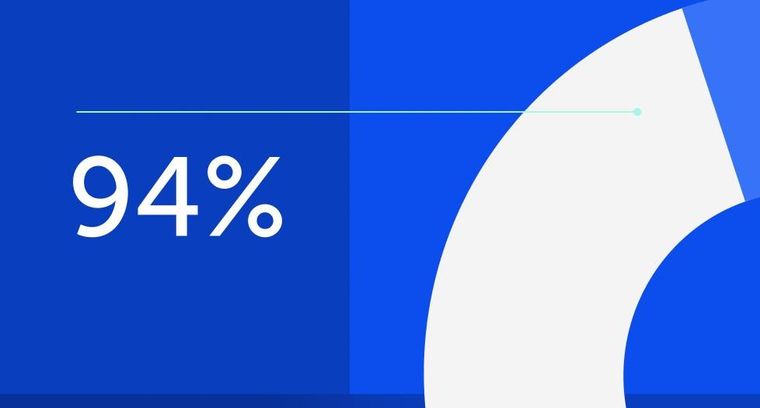
94% of researchers rate our articles as excellent or good
Learn more about the work of our research integrity team to safeguard the quality of each article we publish.
Find out more
ORIGINAL RESEARCH article
Front. Mar. Sci., 11 November 2022
Sec. Global Change and the Future Ocean
Volume 9 - 2022 | https://doi.org/10.3389/fmars.2022.858853
This article is part of the Research TopicThe Philippine Seas: Biodiversity and Ecological Impacts of Natural and Anthropogenic stressors in Tropical Reef SystemsView all 14 articles
Coral reefs are vulnerable to global ocean acidification (OA) and local human activities will continue to exacerbate coastal OA. In Bolinao, Philippines, intense unregulated fish mariculture has resulted in regional eutrophication. In order to examine the coastal acidification associated with this activity and the impact on nearby coral reefs, water quality and carbonate chemistry parameters were measured at three reef sites, a mariculture site and an offshore, minimally impacted control site during both the wet and dry season. Additionally, benthic community composition was characterized at reef sites, and both autonomous carbonate chemistry sampling and high-frequency pH measurements were used to characterize fine-scale (diel) temporal variability. Water quality was found to be poorer at all reefs during the wet season, when there was stronger outflow of waters from the mariculture area. Carbonate chemistry parameters differed significantly across the reef flat and between seasons, with more acidic conditions occurring during the dry season and increased primary production suppressing further acidification during the wet season. Significant relationships of both total alkalinity (TA) and dissolved inorganic carbon (DIC) with salinity across all stations may imply outflow of acidified water originating from the mariculture area where pH values as low as 7.78 were measured. This apparent mariculture-induced coastal acidification was likely due to organic matter respiration as sustained mariculture will continue to deliver organic matter. While TA-DIC vector diagrams indicate greater contribution of net primary production, net calcification potential in the nearest reef to mariculture area may already be diminished. The two farther reefs, characterized by higher coral cover, indicates healthier ecosystem functioning. Here we show that unregulated fish mariculture activities can lead to localized acidification and impact reef health. As these conditions at times approximate those projected to occur globally due to OA, our results may provide insight into reef persistence potential worldwide. These results also underscore the importance of coastal acidification and indicate that actions taken to mitigate OA on coral reefs should address not only global CO2 emissions but also local perturbations, in this case fish mariculture-induced eutrophication.
Approximately 25% of anthropogenic CO2 is absorbed by the oceans, resulting in global declines in seawater pH known as ocean acidification (OA) (Caldeira and Wickett, 2003; Orr et al., 2005; Doney et al., 2009; Friedlingstein et al., 2020). It is widely reported that many species of reef-building corals exhibit reduced calcification under OA, associated with the depressed saturation states (Ω) of calcium carbonate (CaCO3) minerals (Kroeker et al., 2010; Chan and Connolly, 2013). Moreover, OA favors abiotic dissolution and biogenic CaCO3 erosion (bioerosion), leading to the breakdown of important reef framework habitats (Enochs et al., 2016; Schönberg et al., 2017). Since the formation of reef structure via calcification must exceed dissolution and bioerosion in order for reefs to persist and grow, OA-induced alteration of these processes has strong negative implications on the future of coral reef ecosystems (Silverman et al., 2009; Eyre et al., 2018). Ultimately, in conjunction with local (e.g., eutrophication, overfishing) and global (e.g., seawater warming, sea level rise) stressors, OA will facilitate phase shifts towards ecosystem states dominated by macroalgae and seagrass that can persist or even thrive under high pCO2 conditions (Koch et al., 2013; Johnson et al., 2014; Enochs et al., 2015).
Coastal conditions, however, are often more complex than those in the open ocean, where global OA projections are most often applied (Carstensen and Duarte, 2019; Sutton and Newton, 2020). Here, the interactions of land, inland waters, open ocean, and atmosphere create a complex and dynamic coastal carbon system (Aufdenkampe et al., 2011; Duarte et al., 2013). In addition, coastal carbonate chemistry can be strongly affected by anthropogenic activities (e.g., agriculture, mariculture, urban waste) that results in water poorly buffered and rich in CO2, nutrients, and organic matter (Fabricius, 2005; Prouty et al., 2017; Carlson et al., 2019). While coastal eutrophication can enhance primary production that may partially mitigate OA (Borges and Gypens, 2010), the net effect of eutrophication over longer timescales is acidification due to excess organic matter respiration and the subsequent lowering of the seawater’s buffering capacity (Cai et al., 2011; Cai et al., 2021).
In addition to coastal and anthropogenic processes, benthic communities (e.g., coral reefs, seagrass meadows, macroalgal beds) have the capacity to alter their physical and chemical environment (Gutiérrez et al., 2011). Coral reef communities, for example, can modulate the overlying carbonate chemistry through calcification and dissolution, as well as photosynthesis and respiration. Net community calcification (NCC), or the sum of calcification and dissolution, alters total alkalinity (TA) and dissolved inorganic carbon (DIC) in a ratio of 2:1 per mole of CaCO3. Positive NCC (calcification) causes TA and DIC to decrease as well as pH and Ω. Net community production (NCP), or the sum of photosynthesis and respiration, alters DIC with positive NCP (photosynthesis) decreasing DIC and increasing pH and Ω. Using TA-DIC plots, the relative balance of community metabolism (NCP:NCC) can be determined (Suzuki and Kawahata, 2003; Cyronak et al., 2018). The extent to which these metabolic processes can alter carbonate chemistry relies on biological factors, including community composition and health (Kleypas et al., 2011; Albright et al., 2013; Anthony et al., 2013; Page et al., 2016), as well as environmental factors such as depth and light, residence time, waves, and tidal forcing (Falter et al., 2013). Provided that the latter is carefully considered or controlled, TA-DIC plots can serve as a useful tool for evaluating the former, especially within the context of acidification.
The Bolinao reef flat (Figure 1) is located in the municipality of Bolinao, along the western side (37 km of coastline) of the Lingayen Gulf and is part of the most extensive fringing reef (200 km2) in the northwestern Philippines (Cruz-Trinidad et al., 2011). The reef system is characterized by seagrass beds in the reef flat zone, and patches of coral reefs in the reef crest and slope (McManus et al., 1992; Nañola, 2002). The Bolinao reefs are an important source of livelihood to coastal communities through fisheries, mariculture, and tourism (Cruz-Trinidad et al., 2009). In the same manner, the Philippines’ dependence on coral reefs is derived from the reef’s large geographic extent (Gomez et al., 1994; Licuanan et al., 2019), high biodiversity (DeVantier and Turak, 2017), substantial role in food security (Cabral and Geronimo, 2018), and high economic value (Tamayo et al., 2018). Philippine reefs, including those in Bolinao (Lalas et al., 2020; Quimpo et al., 2020), are declining in coral cover (Licuanan et al., 2019) due to multiple disturbances such as pollution (Fabricius, 2005; Kaczmarsky and Richardson, 2011; Panga et al., 2021), overfishing (Hughes et al., 2007; Nañola et al., 2011), seawater warming (Arceo et al., 2001; Hoegh-Guldberg et al., 2007), and disease outbreaks (Harvell et al., 1999; Garren et al., 2009; Raymundo et al., 2009).
Figure 1 Map of Bolinao, Pangasinan showing the sampling locations: mariculture station (circle), three reefs (squares), and offshore station (triangle). Approximate location of mariculture structures (black dots) is also shown.
Adjacent to the Bolinao reef flat is an area of intensive fish mariculture activities, spanning from Guiguiwanen Channel in the north to Caquiputan Channel in the south (Figure 1). The industry generates approximately US$10.8 million annually and is a major source of cultured seafood for the country (David et al., 2014). Mariculture started in the 1970s with the construction of fish pens and cages along the embayment for raising milkfish (Chanos chanos) (Verceles et al., 2000). The number of fish structures, however, rapidly increased, reaching >1600 before a major 2002 fish mortality event (David et al., 2014), but is now below the maximum carrying capacity of 544 structures (San Diego-McGlone et al., 2008). In addition to the unsustainable size of this operation, poor farming practices such as excessive feeding and use of poor low-quality feeds contribute to poor water quality (Holmer et al., 2002; Ferrera et al., 2016). Nutrients and organic matter have accumulated due to wasted food, fish faecal production, and respiration (Wu, 1995; Holmer et al., 2002; Holmer et al., 2003; Holmer, 2010; White, 2013), coupled with restricted water flow due to the overcrowding of pens (San Diego-McGlone et al., 2008). Conditions of eutrophication and hypoxia (San Diego-McGlone et al., 2008; Ferrera et al., 2016) have resulted in fish kills and harmful algal blooms (HABs) (Azanza et al., 2005; Azanza and Benico, 2013; Escobar et al., 2013), with no sign of improvement based on recent monitoring of water quality conditions.
Bolinao has two seasons based on average temperature and rainfall: dry season from December to May and wet season from June to November (PAGASA, 2021). During the wet season there is higher freshwater discharge, primarily from the nearby Bani and Alaminos rivers with 17,000 ha and 21,100 ha of watershed areas, respectively (Rivera, 1997; Yoshikai et al., 2021). Offshore waters are brought in by the prevailing offshore shelf current (Northwest Luzon coastal current) that mix with the waters flowing out of the northeastern end of Guiguiwanen Channel (Altemerano and Villanoy, 2002; Ashikawa et al., 2013). Based on hydrodynamic models, the considerable outflow of eutrophic mariculture waters from Caquiputan and Guiguiwanen Channels reach the reef flat during the wet season (Yoshikai et al., 2016). Poor coral and seagrass health in Bolinao have previously been attributed to water quality issues (Villanueva et al., 2005; Villanueva et al., 2006; Tanaka et al., 2014; Watai et al., 2014). Little is known, however, about the local carbonate chemistry and the potential for mariculture activities to cause localized acidification in the nearby reefs. This study aims to characterize the link between mariculture-driven eutrophication and coastal acidification, describe the seawater carbonate chemistry at nearby reef sites, and ultimately assess the health of these reefs by quantifying the relative balance of metabolic processes (NCC and NCP) that underpin key reef function and relating them to benthic community structure.
Three reef stations (Reef 1: Tomasa, Reef 2: Lucero, Reef 3: East Malilnep) were selected based on their proximity to the mariculture area, whereby Reef 1 is the closest upstream to the mariculture area and located near the open boundary of Guiguiwanen Channel (~1.3 km alongshore), followed by Reef 2 (~3.7 km) and Reef 3 (~10.5 km) (Figure 1). These three stations are located at the shallow reef crest at approximately 4 m depth. A strongly impacted mariculture station (23 m depth, surrounded by fish structures in the channel) and unimpacted distant offshore station (3.8 km northwest of Reef 3; 24 m depth) were sampled to determine the impact of mariculture and offshore oceanographic processes, respectively. Sampling was carried out seasonally in May 2019 (warm dry season; 146.9 mm average monthly rainfall), and August and September 2019 (cool wet season; 495.8 mm average monthly rainfall, data from Philippine Atmospheric, Geophysical and Astronomical Services Administration-PAGASA).
Seasonal sampling for water quality and carbonate chemistry were conducted across all sites. Vertical profiles of in situ temperature (± 0.01 °C), salinity (± 0.003), dissolved oxygen (± 0.4 mg L-1), and turbidity (± 0.3 FTU) were acquired during both seasons at each station using a water quality profiler (AAQ-RINKO, JFE Advantech). Water samples (n = 3-6 per site) for nutrients, chlorophyll-a, and carbonate chemistry were collected from the surface (1 m depth) using 5 L Niskin sampler (General Oceanics). All samples were collected during daytime, between 09:19 and 14:23, and timed to occur during the ebbing phase of neap tide (0.6 m tidal range). Samples for nutrients and chlorophyll-a were immediately stored and kept frozen until analysis. Samples for TA and pH analysis were carefully drawn from the Niskin sampler by attaching a silicone tube from the spigot of the sampler into the bottom of the 250 mL borosilicate glass bottle, minimizing introduction of bubbles and turbulent water movement. Samples were poisoned with 200 μL of saturated HgCl2 (6 g HgCl2/100 mL distilled water) to halt any biological activity. TA and pH samples were stored at room temperature and away from sunlight until analysis.
Sampling for diel variability of carbonate parameters was conducted during dry (1 day per site) and wet (2 days at Reef 1, 3 days at all other sites) seasons across the reef sites. Subsurface automated samplers (SAS) (Enochs et al., 2020) were deployed in each reef station at similar depths (~4 m). SAS were programmed to collect discrete water samples for TA and pH analyses at 3-hour intervals by pumping reef water into pre-poisoned (200 μL of saturated HgCl2 solution) 1 L Tedlar gas sampling bags. The samplers were programmed to collect ~700 mL of seawater, enough to be transferred to a borosilicate glass bottle with rinsing while ensuring that sample bags were not overfilled in the field. An autonomous pH sensor (SeaFET V2 Ocean pH sensor, Sea-Bird Scientific) was co-deployed and set to record pH and temperature at 30-sec intervals throughout the deployment period. Spectrophotometric pH measurements from discrete SAS-collected samples were used to calibrate the SeaFET.
Dissolved inorganic nutrients nitrate (NO3-), nitrite (NO2-), ammonium (NH4+), phosphate (PO43-), and silicate (SiO44-) content were measured using spectrophotometric methods (Shimadzu UV Mini 1240) following Parsons et al. (1984). Nitrate samples were processed before analysis using a modified shaking technique to reduce nitrate to nitrite (Jones, 1984). Chlorophyll-a content was determined using fluorescence method (Trilogy® Laboratory Fluorometer, Turner Designs) following Parsons et al. (1984). Seawater pH (Total scale) at room temperature (~25°C) was measured spectrophotometrically (Shimadzu UV-1900i) with purified m-cresol purple (Byrne Labe, University of South Florida), following standard procedures (Dickson, 2007). The method was calibrated and periodically checked for accuracy and precision during each run with certified reference materials (CRMs) from the laboratory of A. Dickson (Scripps Institution of Oceanography). Seawater pH measurements were determined to have an accuracy of -0.002 ± 0.003 (n = 24), calculated as the average offset (± standard deviation) from the measured CRM pH. TA was measured using a closed-cell potentiometric titration system (Total Alkalinity Analyzer, ATT-05, Kimoto). Measurements were calibrated with CRMs and periodically checked for accuracy and precision during each run. TA measurements were determined to have an accuracy of 3.15 μmol kg-1 ± 3.56 (n = 15). DIC, in situ pH, aragonite saturation state (ΩArag), and partial pressure of CO2 (pCO2) were calculated using CO2SYS (Lewis and Wallace, 1998), taking measured values of in situ temperature, salinity, and nutrients (when nutrient samples were collected alongside TA and pH samples) into consideration. Calculations were made using the carbonic acid dissociation constants (K*1 and K*2) as defined by Mehrbach et al. (1973) and refit by Dickson and Millero (1987), the dissociation constants of bisulfate (KHSO4) by Dickson (1990), and total boron by Uppström (1974).
Benthic communities were characterized at each reef site using phototransects, following the methodologies of van Woesik et al. (2009). Reef surveys were conducted during wet (August 2019, all reef sites) and dry season (February 2021, except for Reef 3 due to rough weather conditions). Briefly, an area (75 m long and 25 m wide, maximum depth of 5 m) was demarcated at each reef and five 50-m transects were randomly placed within this area. The shallower, nearshore side of each transect was photographed at 1-m intervals using a digital camera (Olympus Tough TG-3) mounted on a PVC tetrapod. Photos were analyzed using Coral Point Count with Excel Extension (CPCe 4.1) (Kohler and Gill, 2006), whereby ten random points were overlaid on each photo and classified as one of the following: hard coral, crustose coralline algae (CCA), Halimeda spp., fleshy macroalgae, turf algae, dead coral, octocoral (soft corals and gorgonian corals), other lifeforms, and abiotic (rubble, sand, rock). Fleshy macroalgae are described as any macrophyte with thicker, fleshier appearance and with lamina visible to the naked eye. Turf algae, often growing on dead corals, are described as any filamentous algae, either single species or a multi-species assemblage, with little to no structure observable with the naked eye (Noonan et al., 2018).
Data are presented as mean ± standard deviation. Two-way ANOVAs were used for all physico-chemical parameters to test for differences between reef stations and differences between seasons. All variables, except for pH which is already on a log-scale, were log-transformed prior to ANOVA analysis in order to conform to the assumptions of the test. To examine the relationship of TA and DIC with salinity, two-way ANCOVA was conducted with season and all stations as factors, controlling for salinity.
To account for processes such as precipitation and evaporation, TA and DIC values were normalized (nTA and nDIC) to the annual average salinity (32.2), using a non-zero end member following Friis et al. (2003). To determine the net calcification potential of each reef station during each season, these salinity-normalized values were used to calculate nTA anomalies (ΔnTA, Cyronak et al., 2018). These were calculated by getting the difference between normalized reef and sourcewater TA (i.e., nTAreef – nTAsourcewater ). As there are two potential sources of water incident upon each reef site, we calculate both, using an offshore source (ΔnTAoffshore = reef nTA – offshore nTA) and mariculture source (ΔnTAmariculture = reef TA – mariculture nTA). Mean, range, and mean diurnal ranges (wet season) of pH and temperature were calculated from SeaFET pH measurements.
For diel data, linear relationship of nTA and nDIC were analyzed using Type II linear regressions which assume error for both y and x axes. The slopes produced by the major axis method were used to calculate the NCP:NCC ratios (NCP:NCC ratio = 2/slope – 1) (Suzuki and Kawahata, 2003).
Differences in multivariate community composition were tested between reef sites using permutational multivariate analysis of variance (PERMANOVA) based on Bray-Curtis distances with 999 permutations performed. ANOVA was done to test for differences of the functional groups between the reef stations during the wet season when all reefs were surveyed. Percentage cover data were arcsine-transformed prior to the ANOVA analysis (Sokal and Rohlf, 1995). All analyses and data visualizations were generated using the R statistical software (R Core Team, 2021).
Differences in surface temperature and salinity were observed between the two seasons (Table S1; Figure 2). Averaged across all five stations, temperature and salinity were higher in dry season (31.2 ± 0.2°C and 33.4 ± 0.1) compared to wet season (29.7 ± 0.6°C and 30.7 ± 0.6). The two-way ANOVA (Table S2) yielded a significant effect of season on both parameters, and of reef site on salinity (p < 0.0001, F = 1435). Spatially, a distinguishable trend of increasing salinity from the mariculture station towards the reef flat was observed with lowest mean salinity in the mariculture station during both seasons, and the offshore and Reef 3 stations having the highest mean salinity (Table S1; Figure 2).
Figure 2 Water quality parameters at the sampled stations, grouped by station type (mariculture, three reefs, and offshore). Reefs are arranged from nearest (Reef 1) to farthest (Reef 3) from the mariculture station. Data are divided into dry (red) and wet season (blue) for each station. Value pairs within each station that share a symbol (*p ≤ 0.05; **p ≤ 0.01; ***p ≤ 0.001; ****p ≤ 0.0001) denote significant difference between seasons (t-test of log-transformed values). Circles are outliers > 1.5x the inter-quartile range.
Seasonals trend in nutrients (Table S3; Figure 3) were characterized by, on average, higher phosphate (dry 0.90 ± 0.44 μM vs. wet 0.43 ± 0.04 μM) and nitrite (dry 0.14 ± 0.04 μM vs. wet 0.06 ± 0.03 μM) during the dry season, and higher nitrate (dry 0.57 ± 0.25 μM vs. wet 1.07 ± 0.79 μM), ammonium (dry 6.63 ± 3.14 μM vs. wet 8.45 ± 6.54 μM), and silicate (dry 10.45 ± 2.84 μM vs. wet 18.80 ± 8.54 μM) during the wet season. Overall, this resulted to higher N:P ratios during the wet season (dry 8.54 ± 2.52 vs. wet 22.28 ± 14.97). DO (dry 5.77 ± 1.39 mg L-1 vs. wet 6.56 ± 0.66 mg L-1) was higher during the wet season, with the lowest value in the mariculture station during the dry season (2.96 mg L-1, Table S3; Figure 3). Similarly, chlorophyll-a (dry 0.41 ± 0.34 μg L-1 vs. wet 3.35 ± 4.73 μg L-1) was higher during the wet season, with the highest values obtained in the mariculture station and Reef 1 during the wet season. No clear trend in turbidity was observed between seasons (dry 0.34 ± 0.17 FTU vs. wet 0.43 ± 0.26 FTU). Significant seasonal differences in nutrient levels within the reefs were supported by ANOVA results (p < 0.05, Table S2), but between-reef differences were more nuanced.
Figure 3 Carbonate chemistry parameters at the sampled stations, grouped by station type (mariculture, three reefs, and offshore). Reefs are arranged from nearest (Reef 1) to farthest (Reef 3) from the mariculture station. Data are divided into dry (red) and wet season (blue) for each station. Value pairs within each station that share a symbol (*p ≤ 0.05; **p ≤ 0.01; ***p ≤ 0.001; ****p ≤ 0.0001) denote significant difference between seasons (t-test of log-transformed values). Circles are outliers > 1.5x the inter-quartile range.
Higher TA (dry 2181.8 ± 11.3 μmol kg-1 vs. wet 2147.5 ± 34.4 μg L-1), DIC (dry 1920.2 ± 39.7 μmol kg-1 vs. wet 1886.4 ± 48.8 μmol kg-1) and pCO2 (dry 552 ± 153 μatm vs. wet 459 ± 72 μatm) and lower pH (dry 7.92 ± 0.08 units vs. wet 7.99 ± 0.05) were observed during the dry season (Table S1; Figure 4). ANOVA (p < 0.05) in reefs yielded season as a significant factor for all carbonate chemistry parameters except ΩArag (Table S2).
Figure 4 Predicted tide level, pH and temperature time series recorded in each reef per season. Circles represent the amplitude (lowest and highest) pH values for each reef per season.
Reef location was a significant factor (ANOVA, p < 0.05) for all carbonate chemistry parameters, except for ΩArag and pH. This can be observed by a distinct trend of decreasing TA and DIC from Reef 1 to Reef 3 during both seasons (Figure 4). The mariculture station had the highest levels of TA and DIC (Table S1; Figure 4) and the most acidified waters (highest pCO2 and lowest pH) were during the dry season (953 ± 35 μatm and 7.71 ± 0.01). While all three reefs have significant seasonal TA variations (Figure 4), TA at Reef 1 varied similarly with the mariculture station, while TA at Reefs 2 and 3 have large seasonal fluctuations, similar with the offshore station. ANCOVA results yielded statistically significant difference of all factors (except Season for DIC) for both DIC and TA (Table 1).
Table 1 Summary of analyses (two-way ANCOVA) comparing the effect of station and season to TA and DIC while accounting for salinity.
To determine net calcification potential (Table 2), ΔnTAoffshore was used for the dry season when mariculture outflow is weaker and offshore waters are a more likely source. At Reef 1, a positive ΔnTA (ΔnTAoffshore, dry = 11.2 μmol kg-1) indicated net dissolution. At Reef 2, the ΔnTA value was closer to zero (ΔnTAoffshore, dry = 2.1 μmol kg-1), which may indicate similar calcification and dissolution rates, and likely net accretionary stasis. In Reef 3, a decrease in TA (ΔnTAoffshore, dry = -12.4 μmol kg-1) was observed, suggesting net calcification and potentially more-healthy reef growth. During the wet season when mariculture outflow is more extensive than during dry season, ΔnTAmariculture was considered and all reefs displayed negative values, potentially indicating net calcification.
Table 2 Net calcification potential (ΔnTA in μmol kg-1) of each reef calculated per season and with either mariculture (ΔnTAmariculture) or offshore (ΔnTAoffshore) considered as source water.
The diel range of pH (i.e., diel peak-to-trough amplitude) provided an estimate of the variability in pH at each reef site. The largest diel ranges were recorded during the dry season at Reef 2 (dry 0.35 vs. wet 0.18) and Reef 1 (dry 0.33 vs. wet 0.22), while the smallest range was at Reef 3 during the dry season (dry 0.09 vs. wet 0.22) (Table S4; Figure 5). The lowest pH (7.78) was recorded during nighttime at Reef 1 in the dry season, while the highest pH (8.18) was observed during daytime at Reef 2 also in the dry season. Diel fluctuations in pH and temperature coincided with each other, with the highest values occurring between midday and dusk, and the lowest values between midnight and dawn (Figure 5). Diel fluctuation and amplitudes of the other carbonate chemistry parameters (TA, DIC, pCO2, and ΩArag) were consistent with pH (Table S5; Figure S1). When pH was higher during the day, high ΩArag and low TA, DIC and pCO2 were observed, and at night when pH was depressed, low ΩArag, high TA, DIC and pCO2 were observed.
Figure 5 Relationships between salinity-normalized dissolved inorganic carbon (nDIC) and salinity-normalized total alkalinity (nTA) for the three reef stations, facetted by season. The solid line represents linear regression models (Model II, major axis method) with 95% confidence interval (gray area). Equation of the line, R2 and p-values are given. Non-significant (p>0.05) are marked n.s.
The plots of nTA-nDIC yielded significant relationships (p < 0.05) in Reef 1 during the dry season (slope = 0.31), in Reef 2 both seasons (dry 0.29 and wet 0.32), and in Reef 3 during the wet season (0.24) (Table S6). These slope values were within a narrow range (0.24 – 0.32) corresponding to NCP:NCC ratio range of 5.42 to 7.37. Photosnythesis/respiration over calcification/dissolution were therefore the major contributors to reef metabolism across all Bolinao reefs.
Benthic community composition (Table S7; Figure S2) was significantly different at the three reef stations (PERMANOVA, p < 0.05; Table S8). Some functional groups (hard coral, dead coral, turf algae, and coralline algae) were found to be significantly different between the reef stations during the wet season (ANOVA, p < 0.05) (Table S9). Differences in benthic cover were not tested during the dry season because only two reefs were surveyed. All reefs were dominated by turf algae or dead coral overgrown with algae (Reef 1: dry 58.90 ± 2.25% vs. wet 68.48 ± 12.78%, Reef 2: dry 50.12 ± 4.02% vs. wet 29.08 ± 5.79%, Reef 3: wet 39.08 ± 5.40%) (Table S7). The site furthest from the mariculture area (Reef 3) had the highest hard coral cover (wet 28.49 ± 6.62%) and there was a decrease in coral cover closer to the mariculture site, with Reef 2 having lower coral cover (dry 21.87 ± 3.96% vs. wet 18.96 ± 4.61%), and Reef 1 with no coral cover. In terms of coral community composition, Reef 3 consists of diverse coral morphologies (branching, encrusting, foliose, submassive) dominated by submassive corals, while encrusting corals were dominant at Reef 2.
We utilized two approaches in characterizing the surface carbonate chemistry dynamics of the Bolinao reefs. Seasonal discrete sampling of water quality and carbonate chemistry allowed us to determine any heterogeneity of water chemistry between the reefs and calculate useful metrics (TA and DIC-salinity relationship and ΔnTA). Diel sampling results complemented the discrete sampling data to further evaluate how community metabolism on each reef act on the overlying carbonate chemistry. Here we first describe the environmental setting of Bolinao - the persisting eutrophication in the mariculture area and the significant carbonate chemistry trend among reefs. These observations reveal a water quality gradient, with eutrophied, more-acidic water reaching nearby reefs and influencing overall reef health and function, including community metabolism.
Eutrophic conditions in the mariculture area of Bolinao have continued for years (San Diego-McGlone et al., 2008; Escobar et al., 2013; Ferrera et al., 2016), with poorest water quality in the vicinity of the mariculture area as also seen in this study (Figure 3). The elevated ammonium and phosphate and low DO measured during the dry season can be attributed to high organic matter respiration (Kemp et al., 1990; Slomp and Van Cappellen, 2007; Fennel and Testa, 2019). Aside from a decrease in DO during the dry season when temperatures are warmer (decrease in solubility), there is also a tendency for a temperature-driven increase in metabolic oxygen demand and respiration (Winder and Sommer, 2012; Altieri and Gedan, 2015).
In addition to biogeochemical oxygen consumption, the residence time of the water body contributes to occurrence of low DO conditions (Fennel and Testa, 2019). From hydrodynamic models (San Diego-McGlone et al., 2008; Geček and Legović, 2010), the residence time in the mariculture embayment of Bolinao (12-18 days) is longer than at the open boundaries (< 6 days). Residence time tend to increase during the dry season due to weaker freshwater discharge, southward mass transport of surface currents from offshore (Rivera, 1997), and the direction of residual current flow in the embayment (Ferrera et al., 2016; Yoshikai et al., 2016). A longer residence time may lead to increased biogeochemical oxygen consumption, and consequently to lower DO and higher nutrients.
In addition to elevated phosphate and ammonium content in the water column, the sediments in the mariculture area are enriched with organic matter from fish wastes and uneaten feeds (Holmer et al., 2002; Holmer et al., 2003). When this material is respired by sediment-dwelling microbiota, DO and nitrate are consumed, ultimately resulting in a release of ammonium and phosphate (Holmer et al., 2002; Holmer et al., 2003). Higher nutrients and low DO from deeper layers can be introduced to surface waters during the dry season when vertical density stratification (Figure S3) is weaker due to less freshwater discharge (low precipitation rates, PAGASA, 2021) from rivers to the mariculture embayment (Rivera, 1997; Yoshikai et al., 2021). This is unlike other eutrophic river-dominated estuarine systems where coastal hypoxia occurs seasonally during density stratification from freshwater input that isolates bottom waters from oxygen supply in the surface (Testa and Kemp, 2014; Qian et al., 2018). During the wet season, there was more stratification from increased freshwater discharge driven by higher precipitation rates (PAGASA, 2021). Riverine waters, characterized by high DIN and high N:P ratio (Ferrera et al., 2016) can enhance primary production in the N-limited mariculture embayment as evidenced by the high chlorophyll-a and DO values (Table S3; Figure 3).
The observed trend in carbonate chemistry is likely driven by the same prevailing biogeochemical and hydrodynamic conditions influencing water quality. Vertical mixing during the dry season could introduce low-O2 and high-CO2 bottom waters to the surface (Cai et al., 2010). During the wet season, increased primary production (seen as higher chlorophyll-a content) decreased the DIC, as CO2 is consumed. Simultaneously, the increased influence of freshwater discharge may decrease TA although initial TA measurements in the nearby rivers showed variable results, with the larger Alaminos River having lower values (1610 μmol kg-1) than Bani River (2870 μmol kg-1; unpublished). Nonetheless, TA and DIC in the mariculture area is greater than the reef flat, regardless of season. The mariculture area can therefore be a source of acidified waters (high TA and DIC) to the reef flat. Coastal and estuarine environments with high organic matter respiration such as salt marshes (Cai et al., 2000), seagrass meadows (Turk et al., 2015; Cyronak et al., 2018), mangrove forests (Ho et al., 2017) are also known to be high in DIC. Higher TA in these environments is generally caused by sulfate reduction and CaCO3 dissolution (Wang and Van Cappellen, 1996; Cai et al., 2017). Holmer et al. (2003), for instance, reported that sediments in the Bolinao mariculture area are enriched in organic matter and undergo anaerobic respiration as oxygen is depleted, with consequent high sulfate reduction rates. The contribution of organic alkalinity may also be significant due to the input of dissolved organic matter (DOM) that can be derived from primary production (Kim and Lee, 2009), sediment porewater (Lukawska-Matuszewska et al., 2018), terrestrial input (Kuliński et al., 2014), and possibly from fish food and fecal matter.
Particle tracking simulations show that mariculture outflow, via the open boundaries, can reach the nearby reef flat extensively during the wet season (Yoshikai et al., 2016), which is also seen in the eastward mass transport of surface currents during the southwest monsoon (wet season) (Rivera, 1997). Seasonal circulation patterns can therefore explain why water quality conditions are poorer in the northern part of the reef flat (near Reef 3) during the wet season as also seen from the water quality monitoring in Bolinao, as well as the significant seasonal fluctuations of most nutrients across the reefs (Table S2, Figure 3). Other studies have reported presence of the eutrophication gradient in Bolinao that caused a decrease in seagrass species (Fortes et al., 2012; Tanaka et al., 2014), reduced growth and survivorship of hard corals (Villanueva et al., 2005; Villanueva et al., 2006), and decrease in coral recruitment (Quimpo et al., 2020).
The gradient observed across the reef flat (decreasing TA and DIC from Reefs 1 to 3) reflected the net effect of local biogeochemical processes affecting carbonate chemistry of a water parcel that flows across the reef flat (Page et al., 2018). Reef 1, being closest to the mariculture area (~1 km), received high-TA, high-DIC waters from the mariculture area and has the lowest pH. The reef community in turn can influence the carbonate chemistry through local metabolic processes. During the dry season, DIC decreased and pH increased compared to the mariculture area (Figure 4), indicating active photosynthesis that takes up CO2 which could be driven by the dominant algal community in Reef 1 (Figure S2). Positive ΔnTA values (Table 2) for Reef 1 (except ΔnTAmariculture, wet = -18.1 μmol kg-1) suggest that it is net dissolving given the increase in reef TA relative to both potential source-water sites (mariculture and offshore). This result is supported by benthic surveys in Reef 1 where no visible hard coral cover was seen (Figure S2). Historically, hard coral cover at Reef 1 was 26.13% two decades ago (Nañola, 2002). What remains now are dead corals overgrown with algae, which are likely colonized by bioeroders and experiencing dissolution (de Orte et al., 2021). Nonetheless, considering the extensive outflow of mariculture waters during the wet season, the negative ΔnTAmariculture, wet value suggests a net calcifying state, seen as the persistence of Halimeda spp. (and potentially other calcifying organisms not seen in reef surveys) during wet season. Some species of Halimeda spp. are reported to be tolerant to low-pH conditions (Vogel et al., 2015; Schubert et al., 2022). Reefs 2 and 3 which are further away from the mariculture site exhibited lower TA and DIC which may be due to local metabolic processes (calcification and photosynthesis). This seems to be reflected in the benthic community composition where there is higher cover of calcifying hard coral and CCA. ΔnTA values for Reef 3 were negative across all seasons and source-water indicating its net calcifying state. The negative ΔnTAmariculture, wet value at Reef 2 (net calcifying state) is seen by the presence of hard corals and CCA across both seasons. However, the near-zero, positive ΔnTA values for Reef 2 (except ΔnTAmariculture, wet = -80.5 μmol kg-1) may indicate the reef’s susceptibility towards net dissolution state and should be emphasized in management efforts since it is the next nearest reef to the mariculture area.
The diel range of pH and its amplitude, regardless of season, underscores the capacity of reef metabolism to drive short-term fluctuations in pH (Cyronak et al., 2019). Daytime photosynthesis increases pH and decreases DIC as inorganic carbon is converted to organic carbon. This means that NCP becomes the major contributor to community metabolism, as seen in coral reefs worldwide where net production rates are typically greater than net calcification rates (Davis et al., 2021). The large rhythmic diel patterns in Reefs 1 and 2 during the dry season suggest substantial influence of the reef community on local chemistry, particularly NCP driving changes in DIC, pH and pCO2. Unlike Reef 2, the minor diel fluctuations of TA in Reef 1 during dry season may indicate relatively smaller influence of calcification and dissolution processes (NCC). This should be expected from the dominance of fleshy and turf algae and small cover of calcifying organisms in the benthos. On the other hand, the persistence of calcifying organisms (Halimeda) during wet season may allow for the diel fluctuation of TA to occur, and the negative ΔnTAmariculture, wet may point to their capacity to thrive. In Reef 2 during the dry season, diel TA cycles were also consistent with DIC, suggesting calcification during the daytime and dissolution at night. The calcification of reef-building coral species is strongly dependent on light intensity, as calcification and photosynthesis enhance each other (Gattuso et al., 1999; Erez et al., 2011). Thus, during daytime, elevated saturation states (ΩArag) and NCP are suitable for calcification (Andersson and Gledhill, 2013). Recent studies that measured both NCC and NCP have shown their interactive relationship, with NCP strongly driving NCC (Albright et al., 2015; DeCarlo et al., 2017).
The magnitude of diel fluctuations in reef carbonate chemistry is dependent on the relative balance of community metabolism (NCP and NCC), metabolic rates, depth, and residence time (Takeshita et al., 2018). The extent to which community metabolism can influence carbonate chemistry is dependent on benthic community composition, organismal abundances, health of organisms, and individual metabolic rates, especially in shallow coral reefs where smaller water volumes lead to more pronounced carbonate chemistry alterations (Koweek et al., 2014; Page et al., 2016; Lantz et al., 2017). In Bolinao, benthic composition among the three reefs could potentially contribute to the observed differences in community metabolism, as implied in the diel variability of carbonate chemistry and further supported here by nTA-nDIC relationships. All reefs have slope values within a narrow range of 0.24 to 0.32, indicating greater contribution of NCP over NCC across all reefs, and within values found in most Indo-Pacific reefs (Cyronak et al., 2018). While these values may seem low for Reefs 2 and 3 where there is greater calcifying cover (> 25% combined hard coral and CCA cover) than Reef 1, non-calcifying cover still dominated these reefs. Nonetheless, other similar reefs with extensive coral cover and high NCC rates also yielded low slope values (McMahon et al., 2013; Albright et al., 2015). Such findings are consistent with previous studies that showed differential carbonate chemistry modifications by key reef benthic functional groups due to differences in their metabolic rates (Anthony et al., 2011; Anthony et al., 2013; Page et al., 2016; Page et al., 2017). Moreover, direct measurements of metabolic processes at different functional scales should be done to fully understand the contribution of the benthic community to carbonate chemistry variability. Within the scope of this study, potential metrics (ΔnTA and slope of nTA-nDIC relationships) serve as simple, but effective chemistry-based tools to assess reef health and metabolic state (Cyronak et al., 2018). It is noted that these metrics represent single measurements and may vary over time. Further observations should be collected in order to describe their variance and correlate with the continuing health decline of Bolinao reefs.
Aside from benthic community, the physical setting (e.g., depth, residence time, prevailing current, wave forcing, tides) can strongly influence the magnitude of carbonate chemistry changes. The influence of the mariculture outflow on the carbonate chemistry of nearby reefs is supported by the lowest average pH (7.94) and the lowest single pH value (7.78, dry season) recorded at nearby Reef 1. For reefs that are further away, the capacity of the community to alter the overlying carbonate chemistry may act to partially offset the localized acidification from the mariculture outflow (Anthony et al., 2011; Andersson et al., 2014; Page et al., 2016). For instance, the highest pH recorded (8.18) was on Reef 2 during the dry season, which could be a result of high primary production at this site during the daytime. However, this buffering capacity may be compromised by global OA, warming, and other anthropogenic stressors (Davis et al., 2021).
In addition, the extensive outflow of mariculture waters to the reef flat delivers organic matter and nutrients that can alter rates of NCP and NCC (Kawahata et al., 2000; Silverman et al., 2007; Silbiger et al., 2018). Elevated nutrients could suppress NCC rates as a direct physiological response by calcifying organisms and therefore facilitate transition to net dissolution state (Silbiger et al., 2018). Under future OA, it is likely that diel variability of coastal carbonate chemistry parameters will amplify due to the influence of anthropogenic activities (Cyronak et al., 2014; Torres et al., 2021).
This study reveals the impact of fish mariculture-induced eutrophication on the carbonate chemistry dynamics of Bolinao coral reefs. Extensive outflow of eutrophic and acidified waters has resulted in a decline in reef health and will continue to degrade nearby ecosystems. In Bolinao, although no previous studies have measured community metabolism, the continuing decline in coral cover mainly due to localized eutrophication has caused substantial changes in both net calcification and primary production. This is particularly evident in the reef site closest to the mariculture activity (Reef 1) where no hard coral cover was detected. This reef is likely already in a net erosional state, and a loss of essential reef framework habitat will follow (Eyre et al., 2018). The near-zero values of ΔnTA in Reef 2 may indicate that reefs further downstream will also continue to be affected as mariculture-induced eutrophication persist. Local reef disturbances should therefore be considered and given utmost attention in evaluating future OA conditions, and in developing potential mitigation strategies to locally combat OA in addition to reducing global CO2 emissions. In Bolinao, it is imperative to reduce the number of fish mariculture structures to improve flushing rates and to observe best practices in fish feeding to reduce organic matter and nutrient input.
The raw data supporting the conclusions of this article will be made available by the authors, without undue reservation.
RI and MSD-M conceptualized the study. All authors contributed to the design of the study. RI collected and analysed the data and wrote the first draft of the manuscript. All authors contributed to the article and approved the submitted version.
This study was part of the research program entitled “Coastal Acidification: How it Affects the Marine Environment and Resources in the Philippines” under Project 1, “Spatio-temporal trends in pH, pCO2, and related parameters” (Project Code QMSR-MRRD-MEC-295-1447). The program was funded by the Department of Science and Technology-Philippine Council for Agriculture, Aquatic and Natural Resources Research and Development (DOST-PCAARRD).
This paper is part of the master’s thesis of RI supervised by MS-M entitled “Carbonate chemistry dynamics on the Bolinao reef flat”. The authors are grateful to the Marine Biogeochemistry Laboratory and Bolinao Marine Laboratory of the Marine Science Institute, University of the Philippines for the valuable logistical and laboratory support provided. We thank Jay Burce, Ryan Carl Magyaya, Natasha Tamayo for their tremendous help in field activities and laboratory analyses. We thank Alice Webb for providing insights into improving the manuscript.
The authors declare that the research was conducted in the absence of any commercial or financial relationships that could be construed as a potential conflict of interest.
All claims expressed in this article are solely those of the authors and do not necessarily represent those of their affiliated organizations, or those of the publisher, the editors and the reviewers. Any product that may be evaluated in this article, or claim that may be made by its manufacturer, is not guaranteed or endorsed by the publisher.
The Supplementary Material for this article can be found online at: https://www.frontiersin.org/articles/10.3389/fmars.2022.858853/full#supplementary-material
Albright R., Benthuysen J., Cantin N., Caldeira K., Anthony K. R. N. (2015). Coral reef metabolism and carbon chemistry dynamics of a coral reef flat. Geophys. Res. Lett. 42, 3980–3988. doi: 10.1002/2015GL063488
Albright R., Langdon C. J., Anthony K. R. N. (2013). Dynamics of seawater carbonate chemistry, production, and calcification of a coral reef flat, Central Great Barrier Reef. Biogeosciences 10, 6747–6758. doi: 10.5194/bg-10-6747-2013
Altemerano A. M., Villanoy C. L. (2002). Influence of the Western Luzon Shelf Current on Larval dispersal in Lingayen Gulf based on numerical Lagrangian dispersal experiments. UPV J. Nat. Sci. 1, 103–119.
Altieri A. H., Gedan K. B. (2015). Climate change and dead zones. Glob. Change Biol. 21, 1395–1406. doi: 10.1111/gcb.12754
Andersson A. J., Gledhill D. (2013). Ocean acidification and coral reefs: Effects on breakdown, dissolution, and net ecosystem calcification. Ann. Rev. Mar. Sci. 5, 321–348. doi: 10.1146/annurev-marine-121211-172241
Andersson A. J., Yeakel K. L., Bates N. R., De Putron S. J. (2014). Partial offsets in ocean acidification from changing coral reef biogeochemistry. Nat. Clim. Change 4, 56–61. doi: 10.1038/nclimate2050
Anthony K. R. N., Diaz-Pulido G., Verlinden N., Tilbrook B., Andersson A. J. (2013). Benthic buffers and boosters of ocean acidification on coral reefs. Biogeosciences 10, 4897–4909. doi: 10.5194/bg-10-4897-2013
Anthony K. R. N., Kleypas J. A., Gattuso J.-P. (2011). Coral reefs modify their seawater carbon chemistry – implications for impacts of ocean acidification. Glob. Change Biol. 17, 3655–3666. doi: 10.1111/j.1365-2486.2011.02510.x
Arceo H. O., Quibilan M. C., Aliño P. M., Lim G., Licuanan W. Y. (2001). Coral bleaching in Philippine reefs: Coincident evidences with mesoscale thermal anomalies. Bull. Mar. Sci. 69, 579–593. Availabe at:https://www.ingentaconnect.com/contentone/umrsmas/bullmar/2001/00000069/00000002/art00030
Ashikawa K., Pokavanich T., Kartadikaria A., Nadaoka K., Villanoy C., Herrera E. (2013). Seasonal larval dispersal characteristics and sediment discharge impacts on the coral reefs in Lingayen Gulf, Philippines towards coastal ecosystem conservation. Galaxea J. Coral Reef Stud. 15, 295–299. doi: 10.3755/galaxea.15.295
Aufdenkampe A. K., Mayorga E., Raymond P. A., Melack J. M., Doney S. C., Alin S. R., et al. (2011). Riverine coupling of biogeochemical cycles between land, oceans, and atmosphere. Front. Ecol. Environ. 9, 53–60. doi: 10.1890/100014
Azanza R. V., Benico G. A. (2013). Toxic alexandrium blooms in fish farming sites in Bolinao, Pangasinan. J. Environ. Sci. Manage. 1, 44–49.
Azanza R. V., Fukuyo Y., Yap L. G., Takayama H. (2005). Prorocentrum minimum bloom and its possible link to a massive fish kill in Bolinao, Pangasinan, Northern Philippines. Harmful Algae 4, 519–524. doi: 10.1016/j.hal.2004.08.006
Borges A. V., Gypens N. (2010). Carbonate chemistry in the coastal zone responds more strongly to eutrophication than ocean acidification. Limnol. Oceanogr. 55, 346–353. doi: 10.4319/lo.2010.55.1.0346
Cabral R. B., Geronimo R. C. (2018). How important are coral reefs to food security in the philippines? diving deeper than national aggregates and averages. Mar. Policy 91, 136–141. doi: 10.1016/j.marpol.2018.02.007
Cai W.-J., Feely R. A., Testa J. M., Li M., Evans W., Alin S. R., et al. (2021). Natural and anthropogenic drivers of acidification in large estuaries. Ann. Rev. Mar. Sci. 13, 23–55. doi: 10.1146/annurev-marine-010419-011004
Cai W.-J., Huang W. J., Luther G. W., Pierrot D., Li M., Testa J., et al. (2017). Redox reactions and weak buffering capacity lead to acidification in the Chesapeake Bay. Nat. Commun. 8, 1–12. doi: 10.1038/s41467-017-00417-7
Cai W.-J., Hu X., Huang W.-J., Murrell M. C., Lehrter J. C., Lohrenz S. E., et al. (2011). Acidification of subsurface coastal waters enhanced by eutrophication. Nat. Geosci. 4, 766–770. doi: 10.1038/ngeo1297
Cai W.-J., Luther G. W., Cornwell J. C., Giblin A. E. (2010). Carbon cycling and the coupling between proton and electron transfer reactions in aquatic sediments in Lake Champlain. Aquat. Geochem 16, 421–446. doi: 10.1007/s10498-010-9097-9
Cai W.-J., Wiebe W. J., Wang Y., Sheldon J. E. (2000). Intertidal marsh as a source of dissolved inorganic carbon and a sink of nitrate in the Satilla River-estuarine complex in the southeastern U.S. Limnol. Oceanogr. 45, 1743–1752. doi: 10.4319/lo.2000.45.8.1743
Caldeira K., Wickett M. E. (2003). Anthropogenic carbon and ocean pH. Nature 425, 365. doi: 10.1038/425365a
Carlson R. R., Foo S. A., Asner G. P. (2019). Land use impacts on coral reef health: A ridge-to-reef perspective. Front. Mar. Sci. 6. doi: 10.3389/fmars.2019.00562
Carstensen J., Duarte C. M. (2019). Drivers of pH variability in coastal ecosystems. Environ. Sci. Technol. 53, 4020–4029. doi: 10.1021/acs.est.8b03655
Chan N. C. S., Connolly S. R. (2013). Sensitivity of coral calcification to ocean acidification: A meta-analysis. Glob. Change Biol. 19, 282–290. doi: 10.1111/gcb.12011
Cruz-Trinidad A., Geronimo R. C., Aliño P. M. (2009). Development trajectories and impacts on coral reef use in Lingayen Gulf, Philippines. Ocean Coast. Manage. 52, 173–180. doi: 10.1016/j.ocecoaman.2008.12.002
Cruz-Trinidad A., Geronimo R. C., Cabral R. B., Aliño P. M. (2011). How much are the Bolinao-Anda coral reefs worth? Ocean Coast. Manage. 54, 696–705. doi: 10.1016/j.ocecoaman.2011.07.002
Cyronak T., Andersson A. J., Langdon C. J., Albright R., Bates N. R., Caldeira K., et al. (2018). Taking the metabolic pulse of the world’s coral reefs. PloS One 13, e0190872. doi: 10.1371/journal.pone.0190872
Cyronak T., Schulz K. G., Santos I. R., Eyre B. D. (2014). Enhanced acidification of global coral reefs driven by regional biogeochemical feedbacks. Geophys. Res. Lett. 41, 5538–5546. doi: 10.1002/2014GL060849
Cyronak T., Takeshita Y., Courtney T. A., DeCarlo E. H., Eyre B. D., Kline D. I., et al. (2019). Diel temperature and pH variability scale with depth across diverse coral reef habitats. Limnol. Oceanogr. Lett. 5, 193–203. doi: 10.1002/lol2.10129
David L. T., Pastor-Rengel D., Talaue-McManus L., Magdaong E., Salalila-Aruelo R., Bangi H. G., et al. (2014). The saga of community learning: Mariculture and the Bolinao experience. Aquat. Ecosyst. Heal. Manage. 17, 196–204. doi: 10.1080/14634988.2014.910488
Davis K. L., Colefax A. P., Tucker J. P., Kelaher B. P., Santos I. R. (2021). Global coral reef ecosystems exhibit declining calcification and increasing primary productivity. Commun. Earth Environ. 2, 1–10. doi: 10.1038/s43247-021-00168-w
DeCarlo T. M., Cohen A. L., Wong G. T. F., Shiah F., Lentz S. J., Davis K. A., et al. (2017). Community production modulates coral reef pH and the sensitivity of ecosystem calcification to ocean acidification. J. Geophys. Res. Ocean. 122, 745–761. doi: 10.1002/2016JC012326.Received
de Orte M. R., Koweek D. A., Cyronak T., Takeshita Y., Griffin A., Wolfe K., et al. (2021). Unexpected role of communities colonizing dead coral substrate in the calcification of coral reefs. Limnol. Oceanogr. 66, 1793–1803. doi: 10.1002/lno.11722
De Vantier L., Turak E. (2017). Species richness and relative abundance of reef-building corals in the indo-west pacific. Diversity 9 (3). doi: 10.3390/d9030025
Dickson A. G. (1990). Thermodynamics of the dissociation of boric acid in potassium chloride solutions from 273.15 to 318.15 K. J. Chem. Eng. Data 35, 253–257. doi: 10.1021/je00061a009
Dickson A. G. (2007). Guide to best practices for ocean CO2 measurements. Eds. Dickson A. G., Sabine C. L., Christian J. R. (Sidney, BC: North Pacific Marine Science Organization).
Dickson A. G., Millero F. J. (1987). A comparison of the equilibrium constants for the dissociation of carbonic acid in seawater media. Deep Sea Res. Part A Oceanogr. Res. Pap. 34, 1733–1743. doi: 10.1016/0198-0149(87)90021-5
Doney S. C., Fabry V. J., Feely R. A., Kleypas J. A. (2009). Ocean acidification: The other CO2 problem. Ann. Rev. Mar. Sci. 1, 169–192. doi: 10.1146/annurev.marine.010908.163834
Duarte C. M., Hendriks I. E., Moore T. S., Olsen Y. S., Steckbauer A., Ramajo L., et al. (2013). Is ocean acidification an open-ocean syndrome? understanding anthropogenic impacts on seawater pH. Estuaries Coasts 36, 221–236. doi: 10.1007/s12237-013-9594-3
Enochs I. C., Formel N., Shea L., Chomiak L., Piggot A., Kirkland A., et al. (2020). Subsurface automated samplers (SAS) for ocean acidification research. Bull. Mar. Sci. 96, 735–752. doi: 10.5343/BMS.2020.0018
Enochs I. C., Manzello D. P., Donham E. M., Kolodziej G., Okano R., Johnston L., et al. (2015). Shift from coral to macroalgae dominance on a volcanically acidified reef. Nat. Clim. Change 5, 1083–1088. doi: 10.1038/nclimate2758
Enochs I. C., Manzello D. P., Kolodziej G., Noonan S. H. C., Valentino L., Fabricius K. E. (2016). Enhanced macroboring and depressed calcification drive net dissolution at high-CO2 coral reefs. Proc. R. Soc B Biol. Sci. 283, 1–8. doi: 10.1098/rspb.2016.1742
Erez J., Reynaud S., Silverman J., Schneider K., Allemand D. (2011). “Coral calcification under ocean acidification and global change,” in Coral reefs: An ecosystem in transition. Eds. Dubinsky Z., Stambler N. (London: Springer), 151–177.
Escobar M. T. L., Sotto L. P. A., Jacinto G. S., Benico G. A., San Diego-McGlone M. L., Azanza R. V. (2013). Eutrophic conditions during the 2010 fish kill in Bolinao and Anda, Pangasinan, Philippines. J. Environ. Sci. Manage. 16(Special Issue 1-2013), 29–35.
Eyre B. D., Cyronak T., Drupp P., De Carlo E. H., Sachs J. P., Andersson A. J., et al. (2018). Coral reefs will transition to net dissolving before end of century. Science 359, 908–911. doi: 10.1126/science.aao1118
Fabricius K. E. (2005). Effects of terrestrial runoff on the ecology of corals and coral reefs: review and synthesis. Mar. pollut. Bull. 50, 125–146. doi: 10.1016/j.marpolbul.2004.11.028
Falter J. L., Lowe R. J., Zhang Z., McCulloch M. (2013). Physical and biological controls on the carbonate chemistry of coral reef waters: Effects of metabolism, wave forcing, sea level, and geomorphology. PloS One 8, e53303. doi: 10.1371/journal.pone.0053303
Fennel K., Testa J. M. (2019). Biogeochemical controls on coastal hypoxia. Ann. Rev. Mar. Sci. 11, 105–130. doi: 10.1146/annurev-marine-010318-095138
Ferrera C. M., Watanabe A., Miyajima T., San Diego-McGlone M. L., Morimoto N., Umezawa Y., et al. (2016). Phosphorus as a driver of nitrogen limitation and sustained eutrophic conditions in Bolinao and Anda, Philippines, a mariculture-impacted tropical coastal area. Mar. pollut. Bull. 105, 237–248. doi: 10.1016/j.marpolbul.2016.02.025
Fortes M. D., Go G., Bolisay K., Nakaoka M., Uy W., Lopez M., et al. (2012). “Seagrass response to mariculture-induced physico-chemical gradients in bolinao, northwestern Philippines,” in Proceedings 12th Int. Coral Reef Symp (Cairns, Australia). 9–13. Available at: http://www.icrs2012.com/proceedings/manuscripts/ICRS2012_15B_3.pdf.
Friedlingstein P., O’Sullivan M., Jones M. W., Andrew R. M., Hauck J., Olsen A., et al. (2020). Global carbon budget 2021. Earth Syst. Sci. Data 12, 3269–3340. doi: 10.5194/essd-14-1917-2022
Friis K., Körtzinger A., Wallace D. W. R. (2003). The salinity normalization of marine inorganic carbon chemistry data. Geophys. Res. Lett. 30, 1–4. doi: 10.1029/2002GL015898
Garren M., Raymundo L., Guest J., Harvell C. D., Azam F. (2009). Resilience of coral-associated bacterial communities exposed to fish farm effluent. PloS One 4, 1–9. doi: 10.1371/journal.pone.0007319
Gattuso J.-P., Allemand D., Frankignoulle M. (1999). Photosynthesis and calcification at cellular, organismal and community levels in coral reefs: A review on interactions and control by carbonate chemistry. Am. Zool. 39, 160–183. doi: 10.1093/icb/39.1.160
Geček S., Legović T. (2010). Towards carrying capacity assessment for aquaculture in the Bolinao Bay, Philippines: A numerical study of tidal circulation. Ecol. Modell. 221, 1394–1412. doi: 10.1016/j.ecolmodel.2010.02.005
Gomez E., Aliño P., Yap H., Licuanan W. (1994). A review of the status of philippine reefs. Mar. pollut. Bull. 29 (1-3), 62–68. doi: 10.1016/0025-326X(94)90427-8
Gutiérrez J. L., Jones C. G., Byers J. E., Arkema K. K., Berkenbusch K., Commito A., et al. (2011). “Physical ecosystem engineers and the functioning of estuaries and coasts,” in Treatise on estuarine and coastal science (UK: Elsevier Inc), 53–81. doi: 10.1016/B978-0-12-374711-2.00705-1
Harvell C. D., Kim K., Burkholder J. M., Colwell R. R., Epstein P. R., Grimes D. J., et al. (1999). Emerging marine diseases-climate links and anthropogenic factors. Science 285, 1505–1510. doi: 10.1126/science.285.5433.1505
Hoegh-Guldberg O., Mumby P. J., Hooten A. J., Steneck R. S., Greenfield P., Gomez E., et al. (2007). Coral reefs under rapid climate change and ocean acidification. Science 318, 1737–1742. doi: 10.1126/science.1152509
Ho D. T., Ferrón S., Engel V. C., Anderson W. T., Swart P. K., Price R. M., et al. (2017). Dissolved carbon biogeochemistry and export in mangrove-dominated rivers of the Florida Everglades. Biogeosciences 14, 2543–2559. doi: 10.5194/bg-14-2543-2017
Holmer M. (2010). Environmental issues of fish farming in offshore waters: Perspectives, concerns and research needs. Aquac. Environ. Interact. 1, 57–70. doi: 10.3354/aei00007
Holmer M., Duarte C. M., Heilskov A., Olesen B., Terrados J. (2003). Biogeochemical conditions in sediments enriched by organic matter from net-pen fish farms in the bolinao area, Philippines. Mar. pollut. Bull. 46, 1470–1479. doi: 10.1016/S0025-326X(03)00281-9
Holmer M., Marbá N., Terrados J., Duarte C. M., Fortes M. D. (2002). Impacts of milkfish (Chanos chanos) aquaculture on carbon and nutrient fluxes in the Bolinao area, Philippines. Mar. pollut. Bull. 44, 685–696. doi: 10.1016/S0025-326X(02)00048-6
Hughes T. P., Rodrigues M. J., Bellwood D. R., Ceccarelli D., Hoegh-Guldberg O., McCook L., et al. (2007). Phase shifts, herbivory, and the resilience of coral reefs to climate change. Curr. Biol. 17, 360–365. doi: 10.1016/j.cub.2006.12.049
Johnson M. D., Price N. N., Smith J. E. (2014). Contrasting effects of ocean acidification on tropical fleshy and calcareous algae. PeerJ 2014, 1–28. doi: 10.7717/peerj.411
Jones M. N. (1984). Nitrate reduction by shaking with cadmium: Alternative to cadmium columns. Water Res. 18, 643–646. doi: 10.1016/0043-1354(84)90215-X
Kaczmarsky L., Richardson L. L. (2011). Do elevated nutrients and organic carbon on Philippine reefs increase the prevalence of coral disease? Coral Reefs 30, 253–257. doi: 10.1007/s00338-010-0686-2
Kawahata H., Yukino I., Suzuki A. (2000). Terrestrial influences on the Shiraho fringing reef, Ishigaki Island, Japan: High carbon input relative to phosphate. Coral Reefs 19, 172–178. doi: 10.1007/s003380000093
Kemp W. M., Sampou P., Caffrey J., Mayer M., Henriksen K., Boynton W. R. (1990). Ammonium recycling versus denitrification in Chesapeake Bay sediments. Limnol. Oceanogr. 35, 1545–1563. doi: 10.4319/lo.1990.35.7.1545
Kim H. C., Lee K. (2009). Significant contribution of dissolved organic matter to seawater alkalinity. Geophys. Res. Lett. 36, 1–5. doi: 10.1029/2009GL040271
Kleypas J. A., Anthony K. R. N., Gattuso J. P. (2011). Coral reefs modify their seawater carbon chemistry - case study from a barrier reef (Moorea, French Polynesia). Glob. Change Biol. 17, 3667–3678. doi: 10.1111/j.1365-2486.2011.02530.x
Koch M., Bowes G., Ross C., Zhang X. H. (2013). Climate change and ocean acidification effects on seagrasses and marine macroalgae. Glob. Change Biol. 19, 103–132. doi: 10.1111/j.1365-2486.2012.02791.x
Kohler K. E., Gill S. M. (2006). CCoral Point Count with Excel extensions (CPCe): A Visual Basic program for the determination of coral and substrate coverage using random point count methodology. Comput. Geosci. 32, 1259–1269. doi: 10.1016/j.cageo.2005.11.009
Koweek D., Dunbar R. B., Rogers J. S., Williams G. J., Price N., Mucciarone D., et al. (2014). Environmental and ecological controls of coral community metabolism on Palmyra Atoll. Coral Reefs 34, 339–351. doi: 10.1007/s00338-014-1217-3
Kroeker K. J., Kordas R. L., Crim R. N., Singh G. G. (2010). Meta-analysis reveals negative yet variable effects of ocean acidification on marine organisms. Ecol. Lett. 13, 1419–1434. doi: 10.1111/j.1461-0248.2010.01518.x
Kuliński K., Schneider B., Hammer K., Machulik U., Schulz-Bull D. (2014). The influence of dissolved organic matter on the acid-base system of the Baltic Sea. J. Mar. Syst. 132, 106–115. doi: 10.1016/j.jmarsys.2014.01.011
Lalas J. A. A., Benayahu Y., Baria-Rodriguez M. V. (2020). Community structure and size-frequency distribution of soft corals in a heavily disturbed reef system in northwestern Philippines. Mar. pollut. Bull. 162, 111871. doi: 10.1016/j.marpolbul.2020.111871
Lantz C. A., Carpenter R. C., Comeau S., Edmunds P. J. (2017). Organisms composing an experimental coral reef community from Mo’orea, French Polynesia, exhibit taxon-specific net production: Net calcification ratios. Front. Mar. Sci. 4. doi: 10.3389/fmars.2017.00298
Lewis E., Wallace D. W. R. (1998). “Program developed for CO2 system calculations,” in Carbon dioxide information analysis center (Oak Ridge, TN: Oak Ridge National Laboratory, U.S. Department of Energy).
Licuanan W. Y., Robles R., Reyes M. (2019). Status and recent trends in coral reefs of the Philippines. Mar. pollut. Bull. 142, 544–550. doi: 10.1016/j.marpolbul.2019.04.013
Lukawska-Matuszewska K., Grzybowski W., Szewczun A., Tarasiewicz P. (2018). Constituents of organic alkalinity in pore water of marine sediments. Mar. Chem. 200, 22–32. doi: 10.1016/j.marchem.2018.01.012
McMahon A., Santos I. R., Cyronak T., Eyre B. D. (2013). Hysteresis between coral reef calcification and the seawater aragonite saturation state. Geophys Res. Lett. 40, 4675–4679. doi: 10.1002/GRL.50802
McManus J. W., Nañola C. L., Reyes R. B., Kesner K. N. (1992) Resource ecology of the bolinao coral reef system. Manila: International Center for Living Aquatic Resources Management. Available at: http://pubs.iclarm.net/libinfo/Pdf/PubSR7622.pdf.
Mehrbach C., Culberson C. H., Hawley J. E., Pytkowicx R. M. (1973). Measurement of the apparent dissociation constants of carbonic acid in seawater at atmospheric pressure. Limnol. Oceanogr. 18, 897–907. doi: 10.4319/lo.1973.18.6.0897
Nañola C. J. L. (2002). “Bolinao,” in Atlas of Philippine coral reefs. Eds. Aliño P. M., Miclat E. F. B., Nañola C. J. L., Roa-Quiaoit H. A., Campos R. T. (Quezon City: Goodwill Trading Co), 31–34.
Nañola C. L., Aliño P. M., Carpenter K. E. (2011). Exploitation-related reef fish species richness depletion in the epicenter of marine biodiversity. Environ. Biol. Fishes 90, 405–420. doi: 10.1007/s10641-010-9750-6
Noonan S. H. C., Kluibenschedl A., Fabricius K. E. (2018). Ocean acidification alters early successional coral reef communities and their rates of community metabolism. PloS One 13, e0197130. doi: 10.1371/journal.pone.0197130
Orr J. C., Fabry V. J., Aumont O., Bopp L., Doney S. C., Feely R. A., et al. (2005). Anthropogenic ocean acidification over the twenty-first century and its impact on calcifying organisms. Nature 437, 681–686. doi: 10.1038/nature04095
PAGASA (2021) Climate of the Philippines. Available at: https://www.pagasa.dost.gov.ph/information/climate-philippines.
Page H. N., Andersson A. J., Jokiel P. L., Rodgers K. S., Lebrato M., Yeakel K., et al. (2016). Differential modification of seawater carbonate chemistry by major coral reef benthic communities. Coral Reefs 35, 1311–1325. doi: 10.1007/s00338-016-1490-4
Page H. N., Courtney T. A., Collins A., De Carlo E. H., Andersson A. J. (2017). Net community metabolism and seawater carbonate chemistry scale non-intuitively with coral cover. Front. Mar. Sci. 4. doi: 10.3389/fmars.2017.00161
Page H. N., Courtney T. A., De Carlo E. H., Howins N. M., Koester I., Andersson A. J. (2018). Spatiotemporal variability in seawater carbon chemistry for a coral reef flat in kāne’ohe Bay, hawai’i. Limnol. Oceanogr. 9999, 1–22. doi: 10.1002/lno.11084
Panga F. M., Anticamara J. A., Quibilan M. C. C., Atrigenio M. P., Aliño P. M. (2021). Through the boundaries: Environmental factors affecting reef benthic cover in marine protected areas in the Philippines. Front. Mar. Sci. 8. doi: 10.3389/fmars.2021.702071
Parsons T. R., Maita Y., Lalli C. M. (1984). A manual of chemical and biological methods for seawater analysis. doi: 10.1016/B978-0-08-030287-4.50004-9
Prouty N. G., Cohen A., Yates K. K., Storlazzi C. D., Swarzenski P. W., White D. (2017). Vulnerability of coral reefs to bioerosion from land-based sources of pollution. J. Geophys. Res. Ocean. 122, 9319–9331. doi: 10.1002/2017JC013264
Qian W., Gan J., Liu J., He B., Lu Z., Guo X., et al. (2018). Current status of emerging hypoxia in a eutrophic estuary: The lower reach of the Pearl River Estuary, China. Estuar. Coast. Shelf Sci. 205, 58–67. doi: 10.1016/j.ecss.2018.03.004
Quimpo T. J. R., Ligson C. A., Manogan D. P., Requilme J. N. C., Albelda R. L., Conaco C., et al. (2020). Fish farm effluents alter reef benthic assemblages and reduce coral settlement. Mar. pollut. Bull. 153, 111025. doi: 10.1016/j.marpolbul.2020.111025
Raymundo L. J., Halford A. R., Maypa A. P., Kerr A. M. (2009). Functionally diverse reef-fish communities ameliorate coral disease. Proc. Natl. Acad. Sci. 106, 17067–17070. doi: 10.1073/pnas.0900365106
R Core Team (2021) R: A language and environment for statistical computing. (Vienna, Austria). Available at: http://www.r-project.org/.
Rivera P. C. (1997). ydrodynamics, Sediment Transport and Light Extinction Off Cape Bolinao, Philippines (1st ed.) (Boca Raton: CRC Press). doi: 10.1201/9781003073161
San Diego-McGlone M. L., Azanza R. V., Villanoy C. L., Jacinto G. S. (2008). Eutrophic waters, algal bloom and fish kill in fish farming areas in Bolinao, Pangasinan, Philippines. Mar. pollut. Bull. 57, 295–301. doi: 10.1016/j.marpolbul.2008.03.028
Schönberg C. H. L., Fang J. K. H., Carreiro-Silva M., Tribollet A., Wisshak M. (2017). Bioerosion: The other ocean acidification problem. ICES J. Mar. Sci. 74, 895–925. doi: 10.1093/icesjms/fsw254
Schubert N., Alvarez-Filip L., Hofmann L. C. (2022). Systematic review and meta-analysis of ocean acidification effects in Halimeda: Implications for algal carbonate production. Climate Change Ecol. 4, 100059. doi: 10.1016/J.ECOCHG.2022.100059
Silbiger N. J., Nelson C. E., Remple K., Sevilla J. K., Quinlan Z. A., Putnam H. M., et al. (2018). Nutrient pollution disrupts key ecosystem functions on coral reefs. Proc. R. Soc B Biol. Sci. 285, 2–10. doi: 10.1098/rspb.2017.2718
Silverman J., Lazar B., Cao L., Caldeira K., Erez J. (2009). Coral reefs may start dissolving when atmospheric CO2 doubles. Geophys. Res. Lett. 36, 1–5. doi: 10.1029/2008GL036282
Silverman J., Lazar B., Erez J. (2007). Community metabolism of a coral reef exposed to naturally varying dissolved inorganic nutrient loads. Biogeochemistry 84, 67–82. doi: 10.1007/s10533-007-9075-5
Slomp C. P., Van Cappellen P. (2007). The global marine phosphorus cycle: Sensitivity to oceanic circulation. Biogeosciences 4, 155–171. doi: 10.5194/bg-4-155-2007
Sokal R. R., Rohlf F. J. (1995). Biometry: The principles and practices of statistics in biological research. 3rd ed (New York: W. H. Freeman).
Sutton A., Newton J. (2020). Reaching consensus on assessments of ocean acidification trends. Eos (Washington DC) 101. doi: 10.1029/2020EO150944
Suzuki A., Kawahata H. (2003). Carbon budget of coral reef systems: an overview of observations in fringing reefs, barrier reefs and atolls in the indo-pacific regions. Tellus B 55, 428–444. doi: 10.3402/tellusb.v55i2.16761
Takeshita Y., Cyronak T., Martz T. R., Kindeberg T., Andersson A. J. (2018). Coral reef carbonate chemistry variability at different functional scales. Front. Mar. Sci. 5. doi: 10.3389/fmars.2018.00175
Tamayo N. C. A., Anticamara J. A., Acosta-Michlik L. (2018). National estimates of values of Philippine reefs’ ecosystem services. Ecol. Econ. 146, 633–644. doi: 10.1016/j.ecolecon.2017.12.005
Tanaka Y., Go G. A., Watanabe A., Miyajima T., Nakaoka M., Uy W. H., et al. (2014). 17-year change in species composition of mixed seagrass beds around Santiago Island, Bolinao, the northwestern Philippines. Mar. pollut. Bull. 88, 81–85. doi: 10.1016/j.marpolbul.2014.09.024
Testa J. M., Kemp W. M. (2014). Spatial and temporal patterns of winter–spring oxygen depletion in Chesapeake Bay bottom water. Estuaries Coasts 37, 1432–1448. doi: 10.1007/s12237-014-9775-8
Torres O., Kwiatkowski L., Sutton A. J., Dorey N., Orr J. C. (2021). Characterizing mean and extreme diurnal variability of ocean CO2 system variables across marine environments. Geophys. Res. Lett. 48, 1–12. doi: 10.1029/2020GL090228
Turk D., Yates K. K., Vega-Rodriguez M., Toro-Farmer G., L’Esperance C., Melo N., et al. (2015). Community metabolism in shallow coral reef and seagrass ecosystems, lower Florida keys. Mar. Ecol. Prog. Ser. 538, 35–52. doi: 10.3354/meps11385
Uppström L. R. (1974). The boron/chlorinity ratio of deep-sea water from the Pacific Ocean. Deep. Res. Oceanogr. Abstr. 21, 161–162. doi: 10.1016/0011-7471(74)90074-6
van Woesik R., Gilner J., Hooten A. J. (2009). Standard Operating Procedures for Repeated Measures of Process and State Variables of Coral Reef Environments (The University of Queensland, Melbourne: Coral Reef Targeted Research and Capacity Building for Management Program), 34.
Verceles L. F., McManus L. T., Aliño P. M. (2000). Participatory monitoring and feedback system: An important entry towards sustainable aquaculture in Bolinao, northern Philippines. Sci. Diliman 12, 78–87. Availabe at: https://journals.upd.edu.ph/index.php/sciencediliman/article/view/222
Villanueva R. D., Yap H. T., Montaño M. N. E. (2005). Survivorship of coral juveniles in a fish farm environment. Mar. pollut. Bull. 51, 580–589. doi: 10.1016/j.marpolbul.2005.04.033
Villanueva R. D., Yap H. T., Montaño M. N. E. (2006). Intensive fish farming in the Philippines is detrimental to the reef-building coral Pocillopora damicornis. Mar. Ecol. Prog. Ser. 316, 165–174. doi: 10.3354/meps316165
Vogel N., Fabricius K. E., Strahl J., Noonan S. H. C., Wild C., Uthicke S. (2015). Calcareous green alga Halimeda tolerates ocean acidification conditions at tropical carbon dioxide seeps. Limnol Oceanogr 60, 263–275. doi: 10.1002/LNO.10021
Wang Y., Van Cappellen P. (1996). A multicomponent reactive transport model of early diagenesis: Application to redox cycling in coastal marine sediments. Geochim. Cosmochim. Acta 60, 2993–3014. doi: 10.1016/0016-7037(96)00140-8
Watai M., Nakamura Y., Honda K., Bolisay K. O., Miyajima T., Nakaoka M., et al. (2014). Diet, growth, and abundance of two seagrass bed fishes along a pollution gradient caused by milkfish farming in Bolinao, northwestern Philippines. Fish. Sci. 81, 43–51. doi: 10.1007/s12562-014-0824-9
White P. (2013). “Environmental consequences of poor feed quality and feed management,” in On-farm feeding and feed management in aquaculture. Eds. Hasan M. R., New M. B. (Rome: FAO), 553–564.
Winder M., Sommer U. (2012). Phytoplankton response to a changing climate. Hydrobiologia 698, 5–16. doi: 10.1007/s10750-012-1149-2
Wu R. S. S. (1995). The environmental impact of marine fish culture: Towards a sustainable future. Mar. pollut. Bull. 31, 159–166. doi: 10.1016/0025-326X(95)00100-2
Yoshikai M., Herrera E. C., Tsuchiya T., Watanabe A., Blanco A. C., Nadaoka K. (2016). Numerical modeling analysis of tidal circulation and water quality dynamics in an intensive mariculture area in Bolinao and Anda, Philippines. Prep.
Keywords: coastal acidification, coral reef, Bolinao, mariculture, eutrophication, carbonate chemistry dynamics, Philippine reefs
Citation: Isah RR, Enochs IC and San Diego-McGlone ML (2022) Sea surface carbonate dynamics at reefs of Bolinao, Philippines: Seasonal variation and fish mariculture-induced forcing. Front. Mar. Sci. 9:858853. doi: 10.3389/fmars.2022.858853
Received: 20 January 2022; Accepted: 19 October 2022;
Published: 11 November 2022.
Edited by:
Guang Gao, Xiamen University, ChinaReviewed by:
Pablo P. Leal, Instituto de Fomento Pesquero (IFOP), ChileCopyright © 2022 Isah, Enochs and San Diego-McGlone. This is an open-access article distributed under the terms of the Creative Commons Attribution License (CC BY). The use, distribution or reproduction in other forums is permitted, provided the original author(s) and the copyright owner(s) are credited and that the original publication in this journal is cited, in accordance with accepted academic practice. No use, distribution or reproduction is permitted which does not comply with these terms.
*Correspondence: Raffi R. Isah, cnJpc2FoQHVwLmVkdS5waA==
Disclaimer: All claims expressed in this article are solely those of the authors and do not necessarily represent those of their affiliated organizations, or those of the publisher, the editors and the reviewers. Any product that may be evaluated in this article or claim that may be made by its manufacturer is not guaranteed or endorsed by the publisher.
Research integrity at Frontiers
Learn more about the work of our research integrity team to safeguard the quality of each article we publish.