- 1Department of Paraclinical Sciences, Faculty of Veterinary Medicine, Norwegian University of Life Sciences (NMBU), Ås, Norway
- 2National Aquafeed Safety Assessment Center, Institute of Feed Research, Chinese Academy of Agricultural Sciences, Beijing, China
- 3Cermaq Group AS, Oslo, Norway
- 4Cargill Aqua Nutrition, Stavanger, Norway
Atlantic salmon (Salmo salar L.) undergo great alterations in physiology and gut microbiota composition throughout their life stages. This study assessed gut health and microbiota in out-of-season smolts before and after seawater transfer and modulatory effects of functional feed ingredients under commercial Arctic conditions. The fish were fed two series of diets, one without (Ref diet) and one with a mixture of functional ingredients (Test diet). Both diets varied in nutrient composition as required according to the developmental stage of the fish. For fish in freshwater, the mixture of functional ingredients contained nucleotides and immune stimulants, in seawater nucleotides, DHA, and EPA. Samples were collected four weeks before and four weeks after seawater transfer. Regardless of diet, seawater transfer (seawater compared to freshwater) significantly suppressed fish growth rate, condition factor, plasma nutrient levels, digesta bile acid concentrations, expression of genes related to gut immune functions (i.e., cytokines and T-cell markers), and increased intestinal microbial richness and diversity. Seawater transfer also reduced the symptoms of pyloric caeca lipid malabsorption with a corresponding decreasing effect on perilipin-2 (plin2) expression. In the gut microbiota of fish in freshwater, the unclassified Ruminococcaceae family dominated strongly, accounting for about 85% of the total abundance, whereas in seawater the genera Lactobacillus and Photobacterium were the dominant taxa, accounting for about 90% of the total abundance. Multivariate association analysis showed that relative abundance of certain lactic acid bacteria (LAB) correlated positively with expression of important immune genes. Regarding the effects of functional feed ingredients, Test-fed fish in FW showed higher plasma cholesterol levels, and reduced symptoms of lipid malabsorption, suggesting that the mixture of nucleotides and immune stimulants enhanced digestive and absorptive capacity. However, the inclusion of nucleotides, DHA, and EPA affected the SW-fish only marginally. In conclusion, the outcomes of the present study highlight the great reductions in growth and impact on gut health biomarkers after seawater transfer, in this case observed under commercial Arctic conditions, and the promotion of metabolic capacity of Atlantic salmon fed functional ingredients in freshwater, which may shape future best-practices in salmon industry.
Introduction
The Atlantic salmon (Salmo salar L.) is one of the most produced fish species worldwide with high economic values (FAO, 2018). Salmon is an anadromous species that starts life in freshwater and migrates to the sea when they reach the smolt stage, i.e., when they are physiologically ready for the migration. Currently, salmon production in Norway uses two types of smolts: normal and out-of-season smolts. The normal smolt, or spring smolt, is exposed to a natural light cycle during the freshwater stage and smoltify in the spring; the out-of-season smolt, or autumn smolt, smoltify half a year earlier than the normal smolt when kept under an artificial light regime (Duncan et al., 1998). Studies have indicated that normal and out-of-season smolts have similar growth potential, feed utilization, and fillet quality (Duncan et al., 1998; Mørkøre and Rørvik, 2001). In some cases, out-of-season smolts have showed even better growth performance than normal smolts (Lysfjord et al., 2004; Kristensen et al., 2012) possibly due to better hypo-osmoregulatory ability at higher temperatures (Lysfjord et al., 2004) or reduction in the incidence of early sexual maturation that could reduce the extent of losses due to poor flesh quality (Oppedal et al., 2006). In a study of Johnsen et al. (2013), out-of-season adult salmon were observed to have higher muscle fiber number and density, firmer flesh and pigment content and intensity, and lower fillet fat content than those in normal adult salmon. However, also negative characteristics have been described such as reduced performance due to frequent outbreaks of heart and skeletal muscle inflammation (HSMI) during their first spring at sea (Alne et al., 2009) and higher risk of clinical outbreaks of infectious pancreatic necrosis (IPN) (Jensen and Kristoffersen, 2015).
For both smolt qualities, the most prominent alteration of the physiological functions, such as changes in hormone levels, nutrient metabolism, morphology, and behavior, takes place in the so-called smoltification process ending when they are ready for seawater transfer. The physiological changes taking place are resource demanding and may challenge defense mechanisms in the fish (Hoar, 1988; Johansson et al., 2016; Moore et al., 2018). For example, seawater transfer has been shown to suppress growth, immune responses, and skin barrier functions (Sissener et al., 2009; Tipsmark et al., 2010b; Sundh et al., 2014; Johansson et al., 2016; Karlsen et al., 2018). Moreover, seawater transfer significantly alters gut microbiota profiles in normal as well as out-of-season smolts (Dehler et al., 2017b; Rudi et al., 2018; Jaramillo-Torres et al., 2019; Jin et al., 2019; Lokesh et al., 2019). During this transition, fish sense and respond to various biotic factors (e.g. physiological status) and abiotic factors (e.g. water temperature, water salinity, and adjustments of diet). All these factors, which mostly cannot be controlled under commercial conditions, may influence gut health and microbiota.
Alterations in intestinal bacterial composition may influence host physiological responses such as growth (Bozzi et al., 2020), digestive and absorptive functions (Ray et al., 2012; Semova et al., 2012; Falcinelli et al., 2015), immune functions (Austin, 2006; Gomez and Balcazar, 2008; Merrifield and Ringø, 2014; Parra et al., 2020; Li et al., 2022), flesh pigmentation (Nguyen et al., 2020a; Nguyen et al., 2020b) and lipid metabolism (Dvergedal et al., 2020). Such associations and alterations during the freshwater-to-seawater transition have so far received little attention.
Since the freshwater-to-seawater transition is a challenging period in Atlantic salmon production, functional feed ingredients, such as nucleotides and immune stimulants (e.g. extracts from plants and yeast cell wall), are frequently supplemented to the diets with the aim to enhance fish robustness (Tacchi et al., 2011; Kiron, 2012). Results of earlier studies seem to indicate that inclusion of nucleotides in Atlantic salmon diet stimulates growth performance, increases absorptive capacity by increasing mucosal fold height (Burrells et al., 2001b), and reduces sea lice infection rate and mortality (Burrells et al., 2001a). Increased Na+, K+-ATPase activity in pyloric caeca (Oulad et al., 2009) and enhanced disease resistance against Caligus rogercresseyi infestation (González and Troncoso, 2009) have also been observed. Immune stimulants (e.g. yeast cell wall products), when included in Atlantic salmon diets, may increase feed efficiency (Refstie et al., 2010), alter gut morphology (Sweetman et al., 2008), improve immune responses (Kiron et al., 2016; Carolina, 2018; Wang et al., 2019), modulate gut microbiota (Green et al., 2013) and enhance resistance against sea lice infestation (Refstie et al., 2010; Dimitroglou et al., 2011). The observed improvements in production and health status of fish described above have been suggested to be related to beneficial regulation of both innate and adaptive immune responses, and/or the enhancement of beneficial gut microbiota (Ringø et al., 2010; Song et al., 2014; Ringø et al., 2016; Guerreiro et al., 2018; Mehdinejad et al., 2018; Hossain et al., 2019; Wang et al., 2019).
As the salmon production in the northernmost region in Norway is increasing, it becomes more and more urgent to increase knowledge on the causes of fluctuations of fish health biomarkers and microbiota through the production cycle, to be able to develop feed and management strategies for fish health and welfare in the aquaculture industry in these areas. The work presented here is part of a project investigating the host health and gut microbiota of normal and out-of-season Atlantic salmon smolts from late freshwater to seawater stages in the northernmost regions in Norway. Our previous work with normal smolts revealed that seawater transfer significantly increased the relative abundance of Lactobacillus and Photobacterium (Wang et al., 2021), and at the same time reduced plasma nutrients and expression in the gut of immune and barrier genes (Wang et al., 2020). Out-of-season smolts are exposed to environmental conditions differing from those of the normal smolts before and after seawater transfer. It is highly likely that, due to the different environmental conditions and fish age, the gut microbiota composition and its mechanisms and consequences on host health responses differ from the observation in normal smolts.
The aims of this study were therefore: Firstly, to strengthen knowledge on fluctuations in the gut microbiota and host health responses in out-of-season smolts during the freshwater-to-seawater transition period under commercial Arctic conditions. Secondly, to explore the association between the gut microbiota and host responses in these fish. Finally, to gain an understanding of the effect of dietary functional feed ingredients on the gut microbiota and host health responses.
Material and Methods
Fish Source and Maintenance
The Atlantic salmon used came from the same batch of eggs hatched in the facilities of Cermaq, Norway in March 2015. The fish were kept in two large aluminum tanks (depth: 2.8 m, volume: 450 m3) in Hopen, Norway, for 23 weeks before the seawater transfer. The freshwater was pumped directly from the a nearby lake approved by the Norwegian Food Safety Authorities into the tanks with a drum filter removing large particles. There was no mixing of water from other sources. The temperature of the freshwater followed natural fluctuations and ranged from 9.8 to 16.1°C. The dissolved oxygen level was above 10 mg/L. Each tank contained about 180 000 fish with an average initial body weight of 3.3 ± 0.1 g (Mean ± SD). In September 2015, the fish went through a photoperiod-induced parr-smolt transformation to produce out-of-season smolts before transportation to a sea location in Sommarbukt, near Alta in the far north of Norway. This photoperiod-induced treatment takes 12 weeks from continuous light LD24:0 to LD12:12 for 6 weeks, followed by the day-length from LD12:12 to LD24:0 for 6 more weeks (Duncan et al., 1998). Subsequently, one-day seawater challenge test was conducted to confirm that smolt were all ready for seawater transfer. In saltwater period, the salmon were farmed as is the standard setup for Norwegian salmon farming. This farming was open net pen with no mix of other water qualities than the naturally seasonal hydrodynamics that would occur in a fjord. The water exchange in the net pen would depend on the currents occurring in the period and the growth of fallowing organisms onto the nets. About 55 000 fish were kept in each net pen. A vertical device connected to an automatic winch (HF5000, Belitronics, Lunde, Sweden) was used to monitor the water parameters at 3 m depth in this study. The temperature of saltwater followed natural fluctuations ranged from 6.8 to 9.7°C. The dissolved oxygen level was above 10 mg/L and the salinity levels fluctuated from 20.8 to 40.1 ‰.
Diets
The diets were extruded. As Table 1 shows, the reference (Ref) diets were formulated varying in ingredient composition to meet the variation in nutritional requirements of Atlantic salmon in the freshwater (FW) and seawater (SW) phases. The Test diets (Test) were formulated from the respective reference diets by supplementing with nucleotides and immune stimulants (extracts from plants and yeast cell wall) to the FW-Ref formulation (FW-Test), and nucleotides and EPA and DHA to the SW-Ref formulation (SW-Test). These functional feed ingredients, as dry meal mix, were added to the Test diets according to recommendations from the producers. Their inclusion levels are not specified herein for the need to protect intellectual properties.
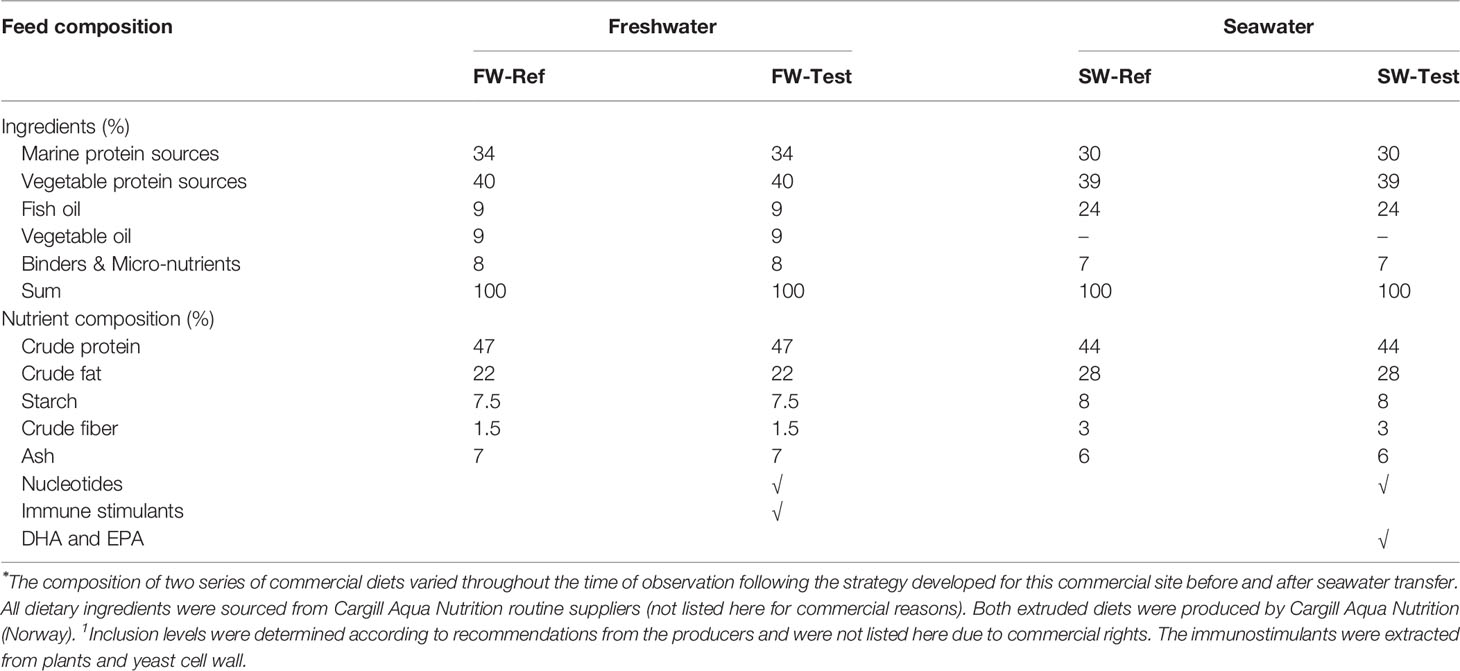
Table 1 Formulation and nutrient composition of Ref and Test diets during freshwater and seawater stages⁎.
Sampling
Fish were sampled four weeks before seawater transfer, in August 2015 and four weeks after seawater transfer in October 2015 as illustrated in Supplementary Figure 1A. The sampling was carried out four hours after the last feeding. Only fish with chyme present along the whole intestine were sampled to ensure exposure to the diets until the point of sampling. Supplementary Figure 1B shows the anatomy of the alimentary tract of Atlantic salmon and the workflow of sampling for analysis based on our previous description in Bakke-McKellep et al. (2000). At the freshwater sampling, 36 fish were taken from each tank which were split into 3 replicates of 12 fish, i.e., a total of 36 fish per treatment. Earlier study has indicated that the variation between the tanks in this facility is negligible making repeated samplings from the same tank acceptable (Wang et al., 2020). For the seawater samplings, triplicate net pens of fish were farmed for each treatment, i.e., a total of 36 fish per treatment. All fish were anesthetized and euthanized using the tricaine methanesulfonate (MS222, Argent Chemical Laboratories) before sampling. The body weight and fork length were recorded. The blood samples were collected from the caudal vein of 36 fish per treatment. Plasma was collected after centrifugation at 2000 g for 10 min at 4°C, and snap-frozen in liquid nitrogen, and then stored at -80°C until analysis. Subsequently, the whole intestine was dissected from each fish, then opened longitudinally after removing attached adipose tissue.
Six fish per replicate were used for collection of digesta samples as follows: the intestinal tract was divided in five sections i.e. the proximal (PI1) and distal (PI2) half of the pyloric intestine, the mid intestine (MI), and the proximal (DI1) and distal (DI2) half of the distal intestine. The digesta from the six fish per tank were pooled, frozen and stored as described above for plasma, until analyses of total bile acid levels and trypsin activities. After removing the digesta, the tissues from PI, MI, and DI were weighed for estimation of intestinal somatic indices, and then collected and stored at -80°C, until analysis of leucine aminopeptidase (LAP) capacity.
Another six fish per replicate were collected for the histological evaluation, gene expression analysis (qPCR), and microbiota profiling (lower left of Supplementary Figure 1). In order to avoid contamination, face masks were used and the samples were handled near a gas burner to reduce bacterial number in the air. All sampling tools were thoroughly cleaned, decontaminated with 70% ethanol sprays and flamed after the sampling of each fish. Tissues from pyloric caeca (PC) and DI were rinsed in sterile phosphate-buffered saline (PBS) three times to remove digesta and cut into pieces for RNA extraction (preserved in RNAlater solution, incubated at 4°C for one day, then stored at -20°C before RNA extraction) and histological evaluation (18 fish per treatment, fixed in 4% phosphate-buffered formaldehyde solution for one day, and transferred to 70% ethanol for storage). For the microbiota profiling, DI digesta was collected into a 10 mL skirted sterile centrifuge tube and mixed thoroughly using a spatula. An aliquot of the homogenate was then transferred into a 1.5 mL sterile Eppendorf tube and snap-frozen in liquid nitrogen, then stored at -80°C before DNA extraction. Between each sampled fish, all tools were decontaminated by an ethanol spray, then sterilized by flaming.
Plasma Biochemistry
Plasma samples were analyzed at Central Laboratory in the Faculty of Veterinary Medicine, Norwegian University of life science according to the standard procedures (Advia 1800, Siemens Healthcare Diagnostics, Erlangen, Germany).
Digestive Functions
Trypsin activity was analyzed according to the method of Kakade et al. (1973) using benzoyl arginine p-nitroanilide (No. B-4875, Sigma Chemical Co., St. Louis, MO) as the substrate. Total bile acid levels were determined at the Central Laboratory of Faculty of Veterinary Medicine of NMBU according to the method of Krogdahl et al. (2020).
The capacity of brush border leucine aminopeptidase (LAP) was analyzed as the description of Bieth et al. (Bieth et al., 1974). The tissue homogenate was prepared using ice-cold Tris−mannitol buffer (1:20, w/v). The four-(two-aminoethyl)-benzene-sulfonyl fluoride hydrochloride (Pefabloc SC, Basel, Switzerland) was used as a serine proteinase inhibitor to prevent protein hydrolysis during sample preparation. The incubations were performed at 37°C according to the method of Krogdahl et al., 2003. The LAP capacity is expressed as mmol substrate hydrolyzed per intestinal section per hour per kg fish.
Evaluation of Histological Sections
Tissues from DI and PC were cut to produce sections of 3-μm thickness and stained with hematoxylin and eosin (H&E) (Penn et al., 2011). The following observations related to typical inflammatory morphological changes were evaluated by light microscopy: the length of mucosal fold, the width of submucosa, cellular infiltration of lamina propria and submucosa, as well as the degree of enterocyte vacuolization. The histological sections were assigned a rank ranging from normal to severe as described in one of our earlier studies (Bakke-McKellep et al., 2007).
Quantitative Real-Time PCR (qPCR)
Three fish per replicate (n = 9 fish per treatment) were randomly selected for qPCR assays, which were carried out following the MIQE guidelines (Bustin et al., 2009). Briefly, total RNA was extracted from distal intestine in a randomized order using a custom made Reliaprep simplyRNA HT protocol (Promega) on the Biomek 4000 laboratory automation workstation (Beckman Coulter). The RNA extraction included a DNase treatment according to the manufacturer’s protocol. The RNA purity and concentration were evaluated by Epoch Microplate Spectrophotometer (BioTeK Instruments, Winooski, VT, USA). The average 260/280 ratio of samples was 2.2 ± 0.02 (Mean ± SD). The RNA integrity was measured by the 2100 Bioanalyzer in combination with an RNA Nano Chip (Agilent Technologies) and 6000 Nano LabChip kit (Agilent Technologies, Palo Alto, CA, USA). The average RNA integrity number (RIN value) of all samples was 9.1 ± 0.4 (Mean ± SD).
The cDNA synthesis and qPCR assays were conducted as described elsewhere (Kortner et al., 2013). Briefly, the cDNA synthesis was conducted using 1.0 μg total RNA from all samples using a Superscript™ IV VILO™ cDNA synthesis kit (Invitrogen, Carlsbad, CA, USA, catalog no., 11756050). The qPCR assays were carried out using the LightCycler 96 (Roche Applied Science, Basel, Switzerland) with a 10-μL DNA amplification reaction including 2 μL of PCR-grade water, 2 μL diluted cDNA template, 5 μL LightCycler 480 SYBR Green I Master (Roche Applied Science) and 0.5 μL (10 μM) of each forward and reverse primer. Samples were run in duplicates in addition to a no-template control for each gene. A three-step qPCR program was performed including an enzyme activation step at 95°C (5 min), 45 cycles of 95°C (10 s), 56–60°C (10 s), as well as 72°C (15 s). The plate pipetting was done using the Biomek® 4000 automation workstation. Details of primer pairs used for the qPCR assays in DI and PC are shown in Supplementary Tables 1, 2, respectively. The RNA polymerase II (rnapoii) and hypoxanthine phosphoribosyl transferase 1 (hprt1) for DI and β-actin (actb) and glyceraldehyde-3-phosphate dehydrogenase (gapdh) for PC using as reference genes were selected based on their overall coefficient of variation (CV) and their interspecific variance (Kortner et al., 2011). The reference genes as an internal normalization factor, the raw quantification cycle (Cq) values were used to calculate the mean normalized levels of target genes in DI and PC (Muller et al., 2002).
Sequencing of Gut Microbiota
DNA extraction: Three fish per replicate (n = 9 fish per treatment) were used for DNA extraction (same fish as qPCR). The sample size was decided here according to previous suggestions (Panteli et al., 2020). One fish per treatment was randomly selected to divide all fish into 9 batches for DNA extraction to avoid batch effect. About 100 mg of DI digesta from each sample was homogenized using the bead beating, then an additional heating step of 95°C for 7 min, was conducted according to the suggestion by Knudsen et al. (2016). Hereafter, the homogenate were used for DNA extraction based on the standard procedure provided by the manufacturer of QIAamp Fast DNA Stool Mini Kit (Qiagen, Hilden, Germany). For quality control purposes, a blank and a mock (Catalog No: D6300, ZymoBIOMICS Mock Community Standard, Zymo Research) were added to the DNA extraction step used as a negative and positive control, respectively.
PCR amplification: The PCR amplification of the 16S rRNA V1-V2 region was performed using the universal primer set 27F (5′-AGA GTT TGA TCM TGG CTC AG-3′) and 338R (5′-GCW GCC WCC CGT AGG WGT-3′) (Roeselers et al., 2011) based on the descriptions of Gajardo et al. (2016). Briefly, total 25μl reaction volume was used for PCR including 2 μl of DNA template, 22.4 μl PCR Master Mix (Thermo Scientific, CA, USA; catalog no: F531L), and 0.6 μl mixture of forward (27F) and reverse 338R primers (50 pM). The PCR was run in duplicate including the molecular grade water as negative PCR controls. The amplification program was set as follows: initial denaturation at 98°C for 3 min; 35 cycles of denaturation at 98°C for 15 s, annealing decreasing from 63°C to 53°C in 10 cycles for 30 s followed by 25 cycles at 53°C for 30 s, and extension at 72°C for 30 s; followed by a final extension at 72°C for 10 min. For samples with invisible bands in the agarose gel after PCR, the PCR condition was optimized by applying serial dilutions to the DNA templates to reduce the influence of PCR inhibitors. After the PCR amplification, the duplicate PCR products were pooled (10 µl) to evaluate the library preparation using 1.5% agarose gels. Samples with a bright band of size between 300bp and 350bp were considered suitable for further processing. As one batch of samples showed poor PCR amplification, we removed these samples and left 8 samples per treatment (n = 8) for final sequencing.
Quantification of 16S rRNA gene by qPCR: The 16S rRNA gene quantity of DNA templates used for the amplicon PCR was measured by qPCR as described in the section of quantitative real-time PCR (qPCR). The qPCR assays were performed using a universal primer set (forward, 5’-CCA TGA AGT CGG AAT CGC TAG-3’; reverse, 5’-GCT TGA CGG GCG GTG T-3’) used for bacterial DNA quantification as described in previous studies (Ramseier et al., 2009; Vandeputte et al., 2017).
Library preparation and sequencing: The PCR products cleanup, library preparation, and sequencing were performed following the Illumina 16S Metagenomic Sequencing Library Preparation Protocol (Illumina, 2013). Briefly, the PCR products were cleaned using the Agencourt AMPure XP system (Catalog No: A63881, Beckman Coulter) multiplexed by dual indexing using the Nextera XT Index Kit (Catalog No: FC-131-1096, Illumina) and purified again using the AMPure beads. The representative libraries were analyzed using the Agilent DNA 1000 Kit (Catalog No: 5067-1505, Agilent Technologies) to verify the library size. Cleaned libraries were quantified using the Invitrogen Qubit™ dsDNA HS Assay Kit (Catalog No: Q32854, Thermo Fisher Scientific), diluted to 4 nM in 10 mM Tris, and pooled in an equal volume. The library was loaded at 6 pM and sequenced using the Miseq Reagent Kit v3 (Catalog No: MS-102-3003, Illumina) according to the manufacturer’s instructions.
Calculations and Statistical Analysis
Calculations
TGC (Thermal growth coefficient) = [sampling body weight (g) 1/3 - initial body weight (g) 1/3] × (∑ day degree)-1;
CF (Condition Factor) = 100 × sampling body weight (g)/sampling body fork length (cm)3;
ISI (Intestinal somatic indices %) = 100 × sampling intestinal section weight/sampling body weight (g).
Statistical Analyses of Data Other Than Microbiota Sequence Data
The Shapiro-Wilk and Barlett’s test were used for the validation of normality and homogeneity of variances. For certain resulting data, log transformation was used to meet normal distribution, then the data were analyzed using one-way ANOVA followed by Tukey multiple comparisons tests. Data not achieving normal distribution after transformation and were analyzed using the Kruskal-Wallis test followed by Dunn’s multiple comparisons test. For the histological features, the scores generated were categorical variables and the differences between the treatments were explored by contingency analysis using the chi-squared test. The statistical analyses and figures were conducted using GraphPad Prism 8 (GraphPad Software, La Jolla, California, United States). Values with the same superscript letter are not significantly different. The level of significance in all analyses was set at p <.05.
Sequence Data Analysis
The demultiplexed raw sequence data were denoised by the DADA2 algorithm (Callahan et al., 2016) in QIIME 2 (version 2019.4) to generate amplicon sequence variants (ASVs) (Bolyen et al., 2019) as described elsewhere (Li et al., 2021). The taxonomy was assigned in QIIME2 against the SILVA database (version 132) (Quast et al., 2013) trained by a naive Bayes machine-learning classifier (Bokulich et al., 2018). The contaminant sequences, including Acinetobacter, Aeromonas, Cutibacterium, Flavobacterium, Leptothrix, Pseudomonas, and Streptophyta, as well as unclassified Chitinophagales (order) and Betaproteobacteriales (class), were removed based on the criteria suggested by Davis et al. (2018). The alpha diversity was evaluated by the Observed species index and Shannon’s index. The beta diversity was assessed by Unweighted UniFrac and Weighted UniFrac Bray-Curtis distances using Primer 7 (version, 7.0.13) followed permutation multivariate analysis of variance (PERMANOVA) (Clarke et al., 2006).
The microbial clades (genus level) were tested for the associations with metadata of interest (the metadata seen in Supplementary Table 3) using the MaAsLin2 (version, 0.99.12) (https://huttenhower.sph.harvard.edu/maaslin2) in R with the default parameters (Mallick et al., 2021). Bacterial taxa of more than 0.1% abundance and at least 25% prevalence of all samples were selected for association testing. The significant association was set at a q-value < 0.25. The metadata of health responses, i.e., plasma nutrients, DI supranuclear vacuolization (histology), and gut immune functions (qPCR in DI), were selected to run the multivariate association testing with fixed factor, i.e., treatment. Since plasma nutrients, including plasma cholesterol, triglyceride, free fatty acid, and glucose, were highly correlated (Supplementary Figure 2), we ran a principal component analysis (PCA) and extracted the first principal component (PC1) for the association testing to avoid multicollinearity and reduce the number of association testing. Regarding the DI supranuclear vacuolization, the skewed five-category degree was collapsed into more balanced binary data, i.e., normal and abnormal (mild, moderate, marked, and severe), to make the data appropriate for the association testing. The gut immune function-related genes selected for the association testing included pro-inflammatory cytokines [interleukin-8 (il8) and interferon gamma (ifnγ)], anti-inflammatory cytokine [transforming growth factor-beta (tgfβ)], as well as T-cell markers [e.g. cluster of differentiation-4 alpha (cd4α), 8 beta (cd8β) and 3γδ (cd3γδ)]. Since the immune-related genes were highly correlated (Supplementary Figure 3), the extracted PC1 of the PCA was also used for association testing.
Results
Growth Performance and Intestinal Indices
Regardless of dietary treatment, transfer to seawater reduced growth rate of the fish, estimated as thermal growth coefficient (TGC) (p <.05, Figures 1A, B). No clear diet effect on growth performance was observed either in FW- or SW-fish (p >.05, Figures 1A, B). Transfer to seawater also decreased condition factor (p <.05, Figure 1C) and this reduction was more pronounced for the Test-fed fish than for those fed the Ref diet (p <.05, Figure 1C).
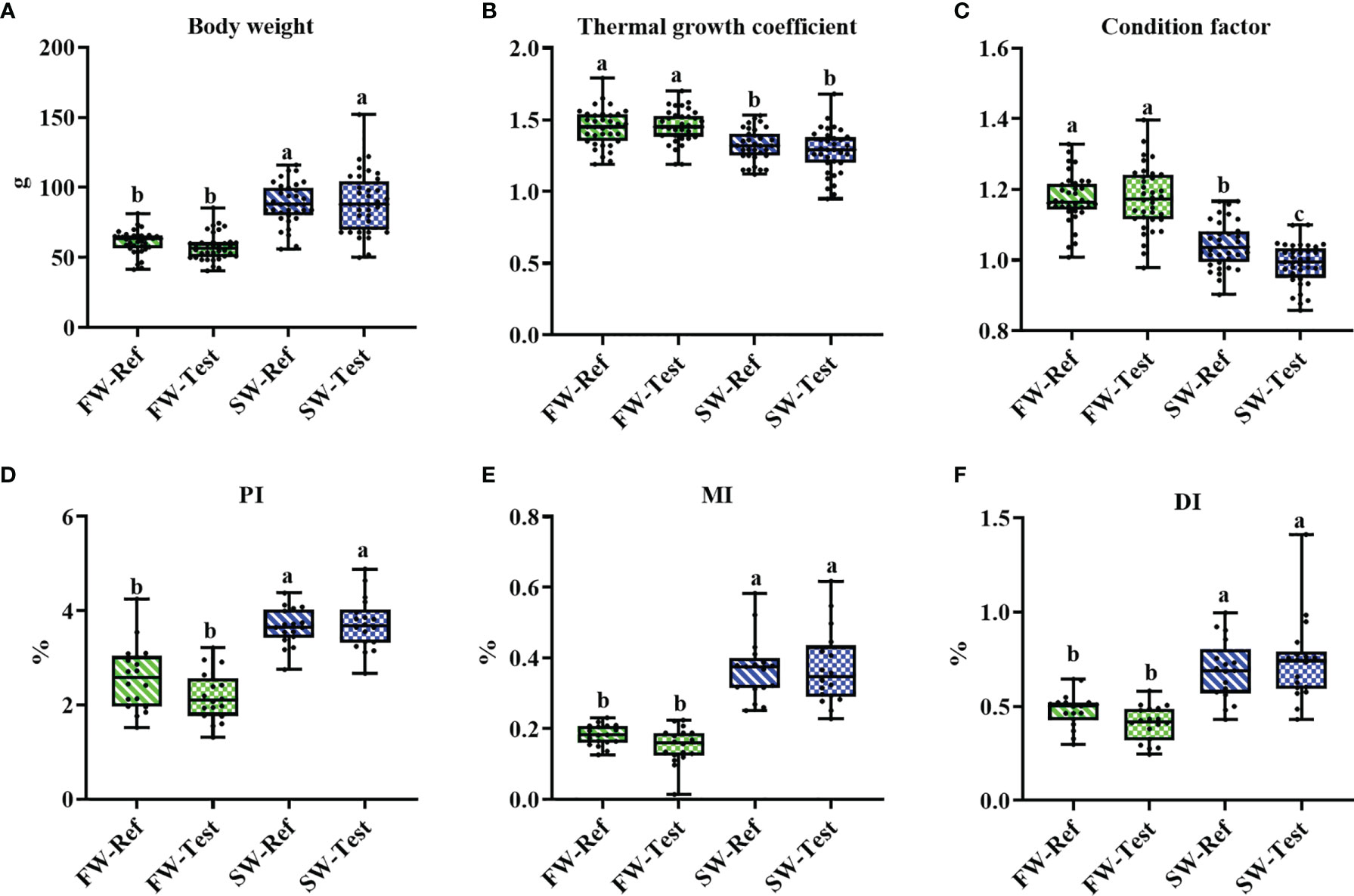
Figure 1 The growth performance (A, B), condition factor (C) and intestinal indices (D–F) of Atlantic salmon among treatments. Different letters among groups denote significant differences (p < .05) and values sharing the same letters are not significantly different. PI, proximal intestine; MI, mid intestine; DI, distal intestine.
Regarding somatic indices of the intestinal segments, seawater transfer significantly increased the values for PI, MI, and DI (p <.05, Figures 1D–F) Diet did not affect the indices either in FW- or in SW-fish (p > .05, Figures 1D–F).
Plasma Biochemistry
Seawater transfer decreased plasma cholesterol, triglyceride, free fatty acid, and glucose levels substantially, in the range from 40% to 70% (p <.05, Figures 2A–D). For plasma chloride and sodium levels, an increase was observed (p <.05, Figures 2E, F), as expected.
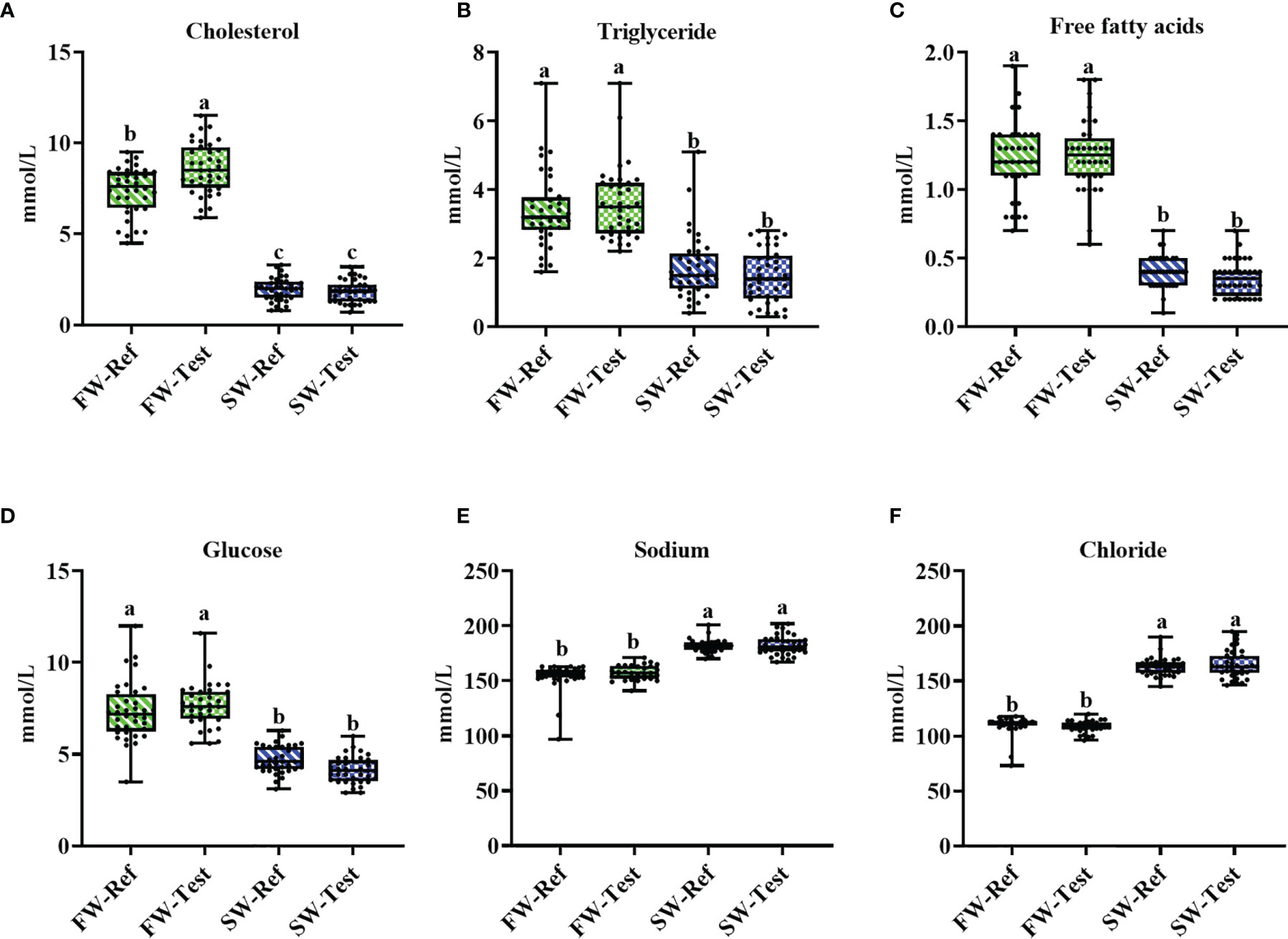
Figure 2 Changes in the measured plasma metabolites in Atlantic salmon fed different diets before and after the seawater transfer. (A-D) plasma nutrients; (E, F) plasma ions. Data are presented from min to max with all points. Different letters among values denote significant differences (p < .05, n = 36) and values sharing the same letters are not significantly different.
At the freshwater stage, but not after seawater transfer, fish fed the Test diet, showed significantly higher cholesterol levels than the Ref fed fish (p <.05, Figure 2A). No significant diet effects were found for other plasma biomarkers (p >.05, Figure 2) whether in FW- or SW-fish.
Intestinal Enzyme Activities and Bile Acid Levels
Fish from the FW-Test treatment, compared to those fed the Ref diet, showed elevated digesta trypsin activity, but only in PI-2 and MI (p <.05, Figure 3A). After sea transfer, no significant diet effects were observed (p >.05, Figure 3A).
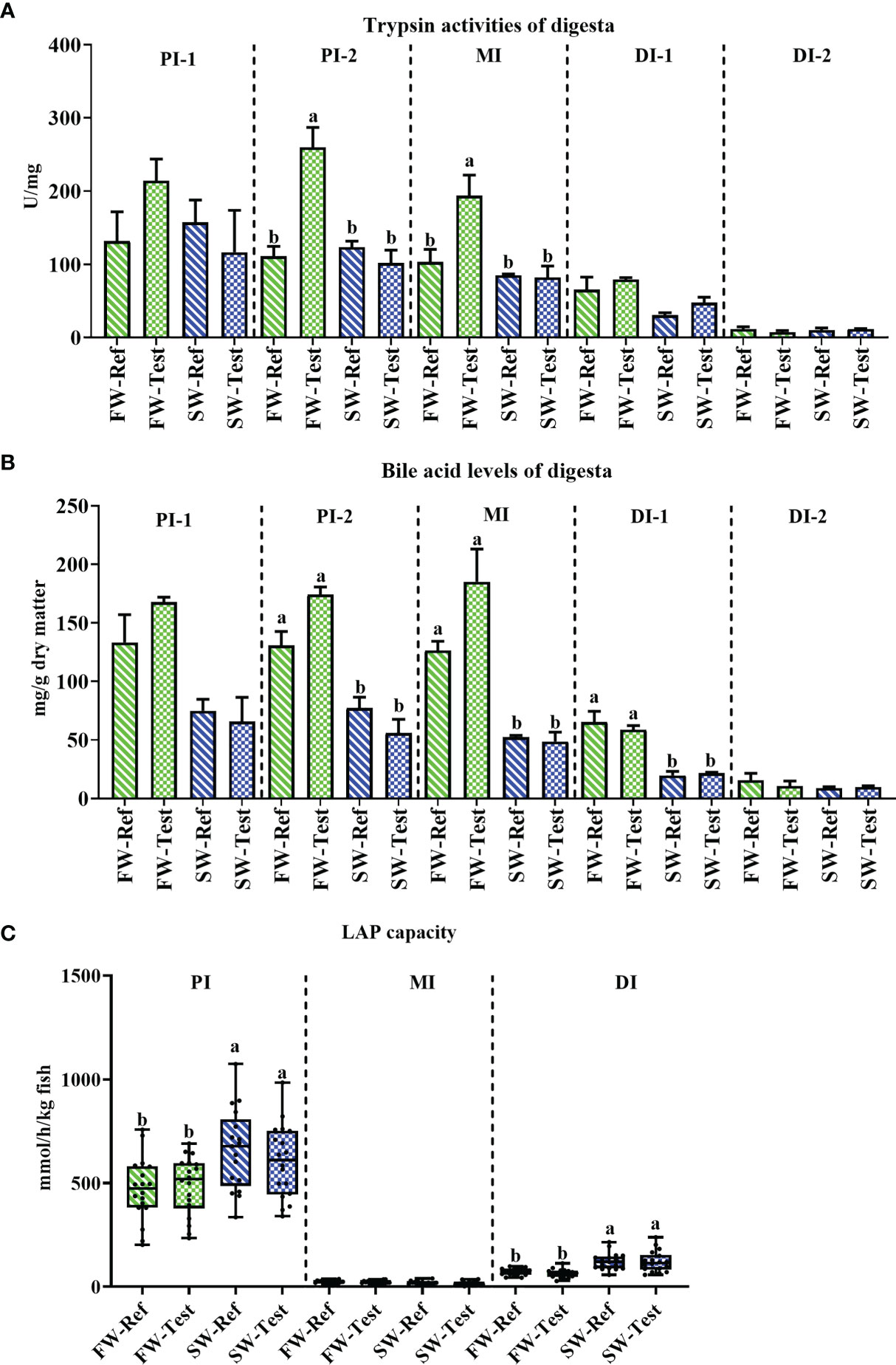
Figure 3 Changes in the digestive enzyme activities and chyme bile salts levels in Atlantic salmon fed different diets before and after the seawater transfer. (A) trypsin activities (n = 3); (B) bile acid level (n = 3); (C) leucine aminopeptidase (LAP) capacity (n = 18). Different letters among values denote significant differences (p <. 05) and values sharing the same letters are not significantly different. PI, proximal intestine; MI, mid intestine DI, distal intestine.
Regarding the chyme bile acid levels, decreased levels were found in PI-2, MI, and DI-1 after seawater transfer (p <.05, Figure 3B), whereas no significant diet effects were observed either in fresh nor seawater (p >.05, Figure 3B).
Compared to fish in FW, fish in SW had significantly higher LAP capacity in PI and DI (p <.05), but not in MI (Figure 3C). There was no significant diet effect on intestinal LAP capacity at any sampling time points or any intestinal segments (p >.05, Figure 3C).
Histological Appearance
Most of the fish showed normal histological characteristics in the DI regarding mucosal fold height, submucosa cellularity and supranuclear vacuolization (Figures 4A–C). Enterocyte hyper-vacuolization, or the so-called steatosis, was observed in the PC in all the sampled fish. The steatosis became significantly less pronounced after the seawater transfer (p <.05, Figure 4D). Moreover, when the fish were kept at FW, the steatosis was less severe in fish fed the Test-diet (p <.05) than in the Ref fed fish (Figure 4D).
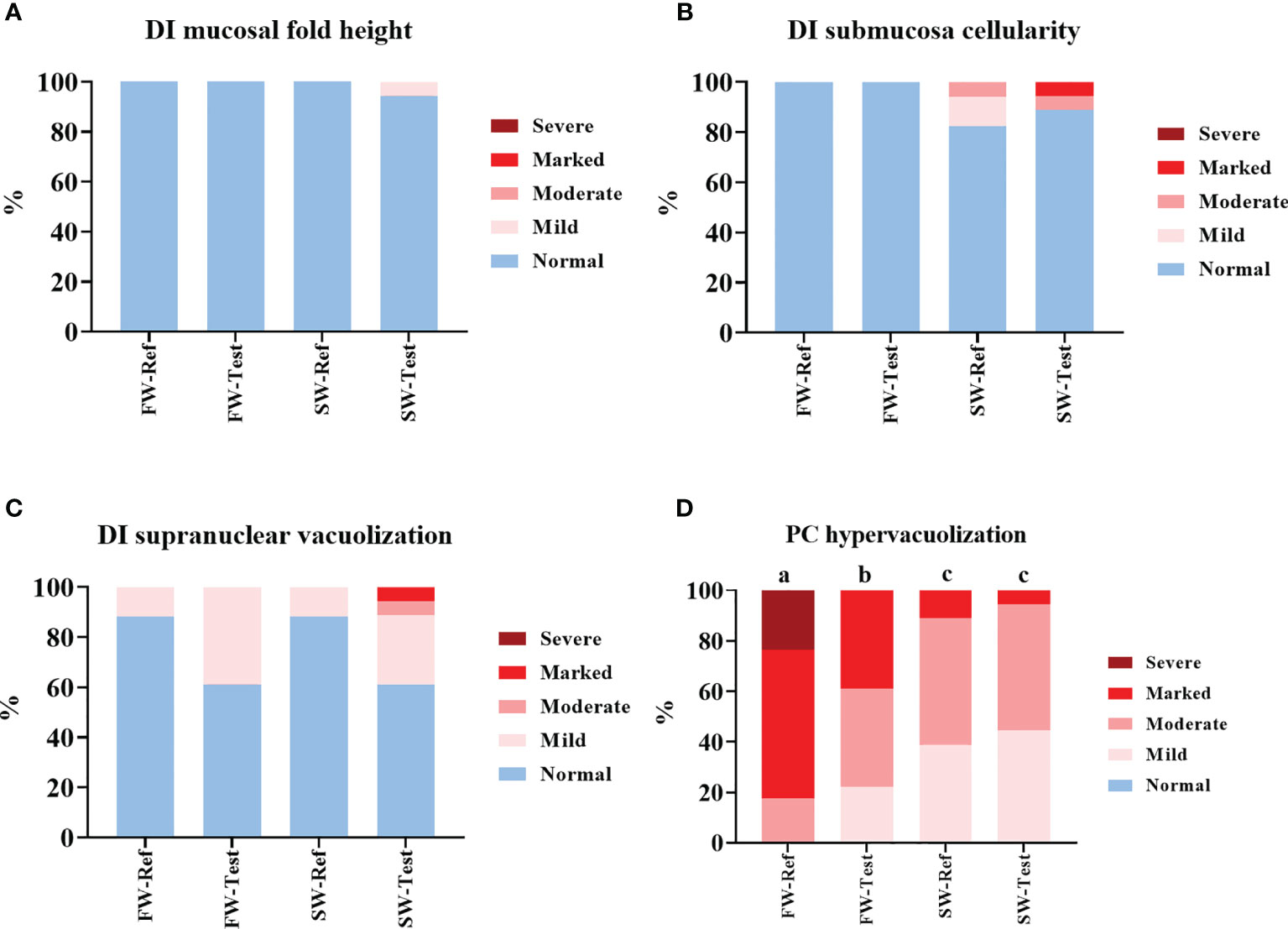
Figure 4 Contingency charts of the distal intestine (A–C) and pyloric caeca (D) morphology results. DI, distal intestine; PC, pyloric caeca.
Gene Expression in DI
Regarding goblet cell markers, lower mucin-2 (muc2) expression was observed in fish in SW compared to those in FW, whereas the mucin-13 (muc13) showed the opposite trend (p <.05, Figures 5A, B).
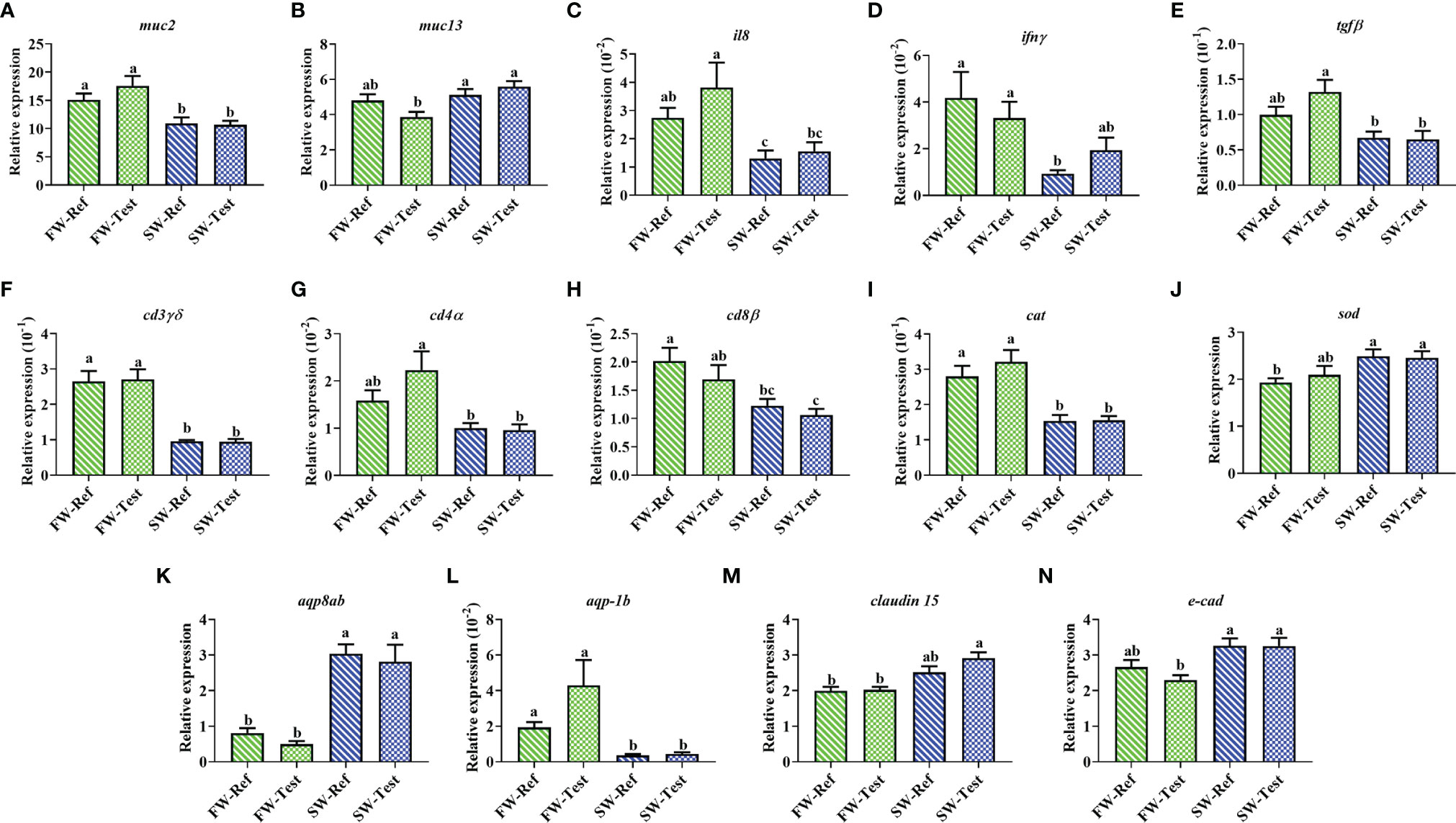
Figure 5 Expression of genes related to distal intestine functions of Atlantic salmon among treatments. (A–H) immunity; (I, J) antioxidation; (K, L) osmoregulation; (M, N) tight junction. Data are presented as mean ± SEM. Different letters among values denote significant differences (p < .05, n = 9) and values sharing the same letters are not significantly different.
A drop in the expression of several immune-related genes was observed after seawater transfer. These genes included pro-inflammatory cytokines (il8 and ifnγ), anti-inflammatory cytokine (tgfβ), and T-cell markers (cd4α, cd8β, and cd3γδ) (p <.05, Figures 5C–H).
Compared to those sampled in FW, fish sampled in SW showed a lower expression in catalase (cat), while the expression of superoxide dismutase (sod) showed an opposite trend (p <.05, Figures 5I, J).
For genes related to osmoregulation, the expression of aquaporin 8ab, aqp8ab, increased significantly after the seawater transfer (p <.05, Figure 5K), whereas an opposite trend was found for aquaporin-1 b (aqp-1b) expression (p <.05, Figure 5L). The claudin-15 (cldn15) and e-cadherin (cdh1) tended to have higher expression in fish at SW compared to those at FW (p <.05, Figures 5M, N).
No significant differences between dietary treatments in FW or in SW were observed for any of the aforementioned genes (p >.05, Figure 5).
The results for other genes showing no clear seawater transfer or diet effect are presented in Supplementary Tables 4, 5.
Gene Expression in PC
Fish in SW showed lower perilipin-2 (plin2) expression than those in FW (p <.05, Figure 6A). In contrast, higher expression of cytochrome P450 51 (cyp51), Niemann-Pick C1 like 1 (npc1l1), and peptide transporter (pept) was observed in SW compared to those in FW (p <.05, Figures 6B–D). No significant diet effects were observed for these genes (p >.05, Figure 6).
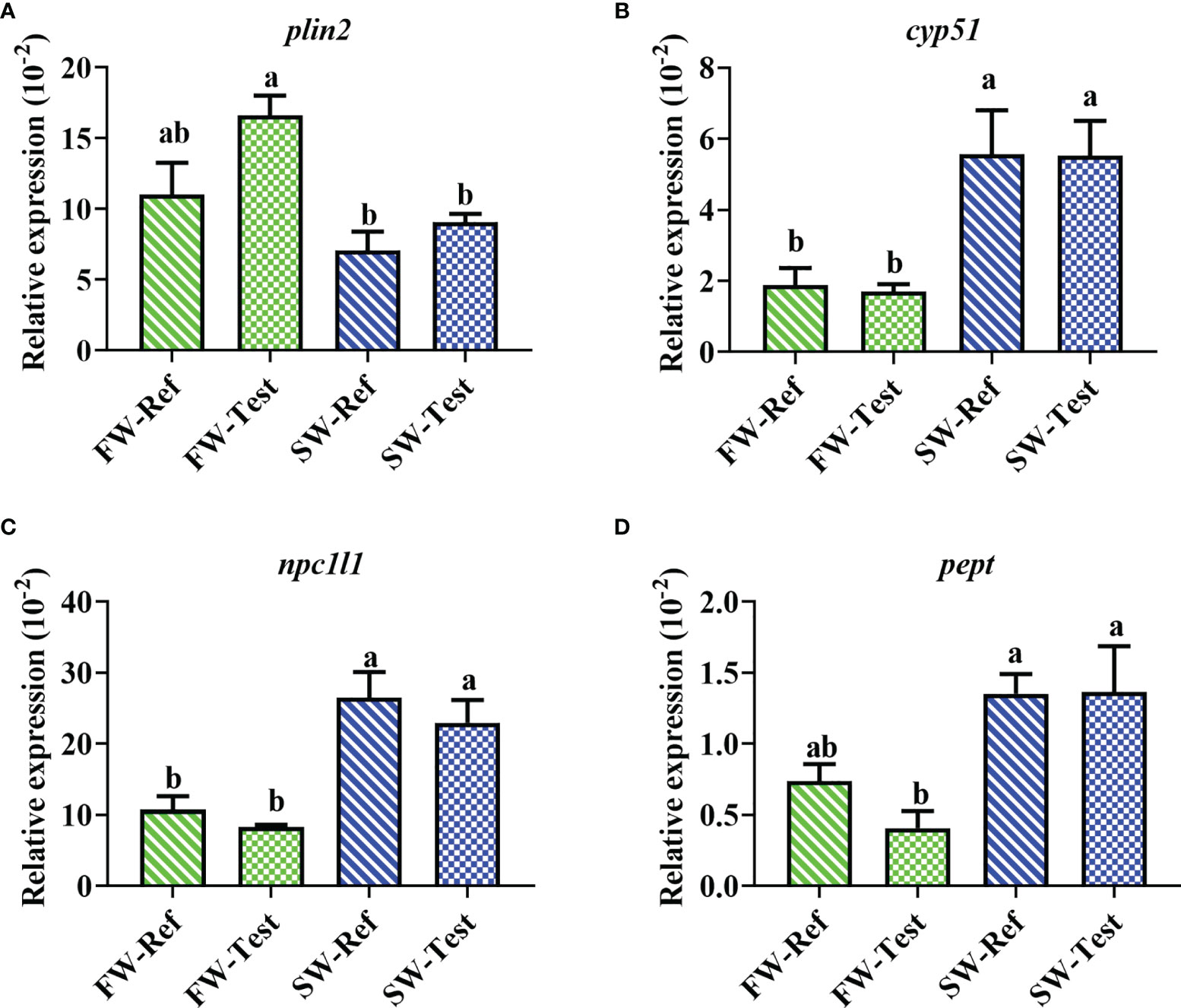
Figure 6 Expression of genes in the pyloric caeca of Atlantic salmon among treatments. (A) surface marker of intestinal lipid droplets; (B) cholesterol biosynthesis; (C) cholesterol transport; (D) peptide transporter. Data are presented as mean ± SEM. Different letters among values denote significant differences (p < .05) and values sharing the same letters are not significantly different.
The results for other genes showing no clear effect of seawater transfer or diet effect are presented in Supplementary Table 6.
Gut Microbiota
The mock from 8 different DNA extraction batches showed a similar microbiota profile indicating good reproducibility (Supplementary Figure 4). Regarding quantification of bacterial 16S rRNA by qPCR, there were no significant differences among treatments (p >.05, Supplementary Figure 5).
Alpha diversity: Compared to fish in FW, significant increases in microbial richness (Observed species index) and diversity (Shannon’s index) were observed in fish in SW. No clear diet effects were observed (Figures 7A, B).
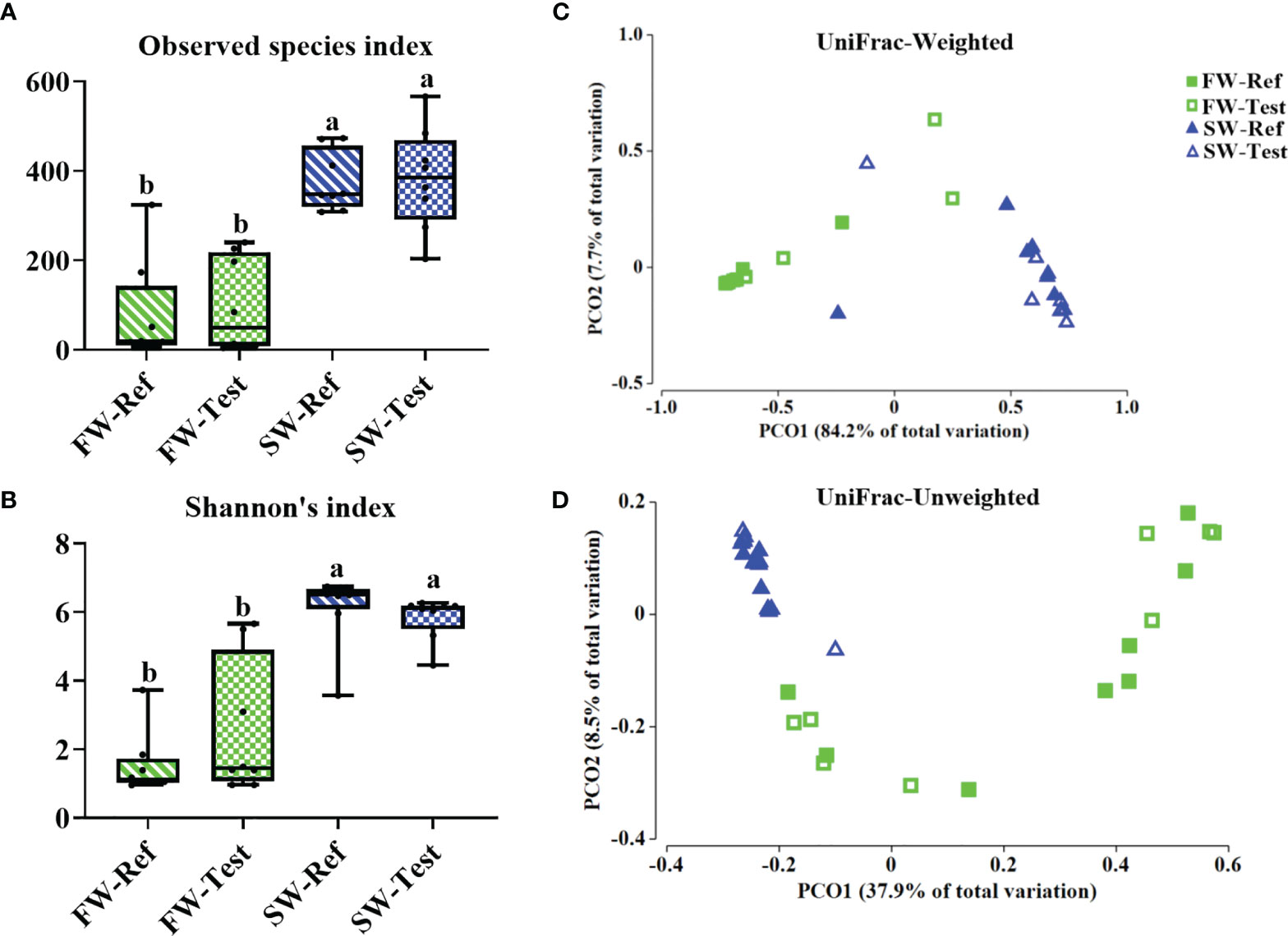
Figure 7 Alpha diversity (A, B) and beta-diversity (C, D) of the microbiota in distal intestinal digesta among treatments. Data are presented from min to max with all points. Different letters among values denote significant differences (p < .05, n = 8).
Beta diversity: PERMANOVA analysis of the gut microbiota showed clear differences in the bacterial composition between FW and SW as illustrated by the PCoA plots based on the UniFrac-Weighted and -Unweighted distances (p <.001, Supplementary Table 7 and Figures 7C, D). A diet effect was observed for the SW samples based on the Pairwise test of UniFrac-Unweighted (p = 0.028, Supplementary Table 7) but not based on the UniFrac-Weighted. In FW, no diet effects were indicated.
Relative abundance of all ASVs for the samples is presented in Supplementary Table 8. The taxonomic compositions of the gut microbiota in Atlantic salmon differed greatly between the FW and SW stage regardless of dietary treatment (Figure 8). More specifically, the microbiota composition of fish in FW was strongly dominated by Firmicutes (98% ± 4%), mainly the unclassified Ruminococcaceae (85% ± 35%). After seawater transfer, Firmicutes (mainly genera Lactobacillus) and Proteobacteria (mainly genera Photobacterium) dominated the gut microbiota, and together they accounted for about 90% of the total abundance, i.e., Firmicutes (63% ± 10%) and Proteobacteria (27% ± 7%), respectively.
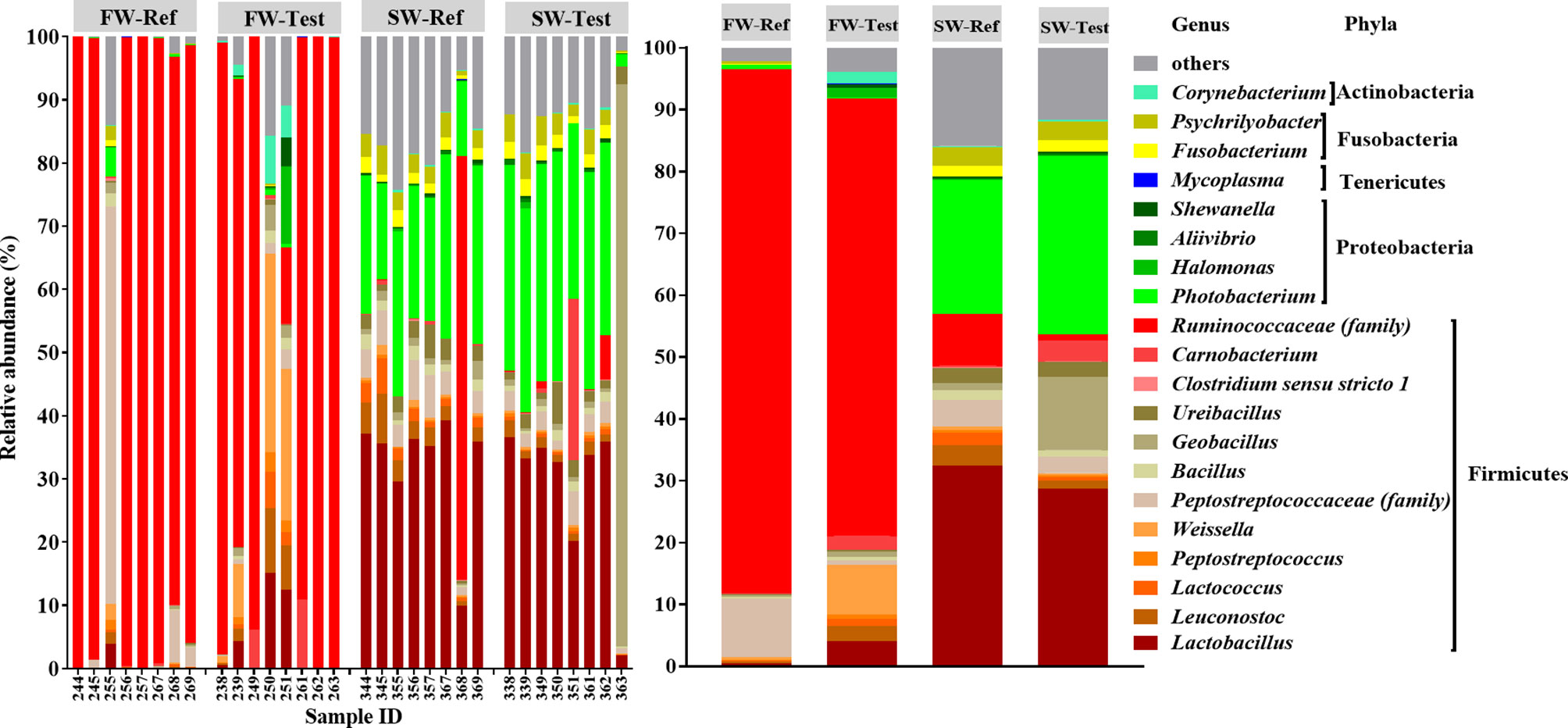
Figure 8 Top 20 most abundant taxa of all samples at the genus level. The top 20 genera accounted for more than 80% of the total abundance in each treatment. The mean relative abundance of each taxon within the same treatment is displayed on the right side. f__, family.
Important Associations Between Microbial Clades and Metadata
The multivariate association analysis identified one taxon, i.e., Clostridium sensu stricto 8, for which the relative abundance was negatively associated with the levels of plasma nutrients (Figure 9 and Supplementary Figure 6), showing an increase as the PC1 of the PCA increased. The relative abundance of unclassified Cyanobiaceae (family) and unclassified SAR86 clade (order) was found to be increased in fish with abnormal DI vacuolization (Figure 9 and Supplementary Figure 7 ). Twelve taxa, including the lactic acid bacteria, e.g. Lactobacillus, Lactococcus, and Leuconostoc, correlated significantly with immune-related gene expression in the DI. Except for the Synechococcus.CC9902, the relative abundance of these taxa was positively associated with expression of immune-related genes (Figure 9 and Supplementary Figure 8), which increased as the PC1 of the PCA increase.
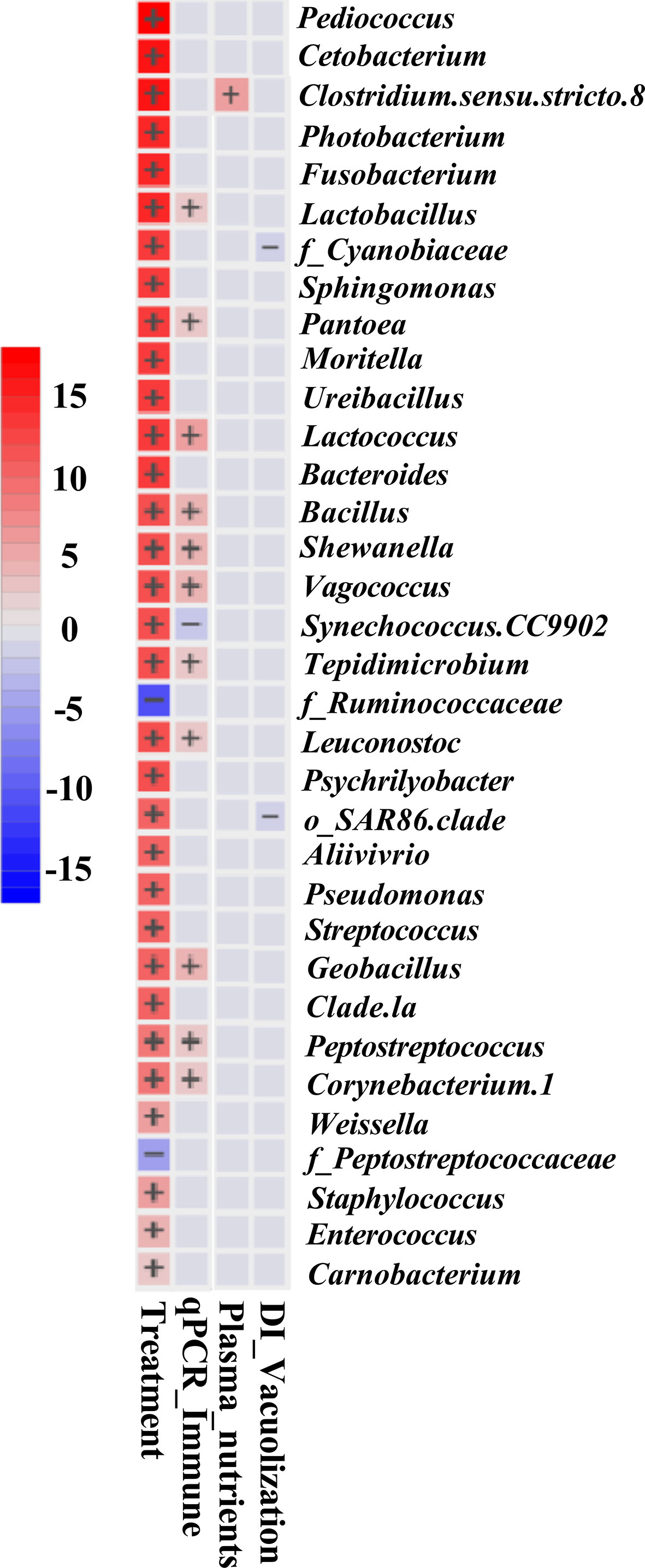
Figure 9 Significant associations between microbial clades with sample metadata of interest. Heatmap summarizing all the significant associations between microbial clades and sample metadata of interest. Color key: -log (q-value) * sign (coefficient). Cells denoting significant associations are colored (red or blue) and overlaid with a plus (+) or minus (−) sign indicating the direction of association. Note that the expression of immune genes increase as the PC1 value of PCA increases, while plasma nutrient values showed the opposite trend (Supplementary Table 3). For immune functions, except the Synechococcus.CC9902, the gut immune gene expressions were found to be positively correlated with the relative abundance of other 11 taxa. Regarding plasma nutrient levels, the plasma nutrient levels were found to be negatively correlated with the relative abundance of Clostridium sensu stricto 8. For DI supranuclear vacuolization, the higher relative abundance of the microbial clade was significantly correlated with abnormal features of distal intestinal supranuclear vacuolization. The significant association was set at FDR (q-value) < 0.25. f__, family; o__, order.
Discussion
The most important findings of our study deserving discussion were the following:
1. Transfer to seawater reduced growth rate, condition factor, plasma nutrient levels, digesta bile acid concentrations, as well as expression of gut immune genes.
2. The unclassified Ruminococcaceae family strongly dominated the gut microbiota of FW-fish, while the genera Lactobacillus and Photobacterium dominated the SW-fish.
3. Multivariate association analysis revealed that relative abundance of certain lactic acid bacteria correlated positively with expression of genes related to gut immune functions.
4. In FW-fish fed the diet supplemented with a mixture of nucleotides and immune stimulants showed a tendency towards an increase in metabolic capacity compared to the FW-fish fed the unsupplemented diet. In SW-fish dietary supplementation with the mixture of nucleotides, DHA, and EPA, affected the fish only marginally.
Effects of Seawater Transfer on Fish Physiology
The reductions in growth rate and condition factor observed after seawater transfer in the present study were most likely due to a combination of reduction in feed intake and increased demand for nutrients and energy the first weeks after seawater transfer. The work of Usher et al. (1991), showing reduced appetite lasting more than one month after seawater transfer, supports this suggestion. Reduction in feed intake and increased energy demand may be related to the physiological alterations taking place in the fish during smoltification, as well as the stressful conditions due to handling, long transport, and environmental changes (Hoar, 1988; Bjornsson et al., 2011). The observation in our study of an increase in LAP activity and capacity may be related to changes in environmental, physiological, diet and nutritional conditions. The physiological transformation required to handle the change in salinity, the increase in workload regarding osmoregulation in saltwater compared to freshwater, and the accompanying increase in need for energy, may induce increase in LAP production. These changes occur in a situation of increased handling and transport, which may take many days, and greatly reduce feed intake. In such situation, the fish may increase its capacity for digestion and utilization of nutrients (Krogdahl et al., 2004). Moreover, the change in environmental temperature, in this case, from a higher to a lower, with inevitable reduction in enzyme activity, may also have triggered higher production of LAP, as well as of other enzymes. The observed reduction in plasma nutrient levels after seawater transfer support the suggestion of deficient nutrient and energy intake (Sheridan, 1989; Sissener et al., 2009), and is also in line with the results of our previous study with normal smolts (Wang et al., 2020).
The lower expression of immune genes including pro-inflammatory cytokines, anti-inflammatory cytokine and T-cell markers after the seawater transfer in our study is in line with earlier findings in both normal and out-of-seasonal smolts (Johansson et al., 2016; Karlsen et al., 2018; Wang et al., 2020) indicating frangible intestinal immune homeostasis after seawater transfer (Secombes et al., 2001; Secombes, 2016). This may be an unavoidable effect of the physiological alterations during smoltification and just after seawater transfer due to the stressful occasions. These effects on immune functions may be related to the state of nutrient deficiencies that the fish supposedly suffer from after transfer to the sea (Moore et al., 2018). Also, the downregulation of muc2 after seawater transfer might indicate the fish’s susceptibility to diseases, since muc2 is highly expressed in the intestinal segments of Atlantic salmon (Sveen et al., 2017) and acts as the first-line defense against pathogens (Van der Marel et al., 2012; Koshio, 2015; Salinas, 2015).
The noticeable hyper-vacuolization in the PC enterocytes independent of diet indicates a situation of limited lipid transport capacity across the gut mucosa, leading to steatosis, also called lipid malabsorption syndrome. The steatosis may be due to high fat intake concomitant with the deficient supply of choline which is necessary for efficient lipid transport (Hansen et al., 2020a; Hansen et al., 2020b). Slower rate of several physiological functions just before and after seawater transfer could be an additional cause of limited lipid transport capacity (Hoar, 1988; Tipsmark et al., 2010a; Tipsmark et al., 2010b; Bjornsson et al., 2011). Seawater transfer reduced PC hyper-vacuolization and decreased the expression of plin2, a marker for intestinal lipid droplets and steatosis (Heid et al., 1998), supposedly as a consequence of reduced feed intake with corresponding reduction in demand for lipid transport capacity. The increased expression of genes related to the synthesis and transport of cholesterol (cyp51 and npc1l1) supports this explanation. On the contrary, normal smolts showed opposite results in our previous study, i.e., exacerbating lipid malabsorption symptoms in fish after seawater transfer (Wang et al., 2020). The explanation for the apparent difference may be differences in the feed intake due to different environmental temperature. The normal smolts in the previous study were transferred from cold (4.5°C) to warmer (9.9°C) waters, which may have stimulated the feed intake (Wang et al., 2020). The situation was the opposite for the out-of-season smolt in the present study, which was transferred from relatively warm (15.6°C) to colder (7.3°C) waters, supposedly reducing feed intake.
Effects of Seawater Transfer on Gut Microbiota
In line with previous studies in out-of-season as well as in normal smolts (Lokesh and Kiron, 2016; Dehler et al., 2017b; Jaramillo Torres, 2017; Rudi et al., 2018; Jaramillo-Torres et al., 2019; Lokesh et al., 2019), we observed that seawater transfer had a substantial effect on the microbiota profile. When in FW the fish were strongly dominated by Ruminococcaceae, suggesting that Ruminococcaceae could be a core taxon in the freshwater life stage, at least under conditions similar to those in the present study. This consideration is supported by a previous study in Atlantic salmon parr showing Ruminococcaceae as one core taxon accounting for about 46% of the total relative abundance in most intestinal digesta samples (Dehler et al., 2017a). Ruminococcaceae can tolerate bile and degrade complex polysaccharides in the mammalian gut (La Reau and Suen, 2018). These traits might have allowed Ruminococcaceae to thrive in the salmon gut. After the seawater transfer, gut microbiota was mainly dominated by Lactobacillus and Photobacterium, which is in line with our previous findings in normal smolts (Wang et al., 2021). Independent of experimental conditions, Lactobacillus is particularly well-adapted to the Atlantic salmon gut environment, especially in the seawater stage (Dehler et al., 2017b; Rudi et al., 2018; Wang et al., 2021). Lactobacillus is resistant to bile acid (Cai et al., 1999; Buntin et al., 2008), which might facilitate their colonization in the salmon gut. Photobacterium has also been reported to be part of the core bacteria in the Atlantic salmon gut (Gajardo, 2016; Gajardo et al., 2017; Wang et al., 2021) and is commonly found in the surrounding water where the experiment was conducted (Rud et al., 2017).
The difference in the microbiota profile before and after seawater transfer may be due to exogenous and/or endogenous factors, which differ substantially between FW and SW. The possible exogenous factors include environmental microbiota, temperature, salinity, as well as the photoperiod under commercial Arctic conditions (Herlemann et al., 2011; Canfora et al., 2014; Dehler et al., 2017a; Dehler et al., 2017b; Rudi et al., 2018; Jin et al., 2019). For instance, salmon migrating from the freshwater to the sea must increase their drinking rate to prevent dehydration in a hyperosmotic environment. This behavioral change increases exposure to the environmental microbiome in the seawater which contains a higher abundance of Photobacterium than in the freshwater (Lorgen-Ritchie et al., 2021). Moreover, the diet compositions differ in protein and fat levels between FM and SW, a factor known to influence the gut microbiota profile (Ringø et al., 2016). Endogenous factors which vary between FW and SW, and which can affect microbiota composition include a range of metabolic, morphological, immunological, and osmoregulatory characteristics (Hoar, 1988; Bjornsson et al., 2011; Johansson et al., 2016; Karlsen et al., 2018), as discussed in more detail above.
Associations Between the Gut Microbiota and Host Responses
The substantially higher microbial diversity and richness observed in fish in SW compared to those in FW, suggest a more diverse microbiota in the SW fish. In mammal, a richer and more diverse microbiota is commonly taken as an indicator of healthier and more normal physiological functions, while relatively low microbial diversity is generally associated with dysbiosis or certain diseases (Mosca et al., 2016). However, whether more diverse microbiota is inherently beneficial for salmon cannot be concluded upon, as our present knowledge basis is still limited regarding the interactions between the microbial diversity and physiological responses (Ray et al., 2012). However, one recent study indicates that relatively low microbial richness and diversity could maintain or even promote growth performance and health of Atlantic salmon (Bozzi et al., 2020), as also may be indicated by the results of the present study.
Our study showed that the relative abundance of twelve taxa, including lactic acid bacteria (e.g. Lactobacillus, Lactococcus, and Leuconostoc), were positively associated with the expression of several gut immune genes. This result agrees with previous observations made in salmonids showing that lactic acid bacteria may affect immune responses through modulation of expression of pro and anti-inflammatory cytokine genes (Panigrahi et al., 2007; Perez-Sanchez et al., 2011). Regarding fish, most previous papers suggest that increased abundance of LAB in the intestine or dietary probiotics which contains LAB strains could exert beneficial actions for the host (Balcázar et al., 2008; Gatesoupe, 2008). From studies of tilapia, it has also been reported that abrupt suspension of probiotic Lactobacillus strains administration could increase host pathogen susceptibility by inducing gut dysbiosis (Liu et al., 2016). However, the presumption that LAB is beneficial, is challenged as there are indications that an increased population of LAB, at least sometimes, accompanies enteritis, suggesting that high LAB may not always be beneficial for Atlantic salmon (Reveco et al., 2014; Schmidt et al., 2016; Gajardo et al., 2017; Krogdahl et al., 2020). Krogdahl et al. (2020) suggest that the increased populations of LAB in fish fed diets containing soybean meal may be due to soybean’s high fiber content, indigestible for the fish, but which can serves as substrate for the microbiota. However, the specific interaction between LAB abundance and host response needs to be further investigated as an increase in abundance of LAB may, under other circumstances, induce detrimental effects on host health. Concerns regarding the efficacy and safety of feeding certain lactic acid bacteria strains have been raised [See reviews by Lerner et al. (2019) and Martinez et al. (2015)]. These concerns are based on studies showing that Lactobacillus plantarum, which is a potent strain of probiotics, may disrupt healthy intestinal tissues in humans (Tsilingiri et al., 2012) and worsen colitis in mice (Mileti et al., 2009). Taken together, it is clear that present knowledge is not sufficient to conclude whether the increase in population of lactic acid bacteria is beneficial or detrimental to Atlantic salmon health. More investigation is required to understand if, when and how the lactic acid bacteria might modulate gut health for the benefit of the fish.
Effects of Functional Feed Ingredients
Our results, showing that the Test-diet fed fish in SW revealed less severe steatosis in the PC compared to those fed the reference diet in the FW, suggest that the mixture of nucleotides and immune stimulants improved lipid transport across the mucosa. The elevation in plasma cholesterol levels, and the similar trend shown for plasma fatty acids in the Test-fed fish support this suggestion (Field and Mathur, 1995)
As in our previous study with normal smolts (Wang et al., 2020), only minor effects of functional feed ingredients were observed regarding most growth and health endpoints, especially for the fish in SW. The same regards the gut microbiota. The explanation may be that effects of functional feed ingredients in general are weak, and that effects of variation in environmental factors under practical farming conditions have greater impact on the fish physiology and gut microbiota and are masking possible effects of functional feed ingredients (Ringø et al., 2010). On the other hand, it is unknown, but likely, that the suppression in feed intake and physiological functions just before and after seawater transfer weakened host responses to these selected functional feed ingredients. However, absence of the intended, beneficial effect of functional feed ingredients deserves attention and further investigation to strengthen the basis for making decisions regarding use of functional feed ingredients in salmon production, at least for economic reasons.
Conclusion
In the out-of-season smolt observed in the present study most growth and health endpoints showed inferior values four weeks after the seawater transfer compared to the values late in the freshwater stage. After the seawater transfer, the intestinal microbiota showed substantial changes in its composition with higher richness and diversity. The relative abundance of several lactic acid bacteria correlated positively with expression of immune genes in the gut. Diets supplemented with functional feed ingredients affected the fish only marginally for most health endpoints and gut microbiota. However, a mixture of nucleotides and immune stimulants seemed to enhance absorptive capacity for lipid and cholesterol for fish in freshwater.
Data Availability Statement
The original contributions presented in the study are included in the article/Supplementary Material, further inquiries can be directed to the corresponding authors.
Ethics Statement
The animal study was reviewed and approved by Norwegian laws and regulations of the experimentation with live animals within the Norwegian Animal Research Authority.
Author Contributions
Experimental design: JW, TK, ÅK, JJ, and ØB. Sampling: JW, TK, ÅK, YL, and AJ-T. Analyses: JW, YL, and AJ-T. Supervision: TK and ÅK. Writing, original draft: JW. Writing, review and editing: JW, TK, ÅK, YL, AJ-T, JJ, and ØB. All authors contributed to the article and approved the submitted version.
Funding
This study was funded by grants from the Norwegian Research council in the north (grant no: 257043) and MABIT, Cermaq Norway (grant no: AF0075). JW was supported by the China Scholarship Council (CSC) to cover his living expenses in Norway.
Conflict of Interest
Author ØB was employed by Cermaq Group AS and author JJ is employed by Cargill Aqua Nutrition.
The remaining authors declare that the research was conducted in the absence of any commercial or financial relationships that could be construed as a potential conflict of interest.
Publisher’s Note
All claims expressed in this article are solely those of the authors and do not necessarily represent those of their affiliated organizations, or those of the publisher, the editors and the reviewers. Any product that may be evaluated in this article, or claim that may be made by its manufacturer, is not guaranteed or endorsed by the publisher.
Acknowledgments
We would like to thank Ellen Hage for her skillful organization of sampling and technical assistance.
Supplementary Material
The Supplementary Material for this article can be found online at: https://www.frontiersin.org/articles/10.3389/fmars.2022.860081/full#supplementary-material
References
Alne H., Thomassen M. S., Takle H., Terjesen B. F., Grammes F., Oehme M., et al. (2009). Increased Survival by Feeding Tetradecylthioacetic Acid During a Natural Outbreak of Heart and Skeletal Muscle Inflammation in S0 Atlantic Salmon, Salmo Salar L. J. Fish Dis. 32 (11), 953–961. doi: 10.1111/j.1365-2761.2009.01078.x
Austin B. (2006). The Bacterial Microflora of Fish, Revised. Sci. World J. 6, 931–945. doi: 10.1100/tsw.2006.181
Bakke-McKellep A. M., Nordrum S., Krogdahl Å., Buddington R. (2000). Absorption of Glucose, Amino Acids, and Dipeptides by the Intestines of Atlantic Salmon (Salmo Salar L.). Fish Physiol. Biochem. 22 (1), 33–44. doi: 10.1023/A:1007872929847
Bakke-McKellep A. M., Penn M. H., Salas P. M., Refstie S., Sperstad S., Landsverk T., et al. (2007). Effects of Dietary Soyabean Meal, Inulin and Oxytetracycline on Intestinal Microbiota and Epithelial Cell Stress, Apoptosis and Proliferation in the Teleost Atlantic Salmon (Salmo Salar L.). Br. J. Nutr. 97 (4), 699–713. doi: 10.1017/S0007114507381397
Balcázar J. L., Vendrell D., de Blas I., Ruiz-Zarzuela I., Muzquiz J. L., Girones O. (2008). Characterization of Probiotic Properties of Lactic Acid Bacteria Isolated From Intestinal Microbiota of Fish. Aquaculture 278, 188–191. doi: 10.1016/j.aquaculture.2008.03.014
Bieth J., Spiess B., Wermuth C. G. (1974). The Synthesis and Analytical Use of a Highly Sensitive and Convenient Substrate of Elastase. Biochem. Med. 11 (4), 350–357. doi: 10.1016/0006-2944(74)90134-3
Bjornsson B. T., Stefansson S. O., McCormick S. D. (2011). Environmental Endocrinology of Salmon Smoltification. Gen. Comp. Endocrinol. 170 (2), 290–298. doi: 10.1016/j.ygcen.2010.07.003
Bokulich N. A., Kaehler B. D., Rideout J. R., Dillon M., Bolyen E., Knight R., et al. (2018). Optimizing Taxonomic Classification of Marker-Gene Amplicon Sequences With QIIME 2’s Q2-Feature-Classifier Plugin 6 (1), 90. doi: 10.1186/s40168-018-0470-z
Bolyen E., Rideout J. R., Dillon M. R., Bokulich N., Abnet C. C., Al-Ghalith G. A., et al. (2019). Reproducible, Interactive, Scalable and Extensible Microbiome Data Science Using QIIME 2. Nat. Biotechnol. 37 (8), 852–857. doi: 10.1038/s41587-019-0209-9
Bozzi D., Rasmussen J. A., Carøe C., Sveier H., Nordøy K., Gilbert M. T. P., et al. (2020). Salmon Gut Microbiota Correlates With Disease Infection Status: Potential for Monitoring Health in Farmed Animals. Anim. Microbiome 3 (1), 1–17. doi: 10.1186/s42523-021-00096-2
Buntin N., Chanthachum S., Hongpattarakere T. (2008). Screening of Lactic Acid Bacteria From Gastrointestinal Tracts of Marine Fish for Their Potential Use as Probiotics. Songklanakarin J. Sci. Technol. 30 (s1), 141–148.
Burrells C., Williams P., Forno P. J. A. (2001a). Dietary Nucleotides: A Novel Supplement in Fish Feeds: 1. Effects on Resistance to Disease in Salmonids. Aquaculture 199 (1-2), 159–169. doi: 10.1016/S0044-8486(01)00577-4
Burrells C., Williams P., Southgate P., Wadsworth S. J. A. (2001b). Dietary Nucleotides: A Novel Supplement in Fish Feeds: 2. Effects on Vaccination, Salt Water Transfer, Growth Rates and Physiology of Atlantic Salmon (Salmo Salar L.). Aquaculture 199 (1-2), 171–184. doi: 10.1016/S0044-8486(01)00576-2
Bustin S. A., Benes V., Garson J. A., Hellemans J., Huggett J., Kubista M., et al. (2009). The MIQE Guidelines: Minimum Information for Publication of Quantitative Real-Time PCR Experiments. Clin. Chem. 55 (4), 611–622. doi: 10.1373/clinchem.2008.112797
Cai Y., Suyanandana P., Saman P., Benno Y. (1999). Classification and Characterization of Lactic Acid Bacteria Isolated From the Intestines of Common Carp and Freshwater Prawns. J. Gen. Appl. Microbiol. 45 (4), 177–184. doi: 10.2323/jgam.45.177
Callahan B. J., McMurdie P. J., Rosen M. J., Han A. W., Johnson A. J. A., Holmes S. P. (2016). DADA2: High-Resolution Sample Inference From Illumina Amplicon Data. Nat. Methods 13 (7), 581–583. doi: 10.1038/nmeth.3869
Canfora L., Bacci G., Pinzari F., Lo Papa G., Dazzi C., Benedetti A. (2014). Salinity and Bacterial Diversity: To What Extent Does the Concentration of Salt Affect the Bacterial Community in a Saline Soil? PloS One 9 (9), e106662. doi: 10.1371/journal.pone.0106662
Carolina A. (2018). Modulation of Selected Inflammatory Responses and non-Specific Defenses in Atlantic Salmon Induced by Beta-1, 3/1, 6-Glucans (Macrogard®) (Ås: Norwegian University of Life Sciences). MS thesis.
Clarke K. R., Clarke K., Gorley K., Clarke K., Gorley R. (2006). PRIMER V6: User Manual/Tutorial Plymouth: Plymouth Routines in Multivariate Ecological Research.
Davis N. M., Proctor D. M., Holmes S. P., Relman D. A., Callahan B. J. (2018). Simple Statistical Identification and Removal of Contaminant Sequences in Marker-Gene and Metagenomics Data. Microbiome 6, 1–14. doi: 10.1186/s40168-018-0605-2
Dehler C. E., Secombes C. J., Martin S. A. M. (2017a). Environmental and Physiological Factors Shape the Gut Microbiota of Atlantic Salmon Parr (Salmo Salar L.). Aquaculture 467, 149–157. doi: 10.1016/j.aquaculture.2016.07.017
Dehler C. E., Secombes C. J., Martin S. A. M. (2017b). Seawater Transfer Alters the Intestinal Microbiota Profiles of Atlantic Salmon (Salmo Salar L.). Sci. Rep. 7 (1), 13877. doi: 10.1038/s41598-017-13249-8
Dimitroglou A., Reynolds P., Ravnoy B., Johnsen F., Sweetman J., Johansen J., et al. (2011). The Effect of Mannan Oligosaccharide Supplementation on Atlantic Salmon Smolts (Salmo Salar L.) Fed Diets With High Levels of Plant Proteins. J. Aquac. Res. Dev. 1, 011. doi: 10.4172/2155-9546.S1-011
Duncan N. J., Auchinachie N., Robertson D., Murray R., Bromage N. (1998). Growth, Maturation and Survival of Out-of-Season 0+ and 1+ Atlantic Salmon (Salmo Salar) Smolts. Aquaculture 168 (1-4), 325–339. doi: 10.1016/S0044-8486(98)00359-7
Dvergedal H., Sandve S. R., Angell I. L., Klemetsdal G., Rudi K. (2020). Association of Gut Microbiota With Metabolism in Juvenile Atlantic Salmon. Microbiome 8 (1), 1–8. doi: 10.1186/s40168-020-00938-2
Falcinelli S., Picchietti S., Rodiles A., Cossignani L., Merrifield D. L., Taddei A. R., et al. (2015). Lactobacillus Rhamnosus Lowers Zebrafish Lipid Content by Changing Gut Microbiota and Host Transcription of Genes Involved in Lipid Metabolism. Sci. Rep. 5, 9336. doi: 10.1038/srep09336
Field F. J., Mathur S. N. (1995). Intestinal Lipoprotein Synthesis and Secretion. Prog. Lipid Res. 34 (2), 185–198. doi: 10.1016/0163-7827(95)00005-K
Gajardo K. (2016). Nutrition and Intestinal Health in Atlantic Salmon (Salmo Salar): Involvement of Antinutrients and Microbiota (Oslo: Norwegian University of Life Sciences). Ph.D thesis.
Gajardo K., Jaramillo-Torres A., Kortner T. M., Merrifield D. L., Tinsley J., Bakke A. M., et al. (2017). Alternative Protein Sources in the Diet Modulate Microbiota and Functionality in the Distal Intestine of Atlantic Salmon (Salmo Salar). Appl. Environ. Microbiol. 83 (5), e02615–16. doi: 10.1128/AEM.02615-16
Gajardo K., Rodiles A., Kortner T. M., Krogdahl Å., Bakke A. M., Merrifield D. L., et al. (2016). A High-Resolution Map of the Gut Microbiota in Atlantic Salmon (Salmo Salar): A Basis for Comparative Gut Microbial Research. Sci. Rep. 6, 30893. doi: 10.1038/srep30893
Gatesoupe F. J. (2008). Updating the Importance of Lactic Acid Bacteria in Fish Farming: Natural Occurrence and Probiotic Treatments. J. Mol. Microbiol. Biotechnol. 14, 107–114. doi: 10.1159/000106089
Gomez G. D., Balcazar J. L. (2008). A Review on the Interactions Between Gut Microbiota and Innate Immunity of Fish. FEMS Immunol. Med. Microbiol. 52 (2), 145–154. doi: 10.1111/j.1574-695X.2007.00343.x
González J., Troncoso J. (2009). “Reducing Caligus Rogercresseyi Infestation in Salmo Salar Fed a Diet Supplemented With Microbial Based Nucleotides and Immunostimulants”. Oral presentation at World Aquaculture 2009, Veracruz, Mexico. Book of abstracts, 25.
Green T. J., Smullen R., Barnes A. C. J. V. m. (2013). Dietary Soybean Protein Concentrate-Induced Intestinal Disorder in Marine Farmed Atlantic Salmon, Salmo Salar is Associated With Alterations in Gut Microbiota. Vet. Microbiol. 166 (1-2), 286–292. doi: 10.1016/j.vetmic.2013.05.009
Guerreiro I., Oliva-Teles A., Enes P. (2018). Prebiotics as Functional Ingredients: Focus on Mediterranean Fish Aquaculture. Rev. Aquac. 10 (4), 800–832. doi: 10.1111/raq.12201
Hansen A. K., Kortner T. M., Denstadli V., Måsøval K., Björkhem I., Grav H. J., et al. (2020a). Dose-Response Relationship Between Dietary Choline and Lipid Accumulation in Pyloric Enterocytes of Atlantic Salmon (Salmo Salar L.) in Seawater. Br. J. Nutr. 123(10), 1–42. doi: 10.1017/S0007114520000434
Hansen A. K. G., Kortner T. M., Krasnov A., Björkhem I., Penn M., Krogdahl Å. J. B. V. R. (2020b). Choline Supplementation Prevents Diet Induced Gut Mucosa Lipid Accumulation in Post-Smolt Atlantic Salmon (Salmo Salar L.). BMC Vet. 16 (1), 32. doi: 10.1186/s12917-020-2252-7
Heid H. W., Moll R., Schwetlick I., Rackwitz H. R., Keenan T. W. (1998). Adipophilin is a Specific Marker of Lipid Accumulation in Diverse Cell Types and Diseases. Cell Tissue Res. 294 (2), 309–321. doi: 10.1007/s004410051181
Herlemann D. P. R., Labrenz M., Jurgens K., Bertilsson S., Waniek J. J., Andersson A. F. (2011). Transitions in Bacterial Communities Along the 2000 Km Salinity Gradient of the Baltic Sea. Isme J. 5 (10), 1571–1579. doi: 10.1038/ismej.2011.41
Hoar W. (1988). The Physiology of Smolting Salmonids. In. Fish Physiol. 11, 275–343. doi: 10.1016/S1546-5098(08)60216-2
Hossain M. S., Koshio S., Kestemont P. (2019). Recent Advances of Nucleotide Nutrition Research in Aquaculture: A Review. Rev. Aquac. 12 (2), 1028–1053. doi: 10.1111/raq.12370
Illumina I. (2013). “16s Metagenomic Sequencing Library Preparation,” in Preparing 16s Ribosomal RNA Gene Amplicons for the Illumina MiSeq System, 1–28.
Jaramillo Torres H. A. (2017). Intestinal Health and Microbiota in Salmonids: The Impact of Probiotics Under Potentially Stressful Conditions (Plymouth: University of Plymouth). Ph.D thesis.
Jaramillo-Torres A., Rawling M., Rodiles A., Mikalsen H. E., Johansen L. H., Tinsley J., et al. (2019). Influence of Dietary Supplementation of Probiotic Pediococcus Acidilactici MA18/5M During the Transition From Freshwater to Seawater on Intestinal Health and Microbiota of Atlantic Salmon (Salmo Salar L.). Front. Microbiol. 10, 2243. doi: 10.3389/fmicb.2019.02243
Jensen B. B., Kristoffersen A. B. (2015). Risk Factors for Outbreaks of Infectious Pancreatic Necrosis (IPN) and Associated Mortality in Norwegian Salmonid Farming. Dis. Aquat. Org. 114 (3), 177–187. doi: 10.3354/dao02867
Jin Y., Angell I. L., Rod Sandve S., Snipen L. G., Olsen Y., Rudi K. (2019). Atlantic Salmon Raised With Diets Low in Long-Chain Polyunsaturated N-3 Fatty Acids in Freshwater Have a Mycoplasma-Dominated Gut Microbiota at Sea. Aquac. Environ. Interact. 11, 31–39. doi: 10.3354/aei00297
Johansson L. H., Timmerhaus G., Afanasyev S., Jorgensen S. M., Krasnov A. (2016). Smoltification and Seawater Transfer of Atlantic Salmon (Salmo Salar L.) is Associated With Systemic Repression of the Immune Transcriptome. Fish Shellfish Immunol. 58, 33–41. doi: 10.1016/j.fsi.2016.09.026
Johnsen C. A., Hagen O., Solberg C., Bjornsson B. T. H., Jonsson E., Johansen S. J. S., et al. (2013). Seasonal Changes in Muscle Structure and Flesh Quality of 0+ and 1+ Atlantic Salmon (Salmo Salar L.): Impact of Feeding Regime and Possible Roles of Ghrelin. Aquac. Nutr. 19 (1), 15–34. doi: 10.1111/j.1365-2095.2011.00927.x
Kakade M. L., Hoffa D. E., Liener I. E. (1973). Contribution of Trypsin Inhibitors to the Deleterious Effects of Unheated Soybeans Fed to Rats. J. Nutr. 103 (12), 1772–1778. doi: 10.1093/jn/103.12.1772
Karlsen C., Ytteborg E., Timmerhaus G., Host V., Handeland S., Jorgensen S. M., et al. (2018). Atlantic Salmon Skin Barrier Functions Gradually Enhance After Seawater Transfer. Sci. Rep. 8, 1–12. doi: 10.1038/s41598-018-27818-y
Kiron V. (2012). Fish Immune System and its Nutritional Modulation for Preventive Health Care. Anim. Feed Sci. Technol. 173 (1-2), 111–133. doi: 10.1016/j.anifeedsci.2011.12.015
Kiron V., Kulkarni A., Dahle D., Vasanth G., Lokesh J., Elvebo O. (2016). Recognition of Purified Beta 1,3/1,6 Glucan and Molecular Signalling in the Intestine of Atlantic Salmon. Dev. Comp. Immunol. 56, 57–66. doi: 10.1016/j.dci.2015.11.007
Knudsen B. E., Bergmark L., Munk P., Lukjancenko O., Prieme A., Aarestrup F. M., et al. (2016). Impact of Sample Type and DNA Isolation Procedure on Genomic Inference of Microbiome Composition. mSystems 1 (5), e00095–16. doi: 10.1128/mSystems.00095-16
Kortner T. M., Gu J., Krogdahl Å., Bakke A. M. (2013). Transcriptional Regulation of Cholesterol and Bile Acid Metabolism After Dietary Soyabean Meal Treatment in Atlantic Salmon (Salmo Salar L.). Br. J. Nutr. 109 (4), 593–604. doi: 10.1017/S0007114512002024
Kortner T. M., Valen E. C., Kortner H., Marjara I. S., Krogdahl Å., Bakke A. M. (2011). Candidate Reference Genes for Quantitative Real-Time PCR (qPCR) Assays During Development of a Diet-Related Enteropathy in Atlantic Salmon (Salmo Salar L.) and the Potential Pitfalls of Uncritical Use of Normalization Software Tools. Aquaculture 318 (3-4), 355–363. doi: 10.1016/j.aquaculture.2011.05.038
Koshio S. (2015). Immunotherapies Targeting Fish Mucosal Immunity - Current Knowledge and Future Perspectives. Front. Immunol. 6. doi: 10.3389/fimmu.2015.00643
Kristensen T., Haugen T. O., Rosten T., Fjellheim A., Atland A., Rosseland B. O. (2012). Effects of Production Intensity and Production Strategies in Commercial Atlantic Salmon Smolt (Salmo Salar L.) Production on Subsequent Performance in the Early Sea Stage. Fish Physiol. Biochem. 38 (1), 273–282. doi: 10.1007/s10695-011-9566-0
Krogdahl Å., Bakke-McKellep A. M., Baeverfjord G. (2003). Effects of Graded Levels of Standard Soybean Meal on Intestinal Structure, Mucosal Enzyme Activities, and Pancreatic Response in Atlantic Salmon (Salmo Salar L.). Aquac. Nutr. 9 (6), 361–371. doi: 10.1046/j.1365-2095.2003.00264.x
Krogdahl Å., Kortner T. M., Jaramillo-Torres A., Gamil A. A. A., Chikwati E., Li Y., et al. (2020). Removal of Three Proteinaceous Antinutrients From Soybean Does Not Mitigate Soybean-Induced Enteritis in Atlantic Salmon (Salmo Salar, L). Aquaculture 514, 734495. doi: 10.1016/j.aquaculture.2019.734495
Krogdahl Å., Sundby A., Olli J. J. (2004). Atlantic Salmon (Salmo Salar) and Rainbow Trout (Oncorhynchus Mykiss) Digest and Metabolize Nutrients Differently. Effects of Water Salinity and Dietary Starch Level. Aquaculture 229 (1-4), 335–360. doi: 10.1016/S0044-8486(03)00396-X
La Reau A. J., Suen G. (2018). The Ruminococci: Key Symbionts of the Gut Ecosystem. J. Microbiol. 56 (3), 199–208. doi: 10.1007/s12275-018-8024-4
Lerner A., Shoenfeld Y., Matthias T. (2019). Probiotics: If it Does Not Help it Does Not do Any Harm. Really? Microorganisms 7 (4), 104. doi: 10.3390/microorganisms7040104
Li Y., Bruni L., Jaramillo-Torres A., Gajardo K., Kortner T. M., Krogdahl Å. (2021). Differential Response of Digesta-and Mucosa-Associated Intestinal Microbiota to Dietary Insect Meal During the Seawater Phase of Atlantic Salmon. Anim. Microbiome 3 (1), 1–18. doi: 10.1186/s42523-020-00071-3
Li Y., Gajardo K., Jaramillo-Torres A., Kortner T. M., Krogdahl Å. (2022). Consistent Changes in the Intestinal Microbiota of Atlantic Salmon Fed Insect Meal Diets. Anim. Microbiome 4 (1), 1–15. doi: 10.1186/s42523-021-00159-4
Liu Z., Liu W., Ran C., Hu J., Zhou Z. (2016). Abrupt Suspension of Probiotics Administration may Increase Host Pathogen Susceptibility by Inducing Gut Dysbiosis. Sci. Rep. 6, 23214. doi: 10.1038/srep23214
Lokesh J., Kiron V. (2016). Transition From Freshwater to Seawater Reshapes the Skin-Associated Microbiota of Atlantic Salmon. Sci. Rep. 6, 19707. doi: 10.1038/srep19707
Lokesh J., Kiron V., Sipkema D., Fernandes J. M. O., Moum T. (2019). Succession of Embryonic and the Intestinal Bacterial Communities of Atlantic Salmon (Salmo Salar) Reveals Stage-Specific Microbial Signatures. Microbiologyopen 8 (4), e00672. doi: 10.1002/mbo3.672
Lorgen-Ritchie M., Clarkson M., Chalmers L., Taylor J. F., Migaud H., Martin S. A. (2021). A Temporally Dynamic Gut Microbiome in Atlantic Salmon During Freshwater Recirculating Aquaculture System (RAS) Production and Post-Seawater Transfer. Front. Mar. Sci. 8, 869. doi: 10.3389/fmars.2021.711797
Lysfjord G., Jobling M., Solberg C. (2004). Atlantic Salmon, Salmo Salar L., Smolt Production Strategy Affects Body Composition and Early Seawater Growth. Aquaculture 237 (1-4), 191–205. doi: 10.1016/j.aquaculture.2004.03.017
Mørkøre T., Rørvik K.-A. (2001). Seasonal Variations in Growth, Feed Utilisation and Product Quality of Farmed Atlantic Salmon (Salmo Salar) Transferred to Seawater as 0+ Smolts or 1+ Smolts. Aquaculture 199 (1-2), 145–157. doi: 10.1016/S0044-8486(01)00524-5
Mallick H., Rahnavard A., McIver L. J., Ma S., Zhang Y., Nguyen L. H., et al. (2021). Multivariable Association Discovery in Population-Scale Meta-Omics Studies. PloS Comput. Biol. 17 (11), e1009442. doi: 10.1371/journal.pcbi.1009442
Martinez R. C., Bedani R., Saad S. M. (2015). Scientific Evidence for Health Effects Attributed to the Consumption of Probiotics and Prebiotics: An Update for Current Perspectives and Future Challenges. Br. J. Nutr. 114 (12), 1993–2015. doi: 10.1017/S0007114515003864
Mehdinejad N., Imanpour M. R., Jafari V. (2018). Combined or Individual Effects of Dietary Probiotic Pedicoccus Acidilactici and Nucleotide on Growth Performance, Intestinal Microbiota, Hemato-Biochemical Parameters, and Innate Immune Response in Goldfish (Carassius Auratus). Probiotics Antimicrob. Proteins 10 (3), 558–565. doi: 10.1007/s12602-017-9297-3
Merrifield D. L., Ringø E. (2014). Gastrointestinal pathogenesis in aquatic animals. Aquaculture Nutrition: Gut Health, Probiotics and Prebiotics0 (Hoboken, NJ: Wiley Blackwell), 53–74.
Mileti E., Matteoli G., Iliev I. D., Rescigno M. (2009). Comparison of the Immunomodulatory Properties of Three Probiotic Strains of Lactobacilli Using Complex Culture Systems: Prediction for In Vivo Efficacy. PloS One 4 (9), e7056. doi: 10.1371/journal.pone.0007056
Moore L. J., Jarungsriapisit J., Nilsen T. O., Stefansson S., Taranger G. L., Secombes C. J., et al. (2018). Atlantic Salmon Adapted to Seawater for 9 Weeks Develop a Robust Immune Response to Salmonid Alphavirus Upon Bath Challenge. Fish Shellfish Immunol. 74, 573–583. doi: 10.1016/j.fsi.2017.12.017
Mosca A., Leclerc M., Hugot J. P. (2016). Gut Microbiota Diversity and Human Diseases: Should We Reintroduce Key Predators in Our Ecosystem? Front. Microbiol. 7, 455. doi: 10.3389/fmicb.2016.00455
Muller P. Y., Janovjak H., Miserez A. R., Dobbie Z. (2002). Processing of Gene Expression Data Generated by Quantitative Real-Time RT-PCR. Biotechniques 32 (6), 1372–1374. 1376, 1378-1379.
Nguyen C. D. H., Amoroso G., Ventura T., Elizur A. (2020a). Assessing the Pyloric Caeca and Distal Gut Microbiota Correlation With Flesh Color in Atlantic Salmon (Salmo Salar L). Microorganisms 8 (8), 1244. doi: 10.3390/microorganisms8081244
Nguyen C. D. H., Amoroso G., Ventura T., Minich J. J., Elizur A. (2020b). Atlantic Salmon (Salmo Salar L) Gut Microbiota Profile Correlates With Flesh Pigmentation: Cause or Effect? Mar. Biotechnol. 22 (6), 786–804. doi: 10.1007/s10126-019-09939-1
Oppedal F., Berg A., Olsen R. E., Taranger G. L., Hansen T. (2006). Photoperiod in Seawater Influence Seasonal Growth and Chemical Composition in Autumn Sea-Transferred Atlantic Salmon (Salmo Salar L.) Given Two Vaccines. Aquaculture 254 (1-4), 396–410. doi: 10.1016/j.aquaculture.2005.10.026
Oulad S., Abedian A. M., Khodabandeh S. (2009). Effect of Dietary Nucleotide on the Osmoregulatory Function of Pyloric Caeca in Caspian Sea Salmon, Salmo Trutta Caspius. Comp. Biochem. Physiol. Part A 2 (153), S81. doi: 10.1016/j.cbpa.2009.04.059
Panigrahi A., Kiron V., Satoh S., Hirono I., Kobayashi T., Sugita H., et al. (2007). Immune Modulation and Expression of Cytokine Genes in Rainbow Trout Oncorhynchus Mykiss Upon Probiotic Feeding. Dev. Comp. Immunol. 31 (4), 372–382. doi: 10.1016/j.dci.2006.07.004
Panteli N., Mastoraki M., Nikouli E., Lazarina M., Antonopoulou E., Kormas K. A. (2020). Imprinting Statistically Sound Conclusions for Gut Microbiota in Comparative Animal Studies: A Case Study With Diet and Teleost Fishes. Comp. Biochem. Physiol. Part D: Genomics Proteomics 36, 100738. doi: 10.1016/j.cbd.2020.100738
Parra M., Espinoza D., Valdes N., Vargas R., Gonzalez A., Modak B., et al. (2020). Microbiota Modulates the Immunomodulatory Effects of Filifolinone on Atlantic Salmon. Microorganisms 8 (9), 1320. doi: 10.3390/microorganisms8091320
Penn M. H., Bendiksen E. Å., Campbell P., Krogdahl Å. (2011). High Level of Dietary Pea Protein Concentrate Induces Enteropathy in Atlantic Salmon (Salmo Salar L.). Aquaculture 310 (3-4), 267–273. doi: 10.1016/j.aquaculture.2010.10.040
Perez-Sanchez T., Balcazar J. L., Merrifield D. L., Carnevali O., Gioacchini G., de Blas I., et al. (2011). Expression of Immune-Related Genes in Rainbow Trout (Oncorhynchus Mykiss) Induced by Probiotic Bacteria During Lactococcus Garvieae Infection. Fish Shellfish Immunol. 31 (2), 196–201. doi: 10.1016/j.fsi.2011.05.005
Quast C., Pruesse E., Yilmaz P., Gerken J., Schweer T., Yarza P., et al. (2013). The SILVA Ribosomal RNA Gene Database Project: Improved Data Processing and Web-Based Tools. Nucleic Acids Res. 41 (Database issue), D590–D596. doi: 10.1093/nar/gks1219
Ramseier C. A., Kinney J. S., Herr A. E., Braun T., Sugai J. V., Shelburne C. A., et al. (2009). Identification of Pathogen and Host-Response Markers Correlated With Periodontal Disease. J. Periodontol. 80 (3), 436–446. doi: 10.1902/jop.2009.080480
Ray A., Ghosh K., Ringø E. J. A. N. (2012). Enzyme-Producing Bacteria Isolated From Fish Gut: A Review. Aquac. Nutr. 18 (5), 465–492. doi: 10.1111/j.1365-2095.2012.00943.x
Refstie S., Baeverfjord G., Seim R. R., Elvebø O. (2010). Effects of Dietary Yeast Cell Wall β-Glucans and MOS on Performance, Gut Health, and Salmon Lice Resistance in Atlantic Salmon (Salmo Salar) Fed Sunflower and Soybean Meal. Aquaculture 305 (1-4), 109–116. doi: 10.1016/j.aquaculture.2010.04.005
Reveco F. E., Øverland M., Romarheim O. H., Mydland L. T. (2014). Intestinal Bacterial Community Structure Differs Between Healthy and Inflamed Intestines in Atlantic Salmon (Salmo Salar L.). Aquaculture 420-421, 262–269. doi: 10.1016/j.aquaculture.2013.11.007
Ringø E., Olsen R. E., Gifstad T. O., Dalmo R. A., Amlund H., Hemre G. I., et al. (2010). Prebiotics in Aquaculture: A Review. Aquac. Nutr. 16 (2), 117–136. doi: 10.1111/j.1365-2095.2009.00731.x
Ringø E., Zhou Z., Vecino J. L. G., Wadsworth S., Romero J., Krogdahl Å., et al. (2016). Effect of Dietary Components on the Gut Microbiota of Aquatic Animals. A Never-Ending Story? Aquac. Nutr. 22 (2), 219–282. doi: 10.1111/anu.12346
Roeselers G., Mittge E. K., Stephens W. Z., Parichy D. M., Cavanaugh C. M., Guillemin K., et al. (2011). Evidence for a Core Gut Microbiota in the Zebrafish. ISME J. 5 (10), 1595–1608. doi: 10.1038/ismej.2011.38
Rudi K., Angell I. L., Pope P. B., Vik J. O., Sandve S. R., Snipen L. G. (2018). Stable Core Gut Microbiota Across the Freshwater-To-Saltwater Transition for Farmed Atlantic Salmon. Appl. Environ. Microbiol. 84 (2), e01974–17. doi: 10.1128/AEM.01974-17
Rud I., Kolarevic J., Holan A. B., Berget I., Calabrese S., Terjesen B. F. (2017). Deep-Sequencing of the Bacterial Microbiota in Commercial-Scale Recirculating and Semi-Closed Aquaculture Systems for Atlantic Salmon Post-Smolt Production. Aquac. Eng. 78, 50–62. doi: 10.1016/j.aquaeng.2016.10.003
Salinas I. (2015). The Mucosal Immune System of Teleost Fish. Biol. (Basel) 4 (3), 525–539. doi: 10.3390/biology4030525
Schmidt V., Amaral-Zettler L., Davidson J., Summerfelt S., Good C. (2016). Influence of Fishmeal-Free Diets on Microbial Communities in Atlantic Salmon (Salmo Salar) Recirculation Aquaculture Systems. Appl. Environ. Microbiol. 82 (15), 4470–4481. doi: 10.1128/AEM.00902-16
Secombes C. J. (2016). What’s New in Fish Cytokine Research? Fish Shellfish Immunol. 53, 1–3. doi: 10.1016/j.fsi.2016.03.035
Secombes C. J., Wang T., Hong S., Peddie S., Crampe M., Laing K. J., et al. (2001). Cytokines and Innate Immunity of Fish. Dev. Comp. Immunol. 25 (8-9), 713–723. doi: 10.1016/s0145-305x(01)00032-5
Semova I., Carten J. D., Stombaugh J., Mackey L. C., Knight R., Farber S. A., et al. (2012). Microbiota Regulate Intestinal Absorption and Metabolism of Fatty Acids in the Zebrafish. Cell Host Microbe 12 (3), 277–288. doi: 10.1016/j.chom.2012.08.003
Sheridan M. A. (1989). Alterations in Lipid-Metabolism Accompanying Smoltification and Seawater Adaptation of Salmonid Fish. Aquaculture 82 (1-4), 191–203. doi: 10.1016/0044-8486(89)90408-0
Sissener N. H., Sanden M., Bakke A. M., Krogdahl Å., Hemre G. I. (2009). A Long Term Trial With Atlantic Salmon (Salmo Salar L.) Fed Genetically Modified Soy; Focusing General Health and Performance Before, During and After the Parr–Smolt Transformation. Aquaculture 294 (1-2), 108–117. doi: 10.1016/j.aquaculture.2009.05.002
Song S. K., Beck B. R., Kim D., Park J., Kim J., Kim H. D., et al. (2014). Prebiotics as Immunostimulants in Aquaculture: A Review. Fish Shellfish Immunol. 40 (1), 40–48. doi: 10.1016/j.fsi.2014.06.016
Sundh H., Nilsen T. O., Lindstrom J., Hasselberg-Frank L., Stefansson S. O., McCormick S. D., et al. (2014). Development of Intestinal Ion-Transporting Mechanisms During Smoltification and Seawater Acclimation in Atlantic Salmon Salmo Salar. J. Fish Biol. 85 (4), 1227–1252. doi: 10.1111/jfb.12531
Sveen L. R., Grammes F. T., Ytteborg E., Takle H., Jorgensen S. M. (2017). Genome-Wide Analysis of Atlantic Salmon (Salmo Salar) Mucin Genes and Their Role as Biomarkers. PloS One 12 (12), e0189103. doi: 10.1371/journal.pone.0189103
Sweetman J., Dimitroglou A., Davies S., Torrecillas S. J. I. A. (2008). Nutrient Uptake: Gut Morphology a Key to Efficient Nutrition. International AquaFeed 11, 27–30.
Tacchi L., Bickerdike R., Douglas A., Secombes C. J., Martin S. A. (2011). Transcriptomic Responses to Functional Feeds in Atlantic Salmon (Salmo Salar). Fish Shellfish Immunol. 31 (5), 704–715. doi: 10.1016/j.fsi.2011.02.023
Tipsmark C. K., Sorensen K. J., Hulgard K., Madsen S. S. (2010a). Claudin-15 and -25b Expression in the Intestinal Tract of Atlantic Salmon in Response to Seawater Acclimation, Smoltification and Hormone Treatment. Comp. Biochem. Physiol. Part A: Mol. Integr. Physiol. 155 (3), 361–370. doi: 10.1016/j.cbpa.2009.11.025
Tipsmark C. K., Sorensen K. J., Madsen S. S. (2010b). Aquaporin Expression Dynamics in Osmoregulatory Tissues of Atlantic Salmon During Smoltification and Seawater Acclimation. J. Exp. Biol. 213 (3), 368–379. doi: 10.1242/jeb.034785
Tsilingiri K., Barbosa T., Penna G., Caprioli F., Sonzogni A., Viale G., et al. (2012). Probiotic and Postbiotic Activity in Health and Disease: Comparison on a Novel Polarised Ex-Vivo Organ Culture Model. Gut 61 (7), 1007–1015. doi: 10.1136/gutjnl-2011-300971
Usher M., Talbot C., Eddy F. (1991). Effects of Transfer to Seawater on Growth and Feeding in Atlantic Salmon Smolts (Salmo Salar L.). Aquaculture 94 (4), 309–326. doi: 10.1016/0044-8486(91)90176-8
Vandeputte D., Kathagen G., D’Hoe K., Vieira-Silva S., Valles-Colomer M., Sabino J., et al. (2017). Quantitative Microbiome Profiling Links Gut Community Variation to Microbial Load. Nature 551 (7681), 507–511. doi: 10.1038/nature24460
Van der Marel M., Adamek M., Gonzalez S. F., Frost P., Rombout J. H., Wiegertjes G. F., et al. (2012). Molecular Cloning and Expression of Two β-Defensin and Two Mucin Genes in Common Carp (Cyprinus Carpio L.) and Their Up-Regulation After β-Glucan Feeding. Fish Shellfish Immunol. 32 (3), 494–501. doi: 10.1016/j.fsi.2011.12.008
Wang J., Jaramillo-Torres A., Li Y., Kortner T. M., Gajardo K., Brevik O. J., et al. (2021). Microbiota in Intestinal Digesta of Atlantic Salmon (Salmo Salar), Observed From Late Freshwater Stage Until One Year in Seawater, and Effects of Functional Ingredients: A Case Study From a Commercial Sized Research Site in the Arctic Region. Anim. Microbiome 3 (1), 14. doi: 10.1186/s42523-021-00075-7
Wang J., Kortner T. M., Chikwati E. M., Li Y., Jaramillo-Torres A., Jakobsen J. V., et al. (2020). Gut Immune Functions and Health in Atlantic Salmon (Salmo Salar) From Late Freshwater Stage Until One Year in Seawater and Effects of Functional Ingredients: A Case Study From a Commercial Sized Research Site in the Arctic Region. Fish Shellfish Immunol. 106, 1106–1119. doi: 10.1016/j.fsi.2020.09.019
Keywords: Atlantic salmon, seawater transfer, commercial Arctic conditions, gut microbiota, gut health, functional feed ingredients
Citation: Wang J, Jaramillo-Torres A, Li Y, Brevik ØJ, Jakobsen JV, Kortner TM and Krogdahl Å (2022) Gut Health and Microbiota in Out-of-Season Atlantic Salmon (Salmo salar L.) Smolts Before and After Seawater Transfer Under Commercial Arctic Conditions: Modulation by Functional Feed Ingredients. Front. Mar. Sci. 9:860081. doi: 10.3389/fmars.2022.860081
Received: 22 January 2022; Accepted: 03 March 2022;
Published: 28 March 2022.
Edited by:
Marty Riche, Florida Atlantic University, United StatesReviewed by:
David Bradshaw, Florida Atlantic University, United StatesAnne Marie Bakke, WALTHAM Centre for Pet Nutrition, United Kingdom
Copyright © 2022 Wang, Jaramillo-Torres, Li, Brevik, Jakobsen, Kortner and Krogdahl. This is an open-access article distributed under the terms of the Creative Commons Attribution License (CC BY). The use, distribution or reproduction in other forums is permitted, provided the original author(s) and the copyright owner(s) are credited and that the original publication in this journal is cited, in accordance with accepted academic practice. No use, distribution or reproduction is permitted which does not comply with these terms.
*Correspondence: Jie Wang, d2FuZ2ppZTAzQGNhYXMuY24=