bottom Seismometers (OBS), 2 pressure gauges, a physical oceanography mooring, a seabed array of four cabled hydrophones that supports microseismicity and marine sound studies at the Tour Eiffel site, a vast array of temperature probes distributed in hot and diffuse vents through the hydrothermal field, several bottom current meters and over 20 autonomous faunal colonization devices. A complementary ship-based field program is implemented during the cruises and contributes to increasing the set of accessible parameters and their temporal and spatial coverage (e.g., fluid and rock sampling, ecological studies, surveys of active and inactive areas, in situ experimentations and repeated high-resolution mapping and 3D imaging of the venting areas). The EMSO-Azores observatory is maintained annually during the MoMARsat cruises (Cannat and Sarradin, 2010). All seafloor system components are recovered, serviced onboard and redeployed by either the Remote Operated Vehicle (ROV) Victor 6000 or the Human Occupied Vehicle (HOV) Nautile. Each dataset is linked to the relevant cruises, which are listed in the data DOI, and each cruise DOI includes a list of the available data with their DOIs (Box 1).
ONC-Endeavour
The hydrothermally active Endeavour Segment, located on the northern part of the intermediate-spreading Juan de Fuca Ridge, is 10 km long, with a 1 km wide axial valley that has rift walls reaching 200 m. Endeavour Segment hosts five major hydrothermal vent fields (Kelley et al., 2012; Kelley et al., 2014) that are underlain by magma lenses at depths of ~2-3 km (e.g., Van Ark et al., 2007) with microseismic activity linked to hydrothermal circulation between the magma lens and the seafloor (e.g., Toomey et al., 2009; Hooft et al., 2010). The Endeavour Hydrothermal Vents (EHV) became Canada’s first MPA, following an act of parliament in 2003. Ocean Networks Canada has been operating real-time observing systems there since September 2010.
The scientific goals of the Endeavour observatory were established during a series of workshops, following which researchers developed a multi-disciplinary community experiment proposal that competed for instrumentation funds with other community experiment proposals during the design and instrumentation phase of the ONC NEPTUNE (NorthEast Pacific Time-series Undersea Networked Experiments) network. The observatory design had to be compliant with the MPA Management Plan, which allowed for two of the vent fields, Mothra and Main Endeavour Field (MEF), to be cabled with scientific instrumentation and experiments. A third vent field, High Rise, was to be left in its natural state, and monitored through ROV visits and occasional deployments of autonomous instruments. The Endeavour Node is sited 5 km east of the axial valley on a relatively flat seafloor. Three fibre optic extension cables provide power and two-way data communications to seafloor junction boxes located in the Mothra and Main Endeavour vent fields, and at a site north of the High Rise field (Figure 3). The junction boxes provide power and communication to sensors and instruments on seafloor platforms and water column moorings. The Main Endeavour Field instrumentation was initially concentrated on a nearly 50 m-across, actively venting sulphide edifice named Grotto (Kelley et al., 2014; Juniper et al., 2019). A seismometer array, designed to precisely locate shallow micro-seismic events in the hydrothermal up-flow zone below the seafloor, supports study of their links to vent fluid discharge. On Grotto, several different temperature sensor technologies monitor hydrothermal discharge at vents that vary from a >335°C black smoker vent to diffuse warm flows. Two camera modules look out onto a tubeworm assemblage and new sulphide worm habitat, and three monitoring systems provide data on chemical properties of the vent environment. Outside of the vent fields, two pairs of water column moorings (RCM), one pair between High Rise and Salty Dawg, the other between Mothra and MEF, monitor water column properties and currents to constrain buoyancy-driven flow in the axial valley (Figure 3). A Junction Box (JB) placed near the northern RCM pair supports a broadband seismometer and bottom pressure recorder on the western flank of the Endeavour segment, about two kilometers west of the MEF, on the Pacific Plate.
A near-tripling of ONC’s observing system at the Endeavour hydrothermal vents is nearing completion. This expansion will enhance the simultaneous, real-time monitoring in the Mothra and Main Endeavor vent fields (Figure 3), with new sensors (including additional cameras and instruments for in situ hydrothermal fluid chemistry), and the completion of the larger scale seismometer network around the segment with deployments at the Endeavour node on the JdF plate. The extension of the observing system to the Mothra vent field provides biologists with new settings to test hypotheses about the coupling of vent communities to habitat dynamics. Expansion of the observing system began in 2016 with the installation of extension optical cables to connect the Endeavour node to the Mothra vent field and a site north of the High Rise field. Installation of new sensors and camera systems began in 2019 and are scheduled for completion in 2022. All data are available for download and visualization through an online query interface linked to the data archive and GIS database (Box 1).
During the deployment of the 840 km backbone NEPTUNE cable, a spur cable (with a cable termination assembly) was extended to the Middle Valley hydrothermal area, to the north of the Endeavour Segment (Figure 3). This installation could support future development of a node and instruments to contrast the hydrothermal activity at the sedimented Middle Valley, with the sediment-starved spreading centre at Endeavour. This would also support extension of the network onto the Pacific Plate to the west, and the Sovanco Fracture Zone to the northeast.
Ocean Observatories Initiative (OOI): Regional Cabled Array on Axial Seamount
Axial Seamount is the largest and most magmatically active volcano along the JdFR (Kelley et al., 2014; Kelley et al., 2016; Smith et al., 2018). It is also the best imaged submarine volcano worldwide, with multiple 3D seismic surveys delineating two magma chambers and a series of stacked feeder sills (West et al., 2001; Arnulf et al., 2014; Arnulf et al., 2018; Carbotte et al., 2020). Repeated high resolution (1 m) bathymetric surveys acquired with deep-sea autonomous vehicles of the caldera and surrounding walls have allowed documentation of the volcanic and hydrothermal geomorphology, and the extent and volume of different volcanic eruptions and of vertical seafloor displacements (e.g., Caress et al., 2012; Clague et al., 2017).
The OOI marine infrastructure was designed and built to meet science requirements developed through community workshops, which identified broad-ranging critical ocean science questions requiring comprehensive and sustained (up to ~25 years) ocean observing infrastructure, enabling examination of processes at multiple spatial (ocean basin to tidal basin) and temporal (short-term, stochastic events and large-scale decadal cycles) scales. The Axial observatory is part of the RCA (Box 1; Figure 4). A 525 km-long, high power (10 kV) and bandwidth (10 gb/s) submarine fiber optic cable provides power and two-way communication from Pacific City, Oregon, to the volcano. In 2014, the RCA observatory was installed at the base and within the caldera of Axial Seamount. Axial Caldera currently hosts a diverse array of 23 core seafloor instruments (Figure 4). In 2016, following commissioning of the RCA, NSF opened the door to Principal Investigators (PI) to add infrastructure onto the RCA through funding outside of OOI. Within Axial Caldera, nine PI instruments are now on the cable and there are three uncabled instruments. Twenty additional cabled and uncabled instruments are funded for installation in the caldera and on its walls. Infrastructure located in Axial Caldera is focused at four sites: The ASHES and International District Hydrothermal Fields, and the Central Caldera and Eastern Caldera sites focused on geophysical investigations (Figure 4).
The geophysical instruments positioned within the caldera that monitor seismic and volcanic activity – including explosive events (Wilcock et al., 2016; Caplan-Auerbach et al., 2017; Chadwick et al., 2022) – include five short-period seismometers, in addition to two pairs of broadband seismometers and low-frequency hydrophones. Four bottom pressure-tilt instruments situated in the Central and Eastern Caldera regions, and the ASHES and International District vent fields, monitor, in real time, changes in seafloor elevation/tilt associated with the inflation/deflation of the magma chamber and are being used for forecast future volcanic eruptions (Box 1). Also distributed within the caldera are three CTDs (Central Caldera, Eastern Caldera, and ASHES) to monitor hydrothermal fluid interactions with crustal and seafloor processes (e.g., diking-eruptive events).
At the ASHES vent field (Figure 4), a 3D-thermistor array and a collocated osmotic-based fluid sampler provide temperature distribution in a diffuse flow site along with physical samples for shore-based chemical analysis of emanating fluids. A HD video camera focused on the 4 m tall actively venting ‘Mushroom’ edifice provides real-time streaming imagery every 3 hrs covering the entire edifice to monitor changes in chimney development and biological communities colonizing the edifice and surrounding seafloor (Box 1). The site also hosts a COVIS multibeam sonar system focused on the 4 m tall edifice Inferno and surrounding diffuse flow sites, and thermistor arrays within diffuse flow.
At the International District vent field (Figure 4), an instrument suite is used for real-time monitoring of temperatures and fluid chemistry of hydrothermal vents and collecting fluids and DNA for later shore-based chemical analyses (Kelley et al., 2014). A high-temperature, resistivity, and redox potential probe (TRHPH/BARS) is installed in the Escargot hydrothermal chimney to examine boiling processes. A high-temperature probe (THSPH) that measures H2, H2S, and pH is installed in the Diva hydrothermal chimney. Instruments positioned at the Tiny Towers site include a mass spectrometer to measure dissolved gases in the venting fluids, coupled microbial DNA (PPS) and hydrothermal fluid (RAS) samplers with temperature co-registered vent fluid temperature. These instruments’ intakes are collocated in a ‘vent cap’ positioned atop emanating diffuse flow thereby providing real-time monitoring of dissolved gas concentrations in hydrothermal fluids while simultaneously allowing for collection of microbial DNA and fluids for post-recovery metagenomic and chemical analyses. A digital still camera at this site allows monitoring of the hydrothermal activity, changes in biological communities, and condition of the previous instrument installations. A 3D single point current meter provides real-time monitoring of currents near the seafloor. A second nearby bare-rock site, includes a short-period seismometer, current meter, and bottom pressure tilt instrument. This site is the focus of an International Ocean Discovery Program proposal to include an array of holes with cabled CORKED observatories to examine the deep biosphere, crustal hydrogeology, and magma injection and crustal deformation (Huber et al., 2021).
The Central Caldera site has become a key site for testing of geodetic instruments to examine seafloor deformation with follow-on applications to monitoring the Cascadia Subduction Zone. Here, the co-registration of two different kinds of self-calibrating pressure sensors and a “flipping” tilt meter along-side Core OOI instrumentation allows cross referencing of these new technologies. Also hosted at this site is a newly developed ADCP to image the entire ~ 1500 m of water column. Finally, two state-of-the-art instrumented cabled profiling moorings at the base of the seamount provide environmental measurements from ~ 2600 m to 125 m to 5 m beneath the ocean’s surface. In concert, the two moorings host a diverse suite of 24 instruments, provide insight into internal waves and may capture the formation-evolution of the next megaplume (Kelley et al., 2016; Devine et al., 2020).
Ten Years of Observatory Research at Vents
Understanding the functioning and dynamics of vent ecosystems requires resolving the range of scales at which processes operate. The three seafloor observatories have supported a decade of multidisciplinary research at hydrothermal environments hosted in a basaltic upper crust, and overlying magma chambers at varying depths. The data and follow-on research have enabled significant advancements in our understanding of processes occurring at multiple scales from the sub-seafloor to the water column. These magmatic sites are visited every year as part of the maintenance cruises allowing for systematic surveys and sampling and integration of the different scales of biological and environmental data. The following section summarizes results obtained using seafloor observatories during the last 10 years, based on both time-series analyses and modeling.
Crustal Processes
While MOR’s represent the world’s largest hydrothermal-volcanic system, we still lack an in-depth understanding of the complex interplay of magmatic, tectonic, and hydrothermal processes that take place along the ~65,000 km of ridge axis. Comprehensive databases acquired in recent decades show that hydrothermal fluids expelled at vents along magmatically robust sections of a MOR have a large spatial and temporal variability in exit fluid velocity, temperature, and chlorinity (e.g., Scheirer et al., 2006; Larson et al., 2007; Sohn, 2007; Sarrazin et al., 2009; Barreyre et al., 2014b; Germanovich et al., 2015; Xu et al., 2017). Monitoring seafloor deformation and seismic activity at active volcanoes provided insights into magma movement at depth (Chadwick et al., 2016; Nooner and Chadwick, 2016; Wilcock et al., 2016) and into the location and time-evolution of the domain of hydrothermal heat extraction (Tolstoy et al., 2006; Crawford et al., 2013; Wilcock et al., 2016). The RCA infrastructure, online for the Axial 2015 eruption, captured the first real-time cabled observatory data of the timing, location, dynamics and volume of eruption-related magma movements, eruptive explosions and fissuring, and egress of fluids out of the subseafloor (Chadwick et al., 2016; Wilcock et al., 2016; Caplan-Auerbach et al., 2017; Spietz et al., 2018). Additionally, an eruption response has been observed in high-temperature vents at Axial instrumented with data logging temperature probes. After the 2011 eruption, temperatures in some high-temperature vents adjacent to the eruptive area dropped to just over 100°C, perhaps as a consequence of rapid recharge of cold seawater that replenished warm fluids injected into the caldera and formation of spectacular snow blowers from which issued microbes and microbial material flowing from the subseafloor (Kelley et al., 2014).
Being on a slow spreading ridge, the Lucky Strike volcano has a significantly lower melt flux than the JdF ridge vent areas, resulting in less frequent eruptions (Rubin et al., 2012). Monitoring full cycles of volcanic activity at a slow spreading ridge is beyond human time scales. Indications for recent magmatic activity at the Lucky Strike volcano include an earthquake swarm interpreted as due to a dike injection in 2001 (Dziak et al., 2004), and increased CO2 content in the vent fluids in 2008 (Pester et al., 2012) and 2010 (Chavagnac et al., 2015; Rommevaux et al., 2019).
One of the most critical crustal accretion variables, and one that is commonly poorly constrained, is indeed permeability, as it is a major control on fluid flow below the seafloor. The use of the natural forcing from ocean tides to constrain crustal dynamics and permeability structure of MOR hydrothermal fields brought new insights in the study of hydrothermal circulation processes and fluxes in the oceanic crust (e.g., Crone and Wilcock, 2005; Barreyre et al., 2018). Using a time series of fluid temperature data, differences in phase lags between tides and the thermal response of exit fluids were shown to correspond to variations in the shallow crustal permeability structure and therefore in mass and heat fluxes (Barreyre et al., 2018). Similar approaches also showed a strong control of shallow spatial variations in permeability on diffuse exit fluids at the ASHES vent field and Lucky Strike (e.g., Mittelstaedt et al., 2016). Recent studies have investigated the feasibility of using models of the poroelastic response to tidal loading manifested in exit-fluid velocity and temperature records to obtain shallow crustal permeability constraints below MOR vent fields (e.g., Jupp and Schultz, 2004; Crone and Wilcock, 2005; Barreyre et al., 2014a; Barreyre and Sohn, 2016; Barreyre et al., 2018). High-resolution seismic studies provide additional constraints into possible subseafloor heterogeneities and related fluid paths between the axial magma chamber and the seafloor (e.g., Marjanović et al., 2019).
While these data sets pertaining to seismic and fluid flow studies provide valuable constraints for coupled geodynamic-hydrothermal models (e.g., Theissen-Krah et al., 2016), these snapshots of the “current state” at a specific location are difficult to interpret in the context of a dynamically evolving hydrothermal and magmatic system. At the same time, numerical models have evolved in recent years and can now handle more realistic thermodynamic fluid properties and two-phase flow phenomena (Coumou et al., 2009; Lewis and Lowell, 2009; Afanasyev et al., 2018; Vehling et al., 2021). An outstanding strength of numerical forward models is their ability to establish process-based causal links between different observations and data sets, including spatial and temporal dependencies. Time series data sets from recurring expeditions to the same geographical location or, ideally, permanent installations, are extremely valuable and enable the stringent design and calibration of models and facilitate non-ambiguous interpretation of transient model results. The combination of state-of-the-art numerical modeling techniques and data from (nearly) continuous monitoring will enhance our understanding of processes and mechanisms taking place at inaccessible locations inside the Earth.
Interface and Biological Response
Integrated studies at the interface of the subseafloor and the water column have contributed to a better understanding of links between geological, geochemical and biological processes. At the Lucky Strike seafloor, the hydrothermal fluid fluxes of 14 monitored vents (Leleu, 2017) have immediate impact on trace element dispersion within the hydrosphere and microbial development. For example, high fluid CO2 contents, induced by replenishment of the magmatic chamber at depth stimulate the thriving of thermophilic and anaerobic Archaea and Bacteria (Archaeoplobales, Nautiliales and Nitratiruptoraceae) (Rommevaux et al., 2019). In addition, the progressive mixing of end-member hydrothermal fluid and surrounding seawater leads to the formation of hydrothermally-derived minerals, in particular sulfate-bearing minerals (anhydrite and barite) which control dissolved rare earth element fluxes and the Nd isotope signature of the hydrothermal plume (Chavagnac et al., 2018b). At Axial Seamount, the past three eruptions provide some insights into subseafloor hydrothermal processes because these eruptions have significantly affected crustal hydrology, fluid geochemistry, and microbial communities. This is exemplified by the development of low-temperature, diffuse snowblower vents formed within ~50 m of eruptive fissures by subsurface circulation of warm, volatile-rich fluids, which results in microbial growth and discharge, indicating a near-instantaneous increase in heat and mass fluxes, followed by exponential decay (Cann et al., 1997; Butterfield et al., 2004; Chadwick et al., 2013; Meyer et al., 2013; Nooner and Chadwick, 2016). Diffuse vent fluids at Axial are hotspots of primary production in the deep ocean, while also providing access points to infer microbial and geochemical processes in the subseafloor (e.g., Butterfield et al., 2004; Huber et al., 2007; Fortunato and Huber, 2016; Olins et al., 2017; Fortunato et al., 2018). Time-series microbial and geochemical studies of these fluids at Axial began in 1998, shortly after the volcano erupted. These multi-year studies at Axial showed that individual vent sites maintained microbial communities and specific populations, with spatially distinct communities and activities mediated by both endemic and unique groups at each site (Opatkiewicz et al., 2009; Fortunato et al., 2018). Such results, combined with cultivation experiments and modeling efforts, suggest that the type and quantity of subseafloor microbes beneath particular vents is controlled by a combination of geochemistry and local geology at individual sites, leading to spatially and temporally stable fluid paths that allow for the persistence of distinct subseafloor microbial communities (Opatkiewicz et al., 2009; Fortunato et al., 2018; Stewart et al., 2019).
In the mixing zone, recurrent in situ sampling with filtration and analysis during maintenance cruises allowed the investigation of the dissolved and particulate partitioning of metals in the early buoyant plume (4–150°C) of smokers at Lucky Strike (Cotte et al., 2020). Noted spatial variability of the chemical composition (Fe and Cu) of the buoyant plume throughout the vent field was limited in the warmest part of the plume. Even though metal partitioning was strongly affected by different precipitation and oxidation processes, hydrothermal iron was almost completely preserved (> 90%) in the dissolved fraction along the mixing gradient (Laës-Huon et al., 2016; Waeles et al., 2017; Cotte et al., 2018). A long-term in situ study of reactive iron (CHEMINI) in a diffuse vent at the base of the Tour Eiffel active edifice showed that reactive iron stabilization over time prevented precipitation and could enhance iron availability to the local biological community structure (Laës-Huon et al., 2016). These studies highlight that metal stabilization processes allow the local export of hydrothermally sourced iron and copper into the deep ocean.
Observatory studies of biological responses to habitat variability combine sensor data on habitat conditions with imagery that documents species presence/absence and behaviour. The few long-term studies at the assemblage-scale have shown faunal communities to be relatively stable in relation to baseline environmental variability (Sarrazin et al., 2014; Van Audenhaege et al., 2022). At the organism-scale, analyses of imagery have highlighted species behavioral responses that correlate with tides and surface storms, both on the MAR and the JdFR (Cuvelier et al., 2014b; Lee et al., 2015; Cuvelier et al., 2017; Lelièvre et al., 2017; Mat et al., 2020). Deep current inversions in response to tides affect the mixing of hydrothermal fluid inputs with surrounding seawater, thus modifying local environmental conditions in vent habitats. Symbiotic vent mussels at Lucky Strike open and close, and tubeworms from MEF extract and retract, following the tidal cycle, probably in response to their physiological/endosymbiont needs (Cuvelier et al., 2014b; Mat et al., 2020). On the JdFR, non-symbiotic species also appear to respond to environmental variability by adjusting their position and behaviour, possibly as a trade-off between resource availability/predation and physiological tolerance (Lelièvre et al., 2017). Daily recording of video imagery has also proved to be a good tool for characterizing the behaviour and interactions of species that could not otherwise be reared in aquaria under controlled conditions (Matabos et al., 2015; Van Audenhaege et al., 2022). It remains a challenge to relate micro environmental changes to life history strategies and physiological tolerances. Combining imagery with multi-variable sensor data should improve our ability to relate recruitment to local environmental cues and broader oceanographic drivers, and consequently better understand regional reproductive patterns.
Maintenance cruises offer opportunities to sample and describe natural communities (e.g., Sarrazin et al., 2015; Husson et al., 2017; Lelièvre et al., 2018; Sarrazin et al., 2020) and perform manipulative and colonizing experiments over time. Extensive experiments conducted during maintenance cruises (e.g., Cannat and Sarradin, 2010) have produced key insights into the biodiversity, functional diversity, and ecology of MAR hydrothermal communities. These include (re-)colonization processes and relationships between vent and cognate communities along a vent gradient and on different types of substrata (Cuvelier et al., 2014a; Zeppilli et al., 2015; Plum et al., 2016; Baldrighi et al., 2018; Alfaro-Lucas et al., 2020; Cowart et al., 2020; Marticorena et al., 2021). Biological samples have contributed to increasing basic knowledge of species biology (Husson et al., 2017; Marticorena et al., 2020), trophic interactions (Lelièvre et al., 2018; Portail et al., 2018; Zeppilli et al., 2019), biological rhythms (Mat et al., 2020) and physiology (Husson et al., 2018), leading to a growing understand of vent faunal functional traits (Chapman et al., 2019; Alfaro-Lucas et al., 2020; Murdock et al., 2021). Post-eruption succession patterns have been studied at Axial, particularly following the 1998 eruption, from repeated visits both pre-eruption as well as 7-, 18-, 30-, and 42-months post-eruption (Marcus et al., 2009).
Water Column: Deep Circulation, Fluid Dispersal and Connectivity
The ocean dynamics above MOR systems and specifically around vents are complex. Broadly, they feature three families of processes with their own phenomenology and impacts. First, the interaction of oscillating tidal currents with the rough seafloor topography generates internal waves at tidal frequencies called internal tides. These waves are unstable and eventually break close to their generation sites, provoking irreversible vertical mixing (St. Laurent and Garrett, 2002; Vic et al., 2018a). Although the astronomical (tidal) forcing is now easily predicted and appears to be remarkably in-phase with the local tidal energy density over ridges (Vic et al., 2021), the spatio-temporal variability of mixing remains poorly quantified, due to sparse data. Studies conducted these last decades highlighted key processes associated with internal tides, including their interactions with seafloor topography and background currents, leading to dynamical modifications of the mean state of the deep ocean (Thomson et al., 1990; Thomson et al., 2003; Lahaye et al., 2019; Lahaye et al., 2020).
Second, low-frequency currents drag on the valley walls and destabilise, generating small-scale swirling structures called submesoscale coherent vortices (SCVs, Vic et al., 2018b). SCVs can feature lifespans of many months and travel thousands of kilometres [recent survey in McCoy et al. (2020)]. Thus, they are effective at trapping and spreading deep-ocean material to the ocean interior (e.g., Bower et al., 2013; Vic et al., 2018b). Observations of SCVs are very sparse in general and even more specifically at depths of MOR.
Third, hydrothermal venting introduces a heat flux that generates mixing of vent fluids with ambient waters near the seabed. In addition, hydrothermal plumes that form over the vent field contain chemical constituents, larvae and microbes. These plumes have buoyant and neutrally-buoyant components. Buoyant plumes rise into the water column, entraining surrounding seawater, until they achieve neutral buoyancy through dilution and cooling. Near the bottom, this upward buoyancy flux drives an inflow towards the vent field and is effective in the retention of plankton (Thomson et al., 2003). Hydrothermal plumes can also carry plankton up into the ambient oceanic currents to be dispersed in along the axis, or off-axis to possibly a less hospitable deep-sea environment. In contrast, organisms with the ability to swim vertically or alter their density can utilise these dynamics and larvae have been shown to actively alter their positions to stay near their preferred environment (Mullineaux et al., 2013). The influence of hydrothermal venting on the chemistry of the deep-sea near the Endeavour segment has been investigated by Coogan et al. (2017). Using tow-yo hydrocasts, sediment traps and push cores they revealed that the plume heat and particulate anomalies indicate a half contribution from the particle-poor diffuse flow and half from (particle rich) high-temperature venting. Coogan et al. (2017) also found that less than 2% of all measured elements accumulate on the seafloor within 1.5 km of the individual vent fields. This implies that most of the chemical flux is transported significant distances away. The variability of plume position at higher frequencies is regulated by the local oscillatory currents in the inertial, tidal and weather bands (Xu and Di Iorio, 2012) and the periodicity of the immediate fallout from the plume, in addition to the direct effects of the local currents, appears to have an effect on the benthic ecology (Lelièvre et al., 2017). Above the neutrally buoyant plume, towed nets along with acoustical methods identified a deep scattering layer associated with the aggregation of zooplankton (e.g., Burd and Thomson, 2012). This deep scattering layer has been shown to have a complex seasonal and higher frequency (20-40d) variability likely due to a combination of both ontogenetic migration and dynamic advective processes (Burd and Thomson, 2019). In the rift valley of the northern MAR, the compilation of different in situ data acquired by CTD-LADCP casts and mooring deployments during repeated visits (Lahaye et al., 2019), combined with modeling studies (Vic et al., 2018b) has revealed consistent north-eastward along-valley currents below 2000 m at sub-inertial time scales. Current variability, dominated by slow time scales (weeks), favors the northward dispersion of tracers. Over the Endeavour segment of the JdFR, data from current meters and tracking of the neutrally buoyant plume, indicate that the long-term mean current tends to the southwest (Thomson et al., 2003; Coogan et al., 2017), with observations suggesting it has been steady for decades.
Integrating ecological/biogeochemical/physical data with modeling approaches can help address dispersion and connectivity questions (e.g., owen and Sponaugle, 2009; Artigue et al., 2021). In coastal studies, sub-kilometer-grid, primitive-equation models have been used to perform Lagrangian dispersion since the 2000’s (‘reef-scale’ models; Werner et al., 2007). No such method has been used in deep-ocean studies until recently. Mesoscale-resolving models (Young et al., 2012; Breusing et al., 2016) and submesoscale-permitting models (Bracco et al., 2016; Cardona et al., 2016; Mitarai et al., 2016; Vic et al., 2018b) have been employed to study connectivity between benthic communities at depths below 1000 m, resulting in improved realism of flow-topography interactions in many ways (Bracco et al., 2016; Vic et al., 2018b). Notably, tidal currents, hitherto absent from high-resolution regional models, are found to increase the vertical spreading of particles and help them cross topographic barriers (Vic et al., 2018b).
Towards a Coordinated Understanding of Mid-Ocean Ridges (MOR) Hydrothermal Vents and Their Ecosystems
The IMOVE working group seeks to refine common process-oriented research questions that can be addressed with data in hand, and identifying what is needed to address questions raised by today’s societal challenges, in order to help and foster interoperability across vent observatory platforms. The locations of the three observatories permits the study of hydrothermal processes in contrasting environments and settings. Each is representative of different dynamics of seafloor spreading and volcanism, from slow (MAR) to intermediate (JdFR) spreading rates, and the relative importance of tectonic (Endeavour) and volcanic (Lucky Strike, Axial Volcano) processes. They also provide contrasting examples of hydrothermal plume behaviour: with episodic megaplume generation during eruptions at Axial volcano, (e.g., Palmer and Ernst, 1998; Baker et al., 2019) a continuously present intense plume generated by multiple hydrothermal vents along the axis of the Endeavour segment (Thomson et al., 2003; Kelley et al., 2012), whereas megaplume events were yet never detected at Lucky Strike. Early international workshops in the 90s and 2000s (National Research Council, 2000) set the scene for a globally-coordinated design of seafloor observatories. Following this collaborative effort, programmatic and funding realities have resulted in each observatory having its own infrastructure design, research goals, and specific scientific questions (Barnes and Tunnicliffe, 2008; Favali and Beranzoli, 2009; Cannat et al., 2011). Since their deployment, large volumes of data have been acquired from all three current observatories, creating opportunities for studies that extend across locations. The first workshop (IMOVE, working group, 2019) defined priority themes and vent processes, establishing a basis for setting common scientific questions. This framework was then used to structure the science-based workshop at which participants developed a set of scientific questions and related societal challenges. This provided a basis for further exploiting existing data, and for identifying EOVs and Essential Biodiversity Variables (EBVs) that need to be measured to improve our understanding of hydrothermal processes (see next Section).
Processes of particular interest include fluid flow geometry, fluxes (heat, mass, chemical), their variability (i.e. periodic and episodic) and how they partition, local oceanographic currents and associated biological responses (Figure 5). These processes can be divided in three domains: the subseafloor, the interface at the ocean floor, and the water column. In the subseafloor, ground deformation, heat transfer, fluid-rock interactions affect fluid flow geometry, fluid composition and crustal stress and permeability, fluid residence times and recharge, and microbial community composition. At the seafloor interface, where the fluid meets the cold background seawater, thermo-chemical exchanges and mixing impact fluid flow characteristics, microbial and vent species distributions and biomass, and energy flow (e.g., carbon flux) (Le Bris et al., 2019). Finally, in the water column, oceanographic forcing, including currents, tides and their interactions with the local topography, drives plume dispersal, hydrothermal fluxes and exports to the ocean, faunal distribution, behaviour and larval dispersal, and influences species connectivity (Table 1 and Figure 5). The multidisciplinary approach enabled by observatories through the concomitant acquisition of environmental and biological variables provides a unique opportunity to integrate this wide range of processes and assess mechanisms driving vent ecosystem function and temporal trajectories. Four key, integrative questions emerged from the two IMOVE workshops:
● How does hydrothermal circulation and associated heat and matter fluxes respond to crustal and oceanographic forces at varying temporal scales?
● How does the interaction between hydrothermal circulation and shallow mixing with seawater control fluid chemistry, flux and temperature in the mixing zone where microbial communities and vent fauna develop?
● How do the hydrothermal biological communities respond to changes in environmental conditions?
● How do vent fluxes, productivity, and communities influence the surrounding deep-sea ecosystems and vice versa?
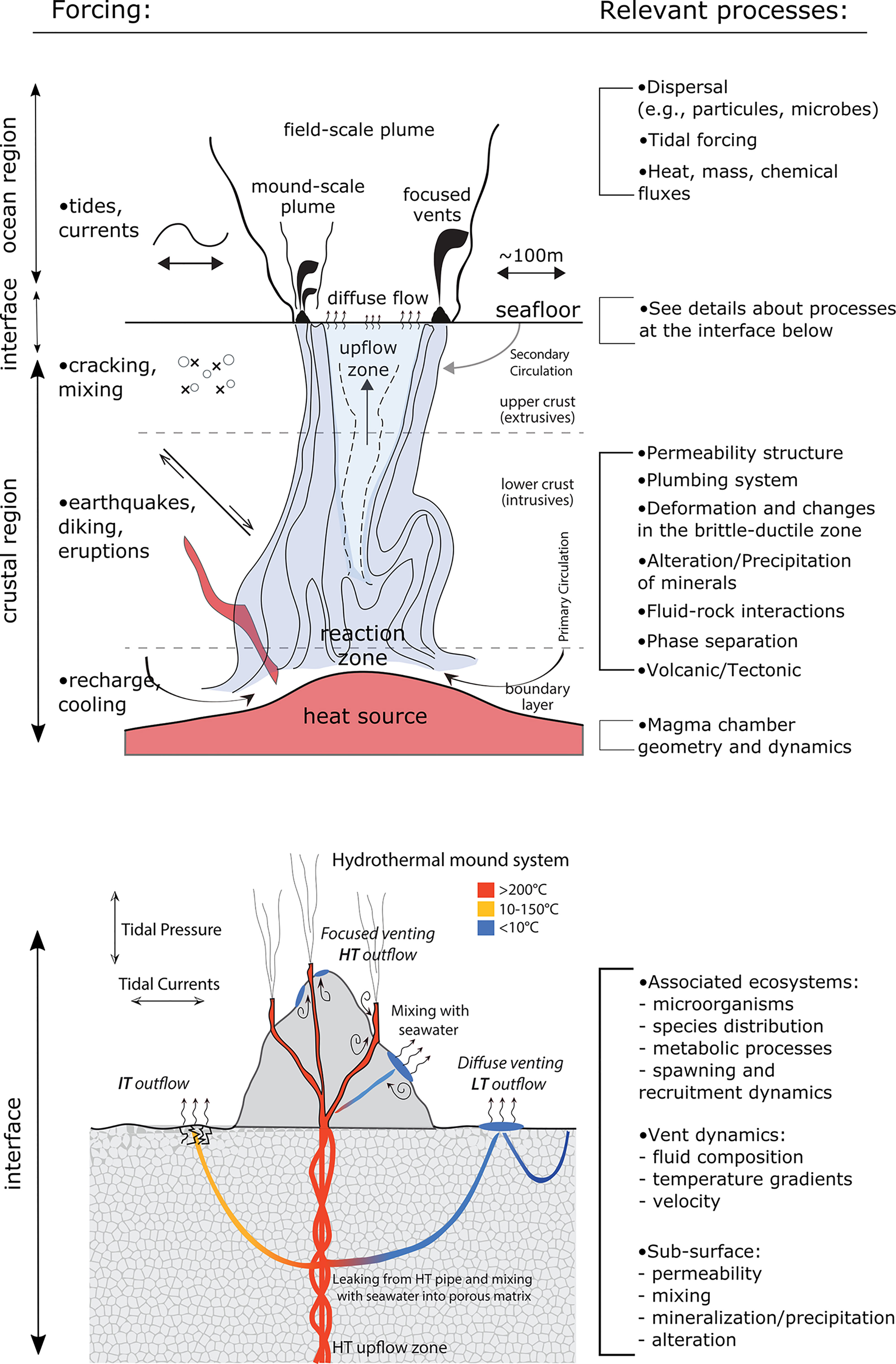
Figure 5 Top Conceptual model of a hydrothermal plumbing system at depth, with the upwelling of a single plume above the magmatic heat source that is focused along high-permeability zones associated with main faults and fractures networks. Bottom Close-up of a hydrothermal mound edifice. High-temperature (HT) outflow is directly fed by the high-temperature up-flow zone (here represented as an anastomosing, interconnected series of conduits), intermediate-temperature (IT) outflow is fed from leakage from the HT pipe and mixed with cold water into the porous matrix [i.e., hypothesis (1)] and low-temperature (LT) outflow is fed from either hypothesis (1) or conductively heated bottom water drawn into the seafloor as part of a secondary circulation system [i.e., hypothesis (2)]. Modified from Barreyre et al. (2014b).
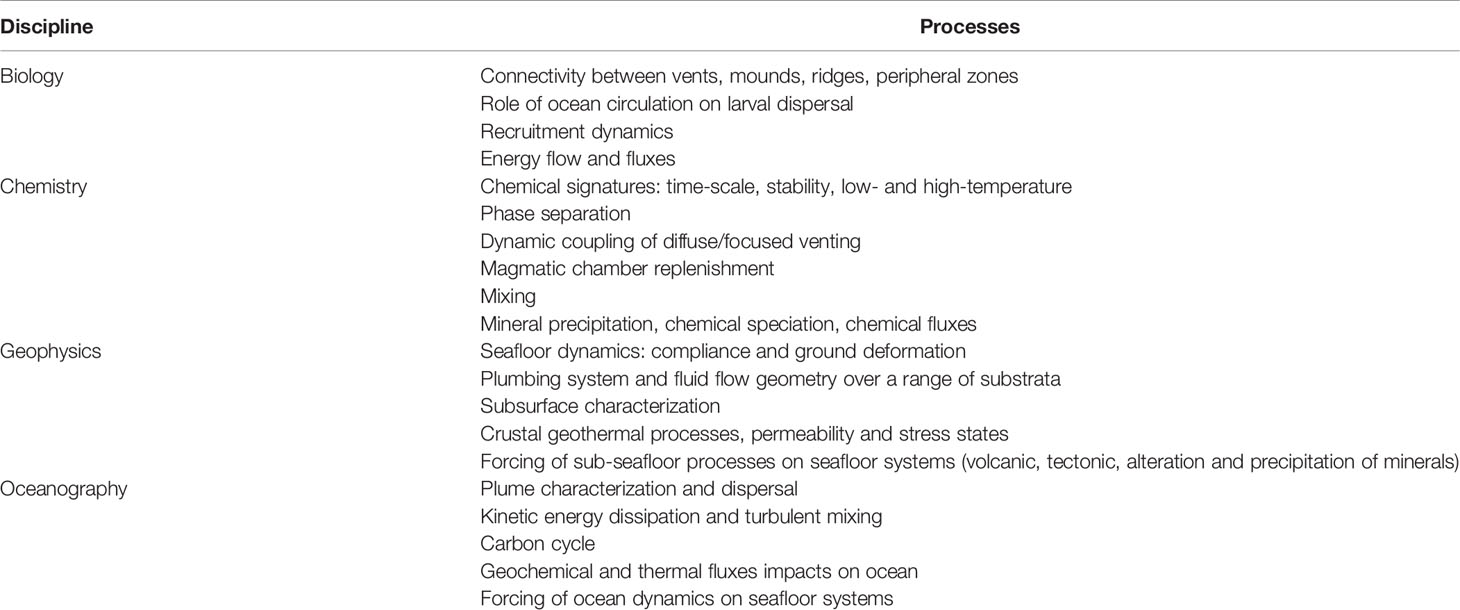
Table 1 Themes and processes to be considered at vents as defined during the first workshop and that can be addressed through observatories and their associated maintenance cruises.
This fundamental knowledge is prerequisite to evaluating faunal responses to anthropogenic disturbances, elaborating conservation strategies, and addressing the societal challenges that mid-ocean ridge systems are increasingly facing.
Anthropogenic Challenges and the Scientific Questions to Address
The three main current or foreseeable anthropogenic challenges to deep-sea vent environments are climate change, pollution (e.g., microplastics, persistent organic pollutants) and deep-sea mining. Fisheries are not discussed since most MOR vents are at (currently) non-fishable depths and do not host significant standing stocks of fish. Observatories contribute to enhancing our fundamental knowledge of ecosystem function, and yearly visits on site offer opportunities for seafloor experiments (Alfaro-Lucas et al., 2020; Marticorena et al., 2021) and collection of biological samples to increase our knowledge of species biology and ecology (e.g., Husson et al., 2017; Lelièvre et al., 2018; Murdock et al., 2021). Directly assessing the potential impacts of deep-sea mining will require similar experimental and sampling approaches. Regular sampling is also needed to characterize the abundance and composition of microplastics in these environments and understand how local and regional hydrography impacts their distribution. Standardized protocols should be defined and shared in order to ensure cross-comparison between observatories and the detection of long-term trends. Future research will also contribute to a new, more environmentally responsible relationship between terrestrial society and the deep sea, in the form of regulations, best practices, environmental ethic, and societal awareness. Section on Observatories and New Societal Approaches to Deep-sea Environments discusses these new research avenues and how they can benefit from observatory infrastructure and associated maintenance cruises.
Essential Variables and Common Best Practices for Mid-Ocean Ridges (MOR) Vent Observatories
The first workshop (IMOVE, working group, 2019) identified all variables currently measured or acquired by the different existing sensors at each established observatory. Each variable was then translated into an observable belonging to a biological, geophysical or geochemical domain (Table 2). As an example, a video camera (the sensor) allows counting the visible species (variable) to measure species distribution and abundance (observable) in the biology (domain) (Table 2). The final table revealed a number of commonalities among the three observatories highlighting the potential for defining common measurable characteristics. Based on this table and the list of common scientific questions, discussions during the second workshop resulted in a list of EOVs to be monitored across the different observatories. Some of the variables measured are mature EOVs, especially the ones related to physics and geochemistry. The biological related EOVs are not yet mature, most of the seabed ones are still at the merging phase, and the specificity of the different environments, require standardization and calibration (e.g., EOVs body size, or biomass or % cover, which are based on imagery, need a standardization towards automation, and calibration of surface observable, calibration size, weight, etc.). This list was built in light of work previously conducted by international networks including The Group on Earth Observations Biodiversity Observation Network (GEO BON; Pereira et al., 2013) and the Deep Ocean Observing Strategy (DOOS; Levin et al., 2019). A number of EOVs recommended by GOOS and DOOS were considered as observables to measure at vents in the context of seafloor observatories and associated maintenance cruises. Table 3 reports these variables that can be measured and provides new ones to be considered when conducting observations at vents. Box 2 summarizes recommendations issued during the second workshop.
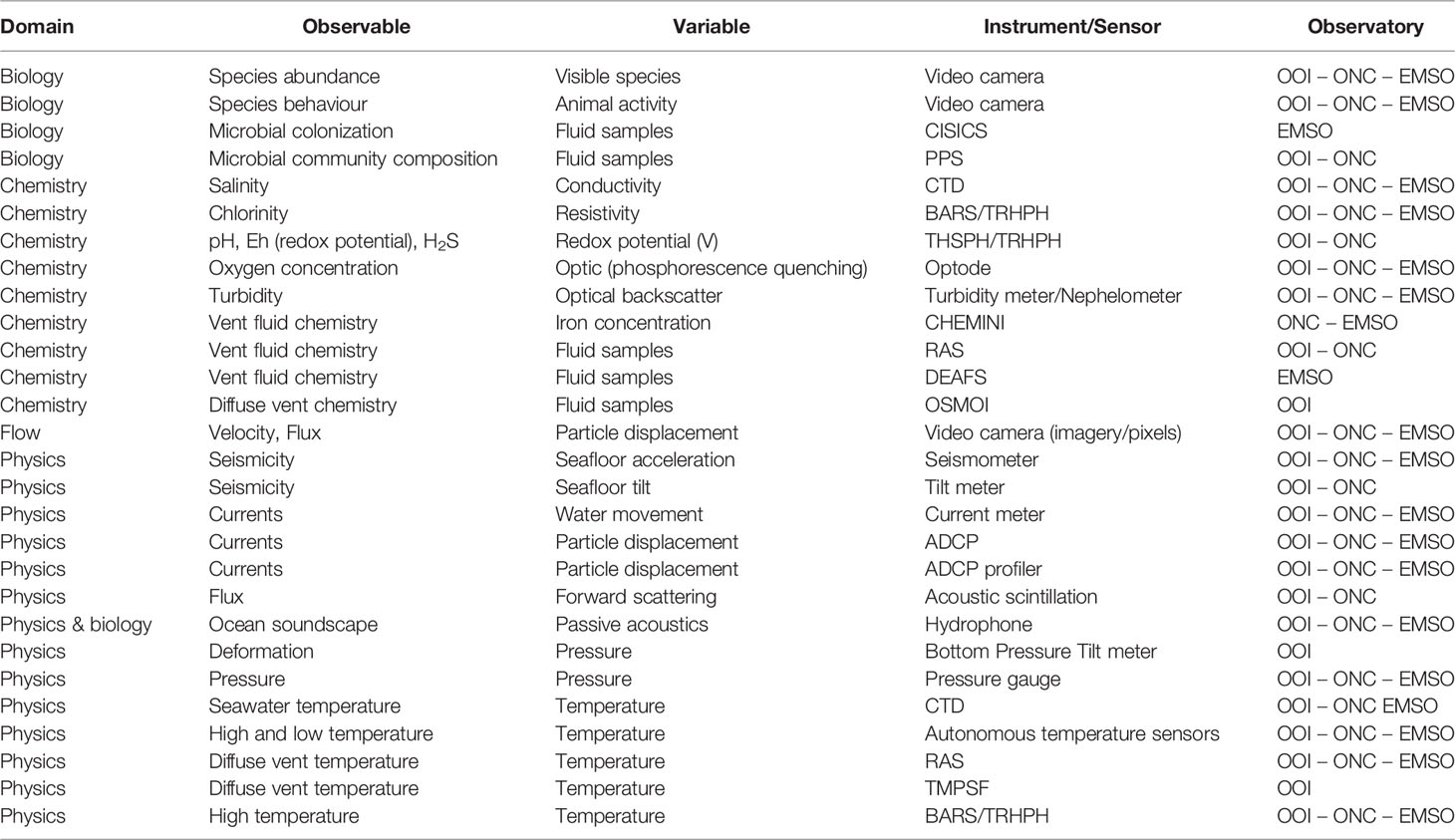
Table 2 List of variables currently measured at the three vent observatory platforms and the corresponding observables.
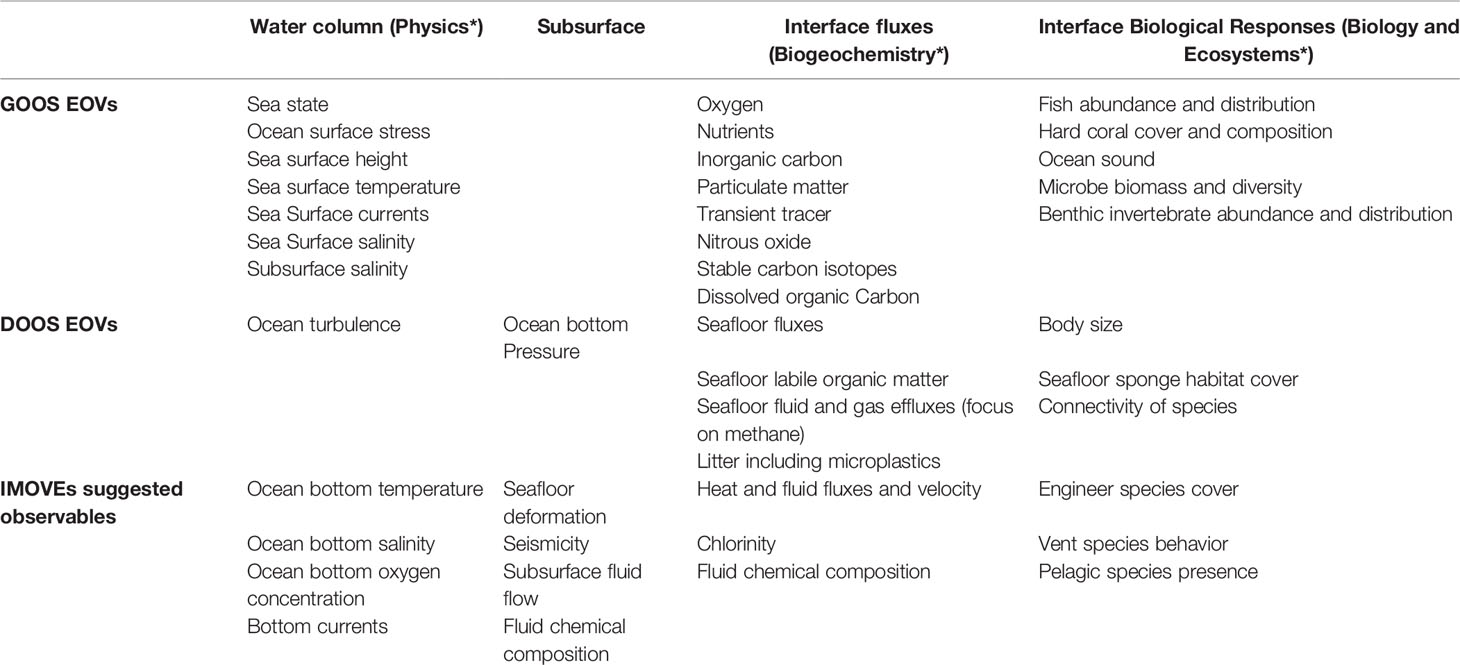
Table 3 Global Ocean Observing System (GOOS) Essential Ocean Variable (EOVs), additional EOVs proposed by the Deep Ocean Observing Strategy (DOOS) and new observables specific to ridges proposed by the InterRidge Integrated Multidisciplinary Observations at Vent Environments that can be measured by seafloor vent observatories, their associated surface buoys and/or during annual maintenance cruises (modified from Levin et al., 2019).
BOX 2 – List of main recommendations from workshop 2
1. Climate change
● Deploy an European Generic Instrument Module -EGIM- (Lantéri et al., 2022), or equivalent, to monitor key regional water mass characteristics at each site
● Sample bottom and full water column seawater to conduct ground truthing chemical measurements and enable sensor calibration
● Install cabled, instrumented profiling moorings for full water column measurements
2. Subsurface processes
● Conduct 2D/3D seismic surveys to image subsurface features that include magma-mush zones, sills, and faults
● Install arrays of broadband and short-period seismometers, low frequency hydrophones, and bottom pressure tilt instruments to monitor magma movement, seafloor deformation
● Install temperature -chemical sensors in vents to inform fluid-rock reactions in the subsurface that are vent fluid sources
● Utilize IODP capabilities for installation of cabled and uncabled CORKed Observatories that allow downhole measurements, sampling (fluid-biological), and cross hole hydrogeological experiments
3. Interface and biological response
● Compile a list of recommended standardized protocols provided by experts to be applied during maintenance including sampling (i.e. microplastics, eDNA), video acquisition (fluid flux estimation)
● Conduct seafloor routine transects with ROVs and AUV’s to map megafauna abundance, geological and chemical features and litter distribution using common protocol acquisition and logging terminology (see "Biological Responses to Hydrothermal Forcing")
● Consistently record vertical video transects during ascent and descent of submersibles
● Quantify the magnitude and distribution of heat, chemical and biological fluxes
4. Water column: deep circulation, megaplume formation, fluid dispersal and connectivity
● Deploy an array of full water column profiling moorings equipped with a diverse suite of instruments at distances of 500 m to 1 km to mimic the scales resolved by numerical models.
● Conduct standardized routine vertical transect with CTD measurements and discrete fluid sampling
The DOOS demonstration projects in the Azores and NE Pacific offer opportunities to feature hydrothermal vents within the concept of integrated observing (Levin et al., 2019). The linkage of multiple platforms and sensors operation both inside (observatories, repeat visits) and outside but proximate to the vents in these regions (e.g., Argo floats, Ocean Sites moorings, GoSHIP tracks, Ocean exploration activities, gliders), can contribute to a powerful multidimensional understanding in space and time of the external forces affecting vent communities and the influence of these ecosystems on surrounding environments.
Monitoring Long-Term Changes in the Context of Climate Change
By enabling sub-daily to multidecadal monitoring, seafloor observatories harbor a great potential to assess long-term changes in the context of climate change. The deep-sea is expected to lose oxygen, increase in temperature and change in pH (Levin and Le Bris, 2015; Sweetman et al., 2017; Levin et al., 2020). One big common question that the scientific community can tackle using an integrated approach is the impact of water column and subseafloor processes on global climate, not only through the emission of gases like CH4 and CO2, which in general are negligible, but also as organic carbon producers, CO2 consumers, and O2 consumption (Le Bris et al., 2019).
Processes identified during the first workshop that should be considered when addressing climate change are the carbon cycle and geochemical and thermal fluxes impacts on the ocean (Table 1). The next paragraphs summarse the recommendations issued during the second workshop.
In an environment characterized by high environmental heterogeneity over limited spatial scales, it appears essential to monitor background seawater characteristics. Although basic, this type of data is not routinely acquired today at all sites. EMSO developed, in the framework of the European Commission H2020 EMSODev project (Grant Agreement 676555), the EGIM, designed to continuously measure long-term series of key variables (Lantéri et al., 2022). EGIM is originally equipped with a CTD, ADCP, oxygen sensor, pressure temperature, turbidity meter and hydrophone to measure conductivity, precise pressure, dissolved O2, turbidity, passive acoustics, and ocean currents but can host additional sensors. The module has been successfully deployed on a number of EMSO-ERIC observatories and can actively contribute to the international effort undertaken by the Global Ocean Observing System on Essential Ocean Variables (Levin et al., 2019; Sloyan et al., 2019). A first recommendation is thus to deploy an EGIM-like module at each observatory site, away from vent influence. This will require the definition of standardized instruments and calibration protocols and the development of new sensors, in particular for long-term pH measurements.
By measuring water mass circulation, dissolved oxygen concentrations, temperature, POC fluxes and chemical and heat exchanges, the vent observatory community can contribute to address some of the key questions raised by DOOS (Levin et al., 2019). However, because yearly maintenance involves recovery and redeployment of platforms, change in sensors and their potential location, some common good practices must be adopted to help make sense of data acquired, and are supported by existing Best Practices guides (Coppola et al., 2016). Groundtruthing measurements and sampling should be performed whenever possible and at every maintenance cruise. This includes oxygen calibration with Winkler measurements, characterizing seawater particulate load by sampling bottom water, in situ temperature measurements using high resolution, reliable and properly calibrated temperature sensors.
The nature of the coupling of water column processes to benthic processes is critical to understanding the ocean’s carbon cycle and its link to climate change and biodiversity (Cathalot et al., 2021 and reference therein). Long term observations of benthic-pelagic coupling can address vent stimulation of photosynthetic productivity or dark carbon fixation, influences on microbes, pelagic larvae and adults of vent and non-vent species, and alteration of food supply for mixotrophic vent species (Levin et al., 2016). These have relevance for the biological pump and opportunities for carbon sequestration. Newly developed seafloor rovers such as RB-II or other benthic crawlers that have potential to track carbon inputs, remineralisation rates and oxygen levels can associate with cabled observatories to obtain power and transmit data in association with other observatory monitoring (Smith et al., 2021).
Crustal and Sub-Surface Processes
During the first IMOVE workshop, several processes were identified as important when addressing subsurface processes: seafloor dynamics including compliance and ground deformation, plumbing system and fluid flow geometry, subsurface characterization, crustal geothermal signature, subseafloor microbial communities, and forcing of sub-seafloor processes on seafloor systems (Figure 5 and Table 1). Similarly, the first workshop identified several processes as important when addressing fluid fluxes and heat exchanges at the interface: forcing of sub-seafloor processes on seafloor systems, chemical signature, dynamic coupling of diffuse/focused venting, crustal plumbing system and fluid flow geometry, phase separation, and sub-surface and post-exit fluid mixing (Figure 5 and Table 1).
During the workshop, several approaches were proposed to better constrain models of fluid circulation and characterize fluid flux dynamics on a local scale. Repeat mapping of smoker vents at certain sites (e.g., Barreyre et al., 2012; Girard et al., 2020) can help better characterize the long-term changes and stability of vent circulation in different ridge settings. Such repeat mapping could occur during maintenance cruises by a submersible or by future drones linked to connected or autonomous benthic docking stations allowing power recharge and data transfer (see section on Ocean Sensing Technology and Future Directions). Taking this standardized approach to fluid flow characterization will foster comparison among different ridge systems and help better understand how temporal and spatial changes in the distribution of hydrothermal venting impacts ecosystem stability and species’ adaptation to their environment (e.g., reproductive biology, growth, mobility). A benefit of the above approach is the ease with which it could be extended to other systems where repeated visits allow for temporal monitoring (e.g., East Pacific Rise, Lau Basin, Guaymas Basin).
As part of repeated monitoring efforts, the use of non-invasive video sequence analyses could be key for volume, heat, and chemical flux estimations at smoker vents. Using pixel-based correlation methods (Particle Image Velocimetry or Diffuse Fluid Velocimetry; Crone et al., 2010; Mittelstaedt et al., 2010; Mittelstaedt et al., 2012; Escartı́n et al., 2015; Puzenat et al., 2021), the flow of high-temperature and low-temperature fluids can be estimated with reasonable accuracy and in relatively short image capture sequences. To allow uniform, inter-comparable results using these techniques requires a common protocol with guidelines for image acquisition (camera distance; use of chessboard for image calibration; Escartin et al., 2013; minimum image resolution and frame rate guidelines), the implementation of a user-friendly computer program to process images as part of routine maintenance visits to vent sites, and sufficiently stable imaging platforms to avoid image motion related to currents and/or vehicle motions. To constrain heat and chemical fluxes, the above techniques for estimating flow rates should be coupled with simultaneous exit-temperature and near-simultaneous fluid sampling data.
Biological Responses to Hydrothermal Forcing
Processes identified during the first workshop that should be considered when addressing biological responses at the interface are: chemical signature, dynamic coupling of diffuse/focused venting, mixing, recruitment dynamics, energy flow and fluxes (Figure 5 and Table 2).
A number of common variables were identified as potential ridge EBVs and were discussed in relation to the work already done by Significant Ecological Components (SECs, DFO, 2006), DOOS (Levin et al., 2019) and GEO BON (Pereira et al., 2013). Variables that can contribute to the ones already defined or proposed by GOOS and DOOS respectively, are presented in Table 3, and new ones are proposed in the context of ridge monitoring. Repeated visits and cameras deployed on the seafloor provide information on species presence/absence, faunal abundance in relation to oceanographic variables. However, because it is not possible to generalize fixed-point observations to regional scale, routine transects should be conducted during cruises when possible to extend the spatial extent of observations. Protocols should be standardized and shared to enable large-scale comparisons and should include the use of a common real-time dive logging strategies and terminology (i.e. annotation of fauna, geological or chemical features, litter) (Schoening et al., 2020). Alternatively, this acquisition could be achieved by resident drones or AUVs in parallel with chemical and geological features mapping (see section on Ocean Sensing Technology and Future Directions). Time-series observations should focus on species and habitats that are indicators of change (e.g., Sarrazin et al., 2015). Deep pelagic ecosystems represent more than 90% of the biosphere but remain the most unexplored and least understood environment (Webb et al., 2010). Maintenance cruises can contribute to enhancing our knowledge of this ecosystem by routinely recording vertical video transect during the ascent and descent of submersibles, coupled with CTD measurements and discrete water samples.
Video imagery collected during observatory maintenance activities is a potentially important and currently undervalued source of information on the spatial distribution and biomass of visible vent and non-vent organisms and on seasonal and inter-annual variability in species abundances and diversity. A structured approach to real-time and post-cruise annotations of video records can greatly increase their usefulness for extraction of biodiversity information. The value of these annotations can be further enhanced by the standardization of vocabularies and their alignment with recognized terms used by international organizations such as the World Registry of Marine Species (WoRMS). Ocean Networks Canada has recently collaborated with WoRMS and the US National Oceanic and Atmospheric Administration (NOAA) Office of Ocean Exploration & Research (OER) to develop a standardized video annotation system. The SeaTube interface allows users at sea or via telepresence to annotate live video streams, and permits post cruise annotation of ROV video recordings. Annotations may be entered as free-format text or structured annotations adhering to custom or standard taxonomies (such as WoRMS, CMECS, etc.). Ancillary data from both ROV and ship mounted sensors are also displayed in conjunction with the video, all synchronised with the video timestamp (Figure 6). Live links to the WoRMS database while annotating allows for near-real-time validation of species identifications against known geographic distributions, and the flagging of potential new occurrences or identification errors.
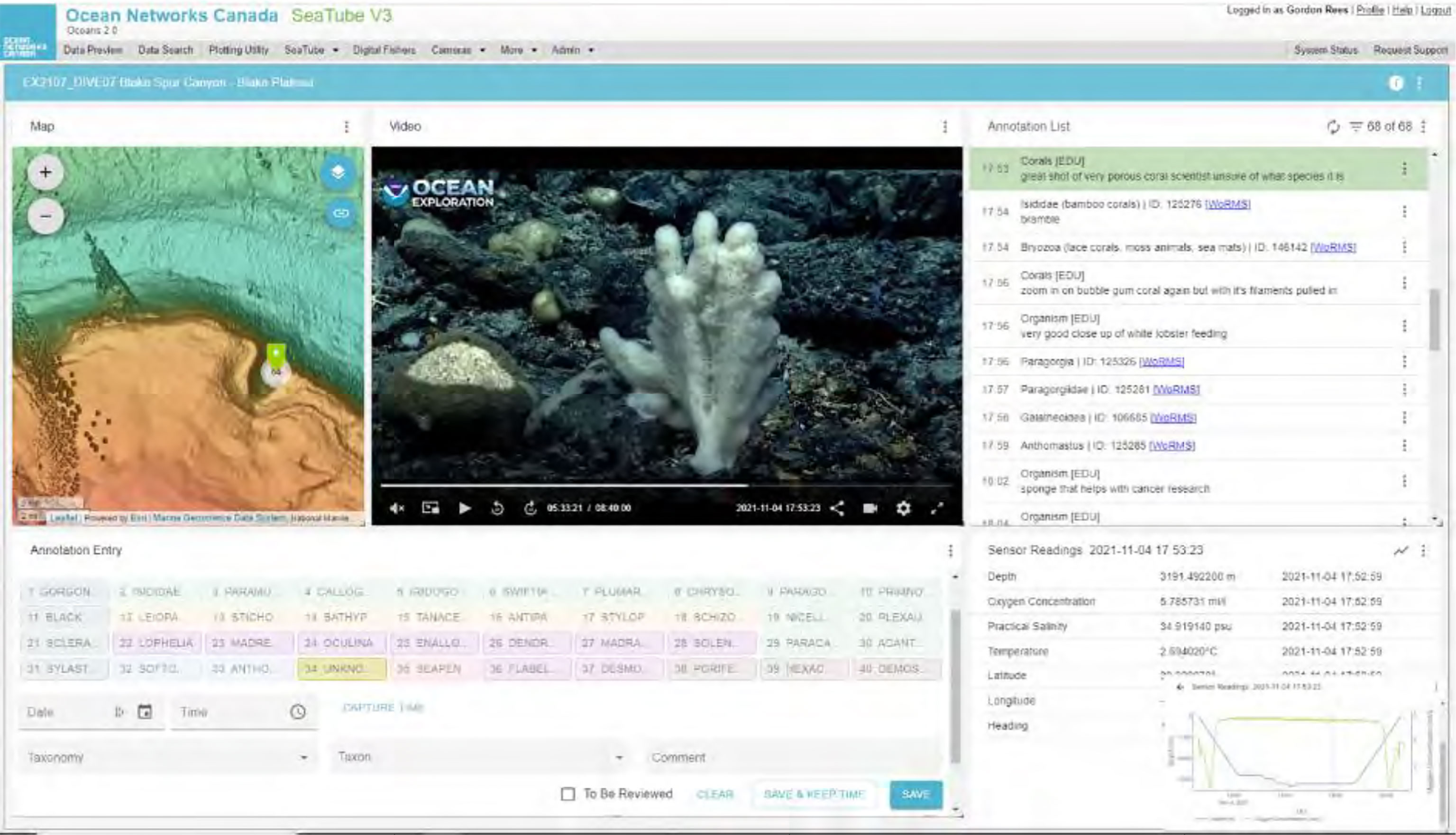
Figure 6 Example of video annotation interface for SeaTube 3.0 showing location of ROV on seafloor bathymetry, a deep-sea coral of interest, and the user-designated annotation options available for identifying the coral. Sensor data from the ROV are also linked to the annotation. Screen capture courtesy of NOAA Office of Ocean Exploration and Research.
Finally, maintenance cruises represent unique opportunities for repeated sampling and have enabled a better characterization of the geological, geochemical and biological settings of vent ecosystems (see section 2). These cruises should be further used to collect opportunistic samples following standardized protocols to monitor microplastics distribution, environmental DNA (eDNA), or other EOVs and pollutants but also to characterize ecosystem function and services (Danovaro et al., 2020). One solution to optimize the acquisition and processing of samples is to involve and offer access to cruises for external researchers which will contribute to fostering a mutualisation of the infrastructures. Such an effort is currently under development within the EMSO-Eric through the establishment of access services including the Transnational Access (TNA) to EMSO ERIC observatories (https://emso.eu/transnational-access/).
Water Column: Deep Circulation, Fluid Dispersal and Connectivity
Processes identified during the first workshop that should be considered when addressing fluid dispersal and connectivity are: plume characterization and dispersal, turbulent mixing and energy dissipation, forcing of ocean dynamics on seafloor systems, connectivity between vents, seafloor topography, mounds, ridges, and role of ocean circulation on larval and free-living microorganisms dispersal (Table 2).
One of the next challenges needed to be addressed in deep-ocean modeling with likely implications for Lagrangian spreading is the representation of the thermal plume dynamics. Due to the lack of sustained measurements in the deep ocean, spatial scale coverage, assessing the realism of numerical models remains arduous. Another challenge is to understand if the mesoscale processes are periodic, or asynchronous, having implications on the connectivity of species, and consequently succession patterns and community resilience. Numerical models of the deep-ocean circulation are now routinely reaching submesoscale-resolving and internal wave-resolving resolutions (dx<1 km). Although the phenomenology of resolved processes is widening, uncovering smaller eddies and turbulent processes, we lack a trustworthy 3-D in situ picture to assess the models’ performances. A rather low-cost solution to address this limitation would be to deploy an array of moorings equipped with deep T-S sensors and current-meters and distant by ca. 1 km to mimic the scales resolved by numerical models. This would allow the measurement of the gradients of the essential properties of oceanic flows, i.e., velocity and density, and a direct comparison with modeled data.
The routine acquisition of EOVs currently not measured on autonomous observing systems requires the development of new technologies and sensors, more particularly for biological and biogeochemical variables that currently require in situ sampling. The collection of samples calls for dedicated time during the yearly maintenance cruises which are already demanding in terms of human and operational resources. The group agreed on the need to reduce and optimize maintenance cruises and several solutions made possible by the current technological developments emerged (see section on Ocean Sensing Technology and Future Directions).
Ocean Sensing Technology and Future Directions
R&D activities on ocean observing sensors is expected to reach USD 128.56 billion by 2025, registering a CAGR of 8.86%, during the period of 2020-2025 (van den Burg et al., 2021). The global underwater drone market integrating these sensors is projected to reach USD 7391 million by 2027, registering a CAGR (i.e. Compouned Annual Growth Rate) of 11.7% (van den Burg et al., 2021). The reason for this growth is the need for precise, reliable, and pervasive sensing technology to measure and follow multiscale spatio-temporal dynamics of ocean physical, geochemical, and biological processes. This technology aims to explore and quantify the role of the ocean and seafloor processes in climate change, in provisioning of rare minerals and unexplored biological resources, and availability of biomass. The future directions on ocean sensors development are within the design of i) novel sensing technology, such as underwater mass spectrometer, eDNA collectors and in situ sequencing, ii) energy efficient and/or resilient solutions to minimise battery consumption and/or harvest ocean energy, iii) use of Artificial Intelligence (AI) to reduce energy/data storage by “pre-filtering” data or allow adaptive sampling, and iv) new networking architectures that integrate the sensor networks at the surface, e.g., as described in Aguzzi et al. (2019) and Mariani et al. (2021) with the underwater sensor networks.
Sensing and Monitoring Platforms
Platforms that integrate ocean sensors and operate over a long time at sea can be fixed, e.g., surface buoys, moorings and landers, or mobile such as Argo floats, crawlers, surface vehicles, gliders and Autonomous Underwater Vehicles (AUV) (Aguzzi et al., 2019; Rountree et al., 2020). These platforms can be cabled with power and data transmission ensured from the shore - but unchangeable location strongly constrains the cable route and price -, or wireless with battery and data storage capabilities dimensioned according to the duration of the foreseen deployment on board the seabed station (Matabos et al., 2016). The future directions on platforms’ development need to address a number of limitations highlighted through the experience acquired over a decade of operation (also see Rountree et al., 2020): i) The operational cost is high, partly because underwater vehicles are required for maintenance operation to place the sensor with precision and perform underwater connection; ii) the time between the maintenance operations is limited due to requirement for sensor calibration and, in case of wireless system, for energy pack and data storage replacement; iii) the spatial coverage is limited to the close surrounding of the seabed station; iv) new regulation will urge for new deployment processes with low environmental footprint; v) a larger number and variety of sensors are fundamental for multidisciplinary approaches and the amount of energy and volume of data storage needed keeps increasing.
These limitations call for technological and operational breakthrough, and the future trends in fixed-point long-term observation are central to developments going on in the field (Figure 7). First, decreasing operational cost requires combining various efforts to reduce the time and means required for maintenance operation at sea. In terms of means, this can be achieved by improving the global robustness of the system to insure the endurance to meteorological and other heavy environmental conditions. Surface buoys relaying the information to the shore, sensitive to storms, can be replaced by autonomous surface vehicle patrolling to recover the data weather permitting. The development of submarine tools specialized and optimized for deployment and recovery of systems could carry out the maintenance from a small ship instead of large multipurpose ROVs. Wireless contacts to recharge batteries and recover data would avoid plugging connectors underwater and can reduce the number of packages to be recovered onboard for refurbishing and contribute to reducing time at sea (Aguzzi et al., 2019). Increasing the intervals between maintenance requires technological developments at the platform and sensor level. For a wireless autonomous system, the amount of energy and data storage volume available are fundamental parameters to determine maintenance frequency considering the growing ambition of observatories (more sensors, better images definitions, etc.). Spare energy and data storage require establishing strategies to collect the data (e.g., optimize multidisciplinary integration and reinforce collaborative strategies), reduce the duration of measurements, and/or trigger measurements on predictable (foreseeable) events detected using sensors with low power needs or using in situ decisions based on IA algorithms for pattern recognition (Aguzzi et al., 2022). An alternative is to harvest energy in small yet decisive quantities from heat exchange occurring at mid-ocean ridges. Technologies are progressing quickly and high capacity energy and data storage are expected in the coming years. As an example, optical fibre cable, supporting fast data transmission, can also power over a few kilometres from the central station low consumption sensors (Matsuura, 2021). This technology by reducing the cable cost also contributes to extending the observations spatial coverage.
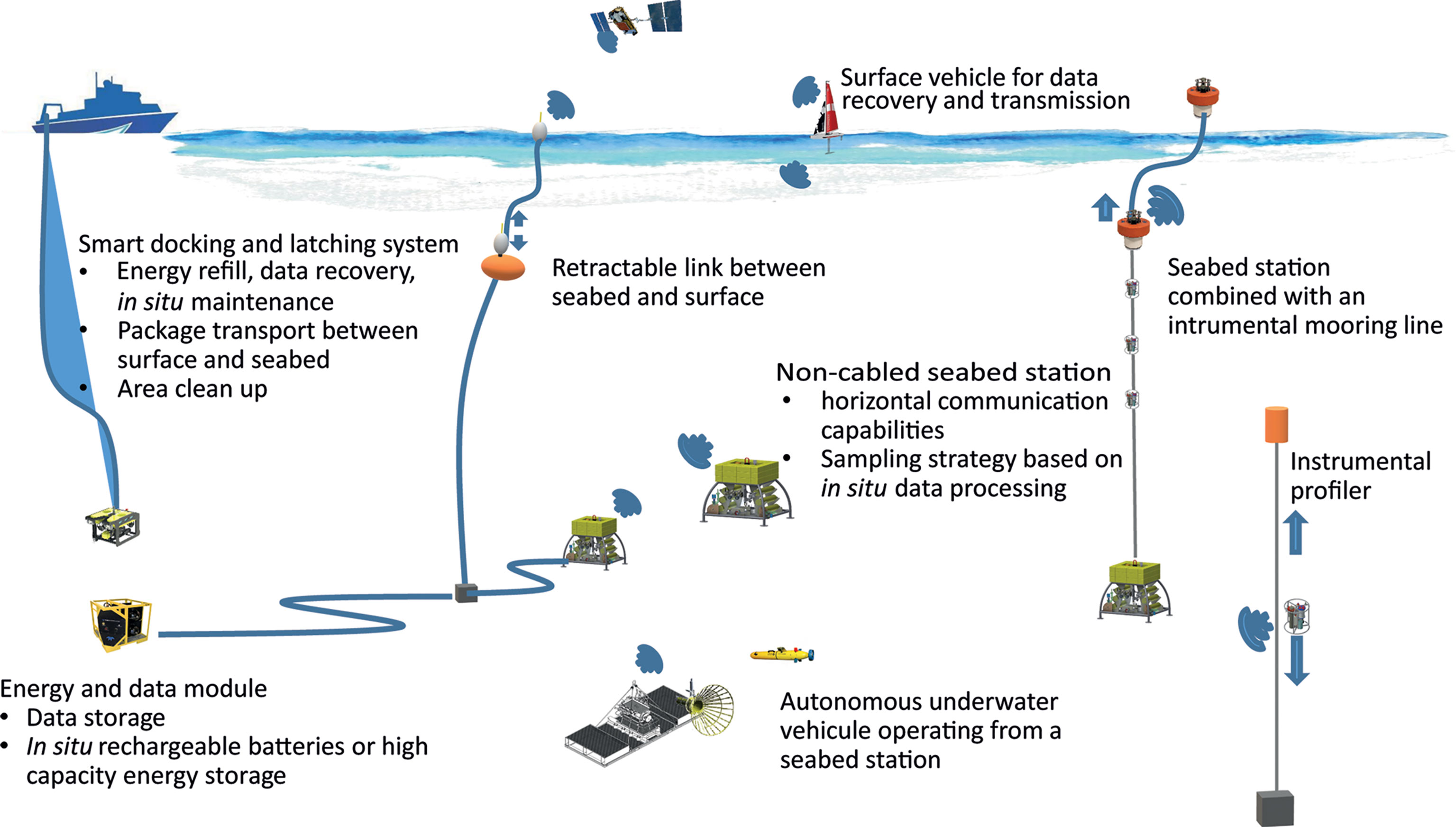
Figure 7 Illustration of future technological and operational breakthroughs to reduce deployment and maintenance cost and reduce environmental footprint.
Extended spatial coverage is a challenge for ocean observation. Fixed observatories, in particular, give a very partial view of the seafloor generally limited to a few meters around the central place (Rountree et al., 2020). There are already a few examples of static or moving devices expanding the scope around the central station, for instance the 100 m thermistance chain deployed on EMSO Azores or the Internet Operated Deep-Sea Crawler Wally (Chatzievangelou et al., 2020) at ONC. Developments are on the way to deep-sea autonomous mobile vehicles operating from a seabed station with large excursion capacities and wireless docking stations to refill batteries and download data (e.g., Costanzi et al., 2020). However with the technologies presently available to store the energy, the feasibility of a vehicle operating around a non-cabled observatory is not conceivable and will be first limited to cabled observatories. Things are however changing very quickly in this field. Fuel cells tomorrow, or quantum batteries in the future, could drastically increase the amount of energy available.
Finally, environmental footprint concerns appeared some 10 years ago and the number of marine protected areas has increased since then. Research activities and observations are a source of disturbance to marine ecosystems (Juniper et al., 2019) and constitute an additional constraint when designing observation systems and operation scenarios.
Internet of Things (IoT)
These platforms can communicate at the surface through IoT protocols, as described in Mariani et al. (2021) or underwater through underwater acoustic, optical, and/or magnetic inductive modems. Acoustic waves propagate over long ranges (orders of km) but can transfer limited data rate (some kpbs) optical modem can reach a few tens of meters with data rates of up to 500 kbps and finally magnetic inductive modems can support contactless data transfer (very short range few cm). Even though the internet of underwater things (Jahanbakht et al., 2021) is a concept introduced a decade ago, and underwater sensor networks have been studied for two decades (Heidemann et al., 2012), it is only recently that in situ underwater networks have been deployed for scientific and industrial use cases (Qiu et al., 2019). Examples of services enabled by this technology are:
(1) Real-time data transfer to a node connected to the end user through IT infrastructure.
(2) Machine-to-machine communication to remotely operate instrumentation, e.g., in hazardous conditions.
(3) Extended spatial coverage data acquisition through resident autonomous drones.
(4) On-demand data offloading of standalone sensors.
The challenges of underwater acoustic communication relate to the impact of environmental factors on acoustic waves propagation in shallow and deep water (Tomasi et al., 2010; Tomasi et al., 2011). In deep waters, variations in sound speed alter the direction of propagation of acoustic waves (i.e., sound refraction). This causes shadow zones and reflected echoes from seabed also affects communication.
The future directions on underwater networks development are within the design of novel networking solutions where both stationary and mobile nodes can connect and coordinate towards a common mission defined by the end user and provide an underwater positioning system. One shift of paradigm that could be enabled by this development is going from ocean observatories to in situ ocean laboratories and virtual laboratories.
Geochemical Sensors
Along with the evolution of the platforms, a larger number and variety of sensors are expected to complement data acquisition. The sensor required for ocean measurements has undergone various phases of technological advancements to meet requirements of robustness, reliability and low maintenance in harsh environments. Some examples of the last technology available to measure chemical properties are based on optical measurement. Optical sensors provide conventional measurements by providing highly stable values for long term applications. As an example, the ECO (Environment Characterization Optics-SBE) technology is used for measuring various parameters such as turbidity, fluorescence, Colored Dissolved Organic Matter (CDOM), Photosynthetically Active Radiation or chlorophyll. For pH, usage of glass electrode-based measurement often poses challenges at remote locations in the ocean and are prone to drift with errors. The recent advancement in pH measurement is by using the state-of- the- art ion-sensitive electronic devices such as ion-sensitive field effect transistors [ISFET type sensor, SeaFET - Seabird Electronics (SBE)] that uses advanced transistor-based technology. The MBARI Submersible Ultraviolet Nitrate Analyzer (SUNA) and In Situ Ultraviolet Spectrophotometer (ISUS) provide a highly stable measurement of nitrate over a wide range of environmental conditions although their sensitivity and accuracy are poorer than those of wet chemical analyzers in conditions of high turbidity and high CDOM (Daniel et al., 2020). These sensors use technology based on the absorption characteristics of nitrate in the UV light spectrum. In situ pCO2 values of seawater are measured using 4 broad approaches such as gas based, electrochemical, wet-chemical or fluorescent. The partial pressure of carbon dioxide in surface ocean waters is a key parameter used to study ocean CO2 absorption, acidification, primary productivity, carbon cycle, source, and sinks of CO2, and biogeochemistry. Finally, better understanding metals transfer from the hydrothermal sources to the open ocean column needs the development of sensors capable of measuring trace metal micronutrients at levels found in the open ocean. There is a clear gap in the development of more sensitive techniques for trace metal analysis and the sensor support infrastructure needs to be redesigned to fully access their dynamic distribution and impact on ocean productivity (Grand et al., 2019).
Biological Sensors
One example of technological development in the last decade that includes these three technological developments is represented by high-throughput sequencing solutions that are increasingly used in ecology and environmental management studies. eDNA studies are also applied in the deep ocean (Laroche et al., 2020). The available technology requires a priori knowledge of deep-sea taxonomy, meaning that a large effort of species barcoding is needed (Cowart et al., 2020). However, in well-known areas observatory site time series where fluid/DNA samplers exist and are coupled to fluid-temperature samplers, we can track changes in microbial communities over time and in response to perturbation events (e.g., Huber et al., 2003; Fortunato et al., 2018). Because lifeforms in the ocean contain or leave behind a biomolecular trace that can be analyzed directly from a seawater sample, 4D seascapes could be a possibility with the development of multi-omic biodiversity sensing capabilities by integrating probes or sensors to existing observing systems (Stefanni et al., 2022). These latter offer the opportunity to integrate additional concomitant biological, biogeochemical and physical parameters to take in consideration the influence of habitat heterogeneity, fluid and larvae dispersion, as well as regional and local oceanography.
If eDNA and other high-throughput sequencing technologies hold great promise to uncover the multiple facets of deep-sea biodiversity, understanding fundamental processes involved in population’s persistence and resilience still requires observations of the organisms themselves, throughout their life cycle, particularly at early life-stages. The development of biophysical modeling to predict connectivity patterns simulating larval trajectories requires in situ observations for model validation and biological parametrization. Taking advantage of the infrastructure of established observatories, where combinations of existing plankton collection tools could be integrated, would most effectively foster rapid advancement in deep-sea larval biology. For example, large volume plankton samplers such as the SyPRID currently deployed on the Woods Hole Oceanographic Institution Sentry AUV (Billings et al., 2017) could be further developed and miniaturized for integration with other platforms, such as small mobile long-range vehicle docking on a seabed station, which could greatly expand the spatial coverage of deep-sea plankton observations. Development of imaging, automated taxonomic identification through machine learning algorithms, and adaptive sampling can also accelerate deep-sea larval research. Recent technological advancements in in situ plankton imaging such as the Underwater Vision Profiler (UVP, Picheral et al., 2010) or holographic cameras (e.g., LISST-Holo2, Sequoia, Lombard et al., 2019) are currently being further developed for deployment on diverse deep-sea observing platforms (e.g., Picheral et al., 2021). New imaging techniques are currently under development to document spawning or larval settlement events in conjunction with multi-parameter sensors to identify environmental cues or oceanographic patterns driving life cycles in the deep sea. Machine-learning can allow for rapid identification of high-volume image samples, as well as enable the collection of higher-resolution samples following detection of an anomaly (adaptive sampling). While such technological innovation could greatly accelerate our knowledge on deep-sea benthic and planktonic communities, most deep-sea species are still undescribed in their adult form, and this is only amplified for their larval forms. Therefore, their identification from images still requires the collection of physical specimens for morphological or genetic analyses, which will feed biodiversity baseline databases and provide training sets for machine learning (e.g., Stefanni et al., 2022).
Observatories and New Societal Approaches to Deep-Sea Environments
Seafloor observatories are a natural platform for collaboration within the ocean science and technology sectors and offer unique opportunity for demonstrations to the public and policy makers about oceanic and ocean floor processes that can stimulate society’s interest in the marine realm and impacts that society is having on oceanic processes. Looking to the future, seafloor observatories have an important role to play in understanding and mitigating the effects of climate change and resource exploitation on deep-sea ecosystems.
A Tool to Inform Environmental Managers and Policy Makers
Because observatories constitute technological development platforms, they can support the design, testing and implementation of environmental monitoring strategies and evolve with new emergent technologies and the innovation of instrumentation for sustained, systematic data acquisition (e.g., Danovaro et al., 2017a). The Endeavour nodes of ONC and EMSO-Azores are both located within an MPA. Together, these seafloor infrastructures constitute an essential tool to inform MPA management and efficiency for the benefits of the local governments but also of international instances such as the International Seafloor Authority (ISA) which is currently developing the standards and guidelines of the international mining code. As an example, ONC has developed SeaTube (Box 1), a software system for assembling ROV dive navigation logs and video records, including real-time annotations, into an online archive and a GIS database. The database can be queried to generate maps for researchers and MPA managers that illustrate the spatial distribution of seafloor features of interest. Juniper et al. (2019) provide examples of how this geodatabase can be used to analyse the distribution of species of interest, debris, and human pressures resulting from research activity. All observatory sensor data and ROV expedition video and navigation records from the Endeavour Hydrothermal Vent MPA are archived and made publicly available by ONC (Box 1). The combined data archive therefore has the potential to support adaptation of the EHV MPA management plan and conservation objectives to a growing understanding of natural rates and scales of environmental and ecosystem change, and the spatial distribution of research activity.
To standardize and implement these conservation objectives at a larger scale, regular workshops should be organized with various stakeholders, including the ISA, State environmental ministries, Non-Governmental Organisations (NGOs), Business/Industry, Science Networks and the civil society to identify their respective needs and work towards the co-design, co-development and co-delivery of commonly defined monitoring approaches and tools. Sustained funding together with governance are required to guarantee future long-term systematic observations.
Outreach and Education
Real-time data available on-line and the associated data archives are an under-tapped resource for educational purposes and for general public communication. Hydrothermal systems are dynamic, and the different links between physical and biological processes could be included in education curricula, at all levels. The associated annual cruises also provide unprecedented opportunities to develop outreach and educational projects. The colossal imagery archive of hydrothermal systems can be exploited and showcased to attract the general public’s interest towards these systems in particular, and oceanography and deep-sea research in general, while increasing the awareness regarding the unknown and endangered ecosystem that the deep ocean still has to reveal fully both to science and society. In the context of increased anthropogenic activities, it is of utmost importance to engage with the next generation and inform the society about our research, especially since they occur in inaccessible areas.
For the last 10 years, various original actions have been proposed in all three observatories to make the science, the people and life onboard accessible to the public. Primary outreach activities are related to maintenance expeditions at sea at all three MOBs (Box 3). At Ocean Networks Canada, live-streaming video with commentary by shipboard personnel allows members of the public to follow ROV dives and on-deck action on the research vessel. The audience for these live streams has been greatly augmented in recent years through a partnership with the Ocean Exploration Trust (OET). ONC has frequently chartered the Exploration Vessel Nautilus with Hercules ROV to perform maintenance activity at the Endeavour observatory. OET has a large global audience for their Nautilus Live program and Education and Outreach staff on the vessel are experienced in interpreting science for the public, providing live commentary during dives and fielding questions from the online audience. OET’s Science Communication Fellowship and Science and Engineering Internship Program allows educators and students to join the outreach staff at sea.
BOX 3 – Outreach activities and materiel related to observatories
EMSO-Azores
Educational resources for the public and researchers:www.deepseaspy.com (see educational resources tab)
Artistic creations: https://www.teatrpiba.bzh/en/
3D immersive visit: https://www.youtube.com/watch?v=hNK1MERlzaY
Endeavour component of ONC
Training and outreach resources: https://www.oceannetworks.ca/learning
Sight and sounds: https://www.oceannetworks.ca/sights-sounds
Regional Cabled Array – Axial Seamount
Educational website https://interactiveoceans.washington.edu/
UW Regional Cabled Array educational Data Portal https://app.interactiveoceans.washington.edu/
Blog to chronicle eruption forecasts at Axial Seamount https://www.pmel.noaa.gov/eoi/axial_blog.html
A well-established University of Washington experiential at-sea learning program ‘VISIONS’ supports outreach at the Axial Seamount component of OOI’s RCA. Since 2010, over 160 undergraduate and graduate students have participated in this uniquely interdisciplinary, hands-on at-sea program that provides training in research and engagement related to many important oceanographic processes operating within the Northeast Pacific ocean and on the seafloor. The oceanographic expeditions are an important component of the National Science Foundations’ OOI RCA operations and maintenance cruises using the global class research ships the R/V Thompson (University of Washington, UW), the R/V Revelle (Scripps Institution of Oceanography, SIO), and the R/V Atlantis (Woods Hole Oceanographic Institution, WHOI). All cruises utilize state-of-the-art underwater robotic vehicles (ROV) that allow students to directly witness some of the most extreme environments on Earth. On all annual expeditions, similar to ONC, live video is continuously streamed from the ship and from the ROV’s to viewers around the globe. In addition, the UW RCA team has developed the interactiveoceans website (Box 3) that provides rich and diverse content about the RCA sites, technologies, expeditions and educational components of the RCA.
In addition to live interactions during cruises, other original actions have been developed including, among others, participative science and art & science projects. AbyssBox, the first permanent public exhibition of live deep-sea hydrothermal fauna maintained at in situ pressure (170 bars, Shillito et al., 2015), is a first step towards maintaining a variety of deep-sea fauna year-round, serving both scientific and public purposes. The Deep Sea Spy (DSS) project (Box 3), developed by Ifremer allows society to be involved in the research process by contributing to the annotation of deep-sea hydrothermal vent imagery acquired by seafloor observatories. To date, over 1300 citizens annotated almost 50,000 images through the DSS platform building a reference database for machine algorithms developments. This alternative approach to the study of ecosystems, by increasing public engagement, also provides scientific transparency which can improve the credibility and relevance of the research process. The SPLUJ and DONVOR (Box 3), two radiophonic theatre plays, co-created in 2018 by Ifremer scientists and the professional theatre company Teatr PIBA, invite the public to explore the unknown and the imaginary by “doing” and “living” in a complete sensitive immersion. This artistic approach helped take up the challenge to reach “different” public: those who are not naturally attracted to science or those living far from the ocean. To date, over 22 000 spectators have assisted to one of the plays and over 50 representations are expected in 2022. Finally, the visit of the Tour Eiffel edifice in an immersive environment using virtual goggles provides a unique way to experience and comprehend an otherwise inaccessible environment (Box 3). This innovative way of accessing the deep ocean significantly increases the outreach potential to raise ocean awareness in the general public. It is essential to develop a wide range of outreach material exploring all five senses to reach the widest range of people. Collaboration with artists, writers and musicians, whose multifaceted visions allow us to explore the unknown in original and diverse fashions, can help meet this objective.
The DSS project supported the development of educational resources for kids from 3 to 11 years old (Box 3), promoting the use of the annotation online platform as a tool in school programs. Similar resources, based on the use of data, can support programs for students in middle school and high-school in several languages. Immersive environments offered by virtual goggles, such as the one developed with the active edifice Tour Eiffel, can allow « field work » in remote environments, including exploration, observation and annotation and harbor valuable application in training by bringing students on a «field trip». More should be achieved with kids and young people in Least Developed Countries (LDCs) and Small Island Developing States (SIDs), more particularly those in the Pacific and the Caribbean Sea that are increasingly concerned with future deep-seabed mining.
While some of these projects result from individual/few scientist initiatives, an important step could be achieved by hiring communication specialists dedicated to each observatory, especially to cope with the numerous media available (e.g., Youtube, Instagram, Twitter). For example, in the EMSO-ERIC European infrastructure, a communication service group exists but is composed of overloaded scientists/engineers and has no targeted budget. At ONC, a communications team supports outreach activities during expeditions that use the Nautilus, and ensures at-sea outreach activities during maintenance expeditions that use other vessels and ROVs.
Conclusions
The InterRidge IMOVE workgroup aimed to internationally coordinate and optimize ridge seafloor infrastructures in terms of technology, scientific questions through a multinational and multi-collaborative endeavor. However, given the variability of hydrothermal systems, particularly at slow-spreading ridges, it is also clear that this limited number of sites, focused in magmatic ridge systems, do not cover key hydrothermal systems such as those that are hosted in peridotite, or displaying only low-temperature hydrothermal outflow and temporal studies at these distinct systems are required.
This review offers an overarching umbrella that can help community-based experiments to react to specific events, and help in designing, as well as coordinating observatory maintenance and complementary samples during cruises. The paper presents the state of the art in terms of results and advances enabled by these observation systems, as well as data, tools and the infrastructure available to address scientific, technological, educational and societal issues. Data acquired the last 10 years enabled great advancement in our understanding of ridges, and more particularly vent ecosystems dynamics. The next step will be to integrate across disciplines to better constrain the overall ecosystem functioning, from geological processes to biological communities’ distribution and responses. This can be achieved by a joint effort in defining common variables, sensors and protocols for complementary systematic samples and data acquisition. Science service groups, such as the ones proposed through the EMSO network can help propagate ocean good practices guidelines of surveying ecosystems from the seafloor to the water column and create a global capacity development initiative that includes data management and accessibility. A wealth of data is being acquired through a number of observing programs and observatories in coastal and global oceans. An effort should be made to link the data to surrounding observations (e.g., Argo, deep Argo, GoSHIP, OceanSITES, OTN) in order to reach an integrated and multidisciplinary understanding of ecosystems functioning at the regional scale. More particularly, the DOOS proposed region-specific interdisciplinary projects to demonstrate the feasibility of sustained deep-ocean observing. Two of the three proposed locations include the Azores Archipelago and the Northeast Pacific from the Cascadian Margin to the Juan de Fuca Ridge. The implementation of such projects provides the opportunity to develop a community-based approach for large-scale interdisciplinary studies, placing the ridge environment and its influence at the heart of the global ocean.
Our ten years’ experience also proved that observatories constitute great means to involve and inform the society about the importance of the deep sea and the challenges we are facing to maintain a healthy ocean and the ecosystem services it provides. However, more should be done to expand our capacity building endeavor and widen the public to be reached. The scientific community needs to imagine new ways to exchange outreach, arts & science experiences across observatories by building dedicated communication plans. The complexity of marine ecosystems in relation to climate change and other anthropogenic impacts confronts us with the need to interact with many scientific disciplines. Researchers from fields in Social and Human Sciences need to be involved to conduct science in the context of sustainability and socio-ecosystems and help develop regulations. They can help in new ways to engage public and policy makers/public administration and advise environmental scientists in the development and management of multi and transdisciplinary studies in order to optimize the societal scope, including the traditional knowledge holders where feasible, and impact of deep-sea research projects in accordance with today’s society mandates.
Author Contributions
MM and TB led the working group, conceived the review and wrote the first draft of the manuscript. All authors contributed the manuscript ideas and text. All authors read and approved the final manuscript.
Funding
The first workshop in Bergen was additionally funded by the K.G. Jebsen Centre for Deep Sea Research and the University of Bergen. The second workshop was supported by ISblue project, Interdisciplinary graduate school for the blue planet (ANR-17-EURE-0015) and co-funded by a grant from the French government under the program “Investissements d’Avenir”. Additional funding was provided by Ifremer, and the départment du Finistère. The operation and maintenance of the EMSO-Azores observatory is funded by the by the EMSO-FR Research Infrastructure (MESR), which is managed by an Ifremer-CNRS collaboration. The operation and maintenance of the Endeavour observatory is funded by the Canada Foundation for Innovation’s Major Science Infrastructure program and the Department of Fisheries and Oceans (Canada). The operation and maintenance of the Axial Seamount observatory is funded by the National Science Foundation as part of the Ocean Observatories Initiative Regional Cabled Array. MM, JS and PMS acknowledge funding from the EU Horizon 2020 iAtlantic project (Grant Agreement No. 818123). AC was supported by the Operational Program AZORES 2020, through the Fund 01-0145-FEDER-1279 000140 “MarAZ Researchers: Consolidate a body of researchers in Marine Sciences in the Azores” of the European Union. She was also supported by FCT – Foundation for Science and Technology, I.P., under the project UIDB/05634/2020 and UIDP/05634/2020 and through the Regional Government of the Azores through the initiative to support the Research Centers of the University of the Azores and through the project M1.1.A/REEQ.CIENTÍFICO UI&D/2021/010.
Conflict of Interest
The authors declare that the research was conducted in the absence of any commercial or financial relationships that could be construed as a potential conflict of interest.
Publisher’s Note
All claims expressed in this article are solely those of the authors and do not necessarily represent those of their affiliated organizations, or those of the publisher, the editors and the reviewers. Any product that may be evaluated in this article, or claim that may be made by its manufacturer, is not guaranteed or endorsed by the publisher.
Acknowledgments
The authors acknowledge the support of the InterRidge office in Paris, Nadine Lebris, Jérôme Dyment and Kamil Szafrański. Thanks to Jina Mousseau (ONC) for creating Figure 3. We thank the captains and crews of the research vessels that enabled infrastructure maintenance: RV Pourquoi Pas?, RV L’Atalante, RV Thalassa, CCGS John P. Tully, RV Thomas G Thompson, EV Nautilus. We are also grateful to the pilots of the submersible Nautile, Victor6000, ROPOS, Hercules, Argus, Odysseus. The work reviewed in this paper would have not been possible without the help of all mechanical, electric and computer engineers and technicians from Ifremer (RDT and RIC), ONC and OOI. The working group was supported by InterRidge (2017-2020). Other participants in IMOVE Working Group: Lise Artigue, Loïc Van Audenhaege, Valérie Ballu, Catherine Borremans, Greace Crystle, Cécile Cathalot, Jerome Dyment, Aida Farough, Anne Godfroy, Sabine Gollner, Zhikui Guo, Orest Kawka, Noé Lahaye, Marie Eide Lien, Anna Lim, Jean-Arthur Olive, Giuliana Panieri, Rolf Birger Pedersen, Eoghan Reeves, Celine Rommevaux, Guillaume Roullet, Lars Rüpke, Ben Snook, Adam Soule, Kamil Szafranski, Julie Tourolle, Benjamin Wheeler. Finally the group would like to thank two reviewers K. Anantharaman and D. M. Toma and Editor Jacopo Aguzzi for their comments.
References
Afanasyev A., Blundy J., Melnik O., Sparks S. (2018). Formation of Magmatic Brine Lenses via Focussed Fluid-Flow Beneath Volcanoes. Earth Planet. Sci. Lett. 486, 119–128. doi: 10.1016/j.epsl.2018.01.013
Aguzzi J., Chatzievangelou D., Marini S., Fanelli E., Danovaro R., Flögel S., et al. (2019). New High-Tech Interactive and Flexible Networks for the Future Monitoring of Deep-Sea Ecosystems. Environ. Sci. Technol. 53, 6616–6631. doi: 10.1021/acs.est.9b00409
Aguzzi J., Flögel S., Marini S., Thomsen L., lbiez J., Weiss P., et al. (2022). Developing Technological Synergies Between Deep-Sea and Space Research. Elementa Sci. Anthropocene 10, 1–9. doi: 10.1525/elementa.2021.00064
Alfaro-Lucas J. M., Pradillon F., Zeppilli D., Michel L. N., Martinez-Arbizu P., Tanaka H., et al. (2020). High Environmental Stress and Productivity Increase Functional Diversity Along a Deep-Sea Hydrothermal Vent Gradient. Ecology 101, 1–13. doi: 10.1002/ecy.3144
Arnulf A. F., Harding A. J., Kent G. M., Carbotte S. M., Canales J. P., Nedimović M. R. (2014). Anatomy of an active submarine volcano. Geology 42, 655–658. doi: 10.1130/G35629.1
Arnulf A. F., Harding A. J., Kent G. M., Wilcock W. S. D. (2018). Structure, Seismicity, and Accretionary Processes at the Hot Spot-Influenced Axial Seamount on the Juan de Fuca Ridge. J. Geophys. Res. Solid Earth 123, 4618–4646. doi: 10.1029/2017JB015131
Artigue L., Wyatt N. J., Lacan F., Mahaffey C., Lohan M. C. (2021). The Importance of Water Mass Transport and Dissolved-Particle Interactions on the Aluminum Cycle in the Subtropical North Atlantic. Global Biogeochem. Cycles 35, e2020GB006569. doi: 10.1029/2020GB006569
Baker E. T., Walker S. L., Chadwick W. W., Butterfield D. A., Buck N. J., Resing J. A. (2019). Posteruption Enhancement of Hydrothermal Activity: A 33-Year, Multieruption Time Series at Axial Seamount (Juan de Fuca Ridge). Geochem. Geophys. Geosystems 20, 814–828. doi: 10.1029/2018GC007802
Baldrighi E., Zeppilli D., Crespin R., Chauvaud P., Pradillon F., Sarrazin J. (2018). Colonization of Synthetic Sponges at the Deep-Sea Lucky Strike Hydrothermal Vent Field (Mid-Atlantic Ridge): A First Insight. Mar. Biodivers. 48, 89–103. doi: 10.1007/s12526-017-0811-3
Ballard R. D., Van Andel T. H. (1977). Project FAMOUS: Operational Techniques and American Submersible Operations. GSA Bull. 88, 495–506. doi: 10.1130/0016-7606(1977)88<495:PFOTAA>2.0.CO;2
Barge L. M., Flores E., Baum M. M., Velde D. G. V., Russell M. J. (2019). Redox and pH Gradients Drive Amino Acid Synthesis in Iron Oxyhydroxide Mineral Systems. Proc. Natl. Acad. Sci. U. S. A. 116, 4828–4833. doi: 10.1073/pnas.1812098116
Barnes C. R., Tunnicliffe V. (2008). “Building the World’s First Multi-Node Cabled Ocean Observatories (NEPTUNE Canada and VENUS, Canada): Science, Realities, Challenges and Opportunities,” in OCEANS 2008 - MTS/IEEE Kobe Techno-Ocean. 1–8 (IEEE). doi: 10.1109/OCEANSKOBE.2008.4531076
Barreyre T., Escartin J., Sohn R., Cannat M. (2014a). Permeability of the Lucky Strike Deep-Sea Hydrothermal System: Constraints From the Poroelastic Response to Ocean Tidal Loading. Earth Planet. Sci. Lett. 408, 146–154. doi: 10.1016/j.epsl.2014.09.049
Barreyre T., Escartı́n J., Garcia R., Cannat M., Mittelstaedt E., Prados R. (2012). Structure, Temporal Evolution, and Heat Flux Estimates From the Lucky Strike Deep-Sea Hydrothermal Field Derived From Seafloor Image Mosaics. Geochemistry, Geophys. Geosystems 13, 4Q04007. doi: 10.1029/2011GC003990
Barreyre T., Escartı́n J., Sohn R. A., Cannat M., Ballu V., Crawford W. C. (2014b). Temporal Variability and Tidal Modulation of Hydrothermal Exit-Fluid Temperatures at the Lucky Strike Deep-Sea Vent Field, Mid-Atlantic Ridge. J. Geophys. Res. Solid Earth. 119(4), 2543–2566. doi: 10.1002/2013JB010478
Barreyre T., Olive J. A., Crone T. J., Sohn R. A. (2018). Depth-Dependent Permeability and Heat Output at Basalt-Hosted Hydrothermal Systems Across Mid-Ocean Ridge Spreading Rates. Geochem. Geophys. Geosystems 19, 1259–1281. doi: 10.1002/2017GC007152
Barreyre T., Parnell-Turner R., Wu J. N., Fornari D. J. (2022). Tracking Crustal Permeability and Hydrothermal Response During Seafloor Eruptions at the East Pacific Rise, 9° 50’n. Geophys. Res. Lett. 49 (3), e2021GL095459. doi: 10.1029/2021GL095459
Barreyre T., Sohn R. A. (2016). Poroelastic Response of Mid-Ocean Ridge Hydrothermal Systems to Ocean Tidal Loading: Implications for Shallow Permeability Structure. Geophys. Res. Lett. 43, 1660–1668. doi: 10.1002/2015GL066479
Barriga F. J. A. S., Colaço A., Escartı́n J., Hooft E., Humphris S. E., Le Bris N., et al. (2005). Long-Term Monitoring of the Mid-Atlantic Ridge: Proceedings of the III MOMAR Workshop. Lisbon (Portugal) 63, 7–9.
Beaulieu S. E., Szafranski K. M. (2020). InterRidge Global Database of Active Submarine Hydrothermal Vent Fields Version 3.4. PANGAEA. doi: 10.1594/PANGAEA.917894
Billings A., Kaiser C., Young C. M., Hiebert L. S., Cole E., Wagner J. K. S., et al. (2017). SyPRID Sampler: A Large-Volume, High-Resolution, Autonomous, Deep-Ocean Precision Plankton Sampling System. Deep Sea Res. Part II Top. Stud. Oceanogr. 137, 297–306. doi: 10.1016/j.dsr2.2016.05.007
Blandin J., Colaço A., Legrand J., Cannat M., Sarradin P., Sarrazin J. (2010). The MoMAR-D Project: A Challenge to Monitor in Real Time the Lucky Strike Hydrothermal Vent Field. ICES J. Mar. Sci. 68, 416–424. doi: 10.1093/icesjms/fsq075
Boschen R. E., Rowden A. A., Clark M. R., Gardner J. P. A. (2013). Mining of Deep-Sea Seafloor Massive Sulfides: A Review of the Deposits, Their Benthic Communities, Impacts From Mining, Regulatory Frameworks and Management Strategies. Ocean Coast. Manage. 84, 54–67. doi: 10.1016/j.ocecoaman.2013.07.005
Bower D. J., Gurnis M., Seton M. (2013). Lower Mantle Structure From Paleogeographically Constrained Dynamic Earth Models. Geochem. Geophys. Geosystems 14, 44–63. doi: 10.1029/2012GC004267
Boyd P. W., Ellwood M. J. (2010). The Biogeochemical Cycle of Iron in the Ocean. Nat. Geosci. 3, 675–682. doi: 10.1038/ngeo964
Bracco A., Choi J., Joshi K., Luo H., McWilliams J. C. (2016). Submesoscale Currents in the Northern Gulf of Mexico: Deep Phenomena and Dispersion Over the Continental Slope. Ocean Model. 101, 43–58. doi: 10.1016/j.ocemod.2016.03.002
Breusing C., Biastoch A., Drews A., Metaxas A., Jollivet D., Vrijenhoek R. C., et al. (2016). Biophysical and Population Genetic Models Predict the Presence of “Phantom” Stepping Stones Connecting Mid-Atlantic Ridge Vent Ecosystems. Curr. Biol. 26, 2257–2267. doi: 10.1016/j.cub.2016.06.062
Burd B. J., Thomson R. E. (2012). Estimating Zooplankton Biomass Distribution in the Water Column Near the Endeavour Segment of Juan De Fuca Ridge Using Acoustic Backscatter and Concurrently Towed Nets. Oceanography 25, 269–276. doi: 10.5670/oceanog.2012.25
Burd B. J., Thomson R. E. (2019). Seasonal Patterns in Deep Acoustic Backscatter Layers Near Vent Plumes in the Northeastern Pacific Ocean. FACETS 4, 183–209. doi: 10.1139/facets-2018-0027
Butterfield D. A., Roe K. K., Lilley M. D., Huber J. A., Baross J. A., Embley R. W., et al. (2004). “Mixing, Reaction and Microbial Activity in the Sub-Seafloor Revealed by Temporal and Spatial Variation in Diffuse Flow Vents at Axial Volcano,” in Subseafloor Biosph. Mid-Ocean Ridges. eds Wilcock W. S., Delong E. F., Kelley D. S., Baross J. A., Craig S. (Geophysical Monograph Series) 144, 269–289. doi: 10.1029/144GM17
Cannat M., Sarradin P., Blandin J., Ballu V., Barreyre T., Chavagnac V., et al. (2016). EMSO-Azores : Monitoring Seafloor and Water Column Processes at the Mid-Atlantic Ridge. Fix03 - Proj. Newsl. Serv. Act. Spec. 3, 11. https://archimer.ifremer.fr/doc/00353/46444/46194.pdf.
Cannat M., Sarradin P., Blandin J., Escartı́n J., Party M. D. S., Aron M., et al (2011). MoMar-Demo at Lucky Strike. A Near-Real Time Multidisciplinary Observatory of Hydrothermal Processes and Ecosystems at the Mid-Atlantic Ridge. AGU Fall Meeting Abstracts 2011, OS22A–05.
Cann J. R., Elderfield H., Laughton A., Butterfield D. A., Jonasson I. R., Massoth G. J., et al. (1997). Seafloor Eruptions and Evolution of Hydrothermal Fluid Chemistry. Philos. Trans. R. Soc Lond. Ser. A Math. Phys. Eng. Sci. 355, 369–386. doi: 10.1098/rsta.1997.0013
Caplan-Auerbach J., Dziak R. P., Haxel J., Bohnenstiehl D. R., Garcia C. (2017). Explosive Processes During the 2015 Eruption of Axial Seamount, as Recorded by Seafloor Hydrophones. Geochem. Geophys. Geosystems 18, 1761–1774. doi: 10.1002/2016GC006734
Carbotte S. M., Arnulf A., Spiegelman M., Lee M., Harding A., Kent G., et al. (2020). Stacked Sills Forming a Deep Melt-Mush Feeder Conduit Beneath Axial Seamount. Geology 48, 693–697. doi: 10.1130/G47223.1
Cardona Y., Ruiz-Ramos D. V., Baums I. B., Bracco A. (2016). Potential Connectivity of Coldwater Black Coral Communities in the Northern Gulf of Mexico. PloS One 11. doi: 10.1371/journal.pone.0156257
Caress D. W., Clague D. A., Paduan J. B., Martin J. F., Dreyer B. M., Chadwick W. W., et al. (2012). Repeat Bathymetric Surveys at 1-Metre Resolution of Lava Flows Erupted at Axial Seamount in April 2011. Nat. Geosci. 5, 483–488. doi: 10.1038/ngeo1496
Cathalot C., Roussel E. G., Perhirin A., Creff V., Donval J. P., Guyader V., et al. (2021). Hydrothermal Plumes as Hotspots for Deep-Ocean Heterotrophic Microbial Biomass Production. Nat. Commun. 12, 4–13. doi: 10.1038/s41467-021-26877-6
Chadwick W. W., Clague D. A., Embley R. W., Perfit M. R., Butterfield D. A., Caress D. W., et al. (2013). The 1998 Eruption of Axial Seamount: New Insights on Submarine Lava Flow Emplacement From High-Resolution Mapping. Geochem. Geophys. Geosystems 14, 3939–3968. doi: 10.1002/ggge.20202
Chadwick W. W., Nooner S. L., Butterfield D. A., Lilley M. D. (2012). Seafloor Deformation and Forecasts of the April 2011 Eruption at Axial Seamount. Nat. Geosci. 5, 474–477. doi: 10.1038/ngeo1464
Chadwick W. W., Paduan J. B., Clague D. A., Dreyer B. M., Merle S. G., Bobbitt A. M., et al. (2016). Voluminous Eruption From a Zoned Magma Body After an Increase in Supply Rate at Axial Seamount. Geophys. Res. Lett. 43, 12,12–63,70. doi: 10.1002/2016GL071327
Chadwick W. W., Wilcock W. S. D., Nooner S. L., Beeson J. W., Sawyer A. M., Lau T.-K. (2022). Geodetic Monitoring at Axial Seamount Since Its 2015 Eruption Reveals a Waning Magma Supply and Tightly Linked Rates of Deformation and Seismicity. Geochem. Geophys. Geosystems 23, e2021GC010153. doi: 10.1029/2021GC010153
Chapman A. S. A., Beaulieu S. E., Colaço A., Gebruk A. V., Hilario A., Kihara T. C., et al. (2019). sFDvent: A Global Trait Database for Deep-Sea Hydrothermal-Vent Fauna. Glob. Ecol. Biogeogr. 28, 1538–1551. doi: 10.1111/geb.12975
Chatzievangelou D., Aguzzi J., Ogston A., Suárez A., Thomsen L. (2020). Visual Monitoring of Key Deep-Sea Megafauna With an Internet Operated Crawler as a Tool for Ecological Status Assessment. Prog. Oceanogr. 184, 102321. doi: 10.1016/j.pocean.2020.102321
Chavagnac V., Leleu T., Boulart C., Barreyre T., Castillo A., Menjot L., et al. (2015). “Deep-Sea Observatory EMSO-Azores (Lucky Strike, 37°17'N MAR): Impact of Fluid Circulation Pathway on Chemical Hydrothermal Fluxes,” in AGU Fall Meeting Abstracts, OS42A-07.
Chavagnac V., Leleu T., Fontaine F. J., Cannat M., Ceuleneer G., Castillo A. (2018a). Spatial Variations in Vent Chemistry at the Lucky Strike Hydrothermal Field, Mid-Atlantic Ridge (37°N): Updates for Subseafloor Flow Geometry From the Newly Discovered Capelinhos Vent. Geochem. Geophys. Geosystems 19, 4444–4458. doi: 10.1029/2018GC007765
Chavagnac V., Saleban Ali H., Jeandel C., Leleu T., Destrigneville C., Castillo A., et al. (2018b). Sulfate Minerals Control Dissolved Rare Earth Element Flux and Nd Isotope Signature of Buoyant Hydrothermal Plume (EMSO-Azores, 37°N Mid-Atlantic Ridge). Chem. Geol. 499, 111–125. doi: 10.1016/j.chemgeo.2018.09.021
Clague D., Paduan J., Caress D., Chadwick W. W., Le Saout M., Dreyer B., et al. (2017). High-Resolution AUV Mapping and Targeted ROV Observations of Three Historical Lava Flows at Axial Seamount. Oceanography 30, 82–89. doi: 10.5670/oceanog.2017.426
Colaço A., Blandin J., Cannat M., Carval T., Chavagnac V., Connelly D. P., et al. (2011). MoMAR-D: A Technological Challenge to Monitor the Dynamics of the Lucky Strike Vent Ecosystem. ICES J. Mar. Sci. J. du Cons. 68, 416–424. doi: 10.1093/icesjms/fsq075
Coogan L. A., Attar A., Mihaly S. F., Jeffries M., Pope M. (2017). Near-Vent Chemical Processes in a Hydrothermal Plume: Insights From an Integrated Study of the Endeavour Segment. Geochem. Geophys. Geosystems 18, 1641–1660. doi: 10.1002/2016GC006747
Coppola L., Ntoumas M., Bozzano R., Bensi M., Hartman S. E., Charcos Llorens M., et al. (2016). Handbook of Best Practices for Open Ocean Fixed Observatories (European Commission, FixO3 project, FP7 Programme 2007-2013 under grant agreement n° 312463). doi: 10.25607/OBP-1488.
Corliss J. B., Dymond J., Gordon L. I., Edmond J. M., Von Herzen R. P., Ballard R. D., et al. (1979). Submarine Thermal Springs on the Galapagos Rift. Science (80-) 203, 1073–1083. doi: 10.1126/science.203.4385.1073
Costanzi R., Fenucci D., Manzari V., Micheli M., Morlando L., Terracciano D., et al. (2020). Interoperability Among Unmanned Maritime Vehicles: Review and First In-Field Experimentation. Front. Robot. AI 7. doi: 10.3389/frobt.2020.00091
Cotte L., Chavagnac V., Pelleter E., Laës-Huon A., Cathalot C., Dulaquais G., et al. (2020). Metal Partitioning After in Situ Filtration at Deep-Sea Vents of the Lucky Strike Hydrothermal Field (EMSO-Azores, Mid-Atlantic Ridge, 37 Degrees N). Deep Sea Res. Part I Oceanogr. Res. Pap. 157, 103204. doi: 10.1016/j.dsr.2019.103204
Cotte L., Omanović D., Waeles M., Laës A., Cathalot C., Sarradin P., et al. (2018). On the Nature of Dissolved Copper Ligands in the Early Buoyant Plume of Hydrothermal Vents. Environ. Chem. 15, 58–73. doi: 10.1071/EN17150
Coumou D., Driesner T., Weis P., Heinrich C. (2009). Phase Separation, Brine Formation, and Salinity Variation at Black Smoker Hydrothermal Systems. J. Geophys. Res. 114, B03212. doi: 10.1029/2008JB005764
Cowart D. A., Matabos M., Brandt M. I., Marticorena J., Sarrazin J. (2020). Exploring Environmental DNA (eDNA) to Assess Biodiversity of Hard Substratum Faunal Communities on the Lucky Strike Vent Field (Mid-Atlantic Ridge) and Investigate Recolonization Dynamics After an Induced Disturbance. Front. Mar. Sci. 6. doi: 10.3389/fmars.2019.00783
Crawford W. C., Rai A., Singh S. C., Cannat M., Escartı́n J., Wang H., et al. (2013). Hydrothermal Seismicity Beneath the Summit of Lucky Strike Volcano, Mid-Atlantic Ridge. Earth Planet. Sci. Lett. 373, 118–128. doi: 10.1016/j.epsl.2013.04.028
Crise A., d’Alcalà M. R., Mariani P., Petihakis G., Robidart J., Iudicone D., et al. (2018). A Conceptual Framework for Developing the Next Generation of Marine OBservatories (MOBs) for Science and Society. Front. Mar. Sci. 5. doi: 10.3389/fmars.2018.00318
Crone T. J., Wilcock W. S. D. (2005). Modeling the Effects of Tidal Loading on Mid-Ocean Ridge Hydrothermal Systems. Geochem. Geophys. Geosystems 6, Q07001. doi: 10.1029/2004GC000905
Crone T. J., Wilcock W. S. D., McDuff R. E. (2010). Flow Rate Perturbations in a Black Smoker Hydrothermal Vent in Response to a Mid-Ocean Ridge Earthquake Swarm. Geochem. Geophys. Geosystems 11, 1–13. doi: 10.1029/2009GC002926
Cuvelier D., Beesau J., Ivanenko V. N., Zeppilli D., Sarradin P., Sarrazin J. (2014a). First Insights Into Macro-and Meiofaunal Colonisation Patterns on Paired Wood/Slate Substrata at Atlantic Deep-Sea Hydrothermal Vents. Deep Sea Res. Part I Oceanogr. Res. Pap. 87, 70–81. doi: 10.1016/j.dsr.2014.02.008
Cuvelier D., Legendre P., Laës-Huon A., Sarradin P., Sarrazin J. (2017). Biological and Environmental Rhythms in (Dark) Deep-Sea Hydrothermal Ecosystems. Biogeosciences 14, 2955–2977. doi: 10.5194/bg-14-2955-2017
Cuvelier D., Legendre P., Laes A., Sarradin P., Sarrazin J. (2014b). Rhythms and Community Dynamics of a Hydrothermal Tubeworm Assemblage at Main Endeavour Field–A Multidisciplinary Deep-Sea Observatory Approach. PloS One 9, e96924. doi: 10.1371/journal.pone.0096924
Cuvelier D., Sarrazin J., Colaço A., Copley J. T. P., Glover A. G., Tyler P. A., et al. (2011). Community Dynamics Over 14 Years at the Eiffel Tower Hydrothermal Edifice on the Mid-Atlantic Ridge. Limnol. Oceanogr. 56, 1624–1640. doi: 10.4319/lo.2011.56.5.1624
Daniel A., Laës-Huon A., Barus C., Beaton A. D., Blandfort D., Guigues N., et al. (2020). Toward a Harmonization for Using in Situ Nutrient Sensors in the Marine Environment. Front. Mar. Sci. 6. doi: 10.3389/fmars.2019.00773
Dañobeitia J. J., Pouliquen S., Johannessen T., Basset A., Cannat M., Gerrit Pfeil B., et al. (2020). Toward a Comprehensive and Integrated Strategy of the European Marine Research Infrastructures for Ocean Observations. Front. Mar. Sci. 7. doi: 10.3389/fmars.2020.00180
Danovaro R., Aguzzi J., Fanelli E., Billet D., Gjerde K., Jamieson A., et al. (2017a). A New International Ecosystem-Based Strategy for the Global Deep Ocean. Science 355, 452–454. doi: 10.1126/science.aah7178
Danovaro R., Corinaldesi C., Dell’Anno A., Snelgrove P. V. R. (2017b). The Deep-Sea Under Global Change. Curr. Biol. 27(11), R461–R465. doi: 10.1016/j.cub.2017.02.046
Danovaro R., Fanelli E., Aguzzi J., Billett D., Carugati L., Corinaldesi C., et al. (2020). Ecological Variables for Developing a Global Deep-Ocean Monitoring and Conservation Strategy. Nat. Ecol. Evol. 4, 181–192. doi: 10.1038/s41559-019-1091-z
Delaney J. R., Kelley D. S., Marburg A., Stoermer M., Hadaway H., Juniper S. K., et al. (2016). “Axial Seamount - Wired and Restless: A Cabled Submarine Network Enables Real-Time, Tracking of a Mid-Ocean Ridge Eruption and Live Video of an Active Hydrothermal System Juan De Fuca Ridge, NE Pacific,” in OCEANS 2016 MTS/IEEE Monterey. 1–8. doi: 10.1109/OCEANS.2016.7761484
Devine B. M., Baker K. D., Edinger E. N., Fisher J. A. D. (2020). Habitat Associations and Assemblage Structure of Demersal Deep-Sea Fishes on the Eastern Flemish Cap and Orphan Seamount. Deep. Res. Part I Oceanogr. Res. Pap. 157, 103210. doi: 10.1016/j.dsr.2019.103210
DFO (2006). “Identification of Ecologically Significant Species and Community Properties,” in DFO Can. Sci. Advis. Sec. Sci. Advis. Rep. 2006/04.
Dick G. J. (2019). The Microbiomes of Deep-Sea Hydrothermal Vents: Distributed Globally, Shaped Locally. Nat. Rev. Microbiol. 17, 271–283. doi: 10.1038/s41579-019-0160-2
Doya C., Aguzzi J., Pardo M., Matabos M., Company J. B., Costa C., et al. (2014). Diel Behavioral Rhythms in Sablefish (Anoplopoma Fimbria) and Other Benthic Species, as Recorded by the Deep-Sea Cabled Observatories in Barkley Canyon (NEPTUNE-Canada). J. Mar. Syst. 130, 69–78. doi: 10.1016/j.jmarsys.2013.04.003
Du Preez C., Fisher C. R. (2018). Long-Term Stability of Back-Arc Basin Hydrothermal Vents. Front. Mar. Sci. 5. doi: 10.3389/fmars.2018.00054
Durden J. M., Bett B. J., Huffard C. L., Pebody C., Ruhl H. A., Smith K. L. (2020). Response of Deep-Sea Deposit-Feeders to Detrital Inputs: A Comparison of Two Abyssal Time-Series Sites. Deep. Res. Part II Top. Stud. Oceanogr. 173, 104677. doi: 10.1016/j.dsr2.2019.104677
Dziak R. P., Smith D. K., Bohnenstiehl D. W. R., Fox C. G., Desbruyeres D., Matsumoto H., et al. (2004). Evidence of a Recent Magma Dike Intrusion at the Slow Spreading Lucky Strike Segment, Mid-Atlantic Ridge. J. Geophys. Res. Solid Earth 109, 1–15. doi: 10.1029/2004JB003141
Escartin J., Garcia R., Barreyre T., Cannat M., Gracias N., Shihavuddin A., et al. (2013). “Optical Methods to Monitor Temporal Changes at the Seafloor: The Lucky Strike Deep-Sea Hydrothermal Vent Field (Mid-Atlantic Ridge),” in 2013 IEEE International Underwater Technology Symposium (UT 2013). 1–6 (Tokyo, France: IEEE). doi: 10.1109/UT.2013.6519838
Escartı́n J., Barreyre T., Cannat M., Garcia R., Gracias N., Deschamps A., et al. (2015). Hydrothermal Activity Along the Slow-Spreading Lucky Strike Ridge Segment (Mid-Atlantic Ridge): Distribution, Heatflux, and Geological Controls. Earth Planet. Sci. Lett. 431, 173–185. doi: 10.1016/j.epsl.2015.09.025
Escartı́n J., Soule S. A., Cannat M., Fornari D. J., Düsünür D., Garcia R. (2014). Lucky Strike Seamount: Implications for the Emplacement and Rifting of Segment-Centered Volcanoes at Slow Spreading Mid-Ocean Ridges. Geochem. Geophys. Geosystems 15, 4157–4179. doi: 10.1002/2014GC005477
Favali P., Beranzoli L. (2009). EMSO: European Multidisciplinary Seafloor Observatory. Nucl. Instrum. Methods Phys. Res. Sect. A Accel. Spectrometers Detect. Assoc. Equip. 602, 21–27. doi: 10.1016/j.nima.2008.12.214
Fitzsimmons J., Boyle E. A., Jenkins W. J. (2014). Distal Transport of Dissolved Hydrothermal Iron in the Deep South Pacific Ocean. Proc. Natl. Acad. Sci. 111, 16654 16661. doi: 10.1073/pnas.1418778111
Fornari D. J., Von Damm K. L., Bryce J. G., Cowen J. P., Ferrini V., Fundis A., et al. (2012). The East Pacific Rise Between 9°N and 10°N: Twenty-Five Years of Integrated, Multidisciplinary Oceanic Spreading Center Studies. Oceanography 25, 18–43. doi: 10.5670/oceanog.2012.02
Fortunato C. S., Huber J. A. (2016). Coupled RNA-SIP and Metatranscriptomics of Active Chemolithoautotrophic Communities at a Deep-Sea Hydrothermal Vent. ISME J. 10, 1925–1938. doi: 10.1038/ismej.2015.258
Fortunato C. S., Larson B., Butterfield D. A., Huber J. A. (2018). Spatially Distinct, Temporally Stable Microbial Populations Mediate Biogeochemical Cycling at and Below the Seafloor in Hydrothermal Vent Fluids. Environ. Microbiol. 20, 769–784. doi: 10.1111/1462-2920.14011
Franke A., Blenckner T., Duarte C. M., Ott K., Fleming L. E., Antia A., et al. (2020). Operationalizing Ocean Health: Toward Integrated Research on Ocean Health and Recovery to Achieve Ocean Sustainability. One Earth 2, 557–565. doi: 10.1016/j.oneear.2020.05.013
Galaasen E. V., Ninnemann U. S., Kessler A., Irvalı N., Rosenthal Y., Tjiputra J., et al. (2020). Interglacial Instability of North Atlantic Deep Water Ventilation. Science 367, 1485–1489. doi: 10.1126/science.aay6381
Germanovich L. N., Hurt R. S., Smith J. E., Genc G., Lowell R. P. (2015). Journal of Geophysical Research : Solid Earth Hydrothermal Environments. J. Geophys. Res. Solid Earth 120, 8031–8055. doi: 10.1002/2015JB012245.Received
Girard F., Sarrazin J., Arnaubec A., Cannat M., Sarradin P., Wheeler B., et al. (2020). Currents and Topography Drive Assemblage Distribution on an Active Hydrothermal Edifice. Prog. Oceanogr. 187. doi: 10.1016/j.pocean.2020.102397
Glover A. G., Gooday A. J., Bailey D., Billet D. S. M., Chevaldonné P., Colaço A., et al. (2010). Temporal Change in Deep-Sea Benthic Ecosystems: A Review of the Evidence From Recent Time-Series Studies. Adv. Mar. Biol. 58, 1–95. doi: 10.1016/B978-0-12-381015-1.00001-0
Grand M. M., Laës-Huon A., Fietz S., Resing J. A., Obata H., Luther G. W. III, et al. (2019). Developing Autonomous Observing Systems for Micronutrient Trace Metals. Front. Mar. Sci. 6, 35. doi: 10.3389/fmars.2019.00035
Hand K. P., German C. R. (2018). Exploring Ocean Worlds on Earth and Beyond. Nat. Geosci. 11, 2–4. doi: 10.1038/s41561-017-0045-9
Hartman S. E., Lampitt R. S., Larkin K. E., Pagnani M., Campbell J., Gkritzalis T., et al. (2012). The Porcupine Abyssal Plain Fixed-Point Sustained Observatory (PAP-SO): Variations and Trends From the Northeast Atlantic Fixed-Point Time-Series. ICES J. Mar. Sci. 69, 776–783. doi: 10.1093/icesjms/fss077
Heidemann J., Stojanovic M., Zorzi M. (2012). Underwater Sensor Networks: Applications, Advances and Challenges. Philos. Trans. R. Soc A 370, 158–175. doi: 10.1098/rsta.2011.0214
Hooft E. E. E., Patel H., Wilcock W., Becker K., Butterfield D., Davis E., et al. (2010). A Seismic Swarm and Regional Hydrothermal and Hydrologic Perturbations: The Northern Endeavour Segment, February 2005. Geochem. Geophys. Geosystems 11, Q12015. doi: 10.1029/2010GC003264
Huber J. A., Butterfield D. A., Baross J. A. (2003). Bacterial Diversity in a Subseafloor Habitat Following a Deep-Sea Volcanic Eruption. FEMS Microbiol. Ecol. 43, 393–409. doi: 10.1016/S0168-6496(02)00451-8
Huber J. A., Crone T. J., Soule D. (2021). Integrating the Regional Cabled Array With Ocean Drilling to Facilitate Observatory-Based Subseafloor Science at Axial Seamount. Ocean Observatories Initiative Facility Board, Ocean Observatories Initiative (OOI) Science Plan: Exciting Science Opportunities Using OOI Data. doi: 10.23860/ooi-science-plan-2021-01
Huber J. A., David B. M. W., Morrison H. G., Huse S. M., Neal P. R., Butterfield D. A., et al. (2007). Microbial Population Structures in the Deep Marine Biosphere. Science (80-. ). 318 (5847), 97–100. doi: 10.1126/science.1146689
Husson B., Sarradin P., Zeppilli D., Sarrazin J. (2017). Picturing Thermal Niches and Biomass of Hydrothermal Vent Species. Deep. Res. Part II Top. Stud. Oceanogr. 137, 6–25. doi: 10.1016/j.dsr2.2016.05.028
Husson B., Sarrazin J., van Oevelen D., Sarradin P., Soetaert K., Menesguen A. (2018). Modelling the Interactions of the Hydrothermal Mussel Bathymodiolus Azoricus With Vent Fluid. Ecol. Modell. 377, 35–50. doi: 10.1016/j.ecolmodel.2018.03.007
IMOVE, working group (2019) IMOVE - Integrating Multidisciplinary Observations in Vent Environments. An InterRidge Working Group. Workshop, Feb. 6-8, Bergen, Norway. Available at: https://archimer.ifremer.fr/doc/00623/73522/.
Jahanbakht M., Xiang W., Hanzo L., Azghadi M. R. (2021). Internet of Underwater Things and Big Marine Data Analytics—A Comprehensive Survey. IEEE Commun. Surv. Tutor. 23, 904–956. doi: 10.1109/COMST.2021.3053118
Juniper S. K., Thornborough K., Douglas K., Hillier J. (2019). Remote Monitoring of a Deep-Sea Marine Protected Area: The Endeavour Hydrothermal Vents. Aquat. Conserv. Mar. Freshw. Ecosyst. 29, 1–19. doi: 10.1002/aqc.3020
Jupp T. E., Schultz A. (2004). Physical Balances in Subseafloor Hydrothermal Convection Cells. J. Geophys. Res. Solid Earth 109, 1–12. doi: 10.1029/2003JB002697
Kelley D. S., Carbotte S. M., Caress D. W., Clague D. A., Delaney J. R., Gill J. B., et al. (2012). Endeavour Segment of the Juan De Fuca Ridge: One of the Most Remarkable Places on Earth. Oceanography 25, 44–61. doi: 10.5670/oceanog.2012.03
Kelley D. S., Delaney J. R., Juniper S. K. (2014). Establishing a New Era of Submarine Volcanic Observatories: Cabling Axial Seamount and the Endeavour Segment of the Juan De Fuca Ridge. Mar. Geol. 352, 426–450. doi: 10.1016/j.margeo.2014.03.010
Kelley D. S., Delaney J. R., Team C. A. (2016). “NSF’s Cabled Array: A Wired Tectonic Plate and Overlying Ocean,” in Ocean. 2016 MTS/IEEE Monterey, OCE 2016. doi: 10.1109/OCEANS.2016.7761398
Kelley D. S., Karson J. A., Früh-Green Gretchen L., Yoerger D. R., Shank T. M., Butterfield D. A., et al. (2005). A Serpentinite-Hosted Ecosystem: The Lost City Hydrothermal Field. Science (80-. ). 307 (5714), 1428–1434. doi: 10.1126/science.1102556
Laës-Huon A., Davy R., Thomas L., Legrand J., Le Piver D., Rousseaux P., et al. (2020). CHEMINI: Chemical Miniaturised Analyser for in situ Monitoring of Macronutrients and Bioactive Metals in Marine Waters. Ocean Science meeting AGU (San Diego), 16-02 / 21-02 2020.
Laës-Huon A., Cathalot C., Legrand J., Tanguy V., Sarradin P. (2016). Long-Term In Situ Survey of Reactive Iron Concentrations at the EMSO-Azores Observatory. IEEE J. Ocean. Eng. 41, 744–752. doi: 10.1109/JOE.2016.2552779
Lahaye N., Gula J., Roullet G. (2020). Internal Tide Cycle and Topographic Scattering Over the North Mid-Atlantic Ridge. J. Geophys. Res. Ocean. 125, 1–21. doi: 10.1029/2020jc016376
Lahaye N., Gula J., Thurnherr A. M., Reverdin G., Bouruet-Aubertot P., Roullet G. (2019). Deep Currents in the Rift Valley of the North Mid-Atlantic Ridge. Front. Mar. Sci. 6. doi: 10.3389/fmars.2019.00597
Langmuir C. H., Humphris S. E., Fornari D. J., Van Dover C. L., Von Damm K., Tivey M. K., et al. (1997). Hydrothermal Vents Near a Mantle Hot Spot: The Lucky Strike Vent Field at 37°N on the Mid-Atlantic Ridge. Earth Planet. Sci. Lett. 148, 69–91. doi: 10.1016/S0012-821X(97)00027-7
Lantéri N., Ruhl H. A., Gates A., Martínez E., del Rio Fernandez J., Aguzzi J., et al. (2022). The EMSO Generic Instrument Module (EGIM): Standardized and Interoperable Instrumentation for Ocean Observation. Front. Mar. Sci., 9. doi: 10.3389/fmars.2022.801033
Laroche O., Kersten O., Smith C. R., Goetze E. (2020). From Sea Surface to Seafloor: A Benthic Allochthonous eDNA Survey for the Abyssal Ocean. Front. Mar. Sci. 7. doi: 10.3389/fmars.2020.00682
Larson B. I., Olson E. J., Lilley M. D. (2007). In Situ Measurement of Dissolved Chloride in High Temperature Hydrothermal Fluids. Geochim. Cosmochim. Acta 71, 2510–2523. doi: 10.1016/j.gca.2007.02.013
Le Bris N., Yücel M., Das A., Sievert S. M., LokaBharathi P. P., Girguis P. R. (2019). Hydrothermal Energy Transfer and Organic Carbon Production at the Deep Seafloor. Front. Mar. Sci. 5. doi: 10.3389/fmars.2018.00531
Lee R. W., Robert K., Matabos M., Bates A. E., Juniper S. K. (2015). Temporal and Spatial Variation in Temperature Experienced by Macrofauna at Main Endeavour Hydrothermal Vent Field. Deep. Res. Part I Oceanogr. Res. Pap. 106, 154–166. doi: 10.1016/j.dsr.2015.10.004
Legrand J., Sarradin P., Cannat M. (2019). EMSO Azores Deep-Sea Observatory: 9 Years of Operations. EGU General Assembly 2019 (Vienna) https://archimer.ifremer.fr/doc/00489/60087/.
Leleu T. (2017). Variabilité Spatio-Temporelle De La Composition Des Fluides Hydrothermaux (Observatoire Fond De Mer EMSO-Açores, Lucky Strike): Traçage De La Circulation Hydrothermale Et Quantification Des Flux Chimiques Associées / Spatial and Temporal Variability of the Composition of Hydrothermal Fluids (Deep Sea Observatory EMSO-Azores, Lucky Strike): Tracing the Hydrothermal Pathway and Quantification of the Associated Chemical Fluxes. PhD Thesis (Université De Toulouse). Available at: https://archimer.ifremer.fr/doc/00692/80380/.
Lelièvre Y., Legendre P., Matabos M., Mihaly S., Lee R. W., Sarradin P., et al. (2017). Astronomical and Atmospheric Impacts on Deep-Sea Hydrothermal Vent Invertebrates. Proc. R. Soc Lond. B Biol. Sci. 284, 20162123. doi: 10.1098/rspb.2016.2123
Lelièvre Y., Sarrazin J., Marticorena J., Schaal G., Day T., Legendre P., et al. (2018). Biodiversity and Trophic Ecology of Hydrothermal Vent Fauna Associated With Tubeworm Assemblages on the Juan De Fuca Ridge. Biogeosciences 15, 2629–2647. doi: 10.5194/bg-15-2629-2018
Levin L. A., Baco A. R., Bowden D. A., Colaço A., Cordes E. E., Cunha M. R., et al. (2016). Hydrothermal Vents and Methane Seeps: Rethinking the Sphere of Influence. Front. Mar. Sci. 3, 766–768. doi: 10.3389/fmars.2016.00072
Levin L. A., Bett B. J., Gates A. R., Heimbach P., Howe B. M., Janssen F., et al. (2019). Global Observing Needs in the Deep Ocean. Front. Mar. Sci. 6. doi: 10.3389/fmars.2019.00241
Levin L. A., Le Bris N. (2015). The Deep Ocean Under Climate Change. Science (80-. ) 350, 766–768. doi: 10.1126/science.aad0126
Levin L. A., Wei C. L., Dunn D. C., Amon D. J., Ashford O. S., Cheung W. W. L., et al. (2020). Climate Change Considerations are Fundamental to Management of Deep-Sea Resource Extraction. Glob. Change Biol. 26, 4664–4678. doi: 10.1111/gcb.15223
Lewis K., Lowell R. P. (2009). Numerical Modeling of Two-Phase Flow in the NaCl-H2O System: Introduction of a Numerical Method and Benchmarking. J. Geophys. Res. 114, B05202. doi: 10.1029/2008JB006029
Lombard F., Boss E., Waite A., Uitz H. M., Stemmann J., Sosik J., et al (2019). Globally Consistent Quantitative Observations of Planktonic Ecosystems. Front. Mar. Sci. 6. doi: 10.3389/fmars.2019.00196
Lutz R. A., Shank T. M., Luther G. W., Vetriani C., Tolstoy M., Nuzzio D. B., et al. (2008). Interrelationships Between Vent Fluid Chemistry, Temperature, Seismic Activity, and Biological Community Structure at a Mussel-Dominated, Deep-Sea Hydrothermal Vent Along the East Pacific Rise. J. Shellfish Res. 27, 177–190. doi: 10.2983/0730-8000(2008)27[177:IBVFCT]2.0.CO;2
Marcus J., Tunnicliffe V., Butterfield D. A. (2009). Post-Eruption Succession of Macrofaunal Communities at Diffuse Flow Hydrothermal Vents on Axial Volcano, Juan De Fuca Ridge, Northeast Pacific. Deep Sea Res. Part II Top. Stud. Oceanogr. 56, 1586–1598. doi: 10.1016/j.dsr2.2009.05.004
Mariani P., Bachmayer R., Kosta S., Pietrosemoli E., Ardelan M. V., Connelly D. P., et al. (2021). Collaborative Automation and IoT Technologies for Coastal Ocean Observing Systems. Front. Mar. Sci. 8. doi: 10.3389/fmars.2021.647368
Marjanović M., Barreyre T., Fontaine F. J., Escartı́n J. (2019). Investigating Fine-Scale Permeability Structure and Its Control on Hydrothermal Activity Along a Fast-Spreading Ridge (the East Pacific Rise, 9°43′–53′N) Using Seismic Velocity, Poroelastic Response, and Numerical Modeling. Geophys. Res. Lett. 46, 11799–11810. doi: 10.1029/2019GL084040
Marticorena J., Matabos M., Ramirez-Llodra E., Cathalot C., Laës-Huon A., Leroux R., et al. (2021). Recovery of Hydrothermal Vent Communities in Response to an Induced Disturbance at the Lucky Strike Vent Field (Mid-Atlantic Ridge). Mar. Environ. Res. 168, 105316. doi: 10.1016/j.marenvres.2021.105316
Marticorena J., Matabos M., Ramirez-Llodra E., Sarrazin J. (2020). Contrasting Reproductive Biology of Two Hydrothermal Gastropods From the Mid − Atlantic Ridge : Implications for Resilience of Vent Communities. Mar. Biol. 167, 1–19. doi: 10.1007/s00227-020-03721-x
Martin W., Baross J., Kelley D. S., Russell M. (2008). Hydrothermal Vents and the Origin of Life. Nat. Rev. Micro. 6 (11), 805–814. doi: 10.1038/nrmicro1991
Matabos M., Best M., Blandin J., Hoeberechts M., Juniper S. K., Pirenne B., et al. (2016). “Seafloor Observatories,” in Biological Sampling in the Deep Sea. Eds. Clark M. R., Consalvey M., Rowden A. A. (Blackwell, Chichester, UK: Wiley), 306–337. doi: 10.1002/9781118332535.ch14
Matabos M., Bui A. O. V., Mihaly S., Aguzzi J., Juniper S. K., Ajayamohan R. S. (2014). High-Frequency Study of Epibenthic Megafaunal Community Dynamics in Barkley Canyon: A Multi-Disciplinary Approach Using the NEPTUNE Canada Network. J. Mar. Syst. 130, 56–68. doi: 10.1016/j.jmarsys.2013.05.002
Matabos M., Cuvelier D., Brouard J., Shillito B., Ravaux J., Zbinden M., et al. (2015). Behavioural Study of Two Hydrothermal Crustacean Decapods: Mirocaris Fortunata and Segonzacia Mesatlantica, From the Lucky Strike Vent Field (Mid-Atlantic Ridge). Deep Sea Res. Part II Top. Stud. Oceanogr. 121, 146–158. doi: 10.1016/j.dsr2.2015.04.008
Mat A. M., Sarrazin J., Markov G. V., Apremont V., Dubreuil C., Eché C., et al. (2020). Biological Rhythms in the Deep-Sea Hydrothermal Mussel Bathymodiolus Azoricus. Nat. Commun. 11, 1–12. doi: 10.1038/s41467-020-17284-4
Matsuura M. (2021). Recent Advancement in Power-Over-Fiber Technologies. Photonics 8, 335. doi: 10.3390/photonics8080335
McCoy D., Bianchi D., Stewart A. L. (2020). Global Observations of Submesoscale Coherent Vortices in the Ocean. Prog. Oceanogr. 189, 102452. doi: 10.1016/j.pocean.2020.102452
Menini E., Van Dover C. L. (2019). An Atlas of Protected Hydrothermal Vents. Mar. Policy 108, 103654. doi: 10.1016/j.marpol.2019.103654
Meyer J., Akerman N., Proskurowski G., Huber J. (2013). Microbiological Characterization of Post-Eruption “Snowblower” Vents at Axial Seamount, Juan De Fuca Ridge. Front. Microbiol. 4. doi: 10.3389/fmicb.2013.00153
Mitarai S., Watanabe H., Nakajima Y., Shchepetkin A. F., McWilliams J. C. (2016). Quantifying Dispersal From Hydrothermal Vent Fields in the Western Pacific Ocean. Proc. Natl. Acad. Sci. U. S. A. 113, 2976–2981. doi: 10.1073/pnas.1518395113
Mittelstaedt E., Davaille A., Van Keken P. E., Gracias N., Escartin J. (2010). A Noninvasive Method for Measuring the Velocity of Diffuse Hydrothermal Flow by Tracking Moving Refractive Index Anomalies. Geochem. Geophys. Geosystems 11, Q10005. doi: 10.1029/2010GC003227
Mittelstaedt E., Escartı́n J., Gracias N., Olive J. A., Barreyre T., Davaille A., et al. (2012). Quantifying Diffuse and Discrete Venting at the Tour Eiffel Vent Site, Lucky Strike Hydrothermal Field. Geochem. Geophys. Geosystems 13, Q04008. doi: 10.1029/2011GC003991
Mittelstaedt E., Fornari D. J., Crone T. J., Kinsey J., Kelley D. S., Elend M. (2016). Diffuse Venting at the ASHES Hydrothermal Field: Heat Flux and Tidally Modulated Flow Variability Derived From in Situ Time-Series Measurements. Geochem. Geophys. Geosystems 17, 1435–1453. doi: 10.1002/2015GC006144
Mullineaux L. S., McGillicuddy D. J., Mills S. W., Kosnyrev V. K., Thurnherr A. M., Ledwell J. R., et al. (2013). Active Positioning of Vent Larvae at a Mid-Ocean Ridge. Deep Sea Res. Part II Top. Stud. Oceanogr. 92, 46–57. doi: 10.1016/j.dsr2.2013.03.032
Mullineaux L. S., Metaxas A., Beaulieu S. E., Bright M., Gollner S., Grupe B. M., et al. (2018). Exploring the Ecology of Deep-Sea Hydrothermal Vents in a Metacommunity Framework. Front. Mar. Sci. 5. doi: 10.3389/fmars.2018.00049
Mullineaux L. S., Mills S. W., Le Bris N., Beaulieu S. E., Sievert S. M., Dykman L. N. (2020). Prolonged Recovery Time After Eruptive Disturbance of a Deep-Sea Hydrothermal Vent Community. Proc. R. Soc B. 287, 20202070. doi: 10.1098/rspb.2020.2070
Murdock S., Tunnicliffe V., Boschen-Rose R. E., Juniper S. K. (2021). Emergent “Core Communities” of Microbes, Meiofauna and Macrofauna at Hydrothermal Vents. ISME Commun. 1, 27. doi: 10.1038/s43705-021-00031-1
National Research Council (2000). Illuminating the Hidden Planet: The Future of Seafloor Observatory Science (Washington, DC, USA: National Academy Press). doi: 10.17226/9920
Nees H. A., Moore T. S., Mullaugh K. M., Holyoke R. R., Janzen C. P., Ma S., et al. (2008). Hydrothermal Vent Mussel Habitat Chemistry, Pre- and Post-Eruption at 9°50′North on the East Pacific Rise. J. Shellfish Res. 27, 169–175. doi: 10.2983/0730-8000(2008)27[169:HVMHCP]2.0.CO;2
Nooner S. L., Chadwick W. W. (2016). Inflation-Predictable Behavior and Co-Eruption Deformation at Axial Seamount. Science (80-. ). 354 (6318), 1399–1403. doi: 10.1126/science.aah4666
Olins H. C., Rogers D. R., Preston C., Ussler W., Pargett D., Jensen S., et al. (2017). Co-Registered Geochemistry and Metatranscriptomics Reveal Unexpected Distributions of Microbial Activity Within a Hydrothermal Vent Field. Front. Microbiol. 8. doi: 10.3389/fmicb.2017.01042
Ondréas H., Cannat M., Fouquet Y., Normand A., Sarradin P., Sarrazin J. (2009). Recent Volcanic Events and the Distribution of Hydrothermal Venting at the Lucky Strike Hydrothermal Field, Mid-Atlantic Ridge. Geochem. Geophys. Geosystems 10, Q02006. doi: 10.1029/2008GC002171
Opatkiewicz A. D., Butterfield D. A., Baross J. A. (2009). Individual Hydrothermal Vents at Axial Seamount Harbor Distinct Subseafloor Microbial Communities. FEMS Microbiol. Ecol. 70, 413–424. doi: 10.1111/j.1574-6941.2009.00747.x
Orcutt B. N., Bradley J. A., Brazelton W. J., Estes E. R., Goordial J. M., Huber J. A., et al. (2020). Impacts of Deep-Sea Mining on Microbial Ecosystem Services. Limnol. Oceanogr. 65, 1489–1510. doi: 10.1002/lno.11403
Palmer M. R., Ernst G. G. J. (1998). Generation of Hydrothermal Megaplumes by Cooling of Pillow Basalts at Mid-Ocean Ridges. Nature 393, 643–647. doi: 10.1038/31397%0A
Pereira H. M., Ferrier S., Walters M., Geller G. N., Jongman R. H. G., Scholes R. J., et al. (2013). Essential Biodiversity Variables. Science (80-. ). 339 (6117), 277–278. doi: 10.1126/science.1229931
Pester N. J., Reeves E. P., Rough M. E., Ding K., Seewald J. S., Seyfried W. E. (2012). Subseafloor Phase Equilibria in High-Temperature Hydrothermal Fluids of the Lucky Strike Seamount (Mid-Atlantic Ridge, 37°17’n). Geochm. Cosmochim. Acta 90, 303–322. doi: 10.1016/j.gca.2012.05.018
Picheral M., Catalano C., Brousseau D., Claustre H., Coppola L., Leymarie E., et al. (2021). The Underwater Vision Profiler 6: An Imaging Sensor of Particle Size Spectra and Plankton, for Autonomous and Cabled Platforms. Limnol. Oceanogr. Methods 20, 115–129. doi: 10.1002/lom3.10475 Association for the Sciences of Limnology and Oceanography.
Picheral M., Guidi L., Stemmann L., Karl D. M., Iddaoud G., Gorsky G. (2010). The Underwater Vision Profiler 5: An Advanced Instrument for High Spatial Resolution Studies of Particle Size Spectra and Zooplankton. Limnol. Oceanogr. Methods 8, 462–473. doi: 10.4319/lom.2010.8.462
Plum C., Pradillon F., Fujiwara Y., Sarrazin J. (2016). Copepod Colonization of Organic and Inorganic Substrata at a Deep-Sea Hydrothermal Vent Site on the Mid-Atlantic Ridge. Deep Sea Res. Part II Top. Stud. Oceanogr. 137, 335–348. doi: 10.1016/j.dsr2.2016.06.008
Portail M., Brandily C., Cathalot C., Colaço A., Gélinas Y., Husson B., et al. (2018). Food-Web Complexity Across Hydrothermal Vents on the Azores Triple Junction. Deep. Res. Part I Oceanogr. Res. Pap. 131, 101–120. doi: 10.1016/j.dsr.2017.11.010
Puzenat V., Escartı́n J., Martelat J. E., Barreyre T., Bauer S. L. M., Nomikou P., et al. (2021). Shallow-Water Hydrothermalism at Milos (Greece): Nature, Distribution, Heat Fluxes and Impact on Ecosystems. Mar. Geol. 438, 106521. doi: 10.1016/j.margeo.2021.106521
Qiu T., Zhao Z., Zhang T., Chen C., Chen C. L. P. (2019). Underwater Internet of Things in Smart Ocean: System Architecture and Open Issues. IEEE Trans. Ind. Inf. 16, 4297–4307. doi: 10.1109/TII.2019.2946618
Ramirez-Llodra E., Brandt A., Danovaro R., De Mol B., Escobar E. G., German C. R., et al. (2010). Deep, Diverse and Definitely Different: Unique Attributes of the World’s Largest Ecosystem. Biogeosciences 7, 2851–2899. doi: 10.5194/bg-7-2851-2010
Rogers A., Brierley A., Croot P., Cunha M., Danovaro R., Devey C., et al. (2015). “Delving Deeper: Critical Challenges for 21st Century Deep-Sea Research,” in Position Paper 22 of the European Marine Board. Eds. Larkin K. E., Donaldson K., McDonough N.(Ostend, Belgium: Position Paper 22 of the European Marine Board), 224 978-94-920431-1-5. doi: 10.13140/RG.2.1.4077.0009
Rommevaux C., Henri P., Degboe J., Chavagnac V., Lesongeur F., Godfroy A., et al. (2019). Prokaryote Communities at Active Chimney and In Situ Colonization Devices After a Magmatic Degassing Event (37°N MAR, EMSO-Azores Deep-Sea Observatory). Geochem. Geophys. Geosystems 20, 3065–3089. doi: 10.1029/2018GC008107
Rountree R. A., Aguzzi J., Marini S., Fanelli E., DE LEO F. C., Del Rio J., et al. (2020). “Towards an Optimal Design for Ecosystem-Level Ocean Observatories,” in Oceanography and Marine Biology 58, 79–105. doi: 10.1201/9780429351495-2
Rubin K. H., Soule S. A., Chadwick W. W., Fornari D. J., Clague D. A., Embley R. W., et al. (2012). Volcanic Eruptions in the Deep Sea. Oceanography 25, 143–157. doi: 10.5670/oceanog.2012.12
Santos R. S., Escartı́n J., Colaço A., Adamczewska (2002). Towards Planning of Seafloor Observatory Programs for the MAR Region: Proceedings of the II MoMAR Workshop. (Azores, Portugal), 14–17 (Suppl. 3), 64.
Sarrazin J., Blandin J., Delauney L., Dentrecolas S., Dorval P., Dupont J., et al. (2007). “TEMPO: A New Ecological Module for Studying Deep-Sea Community Dynamics at Hydrothermal Vents,” in OCEANS 2007-Europe. 1–4 (Ieee). doi: 10.1109/OCEANSE.2007.4302310
Sarrazin J., Cuvelier D., Peton L., Legendre P., Sarradin P. (2014). High-Resolution Dynamics of a Deep-Sea Hydrothermal Mussel Assemblage Monitored by the EMSO-Açores MoMAR Observatory. Deep Sea Res. Part I Oceanogr. Res. Pap. 90, 62–75. doi: 10.1016/j.dsr.2014.04.004
Sarrazin J., Legendre P., de Busserolles F., Fabri M.-C., Guilini K., Ivanenko V. N., et al. (2015). Biodiversity Patterns, Environmental Drivers and Indicator Species on a High-Temperature Hydrothermal Edifice, Mid-Atlantic Ridge. Deep Sea Res. Part II Top. Stud. Oceanogr. 121, 177–192. doi: 10.1016/j.dsr2.2015.04.013
Sarrazin J., Portail M., Legrand E., Cathalot C., Laes A., Lahaye N., et al. (2020). Endogenous Versus Exogenous Factors: What Matters for Vent Mussel Communities? Deep. Res. Part I Oceanogr. Res. Pap. 160, 103260. doi: 10.1016/j.dsr.2020.103260
Sarrazin J., Robigou V., Juniper S. K., Delaney J. R. (1997). Biological and Geological Dynamics Over Four Years on a High-Temperature Sulfide Structure at the Juan De Fuca Ridge Hydrothermal Observatory. Mar. Ecol. Prog. Ser. 153, 5–24. doi: 10.3354/meps153005
Sarrazin J., Rodier P., Tivey M. K., Singh H., Schultz A., Sarradin P. (2009). A Dual Sensor Device to Estimate Fluid Flow Velocity at Diffuse Hydrothermal Vents. Deep. Res. Part I Oceanogr. Res. Pap. 56, 2065–2074. doi: 10.1016/j.dsr.2009.06.008
Scheirer D. S., Shank T. M., Fornari D. J. (2006). Temperature Variations at Diffuse and Focused Flow Hydrothermal Vent Sites Along the Northern East Pacific Rise. Geochem. Geophys. Geosystems 7, Q03002. doi: 10.1029/2005GC001094
Schoening T., Purser A., Langenkämper D., Suck I., Taylor J., Cuvelier D., et al. (2020). Megafauna Community Assessment of Polymetallic-Nodule Fields With Cameras: Platform and Methodology Comparison. Biogeosciences 17, 3115–3133. doi: 10.5194/bg-17-3115-2020
Shank T. M., Fornari D. J., Von Damm K. L., Lilley M. D., Haymon R. M., Lutz R. A. (1998). Temporal and Spatial Patterns of Biological Community Development at Nascent Deep-Sea Hydrothermal Vents (9°50’n, East Pacific Rise). Deep. Res. Part II Top. Stud. Oceanogr. 45, 465–515. doi: 10.1016/S0967-0645(97)00089-1
Shillito B., Ravaux J., Sarrazin J., Zbinden M., Sarradin P., Barthelemy D. (2015). Long-Term Maintenance and Public Exhibition of Deep-Sea Hydrothermal Fauna: The AbyssBox Project. Deep. Res. Part II Top. Stud. Oceanogr. 121, 137–145. doi: 10.1016/j.dsr2.2015.05.002
Singh S. C., Crawford W. C., Carton H., Seher T., Combier V., Cannat M., et al. (2006). Discovery of a Magma Chamber and Faults Beneath a Mid-Atlantic Ridge Hydrothermal Field. Nature 442, 1029–1032. doi: 10.1038/nature05105
Sloyan B. M., Wilkin J., Hill K. L., Chidichimo M. P., Cronin M. F., Johannessen J. A., et al. (2019). Evolving the Global Ocean Observing System for Research and Application Services Through International Coordinatio. Front. Mar. Sci. 6. doi: 10.3389/fmars.2019.00449
Smith L. M., Barth J. A., Kelley D. S., Plueddemann A., Rodero I., Ulses G. A., et al. (2018). The Ocean Observatories Initiative. Oceanography 31, 16–35. doi: 10.5670/oceanog.2018.105
Smith K. L., Sherman A. D., McGill P. R., Henthorn R. G., Ferreira J., Connolly T. P., et al. (2021). Abyssal Benthic Rover, an Autonomous Vehicle for Long-Term Monitoring of Deep-Ocean Processes. Sci. Robot. 6, eabl4925. doi: 10.1126/scirobotics.abl4925
Smith C. R., Tunnicliffe V., Colaço A., Drazen J. C., Gollner S., Levin L. A., et al. (2020). Deep-Sea Misconceptions Cause Underestimation of Seabed-Mining Impacts. Trends Ecol. Evol. 35, 853–857. doi: 10.1016/j.tree.2020.07.002
Sohn R. A. (2007). Stochastic Analysis of Exit Fluid Temperature Records From the Active TAG Hydrothermal Mound (Mid-Atlantic Ridge, 26°N): 1. Modes of Variability and Implications for Subsurface Flow. J. Geophys. Res. 112, B07101. doi: 10.1029/2006JB004435
Spietz R. L., Butterfield D. A., Buck N. J., Larson B. I., Chadwick W. W., Walker S. L., et al. (2018). Deep-Sea Volcanic Eruptions Create Unique Chemical and Biological Linkages Between the Subsurface Lithosphere and the Oceanic Hydrosphere. Oceanography 31, 128–135. doi: 10.5670/oceanog.2018.120
Stefanni S., Mirimin L., Stanković D., Chatzievangelou D., Bongiorni L., Marini S., et al. (2022). Framing Cutting-Edge Integrative Taxonomy in Deep-Sea Biodiversity Monitoring via eDNA and Optoacoustic Augmented Observatories. Front. Mar. Sci. 8, 1914. doi: 10.3389/fmars.2021.797140
Stewart L. C., Algar C. K., Fortunato C. S., Larson B. I., Vallino J. J., Huber J. A., et al. (2019). Fluid Geochemistry, Local Hydrology, and Metabolic Activity Define Methanogen Community Size and Composition in Deep-Sea Hydrothermal Vents. ISME J. 13, 1711–1721. doi: 10.1038/s41396-019-0382-3
St. Laurent L., Garrett C. (2002). The Role of Internal Tides in Mixing the Deep Ocean. J. Phys. Oceanogr. 32, 2882–2899. doi: 10.1175/1520-0485(2002)032<2882:TROITI>2.0.CO;2
Sweetman A. K., Thurber A. R., Smith C. R., Levin L. A., Mora C., Wei C.-L., et al. (2017). Major Impacts of Climate Change on Deep-Sea Benthic Ecosystems. Elem. Sci. Anth. 5, 4. doi: 10.1525/elementa.203
Taylor J., Krumpen T., Soltwedel T., Gutt J., Bergmann M. (2017). Dynamic Benthic Megafaunal Communities: Assessing Temporal Variations in Structure, Composition and Diversity at the Arctic Deep-Sea Observatory HAUSGARTEN Between 2004 and 2015. Deep. Res. Part I Oceanogr. Res. Pap. 122, 81–94. doi: 10.1016/j.dsr.2017.02.008
Taylor J., Staufenbiel B., Soltwedel T., Bergmann M. (2018). Temporal Trends in the Biomass of Three Epibenthic Invertebrates From the Deep-Sea Observatory HAUSGARTEN (Fram Strait, Arctic Ocean). Mar. Ecol. Prog. Ser. 602, 15–29. doi: 10.3354/meps12654
Theissen-Krah S., Rüpke L. H., Hasenclever J. (2016). Modes of Crustal Accretion and Their Implications for Hydrothermal Circulation. Geophys. Res. Lett. 43, 1124–1131. doi: 10.1002/2015GL067335
Thiel H., Kirstein K. O., Luth C., Luth U., Luther G. W., Meyer-Reil L. A., et al. (1994). Scientific Requirements for an Abyssal Benthic Laboratory. J. Mar. Syst. 4, 421–439. doi: 10.1016/0924-7963(94)90019-1
Thomson R. E., Mlhály S. F., Rabinovich A. B., McDuff R. E., Veirs S. R., Stahr F. R. (2003). Constrained Circulation at Endeavour Ridge Facilitates Colonization by Vent Larvae. Nature 424, 545–549. doi: 10.1038/nature01824
Thomson R. E., Roth S. E., Dymond J. (1990). Near-Inertial Motions Over a Mid-Ocean Ridge: Effects of Topography and Hydrothermal Plumes. J. Geophys. Res. 95, 7261–7278. doi: 10.1029/JC095iC05p07261
Tivey M. K., Bradley A. M., Joyce T. M., Kadko D. (2002). Insights Into Tide-Related Variability at Seafloor Hydrothermal Vents From Time-Series Temperature Measurements. Earth Planet. Sci. Lett. 202, 693–707. doi: 10.1016/S0012-821X(02)00801-4
Tolstoy M., Cowen J. P., Baker E. T., Fornari D. J., Rubin K. H., Shank T. M., et al. (2006). A Sea-Floor Spreading Event Captured by Seismometers. Science (80-. ). 314 (5807), 1920–1922. doi: 10.1126/science.1133950
Tomasi B., Preisig J., Deane G. B., Zorzi M. (2011). “A Study on the Wide-Sense Stationarity of the Underwater Acoustic Channel for Non-Coherent Communication Systems,” in 17th European Wireless 2011 - Sustainable Wireless Technologies: Vienna. 1–6.
Tomasi B., Zappa G., McCoy K., Casari P., Zorzi M. (2010). “Experimental Study of the Space-Time Properties of Acoustic Channels for Underwater Communications,” in Ocean. IEEE Sydney, Ocean. 2010. doi: 10.1109/OCEANSSYD.2010.5603667
Toomey D. R., Jousselin D., Dunn R. A., Wilcock W. S. D., Detrick R. S. (2009). Toomey Et Al. Reply. Nature 458, E12–E13. doi: 10.1038/nature07888
Tunnicliffe V. (1991). The Biology of Hydrothermal Vents - Ecology and Evolution. Oceanogr. Mar. Biol. 29, 319–407.
Tunnicliffe V., Juniper S. K., Sibuet M. (2003). “Reducing Environments of the Deep-Sea Floor,” in Ecosystems of the World: The Deep Sea. Chapter 4 (Amsterdam, Netherlands: Elsevier Press), 81–110.
Van Ark E., Detrick R., Canales J. P., Carbotte S. M., Harding A., Kent G., et al. (2007). Seismic Structure of the Endeavour Segment, Juan De Fuca Ridge: Correlations With Seismicity and Hydrothermal Activity. J. Geophys. Res. 112, B02401. doi: 10.1029/2005JB004210
Van Audenhaege L., Matabos M., Brind'Amour A., Drugmand J., Laës-Huon A., Sarradin P. -M., et al. (2022). Long-Term Monitoring Reveals Unprecedented Stability of a Vent Mussel Assemblage on the Mid-Atlantic Ridge. Prog. Oceano. 204. doi: 10.1016/j.pocean.2022.102791
van den Burg S., Visscher M., Sonneveld A., Berges B., Jansen L., Sharp F., et al. (2021). Uptake of New Technology for Ocean Observation. Eur. Marit. Fish. Fund. Publications Office, 2021. doi: 10.2926/37198
Van Dover C. L. (2014). Impacts of Anthropogenic Disturbances at Deep-Sea Hydrothermal Vent Ecosystems: A Review. Mar. Environ. Res. 102, 59–72. doi: 10.1016/j.marenvres.2014.03.008
Van Dover C. L. (2019). Inactive Sulfide Ecosystems in the Deep Sea: A Review. Front. Mar. Sci. 6. doi: 10.3389/fmars.2019.00461
Van Dover C. L., Colaço A., Collins P. C., Croot P., Metaxas A., Murton B. J., et al. (2020). Research is Needed to Inform Environmental Management of Hydrothermally Inactive and Extinct Polymetallic Sulfide (PMS) Deposits. Mar. Policy 121, 104183. doi: 10.1016/j.marpol.2020.104183
Vehling F., Hasenclever J., Rüpke L. (2021). Brine Formation and Mobilization in Submarine Hydrothermal Systems: Insights From a Novel Multiphase Hydrothermal Flow Model in the System H2O–NaCl. Transp. Porous Media 136, 65–102. doi: 10.1007/s11242-020-01499-6
Vic C., Ferron B., Thierry V., Mercier H., Lherminier P. (2021). Tidal and Near-Inertial Internal Waves Over the Reykjanes Ridge. J. Phys. Oceanogr. 51, 419–437. doi: 10.1175/jpo-d-20-0097.1
Vic C., Garabato A. C. N., Green J. A. M., Spingys C., Forryan A., Zhao Z., et al. (2018a). The Lifecycle of Semidiurnal Internal Tides Over the Northern Mid-Atlantic Ridge. J. Phys. Oceanogr. 48, 61–80. doi: 10.1175/JPO-D-17-0121.1
Vic C., Gula J., Roullet G., Pradillon F. (2018b). Dispersion of Deep-Sea Hydrothermal Vent Effluents and Larvae by Submesoscale and Tidal Currents. Deep. Res. Part I Oceanogr. Res. Pap. 133, 1–18. doi: 10.1016/j.dsr.2018.01.001
Waeles M., Cotte L., Pernet-Coudrier B., Chavagnac V., Cathalot C., Leleu T., et al. (2017). On the Early Fate of Hydrothermal Iron at Deep-Sea Vents: A Reassessment After in Situ Filtration. Geophys. Res. Lett. 44, 4233–4240. doi: 10.1002/2017GL073315
Webb T. J., Vanden Berghe E., O’Dor R. (2010). Biodiversity’s Big Wet Secret: The Global Distribution of Marine Biological Records Reveals Chronic Under-Exploration of the Deep Pelagic Ocean. PloS One 5, e10223. doi: 10.1371/journal.pone.0010223
Werner F. E., Cowen R. K., Paris C. B. (2007). Coupled Biological and Physical Models: Present Capabilities and Necessary Developments for Future Studies of Population Connectivity. Oceanography 20, 54–69. doi: 10.5670/oceanog.2007.29
West M., Menke W., Tolstoy M., Webb S., Sohn R. (2001). Magma Storage Beneath Axial Volcano on the Juan De Fuca Mid-Ocean Ridge. Nature 413, 833–836. doi: 10.1038/35101581
Wilcock W. S. D., Tolstoy M., Waldhauser F., Garcia C., Tan Yen J., Bohnenstiehl D. R., et al. (2016). Seismic Constraints on Caldera Dynamics From the 2015 Axial Seamount Eruption. Science 354 (6318), 1395–1399. doi: 10.1126/science.aah5563
Xu G., Di Iorio D. (2012). Deep Sea Hydrothermal Plumes and Their Interaction With Oscillatory Flows. Geochem. Geophys. Geosystems 13, 1–18. doi: 10.1029/2012GC004188
Xu G., Larson B. I., Bemis K. G., Lilley M. D. (2017). A Preliminary 1-D Model Investigation of Tidal Variations of Temperature and Chlorinity at the Grotto Mound, Endeavour Segment, Juan De Fuca Ridge. Geochem. Geophys. Geosystems 18 (1), 75–92. doi: 10.1002/2016GC006537
Young C. M., He R., Emlet R. B., Li Y., Qian H., Arellano S. M., et al. (2012). Dispersal of Deep-Sea Larvae From the Intra-American Seas: Simulations of Trajectories Using Ocean Models. Integr. Comp. Biol. 52, 483–496. doi: 10.1093/icb/ics090
Zeppilli D., Bellec L., Cambon-Bonavita M.-A., Decraemer W., Fontaneto D., Fuchs S., et al. (2019). Ecology and Trophic Role of Oncholaimus Dyvae Sp. Nov. (Nematoda: Oncholaimidae) From the Lucky Strike Hydrothermal Vent Field (Mid-Atlantic Ridge). BMC Zool. 4, 6. doi: 10.1186/s40850-019-0044-y
Keywords: essential ocean variables (EOVs), essential biological variables (EBVs), mid-ocean ridge (MOR), sensors, seabed platforms, vent fluid dynamics, vent communities dynamics
Citation: Matabos M, Barreyre T, Juniper SK, Cannat M, Kelley D, Alfaro-Lucas JM, Chavagnac V, Colaço A, Escartin J, Escobar E, Fornari D, Hasenclever J, Huber JA, Laës-Huon A, Lantéri N, Levin LA, Mihaly S, Mittelstaedt E, Pradillon F, Sarradin P-M, Sarrazin J, Tomasi B, Venkatesan R and Vic C (2022) Integrating Multidisciplinary Observations in Vent Environments (IMOVE): Decadal Progress in Deep-Sea Observatories at Hydrothermal Vents. Front. Mar. Sci. 9:866422. doi: 10.3389/fmars.2022.866422
Received: 31 January 2022; Accepted: 11 April 2022;
Published: 13 May 2022.
Edited by:
Jacopo Aguzzi, Spanish National Research Council (CSIC), SpainReviewed by:
Karthik Anantharaman, University of Wisconsin-Madison, United StatesDaniel Mihai Toma, Universitat Politecnica de Catalunya, Spain
Copyright © 2022 Matabos, Barreyre, Juniper, Cannat, Kelley, Alfaro-Lucas, Chavagnac, Colaço, Escartin, Escobar, Fornari, Hasenclever, Huber, Laës-Huon, Lantéri, Levin, Mihaly, Mittelstaedt, Pradillon, Sarradin, Sarrazin, Tomasi, Venkatesan and Vic. This is an open-access article distributed under the terms of the Creative Commons Attribution License (CC BY). The use, distribution or reproduction in other forums is permitted, provided the original author(s) and the copyright owner(s) are credited and that the original publication in this journal is cited, in accordance with accepted academic practice. No use, distribution or reproduction is permitted which does not comply with these terms.
*Correspondence: Marjolaine Matabos, bWFyam9sYWluZS5tYXRhYm9zQGlmcmVtZXIuZnI=; Thibaut Barreyre, dGhpYmF1dC5iYXJyZXlyZUB1aWIubm8=
†These authors have contributed equally to this work and share first authorship