- 1Department of Geosciences, National Taiwan University, Taipei, Taiwan
- 2Frontiers Science Center for Deep Ocean Multispheres and Earth System, and Key Laboratory of Marine Chemistry Theory and Technology, Ministry of Education, Ocean University of China, Qingdao, China
- 3Department of Earth Sciences, University of Southern California, Los Angeles, CA, United States
A 51-cm core (SBB-8-2012) from the depo-center of Santa Barbara Basin (SBB), California has been dated by 210Pb dating and varve counting, spanning a depositional history during 1815-2011 CE. A total of 89 AMS 14C measurements on samples from 66 horizons, including animal cartilage, shell and total organic carbon (TOC) in the sediments show apparent 14C ages between 500 and 4000 yr BP. Among these AMS dates, D14C values measured in 78 samples from 62 horizons vary in the range of -64.3‰ to -383.8‰. The 14CTOC ages much older than predicted from our sedimentation model are influenced by the input of terrigenous sediments, changes in ocean circulation, biological input and carbon remineralization. Three strong old 14CTOC excursions at 1964~69, 1884~87 and 1819~21 CE indicate higher old carbon input caused by some unusual events (e.g., oil spill, flood event and earthquake). On multi-decadal timescales, the D14CTOC shifts in three zones were mainly caused by changes in fossil carbon emission from the seafloor, fraction of marine productivity to terrigenous input of organic carbon (OC) and the atmospheric nuclear bomb testing input of 14C into the SBB. On interannual to decadal timescales, variations of D14CTOC correspond to El Niño-Southern Oscillation effects. During the La Niña period, stronger upwelling and northerly California Current bring nutrient-enriched water into SBB and lead to higher productivity hence more marine OC with higher D14CTOC. In addition, reduced terrigenous input of OC with lower D14C under less coastal rainfall during La Niña could further elevate the D14CTOC. Lower scanning XRF (K+Ti)/2 (indicating lower terrigenous input), higher scanning XRF Sr/Ti as well as acid-leachable elements (reflecting higher biogenic components), and higher D14CTOC occur during La Niña. During the El Niño period, the phenomena are opposite. Spectrum analyses of the Southern Oscillation Index (SOI) and the D14CTOC as well as the comparison of SOI and SBB-8-2012 records support our scenarios.
1 Introduction
With anoxic conditions on the bottom of Santa Barbara Basin (SBB) offshore of California, laminated sediments have regularly been used to reconstruct high-resolution paleoclimate records (Kennett and Brassell, 1992; Schimmelmann and Lange, 1992; Kennett and Ingram, 1995; Heusser, 1998; Schimmelmann et al., 1998; Schimmelmann et al., 2003; Robert, 2004; Schimmelmann et al., 2006; Barron and Bukry, 2010; Barron et al., 2015; Sarno et al., 2020), and natural disaster signals such as earthquake and flooding events (Schimmelmann et al., 2001; Schimmelmann et al., 2013; Hendy et al., 2015; Du et al., 2018). Numerous investigations of SBB water column, sediment trap and sediment core analyses have been conducted on carbon and nitrogen cycles (e.g., Andrew and Peter, 1977; Pisias, 1978; Lynn and Simpson, 1990; Schimmelmann and Tegner, 1991; Thunell, 1998; Emmer and Thunell, 2000; Thunell, 2003; Tems et al., 2015; Davis et al., 2019; White et al., 2019), biological productivity and chemical conditions in the basin and their linkage to climate and water mixing (e.g., Browne, 1994; Gorsline, 1996; Weinheimer and Cayan, 1997; Warrick et al., 2005; Lund, 2011; Berelson et al., 2019; Napier et al., 2019).
Terrestrial organic carbon input by episodic discharge events from the mountain river systems can survive in coastal processing near Santa Barbara Channel (Kolpack and Drake, 1984; Thunell et al., 1995; Schimmelmann and Lange, 1996). The organic carbon (OC) can be rapidly transported to low-oxygen seafloor sediments in SBB where remineralization is less efficient (Sarno et al., 2020). Due to restricted circulation below the basin sills, oxygen supply to the bottom water of SBB is limited, so a suboxic environment exists at a depth below 480 m (Li et al., 2009). The anoxic condition in SBB is also attributed to the emission of methane and crude oil from oil seeps in the region contribute reduced carbon. For instance, the Coal Oil Point seep field, located at near shore of SBB, is one of the largest natural submarine hydrocarbon emission areas in the world (Allen and Mikolaj, 1970; Hornafius et al., 1999; Valentine et al., 2010) and likely contributes fossil carbon input to SBB. With those exogenous carbons (old and dead carbons), the 14C reservoir age in SBB is older than that of the average world ocean and varies over time (Kennett et al., 1997). Several groups have studied the ΔR (regional differences in the radiocarbon age between sea-surface water and average world surface water, McFadgen and Manning, 1990) in SBB via different methods. Ingram and Southon (1996) used mollusc shell 14C measurements in SBB and found the ΔR of 233±60 years for the Santa Barbara Channel region, close to the average for the coast of California. Kennett et al. (1997) used 14C dates of marine shell and charcoal pairs from finely stratified archaeological deposits at Daisy Cave and Cave of the Chimneys located on San Miguel Island and concluded that the ΔR varied from -360 to 310 years during the Holocene. Hendy et al. (2013) compared 49 mixed planktonic foraminiferal carbonate and 20 terrigenous organic carbon (such as wood, leaves, seeds or charcoal) 14C dates to the varve chronology in a sediment core from SBB and found that the ΔR between 80 and 350 years during the past 2000 years. Therefore, it is necessary to further understand the factors that affect the 14C reservoir ages of SBB.
The 14C reservoir age in SBB is related to oceanic circulation, upwelling of the Pacific deep water, El Niño–Southern Oscillation (ENSO) and Pacific Decadal Oscillation (PDO) (Kennett et al., 1997; Hendy et al., 2013; Du et al., 2018). This is because the regional rainfall of southern California, oceanic circulation and upwelling along the southern California coast are strongly influenced by summer insolation, ENSO and PDO (Kennett et al., 1997 and references therein; Lynn and Bograd, 2002; Jacox et al., 2015). During the warm phases of ENSO (El Niño state) and PDO, rainfall and river discharge increase around SBB, supplying more terrestrial sediments to the depo-center of SBB (Shipe et al., 2002; Warrick and Farnsworth, 2009; Hendy et al., 2015). During this period, the southerly flow of California Counter Current brings warm, nutrient-depleted water from the equatorial Pacific to suppress northerly flow and nutrient-rich California Current as well as upwelling, resulting in low marine productivity (Bray et al., 1999; Bograd and Lynn, 2001; Bograd et al., 2002; Venrick, 2012). On the other hand, increase (decrease) in summer insolation results in warmer air masses over western North America, enhancing (reducing) northwesterly winds and upwelling intensity along the California coast (Kennett et al., 1997). However, up to date, there is no annual to interannual variability of 14C reservoir age in the SBB sediment records to provide evidence for the above scenario. We address this topic in our work here. Furthermore, Hendy et al. (2015) used a 250-yr varved core (SPR0901-04BC) from SBB to identify droughts and floods related to ENSO impact by using changes in elemental contents measured with scanning X-ray fluorescence (XRF), inductively coupled plasma atomic emission spectrometer (ICP-AES) and inductively coupled plasma mass spectrometer (ICPMS). They have found that siliciclastic sediments containing the elements Ti, Fe, K, Al, Si, and Rb have higher scanning XRF intensities during El Niño events and positive (warm) phases of PDO, and biogenic indicators such as Ca/Ti, Sr/Ti and Br/Cl have lower values during El Niño events and negative (cool) phases of the PDO (Hendy et al., 2015). Are we able to reproduce such conclusions?
This study presents results of 14C measurement, scanning XRF elemental content and acid-leachable elemental concentration of the sediments in a 51-cm core from the depo-center of SBB. The core was dated by 210Pb and varve counting. The refined chronology of the core indicates that the sedimentary history of the core covers 1815-2011 CE. The high-resolution (3-yr) D14CTOC record combined with the elemental records allows us to understand sedimentary variations under influence of climatic and oceanographic changes. In this paper, we will discuss the influencing factors of the D14CTOC (i.e., apparent 14C age of total organic carbon) in SBB sediments. The outputs of our results benefit not only the understanding of the variation of the 14C reservoir effect in the Santa Barbara Channel, but also the reconstruction of paleoclimate and paleo-ENSO changes.
2 Materials and Methods
2.1 SBB-8-2012 Core and Sampling
SBB-8-2012 (34°17’N, 120°03’W) was a 51-cm long core collected by a multi-core device in the depo center of the Santa Barbara Basin at a water depth of 580 m by the research team of Prof. William Berelson at the University of Southern California (USC) in August 2012 (Figure 1). The core was split in half and kept in a cold room at 4°C in the Department of Earth Sciences at USC. Half of the core was transported to the Department of Geosciences at the National Taiwan University (NTU) in 2015. During the transportation, the sediments below 12.5 cm separated from the section above, perhaps caused by differential drying due to changes in grain size, density and organic content, etc. (Figure 2A). This shrinkage resulted in a 3-cm gap in the core. Therefore, we have to calibrate the scanned and sampling depths back to the original depths (Figure 2B). Owing to changes in moisture content of the core during the storage (more than 3 years) at USC and transportation from USC to NTU, the water content of the sediments was not measured.
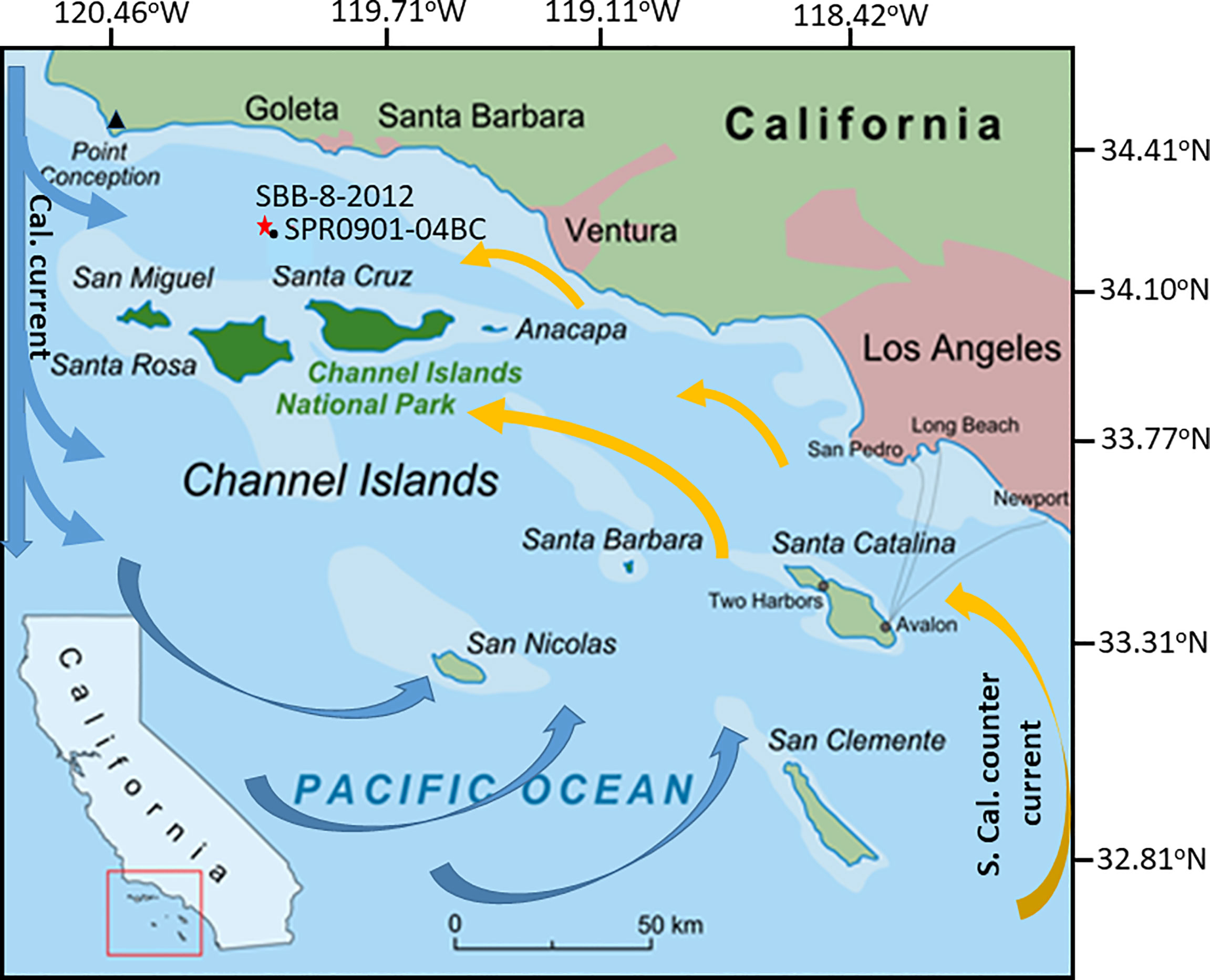
Figure 1 Location map of the study area. The red star shows the location of core SBB-8-2012 site in Santa Barbara Basin and the black dot indicates core SPR0901-04BC location of Hendy et al. (2015). Blue arrows present cold and nutrient-rich current from north, whereas the orange arrows reflect warm and nutrient-depleted current from south.
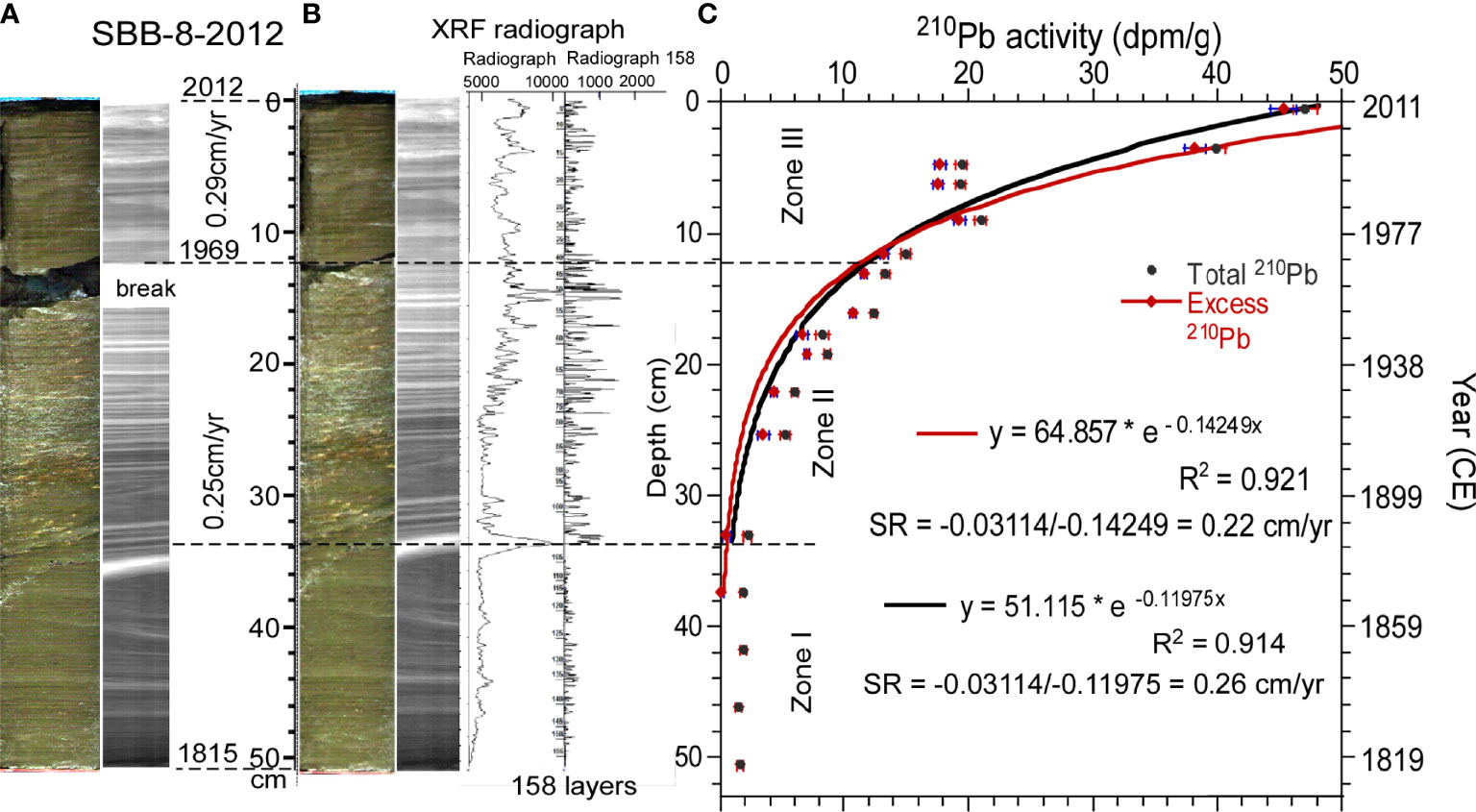
Figure 2 Picture, X-ray radiograph, lamination counting and 210Pb dating of Core SBB-8-2012. (A) Core photo during XRF scanning and sampling. The breaking gap between 12cm and 13cm was formed during the transportation from USA to Taiwan. (B) Core photo after adjusted the depths to remove the breaking gap. The lighter bands in the radiograph reflect the layers with less density. The brightest band at 34 cm indicates an organic rich layer in which all elemental contents by iTRAX XRF scanning are low. (C)210Pb profile of the core. The constructed ages are shown on the right-hand side of the plot. The red curve and black curve fittings are using different ending depths of excess 210Pb, which result different sedimentation rates.
The half core was scanned by an iTRAX core scanner at NTU. After the scan, the core was subsampled at 1-cm interval for 210Pb dating, then subsampled at 2~3-mm interval (roughly 4 samples within one centimeter) with a stainless steel knife for fine resolution analyses. During the sampling, any visible shells, plant or animal remains in the sediments, were picked up individually for 14C dating purposes. Only less than 1/2 of the sediments throughout the half core were taken at NTU, and the rest was sent back to USC where δ13C, δ15N and C/N of the organic matter were planned to be analyzed. Due to the limited amount of subsamples, we did not measure grain size, bulk density and TOC for every sample. All sediment samples were freeze-dried by a Freeze vacuum dryer (model EYELA FDU-1200), then ground and mixed well. The samples were placed in plastic bags and stored in a refrigerator at 8°C until analysis.
2.2 iTRAX XRF Core Scan
The iTRAX XRF scan was performed at NTU with an iTRAX core scanner (Li et al., 2015; Löwemark and Itrax operators, 2019). The core with smooth and flat surface was placed in the XRF scanner which contains a digital optical RBG image, a digital radiographic image, and a μ-XRF elemental profile. The scanning condition was using a Mo tube set at 30 kV and 30 mA, a sampling interval of 200 μm and an exposure time of 40 s. For duplicating checks, the core was scanned twice along two parallel tracks which are 3-mm apart. Elements including Fe, Ca, Sr, K, Ti, Mn, Rb, Zn, Ni, Cu, Si, S, Cl, V, Cr, Co, Y, Zr, Nb, Pd, Cd, In, Sn, Sb, Al, Ba and Pb were measured, but only part of the elements provided meaningful results. The criteria for selecting meaningful data are: 1. The maximum intensity of an element during the entire scan should be greater than 200 counts per second (cps); and 2. The correlation coefficient (R2) of the element along the two duplicated tracks should be greater than 0.6. With such criteria, only Fe, Ca, Sr, K, Ti, Mn, Rb, Zn, Ni, Cu, Si, Cl, Y, Zr and Pb provide meaningful results.
2.3 210Pb Dating
210Pb dating method was originally established by Goldberg (1963). 210Pb profile of a sedimentary sequence can be described by the following equation:
where 210Pb0 and 210Pb are the initial activity and activity at a past time (or depth), respectively. λ is the decay constant of 210Pb (0.0311 yr-1). D stands for depth in a sedimentary profile. SR refers to the linear sedimentation rate. Taking logarithms to Eqn. (1), we have:
If 210Pb0 and SR are relatively constant, the slope of Ln 210Pb — D should yield a linear sedimentation rate SR. This is the fundamental concept of 210Pb dating.
Since the subsample of the core was not enough for our gamma spectrometry, 210Po alpha method was used for the 210Pb dating (Baskaran and Iliffe, 1993; Baskaran and Krishnamurthy, 1993; Li et al., 1996; Paulsen et al., 2003; Li et al., 2011; Kuo et al., 2011; Yin et al., 2014; Zhao et al., 2015; Yin et al., 2017; Zhao et al., 2017a; Yin et al., 2019). As a daughter product of 210Pb decay, 210Po has a half-life of 138.38 days. Hence, within a couple of years, 210Pb and 210Po reach secular equilibrium. The 210Po activity equals that of 210Pb in the measured samples.
A total of 17 horizons from the 1-cm interval samples were selected throughout the core for 210Po dating in the AMS 14C dating Lab at NTU (the NTUAMS Lab) (Table 1). Each dry sample of 0.3~0.5 g was spiked with 209Po in a Teflon beaker and dissolved by aqua regia on a hot plate at 95°C overnight. The sample solution was evaporated to dryness, then added 4N HCl to dissolve residues. The sample solution was transferred into a centrifuge tube for the removal of any detritus. After centrifuge, the supernatant was poured back into the Teflon beaker and evaporated to dryness. Ten ml 1.0 N HCl was added into the Teflon beaker to dissolve the sample. Then, transfer the sample into a 50-ml plastic centrifuge tube. Ascorbic acid powder was added to the solution until it became clear (yellow color would be disappeared) in order to remove the influence of Fe. A silver disc (0.8 cm in diameter) was placed at the bottom of the centrifuge tube. The Po in the sample was self-precipitated onto the silver disc within an 80°C water bath for 6 hours. After washing with deionized water (DIW), the silver disc was counted for 210Po (5304 keV) and 209Po (4884 keV) by an ORTEC alpha spectrometer at NTU. Table 1 lists the 210Po dating results.
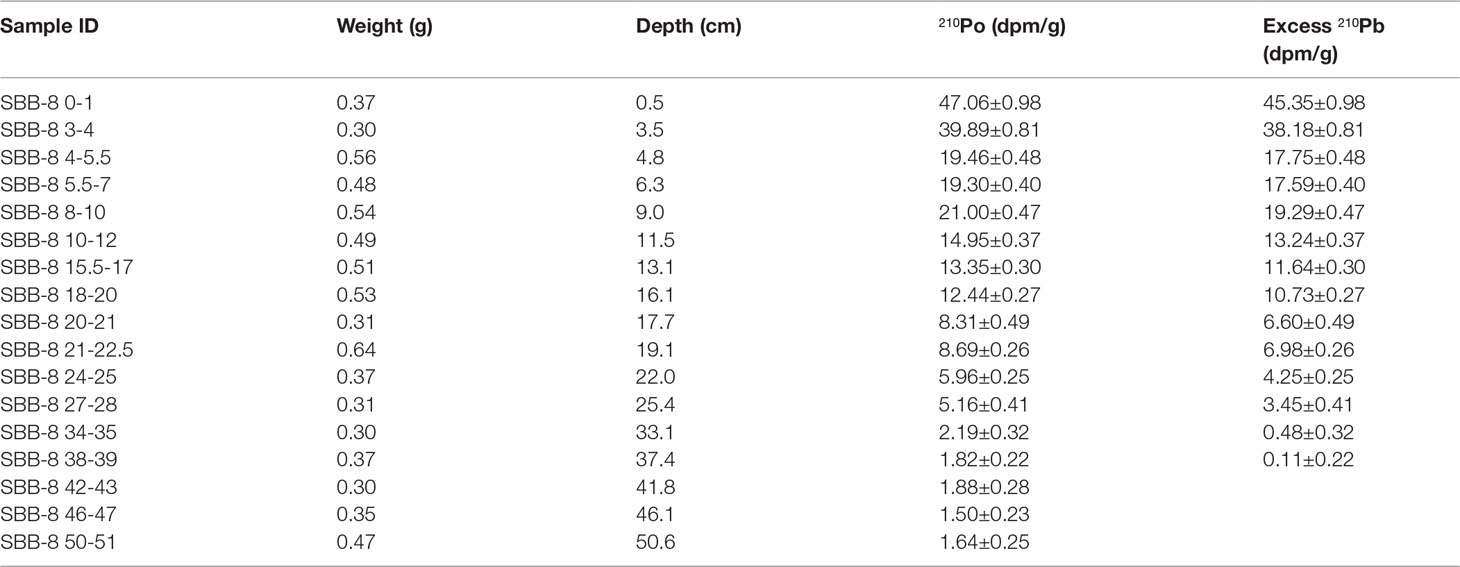
Table 1 210Pb dating results. The excess 210Pb is determined by the 210Po at each layer subtracting the mean 210Po value averaged from the four 210Po values in the deep part.
2.4 AMS 14C Dating
Accelerator mass spectrometry (AMS) 14C dating was performed in the NTUAMS Lab at NTU with a 1.0 MV Tandetron Model 4110 BO AMS. About 50~100 mg of dry sediments were weighted for TOC 14C dating and acid-leachable element analysis. The sample was dissolved in 15 ml 0.5N HCl for removal of carbonates. Using pH paper to check the solution, when pH<3, all inorganic carbons were dissolved by the acid leaching. Centrifuging the sample and washing the residue with DIW, and collecting the supernatant for inductively coupled plasma-optical emission spectrometer (ICP-OES) analysis. The residue was dried at 50°C. The TOC of the dried residue sample was oxidized into CO2 under 850°C. Then, the purified CO2 was made into graphite following the description in Yang et al. (2017) and Zhao et al. (2017b). Six samples (samples with # symbol in Table 2) were used a different acidification treatment for AMS 14C dating. For these samples, the sample was placed in a reaction vessel on a vacuum line for CO2 collection. The sediment sample in the reaction vessel was reacted with 1 ml of 100% H3PO4 on the vacuum line. After the CO2 extraction of the total inorganic carbon (TIC) in the sample was done, the collected CO2 was made into graphite for 14C dating following the description in Zhao et al. (2015). The residue in the reaction vessel was collected into a centrifuge tube and washed by DIW. After centrifuging, the residue was dried at 50°C. The TOC in the dried residue was processed for 14C dating according to the above description. In so doing, we can compare the 14C results of TOC and TIC from the same sediment samples. For every batch of the samples, at least three oxalic acid standards (OXII, SRM 4990C), three organic carbon backgrounds (BKG, made from anthracite), three carbonate backgrounds (NTUB, made from Upper Devonian limestone) and two known-age inter-comparison samples (IRI, distributed by the University of Glasgow) were processed in the same procedures and measured with the sample targets.
One sample is animal cartilage (looks like a fish spine). This sample was just cleaned with DIW, and placed into a 9-mm size quartz tube with pre-combusted CuO powder. The tube was sealed under a vacuum of 10-5 mbar, and then combusted for 6 h at 850°C in a muffle furnace. The carbon from this sample came from mainly organic components, so that we classified its 14C dating as TOC 14C dating. There are four TIC 14C dates of sediment samples (samples with @ symbol in Table 2) and two 14C dates of a conch shell. These samples had the same procedure for TIC 14C dating as described earlier for the samples with # symbol.
The AMS measured both 14C/12C and 13C/12C ratios on all graphite targets. The AMS measurement was setup for four cycles and each cycle contained 50 blocks (30 seconds for every block). When the 14C counts in a measurement cycle reached 40,000, the counting would stop. Therefore, 14C counts of OXII are normally greater than 40,000, with a statistic error<0.5%. In general, the precision of the 14C dating at the NTUAMS Lab is better than 3%.
The percent modern carbon (pMC) was calculated by background corrected and 13C/12C fractionation corrected 14C/12C ratios between sample and OXII following the concept and definition in Stuiver and Polach (1977); Kim et al. (2001) and Stenström et al. (2011):
where 14C/12C with subscripts of s,bkg,corr and oxII,bkg,corr represents background and δ13C corrected 14C/12C value of sample and standard, respectively. The 14C/12C with subscripts of s-bkg and oxII-bkg refers to background corrected 14C/12C value of sample and standard, respectively. Note that δ13C correction for AMS 14C dating must use 13C/12C measurement on the AMS, but not δ13C measured by an isotope ratio mass spectrometry (IRMS) because the isotopic fractionation is different for the two processes. The δ13C value of OXII is -17.88‰ (round to -18‰). δ13C value of a sample [δ(13C)s] determined by the AMS was calculated by equation (4):
By Eqns. (3) and (4), pMC is obtained with background and δ13C (to -25‰) corrections. D14C and apparent age of the sample were calculated by Eqns. (5) and (6), respectively:
We do not use Δ14C value which represents the activity ratio of a sample (As) to the atmospheric CO2 (Aabs) without age correction (Stenström et al., 2011), whereas Aabs refers to the hypothetical specific activity of atmospheric carbon of year 1950. The relationship between Δ14C and D14C can be described by Eqn. (7):
where y in Eqn. (7) is the measurement year. As this study does not focus on the 14C variation in the atmosphere, there is no need to use Δ14C. Furthermore, in order to show the age difference between 14C and 210Pb results, we also calculated the 14C ages. As these 14C dates contain old carbon influences, there is no meaning to provide the calibration dates. We will use the apparent (measured) 14C dates and D14C values for discussion. All D14C values and 14C dates are listed in Table 2.
2.5 Acid-Leachable Elemental Analysis
A total of 72 horizons from the core were subsampled for acid-leachable elemental measurement. The sample weight ranged from 48.45 mg to 325.15 mg with an average and standard deviation of 96.34±51.11 mg (n = 72), implying a very limited sample amount. These samples were leached by 15 ml 0.5N HCl. This procedure is not only for the removal of inorganic carbon during TOC 14C dating, but also for the dissolution of elements that exist in authigenic (precipitated in water column) minerals such as carbonates (both inorganically precipitated in surface water and in biological shells) and oxides as well as absorbed on the particle surface. This procedure does not affect organic components and detrital phases in the sediments. The elements in the solution are considered as an active portion which reflects chemical conditions and productivity in the water column. The sample solution was measured by a PerkinElmer (PE) Optima 8000DV ICP-OES in the NTUAMS Lab. Elements of Ca, Fe, Mg, Ti, Al, Sr, Ba, Mn, Zn, Cr, Cu and Pb were selected for analysis. The concentrations of all elements measured by ICP-OES need to be calculated by the standard curves made from different concentrations of the standard solutions. The standard solutions were made from ICP multi-element standard IV (Certipur® 1.11255.0100) produced by Marck KaGaA, Germany. During the ICP-OES runs, the standard solutions were measured with the sample solutions multiple times. The correlation coefficient (R2) of intensity-concentration relationship for each element is normally greater than 0.98. For the first three batches of ICP-OES runs, 1 ml sample solution was diluted with 9 ml 0.5N HNO3. However, some heavy metal elements such as Zn and Pb were not detectable. In the final run, 5 ml sample solution was diluted with 5 ml 0.5N HNO3. But, the intensity of Ti, Ba, Zn, Cr, Cu and Pb were still low, and might contain some memorial effects from the 100 ppm (mg/L) standard solution during the measurement. Therefore, the measurements of those elemental concentrations may not be reliable. For Ca, Fe, Mg, Al, Sr and Mn which have high concentrations and sensitivities for the ICP-OES measurements, their results should be reliable for discussion. For these elements, the detection limit is about 1 ppb with precision normally better than 2%. Table 3 lists the concentrations of the acid-leachable elements.
3 Results
3.1 Sediment Features of the Core
The sediments of the core are generally fine clay in size with an average TOC of 3.82% and dry bulk density of 0.85 g/cm3. The core sediments show clear laminations on the X-ray radiograph (Figure 2B) with lighter bands reflecting less dense, organic-rich layers. An outstanding light band appears at 34-35 cm depth. The sediments of the core can be classified into three zones: Zone I (35-51 cm depth), the sediments had higher density with thinner lamination. Zone II (12-34 cm depth), the sediments became less dense (increasing light intensity in the radiograph) with clear laminations. Between 26 cm and 27 cm depth, a visible brownish band existed, which was probably caused by a high concentration of Fe oxide (scanning XRF results in Figure 3 show the evidence). In this zone, the sediments contain some white materials (light spots shown in Figure 2B), but they are not carbonates because acid-leachable elements especially Ca, Mg and Sr do not show high concentrations. Zone III (0-12 cm depth), the sediments in this zone have darker color and thick laminations. As we will discuss them in later sections, the criteria for dividing the core into 3 zones are based on four reasons: (1) changes in the D14C trend; (2) changes in the elemental contents; (3) two outstanding depositional layers: one is at 12-13 cm and the other is at 34-35 cm; and (4) changes in the sedimentary features including color and thickness of laminations as described above.
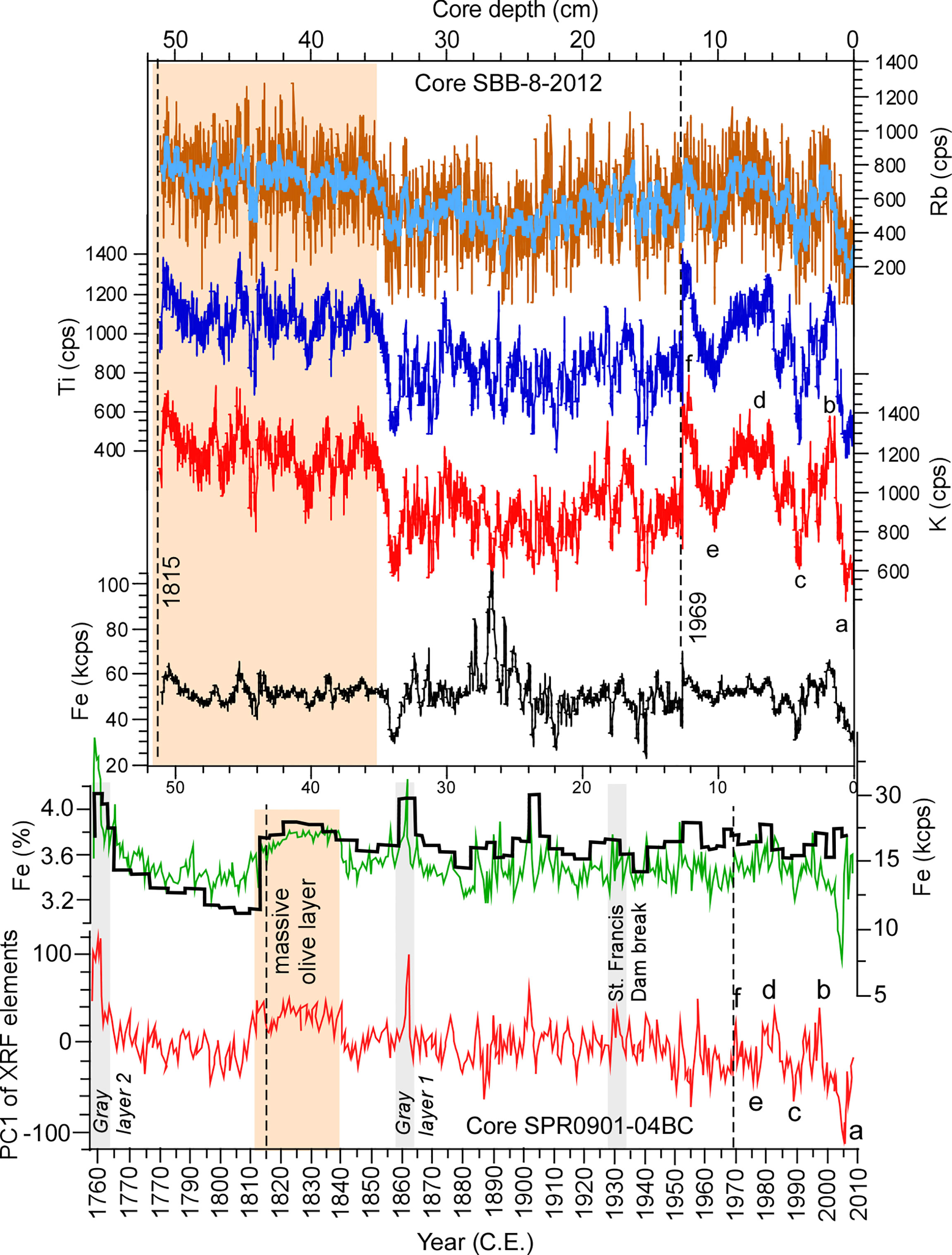
Figure 3 Comparison of scanned XRF elemental contents between Core SBB-8-2012 (this study) and Core SPR0901-04BC (Hendy et al., 2015) from the Santa Barbara Basin. Curves for Fe, K and Ti are 9-point running average of the raw data (roughly monthly resolution). For Rb, the orange curve represents the raw data, and the light blue curve stands for 9-point running average of the raw data.
3.2 Chronology of the Core
The radiograph generated by the iTRAX core scanner allows us to count laminations of the sediments using a computer software called ItraxPlot (https://www.raddec.com/software-itraxplot.php). The counting result indicates that the sediment core has 158 contrast (a dark and light couplet) layers (Figure 2B). If the laminations are annual, then the sediment core contains 158 years with a mean sedimentation rate of 0.32 cm/yr. However, the varve counting may not be accurate and its annual assumption may not be valid. Thus, we applied 210Pb dating to the core.
The total 210Pb activity in the core ranges from 47.06 to 1.50 dpm/g, with an exponential decay trend from the surface toward to bottom (Figure 2C). The 210Pb activities at 4-7 cm depth are slightly low, perhaps due to lower initial 210Pb activity in the input materials. The 210Pb activities below 37 cm depth (ranging from 1.5 to 1.88 dpm/g) are relatively invariant, reflecting supported 210Pb activity. Hence, we use the average of the four deepest 210Pb activities to represent the supported 210Pb which is 1.71 dpm/g. The excess 210Pb value of each layer equals the total 210Pb value subtracting the supported 210Pb (Table 1). Using exponential fitting of the excess 210Pb with depth, we get an equation of y = 64.857*e- 0.14249x . The sedimentation rate (SR) is 0.22 cm/y. Based on this sedimentation rate, the 51-cm long core would cover about 230 years of depositional history, which is significantly longer than the varve counting result (158 years). In fact, 210Pb dating has about 5% uncertainty, especially depending on the determination of the ending point of the excess 210Pb. For example, if we decide the ending point of excess 210Pb is at 33-cm depth instead of 37-cm, the fitting curve (black line in Figure 2C) provides a sedimentation rate of 0.26 cm/yr, which gives 196-year depositional history. Nevertheless, based on the 210Pb dating, the sedimentation rate is around 0.22~0.26 cm/yr. In addition, since the 210Pb dating assumes a constant sedimentation rate and constant initial 210Pb concentration, the conditions may not be valid. Therefore, we need to further refine the chronology of the core.
Hendy et al. (2015) published elemental contents measured by both scanning XRF and ICPMS analyses of bulk elemental concentrations in the sediments of Core SPR0901-04BC (34°16.7’ N, 120°02.49’ W, 588 m) which had a close location to our core (Figure 1). This core covers a sedimentary history of 1755-2008 CE. The chronology of this core was determined by “the distinctive changes in the sedimentary fabric of SBB cores created by the strong El Niño events of 1941, 1957, 1983, and 1997”, “the gray layer at 1761 AD, the turbidite at 1811 AD, and the bioturbated Macoma event at 1841 AD” and varve chronology (Hendy et al., 2015). Although this core did not have direct radiometric dating, the historic events and varve chronology recorded in the sediments made its chronology with an error of ±2 years around those controlling points (Hendy et al., 2015). Comparison of the scanning XRF results between Core SPR0901-04BC and Core SBB-8-2012 can help us to refine the chronology of SBB-8-2012.
In Figure 3, the lower panel shows the profiles of the first principal component (PC1, which explains 45.5% of the elemental variance in the core) of scanning XRF elements which include Al, Fe, K, Rb, Si and Ti) and Fe variations measured by both scanning XRF and ICP-MS during 1755-2008 CE (Hendy et al., 2015). The upper panel displays the profiles of Fe, K, Rb and Ti measured by scanning XRF in Core SBB-8-2012 with depth. First of all, scanning XRF measurement of the elements should be the total elemental content of all types including organic, authigenic and detrital phases in the materials within a few hundred micrometers (μm) (the penetration depth of the X-ray). Since scanning XRF is semi-quantitative in nature, the XRF elemental content cannot represent the total elemental concentration measured by ICP-MS in the sediment, and the latter should be the true elemental concentration. Secondly, the XRF scan has a very high-resolution (200 μm sampling interval), whereas the ICP-MS measurement has much lower resolution in general (1 cm sampling interval for the lower panel in Figure 3). Thirdly, once a core opened to air, oxidation of the surface sediments will allow some oxidation of reduced compounds (e.g., FeS can be oxidized to produce Fe2O3). Therefore, for the scanning XRF elements which can be influenced by the oxidation (e.g., Ca and Fe), discrepancies between XRF and ICP-MS trends are understandable. However, the variation trends of Fe, K, Ti, Rb and Al measured by both scanning XRF and ICP-MS in Core SPR0901-04BC agree with each other in general (Hendy et al., 2015), indicating that the XRF results of these elements reflect variations of the total elemental concentration. The variations of scanning XRF K, Rb, Ti, Mn and Si in Core SBB-8-2012 are very similar each other (Si is plotted in Figure 5). Aluminum content in our core is very low (the maximum intensity is less than 50 cps), so it is excluded in the discussion. Therefore, we compare Fe, K, Rb, Ti and Si contents in Core SBB-8-2012 with the PC1 and Fe in Core SPR0901-04BC.
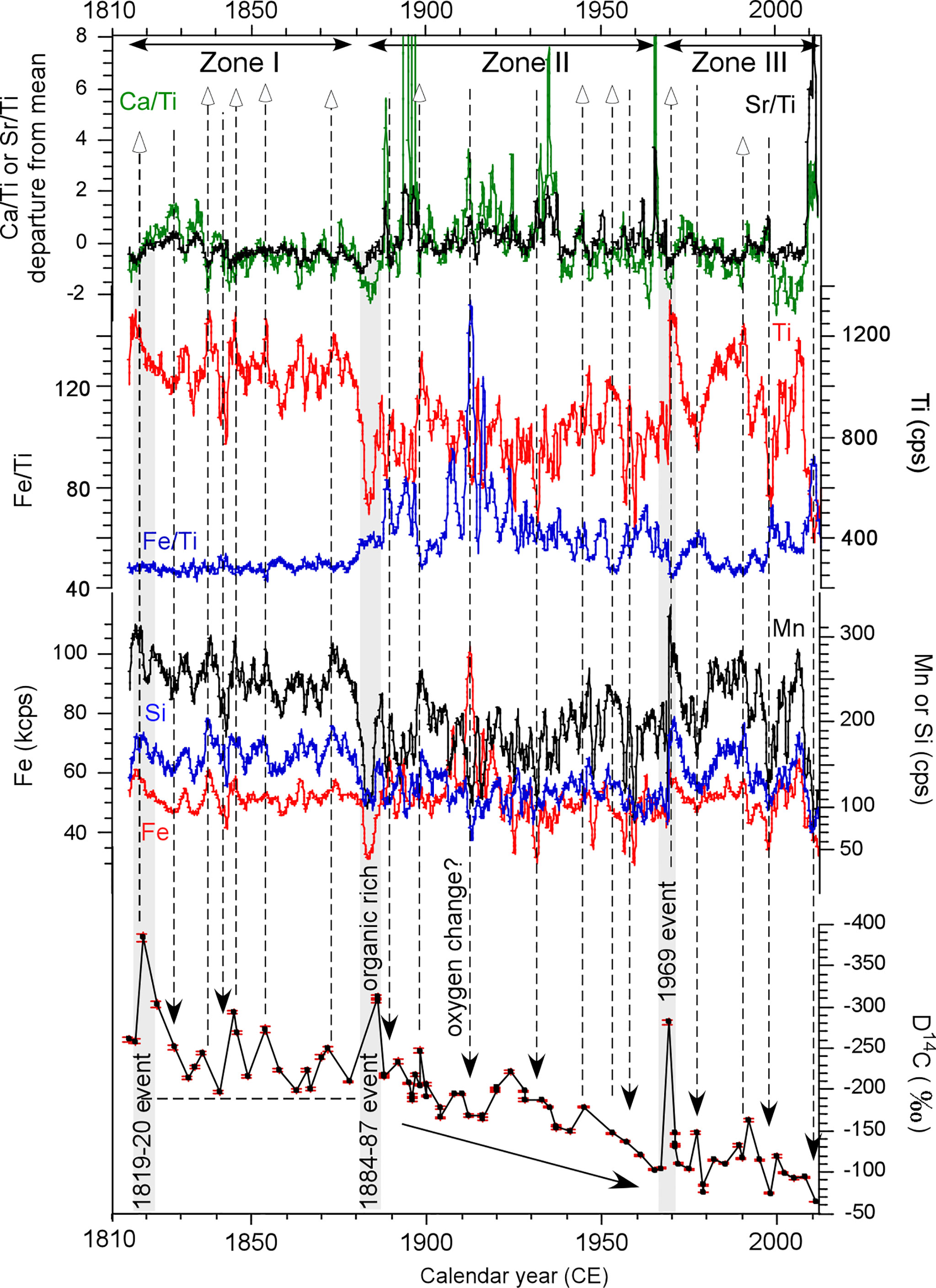
Figure 5 Comparison of the variations in D14CTOC values and the scanning XRF elemental contents in Core SBB-8-2012. In general, more depleted D14C value corresponds to higher siliciclastic elements (K, Ti, Si, Mn and Rb) and lower biogenic proxies (Ca/Ti, Sr/Ti and Fe/Ti); and vice versa. The dashed vertical lines with open arrow refer the periods of more depleted D14C value, whereas the dashed vertical lines with solid arrow show the intervals with less depleted D14C value.
Figure 3 shows the comparison of the two cores. Only two major similarities are certainly identified: (1) variations of K, Rb and Ti in the upper 12 cm (0-12 cm) of SBB-8-2012 are similar to the variations of PC1 during 1969-2008 in SPR0901-04BC; and (2) the high K, Rb and Ti contents below 36 cm depth in SBB-8-2012 are similar to the PC1 XRF elements in the massive olive layer during 1812-1840 in SPR0901-04BC. As we mentioned before, the sediment was broken at 12.5 cm depth during the transportation from USC to NTU, perhaps due to the sudden change in the sedimentary feature at this depth. The flood event of 1969 had been recorded in the sedimentary deposits of SBB (Schimmelmann et al., 2013). According to the report of Santa Barbara County Flood Control & Water Conservation District (https://www.countyofsb.org/uploadedFiles/pwd/Content/Water/1969FloodRpt.pdf), severe flood events occurred in 1964, 1967 and 1969, but “the 1969 12-hour rainfall equaled or exceeded the so-called 100-year storm in the upper Santa Ynez watershed”. In addition, the Santa Barbara oil spill occurred in January and February 1969, which is the third-largest oil spill in the world (Foster et al., 1971). The severe oil spill in the Santa Barbara Channel could have affected sedimentation. Thus, possibly the 12.5-cm depth break may reflect the 1969 flood event and oil spill. If we use 2011 CE for the core top and 1969 CE for 12.5-cm depth, an estimated mean sedimentation rate can be calculated: 12cm/(2011-1969) = 0.29 cm/yr, which is slightly higher than the 0.22-0.26 cm/yr estimated by the 210Pb dating. According to Figure 3, the PC1 XRF elements in SPR0901-04BC during 1770-1810 were very low. A strong drop in the PC1 XRF elements from 1815 to 1805 does not appear in the K, Rb and Ti in SBB-8-2012. If we assume the bottom of SBB-8-2012 is 1815 CE, then a mean sedimentation rate can be calculated: (51-12)/(1969-1815) = 0.25 cm/yr, which agrees with the 0.22-0.26 cm/yr estimated by the 210Pb dating. We did not find gray layers identified in SPR0901-04BC. Although K, Rb and Ti contents in Core SBB-8-2012 show a peak value around 30 cm depth, this peak is not very outstanding and does not exist in Fe content. Thus, the above-mentioned age marks: (A) the 1969 flood event and oil spill at the 12-cm depth break and (B) 1815 CE at the bottom of Core SBB-8-2012 help us to further constrain the chronology. With the lamination and the 210Pb dating estimations, we use a 0.29 cm/yr sedimentation rate for 0-12 cm and 0.25 cm/yr for 12-51 cm for constructing the chronology of the core. According to Inman and Jenkins (1999), the suspended sedimentary flux to SBB during the wet 25 years of 1969-1995 was five times greater than the previous 25 dry years (Schimmelmann et al., 2013). It is reasonable that the sedimentation rate in Zone III was higher. Nevertheless, based on the two sedimentation rates we calculate the chronology of the core which shows on the right-hand side of Figure 2C. The core contains a depositional history from 1815 CE to 2011 CE. We admit that the chronology assumes linear sedimentation rates in the segments and contains about 5% relative uncertainty. The chronology has an error of 1-2 years for the upper 12 cm deposition, and an error of 2-5 years between 12 cm and 51 cm depths.
3.3 14C Age and D14CTOC Variation of the Core
Table 2 lists 89 AMS 14C dates. Amongst these dates, 77 dates are for TOC and 12 dates are for TIC. These 14C ages range from 516±71 to 3889±85 yr BP, much older than the depositional ages of 1815-2011 CE. Of the 77 TOC dates, one is animal cartilage and 13 dates are duplicated measurements on the sediments from 13 horizon samples. The duplicated measurements illustrate that the results are in good agreement within uncertainties, indicating the dating results are reliable. In the 12 TIC dates, 6 TIC dates are coupled with TOC dates from the sediments. They are generally in the same order of their coupled 14CTOC dates, reflecting approximately similar sources of TOC and TIC in the sediments. Two 14CTIC dates are from the same conch shell sample, showing agreement within uncertainties (Table 2). Four 14CTIC dates (marked with @ symbol in Table 2) are from independent layers, showing the 14C ages ranging from 888±66 to 2577±71 yr BP. In this study, we will focus on the discussion of 14CTOC dates. Therefore, the 14CTOC dates from 62 horizons from the core will be used for interpretations of changes in paleoclimate and paleoceanography and the input of different sources of TOC to SBB.
Figure 4 displays the variations of apparent 14C ages throughout the core. The age of the cartilage is the youngest, being 516±71 yr BP without reservoir age correction. However, the true age of this animal remain should be around 1880 CE based on the 210Pb dating and varve counting. This means that if the organism formed in the water column in SBB, the basin water should have a 14C reservoir age of at least 400 years which is much older than the reservoir age (233±60 years) of modern surface water in SBB (Ingram and Southon, 1996). The age of the conch shell is about 980~1010 yr BP, reflecting again the reservoir effect in the Santa Barbara Basin. These two ages imply that the reservoir age in SBB may vary largely on annual-to-decadal time scales. It is interesting that the 14CTOC ages varied generally within the two paralleled dashed lines shown in Figure 4 except for the three layers which have much older ages. The dashed line on the younger side covers the ages from 2000 yr BP at the bottom of the core to 500 yr BP on the core top. As the depositional age of the total core is 200 years, radioactive decay could not cause such a change. If the TOC of the samples on the younger side of the dashed line reflects primarily the organic matters mixed from terrestrial input (allogenic/allochthonous) and from endogenic input (autochthonous) through photosynthesis in the water column of SBB, then the significant age change (~1500 years) implies that either the reservoir age of the SBB water varied a lot during the past 200 years, or the ratio of allochthonous/autochthonous OC input has changed significantly and systematically. Nevertheless, the variations of the 14C ages shown in Figure 4 demonstrate that radiocarbon dating on either organic or inorganic carbon formed in the water column of SBB is problematic. Only terrestrial plant remains in the sediments appear as a valid way to provide “true” depositional ages in SBB.
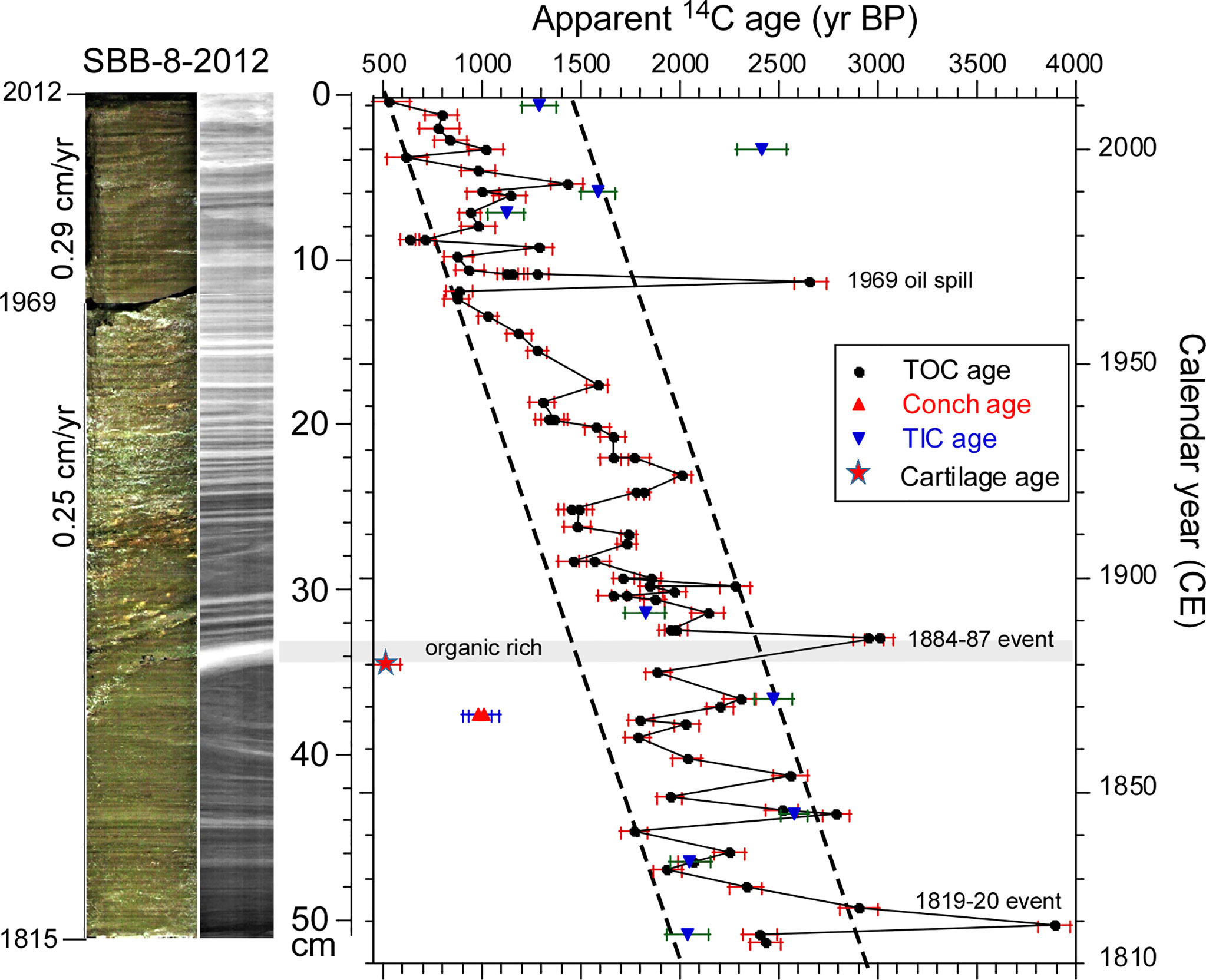
Figure 4 The measured 14C ages of all samples in Core SBB-8-2012. The two parallel dashed lines indicate the envelope of general variations of the measured 14C ages on total organic carbon in the sediments except for three outstanding horizons.
Since the 14C ages do not provide age meaning and our discussions focus on the 14C variation of TOC, we use D14CTOC values for further discussion.
3.4 Elemental Contents Measured by Scanning XRF
As the fore-mentioned scanning XRF elements represent total contents of all phases including organic, authigenic and detrital components. The elements which have meaningful results (by meeting the two criteria) show the intensity in the following order with their average intensity (cps) in the bracket: Fe (51206) > Ca (4771) > Sr (2399) > Cl (1241) > K (1015) > Ti (919) > Rb (597) > Zn (352) > Zr (235) > Mn (213) > Ni (205) > Y (192) > Cu (144) > Si (138) > S (82) > Pb (50). In this study, we only select Fe, Ca, Sr, K, Rb, Ti, Si and Mn for discussion.
The K, Ti, Rb, Si and Mn are lithophile elements that mainly come from terrestrial detrital input (Goldschmidt, 1926). However, Si and Mn can also be influenced by diagenesis and biogenic input. Figures 3, 5 show the variations of K, Ti, Rb, Si and Mn are positively correlated, indicating that they likely have the same sources. The correlation coefficient (R2) between K and Ti, Si, Mn, or Rb are 0.96, 0.84, 0.91 and 0.74, respectively, implying that the scanning XRF Si and Mn were mainly from detrital input. These elements were considered as siliciclastic elements and grouped in PC1 by Hendy et al. (2015). Since Ti is commonly attributed to terrigenous inputs in pelagic sediments, we can use it to normalize for siliciclastic input. The lithophile elements (K, Ti, Si and Mn) appear different average contents: high in Zone I, low in Zone II and moderately high in Zone III. For example, the average intensities (cps) with standard deviation (numbers of data points) of K content are 1190±120 (n = 755), 867±136 (n = 1019) and 1044±208 (n = 633) in Zone I (1815-1880 CE), II (1880-1969 CE) and III (1969-2011 CE), respectively. Similarly, the average intensities of Ti content are 1071±109, 789±139 and 947±212 in Zone I, II and III, respectively. The changes in the average contents of the lithophile elements in three zones indicate that the terrestrial detrital input to the sediments was the highest in Zone I and the lowest in Zone II, which helps us in understanding of the D14CTOC change of the core.
Although Fe, Ca and Sr are also lithophile elements, but their contents in the sediments can be influenced by the chemical and biological conditions in the water column. These elements can have significantly authigenic compositions compared with the detrital compositions. For example, Ca and Sr are easy to co-precipitate into carbonates, resulting a strong positive correlation between the two. However, the scanning XRF Ca and Sr do not have a strong correlation (R2 = 0.4), although the variations of the two are very similar (Figures 5, 6). As mentioned before, oxidation (e.g., FeS2 + O2 → Fe2O3 + H2S) of the scanned surface sediment layer when the core opened to the air for long time could affect some scanning XRF elements including Fe, Ca and Sr. Interpretations of the XRF Fe, Ca and Sr results should be caution. The Ca/Ti and Sr/Ti are considered as indicators of biogenic components to be normalized for the siliciclastic input (Hendy et al., 2015). Their variations are dominated by the variations of Ca and Sr intensities. The higher Ca/Ti and Sr/Ti reflect a stronger productivity.
The variations of Ca/Ti and Sr/Ti shown in Figure 5 vary generally opposite to the siliciclastic elements (K, Ti, Si and Mn), indicating a higher portion of biogenic component corresponding to the lower siliciclastic component. It is interesting to see that less depleted (or less negative) D14C values correspond to higher Ca/Ti and Sr/Ti values; and vice versa (Figure 5). This means that more OC formed in the water column and less old carbon from siliciclastic source lead to smaller 14C reservoir age. In general, Ca/Ti and Sr/Ti values were high in Zone II, especially several high Ca/Ti peaks, reflecting strongly increased biogenic input into the sediments. These changes should reflect variations in the chemical and oceanographic conditions in SBB.
Although Hendy et al. (2015) considered Fe as a siliciclastic element and used Fe/Ti as a proxy of terrigenous input, Fe can be strongly affected by redox conditions in the environment. The Fe profile in Figures 3, 5 shows that the highest content throughout the core was around 1910 CE, whereas other scanning XRF elements were low. The high Fe content in this period is also visible in the core photo, showing the brown band. In Figure 5, Fe/Ti shows totally different patterns with the other siliciclastic elements: low and relatively invariant values in Zone I, high and strongly fluctuated values in Zone II, and moderately high and variant values in Zone III. The Fe/Ti values in Zones II and III clearly co-vary with Ca/Ti and Sr/Ti but opposite to the siliciclastic elements, reflecting biogenic input into the sediments. This situation is quite different from the Fe variations in Core SPR0901-04BC (Hendy et al., 2015). Nevertheless, we are not able to use Fe or Fe/Ti variations as an indicator of siliciclastic input given by Hendy et al. (2015). Instead, the Fe/Ti in Zones II and III together with probably Ca/Ti and Sr/Ti reflected biogenic input into the sediments. Another reason for the Fe profiles discrepancies between our core and Core SPR0901-04BC may be attributed to the oxidation effect of Core SBB-8-2012 as it opened to the air for many years. If it is true, the scanning XRF Fe/Ti and Ca/Ti do not represent the original situation in the sediments. To avoid the oxidation effect, we use Sr/Ti only as an indicator of biogenic input.
Another important phenomenon is that all XRF elements were the lowest in the light band at 34-cm depth (around 1885 CE). This light band showed a strongly depleted D14CTOC value (Figure 5) with the lowest contents of all scanning XRF elements and less density, indicating that this layer may be enriched in organic matter. Although we do not know the cause of this organic rich layer, a few historic references may provide insight on this event. According to the timeline of Santa Barbara (https://www.santabarbaraca.gov/services/community/historic/historysb/timeline.asp), crude oil was first extracted from the Santa Barbara Channel along the coast at Summerland in 1886. Another unusual observation was that a large abundant kelp forest about 300 yards off Santa Barbara coast in 1887 (Fewkes, 1889), which occurred in other places along the coast of Southern California. Schimmelmann et al. (1995) interpreted this as evidence that no severely destructive storm and wave events has occurred in Santa Barbara for a few years prior to 1887 CE. Less terrestrial detrital input due to less surface runoff and highly abundant marine productivity caused by some oceanographic condition changes agree with our results. Whether there was a significant influence from the first extraction of crude oil in the Santa Barbara Channel is unclear. Nevertheless, the scanning XRF elemental contents will help us in understanding the paleoclimate and paleoceanography change on annual-to-multidecadal scales.
In summary, the siliciclastic elements (K, Ti) reflect mainly terrigenous input from the river inflow around SBB, whereas Ca/Ti, Sr/Ti and Fe/Ti reflect chiefly biogenic input from the marine system in SBB. The two groups have generally opposite trend, and higher values of the siliciclastic elements and lower values of biogenic indicators correspond to less depleted (larger) D14CTOC values; and vice versa. In this study, (K+Ti)/2 is used for terrigenous input, whereas Sr/Ti is used for biogenic input. The sediments of Core SBB-8-2012 showed significant changes in terms of geochemical and biological features from Zone I to Zone II after an organic-rich layer was deposited around 1885 CE (34-35 cm depth) with more terrigenous input in Zone I and more biogenic input in Zone II.
3.5 Acid-Leachable Elemental Concentrations Measured by ICP-OES
The ALE concentrations of Ca, Fe, Mg, Al, Sr and Mn were listed in Table 3. Their average concentrations (mg/Kg) with standard deviations are in the following order: Ca (34299±21926) > Fe (18655±13279) > Mg (9685±5738) > Al (6835±6753) > Sr (202±120) > Mn (180±94).
Figure 6 exhibits the ALE concentrations of Ca, Mg, Sr, Fe and Mn and comparisons with the scanning XRF results in the core. The ALE Ca and Sr have a strong correlation (R2 = 0.95), indicating the same source inputs and similar chemical behavior between the two. The same feature does not occur between the scanning XRF Ca and Sr. This is because the ALE Ca and Sr come mainly from authigenic phase, whereas the scanning XRF Ca and Sr count for both authigenic and detrital phases. The correlations (R2) between ALE Fe and ALE Mg, ALE Al or ALE Mn are 0.86, 0.85 and 0.83, respectively, showing that they come from the same sources. The ALE Fe, Mg, Al and Mn have no significant correlations with the ALE Ca (R2< 0.5) except for five high Ca points (>60 g/Kg), indicating that these five layers contain abundant elements from the authigenic input. In addition, Zone I had the lowest ALE Fe, Mg, Al and Mn, but moderately high ALE Ca and Sr. This means that the sediments in Zone I have more siliciclastic feature because detritus contain less ALE Fe, Mg, Al and Mn.
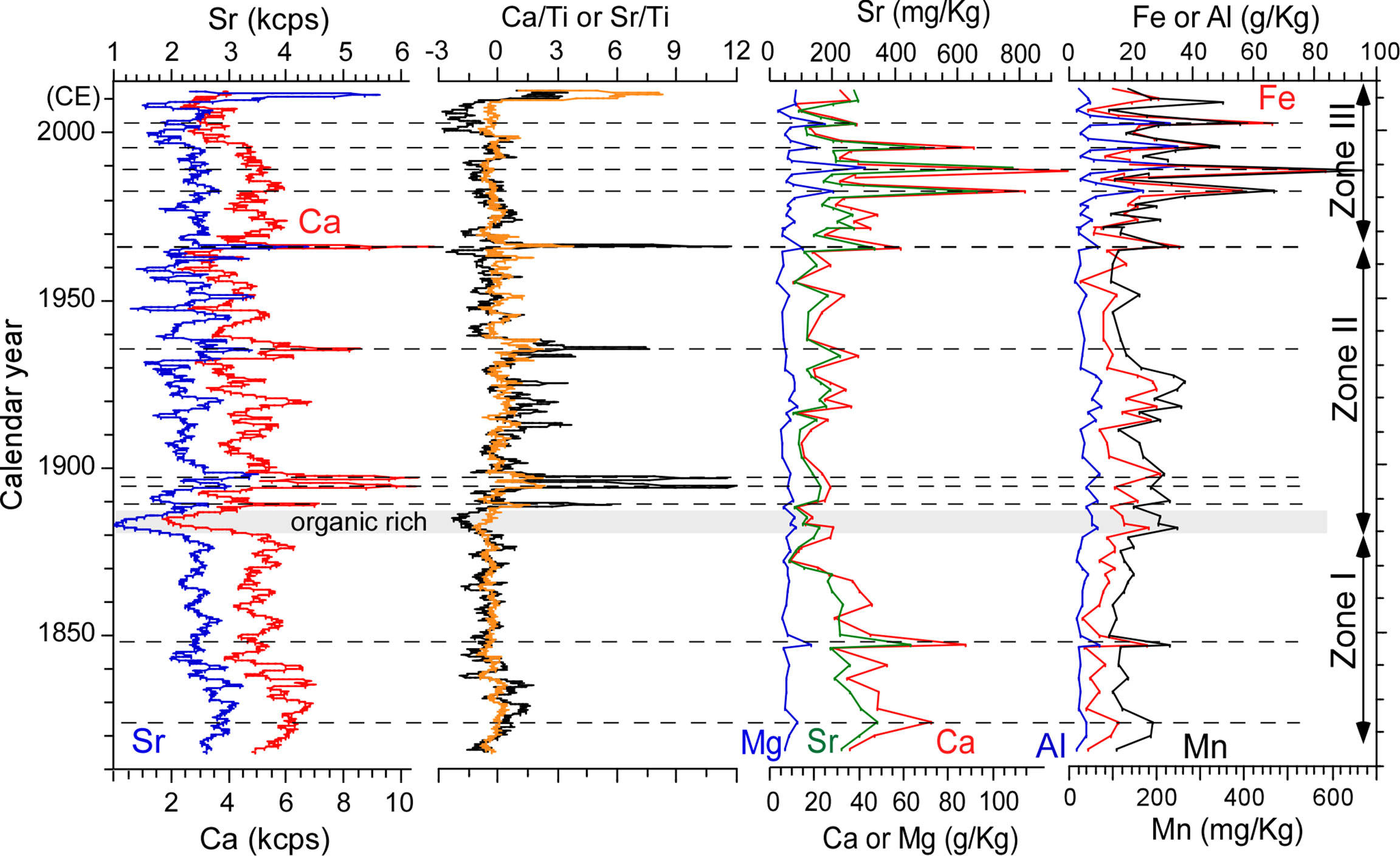
Figure 6 Comparison of the variations in elemental contents measured by XRF scanning and ICP-OES methods in Core SBB-8-2012. Note that elemental concentration has different units, being g/Kg for Fe, Mg and Ca, and mg/Kg for Mn and Sr. The horizontal dashed lines show the comparisons when the acid-leachable elemental concentrations were relatively high.
The comparisons of the scanning XRF contents and the ALE concentrations of Ca and Sr show significant discrepancies in terms on long and short time scales. The ALE concentrations of Ca and Sr were relatively low in Zone II, while the scanning XRF Ca and Sr did not show this. Secondly, several distinct ALE Ca and Sr peaks (i.e., around 1822, 1849, 1982, 1994 and 1999) were not corresponding either to scanning XRF Ca and Sr or Ca/Ti and Sr/Ti. As mentioned before, scanning XRF involves a measured element in all phases, with a matrix effect, very small scan area and semi-quantitative nature. It is understandable that the scanning XRF results may not have relationship with the ALE elements. However, the peaks of ALE elements should reflect oceanic conditions for higher productivity, while the troughs of ALE elements may correspond to low productivity periods. The variations of the ALE concentrations will shed the light on chemical and biological conditions in the water column of SBB.
4 Discussions
4.1 Factors Influencing D14C Variation in Core SBB-8-2012
OC in the sediments of the SBB depo-center comes from three possible major sources: (1) biological products in the marine system of the Santa Barbara Channel (Thunell, 1998; Barron and Bukry, 2010); (2) terrestrial inputs from surface runoff which comes mainly from the Ventura River, Santa Clara River and creeks in the Santa Ynez Mountain drainage basin (Kolpack and Drake, 1984; Thunell et al., 1995; Schimmelmann and Lange, 1996); and (3) fossil OC from leakages in the marine system (e.g., oil spill in surface water; gas and oil are seeping from oil seepage such as from the Coal Oil Point seep field) (Hornafius et al., 1999) and/or lateral transported sediments from other locations inside or outside of SBB (Figure 7).
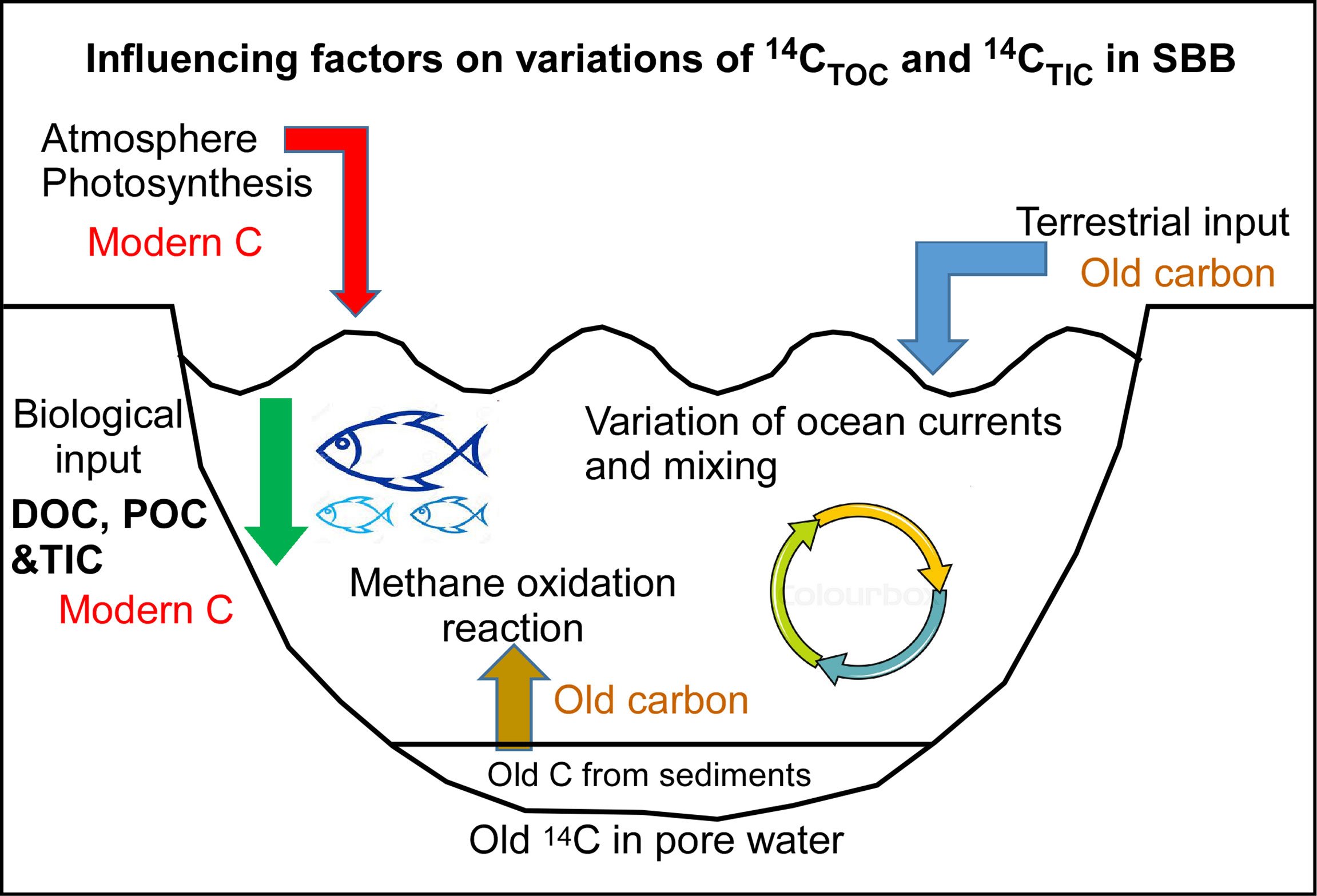
Figure 7 A conceptual box model of the influencing factors on the carbon cycles in Santa Barbara Basin.
The 14C activity of the first OC source which is produced in the SBB water column is influenced by (1) the atmospheric 14C variation and CO2 exchange with the water; (2) variation of oceanic circulation in the SBB channel; and (3) fossil C emission from the seafloor. In general, the D14C of the atmospheric CO2 is much greater than that of the fossil C emission which is -1000‰. The atmospheric D14C at 1950 CE was 0‰ and strongly increased due to nuclear tests (reached to 785‰ in North Hemisphere in 1964) (Hua et al., 2013). The nuclear bomb 14C signal reached a peak during 1970~1976 in the ocean (Grottoli and Eakin, 2007). The highest Δ14C value was ~140‰ in 1974 in the Atlantic coral (Druffel, 1996), and was ~190‰ in 1975 in the Pacific coral (Andrews et al., 2016), showing the bomb 14C significantly affected the 14C reservoir age in oceans.
On the other hand, fossil carbon input in SBB is a well-known phenomenon. The Coal Oil Point seep field located at near shore of SBB is one of the largest natural submarine hydrocarbon emission areas in the world (Allen and Mikolaj, 1970; Valentine et al., 2010). Methane and crude oil are emitted from the seafloor (Hornafius et al., 1999). Most of the emitted hydrocarbons are consumed by methanotrophic bacteria in surface sediments or in the water column (Treude and Ziebis, 2010). The fossil carbon emission from the seafloor in SBB may vary with tidal changes and/or seismic activity (Hornafius et al., 1999; Eichhubl et al., 2000; Boles and Clark, 2001).
According to Kennett et al. (1997), the oceanic circulation in the Santa Barbara Channel influences 14C activity of the marine reservoir as follows: The 14C-depleted California current and associated southern California Eddy flow southward, and bring cold and low salinity water dominated in the winter-spring season (Figure 1). In contrast, the California counter-current flows northward and transports the relatively 14C-rich, warm and saline equatorial water along the southern California coast during the summer-fall season (Figure 1). This annual circulation pattern and upwelling of Pacific intermediate water along the California coast can be modified by summer insolation change (Bakun, 1990; van Geen and Husby, 1996), and ENSO events (McGowan, 1984; Rassmusson, 1984; Ramage, 1986) on interannual scales.
Although many previous studies have found the anomalies of marine 14C reservoir age in SBB (e.g., Ingram and Kennett, 1995; Kennett and Ingram, 1995; Kennett et al., 1997; Roark et al., 2003; Schimmelmann et al., 2006; Hendy et al., 2013; Du et al., 2018), those studies are generally for long time scales (>1000 years). Below, we will discuss the variations of D14CTOC and their causes during 1815~2011 CE. We will focus our discussion on ENSO influences.
4.2 Causes of Strong Depleted D14C Excursions in Core SBB-8-2012
In Figures 4, 5, the three negative D14CTOC (old age) excursions around 1964-69, 1884-87 and 1819-20 CE could be attributed to “extra old carbon influence”. The D14CTOC values of these excursions exceeded significantly the general variation envelope (two paralleled dash lines), so that their old carbon influence should be caused by some abnormal reasons. For the youngest excursion, one reason could be the oil spill event in 1969. During a 10-day period in early 1969, about 4 million gallons of crude oil spilled into the Santa Barbara Channel after a blowout six miles offshore on a Union Oil drilling platform (Foster et al., 1971). This disaster is the third largest oil spill in the world up to date. Organisms and particles could take up the oil to deposit on the seafloor, so that the D14CTOC of this layer could be strongly influenced by the oil. In addition, as we discussed above, flood events in 1964, 1967 and 1969 (especially the 1969 flood) could transport larger portion of old OC in terrigenous sediments in the TOC of this layer. Soil OC from terrestrial input may have 14C age of several thousand years or older than the date of their erosion and deposition. Thus, strong flood events may carry those soil OC into the SBB sediments. The peaks of the lithophile elements in the same layer shown in Figure 5 support the flood impact.
For the 1884-87 excursion, the strongly depleted D14CTOC occurred on the layer of organic rich and minimum concentrations of all scanning XRF elements (Figure 5). The low scanning XRF elements reflect that the depleted D14CTOC should not be attributed to flood events. Two possible events may explain the old carbon influence: 1. Possible sudden short leak of fossil carbon from the Coal Oil Point seep field during the first extraction in 1886; and 2. Strongly increased upwelling from the Pacific deep water entered the basin, which might bring abundant nutrients to cause a surge of biological productivity in SBB, reflected by a large abundant kelp forest along Santa Barbara coast in 1887 (Fewkes, 1889). The 14C activity in the Pacific deep water should be much lower than that of surface water. The abundant OC deposited in the sediments can be preserved due to anoxic conditions on the seafloor of SBB. The above hypothesis needs more direct evidence to support it, especially the reconstruction of upwelling strength during this period. Analysis of the organic compounds in this layer would help reveal its source.
The 1819-20 excursion in the D14CTOC may be explained by the strong input of soil OC into the SBB sediments as the scanning XRF Si, Ti and K (detrital origins) showed high contents in this layer (Figures 5, 9). In addition, if the 1812 Ventura earthquake (also known as the Santa Barbara earthquake) (7.1–7.5 magnitude) caused strongly increased fossil carbon leakage from the Coal Oil Point seep field (Eichhubl et al., 2000), then the leaked fossil carbon could be consumed by methanotrophic bacteria and deposited in the sediments in this layer, given the chronology has an uncertainty of several years in this part.
4.3 The D14CTOC Changes in Different Zones
The D14CTOC variation shown in Figure 5 has experienced three different features. If the D14CTOC value at 35-35.2 cm is excluded as this sample is unlike some sediment samples (organic rich), the mean D14CTOC values and their standard deviations for Zone I, II and III are -247.0±44.4‰ (n = 22), -181.9±34.1‰ (n = 34) and -119.7±45.2‰ (n = 21), respectively. Since the core sediments have only about 200-year history, 14C decay (half-life is 5730 years) should not cause such a large difference. In Zone I (1815~1880 CE), the maxima D14CTOC values (ca. youngest ages) are around -201.8‰ (-197.5‰ at 44.6 cm, -199.4‰ at 39.2 cm, -200.9‰ at 38.1 cm and -209.4‰ at 35.5 cm), no apparent enrichment trend (referred by a horizontal dash line in Figure 5). The much smaller (lower, or lighter) average D14CTOC value and relatively constant maximum D14CTOC value of Zone I indicate that the OC in the sediments during this period contains more old carbon influence and the carbon reservoir effect of the marine system may be larger. The scanning XRF K, Ti, Si and Mn were higher in Zone I, which provides evidence of more soil OC from terrigenous sediments (Figure 5). Other two contributions to the lower mean D14CTOC value in Zone I could be: (1) higher emission flux of fossil carbon from the Coal Oil Point seep field compared to the later periods since the extraction of the fossil fuel began in 1886; and (2) lower marine productivity compared to the later periods. The second hypothesis can be seen in low values of Ca/Ti, Sr/Ti and Fe/Ti which are indicators of biogenic components in the sediments. For the first hypothesis, more studies on the OC budget in SBB are needed.
In Zone II (1885-1969 CE), the D14CTOC value has smaller variations and show an apparently increasing trend. The D14CTOC enrichment trend in Zone II can be explained by two reasons: one is that the fossil carbon emission from the seafloor had been reduced since the extraction beginning in 1886; and another one is increased the biogenic carbon flux into the sediments perhaps due to an enhanced nutrient availability in SBB. As the nutrient supply increased, the primary productivity in SBB produced more OC from endogenic input in the water column. Thus, the portion of OC from terrigenous sediments became smaller. In fact, scanning XRF had relatively lower contents in Zone II, reflecting less contribution of terrigenous sediments. High Ca/Ti, Sr/Ti and Fe/Ti values in Zone II illustrate high biogenic components in the sediments.
The D14CTOC values in Zone III (1969~2011 CE) still show a slightly enrichment trend, and the three maxima values are -75.8‰ in 1977, -74.0‰ in 1998 and -64.3‰ in 2011. Since the nuclear bomb 14C signal from the atmospheric CO2 entered into the ocean in late 1960s and reached its peak in 1975, the enrichment trend of the D14CTOC in Zone III can be influenced by the bomb 14C. However, none of the D14CTOC values are greater than zero in the core (Table 2), indicating that the bomb 14C signal is masked by the 14C reservoir effects in SBB and older terrestrial OC input.
If we assume the cartilage sample at 36 cm depth was formed in the water column, this sample does not contain OC from terrigenous sediments, and has a D14C value of -62.2‰ and 14C age of 516±71 yr BP (Table 2). The calibrated 14C age without reservoir correction of this sample is 92.5% in the range of 454-660 yr BP (2σ) or 560±105 yr BP by using the calibration curve IntCal13. This age is significantly older than the 14C reservoir age (233±60 yr) of modern ocean surface water in SBB (Ingram and Southon, 1996). If we use the D14C value of this sample as the endmember of marine biological carbon in SBB, we may estimate the OC contribution from the marine system and terrigenous input. In Figure 5, the heavier (higher) D14C values are generally corresponding to low scanning XRF K, Ti, Si and Mn (siliciclastic), and high Ca/Ti, Sr/Ti and Fe/Ti (biogenic), whereas the lighter (lower) D14C value are generally corresponding to high siliciclastic elements but low biogenic elements except for the organic-rich layer around 1885 CE.
4.4 ENSO Influence on the D14C Variation of Core SBB-8-2012
The variations of the D14CTOC in the sediments show interannual-to-decadal cycles (Figure 5), reflecting the impact of climatic changes on the terrigenous input of soil TOC and on the productivity of biological marine TOC. Since the precipitation in southern California including Santa Barbara is strongly influenced by ENSO (McGowan, 1984) and oceanic circulation in Santa Barbara Channel is also related to ENSO (Rassmusson, 1984), the D14CTOC variation may be affected by ENSO. For this purpose, we have done spectrum analysis on both the Southern Oscillation Index (SOI) and the D14CTOC record. Figure 8 displays the results. The monthly data of SOI from 1866 to 2021 was downloaded from https://crudata.uea.ac.uk/cru/data/soi/ (Ropelewski and Jones, 1987; Allan et al., 1991). The cycles of 3.6-yr, 5.7-yr and 6.4-yr fall into the 4-7 year band of El Niño/La Niña cycles. The D14C data sets have age intervals on the order of 1-8 years (Table 2). In order to perform the spectrum analysis, the interval should be uniform. Thus, we interpolate the data to annual resolution by using the excel program (linear interpolation), and the spectrum analysis shows that the D14CTOC record has cycles of 4.9-yr, 5.8-yr, 7.3-yr, 8.7-yr, 9.7-yr and 13-yr (Figure 8B). As the resolution of D14C data allows the highest frequent cycle of 6-yr, the 4.9-yr cycle is not considered. The group of 5.8-yr and 7.3-yr cyclicities may reflect the 4~7-year ENSO cycle, whereas the group of 8.7-yr, 9.7-yr and 13-yr cyclicities may represent double of the 4~7-year ENSO cycle.
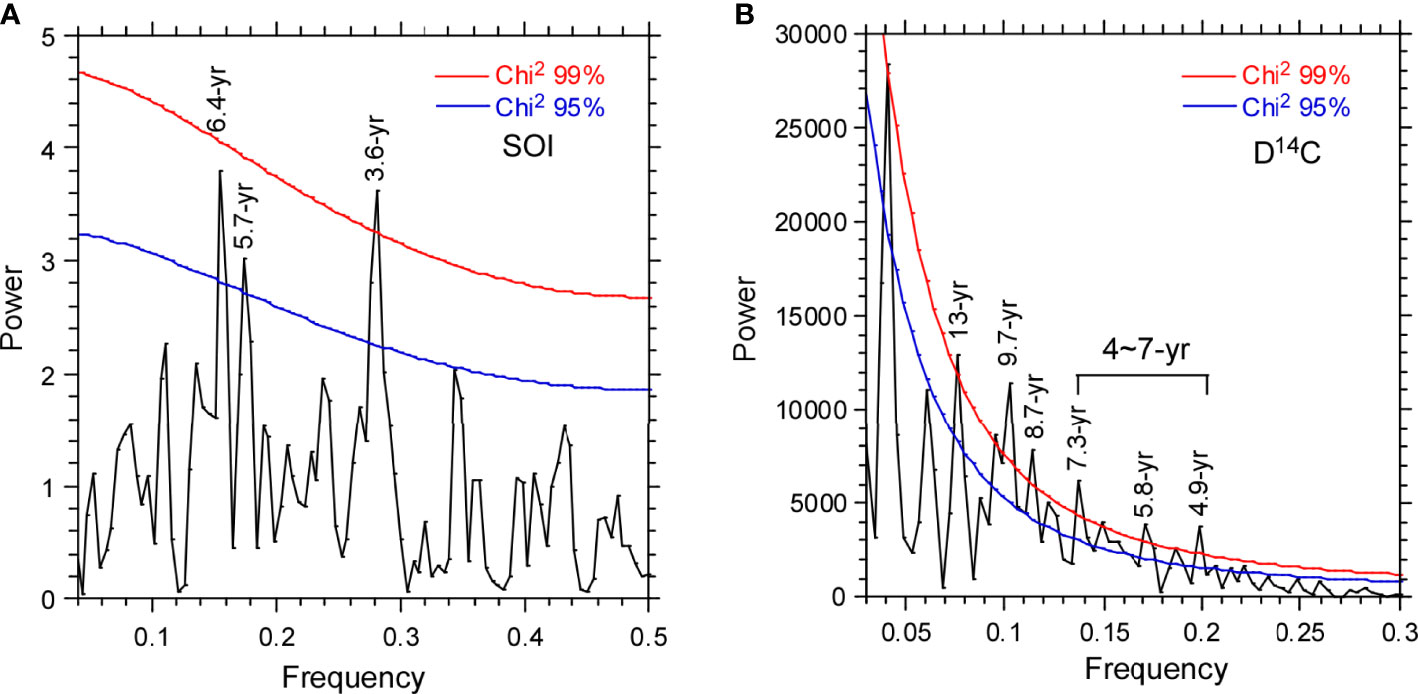
Figure 8 Spectrum analysis for the SOI (data source: https://crudata.uea.ac.uk/cru/data/soi/) (A) and the D14CT OC (B). The blue curve and red curve indicate confidence level and 95% and 99%, respectively. The numbers marked in the plots denote the possible cycles above 95% confidence level.
Since the D14CTOC record has only ~200 years, changes in summer insolation may vary small. Therefore, we focus on the influence of ENSO on the rainfall and oceanic circulation variations in SBB. During El Niño year, the marine biological productivity should decrease (Lange et al., 1987), and heavy rains are frequent along southern California. The ratio of terrigenous soil OC/biogenic OC increases with high input of siliciclastic elements and low ALE in the SBB sediments during El Niño events. In contrast, during La Niña years stronger California Current and upwelling bring abundant nutrients to elevate the marine productivity, resulting higher biological OC content in the sediments and higher D14CTOC.
In Figure 9, we compare the D14CTOC, scanning XRF siliciclastic elements (K+Ti)/2, proxy of biogenic components (Sr/Ti) and ALE contents (Ca, Fe, Mg, Sr and Mn) with the SOI and south coast annual precipitation records. The comparisons show that during strong El Niño years (open head arrows, e.g., 1902, 1941, 1969, 1982-83, 1997 and 2002-05) higher (K+Ti)/2, lower Sr/Ti, minima the ALE contents and decreased D14CTOC were dominant. In contrast, low scanning XRF (K+Ti)/2, higher Sr/Ti, high ALE contents and enriched D14CTOC were corresponding La Niña conditions (solid head arrows). It is worth to mention that our record cannot be accurate on annual scale, so that comparison with low rainfall periods is more reasonable than with individual El Niño or La Niña years. The above comparison between our record with the instrumental records (rainfall and SOI) perhaps also demonstrates that our chronology of the core is reliable as the instrumental records have precise age control.
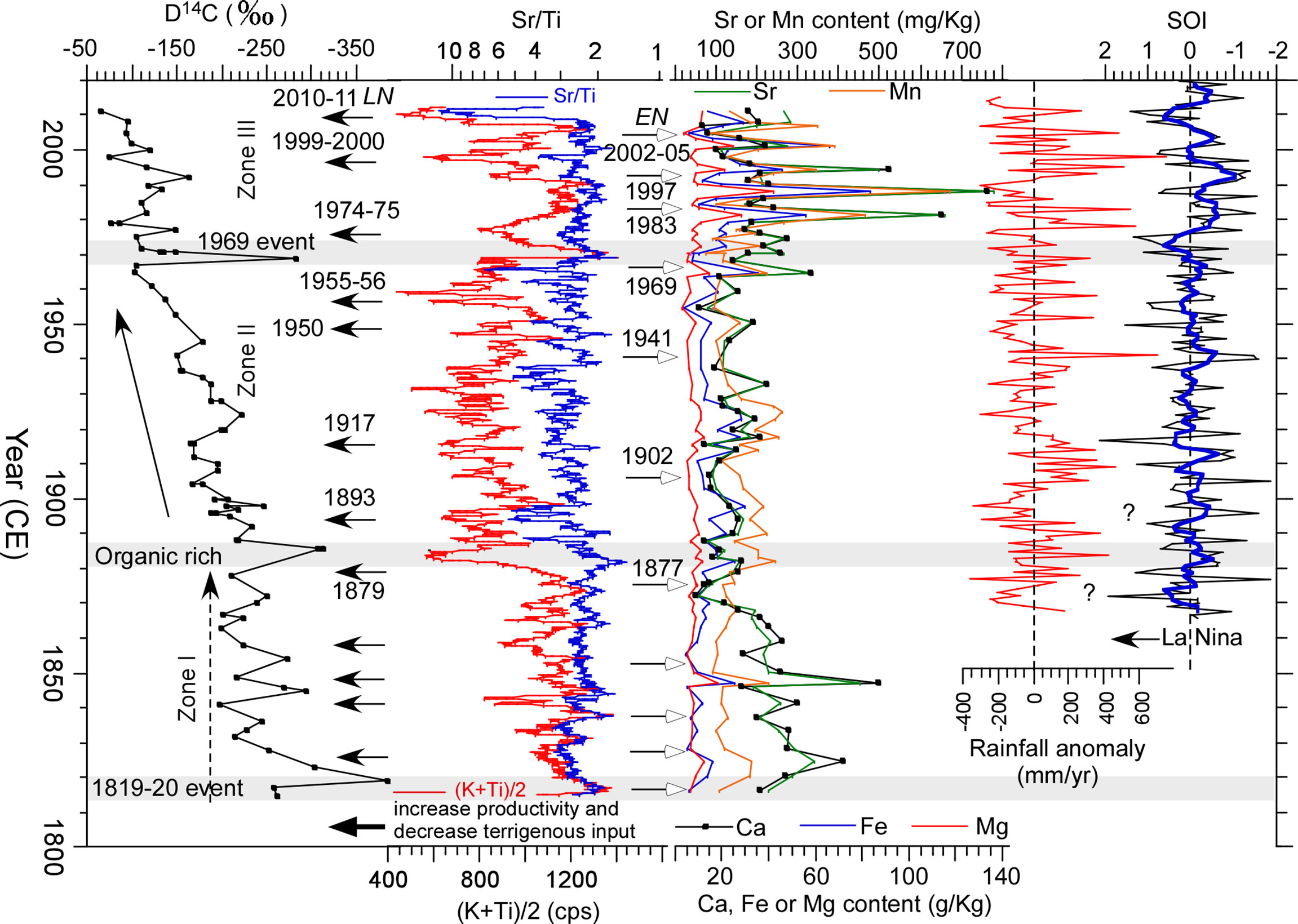
Figure 9 Comparisons of the D14C, scanning XRF elemental content, ICP-OES measured acid-leachable elemental concentration with the historical rainfall (the data was downloaded from https://www.santabarbaraca.gov/SBdocuments/Advisory_Groups/Water_Commission before it was removed.) and SOI records. The open head arrows refer El Niño events, and the solid head arrows reflect La Niña events. Note that the relationship between the rainfall in southern California and ENSO events is not fixed. For example, negative SOI values around late 1890s (shown by a question mark there) did not result in high rainfalls. Another question mark around 1870 shows the comparison is not valid, because low rainfall during this La Niña event would lead to high ALE contents but low scanning XRF siliciclastic elemental contents. This outliner may be caused by chronological uncertainty.
5 Conclusions
A 51-cm long core SBB-8-2012 from SSB was dated by 210Pb and varve counting. The chronology of the core was refined by comparing with a previous well-dated core from the same area, showing the depositional history of 1815-2011 CE with sedimentation rates of 0.25-0.29 cm/yr. The core has been measured for scanning XRF on an ITRAX core scanner, acid-leachable (0.5N HCl) elemental concentrations by an ICP-OES, and a total of 89 14C dates by AMS. Based on the sedimentary features and the above measured results, the core sediments can be classified into three zones: Zone I (1815-1880 CE) contains denser sediments with thinner laminations, higher scanning XRF K, Ti, Si, Mn and Rb, lower Fe/Ti, Ca/Ti and Sr/Ti, and lower D14CTOC values. Zone II (1880-1969 CE) includes less dense sediments with thicker laminations, the lowest scanning XRF K, Ti, Si, Mn and Rb, highest Fe/Ti, Ca/Ti and Sr/Ti, and strongly enriched D14CTOC trends. Zone III (1969-2011 CE) covers well laminated sediments with high CaCO3 contents, high acid-leachable elemental concentrations, strongly fluctuated XRF elemental contents and higher D14CTOC values.
The apparent 14C ages of the 200-year sediment core vary between 500 and 4000 yr BP with D14C values ranging from -62.2‰ to -383.8‰, reflecting influences of terrigenous soil OC input, fossil carbon emission from the Coal Oil Point seep field and 14C reservoir effect controlled by oceanic and atmospheric circulations. The three strongly negative D14CTOC excursions around 1819-20, 1884-87 and 1964-69 CE might be respectively related to the 1812 Santa Barbara earthquake, the first oil extraction in 1886 and unusual marine biological productivity in 1887, and the 1969 oil spill as well as flood events. The long-term mean D14CTOC shifts in three zones were probably caused by relatively high terrigenous soil OC input and fossil carbon emission from the Coal Oil Point seep field in Zone I, reduced fossil carbon emission due to the oil extraction and enhanced biological productivity in Zone II, and nuclear bomb 14C influence in Zone III. Nevertheless, the 14C reservoir effect in Santa Barbara Channel has been changed significantly during the past 200 years.
The ENSO events show strong influences on the D14CTOC, detrital elemental content and acid-leachable elemental concentration in the SBB sediments. During the La Niña period, stronger upwelling and northerly California Current bring nutrient enriched water into SBB and lead to higher productivity. Combining the effect of less terrigenous soil OC input during the La Niña period, lower scanning XRF K, Ti, Si, Mn and Rb, higher acid-leachable elements and higher D14CTOC values can be resulted in the sediments. During the El Nino period, the phenomena are opposite.
Data Availability Statement
Publicly available datasets were analyzed in this study. This data can be found here: SOI (data source: https://crudata.uea.ac.uk/cru/data/soi/).
Author Contributions
H-CL supervised the study, performed sampling of the core, participated lab experiment and wrote the manuscript. YC conducted the study for his MSc thesis. WB provided the core, initiated and discussed the study. MZ discussed the study and helped in revising the manuscript. SM and T-TS helped in data process. All authors contributed to the article and approved the submitted version.
Funding
This study was supported by grants from Ministry of Science and Technology of Taiwan (MOST 106-2116-M-002-012, MOST 107-2116-M-002-005, MOST 108-2116-M-002-012 and MOST 109-2116-M-002-018) to H-CL, and by the National Natural Science Foundation of China (Grant No. 41630966) to MZ.
Conflict of Interest
The authors declare that the research was conducted in the absence of any commercial or financial relationships that could be construed as a potential conflict of interest.
Publisher’s Note
All claims expressed in this article are solely those of the authors and do not necessarily represent those of their affiliated organizations, or those of the publisher, the editors and the reviewers. Any product that may be evaluated in this article, or claim that may be made by its manufacturer, is not guaranteed or endorsed by the publisher.
Acknowledgments
We thank Ms. Su-Chen Kang and Ms. Chun-Yen Chou at the NTUAMS Lab for their help in the AMS 14C dating. The iTRAX Lab in the Department of Geosciences and the NTUAMS Lab in the Instrumentation Center at NTU are acknowledged for their contribution to this study.
Supplementary Material
The Supplementary Material for this article can be found online at: https://www.frontiersin.org/articles/10.3389/fmars.2022.823793/full#supplementary-material
References
Allan R. J., Nicholls N., Jones P. D., Butterworth I. J. (1991). A Further Extension of the Tahiti-Darwin SOI, Early SOI Results and Darwin Pressure. J. Climate 4, 743–749. doi: 10.1175/1520-0442(1991)004<0743:AFEOTT>2.0.CO;2
Allen A. A., Mikolaj P. G. (1970). Natural Oil Seepage at Coal Oil Point, Santa Barbara, California. Science 170, 974–980. doi: 10.1126/science.170.3961.974
Andrew S., Peter A. C. (1977). Sedimentation and Climatic Patterns in the Santa Barbara Basin During the 19th and 20th Centuries. Geolog. Soc. America Bull., 88, (8). 1161–1172. doi: 10.1130/0016-7606(1977)88<1161:SACPIT>2.0.CO;2
Andrews A. H., Asami R., Iryu Y., Kobayashi D. R., Camacho F. (2016). Bomb-Produced Radiocarbon in the Western Tropical Pacific Ocean: Guam Coral Reveals Operation-Specific Signals From the Pacific Proving Grounds, J. Geophys. Res. Oceans 121, 6351–6366. doi: 10.1002/2016JC012043
Bakun A. (1990). Global Climate Change and Intensification of Coastal Ocean Upwelling. Science 247, 198–201. doi: 10.1126/science.247.4939.198
Barron J. A., Bukry D. (2010). Santa Barbara Basin Diatom and Silicoflagellate Response to Global Climate Anomalies During the Past 2200 Years. Quaternary Int., 215, 34–44. doi: 10.1016/j.quaint.2008.08.007
Barron J. A., Bukry D., Hendy I. L. (2015). High-Resolution Paleoclimatology of the Santa Barbara Basin During the Medieval Climate Anomaly and Early Little Ice Age Based on Diatom and Silicoflagellate Assemblages in Kasten Core SPR0901-02kc. Quat. Int. 387, 13–22. doi: 10.1016/j.quaint.2014.04.020
Baskaran M., Krishnamurthy R. V. (1993). Speleothems as Proxy for the Carbon Isotope Composition of Atmospheric CO2. Geophys. Res. Lett. 20 (24), 2905–2908. doi: 10.1029/93GL02690
Baskaran M., Iliffe T.M. (1993). Age Determination of Recent Cave Deposits Using Excess 210Pb—a New Technique. Geophys. Res. Lett. 20, 603–606.
Berelson W. M., Morine L., Sessions A., Rollins N., Fleming J. C., Schwalbach J. (2019). Santa Barbara Basin Flood Layers: Impact on Sediment Diagenesis. In: From the Mountains to the Abyss: The California Borderland as an Archive of Southern California Geologic Evolution. SEPM Special Publ. No. 110 p, 233–240. doi: 10.2110/sepmsp.110.11
Bograd S. J., Lynn R. J. (2001). Physical-Biological Coupling in the California Current During the 1997–99 El Niño-La Niña Cycle. Geophys. Res. Lett. 28 (2), 275–278. doi: 10.1029/2000GL012047
Bograd S. J., Schwing F. B., Castro C. G., Timothy D. A. (2002). Bottom Water Renewal in the Santa Barbara Basin. J. Geophys. Res. 107 (C12). 3216. doi: 10.1029/2001jc001291
Boles J. R., Clark J. F. (2001). Temporal Variation in Natural Methane Seep Rate Due to Tides, Coal Oil Point Area, California. J. Geophys. Res. 106, 27077–27086. doi: 10.1029/2000JC000774
Bray N. A., Keyes A., Morawitz W. M. (1999). The California Current System in the Southern California Bight and the Santa Barbara Channel. J. Geophys. Res. 104 (C4), 7695–7714. doi: 10.1029/1998jc900038
Browne D. R. (1994). Understanding the Oceanic Circulation in and Around the Santa Barbara Channel. Fourth California Islands Symposium: Update Status Resour. ( Santa Barbara Museum of Natural History: Santa Barbara, California), 27–, 34.
Davis C. V., Ontiveros-Cuadras J. F., Benitez-Nelson C., Schmittner A., Tappa E. J., Osborne E., et al. (2019). Ongoing Increase in Eastern Tropical North Pacific Denitrification as Interpreted Through the Santa Barbara Basin Sedimentary δ15n Record. Paleoceanogr. Paleoclimatol. 34, 1554–1567. doi: 10.1029/2019PA003578
Druffel E. M. (1996). Post-Bomb Radiocarbon Records of Surface Corals Tropical Atlantic Ocean. Radiocarbon 38, 563–572. doi: 10.1017/S0033822200030095
Du X. J., Hendy I., Schimmelmann A. (2018). A 9000-Year Flood History for Southern California: A Revised Stratigraphy of Varved Sediments in Santa Barbara Basin. Mar. Geol. 397, 29–42. doi: 10.1016/j.margeo.2017.11.014
Emmer E., Thunell R. C. (2000). Nitrogen Isotope Variations in Santa Barbara Basin Sediments: Implications for Denitrification in the Eastern Tropical North Pacific During the Last 50,000 Years. Paleoceanography 15 (4), 377–387. doi: 10.1029/1999pa000417
Eichhubl P., Greene H. G., Naehr T., Maher N. (2000). Structural Control of Fluid Flow: Offshore Fluid Seepage in the Santa Barbara Basin, California. Geochem. Explor. 69–70, 545–549. doi: 10.1016/S0375-6742(00)00107-2
Fewkes J. W. (1889). Across the Santa Barbara Channel. Am. Nat. 23, 211–217 387-394. doi: 10.1086/274906
Foster M., Charters A. C., Neushul M. (1971). The Santa Barbara Oil Spill. Part 1: Initial Quantities and Distribution of Pollutant Crude Oil. Environ. pollut. 2 (2), 97–113. doi: 10.1016/0013-9327(71)90014-0
Goldberg E. D. (1963). “Geochronology With 210Pb,” in Radioactive Dating (Vienna: . International Atomic Energy Agency), 121–131.
Goldschmidt V. M. (1926). The Laws of Crystal Chemistry. Naturwissenschaften 14, 477–485. doi: 10.1007/BF01507527
Gorsline D. S. (1996). Depositional Events in Santa Monica Basin, California Borderland, Over the Past Five Centuries. Sedimentary Geol. 104, 73–88. doi: 10.1016/0037-0738(95)00121-2
Grottoli A. G., Eakin C. M. (2007). A Review of Modern Coral δ18o and Δ14C Proxy Records. Earth-Science Rev. 81, 67–91. doi: 10.1016/j.earscirev.2006.10.001
Hendy I. L., Dunn L., Schimmelmann A., Pak D. K. (2013). Resolving Varve and Radiocarbon Chronology Differences During the Last 2000 Years in the Santa Barbara Basin Sedimentary Record, California. Quat. Int. 310, 155–168. doi: 10.1016/j.quaint.2012.09.006
Hendy I. L., Napier T. J., Schimmelmann A. (2015). From Extreme Rainfall to Drought: 250 Years of Annually Resolved Sediment Deposition in Santa Barbara Basin, California. Quaternary Int.387, 3–12. doi: 10.1016/j.quaint.2015.01.026
Heusser L. (1998). Direct Correlation of Millennial-Scale Changes in Western North American Vegetation and Climate With Changes in the California Current System Over the Past ~60 Kyr. Paleoceanography 13, 252–262. doi: 10.1029/98PA00670
Hornafius J. S., Quigley D., Luyendyk B. P. (1999). The World’s Most Spectacular Marine Hydrocarbon Seeps (Coal Oil Point, Santa Barbara Channel, California): Quantification of Emissions. J. Geophys. Res. 104, 20703–20711. doi: 10.1029/1999JC900148
Hua Q., Barbetti M., Rakowski A. Z. (2013). Atmospheric Radiocarbon for the Period 1950-2010. Radiocarbon 55, 2059–2072. doi: 10.2458/azu_js_rc.v55i2.16177
Ingram B. L., Kennett D. J. (1995). Radiocarbon Chronology and Planktonic–Benthic Foraminifera 14C Age Differences in Santa Barbara Basin Sediments, Hole 893A. Proc. Ocean. Drill. Program Sci. Results 146 (Pt. 2), 19–27. doi:10.2973/odp.proc.sr.146-2.278.1995
Ingram B. L., Southon J. R. (1996). Reservoir Ages in Eastern Pacific Coastal and Estuarine Waters. Radiocarbon 38, 573–582. doi: 10.1017/S0033822200030101
Inman D. L., Jenkins S. A. (1999). Climate Change and the Episodicity of Sediment Flux of Small California Rivers. J. Geol. 107, 251–270. doi: 10.1086/314346
Jacox M. G., Fiechter J., Moore A. M., Edwards C. A. (2015). ENSO and the California Current Coastal Upwelling Response. J. Geophys. Research: Oceans 120, 1691–1702. doi: 10.1002/2014JC010650
Kennett D. J., Brassell S. C. (1992). Molecular Records of Twentieth Century El Niño Events in Laminated Sediments From Santa Barbara Basin. Nature 357, 62–64. doi: 10.1038/357062a0
Kennett J. P., Ingram B. L. (1995). A 20,000 Year Record of Ocean Circulation and Climate Change From the Santa Barbara Basin. Nature 377, 510–514. doi: 10.1038/377510a0
Kennett D. J., Ingram B. L., Erlandson J. M., Walker P. (1997). Evidence for Temporal Fluctuations in Marine Radiocarbon Reservoir Ages in the Santa Barbara Channel, Southern California. J. Archaeolog Sci. 24, 1051–1059. doi: 10.1006/jasc.1996.0184
Kim J. C., Park J. H., Kim I. C., Lee C., Cheoun M. K., Kang J., et al. (2001). Progress at the Seoul National University AMS Facility. J. Korean Phys. Soc. 39 (4), 23–35. doi: 10.1017/S0033822200037966
Kolpack R. L., Drake D. E. (1984). Transport of Clays in the Eastern Part of Santa-Barbara Channel, California. Geo-Mar. Lett. 4, 191–196. doi: 10.1007/BF02281704
Kuo T.-S., Liu Z.-Q., Li H.-C., Wan N.-J., Shen C.-C., Ku T.-L. (2011). Climate and Environmental Changes During the Past Millennium in Central Western Guizhou Reflected by Stalagmite ZJD-21 Record. J. Asian Earth Sci. 40, 1111–1120. doi: 10.1016/j.jseaes.2011.01.001
Lange C. B., Berger W. H., Burke S. K., Casey R. E., Schimmelmann A., Soutar A., et al. (1987). El Niño in Santa Barbara Basin: Diatom, Radiolarian and Foraminiferan Responses to the “1983 El Niño” Event. Mar. Geol. 78, 153–160. doi: 10.1016/0025-3227(87)90074-0
Li H.-C., Ku T.-L., Chen W.-J., Jiao W.-Q., Zhao S.-S., Chen T.-M., et al. (1996). Isotope Studies of Shihua Cave, Beijing – II: Radiocarbon Dating and Age Correction of Stalagmite. Seismol. Geol. 18, 329–338.
Li H.-C., Lee Z.-H., Wan N.-J., Shen C.-C., Li T.-Y., Yuan D.-X., et al. (2011). Interpretations of 18O and 13C in Aragonite Stalagmites From Furong Cave, Chongqing, China: A 2000-Year Record of Monsoonal Climate, J. Asian Earth Sci. 40, 1121–1130. doi: 10.1016/j.jseaes.2010.06.011
Li C., Sessions A. L., Kinnaman F. S., Valentine D. L. (2009). Hydrogen-Isotopic Variability in Lipids From Santa Barbara Basin Sediments. Geochimica. Cosmochimica. Acta 73 (16), 4803–4823. doi: 10.1016/j.gca.2009.05.056
Li H.-C., Zhao M., Tsai C.-H., Mii H.-S., Wei K.-Y. (2015). The First High-Resolution Stalagmite Record From Taiwan: Climate and Environmental Changes During the Past 1300 Years. J. Asian Earth Sci. 114, 574–587. doi: 10.1016/j.jseaes.2015.07.025
Löwemark L., Itrax operators (2019). Practical Guidelines and Recent Advances in the Itrax XRF Core-Scanning Procedure. Quaternary Int. 514, 16–29. doi: 10.1016/j.quaint.2018.10.044
Lund D. C. (2011). Increased Ventilation Age of the Deep Northeast Pacific Ocean During the Last Deglaciation. Nat. Geosci. 4, 771–774. doi: 10.1038/ngeo1272
Lynn R. J., Bograd S. J. (2002). Dynamic Evolution of the 1997–1999 El Niño–La Niña Cycle in the Southern California Current System. Prog. Oceanogr. 54 (1-4), 59–75. doi: 10.1016/S0079-6611(02)00043-5
Lynn R. J., Simpson J. J. (1990). The Flow of the Undercurrent Over the Continental Borderland Off Southern California. J. Geophys. Res. 95 (C8), 12,995–13,008. doi: 10.1029/JC095iC08p12995
McFadgen B., Manning M. R. (1990). Calibrating New Zealand Radiocarbon Dates of Marine Shells. Radiocarbon 32 (2), 229–232. doi: 10.1017/S0033822200040194
Napier T. J., Hendy I. L., Fahnestock M. F., Bryce J. G. (2019). Provenance of Detrital Sediments in Santa Barbara Basin, California, USA: Changes in Source Contributions Between the Last Glacial Maximum and Holocene. GSA Bull. 132 (1–2), 65–84. doi:10.1130/B32035.1
Paulsen D. E., Li H.-C., Ku T.-L. (2003). Climate Variability in Central China Over the Last 1270 Years Revealed by High-Resolution Stalagmite Records. Quat. Sci. Rev. 22, 691–701. doi: 10.1016/S0277-3791(02)00240-8
Pisias N. G. (1978). Paleoceanography of the Santa Barbara Basin During the Last 8000 Years. Quaternary Res. 10, 366–384. doi: 10.1016/0033-5894(78)90027-3
Roark E. B., Ingram B. L., Southon J. R., Kennett J. P. (2003). Holocene Foraminiferal Radiocarbon Record of Paleocirculation in the Santa Barbara Basin. Geology 31, 379–382. doi: 10.1130/0091-7613(2003)031<0379:HFRROP>2.0.CO;2
Robert C. (2004). Late Quaternary Variability of Precipitation in Southern California and Climatic Implications: Clay Mineral Evidence From the Santa Barbara Basin, ODP Site 893. Quat. Sci. Rev. 23, 1029–1040. doi: 10.1016/j.quascirev.2003.11.005
Ropelewski C. F., Jones P. D. (1987). An Extension of the Tahiti-Darwin Southern Oscillation Index. Monthly Weather Rev. 115, 2161–2165. doi: 10.1175/1520-0493(1987)115<2161:AEOTTS>2.0.CO;2
Sarno C. T., Benitez-Nelson C. R., Ziolkowski L. A., Hendy I. L., Davis C. V., Tappa E. J., et al. (2020). The Impacts of Flood, Drought, and Turbidites on Organic Carbon Burial Over the Past 2,000 Years in the Santa Barbara Basin. California Paleoceanogr. Paleoclimatol. 35 (7), e2020PA00384. doi: 10.1029/2020PA003849
Schimmelmann A., Hendy I. L., Dunn L., Pak D. K., Lange C. B. (2013). Revised ∼2000-Year Chronostratigraphy of Partially Varved Marine Sediment in Santa Barbara Basin, California. GFF 135 (3–4), 258–264. doi: 10.1080/11035897.2013.773066
Schimmelmann A., Lange C. B. (1992). Extreme Climatic Conditions Recorded in Santa Barbara Basin Laminated Sediments: The 1835-1840 Macoma Event. Mar. Geol. pp, 279–299. doi: 10.1016/0025-3227(92)90134-4
Schimmelmann A., Lange C. B. (1996). Tales of 1001 Varves: A Review of Santa Barbara Basin Sediment Studies. Geolog. Soc. London Special Publications 116, 121–141. doi: 10.1144/GSL.SP.1996.116.01.12
Schimmelmann A., Lange C. B., Li H.-C. (2001). Major Flood Events of the Past 2,000 Years Recorded in Santa Barbara Basin Sediment. Paclim. Conf. Proc. pp, 82–103.
Schimmelmann A., Lange C. B., Meggers B. J. (2003). Palaeoclimatic and Archaeological Evidence for a 200-Yr Recurrence of Floods and Droughts Linking California, Mesoamerica and South America Over the Past 2000 Years. Holocene. 13 (5), 763–778. doi: 10.1191/0959683603hl661rp
Schimmelmann A., Lange C. B., Roark E. B., Ingram B. L. (2006). Resources for Paleoceanographic and Paleoclimatic Analysis: A 6,700-Year Stratigraphy and Regional Radiocarbon Reservoir-Age (ΔR) Record Based on Varve Counting and 14C-AMS Dating for the Santa Barbara Basin, Offshore California, U.S.A. J. Sediment. Res. 76, 74–80. doi: 10.2110/jsr.2006.04
Schimmelmann A., Tegner M. J. (1991). Historical Oceanographic Events Reflected in 13C/12C Ratio of Total Organic Carbon in Laminated Santa Barbara Basin Sediment. Global biogeochem. cycles 5, 173–188. doi: 10.1029/91GB00382
Schimmelmann A., Zhao M., Harvey C. C., Lange C. B. (1998). A Large California Flood and Correlative Global Climatic Events 400 Years Ago. Quaternary Res. 49 (1), 51–61. doi: 10.1006/qres.1997.1937
Schimmelmann A., Zhao M., Kuhn G. G., Tegner M. J. (1995). “Sea Surface Temperature and Paleo-El Niño Events in Santa Barbara Basin, AD 1841-1941,” in Proceedings of the Eleventh Annual Pafic Climate (PACLIM) Workshop, April 19-22, 1994. Interagency Ecological Program. Technical Report 40. Eds. Isaacs C. M., Tharp V. L. (California Department of Water Resources), 93–105.
Shipe R. F., Passow U., Brzezinski M. A., Graham W. M., Pak D. K., Siegel D. A., et al. (2002). Effects of the 1997–98 El Niño on Seasonal Variations in Suspended and Sinking Particles in the Santa Barbara Basin. Prog. Oceanogr. 54, 105–127. doi: 10.1016/S0079-6611(02)00045-9
Stenström K. E., Skog G., Georgiadou E., Genberg J., Johansson A. (2011). “A Guide to Radiocarbon Units and Calculations,” in Internal Report LUNFD6(NFFR-3111)/1-17/(2011), Division of Nuclear Physics (Sweden: Department of Physics, Lund University).
Stuiver M., Polach H. A. (1977). Discussion/Reporting of 14C Data. Radiocarbon 19, 355–363. doi: 10.1017/S0033822200003672
Tems C. E., Berelson W. M., Prokopenko M. G. (2015). Particulate δ15n in Laminated Marine Sediments as a Proxy for Mixing Between the California Undercurrent and the California Current: A Proof of Concept. Geophys. Res. Lett. 42, 419–427. doi: 10.1002/2014GL061993
Thunell R. C. (1998). Particle Fluxes in a Coastal Upwelling Zone: Sediment Trap Results From Santa Barbara Basin, California. Deep-Sea Res. Part Ii-Topical Stud. Oceanogr. 45, 1863–1884. doi: 10.1016/S0967-0645(98)80020-9
Thunell R. C. (2003). Distinguishing Between Water Column and Sedimentary Denitrification in the Santa Barbara Basin Using the Stable Isotopes of Nitrate. Geochem. Geophys. Geosyst. 4 (5), 1040. doi: 10.1029/2002gc000384
Thunell R. C., Tappa E., Anderson D. M. (1995). Sediment Fluxes and Varve Formation in Santa Barbara Basin, Offshore California. Geology 23, 1083–1086. doi: 10.1130/0091-7613(1995)023<1083:SFAVFI>2.3.CO;2
Treude T., Ziebis W. (2010). Methane Oxidation in Permeable Sediments at Hydrocarbon Seeps in the Santa Barbara Channel, California. Biogeosciences 7, 3095–3108. doi: 10.5194/bg-7-3095-2010
Valentine D. L., Reddy C. A., Farwell C., Hill T. M., Pizarro O., Yoerger D. R., et al. (2010). Asphalt Volcanoes as Potential Source of Methane to Late Pleistocene Coastal Waters, Nat. Geosci. 3, 345–348. doi: 10.1038/NGEO848
van Geen A., Husby D. M. (1996). Cadmium in the California Current System: Tracer of Past and Present Upwelling. J. Geophys. Res. (Oceans) 101, 3489–3507. doi: 10.1029/95JC03302
Venrick E. L. (2012). Phytoplankton in the California Current System Off Southern California: Changes in a Changing Environment. Prog. Oceanogr. 104, 46–58. doi: 10.1016/j.pocean.2012.05.005
Warrick J. A., Farnsworth K. L. (2009). “Sources of Sediment to the Coastal Waters of the Southern California Bight,” in Earth Science in the Urban Ocean: The Southern California Continental Borderland, Geological Society of America Special Paper, vol. 454 . Eds. Lee H. J., Normark W. R., 39–52). doi: 10.1130/2009.2454(2.2
Warrick J. A., Washburn L., Brzezinski M. A., Siegel D. A. (2005). Nutrient Contributions to the Santa Barbara Channel, California, From the Ephemeral Santa Clara River. Estuarine Coast. Shelf Sci. 62 (4), 559–574. doi: 10.1016/j.ecss.2004.09.033
Weinheimer A. L., Cayan D. R. (1997). Radiolarian Assemblages From Santa Barbara Basin Sediments: Recent Interdecadal Variability. Paleoceanography 12, 658–670. doi: 10.1029/97PA00986
White M. E., Rafter P. A., Stephens B. M., Wankel S. D., Aluwihare L. I. (2019). Recent Increases in Water Column Denitrification in the Seasonally Suboxic Bottom Waters of the Santa Barbara Basin. Geophys. Res. Lett. 46, 6786–6795. doi: 10.1029/2019GL082075
Yang Q. N., Zhao H. Y., Li H.-C., Li H. K., Bu Z. J., Wang S. Z., et al. (2017). Distributions of “Bomb 14C”, Biogeochemistry and Elemental Concentration in Hani Mire Peat Profiles, NE China: Implications of Environmental Change. Quat. Int. 447, 128–143. doi: 10.1016/j.quaint.2017.06.033
Yin J. J., Li H.-C., Rao Z.-G., Shen C.-C., Mii H.-S., Pillutla R. K., et al. (2017). Variations of Monsoonal Rain and Vegetation During the Past Millennium in Tiangui Mountain, North China Reflected by Stalagmite δ18O and δ13C Records From Zhenzhu Cave. Quaternary Int. 447, 89–101. doi: 10.1016/j.quaint.2017.06.039
Yin J. J., Li H.-C., Tang W., Wang Z. J., Mii H.-S., Lin Y. S. (2019). Rainfall Variability and Vegetation Recovery in Rocky Desertification Areas Recorded in Recently-Deposited Stalagmites From Guilin, South China. Quaternary Int. 528, 109–119. doi: 10.1016/j.quaint.2019.01.039
Yin J. J., Yuan D. X., Li H.-C., Cheng H., Li T. Y., Edwards R. L., et al. (2014). Variation in the Asian Monsoon Intensity and Dry–Wet Condition Since the Little Ice Age in Central China Revealed by an Aragonite Stalagmite. Clim. Past 10, 1803–1816. doi: 10.5194/cp-10-1803-2014
Zhao M., Li H.-C., Liu Z.-H., Mii H.-S., Sun H.-S., Shen C.-C. (2015). Changes in Climate and Vegetation of Central Guizhou in Southwest China Since the Last Glacial Reflected by Stalagmite Records From Yelang Cave. J. Asian Earth Sci. 114, 549–561. doi: 10.1016/j.jseaes.2015.07.021
Zhao M., Li H.-C., Shen C.-C., Kang S.-C., Chou C.-Y. (2017a). δ18O, δ13C, Elemental Content and Depositional Features of a Stalagmite From Yelang Cave Reflecting Climate and Vegetation Changes Since Late Pleistocene in Central Guizhou, China. Quaternary Int. 452, 102–115. doi: 10.1016/j.quaint.2016.07.022
Keywords: 14CTOC variation, elemental content, laminated sediment, Santa Barbara basin, El Niño/La Niña, iTRAX XRF scan corer, Icp-oes analysis, acid-leachable elements
Citation: Li H-C, Chang Y, Berelson WM, Zhao M, Misra S and Shen T-T (2022) Interannual Variations of D14CTOC and Elemental Contents in the Laminated Sediments of the Santa Barbara Basin During the Past 200 Years. Front. Mar. Sci. 9:823793. doi: 10.3389/fmars.2022.823793
Received: 28 November 2021; Accepted: 15 June 2022;
Published: 28 July 2022.
Edited by:
Núria Casacuberta, ETH Zürich, SwitzerlandReviewed by:
Fasong Yuan, Cleveland State University, United StatesAmzad Hussain Laskar, Physical Research Laboratory, India
Haiyan Jin, Ministry of Natural Resources, China
Copyright © 2022 Li, Chang, Berelson, Zhao, Misra and Shen. This is an open-access article distributed under the terms of the Creative Commons Attribution License (CC BY). The use, distribution or reproduction in other forums is permitted, provided the original author(s) and the copyright owner(s) are credited and that the original publication in this journal is cited, in accordance with accepted academic practice. No use, distribution or reproduction is permitted which does not comply with these terms.
*Correspondence: Hong-Chun Li, aGNsaTE5NjBAbnR1LmVkdS50dw==