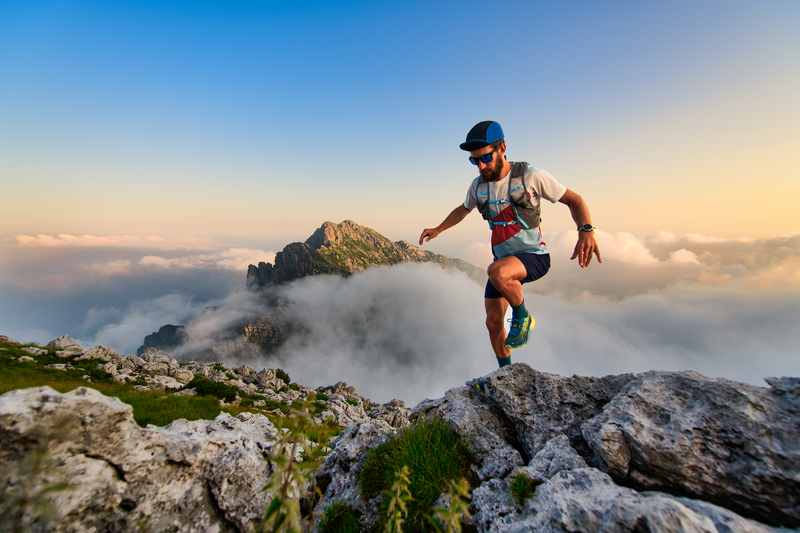
95% of researchers rate our articles as excellent or good
Learn more about the work of our research integrity team to safeguard the quality of each article we publish.
Find out more
ORIGINAL RESEARCH article
Front. Mar. Sci. , 03 June 2022
Sec. Coral Reef Research
Volume 9 - 2022 | https://doi.org/10.3389/fmars.2022.812989
A correction has been applied to this article in:
Corrigendum: Cleaner fish Labroides dimidiatus presence does not indirectly affect demersal zooplankton
Coral reef mutualisms involve complex trophic ecological relationships that produce indirect effects. Excluding mutualistic cleaner fish Labroides dimidiatus from reefs indirectly increases the abundance of many fishes and reduces demersal stages of their ectoparasitic prey (gnathiid isopods). Whether cleaners affect populations of planktivorous fishes that consume demersal zooplankton, and consequently indirectly affect the rest of the demersal zooplankton community — via presumed changes in planktivory — is unknown. Therefore, using a long-term cleaner fish manipulation on patch reefs (July 2000 to December 2012, Great Barrier Reef), we tested whether cleaner treatment (removal or control) affects planktivorous fish abundance and diversity, and demersal zooplankton biomass, abundance, and diversity. Fish surveys, 9 and 12 years after removing cleaners revealed fewer fish on removal compared to control reefs for one of the three most abundant planktivores, but not total abundance (Pomacentridae, 26 species), diversity, and composition. Emerging zooplankton were sampled during the day and night over nine sampling trips across 12 years. There was no effect of cleaner treatment on post-removal values, compared with pre-removal values in July 2000, for zooplankton biomass, abundance, diversity, and composition (34 taxa). Zooplankton abundance showed no diel differences, but diversity, and occasionally biomass, were higher at night. Zooplankton composition also showed diel differences, with three taxa contributing the most to this dissimilarity [Cirripeda nauplii, Facetotecta (Arthopoda), Oikopleuridae (Chordata)]. Zooplankton diversity did not differ among times, abundance was higher in January 2002 relative to July 2000, and composition differed among all times. The lack of detectable indirect effects of cleaner fish presence on zooplankton (non-gnathiid) may partly be due to cleaners’ variable effect on planktivorous fish abundance, but also the result of invertebrate planktivory and other processes that affect zooplankton populations not investigated here. Nevertheless, the pronounced diel and temporal changes in zooplankton observed likely influence coral reef trophic interactions.
Zooplankton are the intermediate trophic level between phytoplankton and higher trophic levels, and they are a food source for a plethora of invertebrate and vertebrate animals (Lewis, 1992; Anthony and Blumstein, 2000). Revealing the linkages between successive trophic levels and the processes involved is essential to understanding the resilience of such food webs. Many fishes, from small fish larvae to large whale sharks and manta rays rely heavily on zooplankton (Hobson, 1975; Hobson and Chess, 1978; Hobson, 1991; Randall et al., 1997; Marnane and Bellwood, 2002; Mann and Sancho, 2007; Couturier et al., 2013; Rohner et al., 2013; Bellwood et al., 2019). Fish predation on zooplankton can reduce zooplankton biomass and prey selectivity can change zooplankton community diversity (O'Brien, 1979; Cury et al., 2000; Maszczyk and Gliwicz, 2014). Mortality in zooplankton corresponds with the known spatial distribution of planktivorous fishes (Acosta and Butler, 1999; Motro et al., 2005). On coral reefs, planktivorous fish can deplete most of the incoming holoplankton (i.e., species that are always floating in the water column) and demersal zooplankton (i.e. species located in or near the substrate that migrate into the water column to feed at different times of the day, usually at night, Ohlhorst, 1982; Hamner et al., 1988; Motro et al., 2005). Therefore, factors that affect the abundance of planktivorous fishes could also indirectly influence the zooplankton community.
The presence of the mutualistic Indo-Pacific cleaner fish Labroides dimidiatus on coral reefs positively affects the abundance of many client fishes, which includes planktivores that consume zooplankton (Bshary, 2003; Grutter et al., 2003; Adam, 2011; Waldie et al., 2011; Adam, 2012; Grutter, 2012; Sun et al., 2015; Wagner et al., 2015; Grutter et al., 2020a). Labroides dimidiatus removes and then consumes ectoparasites, mostly the parasitic stage of blood-sucking gnathiid isopods, but also other isopods, platyhelminths, and copepods infecting cooperating fishes known as clients (Grutter, 1997a). Cleaner fish provide important services such as directly reducing gnathiid parasites (Grutter, 1999) and stress levels, via tactile stimulation (Soares et al., 2011), in individual fish. While cleaner fish are not abundant (Waldie et al., 2011), their effects on fish communities are disproportionately large. On reefs where cleaner fish were experimentally removed for 8.5 y, resident (i.e., site-attached) client fishes, which included planktivorous damselfishes, were negatively affected in many ways: the total abundance of adults and juveniles was reduced; the size frequency distributions of two planktivorous species (Pomacentrus amboinensis, P. moluccensis) were shifted towards smaller individuals; and larger P. moluccensis individuals had reduced growth rates and higher parasite loads (Clague et al., 2011; Waldie et al., 2011; Sun et al., 2015). Cleaners’ effect on planktivorous fishes could result in cascading effects on the food source of these fishes, including demersal zooplankton. A better understanding of the broader community-wide effects of L. dimidiatus is timely. Recently, cleaners experienced a natural reduction in abundance (80%) due to sequential cyclone (2014, 2015) and El Niño (warming) (2016) events at Lizard Island, Great Barrier Reef (GBR) with negative consequences observed in the remaining cleaners’ cleaning interactions (Triki et al., 2018). Furthermore, negative effects of predicted future ocean warming and acidification conditions on cleaners’ neurological and behavior responses have been demonstrated, and L. dimidiatus are also a primary target of the aquarium industry (Rhyne et al., 2017; Paula et al., 2019). To date, cleaners’ indirect effect on the reef community has focused only on the free-living stages of gnathiid isopod parasites that cleaners feed on and farming and grazing fishes’ effect on the benthos they feed upon (Grutter et al., 2019; Sikkel et al., 2019; Grutter et al., 2020a).
Gnathiid isopods are a common component of the demersal zooplankton community, as they live in the benthos when not feeding briefly on fish blood (Jacoby and Greenwood, 1988; Chambers and Sikkel, 2002; Sikkel and Welicky, 2019; Grutter et al., 2020b). The common demersal fish ectoparasites emerge from the benthos to briefly feed only once during each of their three juvenile stages. Individual cleaner fish at Lizard Island consume an average 1200 gnathiids daily (Grutter, 1996b) and this results in a daily reduction of gnathiids on fish (Grutter, 1999). This has been used to calculate an estimated daily gnathiid predation rate per reef based on the cleaners’ abundance per reef (Grutter, 2008). When this high rate was compared with the relatively lower estimated daily emergence rate of gnathiids per reef, the abundance of emerging gnathiids per patch reef was less (66%) than the estimated number of gnathiids that all adult L. dimidiatus per reef ate daily (Grutter, 2008). This suggested that gnathiids may be a limited resource for L. dimidiatus on patch reefs and that cleaners could negatively impact the local demersal gnathiid population. When this was experimentally tested, cleaner treatment (control undisturbed reef vs cleaner-free or removal reef) was repeatedly shown to indirectly reduce the abundance of the demersal stages of gnathiids, both when sampled with emergence traps and with sentinel (i.e., baited with live fish) traps (Grutter et al., 2018; Grutter et al., 2019; Sikkel et al., 2019). Thus, there is robust evidence that variation in the abundance of a fish species (i.e., L. dimidiatus) can indirectly affect at least one of the taxa of the demersal zooplankton community (i.e. gnathiid isopods). This raises the question of whether other fishes (i.e., planktivores), species often many more times abundant than the relatively rare L. dimidiatus (one to four adults per patch reef measuring 61 to 285 m2, Supplementary Table 1), can also affect the rest of the community of demersal zooplankton.
There are many species of planktivorous fishes on the experimental reefs used above, including those in the ray-finned families Apogonidae, Blenniidae, Gobiidae, Pseudochromidae, Sygnathidae, and Pomacentridae; the latter being the most abundant, diurnal, site-attached, highly visible, and speciose family (Waldie et al., 2011). The distribution of planktivorous Pomacentridae fish is affected by habitat preferences at settlement and post-settlement ecological processes such as conspecifics, predation, and shelter availability (Emslie et al., 2019), but the effect of cleaners on this group as a whole is unknown. Predation rate by planktivorous fishes can decrease as fish abundances decrease (O'Brien, 1979; Acosta and Butler, 1999; Motro et al., 2005; Johannsson and Bowen, 2012). Hence, we assumed the predation rate on zooplankton would be influenced by the abundance of planktivorous fishes. We hypothesized that the (non-gnathiid isopod) demersal zooplankton community will be indirectly affected by the presence of cleaner fish, via cleaners’ positive effect on the abundance of planktivorous (Pomacentridae) fishes. Nevertheless, on coral reefs, the abundance, composition, and biomass of demersal zooplankton communities remains relatively little studied (but see, Alldredge and King, 1977; Porter and Porter, 1977; McWilliam et al., 1981; Jacoby and Greenwood, 1988; Amer, 2019).
Specifically, the hypotheses tested were that the removal of cleaner fish indirectly affects the 1) abundance, diversity, and composition of planktivorous fishes, and 2) biomass and abundance, diversity, and composition of demersal zooplankton. This study differs from previous studies on the effects of cleaners on other organisms by focusing specifically on the indirect links between the presence of cleaner fish, the abundance of planktivorous fishes, and the (non-ectoparasite) demersal zooplankton community. We counted the number of planktivorous fish per reef in 2009 and 2012, with a focus on the Pomacentridae. We predicted cleaners’ presence effect on zooplankton will vary on two time-scales: a) between the day and night, as both L. dimidiatus and most planktivores are diurnally active fishes (Hobson, 1991; Grutter, 1996b; Waldie et al., 2011; Leray et al., 2019) and because other studies found that cleaners affect other demersal zooplankton (Gnathiidae, Corallanidae ectoparasitic isopods) during the day only (Grutter, 1999; Grutter and Lester, 2002; Grutter et al., 2018) and b) temporally (months, years), as cleaners affect fishes differently over this time scale (Grutter, 1996a; Grutter, 1997b; Clague et al., 2011; Waldie et al., 2011; Sun et al., 2015). Demersal zooplankton were collected with emergence traps, in the day and the night separately, and over nine trips over 12 y. We used the incidental zooplankton by-catch of the samples used to quantify the effect of cleaner presence on gnathiids in Grutter et al. (2019). Samples were collected from the longest cleaner fish manipulation experiment (12 y long when sampled here), located on Lizard Island. During this time, removal reefs were checked approximately every 3 months during 49 sampling trips, and any new adults or recently-recruited cleaner fish juveniles removed. The removal of cleaners was highly effective, with 90% of all removal-reef inspections revealing they were free of adult cleaners (see Supplementary Methods and Supplementary Table 1).
This study was conducted from July 2000 to December 2012 on isolated patch reefs in open sand at Casuarina Beach (CB, 5 reefs, 111 – 227 m2) and the lagoon (Lagoon, 12 reefs, 61 to 285 m2) off Lizard Island, GBR (14°400’S, 145°280’E; for map, see Figure 2 in Grutter et al. (2019). These experimental reefs are part of an ongoing (as of 2022) long-term cleaner fish (L. dimidiatus) exclusion study (Grutter et al., 2003; Clague et al., 2011; Waldie et al., 2011; Grutter, 2012; Sun et al., 2015; Binning et al., 2018; Grutter et al., 2018; Grutter et al., 2019; Sikkel et al., 2019; Grutter et al., 2020a; Ros et al., 2020; Paula et al., 2021). Cleaner fish were removed in September 2000, with July 2000 representing a baseline period before the impact of removal. Labroides dimidiatus was either removed (1 to 4 adults reef-1) from reefs (removals, CB: n = 2, Lagoon: n = 6), or similarly disturbed but only counted on reefs (controls, CB: n = 3, Lagoon: n = 6) approximately every three months. For cleaner removal details, see Supplementary methods; for date and number of adults and juveniles removed per reef, see Supplementary Table 1.
Fish counts of residents were conducted following Waldie et al. (2011). Fishes were classified as ‘residents’ or ‘visitors’ following lists adapted from Grutter et al. (2003). Residents are smaller and site-attached species (in contrast to so-called visitors, larger species assumed to move regularly among reefs during a day, or over a longer period). Briefly, residents were counted once per reef by a scuba diver (60-120 min, 0930–1430 hrs). Counts during each year were done by different divers, hence differences between years should be interpreted with caution. Surveys alternated between randomly selected control and removal reefs. The diver methodically circled the reef counting one, or several less abundant species, in the same order, during each circle of the reef. All sizes of fish (~≥ 1 cm total length, TL) were counted on 15–21 April 2009 and are sourced from Waldie et al. (2011). Counts in 18 to 22 December 2012 consisted of individuals > 2 cm TL. All the remaining smaller (Pomacentridae) individuals were counted around this time for a separate study on recently recruited juveniles (Sun et al., 2015); the mean abundance of recruits, belonging to 20 planktivorous species, was 229 SE 41, range 25 – 604 reef-1 (unpublished data, Grutter A.S.). Planktivorous nocturnal and mobile (Apogonidae) and cryptic species (Blenniidae, Gobiidae, Pseudochromidae, and Sygnathidae) were not considered. Due to the preponderance of Pomacentridae species categorized as planktivores, identified following (Emslie et al., 2019), we consequently excluded two non-pomacentrid species (present on 1 to 2 reefs per year: Aeoliscus strigatus, Centriscidae; Ptereleotris evides, Ptereleotridae). For final list of 26 species analysed, see Supplementary Figure 1.
Zooplankton samples were collected as part of the study conducted by Grutter et al. (2019) on gnathiid isopod parasites that used the above long-term cleaner fish manipulation experiment. The original focus of the samples was to quantify gnathiids, when emerging from the reef substrate in search of host fish to feed on. Gnathiids were captured using 1 m2 (100 µm mesh) emergence traps (for dimensions see Supplementary methods; for illustration, see supplementary figure 1 in Grutter et al., 2019) between July 2000 and December 2012 from the Casuarina Beach and Lagoon sites. Then, gnathiids were removed from the samples. The sampling method also incidentally captured other demersal zooplankton. In the current study, the effect of cleaner fish (L. dimidiatus) on the biomass and abundance of all remaining non-gnathiid demersal zooplankton emerging from the reef substrate was investigated using the same samples as above, but only from the Lagoon. Between July 2000 and December 2012; samples were collected during nine trips (for dates see Figure 2 legend, Supplementary Table 2). Sampling was intermittent due to logistical constraints. In 2000, sampling was conducted three times; between 2001 and 2003, sampling was done approximately every six months; the subsequent and final sampling was in 2012, 10 y after the previous one.
Sampling was usually conducted over three days, either before (i.e. day 1, 2, 3), or after the full moon (day 4,5,6; but see Supplementary Table 2), as lunar cycles have an effect on emergence rate of gnathiid isopods (Grutter et al., 2000) and other demersal zooplankton (Alldredge and King, 1980). Whether sampling was before or after the full moon was because sampling involved two sites (the CB site was not used here) and this order was randomized. Depending on the size of the reef, each reef had two or three emergence traps (maximum 30 traps in total). Sampling was conducted for both day and night in the same location of the reef.
Traps were placed on the seabed in a manner so as not to damage live coral and where they would remain relatively stable, i.e. not on the edge of a precipice. Every 24 h, traps were moved to a new location on the same reef. During sample collections, 1 L bottles attached to emergence traps were collected either by a snorkeler or scuba diver. A lid was immediately used to close the bottle to reduce loss of zooplankton in the bottle. On the same day, samples were filtered through a 62 µm-mesh filter into a vial followed by the addition of formalin and seawater to create a 10% (v/v) solution (for more details, see Supplementary methods).
For biomass only data, from each reef, one trap was selected randomly out of the two or three placed on the reef per day and night for each of the three days sampled in all nine trips. 551 initial samples were used; of these, nine samples with large amounts of sand were omitted, resulting in a final 542 samples being statistically analyzed. For total traps sampled for biomass, abundance and diversity per day or night by trips, see Supplementary Table 2.
To estimate total abundance, taxa diversity and community composition (taxonomy and abundance per zooplankton type, i.e. taxonomic category) of zooplankton, additional samples were selected. A total of 34 categories of demersal zooplankton were identified (Supplementary Table 3). Because quantifying the community is time consuming, only four trips were analyzed. Trips selected were the first (July 2000) and last (December 2012) ones, and two more representing the same earlier seasons selected (January 2002, July 2002), resulting in two Austral summer and two winter trips. Samples were selected from the last day of the three days sampled, for both day and night, usually one sample per reef for the day and night (n = 3 samples was missing, hence n = 85). To compare abundance with biomass (a destructive procedure) from the same sample, the subsample was divided into two equal parts using a Folsom splitter (McEwen et al., 1954); one subsample was used for biomass and the other for counts; each was multiplied by two to estimate the total sample. The count subsamples were preserved in 10% formalin until counted.
The zooplankton sample was placed onto filter paper (Whatman™ Glass Microfiber Filters, GE Healthcare Life Sciences, Diameter 47 mm, CAT No. 1822-047) and thoroughly rinsed to remove any salt or formalin contamination. Excess moisture was removed using a vacuum filtration apparatus (Millipore, Nalgene®, USA). Prior to their use, labelled filters had been dried in an oven (Thermoline Scientific, TO-152F, Australia) at 60°C for 10 min and pre-weighed to the nearest 0.0001 g (Mettler Toledo, XS204, USA). The filter paper and zooplankton sample were dried in an oven for 60 min and reweighed; a pilot study showed that after this time, the zooplankton sample weight change was negligible (< 0.1% per 10 min increase in duration). Weights were always measured immediately after removing the sample from the oven. Sample biomass was obtained by subtracting the initial pre-weighed filter paper weight from the filter paper plus dried zooplankton weight.
Samples were counted and taxa identified to the narrowest possible taxonomic category, which ranged from phylum to genus, using a stereo microscope (Leica M165 C, 7.3 – 120X) and taxonomic guides [e.g., Swadling et al., 2013)]. Samples were initially filtered through a 100-µm mesh, to remove the formalin from the sample. Collected organisms were placed into a measuring cylinder and diluted with distilled water to make a final volume of 100 mL. A 1 mL volume was obtained using a purpose-built Stempel pipette and placed into a Bogorov sorting tray. A pilot study revealed that approximately 100 organisms were sufficient to sample most of the different taxa per subsample, and often a minimum of 1 mL was sufficient. The volume of the subsample was increased if the number of organisms in the 1 mL subsample did not reach 100 (Eriksen et al., 2019). For example, if 70 organisms were counted in a 1 mL subsample, then another 1 mL subsample would be counted. The median final volume sampled was 5 mL (2, 15, 1 – 100 mL; 25th, 75th quantiles, range). The abundance per original sample was then calculated based on the dilution and number of subsamples.
For fish, we tested total abundance, and abundance of the three most abundant and ubiquitous species (Pomacentrus amboinensis, P. moluccensis (both always present on all reefs), P. nagasakiensis (present on 12 reefs in 2009, and all reefs in 2012), Supplementary Figure 1). Combined, the sum of these three species contributed 47 and 66% of the total abundance of planktivorous Pomacentridae in 2009 and 2012, respectively. We also tested fish species richness index and the Shannon diversity index. For fish abundance measures and diversity indices, the same models with cleaner presence (cleaner treatment: present or absent), site (Casuarina Beach, Lagoon), year (2009 and 2012), all their interactions, and reef area as fixed effects and reef identity as a random effect were tested (Model 1, note all models are abbreviated following R software code format). We used reef area as a fixed continuous effect in the model because it provides information on whether or not it contributes to the patterns observed. All raw data is plotted according to reef area to visualize its role in explaining the patterns observed and how they vary according to the other fixed effects (Figure 1, Supplementary Figure 2).
Figure 1 Abundance of planktivorous Pomacentridae fish (reef-1) according to cleaner fish Labroides dimidiatus presence treatment (Control = yes cleaner fish present, Removal = no cleaners present), Casuarina Beach (small symbols) or Lagoon site (large symbols), reef area, and sampling year for (A) total abundance and (B) Pomacentrus amboinesis.
(Model 1) Fish abundance (or species richness, Shannon species diversity index) ~ Cleaner treatment * Site * Year + Reef Area + (1|Reef identity)
Generalised linear-mixed effects models were used for fish abundances and richness with Poisson or negative binomial distributions tested, as data were counts (Zuur et al., 2009; Brooks et al., 2017). The final distribution was selected by model comparisons using the Aikaike information criterion. Due to the four multiple tests involving fish abundance, the Šidák correction was applied (α = 0.012741) (Abdi, 2007). For species richness, a poisson distribution was assumed, as the variance mean to ratio was close to 1 (0.78), and a negative binomial model did not converge (Zuur et al., 2009). Models with a negative binomial distribution were tested using a linear or quadratic parameterization (Hardin and Hilbe, 2007), with the former selected for all fish abundance models, except for total abundance.
To examine the composition of planktivorous fish using the abundance of fish per species, a Permutational Multivariate Analysis of Variance (PERMANOVA) was used with square-root transformed abundances to downplay the contribution of highly abundant species, and the Bray-Curtis dissimilarity index for the distance measure. Of the 26 fish species, only the 17 species present on > 3 reefs were analyzed. Initially, full three-way models with cleaner treatment, site, and year as fixed effects, with reef identity as a random effect were analyzed, but for the interaction between cleaner treatment and year they returned a negative Pseudo-F value which is not a sensible value (Searle et al., 1992). Therefore, three two-way models with no random effect were constructed, which were successfully modelled. We tested for the effect of site on cleaner treatment within each year in separate analyses (Models 2, 3). We also pooled sites and tested whether there was an interaction between cleaner treatment and year (Model 4). Sums of Squares type used was Type III (partial, for unbalanced designs), with the permutation of residuals under a reduced model, and a maximum number of permutations of 9999, following the recommendations of Anderson et al. (2008). A principal coordinates analysis (PCO) ordination plot was used to visually represent samples in at least two dimensions and aid interpretation of the dissimilarities in the diversity.
(Model 2) Fish abundance per species in 2009 ~ Cleaner treatment * Site
(Model 3) Fish abundance per species in 2012 ~ Cleaner treatment * Site
(Model 4) Fish abundance per species ~ Cleaner treatment * Year
For dried biomass, a linear mixed-effects model (normal distribution) was used to test if biomass was significantly influenced by cleaner presence treatment, sampling trip (month-year, represented by trips numbered 1, 3, 4, 5, 6, 7, 8, 9, 12), and time of day (day or night) as fixed factors (Model 5). We were interested in testing the interaction between cleaner treatment and trip, and how it changed over time. Note that cleaners were removed after Trip 1 (July 2000), during September 2000, and before the following Trip 3 (Trip 2 does not exist). Therefore, Trip 1 was used as the baseline for comparisons. Time of day was included as a predictor because previous studies have shown diel differences in biomass of emergent zooplankton (Ohlhorst, 1982). In addition, the effect of cleaner fish presence (present or removed) has been found to affect several ectoparasitic isopod groups (Gnathiidae, Corallanidae) during the day only, when cleaners are active (Grutter, 1999; Grutter and Lester, 2002; Grutter et al., 2018). Therefore, all interactions of these main effects were included in the model, including the three-way, as a significant three-way could indicate an effect of cleaner during the day only. This three-way interaction for biomass, and 3-ways for the other variables below, were not significant (Tables 2–5) and so are not discussed further. Reef and date were added as random effects predictors because there may be possible spatial and diurnal variation (within a trip), but they were not the focus of this study. Raw plots (separately for day and night) indicated little variation among sampling days and so it was not considered further. Biomass was log10 transformed, as visual inspection of residual and quantile-quantile plots showed that the transformation improved the normality and the homogeneity of variance assumptions. Tests of fixed effects were assessed using analysis of variance (function “anova”) with a conditional F-test and a Kenward-Roger degrees of freedom correction using the R package “pbkrtest” (Halekoh and Højsgaard, 2014).
(Model 5) Log10Biomass ~ Cleaner treatment * Sampling trip * Time of day + (1|Reef identity) + (1|Date)
For zooplankton abundance (normal distribution) and Shannon diversity index (normal distribution), separate linear models as ones for biomass were constructed, except that “Date” was not included since samples were selected from one day only per trip (Model 6). Abundance was log10 transformed, for the same reason as biomass was. A generalised linear model was used for zooplankton taxa richness and a model selected (poisson distribution) as for fish richness. Two outliers were excluded to satisfy the statistical assumptions of the univariate analyses. The two outliers (Trip 1, Day: 225600; Trip 12, Night: 327600) consisted largely of non-Cirripeda nauplii (90%) and gastropod veligers (95%), respectively.
(Model 6) Zooplankton Log10Abundance, richness, or Shannon diversity index ~ Cleaner treatment * Sampling trip * Time of day + (1|Reef identity)
To examine whether the relationship between log10biomass was related to log10abundance, we conducted a linear regression (normal distribution) with log10biomass as the response, with log10abundance as a continuous fixed predictor and cleaner treatment, sampling trip, and time of day as fixed factors, with all interactions included. Reef identity was initially included as a random effects predictor, but it produced a singular fit (i.e., was overfitted), and so was omitted (Model 7). We checked Cook’s distance to ensure there were no highly influential samples.
(Model 7) Log10Biomass ~ Cleaner treatment * Sampling trip * Time of day * Log10Abundance
A PERMANOVA was used to examine the composition of zooplankton, with all 34 taxonomic categories, using the same measures and criteria as for fish counts. A model with cleaner treatment, sampling trip, and time of day as fixed effects was analyzed. We had insufficient replication at the lowest level to include Reef identity as a random effect (Model 8). A PCO of the distances among group centroids was used to detect the underlying patterns for the effect of sampling trip.
(Model 8) Cleaner treatment * Sampling trip * Time of day
Univariate analyses were performed using the software R (4.0.2) (R Core Team, 2020). Multivariate analyses were performed using PERMANOVA+ for PRIMER 7 (Anderson et al., 2008). Species richness was defined as the number of species or taxa reef-1. The Shannon diversity index reef-1 was calculated using the R package “vegan” (Oksanen et al., 2019). Generalised linear-mixed effects models were performed using the R package “glmmTMB” (Brooks et al., 2017). Linear mixed-effects models were used for Shannon diversity indices, zooplankton biomass, and zooplankton abundance using the R package “lme4” (Bates et al., 2015). Analysis of variance tables were calculated using the R package “car” (Fox and Weisberg, 2019). Residual diagnostics was performed using the R package “DHARMa” (Hartig, 2021). Effect plots were used to represent the results, using the R package “Effects” (Fox and Weisberg, 2019) and “lattice” (Sarkar, 2008).
Total abundance of planktivorous fish differed according to an interaction between cleaner treatment and year, but this was no longer significant when the Šidák correction for multiple tests was applied (P = 0.0491 Šidák α = 0.0127), Table 1, Supplementary Table 4A). This interaction showed a tendency of more fish on reefs with, compared to without cleaners, at both sites in 2009 (Figure 1A, Supplementary Figure 2A). Pomacentrus amboinensis abundance was higher on reefs with, compared to without cleaners, at both sites and during both years (P = 0.0012 Šidák α = 0.0127, Table 1, Supplementary Table 4B, Figure 1B). Pomacentrus moluccensis abundance (P = 0.2779Šidák α = 0.0127, Table 1, Supplementary Table 4C, Supplementary Figure 2B) and P. nagasakiensis abundance (P = 0.2905Šidák α = 0.0127, Table 1, Supplementary Table 4D, Supplementary Figure 2C) were not affected by cleaners.
Table 1 Summary of results for fish abundance, diversity, and community composition in relation to the effect of cleaner fish Labroides dimidiatus treatment (presence or absence) reef-1 and or their interactions with other factors (Supplementary Tables 4, 5).
Fish species richness (P = 0.3100, Supplementary Table 4E, Supplementary Figure 2D) and the Shannon diversity index (P = 0.5859, Supplementary Table 4F, Supplementary Figure 2E) were not affected by cleaner treatment (Table 1).
Twenty-six Pomacentridae species were identified, 17 in 2009 (n = 9872 individuals) and 24 in 2012 (n = 8058 individuals). The most abundant planktivorous species across all samples was Pomacentrus moluccensis (171, 107/296), followed P. amboinensis (56, 44/77), Neopomacentrus azysron (34, 10/132), Acanthochromis polyacanthus (14, 0/40), and P. nagasakiensis (14, 5/49, median, 25th/75th quantile reef-1, Supplementary Figure 1). The composition of planktivores did not differ with cleaner treatment when analysed separately by year and including the effect of site (PERMANOVA, 2009: P = 0.6247; 2012: P= 0.9981, (Table 1, Supplementary Figure 3, Supplementary Tables 5A, B) nor when pooled across sites and including the effect of year [PERMANOVA, (Table 1) P = 0.9929; Supplementary Table 5C]. The composition differed between sites in both years and between years (2009: P = 0.0026; 2012: P = 0.0003, year: P =0.0023; Supplementary Table 5).
Zooplankton biomass per trap (1 m2) ranged from 0.0008 to 0.1733 g with a median 0.0074 g (0.0045/0.0138, 25th/75th quantile) (Supplementary Figure 4A). Biomass (log10 g m-2) did not differ with cleaner treatment and sampling trip (Treatment × Trip: P = 0.2433, Table 2). This indicates that zooplankton biomass did not change according to cleaner treatment over time, compared with pre-removal values in Trip 1 (July 2000, Figure 2). There was a difference in biomass between time of day (day or night) and sampling trip (Daynight × Trip: P < 0.0001, Table 2, Supplementary Figure 4B). This difference was due to higher biomass at night compared with the day for Trips 5, 7, 8, and 9 compared with values in the first trip (all P values of Daynight × Trip, relative Trip 1: ≤ 0.0185, Supplementary Table S6).
Figure 2 Effect plot for mean zooplankton biomass per emergence trap (1 m-2) for cleaner fish Labroides dimidiatus presence treatment [Control = yes cleaner fish present (solid line)], Removal = no cleaners present [dashed line] and sampling trip (1 = July 2000, 3 = September 2000, 4 = November 2000, 5 = January 2001*, 6 = August 2001, 7 = January 2002*, 8 = June 2002*, 9 = January 2003*, 12 = December 2012). *P < 0.05 for time of day and trip interaction, relative to trip 1.
The association (slope) between biomass (log10 g m-2) and total abundance (log10 m-2) varied according to the time of day and trip (Daynight × Trip × Total abundance: P = 0.0114, Supplementary Table 7A, Supplementary Figure 5). This was a result of a weak difference in association between the day and night on Trip 7, relative to Trip 1 (P = 0.0494, Supplementary Table 7B). This was due to positive biomass and abundance associations during the day and night on Trip 1 (Pearson’s correlation, Day: r = 0.969, N = 7, P = 0.0003; Night: r = 0.893, N = 10, P = 0.0005) and the day only on Trip 7 (Pearson’s correlation, Day: r = 0.759, N = 12, P = 0.0042; Supplementary Figure 5).
Total zooplankton abundance per trap (1 m-2) analyzed ranged from 428 to 97600 individuals with a median 9760 (3227/2200, 25th/75th quantile) (Supplementary Figure 6A). Total abundance (log10 m-2) did not differ with cleaner treatment and sampling trip (Treatment × Trip: P = 0.5001; Table 3; Figure 3A) and time of day [P = 0.9784; predicted back-transformed values for Day: 9050 (5376–15238), Night: 8212 (4885–13802), mean effect values (95% CI interval), Supplementary Figure 6B]. This suggest that the presence of cleaner fish did not influence zooplankton abundance. Abundance differed among trips (P < 0.0001), due to 4.8 times more zooplankton in Trip 7 [30571 (17610–53070)] compared with Trip 1 [6310 (3491–11406); Trip 8: 4816 (2788–8316); Trip 12: 4921 (2752–8800); predicted back-transformed values, mean effect values (95% CI interval), Supplementary Table 8, Supplementary Figure 6C].
Figure 3 Effect plot for mean zooplankton (A) log10 abundance, (B) richness, and (C) Shannon diversity index per emergence trap (1 m-2) for cleaner fish Labroides dimidiatus presence treatment (Control = yes cleaner fish present [solid line]), Removal = no cleaners present [dashed line] by sampling trip (1 = July 2000, 7 = January 2002*, 8 = June 20002, 12 = December 2012). *P < 0.05 for trip, relative to trip 1. Two outliers were omitted (see Materials and Methods).
Thirty-four taxonomic categories of zooplankton were identified from six phyla (Annelida, Arthropoda, Chordata, Cnidaria, Mollusca, Platyhelminthes; Supplementary Table 3). The richness (Treatment × Trip: P = 0.6536; Table 4, Figure 3B, Supplementary Table 9; Supplementary Figure 7A) and Shannon diversity index of zooplankton (Treatment × Trip: P = 0.8996; Table 5, Supplementary Table 10; Figure 3C, Supplementary Figure 7B) did not differ with cleaner treatment and sampling trip. This suggests that the presence of cleaner fish did not influence zooplankton diversity. Diversity was significantly higher (12%) during the night compared with the day (P = 0.0355; Table 5, Supplementary Table 10, Supplementary Figure 7C; Shannon index: Day: 1.308, 0.160; Night: 1.483, 0.159; mean, 95% CI).
The most abundant zooplankton types were nauplii (unidentified), barnacle nauplii, and some copepods (Oithoidae and Harpactacoida complex), with median abundances per trip for the day and night often reaching several thousand per sample (Supplementary Figure 8). The composition of zooplankton did not differ with cleaner treatment and sampling trip (Treatment × Trip, PERMANOVA, P = 0.5326; Table 6). This indicates that composition did not change according to cleaner treatment over time compared with pre-removal values in Trip 1 (July 2000, see PCO analysis in Figure 4A). There was a difference in composition between time of day (PERMANOVA, P = 0.0012, Table 6) and among sampling trips (PERMANOVA: P = 0.0001, Table 6, Figure 4B). A pairwise analysis comparing all trip pairs indicated that this was due to differences in the composition among all trips, including relative to Trip 1 (comparisons relative to Trip 1: Trip 7, P = 0.0001; Trip 8, P = 0.0184; Trip 12, P = 0.0004; Supplementary Table 11).
Figure 4 Principal coordinates analysis (PCO) ordination plot of zooplankton abundance composition per emergence trap (1 m-2) showing vectors for the three taxa that contributed the most to the dissimilarity (taxa with Pearson’s correlations > 0.5), labelled by the factors (A) cleaner fish Labroides dimidiatus presence treatment (Control = yes cleaner fish present, Removal = no cleaners present) per trip and (B) time of day (day and night) and with (C) segmented balloons representing the relative abundance of above three taxa. Key shows range in abundance per taxa. Data were square root transformed and a Bray-Curtis similarity was calculated for the PCO.
Although the PCO analysis, used to interpret the significant factors, found that the total variation inherent in the resemblance matrix that was explained by the first two PCO axes was modest (44.5%, Figure 4), it showed relatively clear separation of samples among the four trips (Figure 4A), but the separation between day and night was not as strong (Figure 4B). Three taxa contributed the most to the dissimilarity (taxa with Pearson’s correlations > 0.5). The dissimilarity between the day and night was associated with more barnacle (Cirripeda, Arthopoda) nauplii in the day compared with the night (Figures 4B, C, Supplementary Figures 8, 9). The dissimilarity among trips was associated with more Oikopleuridae (a larvacean family, Chordata) during Trip 1 compared with Trips 8 and 12, more Oikopleuridae and Facetotecta larvae (a thecostracan crustacean, Arthopoda) in Trip 7 compared with the other trips, and more barnacle larvae in Trips 7 and 12 (Figures 4A, C, Supplementary Figures 8, 9). Trip 8 and 12 had the most variable composition compared with the other trips.
We tested whether the ecological consequences of the trophic interactions between the cleaner fish L. dimidiatus and their planktivorous fish clients indirectly affects the demersal zooplankton community. We discuss, first, whether cleaner fish presence manipulated over 12 years (July 2000 to December 2012) affected the abundance, diversity, and composition of planktivorous client fishes, second, whether cleaner presence indirectly affected the biomass, abundance, diversity and composition of demersal zooplankton, and third, the diel and temporal patterns in demersal zooplankton.
We focused on planktivorous damselfishes (Pomacentridae) as being the most abundant, diurnal, site-attached, highly visible, abundant, and speciose fish family. Overall, fish surveys after 9 (2009) and 12 y (2012) post-cleaner removal revealed that one species, of a subset of the three most ubiquitous and numerous species, was significantly affected by cleaner presence with abundance higher on reefs with cleaners, compared to without cleaners, at both sites and during both years (Pomacentrus amboinensis, not P. moluccensis, and P. nagasakiensis). All P. amboinensis combined contributed to 11% of the combined total abundance. For the total abundance of planktivorous fishes (Pomacentridae, 26 species), there was a tendency for higher values on control reefs with cleaners, compared to removals without cleaners, at both the Casuarina Beach and Lagoon sites in 2009. The positive effect of cleaners on fish abundance agrees with some cleaner manipulation studies of resident fishes that included planktivores (Bshary, 2003; Waldie et al., 2011; Grutter et al., 2020a) and the Pomacentridae recruits in 2012 not counted here (including a positive effect on P. amboinensis recruits, (Sun et al., 2015), but it does not with others which found no effect (Grutter, 1996a; Grutter, 1997b). It should be noted that all significant effects of cleaner presence on abundance detected to date have involved positive effects.
Planktivore diversity measured as species richness, Shannon diversity index, and composition were not affected by cleaner treatment, like it was not for species richness of all Pomacentridae recruits in December 2012 (Sun et al., 2015), and all resident fishes in April 2002 (Grutter et al., 2003). But for species richness, it is in contrast to all resident (i.e., site-attached) species in 2009, when richness was higher on reefs with cleaners (Waldie et al., 2011). These results further confirm the effect of cleaners is not even across client fishes (Bshary, 2003; Grutter et al., 2003; Grutter, 2012). Pomacentridae are highly site-attached and often territorial (Randall et al., 1997) and thus seeking cleaning services elsewhere is not a viable option. Instead, studies suggest that changes to their populations due to cleaner presence may be influenced by processes that affect their parasite loads, size, growth, cognition, physiology, and recruitment (Clague et al., 2011; Waldie et al., 2011; Binning et al., 2018; Demaire et al., 2020; Ros et al., 2020). However, all the above cleaner manipulations studies, except for Bshary (2003) and Demaire et al. (2020), were done on the same reefs as here and so are not independent of this study, which should be considered when generalizing about the impact of cleaner removal to fish communities. Furthermore, manipulations involving additions of cleaners can also influence fish communities (see also Bshary, 2003). In French Polynesia, L. dimidiatus additions attract corallivorous butterflyfish (Chaetodontidae), and causes increases in local predation pressure on corals at cleaner stations, and indirectly reduces the growth rate of an unpreferred coral (Adam, 2012). Additions in French Polynesia also indicate that L. dimidiatus recruits have access to more resources (clients) at occupied sites, and consequently grow faster despite evidence of competition with resident cleaners (Adam, 2011).
We found no evidence to support the hypothesis that the experimental absence of cleaner fish indirectly affects demersal zooplankton. When sampled with emergence traps placed on the experimentally manipulated reefs, demersal zooplankton biomass, abundance, richness, Shannon diversity index, and community composition did not differ between reefs with and without cleaner fish when sampled up to nine times over 12 years. In contrast, previous studies showed that gnathiid isopods are indirectly affected by the presence of cleaner fish L. dimidiatus on the same reefs sampled here (Grutter et al., 2018; Grutter et al., 2019; Sikkel et al., 2019). Planktivorous damselfish can spend >85% of their time directly feeding on demersal zooplankton (Mann & Sancho, 2007). Elsewhere, the predation rate by planktivorous fishes decreases as fish abundances decreases (O'Brien, 1979; Acosta and Butler, 1999; Motro et al., 2005; Johannsson and Bowen, 2012). However, no evidence of a reduction in such predation was detected here. This has important implications for understanding coral reef food webs, specifically the links between diurnal planktivore abundance, demersal zooplankton, and cleaner fish presence on the reef. Zooplankton are important predators of phytoplankton and other zooplankton and also a food source for so many reef organisms, and so can cause both bottom-up and top-down effects within this food web. Altered phytoplankton and zooplankton abundance and composition are predicted to have cascading effects on higher trophic levels of the GBR (McKinnon et al., 2007). Any change in overall abundance and trophic efficiency of phytoplankton and zooplankton communities may result in a change in higher trophic level populations. This study suggests a certain resilience to the removal of a key mutualist (L. dimidiatus) from this food web, despite a presumed reduction in fish predation on demersal zooplankton.
There are several alternative explanations for why no indirect effect of cleaner fish presence on the non-gnathiid zooplankton was detected here. The effect of cleaners on planktivorous fishes may be too small to influence their predation rate at the times scales sampled. Indeed, we only detected an effect of cleaner presence on a small proportion of the planktivorous fish population. However, it should also be noted that sampling times for planktivorous fish and zooplankton sampling only overlapped in the last sampling trip. Demersal zooplankton may only make up a small fraction of the diet of the planktivorous fishes that were affected by cleaners, as some also eat detritus and algae, holoplankton, and transported oceanic zooplankton (Hamner et al., 1988; Frederich et al., 2009; Hanson et al., 2016; Leray et al., 2019). The predation rate of invertebrates that feed on zooplankton, organisms not likely affected by cleaners, might overwhelm that of planktivorous fishes. Many corals consume zooplankton, as do bivalves, brittle stars, sponges, and tunicates (Ruppert and Barnes, 1994; Houlbreque and Ferrier-Pages, 2009) — all of which occur on the reefs (Grutter A.S., personal observation). Demersal zooplankton might not all originate from or remain on the local patch reef sampled, which could obscure any local reef effect. For example, the prevailing current at the location sampled (Lagoon) is related to the prevailing southeasterly wind (Grutter et al., 2019), which results in a current coming from offshore, across the reef flat, lagoon, and back reef then moving offshore again. However, this current did not appear to obscure the local effect of cleaners on gnathiid isopods at this same site (Grutter et al., 2019).
This study was timely, as L. dimidiatus experienced an 80% reduction in abundance due to sequential cyclone and El Niño (warming) events at other sites in Lizard Island (GBR) between 2014 and 2016 (Triki et al., 2018). At the Lagoon site, all cleaners had disappeared from 4 out of the 6 control reefs by July 2016, with a slow and incomplete recovery by Oct 2018 (Grutter et al., 2019). Such total losses from a control reef during 2000 to 2012 were rare, and when present (four times) only involved one reef per sampling time (Supplementary Table 1). Thus, any studies at this site examining the effect of cleaner presence after 2012 must be interpreted with caution.
While we predicted an effect of cleaner presence on diurnal demersal zooplankton, based on the diurnal habits of cleaners and some planktivores, this effect was not detected here. Occasionally biomass, and in all trips, Shannon diversity index, were higher at night. Zooplankton composition also differed between the day and night during all trips sampled. In contrast, there were no differences between day and night for zooplankton abundance. The latter is likely to be due to the high numbers (yet small size) of nauplii that dominated the data during both the day and night. Nocturnal emergence across a range of taxa tended to be higher, as commonly found elsewhere (Alldredge and King, 1980; Ohlhorst, 1982; Amer, 2019). Emergence rates also varied across taxa, as commonly found elsewhere (Ohlhorst, 1982; Amer, 2019; Leray et al., 2019). Taxa that contributed the most to the dissimilarity in composition between the day and night involved more barnacle (Cirripeda, Arthropoda) nauplii during the day compared to the night. In contrast, gnathiid isopods, which were removed from the same samples quantified here, were less abundant in the day than at night (Grutter et al., 2019).
Diel migratory behavior may be a way to avoid predators (Turner and Mittelbach, 1990; Acosta and Butler, 1999), including diurnal planktivorous damselfishes (Waldie et al., 2011; Leray et al., 2019). The increased diversity observed during the night may also be due to higher zooplankton feeding rates of some taxa at night (Stepien and Brusca, 1985; Roman et al., 1990), such as larger zooplankton species that do so to avoid being detected by diurnal fish (O'Brien, 1979). More information is needed on whether certain diurnal zooplankton taxa are preferentially removed by planktivorous fishes, as well as information on density dependent interactions between planktivorous fish and zooplankton according to taxon.
Our prediction that demersal zooplankton would change after the removal of cleaner fish at the beginning of the experiment in July 2000 was not supported. For biomass, differences among times were due to higher biomass at night (1.7 to 3.2 times, Supplementary Figure 4) compared with the day during some of the trips, relative to the first trip (July 2000). In contrast, for abundance, it was only higher (4.8 times, Supplementary Figure 6C) once during January 2002 (Trip 7), relative to the first trip. Composition varied among all trips. Specifically, composition was characterized by more Oikopleuridae (a larvacean family, Chordata) during July 2000 (Trip 1) compared with June 2002 (Trip 8) and December 2012 (Trip 12) and more Oikopleuridae and Facetotecta larvae (a thecostracan crustacean, Arthropoda) in January 2001 (Trip 7) compared with the other trips. There were also more barnacle larvae in January (Trip 7) and December 2012 (Trip 12), both involving summer months, suggesting the possibility of a seasonal effect on this group. June 2002 and December 2012 (Trips 8 and 12) had the most variable composition (Figures 4A, C). Temporal changes in zooplankton emergence have commonly been demonstrated elsewhere (e.g. The Red Sea, Amer, 2019).
Our finding that the association between zooplankton biomass and biomass per sample per trip was rarely correlated indicates there are differences in the size frequency distribution of organisms across the trips sampled. The only other study on the GBR using the same identical traps, but at night only, was done on Heron Island (Jacoby and Greenwood, 1988). It reveals a similarly diverse zooplankton fauna, with copepod nauplii, various other copepods, decapod larvae, and bivalve veligers being the most abundant groups.
The demersal zooplankton community was clearly diverse and is likely to contribute to the overall energy flux on the reef. Excluding gnathiid isopods, the lack of an effect of the cleaner L. dimidiatus on demersal zooplankton suggests processes associated with predation pressure on zooplankton by non-fish planktivores, as well as processes related to zooplankton behaviour and population dynamics may be more important in explaining variation in demersal zooplankton communities.
Because demersal zooplankton live within the reef substrate, whose composition affects zooplankton community structure (Alldredge and King, 1977; Porter and Porter, 1977; McWilliam et al., 1981; Amer, 2019), they are vulnerable to changes in this habitat. Lizard Island has suffered from two cyclones (2014, 2015) and significant temperature stress resulting in two major mass bleaching events (2016, 2017), leading to high coral mortality and dramatic shifts in reef diversity and habitat structure. Coral cover on reefs around Lizard Island dropped 5-fold from 36% (in 2011) to 7% in 2017 (Madin et al., 2018). Coral species diversity experienced significant declines between 2011 to 2017, and significant recovery from 2017 to 2020 (Richards et al., 2021). The reduction in substrate complexity due to coral death from high water temperatures, has altered the abundance and diversity of many reef species, with significant cascading effects on community structure, food webs, and ecosystem function (Pratchett et al., 2018; Wolfe et al., 2021). Ongoing sampling on zooplankton abundance and biomass since 2012 has continued on the reefs sampled here, with only the abundance of the demersal gnathiid community from 2000 to 2018 published to date which showed significant changes over time (Sikkel et al., 2019). There are few long-term studies on coral reef zooplankton (McKinnon et al., 2007; Carrillo-Baltodano and Morales-Ramirez, 2016), with none to our knowledge on demersal communities. In many communities the demersal zooplankton community and its biomass are poorly described and quantified. Therefore, this study provides a unique insight into this community. It also provides a baseline from a relatively stable period on the GBR to examine temporal changes from this period and onwards.
This study used the longest cleaner fish removal experiment to date, which has provided data for multiple studies trying to investigate the effects of a key-species loss on the rest of the community. The main question asked here was: To what extent does the presence of cleaner fish and its effect on the distribution of their client fish indirectly influence the zooplankton community? Contrary to some of those previous studies which sampled other variables, the present study did not detect an effect of cleaner fish removal on demersal zooplankton as a variable, which indicates that the effect of cleaners is not even across coral reef communities. We found that, at this location, the abundance of fishes that feed on zooplankton was, on occasion, negatively affected by the long-term removal of the cleaner fish L. dimidiatus, nevertheless, this did not indirectly result in changes in zooplankton. We used the longest-running, most intensively studied field study on coral reef demersal zooplankton, which had considerable replication. That we did not detect a signal of an effect of cleaner presence on zooplankton suggests it is not there or it is very weak. Possibly, this may be due to multiple other sources of predation on zooplankton and processes involved in zooplankton population dynamics. This study adds to another similar study at this location which, despite also showing a decrease in the abundance of another group of fishes (algal farmers and grazers, Pomacentridae) in the absence of cleaners, did not detect a subsequent effect on the reef benthos they feed upon (Grutter et al., 2020a). These studies suggest a certain resilience in the reef’s community structure to the loss of cleaner fish at the local scale. Nonetheless, the absence of cleaning services still has numerous adverse consequences for fish physiology and populations. While the zooplankton community was highly variable, we could detect significant effects of time of day and sampling time, suggesting that we have adequately sampled the zooplankton community. This study provides one of longest studies of the composition of zooplankton on a coral reef.
The raw data supporting the conclusions of this article will be made available by the authors, without undue reservation.
The research was approved by The University of Queensland Animal Ethics Committee (permit numbers ZOO/ENT/017/00, 171/01, 320/03, 335/04, 265/05, SIB/269/06, 837/07, 604/08, 821/08, 286/11).
AG was principle investigator. AG, NN, and AR designed the study. AG, NN, and JP collected the data. AG, NN, and AR analyzed the data and wrote the paper. All authors contributed to manuscript drafting and editing. All authors contributed to the article and approved the submitted version.
This work was funded by the Australian Research Council (A00105175, A19937078, ARCFEL010G, DP0557058), CSIRO Oceans and Atmosphere, and The University of Queensland.
The authors declare that the research was conducted in the absence of any commercial or financial relationships that could be construed as a potential conflict of interest.
The reviewer RM declared a past co-authorship with one of the authors AG to the handling Editor.
All claims expressed in this article are solely those of the authors and do not necessarily represent those of their affiliated organizations, or those of the publisher, the editors and the reviewers. Any product that may be evaluated in this article, or claim that may be made by its manufacturer, is not guaranteed or endorsed by the publisher.
We thank R. Sanderson, S. Box, K. Coufal, K. Craig, M.A. Johnson, S. Ivermee, A. O’Toole, B. Fargher, L. Curtis, C. Jones, J. Fenton, R. Bshary, C. Newport, J. Murphy, C. Fury, R. Fogelman, A. Crean, C. Vargas, J. Becker, N. Aurish, G. Clague, T. G. Marsden, R. Slade, T. Brewer, E. Kokkelmans, I. Leiper, T. Sinclair-Taylor, P.A. Waldie, M. De Brauwer, E.C. McClure, Lizard Island Research Station staff, and many volunteers for their assistance in the field, S. P. Blomberg for statistical advice, F. Coman for advice with zooplankton identification and quantification, E.C. McClure for the fish surveys in 2012, and the reviewers.
The Supplementary Material for this article can be found online at: https://www.frontiersin.org/articles/10.3389/fmars.2022.812989/full#supplementary-material
Abdi H. (2007) The Bonferonni and Šidák Corrections for Multiple Comparisons. In: Salkind N(ed) In Encyclopedia of Measurement and Statistics. Sage, Thousand Oaks (CA) pp. 1–9
Acosta C. A., Butler M. I. (1999). Adaptive Strategies That Reduce Predation on Caribbean Spiny Lobster Postlarvae During Onshore Transport. Limnol. Oceanogr. 44 (3), 494–501. doi: 10.4319/lo.1999.44.3.0494
Adam T. C. (2011). High-Quality Habitat and Facilitation Ameliorate Competitive Effects of Prior Residents on New Settlers. Oecologia 166 (1), 121–130. doi: 10.1007/s00442-010-1826-7
Adam T. C. (2012). Mutualistic Cleaner Fish Initiate Trait-Mediated Indirect Interactions by Influencing the Behaviour of Coral Predators. J. Anim. Ecol. 81 (3), 692–700. doi: 10.1111/j.1365-2656.2011.01943.x
Alldredge A. L., King J. M. (1977). Distribution, Abundance, and Substrate Preferences of Demersal Reef Zooplankton at Lizard-Island Lagoon, Great-Barrier-Reef. Mar. Biol. 41 (4), 317–333. doi: 10.1007/bf00389098
Alldredge A. L., King J. M. (1980). Effects of Moonlight on the Vertical Migration Patterns of Demersal Zooplankton. J. Exp. Mar. Biol. Ecol. 44 (2-3), 133–156. doi: 10.1016/0022-0981(80)90150-1
Amer M. A. A. (2019). “Zooplankton of the Red Sea,” in Oceanographic and Biological Aspects of the Red Sea (Charm: Springer), 477–490.
Anderson M. J., Gorley R. N., Clarke K. R. (2008). PERMANOVA+ for PRIMER: Guide to Software and Statistical Methods (UK: PRIMER-E: Plymouth).
Anthony L. L., Blumstein D. T. (2000). Integrating Behaviour Into Wildlife Conservation: Multiple Ways That Behaviour can Reduce Ne. Biol. Conserv. 95 (3), 303–315. doi: 10.1016/S0006-3207(00)00037-9
Bates D., Maechler M., Bolker B., Walker S. (2015). Fitting Linear Mixed-Effects Models Using Lme4. J. Stat. Softw. 67, 1–48. doi: 10.18637/jss.v067.i01
Bellwood D. R., Streit R. P., Brandl S. J., Tebbett S. B. (2019). The Meaning of the Term ‘Function’in Ecology: A Coral Reef Perspective. Funct. Ecol. 33 (6), 948–961. doi: 10.1111/1365-2435.13265
Binning S. A., Roche D. G., Grutter A. S., Colosio S., Sun D., Miest J., et al. (2018). Cleaner Wrasse Indirectly Affect the Cognitive Performance of a Damselfish Through Ectoparasite Removal. Proc. R. Soc. London. Ser. B: Biol. Sci. (London) 285 (1874), 20172447. doi: 10.1098/rspb.2017.2447
Brooks M. E., Kristensen K., van Benthem K. J., Magnusson A., Berg C. W., Nielsen A., et al. (2017). glmmTMB Balances Speed and Flexibility Among Packages for Zero-Inflated Generalized Linear Mixed Modeling. R J. 9 (2), 378–400. doi: 10.32614/rj-2017-066
Bshary R. (2003). The Cleaner Wrasse, Labroides dimidiatus, is a Key Organism for Reef Fish Diversity at Ras Mohammed National Park, Egypt. J. Anim. Ecol. 72, 169–176. doi: 10.1046/j.1365-2656.2003.00683.x
Carrillo-Baltodano A., Morales-Ramirez A. (2016). Changes in Abundance and Composition of a Caribbean Coral Reef Zooplankton Community After 25 Years. Rev. Biol. Trop. 64 (3), 1029–1040. doi: 10.15517/rbt.v64i3.21449
Chambers S. D., Sikkel P. C. (2002). Diel Emergence Patterns of Ecologically Important, Fish-Parasitic, Gnathiid Isopod Larvae on Caribbean Coral Reefs. Caribb. J. Sci. 38 (1-2), 37–43. doi: 10.1007/s00338-006-0154-1
Clague G. E., Cheney K. L., Goldizen A. W., McCormick M. I., Waldie P. A., Grutter A. S. (2011). Long-Term Cleaner Fish Presence Affects Growth of a Coral Reef Fish. Biol. Lett. 7 (6), 863–865. doi: 10.1098/rsbl.2011.0458
Couturier L. I. E., Rohner C. A., Richardson A. J., Marshall A. D., Jaine F. R. A., Bennett M. B., et al. (2013). Stable Isotope and Signature Fatty Acid Analyses Suggest Reef Manta Rays Feed on Demersal Zooplankton. PLoS One 8 (10), 15. doi: 10.1371/journal.pone.0077152
Cury P., Bakun A., Crawford R. J. M., Jarre A., Quinones R. A., Shannon L. J., et al. (2000). Small Pelagics in Upwelling Systems: Patterns of Interaction and Structural Changes in "Wasp-Waist" Ecosystems. ICES J. Mar. Sci. 57 (3), 603–618. doi: 10.1006/jmsc.2000.0712
Demaire C., Triki Z., Binning S. A., Glauser G., Roche D. G., Bshary R. (2020). Reduced Access to Cleaner Fish Negatively Impacts the Physiological State of Two Resident Reef Fishes. Mar. Biol. 167 (4), 1–10. doi: 10.1007/s00227-020-3658-2
Emslie M. J., Logan M., Cheal A. J. (2019). The Distribution of Planktivorous Damselfishes (Pomacentridae) on the Great Barrier Reef and the Relative Influences of Habitat and Predation. Diversity-Basel 11 (3), 23. doi: 10.3390/d11030033
Eriksen R. S., Davies C. H., Bonham P., Coman F. E., Edgar S., McEnnulty F. R., et al. (2019). Australia's Long-Term Plankton Observations: The Integrated Marine Observing System National Reference Station Network. Front. Mar. Sci. 6. doi: 10.3389/fmars.2019.00161
Fox J., Weisberg S. (2019). An {R} Companion to Applied Regression. Second Edition. 3rd ed. (Thousand Oaks: CA: Sage).
Frederich B., Fabri G., Lepoint G., Vandewalle P., Parmentier E. (2009). Trophic Niches of Thirteen Damselfishes (Pomacentridae) at the Grand Récif of Toliara, Madagascar. Ichthyol. Res. 56 (1), 10–17. doi: 10.1007/s10228-008-0053-2
Grutter A. S. (1996a). Experimental Demonstration of No Effect by the Cleaner Wrasse Labroides dimidiatus (Cuvier and Valenciennes) on the Host Fish Pomacentrus moluccensis (Bleeker). J. Exp. Mar. Biol. Ecol. 196 (1-2), 285–298. doi: 10.1016/0022-0981(95)00135-2
Grutter A. S. (1996b). Parasite Removal Rates by the Cleaner Wrasse Labroides dimidiatus. Mar. Ecol. Prog. Ser. 130, 61–70. doi: 10.3354/meps130061
Grutter A. S. (1997a). Spatiotemporal Variation and Feeding Selectivity in the Diet of the Cleaner Fish Labroides Dimidiatus. J. Fish Biol. 50, 1303–1308. doi: 10.1111/j.1095-8649.1997.tb01654.x
Grutter A. S. (1997b). Effect of the Removal of Cleaner Fish on the Abundance and Species Composition of Reef Fish. Copeia 111(1997):346–54. doi: 10.1007/s004420050217
Grutter A. S. (2008). Interactions Between Gnathiid Isopods, Cleaner Fish and Other Fishes on Lizard Island, Great Barrier Reef. J. Fish Biol. 73 (9), 2094–2109. doi: 10.1111/j.1095-8649.2008.02073.x
Grutter A. S. (2012). Enhanced Colonization Success and Competition Associated With Conspecifics in Cleaner Fish Labroides dimidiatus Juveniles. Coral Reefs 31 (4), 1169–1176. doi: 10.1007/s00338-012-0942-8
Grutter A. S., Bejarano S., Cheney K. L., Goldizen A. W., Sinclair-Taylor T., Waldie P. A. (2020a). Effects of the Cleaner Fish Labroides dimidiatus on Grazing Fishes and Coral Reef Benthos. Mar. Ecol. Prog. Ser. 643, 99–114. doi: 10.3354/meps13331
Grutter A. S., Blomberg S. P., Box S., Bshary R., Ho O., Madin E. M. P., et al. (2019). Changes in Local Free-Living Parasite Populations in Response to Cleaner Manipulation Over 12 Years. Oecologia 190 (4), 783–797. doi: 10.1007/s00442-019-04451-8
Grutter A., Brauwer M., Bshary R., Cheney K., Cribb T., Madin E., et al. (2018). Parasite Infestation Increases on Coral Reefs Without Cleaner Fish. Coral Reefs 37 (1), 15–24. doi: 10.1007/s00338-017-1628-z
Grutter A. S., Feeney W. E., Hutson K. S., McClure E. C., Narvaez P., Smit N. J., et al. (2020b). Practical Methods for Culturing Parasitic Gnathiid Isopods. Int. J. Parasitol. 50 (10-11), 825–837. doi: 10.1016/j.ijpara.2020.03.014
Grutter A. S., Lester R. J. G. (2002). Cleaner Fish Labroides dimidiatus Reduce 'Temporary' Corallanid Isopods on the Coral Reef Fish Hemigymnus melapterus. Mar. Ecol. Prog. Ser. 234, 247–255. doi: 10.3354/meps234247
Grutter A. S., Lester R. J. G., Greenwood J. (2000). Emergence Rates From the Benthos of the Parasitic Juveniles of Gnathiid Isopods. Mar. Ecol. Prog. Ser. 207, 123–127. doi: 10.3354/meps207123
Grutter A. S., Murphy J. M., Choat J. H. (2003). Cleaner Fish Drives Local Fish Diversity on Coral Reefs. Curr. Biol. 13 (1), 64–67. doi: 10.1016/s0960-9822(02)01393-3
Hardin J.W., Hilbe J.M. (2007) Generalized Linear Models and Extensions. A Stata Press Publication, StatCorp LP, Texas.
Halekoh U., Højsgaard S. (2014). A Kenward-Roger Approximation and Parametric Bootstrap Methods for Tests in Linear Mixed Models – The R Package Pbkrtest. J. Stat. Softw. 59 (9), 1–30. doi: 10.18637/jss.v059.i09
Hamner W. M., Jones M. S., Carleton J. H., Hauri I. R., Williams D. M. (1988). Zooplankton, Planktivorous Fish, and Water Currents on a Windward Reef Face - Great Barrier-Reef, Australia. Bull. Mar. Sci. 42 (3), 459–479.
Hanson K. M., Schnarr E. L., Leichter J. J. (2016). Non-Random Feeding Enhances the Contribution of Oceanic Zooplankton to the Diet of the Planktivorous Coral Reef Fish Dascyllus flavicaudus. Mar. Biol. 163 (4), 13. doi: 10.1007/s00227-016-2849-3
Hartig F. (2021). DHARMa: Residual Diagnostics for Hierarchical (Multi-Level / Mixed) Regression Models (R package version 0.4.3). Available at: https://CRAN.R-project.org/package=DHARMa.
Hobson E. S. (1991). “Trophic Relationships of Fishes Specialized to Feed on Zooplankters Above Coral Reefs,” in The Ecology of Fishes on Coral Reefs, vol. 69 . Ed. Sale P. F. (San Diego: Academic Press), 95.
Hobson E. S., Chess J. R. (1978). Trophic Relationships Among Fishes and Plankton in Lagoon at Enewetak Atoll, Marshall Islands. Fishery Bull. 76 (1), 133–153.
Houlbreque F., Ferrier-Pages C. (2009). Heterotrophy in Tropical Scleractinian Corals. Biol. Rev. 84 (1), 1–17. doi: 10.1111/j.1469-185X.2008.00058.x
Jacoby C. A., Greenwood J. G. (1988). Spatial, Temporal, and Behavioral Patterns in Emergence of Zooplankton in the Lagoon of Heron Reef, Great Barrier Reef, Australia. Mar. Biol. 97, 309–328. doi: 10.1007/BF00397762
Johannsson O. E., Bowen K. L. (2012). Zooplankton Production in the Bay of Quinte 1975-2008: Relationships With Primary Production, Habitat, Planktivory, and Aquatic Invasive Species (Dreissena Spp. And Cercopagis Pengoi). Can. J. Fish Aquat. Sci. 69 (12), 2046–2063. doi: 10.1139/cjfas-2012-0162
Leray M., Alldredge A. L., Yang J. Y., Meyer C. P., Holbrook S. J., Schmitt R. J., et al. (2019). Dietary Partitioning Promotes the Coexistence of Planktivorous Species on Coral Reefs. Mol. Ecol. 28 (10), 2694–2710. doi: 10.1111/mec.15090
Lewis J. B. (1992). Heterotrophy in Corals - Zooplankton Predation by the Hydrocoral Millepora complanata. Mar. Ecol. Prog. Ser. 90 (3), 251–256. doi: 10.3354/meps090251
Madin J. S., Baird A. H., Bridge T. C. L., Connolly S. R., Zawada K. J. A., Dornelas M. (2018). Cumulative Effects of Cyclones and Bleaching on Coral Cover and Species Richness at Lizard Island. Mar. Ecol. Prog. Ser. 604, 263–268. doi: 10.3354/meps12735
Mann D. A., Sancho G. (2007). Feeding Ecology of the Domino Damselfish, Dascyllus albisella. Copeia 3), 566–576. doi: 10.1643/0045-8511(2007)2007[566:FEOTDD]2.0.CO;2
Marnane M. J., Bellwood D. R. (2002). Diet and Nocturnal Foraging in Cardinalfishes (Apogonidae) at One Tree Reef, Great Barrier Reef, Australia. Mar. Ecol. Prog. Ser. 231, 261–268. doi: 10.3354/meps231261
Maszczyk P., Gliwicz Z. M. (2014). Selectivity by Planktivorous Fish at Different Prey Densities, Heterogeneities, and Spatial Scales. Limnol. Oceanogr. 59 (1), 68–78. doi: 10.4319/lo.2014.59.1.0068
McEwen G. F., Johnson M. W., Folsom T. R. (1954). A Statistical Analysis of the Performance of the Folsom Plankton Splitter, Based Upon Test Observations. Arch. Met. Geophys. Bioklim. Ser. A 6, 502–527. doi: 10.1007/BF02277939
McKinnon A. D., Richardson A. J., Burford M. A., Furnas M. J. (2007). “Chapter 6. Vulnerability of Great Barrier Reef Plankton to Climate Change”, in Book: Climate Change and the Great Barrier Reef: A Vulnerability Assessment (The Great Barier Reef Marine Park Authority). Available at: http://hdl.handle.net/11017/539.
McWilliam P. S., Sale P. F., Anderson D. T. (1981). Seasonal Changes in Resident Zooplankton Sampled by Emergence Traps is One Tree Lagoon, Great Barrier Reef. J. Exp. Mar. Biol. Ecol. 52, 185–203. doi: 10.1016/0022-0981(81)90036-8
Motro R., Ayalon I., Genin A. (2005). Near-Bottom Depletion of Zooplankton Over Coral Reefs: III: Vertical Gradient of Predation Pressure. Coral Reefs 24 (1), 95–98. doi: 10.1007/s00338-004-0451-5
O'Brien W. J. (1979). The Predator-Prey Interaction of Planktivorous Fish and Zooplankton: Recent Research With Planktivorous Fish and Their Zooplankton Prey Shows the Evolutionary Thrust and Parry of the Predator-Prey Relationship. Am. Sci. 67 (5), 572–581.
Ohlhorst S. L. (1982). Diel Migration Patterns of Demersal Reef Zooplankton. J. Exp. Mar. Biol. Ecol. 60 (1), 1–15. doi: 10.1016/0022-0981(81)90176-3
Oksanen J., Blanchet F. G., Friendly M., Kindt R., Legendre P., McGlinn D., et al. (2019). Vegan: Community Ecology Package (R package version 2), 5–6. Available at: https://CRAN.R-project.org/package=vegan.
Paula J. R., Repolho T., Pegado M. R., Thornqvist P. O., Bispo R., Winberg S., et al. (2019). Neurobiological and Behavioural Responses of Cleaning Mutualisms to Ocean Warming and Acidification. Sci. Rep. 9:1–10. doi: 10.1038/s41598-019-49086-0
Paula J. R., Sun D., Pissarra V., Narvaez P., Rosa R., Grutter A. S., et al. (2021). The Role of Corals on the Abundance of a Fish Ectoparasite in the Great Barrier Reef. Coral Reefs 40 (2), 535–542. doi: 10.1007/s00338-021-02051-8
Porter J. W., Porter K. G. (1977). Quantitative Sampling of Demersal Plankton Migrating From Different Coral Reef Substrates. Limnol. Oceanogr. 22 (3), 553–556. doi: 10.4319/lo.1977.22.3.0553
Pratchett M. S., Thompson C. A., Hoey A. S., Cowman P. F., Wilson S. K. (2018). “Effects of Coral Bleaching and Coral Loss on the Structure and Function of Reef Fish Assemblages,” in Coral Bleaching: Patterns, Processes, Causes and Consequences. Eds. van Oppen M. J. H., Lough J. M. (Cham: Springer International Publishing), 265–293.
Randall J. E., Allen G. R., Steene R. C. (1997). Fishes of the Great Barrier Reef and Coral Sea (Bathurst: Crawford House Publishing).
R Core Team (2020). R: A Language and Environment for Statistical Computing (Vienna, Austria: R Foundation for Statistical Computing). Available at: http://www.R-project.org/.
Rhyne A. L., Tlusty M. F., Szczebak J. T., Holmberg R. J. (2017). Expanding Our Understanding of the Trade in Marine Aquarium Animals. Peerj 5, 1–36. doi: 10.7717/peerj.2949
Richards Z. T., Juszkiewicz D. J., Hoggett A. (2021). Spatio-Temporal Persistence of Scleractinian Coral Species at Lizard Island, Great Barrier Reef. Coral Reefs. 40, 1369–1378. doi: 10.1007/s00338-021-02144-4
Rohner C. A., Couturier L. I. E., Richardson A. J., Pierce S. J., Prebble C. E. M., Gibbons M. J., et al. (2013). Diet of Whale Sharks Rhincodon typus Inferred From Stomach Content and Signature Fatty Acid Analyses. Mar. Ecol. Prog. Ser. 493, 219–235. doi: 10.3354/meps10500
Roman M. R., Furnas M. J., Mullin M. M. (1990). Zooplankton Abundance and Grazing at Davies Reef, Great Barrier Reef, Australia. Mar. Biol. 105 (1), 73–82. doi: 10.1007/bf01344272
Ros A. F. H., Nusbaumer D., Triki Z., Grutter A. S., Bshary R. (2020). The Impact of Long-Term Reduced Access to Cleaner Fish on Health Indicators of Resident Client Fish. J. Exp. Biol. 223 (24), 10. doi: 10.1242/jeb.231613
Sikkel P. C., Richardson M. A., Sun D., Narvaez P., Feeney W. E., Grutter A. S. (2019). Changes in Abundance of Fish-Parasitic Gnathiid Isopods Associated With Warm-Water Bleaching Events on the Northern Great Barrier Reef. Coral Reefs 38 (4), 721–730. doi: 10.1007/s00338-019-01835-3
Sikkel N. M., Welicky R. L. (2019). “The Ecological Significance of Parasitic Crustaceans”, in Parasitic Crustacea: State of Knowledge and Future Trends. Eds. Smit N. J., Bruce N. L., Hadfield K. A. (Cham, Switzerland: Springer), 421–477.
Soares M. C., Oliveira R. F., Ros A. F. H., Grutter A. S., Bshary R. (2011). Tactile Stimulation Lowers Stress in Fish. Nat. Commun. 2 (534), ncomms1547. doi: 10.1038/ncomms1547
Stepien C. A., Brusca R. C. (1985). Nocturnal Attacks on Nearshore Fishes in Southern California by Crustacean Zooplankton. Mar. Ecol. Prog. Ser. 25, 91–105. doi: 10.3354/meps025091
Sun D., Cheney K. L., Werminghausen J., Meekan M. G., McCormick M. I., Cribb T. H., et al. (2015). Presence of Cleaner Wrasse Increases the Recruitment of Damselfishes to Coral Reefs. Biol. Lett. 11 (8), 1–5. doi: 10.1098/rsbl.2015.0456
Swadling K., Slotwinski A., Davies C., Beard J., McKinnon A., Coman F., et al. (2013) Australian Marine Zooplankton: A Taxonomic Guide and Atlas [Online]. Available at: https://www.imas.utas.edu.au/zooplankton.
Triki Z., Wismer S., Levorato E., Bshary R. (2018). A Decrease in the Abundance and Strategic Sophistication of Cleaner Fish After Environmental Perturbations. Glob. Change. Biol. 24 (1), 481–489. doi: 10.1111/gcb.13943
Turner A. M., Mittelbach G. G. (1990). Predator Avoidance and Community Structure - Interactions Among Piscivores, Planktivores, and Plankton. Ecology 71 (6), 2241–2254. doi: 10.2307/1938636
Wagner E., Roche D. G., Binning S. A., Wismer S., Bshary R. (2015). Temporal Comparison and Predictors of Fish Species Abundance and Richness on Undisturbed Coral Reef Patches. Peerj 3, 13. doi: 10.7717/peerj.1459
Waldie P. A., Blomberg S. P., Cheney K. L., Goldizen A. W., Grutter A. S. (2011). Long-Term Effects of the Cleaner Fish Labroides dimidiatus on Coral Reef Fish Communities. PLoS One 6 (6), e21201. doi: 10.1371/journal.pone.0021201
Wolfe K., Kenyon T. M., Mumby P. J. (2021). The Biology and Ecology of Coral Rubble and Implications for the Future of Coral Reefs. Coral Reefs 40 (6), 1769–1806. doi: 10.1007/s00338-021-02185-9
Keywords: Pomacentridae, demersal zooplankton, mutualism, indirect effects, cleaning symbiosis, planktivorous fish, coral reef trophic ecology
Citation: Grutter AS, Nishikawa N, Uribe-Palomino J and Richardson AJ (2022) Cleaner Fish Labroides dimidiatus Presence Does Not Indirectly Affect Demersal Zooplankton. Front. Mar. Sci. 9:812989. doi: 10.3389/fmars.2022.812989
Received: 11 November 2021; Accepted: 02 May 2022;
Published: 03 June 2022.
Edited by:
Charles Alan Jacoby, St. Johns River Water Management District, United StatesReviewed by:
Rachel Lynn Welicky, University of Washington, United StatesCopyright © 2022 Grutter, Nishikawa, Uribe-Palomino and Richardson. This is an open-access article distributed under the terms of the Creative Commons Attribution License (CC BY). The use, distribution or reproduction in other forums is permitted, provided the original author(s) and the copyright owner(s) are credited and that the original publication in this journal is cited, in accordance with accepted academic practice. No use, distribution or reproduction is permitted which does not comply with these terms.
*Correspondence: Alexandra S. Grutter, YS5ncnV0dGVyQHVxLmVkdS5hdQ==
†ORCID: Alexandra S. Grutter, orcid.org/0000-0003-1688-2821
Julian Uribe-Palomino, orcid.org/0000-0002-6867-2108
Anthony J. Richardson, orcid.org/0000-0002-9289-7366
Disclaimer: All claims expressed in this article are solely those of the authors and do not necessarily represent those of their affiliated organizations, or those of the publisher, the editors and the reviewers. Any product that may be evaluated in this article or claim that may be made by its manufacturer is not guaranteed or endorsed by the publisher.
Research integrity at Frontiers
Learn more about the work of our research integrity team to safeguard the quality of each article we publish.