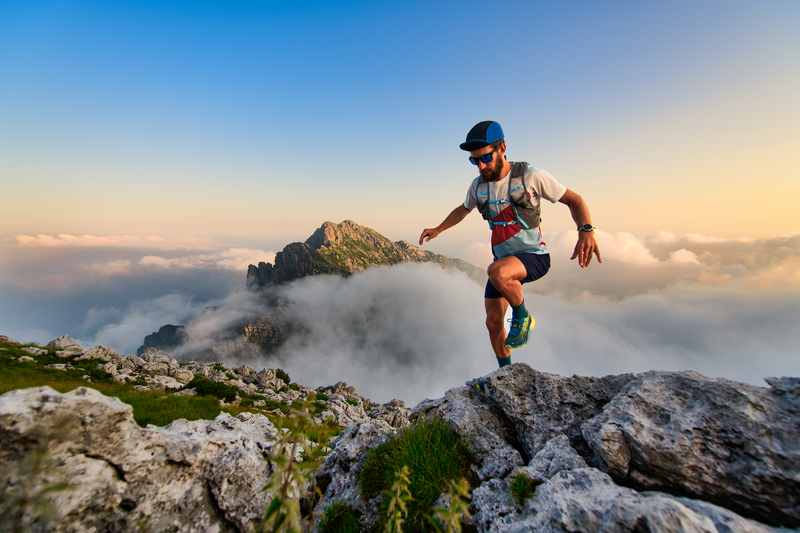
94% of researchers rate our articles as excellent or good
Learn more about the work of our research integrity team to safeguard the quality of each article we publish.
Find out more
ORIGINAL RESEARCH article
Front. Mar. Sci. , 10 March 2022
Sec. Marine Conservation and Sustainability
Volume 9 - 2022 | https://doi.org/10.3389/fmars.2022.841857
This article is part of the Research Topic Science Supporting Management of Eutrophication: Lessons Learned from a Barrier Island Lagoon View all 18 articles
The Indian River Lagoon (IRL) on Florida’s east coast is a biologically diverse estuary and an important habitat to the threatened Florida manatee (Trichechus manatus latirostris). An unusual mortality event (UME) was declared by the Working Group on Marine Mammal Unusual Mortality Events in 2013 after a marked increase in manatee deaths in the IRL of an unknown cause. This UME followed a dramatic reduction of seagrass coverage in the IRL due to chronic non-toxic phytoplankton blooms, with a resultant ecosystem shift to mixed macroalgal dominance. At least 199 manatee deaths fitting the UME case definition were documented in and adjacent to the IRL during 2012–2019; mortality was highest in 2013, when 111 of these deaths were documented. The case definition included carcasses in good nutritional condition, with multiorgan congestion or wet lungs consistent with drowning without trauma. The gastrointestinal compartments of manatee carcasses were filled with diverse macroalga species, and the contents were notably more fluid than usual. Gross intestinal findings included blebbing to segmental thickening of the wall. Microscopic lesions were primarily intestinal, including necrosis, edema, hemorrhage, mucosa-associated lymphoid changes, and inflammation, sometimes associated with Gram-positive bacterial rods. A multidisciplinary approach of environmental and carcass sampling found no causative evidence through tests for micro- and macroalgal biotoxins, trace metals, general toxin screening, or vitreum biochemistry. Microbiological, cytological, immunohistochemical, and molecular analyses of Clostridiales from intestinal samples identified Clostridioides difficile toxin A, toxins A/B and toxin A gene; Paeniclostridium sordellii lethal gene (and other potential virulence factors from a sequenced strain); and Clostridium perfringens alpha and epsilon toxin genes. The results from this 8 year-long investigation are indicative that the cause of death in this manatee UME was associated with clostridial infection, initiated by a shift to a predominantly macroalgal diet.
The Florida manatee (Trichechus manatus latirostris) is a threatened marine mammal inhabiting the southeastern United States, primarily the coastal waters of Florida. Lethal boat-strike injuries, loss of warmwater habitat, and brevetoxicosis are the largest threats to the sustainability of the manatee population (Martin et al., 2017; Runge et al., 2017). Harmful algal blooms (HABs) from Karenia brevis in southwest Florida frequently cause mass mortalities of manatees from brevetoxicosis (Landsberg et al., 2009). Extreme low temperatures in the winters of 2009–2010 and 2010–2011 led to record numbers of cold-related manatee deaths and the declaration of unusual mortality events across the state1 (Hardy et al., 2019). The Marine Mammal Protection Act defines an unusual mortality event (UME) as “a stranding that is unexpected; involves a significant die-off of any marine mammal population; and demands immediate response.”2 These previous two UMEs were associated with high manatee mortality primarily in Brevard County, in east-central Florida. Brevard County’s portion of the Indian River Lagoon (IRL) is the most important area for manatee use on the Atlantic Coast (Deutsch et al., 2003), providing essential habitat to manatees year-round, with counts peaking during the spring and seasonal migration. An aerial abundance survey conducted in March 2012 estimated that 70% (95% CI 60–80%) of the manatees on the east coast of Florida were present in Brevard County at the time (Martin et al., 2015).
The IRL has a long history of diverse HABs (Landsberg et al., 2006; Phlips et al., 2015), which include some toxic species that pose a risk to marine mammals (Fire et al., 2015). In 2011, a series of non-toxic bloom events with direct ecosystem disruption resulted in a chain of effects that ultimately triggered a manatee UME in 2013.3 A dominant spring bloom of mixed microalgae in 2011 reduced light penetration, with resultant seagrass losses, poor water quality, and fish and shellfish kills. The following year, brown tide (Aureoumbra lagunensis) in the northern IRL again impaired seagrass growth, resulting in still more losses (Gobler et al., 2013; Phlips et al., 2015; Breininger et al., 2017; Morris et al., 2018). An estimated 47,000 acres (60%) of seagrass was lost during 2009–2012 between Ponce Inlet, in Volusia County, and Fort Pierce Inlet, in St. Lucie County, covering most of the IRL (Lapointe et al., 2015, 2020; Morris et al., 2018). This loss of seagrass caused dietary shifts in manatees to the then predominant available Rhodophyta, ultimately triggering a manatee mass-mortality event.
In July 2012, the first manatees presenting with sudden death of unknown cause had large volumes of Rhodophyta (e.g., Gracilaria spp.) in the gastrointestinal tract. By the end of March 2013, the total number of manatee deaths from natural and undetermined causes (n = 166) had reached a level of more than six times the previous 5-year average (n = 27; excluding the 2009–2010 and 2010–2011 years with high winter mortalities) for this period in Brevard County. Under two of the UME criteria set forth in procedures of section 404 of the Marine Mammal Protection Act, the Working Group on Marine Mammal Unusual Mortality Events determined a UME occurred from 1 January to 16 December 2013.3 The declaration was based on (1) “a marked increase in the magnitude or a marked change in the nature of morbidity, mortality or strandings when compared with prior records” (UME criterion 1) and (2) “affected animals exhibit[ing] similar or unusual pathological findings, behavior patterns, clinical signs, or general physical condition” (UME criterion 5).4
Manatees are generalist herbivores that feed on an extensive variety of aquatic vegetation, including macroalgae,5,6 which are not normally considered a significant portion of the manatee’s diet (Hartman, 1979). Although macroalgae concentrate and produce a wide range of bioactive compounds with toxic and cytostatic, antiviral, antihelmintic, antifungal, or antibacterial activity (Higa and Kuniyoshi, 2000; de Almeida et al., 2011; Kumar and Sharma, 2021), no negative effects associated with the ingestion of macroalgae have been documented in manatees. Furthermore, other than brevetoxicosis and cold stress syndrome, disease is relatively uncommon in wild manatees (Owen et al., 2018).
The investigation of the 2013 manatee UME described here involved a multidisciplinary approach with the objective of determining the cause of mortality and examining any causal factors that contributed to the broad scale of this event. The dietary change associated with the seagrass die-off in the IRL likely resulted in a novel bacterial pathogenesis and a mass-mortality event previously undescribed in a marine mammal species.
Collection of environmental samples targeted primarily representative macroalgae for identification of potential manatee forage items and to test Gracilaria for toxicity. Four sampling trips were made in March and April 2013, covering the southern Mosquito Lagoon, the northern IRL, the Banana River, and the central and southern IRL at Sebastian River and Crane Creek. The sampling area generally covered the northern, southern, and eastern distribution of known manatee carcasses at the time.
Macroalgae were collected from a boat using a grappling iron or, in shallow areas, by hand. All locations were georeferenced and recorded. Macroalgae were sorted by hand (staff wore sterile gloves) aboard the boat, representative samples photographed, and subsamples placed into glass vials containing 70% ethanol. Voucher specimens were identified using methods outlined in Littler et al. (2008). Gracilaria samples were collected in bulk (∼2 kg), frozen in Ziploc bags at –20°C, shipped on dry ice to the Biotoxin Laboratory, NOAA, Charleston, South Carolina, and processed for polycavernoside A (see Biotoxins).
Florida manatee carcasses were reported by the public to a state-managed wildlife hotline, and biologists transported most carcasses to the Marine Mammal Pathobiology Laboratory of the Florida Fish and Wildlife Conservation Commission’s Fish and Wildlife Research Institute, where carcasses were examined and processed according to standardized procedures (Bonde et al., 1983). Fresh carcasses showed little or no bloating with all internal organs intact, and moderately decomposed carcasses had some bloating and sloughing skin, but all internal organs showed integrity. Badly decomposed carcasses were bloated with loss of organ integrity or complete disintegration and were often necropsied in the field. Carcass information (including sex, length, weight, and cause of death) and recovery data were stored in a relational database, double-verified after entry, and reviewed for consistency. Gastrointestinal contents were immediately submitted to laboratories for analyses and archived at –20 or –80°C. Tissue samples (liver, kidney, lung, fat, brain, gastrointestinal wall, spleen, pancreas, heart, skeletal muscle, hemidiaphragm, lymph nodes, adrenal glands, skin, trachea, ovary, uterus or testis, mammary gland, and urinary bladder wall) were fixed in 10% neutral buffered formalin and archived at –20 or –80°C. The sample analyses described herein summarize diagnostic methods applied in this UME investigation over a course of 8 years, and varying sample numbers reflect limitations related to carcass decomposition, necropsy and laboratory resources, and cost.
Formalin-fixed tissue samples of fresh and moderately decomposed carcasses were embedded in paraffin (Paraplast Plus, Fisher Scientific, Waltham, Pennsylvania) or glycol methacrylate plastic resin (JB-4; Electron Microscopy Sciences, Hatfield, Pennsylvania), sectioned at 5–7 or 3–5 μm, respectively, mounted on frosted glass slides, and stained routinely with Mayer’s hematoxylin and eosin (H&E) for paraffin embedding and Weigert’s H&E for JB4 embedding. Additional stains were used as needed to confirm various microscopic findings, including Brown and Brenn Gram (bacteria) and methenamine–silver nitrate (fungi) (Luna, 1968; Quintero-Hunter et al., 1991).
Tissue imprints of various sites of gastrointestinal tract mucosa (stomach, duodenum, small intestine, or colon) and direct smears of feces from fresh carcasses were prepared and stained with DIFF-QUIK™ (RAL DIFF-QUIK, RAL Diagnostics, Martillac, France) and with Gram stain and Malachite green per standard methods.
For Paeniclostridium sordellii and Clostridium perfringens cell detection, 4.0-μm-thick paraffin sections of small intestine, cecum, and colon from fresh carcasses were processed by indirect immunoperoxidase methods (Ortega et al., 2007; Nyaoke et al., 2020) according to manufacturer’s instructions. Sections were selected based on microscopic observation of enteritis and typhlocolitis (UME cases) and absence of intestinal lesions (control cases, cause of death acute watercraft injury or brevetoxicosis), both without visible autolytic changes.
For detection of Clostridioides difficile toxin A, additional paraffin sections of stomach, small intestine, cecum, and colon from fresh and moderately decomposed carcasses were placed onto charged slides, deparaffinized in two changes of xylene (6 min each), followed by a graded series of alcohols to distilled water, rinsed in Tris-buffered saline (pH 7.6, 3 min), and incubated in dual Endogenous Block™ (DAKO Corporation, Carpinteria, California) (10 min). Slides were covered with Parafilm to ensure even distribution of the blocker, rinsed in Tris-buffered saline (pH 7.6, 3 min), incubated with non-serum-protein block (DAKO Corporation, 15 min), washed twice with Tris-buffered saline (pH 7.6, 3 min), and then the C. difficile toxin A primary antibody (clone PCG4, Novus Biologicals, Centennial, Colorado) (1:100 dilution) was applied, incubated overnight at 4°C, then rinsed in Tris-buffered saline (pH 7.6 × 3, 2 min). Next, the secondary antibody, ultraView Red Multimer (Roche-Ventana, Tucson, Arizona, 12 min), was applied; slides were again covered with Parafilm, rinsed in Tris-buffered saline (pH 7.6, ×3, 2 min), incubated in Permanent Red chromogen (DAKO Corporation, 5 min), and then rinsed in distilled water (3 min), counterstained in Harris hematoxylin (2 dips), rinsed in running tap water for 5 min followed by a graded series of alcohols to xylene, and then coverslipped with Clearmount™. Negative control slides were prepared in parallel for each paraffin section and treated the same way, except no antibodies were applied to the slides. Positive P. sordellii controls were obtained from a horse (Uzal, unpublished data).
For in vitro bacterial culture, sections (about 30 cm long) of the small intestine or colon from fresh carcasses were tied firmly with a string on each end, then the tied ends were excised from the gastrointestinal tract so that the intestine contents in the samples remained intact. This measure minimized cross-contamination of intestine contents or fecal material between samples. Securely tied intestinal samples were immediately transferred in Ziploc bags to a dry laboratory and placed in a clean laminar-flow cabinet. Samples for bacterial culture were taken aseptically from the intestine contents and processed three ways: (1) fecal contents were incubated anaerobically in a brain heart infusion (BHI) broth (Anaerobe Systems, Morgan Hill, California) at 37°C for 24 h and then the broth culture was frozen with 60% sterile glycerol (Thermo Fisher Scientific, Fair Lawn, New Jersey) at –80°C; (2) fecal contents were directly applied onto the general anaerobic blood agar plates (Anaerobe Systems) or various selective culture media; and (3) fecal contents were frozen in sterile cryogenic vials at –80°C. Bacterial culture was also performed in samples taken from outside the gastrointestinal tract (blood, lymph node, heart, kidney, cervix, uterine body, and uterine horn), which were then directly applied onto the media aseptically.
For Clostridia species isolation, the following selective media were used: (1) for Clostridioides difficile, BBL™ Clostridium difficile selective agar [Becton Dickinson and Company (BD), Sparks, Maryland, United States] or cycloserine cefoxitin mannitol broth with taurocholate lysozyme cysteine broth (Anaerobe Systems); (2) for P. sordellii, Brucella agar (BBL™ Brucella Agar, BD); and (3) for Clostridium perfringens, CP Chromo Select Agar (Sigma-Aldrich, Burlington, Massachusetts). All the media were then anaerobically incubated (GasPak™ EZ anaerobe gas generating pouch system, BD) at 37°C for 24 h. The colony isolates were confirmed with Gram stain (BBL™ Gram stain kits, BD), examined under a 100 × oil-immersion objective lens (Olympus BX51). Colonies exhibiting characteristic growth of Clostridia species of interest were further confirmed with molecular analysis. Isolates were identified genetically by polymerase chain reaction (PCR) with species-specific primer sets or 16S rDNA sequencing, described below. Detection of Clostridia species and their toxins were also conducted with culture-independent molecular methods to detect plasmid-encoding toxin genes, which can be lost during multiple transfer of media during culture-dependent isolation processes.
DNA from pure or mixed cultures of bacteria was extracted with UltraClean® Microbial DNA Isolation Kit (Mo Bio Laboratories Inc., Carlsbad, California), eluted in 30 μL of deionized water, and stored at –20°C. DNA from culture-independent frozen intestinal content samples was extracted with Gentra® Puregene® DNA Isolation Kit (Qiagen, Valencia, California) following the manufacturer’s protocol but with an incubation time of 12 h. DNA was eluted in 75 μL of deionized water and stored at –20°C.
Clostridium perfringens, Clostridioides difficile, and P. sordellii were initially screened through PCR primers targeting the 16S rDNA gene, as described by Kikuchi et al. (2002). Clostridium bifermentans was also amplified using the P. sordellii species-specific primer sets. The urea test (P. sordellii, positive; C. bifermentans, negative) was applied to differentiate between these two species. PCR primers targeting the gluD gene (glutamate dehydrogenase) were also used for Clostridioides difficile species confirmation according to the methods described by Paltansing et al. (2007). Clostridium perfringens toxin (alpha, beta, epsilon, iota, beta 2, entero) genes were screened through PCR described by Aschfalk and Müller (2001, 2002). Primers used for PCR are shown in Table 1. Primers targeting P. sordellii toxins [lethal (TcsL) and hemorrhagic (TcsH)] were designed and their PCR reaction (50 μL) was carried out with: 10 pmol of each primer, 10 μL of 50-μM dNTP mix, 15 mM of MgCl2, 1.25 units of Taq polymerase, 5 μL of 10 × buffer (Promega, Madison, Wisconsin), and 2 μL of genomic DNA. Touchdown PCR was run with the following conditions. Initially, the denaturing temperature was set at 94°C for 1.5 min, followed by 40 cycles at 94°C for 45 s, then for 45 s of gradually decreasing annealing temperature from 59 to 55°C, and for 45 s at 72°C. The final extension period was 2 min at 72°C. The PCR product was electrophoresed through 1.5% agarose in a Tris-acetate-EDTA buffer with 1% ethidium bromide. For the P. sordellii toxin PCR products, the targeted DNA bands were excised, and the DNA was extracted with a StrataPrep DNA gel extraction kit (Agilent Technologies, Santa Clara, California), following the manufacturer’s protocol.
Table 1. Specifications of primer sequences (both forward and reverse) used in PCR for the detection of clostridia species (Clostridium perfringens, Paeniclostridium sordellii, and Clostridioides difficile) and their target toxin genes.
Once pure isolates had been identified, their small subunit rRNA genes were amplified using universal primers with standard protocols (Lane, 1991), and PCR products were purified using UltraClean® PCR Clean Kit (Mo Bio Laboratories Inc.). The DNA was sequenced in both directions using a Big Dye Terminator v 1.1 (Applied Biosystems Inc., Waltham, Massachusetts) and visualized with the 3130 Genetic Analyzer (Applied Biosystems Inc.); species were identified through GenBank BLAST analysis.
One of the P. sordellii isolates from the small intestinal contents of a manatee that fit the UME case definition was enriched in BHI broth overnight and pelleted by centrifugation at 3,000 × g for 10 min. DNA extraction from the pelleted bacteria was carried out using a Blood and Cell Culture DNA Midi Kit (Qiagen) according to the manufacturer’s protocol. A DNA-sequencing library was generated using the Ligation Sequencing Kit (Oxford Nanopore Technologies, Oxford, United Kingdom), and sequencing was performed using an R9.4.1 flow cell on a MinION sequencer (Oxford Nanopore Technologies). De novo assembly of the paired-end reads was performed in Canu 1.9 (Koren et al., 2017), with default settings. Additional sequencing was performed on the same DNA extract using an Illumina MiSeq sequencer (Illumina, San Diego, California). The DNA-sequencing library was generated using a NEBNext Ultra II Library prep Kit (New England Biolabs, Ipswich, Massachusetts) and sequenced using a v3 chemistry 600 cycle kit. Genome polishing was performed using Pilon 1.23 (Walker et al., 2014) within OmicsBox v2.0 with the MiSeq reads. The genome was annotated using the National Center for Biotechnology Information Prokaryotic Genome Annotation Pipeline (PGAP) and the virulence factors were predicted based on BLASTP analysis against a custom database created from Clostridium-virulence-factor protein sequences retrieved from the Virulence Factor Database.7
Samples of small intestine or colon contents from fresh carcasses were tested for C. perfringens toxins alpha, beta, and epsilon via a commercial capture ELISA kit (BIO-X, Brussels, Belgium) following manufacturer’s instructions and for enterotoxin via a commercial capture ELISA kit (TECHLAB, Blacksburg, Virginia) following the manufacturer’s instructions.
Intestine content and blood samples from fresh carcasses were analyzed for Clostridioides difficile toxins A/B using a commercial ELISA kit (TECHLAB) according to the manufacturer’s instructions. A mixture of purified toxins A and B was used in the positive control wells; toxins were replaced by buffer in negative control wells. Results were interpreted according to the manufacturer’s instructions. Broth of C. difficile cultured overnight (ATCC BBA-1870™) was also used as a positive control.
Macroalgae, stomach contents, and feces from all carcass conditions were analyzed for polycavernoside A according to the method of Yotsu-Yamashita et al. (2004). Stomach contents were analyzed for microalgal biotoxins as follows: (1) saxitoxins by routine Enzyme Linked Immunosorbent Assay (ELISA) and HPLC methods (Lawrence and Niedzwiadek, 2001; Amaya et al., 2018); (2) brevetoxins by competitive ELISA (Naar et al., 2002; Flewelling, 2008); (3) okadaic acid by colorimetric protein phosphatase assay (modified from Tubaro et al., 1996); and (4) domoic acid by LC-MS/MS (Wang et al., 2007; Fire et al., 2011). Stomach content and feces were also tested for saxitoxins by receptor binding assay (RBA, Doucette et al., 1997; Van Dolah et al., 2012).
For biochemistry analysis, vitreous humor from fresh carcasses was removed from both eyes with a sterile syringe as described for manatees (Varela and Bossart, 2005) and shipped overnight on cold packs to the University of Florida’s College of Veterinary Medicine. Undiluted vitreous humor was analyzed for total protein (TP), calcium (Ca), phosphorus (P), urea nitrogen (UN), sodium (Na), potassium (K), chloride (Cl), ammonia, uric acid, and glucose (Glc) using the Dade Dimension® Xpand® (Siemens, Chicago, Illinois). For general organic-compound toxicology screen and trace-mineral analysis, stomach and liver samples were shipped overnight to Michigan State University’s Veterinary Diagnostic Laboratory. Extracts from stomach contents were run by gas chromatography–mass spectrometry (GC–MS), and the mass-spectral fingerprints of isolated peaks, according to published methods, were compared to libraries containing fingerprints of as many as 500,000 compounds (Bokhart et al., 2015). Liver extracts were analyzed for Tl, Na, SE, K, P, Mo, Hg, Mn, Mg, Pb, Fe, Cu, Co, Cr, B, Ba, As, Sb, and Cd using an ionized coupled plasma mass spectrometry (ICP-MS; see Wahlen et al., 2005).
A case definition was developed to allow standardization of cases in the investigation of the UME and associated carcasses between 2012 and 2019. The definition covered manatees that had adequate body condition; no signs of overt illness or trauma; a full gastrointestinal tract with macroalgae visibly present; and congestion in one or more organs, wet lungs, or mucus-, froth-, or fluid-filled airways.
A total of 201 carcasses fit the case definition of the investigation during 2012–2019. The number of cases was greatest in February–April 2013 (n = 94, Figure 1). Most carcasses were reported in the northern and central IRL (n = 194, Figure 2). Five carcasses were found in the Halifax River just north of the IRL. Two carcasses were found outside the IRL (Tampa Bay and Caloosahatchee River, on Florida’s west coast) but were included for disease investigation purposes because their necropsy findings were consistent with the case definition and provided a rare opportunity for fresh sample collection in this investigation. All size classes except small calves were represented among the cases, with most carcasses (n = 135, 67%) in the adult size category, and fewer in the calf and subadult size categories (n = 31, 15% and n = 35, 17%, respectively) (Table 2). Cases included both sexes (107 males and 94 females), of which 27 females were lactating and 13 females were pregnant.
Figure 1. Heat map chart showing the numbers of manatee carcasses that fit the unusual mortality event (UME) case definition of clostridial infection by month, January 2012–December 2019.
Figure 2. GIS maps showing locations of manatee carcasses (symbols) found in Florida, January 2012–December 2019, from all causes of death (COD), including those fitting the unusual mortality event (UME) case definition. Triangles = UME case definition of clostridial infection; circles = other CODs. (A) Indian River Lagoon, (B) map overview of Florida indicating inset locations shown in (A,C,D). (C) Halifax River. (D) Southwest Florida (UME case-definition cases only).
Table 2. Manatee carcasses that fit the UME case definition suspected of clostridial infection and IRL carcasses of all other causes of death, by sex and size class.
Gross examination of ingested vegetation throughout the gastrointestinal tract of manatee carcasses (n = 201) revealed predominantly miscellaneous macroalgal Rhodophyta, Phaeophyta, and Chlorophyta (Figures 3, 4A), with minimal seagrass (i.e., Halodule, Syringodium, and Halophila). The intestinal content was watery in the small intestine (n = 117), cecum (n = 127) and/or proximal colon (n = 117) (Figure 4B). The mucosa was thickened and lifted off the wall in large sheets, floating loose in the intestinal fluid (n = 89, Figure 4C). The intestinal wall had diffuse congestion and hemorrhage (Figure 4D). In several cases, pronounced blebbed lesions were observed in the mucosa of the cecum (n = 3) and proximal colon (n = 11, Figure 4E). Outside the gastrointestinal tract, congestion was seen in lungs, kidneys, brain, and mesentery. The lung parenchyma was wet indicating edema, and the airways were filled with fluid, mucus, or froth (Figure 4F).
Figure 3. Macroalgae observed in unusual mortality event (UME) manatee carcasses. (A) Gracilaria from the mouth cavity. (B) Undigested Rhodophyta in the stomach. Note purple discoloration of the gastric mucus and fluid. (C) Undigested macroalgal (possibly Phaeophyta) content in the duodenum. (D) Partially masticated macroalgal Chlorophyta taken from stomach.
Figure 4. Gross findings on manatee carcasses that fit the unusual mortality event (UME) case definition of clostridial infection. (A) Macroalgae in the mouth. (B) Fluid-filled proximal colon with masticated pieces of vegetation and sloughed mucosa. (C) Colon with sloughed sheets of thickened mucosa and abundant mesenteric fat. (D) Hemorrhage in the proximal colon. (E) Blebbed lesions in the mucosa of the proximal colon. (F) Froth and fluid exuding from the nares, indicative of asphyxia and drowning.
During 2012–2019, 1,255 dead manatees were reported in the IRL, and necropsies were conducted on 1,221. In addition to the cause under investigation for the mass-mortality event, causes of death in necropsied carcasses were watercraft related (n = 179), trauma from water-control structures (n = 5), other human-related causes such as entanglement, ingested debris, or entrapment (n = 35), cold stress (n = 139), and other natural disease (n = 170). In 499 carcasses, a cause was undetermined because of decomposition or inconclusive necropsy findings. At least 52 of the carcasses with undetermined cause of death had ingested macroalgae and watery intestinal content, but gross observations during necropsy were otherwise obscured by decomposition. The size class distribution of all non-UME causes was 30% small calves < 151 cm, 24% calves, 13% subadults, and 33% adults (Table 2).
Thirty cases that fit the case definition were examined histologically. Microscopic lesions were prominent in the small intestine, cecum, and colon, and included one or more of the following changes: necrosis, edema, inflammation, hemorrhage, and mucosa-associated lymphoid tissue depletion or hyperplasia (Table 3 and Figures 5A–C). These lesions were seen predominantly in the tunica mucosa and tunica submucosa; degenerative lesions in the tunica muscularis were observed primarily in the colon. In addition, intravascular fibrin thrombi and occasional intralesional bacterial rods were observed. Seven cases with grossly evident blebbed lesions in the cecum or colon exhibited marked mucosal expansion by edema, fibrin, and heterophils (Figure 5A). These lesions were associated with superficial mucosal and crypt necrosis, hemorrhage, congestion, and vasculitis. Myofiber vacuolation and degeneration were observed.
Table 3. Types and locations of microscopic lesions in the gastrointestinal tract of manatees that fit the UME case definition which had histopathology performed (n = 30).
Figure 5. Photomicrographs of tissue sections with clostridial infection in the gastrointestinal tract of manatees (hematoxylin and eosin stain). (A) Severe colitis with mucosal expansion by edema, fibrin, and congestion. (B) Enteritis with hemorrhage and crypt hyperplasia. (C) Mucosal necrosis, crypt loss, and lymphatic lacteal dilation (arrow to the dilated villous tip on the upper right) in the distal small intestine.
Brown and Brenn Gram staining was performed in seven cases with enteritis. Gram-positive plump rods were present superficially and within the mucosa (n = 7) and in the submucosa (n = 3). One case had few sporulated Gram-positive rods associated with disrupted crypts, and in another Gram-positive rods formed clusters within or around glands. Gram stain of three cases with blebbed typhlocolitis revealed short Gram-positive rods superficially and within affected areas, rarely with spores.
Other microscopic lesions included mild to moderate multiorgan congestion (lungs, kidneys, liver, brain, heart, lymph nodes, spleen, adrenal glands, hemidiaphragm, and pancreas) and edema (lungs, kidney, brain, heart, and lymph nodes). Other organ-specific findings involving the respiratory, urinary, hepatobiliary, hematopoietic, cardiovascular, and digestive systems are listed in Table 4.
Table 4. Organ-specific extraintestinal microscopic lesions of manatees that fit the UME case definition as confirmed on histopathology (n = 30).
Tissue imprints of gastrointestinal-tract mucosal samples of five manatees were examined. The cytological findings included enteritis (n = 3), colitis (n = 2), hemorrhage (n = 3), or frequent spore-forming bacilli (n = 3) (Figures 6A–C). Direct smears of feces were prepared in six cases, which, in addition to mixed bacteria, also showed variable numbers spore-forming bacilli (n = 4). Malachite green stain was used in one case to confirm the presence of spore formers.
Figure 6. Photomicrographs of small intestine wall imprints from a UME manatee showing representative spore-forming bacteria. (A) Imprint of safety pin–shaped spore formers (black arrowhead); DIFF-QUIK stain. (B) Imprint of matchsticklike spore formers (white arrowheads); DIFF-QUIK stain. (C) Imprint highlighting the comparative morphology of two different spore-forming bacteria; matchsticklike (white arrowheads), safety pin–shaped (black arrowheads); Gram stain.
Four UME manatees were tested for presence of Clostridium perfringens and P. sordellii cells. Either large numbers of both bacteria were observed on the mucosa of the proximal colon (n = 3), or only P. sordellii was positive in the proximal colon (n = 1). No C. perfringens or P. sordellii were detected in the small intestine (n = 1). Three control manatees were either negative for C. perfringens or P. sordellii in the small intestine (n = 1), positive for C. perfringens or P. sordellii in the colon (n = 1), or positive for C. perfringens or P. sordellii (n = 1).
Clostridioides difficile toxin A was detected in 13 of 16 manatees, in multiple gastrointestinal tissues tested: cecum (100%, n = 5, localized staining), mid small intestine (100%, n = 2), distal small intestine (100%, n = 4), distal colon (100%, n = 2), mid colon (100%, n = 1), and proximal colon (73%, n = 11). Stomach (n = 1) and duodenum (n = 1) were negative for C. difficile toxin A. Two control animals (n = 2) were negative. Controls (n = 2) from horses were also positive for C. difficile toxin. Positive toxicity was seen in the basal crypts of the colon and deep in the mucosa of the small intestine (Figures 7A,B). Clostridioides difficile toxin A was also visualized in the cytoplasm of abundant heterophils in the submucosa, near the basal lamina propria, and in blood vessels (Figures 7B,C), as well as in diffuse-tissue areas and in the mucosal lesions of marked edematous expansion (Figure 7A). Clostridioides difficile toxin A was visualized in bacterial cells lining the surface intestinal epithelia and deep in the mucosa in the small intestine (Figures 8A,B).
Figure 7. Photomicrographs of immunohistochemistry of Clostridioides difficile toxin A. (A) Toxin positively visualized in characteristic mucosal edematous expansion lesions in the proximal colon. (B) Toxin positively visualized in the mucosa and crypts of the middle small intestine. (C) Positive toxicity in heterophils infiltrating the mucosa of the proximal colon.
Figure 8. Spore morphology of clostridia in histological sections (A–C) and in pure culture (D–F). (A) Distal small intestine showing positive visualization of Clostridioides difficile toxin A in crypts and in bacterial cells (immunohistochemical staining of Clostridioides difficile toxin A). (B) Middle small intestine showing positive visualization for Clostridioides difficile toxin A in crypt epithelia (arrowhead) and in bacterial cells (arrows) (immunohistochemical staining of Clostridioides difficile toxin A). (C) Proximal small intestine showing gram-positive Paeniclostridium sordellii (Brown and Brenn Gram stain). (D,E) Clostridia isolated from manatees exhibiting clostridial infection (Gram stain). (D) Paeniclostridium sordellii. (E) Clostridium bifermentans. (F) For comparison, an isolate of Clostridioides difficile from the American Type Culture Collection. Arrows show individual cells (Gram stain).
Seventeen UME manatees were screened for isolations of the Clostridium species and their toxins and compared to isolates of Clostridioides difficile obtained from the American Type Culture Collection. Clostridium perfringens was detected in 11 animals, either or both in the small intestine (n = 8) and the colon (n = 6). Among these, the alpha toxin gene was detected in the small intestine (n = 7) and in the colon (n = 6). The epsilon toxin gene was detected with the culture-independent method only in the small intestine (n = 1) and in the colon (n = 2). Clostridium perfringens beta, beta2, iota, and entero toxins were not detected. Paeniclostridium sordellii (Figures 8C,D) was detected in the intestine of seven animals. The lethal toxin gene of P. sordellii was detected from DNA samples extracted directly from contents of the small intestine (n = 1) but not from those identified as P. sordellii isolates. No P. sordellii hemorrhagic toxin gene was detected. Neither culture-dependent nor culture-independent Clostridioides difficile was detected. Clostridium sporogenes, C. disporicum, C. bifermentans (Figure 8E), C. ramosum, C. sardiniense, and C. botulinum were also isolated from the intestinal contents during the bacterial culturing processes. Clostridium perfringens and the alpha toxin gene were detected in the cervix (n = 1), uterine body (n = 1), kidney (n = 1), and uterine horn (n = 1). Paeniclostridium sordellii was detected in an unspecified lymph node (n = 1), heart (n = 1), and cervix (n = 1) but no toxin genes were detected. Clostridioides difficile (Figure 8F) was not detected in any of the non-gastrointestinal tract samples.
The genome of the P. sordellii strain FL-manatee includes a circular chromosome and plasmid of 3,586,821 and 74,500 bp with 28 and 26% of GC content, respectively. A total of 3,352 and 68 open reading frames (ORFs) were predicted in the chromosome and plasmid, respectively. BLASTP searches against a custom Clostridium virulence-factor database identified 12 chromosomally encoded adherence (3 ORFs), exoenzyme (2 ORFs), and toxin (7 ORFs, including hemolysins, phospholipase C and perfringolysin [theta toxin, PFO]) virulence factors (Table 5). The P. sordellii strain FL-manatee genome described in the present study does not encode lethal toxin, hemorrhagic toxin, or other putative virulence factors produced by P. sordellii including neuraminidase and cholesterol-dependent cytolysin sordellilysin. The chromosome and plasmid sequences have been deposited in the National Center for Biotechnology Information (NCBI) GenBank database and are available under accession numbers CP080291 and CP080292, respectively. The MinION and MiSeq sequence data generated during this study are available in the NCBI Sequence Read Archive under accession numbers SRR17734612 and SRR17734613, respectively.
Table 5. Virulence factors identified in the Paeniclostridium sordellii strain FL-manatee chromosome.
Frozen (n = 4) and fresh (n = 6) intestinal contents were tested for Clostridioides difficile toxins A/B. Two cases that were tested within 21 and 23 h of death were both positive for C. difficile toxin A/B. Blood was collected from the heart in one of these cases (tested within 23 h of death) and was also positive for C. difficile toxin A/B. One case with unknown time between death and necropsy tested positive for C. difficile toxin A/B in intestinal contents. Time of death for all cases that tested negative for C. difficile toxin A/B was unknown. Toxins of Clostridium perfringens were not detected in frozen (n = 4) or fresh (n = 2) intestinal contents.
No polycavernoside A was detected in environmental samples of macroalgae. Manatee stomach contents were negative for polycavernoside A (n = 10), brevetoxin (n = 5), saxitoxin (n = 15), okadaic acid (n = 5), and domoic acid (n = 5). Similarly, no polycavernoside A or saxitoxin was found in feces (n = 10).
Vitreous-humor samples from two manatees were processed. Chemistry analysis results for both samples included [with reference ranges (RR by Varela and Bossart, 2005) from moderately decomposed manatees as mean ± SD]: TP 1.0/ < 2.0 g/dL (RR 7.6 ± 2.9 g/dL), Ca 8.4/9.1 mg/dL (RR 4.0 ± 1.3 mg/dL), P 5.6/13.2 mg/dL (RR 18.0 ± 10.0 mg/dL), UN 18/not performed mg/dL (RR 14.5 ± 11.9 mg/dL), Na 162/136 mg/dL (RR 110 ± 19 mg/dL), K > 10/23.4 mg/dL (28.4 ± 8.1 mg/dL), Cl not performed/108 mg/dL (RR 84 ± 17 mg/dL), ammonia 1,341/3,816 (RR N/A), uric acid 1.4/5.5 mg/dL (RR N/A), Glc not performed/ < 20 mg/dL (RR 33 ± 14 mg/dL). No organic compounds were identified by mass-spectral-library match of stomach contents (n = 2). Heavy metals in liver samples were negative or within reference range (n = 3, of which one was a control), and tests of trace minerals were unremarkable.
Manatees in the IRL are at risk from diverse environmental and anthropogenic factors, with documented mass mortalities from algal biotoxins and cold temperatures and deaths associated with watercraft strikes (Stith et al., 2012; Fire et al., 2015; Hardy et al., 2019). As demonstrated here for the first time, the consequential shift in dietary items directly linked to algal blooms, and a seagrass die-off with resultant change in available forage items, posed a new risk to manatees from clostridial toxins. Associated scenarios with this shift in dominance from seagrass to macroalgae could have been a switch to the ingestion by manatees of toxic, bioactive, or contaminant compounds directly from the macroalgae or of epiphytic toxic microalgae (e.g., dinoflagellates) or associated bacterial pathogens. For example, the macroalga Cladophora in the Great Lakes is a significant reservoir for Shiga toxin–producing Escherichia coli, enterococci, and Clostridium botulinum, with the latter linked to outbreaks of avian botulism in Lake Michigan (Whitman et al., 2003; Ishii et al., 2006; Chun et al., 2013, 2015). Natural antimicrobial compounds produced by macroalgae (Ballantine et al., 1987) could also hypothetically cause a shift in the microflora of the manatee digestive tract leading to impaired health or triggering a dysbiosis.
Initial environmental surveys for potentially toxic microalgae or macroalgae in the IRL did not reveal any toxic candidates that could have fit the case definition. Harmful and toxic microalgae in the IRL include the saxitoxin-producing dinoflagellate Pyrodinium bahamense, occasional incursions by Karenia brevis (which produces brevetoxins), and low-toxicity to non-toxic Pseudo-nitszchia spp. (which produce domoic acid) (Landsberg et al., 2006; Phlips et al., 2015), yet there was no evidence that manatees had been exposed to these neurotoxins during the UME. Benthic dinoflagellates Prorocentrum spp., which produce okadaic acid and dinophysistoxins, utilize macroalgae and seagrass as substrate in the IRL (Anderson, 2002), and although these toxins can cause diarrheic shellfish poisoning (DSP) in humans (Murata et al., 1982), manatee gastrointestinal contents were negative for these toxins. Other than diarrhea, the acute toxemia presentation and the gross and microscopic pathological findings in manatees did not parallel the symptoms associated in humans with DSP.
Macroalgae produce a diverse and complex array of secondary metabolites that deter feeding by marine herbivores (Paul and Puglisi, 2004). A shift of manatees to a predominantly macroalgal diet could have resulted in the inadvertent ingestion of toxic or pathogenic compounds. There is a diverse macroalgal population in the IRL, with Gracilaria tikvahiae, Hypnea musciformis, Acanthophora spicifera, Chaetomorpha sp., Ulva lactuca, and Codium decorticatum the major components of the drift algae that sometimes form high-biomass blooms under eutrophic conditions (Virnstein and Carbonara, 1985; Littler et al., 2008; Whitehouse and Lapointe, 2015; Barile, 2018). Qualitative gut-content analysis of manatee carcasses examined during the UME revealed diverse macroalgae and seagrasses, paralleling the known availability of these forage items in the IRL following the major seagrass die-off of Thalassia testudinum, Halodule wrightii, and Syringodium filiforme triggered by superbloom events in 2012 (Morris et al., 2018). During the manatee UME years and following, dominant macroalgal drift communities in the IRL included Rhodophyta G. tikvahiae, Hypnea musciformis, Hydropuntia secunda and the chlorophyte Chaetomorpha linum, as well as cyanobacterial mats (Lapointe et al., 2015, 2020). Such representative macroalgal taxa were found in manatee gut contents.
Because the human consumption of uncooked Rhodophyta Polycavernosa tsudai (= Gracilaria edulis), G. verrucosa, G. chorda, and G. coronopifolia (Gracilariales) has been associated with fatal food-poisoning incidents in the Pacific region (Halstead and Haddock, 1992; Hanne et al., 1995; Yotsu-Yamashita et al., 2004; Kumar and Sharma, 2021), we focused on testing G. tikhaviae, manatee stomach contents, and feces for polycavernoside. Polycavernosides A and B are the primary toxins associated with human intoxications (Yotsu-Yamashita et al., 1993), and, although intoxications were originally postulated to have originated from macroalgae, those polycavernosides might have been produced by cyanobacteria associated with the macroalgae (Navarro et al., 2015). Macroalgae (and any unidentified epiphytes) and manatee stomach contents and feces tested here were negative for polycavernoside A and were not further considered as possible causal factors. Also, the pathological finding of enterocolitis in manatees was different from that resulting from acute toxicity associated with Gracilaria.
Other compounds implicated in Gracilaria-associated poisoning incidents include prostaglandins, aplysiatoxins, and debromoaplysiatoxins (Fusetani and Hashimoto, 1984; Nagai et al., 1996), but these were not further investigated in the present study. Rhodophytes, predominantly species in the Gigartinales (e.g., Hypnea musciformis), also produce the compound carrageenan, which is widely used as a food additive (Tobacman, 2001; Ciancia et al., 2020). Laboratory rodents and lagomorphs fed rhodophytes Chondrus crispus and Eucheuma spinosum over a 1-month to 1-year period initially developed hemorrhagic and ulcerative lesions, cellular infiltrates, and crypt abscesses. Following chronic exposure, there was a loss of mucosal folds and scarring of the colon, leading to the formation of construction rings. Carrageenan was determined to be the primary compound initiating these lesions (Marcus and Watt, 1969). Carrageenan may also alter the intestinal microflora, potentially leading to a state of dysbiosis (see below). Bacteriological studies of carrageenan-exposed guinea pigs with induced cecal ulcerations showed shifts in the microflora with an increase in coliforms and Gram-negative anaerobes (Onderdonk and Bartlett, 1979), as well as a reduction in anti-inflammatory bacteria together with the enrichment of members of the Clostridiales vadin BB60 group, among other Firmicutes and Tenericutes (Shang et al., 2017). Ingestion by manatees of Rhodophyta possibly containing carrageenan could have predisposed them to ulcerative colitis and contributed to a consequential shift in the intestinal microflora. Further study would be required to quantify the carrageenan, prostaglandin, aplysiatoxin, and debromoaplysiatoxin content of the different macroalgae ingested by manatees and to determine whether these compounds could affect gastrointestinal health.
Our investigation did not include a nutritional analysis of macroalgae collected during environmental surveys, but, in general, seaweeds have been reported to be a valuable food source with high levels of carbohydrates as well as minerals, vitamins, and trace elements (MacArtain et al., 2007). To examine any electrolyte imbalances or deficiencies, vitreous humor was analyzed as conceptualized by Varela and Bossart (2005). Findings in UME manatees included lower Glc and TP and higher UN, Na, and Cl. With due consideration of the limitations when interpreting different analytical methodologies, and given the unknown time of death in the previous study, and that data from only two manatees were available in the present study, these findings excluded acute death from hypocalcemia but considerations included septicemia or endotoxemia, reduced production or loss of serum proteins (e.g., protein-losing enteropathy), or dehydration, all of which support the pathological findings in UME manatees. Furthermore, concentrations of trace minerals in manatee livers were within expected range.
Brevard County is a central hub for manatee migration, largely driven by seasonal changes in temperature (Deutsch et al., 2003). At the peak of the UME in March 2013, a distributional aerial survey documented at least 1,719 manatees in the county (Reynolds and Scolardi, 2016). It is likely that most of these manatees were consuming the same, predominantly macroalgal diet as found in carcasses documented during the UME, yet many survived. Nutritional analyses of manatee diets indicate that macroalgae are generally lower in fiber content than are seagrasses (Siegal-Willott et al., 2010; Rodrigues et al., 2021). Gastrointestinal disorders that have been associated with a low-fiber diet in herbivores include gastric acidosis, dysbiosis, diarrhea, poor digestion, bloat, and displacement of the intestines (Dougal et al., 2014).8 An abrupt transition to a macroalgal diet with low fiber content may have made new manatee arrivals in the IRL specifically vulnerable to gastrointestinal dysbiosis, which is supported by the peak in UME carcass numbers during migration in early 2013, the first winter in which manatees encountered predominant Rhodophyta macroalgae. Other hindgut fermenters, such as the horse, can rapidly reverse disruptions in microbial homeostasis following a diet change (Fernandes et al., 2014), and it is plausible that this adaptation eventually occurred in manatees that foraged on macroalgae. Similarly, rumen microbiota of macroalga-consuming North Ronaldsay sheep adapted to efficiently digest this vegetation (Williams et al., 2012). The likely restoration of the gastrointestinal microbial balance after manatees had transitioned to a macroalgal diet is further supported by the decrease in morbidity and mortality cases in the months and years after the peak of the UME.
A multifactorial etiology of a predominant macroalgal diet low in fiber plus the aforementioned macroalgal compounds could have initiated some of the gastrointestinal lesions seen in manatees, with the proximal cause of death being clostridial infection. Macroalgae also produce antimicrobial compounds (de Almeida et al., 2011), so it is possible that the normal intestinal microbiota of manatees was affected by a high macroalgal intake, with an associated increase in Clostridiales. In domestic or captive animal environments and in humans, the initiation of dysbiosis leading to domination by anaerobic clostridia, particularly by Clostridioides difficile, has been linked to antibiotic treatments, hospitalization, and neonatal microbiomes (Antharam et al., 2013; Cassir et al., 2015; Popoff, 2018). In an analogous situation in the wild, dietary shifts or a reduction in forage diversity can contribute to a change in gut microflora, setting up the potential for a dysbiosis and pathobiome (Stecher et al., 2013; Bass et al., 2019). In the horse, a hindgut fermenter similar to the manatee, an abrupt transition from a grain-based diet to pasture grazing caused an immediate change in the relative abundances of the fecal bacteria, including the Clostridiales (Fernandes et al., 2014).
Clostridial gastrointestinal diseases of animals have been well documented, but primarily in domestic livestock, pets, and captive terrestrial mammals (Neiffer, 2001; Bojesen et al., 2006; Rodriguez et al., 2016; Uzal et al., 2016; Capewell et al., 2020; Nyaoke et al., 2020), with few reported cases in terrestrial wildlife (Asaoka et al., 2004; Boujon et al., 2005; Silva et al., 2014), and in captive marine birds (Hines and Dickerson, 1993; Lueders et al., 2017) and marine mammals (Buck et al., 1987; Anderson et al., 2015). Clostridia (e.g., Clostridium perfringens, Clostridioides difficile) are naturally present in the normal gut microbiome and fecal samples of asymptomatic marine mammals, fish, mollusks, and crustaceans (McBee, 1960; Aschfalk and Müller, 2001; Miller et al., 2010; Morris et al., 2011), with ubiquitous distribution in freshwater and marine environments in water, sediment, sewage, and biota and on biological substrates (Miller et al., 2006; Pasquale et al., 2011; Rodriguez et al., 2016). Few cases of lethal clostridial infections have been associated with marine animals, with incidental findings of Clostridium perfringens and P. sordellii suspected in a die-off of the long-spined sea urchin Diadema antillarum (Bauer and Agerter, 1987). Reports of clostridial diseases in free-ranging wild marine animals are less common, with none thus far documented to be the primary cause of mass mortalities in marine mammals. One live-stranded long-beaked common dolphin, Delphinus capensis, beached in La Jolla, California, with systemic infection of C. perfringens type A (Danil et al., 2014), and one stranded sperm whale, Physeter macrocephalus, in Denmark had suspected infection associated with C. septicum (Hansen et al., 2016).
The presence of Clostridia in the hindgut of manatees in the IRL is not unexpected, but no comparative baseline data are available with which to identify shifts to more pathogenic strains or species if manatees are undergoing dysbiosis. Clostridium has been documented to be a dominant bacterial genus in manatees examined from the Crystal River (on Florida’s west coast) and eastern Florida Atlantic (Mao et al., 2011, cited in Merson et al., 2014), but in-depth analysis of clostridial diversity and speciation has not been done. Clostridium butyricum was noted to be one of the 10 most abundant bacterial operational taxonomic units in fecal samples from Crystal River manatees (Merson et al., 2014). In the closely related Antillean manatee, Trichechus manatus manatus, held in captivity in Japan, even under a modified terrestrial herbivorous diet compared to natural conditions, Clostridium spp. were among the top 20 dominant bacteria in fecal samples (Suzuki et al., 2019). Because hindgut fermenters such as manatees have a long intestine, anaerobic conditions suited for Clostridium colonization exist, as most oxygen consumption by microbes takes place in the foregut (Suzuki et al., 2019).
The positive IHC test results for Clostridioides difficile toxin A seen in 13 animals, but the consistent lack of C. difficile PCR detection or isolation, despite repeated efforts using specifically designed primers and specialized agar, may indicate that C. difficile was absent or that the IHC was cross-reacting with P. sordellii hemorrhagic toxin. The chemical similarity between C. difficile toxin A and P. sordellii hemorrhagic toxin has been identified and immunological cross-reactivity recognized, with both species 71% homologous at the genomic level (Martinez and Wilkins, 1988; Scaria et al., 2015). We have not found any cases reporting on this difficulty in interpreting possible false positives of C. difficile IHC toxin A in P. sordellii. But two ELISA tests conducted within 24 h of death confirmed C. difficile toxins A/B, and the clinical presentation, gross and microscopic findings, and the evident positive IHC tests for C. difficile toxin A in 13 manatees (if not cross reacting) is reminiscent of C. difficile–associated disease (CDAD) in horses and pigs (Songer, 2004; Songer and Uzal, 2005; Songer et al., 2009), possibly implicating C. difficile in the pathogenesis of the clostridial infections of manatees. Inflammation, necrosis, and intercryptal exudates of fibrin and neutrophils associated with CDAD (Keel and Songer, 2006; Uzal and Songer, 2019) and P. sordellii described in pigs and horses (Diab et al., 2013; Nyaoke et al., 2020, respectively) were commonly observed microscopically at varying degrees of severity in the present study. Mucosal and submucosal edema, similar to CDAD in horses, was also observed in this study and associated with C. difficile toxin A by IHC in 13 manatees.
Virulence factors determined in the P. sordellii strain FL-manatee genome were diverse, but large clostridial toxin genes (lethal and hemorrhagic) were not detected. In combination with the pathogenic potential of the other clostridial species (with their toxins or toxin genes) detected in symptomatic manatees, this suite of factors could have contributed to the overall clinical presentation of lethal clostridial infection. Numerous Clostridium perfringens toxins including kappa toxin and theta toxins have been implicated in clostridial pathogenicity (Rood, 1998). Perfringolysin O (PFO, theta toxin) in C. perfringens, a cholesterol-dependent cytolysin, synergistically acts with C. perfringens alpha toxin to trigger platelet and leucocyte aggregation in cases of gas gangrene, resulting in the formation of blood vessel obstruction, hypoxia, and tissue destruction (Verherstraeten et al., 2013). It contributes indirectly to toxic shock by releasing inflammatory cytokines, and is involved in bovine enterotoxemia and necrohemorrhagic enteritis (Verherstraeten et al., 2013, 2015; Popoff, 2014). PFO was predicted to be encoded by the ORF_02133 gene in P. sordellii strain FL-manatee. We also sequenced kappa toxin (or collagenase) encoding genes within the P. sordellii strain FL-manatee genome (ORF_01190). Although the role of collagenase in virulence is not known, host collagen remodeling by clostridial bacteria is considered to enhance tissue colonization (Eckhard et al., 2011). An experiment showed that 0.7 μg of purified C. perfringens collagenase produced a definite hemorrhage on rabbit skin, while 30 μg killed mice from associated severe lung hemorrhage (Kameyama and Akama, 1971). The role of hemolysins A and C (ORF_00534, ORF_01294, and ORF_02946) in the P. sordellii isolate from manatees is unknown, but in other bacteria, these are major virulence factors. For example, these hemolysins contribute to mucosal damage in the colon of pigs with swine dysentery (Bellgard et al., 2009), and together with other hemolysins, they have been identified in C. butyricum causing neonatal necrotizing enterocolitis (Dong et al., 2020). In P. sordellii strain FL-manatee, the gene ORF_03500 was predicted to encode phospholipase C (CpPLC), which is homologous to C. perfringens alpha toxin but displays lower enzymatic and hemolytic activity (Vidor et al., 2015). But the role of C. perfringens alpha toxin in enteric disease of mammals and birds is still unknown. Although that toxin might have been involved in the enteric lesions of the manatees studied in the present study, whether it did remains speculative.
The P. sordellii strain FL-manatee gene ORF_01874 was predicted to encode sialidases (neuraminidases), which could play a role in the notable heterophilic and lymphoplasmacytic inflammatory response in manatees. Paeniclostridium sordellii infections are characterized by the profound capillary-leak syndrome, development of a leukemoid reaction (LR), and a marked shift to a granulocytic population; in humans, the LR is a hallmark and predictor of a fatal outcome (Aldape et al., 2007). A leukemoid reaction is also a characteristic of Clostridioides difficile infections (Marinella et al., 2004). Clostridium perfringens strains also typically possess three sialidase-encoding genes. In vitro, the sialidases can enhance binding and cytotoxic effects of epsilon toxin in type C. perfringens type D isolates, contributing to intestinal colonization and increasing toxin action (Li et al., 2011). In vivo, NanI (the main sialidase produced by C. perfringens) increases colonization of C. perfringens type F and the effect of its enterotoxin (CPE) (Li et al., 2021). Sialidase production has also been implicated in the virulence of other pathogenic bacteria, such as Vibrio cholerae (enhancing the activity of cholera toxin by increasing toxin binding levels; Galen et al., 1992) and Streptococcus pneumoniae (assisting in growth, biofilm formation, and exposing adhesion sites; King, 2010).
Adhesion and colonization of animal tissue by bacteria is an important step in establishing infection. Intestinal colonization is a complicated process that involves several colonization factors. The gene that encodes fibronectin-binding protein A (FbpA and Fbp68) was identified in the P. sordellii strain FL-manatee (ORF_03002). FbpA is described as an adhesive protein with a minor but not insignificant role in colonization, implicated in the first step of the intestinal infection by Clostridioides difficile (Barketi-Klai et al., 2011). This role is shared with multiple other adhesins, such as collagen-binding protein (Cna). Cna promotes bacterial adhesion to collagen-rich tissue and therefore contributes to tissue tropism and may influence host species preference due to differences in species-specific collagen-binding affinities (Jost et al., 2006). The Cna protein was implicated as a potential virulence factor in porcine enteritis caused by Clostridium perfringens (Jost et al., 2006) and was correlated with the ability of a C. perfringens strain to cause necrotic enteritis in chickens (Wade et al., 2015). The P. sordellii strain FL-manatee gene ORF_02554 was predicted to encode a Cna protein. Heat-shock GroEL proteins are cell-surface proteins also involved in clostridial cell adhesion and were predicted to be encoded by the P. sordellii strain FL-manatee gene ORF_03377. GroEL has adhesive function in Clostridioides difficile (Hennequin et al., 2001).
Paeniclostridium sordellii, with the two large clostridial toxins, lethal and hemorrhagic, is usually associated with gas gangrene (myonecrosis), sepsis, or fatal toxic shock syndrome in humans (Popoff, 2018), with fewer cases restricted to intestinal disease (de la Fe et al., 2006). In domestic animals, the role of P. sordellii in enteric disease is not fully known, although it has been suggested that it is associated with enterocolitis in horses (Nyaoke et al., 2020). Overall, Clostridia were not detected in manatees in non-digestive-tract lesions, with evidence for three known clostridial pathogens (P. sordellii, C. difficile, and Clostridium perfringens type A), likely working in synergy or by as yet undetermined etiological mechanisms to cause lethal enterocolitis in manatees. A pathogenic role of P. sordellii is proposed based on several lines of evidence, but further research is required. Despite analyses of 17 manatees, we were unable to detect large clostridial toxins in P. sordellii strain FL-manatee but did detect the lethal gene directly from the small intestine of one manatee. Challenges in the detection of lethal (TscL) or hemorrhagic (TscH) toxins in P. sordellii, or evident absence of such toxins, have been described in human cases (Couchman et al., 2015), in hemorrhagic and necrotizing enteropathy in dogs (Capewell et al., 2020), and enterocolitis in horses (Nyaoke et al., 2020), indicating that other virulence factors are involved or that knowledge of virulence factors is incomplete or that diagnostic assays need to be improved for detection. Most strains of P. sordellii do not produce TscL or TscH toxins but are pathogenic (Vidor et al., 2015) and have been associated with necrotizing gastroenteric disease, toxicosis, and mortality in horses, cattle, sheep, dogs, lions, and chickens (Lewis and Naylor, 1998; de la Fe et al., 2006; Rimoldi et al., 2015; Capewell et al., 2020).
New reports and reappraisals of knowledge of the role of Clostridia in animal disease are recognizing that perhaps some virulence factors have been underrecognized for their pathogenesis or that other factors may be at play when known lethal toxins appear to be absent (Goossens et al., 2017). Toxins on plasmids may have been missed, or other factors are significant.
There are few reports of clostridial disease in manatees. A 1-month-old Amazonian manatee, Trichechus inunguis held in captivity, presented with necrohemorrhagic colitis and pneumatosis intestinalis associated with a C. perfringens type A infection (Neto et al., 2016). In managed care of manatees, gastrointestinal disorders from enterocolitis are among the most severe conditions and, undiagnosed, can lead to sepsis, shock, and death9 (Neto et al., 2016). Although C. perfringens type A was detected in 11 cases in the present study, no obvious gross or microscopic lesions with gas bubbles were seen, and the blebbing seen in the cecum of three manatees and colon of 11 manatees, reflecting edema-associated blistering and mucosal lifting similar to CDAD associated with Clostridioides difficile (Songer et al., 2009), was striking. In contrast with previous reports that clostridial infection mostly affected manatee calves, this UME included an unusually high proportion of adults. An investigation into P. sordellii–associated sudden death in flocks of sheep found the most severe lesions in young lambs, but the adults affected had all undergone a change in feeding management (Lewis and Naylor, 1998). Adult manatees may be more sensitive to dietary changes and subsequent dysbiosis due to their increased nutritional demand and larger volume of vegetation ingested. In addition, infants could be refractory to CDAD because they lack toxin receptors (Borriello and Wilcox, 1998; Keel and Songer, 2006).
The suite of clostridial virulence factors and toxins identified here, likely caused by a shift to a predominantly macroalgal diet, appears to play a significant role in the mortality of manatees, primarily in the IRL, but also elsewhere. Concern for manatee health and survival warrants further investigation as to primary causative factors, other as-yet-undetermined contributing factors, bacterial source origins, and links to dramatic ecosystem functional changes and stressors. Potential exogenous sources of Clostridiales are unknown, but numerous wastewater-treatment plants, sewage-disposal systems, agricultural activities, and aquaculture operations near the IRL could be of concern.
The IRL is an ecosystem in distress, and, directly or indirectly, the changes in the stability of a primary food source for manatees has had unforeseen consequences that have persisted for the past decade. Further unprecedented declines in seagrass and macroalgae culminated in a manatee UME related to starvation in December 2020,10 which is ongoing at the time of writing. The findings of Paeniclostridium sordellii, Clostridium perfringens type A, and Clostridioides difficile toxin A in 7, 11, and 13 manatees, respectively, presenting with pathological findings fitting the UME case definition illustrate an important emerging issue for marine animal health and document the expansion of known pathogenesis of Clostridia in marine ecosystems. The risk of clostridial infection to marine mammals is herein highlighted and recognized. Manatees are increasingly at risk from compounding environmental stressors that together pose uncertainties for the long-term stability of this threatened species.
The datasets presented in this study can be found in online repositories. The names of the repository/repositories and accession number(s) can be found below: https://www.ncbi.nlm.nih.gov/genbank/, CP080291 and CP080292; https://www.ncbi.nlm.nih.gov/sra, SRR17734612 and SRR17734613.
Ethical review and approval was not required for the animal study because this research involved diseased animals, not killed for this study, and was conducted by authorized personnel in accordance with section 6 of the Endangered Species Act [implementing regulations 50 CFR 17.21(c) and 50 CFR 17.31(b)] and section 109(h) of the Marine Mammal Protection Act (implementing regulation 50 CFR 18.22).
JL and MW directed the research and investigative component of the UME, and drafted the manuscript. MW conducted and oversaw the manatee necropsies and collected manatee samples. MT prepared and analyzed samples for microbiology and ELISA toxin testing. DR provided histopathological analyses. KS, TR, and TW conducted genome sequencing and annotation. NS performed cytology and biochemistry analyses and coordinated microbiology samples submitted to the University of Florida. PW conducted Clostridium toxin immunohistochemistry. JL evaluated histological and toxin immunohistochemical slides and interpreted results. YK assisted with microbiological analyses and interpretation of toxin immunohistochemical slides. FU conducted and interpreted Clostridium immunohistochemistry and provided ELISA toxin testing. All authors contributed to writing the manuscript.
This research was supported by the State of Florida Manatee Trust Fund and Marine Resources Conservation Trust Fund.
The authors declare that the research was conducted in the absence of any commercial or financial relationships that could be construed as a potential conflict of interest.
All claims expressed in this article are solely those of the authors and do not necessarily represent those of their affiliated organizations, or those of the publisher, the editors and the reviewers. Any product that may be evaluated in this article, or claim that may be made by its manufacturer, is not guaranteed or endorsed by the publisher.
Florida Fish and Wildlife Conservation Commission (FWC) marine mammal staff and partners verified, recovered, and necropsied manatee carcasses. Leslie Ward and Margie Barlas (FWC) were designated UME coordinators communicating with the Working Group on Marine Mammal Unusual Mortality Events (WGMMUME). Andy Garrett, Ann Spellman, Leslie Ward, Margie Barlas, Tom Reinert, and Andrea Mosier (FWC) were critical in mortality event coordination in 2013. Brandon Bassett, Stacie Hardy, and Andrea Krzystan (FWC) were responsible for carcass data management. Andrea Krzystan also created Figures 1, 2 of this manuscript. Patricia Lewis, Monarch Histology Services, prepared histology slides. Sherry Reed and Val Paul from Smithsonian Marine Station, Fort Pierce, assisted with macroalgal collections and identifications. Cathy Beck (U.S. Geological Survey) also provided macroalgal identification. Maggie Broadwater (NOAA) analyzed Gracilaria, manatee stomach contents, and feces for polycavernosides and manatee tissues for saxitoxins by receptor binding assay. Rich Paperno and Doug Adams (FWC) assisted with environmental sampling. Leanne Flewelling (FWC) conducted microalgal biotoxin testing. The WGMMUME provided valuable feedback and guidance on the UME investigation.
Aldape, M. J., Bryant, A. E., Ma, Y., and Stevens, D. L. (2007). The leukemoid reaction in Clostridium sordellii infection: neuraminidase induction of promyelocytic cell proliferation. J. Infect. Dis. 195, 1838–1845. doi: 10.1086/518004
Amaya, O., Quintanilla, R., Stacy, B. A., Dechraoui Bottein, M.-Y., Flewelling, L., Hardy, R., et al. (2018). Large-scale sea turtle mortality events in El Salvador attributed to paralytic shellfish toxin–producing algae blooms. Front. Mar. Sci. 5:e00411. doi: 10.3389/fmars.2018.00411
Anderson, C. E., Haulena, M., Zabek, E., Habing, G., and Raverty, S. (2015). Clinical and epidemiologic considerations of Clostridium difficile in harbor seals (Phoca vitulina) at a marine mammal rehabilitation center. J. Zoo Wildlife Med. 46, 191–197. doi: 10.1638/2014-0048r2.1
Anderson, Y. (2002). The Ecological Relationship Between the Tumor-promoting Dinoflagellate, Prorocentrum spp., and Fibropapillomatosis in Green Turtles (Chelonia mydas) in Hawaii and Florida. Doctoral dissertation. Gainesville, FL: University of Florida.
Antharam, V. C., Li, E. C., Ishmael, A., Sharma, A., Mai, V., Rand, K. H., et al. (2013). Intestinal dysbiosis and depletion of butyrogenic bacteria in Clostridium difficile infection and nosocomial diarrhea. J. Clin. Microbiol. 51, 2884–2892. doi: 10.1128/JCM.00845-13
Asaoka, Y., Yanai, T., Hirayama, H., Une, Y., Saito, E., Sakai, H., et al. (2004). Fatal necrotic enteritis associated with Clostridium perfringens in wild crows (Corvus macrorhynchos). Avian Pathol. 33, 19–24. doi: 10.1080/03079450310001636228
Aschfalk, A., and Müller, W. (2001). Clostridium perfringens toxin types in hooded seals in the Greenland Sea, determined by PCR and ELISA. J. Vet. Med. B 48, 765–769. doi: 10.1046/j.1439-0450.2001.00507.x
Aschfalk, A., and Müller, W. (2002). Clostridium perfringens toxin types from wild-caught Atlantic cod (Gadus morhua L.), determined by PCR and ELISA. Can. J. Microbiol. 48, 365–368. doi: 10.1139/w02-015
Ballantine, D. L., Gerwick, W. H., Velez, S. M., Alexander, E., and Guevara, P. (1987). Antibiotic activity of lipid-soluble extracts from Caribbean marine algae. Hydrobiologia 151, 463–469. doi: 10.1007/BF00046168
Barile, P. J. (2018). Widespread sewage pollution of the Indian River Lagoon system, Florida (USA) resolved by spatial analyses of macroalgal biogeochemistry. Mar. Pollut. Bull. 128, 557–574. doi: 10.1016/j.marpolbul.2018.01.046
Barketi-Klai, A., Hoys, S., Lambert-Bordes, S., Collignon, A., and Kansau, I. (2011). Role of fibronectin-binding protein A in Clostridium difficile intestinal colonization. J. Med. Microbiol. 60, 1155–1161. doi: 10.1099/jmm.0.029553-0
Bass, D., Stentiford, G. D., Wang, H.-C., Koskella, B., and Tyler, C. R. (2019). The pathobiome in animal and plant diseases. Trends Ecol. Evol. 34, 996–1008. doi: 10.1016/j.tree.2019.07.012
Bauer, J. C., and Agerter, C. (1987). Isolation of bacteria pathogenic for the sea urchin Diadema antillarum (Echinodermata: Echinoidea). Bull. Mar. Sci. 40, 161–165.
Bellgard, M. I., Wanchanthuek, P., La, T., Ryan, K., Moolhuijzen, P., Albertyn, Z., et al. (2009). Genome sequence of the pathogenic intestinal spirochete Brachyspira hyodysenteriae reveals adaptations to its lifestyle in the porcine large intestine. PLoS One 4:e4641. doi: 10.1371/journal.pone.0004641
Bojesen, A. M., Olsen, K. E. P., and Bertelsen, M. F. (2006). Fatal enterocolitis in Asian elephants (Elephas maximus) caused by Clostridium difficile. Vet. Microbiol. 116, 329–335. doi: 10.1016/j.vetmic.2006.04.025
Bokhart, M., Lehner, A., Johnson, M., and Buchweitz, J. (2015). Determination of organochlorine pesticides in wildlife liver and serum using gas chromatography tandem quadrupole mass spectrometry. J. Chromatogr. Sep. Tech. 6, 1–11. doi: 10.4172/2157-7064.1000286
Bonde, R. K., O’Shea, T. J., and Beck, C. A. (1983). Manual of Procedures for the Salvage and Necropsy of Carcasses of the West Indian Manatee (Trichechus manatus). Springfield, VA: National Technical Information Service.
Borriello, S. P., and Wilcox, M. H. (1998). Clostridium difficile infections of the gut: the unanswered questions. J. Antimicrob. Chemother. 41 (Suppl. C), 67–69. doi: 10.1093/jac/41.suppl_3.67
Boujon, P., Henzi, M., Penseyres, J. H., and Belloy, L. (2005). Enterotoxaemia involving beta2-toxigenic Clostridium perfringens in a white stork (Ciconia ciconia). Vet. Record. 156, 746–747. doi: 10.1136/vr.156.23.746
Breininger, D. R., Breininger, R. D., and Hall, C. R. (2017). Effects of surrounding land use and water depth on seagrass dynamics relative to a catastrophic algal bloom. Conserv. Biol. 31, 67–75. doi: 10.1111/cobi.12791
Buck, J. D., Shepard, L. L., and Spotte, S. (1987). Clostridium perfringens as the cause of death of a captive Atlantic bottlenose dolphin (Tursiops truncatus). J. Wildl. Dis. 23, 488–491. doi: 10.7589/0090-3558-23.3.488
Capewell, P., Rupp, A., Fuentes, M., McDonald, M., and Weir, W. (2020). Fatal Clostridium sordellii–mediated hemorrhagic and necrotizing gastroenteropathy in a dog: case report. BMC Vet. Res. 16:152. doi: 10.1186/s12917-020-02362-y
Cassir, N., Benamar, S., Khalil, J. B., Croce, O., Saint-Faust, M., Jacquot, A., et al. (2015). Clostridium butyricum strains and dysbiosis linked to necrotizing enterocolitis in preterm neonates. Clin. Infect. Dis. 61, 1107–1115. doi: 10.1093/cid/civ468
Chun, C. L., Kahn, C. I., Borchert, A. J., Byappanahalli, M. N., Whitman, R. L., Peller, J., et al. (2015). Prevalence of toxin-producing Clostridium botulinum associated with the macroalga Cladophora in three Great Lakes: growth and management. Sci. Total Environ. 511, 523–529. doi: 10.1016/j.scitotenv.2014.12.080
Chun, C. L., Ochsner, U., Byappanahalli, M. N., Whitman, R. L., Tepp, W. H., Lin, G., et al. (2013). Association of toxin-producing Clostridium botulinum with the macroalga Cladophora in the Great Lakes. Environ. Sci. Technol. 47, 2587–2594. doi: 10.1021/es304743m
Ciancia, M., Matulewicz, M. C., and Tuvikene, R. (2020). Structural diversity in galactans from red seaweeds and its influence on rheological properties. Front. Plant Sci. 11:559986. doi: 10.3389/fpls.2020.559986
Couchman, E. C., Browne, H. P., Dunn, M., Lawley, T. D., Songer, J. G., Hall, V., et al. (2015). Clostridium sordellii genome analysis reveals plasmid localized toxin genes encoded within pathogenicity loci. BMC Genomics 16:392. doi: 10.1186/s12864-015-1613-2
Danil, K., St. Leger, J. A., Dennison, S., Bernaldo de Quirós, Y., Scadeng, M., Nilson, E., et al. (2014). Clostridium perfringens septicemia in a long-beaked common dolphin Delphinus capensis: an etiology of gas bubble accumulation in cetaceans. Dis. Aquat. Org. 111, 183–190. doi: 10.3354/dao02783
de Almeida, C. L. F., Falcão, H., de, S., Lima, G. R., de, M., Montenegro, C., et al. (2011). Bioactivities from marine algae of the genus Gracilaria. Int. J. Mol. Sci. 12, 4550–4573. doi: 10.3390/ijms12074550
de la Fe, C., Rodríguez, J. M., Ramírez, G. A., Hervás, J., Gil, J., and Poveda, J. B. (2006). Sudden death associated with Clostridium sordellii in captive lions (Panthera leo). Vet. Pathol. 43:370374. doi: 10.1354/vp.43-3-370
Deutsch, C. J., Reid, J. P., Bonde, R. K., Easton, D. E., Kochman, H. I., and O’Shea, T. J. (2003). Seasonal movements, migratory behavior, and site fidelity of West Indian manatees along the Atlantic coast of the United States. Wildl. Monogr. 151, 1–77.
Diab, S. S., Rodriguez-Bertos, A., and Uzal, F. A. (2013). Pathology and diagnostic criteria of Clostridium difficile enteric infection in horses. Vet. Pathol. 50, 1028–1036. doi: 10.1177/0300985813489039
Dong, Y., Li, Y., Zhang, D., Nguyen, S., Maheshwari, N., Hu, Y., et al. (2020). Epidemiological and genetic characterization of Clostridium butyricum cultured from neonatal cases of necrotizing enterocolitis in China. Infect. Control Hosp. Epidemiol. 41, 900–907. doi: 10.1017/ice.2019.289
Doucette, G. J., Logan, M. M., Ramsdell, J. S., and Van Dolah, F. M. (1997). Development and preliminary validation of a microtiter plate-based receptor binding assay for paralytic shellfish poisoning toxins. Toxicon 35, 625–636. doi: 10.1016/S0041-0101(96)00189-4
Dougal, K., de la Fuente, G., Harris, P. A., Girdwood, S. E., Pinloche, E., Geor, R. J., et al. (2014). Characterisation of the faecal bacterial community in adult and elderly horses fed a high fibre, high oil or high starch diet using 454 pyrosequencing. PLoS One 9:e87424. doi: 10.1371/journal.pone.0087424
Eckhard, U., Schönauer, E., Nüss, D., and Brandstetter, H. (2011). Structure of collagenase G reveals a chew-and-digest mechanism of bacterial collagenolysis. Nat. Struct. Mol. Biol. 18, 1109–1114. doi: 10.1038/nsmb.2127
Fernandes, K. A., Kittelmann, S., Rogers, C. W., Gee, E. K., and Bolwell, C. F. (2014). Faecal microbiota of forage-fed horses in New Zealand and the population dynamics of microbial communities following dietary change. PLoS One 9:e112846. doi: 10.1371/journal.pone.0112846
Fire, S. E., Flewelling, L. J., Stolen, M., Durden, W. N., de Wit, M., Spellman, A. C., et al. (2015). Brevetoxin-associated mass mortality event of bottlenose dolphins and manatees along the east coast of Florida, USA. Mar. Ecol. Prog. Ser. 526, 241–251. doi: 10.3354/meps11225
Fire, S. E., Wang, Z., Byrd, M., Whitehead, H. R., Paternoster, J., and Morton, S. L. (2011). Co-occurrence of multiple classes of harmful algal toxins in bottlenose dolphins (Tursiops truncatus) stranding during an unusual mortality event in Texas, USA. Harmful Algae 10, 330–336. doi: 10.1016/j.hal.2010.12.001
Flewelling, L. J. (2008). Vectors of Brevetoxins to Marine Mammals. Ph.D. thesis. Tampa, FL: University of South Florida.
Fusetani, N., and Hashimoto, K. (1984). Prostaglandin E2: a candidate for causative agent of “ogonori” poisoning. Bull. Jpn. Soc. Sci. Fish. 50, 465–469. doi: 10.2331/suisan.50.465
Galen, J. E., Ketley, J. M., Fasano, A., Richardson, S. H., Wasserman, S. S., and Kaper, J. B. (1992). Role of Vibrio cholerae neuraminidase in the function of cholera toxin. Infect. Immun. 60, 406–415. doi: 10.1128/iai.60.2.406-415.1992
Gobler, C. J., Koch, F., Kang, Y., Berry, D. L., Tang, Y. Z., Lasi, M., et al. (2013). Expansion of harmful brown tides caused by the pelagophyte, Aureoumbra lagunensis DeYoe et Stockwell, to the US east coast. Harmful Algae 27, 29–41. doi: 10.1016/j.hal.2013.04.004
Goossens, E., Valgaeren, B. R., Pardon, B., Haesebrouck, F., Ducatelle, R., Deprez, P. R., et al. (2017). Rethinking the role of alpha toxin in Clostridium perfringens–associated enteric diseases: a review on bovine necro-haemorrhagic enteritis. Vet. Res. 48:9. doi: 10.1186/s13567-017-04
Halstead, B. W., and Haddock, R. L. (1992). A fatal outbreak of poisoning from the ingestion of red seaweed Gracilaria tsudae in Guam—a review of the oral marine biotoxicity problem. J. Nat. Tox. 1, 87–115.
Hanne, M., Matsubayshi, H., Vogt, R., Wakida, C., Hau, S., Nagai, H., et al. (1995). Outbreak of gastrointestinal illness associated with consumption of seaweed. MMWR Morb. Mortal. Wkly. Rep. 44, 724–726.
Hansen, M. S., Alstrup, A. K., Hansen, J. H., Al-Sabi, M. N., Nonnemann, B., Jensen, L. F., et al. (2016). Stranding of two sperm whales (Physeter macrocephalus) in the “North Sea Trap” at Henne Strand, Denmark. Aquat. Mammals 42, 35–41. doi: 10.1578/AM.42.1.2016.35
Hardy, S. K., Deutsch, C. J., Cross, T. A., de Wit, M., and Hostetler, J. A. (2019). Cold-related Florida manatee mortality in relation to air and water temperatures. PLoS One 14:e0225048. doi: 10.1371/journal.pone.0225048
Hartman, D. S. (1979). Ecology and Behavior of the Manatee (Trichechus manatus) in Florida. Special Publication 5. Pittsburgh, PA: American Society of Mammologists.
Hennequin, C., Porcheray, F., Waligora-Dupriet, A. J., Collignon, A., Barc, M. C., Bourlioux, P., et al. (2001). GroEL (Hsp60) of Clostridium difficile is involved in cell adherence. Microbiology 147, 87–96. doi: 10.1099/00221287-147-1-87
Higa, T., and Kuniyoshi, M. (2000). Toxins associated with medicinal and edible seaweeds. J. Toxicol. Toxin Rev. 19, 119–137. doi: 10.1081/TXR-100100317
Hines, R. S., and Dickerson, S. (1993). Pseudomembranous enteritis associated with ciprofloxacin and Clostridium difficile in a penguin (Eudyptes chrysolophus). J. Zoo. Wildl. Med. 24, 553–556. doi: 10.2307/20095321
Ishii, S., Yan, T., Shively, D. A., Byappanahalli, M. N., Whitman, R. L., and Sadowsky, M. J. (2006). Cladophora (Chlorophyta) spp. harbor human bacterial pathogens in near shore water of Lake Michigan. Appl. Environ. Microbiol. 72, 4545–4553. doi: 10.1128/AEM.00131-06
Jost, B. H., Billington, S. J., Trinh, H. T., and Songer, J. G. (2006). Association of genes encoding beta2 toxin and a collagen binding protein in Clostridium perfringens isolates of porcine origin. Vet. Microbiol. 115, 173–182. doi: 10.1016/j.vetmic.2006.01.012
Kameyama, S., and Akama, K. (1971). Purification and some properties of kappa toxin of Clostridium perfringens. Jpn. J. Med. Sci. Biol. 24, 9–23. doi: 10.7883/yoken1952.24.9
Keel, M. K., and Songer, J. G. (2006). The comparative pathology of Clostridium difficile–associated disease. Vet. Pathol. 43, 225–240. doi: 10.1354/vp.43-3-225
Kikuchi, E., Miyamoto, Y., Narushima, S., and Itoh, K. (2002). Design of species-specific primers to identify 13 species of Clostridium harbored in human intestinal tracts. Microbiol. Immunol. 46, 353–358. doi: 10.1111/j.1348-0421.2002.tb02706.x
King, S. J. (2010). Pneumococcal modification of host sugars: a major contributor to colonization of the human airway. Mol. Oral Microbiol. 25, 15–24. doi: 10.1111/j.2041-1014.2009.00564.x
Koren, S., Walenz, B. P., Berlin, K., Miller, J. R., Bergman, N. H., and Phillippy, A. M. (2017). Canu: scalable and accurate long-read assembly via adaptive k-mer weighting and repeat separation. Genome Res. 27, 722–736. doi: 10.1101/gr.215087.116
Kumar, M. S., and Sharma, S. A. (2021). Toxicological effects of marine seaweeds: a cautious insight for human consumption. Crit. Rev. Food Sci. Nutr. 61, 500–521. doi: 10.1080/10408398.2020.1738334
Landsberg, J. H., Flewelling, L. J., and Naar, J. (2009). Karenia brevis red tides, brevetoxins in the food web, and impacts on natural resources: decadal advancements. Harmful Algae 8, 598–607. doi: 10.1016/j.hal.2008.11.010
Landsberg, J. H., Hall, S., Johannessen, J. N., White, K. D., Conrad, S. M., Abbott, J. P., et al. (2006). Saxitoxin puffer fish poisoning in the United States, with the first report of Pyrodinium bahamense as the putative toxin source. Environ. Health Perspect. 114, 1502–1507. doi: 10.1289/ehp.8998
Lane, D. J. (1991). “16S/23S rRNA sequencing,” in Nucleic Acid Techniques in Bacterial Systematics, eds E. Stackebrandt and M. Goodfellow (New York, NY: John Wiley and Sons), 115–175.
Lapointe, B. E., Herren, L. W., Brewton, R. A., and Alderman, P. K. (2020). Nutrient over-enrichment and light limitation of seagrass communities in the Indian River Lagoon, an urbanized subtropical estuary. Sci. Total Environ. 699:134068. doi: 10.1016/j.scitotenv.2019.134068
Lapointe, B. E., Herren, L. W., Debortoli, D. D., and Vogel, M. A. (2015). Evidence of sewage-driven eutrophication and harmful algal blooms in Florida’s Indian River Lagoon. Harmful Algae 43, 82–102. doi: 10.1016/j.hal.2015.01.004
Lawrence, J. F., and Niedzwiadek, B. (2001). Quantitative determination of paralytic shellfish poisoning toxins in shellfish by using prechromatographic oxidation and liquid chromatography with fluorescence detection. J. AOAC Int. 84, 1099–1108. doi: 10.1093/jaoac/88.6.1714
Lewis, C. J., and Naylor, R. D. (1998). Sudden death in sheep associated with Clostridium sordellii. Vet. Rec. 142, 417–421. doi: 10.1136/vr.142.16.417
Li, J., Navarro, M. A., Uzal, F. A., and McClane, B. A. (2021). NanI sialidase contributes to the growth and adherence of Clostridium perfringens type F strain F4969 in the presence of adherent mucus. Infect. Immun. 89:e0025621. doi: 10.1128/IAI.00256-21
Li, J., Sayeed, S., Robertson, S., Chen, J., and McClane, B. A. (2011). Sialidases affect the host cell adherence and epsilon toxin-induced cytotoxicity of Clostridium perfringens type D strain CN3718. PLoS Pathog. 7:e1002429. doi: 10.1371/journal.ppat.1002429
Littler, D. S., Littler, M. M., and Hanisak, M. D. (2008). Submersed Plants of the Indian River Lagoon. Washington, DC: Offshore Graphics Inc.
Lueders, I., Ludwig, C., Kasberg, J., Baums, C. G., Klimke, K., Dorner, M. B., et al. (2017). Unusual outbreak of fatal clostridiosis in a group of captive brown pelicans (Pelecanus occidentalis). J. Avian Med. Surg. 31, 359–363. doi: 10.1647/2016-237
Luna, L. G. (1968). Manual of Histologic Staining Methods of the Armed Forces Institutes of Pathology, 3rd Edn. New York, NY: McGraw-Hill.
MacArtain, P., Gill, G. I. R., Brooks, M., Campbell, R., and Rowland, I. R. (2007). Nutritional value of edible seaweeds. Nutr. Rev. 65, 535–543. doi: 10.1111/j.1753-4887.2007.tb00278.x
Mao, Y., Yannarell, A. C., Hong, P., and Mackie, R. I. (2011). Different Groups of Novel Bacteroidetes Dominate the gut Microbial Community of Wild Manatees (Trichechus manatus) from Belize and Florida. Congress on Gastrointestinal Function. Chicago, IL: University of Chicago.
Marcus, R., and Watt, J. (1969). Seaweeds and ulcerative colitis in laboratory animals. Lancet 2, 489–490. doi: 10.1016/s0140-6736(69)90187-1
Marinella, M. A., Burdette, S. D., Bedimo, R., and Markert, R. J. (2004). Leukemoid reactions complicating colitis due to Clostridium difficile. Southern Med. J. 97, 959–964. doi: 10.1097/01.SMJ.0000054537.20978.D4
Martin, J., Edwards, H. H., Fonnesbeck, C. J., Koslovsky, S. M., Harmak, C. W., and Dane, T. M. (2015). Combining information for monitoring at large spatial scales: first statewide abundance estimate of the Florida manatee. Biol. Conserv. 186, 44–51. doi: 10.1016/j.biocon.2015.02.029
Martin, J., Runge, M. C., Flewelling, L. J., Deutsch, C. J., and Landsberg, J. H. (2017). An Expert Elicitation Process to Project the Frequency and Magnitude of Florida Manatee Mortality Events Caused by Red Tide (Karenia brevis). Open-File Report 2017–1132. Reston: VA: U.S. Geological Survey. doi: 10.3133/ofr20171132
Martinez, R. D., and Wilkins, T. D. (1988). Purification and characterization of Clostridium sordellii hemorrhagic toxin and cross-reactivity with Clostridium difficile toxin A (enterotoxin). Infect. Immun. 56, 1215–1221. doi: 10.1128/iai.56.5.1215-1221.1988
McBee, R. H. (1960). Intestinal flora of some Antarctic birds and mammals. J. Bacteriol. 79, 311–312. doi: 10.1128/jb.79.2.311-312.1960
Merson, S. D., Ouwerkerk, D., Gulino, L. M., Klieve, A., Bonde, R. K., Burgess, E. A., et al. (2014). Variation in the hindgut microbial communities of the Florida manatee, Trichechus manatus latirostris over winter in Crystal River, Florida. FEMS Microbiol. Ecol. 87, 601–615. doi: 10.1111/1574-6941.12248
Miller, M. A., Byrne, B. A., Jang, S. S., Dodd, E. M., Dorfmeier, E., Harris, M. D., et al. (2010). Enteric bacterial pathogen detection in southern sea otters (Enhydra lutris nereis) is associated with coastal urbanization and freshwater runoff. Vet. Res. 41, 1–13. doi: 10.1051/vetres/2009049
Miller, W. A., Miller, M. A., Gardner, I. A., Atwill, E. R., Byrne, B. A., Jang, S., et al. (2006). Salmonella spp., Vibrio spp., Clostridium perfringens, and Plesiomonas shigelloides in marine and freshwater invertebrates from coastal California ecosystems. Microb. Ecol. 52, 198–206. doi: 10.1007/s00248-006-9080-6
Morris, L., Hall, L., Chamberlain, R. C., and Jacoby, C. (2018). “Summary report for the northern Indian River Lagoon,” in Seagrass Integrated Mapping and Monitoring Program: Mapping and Monitoring Report No. 3, Technical Report TR-17, version 3, eds L. A. Yarbro and P. R. Carlson Jr. (St. Petersburg, FL: Fish and Wildlife Research Institute). doi: 10.1128/AEM.00888-14
Morris, P. J., Johnson, W. R., Pisani, J., Bossart, G. D., Adams, J., Reif, J. S., et al. (2011). Isolation of culturable microorganisms from free-ranging bottlenose dolphins (Tursiops truncatus) from the southeastern United States. Vet. Microbiol. 148, 440–447. doi: 10.1016/j.vetmic.2010.08.025
Murata, M., Shimatani, M., Sugitani, H., Oshima, Y., and Yasumoto, T. (1982). Isolation and structural elucidation of the causative toxin of the diarrhetic shellfish poisoning. Nipp. Suis. Gakk. 48, 549–552. doi: 10.2331/suisan.48.549
Naar, J., Bourdelais, A., Tomas, C., Kubanek, J., Whitney, P. L., Flewelling, L., et al. (2002). A competitive ELISA to detect brevetoxins from Karenia brevis (formerly Gymnodinium breve) in seawater, shellfish, and mammalian body fluid. Environ. Health. Perspect. 110, 179–185. doi: 10.1289/ehp.02110179
Nagai, H., Yasumoto, T., and Hokama, Y. (1996). Aplysiatoxin and debromoaplysiatoxin as the causative agents of a red alga Gracilaria coronopifolia poisoning in Hawaii. Toxicon 34, 753–776. doi: 10.1016/0041-0101(96)00014-1
Navarro, G., Cummings, S., Lee, J., Moss, N., Glukhov, E., Valeriote, F. A., et al. (2015). Isolation of polycavernoside D from a marine cyanobacterium. Environ. Sci. Technol. Lett. 2, 166–170. doi: 10.1021/acs.estlett.5b00116
Neiffer, D. L. (2001). Clostridium perfringens enterotoxicosis in two Amur leopards (Panthera pardus orientalis). J. Zoo. Wildl. Med. 32, 134–135. doi: 10.1638/1042-7260
Neto, G. G., Bueno, M. G., Silva, R. O. S., Lobato, F. C. F., Guimarães, J. P., Bossart, G. D., et al. (2016). Acute necrotizing colitis with pneumatosis intestinalis in an Amazonian manatee calf. Dis. Aquat. Org. 120, 189–194. doi: 10.3354/dao03019
Nyaoke, A. C., Navarro, M. A., Fresneda, K., Diab, S. S., Moore, J., Lyras, D., et al. (2020). Paeniclostridium (Clostridium) sordellii–associated enterocolitis in 7 horses. J. Vet. Diag. Invest. 32:1040638720903738. doi: 10.1177/1040638720903738
Onderdonk, A. B., and Bartlett, J. G. (1979). Bacteriological studies of experimental ulcerative colitis. Am. J. Clin. Nutrit. 32, 258–265. doi: 10.1093/ajcn/32.1.258
Ortega, J., Daft, B., Assis, R. A., Kinde, H., Anthenill, L., Odani, J., et al. (2007). Infection of internal umbilical remnant in foals by Clostridium sordellii. Vet. Pathol. 44, 269–275. doi: 10.1354/vp.44-3-269
Owen, H., Flint, M. A., and de Wit, M. (2018). “Sirenia,” in Pathology of Wildlife and Zoo Animals, eds K. Terio, D. McAloose, and J. St. Leger (Cambridge, MA: Academic Press), 593–606. doi: 10.1016/B978-0-12-805306-5.00043-2
Paltansing, S., van den Berg, R. J., Guseinova, R. A., Visser, C. E., van der Vorm, E. R., and Kuijper, E. J. (2007). Characteristics and incidence of Clostridium difficile–associated disease in the Netherlands, 2005. Clin. Microbiol. Infect. 13, 1058–1064. doi: 10.1111/j.1469-0691.2007.01793.x
Pasquale, V., Romano, V. J., Rupnik, M., Dumontet, S., Cižnár, I., Aliberti, F., et al. (2011). Isolation and characterization of Clostridium difficile from shellfish and marine environments. Folia Microbiol. 56, 431–437. doi: 10.1007/s12223-011-0068-3
Paul, V. J., and Puglisi, M. P. (2004). Chemical mediation of interactions among marine organisms. Nat. Product. Rep. 21, 189–209. doi: 10.1039/B302334F
Phlips, E. J., Badylak, S., Lasi, M. A., Chamberlain, R., Green, W. C., Hall, L. M., et al. (2015). From red tides to green and brown tides: bloom dynamics in a restricted subtropical lagoon under shifting climatic conditions. Estuaries Coasts 38, 886–904. doi: 10.1007/s12237-014-9874-6
Popoff, M. R. (2014). Clostridial pore-forming toxins: powerful virulence factors. Anaerobe 30, 220–238. doi: 10.1016/j.anaerobe.2014.05.014
Popoff, M. R. (2018). Clostridium difficile and Clostridium sordellii toxins, proinflammatory versus anti-inflammatory response. Toxicon 149, 54–64. doi: 10.1016/j.toxicon.2017.11.003
Quintero-Hunter, I., Grier, H., and Muscato, M. (1991). Enhancement of histological detail using metanil yellow as a counter-stain in periodic acid Schiff’s hematoxylin staining of glycol methacrylate tissue sections. Biotech. Histochem. 66, 169–172. doi: 10.3109/10520299109109964
Reynolds, J. E., and Scolardi, K. M. (2016). Summary Biological Monitoring Report for Activities in Winter 2010–2011, 2011–2012, 2012–2013, 2013–2014, & 2014–2015. Prepared pursuant to Specific Condition of Certification No. V.A.3.a.(5) for Cape Canaveral FPL Energy Center of Document PA08-53A. Juno Beach, FL: Florida Power & Light Company.
Rimoldi, G., Uzal, F., Chin, R. P., Palombo, E. A., Awad, M., Lyras, D., et al. (2015). Necrotic enteritis in chickens associated with Clostridium sordellii. Avian Dis. 59, 447–451. doi: 10.1637/11077-033115-Case.1
Rodrigues, F. M., Marin, A. K. V., Rebelo, V. A., Marmontel, M., Borges, J. C. G., Vergara-Parente, J. E., et al. (2021). Nutritional composition of food items consumed by Antillean manatees (Trichechus manatus manatus) along the coast of Paraíba, northeastern Brazil. Aquat. Bot. 168:103324. doi: 10.1016/j.aquabot.2020.103324
Rodriguez, C., Taminiau, B., Van Broeck, J., Delmée, M., and Daube, G. (2016). Clostridium difficile in food and animals: a comprehensive review. Adv. Microbiol. Infect. Dis. Public Health 932, 65–92. doi: 10.1007/5584_2016_27
Rood, J. I. (1998). Virulence genes of Clostridium perfringens. Annu. Rev. Microbiol. 52, 333–360. doi: 10.1146/annurev.micro.52.1.333
Runge, M. C., Sanders-Reed, C. A., Langtimm, C. A., Hostetler, J. A., Martin, J., Deutsch, C. J., et al. (2017). Status and Threats Analysis for the Florida Manatee (Trichechus manatus latirostris) 2016. Scientific Investigation Report 2017–5030. Reston, VA: U.S. Geological Survey. doi: 10.3133/sir20175030
Scaria, J., Suzuki, H., Ptak, C. P., Chen, J. W., Zhu, Y., Guo, X. K., et al. (2015). Comparative genomic and phenomic analysis of Clostridium difficile and Clostridium sordellii, two related pathogens with differing host tissue preference. BMC Genomics 16:448. doi: 10.1186/s12864-015-1663-5
Shang, Q., Sun, W., Shan, X., Jiang, H., Cai, C., Hao, J., et al. (2017). Carrageenan-induced colitis is associated with decreased population of anti-inflammatory bacterium, Akkermansia muciniphila, in the gut microbiota of c57bl/6j mice. Toxicol. Lett. 279, 87–95. doi: 10.1016/j.toxlet.2017.07.904
Siegal-Willott, J. L., Harr, K., Hayek, L. A. C., Scott, K. C., and Gerlach, T. (2010). Proximate nutrient analyses of four species of submerged aquatic vegetation consumed by Florida manatee (Trichechus manatus latirostris) compared to romaine lettuce. J. Zoo Wild. Med. 41, 594–602. doi: 10.1638/2009-0118.1
Silva, R. O., D’Elia, M. L., Teixeira, E. P. T., Pereira, P. L. L., de Magalhães Soares, D. F., Cavalcanti, A. R., et al. (2014). Clostridium difficile and Clostridium perfringens from wild carnivore species in Brazil. Anaerobe 28, 207–211. doi: 10.1016/j.anaerobe.2014.06.012
Songer, J. G. (2004). The emergence of Clostridium difficile as a pathogen of food animals. Anim. Health Res. Rev. 5, 321–326. doi: 10.1079/AHR200492
Songer, J. G., Trinh, H. T., Dial, S. M., Brazier, J. S., and Glock, R. D. (2009). Equine colitis X associated with infection by Clostridium difficile NAP1/027. J. Vet. Diagn. Invest. 21, 377–380. doi: 10.1177/104063870902100314
Songer, J. G., and Uzal, F. A. (2005). Clostridial enteric infections in pigs. J. Vet. Diagn. Invest. 17, 528–536. doi: 10.1177/104063870501700602
Stecher, B., Maier, L., and Hardt, W.-D. (2013). “Blooming” in the gut: how dysbiosis might contribute to pathogen evolution. Nat. Rev. Microbiol. 11, 277–284. doi: 10.1038/nrmicro2989
Stith, B. M., Slone, D. H., de Wit, M., Edwards, H. H., Langtimm, C. A., Swain, E. D., et al. (2012). Passive thermal refugia provided warm water for Florida manatees during the severe winter of 2009-2010. Mar. Ecol. Prog. Ser. 462, 287–301. doi: 10.3354/meps09732
Suzuki, A., Ueda, K., Segawa, T., and Suzuki, M. (2019). Fecal microbiota of captive Antillean manatee Trichechus manatus manatus. FEMS Microbiol. Lett. 366:fnz134. doi: 10.1093/femsle/fnz134
Tobacman, J. K. (2001). Review of harmful gastrointestinal effects of carrageenan in animal experiments. Environ. Health Perspect. 109, 983–994. doi: 10.1289/ehp.01109983
Tubaro, A., Florio, C., Luxich, E., Sosa, S., DellaLoggia, R., and Yasumoto, T. (1996). A protein phosphatase 2A inhibition assay for a fast and sensitive assessment of okadaic acid contamination in mussels. Toxicon 34, 743–752. doi: 10.1016/0041-0101(96)00027-x
Uzal, F. A., and Songer, J. G. (2019). “Clostridial diseases,” in Diseases of Swine, eds J. J. Zimmerman, L. A. Karriker, A. Ramirez, K. J. Schwartz, G. W. Stevenson, and J. Zhang (Hoboken, NJ: John Wiley and Sons), 792–806. doi: 10.1002/9781119350927
Uzal, F. A., Sentíes-Cué, C. G., Rimoldi, G., and Shivaprasad, H. L. (2016). Non-clostridium perfringens infectious agents producing necrotic enteritis-like lesions in poultry. Avian Pathol. 45, 326–333. doi: 10.1080/03079457.2016.1159282
Van Dolah, F. M., Fire, S. E., Leighfield, T. A., Mikulski, C. M., and Doucette, G. J. (2012). Determination of paralytic shellfish toxins in shellfish by receptor binding assay: collaborative study. J. AOAC Int. 95, 795–812. doi: 10.5740/jaoacint.cs2011_27
Varela, R. A., and Bossart, G. D. (2005). Evaluation of biochemical analytes in vitreous humor collected after death in West Indian manatees. J. Am. Vet. Med. Assoc. 226, 88–92. doi: 10.2460/javma.2005.226.88
Verherstraeten, S., Goossens, E., Valgaeren, B., Pardon, B., Timbermont, L., Haesebrouck, F., et al. (2015). Perfringolysin O: the underrated Clostridium perfringens toxin? Toxins 7, 1702–1721. doi: 10.3390/toxins7051702
Verherstraeten, S., Goossens, E., Valgaeren, B., Pardon, B., Timbermont, L., Vermeulen, K., et al. (2013). The synergistic necrohemorrhagic action of Clostridium perfringens perfringolysin and alpha toxin in the bovine intestine and against bovine endothelial cells. Vet. Res. 44:45. doi: 10.1186/1297-9716-44-45
Vidor, C., Awad, M., and Lyras, D. (2015). Antibiotic resistance, virulence factors and genetics of Clostridium sordellii. Res. Microbiol. 166, 368–374. doi: 10.1016/j.resmic.2014.09.003
Virnstein, R. W., and Carbonara, P. A. (1985). Seasonal abundance and distribution of drift algae and seagrasses in the mid-Indian River Lagoon, Florida. Aquat. Bot. 23, 67–82.
Wade, B., Keyburn, A. L., Seemann, T., Rood, J. I., and Moore, R. J. (2015). Binding of Clostridium perfringens to collagen correlates with the ability to cause necrotic enteritis in chickens. Vet. Microbiol. 180, 299–303. doi: 10.1016/j.vetmic.2015.09.019
Wahlen, R., Evans, L., Turner, J., and Hearn, R. (2005). The use of collision/reaction cell ICP-MS for the determination of elements in blood and serum samples. Spectroscopy 20, 84–89. doi: 10.1016/j.clinbiochem.2014.12.003
Walker, B. J., Abeel, T., Shea, T., Priest, M., Abouelliel, A., Sakthikumar, S., et al. (2014). Pilon: an integrated tool for comprehensive microbial variant detection and genome assembly improvement. PLoS One 9:e112963. doi: 10.1371/journal.pone.0112963
Wang, Z., King, K. L., Ramsdell, J. S., and Doucette, G. J. (2007). Determination of domoic acid in seawater and phytoplankton by liquid chromatography–tandem mass spectrometry. J. Chromatogr. A 1163, 169–176. doi: 10.1016/j.chroma.2007.06.054
Whitehouse, L. N. A., and Lapointe, B. E. (2015). Comparative ecophysiology of bloom-forming macroalgae in the Indian River Lagoon, Florida: Ulva lactuca, Hypnea musciformis, and Gracilaria tikvahiae. J. Exp. Mar. Biol. Ecol. 471, 208–216. doi: 10.1016/j.jembe.2015.06.012
Whitman, R. L., Shively, D. A., Pawlik, H., Nevers, M. B., and Byappanahalli, N. (2003). Occurrence of Escherichia coli and enterococci in Cladophora (Chlorophyta) in nearshore water and beach sand of Lake Michigan. Appl. Environ. Microbiol. 69, 4714–4719. doi: 10.1128/AEM.69.8.4714-4719.2003
Williams, A. G., Withers, S., and Sutherland, A. D. (2012). The potential of bacteria isolated from ruminal contents of seaweed-eating North Ronaldsay sheep to hydrolyse seaweed components and produce methane by anaerobic digestion in vitro. Microb. Biotechnol. 6, 45–52. doi: 10.1111/1751-7915.12000
Yotsu-Yamashita, M., Haddock, R. L., and Yasumoto, T. (1993). Polycavernoside A: a novel glycosidic macrolide from the red alga Polycavernosa tsudai (Gracilaria edulis). J. Am. Chem. Soc. 115, 1147–1148. doi: 10.1021/ja00056a048
Yotsu-Yamashita, M., Yasumoto, T., Yamada, S., Bajarias, F. F., Formeloza, M. A., Romero, M. L., et al. (2004). Identification of polycavernoside A as the causative agent of the fatal food poisoning resulting from ingestion of the red alga Gracilaria edulis in the Philippines. Chem. Res. Toxicol. 17, 1265–1271. doi: 10.1021/tx0498556
Keywords: manatee, mortality, dietary shift, dysbiosis, Paeniclostridium sordellii, Clostridioides difficile, emerging disease, enterocolitis
Citation: Landsberg JH, Tabuchi M, Rotstein DS, Subramaniam K, Rodrigues TCS, Waltzek TB, Stacy NI, Wilson PW, Kiryu Y, Uzal FA and de Wit M (2022) Novel Lethal Clostridial Infection in Florida Manatees (Trichechus manatus latirostris): Cause of the 2013 Unusual Mortality Event in the Indian River Lagoon. Front. Mar. Sci. 9:841857. doi: 10.3389/fmars.2022.841857
Received: 22 December 2021; Accepted: 31 January 2022;
Published: 10 March 2022.
Edited by:
Charles Alan Jacoby, St. Johns River Water Management District, United StatesReviewed by:
Kathy Burek Huntington, Alaska Veterinary Pathology Services, United StatesCopyright © 2022 Landsberg, Tabuchi, Rotstein, Subramaniam, Rodrigues, Waltzek, Stacy, Wilson, Kiryu, Uzal and de Wit. This is an open-access article distributed under the terms of the Creative Commons Attribution License (CC BY). The use, distribution or reproduction in other forums is permitted, provided the original author(s) and the copyright owner(s) are credited and that the original publication in this journal is cited, in accordance with accepted academic practice. No use, distribution or reproduction is permitted which does not comply with these terms.
*Correspondence: Martine de Wit, bWFydGluZS5kZXdpdEBteWZ3Yy5jb20=
†These authors have contributed equally to this work
Disclaimer: All claims expressed in this article are solely those of the authors and do not necessarily represent those of their affiliated organizations, or those of the publisher, the editors and the reviewers. Any product that may be evaluated in this article or claim that may be made by its manufacturer is not guaranteed or endorsed by the publisher.
Research integrity at Frontiers
Learn more about the work of our research integrity team to safeguard the quality of each article we publish.