- Marine Scotland Science, Marine Laboratory, Aberdeen, United Kingdom
There is an urgent need to reduce global greenhouse gas emissions. One method of achieving this is through Carbon Capture and Storage (CCS). Geological structures that lie offshore under continental shelf seas offer huge CCS storage potential. An emerging marine industry is developing to exploit this potential and national marine monitoring agencies will soon need to consider the potential impacts of this emerging industry. This review of published literature is aimed at generalists responsible for the delivery of national marine monitoring, as well as those involved in the management of the marine environment. It briefly summarizes why the emerging offshore CCS industry is needed, how large it may be and what marine infrastructure may be involved. For the purposes of this paper, a hypothetical 20 Mtpa industry has been used to gauge the potential impact of a developing offshore CCS industry. The probability of CO2 leaks from such an industry is low. If they do occur, the spatial scale of impact will be small, and the potential environmental impacts will be low. Irrespective of how CO2 is transported or stored within shelf seas, leaked CO2 will enter the sea as a gas or as a solution dissolved in sediment pore water. CO2 as a gas will dissolve into seawater and/or directly vent to the atmosphere, depending on the initial conditions of the leak. The most probable source of leaks in a developed CCS industry is from pipelines (currently a 2-year event per 1000 km pipeline). The most probable source of leakage from geological storage is through abandoned wells (a 20- to 80-year event for a 20 Mtpa industry). The source of leaks from a CCS scheme with the potential to release the greatest mass of CO2 is through geological faults, as these may go undetected (if they occur) for long periods. The probability of leaks from geological storage, through faults or abandoned wells, is site dependent and minimized by the site selection process. The review concludes with recommended priorities for future marine science development.
Introduction
Marine scientists and managers within national marine agencies are currently required to monitor and assess the environmental impacts on marine ecosystems of many existing industries in order to advise on their sustainable development and management. These include traditional industries such as capture fisheries, dredging and aggregate extraction, shipping and hydrocarbon extraction. In recent years, new industries have become established such as offshore renewable energy and deep sea mining, and these have required the development and implementation of new monitoring and impact assessment techniques and programs. Owing to the growing demand for the reduction of man’s impact on the earth’s climate system, another emerging marine industry which will require national response is offshore carbon capture and storage (CCS).
This review is aimed at generalist marine scientists responsible for the delivery of national marine monitoring, as well as managers of the marine environment who need some degree of scientific understanding of the potential impacts of offshore CCS. It briefly summarizes why the emerging marine industry of offshore carbon capture and storage is needed, how large it may be and what it will consist of. Using a hypothetical case study, it examines the potential processes and scale of the environmental impacts of a regional-scale industry. The review concludes with a series of recommendations we have prepared for the national marine monitoring agency in Scotland. These may be of relevance to other national responses to the development of offshore CCS.
It should be noted that the review is not aimed at developers or operators of offshore CCS schemes, or private sector and academic scientists advising the CCS industry, as experts within these sectors are obviously already fully aware of the literature reviewed here, and indeed are currently pushing forward the boundaries of knowledge to support offshore CCS. This is a rapidly evolving specialist field, and this review aims to update generalists working within national monitoring and/or management agencies who have not yet considered the resources which an offshore CCS industry may require. While the industry will be responsible for monitoring many aspects of the environmental impact of offshore CCS themselves, national monitoring agencies are required to provide objective, independent advice to government for planning and licensing purposes. In the event of an incident involving offshore CCS it is such national agencies who will be asked to assess the environmental impact on behalf of the public.
What Is Carbon Capture and Storage?
Carbon capture and storage, in the context of this review, takes carbon dioxide (CO2) from large industrial sources and stores this in geological formations underground (IPCC, 2005; IOGP, 2019). Hence, a CCS scheme can reduce the quantity of CO2 released into the atmosphere by human activities. As noted by the EU Directive on the geological storage of carbon dioxide (EU, 2009), CCS should be considered as a bridging technology. It can help society move from a high-emission economy to a low-emission economy by dealing with emissions that are currently hard to eliminate or reuse using existing industrial infrastructure. CCS should not be used to reduce efforts to eliminate greenhouse gas emissions or reduce efforts to develop renewable sources of energy (EU, 2009).
Why Is Carbon Capture and Storage Needed?
At the 21st session of the Conference of Parties to the United Nations Framework Convention on Climate Change (UNFCCC), it was agreed that member states would pursue efforts to limit the global average temperature increase to 1.5°C above pre-industrial levels, recognizing that this will significantly reduce the risks and impacts of climate change (UNFCCC, 2015; Article 2, Paris Agreement). In order to achieve this, they additionally agreed to reach global maxima greenhouse gas emissions as soon as possible, and to undertake rapid reductions thereafter. The aim is to achieve a balance between anthropogenic emissions and removals of greenhouse gases by the second half of this century (UNFCCC, 2015; Article 4, Paris Agreement).
This agreement started the global efforts to reduce global warming to 1.5°C and to try to reach net-zero greenhouse gas emissions. In the United Kingdom, the Committee on Climate Change (Committee on Climate Change [CCC], 2019) advised that England and Wales could reach net-zero emissions by 2050, and Scotland by 2045, and these targets were subsequently transposed into law. Similarly, the “Clean Planet for All” strategy (EU, 2018) and the European Green Deal (EU, 2020) committed the European Union to a 2050 target.
The UK Committee on Climate Change (Committee on Climate Change [CCC], 2019) advised that net-zero emissions in the United Kingdom could only be achieved realistically if carbon capture and storage was used to remove hard to eliminate emissions from industries such as natural gas production, cement manufacture and other manufacturing processes (IPCC, 2005; Brownsort et al., 2016). Hydrogen manufacture using many current industrial-scale methods also produces CO2, and so if hydrogen is to provide a transition energy source to help fuel a carbon-free economy, the CO2 released during its production must be sequestered in CCS schemes. Both of these requirements for CCS were reiterated in the EU “Clean Planet for All” strategy (EU, 2018). CCS is also needed as a facilitator of other important developing transition technologies, such as bioenergy with carbon capture and storage (BECCS; Fajardy et al., 2021).
Using CCS to reach net-zero targets also makes economic sense globally, as demonstrated by Davidson et al. (2017). Using economic models of different global energy polices, they found that using CCS reduced the costs of reaching the Paris Agreement emission targets by 22 trillion United States dollars, halving the cost compared to using strategies that did not involve CCS. In summary, carbon capture and storage is clearly needed if countries are to reach net-zero greenhouse gas emissions by the middle of this century, as committed under the United Nations Framework Convention on Climate Change and increasingly by national law.
Why Offshore?
Despite its lower costs, onshore storage of CO2 in geological structures has in the past received public opposition owing to perceived health risks from leaks, effects on local geology, as well as a resistance to what may be seen as waste disposal, and hence many countries have opted to investigate offshore storage initially (Schrag, 2009; Mabon et al., 2014). A review of current energy strategies in 29 countries (IOGP, 2019) found that the Netherlands, Slovenia, Sweden, the United Kingdom and Norway do not permit the onshore sequestration of CO2 in geological storage. For example, the legislative framework for CCS in the United Kingdom, provided by the Energy Act of 2008 (UKGOV, 2008a), only provides a permit system for offshore CCS.
While direct impact on local communities is not a principal concern for offshore CCS schemes, public perception must not be ignored when developing offshore CCS, as emphasized by Mabon et al. (2014). For example, Mabon et al. (2017) describes the concerns of coastal and marine stakeholders in relation to the Tomakomai CCS demonstration project in Hokkaido, Japan (Tanaka et al., 2017). Mabon et al. (2014) warn that public acceptance of offshore CCS may well depend on the scientific evidence presented to the public and the trust they have in the regulatory bodies, and hence the need for national marine monitoring and management agencies to have a sound scientific understanding of the whole system related to offshore CCS.
Another reason to utilize offshore CCS is that much of the technology needed to implement offshore schemes is already well understood and developed, as it utilizes methods and technologies that have been developed by the offshore oil and gas industry. Both ashore and offshore CO2 injection into geological oil and gas bearing structures has been extensively used to enhance oil recovery (e.g., IOGP, 2019). The first use of injected CO2 in an Enhanced Oil Recovery (EOR) scheme occurred in 1972 (IOGP, 2019). Hence, there is over 50 years of experience of transporting CO2 and injecting it into geological features in the oil and gas industries. These industries also have extensive experience of transporting gasses along seabed pressurized pipelines and of building and installing the infrastructure needed to drill into geology deep below the seabed.
How Much Carbon Capture and Storage Is Needed?
Current and future annual sequestration rates of CO2 by CCS required by various international and regional policies and strategies are summarized in Table 1. In order to meet their 2050 net-zero targets, Europe needs to sequester between 80 and 298 Mt CO2 annually, and the United Kingdom between 75 and 175 Mt CO2 annually. Estimates of the capacity of geological storage of CO2 at various geographic scales are given in Table 2. In Europe, the current estimate of geological storage capacity is 300 Gt, hence this could absorb between 1,000 and 3,000 years of CO2 sequestration at the annual rates needed. Even just in the Scottish sector of the North Sea, the 47 Gt of potential geological storage which is available could absorb 150 to 600 years of the annual sequestration needed by the whole of Europe.
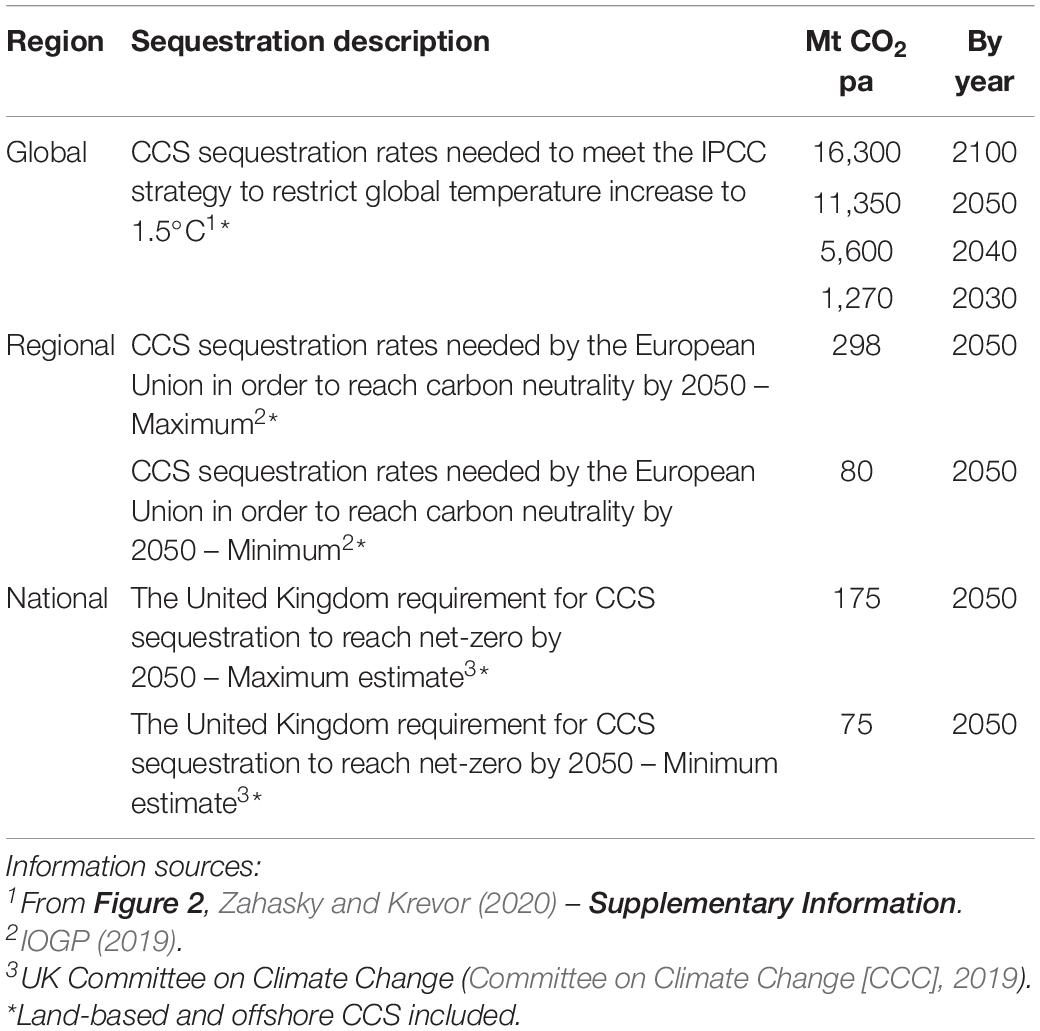
Table 1. Current and future annual sequestration rates of CO2 in millions of tons per annum (Mt CO2 pa) in CCS schemes required by various global/regional/national policies and strategies.
Thus, there is ample potential for the offshore geological storage of CO2. However, in order to assess the scale of new marine science support that may be needed by offshore CCS, we must consider how much CO2 may be needed to be sequestered underground each year in a particular sea region. The annual regional sequestration rate indicates how much CO2 will be transported from CO2 sources to the CO2 injection point in a regional CCS industry, and hence can be used to assess the scale of offshore activities and what potential there is for leakage. Regional CCS industries will be composed of a number of individual schemes, and the scale of some examples of currently existing or planned CCS schemes are given in Table 3.
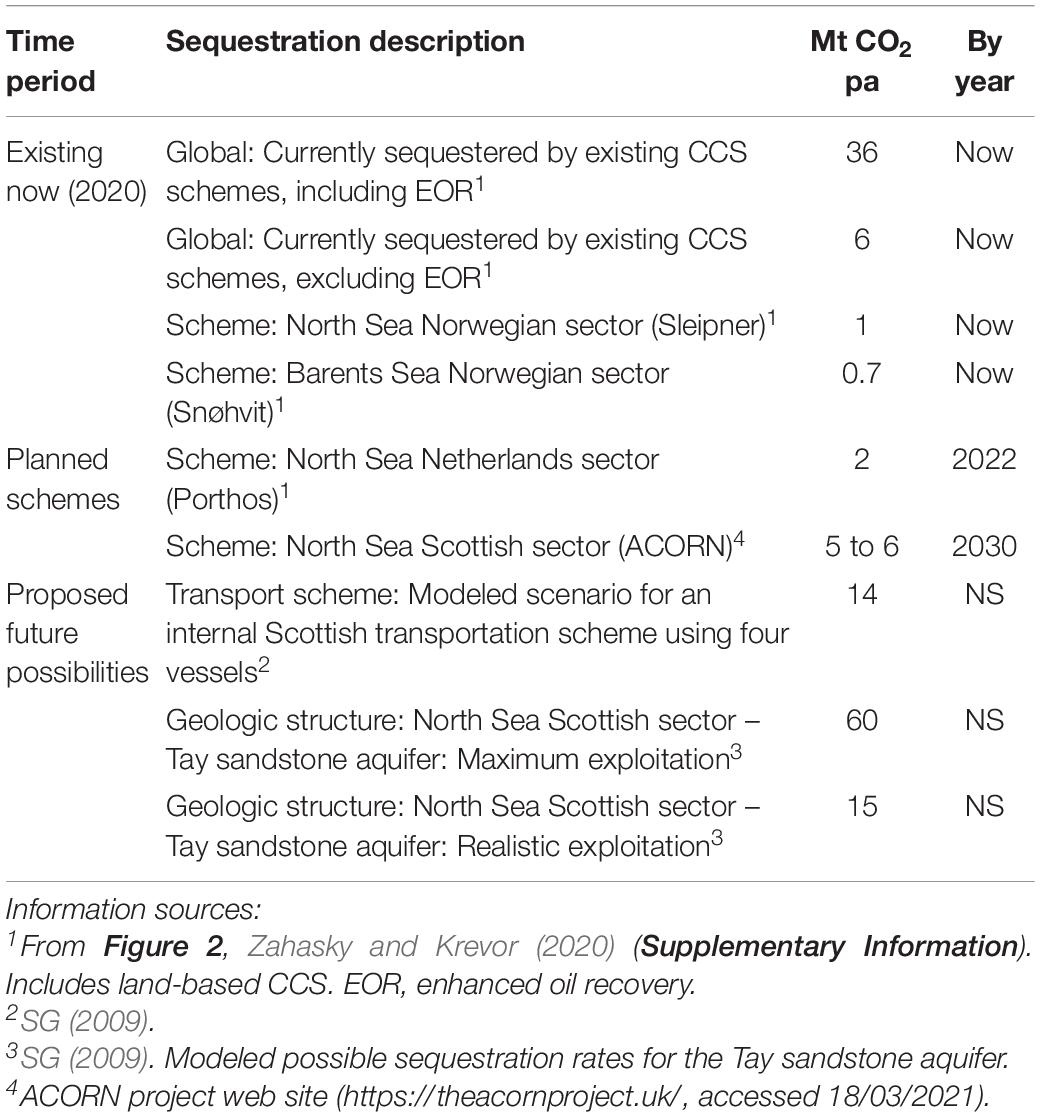
Table 3. Estimated annual sequestration rates (Mt per annum) in existing, planned or future CCS schemes.
A Hypothetical Regional Case Study
From Table 3, we can see that an existing North Sea CCS scheme, at the Sleipner field in the Norwegian North Sea, sequesters about 1 Mtpa (1 Mt per annum) CO2. The Porthos planned offshore scheme in the Netherlands sector of the North Sea aims to sequester 2 Mtpa, and the planned ACORN project in the United Kingdom aims to sequester 5 to 6 Mtpa by 2030. Thus, at the start of a developing offshore CCS industry, pilot schemes are in the range 1 to 6 Mtpa in size. Five individual CCS schemes of 4 Mtpa, or ten schemes of 2 Mtpa would result in a combined industry of 20 Mtpa. These numbers of schemes seem realistic in terms of physical infrastructure when one considers the number of offshore hydrocarbon extraction schemes which currently exist within a region such as the northern North Sea. Hence, for the purposes of this review, a 20 Mtpa scenario has been used to quantify the potential impact of a hypothetical regional CCS industry typical of a shelf sea such as the North Sea.
The Components of an Offshore Carbon Capture and Storage Scheme
A typical offshore CCS scheme has the following components:
Capture
The CO2 is captured from an industrial process. This stage is normally performed at installations ashore, although in some schemes it can be done on offshore platforms (e.g., at the Sleipner field in the Norwegian North Sea).
Pre-conditioning
The CO2 is normally pre-conditioned after capture and prior to transport. Pre-conditioning involves the removal of water content (dehydration) and possibly other impurities, and the pressurization of the CO2 in order to increase its density and to facilitate transport through pipelines. Again, this would normally be performed ashore but might be carried out on an offshore platform if that is the point of capture.
Transport
Liquid CO2 is transported from the capture point to the injection point. This can either be done through seabed pipelines, or by tanked vessel, or by a combination of both. Transport by vessel can occur to a shore-based hub, where the CO2 is injected into the landward end of a pipeline for onward transport to the offshore injection point. Alternatively it can be delivered directly to an offshore platform prior to injection into the geological storage.
Injection
Once transported to the location of the geological storage, the CO2 must be injected underground through wells drilled into the storage rock formation. Operational injection wells will normally be serviced by a platform or by seabed infrastructure. Injection wells in the North Sea need to be between 1 and 2.5 km deep based on the geology of the region and the pressure requirement for CO2 storage (SG, 2009). Injection normally takes place as a supercritical fluid (IPCC, 2005).
Storage
Injected CO2 is subsequently stored in geological structures consisting of permeable rock (e.g., sandstone) lying below an upper layer impermeable (e.g., mudstone caprock) to fluid rising through the permeable component. Such structures often have already captured rising hydrocarbons (i.e., depleted oil and gas fields), or saline water (i.e., saline aquifers) and hence have demonstrated that they lie below an impermeable layer. The IPCC, in their special report on CCS (IPCC, 2005) noted that “observations from engineered and natural analogs as well as models suggest that the fraction retained in appropriately selected and managed geological reservoirs is very likely to exceed 99% over 100 years and is likely to exceed 99% over 1,000 years.” Hence geological storage of CO2 aims to retain the gas for 100 to 1000 years at least.
Each of these different stages must be considered for their potential to impact the environment. Before we proceed with this assessment, we first consider some of the basic properties of the material being processed, i.e., CO2. Readers familiar with the science describing the chemistry of CO2 in seawater may wish to proceed to Section “What Risks Might an Offshore Carbon Capture and Storage Industry Pose?”
Properties of Carbon Dioxide
Figure 1 summarizes the different physical phases of CO2 when subjected to different temperatures and pressures. Also shown are the typical temperatures and pressures that CO2 is transported at in offshore pipelines, and on vessels, as well as typical ambient conditions in the North Sea.
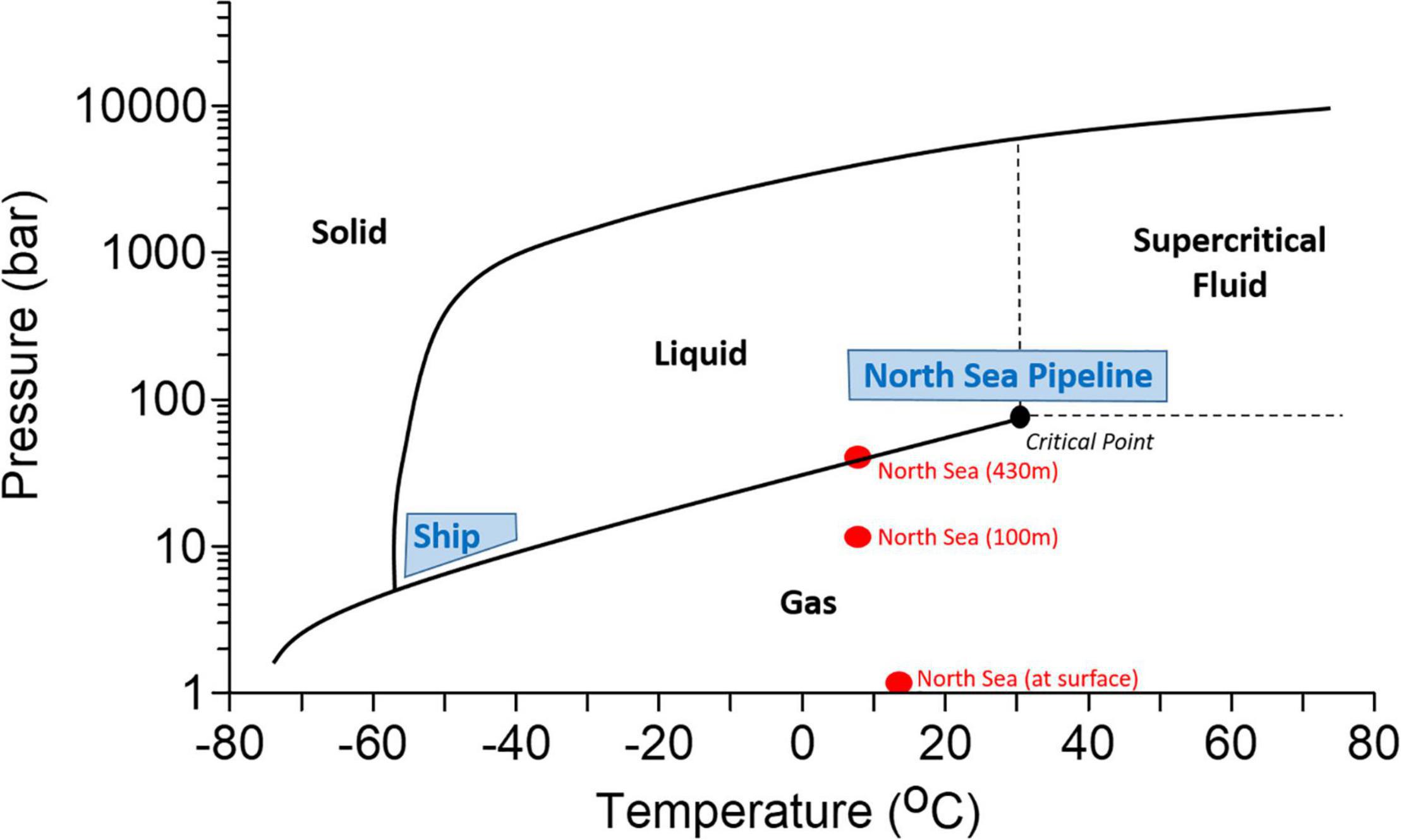
Figure 1. Phase diagram for CO2, including the conditions under which CO2 is transported in ships and pipelines (from Martynov et al., 2012). Typical pressure/temperature conditions for the North Sea are also shown. Supercritical fluid can have properties of both a dense gas and a liquid. Further details given in Supplementary Material S1.
At ambient (atmospheric) temperatures and pressures, pure CO2 is a colorless, odorless, inert and non-combustible gas which can dissolve into water. When being transported to an offshore CCS storage site, it is most common for CO2 to be pumped into a pipeline at the shoreward end as a supercritical fluid (pressure 200 bar, temperature 45°C), but as temperatures decrease along the pipeline due to heat loss, it changes into a liquid. The transport of CO2 in tanks on board ships is most commonly as a liquid (Figure 1).
However, at the temperatures and pressures found within a geological store, CO2 can exist in five phases (dissolved in water, solid, liquid, gas, supercritical fluid), all existing in different parts of the geological structure at the same or different times depending on a range of factors within the rock. This describes CO2 as it is when properly constrained within a CCS transport and storage scheme, but what form might it take once leaked into the sea?
In a detailed study of the thermodynamics of supercritical CO2 during sudden depressurization (for example after being released from a pipeline or a well or the tank of a submerged vessel), Botnen et al. (2013) found that if the release occurs in water depths of about 100 m or less (owing to the pressures there) the CO2 becomes a gas. Below 600 m, the CO2 escapes as a liquid, and between 100 m and 600 m there is both gas and liquid released. Hence for most events in shelf sea waters, it can be assumed that whatever the source, CO2 will enter the sea as a gas. The one exception to this is if CO2 enters the sea from seeping up through seabed sediment and is already fully dissolved in sediment pore water. In this case CO2 solution will enter the sea directly, and then be subject to advection, dispersion and dilution.
If CO2 enters the sea as a gas, it forms bubbles which will start to rise through their own buoyancy, expanding as the surrounding ambient pressure decreases. At the same time the CO2 within them will start to dissolve into the sea, and hence this process shrinks the size of bubbles. The rate bubbles reduce in size due to the dissolution of the CO2 into the water will be a complex function of parameters such as the temperature, pressure, salinity and the existing CO2 content of the seawater, as well as the surface area of the bubbles (e.g., Dewar et al., 2013).
The rate bubbles rise, and hence expand, through the water will be dependent on their size and shape. Bubbles may not be simply spherical, but may take many forms, and may also coalesce and separate in complex ways within a bubble plume (Sellami et al., 2015), especially within a highly energetic bubble stream. To add to the complexity, Dewar et al. (2013) noted that hydrate (i.e., solid CO2) skins may form around CO2 bubbles in waters deeper than 180 m. These skins reduce the dissolution rate and affect the buoyancy of the bubble. Additionally, the CO2 stream can contain impurities, especially water. Impurities can alter the details of the phase diagram seen in Figure 1 in complex ways.
Hence, in summary, CO2 will be transported through the marine environment either as a liquid, a supercritical liquid or a gas. However, if CO2 is leaked into the sea, it will most likely enter the sea as a gas although it may enter the sea already dissolved in sediment pore water if slowly leaked from geological storage. If it enters as a gas, bubbles of gas will form and either fully dissolve into the sea before reaching the sea surface, or will partially dissolve into the sea, and gas will vent from the surface of the sea into the air. The form bubble plumes take is discussed further below.
Shelf Sea Carbonate System
When CO2 dissolves in water (H2O), there is a chemical reaction and the CO2 and water mix to produce carbonic acid (Dewar et al., 2013). The acid and water then start to dissociate into bicarbonate ions, carbonate ions, hydroxide ions, hydrogen ions and small quantities of carbonic acid. Disassociation constants control the balance in the sea between these competing components. These constants must be derived experimentally under ranges of temperature, pressure and salinity and there are a number of different methods to provide these, and hence there are a number of different ways to calculate CO2 concentration and these may vary a little in detail. More details are given in Supplementary Material S2.
When monitoring CO2 concentrations in the sea, one or more of four parameters are often used:
Potential of Hydrogen
As the total concentration of dissolved CO2 increases, so does the number of positive hydrogen ions. This increases the acidity of the water/CO2 solution, and as the number of hydrogen ions increases, acidity increases and the associated value of potential of hydrogen (pH) decreases. In this review, ΔpH is used to describe the reduction of the pH of seawater after the addition of CO2.
Dissolved Inorganic Carbon
The total dissolved inorganic carbon (DIC) is given by the sum of the bicarbonate and carbonate ion concentrations. This quantity is conservative as it is unaffected by temperature, pressure or salinity. Modelers often use this quantity when simulating CO2 dispersion owing to its conservative properties. DIC is consumed during primary production in the sea, which converts it to organic matter (Humphreys et al., 2020), thus “creating space” for the dissolution of more CO2 into the sea. DIC can also increase due to biological respiration and decomposition of organic matter. DIC can be measured directly by acidifying a sample of seawater, extracting the CO2 gas that is produced and measuring its amount (Dickson et al., 2007).
Total Alkalinity
This is another conservative quantity in the sea, which does not change with temperature, pressure or salinity. It is a measure of the number of moles per kilogram of hydrogen ions. As Dewar (2016) notes, the total alkalinity (TA) indicates how rapidly pH changes will occur in seawater.
Partial Pressure (Fugacity) of Carbon Dioxide in Solution
Partial pressure of carbon dioxide (pCO2) of a sample of seawater is the partial pressure that CO2 as an ideal gas would reach if it was allowed to reach an equilibrium with that sample of seawater. In practice, CO2 does not act as an ideal gas, but as a real gas. If CO2 in reality reaches an equilibrium with the sample of sea water, the value of its partial pressure is then called its fugacity (fCO2). However, factors must be applied to correct for the properties of the real gas, in order to calculate pCO2 from the value of fugacity. In practice, fugacity and pCO2 are almost numerically the same value.
Dissolved Inorganic Carbon, Total Alkalinity, Potential of Hydrogen, Partial Pressure of Carbon Dioxide
All four of these quantities involve sums of the concentrations of different sets of ions in seawater, with constants derived experimentally and dependant on external parameters such as temperature, pressure and salinity. There is a complex set of equations linking these four parameters together in such a way that if two are known, the other two may be calculated. Note that auxiliary parameters may also need to be supplied, such as nutrient concentrations (e.g., phosphate, silicate, ammonium). The equations must be solved by iterative computer programs, of which a number are publically available (e.g., Orr et al., 2015).
Potential of hydrogen (pH) and pCO2 can be measured electrically, hence sensors can be mounted on in situ equipment and recorded digitally. TA and DIC are most commonly measured in a chemical laboratory using a sample of seawater. Novel in situ TA and DIC instruments have been developed in the past decade but they are expensive and complex in operation and so are not yet widely used.
Proxy Measure of Ecological Impact – ΔpH
In modeling studies attempting to predict the impact of leaks of CO2 from CCS, changes in pH are often used as a simple proxy measure of biological impact. For example, Blackford et al. (2008) suggested that pH provides a proxy for the “strength of the sum of ecosystem effects.” Different authors have used different ΔpH criteria to describe varying degrees of ecological impact (see Supplementary Material S14). However, a general consensus is as follows:
Note that the pH ranges and phrases used above principally come from Blackford et al. (2008), although they have been slightly modified to reconcile them with those used by Phelps et al. (2015) and Vielstädte et al. (2019). See Supplementary Material S14 for full details.
What Risks Might an Offshore Carbon Capture and Storage Industry Pose?
What might the environmental impact of a regional-scale CCS industry be? To answer this question, the first step is to carry out a brief risk assessment by identifying the source of risks to the marine environment, their probability and their scale.
Risks From Carbon Capture and Storage-Related Engineering
It is clear that the installation and operation of CCS will include processes identical to those conducted in the offshore oil and gas industry; i.e., seismic surveys, pipeline and umbilical laying, pipeline protection and maintenance, platform installation, anchoring, maintenance, decommissioning, well drilling, etc. (e.g., UKGOV, 2008b). Marine scientists in national management agencies are often asked to advise on these in relation to their environmental impact (e.g., discharges, noise, disturbance, seabed impact, migratory species, etc.). The principle difference between the oil and gas industry and the CCS industry is what can potentially be leaked into the marine environment and not what offshore technologies are involved. Hence the engineering associated with an offshore CCS industry will generate more work, but no new skills, knowledge or methodologies will be needed for this aspect of managing the environmental footprint of the industry.
Risks From Leaks
Hence, it is monitoring for leaks and assessing their potential impacts that may drive the requirement for developments in national marine monitoring. It is therefore important to understand what the risk of leaks from an offshore CCS industry is. In a standard risk assessment two parameters are normally considered; the likelihood of a risk happening, and the impacts that may occur if it does happen. This section brings together studies of the likelihood of leaks occurring in the transport (ships and pipelines), injection (wells) and geological storage (abandoned wells, faults, seepage) elements of a future North Sea CCS industry.
What Are the Likelihoods of Leaks?
The likelihood of accidents to shipping has been assessed for safety at sea and insurance purposes (e.g., Cabioc’h et al., 2009; Bužančić Primorac and Parunov, 2016), and the probability of pipeline accidents has been assessed in the oil and gas industry for health and safety and risk assessment purposes (e.g., Borresen et al., 2012; HSE, 2017). For the injection and storage phases of a typical North Sea CCS industry, a report produced for the UK Government by Jewell and Senior (2014) brought together industry, academic and government experts in order to provide quantitative estimates of likelihood and size of potential leaks from wells and faults. The likelihood of leaks from these various sources, assuming an industry size of 20 Mtpa, are summarized in Table 4.
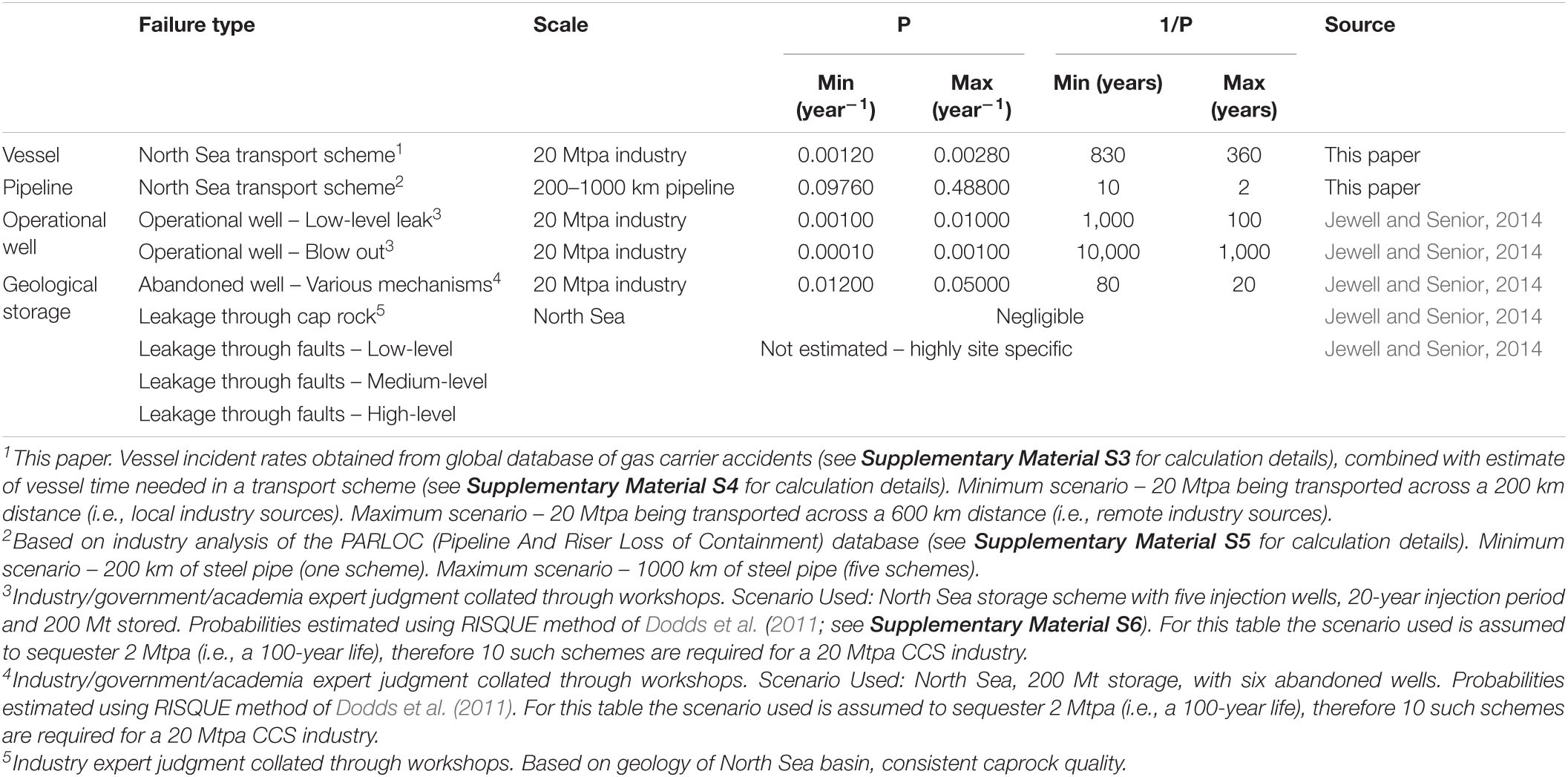
Table 4. Estimated probabilities of CO2 leaks from various sources for a 20 Mtpa offshore CCS industry.
The event that is to be expected most frequently in a 20 Mtpa regional offshore CCS industry is a leak from a pipeline, estimated as a 2- to 10-year event (see Supplementary Material S5.1 for a sense check of this estimate). Leaks from abandoned wells are the next most probable event, in this case a 20- to 80-year event across the industry as a whole. Owing largely to the improvements in safety at sea, a CO2-carrying vessel accident is assessed as being a 360- to 830-year event. At the offshore CCS scheme itself, an operational well low-level leak is estimated as a 100- to 1,000-year event, and an operational well blow-out as a 1,000- to 10,000-year event.
Table 4 gives an indication of how frequent events might be in a 20 Mtpa industry, but how large might those events be in terms of CO2 released? This is discussed in the next section.
What Might the Size of Leaks Be?
Two factors must be considered when attempting to estimate the scale of potential leaks; the leak rate and the leak duration. Table 5 presents some realistic leak scenarios in a 20 Mtpa regional offshore CCS industry, and attempts to quantify their size in terms of mass of CO2 released per day. While published values have been used wherever possible, in order to arrive at realistic values some arbitrary assumptions have to be made. In reality, losses may be smaller or larger than those predicted here, but the aim here is to give an impression of possible order of magnitude scale.
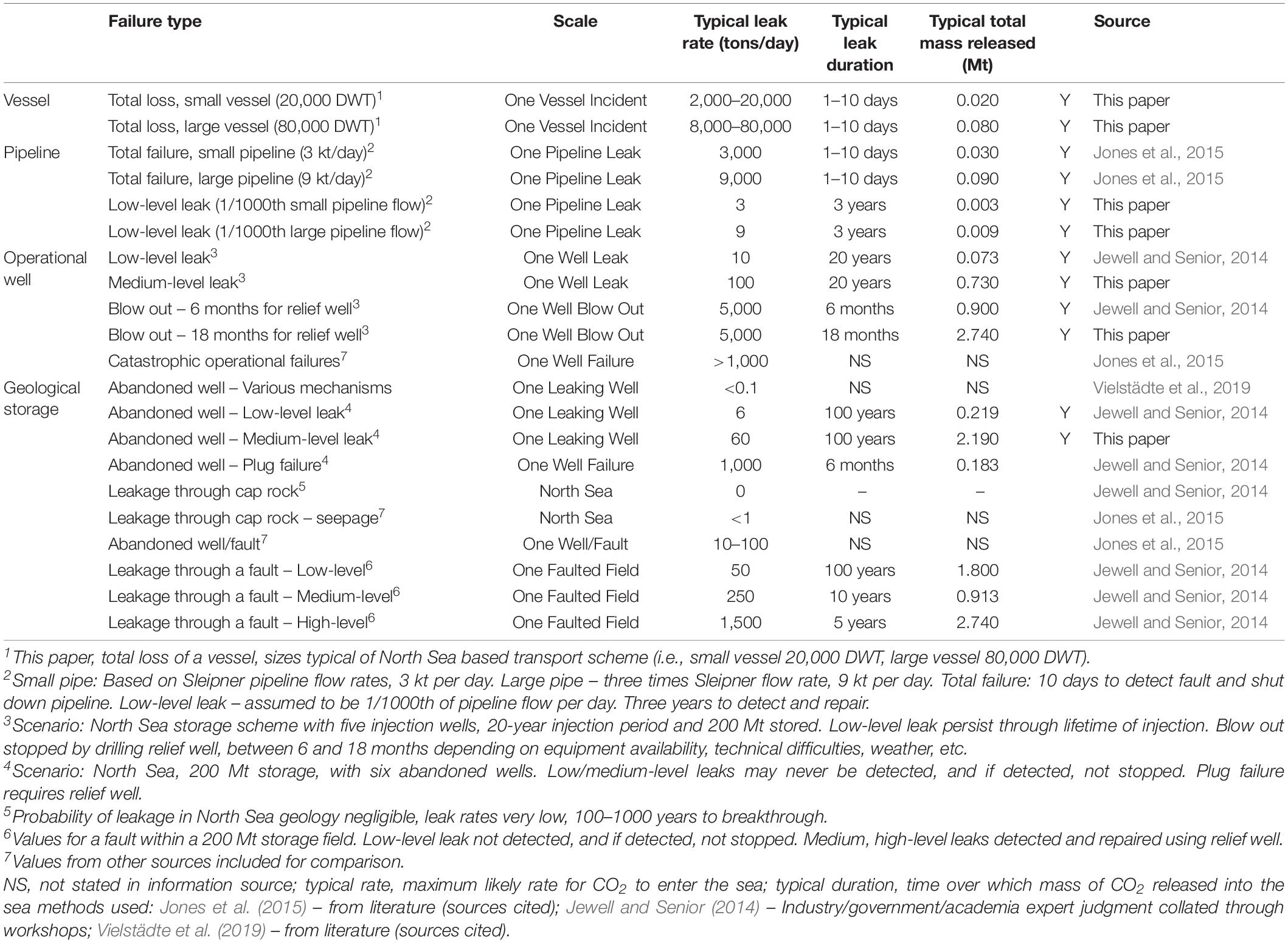
Table 5. Characteristic leak rates, durations and total mass released for North Sea offshore CCS-related leak scenarios.
For a vessel accident, two sizes of vessel were assumed, a smaller 20,000 DWT (deadweight tonnage) CO2 carrier, and a larger 80,000 DWT vessel. The size of loss is considered to be the entire vessel cargo. Here the scenario would be the sinking of a vessel following a collision, for example. CO2 stored in tanks on the vessel would potentially lose their refrigeration and warm up to ambient temperatures. This would lead to pressure excess in the holding tanks, and hence CO2 would vent into the sea through safety mechanisms. The length of time for this to occur is assumed to lie somewhere between 1 and 10 days.
Two modes of pipeline event were assumed; a total failure and a low-level leak. For the total failure, the typical flow rates through an offshore CCS pipeline have been estimated as between 3 and 9 kt per day. For the total failure, 1 day’s worth of this flow is assumed to escape into the sea before the pipeline can be shut down. For the low-level leak, a thousandths of the flow is assumed to be below the monitoring level of pipeline losses, and hence could occur for some time without detection (see Supplementary Material for a discussion of this assumption). Hence, for a pipeline low-level leak, the leak is assumed to discharge between 3 and 9 tons of CO2 per day, but for a period of 3 years before detection and repair is carried out. For the remainder of the leak sources, the industry report authored by Jewell and Senior (2014) has been used. Here industry, academic and government experts considered set scenarios and estimated leak rates and durations.
In Table 5, leaks with leak rates greater than 1,000 tons per day include vessel loss, a total pipeline failure, an operational well blow-out, an abandoned well plug failure and a high-level leak through a geological fault. These could potentially allow gas to escape to the surface of the sea before bubbles of CO2 fully dissolve; i.e., a “fast” release (Figure 2, and explanations below).
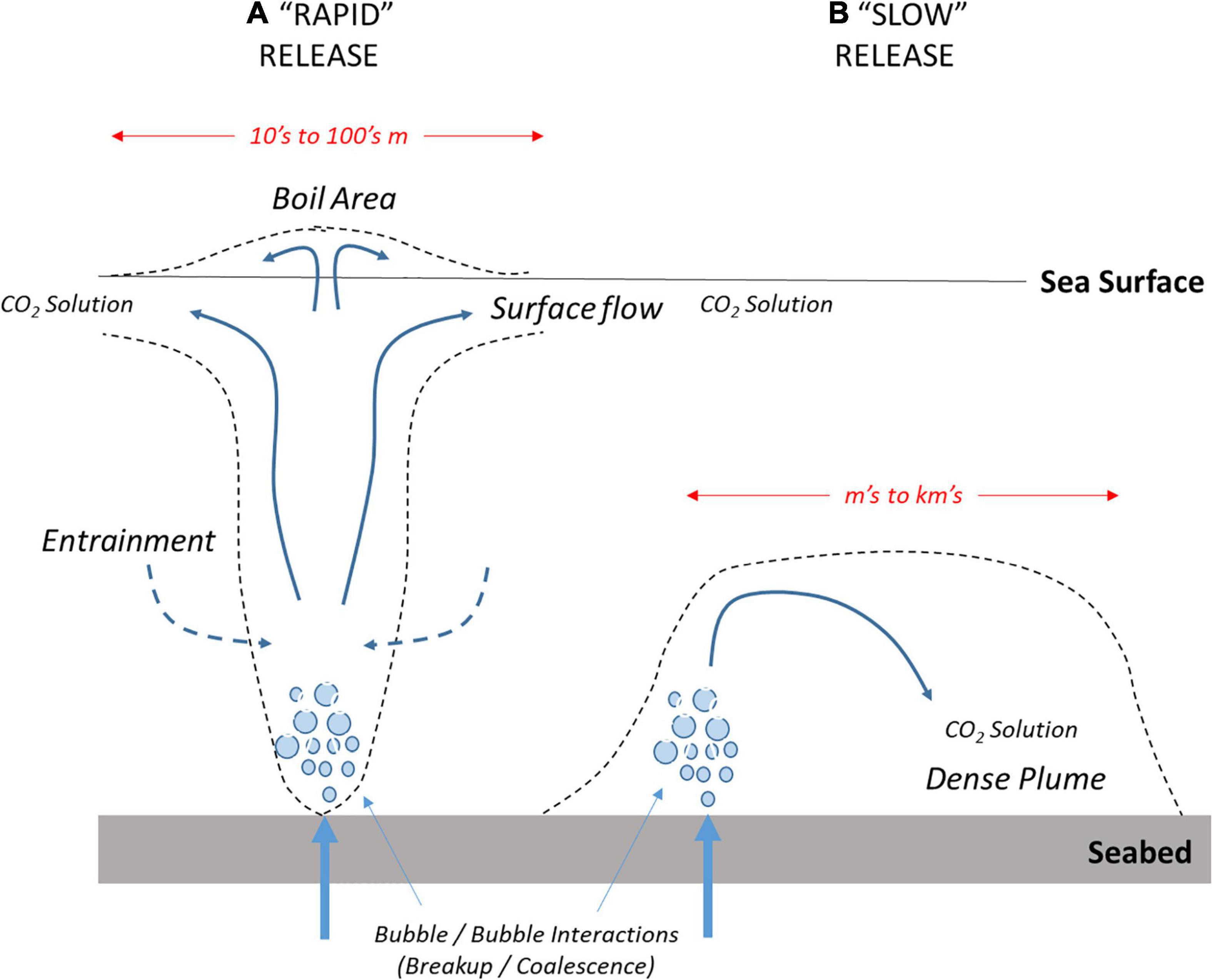
Figure 2. Two models of the fate of a release of CO2. (A) A “fast” release of CO2 where the plume of bubbles reaches the surface (from Chen et al., 2005). (B) A “slow” release of CO2 where the plume of bubbles dissolves into the sea before reaching the surface (from Dewar et al., 2013; Dewar, 2016).
Slow releases (i.e., rates <1,000 tons per day), but with long potential durations and hence the possibility of releasing quite large total quantities of gas, include a medium-level operational well leak which might last for the operational lifetime of a CCS scheme if not detected (i.e., 20 years), and low- and medium-level leaks through geological faults. Jewell and Senior (2014) noted that experts considered high-level leaks from faults would be detected and repaired (e.g., through relief wells) within 5 years.
Finally, from Table 5, leaks which could release more than 0.9 Mt CO2 are an operational well blow-out which takes 6 to 18 months to repair, a medium-level leak from an abandoned well which is never detected or repaired, and leaks through geological faults which last between 5 and 100 years. These leaks may lead to large escapes of CO2 from the storage facility provided by the CCS industry, and hence may be of concern to the integrity of a scheme.
We now have estimates of the probability of leaks, and the amounts of CO2 released into the sea by those leaks, but how does that CO2 spread in the sea?
How Do Leaks Spread Out After They Enter the Sea?
In order to address this question, we consider two spatial scales: (1) The “local” scale immediately in the vicinity of a leak, where the movement and mixing of the CO2 is very much determined by the properties of the CO2 itself and how it has been introduced into the sea. (2) The larger “regional” scale, where advection and dispersion in the sea is largely independent of the initial conditions and determined by the physical oceanography of the region within which the leak occurs.
There have been no published examples of the monitoring of accidental leaks of CO2 in the sea, hence we currently have no direct observations of how escaped CO2 mixes and disperses in the sea at the scale of a real leak. At the local scale, there have been laboratory and tank-based releases of CO2, and these have been used to study the physics of bubble plumes. There have also been three purposeful releases of CO2 in specific experiments in the North Sea and in Scottish coastal waters (listed in Supplementary Material S7) but these have been of limited duration (11 to 37 days) and release rates (0.8 to 1.12 tons/day). While these experiments provide some useful information at the local scale, and can contribute to the validation of models at the regional scale, the principle source of information describing how leaks advect and disperse in the marine environment comes from numerical simulation.
Local Scale
Leaked CO2, leaving a pipeline, leaving a well or leaving the seabed from the geological storage beneath it, will undergo similar processes once it enters the sea irrespective of its source. It is not the type of source that is important once in the sea, rather it is the initial conditions under which the CO2 is released into the water column. The initial conditions of leak rate, initial bubble size, initial gas velocity and pressure, depth of release point, and oceanographic conditions at the release point will combine to determine the ultimate fate of the CO2. This fate determines how we monitor for the results of the leak, and what environmental and ecological effects the leak may have.
As noted above, Botnen et al. (2013) modeled the thermodynamic changes that CO2 will undergo during a leak from a pipeline. They found that the CO2 will remain as a liquid when it enters the sea below depths of about 600 m. Above depths of about 100 m the CO2 will expand almost entirely into a gas. At depths between 600 m and 100 m, the released CO2 can exist as both phases, with the gas component increasing with decreasing depth.
For some leaks, multiple gas bubbles may reach the sea surface, releasing a significant mass of the CO2 directly into the air. Such releases may often be associated with a “fountain” or “boil” of water leaving the surface of the sea (Figure 2A). For this review these will be termed “fast” releases. For other releases, the CO2 will completely dissolve into the seawater before reaching the surface of the sea. For this review these will be termed “slow” releases (Figure 2B). The factors which determine which type of release will occur will be some combination of release rate, release area and total water depth. For example, a release of 100 tons a day, through a 10 cm hole in a pipeline in a water depth of 20 m may result in a “fast” release type, whereas a release of 100 tons a day from a geological fault over an area of 1 square km in a water depth of 100 m may result in a “slow” release type. Currently, there are no simple criteria available to predict whether a release will reach the surface or not.
The two types of release are now discussed further.
“Fast” Carbon Dioxide Releases
There have been several experimental and modeling studies of “fast” releases, as these releases pose most threat to human life and affect offshore health and safety owing to CO2 gas escaping into the atmosphere from the surface of the sea (e.g., Chen et al., 2005; Cloete et al., 2009; Huser et al., 2016).
Blackford et al. (2008) considered that high pressure point releases might create bubble plumes which reached the surface, but noted that some high pressure natural CO2 seeps produced bubble plumes which completely dissolved before reaching the sea surface in water depths >20 m. However, they did not describe the release rates for these examples and hence, while of high pressure, they may have been of low release rate.
Cloete et al. (2009) and Huser et al. (2016) experimentally reproduced “rapid” releases, and also simulated them using dynamical models. They described the plume dynamics as seen in Figure 2A. As bubbles rose toward the surface they transferred momentum to the ambient seawater forming the rising plume of water, which entrained surrounding seawater into it as it rose. Hence, in the Huser et al. (2016) study, CO2-saturated water was discharged from the bubble-plume into the surface waters (Figure 2A). In the experiment, the rising water within the bubble-plume could gain enough momentum to rise above the water surface as a fountain or boil.
Chen et al. (2005) applied a model, validated using experimental CO2 plumes, to pipeline leak scenarios in the South China Sea. Using a leakage rate of 4,300 tons per day and various different leak dimensions and orientations, they found the plume of bubbles reached the surface in a water depth of 90 m, and had a typical diameter of 20 m at the sea surface. A 60 cms–1 current (at the sea surface, decreasing speeds with depth) displaced the surface signature of the bubble plume by up to 30 m from the point vertically above the release point. Hence in the North Sea, under all but extreme conditions, we can expect the surface signature of a “fast” plume to be horizontally close to the point of release.
As an aside, OSPAR (2007a) considered the principle effect of a “fast” release of gas from below the sediment (e.g., failure of caprock due to seismic activity) would be the physical disturbance of the seabed and the violent mixing in the water column caused by rising gas bubble plumes. They noted that “fast” releases could interfere with other users of the sea, including fishing and transport, and may threaten human health (from vented CO2 at the surface).
In summary, “fast” releases will have a surface signature, consisting of bubbles breaking surface or a boil or fountain of water, and therefore will be more easily detected. In addition, large pipeline leaks and well-blow outs will be detected by the operators. Hence, for “fast” releases remedial action may commence quickly, although it will take time to shut down pipelines (hours–days), and much longer times to shut off a blown out well (which may take the drilling of a relief well, i.e., weeks to months). “Fast” releases will also probably attract more media attention, and raise public concern, and may interfere with other users of the sea (through risks posed by dispersing atmospheric CO2). The ultimate fate of the CO2 solution leaving a “fast” plume will be best estimated using regional scale models of advection and diffusion. Finally, note that fast releases, as they may involve gas escaping to the atmosphere, will reduce the rate of CO2 entry into the sea compared to the release rate at the seabed (Blackford et al., 2008).
“Slow” Carbon Dioxide Releases
One example of a modeled “slow” release was presented by Dewar et al. (2013). They simulated releases of approximately 10 and 90 tons per day in conditions typical of the Sleipner field in the North Sea (i.e., 100 m deep, 10 cms–1 bottom current, initial bubble sizes 5–8 mm). Bubbles fully dissolved before reaching 3 m off the seabed, and this occurred within 3 min of entering the sea. A shallow plume of high-CO2 concentration water then moved off as a density current, flowing along the seabed. Within the plume, pH values were decreased by up to 1.5 units compared to the ambient seawater. Dewar (2016) extended the modeling to include leaks of 140 tons per day, and found similar results, but with bubbles reaching 10 to 15 m off the seabed before fully dissolving.
Dewar et al. (2013) also noted the importance of initial bubble size for the plume height during a “slow” release. When gas escapes from the seabed, for example from leakage from geological storage, initial bubble size is set by properties of the sediment (i.e., “channel” width associated with pore size) and near-bed current speed. Their model of conditions similar to the North Sea predicted bubble sizes in the range 1 to 8 mm.
Vielstädte et al. (2019) attempted to simulate a slow release at the Sleipner North Sea CCS scheme. They released CO2 at the seabed, depth 85 m, at a rate equivalent to 0.08 tons per day, over a period of 11.5 h. They found that bubbles completely dissolved within approximately 4 m off the seabed (2 m above the release point), and they measured raised pCO2 levels above the bed out to 20 m horizontally from the source. They went on to numerically simulate a “slow” release at the same site, and made predictions of change in pH. The impacted footprint of a simulated “slow” release is small: the equivalent of a 16 m by 16 m patch of seabed (see Supplementary Material S8). The behavior of “slow” leaks was also described in the RISCS project as reported by Paulley et al. (2013; details in Supplementary Material S9).
Once “slow” releases dissolve into the sea, small scale models of the behavior of the bubble plume itself are no longer useful. Rather the impact of these sources is better represented in models of advection and dispersion, described in the next section.
Summary of the Spread of Carbon Dioxide at the Local Scale
In most shelf seas such as the North Sea, all escapes of CO2 will be as a gas once it enters the sea, or as CO2 in solution from sediment pore water. As a gas, CO2 gas bubbles will rise, expand due to pressure changes, and shrink due to absorption of the CO2 by the seawater. Whether bubbles of CO2 reach the surface will depend on the initial form of the release. A violent rapid release from a small area will see large complex bubbles coalescing and dividing. The rising bubble plume will drag seawater into it, and drive it toward the sea surface, where its momentum may break the surface and form a fountain of water into the air. Such a violent or “rapid” release will emit CO2 solution into the surface waters of the sea.
A second scenario is that CO2 bubbles fully dissolve before reaching the surface of the sea. Some leak rates predicted for geological storage, for example, would result in bubbles fully dissolving within just a few meters above the sea bed.
In both cases, the CO2 solution emitted by the bubble plume will be denser than ambient seawater, but not by very much. Thus, it will have a tendency to sink toward the seabed at first. However, turbulence in the sea will rapidly reduce the density difference, and the solution will become more diluted by ambient sweater, and from that point on the movement of the seawater containing released CO2 will be described by general mixing, advection and diffusion in the sea, as reproduced in the regional models described below.
In terms of changes of pH, its likely that local decreases in pH greater than 1 or 2 units will be present, with ecological impacts rated as “catastrophic” up to 1 km radius from a significant (>1,000 tons per day) leak (Phelps et al., 2015).
Regional Scale
Table 6 summarizes studies which model the advection and dispersion of high CO2 concentration seawater once it leaves the initial “local” zone immediately around the leak source (i.e., >1 km, Blackford et al., 2008). Most models include eddy diffusion, tides and wind-driven residual circulation, and most simulate the dispersion in three dimensions, evolving through time. Some models have routines which describe the carbonate cycle in the sea assuming no interaction with biology, while others use ecosystem models which include interaction between the carbonate system and biological processes. As carbonate cycle models do not permit the internal feedbacks that occur between CO2 concentrations and biological processes in the sea, such as primary and secondary production, full ecosystem models offer greatest accuracy as well as information about changes in the ecosystem due to released CO2. As Table 6 confirms, the ecosystem model that has been used by those studies of relevance to this report is the ERSEM model (e.g., Blackford et al., 2008), and the carbon cycle model is the HALTAFALL model (Ingri et al., 1967).
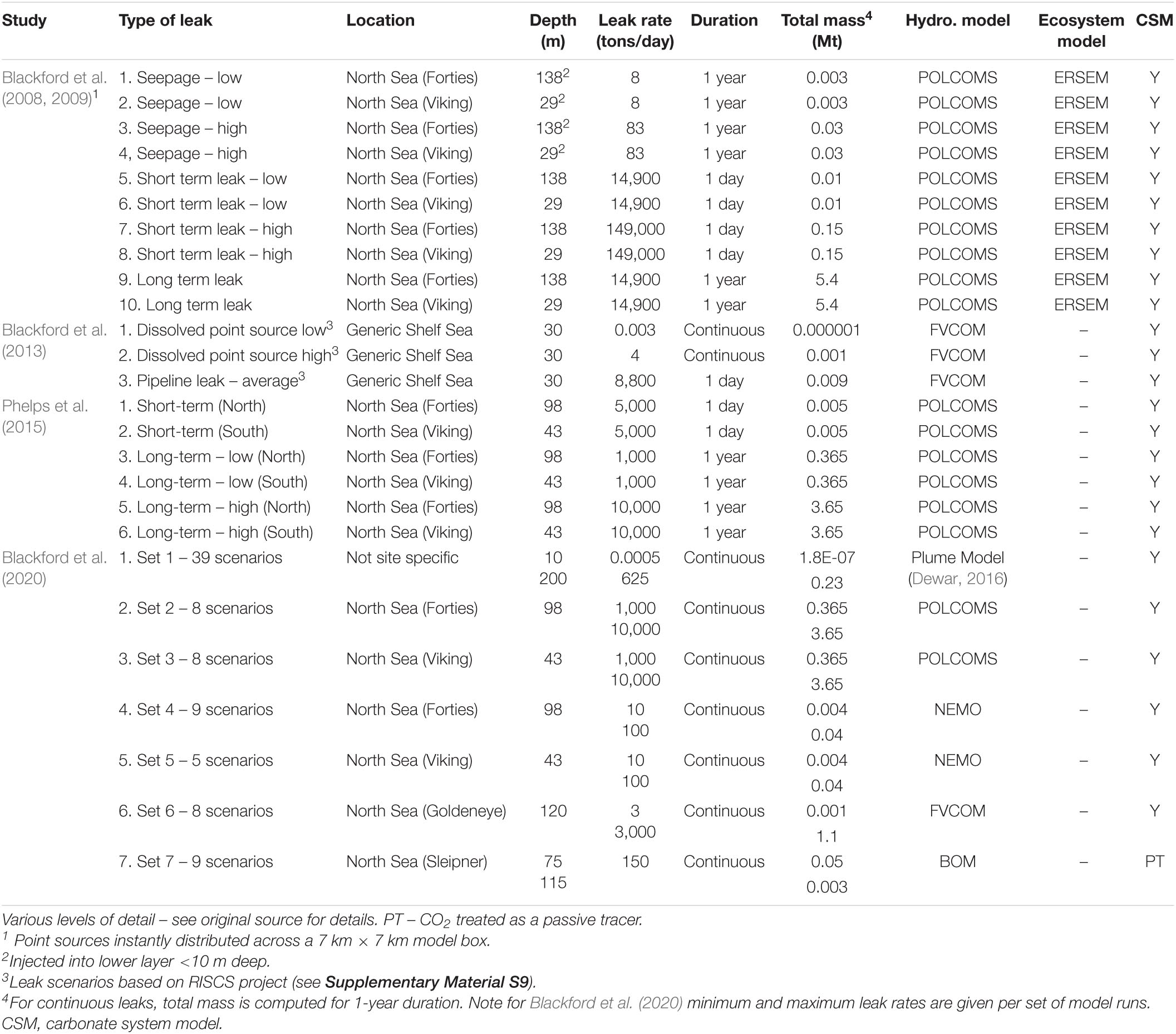
Table 6. Summary of regional scale modeling studies of the advection and dispersion of CO2 in the North Sea or similar environments.
Blackford et al. (2008, 2009) used a coupled hydrodynamic-ecosystem model to examine the dispersion and impact of simulated leaks from the Forties and Viking fields in the North Sea. They noted the possible effect of the local scale interactions described above, and allowed “slow” (low-pressure) releases to enter the near-bed layer of the model, and “fast” (high-pressure) releases to enter already fully mixed throughout the water column. They found the long-term (1 year) low-rate (8 tons per day seepage releases) to have negligible effect on the modeled carbonate system and only very local effects (ΔpH > 0.1) for the high-rate (83 tons/day) long term seepage. Decreases in pH were affected by water depth (greater in shallower water), as well as tidal state (greater decreases during neap tides when mixing and hence dilution was lower).
For the 14,900 tons per day, 1 day leak scenarios, pH declines >0.1 were only evident locally for 1 day, with recovery to background values 9 days after the releases occurred. The 149,000 tons per day scenario resulted in pH declines of up to 0.5, with recovery times of up to 20 days after release. For the long term leak scenarios (1,000 to 10,000 tons per day over 1 year), local pH was decreased by between 0.5 and 1.0. Plumes of low pH then spread away from the leak sites, but pH within the plumes rapidly recovered to background levels as they became diluted when moving away from the leaks.
Using the model, Blackford et al. (2008) were able to examine the out-gassing of CO2 following leaks, i.e., how dissolved CO2 is released from the sea to the atmosphere. Increases in the surface release of CO2 was evident even for the low-level leaks, but were two or three orders of magnitude greater than normal for the larger leak scenarios (see Supplementary Material S10). The variability in the magnitude of out-gassing is determined by several factors, including wind strength. Their model predicted that between 12% and 60% of leaked CO2 is subsequently lost to the atmosphere. Losses are relatively lower in the northern North Sea, as the greater depth results in lower initial concentrations of dissolved CO2 at the surface.
Blackford et al. (2008) go on to use the same model to examine the potential effect on pH in the North Sea of ocean acidification caused by anthropogenic increases in atmospheric CO2. They concluded by noting that even massive leaks from CCS storage would have “minimal” effect on a regional scale (i.e., North Sea scale), and “insignificant” effect compared to the effects of ocean acidification.
This study simulated leaks from the Viking and Forties fields in the North Sea. Both short term (e.g., pipeline failure) and long term (e.g., leakage from geological storage) leaks were simulated (Table 6).
For the short term leaks, they found that ecological impact, as described by ΔpH, was greatest when the sea was vertically stratified (i.e., in late summer), and hence dissolved CO2 was trapped below the seasonal pycnocline. pH decreases of up to 1.92 were recorded. When short-term leaks were stopped, the carbonate system at the leak site quickly returned to background conditions as seawater containing leaked CO2 was advected away from the leak site, and diluted by mixing.
Long term leaks of 10,000 tons per day resulted in local pH reductions of up to 2.67. The models demonstrated that high-CO2 plumes of water streamed away for the leak sites following the general advective flow of the regions modeled. Depression of pH of up to 1 was found up to 30 km away from leak sites “downstream” in relation to the regional residual current pattern.
Tidal flow also affected the shape and dispersion of the CO2 plumes. A “pumping” effect was observed with high CO2 concentrations occurring above a leak site at slack water on a semi-diurnal time scale, and at neap tides on a monthly time scale.
Phelps et al. (2015) went on to examine the effect of vertical stratification on CO2 out-gassing, and found that when stratification occurs (e.g., in the northern North Sea in summer) it greatly reduces the losses of CO2 to the atmosphere from a seabed leak. In conclusion, Phelps et al. (2015) note that leak impacts in the northern North Sea may be greater than in the southern North Sea, as in the south the shallow waters combined with strong tides promote rapid tidal mixing and permit greater out-gassing of CO2. They suggest that the northern North Sea is generally under saturated in CO2 whereas the southern North Sea is fully saturated most of the time.
For the purposes of this review, the summary paper of Blackford et al. (2020) is perhaps the most useful. This paper assembles 86 individual model predictions of the spread of high-CO2 water in the North Sea, and produces simple regressions of the area of seabed, and volume of seawater, impacted for various continuous release rates and under various conditions. The leak scenarios used included release rates ranging from 0.005 tons per day (i.e., very slow geological seeps) up to 10,000 tons per day (i.e., ruptured pipelines or blown operational wells).
The models used were applied to northern (Forties, Goldeneye, Sleipner) and southern (Viking) potential CCS sites in the North Sea. As Phelps et al. (2015) demonstrated, the pattern of dispersion at each site depends strongly on local residual circulation patterns and so to overcome the difficulty of comparing the different shapes of high-CO2 plumes at the different sites, common parameters were used, i.e., the area and volume of sea bounded by different contours of ΔpH. Two values of ΔpH were selected: 0.01 to determine the areas/volumes of sea where the leaked CO2 would be detectable, and 0.1 which defines the “maximum extent of [biological] impact” (Blackford et al., 2020).
In all, five different model systems were used to simulate various leak scenarios (Table 6). The procedure then followed was to use each model system to simulate a continuous leak for 1 year, and at the end of that run to determine the areas and volumes encompassed by the two ΔpH contours (0.01 and 0.1). These were then tabulated and log-log regressions fitted in order to derive summarizing relationships. The final fitted regressions were:
Detectability
The area A (km2) where ΔpH > 0.01 is given by A = 11522.R1.408
The volume V (km3) where ΔpH > 0.01 is given by V = 93331.R1.695
Impact
The area A (km2) where ΔpH > 0.1 is given by A = 629.49.R1.6274
The volume V (km3) where ΔpH > 0.1 is given by V = 1465.6.R1.6929
where R is the leak release rate in tons per day (details in Supplementary Material S11).
It is not a surprise that dispersion in the sea follows such power laws (e.g., Okubo, 1972). The regressions of Blackford et al. (2020) are most likely justified as the high-CO2 plumes are relatively small in comparison to the scale of the circulation patterns within the North Sea. Thus, dispersion and diffusion is dominated by horizontal turbulence rather than advective shear. If patches became much bigger, or took place in a more oceanographically complex region, such power regressions may not be appropriate.
Areas where there will be some biological impact (i.e., ΔpH > 0.1) were predicted to have a characteristic radius of 26 km for a 10,000 tons per day leak, which rapidly decreases to 4 km for a 1,000 tons per day leak. The volumes of the corresponding high-CO2 plumes are 1.3% and 0.03% of the volume of the North Sea. Hence, we can see that the spatial scales of areas affected by CO2 leaks are small in comparison to the North Sea itself.
Summary of How Leaks Spread Out
Figure 3 summarizes the probability of leaks, the size of leaks and the local and regional spread of leaks. In the figure, the scale of leaks has been expressed in four different ways: the total mass released over the leak scenario period (Figure 3A); the release rate expressed in units of tons released per day (Figure 3B); the area of detectability of leaks expressed as the characteristic radius predicted by the Blackford et al. (2020) regressions for ΔpH > 0.01 (Figure 3C); and the area of the impact of leaks expressed as the characteristic radius predicted by the Blackford et al. (2020) regressions for ΔpH > 0.1 (Figure 3D). These four measures of the scale of leaks have been plotted against the probability of leaks occurring, expressed as 1/P (i.e., a 1/P-year event).
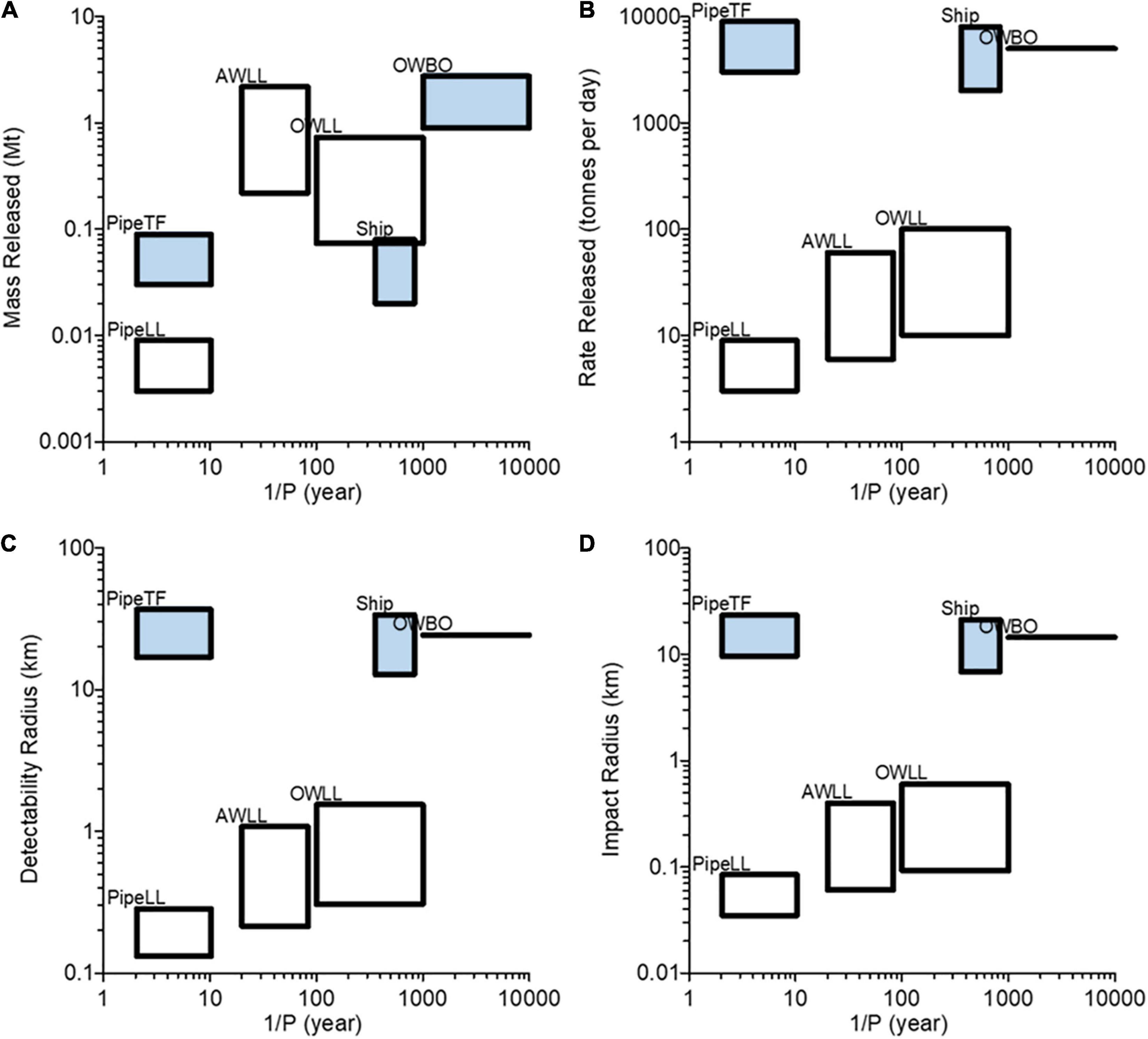
Figure 3. Size (upper two figures) and physical scale (lower two figures) vs. probability diagrams for various sources of leaked CO2 in an offshore CCS industry of approximately 20 Mtpa sequestration capacity. (A) Size, expressed in terms of input rate (tons per day) vs. probability. (B) Size, expressed as total mass released (Mt) vs. probability. (C) Physical scale, in terms of the radius of detectability (km) vs. probability. (D) Physical scale, in terms of radius of impact (km) vs. probability. Detectability defined as ΔpH > 0.01, and impact defined as ΔpH > 0.1. Blue shading: Leaks which will most likely result in “fast” releases. Leak codes: PipeTF, pipeline total failure; PipeLL, pipeline low-level leak; OWLL, operational well low-level leak; OWBO, operational well blow out; AWLL, abandoned well low-level leak. All values used in this diagram are given in Supplementary Material S12.
The most likely leak sources in a 20 Mtpa offshore CCS industry are pipelines, although low-level pipeline leaks have small characteristic impact radii (<100 m). Hence, possibly of most concern is pipeline total failures, which are a 1- to 10-year event, with characteristic impact radii of 10 to 30 km.
The next sources of leaks in terms of significance are low-level leaks from abandoned wells, and operational wells which are 20- to 1000-year events for a 20 Mtpa industry. Low-level leaks from pipeline, abandoned wells and operational wells all have very small regions of detectability, <2 km. Hence, monitoring programs aimed at detecting these must be designed with this small detectability footprint in mind.
Leaks from operational well blow outs may potentially impact the largest areas. However, these areas are still relatively small compared to the dimensions of a shelf sea (e.g., the North Sea), and these events are expected to be rare (i.e., a 1000- to 10,000-year event).
How Might Leaks Affect the Environment of a Regional Shelf Sea?
The aim of this section is to present a brief overview of the possible impacts of CO2 leaks in the sea. It is not a comprehensive review of the subject, which is outside the scope of this initial paper.
Carbon Dioxide in the Sea and Its Biological Effects
Carbon dioxide is a naturally occurring component of the atmosphere/ocean system, and is generated by natural biological processes, such as respiration and decomposition, as well as by anthropogenic activities.
Ocean Acidification
The release of CO2 into the atmosphere by the burning of fossil fuels and other human activities has resulted in more CO2 dissolving into the sea than prior to the industrial revolution. It is estimated that the sea has absorbed about 48% of the CO2 emitted by humans since the industrial age began (Artioli et al., 2012). The addition of excessive CO2 to the sea reduces its pH, i.e., it becomes more acidic (i.e., ocean acidification). Owing to the increase in atmospheric CO2, and the absorption of CO2 by the world’s oceans, the global average ocean surface pH has decreased by 0.1 (Humphreys et al., 2020), referred to as ocean acidification.
Adding Carbon Dioxide From Leaks
Adding CO2 to seawater through leaks from offshore CCS will further alter the sea’s carbonate system. There will be increases in free CO2, free carbonic acid and bicarbonate ion concentrations, while there will be decreases in carbonate ions, pH and carbonate saturation states (Zeebe and Wolf-Gladrow, 2001; Artioli et al., 2012).
In the sea, biological processes also alter the carbonate system. Primary production converts DIC into organic matter, thus “creating space” for more CO2 to dissolve into the sea (Humphreys et al., 2020). CO2 dissolution into the sea is temperature dependent, and increases with cooler temperatures. However, in a regional shelf sea such as the North Sea, biological processes control the seasonal cycle of air-sea CO2 exchange, thus atmospheric CO2 is absorbed into the sea during the productive spring and summer, and “outgassed” from the sea to the atmosphere in winter (Humphreys et al., 2020). Equilibrium of CO2 concentrations between the atmosphere and the sea takes place on the time scale of a year (Humphreys et al., 2020). Acidification caused by uptake of CO2 by the sea reduces its ability to absorb further CO2 (Humphreys et al., 2020).
Changes in the carbonate system in the sea, caused by the addition of dissolved CO2, can impact a range of biological processes such as calcification (e.g., shell production), primary production and reproduction as well as species diversity (Artioli et al., 2012; Humphreys et al., 2020).
Effect on Life in the Sea – Phytoplankton and Microbes
The planktonic and microbial communities are likely to be impacted by changes in the carbonate system. Previous studies looking at the impacts of a controlled CO2 leak on the microbial community found changes in the relative abundance of both major and minor bacterial taxa (Tait et al., 2015). The effect of increased pCO2 on phytoplankton is complex (Sommer et al., 2015; Wells et al., 2015). Some phytoplankton species are poor scavengers of DIC and while the response to increases in pCO2 can vary between functional groups and species it is likely to result in seasonal changes in phytoplankton community structure (Mackey et al., 2015; Bach and Taucher, 2019). Some harmful species can increase toxin production under increased DIC conditions could pose a potential threat to fish in the vicinity of a CO2 release (Riebesell et al., 2018). Studies on shellfish toxin have shown variable results (Raven et al., 2020), however, a leak near areas of aquaculture activity could potentially lead to closures of shellfish harvesting areas to protect human health.
Effect on Life in the Sea – Zooplankton
The corrosive conditions associated with an increase of CO2 will probably have a major impact on zooplankton calcifying species. Short term exposure to extreme acidification conditions is enough to cause significant shell damage and mortalities of pelagic gastropods (Bednaršek et al., 2014; Gardner et al., 2018) and bivalve larvae of commercially important species (Wijsman et al., 2019), resulting in less recruitment (Parker et al., 2013). Given the seasonal patterns of plankton, the intensity of the impact will depend of the time of the year. More damage would be expected in spring and late summer when pelagic gastropod and bivalve larvae are more abundant.
Effect on Life in the Sea – Seabed Organisms
Seabed organisms, including some relevant to commercial fisheries, are likely to perish under extreme acidification scenarios near a leak site, while mortality will reduce for organisms which have the ability to leave the vicinity of a leak. The QICS (Quantifying and monitoring potential ecosystem impacts of geological carbon storage) project (e.g., Blackford et al., 2014) reported rapid seabed community recovery once a small scale leak had ceased. However, impacts to mussel beds, maerl fields, and cold water corals may result in long term damage with potential recovery on a decadal scale.
In summary, owing to concerns about ocean acidification, there is a growing body of literature concerning the effects of changes in the ocean’s carbonate system and its impacts on life in the sea. However, further work is needed in this area, and in more direct studies of the interaction of leaked CO2 with local biota and with ocean acidification, ocean warming and other changes such as eutrophication (where it occurs) and oxygen depletion.
What Science Tasks Need to Be Undertaken by National Monitoring Agencies?
Various national, regional and international policies, guidelines and legislation demand activities which are relevant to the scientific support of an offshore CCS industry (Table 7). These are in addition to policies and regulations relevant to general offshore engineering and transport activities [e.g., Environmental Impact Assessment (EIA) legislation, pipeline safety legislation, vessel safety legislation, general marine pollution legislation]. The aim of this section is to highlight environmental science requirements that are in addition to the general environmental protection statutes, and have arisen specifically due to offshore CCS.
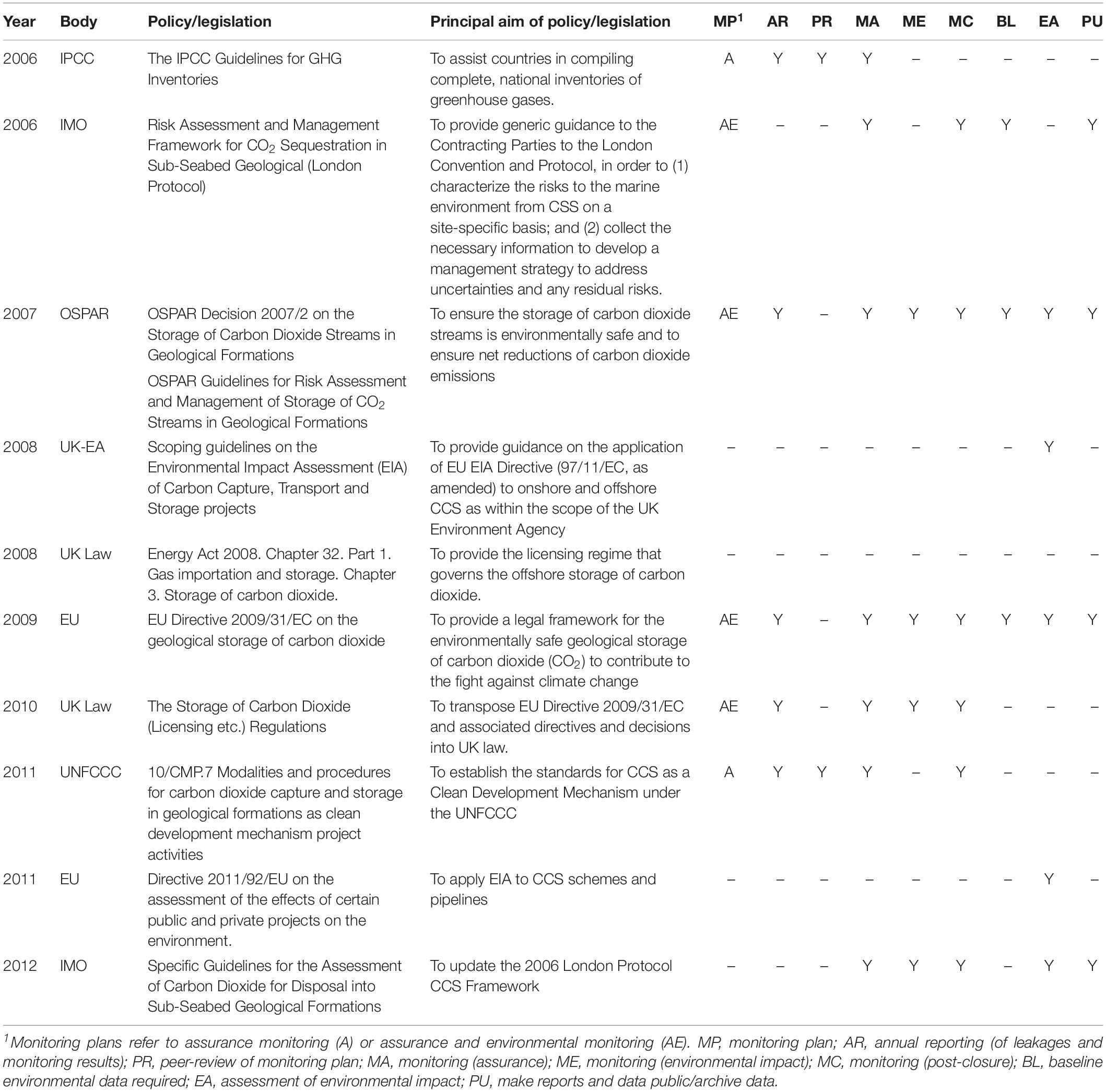
Table 7. A summary of policies, strategies and legislation that require environmental science activities.
While the IPCC (2006) guidelines (Table 7) were essentially focused on the integrity of CCS schemes in terms of retaining the CO2 stored within them, the 2006 IMO guidelines explicitly brought the assessment of the potential environmental impact of CCS schemes to the forefront. It notes that a principle aim of CCS site selection and operation should be that “no impact on human health, the marine environment and other legitimate uses of the sea will occur (OSPAR, 2007a,b).” The 2007 OSPAR Decision and associated guidelines again emphasized that the permitting process for CCS should have the dual objectives of “the avoidance of significant adverse effects on the marine environment” and the “permanent containment of CO2 streams in geological formations.” Hence, risk assessment, site characterization and selection, site operation and site monitoring should aim to meet these two objectives.
In terms of national law, in the United Kingdom for example the 2008 Energy Act (UKGOV, 2008a) provided the legal basis for a permit system for offshore CCS, and the 2010 Storage of Carbon Dioxide (Licensing etc.) Regulations (UKGOV, 2010) added the licensing details which embodied the twin aims of assuring the integrity of a CCS scheme, and ensuring it has minimal environmental impact, transposing details from the EU Directive 2009/31/EC (EU, 2009).
Finally, an update to the London Protocol adopted in 2012 (IMO, 2012) dealt with “risks posed by carbon dioxide sequestration in sub-seabed geological formations over all timescales and primarily at the local and regional scale and thus focus on the potential effects on the marine environment in the proximity of the receiving formations.” It further developed the 2006 London Protocol guidelines by adding the requirement for environmental monitoring and assessment as well as site integrity considerations. A further review of related international policies can be found in de Medeiros Costa and Arlota (2021).
Hence, from the summary of international and regional policies presented in Table 7, scientists and science-policy advisers in national marine monitoring and management organizations should prepare to participate in the following activities:
Monitoring Plans
A Monitoring Plan is a core aspect of the permit system for CCS. The need for a Monitoring Plan was established in the IPCC (2006) guidelines, where it was principally referring to assurance monitoring to demonstrate the integrity of a CCS storage facility. However, the OSPAR (2007a,b) Decision and Guidelines, although they incorporate the Monitoring Plan as a requirement of risk management, introduce the additional aim of ensuring CCS schemes are environmentally safe. The Framework for Risk Assessment and Management of Storage of CO2 Streams in Geological Formations (FRAM) within the OSPAR Guidelines state that an effects assessment, leading to an impact hypothesis, should be used to establish the monitoring program for a CCS scheme. This process must consider “the potential impacts on amenities, sensitive areas, habitat, migratory patterns, biological communities and marketable resources.” The UK 2010 legislation (UKGOV, 2010) notes that the Monitoring Plan must be designed for “the detection of any significant adverse effects on the surrounding environment.” The design and execution of the Monitoring Plan is the responsibility of the CCS scheme operator. Many references are available describing the components of a Monitoring Plan (e.g., Dixon and Romanak, 2015) but this is outside the scope of this review.
Baseline Data
The requirement for baseline data is a prominent feature of the 2007 OSPAR Guidelines (OSPAR, 2007a). Baseline data should include marine environmental data for the purposes of site management and monitoring, and provides “the datum against which change is measured.” However, the development of baselines, particularly in relation to species and habitats, is a complex and rapidly evolving field (e.g., Muller-Karger et al., 2014; Certain and Planque, 2015) outside the scope of this review.
Environmental Impact Assessments
The 2006 London Protocol Guidelines (IMO, 2006) state that “Effects Assessment assembles the information necessary to describe the response of receptors within the marine environment resulting from exposure to the CO2 stream if leakage were to occur. The main effects of concern to such an assessment include effects on human health, marine resources, relevant biological communities, habitats, and ecological processes, and other legitimate uses of the sea.” The 2012 update of the London Protocol added more specific concerns to consider, including fishing and mariculture areas, spawning, nursery and recruitment areas, migration routes, seasonal and critical habitats and coastal and marine areas of environmental, scientific, cultural or historical importance, such as marine protected areas or vulnerable ecosystems. Directive 2011/92/EU on the assessment of the effects of certain public and private projects on the environment placed CCS schemes and pipelines into Annex 1 as projects that required EIAs.
Annual Reporting of Monitoring Data and Results
Annual reporting by the operator of the results of the Monitoring Plan is another key feature of the permit system in the United Kingdom (UKGOV, 2010), enshrining this principle from the IPCC, OSPAR, and IMO guidelines. Other aspects are a 5-yearly review, and a 20-year post-closure extension of the requirement to monitor and report results.
Assurance Monitoring
Regular and comprehensive assurance monitoring by the scheme operator is required to demonstrate the integrity of a CCS storage facility. The IPCC guidelines state that “properly selected geological storage sites are likely to retain greater than 99 percent of the stored CO2 over 1000 years.” There are many types of Assurance Monitoring, mainly geophysical in nature. These are not in the scope of this report, but have been extensively reviewed elsewhere (e.g., Jenkins et al., 2015; de Carvalho Nunes and de Medeiros Costa, 2020). However, as they point out, in-water leak detection may be cheaper and more sensitive than geophysical methods. While Assurance Monitoring is not the role of many national marine monitoring organizations, there will be synergies possible between in-water leak detection for the purposes of assurance monitoring and environmental monitoring.
Environmental Impact Monitoring
Environmental impact monitoring is a requirement of many national licensing schemes. For example, the 2010 Storage of Carbon Dioxide (Licensing etc.) Regulations (UKGOV, 2010) states that the purpose of monitoring includes “the detection of any significant adverse effects on the surrounding environment.”
In addition to impact monitoring, it is important that feedback mechanisms are considered, as well as actions needed to be taken when thresholds, considered to represent significant adverse effects, are breached. Simply detecting effects is largely pointless if the information gained is not used to inform future licensing or to action specific remediation.
Post-closure Monitoring
While post-closure monitoring will in most cases be the responsibility of the operator, it may be handed to national agencies after a certain passage of time. Annex 2 of the EU Directive 2009/31/EC stipulates that a Monitoring Plan must include post-closure monitoring. Article 18 of that Directive states that this should be for a minimum of 20 years post-closure.
Publication of Monitoring and Environmental Impact Assessment Reports and Data
Getting access to monitoring reports, environmental assessment reports and the data which underpins them is vital for marine science and marine scientists to provide relevant and accurate advice concerning CCS schemes. The London Protocol guidelines for CCS (IMO, 2006) put this quite clearly as clause 6.15: “because the aim of [CCS] is to store CO2 permanently, it will be necessary to archive documentation so that future generations are informed of the existence of the CO2 reservoir and its history. This includes keeping records of the authorization and licensing process, together with data of long-term monitoring.”
The OSPAR (2007b) Decision encourages all Contracting Parties to make reports from all phases of a project publically available (Clause 3.4), and to submit reports and their data to OSPAR (Clause 3.5). This is echoed in the preamble of the 2009 EU Directive (EU, 2009), which notes “Member States should make available to the public environmental information relating to geological storage of CO2 in accordance with applicable Community legislation.”
The 2012 update of the London Protocol with respect to CCS noted (Clause 9.5) “Because the aim of disposal of carbon dioxide streams into sub-seabed geological formations is to store CO2 permanently, permits and other supporting documentation, including site location, monitoring results and mitigation or remediation plans should be archived and retained for long periods of time.” This implies publication and archiving data in public databases.
Peer-Review of Monitoring Plans
This aspect is advised by the 2006 IPCC Guidelines which state that “the site operator should at the outset provide the inventory compiler with the results of peer review by a competent third party confirming that […] the monitoring plan is suitable.” While this is aimed at assurance monitoring, and is not repeated by other guidelines or legislation, it is clearly of benefit to environmental monitoring and should perhaps be applied to this for a properly managed CCS industry.
Conclusion
This review suggests that the probability of CO2 leaks from offshore CCS schemes in shelf sea waters is low. If they do occur their spatial scale of impact is small, and the potential environmental impact is low. However, this does not mean marine science should do nothing in preparation for an emerging offshore CCS industry in national or regional shelf seas. For example, international, regional and national legislation is in place that requires marine scientists to participate in the following activities:
• Design of a Monitoring Plan for each CCS scheme
• Review of Monitoring Plans
• Assurance monitoring (i.e., surveillance for leaks)
• Environmental impact monitoring (i.e., surveillance for environmental impact)
• Post-closure monitoring
• Baseline data collection (i.e., prior to scheme commencing)
• Environmental impact assessments
• Archiving and publication of monitoring and EIA reports and data.
Recommendations for Science Priorities in Scotland
The authors of this review provide the science and scientific advice that underpins national marine monitoring and management in Scottish waters, including parts of the North Sea where offshore CCS schemes are being proposed. However, in order to supply these services, some internal developments are needed and this review is an initial step in establishing which developments are a priority for us, taking into account our current capabilities. Further reviews are needed, specifically to review the currently available knowledge concerning (a) current and future environmental monitoring technologies and strategies relevant to the shelf sea carbonate system and its effects on biota, and (b) the potential ecological impacts of leaked CO2. However, we hope these recommendations may help other similar organizations consider their own needs in relation to their current capacities. In this context, our priorities are:
(1) To improve our understanding of the North Sea’s carbonate system, processes that may cause it to change and how North Sea carbonate system change may impact sensitive biota.
(2) To acquire up-to-date methodologies to monitor short and long term changes in the carbonate cycle of the North Sea and their impact on sensitive biota.
(3) To plan and implement a monitoring strategy capable of detecting long term trends in the carbonate system of the North Sea, and in its key sensitive species.
(4) To acquire a modeling capability to assess the impact of possible changes in the carbonate system of the North Sea and their effect on key ecosystem aspects such as primary and secondary production.
(5) Long term change in the oxygen and nutrient content of the North Sea influences the carbonate system, and coupled with carbonate system change may result in cumulative impacts. Hence, long term monitoring of North Sea dissolved oxygen concentrations and nutrients is also needed, combined with the existing monitoring of ocean climate parameters including temperature and salinity.
Fulfilling these priorities will meet the following additional objectives to improve our capacity to provide advice to marine managers:
(1) Improve our understanding of ocean acidification, nutrient cycling, and oxygen depletion, and their ecological impacts. This will contribute to the understanding of the cumulative impacts of climate change and direct anthropogenic inputs, including from an offshore CCS industry.
(2) Ensure Scottish national marine science has the knowledge, methods, skills, equipment and facilities to respond to an incident involving CO2 release.
(3) Ensure Scottish national marine science has the relevant knowledge to provide respected and defensible environmental impact advice to support a growing offshore CCS industry.
Owing to the low probability/small scale/low impact of CCS leakage, understanding ocean acidification, oxygen depletion, nutrient cycling and climate change may well ultimately be an equal if not more urgent driver of new work for ourselves. However, marine science in national marine monitoring and management organizations should consider the priorities needed in their region to support emerging offshore CCS industries.
Author Contributions
WT provided the conception and design of the study and wrote the first draft of the manuscript. BB, EB, PL, SR, LW, PWa, JW, and PWr wrote sections of the manuscript. All authors contributed to manuscript revision, read, and approved the submitted version.
Conflict of Interest
The authors declare that the research was conducted in the absence of any commercial or financial relationships that could be construed as a potential conflict of interest.
Publisher’s Note
All claims expressed in this article are solely those of the authors and do not necessarily represent those of their affiliated organizations, or those of the publisher, the editors and the reviewers. Any product that may be evaluated in this article, or claim that may be made by its manufacturer, is not guaranteed or endorsed by the publisher.
Supplementary Material
The Supplementary Material for this article can be found online at: https://www.frontiersin.org/articles/10.3389/fmars.2022.838309/full#supplementary-material
References
Artioli, Y., Blackford, J. C., Butenschön, M., Holt, J. T., Wakelin, S. L., Thomas, H., et al. (2012). The carbonate system in the North Sea: sensitivity and model validation. J. Mar. Syst. 102-104, 1–13. doi: 10.1016/j.jmarsys.2012.04.006
Bach, L. T., and Taucher, J. (2019). CO2 effects on diatoms: a synthesis of more than a decade of ocean acidification experiments with natural communities. Ocean Sci. 15, 1159–1175. doi: 10.5194/os-15-1159-2019
Bednaršek, N., Feely, R. A., Reum, J. C. P., Peterson, B., Menkel, J., Alin, S. R., et al. (2014). Limacina helicina shell dissolution as an indicator of declining habitat suitability owing to ocean acidification in the California Current Ecosystem. Proc. R. Soc. B Biol. Sci. 281:20140123. doi: 10.1098/rspb.2014.0123
Blackford, J., Alendal, G., Avlesen, H., Brereton, A., Cazenave, P. W., Chen, B., et al. (2020). Impact and detectability of hypothetical CCS offshore seep scenarios as an aid to storage assurance and risk assessment. Int. J. Greenhouse Gas Control 95:102949. doi: 10.1016/j.ijggc.2019.102949
Blackford, J., Jones, N., Proctor, R., Holt, J., Widdicombe, S., Lowe, D., et al. (2009). An initial assessment of the potential environmental impact of CO2 escape from marine carbon capture and storage systems. Proc. Inst. Mech. Eng. A J. Power Energy 223, 269–280. doi: 10.1243/09576509JPE623
Blackford, J., Stahl, H., Bull, J. M., Bergès, B. J. P., Cevatoglu, M., Lichtschlag, A., et al. (2014). Detection and impacts of leakage from sub-seafloor deep geological carbon dioxide storage. Nat. Clim. Change 4, 1011–1016. doi: 10.1038/nclimate2381
Blackford, J. C., Jones, N., Proctor, R., and Holt, J. (2008). Regional scale impacts of distinct CO2 additions in the North Sea. Mar. Pollut. Bull. 56, 1461–1468. doi: 10.1016/j.marpolbul.2008.04.048
Blackford, J. C., Torres, R., Cazanave, P., and Artioli, Y. (2013). Modelling dispersion of CO2 plumes in sea water as an aid to monitoring and understanding ecological impact. Energy Procedia 37, 3379–3386.
Borresen, R., Harton, H., and Bolsover, A. (2012). Pipeline and Riser Loss of Containment 2001-2012 (PARLOC 2012). London: Oil & Gas.
Botnen, H. A., Omar, A. M., Aavatsmark, I., Alendal, G., and Johannessen, T. (2013). PVTx properties of a two-phase CO2 jet from ruptured pipeline. Energy Proc. 37, 3031–3038. doi: 10.1016/j.egypro.2013.06.189
Brownsort, P. A., Scott, V., and Haszeldine, R. S. (2016). Reducing costs of carbon capture and storage by shared reuse of existing pipeline—Case study of a CO2 capture cluster for industry and power in Scotland. Int. J. Greenhouse Gas Control 52, 130–138. doi: 10.1016/j.ijggc.2016.06.004
Bužančić Primorac, B., and Parunov, J. (2016). Review of statistical data on ship accidents. Mar. Technol. Eng. 3, 809–814. doi: 10.1201/b21890-106
Cabioc’h, F., De Castelet, D., Penelon, T., Pagnon, S., Peuch, A., Bonnardot, F., et al. (2009). “Accidents on vessels transporting liquid gases and responder’s concerns: the Galerne Project,” in Proceedings of the 32 AMOP Technical Seminar on Environmental Contamination and Response (Canada: Environment Canada).
Certain, G., and Planque, B. (2015). Biodiversity baseline for large marine ecosystems: an example from the Barents Sea. ICES J. Mar. Sci. 72, 1756–1768. doi: 10.1093/icesjms/fsv040
Chen, B., Song, Y., Nishio, M., Someya, S., and Akai, M. (2005). Modeling near-field dispersion from direct injection of carbon dioxide into the ocean. J. Geophys. Res. Oceans 110:e002567. doi: 10.1029/2004JC002567
Cloete, S., Olsen, J. E., and Skjetne, P. (2009). CFD modeling of plume and free surface behaviour resulting from a sub-sea gas release. Appl. Ocean Res. 31, 220–225. doi: 10.1016/j.apor.2009.09.005
Committee on Climate Change [CCC] (2019). Net Zero: The UK’s Contribution to Stopping Global Warming. London: Committee on Climate Change.
Davidson, C. L., Dahowski, R. T., McJeon, H. C., Clarke, L. E., Iyer, G. C., and Muratori, M. (2017). The value of CCS under current policy scenarios: NDCs and beyond. Energy Proc. 114, 7521–7527. doi: 10.1016/j.egypro.2017.03.1885
de Carvalho Nunes, R., and de Medeiros Costa, H. K. (2020). An overview of international practices for authorization and monitoring CO2 storage facilities. Int. J. Adv. Eng. Res. Sci. 7, 133–142. doi: 10.22161/ijaers.76.16
de Medeiros Costa, H. K., and Arlota, C. (2021). Carbon Capture and Storage in International Energy Policy and Law. Amsterdam: Elsevier.
Dewar, M. (2016). Modelling the Two Phase Plume Dynamics of CO2 Leakage into Open Shallow Waters. Edinburgh: Heriot-Watt University.
Dewar, M., Wei, W., McNeil, D., and Chen, B. (2013). Small-scale modelling of the physiochemical impacts of CO2 leaked from sub-seabed reservoirs or pipelines within the North Sea and surrounding waters. Mar. Pollut. Bull. 73, 504–515. doi: 10.1016/j.marpolbul.2013.03.005
Dickson, A. G., Sabine, C. L., and Christian, J. R. (2007). Guide to Best Practices for Ocean CO2 Measurements. Sydney, NSW: North Pacific Marine Science Organization.
Dixon, T., and Romanak, K. D. (2015). Improving monitoring protocols for CO2 geological storage with technical advances in CO2 attribution monitoring. Int. J. Greenhouse Gas Control 41, 29–40. doi: 10.1016/j.ijggc.2015.05.029
Dodds, K., Watson, M., and Wright, I. (2011). Evaluation of risk assessment methodologies using the In Salah CO2 storage project as a case history. Energy Proc. 4, 4162–4169. doi: 10.1016/j.egypro.2011.02.361
EU (2009). Directive 2009/31/EC of the European Parliament and of the Council on the Geological Storage of Carbon Dioxide. Available online at: https://eur-lex.europa.eu/legalcontent/EN/TXT/PDF/?uri=CELEX:32009L0031&from=EN (accessed March 16, 2021).
EU (2018). A Clean Planet for All: A European Strategic Long-Term Vision for a Prosperous, Modern, Competitive and Climate Neutral Economy. Available online at: https://eur-lex.europa.eu/legal-content/EN/TXT/PDF/?uri=CELEX:52018DC0773&from=EN (accessed March 16, 2021).
EU (2020). A European Green Deal: Striving to be the First Climate-Neutral Continent. Available online at: https://ec.europa.eu/info/strategy/priorities-2019-2024/european-green-deal_en (accessed March 16, 2021).
Fajardy, M., Morris, J., Gurgel, A., Herzog, H., Mac Dowell, N., and Paltsev, S. (2021). The economics of bioenergy with carbon capture and storage (BECCS) deployment in a 1.5 °C or 2 °C world. Glob. Environ. Change 68:102262.
Gardner, J., Manno, C., Bakker, D. C., Peck, V. L., and Tarling, G. A. (2018). Southern Ocean pteropods at risk from ocean warming and acidification. Mar. Biol. 165:8. doi: 10.1007/s00227-017-3261-3
HSE (2017). Offshore Accident and Failure Frequency Data Sources - Review and Recommendations. Prepared by the Health and Safety Executive. Available online at: https://www.hse.gov.uk/research/rrpdf/rr1114.pdf (accessed March 16, 2021).
Humphreys, M. P., Artioli, Y., Bakker, D. C. E., Hartman, S. E., León, P., Wakelin, S., et al. (2020). Air–sea CO2 exchange and ocean acidification in UK seas and adjacent waters. MCCIP Sci. Rev. 2020, 54–75. doi: 10.14465/2020.arc03.oac
Huser, A., Vollestad, P., Rivedal, N., Armstrong, K., Bengherbia, T., Ferrara, G., et al. (2016). Accidental Underwater Release of CO2-CFD Modelling of the Underwater Plume and the Subsequent Above Water Gas Dispersion. DNV GL: Groningen.
IMO (2006). Risk Assessment and Management Framework for CO2 Sequestration in Sub-Seabed Geological Structures (CS-SSGS). (Source LC/SG-CO2 1/7, Annex 3). London: IMO.
IMO (2012). Specific Guidelines for the Assessment of Carbon Dioxide for Disposal into Sub-Seabed Geological Formations. London: IMO.
Ingri, N., Kakolowicz, W., Sillén, L. G., and Warnqvist, B. (1967). High speed computers as a supplement to graphical methods: V. HALTAFALL, a general program for calculating the composition of equilibrium mixtures. Talanta 14:1261. doi: 10.1016/0039-9140(67)80203-0
IOGP (2019). “The potential for CCS and CCU in Europe,” in Report to The Thirty Second Meeting of the European Gas Regulatory Forum, 5-6 June 2019. Coordinated by IOGP. Available online at: https://ec.europa.eu/info/sites/info/files/iogp_-_report_-_ccs_ccu.pdf (accessed March 16, 2021).
IPCC (2005). IPCC Special Report on Carbon Dioxide Capture and Storage. Available online at: https://www.ipcc.ch/site/assets/uploads/2018/03/srccs_wholereport-1.pdf (accessed March 16, 2021).
IPCC (2006). The IPCC Guidelines for GHG Inventories. Volume 3, Chapter 5 - CO2 Transport, Injection and Geological Storage. Available online at: https://www.ipcc-nggip.iges.or.jp/public/2006gl/pdf/2_Volume2/V2_5_Ch5_CCS.pdf (accessed March 16, 2021).
Jenkins, C., Chadwick, A., and Hovorka, S. D. (2015). The state of the art in monitoring and verification—ten years on. Int. J. Greenhouse Gas Control 40, 312–349. doi: 10.1016/j.ijggc.2015.05.009
Jewell, S., and Senior, B. (2014). CO2 Storage Liabilities in the North Sea: An Assessment of Risks and Financial Consequences. London: DECC.
Jones, D., Beaubien, S., Blackford, J., Foekema, E., Lions, J., De Vittor, C., et al. (2015). Developments since 2005 in understanding potential environmental impacts of CO2 leakage from geological storage. Int. J. Greenhouse Gas Control 40, 350–377. doi: 10.1016/j.ijggc.2015.05.032
Mabon, L., Kita, J., and Xue, Z. (2017). Challenges for social impact assessment in coastal regions: a case study of the Tomakomai CCS Demonstration Project. Mar. Policy 83, 243–251.
Mabon, L., Shackley, S., and Bower-Bir, N. (2014). Perceptions of sub-seabed carbon dioxide storage in Scotland and implications for policy: a qualitative study. Mar. Policy 45, 9–15. doi: 10.1016/j.marpol.2013.11.011
Mackey, K. R., Morris, J. J., Morel, F. M., and Kranz, S. A. (2015). Response of photosynthesis to ocean acidification. Oceanography 28, 74–91. doi: 10.5670/oceanog.2015.33
Martynov, S., Mahgerefteh, H., and Brown, S. (2012). CO2 Transportation for CCS. Cambridge, MA: University of Cambridge.
Muller-Karger, F. E., Kavanaugh, M. T., Montes, E., Balch, W. M., Breitbart, M., Chavez, F. P., et al. (2014). A framework for a marine biodiversity observing network within changing continental shelf seascapes. Oceanography 27, 18–23.
Okubo, A. (1972). Some Speculations on Oceanic Diffusion Diagrams (No. COO-3062-4; CONF-720718-1). Baltimore, MD: Johns Hopkins University.
Orr, J., and Epitalon, J.-M., and Gattuso, J.-P. (2015). Comparison of ten packages that compute ocean carbonate chemistry. Biogeosci. Discuss. 12, 1483–1510.
OSPAR (2007a). OSPAR Guidelines for Risk Assessment and Management of Storage of CO2 Streams in Geological Formations. London: OSPAR.
OSPAR (2007b). OSPAR Decision 2007/2 on the Storage of Carbon Dioxide Streams in Geological Formations Adopted: 2007, Ostend. London: OSPAR.
Parker, L. M., Ross, P. M., O’Connor, W. A., Pörtner, H. O., Scanes, E., and Wright, J. M. (2013). Predicting the response of molluscs to the impact of ocean acidification. Biology 2, 651–692. doi: 10.3390/biology2020651
Paulley, A., Metcalfe, R., Egan, M., Maul, P. R., Limer, L., and Grimstad, A. A. (2013). Hypothetical impact scenarios for CO2 leakage from storage sites. Energy Proc. 37, 3495–3502. doi: 10.1016/j.marpolbul.2016.05.071
Phelps, J. J. C., Blackford, J. C., Holt, J. T., and Polton, J. A. (2015). Modelling large-scale CO2 leakages in the North Sea. Int. J. Greenhouse Gas Control 38, 210–220. doi: 10.1016/j.ijggc.2014.10.013
Raven, J. A., Gobler, C. J., and Hansen, P. J. (2020). Dynamic CO2 and pH levels in coastal, estuarine, and inland waters: theoretical and observed effects on harmful algal blooms. Harmful Algae 91:101594. doi: 10.1016/j.hal.2019.03.012
Riebesell, U., Aberle-Malzahn, N., Achterberg, E. P., Algueró-Muñiz, M., Alvarez-Fernandez, S., Arístegui, J., et al. (2018). Toxic algal bloom induced by ocean acidification disrupts the pelagic food web. Nat. Clim. Change 8, 1082–1086.
Schrag, D. P. (2009). Storage of carbon dioxide in offshore sediments. Science 325, 1658–1659. doi: 10.1126/science.1175750
Sellami, N., Dewar, M., Stahl, H., and Chen, B. (2015). Dynamics of rising CO2 bubble plumes in the QICS field experiment: part 1 – the experiment. Int. J. Greenhouse Gas Control 38, 44–51.
SG (2009). Opportunities for CO2 Storage Around Scotland: An Integrated Strategic Research Study. Edinburgh: University of Edinburgh.
Sommer, U., Paul, C., and Moustaka-Gouni, M. (2015). Warming and ocean acidification effects on phytoplankton: from species shifts to size shifts within species in a mesocosm experiment. PLoS One 10:e0125239. doi: 10.1371/journal.pone.0125239
Tait, K., Stahl, H., Taylor, P., and Widdicombe, S. (2015). Rapid response of the active microbial community to CO2 exposure from a controlled sub-seabed CO2 leak in Ardmucknish Bay (Oban, Scotland). Int. J. Greenhouse Gas Control 38, 171–181.
Tanaka, Y., Sawada, Y., Tanase, D., Tanaka, J., Shiomi, S., and Kasukawa, T. (2017). Tomakomai CCS demonstration project of Japan, CO2 injection in process. Energy Proc. 114, 5836–5846.
UKGOV (2008a). Energy Act 2008. Chapter 32. Part 1. Gas Importation and Storage. Chapter 3. Storage of Carbon Dioxide. Available online at: https://www.legislation.gov.uk/ukpga/2008/32/part/1/chapter/3 (accessed March 16, 2021).
UKGOV (2008b). Scoping Guidelines on the Environmental Impact Assessment (EIA) of Carbon Capture, Transport and Storage Projects - GEHO0811BUCQ-E-E. Available online at: https://assets.publishing.service.gov.uk/government/uploads/system/uploads/attachment_data/file/297115/geho0811bucq-e-e.pdf (accessed March 16, 2021).
UKGOV (2010). Statutory Instruments: 2010 no. 2221. Environmental Protection. The Storage of Carbon Dioxide (Licensing etc.) Regulations 2010. Available online at: https://www.legislation.gov.uk/uksi/2010/2221/contents/made (accessed March 16, 2021).
UNFCCC (2015). Paris Agreement. Available online at: https://unfccc.int/process-and-meetings/the-paris-agreement/the-paris-agreement (accessed March 16, 2021).
Vielstädte, L., Linke, P., Schmidt, M., Sommer, S., Haeckel, M., Braack, M., et al. (2019). Footprint and detectability of a well leaking CO2 in the Central North Sea: implications from a field experiment and numerical modelling. Int. J. Greenhouse Gas Control 84, 190–203.
Wells, M. L., Trainer, V. L., Smayda, T. J., Karlson, B. S., Trick, C. G., Kudela, R. M., et al. (2015). Harmful algal blooms and climate change: learning from the past and present to forecast the future. Harmful Algae 49, 68–93. doi: 10.1016/j.hal.2015.07.009
Wijsman, J. W. M., Troost, K., Fang, J., and Roncarati, A. (2019). “Global production of marine bivalves. Trends and challenges,” in Goods and Services of Marine Bivalves, eds Ø Strand, J. G. Ferreira, and J. K. Petersen (Cham: Springer), 7–26.
Zahasky, C., and Krevor, S. (2020). Global geologic carbon storage requirements of climate change mitigation scenarios. Energy Environ. Sci. 13, 1561–1567. doi: 10.1039/D0EE00674B
Keywords: carbon capture and storage, monitoring, impact assessment, CO2, carbonate system
Citation: Turrell WR, Berx B, Bresnan E, León P, Rouse S, Webster L, Walsham P, Wilson J and Wright P (2022) A Review of National Monitoring Requirements to Support Offshore Carbon Capture and Storage. Front. Mar. Sci. 9:838309. doi: 10.3389/fmars.2022.838309
Received: 17 December 2021; Accepted: 31 January 2022;
Published: 08 March 2022.
Edited by:
Roger C. Prince, Stonybrook Apiary, United StatesReviewed by:
Hirdan Costa, University of São Paulo, BrazilWilliam Schmelz, Rutgers, The State University of New Jersey, United States
Copyright © 2022 Turrell, Berx, Bresnan, León, Rouse, Webster, Walsham, Wilson and Wright. This is an open-access article distributed under the terms of the Creative Commons Attribution License (CC BY). The use, distribution or reproduction in other forums is permitted, provided the original author(s) and the copyright owner(s) are credited and that the original publication in this journal is cited, in accordance with accepted academic practice. No use, distribution or reproduction is permitted which does not comply with these terms.
*Correspondence: William R. Turrell, YmlsbC50dXJyZWxsQGdvdi5zY290