- 1Climate Change Cluster, Faculty of Science, University of Technology Sydney, Ultimo, NSW, Australia
- 2Department of Biology, University of Konstanz, Konstanz, Germany
- 3Marine Biology Section, Department of Biology, University of Copenhagen, Helsingør, Denmark
Aquatic deoxygenation has been flagged as an overlooked but key factor contributing to mass bleaching-induced coral mortality. During deoxygenation events triggered by coastal nutrient pollution and ocean warming, oxygen supplies lower to concentrations that can elicit an aerobic metabolic crisis i.e., hypoxia. Surprisingly little is known of the fundamental hypoxia gene set inventory that corals possess to respond to lowered oxygen (i.e., deoxygenation). For instance, it is unclear whether gene copy number differences exist across species that may affect the efficacy of a measured transcriptomic stress response. Therefore, we conducted an ortholog-based meta-analysis to investigate how hypoxia gene inventories differ amongst coral species to assess putative copy number variations (CNVs). We specifically elucidated CNVs for a compiled list of 32 hypoxia genes across 24 protein sets from species with a sequenced genome spanning corals from the robust and complex clade. We found approximately a third of the investigated genes exhibited copy number differences, and these differences were species-specific rather than attributable to the robust-complex split. Interestingly, we consistently found the highest gene expansion present in Porites lutea, which is considered to exhibit inherently greater stress tolerance than other species. Consequently, our analysis suggests that hypoxia stress gene expansion may coincide with increased stress tolerance. As such, the unevenly expanded (or reduced) hypoxia genes presented here provide key genes of interest to target in examining (or diagnosing) coral stress responses. Important next steps will involve determining to what extent such gene copy differences align with certain coral traits.
1 Introduction
Aquatic deoxygenation is one of the most pressing and intensifying concerns worldwide, driving mass mortality to both marine (Diaz and Rosenberg, 2008; Schmidtko et al., 2017; Breitburg et al., 2018) and freshwater biota (Pollock et al., 2007). Since 1960, more than 400 coastal ‘dead zones’ have formed, where nutrient-overloaded waters are deprived of oxygen (O2) exposing aquatic organisms to reduced concentrations – typically < 2mg O2 L-1 – that elicit aerobic metabolic crisis, i.e., hypoxia (Diaz and Rosenberg, 2008; Altieri et al., 2017). Hypoxic regions are considered to be particularly underreported by an order of magnitude for tropical compared to temperate regions (Diaz and Rosenberg, 2008). Where dead zones have been reported in tropical regions to date, over half have been associated with coral reefs (Altieri et al., 2017; Nelson and Altieri, 2019; Hughes et al., 2020). Recent observations have indeed recorded deoxygenation events on shallow reefs off the Caribbean coast of Panama, whereby both chronic and acute hypoxic episodes have resulted in mass coral bleaching-induced mortality (Altieri et al., 2017; Johnson et al., 2021a). As such, the significance and contribution of hypoxia as a driving factor of coral bleaching in tropical reef systems has likely been overlooked (Hughes et al., 2020; Suggett and Smith, 2020; Johnson et al., 2021a).
Of all tropical reef biota, sedentary calcifiers – such as hard corals – have been proposed to suffer the most under hypoxia (Hughes et al., 2020); for example a lethal threshold of 4 mg O2 L-1 has been shown to drive a key reef-building coral species to mortality within 12 hours (Acropora yongei; Haas et al., 2014). However, corals have recently demonstrated the ability to oxy-regulate rather than -conform, showing a large variation between species (Hughes et al., 2022). Other recent studies have also demonstrated that hypoxia responses appear to vary substantially amongst coral taxa. Notably, two evolutionarily distinct Caribbean coral species, Acropora cervicornis and Orbicella faveolata, exhibited stark differences in susceptibility to severe deoxygenation (~0.5 mg L-1): while Acropora cervicornis suffered tissue loss and mortality within a day of exposure, O. faveolata remained unaffected after ~10 days (Johnson et al., 2021b). Also, two Indo-Pacific Acropora species, Acropora tenuis and Acropora selago, demonstrated contrasting bleaching phenotypes to deoxygenation (~2mg O2 L-1), where only A. selago bleached after 12 hours exposure (Alderdice et al., 2021). Further, deep-sea corals in the Red Sea live at remarkably low O2 levels of ~2 mg L-1 (Roder et al., 2013) with putative distinct adaptations, such as mitochondrial hypometabolism, anaerobic glycolysis, and microbiome restructuring (Röthig et al., 2017a; Röthig et al., 2017b; Yum et al., 2017), and show broad capacity for acclimation to high and low oxygen levels (Roik et al., 2015). All studies raise the question whether some coral species have an inherently greater capacity to withstand lower dissolved oxygen (DO) levels predicted for future reefs. Alderdice et al. (2021) demonstrated that differences in stress susceptibility to deoxygenation involved a varied ability to upregulate an array of hypoxia response genes that are transcriptionally controlled by the Hypoxia-inducible Factor (HIF; consisting of α and β subunits). More specifically, HIF transcription is known to be activated under limited O2 when the proteasomal degradation capacity of prolyl hydroxylase domain proteins (PHD) ceases, allowing HIFα proteins to accumulate (Kaelin and Ratcliffe, 2008). The cohort of HIF target genes function to reprogram bioenergetic pathways for anaerobic respiration (e.g., lactate dehydrogenase and pyruvate dehydrogenase kinase) and lipid resourcing (e.g., scavenger receptor, CD36), manage proteome homeostasis (e.g., heat shock protein 70), and finely regulate cell cycling and apoptosis (e.g., BCL2 Interacting Protein 3, BNIP3; Alderdice et al., 2021).
Looking beyond the HIF gene network, a recent study that exposed Acropora selago larvae to 12 hours of deoxygenation (~2mg O2 L-1), reported a significantly greater expression of HIF and non-HIF targeted hypoxia stress genes compared to control samples (Alderdice et al., 2022). The non-HIF targeted genes included melatonin receptors (Yan et al., 2018; Buttar et al., 2020) and universal stress proteins (USPs; Chi et al., 2019) that can function to alleviate cellular stress associated with a disruption in reactive oxygen species (ROS) homeostasis, another known consequence of hypoxia stress in model organisms (Blokhina et al., 2003; Solaini et al., 2010). In corals, excessive ROS levels have mostly been associated with damage to both photosynthetic and mitochondrial membranes and widely implicated to induce symbiosis dysfunction and coral bleaching (Lesser, 2006; Weis, 2008; Suggett and Smith, 2020; Rädecker et al., 2021). However, the contribution of hypoxia stress on such elevated ROS levels and subsequent susceptibility to coral bleaching remains to be determined. Despite HIF-targeting lactate dehydrogenase (LDH), opine dehydrogenases (OpDH) have not yet been defined as targets of HIF. Interestingly, opine dehydrogenases are found to be the preferred glycolytic enzymes for anaerobic respiration in marine invertebrates given that the glucose-opine pathway does not change the intracellular pH to the same extent as the glucose-lactate pathway, therefore allowing for sustained energy production (Harcet et al., 2013). Montipora capitata and Acropora yongei have been previously identified to have greater activity of different OpDHs compared to that of LDH under nighttime hypoxia (Richmond and Murphy 2016; Linsmayer et al., 2020). Together, these findings provide motivation to extend the search for key coral hypoxia stress genes to consider besides the ‘classic’ HIF gene network.
Taken together, the large discrepancies in tolerance to deoxygenation therefore reinforces the need to extend analyses to a greater range of coral species to better understand putative mechanisms underlying the phenotypic variation observed in the field. Whilst hypoxic events can occur naturally during seasonal heating when the water column stratifies and the solubility of O2 in water decreases, climate-induced ocean warming – coupled with eutrophication – is accelerating the frequency and intensity of acute ocean deoxygenation events (Keeling et al., 2010; Schmidtko et al., 2017; Breitburg et al., 2018). Despite the wealth of evidence that ocean acidification and warming can induce coral bleaching (reviewed in Albright, 2018; Cziesielski et al., 2019; Suggett and Smith, 2020), the effect ocean deoxygenation has, either in isolation (Alderdice et al., 2021) or in combination with warming and acidification, on bleaching thresholds remains unclear (Ziegler et al., 2021). Intriguingly, studies on both heat stress (Desalvo et al., 2008; Kenkel et al., 2013; Innis et al., 2021) and acidification stress (Kaniewska et al., 2012; Griffiths et al., 2019) consistently report a reduced oxidative metabolism, an impaired acid-base homeostasis, and an altered lipid content that are common hypoxia responses – a process that is also evident in our recent observations of corals that bleach under deoxygenation (Alderdice et al., 2021). Therefore, it is plausible that a common gene repertoire exists that assists more stress-tolerant corals in sustaining a depressed metabolism under these – and ultimately other – environmental stressors.
Copy number variation (CNV) recently emerged as an important genetic mechanism for phenotypic heterogeneity, outperforming single nucleotide polymorphisms in characterising population structure of non-model species (Dorant et al., 2020). Such structural variants (insertions/deletions) that create CNV can influence distinct expression levels of stress-response proteins through gene-dosage effect, and in turn, may impact the adaptive capacity between different coral to cope with local environmental stressors (Barshis et al., 2013). For example, Acroporids with a greater gene copy number of pigments from the green fluorescent protein (GFP) family, that can provide photoprotective properties under light stress, demonstrated an increased dynamic range over which the corals modulated their transcript levels in response to the light environment (Gittins et al., 2015). Under heat stress, individuals of Pocillopora verrucosa from shallow reefs exhibit greater basal heat shock 70kda (hsp70) gene expression compared to deeper sites and appear able to transcriptionally upregulate hsp70 more rapidly in response to experimental heating – alluding to the potential presence of CNVs for key heat stress genes that are due to environmental selection (Poli et al., 2017). However, at present it remains unknown whether CNV can be observed for key hypoxia stress response genes.
Here we investigate how the hypoxia gene set inventory – many genes of which were recently identified through RNA-Seq analyses of adult and larval Acropora samples exposed to low O2 stress (Alderdice et al., 2021; Alderdice et al., 2022) - differ amongst coral species at large. We specifically characterised CNVs for a compiled list of 32 putative hypoxia homologs across the fraction of protein-coding genes from 24 coral genomes, consisting of species that span the robust and complex clade. Orthologous genes were predicted by OrthoFinder and assigned to categories according to their evolutionary relationship e.g., one-to-one and many-to-one orthologs (Voolstra et al., 2017). We were specifically interested in the presence of unequal gene expansion patterns across coral species and to what extent the distribution of such expansions could align to ascribed differences in coral bleaching susceptibility.
2 Methods
2.1 Coral Genomes
A list of all sequenced coral genomes with protein-coding (i.e., genomic) gene sets available, up until June 2021 (at the time of analysis), was compiled using the following repositories: reefgenomics.org (Liew et al., 2016), NCBI (www.ncbi.nlm.nih.gov/), and marinegenomics.oist.jp (Table 1). Genomes were selected based on their availability, level of genome assembly, level of protein annotation, and taxonomic relatedness. While gene copies can be compared between genomes when present, it is difficult to make unequivocal statements when genes or gene sets are missing, as their absence may be either genuinely biological or methodological in nature. For our analysis, we therefore considered genomic gene sets where the number of predicted genes was ~30,000 to minimise artifacts arising from discrepant genome and gene assemblies (Table 1). Although Acropora echinata deviated from these criteria by having more genes (43886 genes), this species was from a large comparative genomic study of 16 Acropora coral genomes (Shinzato et al., 2021), for which we assumed consistency in methodology used to assemble the genome and predict the genes (information on sequencing platform, genome assembly, and gene annotation methods is available in Table S1). Additionally, to test whether missing genes of interest (GOIs) could be caused by a gene set calling artefact (i.e., the sequences existed in the assembled genome but were not present in the gene set), we searched for the nucleic acid sequences of certain missing proteins. We conducted such searches when the GOI was: 1) known to be highly conserved among metazoans and therefore its absence was unexpected and, 2) missing in only 1 species. In the single case that we tested, the missing gene was not found in the assembled genome, which gave us a higher confidence in the completeness of the gene sets and resultant orthogroups (although we realise that genes could still be missing due to absence in the original assembly).
2.2 Hypoxia Gene Repertoire
A list of candidate GOIs was built based on genes that were 1) previously implicated in the hypoxia stress response in association with both HIF and non-HIF pathways and 2) reported in coral stress response studies. Genes associated with coral stress at large were also included to examine whether or not the suspected commonality of their function to different stressors would result in copy number differences or aligned to coral species considered as more stress-tolerant e.g., Porites lutea (Voolstra et al., 2015; Robbins et al., 2019). The complete list of genes is available in Table 2 with corresponding source references. These criteria resulted in a total of 32 GOIs (Tables 2 and S2), which is by no means comprehensive but enabled us to examine whether different coral species exhibit CNV.
2.3 Orthology Analysis
OrthoFinder v2.5.2, was used to predict orthogroups from the 24 sets of protein sequences derived from 24 coral genomes (Table 1, see Data Availability section). An orthogroup is defined as the set of genes from multiple species descended from a single gene from the last common ancestor (LCA) of that set of species (Emms and Kelly, 2019). Of note, multiple proteins in a single species may be assigned to the same orthogroup due to gene duplication of a given gene after a speciation event. As such, for any given orthogroup, for a given pair of species, one-to-one (one gene per species), one-to-many (one gene in one species, multiple in the other), and many-to-many (multiple genes in both species) categorizations are possible (Voolstra et al., 2017). Accordingly, the multiple proteins in a single species assigned to the same orthogroup are paralogs of each other, but orthologs of the proteins in the other species. Single copy orthologs (i.e., those exhibiting one-to-one relationships across all species) can aid assessing completeness of sequenced genomes, but in this study one-to-many and many-to-many relationship were of most interest as they represent expanding genomic gene content that can reveal important functional differences.
OrthoFinder (Emms and Kelly, 2019) was run under default settings within a Nextflow pipeline using the Docker file davidemms/orthofinder:2.5.4 with the Docker engine. The Nextflow file and associated documentation on its execution are available at the associated GitHub repository (https://github.com/didillysquat/alderdice_2021). For each genomic gene locus, only the longest coding sequence (CDS) was kept, excluding possible splice variants (isoforms), while retaining our ability to determine whether genes were orthologs or paralogs. Consequential to the coral genomic gene sets available, we included a large number of species from the same genus (i.e., Acropora), which in turn could affect the number of orthogroups assigned to the different relationship categories, i.e., lead to an artificially low number of many-to-many (MM) and many-to-one (MO) orthogroups. We tested for such bias by performing OrthoFinder analyses that each contained only one species per genera (Acropora, Astreopora, Montastraea, Montipora, Pocillopora, Stylophora, and Porites) for three different Acropora species (A. acuminata, A. cytherea, A. digitifera). Orthogroups relating to each GOI were identified by searching for the A. millepora representative of each of the GOI based on their KEGG and EggNOG annotations (Alderdice et al., 2021; Alderdice et al., 2022). However, for some GOIs this method of identification was not possible, e.g. melatonin receptors (MTNR), which could have been due to the GOI not having a representative in A. millepora. Further, we found that in 12 cases there were multiple orthogroups that had the same gene name (Table S3). EggNOG mapper results of Montastraea cavernosa, another species considered in our analysis, were also available and so we used this additional EggNOG annotation resource to try to identify missing GOIs or further orthogroups with the same gene name (Table S3). The relationship between these two coral species is phylogenetically characterised by a deep evolutionary divergence and so can possess significant genomic differences in the distribution and number of certain proteins (Ying et al., 2018). Therefore, by incorporating both species there was a greater likelihood in identifying representative orthogroups of the GOIs for all the considered coral species.
As mentioned above, in 12 cases, we identified multiple orthogroups that were annotated with the same gene name. To validate and better understand their placement in distinct orthogroups (i.e., whether this grouping may represent paralogs, or genetically distant orthologs), we performed inter-group alignments of representative sequences from these orthogroups using MAFFT (Katoh et al., 2018; option ‘—auto’). The results demonstrated a considerable dissimilarity between the sequences and so we considered such groupings valid (Data S1). However, given that we had insufficient information to determine these orthogroups to be paralogous or distantly related orthologos, we considered these inter-group genes as distant homologs (Katoh et al., 2018). When investigating these genes for copy number expansions we searched across all identified orthogroups related to the gene name.
The gene names assigned to the orthogroups are based on EggNOG annotations predominantly from model organisms. Thus, the identical naming of certain orthogroups, despite being genetically distinct, likely represents an artefactual similarity and such groups warrant further revision with phylogenetic analyses. Overall, we focused on GOIs that demonstrated expansion of gene families, in particular uneven expansion distributed across species. Of note, gene expansions from certain species may also be a result of a reduction in other species. A heatmap table was created using the R package ‘ztable’ to view the copy number variation of GOI more easily across all species. Of note, in cases where GOIs were represented by multiple orthogroups, the orthogroup with the greatest variance across coral species was selected for the heatmap table. For each GOI we were interested to see whether identified CNVs correlated with either the robust-complex split, or whether these CNVs were specific to a particular species. To do this, we extracted the gene trees for those GOIs that demonstrated significant CNVs for visual examination. The gene trees were annotated using the R package ‘ggtree’.
3 Results
3.1 Overall Gene Distribution
Orthogroups were predicted at the protein level for a total of 24 coral genomic gene sets that consisted of ~25,300 ± 4,700 protein-encoding genes per genome on average (Table 1). OrthoFinder assigned 97.3% of the total number of genes (564,602 out of 589,304) into 24,643 orthogroups, leaving only 2.7% of the genes unassigned (15,702). Of the 24,643 orthogroups, 5,125 contained representatives from all 24 species of which only 879 were single copy orthogroups (i.e., only 1 protein present in all species considered) and 4,246 contained at least one instance of many-to-one or many-to-many relationships (i.e., sets of proteins with a paralogous relation to each other belonging to a single orthogroup and multiple species). When considering the 24,643 orthogroups, those that assigned to an expanded gene set category (many-to-many or many-to-one) were on average consistently lower in Acropora species compared to all other genera examined. To assess whether this finding is potentially biased by the large number of Acropora species in the analysis, we conducted three additional OrthoFinder analyses where we consistently considered one species per coral genus (Acropora, Astreopora, Montastraea, Montipora, Pocillopora, Stylophora, and Porites) for three different Acropora species (A. acuminata, A. cytherea, A. digitifera). These additional analyses showed that the number of many-to-many orthogroups for the Acropora species was no longer significantly lower in comparison to the other genera (Data S2). However, Porites lutea and Montastraea cavernosa still exhibited higher many-to-many and many-to-one orthogroups compared to all other genera. Lastly, 1.1% of genes (6,387) were assigned to species-specific orthogroups, with the smallest and largest proportion of these genes assigned to species of Acropora and Porites lutea (11 and 429, and 1% and 31%, respectively; Figure 1). Thus, the majority of orthogroups exhibited a large disparity in terms of CNV.
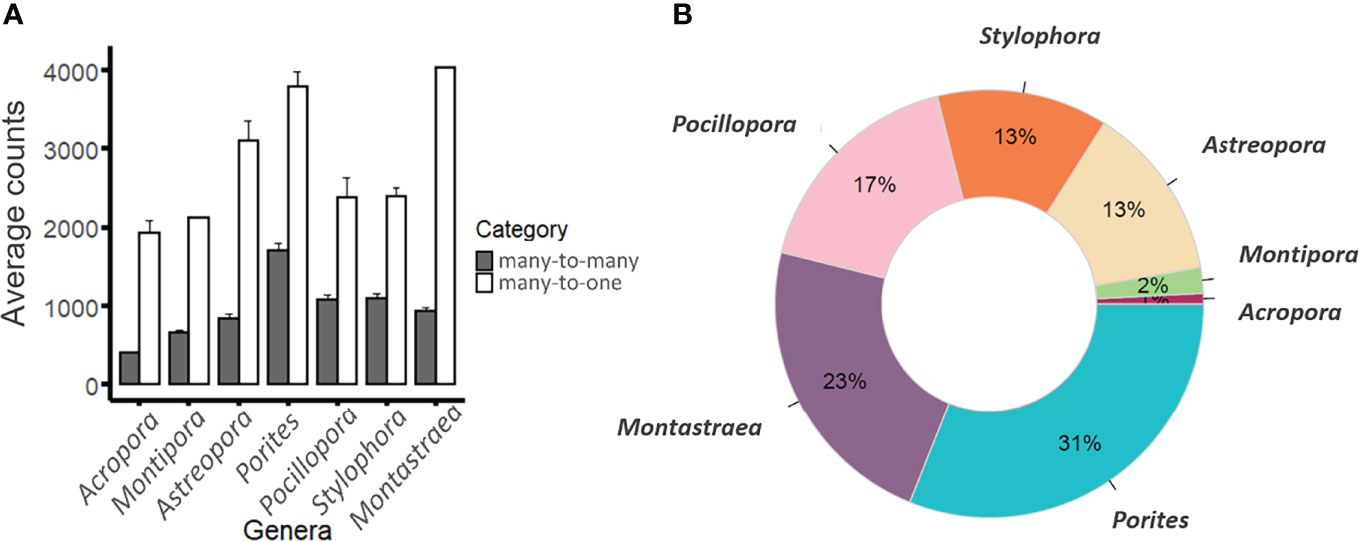
Figure 1 Classification of genomic protein sets. (A) Average counts of genes assigned to many-to-many (grey bars) or many-to-one (white bars) orthogroups across the coral genera examined (sensu Voolstra et al., 2017). Note that most genera consisted of one species except for Montipora (2), Pocillopora (2) species, and Acropora (16). Error bars denote standard error. (B) Average proportions of species-specific orthogroups within each genus.
3.2 Genes Associated With Hypoxia Stress Exhibit Copy Number Variation
Of the 24,643 orthologs considered, we were most interested in a set of 32 hypoxia-related genes (Tables 2 and S2) and in particular copy number differences in these genes that may explain differences in hypoxic stress susceptibility. We examined their CNV from the predicted gene inventory of 24 coral genomes and explored whether expanded gene families correlated to the robust-complex split or certain species.
For 20 out of the 32 GOIs, there were no or minimal predicted gene expansions (i.e., there were at most 1 or 2 copies of each protein per species per orthogroup). Although only 1 of these proteins, the heat shock protein 90kDA alpha class B, hsp90AB1, had a representative in every species. The remaining 12 GOIs exhibited uneven expansion in a subset of the species, falling into the many-to-one or many-to-many orthogroups, and warranted further examination.
Of these 12 unevenly expanded genes, most could be categorised into 3 groups according to their role within the mammalian-based HIF gene network following recent studies (Alderdice et al., 2021; Alderdice et al., 2022): i) HIF transcription factor subunits, ii) HIF suppressors, and iii). HIF target genes. We uses two additional categories to classify remaining genes: iv) Non-HIF targets, which consist of genes associated with hypoxia stress that are not considered to be transcriptionally regulated by HIF in model organisms and, v) ‘General’ stress response genes that have been repeatedly reported in coral studies associated with a ‘general’ stress response to bleaching (Hemond et al., 2014; Cunning et al., 2018). In the following sections we assess the gene trees of the GOIs categorised into one of these 5 categories to determine whether gene expansion aligned to the robust-complex evolutionary split or certain species.
3.2.1 HIF Subunits (HIFα and HIFβ)
The O2-sensitive alpha subunit of the hypoxia-inducible factor (HIFα) exhibited duplication in Montipora efflorescens, Acropora nasta, A. echinata, and two duplication events in A. florida (Figure 2) in the respective orthogroup. Interestingly, the robust-clade species, Montastraea cavernosa, lacked a representative protein in this orthogroup (confirmed by the absence of a match for the A. millepora HIFα sequence in its genomic gene set and assembled genome sequence; see Methods) suggesting a putative loss of the highly conserved metazoan HIFα gene. HIFβ demonstrated uneven expansion with P. lutea, M. cavernosa, and A. muricata exhibiting independent duplication events. While HIFα demonstrated a clear clustering according to the robust-complex split, the stable subunit HIFβ showed no such clustering (Figure S2).
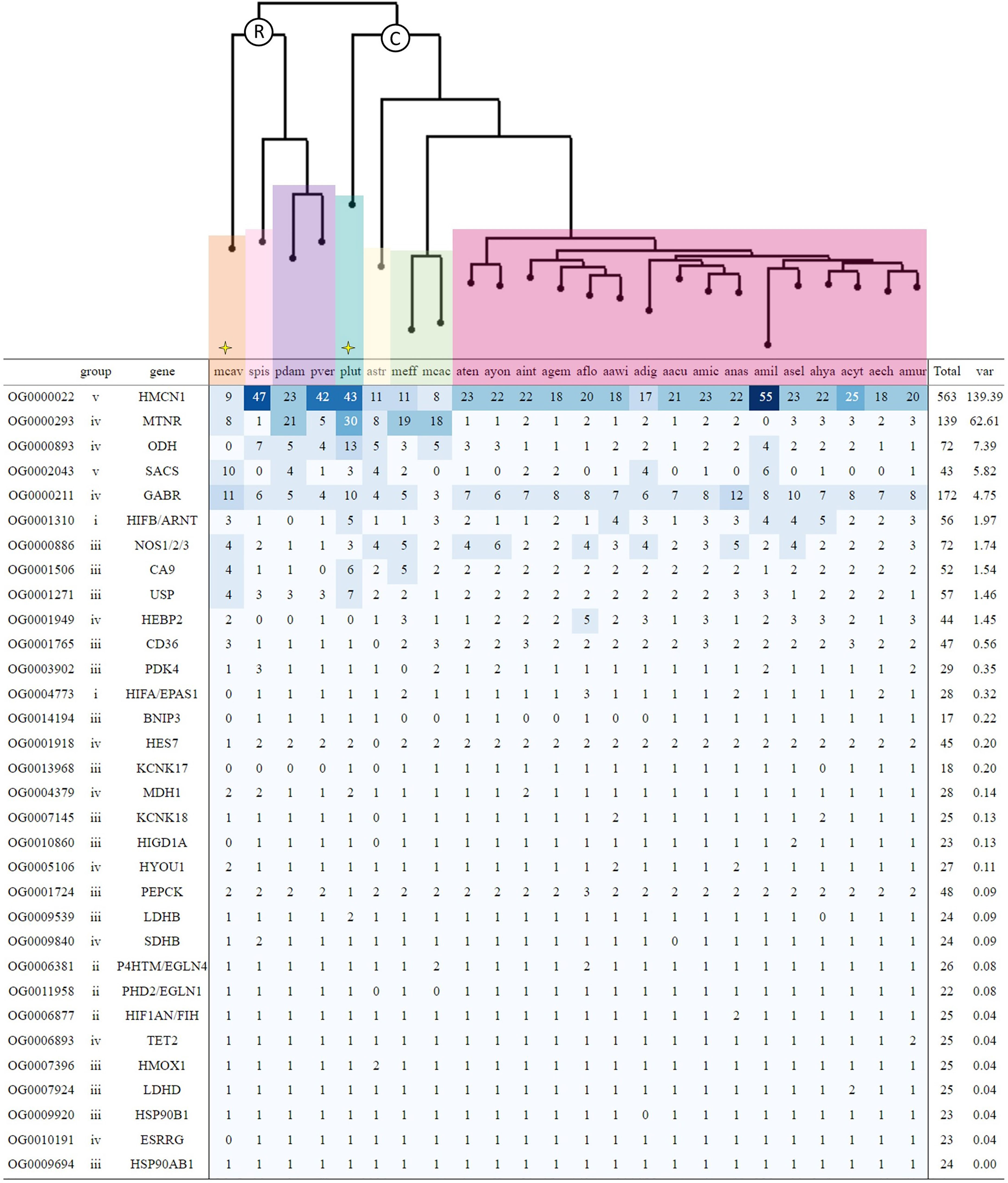
Figure 2 Gene count heatmap table for coral hypoxia genes of interest (GOIs) across 24 coral genomic gene sets ordered by descending variance. The first column denotes the orthogroups that OrthoFinder assigned each gene. Types of genes are grouped according to categories i-v as referred to in Table 2. For genes that had multiple orthogroups, the heatmap details only the orthogroup with the highest variance (see Tables S4–S7 for heatmap tables showing all orthogroups for GOIs with unequal expansion across species). Coral species are grouped according to taxonomy, with R and C indicating Robust and Complex clade species, respectively. Yellow stars flag those coral species that have been reported as more stress-tolerant. Colour gradient from light to dark blue represents increasing variance. Refer to Table 1 for the corresponding full species names.
3.2.2 HIF Suppressors
As opposed to the HIF subunits, Prolyl Hydroxylase Domain 2 (PHD2; also known as EGLN1 or HIF-P4H-2), Prolyl 4 Hydroxylase Transmembrane (P4HTM; also known as EGLN4 or PHD4) and Factor Inhibiting HIF (FIH), which facilitate proteasomal degradation of HIFα when O2 is limited, remained consistent in their copy number presenting a single gene copy across nearly all species and clearly clustered by the robust-complex split (Figures 2 and S2).
3.2.3 HIF Targets
Carbonic anhydrase 9 (CA9), a zinc metalloenzyme that functions in stabilising the intracellular accumulation of acidic metabolic products (e.g., lactic acid) under hypoxia stress, consistently presents 2 gene copies across species (Figure 2). However, P. lutea, M. efflorescens, and M. cavernosa each exhibit independent duplication events, with P. lutea possessing the greatest number of CA9 gene copies at three times of that found in most corals examined (Figure 3). Nitric-oxide synthase (NOS) increases the production of NO, a reactive nitrogen species (RNS), that can promote O2 delivery to cells and also signal to prevent HIFα proteasomal degradation during hypoxia (Poyton and Hendrickson, 2015). Within our analysis, NOS ranged from a single copy to up to 6 across species, with greater copy numbers found in coral species of the Acroporidae family.
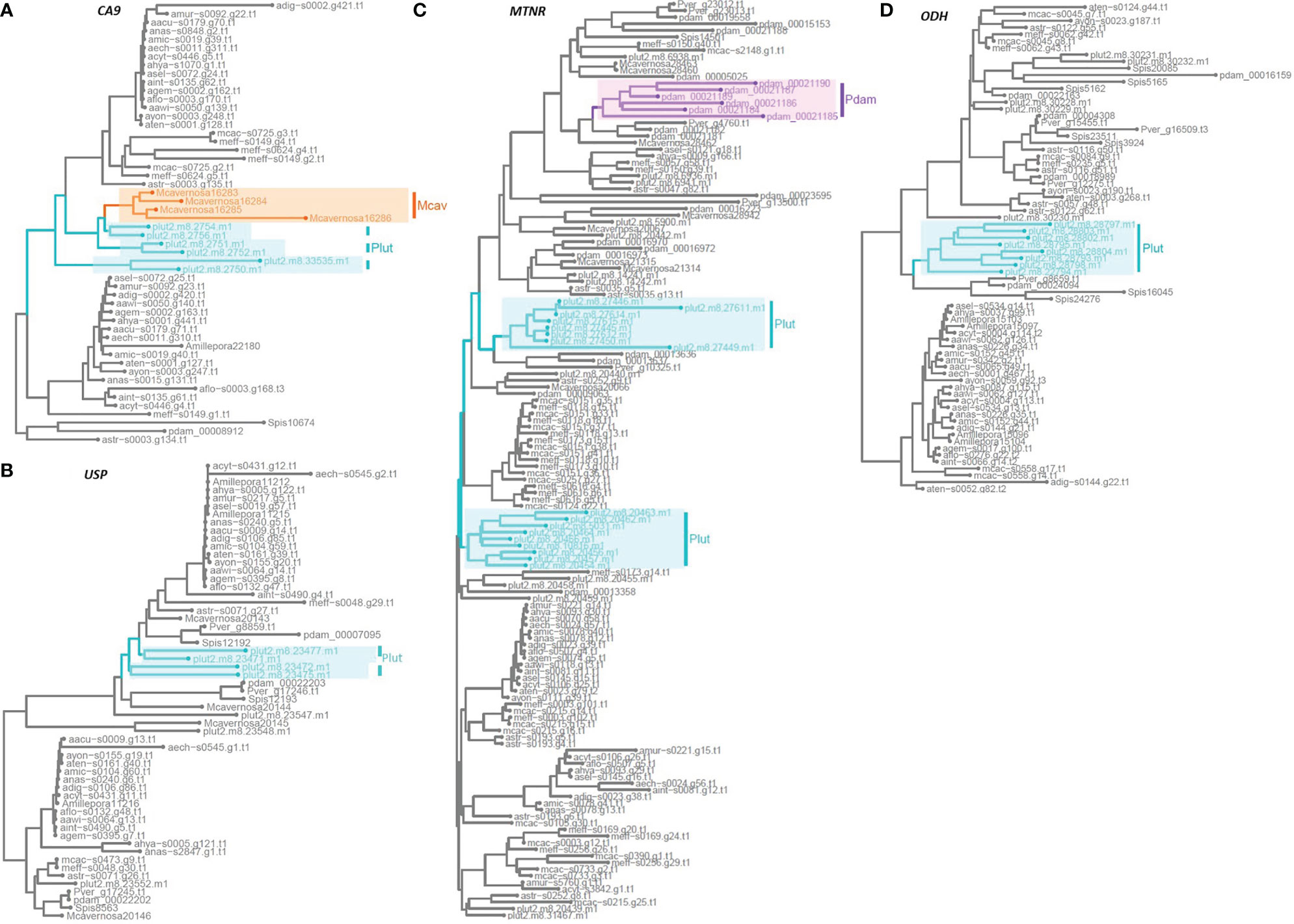
Figure 3 Rooted phylogenetic gene trees across 24 coral species showing species-specific expansions of hypoxia-associated genes that exhibit disparate copy number variation: (A) Carbonic anhydrase 9, CA9; (B) Universal stress protein, USP; (C) Melatonin receptor, MTNR; (D) Octopine dehydrogenase, ODH. Species-specific expansion of > 2 genes in a cluster for each gene family is indicated by the following highlighted boxes: Plut, Porites lutea in turquoise; Pdam, Pocillopora damicornis in purple; and Mcav, Montastraea cavernosa in orange.
3.2.4 Non-HIF Targets
Melatonin receptors (MTNR) and universal stress proteins (USP) are known to signal for, and facilitate, ROS scavenging to alleviate oxidative stress in mammals (Yan et al., 2018; Chi et al., 2019; Buttar et al., 2020). Genes assigned to one of the MTNR orthogroups exhibited one of the greatest variances in distribution of gene copies across species, with the lowest counts between 1-3 found almost exclusively in Acropora species while the highest counts were presented by P. damicornis and P. lutea, which independently expanded up to 21 and 30 gene copies, respectively (Figures 2, 3). Whilst the USP orthogroups showed gene counts to vary randomly across species and the robust-complex split, P. lutea exhibited the greatest number of gene copies (Table S4). In one of the orthogroups, P. lutea demonstrated species-specific duplications, leading to an additional 4 copies of USP (Figure 3) in comparison to the gene complement of the other species in that orthogroup.
Genes encoding for gamma-aminobutyric acid receptor (GABR) that help to restore membrane potential from ROS-induced disruptions and signals to promote the ‘GABA shunt’ that can suppress aerobic respiration during hypoxia (Wu et al., 2021), exhibited unequal distribution with only 3 gene copies in M. cactus and up to 12 gene copies in A. millepora, M. cavernosa, and P. lutea. Gene trees showed species-specific clusters of expanded genes in the latter two species (Figure S3). Genes assigned to the orthogroup annotated as octopine dehydrogenase (ODH), that provide an alternative, more pH-stable anaerobic pathway to glycolysis, had the third greatest variance of all GOIs across species. Interestingly, this variance was driven by an independent expansion in P. lutea where gene copy number was at least double that found in the other species (Figures 2, 3).
3.2.5 ‘General’ Stress Response
Both Hemicentin (HMCN1), which functions in promoting extracellular adhesion during stress, and sacsin (SACS), which acts as a heat shock protein co-chaperone, showed expansions of proteins within their corresponding orthogroups. However, these expansions did not appear to align to those of hypoxia stress associated GOIs or coral species considered more stress-tolerant e.g., P. lutea (Figure 2). Notably, HMCN1 demonstrated the greatest copy number variation across species out of all the GOIs with a range of 8 copies in M. cactus all the way up to 55 copies in A. millepora (Figure 2).
3.3 Genes Assigned to Multiple Orthogroups Exhibit Large Copy Number Differences
Out of the 12 cases of which multiple orthogroups were annotated with the same gene name, SACS, MTNR, and GABR showed a large degree of unequal expansion across the 24 coral species (Tables S5–S7, respectively). Interestingly, more than half of the SACS orthogroups were only present in a few coral species, with few of the represented coral species found in common between the orthogroups. For example, orthogroups OG0023363, OG0022987, and OG0021814 contained a single gene copy in 3 different coral species (Table S5). Within the GOI, the number of orthogroups associated with a given gene name varied considerably, with GABR assigned to the greatest number of different orthogroups (48; Tables S3, S7).
In summary, a high level of disparity existed in the overall proportions of expanding genes (many-to-one or many-to-many) across the 24 coral genomes. Despite most coral species possessing at least one gene copy of the key hypoxia response genes considered, approximately a third of the GOIs exhibited uneven expansions. In particular, P. lutea demonstrated the greatest number of species-specific orthogroups out of all considered coral species and consistently demonstrated a greater number of gene copies for the majority of highly disparate GOIs, including CA9, MTNR, USP, and ODH.
4 Discussion
Despite the growing evidence implicating hypoxia as a key factor contributing to mass coral mortality (Altieri et al., 2017; Suggett and Smith, 2020; Altieri et al., 2021; Johnson et al., 2021a), surprisingly little is known of the responsive gene set inventory corals possess to respond to hypoxic events. Within gene inventories, variation in gene copy number affect gene expression levels with subsequent differences in the effectiveness of any given stress response (Gittins et al., 2015; Żmieńko et al., 2014). Ultimately, such genomic differences can contribute to the acclimation of marine invertebrates to prevailing environments (Dorant et al., 2020). Therefore, analysing copy number differences amongst corals could provide insight into how some populations (Barshis et al., 2013; Palumbi et al., 2014; Voolstra et al., 2020; Voolstra et al., 2021b) or individual coral genotypes (Palumbi et al., 2014; Dixon et al., 2015) with enhanced bleaching resilience exist. Here we considered the extent to which the coral hypoxia stress gene repertoire is variable across species by examining CNV from the protein-coding genes of 24 coral genomes. We followed an ortholog-based approach where proteins were assigned into different categories according to their evolutionary relationship, such as one-to-one and many-to-one ortholog pairwise comparisons. Our meta-analysis of 24 coral genomes of species from both the complex and robust clade complements previous approaches (Voolstra et al., 2017; Cunning et al., 2018; Ying et al., 2018; Shinzato et al., 2021). Our analysis demonstrates comparable numbers of orthogroups that are present in all examined species, i.e. putative coral ‘core’ proteome members, whether the comparison is with only 2 species (6,302 orthogroups; Voolstra et al., 2017) or with 24 coral species as in our study (5,125 orthogroups). Among the 5,125 orthogroups identified here, we found 879 assigned as single copy, similar to reports of comparisons between 18 Acropora spp. gene sets from only the complex clade (818 orthogroups; Shinzato et al., 2021), which can be considered ‘core’ coral genes that have likely retained their function across species.
4.1 Differences in Protein Inventories Between Species at Large
Evidence for high levels of genomic variation was observed. Firstly, there was variation in the total number of genes that were expanded. For example, the number of many-to-many orthogroups was consistently and significantly higher in P. lutea compared to all other genera/species, while a higher number of many-to-one orthogroups was consistently found in A. myriophthalma, M. cavernosa, and P. lutea (Figure 1A, Figure S1, Data S2). In general, the greatest contrast was found between those species that occupy massive (A. myriophthalma, P. lutea, and M. cavernosa) and branching growth forms (remaining species; Figure 1A). Interestingly, species exhibiting massive growth forms have previously been reported to have higher bleaching resilience (Marshall and Baird, 2000; Pratchett et al., 2013; Sutthacheep et al., 2013). Secondly, the massive growth form species from the complex clade, P. lutea, possessed the highest number of genes in species-specific orthogroups compared to all other species examined. This outcome is consistent with Cunning et al. (2018), examining the genomic gene sets across four scleractinians, where another ‘massive’ coral, Orbicella faveolata, exhibited approximately twice the number of species-specific gene families compared to those of the branching corals P. damicornis, S. pistillata, and A. digitifera. Together, these results indicate disparities in gene copy number in line with, but not limited to, growth form. Furthermore, higher gene copies of different heat shock proteins have been observed in coral species reported to have greater bleaching resilience under thermal stress (Poli et al., 2017; Ying et al., 2018). In these cases, sample site depth (Poli et al., 2017) and species coral morphology (Ying et al., 2018), e.g. massive or solitary versus branching growth forms, have similarly been considered factors aligning with gene copy variation. Therefore, the possibility that a higher gene copy number of key genes could be recognisably aligned with specific coral traits, warrants further investigation.
4.2 Orthogroups of Hypoxia-Associated Genes Exhibiting Stark Differences Between Species
We examined copy number differences of 32 hypoxia-associated genes that were compiled based on those 1) previously implicated in the hypoxia response of corals (Acropora sp., see Alderdice et al., 2021), 2) reported as being implicated in coral stress responses such as heat or, 3) associated with hypoxia in other model organisms, e.g. mammals (Table S2). The set of genes activated under varying hypoxia levels can cover a diverse range of critical cellular processes including glycolytic metabolism, decelerated cell proliferation, ROS management, and energy-saving alterations in catabolic processes (Semenza, 2012; Yum et al., 2017). Such processes are also important in the stability of the coral-microalgal symbiosis and ultimate survival of the host (Rädecker et al., 2021) and therefore, expansion of these gene families could be indicative of bleaching resilience differences amongst coral taxa.
Amongst our GOIs, we found that those exhibiting little copy number variation mostly grouped according to the robust-complex split (for examples see Figure S2), while approximately a third of the GOIs demonstrated uneven expansion across species (Figures 2, 3). We particularly focused on those that demonstrated independent expansions of > 2 gene copies. Interestingly, for the majority of these genes the highest copy number was consistently found in P. lutea – a species classified as ‘massive’ in growth form and commonly reported to demonstrate relative greater stress tolerance to bleaching (see Figure 4; Marshall and Baird, 2000; Pratchett et al., 2013). Such genes included the HIF-targeted carbonic anhydrase (CA9) which counteracts the accumulation of acidic metabolites to avoid acidosis during anaerobic-dominated respiration (Swietach et al., 2010; Parks et al., 2011; Zoccola et al., 2016; Osinga et al., 2017). Both P. lutea and M. cavernosa exhibited the greatest independent expansion of CA9 (Figures 2, 3). Interestingly, carbonic anhydrases have also been associated with assisting acid-base balance during coral calcification (Moya et al., 2008; Bertucci et al., 2013; Goff et al., 2016) and studies have shown a greater reliance on CA activity for coral calcification rates during dark conditions (Goreau, 1960) when photosynthesis ceases and coral tissues become hypoxic (Kühl et al., 1995). Therefore, possessing higher CA9 gene copies may enhance a coral’s ability to buffer their intracellular pH and ion exchange, facilitating night-time calcification rates and better yet, surviving longer hypoxic events.
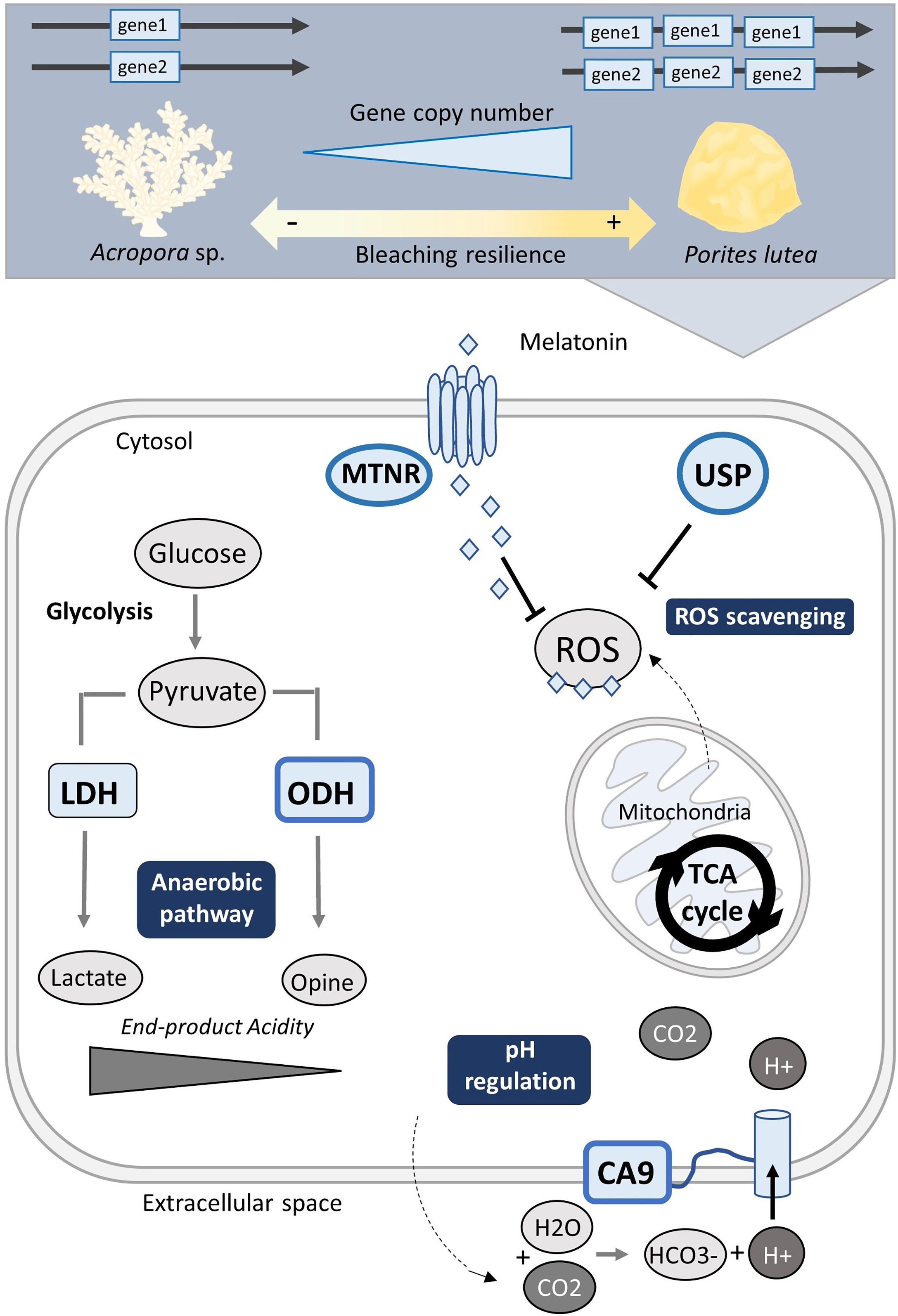
Figure 4 Hypoxia stress gene set expansion may coincide with increased coral stress tolerance and bleaching resilience. Schematic illustration of hypoxia-stress associated genes with > 2 independent expansions: melatonin receptors (MTNR), universal stress protein (USP), octopine dehydrogenase (ODH), and carbonic anhydrase 9 (CA9). Genes represented by a thick blue frame were found expanded in Porites lutea, a species ascribed as more stress tolerant, in contrast to Acropora species.
Two non-HIF targeted GOIs also with the greatest gene expansion in P. lutea, MTNR and USP, function in mammals and plants to alleviate cellular stress associated with ROS (Yan et al., 2018; Chi et al., 2019; Buttar et al., 2020). ROS-handling responses are of particular interest for understanding coral stress tolerance as such oxidative defense strategies become overwhelmed during coral bleaching (Weis, 2008; Rädecker et al., 2021). Interestingly, genes assigned to one of the multiple MTNR orthogroups demonstrated one of the highest CNV across species, ranging from the lowest counts almost exclusively in Acropora species to the highest counts in P. lutea, which independently harboured up to 30 gene copies (Figures 2, 3C). Such an outcome could suggest Acropora species examined here have an inherently lower capacity to ROS scavenging. As for the USP orthogroups, P. lutea solely exhibited gene expansion of the USP gene family (4 copies) in one the orthogroups (Figure 3B). So far neither of the genes have been extensively studied in corals. Although melatonin receptors have been shown to increase during the night in Acropora species in association with the circadian rhythm (Hemond et al., 2014), as per their commonly described function in other invertebrates (Vivien-Roels and Pévet, 1993). However, prolonged hypoxia can also alter circadian activities as the circadian clock is dependent on changes in metabolic rate (Mortola, 2004; Tjong et al., 2006) and therefore melatonin receptor activity in coral is likely not only be bound to the night cycle but also stimulated by a shift to hypometabolism during hypoxia stress. To date, coral USPs have been reported to be expressed under heat stress (Bellantuono et al., 2012), and while we focus on their potential ROS scavenging role, they may also conduct in parallel more diverse roles in resistance to hypoxia stress.
Another non-HIF targeted GOI includes octopine dehydrogenase (ODH), an opine dehydrogenase in the glucose-opine anaerobic pathway, which exhibited an independent expansion in P. lutea where gene copy number was at least double that found in the other species (Figure 2). Opine dehydrogenase (OpDH) rather than lactate dehydrogenase (LDH) activity, often predominates sessile organisms as it generates an end-product with much weaker acidity and so maintains more stable levels of intracellular pH under prolonged low O2 (Zammit, 1978). As previously mentioned, corals have been identified to have greater activity levels of different OpDHs compared to that of LDH under nighttime hypoxia (Richmond and Murphy 2016; Linsmayer et al., 2020), and so may be part of a key hypometabolic pathway in coral during hypoxia stress. Though the types of dominant OpDHs may differ between coral species (e.g., alanopine, octopine, or strombine), our analysis suggests that a higher gene copy number of OpDH may facilitate more stress-tolerant coral to reduce the acidic products of anaerobic respiration and create more favourable conditions for managing prolonged hypoxia. Characterising the nature of these enzymes and determining whether OpDHs are part of the HIF target gene network, or another, will be important next steps for understanding the extent or capacity for corals to sustain a continuous flux of ATP during low O2.
5 Summary
Our study corroborates recent comparative ortholog analyses that support the notion of a conserved coral core proteome on the one hand and high levels of disparity between coral genomes, even for species within the same genus, on the other hand. In examining the genomic protein inventories of 24 coral species, we found that most species possess copies for key hypoxia response genes. However, approximately a third of the orthologs exhibited significant gene copy number differences across species. Notably, such differences did not map onto differences between robust and complex clade corals and were rather species-specific. However, more sequenced genomes are necessary to provide better insight at which phylogenetic level such effects become pre-dominantly apparent, respectively evolutionary emerge. Interestingly, the consistently highest number of gene expansions were found in P. lutea, a coral species that exhibits a massive growth form and is commonly ascribed to exhibit greater stress tolerance. Our analyses suggest that possessing expanded copy numbers of hypoxia stress genes may be associated with increased stress tolerance, in particular hypoxia. As such, the unevenly expanded hypoxia genes presented here provide genes of interest to target in examining or diagnosing coral (thermal) stress thresholds. Ideally, such studies will examine the transcriptional response and corresponding gene copy inventory to elucidate a link between greater gene copy number and greater ability to respond to stress through upregulation of gene expression.
Data Availability Statement
Access to the protein sequence files of each gene set used in the analysis are provided in Table 1. The scripts used to run OrthoFinder and process the outputs can be found at the GitHub repository available at: https://github.com/didillysquat/alderdice_2021. The OrthoFinder output files are found at Zenodo repository available at: https://zenodo.org/record/6396671.
Author Contributions
RA, BH, and CV designed and conceived the comparative analysis. BH performed the OrthoFinder analysis. RA processed OrthoFinder results and generated figures. RA, BH, and CV wrote the paper with input from all authors. All authors reviewed and approved the final manuscript.
Funding
Funding for this work was supported through an Australian Research Council (ARC) Discovery Project (DP180100074) granted to DS, MP, MK and CV. Open access funding enabled and organized by Project DEAL through the University of Konstanz, Germany.
Conflict of Interest
The authors declare that the research was conducted in the absence of any commercial or financial relationships that could be construed as a potential conflict of interest.
Publisher’s Note
All claims expressed in this article are solely those of the authors and do not necessarily represent those of their affiliated organizations, or those of the publisher, the editors and the reviewers. Any product that may be evaluated in this article, or claim that may be made by its manufacturer, is not guaranteed or endorsed by the publisher.
Supplementary Material
The Supplementary Material for this article can be found online at: https://www.frontiersin.org/articles/10.3389/fmars.2022.834332/full#supplementary-material
References
Albright R. (2018). Ocean Acidification and Coral Bleaching (Cham: Springer) 233, 259–323. doi: 10.1007/978-3-319-75393-5_12
Alderdice R., Pernice M., Cárdenas A., Hughes D. J., Harrison P. L., Boulotte N., et al. (2022). Hypoxia as a Physiological Cue and Pathological Stress for Coral Larvae. Mol. Ecol. 31, 1–17. doi: 10.1111/mec.16259
Alderdice R., Suggett D. J., Cárdenas A., Hughes D. J., Kühl M., Pernice M., et al. (2021). Divergent Expression of Hypoxia Response Systems Under Deoxygenation in Reef-Forming Corals Aligns With Bleaching Susceptibility. Glob. Change Biol., gcb.15436, 312–326. doi: 10.1111/gcb.15436
Altieri A. H., Harrison S. B., Seemann J., Collin R., Diaz R. J., Knowlton N. (2017). Tropical Dead Zones and Mass Mortalities on Coral Reefs. Proc. Natl. Acad. Sci. U. S. A. 114, 3660–3665. doi: 10.1073/pnas.1621517114
Altieri A. H., Johnson M. D., Swaminathan S. D., Nelson H. R., Gedan K. B. (2021). Resilience of Tropical Ecosystems to Ocean Deoxygenation. Trends Ecol. Evol. 36, 227–238. doi: 10.1016/j.tree.2020.11.003
Barshis D. J., Ladner J. T., Oliver T. A., Seneca F. O., Traylor-Knowles N., Palumbi S. R. (2013). Genomic Basis for Coral Resilience to Climate Change. Proc. Natl. Acad. Sci. U. S. A. 110, 1387–1392. doi: 10.1073/pnas.1210224110
Bellantuono A. J., Granados-Cifuentes C., Miller D. J., Hoegh-Guldberg O., Rodriguez-Lanetty M. (2012). Coral Thermal Tolerance: Tuning Gene Expression to Resist Thermal Stress. PloS One 7, e50685. doi: 10.1371/journal.pone.0050685
Bertucci A., Moya A., Tambutté S., Allemand D., Supuran C. T., Zoccola D. (2013). Carbonic Anhydrases in Anthozoan Corals - A Review. Bioorg. Med. Chem. 21, 1437–1450. doi: 10.1016/j.bmc.2012.10.024
Blokhina O., Virolainen E., Fagerstedt K. V. (2003). Antioxidants, Oxidative Damage and Oxygen Deprivation Stress: A Review. Ann. Bot. 91, 179–194. doi: 10.1093/aob/mcf118
Breitburg D., Levin L. A., Oschlies A., Grégoire M., Chavez F. P., Conley D. J., et al. (2018). Declining Oxygen in the Global Ocean and Coastal Waters. Sci 359, eaam7240. doi: 10.1126/science.aam7240
Buitrago-López C., Mariappan K. G., Cárdenas A., Gegner H. M., Voolstra C. R. (2020). The Genome of the Cauliflower Coral Pocillopora Verrucosa. Genome Biol. Evol. 12, 1911–1917. doi: 10.1093/GBE/EVAA184
Buttar Z. A., Wu S. N., Arnao M. B., Wang C., Ullah I., Wang C. (2020). Melatonin Suppressed the Heat Stress-Induced Damage in Wheat Seedlings by Modulating the Antioxidant Machinery. Plants 9, 1–17. doi: 10.3390/plants9070809
Chi Y. H., Koo S. S., Oh H. T., Lee E. S., Park J. H., Phan K. A. T., et al. (2019). The Physiological Functions of Universal Stress Proteins and Their Molecular Mechanism to Protect Plants From Environmental Stresses. Front. Plant Sci. 10. doi: 10.3389/fpls.2019.00750
Cunning R., Bay R. A., Gillette P., Baker A. C., Traylor-Knowles N. (2018). Comparative Analysis of the Pocillopora Damicornis Genome Highlights Role of Immune System in Coral Evolution. Sci. Rep. 8, 1–10. doi: 10.1038/s41598-018-34459-8
Cziesielski M. J., Schmidt-Roach S., Aranda M. (2019). The Past, Present, and Future of Coral Heat Stress Studies. Ecol. Evol. 9, 10055–10066. doi: 10.1002/ece3.5576
Desalvo M. K., Voolstra C. R., Sunagawa S., Schwarz J. A., Stillman J. H., Coffroth M. A., et al. (2008). Differential Gene Expression During Thermal Stress and Bleaching in the Caribbean Coral Montastraea Faveolata. Mol. Ecol. 17, 3952–3971. doi: 10.1111/j.1365-294X.2008.03879.x
Diaz R. J., Rosenberg R. (2008). Spreading Dead Zones and Consequences for Marine Ecosystems. 321, 926–929. doi: 10.1126/science.1156401
Dixon G. B., Davies S. W., Aglyamova G. A., Meyer E., Bay L. K., Matz M. V. (2015). Genomic Determinants of Coral Heat Tolerance Across Latitudes. 348, 1460–1462. doi: 10.1126/science.1261224
Dorant Y., Cayuela H., Wellband K., Laporte M., Rougemont Q., Mérot C., et al. (2020). Copy Number Variants Outperform SNPs to Reveal Genotype–Temperature Association in a Marine Species. Mol. Ecol. 29, 4765–4782. doi: 10.1111/mec.15565
Emms D. M., Kelly S. (2019). OrthoFinder: Phylogenetic Orthology Inference for Comparative Genomics. Genome Biol. 20, 1–14. doi: 10.1186/s13059-019-1832-y
Fuller Z. L., Mocellin V. J. L., Morris L. A., Cantin N., Shepherd J., Sarre L., et al. (2020). Population Genetics of the Coroal Acropora Millepora: Toward Genomic Prediction of Bleaching. Sci. 369, eaba4674. doi: 10.1126/science.aba4674
Gittins J. R., D’Angelo C., Oswald F., Edwards R. J., Wiedenmann J. (2015). Fluorescent Protein-Mediated Colour Polymorphism in Reef Corals: Multicopy Genes Extend the Adaptation/Acclimatization Potential to Variable Light Environments. Mol. Ecol. 24, 453–465. doi: 10.1111/mec.13041
Goff C., Le Ganot P., Zoccola D., Caminiti-Segonds N., Allemand D., Tambutté S., et al. (2016). Carbonic Anhydrases in Cnidarians: Novel Perspectives From the Octocorallian Corallium Rubrum. PloS One 11, e0160368. doi: 10.1371/journal.pone.0160368
Goreau T. F. (1960). The Physiology of Skeleton Formation in Corals. IV. On Isotopic Quilibrium Exchanges of Calcium Between Corallium and Environment in Living and Dead Reef Building Corals. Biol. Bull. 119, 416–427. doi: 10.2307/1539259
Griffiths J. S., Pan T.-C. F., Kelly M. W. (2019). Differential Responses to Ocean Acidification Between Populations of Balanophyllia Elegans Corals From High and Low Upwelling Environments. Mol. Ecol. 28, 2715–2730. doi: 10.1111/MEC.15050
Haas A. F., Smith J. E., Thompson M., Deheyn D. D. (2014). Effects of Reduced Dissolved Oxygen Concentrations on Physiology and Fluorescence of Hermatypic Corals and Benthic Algae. PeerJ 2014, e235. doi: 10.7717/peerj.235
Harcet M., Perina D., Pleše B. (2013). Opine Dehydrogenases in Marine Invertebrates. Biochem. Genet. 51, 666–676. doi: 10.1007/s10528-013-9596-7
Hemond E. M., Kaluziak S. T., Vollmer S. V. (2014). The Genetics of Colony Form and Function in Caribbean Acropora Corals. BMC Genomics 15, 1–21. doi: 10.1186/1471-2164-15-1133
Hughes D. J., Alderdice R., Cooney C., Kühl M., Pernice M., Voolstra C. R., et al. (2020). Coral Reef Survival Under Accelerating Ocean Deoxygenation. Nat. Clim. Change 10, 1–12. doi: 10.1038/s41558-020-0737-9
Hughes D. J., Alexander J., Cobbs G., Kühl M., Cooney M., Pernice M., et al. (2022). Widespread oxyregulation in tropical corals under hypoxia. Pollut. Bull 179, 113722. doi: 10.1016/J.MARPOLBUL.2022.113722
Innis T., Allen-Waller L., Brown K. T., Sparagon W., Carlson C., Kruse E., et al. (2021). Marine Heatwaves Depress Metabolic Activity and Impair Cellular Acid–Base Homeostasis in Reef-Building Corals Regardless of Bleaching Susceptibility. Glob. Change Biol. 27, 2728–2743. doi: 10.1111/gcb.15622
Johnson M. D., Scott J. J., Leray M., Lucey N., Bravo L. M. R., Wied W. L., et al. (2021a). Rapid Ecosystem-Scale Consequences of Acute Deoxygenation on a Caribbean Coral Reef. Nat. Commun. 121, 1–12. doi: 10.1038/s41467-021-24777-3
Johnson M. D., Swaminathan S. D., Nixon E. N., Paul V. J., Altieri A. H. (2021b). Differential Susceptibility of Reef-Building Corals to Deoxygenation Reveals Remarkable Hypoxia Tolerance. Sci. Rep. 11, 1–12. doi: 10.1038/s41598-021-01078-9
Kaelin W. G., Ratcliffe P. J. (2008). Oxygen Sensing by Metazoans: The Central Role of the HIF Hydroxylase Pathway. Mol. Cell 30, 393–402. doi: 10.1016/j.molcel.2008.04.009
Kaniewska P., Campbell P. R., Kline D. I., Rodriguez-Lanetty M., Miller D. J., Dove S., et al. (2012). Major Cellular and Physiological Impacts of Ocean Acidification on a Reef Building Coral. PloS One 7, e34659. doi: 10.1371/journal.pone.0034659
Katoh K., Rozewicki J., Yamada K. D. (2018). MAFFT Online Service: Multiple Sequence Alignment, Interactive Sequence Choice and Visualization. Brief. Bioinform. 20, 1160–1166. doi: 10.1093/bib/bbx108
Keeling R. F., Körtzinger A., Gruber N. (2010). Ocean Deoxygenation in a Warming World. Ann. Rev. Mar. Sci. 2, 199–229. doi: 10.1146/annurev.marine.010908.163855
Kenkel C. D., Meyer E., Matz M. V. (2013). Gene Expression Under Chronic Heat Stress in Populations of the Mustard Hill Coral (Porites Astreoides) From Different Thermal Environments. Mol. Ecol. 22, 4322–4334. doi: 10.1111/mec.12390
Kühl M., Revsbech N., Cohen Y., Dalsgaard T., Jørgensen B. (1995). Microenvironment and Photosynthesis of Zooxanthellae in Scleractinian Corals Studied With Microsensors for O2, pH and Light. Mar. Ecol. Prog. Ser. 117, 159–172. doi: 10.3354/meps117159
Lesser M. P. (2006). Oxidative Stress in Marine Environments: Biochemistry and Physiological Ecology. Annu. Rev. Physiol. 68, 253–278. doi: 10.1146/annurev.physiol.68.040104.110001
Liew Y. J., Aranda M., Voolstra C. R. (2016). Reefgenomics.Org - A Repository for Marine Genomics Data. Database (Oxford). 2016, baw152. doi: 10.1093/DATABASE/BAW152
Linsmayer L. B., Deheyn D. D., Tomanek L., Tresguerres M. (2020). Dynamic Regulation Of Coral Energy Metabolism Throughout The Diel Cycle. Sci. Rep. 10 1–11. doi: 10.1038/s41598-020-76828-2
Marshall P. A., Baird A. H. (2000). Bleaching of Corals on the Great Barrier Reef: Differential Susceptibilities Among Taxa. Coral Reefs 19, 155–163. doi: 10.1007/s003380000086
Mortola J. P. (2004). Breathing Around the Clock: An Overview of the Circadian Pattern of Respiration. Eur. J. Appl. Physiol. 91, 119–129. doi: 10.1007/s00421-003-0978-0
Moya A., Tambutté S., Bertucci A., Tambutté E., Lotto S., Vullo D., et al. (2008). Carbonic Anhydrase in the Scleractinian Coral Stylophora Pistillata: Characterization, Localization, and Role in Biomineralization. J. Biol. Chem. 283, 25475–25484. doi: 10.1074/jbc.M804726200
Murphy J. W. A., Richmond R. H.. (2016). Changes To Coral Health And Metabolic Activity Under Oxygen Deprivation. PeerJ 4, 160. doi: 10.7717/peerj.1956
Nelson H. R., Altieri A. H. (2019). Oxygen: The Universal Currency on Coral Reefs. Coral Reefs 38, 177–198. doi: 10.1007/s00338-019-01765-0
Osinga R., Derksen-Hooijberg M., Wijgerde T., Verreth J. A. J. (2017). Interactive Effects of Oxygen, Carbon Dioxide and Flow on Photosynthesis and Respiration in the Scleractinian Coral Galaxea Fascicularis. J. Exp. Biol. 220, 2236–2242. doi: 10.1242/jeb.140509
Palumbi S. R., Barshis D. J., Traylor-Knowles N., Bay R. A. (2014). Mechanisms of Reef Coral Resistance to Future Climate Change. 344, 895–898. doi: 10.1126/science.1251336
Parks S. K., Chiche J., Pouyssegur J. (2011). pH Control Mechanisms of Tumor Survival and Growth. J. Cell. Physiol. 226, 299–308. doi: 10.1002/jcp.22400
Poli D., Fabbri E., Goffredo S., Airi V., Franzellitti S. (2017). Physiological Plasticity Related to Zonation Affects Hsp70 Expression in the Reef-Building Coral Pocillopora Verrucosa. PloS One 12, e0171456. doi: 10.1371/journal.pone.0171456
Pollock M. S., Clarke L. M. J., Dubé M. G. (2007). The Effects of Hypoxia on Fishes: From Ecological Relevance to Physiological Effects. Environ. Rev. 15, 1–14. doi: 10.1139/a06-006
Poyton R., Hendrickson M. (2015). Crosstalk Between Nitric Oxide and Hypoxia-Inducible Factor Signaling Pathways: An Update. Res. Rep. Biochem. 5,147–161. doi: 10.2147/RRBC.S58280
Pratchett M. S., McCowan D., Maynard J. A., Heron S. F. (2013). Changes in Bleaching Susceptibility Among Corals Subject to Ocean Warming and Recurrent Bleaching in Moorea, French Polynesia. PloS One 8, e70443. doi: 10.1371/journal.pone.0070443
Rädecker N., Pogoreutz C., Gegner H. M., Cárdenas A., Roth F., Bougoure J., et al. (2021). Heat Stress Destabilizes Symbiotic Nutrient Cycling in Corals. Proc. Natl. Acad. Sci. U. S. A. 118, e2022653118. doi: 10.1073/pnas.2022653118
Rippe J. P., Dixon G., Fuller Z. L., Liao Y., Matz M. (2021). Environmental Specialization and Cryptic Genetic Divergence in Two Massive Coral Species From the Florida Keys Reef Tract. Mol. Ecol. 30, 3468–84. doi: 10.1111/mec.15931
Robbins S. J., Singleton C. M., Chan C. X., Messer L. F., Geers A. U., Ying H., et al. (2019). A Genomic View of the Reef-Building Coral Porites Lutea and Its Microbial Symbionts. Nat. Microbiol. 4, 2090–2100. doi: 10.1038/s41564-019-0532-4
Roder C., Berumen M. L., Bouwmeester J., Papathanassiou E., Al-Suwailem A., Voolstra C. R. (2013). First Biological Measurements of Deep-Sea Corals From the Red Sea. Sci. Rep. 3, 1–10. doi: 10.1038/srep02802
Roik A., Röthig T., Roder C., Müller P. J., Voolstra C. R. (2015). Captive Rearing of the Deep-Sea Coral Eguchipsammia Fistula Fromthe Red Sea Demonstrates Remarkable Physiological Plasticity. PeerJ 2015, e734. doi: 10.7717/PEERJ.734/FIG-2
Röthig T., Roik A., Yum L. K., Voolstra C. R. (2017a). Distinct Bacterial Microbiomes Associate With the Deep-Sea Coral Eguchipsammia Fistula From the Red Sea and From Aquaria Settings. Front. Mar. Sci. 4, 259. doi: 10.3389/FMARS.2017.00259/BIBTEX
Röthig T., Yum L. K., Kremb S. G., Roik A., Voolstra C. R. (2017b). Microbial Community Composition of Deep-Sea Corals From the Red Sea Provides Insight Into Functional Adaption to a Unique Environment. Sci. Rep. 71, 1–9. doi: 10.1038/srep44714
Schmidtko S., Stramma L., Visbeck M. (2017). Decline in Global Oceanic Oxygen Content During the Past Five Decades. Nature 542, 335–339. doi: 10.1038/nature21399
Semenza G. L. (2012). Hypoxia-Inducible Factors in Physiology and Medicine. Cell 148, 399–408. doi: 10.1016/j.cell.2012.01.021
Shinzato C., Khalturin K., Inoue J., Zayasu Y., Kanda M., Kawamitsu M., et al. (2021). Eighteen Coral Genomes Reveal the Evolutionary Origin of Acropora Strategies to Accommodate Environmental Changes. Mol. Biol. Evol. 38, 16–30. doi: 10.1093/molbev/msaa216
Solaini G., Baracca A., Lenaz G., Sgarbi G. (2010). Hypoxia and Mitochondrial Oxidative Metabolism. Biochim. Biophys. Acta - Bioenerg. 1797, 1171–1177. doi: 10.1016/j.bbabio.2010.02.011
Suggett D. J., Smith D. J. (2020). Coral Bleaching Patterns are the Outcome of Complex Biological and Environmental Networking. Glob. Change Biol. 26, 68–79. doi: 10.1111/gcb.14871
Sutthacheep M., Yucharoen M., Klinthong W., Pengsakun S., Sangmanee K., Yeemin T. (2013). Impacts of the 1998 and 2010 Mass Coral Bleaching Events on the Western Gulf of Thailand. Deep. Res. Part II Top. Stud. Oceanogr. 96, 25–31. doi: 10.1016/j.dsr2.2013.04.018
Swietach P., Hulikova A., Vaughan-Jones R. D., Harris A. L. (2010). New Insights Into the Physiological Role of Carbonic Anhydrase IX in Tumour pH Regulation. Oncogene 29, 6509–6521. doi: 10.1038/onc.2010.455
Tjong Y. W., Chen Y., Liong E. C., Tipoe G. L., Fung M. L. (2006). Chronic Hypoxia Modulates the Function and Expression of Melatonin Receptors in the Rat Carotid Body. J. Pineal Res. 40, 125–134. doi: 10.1111/j.1600-079X.2005.00286.x
Vivien-Roels B., Pévet P. (1993). Melatonin: Presence and Formation in Invertebrates. Experientia 49, 642–647. doi: 10.1007/BF01923945
Voolstra C. R., Buitrago-López C., Perna G., Cárdenas A., Hume B. C. C., Rädecker N., et al. (2020). Standardized Short-Term Acute Heat Stress Assays Resolve Historical Differences in Coral Thermotolerance Across Microhabitat Reef Sites. Glob. Change Biol. 26, 4328–4343. doi: 10.1111/gcb.15148
Voolstra C. R., Li Y., Liew Y. J., Baumgarten S., Zoccola D., Flot J. F., et al. (2017). Comparative Analysis of the Genomes of Stylophora Pistillata and Acropora Digitifera Provides Evidence for Extensive Differences Between Species of Corals. Sci. Rep. 7, 1–14. doi: 10.1038/s41598-017-17484-x
Voolstra C. R., Miller D. J., Ragan M. A., Hoffmann A., Hoegh-Guldberg O., Bourne D., et al. (2015). The ReFuGe 2020 Consortium—Using “Omics” Approaches to Explore the Adaptability and Resilience of Coral Holobionts to Environmental Change. Front. Mar. Sci. 2, 68. doi: 10.3389/FMARS.2015.00068
Voolstra C. R., Valenzuela J. J., Turkarslan S., Cárdenas A., Hume B. C. C., Perna G., et al. (2021b). Contrasting Heat Stress Response Patterns of Coral Holobionts Across the Red Sea Suggest Distinct Mechanisms of Thermal Tolerance. Mol. Ecol. 30, 4466–4480. doi: 10.1111/mec.16064
Weis V. M. (2008). Cellular Mechanisms of Cnidarian Bleaching: Stress Causes the Collapse of Symbiosis. J. Exp. Biol. 211, 3059–3066. doi: 10.1242/jeb.009597
Wu Q., Su N., Huang X., Cui J., Shabala L., Zhou M., et al. (2021). Hypoxia-Induced Increase in GABA Content is Essential for Restoration of Membrane Potential and Preventing ROS-Induced Disturbance to Ion Homeostasis. Plant Commun. 2, 100188. doi: 10.1016/j.xplc.2021.100188
Yan G., Yu L., Jiang S., Zhu J. (2018). Melatonin Antagonizes Oxidative Stress-Induced Mitochondrial Dysfunction in Retinal Pigmented Epithelium Cells via Melatonin Receptor 1 (MT1). J. Toxicol. Sci. 43, 659–669. doi: 10.2131/jts.43.659
Ying H., Cooke I., Sprungala S., Wang W., Hayward D. C., Tang Y., et al. (2018). Comparative Genomics Reveals the Distinct Evolutionary Trajectories of the Robust and Complex Coral Lineages. Genome Biol. 19, 1–24. doi: 10.1186/s13059-018-1552-8
Yum L. K., Baumgarten S., Röthig T., Roder C., Roik A., Michell C., et al. (2017). Transcriptomes and Expression Profiling of Deep-Sea Corals From the Red Sea Provide Insight Into the Biology of Azooxanthellate Corals. Sci. Rep. 7, 6442. doi: 10.1038/s41598-017-05572-x
Zammit V. A. (1978). Possible Relationship Between Energy Metabolism of Muscle and Oxygen Binding Characteristics of Haemocyanin of Cephalopods. J. Mar. Biol. Assoc. UK 58, 421–424. doi: 10.1017/S0025315400028083
Ziegler M. A., Anton A., Klein S. G., Rädecker N., Geraldi N. R., Schmidt-Roach S. (2021). Integrating Environmental Variability To Broaden The Research On Coral Responses To Future Ocean Conditions. Glob. Chang. Biol. 27, 5532–5546. doi: 10.1111/GCB.15840
Żmieńko A., Samelak A., Kozłowski P., Figlerowicz M. (2014). Copy Number Polymorphism In Plant Genomes. Theor. Appl. 127, 1–18. doi: 10.1007/s00122-013-2177-7
Keywords: hypoxia, coral reef, gene expansion, orthology, copy number variation
Citation: Alderdice R, Hume BCC, Kühl M, Pernice M, Suggett DJ and Voolstra CR (2022) Disparate Inventories of Hypoxia Gene Sets Across Corals Align With Inferred Environmental Resilience. Front. Mar. Sci. 9:834332. doi: 10.3389/fmars.2022.834332
Received: 13 December 2021; Accepted: 08 April 2022;
Published: 19 May 2022.
Edited by:
Linda Wegley Kelly, University of California, San Diego, United StatesReviewed by:
Yi Jin Liew, Commonwealth Scientific and Industrial Research Organisation, AustraliaDanwei Huang, National University of Singapore, Singapore
Copyright © 2022 Alderdice, Hume, Kühl, Pernice, Suggett and Voolstra. This is an open-access article distributed under the terms of the Creative Commons Attribution License (CC BY). The use, distribution or reproduction in other forums is permitted, provided the original author(s) and the copyright owner(s) are credited and that the original publication in this journal is cited, in accordance with accepted academic practice. No use, distribution or reproduction is permitted which does not comply with these terms.
*Correspondence: Rachel Alderdice, UmFjaGVsLmFsZGVyZGljZUBzdHVkZW50LnV0cy5lZHUuYXU=; Christian R. Voolstra, Y2hyaXN0aWFuLnZvb2xzdHJhQHVuaS1rb25zdGFuei5kZQ==