- 1Department of Ecology and Coastal Management, Instituto de Ciencias Marinas de Andalucía, Consejo Superior de Investigaciones Científicas, Puerto Real, Spain
- 2Marine Biology Station Piran, National Institute of Biology, Piran, Slovenia
- 3Department of Biology, Faculty of Marine and Environmental Sciences, University of Cádiz, Cádiz, Spain
- 4Instituto Español de Oceanografía, Madrid, Spain
The photosymbiosis with host-specific dinoflagellates is a widespread relationship in marine organisms. Despite the evidenced biodiversity of this kind of mutualism, most research focuses on the study of scleractinian corals, and there is a lack of knowledge about other symbiotic cnidarians such as jellyfishes. The Mediterranean jellyfish Cotylorhiza tuberculata (Rhizostomae, Scyphozoa) harbors an endosymbiotic dinoflagellate of the family Symbiodiniaceae. In this study, we examine the algae distribution within the host body as well as, the pigment content and cell density of the symbiont. Furthermore, the size, morphology and fluorescence of cultured symbionts were studied under light microscopy, Imaging Flow Cytometry (IFC), and Scanning Electron Microscopy (SEM). The C:N composition and optical properties of the medusa tissue were measured to evaluate their role in the symbiosis. The medusae body was divided into two different sections to investigate the distribution of symbionts in hospite: oral arms (OA) and umbrella (UM). C:N composition of C. tuberculata was and symbiont density was significantly higher in the OA section. Mean chlorophyll a concentration of the algae was 1.33 (± 0.83) pg Chl a cell–1. The study of the pigment composition by HPLC (High Performance Liquid Chromatography), revealed the presence of 13 different pigments, being the most representative chlorophyll a, chlorophyll c2, and peridinin typical pigments of Symbiodiniaceae. Cell diameter of algae freshly isolated from the host was 8.71 ± 0.97 μm and cell growth rate was 0.52 (± 0.09) 106 cell ml–1 d–1. The presence of vegetative coccoid cells, doublet and motile mastigotes were revealed within the Symbiodiniaceae cultures. A calcifying matrix typical of Symbiodiniaceae and formed in partner with bacteria, was also observed most cultures. The umbrella tissue of the medusa absorbed at ultraviolet radiation (UVR) region, suggesting that medusae tissue protects photosymbionts from the negative effect of the high energetic UVR and attenuates the light intensity reaching algae inside the host. The presence of a dense Symbiodiniaceae population and the protection to UVR and elevated environmental irradiance provided by medusae tissue, maintain symbionts in optimal light conditions for photosynthesis and may be a reason added to explain the population success of Cotylorhiza tuberculata.
Introduction
The photosymbiosis with host-specialized dinoflagellates is a widespread relationship in marine organisms. This mutualistic association between animal hosts (heterotrophic) and endosymbiotic dinoflagellates (autotrophic) is found in cnidarians, sponges, molluscs, and even in protists (Venn et al., 2008; Decelle et al., 2015). Despite the large diversity in photosymbiosis, most work focus on the study of scleractinian corals, the basis of coral reefs and one of the most important and threatened ecosystems in the world (Done et al., 1996; de Groot et al., 2012; Kennedy et al., 2013). In contrast, other symbiotic cnidarians, such as jellyfishes, have been poorly examined (Djeghri et al., 2019). Nevertheless, due to the rise of jellyfish population in some environments (Arai, 2001; Pérez-Ruzafa et al., 2002; Purcell, 2005), there is a growing interest in studying these symbiotic organisms belonging to the cnidarian group and characterize their role in the ecosystem (Djeghri et al., 2019).
The Mediterranean jellyfish Cotylorhiza tuberculata is one of those symbiotic scyphozoans whose abundance has increased over the past decades. High abundances of this species have been observed in enclosed marine areas such as the Vlyho Bay in Greece (Kikinger, 1992) and the Mar Menor coastal Lagoon in Spain, where annual blooms have been observed since the 1990s (Pérez-Ruzafa et al., 2002). Like most scyphozoans, C. tuberculata has a complex bipartite life cycle with alternation of a pelagic sexual stage (medusa) and a benthic asexual stage (polyp). In this species, the endosymbiotic association occurs early in the jellyfish life cycle, particularly during the polyp stage (Astorga et al., 2012). In addition, the presence of host-specialized symbionts seems to play an important role in the phase transition from sessile polyp to free-living medusae, known as strobilation (Kikinger, 1992; Prieto et al., 2010; Astorga et al., 2012). The symbiotic dinoflagellates are hosted in the jellyfish endodermal tissue (Kikinger, 1992). As in most symbiotic jellyfishes, C. tuberculata polyps acquire their symbionts from the environment by horizontal transmission (Kikinger, 1992; Astorga et al., 2012). Then symbionts are incorporated into their endodermal cells (via lysosomes) and many of those cells containing symbionts become mesogleal amoebocytes filled with algae (Colley and Trench, 1985). Once a polyp strobilates into an ephyra, the algal cell population inside the amoebocytes multiply and accumulate close to the endoderm (Lajeunesse et al., 2009). In adults of C. tuberculata, symbiotic dinoflagellates are distributed along the gastrovascular system indicating nutritional importance (Kikinger, 1992).
Host-specialized dinoflagellates associated with jellyfish species belong to the family Symbiodiniaceae (LaJeunesse et al., 2018) which comprises numerous genera and species with large differences in diversity, ecology, and geographic distribution. Symbiosis between medusozoans and Symbiodiniaceae originated independently at least seven times, with five of these originations involving groups that contain pelagic taxa with three of them having a medusae phase (cubozoa, hydrozoa, and scyphozoan; reviewed in Djeghri et al., 2019). Within the class Scyphozoa, the orders Coronatae (with the family Linuchidae and some Nausithoe species) and Rhizostomeae (in the suborder Kolpophorae) are known to host symbiotic dinoflagellates species, with 20–25% of scyphozoan species being photosymbiotic. However, the majority of the studies about symbiotic jellyfish has concentrated on species of the genus Cassiopea (Fitt and Trench, 1983; Banaszak and Trench, 1995; Verde and McCloskey, 1998; Thornhill et al., 2006; Mortillaro et al., 2009; Lampert et al., 2012; Mellas et al., 2014; Leblond et al., 2015). In fact, the “upside-down” jellyfish Cassiopea spp., has been used as a model organism of cnidarian-dinoflagellate symbiosis (Ohdera et al., 2018) despite having an unusual benthic lifestyle that differs from the rest of zooxanthellate jellyfish, with a pelagic lifestyle. Therefore, there is still a significant lack of knowledge on zooxanthellate jellyfish ecology, and basic information on the role of symbiosis for most species is still scarce.
The symbiosis benefits both, the host and the symbiont. Algae produce organic compounds photosynthetically that are passed to the host medusa. In return, the host provides the symbiont with nitrogen and phosphorus (Ohtsuka et al., 2009). Zooxanthellate jellyfishes in their medusa stage obtain the major part of their nutritional energy from the photosynthesis carried out by the symbiont (Djeghri et al., 2019). Many studies conducted in symbiotic scyphozoans support the idea that photosynthetic rates are often equal or superior to respiration rates (Kremer et al., 1990; Kikinger, 1992; McCloskey et al., 1994; Verde and McCloskey, 1998).
The important role of the symbionts in jellyfish nutrition suggests that the host may present some behavioral and morphological adaptations to maintain their photosynthetic partners under the best light conditions (Djeghri et al., 2019). It is already known that zooxanthellate jellyfish perform complex horizontal and vertical migrations (Kikinger, 1992; Dawson and Hamner, 2003) or circadian regulated tissue contractions to guarantee illumination and optimize photosynthesis. Furthermore, some evidence indicates that jellyfish have developed morphological adaptations to protect the photosynthetic apparatus of the symbiont against the potentially damaging UV radiation (Blanquet and Phelan, 1987) although recent molecular studies do not support this hypothesis (Lampert et al., 2012). Not only the jellyfish tissue protects Symbiodiniaceae, but also the dinoflagellate’s pigments have an important role in the protection of the photosynthetic apparatus (Jeffrey and Haxo, 1968; Jeffrey et al., 2011).
The characteristics of the symbiont population of the medusa C. tuberculata have not often been studied. Symbionts have been genetically identified as Symbiodinium A1 (Lajeunesse et al., 2009) and Symbiodinium Temperate A (Visram et al., 2006). However, specimens isolated from the Mar Menor used in this work, seem to host two genetically distinct Symbiodiniaceae genus: Breviolum and Philozoon (Raspor Dall’Olio, 2016; LaJeunesse et al., 2021).
Here, we examined the distribution and density of Symbiodiniaceae in adult specimens of C. tuberculata. Pigment content, size and morphology, growth and carbon production of the symbiotic population were also characterized after isolation from host and maintenance in culture. The medusa tissue optical properties were also studied to evaluate the symbiont light conditions inside the host and the role played by the jellyfish tissue in the symbiosis.
Materials and Methods
Sampling
C. tuberculata adult specimens were collected at the Mar Menor coastal lagoon (37°44′N 0°47′W), on the southeastern shore of the Iberian Peninsula (Spain) (Figure 1). Samples were captured in October 2010, when the pelagic medusa stage of C. tuberculata thrives in the water column (Prieto et al., 2010). A total of 47 adult medusae were trapped using a net and transferred to large coolers filled with surrounding seawater and aeration. Samples were subsequently transported to the laboratory to be immediately processed.
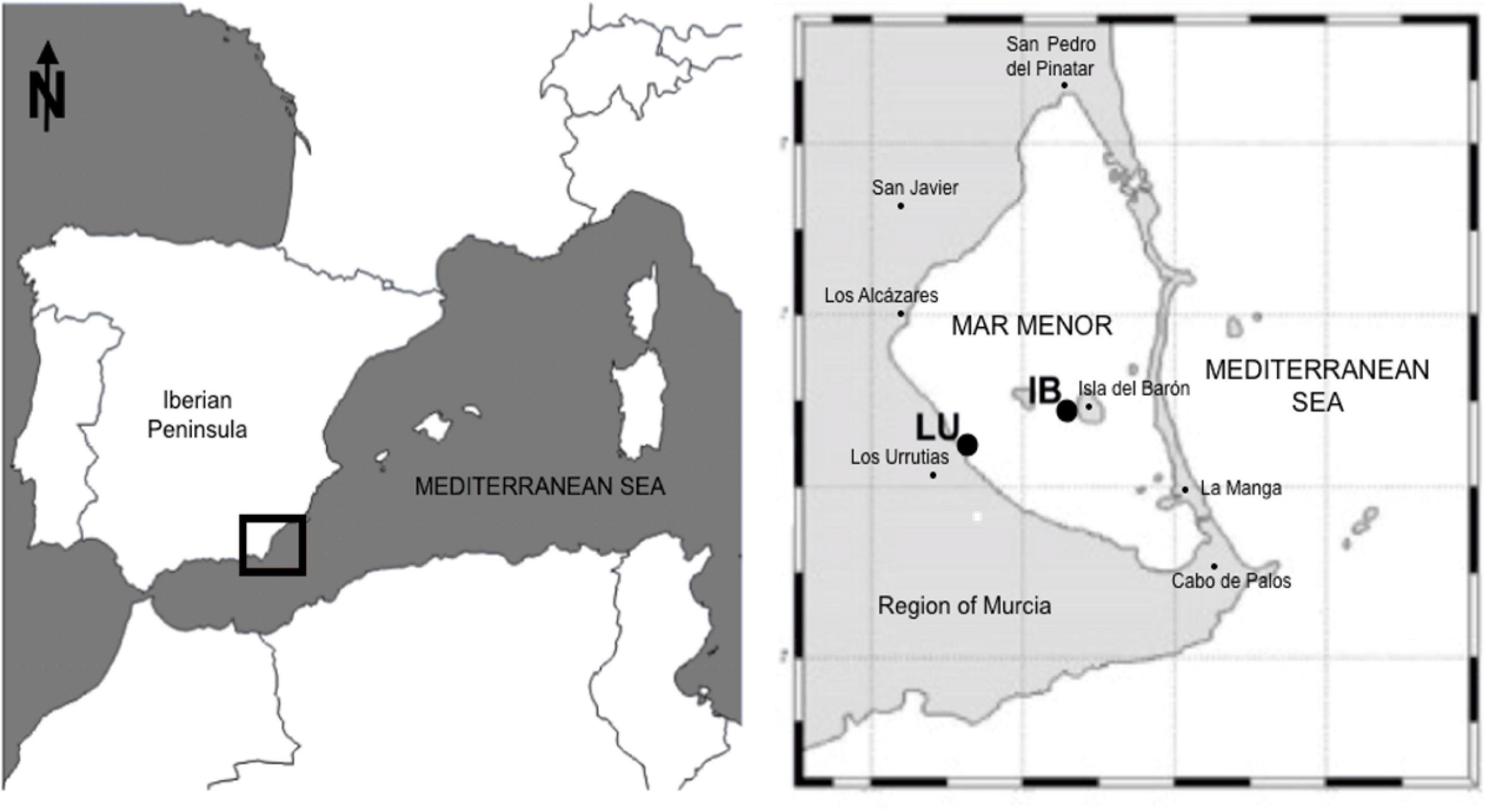
Figure 1. Mar Menor sampling locations within the southwestern Mediterranean Sea (37°44′N 0°47′W). Sample points: Los Urrutias (LU) and Isla del Barón (IB).
Jellyfish Biomass and Elemental Composition
The diameter of the umbrella and wet mass (WM) of each captured individual (N = 47) was measured by carefully extending them and daubing-off excess water. In total, 43 medusae were used to characterize algae distribution within the jellyfish body, pigment content, and C:N composition. The remaining 4 specimens were used for analyses of the tissue absorbance.
Jellyfish specimens (N = 43) were divided into two distinct sections (Figure 2): Umbrella (UM, including umbrella and gastric tissue) and oral arms (OA, which included the moutharm canal and appendages, and the brood-carrying filaments). The total volume of each medusa section was determined by separately homogenizing the jellyfish tissues using a manual mixer. From the known total homogenate volume, 12 samples of 10 ml were taken: six from OA section and six from UM.
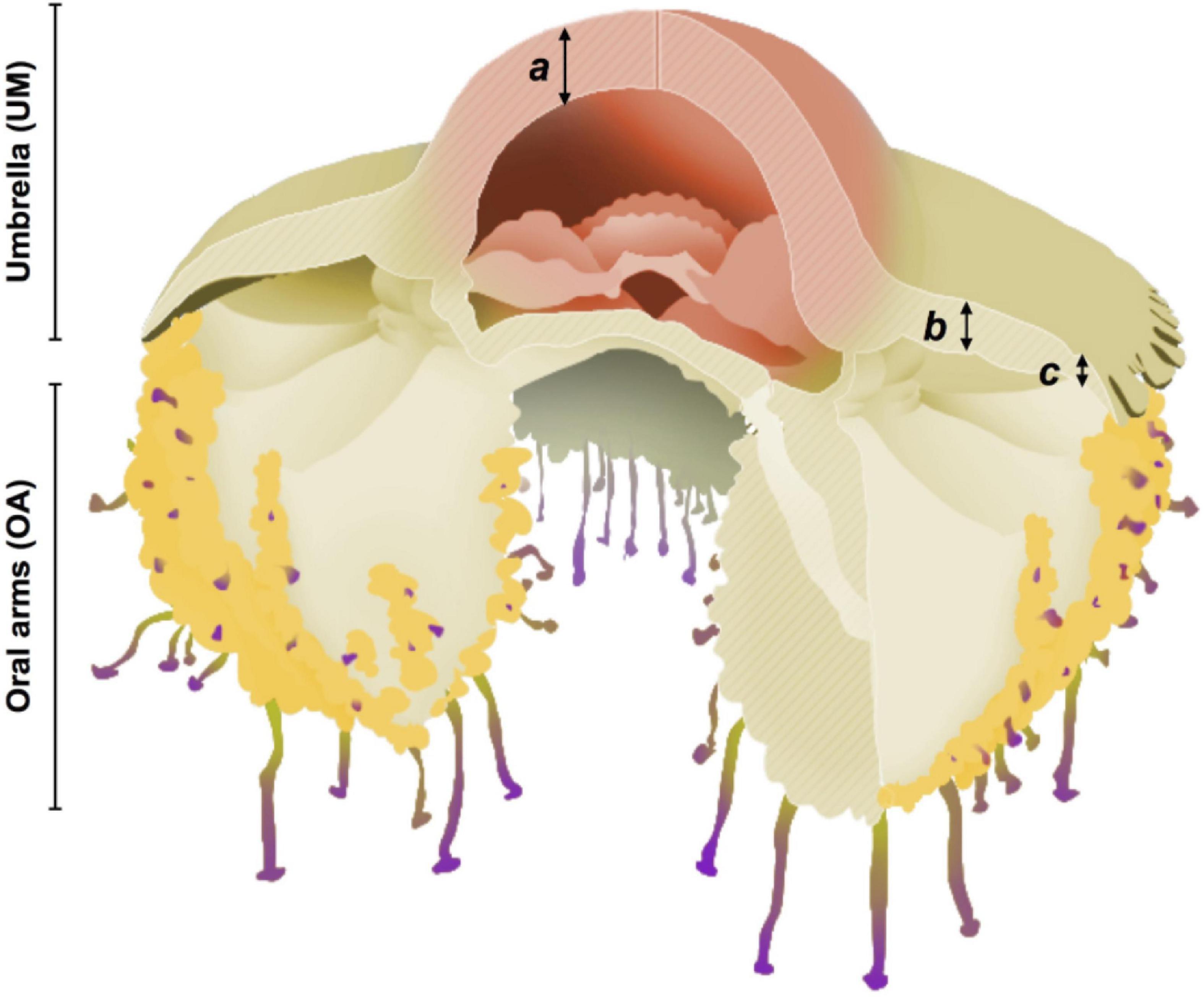
Figure 2. Scheme of Cotylorhiza tuberculata. Umbrella (UM) and oral arms (OA) sections. Bell tissue sections used for absorbance analyses: (a) Central dome, (b) clear section, (c) marginal lappets. Modified and digitalized from Kikinger (1992) by A. Chover Navarro.
To determine the jellyfish biomass and biochemical composition, triplicate samples of 10 ml of each section were used. Samples were transferred to Petri dishes for wet mass (WM) measurements and oven dried at 100°C to a constant dry mass (DM). Then, C:N composition was further determined using a TOC-VCPH/CNP analyzer (Shimadzu) and expressed as percentage of dry mass.
Host-Specialized Dinoflagellates Isolation
Symbiodiniaceae were isolated from the homogenized jellyfish samples with two purposes: (1) analysis of the symbiont distribution and pigments and, (2) assessment of their growth and maintenance in culture. Freshly isolated algae were obtained by three centrifugations at maximum speed (5,000 rpm). After each centrifugation, the jellyfish tissue debris and supernatant were discarded, and the algal pellet was resuspended in sterilized and filtered seawater. Finally, the obtained algal suspension was filtered through a 55 μm mesh, recentrifuged once and resuspended in a final volume of 10 ml filtered seawater to obtain the algal population. The same process was followed for the isolation of symbionts to set up algal cells cultures, although in this case, the pellet was resuspended in sterilized filtered seawater containing f/2 (Guillard and Ryther, 1962) medium. Cultures maintenance and analysis are described in section “Cultured Symbiodiniaceae.”
Symbiont Population and Pigment Content
To characterize the Symbiodiniaceae population, freshly isolated microalgae suspension was used for cell counts and size measurements in the different jellyfish sections. Then, pigment content was analyzed using two different techniques: (1) Fluorometry to measure total Chl a and phaeopigments, and (2) High Performance Liquid Chromatography (HPLC), to quantify and identify pigments content.
Cell counts and size measurements were carried out under optical microscopy. Triplicate samples of 1.5 ml of homogenate volume per medusa and section were used for counts. From these samples, the diameter of 10 randomly chosen cells was measured. After manipulation, algae were fixed in 4% formaldehyde to avoid cell shape damage (McAuley and Smith, 1995).
Chlorophyll a and phaeopigments quantification were measured fluorometrically using a Turner Designs 10-AU Model fluorometer according to the Joint Global Ocean Flux Study (JGOFS) protocol (Knap et al., 1996). Triplicate samples of 1.5 ml of algal suspension per medusa and section were filtered through a GF/F Whatman filter. Then, samples were sonicated into 90% acetone to extract pigments and maintained overnight at dark and 4°C until analyses. The fluorometer was calibrated using a pure Chl a standard from the cyanobacterium Anacystis nibulans (Sigma Chemical Company).
The separation and quantification of photosynthetic pigments were determined using the reverse-phase HPLC method (Mantoura and Llewellyn, 1983; Barlow et al., 1993). Triplicate samples of 10 ml algal suspension from 11 C. tuberculata individuals were analyzed. Samples were filtered (47 mm GF/F Whatman filters), placed in 4 ml of acetone 100% and sonicated for pigment extraction, and finally centrifuged to remove cellular debris and particles. The pigments content was identified by comparing the obtained HPLC profiles and their retention times with those of pure standards (DHI Danmark).
Cultured Symbiodiniaceae
Freshly isolated Symbiodiniaceae were maintained in culture with f/2 as growth medium (Guillard and Ryther, 1962) prepared with sterilized and filtered (0.2 μm) seawater. Cultures were kept in a culturing chamber at 20°C and pH 8.0 under 60 μmol quanta m–2 s–1 and 16 light:8 dark photoperiod. As soon as growth was observed, strains were frequently transferred to a new sterilized culture medium to assure relatively clean algae.
The study of the cultured symbiont population and cell morphology was conducted using different techniques and tools. Light microscopy, differential interference contrast microscopy (DIC) and imaging flow cytometry (IFC), were used to study Symbiodiniaceae in vivo whereas Scanning Electron Microscopy (SEM) was applied to examine the symbiont morphology.
Symbiodiniaceae population was first observed under light microscopy after settling by Utermöhl technique using inverted microscopy at 10×, 20×, and 40× magnification. Then, symbiont samples were analyzed by Image Flow Cytometry (IFC) using an Image Stream X Mark II (Amnis, Seattle, United States) equipped with 20×, 40×, and 60× objectives and two lasers emitting at 488 and 652 nm. Samples were run at low speed acquiring 2,000 cells per sample.
Additionally, Symbiodiniaceae samples for SEM were previously fixed in 2% OsO4 for either 10–15 min and placed on poly-L-lysine coated coverslips or 25 mm black polycarbonate filters of 0.2 μm pore size (GE Water and Process Technologies). After washing in dH2O for 30 min, samples were dehydrated in an EtOH series: 30, 50, 70, 96, and 99.9% (10 min in each change) and two changes of 100% EtOH (30 min in each change), a final change was conducted in acetone 100%. Samples were critical point dried. The filters or coverslips were mounted on stubs, coated with palladium-platinum and finally examined in a Nova NanoSEM 450 (FEI, Field Electron and Ion Company, OR, United States) scanning electron microscope.
Photosynthesis vs. Irradiance
Photosynthesis vs. irradiance (P-I) experiments were carried out using a photosynthetron Lewis and Smith (1983). A volume of 1,000 ml of Symbiodiniaceae culture was spiked with 100 μl 14C-bicarbonate and split into 50 clean glass scintillation vials (20 ml). Then, vials were incubated for 2 h and illuminated with light intensities ranging from a few to ∼1,200 μmol quanta m–2 s–1. Two scintillation vials were maintained in the dark to quantify respiration. Illumination was provided by a tungsten-halogen lamp with a blue-CuSO4 filter mounted between the lamp and the samples. Filters were used to attenuate the light intensity and achieve the desired range of light levels. Light intensity in the photosynthetron sample chambers was measured with a Biospherical PSP-100 sensor. Incubation temperature was 19.5°C. After incubation, samples were filtered through Whatman GF/F glass fiber filters (25 mm) under vacuum pressures < 100 mmHg. Then, labeled organic carbon retained in the filters was measured to estimate the total primary production (PP) (Moran and Hodson, 1990). After 2 h formalin was added to stop the incubation. To eliminate by vaporization all un-incorporated 14C, sample filters were exposed to 750 μl fuming HCl for 12 h prior to the addition of 10 ml of scintillation cocktail (Ultima Gold, Packard). The 14C radioactivity was determined on a liquid scintillation counter (Beckman LS 5000CE). Disintegrations min–1 (dpm; 1 dpm = 0.0167 Bq) were calculated by the external standard method. Before calculations, black vials values were subtracted from the light vials values for correction of non-photosynthetic 14C fixation. Since no photoinhibition was observed, photosynthetic parameters of the P-I curve were determined by non-linear least-squares regression, fitting the data to the model of Webb et al. (1974):
where PB (mgC mg Chl–1 h–1) is the chlorophyll a-normalized photosynthetic rate, PBm (mgC mg Chl–1 h–1) correspond to the maximum chlorophyll a-normalized photosynthetic rate and αB[mg C mg Chl–1 h–1 (μmol quanta m–2 s–1) –1] represents the chlorophyll a-normalized initial slope of the P-I curve.
Optical Properties of the Host Jellyfish Tissue
Four adult specimens of C. tuberculata were maintained alive and destined to measure the tissue optical properties of the intact association. The jellyfish umbrella was divided into three different sections according to coloration (Figure 2): (a) central dome (yellow-brown), (b) clear section (thick and white), (c) marginal lappets (thin and white).
Depending on the medusa size, two or three rectangular pieces of each section were cut from living specimens with a scalpel. Each umbrella section was individually stuck to the lateral side of a quartz cuvette. Then, samples were exposed to the different wavelengths of the light spectrum inside a UV-VIS Perkin Elmer Lambda-5 spectrophotometer (190–2,500 nm). Absorption spectra data were collected with a data collection system and corrected to exclude residual scattering by subtracting the apparent absorbance at 750 nm. Sections were oriented in such a way that spectrophotometer beam of light passed through the jellyfish umbrella tissue layers as in nature: from exumbrella epidermis (ectoderm) to mesoglea and coronal muscle and then to subumbrella epidermis. Considering that the tissue section is scattering light as well as absorbing it, we used a prisma to make a true measurement of absorbance.
To estimate light conditions inside the jellyfish the shade provided by the jellyfish tissue was measured as well as, the light intensity reaching Symbiodiniaceae in each specific part of the umbrella. Light attenuation provided by each umbrella tissue section (a, b, and c, see Figure 2) was measured in 4 living specimens of C. tuberculata. Firstly, surface irradiance in a laboratory aquarium and irradiance under each umbrella tissue on the surface was measured using a QSL-Radiometer (Biospherical instruments Inc.). Finally, the umbrella thickness was measured in each section to estimate the% of light attenuation provided by each tissue as a function of the tissue thickness.
The irradiance reaching the jellyfish tissue at the surface and at 1 m depth was calculated by the following equation: IZ = I0e−kz, where Iz is the light intensity for a given depth (z), Io the light intensity at the surface and k the light extinction coefficient. The surface irradiance measured during the sampling efforts carried out in the present work was approximately 4,000 μmol quanta m–2 s–1, assuming a k of 0.4 as a mean light extinction coefficient in the Mar Menor during summer-autumn (Terrados and Ros, 1991; Lloret et al., 2008), the light intensity reaching the medusa at 1 m depth in the lagoon would be 2,681 μmol quanta m–2 s–1.
Statistical Analyses
Prior to analysis, all data sets were tested for compliance with the statistical test assumptions, normality, and homoscedasticity. If assumptions were violated, the data were retested, or a non-parametric test was utilized. The software package R (R Foundation for Statistical Computing) was utilized to conduct statistical and graphical analysis.
Results
Host Biometrics and Elemental Composition
The diameter and wet mass (WM) (mean ± SD) of C. tuberculata specimens collected from the Mar Menor ranged from 7.0 to 33.5 cm (23.9 ± 6.3) and 39 to 2,780 g (1,332 ± 676), respectively. C and N content of the intact association was expressed as percentage of dry mass. C percentage in the tissues ranged from 1.9 to 10.5% (7.3 ± 1.7) in OA section and from 0.9 to 14.7% (5.3 ± 2.5) in UM section whereas N percentage represented 0.4–2.5% (1.7 ± 0.5) in the OA and from 0.4 to 3.5% (1.4 ± 0.6) in UM. The C:N ratio was significantly higher in OA (4.4 ± 0.30) than in the UM (3.8 ± 0.26) section (Mann-Whitney U-test, U = 1,636, N = 43, P < 0.01).
Symbiont Distribution in Hospite
Symbiodiniaceae were heterogeneously distributed within the host body (Figure 3A). We found significantly (Mann-Whitney U-test, U = 1,789, N = 43, P < 0.05) higher algae densities in the OA section (5.98 ± 3.58⋅106 cell ml–1) than in the UM section (1.07 ± 0.72⋅106 cell ml–1). However, no relationship or tendency was found between Symbiodiniaceae density and host WM. Cell diameter of freshly isolated algae from the host was 8.71 (± 0.97) μm (N = 430).
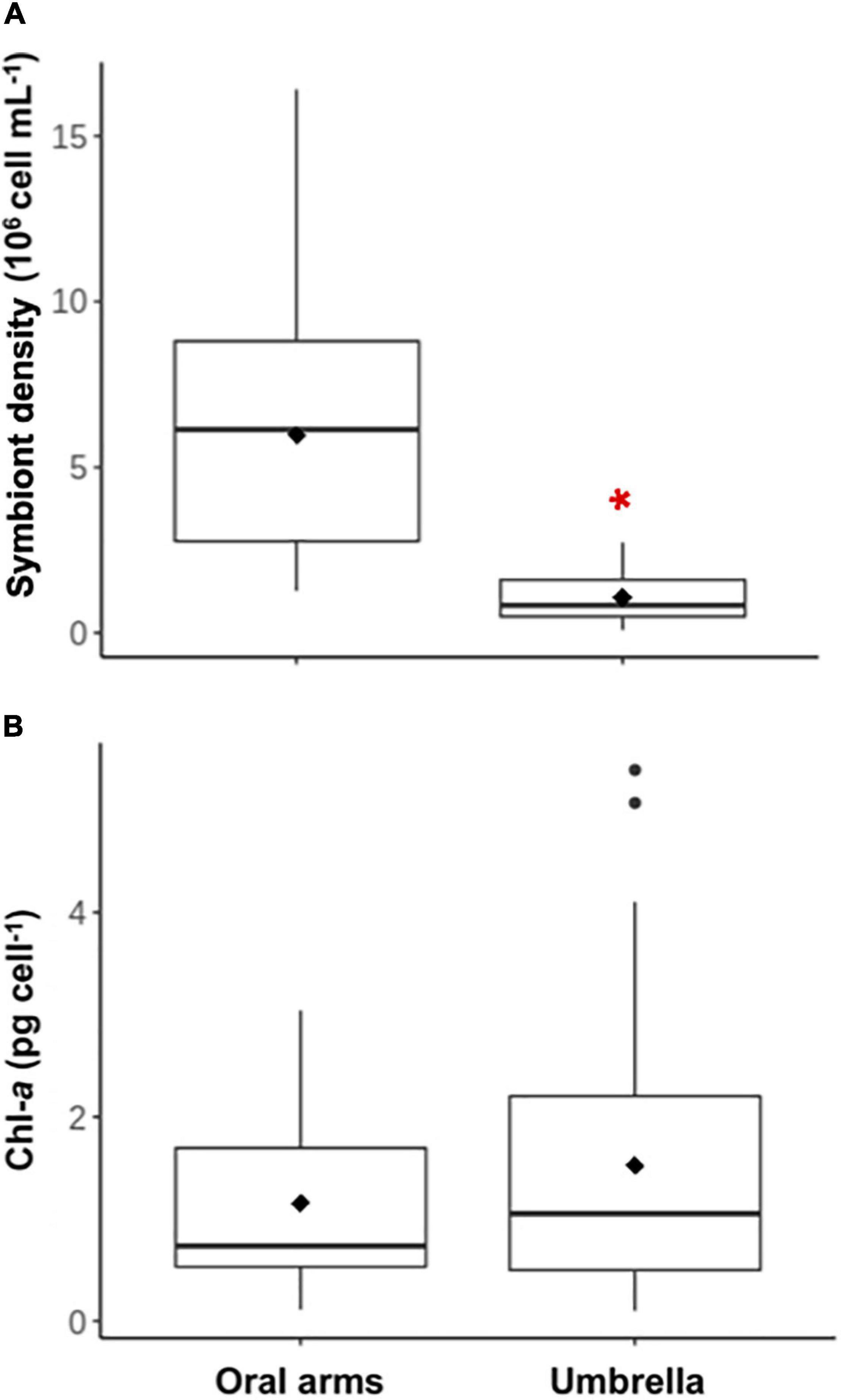
Figure 3. Cell density and chlorophyll a content of Symbiodiniaceae isolated from C. tuberculata. (A) Symbiodiniaceae density within the jellyfish orals arms and umbrella. (B) Chlorophyll a (Chl a) concentration of Symbiodiniaceae isolated from the oral arms and the umbrella. Red asterisk shows significant differences.
Pigment Content
Chlorophyll a content and symbiont diameter were also studied separately in both jellyfish body sections, but no significant differences were found (Mann-Whitney U-test, U = 688, N = 43, P = 0.474, Figure 3B). Average Chl a content per cell was 1.33 (± 0.83) pg Chl a cell–1.
HPLC analysis revealed the presence and quantity of 13 different pigments in C. tuberculata Symbiodiniaceae (Table 1), that were quantified and expressed as percentage (± SD): chlorophyll c2 (31.25% ± 8.90), chlorophyll a (30.12% ± 5.44), peridinin (18.47% ± 3.41), diadinoxanthin (10.10% ± 2.81), fucoxanthin (5.35% ± 6.60), chlorophyll c3 (1.22% ± 0.70), β-carotene (0.88% ± 0.38), 19′-butanoyloxyfucoxanthin (0.86% ± 0.43), alloxanthin (0.72% ± 0.62), 19′-hexanoyloxyfucoxanthin (0.64% ± 0.11), diadinoxanthin (0.33% ± 0.21), zeaxanthin and lutein (0.44% ± 0.01), and chlorophyll b (0.01% ± 0.02).
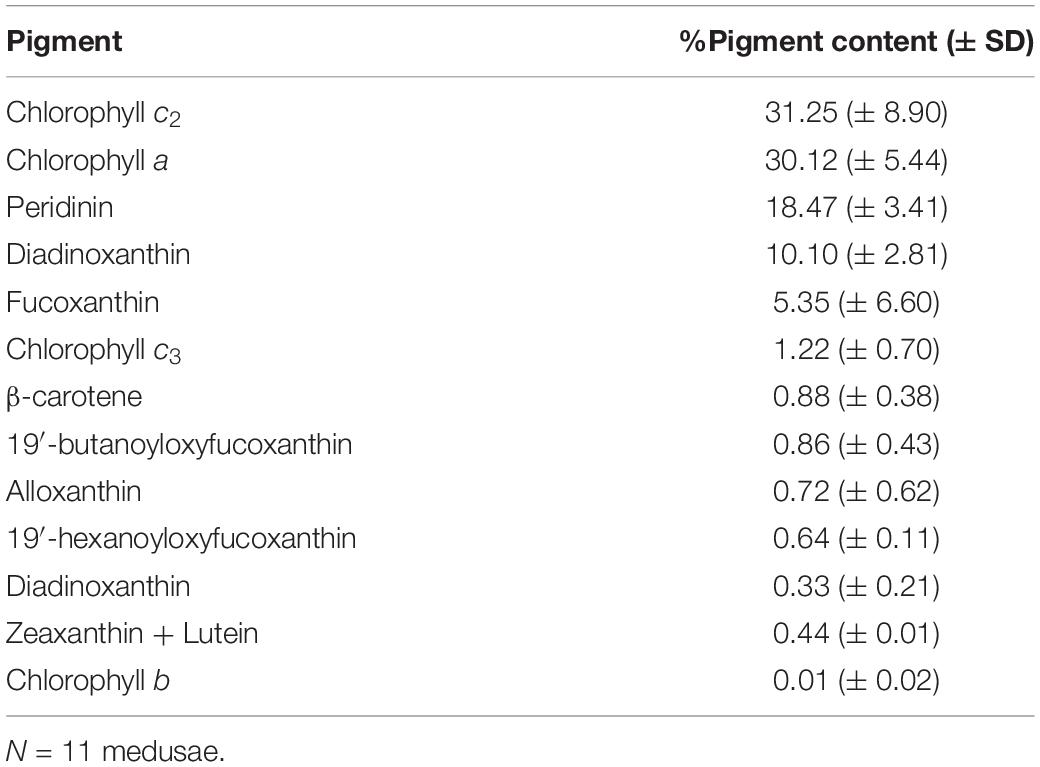
Table 1. Percentage of pigment content (average% ± SD) of Cotylorhiza tuberculata symbionts (Symbiodiniaceae) from High Performance Liquid Chromatography (HPLC) analyses.
Cultured Symbiodiniaceae Study
The growth rate of symbionts in culture was 0.52⋅106 cell ml–1 d–1 (Supplementary Figure 1). In vivo observation of cultures under light microscopy revealed different phases of Symbiodiniaceae. Cultures obtained from recent isolation of symbionts showed the presence of colored coccoid cells (spherical) (Figures 4A, 5A–C), doublet (dividing) (Figures 5D,E), and mastigote (motile zoospore) (Figures 4C, 5F,G). In the most ancient cultures, we only observed coccoid cells (Figures 4A,B), showing the typical mesh-like plastid of Symbiodiniaceae (LaJeunesse et al., 2010), that indicates a reticulated chloroplast located around the entire cell periphery (Figure 4A). Mastigotes were mushroomed-shaped with the hemispherical epistome slightly larger than the hemispherical hyposome (Figures 4C, 5G). The nucleus was in the epistome (Figure 4C). The averaged diameter of coccoid cells was 15.28 (± 1.28) μm for the bigger coccoid morphotype (N = 100) and 4.83 (± 0.678) μm for the smaller morphotype (N = 100). This size ranges have been previously observed in vegetative cysts (Stat et al., 2006). It can be infered that small coccoids might correspond to young vegetative cysts of the symbiont whereas the bigger coccoids represented older cells. Also, doublet cells could be well dividing vegetative cysts giving place to two daughter cells (Figures 5D,E).
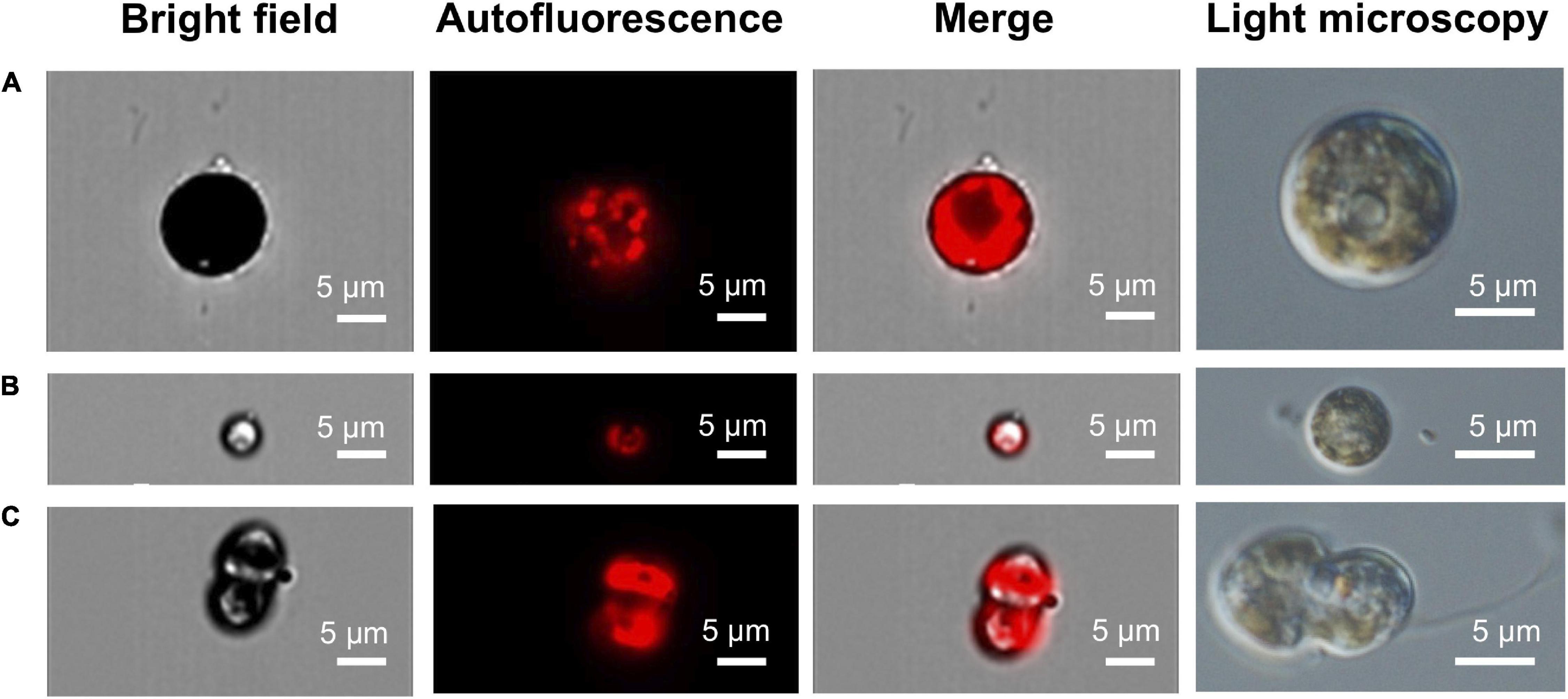
Figure 4. IFC autofluorescence and light microscopy images of in vivo cultures of free living C. tuberculata Symbiodiniaceae. (A) Bigger coccoid stage with a reticulated chloroplast. (B) Small coccoid stage with a parietal chloroplast. (C) Motile flagellated mastigote.
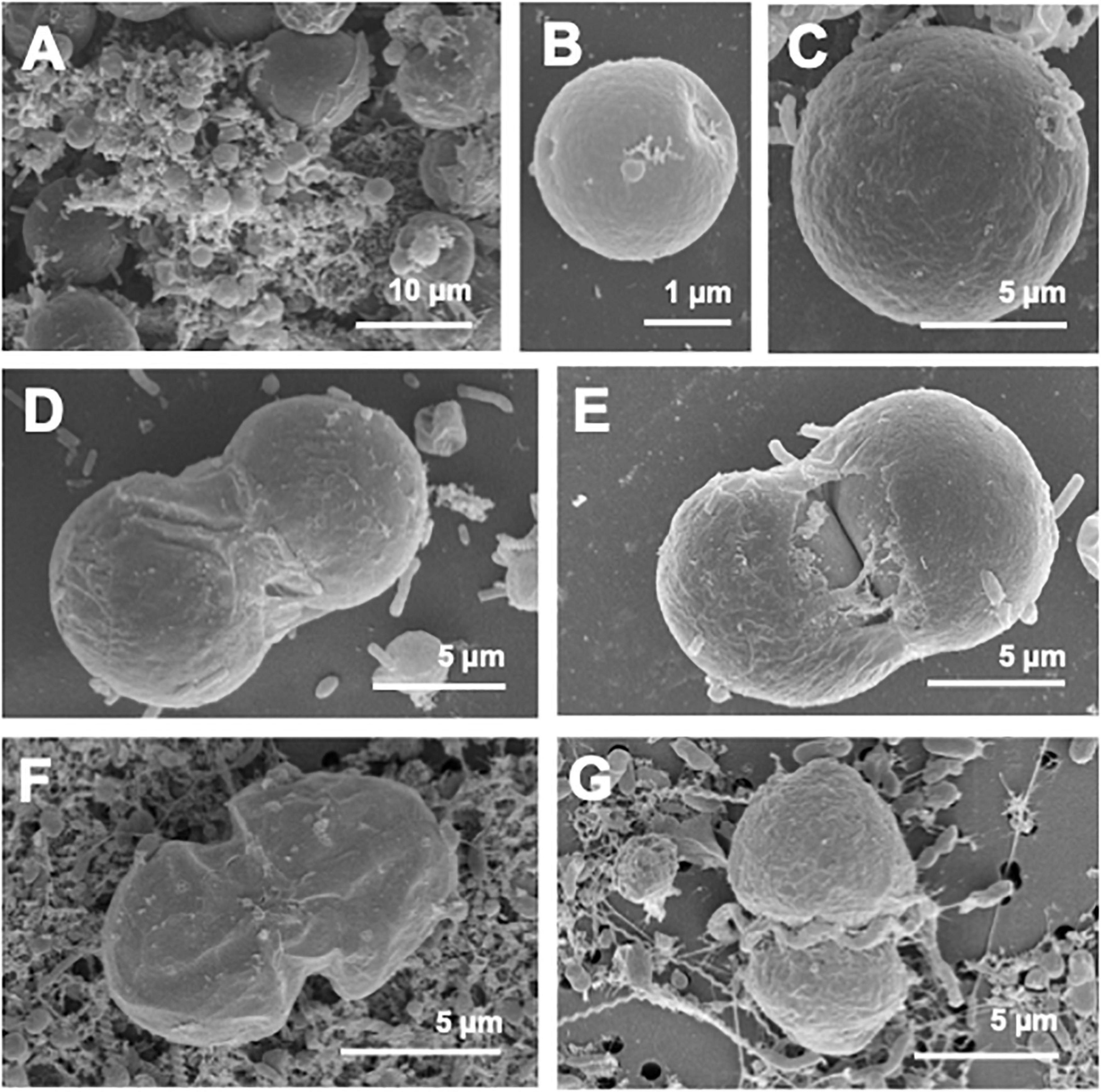
Figure 5. SEM micrographs of different cellular stages observed in Symbiodiniaceae cultures. (A) C. tuberculata symbionts of both sizes. (B) Small coccoid cells observed in cultures. (C) Bigger coccoid stage. (D,E) Doublet, two different phases of bigger coccoid cells undergoing mitosis. (F,G) Motile flagellated mastigote.
In ancient cultures, calcifying biofilm formations were also identified (Figures 6A–D), which were similar to those recently discovered in a diverse range of cultured Symbiodinium strains and their inherent bacterial communities (Frommlet et al., 2015). The association between Symbiodinium and bacteria produces aragonite microbialites, termed “symbiolites,” which encase adjacent symbiotic algae as endolithic cells (Frommlet et al., 2018). In our Symbiodiniaceae cultures, this calcification formed a biofilm matrix (Figure 6) that was attached to the culture flasks, appearing transparent under light microscopy (Figures 6A,B) and containing numerous coccoids cells and associated microbiota (Figures 6C,D). The matrix was indeed composed by calcium carbonate (see Supplementary Figure 2).
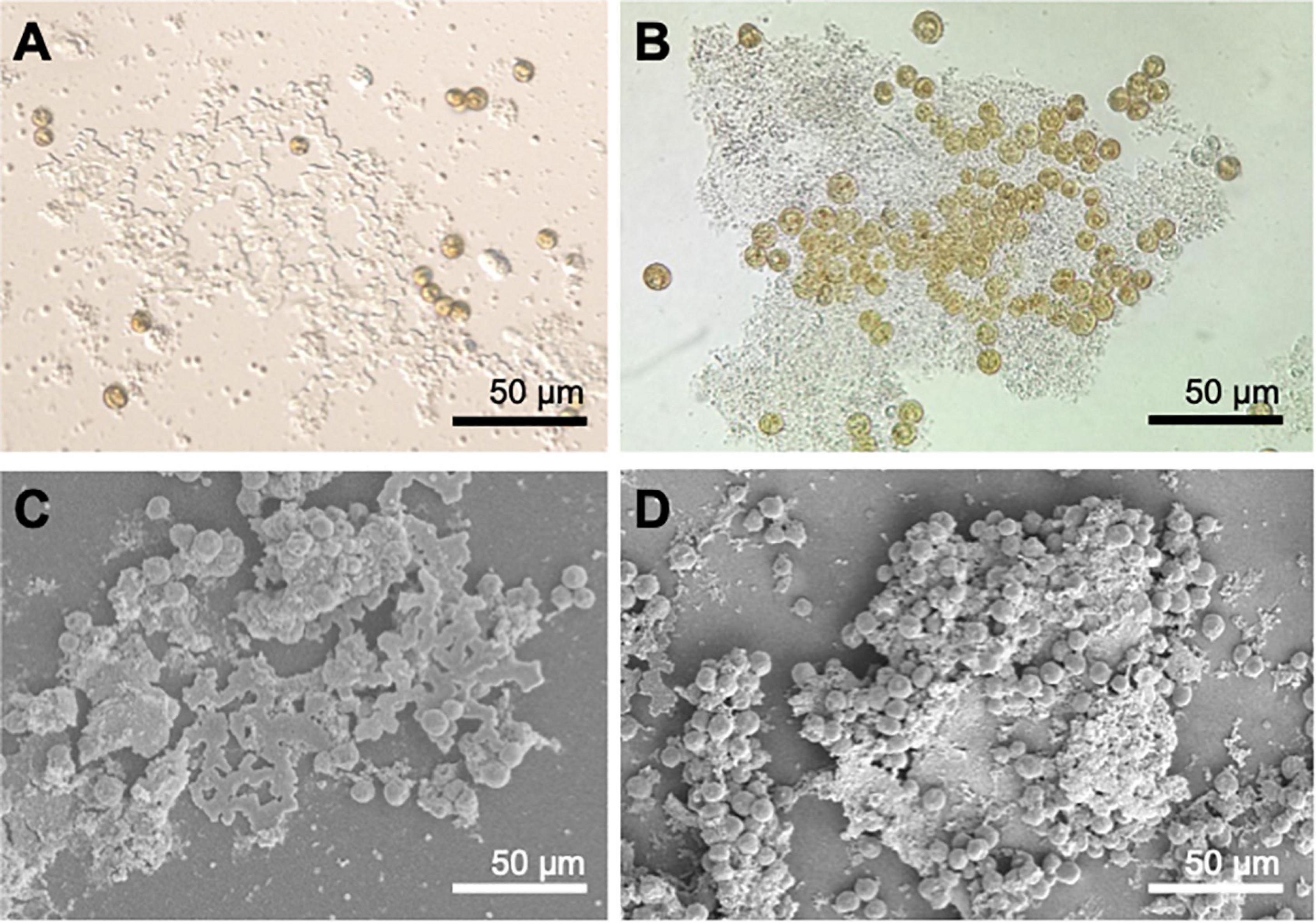
Figure 6. Differential Interference Contrast (DIC) microscopy (A), light microscopy (B) and SEM images (C,D) of calcifying matrix biofilm in Symbiodiniaceae cultures.
Photosynthesis vs. Irradiance
The photosynthetic behavior of cultured Symbiodiniaceae isolated from C. tuberculata was studied by a Photosynthesis-Irradiance (P-I) curve shown in Figure 7. The initial linear part of the P-I curve, where the photosynthetic rate is linearly proportional to the irradiance (light limited region) or the initial slope of the light saturation curve, determined by α, indicated an averaged photosynthetic efficiency of 3.8⋅10–4 (± 1.5⋅10–4) μgC (mg Chl a) –1 h–1 (μmol quanta m–2 s–1). As irradiance increased, the P-I curve showed the typical non-linear tendency reaching a saturation level in which the photosynthetic rate was maximal, Pmax with a value reaching 5.22⋅10–2 mgC (mg Chl a) –1 h–1. The irradiance at the onset of light saturation, Ik, was 142 (± 45) μmol quanta m–2 s–1, calculated as Ik = Pmax/α. No photoinhibition was observed under 1,200 μmol quanta m–2 s–1.
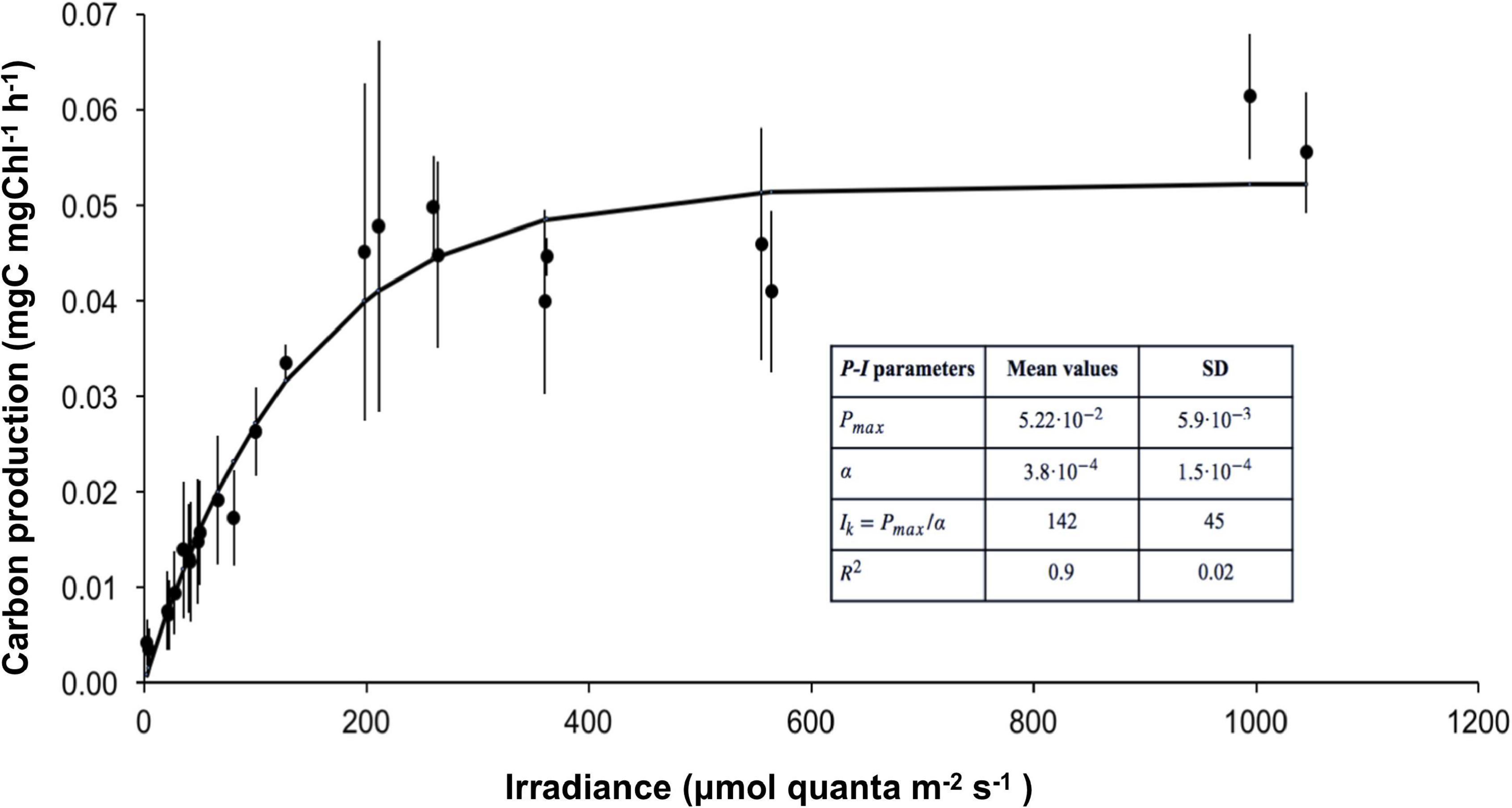
Figure 7. Photosynthesis vs. irradiance (P-I) curve of cultured Symbiodiniaceae isolated from C. tuberculata. The curve was obtained by fitting the data to the model of Webb et al. (1974). Error bars show standard deviation.
Absorbance of Jellyfish Tissue
The UV-VIS absorption spectra of the three sections of the umbrella of C. tuberculata intact association are shown in Figure 8. The absorbance of the tissue sections was relatively low at wavelengths >400 nm (Photosynthetically Active Radiation, PAR, 400–700 nm) and high at wavelengths < 400 nm (Ultraviolet irradiation, UVR, 290–400 nm). Absorption spectrum of the central dome section (a, N = 8), exhibited less accentuated peaks in the UV spectral region and higher absorbance values than tissue b and c. In this section, a high absorbance was also observed in the PAR region, where smooth peaks of 580 and 630 nm approximately were evident. Clear section (b, N = 11) presented two absorption peaks at 270 and 330 nm approximately, but the absorption in the visible light spectrum was higher than in section c. Umbrella edge (c, N = 12), also showed two absorption peaks at 270 and 330 nm approximately. However, the absorption was minimal in the visible light spectrum (400–700 nm).
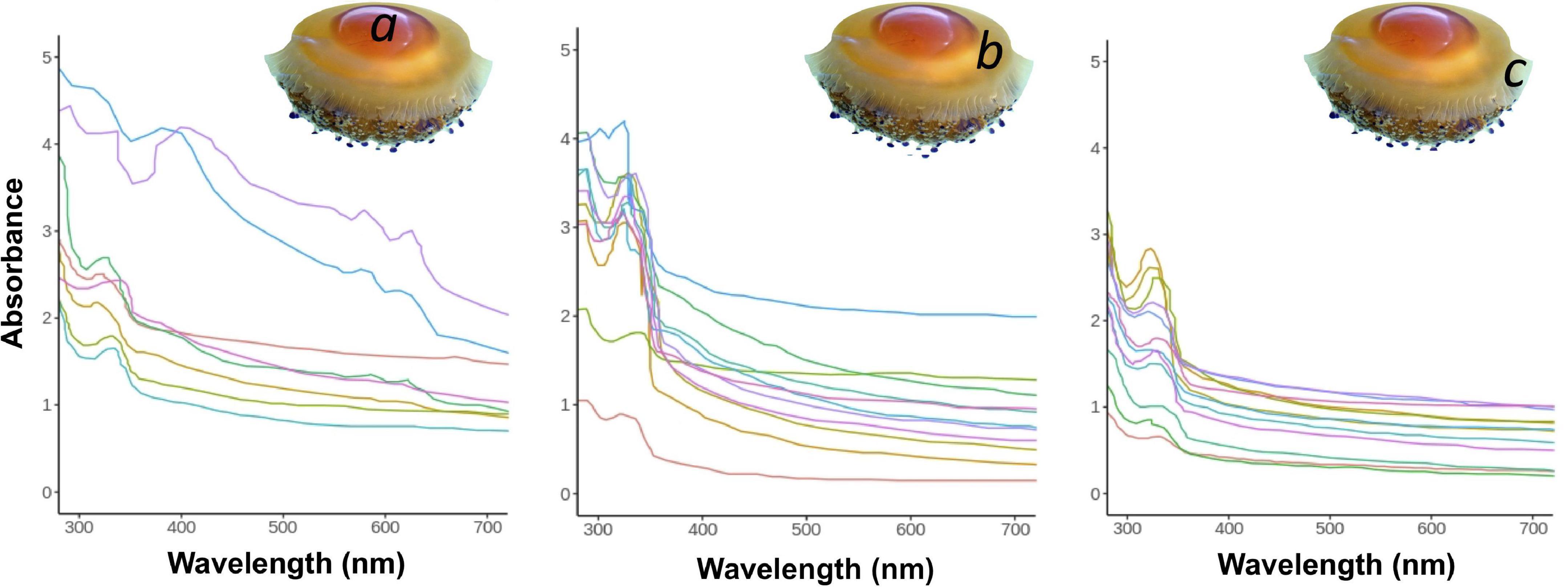
Figure 8. UV-VIS absorption spectra of the three tissue sections of the umbrella of C. tuberculata. (a) Central section, (b) clear section, (c) umbrella edge.
The mean thickness of the umbrella tissue sections of 4 individuals of C. tuberculata (mean umbrella diameter, 20 ± 4.78 cm) were 13 (± 2.89), 22 (± 3.08), and 5 (± 1.65) mm for central dome (a), clear section (b), and umbrella edge (c), respectively. In the laboratory, light attenuation provided by each jellyfish tissue section calculated as percentage of the surface irradiance (100%) was a 75% by central dome, 41% by clear section and 14% by umbrella edge.
Discussion
Role of the Jellyfish in the Symbiotic Association Alga-Cnidarian
The light environment surrounding Symbiodiniaceae is a key factor to understand photosymbiosis. The productivity of the symbiosis depends on the environmental light quantity and quality, in other words, the photon flux and the spectral composition of light reaching the algae (Roth, 2014). Therefore, the depth at which the jellyfish lives and the characteristic of the surrounding seawater, as well as the shade and protection provided by the medusa body itself constitute an important aspect of the Symbiodiniaceae-jellyfish symbiosis.
The spectral composition of the light field inside the medusa tissue might be a key factor regulating photosynthesis of the symbiont. The light field available for symbionts living within the medusa body depends on the optical properties of the host tissues and differs from the incident environmental irradiance due to the scattering and absorption, as demonstrated in scleractinian corals (Kühl et al., 1995). This study shows for the first time the absorption spectrum of a zooxanthellate jellyfish tissue in vivo. The light absorption of C. tuberculata umbrella tissue mostly absorbs on two ultraviolet absorption peaks at approximately 270 and 330 nm. The absorbance of the three umbrella tissue sections (umbrellar edge, clear section, and central section) was high at ultraviolet wavelengths (below 400 nm), indicating that medusae tissue plays an important role protecting Symbiodiniaceae from UV radiation. These results are consistent with those by Higuchi et al. (2010), who found two similar absorption peaks in the UV spectrum using homogenate host tissue extract of the symbiotic coral Galaxea fascicularis and suggested the formation of UV-absorbing compounds in their tissues. Ultraviolet radiation (UVR) is the most damaging portion of the light spectrum, known to have negative effects on physiological and cellular processes, damaging the host tissue and the algae photosynthetic apparatus (Arai, 1997). These high-energy wavelengths cause damage in biologically relevant molecules, chloroplast and photosynthetic apparatus, causing photoinhibition, which leads to the generation of multiple reactive oxygen species (ROS) by the symbionts (Weis, 2008). The effects of UVR have been studied in several Symbiodiniaceae species living in symbiosis with corals and anemones (Lesser and Shick, 1990; Lesser and Lewis, 1996) and, to a lesser extent, in jellyfish (e.g., the scyphozoan Cassiopea sp. Klein, 2016). Under UVR exposition, symbionts of C. xamachana exposed to UVR produced micosporine-like amino acids (MAAs) (Banaszak and Trench, 1995) which are UV-absorbing compounds that act as UV sunscreen and confer protection during heat stress (Banaszak and Lesser, 2009). C. xamachana absorption spectra at UV wavelengths was characterized by a maximum peak at 324 nm and their freshly isolated symbionts at 338 nm (Banaszak and Trench, 1995) similar to those found here for C. tuberculata (330 nm), which may be indicative of the presence of MAAs in this jellyfish tissues. However, despite MAAs accumulate in the host tissues, it is still unclear the symbiotic partner that produces these molecules (Shinzato et al., 2011).
The structure of C. tuberculata umbrella provides different light environments. The dark-colored central dome showed a high absorbance not only in the UV region but also in the PAR region (580–630 nm). Furthermore, light attenuation provided by this tissue section reaches 75% of the environmental irradiance, which may eventually result in light limitation for symbiont photosynthetic activity. Light attenuation provided by host white tissue in the thicker part of the bell was 44%. Symbionts are especially numerous along the radial canals of the gastrovascular system (Kikinger, 1992) in the central dome of the umbrella that provides UVR protection. Finally, the umbrella edge is the thinner part of the jellyfish bell, providing 14% of light attenuation. Here, the absorption is elevated in the UVR region of the spectrum and remains very low in the PAR region, suggesting that no light limitation occurs here, but the light conditions in this region might be damaging the algae. However, no Symbiodiniaceae were found within this tissue.
Light reaching Symbiodiniaceae in hospite will also depend on the host position in the water column, the characteristics of the surrounding seawater and the incident environmental irradiance. Zooxanthellate jellyfish are known to migrate vertically to mitigate UV damage or photoinhibition of their photosymbionts, as well as to maximize their photosynthetic rates (Cimino et al., 2018). C. tuberculata specimens used in this work occur in the Mar Menor, a shallow coastal lagoon (4 m depth on average) (Pérez-Ruzafa et al., 2005). In late summer and early autumn, when the pelagic phase of the jellyfish thrive, the lagoon turns mesotrophic reaching high chlorophyll levels (Pérez-Ruzafa et al., 2005) and irradiance measured at the bottom of the lagoon remains lower than the rest of the year (Terrados and Ros, 1991). C. tuberculata moves in the water column between 1 and 2 m depth (Kikinger, 1992). The calculated light intensity reaching the medusa at 1 and 2 m depth in the Mar Menor was 2,681 and 1,797 μmol quanta m–2 s–1, respectively. Given that the jellyfish umbrella tissue where most Symbiodiniaceae concentrate, attenuates the surrounding irradiation by 41%, the light intensity received by the symbionts at 1 m of the surface would be 1,100 μmol quanta m–2 s–1 and 736 μmol quanta m–2 s–1, at 2 m depth (Figure 9). The study of the photosynthesis-irradiance of C. tuberculata symbionts revealed no photoinhibition at irradiances below 1,200 μmol quanta m–2 s–1, suggesting that Symbiodiniaceae inside their host are protected from the elevated irradiances reached in the surface water. Therefore, the great importance of the jellyfish tissue attenuating the elevated environmental irradiance in the upper water column and absorbing damaging UVR is evidenced. The vertical distribution of zooxanthellate jellyfish occupying a specific depth range to obtain the adequate oxygen for respiration and light for photosynthesis has been previously studied in other zooxanthellate jellyfish (Hamner et al., 1982; Muscatine and Marian, 1982; Dawson and Hamner, 2003). C. tuberculata optimal depth in the Mar Menor may be however influenced by light penetration, water turbidity, seasonal light intensity, and cloud cover. Hence, the jellyfish will respond to changes in the vertical water column by choosing a depth to match their optimal physiological tolerance and maximize photosynthesis.
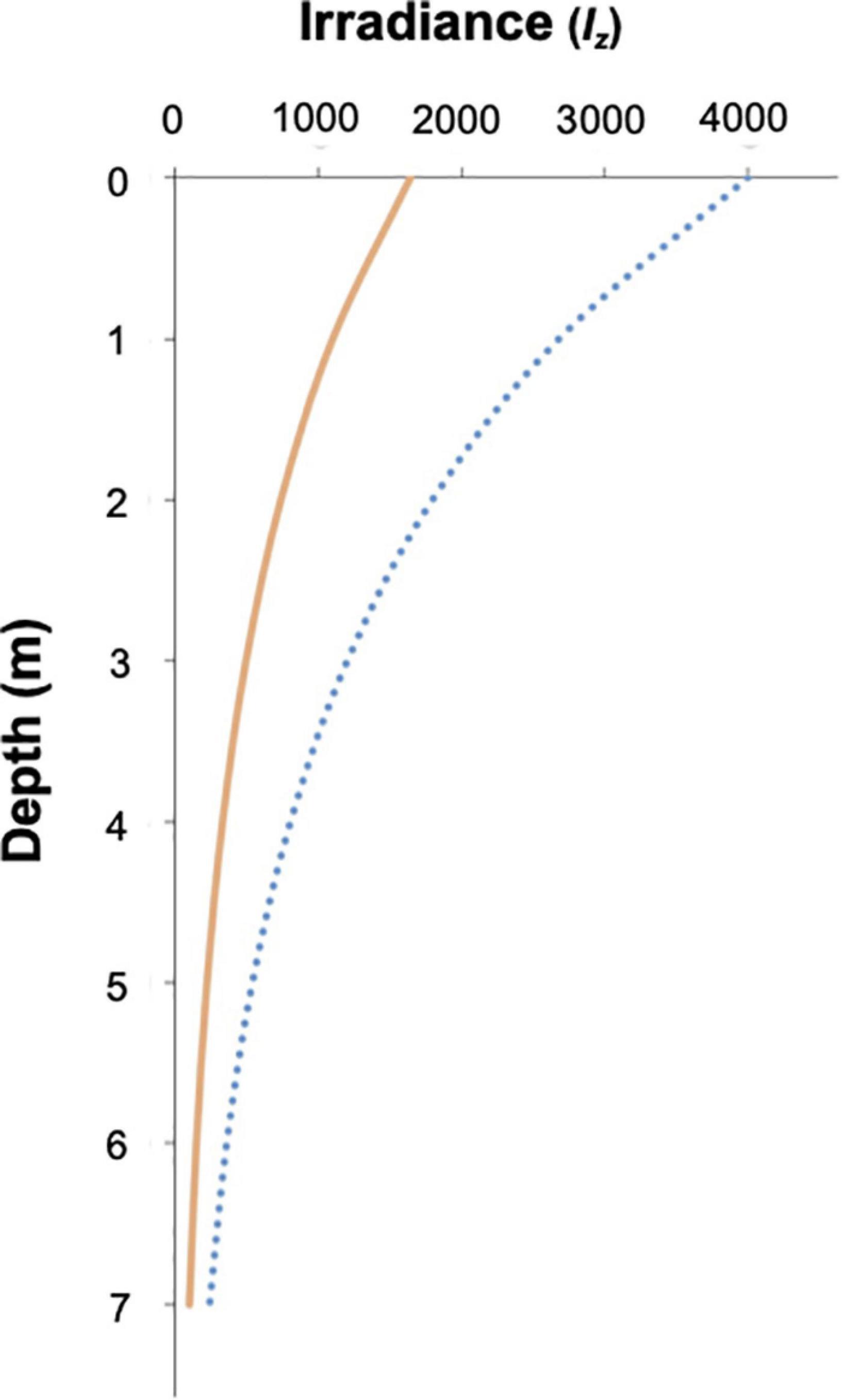
Figure 9. Relative light intensity as function of depth in the Mar Menor coastal lagoon. Blue line represents light intensity reaching the jellyfish body and orange line is the light intensity reaching the symbiont living withing the jellyfish tissue.
Symbiont Distribution Within the Jellyfish Body
The abundance of Symbiodiniaceae is an important feature of the symbiosis because it directly affects the amount of oxygen produced by photosynthesis. In zooxanthellate jellyfish the density of symbionts is affected by their complex life-cycle. In the case of C. tuberculata symbionts are acquired during the polyp stage (Kikinger, 1992; Verde and McCloskey, 1998; Astorga et al., 2012), reaching at this point, algal cell densities between 100 and 300 cells per mm2 (Enrique-Navarro et al., 2021). During strobilation, the algae multiply in the oral region of the polyp where the ephyrae are formed (Ludwig, 1969) hence, releasing zooxanthellate ephyrae (Kikinger, 1992). On the other hand, during medusae growth the abundance of symbionts remains constant or decreases slightly in most species down to the order of 106 cells g–1 wet mass (Muscatine et al., 1986; Kremer et al., 1990; Verde and McCloskey, 1998).
Our results show that symbiotic algae were heterogeneously distributed within the medusa body, being 5.5 fold denser in the oral arms than in the umbrella (Table 2). This heterogeneity might be a consequence of the different body parts roles, functions and morphologies. The jellyfish oral arms act as an uptake organ, thus being a nutrient-rich environment for symbionts nutrition and consequently, for algal primary production (Muscatine et al., 1986). In addition, oral arms and tentacles have a sinuous folding morphology that provides a large area to host microalgae. On the other hand, the bell has a well-developed muscle cell organization to allow active contraction and movements during medusa locomotion. In this part of the animal, the symbionts are concentrated in the radial canals of the gastrovascular system (Kikinger, 1992). The elevated zinc levels measured in C. tuberculata oral arms (4 times higher than umbrella) is also an indication of the higher presence of symbionts in these organs, as zinc is a key element of the enzyme carbonic anhydrase in algae (Furla et al., 2011). In C. tuberculata, the symbiont density was independent of the body size, as previously reported in Mastigiasvsp. (Muscatine and Marian, 1982; Muscatine et al., 1986) and cell density values were similar to those observed in L. unguiculata (Kremer et al., 1990) and C. xamachana (Verde and McCloskey, 1998), in which the symbionts densities were also two to threefold higher in the oral arms than in the bell (Blanquet et al., 1979; Estes et al., 2003; Table 2). This finding is also consistent with the larger values of C measured in the oral arms of C. tuberculata. Overall, jellyfishes are characterized by high water (>95%) and low carbon (<1% of WM) contents, which enables them to increase their size in a much larger way than non-gelatinous animals with an equivalent C content (Pitt et al., 2013). This composition offers many adaptive advantages (Acuña et al., 2011). In our study, biochemical composition of C. tuberculata in terms of carbon and nitrogen was quantitatively similar than that in non-symbiotic (Aurelia aurita, Chrysaora fuscescens, Chrysaora hysoscella, Pelagia noctiluca, and Rhizostoma pulmo) and symbiotic scyphozoans (Cassiopea xamachana, Cyanea capillata, and Mastigias sp.) whose overall C:N ratio falls within the range comprised between 3.2 and 4.5 (Table 3). Even though comparison with the C:N ratios obtained in different parts of the considered jellyfishes is not possible, it is reasonable to assume that the oral arms in zooxanthellate jellyfish would contain a higher amount of carbon than the bell does. This carbon would be the result of the own animal metabolism plus that produced during photosynthesis due to a high accumulation of symbionts. Similarly, this could be the situation encountered in the tentacles that host a higher algal density. On the contrary, the bell or umbrella, with a lower concentration of symbionts, would contain a lower carbon content than the arms but a similar nitrogen proportion that causes in turn, a similar C:N ratio between both parts of the medusa.
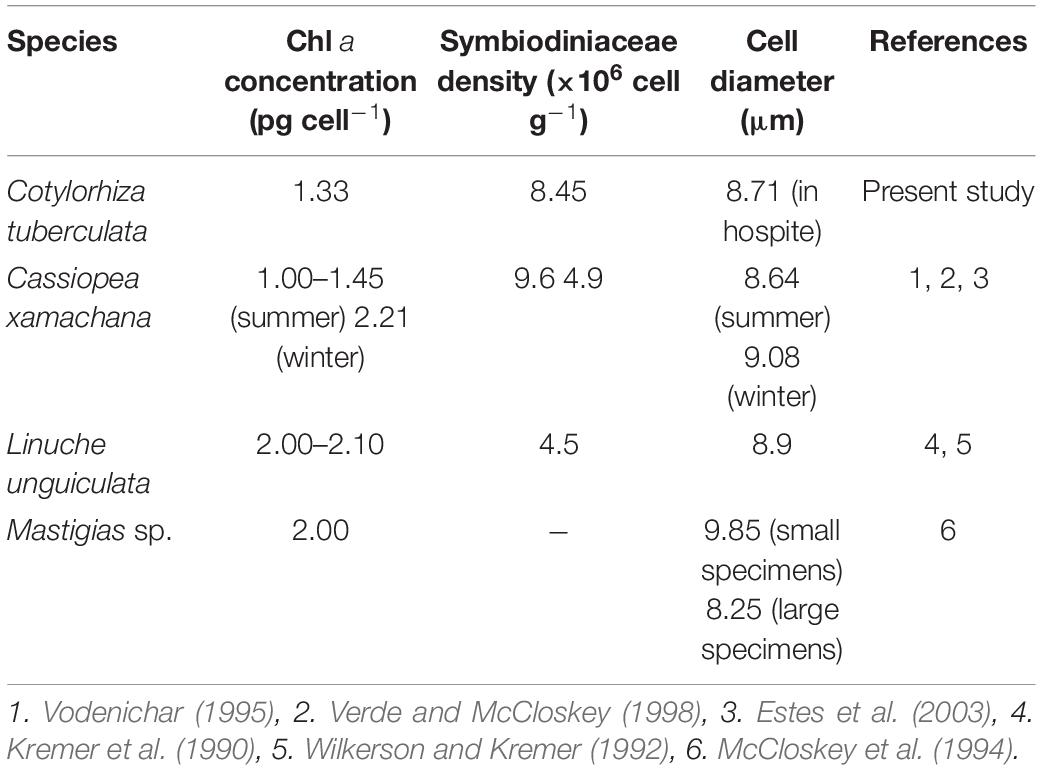
Table 2. Comparison of the Symbiodiniaceae parameters of chlorophyll a concentration, cell density, and diameter of 4 symbiotic jellyfish: Cotylorhiza tuberculata, Cassiopea xamachana, Linuche unguiculata, and Mastigias sp.
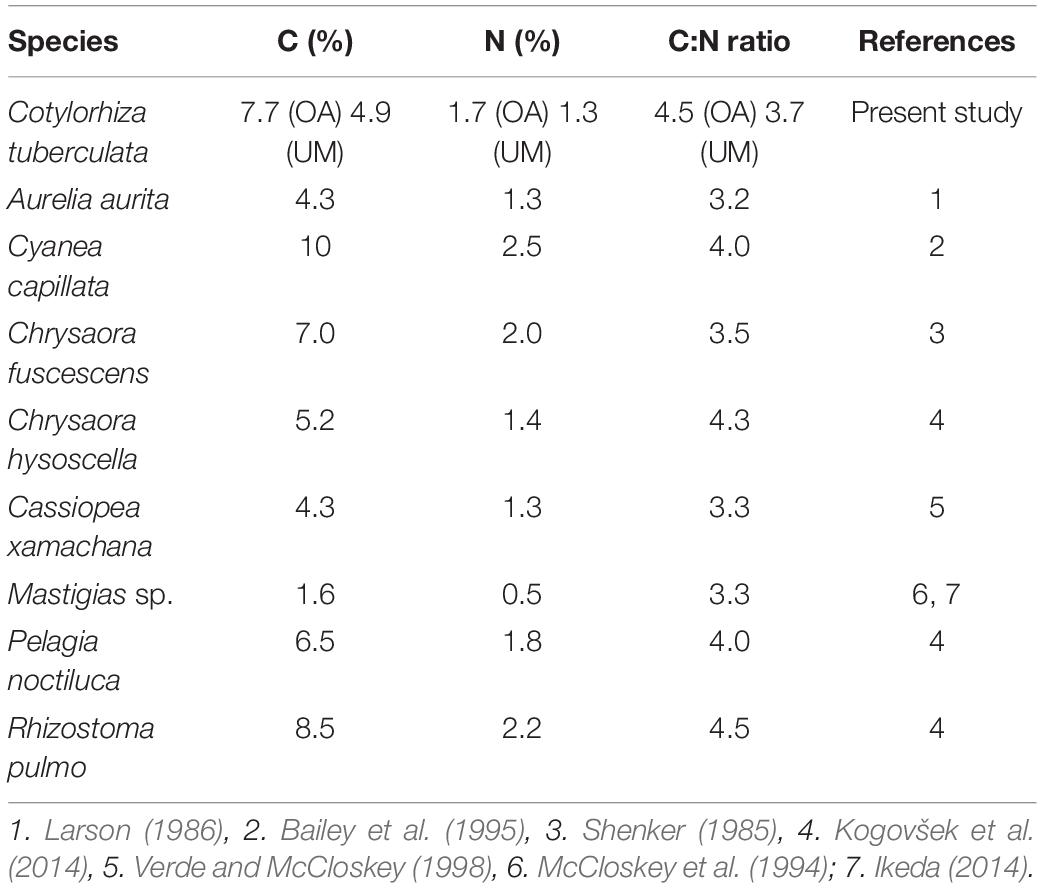
Table 3. Comparison of biochemical C and N composition and C:N ratio expressed as percentage of dry mass (%DM) of symbiotic and non-symbiotic scyphozoan jellyfish: Cotylorhiza tuberculata, Aurelia aurita, Cyanea capillata, Chrysaora fuscescens, Chrysaora hysoscella, Cassiopea xamachana, Mastigias sp., Pelagia noctiluca, and Rhizostoma pulmo.
Symbiodiniaceae Pigments
Photosynthetic dinoflagellates including Symbiodiniaceae have plastids derived from red algae. The most representative photosynthetic pigments in C. tuberculata symbionts are found to be chlorophyll a, chlorophyll c2, and peridinin, the primary photosynthetic pigments of Symbiodiniaceae (Hennige et al., 2009; Roth, 2014). Host-specialized dinoflagellates have different light-harvesting complexes to capture light: the (1) the thylakoid membrane-bound chlorophyll a–chlorophyll c2–peridinin-protein-complex (acpPC) and (2) the water-soluble peridinin–chlorophyll a protein (PCP) (Iglesias-Prieto et al., 1991, 1993). The chlorophylls absorb high-energy blue light (∼430–460 nm), but chlorophyll a absorbs also red light (∼680 nm) (Bricaud et al., 2004). Chl a concentration per cell (1.33 ± 0.83 pg cell–1) of C. tuberculata symbionts was consistent with those reported for other jellyfish symbionts (Table 2). Similar Chl a concentration was found in C. xamachana symbionts (Vodenichar, 1995; Verde and McCloskey, 1998) as well as, L. unguiculata and Mastigias sp. symbionts (Kremer et al., 1990; Wilkerson and Kremer, 1992; McCloskey et al., 1994). Peridinin, which constitutes the typical biomarker pigment on dinoflagellates (Barlow et al., 1993; Jeffrey et al., 2011), has a maximum absorption of blue-green light (∼450–550 nm), expanding the range of photosynthetically usable light of Symbiodiniaceae (Bricaud et al., 2004). Symbiodiniaceae in hospite and cultured photoacclimate to different light conditions by modifying the amount of chlorophyl a, chlorophyl c2, and peridinin per cell. In symbiosis, the pigment ratios are maintained. However, in culture, the proportion of pigments can be modified (Iglesias-Prieto and Trench, 1994; Hennige et al., 2009). This discrepancy between dinoflagellate symbionts in hospite and cultured suggest that the host modulates the light environment of Symbiodiniaceae in symbiosis (Roth et al., 2010).
Carotenoids (carotenes and xanthophylls) are accessory pigments synthesized by photosynthetic organisms. Symbiodiniaceae hosted by C. tuberculata synthesizes β-carotene and xanthophylls peridinin, diadinoxanthin, and diatoxanthin (Table 1). Carotenoids are part of the photoprotective mechanisms of the photosynthetic organisms (Jeffrey and Haxo, 1968) and have a variety of roles, acting as accessory light-harvesting pigments, components of the light-harvesting complexes, antioxidants, and sinks for excess energy (Roth, 2014). Under high light conditions, the xanthophyll cycle is activated and diadinoxanthin is de-epoxidated to diatoxanthin protecting the photosynthetic apparatus against the excess excitation and the consequent oxidative damage (Lavaud et al., 2002). Symbiodiniaceae can increase the capacity for photoprotection by adjusting the amounts of carotenoids (Hennige et al., 2009). Therefore, higher contents of diadinoxanthin in C. tuberculata symbionts may suggest the photoprotection provided by the medusa tissue.
The occurrence of zeaxanthin-lutein and chlorophyll b, present at a very low percentage, may indicate the occurrence of other types of photosynthetic organisms (such as microscopic endolithic algae) present in the samples by accidental contamination (Torres-Pérez et al., 2015) or the presence of photosynthetic bacteria hosted in the gastric cavity of C. tuberculata (Viver et al., 2017). Fucoxanthin and 9′-hexanoyloxifucoxanthin, also found in very low quantity, are not characteristic of dinoflagellates, but it has been previously identified in this group (Jeffrey et al., 2011).
Symbiodiniaceae ex hospite
The growth rate of symbiotic algae is regulated by host factors and growth (Muscatine et al., 1986). Once released from the host regulation factors, cultured Symbiodiniaceae rapidly adapt to free-living conditions (D’Elia and Domotor, 1984). Furthermore, increased nutrient availability outside the host body (where N and P is limited) allows symbionts to reach higher growth rates (Miller et al., 1989; Stoner et al., 2011). The growth rate of C. tuberculata symbionts in culture was consistent with other cultured dinoflagellates isolated from jellyfish hosts (Fitt and Trench, 1983; D’Elia and Domotor, 1984; Taguchi and Kinzie, 2001) and was similar to those of free-living dinoflagellates (Tang, 1996). However, the frequency of mitosis or growth rate of endosymbiotic algae remains much slower (0.05 and 0.1 d–1, respectively, in Wilkerson et al., 1983; Muscatine et al., 1986) than those of ex hospite Symbiodiniaceae.
In our study, the two known associates of C. tuberculata were not genetically distinguished. However, previous genetic analyses on individuals from the Mar Menor (Raspor Dall’Olio, 2016), revealed the presence of the Symbiodiniaceae species Philozoon medusarum (LaJeunesse et al., 2021) and Breviolum psygmophilum (Lajeunesse et al., 2012; LaJeunesse et al., 2018). Even though C. tuberculata may occur symbiotically with distinct Symbiodiniaceae species, its co-occurrence in symbiosis with different species simultaneously has not been demonstrated yet (Raspor Dall’Olio, 2016).
The Symbiodiniaceae life cycle is composed of different stages: (i) coccoid cells are the dominant stage seen inside the host (Supplementary Figure 3), (ii) dividing doublet are coccoid cells undergoing mitosis, (iii) motile mastigotes only occur in free living conditions. Coccoid vegetative cells presented a reticulated chloroplast typical of Symbiodiniaceae (LaJeunesse et al., 2010), the mesh-like plastid is located around the entire cell periphery allowing symbionts to receive photons from any directions. The morphological adaptation of the coccoid cell plastid help in maximizing light absorption efficiency in hospite (LaJeunesse et al., 2010). Vegetative coccoid cells were associated with bacteria in our cultures forming a calcifying bacterial-algal biofilm (Figure 6). This calcification process has been described in ex hospite symbionts of cnidarian hosts (corals and sea anemones) and it is driven by the photosynthetic activity of Symbiodiniaceae but, requires naturally associated bacterial communities to occur (Frommlet et al., 2015, 2018). The process is known as “Photosynthetic Induced Carbonate Precipitation” and is influenced by microbial activity on calcification (Frommlet et al., 2018). Motile flagellated cells or mastigotes were present in cultures during the first month after isolation from the host. Cells with mastigotes possessed seven latitudinal series of amphiesmal vesicles as described by LaJeunesse et al. (2018) and, longitudinal and transverse flagella.
Conclusion
In this study, we examined the characteristics of the symbiosis between the bloom-forming scyphozoan jellyfish Cotylorhiza tuberculata and their photosynthetic partners of the family Symbiodiniaceae. The host-specialized dinoflagellates are heterogeneously distributed within the host body being more concentrated in the medusa oral arms as a consequence of the tissue role, their high metabolic activity and their sinuous folding. The C:N ratio were also elevated in the oral arms indicating lower protein content. The study of the symbionts in hospite revealed no significant differences in size neither chlorophyll a concentration within the oral arms and the umbrella. Pigment composition in symbionts included typical Symbiodiniaceae light-harvesting pigments (chlorophyll a, chlorophyll c2, and peridinin) as well as carotenoids (β-carotene, xanthophylls peridinin, diadinoxanthin, and diatoxanthin), involved in the protection of the photosynthetic apparatus. Furthermore, cultured Symbiodiniaceae behave as free-living dinoflagellates exhibiting elevated growth rates and developing motile mastigotes cells. Symbionts acclimates to a free-living environment acting as a planktonic dinoflagellate when released from host regulation. The morphology of Symbiodiniaceae in culture revealed the presence of vegetative coccoid cells that divided by mitosis (doublet) and motile mastigotes. In addition, we found the typical calcifying matrix synthetized by Symbiodiniaceae and their microbiota in most algal cultures. Finally, the study of the photosymbiosis revealed the light conditions of Symbiodiniaceae in hospite and the protective role played by medusa body. Jellyfish tissue protects the symbionts from the negative effects of ultraviolet radiation and attenuates the elevated environmental light intensity avoiding damages in the algae photosynthetic apparatus. The presence of a dense Symbiodiniaceae population and the protection to UVR provided by medusae tissue maintaining photosymbionts in optimal light conditions to photosynthesis may be a reason added to explain the population success of Cotylorhiza tuberculata.
Despite 20–25% of Scyphozoa being zooxanthellate, basic information on the biology and ecology of most species is still lacking. Therefore, our work may contribute to the scientific knowledge of zooxanthellate jellyfish ecology. Future research on the biology of less studied symbiotic jellyfish and their host-specialized dinoflagellates using more a systematic approach and more recent methods are needed. Most of our knowledge about photosymbiosis comes from scleractinian corals. Consequently, interesting research questions and ideas for future research could be also derived from the extensive knowledge and experience provided by the scientific field of symbiotic corals.
Data Availability Statement
The original contributions presented in the study are included in the article/Supplementary Material, further inquiries can be directed to the corresponding author/s.
Author Contributions
AE-N: data acquisition, data analysis, and writing original draft and editing. EH: supervision, reviewing, and funding acquisition. VF-P and AB: data acquisition, data analysis, and reviewing. GN: data analysis and reviewing. JR and AM: supervision and reviewing. LP: supervision, reviewing, data acquisition, and funding acquisition. All authors contributed to the article and approved the submitted version.
Funding
This work was funded by the Spanish Ministerio de Ciencia, Innovación y Universidades (https://www.ciencia.gob.es) under grant no. CTM2016-75487-R for the project MED2CA. AE-N was financially sustained by a Ph.D. fellowship from the MED2CA project (https://med2ca.csic.es). This work is a contribution to the CSIC interdisciplinary thematic platform, WATER:iOS and the Project Agreement “Sistema de Observación y Predicción de Medusas en el Mar Balear” among Govern des Illes Balears, SOCIB and CSIC.
Conflict of Interest
The authors declare that the research was conducted in the absence of any commercial or financial relationships that could be construed as a potential conflict of interest.
Publisher’s Note
All claims expressed in this article are solely those of the authors and do not necessarily represent those of their affiliated organizations, or those of the publisher, the editors and the reviewers. Any product that may be evaluated in this article, or claim that may be made by its manufacturer, is not guaranteed or endorsed by the publisher.
Acknowledgments
We would like to thank the students, technicians, and researchers who participated in this study for their assistance with field samplings and laboratory analyses.
Supplementary Material
The Supplementary Material for this article can be found online at: https://www.frontiersin.org/articles/10.3389/fmars.2022.817312/full#supplementary-material
References
Acuña, J. L., López-Urrutia, Á, and Colin, S. (2011). Faking Giants: The Evolution of High Prey Clearance Rates in Jellyfishes. Science 333, 1627–1629. doi: 10.1126/science.1205134
Arai, M. N. (1997). A Functional Biology of Scyphozoa. Dordrecht: Springer Netherlands, doi: 10.1007/978-94-009-1497-1
Arai, M. N. (2001). Pelagic coelenterates and eutrophication: A review. Hydrobiologia 451, 69–87. doi: 10.1023/A:1011840123140
Astorga, D., Ruiz, J., and Prieto, L. (2012). Ecological aspects of early life stages of Cotylorhiza tuberculata (Scyphozoa: Rhizostomae) affecting its pelagic population success. Hydrobiologia 690, 141–155. doi: 10.1007/s10750-012-1036-x
Bailey, T. G., Youngbluth, M. J., and Owen, G. P. (1995). Chemical composition and metabolic rates of gelatinous zooplankton from midwater and benthic boundary layer environments off Cape Hatteras, North Carolina, USA. Mar. Ecol. Prog. Ser. 122, 121–134. doi: 10.3354/meps122121
Banaszak, A. T., and Lesser, M. P. (2009). Effects of solar ultraviolet radiation on coral reef organisms. Photochem. Photobiol. Sci. 8, 1276–1294. doi: 10.1039/b902763g
Banaszak, A. T., and Trench, R. K. (1995). Effects of ultraviolet (UV) radiation on marine microalgal-invertebrate symbioses. II. The synthesis of mycosporine-like amino acids in response to exposure to UV in Anthopleura elegantissima and Cassiopeia xamachana. J. Exp. Mar. Bio. Ecol. 194, 233–250. doi: 10.1016/0022-0981(95)00073-9
Barlow, R. G., Mantoura, R. F. C., Gough, M. A., and Fileman, T. W. (1993). Pigment signatures of the phytoplankton composition in the northeastern Atlantic during the 1990 spring bloom. Deep Sea Res. Part II Top. Stud. Oceanogr. 40, 459–477. doi: 10.1016/0967-0645(93)90027-K
Blanquet, R. S., Nevenzel, J. C., and Benson, A. A. (1979). Acetate incorporation into the lipids of the anemone Anthopleura elegantissima and its associated zooxanthellae. Mar. Biol. 54, 185–194. doi: 10.1007/BF00386597
Blanquet, R. S., and Phelan, M. A. (1987). An unusual blue mesogleal protein from the mangrove jellyfish Cassiopea xamachana. Mar. Biol. 94, 423–430. doi: 10.1007/BF00428249
Bricaud, A., Claustre, H., Ras, J., and Oubelkheir, K. (2004). Natural variability of phytoplanktonic absorption in oceanic waters: Influence of the size structure of algal populations. J. Geophys. Res. Ocean. 109, 1–12. doi: 10.1029/2004JC002419
Cimino, M. A., Patris, S., Ucharm, G., Bell, L. J., and Terrill, E. (2018). Jellyfish distribution and abundance in relation to the physical habitat of Jellyfish Lake, Palau. J. Trop. Ecol. 34, 17–31. doi: 10.1017/s0266467418000044
Colley, N. J., and Trench, R. K. (1985). Cellular events in the reestablishment of a symbiosis between a marine dinoflagellate and a coelenterate. Cell Tissue Res. 239, 93–103. doi: 10.1007/BF00214908
Dawson, M., and Hamner, W. M. (2003). Geographic variation and behavioral evolution in marine plankton: the case of Mastigias (Scyphozoa, Rhizostomeae). Mar. Biol. 143, 1161–1174. doi: 10.1007/s00227-003-1155-z
de Groot, R., Brander, L., van der Ploeg, S., Costanza, R., Bernard, F., Braat, L., et al. (2012). Global estimates of the value of ecosystems and their services in monetary units. Ecosyst. Serv. 1, 50–61. doi: 10.1016/j.ecoser.2012.07.005
Decelle, J., Colin, S., and Foster, R. A. (2015). “Photosymbiosis in Marine Planktonic Protists,” in Marine Protists eds S. Ohtsuka, T. Suzaki, T. Horiguchi, N. Suzuki, and F. Not (Tokyo: Springer Japan), 465–500. doi: 10.1007/978-4-431-55130-0_19
D’Elia, C. F., and Domotor, S. L. (1984). Nutrient uptake kinetics and growth of zooxanthellae maintained in laboratory culture. Mar. Biol. 80, 93–101. doi: 10.1007/BF00393132
Djeghri, N., Pondaven, P., Stibor, H., and Dawson, M. N. (2019). Review of the diversity, traits, and ecology of zooxanthellate jellyfishes. Mar. Biol. 166:147. doi: 10.1007/s00227-019-3581-6
Done, T. J., Ogden, J. C., Weibe, W. J., and Rosen, B. R. (1996). “Biodiversity and ecosystem function of coral reefs,” in Functional Roles of Biodiversity: A Global Perspective, eds H. A. Mooney, J. H. Cushman, E. Medina, O. E. Sala, and E.-D. Schulze (New Jersey, NJ: John Wiley & Sons Ltd. U.K), 493.
Enrique-Navarro, A., Emma Huertas, I., Cobo, M. J. L., and Prieto, L. (2021). Impact of ocean warming and ocean acidification on asexual reproduction and statolith formation of the symbiotic jellyfish Cotylorhiza tuberculata. PLoS One 16:e254983. doi: 10.1371/journal.pone.0254983
Estes, A. M., Kempf, S. C., and Henry, R. P. (2003). Localization and quantification of carbonic anhydrase activity in the symbiotic scyphozoan Cassiopea xamachana. Biol. Bull. 204, 278–289. doi: 10.2307/1543599
Fitt, W. K., and Trench, R. K. (1983). The relation of diel patterns of cell division to diel patterns of motility in the symbiotic dinoflagellate Symbiodinium microadriaticum Freudenthal in culture. New Phytol. 94, 421–432. doi: 10.1111/j.1469-8137.1983.tb03456.x
Frommlet, J. C., Sousa, M. L., Alves, A., Vieira, S. I., Suggett, D. J., and Serôdio, J. (2015). Coral symbiotic algae calcify ex hospite in partnership with bacteria. Proc. Natl. Acad. Sci. U.S.A. 112, 6158–6163. doi: 10.1073/pnas.1420991112
Frommlet, J. C., Wangpraseurt, D., Sousa, M. L., Guimarães, B., da Silva, M. M., Kühl, M., et al. (2018). Symbiodinium-induced formation of microbialites: Mechanistic insights from in Vitro experiments and the prospect of its occurrence in nature. Front. Microbiol. 9:998. doi: 10.3389/fmicb.2018.00998
Furla, P., Richier, S., and Allemand, D. (2011). “Physiological adaptation to symbiosis in cnidarians,” in Coral Reefs: An Ecosystem in Transition, eds Z. Dubinsky and N. Stambler (Netherlands: Springer), 187–195. doi: 10.1007/978-94-007-0114-4_12
Guillard, R. R. L., and Ryther, J. H. (1962). Studies of marine planktonic diatoms. I. Cyclotella nana Hustedt, and Detonula confervacea (cleve) Gran. Can. J. Microbiol. 8, 229–239. doi: 10.1139/m62-029
Hamner, W. M., Gilmer, R. W., and Hamner, P. P. (1982). The physical, chemical, and biological characteristics of a stratified, saline, sulfide lake in Palau 1. Limnol. Oceanogr. 27, 896–909. doi: 10.4319/lo.1982.27.5.0896
Hennige, S. J., Suggett, D. J., Warner, M. E., McDougall, K. E., and Smith, D. J. (2009). Photobiology of Symbiodinium revisited: Bio-physical and bio-optical signatures. Coral Reefs 28, 179–195. doi: 10.1007/s00338-008-0444-x
Higuchi, T., Fujimura, H., Hitomi, Y., Arakaki, T., Oomori, T., and Suzuki, Y. (2010). Photochemical formation of hydroxyl radicals in tissue extracts of the coral Galaxea fascicularis. Photochem. Photobiol. 86, 1421–1426. doi: 10.1111/j.1751-1097.2010.00802.x
Iglesias-Prieto, R., Govind, N. S., and Trench, R. K. (1991). Apoprotein composition and spectroscopic characterization of the water-soluble peridinin—Chlorophyll a—Proteins from three symbiotic dinoflagellates. Proc. R. Soc. London. Ser. B Biol. Sci. 246, 275–283.
Iglesias-Prieto, R., Govind, N. S., and Trench, R. K. (1993). Isolation and characterization of three membranebound chlorophyll-protein complexes from four dinoflagellate species. Philos. Trans. R. Soc. London. Ser. B Biol. Sci. 340, 381–392.
Iglesias-Prieto, R., and Trench, R. K. (1994). Acclimation and adaptation to irradiance in symbiotic dinoflagellates. I. Responses of the photosynthetic unit to changes in photon flux density. Mar. Ecol. Prog. Ser. Oldend. 113, 163–175.
Ikeda, T. (2014). Synthesis toward a global model of metabolism and chemical composition of medusae and ctenophores. J. Exp. Mar. Bio. Ecol. 456, 50–64. doi: 10.1016/j.jembe.2014.03.006
Jeffrey, S., Wright, S., and Zapata, M. (2011). “Microalgal classes and their signature pigments,” in Phytoplankton Pigments, eds S. Roy, C. A. Llewellyn, E. S. Egeland, and G. Johnsen (Cambridge: Cambridge University Press), 3–77. doi: 10.1017/CBO9780511732263
Jeffrey, S. W., and Haxo, F. T. (1968). Photosynthetic pigments of symbiotic dinoflagellates (zooxanthellae) from corals and clams. Biol. Bull. 135, 149–165. doi: 10.2307/1539622
Kennedy, E. V., Perry, C. T., Halloran, P. R., Iglesias-Prieto, R., Schönberg, C. H. L., Wisshak, M., et al. (2013). Avoiding Coral Reef Functional Collapse Requires Local and Global Action. Curr. Biol. 23, 912–918. doi: 10.1016/j.cub.2013.04.020
Kikinger, R. (1992). Cotylorhiza tuberculata (Cnidaria: Scyphozoa) - Life History of a Stationary Population. Mar. Ecol. 13, 333–362. doi: 10.1111/j.1439-0485.1992.tb00359.x
Klein, S. (2016). Responses of Jellyfish to Environmental Change. Available online at: https://research-repository.griffith.edu.au/handle/10072/367711
Knap, A., Michaels, A., Close, A., Ducklow, H., and Dickson, A. (1996). Protocols for the Joint Global Ocean Flux Study (JGFOS) Core Measurements. JGOFS Reoprt Nr. 19:198. doi: 10.013/epic.27912
Kogovšek, T., Tinta, T., Klun, K., and Malej, A. (2014). Jellyfish biochemical composition: Importance of standardised sample processing. Mar. Ecol. Prog. Ser. 510, 275–288. doi: 10.3354/meps10959
Kremer, P., Costello, J., Kremer, J., and Canino, M. (1990). Significance of photosynthetic endosymbionts to the carbon budget of the scyphomedusa Linuche unguiculata. Limnol. Oceanogr. 35, 609–624. doi: 10.4319/lo.1990.35.3.0609
Kühl, M., Cohen, Y., Dalsgaard, T., Jørgensen, B. B., and Revsbech, N. P. (1995). Microenvironment and photosynthesis of zooxanthellae in scleractinian corals studied with microsensors for O2, pH and light. Mar. Ecol. Ser. 117, 159–172. doi: 10.3354/meps117159
LaJeunesse, T. C., Fitt, W. K., and Schmidt, G. W. (2010). The reticulated chloroplasts of zooxanthellae (Symbiodinium) and differences in chlorophyll localization among life cycle stages. Coral Reefs 29, 627–627. doi: 10.1007/s00338-010-0635-0
Lajeunesse, T. C., Loh, W., and Trench, R. K. (2009). Do introduced endosymbiotic dinoflagellates “take” to new hosts? Biol. Invasions 11, 995–1003. doi: 10.1007/s10530-008-9311-5
LaJeunesse, T. C., Parkinson, J. E., Gabrielson, P. W., Jeong, H. J., Reimer, J. D., Voolstra, C. R., et al. (2018). Systematic revision of Symbiodiniaceae highlights the antiquity and diversity of coral endosymbionts. Curr. Biol. 28, 2570–2580. doi: 10.1016/j.cub.2018.07.008
Lajeunesse, T. C., Parkinson, J. E., and Reimer, J. D. (2012). A genetics-based description of Symbiodinium minutum sp. nov. and S. psygmophilum sp. nov. (Dinophyceae), two dinoflagellates symbiotic with cnidaria. J. Phycol. 48, 1380–1391. doi: 10.1111/j.1529-8817.2012.01217.x
LaJeunesse, T. C., Wiedenmann, J., Casado-Amezúa, P., D’Ambra, I., Turnham, K. E., Nitschke, M. R., et al. (2021). Revival of Philozoon Geddes for host-specialized dinoflagellates, ‘zooxanthellae’, in animals from coastal temperate zones of northern and southern hemispheres. Eur. J. Phycol. 00, 1–15. doi: 10.1080/09670262.2021.1914863
Lampert, K. P., Bürger, P., Striewski, S., and Tollrian, R. (2012). Lack of association between color morphs of the Jellyfish Cassiopea andromeda and zooxanthella clade. Mar. Ecol. 33, 364–369. doi: 10.1111/j.1439-0485.2011.00488.x
Larson, R. J. (1986). Water content, organic content, and carbon and nitrogen composition of medusae from the northeast Pacific. J. Exp. Mar. Bio. Ecol. 99, 107–120. doi: 10.1016/0022-0981(86)90231-5
Lavaud, J., Rousseau, B., van Gorkom, H. J., and Etienne, A.-L. (2002). Influence of the diadinoxanthin pool size on photoprotection in the marine planktonic diatom Phaeodactylum tricornutum. Plant Physiol. 129, 1398–1406. doi: 10.1104/pp.002014
Leblond, J. D., Khadka, M., Duong, L., and Dahmen, J. L. (2015). Squishy lipids: Temperature effects on the betaine and galactolipid profiles of a C18/C18 peridinin-containing dinoflagellate, Symbiodinium microadriaticum (Dinophyceae), isolated from the mangrove jellyfish, < i > Cassiopea xam. Phycol. Res. 63, 219–230. doi: 10.1111/pre.12093
Lesser, M. P., and Lewis, S. (1996). Action spectrum for the effects of UV radiation on photosynthesis in the hermatypic coral Pocillopora damicornis. Mar. Ecol. Prog. Ser 134, 171–177. doi: 10.3354/meps134171
Lesser, M. P., and Shick, J. M. (1990). Effects of visible and ultraviolet radiation on the ultrastructure of zooxanthellae (Symbiodinium sp.) in culture and in situ. Cell Tissue Res. 261, 501–508. doi: 10.1007/BF00313529
Lewis, M. R., and Smith, J. C. (1983). “Photosynthetron”: a small volume, short-incubation time method for measurement of photosynthesis as a function of incident irradiance. Mar. Ecol. Prog. Ser. 13, 99–102.
Lloret, J., Marín, A., and Marín-Guirao, L. (2008). Is coastal lagoon eutrophication likely to be aggravated by global climate change? Estuar. Coast. Shelf Sci. 78, 403–412. doi: 10.1016/j.ecss.2008.01.003
Ludwig, F.-D. (1969). Die zooxanthellen bei Cassiopea andromeda Eschscholtz 1829 (Polyp-Stadium) und ihre Bedeutung für die strobilation. Zoologische Jahrbuecher 86, 238–277.
Mantoura, R. F. C., and Llewellyn, C. A. (1983). The rapid determination of algal chlorophyll and carotenoid pigments and their breakdown products in natural waters by reverse-phase high-performance liquid chromatography. Anal. Chim. Acta 151, 297–314. doi: 10.1016/S0003-2670(00)80092-6
McAuley, P. J., and Smith, V. J. (1995). Effect of diel photoperiod on nitrogen metabolism of cultured and symbiotic zooxanthellae. Mar. Biol. 123, 145–152. doi: 10.1007/BF00350333
McCloskey, L. R., Muscatine, L., and Wilkerson, F. P. (1994). Daily photosynthesis, respiration, and carbon budgets in a tropical marine jellyfish (Mastigias sp.). Mar. Biol. 119, 13–22. doi: 10.1007/BF00350101
Mellas, R. E., McIlroy, S. E., Fitt, W. K., and Coffroth, M. A. (2014). Variation in symbiont uptake in the early ontogeny of the upside-down jellyfish, Cassiopea spp. J. Exp. Mar. Biol. Ecol. 459, 38–44. doi: 10.1016/j.jembe.2014.04.026
Miller, D. J., Yellowlees, D., and Smith, D. A. (1989). Inorganic nitrogen uptake by symbiotic marine cnidarians: a critical review. Proc. R. Soc. London. B. Biol. Sci. 237, 109–125. doi: 10.1098/rspb.1989.0040
Moran, M. A., and Hodson, R. E. (1990). Contributions of degrading Spartina alterniflora lignocellulose to the dissolved organic carbon pool of a salt marsh. Mar. Ecol. Prog. Ser. Oldend. 62, 161–168.
Mortillaro, J. M., Pitt, K. A., Lee, S. Y., and Meziane, T. (2009). Light intensity influences the production and translocation of fatty acids by zooxanthellae in the jellyfish Cassiopea sp. J. Exp. Mar. Bio. Ecol. 378, 22–30. doi: 10.1016/j.jembe.2009.07.003
Muscatine, L., and Marian, R. E. (1982). Dissolved inorganic nitrogen flux in symbiotic and non-symbiotic medusae. Limnol. Oceanogr. 27, 910–917. doi: 10.4319/lo.1982.27.5.0910
Muscatine, L., Wilkerson, F., and McCloskey, L. (1986). Regulation of population density of symbiotic algae in a tropical marine jellyfish (Mastigias sp.). Mar. Ecol. Prog. Ser. 32, 279–290. doi: 10.3354/meps032279
Ohdera, A. H., Abrams, M. J., Ames, C. L., Baker, D. M., Suescún-Bolívar, L. P., Collins, A. G., et al. (2018). Upside-down but headed in the right direction: Review of the highly versatile Cassiopea xamachana system. Front. Ecol. Evol. 6:35. doi: 10.3389/fevo.2018.00035
Ohtsuka, S., Koike, K., Lindsay, D., Nishikawa, J., Miyake, H., Kawahara, M., et al. (2009). Symbionts of marine medusae and ctenophores. Plankt. Benthos Res. 4, 1–13. doi: 10.3800/pbr.4.1
Pérez-Ruzafa, A., Fernández, A. I., Marcos, C., Gilabert, J., Quispe, J. I., and García-Charton, J. A. (2005). Spatial and temporal variations of hydrological conditions, nutrients and chlorophyll a in a Mediterranean coastal lagoon (Mar Menor, Spain). Hydrobiologia 550, 11–27.
Pérez-Ruzafa, A., Gilabert, J., Gutiérrez, J. M., Fernández, A. I., Marcos, C., and Sabah, S. (2002). Evidence of a planktonic food web response to changes in nutrient input dynamics in the Mar Menor coastal lagoon, Spain. Hydrobiologia 475–476, 359–369. doi: 10.1023/A:1020343510060
Pitt, K. A., Duarte, C. M., Lucas, C. H., Sutherland, K. R., Condon, R. H., Mianzan, H., et al. (2013). Jellyfish Body Plans Provide Allometric Advantages beyond Low Carbon Content. PLoS One 8:e72683. doi: 10.1371/journal.pone.0072683
Prieto, L., Astorga, D., Navarro, G., and Ruiz, J. (2010). Environmental control of phase transition and polyp survival of a massive-outbreaker jellyfish. PLoS One 5:e13793. doi: 10.1371/journal.pone.0013793
Purcell, J. E. (2005). Climate effects on formation of jellyfish and ctenophore blooms: A review. J. Mar. Biol. Assoc. U. K. 85, 461–476. doi: 10.1017/S0025315405011409
Raspor Dall’Olio, L. (2016). SYMBIOSIS ECOLOGY OF SELECTED SCYPHOZOA. Ph.D thesis, Nova Gorica: Raspor Dall’Olio.
Roth, M. S. (2014). The engine of the reef: Photobiology of the coral-algal symbiosis. Front. Microbiol. 5:422. doi: 10.3389/fmicb.2014.00422
Roth, M. S., Latz, M. I., Goericke, R., and Deheyn, D. D. (2010). Green fluorescent protein regulation in the coral Acropora yongei during photoacclimation. J. Exp. Biol. 213, 3644–3655. doi: 10.1242/jeb.040881
Shenker, J. M. (1985). Carbon content of the neritic scyphomedusa Chrysaora fuscescens. J. Plankton Res. 7, 169–173. doi: 10.1093/plankt/7.2.169
Shinzato, C., Shoguchi, E., Kawashima, T., Hamada, M., Hisata, K., Tanaka, M., et al. (2011). Using the Acropora digitifera genome to understand coral responses to environmental change. Nature 476, 320–323. doi: 10.1038/nature10249
Stat, M., Carter, D., and Hoegh-Guldberg, O. (2006). The evolutionary history of Symbiodinium and scleractinian hosts—symbiosis, diversity, and the effect of climate change. Perspect. Plant Ecol. Evol. Syst. 8, 23–43.
Stoner, E. W., Layman, C. A., Yeager, L. A., and Hassett, H. M. (2011). Effects of anthropogenic disturbance on the abundance and size of epibenthic jellyfish Cassiopea spp. Mar. Pollut. Bull. 62, 1109–1114. doi: 10.1016/j.marpolbul.2011.03.023
Taguchi, S., and Kinzie, R. A. (2001). Growth of zooxanthellae in culture with two nitrogen sources. Mar. Biol. 138, 149–155. doi: 10.1007/s002270000435
Tang, E. P. Y. (1996). Why do dinoflagellates have lower growth rates? J. Phycol. 32, 80–84. doi: 10.1111/j.0022-3646.1996.00080.x
Terrados, J., and Ros, J. D. (1991). Production dynamics in a macrophyte-dominated ecosystem: The Mar Menor coastal lagoon (SE Spain). Oecologia Aquat. 10, 255–270.
Thornhill, D. J., Daniel, M. W., LaJeunesse, T. C., Schmidt, G. W., and Fitt, W. K. (2006). Natural infections of aposymbiotic Cassiopea xamachana scyphistomae from environmental pools of Symbiodinium. J. Exp. Mar. Bio. Ecol. 338, 50–56. doi: 10.1016/j.jembe.2006.06.032
Torres-Pérez, J. L., Guild, L. S., Armstrong, R. A., Corredor, J., Zuluaga-Montero, A., and Polanco, R. (2015). Relative pigment composition and remote sensing reflectance of Caribbean shallow-water corals. PLoS One 10:e0143709. doi: 10.1371/journal.pone.0143709
Venn, A. A., Loram, J. E., and Douglas, A. E. (2008). Photosynthetic symbioses in animals. J. Exp. Bot. 59, 1069–1080. doi: 10.1093/jxb/erm328
Verde, E. A., and McCloskey, L. R. (1998). Production, respiration, and photophysiology of the mangrove jellyfish Cassiopea xamachana symbiotic with zooxanthellae: Effect of jellyfish size and season. Mar. Ecol. Prog. Ser. 168, 147–162. doi: 10.3354/meps168147
Visram, S., Wiedenmann, J., and Douglas, A. E. (2006). Molecular diversity of symbiotic algae of the genus Symbiodinium (Zooxanthellae) in cnidarians of the Mediterranean Sea. J. Mar. Biol. Assoc. U. K. 86, 1281–1283. doi: 10.1017/S0025315406014299
Viver, T., Orellana, L. H., Hatt, J. K., Urdiain, M., Díaz, S., Richter, M., et al. (2017). The low diverse gastric microbiome of the jellyfish Cotylorhiza tuberculata is dominated by four novel taxa. Environ. Microbiol. 19, 3039–3058. doi: 10.1111/1462-2920.13763
Vodenichar, J. S. (1995). Ecological Physiology of the Scyphozoan Cassiopea Xamachana. Ph.D thesis, Athens, USA: University of Georgia.
Webb, W. L., Newton, M., and Starr, D. (1974). Carbon dioxideexchange of Alnus rubra: A mathematical model. Oecologia 17, 281–291. doi: 10.1007/BF00345747
Weis, V. M. (2008). Cellular mechanisms of Cnidarian bleaching: stress causes the collapse of symbiosis. J. Exp. Biol. 211, 3059–3066. doi: 10.1242/jeb.009597
Wilkerson, F. P., and Kremer, P. (1992). DIN, DON and PO4 flux by a medusa with algal symbionts. Mar. Ecol. Prog. Ser. 90, 237–250. doi: 10.3354/meps090237
Keywords: Symbiodiniaceae, Chl a, HPLC, microscope (SEM), absorbance, photosymbiosis
Citation: Enrique-Navarro A, Huertas E, Flander-Putrle V, Bartual A, Navarro G, Ruiz J, Malej A and Prieto L (2022) Living Inside a Jellyfish: The Symbiosis Case Study of Host-Specialized Dinoflagellates, “Zooxanthellae”, and the Scyphozoan Cotylorhiza tuberculata. Front. Mar. Sci. 9:817312. doi: 10.3389/fmars.2022.817312
Received: 17 November 2021; Accepted: 07 February 2022;
Published: 08 March 2022.
Edited by:
Tilmann Harder, University of Bremen, GermanyReviewed by:
Allison Helen Kerwin, University of Connecticut, United StatesShelby E. McIlroy, The University of Hong Kong, Hong Kong SAR, China
Copyright © 2022 Enrique-Navarro, Huertas, Flander-Putrle, Bartual, Navarro, Ruiz, Malej and Prieto. This is an open-access article distributed under the terms of the Creative Commons Attribution License (CC BY). The use, distribution or reproduction in other forums is permitted, provided the original author(s) and the copyright owner(s) are credited and that the original publication in this journal is cited, in accordance with accepted academic practice. No use, distribution or reproduction is permitted which does not comply with these terms.
*Correspondence: Angélica Enrique-Navarro, YW5nZWxpY2EuZW5yaXF1ZUBjc2ljLmVz