- 1Alfred Wegener Institute, Helmholtz Centre for Polar and Marine Research, Bremerhaven, Germany
- 2Department of Algal Development and Evolution, Max Planck Institute for Biology Tübingen, Tübingen, Germany
- 3Centre for Marine Sciences (CCMAR)-CIMAR Associated Laboratory, University of Algarve, Faro, Portugal
- 4Medical Faculty, Institute of Biochemistry I, University of Cologne, Cologne, Germany
- 5Institute for Plant Science and Microbiology, University of Hamburg, Hamburg, Germany
- 6Marine Botany, Faculty Biology/Chemistry & Center for Marine Environmental Sciences (MARUM), University of Bremen, Bremen, Germany
Marine forests and kelps as their foundation species are threatened by ocean warming especially at the warm distributional edges. Previously identified genetic divergence and ecotypic differentiation within kelp species may allow to produce more resilient lineages by intraspecific outbreeding among populations. In a mechanistic investigation of heat stress, heterosis (hybrid vigour), and underlying gene expression patterns, we assessed the thermal performance of inbred (selfings) and outbred (reciprocal crosses) sporophytes of the N-Atlantic kelp Laminaria digitata among clonal isolates from two divergent populations; one from the temperate North Sea (Helgoland) and one from the Arctic (Spitsbergen). First, we investigated the upper thermal tolerance of microscopic sporophytes in a 14-day experiment applying sublethal to lethal 20–23°C. The upper survival temperature of microscopic sporophytes was lower for the inbred Arctic selfing (21°C) than for the temperate selfing and the reciprocal crosses (22°C). Only in the temperate selfing, 4.5% of sporophytes survived 23°C. We then subjected 4–7 cm long sporophytes to a control temperature (10°C), moderate (19°C) and sublethal to lethal heat stress (20.5°C) for 18 days to assess gene expression in addition to physiological parameters. Growth and optimum quantum yield decreased similarly in the reciprocal crosses and the temperate selfing at 19 and 20.5°C, while inbred Arctic sporophytes died within seven days at both 19 and 20.5°C. In response to 20.5°C, 252 genes were constitutively regulated across all surviving lineages, which we use to describe metabolic regulation patterns in response to heat stress in kelp. At sublethal 20.5°C, ca. 150 genes were differentially expressed by either crossed lineage in comparison to the temperate selfing, indicating that they maintained a growth response similar to the temperate selfing with differential metabolic regulation during sublethal heat stress. Subtle differences in physiology and the differential expression of nine genes between the reciprocal crosses at 20.5°C indicate that female and male gametophytes may contribute differently to offspring traits. We consider potential inbreeding depression in the Spitsbergen selfing and quantify the better performance of both crosses using heterosis-related parameters. We discuss the potential and risks of outbreeding to produce more resilient crops for mariculture and marine forest restoration.
Introduction
Kelps in the brown algal order Laminariales are the foundation of diverse coastal rocky ecosystems known as kelp forests (Teagle et al., 2017). In addition to their immediate ecosystem functions as shelter and food, they sequester significant amounts of carbon (Krause-Jensen and Duarte, 2016), remove anthropogenic nutrients from coastal waters (Kim et al., 2015) and can be of high direct and indirect economic value (Vásquez et al., 2014; Buschmann et al., 2017). These kelp forests are currently threatened by ocean warming especially at their warm range edges (Voerman et al., 2013; Wernberg et al., 2016; McPherson et al., 2021), which is a manifestation of the predicted poleward shift of kelp forest distributions under climate change (Raybaud et al., 2013; Assis et al., 2018).
In this study, we investigated the kelp Laminaria digitata Hudson J.V. Lamouroux, which grows in the upper sublittoral and infralittoral fringe of cold-temperate and Arctic coasts in the North Atlantic (Lüning, 1990). As for all laminarian kelps, the dioicous, anisogamous life cycle of L. digitata alternates between microscopic haploid gametophytes and macroscopic diploid sporophytes. Sex determination occurs during meiosis, creating the haploid gametophyte stage (UV sexual system; Coelho et al., 2018), whereas the diploid sporophyte is asexual. Life cycle stages differ in their thermal limits, as gametophytes can tolerate higher temperature (23°C over two weeks; Bolton and Lüning, 1982) than sporophytes (21°C over two weeks; Lüning, 1984; tom Dieck, 1992; Franke et al., 2021).
Building on this general knowledge, recent evidence suggests that kelp forest key species are not uniform in their temperature responses, but show signs of adaptation to their local thermal regime (King et al., 2018; Monteiro et al., 2019b; Liesner et al., 2020a; Martins et al., 2020). Two L. digitata populations close to the warm distribution limit, namely the southernmost European population in Quiberon, France, and the population around the North Sea island of Helgoland, showed slightly higher heat resilience of growth and photoprotective responses, respectively, compared to other populations along the species’ latitudinal distribution, including the Arctic (Liesner et al., 2020a). In addition, reduced thermal adaptation was observed in photosynthetic quantum yield of sporophytes (Liesner et al., 2020a) and reproductive traits of gametophytes (Martins et al., 2020) in an Arctic population from Spitsbergen. This performance was in accordance with local maximum sea surface temperatures (SST) reaching > 18°C in Quiberon (Oppliger et al., 2014) and Helgoland (Bartsch et al., 2013), but only 6–7°C in Kongsfjorden, Spitsbergen (Hanelt et al., 2001; Liesner et al., 2020a). Furthermore, the genetic distance between these populations may have facilitated phenotypic divergence (King et al., 2020; Liesner et al., 2020a). First evidence of geographic trait variation on the molecular level shows that heat shock protein expression in L. digitata populations from SW England peaks at higher temperatures than in more northern, Scottish populations (King et al., 2019). However, the mechanisms driving intraspecific variation remain unclear. Therefore, to improve predictions and mitigation guidelines, temperature responses of kelps and their underlying molecular mechanisms are an important field of study (Heinrich et al., 2015; Li et al., 2019; Monteiro et al., 2019b). Isolated and genetically divergent populations may provide a useful test system to investigate thermal trait plasticity and its inheritance, and the associated molecular phenotypes.
The process of thermal trait inheritance is still largely unknown in kelps and macroalgae (Martins et al., 2019), but it is generally known that gene expression variation is an important basis of phenotypic variation and plasticity (e.g., Kliebenstein, 2009; Schoville et al., 2012; Zhao et al., 2015). Recent research has shown that brown algal gametophytes express different transcriptomic regulatory patterns among sexes generally (Lipinska et al., 2015; Pearson et al., 2019; Zhang J. et al., 2021) and in response to temperature (Monteiro et al., 2019a). In the sporophyte stage, there is evidence for beneficial heterosis effects (“hybrid vigour”; Hochholdinger and Hoecker, 2007) in growth and thermal tolerance of hybrid offspring of closely related kelp species (Lüning et al., 1978; tom Dieck and de Oliveira, 1993; Martins et al., 2019). Within species, outbreeding among differentiated genotypes might alleviate the negative effects of mating with closely related individuals (i.e., inbreeding depression; Raimondi et al., 2004) and may even lead to more productive and resilient offspring (Westermeier et al., 2010; Aitken and Whitlock, 2013). Heterosis is a known concept to produce stable and viable cultivars in agriculture and mariculture (Li et al., 2007; Westermeier et al., 2010; Fu et al., 2014), but research on the underlying principles of trait inheritance and heterosis is still scarce even in abundantly used crops (Fujimoto et al., 2018). Therefore, studying reciprocal crosses among different geographical isolates may help in understanding mechanisms of trait inheritance and potential heterosis in kelps.
In this study, we investigated thermal tolerance of juvenile Laminaria digitata sporophytes produced by inbreeding and outbreeding among clonal gametophyte isolates from the geographically distant and genetically distinct populations of Helgoland and Spitsbergen, which evidently differ in their thermal characteristics (Liesner et al., 2020a; Martins et al., 2020). We hypothesised that inbred lineages (selfings) of temperate Helgoland sporophytes are more tolerant to sublethal high temperature than inbred Arctic Spitsbergen sporophytes. Furthermore, we expected outbred crosses between populations to perform better than one or both of the inbred lineages (i.e., heterosis). To investigate the molecular heat stress response and potential underlying mechanisms of differentiation among kelp lineages from different populations, we analysed transcriptome-wide differential expression in addition to physiological measurements in a laboratory heat stress experiment.
Materials and Methods
Experimental Approach
We designed an experiment using sibling clonal gametophyte isolates from the L. digitata populations of Helgoland (North Sea) and Spitsbergen (Arctic), because they represent genetically distinct populations thriving under contrasting thermal conditions along the latitudinal distribution gradient (Liesner et al., 2020a). We induced fertilization of gametophytes, creating clonal lineages of inbred selfings and outbred reciprocal crosses, and defined the upper thermal tolerance and differential survival capacity of these microscopic sporophytes over time (experiment 1). At a later stage, three-month-old and 4–7 cm long sporophytes of the same selfings and crosses were subjected to control (10°C) and sublethal temperature (19 and 20.5°C; based on the results of experiment 1) for 18 days. We measured the physiological traits of growth and photosynthetic optimum quantum yield, and compared transcriptomic responses to the highest sublethal heat stress at 20.5°C among selfings and outbred crosses (experiment 2).
Culture Material, Preparation and Fertilization
We used sibling clonal gametophyte cultures each derived from a single gametophyte isolate in 2015 of one Laminaria digitata sporophyte from Kongsfjorden, Spitsbergen, Norway (AWI seaweed culture collection number: ♀ 3472, ♂ 3471) and one sporophyte from Helgoland, Germany (AWI seaweed culture collection number: ♀ 3436, ♂ 3435). Spitsbergen material had been verified as L. digitata morphologically and by DNA barcoding of the sporophyte (ID 78 in Dankworth et al., 2020) to avoid confusion with the morphologically similar Arctic kelp Hedophyllum nigripes (Franke et al., 2021). Prior to the start of the experiment, cultures were maintained vegetatively under red light (approx. 3 μmol photons m–2 s–1; ProfiLux 3 with LED Mitras daylight 150, GHL Advanced Technology, Kaiserslautern, Germany) in a 16:8 h light:dark cycle at 15°C in a temperature-controlled cooling chamber (error ± 1°C) in sterile Provasoli-enriched seawater (PES; Provasoli, 1968; modifications: HEPES-buffer instead of TRIS, double concentration of Na2glycerophosphate; iodine enrichment following Tatewaki, 1966).
To perform fertilization of selfings and outcrosses, stock suspensions of each gametophyte culture were prepared by gently fragmenting gametophyte material using a mortar and pestle. Suspensions were sieved to obtain a fraction of filaments of length 50–100 μm. Gametophytes were added to petri dishes (Ø 5 cm) containing four glass cover slips and filled with 12 mL half-strength (½) PES. This created the “lineage” treatments (n = 4) of H × H (Helgoland female × Helgoland male), H × S (Helgoland female × Spitsbergen male), S × H (Spitsbergen female × Helgoland male), S × S (Spitsbergen female × Spitsbergen male). Despite efforts to sow gametophytes at identical densities, Spitsbergen gametophytes (f: 227 ± 22 gametophytes cm–2 14 days after sowing; m: 195 ± 10 gametophytes cm–2; mean over all replicates ± SD, n = 8) were about twice as dense as Helgoland gametophytes (f: 120 ± 11 gametophytes cm–2; m: 95 ± 15 gametophytes cm–2). Gametogenesis was induced at 10°C and 15–18 μmol photons m–2 s–1 white light, which is optimal for both populations (tom Dieck, 1992; Martins et al., 2020). After 28 days, means of 75–80% (S × S, S × H, H × S) and 90% (H × H) of female gametophytes had sexually reproduced to generate sporophytes [ANCOVA, F(3,11) = 8.13, p = 0.0039].
Following sporophyte production, the four cover slips per replicate were divided into four plastic dishes (Coria, Ø 6 cm, polystyrol, VKF Renzel, Germany) to conduct an experiment on the upper thermal limit of microscopic sporophytes (experiment 1). The remaining sporophytes in the petri dishes were pooled by lineage and were reared for 73 more days first in glass dishes (Ø 9 cm) at the same conditions. Macroscopic sporophytes were subsequently cultivated in 1 L glass beakers and 5 L bottles with gentle aeration at 10°C under increased irradiance of 30–35 μmol photons m–2 s–1 with weekly changes of ½ PES and were then used in the heat stress experiment on cm-long juvenile sporophytes (experiment 2). Throughout the experiments, 10°C served as the control temperature because it has been described as an optimal growth temperature for temperate and Arctic sporophytes of L. digitata (Bolton and Lüning, 1982; tom Dieck, 1992; Franke et al., 2021).
Experiment 1: Upper Survival Temperature of Microscopic Sporophytes
The four cover slips of one gametogenesis replicate were divided and each assigned to one experimental replicate in each temperature treatment to define the upper thermal limit of microscopic sporophytes (20, 21, 22, 23°C ± 0.1°C). Replicate dishes each containing one coverslip and 100 mL ½ PES were acclimated at 14°C for 11 days, followed by 18°C for two days before reaching the experimental temperatures in water baths controlled by thermostats (Huber Variostat CC + Pilot ONE, Peter Huber Kältemaschinen GmbH, Offenburg, Germany). The number of healthy (fully pigmented), unhealthy (partial bleaching) and dead (fully bleached) sporophytes was counted at the start of the experiment (day 0), after seven and 14 days at the temperature treatments, and after a post-cultivation period of 14 days at the sublethal and growth-suppressing temperature of 20°C (Bolton and Lüning, 1982; tom Dieck, 1992) to identify any delayed treatment effects. In each replicate, ∼300 sporophytes were randomly counted. Medium was changed at the start of the experiment and at the end of the 14-day temperature treatment. Mid-parent heterosis (MPH; hybrid vigour) of survival in the reciprocal crosses was calculated by subtracting the average relative survival over all replicates of both inbred lineages (H × H and S × S) from each single replicate of an outcrossed lineage (either H × S or S × H, respectively), resulting in the difference in percentage of survival for each replicate of the crosses, following Hochholdinger and Hoecker (2007):
where F1 is the trait of a cross (one replicate of either H × S or S × H, respectively), and P1 and P2 are average traits of the parental inbred lineages (all replicates of H × H and S × S).
Experiment 2: Critical Heat Stress on Macroscopic Sporophytes
In the second experiment, 4–7 cm long sporophytes of the same inbred and outcrossed lineages were subjected to temperatures of 10°C (control), 19 and 20.5°C to assess heat stress responses among lineages. These temperatures were chosen based on the results of experiment 1, where the majority of sporophytes survived for 14 days at 20°C and only the Spitsbergen selfing showed reduced tolerance at 21°C (Figure 1), which corresponds to the published upper thermal limit of L. digitata (Bolton and Lüning, 1982; tom Dieck, 1992; Franke et al., 2021). Seven sporophytes were assigned to one replicate plastic container (n = 4; Wide neck containers series 310 PETG, 2000 mL, Kautex GmbH & Co. KG, Bonn-Holzlar, Germany) filled with 1.8 L of ½ PES. Medium was exchanged every 3–4 days. Samples were acclimated for one day at 13.5°C and one day at 17°C before reaching the experimental temperatures of 19 and 20.5°C on (nominal) day 0 of the experiment, while the control treatments remained at 10°C. Two sporophytes per replicate were marked to be used for repeated measurements of growth and quantum yield throughout the experiment by punching small holes in the distal thallus with a Pasteur pipette. Of the unmarked five sporophytes per replicate, three were frozen in liquid nitrogen throughout the experiment (before acclimation, day 1 of temperature treatment, day 18 of temperature treatment). Samples frozen before acclimation and after 18 days of temperature treatment were used for transcriptomic analysis, stored at -80°C and processed within three weeks. The remaining two sporophytes served as backup.
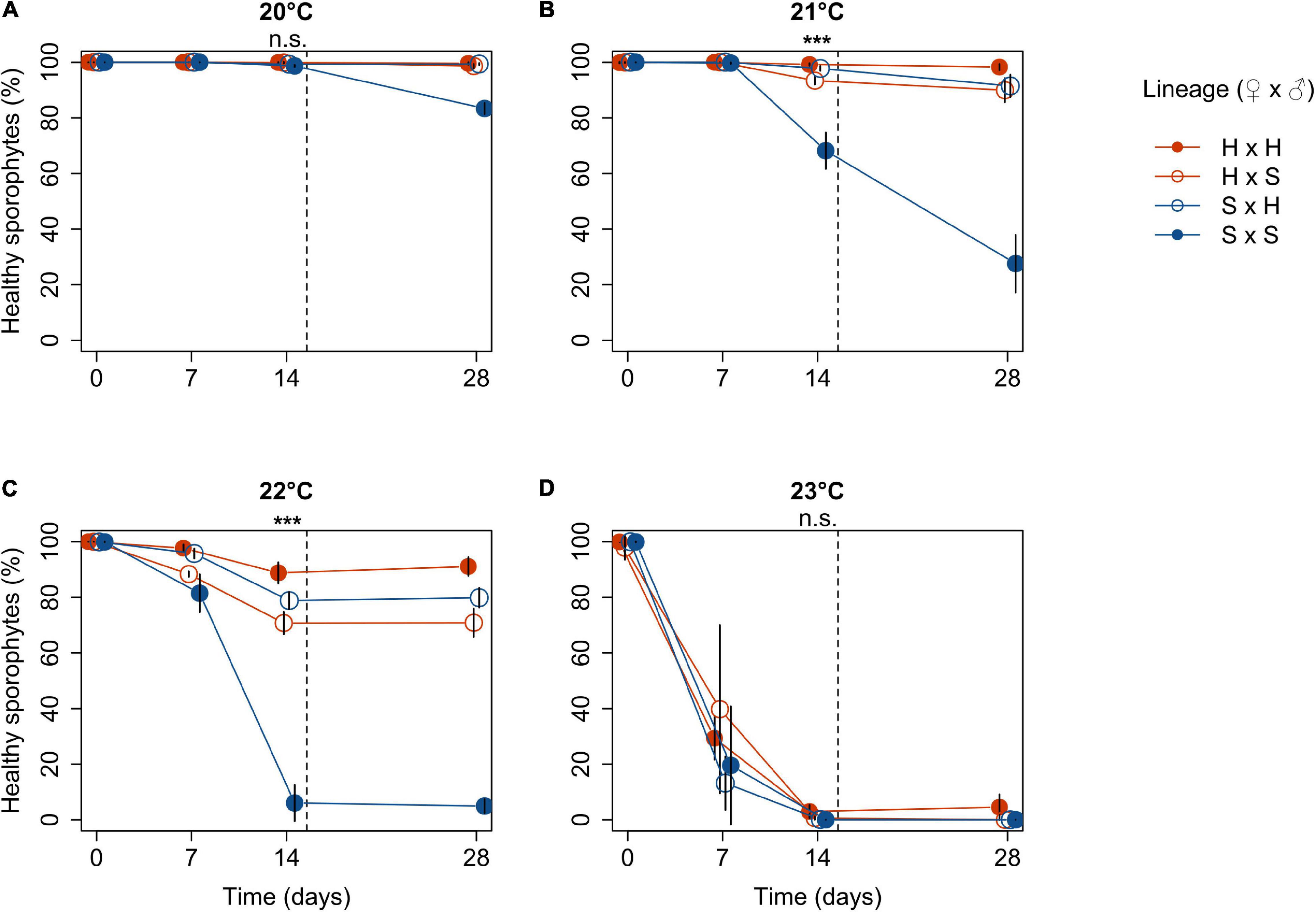
Figure 1. Proportion of fully pigmented (healthy) Laminaria digitata microscopic sporophytes produced by crossing females × males from Helgoland (H) and Spitsbergen (S) in experiment 1 over 14 days in treatments of 20°C (A), 21°C (B), 22°C (C) and 23°C (D) followed by 14 days of post-cultivation at 20°C (separated by dashed line; mean values ± SD, n = 4). Significance of the fixed effect lineage on sporophyte health after 14 days of temperature exposure is indicated above each panel (ANCOVA; ***, p < 0.001; n.s., not significant). For pairwise comparisons, see main text.
Physiological measurements were conducted on days -2 (before acclimation), 0 (beginning of experiment), 3, 7, 10, 14 and 17 on two sporophytes per replicate. Average values per replicate were used for statistical analyses. Fluorometric measurements were conducted with an Imaging-PAM (M-Series, MAXI version, Heinz Walz GmbH, Effeltrich, Germany) following 10 min dark acclimation. Maximum quantum yield (Fv/Fm) was measured in the meristematic region of the sporophytes. Fresh weight was quantified after patting dry sporophytes with paper wipes. Relative growth rates were calculated as
where x1 and x2 are fresh weights at the successive time points t1 and t2, respectively.
Physiological Data Analysis
All analyses were conducted in R 4.0.4 (R Core Team, 2021). To analyse survival of microscopic sporophytes in experiment 1, we calculated fractions of unbleached (defined as healthy) sporophytes in each replicate to produce survival curves. We then modelled percentages of healthy sporophytes after 14 days of exposure against a density covariate and the fixed factor lineage for each experimental temperature using linear models (function “lm”). Relative growth rates and Fv/Fm in experiment 2 were modelled as a function of the fixed factor lineage for each temperature separately on day 3 (all lineages) and day 17 (S × S only included at 10°C because of mortality at 19 and 20.5°C) using linear models. Normal distribution and variance homogeneity of standardised model residuals were verified using Shapiro-Wilk and Levene’s test, respectively. Factor significance was assessed via analyses of variance (ANOVA) and p-values were corrected for multiple testing using the false discovery rate approach (FDR; Benjamini and Hochberg, 1995). Significant differences in MPH were assessed using t-tests.
Sporophyte RNA Extraction and Sequencing
For RNA extraction from frozen sporophyte material, we applied the protocol of Heinrich et al. (2012a) with modifications described by Heinrich (2018). RNA purity was inspected by NanoDrop ND-1000 Spectrophotometer (NanoDrop Technologies LLC, Wilmington, United States) and RNA integrity was confirmed by capillary electrophoresis (Agilent 2100 Bioanalyzer, Agilent Technologies, Santa Clara, United States). The RNA was enriched for polyA+ species and converted to a cDNA sequencing library using a TruSeq Stranded mRNA kit (Illumina, San Diego, United States) according to the manufacturer’s protocol. The cDNA was sequenced as 75 bp paired end libraries on an Illumina HiSeq 4000 at Cologne Center for Genomics, Cologne, Germany. Raw reads were quality trimmed with Trimmomatic (Bolger et al., 2014) and deposited in the NCBI Sequence Read Archive (SRA) under the BioProject accession PRJNA665130.
Assembly, Mapping and Differential Gene Expression Analysis
The L. digitata transcriptome was assembled de novo from 33 libraries (n = 3) sampled on day 0 (10°C, N = 12) and day 18 (10°C, N = 12, and 20.5°C, N = 9) of experiment 2 using rnaSPAdes (Bushmanova et al., 2019) with kmer length 37 on a Linux server (40 cores and 250Gb RAM). Quality assessment of the assembly was performed using Transrate v1.0.3 (Smith-Unna et al., 2016) with read-mapping metrics (SNAP; Zaharia et al., 2011; Salmon; Patro et al., 2015). A reference set of transcripts was built by predicting open reading frames (ORFs) from the high-quality contigs returned by Transrate using FragGeneScan (Rho et al., 2010). Subsequent clustering of predicted ORFs was performed at 97% nucleotide identity using VSEARCH (Rognes et al., 2016). Clustered ORFs were then screened for biological evidence against Stramenopile proteins in the NCBI nr database using local blastx, retaining only those with top hits against Phaeophyceae to produce the final reference transcriptome. The completeness of this reference was evaluated with Benchmarking Universal Single-Copy Orthologs (BUSCO) v2/3 against the OrthoDB v9 eukaryote database (gVolante server1; Nishimura et al., 2017). The reference was annotated by local blastx against the Uniref90 and Swissprot databases2 and by blastp against the KEGG database (Kyoto Encyclopedia of Genes and Genomes; KAAS server3). Accessions for hits to Uniref90 were linked to Gene Ontology, InterPro and Pfam functional terms.
Read pairs for each library were mapped onto the indexed reference transcripts with Bowtie2 (Langmead and Salzberg, 2012) using RSEM (Li and Dewey, 2011), which returned expected count data for each contig. Count data were used for differential gene expression analysis using edgeR (Robinson et al., 2009; McCarthy et al., 2012), following the pipeline described by Smyth et al. (2018) with the requirements for differentially expressed transcripts of log2-fold change (log2FC) > 1 and adjusted p-value < 0.01 (FDR). The analysis was repeated on KEGG orthology (KO) terms, after aggregating read counts where mulitple transcripts were assigned to the same KO entry. Comparisons were made only for samples taken on day 18 to identify (a) the general heat stress response across lineages, (b) differences in gene regulation among lineages at the control temperature of 10°C, and (c) differences in the heat stress response among lineages at 20.5°C. Due to mortality of the Spitsbergen selfed sporophytes at 20.5°C, this treatment could not be included in the comparisons, and one further replicate of the Spitsbergen selfing at 10°C was removed as an outlier (S × S 10 c in Supplementary Figure 1).
Results
Upper Survival Temperature of Microscopic Sporophytes (Experiment 1)
Figure 1 shows the percentage of fully pigmented (“healthy”) microscopic sporophytes for each lineage over 14 days of heat treatment and subsequently 14 days of post-cultivation at 20°C in four panels corresponding to the temperature treatments. While almost all sporophytes survived at 20°C for 14 days, 23°C was lethal for most sporophytes across lineages. At 20°C, there was no significant difference among lineages as 99–100% of sporophytes remained healthy across lineages over the 14-day treatment (p = 0.4164, Table 1), but in the Spitsbergen selfing the fraction of healthy sporophytes decreased to 83% during the subsequent 14 days of post-cultivation at 20°C. Major temperature effects were visible in S × S at 21°C, which significantly decreased the fraction of healthy sporophytes to 68% after 14 days compared to all other lineages, which remained 93–99% healthy (p < 0.0001, Table 1; Tukey tests, p < 0.0001). Again, health of S × S sporophytes further decreased to 28% during post-cultivation at 20°C. At 22°C, health of S × S sporophytes decreased drastically to 6%, while the other crosses remained at 70–80%, and the Helgoland selfing retained 90% healthy sporophytes [p < 0.0001, Table 1; Tukey tests, H × H > (S × H = H × S) > S × S, p < 0.05]. At 23°C, fractions of healthy sporophytes averaged 0% (S × S), 0.08% (S × H), 0.58% (H × S) and 2.96% (H × H), but were not significantly different among lineages (p = 0.0600, Table 1). Only in the Helgoland selfing, 4.5% of healthy sporophytes persisted during post-cultivation. These results led to the decision to perform experiment 2 with a control temperature of 10°C, 19°C as a moderate heat stress treatment and 20.5°C as a critical but sublethal heat stress treatment.
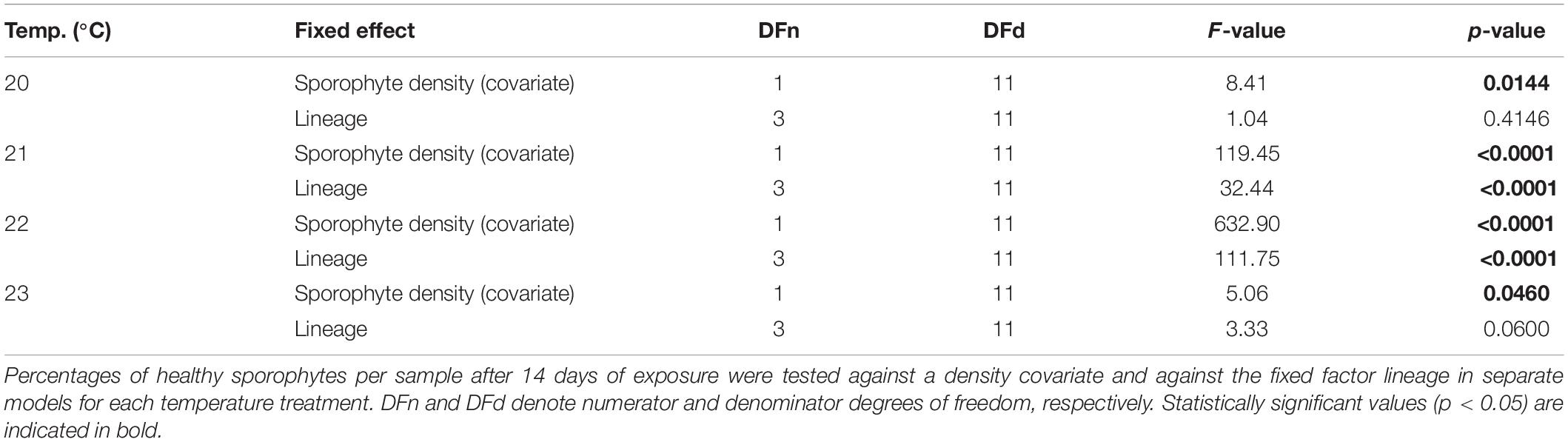
Table 1. Results of linear models to examine fractions of unbleached Laminaria digitata sporophytes in the upper survival temperature experiment on microscopic sporophytes (experiment 1).
The survival of the reciprocal crosses at 21 and 22°C can be related to the survival of the inbred lineages by quantifying mid-parent heterosis (MPH). This parameter describes whether the performance of hybrid offspring is significantly better than the average value of the two parental inbred lines (Hochholdinger and Hoecker, 2007). Mid-parent heterosis was evident in both outcrossed lineages after 14 days of exposure to both 21 and 22°C. Namely, in H × S, relative survival at 21°C was higher by 9.7% ± 1.2% (mean ± SD, n = 4, p = 0.0017, t-test) and by 23.3% ± 3.5% at 22°C (p = 0.0028) compared to the average response of both selfings. In S × H, relative survival at 21°C was higher by 14.1% ± 0.7% (p = 0.0002) and by 31.4% ± 2.7% at 22°C (p = 0.0008) compared to the average selfing response. Contrary to the relative survival rates, MPH was significantly higher in S × H compared to H × S at 21°C (p = 0.0034) and 22°C (p = 0.0209).
Heat Stress Experiment on Macroscopic Sporophytes (Experiment 2)
In experiment 2, we aimed to identify differences among lineages in response to each sublethal temperature treatment to assess whether response patterns differed from those of microscopic sporophytes, and to link physiological responses to gene expression. Most strikingly, all sporophytes of the Spitsbergen selfing bleached at 19 and 20.5°C following day 3. Thus, we analysed differences among lineages within each temperature treatment on day 3 (including the Spitsbergen selfing), and on day 17 (excluding the Spitsbergen selfing at 19 and 20.5°C) to accommodate for the unbalanced design. Relative growth rates of fresh weight (RGR) decreased over time for all lineages at 19 and 20.5°C (Figure 2). We show RGR over the acclimation period (days -2–0) and over 17 days of heat treatment. Each point refers to growth rates over the time period starting from the previous sampling date. Over the first three days at 10°C, growth rates among lineages were not significantly different (p = 0.9521, Table 2) and all achieved > 0.12 g g–1 d–1. Until day 3 at 19°C, growth of the Spitsbergen selfing was significantly reduced to 0.06 g g–1 d–1 compared to all other lineages at 19°C, which ranged around 0.1 g g–1 d–1 (p < 0.0001, Table 2; Tukey tests, p < 0.001). Likewise, at 20.5°C, growth of the Spitsbergen selfing was significantly reduced at < 0.05 g g–1 d–1 compared to the remaining lineages (p = 0.0008, Table 2; Tukey tests, p < 0.01), which showed similar growth rates around 0.07 g g–1 d–1. Between days 14 and 17, growth rates differed significantly among lineages at 10°C (p = 0.0154, Table 2). H × S sporophytes grew significantly faster at 0.09 g g–1 d–1 than S × S at 0.07 g g–1 d–1 [Tukey tests, (H × S > S × S) = (S × H = H × H), p < 0.01]. At 19°C, growth rates among the three remaining lineages did not differ significantly (p = 0.3885, Table 2). At 20.5°C, growth rates differed significantly among lineages (p = 0.0296, Table 2), in that H × S retained slightly higher growth at 0.02 g g–1 d–1 compared to the Helgoland selfing at 0.01 g g–1 d–1 [Tukey tests, (H × S > H × H) = S × H, p < 0.05].
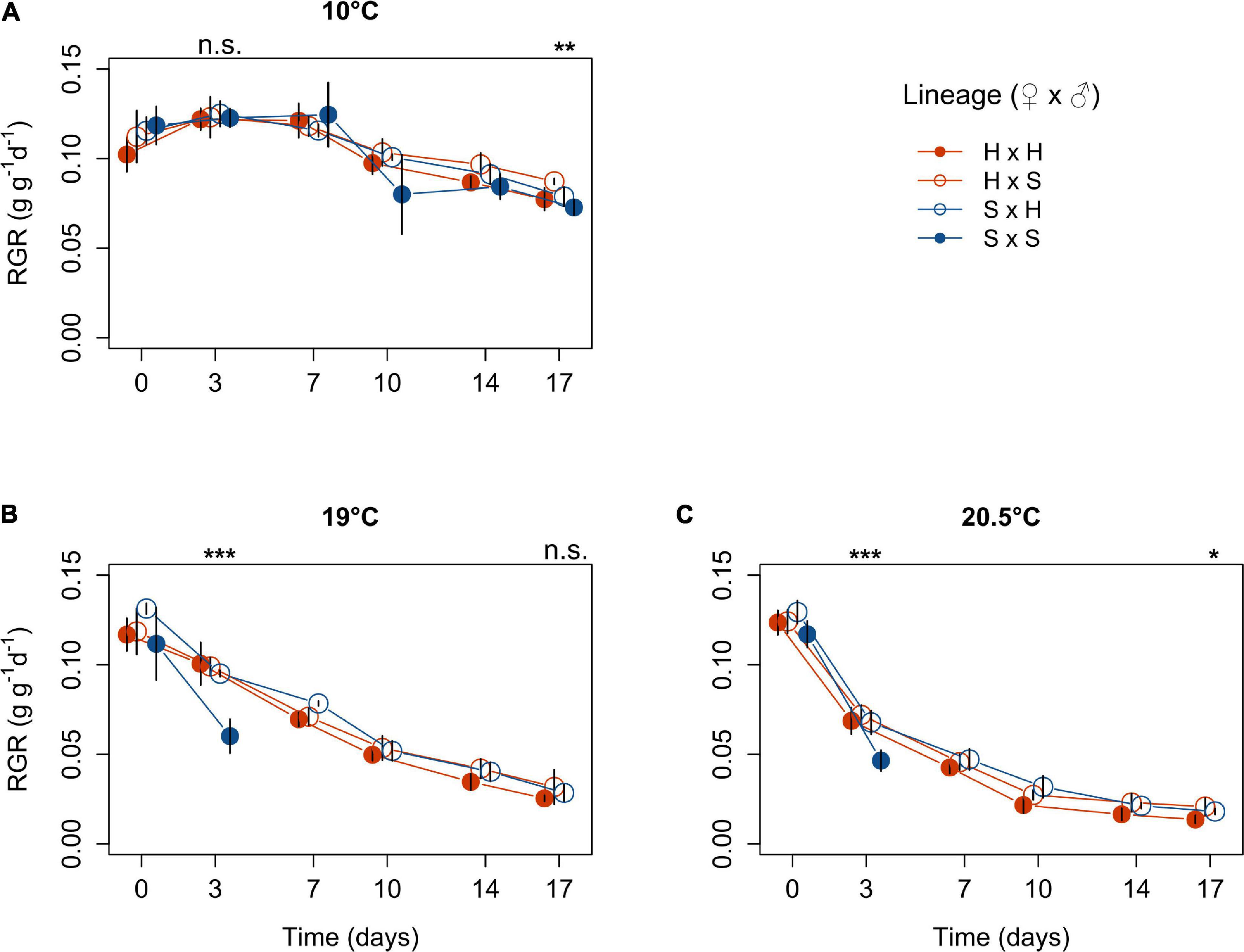
Figure 2. Relative growth rates of Laminaria digitata macroscopic sporophytes produced by crossing females × males from Helgoland (H) and Spitsbergen (S) over time at 10°C (A), 19°C (B) and 20.5°C (C) in experiment 2 (mean ± SD, n = 4). Significance of the fixed effect lineage on sporophyte growth after 3 and 17 days of temperature exposure is indicated above each panel (ANOVA; ***, p < 0.001; **, p < 0.01; *, p < 0.05; n.s., not significant). For pairwise comparisons, see main text.
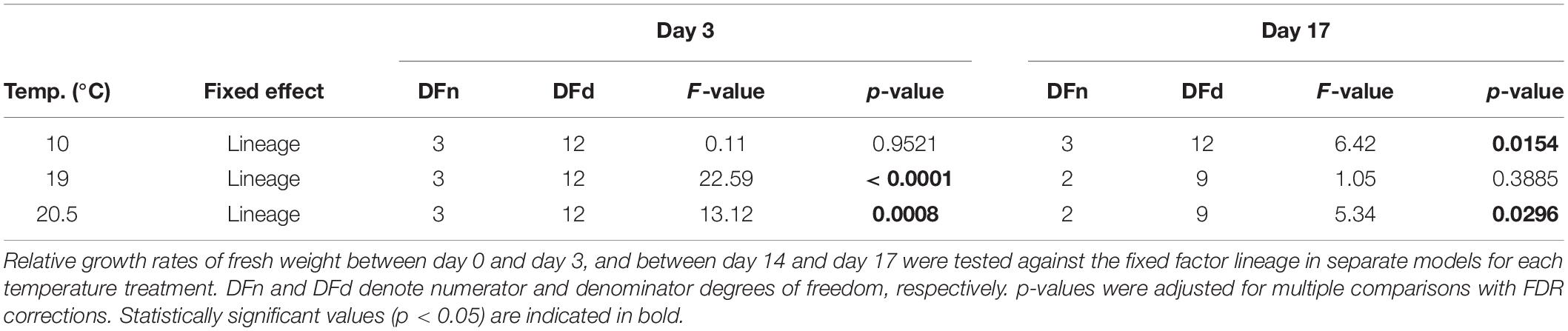
Table 2. Results of linear models to examine relative growth rates among lineages for two time points in the critical heat stress experiment on macroscopic Laminaria digitata sporophytes (experiment 2).
Optimum quantum yield Fv/Fm was relatively stable across lineages and temperatures and was mostly in a healthy range of 0.6–0.7 relative units (Figure 3). On day 3, Fv/Fm differed significantly among lineages at all temperatures. At 10°C, Fv/Fm was at 0.64 in the Spitsbergen selfing and significantly lower than in the other lineages, which ranged from 0.66 to 0.67 (p = 0.0006, Table 3; Tukey tests, p ≤ 0.01). At 19°C, Fv/Fm was highest in the Helgoland selfing at 0.70 and lowest in the Spitsbergen selfing at 0.67, while the reciprocal crosses were intermediate at 0.68 [p = 0.0004, Table 3; Tukey tests, H × H > H × S = (S × H > S × S), p < 0.05]. At 20.5°C, Fv/Fm was at 0.65 in the Spitsbergen selfing and significantly lower than in the Helgoland selfing for which Fv/Fm was 0.70 [p = 0.0414, Table 3; Tukey tests, (H × H > S × S) = (S × H = H × S), p < 0.05]. On day 17, Fv/Fm did not differ significantly among lineages at 10°C (p = 0.0629, Table 3) and ranged between 0.67 and 0.69. At 19°C, Fv/Fm differed significantly among lineages (p = 0.0218, Table 3), in that Fv/Fm was higher in S × H at 0.72 than in H × S and the Helgoland selfing at 0.70 [Tukey tests, S × H > (H × S = H × H), p < 0.05]. At 20.5°C, Fv/Fm ranged between 0.68 and 0.71 and again did not differ significantly among lineages (p = 0.4549, Table 3).
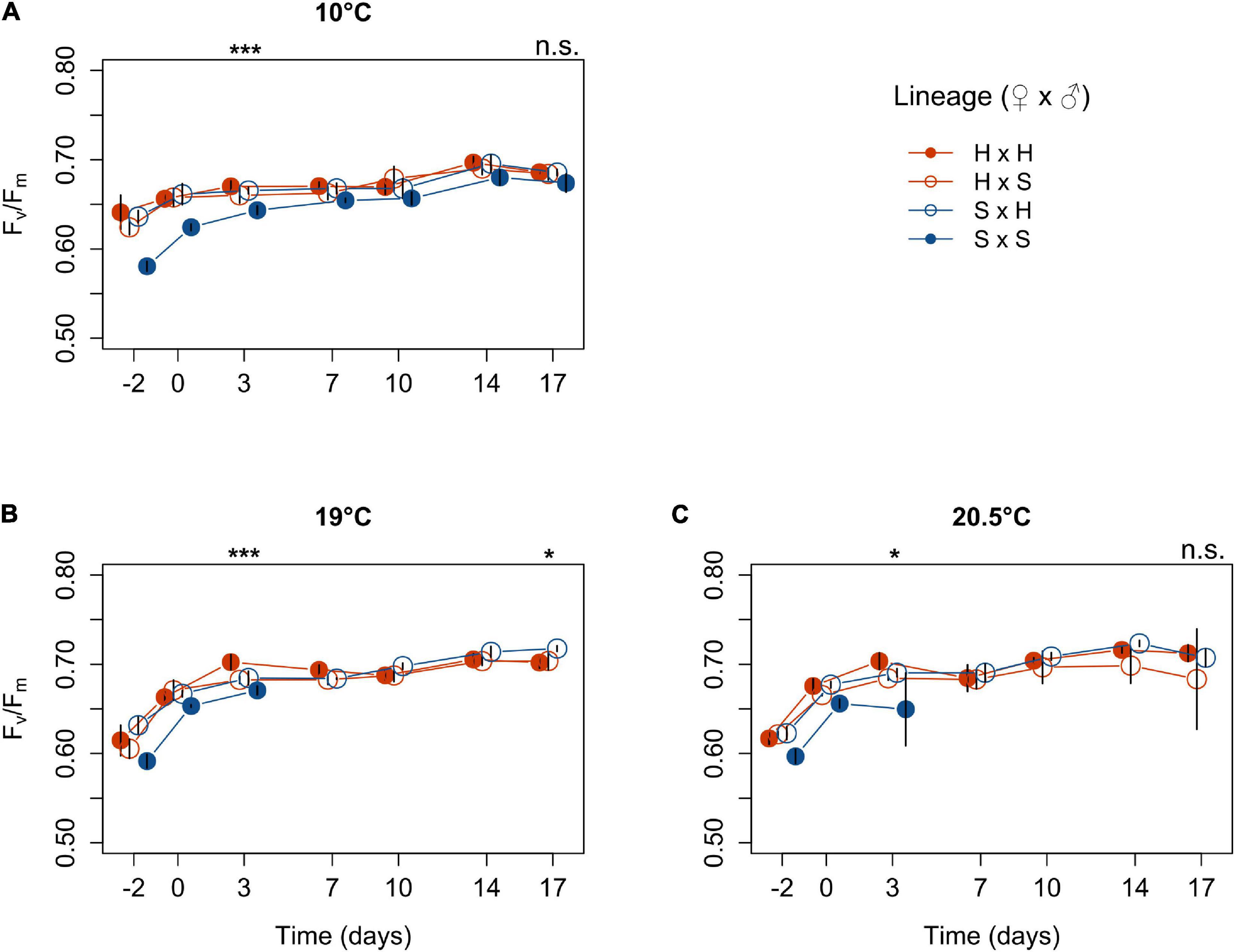
Figure 3. Optimum quantum yield Fv/Fm of Laminaria digitata sporophytes produced by crossing females × males from Helgoland (H) and Spitsbergen (S) over time at 10°C (A), 19°C (B) and 20.5°C (C) in experiment 2 (mean ± SD, n = 4). Significance of the fixed effect lineage on Fv/Fm after 3 and 17 days of temperature exposure is indicated above each panel (ANOVA; ***, p < 0.001; *, p < 0.05; n.s., not significant). For pairwise comparisons, see main text.
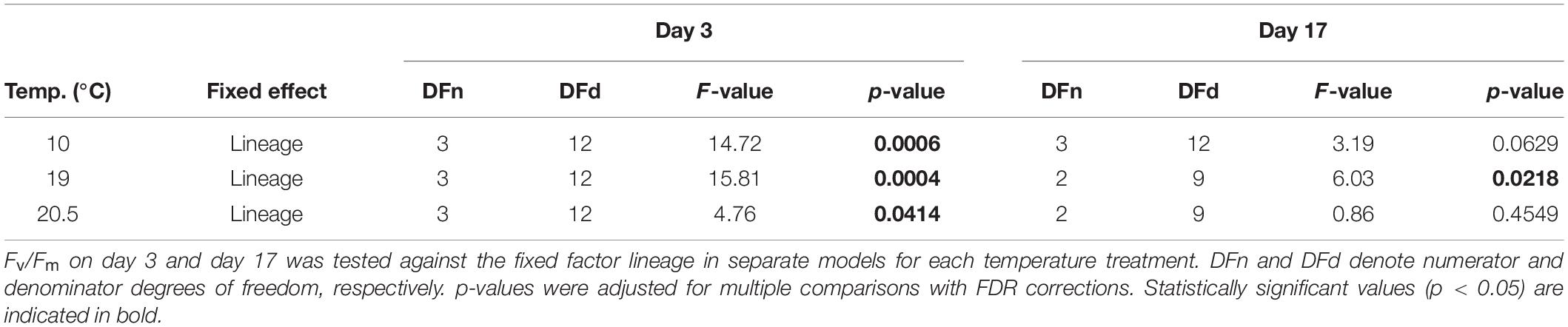
Table 3. Results of linear models to examine optimum quantum yield (Fv/Fm) among lineages for two time points in the critical heat stress experiment on macroscopic Laminaria digitata sporophytes (experiment 2).
Transcriptome Quality
The number of reads per library ranged between 28 million and 44 million with an average of 33.5 million reads per library. The final reference transcriptome based on Phaeophyceae hits contained 34,337 contigs with an average contig size of 928 bp (N50 = 1,230 bp). BUSCO analysis of our reference against the core eukaryotic gene set indicated that 78.22% were present and complete (96.04% complete and partial), with 3.96% missing core genes. The duplication rate was 1.18 orthologs per core gene, indicating a low level of redundancy. A total of 11,519 contigs (33.55%) were annotated using the KEGG database, yielding 3,705 unique KO accessions for which the contig count data were aggregated. Following the removal of lowly expressed KEGG genes, 3,398 were retained to identify differentially expressed genes (DEGs) among the four lineages at 10 and 20.5°C on day 18 of experiment 2.
Differential Gene Expression in Experiment 2
Out of the 3,398 KEGG genes, we extracted 1,111 (32.7%) DEGs among all comparisons in the differential expression analysis. Multidimensional scaling (MDS, Figure 4) of DEG expression revealed that the replicates of the same lineage and temperature treatment clustered closely. Distances between samples can be interpreted as the root-mean-square average (RMS) of the log2-fold-changes for all 1,111 DEGs between each pair of samples (Ritchie et al., 2015). Distribution of data along dimension 1 (66.1% of variance explained) clearly separated the two temperature treatments of 10 and 20.5°C, while dimension 2 (12.5% of variance explained) separated samples based on origin of the parental gametophytes. At 10°C, the Helgoland and Spitsbergen selfings each formed clearly separated clusters while the reciprocal crosses grouped together between the selfings. At 20.5°C, the reciprocal crosses separated slightly from each other. The placement of the reciprocal crosses between both selfings verifies that outcrossing was successful for the sequenced sporophytes.
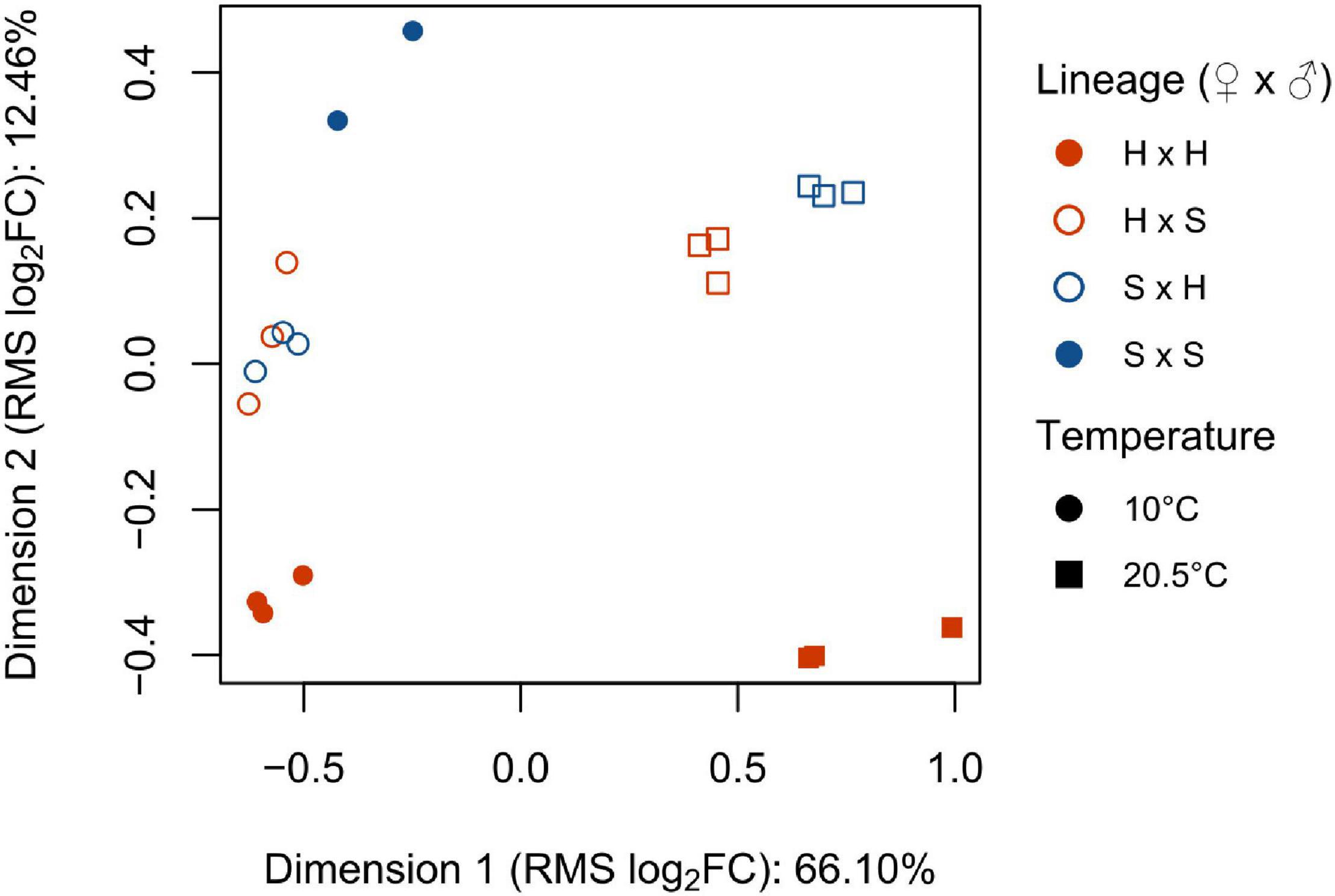
Figure 4. Multidimensional scaling (MDS) plot of gene expression (log counts per million) at 10 and 20.5°C of Laminaria digitata sporophytes produced by crossing females × males from Helgoland (H) and Spitsbergen (S; n = 3; for S × S at 10°C, n = 2). Proportion of variance explained is given for each dimension. Distances on the plot correspond to the root-mean-square average log2-fold-change (RMS log2FC) for all differentially expressed KEGG genes (FDR < 0.01, log2FC ≥ 1).
We assessed general heat responses by analysing differential gene expression between 20.5 and 10°C within each lineage (Figures 5A,B). Gene expression changes in response to 20.5°C was lowest in H × S, where 162 genes were up-regulated and 223 genes were down-regulated compared to the 10°C control. In S × H at 20.5°C, 371 genes were up-regulated and 276 genes were down-regulated, which is in a comparable range to the 388 up-regulated and 355 down-regulated genes in the Helgoland selfing at 20.5°C. Among these, 121 DEGs were constitutively up-regulated across all three lineages, and 131 were constitutively down-regulated (Figure 5B).
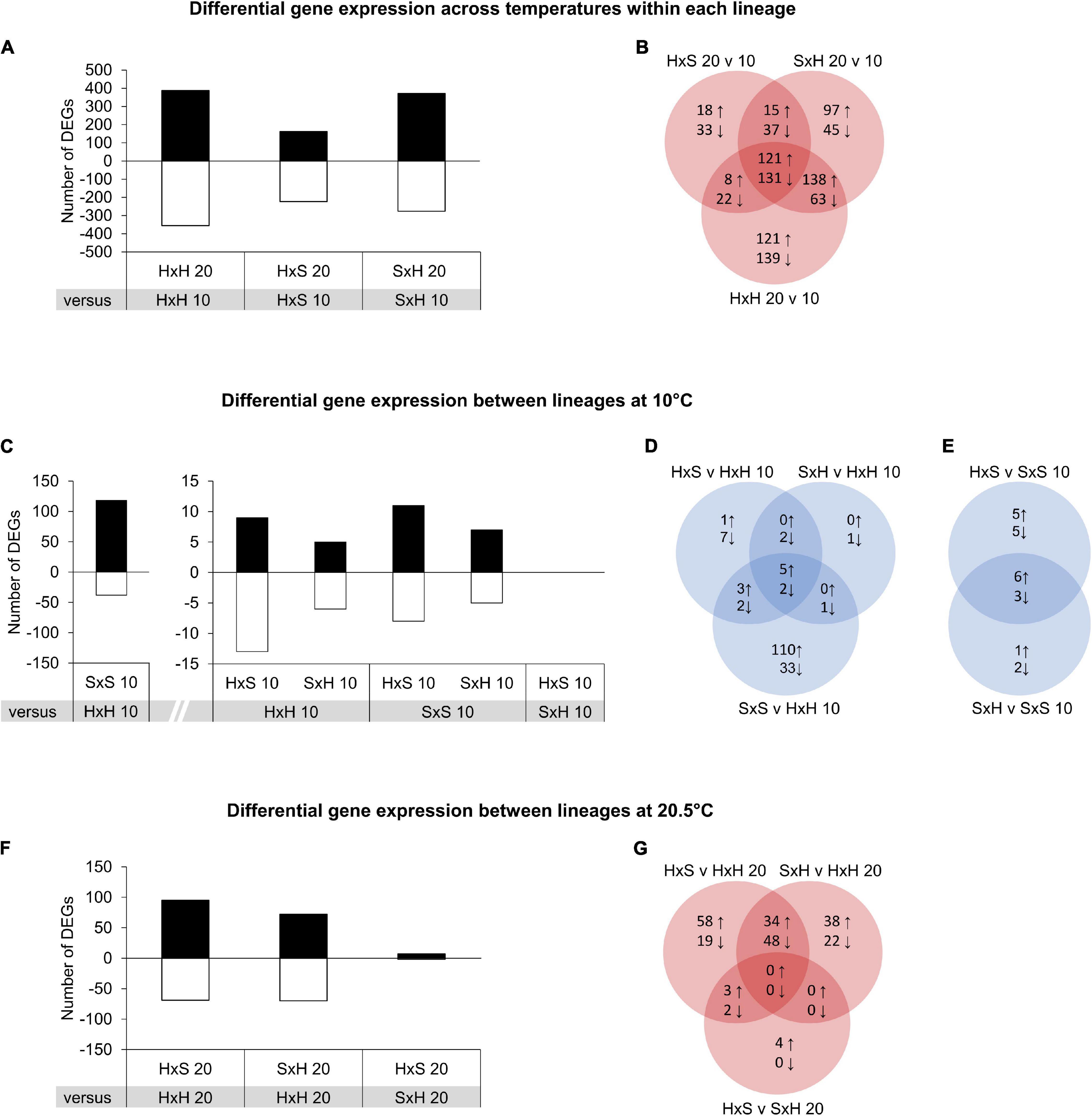
Figure 5. Number of significantly up-regulated (positive, ↑) and down-regulated (negative, ↓) differentially expressed KEGG genes (DEGs; FDR < 0.01, log2FC ≥ 1) in Laminaria digitata sporophytes among comparisons of treatments in experiment 2 displayed as bar plots and Venn diagrams. Treatment abbreviations are composed as female × male from Helgoland (H) and Spitsbergen (S) at 10°C (10) and 20.5°C (20). (A,B) Comparisons within lineages across temperature treatments of 20.5 and 10°C to visualise temperature-dependent gene expression within lineages. (C–E) Comparisons among all lineages at 10°C to visualise baseline differences in gene expression. (F,G) Comparisons of all surviving lineages at 20.5°C to visualise lineage-specific temperature-dependent gene expression.
To identify general gene expression patterns among genotypes of the different locations, we conducted comparisons among all lineages at 10°C (Figures 5C–E). In the Spitsbergen selfing, regulation was strongest as 118 genes were up-regulated and 38 genes were down-regulated compared to the Helgoland selfing (Figure 5C). In contrast, both reciprocal crosses showed reduced gene regulation compared to either selfing, indicating a more generalist response intermediate to the selfings. Compared to the Helgoland selfing at 10°C, H × S up-regulated 9 genes and down-regulated 13 genes at 10°C, whereas S × H up-regulated 5 genes and down-regulated 6 genes at 10°C. When compared to the Spitsbergen selfing at 10°C, H × S up-regulated 11 genes and down-regulated 8 genes, whereas S × H up-regulated 7 genes and down-regulated 5 genes. No genes were differentially expressed among the two reciprocal crosses at 10°C. To investigate potential population-specific regulatory heat responses among the reciprocal crosses, we compared all surviving lineages at 20.5°C (Figures 5F,G). In comparison with the Helgoland selfing, H × S up-regulated 95 genes while 69 genes were down-regulated (Figure 5F). Similarly, in S × H, 72 genes were up-regulated while 70 genes were down-regulated. Among these, both reciprocal crosses shared the up-regulation of 34 KEGG genes and down-regulation of 48 KEGG genes (Figure 5G). In a direct comparison of H × S against S × H at 20.5°C, 7 genes were significantly up-regulated and 2 genes were significantly down-regulated.
Functional Analysis of Differentially Expressed Genes
Constitutive Heat Stress Response Across Lineages
The KEGG genes that were constitutively up- (121 KEGG genes) and down-regulated (131 KEGG genes) at 20.5°C against the 10°C control across the three surviving lineages H × H, H × S and S × H (Figure 5B) corresponded in part to general metabolic pathways shown in Table 4 (for the comprehensive results table, see Supplementary File 1). Expression (expected counts) of single KEGG genes can be investigated by entering the respective KEGG_KO in the interactive Supplementary File 2.
Several KEGG genes related to carbohydrate metabolism were down-regulated (Table 4; in the text, we only refer to the first abbreviation from the “KEGG gene” column for clarity). Expression of key genes of glycolysis (PGK, TPI) and gluconeogenesis (E4.1.1.49) was reduced. Reduced transcription of endo-1,3(4)-beta-glucanase genes indicates a reduced mobilization of the long-term storage compound laminarin. Reduced expression of MNN10 indicates reduced investment into the production of cell wall glycans, which coincides with the reduction of growth rates at high temperature (Figure 2). Across the surviving lineages, six KEGG genes involved with chaperone protein expression were up-regulated at 20.5°C, including two genes related to heat shock protein production (HSP20, HSPBP1). On the other hand, a transcription factor for heat shock proteins (HSF1) was down-regulated along with two genes coding for DnaJ homolog proteins with potential co-chaperone functions. While only two genes involved in cell cycle regulation and DNA structure were up-regulated, taking part in cell division and mitosis (SMC4, CDK7), 23 KEGG genes of this category were down-regulated, most of which are related to mitosis (CDC20, CENPE, WDHD1, YCS4, BRRN1, NCAPD3, NCAPG2, NCAPH2, MCM3, CCNB, MAD2, PLK1, pleD) and/or chromatin and chromosome structure (BRD1, WDHD1, YCS4, BRRN1, NCAPD3, NCAPG2, NCAPH2, HMGB2, H2B, DEK, TTK, SMC2). Several KEGG genes involved in lipid metabolic processes were differentially expressed across lineages at 20.5°C. Eight KEGG genes related to lipid synthesis were down-regulated (fabD, fabZ, AGPS, ELOVL6, GPD1, IPUT1, ppsA, SQD2). Lipid catabolism was affected ambiguously, as the up-regulation of coaE and coaX indicates an increase in Coenzyme A synthesis and an increase in ACAT indicates increased lipid catabolism, but two KEGG genes related to lipid catabolism were down-regulated (HSD17B4, FAO3). The up-regulation of purU indicates a mobilization of lipids into carbohydrates in the glyoxylate cycle. The down-regulation of linoleoyl-CoA desaturase genes indicates decreased fatty acid desaturation and therefore a decrease in polyunsaturated fatty acids (PUFA). Five genes involved in photosynthesis were
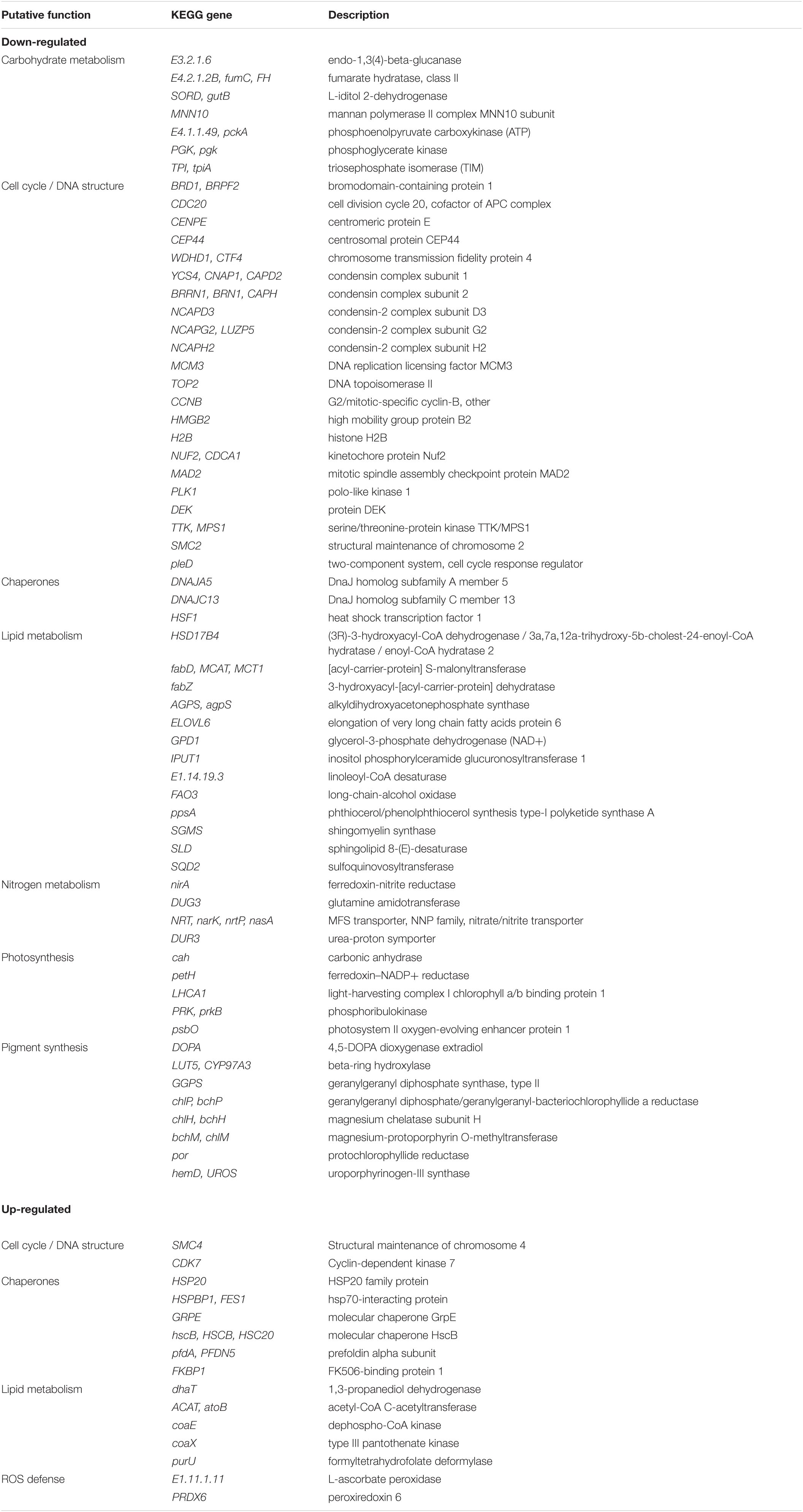
Table 4. Differentially expressed KEGG genes which correspond to functional groups in cellular heat stress responses shared by sporophytes of the Helgoland selfing and both reciprocal crosses among Helgoland and Spitsbergen Laminaria digitata at 20.5°C in comparison to 10°C.
down-regulated. Involved processes include inorganic carbon assimilation (cah), hydrolysis (psbO), light harvesting (LHCA1), photosynthetic electron transfer (petH) and the Calvin cycle (PRK). While expression of genes involved in defense against reactive oxygen species (ROS) were up-regulated, gene expression related to pigment synthesis was down-regulated, especially of genes involved in chlorophyll synthesis (GGPS, chlP, chlH, bchM, por, hemD). Four further KEGG genes related to nitrogen metabolism were down-regulated (Table 4). Additionally, the up-regulation of 29 KEGG genes involved in Ribosome synthesis and 11 genes with a potential function in mitochondrial biogenesis (Supplementary Table 1) indicate increased turnover of proteins and increased metabolic investment into maintenance and repair processes.
Differential Gene Expression Patterns Among Lineages
Conspicuously, in the Spitsbergen selfing at 10°C, 47 KEGG genes related to ribosome biogenesis were up-regulated compared to the Helgoland selfing, whereas only two KEGG genes related to this pathway were down-regulated (Figures 5C,D and Supplementary File 1). In total, 16 DEGs were shared among comparisons of both reciprocal crosses and one selfing against the other (e.g., H × S, S × H, S × S vs. H × H) at the control temperature of 10°C (Figures 5D,E and Supplementary Table 2). These KEGG genes therefore indicate regulation patterns representative of one selfed lineage which may have been inherited by both reciprocal crosses. In comparison to the Helgoland selfing at 20.5°C, both reciprocal crosses shared the up-regulation of 34 KEGG genes and down-regulation of 48 KEGG genes (Figure 5G and Supplementary Table 3). Up-regulated genes were related to functions in DNA structuring and cell cycle (H1_5, SMARCD, TOP2), lipid metabolism (CPT2, ACET6, DGAT2, MGD), photosynthesis (PRK), pigment synthesis (hemL, bchM) and protein metabolism (SPPL2B, PRSS16, NMA111, LRRK2, WDTC1, NTAN1). Down-regulated functions included amino acid metabolism (mmsA, IDO, E3.5.1.1, metE), carbohydrate metabolism (E4.1.3.1, BBOX1) DNA repair and replication (PARP2_3_4, SAMHD1, PRI2), lipid metabolism (PIGL, alkB1_2, THEM4), photosynthetic carbon assimilation (CA) and protein modification (SIL1, ERO1L, FUT8, ALG11). Whether the differential expression of these KEGG genes is specific to the Spitsbergen lineage remains unclear due to the loss of the Spitsbergen selfing at 20.5°C.
Most interestingly, nine KEGG genes were differentially expressed among the two reciprocal crosses at 20.5°C (Figure 5F; Table 5). S × H more highly expressed KEGG genes coding for the meiotic recombination protein DMC1 and for aldehyde dehydrogenase, which can partake in many metabolic pathways related to, e.g., carbohydrates, lipids or amino acids. On the other hand, H × S increased transcription of KEGG genes related to catabolism in the citrate cycle (MDH2), ribosome biogenesis (RRP5) and the production of small heat shock proteins (HSP20). Other KEGG genes were related to the production of secondary metabolites (E2.3.3.10), cytoskeleton proteins (DAW1), protein modification (E.2.7.11) and mitochondrial electron transport (ndh1).
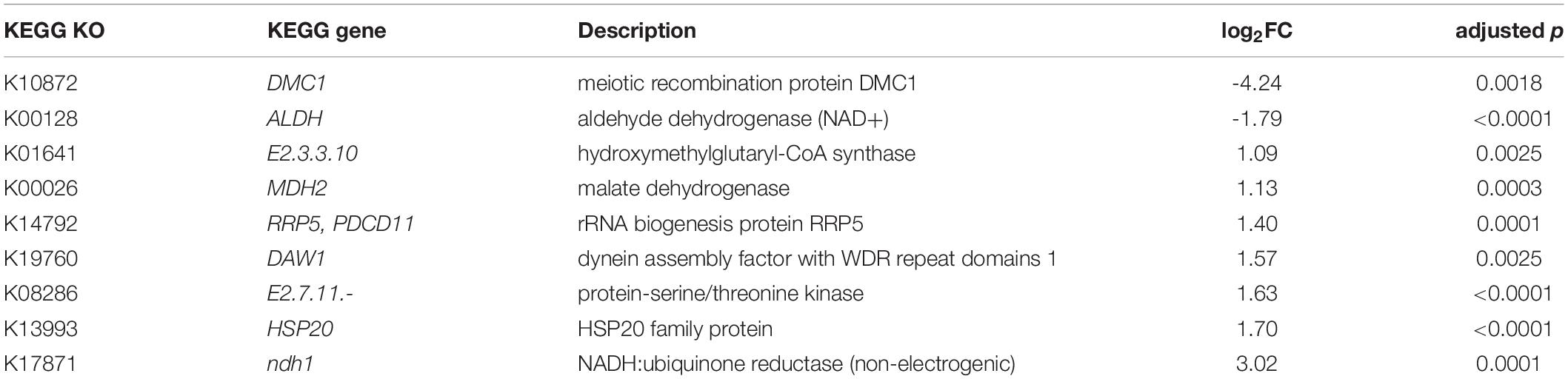
Table 5. Differentially expressed KEGG genes between sporophytes of the two reciprocal crosses among Helgoland and Spitsbergen Laminaria digitata (H × S vs. S × H) at 20.5°C.
Discussion
In this study, we demonstrated the potential beneficial effects of outbreeding among populations within a marine forest key species. Microscopic sporophytes of the inbred Arctic lineage had the lowest thermal tolerance with substantially lower viability at 21 and 22°C and were thereby more susceptible to heat stress than the inbred temperate lineage, which in turn was the only lineage to withstand 23°C over 14 days with marginal survival. This supports earlier studies pointing to ecotypic differentiation between Arctic and temperate populations or may alternatively be explained by inbreeding depression in the Arctic lineage. Interestingly, the heat survival capacity of microscopic sporophytes of both reciprocal crosses among Arctic and temperate L. digitata strains was intermediate to both selfings, and mid-parent heterosis of survival became apparent for the first time in this species. This response was also apparent in growth and optimum quantum yield of macroscopic sporophytes, as both crosses behaved similarly to the inbred temperate lineage, while inbred Arctic sporophytes died within seven days at 19 and 20.5°C. Transcriptomic responses revealed that, despite the similarity of physiological responses, underlying gene regulation differed among lineages. While there were more than 150 DEGs between the selfings at 10°C, both crossed lineages did not differ by more than 22 DEGs from either selfing, indicating a more generalist response. At 20.5°C, the crosses had a specific and substantially different response to the temperate selfing, indicating differential metabolic responses to maintain similar traits in the crosses. Still, the reciprocal crosses did not perform identically during heat stress, as was shown by subtle differences in physiology and by the differential regulation of only nine genes between the outcrossed lineages at 20.5°C.
Inbreeding Depression and Mid-Parent Heterosis
In experiment 1, we identified an upper survival temperature of 21–22°C for microscopic L. digitata sporophytes over 14 days, while we note that a very low percentage of sporophytes survived even at 23°C in all but the Arctic inbred lineage (Figure 1). This is concordant with the published limits for macroscopic, cultivated L. digitata sporophytes (Bolton and Lüning, 1982; tom Dieck, 1992; Martins et al., 2019; Franke et al., 2021). In contrast, the macroscopic sporophytes of the Spitsbergen selfing were even more sensitive to temperatures ≥ 19°C than the reciprocal crosses and the Helgoland selfing. A possible explanation for the reduced tolerance of the Spitsbergen L. digitata sporophytes is inbreeding depression through self-fertilization (Raimondi et al., 2004; Camus et al., 2021). In support of this, the bleaching of all sporophytes of the Spitsbergen selfing after 3 days at 19 and 20.5°C occurred only in experiment 2 and not at comparable temperatures in experiment 1, which complies with the theory that inbreeding depression tends to be more pronounced in later life history phases such as growth and reproduction in routinely selfing species like kelp (Husband and Schemske, 1996; Raimondi et al., 2004). In such species, it is plausible that deleterious alleles affecting the earliest life stages have already been purged by selection (Husband and Schemske, 1996; Raimondi et al., 2004; Camus et al., 2021). For instance, in Macrocystis pyrifera, inbreeding correlated with a decrease of sorus area in fertile sporophytes (Raimondi et al., 2004). In comparison, juvenile macroscopic L. digitata sporophytes obtained by fertilization of several different gametophyte isolates from Spitsbergen survived 22°C over two weeks (Franke et al., 2021), and the upper thermal limit of mature sporophytes along L. digitata’s distribution was recently described as uniform (Liesner et al., 2020a). It has been assumed that genotypic diversity correlates with increased response plasticity among individuals and therefore increased population resilience (Wernberg et al., 2018; Liesner et al., 2020b; Alsuwaiyan et al., 2021), but a potential correlation of intra-individual genetic diversity (i.e., heterozygosity) and environmental tolerance remains to be investigated. An alternative explanation for the reduced thermal tolerance of microscopic and macroscopic S × S sporophytes could be contamination with pathogens (e.g., oomycetes; Gachon et al., 2010; Murúa et al., 2020). However, staining of the affected sporophytes and gametophyte stocks using calcofluor white and DAPI yielded no evidence for the presence of eukaryotic pathogens (C. Gachon, pers. comm.; platform ‘‘My Seaweed Looks Weird’’4). Regardless of the cause of the reduced thermal tolerance of Spitsbergen sporophytes in our experiment, outbreeding with a distantly related genotype (see Liesner et al., 2020a) restored the tolerance of outcrossed sporophytes.
In our study, Arctic L. digitata showed increased gene regulation compared with the cold-temperate Helgoland material at presumed optimum conditions of 10°C (Figure 5C). In contrast, gene regulation in Saccharina latissima from the cold-temperate population of Roscoff was double that of Arctic Spitsbergen samples following gametogenesis, sporophyte recruitment and growth at 8°C for three months (Monteiro et al., 2019b), which indicates different regulation patterns across species. Alternatively, if we interpret the magnitude of gene expression itself as a stress response associated with metabolic costs (Clarke, 2003; Dekel and Alon, 2005; de Nadal et al., 2011), the pattern of increased gene expression in the Spitsbergen selfing might indicate a general disadvantage of this lineage already under control conditions. Local adaptation to cold temperature seems an unlikely explanation, as the growth optimum of juvenile Arctic L. digitata sporophytes was reported at 15°C (Franke et al., 2021). In Arabidopsis lyrata, inbreeding correlated with generally increased gene expression, whereas specifically regulated functions differed between two tested populations (Menzel et al., 2015). A study on Drosophila melanogaster related increased gene expression in inbred individuals to protective responses compensating for inbreeding effects (García et al., 2013), but there are no comparable studies in kelps as yet.
Especially compared to sporophytes from the Spitsbergen selfing, which potentially suffered from inbreeding depression, a benefit of outbreeding may be quantified by applying the concept of mid-parent heterosis on the relatively high survival of the reciprocal crosses in experiment 1 (Figure 1). In experiment 2, the Helgoland selfing and both reciprocal crosses behaved very similarly apart from small, but significant differences in growth rates and optimum quantum yield Fv/Fm (Figures 2, 3). Heterosis has been shown to occur in offspring of closely related kelp species (tom Dieck and de Oliveira, 1993; Martins et al., 2019) and may manifest in different ways. For instance, kelp hybrids of L. digitata and L. pallida showed mid-parent heterosis in optimal growth at 12°C (Martins et al., 2019), but had a 2–3°C higher thermal tolerance than either inbred parent (best-parent heterosis sensu Hochholdinger and Hoecker, 2007). Within a species, outbreeding across populations may alleviate the negative effects of genetic drift and inbreeding depression (Charlesworth and Charlesworth, 1987; Raimondi et al., 2004). Outbreeding among isolates of Saccharina latissima from Helgoland and a less heat tolerant strain from Nova Scotia, Canada, also resulted in offspring with a heat tolerance comparable to the Helgoland strain (Lüning et al., 1978). Beneficial effects of outbreeding have also been shown for the giant kelp Macrocystis pyrifera. For instance, best-parent heterosis significantly increased growth of several lineages of 10-week-old sporophytes produced by crossing gametophyte isolates collected along 250 km of the Chilean coast (Westermeier et al., 2010). However, the potential for outbreeding among natural populations is likely limited in seaweeds due to their low dispersal capacity (Norton, 1992; Billot et al., 2003; King et al., 2018). Still, crossing of few isolates from the same Arctic population of L. digitata as the isolates used here produced offspring sporophytes with a thermal tolerance of 22°C over two weeks (Franke et al., 2021), which supports the theory that for kelps, it is primarily self-fertilization, the most extreme form of inbreeding, that may dramatically lower fitness (Raimondi et al., 2004).
Molecular Heat Stress Response
To investigate how the different lineages maintained a similar phenotype in experiment 2, we examined the genes commonly regulated within all lineages in response to heat stress at 20.5°C compared to 10°C. The functionally annotated genes (Table 4) reflect many known pathways in kelp stress responses (see, e.g., Heinrich et al., 2012b; Salavarría et al., 2018; Li et al., 2019; Monteiro et al., 2019b; Zhang X. et al., 2021). The differential expression of chaperone-related genes such as heat-shock proteins, co-chaperones (GrpE, HscB) and functionally similar proteins (prefoldin alpha, FK506-binding protein) demonstrates that the sporophytes were experiencing stress (e.g., Silberg et al., 1998; Siegert et al., 2000). In contrast, down-regulation of genes with potential heat shock protein or co-chaperone function (DnaJ; Qiu et al., 2006) might be due to a general reduction of HSP expression in longer term temperature acclimation (Heinrich et al., 2015; Monteiro et al., 2019b). The up-regulation of genes coding for two proteins involved in quenching of the reactive oxygen species hydrogen peroxide (H2O2) further supports the expression of cellular stress responses (Collén and Davison, 2001; Rhee et al., 2012). Destructive effects of oxidative stress might have also increased the investment in maintenance and repair processes. For instance, an increased turnover of proteins and mitochondria is indicated by the up-regulation of genes involved in ribosome biogenesis (Supplementary Table 1; Kültz, 2005; Bischof and Rautenberger, 2012) and mitochondrial biogenesis (Supplementary Table 1; Zhang et al., 2009).
Gene expression patterns showed a general reduction of metabolic activity related to carbohydrate metabolism in response to 20.5°C (Table 4). The down-regulation of key genes participating in the citric acid cycle (fumarate hydratase; Nast and Müller-Röber, 1996), and glycolysis and gluconeogenesis (PEP carboxykinase, phosphoglycerate kinase, triosephosphate isomerase; Boldt et al., 1992; Gómez and Huovinen, 2012) indicates that both catabolic and anabolic carbohydrate pathways were down-regulated. Investment in cell wall glycan production was reduced (mannan polymerase II complex MNN10 subunit; Jungmann et al., 1999), as was mobilization of the long-term storage compound laminarin (endo-1,3-beta-glucanase; Chesters and Bull, 1963; Martín-Cuadrado et al., 2008). In combination with the reduction of assimilative processes indicated by the down-regulation of carbonic anhydrase (Raven, 1991; Badger and Price, 1994) and genes related to nitrogen uptake (ferredoxin-nitrite reductase, Lomas and Glibert, 2000; nitrate/nitrite transporter, Fukuda et al., 2015) these results support a regulation of the metabolism in favour of cell maintenance rather than growth (Kültz, 2005), as is supported by the reduced growth rates in experiment 2 (Figure 2) and by the reduced expression of several genes related to mitosis (Table 4).
The regulation of genes involved in lipid metabolism indicates that, in addition to reduction of anabolism, cell and plastid membrane properties were altered in response to heat (Table 4). Neutral lipid contents (i.e., storage lipids) in vegetative kelp sporophyte tissue are generally low, but may still be mobilised for metabolic processes in the meristem (Haug and Jensen, 1954; Velimirov, 1979). The up-regulation of acetyl-CoA C-acetyltransferase and two genes involved in CoA synthesis might indicate an increase of fatty acid degradation (but note the metabolic versatility of CoA; Blanco and Blanco, 2017), while lipid synthesis was strongly down-regulated, which further indicates a reduction of anabolism. As polyunsaturated fatty acids (PUFAs) and high temperature increase cell membrane fluidity, decreasing the expression of a lipid desaturase (linoleoyl-CoA desaturase) might maintain cell membrane rigidity in response to stress (Neidleman, 1987; Tatsuzawa et al., 1996; Maulucci et al., 2016). Modifications to sulfolipid (sulfoquinovosyltransferase) and glycolipid metabolism (1,3-propanediol dehydrogenase) indicate the maintenance of photosynthetic membranes in the chloroplasts (Boudière et al., 2014; Zhan et al., 2019).
Regulation of chlorophyll biosynthesis is a known response to several stressors such as hyposalinity, temperature, PAR and UV radiation in the seaweeds Saccharina latissima (Heinrich et al., 2015; Monteiro et al., 2019b) and Desmarestia anceps (Iñiguez et al., 2017). Here, a reduction of chlorophyll biosynthesis was indicated in response to heat by the down-regulation of, among others, genes coding for the key enzymes magnesium chelatase (Pontier et al., 2007; Stenbaek and Jensen, 2010) and protochlorophyllide reductase (Begley and Young, 1989). Whereas growth at sublethal temperature may increase chlorophyll biosynthesis in kelps (Li et al., 2019), the reduction in our study may be interpreted as a heat stress-induced impairment of chlorophyll synthesis (Tewari and Tripathy, 1998). The thermal optimum of photosynthesis is often higher than that of growth (Davison, 1987; Graiff et al., 2015), so the fact that key processes of photosynthesis were down-regulated in our study indicates that this was not a response to increased temperature, but a general stress response, as has been described for plants and kelps (e.g., Mickelbart et al., 2015; Monteiro et al., 2019b). Still, the stability of optimum quantum yield at 20.5°C in this study suggests that regulation of the above genes may have maintained photosynthetic efficiency even under high thermal stress (Figure 3).
Differential Gene Expression Between the Reciprocal Crosses
KEGG genes linked to a variety of pathways were differentially regulated between the reciprocal crosses at 20.5°C (Figure 5F; Table 5). In combination, these differential regulations may in part be responsible for the subtly increased heat tolerance of only the H × S lineage versus the H × H lineage after 17 days at 20.5°C (Figure 2C). This became evident only under heat stress and not at the control temperature of 10°C (i.e., 0 DEGs among the reciprocal crosses at 10°C; Figure 5C). The increased expression of heat shock protein 20 in H × S (and H × H; Supplementary File 1) compared to S × H at 20.5°C may be related to population-specific responses in HSP expression previously described for HSP70 among British L. digitata populations (King et al., 2019). One of the many functions described for aldehyde dehydrogenase (ALDH) is scavenging of toxic aldehydes produced by interactions of ROS with lipids, proteins and nucleic acids (Sunkar et al., 2003). Reduced expression of ALDH genes in H × S might therefore indicate either low levels of oxidative stress or a high tolerance capacity. In contrast, increased terpene biosynthesis in H × S, as indicated by expression of transcripts coding for hydroxymethylglutaryl-CoA synthase, might indicate defense reactions or increased antioxidant capacity (Fisch et al., 2003; de Oliveira et al., 2017). As protein-serine/threonine kinases are involved in stress-related signaling cascades (Graves et al., 1995; Bischof et al., 2019), the increased expression of related genes in H × S indicates a stronger response to heat stress than in S × H. The increased expression of other KEGG genes in H × S points toward measures of metabolic maintenance. NADH:ubiquinone reductase is involved in cyclic electron transport in photosystem I (Shikanai and Aro, 2016; Zhao et al., 2018), while the expression of RRP5 indicates increased ribosome biogenesis (Missbach et al., 2013). Malate dehydrogenase is a key enzyme in the citric acid cycle (Musrati et al., 1998), the maintenance of which has been shown to be correlated to heat tolerance in land plants (Xu and Huang, 2010). The dynein assembly factor DAW1 has been previously described as sex-biased during fertility of male gametophytes and was linked to flagella production in sperm (Pearson et al., 2019). In H × S at 20.5°C, the higher expression of DAW1 stands in contrast to the reduced expression of DMC1 coding for a meiotic recombination protein, which is reduced under heat stress during fertility in Arabidopsis (Ning et al., 2021). However, we conducted the experiment with juvenile sporophytes which do not produce meiospores, leaving the specific function of these two transcripts unclear.
Female and male gametophytes may contribute differently to sporophyte traits. Martins et al. (2019) suggested a female-specific component to thermal inheritance in kelps, because growth rates of sporophytes from interspecific crosses of L. digitata and the warm-temperate L. pallida in part responded more closely to the female parent at sublethal temperatures. Here, such an effect may explain the subtle differences in physiological and molecular traits between the reciprocal crosses. Sex-biased gene expression has been described in the haploid, sexual gametophytes of the kelp S. latissima (Monteiro et al., 2019a; Pearson et al., 2019). It remains hypothetical whether a female-specific inheritance pattern in diploid, asexual sporophytes may be related to a potential cyto-nuclear compatibility, in which the optimal function of mitochondria and plastids inherited via eggs depends on female-associated nuclear genes.
Implications of Outbreeding for Mariculture and Marine Forest Conservation
Our results imply that outbreeding among differentiated kelp lineages and populations may mitigate effects of genetic drift and may increase stress tolerance in comparison to fully inbred lineages. Interestingly, this became already apparent by combining just two clonal lineages from diverged populations. As shown by the subtly increased performance of the H × S lineage at thermal stress, performance during heat stress may even improve by outbreeding with an Arctic population. This adds to the extensive knowledge from terrestrial agriculture and recently also kelp mariculture, that inter-cultivar crosses may produce stable, healthy descendants with superior characteristics compared to their inbred parental lineages (Li et al., 2007; Westermeier et al., 2010; Fu et al., 2014). However, to reduce response variation, produce stable phenotypes and maximise heterosis, homozygous lines should be inbred over several generations before application in mariculture (Robinson et al., 2013), and investigations on the optimal genetic distance should be conducted (e.g., intra- vs. inter-population crosses; Li et al., 2008). Meanwhile, natural kelp populations, including Laminaria digitata, are threatened by ocean warming especially at the warm range edges (Raybaud et al., 2013; Voerman et al., 2013; Wernberg et al., 2016; Assis et al., 2018; Smale et al., 2019). In addition to gradual ocean warming, increasingly intense and frequent marine heatwaves (Hobday et al., 2016; Oliver et al., 2018) may reduce genetic diversity of persistent populations (Coleman et al., 2020a; Gurgel et al., 2020). An ecologically cautious method for conservation of threatened natural populations may therefore be an introduction of intraspecific cultivars from distant populations in an attempt of assisted evolution (Filbee-Dexter and Smajdor, 2019; Coleman et al., 2020b).
An important caveat regarding assisted evolution is the potential for outbreeding depression in crosses of genetically diverged, locally adapted populations. In contrast to inbreeding depression, outbreeding depression describes the reduced performance of offspring of genetically diverged lineages due to the disruption of interactive gene complexes or local adaptation (McKay et al., 2005; Aitken and Whitlock, 2013). As yet, outbreeding depression could not be identified among distant populations of the fucoid seaweed Hormosira banksii (McKenzie and Bellgrove, 2006), and was also not obvious in performance of the reciprocal crosses here. This suggests that genetic divergence among L. digitata populations is low enough to maintain genetic compatibility, despite the strong spatial structuring and thermal gradient which acts along the species’ distribution gradient (Liesner et al., 2020a). Alternatively, outbreeding depression may manifest later in the life cycle especially with respect to reproduction (Schierup and Christiansen, 1996; McKenzie and Bellgrove, 2006). Therefore, careful assessments of the performance and viability of numerous outbred lineages covering a gradient of genetic differentiation are necessary across several generations before considering an application in mariculture or conservation.
Data Availability Statement
The datasets presented in this study can be found in online repositories. The Illumina sequence reads generated during this study have been published in the NCBI Sequencing Read Archive (SRA) under the accession PRJNA665130. The physiological datasets have been published at PANGAEA (https://doi.pangaea.de/10.1594/PANGAEA.940559).
Author Contributions
DL, IB, SH, GG, and KV planned and designed the experiments. DL conducted the experiments and RNA extraction with help from SR and SH, conducted the physiological data analysis, and the differential gene expression analysis with help from GP, GG, and LH, and wrote the manuscript, which was reviewed and revised by all authors. GP assembled the de novo transcriptome. IB, GP, GG, KB, and KV contributed to the data interpretation and discussion. All authors contributed to the article and approved the submitted version.
Funding
This research was funded through the 2015–2016 BiodivERsA COFUND call for research proposals (program MARFOR), with the national funder German Research Foundation (DFG; grant no. VA 105/25-1). We acknowledge support from the Open Access Publication Funds of Alfred Wegener Institute, Helmholtz Centre for Polar and Marine Research.
Conflict of Interest
The authors declare that the research was conducted in the absence of any commercial or financial relationships that could be construed as a potential conflict of interest.
Publisher’s Note
All claims expressed in this article are solely those of the authors and do not necessarily represent those of their affiliated organizations, or those of the publisher, the editors and the reviewers. Any product that may be evaluated in this article, or claim that may be made by its manufacturer, is not guaranteed or endorsed by the publisher.
Nagoya Protocol Statement
The Norwegian regulation on the access and benefit-sharing of genetic resources has not yet entered into force. We complied with our due diligence by sending an inquiry to the national authorities. In 2020, they replied that no permits are required and this statement was officially confirmed to our institute by the Norwegian National Focal Point in late 2021.
Acknowledgments
We thank Andreas Wagner for culture management, Myriam Valero for discussions about inbreeding depression, Cátia Monteiro and Huiru Li for support in RNA extraction, and Clément Gauci for statistical support.
Supplementary Material
The Supplementary Material for this article can be found online at: https://www.frontiersin.org/articles/10.3389/fmars.2022.838793/full#supplementary-material
Supplementary Figure 1 | Cluster heatmaps based on a distance matrix of KEGG gene expression in replicates (a–c) of Laminaria digitata sporophytes produced by crossing females × males from Helgoland (H) and Spitsbergen (S) in experiment 2 at 10 and 20.5°C (A) including and (B) excluding the outlier replicate S × S 10 c.
Supplementary Table 1 | Differentially expressed KEGG genes which correspond to functional groups of protein metabolism and biogenesis of mitochondria and ribosomes shared by sporophytes of the Helgoland selfing and both reciprocal crosses among Helgoland and Spitsbergen Laminaria digitata at 20.5°C in comparison to 10°C.
Supplementary Table 2 | Differentially expressed KEGG genes common to the comparisons of both reciprocal crosses and one selfing against the remaining selfing at 10°C, which indicates lineage-specific regulation patterns.
Supplementary Table 3 | Differentially expressed KEGG genes shared by both reciprocal crosses at 20.5°C in comparison to the Helgoland selfing at 20.5°C, which indicates regulation patterns specific for the Spitsbergen lineage.
Supplementary File 1 | Comprehensive results table of KEGG gene annotations and differentially expressed genes in all comparisons of Laminaria digitata sporophytes produced by crossing females × males from Helgoland (H) and Spitsbergen (S) in experiment 2 at 10 and 20.5°C.
Supplementary File 2 | Interactive table to plot expression values (expected counts) of each annotated KEGG gene for Laminaria digitata sporophytes produced by crossing females × males from Helgoland (H) and Spitsbergen (S) in experiment 2 at 10 and 20.5°C.
Footnotes
- ^ https://gvolante.riken.jp
- ^ https://www.uniprot.org
- ^ https://www.genome.jp/kegg/kaas/
- ^ https://www.globalseaweed.org/
References
Aitken, S. N., and Whitlock, M. C. (2013). Assisted gene flow to facilitate local adaptation to climate change. Annu. Rev. Ecol. Evol. Syst. 44, 367–388. doi: 10.1146/annurev-ecolsys-110512-135747
Alsuwaiyan, N., Vranken, S., Filbee-Dexter, K., Cambridge, M., Coleman, M., and Wernberg, T. (2021). Genotypic variation in response to extreme events may facilitate kelp adaptation under future climates. Mar. Ecol. Prog. Ser. 672, 111–121. doi: 10.3354/meps13802
Assis, J., Araújo, M. B., and Serrão, E. A. (2018). Projected climate changes threaten ancient refugia of kelp forests in the North Atlantic. Glob. Chang. Biol. 24, e55–e66. doi: 10.1111/gcb.13818
Badger, M. R., and Price, G. D. (1994). The role of carbonic anhydrase in photosynthesis. Annu. Rev. Plant Physiol. Plant Mol. Biol. 45, 369–392. doi: 10.1146/annurev.pp.45.060194.002101
Bartsch, I., Vogt, J., Pehlke, C., and Hanelt, D. (2013). Prevailing sea surface temperatures inhibit summer reproduction of the kelp Laminaria digitata at Helgoland (North Sea). J. Phycol. 49, 1061–1073. doi: 10.1111/jpy.12125
Begley, T. P., and Young, H. (1989). Protochlorophyllide reductase. 1. Determination of the regiochemistry and the stereochemistry of the reduction of protochlorophyllide to chlorophyllide. J. Am. Chem. Soc. 111, 3095–3096. doi: 10.1021/ja00190a071
Benjamini, Y., and Hochberg, Y. (1995). Controlling the false discovery rate: a practical and powerful approach to multiple testing. J. R. Stat. Soc. Ser. B 57, 289–300. doi: 10.2307/2346101
Billot, C., Engel, C. R., Rousvoal, S., Kloareg, B., and Valero, M. (2003). Current patterns, habitat discontinuities and population genetic structure: the case of the kelp Laminaria digitata in the English Channel. Mar. Ecol. Prog. Ser. 253, 111–121. doi: 10.3354/meps253111
Bischof, K., and Rautenberger, R. (2012). “Seaweed responses to environmental stress: reactive oxygen and antioxidative strategies,” in Seaweed Biology: Novel Insights into Ecophysiology, Ecology and Utilization, eds C. Wiencke and K. Bischof (Berlin: Springer), 109–132. doi: 10.1007/978-3-642-28451-9_6
Bischof, K., Buschbaum, C., Fredriksen, S., Gordillo, F. J. L., Heinrich, S., Jiménez, C., et al. (2019). “Kelps and environmental changes in Kongsfjorden: stress perception and responses,” in The Ecosystem of Kongsfjorden, Svalbard, eds H. Hop and C. Wiencke (Cham: Springer International Publishing), 373–422. doi: 10.1007/978-3-319-46425-1_10
Blanco, A., and Blanco, G. (2017). “Lipid metabolism,” in Medical biochemistry, eds A. Blanco and G. Blanco (Cambridge: Academic Press), 325–365. doi: 10.1016/B978-0-12-803550-4.00015-X
Boldt, R., Börner, T., and Schnarrenberger, C. (1992). Repression of the plastidic isoenzymes of aldolase, 3-phosphoglycerate kinase, and triosephosphate isomerase in the barley mutant “albostrians.”. Plant Physiol. 99, 895–900. doi: 10.1104/pp.99.3.895
Bolger, A. M., Lohse, M., and Usadel, B. (2014). Trimmomatic: a flexible trimmer for Illumina sequence data. Bioinformatics 30, 2114–2120. doi: 10.1093/bioinformatics/btu170
Bolton, J. J., and Lüning, K. (1982). Optimal growth and maximal survival temperatures of Atlantic Laminaria species (Phaeophyta) in culture. Mar. Biol. 66, 89–94. doi: 10.1007/BF00397259
Boudière, L., Michaud, M., Petroutsos, D., Rébeillé, F., Falconet, D., Bastien, O., et al. (2014). Glycerolipids in photosynthesis: composition, synthesis and trafficking. Biochim. Biophys. Acta Bioenerg. 1837, 470–480. doi: 10.1016/j.bbabio.2013.09.007
Buschmann, A. H., Camus, C., Infante, J., Neori, A., Israel, Á, Hernández-González, M. C., et al. (2017). Seaweed production: overview of the global state of exploitation, farming and emerging research activity. Eur. J. Phycol. 52, 391–406. doi: 10.1080/09670262.2017.1365175
Bushmanova, E., Antipov, D., Lapidus, A., and Prjibelski, A. D. (2019). rnaSPAdes: a de novo transcriptome assembler and its application to RNA-Seq data. Gigascience 8, 1–13. doi: 10.1093/gigascience/giz100
Camus, C., Solas, M., Martínez, C., Vargas, J., Garcés, C., Gil-Kodaka, P., et al. (2021). Mates matter: gametophyte kinship recognition and inbreeding in the giant kelp, Macrocystis pyrifera (Laminariales, Phaeophyceae). J. Phycol. 57, 711–725. doi: 10.1111/jpy.13146
Charlesworth, D., and Charlesworth, B. (1987). Inbreeding depression and its evolutionary consequences. Annu. Rev. Ecol. Syst. 18, 237–268. doi: 10.1146/annurev.es.18.110187.001321
Chesters, C. G. C., and Bull, A. T. (1963). The enzymic degradation of laminarin. 1. The distribution of laminarinase among micro-organisms. Biochem. J. 86, 28–31. doi: 10.1042/bj0860028
Clarke, A. (2003). Costs and consequences of evolutionary temperature adaptation. Trends Ecol. Evol. 18, 573–581. doi: 10.1016/j.tree.2003.08.007
Coelho, S. M., Gueno, J., Lipinska, A. P., Cock, J. M., and Umen, J. G. (2018). UV chromosomes and haploid sexual systems. Trends Plant Sci. 23, 794–807. doi: 10.1016/j.tplants.2018.06.005
Coleman, M. A., Minne, A. J. P., Vranken, S., and Wernberg, T. (2020a). Genetic tropicalisation following a marine heatwave. Sci. Rep. 10:12726. doi: 10.1038/s41598-020-69665-w
Coleman, M. A., Wood, G., Filbee-Dexter, K., Minne, A. J. P., Goold, H. D., Vergés, A., et al. (2020b). Restore or redefine: future trajectories for restoration. Front. Mar. Sci. 7:237. doi: 10.3389/fmars.2020.00237
Collén, J., and Davison, I. R. (2001). Seasonality and thermal acclimation of reactive oxygen metabolism in Fucus vesiculosus (Phaeophyceae). J. Phycol. 37, 474–481. doi: 10.1046/j.1529-8817.2001.037004474.x
Dankworth, M., Heinrich, S., Fredriksen, S., and Bartsch, I. (2020). DNA barcoding and mucilage ducts in the stipe reveal the presence of Hedophyllum nigripes (Laminariales, Phaeophyceae) in Kongsfjorden (Spitsbergen). J. Phycol. 56, 1245–1254. doi: 10.1111/jpy.13012
Davison, I. R. (1987). Adaptation of photosynthesis in Laminaria saccharina (Phaeophyta) to changes in growth temperature. J. Phycol. 23, 273–283. doi: 10.1111/j.1529-8817.1987.tb04135.x
de Nadal, E., Ammerer, G., and Posas, F. (2011). Controlling gene expression in response to stress. Nat. Rev. Genet. 12, 833–845. doi: 10.1038/nrg3055
de Oliveira, L. S., Tschoeke, D. A., Magalhães Lopes, A. C. R., Sudatti, D. B., Meirelles, P. M., Thompson, C. C., et al. (2017). Molecular mechanisms for microbe recognition and defense by the red seaweed Laurencia dendroidea. mSphere 2:e00094-17. doi: 10.1128/mSphere.00094-17
Dekel, E., and Alon, U. (2005). Optimality and evolutionary tuning of the expression level of a protein. Nature 436, 588–592. doi: 10.1038/nature03842
Filbee-Dexter, K., and Smajdor, A. (2019). Ethics of assisted evolution in marine conservation. Front. Mar. Sci. 6:20. doi: 10.3389/fmars.2019.00020
Fisch, K. M., Böhm, V., Wright, A. D., and König, G. M. (2003). Antioxidative meroterpenoids from the brown alga Cystoseira crinita. J. Nat. Prod. 66, 968–975. doi: 10.1021/np030082f
Franke, K., Liesner, D., Heesch, S., and Bartsch, I. (2021). Looks can be deceiving: contrasting temperature characteristics of two morphologically similar kelp species co-occurring in the Arctic. Bot. Mar. 64, 163–175. doi: 10.1515/bot-2021-0014
Fu, D., Xiao, M., Hayward, A., Fu, Y., Liu, G., Jiang, G., et al. (2014). Utilization of crop heterosis: a review. Euphytica 197, 161–173. doi: 10.1007/s10681-014-1103-7
Fujimoto, R., Uezono, K., Ishikura, W., Osabe, K., Peacock, W. J., and Dennis, E. S. (2018). Recent research on the mechanism of heterosis is important for crop and vegetable breeding systems. Breed. Sci. 68, 145–158. doi: 10.1270/jsbbs.17155
Fukuda, M., Takeda, H., Kato, H. E., Doki, S., Ito, K., Maturana, A. D., et al. (2015). Structural basis for dynamic mechanism of nitrate/nitrite antiport by NarK. Nat. Commun. 6:7097. doi: 10.1038/ncomms8097
Gachon, C. M. M., Sime-Ngando, T., Strittmatter, M., Chambouvet, A., and Kim, G. H. (2010). Algal diseases: spotlight on a black box. Trends Plant Sci. 15, 633–640. doi: 10.1016/j.tplants.2010.08.005
García, C., Ávila, V., Quesada, H., and Caballero, A. (2013). Are transcriptional responses to inbreeding a functional response to alleviate inbreeding depression? Fly 7, 8–12. doi: 10.4161/fly.22559
Gómez, I., and Huovinen, P. (2012). “Morpho-functionality of carbon metabolism in seaweeds,” in Seaweed Biology: Novel Insights into Ecophysiology, Ecology and Utilization, eds C. Wiencke and K. Bischof (Berlin: Springer), 25–46. doi: 10.1007/978-3-642-28451-9_2
Graiff, A., Liesner, D., Karsten, U., and Bartsch, I. (2015). Temperature tolerance of western Baltic Sea Fucus vesiculosus - growth, photosynthesis and survival. J. Exp. Mar. Bio. Ecol. 471, 8–16. doi: 10.1016/j.jembe.2015.05.009
Graves, J. D., Campbell, J. S., and Krebs, E. G. (1995). Protein serine/threonine kinases of the MAPK cascade. Ann. N. Y. Acad. Sci. 766, 320–343. doi: 10.1111/j.1749-6632.1995.tb26684.x
Gurgel, C. F. D., Camacho, O., Minne, A. J. P., Wernberg, T., and Coleman, M. A. (2020). Marine heatwave drives cryptic loss of genetic diversity in underwater forests. Curr. Biol. 30, 1199–1206.e2. doi: 10.1016/j.cub.2020.01.051
Hanelt, D., Tüg, H., Bischof, K., Groß, C., Lippert, H., Sawall, T., et al. (2001). Light regime in an Arctic fjord: a study related to stratospheric ozone depletion as a basis for determination of UV effects on algal growth. Mar. Biol. 138, 649–658. doi: 10.1007/s002270000481
Haug, A., and Jensen, A. (1954). Seasonal Variations in the Chemical Composition of Alaria esculenta, Laminaria saccharina, Laminaria hyperborea and Laminaria digitata From Northern Norway. Nor. Inst. Tang- og Tareforsk., Report No. 4. Oslo: Akademisk Trykningssentral.
Heinrich, S. (2018). “Extraction of high quality RNA from brown algae for transcriptomic analysis,” in Protocols for Macroalgae Research, eds B. Charrier, T. Wichard, and C. R. K. Reddy (Boca Raton, FL: CRC Press), 429–439. doi: 10.1201/b21460-28
Heinrich, S., Frickenhaus, S., Glöckner, G., and Valentin, K. (2012a). A comprehensive cDNA library of light- and temperature-stressed Saccharina latissima (Phaeophyceae). Eur. J. Phycol. 47, 83–94. doi: 10.1080/09670262.2012.660639
Heinrich, S., Valentin, K., Frickenhaus, S., and Wiencke, C. (2015). Temperature and light interactively modulate gene expression in Saccharina latissima (Phaeophyceae). J. Phycol. 51, 93–108. doi: 10.1111/jpy.12255
Heinrich, S., Valentin, K., Frickenhaus, S., John, U., and Wiencke, C. (2012b). Transcriptomic analysis of acclimation to temperature and light stress in Saccharina latissima (Phaeophyceae). PLoS One 7:e44342. doi: 10.1371/journal.pone.0044342
Hobday, A. J., Alexander, L. V., Perkins, S. E., Smale, D. A., Straub, S. C., Oliver, E. C. J., et al. (2016). A hierarchical approach to defining marine heatwaves. Prog. Oceanogr. 141, 227–238. doi: 10.1016/j.pocean.2015.12.014
Hochholdinger, F., and Hoecker, N. (2007). Towards the molecular basis of heterosis. Trends Plant Sci. 12, 427–432. doi: 10.1016/j.tplants.2007.08.005
Husband, B. C., and Schemske, D. W. (1996). Evolution of the magnitude and timing of inbreeding depression in plants. Evolution 50, 54–70. doi: 10.1111/j.1558-5646.1996.tb04472.x
Iñiguez, C., Heinrich, S., Harms, L., and Gordillo, F. J. L. (2017). Increased temperature and CO2 alleviate photoinhibition in Desmarestia anceps: from transcriptomics to carbon utilization. J. Exp. Bot. 68, 3971–3984. doi: 10.1093/jxb/erx164
Jungmann, J., Rayner, J. C., and Munro, S. (1999). The Saccharomyces cerevisiae protein Mnn10p/Bed1p is a subunit of a Golgi mannosyltransferase complex. J. Biol. Chem. 274, 6579–6585. doi: 10.1074/jbc.274.10.6579
Kim, J., Kraemer, G., and Yarish, C. (2015). Use of sugar kelp aquaculture in Long Island sound and the bronx river estuary for nutrient extraction. Mar. Ecol. Prog. Ser. 531, 155–166. doi: 10.3354/meps11331
King, N. G., McKeown, N. J., Smale, D. A., and Moore, P. J. (2018). The importance of phenotypic plasticity and local adaptation in driving intraspecific variability in thermal niches of marine macrophytes. Ecography 41, 1469–1484. doi: 10.1111/ecog.03186
King, N. G., McKeown, N. J., Smale, D. A., Bradbury, S., Stamp, T., Jüterbock, A., et al. (2020). Hierarchical genetic structuring in the cool boreal kelp, Laminaria digitata: implications for conservation and management. ICES J. Mar. Sci. 77, 1906–1913. doi: 10.1093/icesjms/fsaa055
King, N. G., McKeown, N. J., Smale, D. A., Wilcockson, D. C., Hoelters, L., Groves, E. A., et al. (2019). Evidence for different thermal ecotypes in range centre and trailing edge kelp populations. J. Exp. Mar. Bio. Ecol. 51, 10–17. doi: 10.1016/j.jembe.2019.03.004
Kliebenstein, D. (2009). Quantitative genomics: analyzing intraspecific variation using global gene expression polymorphisms or eQTLs. Annu. Rev. Plant Biol. 60, 93–114. doi: 10.1146/annurev.arplant.043008.092114
Krause-Jensen, D., and Duarte, C. M. (2016). Substantial role of macroalgae in marine carbon sequestration. Nat. Geosci. 9, 737–742. doi: 10.1038/ngeo2790
Kültz, D. (2005). Molecular and evolutionary basis of the cellular stress response. Annu. Rev. Physiol. 67, 225–257. doi: 10.1146/annurev.physiol.67.040403.103635
Langmead, B., and Salzberg, S. L. (2012). Fast gapped-read alignment with Bowtie 2. Nat. Methods 9, 357–359. doi: 10.1038/nmeth.1923
Li, B., and Dewey, C. N. (2011). RSEM: accurate transcript quantification from RNA-Seq data with or without a reference genome. BMC Bioinformatics 12:323. doi: 10.1186/1471-2105-12-323
Li, H., Monteiro, C., Heinrich, S., Bartsch, I., Valentin, K., Harms, L., et al. (2019). Responses of the kelp Saccharina latissima (Phaeophyceae) to the warming Arctic: from physiology to transcriptomics. Physiol. Plant. 168, 5–26. doi: 10.1111/ppl.13009
Li, X., Cong, Y., Yang, G., Shi, Y., Qu, S., Li, Z., et al. (2007). Trait evaluation and trial cultivation of Dongfang No. 2, the hybrid of a male gametophyte clone of Laminaria longissima (Laminariales, Phaeophyta) and a female one of L. japonica. J. Appl. Phycol. 19, 139–151. doi: 10.1007/s10811-006-9120-0
Li, X., Yang, G., Shi, Y., Cong, Y., Che, S., Qu, S., et al. (2008). Prediction of the heterosis of Laminaria hybrids with the genetic distance between their parental gametophyte clones. J. Appl. Phycol. 20, 1097–1102. doi: 10.1007/s10811-008-9321-9
Liesner, D., Fouqueau, L., Valero, M., Roleda, M. Y., Pearson, G. A., Bischof, K., et al. (2020a). Heat stress responses and population genetics of the kelp Laminaria digitata (Phaeophyceae) across latitudes reveal differentiation among North Atlantic populations. Ecol. Evol. 10, 9144–9177. doi: 10.1002/ece3.6569
Liesner, D., Shama, L. N. S., Diehl, N., Valentin, K., and Bartsch, I. (2020b). Thermal plasticity of the kelp Laminaria digitata (Phaeophyceae) across life cycle stages reveals the importance of cold seasons for marine forests. Front. Mar. Sci. 7:456. doi: 10.3389/fmars.2020.00456
Lipinska, A., Cormier, A., Peters, A. F., Corre, E., Gachon, C. M. M., Cock, J. M., et al. (2015). Sexual dimorphism and the evolution of sex-biased gene expression in the brown alga Ectocarpus. Mol. Biol. Evol. 32, 1581–1597. doi: 10.1093/molbev/msv049
Lomas, M. W., and Glibert, P. M. (2000). Comparisons of nitrate uptake, storage, and reduction in marine diatoms and flagellates. J. Phycol. 36, 903–913. doi: 10.1046/j.1529-8817.2000.99029.x
Lüning, K. (1984). Temperature tolerance and biogeography of seaweeds: the marine algal flora of Helgoland (North Sea) as an example. Helgoländer Meeresunters. 38, 305–317. doi: 10.1007/BF01997486
Lüning, K. (1990). Seaweeds: Their Environment, Biogeography, and Ecophysiology. New York, NY: John Wiley & Sons.
Lüning, K., Chapman, A. R. O., and Mann, K. H. (1978). Crossing experiments in the non-digitate complex of Laminaria from both sides of the Atlantic. Phycologia 17, 293–298. doi: 10.2216/i0031-8884-17-3-293.1
Martín-Cuadrado, A.-B., Fontaine, T., Esteban, P.-F., del Dedo, J. E., de Medina-Redondo, M., del Rey, F., et al. (2008). Characterization of the endo-β-1,3-glucanase activity of S. cerevisiae Eng2 and other members of the GH81 family. Fungal Genet. Biol. 45, 542–553. doi: 10.1016/j.fgb.2007.09.001
Martins, N., Pearson, G. A., Bernard, J., Serrão, E. A., and Bartsch, I. (2020). Thermal traits for reproduction and recruitment differ between Arctic and Atlantic kelp Laminaria digitata. PLoS One 15:e0235388. doi: 10.1371/journal.pone.0235388
Martins, N., Pearson, G. A., Gouveia, L., Tavares, A. I., Serrão, E. A., and Bartsch, I. (2019). Hybrid vigour for thermal tolerance in hybrids between the allopatric kelps Laminaria digitata and L. pallida (Laminariales, Phaeophyceae) with contrasting thermal affinities. Eur. J. Phycol. 54, 548–561. doi: 10.1080/09670262.2019.1613571
Maulucci, G., Cohen, O., Daniel, B., Sansone, A., Petropoulou, P. I., Filou, S., et al. (2016). Fatty acid-related modulations of membrane fluidity in cells: detection and implications. Free Radic. Res. 50:sup1, S40–S50. doi: 10.1080/10715762.2016.1231403
McCarthy, D. J., Chen, Y., and Smyth, G. K. (2012). Differential expression analysis of multifactor RNA-Seq experiments with respect to biological variation. Nucleic Acids Res. 40, 4288–4297. doi: 10.1093/nar/gks042
McKay, J. K., Christian, C. E., Harrison, S., and Rice, K. J. (2005). “How local is local?” - a review of practical and conceptual issues in the genetics of restoration. Restor. Ecol. 13, 432–440. doi: 10.1111/j.1526-100X.2005.00058.x
McKenzie, P. F., and Bellgrove, A. (2006). No outbreeding depression at a regional scale for a habitat-forming intertidal alga with limited dispersal. Mar. Freshw. Res. 57, 655–663. doi: 10.1071/MF05078
McPherson, M. L., Finger, D. J. I., Houskeeper, H. F., Bell, T. W., Carr, M. H., Rogers-Bennett, L., et al. (2021). Large-scale shift in the structure of a kelp forest ecosystem co-occurs with an epizootic and marine heatwave. Commun. Biol. 4:298. doi: 10.1038/s42003-021-01827-6
Menzel, M., Sletvold, N., Gren, J. A., and Hansson, B. (2015). Inbreeding affects gene expression differently in two self-incompatible Arabidopsis lyrata populations with similar levels of inbreeding depression. Mol. Biol. Evol. 32, 2036–2047. doi: 10.1093/molbev/msv086
Mickelbart, M. V., Hasegawa, P. M., and Bailey-Serres, J. (2015). Genetic mechanisms of abiotic stress tolerance that translate to crop yield stability. Nat. Rev. Genet. 16, 237–251. doi: 10.1038/nrg3901
Missbach, S., Weis, B. L., Martin, R., Simm, S., Bohnsack, M. T., and Schleiff, E. (2013). 40S ribosome biogenesis co-factors are essential for gametophyte and embryo development. PLoS One 8:e54084. doi: 10.1371/journal.pone.0054084
Monteiro, C., Heinrich, S., Bartsch, I., Valentin, K., Corre, E., Collén, J., et al. (2019a). Temperature modulates sex-biased gene expression in the gametophytes of the kelp Saccharina latissima. Front. Mar. Sci. 6:769. doi: 10.3389/fmars.2019.00769
Monteiro, C., Li, H., Bischof, K., Bartsch, I., Valentin, K. U., Corre, E., et al. (2019b). Is geographical variation driving the transcriptomic responses to multiple stressors in the kelp Saccharina latissima? BMC Plant Biol. 19:513. doi: 10.1186/s12870-019-2124-0
Murúa, P., Müller, D. G., Etemadi, M., van West, P., and Gachon, C. M. M. (2020). Host and pathogen autophagy are central to the inducible local defences and systemic response of the giant kelp Macrocystis pyrifera against the oomycete pathogen Anisolpidium ectocarpii. New Phytol. 226, 1445–1460. doi: 10.1111/nph.16438
Musrati, R. A., Kollárová, M., Mernik, N., and Mikulášová, D. (1998). Malate dehydrogenase: distribution, function and properties. Gen. Physiol. Biophys. 17, 193–210.
Nast, G., and Müller-Röber, B. (1996). Molecular characterization of potato fumarate hydratase and functional expression in Escherichia coli. Plant Physiol. 112, 1219–1227. doi: 10.1104/pp.112.3.1219
Neidleman, S. L. (1987). Effects of temperature on lipid unsaturation. Biotechnol. Genet. Eng. Rev. 5, 245–268. doi: 10.1080/02648725.1987.10647839
Ning, Y., Liu, Q., Wang, C., Qin, E., Wu, Z., Wang, M., et al. (2021). Heat stress interferes with formation of double-strand breaks and homolog synapsis. Plant Physiol. 185, 1783–1797. doi: 10.1093/plphys/kiab012
Nishimura, O., Hara, Y., and Kuraku, S. (2017). gVolante for standardizing completeness assessment of genome and transcriptome assemblies. Bioinformatics 33, 3635–3637. doi: 10.1093/bioinformatics/btx445
Norton, T. A. (1992). Dispersal by macroalgae. Br. Phycol. J. 27, 293–301. doi: 10.1080/00071619200650271
Oliver, E. C. J., Donat, M. G., Burrows, M. T., Moore, P. J., Smale, D. A., Alexander, L. V., et al. (2018). Longer and more frequent marine heatwaves over the past century. Nat. Commun. 9:1324. doi: 10.1038/s41467-018-03732-9
Oppliger, L. V., von Dassow, P., Bouchemousse, S., Robuchon, M., Valero, M., Correa, J. A., et al. (2014). Alteration of sexual reproduction and genetic diversity in the kelp species Laminaria digitata at the southern limit of its range. PLoS One 9:e102518. doi: 10.1371/journal.pone.0102518
Patro, R., Duggal, G., and Kingsford, C. (2015). Accurate, fast, and model-aware transcript expression quantification with Salmon. bioRxiv [Preprint]. doi: 10.1101/021592
Pearson, G. A., Martins, N., Madeira, P., Serrão, E. A., and Bartsch, I. (2019). Sex-dependent and -independent transcriptional changes during haploid phase gametogenesis in the sugar kelp Saccharina latissima. PLoS One 14:e0219723. doi: 10.1371/journal.pone.0219723
Pontier, D., Albrieux, C., Joyard, J., Lagrange, T., and Block, M. A. (2007). Knock-out of the magnesium protoporphyrin IX methyltransferase gene in Arabidopsis: effects on chloroplast development and on chloroplast-to-nucleus signaling. J. Biol. Chem. 282, 2297–2304. doi: 10.1074/jbc.M610286200
Provasoli, L. (1968). “Media and prospects for the cultivation of marine algae. in cultures and collections of algae,” in Proceedings of the US-Japan Conference, Hakone, 63–75.
Qiu, X. B., Shao, Y. M., Miao, S., and Wang, L. (2006). The diversity of the DnaJ/Hsp40 family, the crucial partners for Hsp70 chaperones. Cell. Mol. Life Sci. 63, 2560–2570. doi: 10.1007/s00018-006-6192-6
R Core Team. (2021). R: A Language and Environment for Statistical Computing. Available online at: https://www.r-project.org/ (accessed March 15, 2021)
Raimondi, P. T., Reed, D. C., Gaylord, B., and Washburn, L. (2004). Effects of self-fertilization in the giant kelp, Macrocystis pyrifera. Ecology 85, 3267–3276. doi: 10.1890/03-0559
Raven, J. A. (1991). Physiology of inorganic C acquisition and implications for resource use efficiency by marine phytoplankton: relation to increased CO2 and temperature. Plant Cell Environ. 14, 779–794. doi: 10.1111/j.1365-3040.1991.tb01442.x
Raybaud, V., Beaugrand, G., Goberville, E., Delebecq, G., Destombe, C., Valero, M., et al. (2013). Decline in kelp in West Europe and climate. PLoS One 8:e66044. doi: 10.1371/journal.pone.0066044
Rhee, S. G., Woo, H. A., Kil, I. S., and Bae, S. H. (2012). Peroxiredoxin functions as a peroxidase and a regulator and sensor of local peroxides. J. Biol. Chem. 287, 4403–4410. doi: 10.1074/jbc.R111.283432
Rho, M., Tang, H., and Ye, Y. (2010). FragGeneScan: predicting genes in short and error-prone reads. Nucleic Acids Res. 38, e191. doi: 10.1093/nar/gkq747
Ritchie, M. E., Phipson, B., Wu, D., Hu, Y., Law, C. W., Shi, W., et al. (2015). limma powers differential expression analyses for RNA-sequencing and microarray studies. Nucleic Acids Res. 43: e47. doi: 10.1093/nar/gkv007
Robinson, M. D., McCarthy, D. J., and Smyth, G. K. (2009). edgeR: A Bioconductor package for differential expression analysis of digital gene expression data. Bioinformatics 26, 139–140. doi: 10.1093/bioinformatics/btp616
Robinson, N., Winberg, P., and Kirkendale, L. (2013). Genetic improvement of macroalgae: status to date and needs for the future. J. Appl. Phycol. 25, 703–716. doi: 10.1007/s10811-012-9950-x
Rognes, T., Flouri, T., Nichols, B., Quince, C., and Mahé, F. (2016). VSEARCH: a versatile open source tool for metagenomics. PeerJ 4:e2584. doi: 10.7717/peerj.2584
Salavarría, E., Paul, S., Gil-Kodaka, P., and Villena, G. K. (2018). First global transcriptome analysis of brown algae Macrocystis integrifolia (Phaeophyceae) under marine intertidal conditions. 3 Biotech 8:185. doi: 10.1007/s13205-018-1204-4
Schierup, M. H., and Christiansen, F. B. (1996). Inbreeding depression and outbreeding depression in plants. Heredity 77, 461–468. doi: 10.1038/hdy.1996.172
Schoville, S. D., Barreto, F. S., Moy, G. W., Wolff, A., and Burton, R. S. (2012). Investigating the molecular basis of local adaptation to thermal stress: population differences in gene expression across the transcriptome of the copepod Tigriopus californicus. BMC Evol. Biol. 12:170. doi: 10.1186/1471-2148-12-170
Shikanai, T., and Aro, E.-M. (2016). “Evolution of photosynthetic NDH-1: structure and physiological function,” in Cytochrome Complexes: Evolution, Structures, Energy Transduction, and Signaling, eds W. A. Cramer and T. Kallas (Dordrecht: Springer Netherlands), 51–70. doi: 10.1007/978-94-017-7481-9_4
Siegert, R., Leroux, M. R., Scheufler, C., Hartl, F. U., and Moarefi, I. (2000). Structure of the molecular chaperone prefoldin. Cell 103, 621–632. doi: 10.1016/S0092-8674(00)00165-3
Silberg, J. J., Hoff, K. G., and Vickery, L. E. (1998). The Hsc66-Hsc20 chaperone system in Escherichia coli: chaperone activity and interactions with the DnaK-DnaJ-GrpE system. J. Bacteriol. 180, 6617–6624. doi: 10.1128/JB.180.24.6617-6624.1998
Smale, D. A., Wernberg, T., Oliver, E. C. J., Thomsen, M., Harvey, B. P., Straub, S. C., et al. (2019). Marine heatwaves threaten global biodiversity and the provision of ecosystem services. Nat. Clim. Change 9, 306–312. doi: 10.1038/s41558-019-0412-1
Smith-Unna, R., Boursnell, C., Patro, R., Hibberd, J. M., and Kelly, S. (2016). TransRate: reference-free quality assessment of de novo transcriptome assemblies. Genome Res. 26, 1134–1144. doi: 10.1101/gr.196469.115
Smyth, G. K., Ritchie, M. E., Law, C. W., Alhamdoosh, M., Su, S., Dong, X., et al. (2018). RNA-seq analysis is easy as 1-2-3 with limma, Glimma and edgeR. F1000Research 5:1408. doi: 10.12688/f1000research.9005.3
Stenbaek, A., and Jensen, P. E. (2010). Redox regulation of chlorophyll biosynthesis. Phytochemistry 71, 853–859. doi: 10.1016/j.phytochem.2010.03.022
Sunkar, R., Bartels, D., and Kirch, H. H. (2003). Overexpression of a stress-inducible aldehyde dehydrogenase gene from Arabidopsis thaliana in transgenic plants improves stress tolerance. Plant J. 35, 452–464. doi: 10.1046/j.1365-313X.2003.01819.x
Tatewaki, M. (1966). Formation of a crustaceous sporophyte with unilocular sporangia in Scytosiphon lomentaria. Phycologia 6, 62–66. doi: 10.2216/i0031-8884-6-1-62.1
Tatsuzawa, H., Takizawa, E., Wada, M., and Yamamoto, Y. (1996). Fatty acid and lipid composition of the acidophilic green alga Chlamydomonas sp. J. Phycol. 32, 598–601. doi: 10.1111/j.0022-3646.1996.00598.x
Teagle, H., Hawkins, S. J., Moore, P. J., and Smale, D. A. (2017). The role of kelp species as biogenic habitat formers in coastal marine ecosystems. J. Exp. Mar. Bio. Ecol. 492, 81–98. doi: 10.1016/j.jembe.2017.01.017
Tewari, A. K., and Tripathy, B. C. (1998). Temperature-stress-induced impairment of chlorophyll biosynthetic reactions in cucumber and wheat. Plant Physiol. 117, 851–858. doi: 10.1104/pp.117.3.851
tom Dieck, I. (1992). North Pacific and North Atlantic digitate Laminaria species (Phaeophyta): hybridization experiments and temperature responses. Phycologia 31, 147–163. doi: 10.2216/i0031-8884-31-2-147.1
tom Dieck, I., and de Oliveira, E. C. (1993). The section digitatae of the genus Laminaria (Phaeophyta) in the northern and southern Atlantic: crossing experiments and temperature responses. Mar. Biol. 115, 151–160. doi: 10.1007/BF00349397
Vásquez, J. A., Zuñiga, S., Tala, F., Piaget, N., Rodríguez, D. C., and Vega, J. M. A. (2014). Economic valuation of kelp forests in northern Chile: values of goods and services of the ecosystem. J. Appl. Phycol. 26, 1081–1088. doi: 10.1007/s10811-013-0173-6
Velimirov, B. (1979). Fatty acid composition of kelp on the west coast of South Africa and some ecological implications. Bot. Mar. 22, 237–240. doi: 10.1515/botm.1979.22.4.237
Voerman, S. E., Llera, E., and Rico, J. M. (2013). Climate driven changes in subtidal kelp forest communities in NW Spain. Mar. Environ. Res. 90, 119–127. doi: 10.1016/j.marenvres.2013.06.006
Wernberg, T., Bennett, S., Babcock, R. C., de Bettignies, T., Cure, K., Depczynski, M., et al. (2016). Climate-driven regime shift of a temperate marine ecosystem. Science 353, 169–172. doi: 10.1126/science.aad8745
Wernberg, T., Coleman, M. A., Bennett, S., Thomsen, M. S., Tuya, F., and Kelaher, B. P. (2018). Genetic diversity and kelp forest vulnerability to climatic stress. Sci. Rep. 8:1851. doi: 10.1038/s41598-018-20009-9
Westermeier, R., Patiño, D. J., Müller, H., and Müller, D. G. (2010). Towards domestication of giant kelp (Macrocystis pyrifera) in Chile: selection of haploid parent genotypes, outbreeding, and heterosis. J. Appl. Phycol. 22, 357–361. doi: 10.1007/s10811-009-9466-1
Xu, C., and Huang, B. (2010). Differential proteomic response to heat stress in thermal Agrostis scabra and heat-sensitive Agrostis stolonifera. Physiol. Plant. 139, 192–204. doi: 10.1111/j.1399-3054.2010.01357.x
Zaharia, M., Bolosky, W. J., Curtis, K., Fox, A., Patterson, D., Shenker, S., et al. (2011). Faster and more accurate sequence alignment with SNAP. arXiv [Preprint]. arXiv: 1111.5572,Google Scholar
Zhan, X., Shen, Q., Chen, J., Yang, P., Wang, X., and Hong, Y. (2019). Rice sulfoquinovosyltransferase SQD2.1 mediates flavonoid glycosylation and enhances tolerance to osmotic stress. Plant. Cell Environ. 42, 2215–2230. doi: 10.1111/pce.13554
Zhang, J., Li, Y., Luo, S., Cao, M., Zhang, L., and Li, X. (2021). Differential gene expression patterns during gametophyte development provide insights into sex differentiation in the dioicous kelp Saccharina japonica. BMC Plant Biol. 21:335. doi: 10.1186/s12870-021-03117-z
Zhang, L., Li, Y., Xing, D., and Gao, C. (2009). Characterization of mitochondrial dynamics and subcellular localization of ROS reveal that HsfA2 alleviates oxidative damage caused by heat stress in Arabidopsis. J. Exp. Bot. 60, 2073–2091. doi: 10.1093/jxb/erp078
Zhang, X., Fan, X., Wang, Y., Xu, D., Zhang, J., and Ye, N. (2021). Exploring core response mechanisms to multiple environmental stressors via a genome-wide study in the brown alga Saccharina japonica (Laminariales, Phaeophyceae). J. Phycol. 57, 345–354. doi: 10.1111/jpy.13108
Zhao, J., Gao, F., Fan, D. Y., Chow, W. S., and Ma, W. (2018). NDH-1 is important for photosystem I function of Synechocystis sp. strain PCC 6803 under environmental stress conditions. Front. Plant Sci. 8:2183. doi: 10.3389/fpls.2017.02183
Keywords: temperature performance, marine forest, genotype, inbreeding, outbreeding, gene expression, heterosis, heat stress
Citation: Liesner D, Pearson GA, Bartsch I, Rana S, Harms L, Heinrich S, Bischof K, Glöckner G and Valentin K (2022) Increased Heat Resilience of Intraspecific Outbred Compared to Inbred Lineages in the Kelp Laminaria digitata: Physiology and Transcriptomics. Front. Mar. Sci. 9:838793. doi: 10.3389/fmars.2022.838793
Received: 18 December 2021; Accepted: 25 January 2022;
Published: 10 March 2022.
Edited by:
Thomas Wernberg, University of Western Australia, AustraliaReviewed by:
Pamela A. Fernández, Universidad de Los Lagos, ChileSophie J. McCoy, Florida State University, United States
Copyright © 2022 Liesner, Pearson, Bartsch, Rana, Harms, Heinrich, Bischof, Glöckner and Valentin. This is an open-access article distributed under the terms of the Creative Commons Attribution License (CC BY). The use, distribution or reproduction in other forums is permitted, provided the original author(s) and the copyright owner(s) are credited and that the original publication in this journal is cited, in accordance with accepted academic practice. No use, distribution or reproduction is permitted which does not comply with these terms.
*Correspondence: Daniel Liesner, ZGFuaWVsLmxpZXNuZXJAdHVlYmluZ2VuLm1wZy5kZQ==
†Deceased