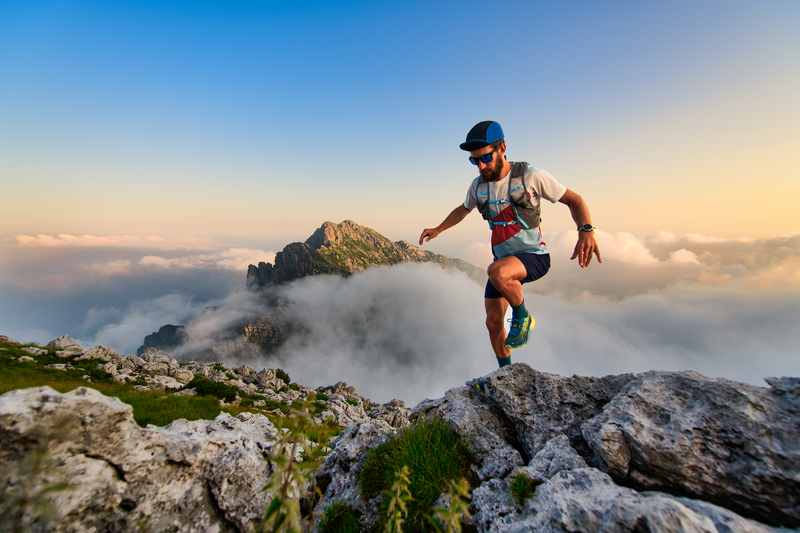
95% of researchers rate our articles as excellent or good
Learn more about the work of our research integrity team to safeguard the quality of each article we publish.
Find out more
ORIGINAL RESEARCH article
Front. Mar. Sci. , 13 May 2022
Sec. Marine Pollution
Volume 9 - 2022 | https://doi.org/10.3389/fmars.2022.863629
This article is part of the Research Topic Marine Pollution - Emerging Issues and Challenges View all 13 articles
Artisanal and small-scale gold mines (ASGMs) have been accompanied by widespread usage of mercury amalgamation to extract gold from ores, putting Indonesia among the top three global emitters of this pollutant and posing potential risks to the marine ecosystem and human health. Although the use of mercury has been largely eliminated following the signature of the Minamata Convention on Mercury, the practice of mercury amalgamation in ASGM has persisted in several regions, including the North Sulawesi. This study assesses how on the contamination of mercury and other trace elements coming from both industrial mines and ASGMs affects marine sediments and their bioaccumulation in two tissues (body wall and guts) of the edible holothurian Holothuria (Halodeima) atra, by comparing samples collected downstream of four mining areas to four control sites in the North Sulawesi province, Indonesia. In sediments, mean concentrations of arsenic, gold, cobalt, chromium, copper, mercury, nickel, lead, antimony, and zinc were significantly higher at sites receiving mine discharges than at control sites. Downstream to gold mines, compared to control sites, significant higher concentrations of As, Au, Cr, Hg, and Ni in holothurians body walls and of As, Au, Co, Cr, Cu, Hg, Pb, Sb, Sn, and Zn in holothurians guts were found. In general, higher contaminations in sediments and tissues were found at the site near the oldest artisanal mine. Trace element levels in H. atra specimens in North Sulawesi were generally higher than those reported in other regions. In the study area, these holothurians significantly bioaccumulate Hg, As, Zn, Cd, Cu, Sn, and biota-sediment accumulation factors were higher in guts than in body walls. From an environmental and human health perspective, Hg is resulted the most concerning element in surface sediment and H. atra specimens. Based on this evidence, further studies are urgently needed to understand better the effect of mercury and other potentially toxic trace elements in marine ecosystems and food webs in mining areas both in North Sulawesi and in many still poorly investigated southeast Pacific areas.
Toxic trace elements including arsenic (As), cadmium (Cd), cobalt (Co), chromium (Cr), copper (Cu), mercury (Hg), nickel (Ni), lead (Pb), antimony (Sb), tin (Sn) and zinc (Zn) can accumulate in marine and coastal environments, derived from natural processes, including rock weathering, volcanic emissions, and aeolian transport, and human activities. Swift industrialization, economic development, and urbanization in coastal regions has contributed to an increase of trace elements in seas, in estuarine and coastal habitats (Jiang et al., 2014). This increasing spread of trace elements and other pollutants from anthropogenic activities threatens local species and humans and has assumed global relevance (Long and Chapman, 1985; Ponti et al., 2021).
Mining activities are among the main causes of mobilization and release of trace elements into the environment (Marmolejo-Rodríguez et al., 2011). In the North Sulawesi province (Indonesia), as in many other areas of the Coral Triangle (sensu Veron et al., 2009), industrial and artisanal gold mining activities are widespread. While industrial gold mines generally use the cyanidation method, artisanal and small-scale gold mines (hereafter ASGMs) still employ Hg amalgamation to extract gold from ores (Bose-O’Reilly et al., 2010; Edinger, 2012). ASGMs are considered a major source for emission and release of Hg worldwide (Velásquez-López et al., 2010; Cordy et al., 2011; Odumo et al., 2014; González-Merizalde et al., 2016; Oke and Vermeulen, 2016), with 410-1040 tons of employed Hg per year corresponding to ca. 37% of total anthropogenic Hg emissions (Futsaeter and Wilson, 2013). Moreover, Hg (as cinnabar, a form of mercury sulfide), As, and Sb can be present in many gold deposits (Edinger, 2012). Mine tailings, even when stored in artificial closed ponds, as occurs in industrial mines, may be dispersed in the catchment basin due to heavy rain and flooding events, frequent in tropical areas (González-Valoys et al., 2022).
After the discovery of the Minamata neurological disease, caused by severe Hg poisoning in the Minamata Bay (Japan) from a local chemical factory in the 1956 (Eto et al., 2010), numerous studies were carried out on the bioaccumulation and marine food web transfers of potentially toxic trace elements (e.g., Bryan and Langston, 1992; Wang, 2002; Gao and Chen, 2012; Li et al., 2017; Murillo-Cisneros et al., 2019). A large number of chemical and biological mediated processes can contribute to trace element mobilization from rocks and soils (e.g., Carrillo‐González et al., 2006; Sessitsch et al., 2013; Sánchez-Donoso et al., 2021). After being released from natural or anthropogenic sources like factories, mines, shipping, dumping, or harbors to the marine environment, soluble trace metal species are accumulated in sediments through physical, chemical, and biological processes. For example, bacterially mediated processes can methylate Hg once it is available at the sediment-water interface as metallic Hg or in metastable phases. Then methylmercury (MeHg) is acutely toxic and readily biomagnifies up marine food webs (Edinger, 2012). The sedimentation of trace elements is quickened thanks to the high salinity of seawater that enhances the aggregation of suspended particles; therefore, sediment is considered the main sink for trace elements (and other pollutants) in urbanized coastal ecosystems (e.g., Du Laing et al., 2009; Jonathan et al., 2011; Qian et al., 2015; Birch et al., 2020).
Given their bioaccumulation and toxic effects on aquatic organisms, trace metals analyses are usually included in marine environmental monitoring programs (e.g., Badr et al., 2009; Greggio et al., 2021). Benthic communities involved in detrital-based food webs are frequently subjected to effluent waste from anthropogenic sources: high levels of trace elements in sediments might mean an ecotoxicological threat for benthic invertebrates (e.g., Bryan and Langston, 1992; Rainbow, 2002; O’Brien and Keough, 2014). In particular, being deposit-feeders and scavengers, many holothurians represent a relevant step in transferring of pollutants from sediments to marine food webs (Aydın et al., 2017). They play major roles in sediment bioturbation (Uthicke, 1999) and are preyed upon by many taxa, thereby transferring accumulated toxic elements to higher trophic levels (Purcell et al., 2016). Some holothurians are also food for humans. They are mainly eaten in Asia, where are regarded as traditional medicine, delicacies and aphrodisiacs (Aydın et al., 2017).
The target species of the present study is Holothuria (Halodeima) atra, commonly known as black sea cucumber or lollyfish. It is an intertidal and shallow-water species widely distributed in the tropical Indo-Pacific region and one of the most frequently encountered in Indonesia (Conand, 1998; Bellchambers et al., 2011). Inshore shallow-water populations are often dense and composed of small individuals (about 20 cm and 0.2 kg live weight, on average) that reproduce mostly by transversal fission (Hartati et al., 2019; Hartati et al., 2020). Individuals of deeper or outer reef populations are more scattered and larger (up to 45 cm and 1 kg live weight) and reproduce sexually more frequently (Conand, 1998). Preferring habitats rich in organic matter, this species plays a relevant role in nutrients recycling and sediment reworking in tropical sea bottom (Conand, 2004). It is considered a deposit-feeders and scavenger species that feeds on bacteria and detritus but may digest microalgae and cyanobacteria as well (Uthicke, 1999; Retno et al., 2020). H. atra also has relevant commercial importance for the Asian markets (Toral-Granda et al., 2008; Purcell et al., 2012; Setyastuti and Purwati, 2015). According to the Red List of threatened species provided by the International Union for the Conservation of Nature (IUCN), the conservation of this species is considered of least concern (Conand et al., 2013).
This study focuses on the contamination of mercury and other trace elements coming from both industrial mines and ASGMs in marine sediments and their bioaccumulation in two tissues (body wall and guts) of the edible holothurian Holothuria (Halodeima) atra, by comparing samples collected in coastal areas downstream of four mines and at four control sites in the North Sulawesi province, Indonesia. Overall, 12 potentially toxic trace elements were investigated (As, Au, Cd, Co, Cr, Cu, Hg, Ni, Pb, Sb, Sn, and Zn), of these four are particularly linked to gold mining and therefore should be more abundant in sediments and holothurian tissues at sites close to the four mines than at the control sites: Hg, used in ASGMs for gold extraction and often associated to gold deposits; As and Sb, abundant in gold deposits in the region; and Au, the target of mining activities.
The present study was carried out in the North Sulawesi (Indonesian: Sulawesi Utara) province and included four putatively impact sites and four control sites randomly interspersed among the impact ones (Figure 1; Supplementary Material S1; Table S1.1; Figure S1.1). This tropical area is affected by monsoon winds and uneven rainfall (mainly from November to April), with annual rates ranging from 1,000 to 5,000 mm. Air temperature ranges from 22 to 30°C (25°C on average), while sea surface temperature ranges from 27 to 29°C1.
Figure 1 Map of the study area and sampling sites (Datum WGS84, Projection UTM zone 51). Included active gold mines: Totok Bay (TB); Talawaan River (TW); Pantai Surabaya (PS); Mining site in preparation: West Bangka mangroves (M1); Control sites: Lihunu Bay (LB); North-west Bangka mangroves (M2); Tumbak Island (TI); Tasik Ria Resort (TR).
Impact sites were chosen as closest as possible to the mouth of streams or rivers flowing through mining areas, identified based on a watershed analysis performed with the GRASS’ “r.watershed” algorithm in QGIS 3.16 (QGIS Development Team, 2021) using the digital elevation model by the Shuttle Radar Topography Mission (SRTM 4.1; horizontal resolution of 90 m at the equator). Maps resulting from the watershed analysis are reported in Supplementary Material S1. These impact sites correspond to different types and periods of gold mining activities.
The first impact site was chosen at Totok Bay (labeled as TB; Figures S1.1a, S1.2), where ASGMs were established in the mid-19th century. Totok Bay and the nearby Buyat Bay (Buyat-Ratatotok district; Turner et al., 1994) are areas already known for pollution from gold mines. From 1996 to 2004, Buyat Bay was affected by a submarine tailing disposal of an industrial gold mine (Blackwood and Edinger, 2007; Lasut and Yasuda, 2008; Edinger, 2012). Totok Bay, separated by a discontinuous peninsula, is mainly affected by the wastewater and surface percolation from the ASGM (Blackwood and Edinger, 2007). Lasut et al. (2010) sampled surface sediments from the beach, river estuary, and marine shallow bottom, and some specimens of soft corals, seaweeds, seagrasses, mollusks, crabs, and fishes from both bays, as well as the scalp hair of residents in the area. Total mercury and MeHg were detected in all samples, with the highest concentrations at Totok Bay and intermediate concentrations at Buyat Bay compared to the control area. The second impact site was chosen at the mouth of Talawaan River (TR; Figures S1.1b, S1.3) downstream of the Talawaan-Tatelu ASGMs, established in 1997. Here, Kambey et al. (2001) found that fish from the affected area had a mercury content 30 times higher than those from the reference site. The third impact site was chosen at Pantai Surabaya (PS; Figures S1.1c, S1.4), downstream to the Toka Tindung industrial mine, operating since 2010. There are no published pollution studies for this area. The fourth impact site was chosen at the mangroves (M1; Figures S1.1d, S1.5) between the Coral Eye resort and an industrial mining site under preparation on the west side of Bangka Island, whose soil handling works started in 2014. The start of mining activities immediately raised concern for local coral reefs’ health (Ponti et al., 2016). The mine was officially closed in 2017 after the Supreme Court of Jakarta accepted a lawsuit filed by residents and tour operators.
Control sites were randomly located at Lihunu Bay (LB; Figure S1.1e), at the North-west Bangka Island mangroves (M2; Figure S1.1f), at Tumbak Island (TI; Figure S1.1g), and close to the Tasik Ria Resort (TR; Figure S1.1h).
All sampling activities took place from June to August 2017, at times chosen around the peak of low tide in a range of depth between -0.9 and 2.8 m below the mean lower low water (Table S1.1). Tide levels were calculated by the freeware software WXTide2, using the closest subordinate harmonic data station available (i.e., Manado or Lembeh Strait).
Three replicate samples of undisturbed surface sediment (top 5 cm) were randomly taken by a plastic scoop at each site to measure grain size and trace element concentration in the sediments. The sampler was washed in deionized water to avoid any contamination after every sampling. Each sample was collected and stored in new low-density polyethylene (LDPE) grip seal bags. All sediment samples were oven-dried at 50°C for 24 h. After a pre-sieving at 2000 μm to remove coral rubbles and large shells, a portion of 10 g from each sample was taken for further chemical analysis. Medium and coarse sand (2000 – 250 µm), fine sand (250 – 63 µm), and mud (silt and clay, < 63 µm) sediment content was measured as dry weight percentage after sieving using certified stainless-steel sieves (Guerra et al., 2009).
At each sites, the population density of H. atra was estimated by counting individuals along three belt transects (100 × 4 m, i.e., 400 m2), carried out at least 10 m apart either by snorkeling or on foot, depending on the depth and tide conditions (Bellchambers et al., 2011). Then, eight specimens of H. atra were collected in the intertidal zone to measure trace element concentrations. To avoid differences in bioconcentration among individuals due to different ages, and therefore time exposures, the collected individuals were of similar size (~ 20 cm in length; mean wet weight: 103.7 ± 8.7 g) (Denton et al., 2009). Specimens were transported alive in plastic grip seal bags with seawater until they were placed in 20-liter aquariums with aerators, four specimens per aquarium, to purge their intestinal sediment. After 24-48 hours in the aquarium, the specimens were put in large beakers with a solution of 8% MgCl2 in seawater for 30 min for tissue relaxing. Later, the samples were washed with tap water and dried on the surface with paper towels, weighted (for wet mass measures), and dissected by a longitudinal incision from the anus to the mouth, in order to separately analyze the body wall (about 4 mm thick; Conand, 1998) and guts (Xing and Chia, 1997). Tissue samples were washed in a stream of distilled water to remove the salt, then they were dried at no more than 50°C for 24 h and individually stored in new LDPE grip seal bags until chemical analysis.
Both dried sediment samples and holothurian tissues were grinded in the laboratory after additional drying for 24 h at no more than 50°C. Approximately 1 g of each sample was powdered in a ceramic mortar and put in a plastic screw-top vial. A sediment subsample (about 0.075 g) was added with 0.35 g of HNO3 (69%) and 0.75 g of HCl (37%, Analytical Reagent). A microwave digestion system (CEM EXPLORER SP-D PLUS) was used for the acid digestion, according to the following protocol: ramp temperature from ambient to 200°C for 4 min, then 200°C for 2 min, and 300 W power with medium stirring and 300 PSI of pressure. Tissue samples (about 0.075 g) were heated in closed polypropylene vials for 3 hours at 100°C and then diluted with Milli-Q water to obtain 5% by weight. All tools, vials and jars were cleaned before every use with 20% nitric acid and deionized water to avoid cross-contamination among samples.
Twelve elements (As, Au, Cd, Co, Cr, Cu, Hg, Ni, Pb, Sb, Sn, and Zn) important for the results of the present study were quantified both in sediment and holothurian tissues by using inductively coupled plasma coupled to a mass spectrometer (ICP-MS, Agilent Technologies 7700x. Agilent Technologies International Japan, Ltd., Tokyo, Japan). In addition, the main rock-forming elements (Ca, Mg, Na, S, Al, Fe, Si, K, Ti) and Li, Ba, and Sr were also quantified to characterize the origin of the sediments. The instrument was equipped with an octupole collision cell operating in kinetic energy discrimination mode, which was used to remove polyatomic and argon-based interferences. The operating conditions and data acquisition parameters were the same as reported in Badocco et al. (2015a). Multielement standard solutions for calibration were prepared in aqua regia (5%) by gravimetric serial dilution at twelve different concentrations - from a minimum of 1 ng L-1 to a maximum of 100 mg L-1. All solutions were prepared in Milli-Q ultrapure water obtained using a Millipore Plus System (Milan, Italy, resistivity 18.2 MOhm cm-1). All regressions were linear with a determination coefficient (R2) larger than 0.9999. The ICP-MS was tuned daily using a tuning solution containing 1 μg L-1 of 140Ce, 7Li, 205Tl, and 89Y (Agilent Technologies, UK). The internal standard mixture (Agilent, 5183-4681) containing Bi, Ge, In, Sc, Tb, Y, and Li (6) at 10 μg mL-1, each in 5% HNO3 was used. The internal standard was added to the sample solution via a T-junction. The Critical Limit (CL), Limit of Detection (LOD) and Limit of Quantification (LOQ) of each element were computed based on the methods described in Lavagnini et al. (2011) and Badocco et al. (2014; 2015a; 2015b; 2015c).
In tissues analysis, the recovery value was assessed using a standard reference material (SRM) 2976 specifically intended for use for the determination of trace elements in marine mollusk tissue or similar matrices. The average recovery obtained from the repetition of 5 measurements was between 93% and 104% for all the elements investigated. The recovery of Au equal to 96% was evaluated by spiking the matrix with suitable amounts of Au solutions. Since the obtained recovery was always close to 100%, the analytical results were not corrected for holothurian tissues as suggested in other works citing recoveries between 80 and 120% as acceptable (Chen and Ma, 2001). In sediment analysis, the recovery of the elements is a variable parameter linked to the nature of the type of rock that originates the sediment. The SRM 2702 Inorganics in Marine Sediment was used as a reference for the tested sediments. The recovery of the elements of interest was between 82% and 112%. The recoveries of Si and Al were lower than 50%. However, the quantification of these elements was used only as an indication of the nature of the sediment.
Trace element concentration values below Critical Limit (CL) were replaced by one-half of the minimum Limit of Detection (LODmin) only for statistical purposes (Paschal et al., 1998). Since there were no reasons to hypothesize that the concentrations of trace elements in sediment and holothurian tissues were affected in the same way in the different basins subject to gold mines, each putatively impacted site was individually compared to the control sites (as in Guerra et al., 2009 and Ponti et al., 2009). To this end, two-way asymmetrical permutational analysis of variance (PERMANOVA; α = 0.05), based on Euclidean distances, was applied to assess the differences in mean concentrations in sediments and tissues (body wall and guts) between Impact and Control, and among sites nested in the interaction Impact × Control, where Impact is represented by a single site while Control has four sites (Anderson and Robinson, 2008). When less than 100 unique permutations were available, the asymptotic Monte Carlo p-value was used instead of the permutational one. When no significant differences in mean trace element concentrations were found among control sites (p > 0.25), site data were pooled to improve the power of the test for differences between Impact and Control (Anderson et al., 2008).
Possible correlations between the concentrations of trace elements in the body wall and guts of individuals of H. atra (n = 64) and between the mean concentrations in the sediments of the different sites and those in the body walls and guts of H. atra (n = 64) were estimated with Pearson’s moment-product correlation coefficient (r), whose difference from zero were assessed by t-test (α = 0.05).
Bioaccumulation is the concentration in the organism of a chemical resulting from the dynamic equilibrium between the uptake rate (by all possible means, including contact, respiration and ingestion) and the elimination rate (Chojnacka and Mikulewicz, 2014). Since H. atra mainly feeds on sediment, for each element, the bioaccumulation in the field can be assessed by the biota-sediment accumulation factor (BSAF), calculated as the ratio between the mean concentrations in tissues (body walls and guts) and sediments at a given site (Thomann et al., 1995). Bioaccumulation in the study area was confirmed when mean BSAF across sites was significantly greater than 1 according to a t-test (α = 0.05, n = 8).
PERMANOVA analyses were done using PRIMER 6 with PERMANOVA+ addon package (Anderson et al., 2008), while linear correlations and t-tests were conducted with the computational language R (R Core Team, 2021). Mean values were reported with their standard errors.
Sandy sediments characterized all sampling sites, but with large variability in medium/coarse sand (12.11-94.83%) and fine sand (5.01-86.16%) contents, while the fraction of mud (silt and clay) was always very low (0.08%-2.59%). Overall, mean grain size at control sites covered the entire range of the putatively impacted sites; however, control M2, TI, and TR sites and the impact site TW showed a higher percentage of medium/coarse sand and a low percentage of fine sand, compared to the other sites (Supplementary Material S2, Figure S2.1). Mean composition of the main rock-forming elements in the sampled sediments reveals a high percentage of calcium (34-43%) at all sites but TB and PS (1.7% and 11.7%, respectively); here, the sediments resulted comparatively richer in aluminum and iron (Supplementary Material S2, Figure S2.2).
Mean concentrations of trace elements in sediment at impact and control sites are shown by bar plots in Figure 2, while tests for significance of differences between mean concentrations at each single impact site to the control ones are reported in the Supplementary Materials (Tables S3.1–4). Mean concentrations of Hg in sediments ranged from below the CL, as occurred at all control sites, to 1.25 ± 0.60 μg g-1 at TB (Figure 2A). At all putatively impacted sites, including M1, where gold extractions should never have started, the mean Hg concentrations in sediments were significantly higher than at the control sites. Mean concentrations of As ranged from 1.53 ± 0.15 μg g-1 at LB to 34.08 ± 3.51 μg g-1 at TB, and at TB and PS they were significantly higher than at the control sites (Figure 2B). Mean Au concentrations were below CL at all sites except TB, where it was 0.07 ± 0.02 μg g-1, clearly significantly higher than at the control sites (Figure 2C). Mean concentrations of Sb ranged from 0.0524 ± 0.0004 μg g-1 at LB to 1.16 ± 0.15 μg g-1 at TB, and at TB and PS they were significantly higher than at the control sites (Figure 2D). Mean concentrations of Cd ranged from 0.023 ± 0.003 μg g-1 at PS to 0.047 ± 0.002 μg g-1 at LB (Figure 2E), resulting significantly heterogeneous between control sites (p < 0.001); since this variability, the concentrations at the impact sites were not significantly different from those of the controls.
Figure 2 Mean concentrations (± s.e.) of Hg (A), As (B), Au (C), Sb (D), Cd (E), Co (F), Cr (G), Cu (H), Ni (I), Pb (J), Sn (K) and Zn (L) in surface sediment at each study site (TB, Totok Bay; TW, Talawaan River; PS, Pantai Surabaya; M1, West Bangka mangroves; LB, Lihunu Bay; M2, North-west Bangka mangroves; TI, Tumbak Island; TR, Tasik Ria Resort). Whenever possible, the default guideline value (DGV), i.e., the concentration below which there is a low risk of unacceptable effects occurring, and the ‘upper’ guideline value (GV-high), i.e., the concentration over which adverse effects are expected, from Simpson et al. (2013), were reported (CL, critical limit). Impact sites that differ significantly from controls are marked with asterisks: *p < 0.05; **p < 0.01; ***p < 0.001.
Mean concentrations of Co ranged from 0.13 ± 0.01 μg g-1 at M2 to 11.74 ± 0.70 μg g-1 at TB (Figure 2F). Mean concentrations of Cr ranged from 2.55 ± 0.41 μg g-1 at M1 to 13.72 ± 2.80 μg g-1 at TB (Figure 2G). Mean concentrations of Cu ranged from 0.76 ± 0.11 μg g-1 at TI to 26.64 ± 1.35 μg g-1 at PS (Figure 2H). Mean concentrations of Ni ranged from 0.80 ± 0.04 μg g-1 at M2 to 8.22 ± 1.16 μg g-1 at TB (Figure 2I). Although with different levels of significance, Co, Cr, Cu, and Ni were all significantly higher at TB and PS than at the control sites.
Mean concentrations of Pb ranged from 0.23 ± 0.02 μg g-1 at TI to 4.25 ± 0.81 μg g-1 at TB, which was the only impact site with significantly enriched compared the control sites (Figure 2J). Mean concentrations of Sn ranged from below the CL at LB and TI to 1.97 ± 1.84 μg g-1 at TR, where it was very variable (Figure 2K). Given this variability, it was not possible to detect significant differences between the individual impact sites and the control sites. Finally, mean concentrations of Zn ranged from 2.49 ± 0.40 μg g-1 at TI to 50.98 ± 4.15 μg g-1 at TB, and, as for many other trace elements, at TB and PS they were significantly higher than at the control sites (Figure 2L).
The mean densities of holothurians ranged from 0.08 ± 0.08 to 3.08 ± 1.18 individuals of H. atra per 100 m2 (Figure 3). The density of holothurians was very variable across control and impact sites. Only at the PS impact site the density was significantly higher (ANOVA p < 0.01) than the average of the control sites.
Figure 3 Mean density (± s.e.) of H. atra at each study site: TB, Totok Bay; TW, Talawaan River; PS, Pantai Surabaya; M1, West Bangka mangroves; LB, Lihunu Bay; M2, North-west Bangka mangroves; TI, Tumbak Island; TR, Tasik Ria Resort.
Mean concentrations of trace elements in body walls and guts, at impact and control sites, are shown by bar plots in Figures 4, 5, respectively, while tests for significance of differences between mean concentrations at each single impact site to the control ones are reported in the Supplementary Materials (Tables S3.5–12). Mean concentrations of Hg in body walls of H. atra specimens ranged from 0.015 ± 0.001 μg g-1 at M2 to 0.25 ± 0.03 μg g-1 at TB, and they were significantly higher at the impact sites TB and TW than at the control sites (Figure 4A). In guts, mean concentrations of Hg ranged from 0.10 ± 0.02 μg g-1 at TR to 1.72 ± 0.37 μg g-1 at TB, and they were significantly higher at all active gold mine sites (TB, TW, and PS) than at the control sites (Figure 5A). Mean concentrations of As in body walls ranged from 10.38 ± 0.59 μg g-1 at TB to 118.72 ± 10.22 μg g-1 at the industrial mine site PS, where it was significantly higher than at the control sites (Figure 4B). In guts, mean concentrations of As ranged from 24.65 ± 1.92 μg g-1 at TB to 219.68 ± 29.09 μg g-1 at PS, and they were significantly higher at TW and PS than at the control sites (Figure 5B). Mean concentrations of Au in body walls ranged from 0.006 ± 0.001 μg g-1 at TR to 0.050 ± 0.002 μg g-1 at PS, where it was significantly higher than at the control sites (Figure 4C). In guts, mean concentrations of Au ranged from below the CL, at control sites M2 and TR, to 0.51 ± 0.06 μg g-1 at PS, and they were significantly higher at all active gold mine sites (TB, TW, and PS) than at the control sites (Figure 5C). Mean concentrations of Sb in body walls ranged from 0.021 ± 0.002 μg g-1 at TB to 0.05 ± 0.001 μg g-1 at PS; however, the mean value at each impact site did not differ significantly from those of the controls (Figure 4D). In guts, mean concentrations of Sb ranged from 0.033 ± 0.004 μg g-1 at M1 to 0.16 ± 0.02 μg g-1 at TB, and they were significantly higher at TB and PS than at the control sites (Figure 5D). Mean concentrations of Cd in body walls ranged from 0.018 ± 0.002 μg g-1 at M1 to 0.053 ± 0.005 μg g-1 at TI (Figure 4E), while in guts, they ranged from 0.25 ± 0.05 μg g-1 at TR to 1.06 ± 0.09 μg g-1 at PS (Figure 5E). Concentrations of this element in holothurian tissues were highly variable between sites, and no impact sites showed mean values significantly higher than controls. Mean concentrations of Co in body walls ranged from 0.054 ± 0.004 μg g-1 at M1 to 0.23 ± 0.03 μg g-1 at TR (Figure 4F), while in guts, they ranged from 0.14 ± 0.01 μg g-1 at LB to 1.14 ± 0.20 μg g-1 at TB (Figure 5F). Only at the old ASGM site TB, the mean concentration in the guts was significantly higher than at the controls. Mean concentrations of Cr in body walls ranged from 0.56 ± 0.08 μg g-1 at M1 to 3.63 ± 1.53 μg g-1 at TB, and they were significantly higher at ASGM sites, TB and TW, than at the control sites (Figure 4G). In guts, mean concentrations of Cr ranged from 1.84 ± 0.38 μg g-1 at M2 to 9.21 ± 1.92 μg g-1 at PS, and they were significantly higher at TB and PS than at the control sites (Figure 5G). Mean concentrations of Cu in body walls ranged from 1.56 ± 0.08 μg g-1 at TB to 2.84 ± 0.54 μg g-1 at TI (Figure 4H), while in guts, they ranged from 4.50 ± 0.60 μg g-1 at LB to 12.84 ± 2.00 μg g-1 at PS (Figure 5H). Only at the industrial mine site PS, the mean concentration in the guts was significantly higher than at the controls. Mean concentrations of Ni in body walls ranged from 0.12 ± 0.01 μg g-1 at M1 to 0.89 ± 0.26 μg g-1 at TB, where the mean value was significantly higher than at the control sites (Figure 4I). In guts, mean concentrations of Ni ranged from 0.40 ± 0.04 μg g-1 at M1 to 5.86 ± 4.87 μg g-1 at control site TI, and no impact sites showed mean values significantly higher than controls (Figure 5I). Mean concentrations of Pb in body walls ranged from 0.04 ± 0.01 μg g-1 at TW to 0.59 ± 0.08 μg g-1 at control site TI (Figure 4J), while in guts, they ranged from 0.08 ± 0.01 μg g-1 at TW to 0.60 ± 0.08 μg g-1 at TB (Figure 5J). Only at the old ASGM site TB, the mean concentration in the guts was significantly higher than at the controls. Mean concentrations of Sn in body walls ranged from 0.11 ± 0.01 μg g-1 at M1 to 1.93 ± 1.40 μg g-1 at control site TI (Figure 4K), while in guts, they ranged from 0.13 ± 0.01 μg g-1 at TI to 0.45 ± 0.09 μg g-1 at TW (Figure 5K). Only at the ASGM site TW, the mean concentration in the guts was significantly higher than at the controls. Finally, mean concentrations of Zn in body walls ranged from 17.72 ± 2.09 μg g-1 at site TB to 25.36 ± 3.15 μg g-1 at TI, and at TB, the mean value was significantly lower than at the controls (Figure 4L). In guts, mean concentrations of Zn ranged from 45.62 ± 6.45 μg g-1 at TR to 94.16 ± 12.34 μg g-1 at M1, where was significantly higher than at the control sites (Figure 5L).
Figure 4 Mean concentrations (± s.e.) in H. atra body wall of Hg (A), As (B), Au (C), Sb (D), Cd (E), Co (F), Cr (G), Cu (H), Ni (I), Pb (J), Sn (K) and Zn (L) at each study site (TB, Totok Bay; TW, Talawaan River; PS, Pantai Surabaya; M1, West Bangka mangroves; LB, Lihunu Bay; M2, North-west Bangka mangroves; TI, Tumbak Island; TR, Tasik Ria Resort). Impact sites that differ significantly from controls are marked with asterisks: *p < 0.05; **p < 0.01; ***p < 0.001.
Figure 5 Mean concentrations (± s.e.) in H. atra guts of Hg (A), As (B), Au (C), Sb (D), Cd (E), Co (F), Cr (G), Cu (H), Ni (I), Pb (J), Sn (K) and Zn (L) at each study site (TB, Totok Bay; TW, Talawaan River; PS, Pantai Surabaya; M1, West Bangka mangroves; LB, Lihunu Bay; M2, North-west Bangka mangroves; TI, Tumbak Island; TR, Tasik Ria Resort). Impact sites that differ significantly from controls are marked with asterisks: *p < 0.05; **p < 0.01; ***p < 0.001.
The relationships between the mean concentrations of trace elements in sediments and holothurian tissues (body wall and guts) are shown in Figure 6, while Pearson’s product-moment correlation coefficients (r) and corresponding t-tests for difference from zero are reported in Table 1. Significant positive correlations were found for the concentrations in the sediment and in H. atra tissues, body walls and guts, both for Hg and Cr, with very high correlation coefficients for Hg. Ni showed a significant, albeit weak, positive correlation between sediments and body walls, but not guts. As, Sb, Co and Cu exhibited significant positive correlations between sediments and guts, but not body walls. Pb had a weak but significant negative correlation between sediment and body wall, and a significant positive correlation between sediments and guts. The correlation between the concentration of gold between sediments and tissues was not significant, probably due to the finding in the sediments only at Totok Bay. Comparing the concentrations of trace elements in body walls and guts of single individuals, strong positive correlations were found for Hg, As, Au; moreover, weak but significant positive correlations were also detected for Sb, Cd, Co, and Ni (Table 1).
Figure 6 Relations between the mean concentrations of Hg (A), As (B), Au (C), Sb (D), Cd (E), Co (F), Cr (G), Cu (H), Ni (I), Pb (J), Sn (K) and Zn (L) in sediments and their accumulation in holothurian tissues (blue: body wall, red: guts). Impact sites are represented by solid symbols (TB, Totok Bay; TW, Talawaan River; PS, Pantai Surabaya; M1, West Bangka mangroves), while control sites by open symbols (LB, Lihunu Bay; M2, North-west Bangka mangroves; TI, Tumbak Island; TR, Tasik Ria Resort). Lines indicate the linear tendencies, and the grey shadow areas the corresponding 95% confidence intervals.
Table 1 Pearson’s product-moment correlation coefficients (r) and corresponding t-tests for difference from zero of trace element concentrations between sediment (Sed) and body walls (BW) or guts (GU), and between body wall (BW) and guts (GU) (n = 64).
Bioaccumulation, assessed in terms of BSAF, largely varied among elements, sites, and tissues from 0.01 to 45.45 (Table 2). In general, higher values were obtained for guts compared to body walls. The most bioaccumulated element was Hg, whose BSAF resulted in greater than 1 at all sites except for body walls at Totok Bay. The second most accumulated element is As, with a BSAF greater than one at all sites except Totok Bay. The highest calculated BSAF is that of Cd in guts at Pantai Surabaya. BSAFs greater than 1 have also generally been found for Zn, especially in guts, and for Sn. The site showing lower BSAFs for the different elements was Totok Bay, while the sites with the highest ones were Tumbak Island for body walls and Pantai Surabaya for guts.
Table 2 Biota-sediment accumulation factors (BSAFs) for each element in body wall (BW) and guts (GU) at each site: Totok Bay (TB); Talawaan River (TW); Pantai Surabaya (PS); West Bangka mangroves (M1); Lihunu Bay (LB); North-west Bangka mangroves (M2); Tumbak Island (TI); Tasik Ria Resort (TR).
By averaging the BSAF values of each element across sites (Table 2; Figure 7), Hg, As and Zn significantly were bioaccumulated both in body walls and in guts in the study region. In addition, cadmium and copper were significantly bioaccumulated in the guts.
Figure 7 Boxplots (median, interquartile, 1.5 interquartile and outliers) of the biota-sediment accumulation factors (BSAFs) for each element in body wall (BW, in blue) and guts (GU, in red) among sites. Mean values are also shown (diamond symbol).
This study provides the first data on the concentration of trace elements in surface sediments and their bioaccumulation in Holothuria (Halodeima) atra in relation to different gold mining activities in North Sulawesi, Indonesia. Impact and control sites were chosen along the coast, analyzing drainage basins with and without mining activities. In particular, Totok Bay was affected by one of the oldest artisanal gold mines in the region, dating back to 1850’s; the mouth of the Talawaan River was downstream to recent and active artisanal gold mines; Pantai Surabaya received drainage waters from a recent industrial gold mine; while the mangroves site at West Bangka was near a mine in preparation, officially closed the year the sampling was carried out, 2017.
At all study sites, surface sediments were mainly sandy with a high content of calcium, which may indicate a prevalent biogenic origin from surrounding coral reefs; however, at Totok Bay and Pantai Surabaya, chemical composition and finer particle size indicate a relevant terrigenous contribution. Control sites, randomly interspersed among impact ones, well represent the variety of sedimentary conditions and coastal habitats in the region. Origin, local depositional environment and mean grain size may have influenced the trace elements regimes in sediments (Li et al., 2017). In general, finer sediments can contain higher concentrations of trace elements than the coarser ones because of the larger surface-to-volume ratio (Amin et al., 2009). Nevertheless, the distribution patterns of some trace elements in the sediment appeared well related to mining activities; contamination generally ranking Totok Bay first, Pantai Surabaya second, Talawaan River third (but see high Hg values here), the mining site in preparation at Bangka Island fourth.
The trace element that deserves more attention and of greatest environmental concern is certainly mercury. Not detectable in the sediments at the control sites, it was found at all impact sites. The most polluted site was Totok Bay, downstream one of the oldest and active ASGM in the area. Here, the mean concentration exceeded that beyond which adverse biological effects on marine life are likely according to Australian guidelines on sediment quality (GV-high in Simpson et al., 2013), which are mostly based on the effects‐range median (ERM) values proposed by Long et al. (1995). Lasut et al. (2010) found similar or even higher concentrations in the surface sediments sampled in this area in 2004.
The second site in order of sediment mercury pollution was at the Talawaan River mouth, downstream of a more recent ASGM. In this case, the concentrations seem less concerning because lower than the default guideline value, therefore with a low risk of unacceptable effects on marine life. However, a previous study conducted along the Talawaan River in 2001 reported a sediment Hg concentration at the river mouth up to 200 times higher than in the present survey (Limbong et al., 2003), suggesting great spatial and/or temporal variability in this area. For both these sites, the release of mercury into the environment is most likely related to the artisanal method of gold mining, which employs Hg amalgamation. Although with lower concentrations than at the sites affected by ASGMs, high levels of mercury were also found in sediments at Pantai Surabaya, downstream of the industrial mine, and at the west Bangka mangroves, the mining site under preparation. Here, not using amalgamation, the mercury could be easily mobilized from the disposal of mining tailings and leaching of soil, probably rich in cinnabar (HgS) often associated with gold deposits in the region (Turner et al., 1994).
Mercury was always found in the holothurian tissues, both body walls and guts, at all study sites, including at the control sites, even where it was not detectable in the sediment. This suggests a high bioaccumulation capacity by H. atra, even at very low environmental concentrations, especially in guts, where the concentrations resulted about an order of magnitude higher than in the body walls. On the other hand, BSAFs values were everywhere greater than 1, except in body walls at Totok Bay, and very high (usually > 20) for guts.
The second trace element of environmental concern is arsenic. Significant sediment enrichments, with values compatible with unacceptable negative biological effects on marine life according to the Australian guidelines on sediment quality (Simpson et al., 2013), primarily adapted from the original effects range‐low (ERL) values provided by Long et al. (1995), were found at Totok Bay and Pantai Surabaya, downstream the two most active mines. Arsenic found in sediment may come from weathering of rocks associated with gold deposits in North Sulawesi, which are often rich in this element (Turner et al., 1994; Edinger et al., 2007). Ore processing for gold extraction can bring rocks disaggregation and the release of As in the river and eventually into the sea. In H. atra, higher concentrations than controls were measured in body walls at Pantai Surabaya and guts at Pantai Surabaya and Talawaan River. In this case, despite a strong correlation between the concentrations of the two tissues, with those of the guts about double, the concentrations in the tissues were not well correlated with those in the sediments, and it was found to be particularly low in the most polluted site (i.e., Totok Bay). Indeed, in terms of BSAF, bioaccumulation was largely greater than 1 both in body walls and guts at all sites except Totok Bay. Again, the bioaccumulation factors are always higher in guts than in body walls.
Although gold is the main target element of mining activities, small quantities can leak into the environment, especially where the extraction processes are less effective and/or have taken place longer. Not surprisingly, gold was found in detectable concentrations only in sediments from Totok Bay. However, H. atra has demonstrated a capacity for gold bioaccumulation in guts at all active mining sites (BSAF up to 40 at Pantai Surabaya), albeit without any correlation between tissue and sediment concentrations and no significant mean bioaccumulation across sites.
Antimony, cobalt, chromium, copper, nickel, and zinc are all consistently associated with gold deposits in North Sulawesi (Turner et al., 1994; Edinger et al., 2007). Significant sediment enrichments of these trace elements were found both at Totok Bay and Pantai Surabaya, downstream the two most active mines. Despite this, none of these trace elements showed concentrations above the Australian guideline values for sediment quality (Simpson et al., 2013), therefore no adverse biological effects on marine life are expected. Their concentration in H. atra tissues was always higher in guts than in body walls but variable case by case. Although Sb, Co, and Cr concentrations in guts were well correlated to those in sediments, they did not show bioaccumulation in the tissues. Ni was also not significantly bioaccumulated, while Cu showed some net bioaccumulation, especially in guts, regardless of mining activities in the area. Although with no correlations among concentrations in sediments and tissues, Zn showed significant bioaccumulation with locally very high BSAF values in guts, disregarding the presence of gold mines. Cadmium and tin didn’t show any distribution pattern in sediment and tissues in relation to mine activities; however, Cd showed net bioaccumulation in guts at all sites with BSAF higher than 45 at Pantai Surabaya, downstream the industrial gold mine. Finally, although relatively abundant at Totok Bay and with concentrations in tissues well correlated with those in sediments, lead was not significantly bioaccumulated either in the body walls or in the guts.
H. atra, like many other holothurians, plays an essential ecological role in sediment bioturbation and mineralization, in the food web, and as a host of several organisms in symbiotic relationships (Purcell et al., 2016). Uthicke (1999) found that a single individual of H. atra daily ingests, on average, 67 g dry wt. of sediment. The author also estimated that a mixed population of H. atra and Stichopus chloronotus Brandt, 1835 was able to rework annually about 4600 kg dry wt. of sediment in 1000 m2, mainly contributing to trace elements mobilization. At the study sites, the densities of H. atra (max 3 individual per 100 m-2) were on average low when compared with other populations reported in Indonesia (up to 15 individual per 100 m-2; Hartati et al., 2020; Luhulima et al., 2020), likely a result of intense local fishing. Holothurians, thanks to their feeding ecology and sediment bioturbation role (Purcell et al., 2016), low-mobility, large size, long lifespan, high abundance, and large geographical distribution, have been proposed as bioindicators and/or biomonitoring species (Storelli et al., 2001; Jinadasa et al., 2014; Aydın et al., 2017). Nevertheless, trace element contamination in holothurian species has been little investigated (see also Laboy-Nieves and Conde, 2001; Noël et al., 2011). This study and the previous ones agree that trace elements concentrations in guts were generally higher than in body walls (Denton et al., 2006; Denton et al., 2009; Ahmed et al., 2017; Gajdosechova et al., 2020). The concentrations of trace elements found in H. atra tissues at the impact sites of this study were higher than in the same tissues of the same species in other areas of the world, subject to different types of pollution (Denton et al., 2006; Denton et al., 2009).
Regardless of the presence of gold mines and sediment pollution levels, in the present study, H. atra has been shown to bioaccumulate some trace elements, mainly mercury, arsenic and zinc, and copper and cadmium in the guts, which had higher overall bioaccumulation factors. The large variability of bioaccumulation factors between sites suggests differences in the bioavailability of the trace elements, which is primarily due to local environmental conditions, water chemistry, and sedimentary regimes, but also the geochemical form of the elements (Thomann et al., 1995; McAloon and Mason, 2003; Sánchez-Donoso et al., 2021). The transport of trace elements from the substrate to the body wall and guts in holothurian is mainly unknown. Holothurian intestines have solubilization and bioaccumulation roles (McAloon and Mason, 2003); a generally lesser degree of accumulations of metals with different profiles in the body wall, compared to guts, could be due to a limited amount of metal-binding proteins (Tunca et al., 2016). Mercury intake in holothurians is probably a complexation process instead of an enzymatic one due to free amino acids produced by guts (Zhong and Wang, 2006). Although there is very little information on the contribution of certain free amino acids to the overall intake by gut fluids of holothurians, cysteine was found to be a predominant binding ligand for Hg, because it extracts far more Hg from ingested sediment than any other amino acid (Zhong and Wang, 2006). Further investigations on the molecular transport pathways in H. atra could highlight the bioaccumulation mechanisms of trace elements in different tissues.
Through bioturbation and being the prey to many marine invertebrates and vertebrates (Purcell et al., 2016), holothurians can play a fundamental role in the transfer of trace elements from sediments to marine organisms, contributing to the biomagnification process in the upper levels of the food web, in which also human sooner or later is involved. In particular, H. atra, even though it has a comparatively low economic value, belongs to Indonesian commercial species (trepang or trīpang, in Indonesian; Purcell et al., 2012; Setyastuti and Purwati, 2015). In a few Pacific Island countries, the body wall, intestines and/or gonads are consumed in traditional diets or in times of hardship. However, most organisms are processed and exported for consumption, above all by Asians (Purcell et al., 2012). The main target markets are China and Hong Kong, but also Ho Chi Minh City in Viet Nam for further export to China. Although it trades at USD 2-20 per dried kg in the countries of origin, its retail price can be up to USD 210 per dried kg in Hong Kong (Purcell et al., 2012).
Although sea cucumbers usually have trace elements concentrations acceptable for human consumption at nutritional and toxicological levels (Wen and Hu, 2010), individuals of H. atra coming from coastal areas of the North Sulawesi impacted by inland mining activities might pose some risks. The toxicity of mercury largely depends on the chemical form in which it is taken; however, the World Health Organization (WHO) guidelines indicate a tolerable human intake of total mercury of 2 µg kg-1 body weight per day (Fisher, 2003), while the Europe Union sets a maximum level of 500 µg kg-1 wet weight in fishery products (Commission Regulation (EC) No 1881/2006). The mean Hg content in guts in individuals of H. atra from Totok Bay and Talawaan River exceeded these values, albeit in terms of dry weight. Unfortunately, neither the FAO/WHO (Codex Alimentarius Commission, 1995) nor the European Commission (EC) has human health-based standards or guidelines for arsenic in seafood. Australia and New Zealand have health-based guidelines providing maximum levels for inorganic arsenic in mollusks and fish (respectively 1000 and 2000 µg kg-1 wet weight; Stewart and Turnbull, 2015), while the US Food and Drug Administration (USFDA) set a less conservative limit of 76000 µg kg-1 dry weight (Wen and Hu, 2010). The mean arsenic content both in body walls and guts in individuals of H. atra exceeded the USFDA values at Pantai Surabaya and Talawaan River. The Europe Union (Commission Regulation (EC) No 1881/2006) also sets maximum levels in different fishery products for lead (500-1500 µg kg-1 wet weight) and cadmium (500-1000 µg kg-1 wet weight), which are higher than concentrations found in the present study. For the North Sulawesi populations, the greatest concern relates to the intake of mercury and arsenic from fish consumption (Bentley and Soebandrio, 2017); however, the risks of consuming other fishery products, including holothurians, should not be overlooked.
In the North Sulawesi, mercury resulted in the most concerning element found in surface sediment and H. atra specimens, followed by arsenic. Both were related to gold mining activities in the region, especially the oldest ASGMs, and were significantly bioaccumulated in the holothurian tissues, mainly in guts. However, further studies are needed to understand better the effect of mercury and other potentially toxic elements in marine ecosystems and trophic chains of impacted areas of North Sulawesi.
Indonesia is recognized by United Nations as the third country globally (after China and India) for Hg emission, with reports warning on mercury usage in ASGMs, which has significantly increased over the past two decades (Spiegel et al., 2018). A global treaty to protect human health and the environment from the adverse effects of Hg was agreed in 2013 – The Minamata Convention on Mercury (http://www.mercuryconvention.org). Indonesia signed this convention in 2013 and ratified it in 2017. However, recent increases in domestic Hg supplies through new cinnabar mining developments have made Hg less expensive and more available in Indonesia, increasing obstacles to implementing the Minamata Convention (Spiegel et al., 2018). Phasing out mercury amalgamation for gold extraction, regulating artisanal mining, and enforcing environmental regulations and regular seafood contamination monitoring must be a priority objective for Indonesia. In this regard, measuring trace elements in H. atra could be adopted in biomonitoring across the Indo-Pacific region.
The raw data supporting the conclusions of this article will be made available by the authors, without undue reservation.
Conceptualization: MP, ET; Formal analysis: MT, DB, ET, FG, LB, RG, PP, MP; Funding acquisition: MP, DB, PP, ML; Resources: MP, DB, PP, ML, FG, LB, RG, DM, JM; Visualization: ET, MT, MP; Writing – original draft: MT, ET, MP; Writing- review & editing: all authors. All authors contributed to the article and approved the submitted version.
ET benefited from a EuroMarine Young Scientist Fellowship by the OYSTER group within its programme for training.
The authors declare that the research was conducted in the absence of any commercial or financial relationships that could be construed as a potential conflict of interest.
All claims expressed in this article are solely those of the authors and do not necessarily represent those of their affiliated organizations, or those of the publisher, the editors and the reviewers. Any product that may be evaluated in this article, or claim that may be made by its manufacturer, is not guaranteed or endorsed by the publisher.
We would like to thank the staff at the Faculty of Fisheries and Marine Science, Sam Ratulangi University (UNSRAT, Manado, Indonesia), who supervised the research activities in Indonesia and supported visa applications for foreign colleagues. In addition, we would like to thank the staff of the Coral Eye (dive resort and marine outpost on Bangka Island) who assisted in the fieldwork. Research was carried out under the research permits from the Ministry of Research, Technology, and Higher Education in Indonesia to MP (n° 357/SIP/FRP/E5/Dit.KI/X/2018), ET (n° 358/SIP/FRP/E5/Dit.KI/X/2018) and GZ (n° 204/SIP/FRP/E5/Dit.KI/VII/2018). We kindly acknowledge the reviewers and the editor for the constructive comments that improved the quality of the manuscript.
The Supplementary Material for this article can be found online at: https://www.frontiersin.org/articles/10.3389/fmars.2022.863629/full#supplementary-material
Ahmed Q., Mohammad Ali Q., Bat L. (2017). Assessment of Heavy Metals Concentration in Holothurians, Sediments and Water Samples From Coastal Areas of Pakistan (Northern Arabian Sea). J. Coast. 5 (5), 191–201. doi: 10.12980/jclm.5.2017J7-56
Amin B., Ismail A., Arshad A., Yap C. K., Kamarudin M. S. (2009). Anthropogenic Impacts on Heavy Metal Concentrations in the Coastal Sediments of Dumai, Indonesia. Environ. Monit. Assess. 148 (1-4), 291–305. doi: 10.1007/s10661-008-0159-z
Anderson M. J., Gorley R. N., Clarke K. R. (2008). PERMANOVA+ for PRIMER: Guide to Software and Statistical Methods (Plymouth, UK: PRIMER-E Ltd).
Anderson M. J., Robinson J. (2008). Permutation Tests for Linear Models. Aust. N. Z. J. Stat. 43 (1), 75–88. doi: 10.1111/1467-842x.00156
Aydın M., Tunca E., Alver Şahin Ü. (2017). Effects of Anthropological Factors on the Metal Accumulation Profiles of Sea Cucumbers in Near Industrial and Residential Coastlines of İzmir, Turkey. Int. J. Environ. Anal. Chem. 97 (4), 368–382. doi: 10.1080/03067319.2017.1315112
Badocco D., Lavagnini I., Mondin A., Favaro G., Pastore P. (2015a). Definition of the Limit of Quantification in the Presence of Instrumental and non-Instrumental Errors. Comparison Among Various Definitions Applied to the Calibration of Zinc by Inductively Coupled Plasma–Mass Spectrometry. Spectrochim. Acta B 114, 81–86. doi: 10.1016/j.sab.2015.10.004
Badocco D., Lavagnini I., Mondin A., Pastore P. (2014). Estimation of the Uncertainty of the Quantification Limit. Spectrochim. Acta B 96, 8–11. doi: 10.1016/j.sab.2014.03.013
Badocco D., Lavagnini I., Mondin A., Pastore P. (2015b). Effect of Multiple Error Sources on the Calibration Uncertainty. Food Chem. 177, 147–151. doi: 10.1016/j.foodchem.2015.01.020
Badocco D., Lavagnini I., Mondin A., Tapparo A., Pastore P. (2015c). Limit of Detection in the Presence of Instrumental and non-Instrumental Errors: Study of the Possible Sources of Error and Application to the Analysis of 41 Elements at Trace Levels by Inductively Coupled Plasma-Mass Spectrometry Technique. Spectrochim. Acta B 107, 178–184. doi: 10.1016/j.sab.2015.03.009
Badr N. B. E., El-Fiky A. A., Mostafa A. R., Al-Mur B. A. (2009). Metal Pollution Records in Core Sediments of Some Red Sea Coastal Areas, Kingdom of Saudi Arabia. Environ. Monit. Assess. 155 (1-4), 509–526. doi: 10.1007/s10661-008-0452-x
Bellchambers L. M., Meeuwig J. J., Evans S. N., Legendre P. (2011). Modelling Habitat Associations of 14 Species of Holothurians From an Unfished Coral Atoll: Implications for Fisheries Management. Aquat. Biol. 14 (1), 57–66. doi: 10.3354/ab00381
Bentley K., Soebandrio A. (2017). Dietary Exposure Assessment for Arsenic and Mercury Following Submarine Tailings Placement in Ratatotok Sub-District, North Sulawesi, Indonesia. Environ. Pollut. 227, 552–559. doi: 10.1016/j.envpol.2017.04.081
Birch G. F., Lee J. H., Tanner E., Fortune J., Munksgaard N., Whitehead J., et al. (2020). Sediment Metal Enrichment and Ecological Risk Assessment of Ten Ports and Estuaries in the World Harbours Project. Mar. Pollut. Bull. 155, 111129. doi: 10.1016/j.marpolbul.2020.111129
Blackwood G. M., Edinger E. N. (2007). Mineralogy and Trace Element Relative Solubility Patterns of Shallow Marine Sediments Affected by Submarine Tailings Disposal and Artisanal Gold Mining, Buyat-Ratototok District, North Sulawesi, Indonesia. Environ. Geol. 52 (4), 803–818. doi: 10.1007/s00254-006-0517-5
Bose-O’Reilly S., McCarty K. M., Steckling N., Lettmeier B. (2010). Mercury Exposure and Children’s Health. Curr. Probl. Pediatr. Adolesc. Health Care 40 (8), 186–215. doi: 10.1016/j.cppeds.2010.07.002
Bryan G. W., Langston W. J. (1992). Bioavailability, Accumulation and Effects of Heavy Metals in Sediments With Special Reference to United Kingdom Estuaries: A Review. Environ. Pollut. 76 (2), 89–131. doi: 10.1016/0269-7491(92)90099-v
Carrillo-González R., Šimůnek J., Sauvé S., Adriano D. (2006). Mechanisms and Pathways of Trace Element Mobility in Soils. Adv. Agron. 91, 111–178. doi: 10.1016/S0065-2113(06)91003-7
Chen M., Ma L. Q. (2001). Comparison of Three Aqua Regia Digestion Methods for Twenty Florida Soils. Soil Sci. Soc. Am. J. 65 (2), 491–499. doi: 10.2136/sssaj2001.652491x
Chojnacka K., Mikulewicz M. (2014). “Bioaccumulation,” in Encyclopedia of Toxicology, 3rd ed. Ed. Wexler P. (Oxford: Academic Press), 456–460.
Codex Alimentarius Commission (1995). General Standard for Contaminants and Toxins in Food and Feed. CXS 193-1995 (Rome: Food and Agriculture Organization & World Health Organization of the United Nations).
Conand C. (1998). “Holothurians (Sea Cucumbers, Class Holothuroidea),” in FAO Species Identification Guide for Fishery Purposes. The Living Marine Resources of the Western Central Pacific. Vol. 2. Cephalopods, Crustaceans, Holothurians and Sharks. Eds. Carpenter K. E., Niem V. H. (Rome: International Union for Conservation of Nature - IUCN), 1157–1190.
Conand C. (2004). Monitoring a Fissiparous Population of Holothuria Atra on a Fringing Reef on Reunion Island (Indian Ocean). SPC Beche-de-mer Inf. Bull. 20, 22–25.
Conand C., Gamboa R., Purcell S. (2013). Holothuria Atra. The IUCN Red List of Threatened Species (Rome: International Union for Conservation of Nature - IUCN).
Cordy P., Veiga M. M., Salih I., Al-Saadi S., Console S., Garcia O., et al. (2011). Mercury Contamination From Artisanal Gold Mining in Antioquia, Colombia: The World’s Highest Per Capita Mercury Pollution. Sci. Total Environ. 410-411, 154–60. doi: 10.1016/j.scitotenv.2011.09.006
Denton G. R. W., Concepcion L. P., Wood H. R., Morrison R. J. (2006). Trace Metals in Marine Organisms From Four Harbours in Guam. Mar. Pollut. Bull. 52 (12), 1784–1804. doi: 10.1016/j.marpolbul.2006.09.010
Denton G. R. W., Morrison R. J., Bearden B. G., Houk P., Starmer J. A., Wood H. R. (2009). Impact of a Coastal Dump in a Tropical Lagoon on Trace Metal Concentrations in Surrounding Marine Biota: A Case Study From Saipan, Commonwealth of the Northern Mariana Islands (CNMI). Mar. Pollut. Bull. 58 (3), 424–431. doi: 10.1016/j.marpolbul.2008.11.029
Du Laing G., Rinklebe J., Vandecasteele B., Meers E., Tack F. M. G. (2009). Trace Metal Behaviour in Estuarine and Riverine Floodplain Soils and Sediments: A Review. Sci. Total Environ. 407 (13), 3972–3985. doi: 10.1016/j.scitotenv.2008.07.025
Edinger E. (2012). Gold Mining and Submarine Tailings Disposal Review and Case Study. Oceanography 25 (2), 184–199. doi: 10.5670/oceanog.2012.54
Edinger E. N., Siregar P. R., Blackwood G. M. (2007). Heavy Metal Concentrations in Shallow Marine Sediments Affected by Submarine Tailings Disposal and Artisanal Gold Mining, Buyat-Ratototok District, North Sulawesi, Indonesia. Environ. Geol. 52 (4), 701–714. doi: 10.1007/s00254-006-0506-8
Eto K., Marumoto M., Takeya M. (2010). The Pathology of Methylmercury Poisoning (Minamata Disease). Neuropathology 30 (5), 471–479. doi: 10.1111/j.1440-1789.2010.01119.x
Fisher J. F. (2003). Elemental Mercury and Inorganic Mercury Compounds: Human Health Aspects (Geneva: World Health Organization & International Programme on Chemical Safety).
Futsaeter G., Wilson S. (2013). The UNEP Global Mercury Assessment: Sources, Emissions and Transport. E3S Web Conf. 1, 36001. doi: 10.1051/e3sconf/20130136001
Gajdosechova Z., Palmer C. H., Dave D., Jiao G., Zhao Y., Tan Z., et al. (2020). Arsenic Speciation in Sea Cucumbers: Identification and Quantitation of Water-Extractable Species. Environ. Pollut. 266, 115190. doi: 10.1016/j.envpol.2020.115190
Gao X., Chen C.-T. A. (2012). Heavy Metal Pollution Status in Surface Sediments of the Coastal Bohai Bay. Water Res. 46 (6), 1901–1911. doi: 10.1016/j.watres.2012.01.007
González-Merizalde M. V., Menezes-Filho J. A., Cruz-Erazo C. T., Bermeo-Flores S. A., Sánchez-Castillo M. O., Hernández-Bonilla D., et al. (2016). Manganese and Mercury Levels in Water, Sediments, and Children Living Near Gold-Mining Areas of the Nangaritza River Basin, Ecuadorian Amazon. Arch. Environ. Con. Tox. 71 (2), 171–182. doi: 10.1007/s00244-016-0285-5
González-Valoys A. C., Arrocha J., Monteza-Destro T., Vargas-Lombardo M., Esbrí J. M., Garcia-Ordiales E., et al. (2022). Environmental Challenges Related to Cyanidation in Central American Gold Mining; the Remance Mine (Panama). J. Environ. Manage. 302, 113979. doi: 10.1016/j.jenvman.2021.113979
Greggio N., Capolupo M., Donnini F., Birke M., Fabbri E., Dinelli E. (2021). Integration of Physical, Geochemical and Biological Analyses as a Strategy for Coastal Lagoon Biomonitoring. Mar. Pollut. Bull. 164, 112005. doi: 10.1016/j.marpolbul.2021.112005
Guerra R., Pasteris A., Ponti M. (2009). Impacts of Maintenance Channel Dredging in a Northern Adriatic Coastal Lagoon. I: Effects on Sediment Properties, Contamination and Toxicity. Estuar. Coast. Shelf Sci. 85 (1), 134–142. doi: 10.1016/j.ecss.2009.05.021
Hartati R., Zainuri M., Ambariyanto A., Redjeki S., Riniatsih I., Azizah R., et al. (2019). Aseksual Reproduction of Black Sea Cucumber From Jepara Waters. ILMU KELAUTAN: Indonesian J. Marine Sci. 24 (3), 121. doi: 10.14710/ik.ijms.24.3.121-126
Hartati R., Zainuri M., Ambariyanto A., Riniatsih I., Endrawati H., Redjeki S., et al. (2020). Density and Population Growth of the Black Sea Cucumber, Holothuria (Halodeima) Atra (Jaege) in Two Different Microhabitats of Jepara Waters, Indonesia. Eco. Env. Cons. 26 (3), 1222–1227.
Jiang X., Teng A., Xu W., Liu X. (2014). Distribution and Pollution Assessment of Heavy Metals in Surface Sediments in the Yellow Sea. Mar. Pollut. Bull. 83 (1), 366–375. doi: 10.1016/j.marpolbul.2014.03.020
Jinadasa B. K. K. K., Samanthi R. I., Wicramsinghe I. (2014). Trace Metal Accumulation in Tissue of Sea Cucumber Species; North-Western Sea of Sri Lanka. Am. J. Public Health Res. 2 (5A), 1–5. doi: 10.12691/ajphr-2-5A-1
Jonathan M. P., Roy P. D., Thangadurai N., Srinivasalu S., Rodríguez-Espinosa P. F., Sarkar S. K., et al. (2011). Metal Concentrations in Water and Sediments From Tourist Beaches of Acapulco, Mexico. Mar. Pollut. Bull. 62 (4), 845–850. doi: 10.1016/j.marpolbul.2011.02.042
Kambey J. L., Farrell A. P., Bendell-Young L. I. (2001). Influence of Illegal Gold Mining on Mercury Levels in Fish of North Sulawesi’s Minahasa Peninsula, (Indonesia). Environ. Pollut. 114 (3), 299–302. doi: 10.1016/s0269-7491(01)00163-4
Laboy-Nieves E. N., Conde J. E. (2001). Metal Levels in Eviscerated Tissue of Shallow-Water Deposit-Feeding Holothurians. Hydrobiologia 459 (1/3), 19–26. doi: 10.1023/a:1012589009243
Lasut M. T., Yasuda Y. (2008). Accumulation of Mercury in Marine Biota of Buyat Bay, North Sulawesi, Indonesia. Coast. Mar. Sci. 32, 33–38.
Lasut M. T., Yasuda Y., Edinger E. N., Pangemanan J. M. (2010). Distribution and Accumulation of Mercury Derived From Gold Mining in Marine Environment and its Impact on Residents of Buyat Bay, North Sulawesi, Indonesia. Water Air Soil Pollut. 208 (1-4), 153–164. doi: 10.1007/s11270-009-0155-0
Lavagnini I., Badocco D., Pastore P., Magno F. (2011). Theil–Sen Nonparametric Regression Technique on Univariate Calibration, Inverse Regression and Detection Limits. Talanta 87, 180–188. doi: 10.1016/j.talanta.2011.09.059
Li H., Kang X., Li X., Li Q., Song J., Jiao N., et al. (2017). Heavy Metals in Surface Sediments Along the Weihai Coast, China: Distribution, Sources and Contamination Assessment. Mar. Pollut. Bull. 115 (1-2), 551–558. doi: 10.1016/j.marpolbul.2016.12.039
Limbong D., Kumampung J., Rimper J., Arai T., Miyazaki N. (2003). Emissions and Environmental Implications of Mercury From Artisanal Gold Mining in North Sulawesi, Indonesia. Sci. Total Environ. 302 (1), 227–236. doi: 10.1016/s0048-9697(02)00397-2
Long E. R., Chapman P. M. (1985). A Sediment Quality Triad: Measures of Sediment Contamination, Toxicity and Infaunal Community Composition in Puget Sound. Mar. Pollut. Bull. 16 (10), 405–415. doi: 10.1016/0025-326x(85)90290-5
Long E. R., Macdonald D. D., Smith S. L., Calder F. D. (1995). Incidence of Adverse Biological Effects Within Ranges of Chemical Concentrations in Marine and Estuarine Sediments. Environ. Manage. 19 (1), 81–97. doi: 10.1007/bf02472006
Luhulima Y., Zamani N. P., Bengen D. G. (2020). Density and Growth Pattern of Sea Cucumbers Holothuria Scabra, Holothuria Atra and Bohadchia Marmorata and Their Association With Seagrass in Coastal Area of Ambon, Saparua, Osi and Marsegu Island, Maluku Province. Jurnal Ilmu Dan Teknol Kelautan Tropis 12 (2), 541–554. doi: 10.29244/jitkt.v12i2.23454
Marmolejo-Rodríguez A. J., Sánchez-Martínez M. A., Romero-Guadarrama J. A., Sánchez-González A., Magallanes-Ordóñez V. R. (2011). Migration of As, Hg, Pb, and Zn in Arroyo Sediments From a Semiarid Coastal System Influenced by the Abandoned Gold Mining District at El Triunfo, Baja California Sur, Mexico. J. Environ. Monit. 13 (8), 2182. doi: 10.1039/c1em10058k
McAloon K. M., Mason R. P. (2003). Investigations Into the Bioavailability and Bioaccumulation of Mercury and Other Trace Metals to the Sea Cucumber, Sclerodactyla Briareus, Using In Vitro Solubilization. Mar. Pollut. Bull. 46 (12), 1600–1608. doi: 10.1016/s0025-326x(03)00326-6
Murillo-Cisneros D. A., O’Hara T. M., Elorriaga-Verplancken F. R., Sánchez-González A., Marín-Enríquez E., Marmolejo-Rodríguez A. J., et al. (2019). Trophic Structure and Biomagnification of Total Mercury in Ray Species Within a Benthic Food Web. Arch. Environ. Con. Tox. 77 (3), 321–329. doi: 10.1007/s00244-019-00632-x
Noël L., Testu C., Chafey C., Velge P., Guérin T. (2011). Contamination Levels for Lead, Cadmium and Mercury in Marine Gastropods, Echinoderms and Tunicates. Food Control 22 (3-4), 433–437. doi: 10.1016/j.foodcont.2010.09.021
O’Brien A. L., Keough M. J. (2014). Ecological Responses to Contamination: A Meta-Analysis of Experimental Marine Studies. Environ. Pollut. 195, 185–191. doi: 10.1016/j.envpol.2014.09.005
Odumo B. O., Carbonell G., Angeyo H. K., Patel J. P., Torrijos M., Rodríguez Martín J. A. (2014). Impact of Gold Mining Associated With Mercury Contamination in Soil, Biota Sediments and Tailings in Kenya. Environ. Sci. Pollut. Res. 21 (21), 12426–12435. doi: 10.1007/s11356-014-3190-3
Oke S., Vermeulen D. (2016). Geochemical Modeling and Remediation of Heavy Metals and Trace Elements From Artisanal Mines Discharge. Soil Sediment Contam. 26 (1), 84–95. doi: 10.1080/15320383.2017.1241216
Paschal D. C., Ting B. G., Morrow J. C., Pirkle J. L., Jackson R. J., Sampson E. J., et al. (1998). Trace Metals in Urine of United States Residents: Reference Range Concentrations. Environ. Res. 76 (1), 53–59. doi: 10.1006/enrs.1997.3793
Ponti M., Fratangeli F., Dondi N., Segre Reinach M., Serra C., Sweet M. J. (2016). Baseline Reef Health Surveys at Bangka Island (North Sulawesi, Indonesia) Reveal New Threats. PeerJ 4, e2614. doi: 10.7717/peerj.2614
Ponti M., Linares C., Cerrano C., Rodolfo-Metalpa R., XXXW H. B. (2021). Editorial: Biogenic Reefs at Risk: Facing Globally Widespread Local Threats and Their Interaction With Climate Change. Front. Mar. Sci. 8. doi: 10.3389/fmars.2021.793038
Ponti M., Pasteris A., Guerra R., Abbiati M. (2009). Impacts of Maintenance Channel Dredging in a Northern Adriatic Coastal Lagoon. II: Effects on Macrobenthic Assemblages in Channels and Ponds. Estuar. Coast. Shelf Sci. 85 (1), 143–150. doi: 10.1016/j.ecss.2009.06.027
Purcell S., Conand C., Uthicke S., Byrne M. (2016). Ecological Roles of Exploited Sea Cucumbers. Oceanogr. Mar. Biol. 54, 367–386. doi: 10.1201/9781315368597
Purcell S. W., Samyn Y., Conand C. (2012). “Commercially Important Sea Cucumbers of the World,” in FAO Species Catalogue for Fishery Purposes No. 6 (Rome: FAO).
QGIS Development Team (2021) QGIS Geographic Information System Version 3.16.5 LTR. Available at: http://qgis.osgeo.org (Accessed March 15, 2021).
Qian Y., Zhang W., Yu L., Feng H. (2015). Metal Pollution in Coastal Sediments. Curr. Pollut. Rep. 1 (4), 203–219. doi: 10.1007/s40726-015-0018-9
Rainbow P. S. (2002). Trace Metal Concentrations in Aquatic Invertebrates: Why and So What? Environ. Pollut. 120 (3), 497–507. doi: 10.1016/S0269-7491(02)00238-5
R Core Team (2021) R: A Language and Environment for Statistical Computing Version 4.0.5. Available at: https://www.r-project.org/ (Accessed March 31, 2021).
Retno H., Ambariyanto A., Widianingsih W., Muhammad Z. (2020). Feeding Selectivity of Holothuria Atra in Different Microhabitat in Panjang Island, Jepara (Java, Indonesia). Biodiversitas 21 (5), 2233–2239. doi: 10.13057/biodiv/d210552
Sánchez-Donoso R., García Lorenzo M. L., Esbrí J. M., García-Noguero E. M., Higueras P., Crespo E. (2021). Geochemical Characterization and Trace-Element Mobility Assessment for Metallic Mine Reclamation in Soils Affected by Mine Activities in the Iberian Pyrite Belt. Geosciences 11 (6), 233. doi: 10.3390/geosciences11060233
Sessitsch A., Kuffner M., Kidd P., Vangronsveld J., Wenzel W. W., Fallmann K., et al. (2013). The Role of Plant-Associated Bacteria in the Mobilization and Phytoextraction of Trace Elements in Contaminated Soils. Soil Biol. Biochem. 60, 182–194. doi: 10.1016/j.soilbio.2013.01.012
Setyastuti A., Purwati P. (2015). Species List of Indonesian Trepang. SPC Beche-de-mer Inf. Bull. 35, 19–25.
Simpson S. L., Batley G. B., Chariton A. A. (2013). “Revision of the ANZECC/ARMCANZ Sediment Quality Guidelines,” in CSIRO Land and Water Science Report 08/07. (Canberra:CSIRO Land and Water)
Spiegel S. J., Agrawal S., Mikha D., Vitamerry K., Le Billon P., Veiga M., et al. (2018). Phasing Out Mercury? Ecological Economics and Indonesia’s Small-Scale Gold Mining Sector. Ecol. Econ. 144, 1–11. doi: 10.1016/j.ecolecon.2017.07.025
Stewart I., Turnbull A. (2015). Arsenic in Australian Seafood: A Review and Analysis of Monitoring Data 2000 - 2013 (Adelaide, Australia: South Australian Research and Development Institute).
Storelli M. M., Storelli A., Marcotrigiano G. O. (2001). Heavy Metals in the Aquatic Environment of the Southern Adriatic Sea, Italy: Macroalgae, Sediments and Benthic Species. Environ. Int. 26 (7-8), 505–509. doi: 10.1016/s0160-4120(01)00034-4
Thomann R. V., Mahony J. D., Mueller R. (1995). Steady-State Model of Biota Sediment Accumulation Factor for Metals in Two Marine Bivalves. Environ. Toxicol. Chem. 14 (11), 1989–1998. doi: 10.1002/etc.5620141121
Toral-Granda V., Lovatelli A., Vasconcellos M. (2008). “Sea Cucumbers. A Global Review of Fisheries and Trade,” in FAO Fisheries and Aquaculture Technical Paper. No. 516 (Rome: FAO).
Tunca E., Aydın M., Şahin Ü. (2016). Interactions and Accumulation Differences of Metal(Loid)s in Three Sea Cucumber Species Collected From the Northern Mediterranean Sea. Environ. Sci. Pollut. Res. 23 (20), 21020–21031. doi: 10.1007/s11356-016-7288-7
Turner S. J., Flindell P. A., Hendri D., Hardjana I., Lauricella P. F., Lindsay R. P., et al. (1994). Sediment-Hosted Gold Mineralisation in the Ratatotok District, North Sulawesi, Indonesia. J. Geochem. Explor. 50 (1-3), 317–336. doi: 10.1016/0375-6742(94)90029-9
Uthicke S. (1999). Sediment Bioturbation and Impact of Feeding Activity of Holothuria (Halodeima) Atra and Stichopus Chloronotus, Two Sediment Feeding Holothurians, at Lizard Island, Great Barrier Reef. Bull. Mar. Sci. 64 (1), 129–141.
Velásquez-López P. C., Veiga M. M., Hall K. (2010). Mercury Balance in Amalgamation in Artisanal and Small-Scale Gold Mining: Identifying Strategies for Reducing Environmental Pollution in Portovelo-Zaruma, Ecuador. J. Clean. Prod. 18 (3), 226–232. doi: 10.1016/j.jclepro.2009.10.010
Veron J. E. N., Devantier L. M., Turak E., Green A. L., Kininmonth S., Stafford-Smith M., et al. (2009). Delineating the Coral Triangle. Galaxea 11 (2), 91–100. doi: 10.3755/galaxea.11.91
Wang W. X. (2002). Interactions of Trace Metals and Different Marine Food Chains. Mar. Ecol. Prog. Ser. 243, 295–309. doi: 10.3354/meps243295
Wen J., Hu C. (2010). Elemental Composition of Commercial Sea Cucumbers (Holothurians). Food Addit. Contam. B 3 (4), 246–252. doi: 10.1080/19393210.2010.520340
Xing J., Chia F.-S. (1997). Heavy Metal Accumulation in Tissue/Organs of a Sea Cucumber, Holothuria Leucospilota. Hydrobiologia 352 (1/3), 17–23. doi: 10.1023/a:1003028601518
Keywords: heavy metals, pollution, lollyfish, trepang, sea cucumbers, artisanal mines, coral reef, coral triangle
Citation: Tamburini M, Badocco D, Ercadi R, Turicchia E, Zampa G, Gasparini F, Ballarin L, Guerra R, Lasut MT, Makapedua DM, Mamuaja J, Pastore P and Ponti M (2022) Bioaccumulation of Mercury and Other Trace Elements in the Edible Holothurian Holothuria (Halodeima) atra in Relation to Gold Mining Activities in North Sulawesi, Indonesia. Front. Mar. Sci. 9:863629. doi: 10.3389/fmars.2022.863629
Received: 27 January 2022; Accepted: 06 April 2022;
Published: 13 May 2022.
Edited by:
Elisabeth Marijke Anne Strain, University of Tasmania, AustraliaReviewed by:
Alberto Sánchez-González, Instituto Politécnico Nacional (IPN), MexicoCopyright © 2022 Tamburini, Badocco, Ercadi, Turicchia, Zampa, Gasparini, Ballarin, Guerra, Lasut, Makapedua, Mamuaja, Pastore and Ponti. This is an open-access article distributed under the terms of the Creative Commons Attribution License (CC BY). The use, distribution or reproduction in other forums is permitted, provided the original author(s) and the copyright owner(s) are credited and that the original publication in this journal is cited, in accordance with accepted academic practice. No use, distribution or reproduction is permitted which does not comply with these terms.
*Correspondence: Eva Turicchia, ZXZhLnR1cmljY2hpYTJAdW5pYm8uaXQ=
†ORCID: Marco Tamburini, orcid.org/0000-0001-7802-3761
Denis Badocco, orcid.org/0000-0001-6270-7966
Eva Turicchia, orcid.org/0000-0002-8952-9028
Greta Zampa, orcid.org/0000-0003-0217-8972
Fabio Gasparini, orcid.org/0000-0002-7574-0834
Loriano Ballarin, orcid.org/0000-0002-3287-8550
Roberta Guerra, orcid.org/0000-0002-7151-6616
Markus T. Lasut, orcid.org/0000-0003-2087-1877
Daisy M. Makapedua, orcid.org/0000-0003-4334-6020
Jane Mamuaja, orcid.org/0000-0002-6496-7582
Paolo Pastore, orcid.org/0000-0003-1448-0175
Massimo Ponti, orcid.org/0000-0002-6521-1330
Disclaimer: All claims expressed in this article are solely those of the authors and do not necessarily represent those of their affiliated organizations, or those of the publisher, the editors and the reviewers. Any product that may be evaluated in this article or claim that may be made by its manufacturer is not guaranteed or endorsed by the publisher.
Research integrity at Frontiers
Learn more about the work of our research integrity team to safeguard the quality of each article we publish.