- Tvärminne Zoological Station, University of Helsinki, Hanko, Finland
Climate change predictions indicate global changes in salinity with negative implications for plankton food webs; an important baseline for functioning of marine ecosystems. Current understanding of how salinity change will impact plankton communities is mostly limited to the salinization of freshwater environments, with little known about the effects of changing salinity in marine systems. In this study, we investigate the effect of salinity change on zooplankton communities under different salinity change scenarios of the Baltic Sea. Projections for future salinity change derived from regional physical-biogeochemical models were used to set-up an outdoor mesocosm experiment in the coastal area of the Gulf of Finland. Each mesocosm was inoculated with natural plankton using a mixture of both marine and freshwater communities, mimicking the natural influx of freshwater species from rivers into the Baltic Sea. Zooplankton diversity and composition changed possibly due to different salinity tolerances among the species. Among zooplankton, rotifers dominated in low salinities (74%) and cladocerans and copepods (69%) in high salinities. Our results suggest that the zooplankton community will shift to a rotifer dominated community in areas with declining salinity due to the intolerance of other zooplankton groups to freshening.
Introduction
The combined effects of climate change, habitat loss and degradation has put substantial strain on aquatic ecosystems (Travis, 2003). One specific pressure on both marine and freshwater environments globally includes changing salinity, as a result of direct human activity such as ‘salting’ roads (Schuler et al., 2017; Hintz et al., 2021) as well as climate change; predicted to affect precipitation and ice melt (Helm et al., 2010; Ross et al., 2021). Salinity change can have a profound impact on aquatic organisms, including altering the distribution, phenology, abundance, composition and trophic interactions of plankton (Intergovernmental Panel Climate Change, 2018).
Zooplankton play a key role in the pelagic food web. They link primary producers (i.e. phytoplankton) to higher trophic levels (such as fish), thus their abundance and community structure directly affect the dynamics of fisheries resources (Sommer et al., 2002) as well as the biodiversity of higher trophic levels such as birds and mammals. How zooplankton will be affected by climate warming has been the subject of great debate (Gyllström et al., 2005; Mackas et al., 2007; Lewandowska et al., 2014; Šorf et al., 2015). However, current understanding of how salinity change in aquatic environments will impact zooplankton communities is mostly limited to the salinization of freshwater environments (e.g. Hintz et al., 2017; Lin et al., 2017; Moffett et al., 2020), estuaries (e.g. Gao et al., 2008) or single-species responses (e.g. Cervetto et al., 1999), while community-level changes and consequences for food web interactions in marine environments remain poorly understood. Studies on the effects of salinization of freshwater environments, have reported a range of responses including, dominance shifts and disruption of trophic interactions (Lin et al., 2017; Gutierrez et al., 2018). These results imply that a similar response to salinity change could be expected in marine environments.
Changes in salinity may directly or indirectly influence zooplankton community composition, leading to the local extinction of some species and the appearance of others. Based on existing literature, zooplankton may be directly affected by salinity depending on their physiology and tolerance to salinity change (Nielsen et al., 2003; Schallenberg et al., 2003; Hall, 2004). Direct responses of zooplankton to stressful salinity conditions include disturbances in reproduction, development and growth (Hart et al., 2003; Santangelo et al., 2014; Bashevkin and Pechenik, 2015). Indirect impacts through trophic interactions are expected because salinity can influence the composition and physiology of phytoplankton (Flameling and Kromkamp, 1994; Bisson and Kirst, 1995; Ayadi, 2004; Chakraborty et al., 2011), affecting the nutritional food quality of zooplankton (van de de Waal et al., 2010) and causing or contributing to food shortages (Perumal et al., 2009). Alterations in zooplankton community composition as a response to salinity change are likely to have negative consequences on food availability and growth of planktivorous fish, such as herring and sprat (Möllmann et al., 2004). This in turn could affect higher trophic levels, such as cod, seabirds and mammals (Alvarez-Fernandez et al., 2015).
Trophic interactions in plankton communities often differ between marine and freshwater environments and thus also their response to environmental perturbations. A recent study by Murphy et al. (2020) showed that warming conditions had no effect on zooplankton density in marine environments, whereas it had a negative effect on zooplankton density and increased grazing by herbaceous zooplankton in freshwaters. Stronger predator-herbivore interactions were also found in freshwater plankton compared to marine plankton communities (Shurin et al., 2002). Sommer and Sommer (2006) argue the difference in the strength of trophic cascades between lentic and marine environments is caused by differences in the zooplankton-phytoplankton link. Cladocerans dominate in freshwaters, whereas copepods dominate in marine ecosystems. Contrary to filter feeders such as cladocerans, copepods can select their food particles based on prey size (Tiselius and Jonsson, 1990), enabling copepod-dominated marine communities to be more resilient to disturbances of trophic cascades due to their selective feeding.
Climate change projections indicate that an increase in precipitation in northern Europe coupled with faster rates of ice-melt will lead to a decline in salinity in northern marine environments (HELCOM, 2013). This includes the Baltic Sea, where salinity is predicted to decrease by 1.5 to 2 psu by the end of the century (Meier et al., 2006). These salinity changes have been shown to be a bottleneck for both marine and freshwater species distribution and diversity, which could have negative impacts on marine ecology, monitoring, modelling and fisheries (Vuorinen et al., 2015). However, these salinity predictions are often uncertain with large variations depending on which general circulation models (GCMs) are used for predictions (Saraiva et al., 2019; Blenckner et al., 2021). Differences in observed data can also depend on region and vertical stratification (Lehmann et al., 2021), such as in the Gulf of Finland, where surface salinity increased by 0.5 psu between 1927-2021 (Merkouriadi and Leppäranta, 2014). Other areas of the world, including the Chesapeake Bay and the Baltic Sea, are predicted to show increasing fluctuations in salinity with climate change (Muhling et al., 2017; Blenckner et al., 2021).
Following these predictions, we designed a salinity change experiment using floating mesocosm platform deployed in the Baltic Sea to investigate the direct and indirect effects of salinity change on zooplankton communities. The use of mesocosms is a well-established tool to explore plankton community responses to environmental change owing to the advantage of manipulation and replication (Benton et al., 2007; Woodward et al., 2010). The brackish environment of the Baltic Sea, where freshwater and marine plankton communities can interact (Telesh et al., 2008), is an ideal study location to investigate the effect of salinity change on natural plankton communities. The general aim of the study was to examine interactions of zooplankton communities under different scenarios of salinity change of the Baltic Sea. We hypothesized that:
(1) Zooplankton abundance will be negatively affected by physiological intolerance to changing salinity,
(2) Salinity change will reduce phytoplankton biomass due to oxidative stress leading to food limitation for zooplankton and altered trophic interactions, and
(3) Salinity change will alter community composition and diversity of zooplankton species depending on their tolerance to salinity; marine species will dominate in higher salinities and brackish and freshwater species in lower ones.
Insights from these findings are important to understand how zooplankton communities may differ under climate driven salinity change and the possible effects it may have on trophic transfer efficiency and overall ecosystem functioning.
Materials and Methods
Experimental Design and Sampling
We conducted a salinity change experiment during summer 2019 (July-September) using outdoor mesocosms to investigate the effect of freshening on phytoplankton abundance and zooplankton community composition in pelagic ecosystems. The experiment was set up offshore the Tvärminne Zoological Station, Finland (59° 50’ 40” N, 23° 14’ 57” E). It consisted of 12 transparent, manually mixed plastic (200 µm thick LDPE) enclosures (1600L volume, diameter 0.9 m, depth 2 m, conical bottom) and combined four salinity scenarios [3.5 (control, no salt addition); 5.5; 7.5; 9.5 psu] with three replicates. Salinity levels were based on predicted salinity change scenarios in the Baltic Sea from Meier et al. (2012) and downscaled to local conditions using data from the MONICOAST monitoring buoy deployed offshore the Tvärminne Zoological Station. On 29 July 2019, we partially filled each mesocosm with 800 L of water taken from Gennarbyviken reservoir (59° 55’ 23” N, 23° 12’ 20” E) due to its low salinity levels (0 psu) and presence of freshwater plankton species despite marine origin (the reservoir was enclosed from the sea in 1957). We then added 800 L of water to each mesocosm taken from the bay in front of the Tvärminne Zoological Station due to its marine conditions (5.5 psu) and associated marine plankton species. The water in the mesocosms was assessed using light microscope to make sure the plankton community had survived the water transport and the initial community composition was similar in all experimental units. On 212 and 213 day of the year (DOY) we added sea salt (Aquarium System Instant Ocean) in batches to the mesocosms to make up four different salinity treatments. We added the salt to each mesocosm by extracting 10 L of water and mixing the salt with the water until it was dissolved. We added the salt to each mesocosm in a slow, circular movement using a Secchi disk to ensure that the mixture was evenly dispersed. The control mesocosm was also disturbed using a Secchi disk to make sure all treatments have received the same agitation. The next day, we measured the salinity to ensure we had reached our salinity goals for each mesocosm. To prevent rainfall and allochthonous nutrient inputs (mainly bird droppings), transparent plastic lids were installed above each mesocosm with gaps to allow air circulation in the mesocosm.
Samples for bacterial and phytoplankton as well as nutrient concentrations were taken every two days. In this article, however, we concentrate on the effects of salinity on chlorophyll-a concentration and zooplankton community composition. We measured all abiotic and biotic parameters at 1m depth from the middle of the mesocosm. Temperature (°C), salinity, dissolved oxygen (mg O2/L) were measured every two days using a portable calibrated digital water meter (MU 6100 H, VWR). 4L of water from each mesocosm was taken every 2 days using a water sampler and placed into a 10L plastic container for transportation to the laboratory. The canister was stored in a cold room before gentle mixing was applied and samples for different uses were syphoned off from the container (e.g. chlorophyll-a, dissolved nutrients, bacteria, etc.). For zooplankton, samples were taken every 6 days as to not over-deplete zooplankton numbers compared to natural conditions. A 50μm mesh-size plankton net was dragged from 1m depth from the centre of each mesocosm and then flushed into 250 ml plastic bottles with 30% ethanol for transportation to the laboratory. Additional in situ measurements were taken to be able to compare experimental data to local conditions and assess the effect of enclosure (data not shown). The experiment was terminated after 30 days due to apparent damage to some of the mesocosm bags.
Response Variables
Chlorophyll-a concentration (μgL-1) was used as a surrogate of phytoplankton biomass. We vacuum-filtered 100-150 ml of water sample for each mesocosm though glass fibre filters (GF/F, Whatman, Inc., Massachusetts, USA). Filters were stored in the dark in -20°C to prevent chlorophyll breakdown. We later measured chlorophyll-a concentration in each filter using a fluorometer (Varian Inc., Cary Eclipse) after ethanol extraction.
To quantify zooplankton abundance, the sample was settled in 50 ml sedimentation chambers and counted using an Olympus CK30 at x10 and x40 magnification using an inverted microscope technique (Lund et al., 1958). Depending on the density of the sample, a Forsblom plankton splitter was used to split the sample into ¼, ½ or ¾ of the original sample. Identification to genus level (and species where possible) was made using Telesh et al. (2008). Abundance was measured as individuals per litre (ind.L-1). Few adult copepods were recorded, therefore we grouped adults and copepodites together in analyses. Nauplii were analysed separately as copepods exhibit different feeding modes during their life cycle (Brandl, 2005) and therefore may differ in their response to treatment effects. Zooplankton richness was quantified as number of species and zooplankton evenness (as opposite of dominance) was quantified using Pielou’s Index (Pielou, 1966). We measured how the community richness changed immediately after salt addition to see if there was an immediate effect of ‘salt shock’ on zooplankton community structure. No statistically significant change in richness was observed (treatment, F= 0.87, p= 0.4963, time, F= 4.20, p= 0.0650). All subsequent analyses are computed from the first sampling after salt addition (DOY 217).
Statistical Analysis
We conducted mixed effects models to test the effect of salinity change on chlorophyll-a concentration, zooplankton community structure (rotifers, cladocerans and copepods) and diversity indices (richness, Pielou’s evenness). Chlorophyll-a concentration and zooplankton group abundances were log transformed to fulfil the normality and variance homogeneity assumptions. A linear mixed effects model fitted using restricted maximum likelihood estimation (REML) was used to determine effect of salinity change over time on response variables. Model selection was made by using Akaike information criteria (AIC) and autocovariance estimates (ACF) (see Supplementary Material). The response variable was x and the fixed effects were salinity treatment, time and their interaction. Mesocosm ID was included as a random factor. Significance levels were set at p<0.05. Model residuals were checked for homogeneity of variances and normality. All analyses were performed in R v4.0.3 (R Core Team, 2020) using package nlme (Pinheiro et al., 2020). Graphs were produced using R package ggplot2 (Wickham, 2016) with a Local Regression (loess) smoother set at the default smoothing span of 0.75.
Results
Food Availability for Zooplankton
Salinity was an important factor for determining chlorophyll-a concentration (a proxy for phytoplankton biomass and therefore also zooplankton food availability) during the experiment (P<0.01, Table 1). Overall, the lowest and highest salinities (3.5 and 9.5 psu) displayed significantly more chlorophyll-a than the intermediate salinities (Figure 1 and Table 1). Chlorophyll-a concentration also varied over time (P=< 0.01, Table 1). In the extreme salinity treatments (3.5 and 9.5 psu) chlorophyll-a concentration peaked in initial stage of the experiment (DOY 213), with a secondary peak during the second half of the experiment (DOY 227 and DOY 229). Chlorophyll then declined for the rest of the experiment (DOY 241). In the 5.5 and 7.5 psu treatments chlorophyll-a was highest in the initial stage of the experiment (DOY 213) before declining for the rest of the experiment (DOY 241). Overall, chlorophyll-a concentration was highest during the first 3 days of the experiment for all treatments.
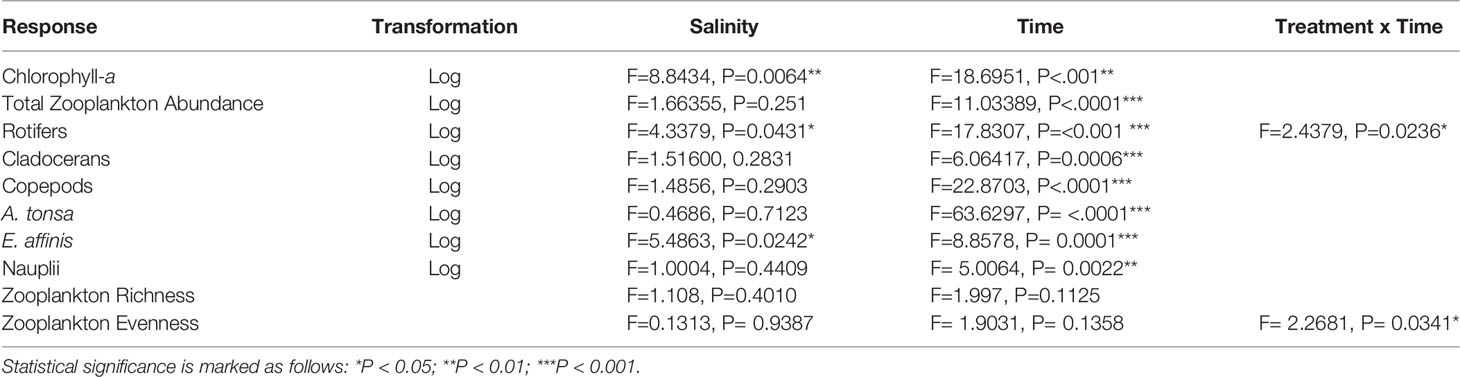
Table 1 Results of the mixed effect models for response variables with salinity, time, and their interaction.
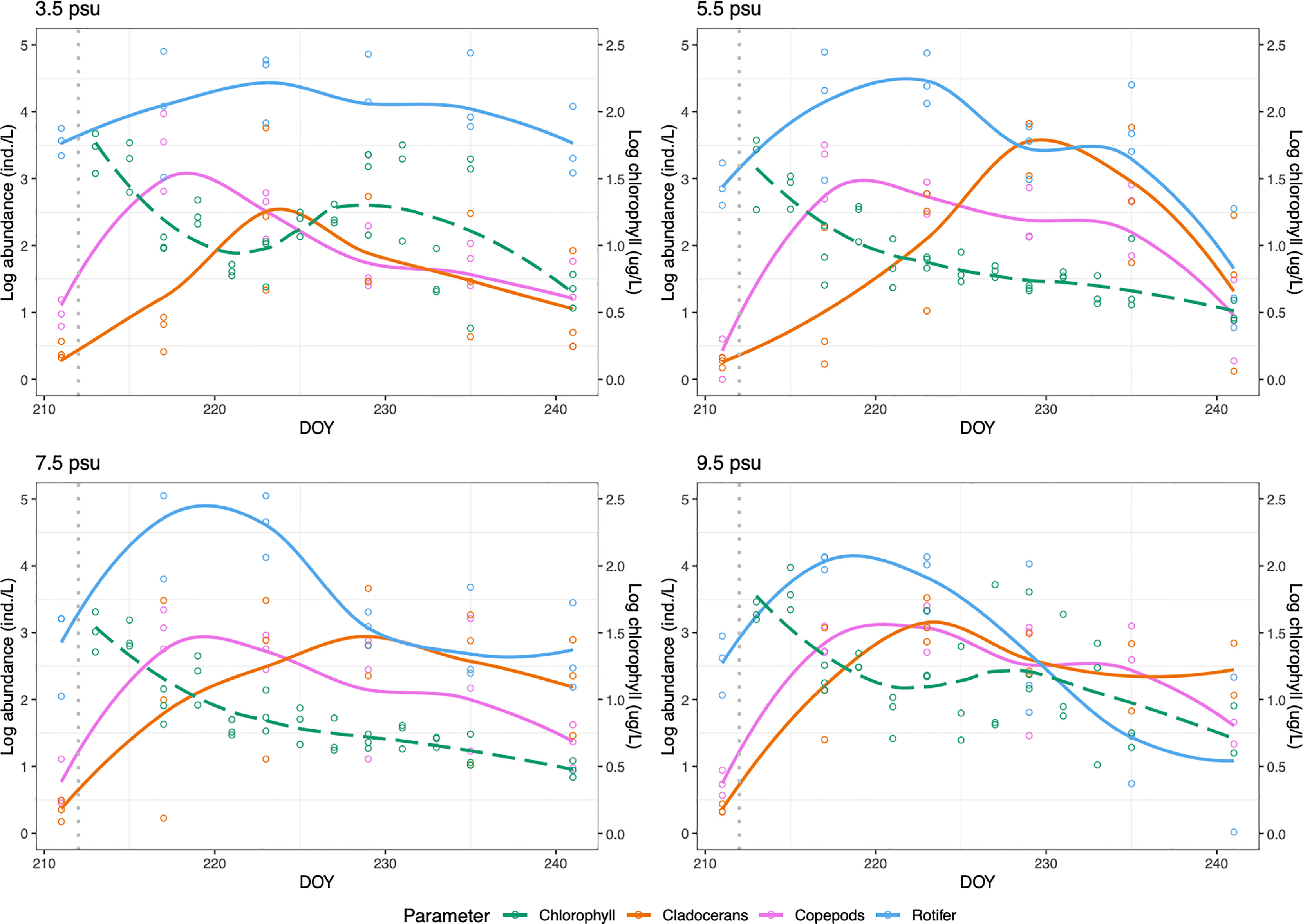
Figure 1 Chlorophyll a concentration (dashed green line) and abundance of zooplankton groups (rotifer, cladoceran and copepod) over time for each treatment. The dashed grey line at DOY 211 indicates salt addition.
Zooplankton Abundance and Community Composition
Total zooplankton abundance was not affected by salinity (P=0.251, Table 1) but varied over time (p<0.001, Table 1). Abundance of zooplankton increased from the start of the experiment and peaked between DOY 217 and 223. Abundance then declined in the last two weeks of the experiment.
Rotifers were the most abundant zooplankton group during the whole course of the experiment. Rotifer assemblages were dominated by Keratella quadrata and Keratella cochlearis in all treatments. A significant effect of salinity treatment over time was found for rotifer abundance (P=<0.05, Table 1). Rotifers were most abundant in 3.5 psu and least abundant in 9.5 psu salinity treatment. Overall, abundance of rotifers increased during the first two weeks of the experiment before declining for the rest of the experiment in all treatments.
The most abundant cladoceran species was Bosmina longirostris in all treatments. Abundance of cladocerans was not affected by salinity (P=0.4891, Table 1) but varied over time (P<0.001, Table 1). Abundance was initially low at the start of the experiment before a rapid increase during the second and third weeks of the experiment.
The most dominant copepod species in all samples were Acartia tonsa and Eurytemora affinis. Total copepod abundance (sum of adults and copepodites) was not affected by salinity (P=0.8371, Table 1) but varied over time (P=<.001, Table 1). Abundance was low at the start of the experiment before exhibiting a rapid increase during the first week of the experiment. The population then declined during the second and third week. Nauplii abundance was not affected by salinity (P=0.8131, Table 1) but varied over time (P<0.01, Table 1). Initially there was a low abundance of nauplii before increasing during the first week of the experiment. Abundance then declined in the second and third weeks of the experiment before increasing again during the fourth and final week of the experiment. Among the two dominant copepod species, E. affinis abundance was affected by salinity change (P=<0.05, Table 1) as well as time (P<0.01, Table 1). They were most abundant in 9.5 psu salinity treatment and least abundant in 3.5 psu. Abundance increased over the first four weeks of the experiment, until the final week when abundance rapidly decreased in all treatments. A. tonsa was not affected by changing salinity but its abundance varied over time (P<.001, Table 1) with a large spike in abundance during the first week of the experiment and population decline thereafter.
Zooplankton Richness and Evenness
A total of 18 zooplankton taxa (9 rotifer, 4 cladoceran, 3 copepod, 1 barnacle larvae and 1 chironomidea larvae) were recorded in the experiment (Supplementary Material, Figure S1). Zooplankton richness was not affected by salinity treatment and did not vary over time (Table 1).
A significant treatment-by-time interaction was found for zooplankton evenness (P=<0.05, Table 1 and Figure 2). In the 3.5 psu treatment, initial low zooplankton evenness was associated with a community dominated by rotifer K. quadrata (72%) and K. cochlearis (18%) (Figure 3). Evenness increased over time when other rotifer species become more abundant and reached the maximum at the end of the experiment (Figure 2) with the greatest contribution of K. cochliaris (48%) followed by Brachionous (18%) and an 8% contribution of K. quadrata.
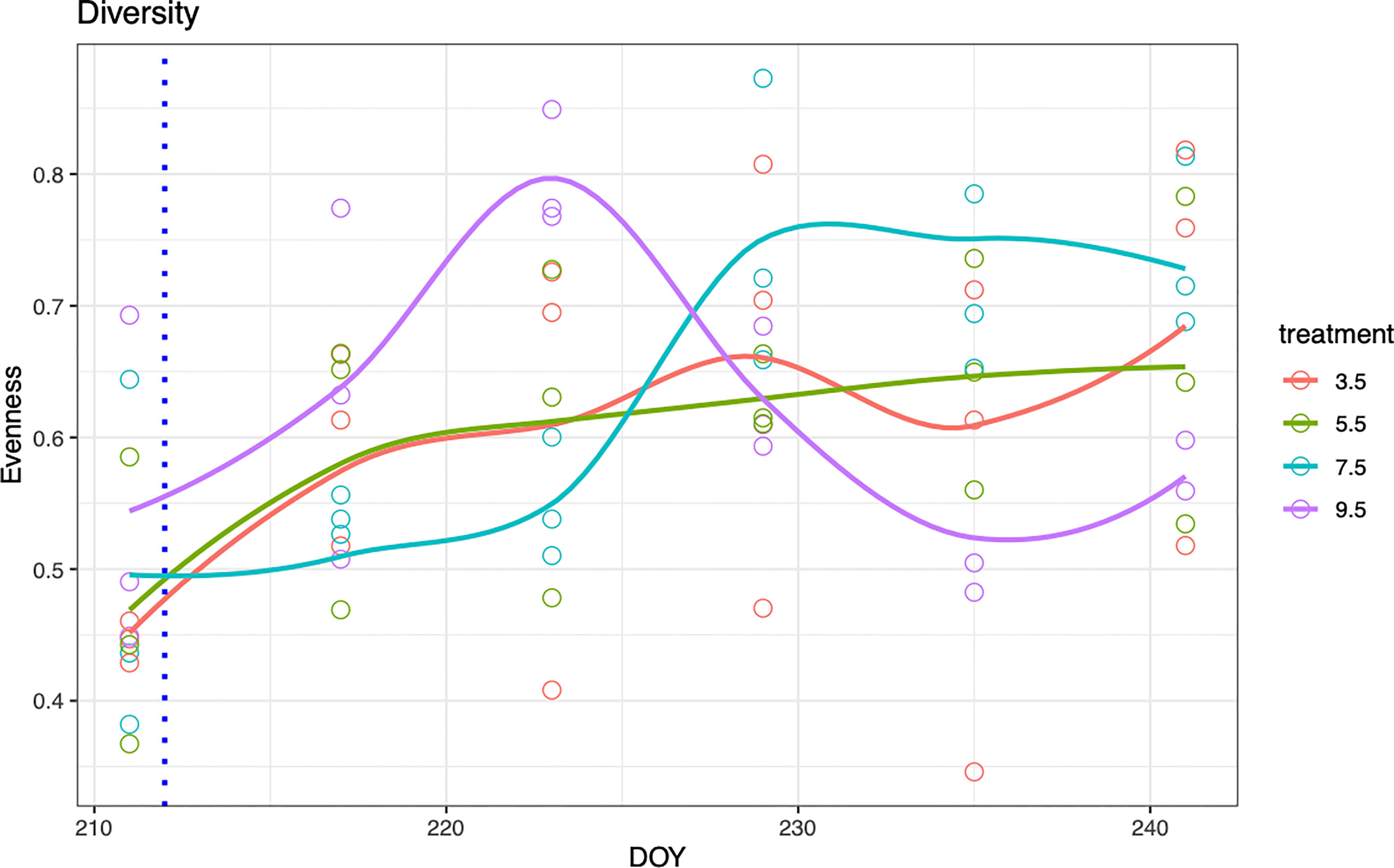
Figure 2 Zooplankton community evenness for each treatment over time. The dashed blue line at DOY 211 indicates salt addition.
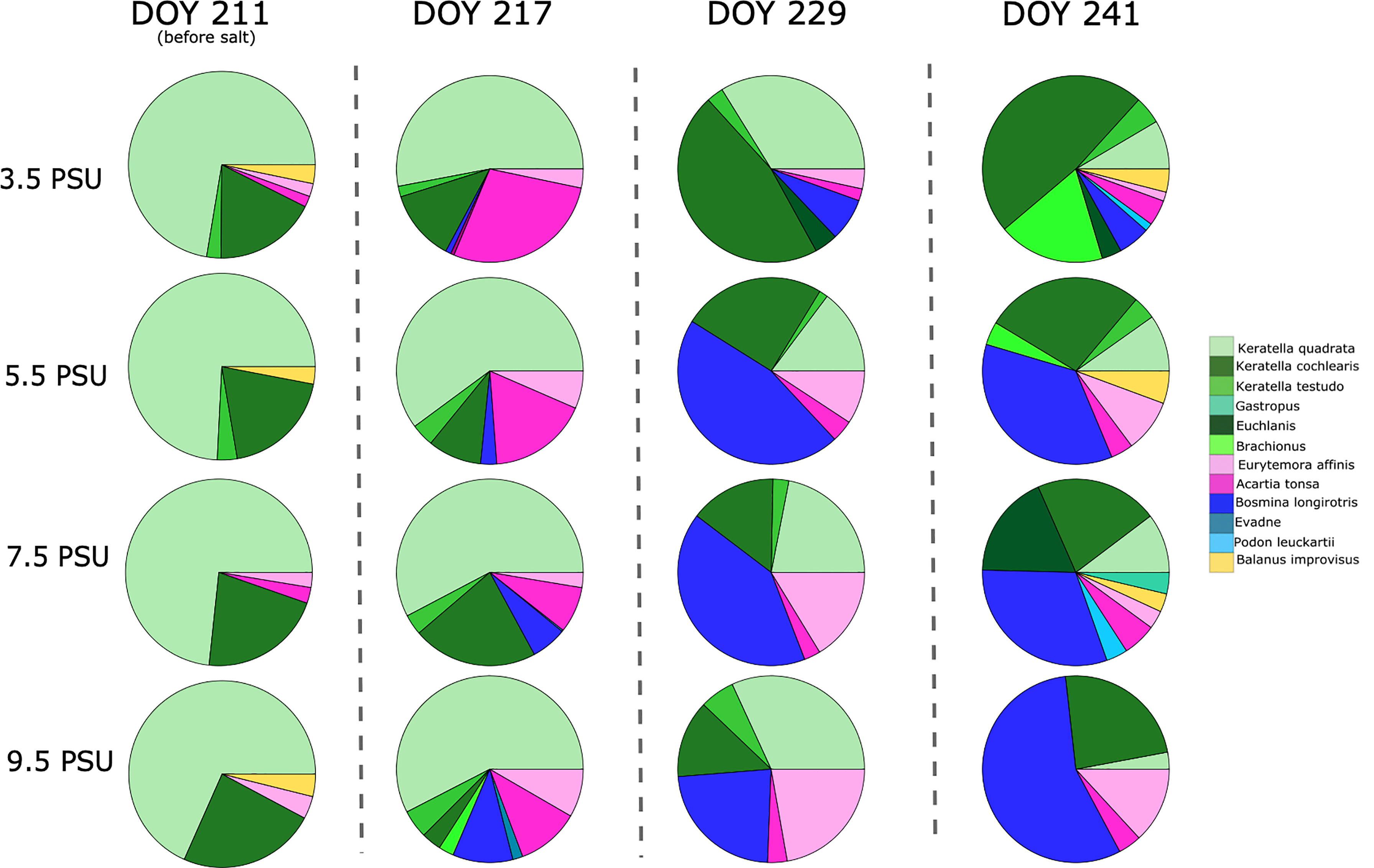
Figure 3 Proportional abundance of taxa on DOY 211, 217, 229 and 241 of the experiment for all salinity treatments. Taxa under 5% abundance are excluded for simplicity. Note that DOY 211 is before salt was added to the treatments and is included to show similarity in community at the start of the experiment.
In the 5.5 psu salinity treatment evenness was initially low and heavily dominated by K. quadrata (74%) and K. cochliaris (19%) (Figure 3). A steady increase in evenness was observed until the end of the experiment with a reduction in the dominance of K. quadrata (10%) and increase in dominance of B. longirostris (36%).
In the 7.5 psu salinity treatment, there was initially low evenness in the community dominated by K. quadrata (73%) and K. cochliaris (21%) (Figure 3). Rapid increase of zooplankton evenness in the third week of the experiment was related to the dominance shift towards B. longirostris (40%) and E. affinis (16%). At the end of the experiment the 7.5 psu treatment was comprised of B. longirostris (31%), K. cochliaris (21%), Euchlanis (18%), and K. quadrata (10%).
In the 9.5 psu salinity treatment, evenness was initially low. The community was dominated by K. quadrata (68%) and K. cochlearis (24%) (Figure 3). Evenness rapidly increased in the first two weeks with a reduction in dominance of K. quadrata (39%) and an increase in B. longirostris (25%), E. affinis (14%), Keratella testudo (10%) and A. tonsa (9%). At the end of the experiment, evenness decreased again after dominance shift to B. longirostris (56%), followed by K. cochliaris (24%) and E. affinis (13%).
Discussion
Our results imply that changing salinity will lead to a re-organisation of the zooplankton community structure (evenness and community composition). Salinity change had a direct impact on zooplankton evenness and caused a dominance shift from rotifers to cladocerans and later to copepods in high salinity treatments (Figure 3). No such shift was observed in low salinity mesocosms, suggesting that rotifers will dominate zooplankton communities in low salinity environments in the future, such as with declining salinity of the Baltic Sea. Rotifers make up a significant proportion of the mesozooplankton community (Telesh and Heerkloss, 2002), are mostly found in freshwater environments and are generally intolerant to salinity increase (Sarma et al., 2006; Medeiros et al., 2010). Jansson et al. (2020) reported that rotifer abundance has been increasing in the Baltic Sea since the 1960s, but this increase was linked to warming and eutrophication and not declining salinity. This may have been due to the relatively narrow salinity range (5.2-6.4 psu) where their study was conducted (Gulf of Riga). In contrast, our experiment, which simulated future salinity change scenarios (Meier et al., 2012), confirms that rotifers are directly affected by salinity change (Table 1) and dominate in low salinities, accounting for 82% of the community composition (Figure 3).
In contrast to the lowest salinity treatment (3.5 psu), where different rotifer species dominated zooplankton community during the whole experimental period, in the higher salinity treatments (7.5 and 9.5 psu) rotifers were outcompeted by cladocerans and copepods (B. longirostris and E. affinis, Figure 3). Hence, we see a reduction in zooplankton evenness at the end of the experiment in 9.5 psu salinity treatment when some zooplankton groups are reduced or lost (e.g. K. quadrata, K. testudo and Brachionus) the shift is complete and the majority of the community is dominated by B. longirostris (Figure 2). In the 7.5 psu salinity treatment the same shift in dominance was found as in 9.5 psu, but it took longer to reach a cladoceran-dominated community (Figure 1). B. longirostris has been found to adapt to a wide range of salinities (Jeppesen et al., 1994; Deasley et al., 2012), thus it was able to outcompete rotifers in 7.5 and 9.5 psu treatments. However, as filter feeders, cladocerans can be negatively affected by toxic cyanobacteria blooms expected to proliferate with projected freshening and warming of the Baltic Sea (Meier et al., 2011) promoting rotifer and copepod abundance. Some rotifers were able to persist in high salinities however, namely K. cochlearis. This could be due to K. cochlaris being able to tolerate fluctuations in salinity (Paturej and Gutkowska, 2015).
Zooplankton richness increased directly after salt addition in all experimental units, with dominant rotifers suffering and copepods benefiting from the disturbance (Figure 3). Copepods dominated by A. tonsa and E. affinis were most abundant in the 9.5 psu salinity treatment. Over the course of the experiment population of A. tonsa declined and was replaced by E. affinis (Figure S1, supplementary online material), indicating competitive exclusion, as both species have similar feeding strategies and prey size preferences (Richman et al., 1977; Engstrom, 2000). A. tonsa exhibit a broad tolerance to salinity, being able to survive salinities as low as 0.5 psu, but with an optimum salinity of between 10 and 20 psu (Cervetto et al., 1999; Calliari et al., 2006). E. affinis, which is generally regarded as a brackish species (Lee and Petersen, 2003), has salinity optimum of 10 psu (Karlsson et al., 2018), which is close to our highest salinity treatment and therefore may be better adapted to this salinity range than A. tonsa.
An alternative reason for the increased abundance of E. affinis found in high salinities could be a potential hormetic response to salt addition whereby, in rapidly producing more nauplii, the population of E. affinis is able to adapt to stressful conditions by guaranteeing the survival of at least a few individuals. Further evidence of this is given by an increase in juvenile and adult copepods found in the second week of the experiment, suggesting the nauplii produced in the first week were able to survive the high salinity environment. A hormetic response is when an environmental stressor (e.g. toxins, herbicides) stimulates an adaptive response that increases the resistance of the organism to a level of stress (Calabrese et al., 2007). This phenomenon has been studied elsewhere in nature (Calabrese and Baldwin, 1998; Beckers et al., 2009; Alyokhin et al., 2013; Vargas-Hernandez et al., 2017).
In intermediate salinities (5.5 and 7.5 psu) copepods were outcompeted by cladocerans, which reflects their different salinity optima, copepods preferring marine and cladocerans freshwater conditions (Sommer and Sommer, 2006). In the lowest salinity treatment (3.5 psu), rotifer abundance did not affect the population dynamics of cladocerans or copepods (Figure 1), with groups tracking the pattern of food availability without apparent competition. This result has also been found elsewhere: a study by MacIsaac and Gilbert (1989) showed that a high abundance of rotifers can be maintained in the presence of small cladocerans such as B. longirostris. We found that in the 3.5 and 9.5 psu salinity treatments an increase in community evenness over the first two weeks of the experiment (Figure 2) corresponded to a reduction in chlorophyll-a concentration. Conversely, when evenness declined in those treatments in the third and fourth week, we saw a recovery in chlorophyll-a concentration. We suggest this could be due to a more diverse zooplankton community using their resources more efficiently and therefore being able to reduce producer biomass (phytoplankton) more greatly (Duffy, 2002). In contrast, constant grazing from multiple zooplankton groups keeps phytoplankton concentrations low in the second half of the experiment in the intermediate salinity treatments (Figure 1). Rotifer dominated communities have also been found to be less efficient in controlling phytoplankton biomass than other zooplankton groups (Jakobsen et al., 2003), which might be a reason why we observed recovery of phytoplankton biomass (measured as chlorophyll a concentration) in low salinity treatments by the end of the experiment (Figure 1). This suggests with declining salinity of the Baltic Sea a shift to a rotifer dominated community may lead to less efficient grazing of phytoplankton and the increased risk of intense algal blooms in summer months.
In conclusion, we show that salinity change results in the restructuring of the zooplankton community. In low salinities, the dominance of rotifers throughout the experiment suggests they are most well adapted to freshwater environments, though some brackish species that are able to tolerate fluctuations in salinity (e.g. A. tonsa copepods and B. longirostris cladocerans) can persist in low abundance. With environments predicted to decline in salinity, such as the Baltic Sea, shifts from marine to brackish conditions will result in reduced food availability and increased resource competition between copepods and cladocerans, benefitting the latter due to their better adaptation to brackish conditions. This will therefore lead to a shift from a community comprised of complex organisms (copepods) to simpler ones (cladocerans and rotifers), potentially strengthening the negative effects of warming and eutrophication on zooplankton (Daufresne et al., 2009; Jansson et al., 2020), and consequently altering the functioning of pelagic food web. Although this study focusses on salinity change, in nature environmental variables are rarely acting in isolation to one another. Other stressors such as warming can affect the metabolic activity of zooplankton (Mayor et al., 2015) and lead to multistressor effects (Souissi et al., 2021). Trophic interactions may also be affected by salinity change and effects on other trophic levels, especially with regards to toxic cyanobacteria and phytoplankton should be considered in future studies (Viitasalo and Bonsdorff, 2022).
Data Availability Statement
The raw data supporting the conclusions of this article will be made available by the authors, without undue reservation.
Author Contributions
Study conception and design: CH and AL. Data collection: CH. Data analysis and interpretation of results: CH and AL. Draft manuscript preparation: CH and AL. All authors have reviewed and approved the final version of this manuscript.
Conflict of Interest
The authors declare that the research was conducted in the absence of any commercial or financial relationships that could be construed as a potential conflict of interest.
Publisher’s Note
All claims expressed in this article are solely those of the authors and do not necessarily represent those of their affiliated organizations, or those of the publisher, the editors and the reviewers. Any product that may be evaluated in this article, or claim that may be made by its manufacturer, is not guaranteed or endorsed by the publisher.
Funding
This research was funded by the Onni Talas Foundation Finland and the University of Helsinki start-up grant of AL. The experimental mesocosm platform is a part of the AQUACOSM (project No 731065) funded by the European Commission EU H2020-INFRAIA.
Acknowledgments
We are grateful for the help of students and technicians at Tvärminne Zoological Station that participated in field and laboratory work supporting this publication. Local firefighters are acknowledged for the water transport.
Supplementary Material
The Supplementary Material for this article can be found online at: https://www.frontiersin.org/articles/10.3389/fmars.2022.861297/full#supplementary-material
Supplementary Figure 1 | Zooplankton abundance with salinity treatment over time for all zooplankton groups. Rare species excluded from analysis are not shown. Statistical tests were performed as described in the main text, methods section.
References
Alvarez-Fernandez S., Licandro P., van Damme C. J. G., Hufnagl M. (2015). Effect of Zooplankton on Fish Larval Abundance and Distribution: A Long-Term Study on North Sea Herring (Clupea Harengus). ICES J. Mar. Sci. 72 (9), 2569–2577. doi: 10.1093/icesjms/fsv140
Alyokhin A., Vincent C., Giordanengo P. (Eds.) (2013). “Insect Pests of Potato,” in Global Perspectives on Biology and Management(Amsterdam; Boston: Academic Press, Elsevier).
Ayadi H. (2004). “Structure of the Phytoplankton Communities in Two Lagoons of Different Salinity in the Sfax Saltern (Tunisia)”. J. Plankton Res. 26 (6), 669–679. doi: 10.1093/plankt/fbh047
Bashevkin S. M., Pechenik J. A. (2015). The Interactive Influence Ssof Temperature and Salinity on Larval and Juvenile Growth in the Gastropod Crepidula Fornicata (L.). J. Exp. Mar. Biol. Ecol. 470, 78–91. doi: 10.1016/j.jembe.2015.05.004
Beckers G. J. M., Jaskiewicz M., Liu Y., Underwood W. R., He S. Y., Zhang S., et al. (2009). Mitogen-Activated Protein Kinases 3 and 6 Are Required for Full Priming of Stress Responses in Arabidopsis Thaliana. Plant Cell 21 (3), 944–953. doi: 10.1105/tpc.108.062158
Benton T. G., Solan M., Travis J. M. J., Sait S. M. (2007). Microcosm Experiments can Inform Global Ecological Problems. Trends Ecol. Evol. 22 (10), 516–521. doi: 10.1016/j.tree.2007.08.003
Bisson M., Kirst G. (1995). Osmotic Acclimation and Turgor Pressure Regulation in Algae. Natur Wissenschaften Aufsätze 82, 461–471. doi: 10.1007/BF01131597
Blenckner T., Ammar Y., Müller-Karulis B., Niiranen S., Arneborg L., Li Q. (2021). The Risk for Novel and Disappearing Environmental Conditions in the Baltic Sea. Front. Mar. Sci. 8, 745722. doi: 10.3389/fmars.2021.745722
Brandl Z. (2005). Freshwater Copepods and Rotifers: Predators and Their Prey. Hydrobiologia 546 (1), 475–489. doi: 10.1007/s10750-005-4290-3
Calabrese E. J., Bachmann K. A., Bailer A. J., Bolger P. M., Borak J., Cai L., et al. (2007). Biological Stress Response Terminology: Integrating the Concepts of Adaptive Response and Preconditioning Stress Within a Hormetic Dose–Response Framework. Toxicol. Appl. Pharmacol. 222 (1), 122–128. doi: 10.1016/j.taap.2007.02.015
Calabrese E. J., Baldwin L. A. (1998). Hormesis as a Biological Hypothesis. Environ. Health Perspect. 106, 6. doi: 10.1289/ehp.98106s1357
Calliari D., Andersen CM, Thor P., Gorokhova E., Tiselius P. (2006). Salinity Modulates the Energy Balance and Reproductive Success of Co-Occurring Copepods Acartia Tonsa and A. Clausi in Different Ways. Mar. Ecol. Prog. Ser. 312, 177–188. doi: 10.3354/meps312177
Cervetto G., Gaudy R., Pagano M. (1999). Influence of Salinity on the Distribution of Acartia Tonsa (Copepoda, Calanoida). J. Of Exp. Mar. Biol. Ecol. 239, 33–45. doi: 10.1016/S0022-0981(99)00023-4
Chakraborty P., Acharyya T., Raghunadh Babu P. V., Bandyopadhyay D. (2011). Impact of Salinity and PH on Phytoplankton Communities in a Tropical Freshwater System: An Investigation With Pigment Analysis by HPLC. J. Environ. Monitoring 13 (3), 614. doi: 10.1039/c0em00333f
Daufresne M., Lengfellner K., Sommer U. (2009). Global Warming Benefits the Small in Aquatic Ecosystems. Proc. Natl. Acad. Sci. 106 (31), 12788–12793. doi: 10.1073/pnas.0902080106
Deasley K., Korosi J. B., Thienpont J. R., Kokelj S. V., Pisaric M. F. J., Smol J. P. (2012). Investigating the Response of Cladocera to a Major Saltwater Intrusion Event in an Arctic Lake From the Outer Mackenzie Delta (Nt, Canada). J. Paleolimnol. 48 (2), 287–296. doi: 10.1007/s10933-012-9577-6
Duffy J. E. (2002). Biodiversity and Ecosystem Function: The Consumer Connection. Oikos 99 (2), 201–219. doi: 10.1034/j.1600-0706.2002.990201.x
Engstrom J. (2000). Feeding Interactions of the Copepods Eurytemora Affinis and Acartia Bifilosa With the Cyanobacteria Nodularia Sp. J. Plankton Res. 22 (7), 1403–1409. doi: 10.1093/plankt/22.7.1403
Flameling I. A., Kromkamp J. (1994). Responses of Respiration and Photosynthesis of Scenedesmus Protuberans Fritsch to Gradual and Steep Salinity Increases. J. Plankton Res. 16 (12), 1781–1791. doi: 10.1093/plankt/16.12.1781
Gao Q., Xu Z., Zhuang P. (2008). The Relation Between Distribution of Zooplankton and Salinity in the Changjiang Estuary. Chin. J. Oceanol. Limnol. 26 (2), 178–185. doi: 10.1007/s00343-008-0178-1
Gutierrez M. F., Tavşanoğlu ÜN., Vidal N., Yu J., Mello F.T.-d., Çakiroglu A. I., et al. (2018). Salinity Shapes Zooplankton Communities and Functional Diversity and Has Complex Effects on Size Structure in Lakes. Hydrobiologia 813 (1), 237–255. doi: 10.1007/s10750-018-3529-8
Gyllström M., Hansson L. A., Jeppesen E., García Criado F., Gross E., Irvine K., et al. (2005). The Role of Climate in Shaping Zooplankton Communities of Shallow Lakes. Limnol. Oceanogr. 50 (6), 2008–2021. doi: 10.4319/lo.2005.50.6.2008
Hall C. J. (2004). Effects of Salinity and Temperature on Survival and Reproduction of Boeckella Hamata (Copepoda: Calanoida) from a Periodically Brackish Lake. J. Plankton Res. 23 (1), 97–104. doi: 10.1093/plankt/23.1.97
Hart B. T., Lake P. S., Angus Webb J., Grace M. R. (2003). Ecological Risk to Aquatic Systems From Salinity Increases. Aust. J. Bot. 51 (6), 689. doi: 10.1071/BT02111
HELCOM (2013). “Climate Change in the Baltic Sea Area: HELCOM Thematic Assessment in 2013,” in Balt. Sea Environ. Proc. No. 137. (Katajanokanlaituri 6 B FI-00160 Helsinki: Helsinki Commission). Available at: http://www.helcom.fi.
Helm K. P., Bindoff N. L., Church J. A. (2010). Changes in the Global Hydrological-Cycle Inferred From Ocean Salinity: HYDROLOGICAL Cycle AND Ocean Salinity. Geophysical Res. Lett. 37 (18), 0094–8276. doi: 10.1029/2010GL044222
Hintz W. D., Fay L., Relyea R. A. (2021). Road Salts, Human Safety, and the Rising Salinity of Our Fresh Waters Frontiers in Ecology and the Environment. Front. Ecol. Environ. 20 (1), 22–30. doi: 10.1002/fee.2433
Hintz W. D., Mattes B. M., Schuler M. S., Jones D. K., Stoler A. B., Lind L., et al. (2017). Salinization Triggers a Trophic Cascade in Experimental Freshwater Communities With Varying Food-Chain Length. Ecol. Appl. 27 (3), 833–844. doi: 10.1002/eap.1487
Intergovernmental Panel Climate Change. (2018). “Global Warming of 1.5 °C,” in An IPCC Special Report on the Impacts of Global Warming of 1.5 °C Above Pre-Industrial Levels and Related Global Greenhouse Gas Emission Pathways, in the Context of Strengthening the Global Response to the Threat of Climate Change, Sustainable Development, and Efforts to Eradicate Poverty (Geneva: World Meteorological Organization).
Jakobsen T. S., Hansen P. B., Jeppesen E., Grønkjær P., Søndergaard M. (2003). Impact of Three-Spined Stickleback Gasterosteus Aculeatus on Zooplankton and Chl a in Shallow, Eutrophic, Brackish Lakes. Mar. Ecol. Prog. Ser. 262, 277–284. doi: 10.3354/meps262277
Jansson A., Klais-Peets R., Grinienė E., Rubene G., Semenova A., Lewandowska A., et al. (2020). Functional Shifts in Estuarine Zooplankton in Response to Climate Variability. Ecol. Evol. 10 (20), 11591–11606. doi: 10.1002/ece3.6793
Jeppesen E., Søndergaard M., Kanstrup E., Petersen B., Eriksen R. B., Hammershøj M., et al. (1994). Does the Impact of Nutrients on the Biological Structure and Function of Brackish and Freshwater Lakes Differ? Hydrobiologia 275 (1), 15–30. doi: 10.1007/BF00026696
Karlsson K., Puiac S., Winder M. (2018). Life-History Responses to Changing Temperature and Salinity of the Baltic Sea Copepod Eurytemora Affinis. Mar. Biol. 165 (2), 30. doi: 10.1007/s00227-017-3279-6
Lee C. E., Petersen C. H. (2003). Effects of Developmental Acclimation on Adult Salinity Tolerance in the Freshwater-Invading Copepod Eurytemora Affinis. Physiol. Biochem. Zool. 76 (3), 296–301. doi: 10.1086/375433
Lehmann A., Myrberg K., Post P., Chubarenko I., Dailidiene I., Hinrichsen H.-H., et al. (2021). Salinity Dynamics of the Baltic Sea. Preprint. Dynamics of the Earth System: Interactions. Earth. Syst. Dyn. 13 (1), 373–392. doi: 10.5194/esd-2021-15
Lewandowska A. M., Boyce D. G., Hofmann M., Matthiessen B., Sommer U., Worm B. (2014). Effects of Sea Surface Warming on Marine Plankton. Ecol. Lett. 17 (5), 614–623. doi: 10.1111/ele.12265
Lin Q., Xu L., Hou J., Liu Z., Jeppesen E., Han B.-P. (2017). Responses of Trophic Structure and Zooplankton Community to Salinity and Temperature in Tibetan Lakes: Implication for the Effect of Climate Warming”. Water Res. 124, 618–629. doi: 10.1016/j.watres.2017.07.078
Lund J. W. G., Kipling C., Le Cren E. D. (1958). “The Inverted Microscope Method of Estimating Algal Numbers and the Statistical Basis of Estimations by Counting”. Hydrobiologia 11 (2), 143–170. doi: 10.1007/BF00007865
MacIsaac H. J., Gilbert J. J. (1989). Competition Between Rotifers and Cladocerans of Different Body Sizes. Oecologia 81 (3), 295–301. doi: 10.1007/BF00377074
Mackas D. L., Batten S., Trudel M. (2007). Effects on Zooplankton of a Warmer Ocean: Recent Evidence From the Northeast Pacific. Prog. Oceanogr. 75 (2), 223–252. doi: 10.1016/j.pocean.2007.08.010
Mayor D. J., Sommer U., Cook K. B., Viant M. R. (2015). The Metabolic Response of Marine Copepods to Environmental Warming and Ocean Acidification in the Absence of Food. Sci. Rep. 5 (1), 13690. doi: 10.1038/srep13690
Medeiros A. M. A., Barbosa J. E. L., Medeiros P. R., Rocha R. M., Silva L. F. (2010). Salinity and Freshwater Discharge Determine Rotifer Distribution at the Mossoró River Estuary (Semiarid Region of Brazil). Braz. J. Biol. 70 (3), 551–557. doi: 10.1590/S1519-69842010000300011
Meier H., Eilola K., Almroth E. (2011). Climate-Related Changes in Marine Ecosystems Simulated With a 3-Dimensional Coupled Physical-Biogeochemical Model of the Baltic Sea. Climate Res. 48 (1), 31–55. doi: 10.3354/cr00968
Meier H. E. M., Hordoir R., Andersson H. C., Dieterich C., Eilola K., Gustafsson B. G., et al. (2012). Modeling the Combined Impact of Changing Climate and Changing Nutrient Loads on the Baltic Sea Environment in an Ensemble of Transient Simulations for 1961–2099. Clim. Dyn. 39 (9), 2421–2441. doi: 10.1007/s00382-012-1339-7
Meier H. E. M., Kjellström E., Graham L. P. (2006). Estimating Uncertainties of Projected Baltic Sea Salinity in the Late 21st Century. Geophysical Res. Lett. 33 (15), 0094–8276. doi: 10.1029/2006GL026488
Merkouriadi I., Leppäranta M. (2014). Long-Term Analysis of Hydrography and Sea-Ice Data in Tvärminne, Gulf of Finland, Baltic Sea. Clim. Change 124 (4), 849–859. doi: 10.1007/s10584-014-1130-3
Moffett E. R., Baker H. K., Bonadonna C. C., Shurin J. B., Symons C. C. (2020). Cascading Effects of Freshwater Salinization on Plankton Communities in the Sierra Nevada. Limnol. Oceanogr. Lett. doi: 10.1002/lol2.10177
Möllmann C., Kornilovs G., Fetter M., Koster F. W. (2004). Feeding Ecology of Central Baltic Sea Herring and Sprat. J. Fish Biol. 65, 1563–1581. doi: 10.1111/j.0022-1112.2004.00566.x
Muhling B. A., Jacobs J., Stock C. A., Gaitan G. F., Saba V. S. (2017). Projections of the Future Occurrence, Distribution, and Seasonality of Three Vibrio Species in the Chesapeake Bay under a High‐emission Climate Change Scenario. GeoHealth 1 (7), 278–296. doi: 10.1002/2017GH000089
Murphy G. E. P., Romanuk T. N., Worm B. (2020). Cascading Effects of Climate Change on Plankton Community Structure. Ecol. Evol. 10 (4), 2170–2181. doi: 10.1002/ece3.6055
Nielsen D. L., Brock M. A., Crossle K., Harris K., Healey M., Jarosinski I. (2003). The Effects of Salinity on Aquatic Plant Germination and Zooplankton Hatching From Two Wetland Sediments. Freshwater Biol. 48 (12), 2214–2223. doi: 10.1046/j.1365-2427.2003.01146.x
Paturej E., Gutkowska A. (2015). The Effect of Salinity Levels on the Structure of Zooplankton Communities. Arch. Biol. Sci. 67 (2), 483–492. doi: 10.2298/ABS140910012P
Perumal N. V., Rajkumar M., Perumal P., Rajasekar K. T. (2009). Seasonal Variations of Plankton Diversity in the Kaduviyar Estuary, Nagapattinam, Southeast Coast of India. J. Environ. Biol. 30 (6), 1035–1046.
Pielou E. C. (1966). “The Measurement of Diversity in Different Types of Biological Collections”. J. Theor. Biol. 13, 131–144. doi: 10.1016/0022-5193(66)90013-0
Pinheiro J., Bates D., DebRoy S., Sarkar D., R Core Team (2020) R Package Version 3.1-150. Available at: https://CRAN.R-project.org/package=nlme.
R Core Team (2020). R: A Language and Environment for Statistical Computing (Vienna, Austria. URL https://www.R-project.org/: R Foundation for Statistical Computing).
Richman S., Heinle D. R., Huff R. (1977). “Grazing by Adult Estuarine Calanoid Copepods of the Chesapeake Bay”. Mar. Biol. 42 (1), 69–84. doi: 10.1007/BF00392015
Roddie B. D., Leakey R. J. G., Berry A. J. (1984). “Salinity-Temperature Tolerance and Osmoregulation in Eurytemora Affinis (Poppe) (Copepoda : Calanoida) in Relation to Its Distribution in the Zooplankton of the Upper Reaches of the Forth Estuary”. J. Exp. Mar. Biol. Ecol. 79 (2), 191–211. doi: 10.1016/0022-0981(84)90219-3
Ross A. C., Najjar R. G., Li M. (2021). A Metamodel-Based Analysis of the Sensitivity and Uncertainty of the Response of Chesapeake Bay Salinity and Circulation to Projected Climate Change. Estuaries Coasts 44 (1), 70–87. doi: 10.1007/s12237-020-00761-w
Santangelo J. M., Francisco de A E., Manca M., Bozelli R. L. (2014). Disturbances Due to Increased Salinity and the Resilience of Zooplankton Communities: The Potential Role of the Resting Egg Bank. Hydrobiologia 722 (1), 103–113. doi: 10.1007/s10750-013-1683-6
Saraiva S., Markus Meier H. E., Andersson H., Höglund A., Dieterich C., Gröger M., et al. (2019). Uncertainties in Projections of the Baltic Sea Ecosystem Driven by an Ensemble of Global Climate Models. Front. Earth Sci. 0. doi: 10.3389/feart.2018.00244
Sarma S. S. S., Nandini S., Morales-Ventura J., Delgado-Martínez I., González-Valverde L. (2006). Effects of NaCl Salinity on the Population Dynamics of Freshwater Zooplankton (Rotifers and Cladocerans). Aquat. Ecol. 40 (3), 349. doi: 10.1007/s10452-006-9039-1
Schallenberg M., Hall C. J., Burns C. W. (2003). Consequences of Climate-Induced Salinity Increases on Zooplankton Abundance and Diversity in Coastal Lakes. Mar. Ecol. Prog. Ser. 251, 181–189. doi: 10.3354/meps251181
Schuler M. S., Hintz W. D., Jones D. K., Lind L. A., Mattes B. M., Stoler A. B., et al. (2017). How Common Road Salts and Organic Additives Alter Freshwater Food Webs: In Search of Safer Alternatives. J. Appl. Ecol. 54 (5), 1353–1361. doi: 10.1111/1365-2664.12877
Shurin J. B., Borer E. T., Seabloom E. W., Anderson K., Blanchette C. A., Broitman B., et al. (2002). A Cross-Ecosystem Comparison of the Strength of Trophic Cascades: Strength of Cascades. Ecol. Lett. 5 (6), 785–791. doi: 10.1046/j.1461-0248.2002.00381.x
Sommer U., Sommer F. (2006). Cladocerans Versus Copepods: The Cause of Contrasting Top–Down Controls on Freshwater and Marine Phytoplankton. Oecologia 147 (2), 183–194. doi: 10.1007/s00442-005-0320-0
Sommer U., Stibor H., Katechakis A., Sommer F., Hansen T. (2002). Pelagic Food Web Configurations at Different Levels of Nutrient Richness and Their Implications for the Ratio Fish Production. Hydrobiologia 484, 11–20. doi: 10.1023/A:1021340601986
Souissi A., Hwang J-S., Souissi S. (2021). “Reproductive Trade-Offs of the Estuarine Copepod Eurytemora Affinis under Different Thermal and Haline Regimes.” Sci. Rep. 11 (1), 20139. doi: 10.1038/s41598-021-99703-0.
Šorf M., Davidson T. A., Brucet S., Menezes R. F., Søndergaard M., Lauridsen T. L., et al. (2015). Zooplankton Response to Climate Warming: A Mesocosm Experiment at Contrasting Temperatures and Nutrient Levels. Hydrobiologia 742 (1), 185–203. doi: 10.1007/s10750-014-1985-3
Telesh I. V., Heerkloss R. (2002). Atlas of Estuarine Zooplankton of the Southern and Eastern Baltic Sea. Part I: Rotifera (Hamburg: Verlag Dr. Kovač), 89 pp.
Telesh I., Postel L., Heerkloss R., Mironova K., Skarlato S. (2008). Zooplankton of the Open Baltic Sea: Atlas. Meereswissenschaftliche Berichte No 73 2008 - Marine Science Reports No 73 2008 The Baltic Marine Biologists Publication No.21(76), 1–290. doi: 10.12754/MSR-2008-0073
Tiselius P., Jonsson P. R. (1990). Foraging Behaviour of Six Calanoid Copepods: Observations and Hydrodynamic Analysis”. Mar. Ecol. Prog. Ser. 66, 23–33. doi: 10.3354/meps066023
Travis J. M. J. (2003). “Climate Change and Habitat Destruction: A Deadly Anthropogenic Cocktail. Proc. R. Soc. London Ser. B: Biol. Sci. 270 (1514), 467–473. doi: 10.1098/rspb.2002.2246
Vargas-Hernandez M., Macias-Bobadilla I., Guevara-Gonzalez R. G., de Romero-Gomez S.J., Rico-Garcia E., Ocampo-Velazquez R. V., et al. (2017). Plant Hormesis Management With Biostimulants of Biotic Origin in Agriculture. Front. Plant Sci. 8, 1762. doi: 10.3389/fpls.2017.01762
Viitasalo M., Bonsdorff E. (2022). Global Climate Change and the Baltic Sea Ecosystem: Direct and Indirect Effects on Species, Communities and Ecosystem Functioning. Earth System Dynamics 13 (2), 711–747. doi: 10.5194/esd-13-711-2022
de Waal D.B.v., Verschoor A. M., Verspagen J. M. H., van Donk E., Huisman J. (2010). Climate-Driven Changes in the Ecological Stoichiometry of Aquatic Ecosystems. Front. Ecol. Environ. 8 (3), 145–152. doi: 10.1890/080178
Vuorinen I., Hänninen J., Rajasilta M., Laine P., Eklund J., Montesino-Pouzols F., et al. (2015). Scenario Simulations of Future Salinity and Ecological Consequences in the Baltic Sea and Adjacent North Sea Areas–Implications for Environmental Monitoring. Ecol. Indic. 50, 196–205. doi: 10.1016/j.ecolind.2014.10.019.
Wickham H. (2016). Ggplot2: Elegant Graphics for Data Analysis (New York: Springer-Verlag). Available at: https://ggplot2.tidyverse.org.
Keywords: salinity, plankton, diversity, Baltic Sea, marine, brackish, habitat loss, climate change
Citation: Hall CAM and Lewandowska AM (2022) Zooplankton Dominance Shift in Response to Climate-Driven Salinity Change: A Mesocosm Study. Front. Mar. Sci. 9:861297. doi: 10.3389/fmars.2022.861297
Received: 24 January 2022; Accepted: 25 April 2022;
Published: 03 June 2022.
Edited by:
Singaraju Sri Subrahmanya Sarma, Universidad Nacional Autónoma de México, MexicoReviewed by:
Brenda Karen González Pérez, Technological University of the Metropolitan Area of Valle de México, MexicoJose Luis Gama Flores, Universidad Nacional Autónoma de México, Mexico
Meral Apaydın Yağcı, Sheep Breeding Research Institute, Turkey
Copyright © 2022 Hall and Lewandowska. This is an open-access article distributed under the terms of the Creative Commons Attribution License (CC BY). The use, distribution or reproduction in other forums is permitted, provided the original author(s) and the copyright owner(s) are credited and that the original publication in this journal is cited, in accordance with accepted academic practice. No use, distribution or reproduction is permitted which does not comply with these terms.
*Correspondence: Clio A. M. Hall, Y2xpby5oYWxsQGhlbHNpbmtpLmZp