- Marine Biological Section, Department of Biology, University of Copenhagen, Helsingør, Denmark
Ocean warming along with nutrient enrichment are major stressors causing global seagrass decline. While the effects of global warming on metabolic parameters in seagrasses are well described, the effect of increasing temperature on the epiphytic overgrowth of seagrass leaves and the consequences for the seagrass plant are poorly understood. Here, we investigated the effects of elevating temperature on the photosynthetic efficiency of the seagrass species Zostera marina L. and its associated epiphytes, to explore how ocean warming might affect epiphytism in seagrasses. Gas exchange and final pH measurements on bare seagrass leaves, leaves with epiphytes, and epiphytes separated from seagrass leaves were used to quantify photosynthesis and respiration rates, and the inorganic carbon extraction capacity of leaves and epiphytes as a function of photon scalar irradiance and temperature (12, 17, 22, and 27°C). Seagrass without epiphytic biofilm had a high ability to exploit the incoming irradiance regardless of the light intensity and temperature, shown as continuously high light use efficiency and maximum net photosynthesis rates. The presence of epiphytic biofilm on the seagrass leaves impaired plant photosynthesis by increasing light requirements and reducing the photosynthetic efficiency (especially at 27°C). Epiphytes showed the lowest respiration rates in darkness and had the highest oxygen surplus over diel cycles up to 22°C, whereas bare leaves had the highest diel oxygen surplus at 27°C. Both bare leaves and epiphytes lost the ability to utilize bicarbonate at 27°C, and epiphytes also did not show use of bicarbonate at 12°C. Our results indicate a competitive advantage for epiphytes in cold CO2-rich environments, whereas seagrass with bare leaves could be less affected under elevated seawater temperatures.
Introduction
Seagrass meadows are among the most productive and diverse coastal benthic ecosystems (Larkum et al., 2006; Unsworth and Cullen-Unsworth, 2017). Seagrasses colonize organic rich and reduced sediments, where the phytotoxin hydrogen sulfide is produced by anaerobic bacterial sulfate reduction (Blaabjerg et al., 1998; Nielsen et al., 2001). Via their internal gas-filled aerenchymal tissue, seagrasses exhibit efficient gas transport to their belowground tissues (Borum et al., 2006; Brodersen et al., 2014, 2018), where it supplies the belowground biomass with oxygen and can protect the seagrass rhizosphere from hydrogen sulfide intrusion, which otherwise can be fatal to the plant (Brodersen et al., 2015a,b, 2017; Brodersen, 2016). Many crucial ecosystem services are provided by seagrass meadows such as fisheries production (Bertelli and Unsworth, 2014; Unsworth and Cullen-Unsworth, 2014, 2017; Unsworth et al., 2019), coastal protection from erosion (Fonseca and Cahalan, 1992), efficient carbon sequestration in the oxygen-depleted seagrass sediments (e.g., Fourqurean et al., 2012; Macreadie et al., 2015; Cullen-Unsworth and Unsworth, 2018) and reduction of microbiological contaminants (Lamb et al., 2017). Seagrass meadows are thus of high ecological and economic value (Costanza et al., 1997; Unsworth et al., 2019) and the ongoing decline in seagrass habitats due to anthropogenic stress can therefore lead to decreasing biodiversity, which further contributes to negative effects induced by climate change.
Anthropogenic impacts, such as coastal development and eutrophication, are major drivers behind the global decline in seagrass meadows (Orth et al., 2006; Waycott et al., 2009). Eutrophication is facilitating epiphytic overgrowth of microalgae and bacteria on the surface of seagrass leaves, which is an increasing problem in coastal waters, due to accelerating nutrient runoff from land (Borum, 1985). Furthermore, nutrient-driven enhanced macroalgal growth can potentially outcompete seagrass species (Ballesteros et al., 2007). While the colonization of microalgae and the presence of plant detritus on the seagrass leaf surface can serve as a food source for small herbivores (Cullen-Unsworth and Unsworth, 2018), the presence of epiphytic biofilms can strongly influence plant fitness (Sand-Jensen, 1977). Epiphytic biofilm growth on seagrass leaves leads to dramatic changes in the seagrass phyllosphere (Brodersen and Kühl, 2022), i.e., the dynamic microhabitat forming at the leaf tissue surface of the seagrass plant (Brodersen et al., 2020a,b; Noisette et al., 2020). The presence of epiphytic biofilms impedes O2 and heat transfer between the leaf area and the ambient water, and both light availability and light quality reaching the seagrass leaves is highly diminished (Brodersen et al., 2015a; Noisette et al., 2020); all negatively affecting seagrass performance and health. However, leaf microorganisms can also be beneficial to seagrasses, as they can facilitate uptake of dissolved organic nitrogen by leaves and thus potentially benefit seagrass growth and productivity (Tarquinio et al., 2018).
The ocean has a high heat capacity and has absorbed > 90% of heat induced by the ongoing increase of greenhouse gases in the atmosphere (Levitus et al., 2000; Resplandy et al., 2019), which drives ongoing ocean warming (Gattuso and Hansson, 2011). Temperature is a primary parameter controlling the distribution of seagrass species from tropical to temperate regions, as evident by the higher temperature optima of photosynthesis in tropical seagrass species (Pedersen et al., 2016), where some species can endure short-term exposure to water temperatures of up to 42°C. Several vital processes within seagrasses are temperature dependent such as the diffusive transport of gases and solutes, rates of photosynthesis and respiration, and the enzymatic capacity of RuBisCO (Staehr and Borum, 2011). The plant’s metabolic rates (e.g., photosynthesis and respiration) and thus nutrient demand increases with increasing temperature toward an optimum temperature, above which increasing deactivation of metabolic processes and/or denaturation of vital metabolic macromolecules (e.g., proteins) lead to increasing plant stress (Staehr and Borum, 2011). Seagrass respiration exhibits a stronger response with increasing temperature than photosynthetic processes (Dennison, 1987). Consequently, a disbalance at high temperatures may affect the light requirements and depth distribution of e.g., eelgrass (Zostera marina L.), since a higher irradiance is needed to compensate the increasing oxygen demands to maintain a positive carbon-balance (Staehr and Borum, 2011). High temperatures, above the plant’s photosynthetic temperature optima, can thus lead to a negative diel O2 balance in the seagrass plant due to reduced leaf photosynthesis and increased tissue respiration, which strongly reduces the internal plant aeration (Staehr and Borum, 2011; Pedersen et al., 2016). The interactions between plant, sediment, and the water column, also strongly depend on the water temperature. As temperature increases, the oxygen solubility in the water decreases, and the intra-plant oxygen content is lowered e.g., due to increasing respiration, which leads to a reduction in the plant tolerance to anoxia (Raun and Borum, 2013). This becomes apparent during night-time or low light levels, when seagrass’ respiration can be limited by the availability of dissolved O2 in the water column, since the supply is sustained solely by the relatively slow diffusion of O2 into the leaf through the diffusive boundary layer (DBL) (Brodersen et al., 2015a; Pedersen et al., 2016). This makes the plant highly susceptible to hydrogen sulfide intrusion (Brodersen et al., 2015b), which can lead to chemical asphyxiation. Especially, seagrass communities inhabiting shallow intertidal waters can experience impeded photosynthetic activity due to extreme light levels, temperatures, O2 fluctuations and pH changes (Pedersen et al., 2016).
The increase in atmospheric CO2 equilibrates with seawater affecting the speciation of inorganic carbon and leading to ocean acidification (Albert et al., 2020). This can influence some marine phototrophs positively since it is favorable to use CO2 as an inorganic carbon source (Riebesell et al., 2018), while calcifying organisms are facing increasing difficulties in depositing calcium carbonate. Different bicarbonate utilization strategies have been detected in temperate seagrasses (Borum et al., 2016; Kim et al., 2018; Larkum et al., 2018). External carbonic anhydrase (CA) can catalyze the conversion of bicarbonate to CO2 at the leaf surface (Borum et al., 2016; Kim et al., 2018; Larkum et al., 2018), while local acidification of the diffusive boundary layer (DBL) via proton pumps on the leaf surface might cause a local decrease in pH and thus enhanced conversion of bicarbonate to CO2 for photosynthesis (Borum et al., 2016). Such CO2 concentrating mechanisms are energy consuming for the plant, which only makes them favorable in CO2 poor environments (Raven et al., 2014). Theoretically, seagrasses are able to use bicarbonate (HCO3–) as a carbon source when they are able to rise pH above 9 using the pH drift approach (Sand-Jensen et al., 1992).
Eelgrass (Z. marina L.) has been shown to exhibit temperature optima at 20–25°C for growth and photosynthesis under saturating light, while light limiting conditions can reduce the optimum temperature by up to 10°C (Beca-Carretero et al., 2018). The photosynthetic saturation level and the compensation photon irradiance of Z. marina generally increase with increasing temperatures (Marsh et al., 1986). Q10 values for light-saturated photosynthesis and dark respiration of 1.5–1.7 and 2.4, respectively, have been determined over the temperature range of 0–35°C (Marsh et al., 1986). Z. marina plants originating from the northern part of their distribution range (i.e., subarctic populations) show higher sensitivity to extreme temperatures with faster declines in photosynthesis and increases in respiration (Beca-Carretero et al., 2018). Compared to other seagrass species such as Ruppia maritima L., Z. marina appears less tolerant to higher temperatures (e.g., Evans et al., 1986), which also corresponds with their respective seasonal community dynamics. Primary productivity cycles of Z. marina and its epiphytes have been suggested to be closely interrelated (Penhale, 1977), where the epiphyte community photosynthesis can double the total primary production of Z. marina leaves (Mazzella and Alberte, 1986), while at the same time having detrimental effects on leaf photosynthesis owing to shading (Sand-Jensen, 1977; Brodersen et al., 2015a) and carbon limitation (Brodersen et al., 2020a). Such knowledge is important to determine critical temperature thresholds for productivity and survival of Z. marina under future climate change conditions. Z. marina thus seems to have relatively high ability to acclimate to environmental change considering its wide latitudinal distribution (Staehr and Borum, 2011; Assis et al., 2020); plants exhibit a higher ability to utilize low irradiance during winter, whereas no difference in optimum temperatures has been found between winter- and summer-acclimated plants (Staehr and Borum, 2011).
Although temperature effects on seagrass leaf photosynthesis are well described, almost nothing is known about how increasing temperature affects leaf/epiphyte interactions and how epiphyte growth on leaves affects the ability of seagrasses to withstand increasing seawater temperatures. In this study, we investigated acute temperature effects on the respiration and photosynthesis of eelgrass (Z. marina) leaves and their epiphytic biofilms using a combination of gas exchange and pH measurements on leaf fragments in closed incubation chambers. We analyze and compare how increasing temperature affects (i) the balance between autotrophy and heterotrophy, (ii) the photosynthetic efficiency, and (iii) the potential to use bicarbonate in photosynthesis in bare seagrass leaves, leaves covered by an epiphytic biofilm, as well as in epiphytes separated from the seagrass leaves. The experiments aimed to obtain a better understanding of how increasing seawater temperature may affect the ability of seagrasses to withstand ongoing ocean warming and increased epiphytic overgrowth.
Materials and Methods
Seagrass and Sediment Sampling
Whole specimens of the common seagrass species Zostera marina L. and marine sediment were collected in early April 2021 at the coast of Julebæk, North Zealand, Denmark (56°03′29.2″N; 12°34′40.7″E). Seagrass plants with and without epiphytic biofilms on their leaves were collected in the shallow (< 2 m) coastal brackish water with a salinity of 18. The average water temperature in April is about 10°C. The specimens were immediately transported to nearby laboratory facilities at the Marine Biology Section (University of Copenhagen) in Elsinore, where the seagrass plants and collected sediment were placed in a large aquarium containing constantly aerated seawater from the sampling site kept at 16–18°C. The seagrass samples were exposed to an incident photon irradiance (400–700 nm) of 100 μmol photons m–2 s–1 as provided by a metal-halide lamp (14:10 h light/dark cyclus; MEGACHROME, Giesemann Aquaristic Gmbh, Nettetal, Germany). The water temperature in the sampling area rapidly increases from about 10–20°C from April to June, and the holding aquarium temperature was adjusted to reflect the in-situ water temperature at the sampling site at the time of the experimental measurements (e.g., Staehr and Borum, 2011). The incubation photon irradiance mimicked the approximate daily average photon irradiance at the sampling site at the depth of the seagrass meadow (e.g., Staehr and Borum, 2011). The plants were acclimated to the aquarium conditions for 1 week prior to the start of the experiments. Leaves with a similar fluffy coating of filamentous epiphytes were selected for the experiments. The epiphytic biofilm community composition was assumed to predominantly consist of green algae, brown algae, diatoms, and bacteria as previously shown by Borum et al. (1984). To minimize dissimilarities between the seagrass leaves selected for the experiment, the youngest and the oldest leaves from each individual plant were excluded (Brodersen et al., 2020a).
Gas Exchange Measurements
We quantified the net O2 exchange in darkness (respiration) and under defined photon irradiance levels (net photosynthesis) by placing seagrass leaf segments of a known area in small custom-made glass chambers (1.8 mL). The chambers were equipped with small oxygen sensor spots (OXSP5, PyroScience GmbH, Aachen, Germany) fixed on the inside with a transparent silicone glue (SPGLUE ELASTOSIL E43, WACKER Chemical Corporation, Adrian MI, United States). An optical fiber, mounted on a holder enclosing each chamber, was pointing at and sending red excitation light to the oxygen sensor spot and collected the resulting O2-dependent infrared luminescence. The optical fibers were connected to a 4-channel optical O2 sensor system (FireSting-O2; FSO2-C4, PyroScience GmbH, Aachen, Germany), which was connected to a PC running Pyroscience Oxygen Logger software (PyroScience GmbH, Germany) that logged and stored the data. A temperature sensor was connected to the system and was used to perform temperature-compensated O2 measurements over the experimental temperature range of 12–22°C. Prior to the measurements, each of the four applied microrespiration chambers was calibrated at two known O2 levels using a sodium sulfite (Na2SO3) solution for the 0% air saturation calibration and air saturated seawater (prepared by aeration with an air pump) for the 100% air saturation calibration. The calibration was repeated before conducting the experiment under 27°C due to drifting calibrations at high temperature.
To manipulate and control the temperature, the closed gas exchange chambers with seawater and seagrass leave sections were placed in an aquarium with thermostated DI-water. To obtain the desired water temperatures in the aquarium and thereby the measuring chambers, we used a combination of a cooling system (Heto Cooling bath type CBN 8-30, HETO-HOLTEN A/S, Allerød, Denmark) and an aquarium heater (Aquael EasyHeater 25W, Suwałki Poland) submerged into the aquarium. A submersed water pump (Universal mini pump 5W, TUNZE, Austin TX, United States) placed in the aquarium ensured sufficient water circulation and homogeneous temperature distribution in the experimental chambers.
The aquarium was placed above two magnetic stirrers (IKA Magnetic Stirrers RCT basic, IKA®-Werke GmbH & Co. KG, Staufen, Germany) and each measuring chamber was equipped with a small magnet to ensure sufficient mixing in the experimental chambers during incubations. Small plankton nets (0.2 mm mask width) were placed above the magnet to protect the specimens from mechanical impact form the stirring magnet. To ensure that the specimens were exposed to equivalent photon irradiances, the three chambers and a control chamber only containing air saturated, filter-sterilized (0.2 μm) seawater and a magnet, were positioned with the same distance to an adjustable fiber-optic tungsten halogen lamp (KL-2500LCD, Schott GmbH, Germany) equipped with a tri-furcated fiber bundle, each mounted with a collimating lens. Photon scalar irradiance in the experimental chambers for defined lamp settings were determined through measurements with a calibrated photon scalar irradiance sensor (3 mm diameter) connected to a calibrated irradiance meter (ULM-500, Walz GmbH, Effeltrich, Germany).
For each experimental run, three leaf segments from one seagrass specimen were used: One leaf segment without visible epiphyte cover (bare leaf, 35 mm lengths), one segment with epiphyte cover (leaf with epiphytes, 30 mm length), and epiphytes which were gently scraped off a leaf segment with a scalpel (epiphytes, 40 mm). All leaf segments originated from the middle part of the leaves to avoid the youngest and oldest regions and thus to minimize age and genetic differences between investigated leaf segments (Brodersen et al., 2020a); where leaf segments with epiphyte cover refer to leaves with 100% areal epiphytic biofilm cover and bare leaves to leaf segments with 0% areal epiphytic biofilm cover. Different leaf segment lengths were used to aim for a similar total photosynthetic biomass between samples (here, determined as a similar DW), while also ensuring a water volume of 1.8 mL in all measuring chambers. The seagrass leaf segments (bare and with epiphytes) and the epiphytes were placed in three custom-made microrespiration chambers containing air saturated and filter-sterilized (0.2 μm) seawater with a salinity of 18.
Four biological replicates were used in the gas exchange experiment (n = 4), one replicate per experimental run. Each replicate was exposed to water temperatures of 12, 17, 22, and 27°C (for a minimum of 30 min prior to onset of measurements); where the high temperature treatment of 27°C represents possible maximal temperatures during summer-time within the investigated meadow under future global warming. Running each temperature treatment, the specimens were alternately exposed to darkness and increasing light irradiances (100, 300, and 600 μmol photons m–2 s–1) for 8 min at each light condition. This time interval resulted in a constant linear change in O2 concentration during incubations. The experiment started out and ended in darkness.
To obtain photosynthesis and respiration rates per gram dry weight (g DW–1), the seagrass leaf segments were placed in an oven and the specimen dry weight was measured after 48 h exposure to 60°C. Respiration and net photosynthesis rates were calculated by multiplying the slope of the linear O2 concentration vs. time curve (nmol L–1 h–1) with the volume of water in the measuring chamber (L) divided by the sample dry weight. Post-illumination respiration (i.e., respiration rates measured after a light period) was measured as the immediate O2 depletion rate in darkness after light exposure to a given photon scalar irradiance and was used as a proxy for respiration in the previous light period. Gross photosynthetic rates were calculated by adding the absolute values of the respective respiration rates (i.e., dark respiration or the post-illumination respiration rates measured immediately after the light period at each experimental photon irradiance level) to the respective net photosynthetic rates: GP (E) = NP (E) + |R (E) |. Control measurements in chambers without leaves or epiphytes did not show any O2 production or consumption under all tested experimental conditions. Such control incubations are performed to allow for potential correction of measurements on samples due to minor microbial contamination in the measuring chambers leading to undesired microbial-mediated O2 production and/or consumption.
pH Drift Measurements
To estimate the dissolved inorganic carbon (DIC) extraction capacity and maximal pH tolerance of the seagrass leaves and the epiphytes, pH drift measurements were performed according to Brodersen et al. (2020a). A final pH of > 9 in such drift experiments indicates the ability of the specimens to utilize bicarbonate as an additional inorganic carbon source in photosynthesis (Pedersen et al., 2016). For each experimental run, a total of four biological replicates were used (n = 4). We used four seagrass leaf segments without visible epiphyte cover (bare leaf, 35 mm length), four leaf segments with epiphyte cover (leaf with epiphytes, 30 mm length), and 4 samples of epiphytes gently scraped off from 4 seagrass leaves with a scalpel (epiphytes, scraped off a 40 mm long leaf segment). Different segment lengths were used to ensure similar photosynthetic biomass (here, determined as a similar g DW). The leaf segments and the epiphyte samples were placed in separate falcon tubes with 35 mL filter-sterilized (0.2 μm) seawater with a salinity of 18, which allowed for a headspace in each tube to minimize photorespiration during the long-term incubations (cf. Pedersen et al., 2016). To manipulate and control the temperature, all tubes were positioned in a DI water-filled thermostated aquarium similar to the one used for gas exchange measurements. A lamp (MIA worldlight, LP300Q-4K.24_RY; MIA Light GmbH, Gronau, Germany) was placed on top of the aquarium to provide the specimens with a constant saturating photon scalar irradiance of 600 μmol photons m–2 s–1. Light levels were quantified with a photon scalar irradiance sensor (3 mm diameter) connected to a calibrated irradiance meter (ULM-500, Walz GmbH, Effeltrich, Germany). The experiment was conducted four times with the following water temperatures: 12, 17, 22, and 27°C. The experiment proceeded 45 h under each temperature before the final pH levels was measured in each sample; this incubation time was chosen after several repetitive measurements at different timescales, i.e., 24, 36, and 48 h showing how pH approached a stable maximum value. The pH measurements were done with a calibrated pH minisensor (BlueLine pH combination electrode; SI Analytics GmbH, Mainz, Germany) connected to a pH meter (PHM220 Lab pH Meter; Radiometer Analytical SAS, Lyon, France) and calibrated with commercial pH buffers of pH 7 and 10 (VWR Chemicals, Pennsylvania, United States).
Data Calculations and Analysis
The software program OriginPro 2020 (OriginLab Corporation, Northampton MA, United States) was used to create photosynthesis vs. irradiance curves, and for fitting and analyzing the experimental data. Non-linear regression fits of the calculated net photosynthesis (NP) and gross photosynthesis (GP) rates normalized to dry weight as a function of photon scalar irradiance (E), were used to obtain photosynthetic parameters (described below). We fitted an exponential saturation model for gross photosynthesis vs. irradiance curves (Webb et al., 1974):
and used a similar model with an additional respiration term for fitting net photosynthesis vs. irradiance curves (Spilling et al., 2010):
Here, PMAX is the calculated photosynthetic rate at saturating photon irradiance, α is the light utilization efficiency related to the photosynthetic activity, i.e., the slope of the P vs. E curve, and R(E) is the respiration rate of the seagrass leaves and/or epiphytes at the respective photon scalar irradiance.
The compensation irradiance, i.e., the photon scalar irradiance above which a sample exhibits net photosynthetic production of O2, EC, was determined from the obtained photosynthetic parameters as:
The photon scalar irradiance at the onset of photosynthesis saturation (Ek) was calculated as:
To evaluate the sensitivity of the different photosynthesis parameters of leaves and epiphytes to temperature changes, a calculation of Q10 was made for NPMAX, αnp (from NP), R, GPMAX, and αgp (from GP):
where ParameterT2 and ParameterT1 are the metabolic parameters of the leaves/epiphytes measured at two given temperatures, i.e., 22 and 12°C, respectively.
The total daily net oxygen production, DP, of the leaves and epiphytes was estimated as a function of increasing temperature assuming 14 h of light exposure at differing light irradiances followed by 10 h of darkness. The 14 h of light exposure were divided into 7 intervals each lasting 2 h (i.e., 5–7, 7–9, 9–11, 11–13, 13–15, 15–17, and 17–19). For each time interval, a photon irradiance average was calculated. The data on diel photon irradiance originated from Staehr et al. (2018).
The net photosynthesis under each averaged photon irradiance interval was calculated with equation 2 using the averaged photon irradiance determined for each of the pre-defined 7 time-intervals (t1-t7).
The daily net oxygen production of the leaves and epiphytes was calculated as:
where NP(E) is the net oxygen production at the given average photon irradiance for a given 2 h time interval, R is the dark respiration, and t represent the respective pre-defined time intervals.
Student’s t-tests were used to statistically analyze data and compare treatments. The significance level was set to p < 0.05. Statistical tests were performed in Microsoft Excel.
Results and Discussion
Our study provides evidence that the seagrass Z. marina L. performs better under elevated temperatures (27°C) in terms of photosynthetic performance (e.g., light use efficiency and maximum net photosynthesis) and diel O2 balance, when no epiphytic biofilm is present on the surface of the leaves. Simultaneously, the seagrass bare leaves also performed better than their associated epiphytes originating from the leaves.
Photosynthesis and Respiration
Net photosynthesis increased with increasing photon scalar irradiance until saturation (Figures 1A–L), and with increasing temperature except for leaves with epiphytes, and for epiphytes originating from Z. marina L. leaves at 27°C. Such response to increasing irradiance and temperature is similar to previous studies on bare seagrass leaves (Ralph et al., 2007; Staehr and Borum, 2011; Pedersen et al., 2016). At 27°C, only bare seagrass leaves showed a positive net photosynthesis at a photon scalar irradiance of 100 μmol photons m–2 s–1. Respiration increased with temperature and varied to a lesser degree with photon scalar irradiance, as determined from comparing dark respiration and post-illumination respiration rates (Figures 1A–L). This is similar to previous studies that also found a strong temperature dependency of the dark respiration rate for seagrass leaves (Staehr and Borum, 2011; Pedersen et al., 2016). Gross photosynthesis rates increased with increasing photon scalar irradiance until saturation and with elevated temperatures (Figures 1A–L). The gross photosynthesis of the bare leaves was highest at all temperatures besides at 17°C, where the gross photosynthesis rate of all samples was very similar and reached a maximum gross photosynthesis rate of ∼150 nmol O2 mg DW–1 h–1 (Figure 1). Epiphytes exhibited the lowest gross photosynthesis rates at 12, 22, and 27°C as compared to bare leaves and leaves with epiphytes.
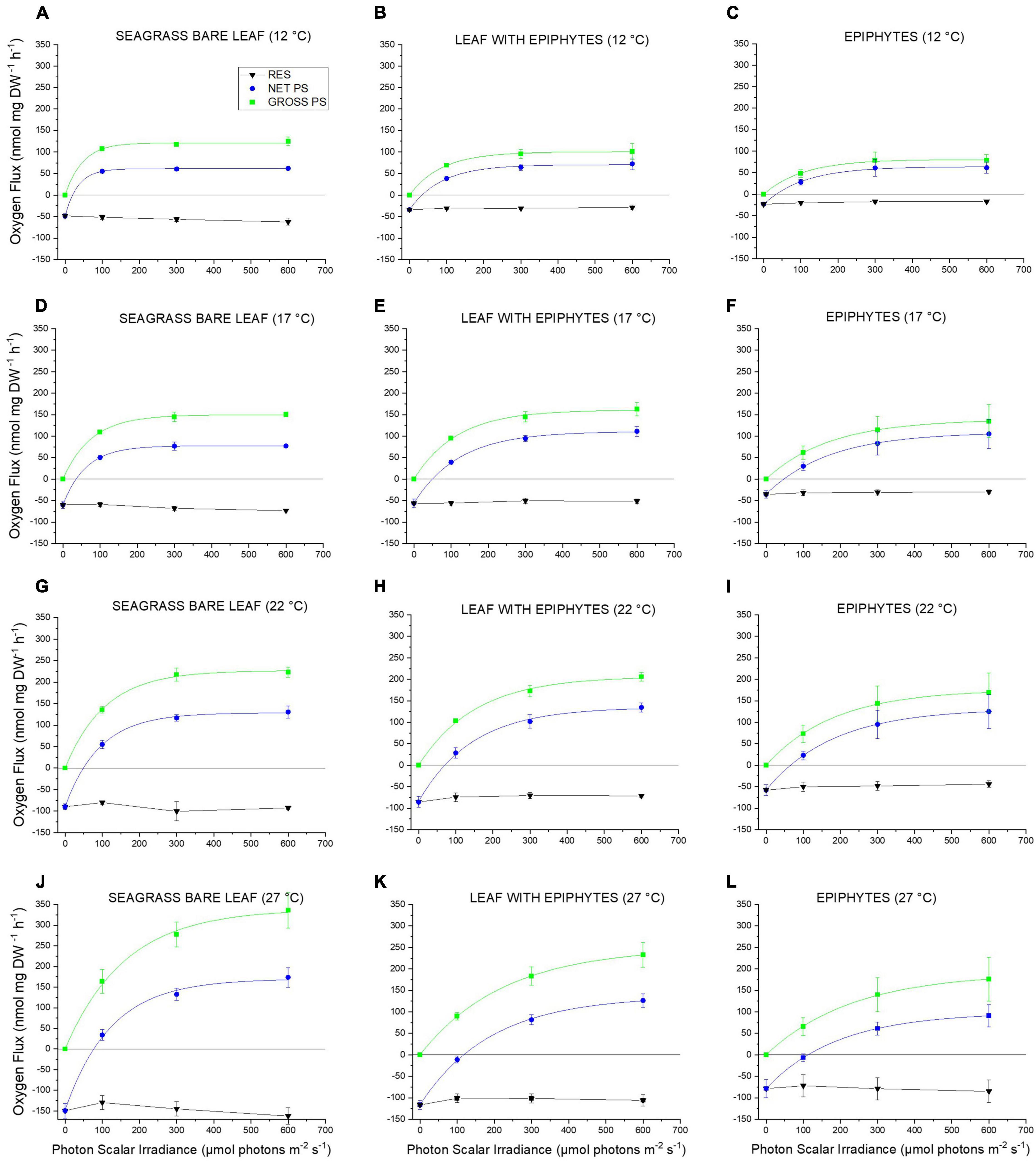
Figure 1. (A–L) Respiration, net and gross O2 production rates as a function of incident photon scalar irradiance for Z. marina L. leaves with and without epiphytes, as well as, for epiphytes originating from Z. marina L. leaves. The respiration, net and gross photosynthesis rates were measured for photon scalar irradiances (PAR, 400–700 nm) of 0, 100, 300, and 600 μmol photons m–2 s–1 and temperatures of 12, 17, 22, and 27°C. Black symbols and lines shows respiration. Blue symbols and lines show net photosynthesis. Zero net O2 production (Y = 0) indicates the compensation photon irradiance of the seagrass leaves and epiphytes. Green symbols and lines show gross photosynthesis. Symbols with error bars represent mean values ± SEM; n = 4, biological replicates. All non-linear regression curve fits had an R2 of ≥ 0.99. Detailed information about our statistical models can be found in Supplementary Notes 1 and Supplementary Tables 1–24.
Maximum net photosynthesis (NPMAX) increased strongly from 12 to 22°C in all samples (Figure 2A). NPMAX for the bare leaves showed a similar increase from 22 to 27°C, while the increase in leaves with epiphytes became less pronounced, and separated epiphytes showed a decrease in NPMAX (Figure 2A). Bare seagrass leaves exhibited higher NPMAX at 27°C compared to leaves with epiphytic cover and epiphytes originating from Z. marina L. leaves. This impediment by epiphytic biofilm of the seagrass metabolic parameters and thus photosynthetic performance is similar to previous studies (Staehr and Borum, 2011; Brodersen et al., 2015a, 2020a; Noisette et al., 2020). Accumulation of gases within the seagrass phyllosphere in light, due to thicker diffusive boundary layers (DBL) and total diffusive distances (TDD) caused by the epiphyte cover, limits the exchange of gases and nutrients (Brodersen et al., 2015a, 2020a; Noisette et al., 2020), and thus the NPMAX.
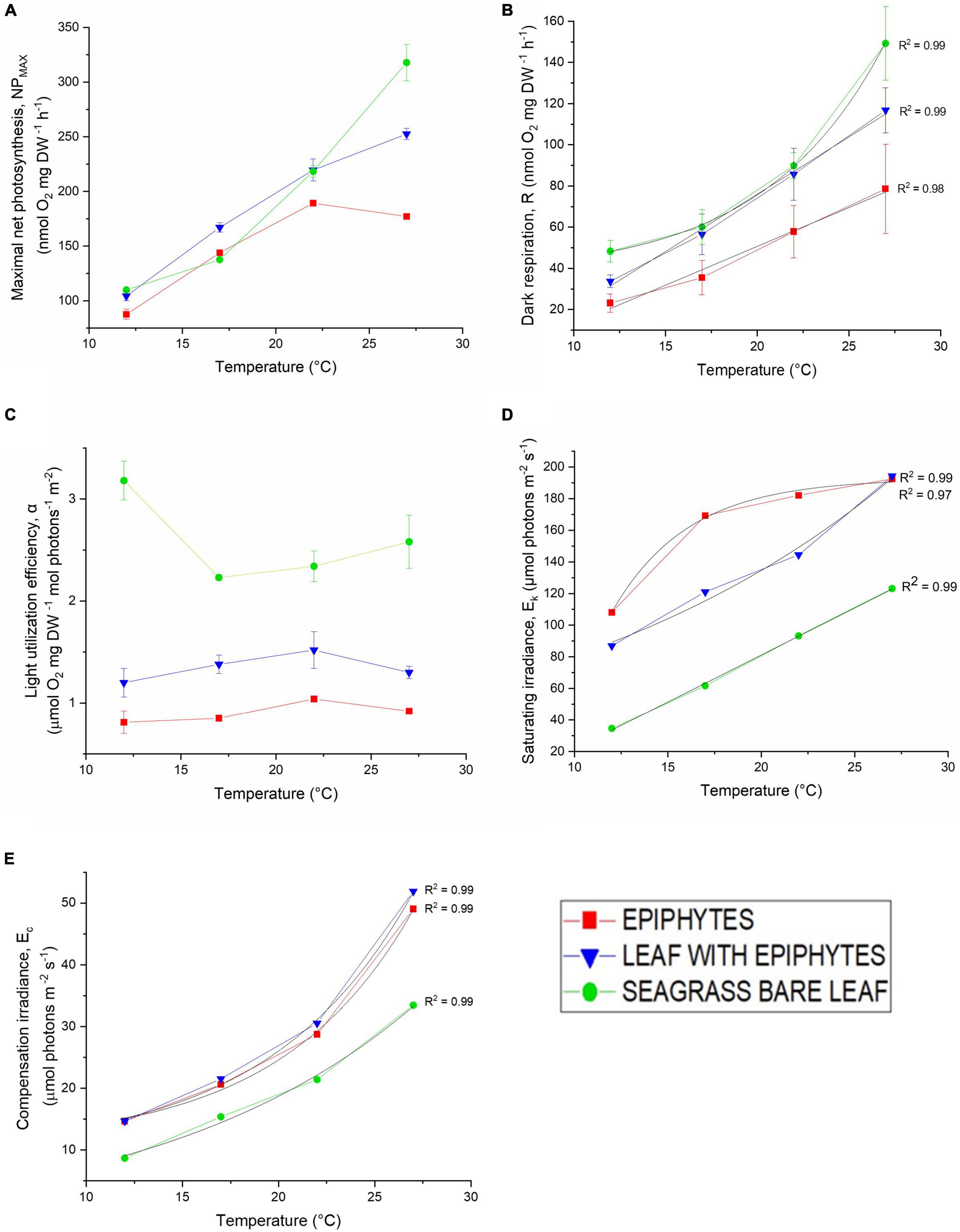
Figure 2. (A) Maximum photosynthesis rate (Pmax), (B) dark respiration (R), (C) light use efficiency (α), (D) photon scalar irradiance at the onset of photosynthesis saturation (Ek), and (E) compensation photon scalar irradiance (Ec) as a function of increasing temperature (12, 17, 22, and 27°C). Red symbols and lines represent epiphytes originating from Z. marina L. leaves. Blue symbols and lines represent Z. marina L. leaves with epiphytes. Green symbols and lines represent bare Z. marina L. leaves. Black lines show fitted exponential or linear function for the photosynthetic parameters: R, Ec and Ek; all with R2 ≥ 0.97. Symbols with error bars represent mean values ± SEM; n = 4, biological replicates.
The dark respiration rate (R) also increased strongly with increasing temperature (Figure 2B). However, while the respiration of leaves with epiphytes and separated epiphytes displayed a quasi-linear increase with temperature (R2 = 0.99 and R2 = 0.98), the respiration of bare seagrass leaves respiration rate increased quasi-exponentially (R2 = 0.99) (Figure 2B). The separated epiphytes exhibited the lowest respiration rate under all measured temperatures (Figure 2B). The respiration rates of bare seagrass leaves exceeded respiration rates of leaves with epiphytes at 12 and 27°C, whereas the respiration rates were similar at 17 and 22°C (Figure 2B). The low dark respiration rate of the epiphytes observed under elevated temperatures (27°C) (Figure 2B), could suggest that the epiphytes are better at adapting to the rising seawater temperature. However, the Q10 relationship between respiration rate and photosynthesis rate of the epiphytes, revealed a negative correlation (Table 1), explaining their low NPMAX values at elevated temperatures (27°C). Such general increase in dark respiration with increasing temperature of seagrass leaves is similar to previous studies (Staehr and Borum, 2011; Pedersen et al., 2016), where Staehr and Borum (2011) found Q10 values for dark respiration ranging from 1.4 to 3.9, which is very similar to our results with Q10 ranging from 1.9 to 2.5 (Table 1).
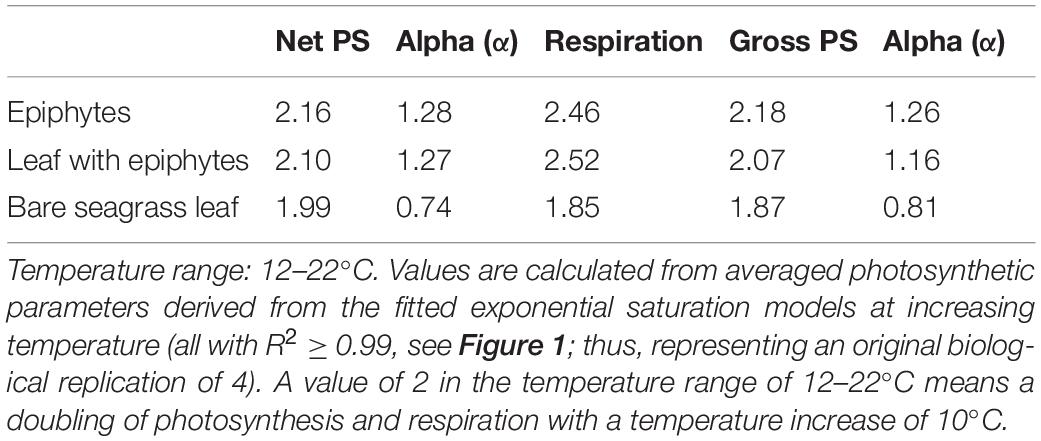
Table 1. Q10 values for photosynthetic parameters: dark respiration, maximum net and gross photosynthesis, and their respective light use efficiencies (α), of Z. marina L. leaves with and without epiphytes, and for epiphytes originating from Z. marina leaves.
The light utilization efficiency (α) of the bare Z. marina L. leaves was higher at all experimental temperatures, as compared to leaves with epiphytic cover and separated epiphytes (Figure 2C). The light use efficiency was lowest in samples with epiphytes separated from the leaves. Leaves with epiphytes and the separated epiphytes light utilization efficiency increased with temperature reaching a maximum at 22°C, followed by a decrease at higher temperature (Figure 2C). Bare leaves exhibited a marked drop in light utilization efficiency from 12°C (maximum) to 17°C (minimum). This decrease of the bare leaves was thus in contrast to leaves with epiphytes and separated epiphytes, and was followed by an increasing efficiency in bare leaves from 22 to 27°C (Figure 2C). An earlier investigation of the seagrass phyllosphere (Brodersen et al., 2015a), concluded that the presence of epiphytic biofilm diminishes both the quantity and quality of light available for the seagrass leaf, which might explain the lower light utilization efficiencies in leaves with epiphytes and separated epiphytes. These observations emphasize the ability of the bare leaves to maintain a higher NPMAX under elevated temperatures. This statement was supported by the positive relation between the Q10 values of photosynthesis rates compared to respiration rates for bare leaves (Table 1). Our estimated light use efficiencies (α) for Z. marina and its epiphytes were the same order of magnitude as previous findings in Danish waters (e.g., Staehr and Borum, 2011). However, we note that the relatively high estimated α value for bare seagrass leaves at 12°C may be overestimated owing to limited measurements of O2 evolution under sub-saturating photon irradiances for bare leaves at this specific temperature treatment (see Figure 1), which challenges the used calculation of α.
The photon scalar irradiance at the onset of photosynthesis saturation (Ek) of the leaves and epiphytes increased with increasing temperature, with the bare leaf showing lowest EK values at all temperatures, as compared to the separated epiphytes and leaves with epiphytes (Figure 2D). At 27°C, separated epiphytes and leaves with epiphytes reached the onset of light saturation at higher light levels (i.e., 193μmol photons m–2 s–1 and 194 μmol photons m–2 s–1), as compared to the bare leaves (123 μmol photons m–2 s–1) (Figure 2D). Similarly, the compensation irradiance (Ec), i.e., the photon scalar irradiance above which leaves/epiphytes exhibited a net O2 production and thus an autotrophic carbon balance, increased quasi-exponentially (R2 = 0.99) with temperature for all samples (Figure 2E). Epiphytes separated from the leaves and leaves with epiphytes displayed a stronger increase in Ec with increasing temperature, as compared to bare leaves exhibiting the lowest Ec values at all temperatures (Figure 2E). Student’s t-tests confirmed significant differences (p < 0.05) between Ec values of the bare leaves, of the epiphytes separated from the leaves, and of the leaves with epiphytes. The temperature dependency of Ec thus increased the light irradiance demand of the specimens to maintain a positive carbon balance. The lower light compensation level (Ec) observed in bare leaves (Figure 2E), also demonstrated by Staehr and Borum (2011), was accompanied by a lower onset of photosynthesis saturation point (Ek) (Figure 2D) indicating that bare leaves were less temperature dependent/affected (e.g., Wieland and Kühl, 2000). In contrast, leaves with epiphytes exhibited the highest Ec levels over all experimental temperatures (Figure 2E). The high irradiance requirement reflects the impediment of the seagrass by the epiphytic cover; that is, the biofilm was shading the incoming irradiance, affecting both the light quality and quantity reaching the leaf surface (Brodersen et al., 2015a), increasing the light requirement of the epiphyte covered leaf. Thus under future warmer ocean conditions, Z. marina might become limited to shallow waters with sufficiently high irradiance. The water clarity in coastal waters is challenged by ongoing eutrophication by accelerating land runoff, which is promoting algal blooms and epiphytic growth on the seagrass leaves (Borum, 1985; Brodersen et al., 2020a). Furthermore, shallow coastal habitats often experience fluctuating temperatures, O2 levels, and pH changes that constitute a threat to seagrass growth and survival both during the day and at nighttime (Greve et al., 2003; Borum et al., 2005; Pulido Pérez and Borum, 2010; Raun and Borum, 2013; Brodersen et al., 2015a,b; Koren et al., 2015; Brodersen et al., 2020a,b; Rasmusson et al., 2020).
Net Daily Oxygen Production
The net daily oxygen production (DP) calculations provided an overview of the net oxygen budget of the bare leaves, leaves with epiphytes and epiphytes separated from the leaves over a diel cycle with 14 h of light exposure followed by 10 h of darkness. The epiphytes had highest oxygen surplus over diel cycles when exposed to 12–22°C, with up to 2.3-fold higher values compared to the seagrass (Figure 3). This dominance by the epiphytes, at the lower temperature, suggests a competitive advantage for the epiphytes in cold waters. Thus, the bare leaves displayed an optimum at about 22°C in the diel oxygen budget (Figure 3), and exceeded the epiphytes and especially the leaves with epiphytes at 27°C, due the lower temperature impact and higher tolerance (Table 1). At 27°C, an oxygen deficit (i.e., negative DP) was observed for all specimens, indicating onset of hypoxic/anoxic stress conditions; with the lowest oxygen deficiency determined for the bare leaves and the highest oxygen deficiency observed on leaves with epiphytes (Figure 3). Bare seagrass leaves were apparently less affected by the increasing temperature. This was supported by our calculated Q10 values over the temperature range of 12–22°C showing a doubling of respiration, maximum gross, and net photosynthesis rates (Table 1). Bare leaves showed a higher Q10 value for photosynthesis than respiration, as compared to leaves with epiphytes and separated epiphytes that both displayed higher Q10 values for respiration as compared to photosynthesis (Table 1). Such negative DP at high seawater temperature can be detrimental to the seagrass plant as H2S intrusion from the surrounding sediment may occur (Pedersen et al., 2004; Borum et al., 2005; Holmer et al., 2009; Holmer and Hasler-Sheetal, 2014). Considering the ability of Z. marina to acclimate to the different seasonal conditions (Staehr and Borum, 2011), seagrass performance is expected to increase, if the experiments described above were conducted with summer acclimated plant tissue. Further investigations of the seasonal epiphytic biofilm composition and performance, especially epiphytes growing on seagrass leaves throughout the summer/autumn season is thus needed (Borum et al., 1984).
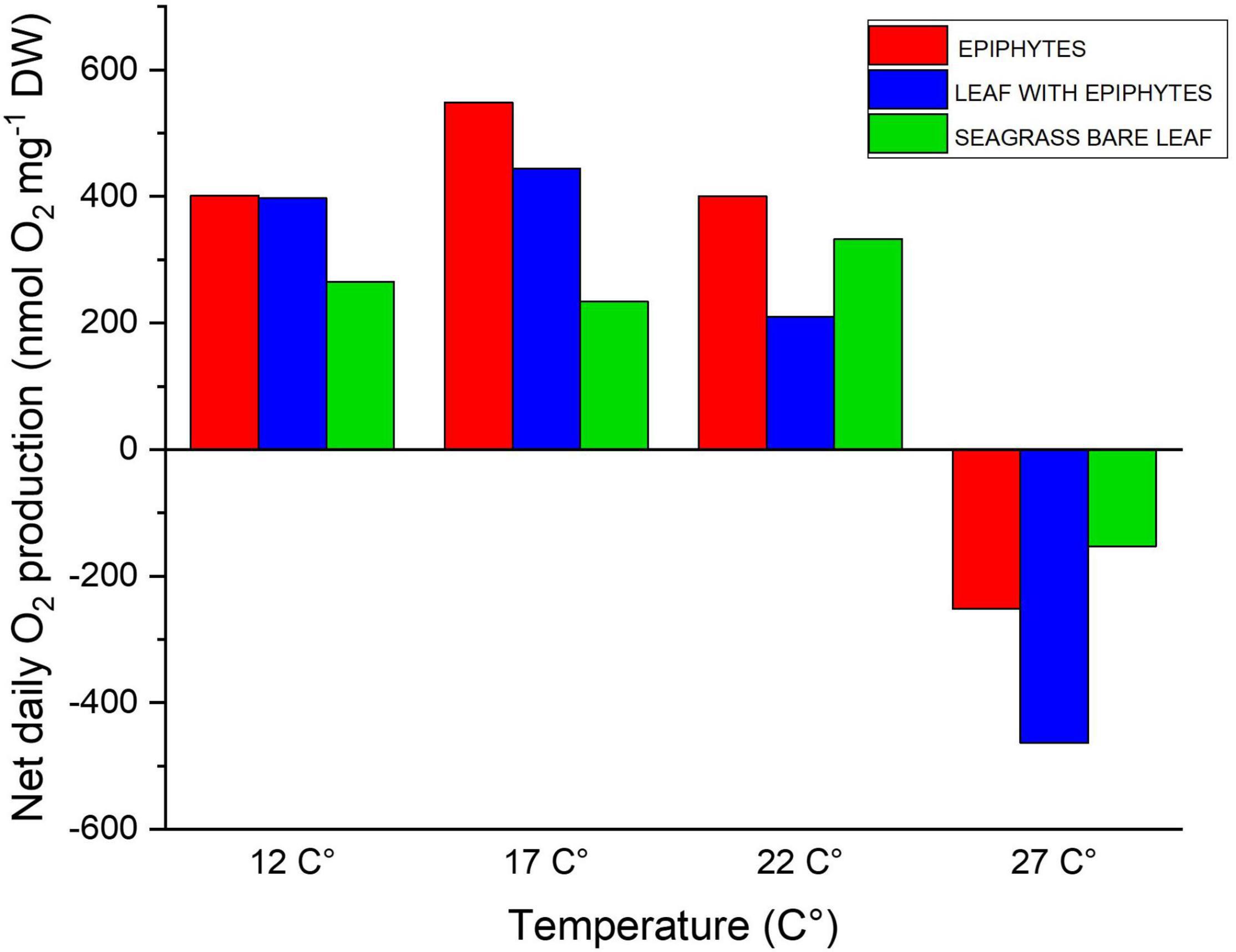
Figure 3. Diel oxygen budget as function of increasing temperatures (12, 17, 22, and 27°C), illustrating the daily net oxygen surplus of the leaf and epiphyte communities. Red bars represent epiphytes originating from Z. marina leaves. Blue bars represent Z. marina leaves with epiphytes. Green bars represent bare Z. marina leaves. The daily net oxygen production was calculated from the fitted exponential saturation models at increasing temperature (see Figure 1; all with a R2 ≥ 0.99) using an in situ measured 14h:10 h light/dark cycle (Staehr et al., 2018). The averaged exponential saturation fits were calculated from a biological replication of 4.
Final pH Measurements: Dissolved Inorganic Carbon Extraction Capacity
A maximal final pH of 9.5 was observed for bare Z. marina L. leaves under 22°C (Figure 4). This result is very similar to recent studies of final pH in other seagrass species, i.e., pH 9.6 in Thalassia hemprichii and Enhalus acoroides (Pedersen et al., 2016) and pH 9.6 in Z. marina L. (Brodersen et al., 2020a). The carbon extraction capacity curves for all specimens (Figure 4) indicate that their CO2 concentration mechanisms (CCMs) are temperature dependent and were mostly affected by the lowest and highest seawater temperatures, corresponding to normal temperature-induced effects on seagrass enzymes like the CO2-concentrating enzyme carbonic anhydrase (CA) (e.g., Staehr and Borum, 2011). The separated epiphytes exhibited the lowest bicarbonate extraction capacity, both under the lowest temperature (12°C; pH 8.9) and the highest temperatures (22 and 27°C), while they exhibited a maximum final pH of 9.4 at 17°C (Figure 4). Leaves with epiphytes and bare leaves reached their maximum final pH of 9.5 at 22°C (Figure 4). A steep decline in final pH was found between 22 and 27°C for all samples, where only leaves with epiphytes reached pH > 9 at temperatures of 27°C (Figure 4). This indicates that bare Z. marina L. leaves and the separated epiphytes apparently lost their ability to utilize bicarbonate in their photosynthesis at 27°C (Figure 4; as the specimens exhibited final pH values < 9). This observation emphasizes that the bicarbonate utilization strategies (CCMs, e.g., Larkum et al., 2017) likely are too energy consuming (Borum et al., 2016) when the temperature is markedly exceeding the normal habitual temperature range (here, at 27°C). Other explanations for the apparent lost or reduced (i.e., for leaves with epiphytes) ability to use bicarbonate at 27°C, are that high temperature-induced increased tissue and/or epiphytic biofilm respiration leads to increased CO2 concentrations in the aerenchyma that can diffuse and thus concentrate CO2 around RuBisCO. Nevertheless, the inorganic carbon extraction capacity was not only specimen specific (i.e., seagrass vs. associated epiphytes) but also temperature dependent (Figure 4). Seagrass and the epiphytes originating from Z. marina L. are therefore expected to experience lower photosynthetic efficiency under future elevated seawater temperatures surpassing 27°C.
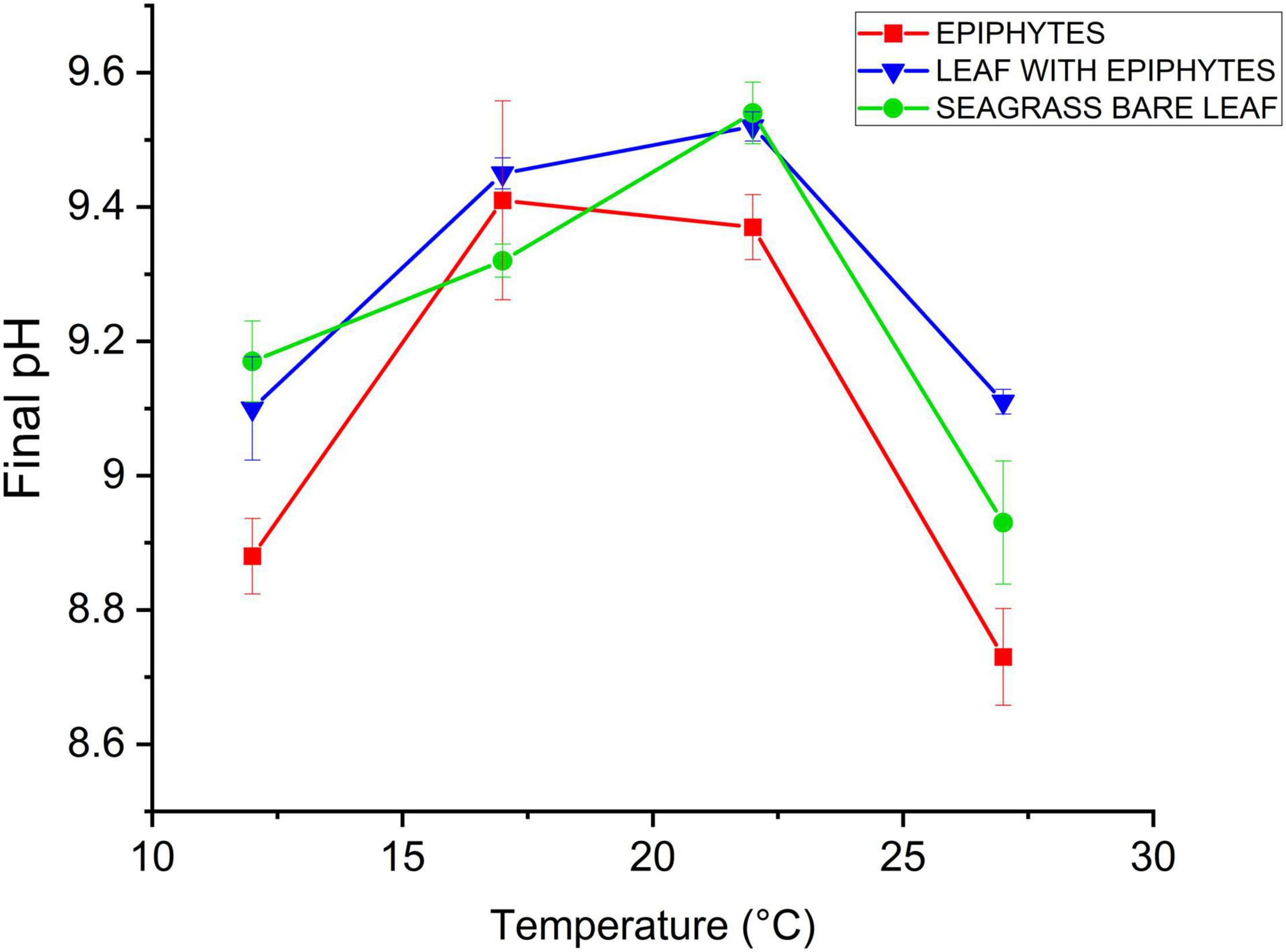
Figure 4. Inorganic carbon extraction capacity as a function of increasing temperature (12, 17, 22, and 27°C). Red symbols and lines represent epiphytes originating from Z. marina leaves. Blue symbols and lines represent Z. marina leaves with epiphytes. Green symbols and lines represent bare Z. marina leaves. Data point with error bars represent means ± SEM (n = 4, biological replicates).
Furthermore, with the lowest inorganic carbon extraction capacities at all temperatures, except from (17°C), the epiphytes originating from Z. marina L. appear to have less efficient CCMs compared to the seagrass leaves. This is inline with previous findings showing that simple filamentous algae often lack effective CCMs (Hepburn et al., 2011). Several members of the epiphytic biofilm community originating from Z. marina L. could thus constitute obligate CO2 users. If this is the case, the epiphytes will be restricted by lack of inorganic carbon sources, as the photosynthetic activity of obligate CO2 users are limited at low CO2 concentrations (Albert et al., 2020). Such epiphytes would benefit from ocean acidification. Whether the seagrass did or did not have epiphytic cover, did not show an indicative difference in the inorganic carbon extraction capacity (Figure 4). However, one must assume that the presence of epiphytic biofilm and consequently a change in the seagrass phyllosphere will have significance for the seagrass’ bicarbonate utilization ability. As demonstrated by Brodersen et al. (2020a), the presence of epiphytic biofilm can lead to a reduced photosynthetic efficiency due to basification of the phyllosphere and increasing DBL thickness; hence, cause inorganic carbon limitation. Furthermore, epiphytes extend the diffusion distance between the leaf and the ambient water, and thus impede gas movement in between, moreover, constitute a competitor for the inorganic carbon sources (Brodersen et al., 2020a). In our study, the observed peak in the final pH at 17°C (Figure 4) along with the results from the gas exchange experiments demonstrate that the epiphytes originating from Z. marina L. exhibited optimal photosynthetic performance at 17–22°C. Hence, this increased dependency on sufficient CO2 availability and supply at high seawater temperatures (27°C), may put seagrass leaves and associated epiphytes at enhanced risk of carbon limitation under future climate conditions.
Here, we investigated eelgrass plants and their epiphyte communities sampled at the beginning of the growth season (April) under the spring epiphyte-bloom (Borum et al., 1984). The results are therefore more likely to reflect how plants and epiphytes may be impacted in the early summer months in northern European waters (here Northern Øresund, Denmark), where the maximal water temperature increases about 10°C over a 3-month period (i.e., from ∼10°C in April to ∼23°C in July), and where the plants generally have higher chlorophyll a and nitrogen content, as compared to the late summer months and the autumn epiphyte-bloom (Borum et al., 1984; Staehr and Borum, 2011). Our results (e.g., the increase in critical light requirement with increasing temperature) might therefore be slightly overestimated as compared to the situation for summer-acclimated plants and epiphyte communities. Furthermore, rates of respiration are expected to be peaking at slightly lower temperatures in our spring-acclimated seagrass and epiphyte communities (Staehr and Borum, 2011). Temperate seagrass meadows growing in more open waters with frequent circulation/mixing of the water body are also less likely to experience such high temperatures as investigated in this study (i.e., 27°C) and the derived negative daily O2 production and loss of the ability to utilize bicarbonate as a response to increasing water temperatures. It is also important to note that the potential role of species adaptation and how species originating from latitudinal gradients respond to climate change was not considered in this study (e.g., Short and Neckles, 1999). To further elucidate and predict if one of the specimens (i.e., seagrass leaf or associated epiphytes) will benefit from the changing environmental conditions induced by global climate change, investigations of metabolic responses in different seasonal epiphytic biofilm communities need to be conducted. Furthermore, experimental temperature treatments above 27°C and with longer-term exposure to different temperatures, could provide more detailed information about Z. marina L. acclimation/adaptation capabilities. However, water temperatures above 27°C are highly unlikely at the seagrass sampling site (open water in Denmark). Another major challenge as a consequence of global warming are marine heat waves (MHW) (Nguyen et al., 2021). MHWs can exceed the average temperature for more than 5 days in a row and may present a bigger threat to marine organisms than the current progressive temperature increase (Nguyen et al., 2021). Thus, investigations of the resilience of seagrass and associated epiphytes to these frequent extreme thermal events could supply crucial information about future changes in seagrass-epiphyte interactions and potential effects on plant fitness. Finally, similar measurements should be performed in tropical environments, where seagrasses may be living at water temperatures closer to their thermal stress thresholds, to further elucidate the global impact of ocean warming on the intricate seagrass/epiphyte relationship.
Conclusion
Based on our measurements of a low light compensation level (Ec), high light utilization efficiency (α), and high maximum net photosynthesis rates (NPMAX values) at all temperatures for bare leaves we conclude that bare seagrass leaves are less affected by elevated temperatures as compared to seagrass leaves with epiphyte cover and the epiphytes themselves. The high Ek values of leaves with epiphytes and separated epiphytes are likely caused by self-shading. The experiments also confirmed impairment of metabolic rates (i.e., photosynthesis and respiration) when the seagrass leaves had epiphytic cover, revealing that the presence of epiphytic biofilm makes the seagrass more vulnerable to elevated temperatures, e.g., due to thicker DBLs and TDDs, as well as diminished availability of light and inorganic carbon. Epiphytes originating from Z. marina L. exhibited the highest temperature dependency, and thus most susceptible to effects of ocean warming. Moreover, epiphytes displayed less efficient CCMs, implying that the epiphytes will benefit from ocean acidification. Seagrass without epiphytes will be less affected by elevated temperatures and marine heat waves. Increasing seawater temperatures in future oceans may thus affect Z. marina leaf-epiphytes interactions as the presence of epiphytes can negatively affect Z. marina plants responses to temperature increases. Hence, we found a ∼2-fold faster decrease in the net daily O2 balance over the temperature range of 17–27°C for leaves with epiphytes as compared to bare leaves, increasing the risk of inadequate internal plant aeration. Such shift in seagrass-epiphyte interactions may induce stress responses in the plant triggering, for example, increased production of zosteric acid with antifouling activity that can reduce the initial colonization of leaves with microorganisms (Villa et al., 2010) and thus likely improve the plants O2 balance.
Data Availability Statement
The original contributions presented in the study are included in the article/Supplementary Material, further inquiries can be directed to the corresponding author/s.
Author Contributions
KEB designed the research and supervised experimental work. MK provided infrastructure, additional supervision, and input to data analysis. AH and AP conducted the experiments, analyzed the data, and wrote the manuscript with editorial help from MK and KEB. All authors contributed to the article and approved the submitted version.
Funding
This study was funded by grants from the Villum Foundation (00028156) to KEB and the Independent Research Fund Denmark (DFF-8021-00308B) to MK.
Conflict of Interest
The authors declare that the research was conducted in the absence of any commercial or financial relationships that could be construed as a potential conflict of interest.
Publisher’s Note
All claims expressed in this article are solely those of the authors and do not necessarily represent those of their affiliated organizations, or those of the publisher, the editors and the reviewers. Any product that may be evaluated in this article, or claim that may be made by its manufacturer, is not guaranteed or endorsed by the publisher.
Acknowledgments
We thank Mary Gonzalez and Victoria Thuesen for technical assistance. We thank Peter A. Staehr for providing the light data used to calculate the diel O2 balances.
Supplementary Material
The Supplementary Material for this article can be found online at: https://www.frontiersin.org/articles/10.3389/fmars.2022.822485/full#supplementary-material
References
Albert, G., Hepburn, C. D., Pajusalu, L., Paalme, T., Pritchard, D. W., and Martin, G. (2020). Could ocean acidification influence epiphytism? A comparison of carbon-use strategies between Fucus vesiculosus and its epiphytes in the Baltic Sea. J. Appl. Phycol. 32, 2479–2487. doi: 10.1007/s10811-019-01953-z
Assis, J., Fragkopoulou, E., Frade, D., Neiva, J., Oliveira, A., Abecasis, D., et al. (2020). A fine-tuned global distribution dataset of marine forests. Sci. Data 7:119. doi: 10.1038/s41597-020-0459-x
Ballesteros, E., Cebrian, E., and Alcoverro, T. (2007). Mortality of shoots of Posidonia oceanica following meadow invasion by the red alga Lophocladia lallemandii. Bot. Mar. 50, 8–13. doi: 10.1515/BOT.2007.002
Beca-Carretero, P., Olesen, B., Marbà, N., and Krause-Jensen, D. (2018). Response to experimental warming in northern eelgrass populations: comparison across a range of temperature adaptations. Mar. Ecol. Prog. Ser. 589, 59–72. doi: 10.3354/meps12439
Bertelli, C. M., and Unsworth, R. K. (2014). Protecting the hand that feeds us: seagrass (Zostera marina) serves as commercial juvenile fish habitat. Mar. Pollut. Bull. 83, 425–429. doi: 10.1016/j.marpolbul.2013.08.011
Blaabjerg, V., Mouritsen, K. N., and Finster, K. (1998). Diel cycles of sulphate reduction rates in sediments of a Zostera marina bed (Denmark). Aquat. Microb. Ecol. 15, 97–102. doi: 10.3354/ame015097
Borum, J. (1985). Development of epiphytic communities on eelgrass (Zostera marina) along a nutrient gradient in a Danish estuary. Mar. Biol. 87, 211–218. doi: 10.1007/BF00539431
Borum, J., Kaas, H., and Wium-Andersen, S. (1984). Biomass variation and autotrophic production of an epiphyte-macrophyte community in a coastal Danish area: II. Epiphyte species composition, biomass and production. Ophelia 23, 165–179. doi: 10.1080/00785326.1984.10426612
Borum, J., Pedersen, O., Greve, T. M., Frankovich, T. A., Zieman, J. C., Fourqurean, J. W., et al. (2005). The potential role of plant oxygen and sulphide dynamics in die-off events of the tropical seagrass, Thalassia testudinum. J. Ecol. 93, 148–158. doi: 10.1111/j.1365-2745.2004.00943.x
Borum, J., Pedersen, O., Kotula, L., Fraser, M. W., Statton, J., Colmer, T. D., et al. (2016). Photosynthetic response to globally increasing CO2 of co-occurring temperate seagrass species. Plant Cell Environ. 39, 1240–1250. doi: 10.1111/pce.12658
Borum, J., Sand-Jensen, K., Binzer, T., Pedersen, O., and Greve, T. (2006). “Oxygen movement in seagrasses,” in Seagrasses: Biology, Ecology and Conservation, eds A. W. D. Larkum, J. R. Orth, and C. M. Duarte (Dordrecht: Springer), 255–270. doi: 10.1007/1-4020-2983-7_10
Brodersen, K. E. (2016). The Seagrass Rhizosphere. Ph.D. thesis. Sydney, NSW: University of Technology Sydney.
Brodersen, K. E., and Kühl, M. (2022). Effects of epiphytes on the seagrass phyllosphere. Front. Mar. Sci. 9:821614. doi: 10.3389/fmars.2022.821614
Brodersen, K. E., Hammer, K. J., Schrameyer, V., Floytrup, A., Rasheed, M. A., Ralph, P. J., et al. (2017). Sediment resuspension and deposition on seagrass leaves impedes internal plant aeration and promotes phytotoxic H2S intrusion. Front. Plant Sci. 8:657. doi: 10.3389/fpls.2017.00657
Brodersen, K. E., Koren, K., Revsbech, N. P., and Kühl, M. (2020a). Strong leaf surface basification and CO2 limitation of seagrass induced by epiphytic biofilm microenvironments. Plant Cell Environ. 43, 174–187. doi: 10.1111/pce.13645
Brodersen, K. E., Kühl, M., Nielsen, D. A., Pedersen, O., and Larkum, A. W. (2018). “Rhizome, root/sediment interactions, aerenchyma and internal pressure changes in seagrasses,” in Seagrasses of Australia, eds A. Larkum, G. Kendrick, and P. Ralph (Cham: Springer), 393–418. doi: 10.1007/978-3-319-71354-0_13
Brodersen, K. E., Kühl, M., Trampe, E., and Koren, K. (2020b). Imaging O2 dynamics and microenvironments in the seagrass leaf phyllosphere with magnetic optical sensor nanoparticles. Plant J. 104, 1504–1519. doi: 10.1111/tpj.15017
Brodersen, K. E., Lichtenberg, M., Paz, L.-C., and Kühl, M. (2015a). Epiphyte-cover on seagrass (Zostera marina L.) leaves impedes plant performance and radial O2 loss from the below-ground tissue. Front. Mar. Sci. 2:58. doi: 10.3389/fmars.2015.00058
Brodersen, K. E., Nielsen, D. A., Ralph, P. J., and Kühl, M. (2014). A split flow chamber with artificial sediment to examine the below-ground microenvironment of aquatic macrophytes. Mar. Biol. 161, 2921–2930. doi: 10.1007/s00227-014-2542-3
Brodersen, K. E., Nielsen, D. A., Ralph, P. J., and Kühl, M. (2015b). Oxic microshield and local pH enhancement protects Zostera muelleri from sediment derived hydrogen sulphide. New Phytol. 205, 1264–1276. doi: 10.1111/nph.13124
Costanza, R., d’Arge, R., de Groot, R., Farber, S., Grasso, M., Hannon, B., et al. (1997). The value of the world’s ecosystem services and natural capital. Nature 387, 253–260. doi: 10.1038/387253a0
Cullen-Unsworth, L. C., and Unsworth, R. (2018). A call for seagrass protection. Science 361, 446–448. doi: 10.1126/science.aat7318
Dennison, W. C. (1987). Effects of light on seagrass photosynthesis, growth and depth distribution. Aquat. Bot. 27, 15–26. doi: 10.1016/0304-3770(87)90083-0
Evans, A. S., Webb, K. L., and Penhale, P. A. (1986). Photosynthetic temperature acclimation in two coexisting seagrasses, Zostera marina L. and Ruppia maritima L. Aquat. Bot. 24, 185–197. doi: 10.1016/0304-3770(86)90095-1
Fonseca, M. S., and Cahalan, J. A. (1992). A preliminary evaluation of wave attenuation by four species of seagrass. Estuar. Coast. Shelf Sci. 35, 565–576. doi: 10.1016/S0272-7714(05)80039-3
Fourqurean, J. W., Duarte, C. M., Kennedy, H., Marba, N., Holmer, M., Mateo, M. A., et al. (2012). Seagrass ecosystems as a globally significant carbon stock. Nat. Geosci. 5, 505–509. doi: 10.1038/ngeo1477
Gattuso, J.-P., and Hansson, L. (2011). Ocean Acidification. Oxford: Oxford University press. doi: 10.1093/oso/9780199591091.001.0001
Greve, T. M., Borum, J., and Pedersen, O. (2003). Meristematic oxygen variability in eelgrass (Zostera marina). Limnol. Oceanogr. 48, 210–216. doi: 10.4319/lo.2003.48.1.0210
Hepburn, C., Pritchard, D., Cornwall, C., McLeod, R., Beardall, J., Raven, J., et al. (2011). Diversity of carbon use strategies in a kelp forest community: implications for a high CO2 Ocean. Glob. Chang. Biol. 17, 2488–2497. doi: 10.1111/j.1365-2486.2011.02411.x
Holmer, M., and Hasler-Sheetal, H. (2014). Sulfide intrusion in seagrasses assessed by stable sulfur isotopes—a synthesis of current results. Front. Mar. Sci. 1:64. doi: 10.3389/fmars.2014.00064
Holmer, M., Pedersen, O., Krause-Jensen, D., Olesen, B., Hedegård Petersen, M., Schopmeyer, S., et al. (2009). Sulfide intrusion in the tropical seagrasses Thalassia testudinum and Syringodium filiforme. Estuar. Coast. Shelf Sci. 85, 319–326. doi: 10.1016/j.ecss.2009.08.015
Kim, M., Brodersen, K. E., Szabó, M., Larkum, A. W., Raven, J. A., Ralph, P. J., et al. (2018). Low oxygen affects photophysiology and the level of expression of two-carbon metabolism genes in the seagrass Zostera muelleri. Photosynth. Res. 136, 147–160. doi: 10.1007/s11120-017-0452-1
Koren, K., Brodersen, K. E., Jakobsen, S. L., and Kühl, M. (2015). Optical sensor nanoparticles in artificial sediments – a new tool to visualize O2 dynamics around the rhizome and roots of seagrasses. Environ. Sci. Technol. 49, 2286–2292. doi: 10.1021/es505734b
Lamb, J. B., van de Water, J. A. J. M., Bourne, D. G., Altier, C., Hein, M. Y., Fiorenza, E. A., et al. (2017). Seagrass ecosystems reduce exposure to bacterial pathogens of humans, fishes, and invertebrates. Science 355, 731–733. doi: 10.1126/science.aal1956
Larkum, A. W. D., Davey, P. A., Kuo, J., Ralph, P. J., and Raven, J. A. (2017). Carbon-concentrating mechanisms in seagrasses. J. Exp. Bot. 68, 3773–3784. doi: 10.1093/jxb/erx206
Larkum, A. W. D., Orth, R. J., and Duarte, C. M. (2006). Seagrasses: Biology, ecology and conservation. Dordrecht: Springer. doi: 10.1007/978-1-4020-2983-7
Larkum, A. W., Pernice, M., Schliep, M., Davey, P., Szabo, M., Raven, J. A., et al. (2018). “Photosynthesis and metabolism of seagrasses,” in Seagrasses of Australia, eds A. W. D. Larkum, G. A. Kendrick, and P. J. Ralph (Cham: Springer), 315–342. doi: 10.1007/978-3-319-71354-0_11
Levitus, S., Antonov, J. I., Boyer, T. P., and Stephens, C. (2000). Warming of the world ocean. Science 287, 2225–2229. doi: 10.1126/science.287.5461.2225
Macreadie, P. I., Trevathan-Tackett, S. M., Skilbeck, C. G., Sanderman, J., Curlevski, N., Jacobsen, G., et al. (2015). Losses and recovery of organic carbon from a seagrass ecosystem following disturbance. Proc. R. Soc. B Biol. Sci. 282:20151537. doi: 10.1098/rspb.2015.1537
Marsh, J. A. Jr., Dennison, W. C., and Alberte, R. S. (1986). Effects of temperature on photosynthesis and respiration in eelgrass (Zostera marina L.). J. Exp. Mar. Biol. Ecol. 101, 257–267. doi: 10.1016/0022-0981(86)90267-4
Mazzella, L., and Alberte, R. S. (1986). Light adaptation and the role of autotrophic epiphytes in primary production of the temperate seagrass, Zostera marina L. J. Exp. Mar. Biol. Ecol. 100, 165–180. doi: 10.1016/0022-0981(86)90161-9
Nguyen, H. M., Ralph, P. J., Marín-Guirao, L., Pernice, M., and Procaccini, G. (2021). Seagrasses in an era of ocean warming: a review – under review in biological reviews. Biol. Rev. 96, 2009–2030. doi: 10.1111/brv.12736
Nielsen, L. B., Finster, K., Welsh, D. T., Donelly, A., Herbert, R. A., De Wit, R., et al. (2001). Sulphate reduction and nitrogen fixation rates associated with roots, rhizomes and sediments from Zostera noltii and Spartina maritima meadows. Environ. Microbiol. 3, 63–71. doi: 10.1046/j.1462-2920.2001.00160.x
Noisette, F., Depetris, A., Kühl, M., and Brodersen, K. E. (2020). Flow and epiphyte growth effects on the thermal, optical and chemical microenvironment in the leaf phyllosphere of seagrass (Zostera marina). J. R. Soc. Interface 17:20200485. doi: 10.1098/rsif.2020.0485
Orth, R. J., Carruthers, T. J. B., Dennison, W. C., Duarte, C. M., Fourqurean, J. W., and Heck, K. L. Jr., et al. (2006). A global crisis for seagrass ecosystems. BioScience 56, 987–999. doi: 10.1641/0006-3568(2006)56[987:AGCFSE]2.0.CO;2
Pedersen, O., Binzer, T., and Borum, J. (2004). Sulphide intrusion in eelgrass (Zostera marina L.). Plant Cell Environ. 27, 595–602. doi: 10.1111/j.1365-3040.2004.01173.x
Pedersen, O., Colmer, T. D., Borum, J., Zavala-Perez, A., and Kendrick, G. A. (2016). Heat stress of two tropical seagrass species during low tides–impact on underwater net photosynthesis, dark respiration and diel in situ internal aeration. New Phytol. 210, 1207–1218. doi: 10.1111/nph.13900
Penhale, P. A. (1977). Macrophyte-epiphyte biomass and productivity in an eelgrass (Zostera marina L.) community. J. Exp. Mar. Biol. Ecol. 26, 211–224. doi: 10.1016/0022-0981(77)90109-5
Pulido Pérez, C., and Borum, J. (2010). Eelgrass (Zostera marina) tolerance to anoxia. J. Exp. Mar. Biol. Ecol. 385, 8–13. doi: 10.1016/j.jembe.2010.01.014
Ralph, P. J., Durako, M. J., Enríquez, S., Collier, C. J., and Doblin, M. A. (2007). Impact of light limitation on seagrasses. J. Exp. Mar. Biol. Ecol. 350, 176–193. doi: 10.1016/j.jembe.2007.06.017
Rasmusson, L. M., Buapet, P., George, R., Gullström, M., Gunnarsson, P. C., and Björk, M. (2020). Effects of temperature and hypoxia on respiration, photorespiration, and photosynthesis of seagrass leaves from contrasting temperature regimes. ICES J. Mar. Sci. 77, 2056–2065. doi: 10.1093/icesjms/fsaa093
Raun, A. L., and Borum, J. (2013). Combined impact of water column oxygen and temperature on internal oxygen status and growth of Zostera marina seedlings and adult shoots. J. Exp. Mar. Biol. Ecol. 441, 16–22. doi: 10.1016/j.jembe.2013.01.014
Raven, J. A., Beardall, J., and Giordano, M. (2014). Energy costs of carbon dioxide concentrating mechanisms in aquatic organisms. Photosynth. Res. 121, 111–124. doi: 10.1007/s11120-013-9962-7
Resplandy, L., Keeling, R. F., Eddebbar, Y., Brooks, M., Wang, R., Bopp, L., et al. (2019). Quantification of ocean heat uptake from changes in atmospheric O2 and CO2 composition. Sci. Rep. 9:20244. doi: 10.1038/s41598-019-56490-z
Riebesell, U., Aberle-Malzahn, N., Achterberg, E. P., Algueró-Muñiz, M., Alvarez-Fernandez, S., Arístegui, J., et al. (2018). Toxic algal bloom induced by ocean acidification disrupts the pelagic food web. Nat. Clim. Change 8, 1082–1086. doi: 10.1038/s41558-018-0344-1
Sand-Jensen, K. (1977). Effect of epiphytes on eelgrass photosynthesis. Aquat. Bot. 3(Suppl. C), 55–63. doi: 10.1016/0304-3770(77)90004-3
Sand-Jensen, K., Pedersen, M. F., and Nielsen, S. L. (1992). Photosynthetic use of inorganic carbon among primary and secondary water plants in streams. Freshw. Biol. 27, 283–293. doi: 10.1111/j.1365-2427.1992.tb00540.x
Short, F. T., and Neckles, H. A. (1999). The effects of global climate change on seagrasses. Aquat. Bot. 63, 169–196. doi: 10.1016/S0304-3770(98)00117-X
Spilling, K., Titelman, J., Greve, T. M., and Kühl, M. (2010). Microsensor measurements of the external and internal microenvironment of Fucus vesiculosus (phaeophyceae). J. Phycol. 46, 1350–1355. doi: 10.1111/j.1529-8817.2010.00894.x
Staehr, P. A., and Borum, J. (2011). Seasonal acclimation in metabolism reduces light requirements of eelgrass (Zostera marina). J. Exp. Mar. Biol. Ecol. 407, 139–146. doi: 10.1016/j.jembe.2011.05.031
Staehr, P. A., Asmala, E., Carstensen, J., Krause-Jensen, D., and Reader, H. (2018). Ecosystem metabolism of benthic and pelagic zones of a shallow productive estuary: spatio-temporal variability. Mar. Ecol. Prog. Ser. 601, 15–32. doi: 10.3354/meps12697
Tarquinio, F., Bourgoure, J., Koenders, A., Laverock, B., Säwström, C., and Hyndes, G. A. (2018). Microorganisms facilitate uptake of dissolved organic nitrogen by seagrass leaves. ISME J. 12, 2796–2800. doi: 10.1038/s41396-018-0218-6
Unsworth, R. K. F., and Cullen-Unsworth, L. C. (2014). “Biodiversity, ecosystem services, and the conservation of seagrass meadows,” in Coastal Conservation, eds B. Maslo and J. Lockwood (Cambridge: Cambridge University Press), 95–130. doi: 10.1017/CBO9781139137089.005
Unsworth, R. K. F., and Cullen-Unsworth, L. C. (2017). Seagrass meadows. Curr. Biol. 27, R443–R445. doi: 10.1016/j.cub.2017.01.021
Unsworth, R. K. F., Nordlund, L. M., and Cullen-Unsworth, L. C. (2019). Seagrass meadows support global fisheries production. Conserv. Lett. 12, 1–8. doi: 10.1111/conl.12566
Villa, F., Albanese, D., Giussani, B., Stewart, P. S., Daffonchio, D., and Cappitelli, F. (2010). Hindering biofilm formation with zosteric acid. Biofouling 26, 739–752. doi: 10.1080/08927014.2010.511197
Waycott, M., Duarte, C. M., Carruthers, T. J. B., Orth, R. J., Dennison, W. C., Olyarnik, S., et al. (2009). Accelerating loss of seagrasses across the globe threatens coastal ecosystems. Proc. Natl. Acad. Sci. U.S.A. 106, 12377–12381. doi: 10.1073/pnas.0905620106
Webb, W. L., Newton, M., and Starr, D. (1974). Carbon dioxide exchange of Alnus rubra. Oecologia 17, 281–291. doi: 10.1007/BF00345747
Keywords: biofilm, CO2, epiphytes, global warming, photosynthesis, respiration, seagrass
Citation: Hansen AB, Pedersen AS, Kühl M and Brodersen KE (2022) Temperature Effects on Leaf and Epiphyte Photosynthesis, Bicarbonate Use and Diel O2 Budgets of the Seagrass Zostera marina L.. Front. Mar. Sci. 9:822485. doi: 10.3389/fmars.2022.822485
Received: 25 November 2021; Accepted: 08 February 2022;
Published: 16 March 2022.
Edited by:
Christian Grenz, UMR 7294 Institut Méditerranéen d’Océanographie (MIO), FranceReviewed by:
Peter Anton Upadhyay Staehr, Aarhus University, DenmarkNadine Schubert, University of Algarve, Portugal
Copyright © 2022 Hansen, Pedersen, Kühl and Brodersen. This is an open-access article distributed under the terms of the Creative Commons Attribution License (CC BY). The use, distribution or reproduction in other forums is permitted, provided the original author(s) and the copyright owner(s) are credited and that the original publication in this journal is cited, in accordance with accepted academic practice. No use, distribution or reproduction is permitted which does not comply with these terms.
*Correspondence: Kasper Elgetti Brodersen, a2FzcGVyLmVsZ2V0dGkuYnJvZGVyc2VuQGJpby5rdS5kaw==
†These authors have contributed equally to this work and share first authorship