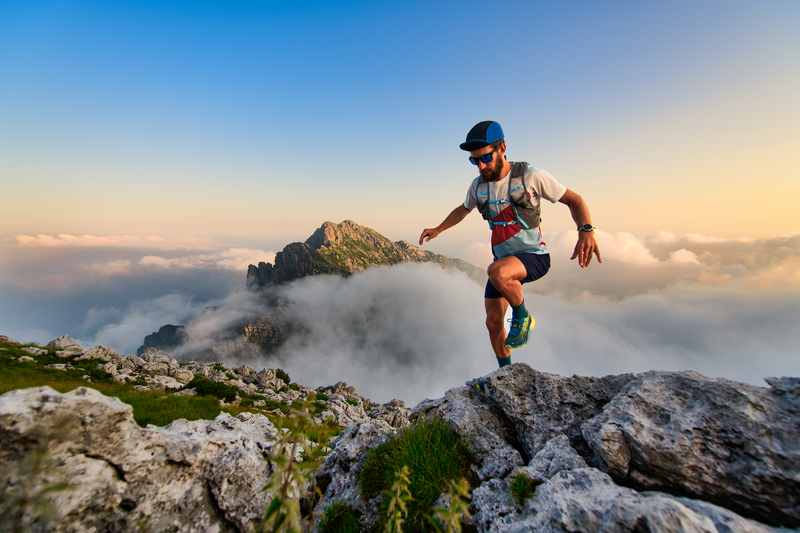
95% of researchers rate our articles as excellent or good
Learn more about the work of our research integrity team to safeguard the quality of each article we publish.
Find out more
MINI REVIEW article
Front. Mar. Sci. , 16 June 2022
Sec. Global Change and the Future Ocean
Volume 9 - 2022 | https://doi.org/10.3389/fmars.2022.864797
This article is part of the Research Topic Influence of Environmental Variability on Climate Change Impacts in Marine Ecosystems View all 12 articles
Environmental variability is an intrinsic characteristic of nature. Variability in factors such as temperature, UV, salinity, and nutrient availability can influence structural and functional properties of marine microbial organisms. This influence has profound implications for biochemical cycles and the ecosystem services provided by the oceans. In this review we discuss some of the most relevant mechanisms underpinning adaptive strategies of microbial organisms in variable and dynamic oceans. We assess the extent to which the magnitude and rate of environmental change influence plastic phenotypic adjustments and evolutionary trajectories of microbial populations. This understanding is fundamental for developing better predictions regarding microbial dynamics at ecological and evolutionary time-scales and in response to climate change.
Oceans are highly variable environments. These systems are characterized by marked fluctuations in environmental conditions (e.g., salinity, pH, temperature, nutrients, irradiance), that operate at different temporal (second to decades) and spatial scales (millimeters to kilometers), creating heterogenous seascapes (Boyd et al., 2016a; Rodríguez-Romero et al., 2022). Such fluctuations modulate the environmental envelope (i.e., a set of environmental variables and conditions that favours species occurrence; Figures 1A, B) of marine microbial communities (Boyd et al., 2010), their distribution and ecological dynamics (Brun et al., 2015), as well as their structure and function across time and space (Tinta et al., 2015; Hutchins and Fu, 2017; Di Pane et al., 2022). However, marine microbes are not static units as they can display a range of biological strategies to short-term changes in environmental conditions such as those occurring at time-scales approximating microbial division times (i.e., within generations; Figure 1C) (Litaker et al., 1993). These strategies mainly involve non-genetic phenotypic adjustments (i.e., phenotypic plasticity) that allow microbes to actively respond to the physiological challenges imposed by environmental fluctuations at such time-scales (Chevin et al., 2013; Schaum et al., 2013; Boyd et al., 2016a; Collins et al., 2020). For instance, short-term fluctuations (e.g., diurnal cycles) in drivers such as underwater irradiance (light intensity/quality), temperature and/or nutrient availability can potentially induce stressful conditions to marine microorganisms, triggering compensatory behavioral (e.g., vertical migration; Aumack et al., 2014) and metabolic (e.g., photosynthetic activity; Gaidarenko et al., 2019) adjustments. The outcome of these phenotypic responses can be adaptive, neutral or maladaptive (i.e., not adjusting adequately to the new environment) depending on the interaction with other environmental drivers (e.g., light x salinity; Sauer et al., 2002) and the overall effect on organismal fitness (Chevin et al., 2013). Although plasticity provides a mechanism for adaptation to changing environments (e.g., Schaum et al., 2013; Kremer et al., 2018), there are limits for plastic responses (DeWitt et al., 1998; Fox et al., 2019; Gill et al., 2022), beyond which genetic adjustments are required to persist (Fox et al., 2019; Gaitán-Espitia and Hobday, 2021). These responses (i.e., adaptive evolution) are particularly relevant if unfavorable environmental changes are difficult to track (unpredictable or occur too rapidly) and persist beyond few generations (Chevin et al., 2013; Bernhardt et al., 2020; Collins et al., 2020). Nevertheless, the ability to undergo these adjustments varies within and across populations (Figures 1B, C) depending on the presence of genetic variation for ecologically important traits (Chevin et al., 2013; Godhe and Rynearson, 2017), and on the strength (e.g., the intensity of selection increases with the number of drivers) and form/direction of natural selection (e.g., directional selection under ocean warming; Figure 1C) (Brennan et al., 2017; Collins et al., 2020; Walworth et al., 2020). These components of selection are ultimately determined by the magnitude and rate of environmental change (Gaitán-Espitia et al., 2017a; Collins et al., 2020). Consequently, inadequate responses (e.g., neutral or maladaptive) to unfavorable conditions (i.e., where selection is strong and induce significant loss of fitness) can drive population declines and local extinctions (Figure 1C). Contrarily, adaptive responses to stress due to environmental change may lead to phenotypic and genetic changes associated to the evolution of tolerance to novel conditions via increased physiological resistance levels, behavioral (e.g., vertical migration) and life-history (e.g., dormancy) avoidance of the stressful conditions. These adaptive evolutionary responses can allow the maintenance of biodiversity and the persistence of populations despite the negative effects on fitness initially induced by environmental change (Figure 1C) (Boyd et al., 2016a; Schaum et al., 2016; Schluter et al., 2016; Fox et al., 2019; Collins et al., 2020; Walworth et al., 2020). In this review, we discuss some of the most relevant mechanisms underpinning the adaptive strategies of microbial organisms and their evolutionary responses and outcomes in variable and dynamic oceans.
Figure 1 Ecological and evolutionary dynamics of marine microbial organisms in variable and dynamic oceans. (A) The overall fitness of a population (green curve) is a function of the tolerances and capacity for plastic adjustments of individual genotypes/strains (colour curves). This function determines the adaptive capacity of a population to persist after rapid environmental changes. (B) These changes involve more than one driver at the time. In a population, the balance between tolerances, plasticity and stress for each driver determines its ecological niche and helps to predict the capacity to respond to future environmental changes. Different populations can be locally adapted as result of differences in the environmental conditions and the variability they experience. This can explain the geographic differences in the capacity for phenotypic plasticity and adaptive potential (e.g., narrower or closer to their tolerance limits). (C) Populations with contrasting differences in the capacity for plastic adjustments, tolerances and potential for adaptive evolution in response to environmental changes show divergent ecological and evolutionary dynamics (within and across generations). Rapid reduction of genetic diversity and high extinction risk is expected for populations with limited phenotypic plasticity inhabiting stable environments where only one driver changes (C.1). If plasticity is present, it can buffer the influence of selection mitigating population decline and extinction (C.2). Fluctuating environments are hypothesised to facilitate plasticity promoting the maintenance of phenotypic/genetic diversity as a consequence of reduced strength of directional selection (C.3). However, if multiple drivers are interacting and changing in these environments, selection is going to be stronger accelerating the fixation of “optimal” phenotypes (more tolerant to directional changes in dominant drivers; C.4).
Phenotypic plasticity offers a fundamental mechanism for marine microbes to cope with short-time environmental fluctuations, allowing them to track environmental changes and escape local extinction (Figure 1C) (Chevin et al., 2013). This capacity for rapid phenotypic adjustments is considered to be shaped by the level of environmental variability experienced by populations (e.g., higher plasticity in organisms from more heterogeneous environments; Boyd et al., 2016a), and the predictability of environmental change (e.g., lower plasticity in less predictable environments; Schaum and Collins, 2014; Bernhardt et al., 2020; Leung et al., 2020; Gill et al., 2022) (Figure 2). Here, we described three common short-term adaptive plastic responses of microbial organisms (i.e. direct environmental sensing-response, anticipatory-memory response, and diversified bet-hedging strategy) to environmental fluctuation (Figure 2). While the mechanisms are described individually in the next section, they are not mutually exclusive, as microbes may combine two or three strategies depending on the magnitude and rate of environmental variability (i.e., the strength and direction of selection).
Figure 2 Adaptive strategies of marine microbes to cope with short-term environmental variability are influenced by the level of predictability (High: A, B; Low: C) of environmental change. Anticipatory and memory mechanisms (D) in which microbes can sense environmental cues (arrows in orange and green linked to predictable environmental changes, (A) that allow them to adjust phenotypically in an anticipatory manner. Direct environmental sensing-respond mechanisms (E) in which microbes exhibit phenotypic responses that correspond to their environmental condition/stimulus (yellow and purple, B). (F) Seed banks representing the diversified bet-hedging (a.k.a. stochastic phenotypic switching) strategy, in which microbes produce long-term resting stages that act as a genetic and phenotypic reservoir that protect populations against unpredictable future conditions (C).
The most common mechanism employed by marine microbes to cope with environmental changes is modulated at the transcriptional level where a specific environmental stimuli/condition triggers the expression of a specific phenotype (sensing-regulatory response) (Figure 2E; Forsman, 2015; Bonamour et al., 2019). For prokaryotes, plasticity in their responses to environmental stimuli is primarily mediated by the two-component signal transduction systems (TCS) (Capra and Laub, 2012; Held et al., 2019). While there are variants to the prokaryotic TCS, the canonical systems are structured by two conserved components called the histidine protein kinases (HPK) and response regulator (RR) (Zschiedrich et al., 2016; Busby, 2019). Although TCS are prevalent in prokaryotes, these signaling elements have also been co-opted to meet the needs of signal transduction in microbial eukaryotes (Schaller et al., 2011). For these organisms, the gene expression/repression activity often involves more complex regulatory networks than those seen in the prokaryotic TCS (Madhani, 2013; Cruz de Carvalho et al., 2016). Nevertheless, in both domains, organisms share some similarity in their plastic responses to environmental stimuli evidenced by the initiation of transcription via RNA polymerase (Andrews, 2017).
A classic system for the study of sensing-regulatory response as an adaptive strategy to short-term scale environmental change comes from the nutrient limitation research. Nutrient limitation or exhaustion is a prevailing environmental challenge often experienced by microbes, rendering them constantly changing between feast and famine states (Suzuki et al., 2004; Rozen and Belkin, 2005; Cruz de Carvalho et al., 2016; Andrews, 2017). One of the solutions “wield” by microbes to cope with fluctuations in nutrients is through transcriptional reprogramming (Brown et al., 2014). For example, upon sensing limitation in the nitrogen (N) supply in surrounding environment, prokaryotic microbes can display a global alteration in gene expression, shifting from growth-associated transcriptomes to growth-arrested stationary-phase transcriptomes (Brown et al., 2014; Switzer et al., 2018). This is an important mechanism for conservation and allocation of resources mainly for cellular maintenance and repairing activities under unfavorable conditions. In photosynthetic microbes, similar adaptive regulatory mechanisms to cope with short-term fluctuations in nutrient availability have been documented (Suzuki et al., 2004; Bender et al., 2014; Cruz de Carvalho et al., 2016; Matthijs et al., 2017). Under these conditions (i.e., fluctuations in phosphate or nitrogen), photosynthetic microbes such as cyanobacteria and diatoms can activate sensory stress response and signaling systems that combine “bacterial CTS” (Suzuki et al., 2004; Cruz de Carvalho et al., 2016) with more complex pathways (e.g., nutrient recycling, carbohydrate and fatty acid metabolism; Bender et al., 2014), and non-coding regulatory systems (e.g., long intergenic nonprotein coding RNAs; Cruz de Carvalho et al., 2016). Comparable signaling-regulatory responses have been documented in marine diatoms exposed to fluctuations in salinity (Krell et al., 2008) and irradiance (Depauw et al., 2012), in which complex signal transduction cascades and regulatory processes, including transcriptional and post-transcriptional networks, second messengers, and chromatin remodeling are activated as part of the adaptive response (Krell et al., 2008; Depauw et al., 2012).
Some microbes have evolved the ability to anticipate future conditions as a result of evolving in highly predictable environments (Figure 2D; Johnson et al., 2008; Tagkopoulos et al., 2008; Gill et al., 2022). For example, several species of marine diatoms can cope with highly variable light conditions, as they possess suitable molecular systems that allow them to perceive, respond to, and anticipate light variations (Sauer et al., 2002; Depauw et al., 2012; Aumack et al., 2014; Serôdio, 2021). The capacity to anticipate daily changes in illumination was explained by the endogenous circadian clock in marine diatoms, a biological system that could be linked to light-driven gene expression regulatory mechanisms (Depauw et al., 2012). The anticipatory mechanism explains behavioural plastic responses of marine microbes to changes in environmental conditions. For instance, photosynthetic microbes in marine sediments exhibit vertical migrations characterized by rhythmic and synchronized movement of cells upward towards the surface of the sediment at the beginning of daytime periods of low tide, followed by the downward migration in anticipation of tidal flood or night (Serôdio, 2021). It has been documented that the disruption of the anticipatory ability can negatively impact the capacity of microbes for physiological adjustments with consequences on ecological dynamics and the fitness of microbial populations (Woelfle et al., 2004).
Another example of anticipatory response of microbes to environmental fluctuations is represented by the capacity of storing “memories” or “past historical experiences” and impart this “knowledge” to the next generation (Casadesús and D’Ari, 2002; Zacharioudakis et al., 2007; Dragosits et al., 2013; Norman et al., 2013; Shimizu, 2013). For example, prokaryotes have shown the existence of a memory-driven anticipatory mechanism that helps microbes to cope with fluctuating carbon sources (e.g. glucose and fructose) (Lambert and Kussel, 2014). When the preferred carbon source (glucose) is exhausted, prokaryotic microbes activate the lac operon (i.e. a set of multiple genes that are responsible for the production of lac protein), in order to use lactose (Östling et al., 1991). However, in normal circumstances, there is a lag phase before these microbes are able to utilize lactose, which can be at an unintended fitness disadvantage in a competitive environment. To overcome this drawback, the bacteria can adopt a phenotypic memory-like mechanism, where the stable intracellular lac protein present in parental cells can be transmitted across dividing daughter cells (Lambert and Kussel, 2014). When prokaryotic cells are adapted to fluctuating carbon sources microbes can continuously express genes required for lactose metabolism (i.e. even after the lac operon inducer is removed), removing the need for regulatory responses (i.e. signal transduction and gene activation/repression) (Lambert and Kussel, 2014). Consequently, this adaptive strategy reduces the metabolic transition in lag phase, thereby increasing microbial fitness in this environment, simply with quicker response for lactose metabolism. Ecological memory is also hypothesized to be important for the tolerance and fitness of photosynthetic microbes to changing oceans, particularly when the environmental signal is cyclic and reliable. In symbiotic dinoflagellates, for example, thermal priming (e.g., past exposure to increased temperatures and heat stress) enhance heat tolerance and photosynthetic performance during heatwaves (Middlebrook et al., 2012). It has been suggested that this type of acquired tolerance through stress memory is modulated by epigenetic modifications that alter gene expression (e.g., DNA methylation, histone modifications and non-coding micro RNAs), and that these can be transmitted across generations (Bossdorf et al., 2008; Walworth et al., 2021a). However, thermal priming does not always enhance performance and fitness for eukaryotic microbes. For instance, in a Southern Ocean diatom, heat-primed populations exhibited higher levels of mortality during heatwaves than populations without pre-exposure to sub-lethal temperatures (Samuels et al., 2021). These findings suggest the existence of more complex mechanisms regulating the ecological memory of past stress in eukaryotic microbes.
Phenotypic plasticity may not always be an effective strategy to cope with environmental change, particularly when fluctuations in environmental conditions are unpredictable (Veening et al., 2008; Grimbergen et al., 2015; Leung et al., 2020; Gill et al., 2022). Under these conditions, natural selection may favour a strategy where a single genotype produces a range of phenotypes each generation but without responding in a specific way to the prevailing conditions but ensuring the survival of sub-populations that will be adaptive to a future condition (Figure 2F; Ackermann, 2015). This mechanism is known as diversified bet-hedging strategy or stochastic phenotypic switching (Veening et al., 2008; Grimbergen et al., 2015; Ellegaard and Ribeiro, 2018). In marine microbes, the maintenance of a seed bank is often hypothesized to constitute a bet-hedging strategy in which long-term resting stages (or propagules) are an insurance against an unpredictable future (Ellegaard and Ribeiro, 2018). For example, some species of diatoms and dinoflagellates are able to survive environmental fluctuations that exceed the tolerance range for vegetative cells through the formation of resting cells (spores/cysts), resuming vegetative growth under favourable conditions (Godhe and Rynearson, 2017; Ellegaard and Ribeiro, 2018). In dinoflagellates, these resting stages are mainly produced as part of their sexual reproduction, which appears to be another form of stress avoidance as it is initiated by unfavourable conditions (e.g., nutrient limitation x suboptimal temperature; Tang and Gobler, 2015; Borowitzka, 2018; Ellegaard and Ribeiro, 2018). Resting cysts of dinoflagellates also contribute to population dynamics influencing rapid shifts in genetic diversity (through genetic recombination) and geographic expansion (Tang and Gobler, 2015). In diatoms, resting cells are not initiated by sexual reproduction but by physiological stress (Borowitzka, 2018; Ellegaard and Ribeiro, 2018). In this case, the formation of resting cells is activated by the upregulation of ferritin (an iron binding protein), a process that triggers the morphological transformation from fusiform to ovoid cells, the decrease in growth rates, the excretion of exopolymeric substances, the upregulation of stress resistance proteins and the increase of nitrate reserves (Liu et al., 2022). Although this adaptive strategy represents a loss of genetic material for marine microbes in the short term (i.e., many of the resting stages never germinate), sacrificing mean fitness for reduced variability in fitness over time, it is described as an effective strategy for risk-spreading, for persistence through longer periods of adverse conditions, and for preparing for an unpredictable future (Ellegaard and Ribeiro, 2018). Despite the important ecological role of the bet-hedging strategy for marine microbes (i.e., influencing population persistence), there is no clear consensus about its role in evolutionary dynamics (Rengefors et al., 2017). Generally, resting stages are considered to weaken the effect of selection and slow down adaptive evolution (Hairston and De Stasio, 1988) by lengthening the generation time of microorganisms, potentially delaying the manifestation of novel mutations (Lennon and Jones, 2011). Contrarily, dormant cells can act as genetic reservoirs that speed up adaptive evolution by increasing phenotypic and genetic variation, which in turn influence the adaptive potential and the response to selection (Kremp et al., 2016; Rengefors et al., 2017).
Predictable fluctuating marine environments are expected to promote adaptive phenotypic plasticity (Figure 2) (Schaum and Collins, 2014; Leung et al., 2020; Gill et al., 2022) at the cost of delaying genetic adaptation (Walworth et al., 2020). However, in environments where changes are less predictable and occur extremely rapidly for organisms to track and adjust phenotypically, plasticity is not promoted (Leung et al., 2020). Under these conditions, genetic adaptation may be the only mechanism that allows species and populations to persist locally (Gaitán-Espitia and Hobday, 2021). Although the link between environmental variability and marine microbial evolution is still poorly understood, in this section we aim to provide glimpses of factors and mechanisms modulating adaptive evolutionary dynamics of microbes in dynamic oceans.
Empirical evidences based on experimental evolution have shown that microbial populations can rapidly adapt to environmental changes (single- and multi-driver environments), throughout, for instance, intraspecific strain sorting and genetic changes (Lohbeck et al., 2012; Hoppe et al., 2018; Wolf et al., 2019). Notwithstanding, the evolutionary responses of microbes to environmental fluctuations are conditioned by the number and identity of the interacting drivers involved (Brennan et al., 2017). Faster rates of adaptation have been documented with increased number of drivers as a result of the increase in the strength of selection (Brennan et al., 2017). However, the effect of drivers on selection is not additive as only few (e.g., temperature, nutrients) explain most of the phenotypic and evolutionary changes observed (Brennan and Collins, 2015; Boyd et al., 2016a; Brennan et al., 2017; Feng et al., 2020). Particularly, temperature has been identified as one of the most dominant drivers modulating phenotypic and genetic responses in marine microorganisms comprising broad evolutionary backgrounds (Boyd et al., 2016b; Brennan et al., 2017; Barton et al., 2020; Cabrerizo et al., 2022). This important and dominant influence across microbial life forms is perhaps explained by the existence of “universal” thermodynamic constraints that limit the pace of life and the thermal window for biochemical performance (Angilletta et al., 2010). Fluctuations in dominant drivers such as temperature can also modulate the rate of adaptation and the evolutionary trajectories of marine microbes. For example, a study by Schaum et al. (2018) showed that marine diatoms can exhibit rapid evolutionary divergence and adaptation to high temperatures when populations have evolved under fluctuating environments. Here, the temporary restoration of benign heat level conditions increased population size and therefore the probability of fixing beneficial mutations required for adaptation (Schaum et al., 2018). These characteristics and their influence on adaptive evolution to changes in temperature have been linked to the “complexity” of microbial organisms in which species with small genomes (e.g., prokaryotes) have higher rates of adaptation (e.g., compensatory evolutionary shifts on metabolic traits) to warming compared to “more complex” microbes with larger genomes (Barton et al., 2020).
The rate of adaptation to changing marine environments is also regulated by evolutionary trade-offs (Gaitán-Espitia J. et al., 2017). These trade-offs reflect the costs and constraints for evolutionary change (O'Donnell et al., 2018; Aranguren-Gassis et al., 2019; Lindberg and Collins, 2020; Walworth et al., 2020), and can alter the trajectories of populations across fitness landscapes, delaying or blocking them to reach a global fitness optimum (Gaitán-Espitia J. et al., 2017; Gaitán-Espitia and Hobday, 2021). Because evolutionary trade-offs are difficult to demonstrate in nature, some proxies are used to asses them. These include selection experiments (e.g., Jin et al., 2022), and the analysis of functional/phenotypic and genetic correlations (e.g., Argyle et al., 2021; Walworth et al., 2021b). In the marine diatom Chaetoceros simplex, a trade-off from a functional correlation between high‐temperature tolerance and increased nitrogen requirements is suggested to underlie the inhibited thermal adaptation under nitrogen limitation conditions (Aranguren-Gassis et al., 2019). Similarly, in the marine diatom Phaeodactylum tricornutum, adaptation to high CO2 was mediated by a functional trade-off in which populations decreased metabolic rates while maintaining the carbon allocation and growth (i.e., fitness; Jin et al., 2022). In marine bacteria, evolutionary trade-offs have been documented when comparing strains growing in natural constant and fluctuating environments. In coastal systems, bacteria carry significantly more environmental stimuli sensing genes than strains in oligotrophic systems (e.g., open ocean; Held et al., 2019). This represents a functional trade-off due to the elevated cost of maintenance of signal transduction systems (TCS) for strains in coastal areas (i.e. dynamic and fluctuating) compared to strains in oceanic (i.e. relatively constant) environment (Mackey et al., 2015). Other evolutionary trade-offs are mostly documented as a result of challenging conditions, these trade-offs can also exist under ameliorated conditions, as evidenced in the study by Lindberg and Collins (2020). In this work, populations of Chlamydomonas reinhardtii evolved to allocate a smaller proportion of carbon to growth while increasing their ability to tolerate and metabolise reactive oxygen species (ROS; Lindberg and Collins, 2020).
Understanding how microbes cope with and adapt to variability in their environments is fundamental for understanding their ecological resilience and adaptive potential in the face of anthropogenic climate change. Unfortunately, most of the mechanistic understanding regarding the environmental-molecular-evolutionary link underpinning the short- and long-term adaptive responses discussed here, have been based on changes in one or two environmental drivers. However, environmental variation and climate change involve multivariate changes (Boyd et al., 2015) that can alter the ecological and evolutionary significance of genetic and non-genetic responses in marine microbes. From the phenotypic plasticity perspective, it is unclear to what extent the number of changing drivers and the type of interactions (i.e., additive, antagonistic or synergetic) can alter the capacity of natural populations to employ plastic strategies such as direct sense-response, anticipatory/memory and bet-hedging. Moreover, we do not know if these responses are differentially modulated across time and space, depending on the level of environmental variation (e.g., higher production of resting cells in more variable environments; lower ecological memory in less variable environments), the existence of physiological constraints (e.g., capacity and costs for activation and maintenance of sensing-regulatory mechanisms), and the signal/duration of the molecular mechanisms underpinning such responses (e.g., different epigenetic factors have different lasting effects). From an evolutionary perspective, we know that fluctuating environments are likely to generate more dynamic adaptive fitness landscapes compared to constant environments. However, in most of the experimental evolution studies, the dynamics have been assessed in stable conditions of one or two drivers, which are characterised by a static local fitness optimum (Steinberg and Ostermeier, 2016). This limits our capacity to understand and predict the tempo and mode of evolution of microbes in fluctuating, multi-driver environments.
JDGE and BA conceived the idea and designed the work. BA led the manuscript writing with feedback and input from ZL and JDGE. All authors contributed to the article and approved the submitted version.
JDGE was supported by the Research Grants Council (ECS 27124318) of Hong Kong.
The authors declare that the research was conducted in the absence of any commercial or financial relationships that could be construed as a potential conflict of interest.
All claims expressed in this article are solely those of the authors and do not necessarily represent those of their affiliated organizations, or those of the publisher, the editors and the reviewers. Any product that may be evaluated in this article, or claim that may be made by its manufacturer, is not guaranteed or endorsed by the publisher.
Ackermann M. (2015). A Functional Perspective on Phenotypic Heterogeneity in Microorganisms. Nat. Rev. Microbiol. 13, 497–508. doi: 10.1038/nrmicro3491
Andrews J. H. (2017). Comparative Ecology of Microorganisms and Macroorganisms. 2nd Edn. New York: Springer Science+Business Media LLC.
Angilletta M. J. Jr, Huey R. B., Frazier M. R. (2010). Thermodynamic Effects on Organismal Performance: Is Hotter Better? Physiol. Biochem. Zool. 83 (2), 197–206. doi: 10.1086/648567
Aranguren-Gassis M., Kremer C. T., Klausmeier C. A., Litchman E. (2019). Nitrogen Limitation Inhibits Marine Diatom Adaptation to High Temperatures. Ecol. Lett. 22, 1860–1869. doi: 10.1111/ele.13378
Argyle P. A., Walworth N. G., Hinners J., Collins S., Levine N. M., Doblin M. A. (2021). Multivariate Trait Analysis Reveals Diatom Plasticity Constrained to a Reduced Set of Biological Axes. ISME Commun. 1 (1), 1–11. doi: 10.1038/s43705-021-00062-8
Aumack C. F., Juhl A. R., Krembs C. (2014). Diatom Vertical Migration Within Land-Fast Arctic Sea Ice. J. Mar. Syst. 139, 496–504. doi: 10.1016/j.jmarsys.2014.08.013
Barton S., Jenkins J., Buckling A., Schaum C. E., Smirnoff N., Raven J. A., et al. (2020). Evolutionary Temperature Compensation of Carbon Fixation in Marine Phytoplankton. Ecol. Lett. 23 (4), 722–733. doi: 10.1111/ele.13469
Bender S. J., Durkin C. A., Berthiaume C. T., Morales R. L., Armbrust E. V. (2014). Transcriptional Responses of Three Model Diatoms to Nitrate Limitation of Growth. Front. Mar. Sci. 1, 1–15. doi: 10.3389/fmars.2014.00003
Bernhardt J. R., O'Connor M. I., Sunday J. M., Gonzalez A. (2020). Life in Fluctuating Environments. Philos. Trans. R. Soc. B 375 (1814), 20190454. doi: 10.1098/rstb.2019.0454
Bonamour S., Chevin L.-M., Charmantier A., Teplitsky C. (2019). Phenotypic Plasticity in Response to Climate Change: The Importance of Cue Variation. Philos. Trans. R. Soc B Biol. Sci. 374, 1–12. doi: 10.1098/rstb.2018.0178
Borowitzka M. A. (2018). The ‘Stress’ Concept in Microalgal Biology—Homeostasis, Acclimation and Adaptation. J. Appl. Phycol. 30 (5), 2815–2825. doi: 10.1007/s10811-018-1399-0
Bossdorf O., Richards C. L., Pigliucci M. (2008). Epigenetics for Ecologists. Ecol. Lett. 11, 106–115. doi: 10.1111j.1461-0248.2007.01130.x
Boyd P., Cornwall C. E., Davison A., Doney S. C., Fourquez M., Hurd C. L., et al. (2016a). Biological Responses to Environmental Heterogeneity Under Future Ocean Conditions. Glob. Change Biol. 22 (8), 2633–2650. doi: 10.1111/gcb.13287
Boyd P. W., Dillingham P. W., McGraw C. M., Armstrong E. A., Cornwall C. E., Feng Y. Y., et al. (2016b). Physiological Responses of a Southern Ocean Diatom to Complex Future Ocean Conditions. Nat. Climate Change 6 (2), 207–213. doi: 10.1038/nclimate2811
Boyd P. W., Lennartz S. T., Glover D. M., Doney S. C. (2015). Biological Ramifications of Climate-Change-Mediated Oceanic Multi-Stressors. Nat. Climate Change 5 (1), 71–79. doi: 10.1038/nclimate2441
Boyd P. W., Strzepek R., Fu F., Hutchins D. A. (2010). Environmental Control of Open-Ocean Phytoplankton Groups: Now and in the Future. Limnol. Oceanogr. 55 (3), 1353–1376. doi: 10.4319/lo.2010.55.3.1353
Brennan G., Colegrave N., Collins S. (2017). Evolutionary Consequences of Multidriver Environmental Change in an Aquatic Primary Producer. Proc. Natl. Acad. Sci. U. S. A. 114, 9930–9935. doi: 10.1073/pnas.1703375114
Brennan G., Collins S. (2015). Growth Responses of a Green Alga to Multiple Environmental Drivers. Nat. Clim. Change. 5 (9), 892–897. doi: 10.1038/nclimate2682
Brown D. R., Barton G., Pan Z., Buck M., Wigneshweraraj S. (2014). Nitrogen Stress Response and Stringent Response are Coupled in Escherichia Coli. Nat. Commun. 5, 1–8. doi: 10.1038/ncomms5115
Brun P., Vogt M., Payne M. R., Gruber N., O'brien C. J., Buitenhuis E. T., et al. (2015). Ecological Niches of Open Ocean Phytoplankton Taxa. Limnol. Oceanogr. 60 (3), 1020–1038. doi: 10.1002/lno.10074
Busby S. J. W. (2019). Transcription Activation in Bacteria: Ancient and Modern. Microbiol. (United Kingdom) 165, 386–395. doi: 10.1099/mic.0.000783
Cabrerizo M., Medina-Sánchez J., González-Olalla J., Sánchez-Gómez D., Carrillo P. (2022). Microbial Plankton Responses to Multiple Environmental Drivers in Marine Ecosystems With Different Phosphorus Limitation Degrees. Sci. Total Environ. 816, 151491. doi: 10.1016/j.scitotenv.2021.151491
Capra E. J., Laub M. T. (2012). Evolution of Two-Component Signal Transduction Systems. Annu. Rev. Microbiol. 66, 325–347. doi: 10.1146/annurev-micro-092611-150039
Casadesús J., D’Ari R. (2002). Memory in Bacteria and Phage. BioEssays 24, 512–518. doi: 10.1002/bies.10102
Chevin L. M., Collins S., Lefèvre F. (2013). Phenotypic Plasticity and Evolutionary Demographic Responses to Climate Change: Taking Theory Out to the Field. Funct. Ecol. 27 (4), 967–979. doi: 10.1111/j.1365-2435.2012.02043.x
Collins S., Boyd P. W., Doblin M. A. (2020). Evolution, Microbes, and Changing Ocean Conditions. Ann. Rev. Mar. Sci. 12, 181–208. doi: 10.1146/annurev-marine-010318-095311
Cruz de Carvalho M. H., Sun H. X., Bowler C., Chua N. H. (2016). Noncoding and Coding Transcriptome Responses of a Marine Diatom to Phosphate Fluctuations. New Phytol. 210 (2), 497–510. doi: 10.1111/nph.13787
Depauw F. A., Rogato A., D’Alcalá M. R., Falciatore A. (2012). Exploring the Molecular Basis of Responses to Light in Marine Diatoms. J. Exp. Bot. 63, 1575–1591. doi: 10.1093/jxb/ers005
DeWitt T. J., Sih A., Wilson D. S. (1998). Costs and Limits of Phenotypic Plasticity. Trends Ecol. Evol. 13 (2), 77–81. doi: 10.1016/S0169-5347(97)01274-3
Di Pane J., Wiltshire K. H., McLean M., Boersma M., Meunier C. L. (2022). Environmentally Induced Functional Shifts in Phytoplankton and Their Potential Consequences for Ecosystem Functioning. Global Change Biol. 28 (8), 2804–2819. doi: 10.1111/gcb.16098
Dragosits M., Mozhayskiy V., Quinones-Soto S., Park J., Tagkopoulos I. (2013). Evolutionary Potential, Cross-Stress Behavior and the Genetic Basis of Acquired Stress Resistance in Escherichia Coli. Mol. Syst. Biol. 9, 1–13. doi: 10.1038/msb.2012.76
Ellegaard M., Ribeiro S. (2018). The Long-Term Persistence of Phytoplankton Resting Stages in Aquatic ‘Seed Banks.’. Biol. Rev. 93, 166–183. doi: 10.1111/brv.12338
Feng Y., Roleda M. Y., Armstrong E., Summerfield T. C., Law C. S., Hurd C. L., et al. (2020). Effects of Multiple Drivers of Ocean Global Change on the Physiology and Functional Gene Expression of the Coccolithophore Emiliania Huxleyi. Global Change Biol. 26 (10), 5630–5645. doi: 10.1111/gcb.15259
Forsman A. (2015). Rethinking Phenotypic Plasticity and its Consequences for Individuals, Populations and Species. Heredity (Edinb). 115, 276–284. doi: 10.1038/hdy.2014.92
Fox R. J., Donelson J. M., Schunter C., Ravasi T., Gaita J. D., Fox R. J. (2019). Beyond Buying Time: The Role of Plasticity in Phenotypic Adaptation to Rapid Environmental Change. Proc. R. Soc B Biol. Sci. 374 (1768), 20180174. doi: 10.1098/rstb.2018.0174
Gaidarenko O., Sathoff C., Staub K., Huesemann M. H., Vernet M., Hildebrand M. (2019). Timing Is Everything: Diel Metabolic and Physiological Changes in the Diatom Cyclotella Cryptica Grown in Simulated Outdoor Conditions. Algal Res. 42, 101598. doi: 10.1016/j.algal.2019.101598
Gaitán-Espitia J. D., Hobday A. J. (2021). Evolutionary Principles and Genetic Considerations for Guiding Conservation Interventions Under Climate Change. Glob. Change Biol. 27, 475–488. doi: 10.1111/gcb.15359
Gaitán-Espitia J. D., Marshall D. J., Dupont S., Bacigalupe L. D., Bodrossy L., Hobday A. J. (2017a). Geographical Gradients in Selection can Reveal Genetic Constraints for Evolutionary Responses to Ocean Acidification. Biol. Lett. 13, 20160784. doi: 10.1098/rsbl.2016.0784
Gaitán-Espitia J. D., Villanueva P. A., Lopez J., Torres R., Navarro J. M., Bacigalupe L. D. (2017b). Spatio-Temporal Environmental Variation Mediates Geographical Differences in Phenotypic Responses to Ocean Acidification. Biol. Lett. 13 (2), 20160865. doi: 10.1098/rsbl.2016.0865
Gill R. L., Collins S., Argyle P. A., Larsson M. E., Fleck R., Doblin M. A. (2022). Predictability of Thermal Fluctuations Influences Functional Traits of a Cosmopolitan Marine Diatom. Proc. R. Soc. B Biol. Sci. 289 (1973), 20212581. doi: 10.1098/rspb.2021.2581
Godhe A., Rynearson T. (2017). The Role of Intraspecific Variation in the Ecological and Evolutionary Success of Diatoms in Changing Environments. Philos. Trans. R. Soc. B: Biol. Sci. 372 (1728), 20160399. doi: 10.1098/rstb.2016.0399
Grimbergen A. J., Siebring J., Solopova A., Kuipers O. P. (2015). Microbial Bet-Hedging: The Power of Being Different. Curr. Opin. Microbiol. 25, 67–72. doi: 10.1016/j.mib.2015.04.008
Hairston N. G. Jr, De Stasio B. T. Jr. (1988). Rate of Evolution Slowed by a Dormant Propagule Pool. Nature 336 (6196), 239–242. doi: 10.1038/336239a0
Held N. A., Mcilvin M. R., Moran D. M., Laub M. T., Saito A. (2019). Unique Patterns and Biogeochemical Relevance of Two- Component Sensing in Marine Bacteria. mSystems 4, 1–16. doi: 10.1128/mSystems.00317-18
Hoppe C. J. M., Wolf K. K. E., Schuback N., Tortell P. D., Rost B. (2018). Compensation of Ocean Acidification Effects in Arctic Phytoplankton Assemblages. Nat. Clim. Change 8, 529–533. doi: 10.1038/s41558-018-0142-9
Hutchins D. A., Fu F. (2017). Microorganisms and Ocean Global Change. Nat. Microbiol. 2, 17058. doi: 10.1038/nmicrobiol.2017.58
Jin P., Ji Y., Huang Q., Li P., Pan J., Lu H., et al. (2022). A Reduction in Metabolism Explains the Trade-Offs Associated With the Long-Term Adaptation of Phytoplankton to High CO2 Concentrations. New Phytol. 233 (5), 2155–2167. doi: 10.1111/nph.17917
Johnson C. H., Mori T., Xu Y. (2008). A Cyanobacterial Circadian Clockwork. Curr. Biol. 18, 816–825. doi: 10.1016/j.cub.2008.07.012
Krell A., Beszteri B., Dieckmann G., Glöckner G., Valentin K., Mock T. (2008). A New Class of Ice-Binding Proteins Discovered in a Salt-Stress-Induced cDNA Library of the Psychrophilic Diatom Fragilariopsis Cylindrus (Bacillariophyceae). Eur. J. Phycol. 43 (4), 423–433. doi: 10.1080/09670260802348615
Kremer C. T., Fey S. B., Arellano A. A., Vasseur D. A. (2018). Gradual Plasticity Alters Population Dynamics in Variable Environments: Thermal Acclimation in the Green Alga Chlamydomonas Reinhartdii. Proc. R. Soc. B: Biol. Sci. 285 (1870), 20171942. doi: 10.1098/rspb.2017.1942
Kremp A., Oja J., LeTortorec A. H., Hakanen P., Tahvanainen P., Tuimala J., et al. (2016). Diverse Seed Banks Favour Adaptation of Microalgal Populations to Future Climate Conditions. Environ. Microbiol. 18 (2), 679–691. doi: 10.1111/1462-2920.13070
Lambert G., Kussel E. (2014). Memory and Fitness Optimization of Bacteria Under Fluctuating Environments. PloS Genet. 10, e1004556. doi: 10.1371/journal.pgen.1004556
Lennon J. T., Jones S. E. (2011). Microbial Seed Banks: The Ecological and Evolutionary Implications of Dormancy. Nat. Rev. Microbiol. 9 (2), 119–130. doi: 10.1038/nrmicro2504
Leung C., Rescan M., Grulois D., Chevin L. M. (2020). Reduced Phenotypic Plasticity Evolves in Less Predictable Environments. Ecol. Lett. 23 (11), 1664–1672. doi: 10.1111/ele.13598
Lindberg R. T., Collins S. (2020). Quality–quantity Trade-Offs Drive Functional Trait Evolution in a Model Microalgal ‘Climate Change Winner.’ Ecol. Lett. 23, 780–790. doi: 10.1111/ele.13478
Litaker W., Duke C. S., Kenney B. E., Ramus J. (1993). Short-Term Environmental Variability and Phytoplankton Abundance in a Shallow Tidal Estuary. II. Spring and Fall. Mar. Ecol. Prog. Ser. 94, 141–154. doi: 10.3354/meps094141
Liu X., Wang L., Wu S., Zhou L., Gao S., Xie X., et al. (2022). Formation of Resting Cells is Accompanied With Enrichment of Ferritin in Marine Diatom Phaeodactylum Tricornutum. Algal Res. 61, 102567. doi: 10.1016/j.algal.2021.102567
Lohbeck K. T., Riebesell U., Reusch T. B. (2012). Adaptive Evolution of a Key Phytoplankton Species to Ocean Acidification. Nat. Geosci. 5 (5), 346–351. doi: 10.1038/ngeo1441
Mackey K. R. M., Post A. F., McIlvin M. R., Cutter G. A., John S. G., Saito M. A., et al. (2015). Divergent Responses of Atlantic Coastal and Oceanic Synechococcus to Iron Limitation. Proc. Natl. Acad. Sci. U. S. A. 112, 9944–9949. doi: 10.1073/pnas.1509448112
Madhani H. D. (2013). The Frustrated Gene: Origins of Eukaryotic Gene Expression. Cell 155, 744. doi: 10.1016/j.cell.2013.10.003
Matthijs M., Fabris M., Obata T., Foubert I., Franco-Zorrilla J. M., Solano R., et al. (2017). The Transcription Factor Bzip14 Regulates the TCA Cycle in the Diatom Phaeodactylum Tricornutum. EMBO J. 36, 1559–1576. doi: 10.15252/embj.201696392
Middlebrook R., Anthony K. R., Hoegh-Guldberg O., Dove S. (2012). Thermal Priming Affects Symbiont Photosynthesis But Does Not Alter Bleaching Susceptibility in Acropora Millepora. J. Exp. Mar. Biol. Ecol. 432, 64–72. doi: 10.1016/j.jembe.2012.07.005
Norman T. M., Lord N. D., Paulsson J., Losick R. (2013). Memory and Modularity in Cell-Fate Decision Making. Nature 503, 481–486. doi: 10.1038/nature12804
O'Donnell D. R., Hamman C. R., Johnson E. C., Kremer C. T., Klausmeier C. A., Litchman E. (2018). Rapid Thermal Adaptation in a Marine Diatom Reveals Constraints and Trade-Offs. Global Change Biol. 24 (10), 4554–4565. doi: 10.1111/gcb.14360
Östling J., Goodman A., Kjelleberg S. (1991). Behaviour of IncP-1 Plasmids and a miniMu Transposon in a Marine Vibrio Sp.: Isolation of Starvation Inducible Lac Operon Fusions. FEMS Microbiol. Lett. 86 (1), 83–93. doi: 10.1111/j.1574-6968.1991.tb04797.x
Rengefors K., Kremp A., Reusch T. B., Wood A. M. (2017). Genetic Diversity and Evolution in Eukaryotic Phytoplankton: Revelations From Population Genetic Studies. J. Plankton Res. 39 (2), 165–179. doi: 10.1093/plankt/fbw098
Rodríguez-Romero A., Gaitán-Espitia J. D., Opitz T., Lardies M. A. (2022). Heterogeneous Environmental Seascape Across a Biogeographic Break Influences the Thermal Physiology and Tolerances to Ocean Acidification in an Ecosystem Engineer. Biodiversity Distributions, 1–12. doi: 10.1111/ddi.13478
Rozen Y., Belkin S. (2005). Survival of Enteric Bacteria in Seawater. Ocean. Heal. Pathog. Mar. Environ. 25, 93–107. doi: 10.1007/0-387-23709-7_4
Samuels T., Rynearson T. A., Collins S. (2021). Surviving Heatwaves: Thermal Experience Predicts Life and Death in a Southern Ocean Diatom. Front. Mar. Sci. 9. doi: 10.3389/fmars.2021.600343
Sauer J., Wenderoth K., Maier U. G., Rhiel E. (2002). Effects of Salinity, Light and Time on the Vertical Migration of Diatom Assemblages. Diatom. Res. 17 (1), 189–203. doi: 10.1080/0269249X.2002.9705538
Schaller G. E., Shiu S. H., Armitage J. P. (2011). Two-Component Systems and Their Co-Option for Eukaryotic Signal Transduction. Curr. Biol. 21, R320–R330. doi: 10.1016/j.cub.2011.02.045
Schaum C. E., Buckling A., Smirnoff N., Studholme D. J., Yvon-Durocher G. (2018). Environmental Fluctuations Accelerate Molecular Evolution of Thermal Tolerance in a Marine Diatom. Nature Communications 9 (1), 1–14. doi: 10.1038/s41467-018-03906-5
Schaum C. E., Buckling A., Smirnoff N., Studholme D. J., Yvon-Durocher G. (2018a). Environmental fluctuations accelerate molecular evolution of thermal tolerance in a marine diatom. Nature Communications 9 (1), 1–14.
Schaum C. E., Rost B., Collins S. (2016). Environmental Stability Affects Phenotypic Evolution in a Globally Distributed Marine Picoplankton. ISME J. 10, 75–84. doi: 10.1038/ismej.2015.102
Schaum E., Rost B., Millar A. J., Collins S. (2013). Variation in Plastic Responses of a Globally Distributed Picoplankton Species to Ocean Acidification. Nat. Climate Change 3 (3), 298–302. doi: 10.1038/nclimate1774
Schluter L., Lohbeck K. T., Gro ger J. P., Riebesell U., Reusch T. B. H. (2016). Long-Term Dynamics of Adaptive Evolution in a Globally Important Phytoplankton Species to Ocean Acidification. Sci. Adv. 2, e1501660–e1501660. doi: 10.1126/sciadv.1501660
Serôdio J. (2021). Diatom Motility: Mechanisms, Control and Adaptive Value. Diatom. Glid. Motil., 159–183. doi: 10.1002/9781119526483.ch7
Shimizu K. (2013). Regulation Systems of Bacteria Such as Escherichia Coli in Response to Nutrient Limitation and Environmental Stresses. Metabolites 4, 1–35. doi: 10.3390/metabo4010001
Steinberg B., Ostermeier M. (2016). Environmental Changes Bridge Evolutionary Valleys. Sci. Adv. 2 (1), e1500921. doi: 10.1126/sciadv.1500921
Suzuki S., Ferjani A., Suzuki I., Murata N. (2004). The SphS-SphR Two Component System is the Exclusive Sensor for the Induction of Gene Expression in Response to Phosphate Limitation in Synechocystis. J. Biol. Chem. 279 (13), 13234–13240. doi: 10.1074/jbc.M313358200
Switzer A., Evangelopoulos D., Figueira R., de Carvalho L. P. S., Brown D. R., Wigneshweraraj S. (2018). A Novel Regulatory Factor Affecting the Transcription of Methionine Biosynthesis Genes in Escherichia Coli Experiencing Sustained Nitrogen Starvation. Microbiol. (United Kingdom) 164, 1457–1470. doi: 10.1099/mic.0.000683
Tagkopoulos I., Liu Y.-C., Tavazoie S. (2008). Predictive Behavior Within Microbial Genetic Networks. Science 320, 1313–1317. doi: 10.1126/science.1154456
Tang Y. Z., Gobler C. J. (2015). Sexual Resting Cyst Production by the Dinoflagellate Akashiwo Sanguinea: A Potential Mechanism Contributing to the Ubiquitous Distribution of a Harmful Alga. J. Phycol. 51 (2), 298–309. doi: 10.1111/jpy.12274
Tinta T., Vojvoda J., Mozetič P., Talaber I., Vodopivec M., Malfatti F., et al. (2015). Bacterial Community Shift is Induced by Dynamic Environmental Parameters in a Changing Coastal Ecosystem (Northern Adriatic, Northeastern Mediterranean Sea)–A 2-Year Time-Series Study. Environ. Microbiol. 17 (10), 3581–3596. doi: 10.1111/1462-2920.12519
Veening J. W., Smits W. K., Kuipers O. P. (2008). Bistability, Epigenetics, and Bet-Hedging in Bacteria. Annu. Rev. Microbiol. 62, 193–210. doi: 10.1146/annurev.micro.62.081307.163002
Walworth N. G., Hinners J., Argyle P. A., Leles S. G., Doblin M. A., Collins S., et al. (2021b). The Evolution of Trait Correlations Constrains Phenotypic Adaptation to High CO2 in a Eukaryotic Alga. Proc. R. Soc. B 288 (1953), 20210940. doi: 10.1098/rspb.2021.0940
Walworth N. G., Lee M. D., Dolzhenko E., Fu F. X., Smith A. D., Webb E. A., et al. (2021a). Long-Term M5c Methylome Dynamics Parallel Phenotypic Adaptation in the Cyanobacterium Trichodesmium. Mol. Biol. Evol. 38 (3), 927–939. doi: 10.1093/molbev/msaa256
Walworth N. G., Zakem E. J., Dunne J. P., Collins S., Levine N. M. (2020). Microbial Evolutionary Strategies in a Dynamic Ocean. Proc. Natl. Acad. Sci. U. S. A. 117, 5943–5948. doi: 10.1073/pnas.1919332117
Woelfle M. A., Ouyang Y., Phanvijhitsiri K., Johnson C. H. (2004). The Adaptive Value of Circadian Clocks: An Experimental Assessment in Cyanobacteria. Curr. Biol. 14, 1481–1486. doi: 10.1016/j.cub.2004.08.023
Wolf K. K. E., Romanelli E., Rost B., John U., Collins S., Weigand H., et al. (2019). Company Matters: The Presence of Other Genotypes Alters Traits and Intraspecific Selection in an Arctic Diatom Under Climate Change. Glob. Change Biol. 25, 2869–2884. doi: 10.1111/gcb.14675
Zacharioudakis I., Gligoris T., Tzamarias D. (2007). A Yeast Catabolic Enzyme Controls Transcriptional Memory. Curr. Biol. 17, 2041–2046. doi: 10.1016/j.cub.2007.10.044
Keywords: phenotypic plasticity, adaptive evolution, phytoplankton, bacteria, climate change, environmental change
Citation: Arromrak BS, Li Z and Gaitán-Espitia JD (2022) Adaptive Strategies and Evolutionary Responses of Microbial Organisms to Changing Oceans. Front. Mar. Sci. 9:864797. doi: 10.3389/fmars.2022.864797
Received: 28 January 2022; Accepted: 23 May 2022;
Published: 16 June 2022.
Edited by:
Michael Raatz, Max Planck Institute for Evolutionary Biology, GermanyReviewed by:
Francisca C. García, King Abdullah University of Science and Technology, Saudi ArabiaCopyright © 2022 Arromrak, Li and Gaitán-Espitia. This is an open-access article distributed under the terms of the Creative Commons Attribution License (CC BY). The use, distribution or reproduction in other forums is permitted, provided the original author(s) and the copyright owner(s) are credited and that the original publication in this journal is cited, in accordance with accepted academic practice. No use, distribution or reproduction is permitted which does not comply with these terms.
*Correspondence: Juan Diego Gaitán-Espitia, anVhZGllZ2FpdGFuQGdtYWlsLmNvbQ==
Disclaimer: All claims expressed in this article are solely those of the authors and do not necessarily represent those of their affiliated organizations, or those of the publisher, the editors and the reviewers. Any product that may be evaluated in this article or claim that may be made by its manufacturer is not guaranteed or endorsed by the publisher.
Research integrity at Frontiers
Learn more about the work of our research integrity team to safeguard the quality of each article we publish.