- 1Marine Geology Department, Faculty of Marine Sciences, King Abdulaziz University, Jeddah, Saudi Arabia
- 2Marine Geology Department, Faculty of Marine Sciences and Environment, Hodeidah University, Al Hudaydah, Yemen
- 3Geology Department, Faculty of Sciences, Mansoura University, Mansoura, Egypt
- 4School of Earth, Atmospheric and Life Sciences, University of Wollongong, Wollongong, NSW, Australia
Contemporary foraminiferal sediment samples were collected from the intertidal sabkha of Al-Kharrar Lagoon, Saudi Arabia, to study the vertical distribution of foraminifers and, based on a modern training set, their potential to develop a predictor of former sea-level changes in the area. Based on hierarchical cluster analysis, the intertidal sabkha is divided into three vertical zones (A, B, and C) represented by three foraminiferal assemblages, where agglutinated species occupied Zone A and calcareous species occupied the other two zones. In Zone A (high intertidal), Agglutinella compressa, Clavulina angularis and C. multicamerata are dominant species with a minor presence of Peneroplis planatus, Coscinospira hemprichii, Sorites orbiculus, Quinqueloculina lamarckiana, Q. seminula, Ammonia convexa and A. tepida. In contrast, in Zone B (middle intertidal) the most abundant species are P. planatus, C. hemprichii, S. orbiculus, Q. lamarckiana, Q. seminula and Q. laevigata, while Zone C (low intertidal) is characterized by C. hemprichii, Q. costata, S. orbiculus, P. planatus, A. convexa, A. tepida, Spiroloculina communis, and S. costigera. A transfer function for sea-level reconstruction was developed using a modern dataset of 75 contemporary sediment samples and 99 species collected from several transects across the sabkha. The model provided an error of 0.12 m, suggesting that intertidal foraminifers can be used to assess past sea-level changes with high precision in Al-Kharrar Lagoon, and adjacent regions.
Introduction
Benthic foraminiferal assemblages in sabkhas display discrete vertical zonation within the intertidal zone controlled by a variety of ecological conditions associated with the duration of tidal inundation (Phleger, 1965; Murray, 1971; Scott et al., 1998; Horton, 1999). Tidal inundation not only affects the distribution of foraminiferal assemblages, but also the distribution of vegetation cover and salinity in the intertidal zone (Leorri et al., 2010; Rogers et al., 2017). Accordingly, the distribution of both foraminiferal assemblages and vegetation cover could provide an indication of the intertidal surface elevation since the elevation is directly related to mean sea-level (msl; De Rijk and Troelstra, 1997; Haslett, 2001; Horton and Edwards, 2006). Several authors (Scott and Medioli, 1980a; Scott and Leckie, 1990; Horton and Edwards, 2003, 2006; Leorri et al., 2010) have indicated a close relationship between the vertical distribution of foraminiferal assemblages and the intertidal surface elevation. However, there are different opinions regarding the effect of elevation on intertidal foraminiferal distributions (Horton et al., 1999; Horton and Edwards, 2006).
Recent studies have suggested that intertidal foraminifers are controlled by environmental variables such as salinity and flooding frequency rather than their elevation with respect to mean-tide level (De Rijk, 1995; De Rijk and Troelstra, 1997; Punniyamoorthy et al., 2021). In some areas, salinity appears to be the most important factor influencing the distribution of foraminifers, while the microfaunal-elevation relationships are not of worldwide significance (De Rijk, 1995; Hayward et al., 2004; Lal et al., 2020). Intertidal foraminifers tolerate a wide variety of daily and seasonal changes in the environmental conditions, which are reflected in their increase or decrease in abundance and diversity (Leorri et al., 2010).
Calcareous and agglutinated taxa are found in intertidal environments and their abundance varies from site to site according to different environmental conditions such as salinity, oxygen and alkalinity (Lal et al., 2020) and elevation (Horton and Murray, 2007). Calcareous species predominated in the lower intertidal sabkha with normal salinity, while agglutinated species predominated in middle and higher intertidal sabkha areas where salinity is more variable (Hayward, 1993; Hayward and Hollis, 1994; De Rijk, 1995; Culver et al., 2012; Strachan et al., 2015; Lal et al., 2020). Alkalinity also plays an essential role in the foraminiferal distribution in the intertidal sabkha, where calcareous tests are susceptible to dissolution at low pH conditions < 7.8 and can completely disappear at ∼7.6 (Dias et al., 2010); however, agglutinated shells are more resistant and dominate the foraminiferal assemblage under conditions where pH ≤ 7.6 (Scott and Medioli, 1978; Edwards and Horton, 2000; Sanders, 2003; Hayward et al., 2004, 2015; Woodroffe et al., 2005; Dias et al., 2010).
Preservation of the foraminiferal assemblages in intertidal sabkhas is of great importance in palaeoenvironmental interpretations of older deposits (Cearreta and Murray, 2000; Leorri and Cearreta, 2004). Moreover, benthic foraminifers have been widely used as bio-indicators for determining intertidal surface elevations and also past sea-level changes, since the foraminifers respond strongly to both changes in elevation above msl and salinity (Gehrels, 1994; Cearreta and Murray, 2000; Horton and Edwards, 2006; Leorri et al., 2008b; Abu-Zied and Bantan, 2013).
Reconstructing intertidal surface elevations is done by developing a transfer function(TF) using a modern training dataset comprising of faunal data (relative abundance within a benthic foraminiferal assemblage) and the environmental data such as elevation and salinity (Imbrie, 1971; Birks, 1995). A better understanding of the nature of the relationship between foraminiferal data and environmental data enhances the precision and accuracy of a sea-level reconstruction and thus to the quality of the study (Horton et al., 1999). This relationship can be quantified in modern intertidal environments using regression model analysis (Cahill et al., 2015). Several models have been used in studies of sea-level changes, including: unimodal (WA-PLS) or linear models (PLS), and the most appropriate model for any study is determined based on the species–environment response model (Birks, 1995).
Over the past four decades, benthic foraminiferal data have been used to better understand and reconstruct past sea-level changes for many areas, including Australia, Europe, Britain, North America, and recently the Saudi Arabian Red Sea coast (Shennan et al., 1996; Horton et al., 1999; Edwards and Horton, 2000; Leorri et al., 2008a,2010; Woodroffe, 2009; Callard et al., 2011; Abu-Zied and Bantan, 2013; Kemp et al., 2013; Strachan et al., 2014; Ghandour et al., 2021). This is because the distribution of foraminifers is controlled by the frequency and duration of inundation, which is fundamentally a function of tidal elevation (Scott and Medioli, 1978; Horton and Edwards, 2006; Kemp et al., 2013). Published studies using foraminifera-based transfer functions in the Red Sea are very scarce, except for the studies by Abu-Zied and Bantan (2013, 2015) at Shuaiba Lagoon, 80 km south of Jeddah city. Previously published works on the Saudi Red Sea coast have not attempted to investigate the vertical zonation of foraminiferal assemblages in the intertidal sabkha zone. Therefore, in this study, we focus on several points: (i) to investigate the distribution of modern foraminifers across the intertidal sabkha of the Al-Kharrar Lagoon (KL) to determine whether the foraminiferal assemblages display evidence of vertical zonation; (ii) to explain the relationship between the foraminifera distribution and environmental variables such as elevation and salinity; and (iii) to present new foraminiferal data to develop a predictor that can be used to assess former sea-level changes in the area and adjacent regions.
Study Area
The Al-Kharrar Lagoon is located 10 km northwest of Rabigh city, Saudi Arabia, on the eastern coast of the Red Sea (Figure 1), between 22.83° to 23°N and 38.81° to 38.97°E. The lagoon occurs in a relatively stable part of the coastal plain, known as the Tihamah plain, where tectonic uplift has been almost negligible or uncertain during the Pliocene and Pleistocene periods (Lambeck, 1995; Plaziat et al., 2008; Manaa et al., 2016). Al-Kharrar Lagoon is a small (74 km2), semi-enclosed shallow basin with an average depth of 5 m (Al-Dubai et al., 2017b), elongated parallel to the sea (about 20 km long and 4 km wide). It probably formed by erosion during the Late Pleistocene glacial sea-level low stand, and was then drowned during the post-glacial sea-level rise, especially in the early Holocene transgression (Braithwaite, 1987; Brown et al., 1989). The southern and eastern sides of the Al-Kharrar Lagoon are bounded by extensive sabkhas, mainly composed of alluvial sand, evaporite deposits, and bioclastic fragments, and are dominated by green cyanobacteria, mangrove trees (Avicennia marina) and macroalgae (Al-Dubai et al., 2017b). In contrast, the western side is bordered by old, raised limestone reef terraces (1–3 m above msl) of Pleistocene age (Al-Sayari and Zötl, 1978; Basaham, 2008). The lagoon connects with the adjacent Red Sea via a very narrow inlet (about 120 m wide and 14 m deep) that allows stratified water bodies to pass through (Al-Dubai et al., 2017b). One layer enters the lagoon as a surface inflow with temperatures between 25 and 30°C in winter and summer, respectively, while the salinity remains constant at 39‰. A second layer leaves the lagoon through its narrow inlet as a subsurface outflow with temperatures of 24.5 and 30°C and salinities of 39.8 and 40.5‰ during winter and summer, respectively (Al-Dubai et al., 2017b). This water exchange with the Red Sea is mainly governed by thermohaline circulation, the tidal force, and the local NNE wind that dominates the area throughout the year (Al-Barakati, 2010; Al-Dubai et al., 2017b). The tidal range on the coast of Rabigh city (0.71 m) varies from the highest astronomical tide (0.39 m HAT above msl) to the lowest astronomical tide (–0.32 m LAT below msl; Saudi Aramco Tide Tables 2014), whereas the daily tidal range in KL is about 0.24 m (Al-Dubai, 2019). The latter is similar to that recorded in the Jeddah area due to its location near the nodal points located in the central Red Sea (Morcos, 1970; Gharbi et al., 2018), with ranges between 0.20 and 0.30 m during a spring-neap cycle (Lisitzin, 1974), that is generated by the semi-diurnal tidal force (Al-Barakati, 2010). The Al-Kharrar Lagoon has a warm, dry tropical climate with scarce rainfall (between 50–100 mm/year), high evaporation rates of up to 2 m/year (Sofianos et al., 2002; Bantan et al., 2020) and no perennial river runoff.
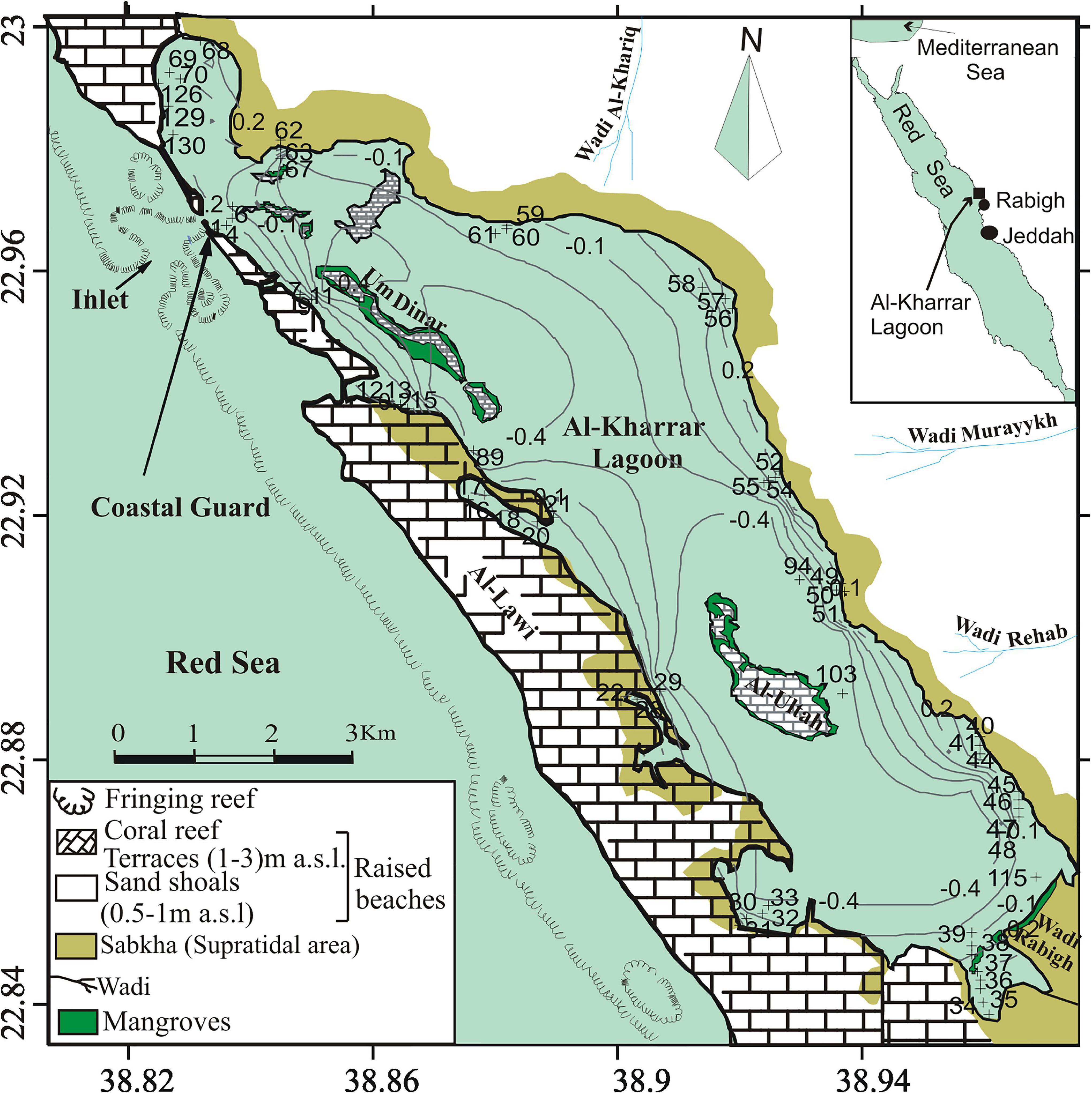
Figure 1. Location map of supratidal–intertidal sabkha, surface sediment sample sites (transects) and elevations in the Al-Kharrar Lagoon during March 2014 (modified after Al-Dubai et al., 2017a).
Materials and Methods
Field Sampling and Elevations
Seventy-five contemporary foraminiferal samples were collected along several elevational transects across the supratidal–intertidal sabkha of KL in March 2014. Sediment samples were collected at ∼50 m horizontal intervals within floral communities using a hand scoop, and were located between 0.6 and –0.5 m with respect to the mean tidal level (Figure 1). The locations of the sediment samples were determined using a Garmin GPS. The physico-chemical parameters temperature, salinity, pH and dissolved oxygen of the bottom waters were measured in situ using a YSI 556 MPS Multi-parameter meter and are listed in Table 1 and presented in Figure 2. Each sediment sample site in the transects was surveyed to a known reference height enabling each sample to be related to the local Saudi tidal datum (local lowest astronomical tide, LAT, which is 32 cm below msl) and the tidal elevation at each site was estimated using the Saudi Tide Tables (Saudi Aramco Tide Tables, 2014; see Table 2). Local LAT is considered as a reference to measure the daily tidal range along the Jeddah coast. Therefore, the elevation of the sample sites relative to LAT is equal to the measured water depth minus the tidal elevation at the time of sampling. Sampling was also focused on the vegetated intertidal zone above mean high water (MHW), since this is the main environment analyzed for sea-level studies (Gehrels and van de Plassche, 1999). Hence, data obtained from all transects were combined to establish a training set of supratidal–intertidal benthic foraminifers.
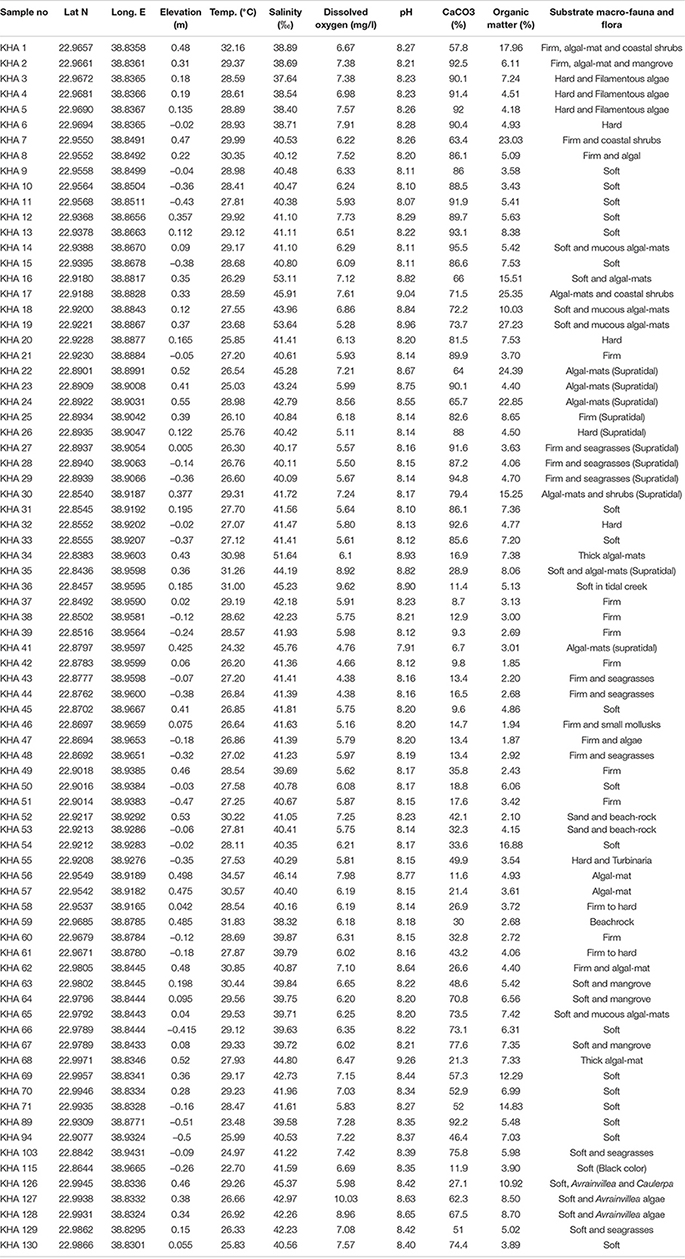
Table 1. Sample sites, elevations, physico-chemical parameters, and carbonate and organic matter contents in surface sediments of Al-Kharrar Lagoon during March 2014 (modified after Al-Dubai et al., 2017a,b).
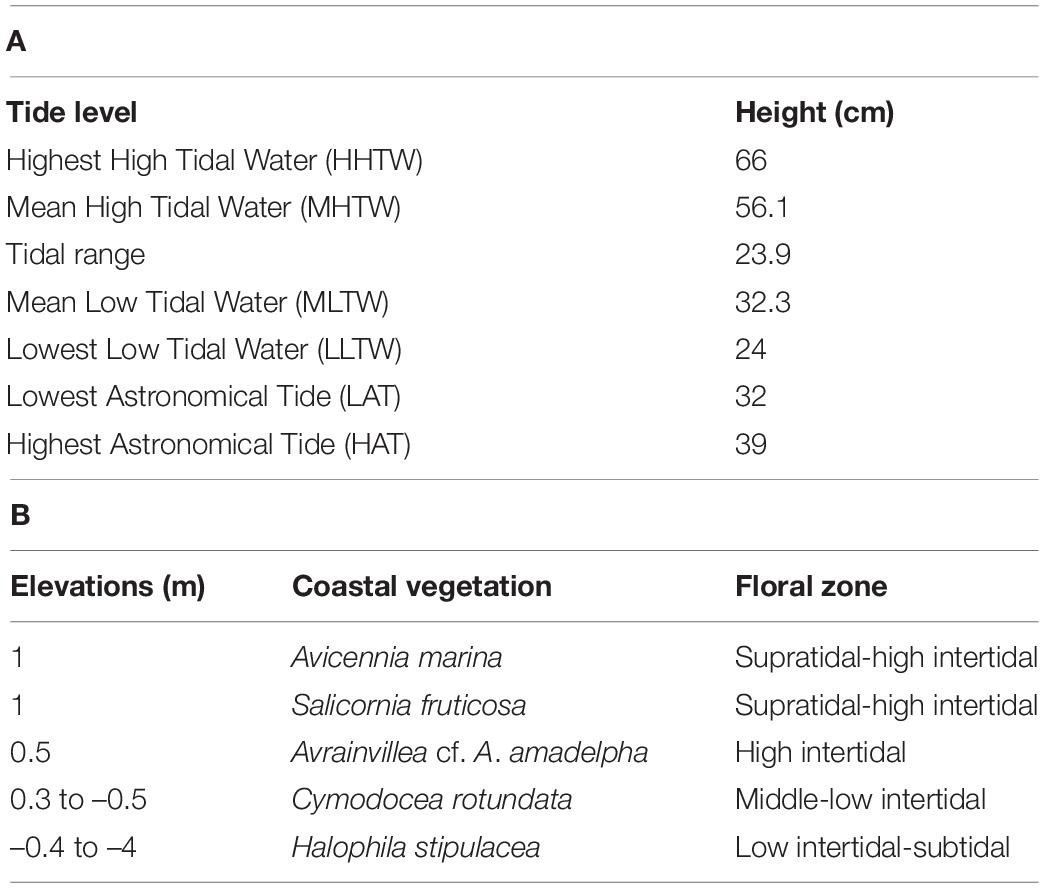
Table 2. (A) Tide levels at Al-Kharrar Lagoon (Rabigh) relative to the Saudi datum (Saudi Aramco Tide Tables, 2014), (B) coastal vegetation on the supratidal intertidal sabkha of Al-Kharrar Lagoon.
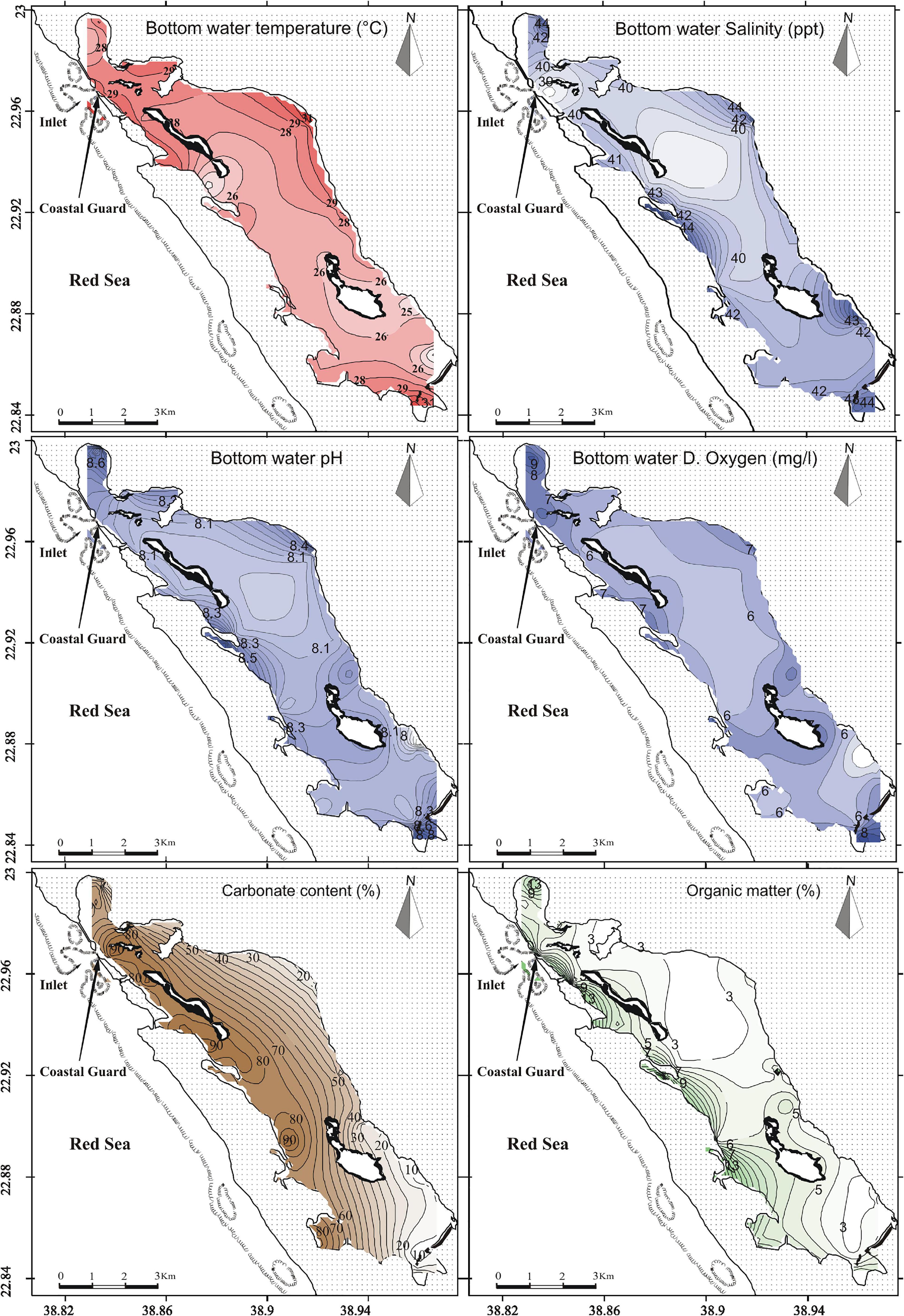
Figure 2. Spatial distribution of physico-chemical parameters (bottom temperature, bottom salinity, bottom pH and bottom dissolved oxygen), carbonate and organic matter contents in the Al-Kharrar Lagoon during winter 2014.
Foraminiferal Analysis
The uppermost layer (∼1 cm) from each sediment sample was scraped off and partitioned into two aliquots: one set of subsampled sediments was stored in numbered polyethylene jars, stained with buffered Rose Bengal solution (1.5 g of Rose Bengal powder for every liter of 95% ethyl alcohol), and then transported to the laboratory and preserved for several days prior to micropaleontologic analysis following (Abu-Zied et al., 2007, 2016). The Rose Bengal was used to distinguish between living (stained) and dead foraminifers (Murray, 1991) as it stains the cytoplasm of foraminifers alive at the time of collection (Walton, 1952), or which died in the last period of weeks to months (Bernhard, 1988; Corliss and Emerson, 1990) while the non-degraded proteins are still stainable (Barras et al., 2014). This makes it difficult to determine which were living at the time of sampling (Horton and Edwards, 2003), and thus overestimates the living assemblages (Schönfeld et al., 2012). Therefore, individuals showing a clear pink (or red) color in the last few chambers were counted as living fauna at time of collection, whereas colorless empty tests were counted as dead (Walton, 1952; Murray, 1991; De Stigter et al., 1998, 1999). The living faunal assemblage was not used in this study because it is influenced by seasonal environmental changes; consequently, they show a seasonal bias when used in palaeoenvironmental reconstructions. In contrast, the dead species represent a time-averaged accumulation of foraminiferal tests, therefore, they are considered to be a better analog for palaeoenvironmental reconstructions (Murray, 1991; Horton and Edwards, 2006).
The second set of subsampled sediments was used for geochemical analysis, such as organic matter and carbonate content. In the laboratory, each sample was oven-dried at 50°C for 24 h and weighted. They were then gently rinsed and washed through 2 and 0.063 mm sieves to remove any excess stain and mud material and to separate gravel (>2 mm) and sand (0.063–2 mm) fractions. The residual fractions obtained in each sieve were re-dried at 50°C and weighed again to determine their percentages using the total dry weight of the sample (Abu-Zied et al., 2007; Frontalini et al., 2013; for further details see Al-Dubai et al., 2017a). The 2–0.063 mm fraction was divided by a splitter to sub-fractions to use in quantitative analysis of benthic foraminifers. Modern benthic foraminiferal tests were picked, counted and identified under a binocular microscope from a known fraction of sampled sediment. Only relative faunal abundances of the death assemblage were used to produce and improve the performance of the transfer function (Leorri et al., 2008b). Several references (Loeblich and Tappan, 1987; Haig, 1988; Abu-Zied et al., 2007, 2013, 2016; Al-Dubai et al., 2017a) were used to aid in taxonomic identifications of benthic foraminiferal species found along the transects. The flora cover in the supratidal–intertidal sabkha along the transects (Figure 3) was identified and listed in Table 2 (for more details about the flora see Al-Dubai et al., 2017a).
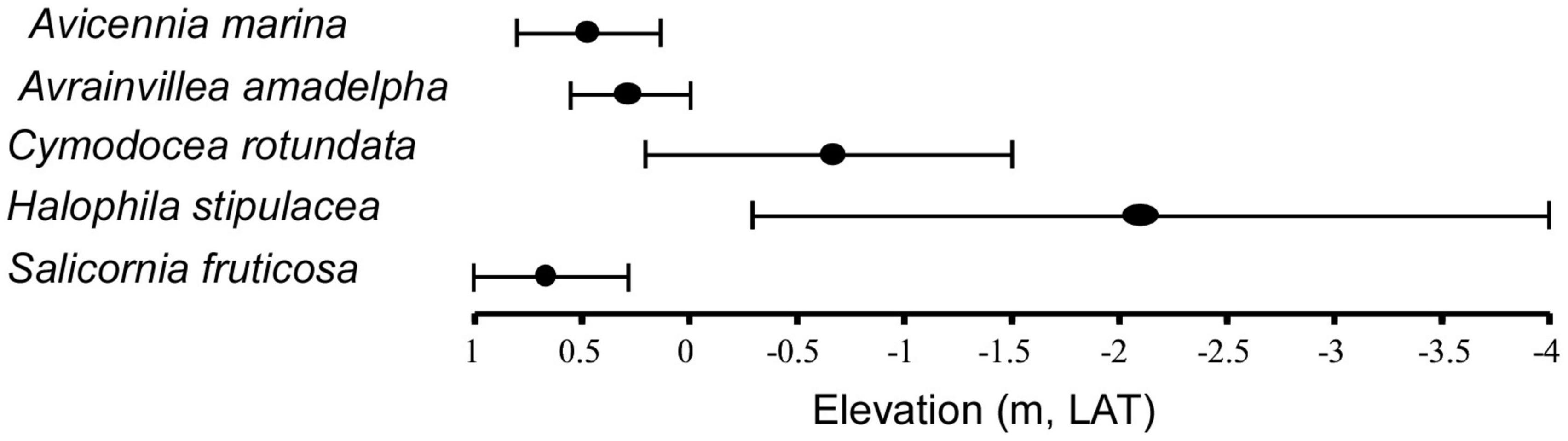
Figure 3. Vegetation zonation along the transects across the intertidal sabkha vs. the elevation (m, LAT) of the Al-Kharrar Lagoon during March 2014.
Organic matter content (LOI) was determined by the loss-on-ignition (LOI) technique on dry bulk sediment samples at 550°C following Heiri et al. (2001) and Abu-Zied et al. (2016). Carbonate content (CaCO3) was determined by loss of weight following Basaham and El-Sayed (1998) and Al-Dubai et al. (2017a).
Statistical Analysis
The relative abundance of modern benthic foraminifers was plotted as percentages against elevation (cm, LAT) above the Saudi datum (Saudi Aramco Tide Tables, 2014). Cluster analysis was applied using Primer v. 5.0 (Clarke and Warwick, 1994), to help interpret the relationship between the modern foraminiferal assemblage and elevation. Hierarchical cluster analysis was applied using group-average linking of Euclidean distance coefficients of Log (x + 1) transformed, standardized species data from the total death assemblage. The transfer function was applied to the relative abundance of modern foraminifers from the KL using the software package C2 (Juggins, 2007), to quantify the relationship of the contemporary benthic foraminifers with site elevations in the intertidal area. Knowledge of environmental gradient lengths is also very important to determine whether linear-based or unimodal-based statistical methods are appropriate in the study (Birks, 1995). This was estimated using Detrended correspondence analysis (DCA) in PAST software version 2 (Hammer et al., 2001). DCA with detrending by segments was applied to determine the elevational gradient lengths of modern foraminiferal training sets in terms of standard deviation (S.D.) units (Birks, 1995). Thus, the training set of death assemblages with elevation has produced a gradient length of 4.8 S.D. units. Hence a unimodal-based method of regression was used to calculate regression statistics, as it is recommended for training datasets with gradient lengths greater than two S.D. units (Birks, 1995). The best regression model for the training datasets was performed using the weighted-averaging partial least squares (WA-PLS) with the leave-one-out cross validation method (“jack-knifing”; Edwards et al., 2004; Callard et al., 2011; Abu-Zied et al., 2013) and log10-transformed species data. Jack-knifing (RMSEPJack) is a measure of the overall predictive abilities of the dataset. Component 3 in the models from the training dataset was chosen for this study, because it is yielded the smallest RMSEP errors in elevation reconstruction, low maximum bias, and the highest coefficients of determination (r2jack) for observed vs. predicted values (Table 4). The overall performance of each transfer function model was assessed in terms of RMSEP, r2 of observed vs. predicted values, and maximum bias since and they are considered to be measures of the overall predictive abilities of the modern training set (Birks, 1995; Horton, 1999; Horton et al., 1999; Horton and Edwards, 2006; Massey et al., 2006; Leorri et al., 2008b; Callard et al., 2011). This allows comparisons among transfer functions (Gasse et al., 1995). In the training set used for the model, outliers were excluded because they showed weak relationships with elevation, and this, in turn, will increase the predictive ability of the training set (Gasse et al., 1995; Jones and Juggins, 1995).
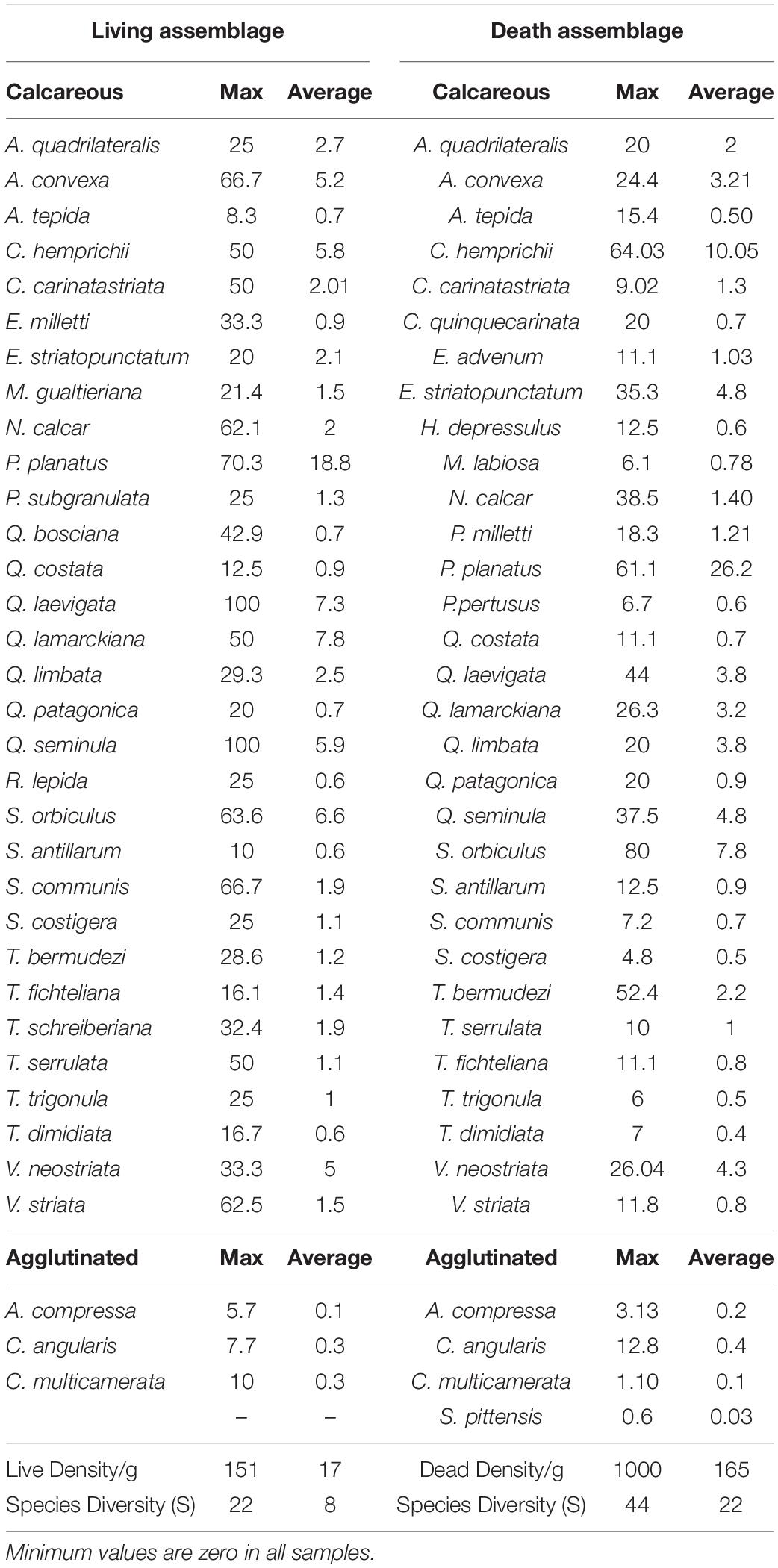
Table 3. Average, density and diversity of the most abundant living/dead foraminifers found on the intertidal sabkha of Al-Kharrar Lagoon.
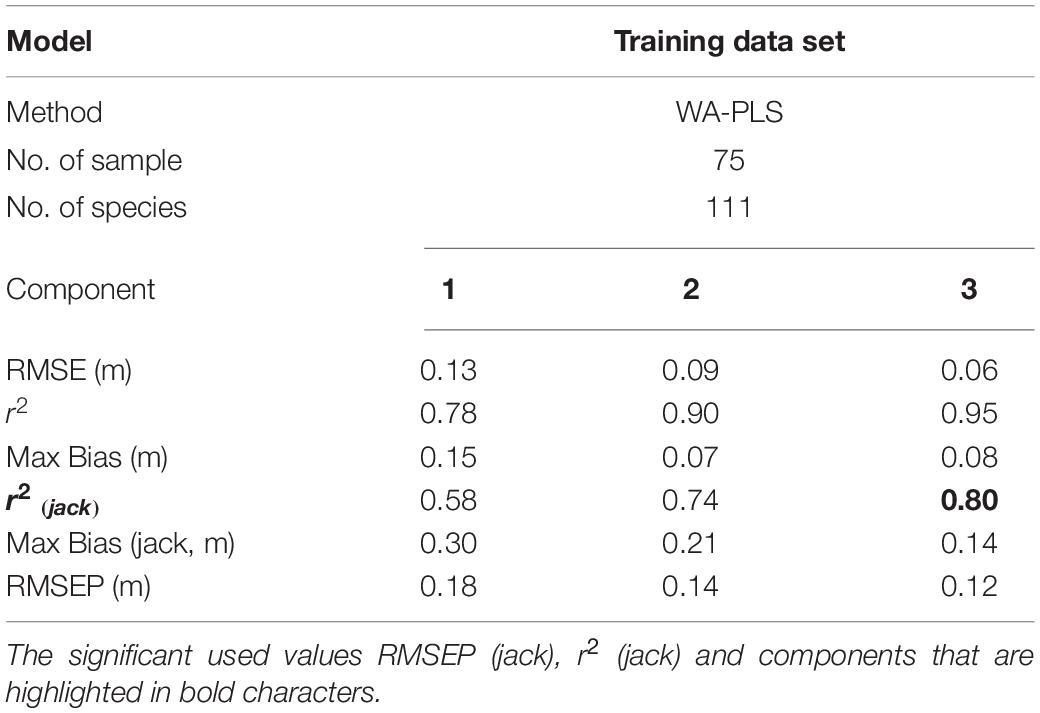
Table 4. Summary statistical parameters of the transfer function of dead foraminiferal training sets using the Weighted Average Partial Least Squares regression (WA-PLS) method for the training data set (N = 75).
Results
Physico-Chemical Parameters
Overall, the water elevation in the KL ranges from 0.5 m in the southern part 0 to –18 m at the inlet (Figure 1), it then deepens gradually to more than –18 m toward the outer part of the lagoon inlet. However, the studied samples were collected from intertidal zones to depths of up to 0.5 (Figure 1). The bottom water temperature decreased from 29°C in the northern part (inlet) to 26°C at the southern part of the lagoon, with an average of 27°C. Then, it increased to 31°C in the supratidal areas of the lagoon that were covered by a thin (∼10 cm) layer of water during the sampling time (Figure 2). In contrast, the bottom water salinity increased from 39 ‰ at the inlet to 42 ‰ in the southern part of the lagoon, with a mean value of 41 ‰. Then, it increased to 44 ‰ in the very shallow areas of the lagoon (Figure 2). The bottom water pH varied from 8.1 at the inlet to 8.3 in the shallowest areas at the southern part of the lagoon, with a mean value of 8.2. Then, it increased to 8.8 in the very shallow areas of the lagoon (Figure 2). The bottom water dissolved oxygen ranged from 6 mg/l at the northern part to 7 mg/l at the southern part of the lagoon (sheltered areas), with an average of 6.4 mg/l. Then, it increased to 9 mg/l in the very shallow areas of the lagoon (Figure 2).
Organic Matter and Carbonate Content
The organic matter predominates in the western and southern parts of the lagoon (up to 13%) where extensive intertidal sabkhas are present; whereas it decreases significantly toward the eastern side of the KL, reaching 3% at the shoreline (Figure 2).
The carbonate content predominates in the western side of the lagoon (up to 85%) where it is bordered by old, raised reefal limestone terraces. It decreases significantly toward both the southern and eastern parts (down to15%), while the clastic materials dominate these parts (Figure 2).
Coastal Vegetation
Al-Kharrar sabkha generally displays several zones, including supratidal, intertidal and subtidal zonations based on the vegetation present along transects across the sabkha flats. However, these zones can be divided into smaller zones based on elevation and vegetation, including supratidal, high intertidal, middle intertidal, low intertidal and high subtidal (Figure 3 and Table 2). The supratidal-high intertidal areas constitute 80% of the KL forming a belt around the lagoon with dense vegetation cover. The supratidal-high intertidal sabkha is covered by a thin layer of seawater at high tide and is dominated by mangrove (Avicennia marina) and Salicornia frulticame, which are more prevalent than other plants and have a moderate tolerance to high salinity (Figure 3). The middle intertidal area is occupied by Avrainvillea cf. A. amadelpha, which forms dense patches growing on coral rubble covered by silt and sand. Low intertidal and high subtidal areas predominantly contain seagrass, such as Cymodocea rotundata and Halophila stipulacea, which represent suitable habitats for many faunas including the larger foraminifer Sorites orbiculus that attaches its test as a white spot on the seagrasses (Figure 4).
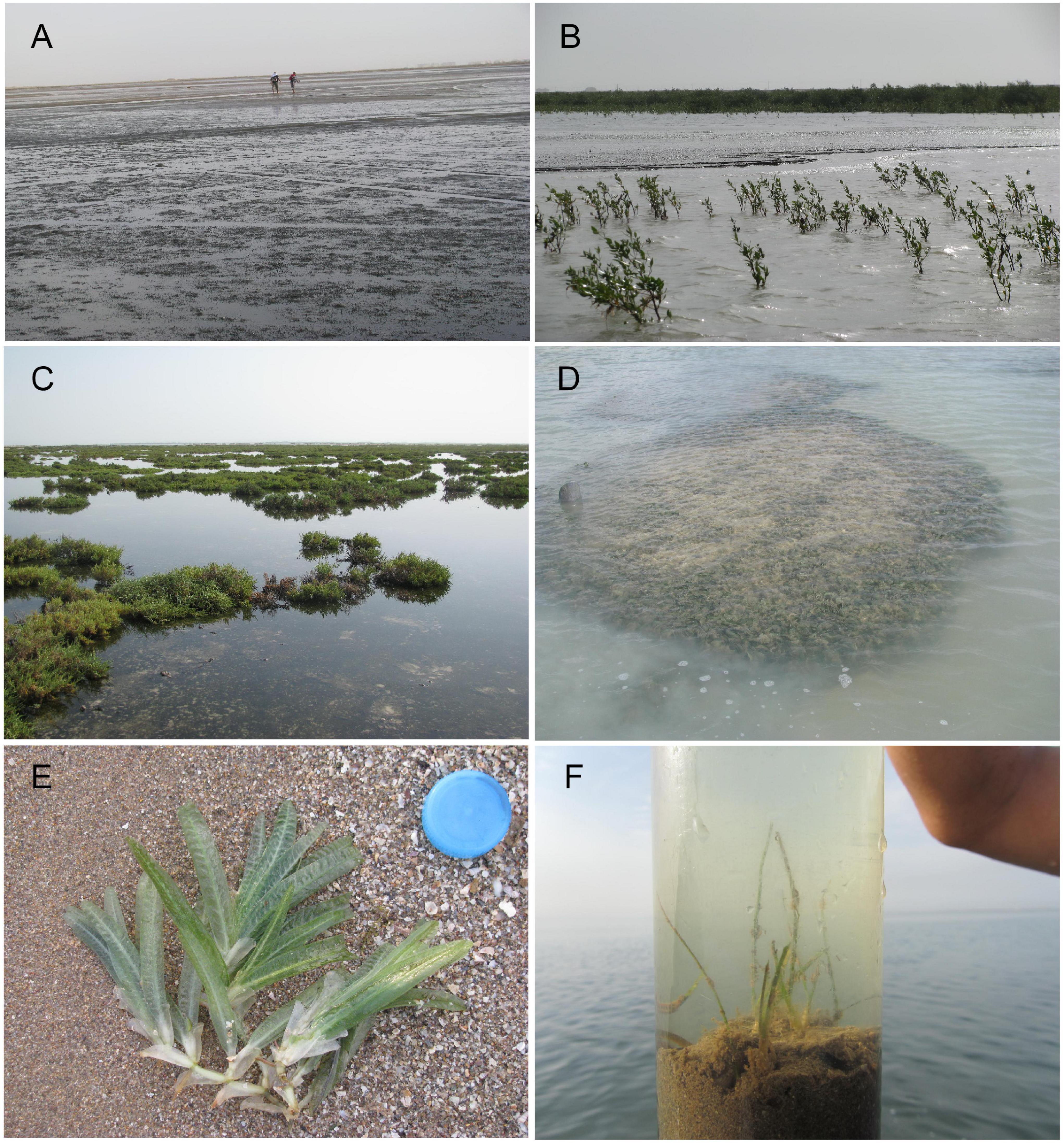
Figure 4. The intertidal sabkha of Al-Kharrar Lagoon during high tide. (A) intertidal sabkha. (B) the mangroves Avicennia marina, (C) the coastal shrubs Salicornia fruticosa, (D) Avrainvillea cf. A. amadelpha, (E) the fan seagrass Halophila stipulacea, (F) the seagrass Cymodocea rotundata (Al-Dubai et al., 2017b).
Dead Foraminiferal Assemblage
Intertidal benthic foraminiferal species were relatively abundant and well-preserved at all elevations on the intertidal sabkha, with the exception of some samples from the high supratidal area where they are characterized by very low faunal abundance and diversity (i.e., only one or two species in each sample), which reflects the marginal marine nature of the upper limit of intertidal-supratidal environments. A total of 99 dead species, exceeding approximately 60% of the total assemblage, were identified across the intertidal sabkha of KL (Figure 5). Only 4 dead agglutinated species were present, and the rest were calcareous (porcelaneous and hyaline) species (Figure 6 and Table 3).
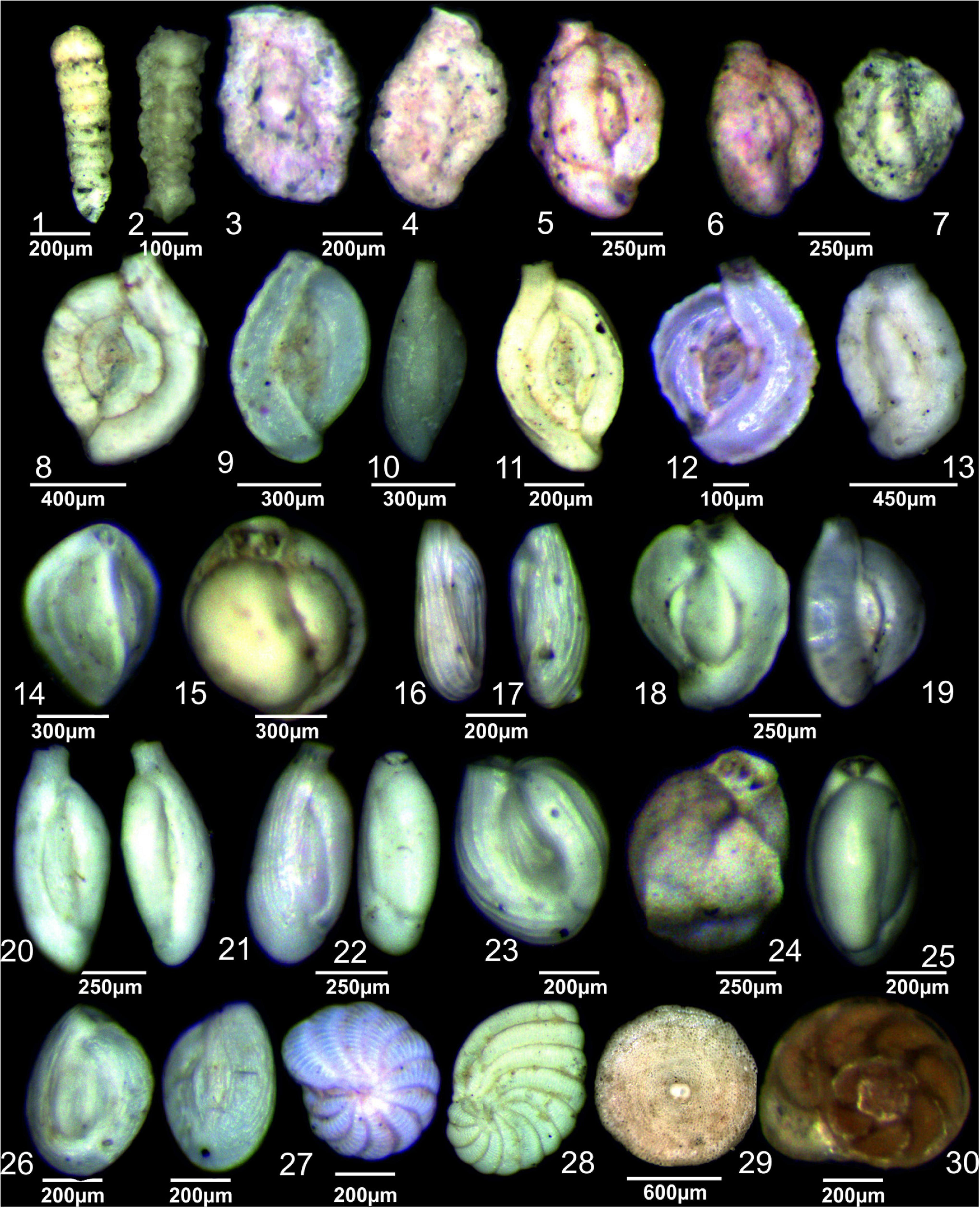
Figure 5. 1 Clavulina multicamerata, side view; 2 Clavulina angularis, side view, 3–4 Agglutinella compressa, side view; 5–6 Siphonaperta pittensis, side view; 7 Siphonaperta cf. S. agglutinans, side view; 8 S. rugosa, side view, 9 Spiroloculina sp. side view; 10 Spiroloculina sulcata, side view; 11 Spiroloculina communis, side view; 12 Spiroloculina costigera, side view; 13 Lachlanella subpolygona, side view; 14 Triloculina tricarinata, side views; 15 Triloculina trigonula, apertural views; 16–17 Q. limbata, side views; 18–19 Quinqueloculina lamarckiana, side views; 20 Cycloforina sulcata, side view; 21–22 Cycloforina carinatastriata, side views; 23 Triloculina fichteliana, side views; 24 Triloculina serrulata, side view; 25 Triloculina cf. T. schreiberiana, side view; 26 Varidentella neostriata, side views; 27 Coscinospira hemprichii, side views; 28 Peneroplis planatus, side view; 29 Sorites orbiculus, side view; 30 Ammonia convexa, spiral views.
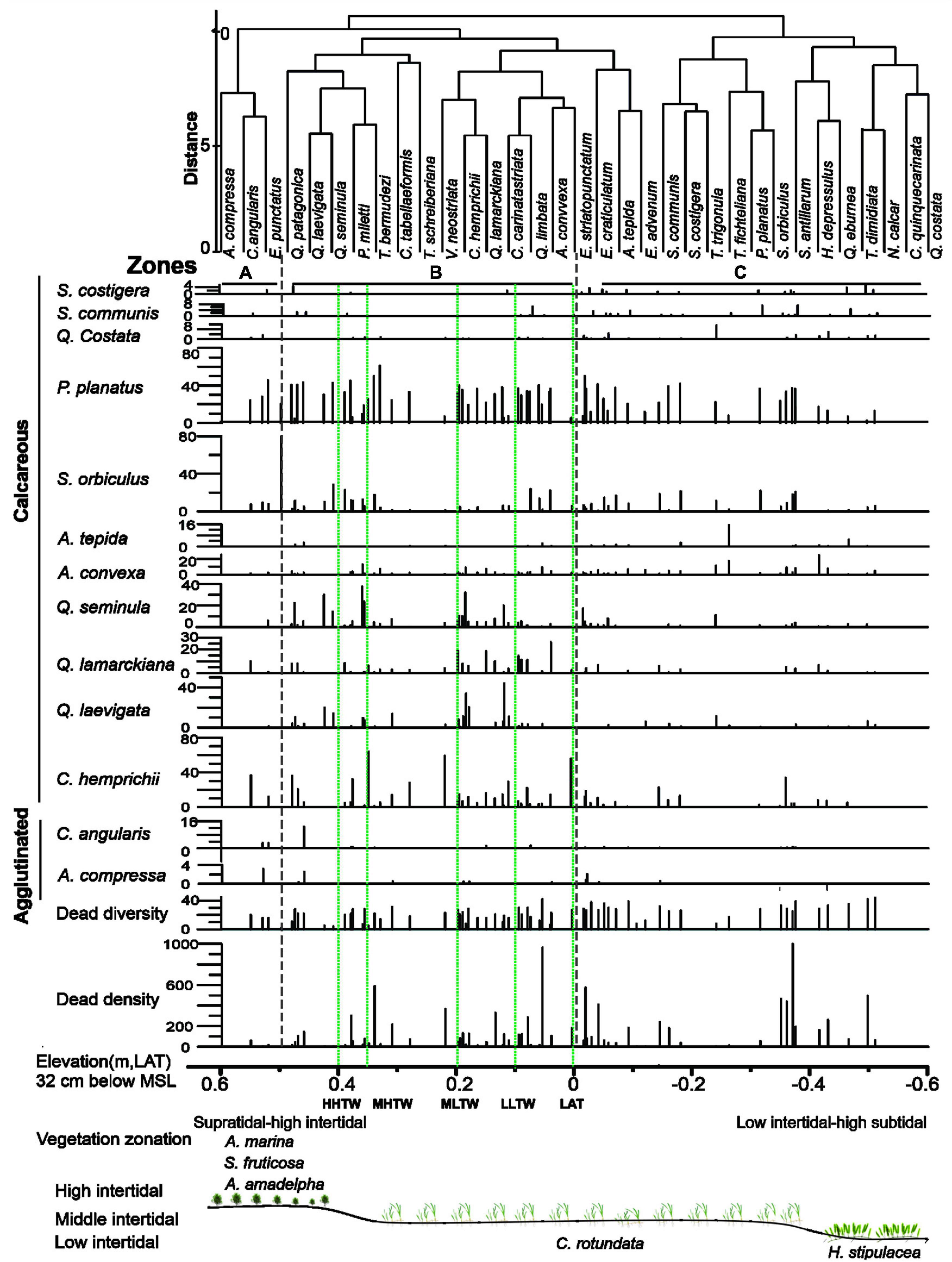
Figure 6. A frequency histogram of the distribution of dead benthic foraminifers across intertidal sabkha vs. the elevation (m, LAT) of the Al-Kharrar Lagoon during March 2014. Cluster analysis of total foraminiferal species based on Log (x + 1) transformed data, and standardized using Bray-Curtis similarity coefficients of group-average. Vegetation zonations based on elevations.
Along several transects across the intertidal sabkha, cluster analysis divided the intertidal sabkha into three zones (A, B, and C) based on the dominant species, and these divisions were remarkably consistent with the geomorphological and vegetative zones. Assemblages in Zone A differ from the assemblages in the rest of the zones. Zone A is characterized by the presence of agglutinated species such as Agglutinella compressa, Clavulina angularis and Clavulina frulticamerate albeit in low abundances. Moreover, calcareous species were present in moderate abundance in this zone, including Peneroplis planatus, Coscinospira hemprichii, Sorites orbiculus, Quinqueloculina lamarckiana, Quinqueloculina seminula, Ammonia convexa, Ammonia tepida and Quinqueloculina laevigata. Zone A extends from 0.6 to 0.5 m above LAT and contains very specific vegetation (e.g., Avicennia marina, Salicornia ?ulticame and Avrainvillea cf. A. amadelpha.
Zone B is dominated by calcareous species of P. planatus, C. hemprichii, S. orbiculus, Q. lamarckiana, Q. seminula and Q. laevigata with very low frequencies of agglutinated species. It is distinct from other zones by having the highest percentages of these species recorded on the intertidal sabkha. Zone B extends from 0.5 to 0 m relative to LAT, and mainly contains seagrass Cymodocea rotundata (Figure 6). Zone C is dominated by numerous calcareous species such as C. hemprichii, Q. costata, S. orbiculus, P. planatus, A. convexa, A. tepida, Spiroloculina communis and Spiroloculina costigera. It differs from other zones by having the highest percentages of deeper water species such as S. communis and S. costigera. This zone is located between 0 and –0.6 m below the LAT and contains the seagrass Halophila stipulacea (Figure 6).
Faunal density of dead foraminifers ranged from 2 to about 1000 tests/g with an average value of 165 tests/g, while the dead diversity ranged from 2 to 44 species/sample with an average value of 22 species (Table 3). Dead-species diversity decreased asymptotically with increasing intertidal sabkha elevation and decreasing tidal inundation, reaching its lowest value in Zone A (Figure 6). The highest abundances of dead foraminifers occurred below MLTW elevation (0.2 m), particularly in Zones B and C, with averages of the most abundant species being P. planatus (26%), C. hemprichii (10%), S. orbiculus (8%), Elphidium striatopunctatum (5%), Q. seminula (5%), Varidentella neostriata (4%) and A. convexa 3%). Calcareous species dominated the death assemblages in all intertidal sabkha samples (average 99%); however, agglutinated foraminifers occurred with very low abundance (average 1%), particularly in Zone A and were represented mainly by C. angularis (average 0.38%), A. compressa (average 0.17%), C. multicamerata (average 0.08%) and Siphonaperta pittensis (average 0.03%; Table 3).
Live Foraminiferal Assemblage
The live species counted in each sample were significantly lower than the dead foraminifers. A total of 68 live species were identified across the intertidal sabkha of KL (Figure 5). Only 5 live agglutinated species were present, and the rest were calcareous (porcelaneous and hyaline) species (Figure 7).
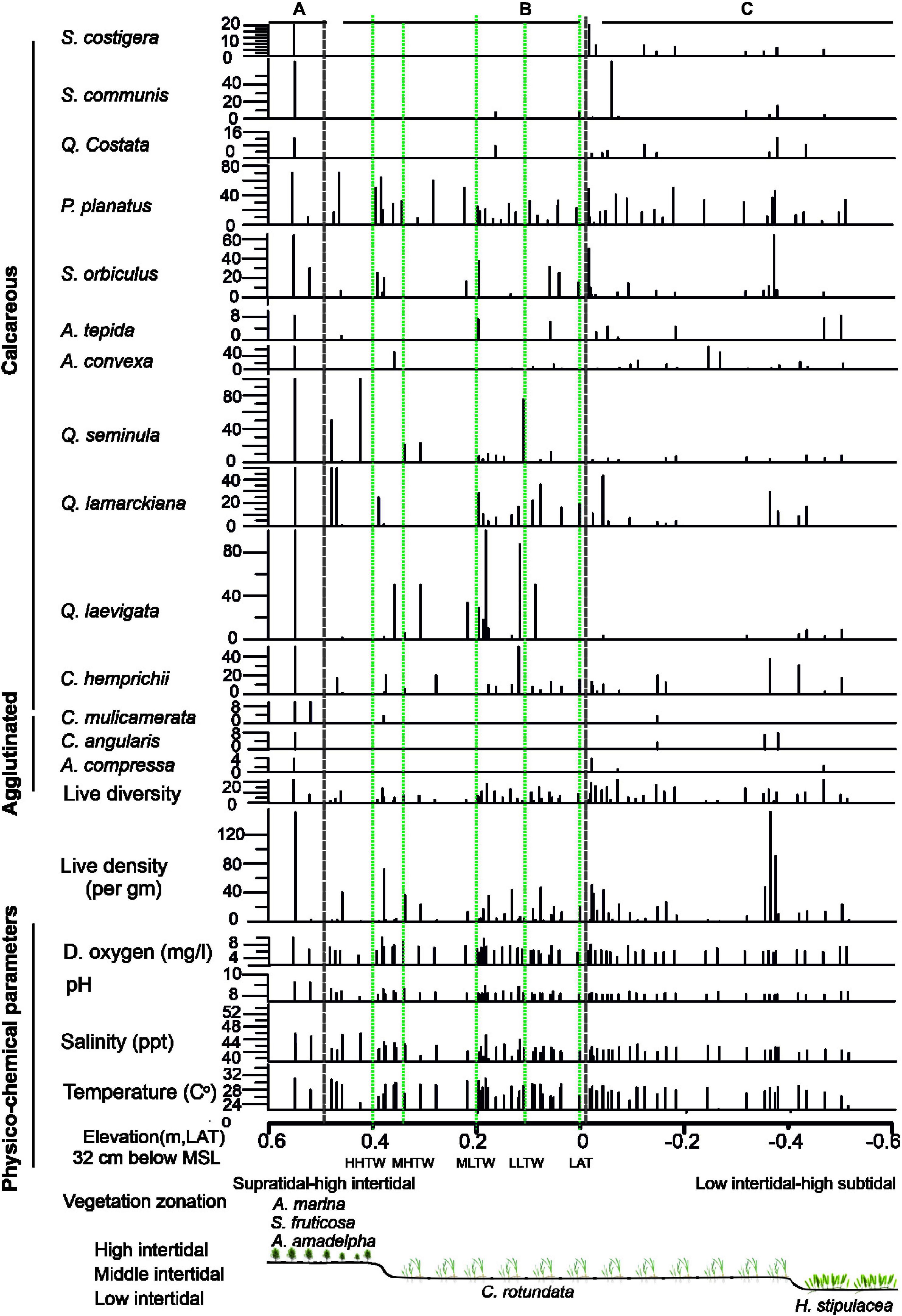
Figure 7. A frequency histogram of the distribution of live benthic foraminifers across intertidal sabkha vs. the elevation (m, LAT) of the Al-Kharrar Lagoon during March 2014. Vegetation zonations based on elevations.
Faunal density of the living assemblage ranged from 1 to 150 tests/g with an average value of 17 tests/g, whereas the living diversity ranged from 1 to 22 species/sample with an average value of 8 species (Table 3). Species diversity showed a close resemblance to the dead-species diversity, whereby it decreased with increasing intertidal sabkha elevation and decreasing tidal inundation, reaching only one species in the samples in the highest supratidal area (Figure 7). The highest abundances of living foraminifers occurred below MLTW elevation (0.2 m), with averages of the most abundant species in the total assemblage being P. planatus (18%), Q. lamarckiana (8%) Q. laevigata (7%), S. orbiculus (7%), Q. seminula (6%), C. hemprichii (6%), A. convexa 5%) and Varidentella neostriata (5%; Table 3). The living assemblage in the whole intertidal sabkha was highly dominated by calcareous foraminifers (average 99%). Whereas, agglutinated foraminifers occurred in Zone A with low abundance (average 1%) and were almost exclusively represented by C. angularis (average 0.3%), C. multicamerata (average 0.3%) and A. compressa (average 0.1%; Table 3).
Elevation and Salinity Relationships of Foraminifers
The distribution of benthic foraminiferal assemblage in the intertidal zone is controlled by dominant factors such as elevation and salinity as indicated in Figure 6. Elevation and salinity are directly related to the duration and frequency of tidal inundation in the area. It appears that the optima for most agglutinated species (Agglutinella compressa, Clavulina angularis and Clavulina multicamerata) occur in the high intertidal zone above HHTW, and decreases toward the lower intertidal zones. Conversely, calcareous species increase toward low intertidal zones, implying that the optimum for most calcareous species is below the HHTW. Therefore, modern benthic foraminifers in the intertidal zones were used to elucidate the relationship between field-observed and model-predicted elevation, which in turn demonstrates the strength and predictive ability of the model. The transfer model was developed from a training set composed of dead benthic foraminifers only; living foraminifers were excluded from this part of the study, since they would be in equilibrium with the environmental conditions prevailing at the time of sampling. This implies that their assemblage changes over time.
A Weighted Averaged Partial Least Squares (WA-PLS) regression model was applied on 75 contemporary sediment samples and 99 species obtained from several transects across the sabkha area accounting for more than 60% of the total death-assemblage of the KL (Table 4). Deeper water samples/species (below –0.5m relative to LAT) were completely omitted from this study since they are always below sea level. The relationship between the observed and foraminiferal-predicted elevation is comparatively strong (r2jack = 0.80 for component 3). This result indicates that the training set composed of death assemblage counts can predict sabkha reconstruction of palaeotidal elevations in the KL with a precision of ± 0.12m (RMSEPjack = 0.12 m; see Table 4). The elevation of samples/species collected from the sabkha ranges between 0.6 and –0.6 (m, LAT) and is shown in the scatter plot of the observed/predicted elevation (m, LAT) with residual errors between 0.2 and –0.15 m (LAT) (Figure 8). In Table 5 we compare our results with some published foraminiferal studies from intertidal/salt marshes intertidal areas of the world.
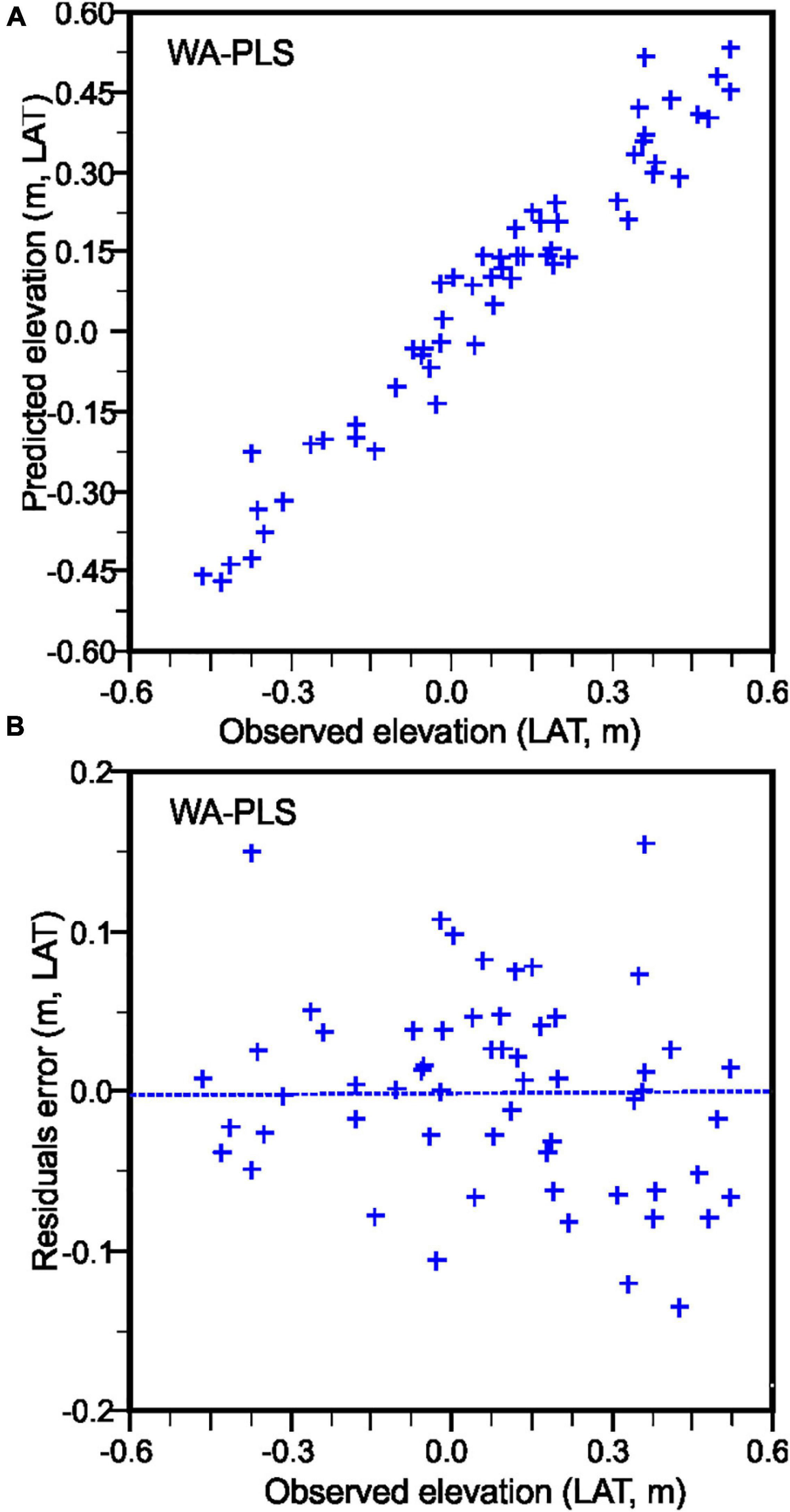
Figure 8. (A) Samples elevations predicted by the WA-PLS regression model, compared with observed elevations. (B) Residual errors of WA-PLS is calculated by (subtracting the observed elevations from the inferred elevations) and using the modern training set data (75 samples and 99 species).
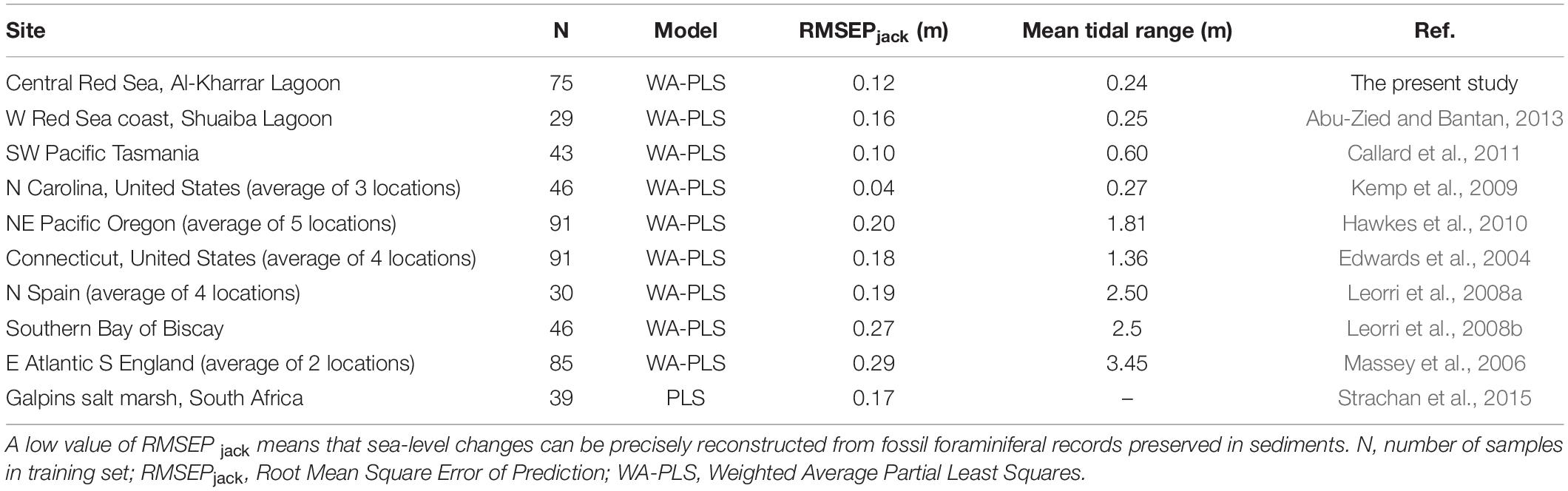
Table 5. Comparisons between our results with modern foraminiferal datasets from other intertidal/salt marshes of the world.
Canonical Correspondence Analysis
The correlation between the living foraminiferal assemblage and environmental variables of KL was assessed by canonical correspondence analysis (CCA; Figure 9). The PCA revealed that ∼89.6% of data variance could be explained by the first three axes (factors). The first axis (eigenvalue = 0.16) explains 41.65% of the variation and is associated with a combination of T. serrulata and H. stipulacea, the second axis (eigenvalue = 0.12) explains 30.94% of the variation, which is largely explained by T. serrulata and A. amadelpha, and the third axis (eigenvalue = 0.07) explains 16.99% of the variation, which is largely due to C. multicamerata and T. serrulata species (Table 6). The CCA biplot displays three vertical zonations of sabkha ranging from 0.6 to –0.5 m relative to LAT, characterized by specific species, vegetation cover, and physico-chemical parameters (Figure 8). For instance, species Q. seminula and C. multicamerata occupy the high intertidal, T. schreiberiana, Q. lamarckiana and C. hemprichii, occupy the high and middle intertidal, while Q. costata, S. costigera, S. communis, A. convexa, A. tepida and N. calcar occur in the low intertidal zone. In the plot of CCA axes (Figure 9), elevation is positive correlated with salinity and the foraminiferal species Q. seminula, S. orbiculus and P. planatus. These species also showed a positive correlation with temperature. A. convexa, A. tepida, N. calcar, S. costigera and S. communis are positively related with dissolved oxygen and pH. In contrast, they are inversely related with elevation. Live density and diversity displayed a positive relationship with dissolved oxygen and pH. However, they showed an inverse relationship with elevation. P. planatus, C. hemprichii and S. orbiculus are positively related with stressors such as temperature and salinity. C. rotundata and H. stipulacea are negatively related with the elevation. The high intertidal vegetation (A. amadelpha, A. marina, and S. fruticosa) zones plot on the left (high elevation, temperature, salinity) and the low intertidal vegetation (C. rotundata and H. stipulacea) zone plots on the right of CCA (low elevation, pH, dissolved oxygen).
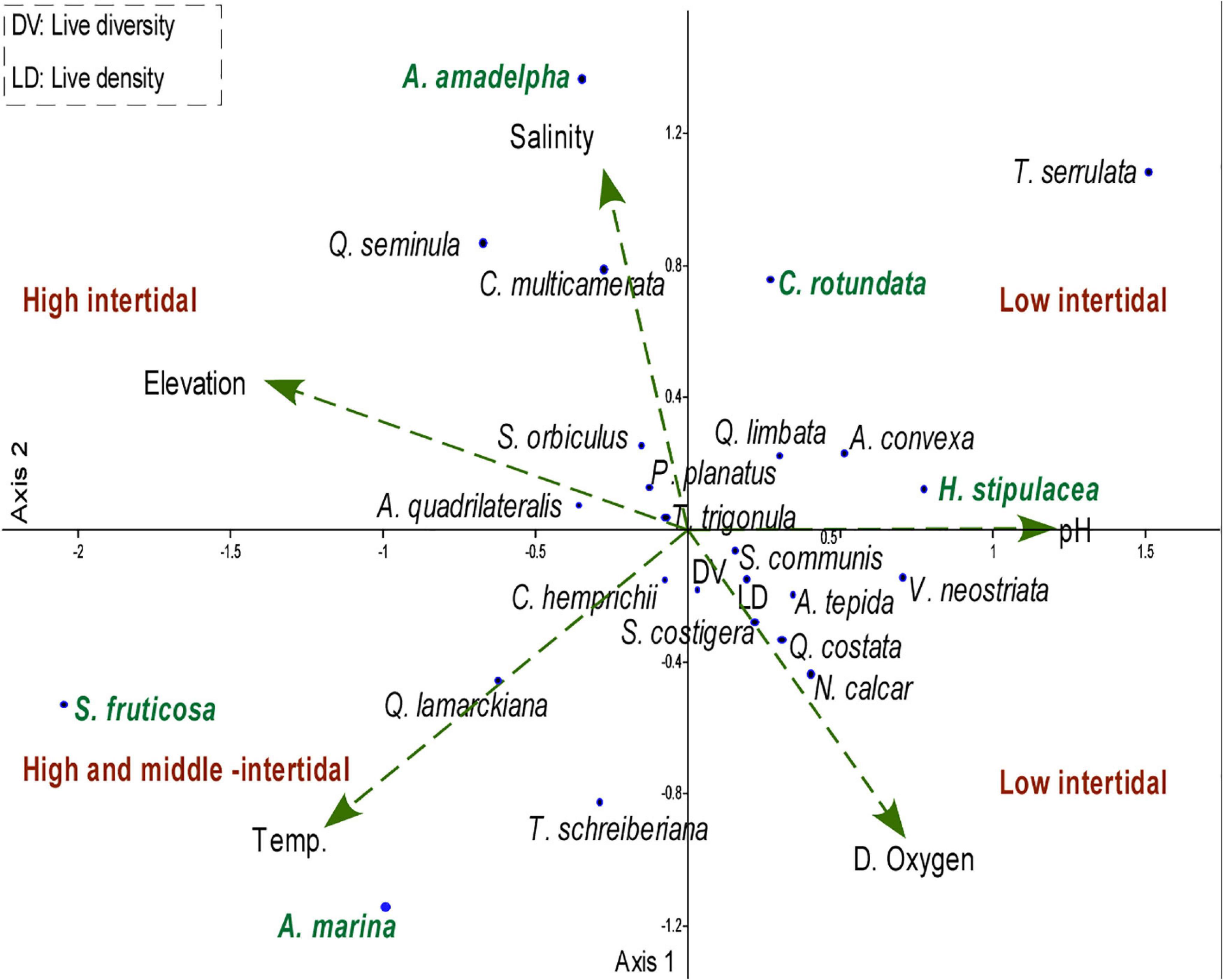
Figure 9. Canonical correspondence analysis (CCA) showing the relationship between of foraminiferal assemblage, density, diversity, intertidal zones and environmental variables (arrows) such as elevation, temperature, salinity, DO, pH in KL.
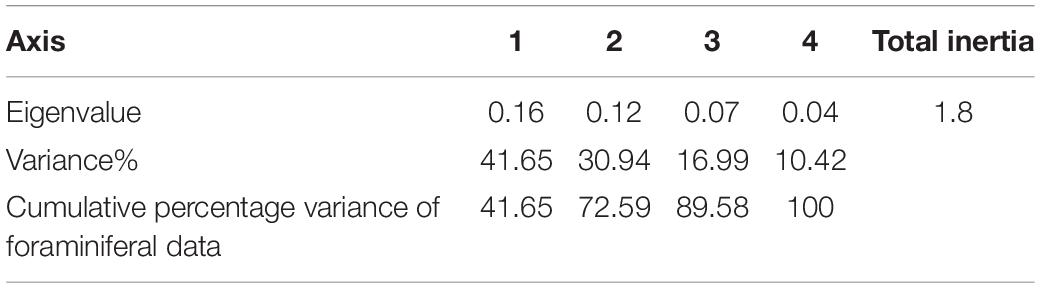
Table 6. Eigenvalues and cumulative percentage of variances of foraminiferal and environmental data obtained by the canonical correspondence analysis (CCA).
Discussion
Faunal Composition, Distribution and Controlling Factors of Benthic Foraminifers
Al-Kharrar Lagoon is surrounded by extensive intertidal sabkha, extending about 4 km toward the land, which is mainly composed of sand alluvium covered with distinctive coastal vegetation (Al-Dubai et al., 2017a,b). The intertidal bottom sediments are dominated by live benthic foraminiferal species, albeit in low percentages, and they generally increased in abundance with decreasing elevation of the sabkha. Only moderate faunal density was recorded in the intertidal zone above 0.2 m elevation at the KL that could be due to the occurrence of frequent stressed conditions accounting for the very low counts of faunal diversity. Salinity and temperature in the study area show a general rising trend from low to high intertidal areas due to the high evaporation and decreasing water cover and depths. Numerous authors (Murray and Alve, 2000; Murray, 2006; Horton and Murray, 2007) reported that the stressful environmental conditions in the intertidal area allow limited species to survive and their reproduction is promoted by their tolerance to the diurnal and seasonal variations in the physiochemical conditions, caused by flood frequency associated with tidal overflow and sea level changes (Eichler, 2019). In contrast, high faunal density accompanied by a relatively high faunal diversity was recorded in the lower intertidal zone below 0.2 m elevation, indicating relatively stable environmental conditions since this elevation is permanently inundated by water and could provide better living conditions. New oxygen-rich water that covers the lower intertidal sabkha during high tide provides good living conditions, which results in a large number of living foraminiferal species (De Rijk and Troelstra, 1999; Berkeley et al., 2007; Scheder et al., 2019). Moreover, the prevalence of coastal vegetation in the lower intertidal area produces oxygen as a result of photosynthesis, which may help promote living foraminifers to reproduce (Al-Dubai et al., 2017a). In addition, many other factors control the abundance and diversity of foraminifers in the intertidal area, including the duration and frequency of intertidal exposure, substrate, temperature, salinity, food (organic matter) and pH (Murray, 1968; Alve and Nagy, 1986; Debenay et al., 2002; Horton and Edwards, 2003; Woodroffe et al., 2005; Abu-Zied and Bantan, 2013). Horton and Edwards (2003) and Eichler (2019) showed that the distribution of surface foraminifers across the intertidal zone of Brazil and the United Kingdom coastlines are controlled by the substrate elevation relative to tidal inundation.
On the other hand, dead foraminifers in intertidal sabkha sediments showed higher faunal density than live foraminifers, indicating a seasonal accumulation of empty tests, or they could be allochthonous and introduced from low intertidal areas by frequent tidal currents (De Rijk and Troelstra, 1999). Murray (1991) and Horton (1999) reported that death assemblages in the intertidal zone are not affected by seasonal variability, and are likely to represent a time-average accumulation for taxa tests. In contrast, live foraminiferal assemblages are in equilibrium with the environment and are affected by seasonal fluctuations and thus vary throughout the year depending on the variation of the environmental conditions. Also, tidal currents are capable of re-suspending foraminiferal tests in the water column, thus causing advective transport of these tests to other places (Eichler, 2019).
Furthermore, the much higher presence of dead than living foraminiferal species could be explained by the good preservation potential of the empty tests without significant dissolution effects.
Most of the dead foraminiferal species in the intertidal zone, especially in the vegetated areas, are not autochthonous in the region, as evidenced by the higher diversity of dead species compared to the living diversity. For example, foraminiferal species Spiroloculina costigera, S. corrugate, S. rugosa, S. antillarum, S. communis, Triloculina trigonula and T. serrulata were present in the intertidal area. However, many authors (e.g., Abu-Zied and Bantan, 2015; Al-Dubai et al., 2017a; Bantan et al., 2019) stated that these species represent deeper water (subtidal) species. Therefore, these species represent allochthonous species, probably transported by flood tide currents from the deeper water region. Also, Bolivina striatula and Amphistegina lessonii were recorded in the intertidal zone, despite their marine habitat. For example, Amphistegina, spp. prefer seagrass and hard substrates such as coral rubble (Murray, 2006) at depths of 0 to 50 m (Hohenegger, 2004; Murray, 2006), while Bolivina striatula prefers coastal marine environments with normal marine salinity (Eichler, 2019). Cibicides lobatula was not present in the intertidal sabkha in either the life or death assemblages, but was reported below LAT (–0.36 m, subtidal) in the KL. Therefore, species that did not occur in the intertidal zone in both life and death assemblages, are probably not autochthonous in this zone and may represent species typical of deeper marine environments that were transported postmortem by tidal currents. In Basque marshes (Spain), especially at mid-tidal elevations, C. lobatula has a distinct presence and was considered as an allochthonous species (Leorri et al., 2010). The same results have also been found on the coasts of Britain (Horton and Edwards, 2006; Massey et al., 2006). Thus, our results corroborate works done by Horton and Edwards (2006), Massey et al. (2006), Leorri et al. (2010). On the other hand, some live species have been recorded along the intertidal sabkha around KL (e.g., Coscinospira hemprichii, Peneroplis planatu, Quinqueloculina laevigata, Q. lamarckiana, Q. seminula, Triloculina fichteliana, T. schreiberiana, Sorites orbiculus, Varidentella neostriata and Ammonia convexa), suggesting that these species are probably autochthonous species in the area. These calcareous (hyaline and porcelaneous) species compose 99% of the total assemblage. In accordance with our expectations, a taphonomic loss of calcareous species could increase due to dissolution in low-pH sediments associated with early diagenesis of organic carbon and the release of CO2, as occurs in many intertidal/salt marshes areas of the world (Scott and Medioli, 1980b; Murray and Alve, 1999; Gómez-León et al., 2018; Eichler, 2019). However, this is not the case in the intertidal sabkha analyzed here, as the calcareous shells are well preserved and do not seem to be affected by dissolution, probably due to the high pH (∼9) values of most Al-Kharrar intertidal regions since these waters are supersaturated with respect to CaCO3 resulting from a high input from surrounding older limestone rocks, which reduces the solubility of the calcareous shells. Photosynthesis consumes CO2 and produces alkalinity, creating precipitation conditions for carbonate, while respiration process causes calcium carbonate dissolution (Moreno et al., 2007). Al-Dubai et al. (2017b) reported that the alkaline (pH ∼9) water in the intertidal zone of KL can be attributed to the consumption of CO2 and production of O2 during photosynthesis by the algal mats covering the intertidal area, or due to degassing of CO2 as a result of rising temperature (>33°C). Hence, the dissolution of calcium carbonate decreases with increasing temperature (Bai and Bai, 2019). In many regions of the world (e.g., Basque marshes, Spain), calcareous species in the total assemblage (living plus dead) constitute an important component in the marshes (Leorri et al., 2010). They concluded that the dissolution of calcareous tests was less prolific, due to the marshes being supplied with carbonate materials (CaCO3) from the surrounding regional limestone rocks (Cearreta and Murray, 2000). This could make the ambient water saturated with calcium carbonate, thereby reducing the solubility of carbonate tests and increasing the calcification rate in the area (Langdon et al., 2000) and thus enhancing the shell preservation potential. On the other hand, agglutinated species (e.g., Agglutinella compressa, Clavulina angularis and C. multicamerata) were found in the intertidal sabkha, but in low percentages (about ∼1%). These species are probably autochthonous species in the intertidal sabkha because they are present in both the living and death assemblages. The intertidal sabkha of KL is characterized by low-energy environments with muddy sand substrates rich in mangrove and coastal vegetation. It also has a salinity of up to ∼44 ‰ and temperature of ∼33°C. Thus it is a sheltered environment that is relatively more suitable for agglutinated species than those subtidal areas that are open to the sea. In the latter areas (unprotected areas), agglutinated tests are subject to abrasion, breakage and transportation due to currents and wave activity, and thus their tests are disintegrated immediately after their death (Murray, 2000; Abu-Zied et al., 2011). On the contrary, a mangrove environment is the preferred site for agglutinated species because mangrove roots create a calm environment that effectively dissipate waves and currents (Berkeley et al., 2007; Hewaidy et al., 2019). Abu-Zied et al. (2011) reported that C. angularis were frequent in the intertidal zone of Khor As Sailah on the Farasan Islands in southern Saudi Arabia but were absent in other parts of the same island. They attributed this to the low-energy conditions at Khor As Sailah that was colonized with mangroves, thus enhancing their preservation potential. However, the low abundance of agglutinated species in Al-Kharrar Lagoon could be well explained by the prevalence of sand substrates, which may preclude agglutinated species, since they generally prefer muddy substrates (Jones and Charnock, 1985; Hohenegger et al., 1993; Haunold et al., 1997; Berkeley et al., 2007; Abu-Zied et al., 2011). Reiss and Hottinger (1984) mentioned that agglutinated species also inhabit warm hypersaline lagoons at water depths of 0–1.5 m. However, these findings are inconsistent with what has already been reported by several authors (Scott and Medioli, 1980a; Sen Gupta, 1999; Kemp et al., 2009; Farouk and Jain, 2018). Eichler (2019) documented that agglutinated species were found in mixohaline and brackish environments in the Bertioga Channel (São Paulo, Brazil) and that their number decreased toward more saline environments.
The distribution of both benthic foraminifers and vegetation in intertidal sabkhas is related to tidal inundation, thus, the foraminiferal assemblage in surface sediments could be used as an indicator of tidal inundation and also intertidal surface elevation relative to msl (Horton and Edwards, 2006; Rogers et al., 2017). Several authors (Horton et al., 1999; Edwards and Horton, 2000; Gehrels, 2000; Edwards et al., 2004; Horton and Edwards, 2006) demonstrated that studies of the distributions of saltmarsh/intertidal foraminifers led to a significant development of the TF used to infer the past elevations of fossil saltmarsh/intertidal deposits. Local transfer functions are therefore needed to reconstruct palaeotidal surface elevations and, thereby, past sea level in the intertidal sabkha in Al-Kharrar lagoon and similar adjacent regions.
Evaluation of the Transfer Function
The contemporary foraminiferal assemblage exhibited three spatial zonations along the intertidal sabkha based on cluster analysis, indicating that it is highly influenced by elevation in the tidal frame, which is consistent with other studies (Horton and Edwards, 2003; Woodroffe et al., 2005; Callard et al., 2011; Strachan et al., 2015). The agglutinated species (Agglutinella compressa, Clavulina angularis, and Clavulina multicamerata) dominate the high intertidal zone, and decrease toward the low intertidal zone, indicating that this assemblage is restricted to a 0.4 m vertical range above HHTW. On the contrary, calcareous species increase toward lower intertidal zones and decrease toward the high intertidal zone, indicating that the optima level is below 0.40 m HHTW. Several other studies also documented that agglutinated species dominated in the high-middle marshes (Strachan et al., 2015; Lal et al., 2020), while calcareous species dominated lower intertidal and subtidal environments (Hayward et al., 1999, 2015).
A transfer function model was developed based on a screened data set comprising 75 contemporary sediment samples and 99 benthic foraminiferal species obtained from the supratidal-high subtidal areas of KL, where elevation ranged from 0.6 to –0.5 m (LAT), since these samples/species showed a good correlation with the tidal elevation. However, the subtidal and deeper water samples/species (below –0.5 m relative to LAT) were omitted to obtain a more accurate training dataset model for the KL. Many authors (e.g., Jones and Juggins, 1995; Edwards and Horton, 2000; Edwards et al., 2004; Hamilton and Shennan, 2005; Woodroffe et al., 2005; Massey et al., 2006; Horton and Murray, 2007) concluded that the incorporation of subtidal and deeper water samples/species with the intertidal agglutinated benthic foraminifers in the model may reduce the predictive ability of the transfer function and, hence, may provide an inaccurate predictor for sea-level elevations. This is because the subtidal and deeper water samples/species displayed no relationship with tidal elevation (Abu-Zied and Bantan, 2013). The precision of the transfer function of model Weighted Averaged Partial Least Squares (WA-PLS) was comparatively strong with the relationship between the observed and predicted elevations in the model being high (r2jack = 0.80 for component 3). Regression modeling of the modern training set indicates intertidal foraminiferal assemblages can predict intertidal surface elevations in the Al-Kharrar Lagoon with a precision of ±0.12 m (RMSEPJack = 0.12 m). Therefore, the WA-PLS regression model is suitable to be used to reconstruct palaeotidal elevations since the estimated predictive measure resulting from the training set was less than the daily tidal range of 0.24 m in Al-Kharrar Lagoon. The results for our study area was comparable with other results of foraminiferal-based transfer functions from other sites in the world. However, at those sites around the world with small tidal ranges (e.g., Tasmania in the southwestern Pacific, and North Carolina) the results were more precise than our result, reaching ± 0.05 m (e.g., Gehrels et al., 2005; Southall et al., 2006; Kemp et al., 2009). A similar study was conducted in South Africa, where benthic foraminifers were used to determine the vertical distribution of the foraminifers and to test its potential for use in studies of sea-level changes (Strachan et al., 2015). The transfer function was applied to benthic foraminifers from Galpins salt-marsh and this study revealed that salt-marsh foraminifers can predict marsh surface elevations with a precision of ±0.17 m. Locally, the present result was slightly more precise than those recorded by Abu-Zied and Bantan (2013) in the intertidal zone of the Shuaiba Lagoon (80 km south Jeddah City, ±0.16 m). Benthic foraminifera from intertidal sabkha on the Saudi Red Sea coast has demonstrated its ability to assess former changes in sea-level, and therefore should be used as an effective tool in reconstruction studies of relative sea-level changes in the region, similar to those published in other regions of the world.
Conclusion
Foraminiferal assemblages obtained from surface sediments along the intertidal sabkha of Al-Kharrar Lagoon show a clear vertical zonation in the study area. Three major intertidal zones (A, B, and C) were identified based on cluster analysis and dominance of particular species. Zone A was found in the high intertidal zone typically above HHTW and is dominated by A. compressa, C. angularis and C. multicamerata. Zone B was common in middle intertidal area and is dominated by P. planatus, C. hemprichii, S. orbiculus, Q. lamarckiana, Q. seminula and Q. laevigata. Zone C was found in low intertidal areas and is dominated by C. hemprichii, Q. costata, S. orbiculus, P. planatus, A. convexa, A. tepida, S. communis, and S. costigera. Factors controlling the vertical distribution of foraminiferal assemblages in the intertidal sabkha are the intertidal elevation and salinity, which are related to the duration and frequency of inundation associated with the tides. Agglutinated species predominantly occurred at higher intertidal elevations where high salinity occurs, whereas calcareous species dominated at lower intertidal elevations and increased in abundance toward lower intertidal zones. The calcareous shells in the intertidal sabkha are well preserved and do not seem to be affected by dissolution due to the waters being oversaturated with respect to calcium carbonate (CaCO3) resulting from the high input from the surrounding older limestone rocks. Dissolution is also inhibited due to the alkaline conditions (∼ pH 9) in the lagoon that reduced the solubility of the shells. The relationship between foraminiferal assemblages and environmental variables (e.g., elevation and salinity) for the intertidal sabkha around Al-Kharrar Lagoon provides greater confidence that these data could be used as a modern analog to assess the past sea-level with high accuracy. The differences between zones suggest that a transfer function is required to obtain a more accurate prediction of sea-level changes.
We developed a transfer function model from a dataset consisting of 75 samples and 99 foraminiferal species using WA-PLS regression. Regression modeling of the modern training set indicates foraminifers can assess sea-level changes with a precision of 0.12 m. We concluded that the modern training set in the sabkha provides great potential to reconstruct past sea level, and thus future assessment of those changes in the area.
Data Availability Statement
The datasets presented in this study can be found in online repositories. The names of the repository/repositories and accession number(s) can be found below: This study uses all publicly available data: Saudi Aramco Tide Tables, 2014 are available (at https://doi.org/10.6084/m9.figshare.15130386.v1). All data used to produce these results can be found (at https://doi.org/10.6084/m9.figshare.15130383.v1) and all figures and tables are available (at https://doi.org/10.6084/m9.figshare.15130296.v1). The software used in this study are free and they are: C2 software version 1.7.7 is available upon registration (at https://www.staff.ncl.ac.uk/stephen.juggins/software/C2Home.htm), while PAST software version 4.03 is available upon registration (at https://past.en.lo4d.com/windows).
Author Contributions
TA-D collected the field samples, performed the methodology, carried out the data analysis, prepared the figures and tables, and wrote the original draft. RB did the conceptualization, supervised the data, and carried out the funding acquisition. RA-Z did the conceptualization, supervised the data, collected the field samples, performed the methodology, and reviewed the manuscript. AA-Z collected the field samples and performed the methodology. BJ reviewed and commented on all the drafts. All authors contributed to the article and approved the submitted version.
Funding
This research was supported by the Deanship of Scientific Research (DSR), King Abdulaziz University (KAU), Jeddah, under Grant No (D-494-150-1443). The authors, therefore, gratefully acknowledge the DSR technical and financial support.
Conflict of Interest
The authors declare that the research was conducted in the absence of any commercial or financial relationships that could be construed as a potential conflict of interest.
Publisher’s Note
All claims expressed in this article are solely those of the authors and do not necessarily represent those of their affiliated organizations, or those of the publisher, the editors and the reviewers. Any product that may be evaluated in this article, or claim that may be made by its manufacturer, is not guaranteed or endorsed by the publisher.
Acknowledgments
We thankful to Ahmed Taqi, Fikry Shaher, and Satria Antoni for help with the fieldwork. We are very grateful for the editor and reviewers for their constructive comments and editorial handling which greatly contributed to the improvement of the manuscript. We express our deep gratitude to anyone who has provided a piece of useful advice.
References
Abu-Zied, R. H., Al-Dubai, T. A., and Bantan, R. A. (2016). Environmental conditions of shallow waters alongside the southern Corniche of Jeddah based on benthic foraminifera, physico-chemical parameters and heavy metals. J. Foraminifer. Res. 46, 149–170. doi: 10.2113/gsjfr.46.2.149
Abu-Zied, R. H., and Bantan, R. A. (2013). Hypersaline benthic foraminifera from the Shuaiba Lagoon, eastern Red Sea, Saudi Arabia: their environmental controls and usefulness in sea-level reconstruction. Mar. Micropaleontol. 103, 51–67. doi: 10.1016/j.marmicro.2013.07.005
Abu-Zied, R. H., and Bantan, R. A. (2015). Palaeoenvironment, palaeoclimate and sea-level changes in the Shuaiba Lagoon during the late Holocene (last 3. 6 ka), eastern Red Sea coast, Saudi Arabia. Holocene 25, 1301–1312. doi: 10.1177/0959683615584204
Abu-Zied, R. H., Bantan, R. A., Basaham, A. S., El Mamoney, M. H., and Al-Washmi, H. A. (2011). Composition, distribution, and taphonomy of nearshore benthic foraminifera of the Farasan Islands, Southern Red Sea, Saudi Arabia. J. Foraminifer. Res. 41, 349–362. doi: 10.2113/gsjfr.41.4.349
Abu-Zied, R. H., Basaham, A. S., and El Sayed, M. A. (2013). Effect of municipal wastewaters on bottom sediment geochemistry and benthic foraminifera of two Red Sea coastal inlets, Jeddah, Saudi Arabia. Environ. Earth Sci. 68, 451–469. doi: 10.1007/s12665-012-1751-7
Abu-Zied, R. H., Keatings, K., and Flower, R. J. (2007). Environmental controls on foraminifera in Lake Qarun, Egypt. J. Foraminifer. Res. 37, 136–149. doi: 10.2113/gsjfr.37.2.136
Al-Barakati, A. M. A. (2010). Some hydrographic features of rabigh lagoon along the Eastern Coast of the Red Sea. Nat. Sci. Med. 21, 123–132.
Al-Dubai, T. A. (2019). Modern and Late Holocene Environmental Conditions of Al-Kharrar Lagoon, Central Red Sea, Saudi Arabia: Palaeoclimatic Inferences. Ph.D. thesis. Jeddah: King Abdulaziz University.
Al-Dubai, T. A., Abu-Zied, R. H., and Basaham, A. S. (2017a). Diversity and distribution of benthic foraminifera in the Al-Kharrar Lagoon, eastern Red Sea coast, Saudi Arabia. Micropaleontology 63, 275–303.
Al-Dubai, T. A., Abu-Zied, R. H., and Basaham, A. S. (2017b). Present environmental conditions of Al-Kharrar Lagoon, centre of the eastern Red Sea coast, Saudi Arabia. Arab J. Geosci. 10, 1–18. doi: 10.1007/s12517-017-3083-0
Al-Sayari, S. S., and Zötl, J. G. (1978). Quaternary Period in Saudi Arabia. V.I. New York: Springer-Verlag. doi: 10.1007/978-3-7091-8494-3
Alve, E., and Nagy, J. (1986). Estuarine foraminiferal distribution in Sandebukta, a branch of the Oslo Fjord. J. Foraminifer. Res. 16, 261–284. doi: 10.2113/gsjfr.16.4.261
Bai, Y., and Bai, Q. (2019). “Subsea corrosion and scale,” in Subsea Engineering Handbook, 2nd Edn, eds Y. Bai and Q. Bai (Amsterdam: Elsevier). doi: 10.1016/b978-0-12-812622-6.00017-8
Bantan, R. A., Abu-Zied, R. H., and Al-Dubai, T. A. (2019). Late holocene environmental changes in a sediment core from Al-Kharrar Lagoon, eastern Red Sea Coast, Saudi Arabia. Arab. J. Sci. Eng. 44, 6557–6570. doi: 10.1007/s13369-019-03958-9
Bantan, R. A., Al-Dubai, T. A., and Al-Zubieri, A. G. (2020). Geo-environmental assessment of heavy metals in the bottom sediments of the Southern Corniche of Jeddah, Saudi Arabia. Mar. Pollut. Bull. 161:111721. doi: 10.1016/j.marpolbul.2020.111721
Barras, C., Jorissen, F. J., Labrune, C., Andral, B., and Boissery, P. (2014). Live benthic foraminiferal faunas from the French Mediterranean Coast: towards a new biotic index of environmental quality. Ecol. Indic. 36, 719–743. doi: 10.1016/j.ecolind.2013.09.028
Basaham, A. S. (2008). Mineralogical and chemical composition of the mud fraction from the surface sediments of Sharm Al-Kharrar, a Red Sea coastal lagoon. Oceanologia 50, 557–575.
Basaham, A. S., and El-Sayed, M. A. (1998). Distribution and phase association of some major and trace elements in the Arabian Gulf sediments. Estuar. Coast. Shelf Sci. 46, 185–194. doi: 10.1006/ecss.1997.0278
Berkeley, A., Perry, C. T., Smithers, S. G., Horton, B. P., and Taylor, K. G. (2007). A review of the ecological and taphonomic controls on foraminiferal assemblage development in intertidal environments. Earth Sci. Rev. 83, 205–230. doi: 10.1016/j.earscirev.2007.04.003
Bernhard, J. M. (1988). Postmortem vital staining in benthic foraminifera; duration and importance in population and distributional studies. J. Foraminifer. Res. 18, 143–146. doi: 10.2113/gsjfr.18.2.143
Birks, H. J. B. (1995). Quantitative palaeoenvironmental reconstructions. Stat. Model. Quat. Sci. Data Tech. Guide 5, 161–254.
Braithwaite, C. J. R. (1987). “Climate and oceanography,” in Red Sea, ed. A. J. Edwards (New York, NY: Pergamon Press). doi: 10.1016/B978-0-08-028873-4.50007-4
Brown, G. F., Schmidt, D. L., and Huffman, A. G. J. (1989). Geology of the Arabian Peninsula Shield Area of Western Saudi Arabia. U.S. Geol. Surv., 560A. Available online at: https://pubs.er.usgs.gov/publication/pp560A (accessed May 6, 2017).
Cahill, N., Kemp, A. C., Horton, B. P., and Parnell, A. C. (2015). A bayesian hierarchical model for reconstructing sea levels: from raw data to rates of change. Clim. Past 12, 525–542. doi: 10.5194/cp-12-525-2016
Callard, S. L., Gehrels, W. R., Morrison, B. V., and Grenfell, H. R. (2011). Suitability of salt-marsh foraminifera as proxy indicators of sea level in Tasmania. Mar. Micropaleontol. 79, 121–131. doi: 10.1016/j.marmicro.2011.03.001
Cearreta, A., and Murray, J. W. (2000). AMS 14C dating of Holocene estuarine deposits: consequences of high-energy and reworked foraminifera. Holocene 10, 155–159. doi: 10.1191/095968300669405262
Clarke, K. R., and Warwick, R. M. (1994). Change in Marine Communities: An Approach to Statistical Analysis and Interpretation. Plymouth: Natural Environment Research Council.
Corliss, B. H., and Emerson, S. (1990). Distribution of Rose Bengal stained deep-sea benthic foraminifera from the Nova Scotian continental margin and Gulf of Maine. Deep Sea Res. Part A Oceanogr. Res. Pap. 37, 381–400. doi: 10.1016/0198-0149(90)90015-N
Culver, S. J., Mallinson, D. J., Corbett, D. R., Leorri, E., Rouf, A. A., Shazili, N. A. M., et al. (2012). Distribution of foraminifera in the Setiu estuary and lagoon, Terengganu, Malaysia. J. Foraminifer. Res. 42, 109–133. doi: 10.2113/gsjfr.42.2.109
De Rijk, S. (1995). Salinity control on the distribution of salt marsh foraminifera (Great Marshes, Massachusetts). J. Foraminifer. Res. 25, 156–166. doi: 10.2113/gsjfr.25.2.156
De Rijk, S., and Troelstra, S. (1999). The application of a foraminiferal actuo-facies model to salt-marsh cores. Palaeogeogr. Palaeoclimatol. Palaeoecol. 149, 59–66.
De Rijk, S., and Troelstra, S. R. (1997). Salt marsh foraminifera from the Great Marshes, Massachusetts: environmental controls. Palaeogeogr. Palaeoclimatol. Palaeoecol. 130, 81–112.
De Stigter, H. C., der Zwaan, G. J., and Langone, L. (1999). Differential rates of benthic foraminiferal test production in surface and subsurface sediment habitats in the southern Adriatic Sea. Palaeogeogr. Palaeoclimatol. Palaeoecol. 149, 67–88. doi: 10.1016/S0031-0182(98)00193-X
De Stigter, H. C., Jorissen, F. J., and der Zwaan, G. J. (1998). Bathymetric distribution and microhabitat partitioning of live (Rose Bengal stained) benthic foraminifera along a shelf to bathyal transect in the southern Adriatic Sea. J. Foraminifer. Res. 28, 40–65.
Debenay, J.-P., Guiral, D., and Parra, M. (2002). Ecological factors acting on the microfauna in mangrove swamps. The case of foraminiferal assemblages in French Guiana. Estuar. Coast. Shelf Sci. 55, 509–533. doi: 10.1006/ecss.2001.0906
Dias, B. B., Hart, M. B., Smart, C. W., and Hall-Spencer, J. M. (2010). Modern seawater acidification: the response of foraminifera to high-CO2 conditions in the Mediterranean Sea. J. Geol. Soc. Lond. 167, 843–846.
Edwards, R. J., and Horton, B. P. (2000). Reconstructing relative sea-level change using UK salt-marsh foraminifera. Mar. Geol. 169, 41–56. doi: 10.1016/S0025-3227(00)00078-5
Edwards, R. J., Van De Plassche, O., Gehrels, W. R., and Wright, A. J. (2004). Assessing sea-level data from Connecticut, USA, using a foraminiferal transfer function for tide level. Mar. Micropaleontol. 51, 239–255. doi: 10.1016/j.marmicro.2003.11.003
Eichler, P. P. B. (2019). Foraminiferal zonation from a subtropical mangrove in Bertioga Channel (São Paulo, SP, Brazil). Reg. Stud. Mar. Sci. 25:100460. doi: 10.1016/j.rsma.2018.100460
Farouk, S., and Jain, S. (2018). Benthic foraminiferal response to relative sea-level changes in the Maastrichtian–Danian succession at the Dakhla Oasis, Western Desert, Egypt. Geol. Mag. 155, 729–746.
Frontalini, F., Margaritelli, G., Francescangeli, F., Rettori, R., and Du Châtelet, E. A. (2013). Benthic foraminiferal assemblages and biotopes in a coastal lake: the case study of Lake Varano (southern Italy). Acta Protozool. 52, 147–160.
Gasse, F., Juggins, S., and Ben Khelifa, L. (1995). Diatom-based transfer functions for inferring past hydrochemical characteristics of African lakes. Palaeogeogr. Palaeoclimatol. Palaeoecol. 117, 31–54. doi: 10.1016/0031-0182(94)00122-O
Gehrels, W. R. (1994). Determining relative sea-level change from salt-marsh foraminifera and plant zones on the coast of Maine, USA. J. Coast. Res. 10, 990–1009.
Gehrels, W. R. (2000). Using foraminiferal transfer functions to produce high-resolution sea-level records from salt-marsh deposits, Maine, USA. Holocene 10, 367–376.
Gehrels, W. R., and van de Plassche, O. (1999). The use of Jadammina macrescens (Brady) and Balticammina pseudomacrescens Brönnimann, Lutze and Whittaker (Protozoa: Foraminiferida) as sea-level indicators. Palaeogeogr. Palaeoclimatol. Palaeoecol. 149, 89–101. doi: 10.1016/S0031-0182(98)00194-1
Gehrels, W. R., Kirby, J. R., Prokoph, A., Newnham, R. M., Achterberg, E. P., Evans, H., et al. (2005). Onset of recent rapid sea-level rise in the western Atlantic Ocean. Quat. Sci. Rev. 24, 2083–2100. doi: 10.1016/j.quascirev.2004.11.016
Ghandour, I. M., Al-Zubieri, A. G., Basaham, A. S., Mannaa, A. A., Al-Dubai, T. A., and Jones, B. G. (2021). Mid-late Holocene paleoenvironmental and sea level reconstruction on the Al Lith Red Sea coast, Saudi Arabia. Front. Mar. Sci. 8:677010. doi: 10.3389/fmars.2021.677010
Gharbi, S. H., Albarakati, A. M., Alsaafani, M. A., Saheed, P. P., and Alraddadi, T. M. (2018). Simulation of tidal hydrodynamics in the Red Sea using COHERENS model. Reg. Stud. Mar. Sci. 22, 49–60.
Gómez-León, A., Rodríguez-Figueroa, G. M., Shumilin, E., Carreño, A. L., and Sánchez, A. (2018). Abundance and distribution of benthic foraminifera as indicators of the quality of the sedimentary environment in a subtropical lagoon, Gulf of California. Mar. Pollut. Bull. 130, 31–39. doi: 10.1016/j.marpolbul.2018.03.013
Haig, D. (1988). Miliolid foraminifera from inner neritic and mud facies of the Papuan Lagoon. J. Foraminifer. Res. 18, 203–236. doi: 10.2113/gsjfr.18.3.203
Hamilton, S., and Shennan, I. (2005). Late Holocene relative sea-level changes and the earthquake deformation cycle around upper Cook Inlet, Alaska. Quat. Sci. Rev. 24, 1479–1498. doi: 10.1016/j.quascirev.2004.11.003
Hammer, Ø, Harper, D. A. T., and Ryan, P. D. (2001). Past: paleontological statistics software package for education and data analysis. Palaeontol. Electron. 4, 1–9.
Haslett, S. K. (2001). The palaeoenvironmental implications of the distribution of intertidal foraminifera in a tropical Australian estuary: a reconnaissance study. Aust. Geogr. Stud. 39, 67–74.
Haunold, T. G., Baal, C., and Piller, W. E. (1997). Benthic foraminiferal associations in the northern Bay of Safaga, Red Sea, Egypt. Mar. Micropaleontol. 29, 185–210. doi: 10.1016/S0377-8398(96)00031-X
Hawkes, A. D., Horton, B. P., Nelson, A. R., and Hill, D. F. (2010). The application of intertidal foraminifera to reconstruct coastal subsidence during the giant Cascadia earthquake of AD 1700 in Oregon, USA. Quat. Int. 221, 116–140. doi: 10.1016/j.quaint.2009.09.019
Hayward, B. W., and Hollis, C. J. (1994). Brackish foraminifera in New Zealand; a taxonomic and ecologic review. Micropaleontology 40, 185–222. doi: 10.2307/1485816
Hayward, B. W., Grenfell, H. R., and Scott, D. B. (1999). Tidal range of marsh foraminifera for determining former sea-level heights in New Zealand. N. Z. J. Geol. Geophys. 42, 395–413. doi: 10.1080/00288306.1999.9514853
Hayward, B. W., Sabaa, A. T., Grenfell, H. R., Cochran, U. A., Clark, K. J., Litchfield, N. J., et al. (2015). Foraminiferal record of Holocene paleo-earthquakes on the subsiding south-western Poverty Bay coastline, New Zealand. N. Z. J. Geol. Geophys. 58, 104–122. doi: 10.1080/00288306.2014.992354
Hayward, B. W., Scott, G. H., Grenfell, H. R., Carter, R., and Lipps, J. H. (2004). Techniques for estimation of tidal elevation and confinement (~ salinity) histories using benthic foraminifera: examples from New Zealand. Holocene 14, 218–232. doi: 10.1191/0959683604hl678rp
Heiri, O., Lotter, A. F., and Lemcke, G. (2001). Loss on ignition as a method for estimating organic and carbonate content in sediments: reproducibility and comparability of results. J. Paleolimnol. 25, 101–110.
Hewaidy, A. G. A., Farouk, S., and Bazeen, Y. S. (2019). Benthic foraminiferal paleoecology of the Maastrichtian succession at the Kharga Oasis, Western Desert, Egypt. Cretac. Res. 94, 152–167. doi: 10.1016/j.cretres.2018.10.007
Hohenegger, J. (2004). Depth coenoclines and environmental considerations of western Pacific larger foraminifera. J. Foraminifer. Res. 34, 9–33. doi: 10.2113/0340009
Hohenegger, J., Piller, W. E., and Baal, C. (1993). Horizontal and vertical spatial microdistribution of foraminifers in the shallow subtidal Gulf of Trieste, northern Adriatic Sea. J. Foraminifer. Res. 23, 79–101. doi: 10.2113/gsjfr.23.2.79
Horton, B. P. (1999). The distribution of contemporary intertidal foraminifera at Cowpen Marsh, Tees Estuary, UK: implications for studies of Holocene sea-level changes. Palaeogeogr. Palaeoclimatol. Palaeoecol. 149, 127–149. doi: 10.1016/S0031-0182(98)00197-7
Horton, B. P., and Edwards, R. J. (2003). Seasonal distributions of foraminifera and their implications for sea-level studies, Cowpen Marsh, UK. SEPM Spec. 75, 21–30. doi: 10.2110/pec.03.75.0021
Horton, B. P., and Edwards, R. J. (2006). Quantifying Holocene sea-level change using intertidal foraminifera: lessons from the British Isles. Cushman Found. Spec. Publ. 40, 1–97.
Horton, B. P., and Murray, J. W. (2007). The roles of elevation and salinity as primary controls on living foraminifera distributions: Cowpen Marsh, Tees Estuary, UK. Mar. Micropaleontol. 63, 169–186. doi: 10.1016/j.marmicro.2006.11.006
Horton, B. P., Edwards, R. J., and Lloyd, J. M. (1999). A foraminiferal-based transfer function: implications for sea-level studies. J. Foraminifer. Res. 29, 117–129. doi: 10.2113/gsjfr.29.2.117
Imbrie, J. (1971). “A new micropaleontological method for quantitative paleoclimatology: application to a late Pleistocene Caribbean core,” in The Late Cenozoic Glacial Ages, ed. K. K. Turekian (New Haven, CT: Yale University Press), 71–181.
Jones, R. W., and Charnock, M. A. (1985). Morphogroups of agglutinating foraminifera. Their life positions and feeding habits and potential applicability in (paleo) ecological studies. Rev. Paléobiol. 4, 311–320.
Jones, V. J., and Juggins, S. (1995). The construction of a diatom-based chlorophyll a transfer function and its application at three lakes on Signy Island (maritime Antarctic) subject to differing degrees of nutrient enrichment. Freshw. Biol. 34, 433–445. doi: 10.1111/j.1365-2427.1995.tb00901.x
Juggins, S. (2007). C2 Version 1.4: Software for Ecological and Palaeoecological Data Analysis and Visualisation. Newcastle upon Tyne: Newcastle University.
Kemp, A. C., Horton, B. P., and Culver, S. J. (2009). Distribution of modern salt-marsh foraminifera in the Albemarle–Pamlico estuarine system of North Carolina, USA: implications for sea-level research. Mar. Micropaleontol. 72, 222–238. doi: 10.1016/j.marmicro.2009.06.002
Kemp, A. C., Horton, B. P., Vane, C. H., Bernhardt, C. E., Corbett, D. R., Engelhart, S. E., et al. (2013). Sea-level change during the last 2500 years in New Jersey, USA. Quat. Sci. Rev. 81, 90–104.
Lal, K. K., Bonetti, C., Woodroffe, C. D., and Rogers, K. (2020). Contemporary distribution of benthic foraminiferal assemblages in coastal wetlands of south-eastern Australia. Estuar. Coast. Shelf Sci. 245:106949. doi: 10.1016/j.ecss.2020.106949
Lambeck, K. (1995). Late Devensian and Holocene shorelines of the British Isles and North Sea from models of glacio-hydro-isostatic rebound. J. Geol. Soc. Lond. 152, 437–448.
Langdon, C., Takahashi, T., Sweeney, C., Chipman, D., Goddard, J., Marubini, F., et al. (2000). Effect of calcium carbonate saturation state on the calcification rate of an experimental coral reef. Glob. Biogeochem. Cycles 14, 639–654.
Leorri, E., and Cearreta, A. (2004). Foraminiferal interpretation of the Holocene environmental development of the Bilbao estuary, N. Spain. Mar. Micropaleontol. 51, 75–94.
Leorri, E., Cearreta, A., and Horton, B. P. (2008a). A foraminifera-based transfer function as a tool for sea-level reconstructions in the southern Bay of Biscay. Geobios 41, 787–797.
Leorri, E., Horton, B. P., and Cearreta, A. (2008b). Development of a foraminifera-based transfer function in the Basque marshes, N. Spain: implications for sea-level studies in the Bay of Biscay. Mar. Geol. 251, 60–74. doi: 10.1016/j.margeo.2008.02.005
Leorri, E., Gehrels, W. R., Horton, B. P., Fatela, F., and Cearreta, A. (2010). Distribution of foraminifera in salt marshes along the Atlantic coast of SW Europe: tools to reconstruct past sea-level variations. Quat. Int. 221, 104–115.
Loeblich, A. R., and Tappan, H. (1987). Foraminiferal Genera and Their classification, Vol. 2. New York, NY: Van Nostrand Reinhold Company. doi: 10.1007/978-1-4899-5760-3
Manaa, A. A., Jones, B. G., McGregor, H. V., Zhao, J.-X., and Price, D. M. (2016). Dating quaternary raised coral terraces along the Saudi Arabian Red Sea coast. Mar. Geol. 374, 59–72. doi: 10.1016/j.margeo.2016.02.002
Massey, A. C., Gehrels, W. R., Charman, D. J., and White, S. V. (2006). An intertidal foraminifera-based transfer function for reconstructing Holocene sea-level change in southwest England. J. Foraminifer. Res. 36, 215–232. doi: 10.2113/gsjfr.36.3.215
Morcos, S. A. (1970). Physical and chemical oceanography of the Red Sea. Oceanogr. Mar. Biol. Annu. Rev 8:202.
Moreno, J., Valente, T., Moreno, F., Fatela, F., Guise, L., and Patinha, C. (2007). Occurrence of calcareous foraminifera and calcite-carbonate equilibrium conditions–a case study in Minho/Coura estuary (Northern Portugal). Hydrobiologia 587, 177–184. doi: 10.1007/s10750-007-0677-7
Murray, J. W. (1968). The living Foraminiferida of Christchurch Harbour, England. Micropaleontology 14, 83–96. doi: 10.2307/1484768
Murray, J. W. (1971). Living foraminiferids of tidal marshes; a review. J. Foraminifer. Res. 1, 153–161. doi: 10.2113/gsjfr.1.4.153
Murray, J. W. (1991). “Chapter 5 – Relationship between living and dead assemblages,” in Ecology and Palaeoecology of Benthic Foraminifera, ed. J. W. Murray (Harlow: Longman Scientific and Technical), 43–54.
Murray, J. W. (2000). The enigma of the continued use of total assemblages in ecological studies of benthic foraminifera. J. Foraminifer. Res. 30, 244–245. doi: 10.2113/0300244
Murray, J. W. (2006). Ecology and Applications of Benthic Foraminifera. Cambridge: Cambridge University Press. doi: 10.1017/CBO9780511535529
Murray, J. W., and Alve, E. (1999). Taphonomic experiments on marginal marine foraminiferal assemblages: how much ecological information is preserved? Palaeogeogr. Palaeoclimatol. Palaeoecol. 149, 183–197.
Murray, J. W., and Alve, E. (2000). Major aspects of foraminiferal variability (standing crop and biomass) on a monthly scale in an intertidal zone. J. Foraminifer. Res. 30, 177–191.
Phleger, F. B. (1965). Patterns of marsh foraminifera, Galveston Bay, Texas. Limnol. Oceanogr. 10, R169–R184. doi: 10.4319/lo.1965.10.suppl2.r169
Plaziat, J.-C., Reyss, J.-L., Choukri, A., and Cazala, C. (2008). Diagenetic Rejuvenation of Raised Coral Reefs and Precision of Dating. The Contribution of the Red Sea Reefs to the Question of Reliability of the Uranium-Series Datings of Middle to Late Pleistocene Key Reef-Terraces of the World. Maintenon: Association Carnets de Géologie. doi: 10.4267/2042/16743
Punniyamoorthy, R., Murugesan, P., Mahadevan, G., and Sanchez, A. (2021). Benthic meiofaunal diversity in four zones of Pichavaram Mangrove Forest, India. J. Foraminifer. Res. 51, 294–307.
Reiss, Z., and Hottinger, L. (1984). The Gulf of Aqaba: Ecological Micropaleontology. Ecological Studies, Vol. 50. Berlin: Springer Verlag. doi: 10.1007/978-3-642-69787-6
Rogers, K., Boon, P., Lovelock, C. E., and Saintilan, N. (2017). “Coastal halophytic vegetation,” in Australian Vegetation, ed. D. A. Keith (Cambridge: Cambridge University Press), 544–569.
Sanders, D. (2003). Syndepositional dissolution of calcium carbonate in neritic carbonate environments: geological recognition, processes, potential significance. J. Afr. Earth Sci. 36, 99–134.
Scheder, J., Frenzel, P., Bungenstock, F., Engel, M., Brueckner, H., and Anna, P. (2019). Vertical and lateral distribution of Foraminifera and Ostracoda in the East Frisian Wadden Sea–developing a transfer function for relative sea-level change. Geol. Belgica 22, 99–110. doi: 10.20341/gb.2019.007
Schönfeld, J., Alve, E., Geslin, E., Jorissen, F., Korsun, S., and Spezzaferri, S. (2012). The FOBIMO (FOraminiferal BIo-MOnitoring) initiative—Towards a standardised protocol for soft-bottom benthic foraminiferal monitoring studies. Mar. Micropaleontol. 94, 1–13.
Scott, D. B., and Medioli, F. S. (1980a). Living vs. total foraminiferal populations: their relative usefulness in paleoecology. J. Paleontol. 54, 814–831.
Scott, D. B., and Medioli, F. S. (1980b). Quantitative studies of marsh foraminiferal distributions in Nova Scotia: their implications for the study of sea-level changes. Cushman Found. Foraminifer. Res. Spec. Publ. 17:58.
Scott, D. K., and Leckie, R. M. (1990). Foraminiferal zonation of Great Sippewissett salt marsh (Falmouth, Massachusetts). J. Foraminifer. Res. 20, 248–266.
Scott, D. S., and Medioli, F. S. (1978). Vertical zonations of marsh foraminifera as accurate indicators of former sea-levels. Nature 272, 528–531.
Scott, G., Thompson, L., Hitchin, R., and Scourse, J. (1998). Observations on selected salt-marsh and shallow-marine species of agglutinating foraminifera: grain size and mineralogical selectivity. J. Foraminifer. Res. 28, 261–267.
Sen Gupta, B. K. (1999). “Foraminifera in marginal marine environments,” in Modern Foraminifera, ed. B. K. Sen Gupta (Dordrecht: Kluwer Academic Publishers), 141–159. doi: 10.1007/0-306-48104-9_9
Shennan, I., Long, A. J., Rutherford, M. M., Green, F. M., Innes, J. B., Lloyd, J. M., et al. (1996). Tidal marsh stratigraphy, sea-level change and large earthquakes, I: a 5000 year record in Washington, USA. Quat. Sci. Rev. 15, 1023–1059.
Sofianos, S. S., Johns, W. E., and Murray, S. P. (2002). Heat and freshwater budgets in the Red Sea from direct observations at Bab el Mandeb. Deep Sea Res. Part II Top. Stud. Oceanogr. 49, 1323–1340. doi: 10.1016/S0967-0645(01)00164-3
Southall, K. E., Gehrels, W. R., and Hayward, B. W. (2006). Foraminifera in a New Zealand salt marsh and their suitability as sea-level indicators. Mar. Micropaleontol. 60, 167–179. doi: 10.1016/j.marmicro.2006.04.005
Strachan, K. L., Finch, J. M., Hill, T., and Barnett, R. L. (2014). A late Holocene sea-level curve for the east coast of South Africa. S. Afr. J. Sci. 110, 1–9.
Strachan, K. L., Hill, T. R., Finch, J. M., and Barnett, R. L. (2015). Vertical zonation of foraminifera assemblages in Galpins salt marsh, South Africa. J. Foraminifer. Res. 45, 29–41.
Walton, W. R. (1952). Techniques for recognition of living foraminifera. Cushman Found. Foraminifer. Res. Contr. 3, 56–60.
Woodroffe, S. A. (2009). Recognising subtidal foraminiferal assemblages: implications for quantitative sea-level reconstructions using a foraminifera-based transfer function. J. Quat. Sci. Publ. Quat. Res. Assoc. 24, 215–223. doi: 10.1002/jqs.1230
Keywords: intertidal sabkha, benthic foraminifera, Red Sea, transfer function, training set, sea-level
Citation: Al-Dubai TA, Bantan RA, Abu-Zied RH, Al-Zubieri AG and Jones BG (2022) Distribution of Benthic Foraminifera in Intertidal Sabkha of Al-Kharrar Lagoon, Saudi Arabia: Tools to Study Past Sea-Level Changes. Front. Mar. Sci. 9:843758. doi: 10.3389/fmars.2022.843758
Received: 26 December 2021; Accepted: 23 February 2022;
Published: 25 March 2022.
Edited by:
Juan Jose Munoz-Perez, University of Cádiz, SpainReviewed by:
Alberto Sánchez-González, Instituto Politécnico Nacional, MexicoSherif Farouk, Egyptian Petroleum Research Institute, Egypt
Copyright © 2022 Al-Dubai, Bantan, Abu-Zied, Al-Zubieri and Jones. This is an open-access article distributed under the terms of the Creative Commons Attribution License (CC BY). The use, distribution or reproduction in other forums is permitted, provided the original author(s) and the copyright owner(s) are credited and that the original publication in this journal is cited, in accordance with accepted academic practice. No use, distribution or reproduction is permitted which does not comply with these terms.
*Correspondence: Talha A. Al-Dubai, dGFsaGFkdWJhaUB5YWhvby5jb20=; dGFsZHViYWlAc3R1LmthdS5lZHUuc2E=