- 1Department of Botany, Abdul Wali Khan University, Mardan, Pakistan
- 2Department of Biotechnology, Abdul Wali Khan University, Mardan, Pakistan
- 3Department of Biotechnology and Genetic Engineering, Hazara University, Mansehra, Pakistan
- 4Institute of Botany, University of the Punjab, Lahore, Pakistan
- 5Department of Botany, University of Education, Lahore, Pakistan
- 6Department of Botany, University of Karachi, Karachi, Pakistan
- 7Department of Biochemistry, Abdul Wali Khan University, Mardan, Pakistan
- 8Department of Applied Biosciences, Kyungpook National University, Daegu, South Korea
Salinity stress has hindered the growth and yield of crops globally. The demands for inducing salt stress tolerance by natural and biological sources with potent antioxidants and growth-promoting metabolites have been the main focus of the recent era. Therefore, the current research was conducted to extract salt stress tolerance-ameliorating metabolites and growth-promoting hormones from the marine brown macroalgae Sargassum wightii Greville ex J. Agardh with maximum antioxidant potential used as a liquid fertilizer for okra (Abelmoschus esculentus L.). In the current study, the biochemical analysis showed that Sargassum aqueous extract (SAE) was rich in growth-promoting metabolites, antioxidants, and hormones. Meanwhile, overaccumulation of glycine betaine attracted the focus of the current research dealing with salt stress tolerance amelioration in A. esculentus. The plants supplemented with SAE (2% and 4%) and 0.04% ascorbic acid (AsA) alone and in combinations were subjected to sodium salt stress (NaCl; 75 mM). Results revealed that SAE efficiently promoted the vegetative and reproductive growth of plants by elevating the growth-promoting metabolites and hormones in comparison to control plants. Ionic contents (Na+, K+, Ca2+, and Mg2+) and ratios (K+/Na+, Mg2+/Na+, and Ca2+/Na+) were modulated in SAE-treated plants. SAE also increased the level of carbohydrates, proteins, lipids, carotenoids, and proline and decreased the level of hydrogen peroxide and abscisic acid in salt-treated plants compared with the control groups. Enzymatic activities of catalase, ascorbate peroxidase, and guaiacol peroxidase were also enhanced by SAE treatment upon salt stress. The SAE-mediated stress tolerance amelioration and the positive growth response of A. esculentus were further accelerated by AsA (0.04%) supplementation used in combination with SAE (2% and 4%). The current study revealed a novel report of the antioxidant and metabolite-rich algal extract (S. wightii) formulation along with AsA that induced salt stress tolerance and promoted the overall growth performance of A. esculentus by rebalancing the ionic and metabolic status.
1 Introduction
Abiotic stresses deteriorate approximately 50% of crop yields. Unexpectedly, drastic fluctuations in the global climate impose numerous environmental stresses (Rane et al., 2021), and due to rapid urbanization, water stress is also associated with salt, drought, and temperature stress and water shortages that prime to various morphological and physiological deformities that cause a reduction in plant growth (Chele et al., 2021). The soil which has greater than 4 dS m−1 electrical conductivity is considered saline soil (Alqahtani et al., 2018). Due to salinity, approximately 4 × 104 ha of land in over 100 countries is becoming unfit for agricultural purposes (FAO and ITPS, 2015). Salt stress adversely impacts plant physiology by affecting metabolic, osmotic, and ionic status, due to extreme uptake of Na+ and Cl− leading to ionic toxicity and deficiency of nutrients, that boost macromolecules’ degradation by reactive oxygen species (ROS) production. Being sessile, it is inevitable for plants to avoid salinity stress imposed by saline soil. Salt-tolerant plants show increased activity of antioxidant enzymes such as catalase, superoxide dismutase, peroxidase, and ascorbate peroxidase (Umar and Siddiqui, 2018), which are called halophytes. Salt-tolerant plants have adapted several other mechanisms to tolerate salt stress such as i) the ability to biosynthesize and accumulate compatible osmolytes that control turgor to prevent ultrastructural damage, maintain ion homeostasis, and regularize water uptake to enhance water use efficiency; ii) adaptation for flexible growth plasticity (morphological and developmental pattern change); iii) enhancement in photosynthetic activity; iv) detoxification of ROS through antioxidant enzymes and non-enzyme molecules; and v) induction of phytohormones and growth-promoting metabolic products, including glycine betaine and proline. However, these adaptive strategies are not sufficient to induce tolerance to rapidly increasing salinity in the soil. Moreover, unfortunately, not all plant species are halophytes; therefore, salt stress has been found to negatively affect the biomass and yield of susceptible plants including Spartina alterniflora, Brassica oleracea, Jerusalem artichoke, Gossypium hirsutum, Helianthus tuberosus, Catapodium rigid, Pistacia vera, Oryza sativa, Lycopersicon esculentum, Fragaria ananassa, Triticum aestivum, and Abelmoschus esculentus (Roy and Chowdhury, 2020). Abelmoschus esculentus is the most popular mallow crop and a common food crop in Asia. Its finger-like fruits are a rich source of vitamins, minerals, and dietary fiber and are low in calories. The mucilage’s properties have enormous medicinal value. The rationale behind the current study was the fact that a 90% loss (6.5 dS m−1) in A. esculentus yield has been reported under high salt levels (Mushtaq et al., 2020). Global food demand requires the production of salt stress-tolerant, nutritionally rich crops including A. esculentus. Therefore, researchers have been exploiting different approaches to ameliorate salt stress tolerance in plants. Scientists have been searching for stress-resistant genes to reconstruct the stress-tolerant response in plants by transgenic approaches. Recently, transcriptome analysis, performed for exploring the molecular mechanism of melatonin-induced salt tolerance in A. esculentus, has revealed the induction of transcription factor genes including MYB, WRKY, and NAC. The genes controlling nitrogen, sulfur, alanine, aspartate, and glutamate metabolism were also significantly induced (Zhan et al., 2021). Although genetic engineering is a powerful strategy for generating ideal plants of desired traits for food production, it is not applicable in agriculture due to its controversial status concerning the risk to human health as well as the agricultural environment. Thus, rather than genetic alteration, understanding the molecular processes for induction of salt stress tolerance-associated pathways provides for enhanced stress resistance efficiency in plants. For example, exogenous application of various priming mediators (trehalose, sodium nitroprusside, manganese sulfate, sodium selenate, sodium silicate, calcium chloride, glycine betaine, proline, nano-silica, α-tocopherol, vitamins, and nanoparticles) has been used for salt stress tolerance amelioration in crops. Recently, glycine betaine has also been found to induce salinity tolerance in plants by modulating the physiological, antioxidant, and ionic status (Dustgeer et al., 2021). The foliar spray of α-tocopherol has been used to mitigate salt stress tolerance in A. esculentus by modulating the antioxidant potential (Naqve et al., 2021). The potential mitigation effect of ZnO nanoparticles has also been found to mitigate the effect of salt stress in A. esculentus (Alabdallah and Alzahrani, 2020). Menadione sodium bisulfite is reported by Akbar et al. (2021) to regulate physiological and biochemical responses for salinity stress alleviation on wheat (T. aestivum L.).
Growth regulators such as polyamine (diamine putrescine, tetramine spermine, triamine spermidine) and gibberellic acid have also received attention from seed priming chemicals due to their strong influence on germination and growth of the plant (Sofy et al., 2020). Growth promotion has also been noticed under salinity stress by exogenously applied gibberellic acid in maize through modulating the morphophysiological, biochemical, and molecular attributes (Shahzad et al., 2021). Exogenous melatonin is reported to mitigate salinity-induced damage in olive seedlings by modulating ion homeostasis, antioxidant defense, and phytohormone balance (Zahedi et al., 2021). Exogenous application of melatonin also proved to be effective for the alleviation of severe salt stress (300 mM NaCl) of okra (Zhan et al., 2021).
However, the use and synthesis of these chemicals are time-consuming, expensive, and mostly not environment-friendly. Furthermore, the efficiency of these chemical fertilizers, priming mediators, and growth regulators differs with different environmental stresses and plant species. Scientists, therefore, are suggesting an alternative promising approach to use biological sources such as seaweeds for inducing salt stress tolerance in various plants. Seaweeds are a group of macroscopic marine algae that are saltwater tolerant and rich in growth regulators and micro- and macronutrients, essential for plant growth promotion (Bulgari et al., 2019).
The application of seaweed extracts in plants has been reported to increase chlorophyll content, photosynthetic activity, transpiration rate, stomatal conductance (Al-Ghamdi and Elansaey, 2018), the electron transfer rates of photosystems I and II (Digruber et al., 2018), plant height, leaf numbers, root width, and length with an overall increase in biomass (Ali et al., 2019).
Previously, foliar application of ascorbic acid significantly increased chlorophyll a, chlorophyll b, carotenoids, and the total photosynthetic pigments at a dose of 400 mg L−1, in common beans under water stress conditions (Gaafar et al., 2020). Therefore, the current research was also planned to use an optimal concentration (0.04%) of ascorbic acid (AsA) solution for foliar spray on A. esculentus under salt stress of 75 mM. Recently, AsA as an antioxidant was also used as a priming agent and mediator for salt stress tolerance amelioration, and foliar application showed powerful potential to reduce salt toxicity in A. esculentus (Saheed, 2020). Thus, AsA (0.04%) was used as a positive control. Meanwhile, Sargassum aqueous extract (SAE, 4%) was used in combination with AsA (0.04%) to evaluate the enhancement in growth response and amelioration of salt stress tolerance response of A. esculentus. It is also well known that salt stress affects A. esculentus plant growth and development as well as its physiological attributes like photosynthesis rate and stomatal conductance and overall growth of plants in a dose-dependent manner, with 25 and 50 mM NaCl being less toxic and 75 mM NaCl being toxic (Shahid et al., 2011). Therefore, under the current study, 75 mM NaCl was supplemented to A. esculentus plants for salt stress induction.
The mechanisms behind positive influences driven by marine macroalgae (seaweed extracts) upon salt stress tolerance have not been studied for A. esculentus so far. Therefore, the current work was designed to investigate the potential of marine algal extract as a biostimulant for growth promotion as well as stress tolerance in A. esculentus. The present study was aimed to explore whether S Sargassum wightii aqueous extract may induce salt stress tolerance in A. esculentus and what could possibly be the essential metabolites, ions, and hormones in SAE to influence the, i) the essential metabolic reshuffling in A. esculentus plants itself upon salt stress, ii) the modulation of growth-controlling hormones, and iii) the antioxidant potential. The findings of the current research allowed us to explore the in-depth physiological, biochemical, metabolic, and ionic reshuffling for salt stress tolerance amelioration in A. esculentus.
2 Methods
2.1 Biological Material and Chemicals
The use of biological material in the current research has fully complied with institutional, national, and international guidelines and legislation. Certified A. esculentus variety (Sabz Pari) was obtained from Early Wood Seed (Pvt) Limited, Multan, Pakistan. Seaweed (marine macroalgae) was collected from the Karachi coast (24°48′N latitude and 66°59′E longitude), Pakistan, in May 2019. The sample was washed first with seawater followed by fresh water thoroughly to remove the epiphytes and other contaminants in the laboratory, Department of Botany, University of Karachi, Pakistan. The sample was air-dried in shade (to avoid thermal degradation of the metabolites) for 7 days. Dried samples were carefully transported to the Plant Physiology and Molecular Biology Laboratory, Department of Botany, Abdul Wali Khan University, Mardan, Pakistan. Marine algal species (seaweed) were identified as S. wightii, according to Chapman and Gellenbeck (1989), and taxonomically classified according to Papenfuss (1955). The identification was based on morphological (external and internal characteristics) as well as ecological (distribution and habitat) features. The chemicals and reagents were bought from Sigma-Aldrich (Taufkirchen, Germany), Merck (Darmstadt, Germany), and Fluka (Buchs, Switzerland).
2.2 Preparation of Sargassum Aqueous Extract
SAE was prepared following the method of Erulan et al. (2009). Fresh sample of collected seaweed was dried and ground to form a fine powder. The fine powder and distilled water were mixed at a ratio of 1 kg:1 L, boiled for an hour, collected in conical flasks using Whatman No. 1 filter paper, and covered with aluminum foil to store at 29°C ± 1°C under dark conditions for 24 h. The filtrate was considered as 100% concentration.
2.3 Compositional Analysis of Sargassum Aqueous Extract
The filtrate (10 ml) was collected, oven-dried at 45°C, and recorded as the total yield. The reconstituted extract (2 mg/ml) was stored at −20°C for composition analysis. Total antioxidant activity was quantified according to Prieto et al. (1999). Total phenolic content was measured following the method of Singleton and Rossi (1965). The method for DPPH radical scavenging activity of SAE was adapted from Yen and Chen (1995). The ferric reducing antioxidant power (FRAP) assay was performed for measuring the reducing power of the SAE following the method of Benzie and Strain (1996). Hormonal quantification from SAE was done according to Benítez García et al. (2020), and glycine betaine quantification was estimated in the Sargassum aqueous extract as mentioned by Grieve and Grattan (1983). Elemental analysis of the SAE was performed by a procedure adapted from Nazarudin et al. (2021). A 10% seaweed extract was used for quantifying the concentrations of macro- (K+, Na+, Mg2+, and Ca2+) and micronutrients (Fe2+, Cu2+, Mn2+, and Zn2+) using the ICE 3000 atomic absorption spectrophotometer (Thermo Scientific, USA).
2.4 Abelmoschus esculentus Growth Conditions and Experimental Setup
The experiments were carried out in the Botanical Garden, Plant Physiology and Molecular Biology Laboratory, Department of Botany, Abdul Wali Khan University, Mardan, Pakistan. Seeds were sterilized for 1 min with 0.1% HgCl2 and then washed with distilled water. Ten seeds per pot were sown; however, the uniformly growing plants were selected and thinned out to five, after 15 days of germination. Plants were irrigated with an equal amount of tap water every day. Each earthen pot with 12 × 12 × 30 cm (length × width × height) size was filled with sandy loam soil. A completely randomized design (CRD) was assigned for all 84 pots which were divided into 12 various groups representing the various treatments such as supplementation of SAE, NaCl salt, and AsA.
The 3-week-old plants were subjected to salt (NaCl) treatments by irrigation with 75 mM salt water with 10.5 dS m−1 electrical conductivity, twice a week regularly. SAE (2% and 4%) + AsA (0.04%) were supplied by foliar spray (4 ml/treatment) on each plant at the 3rd, 6th, and 10th week after germination. The foliar application was applied on the abaxial and adaxial surfaces at sunset to ensure SAE and AsA uptake by the leaves. At the end of the grand period of growth (plants), samples were taken for biochemical analysis (Figure 2).
The experimental setup with treatments is mentioned below.
1. Control (water)
2. SAE (2%)
3. SAE (4%)
4. Control (AsA 0.04%)
5. SAE (2%) + AsA (0.04%)
6. SAE (4%) + AsA (0.04%)
7. NaCl (75 mM)
8. NaCl (75 mM) + SAE (2%)
9. NaCl (75 mM) + SAE (4%)
10. NaCl (75 mM) + AsA (0.04%)
11. NaCl (75 mM) + SAE (2%) + AsA (0.04%)
12. NaCl (75 mM) + SAE (4%) + AsA (0.04%)
2.5 Phenotypic and Physiological Analysis
2.5.1 Measurement of Growth Parameters
At the termination of the experiment, the yield was recorded by measuring vegetative (shoot length, root length, no. of intact leaves, fresh and dry weight of intact leaves) and reproductive attributes (number of pods per plant, pod fresh weight, dry weight of fruit, pod length, number of seeds per pod and weight of seeds per pod, number of seeds per plant, weight of seeds per plant, and weight of 100 seeds).
2.5.2 Relative Water Content and Leaf Water Loss
The relative water content (RWC) of the fresh leaf (leaf number 12 counted from the bottom of each plant) was calculated by using freshly weighed leaves subjected to a rehydration process in deionized water for 2 h and turgor weight was measured. The dry weight of the leaf samples was measured after incubating the samples in a preheated oven. The formula given below was used to calculate RWC:
Leaf water loss was determined by measuring the initial weight (W1) of the fresh-leaf samples (3.0 g) from leaf number 12 of each plant (counted from the base). The sample was kept for 2 h at 30°C and then weighed again (W2). Leaf water loss (LWL) was calculated using the following formula:
2.5.3 Extraction and Estimation of Chlorophyll and Carotenoids
Total chlorophyll and carotenoids in fresh-leaf samples (3.0 g) from leaf number 12 of each plant (counted from the base) were estimated and calculated through a process described by Maclachlan and Zalik (1963).
2.5.4 Analyses of Metabolites
Metabolic quantification was performed by using fresh-leaf samples (3.0 g) from leaf number 12 of each plant (counted from the base). For total sugars, quantification was done by adding phenol and H2SO4 to the sample. The optical density was recorded at 485 nm. Total protein in the leaves was determined according to Lowry et al. (1951). Lipid content was determined as mentioned by Van Handel (1985). Proline quantification was done according to Bates et al. (1973). Total-phenolics were estimated as mentioned by Aziz et al., (2021b). The tannin was quantified using the method described by Makkar et al. (1993). Lycopene and β-carotene were determined following the method of Nagata and Yamashita (1992). Total terpenoid was measured following the method of Fan and He (2006). Indoleacetic acid (IAA) content was measured following the method mentioned by Rauf et al. (2021). SA content was analyzed following the method of Warrier et al. (2013). Endogenous AsA was determined by Jagota and Dani (1982). GA3 and abscisic acid (ABA) content was analyzed by the procedure of Ergun et al. (2002).
2.5.5 Antioxidant Enzyme Activities
Total antioxidant capacity was determined by using fresh samples (3.0 g) from leaf number 12 of each plant (counted from the base), crushed in methanol. Three milliliters of solution with H2SO4 (0.6 mM), sodium phosphate buffer (28 mM), and ammonium molybdate (4 mM) was added to the sample extract. After incubation, absorbance was measured at 695 nm against the blank. Catalase was determined following the method of Luck (1974). Guaiacol peroxidase was determined by the procedure of Haida and Hakiman (2019). Ascorbate peroxidase was determined following the method of Nakano and Asada (1981).
2.5.6 Determination of H2O2
H2O2 determination was found following the method of Mishra and Agrawal (2014). Fresh-leaf samples (3.0 g) from leaf number 12 of each plant (counted from the base) were crushed in trichloroacetic acid (0.1%). After centrifugation, potassium phosphate buffer (10 mM, pH = 7) and potassium iodide (1 M) were added to the samples and taken at an absorbance of 390 nm. ROS detection was performed through the method described by Yokawa et al. (2016).
2.5.7 Ionic Analysis
The concentration of various ions (Ca, Mg, K, Na) were quantified in dried ground plant samples. Approximately, 0.5 g of ground dried plant materials were finely powdered and transferred to digesting vials. For the digestion process, 6.5 ml of acid solution (HNO3, H2SO4, HClO4) with a ratio of 5:1:0.5, was added to each sample. After soaking for 12 h the samples were burned at 300°C for 3 h till the appearance of white fumes. Distilled water was added to the sample for dilution and then filter with Whatman filter paper No. 1. The ionic contents were then determined using an atomic absorption spectrophotometer (ICE 3000; Thermo Scientific, USA) (Aziz et al., 2021a).
2.6 Statistical Analysis
The experiments were carried out in three biological replicates. Two-way ANOVA was used to analyze the data with a significant level of p <0.005. To compare the means of all values, SPSS 20 (SPSS Inc., Chicago, IL, USA) was used for performing DMRT (Duncan multiple range test) at p <0.005.
3 Results
3.1 Characterization of Sargassum wightii
Sargassum wightii composition analysis showed a high level of total sugars (4.3 mg/g FW), proteins (0.145 mg/g FW), lipids (0.15 mg/g FW), lycopene (0.16 mg/g FW), and β-carotene (0.5 mg/g FW). High total phenolics (7 μg/g FW) and flavonoids (8 μg/g FW), proline (0.003 μg/g FW), and tannins (144 μg/g FW) were also produced. A higher content of cytokinin (0.191 mg/100 ml), GA (12.2 mg/100 ml), auxin (0.11 mg/100 ml), and glycine betaine (0.17 µg/g DW) was also noticed. Glycine betaine is an important osmolyte in maintaining osmotic balance and is reported to counter salinity stress by maintaining a high K+/Na+ ratio and antioxidant defense via limiting Na+ uptake. Sargassum wightii also showed high total antioxidant capacity (57 mg AAE/g), DPPH (76%), and FRAP (54 mg AAE/g). Results for the measurement of the mineral composition of S. wightii revealed the following: sodium (Na+) (875 mg/g DW), potassium (K+) (101.05 mg/g DW), calcium (Ca2+) (100 mg/g DW), magnesium (Mg2+) (22 mg/g DW), Fe3+ (16 mg/g DW), Cu2+ (0.5 mg/g DW), Mn2+ (0.7 mg/g DW), and Zn2+ (0.3 mg/g DW) (Figure 1).
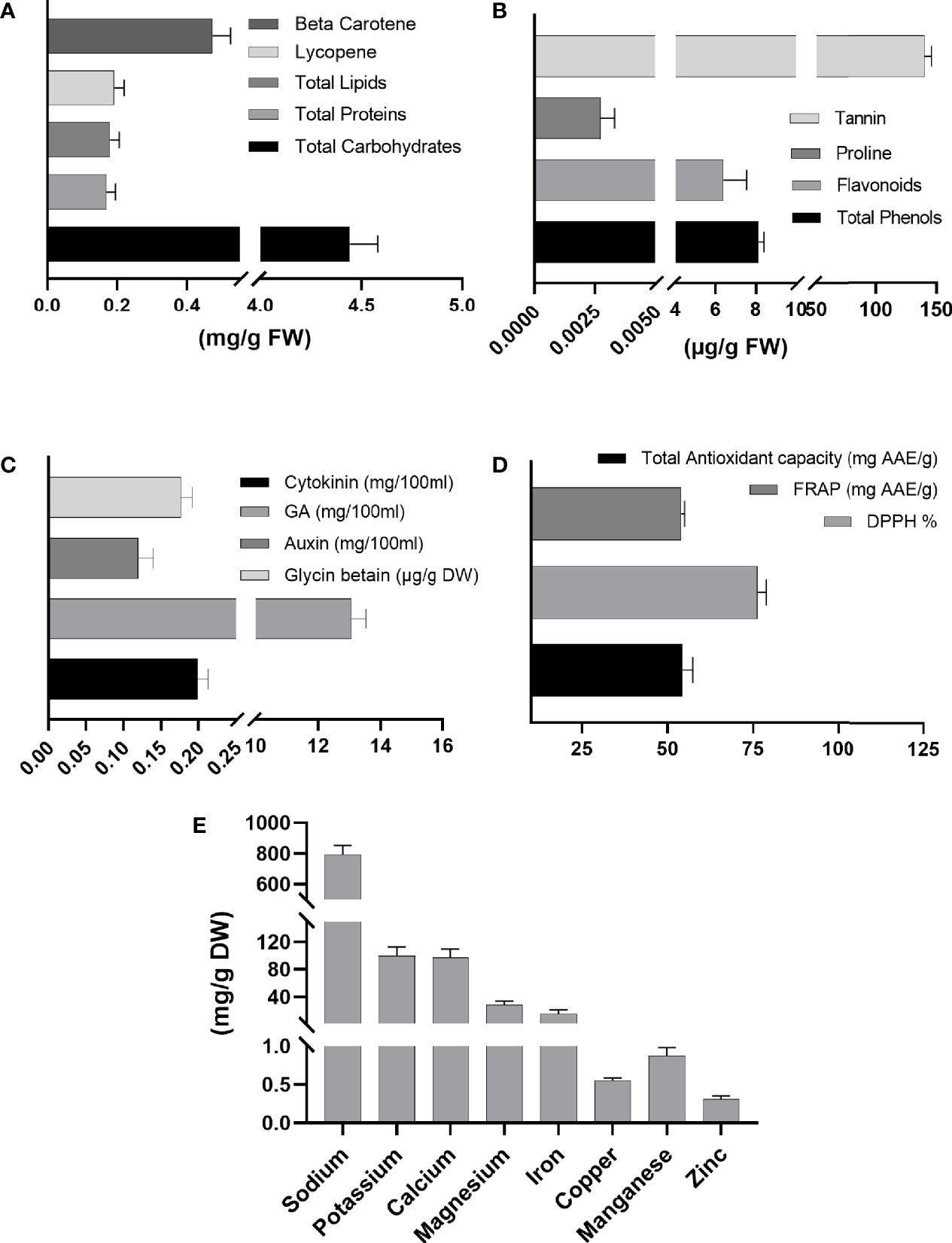
Figure 1 Chemical composition of Sargassum wightii. (A) Primary metabolites; (B) secondary metabolites; (C) hormonal content; (D) antioxidant capacity; (E) ionic analysis. Quantitative data represent means ± SD (n = 7). FW, fresh weight; DW, dry weight.
3.2 Effect of Sargassum wightii Aqueous Extract on the Vegetative Growth of Abelmoschus esculentus Under Salt Stress
Plant height, root length, and leaf number are the main components in plant architecture and play a main role associated with biomass production. The results of the present study displayed that vegetative growth parameters (shoot length, root length, number of intact leaves, fresh and dry weight of intact leaves) were significantly (p < 0.005) reduced in A. esculentus plants after the application of salt stress compared with control plants. Simultaneously, SAE (2% and 4%) supplementation significantly (p < 0.005) increased the growth parameters of A. esculentus plants, compared with control plants as well as those grown under salt stress (Figure 2).
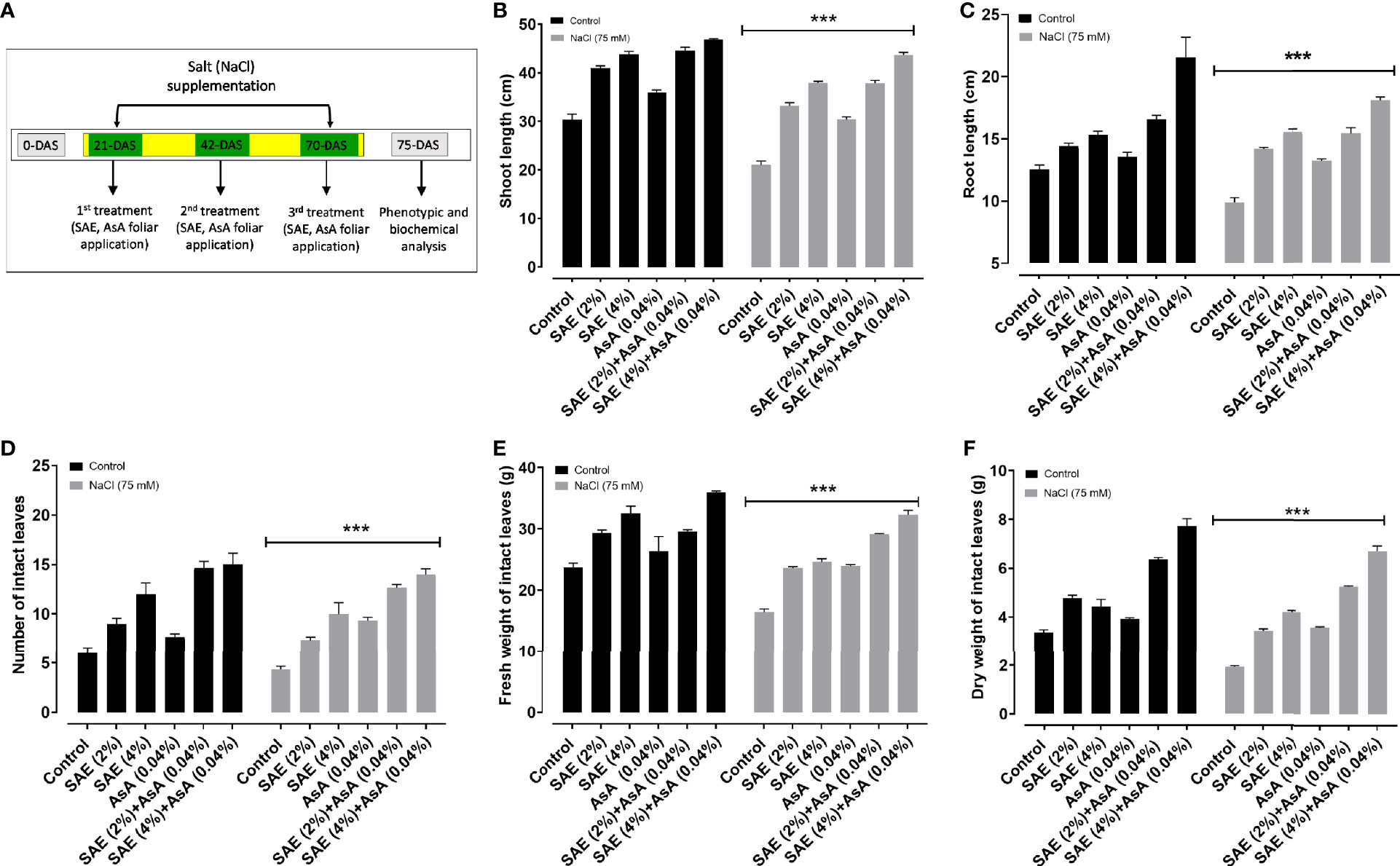
Figure 2 Effect of Sargassum aqueous extract (SAE) on different vegetative growth parameters. (A) Schematic representation of the experimental setup; (B) shoot length; (C) root length; (D) the total number of intact leaves; (E) fresh weight of intact leaves; (F) dry weight. Error bars of quantitative data represent ± SEM (***p < 0.005). FW, fresh weight; DW, dry weight.
3.3 Effect of Sargassum wightii Aqueous Extract on the Reproductive Attributes of Abelmoschus esculentus Under Salt Stress
Current data revealed that salt supplementation exhibited a significant (p < 0.005) decline in different reproductive parameters such as total intact pods/plant, seed number/pod, seed weight/pod, the weight of 100 seeds, total seeds/plant, seed weight/plant, and pod length of A. esculentus plants, compared with control plants. On the contrary, results revealed that SAE (2% and 4%) application improved the reproductive attributes in both control and saline stress conditions. Moreover, it was found that exogenous application of SAE (2% and 4%) proved significantly (p < 0.005) effective in improving different reproductive attributes of A. esculentus plants, compared with control plants as well as those grown under salt stress (Figure 3).
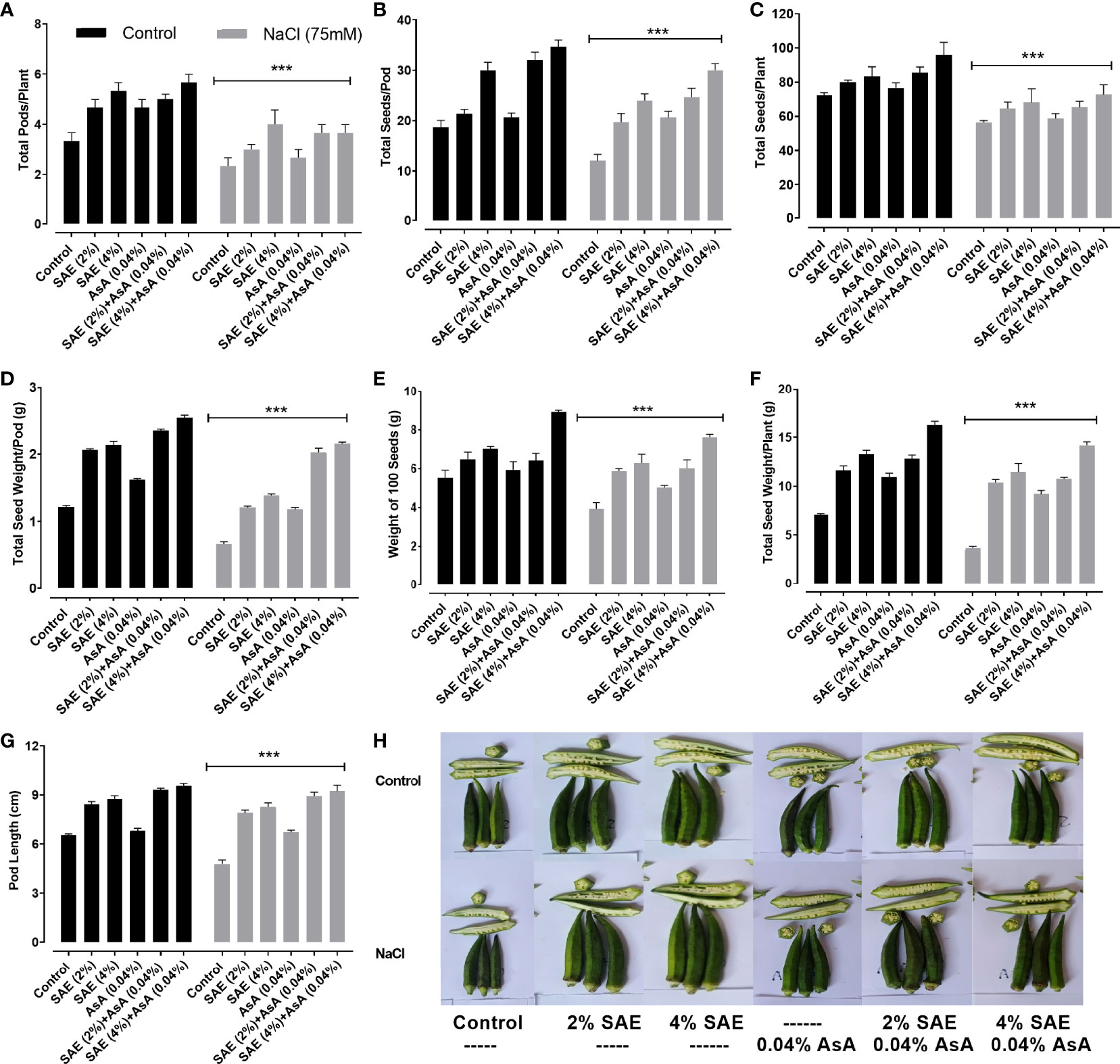
Figure 3 Effect of SAE on different reproductive growth parameters. (A) Total pods/plant; (B) total seed number/pod; (C) total seed number/plant; (D) total seed weight/pod; (E) weight of 100 seeds; (F) total seed weight/plant; (G) average pod length; (H) phenotypes of pods. Error bars of quantitative data represent ± SEM (***p < 0.005).
3.4 Effect of Sargassum wightii Aqueous Extract on the Physiological Attributes of Abelmoschus esculentus Under Salt Stress
Current results revealed that salt-stressed A. esculentus plants showed a significant (p < 0.005) reduction in the level of chlorophyll a, chlorophyll b, and total chlorophyll compared with control plants. On the other hand, foliar application of SAE (2% and 4%) exhibited improvement in chlorophyll a, chlorophyll b, and total chlorophyll contents of A. esculentus plants, compared with control plants as well as those grown under salt stress. Current data showed that salt stress caused a significant (p < 0.005) increase in total carotenoid levels in A. esculentus leaves compared with control. Plants treated with SAE (2% and 4%) and AsA further increased total carotenoid levels and were noticed in both non-saline and saline conditions. Salt stress also caused a significant (p < 0.005) reduction in the relative water content and acceleration in leaf water loss of A. esculentus plants compared with control plants. On the other hand, SAE (2% and 4%) promoted a significant (p < 0.005) accumulation in the relative water content and reduction in leaf water loss of A. esculentus plants, compared with control plants as well as those grown under salt stress (Figure 4).
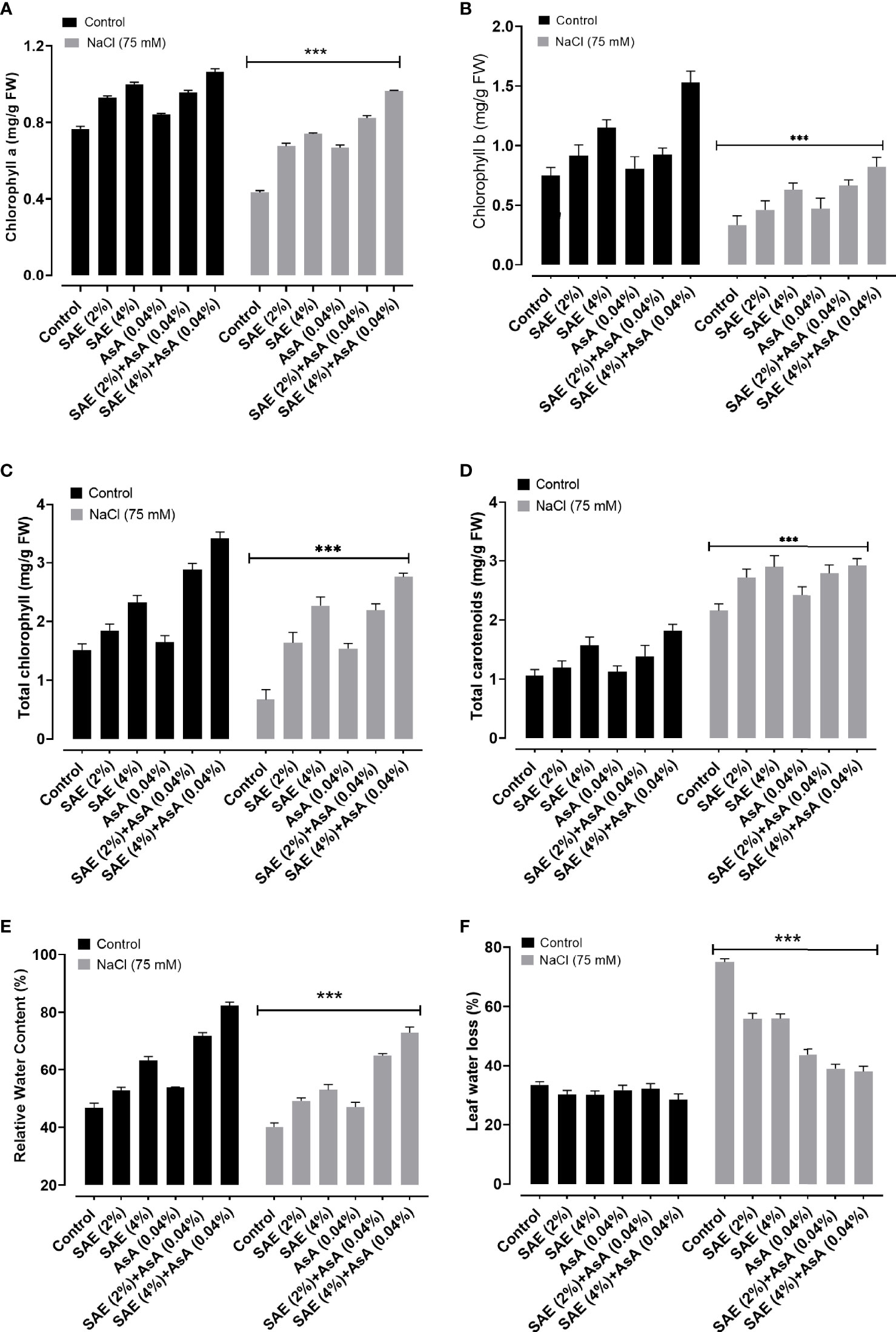
Figure 4 Effect of SAE on pigment composition and water content of Abelmoschus esculentus grown under salt stress. (A) Chlorophyll a; (B) chlorophyll b; (C) total chlorophyll; (D) carotenoids; (E) relative water content; (F) leaf water loss. Error bars of quantitative data represent ± SEM (***p < 0.005). LWL, leaf water loss; FW, fresh weight.
3.5 Effect of Sargassum wightii Aqueous Extract on the Metabolic Attributes of Abelmoschus esculentus Under Salt Stress
The obtained results exhibited that salinity stress significantly (p < 0.005) decreased the total carbohydrates, proteins, lipids, and proline content compared with control plants, while SAE (2% and 4%) significantly (p < 0.005) induced the total carbohydrates, proteins, lipids, and proline content of A. esculentus both under normal and stressed conditions (Figure 5). The obtained data from the current study revealed that 75 mM salt stress caused a significant (p < 0.005) increase in total phenols, flavonoids, tannin, lycopene, β-carotene, and terpenoid contents of A. esculentus compared with control. Simultaneously, SAE (2% and 4%) and AsA supplementation further increased total phenols, flavonoids, tannin, lycopene, β-carotene, and terpenoid contents of A. esculentus plants under both normal and stressed conditions significantly (p < 0.005) (Figure 6).
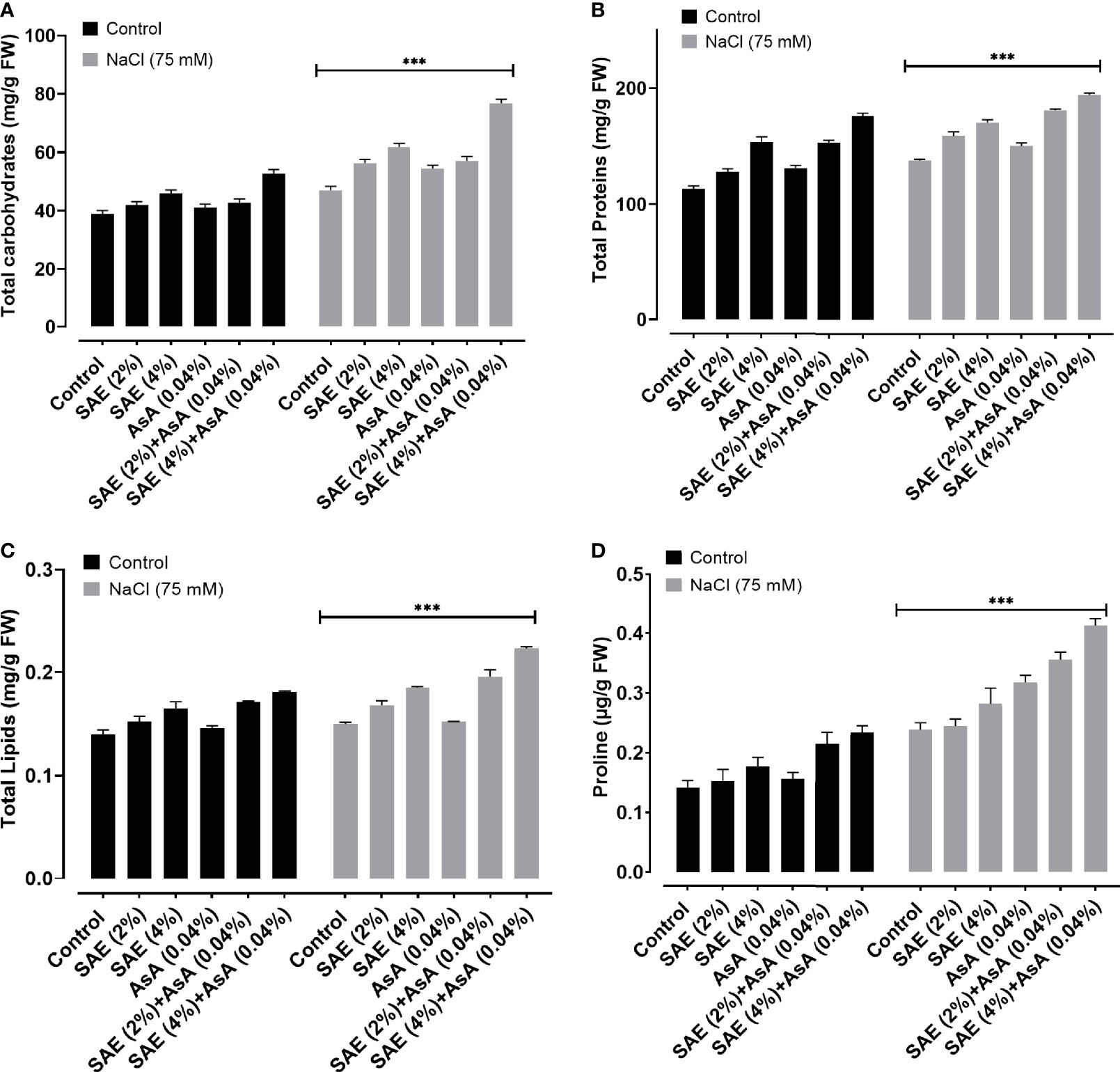
Figure 5 Effect of SAE on primary metabolites of A. esculentus grown under salt stress. (A) Total carbohydrates; (B) total proteins; (C) total lipids; (D) proline content. Error bars of quantitative data represent ± SEM (***p < 0.005). FW, fresh weight.
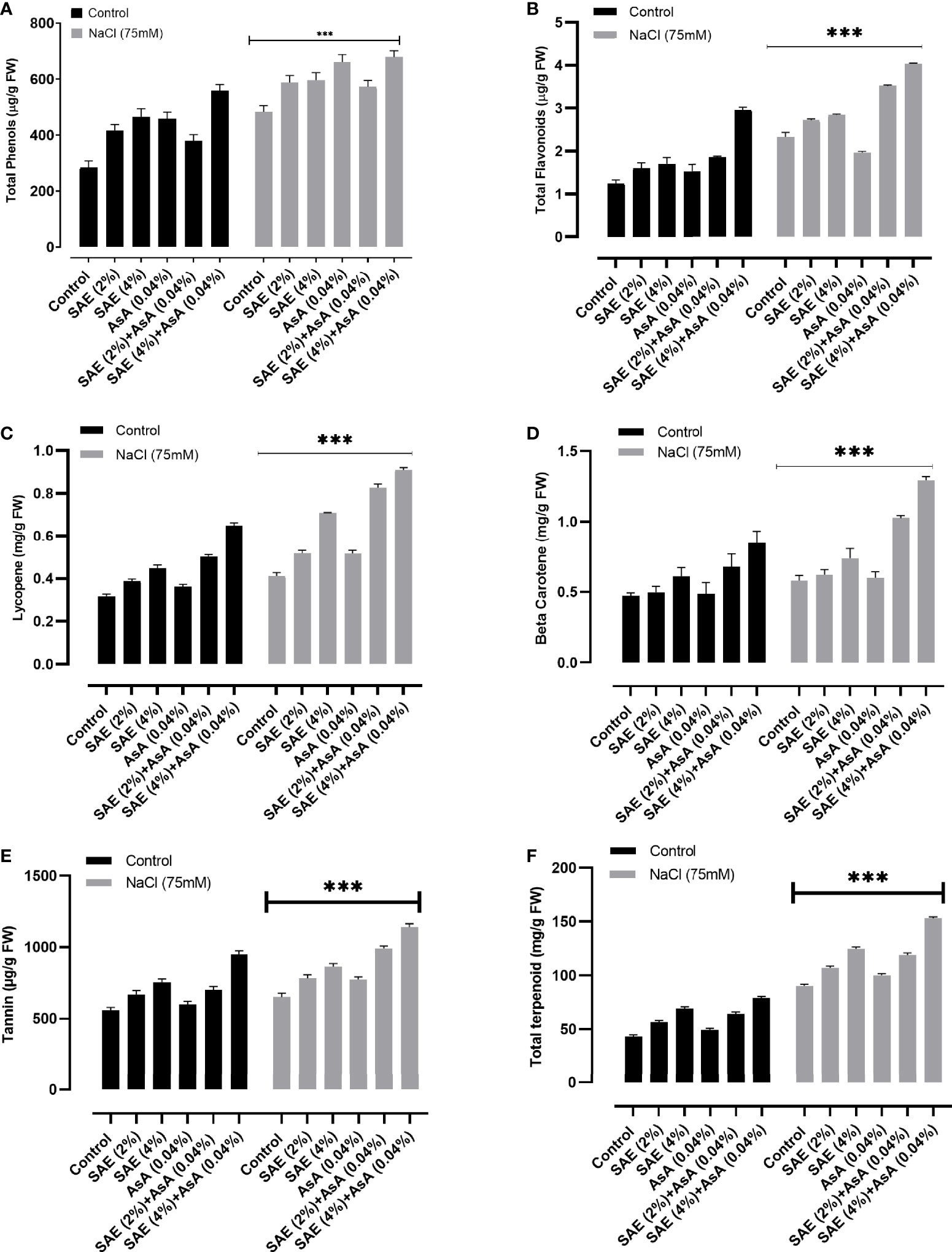
Figure 6 Effect of SAE on secondary metabolites of A. esculentus grown under salt stress. (A) Total phenols; (B) flavonoids; (C) lycopene; (D) β-carotene; (E) tannins; (F) terpenoids. Error bars of quantitative data represent ± SEM (***p < 0.005). FW, fresh weight.
3.6 Effect of Sargassum wightii Aqueous Extract on the Hormonal Attributes of Abelmoschus esculentus Under Salt Stress
The results of the current study indicated that 75 mM salt stress caused a significant (p < 0.005) decrease in IAA, GA, AsA, and salicylic acid levels, while a significant increase was noticed in the ABA level of A. esculentus, compared with the control plants. A reverse pattern of hormonal accumulation was noticed upon SAE (2% and 4%) application compared with control plants (Figure 7).
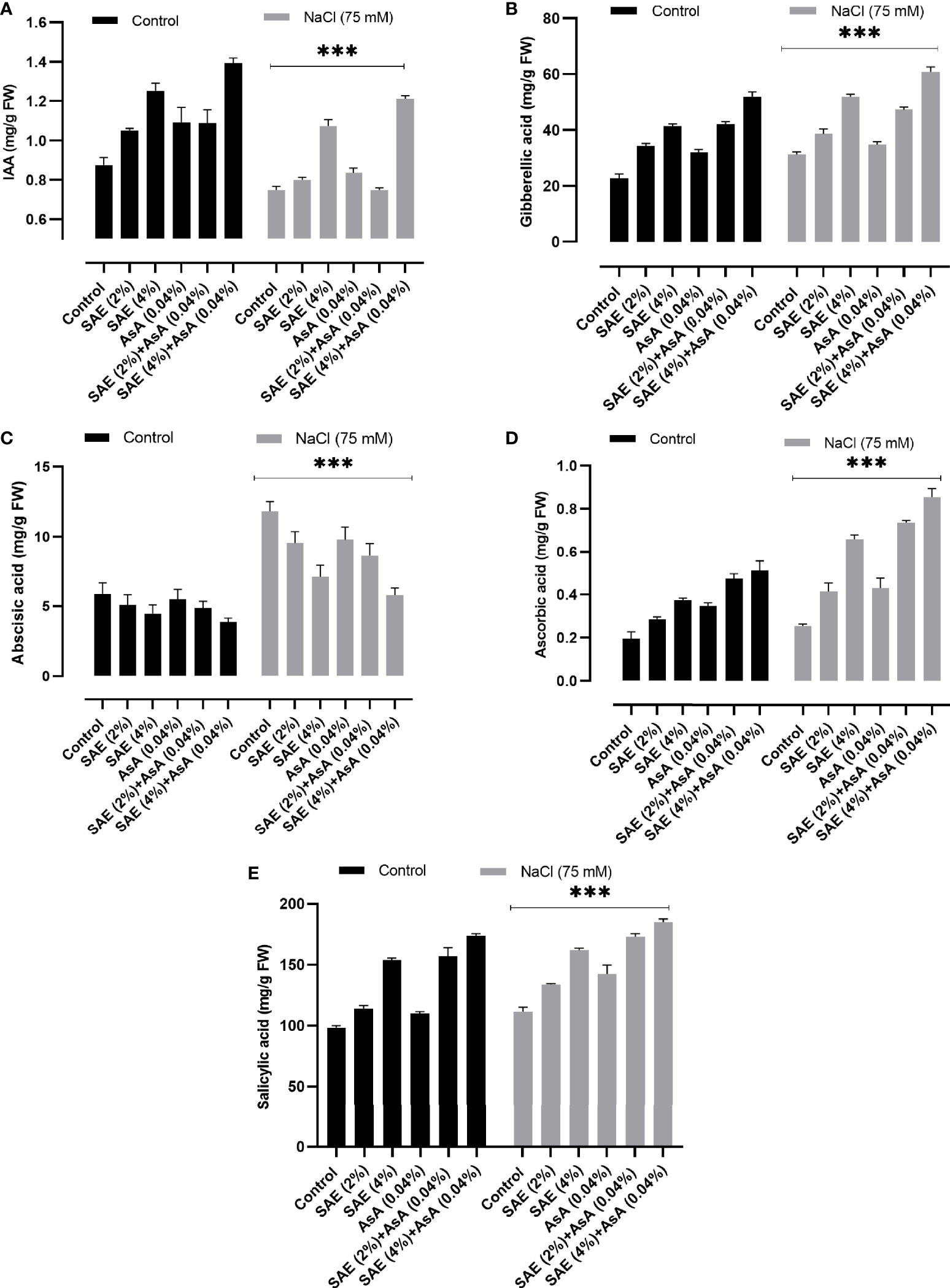
Figure 7 Endogenous hormone levels. (A) IAA; (B) GA3; (C) ABA; (D) AsA; (E) salicylic acid. Error bars of quantitative data represent ± SEM (***p < 0.005). FW, fresh weight.
3.7 Effect of Sargassum wightii Aqueous Extract on the Antioxidant Capacity and ROS Status of Abelmoschus esculentus Under Salt Stress
Soil salinity causes the generation of oxidative stress through an increase in ROS. Different antioxidants especially enzymes play a vital role in protecting the cell by scavenging ROS. In the present study, data indicated that salt stress promoted a significant (p < 0.005) increase in total antioxidants such as catalase, guaiacol peroxidase, and ascorbate peroxidase compared with the control. On the other hand, in SAE (2% and 4%) supplementation, a further significant increase in total antioxidants was noticed in A. esculentus plants, compared with controls as well as those grown under salt stress (Figure 8). The current investigation highlighted that salt stress caused a significant (p < 0.005) increase in hydrogen peroxide levels in A. esculentus plants compared with control. Elevated DAB level further confirmed an overaccumulation of H2O2 in A. esculentus leaf tissue compared with control upon salt supplementation. The current results indicated the positive role of SAE (2% and 4%) in the reduction of hydrogen peroxide level and DAB intensity in A. esculentus under both normal and stressed conditions (Figure 9).
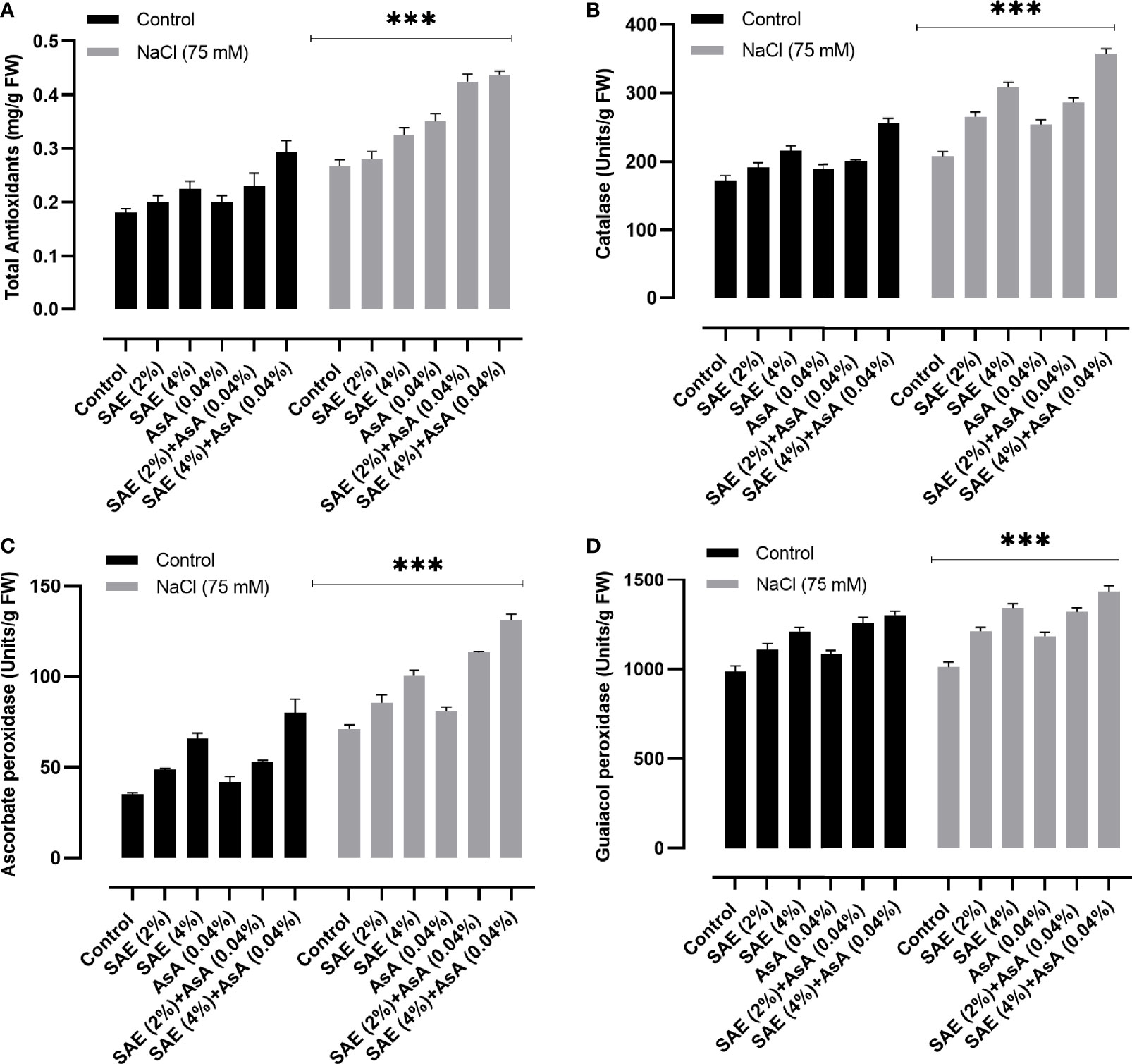
Figure 8 Different antioxidant enzyme activities. (A) Total antioxidants; (B) catalase; (C) ascorbate peroxidase; (D) guaiacol peroxidase. Error bars of quantitative data represent ± SEM (***p < 0.005). FW, fresh weight.
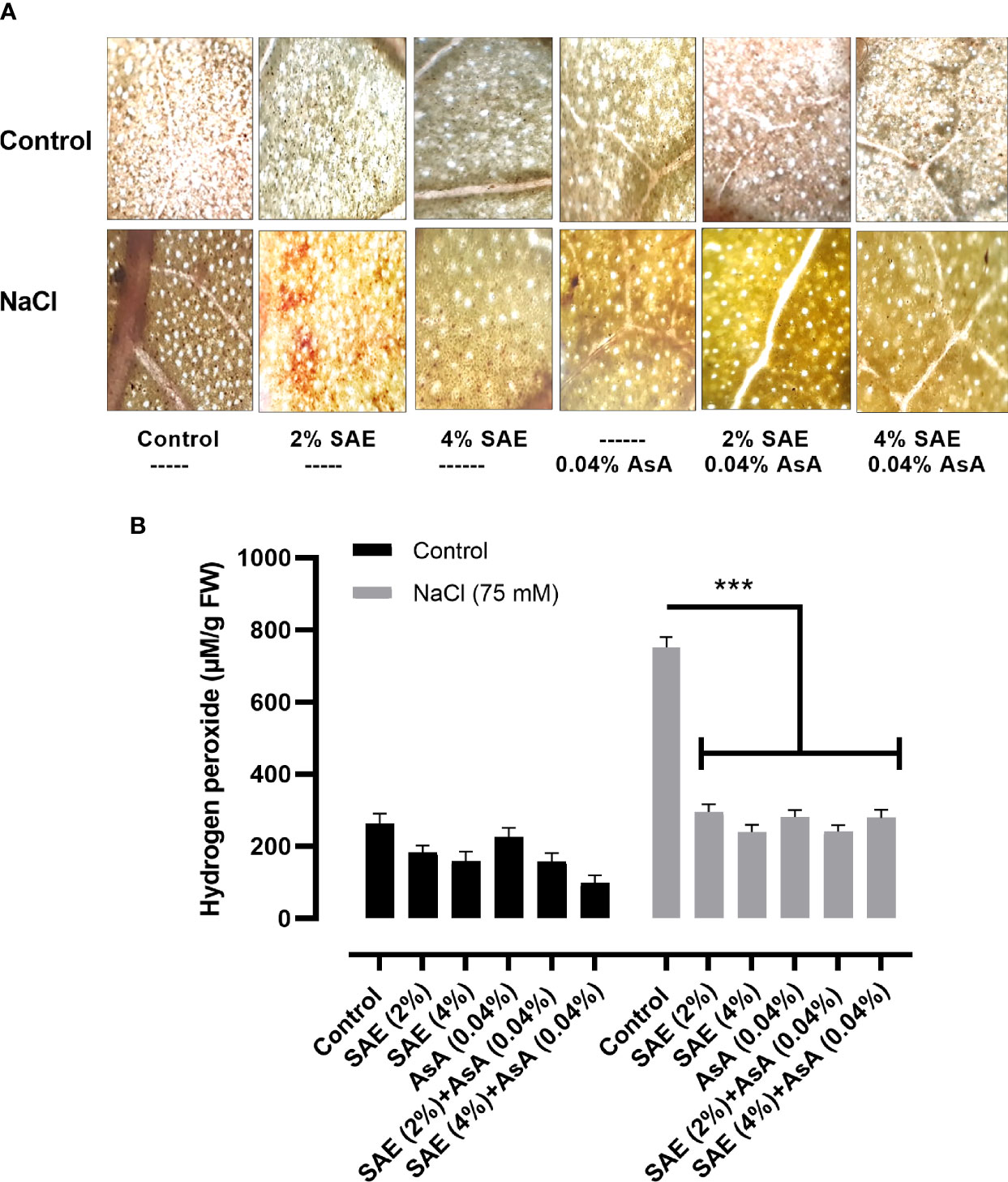
Figure 9 Histochemical analysis for H2O2 quantification. (A) DAB staining for H2O2 detection; (B) H2O2 quantification. Error bars of quantitative data represent ± SEM (***p < 0.005). FW, fresh weight.
3.8 Effect of Sargassum wightii Aqueous Extract on the Mineral Status of Abelmoschus esculentus Under Salt Stress
To cope with salinity-induced water stress, the accumulation of inorganic solutes is the most suitable strategy to maintain its turgor which ultimately balances the cell water potential by enhancing water uptake. During the present investigation, the result showed that salt stress causes a significant (p < 0.005) overaccumulation in Na+ concentration and reduction of K+, Ca2+, and Mg2+ in A. esculentus plants compared with control. The foliar application of SAE (2% and 4%) led to a significant (p < 0.005) increase in K+, Ca2+, and Mg2+ ion concentrations with a reduction in Na+ ions in A. esculentus plants, compared with control plants as well as those grown under salt stress. Thus, increased K+/Na+, Ca2+/Na+, and Mg2+/Na+ ratios were noticed upon foliar application of SAE (2% and 4%) compared with control plants and those treated with salt stress (Figure 10).
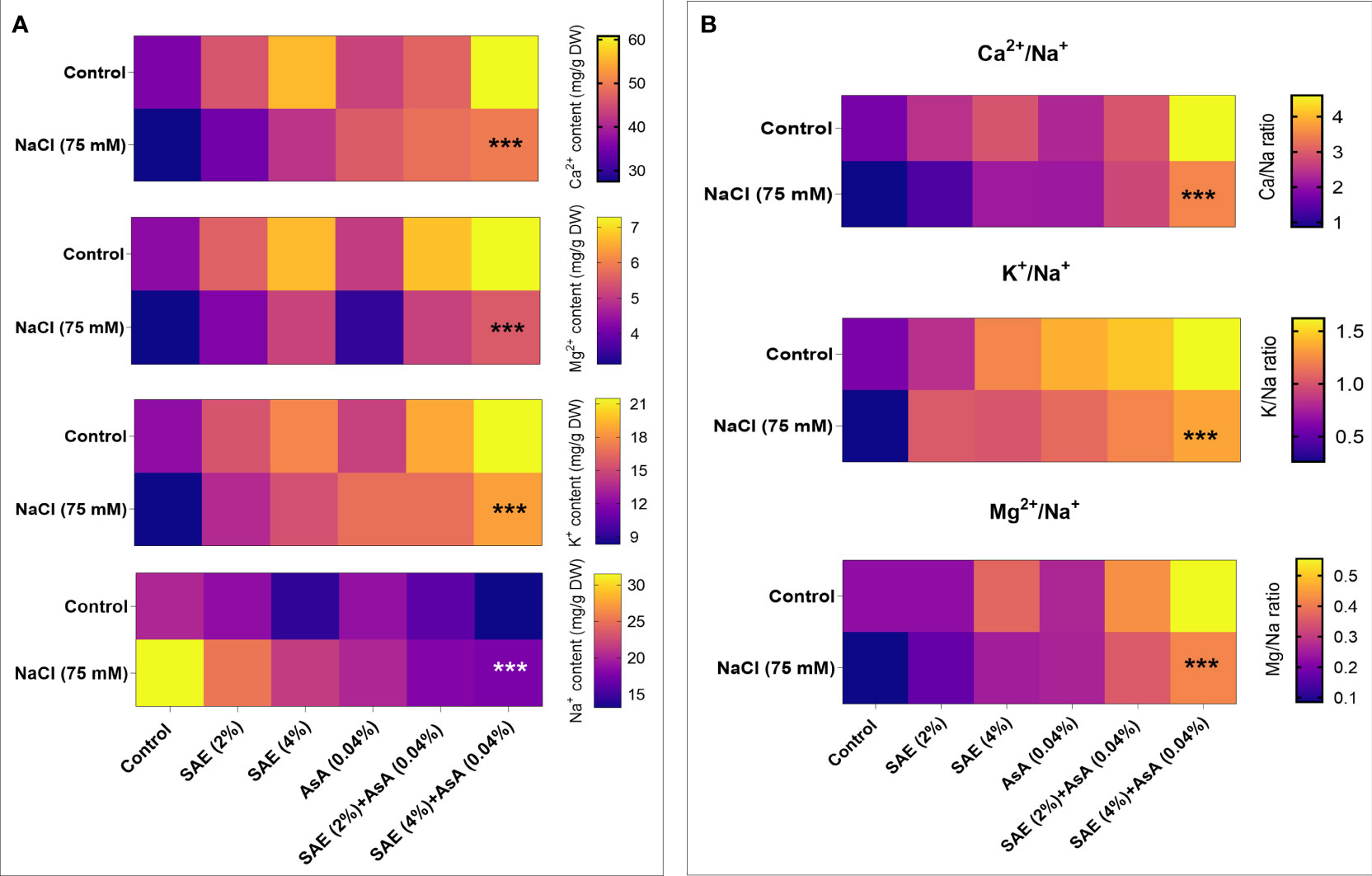
Figure 10 Ionic analysis. (A) sodium, potassium, magnesium; calcium (B) Ca2+/Na+, K+/Na+, and ratios. Error bars of quantitative data represent ± SEM (***p < 0.05). DW, dry wight.
4 Discussion
Salt stress, among the major forms of abiotic stresses in plants, inhibits plant growth due to excessive absorption of Na+ and Cl−, causing ion imbalances and physiological disorders. It causes osmotic stress, which lowers plant development by reducing water uptake, lowering turgor pressure, increasing stomatal closure, and reducing photosynthetic activity. Excessive Na+ generates certain ionic toxicities; therefore, controlling Na+ exclusion as well as conveyance is critical. The lesser the absorption of other nutrients like K+ and Ca2+, the higher the levels of Na+ and Cl, resulting in nutritional imbalance. Plants may produce ROS as a result of salt stress. Increased ROS causes more lipid peroxidation, protein breakdown, and DNA mutations (Mittal et al., 2012; Riaz et al., 2020).
Plants cannot avoid salt stress since they are sessile; thus, they must constantly adapt and evolve a range of ways to mitigate the negative consequences of salt stress. The production of non-enzymatic antioxidants (ascorbic acid) to detoxify ROS, the formation of suitable osmolytes such as proline or glycine betaine, and the accumulation of ABA are only a few of these strategies. The activity of various antioxidant enzymes, including catalase, guaiacol peroxidase, glutathione reductase, and ascorbate peroxidase, is also increased in salt-tolerant cultivars exposed to salt stress. Similar to several salt-sensitive crops, A. esculentus is also salt sensitive as salt supplementation of NaCl 75 mM has been known to negatively affect the growth and development of A. esculentus as well as its physiological attributes like photosynthesis rate and stomatal conductance and its overall growth (Shahid et al., 2011).
Seaweed extracts are used by researchers as low-cost alternatives to synthetic antioxidants with greater efficacy, eco-friendliness, and non-toxic nature that enhance phytohormone production and the moisture-holding capacity of plants as well as the overall growth of cereals, grasses, vegetables, fruits, and spices (Van Oosten et al., 2017; Sohn et al., 2021), and they can also be exploited as soil fertilization as well as plant stress tolerance amelioration. The application of a betaine-rich extract of Ascophyllum nodosum in sweet pepper and tomato showed an increased chlorophyll content. The seaweed extracts with higher betaine compounds showed a reduction in photosynthetic activity and promoted chlorophyll degradation. Similarly, an accelerated photosynthetic and transpiration rate and stomatal conductance were observed in Asparagus plants treated with A. nodosum (Al-Ghamdi and Elansaey, 2018). Treatment of willow plants with an extract of Ecklonia maxima accelerated the electron transfer rates of photosystems I and II (Digruber et al., 2018). Tomato plants supplemented with seaweed extracts showed better plant height and increased leaf numbers, root width, and length with an overall increase in biomass (Ali et al., 2019).
Seaweed extracts have also been proven to promote the performance and quality of horticultural crops by increasing antioxidant capacity such as S. wightii, Kappaphycus alvarezii, A. nodosum, Sargassum johnstonii, and Sargassum latifolium along with Ulva lactuca resulting in a better yield of Vigna sinensis and Phaseolus radiata, L. esculentum, Paspalum vaginatum, and T. aestivum, respectively (Elansaey et al., 2017).
Sargassum spp. are the most common types of brown marine algae naturally growing in the saline environment and are halotolerant. The chemical composition of seaweed extracts relies on the algal species, growth environment, method of preparation, and environmental conditions, as well as its general composition including polysaccharides, minerals, amino acids, and growth hormones affecting the performance and development of plants. Considering their natural adaptation to survive and grow in a saline environment, the marine macroalgae (S. wightii) evaluated in the present study were found to have a strong antioxidant capacity along with a high potential for the production of growth-promoting metabolites and hormones. A pilot-scale pot experiment was conducted under the current research to evaluate and compare the potential of this antioxidant-rich SAE on A. esculentus growth under salt stress. The present observations revealed that foliar spray of SAE significantly improved both vegetative and reproductive growth attributes of A. esculentus, while a clear decline in optimum yield was noticed due to the drastic effects of salt stress. The positive impact of SAE resulting in optimum yield is consistent with those reported for the application of seaweed on other crops (Hernández-Herrera et al., 2018). Previously, foliar application of ascorbic acid was significantly also known to increase the photosynthetic activity and growth in common beans under water stress conditions (Gaafar et al., 2020). AsA as an antioxidant was also used as a priming agent and mediator for salt stress tolerance amelioration, and its foliar application showed a powerful potential to reduce salt toxicity in A. esculentus (Saheed, 2020). In the current research, AsA (a strong antioxidant) was also used alone (as positive control) and in combination with SAE for foliar spray on A. esculentus under salt stress. The evaluation revealed that AsA as an antioxidant further supplemented and amplified the antioxidant potential of SAE on A. esculentus under salt stress, as the foliar application of AsA and SAE showed a powerful potential to reduce salt toxicity in A. esculentus. This complementary enhancement effect was also prominently visible in the growth response as well as stress tolerance response of A. esculentus.
Previously, it was also reported that seaweed extract supplementation by foliar application to the leaves increased the nutrient content of plants, along with the accumulation of metabolites and growth-promoting hormones (Uthirapandi et al., 2018). In a study by Kalaivany et al. (2019) on Vigna unguiculata, they observed a high pod number with Sargassum treatment and considered it as inorganic fertilizer which provides sufficient nutrients to the plant for its proper growth.
Moreover, chemical composition analysis of seaweeds also revealed that seaweed extracts are a rich source of antioxidants that counteract environmental stresses and play an important role in the prevention of toxic free radicals for improved growth and productivity of crops by increasing nutrient availability and uptake (Roy and Chowdhury, 2020). Sargassum extracts have been used as a biostimulant to induce abiotic stress tolerance in Solanum lycopersicum, Capsicum annuum, F. × ananassa, T. aestivum, and O. sativa (reviewed by Ali et al., 2021). The positive effect of algal extracts on photosynthetic pigments has been reported by other authors as well (Uthirapandi et al., 2018). The current research also revealed a high level of total sugars, total proteins, total lipids, lycopene, and β-carotene contents in SAE composition analysis, which is attributed to the positive morphological and physiological effects on A. esculentus plant to such growth regulators. The positive effect of SAE on photosynthetic pigments and promoting photosynthesis machinery may also justify enhanced vegetative growth attributes such as shoot length, root length, fresh biomass, and dry biomass as shown in the results. The sugar accumulation in the SAE could be the reason for the induction of signaling components for biosynthesis pathways of phytohormones for A. esculentus growth promotion under salt stress.
SAE supplementation is known to induce photosynthetic potential by improving the chlorophyll composition of leaves as Ramya et al. (2010) also found that the application of a liquid extract of S. wightii on Cyamopsis tetragonolaba under stress conditions increased the level of photosynthetic pigments due to the high absorption of magnesium ion that is a major constituent for chlorophyll biosynthesis. Generally, seaweeds contain high levels of iron, magnesium, and nitrogen which help increase chlorophyll synthesis. On the other hand, these ions are important in reducing lipase and lipoxygenase enzyme activities, thus protecting membrane breakdown.
The present study revealed that SAE application amplified the carotenoid production of A. esculentus plants under salt stress, consistent with the previous reports where Raphanus sativus treated with salt showed an increase in total carotenoid levels (Kasim et al., 2016). Increased carotenoids are known to protect the chloroplast from photo-oxidative damage induced by ROS production under salinity stress (Latef et al., 2017).
Water status in plants can be best determined through the relative water content of the leaves. In the present research, SAE-treated plants caused an improvement in plant water status and increased relative water content. Previously, a reduction in relative water content was also observed in plants such as Thymus vulgaris and Thymus daenensis Celak. (Bistgani et al., 2019) under salinity stress. In plants under salt stress, negative effects were created on osmotic potential, water potential, and relative water content in the leaves. Elansaey et al. (2017) observed the same results in experimental plants under salt stress due to a reduction in osmotic potential. The favorable impact of AsA on cucumber plants under normal and stressful circumstances was described by Naz et al. (2016), as it promotes water absorption and turgor capacity by maintaining moisture in plant tissues.
High salt concentrations change the water status of plants with a reduction of cell division/elongation with retarded growth due to limited carbon metabolism, leading to cell death by ROS production and deterioration of different biochemical processes. Bioactive compounds of seaweed extract impart a positive impact on plant overall growth by optimizing metabolite production (stress alleviating amino acids), membrane permeability, and transport of osmolytes/ions for enhancing abiotic stress tolerance through modifying the water capacity and turgor pressure in plants. Glycine betaine and proline (osmolytes) perform a vital role in osmoregulation (Annunziata et al., 2019), and they can interchange with molecules to modify the structures, maintain the veracity of membranes upon stresses, conserve the activity of macromolecules, scavenge the ROS, stabilize the photosynthetic activity, and initiate the gene expression responsible for controlling oxidative stress responses in G. hirsutum L. (Hamani et al., 2020), Zea mays L. (Chen et al., 2020), and rice (Hafez et al., 2019).
Consistent with previous reports, the present study also showed that SAE exhibited a high level of glycine betaine and proline which might be one of the reasons for salt stress tolerance amelioration in A. esculentus. Glycine betaine is also reported to counter salinity stress by maintaining a high K+/Na+ ratio and antioxidant defense via limiting Na+ uptake in the common bean (Phaseolus vulgaris L.) (Sofy et al., 2017). Our data also revealed that an increase in K+/Na+ ratio, antioxidant potential, and photosynthetic pigments might be attributed to the effect of glycine betaine supplied to A. esculentus plants via SAE application.
Proline is another important amino acid that is produced in plants under different stresses and helps in plant development. Proline accumulation under salinity stress regulates C and N source, osmosis, plasma membrane regulation, production of proteins, and destruction of free OH radicals. Current data revealed that salt stress drives an increase in proline level in A. esculentus as compared with control, which upon SAE supplementation in salt stress is further increased. According to Sofy et al. (2017), proline level enhancement in barley plants under salinity stress is a stress indicator signal but protects plants as it is involved in the alleviation of lipid peroxidation. The current study also supported the fact as SAE chemical composition has shown higher endogenous proline content too, which justifies the salt stress tolerance amelioration in A. esculentus.
It was previously known that stress-alleviating secondary metabolites and antioxidants act as the first line of defense for plants under different abiotic stresses. Cherry tomatoes with the application of AsA have also shown an increase in lycopene acting as a stress indicator as well as an antioxidant (Abdelgawad et al., 2019). According to different studies, it is evident that AsA is involved in the production of xanthophylls, antioxidants, carotenoids, lycopene, and chlorophyll in plants (Noctor et al., 2012). SAE application with antioxidant potential also showed pronounced influence in improving the lycopene content of A. esculentus plants. Akladious and Mohamed (2018) have also found an increase in lycopene content in pepper plants under salt stress. Researchers reported a rise in phenols, protein, flavonoids, tannins, terpene (particularly camphor and cineole), and antioxidant capacity after applying seaweed and AsA to plants, like chalcone isomerase (flavonoid biosynthesis enzyme) activity. Terpene is a class of secondary metabolites having antioxidant properties proven in different in-vitro assays (Aziz et al., 2018). Tannins have been proven to have a significant impact on plants in terms of inducing ROS-scavenging qualities under stress, and high tannin levels in plants induced by seaweed application improve the uptake of potassium ions, which has a positive influence on plant development and salt stress reduction (Baatour et al., 2018). SAE treatment had a significant impact on the terpene and tannin levels of A. esculentus plants, according to current findings. SAE treatment had a favorable effect on increasing phenolic and flavonoids in A. esculentus in this research, which is consistent with earlier results. For example, an increase in phenols under abiotic stress protected plants and played an important part in the regulation of stress by scavenging the ROS (Naikoo et al., 2019).
Hormones act as signaling elements as well. The hormonal contents present in seaweed extract positively influence the phytohormonal activity of plants that positively drives plant growth (Vinoth et al., 2019; Polat et al., 2021). Hormonal analysis of SAE under the current research showed a high level of auxin, cytokinin, and GA content, while A. esculentus plants treated with SAE also have revealed an increase in endogenous growth-promoting hormones with better overall growth response under salt stress in comparison to control plants. Thus, the growth-promoting impact of seaweed on growth parameters might be attributed to the presence of growth-promoting hormones present in SAE. The cytokinin-rich seaweed extracts are reported to induce photochemical efficiency in Agrostis palustris (Zhang and Ervin, 2004) and heat stress tolerance in Agrostis stolonifera (Zhang et al., 2010). Consistent with current observations, previous reports have indicated that seaweed (Sargassum) extract helped increased leaf number (Kaladharan et al., 2019) and biomass in plants, after the application of seaweed extracts with a higher content of auxin, phosphorus, and other compounds which helped to increase ionic absorption for growth promotion (Jupri et al., 2019).
The chemical composition of SAE used in the current study revealed high total antioxidant potential, with elevated DPPH and FRAP values, and higher total phenolics, flavonoids, proline, and tannins. SAE supplementation induced salt stress tolerance, and it could be due to the presence of such a high level of antioxidants to scavenge the ROS produced upon salt stress. Plants respond to salt stress by activating a complex antioxidant defense system that includes glutathione reductase (GR), superoxide dismutase (SOD), and APX, which generally protects plants from cellular stress, depletes free radicals, and scavenges excessive ROS. Compared with non-supplemented A. esculentus plants under salt stress, SAE-supplemented plants exhibited less H2O2 content due to a reduction in lipid peroxidation of the cellular membrane. Simultaneously, SAE-supplemented plants also showed higher endogenous catalase, guaiacol peroxidase, and ascorbate peroxidase activities upon salt stress. Moreover, total antioxidant activity was also higher upon salt stress exposure in SAE-supplemented A. esculentus plants. The application of SAE to A. esculentus plants during salt stress aided in the decrease of ROS by increasing endogenous antioxidant capacity. Catalase detoxifies ROS by quickly converting H2O2 to H2O, while peroxidase is another powerful antioxidative enzyme that scavenges H2O2 under salt stress (Mehla et al., 2017).
AsA is an important growth regulator which acts positively under abiotic stress and modulates photosynthesis, growth, senescence, cell division, defense, and the yield of plant and hormone biosynthesis. It also plays a vital role as a redox buffer, enzyme cofactor, antioxidant restorer, ROS scavenger, and signal translocator to regulate oxidation–reduction, especially in stress conditions. Exogenous AsA application has promoted plant growth (Hasanuzzaman et al., 2020), minimized the production of H2O2 upon stresses (El Sebai et al., 2016), and increased chlorophyll contents and photosynthetic activity in tomatoes under salt stress (Chem et al., 2021).
An increase in plant height, leaf number, and fresh and dry biomass has been recorded after seaweed application due to the presence of a high amount of nutrients such as phosphorus, in radish (Mahmoud et al., 2019) and V. unguiculata (Kalaivany et al., 2019). Chemical analysis of SAE under the current research also showed a high ionic level of macro- and micronutrients (sodium, potassium, calcium, magnesium, iron, copper, manganese, zinc). The growth enhancement effects of SAE could also be attributed to these macro- and microelements that made up the chemical composition of SAE as found in this study and in agreement with previous investigations as well. It is also known that seaweed extracts contain minerals that plants can readily assimilate to stimulate growth and productivity, such as zinc, copper, boron, and IAA, improving cell division, cell elongation, and root growth in plants. Moreover, consistent with previous reports, SAE supplementation also modulated the ionic status (K+/Na+, Mg2+/Na+, and Ca2+/Na+ ratios) in A. esculentus grown under salt stress that led to a significant reduction of salt-induced toxicity.
5 Conclusion
K+/Na+, Mg2+/Na+, and Ca2+/Na+ ratios in A. esculentus grown under salt stress were increased by foliar application of antioxidant-rich algal extract (S. wightii), noticeably reducing the salt toxicity and providing growth promoting effects. Such growth-promoting and salt stress-alleviating effects induced by SAE are considered a potentially effective strategy for its use as an agricultural biocatalyst and biostimulant. Thus, exploitation of the antioxidant and metabolite-rich algal extract of S. wightii induced salt stress tolerance in A. esculentus by rebalancing the ionic and metabolic status of plants under stress. SAE is found in this research as an eco-friendly, sustainable, feasible, and growth-promoting biological tool for ameliorating salt stress tolerance in A. esculentus. However, future studies are needed to identify the signaling elements driving the salt stress responses in A. esculentus to fully understand the molecular mechanisms activated under salt stress. It is recommended that complete genome, transcriptome, and proteome analyses and characterization of salt stress response genes in A. esculentus be performed to elucidate the molecular basis controlling salt responses.
Data Availability Statement
The raw data supporting the conclusions of this article will be made available by the authors, without undue reservation.
Author Contributions
HG, MR, MH, and MA designed the experiments. ZK, MR, and HG performed the main experiments. AR, AT collected and provided the seaweed sample. ZK, HG, MR, MA, participated in the writing the main manuscript. HG, MR, and MA analyzed the data. ZP, AU-D, SK and ZS assisted in the critical intellectual review of the manuscript. MH and I-JL provided financial and scientific support for the research project. All authors contributed to the article and approved the submitted version.
Funding
The authors declare that current research was supported by the National Research Foundation of Korea (NRF) grant funded by the Korean government (MSIT) (No. 2022R1A2C1008993).
Conflict of Interest
The authors declare that the research was conducted in the absence of any commercial or financial relationships that could be construed as a potential conflict of interest.
Publisher’s Note
All claims expressed in this article are solely those of the authors and do not necessarily represent those of their affiliated organizations, or those of the publisher, the editors and the reviewers. Any product that may be evaluated in this article, or claim that may be made by its manufacturer, is not guaranteed or endorsed by the publisher.
Abbreviations
SAE, Sargassum aqueous extract; AsA, ascorbic acid; GA, gibberellic acid; DPPH, 1,1-diphenyl-2-picryl-hydroxyl; GAE, gallic acid equivalents; AAE, AsA equivalent; FRAP, ferric reducing antioxidant power.
References
Abdelgawad F. K., El-Mogy M. M., IA Mohamed M., Garchery C., Stevens G. R. (2019). Increasing Ascorbic Acid Content and Salinity Tolerance of Cherry Tomato Plants by Suppressed Expression of the Ascorbate Oxidase Gene. Agronomy 9, 51. doi: 10.3390/agronomy9020051
Akbar A., Ashraf M. A., Rasheed R., Ali S., Rizwan M. (2021). Menadione Sodium Bisulphite Regulates Physiological and Biochemical Responses to Lessen Salinity Effects on Wheat (Triticum Aestivum L.). Physiol. Mol. Biol. Plants 27, 1135–1152. doi: 10.1007/s12298-021-01001-6
Akladious S. A., Mohamed H. I. (2018). Ameliorative Effects of Calcium Nitrate and Humic Acid on the Growth, Yield Component and Biochemical Attribute of Pepper (Capsicum Annuum) Plants Grown Under Salt-Stress. Sci. Hortic. 236, 244–250. doi: 10.1016/j.scienta.2018.03.047
Alabdallah N. M., Alzahrani H. S. (2020). The Potential Mitigation Effect of ZnO Nanoparticles on Okra [A. Esculentus Moench] Metabolism Under Salt-Stress Conditions. Saudi. J. Biol. Sci. 27, 3132–3137. doi: 10.1016/j.sjbs.2020.08.005
Al-Ghamdi A. A., Elansaey H. O. (2018). Synergetic Effects of 5-Aminolevulinic Acid and Ascophyllum Nodosum Seaweed Extracts on Asparagus Phenolics and Stress Related Genes Under Saline Irrigation. Plant Physiol. Biochem. 129, 273–284. doi: 10.1016/j.plaphy.2018.06.008
Ali O., Ramsubhag A., Jayaraman J. (2019). Biostimulatory Activities of Ascophyllum Nodosum Extract in Tomato and Sweet Pepper Crops in a Tropical Environment. PloS One 14 (5):e0216710. doi: 10.1371/journal.pone.0216710
Ali O., Ramsubhag A., Jayaraman J. (2021). Biostimulant Properties of Seaweed Extracts in Plants: Implications Towards Sustainable Crop Production. Plants 10, 53. doi: 10.3390/plants10030531
Alqahtani M., Roy S. J., Tester M. (2019). "Increasing Salinity Tolerance of Crops" in Encyclopedia of Sustainability Science and Technology. Switzerland AG: Springer Nature.: 1–24. Available: http://dx.doi.org/10.1007/978-1-4939-2493-6_429-3.
Annunziata M. G., Ciarmiello L. F., Woodrow P., Dell’Aversana E., Carillo P. (2019). Spatial and Temporal Profile of Glycine Betaine Accumulation in Plants Under Abiotic Stresses. Front. Plant Sci. 10, 230–230. doi: 10.3389/fpls.2019.00230
Aziz A., Akram N. A., Ashraf M. (2018). Influence of Natural and Synthetic Vitamin C (Ascorbic Acid) on Primary and Secondary Metabolites and Associated Metabolism in Quinoa (Chenopodium Quinoa Willd.) Plants Under Water Deficit Regimes. Plant Physiol. Biochem. 123, 192–203. doi: 10.1016/j.plaphy.2017.12.004
Aziz L., Hamayun M., Rauf M., Iqbal A., Arif M., Husssin Khan A. S.A., et al (2021a). Endophytic Aspergillus Niger Reprograms the Physicochemical Traits of Tomato Under Cadmium and Chromium Stress. Environmental and Experimental Botany, 186, 104456. doi: 10.1016/j.envexpbot.2021.104456
Aziz L., Hamayun M., Rauf M., Iqbal A., Husssin A., Khan S.A., et al (2021b). Aspergillus Flavus Reprogrammed Morphological and Chemical Attributes of Solanum Lycopersicum Through SlGSH1 and SlPCS1 Genes Modulation Under Heavy Metal Stress. Journal of Plant Interactions, 16(1),104–15. doi: 10.1080/17429145.2021.1903105
Baatour O., Maha Z., Nada B., Zeineb O. A. (2018). Effects of NaCl on Plant Growth and Antioxidant Activities in Fenugreek (Trigonella Foenum Graecum L.). Biosci. J. (Online) 34, 683–696. doi: 10.14393/BJ-v34n3a2018-38069
Bates L. S., Waldren R. P., Teare I. D. (1973). Rapid Determination of Free Proline for Water-Stress Studies. Plant Soil. 39, 205–207. doi: 10.1007/BF00018060
Benítez García I., Dueñas Ledezma A.K., Martínez Montaño E., Salazar Leyva J.A., Carrera E., Osuna Ruiz I., et al (2020). Identification and Quantification of Plant Growth Regulators and Antioxidant Compounds in Aqueous Extracts of Padina durvillaei and Ulva lactuca. Agronomy, 10(6), 866.
Benzie I. F., Strain J. J. (1996). The Ferric Reducing Ability of Plasma (FRAP) as a Measure of “Antioxidant Power”: The FRAP Assay. Anal. Biochem. 239, 70–76. doi: 10.1006/abio.1996.0292
Bistgani Z. E., Hashemi M., DaCosta M., Craker L., Maggi F., Morshedloo M. R. (2019). Effect of Salinity Stress on the Physiological Characteristics, Phenolic Compounds and Antioxidant Activity of Thymus Vulgaris L. And Thymus Daenensis Celak. Ind. Crops Prod. 135, 311–320. doi: 10.1016/j.indcrop.2019.04.055
Bulgari R., Franzoni G., Ferrante A. (2019). Biostimulants Application in Horticultural Crops Under Abiotic Stress Conditions. J. Agron. 9, 306. doi: 10.3390/agronomy9060306
Chapman D. J., Gellenbeck K. W. (1989). An Historical Perspective of Algal Biotechnology. Algal. Cyanobac. Biotechnol. 1, 27.
Chele K. H., Tinte M. M., Piater L. A., Dubery I. A., Tugizimana F. (2021). Soil Salinity, a Serious Environmental Issue and Plant Responses: A Metabolomics Perspective. Metabolites 11, 724. doi: 10.3390/metabo11110724
Chen F., Fang P., Zeng W., Ding Y., Zhuang Z., Peng Y. (2020). Comparing Transcriptome Expression Profiles to Reveal the Mechanisms of Salt Tolerance and Exogenous Glycine Betaine Mitigation in Maize Seedlings. PloS One 15(5):e0233616. doi: 10.1371/journal.pone.0233616
Chen X., Zhou Y., Cong Y., Zhu P., Xing J., Cui J., et al (2021). Ascorbic Acid-Induced Photosynthetic Adaptability of Processing Tomatoes to Salt-Stress Probed by Fast OJIP Fluorescence Rise. Front. Plant Sci. 1650. doi: 10.3389/fpls.2021.594400
Digruber T., Sass L., Cseri A., Paul K., Nagy A. V., Remenyik J., et al. (2018). Stimulation of Energy Willow Biomass With Triacontanol and Seaweed Extract. Ind. Crops Prod. 120, 104–112. doi: 10.1016/j.indcrop.2018.04.047
Dustgeer Z., Seleiman M. F., Imran K. H. A. N., Chattha M. U., Alhammad B. A., JALAL R. S., et al. (2021). Glycine-Betaine Induced Salinity Tolerance in Maize by Regulating the Physiological Attributes, Antioxidant Defense System and Ionic Homeostasis. Not. Bot. Horti. Agrobot. Cluj. Napoca. 49, 12248–12248. doi: 10.15835/nbha49112248
Elansaey H. O., Yessoufou K., Abdel-Hamid A. M., El-Esawi M. A., Ali H. M., Elshikh M. S. (2017). Seaweed Extracts Enhance Salam Turfgrass Performance During Prolonged Irrigation Intervals and Saline Shock. Front. Plant Sci. 8, 830. doi: 10.3389/fpls.2017.00830
El Sebai T. N., Abdallah M. M. S., El-Bassiouny H. M. S., Ibrahim F. M. (2016). Amelioration of the Adverse Effects of Salinity Stress by Using Compost, Nigella Sativa Extract or Ascorbic Acid in Quinoa Plants. Int. J. Pharmtech. Res. 9, 127–144.
Ergun N., Topcuoglu S. F., Yildiz A. (2002). Auxin (Indole-3-Acetic Acid), Gibberellic Acid (GA3), Abscisic Acid (ABA) and Cytokinin (Zeatin) Production by Some Species of Mosses and Lichens. Turk. J. Botany. 26, 13–18.
Erulan V., Soundarapandian P., Thirumaran G., Ananthan G. (2009). Studies on the Effect of Sargassum Polycystum (C. Agardh 1824) Extract on the Growth and Biochemical Composition of Cajanus Cajan (L.) Mill Sp. American-Eur. J. Agric. Environ. Sci. 6, 392–399.
Fan J. P., He C. H. (2006). Simultaneous Quantification of Three Major Bioactive Triterpene Acids in the Leaves of Diospyros Kaki by High-Performance Liquid Chromatography Method. J. Pharm. Biomed. 41, 950–956. doi: 10.1016/j.jpba.2006.01.044
FAO, ITPS (2015). “Status of the World’s Soil Resources (SWSR)–main Report,” in Food and Agriculture Organization of the United Nations and Intergovernmental Technical Panel on Soils, vol. 650. (Rome, Italy). Available at: http://www.fao.org/3/a-i5199e.pdf
Gaafar A. A., Ali S. I., El-Shawadfy M. A., Salama Z. A., Sękara A., Ulrichs C., et al. (2020). Ascorbic Acid Induces the Increase of Secondary Metabolites, Antioxidant Activity, Growth, and Productivity of the Common Bean Under Water Stress Conditions. Plants 9, 627. doi: 10.3390/plants9050627
Grieve C. M., Grattan S. R. (1983). Rapid Assay for Determination of Water-Soluble Quaternary Ammonium Compounds. Plant Soil 70, 303–307. doi: 10.1007/BF02374789
Gul H., Anjum L., Arif Shah M. M. (2018). Effects of Exogeneous Application of Putrescine on Different Biochemical Parameters of Zea Mays L. Under Salinity Stress. FUUAST Journal of Biology, 8(1), 65–72.
Gul H., Muhammad Azeem M., Khan M., Arif M, Yousuf M.A., Shouju , et al (2019) Influence of Inulin on Some Biochemical Aspects of Maize Under Salt Stress Condition. INT. J. BIOL. BIOTECH., 16 (2): 351–62
Hafez E. M., Gowayed S. M., Nehela Y., Sakran R. M., Rady A., Awadalla A., et al (2021). Incorporated Biochar-Based Soil Amendment and Exogenous Glycine Betaine Foliar Application Ameliorate Rice (Oryza Sativa L.) Tolerance and Resilience to Osmotic Stress. Plants 10, 1930.
Haida Z., Hakiman M. (2019). A Comprehensive Review on the Determination of Enzymatic Assay and Nonenzymatic Antioxidant Activities. Food Sci. Nutr. 7, 1555–1563. doi: 10.1002/fsn3.1012
Hamani A. K. M., Wang G., Soothar M. K., Shen X., Gao Y., Qiu R., et al. (2020). Responses of Leaf Gas Exchange Attributes, Photosynthetic Pigments and Antioxidant Enzymes in NaCl-Stressed Cotton (Gossypium Hirsutum L.) Seedlings to Exogenous Glycine Betaine and Salicylic Acid. BMC Plant Biol. 20, 1–14. doi: 10.1186/s12870-020-02624-9
Hasanuzzaman M., Bhuyan M. H. M., Zulfiqar F., Raza A., Mohsin S. M., Mahmud J. A., et al. (2020). Reactive Oxygen Species and Antioxidant Defense in Plants Under Abiotic Stress: Revisiting the Crucial Role of a Universal Defense Regulator. Antioxidants 9, 681. doi: 10.3390/antiox9080681
Hernández-Herrera R. M., Santacruz-Ruvalcaba F., Briceño-Domínguez D. R., Filippo-Herrera D., Andrea D., Hernández-Carmona G. (2018). Seaweed as Potential Plant Growth Stimulants for Agriculture in Mexico. Hidrobiológica 28, 129–140. doi: 10.24275/uam/izt/dcbs/hidro/2018v28n1/HernandezC
Jagota S. K., Dani H. M. (1982). A New Colorimetric Technique for the Estimation of Vitamin C Using Folin Phenol Reagent. Anal. Biochem. 127, 178–182. doi: 10.1016/0003-2697(82)90162-2
Jupri A., Fanani R. A., Syafitri S. M., Mayshara S., Nurijawati, Pebriani S. A., et al. (2019). “Growth and Yield of Rice Plants Sprayed With Sargassum Polycystum Extracted With Different of Concentration,” in AIP Conf Proc. 2199, 1, 070009. AIP Publishing LLC.
Kaladharan P., Sathianandan T. V., Edison S. J., Shahana T. S., Vysakhan P. (2019). Effects of Basal Application of Mulch and Foliar Spray of S. Weightii Extract on Certain Vegetable Crops. Fish. Technol. 56, 44–44.
Kalaivany V., Sutharsan S., Srikrishna S. (2019). Effects of Natural and Commercially Available Seaweed Liquid Extracts on Growth and Yield of Vigna Unguiculata L. Asian J. Biol. Sci. 12, 487–491. doi: 10.3923/ajbs.2019.487.491
Kasim W. A. E. A., Saad-Allah K. M., Hamouda M. (2016). Seed Priming With Extracts of Two Seaweeds Alleviates the Physiological and Molecular Impacts of Salinity Stress on Radish (Raphanus Sativus). Int. J. Agric. Biol. 18, 653–660. doi: 10.17957/IJAB/15.0152
Latef A. A. H. A., Srivastava A. K., Saber H., Alwaleed E. A., Tran L. S. P. (2017). Sargassum Muticum and Jania Rubens Regulate Amino Acid Metabolism to Improve Growth and Alleviate Salinity in Chickpea. Sci. Rep. 7, 1–12.
Lowry O. H., Rosebrough N. J., Farr A. L., Randall R. J. (1951). Protein Measurement With the Folin Phenol Reagent. Int. J. Biol. Chem. 193, 265–275. doi: 10.1016/S0021-9258(19)52451-6
Luck H. (1974). Estimation of Catalase Activity (New York. 885: Methods of enzymology. Academic Press).
Maclachlan S., Zalik S. (1963). Plastid Structure, Chlorophyll Concentration, and Free Amino Acid Composition of a Chlorophyll Mutant of Barley. Can. J. Bot. 41, 1053–1062. doi: 10.1139/b63-088
Mahmoud S. H., Salama D. M., El-Tanahy A. M., Abd El-Samad E. H. (2019). Utilization of Seaweed (Sargassum Vulgare) Extract to Enhance Growth, Yield and Nutritional Quality of Red Radish Plants. Ann. Agric. Sci. 64, 167–175. doi: 10.1016/j.aoas.2019.11.002
Makkar H. P., Blümmel M., Borowy N. K., Becker K. (1993). Gravimetric Determination of Tannins and Their Correlations With Chemical and Protein Precipitation Methods. J. Sci. Food Agric. 61, 161–165. doi: 10.1002/jsfa.2740610205
Mehla N., Sindhi V., Josula D., Bisht P., Wani S. H. (2017). “An Introduction to Antioxidants and Their Roles in Plant Stress Tolerance,” in Reactive Oxygen Species and Antioxidant Systems in Plants: Role and Regulation Under Abiotic Stress (Singapore: Springer), 1–23.
Mishra A. K., Agrawal S. B. (2014). Cultivar Specific Response of CO2 Fertilization on Two Tropical Mung Bean (Vigna Radiata L.) Cultivars: ROS Generation, Antioxidant Status, Physiology, Growth, Yield and Seed Quality. J. Agron. Crop Sci. 200, 273–289. doi: 10.1111/jac.12057
Mittal S., Kumari N., Sharma V. (2012). Differential Response of Salt-Stress on Brassica Juncea: Photosynthetic Performance, Pigment, Proline, D1 and Antioxidant Enzymes. Plant Physiol. Biochem. 54, 17–26. doi: 10.1016/j.plaphy.2012.02.003
Mushtaq Z., Faizan S., Gulzar B. (2020). Salt Stress, its Impacts on Plants and the Strategies Plants are Employing Against it: A Review. J. Appl. Biol. Biotechnol. 8, 81–91. doi: 10.7324/JABB.2020.80315
Nagata M., Yamashita I. (1992). Simple Method for Simultaneous Determination of Chlorophyll and Carotenoids in Tomato Fruit. NSKGA. 39, 925–928.
Naikoo M. I., Dar M. I., Raghib F., Jaleel H., Ahmad B., Raina A., Naushin F. (2019). Role and Regulation of Plants Phenolics in Abiotic Stress Tolerance: An Overview. Plant Signal, edited by M. Iqbal R. Khan, Palakolanu Sudhakar R, Ferrante A. Cambridge, England: Woodhead Publishing; 157–168. doi: 10.1016/B978-0-12-816451-8.00009-5
Nakano Y., Asada K. (1981). Hydrogen Peroxide is Scavenged by Ascorbate-Specific Peroxidase in Spinach Chloroplasts. Plant Cell Physiol. 22, 867–880.
Naqve M., Wang X., Shahbaz M., Fiaz S., Naqvi W., Naseer M., et al. (2021). Foliar Spray of Alpha-Tocopherol Modulates Antioxidant Potential of A. Esculentus Fruit Under Salt-Stress. Plants 10, 1382.
Naz H. I. R. A., Akram N. A., Ashraf M. (2016). Impact of Ascorbic Acid on Growth and Some Physiological Attributes of Cucumber (Cucumis Sativus) Plants Under Water-Deficit Conditions. Pak. J. Bot. 48, 877–883.
Nazarudin M. F., Alias N. H., Balakrishnan S., Wan Hasnan W. N. I., Noor Mazli N. A. I., Ahmad M. I., et al. (2021). Chemical, Nutrient and Physicochemical Properties of Brown Seaweed, Sargassum Polycystum C. Agardh (Phaeophyceae) Collected From Port Dickson, Peninsular Malaysia. Molecules 26, 5216.
Noctor G., Mhamdi A., Chaouch S., Han Y. I., Neukermans J., Marquez-Garcia B.E.L.E.N., et al. (2012). Glutathione in Plants: An Integrated Overview. Plant. Cell Environ. 35, 454–484. doi: 10.1111/j.1365-3040.2011.02400.x
Papenfuss G. (1955). "Classification of the Algae: In a Century of Progress in the Natural Sciences." (San Francisco: California Ac. Sc), 1–125.
Polat S., Trif M., Rusu A., Šimat V., Čagalj M., Alak G., Meral R., Özogul Y., Polat A., Özogul F.. (2021). Recent Advances in Industrial Applications of Seaweeds. Crit. Rev. Food Sci. Nutr., 1–30. doi: 10.1080/10408398.2021.2010646
Prieto P., Pineda M., Aguilar M. (1999). Spectrophotometric Quantitation of Antioxidant Capacity Through the Formation of a Phosphomolybdenum Complex: Specific Application to the Determination of Vitamin E. Anal. Biochem. 269, 337–341. doi: 10.1006/abio.1999.4019
Ramya S. S., Nagaraj S., Vijayanand N. (2010). Biofertilizing Efficiency of Brown and Green Algae on Growth, Biochemical and Yield Parameters of Cyamopsis Tetragonolaba (L.) Taub. Recent Res. Sci. Technol. 2, 45–52.
Rane J., Singh A. K., Kumar M., Boraiah K. M., Meena K. K., Pradhan A., et al. (2021). The Adaptation and Tolerance of Major Cereals and Legumes to Important Abiotic Stresses. Int. J. Mol. Sci. 22, 12970. doi: 10.3390/ijms222312970
Rauf M., Awais M., Din A. U., Ali K., Gul H., Rahman M. M., et al. (2021). Molecular Mechanisms of the 1-Aminocyclopropane-1-Carboxylic Acid (ACC) Deaminase Producing Trichoderma Asperellum MAP1 in Enhancing Wheat Tolerance to Waterlogging Stress. Front. Plant Sci. 11, 2213. doi: 10.3389/fpls.2020.614971
Riaz F., Abbas G., Saqib M., Amjad M., Farooq A., Ahmad S., et al. (2020). Comparative Effect of Salinity on Growth, Ionic and Physiological Attributes of Two Quinoa Genotypes. Pak. J. Agric. Sci. 57, 115–122.
Roy S., Chowdhury N. (2020). Salt-Stress in Plants and Amelioration Strategies: A Critical Review. Abio. Stress Plants, 391.
Saheed S. A. (2020). Impact of Ascorbic Acid and Potassium on Okra (Abelmoschus Esculentus) Growth in Saline Condition. ZJPAS. 32, 144–150.
Shahid M. A., Pervez M. A., Balal R. M., Ahmad R., Ayyub C. M., Abbas T., et al. (2011). Salt-Stress Effects on Some Morphological and Physiological Characteristics of Okra (A. Esculentus). Soil Environ. 30(1), 66–73.
Shahzad K., Hussain S., Arfan M., Hussain S., Waraich E. A., Zamir S., et al. (2021). Exogenously Applied Gibberellic Acid Enhances Growth and Salinity Stress Tolerance of Maize Through Modulating the Morpho-Physiological, Biochemical and Molecular Attributes. Biomolecules 11, 1005. doi: 10.3390/biom11071005
Singleton V. L., Rossi J. A. (1965). Colorimetry of Total Phenolics With Phosphomolybdic-Phosphotungstic Acid Reagents. Am. J. Enol. Vitic. 16, 144–158.
Sofy M. R., Elhawat N., Alshaal T. (2020). Glycine Betaine Counters Salinity Stress by Maintaining High K+/Na+ Ratio and Antioxidant Defense via Limiting Na+ Uptake in Common Bean (Phaseolus Vulgaris L.). Ecotoxicol. Environ. Saf. 200, 110732. doi: 10.1016/j.ecoenv.2020.110732
Sofy M. R., Sharaf A. E. M., Osman M. S., Sofy A. R. (2017). Physiological Changes, Antioxidant Activity, Lipid Peroxidation and Yield Characters of Salt-Stressed Barely Plant in Response to Treatment With Sargassum Extract. Int. J. Biol. Sci. 4, 90–109.
Sohn S. I., Rathinapriya P., Balaji S., Jaya Balan D., Swetha T. K., Durgadevi R., et al. (2021). Phytosterols in Seaweeds: An Overview on Biosynthesis to Biomedical Applications. Int. J. Mol. Sci. 22, 12691. doi: 10.3390/ijms222312691
Umar M., Siddiqui Z. S. (2018). Physiological Performance of Sunflower Genotypes Under Combined Salt and Drought Stress Environment. Acta Bot. Croat. 77, 36–44. doi: 10.2478/botcro-2018-0002
Uthirapandi V., Suriya S., Boomibalagan P., Eswaran S., Ramya S. S., Vijayanand N., et al. (2018). Effects of a Biostimulant Derived From the Brown Seaweed Ascophyllum Nodosum on Ripening Dynamics and Fruit Quality of Grapevines. Sci. Hortic. 232, 97–106. doi: 10.1016/j.scienta.2017.12.054
Van Handel E. (1985). Rapid Determination of Total Lipids in Mosquitoes. J. Am. Mosq. Control. Assoc. 1, 302–304.
Van Oosten M. J., Pepe O., De Pascale S., Silletti S., Maggio A. (2017). The Role of Biostimulants and Bioeffectors as Alleviators of Abiotic Stress in Crop Plants. Chem. Biol. Technol. Agric. 4, 1–12. doi: 10.1186/s40538-017-0089-5
Vinoth S., Gurusaeavanan P., Sivakumar S., Jayabalan N. (2019). Influence of Seaweed Extracts and Plant Growth Regulators on In Vitro Regeneration of Lycopersicon Esculentum From Leaf Explant. J. Appl. Phycol. 31, 2039–2052. doi: 10.1007/s10811-018-1703-z
Warrier R. R., Paul M., Vineetha M. V. (2013). Estimation of Salicylic Acid in Eucalyptus Leaves Using Spectrophotometric Methods. Genet. Plant Physiol. 3, 90–97.
Yen G. C., Chen H. Y. (1995). Antioxidant Activity of Various Tea Extracts in Relation to Their Antimutagenicity. J. Agric. Food Chem. 43, 27–32. doi: 10.1021/jf00049a007
Yokawa K., Kagenishi T., Baluška F. (2016). UV-B Induced Generation of Reactive Oxygen Species Promotes Formation of BFA-Induced Compartments in Cells of Arabidopsis Root Apices. Front. Plant Sci. 6, 1162. doi: 10.3389/fpls.2015.01162
Zahedi S. M., Hosseini M. S., Fahadi Hoveizeh N., Gholami R., Abdelrahman M., Tran L. S. P. (2021). Exogenous Melatonin Mitigates Salinity-Induced Damage in Olive Seedlings by Modulating Ion Homeostasis, Antioxidant Defense, and Phytohormone Balance. Plant Physiol. 173, 1682–1694. doi: 10.1111/ppl.13589
Zhang X., Ervin E. H. (2004). Cytokinin-Containing Seaweed and Humic Acid Extracts Associated With Creeping Bentgrass Leaf Cytokinins and Drought Resistance. Crop Sci. 44, 1737–1745. doi: 10.2135/cropsci2004.1737
Zhang X., Wang K., Ervin E. H. (2010). Optimizing Dosages of Seaweed Extract-Based Cytokinins and Zeatin Riboside for Improving Creeping Bentgrass Heat Tolerance. Crop Sci. 50, 316–320. doi: 10.2135/cropsci2009.02.0090
Keywords: marine macroalgae, seaweed, Abelmoschus esculentus, ion homeostasis, antioxidants, salt stress tolerance, bioactive metabolites, biostimulant
Citation: Khan Z, Gul H, Rauf M, Arif M, Hamayun M, Ud-Din A, Sajid ZA, Khilji S, Rehman A, Tabassum A, Parveen Z and Lee I-J (2022) Sargassum wightii Aqueous Extract Improved Salt Stress Tolerance in Abelmoschus esculentus by Mediating Metabolic and Ionic Rebalance. Front. Mar. Sci. 9:853272. doi: 10.3389/fmars.2022.853272
Received: 12 January 2022; Accepted: 11 April 2022;
Published: 02 June 2022.
Edited by:
Naser A. Anjum, Aligarh Muslim University, IndiaReviewed by:
Kamrun Nahar, Sher-e-Bangla Agricultural University, BangladeshWilson Thau Lym Yong, Universiti Malaysia Sabah, Malaysia
Copyright © 2022 Khan, Gul, Rauf, Arif, Hamayun, Ud-Din, Sajid, Khilji, Rehman, Tabassum, Parveen and Lee. This is an open-access article distributed under the terms of the Creative Commons Attribution License (CC BY). The use, distribution or reproduction in other forums is permitted, provided the original author(s) and the copyright owner(s) are credited and that the original publication in this journal is cited, in accordance with accepted academic practice. No use, distribution or reproduction is permitted which does not comply with these terms.
*Correspondence: Mamoona Rauf, bWFtb29uYUBhd2t1bS5lZHUucGs=; Muhammad Hamayun, aGFtYXl1bkBhd2t1bS5lZHUucGs=; In-Jung Lee, aWpsZWVAa251LmFjLmty
†These authors have contributed equally to this work