- 1Department of Biological and Environmental Sciences, Qatar University, Doha, Qatar
- 2Geochemical and Environmental Research Group, Department of Oceanography, Texas A&M University, College Station, TX, United States
- 3Department of Biology, Texas A&M University, College Station, TX, United States
- 4Institute of Oceanography, National Taiwan University, Taipei, Taiwan
Amphipod crustacean diversity and zonation are described from the large Mississippi Canyon that extends from the continental shelf across the entire continental margin. Benthic amphipods were sampled on four cruises from 2000 to 2004 from six locations in the Mississippi Canyon from depths of 480 through 2,750 m, and compared with five locations in a second transect on the open continental slope approximately 100 km west of the canyon to assess the canyon effect on the structure and composition of amphipod assemblages. Five replicates were collected from each location using 0.2 m–2 GOMEX box corer. Amphipods amounted to 40% of the total faunal abundance within the canyon compared to only 4% in the non-canyon samples. Of the seventy-two species (19 families) collected, 61 were encountered in the canyon compared to 38 on the non-canyon transect. The trough-like head of the canyon (480 m) supported high densities (4,446–26,933 ind./m2) of the filter feeding, tube dwelling ampeliscid amphipod (Ampelisca mississippiana), the highest densities sampled compared to any other single species, at any other location, either within or outside the canyon, reflecting extreme flux of organic detritus from the continental shelf. The dominance by this single species suppressed the within – habitat (alpha) diversity and evenness, compared to relatively high diversity within the 1,000–1,500 m depths interval both in and outside the canyon. The species richness and alpha diversity exhibited mid-depth maxima at ca. 1,100 m both in and out of the canyon. High species richness (61) over the entire length of the canyon is presumed to be a function of greater topographic complexity and intermittent mass wasting of sediment down the canyon axis. The absence of nestedness is attributed to the amphipod reproductive pattern that lacks dispersive larval stages and brooding comparatively small numbers of eggs in this taxon. Despite depressed diversity at the head of the canyon, the fact that the number of amphipod species in the Mississippi Canyon was 1.5 times their numbers on the adjacent slope suggests that this physiographic feature enriches geographic-scale species diversity.
Introduction
The Mississippi Canyon is a prominent topographic feature that incises the continental margin in the north central Gulf of Mexico (GoM) (Shepard and Dill, 1966; Goodwin and Prior, 1989). Canyons are shelf incisions characterized by steep sides and complex topography, which are often associated with increased heterogeneity and distinctive hydrologic regimes (Dorschel et al., 2014). Canyons across geographic scales are distinguished by diverse and complex interacting attributes that may include hydrodynamic conditions, morphological features (Gage et al., 1995; Gunton et al., 2015), proximity to the coast and river system (Bernhardt et al., 2015) and quantity and quality of organic carbon flux (Rowe et al., 1982; Vetter and Dayton, 1998; Duineveld et al., 2001). The interactions between these factors determine distinct levels of canyon activity and varying capacity to transport sediments into the deep sea (Bernhardt et al., 2015). Active canyons, such as the Mississippi, funnel and channel massive amounts of sedimentary materials and particulate organic carbon (POC) derived from the shelf and river drainage basin to greater depths off the continental shelf (Bianchi et al., 2006; Ross et al., 2009). The presence of land derived plant material in the head of the Mississippi Canyon as well as at the abyssal plain below the canyon indicates that it is active conduit for sediments and other debris to the deep-sea (Wei et al., 2012). This high flux of organic carbon in canyons has been assumed to enhance faunal abundance and alter species composition (Rowe, 1971; Rowe et al., 1982; Gage and Tyler, 1991; Gage et al., 1995; Vetter and Dayton, 1998; Puig et al., 2014; Duffy et al., 2016; Fernandez-Arcaya et al., 2017). While high sedimentation in canyons favored colonization by some taxa, they smothered others (Cunha et al., 2011). Although submarine canyons are often reported to harbor distinct and dense faunal assemblages from the adjacent slope areas outside the canyon (Rowe, 1971; Ohta, 1983; Wei et al., 2010b; Oliver et al., 2020), some canyons exhibited depressed densities and insignificant differences from the nearby slope (Maurer et al., 1994; Duineveld et al., 2001; Liao et al., 2017). Inter-canyons variability have been associated with different functional feeding groups from dense masses of polychaetes, bivalves and crustaceans that alternate dominance across different canyons (Cunha et al., 2011; Gunton et al., 2015), but specific conditions underlying these patterns remains poorly understood (Gunton et al., 2015).
Although extensive studies of deep infaunal benthos have been published on the deep northern Gulf of Mexico, including the Mississippi and De Soto canyons (Rowe and Menzel, 1971; Pequegnat, 1983; Pequegnat et al., 1990; Gallaway et al., 2003; Wilson, 2008; Wei et al., 2010b; Carvalho et al., 2013 and others), amphipods remains largely unknown. Amphipods are ubiquitously distributed, even inhabiting the harsh and confining habitats of deep trenches (Dickinson and Carey, 1978; Cartes and Sorbe, 1999; Blankenship et al., 2006). The taxon, however, lacks a dispersive larval phase and thus exhibit a high habitat and niche specificity (Thomas, 1993). Absence of larval stage makes amphipods less susceptible to predators, have less depth and geographic range, and expose them to higher competition for resources (Pechenik, 1999). Therefore, we assume that amphipods offer a very different view of depth related patterns when compared with other taxon studied in the same area (Reish and Barnard, 1979). Amphipods from the northern Gulf of Mexico are only sporadically and fragmentally studied. Studies focused on the taxonomy of species of the littoral zone (Escobar-Briones and Winfield, 2003). The lack of studies on deep-water amphipods is mainly attributable to the absence of adequate sampling and to samples with a limited number of specimens (Cartes and Sorbe, 1999). Our aim has been to explore how the amphipod populations within this large topographic feature differ from amphipod populations outside the canyon. Univariate and multiscale approaches were used to determine which environmental variable best explains distribution patterns as a function of space or depth. We are testing the null hypothesis that the canyon has no effects on the structure and distribution patterns of amphipod assemblages as a function of space or depth. The total densities, diversities (alpha and gamma diversities) and zonation (beta diversity) are compared to illustrate the distinct and rather remarkable differences between the amphipod assemblages in the canyons versus elsewhere on the continental margin. Likewise, we also compared amphipod distribution with other benthic macrofaunal taxa within very similar habitats across the northern Gulf of Mexico (NGoM).
Study Area
The topography of the continental margin of the deep GoM is extremely complex, with large areas of salt domes that are interspersed by basins between the domes (Balsam and Beeson, 2003; Davis, 2017). Seeps of methane nourish communities of reef-like assemblages of vestimentiferan worms, mussels and associated fauna (Brooks et al., 1987; Fisher et al., 2007; Cordes et al., 2009). Carbonate remnants of the seeps support slow growing, cold water coral species (Roberts et al., 2010). The steep Sigsbee and Florida Escarpments stretch around the perimeter of the NGoM and descend to the flat abyssal plain (Martin and Bouma, 1978), referred to as the Sigsbee Deep. The Mississippi Canyon (Transect MT, Figure 1) incises the continental shelf at the center of the NGoM and extends south to southeast across the continental margin into abyssal depths as the Mississippi Fan (MF), a thick wedge of sediments between the east and west NGoM escarpments. The canyon depths range from 50 m on the shelf immediately down into the broad Mississippi Trough, at 400–500 m; from there the canyon extends down the continental slope to over 2,700 m, with a width of about 8–16 km (Shepard and Dill, 1966; Ellwood et al., 2006). Sediments traveling down to the lower continental slope have created massive levees on each side of the channel at depths of 2,000 to almost 3,000 m. Ultimately, the down-canyon sediment has spread out over time to beyond the fan to form the remarkably flat Sigsbee Abyssal Plain at 3,500–3,750 m depth (Shepard, 1981; Davis, 2017). Our analyses extend to a depth of ca. 2,700 m inside and outside the canyon.
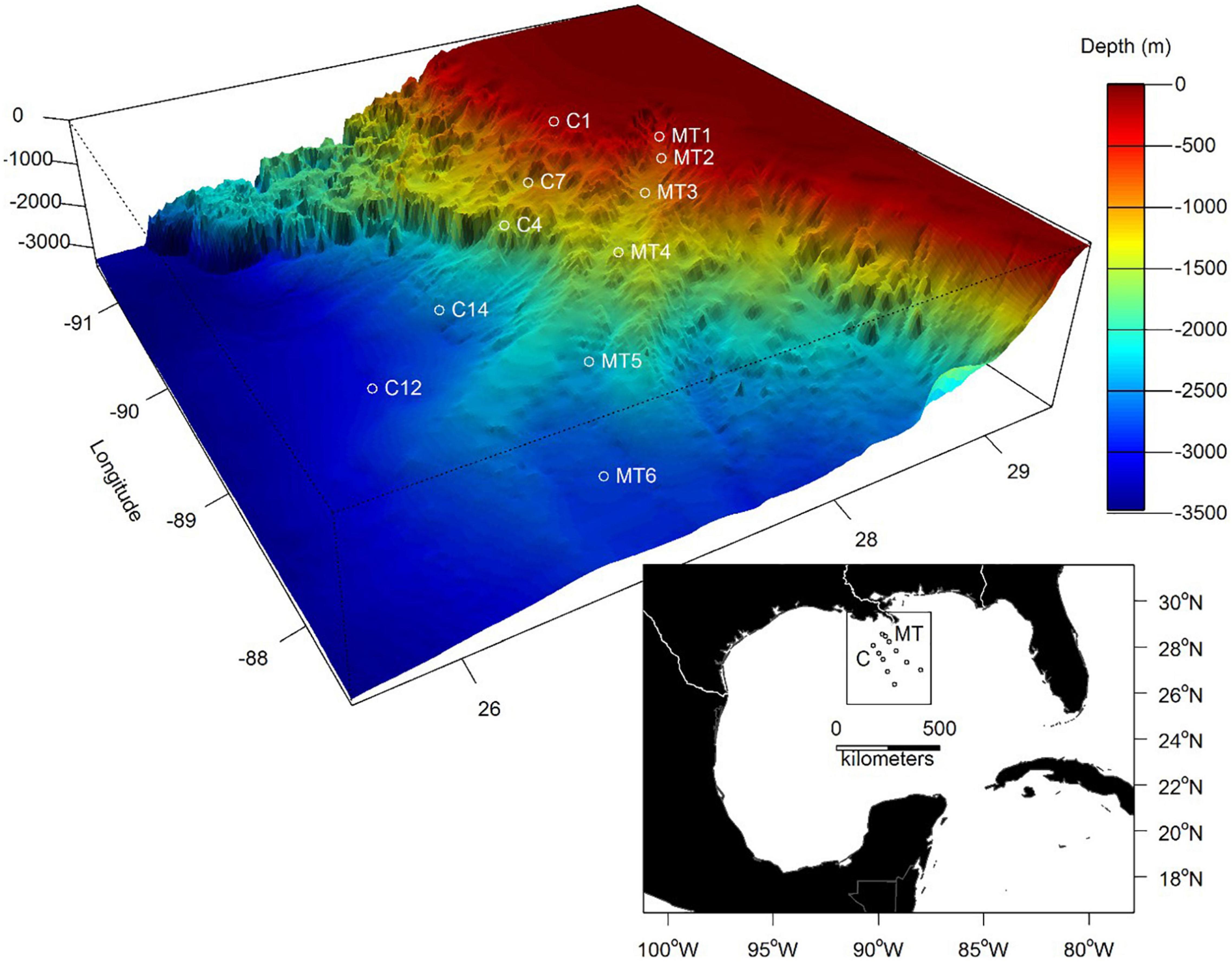
Figure 1. Box core sampling locations along the Mississippi Canyon (MT1-MT6) and a parallel transect (C1–C14). Topographic data were derived from NOAA Geophysical Data Center.
Materials and Methods
Sampling and Analysis of Benthic Amphipods
Benthic macrofauna, including amphipods, was sampled on five cruises from 2000 to 2004 from 11 locations in two transects in the NGoM. Five replicate cores were taken per station/location using the 0.2 m–2 GOMEX or Gray-O‘Hara box core (Boland and Rowe, 1991) deployed from the R.V. Gyre. Six locations (MT1 through MT6) from depths of 480 through 2,750 m represent the Mississippi Canyon (MT) habitat (Figure 1 and Supplementary Table 1). Five adjacent locations were selected from a parallel transect (C transect) as a comparable non-canyon transect to the west of the canyon transect from depth of 300 m through 2,900 m (Figure 1). The top 15 cm of sediment within the box, with a net surface area of 0.17 m–2, was sieved aboard ship through 300 micron sieves using the seawater floatation and sieving through a 300-micron sieve following the approach developed by Howard Sander’s group at the Woods Hole Oceanographic Institution (Rowe and Kennicutt, 2009). The water column samples were collected using the rosette sampler. Salinity was determined with A Guideline Model 8400B salinometer. Dissolved oxygen was measured using micro-Winkler technique. Nutrients (nitrate, nitrite, ammonia, urea, phosphate, and silicate were analyzed with a Technicon Autoanalyzer using standard colorimetric techniques. Two-liters of water were vacuum filtered on pre-weighed filters for total suspended particulate matter (TSPM). Another liter of water was filtered through 25-μm pre-combusted glass fiber filters (GFF) for determination of particulate organic carbon and nitrogen (POC and PON). For pigment analysis, a liter of water was filtered through 0.45 μm Millipore filters. All filters were carefully placed in combusted aluminum foil or plastic bags and properly stored for transport to shore-based laboratories for analysis.
The samples of fauna and any sediment retained with it were fixed in 10% formalin with filtered seawater and the vital stain Rose Bengal. On return to the laboratory, the formalin-Rose Bengal solution was changed to a 70% ethyl alcohol solution. Amphipods were identified to species using the taxonomic works of Barnard (1954a,b, 1960a,b, 1961, 1962, 1964, 1966, 1969, 1971), Mills (1963, 1964, 1965, 1967a,b, 1971), and Lincoln (1979). Individuals that appeared to be undescribed or that could not be identified to species with available resources were given species number designations for consistency between samples and sampling locations.
Measurements for Sea Floor Environmental Parameters
Sediment grain size, total organic carbon, trace metals and organic contaminants were measured in small sub cores taken from each replicate sample. The standard Folk settling method was used to determine sediment grain size (Folk, 1974). A Carlo Erba elemental analyzer was used to determine organic carbon and nitrogen. Dissolved organic carbon (DOC) was measured in pore water, which was collected from the sediment by centrifugation or pressure filtering, by a high-temperature combustion DOC analyzer. Organic and inorganic carbon were determined by standard LECO combustion. The anthropogenic trace organic carbon and polycyclic aromatic hydrocarbons (PAHs) were measured by NOAA Status and Trends methods (Denoux et al., 1998; Qian et al., 1998) using gas chromatography–mass spectrometry. For metal analysis, the upper 2 cm of subcores were sectioned with a teflon-coated spatula and kept stored frozen (−20°C) in a pre-cleaned plastic jars and stored frozen (−20°C). Samples are analyzed for a total of 27 trace metals, including Ba, Cd, Cr, Fe, Hg, Pb, and Zn, were measured (see Wade et al., 2008; Rowe and Kennicutt, 2009). Total suspended matter (TSPM mg l–1) and particulate organic carbon and nitrogen (POC μg.l–1, PON μg.l–1) were measured in the bottom 1–3 m of the water column. Chl-a in the surface water column was estimated from SeaWiFS satellite images. Export POC flux rates (mg C m–2 day–1) arriving the sea floor were estimated from an exponential decay equation based on satellite assessed primary production in Biggs et al. (2008).
Data Analysis
Univariate Methods
Two-way analysis of variance (using SPSS) was used to test the effect of “canyon” and “depth” on the amphipod total abundance. Before the analysis, data of amphipod abundances was transformed using (log N + 1) to meet the assumptions for normality and homogeneity of variance. Olmstead and Tukey’s test for association (Sokal and Rohlf, 2012) was used to measure the ecological representation of each amphipod family and species based on their abundance and frequency of occurrence. Shannon-Wiener diversity index (H’), and Pielou’s Index (Equitability) were calculated using Biodiversity Pro (McAleece et al., 1997) and Primer 6 (Warwick and Clarke, 1991; Clarke and Gorley, 2006). Two non-parametric estimators, Jacknife second order estimator (Burnham and Overton, 1979) and Chao second order estimator (Chao, 1987), were used to estimate the possible number of species present in the study area, had the sampling been more intense.
Multivariate Methods
Amphipod Zonation
The variations in the composition of the amphipod assemblages (bathymetric zonation or Beta diversity) in the canyon (MT) and the non-canyon (C transect) were classified by hierarchical cluster analysis based on Bray-Curtis similarity and the average group linkage method in R 2.15.1 (R Development Core Team, 2019). The mean amphipod species densities at each location were fourth-root transformed prior to the analyses to reduce the influence of over-dominance by numerically abundant species. Rare species (taxa present once at a single site and/or comprised <1% of the total amphipod abundance across all sites), were omitted prior to the analysis as they are arbitrarily distributed across sites and tend to disrupt ordination and clustering patterns (Clarke and Warwick, 2001). Presence/absence transformation was performed to test for indicator species for the two transects. Species presence/absence data were converted to Sorensen dissimilarities and then partitioned into the spatial turnover and nestedness components (Baselga, 2010). Correlations between dissimilarities and depth differences between sites were computed with a Mantel test with 999 permutations (Borcard et al., 2018).
Analysis of Environmental Variables
The abiotic characteristics of the habitats were evaluated using Principal Component Analysis (PCA). The variables included temperature, dissolved oxygen, polycyclic aromatic hydrocarbons (PAHs, without pyrelene), dissolved organic carbon (DOC), particulate organic carbon (POC), nitrate (NO3–), nitrite (NO2–), ammonium (NH4+), sediment grain size (% sand, % silt, and % clay) and a subset of trace metals.
All data were transformed prior to analysis to validate the assumption of normal distribution. The mean of each set of data dimension was subtracted from the total and this was divided by the standard deviation to produce dimensions with a mean equaling zero. Each data set of the environmental data was transformed, with the exception of grain size, which was normalized angularly, x1 = arcsin(sqrt(x)), as recommended for ranges of 30–70% (Ahrens et al., 1990). An initial PCA of the 27 trace metals in the sediment was reduced to a subset that accounted for the highest variance in the main PCA.
Species composition (inter-station faunal resemblance) was regressed against the multivariate environmental data based on distance-based redundancy analysis (MdbRDA). A parsimonious dbRDA model was constructed using backward selection and AIC selection criteria (Akaike, 1973). Distance-based redundancy analysis (dbRDA) was then employed to visualize this parsimonious model through a principal coordinate ordination (PCO). Vector overlays based on multiple correlation coefficients show the direction and strength of environmental predictors on the dbRDA ordination axes. The analyses were performed using R 4.1.0 (R Core Team, 2020) and PRIMER 7.
Results
Amphipod Abundance
Seventy-two species belonging to 19 families were identified across 11 locations in the study area. The number of species (Species richness, or gamma diversity) was higher in the canyon transect (61 species) than in the non-canyon habitats (38 species). The most abundant species was Ampelisca mississippiana, Soliman and Wicksten, 2007, that lives in tubes and create small hillocks that cover the sea floor like a carpet, dominated the head of the canyon (Table 1 and Figure 2). It represented about 89% of the total abundance, with an average abundance of 13,771 ± 6,087 ind.m–2 (Table 1). Ampelisca sp. was the second most abundant species there (average abundance of 1,225 ± 578 ind.m–2) and was characteristic for the canyon’s head, along with a third species (Ericthonius sp., 313 ± 364 ind.m–2), but the latter represented only 2% of the total amphipod abundance. Cephalophoxoides homilis (Barnard, 1960b) was the most abundant species in the canyon’s middle depths, with an average abundance of 550 ± 19 ind.m–2. Other species such as Leptocheirus sp. and Leptophoxoides molaris Barnard, 1962 were also abundant and characteristic for the canyon’s middle slope zone (Table 1). Haploops sp. was characteristic of the lower slope zone (77 ind.m–2).
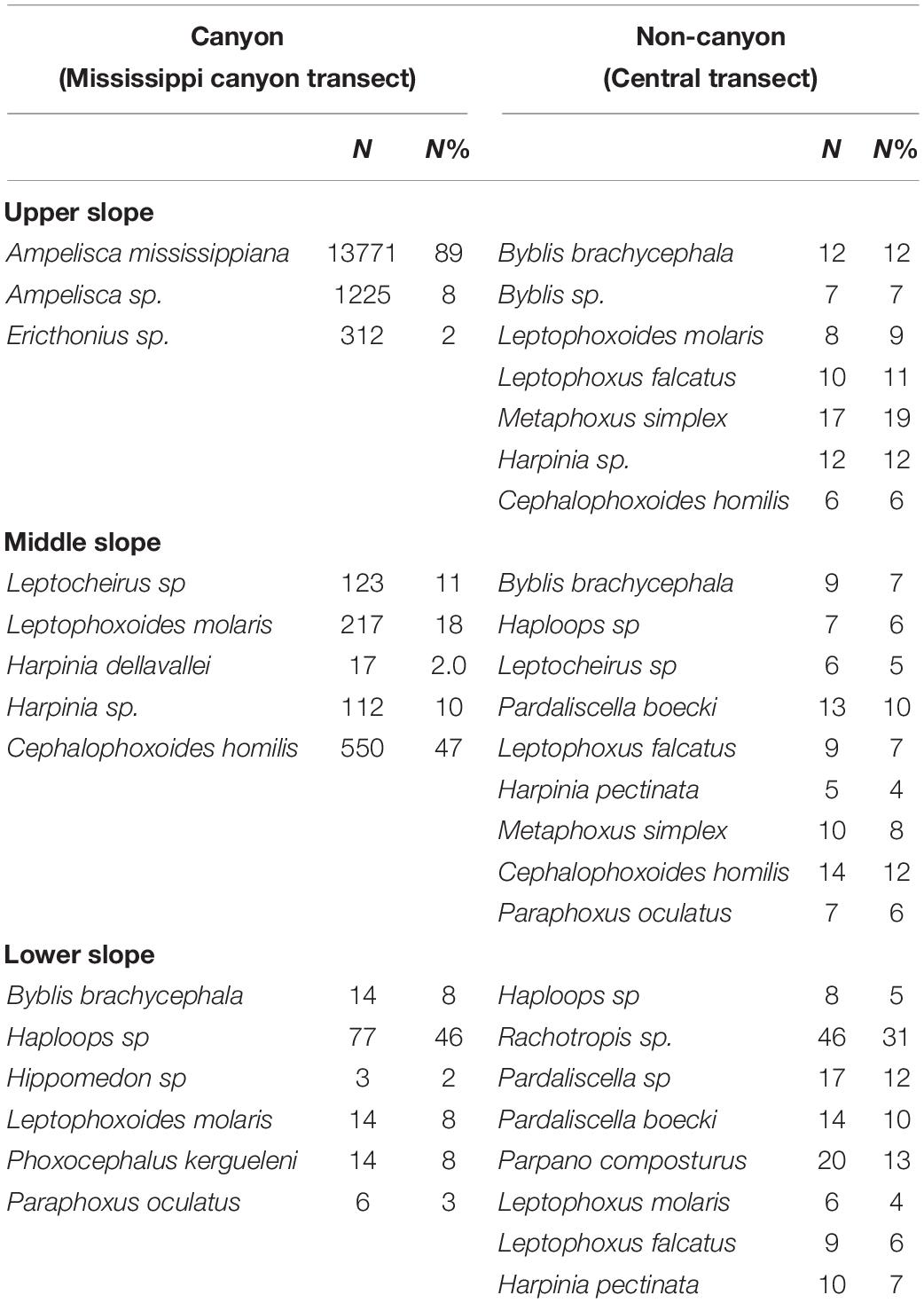
Table 1. Abundance and % contribution of the abundant amphipods to the total abundance at each zone of the slope: The upper (336–500 m), middle (677–990 m), and Lower (>1,000 m) slope.
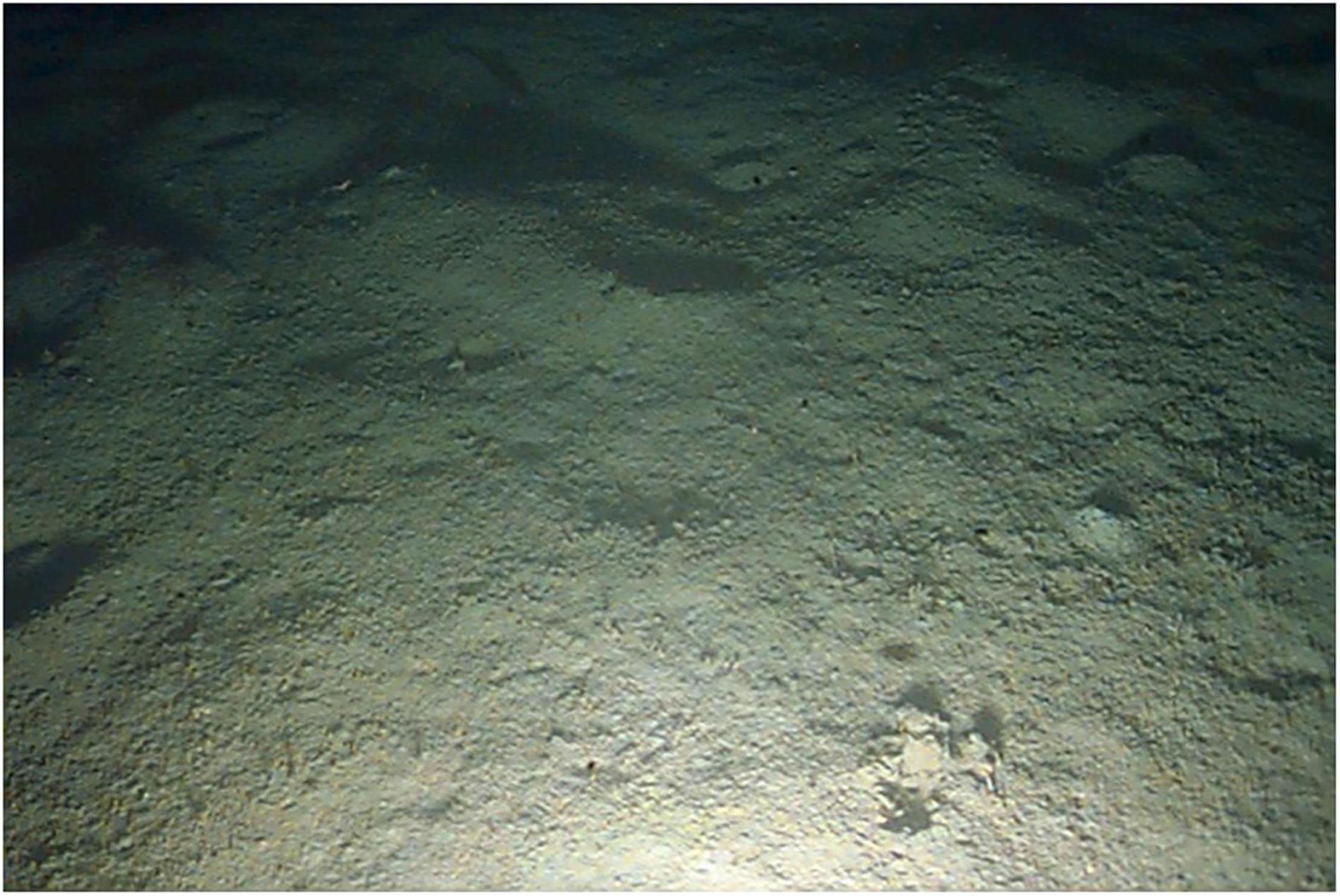
Figure 2. Sea floor photograph in the trough-like head of the Mississippi Canyon, MT1 at 482 m depth. The sediment is mottled by abundant Ampelisca mississippiana tubes.
The non-canyon transect was characterized by a different group of amphipods: Byblis brachycephala Mills, 1971, Leptophoxus falcatus (G.O. Sars, 1883), Metaphoxus simplex (Spence Bate, 1857), Leptophoxoides molaris on the upper slope, Haploops sp., Pardaliscella boecki (Malm, 1870), and Cephalophoxoides homilis on the middle slope, and Haploops sp. and Rachotropis sp. on the lower slope. The densities of these species on the central transect were lower than those in the canyon at any given depth (Table 1).
The total amphipod abundance (mean ± SD) in the canyon was highest in the wide trough at its head at 480 m (15,880 ± 7,003 ind.m–2) and decreased with depth down to 11 ± 1 ind.m–2 at 2,745 m as indicated on a linear regression (Figure 3). The values in the canyon head were thus four and a half orders of magnitude above those in the canyon on the lower continental slope. Abundance also declined on the non-canyon transect, from a maximum of 314 ± 276 ind.m–2) [at 1,017 m (C7)] down to 16 ind.m–2 at the deepest locations (C12, C14, at 2,486–2,920 m, Table 2 and Figure 3).
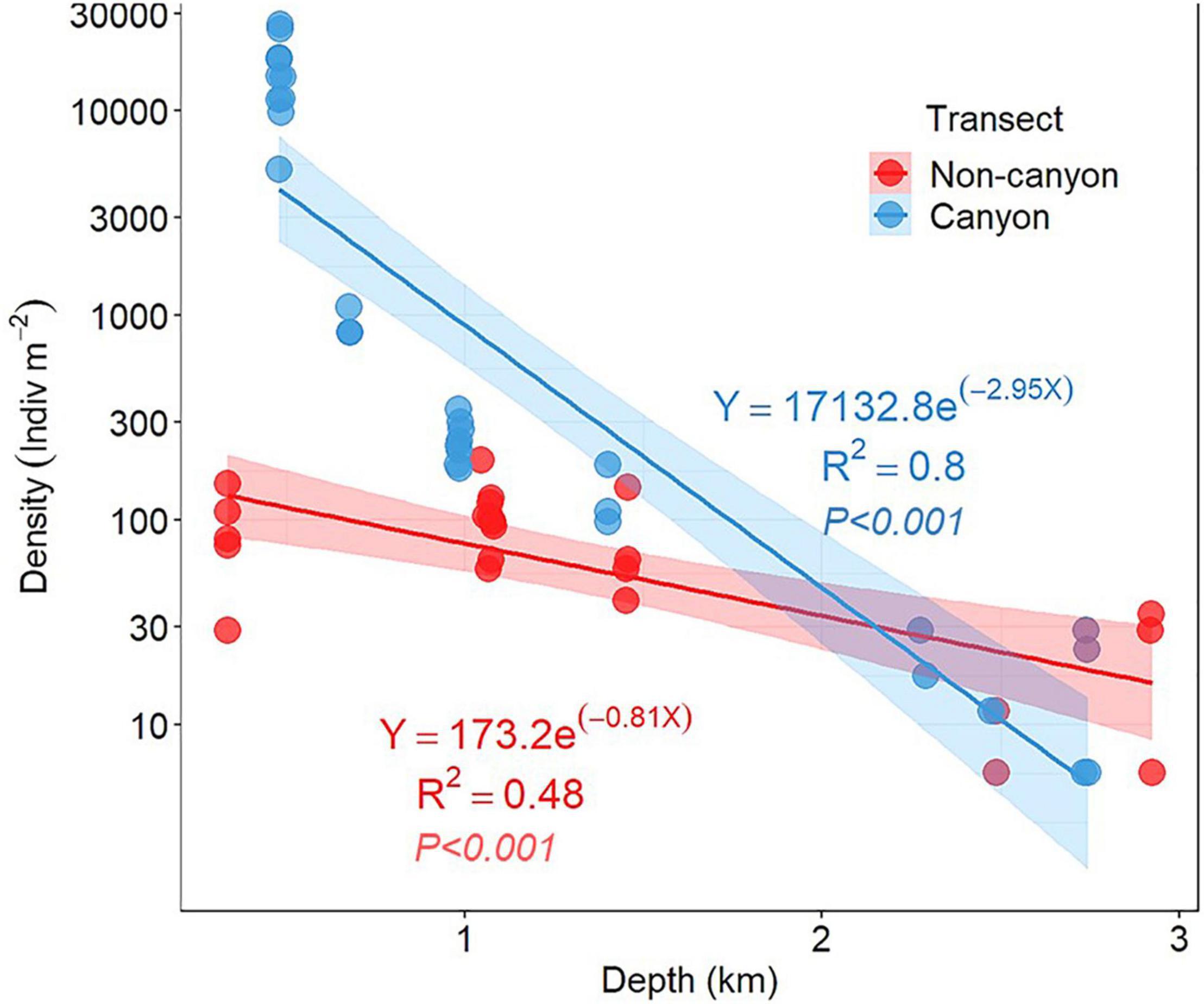
Figure 3. Plot of density (densities per square meter) against depth in the Mississippi Canyon (MT transect) and non-canyon (C for central).
The decline in abundance as a function of depth can be parameterized as follows:
d(densities)/d(depth) = −K (depth), where −K is the “decline rate” constant. The solution of this differential equation is,
The rate of decline in Figure 3, as a function of depth, fit an exponential function where the predicted intercept at the shallowest depth was 17,133 ind./m2 and the rate of decline was −2.95 × (Depth). The intercept and the rate of loss were lower in the non-canyon data: the intercept on the non-canyon intercept was 173.3 and the “decay” rate was –0.8 × (Depth), compared to the canyon (Figure 3).
Amphipods accounted for 41% of the total abundance of all the other macrofaunal invertebrates (isopods, tanaids, cumaceans, bivalve mollusks, polychaetes, and nematodes) in the canyon but represented only 4% of the total community on the non-canyon transect (Figure 4A). The highest contribution by amphipods (90%) was in the canyon head at 480 m (MT1) (Figure 4B). The maximum amphipod contribution outside the canyon was about 10% of the total invertebrate community, and that was at 1,000 m (Figure 4B). The analysis of variance with the two factors (Depth and Canyon) indicated that the mean amphipod abundance significantly changed with depth (F = 99.076, P < 0.001) and significantly varied between canyon and non-canyon (F = 21.018, P < 0.001, Table 3). There was also significant interaction between the two factors (P < 0.001).
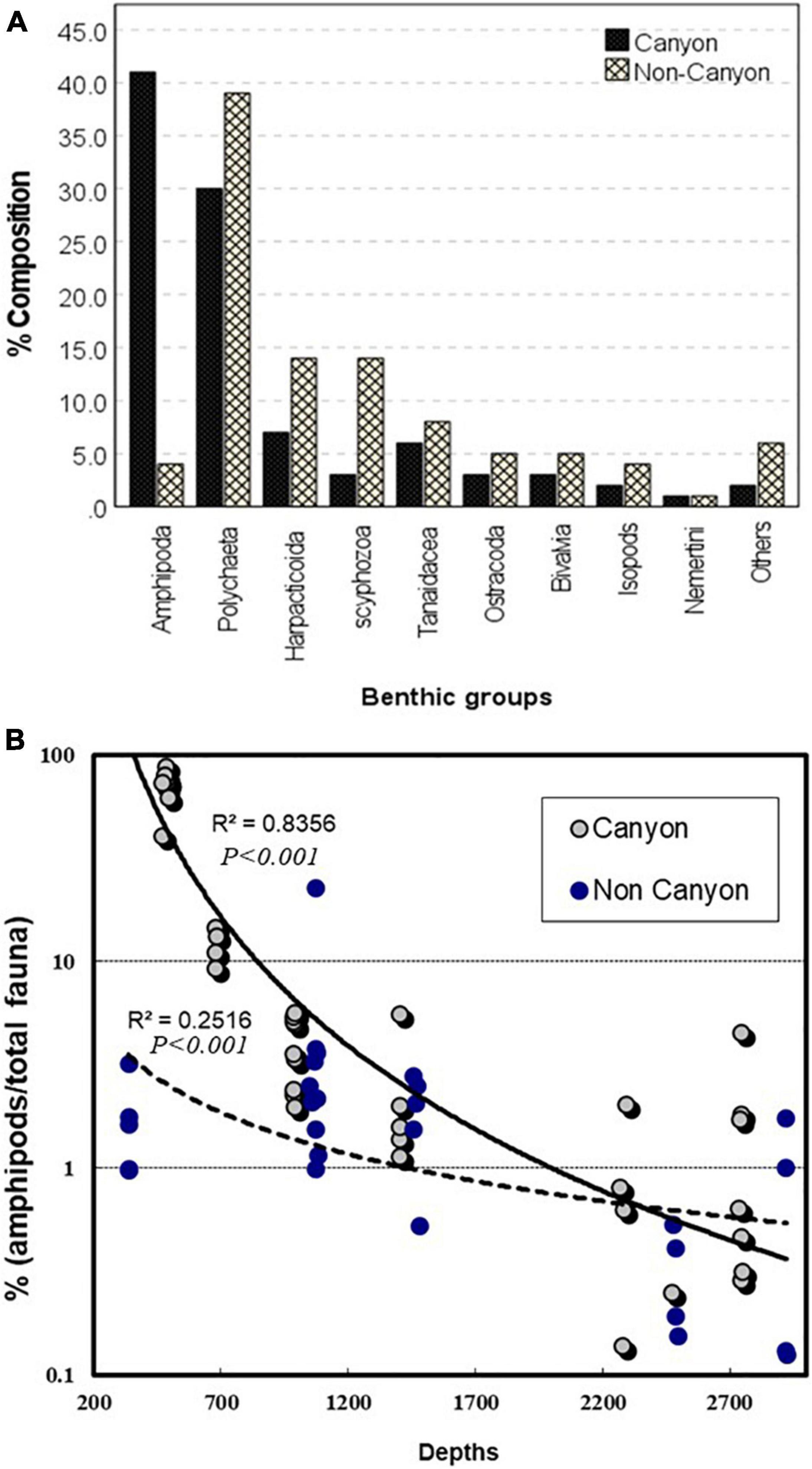
Figure 4. Percentage composition of the major faunal groups collected from the canyon and the non-canyon transect (A) and ratios of amphipods to the total macrofaunal densities at different sampling depth in the two transect (B).
At the family level, the canyon and the open continental slope were very different (Supplementary Figure 1). Of the 19 families collected, the canyon was dominated by Ampeliscidae, followed by Phoxocephalidae, Ischyroceridae, and Aoridae. Lyssianassidae, Melitidae, Synopidae, and Oedicerotidae, along with several others were infrequent families (see Supplementary Figure 1). The Phoxocephalidae was predominant on the non-canyon transect, over the Oedicerotidae, Pardaliscidae, and Synopiidae. The Ampeliscidae, Aoridae and Melitidae occurred in minor numbers outside the canyon (Supplementary Figure 1). Analysis of similarity between the canyon and non-canyon (ANOSIM; R = 0.17, P = 0.001) (Supplementary Figure 2), indicated that the largest differences between canyon and non-canyon species were in the shallow locations with the depths between 500 and 1,000, followed by significant but less profound differences at the deeper locations (Supplementary Figure 2).
Bathymetric Zonation of Amphipod Species
Within the canyon, four assemblages, with little or no overlap, were identified, based on [the 4th root transformed] species abundance (Figure 5). The first group included the canyon head (MT1), with the highest amphipod abundance (Figure 5 and Supplementary Figure 2). There was only minor similarity between MT1 and other canyon or non-canyon locations. The other three canyon groups were located on the upper slope (676–1,000 m), the middle slope (1,077–1,450 m) and on the lower slope, seaward of the latter boundary (Figure 5 and Supplementary Figure 2). The third mid-slope assemblage (1,077–1,450 m) in the canyon was also similar to the group outside the canyon on the upper slope, both in abundance and species composition. The deepest lower-slope canyon group seaward of 1,450 m was characterized by low abundances (Figure 5 and Supplementary Figure 2).
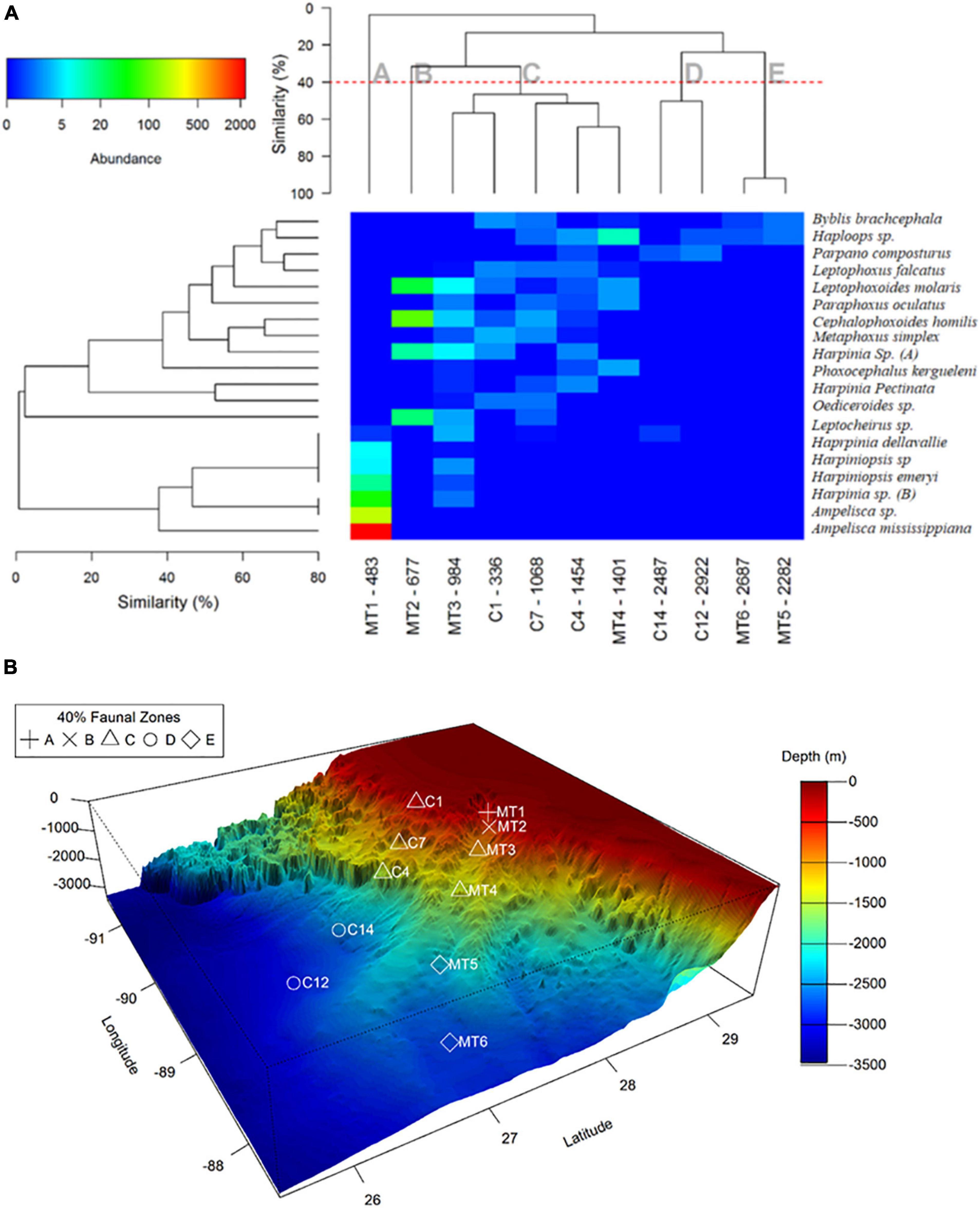
Figure 5. Two-way Cluster analysis using Bray-Curtis similarity between sampling locations (based on 4th root transformation) and for the top-20 most abundant amphipod species (based on presence/absence transformation) (A). The top-20 species accounted for ∼99% of total amphipod abundance. Color image shows the site-by-species matrix for the clustering. The x-axis indicates station name followed by water depth (m) at that location. The y-axis shows the species names. Color key indicates average amphipod abundance per sample (in 0.1725 m2). Geographic distribution of amphipod assemblages along the two transects (B). Symbols indicates assemblages with at least 40% Bray-Curtis similarity.
Cluster analysis of the top 20 most abundant species of both the canyon and non-canyon slope habitats suggests that there were five dissimilar groups (40% similarity or higher within the groups, Figure 5). The first region (Group A) included the canyon’s head (MT1), dominated by tubicolous species with limited bathymetric range (including Ampelisca mississippiana, Ampelisca sp., and Erichthonius sp.). The second group (B) included the middle canyon slope (MT2) dominated by Cephalophoxoides homilis and Leptophoxoides molaris. The third group (C) extended from the upper slope of the non-canyon (C1) down to ∼1,500 m, including both canyon (MT3 and MT4) and non-canyon sites (C7 and C14). This group was characterized by Haploops sp., Leptophoxus falcatus, Leptophoxoides molaris, Paraphoxus oculatus, Cephalophoxoides homilis, Metaphoxus simplex and Harpinia sp. It is also evident that Group C shared a number of species with MT2, at 1,000 m. The two deepest groups (D and E) separated into the non-canyon (C14 and C12) and Canyon habitats (MT5 and MT6). Haploops sp. and Parpano composturus were common in both groups; however, the species in Group D and E appear to be a subset of species from Group B and C (Figure 5).
The majority of amphipod species exhibited narrow depth ranges. More than 82.3% of the species had depth ranges of less than 1,000 m and more than 67.6% of species had depth ranges less than 500 m. Only three and four species had distributions extending from the upper (200–1,000 m) to lower canyon-slope (2,000–3,000 m) and from mid (1,000–2,000 m) to the lower canyon-slope (2,000–3,000 m), respectively (Figure 6A). On the contrary, sets of species that occurred at different minimum depths displayed considerable turnover. Sorensen dissimilarity partitioned among sites also supports strong depth-related patterns in which the total dissimilarity and species turnover correlated significantly with difference in depth between sampling sites (Mantel test, P < 0.05, Figure 6B). The spatial turnover of species contributed much more than nestedness in shaping the amphipods assemblage dissimilarities.
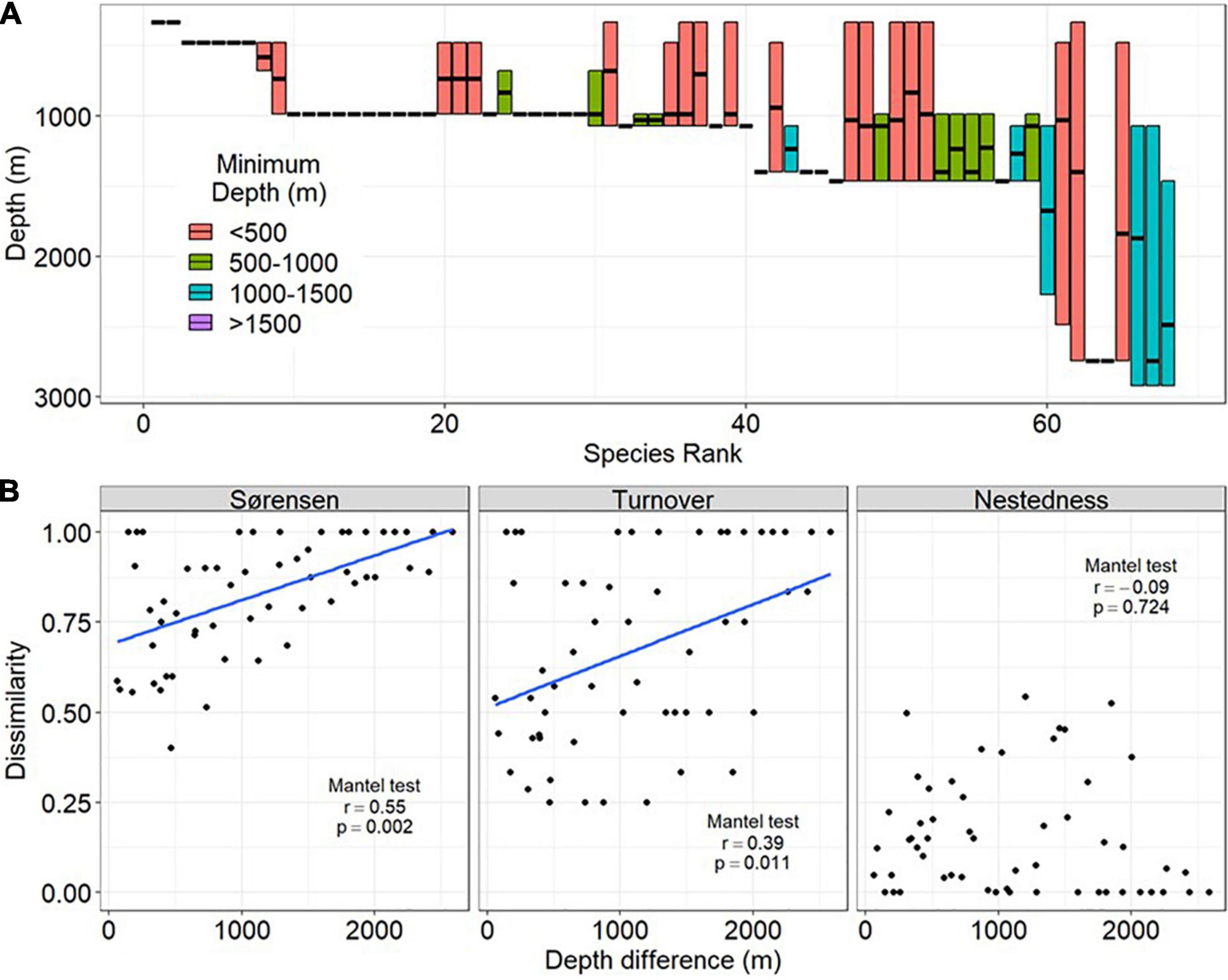
Figure 6. Depth distribution of amphipod species and assemblage dissimilarity as a function of depth difference in the northern Gulf of Mexico. Reported are: (A) Vertical ranges (minimum and maximum depth occurrence) of amphipod species. The horizontal line shows the median depth of occurrence, (B) Sorensen dissimilarity and its turnover and nestedness components as functions of the depth difference between sampling sites.
α and β-Biodiversity Patterns
Generally, the rate of species accumulation was gradual across upper slope depths, rapid at mid-depths, and then gradual again on the lower slope. The rapid species accumulation occurred between depths of 677–1,000 m on both transects. More than 60% of the species collected on the two transects were encountered in the Mississippi Canyon in this depth zone (Figure 7).
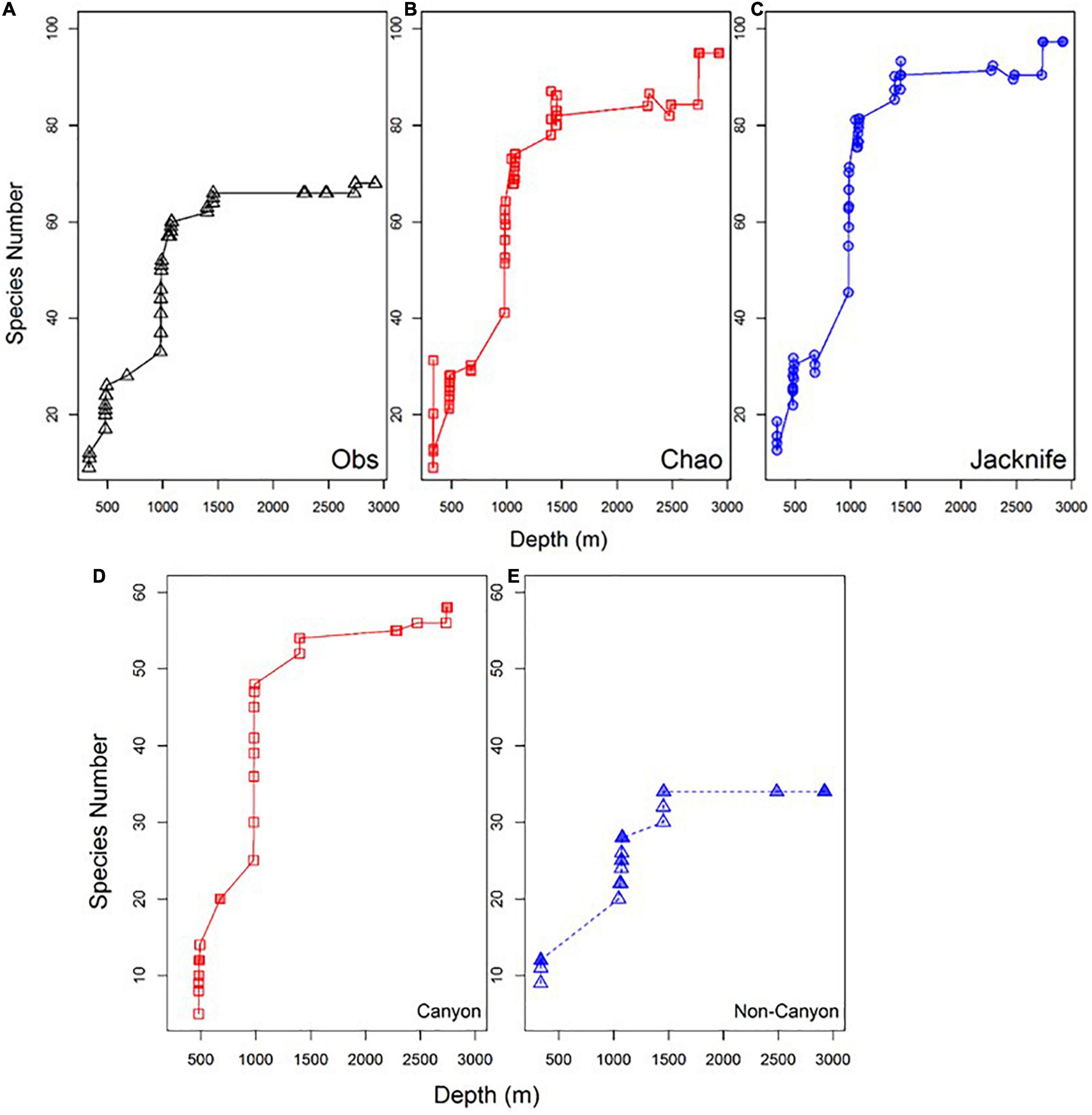
Figure 7. Observed species accumulation (Obs) (A), estimated species accumulation using Chao index (B), Jacknife index estimators (C) and comparison between the accumulations of amphipod species with depth in canyon (D) vs. non-canyon (E) transects.
The Chao and Jacknife estimator curves predicted that the number of species could be close to 100 (96 and 99 for Chao and Jacknife, respectively, Figures 7B,C) if sampling is more intensive. They also predicted that the highest replacement of species would be found between 677 and 1,401 m, with the addition of 65 species that represented about 65% of the total species. Clearly, species are added faster in the canyon than outside the canyon at intermediate depths (Figures 7D,E).
The Shannon diversity index (within habitat or alpha diversity) exhibited a prominent parabolic pattern on the canyon transect (R2 = 0.6, p < 0.001), with a less prominent pattern outside the canyon (R2 = 0.5, P = 0.001) (Figure 8). The maximum diversity for both was at about 1,100 m (Figure 8A). The Equitability was higher on the non-canyon transect than in the Mississippi Canyon (Figure 8B). There was no significant change in Equitability on the non-canyon transect with depth, while the lowest was at MT1 (Figure 8A) in the canyon head (Figure 8B). The MT1 value was extremely low due to high dominance of Ampelisca mississippiana, which represented 89% of the entire amphipod fauna. The dominance disappeared further offshore (MT2 to MT6), with the highest evenness at 1,000 m. The non-canyon transect mimicked the canyon transect, with the highest diversity at the same depth range (1,065 m); however, the non-canyon habitats had lower species diversity at the deepest depths, between 2,487 and 2,921 m (Figure 8B). Generally, the Canyon’s head had the lowest within-habitat species diversity in the whole area, while the mid depth at about 1,000 m (MT3 and C7) had the highest alpha or within-habitat diversity. Although the non-canyon central transect alpha diversity was generally higher than that in the Mississippi Canyon, a greater number of species (see above) characterized the latter.
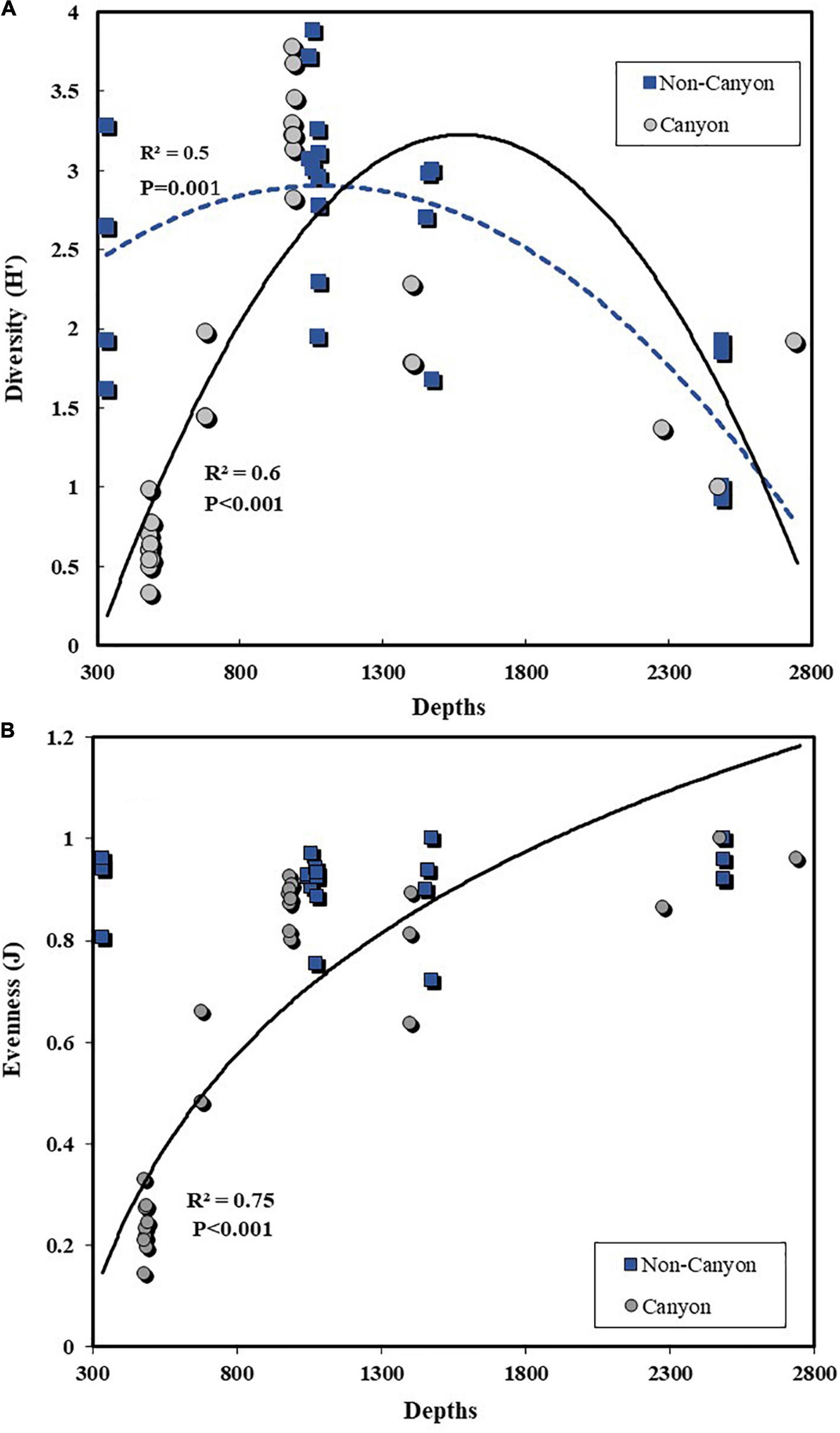
Figure 8. Variation of Shannon (alpha or within habitat) diversity (A) and evenness (B) of amphipod species on the canyon and non-canyon transects.
Amphipod Assemblages as a Function of Habitat Characteristics
Bottom water temperature across the 11 sampled locations ranged between 4.25 and 11.67°C, dissolved oxygen ranged between 2.46 and 5.54 mg/l (Supplementary Table 2). Sediment % clay ranged between 20 and 72% and % sand ranged between 2 and 64% (Supplementary Table 2). Principal Components Analysis (PCA) of an array of 22 environmental parameters has been utilized to identify the variables that explain most of the variation in density and diversity of the amphipod populations between locations (in or out of the canyon) (Supplementary Table 2). At first, the 27 metals have been reduced to four that account for most of the variance in the metals. A PCA for the 27 analyzed trace metals showed that the first principal component (PC1) accounted for about 68% of the total variance in the metals data: these were beryllium (Be), iron (Fe), chromium (Cr), and tin (Sn).
A PCA for the 22 environmental parameters showed that the three first components accounted for 80% of the total variance in the data set. Clay, the four metals (Fe, Cr, Sn, and Be) and % sand were important on the first principal component (PC1), which explained 52.7% of the variation (Figure 9). Percent sand was negatively correlated with PC1, while percent clay, the four metals (Be, Sn, Fe, and Cr) and the bottom water organics (carbon and nitrogen) were positively correlated with PC1, which can be interpreted as “organic-rich” “clay” deposits. Consequently, the high association with metals was in areas close to the head of the canyon, whereas high sand and low metals were in areas far from the canyon. These differences reflect the heterogeneity of the sediment. PC2 accounted for 13.9% of the total variance and it had high positive loading with NH4+, which may indicate high sediment community metabolism associated with high organic carbon flux (Figure 9). PC3 accounted for 9.1% of the total variance and had high positive loading by near bottom TSPM. We suggest this reflects a food source available for suspension feeders.
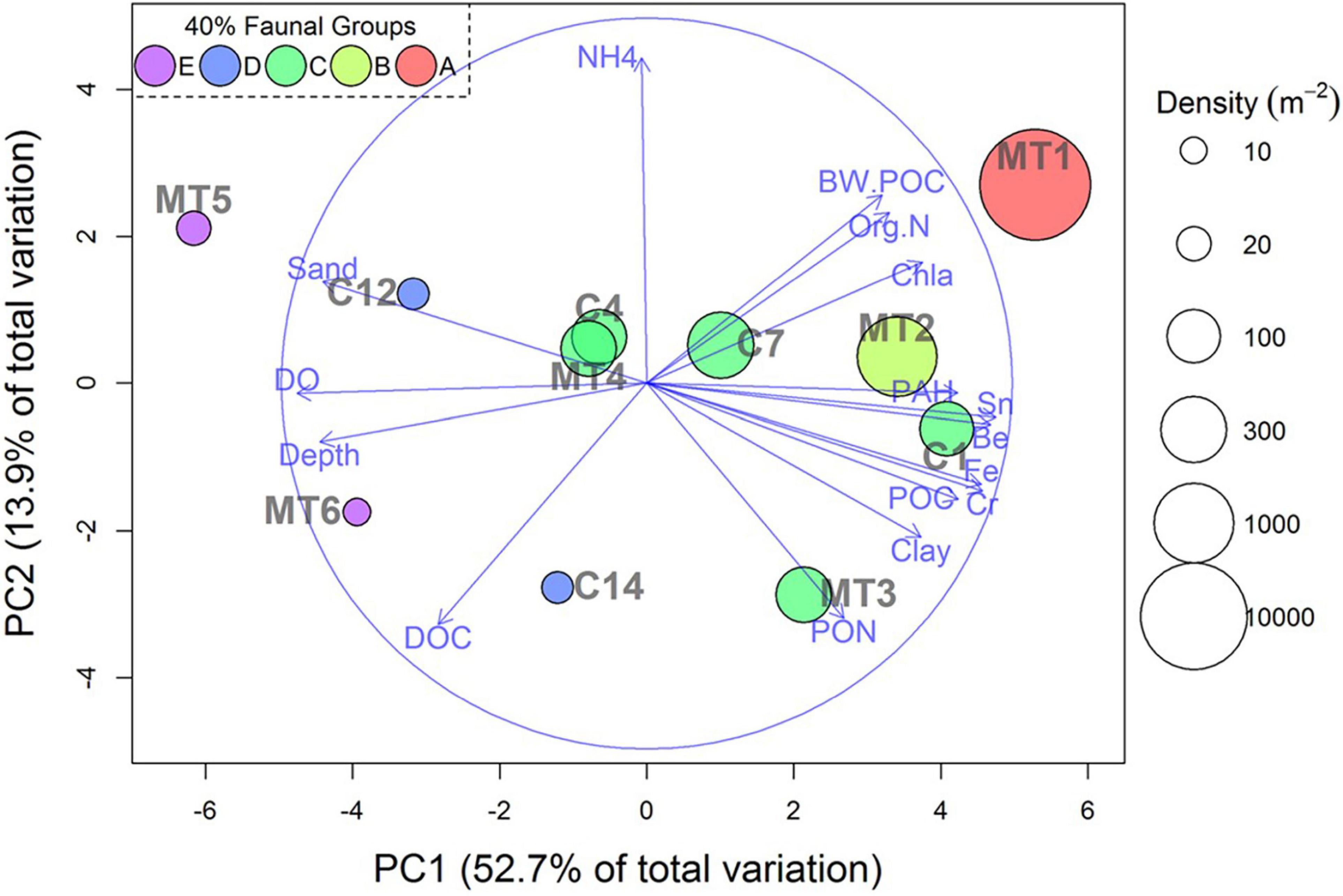
Figure 9. Principal components analysis of 22 environmental variables. Relative distances among samples show environmental ordination on the first PCA axis. Color-coding indicates the five faunal groups with at least 40% Bray-Curtis similarity among samples based on cluster analysis (Figure 5). Bubble sizes are proportional amphipod densities. Vectors are variable loading scores for PC1 vs. PC2 axis. The large circle indicates variable loading = 1.
Because of the peculiarly skewed density and diversity patterns within the canyon, PCA was also carried out with just the canyon’s environmental data. The first three components accounted for 93% of the total variance in the canyon’s data (Supplementary Table 4). Of these, PC1 accounted for 54% of the variance and had high positive loading with % clay, the metals (Fe, Cr, Sn, and Be) and near-bottom POC. It had high negative loading with sand, which we interpret to be a characteristic of enhanced flux of fine sediment-associated organic matter. PC2 accounted for 25% of the variance, with high positive loading with % organic nitrogen and high negative loading with C/N ratio, which we suggest is related to food quality. PC3 accounted for 14% of the variance and had high positive loading with near-bottom suspended materials, which indicate availability of food in suspension. A high correlation between PC3 and amphipod abundances in the canyon (R2 = 0.94, P < 0.001) suggests that high densities are affected by food supply and grain size.
A regression model predicting amphipod abundance as a function of PC1, PC2, PC3, and PC4 as predictors is significant:
Abundance was significantly correlated with the estimated flux of chlorophyll to the sea floor (R2 = 0.73, P < 0.01) (Figure 10). Abundance can also be predicted as a function of carbon flux (POC) to the sea floor. Amphipods (Ind.m–2) = 4594 × Chl a flux – 502.854 (R2 = 0.7, p < 0.001). However, combining the Chl a to the total suspended matter (TSPM) to the model enhances its power (R2 = 0.97, P < 0.001); thus the predicted abundance of amphipods can be calculated as
Where Chl a flux is the amount of chlorophyll settling to deep water sediment and TSPM is the near-bottom suspended materials.
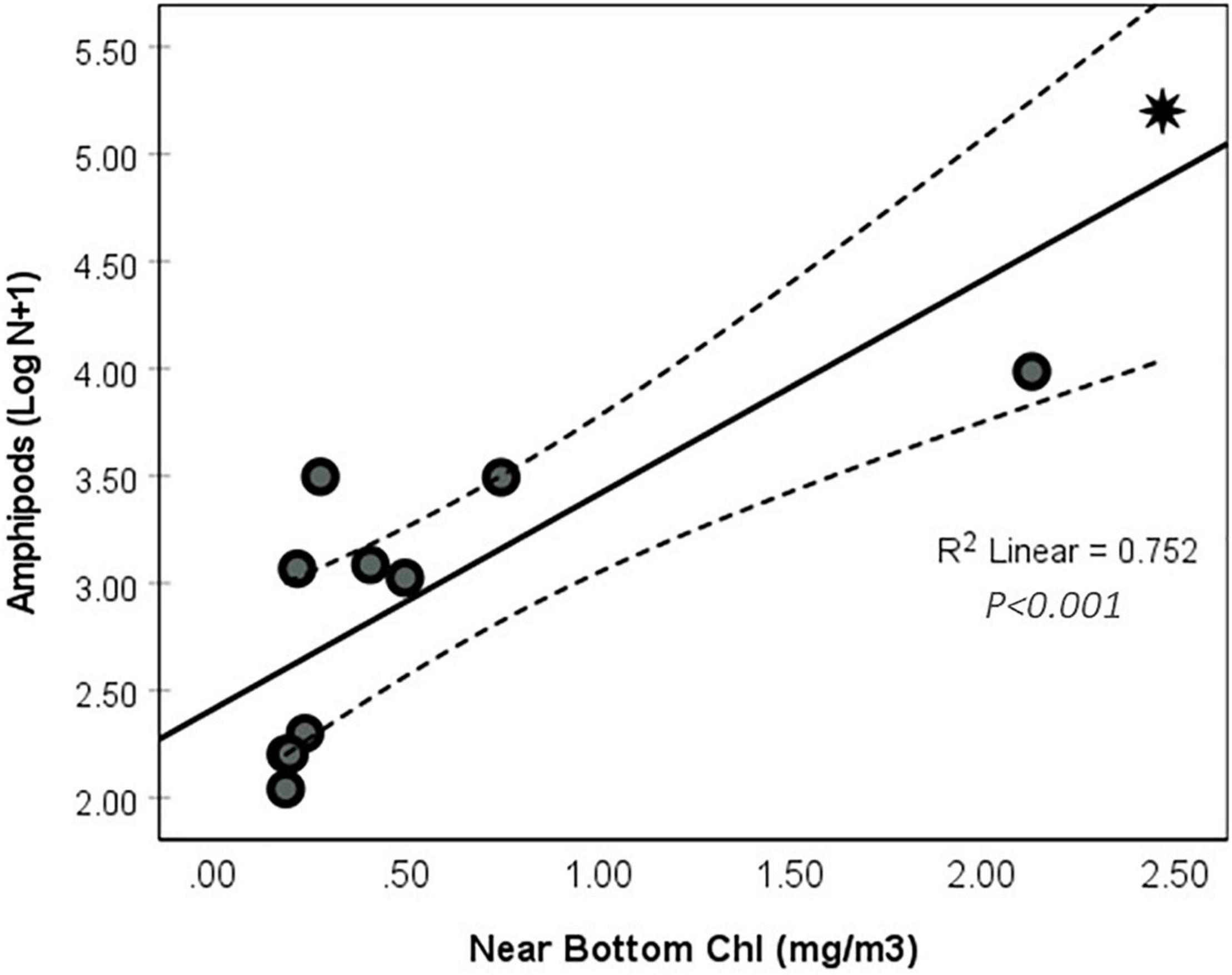
Figure 10. Abundance of amphipod Log (N + 1) as a function of the adjusted chlorophyll as proxy to the flux to the sediment (BW Chl-a) (* MT1).
Discussion
High Densities and Effects of the Canyon Habitat
Ampeliscid populations that are so dense that they “carpet” the sea floor is not unfamiliar in shallow water. However, they were unanticipated in the generally oligotrophic GoM and in deep water. The detected numbers for A. mississippiana (13,771 ± 6,087 ind.m–2) from the Mississippi canyon were similar to densities reported from shallow water (<50 m) (Soliman and Rowe, 2008). Most ampeliscid beds have been observed at high latitudes in extremely cold habitats (Conlan et al., 2018). In cold habitats (<0.5°C), the individuals can be several centimeters in length (Conlan et al., 2018); the GoM specimens were much smaller (Soliman and Rowe, 2008). Beds of tube dwelling amphipods usually occupy areas with strong bottom currents that maintains epi-benthic flow of suspended organic matter on which they feed (Demchenko et al., 2016). Mats can also be encountered at lower latitudes but in organic rich sediments, often being bathed by sewage outfalls (Rhoads et al., 1978; Gallagher and Keay, 1998). We presume that the A. mississippiana mat in the head of the canyon reflect similar conditions with an excessive input of POC, but offshore at the continental shelf margin.
The high abundances of A. mississippiana in the canyon head skewed all the measurements in the canyon habitat compared with the open slope. The densities were so high that they lower the apparent alpha diversity and evenness at the canyon head (MT1). That location by itself was an added “zone” in the depth-related zonation patterns. Without the A. mississippiana at the canyon head, the canyon would still be different because MT2 is still different from the non-canyon and the other canyon locations; the differences, however, would not be so profound.
The steep decline in benthic faunal abundance as a function of depth is a familiar paradigm that reflects the decline of the flux of labile organic matter to the ocean floor (Rowe, 1971, 1983; Rex et al., 2006; Wei et al., 2010a; Montagna et al., 2020). This trend, however, is not uniform and other dense communities are also common that are not necessarily attributed to high POC fluxes (Danovaro et al., 2003; Menot et al., 2010; Rex and Etter, 2010). Higher densities can be associated with elevated accumulation rates caused by topography or water mass (Vetter, 1994; Vetter and Dayton, 1998; Boetius et al., 2000; Brown et al., 2001; Aller et al., 2002). For example, organic carbon depo-centers can also include deep trenches (Rowe, 1983; Pusceddu et al., 2009; Wenzhöfer et al., 2016; Baudin et al., 2017). Because they often concentrate particulate organic matter, canyons are assumed to enhance benthic secondary production (Rowe et al., 1982; Gage and Tyler, 1991; McHugh et al., 1992; Gage et al., 1995; Vetter, 1998; Vetter and Dayton, 1998; Metaxas and Giffin, 2004; Soliman and Rowe, 2008). Similar depth-related patterns have been observed in previous studies on other taxa in the NGoM. For example, densities (or biomass) of macrofauna and meiofauna increased with organic matter but declined to depauperate levels at abyssal depths in the GoM (Baguley et al., 2006; Deming and Carpenter, 2008; Morse and Beazley, 2008; Rowe et al., 2008; Wilson, 2008; Stuart et al., 2016; Montagna et al., 2020). On the other hand, density of bacteria was not correlated to the sedimentary organic carbon indicating the role of other sources of labile carbon (Morse and Beazley, 2008).
Our total amphipod densities in the canyon head were similar to fauna at comparable depths off southern California (about 12,900 ind.m–2) (Vetter, 1998; Vetter and Dayton, 1998). The organic carbon within the sediment in the canyon head (MT1) was not particularly high (Supplementary Table 2), but the C/N ratio (3.6) was lower than the average for the Gulf of Mexico (C/N = 8) (Escobar-Briones and García-Villalobos, 2009), suggesting that the MT1 mud was rich in amino acids and proteins. The flux appears to be coming from vertical and lateral transport of particulate suspended matter from the Mississippi River system (Qian et al., 2003; Bianchi et al., 2006; Biggs et al., 2008). The shallow location (MT1) is thus a “hotspot” for secondary production and the resulting dominance by A. mississippiana reflects a response to high rates of detrital flux (Baguley et al., 2006; Soliman and Rowe, 2008; Conlan et al., 2015; Montagna et al., 2020). Some canyons, however, do not enhance densities (Houston and Haedrich, 1984; Marquiegui and Sorbe, 1999). Perhaps continuous disturbance such as sediment flushing or chronic impacts prevents recruitment (Rowe et al., 1982; Cartes and Sorbe, 1999; Cunha et al., 2011).
The lower slope canyon and non-canyon densities values were both low and not different; suggesting that delivery of detritus on the lower slope was not elevated either within or outside of the canyon due to distances from sources. However, the species composition in and out of the canyon were different (see below).
Amphipod Recurrent Assemblages
The more pronounced vertical, depth-related boundaries between groups of amphipod species in the Mississippi Canyon is related, we suggest, to the peculiar conditions in the canyon’s head (Group A) that supports the dominating populations of A. mississippiana: high concentrations of suspended organic matter and constant physical disturbance that would favor opportunists (Levin et al., 2001). A recent study estimated that about 70% of the sediments delivered to the Mississippi Canyon originated at the shelf from the river plume (Harris et al., 2020). A near-bottom current, storms and internal waves may maintain particles resuspension along the canyon’s floor (Ross et al., 2009; Gardner et al., 2017). Few organisms, such as tube builders, can withstand the shear of the current, which maintain available food in suspension (Demchenko et al., 2016).
The vertical zonation reflects variations in feeding strategies as resources decline with depth (Stora et al., 1999; Cartes et al., 2003; Wei and Rowe, 2006). Both the canyon and non-canyon assemblages between about 900 and 1,500 m (Group C) were characterized by the micro-predator, omnivorous Pardaliscidae and the predatory Phoxocephalidae. The Pardaliscidae are a deep-water family adapted to limited food and extended periods of starvation, with mouthparts that suggest they are predators (Tomikawa et al., 2021). Pardaliscids are powerful swimmers with piercing mouthparts (Lo Brutto et al., 2017). Phoxocephalids are a diverse amphipod family, with 13 genera adapted for burrowing (De Broyer et al., 2003; Chapman, 2007). They prey on meiofauna, polychaete larvae and nematodes (Guerra-García et al., 2014). Phoxocephalids occupied the widest depth range on the two transects because, we suggest, they are able to cope with variations in potential food that would limit suspension and deposit feeders.
The Melitidae and Aoridae are deposit feeders (Guerra-García et al., 2014) in the assemblage at intermediate depths. The deepest amphipod assemblages (Groups C and D on Figure 5) overlapped with the abundant tubicolous ampeliscid, Haploops sp., considered to be a deep-water genus with a wide biogeographic distribution (Bellan-Santini and Dauvin, 1997).
The burrowing phoxocephalids were absent in the trough-like canyon head because the highly variable habitat promoted dominance by the tube builder A. mississippiana. The dense numbers of tubicolous species are known to impede movement of mobile burrowing species (Wilson, 1991), and reduce the abundance of intermediate sized species (see Volkenborn et al., 2009).
The burrowing deposit feeders in the habitats we studied survive at mid-depths with diminished resources. The abrupt displacement on the upper continental slope compared with the lower slope was also observed in bivalves (Wei et al., 2020). The Eurisidae and Pardaliscidae are predominately supra-benthic, mobile predators (Cartes and Sorbe, 1999; Nyssen et al., 2002) that were present only at the lower slope depths along the non-canyon transect.
Alpha and Gamma Diversity Patterns
Offshore of the mid-depth species maximum (at 1,100 m) the diversity declined because fewer species can survive as food supplies dwindle (Rex, 1973), but the decline was not as steep. The mid-depth maximum of within-habitat diversity at 1,100 m depth has also been observed in other invertebrates in the GoM including Isopoda, Polychaeta, and bivalves (Wilson, 2008; Carvalho et al., 2013; Wei and Rowe, 2019; Wei et al., 2020), reinforcing our view that it is related to productivity gradients (Woolley et al., 2016).
This mid-depth maximum (MDM) has now been observed on a number of other continental margins (Rex, 1981, 1983; Allen and Sanders, 1996; Rex et al., 1997; Maciolek and Smith, 2009; Rex and Etter, 2010). The gulf MDM was more prominent in the canyon than on the central transect because of the dominance by A. mississippiana in the canyon head. Disturbances in the canyon head due to increased sedimentation, flushing events or due to chronic inputs of contaminants can reduce species richness and depress biodiversity (Wilson, 2008). The high dominance and low diversity may imply that the canyon head experiences stress. Ampelisca species is listed among the tolerant species that can survive in organically enriched environments and dense ampeliscid mats have been found associated with chronic anthropogenic disturbance (Rhoads et al., 1978; Alcolado, 1992; Paterson and Lambshead, 1995; Gage, 1997; Gardner et al., 2017). The extent to which a dense number of benthos-built tubes stabilize the muddy sediments and the structure of benthic communities has been contradictory. Tube-builders can stabilize sediment with enhanced binding by mucus secreted by associated organisms such as bacteria, whereas other species may destabilize the sediment (Eckman et al., 1981). The tubes enable them to obtain oxygen above the water-sediment interface protection against inward diffusion of toxic anions (Aller, 1982; Gallagher and Keay, 1998). The highest concentrations of polycyclic aromatic hydrocarbons (PAHs) in our sediment samples, as well as other anthropogenic trace metals, were in the canyon head and thus coincident with the dense A. mississippiana mats (Soliman and Wade, 2008; Wade et al., 2008). We attribute the high metals concentration in the sediments of the Mississippi Canyon to sediment fluxes originated from the shelf. However, levels of metals were within the ranges reported in the shelf of the NGoM (Wade et al., 2008).
Maximum species richness (gamma diversity) at about 1,100 m depth was comparable to that reported by to Mediterranean amphipods (Cartes and Sorbe, 1999), but somewhat shallower than that observed on other continental margins characterized by higher primary production. We suspect that the MDM is pushed deeper and further offshore as flux to the sea floor increased [as implied by Wei and Rowe (2019)].
We attribute the higher species richness in the canyon to the complexity of the canyon topography compared to the slope on the central transect (Figure 1). The canyon may also be subjected to intermittent mass wasting of sediment down the canyon axis. Santschi and Rowe (2008) estimated that sediment burial rates at MT3 (ca. 1,000 m depth) were about the same as those at MT6 (ca. 2.7 km depth), but the mean age of the sediments at the shallower canyon location was about 3–5 thousand years compared to about 16–27 thousand years at the deepest canyon site (MT6 at 2,700 m depth). The latter was a mixture of marine and terrestrial organic matter, which again implies that MT6 is subject to mass wasting events.
Nestedness, where the deep faunal species composition is a subset of shallow species, on the other hand, a condition documented for the polychaetes on the oligotrophic northwestern GoM margin (Stuart et al., 2016), was not observed in the amphipods. The deep amphipod species, on the other hand, were entirely different from the shallow assemblage, not just a winnowing portion of the species living in shallow habitats. We would attribute this difference to amphipods’ reproductive pattern, which lacks dispersive larval stages, and brooding relatively small numbers of eggs, as opposed to broadcasting large numbers, as is more common in the polychaete annelids.
Conclusion
The deep Mississippi submarine canyon harbored dense amphipods assemblages. Amphipod species richness and abundances in the canyon were significantly higher than the non-canyon slope. A dense mat of tube dwelling ampeliscids (A. mississippiana) were numerically (4,446–26,933 ind/m2) dominant in the trough-like head of the canyon. This dominance by filter feeders (A. mississippiana) was supported by high concentrations of particulate organic carbon in suspension from the adjacent continental shelf below the Mississippi River plume. A steep decline in abundance as a function of depth reflects a decline in sources of organic matter to the deep ocean floor, both within and outside the canyon. The observed unimodal alpha (within habitat) diversity at a depth of ca. 1,100 m support the widely recognized paradigm of mid-depth maximum (MDM) species richness. The canyon MDM was more pronounced than the MDM on the slope outside the canyon. The dominance by A. mississippiana depressed the alpha diversity value at the near-shore canyon head. Four groups of species were identified at specific depth intervals down the canyon axis. Functional feeding groups changed with depth in response to declining resources and sediment heterogeneity. The deeper canyon and the non-canyon slope included motile predators in low densities. Low nestedness is attributed to the lack of dispersive larval stages, and brooding relatively small numbers of eggs. The high species richness in the canyon suggests that the Mississippi Canyon enriches the regional species diversity. The observed low amphipod diversity on the slope might reflect a need for improved sampling coverage in future studies. Further studies to understand the dynamic of the ampeliscid mat and its role in the fish production and other ecological services is important.
Data Availability Statement
The original contributions presented in the study are included in the article/Supplementary Material, further inquiries can be directed to the corresponding author.
Author Contributions
YS: conceptualization, formal analysis, investigation, visualization, data analysis, writing – original draft, and writing– review and editing. GR: conceptualization, fund acquisition, and writing-review and editing. MW: resources, investigation, and writing – review and editing. C-LW: visualization, data analysis, and writing – review and editing. All authors contributed to the article and approved the submitted version.
Funding
DGoMB study was funded by the U.S. Department of Interior, Minerals Management Service (Contract 30991), now Bureau of Ocean Energy Management (BOEM). The taxonomic and data analysis were partly supported by the Egyptian Ministry of Higher Education through a scholarship to YS.
Conflict of Interest
The authors declare that the research was conducted in the absence of any commercial or financial relationships that could be construed as a potential conflict of interest.
Publisher’s Note
All claims expressed in this article are solely those of the authors and do not necessarily represent those of their affiliated organizations, or those of the publisher, the editors and the reviewers. Any product that may be evaluated in this article, or claim that may be made by its manufacturer, is not guaranteed or endorsed by the publisher.
Supplementary Material
The Supplementary Material for this article can be found online at: https://www.frontiersin.org/articles/10.3389/fmars.2022.822924/full#supplementary-material
References
Ahrens, W., Cox, D., and Budhwar, G. (1990). Use of the Arcsine and Square Root Transformations for Subjectively Determined Percentage Data. Weed Sci. 38, 452–458. doi: 10.1017/S0043174500056824
Akaike, H. (1973). “Information theory and an extension of the maximum likelihood principle,” in Proceedings, 2nd international symposium of information theory, eds B. N. Petrov and F. Caski (Budapest: Akademiai Kiado), 267–281.
Alcolado, M. P. (1992). Sobre la interpretación del ambiente marino mediante el empleo de los índices de diversidad y equitatividad. Cienias Biólógicas 24, 124–127.
Allen, J. A., and Sanders, H. L. (1996). The zoogeography, diversity and origin of the deep-sea protobranch bivalves of the Atlantic: the epilogue. Prog. Oceanogr. 38, 95–153. doi: 10.1016/s0079-6611(96)00011-0
Aller, J. Y., Aller, R. C., and Green, M. A. (2002). Benthic faunal assemblages and carbon supply along the continental shelf/shelf break-slope off Cape Hatteras, North Carolina. Deep-Sea Res. II 49, 4599–4625. doi: 10.1016/S0967-0645(02)00131-5
Aller, R. C. (1982). “The effects of macrobenthos on chemical properties of marine sediment and overlying water,” in Animal-sediment Relations, eds P. L. McCall and M. J. S. Tevesz (New York, NY: Plenum Press), 53–102. doi: 10.1007/978-1-4757-1317-6_2
Baguley, J. G., Montagna, P. A., Hyde, L. J., Kalke, R. D., and Rowe, G. T. (2006). Metazoan meiofauna abundance in relation to environmental variables in the northern Gulf of Mexico deep sea. Deep Sea Res. Part I 53, 1344–1362. doi: 10.1016/j.dsr.2006.05.012
Balsam, W. L., and Beeson, J. P. (2003). Sea-floor sediment distribution in the Gulf of Mexico. Deep-Sea Res. 50, 1421–1444. doi: 10.1016/j.dsr.2003.06.001
Barnard, J. L. (1954a). Amphipoda of the family Ampeliscidae collected by the Velero III in the Caribbean Sea. Allan Hancock Atlant. Exp. Rep. 7, 1–13.
Barnard, J. L. (1954b). Amphipoda of the family Ampeliscidae collected in the eastern Pacific Ocean by the Velero III and Velero IV. Allan Hancock Pacif. Exp. Rep. 18, 1–137.
Barnard, J. L. (1960a). New bathyal and sublittoral ampeliscid amphipods from California, with an illustrated key to Ampelisca. Pacific Natur. 1, 1–36.
Barnard, J. L. (1960b). The amphipod family Phoxocephalidae in the eastern Pacific Ocean, with analyses of other species and notes for a revision of the family. Allan Hancock Pacif. Exp. 18, 175–368.
Barnard, J. L. (1961). Gammaridean Amphipoda from depths of 400 to 6000 metres. Galat. Rep. 5, 23–128.
Barnard, J. L. (1962). Benthic marine Amphipoda of southern California: families Aoridae, Photidae, Ischyroceridae, Corophiidae, Podoceridae. Pacif. Natur. 3, 1–72. doi: 10.3897/zookeys.193.3109
Barnard, J. L. (1964). Deep-sea Amphipoda (Crustacea) collected by the R.V. Vema in the eastern Pacific Ocean and the Caribbean and Mediterranean seas. Bull. Am. Mus. Nat. Hist. 127, 1–46.
Barnard, J. L. (1966). Submarine canyons of southern California. Part V, systematics: Amphipoda. Allan Hancock Pacif. Exp. 27, 1–166.
Barnard, J. L. (1969). The families and genera of marine gammaridean Amphipoda. U S Natl. Mus. Bull. 271, 1–535. doi: 10.3853/j.0812-7387.13.1991.91
Barnard, J. L. (1971). Gammaridean amphipods from a deep-sea transect off Oregon. Smith. Contr. Zool. 61, 1–86. doi: 10.5479/si.00810282.61
Baselga, A. (2010). Partitioning the turnover and nestedness components of beta diversity. Glob. Biogeogr. 19, 134–143. doi: 10.1111/j.1466-8238.2009.00490.x
Baudin, F., Martinez, P., Dennielou, B., Charlier, K., Marsset, T., Droz, L., et al. (2017). Organic carbon accumulation in modern sediments of the Angola basin influenced by the Congo deep-sea fan. Deep-Sea Res. II 142, 64–74. doi: 10.1016/j.dsr2.2017.01.009
Bellan-Santini, D., and Dauvin, J. C. (1997). Ampeliscidae (Amphipoda) from Iceland with a description of a new species (contribution to the BIOICE research programme). J. Nat. Hist. 31, 1157–1173. doi: 10.1080/00222939700770621
Bernhardt, A., Melnick, D., Jara-Muñoz, J., Argandoña, B., González, J., and Strecker, M. R. (2015). Controls on submarine canyon activity during sea-level highstands: The Biobío canyon system offshore Chile. Geosphere 11, 1226–1255. doi: 10.1130/GES01063.1
Bianchi, T. S., Allison, M. A., Canuel, E. A., Corbett, D. R., McKee, B. A., Sampere, T. P., et al. (2006). Rapid export of organic matter to the Mississippi canyon. Mississ. Trans. Am. Geophys. 87, 572–574. doi: 10.1029/2006EO500002
Biggs, D. C., Hu, C., and Muller-Karger, F. E. (2008). Remotely sensed sea-surface chlorophyll and POC flux at deep Gulf of Mexico benthos sampling stations. Deep Sea Res. Part II 55, 2555–2562. doi: 10.1016/j.dsr2.2008.07.013
Blankenship, L. E., Yayanos, A. A., Cadien, D. B., and Levin, L. A. (2006). Vertical zonation patterns of scavenging amphipods from the Hadal zone of the Tonga and Kermadec Trenches. Deep Sea Res. Part I 53, 48–61. doi: 10.1016/j.dsr.2005.09.006
Boetius, A., Ferdelman, T., and Lochte, K. (2000). Bacterial activity in sediments of the deep Arabian Sea in relation to vertical flux. Deep-Sea Res. II 47, 2835–2875. doi: 10.1016/S0967-0645(00)00051-5
Boland, G. S., and Rowe, G. T. (1991). Deep-sea benthic sampling with the GOMEX box corer. Limnol. Oceanogr. 36, 1015–1020. doi: 10.4319/lo.1991.36.5.1015
Borcard, D., Gillet, F., and Legendre, P. (2018). Numerical Ecology with R, Use R!. Cham: Springer International Publishing, doi: 10.1007/978-3-319-71404-2_1
Brooks, J. M., Kennicutt, M. C. II, Fisher, C. R., Macko, S. A., Cole, K., Childress, J. J., et al. (1987). Deep-sea hydrocarbon seep communities: evidence for energy and nutritional carbon sources. Science 238, 1138–1142. doi: 10.1126/science.238.4830.1138
Brown, C. J., Lambshead, P. J. D., Smith, C. R., Hawkins, L. E., and Farley, R. (2001). Phytodetritus and the abundance and biomass of abyssal nematodes in the central, equatorial Pacific. Deep Sea Res. Part I 48, 555–565. doi: 10.1016/S0967-0637(00)00049-2
Burnham, K. P., and Overton, W. S. (1979). Robust estimation of population size when capture probabilities vary among animals. Ecology 60, 927–936. doi: 10.1111/j.1541-0420.2007.00779.x
Cartes, J. E., Jaume, D., and Madurell, T. (2003). Local changes in the composition and community structure of suprabenthic peracarid crustaceans on the bathyal Mediterranean: influence of environmental factors. Mar. Biol. 2003, 745–758. doi: 10.1007/s00227-003-1090-z
Cartes, J. E., and Sorbe, J. C. (1999). Estimating secondary production in bathyal suprabenthic peracarid crustaceans from the Catalan Sea slope (western Mediterranean; 391– 1255 m). J. Exp. Mar. Biol. Ecol. 239, 195–210. doi: 10.1016/s0022-0981(99)00026-x
Carvalho, R., Wei, C.-L., Rowe, G., and Schulze, A. (2013). Complex depth-related patterns in taxonomic and functional diversity of polychaetes in the Gulf of Mexico. Deep Sea Res. Part I 80, 66–77. doi: 10.1016/j.dsr.2013.07.002
Chao, A. (1987). Estimating the population size for capture-recapture data with unequal catchability. Biometrics 43, 783–791. doi: 10.2307/2531532
Chapman, J. W. (2007). Amphipoda: Chapter 39 of The Light and Smith Manual: Intertidal Invertebrates From Central California to Oregon. Fourth Edition, Completely Revised and Expanded. Oakland: UC Press.
Clarke, K. R., and Warwick, R. M. (2001). Change in marine communities: an approach to statistical analysis and interpretation, 2nd Edn. Plymouth: PRIMER-E.
Conlan, K., Hendrycks, E., and Aitken, A. (2018). Dense ampeliscid bed on the Canadian Beaufort shelf: An explanation for species patterns. Polar Biol. 42, 195–215. doi: 10.1007/s00300-018-2417-z
Conlan, K. E., Currie, D. R., Dittmann, S., Sorokin, S. J., and Hendrycks, E. (2015). Macrofaunal Patterns in and around du Couedic and Bonney Submarine Canyons, South Australia. PLoS One 10:e0143921. doi: 10.1371/journal.pone.0143921
Cordes, E. E., Bergquist, D. C., and Fisher, C. R. (2009). Macro-ecology of Gulf of Mexico cold seeps. Ann. Rev. Mar. Sci. 1, 143–168. doi: 10.1146/annurev.marine.010908.163912
Cunha, M. R., Paterson, G. L. J., Amaro, T., Blackbird, S., de Stigter, H. C., Ferreira, C., et al. (2011). Biodiversity of macrofaunal assemblages from three Portuguese submarine canyons (NE Atlantic). Deep-Sea Res. II 58, 2433–2447. doi: 10.1016/j.dsr2.2011.04.007
Danovaro, R., Dell Croce, N., Dell Anno, A., and Pusceddu, A. (2003). A depocenter of organic matter at 7800 m depth in the SE Pacific Ocean. Deep-Sea Res. I 50, 1411–1420. doi: 10.1016/j.dsr.2003.07.001
Davis, R. A. (2017). “Sediments of the Gulf of Mexico,” in Habitats and Biota of the Gulf of Mexico: Before the Deep Water Horizon Oil Spill, ed. H. Ward (New York, NY: Springer), 165–215. doi: 10.1007/978-1-4939-3447-8_3
De Broyer, C., Jazdzewski, K., and Dauby, P. (2003). “Biodiversity in the Southern Ocean: lessons from Crustacea,” in Antarctic biology in a global context, eds A. H. L. Huiskes, W. W. C. Gieskes, J. Rozema, R. M. L. Schorno, S. M. van der Vies, and W. J. Wolff (Leiden: Backhuys Publishers).
Demchenko, N. L., Chapman, J. W., Durkina, V. B., and Fadeev, V. I. (2016). Life History and Production of the Western Gray Whale’s Prey, Ampelisca eschrichtii Krøyer, 1842 (Amphipoda, Ampeliscidae). PLoS One 11:e0147304. doi: 10.1371/journal.pone.0147304
Deming, J., and Carpenter, S. (2008). Factors influencing benthic bacterial abundance, biomass and activity on the northern continental margin and deep basin of the Gulf of Mexico. Deep-Sea Res. II 2008:9. doi: 10.1016/j.dsr2.2008.07.009
Denoux, G., Gardinali, P., and Wade, T. L. (1998). Quantitative determination of polynuclear aromatic hydrocarbons by gas chromatography/mass spectrometry—selected ion monitoring (SIM) mode. in: Sampling and Analytical Methods of the National Status and Trends Program, Mussel Watch Project: 1993–1996 Update, Vol. 130. Washington, D.C.: NOAA Technical Memorandum NOS ORCA, 129–139.
Dickinson, J. J., and Carey, A. G. (1978). Distribution of gammarid Amphipoda (Crustacea) on Cascadia Abyssal Plain (Oregon). Deep-Sea Res. I 25, 97–106. doi: 10.1016/s0146-6291(21)00008-4
Dorschel, B., Gutt, J., Piepenburg, D., Schröder, M., and Arndt, J.-E. (2014). The influence of the geo-morphological and sedimentological settings on the distribution of epibenthic assemblages on a flat topped hill on the over-deepened shelf of the western Weddell Sea. Biogeosciences 11, 3797–3817. doi: 10.5194/bg-11-3797-2014
Duffy, G., Gutteridge, Z., Thurston, M., and Horton, T. (2016). A comparative analysis of canyon and non-canyon populations of the deep-sea scavenging amphipod Paralicella caperesca. J. Mar. Biol. Assoc. U K 96, 1687–1699. doi: 10.1017/S0025315415002064
Duineveld, G., Lavaleye, M., Berghuis, E., and de Wilde, P. (2001). Activity and composition of the benthic fauna in the Whittard Canyon and the adjacent continental slope (NE Atlantic). Oceanol. Acta 24, 69–83. doi: 10.1016/s0399-1784(00)01129-4
Eckman, J. E., Nowel, A. R. M., and Jumars, P. A. (1981). Sediment destabilization by animal tubes. J. Mar. Res. 39, 361–374.
Ellwood, B. B., Balsam, W. L., and Roberts, H. H. (2006). Gulf of Mexico sediment sources and sediment transport trends from magnetic susceptibility measurements of surface samples. Mar. Geol. 230, 237–248. doi: 10.1016/j.margeo.2006.05.008
Escobar-Briones, E., and García-Villalobos, F. (2009). Distribution of total organic carbon and total nitrogen in deep-sea sediments from the southwestern Gulf of Mexico. Boletin de la Sociedad Geologica Mexicana 61, 73–86. doi: 10.18268/bsgm2009v61n1a7
Escobar-Briones, E., and Winfield, I. (2003). Checklist of the Benthic Gammaridea and Caprellidea (Crustacea: Peracarida: Amphipoda) from the Gulf of Mexico Continental Shelf and Slope. Belg. J. Zool. 133, 37–44.
Fernandez-Arcaya, U., Ramirez-Llodra, E., Aguzzi, J., Allcock, A. L., Davies, J. S., Dissanayake, A., et al. (2017). Ecological Role of Submarine Canyons and Need for Canyon Conservation, 2017. Front. Mar. Sci. 4:5. doi: 10.3389/fmars.2017.00005
Fisher, C., Robers, H., Cordes, E., and Bernard, B. (2007). Cold seeps and associated communities of the Gulf of Mexico. Oceanography 20:4. doi: 10.5670/oceanog.2007.12
Gage, J. D. (1997). “High benthic species diversity in deep-sea sediments: the importance of hydrodynamics,” in Marine Biodiversity, eds R. F. G. Ormond, J. D. Gage, and M. V. Angel (Cambridge, MA: Cambridge University Press), 148–177. doi: 10.1017/cbo9780511752360.008
Gage, J. D., Lamont, P. A., and Tyler, P. A. (1995). Deep-sea macrobenthic community at contrasting sites off Portugal, preliminary results: I. Introduction and diversity comparisons. Internationale Revue der gesamten. Hydrobiologie 80, 235–250. doi: 10.1002/iroh.19950800211
Gage, J. D., and Tyler, P. A. (1991). Deep-sea biology: A natural history of organisms at the deep-sea floor. Cambridge, MA: Cambridge University Press. doi: 10.1017/CBO9781139163637
Gallagher, E. D., and Keay, K. K. (1998). “Organism-sediment-contaminant interactions in Boston Harbor,” in Contaminated Sediments in Boston Harbor, eds K. D. Stolzenbach and E. E. Adams (Cambridge, MA: MIT Sea Grant Press), 89–132.
Gallaway, B. J., Cole, J. G., and Fechhelm, R. G. (2003). Selected aspects of the ecology of the Continental Slope Fauna of the Gulf of Mexico: A Synopsis of the Northern Gulf of Mexico Continental Slope Study, 1983-1988. OCS Study MMS 2003-072. New Orleans, LA: USDOI/MMS, Gulf of Mexico OCS Region, 44.
Gardner, W. D., Tucholke, B. E., Richardson, M. J., and Biscaye, P. E. (2017). Benthic storms, nepheloid layers, and linkage with upper ocean dynamics in the western North Atlantic. Mar. Geol. 385, 304–327. doi: 10.1016/j.margeo.2016.12.012
Goodwin, R. H., and Prior, D. B. (1989). Geometry and depositional sequences of the Mississippi canyon, Gulf of Mexico. J. Sed. Res. 59, 318–329. doi: 10.1306/212F8F85-2B24-11D7-8648000102C1865D
Guerra-García, J. M., de Figueroa, T., de Figueroa, J. T., Navarro-Barranco, C., Navarro-Barranco, C., Ros, M. R., et al. (2014). Dietary analysis of the marine Amphipoda (Crustacea: Peracarida) from the Iberian Peninsula. J. Sea Res. 85, 508–517. doi: 10.1016/j.seares.2013.08.006
Gunton, L. M., Gooday, A. J., Glover, A. G., and Bett, B. J. (2015). Macrofaunal abundance and community composition at lower bathyal depths in different branches of the Whittard Canyon and on the adjacent slope (3500 m; NE Atlantic). Deep Sea Res. Part I 97, 29–39. doi: 10.1016/j.dsr.2014.11.010
Harris, C. K., Syvitski, J., Arango, H. G., Meiburg, E. H., Cohen, S., Jenkins, C. J., et al. (2020). Data-Driven, Multi-Model Workflow Suggests Strong Influence from Hurricanes on the Generation of Turbidity Currents in the Gulf of Mexico. J. Mar. Sci. Eng. 8:586. doi: 10.3390/jmse8080586
Houston, K. A., and Haedrich, R. L. (1984). Abundance and biomass of macrobenthos in the vicinity of Carson Canyon, northwest Atlantic ocean. Mar. Biol. 82, 301–305. doi: 10.1007/bf00392410
Levin, L. A., Etter, R. J., Rex, M. A., Gooday, A. J., Smith, C. R., Pineda, J., et al. (2001). Environmental influences on regional deepsea species diversity. Ann. Rev. Ecol. Syst. 132, 51–93. doi: 10.1146/annurev.ecolsys.32.081501.114002
Liao, I., Chen, G., Chiou, M., Jan, S., and Wei, C. (2017). Internal tides affect benthic community structure in an energetic submarine canyon off SW Taiwan. Deep Sea Res. Part I 125, 147–160. doi: 10.1016/j.dsr.2017.05.014
Lincoln, R. (1979). British Marine Amphipoda: Gammaridea, British Museum. London: Natural History, 657.
Lo Brutto, S., Schimmenti1, E., and Iaciofano, D. (2017). The 17th International Colloquium on Amphipoda. Biodiv. J. 8:2.
Maciolek, N. J., and Smith, W. K. (2009). Benthic species diversity along a depth gradient: Boston Harbor to Lydonia canyon. Deep Sea Res. Part II 56, 1763–1774. doi: 10.1016/j.dsr2.2009.05.031
Malm, A. W. (1870). Om tva for Vetenskapen nya Amfipod-Species fran Bohuslan, af Hvilka det Ena ar Typ for ett nytt Genus inom Pontoporeinernas Grupp. Ofversigt af Kongliga Vetenskaps-Akademiens Forhandlingar 27, 543–548.
Marquiegui, M. A., and Sorbe, J. C. (1999). Influence of near-bottom environmental conditions on the structure of bathyal macrobenthic crustacean assemblages from the Cap Breton canyon (Bay of Biscay, NE Atlantic). Acta Oecologica 20, 353–362. doi: 10.1016/s1146-609x(99)00131-9
Martin, R. G., and Bouma, A. H. (1978). “Physiography of Gulf of Mexico,” in Framework, Facies, and Oil Trapping Characteristics of the Upper Continental Margin, Vol. 7, eds A. H. Bouma, G. T. Moore, and J. M. Coleman (Tulsa, OK: American Association of Petroleum Geologists Studies in Geology), 3–19.
Maurer, D., Diener, D., Robertson, G., and Gerlinger, T. (1994). Comparison of community structure of soft-bottom macrobenthos of the Newport Submarine Canyon, California and adjoining shelf. Internat. Revue der Gesamten Hydrobiol. Hydr. 79, 519–603. doi: 10.1002/iroh.19940790409
McAleece, N., Gage, J. D. G., Lambshead, P. J. D., and Paterson, G. L. J. (1997). “Biodiversity professional statistics analysis software,” in Jointly Developed by the Scottish Association for Marine Science and the Natural History Museum London.
McHugh, C. M., Ryan, W. B. F., and Hecker, B. (1992). Contemporary sedimentary processes in the Monterey Canyon-fan system. Mar. Geol. 107, 35–50. doi: 10.1016/0025-3227(92)90067-R
Menot, L., Galeron, J., Olu, K., Caprais, J.-C., Crassous, P., Khripounoff, A., et al. (2010). Spatial heterogeneity of macrofaunal communities in and near a giant pockmark area in the deep Gulf of Guinea. Mar. Ecol. Evol. Perspect. 31, 78–93. doi: 10.1111/j.1439-0485.2009.00340.x
Metaxas, A., and Giffin, B. (2004). Dense beds of the ophiuroid Ophiacantha abyssicola on the continental slope off Nova Scotia, Canada. Deep-Sea Res. Part I 51, 1307–1317. doi: 10.1016/j.dsr.2004.06.001
Mills, E. (1963). A new species of Ampelisca (Crustacea: Amphipoda) from eastern North America, with notes on other species in the genus. Can. J. Zool. 41, 971–989. doi: 10.1139/z63-073
Mills, E. (1964). Ampelisca abdita, a new amphipod crustacean from eastern North America. Can. J. Zool. 42, 559–575. doi: 10.1139/z64-049
Mills, E. (1965). The zoogeography of North Atlantic and North Pacific ampeliscid amphipod crustaceans. Syst. Zool. 14, 119–130. doi: 10.2307/2411735
Mills, E. (1967a). The biology of an amphipod ampeliscid crustacean sibling species pair. J. Fish. Res. Board Can. 24, 305–355. doi: 10.1139/f67-030
Mills, E. (1967b). A reexamination of some species of Ampelisca (Crustacea: Amphipoda) from the east coast of North America. Can. J. Zool. 45, 635–652. doi: 10.1139/z67-080
Mills, E. (1971). Deep-sea Amphipoda from the western North Atlantic Ocean. The family Ampeliscidae. Limnol. Oceanogr. 16, 357–386.
Montagna, P., Baguley, J., Reuscher, M., Rowe, G., and Wade, T. (2020). Linking abiotic variables with macrofaunal and meiofaunal abundance and community structure patterns on the Gulf of Mexico continental margin. Scen. Resp. Fut. Deep Oil Spills 2020:7. doi: 10.1007/978-3-030-12963-7
Morse, J. W., and Beazley, M. J. (2008). Organic matter in deep water sediments of the Northern Gulf of Mexico and its relationship to the distribution of benthic organisms. Deep Sea Res. II 55, 2563–2571. doi: 10.1016/j.dsr2.2008.07.004
Nyssen, F., Brey, T., Lepoint, G., Bouquegneau, J., De Broyer, C., and Dauby, P. (2002). A stable isotope approach to the eastern Weddell Sea trophic web: focus on benthic amphipods. Polar Biol. 25, 280–287. doi: 10.1007/s00300-001-0340-0
Ohta, S. (1983). Photographic census of large-sized benthic organisms in the bathyal zone of Suruga Bay, central Japan. Bull. Ocean Res. Inst. 15:244.
Oliver, J., Hammerstrom, K., Kuhnz, L., Slattery, P., Oakden, J., and Kim, S. (2020). Benthic invertebrate communities in the continental margin sediments of the Monterey Bay area. Deep-Sea Pycnog. Crust. Am. 2020:8. doi: 10.1007/978-3-58410-8_8
Paterson, G. L. J., and Lambshead, P. J. D. (1995). Bathymetric patterns of polychaete diversity in the Rockall Trough, north-east Atlantic. Deep-Sea Res. 42, 1199–1214. doi: 10.1016/0967-0637(95)00041-4
Pechenik, J. A. (1999). On the advantages and disadvantages of larval stages in benthic marine invertebrate life cycles. Mar. Ecol. Prog. Ser. 177, 269–297. doi: 10.1016/bs.amb.2015.07.001
Pequegnat, W. E. (1983). The ecological communities of the continental slope and adjacent regimes of the northern Gulf of Mexico. Final report to the U.S. Dept. of the Interior, Minerals Management Service. New Orleans, LA: Gulf of Mexico OCS Region.
Pequegnat, W. E., Gallaway, B. J., and Pequegnat, L. H. (1990). Aspects of the ecology of the deep-water fauna of the Gulf of Mexico. Am. Zool. 30, 45–64. doi: 10.1093/icb/30.1.45
Puig, P., Palanques, A., and Martín, J. (2014). Contemporary sediment-transport processes in submarine canyons. Annu. Rev. Mar. Sci. 6:53. doi: 10.1146/annurev-marine-010213-135037
Pusceddu, A., Bianchelli, S., Canals, M., Sanchez-Vidal, A., Currieu de Madron, X., Hekussner, S., et al. (2009). Organic matter in sediments of canyons and open slopes of the Portuguese, Catalan, Southern Adriatic and Cretan Sea Margins. Deep-Sea Res. 2009:008. doi: 10.1016/j.dsr.2009.11.008
Qian, Y., Jochens, A. E., Kennicutt, M. C. II, and Biggs, D. C. (2003). Spatial and temporal variability of phytoplankton blooms and community structure over the continental margin of the northeast Gulf of Mexico based on pigment analysis. Contin. Shelf Res. 23, 1–17.
Qian, Y., Sericano, J. L., and Wade, T. L. (1998). Extraction and Clean-up of Sediments for Trace Organic Analysis. In: Sampling and Analytical Methods of the National Status and Trends Program, Mussel Watch Project: 1993-1996 Update, Vol. 130. Washington, D.C: NOAA Technical Memorandum NOS ORCA, 94–97.
R Core Team (2020). R: A Language and Environment for Statistical Computing. Vienna: R Foundation for Statistical Computing. Available online at: https://www.R-project.org/
R Development Core Team (2019). R: A language and environment for statistical computing. Vienna: R foundation for statistical computing.
Reish, D. J., and Barnard, J. L. (1979). “Amphipods,” in Pollution Ecology of Estuarine Invertebrates, eds C. W. Hart and S. L. H. Fuller Jr. (New York, NA: Academic Press), 345–370.
Rex, M. A. (1973). Deep-sea species diversity: Decreased gastropod diversity at abyssal depths. Science 181, 1051–1053. doi: 10.1126/science.181.4104.1051
Rex, M. A. (1981). Community structure in the deep-sea benthos. Ann. Rev. Ecol. Syst. 12, 331–353. doi: 10.1146/annurev.es.12.110181.001555
Rex, M. A. (1983). Geographic patterns of species diversity in the deep-sea benthos. Deep-Sea Biol. 453–472. doi: 10.1073/pnas.050589497
Rex, M. A., and Etter, R. J. (2010). Deep-sea biodiversity: Pattern and scale. Cambridge, MA: Harvard University Press.
Rex, M. A., Etter, R. J., Morris, J. S., Crouse, J., McClain, C. R., Johnson, N. A., et al. (2006). Global bathymetric patterns of standing stock and body size in the deep-sea benthos. Mar. Ecol. Prog. Ser. 317, 1–8.
Rex, M. A., Etter, R. J., and Stuart, C. T. (1997). “Large-scale patterns of species diversity in the deep-sea benthos,” in Marinebiodiversity Eds RFG Ormond, JD Gage, and MV. Angel (Cambridge, MA: Cambridge University Press). p 94–121 doi: 10.1017/cbo9780511752360.006
Rhoads, D. C., McCall, P. L., and Yingst, J. L. (1978). Disturbance and production on the estuarine seafloor. Am. Sci. 66, 577–586.
Roberts, H. H., Feng, D., and Joye, S. B. (2010). Cold-seep carbonates of the middle and lower continental slope, northern Gulf of Mexico. Deep-Sea Res. II 57, 2040–2054.
Ross, C. B., Gardner, W. D., Richardson, M. J., and Asper, V. L. (2009). Currents and sediment transport in the Mississippi canyon and effects of hurricane Georges. Contin. Shelf Res. 29, 1384–1396. doi: 10.1016/j.csr.2009.03.002
Rowe, G. (1983). “Biomass and production of the deep-sea benthos,” in Deep Sea Biology, Wiley-Interscience, Vol. 8, ed. G. Rowe (New York, NY: The Sea), 97–121.
Rowe, G. T. (1971). “Benthic biomass and surface productivity,” in Fertility of the Sea, Vol. 2, ed. J. D. Costlow Jr. (New York, NY: Gordon and Breach), 441–454.
Rowe, G. T., and Kennicutt, M. C. II (eds) (2009). Northern Gulf of Mexico continental slope habitats and benthic ecology study: Final report. U.S. Dept, of the Interior, Minerals Management. Service. New Orleans, LA: Gulf of Mexico OCS Region, 456.
Rowe, G. T., and Menzel, D. W. (1971). Quantitative benthic samples from the deep Gulf of Mexico with some comments on the measurement of deep-sea biomass. Bull. Mar. Sci. 21, 556–566.
Rowe, G. T., Morse, J., Nunnally, C., and Boland, G. S. (2008). Sediment community oxygen consumption in the deep Gulf of Mexico. Deep Sea Res. Part II 55, 2686–2691.
Rowe, G. T., Polloni, P. T., and Haedrich, R. L. (1982). The deep-sea macrobenthos on the continental margin of the northwest Atlantic Ocean. Deep Sea Res. Part A. 29, 257–278. doi: 10.1016/0198-0149(82)90113-3
Santschi, P., and Rowe, G. (2008). Radiocarbon-derived sedimentation rates in the Gulf of Mexico. Deep-Sea Res. II. 55, 2572–2576. doi: 10.1016/j.dsr2.2008.07.005
Sars, G. O. (1883). Oversigt af Norges Crustaceer med foreløbige Bemærkninger over de nye eller mindre bekjendte Arter. I. (Podophthalmata - Cumacea - Isopoda - Amphipoda). Videnskabs-Selskabet i Christiana 18, 1–124.
Shepard, F. P. (1981). Submarine canyons: multiple causes and long-time persistence. AAPG Bull. 65, 1062–1077. doi: 10.1306/03B59459-16D1-11D7-8645000102C1865D
Shepard, F. P., and Dill, R. F. (1966). “Summary characteristics and dimensions of submarine canyons,” in Submarine Canyons and Other Sea Valleys, eds F. P. Shepard and R. F. Dill (Chicago: Rand McNally), 223–231.
Soliman, Y., and Rowe, G. (2008). Secondary production of Ampelisca mississippiana Soliman and Wicksten, 2007 (Amphipoda, Crustacea) in the head of the Mississippi Canyon, northern Gulf of Mexico. Deep-Sea Res. II 55, 2692–2698. doi: 10.1016/j.dsr2.2008.07.019
Soliman, Y., and Wicksten, M. (2007). Ampelisca mississippiana: a new species (Crustacea: Amphipoda: Gammaridea) from the Mississippi canyon (northern Gulf of Mexico). Zootaxa 1389, 45–54.
Soliman, Y. S., and Wade, T. L. (2008). Estimates of PAHs burdens in a population of ampeliscid amphipods at the head of the Mississippi Canyon (N. Gulf of Mexico). Deep-Sea Res. II Top. Stud. Oceanogr. 55, 2577–2584. doi: 10.1016/j.dsr2.2008.07.007
Spence Bate, C. (1857). British Edriophthalma. Ann. Mag. Nat. Hist. 20, 524–525. doi: 10.1080/00222935708693983
Stora, G., Bourcier, M., Arnoux, A., Ge’rino, M., Le Campion, J., Gilbert, F., et al. (1999). The deep-sea macrobenthos on the continental slope of the nothwestern Mediterranean Sea: a quantitative approach. Deep Sea Res. Part I 46, 1349–1368.
Stuart, C., Braudt, S., Rowe, G., Wei, C.-L., Wagstaff, M., McClain, C., et al. (2016). Nestedness and species replacement along bathymetric gradients in the deep sea reflect productivity: a test with polychaete assemblages in the oligotrophic north-west Gulf of Mexico. J. Biogeogr. 2016:12810. doi: 10.1111/jbi.12810
Thomas, J. D. (1993). Biological monitoring and tropical biodiversity in marine environments: a critique with recommendations, and comments on the use of amphipods as bioindicators. J. Nat. Hist. 27, 795–806. doi: 10.1080/00222939300770481
Tomikawa, K., Watanabe, H. K., Tanaka, K., and Ohara, Y. (2021). A new species of Princaxelia from Shinkai Seep Field, Mariana Trench (Crustacea, Amphipoda, Pardaliscidae). Zookeys 1015, 115–127. doi: 10.3897/zookeys.1015.59683
Vetter, E. W. (1998). Population dynamics of a dense assemblage of marine detritivores. J. Exp. Mar. Biol. Ecol. 226, 131–161. doi: 10.1016/s0022-0981(97)00246-3
Vetter, E. W., and Dayton, P. K. (1998). Macrofaunal communities within and adjacent to a detritus-rich submarine canyon system. Deep-Sea Res. Part II 45, 25–54. doi: 10.1016/S0967-0645(97)00048-9
Volkenborn, N., Robertson, D. M., and Reise, K. (2009). Sediment destabilizing and stabilizing bio-engineers on tidal flats: cascading effects of experimental exclusion. Helgol. Mar. Res. 63, 27–35. doi: 10.1007/s10152-008-0140-9
Wade, T. L., Soliman, Y., Sweet, S. T., Wolff, G. A., and Presley, B. J. (2008). Trace elements and polycyclic aromatic hydrocarbons (PAHs) concentrations in deep Gulf of Mexico sediments. Deep Sea Res. Part II 55, 2585–2593. doi: 10.1016/j.dsr2.2008.07.006
Warwick, R. M., and Clarke, K. R. (1991). A comparison of some methods for analyzing changes in benthic community structure. J. Mar. Biol. Assoc. U K 71, 225–244. doi: 10.1017/S0025315400037528
Wei, C. L., Chen, M., Wicksten, M. K., and Rowe, G. T. (2020). Macrofauna bivalve diversity from the deep northern Gulf of Mexico. Ecol. Res. 35, 343–361. doi: 10.1111/1440-1703.12077
Wei, C. L., and Rowe, G. (2006). “The bathymetric zonation and community structure of deep-sea macrobenthos in the northern Gulf of Mexico,” in International Commission on the Exploration of the Seas Annual Science Conference, Vol. 0508, (ICES), 77.
Wei, C. L., and Rowe, G. T. (2019). Productivity controls macrofauna diversity in the deep northern Gulf of Mexico. Deep Sea Res. Part I 143, 17–27. doi: 10.1016/j.dsr.2018.12.005
Wei, C. L., Rowe, G. T., Escobar-Briones, E., Boetius, A., Soltwedel, T., Caley, M. J., et al. (2010a). Global patterns and predictions of seafloor biomass using random forests. PLoS One 5:e15323. doi: 10.1371/journal.pone.0015323
Wei, C.-L., Rowe, G. T., Hubbard, G. F., Scheltema, A. H., Wilson, G. D. F., Petrescu, I., et al. (2010b). Bathymetric zonation of deep-sea macrofauna in relation to export of surface phytoplankton production. Mar. Ecol. Prog. Ser. 399, 1–14. doi: 10.3354/meps08388
Wei, C.-L., Rowe, G. T., Escobar-Briones, E., Nunnally, C., Soliman, Y., and Ellis, N. (2012). Standing stocks and body size of Deep-Sea macrofauna: Predicting the baseline of 2010 Deep water horizon oil spill in the northern Gulf of Mexico. Deep Sea Res. Part I 69, 82–99. doi: 10.1016/j.dsr.2012.07.008
Wenzhöfer, F., Oguri, K., Middelboe, M., Turnewitsch, R., Toyofuku, T., Kitazato, H., et al. (2016). Benthic carbon mineralization in hadal trenches: Assessment by in situ O2 microprofile measurements. Deep-Sea Res. Part I Oceanogr. Res. Pap. 116, 276–286. doi: 10.1016/j.dsr.2016.08.013
Wilson, G. D. F. (2008). Local and regional species diversity of benthic isopoda (Crustacea) in the deep Gulf of Mexico. Deep Sea Res. Part II: Top. Stud. Oceanogr. 55, 2634–2649.
Wilson, W. H. Jr. (1991). Competition and predation in marine soft-sediment communities. Annu. Rev. Ecol. Syst. 21, 221–241. doi: 10.1890/07-1155.1
Keywords: canyons, amphipods, biodiversity, zonation, organic carbon, sediments
Citation: Soliman YS, Rowe GT, Wicksten M and Wei C-L (2022) Diversity and Zonation of Benthic Amphipod Crustaceans Affected by the Mississippi Submarine Canyon in the Northern Gulf of Mexico. Front. Mar. Sci. 9:822924. doi: 10.3389/fmars.2022.822924
Received: 26 November 2021; Accepted: 31 January 2022;
Published: 10 March 2022.
Edited by:
Elva G. Escobar-Briones, National Autonomous University of Mexico, MexicoReviewed by:
Pierluigi Strafella, National Research Council (CNR), ItalyLenaick Menot, Institut Français de Recherche pour l’Exploitation de la Mer (IFREMER), France
Javier Sellanes, Catholic University of the North, Chile
Copyright © 2022 Soliman, Rowe, Wicksten and Wei. This is an open-access article distributed under the terms of the Creative Commons Attribution License (CC BY). The use, distribution or reproduction in other forums is permitted, provided the original author(s) and the copyright owner(s) are credited and that the original publication in this journal is cited, in accordance with accepted academic practice. No use, distribution or reproduction is permitted which does not comply with these terms.
*Correspondence: Yousria S. Soliman, eW91c3JhLnNvbGltYW5AZ21haWwuY29t