- 1Department of Biological Sciences, Pusan National University, Busan, South Korea
- 2College of Marine Science, Hainan University, Haikou, China
Because sexual reproduction is essential for the establishment and persistence of seagrass meadows, flowering intensity is an important trait that influences the resilience and stability of seagrass populations. Although the effects of excessive coastal nutrient loading on seagrass vegetative growth have been extensively documented, the effects on seagrass reproductive phenology and intensity remain unclear. To examine the reproductive responses of seagrass populations to increased coastal nutrient loading, the flowering phenology and intensity of Zostera marina were compared between sites with high-nutrient, low-light conditions (Deukryang Bay and Dongdae Bay) and low-nutrient, high-light conditions (Koje Bay) on the southern coast of Korea. Nutrient contents of the above- and below-ground tissues of Z. marina reflected in situ nutrient and light availability at the study sites. Reproductive shoot density and biomass, as well as flowering frequency and reproductive effort, were much higher (1.5–4.6-fold) at the high-nutrient, low-light study sites of Deukryang Bay and Dongdae Bay than at the low-nutrient, high-light site of Koje Bay. Consequently, potential seed production was higher in Deukryang Bay and Dongdae Bay than in Koje Bay. Chronic high-nutrient and low-light conditions significantly increased the reproductive intensity of Z. marina, supporting the persistence and resilience of Z. marina populations. The results of this study could provide insights into the conservation and management of seagrass meadows under increased coastal nutrient loading.
Introduction
Seagrasses are marine flowering plants forming persistent meadows in shallow coastal areas, which provide numerous ecological functions in the marine environment (Duarte, 2002; Orth et al., 2006; Fourqurean et al., 2012; Olsen et al., 2016; Yue et al., 2020; Johnson et al., 2021; Pazzaglia et al., 2021). However, seagrass meadows are among the most threatened ecosystems on the planet, because they have been disappearing worldwide at an accelerating loss rate of approximately 7% per year since 1990 (Waycott et al., 2009). Water quality degradation, driven by anthropogenic disturbance such as coastal development or nutrient input, has been largely responsible for the losses thus far (Hemminga and Duarte, 2000; Duarte, 2002; Orth et al., 2006; Fraser and Kendrick, 2017; Moreno-Marín et al., 2018; Thomsen et al., 2020; Rodemann et al., 2021). Coastal eutrophication caused by anthropogenic nutrient overloading via increased anthropogenic N deposition, benthic nutrient release from aquacultural sediments, and nutrient runoff from agricultural lands, can lead to the deterioration of seagrass ecosystems via increased light attenuation and harmful algae blooms (Duarte, 2002; Lee, 2004; Orth et al., 2006; Burkholder et al., 2007; Lee et al., 2007b; Waycott et al., 2009; Fraser and Kendrick, 2017; Lee et al., 2018; Qin et al., 2021). Although excessive nutrient enrichment in coastal areas leads to reduced underwater light availability through the overgrowth of epiphytes, macroalgae, and phytoplankton, consequently decreasing the growth and survival of seagrasses (Short et al., 1995; Lee et al., 2007b; Fraser and Kendrick, 2017; Moreno-Marín et al., 2018), moderate increases in surrounding nutrient availability may stimulate seagrass performance under pristine conditions (Udy and Dennison, 1997; Armitage et al., 2005; Burkholder et al., 2007; Moreno-Marín et al., 2018; Qin et al., 2021).
Seagrasses can reproduce both asexually via clonal growth and sexually via flowering and seed production (den Hartog, 1970). Although asexual reproduction is the dominant process that sustains seagrass populations, sexual reproduction through seedling recruitment (via germination of the sediment seed bank) is critical for rapid recolonization and meadow extension after natural and anthropogenic disturbances (Inglis, 2000; Plus et al., 2003; Greve et al., 2005; Lee et al., 2007a; Jarvis and Moore, 2010; Kendrick et al., 2012; Jarvis et al., 2014; Kim et al., 2014). Recent molecular studies have highlighted the essential role of sexual reproduction in the maintenance and persistence of seagrass habitat through genetic diversity enhancement in seagrass populations (Kendrick et al., 2012; Kendrick et al., 2017; Kim et al., 2019; Zhang et al., 2020a; Pazzaglia et al., 2021). Thus, sexual reproduction plays significant roles in assuring the stability and resilience of seagrass populations in dynamic coastal environments (Rasheed, 2004; Lee et al., 2007a; Macreadie et al., 2014; Suonan et al., 2017; Zhang et al., 2020b; Johnson et al., 2021). Because ecosystem services provided by seagrass meadows are threatened by increasing levels of natural and anthropogenic disturbance, it is evolutionarily and ecologically important to understand the dependencies of sexual reproduction, especially flowering intensity and seed production, on the resistance of seagrass populations to disturbance (Johnson et al., 2020; Qin et al., 2021). Reproductive intensity—assessed by measuring flowering phenology, flowering frequency, and seed production—could influence the health and resilience of seagrass populations by supporting seedling recruitment and increasing genetic diversity (Kendrick et al., 2012; Smith et al., 2016; Kim et al., 2019; Zhang et al., 2020b). Flowering phenology and relative investment in asexual versus sexual reproduction of seagrasses vary significantly within and among geographic locations depending on local environmental conditions (e.g., water temperature, nutrient availability, and light intensity) (Phillips et al., 1983; Cabaço and Santos, 2012; Kendrick et al., 2012; Kim et al., 2014; Suonan et al., 2017; Blok et al., 2018; Qin et al., 2020a; Qin et al., 2020b; Qin et al., 2021). Consequently, seagrass generally displays variability in reproductive intensity across environmental gradients (Darnell and Dunton, 2017; Johnson et al., 2017; Johnson et al., 2020; von Staats et al., 2020).
Reproductive plasticity, which usually pronounced under contrasting environmental conditions, is an important trait for the maintenance of seagrass meadows (Harper, 1977; Darnell and Dunton, 2017; Johnson et al., 2020). Water temperature has been considered the major factor that controls flowering phenology and intensity in seagrass populations; seasonal temperature anomalies, latitude-induced temperature differences, elevated sea surface temperature, and other thermal stress conditions affect the formation and development of seagrass flowering shoots (Diaz-Almela et al., 2007; Potouroglou et al., 2014; Zimmerman et al., 2017; Blok et al., 2018; Moreno-Marín et al., 2018; Qin et al., 2020a; Qin et al., 2020b). According to the results of sediment nutrient enrichment experiments, nutrient availability may affect the reproductive strategies of seagrasses, but the patterns of flowering intensity and potential seed production along nutrient gradients are unclear (van Lent et al., 1995; Darnell and Dunton, 2017; Jackson et al., 2017; Johnson et al., 2017; Qin et al., 2021). Although numerous nutrient enrichment experiments have been performed in situ or in mesocosms to examine the relationships between seagrass vegetative growth and nutrient conditions (Peralta et al., 2003; Lee et al., 2007b; Carroll et al., 2008; Leoni et al., 2008), few studies have provided observational evidence of reproductive plasticity in response to in situ nutrient conditions. Furthermore, light availability may regulate sexual reproduction of seagrasses (Kim et al., 2014; Johnson et al., 2017; Peñalver et al., 2020), but few studies have demonstrated reproductive responses of seagrasses to chronic low-light conditions. Thus, the roles of these environmental factors in controlling seagrass reproductive phenology and intensity continue to be debated. Since increased nutrient availability and reduced light may directly influence the maintenance and resilience of seagrass populations by altering the growth and reproduction of seagrasses, the examination of seagrass reproductive responses to high-nutrient and low-light conditions (observable at early stages of coastal eutrophication) is essential for effective management and protection of seagrass ecosystems affected by ongoing anthropogenic disturbance.
The goal of this study was to investigate changes in sexual reproduction of the seagrass Zostera marina in response to high-nutrient and low-light conditions at natural ecosystems with increased coastal nutrient loading. Flowering phenology, flowering frequency, reproductive effort, and potential seed production of Z. marina populations at study sites with higher nutrient and lower light levels (Deukryang Bay and Dongdae Bay) were compared with those characteristics measured at a study site with lower nutrient and higher light levels (Koje Bay) on the southern coast of Korea. We hypothesized that Z. marina populations grown under high-nutrient and low-light conditions, representing an early stage of coastal eutrophication, would increase their reproductive intensity to ensure the persistence of seagrass meadows through enhancing population resilience under these stressful conditions.
Materials and Methods
Study Site
Three study sites were selected in Deukryang Bay (34°26′N, 126°54′E), Dongdae Bay (34°53′N, 128°01′E), and Koje Bay (34°48′N, 128°35′E) on the southern coast of Korea (Figure 1). Z. marina was the dominant seagrass species at all three study sites. In Deukryang Bay and Dongdae Bay, Z. marina formed monospecific meadows covering areas of approximately 4.5 km2 and 1.6 km2, respectively. In contrast, three seagrass species (Zostera japonica, Z. marina, and Zostera caespitosa) were distributed sequentially from the intertidal zone to a depth of approximately 4 m below mean lower low water (MLLW) in Koje Bay, with Z. marina coverage of 0.8 km2. Z. marina was distributed at water depths of 2–3 m in Deukryang Bay and 1.5–2 m at the other two study sites. All sampling was carefully conducted in selected monospecific Z. marina meadows at the three study sites. The sediments in Deukryang Bay and Dongdae Bay were characterized by high mud content (11.8% sand and 88.2% silt + clay in Deukryang Bay; 7.6% sand and 92.4% silt + clay in Dongdae Bay), while the sediments in Koje Bay had a high sand content (87.9% sand and 12.1% silt + clay). Large-scale shellfish and seaweed farming is present in the shallow waters of Deukryang Bay, where local residents also participate in seagrass-dependent fisheries (Cho et al., 1982; Kim et al., 2015). Deukryang Bay is in an early stage of eutrophication caused by excessive nutrient loading from land sources since the 1990s (Cho et al., 1982; Cho, 1991; Kang et al., 1999). Many oyster and fish farms are located in adjacent waters of the Dongdae Bay site, where discharges of sewage from local residences and freshwater from land also occur (Kwak and Cho, 2020; Choi et al., 2020). The Z. marina meadow in Koje Bay is well protected, and no sewage or industrial waste inputs were observed in this area (Lee et al., 2019).
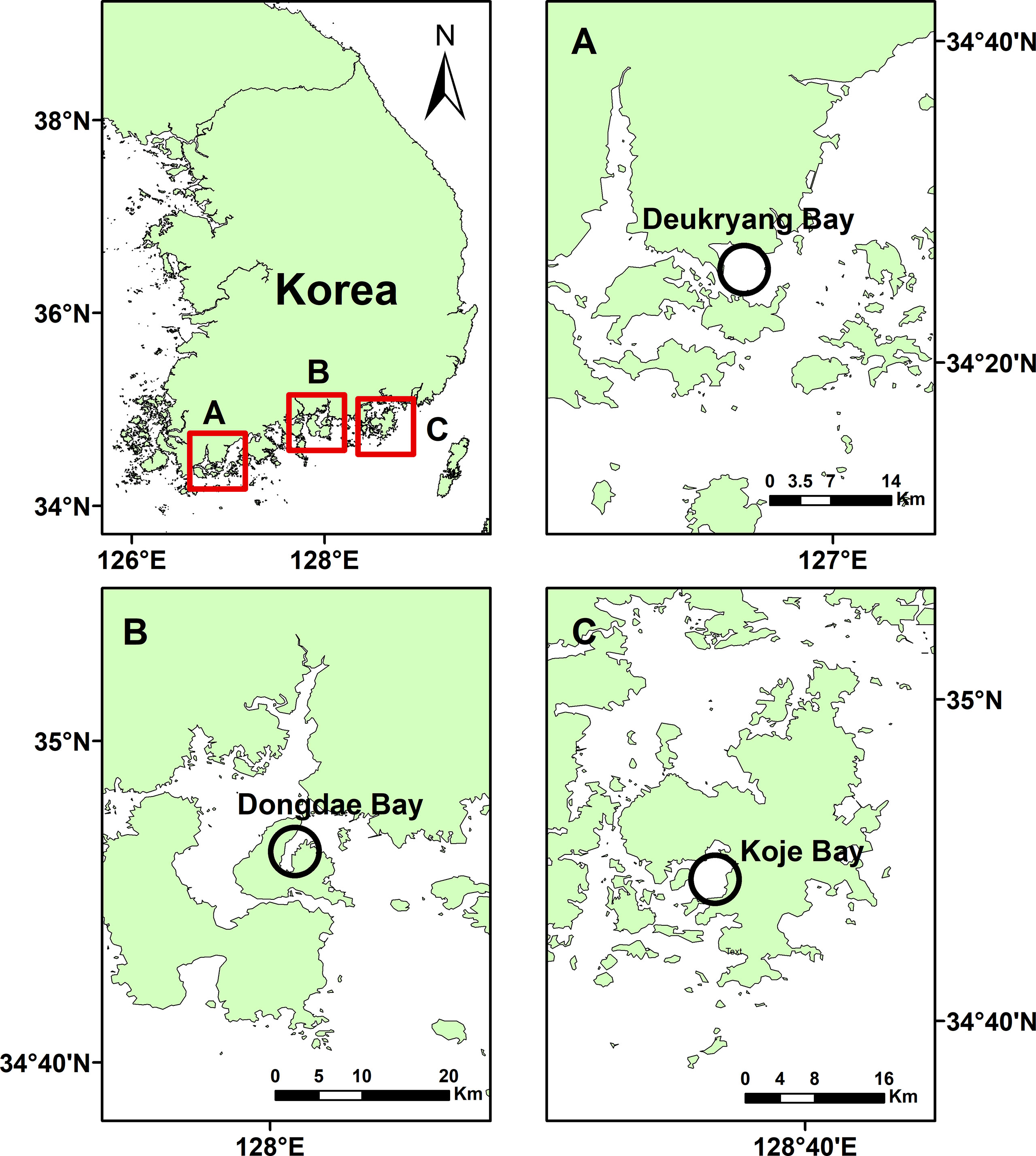
Figure 1 Map showing the three study sites in Deukryang Bay (A), Dongdae Bay (B), and Koje Bay (C) on the southern coast of Korea.
Environmental Parameters
Water temperature was monitored every 15-min interval at each study site using a Hobo data logger (Onset Computer Corp., Bourne, MA, USA) during the experimental period from January 2018 to December 2019. Underwater photosynthetic photon flux density (PPFD) was monitored at 15-min intervals using an Odyssey photosynthetic irradiance recording system (Dataflow Systems Ltd., Christchurch, New Zealand). Water temperature was averaged daily. The Odyssey logger was calibrated using an LI-1400 data logger and an LI-193SA spherical quantum sensor (Li-Cor, Lincoln, NE, USA). Daily irradiance (mol photons m−2 d−1) was calculated as the sum of the quantum flux over each 24-h period. The duration of irradiance above the compensation point (Hcomp) and duration of irradiance above the light saturation point (Hsat) were estimated based on the compensation irradiance of 20 μmol photons m−2 s−1 and saturation irradiance of 100 μmol photons m−2 s−1 required for photosynthesis of Z. marina (Lee et al., 2007b).
In each sampling month, four water column samples were collected using 50-mL polypropylene bottles from each study site for analysis of nutrient concentrations. Sediment samples were collected in the vicinity of a randomly thrown quadrat for Z. marina shoot sampling using a plexiglass corer (5-cm diameter, 10-cm length; n = 4) at each study site for determination of nutrient concentrations in sediment pore water. Sediment pore water was obtained through centrifugation (8000 × g for 20 min), and pore water nutrient concentrations were determined after dilution with low-nutrient seawater (< 0.1 μM). Dissolved inorganic nitrogen (i.e., and + ) and concentrations in both the water column and sediment pore water were measured using standard colorimetric techniques (Parsons et al., 1984). The + concentration was determined after running samples through a column containing copper-coated cadmium, which reduces to . Annual occurrences of red tide events in adjacent water of the three study sites were obtained from annual reports by the National Institute of Fisheries Science (www.nifs.go.kr); these data are represented as the cumulative frequency of red tide events in each year for 2010–2019.
Biological Measurements
All Z. marina shoots, including above- and below-ground tissues, were collected within four randomly thrown quadrats (30 × 30 cm) at each study site, which were used to measure shoot density, biomass, and reproductive phenology from January 2018 to December 2019. Z. marina tissues from each quadrat sample were thoroughly cleaned of sediments, then separated into vegetative (lateral shoots + terminal shoots) and reproductive shoots. The numbers of vegetative and reproductive shoots were counted to estimate shoot density (shoots m−2). Flowering frequency was estimated as the percentage of reproductive shoot density relative to total shoot density. All vegetative and reproductive tissues in the quadrat were cleaned of epiphytes using a razor blade and oven-dried separately at 60°C to a constant weight. Biomass was expressed on a dry weight per unit area basis (g DW m−2). Reproductive effort (RE) was estimated as the percentage of reproductive shoot biomass to total shoot biomass. The proportion of lateral shoot density to vegetative shoot density and the proportion of lateral shoot biomass to vegetative shoot biomass were calculated to represent the relative contribution of asexual reproduction.
To measure the morphological characteristics of reproductive shoots and potential seed production, five reproductive shoots were randomly chosen from each quadrat sample collected when the reproductive shoots were mature. The numbers of spathes per shoot and seeds per spathe were measured for each reproductive shoot. The number of seeds per shoot was estimated by multiplying the number of spathes per shoot by the number of seeds per spathe. Potential seed production per unit area was determined by multiplying the number of seeds per shoot by the maximum reproductive shoot density. To estimate the relative contribution of seedling recruitment to the maintenance of Z. marina populations at the study sites, Z. marina seedlings within four randomly thrown quadrats (30 × 30 cm) at each study site were carefully collected in January 2019, then counted to estimate seedling density (shoots m−2).
To measure the morphological characteristics of vegetative shoots, 2 to 3 vegetative shoots were collected in the vicinity of the four randomly thrown quadrats at each study site (n = 8–12 per study site) from January 2018 to December 2019. The number of leaves per shoot was counted. Shoot height was measured from the meristem to the tip of the longest leaf to the nearest 1.0 mm; the width of the longest leaf was measured to the nearest 0.1 mm. Sheath length was measured from the meristem to the top of the sheath. The diameter of the third rhizome internode was measured to nearest 0.1 mm; rhizome internode length was estimated as the mean of the first 6 internode lengths.
Nutrient Contents of Z. marina Tissues
To determine carbon (C), nitrogen (N), and phosphorous (P) contents of above- and below-ground tissues, 2 to 3 vegetative shoots were randomly collected in the vicinity of the four quadrats in each site (n = 8–12 per study site) biannually before (January) and after (July) the flowering season from 2018 to 2019. The second or third youngest leaf (with sheath) and rhizome internode (with roots) were dried at 60°C to constant weight. Dried tissues were ground using an agate mortar and pestle; approximately 2 mg of ground tissue was placed in a tin capsule for determination of tissue C and N contents using a CHN elemental analyzer (Fisons NA1500). Approximately 10 mg of ground tissue was subjected to determination of tissue P content using a modified version of the method described by Fourqurean et al., 1992. The C, N, and P contents of above- and below-ground tissues were calculated on a dry weight basis. C:N, C:P, and N:P ratios were calculated on a molar basis.
Statistical Analyses
Significant differences in physical and chemical environmental factors, shoot density, biomass, morphological characteristics of vegetative shoots, and nutrient contents of seagrass tissues among study sites and sampling months were tested using two-way ANOVAs; differences in the frequencies of red tide events among study sites and years were tested using a two-way ANOVA without replication. When significant differences were found among study sites, a post hoc Tukey’s test was performed to determine where the differences occurred. Differences in the morphological characteristics of reproductive shoots, potential seed production, and seedling density among study sites were identified using one-way ANOVAs. Separated one-way ANOVA, followed by Tukey’s test, was performed to test for differences in above- and below-ground tissue nutrient contents among study sites at each sampling time. Prior to analysis, all variables were individually checked for normality and homogeneity of variance using Q-Q plots and residual plots, respectively. If the assumptions of parametric statistics were not satisfied, the measurements were either square root- or log-transformed. A significance level of 0.05 was used in all cases. All statistical analyses were performed with SPSS software, ver. 23.0.
Results
Environmental Parameters
Differences in mean water temperature among the three study sites were < 1°C (Table 1, Supplementary Figure 1). Daily water temperature exhibited distinct seasonal variations at all three study sites (Supplementary Figure 1, Supplementary Table 1). The water temperature in Dongdae Bay exhibited stronger seasonal variations, such that it was colder in winter and warmer in summer than Deukryang Bay and Koje Bay (Supplementary Figure 1). Mean daily PPFD was highest in Koje Bay, where it was approximately three-fold higher than in Deukryang Bay and two-fold higher than in Dongdae Bay throughout the sampling period (Table 1). Underwater PPFD at the Z. marina canopy level showed significant (ANOVA, p < 0.001) temporal variations with high degree of fluctuations at all three study sites; it was higher in winter and spring than in summer and fall (Figures 2A–C; Supplementary Table 1). Hcomp and Hsat were significantly longer in Koje Bay than in Deukryang Bay and Dongdae Bay (Table 1). Hcomp and Hsat displayed temporal patterns similar to the patterns of PPFD (Figures 2D–I; Supplementary Table 1).
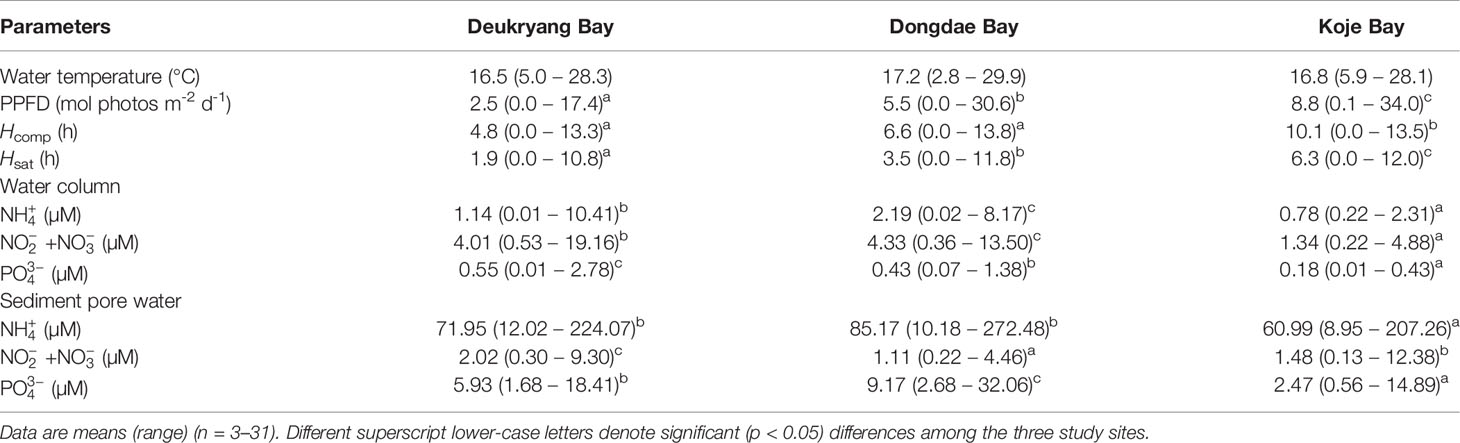
Table 1 Water temperature, underwater photosynthetic photon flux density (PPFD), daily period of light compensation (Hcomp), daily period of light saturation (Hsat), and nutrient concentrations in Deukryang Bay, Dongdae Bay, and Koje Bay on the southern coast of Korea.
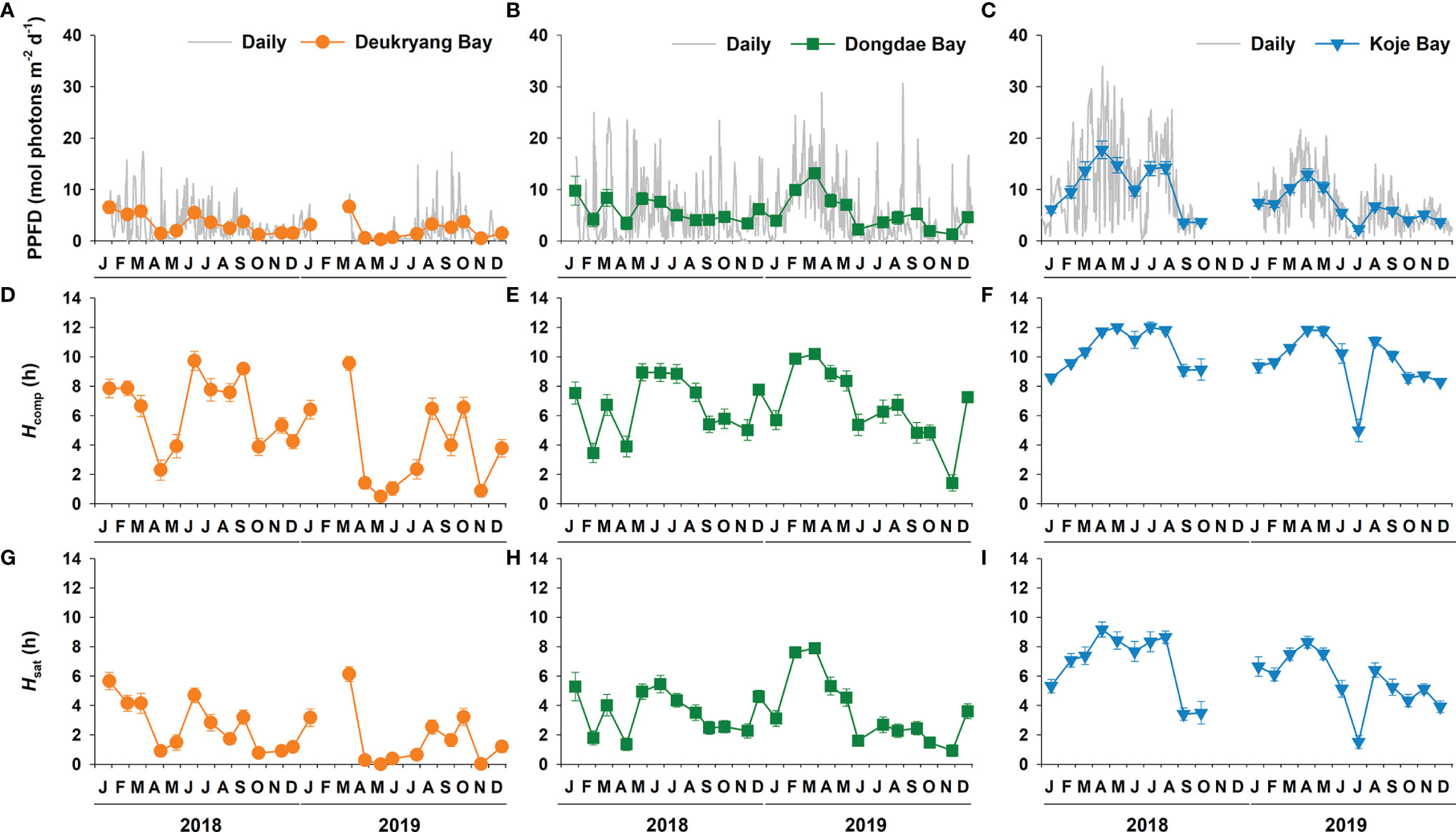
Figure 2 Seasonal variations in underwater photosynthetic photon flux density (PPFD; A–C), daily period of light compensation (Hcomp; D–F), and daily period of light saturation (Hsat; G–I) in Deukryang Bay, Dongdae Bay, and Koje Bay on the southern coast of Korea from January 2018 to December 2019.
All nutrient concentrations in the water column and sediment pore water significantly (ANOVA, all p < 0.001) varied among study sites and over time (Figure 3; Supplementary Table 1). Mean water column , + , and concentrations were significantly (Tukey’s test, all p < 0.05) higher in Deukryang Bay and Dongdae Bay than in Koje Bay (Table 1). Sediment and concentrations were also higher in Dongdae Bay and Deukryang Bay than in Koje Bay throughout the experimental period (Figure 3; Table 1). The mean sediment + concentration was highest in Deukryang Bay (2.02 µM), followed by Koje Bay (1.48 µM), and lowest in Dongdae Bay (1.11 µM). No nutrient concentrations in the water column and sediment pore water exhibited clear seasonal trends, with the exception of sediment concentrations (Figure 3). Sediment concentrations were higher in summer and fall than in winter and early spring. Annual occurrences of red tide events during 2010–2019 in adjacent waters of the study sites significantly (ANOVA, all p < 0.05) varied among study sites. The total occurrence of red tide events was highest in Dongdae Bay, followed by Deukryang Bay, and lowest in Koje Bay (Supplementary Table 2).
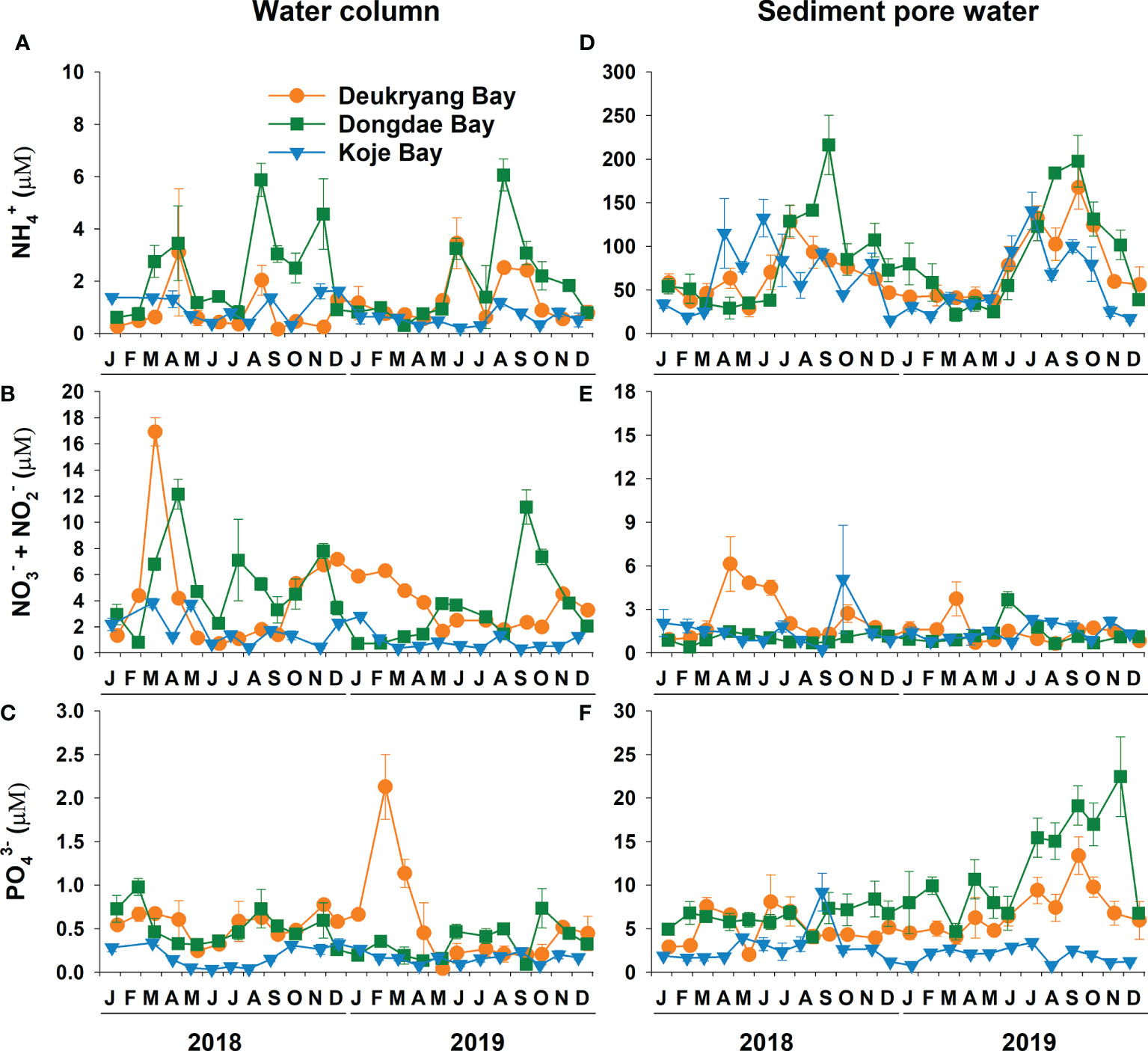
Figure 3 Seasonal variations in , +, and concentrations of the water column (A–C) and sediment pore water (D–F) in Deukryang Bay, Dongdae Bay, and Koje Bay on the southern coast of Korea from January 2018 to December 2019. Values are means ± SE (n = 3–4).
Seagrass Shoot Density and Biomass
Reproductive and total shoot density, as well as the corresponding flowering frequency, significantly (ANOVA, all p < 0.05) differed among the three study sites and markedly (ANOVA, all p < 0.001) varied among sampling months (Figures 4A–C, Table 2; and Supplementary Table 3). Mean reproductive shoot density was higher at the high-nutrient and low-light sites in Dongdae Bay (20 shoots m-2) and Deukryang Bay (16 shoots m-2) than at the low-nutrient and high-light site in Koje Bay (10 shoots m-2) during the flowering period, whereas mean total shoot density was significantly (Tukey’s test, p < 0.001) higher in Koje Bay than in Dongdae Bay and Deukryang Bay (Table 2). Consequently, mean flowering frequency was higher in Deukryang Bay (10.5%) and Dongdae Bay (9.5%) than in Koje Bay (3.7%; Table 2). The maximum flowering frequency was 2.5- and 4.6-fold higher in Deukryang Bay than in Koje Bay during 2018 and 2019, respectively; it was 3.7- and 1.5-fold higher in Dongdae Bay than in Koje Bay during 2018 and 2019, respectively (Figure 4C). No significant differences in reproductive shoot density or flowering frequency were found between Deukryang Bay and Dongdae Bay (Table 2). The highest reproductive shoot density and flowering frequency were observed from March to April at all three study sites (Figures 4A, C). Total shoot density usually peaked in late winter and spring, with the lowest values in fall and early winter (Figure 4B). In 2019, low reproductive shoot density and flowering frequency occurred in Dongdae Bay because young lateral shoots comprised the majority of Z. marina shoots during the period of flowering shoot formation (Figures 4A, C, and Supplementary Figure 2).
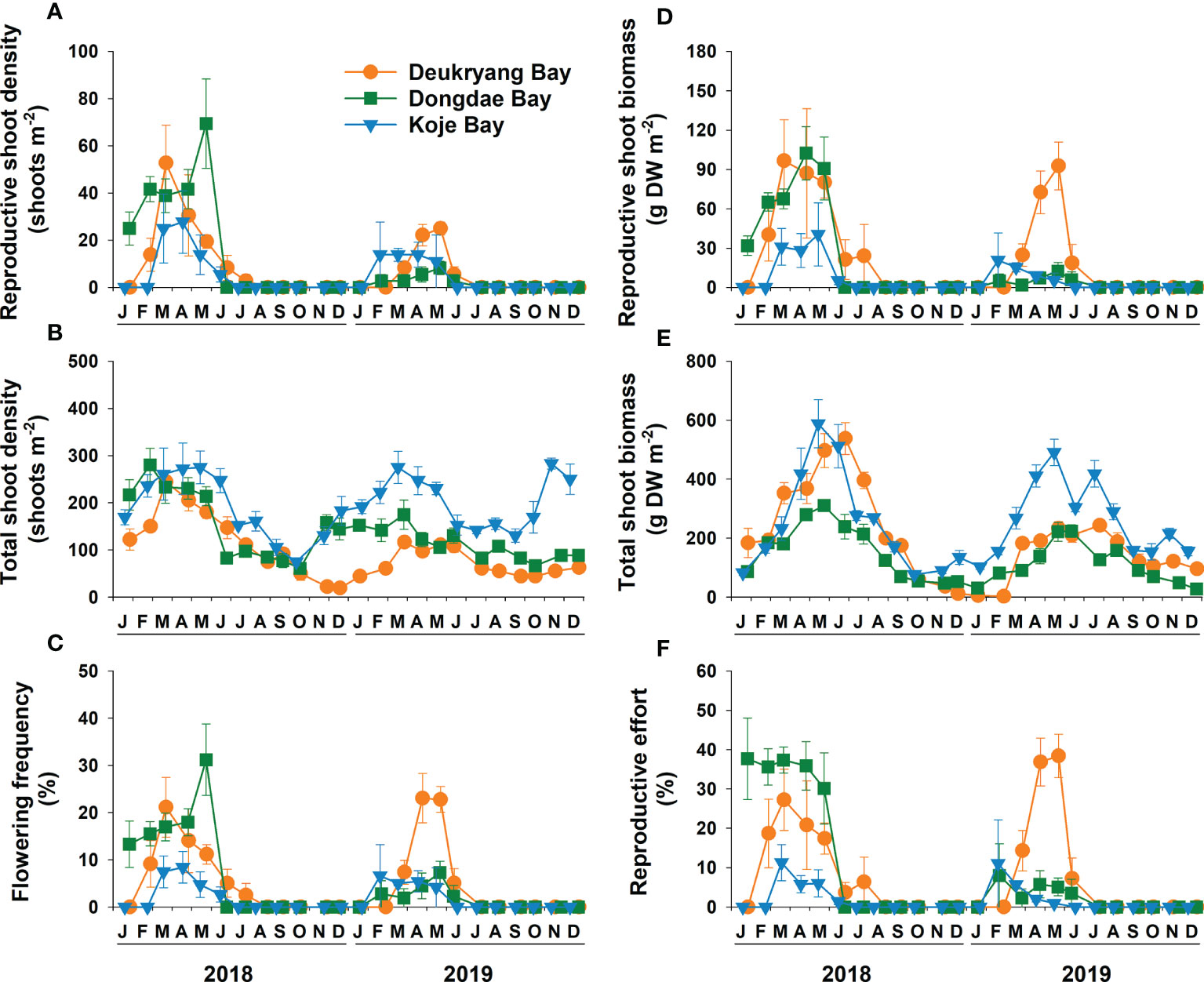
Figure 4 Zostera marina. Seasonal changes in reproductive (A) and total (B) shoot density, flowering frequency (C), reproductive (D) and total (E) shoot biomass, and reproductive effort (F) in Deukryang Bay, Dongdae Bay, and Koje Bay on the southern coast of Korea from January 2018 to November 2019. Values are means ± SE (n = 4).
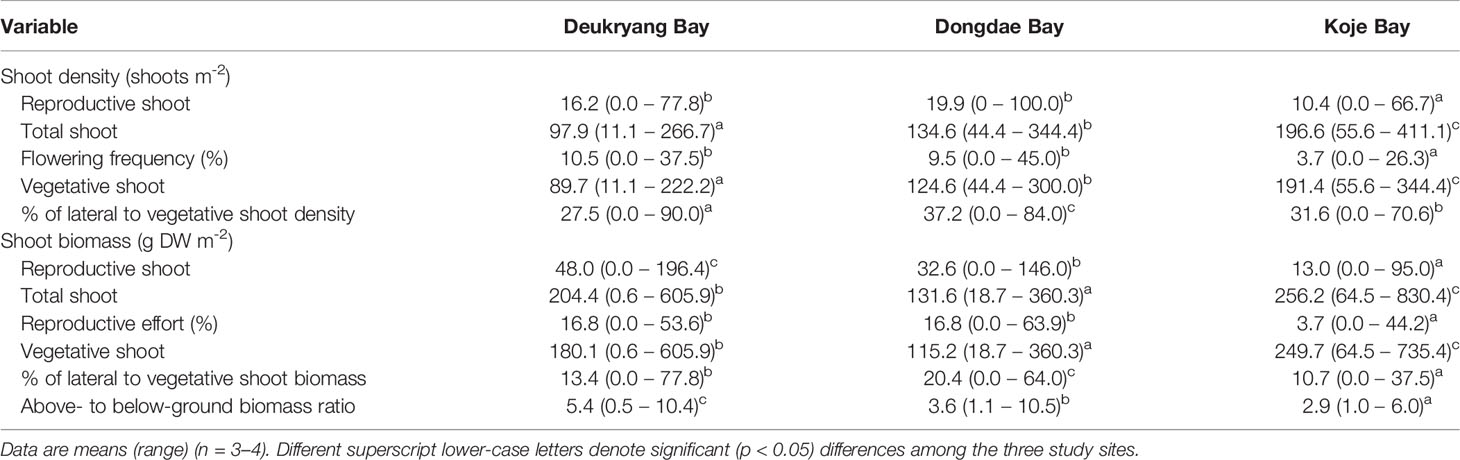
Table 2 Zostera marina. Mean values of shoot density and biomass and the corresponding flowering frequency and reproductive effort, the percentage of lateral shoot density to vegetative shoot density, the percentage of lateral shoot biomass to vegetative shoot biomass, and above- to below-ground biomass ratio in Deukryang Bay, Dongdae Bay, and Koje Bay on the southern coast of Korea.
Reproductive and total shoot biomass, as well as the corresponding reproductive effort, significantly (ANOVA, all p < 0.001) differed among the three study sites and markedly (ANOVA, all p < 0.001) varied among sampling months (Figures 4D–F; Table 2, and Supplementary Table 3). Mean reproductive shoot biomass was highest in Deukryang Bay (48.0 g DW m-2), followed by Dongdae Bay (32.6 g DW m-2), and lowest in Koje Bay (13.0 g DW m-2; Table 2). Mean total shoot biomass was lowest in Dongdae Bay and highest in Koje Bay (Table 2). Consequently, mean values of reproductive effort were higher in Deukryang Bay and Dongdae Bay than in Koje Bay (Table 2). Maximum reproductive effort was greatest in Dongdae Bay (37.7%), followed by Deukryang Bay (27.7%), and lowest in Koje Bay (11.3%) during the flowering period of 2018. In contrast, maximum reproductive effort was highest in Deukryang Bay (38.4%), followed by Koje Bay (11.1%), and lowest in Dongdae Bay (8.0%) during the flowering period of 2019 (Figure 4F). The highest values of reproductive effort were observed during March or May in Deukryang Bay and Dongdae Bay, whereas they were observed during February or March in Koje Bay (Figure 4F).
Vegetative shoot density and biomass, the percentage of lateral shoot density to vegetative shoot density, and the percentage of lateral shoot biomass to vegetative shoot biomass significantly (ANOVA, all p < 0.001) differed among the three study sites and markedly (ANOVA, all p < 0.001) varied among sampling months (Table 2; Supplementary Figure 2, and Supplementary Table 3). Mean vegetative shoot density and biomass were significantly (Tukey’s test, p < 0.001) higher in Koje Bay than in Deukryang Bay and Dongdae Bay (Table 2). The percentage of lateral shoot density to vegetative shoot density and the percentage of lateral shoot biomass to vegetative shoot biomass were significantly (Tukey’s test, p < 0.001) higher in Dongdae Bay than in Deukryang Bay and Koje Bay (Table 2). The above- to below-ground biomass ratio was highest in Deukryang Bay and lowest in Koje Bay (Table 2). Vegetative shoot density and biomass generally peaked in late winter and spring; they were lowest in fall and early winter (Supplementary Figure 2). The highest vegetative shoot density and biomass occurred in late winter and spring, primarily due to highest lateral shoot formation during that period (Supplementary Figure 2).
Flowering Phenology
The duration of flowering events was not consistent among the three study sites (Figure 4A). In 2018, the flowering duration was longest in Deukryang Bay, followed by Dongdae Bay, and shortest in Koje Bay (Figure 4A). The flowering season lasted from February to July in Deukryang Bay and from March to June in Koje Bay (Figure 4A). Reproductive shoots were observed first in January, then persisted until May in Dongdae Bay (Figure 4A). In 2019, reproductive shoots were observed from March to June in Deukryang Bay, while they occurred from February to June in Dongdae Bay and from February to May in Koje Bay (Figure 4A). The mean flowering period was 5 months in both Deukryang Bay and Dongdae Bay; it was 4 months in Koje Bay. Despite the difference in flowering period among study sites, water temperature during flowering events was relatively consistent (10.4–15.5°C) among the three study sites.
Reproductive Shoot Morphology, Seed Production, and Seedling Density
The number of spathes per shoot did not significantly (ANOVA, p = 0.425) differ among study sites in 2018, but it significantly (ANOVA, p < 0.05) varied among study sites in 2019. The number of spathes per shoot was higher in Deukryang Bay than in Dongdae Bay and Koje Bay during the flowering period of 2019 (Table 3). The number of seeds per spathe was significantly (Tukey’s test, p < 0.05) higher in Deukryang Bay than in Dongdae Bay and Koje Bay during the flowering periods of both 2018 and 2019 (Table 3). Thus, the estimated number of seeds per shoot was highest in Deukryang Bay, followed by Dongdae Bay, and lowest in Koje Bay in both 2018 and 2019 (Table 3). Potential seed production was highest in Deukryang Bay during the flowering periods of both 2018 and 2019; it was lowest in Koje Bay during 2018 and in Dongdae Bay during 2019 (Table 3). Annual mean potential seed production was over 4-fold higher in Deukryang Bay than in Koje Bay; it was over 2-fold higher in Dongdae Bay than in Koje Bay. In January 2019, seedling density was significantly (Tukey’s test, p < 0.05) higher in Dongdae Bay and Deukryang Bay than in Koje Bay; no significant (Tukey’s test, p = 0.892) difference in seedling density was observed between Dongdae Bay and Deukryang Bay (Table 3).
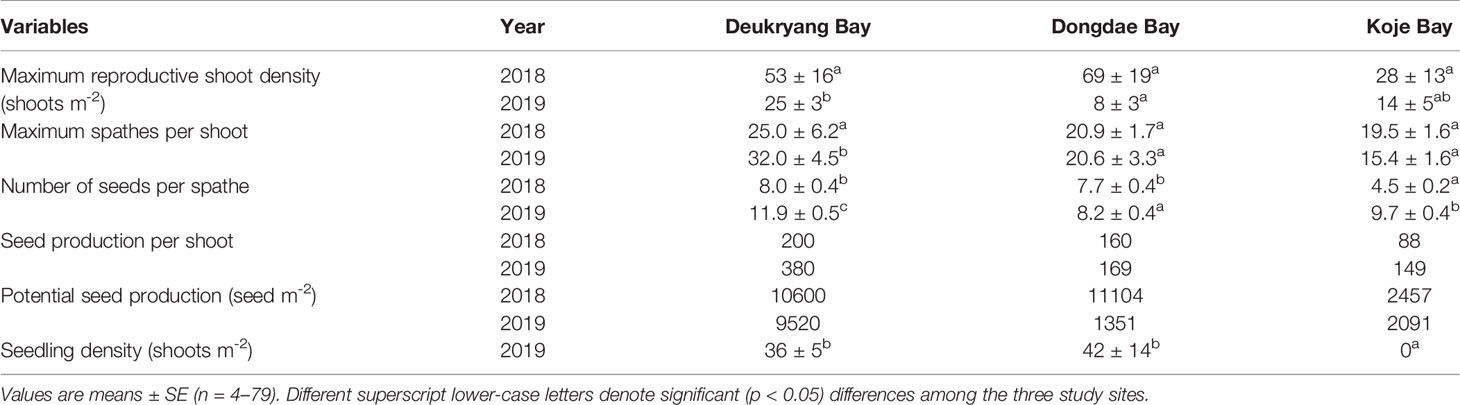
Table 3 Zostera marina. Mean values of maximum reproductive shoot density, maximum spathes per shoot, number of seeds per spathe, seed production per shoot, and potential seed production during 2018 and 2019, and seedling density during January 2019 in Deukryang Bay, Dongdae Bay, and Koje Bay on the southern coast of Korea.
Vegetative Shoot Morphological Characteristics
All morphological characteristics of vegetative shoots significantly (ANOVA, all p < 0.001) differed among the three study sites and markedly (ANOVA, all p < 0.001) varied over the study period (Supplementary Figure 3; Supplementary Table 4, and Supplementary Table 5). The number of leaves per shoot was higher in Dongdae Bay than in Deukryang Bay and Koje Bay, while leaf width was greater in Deukryang Bay and Dongdae Bay than in Koje Bay (Supplementary Table 4). Mean shoot height and sheath length were highest in Deukryang Bay, followed by Koje Bay, and lowest in Dongdae Bay (Supplementary Table 4). Rhizome diameter was higher in Deukryang Bay and Koje Bay than in Dongdae Bay (Supplementary Table 4). Rhizome internode length was longest in Koje Bay, followed by Dongdae Bay, and lowest in Deukryang Bay (Supplementary Table 4).
Nutrient Contents of Zostera marina Tissues
All nutrient contents and elemental ratios in above- and below-ground tissues of Z. marina shoots significantly (ANOVA, all p < 0.05) differed among the three study sites and markedly (ANOVA, all p < 0.05) varied among sampling months (Figure 5; Table 4, and Supplementary Table 6). In above-ground tissues, C, N, and P contents were significantly (Tukey’s test, all p < 0.05) higher in Deukryang Bay and Dongdae Bay than in Koje Bay; no significant (Tukey’s, all p > 0.05) difference was found between Deukryang Bay and Dongdae Bay (Table 4). In below-ground tissues, the C content was higher in Deukryang Bay than in Dongdae Bay and Koje Bay; no significant (Tukey’s test, p = 0.989) difference was found between Dongdae Bay and Koje Bay (Table 4). N and P contents of below-ground tissues exhibited spatial variations similar to the variations in above-ground tissues (Table 4). In both above- and below-ground tissues, C:N and C:P ratios were significantly (Tukey’s test, all p < 0.05) higher in Koje Bay than in Deukryang Bay and Dongdae Bay; the N:P ratio was higher in Deukryang Bay than in Dongdae Bay and Koje Bay (Table 4).
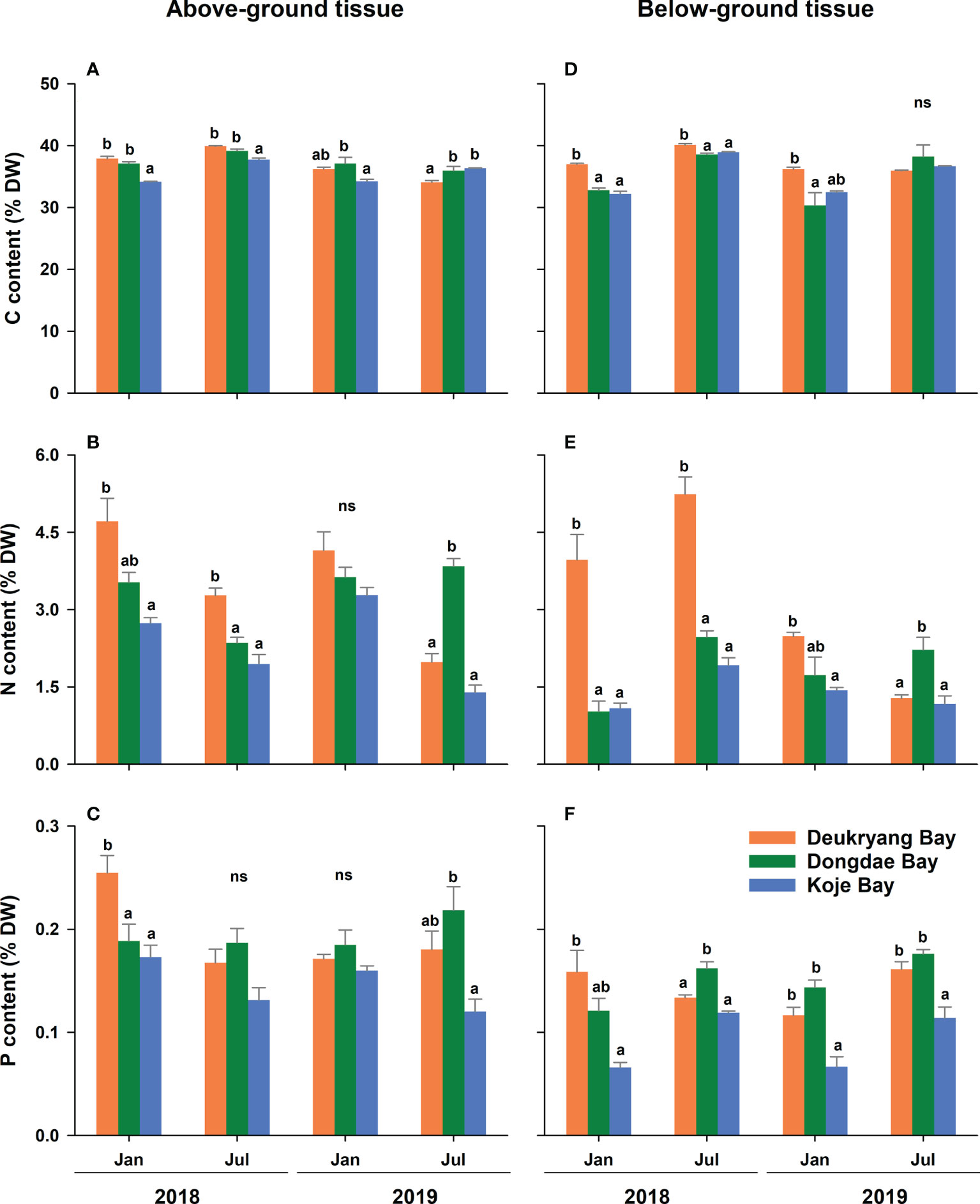
Figure 5 Carbon (C), nitrogen (N), and phosphorous (P) contents in above- (A–C) and below-ground (D–F) tissues of Zostera marina in Deukryang Bay, Dongdae Bay, and Koje Bay on the southern coast of Korea. Values are means ± SE (n = 3–4). Different letters denote significant (p < 0.05) differences among the three study sites. ns, not significant.
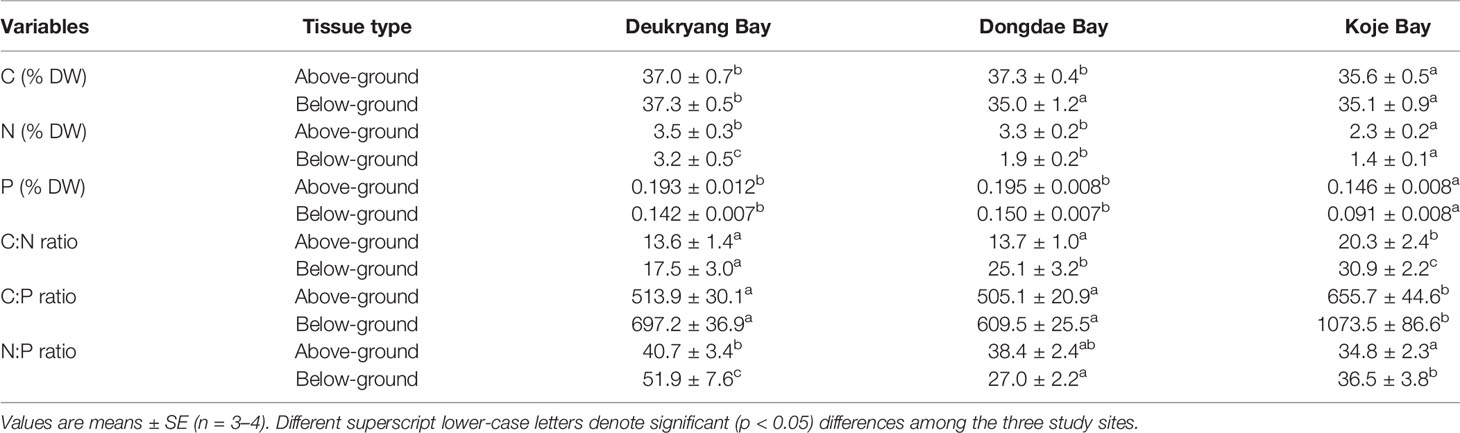
Table 4 Carbon (C), nitrogen (N), and phosphorous (P) contents, as well as their elemental ratios, in above- and below-ground tissues of Zostera marina in Deukryang Bay, Dongdae Bay, and Koje Bay on the southern coast of Korea.
Discussion
Influences of Increased Nutrient Availability on Seagrass Reproductive Strategy
Inorganic nutrient concentrations in both the water column and sediments, with the exception of nitrite and nitrate concentrations in sediments, were significantly higher in Deukryang Bay and Dongdae Bay than in Koje Bay in this study. Tissue nutrient contents of Z. marina were also significantly higher in Deukryang Bay and Dongdae Bay than in Koje Bay; the C:N and C:P ratios of Z. marina tissues were significantly lower in Deukryang Bay and Dongdae Bay than in Koje Bay. The nutrient contents of seagrass tissues can be used as a bioindicator of environmental nutrient conditions, allowing for assessment of in situ nutrient availability (Fourqurean and Zieman, 2002; Lee et al., 2004; Fourqurean et al., 2005; Campbell and Fourqurean, 2009; Yang et al., 2018; Xu et al., 2021). Seagrasses have significantly higher C:N and C:P ratios when grown under low-nutrient conditions than when grown under high-nutrient conditions (Fourqurean et al., 1997; Lee and Dunton, 1999; Campbell and Fourqurean, 2009; Xu et al., 2021). Although in situ inorganic nutrient measurements represent the instantaneous nutrient status after dilution or rapid uptake by primary producers, seagrass tissue nutrient contents have been suggested as an indicator of environmental nutrient history (Lee and Dunton, 1999; Fourqurean and Zieman, 2002; Lee et al., 2004; Qin et al., 2020b). Thus, measurement of seagrass tissue nutrient contents in this study indicated that Z. marina shoots have been exposed to more enriched nutrient conditions in Deukryang Bay and Dongdae Bay than in Koje Bay. Red tide algal bloom events have also occurred more frequently in adjacent waters of the study sites in Deukryang Bay and Dongdae Bay than in such areas of Koje Bay over the past decade, suggesting that the study sites in Deukryang Bay and Dongdae Bay are more nutrient-enriched than the site in Koje Bay. Reproductive shoot density, biomass, as well as the corresponding flowering frequency and reproductive effort of Z. marina were 1.5–5.0-fold higher under high-nutrient conditions in Deukryang Bay and Dongdae Bay than under the comparatively low-nutrient conditions of Koje Bay. The flowering period of Z. marina was longer and consequently seed production was higher in Deukryang Bay and Dongdae Bay than in Koje Bay. Abundant seedlings were observed at the study sites with high flowering intensity and seed production in Deukryang Bay and Dongdae Bay, whereas no seedlings were observed in Koje Bay during the seed germination period of 2019. These results suggest that Z. marina populations under more nutrient-enriched conditions exhibit increased reproductive intensity and investment in sexual reproduction, leading to increased seedling recruitment via seed germination, which may enhance the resilience of seagrass meadows.
In many clonal species of terrestrial plants, environmental nutrient availability has been known to influence relative investments in sexual and asexual reproduction (Burkle and Irwin, 2009; Liu et al., 2009; Grainger and Turkington, 2013). However, changes in nutrient availability can lead to conflicting results regarding reproductive investment in both terrestrial and seagrass ecosystems (Grainger and Turkington, 2013; Johnson et al., 2017; Qin et al., 2021). Plants can invest more resources into sexual reproduction when nutrient availability is high because of high metabolic costs incurred during construction of the flowering shoots, fruits, and seeds needed for sexual reproduction (Loehle, 1987; Grainger and Turkington, 2013). Thus, when nutrients are abundant and increasing, plants would allocate more resources to sexual reproduction (Gardner and Mangel, 1999; Burkle and Irwin, 2009; Liu et al., 2009; Grainger and Turkington, 2013). Conversely, decreased investment in sexual reproduction has been reported when environmental nutrients are abundant and increasing, since asexual reproduction produces genetically identical individuals with a proven genotype that can exploit favorable local conditions (Sakai, 1995; Grainger and Turkington, 2013).
In the present study, dissolved inorganic nitrogen concentrations in the water column at all study sites were consistently above the concentrations required for Z. marina growth (Zimmerman et al., 1987; Lee et al., 2007b); in contrast, the mean values of sediment ammonium concentrations were < 100 µM, which has been suggested as the threshold concentration for nitrogen limitation of seagrass growth (Dennison et al., 1987; Zimmerman et al., 1987; Lee et al., 2007b; Qin et al., 2021). Because Z. marina growth in Koje Bay has been described as nitrogen-limited (Qin et al., 2021), Z. marina in Koje Bay may benefit from greater resource investments into vegetative growth and clonal reproduction, which is less energetically expensive than sexual reproduction (Philbrick and Les, 1996; Johnson et al., 2020). However, with the comparatively higher nutrient availability of Deukryang Bay and Dongdae Bay, Z. marina may invest more resources into sexual reproduction, allowing production of more flowering shoots and seeds. Increased Z. marina flowering intensity has been reported under high sediment nutrient conditions in the Chesapeake Bay in Virginia, USA (Johnson et al., 2017). In addition, significant increases in flowering shoot height, number of spathes, and seed production per shoot in response to nutrient fertilization have been observed in Z. marina meadows in Shinnecock Bay, New York, USA and Koje Bay, South Korea (Jackson et al., 2017; Qin et al., 2021). Thus, the increased coastal nutrient loading may be advantageous in enhancing seagrass sexual reproduction by providing resources to support the production of more flowering shoots and seeds.
Negative or null relationships between in situ environmental nutrient conditions and flowering intensity of seagrass also have been reported in previous studies (Short, 1983; Van Dijk and van Tussenbroek, 2010). Environmental variables such as water temperature and light intensity may confound the effects of nutrient availability on sexual reproduction of seagrass (Johnson et al., 2017; Qin et al., 2021). Additionally, because of differences in nutrient acquisition capacity among seagrass species (Lee et al., 2007b; Vonk et al., 2008), the reproductive responses of seagrasses to increased nutrient availability are presumably species-specific. Studies using manipulated nutrient enrichment have generally shown only weak effects of increased nutrient availability on sexual reproduction of seagrass, probably because of short-term exposure to increased nutrients (Darnell and Dunton, 2017; Johnson et al., 2017), suggesting that the duration of exposure to increased nutrient levels may have a critical effect on reproductive investment in seagrasses (Johnson et al., 2017).
Influences of Light and Temperature on Seagrass Reproduction
The duration and intensity of underwater irradiance have been demonstrated to regulate the investments in sexual reproduction of many seagrass species (McMillan, 1976; van Lent et al., 1995; Kim et al., 2014; Johnson et al., 2017; Olesen et al., 2017). Z. marina generally requires a minimum of 7 mol photons m-2 d-1 for non-light-limited growth (Thom et al., 2008). In the present study, underwater PPFD in Koje Bay was > 7 mol photons m-2 d-1 during winter to spring, which is the season for growth and colonization of Z. marina on the south coast of Korea (Lee et al., 2005; Kim et al., 2008; Kim et al., 2014). Underwater PPFD in Deukryang Bay and Dongdae Bay was lower than the light requirement for non-light-limited growth of Z. marina during winter and spring. The lengths of Hcomp and Hsat have been closely correlated with the growth and survival of seagrasses (Dennison and Alberte, 1982; Dennison and Alberte, 1985; Dennison, 1987; Zimmerman et al., 1991; Lee et al., 2007b; Kim et al., 2014). Monthly mean Hcomp and Hsat values were shorter in Deukryang Bay and Dongdae Bay than in Koje Bay throughout the experimental period. The C:N and C:P ratios of seagrass above-ground tissues have been used as indicators of in situ light availability (Fourqurean et al., 1997; Campbell and Fourqurean, 2009). Higher C:N and C:P ratios in leaf tissues have been observed under high-light conditions (Abal et al., 1994; Grice et al., 1996; Fourqurean et al., 1997). In the present study, the C:N and C:P ratios of leaf tissues were significantly higher in the high underwater light conditions of Koje Bay than in Deukryang Bay and Dongdae Bay. These results indicate that Z. marina populations in Deukryang Bay and Dongdae Bay may have experienced greater stress because of low-light conditions. Seagrasses generally allocate a greater proportion of their resources to sexual reproduction in more stressful or disturbed environments (Cabaço and Santos, 2012; Kim et al., 2014; Suonan et al., 2017). Repetitive and acute light reduction combined with high summer temperature caused approximately 95% of Z. marina shoots to flower in a deep-water population (Kim et al., 2014). Much higher reproductive effort and potential seed production were observed under low underwater light conditions in Deukryang Bay and Dongdae Bay than under high underwater light conditions in Koje Bay, indicating possible adaptation to improve the persistence and maintenance of seagrass populations under chronic low-light conditions. These results suggest that underwater light conditions may have an important role in determining sexual reproduction investment by Z. marina. In addition, Z. marina population in Koje Bay may have reached apparent carrying capacity with high shoot density (ca. 230–270 shoots m-2) during March through May, when the other Z. marina populations in Dongdae Bay and Deukryang Bay flowered and reached the maximum reproductive shoot density. The high shoot density in Koje Bay during the flowering period may limit light and resources for the flowering shoots, consequently leading to lower sexual reproduction.
Variability in regional water temperature also can affect Z. marina flowering phenology and reproductive strategies (Qin et al., 2020b). Z. marina at study sites with milder winter and summer water temperatures had an extended flowering period and enhanced sexual reproduction, whereas Z. marina exposed to colder winter and warmer summer temperatures had a shorter flowering period and reduced sexual reproduction (Qin et al., 2020b). In the present study, differences in mean water temperature were < 1°C among the three study sites, and the reproductive intensity of Z. marina significantly differed. Thus, the prolonged flowering duration and high investment in sexual reproduction at the study sites in Deukryang Bay and Dongdae Bay were probably not driven by differences in water temperature.
Resilience of Seagrass Populations
Differences in reproductive strategies among seagrass populations may facilitate seagrass colonization in diverse environments and habitats, thus enhancing the resilience of seagrass populations to natural and anthropogenic stressors and disturbances (Kim et al., 2014; Unsworth et al., 2015; Connolly et al., 2018; Johnson et al., 2020; Qin et al., 2021). Seagrass ecosystem resilience is related to the capacity to resist disturbances or stressors, as well as the ability to recover from loss or degradation (Unsworth et al., 2015; Johnson et al., 2021). The relative contributions of sexual and asexual reproduction to the maintenance of established seagrass meadows significantly vary with the magnitude of disturbances or stressors (Olesen et al., 2017; von Staats et al., 2020). Asexual reproduction is the main contributor to the preservation and recolonization of seagrass meadows after minor or small-scale disturbances through rhizome elongation and the regrowth of surviving shoots (Duarte et al., 2007; Boese et al., 2009; Macreadie et al., 2014; Olesen et al., 2017). However, seagrass populations rely primarily on sexual reproduction through seedling recruitment via seed germination to recover from intense and large-scale disturbances (Plus et al., 2003; Lee et al., 2007a; Park et al., 2011; Kim et al., 2014; Potouroglou et al., 2014; Johnson et al., 2021). In seagrass meadows that have become denuded by repetitive and acute unfavorable environmental conditions, recolonization and recovery have been reported to primarily depend on sexual reproduction through seedling recruitment (Inglis, 2000; Santamarı́a-Gallegos et al., 2000; Lee et al., 2007a; Jarvis et al., 2012; Kim et al., 2014). Thus, sexual reproduction of seagrasses can enhance the capability of seagrass populations to resist successive disturbances and stresses caused by anthropogenic activities and climate change, thereby increasing seagrass meadow resilience (Park et al., 2009; Kim et al., 2014; Macreadie et al., 2014; Johnson et al., 2020; Zhang et al., 2020b; Qin et al., 2021).
The high contribution of sexual reproduction to the persistence and maintenance of seagrass populations could lead to increased genetic diversity in established seagrass populations through the production of genetically distinct new shoots via seedling recruitment (Reusch, 2006; Procaccini et al., 2007; Kim et al., 2019). The genetic diversity of seagrass meadows may play a crucial role in enhancing the resilience of degraded seagrass populations (Ackerman, 2007; Ehlers et al., 2008; Kim et al., 2019; Qin et al., 2020a). Severe coastal eutrophication caused by excessive nutrient loading could result in extensive declines or die-offs of seagrass populations in coastal areas (van Katwijk et al., 2010). Thus, increments of reproductive intensity and seed production under high-nutrient and low-light conditions may be essential for enhancing the resilience of degraded seagrass meadows under the stress of coastal eutrophication.
Conclusion
The reproductive intensity of Z. marina varied in response to changes in nutrient and light availability. Z. marina populations at study sites with high-nutrient and low-light conditions showed higher flowering shoot density, biomass, flowering frequency, and reproductive effort than did populations at the study site with low-nutrient and high-light conditions (Figure 6). Thus, Z. marina populations under high-nutrient and low-light conditions increased their reproductive intensity and seed production; these changes are likely to improve the persistence and survival of their populations under stressful environmental conditions. Because the effects of nutrient and light availability on the reproductive strategies of Z. marina were not independently investigated in this study, high-nutrient and low-light stresses may simultaneously and/or interactively affect the sexual reproduction of Z. marina. Thus, the variation of reproductive intensity under differing nutrient and light conditions is an adaptation that may improve resilience and stability of seagrass populations, helping them to withstand the stresses of excessive coastal nutrient loading and light reduction.
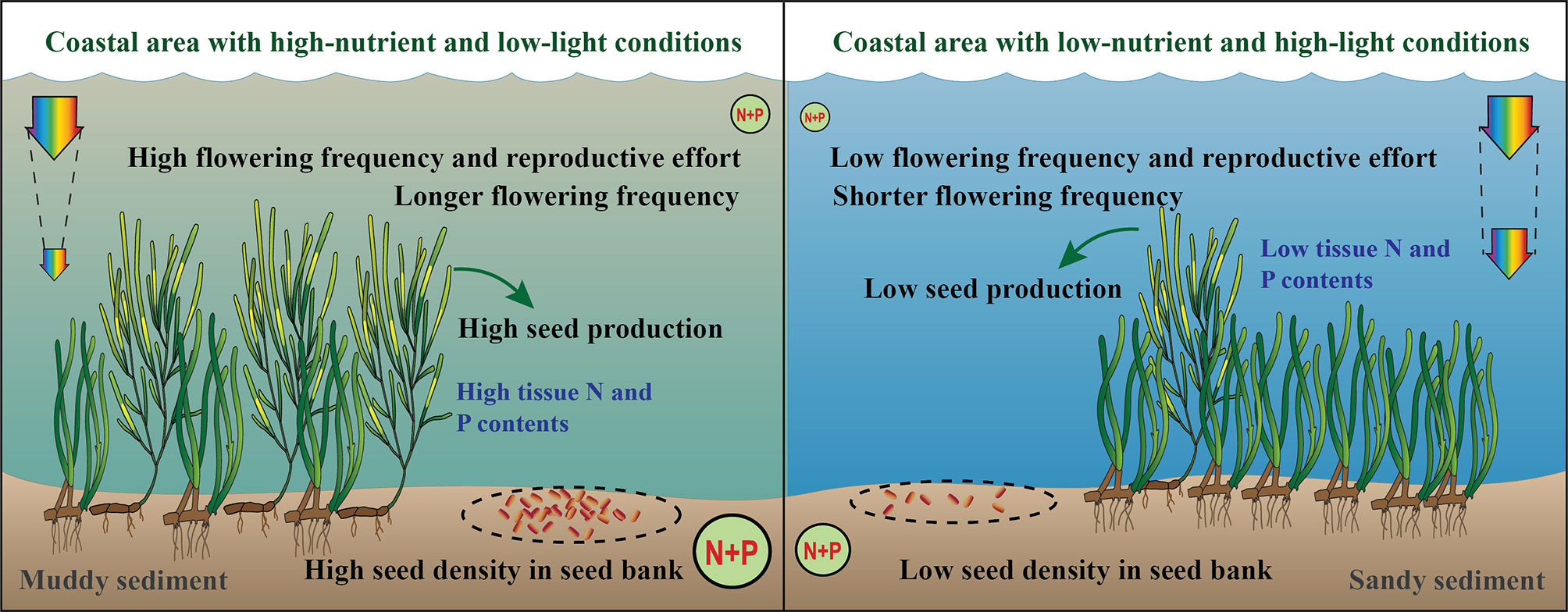
Figure 6 A summary of the reproductives responses of Z. marina to changes in nutrient and light availability. Diagram symbols are from the Integration and Application Network, University of Maryland Center for Environmental Science (http://ian.umces.edu/imagelibrary/).
Data Availability Statement
The raw data supporting the conclusions of this article will be made available by the authors, without undue reservation.
Author Contributions
ZS, SK, L-ZQ, and K-SL conceptualized the experiments. ZS, SK, L-ZQ, HK, and FZ performed the experiments. ZS, SK, L-ZQ, and K-SL analyzed the results. All authors contributed to writing the manuscript and approved the submission.
Funding
This research was supported by “Responses of species-populations to climate change scenario” program of Korea institute of Marine Science & Technology Promotion (KIMST) funded by the Ministry of Oceans and Fisheries (Funding number: KIMST-20220559); and the National Research Foundation of Korea (NRF) grant funded by the Korean government (Grant number: NRF-2019R1A2C1090641 and NRF-2021R1I1A1A01049507).
Conflict of Interest
The authors declare that the research was conducted in the absence of any commercial or financial relationships that could be construed as a potential conflict of interest.
Publisher’s Note
All claims expressed in this article are solely those of the authors and do not necessarily represent those of their affiliated organizations, or those of the publisher, the editors and the reviewers. Any product that may be evaluated in this article, or claim that may be made by its manufacturer, is not guaranteed or endorsed by the publisher.
Acknowledgments
We thank H. J. Song, J. Y. Kim, and D. H. Im for their many hours of field and laboratory assistance.
Supplementary Material
The Supplementary Material for this article can be found online at: https://www.frontiersin.org/articles/10.3389/fmars.2022.832035/full#supplementary-material
References
Abal E., Loneragan N., Bowen P., Perry C., Udy J., Dennison W. (1994). Physiological and Morphological Responses of the Seagrass Zostera Capricorni Aschers, to Light Intensity. J. Exp. Mar. Biol. Ecol. 178 (1), 113–129. doi: 10.1016/0022-0981(94)90228-3
Ackerman J. D. (2007). “"Sexual Reproduction of Seagrasses: Pollination in the Marine Context,",” in Seagrasses: Biology, Ecologyand Conservation. Eds. Larkum A., Orth R. J., Duarte C. M. (Dordrecht, Netherlands: Springer), 89–109.
Armitage A. R., Frankovich T. A., Heck K. L., Fourqurean J. W. (2005). Experimental Nutrient Enrichment Causes Complex Changes in Seagrass, Microalgae, and Macroalgae Community Structure in Florida Bay. Estuaries 28 (3), 422–434. doi: 10.1007/BF02693924
Blok S. E., Olesen B., Krause-Jensen D. (2018). Life History Events of Eelgrass Zostera Marina L. Populations Across Gradients of Latitude and Temperature. Mar. Ecol. Prog. Ser. 590, 79–93. doi: 10.3354/meps12479
Boese B. L., Kaldy J. E., Clinton P. J., Eldridge P. M., Folger C. L. (2009). Recolonization of Intertidal Zostera Marina L.(eelgrass) Following Experimental Shoot Removal. J. Exp. Mar. Biol. Ecol. 374 (1), 69–77. doi: 10.1016/j.jembe.2009.04.011
Burkholder J. M., Tomasko D. A., Touchette B. W. (2007). Seagrasses and Eutrophication. J. Exp. Mar. Biol. Ecol. 350 (1-2), 46–72. doi: 10.1016/j.jembe.2007.06.024
Burkle L. A., Irwin R. E. (2009). The Effects of Nutrient Addition on Floral Characters and Pollination in Two Subalpine Plants, Ipomopsis Aggregata and Linum Lewisii. Plant Ecol. 203 (1), 83–98. doi: 10.1007/s11258-008-9512-0
Cabaço S., Santos R. (2012). Seagrass Reproductive Effort as an Ecological Indicator of Disturbance. Ecol. Indic. 23, 116–122. doi: 10.1016/j.ecolind.2012.03.022
Campbell J. E., Fourqurean J. W. (2009). Interspecific Variation in the Elemental and Stable Isotope Content of Seagrasses in South Florida. Mar. Ecol. Prog. Ser. 387, 109–123. doi: 10.3354/meps08093
Carroll J., Gobler C. J., Peterson B. J. (2008). Resource-Restricted Growth of Eelgrass in New York Estuaries: Light Limitation, and Alleviation of Nutrient Stress by Hard Clams. Mar. Ecol. Prog. Ser. 369, 51–62. doi: 10.3354/meps07593
Cho C.-H. (1991). Mariculture and Eutrophication in Jinhae Bay, Korea. Mar. Pollut. Bull. 23, 275–279. doi: 10.1016/0025-326X(91)90687-N
Choi A., Kim B., Mok J.-S., Yoo J., Kim J. B., Lee W.-C., et al. (2020). Impact of Finfish Aquaculture on Biogeochemical Processes in Coastal Ecosystems and Elemental Sulfur as a Relevant Proxy for Assessing Farming Condition. Mar. Pollut. Bull. 150, 110635. doi: 10.1016/j.marpolbul.2019.110635
Cho C.-H., Park K.-Y., Yang H.-S., Hong J.-S. (1982). Eutrophication of Shellfish Farms in Deukryang and Gamagyang Bays. Kor. J. Fish. Aquat. Sci. 15 (3), 233–240.
Connolly R. M., Smith T. M., Maxwell P. S., Olds A. D., Macreadie P. I., Sherman C. D. (2018). Highly Disturbed Populations of Seagrass Show Increased Resilience But Lower Genotypic Diversity. Front. Plant Sci. 9. doi: 10.3389/fpls.2018.00894
Darnell K. M., Dunton K. H. (2017). Plasticity in Turtle Grass (Thalassia Testudinum) Flower Production as a Response to Porewater Nitrogen Availability. Aquat. Bot. 138, 100–106. doi: 10.1016/j.aquabot.2017.01.007
Dennison W. C. (1987). Effects of Light on Seagrass Photosynthesis, Growth and Depth Distribution. Aquat. Bot. 27 (1), 15–26. doi: 10.1016/0304-3770(87)90083-0
Dennison W. C., Alberte R. S. (1982). Photosynthetic Responses of Zostera Marina L.(eelgrass) to in Situ Manipulations of Light Intensity. Oecologia 55 (2), 137–144. doi: 10.1007/BF00384478
Dennison W. C., Alberte R. S. (1985). Role of Daily Light Period in the Depth Distribution of Zostera Marina (Eelgrass). Mar. Ecol. Prog. Ser. 25 (1), 51–61. doi: 10.3354/meps025051
Dennison W., Aller R., Alberte R. (1987). Sediment Ammonium Availability and Eelgrass (Zostera Marina) Growth. Mar. Biol. 94 (3), 469–477. doi: 10.1007/BF00428254
Diaz-Almela E., Marbà N., Duarte C. M. (2007). Consequences of Mediterranean Warming Events in Seagrass (Posidonia Oceanica) Flowering Records. Global Change Biol. 13 (1), 224–235. doi: 10.1111/j.1365-2486.2006.01260.x
Duarte C. M. (2002). The Future of Seagrass Meadows. Environ. Conserv. 29 (2), 192–206. doi: 10.1017/S0376892902000127
Duarte C. M., Fourqurean J. W., Krause-Jensen D., Olesen B. (2007). “Dynamics of Seagrass Stability and Change,” in Seagrasses: Biology, Ecologyand Conservation (Dordrecht, Netherlands: Springer), 271–294.
Ehlers A., Worm B., Reusch T. B. (2008). Importance of Genetic Diversity in Eelgrass Zostera Marina for its Resilience to Global Warming. Mar. Ecol. Prog. Ser. 355, 1–7. doi: 10.3354/meps07369
Fourqurean J. W., Duarte C. M., Kennedy H., Marbà N., Holmer M., Mateo M. A., et al. (2012). Seagrass Ecosystems as a Globally Significant Carbon Stock. Nat. Geosci. 5 (7), 505–509. doi: 10.1038/ngeo1477
Fourqurean J. W., Escorcia S. P., Anderson W. T., Zieman J. C. (2005). Spatial and Seasonal Variability in Elemental Content, δ 13 C, and δ 15 N of Thalassia Testudinum From South Florida and its Implications for Ecosystem Studies. Estuaries 28 (3), 447–461. doi: 10.1007/BF02693926
Fourqurean J. W., Moore T. O., Fry B., Hollibaugh J. T. (1997). Spatial and Temporal Variation in C: N: P Ratios, δ15n, and δ13c of Eelgrass Zostera Marina as Indicators of Ecosystem Processes, Tomales Bay, California, USA. Mar. Ecol. Prog. Ser. 157, 147–157. doi: 10.3354/meps157147
Fourqurean J. W., Zieman J. C. (2002). Nutrient Content of the Seagrass Thalassia Testudinum Reveals Regional Patterns of Relative Availability of Nitrogen and Phosphorus in the Florida Keys USA. Biogeochemistry 61 (3), 229–245. doi: 10.1023/A:1020293503405
Fourqurean J. W., Zieman J. C., Powell G. V. (1992). Phosphorus Limitation of Primary Production in Florida Bay: Evidence From C: N: P Ratios of the Dominant Seagrass Thalassia Testudinum. Limnol. Oceanogr. 37 (1), 162–171. doi: 10.4319/lo.1992.37.1.0162
Fraser M. W., Kendrick G. A. (2017). Belowground Stressors and Long-Term Seagrass Declines in a Historically Degraded Seagrass Ecosystem After Improved Water Quality. Sci. Rep. 7 (1), 1–11. doi: 10.1038/s41598-017-14044-1
Gardner S. N., Mangel M. (1999). Modeling Investments in Seeds, Clonal Offspring, and Translocation in a Clonal Plant. Ecology 80 (4), 1202–1220. doi: 10.1890/0012-9658(1999)080[1202:MIISCO]2.0.CO;2
Grainger T. N., Turkington R. (2013). Long-Term Nutrient Enrichment Differentially Affects Investment in Sexual Reproduction in Four Boreal Forest Understory Species. Plant Ecol. 214 (8), 1017–1026. doi: 10.1007/s11258-013-0227-5
Greve T. M., Krause-Jensen D., Rasmussen M. B., Christensen P. B. (2005). Means of Rapid Eelgrass (Zostera Marina L.) Recolonisation in Former Dieback Areas. Aquat. Bot. 82 (2), 143–156. doi: 10.1016/j.aquabot.2005.03.004
Grice A., Loneragan N., Dennison W. (1996). Light Intensity and the Interactions Between Physiology, Morphology and Stable Isotope Ratios in Five Species of Seagrass. J. Exp. Mar. Biol. Ecol. 195 (1), 91–110. doi: 10.1016/0022-0981(95)00096-8
Hemminga M. A., Duarte C. M. (2000). Seagrass Ecology (Cambridge, United Kingdom: Cambridge University Press).
Inglis G. J. (2000). Disturbance-Related Heterogeneity in the Seed Banks of a Marine Angiosperm. J. Ecol. 88 (1), 88–99. doi: 10.1046/j.1365-2745.2000.00433.x
Jackson L. J., Furman B. T., Peterson B. J. (2017). Morphological Response of Zostera Marina Reproductive Shoots to Fertilized Porewater. J. Exp. Mar. Biol. Ecol. 489, 1–6. doi: 10.1016/j.jembe.2017.01.002
Jarvis J. C., Brush M. J., Moore K. A. (2014). Modeling Loss and Recovery of Zostera Marina Beds in the Chesapeake Bay: The Role of Seedlings and Seed-Bank Viability. Aquat. Bot. 113, 32–45. doi: 10.1016/j.aquabot.2013.10.010
Jarvis J. C., Moore K. A. (2010). The Role of Seedlings and Seed Bank Viability in the Recovery of Chesapeake Bay, USA, Zostera Marina Populations Following a Large-Scale Decline. Hydrobiologia 649 (1), 55–68. doi: 10.1007/s10750-010-0258-z
Jarvis J. C., Moore K. A., Kenworthy W. J. (2012). Characterization and Ecological Implication of Eelgrass Life History Strategies Near the Species’ Southern Limit in the Western North Atlantic. Mar. Ecol. Prog. Ser. 444, 43–56. doi: 10.3354/meps09428
Johnson A. J., Moore K. A., Orth R. J. (2017). The Influence of Resource Availability on Flowering Intensity in Zostera Marina (L.). J. Exp. Mar. Biol. Ecol. 490, 13–22. doi: 10.1016/j.jembe.2017.02.002
Johnson A. J., Orth R. J., Moore K. A. (2020). The Role of Sexual Reproduction in the Maintenance of Established Zostera Marina Meadows. J. Ecol. 108 (3), 945–957. doi: 10.1111/1365-2745.13362
Johnson A., Shields E., Kendrick G., Orth R. (2021). Recovery Dynamics of the Seagrass Zostera Marina Following Mass Mortalities From Two Extreme Climatic Events. Estuar. Coast. 44 (2), 535–544. doi: 10.1007/s12237-020-00816-y
Kang C.-K., Kim P.-J., Lee W.-C., Lee P.-Y. (1999). Nutrients and Phytoplankton Blooms in the Southern Coastal Waters of Korea: I. The Elemental Composition of C, N, and P in Particulate Matter in the Coastal Bay Systems. J. Kor. Soc Oceanogr. 34 (2), 86–94.
Kendrick G. A., Orth R. J., Statton J., Hovey R., Ruiz Montoya L., Lowe R. J., et al. (2017). Demographic and Genetic Connectivity: The Role and Consequences of Reproduction, Dispersal and Recruitment in Seagrasses. Biol. Rev. 92 (2), 921–938. doi: 10.1111/brv.12261
Kendrick G. A., Waycott M., Carruthers T. J., Cambridge M. L., Hovey R., Krauss S. L., et al. (2012). The Central Role of Dispersal in the Maintenance and Persistence of Seagrass Populations. Bioscience 62 (1), 56–65. doi: 10.1525/bio.2012.62.1.10
Kim K., Choi J.-K., Ryu J.-H., Jeong H. J., Lee K., Park M. G., et al. (2015). Observation of Typhoon-Induced Seagrass Die-Off Using Remote Sensing. Estuar. Coast. Shelf. Sci. 154, 111–121. doi: 10.1016/j.ecss.2014.12.036
Kim S. H., Kim J.-H., Park S. R., Lee K.-S. (2014). Annual and Perennial Life History Strategies of Zostera Marina Populations Under Different Light Regimes. Mar. Ecol. Prog. Ser. 509, 1–13. doi: 10.3354/meps10899
Kim Y. K., Kim S. H., Yi J. M., Park S. R., Lee K. S. (2019). Influence of Environmental Disturbances and Reproductive Strategy on Genetic Diversity and Differentiation of Zostera Marina Populations on the Southern Coast of Korea. Mar. Ecol. 40 (1), e12537. doi: 10.1111/maec.12537
Kim T.-H., Rark S.-R., Kim Y.-K., Kim J.-H., Kim S.-H., Kim J.-H., et al. (2008). Growth Dynamics and Carbon Incorporation of the Seagrass, Zostera Marina L. @ in Jindong Bay and Gamak Bay on the Southern Coast of Korea. Algae 23 (3), 241–250. doi: 10.4490/ALGAE.2008.23.3.241
Kwak M.-T., Cho Y.-K. (2020). Seasonal Variation in Residence Times of Two Neighboring Bays With Contrasting Topography. Estuar. Coast. 43 (3), 512–524. doi: 10.1007/s12237-019-00644-9
Lee K.-S. (2004). Development of Indicator for Coastal and Estuarine Eutrophication Using Morphological Characteristics and Tissue N Content of Eelgrass, Zostera Marina. Algae 19 (2), 129–137. doi: 10.4490/ALGAE.2004.19.2.129
Lee K.-S., Dunton K. (1999). Influence of Sediment Nitrogen-Availability on Carbon and Nitrogen Dynamics in the Seagrass Thalassia Testudinum. Mar. Biol. 134 (2), 217–226. doi: 10.1007/s002270050540
Lee K.-S., Kim S. H., Kim Y. K. (2018). “Current Status of Seagrass Habitat in Korea,” in The Wetland Book II: Distribution, Description, and Conservation. Eds. Finlayson C. M., Milton G. R., Prentice R. C., Davidson N. C. (Dordrecht, Netherlands: Springer), 1589–1596.
Lee K.-S., Park S. R., Kim J.-B. (2005). Production Dynamics of the Eelgrass, Zostera Marina in Two Bay Systems on the South Coast of the Korean Peninsula. Mar. Biol. 147 (5), 1091–1108. doi: 10.1007/s00227-005-0011-8
Lee K.-S., Park S. R., Kim Y. K. (2007b). Effects of Irradiance, Temperature, and Nutrients on Growth Dynamics of Seagrasses: A Review. J. Exp. Mar. Biol. Ecol. 350 (1-2), 144–175. doi: 10.1016/j.jembe.2007.06.016
Lee K.-S., Park J.-I., Kim Y. K., Park S. R., Kim J.-H. (2007a). Recolonization of Zostera Marina Following Destruction Caused by a Red Tide Algal Bloom: The Role of New Shoot Recruitment From Seed Banks. Mar. Ecol. Prog. Ser. 342, 105–115. doi: 10.3354/meps342105
Lee K.-S., Short F. T., Burdick D. M. (2004). Development of a Nutrient Pollution Indicator Using the Seagrass, Zostera Marina, Along Nutrient Gradients in Three New England Estuaries. Aquat. Bot. 78 (3), 197–216. doi: 10.1016/j.aquabot.2003.09.010
Lee G., Suonan Z., Kim S. H., Hwang D.-W., Lee K.-S. (2019). Heavy Metal Accumulation and Phytoremediation Potential by Transplants of the Seagrass Zostera Marina in the Polluted Bay Systems. Mar. Pollut. Bull. 149, 110509. doi: 10.1016/j.marpolbul.2019.110509
Leoni V., Vela A., Pasqualini V., Pergent-Martini C., Pergent G. (2008). Effects of Experimental Reduction of Light and Nutrient Enrichments (N and P) on Seagrasses: A Review. Aquat. Conserv.: Mar. Freshwat. Ecosyst. 18 (2), 202–220. doi: 10.1002/aqc.842
Liu F., Chen J.-M., Wang Q.-F. (2009). Trade-Offs Between Sexual and Asexual Reproduction in a Monoecious Species Sagittaria Pygmaea (Alismataceae): The Effect of Different Nutrient Levels. Plant Syst. Evol. 277 (1), 61–65. doi: 10.1007/s00606-008-0103-2
Loehle C. (1987). Partitioning of Reproductive Effort in Clonal Plants: A Benefit-Cost Model. Oikos 49 (2), 199–208. doi: 10.2307/3566027
Macreadie P. I., York P. H., Sherman C. D. (2014). Resilience of Zostera Muelleri Seagrass to Small-Scale Disturbances: The Relative Importance of Asexual Versus Sexual Recovery. Ecol. Evol. 4 (4), 450–461. doi: 10.1002/ece3.933
McMillan C. (1976). Experimental Studies on Flowering and Reproduction in Seagrasses. Aquat. Bot. 2, 87–92. doi: 10.1016/0304-3770(76)90011-5
Moreno-Marín F., Brun F. G., Pedersen M. F. (2018). Additive Response to Multiple Environmental Stressors in the Seagrass Zostera Marina L. Limnol. Oceanogr. 63 (4), 1528–1544. doi: 10.1002/lno.10789
Olesen B., Krause-Jensen D., Christensen P. B. (2017). Depth-Related Changes in Reproductive Strategy of a Cold-Temperate Zostera Marina Meadow. Estuar. Coast. 40 (2), 553–563. doi: 10.1007/s12237-016-0155-4
Olsen J. L., Rouzé P., Verhelst B., Lin Y.-C., Bayer T., Collen J., et al. (2016). The Genome of the Seagrass Zostera Marina Reveals Angiosperm Adaptation to the Sea. Nature 530 (7590), 331–335. doi: 10.1038/nature16548
Orth R. J., Carruthers T. J., Dennison W. C., Duarte C. M., Fourqurean J. W., Heck K. L., et al. (2006). A Global Crisis for Seagrass Ecosystems. Bioscience 56 (12), 987–996. doi: 10.1641/0006-3568(2006)56[987:AGCFSE]2.0.CO;2
Park S. R., Kim J.-H., Kang C.-K., An S., Chung I. K., Kim J. H., et al. (2009). Current Status and Ecological Roles of Zostera Marina After Recovery From Large-Scale Reclamation in the Nakdong River Estuary, Korea. Estuar. Coast. Shelf. Sci. 81 (1), 38–48. doi: 10.1016/j.ecss.2008.10.003
Park S. R., Kim Y. K., Kim J.-H., Kang C.-K., Lee K.-S. (2011). Rapid Recovery of the Intertidal Seagrass Zostera Japonica Following Intense Manila Clam (Ruditapes Philippinarum) Harvesting Activity in Korea. J. Exp. Mar. Biol. Ecol. 407 (2), 275–283. doi: 10.1016/j.jembe.2011.06.023
Parsons T., Maita Y., Lalli C. (1984). A Manual of Biological and Chemical Methods for Seawater Analysis (Oxford: Pergamon Press).
Pazzaglia J., Reusch T. B., Terlizzi A., Marín-Guirao L., Procaccini G. (2021). Phenotypic Plasticity Under Rapid Global Changes: The Intrinsic Force for Future Seagrasses Survival. Evol. Appl. 14 (5), 1181–1201. doi: 10.1111/eva.13212
Peñalver M. M., Durako M. J., Furman B. T., Hall M. O. (2020). Multiple Stressors Result in Reduced Reproductive Effort by Thalassia Testudinum in Florida Bay, USA. Mar. Ecol. Prog. Ser. 647, 65–78. doi: 10.3354/meps13395
Peralta G., Bouma T. J., van Soelen J., Pérez-Lloréns J. L., Hernández I. (2003). On the Use of Sediment Fertilization for Seagrass Restoration: A Mesocosm Study on Zostera Marina L. Aquat. Bot. 75 (2), 95–110. doi: 10.1016/S0304-3770(02)00168-7
Philbrick C. T., Les D. H. (1996). Evolution of Aquatic Angiosperm Reproductive Systems. Bioscience 46 (11), 813–826. doi: 10.2307/1312967
Phillips R. C., Grant W. S., McRoy C. P. (1983). Reproductive Strategies of Eelgrass (Zostera Marina L.). Aquat. Bot. 16 (1), 1–20. doi: 10.1016/0304-3770(83)90047-5
Plus M., Deslous-Paoli J.-M., Dagault F. (2003). Seagrass (Zostera Marina L.) Bed Recolonisation After Anoxia-Induced Full Mortality. Aquat. Bot. 77 (2), 121–134. doi: 10.1016/S0304-3770(03)00089-5
Potouroglou M., Kenyon E. J., Gall A., Cook K. J., Bull J. C. (2014). The Roles of Flowering, Overwinter Survival and Sea Surface Temperature in the Long-Term Population Dynamics of Zostera Marina Around the Isles of Scilly, UK. Mar. Pollut. Bull. 83 (2), 500–507. doi: 10.1016/j.marpolbul.2014.03.035
Procaccini G., Olsen J. L., Reusch T. B. (2007). Contribution of Genetics and Genomics to Seagrass Biology and Conservation. J. Exp. Mar. Biol. Ecol. 350 (1-2), 234–259. doi: 10.1016/j.jembe.2007.05.035
Qin L.-Z., Kim S. H., Song H.-J., Kim H. G., Suonan Z., Kwon O., et al. (2020a). Long-Term Variability in the Flowering Phenology and Intensity of the Temperate Seagrass Zostera Marina in Response to Regional Sea Warming. Ecol. Indic. 119, 106821. doi: 10.1016/j.ecolind.2020.106821
Qin L.-Z., Kim S. H., Song H.-J., Suonan Z., Kim H., Kwon O., et al. (2020b). Influence of Regional Water Temperature Variability on the Flowering Phenology and Sexual Reproduction of the Seagrass Zostera Marina in Korean Coastal Waters. Estuar. Coast. 43 (3), 449–462. doi: 10.1007/s12237-019-00569-3
Qin L.-Z., Suonan Z., Kim S. H., Lee K.-S. (2021). Growth and Reproductive Responses of the Seagrass Zostera Marina to Sediment Nutrient Enrichment. ICES J. Mar. Sci. 78 (3), 1160–1173. doi: 10.1093/icesjms/fsab031
Rasheed M. A. (2004). Recovery and Succession in a Multi-Species Tropical Seagrass Meadow Following Experimental Disturbance: The Role of Sexual and Asexual Reproduction. J. Exp. Mar. Biol. Ecol. 310 (1), 13–45. doi: 10.1016/j.jembe.2004.03.022
Reusch T. B. (2006). Does Disturbance Enhance Genotypic Diversity in Clonal Organisms? A Field Test in the Marine Angiosperm Zostera Marina. Mol. Ecol. 15 (1), 277–286. doi: 10.1111/j.1365-294X.2005.02779.x
Rodemann J. R., James W. R., Santos R. O., Furman B. T., Fratto Z. W., Bautista V., et al. (2021). Impact of Extreme Disturbances on Suspended Sediment in Western Florida Bay: Implications for Seagrass Resilience. Front. Mar. Sci. 8, 633240. doi: 10.3389/fmars.2021.633240
Sakai S. (1995). Optimal Resource Allocation to Vegetative and Sexual Reproduction of a Plant Growing in a Spatially Varying Environment. J. Theor. Biol. 175 (3), 271–282. doi: 10.1006/jtbi.1995.0141
Santamarı́a-Gallegos N. A., Sánchez-Lizaso J. L., Félix-Pico E. F. (2000). Phenology and Growth Cycle of Annual Subtidal Eelgrass in a Subtropical Locality. Aquat. Bot. 66 (4), 329–339. doi: 10.1016/S0304-3770(99)00082-0
Short F. T. (1983). The Seagrass, Zostera Marina L.: Plant Morphology and Bed Structure in Relation to Sediment Ammonium in Izembek Lagoon, Alaska. Aquat. Bot. 16 (2), 149–161. doi: 10.1016/0304-3770(83)90090-6
Short F. T., Burdick D. M., Kaldy III, J.E. (1995). Mesocosm Experiments Quantify the Effects of Eutrophication on Eelgrass, Zostera Marina. Limnol. Oceanogr. 40 (4), 740–749. doi: 10.4319/lo.1995.40.4.0740
Smith T. M., York P. H., Macreadie P. I., Keough M. J., Ross D. J., Sherman C. D. (2016). Spatial Variation in Reproductive Effort of a Southern Australian Seagrass. Mar. Environ. Res. 120, 214–224. doi: 10.1016/j.marenvres.2016.08.010
Suonan Z., Kim S. H., Qin L.-Z., Lee K.-S. (2017). Reproductive Strategy of the Intertidal Seagrass Zostera Japonica Under Different Levels of Disturbance and Tidal Inundation. Estuar. Coast. Shelf. Sci. 197, 185–193. doi: 10.1016/j.ecss.2017.08.031
Thomsen E., Herbeck L. S., Jennerjahn T. C. (2020). The End of Resilience: Surpassed Nitrogen Thresholds in Coastal Waters Led to Severe Seagrass Loss After Decades of Exposure to Aquaculture Effluents. Mar. Environ. Res. 160, 104986. doi: 10.1016/j.marenvres.2020.104986
Thom R. M., Southard S. L., Borde A. B., Stoltz P. (2008). Light Requirements for Growth and Survival of Eelgrass (Zostera Marina L.) in Pacific Northwest (USA) Estuaries. Estuar. Coast. 31 (5), 969–980. doi: 10.1007/s12237-008-9082-3
Udy J. W., Dennison W. C. (1997). Growth and Physiological Responses of Three Seagrass Species to Elevated Sediment Nutrients in Moreton Bay, Australia. J. Exp. Mar. Biol. Ecol. 217 (2), 253–277. doi: 10.1016/S0022-0981(97)00060-9
Unsworth R. K., Collier C. J., Waycott M., Mckenzie L. J., Cullen-Unsworth L. C. (2015). A Framework for the Resilience of Seagrass Ecosystems. Mar. Pollut. Bull. 100 (1), 34–46. doi: 10.1016/j.marpolbul.2015.08.016
Van Dijk J. K., van Tussenbroek B. I. (2010). Clonal Diversity and Structure Related to Habitat of the Marine Angiosperm Thalassia Testudinum Along the Atlantic Coast of Mexico. Aquat. Bot. 92 (1), 63–69. doi: 10.1016/j.aquabot.2009.10.005
van Katwijk M. M., Bos A. R., Kennis P., de Vries R. (2010). Vulnerability to Eutrophication of a Semi-Annual Life History: A Lesson Learnt From an Extinct Eelgrass (Zostera Marina) Population. Biol. Conserv. 143 (1), 248–254. doi: 10.1016/j.biocon.2009.08.014
van Lent F., Verschuure J. M., van Veghel M. L. (1995). Comparative Study on Populations of Zostera Marina L.(eelgrass): In Situ Nitrogen Enrichment and Light Manipulation. J. Exp. Mar. Biol. Ecol. 185 (1), 55–76. doi: 10.1016/0022-0981(94)00131-V
Vonk J. A., Middelburg J. J., Stapel J., Bouma T. J. (2008). Dissolved Organic Nitrogen Uptake by Seagrasses. Limnol. Oceanogr. 53 (2), 542–548. doi: 10.4319/lo.2008.53.2.0542
von Staats D. A., Hanley T. C., Hays C. G., Madden S. R., Sotka E. E., Hughes A. R. (2020). Intra-Meadow Variation in Seagrass Flowering Phenology Across Depths. Estuar. Coast. 44, 1–14. doi: 10.1007/s12237-020-00814-0
Waycott M., Duarte C. M., Carruthers T. J., Orth R. J., Dennison W. C., Olyarnik S., et al. (2009). Accelerating Loss of Seagrasses Across the Globe Threatens Coastal Ecosystems. Proc. Natl. Acad. Sci. U.S.A. 106 (30), 12377–12381. doi: 10.1073/pnas.0905620106
Xu S., Zhou Y., Wang P., Wang F., Zhang X., Yue S., et al. (2021). Temporal-Spatial Variations in the Elemental and Stable Isotope Contents of Eelgrass (Zostera Marina L.) in the Bohai Sea and Yellow Sea, Northern China: Sheath as a Novel Ecological Indicator for Geochemical Research. Ecol. Indic. 121, 107181. doi: 10.1016/j.ecolind.2020.107181
Yang X., Zhang P., Li W., Hu C., Zhang X., He P. (2018). Evaluation of Four Seagrass Species as Early Warning Indicators for Nitrogen Overloading: Implications for Eutrophic Evaluation and Ecosystem Management. Sci. Tot. Environ. 635, 1132–1143. doi: 10.1016/j.scitotenv.2018.04.227
Yue S., Zhang X., Xu S., Zhang Y., Zhao P., Wang X., et al. (2020). Reproductive Strategies of the Seagrass Zostera Japonica Under Different Geographic Conditions in Northern China. Front. Mar. Sci. 7. doi: 10.3389/fmars.2020.574790
Zhang Y.-H., Li C., Zhao J.-S., Li W.-T., Zhang P.-D. (2020b). Seagrass Resilience: Where and How to Collect Donor Plants for the Ecological Restoration of Eelgrass Zostera Marina in Rongcheng Bay, Shandong Peninsula, China. Ecol. Eng. 158, 106029. doi: 10.1016/j.ecoleng.2020.106029
Zhang X., Zhou Y., Xu S., Wang P., Zhao P., Yue S., et al. (2020a). Differences in Reproductive Effort and Sexual Recruitment of the Seagrass Zostera Japonica Between Two Geographic Populations in Northern China. Mar. Ecol. Prog. Ser. 638, 65–81. doi: 10.3354/meps13248
Zimmerman R. C., Hill V. J., Jinuntuya M., Celebi B., Ruble D., Smith M., et al. (2017). Experimental Impacts of Climate Warming and Ocean Carbonation on Eelgrass Zostera Marina. Mar. Ecol. Prog. Ser. 566, 1–15. doi: 10.3354/meps12051
Zimmerman R. C., Reguzzoni J. L., Wyllie-Echeverria S., Josselyn M., Alberte R. S. (1991). Assessment of Environmental Suitability for Growth of Zostera Marina L.(eelgrass) in San Francisco Bay. Aquat. Bot. 39 (3-4), 353–366. doi: 10.1016/0304-3770(91)90009-T
Keywords: coastal eutrophication, flowering intensity, nutrient loading, population resilience, seagrass, Zostera marina
Citation: Suonan Z, Kim SH, Qin L-Z, Kim H, Zhang F and Lee K-S (2022) Increased Coastal Nutrient Loading Enhances Reproductive Intensity of Zostera marina: Implications for Seagrass Meadow Resilience. Front. Mar. Sci. 9:832035. doi: 10.3389/fmars.2022.832035
Received: 09 December 2021; Accepted: 18 May 2022;
Published: 09 June 2022.
Edited by:
Suzanne Jane Painting, Centre for Environment, Fisheries and Aquaculture Science (CEFAS), United KingdomReviewed by:
Peter M. J. Herman, Delft University of Technology, NetherlandsJon Barry, Centre for Environment, Fisheries and Aquaculture Science (CEFAS), United Kingdom
Copyright © 2022 Suonan, Kim, Qin, Kim, Zhang and Lee. This is an open-access article distributed under the terms of the Creative Commons Attribution License (CC BY). The use, distribution or reproduction in other forums is permitted, provided the original author(s) and the copyright owner(s) are credited and that the original publication in this journal is cited, in accordance with accepted academic practice. No use, distribution or reproduction is permitted which does not comply with these terms.
*Correspondence: Kun-Seop Lee, a2xlZUBwdXNhbi5hYy5rcg==