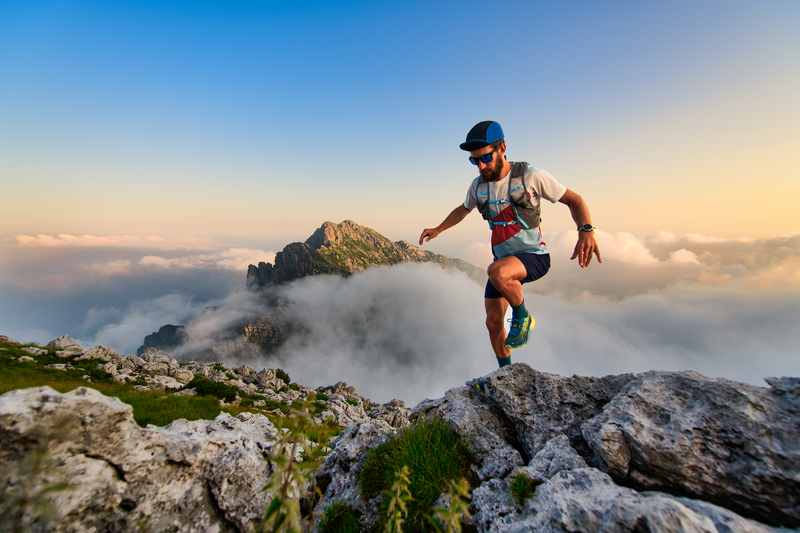
95% of researchers rate our articles as excellent or good
Learn more about the work of our research integrity team to safeguard the quality of each article we publish.
Find out more
ORIGINAL RESEARCH article
Front. Mar. Sci. , 29 August 2022
Sec. Ocean Observation
Volume 9 - 2022 | https://doi.org/10.3389/fmars.2022.833617
This article is part of the Research Topic The Discovery of the Unknown Planet: The Ocean View all 17 articles
Scientific, industrial and societal needs call urgently for the development and establishment of intelligent, cost-effective and ecologically sustainable monitoring protocols and robotic platforms for the continuous exploration of marine ecosystems. Internet Operated Vehicles (IOVs) such as crawlers, provide a versatile alternative to conventional observing and sampling tools, being tele-operated, (semi-) permanent mobile platforms capable of operating on the deep and coastal seafloor. Here we present outstanding observations made by the crawler “Wally” in the last decade at the Barkley Canyon (BC, Canada, NE Pacific) methane hydrates site, as a part of the NEPTUNE cabled observatory. The crawler followed the evolution of microhabitats formed on and around biotic and/or abiotic structural features of the site (e.g., a field of egg towers of buccinid snails, and a colonized boulder). Furthermore, episodic events of fresh biomass input were observed (i.e., the mass transport of large gelatinous particles, the scavenging of a dead jellyfish and the arrival of macroalgae from shallower depths). Moreover, we report numerous faunal behaviors (i.e., sablefish rheo- and phototaxis, the behavioral reactions and swimming or resting patterns of further fish species, encounters with octopuses and various crab intra- and interspecific interactions). We report on the observed animal reactions to both natural and artificial stimuli (i.e., crawler’s movement and crawler light systems). These diverse observations showcase different capabilities of the crawler as a modern robotic monitoring platform for marine science and offshore industry. Its long deployments and mobility enable its efficiency in combining the repeatability of long-term studies with the versatility to opportunistically observe rarely seen incidents when they occur, as highlighted here. Finally, we critically assess the empirically recorded ecological footprint and the potential impacts of crawler operations on the benthic ecosystem of the Barkley Canyon hydrates site, together with potential solutions to mitigate them into the future.
Direct and indirect human pressures on marine ecosystems will intensify rapidly during the coming decade. This will result in an even more severe loss of marine biodiversity and ecosystem services (Danovaro et al., 2008; Danovaro et al., 2017). To support conservation efforts for these ecosystems, it is important to adapt and integrate new monitoring guidelines, data acquisition protocols and cost-effective technologies (Gann et al., 2019; Aguzzi et al., 2020b; Fanelli et al., 2021). Intelligent (i.e., highly autonomous and robotic-sustained) monitoring, not only on a scientific and logistical level but also from the point of view of operational sustainability, is seen as increasing in necessity within the EU’s Marine Strategy Framework Directive (Heiskanen et al., 2016) and the UN’s Decade of Ocean Science for Sustainable Development (Ryabinin et al., 2019). The importance of this automated approach is highlighted by entities such as the Intergovernmental Panel on Climate Change - ICCP (Bindoff et al., 2020) and the Intergovernmental Science-Policy Platform on Biodiversity and Ecosystem Services - IPBES (Díaz et al., 2019), rendering the development of automation as one of the grand upcoming challenges for ocean science (Borja et al., 2020).
The increasing interest in spatiotemporally structured monitoring programs has resulted in the rapid and spectacular development of marine robotics in this field during this past decade. Additionally, ecological monitoring has been supported with a plethora of developing platforms designs (Aguzzi et al., 2021). A new CONcept of OPerationS (CONOPS) needs to be developed, allowing the implementation of cost-effective technology solutions which rely to a much lesser degree on expensive ship-time, allowing personnel to remain on shore to remotely monitor and support research missions and monitoring plans (Jones et al., 2019; Howell et al., 2020). In this paradigm shift, semi- or fully resident underwater monitoring vehicles are needed to regularly cover large areas of the seafloor under acceptable operational costs and with a minimum environmental impact (Marini et al., 2020; Smith et al., 2021; Zhang et al., 2021). Following this approach, cabled observatories are becoming the core of in situ marine ecological laboratories, defining operational control fields for docked mobile platforms, which can even manipulate the environment (Aguzzi et al., 2019).
Here we provide data and examples of use from one such resident robot; the crawler “Wally”, an Internet Operated Vehicle (IOV) in use at the cabled Ocean Networks Canada’s (ONC) NEPTUNE observatory (Barkley Canyon, off the west coast of Vancouver Island, Canada, NE Pacific) 1. Operational since 2010 (Purser et al., 2013), this tele-operated robot has gained interest from both industry and science, with additional deployment sites planned for the Mediterranean, the Atlantic, the Norwegian Sea, China, and along the coastlines of Germany, either cabled or untethered to the hosting platforms. A preliminary use of a coastal prototype is foreseen for European Multidisciplinary Seafloor and water column Observatory (EMSO) testing sites such as the OBSEA (NW Mediterranean) and SmartBay (N Atlantic) (e.g., Falahzadeh et al., in review). In parallel, autonomous versions (i.e., “Rossia” advanced model) have been developed for deployment at LoVe (Lofoten) and the Hausgarten site of AWI off Svalbard, or connected to a surface buoy for the detection of UXO (unexploded ordnance) in the Baltic Sea and North Sea (e.g., Aguzzi et al., 2020a). In the untethered mode, a dragged communications buoy is towed behind the vehicle on the surface, connected by a cable which facilitates allows fast radio-frequency or WiFi communication with an operational center located kilometers away.
Within the first 7 years of deployment, this resident crawler has improved our understanding of biogeochemical, oceanographic and ecological processes at the deep-seafloor of a methane cold seep in a submarine canyon. In particular, we have gained insight on the spatial and temporal variability of methane seepage into bottom waters under varying flow conditions, the importance of benthopelagic coupling in the form of carbon fluxes to the deep sea during winter (i.e., fresh chlorophyll reaching the seep site), the effect of oceanographic conditions on the diel rhythmic activity and seasonality of megafauna, the spatial distribution of fauna in relation to seepage and chemosynthetic food supply, and aspects of benthic animals’ reproductive behavior (Thomsen et al., 2012; Chatzievangelou et al., 2016; Thomsen et al., 2017; Doya et al., 2017; Chatzievangelou et al., 2020a). In addition, technical crawler performance assessments were carried out in parallel; including the design and integration of imaging and data treatment protocols, scientific bias and ecological footprint assessment (Purser et al., 2013; Doya et al., 2017; Chatzievangelou et al., 2020a; Chatzievangelou et al., 2020b).
Discoveries and observations were made during the 24/7 deployments, by pilots operating the crawler in its environment in Barkley Canyon from diverse locations worldwide. A number of these observations have not been published as part of scientific papers to date. Nevertheless, they can provide important information on animal behavior, distribution and the site’s morphology, which should be considered when designing upcoming monitoring projects with the crawler in this and other sites, and in guiding future research endeavors. We will present a selection of these observations, many of which may grow into statistically significant datasets in future years, to serve as an example on how to improve protocols for deep-sea ecosystem monitoring. We also critically evaluate the footprint of such resident robots on the faunal and physical composition of deep-sea sites monitored with crawler platforms. This knowledge can be used to support the development of a new framework for the interpretation of animal behavior, considering the influence of the monitoring platform, to improve monitoring efficiency (e.g., sensu Ayma et al., 2016).
The Barkley Canyon hydrates site is located on a plateau of the west wall of the canyon, at a depth of approximately 870 m. The monitored surface extends over approximately 400 m2 and is characterized by soft seabed and the presence of short (i.e., ~ 2 m) mounds with visible hydrate outcrops on the west side. The depth differences across the site are < 10 m from the highest peak to the lowest trough, with recorded observations not spread across a particular bathymetric gradient of significance within these depths. Figure 1 shows a schematic representation of the site, the main morphological features and positions of several selected observation spots for which the exact position may hold an ecological importance.
Figure 1 Schematic map of the study site (Barkley Canyon hydrates, 870 m depth) with marked locations of several observations. The main transect lines are represented in light cyan. Observations of jellyfish carcasses; decaying macroalgae; sablefish positioning themselves facing against the current; Opisthoteuthidae octopus encounters; aggregations of small crabs; adult crabs with severed limbs; recorded crab aggression; and crab mating behavior are represented by dark red circles; olive-colored stars; green triangles; dark blue squares; orange diamonds; purple, thick flower symbols; brown, thin flower symbols; and black empty flower symbols, respectively.
The “Wally” crawler is an approximately 1 m3, cubic-shaped IOV, which moves on caterpillar tracks. It is connected to an in situ central junction box through a 70 m long tether cable, although a further limitation on operational range is the morphology and characteristics of the seabed, preventing full cable extension due to seafloor elevations, or suitability of seafloor for movement (i.e., not too steep, or too soft). The crawler is occasionally removed for servicing and to swap instrumentation, and sensor payloads have varied by deployments in terms of specific sensor models, but in all cases the payload has included one or two cameras (i.e., a forward facing camera for driving and at times a lateral facing camera of higher quality, for observations during transits), a CTD, an ADCP, and sensors for turbidity and concentration of chlorophyll and methane. As marine camera technologies have advanced throughout the last decade, the quality of the footage has varied from 480 pixels to full HD 1080 pixels between deployments, while different types of lasers (i.e., point-lasers and line-lasers) have been used for sizing and 3D scanning.
The crawler weighs ~ 50 Kg in fresh water, including the cable. In practice, this translates to a 0.35 m2 footprint on the seafloor, applying a weight of ~ 10 g/cm2 (Purser et al., 2013). These values are comparable to those reported for the abyssal benthic rover deployed at Station M (Smith et al., 2021). Depending on the number of cameras, illumination is provided by either two or three 33 W LED lamps (i.e., two facing forward for driving and the third one serving as light for the lateral facing camera).
All data (numeric output of the instruments, images and video recordings) are stored on collection in an onshore database, and made publicly available via the Ocean Networks Canada Oceans 3.0 Portal2.
The crawler has been deployed to traverse specific paths within its operational radius, primarily along an E-W axis, and also to observe the various aspects of the hydrate mound within the western survey area (Figure 1). As precise odometry and navigation are still in development, numerous numbered markers were deployed across the site to provide positional information of the crawler, distances or the precise location of landmark observations.
A southern path, shorter (i.e., ~ 20 m) and straight, was used for linear imaging transects in the first half of the decade. The northern path was longer and partly winding (following the morphology of the seabed and the presence of features of interest such as the hydrate mounds), and it was mostly used for transects during the later deployments.
Transects were performed at a constant speed of ~ 5 cm/s and the imaging mode varied between missions, depending on the specific objectives of each experimental survey (e.g., continuous, forward-looking video recording or time-lapse sideways-looking imaging). As an alternative to transecting, at times the crawler moved to survey spots of interest, where it remained stationary for periods to capture still images or rotating video-scans.
As a mobile, resident monitoring vehicle performing transects along marked seafloor stations, the crawler has been able to make spatially geo-referenced observations and record features on the seabed which might have been missed by other mobile, sporadically deployed vehicles (e.g., ROVs, AUVs, towed or drift-cameras) which are vessel-dependent and are not capable of spatiotemporally repetitive and intensive monitoring across the extended temporal scales achievable with the crawler (Bicknell et al., 2016; Dominguez-Carrió et al., 2021). Similarly, fixed platforms (e.g., lander cameras) have a restricted field of view which limits the ecological representation capability of acquired data spatially, though achieving the temporality capable with the crawler platform (Rountree et al., 2020).
Reproductive strategies and phenology in many deep-sea animals are still largely unknown (Danovaro et al., 2014; Danovaro et al., 2020). In gastropods in particular, food availability may trigger specific strategies or aggregations which are otherwise inhibited as too expensive from an energetic point of view (McClain et al., 2014). In April 2014, a dense aggregation of buccinid gastropods was encountered on the western flank of the gas hydrate mound monitored by the crawler (Figure 2A). Close examination showed that these gastropods were in the process of secreting egg towers – defensive nursery structures from which snail juveniles would eventually hatch, also reported in other NE Pacific seeps3. In this initial visit, towers were no more than 5 cm in height.
Figure 2 Egg towers of buccinid snails, located between two hydrate mounds. (A) Snail aggregation covering the seafloor from the point at which bacterial mats were visible on the hydrate mound, and down the flank (image taken on April 22 2014). (B) Fully developed and dense egg towers, with only a handful of snails present (image taken on November 06 2014). (C) Remnants of the tower field (image taken on August 31 2021). Note the patchy distribution, as well as the varying decaying state of some of the towers.
In November 2014 the egg tower site was revisited, and the 5 m2 area was now abundant with ~ 100 perpendicular, ~ 20 cm tall snail towers (Figure 2B, Supplementary Video 1). Only occasional gastropod shells and fish of the family Sebastidae (i.e., rockfish Sebastes and thornyheads Sebastolobus) were observed amongst the tower matrix, contrasting with the hundreds of snails observed in April. These egg towers added local structural complexity and habitat niches to the environment (Levin and Dayton, 2009). A subsequent survey in January 2015 showed no observable change in the tower matrix, with occasional adult gastropods and Sebastidae still observed between the towers.
The area was revisited in late summer of 2021, and patches of egg towers were observed and recorded on video (Figure 2C and Supplementary Video 2). Many of the towers were no longer present, and those still present were thickly covered with filter feeding fauna, potentially bryzoans and/or hydroids. Sebastidae again dominated the terrain between the towers, with the occasional pacific hagfish Eptatretus stoutii and crabs (i.e., grooved tanner crabs Chionoecetes tanneri and, more rarely, big scarlet king crab Lithodes couesi individuals).
The comparison of footage from the different deployments is expected to shed more light on various aspects of the reproductive behavior of buccinid snails in Barkley Canyon. Observations to date indicate that snails are abundant in the study area. From these initial observations, it would appear that gastropod spawning is occasional across the region, and not repeated on an annual cycle. Continued, long-term observation will allow us to determine the timing of their egg deposition and hatching, and the spatial distribution of the tower fields. Remnant material from the reproductive event increased the habitat complexity of the gas hydrate mound and provided both a habitat for mobile fish and crab fauna, as well as a suitable hard substrate for colonization by filter-feeders. By analyzing the abundance, distribution and overall diversity of other fauna species, we can obtain information on the effect of the presence of the towers on a community level.
Approximately 40 m away from the main hydrate mound, a boulder of approximately 50 cm diameter was first imaged on March 27 2014. A selection of the images from repeated visits made to the boulder over the following months (and years) are given in Figure 3. As a rare example of hard substrate in a site otherwise characterized by soft sediment, the boulder was clearly colonized by numerous encrusting sponges, with the hard, cracked surface providing habitat niches for shrimp and galatheids (Figure 3A). Closer examination showed the boulder to be fringed with unidentified harpacticoid shrimps (Figure 3B).
Figure 3 Boulder providing several niches colonized by galatheids, rockfish and harpacticoids, and the short (i.e., weeks) and long (i.e., years) term evolution of this small ecosystem. Two images (i.e., a view of the entire boulder -letters a, c, e, and g- and a zoomed part defined by a red rectangle -letters b, d, f, and h-) are provided for each observation. The red arrow points towards a harpacticoid shrimp on top of biogenic (bryozoan) substrate, while the red “N” indicates a nudibranch.
As a site of interest, the boulder was revisited after 4 days. In Figures 3C, D (images taken 4 days after Figures 3A, B), a nudibranch was also present on the rock encrusting bryozoan/hydrozoan epifauna, beneath the swaying harpacticoid shrimp individuals. In Figure 3D a chain of eggs has been attached to the bryozoan colony, although it is unclear if it belongs to the nudibranch. Just over a week later (April 08 2014), in Figures 3E, F, the nudibranch has left the bryozoans and moved some cm away. The eggs however were still evident, surrounded by a slightly reduced number of harpacticoids, possibly the result of nudibranch predation. In Figure 3E a rockfish individual was observed utilizing the boulder as refuge or hydrodynamic trap.
From this brief time-series numerous interesting observations were apparent. The harpacticoid species’ behavior deep within the canyon was similar to that observed at shallower sub-littoral depths (Caine, 1980). Though caprellid harpacticoid amphipods have been reported within the deep sea, and indeed at methane seep sites, observations have been ‘snapshots’ rather than time-series behavioral studies (Sibuet et al, 1988). The timing of the egg deposition is also of interest – corresponding with annual surface productivity blooms within the area (Juniper et al., 2013). Reports on interspecific interactions between nudibranchs and caprellids up to now have described mainly antagonistic/competitive relationships (e.g., Caine, 1998; Ros and Guerra-García, 2012), therefore further data are needed in order to determine whether the eggs deposited in the caprellid rich bryozoan/hydrozoan branches rather than the unoccupied thickets elsewhere on the boulder were indeed nudibranch eggs.
On a different temporal scale, revisits to the boulder feature in September 2021 indicated the long-term use of the boulder by both rockfish and galatheid squat lobsters, occupying the same spots as individuals of their species in 2014 (Figure 3G). Likewise, the bryzoa/hydrozoa and caprellid relationship was still evident on the fringes of the boulder (Figure 3H). Additional information on sponge growth and longevity may also be gleaned from this time-series data if monitored into the future, with any new settling sponges being observed over time as they develop and grow on the boulder surface.
The input of fresh biomass in the form of phytodetritus or carrion is an important carbon source that contributes to deep-sea ecosystem functioning (e.g., Van Nugteren et al., 2009; Dunlop et al., 2016; Stratmann et al., 2018). The continuous operations of the crawler allowed for the observation and recording of multiple episodic events (see below) unlikely to have been recorded by any single research cruise. Long-term observations during winter with the crawler revealed the importance of winter storms and downwelling on the export of fresh phytodetritus, showing that winter can be as important for its transfer to deep-sea areas as the period from spring to autumn, despite much lower primary production rates (Thomsen et al., 2017). These phytoplankton pulses disappeared already during and up to the 2 days after bad weather affected the area, and reached the study site at 870 m water depth with a delay of up to two days.
For ~ 20 hours in October 2010, a lateral advection of disintegrated gelatinous particles which resembled shredded jellyfish or salps at 0–2 m height above the seafloor was recorded during a downwelling event (Figure 4). The observations underline the fact that canyons can act as conduits for the transport of organic carbon to the deep sea, either as marine snow, macroalgae (kelp) or carcasses and have been reported elsewhere (e.g., Sweetman and Chapman, 2011; Henschke et al., 2013; Sweetman et al., 2014) but rarely the event itself (e.g., Smith et al., 2014). While turbidity meters can detect such events, the actual shape and size of these particles cannot be determined unless particle cameras (e.g., Nowald et al., 2009) become available in future deployments.
Figure 4 Lateral advection of disintegrated gelatinous particles, as an example of a rare biomass input from shallower waters. Note the technical difficulties in determining the depth of view and the shape of the particles, and as a result, the challenging estimation of their size in order to calculate fluxes.
Closer observations of the sedimented seafloor during the October 2010 event revealed that decapod crustaceans were feeding on jelly carcasses in different phases of decomposition. On several occasions, even entire jellyfish were observed laying on the seabed, either in deteriorating state (Supplementary Video 3) or actively being consumed by scavenging decapods such as grooved tanner crabs (Figure 5A). On December 08 2021 another entire jellyfish was observed being transported by currents along the seabed, before settling (Figure 5B). Due to this displacement, the carcass had not yet started to be consumed by benthic scavengers. Future research can reveal the decomposition time of this biomass input, in relation to hydrodynamic conditions (does the carcass settle or gets dragged by currents)?, scavenging by the resident benthic community or transient, opportunistic and possibly attracted species (e.g., Aguzzi et al., 2012), and finally natural decomposition times.
Figure 5 Jellyfish laying on the seabed. (A) The carcass is being scavenged by numerous small individuals of grooved tanner crab (image taken in October 2010). (B) Consumption of the carcass has not started yet, although a tanner crab individual is visible (image taken on December 08 2021).
During a transect on August 28 2021, a vibrant, orange-colored piece of surface-originated macroalgae, was observed on the seabed. The crawler did not approach too close initially, as the mass was relatively bulky and we could not determine whether it consisted of fish eggs or some other biogenic feature. Thus, the pilot followed a policy of minimum disturbance by staying at a safe distance. The algae in contact with the seabed and rippled in response to the movement of bottom waters without being displaced for some minutes, before finally detaching from the seafloor and being transported for a short (i.e., < 1 m) distance by the current (Supplementary Video 4).
Some days later (August 31 2021), close up images were taken from distance nearer proximity and stages of the decaying process were monitored, until the algae disappeared a week after its first appearance (Figure 6). It is reasonable to assume that, following removal of much of its mass, the remains were detached from the seabed and transported out of the monitoring area by the ambient seafloor currents, at a minute-averaged down-canyon velocity of ~ 11 cm/s (maximum > 50 cm/s), as recorded for the period of time between the last sighting of the algae and the next visit of the resting spot, at which point its absence was observed.
Figure 6 Piece of macroalgae, vertically transported to the hydrates site from the surface layers of Barkley Canyon. (A) Three days after the first sighting (image taken on August 31 2021). (B) Five days after the first sighting on the seabed (image taken on September 02 2021).
Pieces of brown macroalgae have also been observed on various occasions (e.g., Supplementary Figure 1). Opportunistic observations like these, hard to be specifically targeted for studies in deep-sea research, can illuminate questions on the triggers (e.g., surface storms) of vertical transport of fresh plant biomass to deeper waters through canyons during downwelling events (e.g., Zheng et al., 2017), and the role of such material and occasional events in supporting deep ecosystems alongside the regular and/or episodic input of smaller phytodetritus (Baker et al., 2018). Such data are rare; nevertheless, they provide observations of down-canyon transfer of macroalgae which enable discussions on their potential role in carbon sequestration (Pedersen et al., 2021). No direct consumption by fauna was observed with the crawler, underlining the importance of such studies on sequestration. Conventional ROV operations would not allow such observations over extended time, which only resident ROVs would do (McLean et al., 2020).
Ethological information on displacement rhythms of fauna, bioturbation, feeding rates or predator-prey interactions is of pivotal importance for better understanding the life traits of deep-sea species (Danovaro et al., 2014; Melo-Merino et al., 2020). Unfortunately, this information still eludes standard survey practices (e.g., behavioral interactions beyond the mere time-series of animal counts). Several different behaviors of benthic fauna have been recorded by the crawler throughout the years, including various sorts of interaction between animals and the crawler. These types of observations can increase our knowledge on the impact of a monitoring platform such as the crawler may have on animal behavior. The crawler does not have acoustic imaging or passive acoustic instruments at the moment. Nevertheless, two imaging rotary sonars are deployed further away, outside its operational range (approximately 20 m away towards the east and west extremes). The sonars scan a circular area of 10 m radius (Thomsen et al., 2017). Data from these can be contrasted to, for instance, the fish counts in the crawler’s footage, as there is no reason to assume that expected fish densities should differ between the three platforms other than a potential effect of the crawler.
Large groups of sablefish (~ 10 ind.) were observed resting on – or hovering above – the seabed, facing into the current (Figure 7 and Supplementary Video 5). Sablefish utilize odor plumes in their search for food (Løkkeborg et al., 1995; Sigler, 2000; Bailey and Priede, 2002), plumes which are directed by the current regime and circulation within the canyon. More data on their tactic behavior (i.e., response to a stimulus by modifying their orientation and/or locomotion) could be used as an indicator for the origin of food, and also to explain their reported migration patterns across different Barkley Canyon depths (Doya et al., 2014; Chatzievangelou et al., 2016).
Figure 7 Sablefish resting on the seabed or hovering right above it, while all facing against the current (current direction from the right towards the left of the images). Images taken on August 24 2021.
Sablefish have been observed interacting with the crawler in different ways. Indicatively, sablefish individuals within the range of the crawler’s lights (a few 10s of m, depending on the turbidity of the water, which influences light transmission) have approached the vehicle in a check-and-go act of curiosity. On rare occasions, sablefish approached the crawler at speed and shifted their direction in the last moment to avoid collision, although in one instance, a sablefish swam into the sphere containing the camera. Figure 8 provides evidence that specific sensor lights, e.g., a blinking blue light of a fluorometer, can attract sablefish when pointed at and reflected from the seafloor. Possibly, sablefish attraction to blue light reflects its visual hunting capability to spot and prey upon common bioluminescent organisms, being visual predators (Ryer and Olla, 1999). In this particular case in 2012, a WetLabs fluorescence sensor was used for redundancy and emitted a light beam into the water column. This may have impacted on the interpretation of turbidity sensor data, as fish are attracted after several hours of recurrent blinking of the sensor’s light. During their inspection of the light source they resuspend the sediments, thus sensors of this type should preferably be used only in the water column.
Figure 8 Group of sablefish, resting in front of the crawler. Fish are attracted by the blue light of a WetLabs fluorometer, which creates a light stream onto the sediments.
Hagfish were observed primarily prone on the seafloor, flat, coiled or semi-coiled, though they were sometimes observed swimming (parallel or even perpendicular to the seafloor). Interestingly, the angle of the animals’ orientation in relation to the crawler (diagonal; Supplementary Video 6), indicated they were (to some degree) aware of the presence of the crawler, even when they did not display an explicit reaction to it. Another common behavior of this species was to swim statically and in a vertical position while maintaining the mouth fixed on the same seabed spot.
Both the deep-sea sole (Embassichthys bathybius) and the pacific halibut (Hippoglossus stenolepis) seemed barely affected by the presence of the crawler, not moving even when the crawler was at a distance of only a few centimeters, or moving away from them following a period of inactivity. Once though, a deep-sea sole followed the movement of the crawler swimming beside it for a few meters (Supplementary Video 7).
Eelpouts (Lycenchelis sp.) were commonly seen crossing the camera’s field of view with quick changes of direction, sometimes touching the seafloor during their transit. Commonly they were observed laying on the seafloor and then suddenly burst away when the crawler got too close. At times they were observed drifting vertically, with the head facing towards either the water column above or the seafloor below. In very rare occasions these animals appeared laying on the seafloor flapping their pectoral and caudal fins, independently of their orientation in relation to the crawler.
Rockfish and thornyheads were always observed laying on the seafloor, occasionally moving several centimeters to one meter away if the crawler got too close. Many individuals showed an agonistic behavior towards the crawler (i.e., wide open mouth, flapping their fins).
Over the span of 9 years, several encounters with octopus of the family Opisthoteuthidae were made. Given the time span between observations, it is unlikely that all these octopod observations were made of the same individual.
During transect missions in June 2012, the first individual was spotted laying on the seabed right on a previous track depression made by the crawler (Supplementary Video 8). The area was at the time not stable for the crawler to approach, so the pilot circumnavigated the octopus, which showed no sign of stress response.
A second individual was again detected resting in the same position during several days in November 2013 (Supplementary Figure 2). Although the animal’s behavior between the periodic transects is unknown, it is possible that the octopus did not move over the intervening days. Time-intensive observations may address questions on the behavior and bioenergetics of Opisthoteuthidae octopods which are otherwise understudied, such as their preying strategies (i.e., are they actively hunting for prey or deploying a waiting strategy)?, or if they are following any circadian, infradian or ultradian activity rhythm.
Later in November 2016, there was a third encounter in similar settings (Figure 9A and Supplementary Video 9). The crawler approached as close as possible, with minimum speed, and came to touch the octopus with the caterpillar. No avoidance behavior was observed from the side of the animal. On the contrary, when the crawler moved slightly backwards, it actively returned to the vicinity of the caterpillar, possibly identifying the crawler as a potential shelter against predation, rare in soft bottoms (Katsanevakis and Verriopoulos, 2004).
Figure 9 Octopus resting on the seabed in a flat-spreading position, on top of the crawler’s tracks. (A) Note the opened eyes during the octopus’ interaction with the crawler’s caterpillars (image taken on November 24 2016). (B) Note the closed eyes while the crawler remained close to the octopus (image taken on September 13 2021).
Finally, another specimen was recorded several times in September 2021 (Figure 9B and Supplementary Video 10), and then again on November 24 2021. The octopus did not react to the crawler’s approach. On all occasions, the initial posture of the octopus was a non-threatening, flat-spreading position (i.e., horizontally extended on the seabed; Villanueva, 2000).
Aggregations of small crabs, presumably small individuals of grooved tanner crab, were observed in several occasions on small elevations of the seabed or on a short hydrate mound (Supplementary Figure 3; Supplementary Video 11). Further studies are needed to understand this behavior, with small decapods previously observed climbing on seabed structures to escape the anoxic first few cm of the BBL (Doya et al., 2016), and generally aggregating as a defense mechanism (Dew, 2010).
Doya et al. (2017) reported an agonistic interaction between a grooved tanner crab and a scarlet king crab over a piece of food. Aggression between various larger crabs was observed in 2021, although the motives were not clear in the video (Supplementary Video 12). Contrary to big burrowing and territorial decapods (e.g., Norway lobster Nephrops norvegicus; Sbragaglia et al., 2017), grooved tanner crabs and scarlet king crabs have not been observed defending a particular spot. Overall, many individuals lacked one or several walking legs or claws, while in 2013 a scarlet red leg was observed on the seafloor (Supplementary Figures 4 and 5, respectively). In any case, limb loss can be a common phenomenon within the Chionoecetes genus (Edwards, 1972), while it can be a potential sign of aggressive, density-dependent interactions (e.g., dominance hierarchies) leading to autotomy (Fleming et al., 2007).
During a transect in 2021, a couple of grooved tanner crabs were observed displaying the same mating behavior previously described in Doya et al. (2017), with one individual grabbing the other, lifting it and transporting it nearby, farther away from the crawler (Supplementary Video 13). Sometimes an extra conspecific individual can be present nearby during mating, in agonistic posture (Supplementary Video 14).
Small shrimp individuals have been observed being “carried” by the crawler for various meters, laying or walking either on its frame or/and on its floating foam blocks (Figure 10), or feeding from the biofilm on the camera’s glass sphere.
Figure 10 (A) Shrimp on the protective frame of an instrument (center). (B) Shrimps on the crawler’s frame (bottom left), being carried by the crawler during a transect mission (image taken on November 11 2016). Unfortunately, as they were observed a posteriori in images, the camera was focusing further away on the mission’s objective (i.e., hydrate mound’s surface).
What it looked to be a rusty battery was found over one of the periodic transects of the crawler in 2013 (Supplementary Video 15). During the same deployment period and at a nearby spot, a white plastic object was observed (Supplementary Video 16). The eventual fate of these pieces of debris was not tracked in this case. Canyon circulation dynamics favor the flushing of deep-sea macro-litter (Pierdomenico et al., 2019). This is relevant to the debate of either letting deep-sea litter shelf-bury into the sediment or whether enforcing an active removal is preferable (Madricardo et al., 2020). The crawler can be innovatively used to identify litter debris as is currently done by ROV imaging (e.g., Mecho et al., 2020; Mecho et al., 2021) with the advantage of being able to track the integrity dynamics of the litter and how species interact with it.
Examples of observations such as those presented above are indicative of the potential of crawlers as deep-sea monitoring platforms, capable of encountering events and phenomena that would possibly be missed by short-term surveying or by fixed cabled observatory platforms, or at least highly complementary to their observations. At the same time, these observations provide valuable information useful for critically assessing the monitoring footprint of the presence and operations of such a platform on deep benthic ecosystems (summarized in Table 1 below). In the next sections, we will expand on some of these aspects.
Table 1 Selected observations made with the crawler during a decade-long period (i.e., 2009–2021), alongside their ecological importance and/or operational impact.
Contrary to more traditional methods (e.g., trawling, dredging, traps and box-corers), imaging transects by crawlers are a non-extractive monitoring methodology (Cappo et al., 2006; Jamieson et al., 2013) which resembles the classic underwater visual censing by SCUBA divers (e.g., Assis et al., 2013). While selectivity of extractive gears has been improving with time (Feekings et al., 2019), imaging is a less invasive alternative capable of providing reliable data on epibenthic megafauna (Ayma et al., 2016; Bicknell et al., 2016) while also representing an overall positive step towards true non-invasive monitoring (Pauli et al., 2010). Nevertheless, some level of physical disturbance has to be assumed, as the crawler leaves tracks on soft sediments which may take from months to years to eradicate, depending on the hydrodynamic conditions and sediment flux to the seafloor (Thomsen and Gust, 2000; Ogston et al., 2008), sediment resuspension by fauna (i.e., bioturbation; Katz et al., 2012; Robert and Juniper, 2012), with artificial heterogeneity potentially affecting beta diversity at small spatial scales (Hewitt et al., 2005). This particular aspect could be tackled with resident ROVs (e.g., Zhang et al., 2021), although potentially compromising the stability during and repeatability among missions.
Differences in the diversity and abundances of the most abundant megafauna species at the site were observed by the crawler, between an area with no previous tracks and an area where crawler missions had taken place before (Chatzievangelou et al., 2020a). In the latter, remnants of the old (i.e., ~ 2 years) tracks were still visible at some spots. The same is true for the tracks left after the late 2016 deployment, with some being visible during an ROV maintenance dive in August 2018. On the other hand, these 2016 tracks were completely covered by a thick layer of soft sediment by the time the area was revisited in summer 2021. In that sense, the crawler can be utilized as a monitoring platform for small-scale ecosystem restoration and recovery experiments with operation terrains revisited at later stages (e.g., after 3 years) and footprint assessed in a similar way as, e.g., in previously trawled areas (Amoroso et al., 2018). Common practice during crawler operations during the last years is to drive the vehicle on the same tracks during transect missions, thus keeping sediment disturbance at a minimum. This approach can be enhanced in the future by the development of increased navigational autonomy and precise repetition of pre-established missions (as, for instance, the more biogeochemistry-orientated abyssal benthic rover; Smith et al., 2021), although this may come at the expense of the ability to avoid slow fauna (see below).
Additionally, some species showed a particular affinity for the crawler’s tracks, with the exact reasons currently unclear (e.g., potential shelter from visual predators, grazing in the resuspended sediment or even preferring the distinct micro-circulating patterns). Larger animals (e.g., rockfish, hagfish, halibuts, soles, etc.) very rarely occupied the tracks transversally, but rather along the track axis (i.e., facing forwards or backwards in relation to the crawler). Such species could be incommoded by the bumps of the tracks, or plainly not fit in the width of the tracks due to their length. These are examples of the potential for high local physical heterogeneity originating from crawler actions to influence seafloor community structure and use of space.
Due to the small lag in communications (i.e., ~ 2s, which can increase depending on the ONC system’s traffic) between the user and the vehicle, it is possible that animals moving with intermediate-speed can be run over by the caterpillars if they enter the tracks after a move order has been confirmed. This would not be expected to affect fish, as they are quick to burst and swim when they perceive the crawler approaching, nor snails which cannot enter the field of view at high speed and are easy to avoid. The most probable casualties would include medium- to large-sized crabs, which could theoretically move fast enough to appear suddenly on the tracks but not fast enough to avoid the crawler if even an emergency stop is carried out too late. In one case, this lead to an aggregation of a numerous small crabs and buccinid snails that scavenged on the crushed carcass (Supplementary Figure 6). On another occasion, the tip of a scarlet king crab’s leg got under the crawler’s caterpillar and sank into the muddy seafloor, with this softness preventing the animal from being hurt. The crab reacted by jumping forward at a surprisingly high speed for what is normally observed for this species (Supplementary Video 17). Such rare incidents may result in punctual stress for the animal, but thanks to their generally rapid reactions and the cushioning effect of the muddy seafloor, potential risks remain low. In any case, the percentage of such incidents occurring is minimal in comparison to the total distance covered, the total time of operations and the recorded faunal abundances, while the crawler’s speed is maintained low (~ 5 cm/s).
Photic and sound contamination is another aspect of a potential effect of the monitoring platform (Ryer et al., 2009; Lin et al., 2019; Chen et al., 2021). ONC regulations do not allow the lights to be on for more than 2 hours per day, in an attempt to control the effect of artificial light in aphotic depths. Startling, approaching and evasion have all been observed by different species or individuals of a particular species (see section “4.3 Adaptation of fauna” below). Photic contamination can be diminished consistently by using optoacoustic imaging technologies which identify animals without light sources, but based on multi-beam sound frequency emission (e.g., Jones et al., 2019). This solution is especially suited for increasing imaging monitoring penetrability of crawlers of several meters beyond the usual reach of HD camera systems (Aguzzi et al., 2019). Additional solutions may include the use of new, low-light cameras and motors with reduced sound emissions could tackle the issue of light and noise in the future (Phillips et al., 2016; Rountree et al., 2020), though the crawler is already a far quieter system than thruster equipped AUVs or ROVs.
Finally, residual litter can be generated during operations and maintenance (Figure 11). A next generation of crawlers with conventional grippers/robotic arms (Wehde et al., 2019) or based on biomimicking design (e.g., soft robotics) could detect in footage (e.g., Watanabe et al., 2019), collect and store litter items resulting from deployment, in addition to litter reaching the seafloor from the surface (e.g., Pham et al., 2014; Pierdomenico et al., 2019) to reduce this effect. However, the main purpose of such manipulators would be to carry out additional experiments and deploy/recover small experimental chambers, microprofilers or to recalibrate/relocate other sensors. This was evidenced in May 2010, when a comparison with data from a temperature probe with the CTD of the crawler was necessary (Supplementary Video 18).
Figure 11 Zip tie on the seabed, as an example of marine litter encountered by the crawler during different missions (image taken on September 27 2016).
Throughout the various deployments of the crawler since 2009, the spatial range of monitoring operations was ultimately limited by operational autonomy (i.e., the need of an umbilical cable to provide power and communication; Brandt et al., 2016) and, equally importantly, by seabed morphology, such as the steep flanks of one side of the hydrates plateau and the hydrate mounds. In the future, the use of crawlers in unison with faster/more mobile vehicles and crab-like robots (Picardi et al., 2020) may allow the expansion of the spatial representativeness of the results (Aguzzi et al., 2021).
The aforementioned ONC’s lighting restrictions may limit the temporal resolution of monitoring plans during the 24h cycle (see previous section “4.1 Impact of operations on the ecosystem” above), with no current capability of multiple transects on a daily basis in the long-term. This issue could be tackled by the development of adequate artificial intelligence, possibly in collaboration with space research scientists, enabling highly automated/pre-programmed missions with optimized duration (Flögel et al., 2018; Aguzzi et al., 2022). Laser-scanning and modelling of 3D point-clouds of the seabed within the crawler field of view may be processed in near real-time, to allow adaptive driving capabilities (Aguzzi et al., 2020a).
The crawler is endowed with a high-resolution camera and even very small animals can be detected from and appropriate distance given suitable illumination. Yet, another potential limitation would be the detection of smaller animal sizes such as macro- and meiofauna, for whom, as well as for endobenthic animals, imaging might underestimate or even completely miss individuals or species (McLean et al., 2020), especially when the crawler is moving and the water is turbid. Thus, a combination of regular imaging, hyperspectral imaging, pin-pointed physical sampling and environmental DNA (eDNA) approaches could be the way to go for comprehensive biodiversity studies (Dumke et al., 2018). Equipping crawlers with eDNA sampling tools may allow the identification of molecular markers for species in a much wider range of sizes in comparison to those targeted by optoacoustic systems (Mirimin et al., 2021, Stefanni et al., submitted).
As mentioned in the previous section, artificial light may attract individuals from outside the current operational range (Widder et al., 2005; Doya et al., 2014) or, on the contrary, repel the ones present, with complex inter- and intraspecific variability making behavioral reactions hard to determine and predict (Geoffroy et al., 2021). Scientists at the French National Centre for Scientific Research (CNRS) who prepare a crawler for long-term deployments at 2500 m water depth in the NW Mediterranean will apply a pulsed, multi-color (i.e., of adjustable wavelength) LED light which can be also dimmed on demand, in an attempt to communicate with deep-sea fauna. The headlights of the crawler are multi-color and can be dimmed on demand (Tamburini et al., pers. comm.). Less is known on the effect of anthropogenic sound on deep-sea fish (Bolgan et al., 2020), however it can disturb their communication and orientation (Hawkins et al., 2015). Raw abundances and densities of fauna may have to be adjusted to account for this type of effect, as shown in the case of strong swimmers such as sablefish, with methodologies similar to the ones applied for the estimation of true densities in baited camera experiments (Priede and Merrett, 1996).
Finally, the platform itself can act as a morphologically complex 3D structure, simulating a small reef as in the case of the octopus and the shrimps, similar to offshore structures industry structures (Gates et al., 2019). This property may affect the counting of animals, and has to be taken into account on a case-by-case basis when estimating faunal abundances.
Generally, differences in the reactions of the benthic fauna of the Barkley Canyon hydrates towards the presence and operations of the crawler have been observed, both among species and individuals of the same species. These included evasive or aggressive behavior, with animals moving away in order to escape as the crawler approached, attraction, with animals approaching the crawler either out of curiosity or for practical reasons, and indifference, with animals not reacting to the crawler on a visible level. Potential physiological reactions due to stress would be very hard to be accounted for with conventional in situ methodologies, though potentially the microfluidic detection of hormones with a lab-on-a-chip approach may elucidate such responses (Ozhikandathil et al., 2017). Different reactions reflect a combination of the sensory ecology of the respective species (the way they perceive the world; Burnett, 2011), the level of familiarization with the crawler as a semi-permanent monitoring platform, and other ecological characteristics briefly discussed below.
The use of space may play a role in the level of familiarization reached by each animal. An acceptance/indifference towards biomimetic robots has been observed for many species with increasing coexisting time (Bohlen, 1999; Garnier et al., 2008; Polverino and Porfiri, 2013), which in the case of the crawler can apply to resident individuals/species. Conversely, species known for their territoriality or individuals defending their prey/mate might display agonistic posture (e.g., grooved tanner crabs; Doya et al., 2017).
In general, time spent in the vicinity of the crawler can be expected to reduce reactions, as for instance for sablefish which did not generally avoid it in an active way. For many fish species, as has already been reported for ROV studies, the platform’s presence may not represent a meaningful evolutionary signal in the context of their commonly experienced ecological niche (Ayma et al., 2016). On the contrary, Krieger (1997) reported that while sedentary sablefish did not react to the first contact with a submersible, active ones would rapidly turn away (this probably being the first time they encountered an underwater vehicle). The same behavior (rapid avoidance) was observed in simulations of underwater vehicle lighting with sablefish in captivity (Ryer et al., 2009). Behavioral responses of fish towards mobile monitoring platforms can vary in intensity by various external (e.g., vehicle type and operational range, altitude from the seabed etc.), and ecological (e.g., habitat complexity) parameters (Campbell et al., 2021). Nonetheless, risk assessment in animals can vary upon different circumstances and fear can be amplified when, for instance, the crawler’s lights trigger curiosity, or when fish in larger schools tolerate more its approach, in ways that a different need can prevail and balance out the predator-induced fear component (Stankowich and Blumstein, 2005; Clinchy et al., 2013). Similarly, many crustaceans reportedly prioritize getting access to more oxygenated water over the instinctive fear of predators that shapes their cryptic behavior (Aguzzi and Sardà, 2008; Haselmair et al., 2010; Riedel et al., 2014; Doya et al., 2016).
For shrimps, the crawler represented an artificial reef that offered the possibility to graze on of bio-fouling layers developed on various parts of its parts, as well as physical protection from potential predators by its structure. This interaction resembles the symbiosis of small parasitic organisms “cleaning” bigger animals and being tolerated by them (Côté, 2000; Bshary and Côté, 2008).
Finally, more motile animals reacted predictably to the presence of the crawler. Long distance swimming predators (e.g., sablefish) could detect the crawler from afar, approach to check the source of the signal and depart, being considerably faster than the crawler. On a larger scale, fishing pressure and the approach of gear may modify fish shoaling tendency and overall collective behavior (Sbragaglia et al., 2021). On the other hand, burst swimmers (e.g., pacific hagfish, soles and rockfish/thornyheads individuals) that normally lay on the seabed, did not react unless the crawler was heading directly towards them and getting too close (e.g., when they were laying on the tracks). Finally, epibenthic animals (e.g., crabs, snails, bivalves, etc.) that could crawl or move slowly were either not reacting, or reacting at speeds not detectable (i.e., minimal in comparison to the already low operating speed of the crawler of ~ 5 cm/s), except for the case of some big crab individuals (i.e., > 20 cm total width – including legs – as calculated by comparing them to the width of the tracks) which would move at relatively fast (e.g., crossing the field of view in a few 10s of s).
The examples presented here indicate the need to perform measurements at specific stations which should be revisited across different time spans. Such examples should be particularly considered in the light of restoration needs and the quantification of relevant ecological indicators. This is especially relevant as such observations can directly address the attributes that characterize the integrity of an ecosystem (in this case an impacted, recovering or restored deep-sea ecosystem), such as threats, physical conditions, species composition, structural complexity, ecosystem function and external exchanges (Gann et al., 2019).
We list a series of advances which have to occur in order to push intelligent monitoring a level above the current technological and methodological thresholds. In particular:
● Autonomous operational capabilities, in order to disengage specialized personnel from manual operations.
● Increase in intelligent algorithms to enable animal tracking and classification, to increase the percentage of biologically-meaningful information stored on-board.
● Increase in intelligent algorithms for posterior data processing and analysis, to reduce manual human intervention and efficiently translate between crawler-generated data and already available, decades-long datasets derived by long-established methodologies based on physical sampling.
● Minimization of the ecological footprint of crawlers, through continuous assessment and integration of new knowledge into monitoring protocols.
The aforementioned forward steps can advance the characterization of deep-sea species behavior and use of space in small scales, the identification of resident individuals with insights into their life expectancy, as well as community dynamics, biodiversity and habitat quality, in ways that would not be possible with traditional sampling. We believe that the development in crawler technologies can boost deep-sea benthic research and monitoring, in a similar way that mid-water research coevolved with ROVs in previous decades (Robison et al., 2017).
The datasets presented in this study can be found in online repositories. The names of the repository/repositories and accession number(s) can be found in the article/Supplementary Material. The Supplementary Videos containing the material described in this study can be found in the figshare repository doi: 10.6084/m9.figshare.17162063. All data (numeric output of the instruments, images and video recordings) are stored on collection in an onshore database, and made publicly available via the Ocean Networks Canada Oceans 3.0 Portal.
DC, CD, and AP: conceptualization, methodology, investigation, writing—original draft, writing—review and editing, visualization. LT: conceptualization, methodology, investigation, resources, writing—original draft, writing—review and editing, supervision, project administration, funding acquisition. JA: conceptualization, writing—original draft, writing—review and editing, supervision, project administration, funding acquisition. All authors contributed to the article and approved the submitted version.
This research has been funded by the within the framework of the project Robotic Exploration of Extreme Environments (ROBEX) project by the Helmholtz Alliance (HA-304; DC and AP). CD was funded by the project RITFIM (ref. CTM2010-16274) of the Spanish national RTD program. In addition, funding was provided through the Tecnoterra Associate Research UNIT (ICM-CSIC/UPC) and the following project activities: ARIM (Autonomous Robotic sea-floor Infrastructure for benthopelagic Monitoring; MarTERA ERA-Net Cofund) plus RESBIO (TEC2017-87861-R) and PLOME (PELC2021-007525), both funded by Spanish MICINN (Ministerio de Ciencia, Innovación y Universidades). We also profited from the funding from the Spanish Government through the ‘Severo Ochoa Centre of Excellence’ accreditation (CEX2019-000928-S).
The authors would like to thank Ocean Networks Canada staff and scientists for their support and counsel, as well as the numerous technical and logistical staff, ship and ROV crews of all deployment and maintenance cruises along this decade.
The authors declare that the research was conducted in the absence of any commercial or financial relationships that could be construed as a potential conflict of interest.
All claims expressed in this article are solely those of the authors and do not necessarily represent those of their affiliated organizations, or those of the publisher, the editors and the reviewers. Any product that may be evaluated in this article, or claim that may be made by its manufacturer, is not guaranteed or endorsed by the publisher.
The Supplementary Material for this article can be found online at: https://www.frontiersin.org/articles/10.3389/fmars.2022.833617/full#supplementary-material
Aguzzi J., Albiez J., Flögel S., Godø O. R., Grimsbø E., Marini S., et al. (2020a). A flexible autonomous robotic observatory infrastructure for bentho-pelagic monitoring. Sensors 20, 1614. doi: 10.3390/s20061614
Aguzzi J., Chatzievangelou D., Company J. B., Thomsen L., Marini S., Bonofiglio F., et al. (2020b). The potential of video imagery from worldwide cabled observatory networks to provide information supporting fish-stock and biodiversity assessment. ICES J. Mar. Sci. 77, 2396–2410. doi: 10.1093/icesjms/fsaa169
Aguzzi J., Chatzievangelou D., Marini S., Fanelli E., Danovaro R., Flögel S., et al. (2019). New high-tech flexible networks for the monitoring of deep-sea ecosystems. Environ. Sci. Technol. 53, 6616–6631. doi: 10.1021/acs.est.9b00409
Aguzzi J., Costa C., Calisti M., Funari V., Stefanni S., Danovaro R., et al. (2021). Research trends and future perspectives in marine biomimicking robotics. Sensors 21, 3778. doi: 10.3390/s21113778
Aguzzi J., Flögel S., Marini S., Thomsen L., Albiez J., Weiss P., et al. (2022). Developing technological synergies between deep-sea and space research. Elem. Sci. Anth. 10, 00064. doi: 10.1525/elementa.2021.00064
Aguzzi J., Jamieson A. J., Fujii T., Sbragaglia V., Costa C., Menesatti P., et al. (2012). Shifting feeding behaviour of deep-sea buccinid gastropods at natural and simulated food falls. Mar. Ecol. Prog. Ser. 458, 247–253. doi: 10.3354/meps09758
Aguzzi J., Sardà F. (2008). A history of recent advancements on Nephrops norvegicus behavioral and physiological rhythms. Rev. Fish. Biol. Fisheries 18, 235–248. doi: 10.1007/s11160-007-9071-9
Amoroso R. O., Pitcher C. R., Rijnsdorp A. D., McConnaughey R. A., Parma A. M., Suuronen P., et al. (2018). Bottom trawl fishing footprints on the world’s continental shelves. Proc. Natl. Acad. Sci. U.S.A. 115, E10275–E10282. doi: 10.1073/pnas.1802379115
Assis J., Claro B., Ramos A., Boavida J., Serrão E. A. (2013). Performing fish counts with a wide-angle camera, a promising approach reducing divers' limitations. J. Exp. Mar. Biol. Ecol. 45, 93–98. doi: 10.1016/j.jembe.2013.04.007
Ayma A., Aguzzi J., Canals M., Lastras G., Bahamon N., Mecho A., et al. (2016). Comparison between ROV video and agassiz trawl methods for sampling deep water fauna of submarine canyons in the northwestern Mediterranean Sea with observations on behavioural reactions of target species. Deep-Sea Res. I 114, 149–159. doi: 10.1016/j.dsr.2016.05.013
Bailey D. M., Priede I. G. (2002). Predicting fish behaviour in response to abyssal food falls. Mar. Biol. 141, 831–840. doi: 10.1007/s00227-002-0891-9
Baker P., Minzlaff U., Schoenle A., Schwabe E., Hohlfeld M., Jeuck A., et al. (2018). Potential contribution of surface-dwelling Sargassum algae to deep-sea ecosystems in the southern north Atlantic. Deep-Sea Res. II 148, 21–34. doi: 10.1016/j.dsr2.2017.10.002
Bicknell A. W. J., Godley B. J., Sheehan E. V., Votier S. C., Witt M. J. (2016). Camera technology for monitoring marine biodiversity and human impact. Front. Ecol. Environ. 14, 424–432. doi: 10.1002/fee.1322
Bindoff N. L., Cheung W. W. L., Kairo J. G., Arístegui J., Guinder V. A., Hallberg R., et al. (2020). ““Changing ocean, marine ecosystems, and dependent communities“,” in IPCC special report on the ocean and cryosphere in a changing climate. Eds. Pörtner H. O., Roberts D. C., Masson-Delmotte V., Zhai P., Tignor M., Poloczanska E., et al (Geneva, Switzerland: IPCC).
Bohlen M. (1999). “"A robot in a cage-exploring interactions between animals and robots",” in Proceedings of the 1999 IEEE international symposium on computational intelligence in robotics and automation, CIRA'99 (Cat. No.99EX375) (Monterey, CA, USA: IEEE), 214–219. doi: 10.1109/CIRA.1999.810051
Bolgan M., Gervaise C., Di Iorio L., Lossent J., Lejeune P., Raick X., et al. (2020). Fish biophony in a Mediterranean submarine canyon. J. Acoust. Soc Am. 147, 2466–2477. doi: 10.1121/10.0001101
Borja A., Andersen J. H., Arvanitidis C. D., Basset A., Buhl-Mortensen L., Carvalho S., et al. (2020). Past and future grand challenges in marine ecosystem ecology. Front. Mar. Sci. 7. doi: 10.3389/fmars.2020.00362
Brandt A., Gutt J., Hildebrandt M., Pawlowski J., Schwendner J., Soltwedel T., et al. (2016). Cutting the umbilical: new technological perspectives in benthic deep-sea research. J. Mar. Sci. Eng. 4, 36. doi: 10.3390/jmse4020036
Bshary R., Côté I. M. (2008). ““New perspectives on marine cleaning mutualism“,” in Fish behaviour. Ed. Magnhagen C. (Boca Raton, FL, USA: CRC Press), 563–592. doi: 10.1201/b10757
Caine E. A. (1980). Ecology of two littoral species of caprellid amphipods (Crustacea) from Washington, USA. Mar. Biol. 56, 327–335. doi: 10.1007/BF00386871
Caine E. A. (1998). First case of caprellid amphipod-hydrozoan mutualism. J. Crustac. Biol. 18, 317–320. doi: 10.2307/1549325
Campbell M. D., Huddleston A., Somerton D., Clarke M. E., Wakefield W., Murawski S., et al. (2021). Assessment of attraction and avoidance behaviors of fish in response to the proximity of transiting underwater vehicles. Fish. Bull. 119, 216–230. doi: 10.7755/FB.119.4.2
Cappo M., Harvey E., Shortis M. (2006). “Counting and measuring fish with baited video techniques – an overview,” in Cutting-edge technologies in fish and fisheries science, ASFB 2006 workshop proceedings. Eds. Lyle J. M., Furlani D. M., Buxton C. D. (Hobart, Tasmania: Australian Society for Fish Biology), 101–144.
Chatzievangelou D., Aguzzi J., Ogston A., Suárez A., Thomsen L. (2020a). Visual monitoring of key deep-sea megafauna with an Internet operated crawler as a tool for ecological status assessment. Prog. Oceanogr. 184, 102321. doi: 10.1016/j.pocean.2020.102321
Chatzievangelou D., Aguzzi J., Scherwath M., Thomsen L. (2020bs). Quality control and pre-analysis treatment of the environmental datasets collected by an Internet operated deep-sea crawler during its entire 7-year long deployment, (2009–2016). Sensors 20, 2991. doi: 10.3390/s20102991
Chatzievangelou D., Doya C., Thomsen L., Purser A., Aguzzi J. (2016). High-frequency patterns in the abundance of benthic species near a cold-seep - an Internet operated vehicle application. PloS One 11, e0163808. doi: 10.1371/journal.pone.0163808
Chen C., Lin T.-H., Watanabe H. K., Akamatsu T., Kawagucci S. (2021). Baseline soundscapes of deep-sea habitats reveal heterogeneity among ecosystems and sensitivity to anthropogenic impacts. Limnol. Oceanogr. 66, 3714–3727. doi: 10.1002/lno.11911
Clinchy M., Sheriff M. J., Zanette L. Y. (2013). Predator-induced stress and the ecology of fear. Funct. Ecol. 27, 56–65. doi: 10.1111/1365-2435.12007
Côté I. M. (2000). “Evolution and ecology of cleaning symbioses in the sea,” in Oceanography and marine biology: An annual review, 38. Eds. Gibson R. N., Barnes M. (London: Taylor & Francis), 311–356. doi: 10.1201/b12589
Danovaro R., Corinaldesi C., Dell’Anno A., Snelgrove P. V. R. (2017). The deep-sea under global change. Curr. Biol. 27, R461–R465. doi: 10.1016/j.cub.2017.02.046
Danovaro R., Fanelli E., Aguzzi J., Billett D., Carugati L., Corinaldesi C., et al. (2020). Ecological variables for developing a global deep-ocean monitoring and conservation strategy. Nat. Ecol. Evol. 4, 181–192. doi: 10.1038/s41559-019-1091-z
Danovaro R., Gambi C., Dell'Anno A., Corinaldesi C., Fraschetti S., Vanreusel A., et al. (2008). Exponential decline of deep-sea ecosystem functioning linked to benthic biodiversity loss. Curr. Biol. 18, 1–8. doi: 10.1016/j.cub.2007.11.056
Danovaro R., Snelgrove P. V. R., Tyler P. (2014). Challenging the paradigms of deep-sea ecology. Trends Ecol. Evol. 29, 465–475. doi: 10.1016/j.tree.2014.06.002
Dew C. B. (2010). Podding behavior of adult king crab and its effect on abundance-estimate precision. In: Biology and management of exploited crab populations under climate change (Fairbanks, AK: Alaska Sea Grant, University of Alaska Fairbanks). Available at: http://www.alaskaseagrant.org/bookstore/pubs/AK-SG-10-01.html (Accessed October 28, 2021).
Díaz S., Settele J., Brondízio E. S., Ngo H. T., Guèze M., Agard J., et al. (2019). Summary for policymakers of the global assessment report on biodiversity and ecosystem services of the intergovernmental science-policy platform on biodiversity and ecosystem services (Bonn, Germany: IPBES Secretariat).
Dominguez-Carrió C., Fontes J., Morato T. (2021). A cost-effective video system for a rapid appraisal of deep-sea benthic habitats: The azor drift-cam. Methods Ecol. Evol. 12, 1379–1388. doi: 10.1111/2041-210X.13617
Doya C., Aguzzi J., Chatzievangelou D., Costa C., Company J. B., Tunnicliffe V. (2016). The seasonal use of small-scale space by benthic species in a transiently hypoxic area. J. Mar. Syst. 154, 280–290. doi: 10.1016/j.jmarsys.2015.09.005
Doya C., Aguzzi J., Pardo M., Matabos M., Company J. B., Costa C., et al. (2014). Diel behavioral rhythms in sablefish (Anoplopoma fimbria) and other benthic species as recorded by the deep-sea cabled observatories in barkley canyon (NEPTUNE-Canada). J. Mar. Syst. 130, 69–78. doi: 10.1016/j.jmarsys.2013.04.003
Doya C., Chatzievangelou D., Bahamon N., Purser A., De Leo F., Juniper K., et al. (2017). Seasonal monitoring of deep-sea cold-seep benthic communities using an Internet operated vehicle (IOV). PloS One 12, e0176917. doi: 10.1371/journal.pone.0176917
Dumke I., Purser A., Marcon Y., Nornes S. M., Johnsen G., Ludvigsen M., et al. (2018). Underwater hyperspectral imaging as an in situ taxonomic tool for deep-sea megafauna. Sci. Rep. 8, 12860. doi: 10.1038/s41598-018-31261-4
Dunlop K. M., Van Oevelen D., Ruhl H. A., Huffard C. L., Kuhnz L. A., Smith K. L. Jr. (2016). Carbon cycling in the deep eastern north pacific benthic food web: Investigating the effect of organic carbon input. Limnol. Oceanogr. 61, 1956–1968. doi: 10.1002/lno.10345
Edwards J. S. (1972). Limb loss and regeneration in two crabs: The king crab Paralithodes camtschatica and the tanner crab Chionoecetes bairdi. Acta Zool. 53, 105–112. doi: 10.1111/j.1463-6395.1972.tb00577.x
Falahzadeh A., Aguzzi J., Chatzievangelou D., Nogueras M., Martínez E., Toma D., et al. in review. A new coastal crawler prototype for the internet-operated ecological monitoring. Sensors.
Fanelli E., Bianchelli S., Foglini F., Canals M., Castellan G., Güell-Bujons Q., et al. (2021). Identifying priorities for the protection of deep Mediterranean Sea ecosystems through an integrated approach. Front. Mar. Sci. 8. doi: 10.3389/fmars.2021.698890
Feekings J., O’Neill F. G., Krag L., Ulrich C., Veiga Malta T. (2019). An evaluation of European initiatives established to encourage industry-led development of selective fishing gears. Fish. Manage. Ecol. 26, 650–660. doi: 10.1111/fme.12379
Fleming P. A., Muller D., Bateman P. W. (2007). Leave it all behind: a taxonomic perspective of autotomy in invertebrates. Biol. Rev. 82, 481–510. doi: 10.1111/j.1469-185X.2007.00020.x
Flögel S., Ahrns I., Nuber C., Hildebrandt M., Duda A., Schwendner J., et al. (2018). “A new deep-sea crawler system - MANSIO-VIATOR,” in 2018 OCEANS - MTS/IEEE Kobe techno-oceans (OTO) (Kobe, Japan: IEEE), 1–10. doi: 10.1109/OCEANSKOBE.2018.8559368
Gann G. D., McDonald T., Walder D., Aronson J., Nelson C. R., Jonson J., et al. (2019). International principles and standards for the practice of ecological restoration. second edition. Restor. Ecol. 27, S1–S46. doi: 10.1111/rec.13035
Garnier S., Jost C., Gautrais J., Asadpour M., Caprari G., Jeanson R., et al. (2008). The embodiment of cockroach aggregation behavior in a group of micro-robots. Artif. Life 14, 387–3408. doi: 10.1162/artl.2008.14.4.14400
Gates A. R., Horton T., Serpell-Stevens A., Chandler C., Grange L. J., Robert K., et al. (2019). Ecological role of an offshore industry artificial structure. Front. Mar. Sci. 6. doi: 10.3389/fmars.2019.00675
Geoffroy M., Langbehn T., Priou P., Varpe Ø., Johnsen G., Le Bris A., et al. (2021). Pelagic organisms avoid white, blue, and red artificial light from scientific instruments. Sci. Rep. 11, 14941. doi: 10.1038/s41598-021-94355-6
Haselmair A., Stachowitsch M., Zuschin M., Riedel B. (2010). Behaviour and mortality of benthic crustaceans in response to experimentally induced hypoxia and anoxia in situ. Mar. Ecol. Prog. Ser. 414, 195–208. doi: 10.3354/meps08657
Hawkins A. D., Pembroke A. E., Popper A. N. (2015). Information gaps in understanding the effects of noise on fishes and invertebrates. Rev. Fish Biol. Fisheries 25, 39–64. doi: 10.1007/s11160-014-9369-3
Heiskanen A.-S., Berg T., Uusitalo L., Teixeira H., Bruhn A., Krause-Jensen D., et al. (2016). Biodiversity in marine ecosystems–European developments toward robust assessments. Front. Mar. Sci. 3. doi: 10.3389/fmars.2016.00184
Henschke N., Bowden D. A., Everett J. D., Holmes S. P., Kloser R. J., Lee R. W., et al. (2013). Salp-falls in the Tasman Sea: a major food input to deep-sea benthos. Mar. Ecol. Prog. Ser. 491, 165–175. doi: 10.3354/meps10450
Hewitt J. E., Thrush S. F., Halliday J., Duffy C. (2005). The importance of small-scale habitat structure for maintaining beta diversity. Ecology 86, 1619–1626. doi: 10.1890/04-1099
Howell K. L., Hilário A., Allcock A. L., Bailey D. M., Baker M., Clark M. R., et al. (2020). A blueprint for an inclusive, global deep-sea ocean decade field program. Front. Mar. Sci. 7. doi: 10.3389/fmars.2020.584861
Jamieson A. J., Boorman B., Jones D. O. B. (2013). “Deep-sea benthic sampling,” in Methods for the study of marine benthos, 4th Edn. Ed. Eleftheriou A. (Oxford: Wiley Blackwell), 285–347. doi: 10.1002/9781118542392.ch7
Jones D. O. B., Gates A. R., Huvenne V. A. I., Phillips A. B., Bett B. J. (2019). Autonomous marine environmental monitoring: Application in decommissioned oil fields. Sci. Total Environ. 668, 835–853. doi: 10.1016/j.scitotenv.2019.02.310
Juniper S. K., Matabos M., Mihály S., Ajayamohan R. S., Gervais F., Bui A. O. V. (2013). A year in barkley canyon: A time-series observatory study of mid-slope benthos and habitat dynamics using the NEPTUNE Canada network. Deep-Sea Res. II 92, 114–123. doi: 10.1016/j.dsr2.2013.03.038
Katsanevakis S., Verriopoulos G. (2004). Den ecology of Octopus vulgaris cuvier 1797, On soft sediment: availability and types of shelter. Sci. Mar. 68, 147–157. doi: 10.3989/scimar.2004.68n1147
Katz T., Yahel G., Reidenbach M., Tunnicliffe V., Herut B., Crusius J., et al. (2012). Resuspension by fish facilitates the transport and redistribution of coastal sediments. Limnol. Oceanogr. 57, 945–958. doi: 10.4319/lo.2012.57.4.0945
Krieger K. J. (1997). “Sablefish, anoplopoma fimbria, observed from a manned submersible,” in Biology and management of sablefish, anoplopoma fimbria, papers from the international symposium on the biology and management of sablefish, NOAA tech. rep. NMFS, 130. Eds. Wilkins M. E., Saunders M. W. (Seattle, WA, USA: NOAA), 39–43.
Løkkeborg S., Olla B. L., Pearson W. H., Davis M. W. (1995). Behavioural responses of sablefish, Anoplopoma fimbria, to bait odour. J. Fish Biol. 46, 142–155. doi: 10.1111/j.1095-8649.1995.tb05953.x
Levin L. A., Dayton P. K. (2009). Ecological theory and continental margins: where shallow meets deep. Trends Ecol. Evol. 24, 606–617. doi: 10.1016/j.tree.2009.04.012
Lin T. H., Chen C., Watanabe H. K., Kawagucci S., Yamamoto H., Akamatsu T. (2019). Using soundscapes to assess deep-sea benthic ecosystems. Trends Ecol. Evol. 34, 1066–1069. doi: 10.1016/j.tree.2019.09.006
Madricardo F., Ghezzo M., Nesto N., McKiver W. J., Faussone G. C., Fiorin R., et al. (2020). How to deal with seafloor marine litter: An overview of the state-of-the-art and future perspectives. Front. Mar. Sci. 7. doi: 10.3389/fmars.2020.505134
Marini S., Gjeci N., Govindaraj S., But A., Sportich B., Ottaviani E., et al. (2020). ENDURUNS: An integrated and flexible approach for seabed survey through autonomous mobile vehicles. J. Mar. Sci. Eng. 8, 633. doi: 10.3390/jmse8090633
McClain C. R., Filler R., Auld J. R. (2014). Does energy availability predict gastropod reproductive strategies? Proc. R. Soc B 281, 20140400. doi: 10.1098/rspb.2014.0400
McLean D. L., Parsons M. J. G., Gates A. R., Benfield M. C., Bond T., Booth D. J., et al. (2020). Enhancing the scientific value of industry remotely operated vehicles (ROVs) in our oceans. Front. Mar. Sci. 7. doi: 10.3389/fmars.2020.00220
Mecho A., Francescangeli M., Ercilla G., Fanelli E., Estrada F., Valencia J., et al. (2020). Deep-sea litter in the gulf of cadiz (Northeastern Atlantic, Spain). Mar. Pollut. Bull. 153, 110969. doi: 10.1016/j.marpolbul.2020.110969
Mecho A., Sellanes J., Aguzzi A. (2021). Seafloor litter at oceanic islands and seamounts of the southeastern pacific. Mar. Pollut. Bull. 170, 112641. doi: 10.1016/j.marpolbul.2021.112641
Melo-Merino S. M., Reyes-Bonilla H., Lira-Noriega A. (2020). Ecological niche models and species distribution models in marine environments: A literature review and spatial analysis of evidence. Ecol. Modell. 415, 108837. doi: 10.1016/j.ecolmodel.2019.1088
Mirimin L., Desmet S., López Romero D., Fernandez Fernandez S., Miller D. L., Mynott S., et al. (2021). Don't catch me if you can – using cabled observatories as multidisciplinary platforms for marine fish community monitoring: An in situ case study combining underwater video and environmental DNA data. Sci. Total Environ. 773, 145351. doi: 10.1016/j.scitotenv.2021.145351
Nowald N., Fischer G., Ratmeyer V., Iversen M., Reuter C., Wefer G. (2009). “In-situ sinking speed measurements of marine snow aggregates acquired with a settling chamber mounted to the Cherokee ROV,” in 2009 OCEANS – EUROPE (Bremen, Germany: IEEE), 1–6. doi: 10.1109/OCEANSE.2009.5278186
Ogston A. S., Drexler T. M., Puig P. (2008). Sediment delivery, resuspension, and transport in two contrasting canyon environments in the southwest gulf of lions. Cont. Shelf Res. 28, 2000–2016. doi: 10.1016/j.csr.2008.02.012
Ozhikandathil J., Badilescu S., Packirisamy M. (2017). A brief review on microfluidic platforms for hormones detection. J. Neural Transm. 124, 47–55. doi: 10.1007/s00702-016-1610-x
Pauli J. N., Whiteman J. P., Riley M. D., Middleton A. R. (2010). Defining noninvasive approaches for sampling of vertebrates. Conserv. Biol. 24, 349–352. doi: 10.1111/j.1523-1739.2009.01298.x
Pedersen M. F., Filbee-Dexter K., Frisk N. L., Sárossy Z., Wernberg T. (2021). Carbon sequestration potential increased by incomplete anaerobic decomposition of kelp detritus. Mar. Ecol. Prog. Ser. 660, 53–67. doi: 10.3354/meps13613
Pham C. K., Ramirez-Llodra E., Alt C. H. S., Amaro T., Bergmann M., Canals M., et al. (2014). Marine litter distribution and density in European seas, from the shelves to deep basins. PloS One 9, e95839. doi: 10.1371/journal.pone.0095839
Phillips B. T., Grubber D. F., Vasan G., Roman C. N., Pieribone V. A., Sparks J. S. (2016). Observations of in situ deep-sea marine bioluminescence with a high-speed, high-resolution sCMOS camera. Deep-Sea Res. I 111, 102–109. doi: 10.1016/j.dsr.2016.02.012
Picardi G., Chellapurath M., Iacoponi S., Stefanni S., Laschi C., Calisti M. (2020). Bioinspired underwater legged robot for seabed exploration with low environmental disturbance. Sci. Robot. 5, eaaz1012. doi: 10.1126/scirobotics.aaz1012
Pierdomenico M., Casalbore D., Chiocci F. L. (2019). Massive benthic litter funnelled to deep sea by flash-flood generated hyperpycnal flows. Sci. Rep. 9, 5330. doi: 10.1038/s41598-019-41816-8
Polverino G., Porfiri M. (2013). Zebrafish (Danio rerio) behavioural response to bioinspired robotic fish and mosquitofish (Gambusia affinis). Bioinspir. Biomim. 8, 44001. doi: 10.1088/1748-3182/8/4/044001
Priede I. G., Merrett N. R. (1996). Estimation of abundance of abyssal demersal fishes; a comparison of data from trawls and baited cameras. J. Fish Biol. 49, 207–216. doi: 10.1111/j.1095-8649.1996.tb06077.x
Purser A., Thomsen L., Hofbauer M., Menzel M., Wagner H., Chapman R., et al. (2013). Temporal and spatial benthic data collection via Internet operated deep Sea crawler. Methods Oceanogr. 5, 1–18. doi: 10.1016/j.mio.2013.07.001
Riedel B., Pados T., Pretterebner K., Schiemer L., Steckbauer A., Haselmair A., et al. (2014). Effect of hypoxia and anoxia on invertebrate behaviour: ecological perspectives from species to community level. Biogeosciences 11, 1491–1518. doi: 10.5194/bg-11-1491-2014
Robert K., Juniper S. K. (2012). Surface-sediment bioturbation quantified with cameras on the NEPTUNE Canada cabled observatory. Mar. Ecol. Prog. Ser. 453, 137–149. doi: 10.3354/meps09623
Robison B. H., Reisenbichler K. R., Sherlock R. E. (2017). The coevolution of midwater research and ROV technology at MBARI. Oceanography 30, 26–37. doi: 10.5670/oceanog.2017.421
Ros M., Guerra-García J. M. (2012). On the occurrence of the tropical caprellid paracaprella pusilla mayer 1890 (Crustacea: Amphipoda) in Europe. Mediterr. Mar. Sci. 13, 134–139. doi: 10.12681/mms.30
Rountree R. A., Aguzzi J., Marini S., Fanelli E., De Leo F. C., Del Rio J., et al. (2020). ““Towards an optimal design for ecosystem level ocean observatories,”,” in Oceanography and marine biology: An annual review, 58. Eds. Hawkins S. J., Allcock A. L., Bates A. E., Evans A. J., Firth L. B., McQuaid C. D., et al (London: Taylor & Francis CRC Press), 79–106. doi: 10.1201/9780429351495-2
Ryabinin V., Barbière J., Haugan P., Kullenberg G., Smith N., McLean C., et al. (2019). The UN decade of ocean science for sustainable development. Front. Mar. Sci. 6. doi: 10.3389/fmars.2019.00470
Ryer C. H., Olla B. L. (1999). Light-induced changes in the prey consumption and behavior of two juvenile planktivorous fish. Mar. Ecol. Prog. Ser. 181, 41–51. doi: 10.3354/meps181041
Ryer C. H., Stoner A. W., Iseri P. J., Spencer M. L. (2009). Effects of simulated underwater vehicle lighting on fish behavior. Mar. Ecol. Prog. Ser. 391, 97–106. doi: 10.3354/meps08168
Sbragaglia V., Jolles J. W., Coll M., Arlinghaus R. (2021). Fisheries-induced changes of shoaling behaviour: mechanisms and potential consequences. Trends Ecol. Evol. 36, 885–888. doi: 10.1016/j.tree.2021.06.015
Sbragaglia V., Leiva D., Arias A., García J. A., Aguzzi J., Breithaupt T. (2017). Fighting over burrows: the emergence of dominance hierarchies in the Norway lobster (Nephrops norvegicus). J. Exp. Biol. 220, 4624–4633. doi: 10.1242/jeb.165969
Sibuet M., Juniper K. S., Pautot G. (1988). Cold-seep benthic communities in the Japan subduction zones: Geological control of community development. J. Mar. Sci. 46, 333–348. doi: 10.1357/002224088785113595
Sigler M. F. (2000). Abundance estimation and capture of sablefish (Anoplopoma fimbria) by longline gear. Can. J. Fish. Aquat. Sci. 57, 1270–1283. doi: 10.1139/f00-066
Smith K. L. Jr., Sherman A. D., Huffard C. L., McGill P. R., Henthorn R. G., Von Thun S., et al. (2014). Large Salp bloom export from the upper ocean and benthic community response in the abyssal northeast pacific: Day to week resolution. Limnol. Oceanogr. 59, 745–757. doi: 10.4319/lo.2014.59.3.0745
Smith K. L. Jr., Sherman A. D., McGill P. R., Henthorn R. G., Ferreira J., Connolly T. P., et al. (2021). Abyssal benthic rover, an autonomous vehicle for long-term monitoring of deep-ocean processes. Sci. Robot. 6, eaaz1012. doi: 10.1126/scirobotics.abl4925
Stankowich T., Blumstein D. T. (2005). Fear in animals: a meta-analysis and review of risk assessment. Proc. R. Soc B 272, 2627–2634. doi: 10.1098/rspb.2005.3251
Stratmann T., Mevenkamp L., Sweetman A. K., Vanreusel A., Van Oevelen D. (2018). Has phytodetritus processing by an abyssal soft-sediment community recovered 26 years after an experimental disturbance? Front. Mar. Sci. 5. doi: 10.3389/fmars.2018.00059
Sweetman A. K., Chapman A. (2011). First observations of jelly-falls at the seafloor in a deep-sea fjord. Deep-Sea Res. I 58, 1206–1211. doi: 10.1016/j.dsr.2011.08.006
Sweetman A. K., Smith C. R., Dale T., Jones D. O. B. (2014). Rapid scavenging of jellyfish carcasses reveals the importance of gelatinous material to deep-sea food webs. Proc. R. Soc B. 281, 20142210. doi: 10.1098/rspb.2014.2210
Thomsen L., Aguzzi J., Costa C., De Leo F., Ogston A., Purser A. (2017). The oceanic biological pump: rapid carbon transfer to depth at continental margins during winter. Sci. Rep. 7, 10763. doi: 10.1038/s41598-017-11075-6
Thomsen L., Barnes C., Best M., Chapman R., Pirenne B., Thomson R., et al. (2012). Ocean circulation promotes methane release from gas hydrate outcrops at the NEPTUNE Canada barkley canyon node. Geophys. Res. Lett. 39, L16605. doi: 10.1029/2012GL052462
Thomsen L., Gust G. (2000). Sediment erosion thresholds and characteristics of resuspended aggregates on the western European continental margin. Deep-Sea Res. I 47, 1881–1897. doi: 10.1016/S0967-0637(00)00003-0
Van Nugteren P., Moodley L., Brummer G. J., Heip C. H. R., Herman P. M. J., Middelburg J. J. (2009). Seafloor ecosystem functioning: the importance of organic matter priming. Mar. Biol. 156, 2277–2287. doi: 10.1007/s00227-009-1255-5
Villanueva R. (2000). Observations on the behaviour of the cirrate octopod Opisthoteuthis grimaldii (Cephalopoda). J. Mar. Biol. Ass. UK 80, 555–556. doi: 10.1017/s0025315400002307
Watanabe J., Shao Y., Miura N. (2019). Underwater and airborne monitoring of marine ecosystems and debris. J. Appl. Remote Sens. 13, 44509. doi: 10.1117/1.JRS.13.044509
Wehde H., Thomsen L., Pfannkuche O., Albiez J., Flögel S., Godø O. R., et al. (2019). “A flexible autonomous bottom resident infrastructure for bentho-pelagic monitoring,” in Proceedings of the 2019 IMEKO TC-19 international workshop on metrology for the Sea (Genoa, Italy. Budapest, Hungary: IMEKO), 156–160.
Widder E. A., Robison B. H., Reisenbichler K. R., Haddock S. H. D. (2005). Using red light for in situ observations of deep-sea fishes. Deep-Sea Res. I 52, 2077–2085. doi: 10.1016/j.dsr.2005.06.007
Zhang Y., Zhang Q., Zhang A., Sun Y., He Z. (2021). ““Development and experiments of a novel deep-sea resident ROV”,” in Proceedings of the 2021 6th international conference on control and robotics engineering (ICCRE) (Beijing, China: IEEE), 129–134. doi: 10.1109/ICCRE51898.2021.9435653
Zheng L. W., Ding X., Liu J. T., Li D., Lee T. Y., Zheng X., et al. (2017). Isotopic evidence for the influence of typhoons and submarine canyons on the sourcing and transport behavior of biospheric organic carbon to the deep sea. Earth Planet. Sci. Lett. 465, 103–111. doi: 10.1016/j.epsl.2017.02.037
Keywords: internet operated vehicle, crawler, intelligent marine monitoring, (semi-) permanent mobile robotic platforms, remarkable observations, animal behavior, ecological footprint
Citation: Chatzievangelou D, Thomsen L, Doya C, Purser A and Aguzzi J (2022) Transects in the deep: Opportunities with tele-operated resident seafloor robots. Front. Mar. Sci. 9:833617. doi: 10.3389/fmars.2022.833617
Received: 11 December 2021; Accepted: 01 August 2022;
Published: 29 August 2022.
Edited by:
Juan José Dañobeitia, ERIC foundation, ItalyReviewed by:
Jan Marcin Weslawski, Institute of Oceanology, Polish Academy of Sciences, PolandCopyright © 2022 Chatzievangelou, Thomsen, Doya, Purser and Aguzzi. This is an open-access article distributed under the terms of the Creative Commons Attribution License (CC BY). The use, distribution or reproduction in other forums is permitted, provided the original author(s) and the copyright owner(s) are credited and that the original publication in this journal is cited, in accordance with accepted academic practice. No use, distribution or reproduction is permitted which does not comply with these terms.
*Correspondence: Damianos Chatzievangelou, ZGFtaWFub3NAaWNtLmNzaWMuZXM=; Laurenz Thomsen, bGF1cmVuei50aG9tc2VuQGd1LnNl
†Last active affiliation
Disclaimer: All claims expressed in this article are solely those of the authors and do not necessarily represent those of their affiliated organizations, or those of the publisher, the editors and the reviewers. Any product that may be evaluated in this article or claim that may be made by its manufacturer is not guaranteed or endorsed by the publisher.
Research integrity at Frontiers
Learn more about the work of our research integrity team to safeguard the quality of each article we publish.