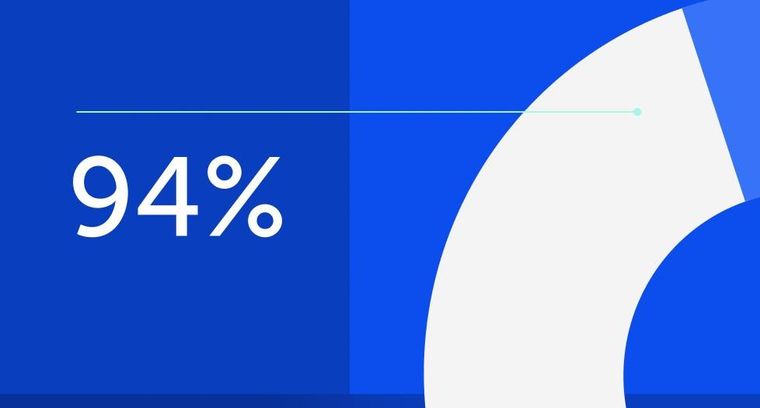
94% of researchers rate our articles as excellent or good
Learn more about the work of our research integrity team to safeguard the quality of each article we publish.
Find out more
ORIGINAL RESEARCH article
Front. Mar. Sci., 03 March 2022
Sec. Aquatic Physiology
Volume 9 - 2022 | https://doi.org/10.3389/fmars.2022.846566
Although the low temperature is a critical growth constraint on plants, the physiological mechanism remains unclear, especially in mangrove plants. Hence, the morphological characteristics of five mangrove plants (Bruguiera gymnorrhiza, Rhizophora stylosa, Aegiceras corniculatum, Avicennia marina, and Kandelia obovata) were compared under chilling stress. The contents of hydrogen peroxide (H2O2), malondialdehyde (MDA), and proline were tested. Activities of reactive oxygen species (ROS)-scavenging enzyme [superoxide dismutase (SOD), peroxidase (POD), and catalase (CAT)] were also measured after chilling stress. It was concluded that K. obovata can well tolerate chilling stress, and B. gymnorrhiza suffered the most severe chilling damage. Leaf-morphology observation exhibited that K. obovata and A. corniculatum can sustain chilling stress, while B. gymnorrhiza wilted and A. marina turned brown. The content of H2O2 increased at first and subsequently decreased in all plants. MDA increased instantaneously in B. gymnorrhiza and R. stylosa but changed slowly in K. obovata and A. corniculatum. The high content of proline accumulated in B. gymnorrhiza and K. obovata. The activities of the SOD, POD, and CAT increased at first and then decreased in all mangrove species. The antioxidants maintained high activity in K. obovata while decreasing earliest in A. marina exposed to the long-term chilling stress. Principal component analysis (PCA) indicated that high antioxidant enzyme activities play key roles in chilling tolerance for mangrove plants. The longer-term chilling tolerance of K. obovata may be related to the high antioxidant enzyme activities and proline accumulation. Lower H2O2 and MDA contents strengthen the anti-chilling ability of A. corniculatum. Further investigation on the molecular mechanisms will facilitate the understanding of the anti-chilling ability of mangrove plants.
Mangrove forest distributes in the intertidal coastal areas of the tropics and subtropics, which possesses four high characteristics, namely, high productivity, high return rate, high decomposition rate, and high resistance as one of the unique marine ecosystems (Wang, 2019), and plays a pivotal role in the global climate change due to its special ecological characteristics in the world (Wang and Gu, 2021). Global climate change has a strong impact on the stability of mangrove ecosystems (Alongi, 2015). Since 1950, it has been observed in a number of climate changes and extreme weather events associated with low or high temperatures (Pachauri et al., 2014). Temperature is one of the key abiotic factors, which can affect the growth and development of mangrove ecosystems (Hatfield and Prueger, 2015).
Climate warming leads to early phenol-logical development, making plants more susceptible to the subsequent low-temperature damage in the spring (Gu et al., 2008). Chilling stress (0–12°C) of plants results from low temperature (Allen and Ort, 2001). It has been observed that chilling stress can affect the leaf morphology of plants. Most studies that focused on maize, rice, and other plants confirmed that cold temperature exposure reduced leaf expansion and growth, resulting in browning, wilting, and chlorosis (Boese and Huner, 1990; Yoshida et al., 1996). Low temperature can result in sudden leaf loss, branch and stem reductions, and even mortality of various plants including mangrove plants (Peng et al., 2013, 2015; Fei et al., 2015; Lovelock et al., 2016).
Because of the great reactive oxygen species (ROS) produced by chilling-exposed plant tissues, from the perspective of plant physiology, oxidative stress is one of the earliest challenges facing the plant (Finkel and Holbrook, 2000; Mittler, 2002).
The imbalance between ROS production and removal could induce oxidative stress (Finkel and Holbrook, 2000). Chilling as the abiotic stress would break the balance of ROS in plants such as maize and winter wheat (Okuda et al., 1991; Prasad et al., 1994). Accumulation of a certain quantity of ROS would activate the antioxidase system to alleviate the damage from overoxidation (Mittler, 2002; Foyer and Noctor, 2005). For example, the activity of ascorbate peroxidase (APX) increases the hydrogen peroxide (H2O2) in spinach chloroplasts, and ROS also enhances the enzyme activity [superoxide dismutase (SOD), peroxidase (POD), and catalase (CAT)] in mangrove plants (Peng et al., 2015). The accumulation of low-molecular-weight osmotic adjustment substances (e.g., proline, betaine, soluble sugars) is considered to enhance cold tolerance and had been confirmed in citrus and two coffee species (Kushad and Yelenosky, 1987; Jouve et al., 1993). Chilling exposure induces the movements of the membrane (Örvar et al., 2000) and the change in malondialdehyde (MDA) contents (Campos et al., 2003). Low temperature affects the balance between metabolic reactions, the fluidity of cell membranes, and the activity of antioxidant enzymes; it also leads to cell oxidation, photosynthesis impairment, DNA strand disruption, and protein instability (Ruelland et al., 2009). Mangrove plants are sensitive to low temperatures and can hardly survive the long-term chilling stress, but they still show different tolerance to low temperatures (Lovelock et al., 2016).
In this study, the response mechanisms of five mangrove species (Rhizophora Stylosa, Bruguiera Gymnorrhiza, Aegiceras Corniculatum, Avicennia Marina, and Kandelia obovata) exposed to a long-term chilling treatment are elaborated, the ability of cold tolerance is analyzed, and the understanding of ecophysiological effects on mangrove plants is mentioned under global climate change.
Mangrove seedlings of B. gymnorrhiza, R. stylosa, A. corniculatum, A. marina, and K. obovata were collected from Zhanjiang City, Guangdong Province, China, and the seedlings were grown indoors at 5°C during the experiment (Mcwilliam et al., 1982; Kishitani et al., 1994; Trunova et al., 2003). The test soil was collected from the locally grown seedlings, rinsed with tap water, and dried before potting experiments to ensure that the experimental materials lived in the same soil type. After the embryonic axis germinated, it was transferred to artificial climate boxes for cultivation and fertilized with half Hoagland regularly. The parameters were as follows: 25°C, 14/10 h light/dark cycle, 20,000 light density daytime, and 70% humidity.
The five mangrove plants with basically the same level of growth were selected as experimental stress materials in artificial climate boxes to simulate low temperature, in which at least five pairs of mature leaves have grown. The temperature was controlled under 5°C, and the other parameters remained the same. Regular watering with half Hoagland to supplement the nutrients was needed for the growth of mangrove plants. During the experiment, the growth status of each mangrove seedling was observed. Leaf samples were taken at 0, 3, 5, 7, 10, 13, 15, and 20 days under 5°C chilling stress. The conductivity was measured immediately, and other samples were quick-frozen with liquid nitrogen and stored at −80°C for measuring related physiological parameters. After 20 days, the experimental materials were relieved of chilling stress and rewarmed to 25°C to observe growth.
The amount of hydrogen peroxide can be detected by measuring the reaction product of H2O2 and molybdic acid (Zhang et al., 2008). The H2O2 in the sample was assayed using a commercial kit (Nanjing Jiancheng Bioengineering Institute), and the absorbance was measured at 405 nm.
Lipid peroxidation was reflected by the contents of MDA. MDA concentration was measured by using the thiobarbituric acid chromogenic method (Heath and Packer, 1968). It is a product of unsaturated fatty acid peroxidation. Samples (0.5 g) were ground in trichloroacetic acid (4.5 ml, 5%), ground, and centrifuged. The reaction mixture contained the supernatant and 2 ml of 0.67% of thiobarbituric acid, which was boiled for 20 min and centrifuged. The absorbance was measured at 450, 532, and 600 nm. The MDA content was calculated based on the following formula: C (μmol L–1) = 6.452 × (A532 − A600) − 0.559 × A450.
Free proline was estimated from the product derivatized with acid ninhydrin and proline. The reaction mixture consisted of acid ninhydrin (2 ml), glacial acetic acid (2 ml), and supernatant, which was extracted from leaf (0.5 g) using aqueous sulfosalicyclic acid (5 ml, 3%), boiled for 45 min, and extracted by toluene. Absorbance was measured at 520 nm, and the concentration of proline was calculated according to the standardized proline curve (Bates et al., 1973).
Samples (0.5 g) were homogenized at 4.5 ml of phosphate buffer solution at 4°C. The homogenate was centrifuged at 12,000 g for 20 min to obtain a crude enzyme extract, which was to assay the SOD, activities POD, and CAT.
Superoxide dismutase activity was determined according to the method of the photoreduction of nitroblue tetrazolium (Beauchamp and Fridovich, 1971). The reaction mixture contained 0.5 ml of leaf extract, 50 mM of phosphate buffer (pH 7.8), 2 mM of riboflavin, 75 μM of nitroblue tetrazolium, and 13 mM of methionine, and reacted with 120 μmol m–2 s–1 light intensity for 20 min, and the absorbance was measured at 560 nm.
The POD was exposed to organic solvents and assayed by using the peroxidase guaiacol method (Ryu and Dordick, 1992). Leaf extract (50 μl) was mixed with 20 mM of guaiacol and 10 mM of H2O2. One unit of POD activity was defined as catalytic production of 0.01 absorbance unit with the amount of the enzyme at 470 nm min–1 g–1 tissue sample.
The CAT was measured by using the UV absorption method (Beers and Sizer, 1952). Leaf extract was added in mixed liquor of 50 mM of phosphate buffer (pH: 7.0), 0.1 mM of ethylenediaminetetraacetic acid, and 10 mM of H2O2. One unit of CAT activity was defined as catalytic production of 0.01 absorbance unit with the amount of the enzyme at 240 nm min–1 mg–1 protein.
The experimental data were expressed using mean ± SEs, and Excel 2010 (Microsoft Company, Washington, DC, United States) and Origin 2021 (Origin Lab Company, MA, United States) were used for routine calculation and graph processing of the data. The Shapiro-Wilk test for normality of data was used, conforming to the normal distribution. One-way ANOVA statistical analysis and Pearson correlation coefficient were carried out by using the SPSS version 22.0 (IBM Company, Armonk, NY, United States). Principal component analysis (PCA) was conducted using the origin 2021 to reveal the response differences between parameters in the five mangrove plants. In the results, asterisks presented the levels of significance: ∗P < 0.05 and ∗∗P < 0.01.
It has been observed that the leaf morphology of the five mangrove species had changed significantly after exposure to low temperatures (Figure 1). Under short-term stress (7th day), the shape and color of the leaves for the five mangrove plants remained normal. However, there were obvious differences in the late stage of low-temperature stress. In A. marina and R. stylosa, the color of the leaves had turned brown from part of the leaf veins and gradually developed to the entire leaf, and the browning area was larger than 50% on the 15th day. The browning trend of A. marina was heavier than R. stylosa. Besides, leaves of B. gymnorrhiza obviously turned yellow, curled, and wilted. The changes were distinct on the 13th day and more obvious with elongated treated time. Some leaves of A. corniculatum curled, while most of the leaves were in good condition.
Figure 1. Morphological changes under 5°C treatment of five mangrove plants, Bruguiera gymnorhiza (A), Rhizophora stylosa (B), Aegiceras corniculatum (C), Avicennia marina (D), and Kandelia obovata (E).
The content of H2O2 can reflect the accumulation of ROS in the plants (Mittler, 2002). Generally, there was a trend of increased H2O2 content and subsequently decreased in all mangrove plants exposed to chilling stress (Figure 2A). In B. gymnorrhiza, A. marina, and K. obovata, the accumulation of H2O2 reached the maximum on the 3rd day, with 3.37-fold, 3.48-fold, and 3.68-fold increase, respectively. In R. stylosa and A. corniculatum, the content of H2O2 peaked on the 7th day with 2.45-fold and 3.51-fold increase each. After 15 days of treatment at 5°C, H2O2 decreased near the control level.
Figure 2. Oxidative damage characterization in H2O2 (A) and MDA (B) of five mangrove leaves after 5°C treatment. The sample sizes per mangrove species were 27. The ∗presents the difference is statistically significant (P < 0.05).
The fluctuation of MDA in five mangrove plants is mapped in Figure 2B. In B. gymnorrhiza, MDA suddenly peaked (5.1 times the control) on the 15th day and quickly fell back to the control value on the 20th day. In A. marina and K. obovata, MDA exhibited a similar trend but different timing of early and late stages. In R. stylosa, it was high on the 3rd and 10th days. In A. corniculatum, the accumulation of MDA roughly increased over time.
The content of proline in mangrove plants was different under the chilling stresses (Figure 3A). It was observed that the amount of free proline increased in all mangrove species. In B. gymnorrhiza, the free proline rose with stress time. However, the fluctuation of proline appeared in R. stylosa, A. corniculatum, and A. marina. In K. obovata, the value was high on the 15th day, which was 4.3-fold as high as the control group.
Figure 3. Antioxidant parameters change in proline (A), SOD (B), POD (C), and CAT (D) of five mangrove leaves after 5°C treatment. The sample sizes per mangrove species were 27. The ∗presents the difference is statistically significant (P < 0.05).
The data (Figure 3B) indicated that the initial SOD activity of A. marina was higher than other species, whereas B. gymnorrhiza had the largest change, followed by R. stylosa, and increased by 1,327.1% (5th day) and 1,013.4% (5th day) in comparison with the control group, respectively. On the 1st and 3rd days of cold exposure, the differences between R. stylosa and A. corniculatum were insignificant, but the SOD activity was drastically higher than the control group on the 3rd day of stress in B. gymnorrhiza, A. marina, and K. obovata. All five mangrove plants showed an initial increase and subsequent decrease. When the chilling stress was applied for 7 days, the SOD activity of A. marina had decreased, while other mangrove plants maintained a high level up to the 10th day under cold treatments.
An increase in the activity of POD (Figure 3C) was presented after the 3rd day of cold treatment in A. marina and K. obovata. The highest increase was presented in A. corniculatum on the 5th day, which has increased by 4,097.2%. It is evident that the initial POD activities of A. marina and K. obovata were higher than the other three species. Although the activity of POD was increased in B. gymnorrhiza with the increase in stress time, it obviously lagged behind (13th day) other species. In R. stylosa, it was lower than A. marina and K. obovata, but higher than B. gymnorrhiza and A. corniculatum whether the initial activity or change throughout the low temperature. Nevertheless, the trend of reduction in the POD activity was displayed in all mangrove plants on the 13th day.
The results of CAT activity under low temperature are shown in Figure 3D. Although the activity of CAT significantly fluctuated during various periods, the overall trend of change was initially increasing and subsequently decreasing as the stress time extended. The response time of CAT activity varied under low-temperature stress, and R. stylosa increased instantaneously from 0.91 to 13.91 U mg–1 protein on the 5th day. The peak activity usually appeared from the 3rd to 7th days, whereas the CAT activity was the highest on the 20th day in A. marina.
The results of six physiological parameters in five mangrove plants are documented in Figure 4A (Pearson correlation coefficient) and Figure 4B (PCA), respectively. Pearson correlation analysis revealed a significant positive correlation between H2O2 and SOD, POD, and CAT (P < 0.01). The correlation among enzyme activities (POD, SOD, and CAT) was significant (P < 0.01). A significant positive correlation between MDA and SOD was observed (P < 0.05). MDA showed a negative correlation with CAT (insignificant), and proline showed a negative correlation with SOD, POD, CAT, and H2O2 (insignificant).
Figure 4. Pearson correlation coefficient (A) and principal component analysis (B) of physiological and biochemical parameters in five mangrove leaves after 5°C treatment.
Two principal components were obtained through dimensionality reduction using PCA, which explained 67.3% of the variables. First, principal component one (PC1) explained 46.7% of the variables, which was positively correlated with the MDA and H2O2 contents and antioxidant enzyme activities (SOD, POD, and CAT), and negatively correlated with the content of proline. Second, principal component two (PC2) explained 20.6% of the variables, which was positively correlated with the H2O2 and MDA contents and SOD activity, and negatively correlated with the activities of POD and CAT. These physiological and biochemical parameters can be divided into two parts as follows: one part was composed of antioxidant enzymes (SOD, POD, and CAT) and ROS (H2O2), and the other part was composed of characteristic membrane levels (MDA and proline).
Plants are subjected to temperature fluctuations and have evolved mechanisms to minimize the negative effects at temperature extremes (Chinnusamy et al., 2003). Generally, different species of plants exposed to low temperatures enable to induce different metabolic patterns (Farooq et al., 2009). This study suggested that chilling stress affected leaf morphology, antioxidant enzymes, lipid peroxidation, hydrogen peroxide, and proline content, and the tested mangrove plants responded in different ways.
This study was carried out for the morphological recording of the five mangrove plants under low-temperature stress. Leaves are sensitive to low temperature, and the morphological changes would be more obvious when the low-temperature stress is enhanced (Boese and Huner, 1990). According to the morphological characteristics of the plants under chilling stress, the cold tolerance of different mangrove species was revealed. In this work, the leaf morphology of K. obovata was the best and A. corniculatum was the second, while the damage to B. gymnorrhiza and A. marina was more serious, and it was irreversible damage. Previous studies also compared the leaf morphology of three mangrove species (K. obovata, A. corniculatum, and A. marina), but the difference in leaf morphology was not obvious in short-term chilling exposure (Peng et al., 2015). Nevertheless, the impaired symptom caused by low temperature was different, the leaves of A. marina were browned, and the leaves of B. gymnorrhiza were curled and wilted. During the 20-day chilling exposure, five mangrove plants were damaged to a certain extent.
In addition to leaf morphology, physiological and biochemical indicators of five mangrove species were studied. It was found that cold exposure affected H2O2, MDA, proline, SOD, POD, and CAT activities. Chilling has been proven to induce the accumulation of ROS and impair the normal functioning of the plants at the cellular level (Apel and Hirt, 2004; Ruelland et al., 2009). The H2O2 is a ROS and leads to oxidative injuries due to chilling stress (Prasad et al., 1994). The high H2O2 accumulation in the leaves indicates that cells are beginning to be damaged by chilling stress and is also an antioxidant signal (Penfield, 2008). In the experiment, an increase in H2O2 was shown in all mangrove plants, but more evident in A. marina. Although K. obovata had also high H2O2 contents, it showed slight damage due to its same high antioxidant enzyme activity.
Furthermore, the chilling application causes greater membrane injury as assessed by lipid peroxidation due to ROS production (Apel and Hirt, 2004). MDA is the product of lipid peroxidation and is used to evaluate the stability of the cell membrane (Bonnes-Taourel et al., 1992). It is also considered a cold sensitivity marker (Campos et al., 2003). H2O2 accumulation and consequently lipid peroxidation occurred. The obtained results indicated that lower values of the H2O2 and MDA contents in A. corniculatum reflected higher antioxidative capability in comparison to A. marina and R. stylosa. It seemed likely that the double cumulative pressure of H2O2 and MDA induced more severe chilling injury in A. marina. The response in cold-tolerant plants was more pronounced than in cold-sensitive plants, consistent with the results reported in previous studies. These studies also reflected strong membrane damage in cold-sensitive plants (Campos et al., 2003). The contents of proline in A. marina and R. stylosa were higher than in A. corniculatum and K. obovata. Drastic MDA changes on the 15th day in B. gymnorrhiza were probably a sign that the injury has exceeded the reasonable tolerance.
In contrast, ROS is thought to act as a signal and to be regulated by antioxidants that could scavenge the reactive oxygen to alleviate the injury (Mittler, 2002). The accumulation of H2O2 increased earlier than the change in enzyme activity, which may also explain the signal function of ROS. Previous studies have reported that the first defensive line for ROS is constituted by the SOD, removing O2– radical and producing H2O2 within cells (Alscher et al., 2002). The elimination of H2O2 is inseparable from the participation of POD and CAT. In mangrove plants subjected to chilling stress, SOD, POD, and CAT activities were stimulated. In this study, the continuously increased POD activity cooperated with CAT to scavenge the H2O2. Comparing the POD and CAT activities, CAT was the most effective enzyme in cell protection against H2O2 exposed to chilling stress. However, other enzymes may cooperate or replace CAT activity in longer chilling stress. It may be deduced that higher CAT activity was the effective way against chilling stress in R. stylosa. Although the enzyme activity of A. marina was high in the early stage, the decline time was earlier than other species. This may be the reason why A. marina had better cold tolerance in the early stage, and the damage was serious in the later stage. B. gymnorrhiza was sensitive and accumulated active oxygen to low-temperature stress, and its antioxidant enzyme activity was negative, thus, the excessive active oxygen could not be cleared in time. K. obovata had a higher antioxidative capability, reflecting a higher tolerance to cold stress, which was associated with the high activities of POD and CAT when chilling stress was applied.
In addition to antioxidant enzymes, it has been noted that proline accumulation is higher in plants exposed to abiotic stresses (Jouve et al., 1993). The accumulation of proline is effective to scavenge hydroxyl radicals, protect and stabilize ROS scavenging enzymes and has regulatory functions as a signal molecule (Szabados and Savoure, 2010). In previous studies, the accumulation of proline could influence chilling tolerance in A. corniculatum (Peng et al., 2015). In this study, B. gymnorrhiza intended to accumulate more proline, which might be the main method to regulate oxidative injury. Higher proline content and CAT activity seemed to play a key role in the detoxification of oxidative stress in K. obovata.
In the five mangrove species, the significant correlation between antioxidant enzymes (SOD, POD, and CAT) and H2O2 also revealed its inherent correlation mechanism encountered due to chilling stress. POD and CAT are important enzymes for removing H2O2 and alleviating the damage caused by ROS such as H2O2 to cells. Proline also plays an important role in regulating the redox state of cells. The level of cold tolerance is a complex process of multifactor coordination.
The five mangrove species differed in their morphological, physiological, and biochemical characteristics under chilling treatment. Lower H2O2 and MDA contents were the chilling tolerance performance of A. corniculatum. The results indicate that the chilling tolerance mechanisms of five mangrove plants are the increasing activity of antioxidant enzymes and the content of proline. Moreover, proline may play a more important role in alleviating the effect of chilling stress in B. gymnorrhiza and K. obovata. The stronger active oxygen scavenging mechanism explained the ability of R. stylosa, A. marina, and K. obovata to resist low-temperature stress, and meanwhile, it shows that the level of active oxygen and the activity of antioxidant enzymes are important physiological parameters for the evaluation of chilling tolerance. There is a synergistic competition mechanism in the antioxidant system, which is more conducive to enhancing the ability of mangrove plants to withstand low temperatures. Compared with other mangrove species, K. obovata has better performance exposed to chilling stress and is an alternative plant for planting in the higher latitude region or preparing for the impact of chilling stress for climate change.
The original contributions presented in the study are included in the article/supplementary material, further inquiries can be directed to the corresponding author.
S-MW wrote the manuscript and undertook the experiments. Y-SW designed, conceptualized, and supervised the manuscript. B-YS modified the manuscript and undertook the experiments. Y-YZ, L-FC, X-YM, and X-ML undertook the experiments. All authors contributed to the article and approved the submitted version.
This research was supported by the National Natural Science Foundation of China (Nos. U1901211 and 41876126), the Strategic Priority Research Program of the Chinese Academy of Sciences (Nos. XDA23050200 and XDA19060201), the International Partnership Program of the Chinese Academy of Sciences (No. 133244KYSB20180012), the National Key Research and Development Plan (No. 2017FY100700), and the Key Special Project for Introduced Talents Team of Southern Marine Science and Engineering Guangdong Laboratory (Guangzhou) (No. GML2019ZD0305).
The authors declare that the research was conducted in the absence of any commercial or financial relationships that could be construed as a potential conflict of interest.
All claims expressed in this article are solely those of the authors and do not necessarily represent those of their affiliated organizations, or those of the publisher, the editors and the reviewers. Any product that may be evaluated in this article, or claim that may be made by its manufacturer, is not guaranteed or endorsed by the publisher.
Allen, D., and Ort, D. (2001). Impacts of chilling temperatures on photosynthesis in warm-climate plants. Trends Plant Sci. 6, 36–42. doi: 10.1016/s1360-1385(00)01808-2
Alongi, D. M. (2015). The Impact of Climate Change on Mangrove Forests. Curr. Clim. Change Rep. 1, 30–39. doi: 10.1007/s40641-015-0002-x
Alscher, R. G., Erturk, N., and Heath, L. S. (2002). Role of superoxide dismutases (SODs) in controlling oxidative stress in plants. J. Exp. Bot. 53, 1331–1341. doi: 10.1093/jexbot/53.372.1331
Apel, K., and Hirt, H. (2004). Reactive oxygen species: metabolism, oxidative stress, and signal transduction. Annu. Rev. Plant Biol. 55, 373–399. doi: 10.1146/annurev.arplant.55.031903.141701
Bates, L. S., Waldren, R. P., and Teare, I. D. (1973). Rapid determination of free proline for water-stress studies. Plant Soil 39, 205–207. doi: 10.1007/BF00018060
Beauchamp, C., and Fridovich, I. (1971). Superoxide dismutase: Improved assays and an assay applicable to acrylamide gels. Analyt. Biochem. 44, 276–287. doi: 10.1016/0003-2697(71)90370-8
Beers, R. F., and Sizer, I. W. (1952). A spectrophotometric method for measuring the breakdown of hydrogen peroxide by catalase. J. Biol. Chem. 195, 133–140. doi: 10.1016/s0021-9258(19)50881-x
Boese, S. R., and Huner, N. P. A. (1990). Effect of Growth Temperature and Temperature Shifts on Spinach Leaf Morphology and Photosynthesis 1. Plant Physiol. 94, 1830–1836. doi: 10.1104/pp.94.4.1830
Bonnes-Taourel, D., Guérin, M., and Torreilles, J. (1992). Is malonaldehyde a valuable indicator of lipid peroxidation? Biochem. Pharmacol. 44, 985–988. doi: 10.1016/0006-2952(92)90132-3
Campos, P. S., Quartin, V. N., Ramalho, J. C., and Nunes, M. A. (2003). Electrolyte leakage and lipid degradation account for cold sensitivity in leaves ofCoffea sp. plants. J. Plant Physiol. 160, 283–292. doi: 10.1078/0176-1617-00833
Chinnusamy, V., Ohta, M., Kanrar, S., Lee, B. H., Hong, X., Agarwal, M., et al. (2003). ICE1: a regulator of cold-induced transcriptome and freezing tolerance in Arabidopsis. Genes Dev. 17, 1043–1054. doi: 10.1101/gad.1077503
Farooq, M., Aziz, T., Wahid, A., Lee, D.-J., and Siddique, K. H. M. (2009). Chilling tolerance in maize: agronomic and physiological approaches. Crop Pasture Sci. 60, 501–516. doi: 10.1071/cp08427
Fei, J., Wang, Y. S., Jiang, Z. Y., Cheng, H., and Zhang, J. D. (2015). Identification of cold tolerance genes from leaves of mangrove plant Kandelia obovata by suppression subtractive hybridization. Ecotoxicology 24, 1686–1696. doi: 10.1007/s10646-015-1486-9
Finkel, T., and Holbrook, N. J. (2000). Oxidants, oxidative stress and the biology of ageing. Nature 408, 239–247. doi: 10.1038/35041687
Foyer, C. H., and Noctor, G. (2005). Redox Homeostasis and Antioxidant Signaling: A Metabolic Interface between Stress Perception and Physiological Responses. Plant Cell 17, 1866–1875. doi: 10.1105/tpc.105.033589
Gu, L., Hanson, P. J., Post, W. M., Kaiser, D. P., Yang, B., Nemani, R., et al. (2008). The 2007 Eastern US Spring Freeze: Increased Cold Damage in a Warming World? BioScience 58, 253–262. doi: 10.1641/b580311
Hatfield, J. L., and Prueger, J. H. (2015). Temperature extremes: Effect on plant growth and development. Weather Clim. Extremes 10, 4–10. doi: 10.1016/j.wace.2015.08.001
Heath, R. L., and Packer, L. (1968). Photoperoxidation in isolated chloroplasts. Archiv. Biochem. Biophys. 125, 189–198. doi: 10.1016/0003-9861(68)90654-1
Jouve, L., Engelmann, F., Noirot, M., and Charrier, A. (1993). Evaluation of biochemical markers (sugar, proline, malonedialdehyde and ethylene) for cold sensitivity in microcuttingsof two coffee species. Plant Sci. 91, 109–116. doi: 10.1016/0168-9452(93)90194-5
Kishitani, S., Watanabe, K., Yasuda, S., Arakawa, K., and Takabe, T. (1994). Accumulation of glycinebetaine during cold acclimation and freezing tolerance in leaves of winter and spring barley plants. Plant Cell Environ. 17, 89–95. doi: 10.1111/j.1365-3040.1994.tb00269.x
Kushad, M. M., and Yelenosky, G. (1987). Evaluation of Polyamine and Proline Levels during Low Temperature Acclimation of Citrus 1. Plant Physiol. 84, 692–695. doi: 10.1104/pp.84.3.692
Lovelock, C. E., Krauss, K. W., Osland, M. J., Reef, R., and Ball, M. C. (2016). “The physiology of mangrove trees with changing climate,” in Tropical Tree Physiology. Tree Physiology, G. Goldstein. and L. Santiago, (Cham: Springer). doi: 10.1007/978-3-319-27422-5_7
Mcwilliam, J., Kramer, P., and Musser, R. (1982). Temperature-Induced Water Stress in Chilling-Sensitive Plants. Funct. Plant Biol. 9, 343–352. doi: 10.1071/PP9820343
Mittler, R. (2002). Oxidative stress, antioxidants and stress tolerance. Trends Plant Sci. 7, 405–410. doi: 10.1016/S1360-1385(02)02312-9
Okuda, T., Matsuda, Y., Yamanaka, A., and Sagisaka, S. (1991). Abrupt Increase in the Level of Hydrogen Peroxide in Leaves of Winter Wheat Is Caused by Cold Treatment 1. Plant Physiol. 97, 1265–1267. doi: 10.1104/pp.97.3.1265
Örvar, B. L., Sangwan, V., Omann, F., and Dhindsa, R. S. (2000). Early steps in cold sensing by plant cells: the role of actin cytoskeleton and membrane fluidity. Plant J. 23, 785–794. doi: 10.1046/j.1365-313x.2000.00845.x
Pachauri, R. K., Allen, M. R., Barros, V. R., Broome, J., Cramer, W., Christ, R., et al. (2014). “Climate change 2014: synthesis report,” in Contribution of Working Groups I, II and III to the fifth assessment report of the Intergovernmental Panel on Climate Change, eds R. K. Pachauri and L. A. Meyer (Geneva: IPCC), 151.
Penfield, S. (2008). Temperature perception and signal transduction in plants. New Phytol. 179, 615–628. doi: 10.1111/j.1469-8137.2008.02478.x
Peng, Y. L., Wang, Y. S., Cheng, H., Sun, C. C., Wu, P., Wang, L. Y., et al. (2013). Characterization and expression analysis of three CBF/DREB1 transcriptional factor genes from mangrove Avicennia marina. Aquat. Toxicol. 14, 68–76. doi: 10.1016/j.aquatox.2013.05.014
Peng, Y. L., Wang, Y. S., Fei, J., Sun, C. C., and Cheng, H. (2015). Ecophysiological differences between three mangrove seedlings (Kandelia obovata, Aegiceras corniculatum, and Avicennia marina) exposed to chilling stress. Ecotoxicology 24, 1722–1732. doi: 10.1007/s10646-015-1488-7
Prasad, T. K., Anderson, M. D., Martin, B. A., and Stewart, C. R. (1994). Evidence for Chilling-Induced Oxidative Stress in Maize Seedlings and a Regulatory Role for Hydrogen Peroxide. Plant Cell 6, 65–74. doi: 10.1105/tpc.6.1.65
Ruelland, E., Vaultier, M.-N., Zachowski, A., and Hurry, V. (2009). Chapter 2 Cold Signalling and Cold Acclimation in Plants. Adv. Bot. Res. 49, 35–150. doi: 10.1016/s0065-2296(08)00602-2
Ryu, K., and Dordick, J. (1992). How do organic solvents affect peroxidase structure and function? Biochemistry 31, 2588–2598. doi: 10.1021/bi00124a020
Szabados, L., and Savoure, A. (2010). Proline: a multifunctional amino acid. Trends Plant Sci. 15, 89–97. doi: 10.1016/j.tplants.2009.11.009
Trunova, T., Astakhova, N., Deryabin, A., and Sabel’nikova, E. (2003). Ultrastructural Organization of Chloroplasts of the Leaves of Potato Plants Transformed with the Yeast Invertase Gene at Normal and Low Temperature. Netherland: Kluwer Academic Publishers-Plenum Publishers, 176–179.
Wang, Y.-S., and Gu, J.-D. (2021). Ecological responses, adaptation and mechanisms of mangrove wetland ecosystem to global climate change and anthropogenic activities. Int. Biodeter. Biodegr. 162:105248. doi: 10.1016/j.ibiod.2021.105248
Yoshida, R., Kanno, A., and Kameya, T. (1996). Cool Temperature-Induced Chlorosis in Rice Plants (II. Effects of Cool Temperature on the Expression of Plastid-Encoded Genes during Shoot Growth in Darkness). Plant Physiol. 112, 585–590. doi: 10.1104/pp.112.2.585
Keywords: mangrove plants, chilling stress, oxidative injury, osmoregulation, ecophysiological responses
Citation: Wang S-M, Wang Y-S, Su B-Y, Zhou Y-Y, Chang L-F, Ma X-Y and Li X-M (2022) Ecophysiological Responses of Five Mangrove Species (Bruguiera gymnorrhiza, Rhizophora stylosa, Aegiceras corniculatum, Avicennia marina, and Kandelia obovata) to Chilling Stress. Front. Mar. Sci. 9:846566. doi: 10.3389/fmars.2022.846566
Received: 31 December 2021; Accepted: 17 January 2022;
Published: 03 March 2022.
Edited by:
Youji Wang, Shanghai Ocean University, ChinaReviewed by:
Wenyue Su, Western University of Health Sciences, United StatesCopyright © 2022 Wang, Wang, Su, Zhou, Chang, Ma and Li. This is an open-access article distributed under the terms of the Creative Commons Attribution License (CC BY). The use, distribution or reproduction in other forums is permitted, provided the original author(s) and the copyright owner(s) are credited and that the original publication in this journal is cited, in accordance with accepted academic practice. No use, distribution or reproduction is permitted which does not comply with these terms.
*Correspondence: You-Shao Wang, eXN3YW5nQHNjc2lvLmFjLmNu
Disclaimer: All claims expressed in this article are solely those of the authors and do not necessarily represent those of their affiliated organizations, or those of the publisher, the editors and the reviewers. Any product that may be evaluated in this article or claim that may be made by its manufacturer is not guaranteed or endorsed by the publisher.
Research integrity at Frontiers
Learn more about the work of our research integrity team to safeguard the quality of each article we publish.