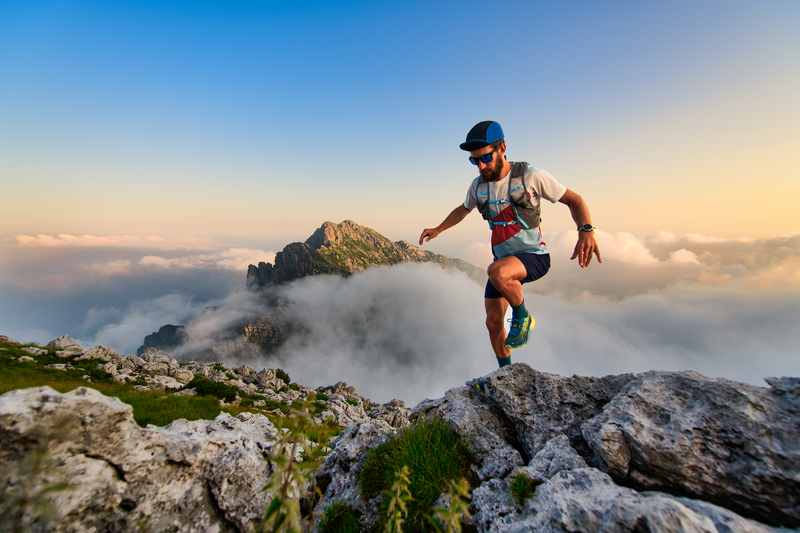
95% of researchers rate our articles as excellent or good
Learn more about the work of our research integrity team to safeguard the quality of each article we publish.
Find out more
ORIGINAL RESEARCH article
Front. Mar. Sci. , 29 March 2022
Sec. Marine Biology
Volume 9 - 2022 | https://doi.org/10.3389/fmars.2022.818083
This article is part of the Research Topic Effects of Environmental Stress on Marine Mollusks: Biomarkers, Physiological Responses and Underlying Mechanisms View all 7 articles
The bay scallop southern subspecies, Argopecten irradians concentricus, which is widely cultured in southern China waters, is a eurythermal animal that is more adaptive to high-temperature waters but less tolerant to low-temperature waters. Despite that temperature is one of the most dominant environmental factors affecting dramatically its growth, survival, and hence production, the mechanism underlying the responses to thermal stress has not been explored in this animal. In this study, transcriptomic and metabolomic analyses were carried out in the adductor muscles of the bay scallop southern subspecies exposed to low and high thermal stresses. Transcriptomic analyses revealed that differentially expressed genes (DEGs) were enriched in the calcium ion, kinase activity, phosphatase activity, and lipid-related pathways in the group exposed to thermal stress, while most DEGs were enriched in the RNA processing pathways in the group exposed to low-temperature thermal stress. A large number of differentially expressed transcription factors involved in oxidation-reduction process, membrane-related pathways, transmembrane signaling receptor activity, and transduction-related pathways were induced by exposure to thermal stress. Results from metabolomic analyses showed that the retinol metabolism, inositol phosphate metabolism, and phosphatidylinositol pathways may be involved in the responses to high thermal stress, while more signaling pathways were enriched in the group exposed to low thermal stress. Integrated analyses of the transcriptomic and metabolomic data indicated that the degradation of valine, leucine, and isoleucine and the tricarboxylic acid cycle may be the major events induced by low and high thermal stress, and the retinol pathway may play critical roles in the responses of the scallops to high thermal stress. It seems that the bay scallop southern species have evolved distinct pathways in dealing with low and high thermal stress. Our results may provide useful information for marker-assisted selection of high-resistant strains in this scallop.
Water temperature is one of the most dominant environmental factors affecting the success of aquaculture (Valente et al., 2013). It is well known that water temperature fluctuation has a great influence on the life cycle, growth, and survival of aquatic animals (Takahara et al., 2011). When exposed to extreme temperatures, the aquatic animals tend to alleviate stress through a series of stress responses (Soyano and Mushirobira, 2018). Therefore, exploring the responses of aquatic animals to temperature fluctuations may provide a better understanding on how organisms cope with environmental challenges.
Various genes and pathways have been suggested to be involved in the responses of aquatic animals to thermal stresses. Immunity-associated genes, such as mucin genes (Wang et al., 2020), mannan-binding lectin-associated serine protease genes (Gao et al., 2021), heat shock protein (Lin et al., 2018; Wang et al., 2020), and ferritin (Zhao et al., 2015), have been found to express differentially under temperature stress, suggesting the possible involvement of immune system in stress response in aquatic animals. Similarly, differential expression of many antioxidant-related genes, such as catalase unigenes (Dadras et al., 2016; Wen et al., 2021), superoxide dismutases (Li et al., 2021), and glutathione peroxidase unigenes (Sun et al., 2020a) under temperature stress, may indicate the activation of antioxidant system in thermal stress response. Pathways, such as steroid hormones (Takahara et al., 2011; Gao et al., 2021), RNA splicing (Tan et al., 2019), energy metabolism (Artigaud et al., 2015; Gao et al., 2021), and ubiquitin-mediated proteolysis (Chen et al., 2018), have also been reported to be involved in responses to temperature stress in aquatic animals. In addition, temperature stress may also alter the metabolism of some important substances, such as fatty acids and lipids, in aquatic animals (Child and Laing, 1998; Mininni et al., 2014; Artigaud et al., 2015).
The bay scallop southern subspecies, Argopecten irradians concentricus (Say, 1,822) (A. i. concentricus), naturally distributed along the Atlantic coasts of the United States and the Gulf of Mexico, is a eurythermal species with a temperature range of 13–33°C (Zhang et al., 2007). It was introduced to China in 1991 and is now widely cultured in southern China waters (Zhang et al., 2007). Unlike the bay scallop northern subspecies, A. i. concentricus, whose temperature range is –1 to 31°C (Zhang et al., 2007), this subspecies is more adaptive to high-temperature waters but stops growing at temperatures lower than 13°C. As an economically important species with great aquaculture potential as well as a species with special adaptation to high temperatures, it is thus interesting to examine its responses to both low and high thermal stresses. The findings may provide new insights into temperature adaptation in this species.
Argopecten irradians concentricus were collected from a scallop farm in Laizhou, Shandong Province, China, and transported to the laboratory. The scallops were kept at 25°C and acclimatized to the laboratory conditions for 3 days before the experiments. Ninety scallops were randomly divided into three groups and transferred to three different tanks filled with water at 25°C. The water in the tanks was either kept at 25°C (control group) or cooled to 12°C (low thermal stress group) or heated to 32°C (high thermal stress group) at a rate of 1°C/h. The animals were held at the designated temperature for 72 h, and 15 animals were randomly sampled for each group at the end of the experiment. The sampled animals were dissected, and their adductor muscles were frozen immediately in liquid nitrogen and stored at –80°C for subsequent RNA or metabolite extraction. A total of 15 samples were collected for each group, of which 9 samples were used for transcriptomic analysis and 6 replicates were used for metabolomic analysis.
Total RNA was extracted from the adductor muscles using a TRIzol reagent (Invitrogen, United Kingdom) following the manufacturer’s protocols. Three adductor muscles from each group were pooled together for RNA extraction. Then, three biological replicates for transcriptomic analysis were used for Illumina RNA-Seq library construction and sequencing. After removing low-quality sequences, the transcripts were mapped to the genome of the bay scallop southern subspecies (Liu et al., 2020b). Fragments per kilobase of transcript per million mapped reads (FPKM) were used to calculate the gene expression levels. The differentially expressed genes (DEGs) were detected by comparing FPKM values of the experimental groups (high-temperature stress group and low thermal stress group) with those of the control group. DEGSeq R package was used to analyze the differential expression of genes. Genes with | log2FoldChange| > 0 and at false discovery rate (padj) < 0.05 were defined as DEGs. For further functional analysis, Gene Ontology (GO) terms and the Kyoto Encyclopedia of Genes and Genomes (KEGG) database pathways were utilized to identify the enriched pathways of DEGs with P < 0.05.
Metabolite extractions were performed following the protocol suggested by Hao et al. (2019). LC-MS/MS analysis was performed using a Vanquish UHPLC system (Thermo Fisher Scientific) equipped with an Orbitrap Q Exactive series mass spectrometer (Thermo Fisher Scientific). This system was installed with a Hypersil Gold column (100 × 2.1 mm, 1.9 μm) for sample injection. The gradient elution mode was applied in chromatographic separation. Polarity mode was performed with 35 arb of sheath gas flow rate and 10 arb of aux gas flow rate at 320°C by a Q Exactive series mass spectrometer. Compound Discoverer 3.0 (CD3.0, Thermo Fisher Scientific) software was used to analyze the raw data files. Metabolites were annotated by Lipid Maps database,1 KEGG database,2 and HMDB database.3 Principal component analysis (PCA) was performed using the software metaX. Metabolites with P-value < 0.05, VIP > 1, and FC ≤ 0.5 or fold change ≥ 2 were considered as the differential metabolites (DMs).
MetScape 3 for Cytoscape4 was utilized to visualize and integrate networks from enriched DEGs and DMs from transcriptome and metabolome.
A total of nine libraries from the high thermal group (H), the low thermal group (L), and the control group (C) were sequenced, and 43,476,657 (95.77% of total reads), 42,631,564 (96.10%), and 47,198,361 (96.56%) clean reads (GEO accession number: GSE178138) were obtained. Of all the gene-mapped reads, 95.29% from L, 95.18% from H, and 95.49% from C were mapped onto reference genes (Supplementary Table 1).
The number of DEGs for H vs. C was 1,294, with 791 upregulated and 503 downregulated in H compared with C (Figure 1A). The number of DEGs for L vs. C was 1,276, with 613 upregulated and 663 downregulated (Figure 1B). The number of DEGs for H vs. L was 1,550, with 980 upregulated and 570 downregulated (Figure 1C). Based on the GO analysis, GO terms were categorized into three major functional groups. The top 30 GO terms in each major functional group were shown in Supplementary Tables 2–4. The potential pathways that may be related to thermal stress response were analyzed. In summary, 14 DEGs in calcium ion binding, 41 DEGs in kinase activity, 9 DEGs in phosphatase activity, 21 DEGs in RNA biosynthetic process, and 28 DEGs in RNA metabolic process were enriched for H vs. C. In addition, 29 DEGs were assigned to lipid-related pathways, such as lipid transport, lipid localization, response to lipid, lipid biosynthetic process, and lipid metabolic process (Figure 2A). For L vs. C, 21 DEGs in calcium ion binding, 47 DEGs in kinase activity, 7 DEGs in phosphatase activity process, and 40 DEGs in lipid-related pathways were enriched (Figure 2B). Notably, the RNA-related pathways were the prominent enrichment, including RNA metabolic process with 40 genes enriched and RNA biosynthetic process with 51 genes enriched. For H vs. L, 20 DEGs in calcium ion binding, 52 DEGs in kinase activity, 6 DEGs in phosphatase activity, 50 DEGs in lipid-related pathways, 46 DEGs in RNA biosynthetic process, and 52 DEGs in RNA metabolic process were enriched (Figure 2C).
Figure 2. Scatterplot of the GO pathway related to temperature stress response enriched the differentially expressed genes in H vs. C (A), L vs. C (B), and H vs. L (C).
All DEGs were also subjected to KEGG functional analysis (Supplementary Tables 5–7). Potential temperature stress response pathway; valine, leucine, and isoleucine degradation; retinol metabolism; inositol phosphate metabolism; and fatty acid-related pathways were enriched by DEGs under high temperature (Figure 3A). Low thermal stress led to the activation of inositol phosphate metabolism, phosphatidylinositol signaling system, pentose phosphate pathway, fatty acid-related pathways, and RNA degradation pathways (Figure 3B). For H vs. L, stress response pathways include pentose phosphate pathway; valine, leucine, and isoleucine degradation; inositol phosphate metabolism; RNA degradation; and fatty acid-related pathways (Figure 3C).
Figure 3. Scatterplot of the KEGG pathway related to temperature stress response enriched the differentially expressed genes in the H vs. C (A), L vs. C (B), and H vs. L (C).
Under high-temperature stress, 434 transcription factors (TFs) were differentially expressed, representing 33.53% of the total DEGs. Under low-temperature stress, the number of differentially expressed TFs was 499, accounting for 39.1% of the total DEGs. A total of 600 differentially expressed TFs were identified in H vs. L, constituting 38.7% of the total DEGs. The GO and KEGG analyses were also applied to enrich the differentially expressed TFs. GO analyses showed that these genes were enriched in similar pathways, including signal transduction-related pathways, membrane-related pathways, transmembrane transporter activity-related pathways, G-protein-coupled receptor activity, and oxidation/reduction (redox) reactions (Supplementary Figure 1). Based on KEGG analyses, high thermal stress response-related pathways involve retinol metabolism; valine, leucine, and isoleucine degradation; fatty acid-related pathways; and signal transduction-related pathways. Moreover, RNA-related pathways, tricarboxylic acid cycle (TCA cycle), and signal transduction-related pathways were enriched under cold stress by KEGG. In H vs. C, signal transduction-related pathways; fatty acid-related pathways; and valine, leucine, and isoleucine degradation pathways were identified by KEGG enrichment analyses (Supplementary Figure 2).
In this study, a total of 63 DMs in H vs. C (Figure 4A), 121 DMs in L vs. C (Figure 4B), and 89 DMs in H vs. L were identified (Figure 4C). PCAs effectively distinguished DMs into three groups (Figure 5). A total of 30 pathways were identified in the high thermal stress group, which were linked to retinol metabolism, cAMP signaling pathway, inositol phosphate metabolism, sphingolipid signaling pathways, phosphatidylinositol signaling system, and cGMP-PKG signaling pathway (Figure 6A). A total of 60 pathways were identified in the low thermal stress group, including calcium signaling; valine, leucine, and isoleucine degradation; regulation of lipolysis in adipocytes; cGMP-PKG signaling; cAMP signaling; sphingolipid signaling; PI3K-Akt signaling; mTOR signaling; AMPK signaling; and FoxO signaling, as the major altered signaling pathways (Figure 6B). For H vs. L, 40 pathways were enriched, with many DMs involved in valine, leucine, and isoleucine degradation; phosphate-related pathways; fatty acid-related pathways; and metabolic pathways (Figure 6C).
Figure 5. PCA score plots of differential metabolites in the H vs. C (A), L vs. C (B), and H vs. L (C).
Figure 6. Scatterplot of the KEGG pathway related to temperature stress response enriched the differential metabolites in the H vs. C (A), L vs. C (B), and H vs. L (C).
Integrated analysis of DEGs and DMs responsive to temperature stress revealed several different enriched pathways. The results showed that the pentose phosphate pathway; fatty acid biosynthesis; TCA cycle; and valine, leucine, and isoleucine degradation may have participated in responses to high and low thermal stress, while the retinol pathway is likely involved in the responses to high-temperature stress (Supplementary Figures 3, 4).
Water temperature is one of the major factors affecting the growth, metabolic rate, and survival of aquatic animals. Temperature stress reduces the fitness of the aquatic organisms, which will have to make adaptive responses to maintain physiological homeostasis. Acting as a stressor, the water temperature can induce various complicated responsive processes in aquatic organisms. An overview of signaling pathways in the responses of animals to temperature stress is shown in Figure 7. Initially, the stress is perceived by the receptors on the cell membranes, followed by the activation of phospholipase to generate signaling molecules (Mahajan and Tuteja, 2005), including second messengers, especially calcium. Second messengers generated by different types of stresses are similar (Bjornson et al., 2016). These second messengers transduce signals to effectors by initiating an array of downstream cascades, which may be the activation of kinases and/or phosphatases (Schmidt et al., 2018). These signals target the transcription factors or the major stress genes controlling these genes (Soyano and Mushirobira, 2018). The products of stress-responsive genes/pathways help the organisms in adapting to unfavorable environments and ultimately lead to survival. In this study, an integrative analysis of the transcriptome and metabolome displayed a comprehensive perspective between the DEGs and the DMs responding to temperature stress in A. i. concentricus.
In this study, the calcium ion binding pathway and lipid metabolism pathways were enriched in both H and L thermal stress groups, indicating again that scallops employed similar second messengers in both high and low thermal stress responses.
As a second messenger, calcium plays an important role in signal transduction pathways, mediating various defensive responses under external stresses. It is noticeable that the DEGs enriched in calcium ion binding pathways were different in the high and low thermal stress groups. Notably, 14 DEGs in the high thermal stress group and 21 DEGs in the low thermal stress group were found to be involved in the calcium ion binding pathway. The calcium signals produced by different stimuli differ in kinetic and spatial features (Delian et al., 2014), and therefore, the subsequent responses induced were also different, as observed in this study. As a DEG, calmodulin in calcium ion binding pathways was upregulated in both thermal stress groups. Calmodulin is a primary intracellular mediator of calcium signaling (Kudla et al., 2010). Recently, it has been demonstrated that calmodulin was a key component in the heat-shock signal transduction pathway in Arabidopsis (Arabidopsis thaliana) (Zhang et al., 2009). Studies in papaya (Carica papaya L.) showed that calmodulin also responded to high and cold temperature stresses (Ding et al., 2018). In this study, it is likely that calmodulin acting as a calcium sensor may participate in thermal stress response.
It is well known that lipids are major components of membranes. The membrane fluidity or permeability can be altered through membrane lipids to deal with diverse stresses (Jain et al., 2018). In our study, it was notable that changes in lipid metabolism, such as lipid transport, lipid localization, response to lipid, lipid biosynthetic process, and lipid metabolic process, were observed in both high and low thermal groups. These results indicated that some properties of membranes may be changed by temperature stress, which was also confirmed by the enrichment of TFs. Membrane is the first target influenced by temperature change, and lipid components in membrane formulation immediately respond to this challenge (Farkas et al., 2001). Temperature stress causes alterations in the fluidity of the membranes by altering the proportion of unsaturated fatty acids in the membrane (Losa and Murata, 2004). In animals, changes in membrane fluidity can modulate the activity of G protein, which is a component of signal-transduction systems and is related to transmembrane receptors (Gudi et al., 1998). In addition, lipid metabolism, including phospholipases, lipid kinases, or phosphatases, may generate specific second messenger molecules from membrane lipids in response to thermal stress. Phospholipids may be hydrolyzed by phospholipases, which may generate a number of lipophilic molecules to mediate calcium signals under stress (Barman et al., 2018). It has been proved that kinases and phosphatases may function in calcium-signaling pathways to amplify and mediate the signal transduction of the cascade in response to temperature stress (Jonak et al., 1996; Sopory and Munshi, 2010). In our results, the phosphatases pathway, the phospholipases pathway, and the kinase pathway were enriched in both the high and low thermal stress groups, although the DEGs were different. Specific responses may be induced by different lipid signals in the high and low thermal stress groups. It is thus possible that temperature sensors detect various changes in the membrane and initiate different signal transduction machinery.
The second messengers target the transcription factors or the major stress-responsive genes controlling responsive genes. Upstream transcriptional factors may regulate the stress-responsive genes with the promoters in stress genes (Mahajan and Tuteja, 2005). Previous studies in plants showed that transcription factors control a series of enzymes, proteins, and pathways to defend against thermal stress (Naohiko et al., 2017; Yu et al., 2020). In our study, a functional enrichment analysis indicated that numerous TFs from DEGs were enriched in the signal transduction-related pathways, membrane-related pathways, transmembrane transporter activity-related pathways, G-protein-coupled receptor activity, and oxidation/reduction (redox) reactions. It has been reported that redox reaction was enriched in both cold stress-induced and heat stress-induced genes in zebrafish (Danio rerio) (Dhanasiri et al., 2013), which is consistent with our results. It was speculated that thermal stress (cold or heat) can lead to alterations in the redox reaction. It was shown that redox activated the responses of stress through the control signal pathway (Nobuhiro et al., 2012). In addition, it is known that the intracellular redox state can modulate gene expression by activating the TFs or by binding the TF to the modular promoter elements of the target gene (Liu et al., 2005). This may explain the enrichment of transcription factors in this pathway. Thus, the redox state can regulate signaling pathways and the expression of TFs, thereby enabling the adjustment of metabolism and development in response to stress (Liu et al., 2005).
Besides their crucial roles as molecular switches for gene expression, TFs may also act as terminal points of signal transduction in response to thermal stress (Chen et al., 2008). The result of Lilium lancifolium also supported this point (Wang et al., 2014). This could possibly explain the enrichment of the transmembrane signaling receptor activity and protein phosphorylation pathway for TFs.
Notably, our results of integrated network analysis of transcriptomics and metabolomics showed that valine, leucine, and isoleucine degradation pathway was enriched in both thermal stress groups. DEGs in the valine, leucine, and isoleucine degradation were downregulated. Similar observations were observed in hybrid grouper under low thermals (Fan et al., 2019). Valine, leucine, and isoleucine are branched-chain amino acids (BCAAs), and they are also essential amino acids (Holeček, 2018). The degradation of BCAAs can be glucogenic (valine), ketogenic (leucine and isoleucine), or both (isoleucine). The end products of degradation are the main substrate for the TCA cycle for energy generation (Banerjee et al., 2020). Therefore, BCAAs are important in the antifreeze reaction (Soyano and Mushirobira, 2018). Consistent with this possibility, the downregulated valine, leucine, and isoleucine degradation pathway may indicate that BCAAs were employed to resist the cold stress via energy production.
It is notable that the valine, leucine, and isoleucine degradation pathway was the only significantly enriched upregulated KEGG pathway in the H group. Similar results were also reported in pearl millet (Pennisetum glaucum L.) (Sun et al., 2020b). As the major component of tissue proteins, BCAA supplementation has been shown to decrease muscle protein breakdown and increase protein synthesis (Zhang et al., 2017). It was found that feeding of leucine improved thermotolerance in chicks (Chowdhury et al., 2021). It has also been reported that the supplementation of BCAAs accelerated recovery following injury by heat and synthesis of related protein (Hsu-Ming et al., 2012). Therefore, it was speculated that increased valine, leucine, and isoleucine degradation may have positive effects on immune response and protection against muscle injury caused by high thermal stress.
Integrated analysis of transcriptomics and metabolomics in energy metabolism perspective in H and L groups showed similar results. It is interesting to note that most DEGs related to lipids were upregulated. However, most DMs related to fatty acids and lipids were downregulated. Integrated analysis revealed that TCA was enriched under both thermal stresses. As energy metabolism, TCA is the main source of energy for the organism. These results suggested that stored energy reserves were mobilized under thermal stress. Similar observations were found in thermally stressed organisms, such as sea bream (Sparus aurata) (Mininni et al., 2014) and king scallop (Pecten maximus) (Artigaud et al., 2015). Based on the concept of energy-limited tolerance to stress, relatively more energy is put into physical function maintenance and the energy stored is reduced or even depleted under non-optimal conditions (Ibarz et al., 2010). Exposure to non-optimal temperatures might impose on additional energetic costs. In addition, depletion of fat reserves is more significant at low temperatures than that at high temperatures by metabolomic analysis. One possible explanation is that more energy was consumed under low thermal stress.
According to the KEGG analysis of DEGs and DMs from H vs. C, the retinol metabolism may be involved in the response to high thermal stress. This result was consistent with the integrated network analysis. Most DEGs and DMs enriched in the retinol metabolism pathway were upregulated in the H group. Retinol is the main existing form of vitamin A, which is an essential nutrient for maintaining the physiological metabolism of aquatic animals (Gonçalves et al., 2016). In marine fish, retinol has been shown to protect the skeleton from abnormality and modulate immune response (Takeuchi et al., 1998; Fernández and Gisbert, 2011; Negm et al., 2013; Sharifian et al., 2017). In addition, a few studies showed that retinol may provide protection from heat stress. It was reported that retinol may stabilize sperm acrosomal membrane under oxidative stress caused by high temperatures (Maya-Soriano et al., 2013b). Similarly, retinol has been proved to be valuable in heat-stressed oocyte protection (Maya-Soriano et al., 2013a). As the precursors of vitamin A, carotenoids are the main sources of retinol in animals (Olson, 1992). It was shown that high content carotenoids improved survival rates under high-temperature stress by enhancing the antioxidant system in pearl oyster (Pinctada fucata) (Meng et al., 2017). Thus, the upregulated retinol pathway may be one of the reasons for the high-temperature tolerance of A. i. concentricus. This is possible because retinol acts as an antioxidant agent against peroxidation in living cells (Sinbad et al., 2019).
A broad array of genes related to RNA processing were active at low thermal stress. At low temperature, the RNA folding problem becomes more dominant because non-native structures are more stable (Rajkowitsch et al., 2007). Numerous studies have demonstrated that RNA processing is altered by cold stimuli in maize (Zea mays) (Liu et al., 2020a), Drosophila (Colinet et al., 2017), and sea bream (Sparus aurata) (Ibarz et al., 2010). In general, alternation of RNA processing is suggested to require compensation for the enhanced stability of RNA secondary structures at cold temperatures.
This study aimed to examine the mechanisms underlying the responses of the scallops to heat and cold stress. Combining metabolomic and transcriptomic approaches, we identified differential expression in a number of pathways. Several DEGs and DMs were found to be involved in calcium pathway and lipid-related metabolism pathways, suggesting that the second messengers may participate in thermal stress response. A large number of TFs from DEGs were enriched in membrane and redox reactions, suggesting that TFs play important roles in response to thermal stress. The results also showed that both high and cold temperature stress modified valine, leucine, and isoleucine degradation pathway and energy reserves. Despite the enrichment of common pathways, DEGs and DMs, different genes and metabolites appear to be responsible for pathway activation in both the high and cold temperature stress groups. Moreover, the retinol pathway was revealed to be involved in response to heat stress. And low temperature could induce RNA processing.
The results in this study may have immediate application in scallop breeding. For example, retinol metabolism-related genes may be used as markers for the selection of heat-resistant strains of scallops.
The datasets presented in this study can be found in online repositories. The names of the repository/repositories and accession number(s) can be found in the article/Supplementary Material.
Ethical review and approval was not required for the animal study because the scallops used in the current study were cultured by us, and we are allowed to use these materials for research. Since no endangered or protected species were involved in the experiments of this study, no specific permission was required for the animal material.
JS designed the experiment, performed the analyses, and wrote the manuscript. BL contributed to the experimental samples. CW supervised the project and corrected the manuscript. All authors contributed to the article and approved the submitted version.
This work was funded by the National Natural Science Foundation (Grant No. 3197210450), the Earmarked Fund for Agriculture Seed Improvement Project of Shandong Province (No. 2020LZGC016), and the Earmarked Fund for Shandong Modern Agro-Industry Technology Research System (No. SDAIT-14) granted to CW, and Qingdao Agricultural University Foundation (No. 663-1119015) and Shandong Province Natural Science Foundation (Grant No. ZR2020QC203) granted to JS.
The authors declare that the research was conducted in the absence of any commercial or financial relationships that could be construed as a potential conflict of interest.
All claims expressed in this article are solely those of the authors and do not necessarily represent those of their affiliated organizations, or those of the publisher, the editors and the reviewers. Any product that may be evaluated in this article, or claim that may be made by its manufacturer, is not guaranteed or endorsed by the publisher.
The Supplementary Material for this article can be found online at: https://www.frontiersin.org/articles/10.3389/fmars.2022.818083/full#supplementary-material
Artigaud, S., Richard, J., Thorne, M. A., Lavaud, R., Flye-Sainte-Marie, J., Jean, F., et al. (2015). Deciphering the molecular adaptation of the king scallop (Pecten maximus) to heat stress using transcriptomics and proteomics. BMC Genomics 16:988. doi: 10.1186/s12864-015-2132-x
Banerjee, P., Carmelo, V. A. O., and Kadarmideen, H. N. (2020). Integrative analysis of metabolomic and transcriptomic profiles uncovers biological pathways of feed efficiency in pigs. Metabolites 10, 275–292. doi: 10.3390/metabo10070275
Barman, A., Gohain, D., Bora, U., and Tamuli, R. (2018). Phospholipases play multiple cellular roles including growth, stress tolerance, sexual development, and virulence in fungi. Microbiol. Res. 209, 55–69. doi: 10.1016/j.micres.2017.12.012
Bjornson, M., Dandekar, A., and Dehesh, K. (2016). Determinants of timing and amplitude in the plant general stress response. J. Integr. Plant Biol. 58, 119–126. doi: 10.1111/jipb.12373
Chen, N., Huang, Z., Lu, C., Shen, Y., Luo, X., Ke, C., et al. (2018). Different transcriptomic responses to thermal stress in heat-tolerant and heat-sensitive pacific abalones indicated by cardiac performance. Front. Physiol. 9:1895. doi: 10.3389/fphys.2018.01895
Chen, Y.-N., Erin, S., and Federica, B. (2008). Membrane-tethered transcription factors in Arabidopsis thaliana: novel regulators in stress response and development. Curr. Opin. Plant Biol. 11, 695–701. doi: 10.1016/j.pbi.2008.10.005
Child, A. R., and Laing, I. (1998). Comparative low temperature tolerance of small juvenile european, Ostrea edulis L., and pacific oysters, Crassostrea gigas thunberg. Aquac. Res. 29, 103–113. doi: 10.1111/j.1365-2109.1998.tb01114.x
Chowdhury, V. S., Han, G., Eltahan, H. M., Haraguchi, S., Gilbert, E. R., Cline, M. A., et al. (2021). Potential role of amino acids in the adaptation of chicks and market-age broilers to heat stress. Front. Vet. Sci. 7:610541. doi: 10.3389/fvets.2020.610541
Colinet, H., Pineau, C., and Com, E. (2017). Large scale phosphoprotein profiling to explore Drosophila cold acclimation regulatory mechanisms. Sci. Rep. 7:1713. doi: 10.1038/s41598-017-01974-z
Dadras, H., Dzyuba, V., Cosson, J., Golpour, A., and Dzyuba, B. (2016). The in vitro effect of temperature on motility and antioxidant response of common carp Cyprinus carpio spermatozoa. J. Therm. Biol. 59, 64–68. doi: 10.1016/j.jtherbio.2016.05.003
Delian, E., Chira, A., Bădulescu, L., and Chira, L. (2014). Calcium alleviates stress in plants: insight into regulatory mechanisms. Agrolife Sci. J. 3, 19–28.
Dhanasiri, A. K. S., Fernandes, J. M. O., and Kiron, V. (2013). Liver transcriptome changes in zebrafish during acclimation to transport-associated stress. PLoS One 8:e65028. doi: 10.1371/journal.pone.0065028
Ding, X., Zhang, L., Hao, Y., Xiao, S., Wu, Z., Chen, W., et al. (2018). Genome-wide identification and expression analyses of the calmodulin and calmodulin-like proteins reveal their involvement in stress response and fruit ripening in papaya. Postharvest Biol. Tec. 143, 13–27.
Fan, X., Qin, X., Zhang, C., Zhu, Q., Chen, J., and Chen, P. (2019). Metabolic and anti-oxidative stress responses to low temperatures during the waterless preservation of the hybrid grouper (Epinephelus fuscogutatus ♀×♂ Epinephelus lanceolatus?). Aquaculture 508, 10–18.
Farkas, T., Fodor, E., Kitajka, K., and Halver, J. E. (2001). Response of fish membranes to environmental temperature. Aquac. Res. 32, 645–655. doi: 10.1046/j.1365-2109.2001.00600.x
Fernández, I., and Gisbert, E. (2011). The effect of vitamin a on flatfish development and skeletogenesis. Aquaculture. 315, 34–48. doi: 10.1016/j.aquaculture.2010.11.025
Gao, Y., Wei, Y., Cao, D., Ge, Y., and Gong, S. (2021). Transcriptome analysis reveals decreased immunity under heat stress in Mauremys mutica. Aquaculture 531:735894. doi: 10.1016/j.aquaculture.2020.735894
Gonçalves, A., Estevinho, B. N., and Rocha, F. (2016). Microencapsulation of vitamin a. Trends. Food Sci. Tech. 51, 76–87. doi: 10.1081/ddc-100105181
Gudi, S., Nolan, J. p, and Frangos, J. a. (1998). Modulation of GTPase activity of G proteins by fluid shear stress and phospholipid composition. Proc. Natl. Acad. Sci. U.S.A. 95, 2515–2519. doi: 10.1073/pnas.95.5.2515
Hao, R., Du, X., Yang, C., Deng, Y., Zheng, Z., and Wang, Q. (2019). Integrated application of transcriptomics and metabolomics provides insights into unsynchronized growth in pearl oyster Pinctada fucata martensii. Sci. Total Environ. 666, 46–56. doi: 10.1016/j.scitotenv.2019.02.221
Holeček, M. (2018). Branched-chain amino acids in health and disease: metabolism, alterations in blood plasma, and as supplements. Nutr. Metab. 15:33. doi: 10.1186/s12986-018-0271-1
Hsu-Ming, W., Naito, K., Kinoshita, Y., Kobayashi, H., Honjoh, K., Tashiro, K., et al. (2012). Changes in transcription during recovery from heat injury in Salmonella typhimurium and effects of BCAA on recovery. Amino. Acids 42, 2059–2066. doi: 10.1007/s00726-011-0934-y
Ibarz, A., Blasco, J., Gallardo, M. A., and Fernández-Borràs, J. (2010). Energy reserves and metabolic status affect the acclimation of gilthead sea bream (Sparus aurata) to cold. Comp. Biochem. Phys. 155, 319–326. doi: 10.1016/j.cbpa.2009.11.012
Jain, M., Nagar, P., Goel, P., Singh, A. A., Kumari, S., and Mustafiz, A. (2018). “Second messengers: central regulators in plant abiotic stress response,” in Abiotic Stress–Mediated Sensing and Signaling in Plants: An Omics Perspective, eds S. M. Zargar and M. Y. Zargar (Singapore: Springer), 47–94. doi: 10.1007/978-981-10-7479-0_2
Jonak, C., Kiegerl, S., Ligterink, W., Barker, P. J., Huskisson, N. S., and Hirt, H. (1996). Stress signaling in plants: a mitogen-activated protein kinase pathway is activated by cold and drought. Proc. Natl. Acad. Sci. U.S.A. 93, 11274–11279. doi: 10.1073/pnas.93.20.11274
Kudla, J., Batistic, O., and Hashimoto, K. (2010). Calcium signals: the lead currency of plant information processing. Plant Cell 22, 541–563. doi: 10.1105/tpc.109.072686
Li, P., Li, Z., and Wu, Y. (2021). Interactive effects of temperature and mercury exposure on the stress-related responses in the freshwater fish Ctenopharyngodon idella. Aquac. Res. 52, 2070–2077. doi: 10.1111/are.15058
Lin, X., Wu, X., and Liu, X. (2018). Temperature stress response of heat shock protein 90 (Hsp90) in the clam Paphia undulata. Aquac. Fish. 3, 106–114. doi: 10.1016/j.aaf.2018.04.003
Liu, H., Colavitti, R., Rovira, I. I., and Finkel, T. (2005). Redox-dependent transcriptional regulation. Cir. Res. 97, 967–974. doi: 10.1161/01.res.0000188210.72062.10
Liu, X., Li, C., Chen, M., Liu, B., Yan, X., Ning, J., et al. (2020b). Draft genomes of two Atlantic bay scallop subspecies Argopecten irradians irradians and A. i. concentricus. Sci. Data 7:99. doi: 10.1038/s41597-020-0441-7
Liu, G., Yan, P., Du, Q., Wang, Y., Guo, Y., Fu, Z., et al. (2020a). Pre-rRNA processing and its response to temperature stress in maize. J. Exp. Bot. 71, 1363–1374. doi: 10.1093/jxb/erz488
Losa, D. A., and Murata, N. (2004). Membrane fluidity and its roles in the perception of environmental signals. BBA 1666, 142–157. doi: 10.1016/j.bbamem.2004.08.002
Mahajan, S., and Tuteja, N. (2005). Cold, salinity and drought stresses. Arch. Biochem. Biophys. 444, 139–158. doi: 10.1016/j.abb.2005.10.018
Maya-Soriano, M. J., Taberner, E., Sabés-Alsina, M., and López-Béjar, M. (2013b). Retinol might stabilize sperm acrosomal membrane in situations of oxidative stress because of high temperatures. Theriogenology 79, 367–373. doi: 10.1016/j.theriogenology.2012.10.009
Maya-Soriano, M. J., Taberner, E., and López-Béjar, M. (2013a). Retinol improves in vitro oocyte nuclear maturation under heat stress in heifers. Zygote 21, 377–384. doi: 10.1017/S0967199412000135
Meng, Z., Zhang, B., Liu, B., Li, H., Fan, S., and Yu, D. (2017). High carotenoids content can enhance resistance of selected Pinctada fucata families to high temperature stress. Fish Shellfish Immunol. 61, 211–218. doi: 10.1016/j.fsi.2016.12.032
Mininni, A. N., Milan, M., Ferraresso, S., Petochi, T., Di Marco, P., Marino, G., et al. (2014). Liver transcriptome analysis in gilthead sea bream upon exposure to low temperature. BMC Genomics 15:765. doi: 10.1186/1471-2164-15-765
Naohiko, O., Hikaru, S., Kazuo, S., and Yamaguchi-Shinozaki, K. (2017). Transcriptional regulatory network of plant heat stress response. Trends Plant Sci. 22, 53–65.
Negm, R. K., Cobcroft, J. M., Brown, M. R., Nowak, B. F., and Battaglene, S. C. (2013). The effects of dietary vitamin a in rotifers on the performance and skeletal abnormality of striped trumpeter Latris lineata larvae and post larvae. Aquaculture 404-405, 105–115.
Nobuhiro, S., Shai, K., Ron, M., and Miller, G. (2012). ROS and redox signalling in the response of plants to abiotic stress. Plant Cell Environ. 35, 259–270. doi: 10.1111/j.1365-3040.2011.02336.x
Olson, J. A. (1992). Lipid–Soluble Antioxidants: Biochemistry and Clinical Applications. Basel: Birkhäuser Verlag.
Rajkowitsch, L., Chen, D., Stampfl, S., Semrad, K., Waldsich, C., Mayer, O., et al. (2007). RNA Chaperones, RNA Annealers and RNA helicases. RNA Biol. 4, 118–130. doi: 10.4161/rna.4.3.5445
Schmidt, R. R., Weits, D. A., Feulner, C. F. J., and van Dongen, J. T. (2018). Oxygen sensing and integrative stress signaling in plants. Plant Phys. 176, 1131–1142. doi: 10.1104/pp.17.01394
Sharifian, M., Hajimoradloo, A., Ghorbani, R., and Hoseinifar, S. H. (2017). Effects of dietary retinol acetate on growth performance, skin mucus immune responses and haematological parameters of Caspian roach (Rutilus caspicus). Aquacult. Nutr. 23, 893–898.
Sinbad, O. O., Folorunsho, A. A., Olabisi, O. L., Ayoola, A. O., and Temitope, J. (2019). Vitamins as antioxidants. J. Food Sci. Nutr. Res. 3, 214–235.
Sopory, S. K., and Munshi, M. (2010). Protein kinases and phosphatases and their role in cellular signaling in plants. Crit. Rev. Plant Sci. 17, 245–318. doi: 10.1080/07352689891304230
Soyano, K., and Mushirobira, Y. (2018). The mechanism of low-temperature tolerance in fish. Adv. Exp. Med. Biol. 1081, 149–164. doi: 10.1007/978-981-13-1244-1_9
Sun, J. L., Zhao, L. L., Liao, L., Tang, X.-H., Cui, C., Liu, Q., et al. (2020a). Interactive effect of thermal and hypoxia on largemouth bass (Micropterus salmoides) gill and liver: aggravation of oxidative stress, inhibition of immunity and promotion of cell apoptosis. Fish Shellfish Immunol. 98, 923–936. doi: 10.1016/j.fsi.2019.11.056
Sun, M., Huang, D., Zhang, A., Khan, I., Yan, H., Wang, X., et al. (2020b). Transcriptome analysis of heat stress and drought stress in pearl millet based on Pacbio full-length transcriptome sequencing. BMC Plant Biol. 20:323. doi: 10.1186/s12870-020-02530-0
Takahara, T., Yamanaka, H., Suzuki, A. A., Honjo, M., Minamoto, T., Yonekura, R., et al. (2011). Stress response to daily temperature fluctuations in common carp, Cyprinus carpio L. Hydrobiologia 675, 65–73.
Takeuchi, T., Dedi, J., Haga, Y., Seikai, T., and Watanabe, T. (1998). Effect of vitamin A compounds on bone deformity in larval Japanese flounder (Paralichthys oliÕaceus). Aquaculture 169, 155–165. doi: 10.1016/s0044-8486(98)00373-1
Tan, S., Wang, W., Tian, C., Niu, D., Zhou, T., Jin, Y., et al. (2019). Heat stress induced alternative splicing in catfish as determined by transcriptome analysis. Comp. Biochem. Phys. 29, 166–172. doi: 10.1016/j.cbd.2018.11.008
Valente, L. M. P., Moutou, K. A., Conceição, L. E. C., Engrola, S., Fernandes, J. M., and Johnston, I. A. (2013). What determines growth potential and juvenile quality of farmed fish species? Rev. Aquacult. 5, S168–S193.
Wang, J., Yang, Y., Liu, X., Huang, J., Wang, Q., Gu, J., et al. (2014). Transcriptome profiling of the cold response and signaling pathways in Lilium lancifolium. BMC Genomics 15:203. doi: 10.1186/1471-2164-15-203
Wang, Z., Zhou, J., Li, J., and Fan, L. (2020). The immune defense response of Pacific white shrimp (Litopenaeus vannamei) to temperature fluctuation. Fish Shellfish Immunol. 103, 103–110. doi: 10.1016/j.fsi.2020.04.053
Wen, X., Chu, P., Xu, J. J., Wei, X. Z., Fu, D. Y., Wang, T., et al. (2021). Combined effects of low temperature and salinity on the immune response, antioxidant capacity and lipid metabolism in the pufferfish (Takifugu fasciatus). Aquaculture 531:735866. doi: 10.1016/j.aquaculture.2020.735866
Yu, H., Kong, X., Huang, H., Wu, W., Park, J., Yun, D. J., et al. (2020). STCH4/REIL2 confers cold stress tolerance in Arabidopsis by PRomoting rRNA processing and CBF protein translation. Cell Rep. 30, 229-242.e5. doi: 10.1016/j.celrep.2019.12.012
Zhang, H., Liu, X., Zhang, G., and Wang, C. (2007). Growth and survival of reciprocal crosses between two bay scallops, argopecten irradians concentricus say and A. irradians irradians lamarck. Aquaculture 272, S88–S93.
Zhang, S., Zeng, X., Ren, M., Mao, X., and Qiao, S. (2017). Novel metabolic and physiological functions of branched chain amino acids. J. Anim. Sci. Biotechnol. 8:10. doi: 10.1186/s40104-016-0139-z
Zhang, W., Zhou, R. G., Gao, Y. J., Zheng, S. Z., Xu, P., Zhang, S. Q., et al. (2009). Molecular and genetic evidence for the key role of AtCaM3 in heat-shock signal transduction in Arabidopsis. Plant Physiol. 149, 1773–1784. doi: 10.1104/pp.108.133744
Keywords: high thermal stress, low thermal stress, Argopecten irradians concentricus, transcriptomic analysis, metabolomic analysis
Citation: Song J, Liu B and Wang C (2022) Metabolomic and Transcriptomic Responses of Argopecten irradians concentricus to Thermal Stresses. Front. Mar. Sci. 9:818083. doi: 10.3389/fmars.2022.818083
Received: 19 November 2021; Accepted: 03 February 2022;
Published: 29 March 2022.
Edited by:
Pengzhi Qi, Zhejiang Ocean University, ChinaCopyright © 2022 Song, Liu and Wang. This is an open-access article distributed under the terms of the Creative Commons Attribution License (CC BY). The use, distribution or reproduction in other forums is permitted, provided the original author(s) and the copyright owner(s) are credited and that the original publication in this journal is cited, in accordance with accepted academic practice. No use, distribution or reproduction is permitted which does not comply with these terms.
*Correspondence: Chunde Wang, Y2h1bmRld2FuZzIwMDdAMTYzLmNvbQ==
Disclaimer: All claims expressed in this article are solely those of the authors and do not necessarily represent those of their affiliated organizations, or those of the publisher, the editors and the reviewers. Any product that may be evaluated in this article or claim that may be made by its manufacturer is not guaranteed or endorsed by the publisher.
Research integrity at Frontiers
Learn more about the work of our research integrity team to safeguard the quality of each article we publish.