- 1Department of Ocean Science and Engineering, Southern University of Science and Technology, Shenzhen, China
- 2Southern Marine Science and Engineering Guangdong Laboratory (Guangzhou), Guangzhou, China
- 3State Key Laboratory of Tropical Oceanography, South China Sea Institute of Oceanology, Chinese Academy of Sciences, Guangzhou, China
Estuaries have experienced significant changes due to global climate change and human perturbations since the last century. However, the climate and anthropogenic influence on the burial of sedimentary organic carbon (OC) in estuaries is still not understood well yet. Here, a 3-meter sediment core was taken from the Pearl River Estuary (PRE) in China. Depth profiles of both bulk OC and lignin biomarker data indicated three stages with different features of buried OC during the 130-year sediment deposition. The 1893-1957 stage showed 20% more burial of marine derived OC, which was mostly adsorbed on finer minerals compared to the years after 1957. The 1957-1980 period witnessed 4.6 times higher burial rate of petrogenic OC, which made the radiocarbon age of total organic carbon 42% older than before due to soil erosion and carbonate rock weathering. The 7-year running average variation of terrestrial OC input based on endmember mixing model was correlated with the Pacific Decadal Oscillation index before 1957, but correlated with the Atlantic Multidecadal Oscillation between 1957 and 1980 in the region. The reduction of land derived OC content after 1980s was mostly affected by human perturbations such as deforestation and dam construction which corresponded to the beginning of Economic Reform and Open Up in China. The overall increase of lignin content from bottom to surface sediment indicated increased vascular plant derived OC due to deforestation activities during the urbanization process. The study suggested different time periods when climate or human disturbance dominantly affected the OC burial in the PRE, which have significant indications for local and global carbon cycling and environmental ecology.
1 Introduction
The estuaries are major components of the global carbon cycle (Canuel and Hardison, 2016). Estuaries are hotspots for organic carbon (OC) burial (Bianchi et al., 2018) that is a global benefit for warming (Breithaupt et al., 2020). However, the estuaries are also “carbon incinerators” (Aller and Blair, 2006) with high OC remineralization rates (Chen et al., 2022) which can be affected by local and global environmental changes, as well as human perturbations (Syvitski et al., 2022). Therefore, understanding changes in OC biogeochemistry during sediment burial in the estuaries is essential to better understand the role they play in global climate change (Bianchi and Allison, 2009).
Stratigraphic change of OC burial can result from the combined effect of climate change and human perturbations. Sediment records provide an alternative method to analyze the impact of extreme events on sediment deposition and OC burial in coastal estuaries (e.g. Wheatcroft et al., 2010; Swindles et al., 2018) over extended time periods. For example, the stable carbon isotopes and terrestrial biomarkers have been applied as efficient indicators (Dalzell et al., 2005; Clark et al., 2013; Li et al., 2013; Li et al., 2020) of transportation and deposition of flooding induced terrestrial OC in coastal sediment cores in China (Wu et al., 2007). The rainfall frequency in China has been reported to be affected by the Pacific Decadal Oscillation (PDO) (Qian and Zhou, 2014; Wu and Mao, 2017). The Atlantic Multidecadal Oscillation (AMO) may also control the extreme weather and climate events including monsoon occurrence, runoff, and rainfall in China (Li and Bates, 2007; Qian et al., 2014) which affected the delivery of sediment and terrestrial OC to estuaries. Moreover, human activities have increasingly affected soil erosion and the delivery of terrestrial OC (Wang et al., 2018; Ye et al., 2021) to estuaries in China. Therefore, the organic carbon burial in coastal sediment of China is controlled by multiple processes.
The Pearl River Estuary (PRE) acts significantly in the “source to sink” process of OC cycling by linking the Pearl River to the South China Sea. With a population of ~100 million, the region of the PRE has become one of the fastest developing regions in China over the past decades. The human activities such as dam constructions (Wu et al., 2016), deforestation (Liu et al., 2014) has influenced the PRE sedimentation (Owen and Lee, 2004; Ye et al., 2021), subaqueous topography, ecological environment (Wu et al., 2016; Wu et al., 2018), and potentially the delivery of OC to the of PRE. Meanwhile, the climate effect on the local and national precipitation and drought have been widely studied (Duan et al., 2013; Drinkwater et al., 2014; Yang et al., 2017b). However, the climate effect on the variation of sediment deposition and OC burial in the PRE has rarely been well studied on decadal to centennial scales. Considering climate change and human perturbation have become significant drivers that may translate to simultaneous responses in sediments records, delineating their influences on sediment and OC burial is becoming significant to understand the changes of OC cycling to more intensified extreme human and climate events in recent years.
This study aimed to address this knowledge gap using multiple proxies to identify how the OC burial in the sediment record responded to human perturbations and climate effects. Bulk carbon proxies including total organic carbon (TOC) and total nitrogen (TN), stable isotopes (δ13C), radiocarbon (Δ14C), and terrestrial organic biomarker of lignin were analyzed in a 210Pb-dated core to examine the sources and composition changes of OC in the PRE over the last century. The variation of terrestrial OC input was then compared with the climate oscillation index, human activities, and sediment mineralogy to explore the mechanisms for OC burial during the sedimentation process in the PRE.
2 Sampling and Methods
2.1 Site Description and Sample Collection
Nearly half of the Pearl River water discharges into the SCS through the PRE via three main tributaries in the lower drainage basin: North River, West River, and East River (Figure 1). A 3-meter gravity core was collected at 21-m water depth off Guishan Island in the PRE (22.1315°N, 113.8055°E) in October 2017. The core was sectioned at an interval of 5cm for 0–100 cm and 10cm for 100–300 cm. The samples were immediately stored at -80°C until further analysis.
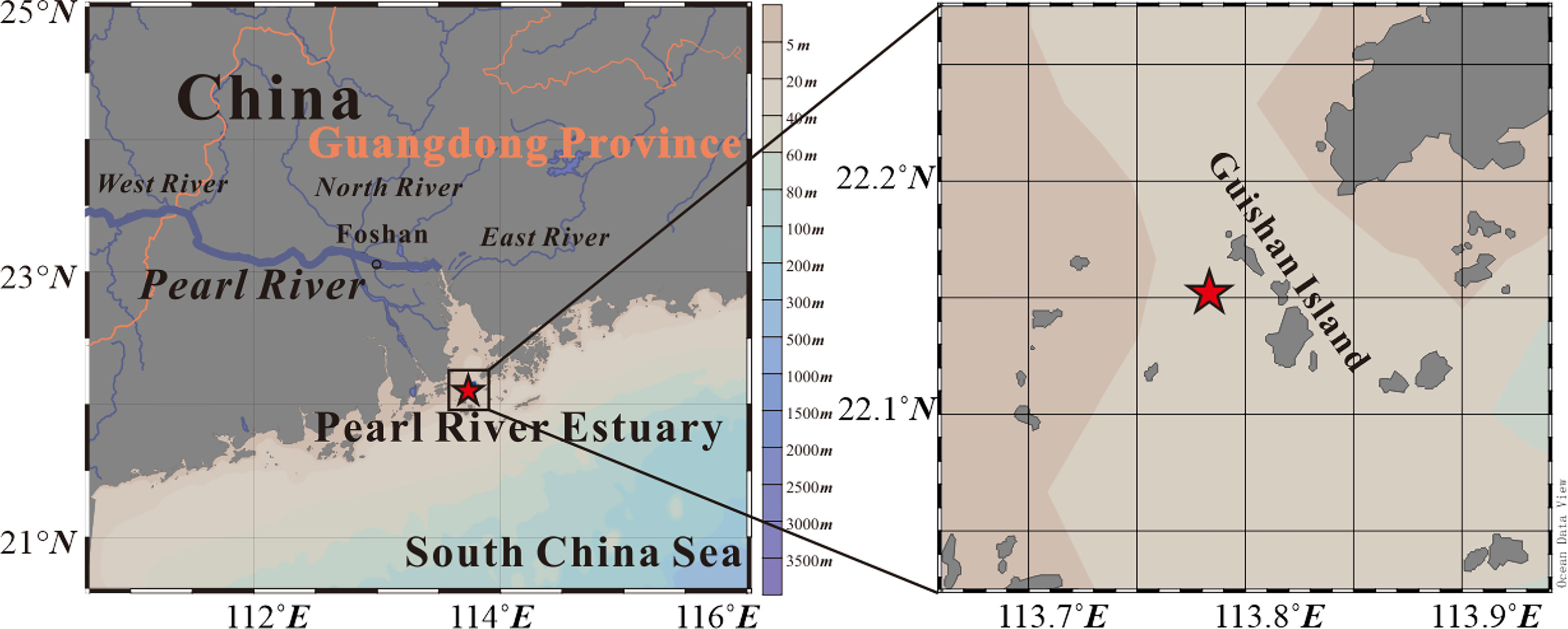
Figure 1 Sampling site of the sediment core from Guishan Island, Pearl River Estuary. The three main tributaries of North River, West River, and East River are labeled.
2.2 210Pb Chronology
The total 210Pb (210Pbt), 226Ra, and 137Cs activities were analyzed in dry samples (6∼9g) with a low background high-purity germanium (HPGe) γ-ray detector (EG& G Ortec Ltd., USA) at the State Key Laboratory of Marine Geology, Tongji University. The samples were sealed in polyethylene tubes to allow for radioactive equilibration for 30 days before analysis (Ye et al., 2020).
The activities of 137Cs were too low to obtain any confident results, therefore only 210Pb dating was used in this study. 226Ra was used as an index of supported 210Pb (210Pbsu), and excess 210Pb (210Pbex) activities were calculated by subtracting 210Pbsu activities from 210Pbt activities. The sediment accumulation rate (SAR) was then calculated with the constant rate of supply (CRS) model which assumes that the flux of 210Pb to the accumulating sediment is constant during a timescale of 100–200 years. The chronology was derived by fitting the exponential 210Pb decay profiles with the cumulative dry mass (Appleby and Oldfield, 1978; Zhang et al., 2014; Li et al., 2021). Fitting analysis was done using the ‘Exp2PMod1’ function in the Origin 2021 program.
2.3 Bulk Carbon/Nitrogen, Stable and Radiocarbon Isotope Analysis
Sediment samples are first floated in deionized water, dispersed through agitation, and then sieved to <180 microns to remove any root materials. Then the <180 microns organic sediment size fraction is treated with a series of hot (~ 70-90°C) acid leaches with HCl at a concentration of 0.1N for a period of 4-12 hours. Additional applications of HCl are provided until any carbonate presence has been completely removed. Samples are then rinsed to neutral with deionized water and dried at 100°C until dry. The sediments are then homogenized to insure an equal dispersion of the available carbon. A small aliquot is then tested with concentrated HCl to check for completion of carbonate removal. The sample was then measured either in whole or where applicable appropriately sub-sampled for combustion and analysis. The TOC (%), TN (%) content was determined on an elemental analyzer (Vaio EL Cube, Germany) after decarbonation. Stable isotopes of TOC (δ13C) were measured using an isotope ratio mass spectrometer (Thermo Science Delta Plus, USA) connected on-line to an elemental analyzer (Carlo Erba Instruments Flash 1112, USA). The C/N ratio was calculated as the atomic ratio of TOC and TN. The 14C of these samples was measured by accelerator mass spectrometer (AMS) interfaced with an elemental analyzer at the Beta Analytic testing laboratory, USA. Radiocarbon data were expressed as Δ14C values and fraction modern (Fm).
2.4 Grain Size and Porosity
The grain size of the sediments was analyzed by a laser grain-size analyzer (Mastersizer 3000, Malven Instruments Ltd., UK) following the methodology described by Jiang et al. (2016). Briefly, about 0.2 g of the samples were treated with 15ml 10% (v/v) hydrogen peroxide to remove the organic matter. Carbonates were then removed by gradual addition of 15 ml of 10% HCl. The sample residue was dispersed with 10 ml of 10% (NaPO3)6 on an ultrasonic vibrator for 10 min before instrumental analysis. The particle sizes less than 4 μm were defined as clay, 4-63 μm as silt, and > 63 μm as sand. To sort the grain-size distribution into valuable information on geological processes and palaeo-environmental changes, end-member analysis (EMA) was applied to estimate end-member variations according to the methods by Prins et al. (2000). In this study, we used a newly developed GUI software of AnalySize for processing and unmixing grain size data (Paterson and Heslop, 2015) to determine the grain-size distributions of the detrital fraction in our sediment core. Porosity of each sample was calculated from the water content (wet-dry weight) prior to and after freeze-drying.
2.5 Lignin-Phenols Analyses
Lignin analyses were performed using CuO oxidation method of Hedges and Ertel (1982), as modified by Bianchi et al. (2002). Homogenized sediments containing ca. 3 to 5 mg of organic carbon were transferred to stainless-steel reaction vials with 330 ± 4 mg CuO and 3 to 5 ml 2 N NaOH in glove box purged by nitrogen and then digested at 150°C for 3 h. Reaction products were neutralized and extracted with diethyl ether (peroxides removed), filtered through combusted glass-fiber filled with glass wool, dried under N2, and converted to trimethylsilyl derivatives using bis-(trimethylsilyl)-trifluoroacetamide (BSTFA). Lignin-phenol derivatives were analyzed with an Agilent 7890-Gas Chromatograph/5977-Mass Spectrometric Detector (GC–MS).
Quantification of lignin-phenols was based on a mixed standard calibration curve containing known amounts of 12 lignin reaction products of interest as well as the internal standard ethyl vanillin. Eight lignin-phenol oxidation monomer products (LOPs): C (ferulic acid+cinnamic acid), V (vanillin+acetovanillone+vanillic acid) and S (syringealdehyde+ acetosyringone+syringic acid) were quantified and used as molecular indicators for source and diagenetic state of terrestrial vascular plant tissue (Hedges and Parker, 1976). Compound of 3,5-dihydroxybenzoic acid (3,5Bd) was also derived after cupric oxidation and quantified (Goñi and Hedges, 1995). The precision for the total lignin phenols was within ±10%, while that for individual compound ranged from ±5 to ±15% based on triplicate analysis.
Lambda-8 (Λ8),which is defined as the total weight in milligrams of the sum of C, V, and S phenols, normalized to 100 mg of organic carbon (Hedges and Parker, 1976), is used as a biomarker for terrestrial vascular plants. The acid-to-aldehyde ratios of both V and S phenols: (Ad/Al)v, (Ad/Al)s, were used as indicators of lignin degradation state prior to burial (Hedges et al., 1988). The C/V (woody/non-woody) and S/V (gymnosperm/angiosperm) ratios were plotted as indicators of the source of vascular plant (Hedges and Mann, 1979). The lignin-phenol vegetation index (LPVI) was also applied to study sources of vascular plant materials (Tareq et al., 2004; Sánchez-García et al., 2009). The 3,5Bd is used as an index of soil degradation processes, while the 3,5Bd/V indicated inputs of organic matter humification products sorbed to fine particles in soils (Houel et al., 2006).
2.6 Modelling to Distinguish Sources of OC
A binary mixing model was used to resolve the non-rock-derived biospheric (OCbio) and petrogenic (OCpetro) OC (Galy et al., 2008). Then the radiocarbon composition of the bulk OC can be expressed as follows:
where, Fm, Fmbio, and Fmpetro are fraction modern values of bulk TOC, OCbio, and OCpetro, respectively. OCbio has different quantities of radioactive carbon (Fm> 0), while OCpetro does not contain radioactive carbon (Fm = 0) (Galy et al., 2008). So, equation (1) is further modified as:
Thus, Fmbio (slope) and OCpetro (intercept/slope) can be estimated by plotting Fm ×TOC versus TOC on an X–Y plot, while OCbio is estimated by the offset between bulk TOC and OCpetro.
A Monte Carlo simulation model was applied to track the sources of the sedimentary TOC from C3, C4 plants, riverbank soil, river phytoplankton, and marine algae. Assuming that the endmember parameters (δ13C and N/C) followed a normal distribution (mean ± standard deviation) for different OC sources in the study system (Table S1), the program was run in Python 3.8.2. Briefly, 4000 out of 1,000,000 random samples from the normal distribution of each end‐member were taken in order to simultaneously optimize the following underdetermined system of linear equations:
where Fi is the fraction of the i end‐member and i = vascular C3 plant, C4 plant, soil OC, marine, and river phytoplankton, respectively. N/C, the inverse of C/N ratio, is used as a more sensitive end-member of terrestrial OC (Perdue and Koprivnjak, 2007; Li et al., 2017). The variation of the mean value for each end‐member was less than 0.2‰ by randomly sampling each parameter value five times, ensuring the statistical stability of the model.
2.7 Carbon Burial Rate
The bulk carbon burial rate is calculated by the following equation:
where, SAR was determined from 210Pb chronology; the bulk density was assumed to be 1.5 g cm-3 in this region (Chen et al., 2006); TOC and porosity were the average values of 20-110 cm, 120-170 cm, 180-300 cm and the entire core.
The burial rate of each end-member was calculated by multiplying the bulk carbon burial rate and the fraction of each end-members from the mixing model. For example, the burial rate of lignin is calculated as:
2.8 Data Analyses
The Origin 2021 software was used to graph the figures. Statistical differences were calculated using one-way ANOVA. Statistically significant differences were discussed within the 95% confidence interval. Principle component analysis (PCA) was performed to discriminate for any other controlling variables linked with bulk and biomarker patterns in sediment samples with all parameters being mean-normalized.
3 Results
3.1 Sediment Chronology
The cores displayed relatively low excess activities of 210Pb ranging from 0.22 dpm g−1 to 2.62 dpm g−1 (1.33 ± 0.65 dpm g−1, n=14) (Figure 2 and Table S2). While grain size variation can generate an error in the downcore decay trend in relatively low excess activity samples, the core showed a supported 210Pb level (from 226Ra activity) that were relatively invariant downcore (2.16–2.86 dpm g−1) (Figure 2). The surface mixed layer was around 20 cm with the depth below showing the sediment accumulation. The best fit exponential regression of excess 210Pb activity (R2 = 0.69, p<0.01) yielded a varied sedimentation rate (LSR) ranging from 1.71 cm yr-1 (210-250 cm) to 3.18 cm yr−1 (20-30 cm). The calculated geochronology dated back to 1893 for the deepest sample. Therefore, the core represents about 130 years of sediment deposition.
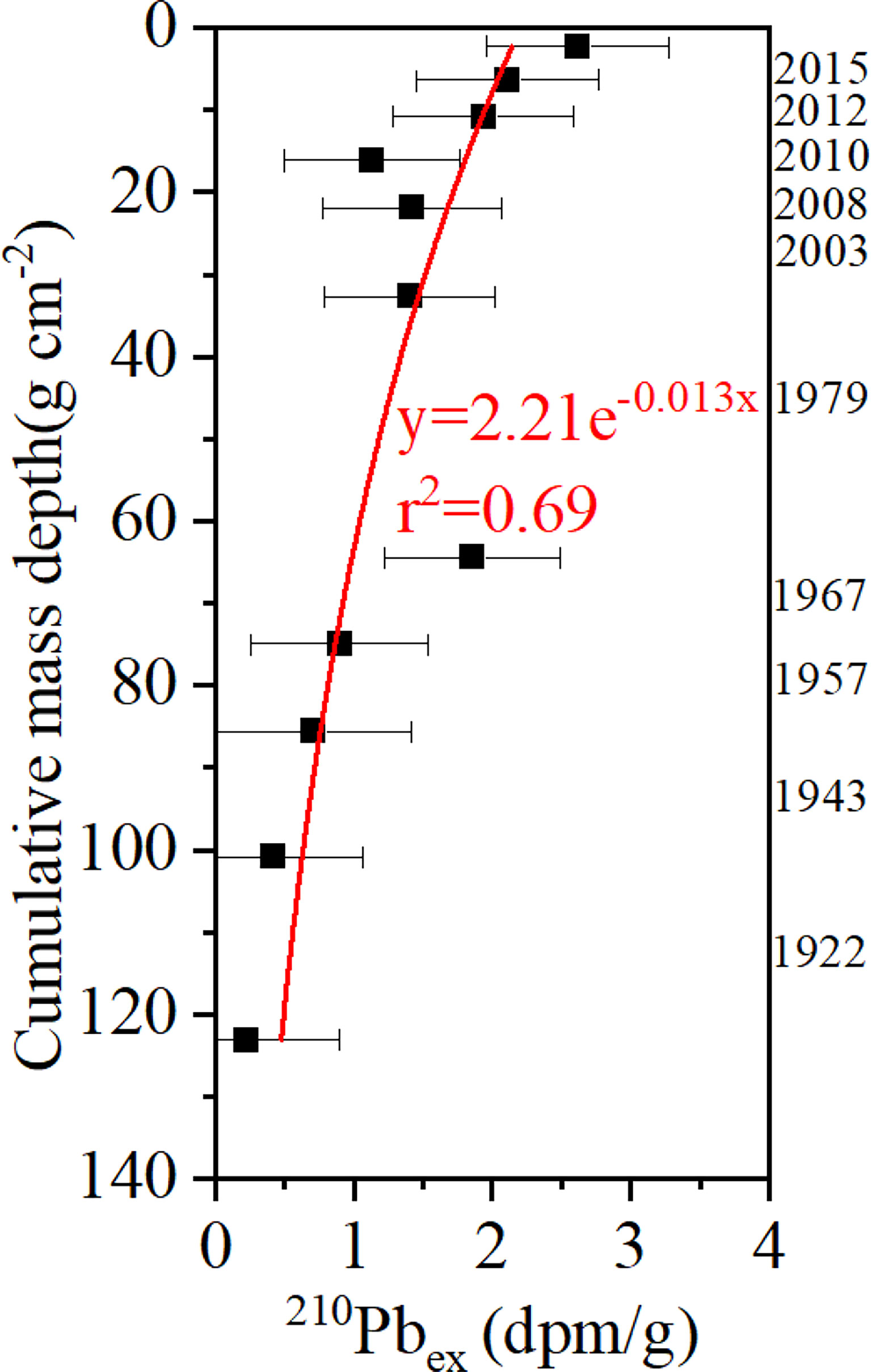
Figure 2 The cumulative mass depth profile of excess 210Pb (210Pbex) of the sediment core. The right scale labeled the chronology.
3.2 Bulk Organic Carbon/Nitrogen, Stable and Radiocarbon Isotopes
The TOC (%) varied from 0.43% to 1.43% (0.96 ± 0.22%, n=40) (Figure 3A). The TN ranged from 0.11% to 0.28% (0.24 ± 0.03%, n=40) (Figure 3B). A significant linear relationship between TN and TOC (R2 = 0.49, p < 0.01) suggested that TN was derived predominantly from the organic origin. The C/N ratio varied from 2.27 to 9.33 (4.69 ± 1.18, n=40) (Figure 3D). The δ13C ranged from -24.54 to -22.89‰ and showed three stages with different δ13C features (Figure 3C and Figure S1). Between 1893 and 1957, the average δ13C value was -23.08 ± 0.16‰. Between 1957 and 1980, the value showed a large variability (-23.59 ± 0.29‰), while after 1980, the average δ13C decreased to -23.86 ± 0.34‰ (Figure S1), indicating an increased proportion of terrestrial OC. The Δ14C value varied from -448.40‰ to -209.55‰ (-299.26 ± 66.89‰, n=20) (Figure 3E and Figure S1). The average Δ14C value was -226.84‰ (n=6), -399.04‰ (n=3), and -316.88‰ (n=9) (Figure S1) in stage of 1893 to 1957, 1957 to 1980, and after 1980, respectively. Correspondingly, the average Fm in stages of 1893 to 1957, 1957 to 1980, and after 1980 were 0.78 (n=6), 0.61 (n=3), and 0.69 (n=9) (Figure S1), respectively.
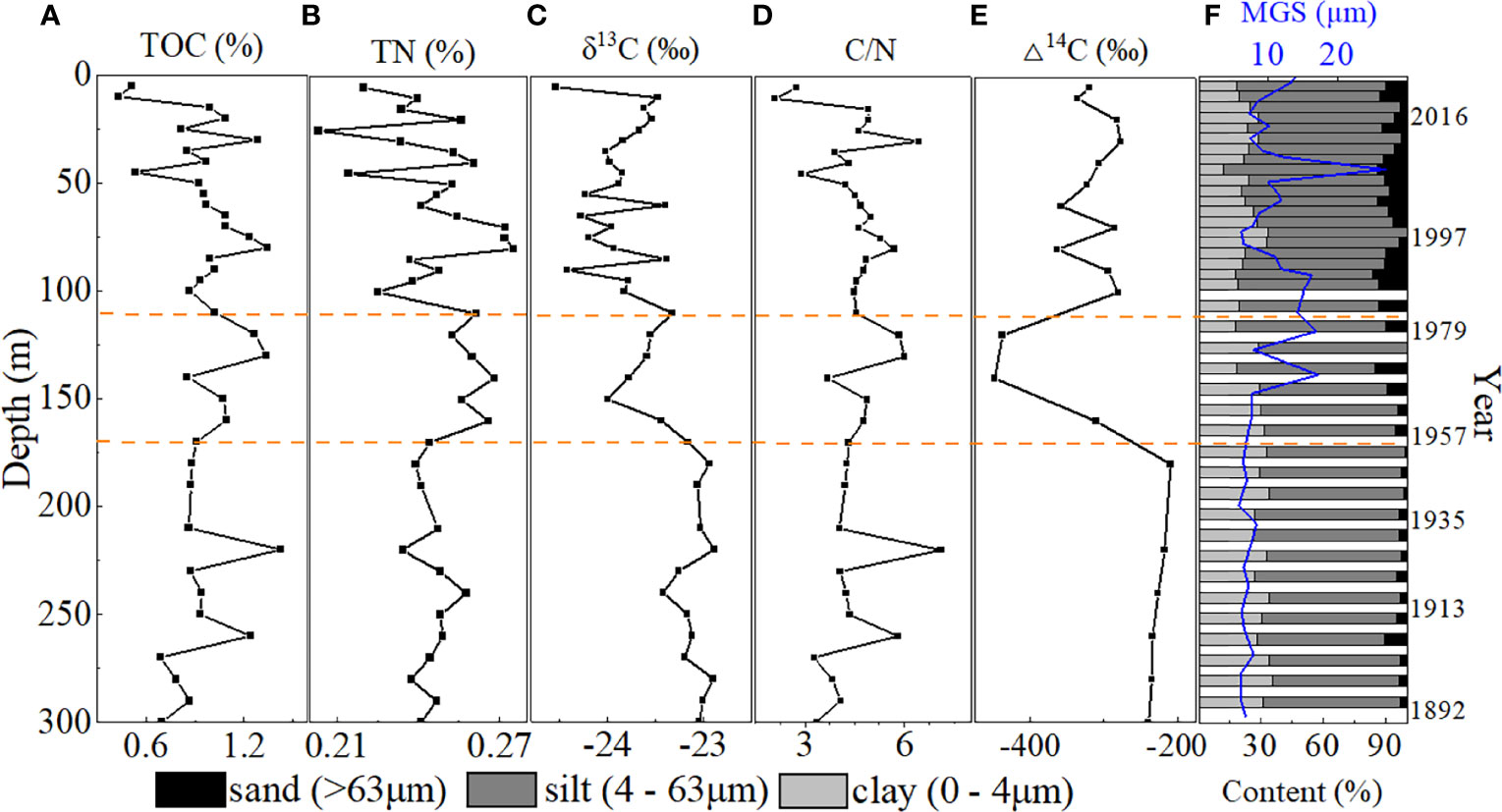
Figure 3 Depth profiles of (A) TOC(%), (B) TN(%), (C) δ13C(‰), (D)C/N ratio, (E) Δ14C(‰), and (F) median grain size (MGS) and mineral content (%).
3.3 Grain Size
The clay, silt and sand content of the core ranged from 11.58 to 35.6% (26.24 ± 5.82%, n=40), 60.36 to 74.56% (66.54 ± 3.34%, n=40), 0 to 16.2% (7.22 ± 4.63%, n=40), respectively (Figure 3F and Figure S1). The sediments were predominately fine-grained (<63 μm) that the sum of clay and silt ranged from 83.8% to 100%. The proportion of clay and silt were negatively correlated in all layers (R2 = 0.61, p<0.01).
The median grain size (MGS) ranged from 5.83 to 26.9μm (9.88 ± 4.33μm, n=40) (Figure 3F). Before the 1960s, the MGS was relatively constant at a low value (6.90 ± 0.74, n=15), while they became more variable and showed relatively higher values (11.83 ± 4.61, n=25) after the 1960s. The MGS has a peak value around 2008. The correlation map between multiple correlation coefficient and numbers of end-member (EM) indicated that two EMs could fulfill the observed compositional variation required in EMA (Jiang et al., 2017) and explain the grain-size distribution pattern that the peak values were concentrated at 6.72 μm (EM 1), and 40.14 μm (EM 2), respectively. The EM1 and EM2 ranged from 36.91% to 97.42% (78.43 ± 14.78%, n=40), 2.58% to 63.09% (21.57 ± 14.78%, n=40), respectively (Figure S2).
3.4 Lignin-Phenols
The Λ8 values ranged from 0.43 to 2.84 mg 100mg-1 OC (1.39 ± 0.54, n=40) (Figure 4A). There was a general increase in Λ8 from the bottom to the surface sediment, that the average Λ8 in stages of 1893 to 1957, 1957 to 1980, and after 1980 were 1.04 (n=13), 1.18 (n=6), 1.68 (n=21) mg 100mg-1 OC, respectively. This trend indicated an increasing accumulation of vascular plant derived OC. The 3,5Bd showed no significant changes with depth (p > 0.01) (Figure 4B). The average (Ad/Al)v values were 0.38 (n=13), 0.35 (n=6) and 0.26 (n=21), while the average (Ad/Al)s were 0.35 (n=13), 0.36 (n=6) and 0.23 (n=21) from 1893 to 1957, 1957 to 1980, and after 1980, respectively (Figures 4C, D). Their insignificant depth variations (p<0.01) indicated insignificant degradation, or that the majority of bioavailable lignin has been consumed prior to delivery to the ocean (Seidel et al., 2015). The ratios of 3,5Bd/V and P/(S+V) ranged from 0.03 to 0.17 (0.08 ± 0.03, n=40), 0.11 to 0.3 (0.17 ± 0.05, n=40) with slight increase with depths (Figures 4E, F).
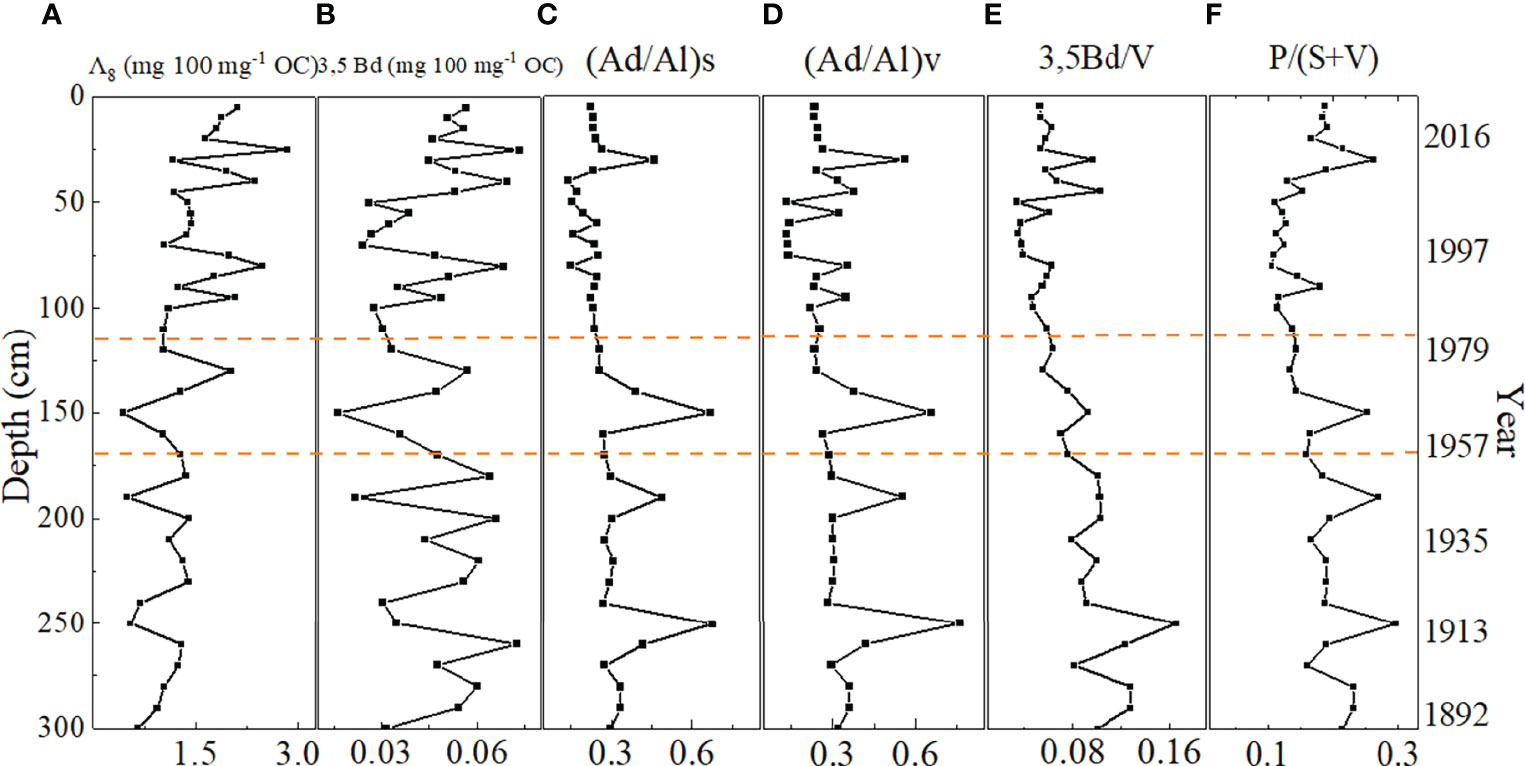
Figure 4 Depth profiles of (A) Λ8(mg 100mg-1 OC), (B) 3,5Bd (mg 100mg-1 OC), (C) the (Ad/Al)s ratio, (D) (Ad/Al)v ratio, (E) 3,5Bd/V ratio, and (F) P/(S+V) ratio in sediment cores of the PRE.
3.5 Modelling Results and Carbon Burial Rates
The modelling results showed historical variability in the Fmbio, OCpetro (%), OCbio (%), OCbio/OC (%) in the PRE sediments (Figure 5A and Table 1). The Fmbio (0.79) values in 1957-1980 were smaller than before 1980 (0.63). Conversely, the OCpetro value (0.03) in 1957-1980 was three times higher than before 1980 (0.01), indicating more petrogenic and less biospheric OC input during 1957-1980.
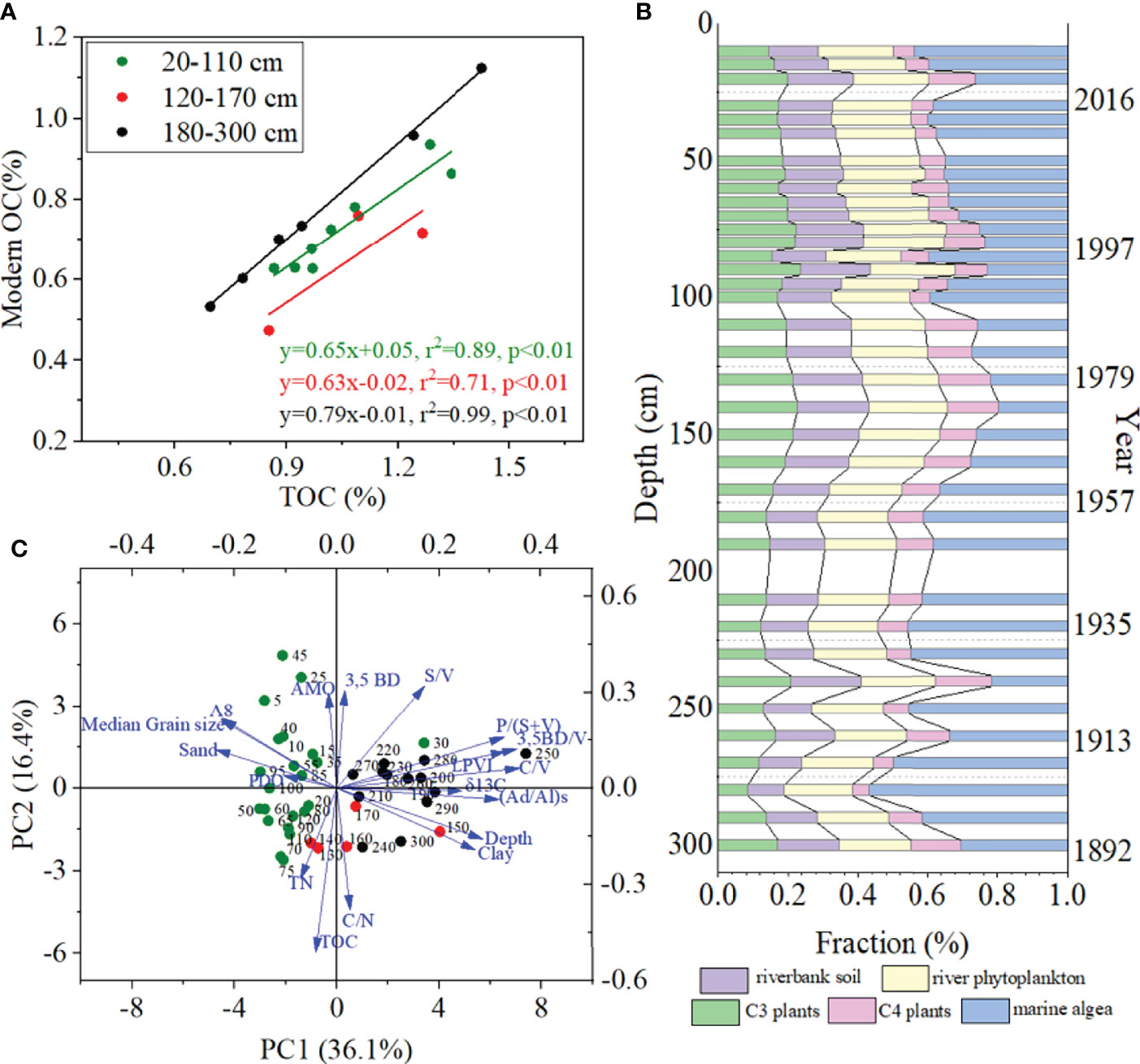
Figure 5 (A) A binary plot used to determine OCpetro concentration in bulk sediments by plotting Fm of bulk TOC × TOC (%) vs. TOC (%). The solid lines represented the best linear fit of the three periods. The slope represented the fraction of modern value of biospheric OC (Fmbio), while the intercept on x-axis represented the content of petrogenic OC (OCpetro) in sediments. (B) Fraction of OC sources from five end-members based on Monte Carlo simulation of the PRE sediment core. (C) Principal component analyses of parameters in this study.
The comprehensive five-end-member simulation showed that the fraction of C3 plants (FC3), riverbank soil (Fsoil), riverine phytoplankton (Friverine phytoplankton), marine algae (Fmarine) and, C4 plants (FC4) were 17 ± 3%, 17 ± 2%, 22 ± 1%, 35 ± 10%, 10 ± 3%, respectively (Figure 5B and Table 2). The fraction of total terrestrial OC (Fterr= FC3+Fsoil+Friverine phytoplankton+FC4) was 65 ± 10%. The FC3 in 1893-1957 was 14 ± 3%, which was smaller than 1957-1980 (20 ± 2%) and 1980-2016 (19 ± 2%). The FC4 in 1980-2016 was lower than 1957-1980(13 ± 2%) and 1897-1957(10 ± 4%). This indicated a change in plant contribution from C4 plants to C3 plants from the bottom to the surface sediment. The Fsoil (19 ± 2%) in 1957-1980 were larger than the average value (17 ± 2%), while the Fmarine (27 ± 6%) in 1957-1980 was smaller than the average value (35 ± 10%) in the entire core.

Table 2 Fraction of C3 plants, riverbank soil, riverine phytoplankton, C4 plants, marine algae and total terrestrial OC in PRE sediments.
The PCA biplot included 17 normalized variables (Figure 5C). The first two components explained 52.5% of the sedimentary OC (PC1: 36.1% and PC2: 16.4%). The plot showed significant depth variations of the distribution and preservation of sedimentary OC across different temporal scales (Figure 5B). Most of the samples above 110cm (exclude 30cm) were located in Quadrant II and III, and the samples between 120 and 180cm (exclude 180cm) were located in Quadrants III and IV, while most of the deeper (190-300cm) samples were located in Quadrants I and IV.
The bulk OC burial rate increased from 1893-1957 (94 ± 22 gC m-2 yr-1) to 1957-1980 (138 ± 24 gC m-2 yr-1), but decreased after 1980 (151 ± 44 gC m-2 yr-1) (Table 3). The trends of the end-member burial rates were similar to the bulk OC, which were increased first and then decreased except the burial rate of marine algae (Table 3).
4 Discussion
4.1 Historical Changes of OC Sources in the PRE Sediment
The significant correlation between δ13C and C/N (R2 = 0.55, p< 0.01, Figure 6A) suggested mixed source input from terrestrial and marine OC. The MGS and δ13C signatures were invariable in the bottom of the sediment core (> 150cm), but became coarser and more negative at discrete intervals in upper parts of the core (above 150cm) (Figures 3C, F) indicating a shift from marine plankton with younger 14C age to terrestrial derived OC which was older (Figures 3E, 5A). This was likely due to a transition (~ the 1980) from steady-state deposition with lower sediment accumulation rate (Figure 2 and Table S2), to an environment influenced by both stronger anthropogenic and climate disturbance in the modern PRE (Yuan et al., 2019) with enhanced sediment accumulation rate (Figure 2 and Table S2) (Owen and Lee, 2004).
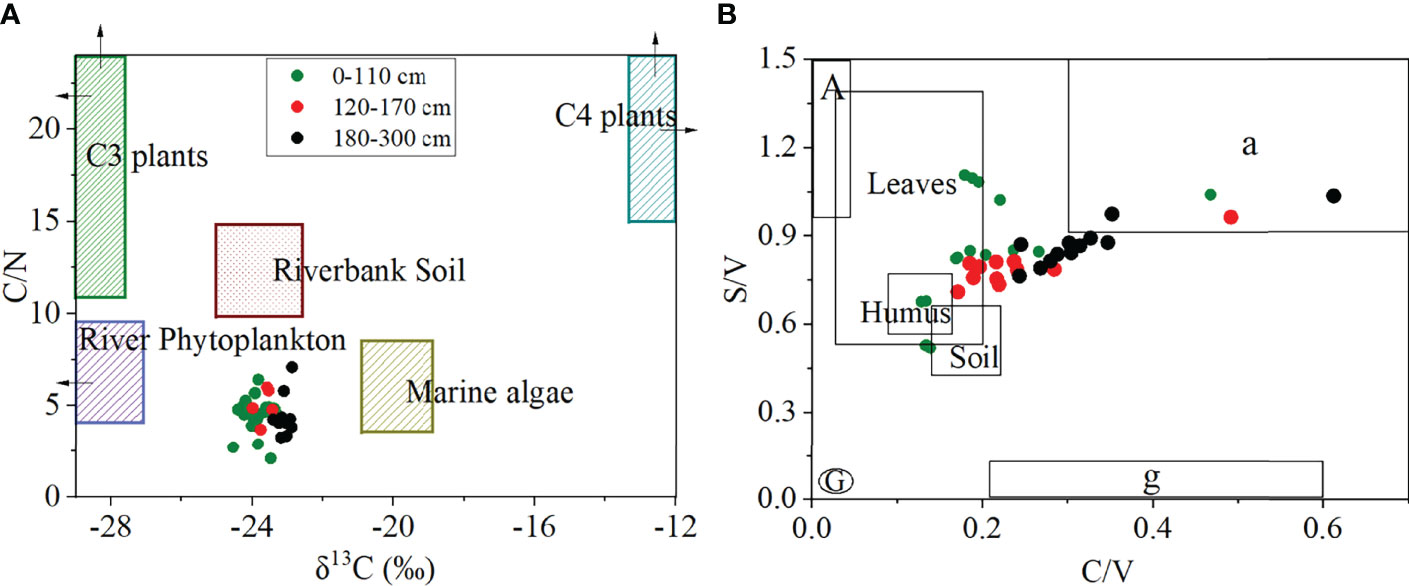
Figure 6 Source plot of the sedimentary OC in the PRE based on (A) δ13C and C/N; (B) C/V and S/V. G=woody gymnosperm, g=nonwoody gymnosperm, A=woody angiosperm and a=nonwoody angiosperm.
More than 100 red tides have been reported in the PRE since the 1970s, and their frequency has increased in recent years due to human influences (Jia and Peng, 2003; Hu et al., 2008). However, the average marine sourced OC derived from the Monte Carlo model decreased by 20% during 1980-2016 than that from 1893-1957 (Table 2). This may be related to the high degradation rates of marine algae enriched in labile compositions such as carbohydrates, sugars, amino acids, and low-molecular-weight organic acids (Hardison et al., 2013). Instead, the vascular plant biomarker of lignin did not show much degradation during the burial process (Figures 4C, D) that the variations were more induced by concentration changes with continuous input of relatively stable terrestrial OC, which were partly diluted by the marine-derived OC as was indicated from a lack of correlation between lignin‐phenol abundance and TOC (R2 = 0.02, p<0.01).
Sources of vascular plant derived OC changed during the sediment accumulation. In general, the angiosperms (woody or non-woody tissues) contain a large amount of syringyl phenols. Therefore, the bottom depths with relatively high S/V values (0.83 ± 0.14, n=40) (Figure 6B) suggested an important fraction of lignin originating from angiosperm plants with higher LPVI (Table S3) than that of gymnosperms (Tareq et al., 2004). The surface sediments, instead, tended to be sourced from leaves, humus, and soil (Figure 6B) as a result of increased soil erosion induced terrestrial plant input. The average LPVI decreased from the bottom to the surface sediment also supported a change from angiosperms to gymnosperms (Tareq et al., 2004; Li et al., 2017) that rapid urbanization has caused such loss in the angiosperm-rich farmland since the 1980s (Zhang et al., 2008) (Table S3).
4.2 Biospheric and Petrogenic OC in the PRE Sediment
The sediment record showed a significant input of old carbon during 1957-1980 (Figure 3; Figure 7), which corresponded to lower Fm and OCbio values and higher Δ14C and OCpetro values (Table 1). It happened that this period had a higher TOC value (Figure S1) and terrestrial input (73%, Table 2). This may be caused by hydrodynamic sorting of riverine OC containing significant eroded soil with more negative Δ14C and older apparent ages (Wei et al., 2010). The significant correlation between MGS and Δ14C values (R2 = 0.52, p<0.01, Figure S3) illustrated the importance of hydrodynamics on the transport and sorting of different grain size fractions, which should be considered in the aging processes, particularly in the estuary and continental shelf characterizing by strong hydrodynamic gradients (Bao et al., 2018a). Specifically, OC in the finer fraction was the youngest, with 14C ages and OCpetro increasing in the coarser fraction. So, the OC was preferentially associated with fine-grained, large-surface-area minerals providing stronger protection against degradation (Ausín et al., 2021). The young OC with less negative δ13C values (Figure S1) were mainly from marine inputs during 1893-1957. Selective removal of labile marine OC was expected leading to more negative Δ14C and δ13C values, via preferential degradation of 13C-enriched, labile organic components afterwards. Correspondingly, the finer EM1 (6.72 μm) was dominant (78.4 ± 14.8%, n=40) (Figure S2) in the bottom of the PRE sediments, which was beneficial to the OC preservation. Thus, the 14C age was younger with higher EM1.
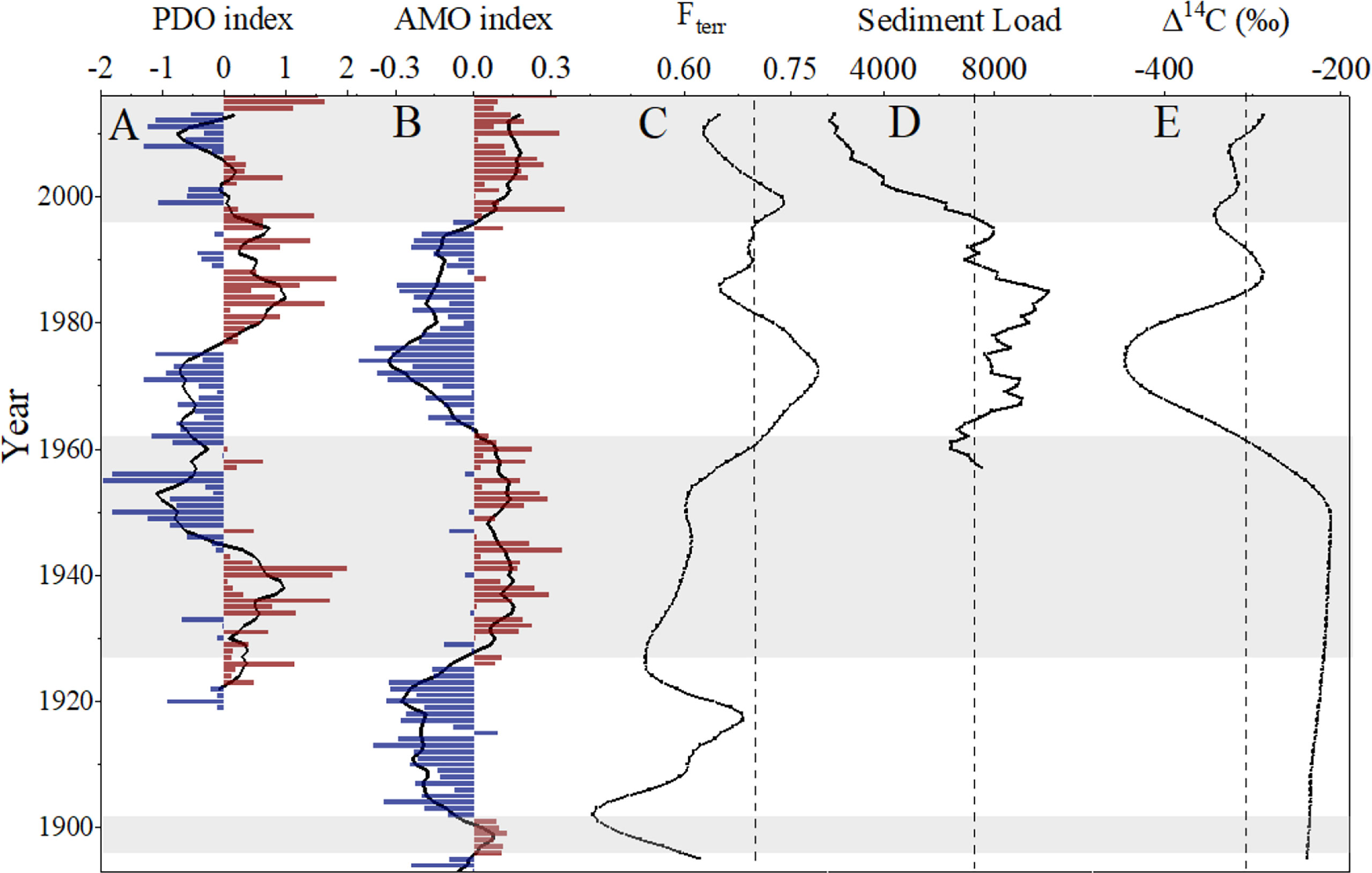
Figure 7 (A)Pacific Decadal Oscillation (PDO) index; (B) Atlantic Multidecadal Oscillation (AMO) index; (C) Fraction of total terrestrial OC (Fterr, %) from Monte Carlo simulation; (D) Sediment load (104 t yr-1) of Pearl River, modified from Wei et al. (2020); and (E) Δ14C (‰). The interpolation method was applied for data processing. The values for the PDO index were taken from University of Washington (http://jisao.washington.edu/pdo/PDO.latest) (Duan et al., 2013). The AMO index is downloaded from Earth System Research Laboratory (http://www.esrl.noaa.gov/psd/data/correlation/amon.sm.data). The bars were annual index value. The black curves represented 7 year running mean value in (A, B) The monthly mean global average sea surface temperature (SST) anomalies have been removed to separate this pattern of variability from any global warming signal that may be present in the data (Mantua et al., 1997).
The average Fmbio value (0.71) of the PRE is the lowest in coastal China among Bohai, Yellow Sea, and East China Sea (Table 4), but closer to that of the Yellow River POC (0.61), indicating older ages of biogenic OC in the PRE. The Yellow River generally has a large amount of pre-aged POC sourced from the Loess Plateau where distributes thick loess-paleosol deposits, with serious soil erosion and sparse vegetation (Eliassen, 2020). In comparison, the upper and middle reaches of the PRE drain the karst morphology areas with the carbonate rocks being the dominant lithology (Wu et al., 2020). The weathering rate of carbonate rocks is more than an order of magnitude higher than that of silicate rocks (Meybeck, 1987). As the carbonate rock weathers, the pre-aged materials including dissolved inorganic carbon are released into the river (Liu et al., 2017). Phytoplankton thus synthesize such old dissolved inorganic carbon resulting in biogenic OC with older ages and low Fmbio values (Table 4). The relatively lower Fmbio values have also been found in some other fluvial systems such as in the Mackenzie River shelf (Table 4). Warming and associated permafrost thaw exposed older biogenic OC with less OCpetro and lower Fmbio accumulated in marine sediments (Hilton et al., 2015). Therefore, the relatively low Fmbio composition in these aquatic ecosystems were attributed to the contribution from pre-aged OC in the drainage basin. As a result, the OCpetro contributed to 2% of TOC in the PRE. The ratio can be as low as 1% in the East China Sea sand area, and as high as 87% in the southeast Alaskan fjords (Table 4). But on average, the OCpetro/TOC in the PRE was lower than the mean values of 13 ± 18% in global aquatic systems.
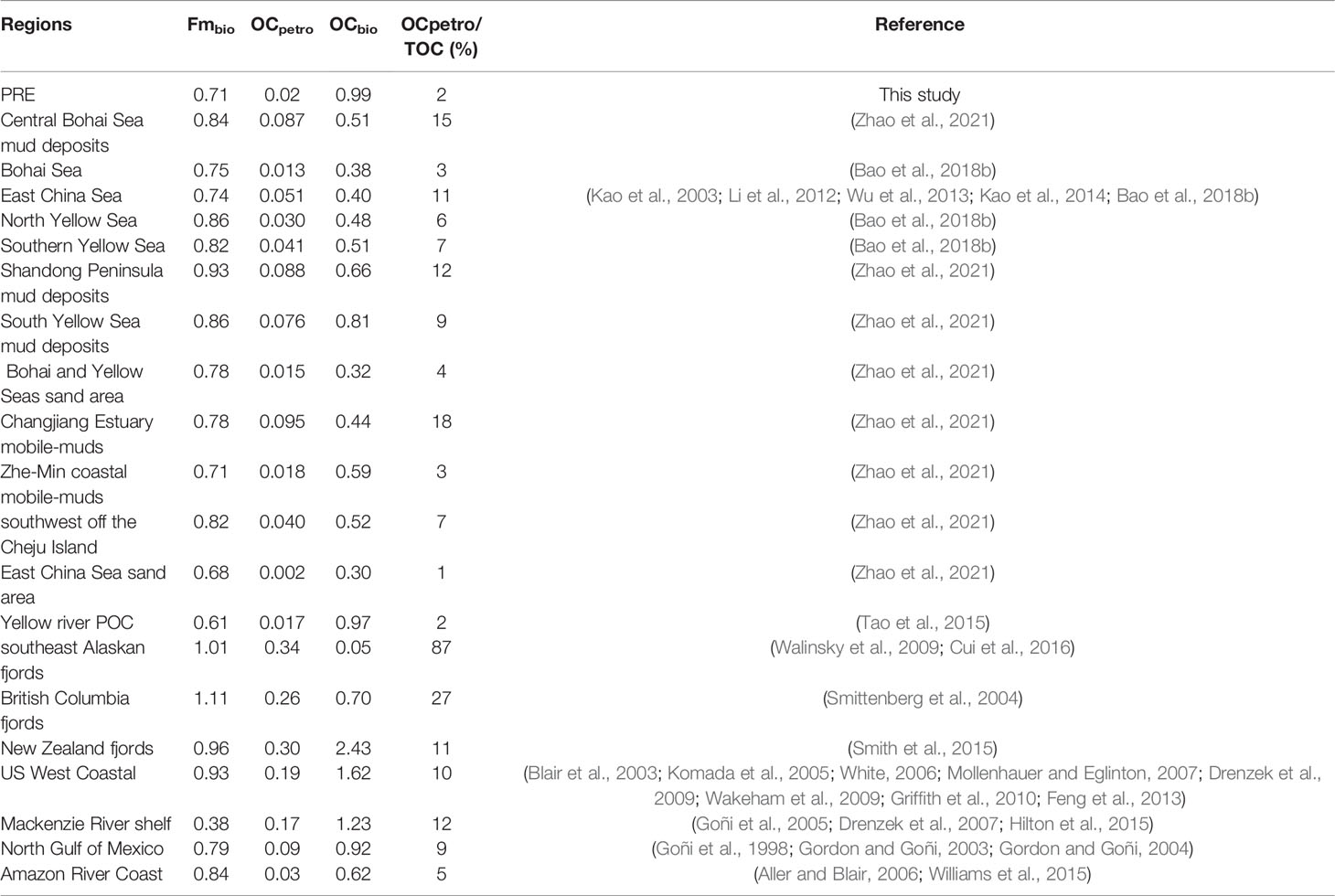
Table 4 Comparison of Fmbio, OCpetro, OCbio and OCpetro/TOC values in the sediments between the PRE and other studies.
4.3 Drivers for the Historical Changes of OC Burial Rate
4.3.1 The Climate Oscillation Effect
The climate oscillation associated with rainfall intensity affected the frequency and magnitude of soil erosion (Starkloff and Stolte, 2014; Li and Fang, 2016; Zhang et al., 2022) and sediment accumulation rate. A significant increase in the sedimentary grain size in the upper core around 2008 (Figure 3F) suggested stronger hydrodynamic conditions due to pulsed flooding events this year with significant sediment delivery (Owen and Lee, 2004). In fact, a big flooding event occurred in almost every other year between 2000 and 2011 due to typhoon and flood impacts in the PRE (Yang et al., 2015). Strong rainfall-runoff processes would in turn erode deep soil and vascular plant OC from the drainage basin in pulses (Hao and Lu, 2021), which cause a large amount of old OC to enter the river and further the PRE. As a result, the riverine POC was dominated by aquatic organisms during the low-flow periods, while the terrigenous POC (mainly from soil minerals and degraded plant debris) became dominant during high-flow periods. The pulsed (Ad/Al)v and (Ad/Al)s ratio that appeared during this time proved the input of highly degraded vascular plant materials during the flooding erosion. The increased transportation capacity of rivers could then accelerate POC transportation with less residence time for POC oxidation and high burial efficiency (Blair and Aller, 2012). Therefore, the export of OCpetro and the escape of OCbio from oxidation by rapid transport along rivers resulted in subsequent increase in OC burial on adjacent margins in the PRE. In fact, the 1957-1980 period witnessed 4.6 times higher petrogenic OC burial rate than before with a significant decrease of Δ14C from -218.6 ± 81.8‰ (1893-1957) to -395.3 ± 31.8 ‰ (1957-1980) (Figure 7 and Table 3).
The large variability of summer precipitation may easily trigger floods and droughts in the Pearl River basin that a correlation (R2 = 0.89) has been found between precipitation and surface runoff (Luo et al., 2016). As global climate phenomenon, the PDO have been reported to be closely related to precipitation and droughts frequency in China over the last century (Chan and Zhou, 2005; Duan et al., 2013; Yang et al., 2017a; Yang et al., 2017b). However, the AMO may act as a key pacemaker that the western tropical Pacific multidecadal climate variability is forced by the AMO instead of PDO in interdecadal time scales over the last century (Sun et al., 2017; Zheng and Wang, 2021). In this study, the correlations between terrestrial OC parameters (e.g., Fterr, Table 5) and both 7-year running mean of climate oscillation index were not significantly correlated (AMO, R = 0.28, p <0.01; PDO, R=0.27, p <0.01) (Figure 7 and Table 5) through the whole core. However, there is a significant correlation between Fterr and PDO than that with AMO before 1957 (R=0.43, p<0.01). Instead, the AMO and Fterr were significantly correlated between 1957-1980 (R=-0.93, p<0.01), but not before 1957 (R=-0.10, p=0.43) and after 1980 (R=-0.20, p=0.27), indicating that the climate effect on the OC burial has changed from PDO before 1957, to AMO between 1957 and 1980 in the PRE. The reason might be that during the negative phase of AMO (e.g., 1957-1980), humid climate condition and more typhoon events in PRE intensified the washout of riverbanks and surrounding soils, hence more terrestrial (Fterr) including petrogenic OC were transported to the sediments (Figure 7 and Table 5). At the positive phase of AMO, cold climate and less precipitation, in contrast, would result in less soil erosion, delivery and burial of terrestrial and petrogenic OC. There was no significant correlation between the Fterr and the climate oscillation index (AMO, R = 0.20, p =0.27; PDO, R=0.31, p=0.08) after 1980, which is mostly related to the beginning of Economic Reform and Open Up, suggesting the dominate influence from human perturbations.
4.3.2 The Impact From Human Perturbations
Deforestation tended to destabilize slopes and increased soil erosion rates (Owen and Lee, 2004). With the explosive growth of population and gross domestic product (GDP) in the Pearl River basin (Figure 8), large areas have been deforested since the 1950s (Liu et al., 2014). Correspondingly, the average Λ8 value increased from 1.02 (n=10) before 1950 to 1.52 (n=30) mg 100 mg-1 OC after 1950 (Figure 8) with 1.6 times increase in the lignin burial rates from 1893-1957 (1.0 g lignin m-2 yr-2) to 1980-2016 (2.4 g lignin m-2 yr-2) (Table 3). The obvious discontinuities displayed on Λ8 were also evidence of deforestation activities (Bélanger et al., 2017). In Foshan, a city on the coast of PRE, approximately 60% of the newly built-up land was converted from pond, farmland, forest, and shrub during 1988–2003, and the forest and shrub were then changed to farmland to compensate the farmland loss (Yang et al., 2015). Eventually, a shift from marine plankton to terrestrial plants was observed from the bottom to surface sediments, in agreement with significant older radiocarbon signature (Figure 5A) with increased MGS in the study (Figure 3F). The dam construction on Pearl rivers have greatly modified the transport of sediment since mid-1980s (Dai et al., 2008; Wei et al., 2020). The decrease of TOC (Figure 3A) and Fterr (Figure 7C), as well as significant correlation between sediment load and Fterr (R=0.64, p<0.01) supported that dam construction has reduced delivery of terrestrial derived OC since the 1980s. The unpredictable human disturbance also likely resulted in dynamic source and fate of OC and unresolved OCpetro(Figure 5A and Table 1). So, the OC cycle under the varied extent of human perturbations in the PRE is definitely important to monitor in the future.
5 Conclusions
The study synthesized marine sediment records spanning the past 130 yr to decipher the sources and burial rate of OC in the PRE. The results suggested three stages of 1893-1957, 1957-1980 and 1980-2016 with distinct OC features. The 1893-1957 stage was more affected by the PDO with burial of younger marine derived OC. The input of petrogenic OC is increasing during the 1957-1980 that the burial rate of OCpetro was 4.6 times higher than before due to input of eroded older soil OC and marine derived OC assimilated from weathered old dissolved inorganic carbon. Additionally, a significant correlation between the Fterr and AMO was observed that the increasing frequency of the negative AMO events. After 1980, there was no significant relationship between Fterr and the two climate indices suggesting a shift to human perturbation such as deforestation and dam construction likely affecting the OC burial in the region. A transition stage from low sediment accumulation rate to a relatively higher deposition environment was observed after ca. ~1980s in the PRE. Therefore, it is important to understand the effects of climate oscillation and human perturbation on the OC burial in the dynamic PRE to better understand its role in current climate change.
Data Availability Statement
The original contributions presented in the study are included in the article/Supplementary Material. Further inquiries can be directed to the corresponding author.
Author Contributions
Conceptualization: XL and CW. Methodology: WL, XL, and CW. Investigation: WL, XZ, CS, TN, and YH. Visualization: WL and XL. Funding acquisition: XL. Supervision: XL and CW. Writing – original draft: WL, XL, and CW. Writing – review and editing: WL, XL, XZ, CS, TN, YH, and CW. All authors contributed to the article and approved the submitted version.
Funding
This work was supported by Southern Marine Science and Engineering Guangdong Laboratory (Guangzhou) (GML2019ZD0210, K19313901), National Natural Science Foundation of China (42076029, 41720104001), Shenzhen Key Laboratory of Marine Archaea Geo-Omics, Southern University of Science and Technology (ZDSYS20180208184349083) and Guangxi Key Laboratory of Marine Disaster in the Beibu Gulf, Beibu Gulf University (No.2020KF01).
Conflict of Interest
The authors declare that the research was conducted in the absence of any commercial or financial relationships that could be construed as a potential conflict of interest.
Publisher’s Note
All claims expressed in this article are solely those of the authors and do not necessarily represent those of their affiliated organizations, or those of the publisher, the editors and the reviewers. Any product that may be evaluated in this article, or claim that may be made by its manufacturer, is not guaranteed or endorsed by the publisher.
Acknowledgments
We would like to thank Wenxiu Wang and Chuanlun Zhang for providing sediment samples, Hanchao Jiang and Qiaoqiao Guo for grain size measurement and analysis, Daidu Fan and Yijing Wu for the 210 Pb measurement and analysis, and the reviewers for the constructive comments.
Supplementary Material
The Supplementary Material for this article can be found online at: https://www.frontiersin.org/articles/10.3389/fmars.2022.848757/full#supplementary-material
References
Aller R. C., Blair N. E. (2006). Carbon Remineralization in the Amazon–Guianas Tropical Mobile Mudbelt: A Sedimentary Incinerator. Continent. Shelf. Res. 26, 2241–2259. doi: 10.1016/j.csr.2006.07.016
Appleby P. G., Oldfield F. (1978). The Calculation of Lead-210 Dates Assuming a Constant Rate of Supply of Unsupported 210Pb to the Sediment. CATENA 5, 1–8. doi: 10.1016/S0341-8162(78)80002-2
Ausín B., Bruni E., Haghipour N., Welte C., Bernasconi S. M., Eglinton T. I. (2021). Controls on the Abundance, Provenance and Age of Organic Carbon Buried in Continental Margin Sediments. Earth Planet. Sci. Lett. 558, 1–10. doi: 10.1016/j.epsl.2021.116759
Bao R., Uchida M., Zhao M., Haghipour N., Montlucon D., McNichol A., et al. (2018a). Organic Carbon Aging During Across-Shelf Transport. Geophys. Res. Lett. 45, 8425–8434. doi: 10.1029/2018GL078904
Bao R., van der Voort T. S., Zhao M., Guo X., Montluçon D. B., McIntyre C., et al. (2018b). Influence of Hydrodynamic Processes on the Fate of Sedimentary Organic Matter on Continental Margins. Global Biogeochem. Cycle. 32, 1420–1432. doi: 10.1029/2018GB005921
Bélanger É, Lucotte M., Moingt M., Paquet S., Oestreicher J., Rozon C. (2017). Altered Nature of Terrestrial Organic Matter Transferred to Aquatic Systems Following Deforestation in the Amazon. Appl. Geochem. 87, 136–145. doi: 10.1016/j.apgeochem.2017.10.016
Bianchi T. S., Allison M. A. (2009). Large-River Delta-Front Estuaries as Natural "Recorders" of Global Environmental Change. Proc. Natl. Acad. Sci. 106, 8085–8092. doi: 10.1073/pnas.0812878106
Bianchi T. S., Cui X. Q., Blair N. E., Burdige D. J., Eglinton T. I., Galy V. (2018). Centers of Organic Carbon Burial and Oxidation at the Land-Ocean Interface. Organ. Geochem. 115, 138–155. doi: 10.1016/j.orggeochem.2017.09.008
Bianchi T. S., Mitra S., McKee B. A. (2002). Sources of Terrestrially-Derived Organic Carbon in Lower Mississippi River and Louisiana Shelf Sediments: Implications for Differential Sedimentation and Transport at the Coastal Margin. Mar. Chem. 77, 211–223. doi: 10.1016/S0304-4203(01)00088-3
Blair N. E., Aller R. C. (2012). The Fate of Terrestrial Organic Carbon in the Marine Environment. Ann. Rev. Mar. Sci. 4, 401–423. doi: 10.1146/annurev-marine-120709-142717
Blair N. E., Leithold E. L., Ford S. T., Peeler K. A., Holmes J. C., Perkey D. W. (2003). The Persistence of Memory: The Fate of Ancient Sedimentary Organic Carbon in a Modern Sedimentary System. Geochim. Cosmochim. Acta 67, 63–73. doi: 10.1016/S0016-7037(02)01043-8
Breithaupt J. L., Smoak J. M., Bianchi T. S., Vaughn D. R., Sanders C. J., Radabaugh K. R., et al. (2020). Increasing Rates of Carbon Burial in Southwest Florida Coastal Wetlands. J. Geophys. Res.: Biogeosci. 125 (2), 1–25. doi: 10.1029/2019jg005349
Canuel E. A., Hardison A. K. (2016). Sources, Ages, and Alteration of Organic Matter in Estuaries. Ann. Rev. Mar. Sci. 8, 409–434. doi: 10.1146/annurev-marine-122414-034058
Chan J. C. L., Zhou W. P. D. O. (2005). ENSO and the Early Summer Monsoon Rainfall Over South China. Geophys. Res. Lett. 32 (8), 1–5. doi: 10.1029/2004GL022015
Chen S.-J., Luo X.-J., Mai B.-X., Sheng G.-Y., Fu J.-M., Zeng E. Y. (2006). Distribution and Mass Inventories of Polycyclic Aromatic Hydrocarbons and Organochlorine Pesticides in Sediments of the Pearl River Estuary and the Northern South China Sea. Environ. Sci. Technol. 40, 709–714. doi: 10.1021/es052060g
Chen Z., Nie T., Zhao X., Li J., Yang B., Cui D., et al. (2022). Organic Carbon Remineralization Rate in Global Marine Sediments: A Review. Reg. Stud. Mar. Sci. 49, 102112. doi: 10.1016/j.rsma.2021.102112
Clark K. E., Hilton R. G., West A. J., Malhi Y., Gröcke D. R., Bryant C. L., et al. (2013). New Views on “Old” Carbon in the Amazon River: Insight From the Source of Organic Carbon Eroded From the Peruvian Andes. Geochem. Geophys. Geosyst. 14, 1644–1659. doi: 10.1002/ggge.20122
Cui X. Q., Bianchi T. S., Jaeger J. M., Smith R. W. (2016). Biospheric and Petrogenic Organic Carbon Flux Along Southeast Alaska. Earth Planet. Sci. Lett. 452, 238–246. doi: 10.1016/j.epsl.2016.08.002
Dai S. B., Yang S. L., Cai A. M. (2008). Impacts of Dams on the Sediment Flux of the Pearl River, Southern China. CATENA 76 (1), 36–43. doi: 10.1016/j.catena.2008.08.004
Dalzell B. J., Filley T. R., Harbor J. M. (2005). Flood Pulse Influences on Terrestrial Organic Matter Export From an Agricultural Watershed. J. Geophys. Res.: Biogeosci. 110 (G2), 1–14. doi: 10.1029/2005jg000043
Drenzek N. J., Hughen K. A., Montluçon D. B., Southon J. R., Dos Santos G. M., Druffel E. R. M., et al. (2009). A New Look at Old Carbon in Active Margin Sediments. Geology 37, 239–242. doi: 10.1130/G25351A.1
Drenzek N. J., Montluçon D. B., Yunker M. B., Macdonald R. W., Eglinton T. I. (2007). Constraints on the Origin of Sedimentary Organic Carbon in the Beaufort Sea From Coupled Molecular 13C and 14C Measurements. Mar. Chem. 103, 146–162. doi: 10.1016/j.marchem.2006.06.017
Drinkwater K. F., Miles M., Medhaug I., Otterå O. H., Kristiansen T., Sundby S., et al. (2014). The Atlantic Multidecadal Oscillation: Its Manifestations and Impacts With Special Emphasis on the Atlantic Region North of 60°N. J. Mar. Syst. 133, 117–130. doi: 10.1016/j.jmarsys.2013.11.001
Duan W. S., Song L. Y., Li Y., Mao J. Y. (2013). Modulation of PDO on the Predictability of the Interannual Variability of Early Summer Rainfall Over South China. J. Geophys. Res-Atmos. 118, 13008–13021. doi: 10.1002/2013JD019862
Eliassen S. (2020). Soil Erosion and River Regulation With Special Reference to the Yellow River. Environ. Geomorph. Landscape Conserv. Routled. 17, 325–344. doi: 10.4324/9781003026563-18
Feng X., Benitez-Nelson B. C., Montluçon D. B., Prahl F. G., McNichol A. P., Xu L., et al. (2013). 14C and 13C Characteristics of Higher Plant Biomarkers in Washington Margin Surface Sediments. Geochim. Cosmochim. Acta 105, 14–30. doi: 10.1016/j.gca.2012.11.034
Galy V., Beyssac O., France-Lanord C., Eglinton T. (2008). Recycling of Graphite During Himalayan Erosion: A Geological Stabilization of Carbon in the Crust. Science 322, 943–945. doi: 10.1126/science.1161408
Goñi M. A., Hedges J. I. (1995). Sources and Reactivities of Marine-Derived Organic Matter in Coastal Sediments as Determined by Alkaline CuO Oxidation. Geochim. Cosmochim. Acta 59, 2965–2981. doi: 10.1016/0016-7037(95)00188-3
Goñi M. A., Ruttenberg K. C., Eglinton T. I. (1998). A Reassessment of the Sources and Importance of Land-Derived Organic Matter in Surface Sediments From the Gulf of Mexico. Geochim. Cosmochim. Acta 62, 3055–3075. doi: 10.1016/S0016-7037(98)00217-8
Goñi M. A., Yunker M. B., Macdonald R. W., Eglinton T. I. (2005). The Supply and Preservation of Ancient and Modern Components of Organic Carbon in the Canadian Beaufort Shelf of the Arctic Ocean. Mar. Chem. 93, 53–73. doi: 10.1016/j.marchem.2004.08.001
Gordon E. S., Goñi M. A. (2003). Sources and Distribution of Terrigenous Organic Matter Delivered by the Atchafalaya River to Sediments in the Northern Gulf of Mexico. Geochim. Cosmochim. Acta 67, 2359–2375. doi: 10.1016/S0016-7037(02)01412-6
Gordon E. S., Goñi M. A. (2004). Controls on the Distribution and Accumulation of Terrigenous Organic Matter in Sediments From the Mississippi and Atchafalaya River Margin. Mar. Chem. 92, 331–352. doi: 10.1016/j.marchem.2004.06.035
Griffith D. R., Martin W. R., Eglinton T. I. (2010). The Radiocarbon Age of Organic Carbon in Marine Surface Sediments. Geochim. Et. Cosmochim. Acta 74, 6788–6800. doi: 10.1016/j.gca.2010.09.001
Hao Y., Lu J. (2021). Teleconnection Between Climate Oscillations and Riverine Nutrient Dynamics in Southeast China Based on Wavelet Analysis. Environ. Sci. Pollu. Res 28, 41807–41820. doi: 10.1007/s11356-021-13715-x
Hardison A. K., Canuel E. A., Anderson I. C., Tobias C. R., Veuger B., Waters M. N. (2013). Microphytobenthos and Benthic Macroalgae Determine Sediment Organic Matter Composition in Shallow Photic Sediments. Biogeosciences 10, 5571–5588. doi: 10.5194/bg-10-5571-2013
Hedges J. I., Blanchette R. A., Weliky K., Devol A. H. (1988). Effects of Fungal Degradation on the CuO Oxidation Products of Lignin: A Controlled Laboratory Study. Geochim. Cosmochim. Acta 52, 2717–2726. doi: 10.1016/0016-7037(88)90040-3
Hedges J. I., Ertel J. R. (1982). Characterization of Lignin by Gas Capillary Chromatography of Cupric Oxide Oxidation Products. Anal. Chem. 54, 174–178. doi: 10.1021/ac00239a007
Hedges J. I., Mann D. C. (1979). The Characterization of Plant Tissues by Their Lignin Oxidation Products. Geochim. Cosmochim. Acta 43, 1803–1807. doi: 10.1016/0016-7037(79)90028-0
Hedges J. I., Parker P. L. (1976). Land-Derived Organic Matter in Surface Sediments From the Gulf of Mexico. Geochim. Cosmochim. Acta 40, 1019–1029. doi: 10.1016/0016-7037(76)90044-2
Hilton R. G., Galy V., Gaillardet J., Dellinger M., Bryant C., O'Regan M., et al. (2015). Erosion of Organic Carbon in the Arctic as a Geological Carbon Dioxide Sink. Nature 524, 84–87. doi: 10.1038/nature14653
Houel S., Louchouarn P., Lucotte M., Canuel R., Ghaleb B. (2006). Translocation of Soil Organic Matter Following Reservoir Impoundment in Boreal Systems: Implications for in Situ Productivity. Limnol. Oceanog. 51, 1497–1513. doi: 10.4319/lo.2006.51.3.1497
Hu J., Zhang G., Li K., Peng PA, Chivas A. R. (2008). Increased Eutrophication Offshore Hong Kong, China During the Past 75 Years: Evidence From High-Resolution Sedimentary Records. Mar. Chem. 110, 7–17. doi: 10.1016/j.marchem.2008.02.001
Jiang H., Guo G., Cai X., Thompson J. A., Xu H., Zhong N. (2016). Geochemical Evidence of Windblown Origin of the Late Cenozoic Lacustrine Sediments in Beijing and Implications for Weathering and Climate Change. Palaeogeograph. Palaeoclimatol. Palaeoecol. 446, 32–43. doi: 10.1016/j.palaeo.2016.01.017
Jiang H., Wan S., Ma X., Zhong N., Zhao D. (2017). End-Member Modeling of the Grain-Size Record of Sikouzi Fine Sediments in Ningxia (China) and Implications for Temperature Control of Neogene Evolution of East Asian Winter Monsoon. PloS One 12, e0186153. doi: 10.1371/journal.pone.0186153
Jia G.-D., Peng P.-A. (2003). Temporal and Spatial Variations in Signatures of Sedimented Organic Matter in Lingding Bay (Pearl Estuary), Southern China. Mar. Chem. 82, 47–54. doi: 10.1016/S0304-4203(03)00050-1
Kao S. J., Hilton R. G., Selvaraj K., Dai M., Zehetner F., Huang J. C., et al. (2014). Preservation of Terrestrial Organic Carbon in Marine Sediments Offshore Taiwan: Mountain Building and Atmospheric Carbon Dioxide Sequestration. Earth Surf. Dynamic. 2, 127–139. doi: 10.5194/esurf-2-127-2014
Kao S. J., Lin F. J., Liu K. K. (2003). Organic Carbon and Nitrogen Contents and Their Isotopic Compositions in Surficial Sediments From the East China Sea Shelf and the Southern Okinawa Trough. Deep. Sea. Res. Part II: Top. Stud. Oceanog. 50, 1203–1217. doi: 10.1016/S0967-0645(03)00018-3
Komada T., Druffel E. R. M., Hwang J. (2005). Sedimentary Rocks as Sources of Ancient Organic Carbon to the Ocean: An Investigation Through Δ14C and δ13c Signatures of Organic Compound Classes. Global Biogeochem. Cycle. 19 (2), 1–10. doi: 10.1029/2004GB002347
Li S., Bates G. T. (2007). Influence of the Atlantic Multidecadal Oscillation on the Winter Climate of East China. Adv. Atmos. Sci. 24, 126–135. doi: 10.1007/s00376-007-0126-6
Li X. X., Bianchi T. S., Allison M. A., Chapman P., Mitra S., Zhang Z. R., et al. (2012). Composition, Abundance and Age of Total Organic Carbon in Surface Sediments From the Inner Shelf of the East China Sea. Mar. Chem. 145, 37–52. doi: 10.1016/j.marchem.2012.10.001
Li X. X., Bianchi T. S., Allison M. A., Chapman P., Yang G. P. (2013). Historical Reconstruction of Organic Carbon Decay and Preservation in Sediments on the East China Sea Shelf. J. Geophys. Res-Biogeosci. 118, 1079–1093. doi: 10.1002/jgrg.20079
Li X. X., Zhang Z. R., Wade T. L., Knap A. H., Zhang C. L. L. (2017). Sources and Compositional Distribution of Organic Carbon in Surface Sediments From the Lower Pearl River to the Coastal South China Sea. J. Geophys. Res-Biogeosci. 122, 2104–2117. doi: 10.1002/2017JG003981
Li W., Li X., Mei X., Zhang F., Xu J., Liu C., et al. (2021). A Review of Current and Emerging Approaches for Quaternary Marine Sediment Dating. Sci. Total. Environ. 780, 1–19. doi: 10.1016/j.scitotenv.2021.146522
Li Z., Fang H. (2016). Impacts of Climate Change on Water Erosion: A Review. Earth-Sci. Rev. 163, 94–117. doi: 10.1016/j.earscirev.2016.10.004
Li Z., Wu Y., Yang L., Du J., Deng B., Zhang J. (2020). Carbon Isotopes and Lignin Phenols for Tracing the Floods During the Past 70 Years in the Middle Reach of the Changjiang River. Acta Oceanologica. Sin. 39, 33–41. doi: 10.1007/s13131-020-1543-y
Liu F., Yuan L., Yang Q., Ou S., Xie L., Cui X. (2014). Hydrological Responses to the Combined Influence of Diverse Human Activities in the Pearl River Delta, China. CATENA 113, 41–55. doi: 10.1016/j.catena.2013.09.003
Liu Z., Zhao M., Sun H., Yang R., Chen B., Yang M. (2017). “Old” Carbon Entering the South China Sea From the Carbonate-Rich Pearl River Basin: Coupled Action of Carbonate Weathering and Aquatic Photosynthesis. Appl. Geochem. 78, 96–104. doi: 10.1016/j.apgeochem.2016.12.014
Luo Z., Yao C., Li Q., Huang Z. (2016). Terrestrial Water Storage Changes Over the Pearl River Basin From GRACE and Connections With Pacific Climate Variability. Geodes. Geodynamic. 7, 171–179. doi: 10.1016/j.geog.2016.04.008
Mantua N. J., Hare S. R., Zhang Y., Wallace J. M., Francis R. C. (1997). A Pacific Interdecadal Climate Oscillation With Impacts on Salmon Production**. Bull. Am. Meteorological Soc. 78, 1069–1080. doi: 10.1175/1520-0477(1997)078<1069:APICOW>2.0.CO;2
Meybeck M. (1987). Global Chemical Weathering of Surficial Rocks Estimated From River Dissolved Loads. Am. J. Sci. 287, 401–428. doi: 10.2475/ajs.287.5.401
Mollenhauer G., Eglinton T. I. (2007). Diagenetic and Sedimentological Controls on the Composition of Organic Matter Preserved in California Borderland Basin Sediments. Limnol. Oceanog. 52, 558–576. doi: 10.4319/lo.2007.52.2.0558
Owen R. B., Lee R. (2004). Human Impacts on Organic Matter Sedimentation in a Proximal Shelf Setting, Hong Kong. Continent. Shelf. Res. 24, 583–602. doi: 10.1016/j.csr.2003.11.004
Paterson G. A., Heslop D. (2015). New Methods for Unmixing Sediment Grain Size Data. Geochem. Geophys. Geosyst. 16, 4494–4506. doi: 10.1002/2015GC006070
Perdue E. M., Koprivnjak J.-F. (2007). Using the C/N Ratio to Estimate Terrigenous Inputs of Organic Matter to Aquatic Environments. Estua. Coast. Shelf. Sci. 73, 65–72. doi: 10.1016/j.ecss.2006.12.021
Prins M. A., Postma G., Weltje G. J. (2000). Controls on Terrigenous Sediment Supply to the Arabian Sea During the Late Quaternary: The Makran Continental Slope. Mar. Geo. 169, 351–371. doi: 10.1016/S0025-3227(00)00087-6
Qian C., Yu J.-Y., Chen G. (2014). Decadal Summer Drought Frequency in China: The Increasing Influence of the Atlantic Multi-Decadal Oscillation. Environ. Res. Lett. 9, 124004. doi: 10.1088/1748-9326/9/12/124004
Qian C., Zhou T. J. (2014). Multidecadal Variability of North China Aridity and Its Relationship to PDO During 1900-2010. J. Climate 27, 1210–1222. doi: 10.1175/JCLI-D-13-00235.1
Sánchez-García L., de Andrés J. R., Martín-Rubí J. A., Louchouarn P. (2009). Diagenetic State and Source Characterization of Marine Sediments From the Inner Continental Shelf of the Gulf of Cádiz (SW Spain), Constrained by Terrigenous Biomarkers. Organ. Geochem. 40, 184–194. doi: 10.1016/j.orggeochem.2008.11.001
Seidel M., Yager P. L., Ward N. D., Carpenter E. J., Gomes H. R., Krusche A. V., et al. (2015). Molecular-Level Changes of Dissolved Organic Matter Along the Amazon River-To-Ocean Continuum. Mar. Chem. 177, 218–231. doi: 10.1016/j.marchem.2015.06.019
Smith R. W., Bianchi T. S., Allison M., Savage C., Galy V. (2015). High Rates of Organic Carbon Burial in Fjord Sediments Globally. Nat. Geosci. 8, 450–453. doi: 10.1038/ngeo2421
Smittenberg R. H., Hopmans E. C., Schouten S., Hayes J. M., Eglinton T. I., Damste J. S. S. (2004). Compound-Specific Radiocarbon Dating of the Varved Holocene Sedimentary Record of Saanich Inlet, Canada. Paleoceanography 19 (2), 1–16. doi: 10.1029/2003PA000927
Starkloff T., Stolte J. (2014). Applied Comparison of the Erosion Risk Models EROSION 3D and LISEM for a Small Catchment in Norway. CATENA 118, 154–167. doi: 10.1016/j.catena.2014.02.004
Sun C., Kucharski F., Li J., Jin F.-F., Kang I.-S., Ding R. (2017). Western Tropical Pacific Multidecadal Variability Forced by the Atlantic Multidecadal Oscillation. Nat. Commun. 8 (1), 1–10. doi: 10.1038/ncomms15998
Swindles G. T., Galloway J. M., Macumber A. L., Croudace I. W., Emery A. R., Woulds C., et al. (2018). Sedimentary Records of Coastal Storm Surges: Evidence of the 1953 North Sea Event. Mar. Geo. 403, 262–270. doi: 10.1016/j.margeo.2018.06.013
Syvitski J., Ángel J. R., Saito Y., Overeem I., Vörösmarty C. J., Wang H., et al. (2022). Earth’s Sediment Cycle During the Anthropocene. Nat. Rev. Earth Environ. 3, 179–196. doi: 10.1038/s43017-021-00253-w
Tao S., Eglinton T. I., Montluçon D. B., McIntyre C., Zhao M. (2015). Pre-Aged Soil Organic Carbon as a Major Component of the Yellow River Suspended Load: Regional Significance and Global Relevance. Earth Planet. Sci. Lett. 414, 77–86. doi: 10.1016/j.epsl.2015.01.004
Tareq S. M., Tanaka N., Ohta K. (2004). Biomarker Signature in Tropical Wetland: Lignin Phenol Vegetation Index (LPVI) and its Implications for Reconstructing the Paleoenvironment. Sci. Total. Environ. 324, 91–103. doi: 10.1016/j.scitotenv.2003.10.020
Wakeham S. G., Canuel E. A., Lerberg E. J., Mason P., Sampere T. P., Bianchi T. S. (2009). Partitioning of Organic Matter in Continental Margin Sediments Among Density Fractions. Mar. Chem. 115, 211–225. doi: 10.1016/j.marchem.2009.08.005
Walinsky S. E., Prahl F. G., Mix A. C., Finney B. P., Jaeger J. M., Rosen G. P. (2009). Distribution and Composition of Organic Matter in Surface Sediments of Coastal Southeast Alaska. Continent. Shelf. Res. 29, 1565–1579. doi: 10.1016/j.csr.2009.04.006
Wang S., Zhuang Q., Lähteenoja O., Draper F. C., Cadillo-Quiroz H. (2018). Potential Shift From a Carbon Sink to a Source in Amazonian Peatlands Under a Changing Climate. Proc. Natl. Acad. Sci. 115, 12407–12412. doi: 10.1073/pnas.1801317115
Wei X., Cai S., Ni P., Zhan W. (2020). Impacts of Climate Change and Human Activities on the Water Discharge and Sediment Load of the Pearl River, Southern China. Sci. Rep. 10 (1), 1–11. doi: 10.1038/s41598-020-73939-8
Wei X., Yi W., Shen C., Yechieli Y., Li N., Ding P., et al. (2010). 14C as a Tool for Evaluating Riverine POC Sources and Erosion of the Zhujiang (Pearl River) Drainage Basin, South China. Nucl. Instru. Methods Phys. Res. Sec. B.: Beam. Interact. Material. Atoms. 268, 1094–1097. doi: 10.1016/j.nimb.2009.10.107
Wheatcroft R. A., Goñ I M. A., Hatten J. A., Pasternack G. B., Warrick J. A. (2010). The Role of Effective Discharge in the Ocean Delivery of Particulate Organic Carbon by Small, Mountainous River Systems. Limnol. Oceanog. 55, 161–171. doi: 10.4319/lo.2010.55.1.0161
White H. K. (2006). Isotopic Constraints on the Sources and Associations of Organic Compounds in Marine Sediments. Massachu. Insti. Technol 1, 134–166. doi: 10.1575/1912/1512
Williams E. K., Rosenheim B. E., Allison M., McNichol A. P., Xu L. (2015). Quantification of Refractory Organic Material in Amazon Mudbanks of the French Guiana Coast. Mar. Geo. 363, 93–101. doi: 10.1016/j.margeo.2015.02.009
Wu Y., Eglinton T., Yang L., Deng B., Montluçon D., Zhang J. (2013). Spatial Variability in the Abundance, Composition, and Age of Organic Matter in Surficial Sediments of the East China Sea. J. Geophys. Res.: Biogeosci. 118, 1495–1507. doi: 10.1002/2013JG002286
Wu X. F., Mao J. Y. (2017). Interdecadal Variability of Early Summer Monsoon Rainfall Over South China in Association With the Pacific Decadal Oscillation. Int. J. Climatol. 37, 706–721. doi: 10.1002/joc.4734
Wu Z. Y., Milliman J. D., Zhao D. N., Cao Z. Y., Zhou J. Q., Zhou C. Y. (2018). Geomorphologic Changes in the Lower Pearl River Delta 1850-2015, Largely Due to Human Activity. Geomorphology 314, 42–54. doi: 10.1016/j.geomorph.2018.05.001
Wu W., Qu S., Nel W., Ji J. (2020). The Impact of Natural Weathering and Mining on Heavy Metal Accumulation in the Karst Areas of the Pearl River Basin, China. Sci. Total. Environ. 734, 139480. doi: 10.1016/j.scitotenv.2020.139480
Wu Z. Y., Saito Y., Zhao D. N., Zhou J. Q., Cao Z. Y., Li S. J., et al. (2016). Impact of Human Activities on Subaqueous Topographic Change in Lingding Bay of the Pearl River Estuary, China, During 1955-2013. Sci. Rep. 6, 37742. doi: 10.1038/srep37742
Wu Y., Zhang J., Liu S. M., Zhang Z. F., Yao Q. Z., Hong G. H., et al. (2007). Sources and Distribution of Carbon Within the Yangtze River System. Estua. Coast. Shelf. Sci. 71, 13–25. doi: 10.1016/j.ecss.2006.08.016
Yang Q., Ma Z., Fan X., Yang Z.-L., Xu Z., Wu P. (2017a). Decadal Modulation of Precipitation Patterns Over Eastern China by Sea Surface Temperature Anomalies. J. Climate 30, 7017–7033. doi: 10.1175/JCLI-D-16-0793.1
Yang Q., Ma Z. G., Xu B. L. (2017b). Modulation of Monthly Precipitation Patterns Over East China by the Pacific Decadal Oscillation. Climat. Change 144, 405–417. doi: 10.1007/s10584-016-1662-9
Yang L., Scheffran J., Qin H., You Q. (2015). Climate-Related Flood Risks and Urban Responses in the Pearl River Delta, China. Reg. Environ. Change 15, 379–391. doi: 10.1007/s10113-014-0651-7
Ye Y., Bo L., Li S., Hu J. (2021). A Study on the Response of Carbon Cycle System in the Pearl River Estuary to Riverine Input Variations. J. Mar. Syst. 215, 103498. doi: 10.1016/j.jmarsys.2020.103498
Ye Z., Chen J., Gao L., Liang Z., Li S., Li R., et al. (2020). (210)Pb Dating to Investigate the Historical Variations and Identification of Different Sources of Heavy Metal Pollution in Sediments of the Pearl River Estuary, Southern China. Mar. Pollut. Bull. 150, 110670. doi: 10.1016/j.marpolbul.2019.110670
Yuan X. Q., Yang Q. S., Luo X. X., Yu F. L., Liu F., Li J. Y., et al. (2019). Distribution of Grain Size and Organic Elemental Composition of the Surficial Sediments in Lingding Bay in the Pearl River Delta, China: A Record of Recent Human Activity. Ocean. Coast. Manage. 178, 1–11. doi: 10.1016/j.ocecoaman.2019.104849
Zhang Z., Leduc G., Sachs J. P. (2014). El Niño Evolution During the Holocene Revealed by a Biomarker Rain Gauge in the Galápagos Islands. Earth Planet. Sci. Lett. 404, 420–434. doi: 10.1016/j.epsl.2014.07.013
Zhang F., Li S., Sun C., Li W., Zhao X., Chen Z., et al. (2022). Human Impacts Overwhelmed Hydroclimate Control of Soil Erosion in China 5,000 Years Ago. Geophys. Res. Lett. 49 (5), 1–10. doi: 10.1029/2021GL096983
Zhang H., Ma W.-C., Wang X.-R. (2008). Rapid Urbanization and Implications for Flood Risk Management in Hinterland of the Pearl River Delta, China: The Foshan Study. Sensors 8, 2223–2239. doi: 10.3390/s8042223
Zhao B., Yao P., Bianchi T. S., Yu Z. G. (2021). Controls on Organic Carbon Burial in the Eastern China Marginal Seas: A Regional Synthesis. Global Biogeochem. Cycle. 35 (4), 1–27. doi: 10.1029/2020GB006608
Keywords: Pearl River Estuary, organic carbon, lignin, Atlantic Multidecadal Oscillation, deforestation, hydrodynamics
Citation: Li W, Li X, Zhao X, Sun C, Nie T, Hu Y and Wang C (2022) Impacts of Climate Change and Human Perturbations on Organic Carbon Burial in the Pearl River Estuary Over the Last Century. Front. Mar. Sci. 9:848757. doi: 10.3389/fmars.2022.848757
Received: 05 January 2022; Accepted: 28 March 2022;
Published: 22 April 2022.
Edited by:
Zhaohui Zhang, Zhejiang University, ChinaReviewed by:
Rienk H. Smittenberg, Stockholm University, SwedenRui Bao, Ocean University of China, China
Karl Ljung, Lund University, Sweden
Copyright © 2022 Li, Li, Zhao, Sun, Nie, Hu and Wang. This is an open-access article distributed under the terms of the Creative Commons Attribution License (CC BY). The use, distribution or reproduction in other forums is permitted, provided the original author(s) and the copyright owner(s) are credited and that the original publication in this journal is cited, in accordance with accepted academic practice. No use, distribution or reproduction is permitted which does not comply with these terms.
*Correspondence: Xinxin Li, bGl4aW54aW5Ac3VzdGVjaC5lZHUuY24=