- 1Alfred-Wegener-Institute, Helmholtz Center for Polar and Marine Research, Bremerhaven, Germany
- 2Algae and Microbial Biotechnology Division, Faculty of Life Sciences and Aquaculture Nord University, Bodø, Norway
- 3Centre for Marine Sciences (CCMAR)-CIMAR Associated Laboratory, University of Algarve, Faro, Portugal
- 4Department of Algal Development and Evolution, Max Planck Institute for Biology Tübingen, Tübingen, Germany
Thermal characteristics of kelp species have been studied in many ways, but potentially persistent effects of temperature across generations are yet poorly understood. In this context, the effect of thermal priming on fertility and growth of the N-Atlantic kelp species Laminaria digitata was investigated within and across life cycle generations in a two-step common garden experiment. Using vegetative clonal gametophytes from cold (5°C) and warm (15°C) pre-experimental cultivation (3 years), we first quantified gametogenesis and recruitment over two weeks at a common temperature of 10°C. Then, recruited sporophytes were transferred to a temperature gradient spanning the tolerance range of the species from 0°C to 20°C. We hypothesized that a warm gametophyte pre-experimental cultivation promotes performance of sporophytes at warm temperatures and vice versa. Interestingly, gametogenesis speed and sporophyte recruitment were higher in gametophytes following cold compared to warm pre-experimental cultivation, which indicates carry-over effects of temperature within the gametophyte generation. Compared to warm pre-experimental cultivation of gametophytes, a cold pre-experimental cultivation enhanced growth of juvenile Laminaria digitata sporophytes by more than 69% at the extreme low and high temperatures of 0 and 20°C. This is the first evidence for a cross-generational effect between gametophyte parents and offspring sporophytes. As cold gametophyte cultivation increased the trait performance of gametogenesis, recruitment and thermal tolerance of juvenile sporophytes, priming of early life cycle stages may be used to increase resilience and productivity of kelps in marine forest restoration efforts and kelp mariculture.
Introduction
Kelps, brown algae of the order Laminariales, form the basis of one of the most productive ecosystems on earth (Mann, 1973; Schiel and Foster, 2015), but these marine forests are currently threatened by global warming. A systematic decline of kelp populations attributed to global warming has already been reported globally (reviewed by Smale, 2020). As sessile organisms are unable to migrate, their stability at their warm range edge is dependent on their thermal adaptation and plasticity towards warm events. While adaptation occurs over many generations of natural selection of heritable traits that increase fitness (Goodnight, 2000), trait acclimation to a specific environment may be inherited across few generations without permanent changes in DNA sequence. When environmental changes occur during individual development, the phenotypic traits are altered in such a way that trait expression at a later time point differs from that of an identical genotype which experienced a constant environment (carry-over effects sensu Byrne et al., 2020). Furthermore, recent research shows that trait plasticity of an individual is not only influenced by the immediate environment but can also be shaped by the environment experienced by the parents (cross-generational plasticity sensu Byrne et al., 2020).
The kelp life cycle offers an intriguing experimental system for the study of cross-generational effects. Laminariales exhibit a haplo-diplontic heteromorphic life cycle alternating between a macroscopic diploid sporophyte phase and, following meiosis, a microscopic phase of free-living haploid male and female gametophytes (Kain, 1979; Schiel and Foster, 2006). Kelp life cycle stages have different physiological requirements for growth and reproduction (Bolton and Lüning, 1982; Wiencke et al., 1994) and they experience different environmental conditions due to the alternation of generations, their seasonality, their contrasting size and life span, which results in different micro-habitats for each stage. Byrne et al. (2020) suggest that cross-generational studies shall be designed in a way that the parental treatment is administered during germline development before investigating trait differences in offspring created by gamete fusion. Following the definition of germlines in plants (Grossniklaus, 2011), kelp gametophytes can be viewed as part of the parental germline (Liesner et al., 2020b), which is initiated by meiosis and ends with the production of eggs and spermatozoids. Following these concepts, gametophytes belong to the parental generation whereas the offspring generation is initiated by the formation of the zygote which develops into a diploid sporophyte. The kelp system therefore allows to experimentally treat only the haploid phase of the life cycle to investigate generation-spanning effects in the diploid offspring which is not possible in animal models (Byrne et al., 2020).
The kelp Laminaria digitata (Hudson) J.V. Lamouroux is a temperate to Arctic North-Atlantic species, distributed from Svalbard (Norway; Lüning et al., 1990) to southern Brittany (France; Burel et al., 2019) in the East Atlantic and from Greenland (Lund, 1959) to Long Island Sound (USA; Taylor, 1957) in the West Atlantic. L. digitata is a perennial species, growing in the infralittoral fringe and upper sublittoral on rocky substrates. The abundance of this habitat-forming species with an high economical value (especially for the iodine and alginate industry; Bartsch et al., 2008) has already declined at its warm distributional edge in Brittany (Davoult et al., 2011) and in Normandy (Cosson, 1999), which was in part attributed to harvesting of wild populations, eutrophication, and to increasing temperatures. Models have predicted a northward shift of L. digitata’s range distribution under intermediate climate change scenarios (RCP 4.5) before the end of the century with local extinctions in Britany and an expansion into Arctic regions (Raybaud et al., 2013; Assis et al., 2018).
Thermal tolerance and reproductive requirements of each life cycle stage are well-known in L. digitata. Gametophytes survive between -1.5°C and 23-24°C with a growth optimum at 10-18°C (Lüning, 1980; Bolton and Lüning, 1982; tom Dieck, 1993; Martins et al., 2017). Sporophytes on the other hand have a narrower temperature window for survival, between 0°C (lower temperatures were not tested) and 20-21°C with a growth optimum at 10-15°C (Perez, 1971; Bolton and Lüning, 1982; tom Dieck, 1992; Wilson et al., 2015; Franke et al., 2021; Liesner et al., 2022). Gametogenesis is optimal at temperatures between 5 and 15°C (Lüning, 1980; Martins et al., 2017). Although gametogenesis was fastest around 10-15°C in a laboratory setting, the number of recruited sporophytes after 20 days was highest at 5°C (Martins et al., 2017). While these thermal characteristics of L. digitata’s life cycle have been studied thoroughly (Bolton and Lüning, 1982; Delebecq et al., 2015; Martins et al., 2017), any potential persistent effects of temperature across generations and ontogeny are yet poorly investigated in macroalgae in general (Liesner et al., 2020b).
Sporogenesis and release of meiospores in L. digitata mainly occur from early summer to late autumn during periods of reduced sporophyte growth (Bolton and Lüning, 1982; Sjøtun and Schoschina, 2002; Bartsch et al., 2013). It is thus possible that gametogenesis takes place in late autumn directly after meiospore germination (Lüning, 1980; Sjøtun and Schoschina, 2002; Martins et al., 2017). However, under limiting conditions of, e.g., temperature or irradiance, gametophytes can grow vegetatively (tom Dieck, 1993; Edwards, 2000; Martins et al., 2017; Martins et al., 2020) and may therefore potentially become fertile year-round under a wide range of temperatures. They may also overwinter as unicellular gametophytes and initiate reproduction as early as sufficient blue quanta reach their habitat in late winter/early spring; i.e., during the cold season (Lüning, 1980). As a consequence, development of young sporophytes may be influenced by the seasonally changing thermal environment after their formation (carry-over effects; Byrne et al., 2020) and also by the thermal history of the gametophyte (cross-generational plasticity sensu Donelson et al., 2018; Byrne et al., 2020). If these effects increase trait performance (e.g., temperature tolerance), controlled priming of early life cycle stages may provide a tool to increase resilience or productivity in cultivation and restoration efforts (Jueterbock et al., 2021).
The purpose of this study was to investigate trait acclimation of L. digitata gametophytes and the inheritance of acclimated traits to sporophytes. Clonal gametophytes grown at 5°C and 15°C over 3 years were fertilized in identical conditions at 10°C. We hypothesized that the pre-experimental cultivation of gametophytes impacts the speed and success of gametophyte reproduction (i.e., carry-over effects within the gametophyte generation) as well as the thermal plasticity of the recruited sporophytes in response to a temperature gradient (ranging from 0°C to 20°C; i.e., cross-generational plasticity across gametophyte and sporophyte generations). We expected that sporophytes recruited from warm-cultivated (15°C) gametophyte grow faster at higher temperatures while sporophytes from cold-cultivated (5°C) gametophyte grow faster at low temperatures.
Material and Methods
To investigate the effect of pre-experimental cultivation (PEC) on the ontogeny of L. digitata across haploid gametophytes and diploid sporophytes, we designed a two-step common garden experiment (Figure 1). Step 1: Clonal vegetative gametophytes were grown at 5°C and 15°C for 3 years (PEC). These temperatures reflect the lower and upper temperature range for optimal growth and reproduction of L. digitata gametophytes (tom Dieck, 1993; Bartsch et al., 2013; Martins et al., 2017). Gametophytes from both priming temperatures were fertilized at a common temperature of 10°C over two weeks, during which developmental stages of gametogenesis and sporophyte formation were quantified. Step 2: recruited sporophytes from step 1 were transferred to a temperature gradient spanning the tolerance of the species from 0°C to 20°C for two weeks. We thereby investigated the potential differences in response to temperature based on the PEC of their parental gametophytes using growth rates and optimum quantum yield of offspring sporophytes as response parameters.
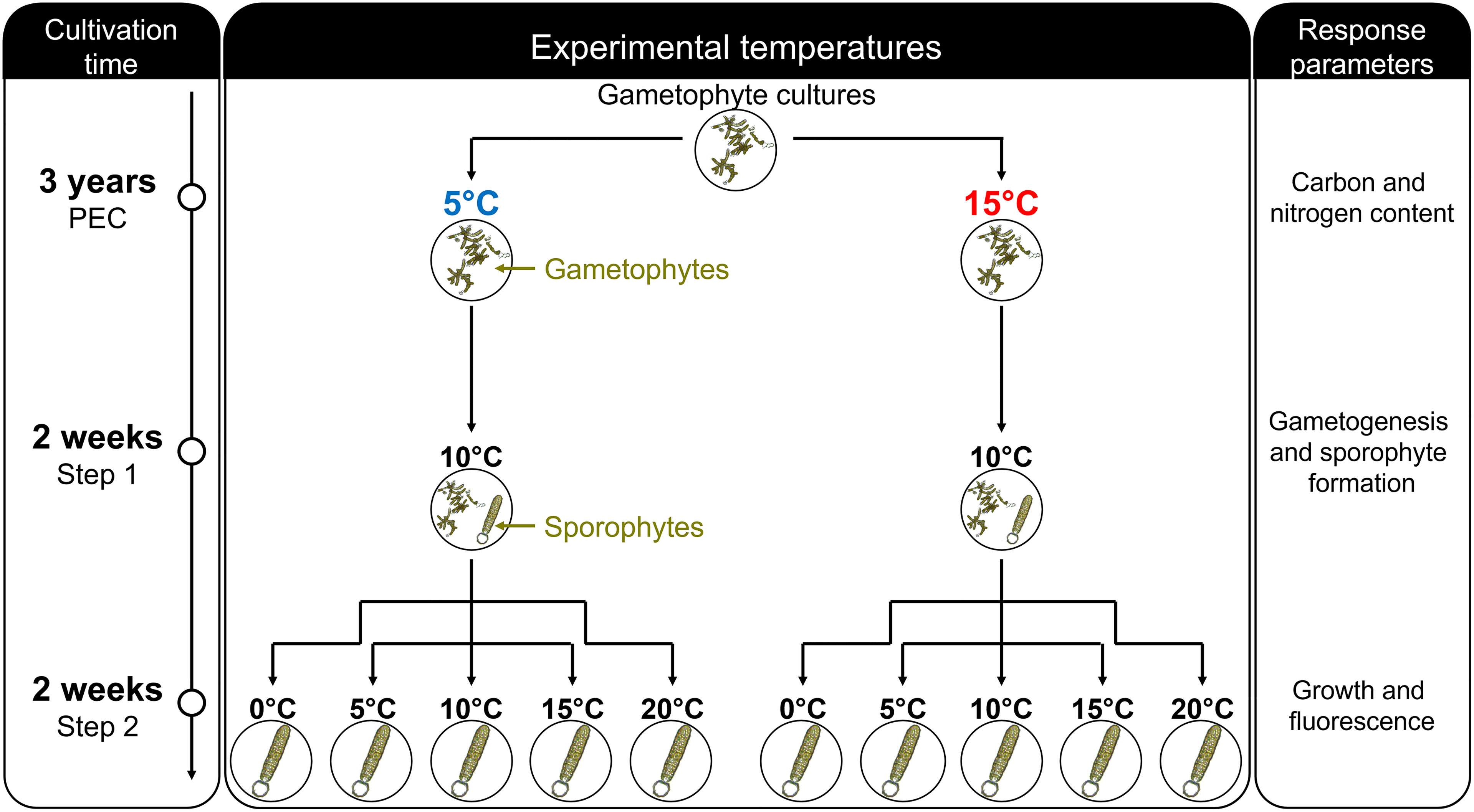
Figure 1 Scheme of the two-step common garden experiment to investigate the effects of pre-experimental cultivation (PEC) across haploid gametophyte parents and diploid sporophyte offspring. Clonal gametophytes were cultivated for 3 years in parallel at 5°C and 15°C (pre-experimental cultivation), after which carbon and nitrogen contents of gametophytes were measured. Gametogenesis was induced simultaneously in both sets of gametophytes at 10°C for two weeks, during which gametogenesis and sporophyte formation were assessed (step 1). Differences in thermal tolerance of the resulting sporophytes were then investigated by measuring sporophyte growth and chlorophyll fluorescence in a temperature gradient spanning 0–20°C over two weeks (step 2).
Preparation of Experimental Material
Clonal isolates of four pairs of sibling male and female gametophytes from Helgoland, North Sea (male culture numbers: 3435, 3437, 3443 and 3447; female culture numbers: 3436, 3438, 3444 and 3448; isolated from four individual sporophytes in 2015) were maintained in parallel at both 5°C and 15°C for three years in a cooling chamber. They were kept under an irradiance of 2 µmol photons m-2 s-1 red light, in a 16 h: 8 h light: dark (LD) cycle (Mitras Lightbar 2 Daylight, controlled by a Profilux 3 computer, GHL Advanced Technology GmbH & Co. KG, Kaiserslautern, Germany), in 50% Provasoli-enriched seawater (1/2 PES; Provasoli, 1968) without iron to prevent gametogenesis (Lüning and Dring, 1975; Lewis et al., 2013). To control for similar densities per gametophyte sex in the experiment, stock solutions were prepared by pooling similar amounts of the four female and male clonal gametophyte cultures (following the protocol of Bartsch, 2018. Gametophytes were gently crushed in a mortar and filtered through a 100 µm sieve with 100 mL of sea water to obtain gametophytes fragments of similar size.
Twenty-five plastic dishes (diameter: 6 cm, Coria, polystyrol, VKF Renzel, Germany) containing four thick microscope cover slips (No. 3, Menzel, Germany) as settling substrate and 100 mL of 1/2 PES were inoculated per PEC with a target density of approximately 300 gametophytes cm-2 of each sex for assessments of gametogenesis and sporophyte growth. This number of dishes was chosen to ensure n = 5 replicates per experimental temperature for step 2 of the experiment. A parallel set of 25 dishes containing 100 mL of 1/2 PES were inoculated per PEC with a target density of 600 gametophytes per cm-² of each sex to ensure a sufficient density for measuring chlorophyll fluorescence of juvenile sporophytes in step 2 (n = 5 replicates per experimental temperature).
Carbon and Nitrogen Content
After inoculating the experimental replicates, the remaining biomass of vegetative male and female gametophyte material from both PECs was sampled for the analysis of cellular carbon and nitrogen content to relate potential cross-generational effects to the nutrient content of their parental gametophytes (n = 4). The gametophytes were rinsed with fresh water, transferred into 1.5 mL Eppendorf tubes, flash-frozen in liquid nitrogen and stored at -80°C prior to analysis. Samples were lyophilized (ALPHA 1-4 LSC plus, CHRIST, Osterode am Harz, Germany) for 24 hours and ground in a ball mill (Mixer Mill MM400, Retsch, Haan, Germany). From each sample, between 2-6 mg of dry weight were combusted at 1000°C in an elemental analyzer (EURO EA, HEKAtech GmbH, Wegberg, Germany) with acetanilide as standard.
Step 1: Gametogenesis and Sporophyte Formation
Before the start of the experiment, gametophytes were allowed to recover from mechanical stress due to grinding at 10°C in water baths tempered by a Huber Variostat with Pilot ONE (Peter Huber Kältemaschinenbau AG, Offenburg, Germany) under 2 µmol photons m-2 s-1 of white light, in a 16 h: 8 h LD cycle for 3 days. Gametogenesis was then induced by increasing the irradiance to 15 µmol photons m-2 s-1 white light for two weeks.
Gametophyte density and ontogenetic stages of gametogenesis at 10°C were quantified on day 0, 3, 7, 10 and 14 following a subset of replicates over time (n = 10 per PEC inoculated at a target density of 300 gametophytes cm-2 per sex). All counts were performed using an inverted microscope (CKX41, Olympus, Tokyo, Japan) at 100x magnification with a 1 mm² ocular grid. For each replicate, a minimum of 40 fields of view was considered, representing at least 100 female gametophytes. Males and females were differentiated from day 3 onwards when sexual dimorphism became evident. Images were taken with a microscope camera (Axiocam ERc5s, Carl Zeiss, Oberkochen, Germany) on the inverted microscope on day 3 to estimate the number of female cells per cm² as a control parameter for the potential mean number of eggs that can be produced. Four ontogenetic stages of female gametophytes were considered from day 3 onwards: vegetative gametophytes, gametophytes bearing oogonia, egg release, and gametophytes with offspring sporophytes (Martins et al., 2017). For each female gametophyte, the most advanced stage of development was noted, i.e., when at least one of the gametophyte cells had entered the respective stage of development. Absolute numbers of recruited sporophytes were counted on day 14. The medium in the Petri dishes was changed once after 14 days.
Step 2: Thermal Plasticity of Juvenile Sporophytes
After 14 days of gametogenesis at 10°C, dishes were acclimated to a temperature gradient of 0°C to 20°C in order to follow growth and photosynthetic fluorescence in the microscopic sporophytes. Samples for the 0°C and 20°C treatments first stayed at 5 and 15°C respectively for three days before reaching the experimental temperatures, whereas samples for the 5°C, 10°C and 15°C treatments remained at 10°C. On day 0 of the experiment, all samples were transferred to the experimental temperatures. Twenty images were taken per replicate (n = 5) on day 0, 7 and 14. On day 7, images of sporophytes growing on the cover slips were taken using a compound microscope at 50x magnification (Axiophot, Carl Zeiss, Oberkochen, Germany) to accommodate for sporophyte growth. On day 14, sporophyte images of material from 5°C, 10°C and 15°C were taken under a stereo microscope at 25x magnification (SZX10, OLYMPUS, Tokyo, Japan) while images of 0°C and 20°C material were taken using the compound microscope at 50x magnification. To enhance the probability that only the sporophytes recruited during 10°C were evaluated, the length and width of the 5 biggest sporophytes per image were measured using ZEN software (Carl Zeiss, Oberkochen, Germany).
Linear length and width growth rates were calculated over the first week, over the second week, and over the entire 14 days, from mean values per replicate with the formula:
Where xf is the length or width (in µm) at final time; xi is the length or width at initial time; tf is final time (in days); ti is initial time.
Maximum quantum yield of photosystem II (Fv/Fm) of sporophytes was measured in the center of each dish (n = 5 dishes inoculated at a target density of 600 gametophytes cm-2 per sex) after 10 minutes dark acclimation on day 0, 7 and 14 with an Imaging PAM (Walz, Effeltrich, Germany).
Statistical Analysis
All analyses were performed in the R statistical environment version 4.0.3 (R Core Team, 2020). Carbon, nitrogen, and carbon to nitrogen (C:N) ratio were analyzed with a two-way ANOVA using PEC and sex as fixed factors. Step 1: Differences in proportions of ontogenetic stages (vegetative, oogonia, egg release and sporophytes) between PECs (5°C and 15°C) were investigated for each time point using a chi-square test. Gametophyte densities on day 0 were not significantly different between PECs (5°C PEC: 739 ± 45; 15°C PEC: 774 ± 96, t18 = 1.05, p = 0.309, Student t-test). Neither was the number of female cells cm-² on day 3 significantly different between PECs (5°C PEC: 3040 ± 128; 15°C PEC: 3088 ± 129, t18 = -0.26, p = 0.795). Therefore, both PECs had the same chance to produce sporophytes and thus the absolute density of sporophytes developed until day 14 from both PECs could be directly compared using t-tests without normalization. Step 2: The mean length of sporophytes on day 0 of the growth experiment was significantly higher in the 5°C PEC (342 ± 15 µm) than in the 15°C PEC (320 ± 19 µm, t48 = 4.59, p < 0.001). Therefore, initial length was used as a covariate in an analysis of covariance (ANCOVA) to assess the interactive effect of PEC and experimental temperature (i.e., cross-generational plasticity) on length growth rates. Pairwise comparisons of growth rates between PEC within each experimental temperature were conducted using Tukey’s test. Length growth rates during week 1 were compared with week 2 for each experimental temperature within a PEC using paired t-tests. The mean width of sporophytes on day 0 of the growth experiment was also significantly larger in the 5°C PEC (70 ± 4 μm) than in the 15°C PEC (64 ± 4 μm, t48 = 5.43, p < 0.001). Therefore, initial width was used as a covariate in an ANCOVA to investigate the interactive effect of PEC and experimental temperature on width growth rates. Maximum quantum yield of photosystem II (Fv/Fm) was analyzed with a two-way ANOVA using PEC and experimental temperature as interactive fixed factors.
Results
Carbon and Nitrogen Content in Vegetative Gametophytes
No interactive effect of PEC and sex affected the nitrogen content, carbon content or C:N ratio (Tables 1; 2). Nitrogen concentrations were significantly higher in male (2.53 ± 0.41 mmol g-1) than in female (1.96 ± 0.48 mmol g-1) gametophytes but carbon content and C:N ratio did not differ significantly between sexes (Table 2). No significant differences were observed between PECs.

Table 1 Molar concentration of nitrogen (N), carbon (C) and carbon: nitrogen ratio (C:N) of vegetative male and female gametophytes of Laminaria digitata kept in long-term pre-experimental cultivation (PEC) at 5°C and 15°C (mean ± standard deviation, n = 4).

Table 2 Results of two-way ANOVA to investigate the effects of pre-experimental cultivation (PEC) and sex on nitrogen content, carbon content and carbon: nitrogen (C:N) ratio of vegetative Laminaria digitata gametophytes kept in long-term cultivation at 5°C and 15°C.
Step 1: Gametogenesis and Sporophyte Formation
Gametophytes with a PEC of 5°C completed gametogenesis at 10°C faster than gametophytes with a PEC of 15°C (Figure 2). There were significant differences in proportions of ontogenetic stages between PECs at each time point (Table 3). On day 7, 28% of female gametophytes from the 5°C PEC had undergone full development (gametophytes with sporophytes), whereas only 2% of the gametophytes from the 15°C PEC had recruited sporophytes yet. On day 14, only 1% of female gametophytes remained vegetative in material from both PECs. However, gametophytes with a PEC of 5°C more rapidly completed gametogenesis, as 57% of the respective female gametophytes had reached the sporophyte stage while only 33% of female gametophytes with a PEC of 15°C had recruited sporophytes within 14 days.
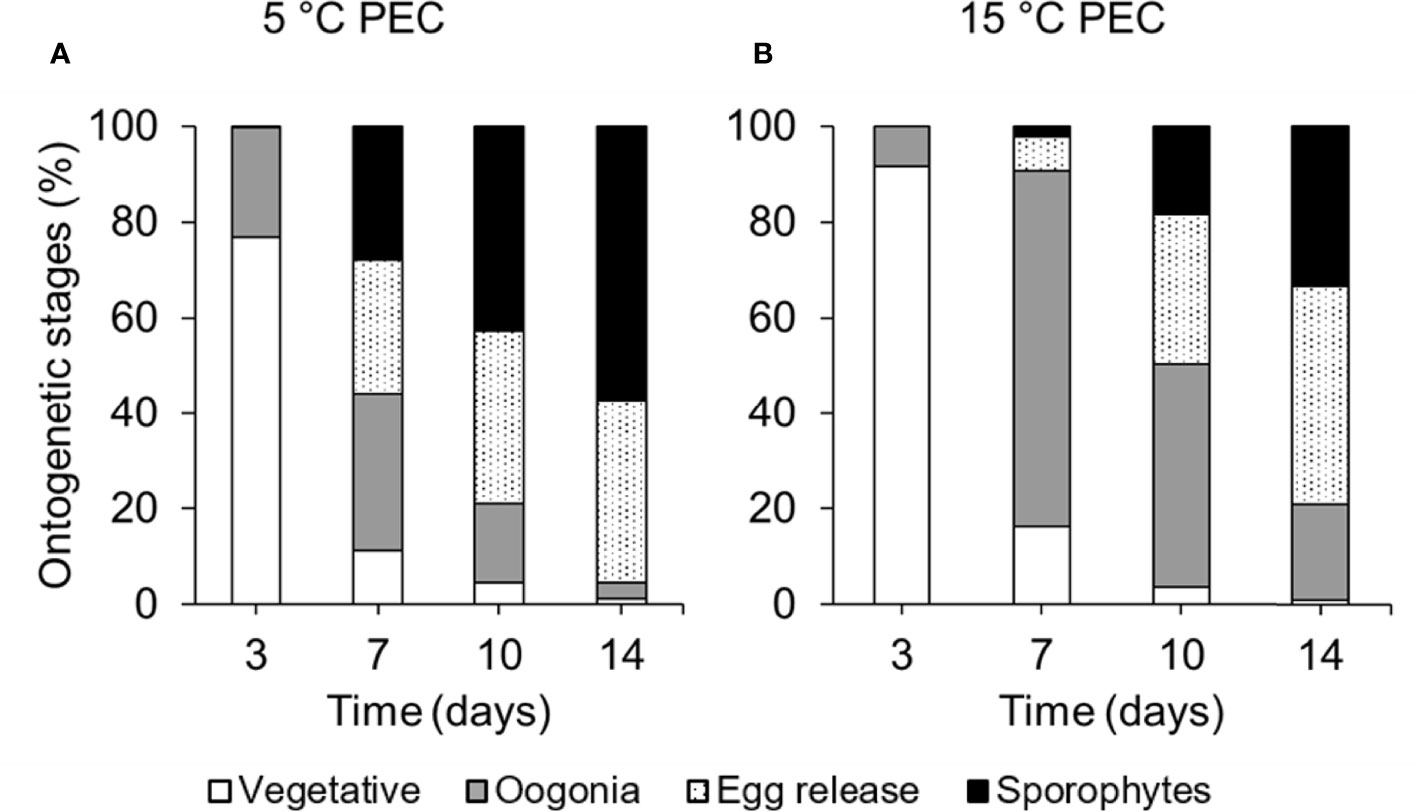
Figure 2 Development of ontogenetic stages of female Laminaria digitata gametophytes over time at 10°C (step 1) after long-term pre-experimental cultivation at (A) 5°C and (B) 15°C (mean values, standard errors omitted for clarity; n = 10).

Table 3 Results of chi-square test of homogeneity of variances to investigate differences in proportions of ontogenetic stages of Laminaria digitata gametophytes between pre-experimental cultivation temperatures of 5°C and 15°C at each time point.
Sporophyte production (Figure 3) after 14 days at 10°C was significantly higher in material from the 5°C PEC (278 ± 70 sporophytes cm-2) than in material from the 15°C PEC (194 ± 36 sporophytes cm-2, t18, p < 0.01, Student t-test). Therefore, the faster gametogenesis of gametophytes with a 5°C PEC is associated with an increased recruitment of sporophytes over 14 days.
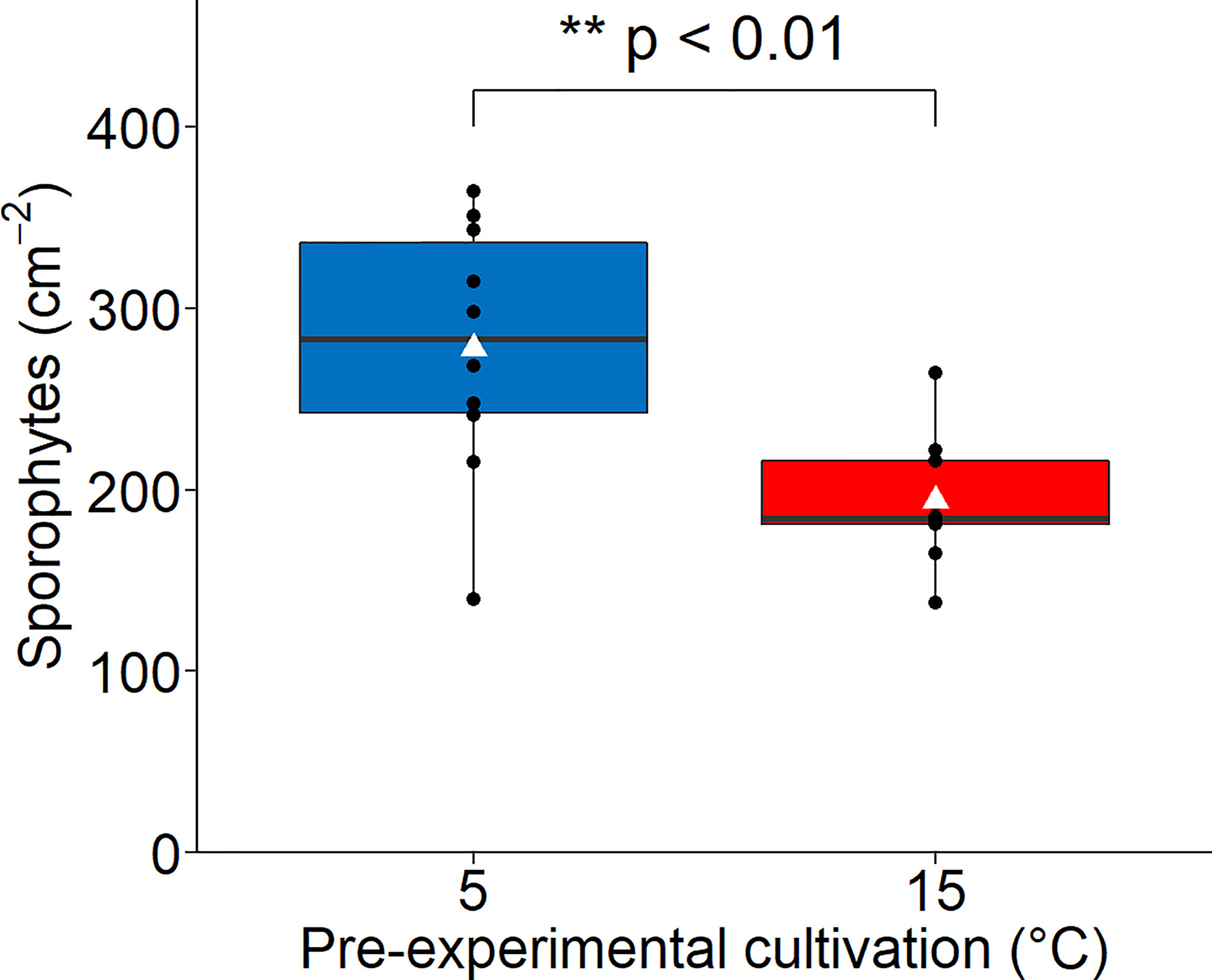
Figure 3 Absolute number of Laminaria digitata microscopic sporophytes after 14 days of gametophyte fertilization at 10°C (step 1) following pre-experimental cultivation (PEC) of parental gametophytes at 5°C and 15°C (n = 10). Boxplots represent the median as a line; triangle as the mean density of sporophytes; interquartile range as a box; minimum and maximum values as whiskers; dots as single replicates. Asterisks represent significant differences between PECs (p < 0.01, Student t-test).
Step 2: Thermal Plasticity of Juvenile Sporophytes
Sporophytes from both parental PECs showed a broad growth optimum between 5°C and 15°C (Figure 4A), while growth at 0°C and 20°C decreased considerably by more than 48% for the 5°C PEC and 68% for the 15°C PEC compared to their respective optimum. Length growth rates were interactively affected by parental PEC and experimental temperature (Table 4), indicating that temperatures experienced by the parental gametophytes influenced thermal responses of juvenile sporophytes (i.e., cross-generational plasticity). Growth rates in the temperature range of 5°C to 10°C did not differ significantly between PECs over two weeks. However, compared to sporophytes with a parental PEC of 15°C, sporophytes with a PEC of 5°C grew 94% better at 0°C (p < 0.001, Tukey test) and 69% better at 20°C (p = 0.001, Tukey test).
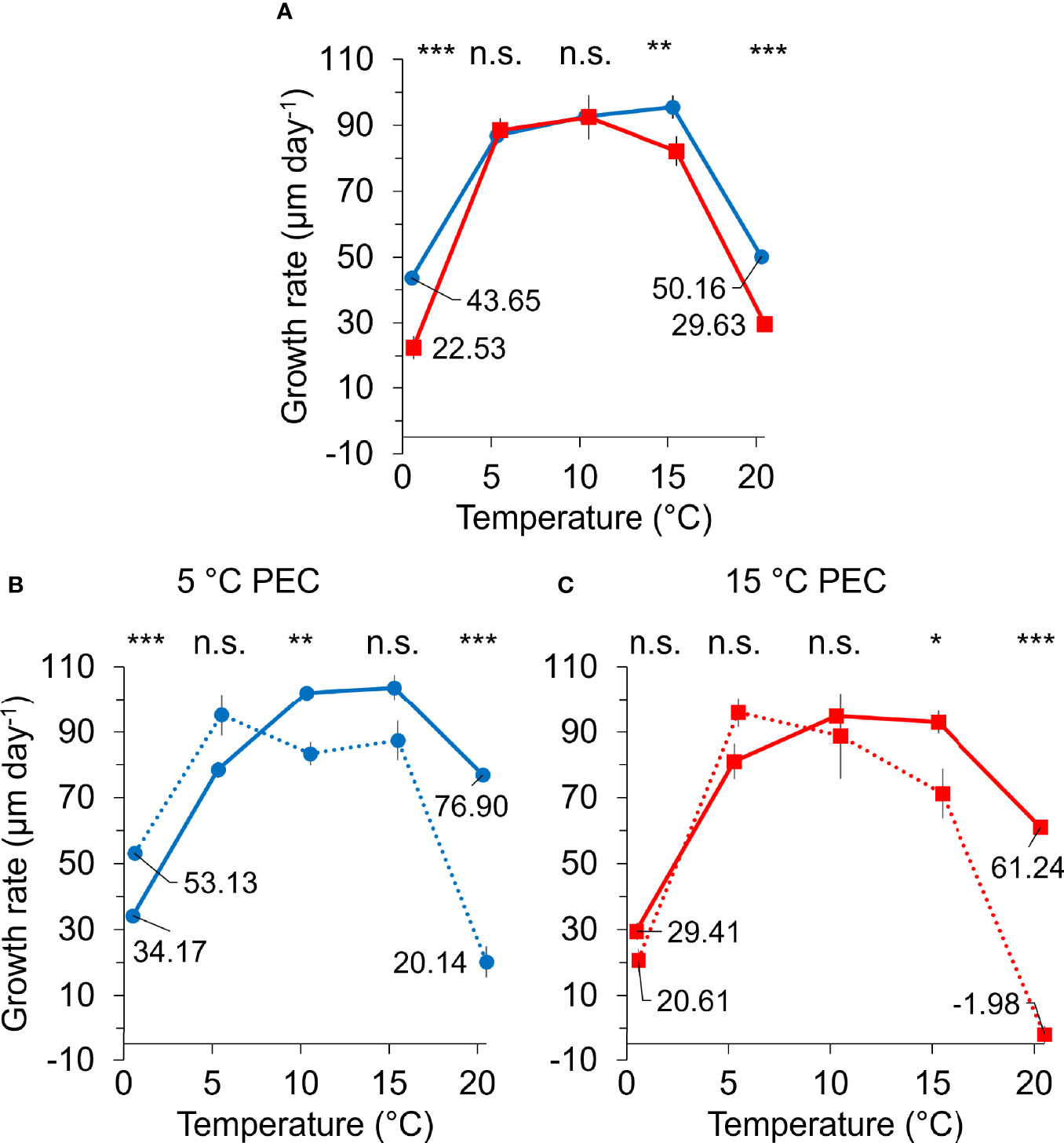
Figure 4 Length growth rates of juvenile Laminaria digitata sporophytes in a temperature gradient (step 2) following pre-experimental cultivation (PEC) of parental gametophytes at 5°C (blue) and 15°C (red; mean ± standard error, n = 5). (A) Growth rates over 2 weeks following 5 and 15°C PEC. Asterisks represent significant differences between PECs (*** p < 0.001, ** p < 0.01, n.s. not significant; Tukey test). (B, C) Growth rates following (B) 5°C and (C) 15°C PEC between day 0 and 7 (full line) and day 7 and 14 (dotted line). Asterisks represent significant differences between day 0 and 7 and day 7 and 14 within an experimental temperature (*** p < 0.001, ** p < 0.01, * p < 0.05, n.s. not significant; paired t-tests).

Table 4 Results of two-way ANCOVA to investigate effects of pre-experimental cultivation (PEC) of parental gametophytes and experimental temperature on length growth rates of juvenile Laminaria digitata sporophytes over 2 weeks in a temperature gradient with length at day 0 as a covariate.
In addition, growth rates changed over time (Figures 4B, C). At 0°C, growth rates of juvenile sporophytes with a parental PEC of 5°C had significantly increased by 55% during the second week compared to the first week (p < 0.001, paired t-test). In contrast, the reduction in growth of sporophytes with a parental PEC of 15°C at 0°C by 30% in the second week was not significant. At 20°C, the sporophytes with a parental PEC of 15°C ceased growth completely during the second week while growth rates were low but still positive in sporophytes with a PEC of 5°C (Figures 4B, C).
Growth rates based on the width of juvenile sporophytes showed a growth optimum at 15°C, a sharp decline towards 20°C and receding growth rates towards 0°C (Figure S1). This response pattern was more expressed for sporophytes with a parental PEC of 5°C. Similarly as for length growth, width growth was interactively affected by the parental PEC and experimental temperature (Table S1). This differential pattern of length and width growth rates resulted in a different morphology of offspring sporophytes from both parental PECs. Sporophytes with a parental PEC of 5°C grew significantly wider than sporophytes with a parental PEC of 15°C for all experimental temperatures except 5°C (p < 0.05, Tukey test).
A broad temperature acclimation spectrum became evident in photosynthetic optimum quantum yield Fv/Fm with an optimum at 10-15°C but clear indications for stress occurred at 20°C (Table 5). Fv/Fm differed significantly between the experimental temperatures, but not between PECs (Table 6). Fv/Fm of sporophytes at 20°C reached 0.36–0.37 and was thereby significantly lower than at all other temperatures (p < 0.001, Tukey test). Across both PECs, Fv/Fm of sporophytes at 0°C (PEC 5°C: 0.49; PEC 15°C: 0.45) were significantly higher than at 20°C (p < 0.001, Tukey test) but significantly lower than at the other temperatures (p < 0.01 for 5°C, p < 0.001 for 10 and 15°C, Tukey test).

Table 5 Photosynthetic optimum quantum yield (Fv/Fm) of juvenile Laminaria digitata sporophytes following pre-experimental cultivation of parental gametophytes and sporophytes growth for 2 weeks in a temperature gradient (mean ± standard deviation, n = 5).

Table 6 Two-way ANOVA to investigates effects of pre-experimental cultivation (PEC) and experimental temperature on optimum quantum yield Fv/Fm of juvenile Laminaria digitata sporophytes after 2 weeks in a temperature gradient following PEC of parental gametophytes.
Discussion
This study demonstrates that the thermal environment experienced by vegetative kelp gametophytes, which we consider to be parents to offspring sporophytes sensu Byrne et al. (2020), might have significant effects on the persistence of marine forests and may be applicable in mariculture and restoration efforts. The pre-experimental cultivation of the gametophytes altered their reproductive capacity and the growth performance of next-generation juvenile sporophytes in a common-garden laboratory approach. Gametogenesis at 10°C was faster in parents following a three-year pre-experimental cultivation at 5°C compared to 15°C, and resulted in a higher number of sporophytes despite no difference in CN content across PEC. Another major result of this study was that gametophyte cultivation at a cold temperature of 5°C promotes growth of offspring sporophytes at extreme temperatures (0°C and 20°C) which provides first evidence for cross-generational plasticity (Donelson et al., 2018; Byrne et al., 2020) in a kelp species. It furthermore indicates that short term resilience of juvenile offspring sporophytes is higher if their gametophytes experienced a cool temperature environment. This refutes our hypothesis that cultivation of gametophytes in warm or cold conditions promotes offspring sporophyte growth at matching warm or cold temperature, respectively. In contrast, there was an overall beneficial effect of cold cultivation on the performance of microscopic and juvenile L. digitata life cycle stages.
The fact that a cold temperature enhances performance of L. digitata has been observed in a similar study (Liesner et al., 2020b), where gametogenesis and recruitment at 5°C enhanced growth of juvenile sporophytes even three months after thermal priming compared to sporophytes with a warm (15°C) temperature history. This was considered to represent a potential “silver-spoon” parental effect (Uller et al., 2013; Baker et al., 2019; Germain et al., 2019), which describes a physiological advantage for individuals whose parents had access to abundant resources. As the amount and composition of storage products in sporophytes vary between regions and over seasons (Haug and Jensen, 1954; Schiener et al., 2015), environmental gradients might theoretically also affect the energy or nutrient provisioning of gametes by gametophytes, but respective investigations are lacking as yet. We hypothesized that carry-over effects and cross-generational plasticity might be related to the nutrient content of parental gametophytes. In our experiment, however, we could not explain the effects by initial carbon and nitrogen contents in gametophytes as they did not differ between PECs but only between sex for nitrogen content. Generally, a C:N ratio < 20 indicates that gametophytes were not nutrient-limited for growth and reproductive processes (Hurd et al., 2016).
Because we used the same genetic pool of gametophytes in both PECs, they were genetically identical at the beginning of the pre-cultivation. Therefore, we assumed that the observed differences in trait performance are not related to permanent changes in DNA sequence, as these would have to result from fixation of random mutations, which is unlikely considering the slow growth of gametophytes and the relatively short time scale of the experiment. In contrast, epigenetic mechanisms such as DNA methylation or histone modifications may explain the patterns highlighted in this study, as they may alter gene expression resulting in phenotypic differentiation without changing the DNA sequence. Several studies on different phyla (seagrass: Jueterbock et al., 2020; cyanobacteria: Hu et al., 2018; corals: Liew et al., 2020) suggested that DNA methylation can be inherited across generations and might play a role in the acclimation towards changing environments. Heritable DNA methylation patterns in plants may vary by genotype, developmental stage and parental environment (Herman and Sultan, 2016). A previous study on the kelp Saccharina japonica highlighted that differential DNA methylation may contribute to the transition between life stages (Fan et al., 2020). Methylation is also positively correlated with temperature response in kelp (Scheschonk et al., in review), but epigenetic inheritance in kelps has not been demonstrated yet.
Furthermore, we show that a nutritional “silver-spoon” parental effect, which would provide benefits across all experimental environments, does not provide an explanation for the pattern observed here, as growth was not generally higher in sporophytes from the 5°C PEC but only at the extreme temperatures. In both PECs, a temperature of 20°C led to a decrease of optimum quantum yield by 34% compared to the optimum, which indicates physiological stress (Hanelt, 2018). However, sporophytes with a 5°C PEC were able to maintain growth at 20°C while those with a 15°C PEC ceased growth after two weeks. Only sporophytes with a 5°C PEC even increased their growth rates at 0°C between week 1 and week 2. In consequence, the environment experienced by parental gametophytes influenced the response plasticity of offspring sporophytes, in this case by modulating their thermal performance at sub-optimal temperatures. Therefore, the relatively faster growth of sporophytes with a cold temperature history at the extreme temperatures 0°C and 20°C is evidence for cross-generational plasticity (Byrne et al., 2020).
While immediate temperature exposure has been known to affect gametogenesis and sporophyte recruitment in L. digitata (tom Dieck, 1992; Müller et al., 2008; Martins et al., 2017) we demonstrated a carry-over effect on gametogenesis speed following long-term thermal priming. Because all gametophyte cells may develop gametangia (Bartsch et al., 2008), a similar amount of gametophytes cells cm-2 (see Statistical Analysis) in both conditions provided the same potential recruitment capacity. However, gametogenesis was faster and more sporophytes were recruited by gametophytes following a cold PEC. One explanation is that a higher proportion of eggs may have been released at once in the 5°C PEC and thus a synchronous release of spermatozoids triggered by the pheromone Lamoxirene (Maier and Müller, 1986) may have resulted in a massive fertilization event and subsequent enhanced reproduction success. In the warm PEC, where gametogenesis stretched over a longer time span, most spermatozoids might have been released already during the first egg release as male reproduction is protandtric (Hsiao and Druehl, 1971). So eggs released at a later time point may have had lower chances of fertilization (for a detailed discussion see Martins et al., 2017). In line with the description of sporogenesis being a cold adapted process (Bartsch et al., 2013), our results of gametogenesis further support that L. digitata is a cold-temperate to Arctic species (Lüning et al., 1990) with some ability to tolerate warm summer temperature around 20°C.
The time of the year when gametogenesis occurs in nature has been poorly documented due to the difficulty of studying microscopic processes in the field, but experimental data suggest that recruitment of juvenile L. digitata sporophytes occurs from autumn to early spring (Lüning, 1980; Martins et al., 2017). Our experiment represented temperature successions of early spring (5°C ➔ 10°C) and autumn conditions (15°C ➔ 10°C) at Helgoland, North Sea (Bartsch et al., 2013) which is the location of the source population used in this study. In our experiment, sporophyte recruitment was higher in temperature successions representing early spring compared to autumn conditions. However, this should be verified using a more realistic treatment duration for thermal priming and in combination with in situ studies. The temperature conditions used in this study are not specific for Helgoland but exist along wide stretches of the latitudinal distribution gradient of the species. In Kongsfjorden (Spitsbergen) which is near to the cold distributional edge, sea surface temperatures (SST) may meanwhile reach 6-7°C during summer (Hanelt et al., 2001; Liesner et al., 2020a). Therefore, in the northern regions of the species’ distribution, gametogenesis might take place directly after meiospore release in late summer or autumn during long daylength and cold temperature, which has been shown to lead to a high recruitment of sporophytes (Martins et al., 2017; Silva et al., 2022). However, in southern Brittany, which is the warm distributional limit of L. digitata, SST vary between 7°C in winter and 18°C in summer (Oppliger et al., 2014). Therefore, the potentially best recruitment period at the warm range edge is not in autumn like at the cold range edge, but months later during the cold season between the end of autumn and spring (see also Liesner et al., 2020b).
Sporophytes with a parental thermal history of 15°C where not only fewer in numbers but were also more vulnerable to thermal sub-lethal stress. In summer, gametogenesis is thermally inhibited at temperatures higher than 17°C (tom Dieck, 1992; Martins et al., 2017). As periods of inhibiting temperatures may increase in duration, intensity and frequency under global warming conditions at southern locations (IPCC, 2014; Oppliger et al., 2014; Hobday et al., 2016), the fertile window of L. digitata during colder seasons will become reduced. Similarly, the fraction of time in which L. digitata can recruit at “cold” temperatures (e.g., < 10°C) will become shorter especially at southern locations. Consequently, relatively more sporophytes with a warm parental temperature history will be formed, which may result in reduced tolerance to high temperature of the sporophyte population. This highlights the importance of a cold season for the persistence of L. digitata populations especially at their warm range edges. In addition to the increasing frequency and intensity of marine heatwaves (Hobday et al., 2016; Oliver et al., 2018), the projected increase of North Atlantic SST by 1-3°C (Müller et al., 2009; Schrum et al., 2016) could progressively reduce the persistence of cold temperate kelp populations across generations by reducing sporophyte plasticity (Liesner et al., 2020b) and sporophyte recruitment and tolerance to future warm events, as shown in our study.
Not only does ocean warming threaten wild kelp populations, but also cultivated marine crops. In Japan, an average temperature increase of just 1°C during winter significantly reduced growth and increased blade erosion of Saccharina japonica cultivars (Suzuki et al., 2009). Selective breeding over years across many generations resulted in more productive and heat resistant cultivars that are able to cope with new environmental challenges (e.g., Liu et al., 2014). Here, we provide evidence that priming of gametophytes, a common strategy to enhance crop production (Wang et al., 2017; Mercé et al., 2020), can additionally modulate performance of their offspring sporophytes in the short term. In contrast to findings from higher plants and seagrass (e.g., Fan et al., 2018; Nguyen et al., 2020), thermal priming of kelp may not be effective through heat stress exposure of early life cycle stages, but rather through the application of cold temperature. However, our current knowledge is based on the priming effects of temperatures at either end of L. digitata’s growth optimum (this study; Liesner et al., 2020b), so further research on priming using sublethal high temperatures is necessary to support this hypothesis. As cold priming increased trait performance of gametogenesis, recruitment and enhanced sporophyte growth, priming of gametophyte stocks may be developed into a valuable tool applicable to increase productivity in kelp mariculture. Cold gametophyte priming also increased heat resilience of offspring sporophytes in our study, making priming a promising strategy not only for kelp cultivation but also for kelp forest restoration efforts (e.g., Wood et al., 2019; Coleman et al., 2020) and resilience under climate change.
Data Availability Statement
The raw data supporting the conclusions of this article will be made available by the authors, without undue reservation.
Author Contributions
NM proposed the idea of long-term gametophyte priming. DL and IB planned and designed the experiment. CG conducted the experiment and analyzed the data with the help of DL. CG, DL, IB, and NM contributed to the data interpretation and discussion. CG wrote the manuscript, which was reviewed and revised by all authors. All authors contributed to the article and approved the submitted version.
Funding
DL was funded through the 2015-2016 BiodivERsA COFUND call for research proposals (program MARFOR), with the national funder German Research Foundation (DFG; grant no. VA 105/25-1). NM was funded by FCT - Foundation for Science and Technology (Portugal) through SFRH/BPD/122567/2016 (in transitional norm DL 57/2016/CP1361/CT0039).
Conflict of Interest
The authors declare that the research was conducted in the absence of any commercial or financial relationships that could be construed as a potential conflict of interest.
Publisher’s Note
All claims expressed in this article are solely those of the authors and do not necessarily represent those of their affiliated organizations, or those of the publisher, the editors and the reviewers. Any product that may be evaluated in this article, or claim that may be made by its manufacturer, is not guaranteed or endorsed by the publisher.
Acknowledgments
We thank Andreas Wagner for culture maintenance, Claudia Daniel for image analysis and lyophilization, and Sandra Murawski for the CN measurements. We thank Alexander Jueterbock for valuable discussions of terminology. We thank two reviewers for their critical comments that improved the quality of the manuscript and the university of Bodø for financing open access.
Supplementary Material
The Supplementary Material for this article can be found online at: https://www.frontiersin.org/articles/10.3389/fmars.2022.862923/full#supplementary-material
References
Assis J., Araújo M. B., Serrão E. A. (2018). Projected Climate Changes Threaten Ancient Refugia of Kelp Forests in the North Atlantic. Glob. Change Biol. 24, e55–e66. doi: 10.1111/gcb.13818
Baker B. H., Sultan S., Lopez-Ichikawa M., Waterman R. (2019). Transgenerational Effects of Parental Light Environment on Progeny Competitive Performance and Lifetime Fitness. Philos. Trans. R. Soc B 374, 20180182. doi: 10.1098/rstb.2018.0182
Bartsch I. (2018). “Derivation of Clonal Stock Cultures and Hybridization of Kelps: A Tool for Strain Preservation and Breeding Programs”, in Protocols for Macroalgae Research. Eds. Charrier B., Wichard T., Reddy C. R. K. (Boca Raton, USA: CRC Press), 61–78. doi: 10.1201/b21460-3
Bartsch I., Vogt J., Pehlke C., Hanelt D. (2013). Prevailing Sea Surface Temperatures Inhibit Summer Reproduction of the Kelp Laminaria Digitata at Helgoland (North Sea). J. Phycol. 49, 1061–1073. doi: 10.1111/jpy.12125
Bartsch I., Wiencke C., Bischof K., Buchholz C. M., Buck B. H., Eggert A., et al. (2008). The Genus Laminaria Sensu Lato: Recent Insights and Developments. Eur. J. Phycol. 43, 1–86. doi: 10.1080/09670260701711376
Bolton J. J., Lüning K. (1982). Optimal Growth and Maximal Survival Temperatures of Atlantic Laminaria Species (Phaeophyta) in Culture. Mar. Biol. 66, 89–94. doi: 10.1007/BF00397259
Burel T., le Duff M., Ar Gall E. (2019). Updated Check-List of the Seaweeds of the French Coasts, Channel and Atlantic Ocean. Cah. Nat. L’Observ. Mar. 7, 1–38.
Byrne M., Foo S. A., Ross P. M., Putnam H. M. (2020). Limitations of Cross- and Multigenerational Plasticity for Marine Invertebrates Faced With Global Climate Change. Glob. Change Biol. 26, 80–102. doi: 10.1111/gcb.14882
Coleman M. A., Wood G., Filbee-Dexter K., Minne A. J. P., Goold H. D., Vergés A., et al. (2020). Restore or Redefine: Future Trajectories for Restoration. Front. Mar. Sci. 7. doi: 10.3389/fmars.2020.00237
Cosson J. (1999). Sur La Disparition Progressive De Laminaria Digitata Sur Les Côtes Du Calvados (France). Cryptogam. Algol. 20, 35–42. doi: 10.1016/S0181-1568(99)80005-1
Davoult D., Engel C. R., Arzel P., Knoch D., Laurans M. (2011). Environmental Factors and Commercial Harvesting: Exploring Possible Links Behind the Decline of the Kelp Laminaria Digitata in Brittany, France. Cah. Biol. Mar. 52, 429–435.
Delebecq G., Davoult D., Janquin M.-A., Oppliger L., Menu D., Dauvin J.-C., et al. (2015). Photosynthetic Response to Light and Temperature in Laminaria Digitata Gametophytes From Two French Populations. Eur. J. Phycol. 51, 1–12. doi: 10.1080/09670262.2015.1104556
Donelson J. M., Salinas S., Munday P. L., Shama L. N. S. (2018). Transgenerational Plasticity and Climate Change Experiments: Where do We Go From Here? Glob. Change Biol. 24, 13–34. doi: 10.1111/gcb.13903
Edwards M. S. (2000). The Role of Alternate Life-History Stages of a Marine Macroalga: A Seed Bank Analogue? Ecology 81, 2404–2415. doi: 10.2307/177463
Fan X., Han W., Teng L., Jiang P., Zhang X., Xu D., et al. (2020). Single-Base Methylome Profiling of the Giant Kelp Saccharina Japonica Reveals Significant Differences in DNA Methylation to Microalgae and Plants. New Phytol. 225, 234–249. doi: 10.1111/nph.16125
Fan Y., Ma C., Huang Z., Abid M., Jiang S., Dai T., et al. (2018). Heat Priming During Early Reproductive Stages Enhances Thermo-Tolerance to Post-Anthesis Heat Stress via Improving Photosynthesis and Plant Productivity in Winter Wheat (Triticum Aestivum L.). Front. Plant Sci. 9. doi: 10.3389/fpls.2018.00805
Franke K., Liesner D., Heesch S., Bartsch I. (2021). Looks can be Deceiving: Contrasting Temperature Characteristics of Two Morphologically Similar Kelp Species Co-Occurring in the Arctic. Bot. Mar. 64, 163–175. doi: 10.1515/bot-2021-0014
Germain R. M., Grainger T. N., Jones N. T., Gilbert B. (2019). Maternal Provisioning is Structured by Species’ Competitive Neighborhoods. Oikos 128, 45–53. doi: 10.1111/oik.05530
Goodnight C. J. (2000). Heritability at the Ecosystem Level. Proc. Natl. Acad. Sci. 97, 9365–9366. doi: 10.1073/pnas.97.17.9365
Grossniklaus U. (2011). Plant Germline Development: A Tale of Cross-Talk, Signaling, and Cellular Interactions. Sex Plant Reprod. 24, 91–95. doi: 10.1007/s00497-011-0170-3
Hanelt D. (2018). Photosynthesis Assessed by Chlorophyll Fluorescence,” in Bioassays, Advanced Methods and Applications. 169–198.
Hanelt D., Tüg H., Bischof K., Groß C., Lippert H., Sawall T., et al. (2001). Light Regime in an Arctic Fjord: A Study Related to Stratospheric Ozone Depletion as a Basis for Determination of UV Effects on Algal Growth. Mar. Biol. 138, 649–658. doi: 10.1007/s002270000481
Haug A., Jensen A. (1954). Seasonal Variations in the Chemical Composition of Alaria Esculenta, Laminaria Saccharina, Laminaria Hyperborea and Laminaria Digitata From Northern Norway. Nor. Inst. tang-og. Tareforsk. Oslo.
Herman J. J., Sultan S. E. (2016). DNA Methylation Mediates Genetic Variation for Adaptive Transgenerational Plasticity. Proc. R. Soc B Biol. Sci. 283, 20160988. doi: 10.1098/rspb.2016.0988
Hobday A. J., Alexander L. V., Perkins S. E., Smale D. A., Straub S. C., Oliver E. C. J., et al. (2016). A Hierarchical Approach to Defining Marine Heatwaves. Prog. Oceanogr. 141, 227–238. doi: 10.1016/j.pocean.2015.12.014
Hsiao S. I. C., Druehl L. D. (1971). Environmental Control of Gametogenesis in Laminaria Saccharina. I. The Effects of Light and Culture Media. Can. J. Bot. 49, 1503–1508. doi: 10.1139/b71-211
Hurd C. L., Harrison P. J., Bischof K., Lobban C. S. (2016). Seaweed Ecology and Physiology. Cambridge University Press, Cambridge, UK, 551 Pp. J. Phycol. 52, 315–316. doi: 10.1111/jpy.12398
Hu L., Xiao P., Jiang Y., Dong M., Chen Z., Li H., et al. (2018). Transgenerational Epigenetic Inheritance Under Environmental Stress by Genome-Wide DNA Methylation Profiling in Cyanobacterium. Front. Microbiol. 9. doi: 10.3389/fmicb.2018.01479
IPCC (2014). Climate Change 2014: Synthesis Report. Contribution of Working Groups I, II and III to the Fifth Assessment Report of the Intergovernmental Panel on Climate Change Vol. 151. Eds. Core Writing Team, Pachauri R. K., Meyer L. A. (Geneva, Switzerland: IPCC), 169.
Jueterbock A., Boström C., Coyer J. A., Olsen J. L., Kopp M., Dhanasiri A. K. S., et al. (2020). The Seagrass Methylome Is Associated With Variation in Photosynthetic Performance Among Clonal Shoots. Front. Plant Sci. 11. doi: 10.3389/fpls.2020.571646
Jueterbock A., Minne A. J. P., Cock J. M., Coleman M. A., Wernberg T., Scheschonk L., et al. (2021). Priming of Marine Macrophytes for Enhanced Restoration Success and Food Security in Future Oceans. Front. Mar. Sci. 8. doi: 10.3389/fmars.2021.658485
Lewis R. J., Green M. K., Afzal M. E. (2013). Effects of Chelated Iron on Oogenesis and Vegetative Growth of Kelp Gametophytes (Phaeophyceae): Effects of Iron on Kelp Gametophytes. Phycol. Res. 61, 46–51. doi: 10.1111/j.1440-1835.2012.00667.x
Liesner D., Fouqeau L., Valero M., Roleda M., Pearson G., Bischof K., et al. (2020a). Heat Stress Responses and Population Genetics of the Kelp Laminaria Digitata (Phaeophyceae) Across Latitudes Reveal Differentiation Among North Atlantic Populations. Ecol. Evol. 10, 9144–9177. doi: 10.1002/ece3.6569
Liesner D., Pearson G. A., Bartsch I., Rana S., Harms L., Heinrich S., et al. (2022). Increased Heat Resilience of Intraspecific Outbred Compared to Inbred Lineages in the Kelp Laminaria Digitata: Physiology and Transcriptomics. Front. Mar. Sci. 9. doi: 10.3389/fmars.2022.838793
Liesner D., Shama L. N. S., Diehl N., Valentin K., Bartsch I. (2020b). Thermal Plasticity of the Kelp Laminaria Digitata (Phaeophyceae) Across Life Cycle Stages Reveals the Importance of Cold Seasons for Marine Forests. Front. Mar. Sci. 7. doi: 10.3389/fmars.2020.00456
Liew Y. J., Howells E. J., Wang X., Michell C. T., Burt J. A., Idaghdour Y., et al. (2020). Intergenerational Epigenetic Inheritance in Reef-Building Corals. Nat. Clim. Change 10, 254–259. doi: 10.1038/s41558-019-0687-2
Liu F., Sun X., Wang F., Wang W., Liang Z., Lin Z., et al. (2014). Breeding, Economic Traits Evaluation, and Commercial Cultivation of a New Saccharina Variety “Huangguan No. 1’’. Aquac. Int. 22, 1665–1675. doi: 10.1007/s10499-014-9772-8
Lund S. (1959). The Marine Algae of East Greenland. I. Taxonomic Part. Meddelser. om Grønland. 156 (1), 1–247.
Lüning K. (1980). Critical Levels of Light and Temperature Regulating the Gametogenesis of Three Laminaria Species (Phaeophyceae). J. Phycol. 16, 1–15. doi: 10.1111/j.1529-8817.1980.tb02992.x
Lüning K., Dring M. J. (1975). Reproduction, Growth and Photosynthesis of Gametophytes of Laminaria Saccharina Grown in Blue and Red Light. Mar. Biol. 29, 195–200. doi: 10.1007/BF00391846
Lüning K., Yarish C., Kirkman H. (1990). Seaweeds: Their Environment, Biogeography, and Ecophysiology. New York: John Wiley & Sons. doi: 10.2307/2260740
Maier I., Müller D. G. (1986). Sexual Pheromones in Algae. Biol. Bull. 170, 145–175. doi: 10.2307/1541801
Mann K. (1973). Seaweeds: Their Productivity and Strategy for Growth. Science 182, 975–981. doi: 10.1126/science.182.4116.975
Martins N., Pearson G. A., Bernard J., Serrão E. A., Bartsch I. (2020). Thermal Traits for Reproduction and Recruitment Differ Between Arctic and Atlantic Kelp Laminaria Digitata. PloS One 15, e0235388. doi: 10.1371/journal.pone.0235388
Martins N., Tanttu H., Pearson G. A., Serrão E. A., Bartsch I. (2017). Interactions of Daylength, Temperature and Nutrients Affect Thresholds for Life Stage Transitions in the Kelp Laminaria Digitata (Phaeophyceae). Bot. Mar. 60, 109–121. doi: 10.1515/bot-2016-0094
Mercé C., Bayer P. E., Tay Fernandez C., Batley J., Edwards D. (2020). Induced Methylation in Plants as a Crop Improvement Tool: Progress and Perspectives. Agronomy 10, 1484. doi: 10.3390/agronomy10101484
Müller R., Laepple T., Bartsch I., Wiencke C. (2009). Impact of Oceanic Warming on the Distribution of Seaweeds in Polar and Cold-Temperate Waters. Bot. Mar. 52, 617–638. doi: 10.1515/BOT.2009.080
Müller R., Wiencke C., Bischof K. (2008). Interactive Effects of UV Radiation and Temperature on Microstages of Laminariales (Phaeophyceae) From the Arctic and North Sea. Clim. Res. 37, 203–213. doi: 10.3354/cr00762
Nguyen H. M., Kim M., Ralph P. J., Marín-Guirao L., Pernice M., Procaccini G. (2020). Stress Memory in Seagrasses: First Insight Into the Effects of Thermal Priming and the Role of Epigenetic Modifications. Front. Plant Sci. 11. doi: 10.3389/fpls.2020.00494
Oliver E. C. J., Donat M. G., Burrows M. T., Moore P. J., Smale D. A., Alexander L. V., et al. (2018). Longer and More Frequent Marine Heatwaves Over the Past Century. Nat. Commun. 9, 1324. doi: 10.1038/s41467-018-03732-9
Oppliger L. V., von Dassow P., Bouchemousse S., Robuchon M., Valero M., Correa J. A., et al. (2014). Alteration of Sexual Reproduction and Genetic Diversity in the Kelp Species Laminaria Digitata at the Southern Limit of its Range. PloS One 9, e102518. doi: 10.1371/journal.pone.0102518
Perez R. (1971). Ecologie, Croissance Et Régénération, Teneurs an Acide Alginique De Laminaria Digitata Sur Les Côtes Françaises De La Manche. Rev. Trav. Inst. Pêch. Marit. 35, 287–346.
Provasoli L. (1968). Media and Prospects for the Cultivation of Marine Algae. Cult. Collect. Algae. Proc. US-Jpn. Conf. Hakone. Sept., 1966.
R Core Team. (2020). R: A Language and Environment for Statistical Computing Vienna: Austria: R Foundation for Statistical Computing. Available at: https://www.r-project.org/.
Raybaud V., Beaugrand G., Goberville E., Delebecq G., Destombe C., Valero M., et al. (2013). Decline in Kelp in West Europe and Climate. PloS One 8, e66044. doi: 10.1371/journal.pone.0066044
Scheschonk L., Bischof K., Jueterbock A. (in reviewe). Differences by Origin in Methylome Suggest Eco-Phenotypes in the Kelp Saccharina Latissima.
Schiel D. R., Foster M. S. (2006). The Population Biology of Large Brown Seaweeds: Ecological Consequences of Multiphase Life Histories in Dynamic Coastal Environments. Annu. Rev. Ecol. Evol. Syst. 37, 343–372. doi: 10.1146/annurev.ecolsys.37.091305.110251
Schiel D. R., Foster M. S. (2015). The Biology and Ecology of Giant Kelp Forests. J. Phycol. 53, 462–465. doi: 10.1111/jpy.12516
Schiener P., Black K. D., Stanley M. S., Green D. H. (2015). The Seasonal Variation in the Chemical Composition of the Kelp Species Laminaria Digitata, Laminaria Hyperborea, Saccharina Latissima and Alaria Esculenta. J. Appl. Phycol. 27, 363–373. doi: 10.1007/s10811-014-0327-1
Schrum C., Lowe J., Meier H. E. M., Grabemann I., Holt J., Mathis M., et al. (2016). “Projected Change—North Sea,” in North Sea Region Climate Change Assessment, eds. Quante M., Colijn F. (Cham: Springer International Publishing), 175–217. doi: 10.1007/978-3-319-39745-0_6
Silva C. F., Pearson G. A., Serrão E. A., Bartsch I., Martins N. (2022)Microscopic Life Stages of Arctic Kelp Differ in Their Resilience and Reproductive Output in Response to Arctic Seasonality Eur. J. Phycol. doi: 10.1080/09670262.2021.2014983
Sjøtun K., Schoschina E. V. (2002). Gametophytic Development of Laminaria Spp. (Laminariales, Phaeophyta) at Low Temperature. Phycologia 41, 147–152. doi: 10.2216/i0031-8884-41-2-147.1
Smale D. A. (2020). Impacts of Ocean Warming on Kelp Forest Ecosystems. New Phytol. 225, 1447–1454. doi: 10.1111/nph.16107
Suzuki S., Furuya K., Kawai T., Takeuchi I. (2008). Effect of Seawater Temperature on the Productivity of Laminaria japonica in the Uwa Sea, Southern Japan. J. Appl. Phycol. 20, 833–844. doi: 10.1007/s10811-007-9283-3
Taylor W. R. (1957)Marine Algae of the Northeastern Coast of North America. In: Ann Arbor: The University of Michigan Press. Available at: https://www.algaebase.org/search/bibliography/detail/?biblio_id=12262 (Accessed October 21, 2021).
tom Dieck I. (1992). North Pacific and North Atlantic Digitate Laminaria Species (Phaeophyta): Hybridization Experiments and Temperature Responses. Phycologia 31, 147–163. doi: 10.2216/i0031-8884-31-2-147.1
tom Dieck I. (1993). Temperature Tolerance and Survival in Darkness of Kelp Gametophytes (Laminariales, Phaeophyta): Ecological and Biogeographical Implications. Mar. Ecol. Prog. Ser. 100, 253–264. doi: 10.3354/meps100253
Uller T., Nakagawa S., English S. (2013). Weak Evidence for Anticipatory Parental Effects in Plants and Animals. J. Evol. Biol. 26, 2161–2170. doi: 10.1111/jeb.12212
Wang X., Liu F., Jiang D. (2017). Priming: A Promising Strategy for Crop Production in Response to Future Climate. J. Integr. Agric. 16, 2709–2716. doi: 10.1016/S2095-3119(17)61786-6
Wiencke C., Bartsch I., Bischoff B., Peters A. F., Breeman A. M. (1994). Temperature Requirements and Biogeography of Antarctic, Arctic and Amphiequatorial Seaweeds. Bot. Mar. 37, 247–260. doi: 10.1515/botm.1994.37.3.247
Wilson K. L., Kay L. M., Schmidt A. L., Lotze H. K. (2015). Effects of Increasing Water Temperatures on Survival and Growth of Ecologically and Economically Important Seaweeds in Atlantic Canada: Implications for Climate Change. Mar. Biol. 162, 2431–2444. doi: 10.1007/s00227-015-2769-7
Keywords: kelp, life cycle, gametogenesis, thermal response, cross-generational plasticity, carry-over effects
Citation: Gauci C, Bartsch I, Martins N and Liesner D (2022) Cold Thermal Priming of Laminaria digitata (Laminariales, Phaeophyceae) Gametophytes Enhances Gametogenesis and Thermal Performance of Sporophytes. Front. Mar. Sci. 9:862923. doi: 10.3389/fmars.2022.862923
Received: 26 January 2022; Accepted: 22 March 2022;
Published: 19 April 2022.
Edited by:
Bernardo Antonio Perez Da Gama, Fluminense Federal University, BrazilCopyright © 2022 Gauci, Bartsch, Martins and Liesner. This is an open-access article distributed under the terms of the Creative Commons Attribution License (CC BY). The use, distribution or reproduction in other forums is permitted, provided the original author(s) and the copyright owner(s) are credited and that the original publication in this journal is cited, in accordance with accepted academic practice. No use, distribution or reproduction is permitted which does not comply with these terms.
*Correspondence: Clément Gauci, Z2F1Y2ljbGVtZW50QGdtYWlsLmNvbQ==