- 1Marine Ecophysiology Group (EOMAR), IU-ECOAQUA, Universidad de Las Palmas de Gran Canaria, Las Palmas de Gran Canaria, Spain
- 2Biology, Western Washington University, Bellingham, WA, United States
Physalia physalis and Velella velella, are among the few marine organisms that harness the wind for their locomotion, whereas other cnidarian jellyfish make use of their pulsating bell-shaped bodies to propel themselves through the seas. We investigate their composition and metabolism compared with two species of pulsating scyphozoan jellyfish, Aurelia aurita and Pelagia noctiluca. Protein (P), lipid (L), carbohydrate (K), and derived energy content (Ec), provided information on the biochemical composition of these species and their relevance as prey. Physiological respiration (R) from oxygen consumption. As well as potential respiration (Φ) from the electron transport system (ETS) activity and the derived respiratory carbon demand (RCD) and heterotrophic energy transformation (HET), allow the comparison of the impact of these two types of propulsion on the metabolism, along with the impact of these organisms as predators. In this study it was found that these hydrozoans depicted a different biochemical composition relative to other gelatinous zooplankton. Lower water content at around 90% was observed, while WM-specific P, L, K, and Ec were higher, showcasing new aspects of these species as prey. The lower R/P in P. physalis and V. velella (1.8 ± 0.7 and 2.9 ± 1.1 μL O2 h–1 mg Prot–1, respectively) and the low R/Φ, around 0.1, indicate lower respiration in wind-driven propulsion compared to pulsation-driven propulsion. Additionally, these results encourage the use and research on enzymatic techniques that are particularly useful for gelatinous research, and the calculation of RCD and HET helps in understanding the physiology and role played by the organisms as predators from carbon and energy perspectives.
Introduction
There is a growing interest in gelatinous zooplankton in ocean ecosystems (Chiaverano et al., 2018; Ruzicka et al., 2020; Wright et al., 2021). It is known that their role as predator and prey extends from the deep ocean (Choy et al., 2017) to the neuston (Bieri, 1966). The neuston, also known as pleuston, is the ecosystem located on the ocean surface, comprising of a unique community of floating organisms, such as Sargassum-type seaweeds, wind-propelled cnidarians, and other floating invertebrates. This highly dynamic surface layer fosters the exploration of various lifestyles and locomotion strategies and connects disparate atmospheric and oceanic habitats (Helm, 2021). However, it is also, heavily impacted by human waste (Egger et al., 2021).
Research conducted in various ecosystems has demonstrated jellyfish consumption of fish eggs, fish larvae, other fish life-stages, multiple zooplankton species, and other members of pelagic environments (Bieri, 1966; Purcell, 1984, 1997, 2003; Purcell and Arai, 2001; Hansson et al., 2005; Boero, 2013; Purcell et al., 2015; Choy et al., 2017; Zeman et al., 2018). Jellyfish have been described as the main competitor of zooplanktivorous fish (Pauly et al., 2009), thereby known as predators as well as competitors of several commercial fish species. Substantial efforts have been made to quantify and assess the impact of this consumption (Larson, 1987; Morand et al., 1987; Schneider, 1989; Purcell, 1997; Uye and Shimauchi, 2005; Ishii and Tanaka, 2006; Han et al., 2012; Iguchi et al., 2017; Nagata and Morandini, 2018). Furthermore, several studies have investigated the formation of dense aggregations by some jellyfish and their impact on prey populations (Malej, 1989a; Mills, 1995; Schneider and Behrends, 1998; Hansson et al., 2005; Ishii and Tanaka, 2006; Condon et al., 2011; Boero, 2013; Iguchi et al., 2017; Schiariti et al., 2018). Quantifying consumption rates of these organisms will aid in the assessment of their effect on the recovery of populations in areas where jellyfish are proliferating (Purcell et al., 2007; Richardson et al., 2009; Boero, 2013). Carbon demand, associated with the respiratory activity can be calculated from the respiration measurements, which can help in the estimation of the impact on prey populations (Purcell et al., 2010; Lilley et al., 2014; Iguchi et al., 2017).
However, the trophic importance of gelatinous zooplankton as prey is presently experiencing a paradigm shift (Hays et al., 2018). In some cases, the energy content in jellyfish is insufficient to explain the predation observed. It is hypothesized that this may occur due to their high water content (Thiebot and McInnes, 2020). Yet, multiple organisms predate on them, such as turtles, nudibranchs, cirripeds, crustaceans, birds, cephalopods, sharks and fish (especially sunfish), which have been known to associate with cnidarian jellyfish by either living symbiotically, feeding upon them, or both (Bieri, 1966; Jenkins, 1983; Arai, 1988, 2005; Frick et al., 2009; Phillips et al., 2017; Griffin et al., 2019; Thiebot and McInnes, 2020). This begs further research on capture practices, opportunism, and self-medication using jellyfish. Hays et al. (2018) and Thiebot and McInnes (2020) agree that further studies on the gelatinous prey’s dietary “value” are needed.
Jellyfish, or planktonic cnidarians, are usually envisioned as umbrella-shaped (bell), gelatinous organisms, such as Aurelia aurita or Pelagia noctiluca that efficiently propel themselves across the ocean by contracting their bells (Gemmell et al., 2014, 2020; Hoover and Miller, 2015; Costello et al., 2021). Yet, their hydrozoan relatives, Physalia physalis and Velella velella, use their pneumatophore to exploit the wind for their locomotion with a much higher propulsion efficiency (Ferrer and González, 2021; Lee et al., 2021). These two wind-propelled hydrozoans belong to the neuston.
Attention is being paid to the distribution and mass stranding of these two colonial organisms, V. velella and P. physalis, that travel the neuston (Canepa et al., 2020; Headlam et al., 2020; Fierro et al., 2021; Jones et al., 2021; Macías et al., 2021). The life cycle of P. physalis was recently revised (Munro et al., 2019), although V. velella’s life cycle requires further study (Schuchert, 2010; Duarte et al., 2019). Despite this recent interest, the metabolism of these wind-propelled hydrozoans has been meagerly studied. In this paper, the respiratory metabolism of these organisms is measured and compared with the pulsating scyphozoans A. aurita and P. noctiluca, thereby comparing between different types of locomotion.
Taking advantage of the chance-beaching of smacks of P. physalis and V. velella on the north coast of Gran Canaria Island (Canary Islands, Spain) and aquarium A. aurita and P. noctiluca, we were able to measure and examine the respiratory nature and biochemical composition in both hydrozoans and scyphozoans. Respiration was the metabolism proxy used to investigate the metabolic difference between these two types of locomotion (Webb, 1971; Cowen, 1996; Gemmell et al., 2013; Fu and Uye, 2021). In particular, we analyzed the oxygen consumption rate, i.e., physiological respiration (R), and the respiratory electron transport system (ETS) activity, i.e., potential respiration (Φ), which is the enzyme activity at the cellular level responsible for the physiological macroscopic response (Packard, 1985). Additionally, this work describes the application of enzymatic ETS analysis to study the respiratory metabolism of these fragile cnidarians that are often cumbersome to sample and incubate without damage (Raskoff et al., 2003). These respiratory results were also used to investigate the impact of these organisms as predators through the calculation of the associated carbon demand. On the other hand, measurements of the basic biochemical composition and energy content provided insight into the role of these species as prey. This work helps us to understand the feeding strategies of gelativore predators and further deconstruct the viewpoint that jellyfish are trophic-dead ends (Hamilton, 2016).
Therefore, we hypothesized that the biochemical and energy content per wet mass (WM) of the two hydrozoan species may be higher since these species have chitinous structures that are absent in other, more fragile gelatinous zooplankton. We also hypothesized that R, Φ, and derived heterotrophic energy transformation (described as heterotrophic energy production in Packard et al., 2015), as well as the R:Φ ratio (Hernández-León and Gómez, 1996), should be lower in P. physalis and V. velella than in pulsating hydrozoans or scyphozoans. The wind-driven hydrozoans would require less respiration-derived energy to cover the same distance compared to their scyphozoan relatives. Our objective was to investigate the physiology of these understudied organisms resulting in the first measurements of the respiratory metabolism and biochemical composition (protein, lipid, carbohydrate, and associated energy content) in P. physalis and V. velella and comparing them with measurements in A. aurita and P. noctiluca with differing locomotion. This information provides novel insight into the impact of hydrozoans as both predator and prey, i.e., insight on integral members of mostly overlooked food webs (Helm, 2021).
Materials and Methods
Sampling and Storage
Samples of A. aurita and P. noctiluca were provided by the Loro Parque Foundation’s in Tenerife (Canary Islands, Spain). The A. aurita culture traced its origins to a stock culture from the Madrid aquarium (Spain). Specimens of P. noctiluca were captured during a jellyfish bloom that occurred in the Canary Islands in 2012. Generations of these two species have been cultivated at Loro Parque since their capture. The culturing conditions included filtered seawater at 18–20°C, 33 PSU (‰), and a pH of 8.2. A. aurita was fed thrice daily with Artemia nauplii and P. noctiluca was fed both A. aurita portions and Artemia nauplii. Prior to the experiment, A. aurita was fed Artemia nauplii, ad libitum, while P. noctiluca was not provided with its feed due to the requirements of the aquarium.
In the case of V. velella and P. physalis, both specimens were collected in the northeastern beaches of Gran Canaria (Canary Island, Spain). These organisms arrived naturally as smacks in January and February 2017. They were carefully collected by gloved hands as they beached by the tide, after which they were transported live in containers half full of seawater. The samples were separated to be used for respiratory analysis as well as wet mass (WM) and dry mass (DM) determination.
The physiological respiration was first measured in organisms used to study respiratory metabolism, followed by the determination of WM. Next, the samples were then stored at –80°C to prevent enzyme degradation until enzymatic analysis was performed (Ahmed et al., 1976; Thuesen and Childress, 1994).
To determine the relation between WM and DM in V. velella and P. physalis individual colonies of each species were rinsed with distilled water, placed on several dried petri dishes to remove the excess water, weighed inside pre-weighed aluminum foil, and then left in the drying oven at 60°C until constant DM was observed (∼7 days). The samples from respiration experiments followed a similar weighing procedure; however, they were stored in a bag at a temperature of –80°C instead of the drying oven.
Oxygen Consumption Measurement (Physiological Respiration)
Jellyfish R was determined using oxygen-sensitive optodes to monitor oxygen (O2) consumption [Fibox-4 by Presens (Regensburg, Germany) (Lilley and Lombard, 2015)]. The organisms, submerged in seawater, were carefully transferred to containers ready for incubation and O2 monitoring. Great care was taken during manipulation to avoid harm and stress to the organisms. A pre-calibrated optode spot was already installed inside the containers. The calibration consisted of a two-point calibration of 0 and 100% oxygen saturation using a saturated solution of NaSO3 and vortexed seawater at the incubation temperature, respectively. The container size was dependent on the organism’s size and was selected to maintain a container volume (CV)-to-wet mass (WM) ratio above 30 mL g–1. Such containers included 60 mL glass BOD bottles, 350 mL glass jars, 600 mL glass jars, and 1 L glass jars. In the case of V. velella and P. physalis, their floating nature posed a challenge for the incubation. R was measured with the organism completely submerged. An identical-sized vessel, filled only with filtered seawater, was used as a control.
All incubations took place in the dark at 20°C for 3–6 h with measurements every 10–20 min, and bubbles were rigorously avoided throughout the incubations. The seawater in the container was gently mixed prior to measurement of O2 concentration ([O2]) in μmol L–1.
Electron Transport System Analysis (Enzymatic Respiration)
The ETS assay used was performed kinetically (an absorbance time-course) as described by Packard and Christensen (2004). The chemistry was based on Packard’s first experiments (Packard et al., 1974), which was improved by several authors (Owens and King, 1975; Gómez et al., 1996; Purcell et al., 2019). The A. aurita samples and smallest P. noctiluca were homogenized using an ultrasonic probe (Cole Parmer) with a Vibracell VCX 130 ultrasonic processor in cold 0.1 M phosphate buffer [0.1 M Na2HPO4, 0.1 mM KH2PO4, 75 μM MgSO4⋅7H2O, polyvinylpyrrolidone (1.5 mg mL–1), TRITON X-100 (2 mL L–1)] at 8.5 pH. P. noctiluca medusa, V. velella, and P. physalis samples were homogenized with a motor-driven Teflon-glass tissue-grinder at 4,000 rpm for 2–3 min, in cold 0.1 M phosphate buffer. A glass microfiber filter (GF/F Circle, 25 mm) was inserted into the tissue grinder to serve as an abrasive (Packard, 1971). The largest specimens were first blended, in a mechanical liquefier, with a known volume of cold deionized water (Puranity TU3), that was, sufficient to macerate the sample (Packard, 1971; King et al., 1978). Then, a known aliquot of that blended homogenate was blended in cold buffer with a GF/F filter in the Teflon-glass tissue-grinder as described earlier. In the case of the hydrozoans, a known aliquot of that buffered homogenate was also diluted to achieve a measurable concentration. The maximum final volume-to-mass ratio for A. aurita was kept close to 10 mL homogenate per g sample, for P. noctiluca close to 15 mL homogenate per g sample, and for V. velella and P. physalis, it was kept between 30 and 45 mL of homogenate per g sample. These homogenization steps were always performed at 0–4°C in ice baths to retard enzyme degradation (Packard, 1971). The crude homogenate was then centrifuged at 4,000 rpm (1,500 g) for 10 min at 0–4°C using a refrigerated microcentrifuge. The resulting supernatant contained the enzymes for analysis. The enzyme analysis consisted of mixing, in a cuvette, 0.1 mL of the supernatant, 0.3 mL of the buffered substrates [NADH (1.7 mM) and NADPH (1.7 mM)] and 0.1 mL of INT solution [INT tetrazolium salt 98%, 3-(4-Iodophenyl)-2-(4-nitrophenyl)-5-phenyl-2H-tetrazol-3-ium chloride (Acros Organics, Geel, Belgium) 2 mg per mL deionized H2O (3.88 mM)]. The reaction in the cuvette was monitored in a Cary100 UV-Vis Spectrophotometer [Agilent Technologies (Santa Clara, CA, United States)] for 8 min at 490 nm until INT was reduced to a dark-red formazan. From the evolution of this absorbance, the rate of formazan production can be determined with the extinction coefficient of that batch of INT (13.76 mM–1 cm–1). The potential respiration (Φ) associated with this enzymatic system was calculated, knowing that 4 moles of electrons transferred down the ETS reduced 2 moles of INT and would normally reduce, at cytochrome oxidase, 1 mole of O2. This implies that an ETS activity of 4 μmol e– h–1 is stoichiometrically equivalent to 1 μmol O2 h–1 of Φ (Packard, 1985). Blanks without homogenate were required to control any non-enzymatic INT reduction (Maldonado et al., 2012). The residual crude homogenate was stored at –20°C for biochemical determinations.
Biochemical Composition Measurements and Derived Energy Content
The protein content was measured in the samples homogenized for the ETS assay. These samples were diluted with buffer, when necessary, to reach a ratio of 120 mL of homogenate per g of sample. The analysis was based on the Biuret reaction as described by Lowry et al. (1951) and modified by Rutter (1967). It was further modified in this study by the addition of Dodecyl sulfate sodium salt (SDS) as suggested by Markwell et al. (1978) and Martínez et al. (2020). The lipid content was measured in samples homogenized previously without any further dilution. The content was determined based on the “index of total lipids,” as defined by Knight (Marsh and Weinstein, 1966; Knight et al., 1972; Barnes and Blackstock, 1973; De Coen and Janssen, 1997). We followed the methodology described by Bligh and Dyer (1959) for the extraction of lipids. A standard curve, ranging from 0 to 4.8 mg mL–1 of olive oil dissolved in chloroform, was utilized for calculations. The carbohydrate content was measured in homogenized samples as previously described, without any further dilution. The content was studied using the method proposed by Dubois et al. (1956). A standard curve of glucose dissolved in homogenization buffer ranging between 0 and 1.5 mg mL–1 was used. In the case of the smallest A. aurita and P. noctiluca, a more concentrated solution would have been desirable for the analysis, as in some cases, the biochemical components were below the limit of detection. The protein, lipid, and carbohydrate contents were used to calculate composition-based energy-contents and energy-densities (Ec) (Doyle et al., 2007) as proposed in Clarke et al. (1992). The average enthalpies of combustion are 23.9, 39.5, and 17.5 KJ g–1 for proteins, lipids, and carbohydrates, respectively.
Carbon Demand Calculations and Heterotrophic Energy Transformation
The daily respiratory carbon demand (RCD) in mg C d–1 g WM–1 can be calculated using the following equation (Uye and Shimauchi, 2005):
where: R represents respiration in μL O2 h–1 g WM–1, RQ represents the unitless ratio of CO2-produced to O2-consumed (0.85) (Uye and Shimauchi, 2005); F is the C:CO2 conversion 0.536 μg C (μL CO2)–1, t is 24 to convert hours to days; and AE is the assimilation efficiency described by Schneider (1989). This is the percentage of assimilated carbon consumed, in this case, 0.8 for the scyphozoans (Schneider, 1989) and 0.9 for the hydrozoans (Purcell and Kremer, 1983). The equation is divided by 103 to convert micrograms to milligrams. The same respiration rates were used to calculate the heterotrophic energy transformation (HET) in units of J d–1 g WM–1, using the associated energetics of the adenosine triphosphate (ATP) production (Packard et al., 2015; Azzaro et al., 2019). We prefer to use the term, “Heterotrophic Energy Transformation,” rather than the original term, “Heterotrophic Energy Production.” This is because according to the Law of Conservation of Energy (physics) and the First Law of Thermodynamics (chemistry), energy is neither produced nor destroyed. It is constant and can only be transformed from one form to another (Feynman et al., 1970). It would be calculated using R, in units of μmol O2 h–1 g WM–1, based on Equation (2):
where HET was reported as J d–1 g WM–1. Oe is 2, the number of electron pairs that participate in the reduction of one mole of O2 to 2 moles of H2O; PO equals 2.5 the modified P/O ratio (the ATP produced by the flow of one electron pair to atomic oxygen); ΔGATP equals to 0.048, the Gibbs free energy associated with ATP in J (μmol ATP)–1, and t is again 24 to convert hours to days. The original concept can be found in Ochoa’s work (Ochoa, 1943). The constants and ratios are explained by several authors (Alberty and Goldberg, 1992; Ferguson, 2010; Moran et al., 2012; Packard et al., 2015). To calculate potential HET (p-HET) from ETS activity, the calculation uses Φ instead of R.
Statistical Analysis
The boxplots were built using Microsoft excel (2016) (Microsoft-Corporation, 2016). R software was used to perform the statistical analysis (R Core Team, 2017) to compare the results for each species. The data for species have an unequal size and significantly unequal variances (p < 0.05) according to the Levene and Brown-Forsythe tests (Brown and Forsythe, 1974). Therefore, multiple comparisons were performed using Games-Howell post-hoc tests, which are known as a non-parametric approach based on Welch’s degrees of freedom correction and do not require equal variances or equal sample size (Games and Howell, 1976; Lee and Lee, 2018).
Results
Wet Mass
The wet masses of the samples and the number of samples (in parentheses) used in the respiration and composition studies ranged from 0.14 to 59.8 g for A. aurita (n = 38), 0.6–70.7 g for P. noctiluca (n = 20), 0.17–5.8 g for P. physalis (n = 11), and 0.19–1.1 g for V. velella (n = 5).
Respiratory Metabolism
The respiration results (R & Φ) were normalized by WM and protein (Table 1 and Figure 1). R, normalized by WM, was observed to be higher in the hydrozoans than the scyphozoans (Table 1 and Figure 1A). Games-Howell statistical analysis showed a significant difference between the results of the scyphozoans and P. physalis (p < 0.01); however, it did not show a significant difference between the scyphozoans and V. velella. The WM normalized Φ (Figure 1B) were even higher by a factor of 10. Here, the hydrozoans had a significant difference from the scyphozoans, but not among each other (p < 0.0001 for the test with P. physalis and p < 0.05 for the test with V. velella). However, a higher water content was also observed for scyphozoans. Therefore, when the results were normalized by protein (P) (Figures 1C,D), R/P in P. physalis and V. velella (1.81 ± 0.73 and 2.94 ± 1.12 μL O2 h–1 mg P–1, respectively) were below and significantly different (p < 0.0001) from the P-specific R of A. aurita and P. noctiluca (15.82 ± 13.5 and 7.69 ± 1.22 μL O2 h–1 mg P–1, respectively). P-specific Φ (Figure 1D) was similar in all species with only P. physalis being significantly different from A. aurita with a p = 0.041.
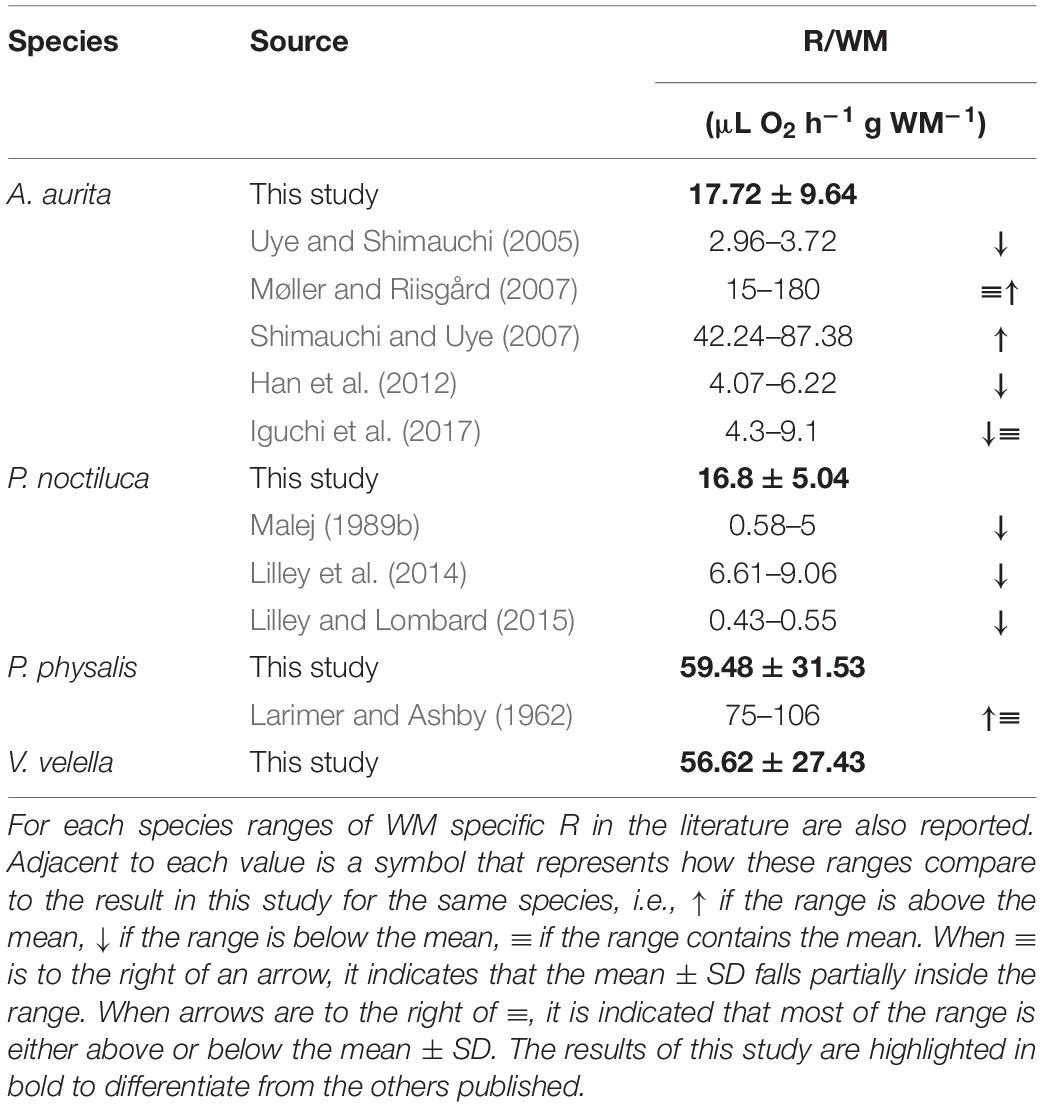
Table 1. Wet mass (WM) specific physiological respiration (R) results for each of the species studied here in the format of mean ± SD.
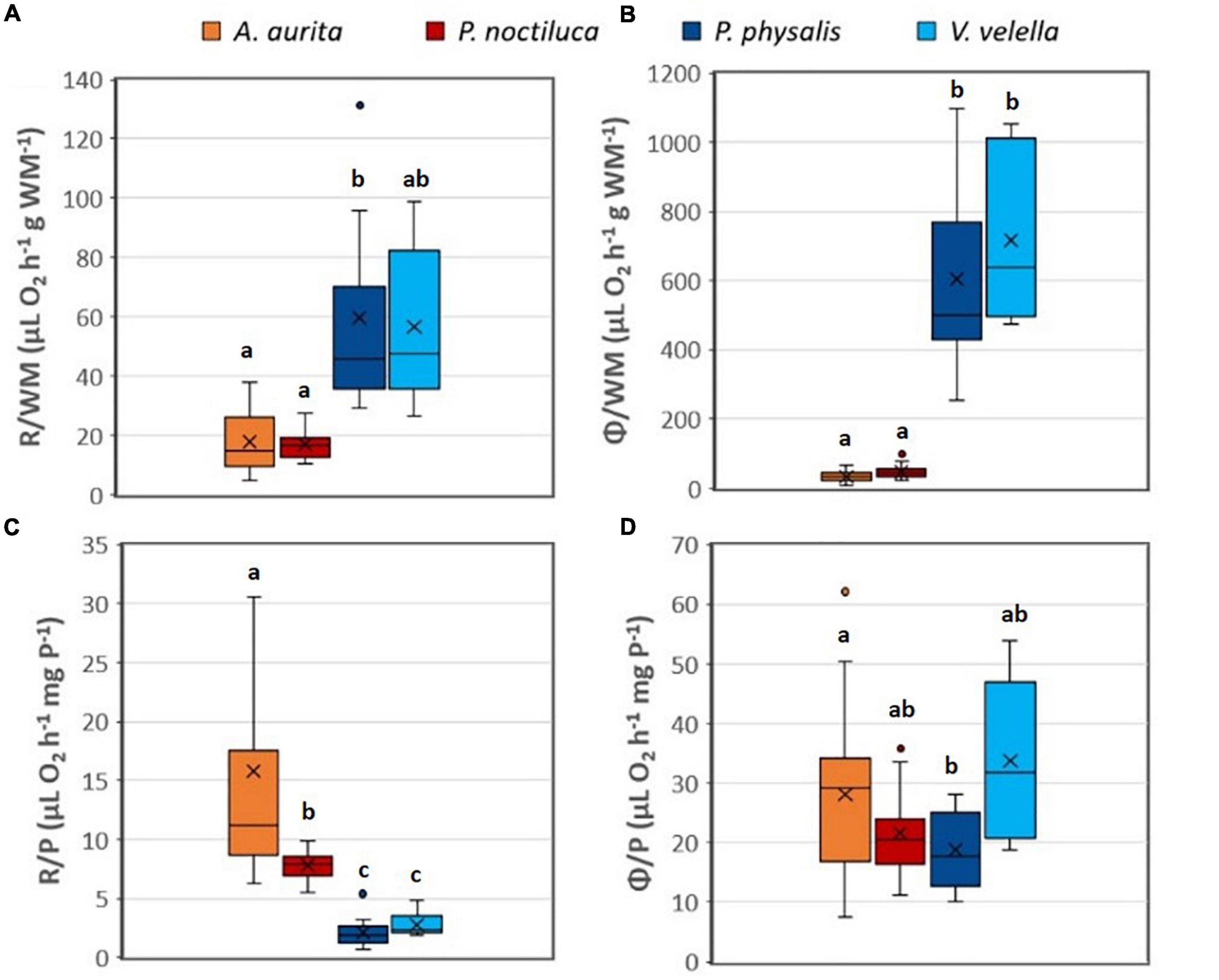
Figure 1. Boxplots summarizing the results of physiological (R) and potential (Φ) respiration normalized by wet mass (WM) (A,B) and by protein content (P) (C,D) for each species. (A,C) Are for R while (B,D) are for Φ results. Small dots mark the outliers and “x,” the average. A. aurita, n = 38; P. noctiluca, n = 20; P. physalis, n = 11; V. velella, n = 5. A significant difference (p < 0.05) in Games-Howell post hoc analysis is represented with the letters “abcd.” If the boxplots share a letter, there was no significant difference found when their data was compared.
The ratio R/Φ representing the fraction of the maximum enzymatic activity used by the physiological respiration varied as seen while comparing the hydrozoans and the scyphozoans. A. aurita and P. noctiluca showed an R/Φ of 0.61 ± 0.38 and 0.40 ± 0.15, respectively, which were significantly different from each other (p < 0.05), whereas the ratios in P. physalis and V. velella were significantly lower (p < 0.0001) than those of the scyphozoans by about a factor of 5 (0.11 ± 0.08 and 0.09 ± 0.04, respectively). Hence, the wind-driven hydrozoans from this study used less of their potential metabolism, as hypothesized. Earlier zooplankton studies found ratios around 0.5 (Packard, 1985; Hernández-León and Gómez, 1996), which was the expected ratio between the in vivo enzyme activity and the maximum enzyme activity (Cleland, 1967).
Composition
The protein (P), lipid (L), and carbohydrate (K) reported in this study, are the first such measurements in P. physalis and V. velella. These results are showcased per WM in Figure 2. The average content per WM for A. aurita, P. noctiluca, P. physalis, and V. velella for P were 0.12 ± 0.04%, 0.21 ± 0.07%, 3.72 ± 0.97%, 2.39 ± 0.76% (Figure 2), showing significant differences from each other (p < 0.005). The results for L/WM were 0.11 ± 0.14%, 0.07 ± 0.06%, 0.62 ± 0.19%, 0.24 ± 0.15% (Figure 2), where only P. physalis varied from the other species (p < 0.05). In the case of K, the scyphozoans demonstrated a few cases below the detection limit, showing the resulting values of 0.05 ± 0.05, 0.02 ± 0.01, 0.3 ± 0.05, 0.17 ± 0.08, respectively (Figure 2). The K content in scyphozoans was significantly different from hydrozoans (p < 0.05) with the exception of A. aurita and V. velella (p = 0.053). The resulting WM-specific Ec in the hydrozoans was significantly higher than in the scyphozoans (p < 0.0001) and significantly different between the two hydrozoan colonial species (p < 0.0001).
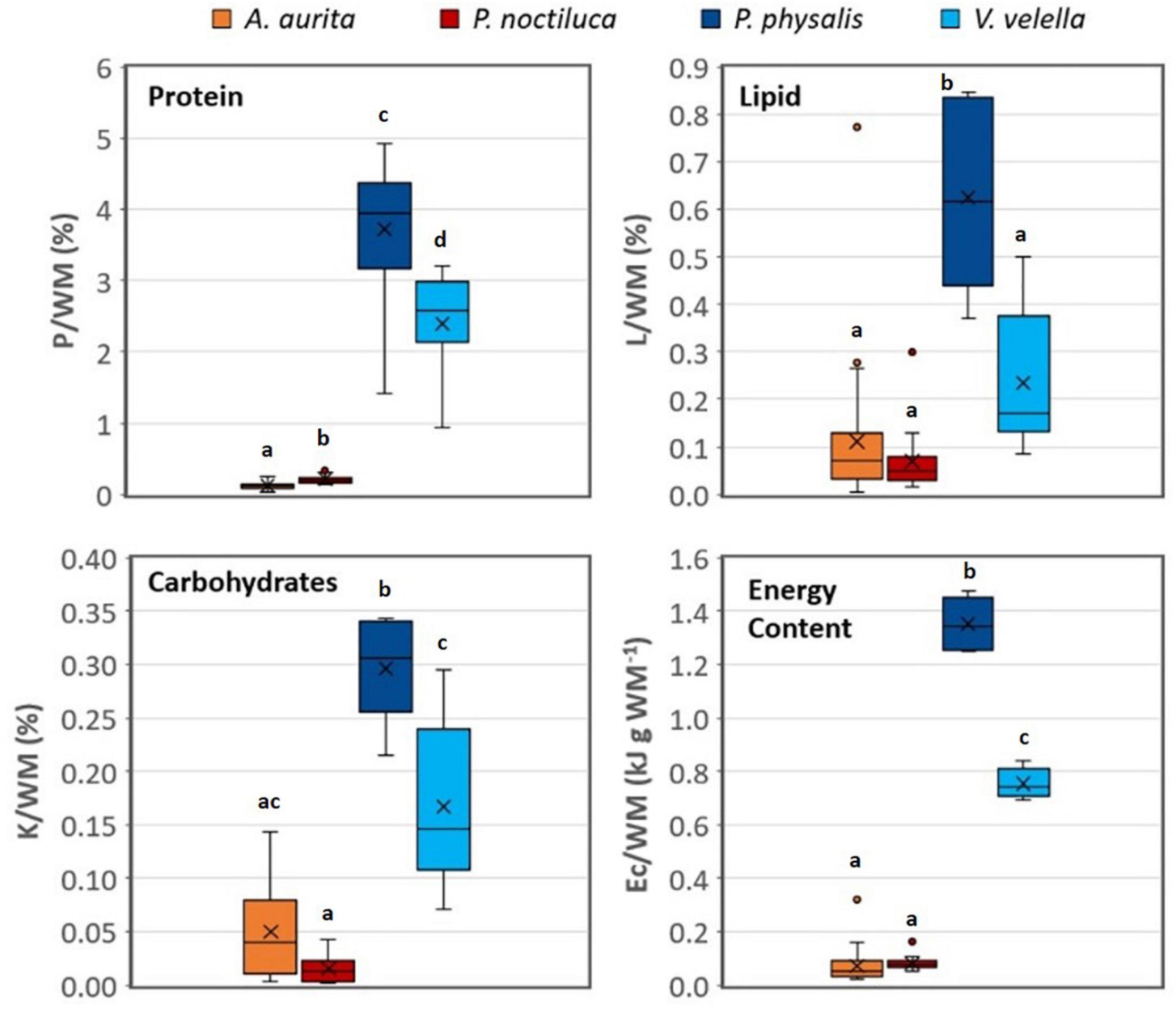
Figure 2. Boxplots summarizing the protein (P), lipid (L), carbohydrate (K) and energy content (Ec) per wet mass (WM) in the four species. Small dots mark the outliers and “x,” the average. A. aurita, n = 38; P. noctiluca, n = 20; P. physalis, n = 17; V. velella, n = 11. A significant difference (p < 0.05) in Games-Howell post hoc analysis represented with the letters “abcd.” If the boxplots share a letter, there was no significant difference found when their data was compared.
The P, L, and K measurements were normalized by dry mass (DM) based on the DM:WM ratio of 51 samples of P. physalis (0.04–13.1 g) and 24 of V. velella (0.02–0.6 g). The resulting percentages of DM per WM were 11.7 ± 1.6% and 9.3 ± 1.1% for P. physalis and V. velella, respectively. These values were higher than those of A. aurita and P. noctiluca (3.6 and 6%, respectively) and other gelatinous organisms (Morand et al., 1987; Malej et al., 1993; Lucas, 1994; Uye and Shimauchi, 2005). This is consistent with the water content in these two hydrozoans (89.3 and 90.7%) below the range expected for gelatinous zooplankton (94–97.6%), including pyrosomes (92.8%) (Bailey et al., 1995; Lucas et al., 2011; Ikeda, 2014). With respect to WM, the P, L, and K content of every component were higher in the two hydrozoans since the water content in these two is lower. However, for DM (Table 2), the P content was higher in the hydrozoans compared to scyphozoans measured, while lipid and carbohydrate contents in scyphozoans were closer to the content measured in hydrozoans, yet still lower.
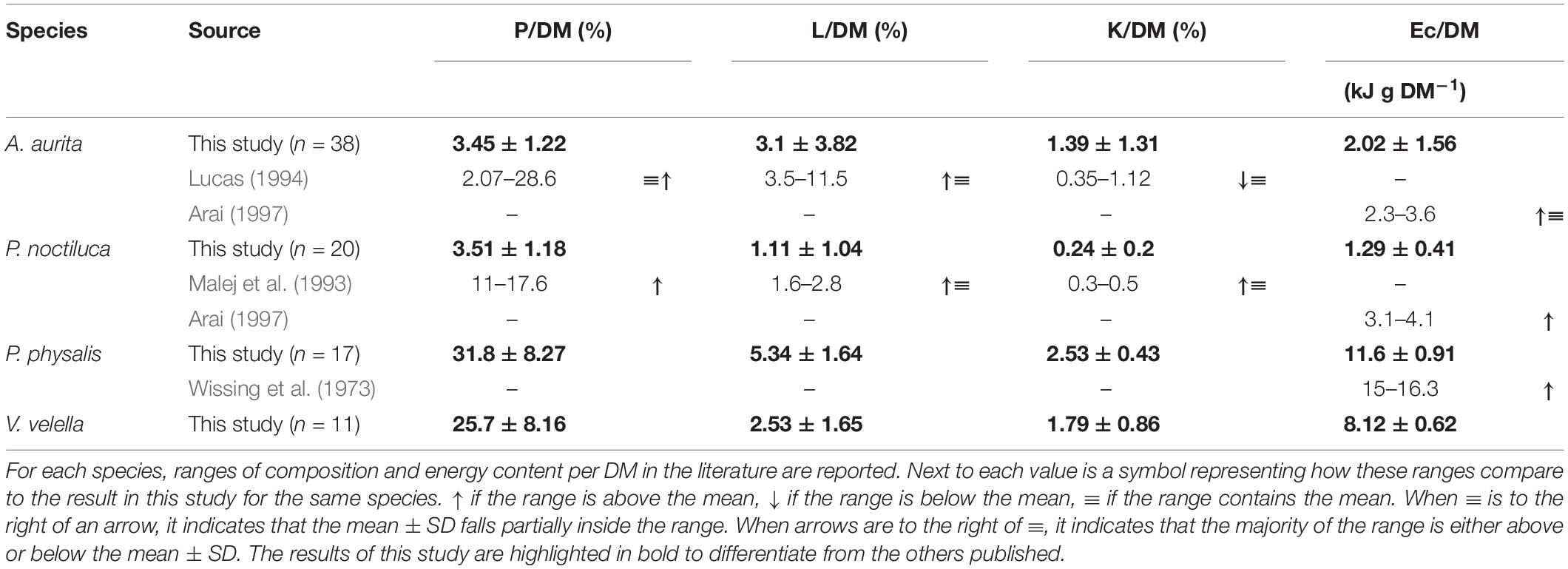
Table 2. Protein (P), Lipid (L), Carbohydrate (K) and energy content (Ec) per dry mass (DM) for each species as mean ± SD.
Respiratory Carbon Demand and Heterotrophic Energy Transformation
The results of respiratory carbon demand (RCD) and heterotrophic energy transformation (HET), calculated from R and Φ, are shown in Tables 3, 4. RCD and HET per WM were higher in the hydrozoans than in the scyphozoans, particularly the potential parameters derived from Φ.
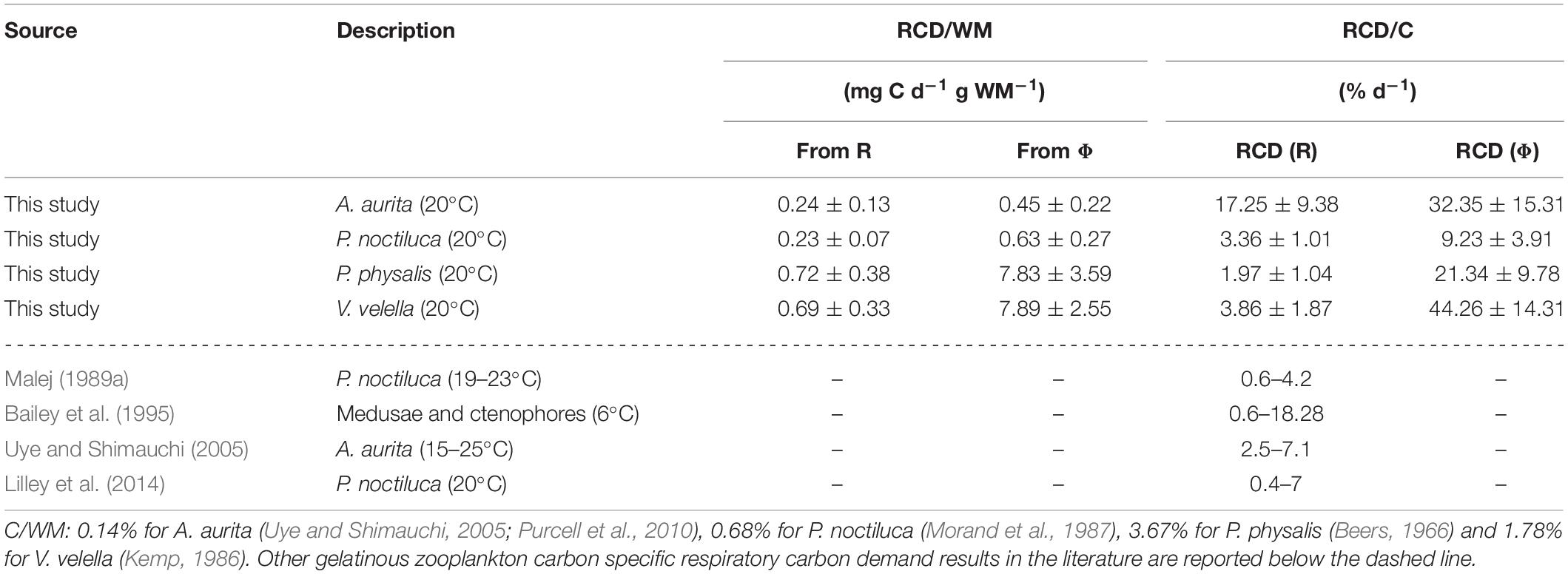
Table 3. Compilation of the RCD (mean ± SD) for our four species of planktonic cnidarians normalized by wet mass (WM) and by carbon content (C).
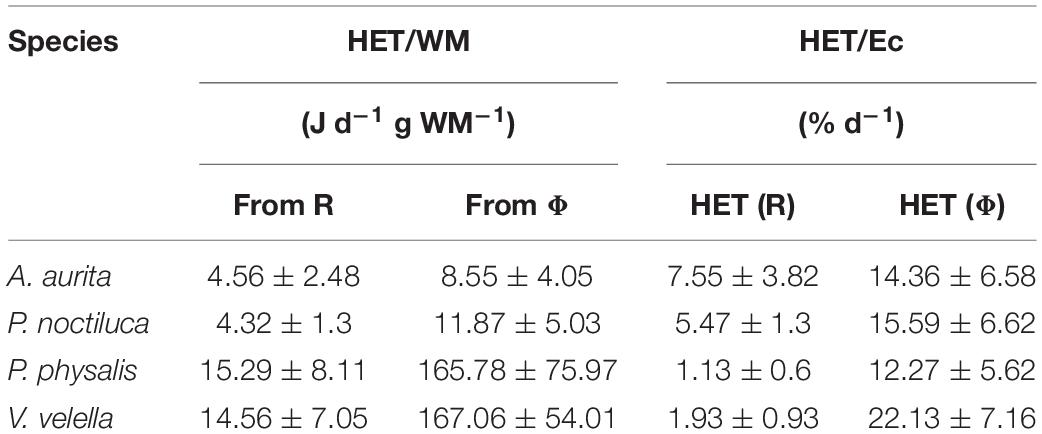
Table 4. Compilation of the HET (mean ± SD) for our four species of planktonic cnidarians normalized by wet mass and by energy content (Ec).
The RCD in Table 3 was further normalized by carbon (C) to showcase the percentage of each organism’s C content required daily for survival. The resulting C-specific RCD illustrates that the daily respiratory C requirements fall below 5% of their own body-C mass except for A. aurita, which required an estimated 17% d–1. This high percentage in A. aurita has been described previously (Table 3; Uye and Shimauchi, 2005). C ingested can sometimes even exceed its own body-C mass as reported by ingestion experiments with different types of preys (Båmstedt et al., 1994). In the case of P. noctiluca, the results presented (Table 3) are fairly close to the percent of body-C respired reported by Malej (1989a). However, Lilley et al. (2014) described this percentage as closer to 7%. These values fit into the range found in other gelatinous zooplankton (Bailey et al., 1995; Table 3).
Similarly, HET was normalized by the Ec calculated from the P, L, K composition to determine the daily percentage of its own Ec required by the organism to survive. Here, all organisms required less than 10% of their own Ec daily with P. physalis and V. velella requiring less than 2% (Table 4).
The inverse of C-specific RCD and Ec-specific HET serve to estimate the number of days that the organism can survive with only its own carbon or Ec as sources to satisfy only their respiratory requirements. In this case, based on R-derived RCD, A. aurita would last for almost 5 days, P. noctiluca for 29, P. physalis for almost 50, and V. velella around 25. Based on Ec-specific HET derived from R, A. aurita and P. noctiluca would last 13 and 18 days, respectively, while P. physalis and V. velella would survive, 88 and 51 days, respectively. It must be noted that these are rough estimations. Nevertheless, these estimations help in achieving a better understanding of both carbon- and energy-based metabolism.
Discussion
Comparison and Implications of Derived Respiratory Carbon Demand and Heterotrophic Energy Transformation
The RCD reported here can be compared with estimations of carbon (C) ingestion rates to determine the percentage of C ingested that is approximately destined for respiratory metabolism. Uye and Shimauchi (2005) published an ingestion rate between 0.06 and 0.30 mg C d–1 gWM–1, whereas Møller and Riisgård (2007) reported a range between 0.58 and 0.69 mg C d–1 gWM–1 for A. aurita. Lilley et al. (2014) estimated the necessary carbon ingestion for an 8 cm P. noctiluca to be 0.57 mg C d–1 g WM–1. Based on these values and our RCD results (Table 3), we can infer that respiration in the scyphozoans requires a significant percentage of the carbon ingested with the potential of requiring further carbon. Uye and Shimauchi (2005) concluded that around one-half of the carbon ingested was used for respiration, which implies an even higher percentage of the carbon assimilated.
Fish larvae have been identified as the main prey of P. physalis (Purcell, 1984). It was documented that P. physalis, with floats between 1 and 20 cm, captured fish larvae between 2 and 20 mm in length (Purcell, 1984). It was estimated that about 120 fish larvae were consumed daily by each colony (Purcell, 1984). The carbon content (g C) per fish larva can be estimated using the equation, C = 0.44⋅ 10−3⋅L3.272 (where C is carbon in grams and L is the length, in cm, for each larva) (Harris et al., 1986) for Gadus chalcogramma from the Gadidae family, one of the fish families sampled by Purcell (1984). This was followed by a calculation of the range of carbon content based on the fish length ranges published by Purcell (1984) (0.002–4.25 mg C) and determination of the associated C demand based on the amounts of fish consumed (about 120 individuals). Hence, it was found the C demand per individual colony associated with those predation rates, ranged between 0.27 and 510.04 mg C d–1 ind–1.
In the P. physalis colonies, the RCD per colony and potential RCD per colony, in mg C d–1 ind–1, ranged from 0.19 to 3.51 and 0.90–50.49, respectively (Table 3). Considering the fact that the float length of our study’s siphonophores ranged from 2.39 to 6.77 cm, it is reasonable to propose that our results are closer to the lower values stated by Purcell (1984). Our results are consistent with predation observations, considering that the amount of the ingested C destined for respiration will be lower than the total C ingested. Hence, RCD would be the minimum C requirement. For example, Purcell and Kremer (1983) found that the siphonophore, Sphaeronectes gracilis, at low food levels, ingested 5.5 times more C than necessary to meet metabolic demands and even 9 times more at high food levels. This observation agrees with the low metabolic strategy hypothesized but begs to question if the rest of the assimilated C is invested in growth, reproduction, or the generation of specific organic substances in these colonial pelagic hydrozoans.
Regarding V. velella, there has been some discussion regarding its main prey. According to the most recent findings (Bieri, 1966; Purcell et al., 2012, 2015; Zeman et al., 2018), its main prey include cladocerans, euphausid larvae, copepods, and fish eggs. For example, it has been found that V. velella gut contained 43% cladocerans, 19% copepods (6% cyclopoida, 13% calanoid), 28% fish eggs, and 5% invertebrate eggs (Zeman et al., 2018). In other examples (Purcell et al., 2015), the prey found in V. velella’s gastrozoids mainly included calanoid copepods and euphausid larvae (calycopsis and furcilia). By converting the ingestion rates that appear in these articles n° of prey consumed colony–1 d–1 to carbon mass consumed colony–1 d–1, we can compare the values obtained from ingestion studies with our metabolic results. To achieve this, we needed the approximate carbon content for the different types of prey ingested by V. velella (μg C ind–1). These carbon content values were for the calanoid copepod, 1.55 and for the cladoceran, 0.72 (Walve and Larsson, 1999); for the fish eggs, 30 (Pereira et al., 2014); for the euphausid larvae it depended on the stage, 10 for calycopsis, 984 for furcilia (Lindley, 1999; Guglielmo et al., 2015). Based on the ingestion rates we obtained the range 4.10–13.09 mg C ind–1 d–1 for 94.9% of the prey ingested (Purcell et al., 2015) as well as the range 0.02–7.62 mg C ind–1 d–1 for 84% of the prey ingested (Zeman et al., 2018). From our results (Table 3), the RCD per colony ranged from 0.21 to 0.35 mg C ind–1 d–1 and potential RCD per colony ranged from 1.4 to 6.2 mg C ind–1 d–1. It must also be considered that V. velella colonies used for our research had a sail length between 1.16 and 3.39 cm. Sail lengths from Purcell et al. (2015) ranged between 1.7 and 5.2 cm and between 1.9 and 3.3 cm from Zeman et al. (2018). Our results are in the lower end of the range compared to previous ingestion studies. These observations are similar to the P. physalis comparison. The ingestion may be higher to fulfill the demand for biological processes other than respiration, such as V. velella’s 0.5 mm daily growth (in length) (Bieri, 1977). Low carbon destined for respiratory metabolism is in line with our hypothesis of the low metabolic strategy of these neuston wanderers.
The measurement of HET presents great potential. If HET were measured in other organisms, we may be able to assess and compare their energy transforming capabilities and study energy circulation in the future (Karl, 2014). This may also be useful to address Margalef’s suggested unifying principle in ecology regarding complexity and energy expenditure: “The energy required to maintain an ecosystem is inversely related to complexity, with the natural trend toward decreasing flow of energy per unit biomass; that is, increased maturity” (Margalef, 1963). Furthermore, HET also allows for calculations such as the one in Lane (2015) which described that the energy release, in terms of ATP synthesis, for an average person per gram was greater than that of the sun by a factor of 10,000. This inspired our calculation where we compared the HET by scyphozoan and hydrozoan mitochondria with the mass-specific energy-release rate of the sun at 0.017 J d–1 g–1 (Williams, 2018). From Table 4, the resulting ranges of average HET were between 4 and 15 J d–1 g WM–1, approximately. The calculation based on potential-HET was approximately between 8 and 170 J d–1 g WM–1 considering the four species in this study. Consequently, the WM-specific heterotrophic energy transformation in these jellyfish is between 500 and 10,000 times greater, on a per mass basis, than the sun’s energy release rate. If jellyfish dry-mass is used in this calculation, the difference would be at least more than a thousand-fold greater. This is consistent with the findings presented by Lane (2015).
Composition
Regarding the scyphozoan composition measurements, our results for A. aurita can be compared with those of Lucas (1994). Protein and lipid content per DM in this study (Table 2) were found to be within the ranges presented by Lucas, but on the lower side. Carbohydrate measurements per DM were slightly above those illustrated by Lucas. For P. noctiluca, we compared our results to the results by Malej et al. (1993), which were generally higher than the percentages reported in this study (Table 2).
There is much less information about the general biochemical composition of P. physalis and V. velella. The explanation behind the high DM per WM percentage in the two hydrozoans compared to the other gelatinous organism could be related to the presence of chitin in the formation of the pneumatophore (Kirkpatrick and Pugh, 1984). The chitin gives both P. physalis and V. velella a more rigid structure that supports the changing interior gas pressure of the pneumatophore used to maintain the colony floating through the neuston. These harder structures are absent in other gelatinous zooplankton. However, the DM/WM of V. velella in this study (9.3 ± 1.1%) is higher than previously described 5.93 ± 1.56% (Kemp, 1986).
Comparing the P, L, K content per WM in this study (Figure 2) with the gelatinous zooplankton composition analyses by several authors (Arai et al., 1989; Clarke et al., 1992; Malej et al., 1993; Lucas, 1994, 2009; Bailey et al., 1995; Dubischar et al., 2006; De Barba et al., 2016), we can observe that the content of these components is higher in P. physalis and V. velella with certain minor exceptions. Hence, for a similar WM, these two could be a better source of some of these components due to their low water content.
When comparing the biochemical content per DM (Table 5) we observed that the range in the literature for protein was generally below the mean content determined in P. physalis and V. velella (Arai et al., 1989; Clarke et al., 1992; Malej et al., 1993; Lucas, 1994, 2009; Bailey et al., 1995; Dubischar et al., 2006; De Barba et al., 2016). Table 5 also depicts several exceptions, for example, the two cubozoans, Chiropsalmus quadrumanus and Tamoya haplonema (De Barba et al., 2016); as well as a few arctic species (Percy and Fife, 1981); Aglantha digitale (Ikeda, 1972); certain edible species (Khong et al., 2016); and the gonad tissue of A. aurita and Cyanea capillata (Lucas, 1994; Doyle et al., 2007). The higher presence of protein in the neustonic hydrozoans may be due to the protein-based blue pigment, protein-based venoms, chitin-protein complexes associated with the pneumatophore, or the protein in extracellular fluids (Lane et al., 1965; Gainey, 1972; Zagalsky and Herring, 1977; Tamkun and Hessinger, 1981; Roberts, 1992).
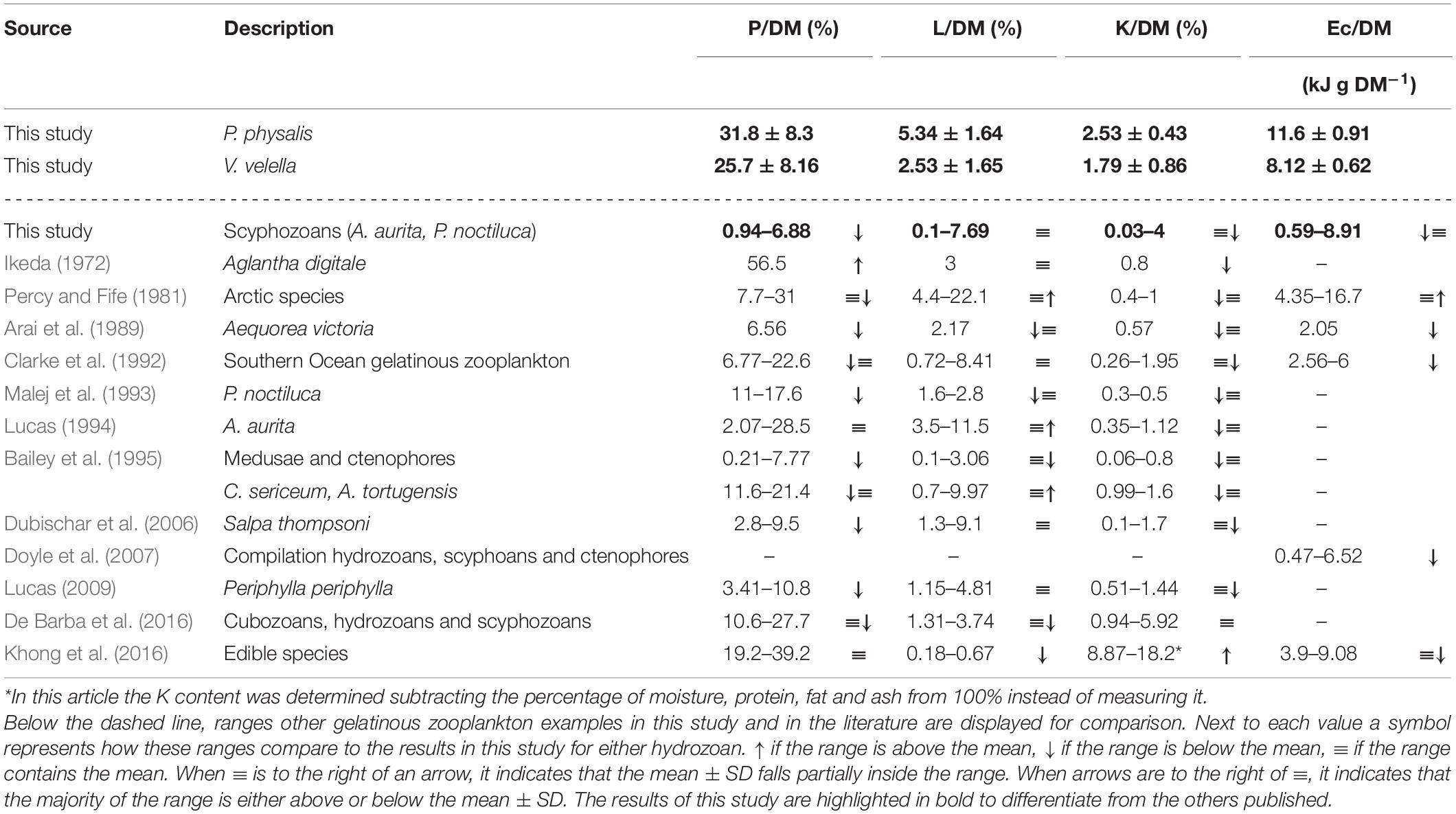
Table 5. Protein (P), Lipid (L), Carbohydrate (K) and energy content (Ec) per dry mass (DM) for P. physalis and V. velella presented as mean ± SD.
The gelatinous zooplankton L/DM range in the literature varied widely (Table 5; Arai et al., 1989; Clarke et al., 1992; Malej et al., 1993; Lucas, 1994, 2009; Bailey et al., 1995; Dubischar et al., 2006; De Barba et al., 2016). P. physalis presents above most measurements in the other species of this article (Figure 2). This could be due to their different diets (Lopes et al., 2016), which, in the case of P. physalis, is mainly fish-focused, or it could be related to the lipid content associated with nematocyst capsules and toxins (Stillway, 1974, 1976; Joseph, 1979). The exceptions to the lipid content in gelatinous species were the higher values in the arctic species described by Percy and Fife (1981; Table 5). These values will have implications on the energy content explored later. The arachidonic acid (20:4ω6), eicosapentaenoic acid (20:5ω3), and docosahexaenoic acid (22:6ω3) are proposed to be essential fatty acids (EFAs) (Cater et al., 2021), which may explain the reason jellyfish are in demand by some predators (Arai et al., 1989; Stenvers et al., 2020). These EFAs have often been found at high levels in both hydrozoans and scyphozoans (Stillway, 1976; Lopes et al., 2016; Tilves et al., 2016; Stenvers et al., 2020).
The K/DM range in the literature was normally between 0.1 and 1.7% (Table 5; Larson and Harbison, 1989; Clarke et al., 1992; Malej et al., 1993; Lucas, 1994, 2009; Bailey et al., 1995; Doyle et al., 2007). The results here for A. aurita and P. noctiluca were within this range while the K/DM ratios for the two hydrozoans were, in general, above this range (Table 5). The only exceptions were the K content described in the previously mentioned cubozoans, in the hydrozoan, Olindias sambaquiensis (De Barba et al., 2016), and in edible jellyfish (Khong et al., 2016). However, this was mainly due to the K content being calculated from other components instead of being measured. Carbohydrates are, in general, the least abundant of the three biochemical components (Lucas, 2009). Chitin intermediates present in both V. velella and P. physalis, as well as hexose associated with the float in P. physalis (Gross et al., 1958), were likely to be responsible for the K concentrations in their tissue.
Based on composition, the resulting Ec/WM (Figure 2) in A. aurita (0.07 ± 0.06 kJ g WM–1) and P. noctiluca (0.08 ± 0.02 kJ g WM–1) were within the range of the compiled observations in gelatinous zooplankton, as shown by Doyle et al. (2007), at 0.07–0.3 KJ g WM–1, excluding the arctic species. Since arctic species such as those analyzed by Percy and Fife (1981), have a high lipid content, their maximum Ec/WM would be 0.85 kJ g WM–1. The results for P. physalis (1.35 ± 0.11 kJ g WM–1) and V. velella (0.75 ± 0.06 kJ g WM–1) were close or above this maximum Ec/WM. Hence, for the same WM, the Ec in P. physalis was the highest. The Ec/DM for the hydrozoans (Tables 2, 4) was slightly above the results compiled by Doyle et al. (2007), except for the Ec/DM in high lipid arctic hydrozoans, which was double the maximum Ec/DM reported (Percy and Fife, 1981; Table 5). The Ec/DM values in A. aurita and P. noctiluca were slightly below the ranges for these species published by Arai (1997; Table 2). In the tables of Ec compiled by Arai (1988), the Ec/WM for siphonophores was 0.67 kJ g WM–1 (Musayeva and Sokolova, 1979), and the Ec/DM in P. physalis, based on bomb calorimetry, was 15–16.3 kJ g DM–1 (Wissing et al., 1973). The first value agrees with our observations while the second, based on calorimetry, suggests a higher Ec than the one calculated here based on composition (Table 2).
Respiratory Metabolism
Due to logistical problems associated with incubations, we encourage research on enzyme-based alternatives. Enzyme-based techniques are also useful when working with fragile gelatinous zooplankton species that are often damaged during sampling, hindering live measurements (Purcell et al., 2010).
Comparing our WM-specific R results with similar studies in the literature (Table 1), we observe that, with regards to A. aurita, the average R/WM presented in this article (Table 1) was below some of the recently published results (Møller and Riisgård, 2007; Shimauchi and Uye, 2007) and above some others (Uye and Shimauchi, 2005; Han et al., 2012; Iguchi et al., 2017).
Iguchi et al. (2017) reported ETS activities in 10 A. aurita medusae (Table 6). They used this information to estimate R in the giant jellyfish Nemopilema nomurai, observing great potential in the application of the ETS assay. However, they were cautious about their results and advocated further studies in their final paragraphs. The results in this work for the 6 A. aurita medusae were closest in size to A. aurita in their study (Table 6), showing a considerable difference. The difference between studies may have several explanations. We measured R with optodes, whereas the researchers used the Winkler technique that only measured the initial and final O2 concentrations from the incubations. This technique is known to be an endpoint-type of analysis. Furthermore, their long (24 h) incubations may have led to O2-limited respiration (Gnaiger, 2001; Purcell et al., 2010).
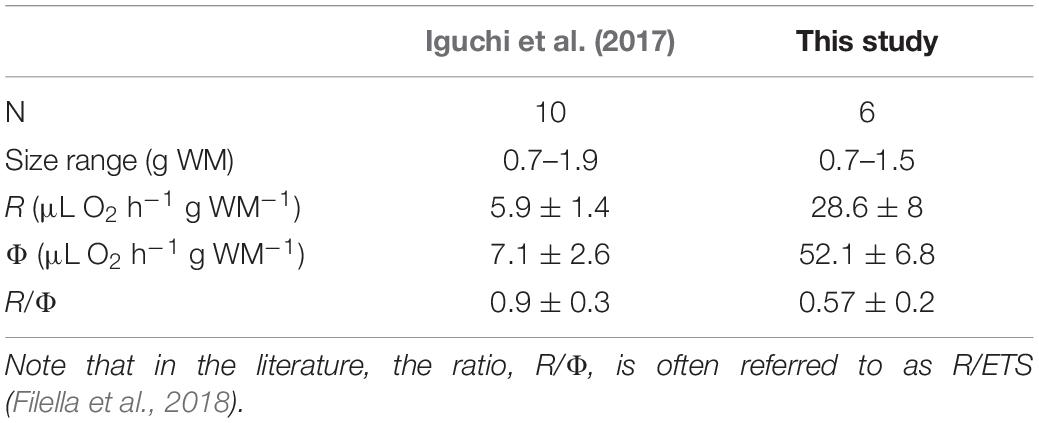
Table 6. Comparison between ETS results (mean ± SD) on A. aurita medusae from Iguchi et al. (2017) and from our study.
Apart from the deterioration mentioned in their article, there are other factors in the study (Iguchi et al., 2017) that may have led to a loss in enzymatic activity. They describe freezing the homogenates, instead of the samples, with liquid nitrogen at –196°C, followed by storing the frozen homogenate at –80°C. From our experience, this leads to a significant loss in enzyme activity within 24 h (Ahmed et al., 1976). Furthermore, they also used the previous substrate concentrations (Owens and King, 1975), which might have slightly underestimated the ETS activity in scyphozoans. They also used the extinction coefficient of INT, 15.9 mM–1 cm–1, from Packard and Williams (1981) rather than determining the INT-formazan extinction coefficient for their own solutions. Most research teams do the same; however, we have found that this practice can lead to underestimations of ETS activity. In summary, Iguchi et al. (2017) inadvertently, may have underestimated both respiration and ETS activity. To resolve this type of discrepancy in the literature, an international intercalibration workshop or program should be organized for ETS analysis.
In the case of P. noctiluca, the R/WM results here (Figure 1 and Table 1) are higher than those reported in the literature (Table 1; Malej, 1989b; Lilley et al., 2014; Lilley and Lombard, 2015). For these two hydrozoans, the results are one of the first regarding their respiratory metabolism. No other study of R in V. velella was found. R measurements in P. physalis tissue were reported in Larimer and Ashby (1962). Their results at 25°C were slightly above but close to our results (Table 1).
While comparing P. physalis and V. velella with other jellyfish, WM-specific results here (Figure 1 and Table 1) were generally higher due to the lower water content compared to other gelatinous zooplankton. Therefore, the following comparisons are DM- or P-specific (Table 7). Compared to the study from Bailey et al. (1995), the R/DM results here (Table 7) are higher than in most gelatinous organisms except the Narcomedusa, Aeginura grimaldii and in situ incubations of other hydrozoan and scyphozoan species (Table 7). However, considering that these measurements were at temperatures between 4 and 7°C it is reasonable to conclude that the R/DM results here could be closer to or below those in the literature (Table 7).
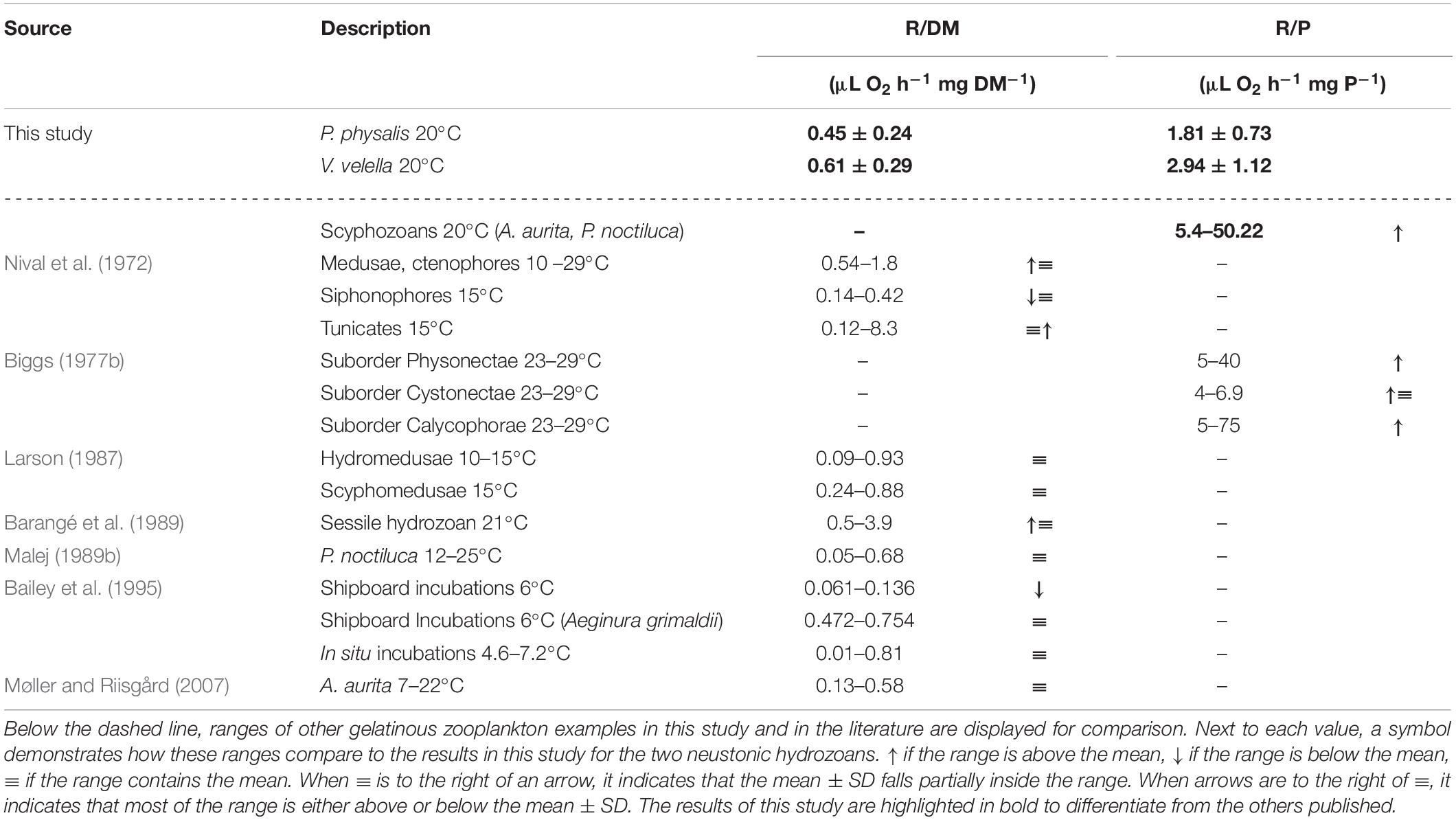
Table 7. Dry mass (DM) specific and protein (P) specific physiological respiration (R) results for P. physalis and V. velella studied are presented as mean ± SD.
On the contrary, our protein-specific results (Figure 1 and Table 7) are lower than similar results from a variety of hydrozoan species, including several siphonophores (Table 7; Biggs, 1977b). However, these measurements taken by Biggs (1977b) were performed at 26 ± 3°C, thus, it is also reasonable to assume that our R results are low but within the range. A detailed comparison between the siphonophore species present in Biggs (1977b) showed that individuals of the suborder Cystonectae, Bathyphysa sibogae, and Rhizophysa filiformis had the lowest respiratory rates. Both species are from the same suborder as P. physalis, and neither are observed to pulsate predominantly. This was consistent with our hypothesis that using wind (or currents) for movement through the ocean permits life at low levels of respiration, which can be detected by a low R:Φ ratio. Meanwhile, the genus from the suborder Physonectae, such as Forskalia, Agalma, or Cordagalma and suborder Calycophorae Sulculeolaria or Stephanophyes had the highest respiratory values, and were observe to pulsate frequently (Bone and Trueman, 1982; Costello et al., 2015). However, exceptions, such as the results from species of the genus Diphyes and Abyla, showed low respiration regardless of their pulse. This was not in line with our hypothesis. Similarly, our respiration results were lower than those compiled by Barangé et al. (1989) for sessile hydrozoans. In addition, Nival et al. (1972) presented very similar respiration rates in pulsating hydrozoans and much higher ranges in medusae and tunicates at 15°C (Table 7).
Low R:Φ ratios may support our hypothesis that, from a respiratory standpoint, these neuston dwellers should have a low R:Φ, due to their drifting nature. Few studies have measured both R and Φ in a cnidarian. In this study, P. noctiluca and A. aurita showed R:Φ ratios of 0.40 ± 0.15 and 0.61 ± 0.38, respectively. Iguchi et al. (2017) observed a high R:Φ ratio of 0.9 ± 0.3 in A. aurita. Three pulsating hydrozoans (Leuckartiara octona, Clytia gregaria, and Stomatoca atra) showed R:Φ ratios between 0.5 and 0.92 (King and Packard, 1975). The exception amongst pulsating jellyfish may be the results in Cassiopea sp., which presented ratios R:Φ of approximately 0.17 ± 0.02 (Aljbour et al., 2017). These upside-down jellyfish have a more benthic lifestyle with a strong reliance for food on its symbiotic dinoflagellate (Lampert, 2016). Hence, their pulsation is less locomotive and the presence of these autotrophs may increase the ETS activity measured, leading to a higher Φ (Bondyale-Juez et al., 2017). A low R:Φ (0.19 ± 0.10) was also found in sessile polyps of A. aurita (Purcell et al., 2019). The low R:Φ in this study (0.11 ± 0.08 and 0.09 ± 0.04 in P. physalis and V. velella, respectively) resembled the low ratio of A. aurita polyps. Since both hydrozoans in this study are wind-propelled polyp colonies, there may be a low ratio amongst sessile life forms that do not require ATP for locomotion as readily. In the case of the free-floating, wind-driven hydrozoans in this study, a low metabolism may allow them to survive prolonged periods without prey consumption as they sail along the surface of pelagic oligotrophic waters (Biggs, 1977a).
In the case of V. velella, the presence of endosymbiotic photosynthetic dinoflagellates (Blank and Trench, 1986) may allow it to survive without prey and live with an even lower R:Φ ratio (Bondyale-Juez et al., 2017). However, we realize that our measurements need confirmation and replication in order to strengthen them (Schmidt, 2009). For example, the hydrozoan samples were close to being stranded, and there is a possibility that the colonies near the shore did not feed as much as in the open ocean. Lane et al. (1965) suggested that P. physalis could not provide reliable physiological data after being maintained for more than a few hours due to problems with interaction with aquariums (Lane et al., 1965). In our experiments, no specimen that clouded the water or deflated abnormally was used.
However, gases from the floats from both organisms could cause an increase in O2 concentration during incubation. Munro et al. (2019) described gas release in young Physalia and the unlikely release of gas naturally in mature colonies. Carter (1931) suggested there is gas exchange between pneumatophores of Physalia, Velella, and Porpita species with the exterior. However, Larimer and Ashby (1962) tested that CO was the main gas secreted, while O2 and N2 were diffused inward. The possibility of pneumatophores with a respiratory function has been speculated (Carter, 1931), which could also reduce the consumption of the O2 dissolved measured in the water if it was obtained it from the gases in their pneumatophore. The idea of gas exchange between the pneumatophore and the exterior with a production of bubbles in the case of vertical migrating siphonophores has also been proposed (Pickwell, 1966), which is presumably not the case for P. physalis. No abnormal deflation, production of bubbles, or sudden increase in O2 was observed. Nevertheless, these issues should be considered. Research on enzymatic alternatives is also encouraged, and hence, we tentatively considered low R:Φ ratios to support our hypothesis; however, we realize that future research addressing alternative explanations for the high Φ is needed.
In both cases, it is also possible that nearshore samples may not exhibit the same healthy metabolism demonstrated by open ocean samples. Regardless, these novel respiration and composition measurements bring us closer to understanding trophic exchanges and physiological strategies occurring in the neuston ecosystem.
Conclusion
The composition of P. physalis and V. velella varies from other gelatinous zooplankton. Their lower water content (approximately between 85 and 91%) leads to a higher concentration of protein, lipid, carbohydrate, and energy content per wet mass.
Enzymatic ETS analysis serves as a complementary or alternative measurement to study the metabolism of fragile and difficult-to-incubate zooplankton. In this study, we propose the optimum conditions for assaying ETS activity in cnidarian zooplankton. These respiratory metabolism measurements facilitate RCD and HET calculations.
We hypothesized that the respiratory metabolism of wind-driven, sail-based, V. velella and P. physalis would be lower compared to that of A. aurita or P. noctiluca with their muscular-based pulsation-driven propulsion. This hypothesis was supported by the respiratory results in these four jellyfish. A lower R:Φ ratio (∼0.1) was found in these hydrozoans. A lower protein-specific R (1.81 and 2.94 μL O2 h–1 mg P–1, respectively) in P. physalis and V. velella was measured compared to results here and in the literature. The respiratory carbon demand compared to their carbon ingestion rates in the literature was below that of scyphozoans. The novel HET/Ec was approximately 2%. All these are consistent with our hypothesis.
Data Availability Statement
The raw data supporting the conclusions of this article will be made available by the authors, without undue reservation.
Author Contributions
JP, TP, and MG conceived of the presented idea. DB-J, VR-K, and IM designed and performed the experimental analysis supervised by TP and MG. DB-J and VR-K processed the data in consultation with TP and IM. DB-J and TP wrote the manuscript in consultation with all the other co-authors. All authors contributed to the article and approved the submitted version.
Funding
This work was supported by the PERSEO project (ProID201710051) granted to MG, the Canary Islands Government and the European Regional Development Fund (“Canarias Avanza con Europa”). JP was supported by the Commission for Cultural, Educational and Scientific Exchange between the United States of America and Spain as a Fulbright United States. Senior Researcher in the 2016–2017 Scholar Program. Other authors in this work received financial support from the Spanish Ministry of Education Formación de Profesorado Universitario grants (FPU2015-036973) (DB-J); Canary Islands Government (Agencia Canaria de Investigación, Innovación y Sociedad de la información) (VR-K); and TIAA-CREF and Social Security-United States (to TP).
Conflict of Interest
The authors declare that the research was conducted in the absence of any commercial or financial relationships that could be construed as a potential conflict of interest.
Publisher’s Note
All claims expressed in this article are solely those of the authors and do not necessarily represent those of their affiliated organizations, or those of the publisher, the editors and the reviewers. Any product that may be evaluated in this article, or claim that may be made by its manufacturer, is not guaranteed or endorsed by the publisher.
Acknowledgments
We are grateful to Loro Parque Foundation for their collaboration, especially to Ester Alonso and Javier Almunia. We also thank Rosa Caprioli and Cristina Villanova Solano for her contributions during her sojourn in our lab. This work was accomplished thanks to the Spanish Ministry of Economy and Competitiveness and the Spanish Ministry of Education, Culture and Sports. The research was completed while the senior author (DB-J) was a Ph.D. student in the Doctoral Programme in Oceanography and Global Change at the University of Las Palmas de Gran Canaria.
Abbreviations
AE, Assimilation efficiency; ATP, Adenosine triphosphate; C, Carbon; DM, Dry mass; Ec, Energy content; ETS, Electron transport system; F, C:CO2 conversion; HET, Heterotrophic energy transformation; INT, 2-p-iodophenyl-3-p-nitrophenyl-5-phenyltetrazolium chloride; K, Carbohydrate; L, Lipid; NADH, Nicotinamide adenine dinucleotide reduced; NADPH, Nicotinamide adenine dinucleotide phosphate reduced; Oe, Number of electron pairs needed to reduce one mole of O2; P, Protein; p-HET, Potential HET; PO, The modified P/O ratio, ATP produced per O; R, Aerobic respiration; RCD, Respiratory carbon demand; RQ, unitless ratio of CO2 to O2 during R; t, Number of hours in a day; WM, Wet mass; ΔGATP, Gibbs free energy asociated with ATP; Φ, Potential respiration.
References
Ahmed, S. I., Kenner, R. A., and King, F. D. (1976). Preservation of enzymic activity in marine plankton by low-temperature freezing. Mar. Chem. 4, 133–139. doi: 10.1016/0304-4203(76)90002-5
Alberty, R. A., and Goldberg, R. N. (1992). Standard thermodynamic formation properties for the adenosine 5′-triphosphate series. Biochemistry 31, 10610–10615. doi: 10.1021/bi00158a025
Aljbour, S. M., Zimmer, M., and Kunzmann, A. (2017). Cellular respiration, oxygen consumption, and trade-offs of the jellyfish Cassiopea sp. in response to temperature change. J. Sea Res. 128, 92–97. doi: 10.1016/j.seares.2017.08.006
Arai, M. N. (1988). Interactions of fish and pelagic coelenterates. Can. J. Zool. 66, 1913–1927. doi: 10.1139/z88-280
Arai, M. N. (2005). Predation on pelagic coelenterates: a review. J. Mar. Biol. Assoc. U. K. 85, 523–536. doi: 10.1017/S0025315405011458
Arai, M. N., Ford, J. A., and Whyte, J. N. C. (1989). Biochemical composition of fed and starved Aequorea victoria (Murbach et Shearer, 1902) (Hydromedusa). J. Exp. Mar. Bio. Ecol. 127, 289–299. doi: 10.1016/0022-0981(89)90080-4
Azzaro, M., Packard, T. T., Monticelli, L. S., Maimone, G., Rappazzo, A. C., Azzaro, F., et al. (2019). Microbial metabolic rates in the Ross Sea: the ABIOCLEAR Project. Nat. Conserv. 34, 441–475. doi: 10.3897/natureconservation.34.30631
Bailey, T. G., Youngbluth, M. J., and Owen, G. P. (1995). Chemical composition and metabolic rates of gelatinous zooplankton from midwater and benthic boundary layer environments off Cape Hatteras, North Carolina, USA. Mar. Ecol. Prog. Ser. 122, 121–134. doi: 10.3354/meps122121
Båmstedt, U., Martinussen, M. B., and Matsakis, S. (1994). Trophodynamics of the two scyphozoan jellyfishes, Aurelia aurita and Cyanea capillata, in western Norway. ICES J. Mar. Sci. 51, 369–382.
Barangé, M., Zabala, M., Riera, T., and Gili, J. M. (1989). A general approach to the in situ energy budget of Eudendrium racemosum (Cnidaria, Hydrozoa) in the Western Mediterranean. Sci. Mar. 53, 423–427.
Barnes, H., and Blackstock, J. (1973). Estimation of lipids in marine animals and tissues: detailed investigation of the sulphophosphovanilun method for ‘total’lipids. J. Exp. Mar. Biol. Ecol. 12, 103–118.
Beers, J. R. (1966). Studies on the chemical composition of the major zooplankton groups in the Sargasso Sea off Bermuda. Limnol. Oceanogr. 11, 520–528. doi: 10.4319/lo.1966.11.4.0520
Bieri, R. (1966). Feeding preferences and rates of the snail, Ianthina prolongata, the barnacle, Lepas anserifera, the nudibranchs, Glaucus atlanticus and Fiona pinnata, and the food web in the marine neuston. Publ. Seto Mar. Biol. Lab. 14, 161–170. doi: 10.5134/175429
Bieri, R. (1977). The ecological significance of seasonal occurrence and growth rate of Velella (Hydrozoa). Publ. Seto Mar. Biol. Lab. 24, 63–76. doi: 10.5134/175957
Biggs, D. C. (1977b). Respiration and ammonium excretion by open ocean gelatinous zooplankton. Limnol. Oceanogr. 22, 108–117. doi: 10.4319/lo.1977.22.1.0108
Biggs, D. C. (1977a). Field studies of fishing, feeding, and digestion in siphonophores. Mar. Behav. Physiol. 4, 261–274. doi: 10.1080/10236247709386958
Blank, R. J., and Trench, R. K. (1986). Nomenclature of endosymbiotic dinoflagellates. Taxon 35, 286–294. doi: 10.2307/1221270
Bligh, E. G., and Dyer, W. J. (1959). A rapid method of total lipid extraction and purification. Can. J. Biochem. Physiol. 37, 911–917. doi: 10.1139/y59-099
Boero, F. (2013). Review of Jellyfish Blooms in the Mediterranean and Black Sea. Rome. Available online at: http://www.vliz.be/en/imis?refid=226251 (accessed November 13, 2018).
Bondyale-Juez, D. R., Packard, T. T., Viera-Rodríguez, M. A., and Gómez, M. (2017). Respiration: comparison of the Winkler technique, O2 electrodes, O2 optodes and the respiratory electron transport system assay. Mar. Biol. 164, 1–11. doi: 10.1007/s00227-017-3271-1
Bone, Q., and Trueman, E. R. (1982). Jet propulsion of the calycophoran siphonophores chelophyes and abylopsis. J. Mar. Biol. Assoc. U. K. 62, 263–276. doi: 10.1017/S0025315400057271
Brown, M. B., and Forsythe, A. B. (1974). Robust tests for the equality of variances. J. Am. Stat. Assoc. 69, 364–367. doi: 10.1080/01621459.1974.10482955
Canepa, A., Purcell, J. E., Córdova, P., Fernández, M., and Palma, S. (2020). Massive strandings of Pleustonic Portuguese man-of-war (Physalia physalis) related to ENSO events along the Southeastern Pacific ocean. Lat. Am. J. Aquat. Res. 48, 806–817. doi: 10.3856/vol48-issue5-fulltext-2530
Carter, G. S. (1931). Aquatic and aerial respiration in animals. Biol. Rev. 6, 1–35. doi: 10.1111/j.1469-185X.1931.tb01020.x
Cater, R. J., Chua, G. L., Erramilli, S. K., Keener, J. E., Choy, B. C., Tokarz, P., et al. (2021). Structural basis of omega-3 fatty acid transport across the blood–brain barrier. Nature 595, 315–319. doi: 10.1038/s41586-021-03650-9
Chiaverano, L. M., Robinson, K. L., Tam, J., Ruzicka, J. J., Quiñones, J., Aleksa, K. T., et al. (2018). Evaluating the role of large jellyfish and forage fishes as energy pathways, and their interplay with fisheries, in the Northern Humboldt Current System. Prog. Oceanogr. 164, 28–36. doi: 10.1016/j.pocean.2018.04.009
Choy, C. A., Haddock, S. H. D., and Robison, B. H. (2017). Deep pelagic food web structure as revealed by in situ feeding observations. Proc. Biol. Sci. 284:20172116. doi: 10.1098/rspb.2017.2116
Clarke, A., Holmes, L. J., and Gore, D. J. (1992). Proximate and elemental composition of gelatinous zooplankton from the Southern Ocean. J. Exp. Mar. Biol. Ecol. 155, 55–68. doi: 10.1016/0022-0981(92)90027-8
Cleland, W. W. (1967). Enzyme kinetics. Annu. Rev. Biochem. 36, 77–112. doi: 10.1017/cbo9780511608438.007
Condon, R. H., Steinberg, D. K., del Giorgio, P. A., Bouvier, T. C., Bronk, D. A., Graham, W. M., et al. (2011). Jellyfish blooms result in a major microbial respiratory sink of carbon in marine systems. Proc. Natl. Acad. Sci. U.S.A. 108, 10225–10230. doi: 10.1073/pnas.1015782108
Costello, J. H., Colin, S. P., Dabiri, J. O., Gemmell, B. J., Lucas, K. N., and Sutherland, K. R. (2021). The hydrodynamics of jellyfish swimming. Ann. Rev. Mar. Sci. 13, 375–396. doi: 10.1146/annurev-marine-031120-091442
Costello, J. H., Colin, S. P., Gemmell, B. J., Dabiri, J. O., and Sutherland, K. R. (2015). Multi-jet propulsion organized by clonal development in a colonial Siphonophore. Nat. Commun. 6:8158. doi: 10.1038/ncomms9158
Cowen, R. (1996). “Locomotion and respiration in aquatic air-breathing vertebrates,” in Evolutionary Paleobiology, eds D. Jablonski, D. H. Erwin, and J. H. Lipps (Chicago, IL: University of Chicago Press), 337–354.
De Barba, F. F. M., Bazi, C. C., Pessatti, M. L., and Resgalla, C. (2016). Macromedusae of Southern Brazil: temporal variation, population structure and biochemical composition. Braz. J. Oceanogr. 64, 127–136. doi: 10.1590/S1679-87592016101806402
De Coen, W. M., and Janssen, C. R. (1997). The use of biomarkers in Daphnia magna toxicity testing. IV. Cellular energy allocation: a new methodology to assess the energy budget of toxicant-stressed Daphnia populations. J. Aquat. Ecosyst. Stress Recovery 6, 43–55.
Doyle, T. K., Houghton, J. D. R., McDevitt, R., Davenport, J., and Hays, G. C. (2007). The energy density of jellyfish: estimates from bomb-calorimetry and proximate-composition. J. Exp. Mar. Biol. Ecol. 343, 239–252. doi: 10.1016/j.jembe.2006.12.010
Duarte, I., Leandro, S., Ferreira, M., Pinto, H., Falcão, J., Reis, J., et al. (2019). “Early development of Velella velella medusae in laboratory,” in Poster Presented at the IMMR’18 — International Meeting on Marine Research 2018 Front. Mar. Sci., Vol. 5, Peniche. doi: 10.3389/conf.FMARS.2018.06.00080
Dubischar, C. D., Pakhomov, E. A., and Bathmann, U. V. (2006). The tunicate Salpa thompsoni ecology in the Southern Ocean. II. Proximate and elemental composition. Mar. Biol. 149, 625–632. doi: 10.1007/s00227-005-0226-8
Dubois, M., Gilles, K., Hamilton, J. K., Rebers, P. A., and Smith, F. (1956). A colorimetric method for the determination of sugars. Nature 168, 350–356. doi: 10.1038/168167a0
Egger, M., Quiros, L., Leone, G., Ferrari, F., Boerger, C. M., and Tishler, M. (2021). Relative abundance of floating plastic debris and neuston in the Eastern North Pacific Ocean. Front. Mar. Sci. 8:626026. doi: 10.3389/fmars.2021.626026
Ferguson, S. J. (2010). ATP synthase: from sequence to ring size to the P/O ratio. Proc. Natl. Acad. Sci. U.S.A. 107, 16755–16756. doi: 10.1073/pnas.1012260107
Ferrer, L., and González, M. (2021). Relationship between dimorphism and drift in the Portuguese man-of-war. Cont. Shelf Res. 212:104269. doi: 10.1016/j.csr.2020.104269
Feynman, R. P., Leighton, R. B., and Sands, M. (1970). The Feynman Lectures on Physics, 5th Printing. (1969a). The Feynman Lectures on Physics, Vol. 1, Reading, MA: Addison-Wesley.
Fierro, P., Arriagada, L., Piñones, A., and Araya, J. F. (2021). New insights into the abundance and seasonal distribution of the Portuguese man-of-war Physalia physalis (Cnidaria: Siphonophorae) in the southeastern Pacific. Reg. Stud. Mar. Sci. 41:101557. doi: 10.1016/j.rsma.2020.101557
Filella, A., Baños, I., Montero, M. F., Hernández-Hernández, N., Rodríguez-Santos, A., Ludwig, A., et al. (2018). Plankton community respiration and ETS activity under variable CO2 and nutrient fertilization during a mesocosm study in the subtropical North Atlantic. Front. Mar. Sci. 5:310. doi: 10.3389/fmars.2018.00310
Frick, M. G., Williams, K. L., Bolten, A. B., Bjorndal, K. A., and Martins, H. R. (2009). Foraging ecology of oceanic-stage loggerhead turtles Caretta caretta. Endanger. Species Res. 9, 91–97. doi: 10.3354/esr00227
Fu, Z., and Uye, S.-I. (2021). Starvation of the respiratory metabolism and locomotion of Aurelia aurita s.l. ephyrae. Open J. Mar. Sci. 11, 1–16. doi: 10.4236/ojms.2021.111001
Gainey, R. L. (1972). The Chemical Analysis of Velella lata Float. M.Sc. thesis. Stockton, CA: University of the Pacific.
Games, P. A., and Howell, J. F. (1976). Pairwise multiple comparison procedures with unequal N’s and/or variances: a Monte Carlo study. J. Educ. Stat. 1, 113–125. doi: 10.3102/10769986001002113
Gemmell, B. J., Costello, J. H., and Colin, S. P. (2014). Exploring vortex enhancement and manipulation mechanisms in jellyfish that contributes to energetically efficient propulsion. Commun. Integr. Biol. 7:e29014. doi: 10.4161/cib.29014
Gemmell, B. J., Costello, J. H., Colin, S. P., Stewart, C. J., Dabiri, J. O., Tafti, D., et al. (2013). Passive energy recapture in jellyfish contributes to propulsive advantage over other metazoans. Proc. Natl. Acad. Sci. U.S.A. 110, 17904–17909. doi: 10.1073/pnas.1306983110
Gemmell, B. J., Du Clos, K. T., Colin, S. P., Sutherland, K. R., and Costello, J. H. (2020). The most efficient metazoan swimmer creates a ‘virtual wall’ to enhance performance. Anim. Behav. Cogn. 288:20202494. doi: 10.1101/2020.05.01.069518
Gnaiger, E. (2001). Bioenergetics at low oxygen: dependence of respiration and phosphorylation on oxygen and adenosine diphosphate supply. Respir. Physiol. 128, 277–297. doi: 10.1016/S0034-5687(01)00307-3
Gómez, M., Torres, S., and Hernzández-León, S. (1996). Modification of the electron transport system (ETS) method for routine measurements of respiratory rates of zooplankton. South Afr. J. Mar. Sci. 17, 15–20. doi: 10.2989/025776196784158446
Griffin, D. C., Harrod, C., Houghton, J. D. R., and Capellini, I. (2019). Unravelling the macro-evolutionary ecology of fish-jellyfish associations: life in the “gingerbread house.” Proc. Biol. Sci. 286, 1–9. doi: 10.1098/rspb.2018.2325
Gross, J., Dumsha, B., and Glazer, N. (1958). Comparative biochemistry of collagen some amino acids and carbohydrates. BBA Biochim. Biophys. Acta 30, 293–297. doi: 10.1016/0006-3002(58)90053-2
Guglielmo, L., Granata, A., and Guglielmo, R. (2015). Orden euphausiacea. Ibero Divers. Etimol. 86A, 1–20.
Han, C., Chae, J., Jin, J., and Yoon, W. (2012). Estimation of the minimum food requirement using the respiration rate of medusa of Aurelia aurita in Sihwa Lake. Ocean Sci. J. 47, 155–160. doi: 10.1007/s12601-012-0015-5
Hansson, L. J., Moeslund, O., Kiørboe, T., and Riisgård, U. (2005). Clearance rates of jellyfish and their potential predation impact on zooplankton and fish larvae in a neritic ecosystem (Limfjorden, Denmark). Mar. Ecol. Prog. Ser. 304, 117–131. doi: 10.3354/meps304117
Harris, R., Nishiyama, T., and Paul, J. (1986). Carbon, nitrogen and caloric content of eggs, larvae, and juveniles of the walleye pollock, Theragra chalcogramma. J. Fish Biol 29, 87–98. doi: 10.1111/j.1095-8649.1986.tb04928.x
Hays, G. C., Doyle, T. K., and Houghton, J. D. R. (2018). A paradigm shift in the trophic importance of jellyfish? Trends Ecol. Evol. 33, 874–884. doi: 10.1016/j.tree.2018.09.001
Headlam, J. L., Lyons, K., Kenny, J., Lenihan, E. S., Quigley, D. T. G., Helps, W., et al. (2020). Insights on the origin and drift trajectories of Portuguese man of war (Physalia physalis) over the Celtic Sea shelf area. Estuar. Coast. Shelf Sci. 246:107033. doi: 10.1016/j.ecss.2020.107033
Helm, R. R. (2021). The mysterious ecosystem at the ocean’s surface. PLoS Biol. 19:e3001046. doi: 10.1371/journal.pbio.3001046
Hernández-León, S., and Gómez, M. (1996). Factors affecting the respiration/ETS ratio in marine zooplankton. J. Plankton Res. 18, 239–255. doi: 10.1093/plankt/18.2.239
Hoover, A., and Miller, L. (2015). A numerical study of the benefits of driving jellyfish bells at their natural frequency. J. Theor. Biol. 374, 13–25. doi: 10.1016/j.jtbi.2015.03.016
Iguchi, N., Iwatani, H., Sugimoto, K., Kitajima, S., Honda, N., and Katoh, O. (2017). Biomass, body elemental composition, and carbon requirement of Nemopilema nomurai (Scyphozoa: Rhizostomeae) in the southwestern Japan Sea. Plankton Benthos Res. 12, 104–114. doi: 10.3800/pbr.12.104
Ikeda, T. (1972). “Chemical composition and nutrition of zooplankton in the Bering Sea,” in Biological Oceanography of the Northern North Pacific Ocean. Idemitsu Shoten, Tokyo, ed. A. Y. Takenouti (Tokyo: Idemitsu Shoten), 433–442.
Ikeda, T. (2014). Synthesis toward a global model of metabolism and chemical composition of medusae and ctenophores. J. Exp. Mar. Biol. Ecol. 456, 50–64. doi: 10.1016/j.jembe.2014.03.006
Ishii, H., and Tanaka, F. (2006). Respiration rates and metabolic demands of Aurelia aurita in Tokyo Bay with special reference to large medusae. Plankton Benthos Res. 1, 64–67. doi: 10.3800/pbr.1.64
Jenkins, R. L. (1983). Observations on the commensal relationship of Nomeus gronovii with Physalia physalis. Am. Soc. Ichthyol. Herpetol. 1983, 250–252.
Jones, T., Parrish, J., and Burgess, H. (2021). Long-term patterns of mass stranding of the colonial cnidarian Velella velella: influence of environmental forcing. Mar. Ecol. Prog. Ser. 662, 69–83. doi: 10.3354/meps13644
Joseph, J. D. (1979). Lipid composition of marine and estuarine invertebrates: porifera and cnidaria. Prog. Lipid Res. 18, 1–30. doi: 10.1016/0163-7827(79)90002-X
Karl, D. M. (2014). Solar energy capture and transformation in the sea. Elem. Sci. Anthr. 2, 1–6. doi: 10.12952/journal.elementa.000021
Kemp, P. F. (1986). Deposition of organic matter on a high-energy sand beach by a mass stranding of the cnidarian Velella velella (L.). Estuar. Coast. Shelf Sci. 23, 575–579. doi: 10.1016/0272-7714(86)90010-7
Khong, N. M. H., Yusoff, F. M., Jamilah, B., Basri, M., Maznah, I., Chan, K. W., et al. (2016). Nutritional composition and total collagen content of three commercially important edible jellyfish. Food Chem. 196, 953–960. doi: 10.1016/j.foodchem.2015.09.094
King, F. D., and Packard, T. T. (1975). Respiration and the activity of the respiratory electron transport system in marine zooplankton. Limnol. Oceanogr. 20, 849–854. doi: 10.4319/lo.1975.20.5.0849
King, F. D., Devol, A. H., and Packard, T. T. (1978). Plankton metabolic activity in the eastern tropical North Pacific. Deep. Sea Res 25, 689–704. doi: 10.1016/0146-6291(78)90624-0
Kirkpatrick, P. A., and Pugh, P. R. (1984). “Siphonophores and Velellids: keys and notes for the identification of the species,” in Synopsis of the British Fauna, eds D. M. Ker-mack and R. S. K. Barnes (Great Britain: Pitman Press), 1–155.
Knight, J. A., Anderson, S., and Rawle, J. M. (1972). Chemical basis of the sulfo-phospho-vanillin reaction for estimating total serum lipids. Clin. Chem. 18, 199–202.
Lampert, K. P. (2016). “Cassiopea and its zooxanthellae,” in The Cnidaria, past, present and future, eds S. Goffredo and Z. Dubinsky (Cham: Springer), 415–423. doi: 10.1007/978-3-319-31305-4_26
Lane, C. E., Pringle, E., and Bergere, A. M. (1965). Amino acids in extracellular fluids of Physalia physalis and Aurelia aurita. Comp. Biochem. Physiol. 15, 259–262. doi: 10.1016/0010-406x(65)90350-6
Lane, N. (2015). The Vital Question: Energy, Evolution, and the Origins of Complex Life. New York, NY: WW Norton & Company.
Larimer, J. L., and Ashby, E. A. (1962). Float gases, gas secretion and tissue respiration in the Portuguese man-of-war, Physalia. J. Cell. Comp. Physiol. 60, 41–47. doi: 10.1002/jcp.1030600106
Larson, R. J. (1987). Respiration and carbon turnover rates of Medusae from the NE pacific. Comp. Biochem. Physiol. Part A Physiol. 87, 93–100. doi: 10.1016/0300-9629(87)90430-0
Larson, R. J., and Harbison, G. R. (1989). Source and fate of lipids in polar gelatinous zooplankton. Arctic 42, 339–346. doi: 10.14430/arctic1675
Lee, D., Schaeffer, A., and Groeskamp, S. (2021). Drifting dynamics of the bluebottle (Physalia physalis). Ocean Sci. 17, 1341–1351. doi: 10.5194/os-17-1341-2021
Lee, S., and Lee, D. K. (2018). What is the proper way to apply the multiple comparison test? Korean J. Anesthesiol. 71, 353–360. doi: 10.4097/kja.d.18.00242
Lilley, M. K. S., and Lombard, F. (2015). Respiration of fragile planktonic zooplankton: extending the possibilities with a single method. J. Exp. Mar. Biol. Ecol. 471, 226–231. doi: 10.1016/j.jembe.2015.06.013
Lilley, M. K. S., Elineau, A., Ferraris, M., Thiéry, A., Stemmann, L., Gorsky, G., et al. (2014). Individual shrinking to enhance population survival: quantifying the reproductive and metabolic expenditures of a starving jellyfish, Pelagia noctiluca. J. Plankton Res. 36, 1585–1597. doi: 10.1093/plankt/fbu079
Lindley, J. A. (1999). Dry weight carbon and nitrogen content of some Euphausiids from the North Atlantic Ocean and the Celtic Sea. J. Plankton Res. 21, 2053–2066. doi: 10.1093/plankt/21.11.2053
Lopes, A. R., Baptista, M., Rosa, I. C., Dionísio, G., Gomes-Pereira, J., Paula, J. R., et al. (2016). “Gone with the wind”: fatty acid biomarkers and chemotaxonomy of stranded pleustonic hydrozoans (Velella velella and Physalia physalis). Biochem. Syst. Ecol. 66, 297–306. doi: 10.1016/j.bse.2016.03.016
Lowry, O. H., Rosebrough, N. J., Farr, A. L., and Randall, R. J. (1951). Protein measurement with the folin phenol reagent. J. Biol. Chem. 193, 265–275. doi: 10.1016/s0021-9258(19)52451-6
Lucas, C. H. (1994). Biochemical composition of Aurelia aurita in relation to age and sexual maturity. J. Exp. Mar. Biol. Ecol. 183, 179–192. doi: 10.1016/0022-0981(94)90086-8
Lucas, C. H. (2009). Biochemical composition of the mesopelagic coronate jellyfish Periphylla periphylla from the Gulf of Mexico. J. Mar. Biol. Assoc. U. K. 89, 77–81. doi: 10.1017/S0025315408002804
Lucas, C. H., Pitt, K. A., Purcell, J. E., Lebrato, M., and Condon, R. H. (2011). What’s in a jellyfish? Proximate and elemental composition and biometric relationships for use in biogeochemical studies. Ecology 92:1704. doi: 10.1890/11-0302.1
Macías, D., Prieto, L., and García-Gorriz, E. (2021). A model-based management tool to predict the spread of Physalia physalis in the Mediterranean Sea. Minimizing risks for coastal activities. Ocean Coast. Manag. 212:105810. doi: 10.1016/j.ocecoaman.2021.105810
Maldonado, F., Packard, T. T., and Gómez, M. (2012). Understanding tetrazolium reduction and the importance of substrates in measuring respiratory electron transport activity. J. Exp. Mar. Biol. Ecol. 434–435, 110–118. doi: 10.1016/j.jembe.2012.08.010
Malej, A. (1989a). Behaviour and trophic ecology of the jellyfish Pelagia noctiluca (Forsskål, 1775). J. Exp. Mar. Biol. Ecol. 126, 259–270. doi: 10.1016/0022-0981(89)90191-3
Malej, A. (1989b). “Respiration and excretion rates of Pelagia noctiluca (Semaeostomeae, Scyphozoa),” in Proceedings of the 21st EMBS Polish Academy of Sciences, (Gdansk: Polish Academy of Sciences, Institute of Oceanology), 107–113.
Malej, A., Faganeli, J., and Pezdič, J. (1993). Stable isotope and biochemical fractionation in the marine pelagic food chain: the jellyfish Pelagia noctiluca and net zooplankton. Mar. Biol. Int. J. Life Ocean. Coast. Waters 116, 565–570. doi: 10.1007/BF00355475
Markwell, M. A. K., Haas, S. M., Bieber, L. L., and Tolbert, N. E. (1978). A modification of the Lowry procedure to simplify protein determination in membrane and lipoprotein samples. Anal. Biochem. 87, 206–210. doi: 10.1016/0003-2697(78)90586-9
Marsh, J. B., and Weinstein, D. B. (1966). Simple charring method for determination of lipids. J. Lipid Res. 7, 574–576. doi: 10.1016/s0022-2275(20)39274-9
Martínez, I., Herrera, A., Tames-espinosa, M., Bondyale-juez, D. R., Romero-kutzner, V., Packard, T. T., et al. (2020). Protein in marine plankton: a comparison of spectrophotometric methods. J. Exp. Mar. Biol. Ecol. 526:151357. doi: 10.1016/j.jembe.2020.151357
Microsoft-Corporation (2016). Microsoft Excel. Available online at: https://office.microsoft.com/excel (accessed January 22, 2018).
Mills, C. E. (1995). Medusae, siphonophores, and ctenophores as planktivorous predators in changing global ecosystems Introduction Observations from blue-water diving and. ICES J. Mar. Sci 52, 575–581. doi: 10.1016/1054-3139(95)80072-7
Møller, L. F., and Riisgård, H. U. (2007). Respiration in the scyphozoan jellyfish Aurelia aurita and two hydromedusae (Sarsia tubulosa and Aequorea vitrina): effect of size, temperature and growth. Mar. Ecol. Prog. Ser. 330, 149–154. doi: 10.3354/meps330149
Moran, L. A., Horton, H. R., Scrimgeour, K. G., and Perry, M. D. (2012). Principles of Biochemistry. Boston, MA: Pearson.
Morand, P., Carré, C., and Biggs, D. C. (1987). Feeding and metabolism of the jellyfish Pelagia noctiluca (scyphomedusae, semaeostomae). J. Plankton Res. 9, 651–665. doi: 10.1093/plankt/9.4.651
Munro, C., Vue, Z., Behringer, R. R., and Dunn, C. W. (2019). Morphology and development of the Portuguese man of war, Physalia physalis. Sci. Rep. 9:15522. doi: 10.1038/s41598-019-51842-1
Musayeva, E. I., and Sokolova, I. A. (1979). Caloricity (caloric value) of planktonic animals from the Pacific Ocean. Oceanology 19, 90–92.
Nagata, R. M., and Morandini, A. C. (2018). Diet, prey selection, and individual feeding rates of the jellyfish Lychnorhiza lucerna (Scyphozoa, Rhizostomeae). Mar. Biol. 165, 1–17. doi: 10.1007/s00227-018-3445-5
Nival, P., Nival, S., and Palazzoli, I. (1972). Données sur la respiration de différents organismes communs dans le plancton de Villefranche-sur-Mer. Mar. Biol. 17, 63–76. doi: 10.1007/BF00346954
Ochoa, S. (1943). Efficiency of aerobic phosphorylation in cell-free heart extracts. J. Biol. Chem. 151, 493–505.
Owens, T. G., and King, F. D. (1975). The measurement of respiratory electron-transport-system activity in marine zooplankton. Mar. Biol. 30, 27–36. doi: 10.1007/BF00393750
Packard, T. T. (1971). The measurement of respiratory electron-transport activity in marine phytoplankton. J. Mar. Res. 29, 235–244.
Packard, T. T. (1985). “Measurement of electron transport activity of marine microplankton,” in Advances in Aquatic Microbiology, eds P. J. Williams and H. W. Jannasch (New York, NY: Academic Press), 207–261.
Packard, T. T., and Christensen, J. P. (2004). Respiration and vertical carbon flux in the Gulf of Maine water column. J. Mar. Res. 62, 93–115. doi: 10.1357/00222400460744636
Packard, T. T., and Williams, P. J. (1981). Rates of respiratory oxygen consumption and electron transport in surface seawater from the Northwest Atlantic. Oceanol. Acta 4, 351–358.
Packard, T. T., Harmon, D., and Boucher, J. (1974). Respiratory electron transport activity in plankton from upwelled waters. Tethys 6, 213–222.
Packard, T. T., Osma, N., Fernández-Urruzola, I., Codispoti, L. A., Christensen, J. P., and Gómez, M. (2015). Peruvian upwelling plankton respiration: calculations of carbon flux, nutrient retention efficiency, and heterotrophic energy production. Biogeosciences 12, 2641–2654. doi: 10.5194/bg-12-2641-2015
Pauly, D., Graham, W., Libralato, S., Morissette, L., and Deng Palomares, M. L. (2009). Jellyfish in ecosystems, online databases, and ecosystem models. Hydrobiologia 616, 67–85. doi: 10.1007/s10750-008-9583-x
Percy, J. A., and Fife, F. J. (1981). The biochemical composition and energy content of arctic marine Macrozooplankton. Arctic 34, 307–313. doi: 10.14430/arctic2533
Pereira, R., Teodósio, M. A., and Garrido, S. (2014). An experimental study of Aurelia aurita feeding behaviour: inference of the potential predation impact on a temperate estuarine nursery area. Estuar. Coast. Shelf Sci. 146, 102–110. doi: 10.1016/j.ecss.2014.05.026
Phillips, N., Eagling, L., Harrod, C., Reid, N., Cappanera, V., and Houghton, J. (2017). Quacks snack on smacks: mallard ducks (Anas platyrhynchos) observed feeding on hydrozoans (Velella velella). Plankton Benthos Res. 12, 143–144. doi: 10.3800/pbr.12.143
Pickwell, G. V. (1966). Physiological Dynamics of Siphonophores from Deep Scattering Layers: Size of Gas-Filled Floats and Rate of Gas Production. San Diego, CA: US Navy Electronics Laboratory.
Purcell, J. E. (1984). Predation on fish larvae by Physalia physalis, the Portuguese man of. Mar. Ecol. Prog. Ser. 19, 189–191.
Purcell, J. E. (1997). Pelagic cnidarians and ctenophores as predators : selective predation, feeding rates, and effects on prey populations. Ann. l’Inst. océanogr. 73, 125–137.
Purcell, J. E. (2003). Predation on zooplankton by large jellyfish, Aurelia labiata, Cyanea capillata and Aequorea aequorea, in Prince William Sound, Alaska. Mar. Ecol. Prog. Ser. 246, 137–152.
Purcell, J. E., and Arai, M. N. (2001). Interactions of pelagic cnidarians and ctenophores with fish: a review. Hydrobiologia 451, 27–44.
Purcell, J. E., and Kremer, P. (1983). Feeding and metabolism of the siphonophore Sphaeronectes gracilis. J. Plankton Res. 5, 95–106. doi: 10.1093/plankt/5.1.95
Purcell, J. E., Bondyale-Juez, D. R., Romero-Kutzner, V., Martínez, I., Caprioli, R., Tames-Espinosa, M., et al. (2019). Food supply effects on the asexual reproduction and respiratory metabolism of Aurelia aurita polyps. Hydrobiologia 846, 135–146. doi: 10.1007/s10750-019-04057-4
Purcell, J. E., Clarkin, E., and Doyle, T. K. (2012). Foods of Velella velella (Cnidaria: Hydrozoa) in algal rafts and its distribution in Irish seas. Hydrobiologia 690, 47–55. doi: 10.1007/s10750-012-1052-x
Purcell, J. E., Fuentes, V., Atienza, D., Tilves, U., Astorga, D., Kawahara, M., et al. (2010). Use of respiration rates of scyphozoan jellyfish to estimate their effects on the food web. Hydrobiologia 645, 135–152. doi: 10.1007/s10750-010-0240-9
Purcell, J. E., Milisenda, G., Rizzo, A., Carrion, S. A., Zampardi, S., Airoldi, S., et al. (2015). Digestion and predation rates of zooplankton by the pleustonic hydrozoan Velella velella and widespread blooms in 2013 and 2014. J. Plankton Res. 37, 1056–1067. doi: 10.1093/plankt/fbv031
Purcell, J. E., Uye, S. I., and Lo, W. T. (2007). Anthropogenic causes of jellyfish blooms and their direct consequences for humans: a review. Mar. Ecol. Prog. Ser. 350, 153–174. doi: 10.3354/meps07093
R Core Team (2017). R: A Language and Environment for Statistical Com-puting. Vienna: R Foundation for Statistical Computing.
Raskoff, K., Sommer, F., Hamner, W., and Cross, K. (2003). Collection and culture techniques for gelatinous zooplankton. Biol. Bull. 204, 68–80. doi: 10.2307/1543497
Richardson, A. J., Bakun, A., Hays, G. C., and Gibbons, M. J. (2009). The jellyfish joyride: causes, consequences and management responses to a more gelatinous future. Trends Ecol. Evol. 24, 312–322. doi: 10.1016/j.tree.2009.01.010
Rutter, W. J. (1967). “Protein determinations in embryos,” in Methods in Developmental Biology, eds F. H. Wilt and N. K. Wessels (New York, NY: Academic Press), 671–683.
Ruzicka, J., Brodeur, R. D., Cieciel, K., and Decker, M. B. (2020). Examining the ecological role of jellyfish in the Eastern Bering Sea. ICES J. Mar. Sci. 77, 791–802. doi: 10.1093/icesjms/fsz244
Schiariti, A., Dutto, M. S., Carrara Morandini, A., Nagata, R. M., Pereyra, D. Y., Puente Tapia, F. A., et al. (2018). “An overview of the Medusozoa from the Southwestern Atlantic,” in Plankton Ecology of the Southwestern Atlantic, eds M. S. Hoffmeyer, M. E. Sabatini, F. P. Brandini, D. L. Calliari, and N. H. Santinelli (Gewerbestrasse: Springer Nature), 413–449. doi: 10.1007/978-3-319-77869-3
Schmidt, S. (2009). Shall we really do it again? The powerful concept of replication is neglected in the social sciences. Rev. Gen. Psychol. 13, 90–100. doi: 10.1037/a0015108
Schneider, G. (1989). Estimation of food demands of Aurelia aurita medusae populations in the Kiel bight/western Baltic. Ophelia 31, 17–27. doi: 10.1080/00785326.1989.10430848
Schneider, G., and Behrends, G. (1998). Top-down control in a neritic plankton system by Aurelia aurita medusae — a summary. Ophelia 48, 71–82. doi: 10.1080/00785236.1998.10428677
Schuchert, P. (2010). The European athecate hydroids and their medusae (Hydrozoa, Cnidaria): capitata Part 2. Rev. Suisse Zool. 117, 337–555. doi: 10.5962/bhl.part.117793
Shimauchi, H., and Uye, S. I. (2007). Excretion and respiration rates of the scyphomedusa Aurelia aurita from the Inland Sea of Japan. J. Oceanogr. 63, 27–34. doi: 10.1007/s10872-007-0003-z
Stenvers, V., Chi, X., and Javidpour, J. (2020). Seasonal variability of the fatty acid composition in Aurelia aurita (Cnidaria: Scyphozoa): implications for gelativore food web studies. J. Plankton Res. 00, 1–13. doi: 10.1093/plankt/fbaa026
Stillway, L. W. (1974). Nematocyst lipids of the Portuguese man-of-war Physalia physalis. Comp. Biochem. Physiol. Part B Biochem. 48, 35–38. doi: 10.1016/0305-0491(74)90039-X
Stillway, L. W. (1976). Fatty acids of the portuguese man-of-war Physalia physalis. Comp Biochem Physiol. B 53, 535–537.
Tamkun, M. M., and Hessinger, D. A. (1981). Isolation and partial characterization of a hemolytic and toxic protein from the nematocyst venom of the Portuguese man-of-war, Physalia physalis. Biochim. Biophys. Acta (BBA) Protein Struct. 667, 87–98. doi: 10.1016/0005-2795(81)90069-6
Thiebot, J. B., and McInnes, J. C. (2020). Why do marine endotherms eat gelatinous prey? ICES J. Mar. Sci. 77, 58–71. doi: 10.1093/icesjms/fsz208
Thuesen, E. V., and Childress, J. J. (1994). Oxygen consumption rates and metabolic enzyme activities of oceanic California Medusae in relation to body size and habitat depth. Biol. Bull. 187, 84–98. doi: 10.2307/1542168
Tilves, U., Purcell, J. E., Fuentes, V. L., Torrents, A., Pascual, M., Raya, V., et al. (2016). Natural diet and predation impacts of Pelagia noctiluca on fish eggs and larvae in the NW Mediterranean. J. Plankton Res. 38, 1243–1254. doi: 10.1093/plankt/fbw059
Uye, S., and Shimauchi, H. (2005). Population biomass, feeding, respiration and growth rates, and carbon budget of the scyphomedusa Aurelia aurita in the Inland Sea of Japan. J. Plankton Res. 27, 237–248. doi: 10.1093/plankt/fbh172
Walve, J., and Larsson, U. (1999). Carbon, nitrogen and phosphorus stoichiometry of crustacean zooplankton in the Baltic Sea: implications for nutrient recycling. J. Plankton Res. 21, 2309–2321. doi: 10.1093/plankt/21.12.2309
Webb, P. W. (1971). The swimming energetics of trout. II. Oxygen consumption and swimming efficiency. J. Exp. Biol. 55, 521–540. doi: 10.1242/jeb.55.2.521
Williams, D. R. (2018). Sun Fact Sheet. Nasa Off. Available online at: https://nssdc.gsfc.nasa.gov/planetary/factsheet/sunfact.html (accessed March 23, 2018).
Wissing, T. E., Darnell, R. M., Ibrahim, M. A., and Berner, L. (1973). Caloric values of marine animals from Gulf of Mexico. Contrib. Mar. Sci. 17, 1–7.
Wright, R. M., Le Quéré, C., Buitenhuis, E., Pitois, S., and Gibbons, M. J. (2021). Role of jellyfish in the plankton ecosystem revealed using a global ocean biogeochemical model. Biogeosciences 18, 1291–1320. doi: 10.5194/bg-18-1291-2021
Zagalsky, P. F., and Herring, P. J. (1977). Studies on the blue astaxanthin-proteins of Velella velella (Coelenterata: Chondrophora). Philos. Trans. R. Soc. Lond. B Biol. Sci. 279, 289–326. doi: 10.1098/rstb.1977.0092
Zeman, S. M., Corrales-Ugalde, M., Brodeur, R. D., and Sutherland, K. R. (2018). Trophic ecology of the neustonic cnidarian Velella velella in the northern California Current during an extensive bloom year: insights from gut contents and stable isotope analysis. Mar. Biol. 165, 1–13. doi: 10.1007/s00227-018-3404-1
Keywords: hydrozoa, scyphozoa, neuston, oxygen consumption, ETS, respiration, jellyfish locomotion
Citation: Bondyale-Juez DR, Romero-Kutzner V, Purcell JE, Martínez I, Packard TT and Gómez M (2022) Wind Drifting vs. Pulsating Swimming Jellyfish: Respiratory Metabolism and Composition Differences in Physalis physalis, Velella velella, Aurelia aurita, and Pelagia noctiluca. Front. Mar. Sci. 9:817196. doi: 10.3389/fmars.2022.817196
Received: 17 November 2021; Accepted: 16 February 2022;
Published: 10 March 2022.
Edited by:
Anna Di Cosmo, University of Naples Federico II, ItalyReviewed by:
Elisa Costa, Institute for the Study of Anthropogenic Impacts and Sustainability in Marine Environment (CNR), ItalyNelly Tremblay, Fisheries and Oceans Canada, Canada
Cathy Lucas, University of Southampton, United Kingdom
Copyright © 2022 Bondyale-Juez, Romero-Kutzner, Purcell, Martínez, Packard and Gómez. This is an open-access article distributed under the terms of the Creative Commons Attribution License (CC BY). The use, distribution or reproduction in other forums is permitted, provided the original author(s) and the copyright owner(s) are credited and that the original publication in this journal is cited, in accordance with accepted academic practice. No use, distribution or reproduction is permitted which does not comply with these terms.
*Correspondence: Daniel R. Bondyale-Juez, ZGFuaWVsLmJvbmR5YWxlQHVscGdjLmVz