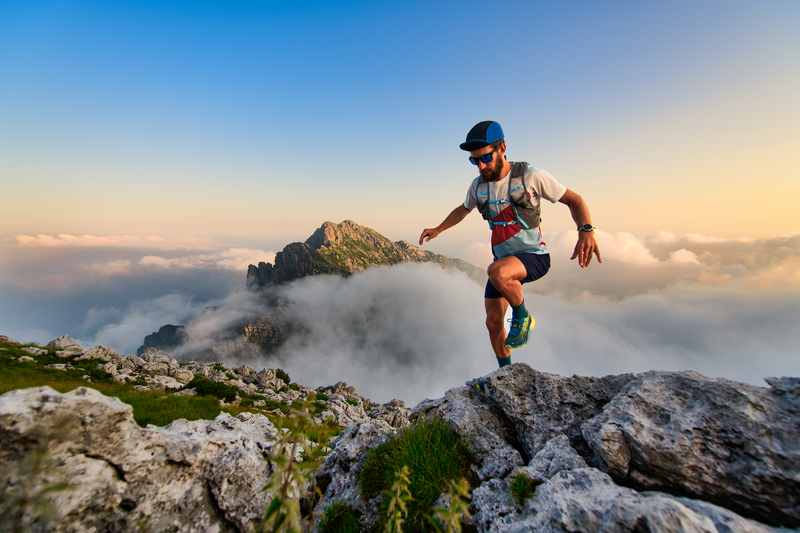
94% of researchers rate our articles as excellent or good
Learn more about the work of our research integrity team to safeguard the quality of each article we publish.
Find out more
ORIGINAL RESEARCH article
Front. Mar. Sci. , 10 March 2022
Sec. Marine Molecular Biology and Ecology
Volume 9 - 2022 | https://doi.org/10.3389/fmars.2022.831286
This article is part of the Research Topic Deep-sea Chemosynthetic Ecosystems: Living in Extreme Environments View all 13 articles
Invertebrates living at methane seeps such as mussels and clams gain nutrition through symbiosis with chemosynthetic, chiefly methanotrophic and thiotrophic bacteria. Lipid biomarkers, including their compound-specific carbon stable isotope compositions, extracted from the host tissues are predestined for deciphering the various sources of diets and the associations among varying environments, endosymbionts, and hosts. Here, we investigated lipid inventories of soft tissues of two bathymodiolin mussel species hosting aerobic methanotrophic bacteria (Gigantidas platifrons from Site F and Gigantidas haimaensis from Haima seeps), one bathymodiolin mussel with thiotrophic bacteria (Bathymodiolus aduloides from Haima seeps), and one vesicomyid clam (Archivesica marissinica from Haima seeps) from the South China Sea. The gills of mussels hosting methanotrophic symbionts were found to contain high amounts of lipids of aerobic methanotrophic bacteria, such as the 4,4-dimethyl lanosterol, and other 4-methyl sterols, and the type I methanotroph-specific monounsaturated fatty acids (MUFAs) C16:1ω9 and C16:1ω8. Production of methyl-sterols is favored over fatty acids at low oxygen concentrations, as demonstrated in culture experiments with Methylococcus capsulatus. Since lesser fatty acids and abundant sterols are found in G. haimaensis compared to G. platifrons, G. haimaensis apparently lived at very low oxygen levels. Extremely high levels of MUFAs C16:1ω7 and C18:1ω7 were found in gill tissue of both B. aduloides and the vesicomyid clam A. marissinica. Given the absence of ω8 fatty acids, both B. aduloides and the vesicomyid clam contain thiotrophic bacteria only. The occurrence of 13C-enriched 24-methylenecholesterol in B. aduloides indicates that the animal complemented its diet by filter-feeding (ca. 3% of the total sterol inventory) on photosynthetically derived carbon, whereas the majority of sterols are pointing to a diet relying on endosymbionts. Different types of 4-methyl sterols were observed between the thiotroph-containing mussel and methanotroph-containing mussels, suggesting different biosynthetic steps are present from lanosterol to cholesterol between animal hosts and aerobic methanotrophs. Among the four bivalve species, specific lipid biomarker patterns diagnostic for either the symbionts or the hosts yielded similar δ13C values in each species, indicating that the host obtained its nutrition either directly from the symbionts or derived at least most of its carbon in this way. The information derived from lipid biomarkers of bivalves and their corresponding symbionts in modern environments is vital to interpret data from the rock record, where most other methods to study microbial community composition are not applicable.
Chemosymbiotic invertebrates from hydrothermal vents and methane seeps, including mussels and clams, gain nutrition through chemosynthetic bacterial symbionts (Childress et al., 1986; Brooks et al., 1987; Fisher et al., 1987; Duperron et al., 2009, 2014). Such symbionts use either reduced sulfur compounds like hydrogen sulfide or methane as electron donors, with oxygen as electron acceptor, providing the invertebrate hosts with nutrition (Petersen and Dubilier, 2010). The host animal provides a stable environment, electron donors, and electron acceptors for the symbionts (Petersen and Dubilier, 2010).
The first report of mussels living in symbiosis with thiotrophic bacteria was from a hydrothermal vent site (Rau and Hedges, 1979), followed by reports from methane seeps, where mussels were found to harbor abundant intracellular methanotrophic bacteria in their gills (Childress et al., 1986). To date, more than 10 species of chemosymbiotic bathymodiolin mussels and clams have been reported from methane seep habitats and whale falls (Cordes et al., 2009; Faure et al., 2015; Duperron and Gros, 2016; Feng et al., 2018; Lan et al., 2019; Xu et al., 2019). It has been demonstrated that some bathymodiolin mussels host either thiotrophic (Cavanaugh, 1983; Distel et al., 1988) or aerobic methanotrophic bacteria, others harbor both types of symbionts, a phenomenon referred to as dual symbiosis (Childress et al., 1986; Fisher et al., 1993; Fiala-Médioni et al., 2002; Cordes et al., 2009; Xu et al., 2019). Putative chemosymbiotic invertebrates were also common at ancient seeps (Kiel, 2010; Kiel and Peckmann, 2019; Kiel et al., 2021).
The phylogeny of bacterial symbionts varies with chemosymbiotic invertebrate species, habitat characteristics, and locations (Nelson and Fisher, 2000; Thornhill et al., 2008; Lorion et al., 2013; Laming et al., 2015; Szafranski et al., 2015; Duperron and Gros, 2016), but can also vary with symbiont populations in response to environmental change (Kádár et al., 2005; Bettencourt et al., 2008). For example different clades of symbionts were found for Escarpia laminata from Alaminos Canyon and the Florida Escarpment, both Gulf of Mexico (Nelson and Fisher, 2000; Thornhill et al., 2008). Furthermore, gradual loss and re-establishment of symbiosis in the gills of Bathymodiolus azoricus was observed when keeping the host in sulfide-free or sulfide-containing seawater, respectively (Kádár et al., 2005; Bettencourt et al., 2008). Fiala-Médioni et al. (2002) suggested the existence of dual symbiosis, providing the host with greater environmental tolerance and increased niche space. Unfortunately, symbiotic bacteria from hydrothermal vents and hydrocarbon seeps have not been cultured successfully to date (Franke et al., 2021). Symbiotic bacteria and their symbiotic associations between hosts and symbionts are typically studied with electron microscopy, enzymatic analyses, 16S rRNA work, as well as lipid biomarkers and their carbon stable isotope compositions (Duperron et al., 2009; Petersen and Dubilier, 2010; Feng et al., 2015).
The analysis of lipid biomarkers is not only a powerful tool to identify bacterial signatures in symbiotic invertebrates, but also to quantify the abundance of bacterial symbionts. Typically, the most abundant lipids are fatty acids. The fatty acid inventory of aerobic methanotrophic bacteria is characterized by the monounsaturated fatty acid (MUFA) C16:1ω9, but especially by the group-specific MUFAs C16:1ω8, and C18:1ω8. In contrast, thiotrophic symbionts produce the ubiquitous MUFAs C16:1ω7 and C18:1ω7 (Jannasch, 1985; Nichols et al., 1985; Jahnke and Nichols, 1986; Bowman et al., 1991; Guezennec and Fiala-Medioni, 1996), which are common constituents of bacteria and eukaryotes, and are also produced or taken up by the host animals. Polyunsaturated fatty acids (PUFAs) n-C18, n-C20, and n-C22 are most likely taken up and stored by the host after heterotrophic consumption (Fullarton et al., 1995; Pond et al., 2000) rather than produced by endosymbiotic bacteria (Dunstan et al., 1993; Kawashima and Ohnishi, 2003). Besides fatty acids, chemosymbiotic mussels contain bacteriohopanepolyols (aminotriol and aminotetrol), and various 4,4-dimethyl sterols and 4-methyl sterols, exclusively derived from symbiotic aerobic methanotrophic bacteria (Fang et al., 1993; Jahnke et al., 1995; Pond et al., 1998; Riou et al., 2010; Kellermann et al., 2012).
Apart from the lipids of endosymbiotic bacteria, lipid biomarkers and compound-specific carbon stable isotope compositions of free-living aerobic methanotrophic bacteria have been studied intensively (Summons et al., 1994; Jahnke et al., 1999; Schouten et al., 2000; Talbot et al., 2001; Cordova-Gonzalez et al., 2020). In contrast to the ubiquitous occurrence of Type I and X methanotrophs in diverse natural environments, including the marine realm, type II methanotrophs seem to be confined to terrestrial habitats (Knief, 2015). The identification of the different types of aerobic methanotrophs (Type I, X, and II) is commonly possible with biomarkers, since the different types of methanotrophs contain different biomarkers. For example, a high abundance of the MUFA C16:1ω8 was found in Type I and X methanotrophs, whereas MUFA C18:1ω8 tends to be predominant in Type II methanotrophs (Nichols et al., 1985; Bowman et al., 1991). On the other hand, 4-methyl steroids are highly specific biomarkers of Type I methanotrophs (e.g., Bouvier et al., 1976; Schouten et al., 2000). Information from lipid biomarkers can be complemented by their carbon stable isotope patterns, reflecting different carbon assimilation pathways of aerobic methanotrophs. Type-I and -X methanotrophs use the ribulose monophosphate (RuMP) pathway, whereas Type-II methanotrophs use the serine pathway (Anthony, 1982; Summons et al., 1994; Jahnke et al., 1999). The different assimilation pathways lead to distinct carbon isotopic fractionation (Jahnke et al., 1999; Cordova-Gonzalez et al., 2020). For example, Type I methanotrophs produce more highly 13C-depleted lipids relative to Type II methanotrophs (Summons et al., 1994; Jahnke et al., 1999; Cordova-Gonzalez et al., 2020). Moreover, the δ13C values of hopanoids and steroids synthesized by Type I methanotrophs are as much as 5–7‰ more negative than fatty acids, whereas in Type II methanotrophs, fatty acids are more depleted in 13C than hopanoids (Summons et al., 1994; Jahnke et al., 1999). Therefore, lipid biomarkers alongside compound-specific carbon stable isotopes are primed to become part of the standard toolkit to discriminate the different types of symbionts and evaluate the nutritional status and associations between symbionts and hosts in chemosymbiosis.
We studied seep bivalves collected from two active seep sites of the South China Sea (Figures 1, 2). The mussel Gigantidas platifrons was collected at Site F seep (also called Formosa ridge) at 1120 m water depth (Feng et al., 2015). One of the mussels and a clam retrieved from the Haima seep at 1390 m water depth were identified as new species and described as G. haimaensis and A. marissinica (Liang et al., 2017). The other mussel, Bathymodiolus aduloides, was collected at the Haima seep at 1390 m water depth. Transmission electron microscopy and 16S rRNA-encoding gene sequence analyses indicated that G. platifrons and G. haimaensis contain mainly aerobic methanotrophic bacteria as symbionts in their gills (Barry et al., 2002; Xu et al., 2019), whereas B. aduloides and A. marissinica were reported to live in symbiosis with thiotrophic bacteria (Feng et al., 2018; Lan et al., 2019). In this study, lipid biomarkers and compound-specific carbon stable isotope compositions were used to examine carbon assimilation pathways, nutritional status, and different host–symbiont associations in various environments, hosts, and symbionts of the four species of seep-dwelling bivalves from the South China Sea.
Figure 1. Map showing the location of the sampling sites. Site F and Haima are active methane seeps (highlighted by red circles); orange circles indicate inactive methane seeps on the northern and western continental slope of the South China Sea.
Figure 2. Seafloor images from Site F (a) and Haima (b–d) methane seeps and the four species of bivalves Gigantidas platifrons (a), Gigantidas haimaensis (b), Archivesica marissinica (c), and Bathymodiolus aduloides (d).
Specimens of G. platifrons were collected in May 2018 using the ROV ROPOS during a cruise with the Research Vessel “Tan Kah Kee” to Site F. Specimens of G. haimaensis, A. marissinica, and B. aduloides were retrieved in April 2018 and May 2019 from the Haima seep using the ROV Haima (Table 1). At the sampling sites, dense G. platifrons, G. haimaensis, and A. marissinica clusters were observed, respectively, covering large areas of the seabed. Several B. aduloides were scattered among carbonate blocks. Bottom water temperatures at both Site F and Haima seep were 3.0 to 3.5°C. After sampling, bivalves were stored onboard at −80°C and shipped to the home laboratory and kept at −25°C. The bivalves were dissected into gill, foot, and remaining tissue, immediately freeze-dried, ground, homogenized and kept at −25°C until further analysis.
Lipids were extracted from the gill and foot tissues of G. platifrons, G. haimaensis, A. marissinica, and B. aduloides. The homogenate was extracted with the solvents dichloromethane (3×), methanol:dichloromethane (1:1, v:v) (3×), and methanol (3×) using an ultrasonic bath to obtain the total lipid extracts (TLE). An aliquot of the TLE was saponified after addition of internal standards (19-methylarachidic acid, and tridecyl alcohol) with 6% KOH (w/v) in methanol (2 h at 70°C). Unfortunately, the concentration of the 19-methylarachidic acid standard in the fatty acid fraction was too low in comparison with the target compounds, resulting in a potential error in the quantification of fatty acids. The absolute contents of fatty acids should consequently not be taken at face value. Therefore, the relative portions of carboxylic acids and alcohols of the overall compound inventory of the respective fractions are provided in Tables 2, 3 in addition to absolute contents. The neutral fractions were extracted from the saponified TLE with n-hexane. To obtain carboxylic acids, the residuals were treated with 10% HCl to pH = 2 and extracted using n-hexane. It needs to be stressed that bacteriohopanepolyols (BHPs) are commonly present in aerobic methanotrophic bacteria; however, we decided to focus on fatty acids and sterols and did not analyze BHPs in this study. The neutral fractions were silylated with bis(trimethylsilyl) trifluoroacetamide (BSTFA) for 3 h at 70°C, and the acid fractions were methylated with 14% BF3-methanol in a screwcap vial (2 h at 60°C). To determine double bond positions of monounsaturated fatty acids, fatty acid methyl esters in n-hexane (50 μl) were treated with dimethyl disulfide (DMDS, 100 μ1) and iodine solution (500 μl; 6% w/v in diethyl ether), filled with argon and stored at 50°C for 48 h. After cooling, iodine was removed by shaking with drops of 5% (w:v) aqueous sodium thiosulfate (Nichols et al., 1986; Guan et al., 2021). The organic phase was removed and the aqueous phase was extracted with n-hexane to obtain the DMDS adducts. All fractions were analyzed with a Thermo Electron Trace GC–MS equipped with a 60-m DB-5 MS fused silica capillary column (0.32 mm i.d., 0.25 μm film thickness). Helium was used as carrier gas at a flow rate of 1.2 ml/min. Compound-specific carbon stable isotope analysis was performed on a GV Isoprime system interfaced to a Hewlett-Packard 6890 gas chromatograph at the Guangzhou Institute of Geochemistry, Chinese Academy of Sciences (GIG, CAS). The same chromatographic column was used for GC-MS analysis. The following GC temperature program was applied for GC-MS and GC-IRMS: initial temperature was held at 60°C for 2 min, from 60°C to 160°C at a ramp of 10°C/min; from 160 to 320°C at a ramp of 1°C/min, and then held at 320°C for 30 min. The analytes were identified by their retention times and comparison with published mass spectra. The carbon isotopic composition is expressed in the standard δ notation in per mil (‰) relative to Vienna-PeeDee Belemnite (V-PDB) standard. The fatty acid methyl esters (FAMEs) and trimethylsiloxyl (TMS) derivatives were corrected for the addition of carbon during the derivatization. The fatty acids were measured as FAMEs and were corrected for the addition of an extra carbon due to the derivatization according to the following equation:
Table 3. Contents and isotopic compositions of sterols, hopanoid, and n-alcohols from bivalve tissues.
The δ13CFA indicates the δ13C value of the fatty acid, Cn represents the number of carbons in the fatty acid, δ13CFAME is the δ13C value of the FAME, and δ13CMEOH is the δ13C value of the methanol used for the methylation reaction.
The sterols or n-alcohols were measured as TMS-derivatives and were corrected for the addition of three extra carbons according to the following equation:
The δ13Calcohol and Cn denotes the δ13C value and the number of carbons in the target biomarker, respectively; δ13CTMS is the δ13C value of the TMS in BSTFA, and δ13CTMS–derivatives is the δ13C value for the TMS-derivative.
Each sample was measured at least in duplicate and the average values were used. Measurements were calibrated by several pulses of carbon dioxide with known δ13C value before and after each run. An n-alkane mixture (C12 to C35) of known isotopic composition was used to check instrument precision. All measured fractions and their compounds were diluted to concentrations of about 20 μg/ml. The injection volume was 1 to 2 μl. Generally, δ13C values of target compounds were only collected when peak heights were higher than one third of the reference carbon dioxide peaks (see Supplementary Figure S1). The standard deviation of the compound-specific carbon isotope measurement was <0.8‰.
Generally, the net contents of fatty acids were much higher in gill than in foot tissues of G. platifrons, A. marissinica, and B. aduloides. Only for G. haimaensis, the contents of fatty acids were similar in gill and foot tissues. In foot tissues, PUFAs C18, C20, and C22 were the most abundant fatty acids of G. platifrons, G. haimaensis, and B. aduloides with contents varying from 4 to 10 mg/g dry weight (dw). In contrast, both gill and foot tissues of A. marissinica were dominated by MUFAs. Among the MUFAs, C16:1ω7 was the most abundant fatty acid, corresponding to 24% of total fatty acids in the gill tissues of G. platifrons, 30% of total fatty acids in B. aduloides, and 43% of total fatty acids in A. marissinica. However, PUFA C22:2, accounting for 23% of total fatty acids, dominated in the gill tissue of G. haimaensis. The fatty acids C16:1ω8 (12% and 9% of total fatty acids, respectively) and C18:1ω8 (3% and 1% of total fatty acids, respectively) were only observed in the gill tissues of G. platifrons and G. haimaensis. Generally, the fatty acids C16:1ω7, C18:1ω7, and C20:1ω7 were among the most abundant compounds of total fatty acids in gill and foot tissues of the four species, making up 32% and 22% for G. platifrons, 24% and 19% for G. haimaensis, and 58% and 28% for A. marissinica, and 44% and 17% for B. aduloides, respectively, with significantly higher relative abundances in gill tissues than in foot tissues. All relative percentages are referring to total fatty acids. Furthermore, C16:1ω5 was found in the gill tissue of A. marissinica in trace amounts (<1% of total fatty acids), while C16:1ω5 and C18:1ω5 were present in both gill and foot tissues of B. aduloides (Figures 3, 4).
Figure 3. Partial gas chromatograms of fatty acids in gill tissues of (A) Gigantidas platifrons and (B) Bathymodiolus aduloides. The ω indicates the double bond position of monounsaturated fatty acids (MUFAs), the suffix c is for cis and t for trans, referring to stereoisomers of MUFAs. Double bond positions of polyunsaturated fatty acids (PUFAs) have not been determined.
Figure 4. Contents and carbon isotopic compositions of selected fatty acids and sterols for (A) Gigantidas platifrons and (B) Gigantidas haimaensis. Blue bars = contents of gill tissues; orange bars = contents of foot tissues; blue diamonds = δ13C values of biomarkers from gill tissues; orange diamonds = δ13C values of biomarkers from foot tissues.
The fatty acids from the gill and foot tissues of G. platifrons and G. haimaensis were strongly depleted in 13C, with δ13C values ranging from −75‰ to −59‰ and −72‰ to −54‰, respectively. Compared to G. platifrons and G. haimaensis, A. marissinica and B. aduloides yielded moderately negative δ13C values for fatty acids from −51‰ to −37‰ and −52‰ to −36‰, respectively (Table 2).
Various sterols were found in the gill and foot tissues of G. platifrons, G. haimaensis, A. marissinica, and B. aduloides (Table 3 and Figure 5). Even though the inventory of sterols was generally similar for G. platifrons and G. haimaensis, the contents of individual compounds varied significantly between the two species and types of tissues (Figure 4). The total content of sterols in the gill tissue of G. haimaensis was 60 mg/g dw, whereas the gill tissue content of sterols of G. platifrons was very low (2.6 mg/g dw). In B. aduloides, the sterol contents were even below 2 mg/g dw. Lanosterol (IX, see Figure 6 for structure) and cholesterol (I) were the most abundant sterols in the gill tissue of both G. haimaensis (16 mg/g dw) and G. platifrons (1.1 mg/g dw), representing 25% and 44% of the total alcohols, respectively. In B. aduloides, cholesterol (I) was the most abundant sterol, accounting for 46% and 53% of the total alcohols in gill and foot tissues, respectively, followed by lanost-8(9)-en-3β-ol (VIII), lanosterol, cholestanol, 24-methylenecholesterol (IV), and cholest-7-en-3β-ol (Table 3 and Figure 7). In the foot tissue, cholesterol (I) was most abundant in G. platifrons and G. haimaensis (0.4 mg/g dw and 12 mg/g dw, respectively), but was slightly more abundant in the gills (1.1 mg/g dw and 13 mg/g dw, respectively). Lanosterol, though, was not found in any of the foot tissue samples.
Figure 5. Gas chromatograms of alcohols in gill tissues of (A) Gigantidas platifrons and (B) Gigantidas haimaensis. Roman numerals indicate n-alcohols; ? denotes presently unknown; Cont. = contaminant.
Figure 7. Contents and carbon isotopic compositions of selected fatty acids and sterols for (A) Archivesica marissinica and (B) Bathymodiolus aduloides. Blue bars = contents of gill tissues; orange bars = contents of foot tissues; blue diamonds = δ13C values of biomarkers from gill tissues; orange diamonds = δ13C values of biomarkers from foot tissues.
Other sterols, such as nor-lanosterol (X), cholestanol (II), cholesta-5,24-dien-3β-ol (III), cholest-7-en-3β-ol (V), 4α-methylcholesta-8(14),24-dien-3β-ol (VI), and 4α-methylcholesta-8(14)-en-3β-ol (VII), were as well more prominent in the gill tissues than in foot tissues of G. platifrons and G. haimaensis. In B. aduloides, the composition and contents of sterols in the foot tissue are similar to gill tissue. The single hopanoid, found only in traces in the gills of G. platifrons, was diplopterol (Figure 5). Minute amounts of cholesterol (I), cholestanol (II), and stigmast-5-en-3β-ol (XI) were found in A. marissinica. The total sterols accounted for 17% and 6% of all alcohols in the gill and foot tissues, respectively, with relatively higher contents in the foot than in the gill.
The n-alcohols C22:0, C28:0, and C30:0 were found in gill and foot tissues of G. haimaensis, A. marissinica, and B. aduloides. The gill and foot tissues revealed similar 13C depletion for sterols, with δ13C values varying from −84‰ to −78‰ and −80‰ to −74‰ for G. platifrons and G. haimaensis, respectively. For B. aduloides, cholesterol, cholestanol, cholest-7-en-3β-ol, and the 4,4-dimethyl sterols (lanosterol and lanost-8(9)-en-3β-ol) in both gill and foot tissues yielded δ13C values from −46‰ to −40‰, whereas the δ13C values of 24-methylenecholesterol in gill and foot tissues ranged from −32‰ to −30‰.
Both G. platifrons (Site F) and G. haimaensis (Haima seeps) were referred to as B. platifrons previously (Feng et al., 2015, 2018) and were reported to host methanotrophs (Duperron et al., 2009; Feng et al., 2015; Assié et al., 2016; Xu et al., 2019). Xu et al. (2019) analyzed the microbial 16S rRNA genes in gill tissue of G. haimaensis collected from the Haima seep site, and found that the bacteria were dominated by three phylotypes of symbiotic Gammaproteobacteria and three phylotypes of symbiotic Epsilonproteobacteria. The most abundant gammaproteobacterial phylotype of G. haimaensis clustered close to methanotrophic Gammaproteobacteria of other Gigantidas species and were assigned to Type I aerobic methanotrophic bacteria (Xu et al., 2019). Given Type II methanotrophs have never been reported in the marine realm (Knief, 2015), the symbionts harbored in G. platifrons are likely to be Type I methanotrophs too. Type I methanotrophs are the most prominent symbionts in the gills of both G. platifrons and G. haimaensis, which is supported by characteristic fatty acid inventories, including cis and trans isomers of C16:1 with double bonds at ω6, ω7, ω8, and ω9 (Bowman et al., 1993) and a predominance of C16:1ω8 fatty acid over C18:1ω8 fatty acid (cf. Nichols et al., 1985; Bowman et al., 1991; Niemann et al., 2006; Riou et al., 2010).
In addition to fatty acids, 4,4-dimethyl sterols (lanosterol and nor-lanosterol), 4-methyl sterols (4α-methylcholesta-8(14),24-dien-3β-ol and 4α-methylcholesta-8(14)-en-3β-ol), and desmethyl sterols (cholesterol, cholestanol, cholesta-5,24-dien-3β-ol, and cholest-7-en-3β-ol) were observed in soft tissues of G. platifrons and G. haimaensis. The absolute and relative contents of sterols are generally different, for example lanosterol accounted for 15% and 25% of the total alcohols in gills of G. platifrons and G. haimaensis, respectively. However, the overall inventory of sterols of G. platifrons and G. haimaensis is similar (Table 3). Cholesterol is the most abundant sterol in the gill of G. platifrons (44%), whereas in G. haimaensis lanosterol dominates (25%; Figure 4). Cholesterol and other desmethyl sterols are common in eukaryotes, but lanosterol-like 4,4-dimethyl sterols and 4-methyl sterols are only present in free-living (Jahnke and Nichols, 1986; Cordova-Gonzalez et al., 2020) and endosymbiotic aerobic methanotrophs among bacteria (Jahnke et al., 1995; Kellermann et al., 2012). Both 4,4-dimethyl sterols and 4-methyl sterols are demethylation products of lanosterol in aerobic methanotrophic bacteria (Methylococcus capsulatus; Bouvier et al., 1976; Summons et al., 1994). Based on similar 13C-depletions and the co-occurrence of 4-methyl sterol intermediates and desmethylated cholesterol in soft tissues other than gills, cholesterol was supposed to be synthesized by the animal host using bacterial 4-methyl sterols as diet (Jahnke et al., 1995). For G. platifrons and G. haimaensis, 4-methyl sterols and cholesterol occurred in both gill and foot tissues and yielded similar δ13C values, indicating that 4-methyl sterols are most likely biosynthetic intermediates and represent metabolic precursors in cholesterol biosynthesis. The high abundance of 4,4-dimethyl sterols and 4-methyl sterols agrees with the presence of Type I methanotrophs, which are the only bacteria known to synthesize these source-specific compounds in noteworthy amounts (Bird et al., 1971; Schouten et al., 2000; Wei et al., 2016; Cordova-Gonzalez et al., 2020). In culture, Methylococcus capsulatus biosynthesizes fatty acids with significant 13C-depletion compared to methane, with Δ values ranging from −25‰ to −17‰ (Jahnke et al., 1999), whereas the fatty acids in the gills of type I methanotroph-containing mussels were more 13C-depleted by ca. −16‰ (Jahnke et al., 1995). At site F and Haima seeps, available δ13C values of methane approximately correspond to −60‰ (Feng et al., 2015; Fang et al., 2019). In the gills of G. platifrons and G. haimaensis, the symbiont-specific C16:1ω8 fatty acid and most fatty acids yielded δ13C values from −75‰ to −72‰ and −75‰ to −65‰, respectively, which is consistent with fractionation patterns of fatty acids in type I/X methanotrophs and mussels that live in symbiosis with type I methanotrophs (Jahnke et al., 1995; Kellermann et al., 2012). Further, both 4,4-dimethyl sterols and 4-methyl sterols were more 13C-depleted than fatty acids, with an average Δδ13Csterols–fatty acids between −13‰ and −8‰, similar to values reported from laboratory cultures (Summons et al., 1994; Jahnke et al., 1999; Cordova-Gonzalez et al., 2020). As for fatty acids, the isotopic pattern of sterols confirms a predominance of Type I and/or X methanotrophs (cf. Cordova-Gonzalez et al., 2020).
Although δ13C values of fatty acids and sterols were uniform for G. platifrons and G. haimaensis, contents of the various lipids varied in the two mussels. While the same fatty acid inventory was found in gill and foot tissues of both mussels, contents of fatty acids were much higher in G. platifrons (see Figure 4), whereas both 4,4-dimethyl and 4-methyl sterols were more abundant in G. haimaensis. The strong variation in the membrane lipid composition, especially the ratio of fatty acids over sterols, is most likely caused by variation of oxygen levels in the environment. Such an effect was shown by Jahnke and Nichols (1986) for Methylococcus capsulatus grown under varying oxygen tensions, revealing that sterols (4-methyl and 4,4-dimethyl sterols) and phospholipids were most abundant in cells grown with 0.5 and 1.1% oxygen. As oxygen was reduced, the amount of MUFA C16:1 decreased but the 4,4-dimethyl sterols were predominantly produced at very low oxygen tensions (0.1%). Except for type X methanotrophs, oxygen level affecting membrane lipid composition was also reported for Methylobacterium organophilum (Type II methanotroph; Patt and Handon, 1978). Bathymodiolin mussels acquire symbionts horizontally and may take up various types and different amounts of symbionts in response to varying environmental conditions (Won et al., 2003; Kádár et al., 2005; Franke et al., 2021), which may also affect the biosynthesis of membrane lipids. In the present study, G. platifrons yielded significantly higher contents of MUFA C16:1 than G. haimaensis, which may reflect a higher oxygen level for G. platifrons. Although no oxygen concentrations have been reported for the study sites, applying these observations to the biomarker patterns in G. haimaensis suggests that G. haimaensis most likely lived at a low level of dissolved oxygen.
Apart from the symbiont-specific fatty acids, the MUFAs C20 and C22, as well as PUFAs C20 and C22 of the animal host (Conway and McDowell-Capuzzo, 1991; Pond et al., 1998) were present in both G. platifrons and G. haimaensis. In chemotrophic symbioses, some bivalves are highly dependent on the metabolic intermediates transferred by the symbionts, which leads to a similar range of δ13C values for the lipids produced by hosts and symbionts (Jahnke et al., 1995; Pond et al., 1998; Kellermann et al., 2012). With respect to the seep-dwelling bivalves from the South China Sea, most symbiont- and host-specific fatty acids of G. platifrons and G. haimaensis yielded δ13C values from −75‰ to −68‰ and −72‰ to −60‰, respectively, indicating that the host obtained most of its nutrition either directly from the symbionts or consumes symbiont-derived carbon.
Membrane lipids of free-living sulfide-oxidizing bacteria are usually characterized by prominent ω7 fatty acids with either C16:1ω7 or C18:1ω7 predominating (Jannasch, 1985; Guezennec and Fiala-Medioni, 1996; Arning et al., 2008). These compounds were also found to be abundant in thiotrophic bacteria living in mussels (Pond et al., 1998; Kellermann et al., 2012) and mussels cultured in the presence of hydrogen sulfide (Riou et al., 2010). The high abundance of MUFAs C16:1ω7 and C18:1ω7 and the absence of 4-methyl sterols in A. marissinica (yielding cholesterol, cholestanol, and stigmast-5-en-3β-ol instead) agrees with thiotrophic bacteria as only endosymbionts.
For B. aduloides, in addition to the abundant MUFAs C16:1ω7 and C18:1ω7, a range of sterols, including cholesterol, cholestanol, 24-methylenecholesterol, cholest-7-en-3β-ol, lanost-8(9)-en-3β-ol, and lanosterol, were found in both gill and foot tissues. It has been demonstrated that some mussels are capable of synthesizing sterols (Teshima and Kanazawa, 1974; Teshima and Patterson, 1981). Sterols in soft tissues of symbiont-containing mussels may originate from its symbionts, diets from ingested plant and algal material, or synthesis by the animal host. For B. aduloides, a derivation of sterols except for 24-methylenecholesterol from filter-feeding is unlikely, because sterols synthesized by plants and algae are characterized by high abundance of sterols alkylated at C-24 containing either a methyl group or ethyl group (Jarzebski and Popov, 1985; Volkman, 2003, 2005). Furthermore, such photosynthetically derived carbon reveals δ13C values around −30‰ to −20‰ (Conway and McDowell-Capuzzo, 1991; Kellermann et al., 2012), which is inconsistent with the carbon stable isotopic pattern of the B. aduloides sterols (from −46‰ to −40‰). Feng et al. (2018) reported δ13Cbiomass values (−37‰ to −33‰) in soft tissues of B. aduloides from Site F of the South China Sea and concluded that the mussels host thiotrophic bacteria. However, the thiotrophic symbionts are not a potential source candidate for sterols, since no sulfide-oxidizing bacteria have been shown to synthesize sterols to date (McCaffrey et al., 1989; Conway and McDowell-Capuzzo, 1991). Given the absence of ω8 fatty acids, a derivation of 4,4-dimethyl sterols (lanosterol and lanost-8(9)-en-3β-ol) from aerobic methanotrophic bacteria is highly unlikely as well.
Apart from the bacterial domain, eukaryotes including animals are known to produce lanosterol. Using 14C-labeling in cultures revealed that the oyster Crassostrea virginica incorporated acetate to form squalene, 4,4-dimethylsterols, and 4-methylsterols, which can also convert lanosterol to cholesterol (Teshima and Patterson, 1981). These results suggest that the lanosterol in B. aduloides was most likely synthesized by the animal host. However, the lanost-8(9)-en-3β-ol as an intermediate has never been reported in aerobic methanotrophs and symbiont-containing invertebrates before, but it was found to co-occur with lanosterol in hyperlipidemic serum and rat livers (Gray et al., 1969; Scallen et al., 1971). As one of the intermediates in the conversion from lanosterol to cholesterol, the production of lanost-8(9)-en-3β-ol may occur as the first step when the side chain of lanosterol is reduced (Gray et al., 1969; Scallen et al., 1971). Although uncertainties are present, the absence of lanost-8(9)-en-3β-ol in aerobic methanotrophs is most likely caused by differences in biosynthetic steps from lanosterol to cholesterol between aerobic methanotrophs and animals.
In addition to nutrients supplied by the endosymbionts, B. aduloides complemented its diet by filter-feeding on photosynthetically derived carbon, as indicated by the presence of 13C-enriched 24-methylenecholesterol (δ13C values from −32‰ to −30‰) in both gill and foot tissues. Since consumers are generally 13C-enriched by only 0.4‰ to 1‰ compared to their diet (McCutchan et al., 2003), these δ13C values suggest higher plants or marine phytoplankton as sources (Goericke et al., 1994). Similar observations were made for the Pacific-Antarctic Ridge and the Gulf of Mexico. At the Pacific-Antarctic Ridge, a chemosynthesis-based community was observed to progressively shift its nutritional lifestyle to filter-feeding when diffuse hydrothermal venting vanished (Stecher et al., 2002). Reduction in symbiont abundances and changes in energy sources induced by decrease of hydrogen sulfide concentration were also reflected by very low contents of symbiont-specific lipids and relatively 13C-enriched cholesterol (−22‰) in Bathymodiolus cf. thermophilus from seeps of the Gulf of Mexico (Kellermann et al., 2012). Although both A. marissinica and B. aduloides contain thiotrophic bacteria, there are major differences when it comes to their symbionts. A. marissinica transmits the symbionts from parent to offspring (Ohishi et al., 2016; Lan et al., 2019), whereas B. aduloides acquires symbionts from the ambient environments for each new generation (Franke et al., 2021). Such differences in symbiont clades and amounts may lead to variations in the biomarker inventories of both hosts and symbionts. The above findings including our study confirm that lipid biomarkers and their isotope signatures are excellent tracers to decode varying environmental conditions and carbon and energy pathways in chemosynthesis-based ecosystems.
Four species of seep bivalves collected from Site F (Gigantidas platifrons) and Haima seeps (Gigantidas haimaensis, Bathymodiolus aduloides, and Archivesica marissinica) in the South China Sea were analyzed for lipid biomarkers and compound-specific carbon stable isotope patterns. Gigantidas platifrons and Gigantidas haimaensis were demonstrated to live in symbioses with methanotrophic bacterial symbionts as revealed by abundant 13C-depleted lanosterol, nor-lanosterol, 4-methylsterols, and monounsaturated fatty acids C16:1ω9 and C16:1ω8 extracted from gill tissues. The relatively higher abundance of C16:1ω8 relative to C18:1ω8 and the occurrence of 4,4-dimethylsterols and 4-methylsterols agree with an assignment to type I methanotrophs. The much higher sterol contents and the less abundant fatty acids in G. haimaensis compared to G. platifrons probably reflect low oxygen levels during the growth of G. haimaensis. The gill tissues of both B. aduloides and A. marissinica contained significant amounts of C16:1ω7 and C18:1ω7 fatty acids, and their nutritional status was found to be different from G. platifrons and G. haimaensis. A. marissinica apparently contains only thiotrophic bacteria, indicated by the lack of ω8 fatty acids, 4,4-dimethylsterols, and 4-methylsterols. Based on the biomarker patterns, B. aduloides is also suggested to contain thiotrophic bacteria, but the animal host complemented its diet through filter-feeding as revealed by relatively 13C-enriched 24-methylenecholesterol. The different types of 4-methyl sterols between the thiotroph-containing mussel (B. aduloides) and the methanotroph-containing mussels (G. platifrons and G. haimaensis) are likely caused by different biosynthetic steps from lanosterol to cholesterol between animal hosts and aerobic methanotrophs. Furthermore, the host-specific polyunsaturated fatty acids C20 and C22 in all four bivalve species yielded δ13C values close to those of symbiont-specific fatty acids, suggesting that the hosts acquired most of their nutrition from the symbionts.
The original contributions presented in the study are included in the article/Supplementary Material, further inquiries can be directed to the corresponding authors.
Ethical review and approval was not required for the animal study because invertebrate studies at seeps are common.
HG, DF, and JT collected the samples. HG did the experiments. HG, DB, and JP analyzed the biomarker data. All authors contributed to the preparation of the manuscript.
This research was supported by NSF of China (Grant Number 91958105) and the Shandong Special Fund of Pilot National Laboratory for Marine Science and Technology (Qingdao; Grant Number 2021QNLM020002).
The authors declare that the research was conducted in the absence of any commercial or financial relationships that could be construed as a potential conflict of interest.
All claims expressed in this article are solely those of the authors and do not necessarily represent those of their affiliated organizations, or those of the publisher, the editors and the reviewers. Any product that may be evaluated in this article, or claim that may be made by its manufacturer, is not guaranteed or endorsed by the publisher.
We express our sincere appreciation to the crews of the Haima, ROPOS, Haiyang-06, and “Tan Kah Kee” Cruises for their professionalism in sampling. We thank S. Gao (GIG, CAS) for sample preparation and technical assistance. The manuscript benefited from insightful comments of the reviewers.
The Supplementary Material for this article can be found online at: https://www.frontiersin.org/articles/10.3389/fmars.2022.831286/full#supplementary-material
Arning, E. T., Birgel, D., Schulz-Vogt, H. N., Holmkvist, L., Jørgensen, B. B., Larson, A., et al. (2008). Lipid biomarker patterns of phosphogenic sediments from upwelling regions. Geomicrobiol. J. 25, 69–82.
Assié, A., Borowski, C., van der Heijden, K., Raggi, L., Geier, B., Leisch, N., et al. (2016). A specific and widespread association between deep-sea Bathymodiolus mussels and a novel family of Epsilonproteobacteria. Environ. Microbiol. Rep. 8, 805–813. doi: 10.1111/1758-2229.12442
Barry, J. P., Buck, K. R., Kochevar, R. K., Nelson, D. C., Fujiwara, Y., Goffredi, S. K., et al. (2002). Methane-based symbiosis in a mussel, Bathymodiolus platifrons, from cold seeps in Sagami Bay, Japan. Invertebrate Biol. 121, 47–54. doi: 10.1111/j.1744-7410.2002.tb00128.x
Bettencourt, R., Dando, P., Rosa, D., Riou, V., Colaço, A., Sarrazin, J., et al. (2008). Changes of gill and hemocyte-related bio-indicators during long term maintenance of the vent mussel Bathymodiolus azoricus held in aquaria at atmospheric pressure. Comparat. Biochem. Physiol. Part A 150, 1–7. doi: 10.1016/j.cbpa.2008.02.020
Bird, C. W., Lynch, J. M., Pirt, S. J., and Reid, W. W. (1971). Steroids and squalene in Methylococcus capsulatus grown on methane. Nature 230, 473–474. doi: 10.1038/230473a0
Bouvier, P., Rohmer, M., Benveniste, P., and Ourisson, G. (1976). Δ8(14)-Steroids in the bacterium Methylococcus capsulatus. Biochem. J. 159, 267–271. doi: 10.1042/bj1590267
Bowman, J. P., Skerrat, J. H., Nichols, P. D., and Sly, L. I. (1991). Phospholipid fatty acid and lipopolysaccharide fatty acid signature lipids in methane-utilizing bacteria. FEMS Microbiol. Lett. 85, 15–21.
Bowman, J. P., Sly, L. I., Nichols, P. D., and Hayward, A. C. (1993). Revised taxonomy of the methanotrophs: description of Methylobacter gen. nov., emendation of Methylococcus, validation of Methylosinus and Methylocystis species, and a proposal that the family Methylococcaceae includes only the group I methanotrophs. Int. J. Systematic Evol. Microbiol. 43, 735–753.
Brooks, J. M., Kennicutt, M. C., Fisher, C. R., Macko, S. A., Cole, K., Childress, J. J., et al. (1987). Deep-sea hydrocarbon seep communities: evidence for energy and nutritional carbon sources. Science 238, 1138–1142. doi: 10.1126/science.238.4830.1138
Cavanaugh, C. M. (1983). Symbiotic chemoautotrophic bacteria in marine invertebrates from sulfide-rich habitats. Nature 302, 58–61. doi: 10.1016/j.scitotenv.2021.150054
Childress, J. J., Fisher, C. R., Brooks, J. M., Kennicutt, M. C., Bidigare, R., and Anderson, A. E. (1986). A methanotrophic marine molluscan (Bivalvia: Mytilidae) symbiosis: mussels fueled by gas. Science 233, 1306–1308. doi: 10.1126/science.233.4770.1306
Conway, N., and McDowell-Capuzzo, J. (1991). Incorporation and utilization of bacterial lipids in the Solemya velum symbiosis. Mar. Biol. 108, 277–291.
Cordes, E. E., Bergquist, D. C., and Fisher, C. R. (2009). Macro-ecology of Gulf of Mexico cold seeps. Annual Rev. Mar. Sci. 1, 143–168. doi: 10.1146/annurev.marine.010908.163912
Cordova-Gonzalez, A., Birgel, D., Kappler, A., and Peckmann, J. (2020). Carbon stable isotope patterns of cyclic terpenoids: a comparison of cultured alkaliphilic aerobic methanotrophic bacteria and methane-seep environments. Organ. Geochem. 139:103940.
Distel, D., Lane, D., Olsen, G., Giovannoni, S., Pace, B., Pace, N., et al. (1988). Sulfur-oxidizing bacterial endosymbionts–analysis of phylogeny and specificity by 16S ribosomal RNA sequences. J. Bacteriol. 170, 2506–2510.
Dunstan, G. A., Volkman, J. K., and Barrett, S. M. (1993). The effect of lyophilization on the solvent extraction of lipid classes, fatty acids and sterols from the oyster Crassostrea gigas. Lipids 28, 937–944. doi: 10.1007/bf02537504
Duperron, S., and Gros, O. (2016). Colwellia and sulfur-oxidizing bacteria: an unusual dual symbiosis in a Terua mussel (Mytilidae: Bathymodiolinae) from whale falls in the Antilles arc. Deep-Sea Res. Part I 115, 112–122. doi: 10.1016/j.dsr.2016.05.012
Duperron, S., Gaudron, S. M., Lemaitre, N., and Bayon, G. (2014). A microbiological and biogeochemical investigation of the cold seep tubeworm Escarpia southwardae (Annelida: Siboglinidae): symbiosis and trace element composition of the tube. Deep-Sea Res. Part I 90, 105–114.
Duperron, S., Lorion, J., Samadi, S., Gros, O., and Gaill, F. (2009). Symbioses between deep-sea mussels (Mytilidae: Bathymodiolinae) and chemosynthetic bacteria: diversity, function and evolution. C. R. Biol. 332, 298–310. doi: 10.1016/j.crvi.2008.08.003
Fang, J., Abrajano, T. A., Comet, P. A., Brooks, L. M., Sassen, R., and MacDonald, I. R. (1993). Gulf of Mexico hydrocarbon seep communities XI. Carbon isotopic fractionation during fatty acid biosynthesis of seep organisms and its implication for chemosynthetic processes. Chem. Geol. 109, 271–279.
Fang, Y., Wei, J., Lu, H., Liang, J., Lu, J., Fu, J., et al. (2019). Chemical and structural characteristics of gas hydrates from the Haima cold seeps in the Qiongdongnan Basin of the South China Sea. J. Asian Earth Sci. 182:103924. doi: 10.1016/j.jseaes.2019.103924
Faure, B., Schaeffer, S. W., and Fisher, C. R. (2015). Species distribution and population connectivity of deep-sea mussels at hydrocarbon seeps in the Gulf of Mexico. PLoS One 10:e0118460. doi: 10.1371/journal.pone.0118460
Feng, D., Cheng, M., Kiel, S., Qiu, J.-W., Yang, Q., Zhou, H., et al. (2015). Using Bathymodiolus tissue stable carbon, nitrogen and sulfur isotopes to infer biogeochemical process at a cold seep in the South China Sea. Deep-Sea Res. Part I 104, 52–59. doi: 10.1016/j.dsr.2015.06.011
Feng, D., Peckmann, J., Li, N., Kiel, S., Qiu, J.-W., Liang, Q., et al. (2018). The stable isotope fingerprint of chemosymbiosis in the shell organic matrix of seep-dwelling bivalves. Chem. Geol. 479, 241–250. doi: 10.1016/j.chemgeo.2018.01.015
Fiala-Médioni, A., McKiness, Z. P., Dando, P., Boulegue, J., Mariotti, A., Alayse-Danet, A. M., et al. (2002). Ultrastructural, biochemical, and immunological characterization of two populations of the mytilid mussel Bathymodiolus azoricus from the Mid-Atlantic Ridge: evidence for a dual symbiosis. Mar. Biol. 141, 1035–1043. doi: 10.1007/s00227-002-0903-9
Fisher, C. R., Brooks, J. M., Vodenichar, J. S., Zande, J. M., Childress, J. J., and Burke, R. A. Jr. (1993). The co-occurrence of methanotrophic and chemoautotrophic sulfur-oxidizing bacterial symbionts in a deep-sea mussel. Mar. Ecol. 14, 277–289.
Fisher, C. R., Childress, J. J., Oremland, R. S., and Bidigare, R. R. (1987). The importance of methane and thiosulfate in the metabolism of the bacterial symbionts of two deep-sea mussels. Mar. Biol. 96, 59–71. doi: 10.1007/bf00394838
Franke, M., Geier, B., Hammel, J. U., Dubilier, N., and Leisch, N. (2021). Coming together—symbiont acquisition and early development in deep-sea bathymodioline mussels. Proc. R. Soc. B 288:20211044. doi: 10.1098/rspb.2021.1044
Fullarton, J. G., Dando, P. R., Sargent, J. G., Southward, A. J., and Southward, E. C. (1995). Fatty acids of hydrothermal vent Ridgeia piscesae and inshore bivalves containing symbiotic bacteria. J. Mar. Biol. Assoc. U K. 75, 455–468. doi: 10.1017/s0025315400018300
Goericke, R., Montoya, J. P., and Fry, B. (1994). “Physiology of isotopic fractionation in algae and cyanobacteria,” in Stable Isotopes in Ecology and Environmental Science, eds K. Lajtha and R. H. Michener (Oxford: Blackwell). doi: 10.1073/pnas.70.10.2727
Gray, M. F., Morrison, A., Farish, E., Lawrie, T. D. V., and Brooks, C. J. W. (1969). Characterization of 5α-lanost-8(9)-en-3β-ol in hyperlipaemic serum. Biochim. Biophys. Acta 187, 163–165. doi: 10.1016/0005-2760(69)90147-7
Guan, H., Birgel, D., Feng, D., Peckmann, J., Liu, L., Liu, L., et al. (2021). Lipids and their δ13C values reveal carbon assimilation and cycling in the methane-seep tubeworm Paraescarpia echinospica from the South China Sea. Deep–Sea Res. I 174:103556. doi: 10.1016/j.dsr.2021.103556
Guezennec, J., and Fiala-Medioni, A. (1996). Bacterial abundance and diversity in the Barbados Trench determined by phospholipid analysis. FEMS Microbiol. Ecol. 19, 83–93. doi: 10.1111/j.1574-6941.1996.tb00201.x
Jahnke, L. L., and Nichols, P. D. (1986). Methyl sterol and cyclopropane fatty acid composition of Methylococcus capsulatus grown at low oxygen tensions. J. Bacteriol. 167, 238–242. doi: 10.1128/jb.167.1.238-242.1986
Jahnke, L. L., Summons, R. E., Dowling, L. M., and Zahiralis, K. D. (1995). Identification of methanotrophic lipid biomarkers in cold seep mussel gills: chemical and isotopic analysis. Appl. Environ. Microbiol. 61, 576–582. doi: 10.1128/aem.61.2.576-582.1995
Jahnke, L. L., Summons, R. E., Hope, J. M., and Des Marais, D. J. (1999). Carbon isotopic fractionation in lipids from methanotrophic bactria II: the effects of physiology and environmental parameters on the biosynthesis and isotopic signatures of biomarkers. Geochimica Cosmochimica Acta 63, 79–93. doi: 10.1016/s0016-7037(98)00270-1
Jannasch, H. W. (1985). The chemosynthetic support of life and the microbial diversity at deep-sea hydrothermal vents. Proc. R. Soc. London 225, 277–297.
Jarzebski, A., and Popov, S. (1985). Comparison of sterol composition of some Baltic Sea and Black Sea mussels. Mar. Biol. 86, 159–161.
Kádár, E., Bettencourt, R., Costa, V., Serrão Santos, R., Lododa-Cunha, A., and Dando, P. R. (2005). Experimentally induced endosymbiont loss and re-acquirement in the hydrothermal vent bivalve Bathymodiolus azoricus. J. Exp. Mar. Biol. Ecol. 318, 99–110.
Kawashima, H., and Ohnishi, M. (2003). Fatty acid compositions of various tissue lipids in the marine bivalves, Megangulus venulosus and Megangulus zyonoensis, from coastal waters of Hokkaido, Northern Japan. J. Oleo Sci. 52, 309–315.
Kellermann, M. Y., Schubotz, F., Elvert, M., Lipp, J. S., Birgel, D., Prieto-Mollar, X., et al. (2012). Symbiont–host relationships in chemosynthetic mussels: a comprehensive lipid biomarker study. Organ. Geochem. 43, 112–124.
Kiel, S. (2010). “The fossil record of vent and seep mollusks,” in The Vent and Seep Biota. Topics in Geobiology, ed. S. Kiel (Heidelberg: Springer), 255–278. doi: 10.1098/rspb.2014.2908
Kiel, S., and Peckmann, J. (2019). Resource partitioning among brachiopods and bivalves at ancient hydrocarbon seeps: a hypothesis. PLoS One 14:e0221887. doi: 10.1371/journal.pone.0221887
Kiel, S., Birgel, D., Lu, Y., Wienholz, D., and Peckmann, J. (2021). A thyasirid-dominated methane-seep deposit from Montañita, southwestern Ecuador, from the Oligocene-Miocene boundary. Palaeogeography Palaeoclimatol. Palaeoecol. 575:110477.
Knief, C. (2015). Diversity and habitat preferences of cultivated and uncultivated aerobic methanotrophic bacteria evaluated based on pmoA as molecular marker. Front. Microbiol. 6:1346. doi: 10.3389/fmicb.2015.01346
Laming, S. R., Szafranski, K. M., Rodrigues, C. F., Gaudron, S. M., Cunha, M. R., Hilário, A., et al. (2015). Fickle or faithful: the roles of host and environmental context in determining symbiont composition in two bathymodioline mussels. PLoS One 10:e0144307. doi: 10.1371/journal.pone.0144307
Lan, Y., Sun, J., Zhang, W., Xu, T., Zhang, Y., Chen, C., et al. (2019). Host–symbiont interactions in deep-sea chemosymbiotic vesicomyid clams: insights from transcriptome sequencing. Front. Mar. Sci. 6:680. doi: 10.3389/fmars.2019.00680
Liang, Q., Hu, Y., Feng, D., Peckmann, J., Chen, L., Yang, S., et al. (2017). Authigenic carbonates from newly discovered active cold seeps on the northwestern slope of the South China Sea: constraints on fluid sources, formation environments, and seepage dynamics. Deep-Sea Res. Part I 124, 31–41. doi: 10.1016/j.dsr.2017.04.015
Lorion, J., Kiel, S., Faure, B. M., Kawato, M., Ho, S. Y. W., Marshall, B. A., et al. (2013). Adaptive radiation of chemosymbiotic deep-sea mussels. Proc. R. Soc. B 280:20131243. doi: 10.1098/rspb.2013.1243
McCaffrey, M. A., Farrington, J. W., and Repeta, D. (1989). Geochemical implications of the lipid composition of Thioploca spp. from the Peru upwelling region–15°S. Organ. Geochem. 14, 61–68.
McCutchan, J. H., Lewis, W. M., Kendall, C., and McGrath, C. C. (2003). Variation in trophic shift for stable isotope ratios of carbon, nitrogen, and sulfur. Oikos 102, 378–390. doi: 10.1034/j.1600-0706.2003.12098.x
Nelson, K., and Fisher, C. R. (2000). Absence of cospeciation in deep-sea vestimentiferan tube worms and their bacterial endosymbionts. Symbiosis 28, 1–15. doi: 10.1002/jmor.1054
Nichols, P. D., Guckert, J. B., and White, D. C. (1986). Determination of monounsaturated fatty acid double-bond position and geometry for microbial monocultures and complex consortia by capillary GC–MS of their dimethyl disulphide adducts. J. Microbiol. Methods 5, 49–55.
Nichols, P. D., Smith, G. A., Antworth, C. P., Hanson, R. S., and White, D. C. (1985). Phospholipid and lipopolysaccharide normal and hydroxy fatty acids as potential signatures for methane-oxidizing bacteria. FEMS Microbiol. Ecol. 31, 327–335.
Niemann, H., Lösekann, T., de Beer, D., Elvert, M., Nadalig, T., Knittel, K., et al. (2006). Novel microbial communities of the Haakon Mosby mud volcano and their role as a methane sink. Nature 443, 854–858. doi: 10.1038/nature05227
Ohishi, K., Yamamoto, M., Tame, A., Kusaka, C., Nagai, Y., Sugimura, M., et al. (2016). Long-term cultivation of the deep-sea clam Calyptogena okutanii: changes in the abundance of chemoautotrophic symbiont, elemental sulfur, and mucus. Biol. Bull. 230, 257–267. doi: 10.1086/BBLv230n3p257
Patt, T. E., and Handon, R. S. (1978). Intracytoplasmic membrane, phospholipid, and sterol content of Methylobacterium organophilum cells grown under different conditions. J. Bacteriol. 134, 636–644.
Petersen, J. M., and Dubilier, N. (2010). “Symbiotic methane oxidizers,” in Handbook of Hydrocarbon and Lipid Microbiology, ed. K. N. Timmis (Berlin and Heidelberg: Springer-Verlag).
Pond, D. W., Bell, M. V., Dixon, D. R., Fallick, A. E., Segonzac, M., and Sargent, J. R. (1998). Stable-carbon-isotope composition of fatty acids in hydrothermal vent mussels containing methanotrophic and thiotrophic bacterial endosymbionts. Appl. Environ. Microbiol. 64, 370–375. doi: 10.1128/AEM.64.1.370-375.1998
Pond, D. W., Gebruk, A., Southward, E. C., Southward, A. J., Fallick, A. E., Bell, M. V., et al. (2000). Unusual fatty acid composition of storage lipids in the bresiloid shrimp, Rimicaris exoculata, couples the photic zone with MAR hydrothermal vent sites. Mar. Ecol. Prog. Series 198, 171–179.
Rau, G. H., and Hedges, J. I. (1979). Carbon-13 depletion in a hydrothermal vent mussel: suggestion of a chemosynthetic food source. Science 203, 648–649. doi: 10.1126/science.203.4381.648
Riou, V., Bouillon, S., Serrão Santos, R., Dehairs, F., and Colaco, A. (2010). Tracing carbon assimilation in endosymbiotic deep-sea hydrothermal vent mytilid fatty acids by 13C-fingerprinting. Biogeosci. Discussions 7, 3453–3475.
Scallen, T. J., Dhar, A. K., and Loughran, E. D. (1971). Isolation and characterization of C-4 methyl intermediates in cholesterol biosynthesis after treatment of rat liver in vitro with cholestan-3β,5α,6β-triol. J. Biol. Chem. 246, 3168–3174.
Schouten, S., Bowman, J. P., Irene, W., Rijpstra, C., and Sinninghe-Damsté, J. S. (2000). Sterols in a psychrophilic methanotroph, Methylosphaera hansonii. FEMS Microbiol. Lett. 186, 193–195. doi: 10.1111/j.1574-6968.2000.tb09103.x
Stecher, J., Türkay, M., and Borowski, C. (2002). Faunal assemblages on the Pacific– Antarctic ridge near the foundation seamount chain (37 30’S, 110 30’W). Cahiers Biol. Mar. 43, 271–274.
Summons, R. E., Jahnke, L. L., and Rocsandic, T. (1994). Carbon isotopic fractionation in lipids from methanotrophic bacteria: relevance for interpretation of the geochemical record of biomarkers. Geochimica Cosmochimica Acta 58, 2853–2863. doi: 10.1016/0016-7037(94)90119-8
Szafranski, K. M., Piquet, B., Shillito, B., Lallier, F. H., and Duperron, S. (2015). Relative abundances of methane- and sulfur-oxidizing symbionts in gills of the deep-sea hydrothermal vent mussel Bathymodiolus azoricus under pressure. Deep-Sea Res. Part I 101, 7–13. doi: 10.1016/j.dsr.2015.03.003
Talbot, H. M., Watson, D. F., Murrel, J. C., Carter, J. F., and Farrimond, P. (2001). Analysis of intact bacteriohopanepolyols from methanotrophic bacteria by reversed-phase high-performance liquid chromatography–atmospheric pressure chemical ionization mass spectrometry. J. Chromatogr. A 921, 175–185. doi: 10.1016/s0021-9673(01)00871-8
Teshima, S.-I., and Kanazawa, A. (1974). Biosynthesis of sterols in abalone, Haliotis gurneri, and mussel, Mytilus edulis. Comp. Biochem. Physiol. Part B: Comp. Biochem. 47B, 555–561. doi: 10.1016/0305-0491(74)90004-2
Teshima, S.-I., and Patterson, S. W. (1981). Sterol biosynthesis in the oyster Crassostrea virginica. Lipids 16, 234–239.
Thornhill, D. J., Wiley, A. A., Campbell, A. L., Bartol, F. F., Teske, A., and Halanych, K. M. (2008). Endosymbionts of Siboglinum fiordicum and the phylogeny of bacterial endosymbionts in Siboglinidae (Annelida). Biol. Bull. 214, 135–144. doi: 10.2307/25066670
Volkman, J. K. (2005). Sterols and other triterpenoids: source specificity and evolution of biosynthetic pathways. Organ. Geochem. 36, 139–159.
Wei, J. H., Yin, X. C., and Welander, P. V. (2016). Sterol synthesis in diverse bacteria. Front. Microbiol. 7:990. doi: 10.3389/fmicb.2016.00990
Won, Y.-J., Hallam, S. J., O’Mullan, G. D., Pan, I. L., Buck, K. R., and Vrijenhoek, R. C. (2003). Environmental acquisition of thiotrophic endosymbionts by deep-sea mussels of the genus Bathymodiolus. Appl. Environ. Microbiol. 69, 6785–6792. doi: 10.1128/AEM.69.11.6785-6792.2003
Keywords: chemosynthesis, symbiosis, aerobic methanotrophic bacteria, thiotrophic bacteria, lipid biomarkers, compound-specific carbon isotopes, South China Sea
Citation: Guan H, Feng D, Birgel D, Kiel S, Peckmann J, Li S and Tao J (2022) Lipid Biomarker Patterns Reflect Nutritional Strategies of Seep-Dwelling Bivalves From the South China Sea. Front. Mar. Sci. 9:831286. doi: 10.3389/fmars.2022.831286
Received: 08 December 2021; Accepted: 15 February 2022;
Published: 10 March 2022.
Edited by:
Fengping Wang, Shanghai Jiao Tong University, ChinaReviewed by:
Wang Minxiao, Institute of Oceanology (CAS), ChinaCopyright © 2022 Guan, Feng, Birgel, Kiel, Peckmann, Li and Tao. This is an open-access article distributed under the terms of the Creative Commons Attribution License (CC BY). The use, distribution or reproduction in other forums is permitted, provided the original author(s) and the copyright owner(s) are credited and that the original publication in this journal is cited, in accordance with accepted academic practice. No use, distribution or reproduction is permitted which does not comply with these terms.
*Correspondence: Hongxiang Guan, Z3Vhbmhvbmd4aWFuZ0BvdWMuZWR1LmNu; Daniel Birgel, ZGFuaWVsLmJpcmdlbEB1bmktaGFtYnVyZy5kZQ==
Disclaimer: All claims expressed in this article are solely those of the authors and do not necessarily represent those of their affiliated organizations, or those of the publisher, the editors and the reviewers. Any product that may be evaluated in this article or claim that may be made by its manufacturer is not guaranteed or endorsed by the publisher.
Research integrity at Frontiers
Learn more about the work of our research integrity team to safeguard the quality of each article we publish.