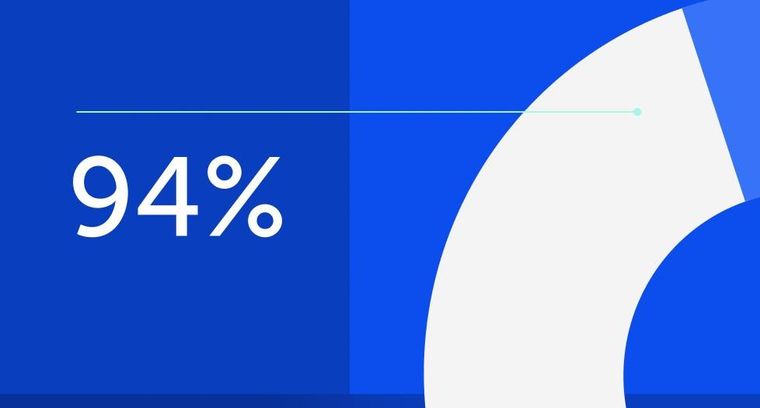
94% of researchers rate our articles as excellent or good
Learn more about the work of our research integrity team to safeguard the quality of each article we publish.
Find out more
ORIGINAL RESEARCH article
Front. Mar. Sci., 28 March 2022
Sec. Marine Biogeochemistry
Volume 9 - 2022 | https://doi.org/10.3389/fmars.2022.861998
This article is part of the Research TopicEcophysiology and Biogeochemistry of Marine Plants in the AnthropoceneView all 15 articles
We assessed the potential of dead seagrass Posidonia oceanica matte to act as a biogeochemical sink and provide a coherent archive of environmental change in a degraded area of the Mediterranean Sea (Augusta Bay, Italy). Change in sediment properties (dry bulk density, grain size), concentration of elements (Corg, Cinorg, N, Hg) and stable isotope ratios (δ13C, δ 15N) with sediment depth were measured in dead P. oceanica matte and unvegetated (bare) sediments in the polluted area, and an adjacent P. oceanica meadow. Principal Component Analysis (PCA) revealed a clear clustering by habitat, which explained 72% of variability in our samples and was driven mainly by the accumulation of N and Hg in finer sediments of the dead matte. Assessment of the temporal trends of Corg, N and Hg concentrations in the dead matte revealed changes in the accumulation of these elements over the last 120 years, with an increase following the onset of industrial activities 65 y BP (i.e., yr. 1950) that was sustained even after seagrass loss around 35 y BP. Despite a decrease in Hg concentrations in the early 1980s following the onset of pollution abatement, overall Hg levels were 2-fold higher in the local post-industrial period, with a Hg enrichment factor of 3.5 in the dead matte. Mean stocks of Corg, N and Hg in 25 cm thick sediment deposits (4.08 ± 2.10 kg Corg m-2, 0.14 ± 0.04 kg N m-2, 0.19 ± 0.04 g Hg m-2) and accumulation in the last 120 yr (35.3 ± 19.6 g Corg m-2 y-1, 1.2 ± 0.4 g N m-2 y-1, 0.0017 ± 0.0004 g Hg m-2 y-1) were higher in the dead matte than bare sediment or adjacent P. oceanica meadow. Our results indicate that dead P. oceanica matte maintained its potential as a biogeochemical sink and, like its living counterpart, dead matte can serve as an effective archive to allow for reconstructing environmental change in coastal areas of the Mediterranean where severe perturbations have led to P. oceanica loss. Appropriate management for contaminated areas should be prioritized to prevent release of pollutants and carbon from dead mattes.
There is an increasing appreciation of the role of seagrass ecosystems as nature-based climate solutions due to their effective carbon sequestration (Macreadie et al., 2021). As blue carbon (BC) sinks, seagrass meadows sequester organic carbon through metabolism (Duarte et al., 2010) and trapping of allochthonous particles (Gacia and Duarte, 2001), which results in the formation of biogenic reefs comprised of senescent plant tissue and sediment. These reefs show increased carbon preservation as their sub-surface anoxic conditions favor slow decomposition and effectively retain buried carbon (Duarte et al., 2013). This is particularly impressive in the case of the Mediterranean endemic seagrass species, Posidonia oceanica (L.) Delile, which can form extensive deposits called ‘matte’ (Boudouresque et al., 2015) that can be up to 6.5 m thick (Lo Iacono et al., 2008) and 9,000 yr. old (Monnier et al., 2021). P. oceanica is a slow-growing, perennial, long-lived seagrass that owes the capacity to form these mattes to the vertical growth of its rhizomes that raise the matte over time, while the highly recalcitrance nature of its modules (Kaal et al., 2018) favors slow decay rates (Mateo et al., 1997). It has been well documented that these mattes act as significant sinks for carbon, storing up to 88 kg Corg m-2 in their top meter of sediment (Serrano et al., 2016b; Mazarrasa et al., 2017b; Apostolaki et al., 2019; Monnier et al., 2021), which places P. oceanica meadows among the top blue carbon sinks compared to other seagrass species around the world (Lavery et al., 2013; Thorhaug et al., 2017; Röhr et al., 2018; Serrano et al., 2018; Sousa et al., 2019).
Less studied, yet seemingly equally important, is the capacity of P. oceanica mattes to sequester and retain nutrients and contaminants, and the consequent role of seagrass meadows in the mitigation of coastal eutrophication and contamination in the Mediterranean Sea. Anthropogenic activities around Mediterranean coasts and industrialization have increased the levels of nutrients, such as nitrogen (N), in the water column and studies, albeit very limited so far, have documented that part of the N incorporated in P. oceanica tissues is stored in their underlying sediments (Mateo et al., 1997; Apostolaki et al., 2019), supplemented also by the high capacity of these meadows to trap suspended N-bearing particles from the bottom water above the canopy (Gacia et al., 2002). Similarly, P. oceanica shoots tend to accumulate trace elements in their tissues (Bonanno and Orlando-Bonaca, 2017), while the meadows enhance the deposition of fine organic-rich particles that have strong binding affinity with trace elements and, thereby, immobilizing a large quantity of pollutants in their substrates (Serrano et al., 2011). As such, the P. oceanica matte represents an important archive of anthropogenic disturbances that has helped to reconstruct environmental change and identify thresholds of ecosystem processes following perturbations (Serrano et al., 2011; Serrano et al., 2013; Leiva-Dueñas et al., 2021).
Intensification of anthropogenic activities around the coasts of the Mediterranean has resulted in an accelerating degradation of P. oceanica ecosystems (de los Santos et al., 2019), with important implications for the role of this species in the biogeochemical cycles of the Mediterranean Sea. The loss of P. oceanica foliar canopy results in the exposure of matte, which is then comprised only of the underlying rhizomes and roots, and sediment particles and is often called ‘dead matte’. Being indicative of seagrass degradation, dead P. oceanica mattes have been traditionally considered a habitat of poor ecological quality, failing to attract considerable research attention or management. However, there has been early evidence of the importance of these ecosystems (Borg et al., 2006) and their very recent use as a substrate for successful transplantation and regeneration of P. oceanica meadows (Calvo et al., 2021) could revalorize dead mattes in the current consensus for ocean restoration. In fact, dead mattes may persist for several years or even decades (Boudouresque et al., 2015). This feature could allow the assessment of historical fluctuations in areas with unknown local history, where severe pressure has resulted in complete disappearance of the meadows. This would advocate an implementation of relative conservation actions, but at present we lack a generalized understanding of the fate of stocks in dead mattes that is necessary to build a comprehensive framework to support environmental management of these degraded matte areas.
Currently it is unclear whether dead mattes maintain their chrono-stratigraphy coherently and their biogeochemical sinks intact, and there is still a significant knowledge deficit in the fate of carbon and nutrient following cover loss. Apparently, in the absence of leaves there would be no seagrass metabolic capture, as seagrass productivity is a key factor regulating the magnitude of carbon storage (Mazarrasa et al., 2018), while a potential decrease in trapping of allochthonous matter is also expected to occur. Seagrass loss may pose a risk of erosion and subsequent remineralisation of historic stores of organic matter which have been built-up in seagrass sediments over centuries, or even millennia in the case of P. oceanica, as the upper, previously anoxic layers of sediment can become exposed to oxic conditions (Pendleton et al., 2015; Lovelock et al., 2017). However, the vulnerability to decomposition depends on the microbial activity and recalcitrance of the residual organic matter (Spivak et al., 2019), which in turn varies among seagrass species. P. oceanica cover loss does not necessarily lead to erosion, but it can result in increase in mineralization of organic matter at least in the rhizosphere (Piñeiro-Juncal et al., 2021) and it is the balance between the decomposition and preservation and the environmental conditions that would ultimately determine the magnitude of elemental loss.
Here, we study the potential of dead seagrass P. oceanica matte to act as a biogeochemical sink. We do so by reconstructing the historical trends in concentration and accumulation of carbon, nitrogen and mercury in sediments beneath dead P. oceanica matte and unvegetated (bare) sediments in a highly contaminated coastal marine area in the Mediterranean Sea (Augusta Bay, Italy), and in sediments beneath an adjacent P. oceanica meadow. More widely, we address the capacity of dead mattes to provide a coherent archive of environmental change in degraded areas along the Mediterranean coastlines.
The study was performed in Augusta Bay, a semi-enclosed basin located in eastern Sicily (Italy), delimited to the north by Santa Croce Cape and to the south by Santa Panagia Cape (Figure 1). The north-eastern side of the bay is occupied by Augusta Harbour, where major commercial and industrial activities commenced after World War II. The area hosts the largest European petrochemical complex and the most important chloro-alkali plant in Italy, which was active from 1954 to 2005, and until the early 1980s had been discharging waste waters into the sea across the Vallone della Neve River without treatment. Consequently, high accumulations of mercury (Hg) have been reported in the marine environment of Augusta Bay (Bellucci et al., 2012), with major Hg discharges continuing until the construction of a demercurization and waste treatment plant in 1983, in accordance with the national law (G.U.R.I, L.319/1976). Two breakwaters built in the 1960s have delimited Augusta Harbour from the open sea, and the presence of two narrow inlets (Levante, which is 400 m wide and 40 m deep, and Scirocco, which is 300 m wide and 13 m deep) together with a general cyclonic water circulation (ICRAM, 2008) (Figure 1) prevented higher water exchange and facilitated further accumulation of contaminants (Sprovieri et al., 2011).
Figure 1 Map of Augusta Bay (Ionian Sea, southern Italy, eastern Sicily). Arrows indicate the main currents (modified from ICRAM, 2008 and Di Leonardo et al., 2014). The grey segments indicate the breakwaters built in the 1960s that delimit connection of Augusta Harbour from the open sea through the Scirocco and Levante inlets. The chloro-alkali plant (indicated with a star) and the mouth of the river Vallone della Neve are also shown. The sampling stations (dead matte and bare sediment at Priolo Bay, and Posidonia oceanica at Xifonio Gulf) are reported.
Through the Scirocco Inlet, Augusta Harbour connects with the coastal area of Priolo Bay, defined by the European Commission Habitat Service (94/43/EEC) as a Site of Community Importance (SCI). The outflows of bottom water export contaminants from the Harbour to Priolo Bay through this inlet (Di Leonardo et al., 2014; Signa et al., 2017). Consequently, high contents of Hg (1.3 ± 0.2 mg kg-1), Cd (0.2 ± 0.0 mg kg-1), Ni (46.2 ± 7.2 mg kg-1) and PAHs (ΣPAHs18 164.4 ng g-1), exceeding sediment quality guidelines, have been detected in the surface sediments of Priolo Bay (Di Leonardo et al., 2014). Priolo Bay shows complex current dynamics with a general water flow moving from NE to SW and a local current parallel to the coast (Di Leonardo et al., 2014). The large input of contaminants during the last century caused gradual regression of the Posidonia oceanica seagrass meadow extending in the area until its complete loss in the late 1970s (Costantini, 2015; Di Leonardo et al., 2017). Currently, the seabed is characterized by sand and dead seagrass matte (Di Leonardo et al., 2014).
The north-western sector of Augusta Bay is covered by extensive and abundant P. oceanica meadows in Xifonio Gulf, around 3 - 7 km away from August Harbour and the petrochemical industries. The meadow expands at 13 m depth, with shoot density of 363 ± 20 shoots m-2 and leaf area index of 6.6 m2 leaves m-2 (Costantini, 2015). Hg concentration in P. oceanica leaves is 0.09 ± 0.02 mg kg-1 (S. Vizzini, unpubl. data), close to the Hg mean reported from areas with minimal contamination (0.04 ± 0.00 mg kg-1, (Lafabrie et al., 2008).
Three stations were selected, each one characterised by a different habitat but at similar water depth (ca. 12 m). Two stations were located at Priolo Bay: one characterized by the presence of dead P. oceanica matte, hereafter called ‘dead matte’, and the other characterized by unvegetated sediments, hereafter called ‘bare sediment’ (Figure 1). Another station was sampled at Xifonio Gulf, within the P. oceanica meadow, hereafter called ‘P. oceanica meadow’. Sediment cores were collected at each station in July 2015 by scuba-divers in duplicate (around 10 m apart from each other) to allow the assessment of spatial and temporal variability, using a hand-corer consisted of a 60 cm long and 7.4 cm wide PVC pipe. Compression was estimated by measuring the outer, inner, and total length of the core. Prior to any calculation, the thickness of each sediment slice was corrected by applying a compression correction factor. The compression factor was on average 0.76 for dead matte cores, 0.86 for bare sediment cores and 0.65 for P. oceanica cores. Sediment samples were immediately frozen at -20°C.
The cores were sliced into 1 cm sections for the first 10 cm and every 2 cm for the remaining sediment. Subsamples of each slice from the first ten cm of sediment were homogenized to correspond to a slice thickness of 0 – 10 cm of sediment, which was used for granulometry analysis. Wet samples were previously left in a H2O2 solution (3 - 10%) to eliminate organic matter. Subsequently, sediment fractions (gravel > 2 mm, sand 2 mm - 63 μm, silt/clay < 63 μm) were oven-dried at 60°C, separated by dry sieving using a set of sieves up to 63 µm, and weighed.
The remaining sediment of each slice was freeze-dried for 48 hours and powdered for the determination of dry bulk density, elemental concentration and isotopic composition of carbon and nitrogen and mercury concentration per slice. For the determination of concentration of total carbon and nitrogen (Ctot, N) and nitrogen isotopic composition (δ15N), sediment aliquots were weighed in tin capsules and analysed using an isotope ratio mass spectrometer (Thermo IRMS Delta Plus XP) coupled with an elemental analyser (Thermo EA-1112). For the determination of organic carbon (Corg) a fraction of each sediment sample was acidified in silver capsules prior to the analysis in the EA-IRMS system according to Nieuwenhuize et al. (1994) to eliminate carbonates. For carbon stable isotope (δ13C) determination, each sample was acidified with HCl 2N until bubbling stopped to remove carbonates, centrifuged and the supernatant removed by pipette; sample was then washed with Milli-Q water, centrifuged, oven dried after supernatant removal and weighed in tin capsules. Analytical uncertainty based on replicate measurements of internal standards (Acetanilide for C and N, International Atomic Energy Agency IAEA- CH-6 for δ13C and IAEA-NO-3 for δ15N) was 0.02%, 0.05%, 0.1‰ and 0.2‰ for C, N, δ13C and δ15N, respectively. The concentration of inorganic carbon (Cinorg) was calculated as the difference between the concentration of Ctot and Corg.
To quantify aluminium (Al) and mercury (Hg) concentration, dry sediment sub-samples (0.2 g each) were mineralised using an automatic microwave digestion system (MARS 5, CEM) with a solution of 67–70% HNO3, 30% HF, 30% H2O2 and Milli-Q water at a ratio of 6:2:0.4:1.6 according to the USEPA (1996) method. Hg concentration was determined using a hydride generation system linked to ICP-OES with a reductant solution, consisting of 0.2% sodium (Na) borohydride and 0.05% Na hydroxide. Samples were analysed by inductively coupled plasma optical emission spectrometry (ICP-OES, Optima 8000, PerkinElmer). Analytical quality control was performed using Marine sediment NIST 2702 (National Institute of Standards and Technology) as the Certified Reference Material (CRM) (recovery percentage was between 88% and 101%). RSD was lower than 10%; LOQ was 0.003 mg kg-1. Hg data were normalised to Al measured at the corresponding slices to account for the difference in grain size and minerology (e.g., Ackermann, 1980; Di Leonardo et al., 2007).
The activity of 210Pb was determined in selected sub-samples at dead matte core 1 and 2 and P. oceanica core 1. P. oceanica core 2 and both bare sediment cores thought to be unsuitable for dating due to their sandy nature and high bioturbation and the potential to be highly mixed. The activity was determined through the measurement of its granddaughter 210Po, using alpha spectrometry. This method is based on that outlined in (Flynn, 1968) and used double acid leaching (with freshly prepared aqua regia, 3:1 conc. HCl: conc. HNO3) of the dried sediment sample followed by auto-deposition of the Po in the leachate onto silver polished discs. 209Po was added as an isotopic yield tracer. Samples were counted over three days on a Canberra Alpha spectrometry Quad system. The limit of detection was 0.1 Bq kg 1.
Mass and sediment accumulation rates (MAR, g cm-2 y-1 and SAR, cm y-1) were calculated from 210Pb data using the Constant Flux: Constant Sedimentation CF: CS model (Robbins, 1978) of 210Pb dating, which gives an average sediment accumulation rate over the datable cumulative mass or core depth, respectively, and via the Constant Rate of Supply (CRS) model (Appleby and Oldfield, 1978). While the CF:CS model has been shown to be robust in many marine settings, the CRS model allows for the assessment of variations in sediment accumulation rate over time, although it makes the fundamental assumption that 210Pb supply is dominantly atmospheric (rather than supplied on sediment particles), which may not apply in dynamic sedimentary environments such as Augusta Bay where significant sediment in-wash or remobilization occurs. Application of the CRS model here indicated no large-scale inflections or variations in sediment accumulation rate in the cores dated, in line with the assumptions used in the CF:CS model. So, while we used both methods here to evaluate sediment accumulation rates, we used the CF:CS model as the primary model for age control. Supported 210Pb activities (ca. 12 Bq kg-1) were estimated using average activities in older, deeper sediments, effectively the activity at which 210Pb declines to near-constant values at depth in older core material (Cundy and Croudace, 1996). For the simple or CF: CS model, the sedimentation rate was calculated by plotting the natural logarithm of the unsupported 210Pb activity (210Pbexcess) against depth and determining the least-squares fit. Inventories of excess 210Pb (in Bq m-2) per core were calculated as the sum of excess 210Pb activity multiplied by the DBD and slice thickness of each core slice.
Analysis of Covariance (ANCOVA) was used to examine whether there were significant differences in the temporal profiles of geochemical variables among dead matte and P. oceanica habitat. Given the low number of cores per habitat, we used ‘core’ as the categorical variable instead of ‘habitat’, while ‘age’ was the continuous explanatory variable. The residuals were checked for normality using the Shapiro-Wilk test and for homogeneity of variance using the Levene’ s test. When necessary, data were log10 transformed. When a significant interaction occurred among the explanatory variables, a linear regression was used to assess the change in geochemical variables along the years for each core separately. A linear regression was also used to estimate possible variation in the distribution of elements downcore in the case of bare sediment cores, as we were not able to ascribe dates to those cores (see below).
A paired t-test was used to identify the impact of contamination in Priolo Bay, by comparing the mean concentrations of elements before and after the onset of industrial activities in dead matte cores, based on the 210Pb geochronology. Prior to the analysis, the data were checked for normality using the Shapiro-Wilk test.
Principal component analysis (PCA) was performed to identify patterns of variance between habitats. The PCA was performed using an Euclidean distance matrix considering the vertical profile of DBD, Corg, Cinorg, N, Hg:Al, δ13C and δ15N per core, while the granulometric data of the 0 – 10 cm sediment slice were extened to the whole core. Compositional data (grain size fractions and elemental concentrations) were transformed using the log center ratio (Aitchison, 1986) and all data were standardized using z-scores. Permutational multivariate analysis of variance was used (PERMANOVA, n perm. = 999) (Anderson, 2001) to detect potential significant differences in the ordination of habitats based on their vertical profiles.
Elemental stocks were estimated as the cumulative product of element concentration, DBD and decompressed sediment slice thickness and were standardized to 25 cm of sediment thickness. We also standardized the estimates of stocks to sediment thickness of one meter to allow comparisons, by fitting a linear regression of cumulative stock per slice against sediment depth. Accumulation rate of elements were standardized to the last 120 years (the effective age limit of 210Pb dating) by multiplying the corresponding stock with SAR.
Mixing models using δ13C were applied [R package simmr: Stable Isotope Mixing Models in R (Parnell et al., 2013)] to estimate the contribution of potential sources of organic matter to the sediment Corg in the three different habitats. As the deeper layers may have undergone post-depositional diagenesis processes, mixing models were applied just for the first 5 cm of sediment depth. The end-members included in the model were: particulate organic matter (POM; δ13C = -22.9 ± 0.3‰), seagrass leaves (P. oceanica; δ13C = -12.0 ± 1.0‰) and the macroalgae (Caulerpa prolifera and Dictyopteris polypodioides; averaged δ13C = -16.5 ± 5.6‰). δ13C data for POM and macroalgae were retrieved from Signa et al. (2017), while those of P. oceanica leaves are unpublished (S. Vizzini data).
All the analyses were performed using R version 3.6.1 (R Core Team, 2021).
The total activity of 210Pb showed a quasi-exponential decline with sediment depth in dead matte core 1, reaching supported activities (ca. 12 Bq kg-1) at 19 - 20 cm of sediment depth (Figure 2). The application of the CF:CS rate model yielded a SAR of 0.19 ± 0.03 cm y-1 (MAR = 0.14 g cm-2 y-1). We did not reach these background activities at the base of the core material available for dating (11 - 12 cm) in dead matte 2, as activities were still higher than supported activities in this shorter core, and SAR was estimated at 0.19 ± 0.21 cm y-1 (MAR = 0.11 g cm-2 y-1). SAR of the P. oceanica core 1 was 0.21 ± 0.77 cm y-1 (MAR = 0.12 g cm-2 y-1). Mixing of sediment profiles (and the very sandy composition of the sediment, see below) precluded the estimation of SAR by 210Pb in bare sediment cores and P. oceanica core 2. For the latter, we used the SAR of P. oceanica core 1 to calculate the corresponding accumulation rates. The 210Pbexcess inventories of dead matte 1 (3,605 ± 469 Bq m-2) and dead matte 2 (2,884 ± 490 Bq m-2) were higher than that of P. oceanica 1 (2,711 ± 461 Bq m-2) (Figure 3).
Figure 2 Total 210Pb activity (Bq kg−1 ± SE) with sediment depth. Shaded area represents the supported activity (12 Bq kg-1).
Figure 3 Inventories of 210Pbexcess (Bq m-2) at each core and habitat. Errors are based on uncertainties on the 210Pb measurements used in the inventory calculations.
The sediments beneath each habitat were mainly sandy, but those of dead matte had two to three-fold higher silt/clay content (Table 1). The vertical distribution of elemental concentrations and isotopic composition differed between habitats, with dead matte cores showing a higher range and larger variability within the sediment profile. The temporal distribution of geochemical variables studied over the last 120 years differed between dead matte and P. oceanica cores, although the way age affected the variation depended on the core studied and not all cores showed a significant variation with time (Figures 4A, B and Table S1). The range of DBD in dead matte cores (0. 29 - 0.78 g cm-3 with mean 0.52 ± 0.16 g cm-3) was similar to that in P. oceanica cores (0.15 - 0.91 g cm-3 and mean 0.49 ± 0.16 g cm-3), but P. oceanica cores showed a decrease in DBD towards the present day (Figure 4A). Overall, high variation in the investigated variables was evident for dead matte cores from 12 cm sediment depth up to the sediment surface, which, based on the chrono-stratigraphy of the cores, corresponds to the period of 1950 (i.e., the onset of industrial activity) to present (i.e., 2015, when cores were collected). A significant increase towards present was measured for Corg and N in dead matte 1, while both dead matte cores showed significantly more 15N-enriched and 13C-depleted values. Overall, the range and mean (± STDEV) of Corg (1.65 – 3.50 and 2.28 ± 0.44% DW) and N (0.02 - 0.2 and 0.08 ± 0.05% DW) in P. oceanica cores showed generally lower values than in the dead matte ones, with only the N concentration of P. oceanica core 1 showing a significant increase towards the present (Figures 4A, B). The δ13C and δ15N ranges were similar between habitats, with P. oceanica core 1 showing higher δ13C and δ15N values and core 2 lower δ15N values towards the present. The range of Hg concentration (0.28 – 3.18 mg kg-1) was above the local background value (BGV 0.28 mg kg-1; Romano et al. (2021)) almost along the whole sediment profile of the dead matte cores. Hg was marginally at the BGV only at the base of the cores. Hg and Hg:Al showed a major increase starting at around 65 y BP (after yr. 1950) and high accumulation during the 1960s and 1970s. On the contrary, Hg concentration was constantly below the BGV in the P. oceanica cores, with 0.02 – 0.20 mg kg-1 range and a 24-fold lower mean (0.08 ± 0.05 mg kg-1) than in the dead matte cores (1.84 ± 0.92 mg kg-1). Paired t-tests between pre- and post-industrial mean values for dead matte revealed a significant increase in Hg (t = 6.5, P < 0.05) and Hg:Al (t = -488, P < 0.001) and a significant depletion in the δ13C signal (t = − 10.6, P < 0.05) after 1950 (post-industrial period). By dividing the maximum Hg concentration in the post-industrial period with the median one during the pre-industrial period, we calculated an enrichment factor for Hg of 3.5 at the dead matte habitat.
Table 1 Range (min – max) and mean (± STDEV) values of sediment properties over the top 25 cm of substrate per habitat.
Figure 4 Vertical profiles of (A) dry bulk density, Corg, Cinorg and δ13C and (B) N, δ15N, Hg, Hg:Al with sediment depth for each core collected per habitat. Temporal trend for the last 120 years is also provided for dead matte and P. oceanica cores (only distribution with sediment depth is given after 120 yr. BP). The grey shaded area in the Hg profile indicates the local background value (BGV) of 0.28 mg kg-1 (Romano et al., 2021). The brown line indicates the onset of industrial activities in Augusta Bay, while the blue one indicates the commissioning of the demercurization plant and waste treatment. The green shaded area indicates the period of seagrass loss. Red asterisks indicate significant linear relationship with age for dead matte and P. oceanica cores and with depth for bare sediment cores (D, dead matte; B, bare sediment, P. oceanica meadow).
The range of DBD in bare sediment cores (0.40 – 1.18 g cm-3 and mean 0.83 ± 0.23 g cm-3) was higher than that in dead matte cores (Table 1). Both Corg and N concentrations showed an increase towards the surface of the bare sediment cores, but this increase was not as pronounced as in the case of the dead matte cores (Figure 4A). Hg concentration showed a different pattern from that found in the dead matte cores, with decreasing values towards the surface. Dead matte cores contained, on average, 12 times higher Corg (3.48 ± 1.15% DW), 5 times higher N (0.13 ± 0.02% DW), 5 times higher Hg (1.84 ± 0.92 mg kg-1), and 2 times higher δ15N values (2.25 ± 0.6 ‰) than bare sediments (Corg 0.30 ± 0.09% DW, N 0.03 ± 0.01% DW, Hg 0.36 ± 0.19 mg kg-1, δ15N 1.00 ± 0.6 ‰) (Figures 4A, B). Only Cinorg was higher in bare sediments (8.25 – 13. 37% DW, 11.03 ± 1.28% DW) than in dead matte (6.23 – 13.46% DW, 8.25 ± 1.51% DW).
Two principal components explained 72% of the total variability in the vertical profiles among the habitats (Figure 5). The three habitats are well separated along the PC1 axis, which accounted for 48% of total variability, while PC2, explaining 24% of total variability, separated P. oceanica meadow from the other two habitats. Dead matte was characterised by a higher silt/clay, Hg:Al and N content, while bare sediment had higher DBD and Cinorg content. PERMANOVA showed a strong clustering of habitats based on their corresponding profiles (Table S2, P < 0.001).
Figure 5 PCA biplot of the vertical profiles (standardized to the top 25 cm) of dry bulk density, grain size fractions (gravel, sand, silt/clay), and elements (Corg, Cinorg, N, δ13C, δ15N, Hg:Al) for all habitats.
Dead matte supported on average 7 times higher Corg stock (4.08 ± 2.10 kg Corg m-2) and 3 times higher N stock (0.14 ± 0.04 kg N m-2) than bare sediment (0.55 ± 22.13 kg Corg m-2, 0.05 ± 0.01 kg N m-2) in the top 25 cm of sediment (Table 2). Dead matte also supported higher Corg and N stocks than P. oceanica (2.88 ± 0.53 kg Corg m-2, 0.07 ± 0.01 kg N m-2), but the difference was not that pronounced. Bare sediments supported the highest Cinorg stocks (22.13 ± 3.04 kg Cinorg m-2), with 3-fold higher values than in both dead matte (8.85 ± 1.97 kg Cinorg m-2) and P. oceanica (10.89 ± 4.7 kg Cinorg m-2). Mean stocks extrapolated in the top meter in dead matte were 179 ± 118 Mg Corg ha-1, 399 ± 113 Mg Cinorg ha-1 and 6.1 ± 2.3 Mg N ha-1, in P. oceanica were 124 ± 20 Mg Corg ha-1, 457 ± 178 Mg Cinorg ha-1 and 3.1 ± 0.7 Mg N ha-1 and in bare sediments were 24 ± 6 Mg Corg ha-1, 977 ± 164 Mg Cinorg ha-1 and 2.1 ± 0.2 Mg N ha-1. The difference in Hg stock between habitats was very high, with dead matte (0.19 ± 0.04 g Hg m-2) having 2-fold higher mean than that in bare sediment (0.09 ± 0.01 g Hg m-2) and 26-fold higher than that in P. oceanica (0.007 ± 0.004 g Hg m-2).
The mixing model outcome indicated that the contribution of different sources to the organic carbon was similar between the dead matte and P. oceanica meadow, while it differed at the bare sediment (Figure S1). Seagrass detritus contributed on average 32% and 36% in dead matte and P. oceanica meadow, respectively. The overall contribution of other sources (i.e., macroalgae D. polyploides and C. prolifera, and POM) to the organic carbon pool was higher than that of seagrass; macroalgae contributed in total 52% and 51% in dead matte and P. oceanica meadow, respectively, while POM contributed the remaining 16% and 12%, respectively. Macroalgae (D. polyploides and C. prolifera) and POM were the main contributors to the bare sediment (44% and 38%, respectively).
Over the last 120 years, the rate of accumulation of Corg and N was higher in dead matte (35.3 ± 19.6 g Corg m-2 y-1, 1.2 ± 0.4 g N m-2 y-1) than in P. oceanica (24.3 ± 3.7 g Corg m-2 y-1, 0.6 ± 0.1 g N m-2 y-1). The accumulation rate of Hg was especially higher in dead matte than that in P. oceanica (i.e., by 27-fold; 0.0017 ± 0.0004 g Hg m-2 y-1 and 0.00006 ± 0.00003 g Hg m-2 y-1 in dead matte and P. oceanica, respectively). The Cinorg accumulation rate was lower in the dead matte than in P. oceanica (74.4 ± 22.8 g Cinorg m-2 y-1 and 91.6 ± 33.9 g Cinorg m-2 y-1 in dead matte and P. oceanica, respectively). More than half of the stocks in the dead matte were accumulated after the onset of industrial activities, with a large part even after seagrass loss (Figure 6).
Figure 6 Temporal trend of Corg, Cinorg, N and Hg stocks in dead matte and P. oceanica cores over approx. the last 120 yr. Note the different scale in Hg stock.
Our data analysis revealed that dead P. oceanica matte is an important biogeochemical sink and a valid archive for reconstructing Hg contamination in Augusta Bay. The vertical and temporal trends in geochemical variables studied here reflect the change in environmental conditions following the onset of industrial development in Augusta Harbour after World War II. The release of contaminants from 1950 (Bellucci et al., 2012) and the concurrent human intervention in water circulation with the building of breakwaters in the early 1960s resulted in the accumulation of elements in the southern part of the bay (Sprovieri et al., 2011) and the subsequent higher post-industrial contamination levels in Priolo Bay through export of contaminants from Augusta Harbour (Di Leonardo et al., 2014).
Dead matte had high capacity to accumulate elements, as seen by the increase of Corg, N and δ15N values over the past 120 years and the higher N and Hg association with fine sediments shown by the PCA. Post-industrial concentrations in P. oceanica cores remained at pre-industrial levels, despite slight and occasional (i.e., not consistent among cores) increases in N, δ15N and Hg, which fall inside the natural temporal and spatial variability within seagrass meadows (Kennedy et al., 2010; Bonanno and Orlando-Bonaca, 2017). The strong separation of habitats shown in statistical analyses reflected their corresponding geochemical profiles, with organic-rich sediments of both Posidonia mattes supporting higher organic stocks, as opposed to the coarse, high density and inorganic-rich bare sediment. The concentration of Cinorg does not necessarily differ among seagrass and adjacent bare sediments (Mazarrasa et al., 2015) and the magnitude of Cinorg accumulation varies considerably among habitats and seagrass species, depending on the balance between carbonates precipitation, dissolution and sedimentation and the environmental conditions, implying that in the given study the lower Cinorg stocks in Posidonia mattes should reflect enhanced dissolution of carbonates in seagrass sediments (Saderne et al., 2019). The capacity of seagrass sediments to support larger organic stocks than bare sediments has been shown previously (e.g., (Serrano et al., 2016c; Thorhaug et al., 2017; Gullström et al., 2018), including P. oceanica meadows (Apostolaki et al., 2019). That capacity has been related to the intense metabolism (Duarte et al., 2010) and high trapping capacity of seagrass shoots (Gacia and Duarte, 2001) and the slow decay of seagrass sediments due to the recalcitrant nature of P. oceanica detritus (Mateo et al., 1997; Kaal et al., 2018). This is the first study though to show that, even when dead, the P. oceanica matte maintained high Corg and N stocks. The dead matte accumulated sediments at a rate (1.9 ± 0.9 mm y-1, on average) close to the mean reported for the species (2.1 ± 0.4 mm y-1; Serrano et al. (2016b)) and supported stocks in the top meter that were inside the range reported for other P. oceanica meadows in the western and eastern parts of the Mediterranean basin (Mazarrasa et al., 2017b; Apostolaki et al., 2019). The stocks were standardized to 1 m sediment thickness for the sake of comparison between habitats studied here and elsewhere, but normalization by a period of accumulation rather than depth should be more effective in providing robust comparisons. Unfortunately, the absence of a valid chronological record from bare sediment cores precluded unvegetated sediments from this comparison. The upper ~10 cm of sediment in bare sediment cores seemed to be eroded or mixed rapidly, indicative of low retention capacity of superficial sandy unvegetated sediments (Thorhaug et al., 2017). However, the comparison of Posidonia mattes for the past 120 years revealed that dead matte retained its sink capacity even 30 years after the death of the shoots.
Other studies that examined the effect of decline or complete loss of other seagrass species on BC storage have reported extensive decreases in stocks (Marbà et al., 2015; Dahl et al., 2016; Thorhaug et al., 2017; Arias-Ortiz et al., 2018; Trevathan‐Tackett et al., 2017; Githaiga et al., 2019; Salinas et al., 2020; Moksnes et al., 2021). These decreases are usually attributed to erosion following canopy loss and the subsequent enhancement in mineralization of previously buried Corg under newly oxic conditions. In our case, however, erosion of dead matte had been minimal, as seen by the comparison of 210Pbexcess inventories across Posidonia matte cores. It should be noted here that differences in sedimentary setting and sediment composition, and possibly in 210Pb supply dynamics between Priolo Bay and Xifonio Gulf presumably yielded different 210Pbexcess inventories and therefore the use of the 210Pbexcess inventory of Xifonio Gulf as a reference for assessing possible erosion should be considered with caution. Nevertheless, the sedimentary record stretches to the present day, and, based on elemental and isotopic compositions and profiles, it seems that there has been continuous sediment accumulation in dead matte. In fact, there have been other occasions where no erosion was detected following cover loss of several seagrass species, including P. oceanica (Macreadie et al., 2014; Salinas et al., 2020; Piñeiro-Juncal et al., 2021). The fate of stocks following disturbance in BC ecosystems is not thoroughly understood (Spivak et al., 2019) and the magnitude of elemental loss will ultimately depend on the interplay between local environmental settings and biogeochemical conditions that drive the balance between decomposition and preservation of organic matter. Here, it appears that the chronic loading of effluents from intensive industrial activities increased the sediment pools and gradually resulted in loss of foliar canopy but did not disrupt the matte in the given short time period. The specific geomorphology of the dead matte area with mild hydrodynamics (ICRAM, 2008) and moderate depth, where the wave-orbital motions potentially causing sediment resuspension and erosion are expected to be limited (Fonseca and Bell, 1998; Samper‐Villarreal et al., 2016), and the establishment of the breakwaters, which further attenuated water movement (Sprovieri et al., 2011), provided protection from physical disturbance and significant erosion. The absence of mechanical damage such as anchoring, that can lead to extensive erosion of dead matte patches (Abadie et al., 2016), also provided a degree of physical protection. Although we have not measured the mineralization rate of sedimentary organic pools, available data from the area show microbial degradation of organic matter (Oliveri et al., 2016). It appears that any microbial priming and increase in efflux of CO2 (Trevathan-Tackett et al., 2018) must have been counterbalanced by the continuous deposition of organic matter and the recalcitrant nature of seagrass detritus, limiting the long-term loss of sediment Corg. A similar situation has been described in fish-farm impacted seagrass sediments, where continuous deposition of fish farm effluents had led to enhancement of mineralization and efflux of CO2 and dissolved nitrogen (Apostolaki et al., 2010) and at the same time to increase in the sedimentary organic matter pools (Apostolaki et al., 2011), suggesting that continuous deposition may outpace the deriving effluxes or that there may be a time lag between efflux and considerable decrease in organic matter pools. Perhaps this could be a unique characteristic of P. oceanica dead matte, owing to its intrinsic properties where rhizomes and roots promote stability (Dahl et al., 2021; Piñeiro-Juncal et al., 2021) while slow decay rates (Mateo et al., 1997) and refractory sediment organic matter (Kaal et al., 2018) limit the loss of stored elements even for several decades after seagrass loss, at least where the interaction between the environmental conditions and the physical setting does not induce the erosional process.
The colonization of the dead matte by macroalgae may have partly contributed to carbon preservation and further sequestration in dead matte cores. Dead P. oceanica mattes are often colonized by other seagrass (Cymodocea nodosa and Halophila stipulacea) and/or macroalgae with root-like rhizoids (e.g., Caulerpa spp.) (Boudouresque et al., 2015), that may contribute both to metabolic production and trapping of elements through their leaves, and to the stabilization of the upper layers of the matte through their roots and rhizoids. Indeed, macroalgae contributed around 50% to the organic matter pool in dead matte, and macroalgae like C. prolifera found here possess a high capacity to trap allochthonous particles (Hendriks et al., 2009). The depletion of δ13C signal found in post-industrial period also suggests enhancement in trapping of allochthonous material towards the present, which typically has more 13C-depleted values than seagrasses, and reflects nutrient enrichment in the area, as also seen in other meadows under human pressure (Mazarrasa et al., 2017a). Additional studies are necessary to assess the contribution of other macrophytes to the storage capacity and fate of carbon following colonization of dead matte by different macrophytes, especially in the context of changing seagrass biogeography of the Mediterranean where C. nodosa and H. stipulacea are expected to expand (Chefaoui et al., 2016; Wesselmann et al., 2021a) contributing to the BC storage in the region (Apostolaki et al., 2019; Wesselmann et al., 2021b).
The 2.5-fold increase in Hg levels of the dead matte and the enrichment factor of 3.5 in the post-industrial period indicates the severe contamination by Hg in Priolo Bay and reflects the capacity of dead matte to serve as an environmental archive, similar to the case of living Posidonia mattes (Serrano et al., 2011; Serrano et al., 2016a; Lafratta et al., 2019). The vertical resolution of the dead matte cores was 6 – 9 years which provided a sufficient record to reconstruct the history of contamination. The temporal variation of Hg aligns well with the described pattern of Hg in the area from unvegetated sediments (Sprovieri et al., 2011; Bellucci et al., 2012; Di Leonardo et al., 2014; Romano et al., 2021), showing an initial increase after the 1950s, and a further increment between the 1960s and 1980s with a peak around 1970 – 1975. A declining concentration trend was seen after the 1980s which followed the establishment of demercurization and waste treatment plants in 1983 (Bellucci et al., 2012). Hg and Corg concentrations were positively interrelated (P < 0.01) in dead matte, showing the strong binding of Hg in organic-rich superficial seagrass sediments (Serrano et al., 2013). The capacity of bioaccumulation by seagrasses has been well documented (Sanz-Lazaro et al., 2012; Malea et al., 2019) and it is believed to be the result of active uptake from leaves and roots and absorption in seagrass detritus after burial, as well as trapping of metal-bearing particles from the water column. However, despite the correlation between the two elements, normalization of Hg to Corg clearly indicates the high accumulation of Hg during the 1960 – 1980 period, suggesting that the increase in Hg is not merely controlled by the availability of organic matter. In fact, Hg in the area is mainly immobilized and is available predominantly as Hg0 and secondarily as HgS, while only a minor part (< 2%) accounts for the labile fraction (Oliveri et al., 2016). The disproportion of Hg with organic matter is indicative of highly contaminated areas with large quantities of anthropogenically derived Hg, such as Augusta Bay (Di Leonardo et al., 2007; Oliveri et al., 2016). High values of Hg have been reported from other industrial-impacted P. oceanica meadows [0.56 ± 0.14 mg kg-1, Lafabrie et al. (2007); 1.79 ± 0.01 mg kg-1, Di Leo et al. (2013)]. However, in our case even after remedial measures the Hg levels remained high and certainly higher than the BGV of 0.28 mg kg-1 (Romano et al., 2021), with a mean value of 2.55 ± 0.44 mg kg-1. Recent increases or still high Hg concentrations locally in Augusta Harbour have been measured before, mainly in unvegetated sediments (Bellucci et al., 2012; Romano et al., 2021) but also in a dead matte core once (Di Leonardo et al., 2017) and have been attributed to the mobilization of polluted sediment caused by dredging and ship traffic (Bellucci et al., 2012). It is important to note here that pre-industrial Hg levels in Priolo Bay (dead matte area) were already higher than BGV (1.02 ± 0.51 mg kg-1), which is in accordance with previous measurements in unvegetated sediments (Sprovieri et al., 2011; Bellucci et al., 2012; Di Leonardo et al., 2014; Romano et al., 2021) and dead matte (Di Leonardo et al., 2017) from the area. This suggests that there had already been human development in the area and that the pre-industrial period considered here is relatively short (~ 55 years) and certainly not representative of baseline conditions, as shown in samples older than 2800 14C yr BP in P. oceanica matte from the northwest Mediterranean, where Hg concentration was as low as 0.007 ± 0.001 mg kg-1 (Serrano et al., 2013).
The coarse, high density and organic-poor unvegetated sediments showed limited capacity to retain Hg, as seen by the Hg profile and the 5-fold lower mean value in bare sediments compared to that in dead matte. Hg was at the BVG throughout the top 10 cm while it increased above BGV at the 10 – 20 cm sediment thickness (mean 0.57 ± 0.2 mg kg-1, median 0.47 mg kg-1), indicating the resuspension of surface sediments and the subsequent accumulation of Hg in deeper sediments. Nevertheless, the 5-fold higher Hg concentration of bare sediment compared to P. oceanica meadow in Xifonio Gulf showed the severe contamination of Priolo Bay and the lack of correlation between Corg and Hg in bare sediments points to the industrially derived loading of Hg. The analysis of Hg content in P. oceanica leaves from Xifonio Gulf clearly shows that the meadow is not subjected to contamination by Hg, with a mean content of 0.09 ± 0.016 mg kg-1 (S. Vizzini, unpubl. data) close to the mean reported for leaves from areas with minimal contamination (0.04 ± 0.00 mg kg-1, Lafabrie et al. (2008)) and generally close to the low end of the range reported for P. oceanica leaves in the Mediterranean Sea (0.01 – 0.27 mg kg-1, Bonanno and Orlando-Bonaca (2017)). Similarly, the mean Hg concentration in the P. oceanica sediments (0.08 ± 0.05 mg kg-1) fell closer to the lower values reported from P. oceanica rhizosphere (0.02 – 1.79 mg kg-1, Bonanno and Orlando-Bonaca (2017)), albeit, as also seen in the case of dead matte, this is higher than what are considered pre-anthropogenic levels (Serrano et al., 2013).
The two dead matte cores showed small differences in concentrations, stocks, and accumulation rates of elements, possibly reflecting the spatial variation in deposition within the dead matte. Nevertheless, the variables that are indicative of nutrient enrichment (i.e., Corg, N, δ15N) had similar temporal trends between the replicate cores, with increasing values towards the present, while the temporal trends in Hg followed the well described pattern of Hg accumulation in the Bay. Furthermore, their mean values were higher than the corresponding means in P. oceanica and bare sediment cores, indicating that the changes observed in the dead matte over the last 65 years were related mainly to the increase in nutrient and Hg deposition from human activities in Augusta Harbour and less to changes in the composition of sediment.
The interpretation of our data suggests that dead P. oceanica mattes act as biogeochemical sinks and may be used as archives to identify environmental and ecosystem shifts in areas with unknown environmental history, reinforcing the paramount importance of seagrasses, and P. oceanica in particular, also after their death. Potential losses of Corg and N due to an increase in mineralization following seagrass loss must have been decelerated by the physical setting and/or counterbalanced by chronic loading from industrial activities and allochthonous sequestration. However, detailed studies are needed to elucidate the fate of carbon following natural recolonization of the matte by different macrophytes and, therefore, to evaluate the opportunity for active recolonization actions (i.e., plant transplantation) aimed at the stabilisation of sediment and associated nutrients and contaminants. Further studies are also necessary to determine the decomposition rate, and CO2 and nutrient fluxes of dead matte and consequent risk of release of stored Corg and pollutants following possible element mobilization. In this framework, new management schemes for highly contaminated areas should be elaborated and prioritized to prevent pollutant and carbon release, including the protection and conservation of dead mattes, and the development of adequate remediation actions (e.g., seagrass restoration) avoiding sediment dredging and mobilization, practices widely advocated and adopted so far.
The raw data supporting the conclusions of this article will be made available by the authors, without undue reservation.
SV and AM conceived the research idea and designed the sampling. SV and CT conducted field work. AC and CT performed the laboratory analyses. EA and LC analyzed the data. EA wrote the first draft of the manuscript. All authors contributed to the article and approved the submitted version.
The study was funded by the project TETIDE, PON01_03112, Italian Ministry of University and Research (MIUR) and by the project Marine Hazard, PON03PE_00203_1, Italian Ministry of Education, University and Research (MIUR).
The authors declare that the research was conducted in the absence of any commercial or financial relationships that could be construed as a potential conflict of interest.
All claims expressed in this article are solely those of the authors and do not necessarily represent those of their affiliated organizations, or those of the publisher, the editors and the reviewers. Any product that may be evaluated in this article, or claim that may be made by its manufacturer, is not guaranteed or endorsed by the publisher.
We thank Andrea Savona, Antonino M. Vaccaro and Rossella Di Leonardo for sampling, Adele E. Aleo for helping with laboratory analyses, and Victoria Litsi Mizan for helping with the graphical representation of the results. AC is grateful to colleagues in GAU-Radioanalytical, University of Southampton, for radiochemical analysis. We highly acknowledge constructive criticism by the three reviewers.
The Supplementary Material for this article can be found online at: https://www.frontiersin.org/articles/10.3389/fmars.2022.861998/full#supplementary-material
Abadie A., Lejeune P., Pergent G., Gobert S. (2016). From Mechanical to Chemical Impact of Anchoring in Seagrasses: The Premises of Anthropogenic Patch Generation in Posidonia Oceanica Meadows. Mar. Pollut. Bull. 109, 61–71. doi: 10.1016/j.marpolbul.2016.06.022
Ackermann F. (1980). A Procedure for Correcting Grain Size Effect in Heavy Metal Analysis of Estuarine and Coastal Sediments. Env. Tech. Lett. 1, 518–527. doi: 10.1080/09593338009384008
Aitchison J. (1986). The Statistical Analysis of Compositional Data (London: Chapman and Hall Ltd.), 416.
Anderson M. J. (2001). A New Method for Nonparametric Multivariate Analysis of Variance. Austral Ecol. 2632, 46. doi: 10.1111/j.1442-9993.2001.01070.pp.x
Apostolaki E. T., Holmer M., Marba N., Karakassis I. (2010). Degrading Seagrass (Posidonia Oceanica) Ecosystems: A Source of Dissolved Matter to the Mediterranean. Hydrobiologia 649, 13–23. doi: 10.1007/s10750-010-0255-2
Apostolaki E. T., Holmer M., Marba N., Karakassis I. (2011). Reduced Carbon Sequestration in a Mediterranean Seagrass (Posidonia Oceanica) Ecosystem Impacted by Fish Farming. Aquac. Environ. Interact. 2, 49–59. doi: 10.3354/aei00031
Apostolaki E. T., Vizzini S., Santinelli V., Kaberi H., Andolina C., Papathanassiou E. (2019). Exotic Halophila Stipulacea is an Introduced Carbon Sink for the Eastern Mediterranean Sea. Sci. Rep-uk. 9, 9643. doi: 10.1038/s41598-019-45046-w
Appleby P. G., Oldfield F. (1978). The Calculation of Lead-210 Dates Assuming a Constant Rate of Supply of Unsupported 210Pb to the Sediment. Catena 5, 1–8. doi: 10.1016/S0341-8162(78)80002-2
Arias-Ortiz A., Serrano O., Masque P., Lavery P. S., Mueller U., Kendrick G. A., et al. (2018). A Marine Heatwave Drives Massive Losses From the World’s Largest Seagrass Carbon Stocks. Nat. Clim. Change 81, 9. doi: 10.1038/s41558-018-0096-y
Bellucci L. G., Giuliani S., Romano S., Albertazzi S., Mugnai C., Frignani M. (2012). An Integrated Approach to the Assessment of Pollutant Delivery Chronologies to Impacted Areas: Hg in the Augusta Bay (Italy). Environ. Sci. Technol. 46, 2040–2046. doi: 10.1021/es203054c
Bonanno G., Orlando-Bonaca M. (2017). Trace Elements in Mediterranean Seagrasses: Accumulation, Tolerance and Biomonitoring. A Review. Mar. Pollut. Bull. 125, 8–18. doi: 10.1016/j.marpolbul.2017.10.078
Borg J. A., Rowden A. A., Attrill M. J., Schembri P. J., Jones M. B. (2006). Wanted Dead or Alive: High Diversity of Macroinvertebrates Associated With Living and “Dead” Posidonia Oceanica Matte. Mar. Biol. 149, 667–677. doi: 10.1007/S00227-006-0250-3
Boudouresque C. F., Pergent G., Pergent-Martini C., Ruitton S., Thibaut T., Verlaque M. (2015). The Necromass of the Posidonia Oceanica Seagrass Meadow: Fate, Role, Ecosystem Services and Vulnerability. Hydrobiologia 781, 1–18. doi: 10.1007/s10750-015-2333-y
Calvo S., Calvo R., Luzzu F., Raimondi V., Assenzo M., Cassetti F. P., et al. (2021). Performance Assessment of Posidonia Oceanica (L.) Delile Restoration Experiment on Dead Matte Twelve Years After Planting—Structural and Functional Meadow Features. Water-sui 13, 724. doi: 10.3390/w13050724
Chefaoui R. M., Assis J., Duarte C. M. (2016). And Serrão, ELarge-Scale Prediction of Seagrass Distribution Integrating Landscape Metrics and Environmental Factors: The Case of Cymodocea Nodosa (Mediterranean–Atlantic). A. Ecol. Model 39, 123–137. doi: 10.1016/s0304-3800(02)00199-0
Costantini C. (2015). Ripristino Dei Fondali Degradati E Recupero Del Paesaggio Sommerso Nell’ Area Mediterranea. PhD Thesis (Italy: Univerisita di Palermo).
Cundy A. B., Croudace I. W. (1996). Sediment Accretion and Recent Sea-Level Rise in the Solent, Southern England: Inferences From Radiometric and Geochemical Studies. Estuar. Coast. Shelf. Sci. 43, 449–467. doi: 10.1006/ecss.1996.0081
Dahl M., Björk M., Gullström M. (2021). Effects of Seagrass Overgrazing on Sediment Erosion and Carbon Sink Capacity: Current Understanding and Future Priorities. Limnol. Oceanogr. Lett. 6, 309–319. doi: 10.1002/lol2.10211
Dahl M., Deyanova D., Lyimo L. D., Näslund J., Samuelsson G. S., Mtolera M. S. P., et al. (2016). Effects of Shading and Simulated Grazing on Carbon Sequestration in a Tropical Seagrass Meadow. J. Ecol. 104, 654–664. doi: 10.1111/1365-2745.12564
de los Santos C. B., Krause-Jensen D., Alcoverro T., Marbà N., Duarte C. M., Katwijk M. M., et al. (20193356). Recent Trend Reversal for Declining European Seagrass Meadows. Nat. Commun. 10, 3356. doi: 10.1038/s41467-019-11340-4
Di Leo A., Annicchiarico C., Cardellichio N. (2013). Trace Metal Distributions in Posidonia Oceanica and Sediments From Taranto Gulf (Ionian Sea, Southern Italy). Mediterr. Mar. Sci. 14, 204–213. doi: 10.12681/mms.316
Di Leonardo R., Bellanca A., Capotondi L., Cundy A., Neri R. (2007). Possible Impacts of Hg and PAH Contamination on Benthic Foraminiferal Assemblages: An Example From the Sicilian Coast, Central Mediterranean. Sci. Total Environ. 388, 168–183. doi: 10.1016/j.scitotenv.2007.08.009
Di Leonardo R., Mazzola A., Cundy A. B., Tramati C. D., Vizzini S. (2017). Trace Element Storage Capacity of Sediments in Dead Posidonia Oceanica Mat From a Chronically Contaminated Marine Ecosystem. Environ. Toxicol. Chem. 36, 49–58. doi: 10.1002/etc.3539
Di Leonardo R., Mazzola A., Tramati C. D., Vaccaro A., Vizzini S. (2014). Highly Contaminated Areas as Sources of Pollution for Adjoining Ecosystems: The Case of Augusta Bay (Central Mediterranean). Mar. Pollut. Bull. 89, 417–426. doi: 10.1016/j.marpolbul.2014.10.023
Duarte C. M., Kennedy H., Marbà N., Hendriks I. (2013). Assessing the Capacity of Seagrass Meadows for Carbon Burial: Current Limitations and Future Strategies. Ocean Coast. Manage 83, 32–38. doi: 10.1016/j.ocecoaman.2011.09.001
Duarte C. M., Marba N., Gacia E., Fourqurean J. W., Beggins J., Barrón C., et al. (2010). Seagrass Community Metabolism: Assessing the Carbon Sink Capacity of Seagrass Meadows. Global Biogeochem. Cy 24, GB4032. doi: 10.1029-2010GB003793
Flynn W. W. (1968). The Determination of Low Levels of Polonium-210 in Environmental Materials. Anal. Chim. Acta 43, 221–227. doi: 10.1016/s0003-2670(00)89210-7
Fonseca M., Bell S. (1998). Influence of Physical Setting on Seagrass Landscapes Near Beaufort, North Carolina, USA. Mar. Ecol. Prog. Ser. 171, 109–121. doi: 10.3354/meps171109
Gacia E., Duarte C. M. (2001). Sediment Retention by a Mediterranean Posidonia Oceanica Meadow: The Balance Between Deposition and Resuspension. Estuar. Coastal Shelf. Sci. 52, 505–514. doi: 10.1006/ecss.2000.0753
Gacia E., Duarte C. M., Middelburg J. J. (2002). Carbon and Nutrient Deposition in a Mediterranean Seagrass (Posidonia Oceanica) Meadow. Limnol. Oceanogr. 47, 23–32. doi: 10.4319/lo.2002.47.1.0023
Githaiga M. N., Frouws A. M., Kairo J. G., Huxham M. (2019). Seagrass Removal Leads to Rapid Changes in Fauna and Loss of Carbon. Front. Ecol. Evol. 7. doi: 10.3389/fevo.2019.00062
Gullström M., Lyimo L. D., Dahl M., Samuelsson G. S., Eggertsen M., Anderberg E., et al. (2018). Blue Carbon Storage in Tropical Seagrass Meadows Relates to Carbonate Stock Dynamics, Plant–Sediment Processes, and Landscape Context: Insights From the Western Indian Ocean. Ecosystems 21551, 566. doi: 10.1007/s10021-017-0170-8
Hendriks I. E., Bouma T. J., Morris E. P. (2009). Effects of Seagrasses and Algae of the Caulerpa Family on Hydrodynamics and Particle-Trapping Rates. Mar. Biol. 157, 473–481. doi: 10.1007/S00227-009-1333-8
Lo Iacono C., Mateo M. A., Gràcia E., Guasch L., Carbonell R., Serrano L., et al. (2008). Very High-Resolution Seismo-Acoustic Imaging of Seagrass Meadows (Mediterranean Sea): Implications for Carbon Sink Estimates. Geophys. Res. Lett. 35, L18601. doi: 10.1029/2008gl034773
ICRAM-Istituto Centrale per la Ricerca scientifica e tecnologica Applicata al Mare (2008). Progetto Preliminare Di Bonifica Dei Fondali Della Rada Di Augusta Nel Sito Di Interesse Nazionale Di Priolo—Elaborazione Definitiva. (Rome: ICRAM) 182, BoI-Pr-SI-PR-Rada di Augusta-03.22.
Kaal J., Serrano O., del Río J. C., Rencoret J. (2018). Radically Different Lignin Composition in Posidonia Species may Link to Differences in Organic Carbon Sequestration Capacity. Org. Geochem. 124, 247–256. doi: 10.1016/j.orggeochem.2018.07.017
Kennedy H., Beggins J., Duarte C. M., Fourqurean J. W., Holmer M., Marba N., et al. (20104026). Seagrass Sediments as a Global Carbon Sink: Isotopic Constraints. Global Biogeochem. Cy 24, GB4026. doi: 10.1029/2010GB003848
Lafabrie C., Pergent G., Kantin R., Pergent-Martini C., Gonzalez J. L. (2007). Trace Metals Assessment in Water, Sediment, Mussel and Seagrass Species - Validation of the Use of Posidonia Oceanica as a Metal Biomonitor. Chemosphere 68, 2033–2039. doi: 10.1016/j.chemosphere.2007.02.039
Lafabrie C., Pergent-Martini C., Pergent G. (2008). Metal Contamination of Posidonia Oceanica Meadows Along the Corsican Coastline (Mediterranean). Environ. Pollut. 151, 262–268. doi: 10.1016/j.envpol.2007.01.047
Lafratta A., Serrano O., Masqué P., Mateo M. A., Fernandes M., Gaylard S., et al. (2019). Seagrass Soil Archives Reveal Centennial-Scale Metal Smelter Contamination While Acting as Natural Filters. Sci. Total Environ. 649, 1381–1392. doi: 10.1016/j.scitotenv.2018.08.400
Lavery P. S., Mateo M.-Á., Serrano O., Rozaimi M. (2013). Variability in the Carbon Storage of Seagrass Habitats and Its Implications for Global Estimates of Blue Carbon Ecosystem Service. PloS One 8, e73748. doi: 10.1371/journal.pone.0073748.t008
Leiva-Dueñas C., Cortizas A. M., Piñeiro-Juncal N., Díaz-Almela E., Garcia-Orellana J., Mateo M. A. (2021). Long-Term Dynamics of Production in Western Mediterranean Seagrass Meadows: Trade-Offs and Legacies of Past Disturbances. Sci. Total Environ. 754, 142117. doi: 10.1016/j.scitotenv.2020.142117
Lovelock C. E., Atwood T., Baldock J., Duarte C. M., Hickey S., Lavery P. S., et al. (2017). Assessing the Risk of Carbon Dioxide Emissions From Blue Carbon Ecosystems. Mar. Policy 15257, 265. doi: 10.1016/j.marpol.2015.12.020
Macreadie P. I., Anton A., Raven J. A., Beaumont N., Connolly R. M., Friess D. A., et al. (2019). The Future of Blue Carbon Science. Nat. Commun. 10, 3998. doi: 10.1038/s41467-019-11693-w
Macreadie P. I., Costa M. D. P., Atwood T. B., Friess D. A., Kelleway J. J., Kennedy H., et al. (2021). Blue Carbon as a Natural Climate Solution. Nat. Rev. Earth Environ. 2, 826–839. doi: 10.1038/s43017-021-00224-1
Macreadie P. I., York P. H., Sherman C. D. H., Keough M. J., Ross D. J., Ricart A. M., et al. (2014). No Detectable Impact of Small-Scale Disturbances on ‘Blue Carbon’ Within Seagrass Beds. Mar. Biol. 161, 2939–2944. doi: 10.1007/s00227-014-2558-8
Malea P., Mylona Z., Kevrekidis T. (2019). Improving the Utility of the Seagrass Posidonia Oceanica as a Biological Indicator of Past Trace Element Contamination. Ecol. Indic. 107, 105596. doi: 10.1016/j.ecolind.2019.105596
Marbà N., Arias-Ortiz A., Masqué P., Kendrick G. A., Mazarrasa I., Bastyan G. R., et al. (2015). Impact of Seagrass Loss and Subsequent Revegetation on Carbon Sequestration and Stocks. J. Ecol. 103, 296–302. doi: 10.1111/1365-2745.12370
Mateo M. A., Romero J., Perez M., Littler M. M., Littler D. S. (1997). Dynamics of Millenary Organic Deposits Resulting From the Growth of the Mediterranean Seagrass Posidonia Oceanica. Estuar. Coastal Shelf. Sci. 44, 103 110. doi: 10.1006/ecss.1996.0116
Mazarrasa I., Marbà N., Garcia-Orellana J., Masqué P., Arias-Ortiz A., Duarte C. M. (2017a). Dynamics of Carbon Sources Supporting Burial in Seagrass Sediments Under Increasing Anthropogenic Pressure. Plant Physiol. 62, 1451–1465. doi: 10.1104/pp.115.2.599
Mazarrasa I., Marbà N., Garcia-Orellana J., Masqué P., Arias-Ortiz A., Duarte C. M. (2017b). Effect of Environmental Factors (Wave Exposure and Depth) and Anthropogenic Pressure in the C Sink Capacity of Posidonia Oceanica Meadows. Proc. Natl. Acad. Sci. 62, 1436–1450. doi: 10.1073/pnas.0905620106
Mazarrasa I., Marba N., Lovelock C. E., Serrano O., Lavery P. S., Fourqurean J. W., et al. (2015). Seagrass Meadows as a Globally Significant Carbonate Reservoir. Biogeosci. Discussions 124107, 4138. doi: 10.5194/bgd-12-4993-2015
Mazarrasa I., Samper-Villarreal J., Serrano O., Lavery P. S., Lovelock C. E., Marbà N., et al. (2018). Habitat Characteristics Provide Insights of Carbon Storage in Seagrass Meadows. Mar. Pollut. Bull. 134106, 117. doi: 10.1016/j.marpolbul.2018.01.059
Moksnes P., Röhr M. E., Holmer M., Eklöf J. S., Eriander L., Infantes E., et al. (2021). Major Impacts and Societal Costs of Seagrass Loss on Sediment Carbon and Nitrogen Stocks. Ecosphere 12, e03658. doi: 10.1002/ecs2.3658
Monnier B., Pergent G., Mateo M.Á., Carbonell R., Clabaut P., Pergent-Martini C. (2021). Sizing the Carbon Sink Associated With Posidonia Oceanica Seagrass Meadows Using Very High-Resolution Seismic Reflection Imaging. Mar. Environ. Res. 170, 105415. doi: 10.1016/j.marenvres.2021.105415
Nieuwenhuize J., Maas Y. E. M., Middelburg J. J. (1994). Rapid Analysis of Organic Carbon and Nitrogen in Particulate Materials. Mar. Chem. 45, 217–224. doi: 10.1016/0304-4203(94)90005-1
Oliveri E., Manta D. S., Bonsignore M., Cappello S., Tranchida G., Bagnato E., et al. (2016). Mobility of Mercury in Contaminated Marine Sediments: Biogeochemical Pathways. Mar. Chem. 186, 1–10. doi: 10.1016/j.marchem.2016.07.002
Parnell A. C., Phillips D. L., Bearhop S., Semmens B. X., Ward E. J., Moore J. W., et al. (2013). Bayesian Stable Isotope Mixing Models. Environmetrics 24, 387–399. doi: 10.1002/env.2221
Pendleton L., Donato D. C., Murray B. C., Crooks S., Jenkins W. A., Sifleet S., et al. (2015). Estimating Global “Blue Carbon” Emissions From Conversion and Degradation of Vegetated Coastal Ecosystems. PloS One 7, e43542. doi: 10.1371/journal.pone.0043542
Piñeiro-Juncal N., Kaal J., Moreira J. C. F., Cortizas A. M., Lambais M. R., Otero X. L., et al. (2021). Cover Loss in a Seagrass Posidonia Oceanica Meadow Accelerates Soil Organic Matter Turnover and Alters Soil Prokaryotic Communities. Org. Geochem. 151, 104140. doi: 10.1016/j.orggeochem.2020.104140
R Core Team (2021) R: A Language and Environment for Statistical Computing. Available at: https://www.R-project.org/.
Robbins J. A. (1978). “Geochemical and Geophysical Applications of Radioactive Lead,” in The Biogeochemistry of Lead in the Environment Part a. Ed. Nriagu J. O. (Amsterdam: Elsevier Scientific), 285–293.
Röhr M. E., Holmer M., Baum J. K., Björk M., Boyer K., Chin D., et al. (2018). Blue Carbon Storage Capacity of Temperate Eelgrass (Zostera Marina) Meadows. Global Biogeochem. Cy 32, 1457–1475. doi: 10.1029/2018gb005941
Romano E., Bergamin L., Croudace I. W., Pierfranceschi G., Sesta G., Ausili A. (2021). Measuring Anthropogenic Impacts on an Industrialised Coastal Marine Area Using Chemical and Textural Signatures in Sediments: A Case Study of Augusta Harbour (Sicily, Italy). Sci. Total Environ. 755, 142683. doi: 10.1016/j.scitotenv.2020.142683
Saderne V., Geraldi N. R., Macreadie P. I., Maher D. T., Middelburg J. J., Serrano O., et al. (20191106). Role of Carbonate Burial in Blue Carbon Budgets. Nat. Commun. 10, 1106. doi: 10.1038/s41467-019-08842-6
Salinas C., Duarte C. M., Lavery P. S., Masque P., Arias-Ortiz A., Leon J. X., et al. (2020). Seagrass Losses Since Mid-20th Century Fuelled CO2 Emissions From Soil Carbon Stocks. Global Change Biol. 26, 4772–4784. doi: 10.1111/gcb.15204
Samper-Villarreal J., Lovelock C. E., Saunders M. I., Roelfsema C., Mumby P. J. (2016). Organic Carbon in Seagrass Sediments is Influenced by Seagrass Canopy Complexity, Turbidity, Wave Height, and Water Depth. Limnol. Oceanogr. 61, 938–952. doi: 10.1002/lno.10262
Sanz-Lazaro C., Malea P., Apostolaki E. T., Kalantzi I., Marin A., Karakassis I. (2012). The Role of the Seagrass Posidonia Oceanica in the Cycling of Trace Elements. Biogeosciences 9, 2497–2507. doi: 10.5194/bg-9-2497-2012
Serrano O., Almahasheer H., Duarte C. M., Irigoien X. (2018). Carbon Stocks and Accumulation Rates in Red Sea Seagrass Meadows. Sci. Rep-uk. 8, 1 13. doi: 10.1038/s41598-018-33182-8
Serrano O., Davis G., Lavery P. S., Duarte C. M., Martinez-Cortizas A., Mateo M.-Á., et al. (2016a). Reconstruction of Centennial-Scale Fluxes of Chemical Elements in the Australian Coastal Environment Using Seagrass Archives. Sci. Total Environ. 541, 883–894. doi: 10.1016/j.scitotenv.2015.09.017
Serrano O., Lavery P. S., López-Merino L., Ballesteros E., Mateo M. A. (2016b). Location and Associated Carbon Storage of Erosional Escarpments of Seagrass Posidonia Mats. Front. Mar. Sci. 3. doi: 10.3389/fmars.2016.00042
Serrano O., Martínez-Cortizas A., Mateo M. A., Biester H., Bindler R. (2013). Millennial Scale Impact on the Marine Biogeochemical Cycle of Mercury From Early Mining on the Iberian Peninsula. Global Biogeochem. Cy 27, 21–30. doi: 10.1029/2012gb004296
Serrano O., Mateo M. A., Dueñas-Bohórquez A., Renom P., López-Sáez J. A., Cortizas A. M. (2011). The Posidonia Oceanica Marine Sedimentary Record: A Holocene Archive of Heavy Metal Pollution. Sci. Total Environ. 409, 4831 4840. doi: 10.1016/j.scitotenv.2011.08.001
Serrano O., Ricart A. M., Lavery P. S., Mateo M.-Á., Arias-Ortiz A., Masqué P., et al. (2016c). Key Biogeochemical Factors Affecting Soil Carbon Storage In Posidonia Meadows. Biogeosciences 134581, 4594. doi: 10.4225/75/56b2fd48b92d5
Signa G., Mazzola A., Tramati C. D., Vizzini S. (2017). Diet and Habitat Use Influence Hg and Cd Transfer to Fish and Consequent Biomagnification in a Highly Contaminated Area: Augusta Bay (Mediterranean Sea). Environ. Pollut. 230, 394–404. doi: 10.1016/j.envpol.2017.06.027
Sousa A. I., da Silva J. F., Azevedo A., Lillebø A. I. (2019). Blue Carbon Stock in Zostera Noltei Meadows at Ria De Aveiro Coastal Lagoon (Portugal) Over a Decade. Sci. Rep-uk. 9, 14387. doi: 10.1038/s41598-019-50425-4
Spivak A. C., Sanderman J., Bowen J. L., Canuel E. A., Hopkinson C. S. (2019). Global-Change Controls on Soil-Carbon Accumulation and Loss in Coastal Vegetated Ecosystems. Nat. Geosci. 12, 685–692. doi: 10.1038/s41561-019-0435-2
Sprovieri M., Oliveri E., Leonardo R. D., Romano E., Ausili A., Gabellini M., et al. (2011). The Key Role Played by the Augusta Basin (Southern Italy) in the Mercury Contamination of the Mediterranean Sea. J. Environ. Monitor 13, 1753–1760. doi: 10.1039/c0em00793e
Thorhaug A., Poulos H. M., López-Portillo J., Ku T. C. W., Berlyn G. P. (2017). Seagrass Blue Carbon Dynamics in the Gulf of Mexico: Stocks, Losses From Anthropogenic Disturbance, and Gains Through Seagrass Restoration. Sci. Total Environ., 605–606, 626–636. doi: 10.1016/j.scitotenv.2017.06.189
Trevathan-Tackett S. M., Thomson A. C. G., Ralph P. J., Macreadie P. I. (2018). Fresh Carbon Inputs to Seagrass Sediments Induce Variable Microbial Priming Responses. Sci. Total Environ. 621, 1–7. doi: 10.1016/j.scitotenv.2017.11.193
Trevathan-Tackett S. M., Wessel C., Cebrián J., Ralph P. J., Masqué P., Macreadie P. I. (2017). Effects of Small-Scale, Shading-Induced Seagrass Loss on Blue Carbon Storage: Implications for Management of Degraded Seagrass Ecosystems. I. J. Appl. Ecol. 55, 1351–1359. doi: 10.1111/1365-2664.13081
USEPA - United States Environmental Protection Agency (1996). Method 3052. Microwave Assisted Acid Digestion of Siliceous and Organically Based Matrices (Washington, DC: USEPA).
Wesselmann M., Chefaoui R. M., Marbà N., Serrao E. A., Duarte C. M. (2021a). Warming Threatens to Propel the Expansion of the Exotic Seagrass Halophila Stipulacea. Front. Mar. Sci. 8. doi: 10.3389/fmars.2021.759676
Keywords: blue carbon, nutrient filters, contamination, seagrass degradation, anthropogenic impact, seagrass archives
Citation: Apostolaki ET, Caviglia L, Santinelli V, Cundy AB, Tramati CD, Mazzola A and Vizzini S (2022) The Importance of Dead Seagrass (Posidonia oceanica) Matte as a Biogeochemical Sink. Front. Mar. Sci. 9:861998. doi: 10.3389/fmars.2022.861998
Received: 25 January 2022; Accepted: 07 March 2022;
Published: 28 March 2022.
Edited by:
Stacey Marie Trevathan-Tackett, Deakin University, AustraliaReviewed by:
Mohammad Rozaimi, Universiti Kebangsaan Malaysia, MalaysiaCopyright © 2022 Apostolaki, Caviglia, Santinelli, Cundy, Tramati, Mazzola and Vizzini. This is an open-access article distributed under the terms of the Creative Commons Attribution License (CC BY). The use, distribution or reproduction in other forums is permitted, provided the original author(s) and the copyright owner(s) are credited and that the original publication in this journal is cited, in accordance with accepted academic practice. No use, distribution or reproduction is permitted which does not comply with these terms.
*Correspondence: Eugenia T. Apostolaki, ZWFwb3N0QGhjbXIuZ3I=
Disclaimer: All claims expressed in this article are solely those of the authors and do not necessarily represent those of their affiliated organizations, or those of the publisher, the editors and the reviewers. Any product that may be evaluated in this article or claim that may be made by its manufacturer is not guaranteed or endorsed by the publisher.
Research integrity at Frontiers
Learn more about the work of our research integrity team to safeguard the quality of each article we publish.