- 1Key Laboratory of Biofuels, Shandong Provincial Key Laboratory of Energy Genetics, Qingdao Institute of Bioenergy and Bioprocess Technology, Chinese Academy of Sciences, Qingdao, China
- 2University of Chinese Academy of Sciences, Beijing, China
- 3Laboratory of Marine Organism Taxonomy and Phylogeny, Shandong Province Key Laboratory of Experimental Marine Biology, Institute of Oceanology, Center for Ocean Mega-Science, Chinese Academy of Sciences, Qingdao, China
Phytoplankton have been observed to be widely distributed in the oceanic vertical water columns and have an important contribution to carbon sequestration via biological pump mechanism. However, in seamount areas with strong hydrodynamics, their vertical export is still unclear. Moreover, considering phyto- and bacterioplankton are inseparable in the ocean, their correlation in the water columns is also an important scientific issue to be understood, which is related to the microbial ecological process in the aphotic zone. Here, we revealed that there were various phytoplankton (< 20 µm) along a deep-sea seamount (M5 seamount) in the Western Pacific Ocean, and their molecular community structures had no significant difference in different water layers, which were all dominated by Dinophyceae, Syndiniales, and Baciilariophyta. In contrast, the vertical distribution of bacterioplankton community structure showed great variation. Notably, distinct species-specific algae-bacteria relationships occurred in different water layers, and relatively more prominent algae-bacteria relationships occurred in the mesopelagic and bathypelagic zones than the euphotic zone. In laboratory experiments, after co-cultivating the significantly different bacterial communities from different water layers with Synechococcus sp. PCC7002 and Phaeodactylum tricornutum CCMP2561, respectively, the distinct bacterial community structures from different water layers turned similar, reflecting the strong reshaping effect of phytoplankton on the structure of bacterial communities. However, the reshaping effects on bacterial communities by the two algae differed significantly. Overall, the vertically transported phytoplankton in the seamount area not only contribute importantly to carbon sequestration via biological pump, but also may have an important reshaping effect on the bacterioplankton community structure in different water layers.
Introduction
As main primary producers, phytoplankton communities in pelagic oceans are dominated by picophytoplankton (< 2 ~ 3 µm) and nanophytoplankton (3 ~ 20 µm), which play a vital role in marine carbon sequestration and form the basis of marine food webs within euphotic zones (Buchan et al., 2014; de Vargas et al., 2015; Guidi et al., 2016). Meanwhile, more and more investigations show that phytoplankton are widely distributed throughout the water column, even in the challenger deep of Mariana Trench (Worden et al., 2015; Guo et al., 2018; Treguer et al., 2018). Strikingly, living phytoplankton cells have also been observed in the deep sea. For example, driven by internal solitary waves, abundant living Prochlorococcus cells were exported to up to 1,000 m depth at the Luzon Strait in the western Pacific Ocean (Jiao et al., 2014). Microscopy photos of various healthy diatom cells from the deep sea at depths of 2,000−4,000 m had ever been successfully obtained (Agusti et al., 2015). Indeed, the transfer of phytoplankton to deep sea (i.e., the sinking biological pump process) is affected by multiple factors that vary with different water current conditions or geographic locations (Schallenberg et al., 2018; Rocke et al., 2020; Dai et al., 2022). The distribution of phytoplankton in the whole water column is an important ecological issue that deserves in-depth study. On the one hand, it is related to the supply of organic nutrients to the microbial communities in the whole water columns (Amin et al., 2012; Turner, 2015; Durkin et al., 2016). 10%−50% of phytoplankton-derived organic carbon can be utilized by bacterioplankton and transformed to bacterial biomass for fueling microbial food web (Azam et al., 1983). On the other hand, since phyto- and bacterioplankton are inseparable in the ocean (Zhang et al., 2021a; Zhang et al., 2021b), it may have an important effect on the structure of microbial communities in the entire water columns. However, there is little knowledge about this aspect at present.
The photosynthetic nature of phytoplankton determines that they are mainly distributed in the euphotic zone of the ocean (Treguer et al., 2018; Karlusich et al., 2020), despite that living phytoplankton cells have been sporadically observed in the deep sea (Agusti et al., 2015). Compared with phytoplankton, the metabolic versatility of bacteria makes them ubiquitous in the ocean (Worden et al., 2015; Cuil et al., 2019; Alcolombri et al., 2021). As is known, phytoplankton in the epipelagic ocean can be transported to mesopelagic and deeper ocean via sinking biological pump as carbon sources for deep-sea microorganisms or long-term sequestered carbon (i.e., carbon sink) (Turner, 2015; Treguer et al., 2018). It was reported that the organic matter in the deep sea supporting bacterial productivity is mainly from phytoplankton debris (Luo et al., 2017). While in the euphotic zone, photosynthesis phytoplankton contributes to a significant proportion of organic matter by primary production, these organic matters through cascading trophic interactions are utilized by bacteria and support their growth (Thornton, 2014). It hints that no matter in the euphotic zone or the deep sea, there is a close correlation between phytoplankton and bacteria, and their correlation may vary in different depths of a water column and be influenced by the distribution of plankton communities.
Seamounts represent unique marine environments characterized by substantially enhanced currents and hydrodynamics changes (Becker et al., 2014; Ma et al., 2020). For example, eddies, isopycnal doming, and turbulent mixing occur frequently in the seamount area (Mendonca et al., 2012; Rocke et al., 2020; Sergi et al., 2020; Mohn et al., 2021). The specific topographic characteristics and complex hydrodynamics of the seamounts create distinct plankton habitats, by increasing vertical fluxes of nutrient-rich waters, enhancing primary productivity and supporting high biodiversity, especially around seamount of the oligotrophic oceans, known as “seamount effects” (Clark et al., 2010; Mendonca et al., 2012; Ma et al., 2021). In particular, the vertical flux of seawater (up-or-down-wellings) will affect the redistribution of plankton communities in the entire water columns of the seamount area (Clark et al., 2010; Levin and Sibuet, 2012; Santos et al., 2013; Xu, 2021).
Recently, a deep seamount (M5, 10.1°N, 140.2°E, the summit depth of 810 m) in the Western Pacific Ocean was discovered (Sun et al., 2020; Sun et al., 2021). We speculate that the vertical distribution of phytoplankton in this special area may have certain particularities. Meanwhile, there are usually species-specific relationships between phytoplankton and bacterial communities (Teeling et al., 2016; Kruger et al., 2019), e.g., the algae-associated bacterial communities of the diatom Nitzschia longissima were dominated by Neptuniibacter, Mesorhizobium, and Sphingorhabdus, while that of another diatom Pseudo-nitzschia were dominated by Alteromonas, Flavobacterium, and Roseobacter (Guannel et al., 2011; Behringer et al., 2018). Moreover, different Synechococcus strains in the same environment were surrounded by different microbial communities (Zhang et al., 2021b). Therefore, the vertically distributed phytoplankton may in turn have a certain regulatory effect on the bacterial community structure in the water columns. To this end, we investigated the vertical distribution of both phytoplankton (< 20 µm) and bacterioplankton communities in four water columns in this seamount area and studied their correlation networks at different depths. The results shed light on the vertical distribution and carbon sink of phytoplankton and their relationship with bacterioplankton in the deep-sea seamount.
Materials and Methods
Sample Collection
Sampling was carried out at four stations in the M5 Seamount of the Western Pacific Ocean (9°−16°N, 135°−145°E) on board of RV “Kexue No.3” during May 2019 (Figure 1). Seawater was collected at the surface (5 m), deep chlorophyll maximum layer (DCM, 90−106 m), 200 m, 500 m, 1,000 m, and bottom layer (1,400–2,222 m) using Niskin bottles (KC-Denmark, Denmark) with CTD sensors. Each water sample was passed through a 20 μm pore-sized filter to remove the large particles, and then a 0.22 μm polycarbonate membrane (Millipore, Ireland) to trap picophytoplankton and nanophytoplankton and bacterioplankton cells. The collected samples were stored at −80°C immediately after flash-freezing in liquid nitrogen for subsequent DNA extraction.
Phyto- and Bacterioplankton Abundance
The abundance of phytoplankton (< 20 µm) and bacterioplankton was measured by a BD FACSAria II Flow Cytometer (BD Bioscience, CA, United States) following the protocol described previously (Liang et al., 2017). In brief, the 20 μm pre-filtered seawater samples were fixed with glutaraldehyde (final concentration: 0.5%), kept in dark for 30 min at room temperature, and stored at −80°C immediately after flash-freezing in liquid nitrogen. The abundance of picoeukaryotes, Prochlorococcus, and Synechococcus were identified and measured based on light scatter red chlorophyll fluorescence (692 nm), and yellow phycoerythrin (585 nm). Bacteria were stained with SYBR Green I and kept in dark at room temperature for 15 minutes before detection.
DNA Extraction and High-Throughput Sequencing
Total DNA was extracted from water samples using Qiagen’s Dneasy PowerSoil Kit (Qiagen, Duesseldorf, Germany) following the manufacturer’s instructions. The V3~V4 variable region of 16S rRNA gene was amplified using primers 343F (5’−TACGGRAGGCAGCAG−3’) and 798R (5’−AGGGTATCTAATCCT−3’) (Nossa et al., 2010). The V4 region of 18S rRNA gene was amplified using primers 528F (5’− GCGGTAATTCCAGCTCCAA−3’) and 706R (5’−AATCCRAGAATTTCACCTCT−3’) (Cheung et al., 2010). Sequencing was performed using the Illumina MiSeq platform with 250-bp pair-ends reads (OE Biotech, Shanghai, China). The raw sequences were deposited in the National Center for Biotechnology Information (NCBI) Sequence Read Archive (SRA) database under BioProject PRJNA779616.
Phyto- and Bacterioplankton Community Structure Analysis
For 16S rRNA genes, raw sequence data were processed using the package USEARCH by UPARSE algorithms (Edgar, 2013). Chimeric sequences were identified and removed using Vsearch v2.12.z0 (Rognes et al., 2016). Filtered sequences were clustered into amplicon sequence variants (ASVs) using UCLUST in QIIME at 100% similarity level and assigned against the SILVA138 database (Quast et al., 2013). For 18S rRNA genes, sequencing data were processed using the package DADA2 in R following the default settings (Callahan et al., 2016). Here, the reads were filtered with the following parameters: maxN = 0, maxEE = c (5, 5), truncQ = 2, minBoot = 80. Taxonomic assignment of ASVs of 18S rRNA genes was performed using the Protist Ribosomal Reference (PR2) database (Guillou et al., 2013), with 80% minimum bootstrap confidence threshold. Sequences classified as mitochondrion, chloroplast, and unknown taxa were excluded from the datasets. The sequences of fungi, protozoa, and other eukaryotic taxa were removed, and the eukaryotic phytoplankton reads were further confirmed by the NCBI’s NT database. Furthermore, singleton sequences were eliminated before statistical analysis.
All analyses were performed with the software R and the specified package. For alpha diversity analysis, Chao1 and Shannon indices were calculated using the package vegan (Oksanen et al., 2018). For Beta diversity analysis, non-metric multidimensional scaling (NMDS) based on Bray-Curtis distance was performed with the package vegan. One-way analysis of Variance (ANOVA) and Permutational multivariate analysis of variance (PERMANOVA) was used to statistically test significant differences in phyto- and bacterioplankton communities at different depths. Linear discriminant analysis effect size (LEfSe) was performed at the genus level (http://huttenhower.sph.harvard.edu/galaxy/) to identify phytoplankton and bacteria taxa with significantly different relative abundance among the different water layers (Segata et al., 2011). Significant differences (p < 0.05) in the bacterial community structure after co-cultivation of microalgae with bacterial communities from different water layers were further analyzed using Welch’s t-test, through the STAMP software (Parks et al., 2014).
Phyto- and Bacterioplankton Network Analysis
Spearman correlation coefficients between the relative abundance of bacterioplankton ASVs and phytoplankton ASVs were calculated using the package Hmisc (Liu et al., 2020). To eliminate the spurious correlation of low abundance taxa and reduce the complexity of the networks, only the phytoplankton ASVs observed in more than 20% of the samples; and the bacterioplankton ASVs observed in more than 70% of the samples were retained, the ASVs with an average relative abundance of less than 0.05% were removed. The co-occurrence networks in different water layers were constructed based on Spearman correlation coefficients, including 0−200 m (epipelagic), 200−1,000 m (mesopelagic), 1,000 m−bottom (bathypelagic), 200 m−bottom (dark water column), and 0−bottom (entire water column). Positive (ρ > 0.6, p < 0.05) and negative (ρ < −0.6, p < 0.05) correlation were selected and visualized in network using Gephi 0.9.2 (Bastian et al., 2009). The p-values were corrected for the false discovery rate using the Benjamini-Hochberg method (Benjamini and Hochberg, 1995). The topological properties, i.e., nodes, edges, average clustering coefficient, average path distance, diameter, density, and modularity, were characterized.
Co-Cultivation of Microalgae With Bacterial Communities From Different Water Layers
To investigate the potential influences of different phytoplankton on bacterial communities from different water layers, axenic Synechococcus sp. PCC7002 and Phaeodactylum tricornutum CCMP2561 were co-cultivated on board with the bacterial communities collected on site B2 at different sampling depths (i.e., 5 m, 106 m, 200 m, 500 m, 1,000 m, 2,222 m) along a water column. Both Synechococcus and Phaeodactylum, universally known as model organisms of marine cyanobacteria and diatom groups, respectively, have been extensively studied in the laboratory (Nymark et al., 2013; Zhang et al., 2021a). Axenic Synechococcus sp. PCC7002 and Phaeodactylum tricornutum CCMP2561 were purified and maintained in an illumination incubator at 22 ± 1°C with a light intensity of 3,000 lx in a 12:12 light-dark cycle and cultured in A+ and f/2 medium (Ryther and Guillard, 1962; Mou et al., 2017), respectively. The purity of the two algal strains was confirmed by microscopic observation after fluorescent staining. Seawater was passed through a 1.0 μm membrane to remove large particles and phytoplankton cells, and then 20 ml of the filtrate was added to an equal volume of algal culture. The co-culture systems were placed under the above-mentioned culture conditions until the end of the voyage (~ three weeks). Back to the laboratory, these cultures were filtered onto a 0.2 μm-pore-size polycarbonate membrane and washed with sterile bacteria-free seawater to remove free-living bacterial cells. The bacterial communities trapped on the 0.2 μm filters were regarded as the total bacterial community for further analysis.
Results
Vertical Distribution of Phytoplankton (< 20 µm) Community
Phytoplankton (< 20 µm) is mainly distributed in the ocean at depth < 200 m, and very rare phytoplankton cells could be detected by flow cytometry at depths > 200 m due to the detection limit. The abundance of picoeukaryote, Prochlorococcus, and Synechococcus were 0.04 × 103 to 3.35 × 103 cells ml-1, 0.21 × 103 to 2.04 × 104 cells ml-1, and 0.03 × 103 to 0.70 × 103 cells ml-1, respectively (Table 1). The highest abundance of them all occurred at the DCM layer (90−106 m).
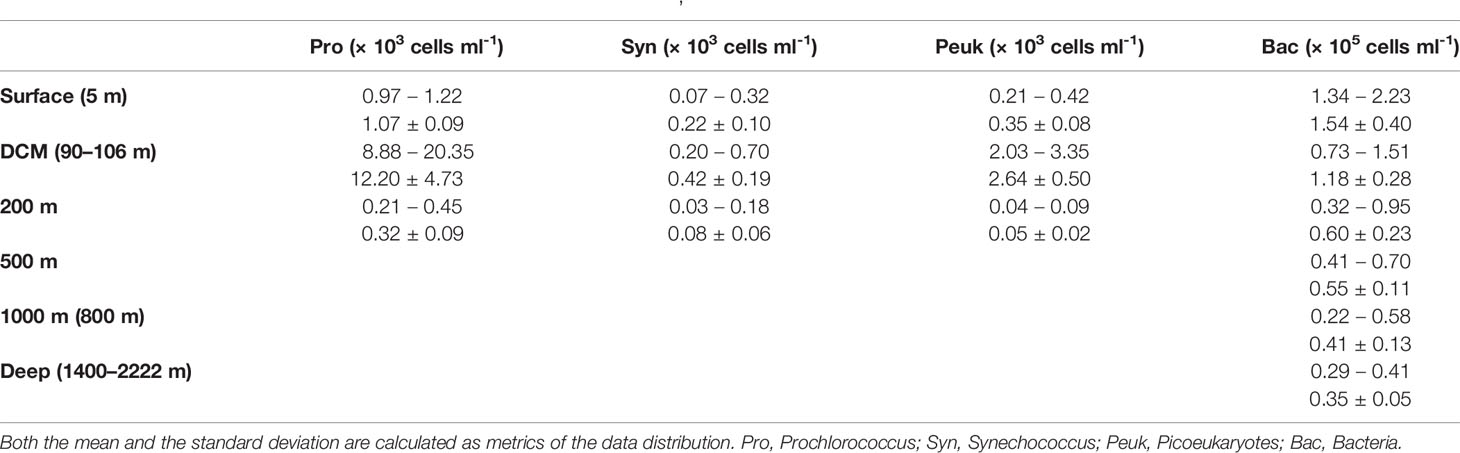
Table 1 The abundance of picoplankton (picoeukaryotes, Prochlorococcus, Synechococcus, and bacteria) at different depths in the M5 seamount.
Although phytoplankton abundance at depth > 200 m could not be detected by flow cytometry, molecular analysis revealed the presence of various phytoplankton throughout the water columns. A total of 42 classes of phytoplankton were detected, which are affiliated to Dinoflagellata, Ochrophyta, Chlorophyta, Haptophyta, Cryptophyta, Sagenista, and Katablepharidophyta (Supplementary Table S1). Interestingly, no significant difference was observed in the community structure and diversity of phytoplankton in different water layers, reflected by the α-diversity (Figures 2A, B; ANOVA, p > 0.05) and β-diversity analyses (Figure 2E and Supplementary Figure S1; PERMANOVA, p > 0.05). The most dominant taxa were all Dinophyceae, Syndiniales, and Bacillariophyta, which accounted for 79.7%−80.1%, 67.3%−88.6%, and 76.7%−90.9% of the total community structure in the epipelagic, mesopelagic, and bathypelagic layers, respectively (Figure 3). At the genus level, the phytoplankton communities in most water layers were dominated by Lepidodinium, Gyrodinium, Polykrikos, and Gymnodinium affiliated to Dinophyceae, Dino-Group-I and Dino-Group-II affiliated to Syndiniales, and Thalassiosira and Chaetoceros affiliated to Bacillariophyta (Supplementary Figure S2 and Supplementary Table S1).
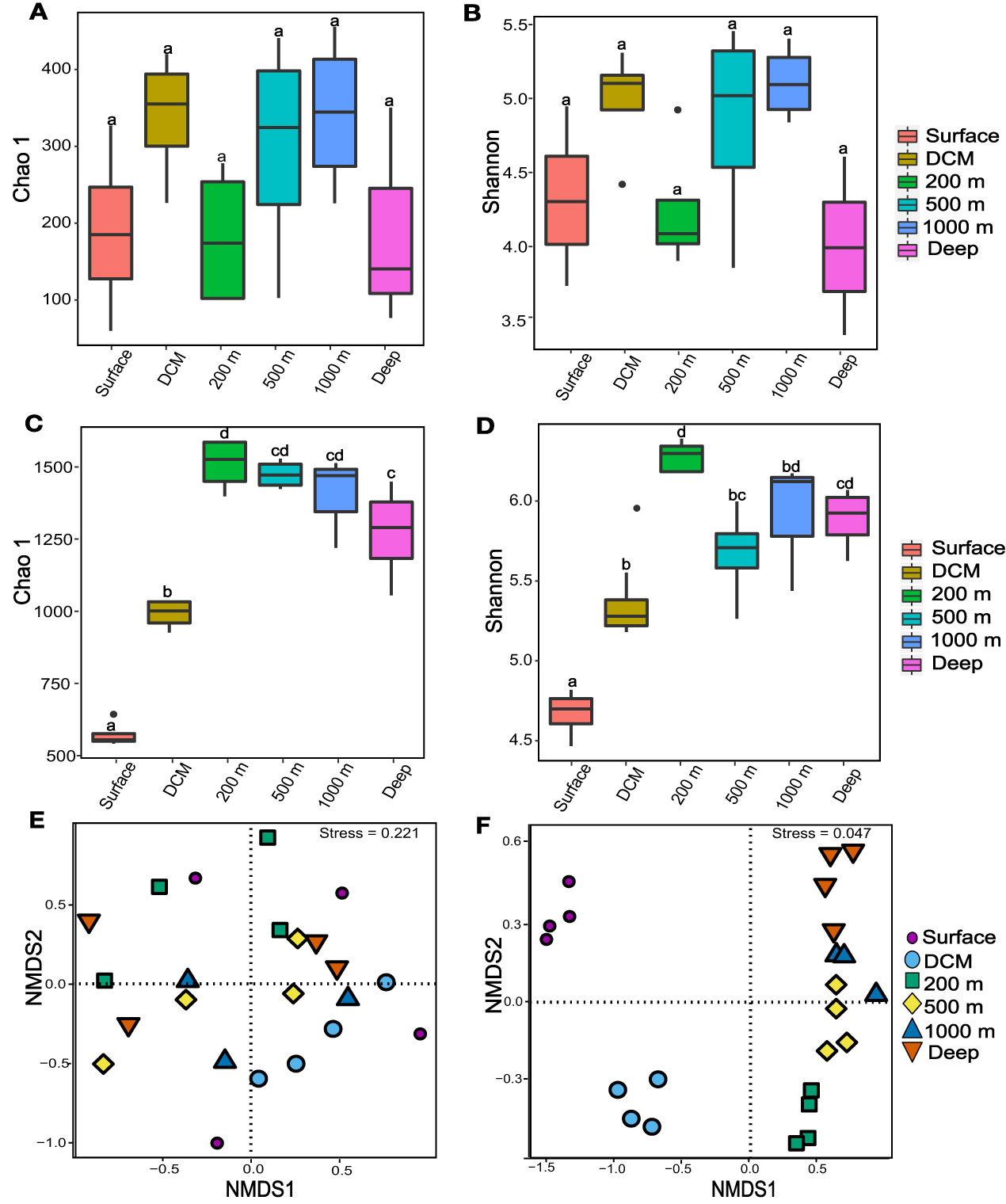
Figure 2 Characteristics of phytoplankton and bacterioplankton communities in the M5 seamount. The alpha diversity of the phyto- and bacterioplankton community at each depth of the seamount: Chao 1 and Shannon of phytoplankton (A, B); Chao 1 and Shannon of bacterioplankton (C, D). Comparison of phytoplankton and bacterial community structure based on NMDS analysis (E, F), respectively. Box marked with the different letters a–c show the statistically significant differences (ANOVA, p < 0.05) and the same letter indicates no significant differences.
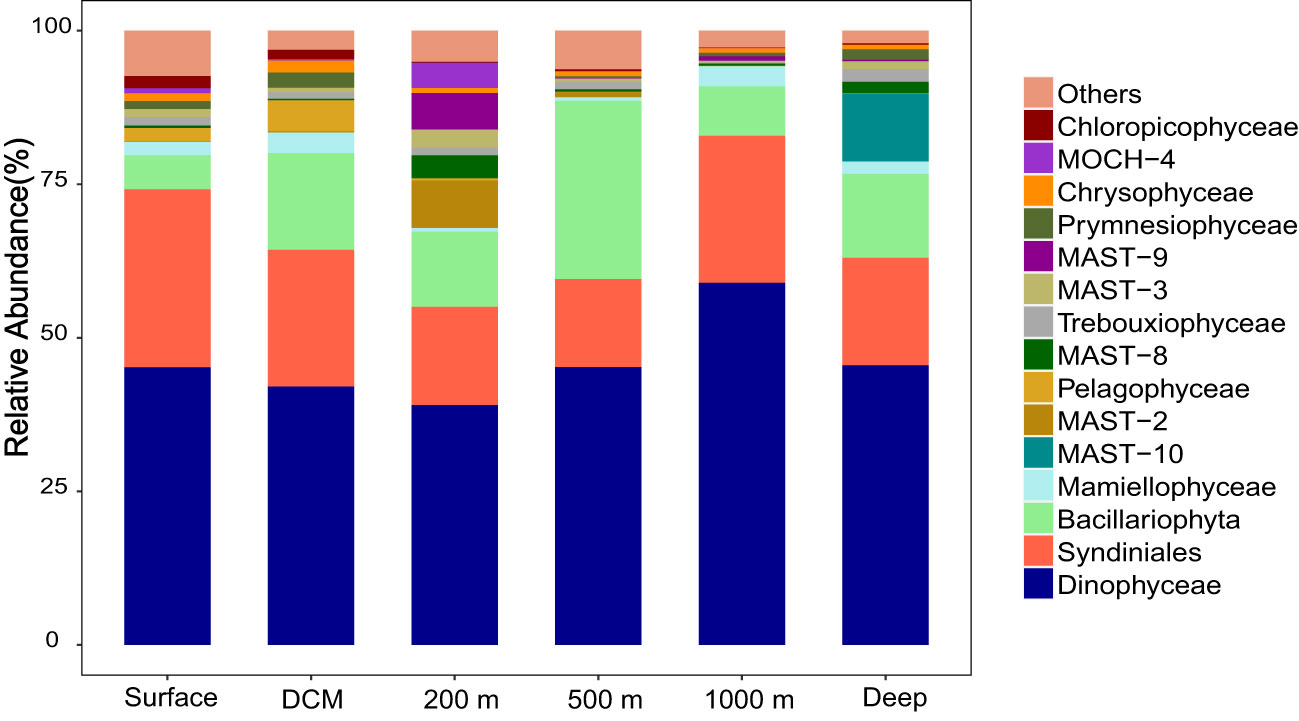
Figure 3 The composition of phytoplankton communities in different depths of the M5 seamount at the phylum level.
Although in the molecular diversity level, the dominant groups of phytoplankton were similar in different water layers, some specific phytoplankton groups (such as the different MAST clades affiliated to Marine Stramenopiles) had quite different relative abundances at different water depths. The relative abundance of MAST-10 clade increased significantly in the deep sea at depth of 1,400−2,222 m, while the relative abundance of MAST-2, MAST-8, and MAST-9 groups were much higher at depth of 200 m (Figure 3; p < 0.05). In contrast, the relative abundance of Pelagophyceae (Pelagomonas) was highest in the DCM layer (90−106 m) (Figure 3 and Supplementary Figure S2). As expected, picocyanobacteria dominated by Prochlorococcus existed in all water layers but were relatively abundant in the euphotic waters (Figure 4 and Supplementary Table S3).
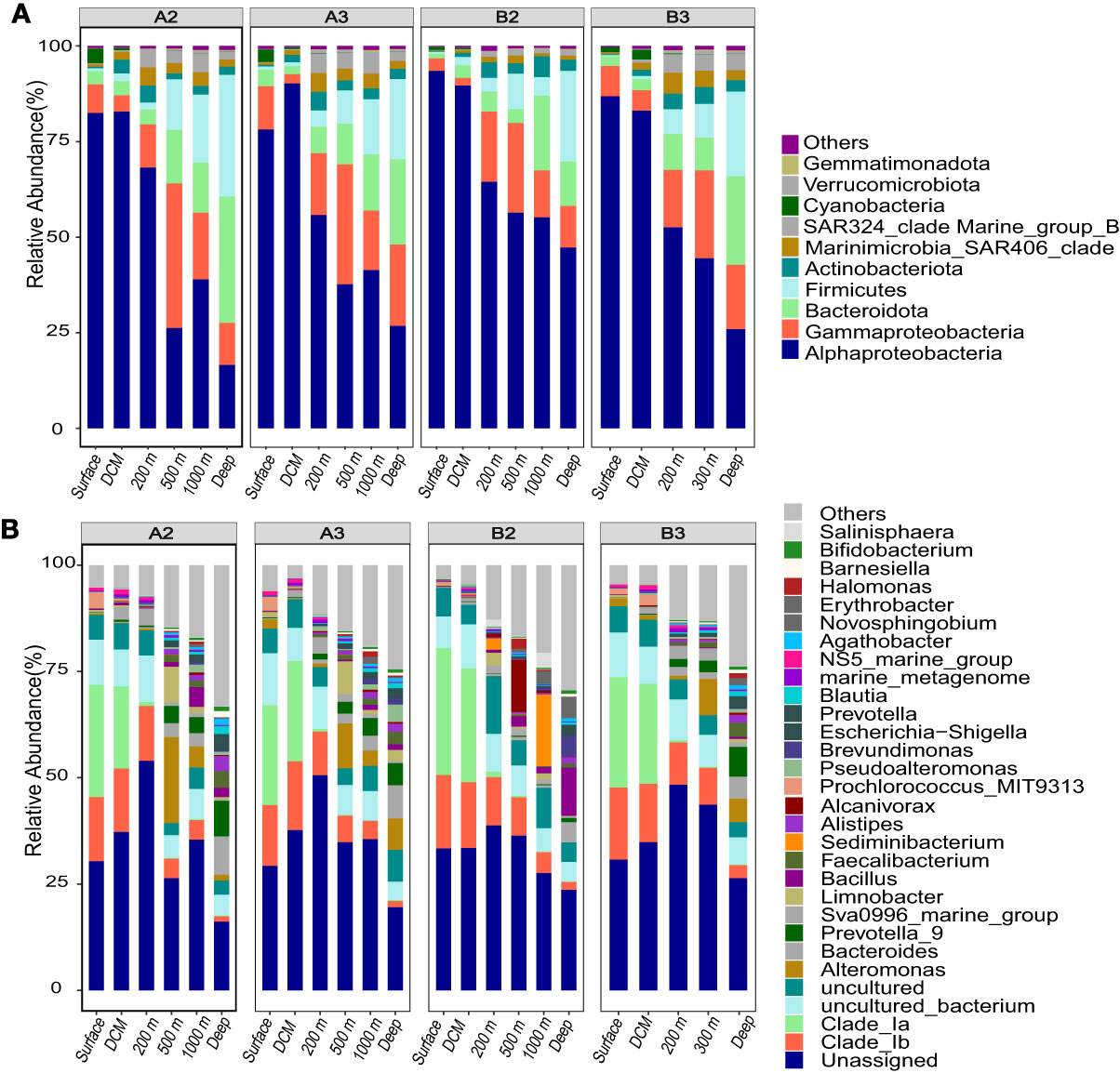
Figure 4 The composition of bacterioplankton communities in four stations along the M5 seamount. (A) phylum level (Proteobacteria at class level); (B) genus level.
Vertical Distribution of Bacterial Community
Bacterial abundance gradually decreased along with depth from 2.23 × 105 cells ml-1 in the surface layer to 2.15 × 104 cells ml-1 in the deep sea (Table 1). Meanwhile, bacterial community structure showed obvious heterogeneity at different depths, e.g., bacterial communities in the same water layers were clustered together, while those in different water layers were significantly separated (Figure 2F; PERMANOVA, p < 0.01). The α-diversity of the bacterial community increased significantly from surface to deep sea (Figures 2C, D; ANOVA, p < 0.05).
A total of 23 bacterial phyla were detected, among which Proteobacteria (mainly composed of Alphaproteobacteria and Gammaproteobacteria) was the most dominant phylum (27.6%−94.7%) in all water layers (Figure 4A). Whereas Bacteroidetes and Firmicutes had a higher relative abundance in the deep layers (> 1,000 m) than the epipelagic layer, with a maximum relative abundance of 33% and 31.9%, respectively. Actinobacteria and Marinimicrobia were also relatively abundant in the aphotic zones, accounting for 0.7%−5.5% of the proportions. At the class level, Alphaproteobacteria was the most abundant class (26%−86.9%) in the entire water columns and showed a decreasing trend in relative abundance along depth, with the largest proportion in the epipelagic zone. The relative abundance of Gammaproteobacteria changed significantly with depth, where they accounted for 2%−37.8% across all depth samples (Figure 4A). The SAR324 clade of Deltaproteobacteria was more abundant in the aphotic zones (Figure 4A).
Furthermore, bacterial community variations among different layers were analyzed at the genus level (Figure 4B and Supplementary Figure S3). In the epipelagic layer, SAR11 clade Ia and clade Ib affiliated to Alphaproteobacteria were the two most dominant genera (with the maximum relative abundance of up to 34.2%−47.1%) and were significantly more abundant than that in other layers. In the mesopelagic layer, SAR11 clade_Ib, Alteromonas, Sva0996_marine_group, and Limnobacter were the most dominant genera (with the relative abundance of more than 2%), and the relative abundance of genera Alcanivorax (Gammaproteobacteria), Sva0996_marine_group (Actinobacteriota), and NS5_marine_group (Bacteroidetes) were significantly higher in the mesopelagic layer than in the other layers (Supplementary Figure S3; p < 0.05). While in the bathypelagic layer, Prevotella_9, and Bacteroides, Bacillus, and Faecalibacterium were predominant genera (with the relative abundance of more than 2%) and were also significantly more abundant (Supplementary Figure S3; p < 0.05) in this water layer.
Phyto- and Bacterioplankton Correlations on a Vertical Scale
Phytoplankton and bacterioplankton communities established more prominent connections in the mesopelagic and bathypelagic zones than that in the epipelagic zone. The phyto- and bacterioplankton networks in mesopelagic and bathypelagic zones had 188−190 nodes and 486−1,077 edges, which were nearly 2.5 times and 5−11 times that in the epipelagic zone, respectively (Supplementary Table S2). If combining the samples from the mesopelagic and bathypelagic zones (i.e., aphotic layer), the phyto- and bacterioplankton network in the aphotic layer had much more connections (about 30 times) than that in the euphotic zone (Supplementary Figure S4).
The phytoplankton and bacterioplankton network exhibited obvious species-specific characteristics, which varied in different water layers. In the epipelagic zone, the most obvious connections were between the phytoplankton Pelagophyceae (Pelagomonas) and bacterioplankton Proteobacteria (mainly Algiphilus and Rhodobacteraceae) (Table 2). While in the mesopelagic and bathypelagic zones, different genera of phytoplankton belonging to Dinophyceae, Syndinales, and Bacillariophyta established strong links with the bacterioplankton of Proteobacteria, Firmicutes, and Bacteriodetes (Figure 5 and Table 2). For example, several genera affiliated to Dinophyceae and Syndinales, which include Testudodnium, Warnowia, Gyrodinium, Torodinum, Dino-Group-II-clade, and Dino-Group-III, etc., established close links with the bacterial genera SAR86_clade (Gammaproteobacteria), UBA10353_marine_group (Gammaproteobacteria), NS9_marine_group (Bacteroidetes), and P3OB-42 (Myxococcia) (Figure 5A). Each of these bacterial genera had a strong correlation with three to six phytoplankton genera belonging to Dinophyceae and Syndinales. In the bathypelagic zone, the closest connections between phyto- and bacterioplankton occurred between Syndinales and the bacteria of Bacteroidales and Clostridia. Nine bacterial genera affiliated to Clostridia and three bacterial genera affiliated to Bacteroidales together formed a close cluster with the Dino-Group-II and Dino-Group-V (Syndinales) in the bathypelagic zone (Figure 5B).
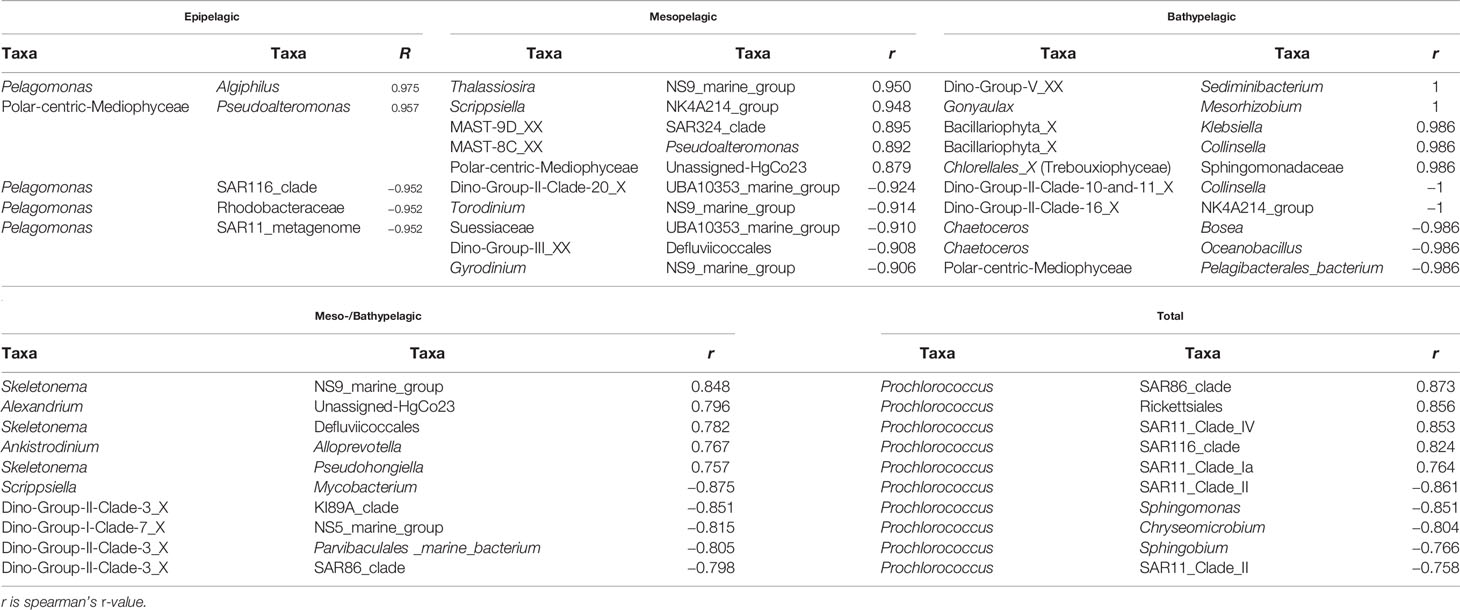
Table 2 The significant positive and negative correlations between phytoplankton and bacteria in different water layers of the seamount.
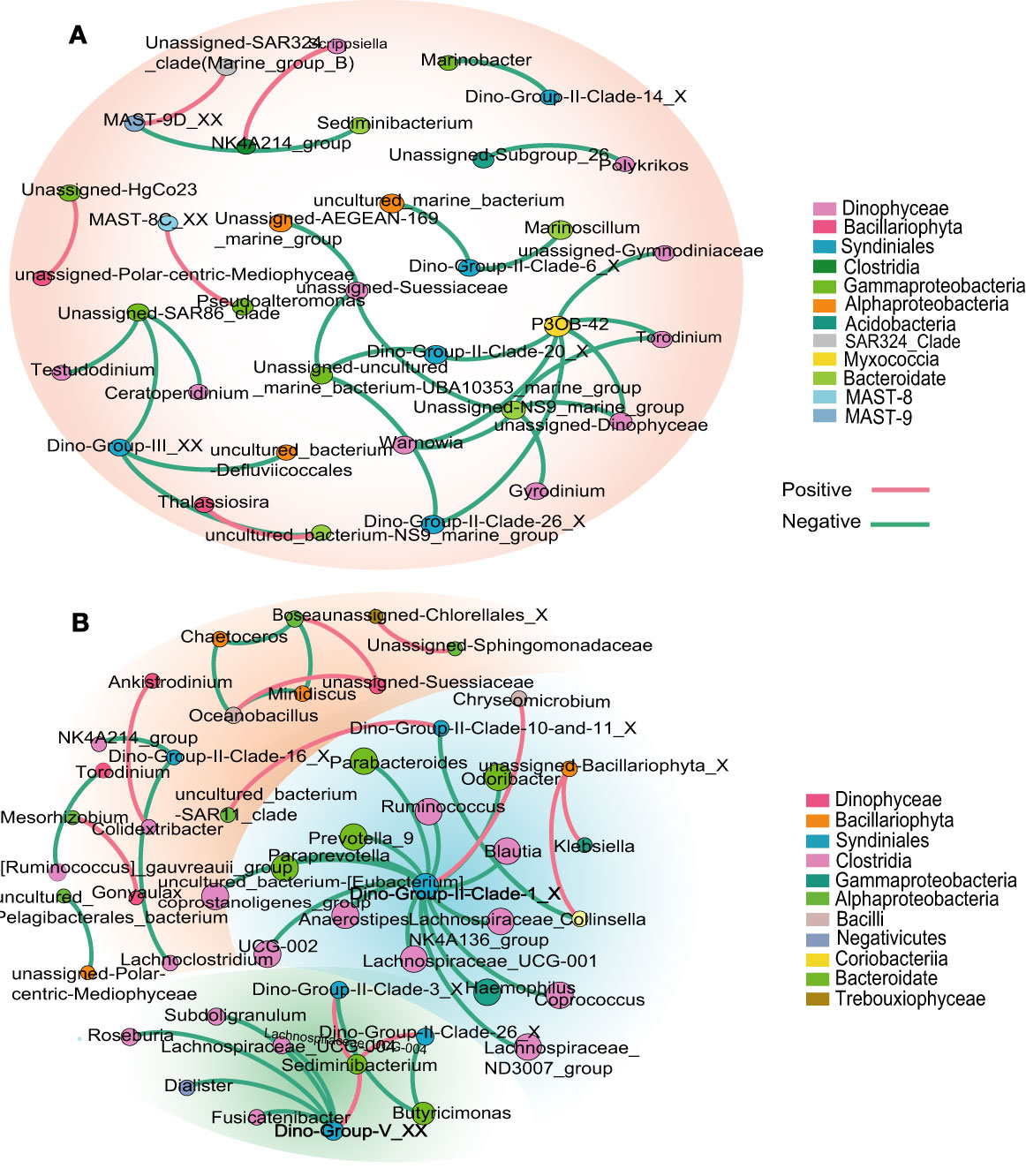
Figure 5 Co-occurrence networks highlighting the phytoplankton and bacterioplankton associations in the mesopelagic (A) and bathypelagic (B) zones of the seamount. Each node represents an ASV, size of the node corresponds to the proportional abundance of each ASV. Red lines show positive correlations (r > 0.6, p < 0.05), while green lines show negative correlations (r < −0.6, p < 0.05).
It is worth mentioning that among the complex correlation networks between different phyto- and bacterioplankton, only the correlation between Bacillariophyta and Gammaproteobacteria occurred in the whole water columns, although their dominant groups also varied in different water layers. Bacillariophyta-Gammaproteobacteria correlation network mainly occurred between Polar-centric-Mediophyceae and Pseudoalteromonas, Polar-centric-Mediophyceae and Unassigned-HgCo23, Bacillariophyta_X and Klebsiella in the epipelagic, mesopelagic, and bathypelagic zones, respectively (Figure 5 and Table 2).
Shaping Effect of Typical Phytoplankton on Their Associated Bacterial Community
Although the bacterial community structure varied significantly at different water layers of the M5 seamount (Figure 4), after co-cultivation with Synechococcus sp. PCC7002 and Phaeodactylum tricornutum CCMP2561, the bacterial communities from different depths that turned to be associated with algae became similar, but the algae-associated bacterial communities of Synechococcus sp. PCC7002 and P. tricornutum CCMP2561 differed significantly between each other (Figure 6 and Supplementary Figure S5; PERMANOVA, p < 0.05). After cocultivation, the Synechococcus-associated bacterial communities from different depths were all dominated by the families of Rhodobacteraceae, Cyclobacteriaceae, Hyphomonadaceae, and Marinobacteraceae, or the genera of Algoriphagus, Dinoroseobacter, Hyphobacterium, and Marinobacter (Figure 6 and Supplementary Figure S6). In comparison, the closely associated bacterial communities with P. tricornutum were all dominated by the families of Rhodobacteraceae, Hyphomonadaceae, NS9_marine_group, and Rhizobiaceae, or the genera of Oceanicaulis, Cohaesibacter, Porphyrobacter, and Bacteroides (Figure 6 and Supplementary Figure S6).
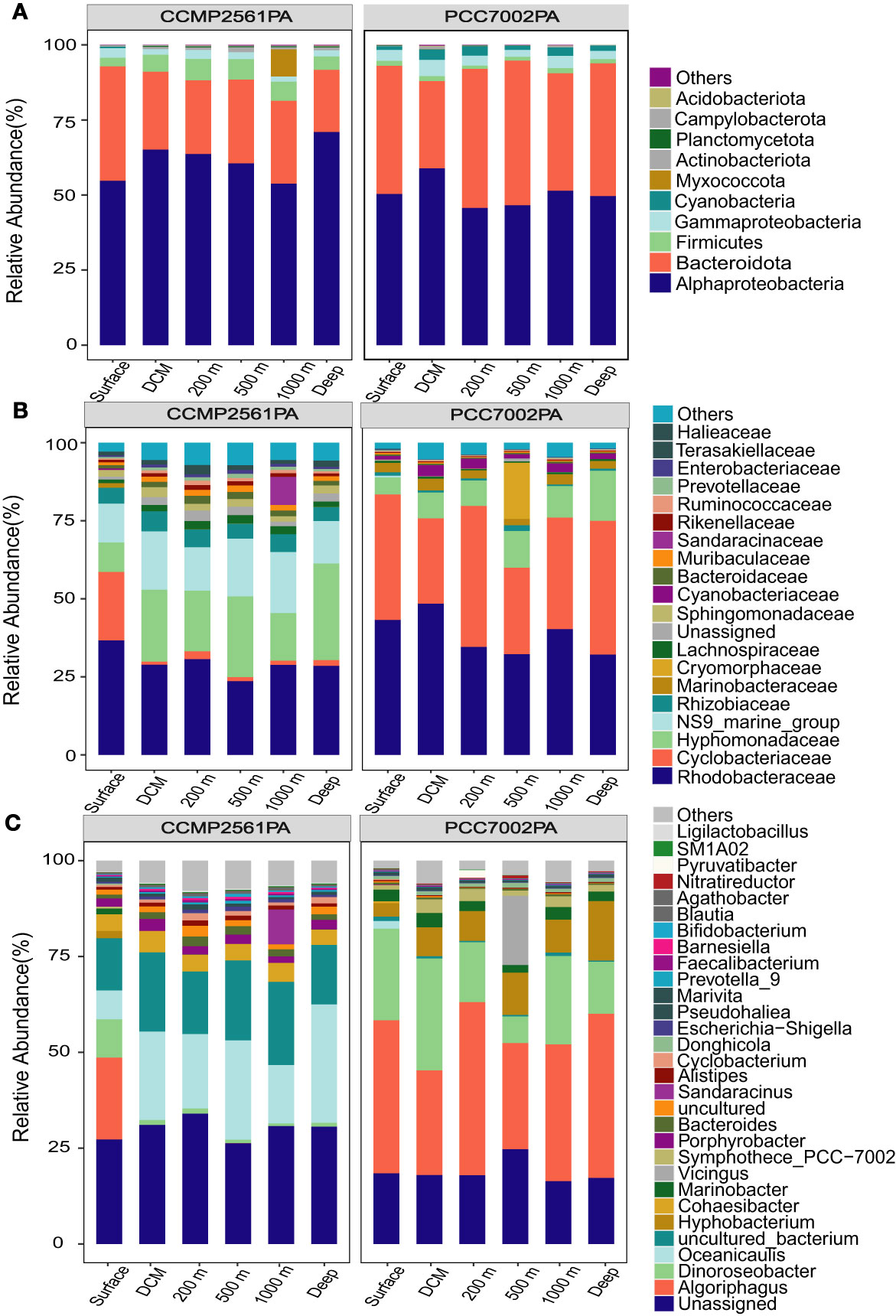
Figure 6 The structure of bacterial communities in different water layers after co-cultivation with Synechococcus sp. PCC7002 and Phaeodactylum tricornutum CCMP2561, respectively, at the phylum (A), family (B), and genus (C) level.
Discussion
Phytoplankton are the main primary producers in marine euphotic ecosystems (Durkin et al., 2016; Guidi et al., 2016; Treguer et al., 2018). Meanwhile, they are reported to be the most important source of organic matter in the deep sea (Luo et al., 2017). Previous sporadic studies have observed that there were diverse phytoplankton in the oceanic vertical water column (Guo et al., 2018; Richert et al., 2019), while under the influence of multiple factors, the vertical distribution of phytoplankton should have obvious geographic and temporal variations, which is poorly understood so far. Moreover, since phyto- and bacterioplankton are inseparable in the ocean, and the main organic matter required by bacterioplankton in the ocean is also mainly derived from phytoplankton (Zhang et al., 2021a; Zhang et al., 2021b), it is speculated that phytoplankton should have important impacts on the bacterioplankton community in the water columns from surface to deep oceans. In this study, we uncovered the vertical distribution of phytoplankton and bacterioplankton communities and revealed their potential associations in the water column of seamounts.
Although phytoplankton cells were only detected by flow cytometry in the water layer of < 200 m, the molecular investigation revealed that diverse phytoplankton existed throughout the water column of the seamount area (Figure 3). Interestingly, there was no significant difference in the molecular community structure of phytoplankton among different water layers, which was reflected by their comparable β-diversity and similar dominant taxa in different layers (Figure 2). The sinking biological carbon pump was reported to be the major force for the output of phytoplankton (including intact algal cells, algal aggregates, phytodetritus, etc.) from the euphotic zone to the deep sea and contributes significantly to oceanic carbon sinks (Agusti et al., 2015; Turner, 2015; Treguer et al., 2018; Giner et al., 2020). In the M5 seamount area, diatoms and dinoflagellates (Dinophyceae and Syndinales) had the highest relative abundance in the whole water column (Figure 3), which is consistent with the previous observation in the Western Pacific Ocean and the Tara Ocean (Karlusich et al., 2020; Zhao et al., 2020). This may be due to the relatively larger cell size of diatoms and dinoflagellates than other phytoplankton in oligotrophic oceans, making them easier to sink into the deep sea (Crombet et al., 2011; Durkin et al., 2016). Furthermore, many diatoms and dinoflagellates also have mixotrophic metabolic characteristics, which can uptake organic matter and parasitize other plankton (Guillou et al., 2008; Sherr and Sherr, 2007; Marella et al., 2021), thus helping them to tolerate or survive in the dark environment. For example, a diatom (Chaetoceros sp. DS1) with the facultative heterotrophic function that was cultured from 1000m deep sea showed a very strong ability to tolerate darkness (Mou et al., 2022). Moreover, there are strong westward North Equatorial Current, reverse subcurrent, and possible upwelling in the M5 seamount area (Sun et al., 2020), the strong hydrodynamics in the seamount environment may strengthen the “biological pump” process and intensify the export of phytoplankton (even the very small picocyanobacteria cells) to the deep sea (Jiao et al., 2014), which led to the wide distribution of phytoplankton in the whole water column and the similarity of phytoplankton community structure in different water layers. The strong hydrodynamics around the deep-sea seamount regions are naturally similar to those created by the geoengineering of artificial upwelling or downwelling to increase carbon sinks (Bach and Boyd, 2021; NASEM, 2021), promoting vertical mixing of nutrients on the one hand to increase primary production, and improve the efficiency of biological carbon pump on the other hand (Casareto et al., 2017; Zhang et al., 2017; Ma et al., 2021).
However, there were also some phytoplankton groups (e.g., MAST clade belonging to Marine Stramenopile) that were only distributed in certain water layers under the impact of strong hydrodynamics. Currently, the underlying mechanism is still unclear. MAST clades have been reported to play multiple roles in microbial food webs and were driven by environmental factors, for example as symbionts with diatoms and cyanobacteria, and as heterotrophic grazers on bacteria and algae (Lin et al., 2012; Pernice et al., 2016). It was reported that the MAST clade possesses a variety of metabolic strategies adapted to different environments, making some of them to be relatively abundant in epipelagic water, while some are abundant in the deep sea (Logares et al., 2012; Massana et al., 2014; Giner et al., 2020; Obiol et al., 2020).
Unlike phytoplankton, from the epipelagic zone to deeper layers, an increase of bacterial diversity with depth have been observed in the Tara Ocean at a global scale (Sunagawa et al., 2015). The trend observed in this study is in accordance with the observation in the North Atlantic (Agogue et al., 2011) and the NW Mediterranean Sea (Mestre et al., 2017). The molecular community structure of bacterioplankton in different water layers showed great variation (Figure 4), which is consistent with the phenomena observed in many previous deep-sea investigations (Li et al., 2018; Reji et al., 2020; Wang et al., 2020). Compared with phytoplankton, the cell size of bacterioplankton is much smaller, therefore their vertical output can be attached to particles and sank into the deep ocean (Mestre et al., 2018). Moreover, the bacterial community can respond more quickly to environmental changes, therefore the bacterial community is often used as an ideal biological indicator to reflect environmental disturbances (Zhang et al., 2014; Zorz et al., 2019). The huge environmental variation in different layers along the water column (Liang et al., 2017; McNichol et al., 2018; Wang et al., 2020) should be the main reason for the big differences in bacterial communities in different water layers.
From the bacterial classification, taxonomic classification showed that SAR11 clade (Alphaproteobacteria) was one of the most abundant heterotrophic microbial groups in the surface zone of this seamount (Figure 4) (DeLong et al., 2006; Wang et al., 2020). However, different subclades of SAR11 showed a significantly different distribution pattern across the vertical water column of the seamount in this study. SAR11 clade Ia was overwhelmingly dominated (mean relative abundance of 24.85%) in the upper ocean (< 200 m), whereas SAR11 clade Ib had a wider distribution compared to SAR11 clade Ia and was observed in the whole water column, which mainly because these subclades of SAR11 had broader light intensity adaptabilities and nutrient metabolic capabilities (DeLong et al., 2006; Vergin et al., 2013; Traving et al., 2021). By comparison, Gammaproteobacteria, Bacteroidetes, and Firmicutes had higher relative abundance in the deep-sea compared to epipelagic waters (Figure 4A), similar to the investigation on deep sea of the Western Pacific (Wang et al., 2020), which may be due to the better metabolic adaptability of these taxa than others in the extreme environment of deep sea (Wu et al., 2013). For example, Alteromonas (affiliated to Gammaproteobacteria) was dominant in the mesopelagic zone of the seamount. It was reported that a member of Alteromonas (one strain of the “deep ecotype” isolated from 1,000 m deep sea) was more suitable for microaerophilic conditions, capable of degrading recalcitrant compounds and attached to relatively large particles that sink rapidly to mesopelagic depths (Ivars-Martinez et al., 2008). Bacillus (Firmicutes) and Bacteroides (Bacteroidetes) were significantly more abundant in the bathypelagic layer (Figure 4B and Supplementary Figure S3). Among them, most Bacillus strains were able to form spores serving as an essential mechanism for survival in extreme environments (Cote et al., 2015; Lakhal et al., 2015). While certain Bacteroides strains could degrade difficult-to-breakdown polysaccharides and acquire carbohydrate substrates through a ‘selfish uptake’ mechanism that contributes to the efficient utilization of organic carbon due to avoiding the liberation of low molecular weight carbon (Cuskin et al., 2015; Lidbury et al., 2021; Zheng et al., 2021). This may help Bacteroides to survive in the bathypelagic layer where organic matter is barren and highly recalcitrant. Overall, the bacterial community in the seamount environment showed significant vertical heterogeneity, which was closely related to the metabolic characteristics of different bacterial groups.
Usually, phyto- and bacterioplankton have species-specific relationships due to the strong shaping effects of phytoplankton on the associated bacterial communities (Amin et al., 2012; Seymour et al., 2017). For example, when the same seawater bacterial community was co-cultivated with two different Synechococcus strains (i.e., PCC7002 and CCMP1334), the bacterial community was reshaped by the two Synechococcus strains into significantly distinct community structures (Zhang et al., 2021b). Here, a similar phenomenon occurred in Synechococcus sp. PCC7002 and P. tricornutum CCMP2561 (diatom). The same heterotrophic bacterial community in seawater was shaped by Synechococcus sp. PCC7002 or P. tricornutum CCMP2561 into significantly divergent community structures (Figure 6). The difference in organic matter released by the two algae may be the reason for the difference in the bacterial community associated with the algae. Finally, at the genus level, the Synechococcus-associated bacterial communities were dominated by Algoriphagus, Dinoroseobacter, and Hyphobacterium, while the associated bacterial communities with the diatom P. tricornutum were dominated by Oceanicaulis and Cohaesibacter. (Supplementary Figure S6).
Since phytoplankton can be distributed widely in the water columns and is an important organic source for bacterioplankton in both euphotic zone and deep sea (Buchan et al., 2014; Luo et al., 2017), we speculate that the close species-specific relationships between phyto- and bacterioplankton should occur in the entire water columns. Just as we expected, this phenomenon was observed in the vertical water columns of the M5 seamount area (Figure 5 and Supplementary Figure S4). Notably, distinct species-specific algae-bacteria relationships occurred on different phyto- and bacterioplankton groups in different water layers (Figure 5 and Supplementary Figure S4). While in the deep sea (i.e., mesopelagic and bathypelagic layers), there were more prominent algae-bacteria relationships than that in the euphotic layer. This is likely because phytoplankton-derived organic matter is the major carbon source supporting the survival of heterotrophic bacteria in the deep sea, while in the euphotic zone, the complex organic matters also come from a variety of other sources (such as protists, fungi, and zooplankton) (Netotea and Pongor, 2006; Durkin et al., 2016; Luo et al., 2017; Stock et al., 2019) and may mask the phyto- and bacterioplankton relationships, therefore, more prominent and diverse relationships were observed between phyto- and bacterioplankton in the deep sea. Furthermore, except for organic sources, the extreme physicochemical conditions in the deep sea, such as cold temperature and high pressure, can also significantly impact the microbial community structures (Hu et al., 2015; Zheng et al., 2020). It is worth mentioning that the dominant phytoplankton Bacillariophyta exhibited a species-specific relationship with Gammaproteobacteria throughout the entire water column (Table 2), suggesting Bacillariophyta may have a strong enrichment effect on Gammaproteobacteria during their sinking process from the surface to the deep sea.
Here, it is difficult to define whether there is a positive or negative relationship between algae and bacteria in the vertical column, because when bacteria feed on organic matter from phytoplankton, there should be a positive correlation between phyto- and bacterioplankton, while bacterial growth comes at the expense of algal cell rupture and algal DNA loss, reflecting the negative correlation between phyto- and bacterioplankton (Samo et al., 2018; Mikhailov et al., 2019). In addition, the algae-bacteria interactions also include other aspects, such as competition due to heterotrophic growth of algae (Langwig et al., 2021), and bacterivory by some phytoplankton (Rii et al., 2016). The detailed relationship between phyto- and bacterioplankton in oceanic water columns need further in-depth study in the future. Even so, considering the species-specific relationships between phyto- and bacterioplankton that occurred throughout the water columns, we speculate that the various phytoplankton transported by biological pump to the deep sea may have an important role in shaping the structure of the bacterioplankton community in the entire water columns of the M5 seamount area.
To further verify the shaping effect of phytoplankton on bacterial communities, the quite different bacterial communities collected from different water layers were co-cultivated with Synechococcus PCC7002 and P. tricornutum CCMP2561, respectively. Interestingly, the originally distinct bacterial communities from different water layers all turned into similar bacterial communities under the impact of Synechococcus PCC7002 or P. tricornutum CCMP2561 (Figure 6). However, as mentioned above, due to the species-specific relationship between algae and bacteria, the final bacterial community structures formed in the two algal cultures became significantly different (Figure 6 and Supplementary Figure S6; PERMANOVA, p < 0.05). The observed phenomena in the laboratory further imply that during the export of various phytoplankton to the deep sea, the species-specific shaping effect of phytoplankton on bacterial communities (Monnich et al., 2020) may have an important impact on the community structures of bacterioplankton in the entire water columns. Here, due to the similarity in phytoplankton community structures at different water layers along the M5 seamount, we speculate that the vertically distributed phytoplankton communities may have the potential to weaken the variation of bacterial communities caused by the difference in physicochemical conditions of different water layers.
Conclusion
Seamount environments with strong hydrodynamics are naturally similar to those created by artificial upwelling or downwelling engineering to increase carbon sinks. Investigating such scenarios has certain implications for assessing the effects of these geoengineering strategies. The strong hydrodynamics in the seamount area may facilitate the export of diverse phytoplankton from the surface to the deep sea, i.e., increase carbon sinks through the biological pump mechanism, and lead to the similarity in the molecular community structure of phytoplankton in different water layers. The exported variety of phytoplankton may have important reshaping effects on the bacterioplankton communities in different water layers and weaken the divergence of bacterioplankton community structures caused by the variation of environmental conditions in different water layers. There is a more prominent and diverse species-specific relationship between phyto- and bacterioplankton in the deep sea as compared to the euphotic zone.
Data Availability Statement
The datasets presented in this study can be found in online repositories. The names of the repository/repositories and accession number(s) can be found below: https://www.ncbi.nlm.nih.gov/, PRJNA779616.
Author Contributions
YZ designed this study and wrote the manuscript. HZ and ZZ performed the experiments, analyzed the data, and wrote the manuscript. JZ assisted with the sample collecting. SN and SM assisted data analysis. YZ revised the manuscript. All authors contributed to the article and approved the submitted version.
Funding
This work was supported by the Senior User Project of RV KEXUE (KEXUE2019GZ03) supported by the Center for Ocean Mega-Science, Chinese Academy of Sciences, the NSFC project (No. U1906216, 31700104, 41806172), the National Key R & D Program of China (2020YFA0607603), the DICP&QIBEBT (DICP&QIBEBT UN201803), the QIBEBT (QIBEBT ZZBS 201805), Dalian National Laboratory For Clean Energy (DNL), CAS.
Conflict of Interest
The authors declare that the research was conducted in the absence of any commercial or financial relationships that could be construed as a potential conflict of interest.
Publisher’s Note
All claims expressed in this article are solely those of the authors and do not necessarily represent those of their affiliated organizations, or those of the publisher, the editors and the reviewers. Any product that may be evaluated in this article, or claim that may be made by its manufacturer, is not guaranteed or endorsed by the publisher.
Acknowledgments
We would like to thank all of the scientists and crew of the R/V “Kexue No.3” for their professional sampling assistance during cruises.
Supplementary Material
The Supplementary Material for this article can be found online at: https://www.frontiersin.org/articles/10.3389/fmars.2022.862494/full#supplementary-material
References
Agogue H., Lamy D., Neal P. R., Sogin M. L., Herndl G. J. (2011). Water Mass-Specificity of Bacterial Communities in the North Atlantic Revealed by Massively Parallel Sequencing. Mol. Ecol. 20, 258–274. doi: 10.1111/j.1365-294X.2010.04932.x
Agusti S., Gonzalez-Gordillo J. I., Vaque D., Estrada M., Cerezo M. I., Salazar G., et al. (2015). Ubiquitous Healthy Diatoms in the Deep Sea Confirm Deep Carbon Injection by the Biological Pump. Nat. Commun. 6, 7608. doi: 10.1038/ncomms8608
Alcolombri U., Peaudecerf F. J., Fernandez V. I., Behrendt L., Lee K. S., Stocker R. (2021). Sinking Enhances the Degradation of Organic Particles by Marine Bacteria. Nat. Geoscience. 14, 775–780. doi: 10.1038/s41561-021-00817-x
Amin S. A., Parker M. S., Armbrust E. V. (2012). Interactions Between Diatoms and Bacteria. Microbiol. Mol. Biol. Rev. 76, 667–684. doi: 10.1128/MMBR.00007-12
Azam F., Fenchel T., Field J. G., Gray J. S., Meyerreil L. A., Thingstad F. (1983). The Ecological Role of Water-Column Microbes in the Sea. Mar. Ecol. Prog. Ser. 10, 257–263. doi: 10.3354/meps010257
Bach L. T., Boyd P. W. (2021). Seeking Natural Analogs to Fast-Forward the Assessment of Marine CO2 Removal. Proc. Natl. Acad. Sci. U.S.A. 118, e2106147118. doi: 10.1073/pnas.2106147118
Bastian M., Heymann S., Jacomy M. (2009). Gephi: An Open Source Software for Exploring and Manipulating Networks. ICWSM 8, 361–362.
Becker J. W., Berube P. M., Follett C. L., Waterbury J. B., Chisholm S. W., DeLong E. F., et al. (2014). Closely Related Phytoplankton Species Produce Similar Suites of Dissolved Organic Matter. Front. Microbiol. 5. doi: 10.3389/fmicb.2014.00111
Behringer G., Ochsenkühn M. A., Fei C., Fanning J., Koester J. A., Amin s. A. (2018). Bacterial Communities of Diatoms Display Strong Conservation Across Strains and Time. Front. Microbiol. 9. doi: 10.3389/fmicb.2018.00659
Benjamini Y., Hochberg Y. (1995). Controlling the False Discovery Rate: A Practical and Powerful Approach to Multiple Testing. J. R. Stat. Soc 57, 289–300. doi: 10.2307/2346101
Buchan A., LeCleir G. R., Gulvik C. A., Gonzalez J. M. (2014). Master Recyclers: Features and Functions of Bacteria Associated With Phytoplankton Blooms. Nat. Rev. Microbiol. 12, 686–698. doi: 10.1038/nrmicro3326
Callahan B. J., McMurdie P. J., Rosen M. J., Han A. W., Johnson A. J. A., Holmes S. P. (2016). DADA2: High-Resolution Sample Inference From Illumina Amplicon Data. Nat. Methods 13, 581–583. doi: 10.1038/nmeth.3869
Casareto B. E., Niraula M. P., Suzuki Y. (2017). Marine Planktonic Ecosystem Dynamics in an Artificial Upwelling Area of Japan: Phytoplankton Production and Biomass Fate. J. Exp. Mar. Bio. Ecol 487, 1–10. doi: 10.1016/j.jembe.2016.11.002
Cheung M. K., Au C. H., Chu K. H., Kwan H. S., Wong C. K. (2010). Composition and Genetic Diversity of Picoeukaryotes in Subtropical Coastal Waters as Revealed by 454 Pyrosequencing. ISME J. 4, 1053–1059. doi: 10.1038/ismej.2010.26
Clark M. R., Rowden A. A., Schlacher T., Williams A., Consalvey M., Stocks K. I., et al. (2010). The Ecology of Seamounts: Structure, Function, and Human Impacts. Ann. Rev. Mar. Sci. 2, 253–278. doi: 10.1146/annurev-marine-120308-081109
Cote C. K., Heffron J. D., Bozue J. A., Welkos S. L. (2015). “Bacillus Anthracis and Other Bacillus Species”, in Molecular Medical Microbiology. Ed. Sussman M. (London: Academic Press), 1789–1844.
Crombet Y., Leblanc K., Queguiner B., Moutin T., Rimmelin P., Ras J., et al. (2011). Deep Silicon Maxima in the Stratified Oligotrophic Mediterranean Sea. Biogeosciences 8, 459–475. doi: 10.5194/bg-8-459-2011
Cuil G., Li J., Gaol Z., Wang Y. (2019). Spatial Variations of Microbial Communities in Abyssal and Hadal Sediments Across the Challenger Deep. PeerJ 7, e6961. doi: 10.7717/peerj.6961
Cuskin F., Lowe E. C., Temple M. J., Zhu Y. P., Cameron E. A., Pudlo N. A., et al. (2015). Human Gut Bacteroidetes can Utilize Yeast Mannan Through a Selfish Mechanism. Nature 517, 165–169. doi: 10.1038/nature13995
Dai S., Zhao Y. F., Li X. G., Wang Z. Y., Zhu M. L., Liang J. H., et al. (2022). Seamount Effect on Phytoplankton Biomass and Community Above a Deep Seamount in the Tropical Western Pacific. Mar. Pollut. Bull. 175, 113354. doi: 10.1016/j.marpolbul.2022.113354
DeLong E. F., Preston C. M., Mincer T., Rich V., Hallam S. J., Frigaard N.-U., et al. (2006). Community Genomics Among Stratified Microbial Assemblages in the Ocean’s Interior. Science 311, 496–503. doi: 10.1126/science.1120250
de Vargas C., Audic S., Henry N., Decelle J., Mahe F., Logares R., et al. (2015). Eukaryotic Plankton Diversity in the Sunlit Ocean. Science 348, 11. doi: 10.1126/science.1261605
Durkin C. A., Van Mooy B. A. S., Dyhrman S. T., Buesseler K. O. (2016). Sinking Phytoplankton Associated With Carbon Flux in the Atlantic Ocean. Limnol. Oceanogr. 61, 1172–1187. doi: 10.1002/lno.10253
Edgar R. C. (2013). UPARSE: Highly Accurate OTU Sequences From Microbial Amplicon Reads. Nat. Methods 10, 996–998. doi: 10.1038/nmeth.2604
Giner C. R., Pernice M. C., Balague V., Duarte C. M., Gasol J. M., Logares R., et al. (2020). Marked Changes in Diversity and Relative Activity of Picoeukaryotes With Depth in the World Ocean. ISME J. 14, 437–449. doi: 10.1038/s41396-019-0506-9
Guannel M. L., Horner-Devine M. C., Rocap G. (2011). Bacterial Community Composition Differs With Species and Toxigenicity of the Diatom Pseudo-Nitzschia. Aquat. Microb. Ecol. 64, 117–133. doi: 10.3354/ame01513
Guidi L., Chaffron S., Bittner L., Eveillard D., Larhlimi A., Roux S., et al. (2016). Plankton Networks Driving Carbon Export in the Oligotrophic Ocean. Nature 532, 465–470. doi: 10.1038/nature16942
Guillou L., Bachar D., Audic S., Bass D., Berney C., Bittner L., et al. (2013). The Protist Ribosomal Reference Database (PR2): A Catalog of Unicellular Eukaryote Small Sub-Unit rRNA Sequences With Curated Taxonomy. Nucleic Acids Res. 41, D597–D604. doi: 10.1093/nar/gks1160
Guillou L., Viprey M., Chambouvet A., Welsh R. M., Kirkham A. R., Massana R., et al. (2008). Widespread Occurrence and Genetic Diversity of Marine Parasitoids Belonging to Syndiniales (Alveolata). Environ. Microbiol. 10, 3349–3365. doi: 10.1111/j.1462-2920.2008.01731.x
Guo R., Liang Y., Xin Y., Wang L., Mou S., Cao C., et al. (2018). Insight Into the Pico- and Nano-Phytoplankton Communities in the Deepest Biosphere, the Mariana Trench. Front. Microbiol. 9. doi: 10.3389/fmicb.2018.02289
Hu D., Wu L., Cai W., Sen Gupta A., Ganachaud A., Qiu B., et al. (2015). Pacific Western Boundary Currents and Their Roles in Climate. Nature 522, 299–308. doi: 10.1038/nature14504
Ivars-Martinez E., Martin-Cuadrado A. B., D’Auria G., Mira A., Ferriera S., Johnson J., et al. (2008). Comparative Genomics of Two Ecotypes of the Marine Planktonic Copiotroph Alteromonas Macleodii Suggests Alternative Lifestyles Associated With Different Kinds of Particulate Organic Matter. ISME J. 2, 1194–1212. doi: 10.1038/ismej.2008.74
Jiao N., Luo T., Zhang R., Yan W., Lin Y., Johnson Z. I., et al. (2014). Presence of Prochlorococcus in the Aphotic Waters of the Western Pacific Ocean. Biogeosciences 11, 2391–2400. doi: 10.5194/bg-11-2391-2014
Karlusich J. J. P., Ibarbalz F. M., Bowler C. (2020). Phytoplankton in the Tara Ocean. Ann. Rev. Ma.r Sci. 12, 233–265. doi: 10.1146/annurev-marine-010419-010706
Kruger K., Chafee M., Ben Francis T., Glavina Del Rio T., Becher D., Schweder T., et al. (2019). In Marine Bacteroidetes the Bulk of Glycan Degradation During Algae Blooms is Mediated by Few Clades Using a Restricted Set of Genes. ISME J. 13, 2800–2816. doi: 10.1038/s41396-019-0476-y
Langwig M. V., De Anda V., Dombrowski N., Seitz K. W., Rambo I. M., Greening C., et al. (2021). Large-Scale Protein Level Comparison of Deltaproteobacteria Reveals Cohesive Metabolic Groups. ISME J 16, 307–320. doi: 10.1038/s41396-021-01057-y
Levin L. A., Sibuet M. (2012). Understanding Continental Margin Biodiversity: A New Imperative. Ann. Rev. Mar. Sci. 4, 79–112. doi: 10.1146/annurev-marine-120709-142714
Liang Y., Zhang Y., Zhang Y., Luo T., Rivkin R. B., Jiao N. (2017). Distributions and Relationships of Virio- and Picoplankton in the Epi-, Meso and Bathypelagic Zones of the Western Pacific Ocean. FEMS Microbiol. Ecol. 93, fiw238. doi: 10.1093/femsec/fiw238
Lidbury I., Borsetto C., Murphy A. R. J., Bottrill A., Jones A. M. E., Bending G. D., et al. (2021). Niche-Adaptation in Plant-Associated Bacteroidetes Favours Specialisation in Organic Phosphorus Mineralisation. ISME J. 15, 1040–1055. doi: 10.1038/s41396-020-00829-2
Lin Y. C., Campbell T., Chung C. C., Gong G. C., Chiang K. P., Worden A. Z. (2012). Distribution Patterns and Phylogeny of Marine Stramenopiles in the North Pacific Ocean. Appl. Environ. Microbiol. 78, 3387–3399. doi: 10.1128/AEM.06952-11
Li Y., Sun L. L., Sun M. L., Su H. N., Zhang X. Y., Xie B. B., et al. (2018). Vertical and Horizontal Biogeographic Patterns and Major Factors Affecting Bacterial Communities in the Open South China Sea. Sci. Rep. 8, 8800. doi: 10.1038/s41598-018-27191-w
Liu J., Hu Z., Liu G. Y., Huang Y. G., Zhang Z. Y. (2020). Selective Flotation of Copper Oxide Minerals With a Novel Amino-Triazole-Thione Surfactant: A Comparison to Hydroxamic Acid Collector. Min. Proc. Ext. Met. Rev. 41, 96–106. doi: 10.1080/08827508.2019.1575214
Logares R., Audic S., Santini S., Pernice M. C., de Vargas C., Massana R. (2012). Diversity Patterns and Activity of Uncultured Marine Heterotrophic Flagellates Unveiled With Pyrosequencing. ISME J. 6, 1823–1833. doi: 10.1038/ismej.2012.36
Luo M., Gieskes J., Chen L., Shi X., Chen D. (2017). Provenances, Distribution, and Accumulation of Organic Matter in the Southern Mariana Trench Rim and Slope: Implication for Carbon Cycle and Burial in Hadal Trenches. Mar. Geol. 386, 98–106. doi: 10.1016/j.margeo.2017.02.012
Marella T. K., Bhattacharjya R., Tiwari A. (2021). Impact of Organic Carbon Acquisition on Growth and Functional Biomolecule Production in Diatoms. Microb. Cell. Fact. 20, 135. doi: 10.1186/s12934-021-01627-x
Ma J., Song J. M., Li X. G., Wang Q. D., Yuan H. M., Li N., et al. (2021). Analysis of Differences in Nutrients Chemistry in Seamount Seawaters in the Kocebu and M4 Seamounts in Western Pacific Ocean. J. Oceanol. Limnol. 39, 1662–1674. doi: 10.1007/s00343-020-0239-7
Ma J., Song J. M., Li X. G., Yuan H. M., Li N., Duan L. Q., et al. (2020). Control Factors of DIC in the Y3 Seamount Waters of the Western Pacific Ocean. J. Oceanol. Limnol. 38, 1215–1224. doi: 10.1007/s00343-020-9314-3
Massana R., del Campo J., Sieracki M. E., Audic S., Logares R. (2014). Exploring the Uncultured Microeukaryote Majority in the Oceans: Reevaluation of Ribogroups Within Stramenopiles. ISME J. 8, 854–866. doi: 10.1038/ismej.2013.204
McNichol J., Stryhanyuk H., Sylva S. P., Thomas F., Musat N., Seewald J. S., et al. (2018). Primary Productivity Below the Seafloor at Deep-Sea Hot Springs. Proc. Natl. Acad. Sci. U.S.A. 115, 6756–6761. doi: 10.1073/pnas.1804351115
Mendonca A., Aristegui J., Vilas J. C., Montero M. F., Ojeda A., Espino M., et al. (2012). Is There a Seamount Effect on Microbial Community Structure and Biomass? The Case Study of Seine and Sedlo Seamounts (Northeast Atlantic). PloS One 7, e29526. doi: 10.1371/journal.pone.0029526
Mestre M., Ferrera I., Borrull E., Ortega-Retuerta E., Mbedi S., Grossart H. P. (2017). Spatial Variability of Marine Bacterial and Archaeal Communities Along the Particulate Matter Continuum. Mol. Ecol. 26, 6827–6840. doi: 10.1111/mec.14421
Mikhailov I. S., Bukin Y. S., Zakharova Y. R., Usoltseva M. V., Galachyants Y. P., Sakirko M. V., et al. (2019). Co-Occurrence Patterns Between Phytoplankton and Bacterioplankton Across the Pelagic Zone of Lake Baikal During Spring. J. Microbiol. 57, 252–262. doi: 10.1007/s12275-019-8531-y
Mohn C., White M., Denda A., Erofeeva S., Springer B., Turnewitsch R., et al. (2021). Dynamics of Currents and Biological Scattering Layers Around Senghor Seamount, a Shallow Seamount Inside a Tropical Northeast Atlantic Eddy Corridor. Deep Sea Res. Part. I Oceanogr. Res. Pap. 171, 103497. doi: 10.1016/j.dsr.2021.103497
Monnich J., Tebben J., Bergemann J., Case R., Wohlrab S., Harder T. (2020). Niche-Based Assembly of Bacterial Consortia on the Diatom Thalassiosira Rotula is Stable and Reproducible. ISME J. 14, 1614–1625. doi: 10.1038/s41396-020-0631-5
Mou S. L., Zhang Y. Y., Li G., Li H. M., Liang Y. T., Tang L. L., et al. (2017). Effects of Elevated CO2 and Nitrogen Supply on the Growth and Photosynthetic Physiology of a Marine Cyanobacterium, Synechococcus Sp PCC7002. J. Appl. Phycol. 29, 1755–1763. doi: 10.1007/s10811-017-1089-3
Mou S. L., Zhang Z. H., Zhao H. S., Shailesh N., Li Y. H., Xu K. D., et al. (2022). A Dark-Tolerant Diatom (Chaetoceros) Cultured From the Deep Sea. J. Phycol. doi: 10.1111/jpy.13240
NASEM (National Academies of Sciences, Engineering, and Medicine) (2021). A Research Strategy for Ocean-Based Carbon Dioxide Removal and Sequestration (Washington, DC: The National Academies Press).
Netotea S., Pongor S. (2006). Evolution of Robust and Efficient System Topologies. Cell. Immunol. 244, 80–83. doi: 10.1016/j.cellimm.2006.12.007
Nossa C. W., Oberdorf W. E., Yang L. Y., Aas J. A., Paster B. J., DeSantis T. Z., et al. (2010). Design of 16S rRNA Gene Primers for 454 Pyrosequencing of the Human Foregut Microbiome. World. J. Gastroenterol. 16, 4135–4144. doi: 10.3748/wjg.v16.i33.4135
Nymark M., Valle K. C., Hancke K., Winge P., Andresen K., Johnsen G., et al. (2013). Molecular and Photosynthetic Responses to Prolonged Darkness and Subsequent Acclimation to Re-Illumination in the Diatom Phaeodactylum Tricornutum. PloS One 8, e58722. doi: 10.1371/journal.pone.0058722
Obiol A., Giner C. R., Sanchez P., Duarte C. M., Acinas S. G., Massana R. (2020). A Metagenomic Assessment of Microbial Eukaryotic Diversity in the Global Ocean. Mol. Ecol. Resour. 20, 718–731. doi: 10.1111/1755-0998.13147
Oksanen J., Friendly M., Kindt R., Legendre P., McGlinn D., Minchin P., et al. (2018) Vegan: Community Ecology Package. Available at: https://CRAN.Rproject.org/package=vegan.
Parks D. H., Tyson G. W., Hugenholtz P., Beiko R. G. (2014). STAMP: Statistical Analysis of Taxonomic and Functional Profiles. Bioinformatics 30, 3123–3124. doi: 10.1093/bioinformatics/btu494
Pernice M. C., Giner C. R., Logares R., Perera-Bel J., Acinas S. G., Duarte C. M., et al. (2016). Large Variability of Bathypelagic Microbial Eukaryotic Communities Across the World’s Oceans. ISME J. 10, 945–958. doi: 10.1038/ismej.2015.170
Quast C., Pruesse E., Yilmaz P., Gerken J., Schweer T., Yarza P., et al. (2013). The SILVA Ribosomal RNA Gene Database Project: Improved Data Processing and Web-Based Tools. Nucleic Acids Res. 41, D590–D596. doi: 10.1093/nar/gks1219
Reji L., Tolar B. B., Chavez F. P., Francis C. A. (2020). Depth-Differentiation and Seasonality of Planktonic Microbial Assemblages in the Monterey Bay Upwelling System. Front. Microbiol. 11. doi: 10.3389/fmicb.2020.01075
Richert I., Yager P. L., Dinasquet J., Logares R., Riemann L., Wendeberg A., et al. (2019). Summer Comes to the Southern Ocean: How Phytoplankton Shape Bacterioplankton Communities Far Into the Deep Dark Sea. Ecosphere 1, e02641. doi: 10.1002/ecs2.2641
Rii Y. M., Duhamel S., Bidigare R. R., Karl D. M., Repeta D. J., Church M. J. (2016). Diversity and Productivity of Photosynthetic Picoeukaryotes in Biogeochemically Distinct Regions of the South East Pacific Ocean. Limnol. Oceanogr. 61, 806–824. doi: 10.1002/lno.10255
Rocke E., Noyon M., Roberts M. (2020). Picoplankton and Nanoplankton Composition on and Around a Seamount, Affected by an Eddy Dipole South of Madagascar. Deep Sea Res. Part II Top. Stud. Oceanogr. 176, 104744. doi: 10.1016/j.dsr2.2020.104744
Rognes T., Flouri T., Nichols B., Quince C., Mahé F. (2016). VSEARCH: A Versatile Open Source Tool for Metagenomics. PeerJ 4, e2584. doi: 10.7717/peerj.2584
Ryther J. H., Guillard R. (1962). Studies of Marine Planktonic Diatoms:II. Use of Cyclotella Nana Hustedt for Assays of Vitamin B12 in Sea Water. Can. J. Microbiol. 8, 437–445. doi: 10.1128/AEM.02380-14
Samo T. J., Kimbrel J. A., Nilson D. J., Pett-Ridge J., Weber P. K., Mayali X. (2018). Attachment Between Heterotrophic Bacteria and Microalgae Influences Symbiotic Microscale Interactions. Environ. Microbiol. 20, 4385–4400. doi: 10.1111/1462-2920.14357
Santos M., Moita M. T., Bashmachnikov I., Menezes G. M., Carmo V., Loureiro C. M., et al. (2013). Phytoplankton Variability and Oceanographic Conditions at Condor Seamount, Azores (NE Atlantic). Deep Sea Res. Part II Top. Stud. Oceanogr. 98, 52–62. doi: 10.1016/j.dsr2.2013.05.037
Schallenberg C., Bestley S., Klocker A., Trull T. W., Davies D. M., Gault-Ringold M., et al. (2018). Sustained Upwelling of Subsurface Iron Supplies Seasonally Persistent Phytoplankton Blooms Around the Southern Kerguelen Plateau, Southern Ocean. J. Geophys. Res. Oceans. 123, 5986–6003. doi: 10.1029/2018JC013932
Segata N., Izard J., Waldron L., Gevers D., Miropolsky L., Garrett W. S., et al. (2011 Metagenomic Biomarker Discovery and Explanation. Genome Biol. 12, 1–18. doi: 10.1186/gb-2011-12-6-r6
Sergi S., Baudena A., Cotté C., Ardyna M., Blain S., d’Ovidio F. (2020). Interaction of the Antarctic Circumpolar Current With Seamounts Fuels Moderate Blooms But Vast Foraging Grounds for Multiple Marine Predators. Front. Mar. Sci. 7. doi: 10.3389/fmars.2020.00416
Seymour J. R., Amin S. A., Raina J. B., Stocker R. (2017). Zooming in on the Phycosphere: The Ecological Interface for Phytoplankton-Bacteria Relationships. Nat. Microbiol. 2, 17065. doi: 10.1038/nmicrobiol.2017.65
Sherr E. B., Sherr B. F. (2007). Heterotrophic Dinoflagellates: A Significant Component of Microzooplankton Biomass and Major Grazers of Diatoms in the Sea. Mar. Ecol. Prog. Ser. 352, 187–197. doi: 10.3354/meps07161
Stock C. A., Cheung W. W. L., Sarmiento J. L., Sunderland E. M. (2019). Changing Ocean Systems: A Short Synthesis. Predicting. Future. Oceans. 2, 19–34. doi: 10.1016/B978-0-12-817945-1.00002-2
Sunagawa S., Coelho L. P., Chaffron S., Kultima J. R., Labadie K., Salazar G., et al. (2015). Structure and Function of the Global Ocean Microbiome. Science 348, 1261359. doi: 10.1126/science.1261359
Sun Q. Q., Song J. M., Li X. G., Yuan H. M., Ma J., Wang Q. D. (2020). Bacterial Vertical and Horizontal Variability Around a Deep Seamount in the Tropical Western Pacific Ocean. Mar. Pollut. Bull. 158, 111419. doi: 10.1016/j.marpolbul.2020.111419
Sun Q. Q., Song J. M., Li X. G., Yuan H. M., Wang Q. D. (2021). The Bacterial Diversity and Community Composition Altered in the Oxygen Minimum Zone of the Tropical Western Pacific Ocean. J. Oceanol. Limnol. 39, 1690–1704. doi: 10.1007/s00343-021-0370-0
Teeling H., Fuchs B. M., Bennke C. M., Kruger K., Chafee M., Kappelmann L., et al. (2016). Recurring Patterns in Bacterioplankton Dynamics During Coastal Spring Algae Blooms. eLife 5, e11888. doi: 10.7554/eLife.11888
Thornton D. C. O. (2014). Dissolved Organic Matter (DOM) Release by Phytoplankton in the Contemporary and Future Ocean. Eur. J. Phycol. 49, 20–46. doi: 10.1080/09670262.2013.875596
Traving S. J., Kellogg C. T. E., Ross T., McLaughlin R., Kieft B., Ho G. Y., et al. (2021). Prokaryotic Responses to a Warm Temperature Anomaly in Northeast Subarctic Pacific Waters. Commun. Biol. 4, 1217. doi: 10.1038/s42003-021-02731-9
Treguer P., Bowler C., Moriceau B., Dutkiewicz S., Gehlen M., Aumont O., et al. (2018). Influence of Diatom Diversity on the Ocean Biological Carbon Pump. Nat. Geosci. 11, 27–37. doi: 10.1038/s41561-017-0028-x
Turner J. T. (2015). Zooplankton Fecal Pellets, Marine Snow, Phytodetritus and the Ocean’s Biological Pump. Prog. Oceanogr. 130, 205–248. doi: 10.1016/j.pocean.2014.08.005
Vergin K. L., Beszteri B., Monier A., Thrash J. C., Temperton B., Treusch A. H., et al. (2013). High-Resolution SAR11 Ecotype Dynamics at the Bermuda Atlantic Time-Series Study Site by Phylogenetic Placement of Pyrosequences. ISME J. 7, 1322–1332. doi: 10.1038/ismej.2013.32
Wang M., Ma Y., Feng C., Cai L., Li W. (2020). Diversity of Pelagic and Benthic Bacterial Assemblages in the Western Pacific Ocean. Front. Microbiol. 11. doi: 10.3389/fmicb.2020.01730
Worden A. Z., Follows M. J., Giovannoni S. J., Wilken S., Zimmerman A. E., Keeling P. J. (2015). Rethinking the Marine Carbon Cycle: Factoring in the Multifarious Lifestyles of Microbes. Science 347, 1257594. doi: 10.1126/science.1257594
Wu J., Gao W., Johnson R. H., Zhang W., Meldrum D. R. (2013). Integrated Metagenomic and Metatranscriptomic Analyses of Microbial Communities in the Meso- and Bathypelagic Realm of North Pacific Ocean. Mar. Drugs 11, 3777–3801. doi: 10.3390/md11103777
Xu K. D. (2021). Exploring Seamount Ecosystems and Biodiversity in the Tropical Western Pacific Ocean. J. Oceanol. Limnol. 39, 1585–1590. doi: 10.1007/s00343-021-1585-9
Zhang Z. H., Nair S., Tang L. L., Zhao H. S., Hu Z. Z., Chen M. M., et al. (2021a). Long-Term Survival of Synechococcus and Heterotrophic Bacteria Without External Nutrient Supply After Changes in Their Relationship From Antagonism to Mutualism. mBio 12:e0161421. doi: 10.1128/mBio.01614-21
Zhang Z. H., Tang L. L., Liang Y. T., Li G., Li H. M., Rivkin R. B., et al. (2021b). The Relationship Between Two Synechococcus Strains and Heterotrophic Bacterial Communities and its Associated Carbon Flow. J. Appl. Phycol. 33, 953–966. doi: 10.1007/s10811-020-02343-6
Zhang Y., Zhao M. X., Cui Q., Fan W., Qi J. G., Chen Y., et al. (2017). Processes of Coastal Ecosystem Carbon Sequestration and Approaches for Increasing Carbon Sink. Sci. China. Earth. Sci. 60, 809–820. doi: 10.1007/s11430-016-9010-9
Zhang Y., Zhao Z. H., Dai M. H., Jiao N. Z., Herndl G. J. (2014). Drivers Shaping the Diversity and Biogeography of Total and Active Bacterial Communities in the South China Sea. Mol. Ecol. 23, 2260–2274. doi: 10.1111/mec.12739
Zhao F., Filker S., Xu K. D., Huang P. P., Zheng S. (2020). Microeukaryote Communities Exhibit Phyla-Specific Distance-Decay Patterns and an Intimate Link Between Seawater and Sediment Habitats in the Western Pacific Ocean. Deep Sea Res. Part. I Oceanogr. Res. Pap. 160, 103279. doi: 10.1016/j.dsr.2020.103279
Zheng R. K., Cai R. N., Liu R., Liu G., Sun C. M. (2021). Maribellus Comscasis Sp. Nov., a Novel Deep-Sea Bacteroidetes Bacterium, Possessing a Prominent Capability of Degrading Cellulose. Environ. Microbiol. 23, 4561–4575. doi: 10.1111/1462-2920.15650
Zheng Y. F., Wang J. Y., Zhou S., Zhang Y. H., Liu J., Xue C. X., et al. (2020). Bacteria are Important Dimethylsulfoniopropionate Producers in Marine Aphotic and High-Pressure Environments. Nat. Commun. 11, 4658. doi: 10.1038/s41467-020-18434-4
Keywords: seamount, phytoplankton, bacterioplankton, vertical distribution, co-occurrence network
Citation: Zhao H, Zhang Z, Nair S, Zhao J, Mou S, Xu K and Zhang Y (2022) Vertically Exported Phytoplankton (< 20 µm) and Their Correlation Network With Bacterioplankton Along a Deep-Sea Seamount. Front. Mar. Sci. 9:862494. doi: 10.3389/fmars.2022.862494
Received: 26 January 2022; Accepted: 14 March 2022;
Published: 07 April 2022.
Edited by:
Lu Lin, Shandong University, ChinaCopyright © 2022 Zhao, Zhang, Nair, Zhao, Mou, Xu and Zhang. This is an open-access article distributed under the terms of the Creative Commons Attribution License (CC BY). The use, distribution or reproduction in other forums is permitted, provided the original author(s) and the copyright owner(s) are credited and that the original publication in this journal is cited, in accordance with accepted academic practice. No use, distribution or reproduction is permitted which does not comply with these terms.
*Correspondence: Yongyu Zhang, zhangyy@qibebt.ac.cn
†These authors have contributed equally to this work and share first authorship