- 1College of Geography and Environment, Shandong Normal University, Jinan, China
- 2Third Institute of Oceanography, Ministry of Natural Resources, Xiamen, China
- 3Chinese Research Academy of Environmental Sciences, Beijing, China
- 4College of Ocean and Earth Sciences, Xiamen University, Xiamen, China
- 5State Key Laboratory of Marine Pollution, City University of Hong Kong, Kowloon, Hong Kong SAR, China
Accumulating evidence indicates that aquatic organisms ingest microplastics (MPs), which may be a threat to essentially the entire global ecosystem. In current detection methods, even in cutting-edge nanoplastic technology, a major challenge for detecting microplastics (MPs) in aquatic organisms is removing complex biological matrices, such as fat. Herein we report combining HNO3 and H2O2 to form a binary digestive reagent system to determine MPs in biological tissue. With insights obtained from a Gaussian model, the adding manners of two reagents were discussed. Thus, in the final protocol, we mixed MPs and tissue with 20 mL of 30% (v/v) aqueous H2O2, 10 mL 0.5 M NaOH,1 mL 5 mM Fe2+, and 40 mL 11.5% (v/v) aqueous HNO3, in sequence at different time intervals. What’s more, sodium dodecyl sulfate (SDS) and ultrasound—alone or together—were explored to solve the problem of removing fat residues and thus membrane blockage during filtration. In this paper, we used the O-PTIR microscope to verify the feasibility of the protocol. Compared with traditional detection methods, the O-PTIR spectroscopy can significantly improve the lateral resolution, down to sub and super-micrometer, and the ability to quickly obtain high spatial resolution far-field non-contact infrared spectra, which provide a novel method for qualitative analysis of MPs. In field applications, in our attempt, the fixed wavenumber image by O-PTIR can realize sub and super-micrometer MPs in situ, far-field measurements. The present method is highly efficient, and facilitates the identification of plastic particles.
Introduction
Discarded bulk plastic products can eventually yield microplastics (MPs; < 5 mm in diameter), which are hazardous to aquatic environments (Collard et al., 2019; Gedik and Eryasar, 2020). Accumulating evidence indicates that there are plastic debris in hydrobiontes, such as zooplankton, fish, mussel, and crab (Wright et al., 2013; Watts et al., 2014; Hossain et al., 2019; Süssmann et al., 2021). MPs may essentially threaten the entire global ecosystem, including human consumers, through the consumption of seafood products (Dehaut et al., 2016; Gedik and Eryasar, 2020; Süssmann et al., 2021).
To determine the ecological threat of MPs, the quantity of MPs in marine organisms should be known. Over the last 2 years, the detection and extraction of nanoplastics have been an especially active line of research (Nguyen et al., 2019; Zhou et al., 2019; Merzel et al., 2020; Ribeiro et al., 2020). Combining pyrolysis with gas chromatography–mass spectrometry (PY–GC/MS) has facilitated quantitation of nanoplastics in complex biological tissues for the first time (Zhou et al., 2021). However, the abundance of organic matter in biological matrices interferes with the extraction and PY–GC/MS determination of nanoplastics, necessitating adequate digestion of the biological matrices (Zhou et al., 2021).
Field investigations of MPs remain challenging. Although it is worth mentioning that nanoplastics are still in the research and development stage (Merzel et al., 2020), far from field applications, yet MPs detection is a part of routine environmental monitoring by relevant departments at home and abroad (Gorokhova, 2015). However, current detection methods are low-efficiency and high-loss, which substantially affect the efficiency of the work and the reliability of the experimental results (Eerkes-Medrano et al., 2015; Rodrigues et al., 2018; Süssmann et al., 2021). Both nanoplastics and MPs quantitation in environmental samples would benefit from an efficient means of digesting the biological matrix, especially the fat (Collard et al., 2019). However, to date, no highly efficient, standard method is available that completely removes tissues without affecting the integrity of the plastic polymers.
To improve the efficiency of quantitating MPs in fish, researchers have developed and applied various methods for digesting the soft tissues of marine organisms (Loder et al., 2017). The basic idea of such methods is to eliminate the biological materials and tissues that mask the MPs (Budimir et al., 2018; Collard et al., 2019; Nguyen et al., 2019). Four major classes of digestion agents to eliminate organic materials are acids (Dehaut et al., 2016; Karami et al., 2017), alkalis (Karami et al., 2017; Schirinzi et al., 2020), oxidative agents (Avio et al., 2015; Collard et al., 2015; Karami et al., 2017), and enzymes (Cole et al., 2014; Loder et al., 2017).
Some limitations of digestion agents are as follows. In acid treatment, the acid is generally in an especially high concentration, and the temperature is high, which may eliminate the organic tissue but may not be compatible with plastic preservation (Cole et al., 2014; Nguyen et al., 2019). For example, we demonstrate herein that polyamides in HClO4 exhibit various degrees of corrosion or even complete degradation (Claessens et al., 2013) used HNO3 for MPs extraction from mussels, wherein the treatment destroyed some of the fibers. The use of H2O2 has been limited because of the incomplete digestion of soft tissue fractions (Mathalon and Hill, 2014). Enzymatic digestion has been impeded by high prices and poor bone digestion (Simonsen et al., 2011). In summary, the research indicates that digestion based on a single type of digestive agent is insufficient.
In this paper, changes in the molecular integrity of plastic polymers across various treatments and field sample detection are analyzed by Optical Photothermal Infrared (O-PTIR) microscope. Traditional microplastics detection mostly uses Fourier transform micro-infrared (μFTIR) and micro-Raman spectroscopy (μRM). The μFTIR or μRM, in actual operation, requires complicated sample processing. The boundedness of μFTIR depends on the infrared wavelength, which is only 10–20 μm. μRM is impressionable to fluorescence interference, and the resolution is at least 1 μm (Kansiz et al., 2020; Olson et al., 2020). It can’t characterize the chemical changes of the plastic surface at the sub-micron scale, nor can it identify individual nanoplastics (<1 μm) meanwhile making it very difficult to comprehensively detect and identify the type and composition of microplastics. A recent development on the O-PTIR, can significantly improve the lateral resolution, down to sub and super-micrometer. The unique pump-probe system architecture also facilitates submicron simultaneous μFTIR + μRM from the same spot with the same spatial resolution (Kansiz et al., 2020). O-PTIR is a still-emerging technique, the ability to rapidly obtain far-field non-contact μFTIR spectra at high spatial resolution facilitates the chemical identification of small organic contaminants that are not possible to measure with conventional μFTIR micro spectroscopy, which has been applied in such atmospheric particles, organic particles analysis and formation mechanism of micro-nano plastics (Olson et al., 2020; Fang et al., 2021; Su et al., 2021). In the field of microbiology, O-PTIR designed for (3D-) imaging living cells and organisms (Zhang et al., 2016), especially regarding fixed wavelengths images with the ultra-high image resolution (Ivleva, 2021). However, it has relatively few applications in MPs detection, limited studies indicator may be a better technique for detecting environmental plastics (nano) than other μFTIR techniques (Barrett et al., 2020; Merzel et al., 2020).
Thus, we optimized the fish tissue digestion protocol by designing a unitary digestion system composed of a series of single solutions (an acid, or a base, or an oxidizer) to find the most suitable digestion candidates and concentrations. On the basis of the corresponding results, we chose HNO3 and H2O2 to form a binary digestion system. We performed chemical calculations with a Gaussian model to explain the reaction mechanism of digestion, guiding the design of a binary digestion system. To solve the problem of fat digestion, we tested the efficacy of ultrasonic treatment and sodium dodecyl sulfate (SDS). The O-PTIR was conducted to verify the feasibility of the protocol. What’s more, we apply the fixed wavenumber image by O-PTIR in recovery experiments and in the field samples to explore its application in non-contact, far-field measurements that down to sub and super-micrometer plastic detection.
Materials and Methods
Samples and Reagents
Fish Samples
Fish were brought from a local market in Shandong Province, collected from the Yellow Sea (detailed information of the fish species is provided as Supplementary Material). The digestive tract characteristics of the fish we used are different, such as the fat content and the thickness of the digestive tract. Furthermore, because of different feeding habits the intestinal contents were quite different. For example, some contents were mainly small fish, shrimp, crab, and other arthropods; and some were mainly aquatic algae. Because of these differences, even if one uses the same experimental protocol, each kind of fish’s digestion effect (the experimental result of the designed digestion experiment) is quite different. To eliminate such errors as much as possible, the tissues used in our study were derived from a mixture of all the fish digestive tracts and subsequently stored at −20°C.
Plastic Preparation
The 11 types of plastics tested in this study were supplied by Shanghai Yichen Industrial Co., Ltd. These include acrylonitrile–butadiene–styrene (ABS), acrylonitrile–styrene copolymer (AS), high-impact polystyrene (HIPS), high- and low-density polyethylene (HDPE and LDPE, respectively), polyamide (PA), polyethylene (PE), polyethylene phthalate (PET), polypropylene (PP), polystyrene (PS), and polyvinyl chloride (PVC). The following plastic samples differed in color: milky white (ABS, PE, LDPE, and HDPE), transparent (AS, PS, and PVC), semitransparent (PP, HIPS, and PA), and white (PET).
Our initial investigations used particle sizes from ∼3 to 4 mm in diameter. After confirming the digestion protocol, we used MPs (>60–500 μm in diameter) for the recovery experiments.
Subtle effects of the digestion system on the plastics were taken into consideration. A cutting knife was used to make an irregular cross-shaped incision on the surface of the particles. After marking, the particles were rinsed with Milli-Q water and dried to a constant mass at room temperature (RT).
For the recovery rate experiments, the aforementioned 11 plastic particles were polished with a pulverizer (HCP-100) for ∼1 min and a temperature below 40°C. The ground plastic particles were passed through a series of sieves with different mesh sizes (60, 100, 200, and 500 μm) and sorted into four grain size classes (<60, 60–100, 100–200, and 200–500 μm). The samples were measured with a microscope for further size determinations.
Chemicals
Hydrogen peroxide (H2O2), nitric acid (HNO3), perchloric acid (HClO4), sodium hydroxide (NaOH), and ferrous sulfate (FeSO4) were purchased from Sinopharm Chemical Reagent Co., Ltd. SDS was supplied by Kalmar-reagent. Aqueous solutions of NaOH (0.5 M), HNO3 (11.5% v/v), FeSO4 (5 mM), and SDS (150 g/L) were prepared in Milli-Q water. All the digestion solutions, including the acid digests (HClO4 and HNO3) and oxidizing solutions (H2O2), were mixed with Milli-Q water to obtain the following proportions solutions: 1:0, 4:1, 2:1, and 1:1 (v/v), which is the high-concentration group [69, 55.2, 46, and 34.5% (v/v), respectively]; and 1:2, 1:3, 1:4, and 1:5 (v/v), which is the low-concentration group [23, 17.25, 13.8, and 11.5% (v/v), respectively]. The filter was obtained from Yancheng Prich Laboratory Instrument Co., Ltd. We used a filter of the following specifications: pore diameter, 5 μm; and filter diameter, 50 mm.
Digestion Protocol
To efficiently digest fish tissue without destroying the plastic polymers, we systematically investigated various protocols. In this paper, we investigated the effect of different kinds of digestion solution systems. We first studied a typical reagent at various concentrations; the results were not satisfactory. On the basis of the preliminary conclusions from typical single-reagent experiments, we continued to investigate the experimental effects of various combinations of digestion reagents. We accordingly differentiated the experimental batches as unitary and binary systems, respectively. We also optimized the experimental protocol by supplementing it with surfactants and sonication to overcome the fat-digestion problem to improve the experimental efficiency. We also used recovery experiments and field application to test whether the research protocol developed in this paper is feasible.
Validation of Method
Mixtures of fish digestive tracts were thawed at RT for 30 min. Samples of fish tissues (3 g ± 0.01 g) were weighed and spiked with three plastic particles each of ABS, AS, PA, PP, PE, PET, PS, PVC, HIPS, HDPE, and LDPE, the experiment was triplicate for each group. Our research investigated a series of digestion protocols to establish a high-efficiency digestion method, evaluated in four aspects: efficacy of digestion, impact on plastic particles, recovery rate, and field application.
Efficacy of Digestion
The effects of the digestion were determined by comparing photos taken before and after the reaction. Note that because the digestion solution bubbled as soon as the reagent was added, it was not possible to photograph the solution. Therefore, an equal volume of Milli-Q water was used to represent the digestive reagent. A corresponding photo was taken to represent the initial state. Then the Milli-Q water was poured out and replaced with a digestive reagent. At the end of the digestion, a photo was taken of the final state. Specifically, the mixtures were first added to 60 mL of Milli-Q water (start point) in a breaker to take photos to represent the state of the starting point of digestion. Next, the water was replaced by the same volume of various types of digestion solutions, and the digestion was maintained at RT for 12 h, if necessary, through ultrasonic (JP-040S, 180W) and/or SDS treatment (end point). At the ending point of digestion, the visual examination was performed and photographed, comparing with the starting point photo the initial digestion effect can be recorded. And the efficiency of the digestions was measured by the frequency of changing the filter membrane and the filtration duration.
Impact on Plastic Particles
The impact of digestion on the plastic particles was investigated in terms of their shape and chemical composition. The MPs were removed from the beaker, washed with Milli-Q water 3 × to remove the impurities attached to the surface, and dried at RT for further examination.
In the initial investigation (sections “Unitary Digestion System,” “Binary Digestion System,” and “Protocol Optimization for Dispersion of Fat Problem”), the particles before and after the tests for the various digestion protocols were analyzed by a visual examination to check for signs of subtle changes in the cross marker (section “Plastic Preparation”) which used by the digestion treatments. For unbiased comparisons, the observation point—set exactly at the intersection—was analyzed with a Raman spectrometer (Horiba, LabRAM, HR Evolution) with a 5 × Olympus objective.
The O-PTIR spectra were collected on the MP surface with an effective spectral resolution of 2 cm–1 and co-averaged for five spectral scans.
In field application investigations, the O-PTIR microscope was used to identify plastic particles. It is worth noting that the O-PTIR images were obtained at a 500-nm step size and 100-nm step resolution by tuning the QCL device to the frequencies that correspond to the specific vibrations of extraneous materials at various wavenumbers (1,023, 1,075, 1,641, and 1,725 cm–1). The system was equipped with IR laser settings where the wavenumber was 1,247 cm–1, the IP power was 4%, the pulse rate was 104.63 kHz, the duty cycle was 5%, and the pulse width was 480 ns. Regarding the probe settings, the probe power was 5% and the detector gain was 2 ×.
Determination of the Recovery Rate
In the recovery experiments, we counted the recovery rates for four particle sizes, and each particle size was spiked with 30 particles. The digests were then filtered with filter paper. The quantity of the MPs on the filter membrane was used to calculate recovery percentages using Equation 1:
where Ra = number of MPs recovered; and Ri = initial number of MPs.
Field Application
Fish species were collected from the Bohai Sea north of the Shandong Peninsula, and processed with the digestion method previously described and analyzed for MPs. The O-PTIR microscope was used to identify plastic particles.
Contamination Prevention
During all sample treatment and analysis steps, precautions were taken to avoid background plastic contamination. All equipment and glassware were washed with a commercial dishwashing liquid, and thoroughly rinsed with Milli-Q water before use. To avoid contamination of the samples by airborne fibers and other particles, vials were capped with aluminum foil during digestion. Within the laboratory, a cotton lab coat, nitrile gloves, and face masks were worn throughout the experiments.
Results and Discussion
Unitary Digestion System
We investigated the digestion of 11 types of plastic particles—including ABS, PE, LDPE, HDPE, AS, PS, PVC, PP, HIPS, PA, and PET—and the effects of the plastic. Fish tissues were treated with a single solution at various concentrations (HClO4, HNO3, and H2O2; individually) at RT. Different protocols had different effects on the plastic particle integrity and fish tissue digestion. In terms of fish tissue digestion, high concentrations of acids were superior to those of low concentrations. Different types of digestion solutions had different effects on plastic particles (Supplementary Figures 1–3); we next elaborated on the effects of various treatments.
Acid Treatment
We explored each digestion reagent separately. Accordingly, we mixed HClO4 (70% v/v) and HNO3 (69% v/v) with water separately in various proportions, as detailed in section “Chemicals.” One can visually observe the digestion of fish tissue and plastic particles in an aqueous acid solution. During digestion as per HClO4, most of the plastic particles corroded yet the tissues were completely dissolved at various acid concentrations (Supplementary Figure 1). The use of HClO4 in high concentrations is detrimental to nylon fibers (Claessens et al., 2013). We suggested that HClO4 strongly corrodes plastics, rendering it unsuitable as a digestive agent. Regarding HNO3, the corrosion of plastic particles by high concentrations of HNO3 was obvious; the damage to PA was the most drastic. Low concentrations of HNO3 had no obvious effects on the plastic particles except for PVC; only PVC had bubbles on its surface. However, fish tissue was poorly digested (Supplementary Figure 2). Among the plastics, we observed the most destruction for PA, which was completely destroyed by aqueous HNO3 at proportions of 1:0, 2:1 and 4:1 v/v. As the concentration of HNO3 decreased, PA initially dissolved, softened at lower concentrations, and at sufficiently low concentrations was undamaged. The results for other plastics were similar; that is, they retained their original shape when the concentration of HNO3 was sufficiently low.
Our results are consistent with the literature of completely dissolved PA by acid treatment (Enders et al., 2017). Other polymers were affected to lesser extents, mainly color leaching and softening. Karami et al. (2017) digested biological materials in concentrated HNO3 and produced optimum digestion efficiency only at RT. Concentrated HNO3 had the most destructive effect on melted LDPE and PP fragments, and altered the color of most of the tested plastic polymers. Dehaut et al. (2016) recommended against using acid for studies of MPs because of its degradation of polyamide and its yellowing tendency to plastics; acid digestion may result in miscataloging of MPs during the optical examination. Thus, the use of acid to digest fish tissues for MP surveys is not straightforward. If acid treatment is to be implemented in practice, the experimental parameters (such as concentration and temperature) should be optimized or refer to Enders et al. (2017) to combine the treatment with other digestion solutions.
Oxidizing Treatment
Compared with the results of acid treatment, the results from H2O2 treatment were mild. The fish tissue gradually dissolved as per visual observations at the H2O2 proportions of 1:0 and 4:1 (v/v). The tissue was less well digested in accordance with decreasing H2O2 concentrations. Karami et al. (2017) reported that H2O2 did not completely dissolve biological material at either RT or 40°C. Li et al. (2015) dissolved bivalve tissues in 30% (v/v) aqueous H2O2, incubated the mixture at 65°C for 24 h, and then digested the mixture at RT for 24∼48 h. The tissues dissolved but the quantity of tissues is an important parameter. Cole et al. (2014) used H2O2 (35% v/v) at RT for 7 days, but did not observe much digestion. Avio et al. (2015) reported incubation with H2O2 (15% v/v) at 50°C overnight. Thus, temperature is an important parameter in digestion. Although increasing the temperature results in a greater extent of tissue digestion, there will be deterioration of the plastic particles.
In summary, the use of various concentrations of H2O2 was promising in that there was almost no effect on the plastics. Nevertheless, low concentrations of H2O2 poorly digested fish tissues, especially the digestive tract (Supplementary Figure 3). Therefore, the application of H2O2 as a digestive solution for extracting MPs from fish tissues is limited and requires improvement.
Binary Digestion System
In aforementioned unitary digestion system, HClO4 dissolves fish tissue yet also deteriorates plastics. Low concentrations of HNO3 should be used to maintain plastic integrity. H2O2 is comparatively mild and less corrosive to plastics than the aforementioned acids but poorly digests fish tissue. We also investigated experimental parameters such as time and temperature with a single digestion solution system, but the results were still unsatisfactory. Thus, a binary digestion system was necessary.
On the basis of the aforementioned results of the unitary digestion systems, considering the corrosivity of HClO4, we chose HNO3 and H2O2 for investigations of a binary digestive system (Supplementary Figures 4, 5). In accordance with the experimented results under a single condition, we chose an 11.5% (v/v) concentration of HNO3 (not expected to damage plastics). We thus added 11.5% (v/v) HNO3 and 30% (v/v) H2O2 in accordance with the following proportions (all v/v) 1:0, 4:1, 2:1, 1:1, 1:2, 1:3, 1:4, and 1:5. We added the reagents either in combination or in sequence.
In accordance with the method of adding the two digestion reagents—i.e., whether to combine or separate, the results are discussed separately. The difference between these two methods of addition is whether the reagents are mixed before digestion or instead added in sequence.
Added in Combination
We first mixed 11.5% (v/v) HNO3 with 30% (v/v) H2O2 in various proportions to form a mixture, and the tissue and plastic particles were digested by the added mixture. The digestion protocol did not substantially affect the plastic polymers; we observed no changes in shape. In accordance with increasing proportions of HNO3, the digestion of the fish digestive tract epidermis gradually worsened. There were tissue residues in all the solutions except at the proportion of 1:0 v/v, especially in the low-concentration groups (Supplementary Figure 5).
It is odd that none of the binary digestive systems (HNO3 and H2O2 added in combination) were as effective as any of the unitary digestion systems (Supplementary Figures 1–3). We hypothesized that these results for the binary digestion system may be because of a chemical reaction between HNO3 and H2O2; after the reaction, the concentrations of H2O2 and HNO3 decrease, resulting in an inferior digestion. To test this hypothesis, we calculated the reaction between H2O2 and HNO3 (Equation 2) using Gaussian modeling. Figure 1 shows the change in energy and material over the course of the reaction. The hypothetical reaction process and intermediate products in the reaction are shown in Figures 1A,B. As for Figure 1A, the A represents reactants HNO3 and H2O2, which are optimized before calculation; as the reaction progresses, HNO3 bond breaks and formed intermediate product B; the C represents the TS1 (NO2, H⋅, OH⋅, and -O2H) which was the transition state of the reaction, the D was the product of phase 1 which forming the final product NO2, H2O and the reactant of phase 2. For Figure 1B, the A represents the reactants OH⋅ and -O2H, which undergo chemical bond breaking and recombination (B, C) to form the final products O2 and H2O. The specific reaction path is shown in Figure 1C. The modeling results indicate that H2O2 and HNO3 react under certain conditions, which will affect the concentration of the solution in the digestion system and ultimately affect the experimental results.
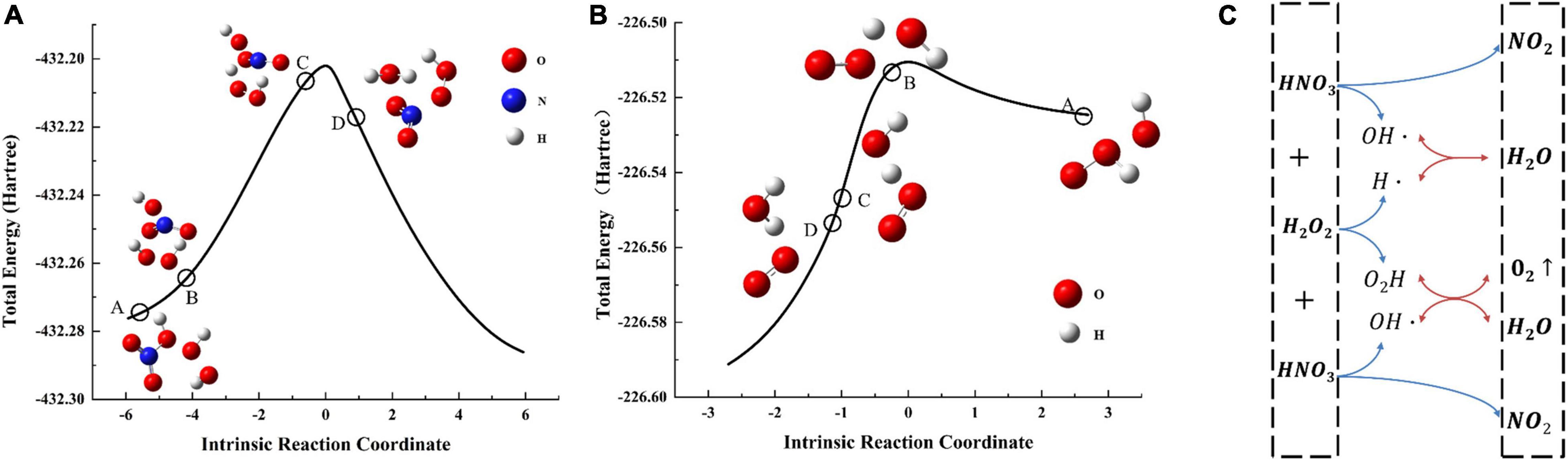
Figure 1. Energy change over the course of the reaction: (A) reaction path 1, (B) reaction path 2. Bonds are broken and formed, affording substances in different reaction phases which shown in A, B, C, D of panels (A,B). Panel (C) showed the specific reaction process of two phases and the intermediate products in the reaction process. (blue line) Reaction path 1, (red line) reaction path 2.
Added in Sequence
Accordingly, to avoid a chemical reaction between HNO3 and H2O2 that hinders digestion, we added 11.5% (v/v) HNO3 and 30% (v/v) H2O2 in succession, in various proportions for tissue digestion. We first added H2O2 and then HNO3. These treatments did not affect the polymers, whereas compared with the results in section “Added in Combination” the efficiency of the tissue digestion improved; large masses of tissue were not evident (Supplementary Figure 6). The proportion of 2:1 (v/v) afforded the best experimental results.
Although 11.5% HNO3 and 30% H2O2 separately added in two steps can partly avoid both direct mixing reactions. The experimental results also indicated that, compared with directly adding, the digestion effect of tissues was significantly improved (Supplementary Figure 6), the fish tissues were not completely dissolved. The digestion efficiency was not as ideal as possible. This may be because of residual H2O2 in the digestion system, similarly to why the combination treatments were ineffective. Thus, we sought to decrease the quantity of residual H2O2. Accordingly, on the basis of the Fenton reaction principle, we added Fe2+ and NaOH after digestion by H2O2, yet before digestion by HNO3. Other studies have also shown evidence that both enzymes and Fenton’s reagent could achieve efficient, rapid, and inexpensive digestion of organic matter and other materials. Dissolving biological matrices to analyze the gut contents of freshwater fish has literature precedent (Rodrigues et al., 2018; Collard et al., 2019).
After adding Fe2+ and NaOH, digestion was slightly improved compared with simply adding H2O2, perhaps because NaOH and Fe2+ catalyze digestion. Subsequent digestion as per H2O2–HNO3 was considerably improved compared with the simple sequential H2O2/HNO3 digestions, in which HNO3 is used to react with the residual H2O2 rather than digestion. The plastics were not damaged.
Thus far, we had obtained optimum results for the following binary digestion: add 30% (v/v) H2O2 and allow to stand for 1 h; then add 10 mL of 0.5 M NaOH and 1 mL of 5 mM Fe2+, and allow to stand for 4 h; then add 11.5% (v/v) HNO3 and allow to stand for 6 h. Nevertheless, residual fat remained in the filter membrane, which decreased the filtration efficiency and recovery. We thus needed to optimize the protocol for dispersion of fat problem.
Protocol Optimization for Dispersion of Fat Problem
As aforementioned, sometimes the fat residue clearly affected the filtration speed. Previous study methods for overcoming the fat problem include high-temperature treatment, surface activation, and ultrasound. We studied only ultrasound and surface activation in this work because of corrosivity that resulted from our preliminary high-temperature studies. Sometimes, our initial experiments with ultrasound were unsuccessful. We observed granular fat residues after 1 h (Supplementary Figure 7A), despite the positive results reported by Lenz et al. (2015) within 10 min. This may be because of differences in the type of fish.
Sodium dodecyl sulfate is a common surfactant that has been used to disrupt organic matrices in marine MP research (Loder et al., 2017; Rodrigues et al., 2018). Lenz et al. (2015) revealed that applying SDS can facilitate the dissolution of coatings from hydrophobic surfaces. Budimir et al. (2018) studied Baltic herring purchased from their local supermarket as a fish sample. They added NaOH and SDS to a glass jar of fish tissue, and kept the mixture at 50°C for 24 h; their good dissolution results may be because of the low-fat content of herring. Our experiments indicate that the protocol of Budimir et al. (2018) is sufficient for the digestion of most fish samples, but not for fish that are high in fat (Supplementary Figure 7B).
Ultrasound by itself was insufficient and SDS in itself was insufficient, and therefore we combined the two methods for disrupting fat. We conducted experiments on the digestive tract, which contains a substantial quantity of fats. The fat gradually dispersed and the droplets’ volume decreased. The effect was remarkable (Supplementary Figure 8). Thus, we combined our results into a binary resolution system.
Accordingly, we obtained an optimal protocol for extracting spiked MPs from tissues. First, thaw fish tissue to RT, then weigh ∼3 g ± 0.01 g on an analytical balance. Dissolve the tissue and MPs in 20 mL of 30% (v/v) aqueous H2O2, and allow to stand at RT for 1 h. Then, add 10 mL 0.5 M of aqueous NaOH and 1 mL of 5 mM aqueous Fe2+, and let stand for 4 h. Next, add 40 mL of 11.5% (v/v) aqueous HNO3 and let stand for 6 h. The final step is to remove the remaining fat particles: first add 16 mL of 150 g/L SDS, then apply ultrasound for 1 h, and then let stand for a few minutes for subsequent filtration (Figure 2).
Verifying the Protocol
Evaluation of the Digestion Efficacy
We developed an efficient fish digestion protocol by visual inspection; it is difficult to conceive of a clear-cut quantitative metric. The literature indicates that the gravimetric method is pertinent to evaluating the efficiency of digestion, but compared with our method the weight change is not the most important factor. We found that the most important factor was the type of residue rather than the weight that affected the final test result, such as digestive tract dyspepsia. However, compared with the residual fat particles after digestion, the undigested digestive tract did not considerably interfere with the test results; the residual digestive tract can be cleaned on its surface and removed without participating in filtration. Therefore, by observing the digestion of fish tissues with unaided eyes, we can preliminarily test whether our digestion plan of fish tissues is feasible.
We applied our protocol to the detection of 368 fish samples. We greatly improved the filtration speed. The last step of our experiment was to filter the digestion solution and observe the types of plastic particles on the filter membrane; thus, the filtration time is an important indicator to evaluate our method. After the application of our protocol, 40% roughly of the sample filtration time was controlled within 20 min, followed by 10 min (30%), 30–60 min (20%), and longer than 60 min (10%), thus proving that our binary digestion system improves efficiency and saves time.
The Impact of the Protocol on Plastic Particles
To further verify the feasibility of our protocol, its impact on MPs (micromorphology and chemical structure) should be evaluated. In order to improve the efficiency of the experiment, we used different methods to evaluate plastics in different experimental exploration stages. In initial investigations we used microscopes to observe changes in the details of the plastic and rule out methods that clearly affected the plastic. After obtaining a clear experimental protocol, we confirmed that the digestion process had no effect on the chemical structure of plastics by O-PTIR. The results for the recovery rate and field application finally proved that the method is feasible.
In initial investigations, we performed particle analyses by static image analyses with a Raman spectrometer. Artificial marks provide more details than smooth plastic particles; we made cross marks with a knife in each plastic particle. We used the position of the mark to compare readily identifiable changes before and after digestion. The cross marks on the surface of 11 types of plastic particles did not change appreciably after digestion compared with before digestion (Figure 3). The digestion solution did not corrode or dissolve the plastic particles.
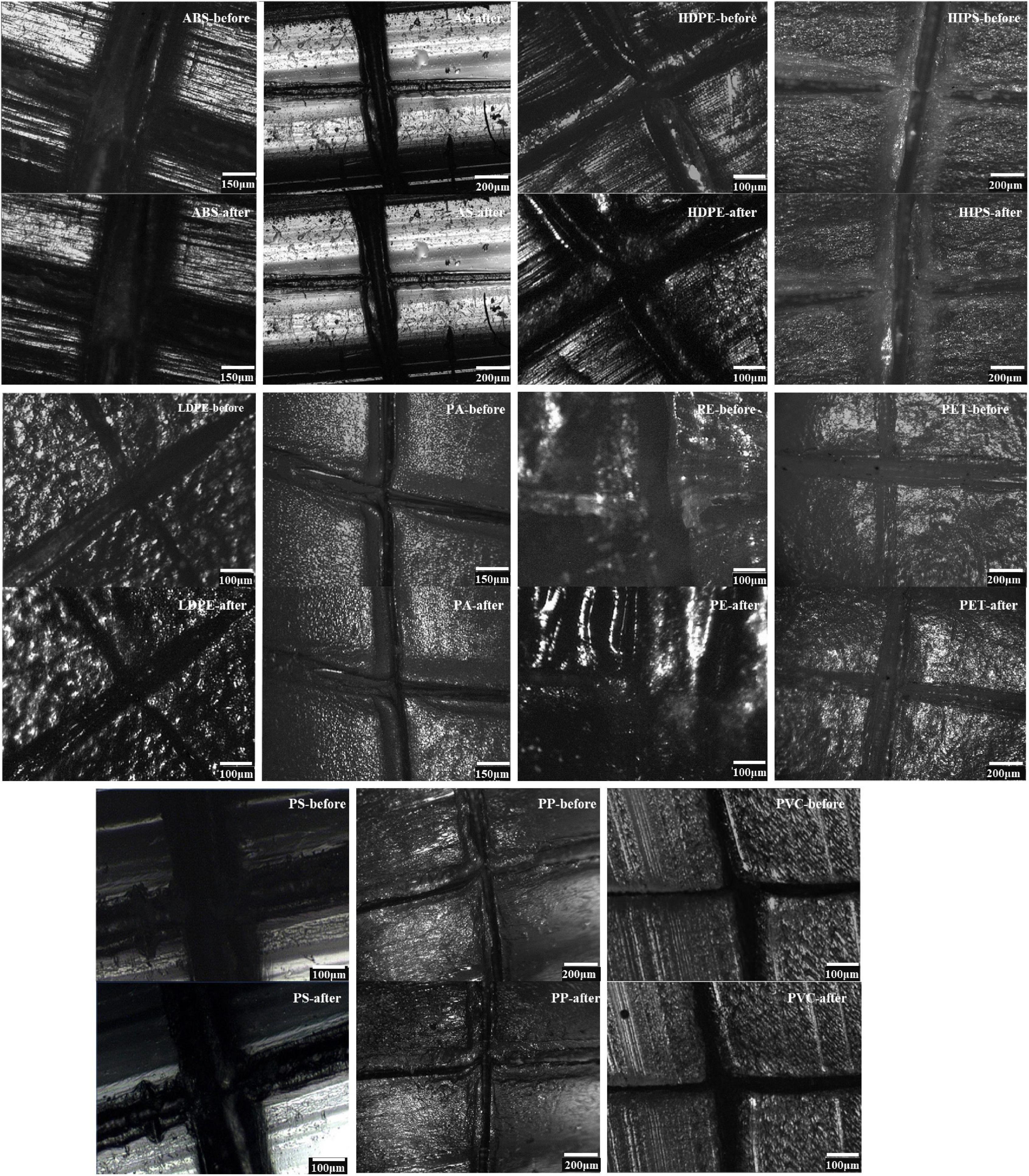
Figure 3. Spectrometer photographs of 11 types of plastic particles before and after digestion, as per the final protocol. Microphotography of the following surface morphology: (upper) before particle digestion, and (under) post-digestion.
We observed the changes in the chemical composition of the polymers by comparing the O-PTIR spectra before and after the digestion (Figure 4). The peak positions of the 11 plastics before and after the reaction were not obviously changed, especially the HIPS, LDPE, PET, ABS, AS, and PVC particles. However, we observed a few differences in the spectra of individual plastic particles compared with the standard sample, such as for PA, PE, and PS. For PE, the peak intensity at the carbonyl stretching region around 1,720–1,770 cm–1 or 1,730–1,750 cm–1 was lower than that of the standard spectrum. Using O-PTIR Studio software, we determined that the corresponding stretching peak of these three positions represents two residues. One is attributable to the C = O stretching peak in ClCOOC and the other is attributable to the C = O stretching peak in COO. For PA, although an expected peak was not evident at 1,372 cm–1, this peak represents the C = O stretching peak of RCOO; which indicates that the plastic has been reduced to produce carboxylic acids. Similarly, as for PS, the corresponding peak change was at 1,638 cm–1, which indicates a C = O or C = N stretching peak. The functional group represented by the changed characteristic peak is either C = O or C = N. These two kinds of chemical bonds indicate that the plastic particles underwent a redox reaction over the course of digestion. This is an acceptable experimental error; the digestion reagents had no obvious effect on the molecular structure of the plastics and did not affect the particle quantitation results. Therefore, the structures of the plastics did not change appreciably. The digestion protocol had little impact on the plastic particles and did not affect the detection of MPs in fish tissue.
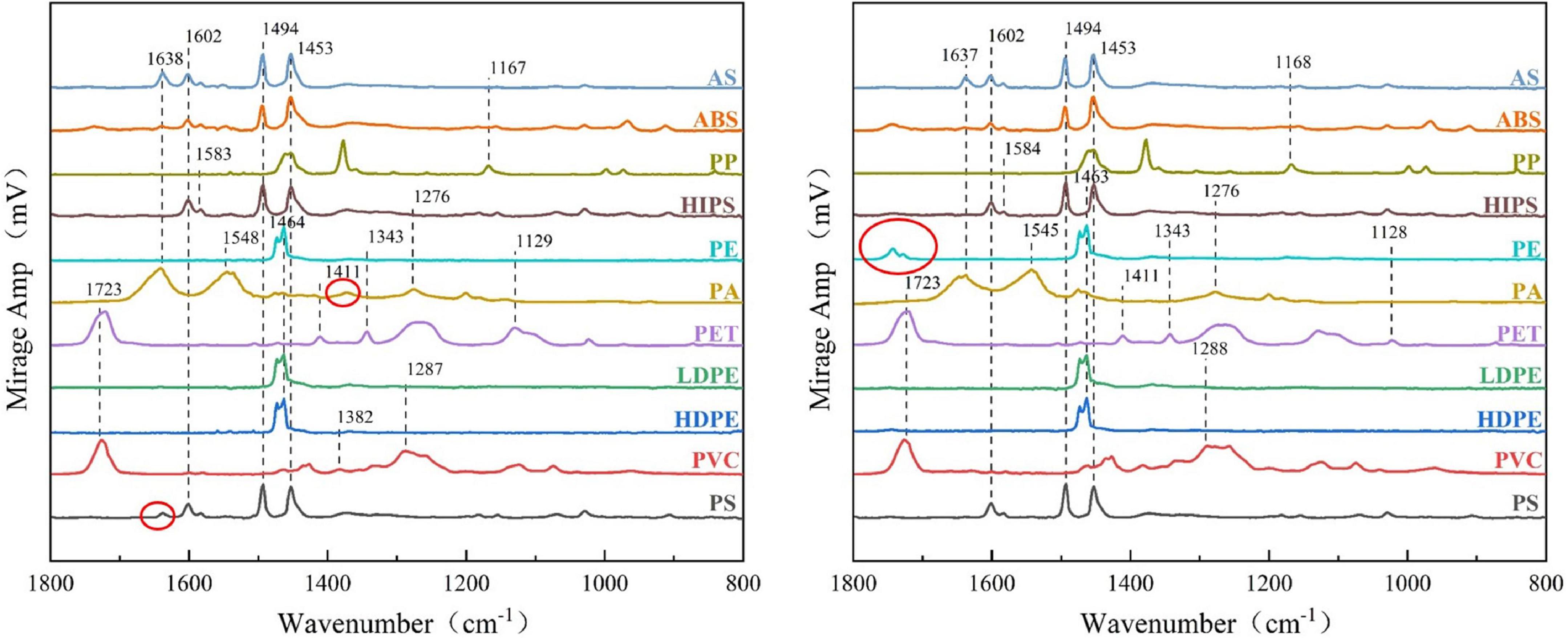
Figure 4. Comparison of spectroscopy measured by O-PTIR spectra before and after digestion of 11 types of plastic particles, (left) before particle digestion, and (right) post-digestion.
Recovery Rate
In our study (Figure 5), the recoveries of 11 types of plastic particles larger than 60 μm in diameter each reached more than 87%, as same as diameter < 60 μm. Larger-sized plastics (200–500 μm) each reached 90%; the recoveries of ABS, PE, PET, HIPS, and LDPE (200–500 μm) all reached 100%; PP reached 103% and PET reached 110%. The recoveries of PP, PVC, PE, and HDPE (100–200 μm) also each reached 100%; PE reached 103% and PET reached 107%. In the small size of 60–100-μm plastic particles, only ABS and HIPS reached 100%. Regarding sizes less than 60 μm, the maximal recovery rate was 97%, which refers to PE and PVC. A recovery rate greater than 100% may be because of the influence of preexisting microplastics in the digestive glands of the fish. Similar results also appeared in Maes et al. (2017); Fraissinet et al. (2021) which is a relatively common situation. The recovery rate ranged from 87 to 100%, which indicated that the digestion protocol used in our experiments generally had relatively little influence on the recovery rate of MPs as a function of the type of plastic.
Field Application
Based on the verification of the protocol, we next analyzed field-collected fish from Shandong Tantai.
As shown in Figure 6, we obtained single-frequency O-PTIR images at a 500-nm step size and 100-nm step resolution by tuning the QCL device to the frequencies that correspond to the specific vibrations of extraneous materials at various wavenumbers (1,023, 1,075, 1,641, and 1,725 cm–1); detailed analysis of eight materials in Figure 6. In addition to PA, PET, and other plastic particles, we detected some crustacean bones and plastic additives.
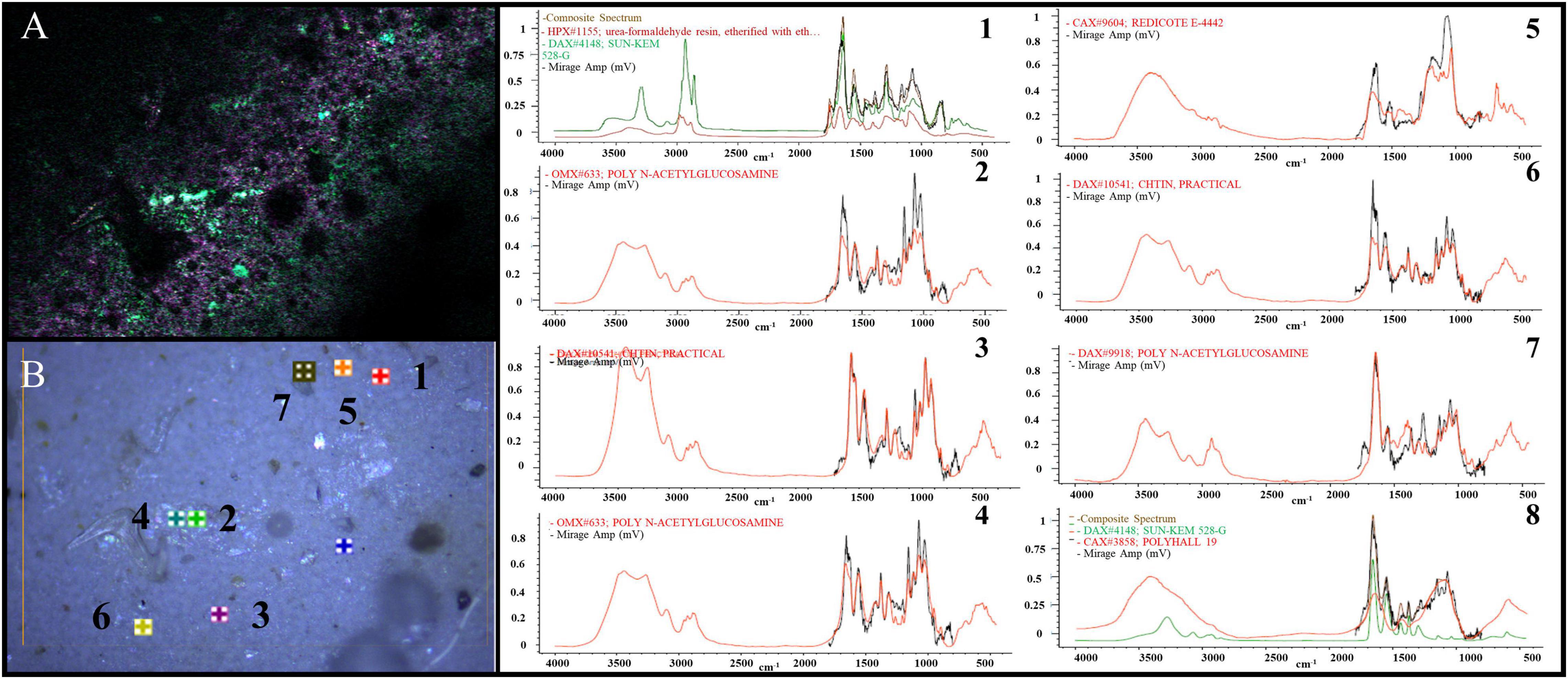
Figure 6. Images of specific vibrations (1,023, 1,075, 1,641, and 1,725 cm–1) of extraneous materials in various regions imaged by single-frequency O-PTIR was on the left (A,B), the detailed analysis of eight materials in panel (B) was showed 1∼8.
We thus comprehensively confirmed the feasibility of our protocol. Our method is not only applicable to the digestion of fish tissues in the laboratory, but also applicable to practical analysis of real fish samples from the field, which has a practical value and promotion significance.
Conclusion
Because of the presence of MPs in many fish, albeit at low concentrations, it is critical to employ an extraction protocol that efficiently digests fish tissue without affecting the integrity of the plastic polymers. No single-component digestion solution was suitable for extracting MPs from fish tissues in our research because of inefficient digestion of fish tissues or corrosivity to plastics. Although HClO4 had a high efficiency toward tissue digestion, it was highly corrosive to plastic particles. HNO3 was effective toward tissue digestion but was strongly corrosive to plastic particles. The digestion protocol of H2O2 did not damage the tested particles but had poor digestion efficiency.
Using thorough experimentation, we obtained a plastic-safe and high-efficiency protocol (Figure 2): a synergistic binary digestion system that consists of 30% (v/v) H2O2 and 11.5% (v/v) HNO3. In accordance with insights obtained from a Gaussian model, Fenton’s reagent (Fe2+ and NaOH) should be added after digestion by H2O2, yet before digestion by HNO3, to avoid the reaction between H2O2 and HNO3. Based on the results of a single condition, the single-digestion reagent experiment confirmed that the reagents with a good digestion effect were 11.5% HNO3 and 30% H2O2; thus, we combined the two reagents to construct a new binary digestion system. Under the guidance of a chemical calculation theory based on a Gaussian model, we successively added 11.5% HNO3 and 30% H2O2 to solve the phenomenon of the synergistic reaction of HNO3 and H2O2 that affects the digestion. We used SDS and ultrasound together to solve the problem of fat residue blockage of the membrane during filtration. We tentatively propose this protocol for the extraction and characterization of MPs from fish tissues.
We demonstrated the feasibility of our protocol by calculating the MPs recovery rate (Figure 5). We applied our protocol to field-collected fish samples and obtained considerable digestion results, which we verified by the mapping function of O-PTIR, and confirmed the practical applicability of this protocol. The results of our protocol may vary in accordance with the species of fish, but the protocol is efficient and reliable.
Data Availability Statement
The original contributions presented in the study are included in the article/Supplementary Material, further inquiries can be directed to the corresponding author/s.
Author Contributions
FfY: writing-original draft and confirmed the experimental results. XcW: experimental scheme design and completed the digestion experiment. HrS: field sampling. WhS: field investigation. ZxZ: O-PTIR analysis. XlS: Gaussian model analysis. JpZ: statistical analysis. LZ: pretreatment. XfW: instrumental analysis. MyL: editing. MgC: supervision. YZ: conceptualization, experimental scheme design, writing-review, and supervision. All authors contributed to the article and approved the submitted version.
Funding
This work was supported by the National Natural Science Foundation of China (41606125, 52070125, and 41961144011) and Natural Science Foundation of Fujian Province (2020J0141).
Conflict of Interest
The authors declare that the research was conducted in the absence of any commercial or financial relationships that could be construed as a potential conflict of interest.
Publisher’s Note
All claims expressed in this article are solely those of the authors and do not necessarily represent those of their affiliated organizations, or those of the publisher, the editors and the reviewers. Any product that may be evaluated in this article, or claim that may be made by its manufacturer, is not guaranteed or endorsed by the publisher.
Acknowledgments
We would like to thank Chengxiu Lu and Yingxue Sun from College of Geography and Environment of Shandong Normal University for their supporting in instrumental analysis and testing. We would also like to thank Michael Scott Long, from Liwen Bianji (Edanz) (www.liwenbianji.cn/), for editing the English text of a draft of this manuscript. We would also further like to thank Xi Hu and Hongjie Tang from Quantum Design China for helping the O-PTIR measurements.
Supplementary Material
The Supplementary Material for this article can be found online at: https://www.frontiersin.org/articles/10.3389/fmars.2022.845062/full#supplementary-material
References
Avio, C. G., Gorbi, S., and Regoli, F. (2015). Experimental development of a new protocol for extraction and characterization of microplastics in fish tissues: first observations in commercial species from Adriatic Sea. Mar. Environ. Res. 111, 18–26. doi: 10.1016/j.marenvres.2015.06.014
Barrett, J., Chase, Z., Zhang, J., Holl, M. M. B., Willis, K., Williams, A., et al. (2020). Microplastic Pollution in Deep-Sea Sediments From the Great Australian Bight. Front. Mar. Sci. 7:576170. doi: 10.3389/fmars.2020.576170
Budimir, S., Setala, O., and Lehtiniemi, M. (2018). Effective and easy to use extraction method shows low numbers of microplastics in offshore planktivorous fish from the northern Baltic Sea. Mar. Pollut. Bull. 127, 586–592. doi: 10.1016/j.marpolbul.2017.12.054
Claessens, M., Van Cauwenberghe, L., Vandegehuchte, M. B., and Janssen, C. R. (2013). New techniques for the detection of microplastics in sediments and field collected organisms. Mar. Pollut. Bull. 70, 227–233. doi: 10.1016/j.marpolbul.2013.03.009
Cole, M., Webb, H., Lindeque, P. K., Fileman, E. S., Halsband, C., and Galloway, T. S. (2014). Isolation of microplastics in biota-rich seawater samples and marine organisms. Sci. Rep. 4:4528. doi: 10.1038/srep04528
Collard, F., Gasperi, J., Gabrielsen, G. W., and Tassin, B. (2019). Plastic Particle Ingestion by Wild Freshwater Fish: a Critical Review. Environ. Sci. Technol. 53, 12974–12988. doi: 10.1021/acs.est.9b03083
Collard, F., Gilbert, B., Eppe, G., Parmentier, E., and Das, K. (2015). Detection of Anthropogenic Particles in Fish Stomachs: an Isolation Method Adapted to Identification by Raman Spectroscopy. Arch. Environ. Contam. Toxicol. 69, 331–339. doi: 10.1007/s00244-015-0221-0
Dehaut, A., Cassone, A. L., Frere, L., Hermabessiere, L., Himber, C., Rinnert, E., et al. (2016). Microplastics in seafood: benchmark protocol for their extraction and characterization. Environ. Pollut. 215, 223–233. doi: 10.1016/j.envpol.2016.05.018
Eerkes-Medrano, D., Thompson, R. C., and Aldridge, D. C. (2015). Microplastics in freshwater systems: a review of the emerging threats, identification of knowledge gaps and prioritisation of research needs. Water Res. 75, 63–82. doi: 10.1016/j.watres.2015.02.012
Enders, K., Lenz, R., Beer, S., Stedmon, C. A., and Browman, H. (2017). Extraction of microplastic from biota: recommended acidic digestion destroys common plastic polymers. ICES J. Mar. Sci. 74, 326–331. doi: 10.1093/icesjms/fsw173
Fang, C., Sobhani, Z., Zhang, X., McCourt, L., Routley, B., Gibson, C. T., et al. (2021). Identification and visualisation of microplastics / nanoplastics by Raman imaging (iii): algorithm to cross-check multi-images. Water Res. 194:116913. doi: 10.1016/j.watres.2021.116913
Fraissinet, S., Pennetta, A., Rossi, S., De Benedetto, G. E., and Malitesta, C. (2021). Optimization of a new multi-reagent procedure for quantitative mussel digestion in microplastic analysis. Mar. Pollut. Bull. 173:112931. doi: 10.1016/j.marpolbul.2021.112931
Gedik, K., and Eryasar, A. R. (2020). Microplastic pollution profile of Mediterranean mussels (Mytilus galloprovincialis) collected along the Turkish coasts. Chemosphere 260:127570. doi: 10.1016/j.chemosphere.2020.127570
Gorokhova, E. (2015). Screening for microplastic particles in plankton samples: how to integrate marine litter assessment into existing monitoring programs? Mar. Pollut. Bull. 99, 271–275. doi: 10.1016/j.marpolbul.2015.07.056
Hossain, M. S., Sobhan, F., Uddin, M. N., Sharifuzzaman, S. M., Chowdhury, S. R., Sarker, S., et al. (2019). Microplastics in fishes from the Northern Bay of Bengal. Sci. Total Environ. 690, 821–830. doi: 10.1016/j.scitotenv.2019.07.065
Ivleva, N. P. (2021). Chemical Analysis of Microplastics and Nanoplastics: challenges, Advanced Methods, and Perspectives. Chem Rev. 121, 11886–11936. doi: 10.1021/acs.chemrev.1c00178
Kansiz, M., Prater, C., Dillon, E., Lo, M., Anderson, J., Marcott, C., et al. (2020). Optical Photothermal Infrared Microspectroscopy with Simultaneous Raman - A New Non-Contact Failure Analysis Technique for Identification of <10 mum Organic Contamination in the Hard Drive and other Electronics Industries. Micros. Today 28, 26–36. doi: 10.1017/s1551929520000917
Karami, A., Golieskardi, A., Choo, C. K., Romano, N., Ho, Y. B., and Salamatinia, B. (2017). A high-performance protocol for extraction of microplastics in fish. Sci. Total Environ. 578, 485–494. doi: 10.1016/j.scitotenv.2016.10.213
Lenz, R., Enders, K., Stedmon, C. A., Mackenzie, D. M. A., and Nielsen, T. G. (2015). A critical assessment of visual identification of marine microplastic using Raman spectroscopy for analysis improvement. Mar. Pollut. Bull. 100, 82–91. doi: 10.1016/j.marpolbul.2015.09.026
Li, J., Yang, D., Li, L., Jabeen, K., and Shi, H. (2015). Microplastics in commercial bivalves from China. Environ. Pollut. 207, 190–195. doi: 10.1016/j.envpol.2015.09.018
Loder, M. G. J., Imhof, H. K., Ladehoff, M., Loschel, L. A., Lorenz, C., Mintenig, S., et al. (2017). Enzymatic Purification of Microplastics in Environmental Samples. Environ. Sci. Technol. 51, 14283–14292. doi: 10.1021/acs.est.7b03055
Maes, T., Jessop, R., Wellner, N., Haupt, K., and Mayes, A. G. (2017). A rapid-screening approach to detect and quantify microplastics based on fluorescent tagging with Nile Red. Sci. Rep. 7:44501. doi: 10.1038/srep44501
Mathalon, A., and Hill, P. (2014). Microplastic fibers in the intertidal ecosystem surrounding Halifax Harbor, Nova Scotia. Mar. Pollut. Bull. 81, 69–79. doi: 10.1016/j.marpolbul.2014.02.018
Merzel, R. L., Purser, L., Soucy, T. L., Olszewski, M., Colon-Bernal, I., Duhaime, M., et al. (2020). Uptake and Retention of Nanoplastics in Quagga Mussels. Glob. Chall. 4:1800104. doi: 10.1002/gch2.201800104
Nguyen, B., Claveau-Mallet, D., Hernandez, L. M., Xu, E. G., Farner, J. M., and Tufenkji, N. (2019). Separation and Analysis of Microplastics and Nanoplastics in Complex Environmental Samples. Acc. Chem. Res. 52, 858–866. doi: 10.1021/acs.accounts.8b00602
Olson, N. E., Xiao, Y., Lei, Z., and Ault, A. P. (2020). Simultaneous Optical Photothermal Infrared (O-PTIR) and Raman Spectroscopy of Submicrometer Atmospheric Particles. Anal. Chem. 92, 9932–9939. doi: 10.1021/acs.analchem.0c01495
Ribeiro, F., Okoffo, E. D., O’Brien, J. W., Fraissinet-Tachet, S., O’Brien, S., Gallen, M., et al. (2020). Quantitative Analysis of Selected Plastics in High-Commercial-Value Australian Seafood by Pyrolysis Gas Chromatography Mass Spectrometry. Environ. Sci. Technol. 54, 9408–9417. doi: 10.1021/acs.est.0c02337
Rodrigues, M. O., Gonçalves, A. M. M., Gonçalves, F. J. M., Nogueira, H., Marques, J. C., and Abrantes, N. (2018). Effectiveness of a methodology of microplastics isolation for environmental monitoring in freshwater systems. Ecol. Indic. 89, 488–495. doi: 10.1016/j.ecolind.2018.02.038
Schirinzi, G. F., Peda, C., Battaglia, P., Laface, F., Galli, M., Baini, M., et al. (2020). A new digestion approach for the extraction of microplastics from gastrointestinal tracts (GITs) of the common dolphinfish (Coryphaena hippurus) from the western Mediterranean Sea. J. Hazard. Mater. 397:122794. doi: 10.1016/j.jhazmat.2020.122794
Simonsen, K. P., Rasmussen, A. R., Mathisen, P., Petersen, H., and Borup, F. (2011). A fast preparation of skeletal materials using enzyme maceration. J. Forensic. Sci. 56, 480–484. doi: 10.1111/j.1556-4029.2010.01668.x
Su, Y., Hu, X., Tang, H., Lu, K., Li, H., Liu, S., et al. (2021). Steam disinfection releases micro(nano)plastics from silicone-rubber baby teats as examined by optical photothermal infrared microspectroscopy. Nat. Nanotechnol. 17, 76–85. doi: 10.1038/s41565-021-00998-x
Süssmann, J., Krause, T., Martin, D., Walz, E., Greiner, R., Rohn, S., et al. (2021). Evaluation and optimisation of sample preparation protocols suitable for the analysis of plastic particles present in seafood. Food Control 125:107969. doi: 10.1016/j.foodcont.2021.107969
Watts, A. J., Lewis, C., Goodhead, R. M., Beckett, S. J., Moger, J., Tyler, C. R., et al. (2014). Uptake and retention of microplastics by the shore crab Carcinus maenas. Environ. Sci. Technol. 48, 8823–8830. doi: 10.1021/es501090e
Wright, S. L., Thompson, R. C., and Galloway, T. S. (2013). The physical impacts of microplastics on marine organisms: a review. Environ. Pollut. 178, 483–492. doi: 10.1016/j.envpol.2013.02.031
Zhang, D., Li, C., Zhang, C., Slipchenko, M. N., Eakins, G., and Cheng, J. X. (2016). Depth-resolved mid-infrared photothermal imaging of living cells and organisms with submicrometer spatial resolution. Sci. Adv. 2:e1600521. doi: 10.1126/sciadv.1600521
Zhou, X. X., Hao, L. T., Wang, H. Y., Li, Y. J., and Liu, J. F. (2019). Cloud-Point Extraction Combined with Thermal Degradation for Nanoplastic Analysis Using Pyrolysis Gas Chromatography-Mass Spectrometry. Anal. Chem. 91, 1785–1790. doi: 10.1021/acs.analchem.8b04729
Keywords: microplastics, binary digestion system, O-PTIR, detection, extraction method
Citation: Yan F, Wang X, Sun H, Zhu Z, Sun W, Shi X, Zhang J, Zhang L, Wang X, Liu M, Cai M and Zhang Y (2022) Development of a Binary Digestion System for Extraction Microplastics in Fish and Detection Method by Optical Photothermal Infrared. Front. Mar. Sci. 9:845062. doi: 10.3389/fmars.2022.845062
Received: 29 December 2021; Accepted: 20 January 2022;
Published: 11 February 2022.
Edited by:
Jun Wang, South China Agricultural University, ChinaReviewed by:
Licheng Peng, Hainan University, ChinaBin Xia, Chinese Academy of Fishery Sciences (CAFS), China
Sha-Yen Cheng, National Taiwan Ocean University, Taiwan
Copyright © 2022 Yan, Wang, Sun, Zhu, Sun, Shi, Zhang, Zhang, Wang, Liu, Cai and Zhang. This is an open-access article distributed under the terms of the Creative Commons Attribution License (CC BY). The use, distribution or reproduction in other forums is permitted, provided the original author(s) and the copyright owner(s) are credited and that the original publication in this journal is cited, in accordance with accepted academic practice. No use, distribution or reproduction is permitted which does not comply with these terms.
*Correspondence: Minggang Cai, bWdjYWlAeG11LmVkdS5jbg==; Ying Zhang, emhhbmd5aW5nQHNkbnUuZWR1LmNu
†These authors have contributed equally to this work