- 1ENTROPIE – UMR 9220 (CNRS, IRD, UR, UNC, IFREMER), Institut de Recherche pour le Développement, Nouméa, New Caledonia
- 2LEMAR – UMR 6539 (CNRS, IRD, UBO, IFREMER), Institut Universitaire Européen de la Mer, Plouzané, France
- 3Red Sea Research Center (RSRC), King Abdullah University of Science and Technology (KAUST), Thuwal, Saudi Arabia
The crucial role of crustose coralline algae (CCA) in inducing hard coral larval settlement and ensuring the replenishment of coral reefs is widely accepted, and so are the negative effects of anthropogenic CO2 emissions on both CCA abundance and coral development. However, diversified and well-developed coral reef communities have been recently discovered in natural conditions where CCA and corals would not be expected to thrive. Back-reef pools, volcanic CO2 vents, mangrove estuaries, and semi-enclosed lagoons systems can present seawater pH, temperature, and dissolved oxygen values reaching or even exceeding the conditions currently predicted by the Inter Panel on Climate Change (IPCC) for 2100. In the semi-enclosed lagoon of Bouraké (New Caledonia, southwest Pacific Ocean), seawater pHT, dissolved oxygen, and temperatures regularly fluctuate with the tide reaching respectively minimum values of 7.23 pHT units, 2.28 mg O2 L-1, and maximum of 33.85°C. This study reports the effect of such extreme environmental conditions on hard coral recruitment and CCA originally settled at a forereef on artificial substrates that were transplanted over two years in two fringing reef and at the Bouraké lagoon. Our data emphasize the negative effects of the extreme conditions in our study sites on the CCA, which decreased in cover by ca. 80% and lost in the competition with turf algae, which, in turn, increased up to 162% at the end of the two years. Conversely, hard coral recruitment remained high at Bouraké throughout the study, three-fold higher than at two sites located outside Bouraké where environmental conditions were typical for coastal fringing reefs. Our findings show that while such extreme, climate change like-conditions have a direct and adverse effect on CCA abundance, and despite a certain persistence, coral larvae settlement was not affected. Based on previous findings from Bouraké, and the present observations, both coral recruits and adults seem to be unaffected despite the extreme environmental conditions. This study supports previous research illustrating how extreme natural and variable environments may reveal unexpected and positive insights on the processes underlying coral acclimatization and adaptation to global change.
Introduction
Scleractinian corals begin their life as pelagic larvae eventually settling to the reef, growing and establishing as adult, long-lasting colonies (Harrison and Wallace, 1990). Healthy and functional coral reef communities directly depend on coral recruitment success that, in turn, depends on the abundance of their preferred settling substrate i.e., crustose coralline algae (CCA, family Corallinaceae, Lithophyllaceae, and Porolithaceae) (Heyward and Negri, 1999). Indeed, CCA are known as framework binders and act as a preferential settlement substrate for coral larvae (Morse et al., 1988; Heyward and Negri, 1999; Harrington et al., 2004; Nelson, 2009; Price, 2010). Whilst, CCA thallus surface topography differences imply biotic interaction changes (Figueiredo et al., 1996), this preferred substrate is most likely induced by chemical cues from the CCA wall-associated compounds and to a lesser extent from their bacterial biofilm (Tebben et al., 2015; Gómez-Lemos et al., 2018). The early-life stage is a harsh period for coral survival since a multitude of biotic and environmental factors such as light (Bessell-Browne et al., 2017), smothering from sedimentation (Fabricius and Wolanski, 2000), and several complex trophic interactions (Fabricius et al., 2015) including competition with turf algae (Swierts and Vermeij, 2016; Jorissen et al., 2020), suspension-feeders (Elmer et al., 2018), grazers and predators (Penin et al., 2011; O’Leary et al., 2013), cause high mortality rates (67-99%) in coral recruits (Wilson and Harrison, 2005). In particular, competitive interactions play a significant role in the survival of both coral recruits and adult colonies (Penin et al., 2011; Swierts and Vermeij, 2016). In this context, the complex and only partially studied interactions between CCA and coral larvae and their consequences on larval settlement, have been shown to play a fundamental role in the replenishment of the reef (O’Leary et al., 2012). Likely, this complex ecological framework could be furthermore compromised in the future when considering climate changes, in particular rising temperatures and ocean acidification as it has been widely described across several studies (e.g., Mccoy and Kamenos, 2015; Cornwall et al., 2021a; Page et al., 2021).
Ocean acidification (OA) is generally described as the rapid and global decline of pH and calcium carbonate saturation in surface seawater (Orr et al., 2005). Several lab-based experiments addressing the effects of OA on CCA have demonstrated reduced calcification and growth rates (Kuffner et al., 2008; Comeau et al., 2013), increased dissolution (Anthony et al., 2008; Diaz-Pulido et al., 2012), and reduced recruitment (Kuffner et al., 2008; Bradassi et al., 2013). Field studies have shown as well reduced recruitment (Fabricius et al., 2011; Fabricius et al., 2015) or impaired capacity to attract coral larval settlement (Doropoulos et al., 2012). However, it has been recently shown that lab-based versus field experiments tend to conclude that OA has a stronger impact on CCA (Page et al., 2022). Indeed, CCA and corals persist at CO2 seep sites (Kamenos et al., 2016; Enochs et al., 2020; but see also Fabricius et al., 2011 for an opposite finding) and at low pH environments in Palau (Barkley et al., 2015). For coral recruits, OA has been shown to cause skeletal malformations (Foster et al., 2016), and decrease in availability of settlement cues (Albright et al., 2010; Albright and Langdon, 2011). Thus, in general, OA could affect coral recruitment by dissolving CCA or changing their microbial biofilms compounds, hence decreasing the availability of a supposedly ideal substrate for coral recruits (Webster et al., 2013) and finally affecting the recruit survival (Doropoulos et al., 2012; Doropoulos and Diaz-Pulido, 2013). However, OA is only one of the ongoing threats to coral reefs, and its combination with both ocean warming (OW) and deoxygenation (OD) will likely cause CCA bleaching and mortality (Diaz-Pulido et al., 2012; Cornwall et al., 2019; Hughes et al., 2020), and impairment of coral larvae settlement (Jorissen and Nugues, 2021). Combining all those environmental parameters and testing their effects using short-term lab-based experiments is challenging. Natural systems that are exposed to natural in-situ gradients can be used to study their integrated effect (Page et al., 2022). Despite several limitations, few study sites have been recognized as natural laboratories to study the response of species and ecosystem levels to future extreme environmental conditions (e.g., Hall-Spencer et al., 2008; Fabricius et al., 2011; Kroeker et al., 2013; Barkley et al., 2015; Agostini et al., 2018). The semi-enclosed lagoon of Bouraké in New Caledonia is one of these natural laboratories; it hosts well-diversified and complex coral assemblages despite fluctuating acidified, warm, and deoxygenated environments (Camp et al., 2017; Maggioni et al., 2021). In this study, the Bouraké site provided the opportunity to test the long-term responses of CCA and coral recruitment to multiple stressors in a natural setting, which would be hard to reproduce in tank experiments artificially mimicking future climatic conditions.
Here, we assessed the effects of extreme and fluctuating environmental conditions (i.e., low pH, O2, and high temperature) on the abundance of CCA, algal turf, and coral recruits. To address this objective, we obtained CCA-covered tiles from a forereef and moved them to three fringing coastal reef sites with distinct environmental conditions. We investigated the effect of the environmental conditions typical of coastal fringing reefs on the CCA cover twice during the two years experiment and we quantified and identified the coral family recruited on the tiles at the end. Based on the reported effect of OA on CCA cover and coral recruitment rates, we hypothesized that in Bouraké both will be heavily affected by the extreme conditions.
Materials and Methods
Study Sites, Coral Community Characterization, and Environmental Assessment
This study was conducted from September 2017 to October 2019 at a semi-enclosed lagoon within a mangrove ecosystem in Bouraké, New Caledonia (St B3, Figure 1B), and at two fringing reefs, one adjacent to Bouraké (St R2 in Figure 1C, 4 km away) and another one (St M1) located 80 km south in the Maa’s Bay (Figure 1D). Station R2 is a large bay-sheltered reef that is enclosed between two islets and has an abundant and scattered reef. Station M1 is a typical fringing reef that is open to the lagoon.
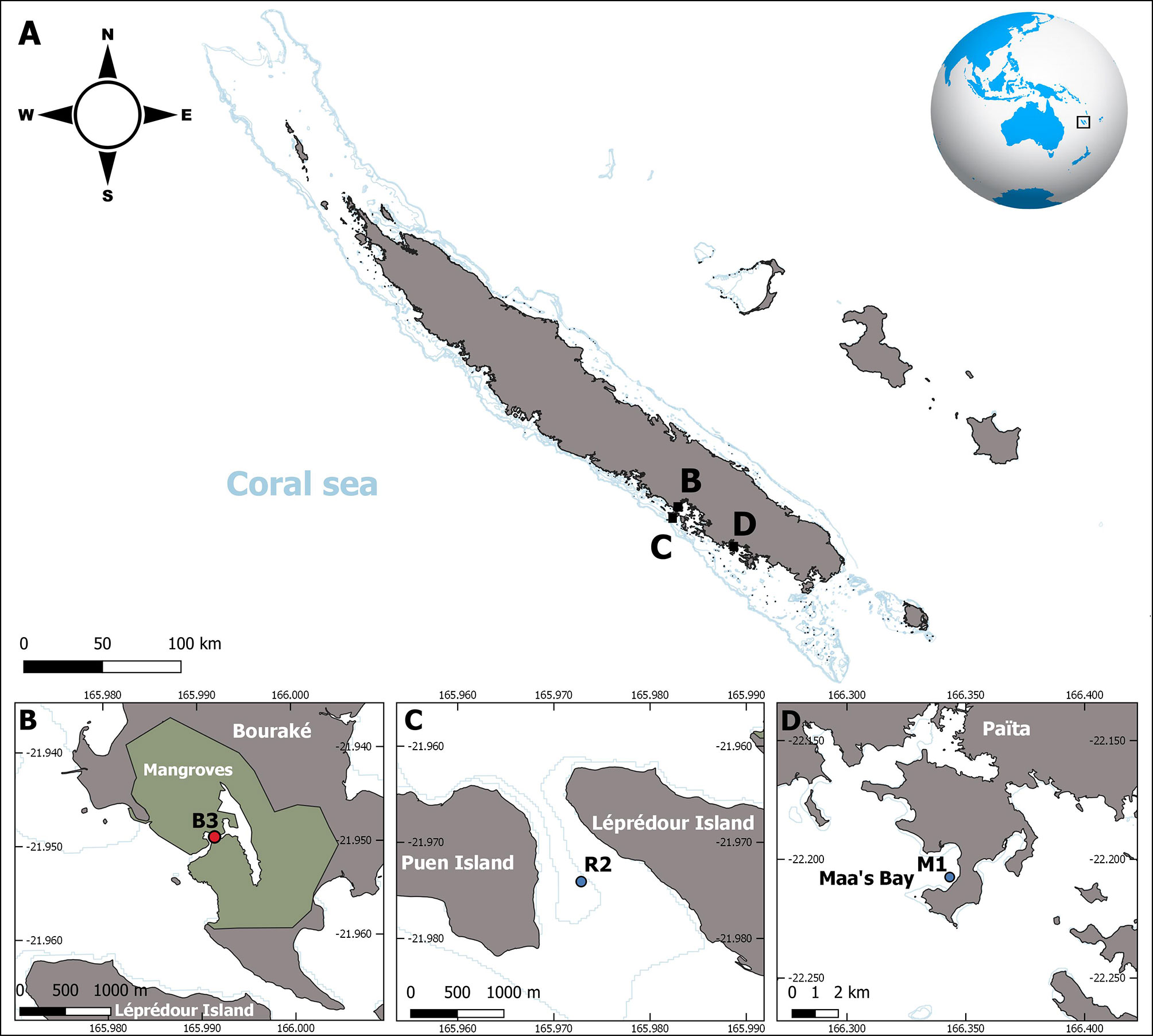
Figure 1 Map showing the location of the study sites in New Caledonia (A) and the position of the stations where the 2-year experiment took place (B-D). Station B3 was located in the semi-enclosed lagoon of Bouraké (B), while two other stations were located either near Bouraké (St R2) (C) or in Maa’s Bay (St M1) (D).
In Bouraké extreme conditions are recorded in seawater temperature (range 17.5 - 33.8°C), dissolved oxygen (range 1.87 - 7.24 mg L-1), and pH (range 7.23 - 7.92 pHT units) (Maggioni et al., 2021). Those environmental parameters fluctuate regularly according to the tide (ca. 1 m high) and only return close to coastal values during the high tide of spring tides when seawater of the semi-enclosed lagoon is partially renewed with seawater entering from the external lagoon. As a result, daily fluctuations of dissolved oxygen (DO), temperature, and pH fluctuate can reach 4.91 mg O2 L-1, 6.50°C, and 0.69 pHT units, respectively. Despite these harsh conditions, at least 66 coral species form compact and wealthy reefs all along the lagoon (Maggioni et al., 2021). In the first assessment of the Bouraké lagoon (Camp et al., 2017), the authors found that these coral species spent 44% of the time at pHT 7.7–7.8, thus reaching the worst scenarios RCP7.0 and RCP8.5 predicted in global ocean by the Inter Panel on Climate Change for the end of the century (IPCC Report, 2021). During particularly warm years, such as the El Niño event in 2016 (Camp et al., 2017), they experienced temperatures predicted for the end of this century 71% of the time, while facing now the best case scenarios RCP2.6 and RCP1.9 (IPCC Report, 2021).
The composition of the reef communities was assessed at each station visually, by taking photographs of the corals found along three 30-m line transects, and when necessary by collecting fragments of corals and further observing their skeletal structure under a stereomicroscope for final identification. Most of the corals, which are common fringing reef species, were recognized at the genus and species level, with reference to a species list previously established for B3 and R2 (Maggioni et al., 2021). Because the reef community composition might affect coral recruitment rates and the comparison between sites, we verified that the reef diversity was similar among the three stations using the Shannon’s diversity index (H) on identified species trough the stations (Supplementary Tables S1, S4). The three stations were chosen because in addition to have similar reef composition, they allow to compare the effect of a gradient of seawater carbonate chemistry on the larvae and CCA settlement. In fact, as previously found (Maggioni et al., 2021) and also during our measurements (see results section), R2 could be considered as an intermediate station between B3 and M1, regarding pH absolute value. Therefore, this continuum in the pH experienced by the organisms allows us to evaluate the impact of three different pH scenarios: the end of the century, future 30 years, and the actual conditions (i.e., B3, R2 and M1, respectively).
For stations, B3 and R2, total scale seawater pHT, dissolved oxygen, and temperature were periodically undertaken since 2016 (Maggioni et al., 2021) using Seabird SeaFET pH loggers, YSI 600 OMS-M, and Hobo water temperature Pro V2, all settled at 10-min logging intervals. For station M1, seawater pHT and dissolved oxygen content (DO, mg L-1) were measured during three 7-14 day periods (August 2017, June 2018, and January 2019). Unfortunately, the temperature probe was lost twice, therefore the temperature variations could not be assessed for this site.
Terracotta Tiles Set-Up and Settlement Analyses
A total of 60 red terracotta tiles (11 x 11 x 1 cm) were deployed after being soaked for 7 months at a forereef (22°17.152’S, 166°10.992’E) at 12 meters depth. In September 2017, they were transferred and randomly deployed at the three stations B3, R2, and M1. This experimental approach was necessary to obtain 60 tiles having both the same CCA community and a similar percentage cover that could be difficult to obtain if the tiles were pre-soaked directly at the stations. This same basis cover allowed us to consider in particular the effects of environmental changes. At each station, 20 tiles were secured to the reef with metal rods at 1.5-2 m depth. Tiles were arranged with a 30-degree inclination at approximately 10 cm from the substrate. They were positioned ca. 3 m distant along a transect of ca. 60 m. The tiles were therefore photographed underwater before moving them to the three stations (T0), two months after their deployment (T2), and two years after deployment (T24/26). The top sides of 39 (off 60) randomly chosen tiles were photographed in situ at T0, using an underwater Canon G16 camera. The top sides of all the tiles were imaged at T2. Tiles from B3 and R2 were retrieved in September 2019 (T24). Tiles from M1 were collected in October 2019 (T26). Of the 20 tiles initially installed at St B3 and M1, only 15 and 19 were found, respectively. All the deployed tiles were recovered at R2. In the laboratory, both top and bottom sides were photographed under a dissecting stereomicroscope (tiles were immersed in seawater).
All photographs were analyzed using the photoQuad software (version 1.4) as in Trygonis and Sini (2012), and the percent cover of sessile organisms was assessed. Each photograph was calibrated for size and pixels-segmented to the highest level possible (segments ignored < 10 pixels). PhotoQuad’s image segmentation is based on the SRM algorithm developed by Nock and Nielsen (2004). The photograph’s size was usually comprised between 3x106 pixels to 9x106 pixels for a surface area typically around 125 cm2. For top sides, three main categories describing the settled organisms were identified: (i) Algal turf, (ii) CCA, and (iii) empty (i.e., tile surface devoid of any recognizable benthic organisms). For bottom tile sides, one more category was assessed, namely: (iv) suspension-feeders (i.e., ascidians, sponges, serpulid worms, and bryozoans). At the end of the experiment, tiles were cleaned for 24 h in a 10% NaCl solution to remove all organic material, rinsed, and dried. The coral recruits settled on the top, bottom, and the four lateral sides (all sides pooled) of the tiles were counted under a stereomicroscope and identified to the lowest possible taxonomic level. We were able to categorize the coral recruits into three families: Acroporidae, Poritidae, and Pocilloporidae.
Statistical Analyses and Data Presentation
The database for the map (Figure 1) was collected from map tiles at www.georep.nc (©Georep contributors) and customized in QGIS (version 3.4.14). Statistical analyses were conducted and figures were produced using RStudio (R Development Core Team, version 4.1.0, 2021), including the packages: “ggplot2”, “stats”, “dunn.test”, “FSA”. Homogeneity of variance was tested using the Levene test and the normality of variance distribution was tested using the Shapiro-Wilk test and checked graphically with a Q-Q plot. When data did not conform to normality or homogeneity of variance, we used the non-parametric Wilcoxon test or the Kruskal-Wallis followed by the Dunn’s multiple comparisons test (Bonferroni-adjusted). First, for each site separately we used two factors Wilcoxon test (two groups) for each of the three categories of organisms (CCA, Turf, and Empty) settled on the top sides of tiles to test for statistical differences first after two months of incubation (i.e., T0 vs T2), and then after two years (i.e., T2 vs T24/26). To compare significant differences between stations (i.e., B3, R2, and M1) at the start of the study (T2), we used the non-parametric Kruskal-Wallis followed by the Dunn’s multiple comparisons test (Bonferroni-adjusted). The latter was also used for each of the four categories identified on the bottom sides of tiles (i.e., Turf, CCA, Suspension-feeders, and Empty) and the three categories identified on the top sides of tiles (i.e., Turf, CCA and Empty) at the end of the experiment (T24/26) to test for statistical differences between stations (B3, R2, M1). The same tests were also used for statistical differences in coral recruits between stations (B3, R2, and M1) from all tiles sides pooled at T24/26. Data were graphically reported as median values ± 25th and 75th percentiles (box), minimum and maximum values (whiskers), otherwise specified.
Results
Environmental Parameters
Our results showed differences in the main environmental parameters between stations with lower values at B3, intermediates at R2, and normal at M1 (Table 1). At B3, pH was strongly correlated with the tidal cycle decreasing and increasing with the ebb and rising tide, respectively (Figure 2). Within a tidal cycle, the pH reached a minimum of 7.23 and a maximum of 8.06 pHT units at B3 (Table 1) and it changed on average by about 0.15 units per hour. At stations R2 and M1, pH fluctuated between 7.69 and 8.12 pHT units for R2, and from 7.93 to 8.18 pHT units for M1. Similar to pH values, the mean dissolved oxygen concentration (DO) was lower in the Bouraké lagoon than at the other stations. DO concentrations were lower in the early morning at R2 and M1 and higher at mid-day, while they were tidally driven at B3 with low and high values coincident with low and high tide, respectively. Over a 48-hour cycle, DO fluctuates up to 4.25 mg L-1 at station R2 and 3.75 mg L-1 at stations M1 and B3.
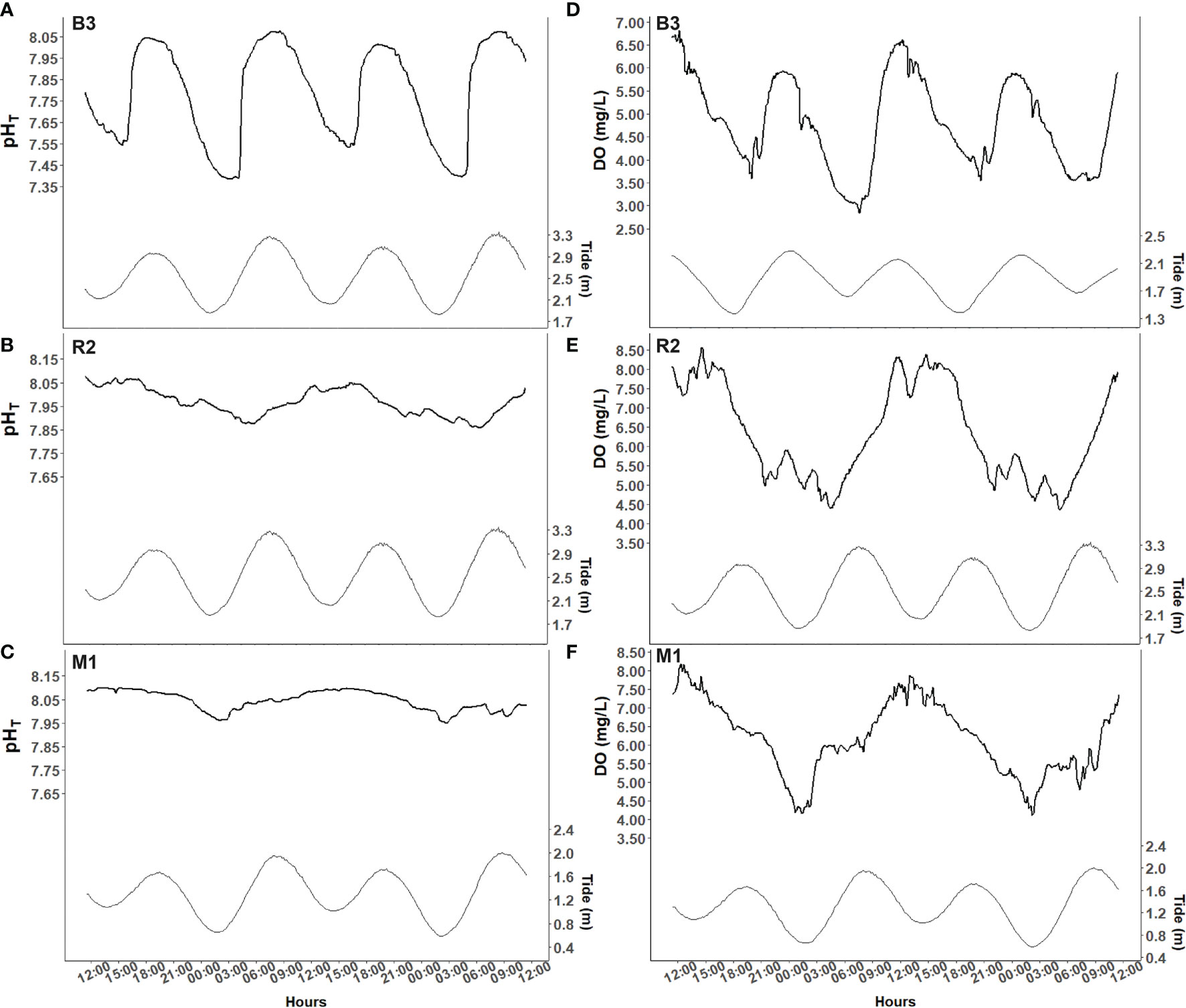
Figure 2 Seawater pHT (A–C) and dissolved oxygen (DO) (D–F) variations (black lines) recorded during 48 hours at Bouraké (St B3) and stations R2 and M1. Data were collected at the end of March 2019 at St R2 for pH (B) and DO (E) and at St B3 for pH (A). DO was measured at St B3 at the beginning of April 2019 (D). At St M1, data were collected at the end of January 2019 (C, F). Tidal variations (grey lines) are on the right y-axis.
Changes in Crustose Coralline Algae and Turf Covers
After 7 months of conditioning at a forereef, i.e., just before they were moved and randomly shared between the three study stations (T0), the top sides of the tiles were predominantly covered in CCA (median percent cover of 69.21%, all tiles pooled), while algal turf was rare (median percent cover of 11.95%). Two main CCA genera were recognized on the tiles on a simple visual check regarding the conceptacles size and disposal: Porolithon and Neogoniolithon. After two months (T2) at the three stations, the CCA median percent cover was significantly different among stations and significantly decreased at B3 from 69.21% to 41.4% (Figure 3; Table 2). CCA cover significantly increased at the two stations R2 and M1, respectively 64.4% and 81.3%. In contrast, turf cover was significantly different between stations, reaching the highest values in B3 (20.4%), and only 6.4% and 9.7% at R2 and M1, respectively. After two years of deployment (T24/26), CCA median percent cover on the top sides of the tiles was lower than the one at T2 at all stations: this decrease was dramatic at B3 (from 41.4% to 8.4%) and M1 (from 81.3% to 1.5%), but to a much lower extent at R2 (from 64.4% to 49.3%).
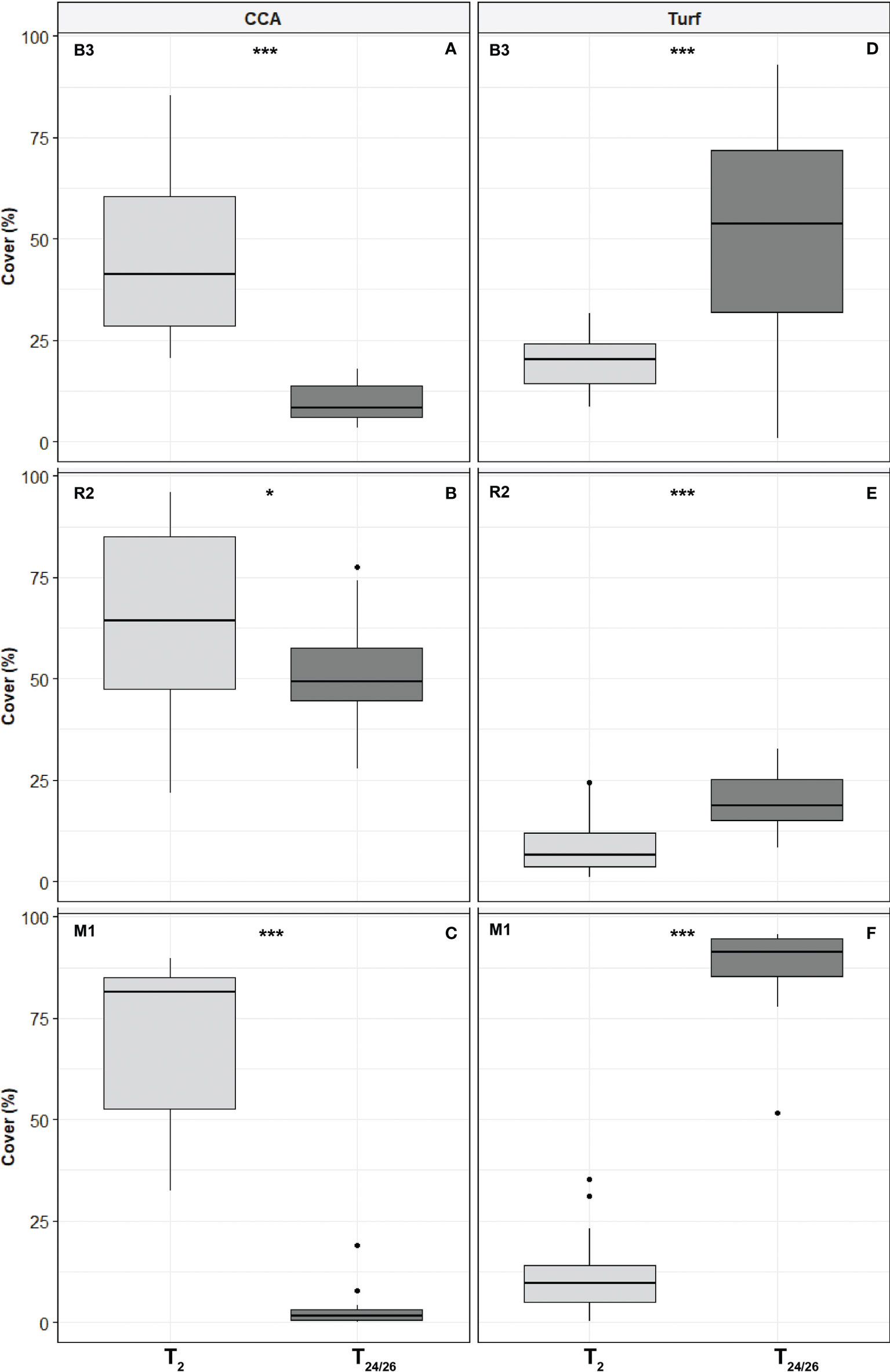
Figure 3 Change in crustose coralline algae (CCA) (A–C) and Turf percent cover (D–F) on top sides of the tiles between the beginning (two months after the time of deployment, T2) and the end (after ca two years of deployment, T24/26) of collector deployment at study stations B3, R2 and M1 (number of tiles n = 15, 20 and 19, respectively). Data are median values ± 25th and 75th percentiles (box), minimum and maximum values (whiskers), and outliers (dots). Stars represent statistical significance (see Table 2).
Turf cover on the top significantly increased at all stations, dramatically at both B3 and M1 (from 20.4% to 53.5%, and from 9.7% to 91.2%, respectively), to a much lower extent at R2 (from 6.4% to 18.7%). Similarly, Turf cover on the bottom was significantly different between stations (Table S3; Figure S3), low at B3 (31.89%) and high at R2 and M1 (71.79% and 71.56%, respectively). Crustose coralline algae median percent cover on the bottom was similar at B3 (16.12%) and R2 (21.21%), and significantly lower at M1 (3.42%) after two years of immersion
Suspension-feeder percent covers on the bottom of tiles in B3 and R2 were as low as 2.74% and 9.57% for B3 and M1, respectively, and rare for R2 (Table S3). Tile surface areas devoid of settlers significantly differed between stations. They were similar at M1 and R2 (6.78% and 6.26%, respectively) and higher at B3 (39.47%).
Spatial Variability in Coral Recruitment
Coral recruitment significantly differed among stations and sides after two years of tile immersion (Table S3). It was found to be ca. 3-fold higher at B3 than R2 and M1 with 18.4, 4.6, and 5.5 recruits per tile (all tiles pooled per station), respectively. Regarding where the larvae preferentially settled on the tile, the number of recruits was always higher on the bottom than on the top, and scarce on the lateral sides (Figure 4).
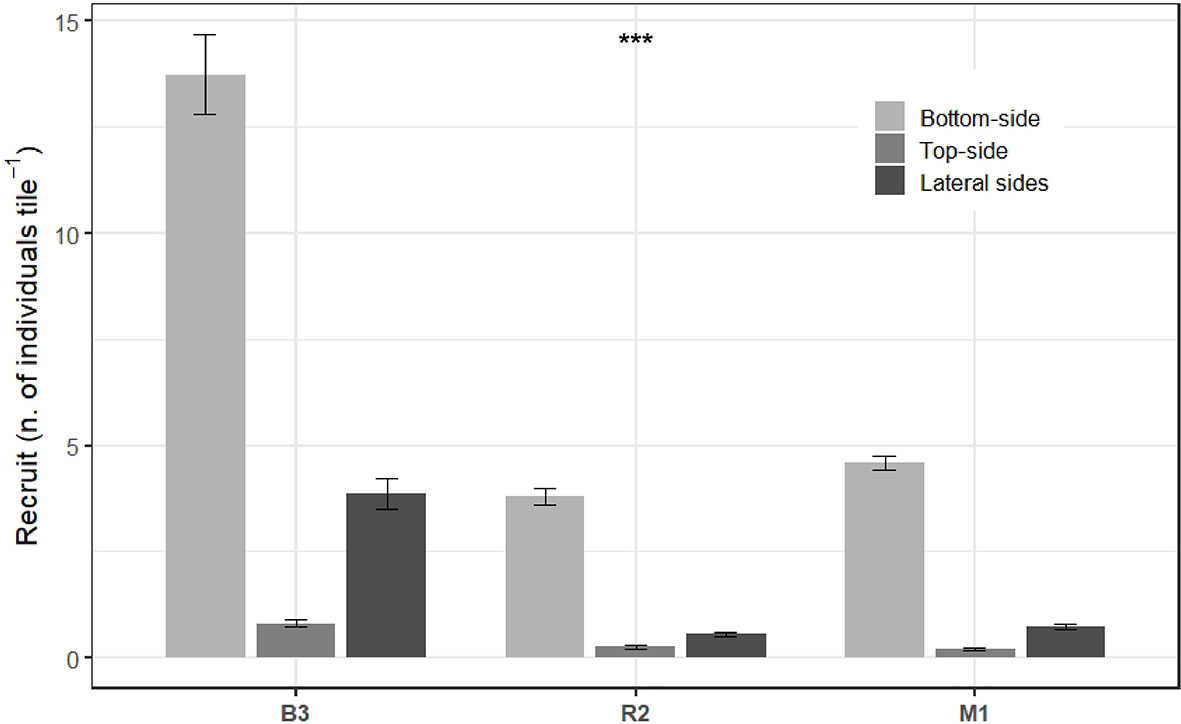
Figure 4 Number of coral recruits (per tile) found on the different sides of the tiles immersed during ca. two years at Bouraké (B3) and stations R2 and M1. Data are means ± SD (number of tiles n = 15, 20, and 19, for B3, R2, and M1, respectively). Data from the lateral sides were pooled. Stars represent statistical significance (see Table S3).
Acroporidae was the dominant family of coral recruits settled in our experiment (Figure S4), with similar relative abundances between stations (65.58%, 73.91%, and 67.33%, at B3, R2, and M1, respectively). Pocilloporidae was the second most abundant family, with recruits who equally settled at all three stations in terms of relative abundance (14.85%, 17.39%, and 16.83% at B3, R2, and M1, respectively). Only a few Poritidae were found at B3 and M1 (17.39%, and 6.52%), and none at M1 which exhibit more unknown genera.
Discussion
Coral larvae are tied to preferred settlement substrates such as CCA (Harrington et al., 2004; Price, 2010), which in turn can be severely affected by environmental conditions, such as OA. In fact, reducing both the availability of preferred substrate for coral settlement, such as CCA and biofilms and increasing turf algae cover, may negatively reduce recruitment rates and coral population replenishment and/or recovery (Doropoulos et al., 2012; O’Leary et al., 2012; Fabricius et al., 2017). Although the CCA we transplanted at study sites suffered from moderate bleaching, partially confounding the effect of environmental conditions on their survival, the present study corroborates previous findings reporting a decline in CCA cover and a shift towards an increase in turf algae under extreme conditions (Kuffner et al., 2008; Martin et al., 2008; Barott et al., 2012; Kroeker et al., 2013; Porzio et al., 2013; Enochs et al., 2015). While the CCA abundance decline was expected, surprisingly, coral recruitment rates in Bouraké were higher than outside, at both the two fringing reefs. Our study sites differed in several ways, one is tidally driven and exhibits variable and extreme pH, temperature and hypoxia while the two others are far less variable and close to ambient values. While our findings appear to contradict results from several OA laboratory-based experiments (e.g., Doropoulos et al., 2012; Webster et al., 2013; Jorissen et al., 2020), they are in accordance with some field experiments (Kamenos et al., 2016; Enochs et al., 2020) showing in general, a lesser impact of OA (Page et al., 2022). All these observations together reinforce the idea that coral recruitment is possible even under extreme conditions and that, particularly for the Bouraké site, corals may have adapted to such variable and extreme environmental conditions.
Shift Between CCA and Turf Algae
CCA cover on the top sides of the tiles remarkably declined at Bouraké after only 2 months of immersion, while remaining stable or slightly higher at the two other reef stations. This suggests that ca. 40% of CCA settled during the tiles pre-deployment, dissolved during a short-term exposure, therefore further indicating a strong negative effect of the Bouraké natural environmental conditions on CCA. At the same time, turf algae cover was significantly higher at Bouraké than at other reef stations. It could be argued that our findings were mostly due to the effect of an abrupt change of environmental conditions, including light exposure, from those characterizing the forereef where the tiles were soaked before deployment to the three study sites. The abrupt deployment could have impacted the benthic organisms present on tiles and indeed, moderate bleaching was evident after the first two months at the study sites (see Figure 5A–C), potentially accelerating a decline in the CCA abundance. However, such deployment effect, should be common across the three stations, and this has not been observed. Indeed, while CCA cover decreased in Bouraké, it was stable or slightly increased at the two stations R2 and M1, after two months (Table 2), which we believe to be a long enough time for any consequence of an abrupt displacement to occur. Furthermore, the initial phase-shift between CCA and turf seen after the first two months at Bouraké, was still confirmed after our two-years in situ experiment: a significant loss in CCA cover (ca. 80%) and a significant increase of turf algae (up to 162%) were measured (Figure 5D–F). While we cannot fully dismiss the hypothesis that the initial deployment has affected the tiles, our 2-years observation did not show a substantial recovery in the color of the settled CCA and we are unable to discern the effect of bleaching from that environmental conditions. A potential caveat of our experimental design is that forereef where the CCA community (mostly Porolithon and Neogoniolithon) had settled on the tiles was characterized by different environmental condition compared to the three study sites where the tiles were kept during the 2-year incubation. Although both CCA families are also commonly found on coastal fringing reefs (Claude Payri, personal communication), and the same families were also found at our study sites, we are unable to verify whether the CCAs we transplanted were the same as those that would be found naturally at the study sites.
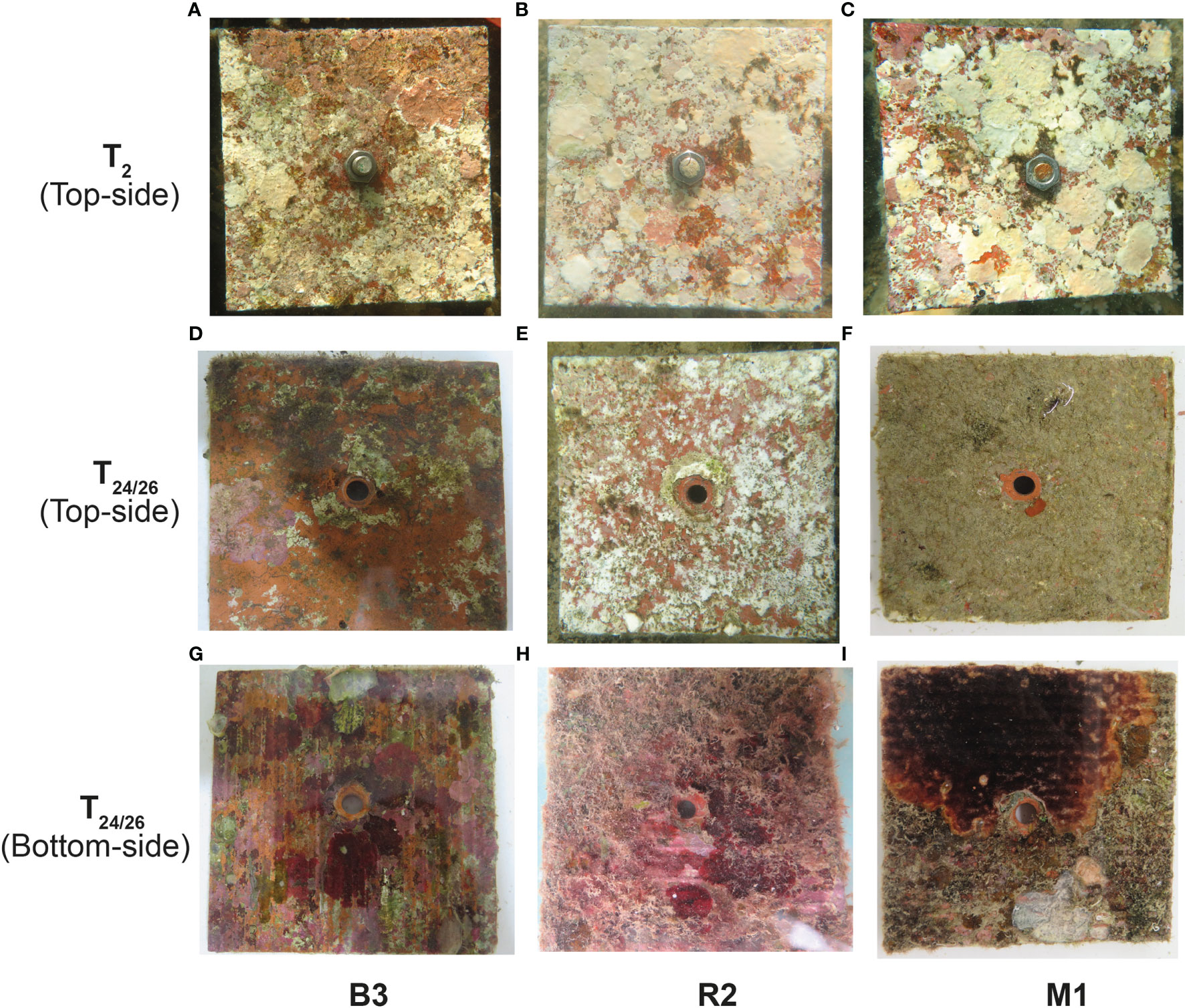
Figure 5 Photos of the tiles (randomly selected) deployed at Bouraké B3 (left), station R2 (middle), and station M1 (right). Pictures of the top sides were taken after two months of deployment (T2) (A–C), and after 24 (D, E) and 26 months of deployment (F). Pictures of bottom sides of tiles after 24 months (G, H) and ca. 26 months of deployment (I).
Inter-species interactions, in combination with a direct effect of acidification and the extreme environmental conditions in the Bouraké system on CCA skeletons, could partially explain this cover drop-off despite a certain persistence obtained by altering skeletal mineralogy, although it remains difficult to assess with certainty (Goffredo et al., 2014; Kamenos et al., 2016). In Bouraké, seawater pH decreased down to 7.23 and exhibited a mean value of 7.67, thus reducing carbonate ion concentrations ([]) available and the saturation state of seawater with respect to aragonite and calcite (Ωarag). Low values of seawater pH have been experimentally shown to cause a decrease in CCA growth and an increase in skeletal dissolution (Kuffner et al., 2008; Comeau et al., 2013; Fabricius et al., 2015; Foster et al., 2016), and are known to reduce their skeletal density (Chan et al., 2020; Williams et al., 2021). Our findings are in agreement with several reports of overall CCA cover reduction ranging from 43% to 92% under low pH (Jokiel et al., 2008; Kuffner et al., 2008; Doropoulos et al., 2012; Fabricius et al., 2015), and from 60% to 100% in combination with high temperature (Martin and Gattuso, 2009; Diaz-Pulido et al., 2012; Vogel et al., 2016). Dissolved oxygen concentration in Bouraké exhibits a mean value of 5.23 mg L-1 with a minimum of 2.28 mg L-1 (Table 1) which is equivalent to depletion by ca. 30% compared to both stations R2 and M1. Despite the lack of research on hypoxia effects, we could hypothesize an additional negative effect from low dissolved oxygen on CCA growth as it has been previously reported (Altieri et al., 2017; Nelson and Altieri, 2019).
The decline of CCA cover could also be due to the combined effect of decreased light exposure and high sedimentation. Indeed, under OA conditions, CCA growth significantly decreases under the combination of low light and low flow conditions (Comeau et al., 2019), and faces dissolution in shade environments (Martin et al., 2013; Bessell-Browne et al., 2017; McNicholl and Koch, 2021). Although light irradiance was not measured and sedimentation rates in the Bouraké lagoon have yet to be comprehensively measured, it has been suggested that high turbidity in Bouraké could attenuate solar radiation (Jacquemont et al., 2022). Indeed, the Bouraké lagoon is inside a mangrove forest, and when the sediment interstitial water is sucked by the current that empties the mangrove and arrives in the lagoon during ebb tide (spring ebb tide in particular), turbidity temporally increases because of the organic compounds released from the mangrove. This, in turn, and either alone or combined with the high pCO2 and the warm temperatures in Bouraké may enhance turf algae productivity by alleviating carbon limitation (Johnson et al., 2017). Although such potential negative mechanisms, in Bouraké more than 66 species of corals, and also some CCA on coral rubbles were found (Maggioni et al., 2021). Further measurements of light availability to CCA along diel and seasonal cycles, as well as specific experiments will be necessary to disentangle the contribution of turbidity in combination with high pCO2 on the CCA growth and dissolution. However, CCA and turf algae cover on the bottom sides of the tiles i.e., the CCA cover median was low in B3 than in R2, and quasi absent in M1, partially confirmed data found on the top sides (Figure 5G–I). While the quasi absence of CCA at M1 could be due to both the high turf algae cover (ca. 75%) and the presence of suspension feeders such as bryozoans, known to be efficient competitors for space (Glassom et al., 2004; Elmer et al., 2018), the presence of CCA at both B3 and R2 at bottom side support the hypothesis that the decline of CCA found at the top side is due to the combination of the high pCO2 and other factors such as light and sedimentation.
Our results emphasized the extent to which CCA growth is limited at Bouraké when compared to the station R2 where CCA cover decreased by 24% during the two years of deployment. This result was a bit unexpected because although previous measurements suggested that R2 could show averaged pH values lower than for a typical reef (Maggioni et al., 2021), the study stations were selected having in mind that R2 and M1 were two reef stations having the main environmental parameters (e.g., seawater pH) close to ambient values typical for a coastal fringing reef. However, in terms of seawater pH, R2 turned out to be intermediate between Bouraké and M1 according to recent data on the same site (Maggioni et al., 2021). With regard to R2, the low seawater pH (mean of 7.89 and a minimum of 7.69 pHT units) might explain why we measured some decrease in CCA cover, since similar pH values have led experimentally to a significant loss in CCA cover (Kuffner et al., 2008; Doropoulos et al., 2012; Comeau et al., 2013; Fabricius et al., 2015). In fact, this presupposed reference station actually exhibited a pH close to values expected in global ocean from the RCP7.0 scenario in 30-40 years (IPCC Report, 2021). These unexpected environmental conditions widened our opportunities to study the effect of OA on CCA and coral recruitment. The low pH was likely due to the site topography. Indeed, R2 is located in a “cul-de-sac” between two islets with sparse but abundant reefs. It is characterized by a fine sediment bottom, and a low seawater renewal rate, likely such as for the Rock Islands in Palau (Micronesia) (Barkley et al., 2015; Shamberger et al., 2018; Kurihara et al., 2021). The combined effect of biological activities and carbonate disequilibrium likely caused such low pH values which are frequent and typical for many coastal fringing reefs with limited seawater exchanges with the lagoon. In contrast to Bouraké, turbid waters were never observed at R2 that benefits from greater light intensity; despite low pH, environmental conditions would still allow CCA persistence.
At station M1, CCA was completely dissolved after two years and tiles were recovered by turf algae. While the effect of pH, in this case, can be discarded (ambient pH of ca. 8.07 pHT units), the combined effect of lower grazing pressure and well-established wave exposure may partially explain this evolution. It has been shown that grazer presence depends directly on factors such as overfishing, tidal height and wave exposure (Doropoulos et al., 2017). In particular, turf algae seems more abundant where wave action is greater and grazers are less present. Conversely, turf algae are less abundant in sheltered areas where grazers are more active (Underwood and Jernakoff, 1984; Doropoulos et al., 2017). While Bouraké and R2 are sheltered from wave action and quite isolated sites, M1 is an open bay much more subject to wave action and a more intense fishing pressure due to its proximity to Nouméa. Our findings are in agreement with previous studies reporting both limited CCA growing on the top of the tiles (O’Leary et al., 2012; O’Leary et al., 2013) and an increase in the turf algal cover (Penin et al., 2011). In addition, we observed a high sedimentation rate in M1 with fine particles being trapped by the dense algal cover. This accumulation of sediment certainly contributed to smothering the underlying CCA.
Coral Recruitment Seems to be Maintained in Bouraké
Ocean acidification, warming and deoxygenation have been shown to negatively affect coral larvae settlement by reducing the growth of favorite substrate (e.g., Diaz-Pulido et al., 2012; Doropoulos et al., 2012) and altering chemical biofilm and microbiological cues for settlement onto CCA (Albright et al., 2010; Albright and Langdon, 2011; Webster et al., 2013; Fabricius et al., 2017; Jorissen et al., 2021). Our findings seem to provide an alternative scenario using a natural extreme environment where coral recruits survived. In fact, although the extreme conditions reduced the CCA cover in Bouraké, after two years of exposure we observed a 3-fold increase in coral recruits when compared with the stations R2 and M1. Recruitment rates recorded in this study are difficult to compare with the literature because values are quite variable. For instance, in French Polynesia, it varies from 0 to 10.8 recruits tile-1 (Gleason, 1996; Adjeroud et al., 2007; Bramanti and Edmunds, 2016), and it was less in the Virgin Islands (average of 3 recruits tile-1; Edmunds, 2021) and in Palau (0.3 to 3.9 recruits tile-1; Victor, 2008), while no data is available for New Caledonia. There might be several explanations for our uncommon and counterintuitive findings. Firstly, because the whole set of tiles was maintained in situ for two years, potentially acted as substrates during two consecutive spawning events. The latter follows the full moon in November, coincident with the mass spawning period on the Great Barrier Reef in Australia (Baird et al., 2010). During the first spawning event, which happened 1-2 months after deployment, the CCA cover was still quite high (41.4%) and still may have allowed tiles to attract coral larvae. If coral recruitment intensity on tiles was the result of a single spawning event, it was quite massive and unusual. According to the disruptive effect of OA on the coral skeleton, especially during early life stages (Cohen et al., 2009; Foster et al., 2016), coral recruits would have been dissolved after two years or at least would have shown important malformation which was not the case (see below some considerations about the site M1). Most likely, our tiles received recruits also during the second spawning event, in spite of the low CCA cover. The role of biofilm acting as an ideal chemical cue for coral larvae settlement (Tebben et al., 2015) could be crucial in this case. Alternatively, coral spawning was either low during two consecutive years at R2 and M1 or recruits did not survive and were covered by competitors, such as bryozoans. The unexpected higher recruitment in B3 could also be due to high concentrations of organic carbon and high levels of nutrients such as [Si(OH)4], [NOx] and [PO4]3- which were 8.88, 0.54 and 0.19 µmol L-1, respectively, in Bouraké (Maggioni et al., 2021) and were, in general, more concentrated (up to 5 times) than at station R2. These concentrations coupled together would have increased coral heterotrophic rates and helped recruits to cope with the extreme conditions (Anthony, 2006; Houlbrèque and Ferrier-Pagès, 2009; Ragazzola et al., 2013; Goldberg, 2018). Increasing the energy budget through heterotrophic nutrition could enhance juvenile growth and facilitate coral recruitment under high temperatures (Huffmyer et al., 2021). This energy budget enhancement could also benefit coral larvae under deoxygenation which has recently been shown to increase the coral larvae energy needs at a dissolved oxygen concentration of around 2 mg L-1 (Alderdice et al., 2021).
The role of water circulation in the semi-enclosed lagoon of Bouraké has also to be considered since a close water circulation could enhance coral larvae settlement via a decrease in larval dispersal (O’Connor et al., 2007). The particular morphology and hydrodynamics of Bouraké could make this lagoon a real recruitment hotspot as recently observed in St. John, US Virgin Islands (Edmunds, 2021). Recruitment was especially noticeable on the bottom sides of the tiles for each station, likely because tiles act as a shelter against grazing and predation and enhance coral recruitment compared with the top or lateral sides (Penin et al., 2011). Indeed, the top and lateral sides are prone to a more intense grazing activity. In addition, recruits face high levels of sedimentation on top sides which induces smothering (Fabricius and Wolanski, 2000), settlement limitation (Ricardo et al., 2017) and decreases in fertilization, embryogenesis and larval development (Jones et al., 2015). This response was similar among the three stations despite the different environmental conditions.
Our recruit identification revealed the same proportions of corals taxa settled between stations with the Acroporidae as the dominant family (70% of all recruits) followed by the Pocilloporidae (ca. 16%) and only a few Poritidae. This is in agreement with previous studies showing that Acroporidae and other broadcasting corals have high recruitment rates, especially in low water-circulation systems like Bouraké (Oprandi et al., 2019). The dominance of branching morphologies (Acroporidae and Pocilloporidae) may be due to their ability to compete with vertical CCA growth for space in light-limited environments (Jorissen et al., 2020). On the contrary, recruits from massive species such Poritidae, which mainly have a planar growth form, were rare, likely because they are unable to face competitive interactions with other coral species, CCA or suspension feeders. In agreement with this hypothesis, we found only a few recruits of Poritidae at R2 (6.52%) and zero at M1, the latter exhibiting a high density of bryozoans. At site M1 some recruit malformations were found and most of the recruits were classified as unknown genera. Such skeleton malformations are likely to be due to the competition with other organisms recovering the recruits, than to the effect of dissolving seawater carbonate values.
In this study, we used one of the few natural sites exhibiting extreme and highly variable environmental conditions including high temperature, low DO and low pH. Therefore, our experimental setting benefits from the natural conditions, but in a unique setting where CCA, adult corals and their recruits have been exposed to extreme conditions for several generations over likely hundreds of years. In particular, high pCO2 and low dissolved oxygen in Bouraké were even worse than what is expected for 2100. Surprisingly, healthy and diversified coral populations still persist as likely because of unexpected high coral recruitment (this study) that gives a glimpse of hope for the future of coral reefs facing climate change. However, our findings need to be used with caution as the Bouraké system is not a typical reef ecosystem. In fact, although our results showed that recruitment is possible even under extreme conditions, it does not mean that it might be the case for other reefs in the future. For instance, it has been shown that OA can cause morphological changes to the coral skeleton and thus, reduce their bulk density and wave resistance (Fantazzini et al., 2015; Tambutté et al., 2015). Further measurements on the skeleton of coral recruits and adults (i.e., SEM observations) from Bouraké are underway to shed light on this possibility.
Importantly, habitats displaying natural variability like Bouraké have been hypothesized to be populated by resilient coral reef organisms (Padilla-Gamiño et al., 2016; Vargas et al., 2017; Kapsenberg and Cyronak, 2019; Enochs et al., 2020). Coral recruits could as well benefit from such high natural variability (Jiang et al., 2017; Jiang et al., 2020), although some studies described small influences from variability on some corals and CCA (Cornwall et al., 2018), increased negative effect of OA on CCA calcification (Johnson et al., 2019), or no systematic coral tolerance to warming (Klepac and Barshis, 2020). Defining how variability in multiple co-varying parameters could influence tolerance in biological responses of coral reef organisms remains a significant challenge for the scientific community (Kapsenberg et al., 2015; Boyd et al., 2016; Rivest et al., 2017). Even though the effect of environmental parameters has not been fully deciphered yet, we could assume that Bouraké’s corals could have acquired specific mechanisms providing recruits with the ability to face extreme environmental conditions (Putnam et al., 2016; Putnam et al., 2017). Which mechanism is used and to what extent acclimation vs adaptation is involved was not the objective of this study and would need further experiments using genomic approaches and reciprocal transplantations between Bouraké and reference stations. However, the present study provided evidence that survival and specific high coral recruitment are possible in natural and extreme habitats such as Bouraké leading to lifelong adapted coral reefs.
Data Availability Statement
The original contributions presented in the study are included in the article/Supplementary Material. Further inquiries can be directed to the corresponding author.
Author Contributions
RR-M conceived and designed the project. CT, RR-M and FB collected the data. FB identified the corals. CP counted coral recruits and identified them. CT performed the data analysis and drafted the manuscript in collaboration with RR-M. All co-authors read and edited the final version of the manuscript.
Funding
This study was supported by the French grant scheme Fonds Pacifique (no. 1976; project SuperCoraux). CT received a PhD fellowship from the University of Western Brittany (UBO) and the Brittany region (France). This study has also received financial support from the CNRS through the MITI interdisciplinary programs and was supported by ISblue project, Interdisciplinary graduate school for the blue planet (ANR-17-EURE-0015) and co-funded by a grant from the French government under the program “Investissements d’Avenir”. Funding for FB was provided by KAUST baseline research funding and award FCC/1/1973-49-01.
Conflict of Interest
The authors declare that the research was conducted in the absence of any commercial or financial relationships that could be construed as a potential conflict of interest.
Publisher’s Note
All claims expressed in this article are solely those of the authors and do not necessarily represent those of their affiliated organizations, or those of the publisher, the editors and the reviewers. Any product that may be evaluated in this article, or claim that may be made by its manufacturer, is not guaranteed or endorsed by the publisher.
Acknowledgments
We wish to express our thanks to the captains of the IRD vessels and the diving technical staff for their fieldwork assistance. We thank Dr. Mehdi Adjeroud for providing us with the tiles used and for scientific advice on coral recruitment. We thank Dr. Claude Payri and Dr. Christopher Cornwall for their help and expertise regarding the assessment of organisms percentage cover and for CCA taxonomy and health review. We also thank the Province Sud of New-Caledonia for sample collection permits (no. 3413-2019). We are indebted to Federica Maggioni for environmental data sharing. We thank Yuri Artioli for his help throughout the reviewing and editing process of this article.
Supplementary Material
The Supplementary Material for this article can be found online at: https://www.frontiersin.org/articles/10.3389/fmars.2022.837877/full#supplementary-material
References
Adjeroud M., Penin L., Carroll A. (2007). Spatio-Temporal Heterogeneity in Coral Recruitment Around Moorea, French Polynesia: Implications for Population Maintenance. J. Exp. Mar. Bio. Ecol. 341, 204–218. doi: 10.1016/j.jembe.2006.10.048
Agostini S., Harvey B. P., Wada S., Kon K., Milazzo M., Inaba K., et al. (2018). Ocean Acidification Drives Community Shifts Towards Simplified non-Calcified Habitats in a Subtropical–Temperate Transition Zone. Sci. Rep. 8, 5–10. doi: 10.1038/s41598-018-29251-7
Albright R., Langdon C. (2011). Ocean Acidification Impacts Multiple Early Life History Processes of the Caribbean Coral Porites Astreoides. Glob. Change Biol. 17, 2478–2487. doi: 10.1111/j.1365-2486.2011.02404.x
Albright R., Mason B., Miller M., Langdon C. (2010). Ocean Acidification Compromises Recruitment Success of the Threatened Caribbean Coral Acropora Palmata. Proc. Natl. Acad. Sci. U. S. A. 107, 20400–20404. doi: 10.1073/pnas.1007273107
Alderdice R., Pernice M., Cárdenas A., Hughes D. J., Harrison P. L., Boulotte N., et al. (2021). Hypoxia as a Physiological Cue and Pathological Stress for Coral Larvae. Mol. Ecol. 31 (2), 1–17. doi: 10.1111/mec.16259
Altieri A. H., Harrison S. B., Seemann J., Collin R., Diaz R. J., Knowlton N. (2017). Tropical Dead Zones and Mass Mortalities on Coral Reefs. Proc. Natl. Acad. Sci. U. S. A. 114, 3660–3665. doi: 10.1073/pnas.1621517114
Anthony K. R. N. (2006). Enhanced Energy Status of Corals on Coastal, High-Turbidity Reefs. Mar. Ecol. Prog. Ser. 319, 111–116. doi: 10.3354/meps319111
Anthony K. R. N., Kline D. I., Diaz-Pulido G., Dove S., Hoegh-Guldberg O. (2008). Ocean Acidification Causes Bleaching and Productivity Loss in Coral Reef Builders. Proc. Natl. Acad. Sci. U. S. A. 105, 17442–17446. doi: 10.1073/pnas.0804478105
Baird A. H., Kospartov M. C., Purcell S. (2010). Reproductive Synchrony in Acropora Assemblages on Reefs of New Caledonia. Pacific. Sci. 64, 405–412. doi: 10.2984/64.3.405
Barkley H. C., Cohen A. L., Golbuu Y., Starczak V. R., DeCarlo T. M., Shamberger K. E. F. (2015). Changes in Coral Reef Communities Across a Natural Gradient in Seawater pH. Sci. Adv. 1, 1–8. doi: 10.1126/sciadv.1500328
Barott K. L., Williams G. J., Vermeij M. J. A., Harris J., Smith J. E., Rohwer F. L., et al. (2012). Natural History of Coral-Algae Competition Across a Gradient of Human Activity in the Line Islands. Mar. Ecol. Prog. Ser. 460, 1–12. doi: 10.3354/meps09874
Bessell-Browne P., Negri A. P., Fisher R., Clode P. L., Jones R. (2017). Impacts of Light Limitation on Corals and Crustose Coralline Algae. Sci. Rep. 7, 1–12. doi: 10.1038/s41598-017-11783-z
Boyd P. W., Cornwall C. E., Davison A., Doney S. C., Fourquez M., Hurd C. L., et al. (2016). Biological Responses to Environmental Heterogeneity Under Future Ocean Conditions. Glob. Change Biol. 22, 2633–2650. doi: 10.1111/gcb.13287
Bradassi F., Cumani F., Bressan G., Dupont S. (2013). Early Reproductive Stages in the Crustose Coralline Alga Phymatolithon Lenormandii are Strongly Affected by Mild Ocean Acidification. Mar. Biol. 160, 2261–2269. doi: 10.1007/s00227-013-2260-2
Bramanti L., Edmunds P. J. (2016). Density-Associated Recruitment Mediates Coral Population Dynamics on a Coral Reef. Coral. Reef. 35, 543–553. doi: 10.1007/s00338-016-1413-4
Camp E. F., Nitschke M. R., Rodolfo-Metalpa R., Houlbreque F., Gardner S. G., Smith D. J., et al. (2017). Reef-Building Corals Thrive Within Hot-Acidified and Deoxygenated Waters. Sci. Rep. 7, 1–9. doi: 10.1038/s41598-017-02383-y
Chan P.T.W., Halfar J., Adey W.H., Lebednik P.A., Steneck R., Norley C.J.D., et al. (2019). Recent Density Decline in Wild-Collected Subarctic Crustose Coralline Algae Reveals Climate Change Signature. Geology, 48 (3), 226–230. doi: 10.1130/G46804.1
Cohen A. L., McCorkle D. C., De Putron S., Gaetani G. A., Rose K. A. (2009). Morphological and Compositional Changes in the Skeletons of New Coral Recruits Reared in Acidified Seawater: Insights Into the Biomineralization Response to Ocean Acidification. Geochem. Geophys. Geosyst. 10 (7), 1–12. doi: 10.1029/2009GC002411
Comeau S., Cornwall C. E., Pupier C. A., DeCarlo T. M., Alessi C., Trehern R., et al. (2019). Flow-Driven Micro-Scale pH Variability Affects the Physiology of Corals and Coralline Algae Under Ocean Acidification. Sci. Rep. 9, 1–12. doi: 10.1038/s41598-019-49044-w
Comeau S., Edmunds P. J., Spindel N. B., Carpenter R. C. (2013). The Responses of Eight Coral Reef Calcifiers to Increasing Partial Pressure of CO2 do Not Exhibit a Tipping Point. Limnol. Oceanogr. 58, 388–398. doi: 10.4319/lo.2013.58.1.0388
Cornwall C. E., Comeau S., DeCarlo T. M., Moore B., D’Alexis Q., McCulloch M. T. (2018). Resistance of Corals and Coralline Algae to Ocean Acidification: Physiological Control of Calcification Under Natural pH Variability. Proc. R. Soc B. Biol. Sci. 285, 1168–1176. doi: 10.1098/rspb.2018.1168
Cornwall C. E., Comeau S., Kornder N. A., Perry C. T., van Hooidonk R., DeCarlo T. M., et al. (2021). Global Declines in Coral Reef Calcium Carbonate Production Under Ocean Acidification and Warming. Proc. Natl. Acad. Sci. U. S. A. 118, 1–10. doi: 10.1073/pnas.2015265118
Cornwall C. E., Diaz-Pulido G., Comeau S. (2019). Impacts of Ocean Warming on Coralline Algae: Knowledge Gaps and Key Recommendations for Future Research. Front. Mar. Sci. 6. doi: 10.3389/fmars.2019.00186
Diaz-Pulido G., Anthony K. R. N., Kline D. I., Dove S., Hoegh-Guldberg O. (2012). Interactions Between Ocean Acidification and Warming on the Mortality and Dissolution of Coralline Algae. J. Phycol. 48 (1), 32–39. doi: 10.1111/j.1529-8817.2011.01084.x
Doropoulos C., Diaz-Pulido G. (2013). High CO2 Reduces the Settlement of a Spawning Coral on Three Common Species of Crustose Coralline Algae. Mar. Ecol. Prog. Ser. 475, 93–99. doi: 10.3354/meps10096
Doropoulos C., Roff G., Visser M. S., Mumby P. J. (2017). Sensitivity of Coral Recruitment to Subtle Shifts in Early Community Succession. Ecology 98, 304–314. doi: 10.1002/ecy.1663
Doropoulos C., Ward S., Diaz-Pulido G., Hoegh-Guldberg O., Mumby P. J. (2012). Ocean Acidification Reduces Coral Recruitment by Disrupting Intimate Larval-Algal Settlement Interactions. Ecol. Lett. 15, 338–346. doi: 10.1111/j.1461-0248.2012.01743.x
Edmunds P. J. (2021). Recruitment Hotspots and Bottlenecks Mediate the Distribution of Corals on a Caribbean Reef. Biol. Lett. 17 (7), 149–155. doi: 10.1098/rsbl.2021.0149
Elmer F., Bell J. J., Gardner J. P. A. (2018). Coral Larvae Change Their Settlement Preference for Crustose Coralline Algae Dependent on Availability of Bare Space. Coral. Reef. 37, 397–407. doi: 10.1007/s00338-018-1665-2
Enochs I. C., Formel N., Manzello D., Morris J., Mayfield A. B., Boyd A., et al. (2020). Coral Persistence Despite Extreme Periodic pH Fluctuations at a Volcanically Acidified Caribbean Reef. Coral. Reef. 39, 523–528. doi: 10.1007/s00338-020-01927-5
Enochs I. C., Manzello D. P., Donham E. M., Kolodziej G., Okano R., Johnston L., et al. (2015). Shift From Coral to Macroalgae Dominance on a Volcanically Acidified Reef. Nat. Clim. Change 5, 1083–1088. doi: 10.1038/nclimate2758
Fabricius K. E., Kluibenschedl A., Harrington L., Noonan S., De’Ath G. (2015). In Situ Changes of Tropical Crustose Coralline Algae Along Carbon Dioxide Gradients. Sci. Rep. 5, 1–7. doi: 10.1038/srep09537
Fabricius K. E., Langdon C., Uthicke S., Humphrey C., Noonan S., De’ath G., et al. (2011). Losers and Winners in Coral Reefs Acclimatized to Elevated Carbon Dioxide Concentrations. Nat. Clim. Change 1, 165–169. doi: 10.1038/nclimate1122
Fabricius K. E., Noonan S. H. C., Abrego D., Harrington L., De’Ath G. (2017). Low Recruitment Due to Altered Settlement Substrata as Primary Constraint for Coral Communities Under Ocean Acidification. Proc. R. Soc B. Biol. Sci. 184(1862), 1536–1545. doi: 10.1098/rspb.2017.1536
Fabricius K. E., Wolanski E. (2000). Rapid Smothering of Coral Reef Organisms by Muddy. Mangroves. Salt. Marshes. 50 (1), 115–120. Estuarine, Coastal and Shelf Science. http://www.idealibrary.comon. doi: 10.1006/ecss.1999.0538
Fantazzini P., Mengoli S., Pasquini L., Bortolotti V., Brizi L., Mariani M., et al. (2015). Gains and Losses of Coral Skeletal Porosity Changes With Ocean Acidification Acclimation. Nat. Commun. 6, 77-85. doi: 10.1038/ncomms8785
Figueiredo M. A., Kain J. M., Norton T. A. (1996). Biotic Interactions in the Colonization of Crustose Coralline Algae by Epiphytes. J. Exp. Mar. Bio. Ecol. 199, 303–318. doi: 10.1016/0022-0981(96)00018-4
Foster T., Falter J. L., McCulloch M. T., Clode P. L. (2016). Climate Science: Ocean Acidification Causes Structural Deformities in Juvenile Coral Skeletons. Sci. Adv. 2, 1–8. doi: 10.1126/sciadv.1501130
Glassom D., Zakai D., Chadwick-Furman N. E. (2004). Coral Recruitment: A Spatio-Temporal Analysis Along the Coastline of Eilat, Northern Red Sea. Mar. Biol. 144, 641–651. doi: 10.1007/s00227-003-1243-0
Gleason M. G. (1996). Coral Recruitment in Moorea, French Polynesia: The Importance of Patch Type and Temporal Variation. J. Exp. Mar. Bio. Ecol. 207, 79–101. doi: 10.1016/S0022-0981(96)02647-0
Goffredo S., Prada F., Caroselli E., Capaccioni B., Zaccanti F., Pasquini L., et al. (2014). Biomineralization Control Related to Population Density Under Ocean Acidification. Nat. Clim. Change 4, 593–597. doi: 10.1038/nclimate2241
Goldberg W. M. (2018). Coral Food, Feeding, Nutrition, and Secretion: A Review. In: Kloc M., Kubiak J. (eds). Marine Organisms as Model Systems in Biology and Medicine. Results and Problems in Cell Differentiation, vol 65. Springer, Cham. doi: 10.1007/978-3-319-92486-1_18
Gómez-Lemos L. A., Doropoulos C., Bayraktarov E., Diaz-Pulido G. (2018). Coralline Algal Metabolites Induce Settlement and Mediate the Inductive Effect of Epiphytic Microbes on Coral Larvae. Sci. Rep. 8, 1–11. doi: 10.1038/s41598-018-35206-9
Hall-Spencer J. M., Rodolfo-Metalpa R., Martin S., Ransome E., Fine M., Turner S. M., et al. (2008). Volcanic Carbon Dioxide Vents Show Ecosystem Effects of Ocean Acidification. Nature 454, 96–99. doi: 10.1038/nature07051
Harrington L., Fabricius K., De’Ath G., Negri A. (2004). Recognition and Selection of Settlement Substrata Determine Post-Settlement Survival in Corals. Ecology 85, 3428–3437. doi: 10.1890/04-0298
Harrison P., Wallace C. (1990). Reproduction, Dispersal and Recruitment of Scleractinian Corals. Coral Reefs 25, 133–207. https://www.researchgate.net/publication/304784746
Heyward A. J., Negri A. P. (1999). Natural Inducers for Coral Larval Metamorphosis. Coral. Reef. 18, 273–279. doi: 10.1007/s003380050193
Houlbrèque F., Ferrier-Pagès C. (2009). Heterotrophy in Tropical Scleractinian Corals. Biol. Rev. 84, 1–17. doi: 10.1111/j.1469-185X.2008.00058.x
Huffmyer A. S., Johnson C. J., Epps A. M., Lemus J. D., Gates R. D. (2021). Feeding and Thermal Conditioning Enhance Coral Temperature Tolerance in Juvenile Pocillopora Acuta. R. Soc Open Sci. 8(5), 1–13. doi: 10.1098/rsos.210644
Hughes D. J., Alderdice R., Cooney C., Kühl M., Pernice M., Voolstra C. R., et al. (2020). Coral Reef Survival Under Accelerating Ocean Deoxygenation. Nat. Clim. Change 10, 296–307. doi: 10.1038/s41558-020-0737-9
IPCC Report, I (2021). “Climate Change 2021: The Physical Science Basis Summary for Policymakers,” in Contribution of Working Group I to the Sixth Assessment Report of the Intergovernmental Panel on Climate Change pp. 3–32. (United Kingdom and New York, NY, USA: United Nations Environ. Program. UNEP AR6). doi: 10.1017/9781009157896.001
Jacquemont J., Houlbrèque F., Tanvet C., et al. (2022). Long-term exposure to an extreme environment induces species-specific responses in corals’ photosynthesis and respiration rates. Mar Biol 169, 82. doi: 10.1007/s00227-022-04063-6
Jiang L., Guo M. L., Zhang F., Zhang Y. Y., Zhou G. W., Lei X. M., et al. (2020). Impacts of Elevated Temperature and Pco2 on the Brooded Larvae of Pocillopora Damicornis From Luhuitou Reef, China: Evidence for Local Acclimatization. Coral. Reef. 39, 331–344. doi: 10.1007/s00338-020-01894-x
Jiang L., Sun Y. F., Zhang Y. Y., Zhou G. W., Li X. B., McCook L. J., et al. (2017). Impact of Diurnal Temperature Fluctuations on Larval Settlement and Growth of the Reef Coral Pocillopora Damicornis. Biogeosciences 24, 5741–5752. doi: 10.5194/bg-14-5741-2017
Johnson M. D., Bravo L. M. R., O’Connor S. E., Varley N. F., Altieri A. H. (2019). pH Variability Exacerbates Effects of Ocean Acidification on a Caribbean Crustose Coralline Alga. Front. Mar. Sci. 6. doi: 10.3389/fmars.2019.00150
Johnson M. D., Comeau S., Lantz C. A., Smith J. E. (2017). Complex and Interactive Effects of Ocean Acidification and Temperature on Epilithic and Endolithic Coral-Reef Turf Algal Assemblages. Coral. Reef. 36, 1059–1070. doi: 10.1007/s00338-017-1597-2
Jokiel P. L., Rodgers K. S., Kuffner I. B., Andersson A. J., Cox E. F., Mackenzie F. T. (2008). Ocean Acidification and Calcifying Reef Organisms: A Mesocosm Investigation. Coral. Reef. 27, 473–483. doi: 10.1007/s00338-008-0380-9
Jones R., Ricardo G. F., Negri A. P. (2015). Effects of Sediments on the Reproductive Cycle of Corals. Mar. Pollut. Bull. 100, 13–33. doi: 10.1016/j.marpolbul.2015.08.021
Jorissen H., Galand P. E., Bonnard I., Meiling S., Raviglione D., Meistertzheim A. L., et al. (2021). Coral Larval Settlement Preferences Linked to Crustose Coralline Algae With Distinct Chemical and Microbial Signatures. Sci. Rep. 11, 1–11. doi: 10.1038/s41598-021-94096-6
Jorissen H., Nugues M. M. (2021). Coral Larvae Avoid Substratum Exploration and Settlement in Low-Oxygen Environments. Coral. Reef. 40, 31–39. doi: 10.1007/s00338-020-02013-6
Jorissen H., Nugues M. M., Steneck R. S. (2020). Contrasting Effects of Crustose Coralline Algae From Exposed and Subcryptic Habitats on Coral Recruits. Coral Reefs 39, 1767–1778. doi: 10.1007/s00338-020-02002-9
Kamenos N. A., Perna G., Gambi M. C., Micheli F., Kroeker K. J. (2016). Coralline Algae in a Naturally Acidified Ecosystem Persist by Maintaining Control of Skeletal Mineralogy and Size. Proc. R. Soc B. Biol. Sci. 283, 1–8. doi: 10.1098/rspb.2016.1159
Kapsenberg L., Cyronak T. (2019). Ocean Acidification Refugia in Variable Environments. Glob. Change Biol. 25, 3201–3214. doi: 10.1111/gcb.14730
Kapsenberg L., Kelley A. L., Shaw E. C., Martz T. R., Hofmann G. E. (2015). Near-Shore Antarctic pH Variability has Implications for the Design of Ocean Acidification Experiments. Sci. Rep. 5, 1–10. doi: 10.1038/srep09638
Klepac C. N., Barshis D. J. (2020). Reduced Thermal Tolerance of Massive Coral Species in a Highly Variable Environment: Reduced Heat Tolerance of Massive Corals. Proc. R. Soc B. Biol. Sci. 287, 19–21. doi: 10.1098/rspb.2020.1379rspb20201379
Kroeker K. J., Micheli F., Gambi M. C. (2013). Ocean Acidification Causes Ecosystem Shifts via Altered Competitive Interactions. Nat. Clim. Change 3, 156–159. doi: 10.1038/nclimate1680
Kuffner I. B., Andersson A. J., Jokiel P. L., Rodgers K. S., MacKenzie F. T. (2008). Decreased Abundance of Crustose Coralline Algae Due to Ocean Acidification. Nat. Geosci. 1, 114–117. doi: 10.1038/ngeo100
Kurihara H., Watanabe A., Tsugi A., Mimura I., Hongo C., Kawai T., et al. (2021). Potential Local Adaptation of Corals at Acidified and Warmed Nikko Bay, Palau. Sci. Rep. 11, 1–10. doi: 10.1038/s41598-021-90614-8
Maggioni F., Pujo-Pay M., Aucan J., Cerrano C., Calcinai B., Payri C., et al. (2021). The Bouraké Semi-Enclosed Lagoon (New Caledonia) - A Natural Laboratory to Study the Life-Long Adaptation of a Coral Reef Ecosystem to Climate Change-Like Conditions. Biogeosci. Discuss. 18, 5117–5140. doi: 10.5194/bg-2021-90
Martin S., Charnoz A., Gattuso J. P. (2013). Photosynthesis, Respiration and Calcification in the Mediterranean Crustose Coralline Alga Lithophyllum Cabiochae (Corallinales, Rhodophyta). Eur. J. Phycol. 48, 163–172. doi: 10.1080/09670262.2013.786790
Martin S., Gattuso J. P. (2009). Response of Mediterranean Coralline Algae to Ocean Acidification and Elevated Temperature. Glob. Change Biol. 15, 2089–2100. doi: 10.1111/j.1365-2486.2009.01874.x
Martin S., Rodolfo-Metalpa R., Ransome E., Rowley S., Buia M. C., Gattuso J. P., et al. (2008). Effects of Naturally Acidified Seawater on Seagrass Calcareous Epibionts. Biol. Lett. 4, 689–692. doi: 10.1098/rsbl.2008.0412
Mccoy S. J., Kamenos N. A. (2015). Coralline Algae (Rhodophyta) in a Changing World: Integrating Ecological, Physiological, and Geochemical Responses to Global Change. J. Phycol. 51, 6–24. doi: 10.1111/jpy.12262
McNicholl C., Koch M. S. (2021). Irradiance, Photosynthesis and Elevated Pco2 Effects on Net Calcification in Tropical Reef Macroalgae. J. Exp. Mar. Bio. Ecol. 535, 151489. doi: 10.1016/j.jembe.2020.151489
Morse D. E., Hooker N., Morse A. N. C., Jensen R. A. (1988). Control of Larval Metamorphosis and Recruitment in Sympatric Agariciid Corals. J. Exp. Mar. Bio. Ecol. 116, 193–217. doi: 10.1016/0022-0981(88)90027-5
Nelson W. A. (2009). Calcified Macroalgae Critical to Coastal Ecosystems and Vulnerable to Change: A Review. Mar. Freshw. Res. 60, 787–801. doi: 10.1071/MF08335
Nelson H. R., Altieri A. H. (2019). Oxygen: The Universal Currency on Coral Reefs. Coral. Reef. 38, 177–198. doi: 10.1007/s00338-019-01765-0
Nock R., Nielsen F. (2004). Statistical Region Merging. IEEE Trans. Pattern Anal. Mach. Intell. 26, 1452–1458. doi: 10.1109/TPAMI.2004.110
O’Connor M. I., Bruno J. F., Gaines S. D., Halpern B. S., Lester S. E., Kinlan B. P., et al. (2007). Temperature Control of Larval Dispersal and the Implications for Marine Ecology, Evolution, and Conservation. Proc. Natl. Acad. Sci. U. S. A. 104, 1266–1271. doi: 10.1073/pnas.0603422104
O’Leary J. K., Potts D. C., Braga J. C., McClanahan T. R. (2012). Indirect Consequences of Fishing: Reduction of Coralline Algae Suppresses Juvenile Coral Abundance. Coral. Reef. 31, 547–559. doi: 10.1007/s00338-012-0872-5
O’Leary J. K., Potts D., Schoenrock K. M., McClahanan T. R. (2013). Fish and Sea Urchin Grazing Opens Settlement Space Equally But Urchins Reduce Survival of Coral Recruits. Mar. Ecol. Prog. Ser. 493, 165–177. doi: 10.3354/meps10510
Oprandi A., Montefalcone M., Morri C., Benelli F., Bianchi C. N. (2019). Water Circulation, and Not Ocean Acidification, Affects Coral Recruitment and Survival at Shallow Hydrothermal Vents. Estuar. Coast. Shelf. Sci. 217, 158–164. doi: 10.1016/j.ecss.2018.11.017
Orr J. C., Fabry V. J., Aumont O., Bopp L., Doney S. C., Feely R. A., et al. (2005). Anthropogenic Ocean Acidification Over the Twenty-First Century and its Impact on Calcifying Organisms. Nature 437, 681–686. doi: 10.1038/nature04095
Padilla-Gamiño J. L., Gaitán-Espitia J. D., Kelly M. W., Hofmann G. E. (2016). Physiological Plasticity and Local Adaptation to Elevated Pco2 in Calcareous Algae: An Ontogenetic and Geographic Approach. Evol. Appl. 9, 1043–1053. doi: 10.1111/eva.12411
Page H. N., Hewett C., Tompkins H., Hall E. R. (2021). Ocean Acidification and Direct Interactions Affect Coral, Macroalga, and Sponge Growth in the Florida Keys. J. Mar. Sci. Eng. 9 (7), 739. doi: 10.3390/jmse9070739
Page H. N., Mccoy S. J., Bahr K. D., Cyronak T., Jewett E. B., Johnson M. D., et al. (2022). Responses of Benthic Calcifying Algae to Ocean Acidification Differ Between Laboratory and Field Settings. ICES Journal of Marine Science 79, 1–11. doi: 10.1093/icesjms/fsab232
Penin L., Michonneau F., Carroll A., Adjeroud M. (2011). Effects of Predators and Grazers Exclusion on Early Post-Settlement Coral Mortality. Hydrobiologia 663, 259–264. doi: 10.1007/s10750-010-0569-0
Porzio L., Garrard S. L., Buia M. C. (2013). The Effect of Ocean Acidification on Early Algal Colonization Stages at Natural CO2 Vents. Mar. Biol. 160, 2247–2259. doi: 10.1007/s00227-013-2251-3
Price N. (2010). Habitat Selection, Facilitation, and Biotic Settlement Cues Affect Distribution and Performance of Coral Recruits in French Polynesia. Oecologia 163, 747–758. doi: 10.1007/s00442-010-1578-4
Putnam H. M., Barott K. L., Ainsworth T. D., Gates R. D. (2017). The Vulnerability and Resilience of Reef-Building Corals. Curr. Biol. 27, R528–R540. doi: 10.1016/j.cub.2017.04.047
Putnam H. M., Davidson J. M., Gates R. D. (2016). Ocean Acidification Influences Host DNA Methylation and Phenotypic Plasticity in Environmentally Susceptible Corals. Evol. Appl 9 (9), 1165–1178. doi: 10.1111/eva.12408
Ragazzola F., Foster L. C., Form A. U., Büscher J., Hansteen T. H., Fietzke J. (2013). Phenotypic Plasticity of Coralline Algae in a High CO2 World. Ecol. Evol. 3, 3436–3446. doi: 10.1002/ece3.723
Ricardo G. F., Jones R. J., Nordborg M., Negri A. P. (2017). Settlement Patterns of the Coral Acropora Millepora on Sediment-Laden Surfaces. Sci. Tot. Environ. 609, 277–288. doi: 10.1016/j.scitotenv.2017.07.153
Rivest E. B., Comeau S., Cornwall C. E. (2017). The Role of Natural Variability in Shaping the Response of Coral Reef Organisms to Climate Change. Curr. Clim. Change Rep. 3, 271–281. doi: 10.1007/s40641-017-0082-x
Shamberger K. E. F., Lentz S. J., Cohen A. L. (2018). Low and Variable Ecosystem Calcification in a Coral Reef Lagoon Under Natural Acidification. Limnol. Oceanogr. 63, 714–730. doi: 10.1002/lno.10662
Swierts T., Vermeij M. J. A. (2016). Competitive Interactions Between Corals and Turf Algae Depend on Coral Colony Form. PeerJ 2016, 1–18. doi: 10.7717/peerj.1984
Tambutté E., Venn A. A., Holcomb M., Segonds N., Techer N., Zoccola D., et al. (2015). Morphological Plasticity of the Coral Skeleton Under CO2-Driven Seawater AcidificationNat. Commun. 6, 7368. doi: 10.1038/ncomms8368
Tebben J., Motti C. A., Siboni N., Tapiolas D. M., Negri A. P., Schupp P. J., et al. (2015). Chemical Mediation of Coral Larval Settlement by Crustose Coralline Algae. Sci. Rep. 5, 1–11. doi: 10.1038/srep10803
Trygonis V., Sini M. (2012). PhotoQuad: A Dedicated Seabed Image Processing Software, and a Comparative Error Analysis of Four Photoquadrat Methods. J. Exp. Mar. Bio. Ecol. 424–425, 99–108. doi: 10.1016/j.jembe.2012.04.018
Underwood A. J., Jernakoff P. (1984). The Effects of Tidal Height, Wave-Exposure, Seasonality and Rock-Pools on Grazing and the Distribution of Intertidal Macroalgae in New South Wales. J. Exp. Mar. Bio. Ecol. 75, 71–96. doi: 10.1016/0022-0981(84)90024-8
Vargas C. A., Lagos N. A., Lardies M. A., Duarte C., Manríquez P. H., Aguilera V. M., et al. (2017). Species-Specific Responses to Ocean Acidification Should Account for Local Adaptation and Adaptive Plasticity. Nat. Ecol. Evol. 1. doi: 10.1038/s41559-017-0084
Victor S. (2008). Stability of Reef Framework and Post Settlement Mortality as the Structuring Factor for Recovery of Malakal Bay Reef, Palau, Micronesia: 25 Years After a Severe COTS Outbreak. Estuar. Coast. Shelf. Sci. 77, 175–180. doi: 10.1016/j.ecss.2007.09.009
Vogel N., Cantin N. E., Strahl J., Kaniewska P., Bay L., Wild C., et al. (2016). Interactive Effects of Ocean Acidification and Warming on Coral Reef Associated Epilithic Algal Communities Under Past, Present-Day and Future Ocean Conditions. Coral. Reef. 35, 715–728. doi: 10.1007/s00338-015-1392-x
Webster N. S., Uthicke S., Botté E. S., Flores F., Negri A. P. (2013). Ocean Acidification Reduces Induction of Coral Settlement by Crustose Coralline Algae. Glob. Change Biol 19, 303–315. doi: 10.1111/gcb.12008
Williams B., Chan P. T. W., Westfield I. T., Rasher D. B., Ries J. (2021). Ocean Acidification Reduces Skeletal Density of Hardground-Forming High-Latitude Crustose Coralline Algae. Geophys. Res. Lett. 48, 1–10. doi: 10.1029/2020GL091499
Keywords: coral recruitment, crustose coralline algae (CCA), extreme environmental conditions, persistence, natural variability
Citation: Tanvet C, Benzoni F, Peignon C, Thouzeau G and Rodolfo-Metalpa R (2022) High Coral Recruitment Despite Coralline Algal Loss Under Extreme Environmental Conditions. Front. Mar. Sci. 9:837877. doi: 10.3389/fmars.2022.837877
Received: 17 December 2021; Accepted: 17 May 2022;
Published: 16 June 2022.
Edited by:
Yuri Artioli, Plymouth Marine Laboratory, United KingdomReviewed by:
Nick Kamenos, University of Glasgow, United KingdomMaggy Nugues, Université de Sciences Lettres de Paris, France
Copyright © 2022 Tanvet, Benzoni, Peignon, Thouzeau and Rodolfo-Metalpa. This is an open-access article distributed under the terms of the Creative Commons Attribution License (CC BY). The use, distribution or reproduction in other forums is permitted, provided the original author(s) and the copyright owner(s) are credited and that the original publication in this journal is cited, in accordance with accepted academic practice. No use, distribution or reproduction is permitted which does not comply with these terms.
*Correspondence: Clément Tanvet, Y2xlbWVudC50YW52ZXRAaXJkLmZy